- 1PET Center, Huashan Hospital, Fudan University, Shanghai, China
- 2Center for Molecular Imaging and Translational Medicine, State Key Laboratory of Molecular Vaccinology and Molecular Diagnostics, School of Public Health, Xiamen University, Xiamen, China
- 3Department of Radiology, The University of Texas Southwestern Medical Center, Dallas, TX, United States
Background: Brain development and maturation in adolescence is a complex process with active changes of metabolic and neurotransmission pathways. Positron emission tomography (PET) is a useful imaging modality for tracking metabolic and functional changes in adolescent brain. In this study, changes of glucose metabolism, expression of vesicular monoamine transporter 2 and dopamine transporter during adolescent brain development in rats were investigated with PET/CT.
Methods: A longitudinal PET/CT study of age-dependent changes of VMAT2, DAT and glucose metabolism in adolescent brain was conducted in a group of Wistar rats (n = 6) post sequential intravenous injection of 18F-PF-(+)-DTBZ, 11C-CFT, and 18F-FDG, respectively. PET acquisition was performed at 2, 4, 9, and 12 months of age. Radiotracer uptake in different brain regions, including the striatum, cerebellum, and hippocampus, were quantified and recorded as Standardized uptake value (SUV) and striatal specific uptake ratio (SUVR: SUV in brain regions/SUV in cerebellum).
Results: Variable uptake of 18F-PF-(+)-DTBZ and 11C-CFT were detected, with highest level uptake in the striatum and accumbens. There was significant age-dependent increase of 18F-PF-(+)-DTBZ and 11C-CFT uptake in the striatum from 2 months of age (SUV: 1.36 ± 0.22, 1.37 ± 0.39, respectively), to 4 months (SUV: 2.22 ± 0.29, 2.04 ± 0.33), 9 months (1.98 ± 0.34, 2.09 ± 0.18), 12 months (SUV: 1.93 ± 0.19, 2.00 ± 0.17) of age, SUV of 18F-FDG also increased from 2 months of age to older ages (SUV in the striatum: 3.71 ± 0.78 at 2 month, 5.28 ± 0.81, 5.14 ± 0.73, 4.94 ± 0.50 at 4, 9, 12 month, respectively).
Conclusion: Age-dependent increases of striatal of 18F-FDG, 18F-PF-(+)-DTBZ, and 11C-CFT uptake were detected in rats from 2 to 4 month of age, demonstrating striatal development presents over the first 4 months of age. Four months of age can be considered a safe threshold to launch brain disease studies for exclusion of confusion of continuing tissue development. These findings support further investigation of age-dependent changes in expression of DAT, VMAT2, and glucose metabolism for their potential use as a new imaging biomarker for study of brain development and functional maturation.
Introduction
Brain development is a dynamic and complex process, including the change of brain volume and morphology, metabolism, expression of receptors and transporters, and function (Chugani and Phelps, 1986; Chugani, 1998; Khundrakpam et al., 2016). A number of investigations indicated brain regional growth with changes of cortical thickness during brain development and maturation using MR (Sowell et al., 2004; Aljabar et al., 2008; Mengler et al., 2014). However, metabolic and functional changes associated with brain development and maturation remain largely unknown or poorly understood, particularly in adolescent population. Because of vulnerability caused by un-mature status of brain development, the adolescent population is at a high risk for development of various mental health problems, such as uncontrolled violence, depression, and drug abuse. It is important to explore metabolic changes and changes of receptors or neurotransmission pathways during brain development and maturation process in adolescent population.
Positron emission tomography (PET) is a particularly useful functional imaging tool for tracking metabolic changes or changes of neuro-receptor expression during brain development using a radiotracer. PET imaging with [18F]fluoro-2-deoxy-D-glucose (18F-FDG) is widely used to detect alterations of cerebral glucose metabolism in brain development, aging, and various brain disorders (Mosconi et al., 2008; Smailagic et al., 2015). Some study present the glucose metabolism change in brain maturation using 18F-FDG/PET (Choi et al., 2015). Using radioligands binding to brain receptors and transporters, such as dopamine transporter (DAT) and vesicle monoamine transporter 2 (VMAT2), PET is also a useful tool for studying of changes of the expression and function of brain receptors and transporters during brain development and maturation (Alexander et al., 2017).
It is well-known that there are changes of glucose metabolism that have some inherent connections with the development of brain transporters and 18F-FDG PET may be used to evaluate changes of glucose metabolism (Chugani and Phelps, 1986; Goyal and Raichle, 2018). There are continued efforts to explore changes of other metabolic pathways in brain development such as those related to synapse activity and biometals. DAT are protein molecules located in the dopaminergic nerve terminals, which mediate neuronal uptake of dopamine from extracellular space into extravesicular cytoplasmic compartments. [11C] 2-β-carbomethoxy-3-β- (4-fluorophenyl)tropane (11C-CFT) is a radioligand with high affinity binding to DAT (Brownell et al., 1996). VMAT2 are responsible for packaging and transporting neurotransmitters, such as dopamine, into synaptic vesicles (Eiden and Weihe, 2011). [18F]9-fluoropropyl-(+)-dihydrotetrabenazine (18F-FP-(+)-DTBZ) can bind to VMAT2 with high affinity. Both DAT and VMAT2 regulate the synaptic concentration of neurotransmitters in the brain (Hall et al., 2014). Expression level of DAT and VMAT2 reflect the density of dopamine terminals in brain regions, which may change during brain development and pathogenesis of brain disorders such as Parkinson’s disease. It was demonstrated that 11C-CFT and 18F-FP-(+)-DTBZ could be used for quantification of the density of DAT and VMAT2 in brain (Hsiao et al., 2014; Wood, 2014).
Rat adulthood is defined as full sexual maturity in many studies, and the time window is typically described by age and body weight, which lack clear and unambiguous boundaries. Mengler et al. (2014) found clear indications of ongoing developmental changes of cortical thickness, myelination in the rat brain, which point to the time window of maturity within 3 months of age. In this study, to better characterize the brain development of glucose metabolism, DAT and VMAT2, three radiotracers were utilized to observe the development of rat brains in maturation. We recorded the PET signals of these three radiotracers in Wistar rats from 2 to 12 months of age in a longitudinal study. with this multi-tracer approach, we undertook to chart the interrelations of DAT and VMAT2 in vivo during the early life of rats, and find a time window of brain maturation.
Materials and Methods
Animals and Radiopharmaceuticals
Wistar rats were purchased from Shanghai Laboratory Animal Center (Shanghai, China) and housed in the animal housing facility, with free access to food and drinking water. 18F-PF-(+)-DTBZ, 11C-CFT, and 18F-FDG were prepared in the radiochemistry facility of PET center, Huashan Hospital, Fudan University for clinical use under requirement of GMP. All small animal experiments were conducted according to a protocol approved by Huashan Hospital, Shanghai, China.
Study Design
A longitudinal, sequential micro-PET/CT study was performed to assess age-dependent changes of VMAT2, DAT, and glucose metabolism in a group of Wistar rats (male, n = 6) from 2 to 12 months of age (equivalent to 12 to 30 years in humans) using 18F-PF-(+)-DTBZ, 11C-CFT, and 18F-FDG, respectively, (Figure 1). Small animal imaging was conducted at 2, 4, 9 months and approximately 12 months of age. Rat ages were defined as the human age equivalents outlined below according to literature (Quinn, 2005).
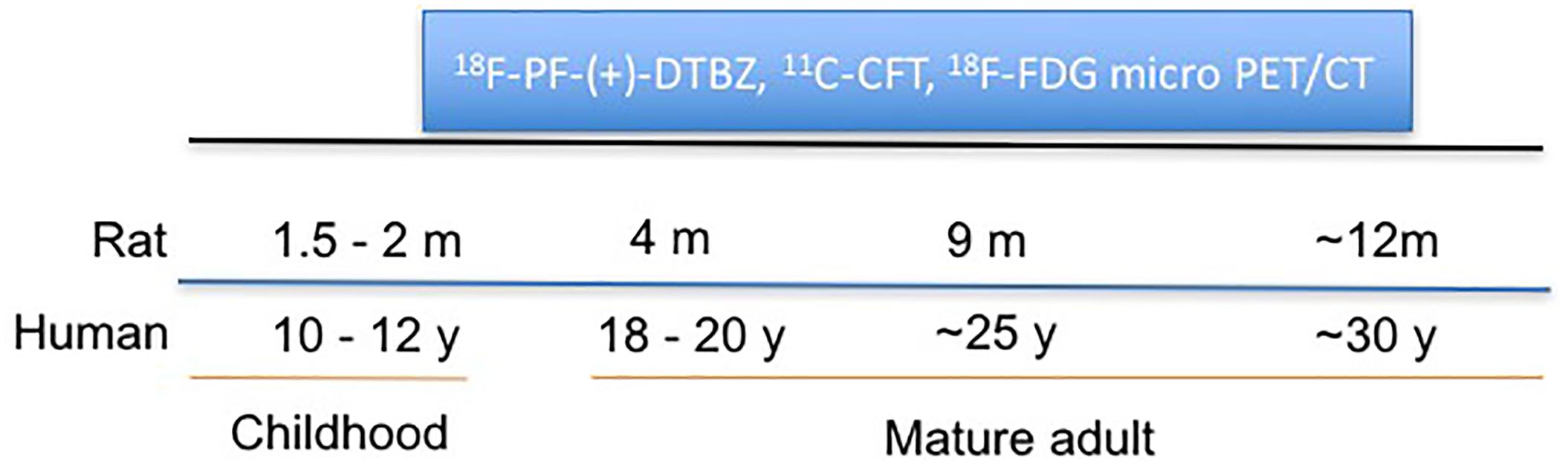
Figure 1. Schematic representation of longitudinal study of change of VMAT2, DAT, and glucose metabolism in rat brains with PET/CT at different ages.
Micro-PET/CT Imaging
Micro-PET/CT imaging of Wistar rats was conducted by a method described previously using Inveon microPET-CT scanner (Siemens Inc., United States) (Zilliox et al., 2016). Briefly, the rats were anesthetized using 2–3% isoflurane in medical oxygen (1 L/min) at room temperature with an isoflurane vaporizer (Molecular Imaging Products Company, United States). The rats were positioned in a spread-supine position on the imaging bed and subjected to inhalation of the anesthetic during the PET/CT procedure. Static PET/CT imaging was obtained for 10 min at 60 min post intravenous administration of 18F-FDG (∼10 μCi/g body weight), at 60 min post intravenous administration of 18F-PF-(+)-DTBZ (∼10 μCi/g body weight), and 45 min post administration of 11C-CFT (∼20 μCi/g body weight). The imaging time is based on previous dynamic study we performed. PET/CT images were reconstructed using the ordered subsets expectation maximization 3D algorithm (OSEM3D), and data was reviewed using the Inveon Research Workplace (IRW) software (Siemens) and processed by PMOD software (version 3.4, PMOD Technologies Ltd., Zurich, Switzerland).
Quantification of Radiotracer Activity in the Brain Regions of Rats
18F-FDG PET images were automatically fused using the PMOD FDG rat brain template (Schiffer et al., 2006) while 18F-PF-(+)-DTBZ and 11C-CFT PET images were manually fused with the T2-MRI template. 58 brain regions of interest (ROIs) were collected on the PET/CT images with reference to the MR imaging-based atlas (Schiffer atlas) (Schiffer et al., 2006). The regions analyzed included: cerebellum, accumbens (left and right), amygdala (left and right), striatum (left and right), hippocampus posterior (left and right), hypothalamus (left and right), olfactory (left and right), midbrain (left and right), thalamus (left and right), and cortex etc., All collected brain regions with a sample of 18F-FDG are present in Figure 2. The quantity of 18F-PF-(+)-DTBZ, 11C-CFT, and 18F-FDG activity was obtained and recorded as a standardized uptake value (SUV). SUV ratio (SUVR) was calculated by SUV uptake in the specific brain regions compared to that of the cerebellum.
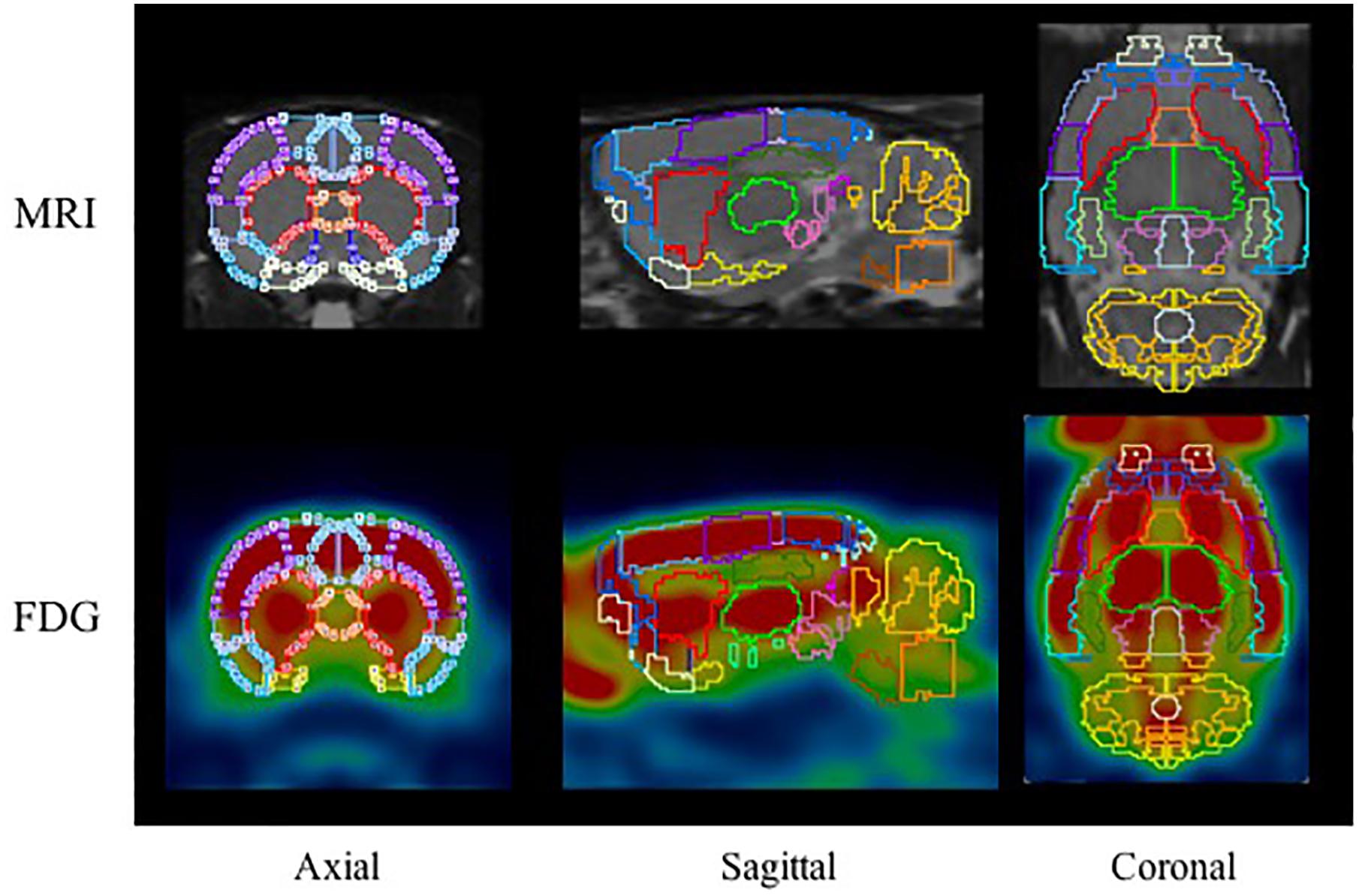
Figure 2. The ROIs applied to the PET images using MR imaging-based atlas by PMOD (top) and corresponding example for FDG (bottom). The main brain regions included: cerebellum (marigold), accumbens (blue), amygdala (yellow), striatum (red), hippocampus posterior (green), midbrain (purple), thalamus (lime), and cortex etc.
Statistical Analysis
In order to determine whether the SUV and SUVR of [18F]FDG, [11C]CFT, [18F]DTBZ in these rats differ among different ages, we applied two-way analysis of variance (ANOVA), followed by Tukey multiple comparison post-test, with the between-subjects factor representing the different age groups and the within-subjects factor representing the brain regions. Statistical analyses was performed in Prism (version 7.0, GraphPad Software, San Diego, CA, United States). A P-value of less than 0.05 was considered statistically significant.
Results
Comparison of 18F-PF-(+)-DTBZ, 11C-CFT, and 18F-FDG Uptake in Rat Brains
Bodyweight of these rats increased from 222.0 ± 14.6 to 424.5 ± 7.2 from 2 to 12 months of age, and kept in a relatively stable range between 371.7 ± 32.9 and 424.5 ± 7.2 from 4 to 12 months of age (Supplementary Figure S1). 18F-PF-(+)-DTBZ and 11C-CFT displayed similar distribution patterns in rats after intravenous administration. Both radiotracers mainly accumulated in the striatum and accumbens, yet uptake of 18F-PF-(+)-DTBZ could also be found in the skull (Figure 3). The pattern of 18F-FDG uptake in rat brains was different from these two tracers, as the cortex, striatum and accumbens had the highest FDG uptake, followed by the cerebellum, while the pituitary gland demonstrated the lowest uptake (Figure 3 and Supplementary Tables S1–S6). Additionally, age-dependent increase of whole brain radiotracer uptake were observed, radioactivity uptake of these 3 radiotracers were higher in the rat brains at 4, 9, 12 months of age than that at 2 months of age.
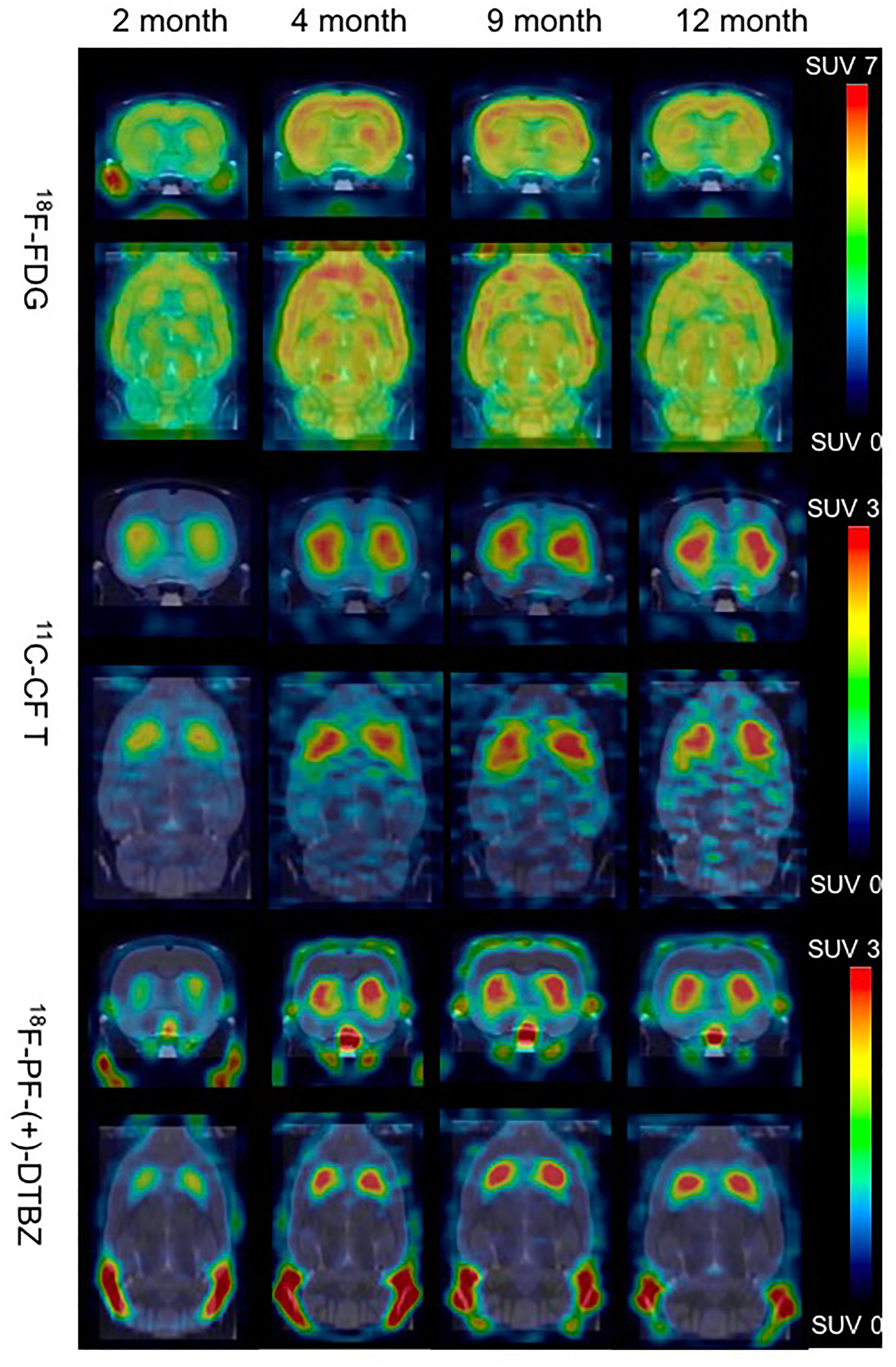
Figure 3. Biodistribution of radiotracer activity in rat brains by PET/CT imaging at different ages, post intravenous administration of 18F-PF-(+)-DTBZ, 11C-CFT and 18F-FDG, respectively. There was increase of brain uptake of these 3 radiotracers increased from 2 months to 4, 9, 12 months of age by PET quantification.
Age-Dependent Changes of 11C-CFT Uptake in Rat Brains
A decrease in DAT expression was observed in human and small animal brain aging (Hall et al., 2014). In order to determine whether there are age-dependent changes of DAT expression in brain development and maturation, a longitudinal PET/CT study was performed to measure 11C-CFT in the brains of rats at 2, 4, 9, and 12 months of age.
11C-CFT uptake (SUV) reached a slightly descending plateau at 4 months of age in the striatum and accumbens (Figure 4). The striatum displayed a significantly lower 11C-CFT uptake at 2 months of age (SUV: 1.37 ± 0.39) compared to 4 months (SUV: 2.04 ± 0.33, P < 0.0001), 8 months (SUV: 2.09 ± 0.18, P < 0.0001 ) and 12 months (SUV: 2.00 ± 0.17, P < 0.0001). The same trend was found in the accumbens, as increased SUV was observed from 2 months (1.01 ± 0.32) to 4 months (1.38 ± 0.22, P = 0.0021), 9 months (1.36 ± 0.24, P = 0.0033), and 12 months (1.19 ± 0.25, P = 0.3705). SUV uptake in nearly all other brain regions did not demonstrate significant change, aside from the thalamus from 2 to 4 months (P = 0.02) (Supplementary Table S5).
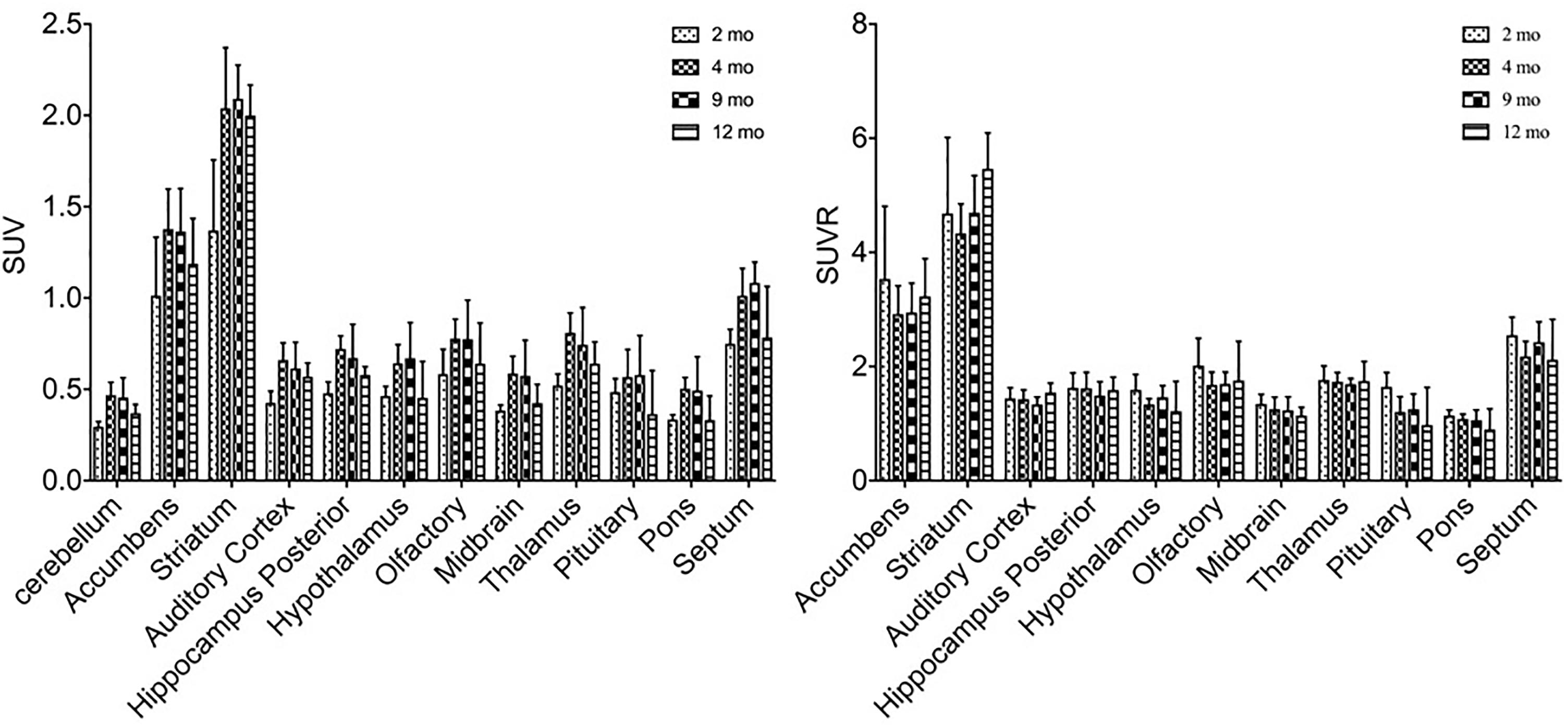
Figure 4. Alterations of SUV and SUVR of 11C-CFT in brain regions from 2 to 12 months of age. SUV (left) increased from 2 to 4 months of age in the striatum and accumbens, along with other regions. SUVR (right) did not fluctuate in most brain regions, aside from varying patterns in the striatum and accumbens. All data were presented by mean ±SD.
SUVR of 11C-CFT displayed a different pattern compared to that of SUV uptake. Striatum SUVR did not change significantly from 2 to 4 months of age (4.68 ± 1.33 to 4.33 ± 0.52), but increased from 2 to 12 months (4.68 ± 1.33 to 5.47 ± 0.63, P = 0.04) and also from 4 to 12 months (+26.3%, P = 0.0017) (Supplementary Table S6).
Age-Dependent Changes of 18F-PF-(+)-DTBZ Radioactivity in Rat Brain
Similar alterations of VMAT2 expression were found in these rats by PET imaging using 18F-PF-(+)-DTBZ compared to 11C-CFT, when quantifying by SUV (Figure 5). Highest 18F-PF-(+)-DTBZ activity accumulated in the striatum, accumbens, and septum. Additionally, the striatum displayed significantly low 18F-PF-(+)-DTBZ uptake at 2 months of age (SUV: 1.36 ± 0.22) compared to 4 months (SUV: 2.22 ± 0.29, P < 0.0001 ), 9 months (SUV: 1.98 ± 0.34, P = 0.0005), and 12 months (SUV: 1.93 ± 0.19, P = 0.0015) of age. Again, the same pattern in the accumbens that was found using 11C-CFT was observed, as there was significantly low 18F-PF-(+)-DTBZ uptake at 2 months of age (SUV: 1.24 ± 0.18) compared with 4 months (SUV: 1.96 ± 0.17, P < 0.0001), 9 months (SUV: 1.68 ± 0.31, P = 0.0273), and 12 months (SUV: 1.65 ± 0.19, P = 0.0442) of age. Note that uptake in certain brain regions, such as cortex, were not included here because they were affected by high bone uptake (Supplementary Table S3).
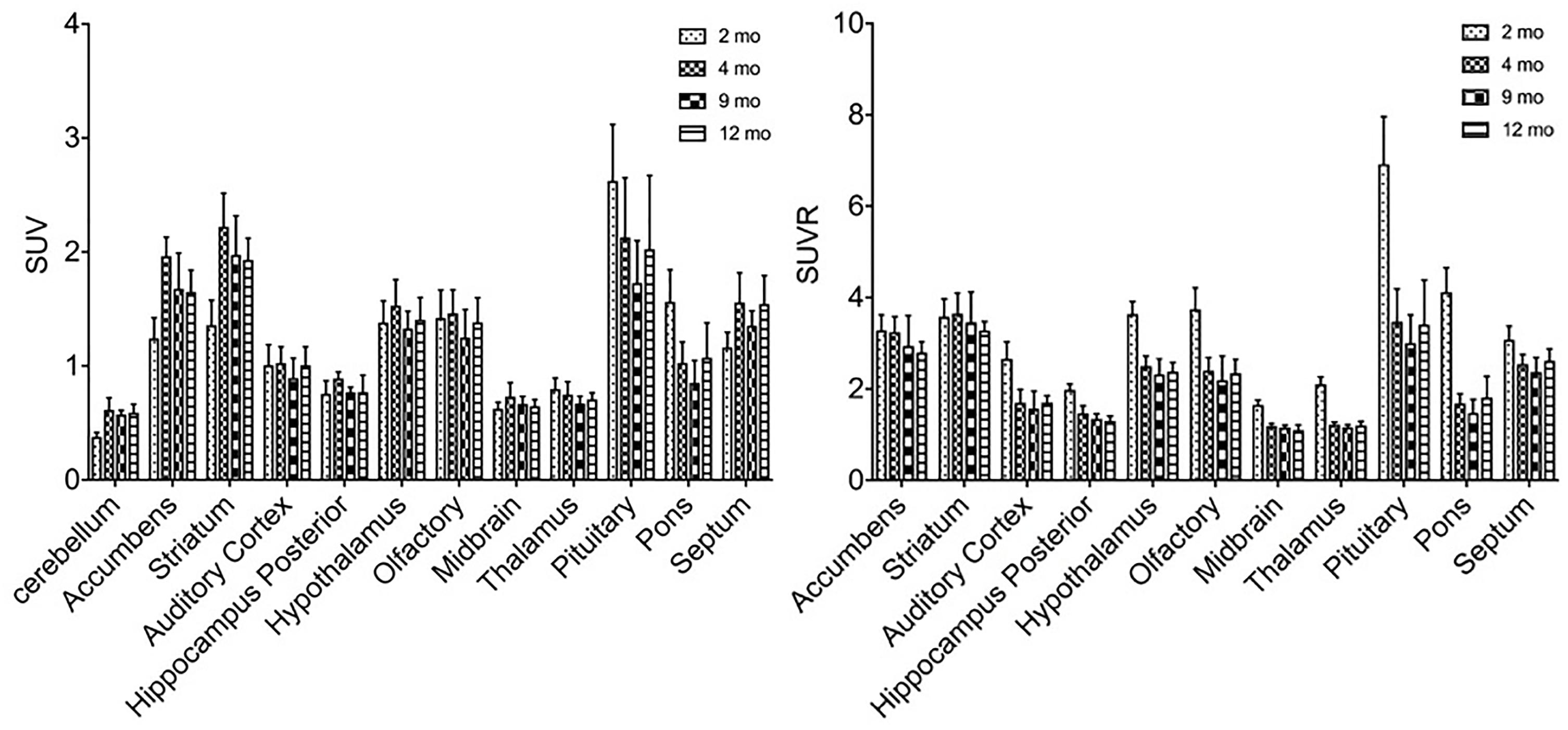
Figure 5. SUV and SUVR of 18F-PF-(+)-DTBZ in brain regions of rats at different ages. Increased SUV (left) and decreased SUVR (right) 18F-PF-(+)-DTBZ uptake was observed from 2 months of age onward. All data were presented by mean ±SD.
A slight, non-significant decline SUVR of 18F-PF-(+)-DTBZ was observed from 2 to 12 months of age at the striatum (-8.4%) and accumbens (-14.7%). Yet, significant decreases of 18F-PF-(+)-DTBZ SUVR were observed in other brain regions in this period, such as in the hippocampus (P = 0.0463) and auditory cortex (P = 0.0021) (Supplementary Table S4).
Age-Dependent Changes of Glucose Metabolism in Brains of Rats
In order to study the role of glucose metabolism during brain growth and the relationship among glucose metabolism and DAT and VMAT2 expression, we also examined glucose changes in the brain using 18F-FDG (Figure 6). A similar increased age-dependent FDG SUV uptake pattern was discovered from 2 months to 4, 9, and 12 months of age, as seen in both 11C-CFT and 18F-PF-(+)-DTBZ. However, high accumulations were found in the cortex, striatum, and accumbens, while the lowest uptake was observed in the pituitary gland. SUV in the striatum at 2 months of age increased significantly from 3.71 ± 0.78 to 5.28 ± 0.81 (P < 0.0001) at 4 months of age, and then decreased gradually from 4 to 9 and 12 months of age. Almost all of the brain regions demonstrated a significantly lower FDG SUV uptake at 2 months compared to older ages, except in the pituitary gland, which possessed the lowest uptake and displayed no significant change of uptake at different ages. SUVR uptake decreased significantly from 2 months to older ages in most brain regions, including the striatum, accumbens and cortex etc., this decrease was not observed in the thalamus, pons, and auditory cortex (Supplementary Tables S1, S2).
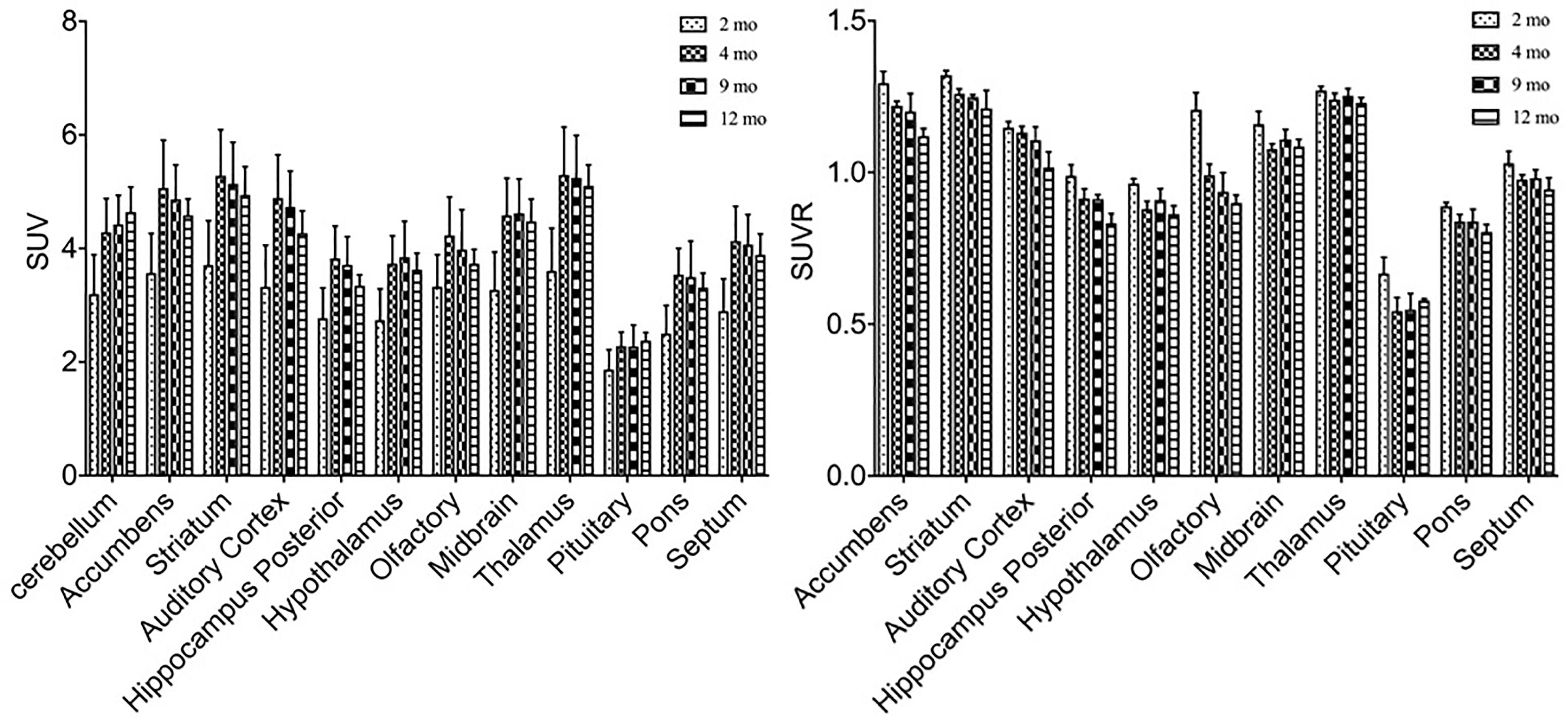
Figure 6. Age-dependent changes of SUV and SUVR uptake of 18F-FDG in brain regions of rats at different ages by a longitudinal PET study after intravenous administration. Increased SUV (left) and decreased SUVR (right) of 18F-FDG was observed from 2 months of age to older ages. All data were presented by mean ± SD.
Relationship Between Age-Dependent Changes of 18F-FDG, 18F-PF-(+)-DTBZ, and 11C-CFT Uptake in Brains
In order to determine the relationship of glucose metabolism, DAT, and VMAT2 during brain development, we compared the age-dependent change of striatal uptake of these three radiotracers. There existed a similar pattern of age-dependent change in regional brain SUV uptake among these three radiotracers from 2 to 12 month of age, but no correlation of SUVR uptake in these brain regions. As shown in Figure 7, 18F-FDG, 18F-PF-(+)-DTBZ, and 11C-CFT SUV in the striatum displayed similar patterns by increasing from 2 to 4 months and then reaching a slightly descending plateau until 12 months of age. Interestingly, SUV of 18F-PF-(+)-DTBZ, and 11C-CFT were similar at different individual ages (P > 0.05 at 2, 4, 9, and 12 months). However, SUVR demonstrated different patterns in striatum for these three radiotracers. The striatum 11C-CFT SUVR was higher than 18F-PF-(+)-DTBZ SUVR at all ages.
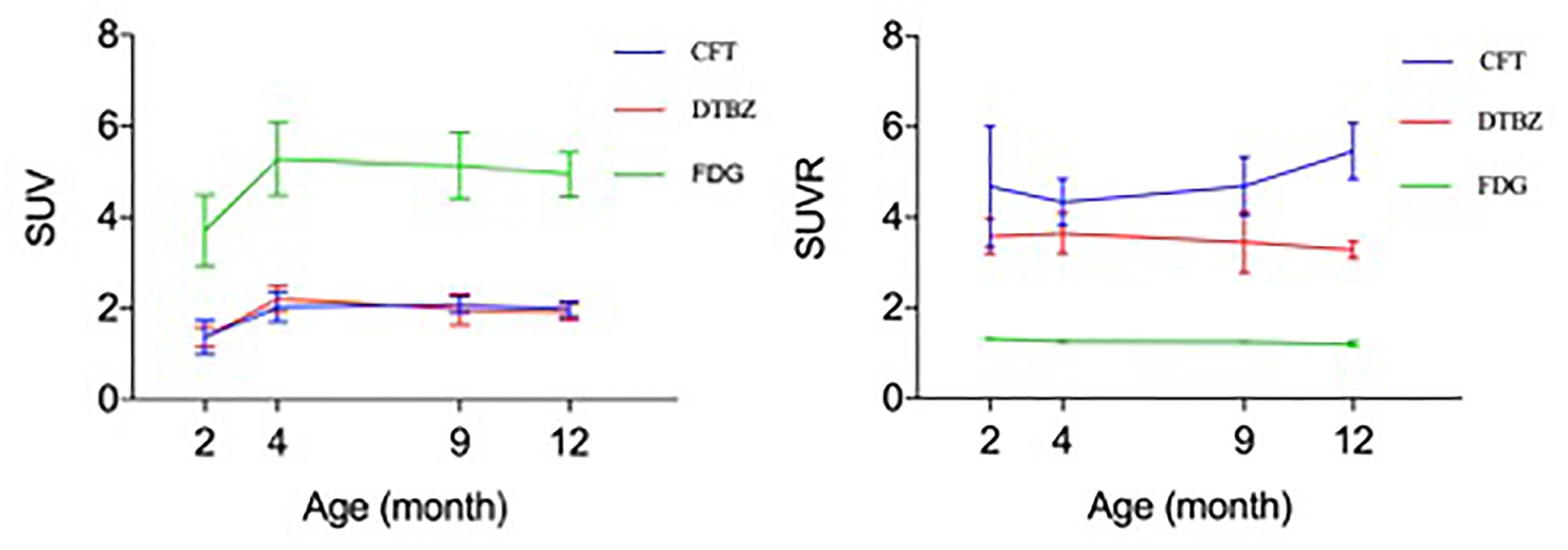
Figure 7. Correlation of 18F-FDG, 18F-PF-(+)-DTBZ and 11C-CFT uptake in the striatum of rats. A similar trend of SUV (left) at different ages for these 3 radiotracers, age-dependent increase uptake was observed from 2 months of age to an older age. And a coincidence of SUV uptake pattern for 18F-PF-(+)-DTBZ and 11C-CFT, but no correlation was observed for SUVR uptake (right) of these radiotracers. All data were presented by mean ±SD.
Discussion
Investigation of age-dependent changes of various metabolic pathways or expression or function of receptors or transporters is critical for a better understanding regulation of brain development and maturation. Many studies revealed brain function alterations in brain aging, such as fluctuations in DAT, glucose levels, and copper metabolism (Kawamura et al., 2003; Peng et al., 2018). However, few studies were dedicated to study of morphological and functional changes in brain development and maturation at adolescent population, partly due to technical limitation and other factors such as access to adolescent human subject population. In vivo imaging techniques, such as MRI and PET, are useful tools for monitoring disease progression along with changes in biological pathways and metabolism. The definition of brain maturity is divided into many types, such as social, cognitive, and neurotransmitter system etc., Previous studies using MRI found brain structures, especially the rat brain cortex, are not fully developed at 3 months of age. Therefore, they recommend careful examination of the brain structures before longitudinal examinations (Mengler et al., 2014). However, the function examination of brain development is rarely considered, particularly the examination of expression of dopamine-related receptors and transporters in brain maturation.
Dopamine levels are considered to represent the density of dopamine terminals. DAT and VMAT2 can reflect dopamine levels, and they are biomarkers brain disease and used to study brain aging (Colebrooke et al., 2006; Troiano et al., 2010; Oh et al., 2012). DAT and VMAT2 in the striatum were found to be decreased in the brains of aging rats and in human brains using 11C-CFT and 18F-PF-(+)-DTBZ as radiotracers (Kawamura et al., 2003; Hall et al., 2014). More importantly, DAT and VMAT2 were biomarkers for Parkinson’s Disease (PD). 11C-CFT and 18F-PF-(+)-DTBZ were applied to diagnose PD and evaluate the severity of PD (Nurmi et al., 2000; Hsiao et al., 2014). The increased expression of dopamine terminals can reflect the process of brain maturation (O’Donnell, 2010). Age-dependent changes of the DAT and VMAT2 biomarkers could be useful in the assessment of density changes of dopamine terminals associated with maturation.
In this study, we found age-dependent increases in FDG uptake in rat brains from 2 to 4 months of age, and a slightly non-significant decline of glucose metabolism was observed from 4 to 12 month. This finding is different from decreased glucose levels in aging brains described in previous reports (Chetelat et al., 2013; Ewers et al., 2014; Nugent et al., 2014). The increased glucose metabolism may be caused by the increase in glucose demand in brain maturation. In addition to the increase in glucose metabolism, expression of DAT and VMAT2 was also elevated from 2 to 4 months of age within the striatum. This increased density of dopamine terminals in the striatum and suggests an increase in dopamine level or concentration. Mechanisms of concurrent increase of FDG uptake and elevated expression of DAT and VMAT2 are not clear.
Aside from the measurement of SUV, we also calculated the SUVR of these three radiotracers. Calculation of SUVR is considered standard practice in clinical 18F-FDG PET brain imaging. Interestingly, the pattern of SUVR is different from the pattern of SUV among these three radiotracers. SUV of 18F-FDG increased from 2 to 4 months of age in most brain regions, while SUVR declined slightly, which is caused by global increased glucose uptake in rat brains and faster increase of FDG uptake in the cerebellum. For SUVR of 11C-CFT and 18F-PF-(+)-DTBZ, no significant changes were observed in these brain regions, except in the stratum (2 m vs. 12 m, P = 0.04; 4 m vs. 12 m, P = 0.002) for 11C-CFT. This may be induced by the global brain increase of 11C-CFT uptake. The age-dependent higher and increased SUVR of 11C-CFT in the striatum compared with a decline in SUVR of 18F-PF-(+)-DTBZ showed the potential of 11C-CFT in the diagnosis of brain disease in the striatum, such as PD (Yagi et al., 2010).
Owing to the high concentrations of dopamine in the striatal regions, several studies of dopamine in aging have been focused on this region. Different from those studies in aged animals, we found a correlation in the SUV uptake curves of 11C-CFT and 18F-PF-(+)-DTBZ in the striatum (Figure 7). Both radiotracers could reflect the expression of dopamine levels. Our results indicated the positive correlation of absolute quantification (SUV) of DAT and VMAT2 in the striatum in maturation, which demonstrates growth of dopamine terminals in the striatum, and the numbers of DAT and VMAT2 may also be similar within the striatum. 18F-FDG possessed a higher SUV uptake and similar SUV pattern compared with the other two radiotracers within the stratum, indicating the significance of glucose metabolism in the development of DAT and VMAT2 in this region.
The age of small animals is a key impact factor in the study of brain disease, yet many brain studies are based on the assumption of matured brains. When the animals are too young with undeveloped brain-blood barrier (BBB), radiotracers will not need to penetrate the BBB to induce high brain uptake (Patel and Gibson, 2008). Additionally, immature brains will introduce deviation in longitudinal studies. Therefore, mature mice or rats are essential in the evaluation of new radiotracers targeting the brain and longitudinal studies examining brain disease and brain functions. However, about 300 g of body weight or 2 to 3 months of age have often been applied to define the time window of maturity in rats.
In previous studies, volume change, cortical thickness, and cell density in the striatum were found to be stabilized in 3 months of age, but myelination in the striata continued up to 3 months of age and in the cortex up to 6 months (Mengler et al., 2014). Our study confirms that brain synaptic development is not stabilized within the first 4 months, as the densities of DAT and VMAT2 continue grow at 4 months of age, which coincided with previous study. Therefore, 4 months of age may be considered as the window time for brain maturation of dopamine. Longitudinal study for brain, especially PET imaging study, should not start before 4 months of age in rats. And future studies need to better define the end of the developmental phase before a related study can begin.
There were several limitations in this study. Brain size could be influent factor for the measurement of brain uptake. In previous study, brain size did not change significantly after 2 month of age in Wistar rats (Mengler et al., 2014). Brain uptake pattern of these rats at 2 and 12 month matched PMOD template (Supplementary Figure S2), which means brain uptake from PMOD can be used for comparison. That conclusion supported us to start our study from 2 month of age. However, Lack of MRI study for brain size still limit this study. Perfusion of the brain uptake as a consequence of brain development and differential response to anesthetization is another limitation. Different type of anesthetization brought different response to brain uptake. Brain uptake of 18F-FDG decreased in condition of isoflurane and ketamine/xylazine anesthetization, no change of striatal 18F-PF-(+)-DTBZ uptake was observed in isoflurane but ketamine increased 18F-PF-(+)-DTBZ striatal uptake (Toyama et al., 2004; Chen et al., 2016). All of these results indicated anesthetization is one of the confounding factor to brain uptake. Especially at different age of rats, differential response to anesthetization may cause different perfusion pattern, relative proportion of the tracer in the brain may alter. This is also one of the main limitation in this study. All of these limitations desired further study to investigate the infections.
Conclusion
Age-dependent increase of striatal 18F-FDG, 18F-PF-(+)-DTBZ and 11C-CFT radiotracer uptake was detected in Wistar rats from 2 to 4 months of age, reaching plateau of uptake in matured rats at 4 month of age. The findings suggest that 4 months of age may represent a desirable time point for conducting neurological study of brain disease to avoid confounding effects of ongoing changes of glucose metabolism and neuroreceptor expression on neurological studies involving use of PET and radiopharmaceuticals. The findings support further investigation of age-dependent changes of DAT and VMAT2 expression during brain development and maturation with PET using 18F-PF-(+)-DTBZ and 11C-CFT radiotracer, respectively.
Author Contributions
DJ and FX designed and performed the experiments. XL and FX produced the radiotracers. DJ, ZL, FH, CZ, YG, and FX contributed to conception and designed the study. DJ, FX, and FH contributed to analysis and interpretation of data. FX wrote the draft of this manuscript. DJ, NR, FP, and FX contributed to critical review and revision of the manuscript for this article.
Funding
This research project was supported by the National Science Foundation of China (81571345), National Key Research and Development Program Foundation of China (2016YFC1306403), Natural Science Foundation and Major Basic Research Program of Shanghai (16JC1420502), program of the Shanghai Science and Technology Commission (17511107103 and 17411953500), and Fudan University-SIBET Medical Engineering Joint Fund (yg2017-003) to YG. This research was also sponsored by the Shanghai Sailing Program (18YF1403200) and startup fund of Huashan Hospital, Fudan University (2017QD081) to FX.
Conflict of Interest Statement
The authors declare that the research was conducted in the absence of any commercial or financial relationships that could be construed as a potential conflict of interest.
Acknowledgments
The authors want to thank the generous assistance from Jianfei Xiao.
Supplementary Material
The Supplementary Material for this article can be found online at: https://www.frontiersin.org/articles/10.3389/fnins.2018.01052/full#supplementary-material.
References
Alexander, P. K., Lie, Y., Jones, G., Sivaratnam, C., Bozinvski, S., Mulligan, R. S., et al. (2017). Management impact of imaging brain vesicular monoamine transporter type 2 in clinically uncertain parkinsonian syndrome with 18F-AV133 and PET. J. Nucl. Med. 58, 1815–1820. doi: 10.2967/jnumed.116.189019
Aljabar, P., Bhatia, K. K., Murgasova, M., Hajnal, J. V., Boardman, J. P., Srinivasan, L., et al. (2008). Assessment of brain growth in early childhood using deformation-based morphometry. Neuroimage 39, 348–58. doi: 10.1016/j.neuroimage.2007.07.067
Brownell, A. L., Elmaleh, D. R., Meltzer, P. C., Shoup, T. M., Brownell, G. L., Fischman, A. J., et al. (1996). Cocaine congeners as PET imaging probes for dopamine terminals. J. Nucl. Med. 37, 1186–1192.
Chen, Z., Tang, J., Liu, C., Li, X., Huang, H., Xu, X., et al. (2016). Effects of anesthetics on vesicular monoamine transporter type 2 binding to 18F-FP-(+)-DTBZ: a biodistribution study in rat brain. Nucl. Med. Biol. 43, 124–129. doi: 10.1016/j.nucmedbio.2015.09.009
Chetelat, G., Landeau, B., Salmon, E., Yakushev, I., Bahri, M. A., Mezenge, F., et al. (2013). Relationships between brain metabolism decrease in normal aging and changes in structural and functional connectivity. Neuroimage 76, 167–177. doi: 10.1016/j.neuroimage.2013.03.009
Choi, H., Choi, Y., Kim, K. W., Kang, H., Hwang, D. W., Kim, E. E., et al. (2015). Maturation of metabolic connectivity of the adolescent rat brain. eLife 4:e11571. doi: 10.7554/eLife.11571
Chugani, H. T. (1998). A critical period of brain development: studies of cerebral glucose utilization with PET. Prev. Med. 27, 184–188. doi: 10.1006/pmed.1998.0274
Chugani, H. T., and Phelps, M. E. (1986). Maturational changes in cerebral function in infants determined by 18FDG positron emission tomography. Science 231, 840–843. doi: 10.1126/science.3945811
Colebrooke, R. E., Humby, T., Lynch, P. J., McGowan, D. P., Xia, J., Emson, P. C. (2006). Age-related decline in striatal dopamine content and motor performance occurs in the absence of nigral cell loss in a genetic mouse model of Parkinson’s disease. Eur. J. Neurosci. 24, 2622–2630. doi: 10.1111/j.1460-9568.2006.05143.x
Eiden, L. E., and Weihe, E. (2011). VMAT2: a dynamic regulator of brain monoaminergic neuronal function interacting with drugs of abuse. Ann. N. Y. Acad. Sci. 1216, 86–98. doi: 10.1111/j.1749-6632.2010.05906.x
Ewers, M., Brendel, M., Rizk-Jackson, A., Rominger, A., Bartenstein, P., Schuff, N., et al. (2014). Reduced FDG-PET brain metabolism and executive function predict clinical progression in elderly healthy subjects. Neuroimage Clin. 4, 45–52. doi: 10.1016/j.nicl.2013.10.018
Goyal, M. S., and Raichle, M. E. (2018). Glucose requirements of the developing human brain. J. Pediatr. Gastroenterol. Nutr. 66(Suppl. 3), S46–S49. doi: 10.1097/mpg.0000000000001875
Hall, F. S., Itokawa, K., Schmitt, A., Moessner, R., Sora, I., Lesch, K. P., et al. (2014). Decreased vesicular monoamine transporter 2 (VMAT2) and dopamine transporter (DAT) function in knockout mice affects aging of dopaminergic systems. Neuropharmacology 76, 146–155. doi: 10.1016/j.neuropharm.2013.07.031
Hsiao, I. T., Weng, Y. H., Hsieh, C. J., Lin, W. Y., Wey, S. P., Kung, M. P., et al. (2014). Correlation of parkinson disease severity and 18F-DTBZ positron emission tomography. JAMA Neurol. 71, 758–766. doi: 10.1001/jamaneurol.2014.290
Kawamura, K., Oda, K., Ishiwata, K. (2003). Age-related changes of the [11C]CFT binding to the striatal dopamine transporters in the Fischer 344 rats: a PET study. Ann. Nucl. Med. 17, 249–253.
Khundrakpam, B. S., Lewis, J. D., Zhao, L., Chouinard-Decorte, F., Evans, A. C. (2016). Brain connectivity in normally developing children and adolescents. Neuroimage 134, 192–203. doi: 10.1016/j.neuroimage.2016.03.062
Mengler, L., Khmelinskii, A., Diedenhofen, M., Po, C., Staring, M., Lelieveldt, B. P., et al. (2014). Brain maturation of the adolescent rat cortex and striatum: changes in volume and myelination. Neuroimage 84, 35–44. doi: 10.1016/j.neuroimage.2013.08.034
Mosconi, L., Tsui, W. H., Herholz, K., Pupi, A., Drzezga, A., Lucignani, G., et al. (2008). Multicenter standardized 18F-FDG PET diagnosis of mild cognitive impairment, Alzheimer’s disease, and other dementias. J. Nucl. Med. 49, 390–8. doi: 10.2967/jnumed.107.045385
Nugent, S., Tremblay, S., Chen, K. W., Ayutyanont, N., Roontiva, A., Castellano, C. A., et al. (2014). Brain glucose and acetoacetate metabolism: a comparison of young and older adults. Neurobiol. Aging 35, 1386–1395. doi: 10.1016/j.neurobiolaging.2013.11.027
Nurmi, E., Ruottinen, H. M., Kaasinen, V., Bergman, J., Haaparanta, M., Solin, O., et al. (2000). Progression in Parkinson’s disease: a positron emission tomography study with a dopamine transporter ligand [18F]CFT. Ann. Neurol. 47, 804–808.
O’Donnell, P. (2010). Adolescent maturation of cortical dopamine. Neurotox. Res. 18, 306–312. doi: 10.1007/s12640-010-9157-9153
Oh, M., Kim, J. S., Kim, J. Y., Shin, K. H., Park, S. H., Kim, H. O., et al. (2012). Subregional patterns of preferential striatal dopamine transporter loss differ in Parkinson disease, progressive supranuclear palsy, and multiple-system atrophy. J. Nucl. Med. 53, 399–406. doi: 10.2967/jnumed.111.095224
Patel, S., and Gibson, R. (2008). In vivo site-directed radiotracers: a mini-review. Nucl. Med. Biol. 35, 805–815. doi: 10.1016/j.nucmedbio.2008.10.002
Peng, F., Xie, F., Muzik, O. (2018). Alteration of copper fluxes in brain aging: a longitudinal study in rodent using 64CuCl2-PET/CT. Aging Dis. 9, 109–118. doi: 10.14336/ad.2017.1025
Quinn, R. (2005). Comparing rat’s to human’s age: how old is my rat in people years? Nutrition 21, 775–777. doi: 10.1016/j.nut.2005.04.002
Schiffer, W. K., Mirrione, M. M., Biegon, A., Alexoff, D. L., Patel, V., Dewey, S. L. (2006). Serial microPET measures of the metabolic reaction to a microdialysis probe implant. J. Neurosci. Methods 155, 272–284. doi: 10.1016/j.jneumeth.2006.01.027
Smailagic, N., Vacante, M., Hyde, C., Martin, S., Ukoumunne, O., Sachpekidis, C. (2015). 18F-FDG PET for the early diagnosis of Alzheimer’s disease dementia and other dementias in people with mild cognitive impairment (MCI). Cochrane Database Syst. Rev. 1:CD010632. doi: 10.1002/14651858.CD010632.pub2
Sowell, E. R., Thompson, P. M., Leonard, C. M., Welcome, S. E., Kan, E., Toga, A. W. (2004). Longitudinal mapping of cortical thickness and brain growth in normal children. J. Neurosci. 24, 8223–8231. doi: 10.1523/jneurosci.1798-04.2004
Toyama, H., Ichise, M., Liow, J. S., Vines, D. C., Seneca, N. M., Modell, K. J., et al. (2004). Evaluation of anesthesia effects on [18F]FDG uptake in mouse brain and heart using small animal PET. Nucl. Med. Biol. 31, 251–256. doi: 10.1016/s0969-8051(03)00124-120
Troiano, A. R., Schulzer, M., de la Fuente-Fernandez, R., Mak, E., McKenzie, J., Sossi, V., et al. (2010). Dopamine transporter PET in normal aging: dopamine transporter decline and its possible role in preservation of motor function. Synapse 64, 146–151. doi: 10.1002/syn.20708
Wood, H. (2014). Parkinson disease: 18F-DTBZ PET tracks dopaminergic degeneration in patients with Parkinson disease. Nat. Rev. Neurol. 10:305. doi: 10.1038/nrneurol.2014.81
Yagi, S., Yoshikawa, E., Futatsubashi, M., Yokokura, M., Yoshihara, Y., Torizuka, T., et al. (2010). Progression from unilateral to bilateral parkinsonism in early parkinson disease: implication of mesocortical dopamine dysfunction by PET. J. Nucl. Med. 51, 1250–1257. doi: 10.2967/jnumed.110.076802
Keywords: positron emission tomography, brain development, vesicular monoamine transporter 2 (VMAT2), dopamine transporter (DAT), glucose metabolism
Citation: Jiang D, Lu X, Li Z, Rydberg N, Zuo C, Peng F, Hua F, Guan Y and Xie F (2019) Increased Vesicular Monoamine Transporter 2 (VMAT2) and Dopamine Transporter (DAT) Expression in Adolescent Brain Development: A Longitudinal Micro-PET/CT Study in Rodent. Front. Neurosci. 12:1052. doi: 10.3389/fnins.2018.01052
Received: 25 June 2018; Accepted: 27 December 2018;
Published: 15 January 2019.
Edited by:
Yun Zhou, Washington University School of Medicine in St. Louis, United StatesCopyright © 2019 Jiang, Lu, Li, Rydberg, Zuo, Peng, Hua, Guan and Xie. This is an open-access article distributed under the terms of the Creative Commons Attribution License (CC BY). The use, distribution or reproduction in other forums is permitted, provided the original author(s) and the copyright owner(s) are credited and that the original publication in this journal is cited, in accordance with accepted academic practice. No use, distribution or reproduction is permitted which does not comply with these terms.
*Correspondence: Fengchun Hua, aHVhZmNAaG90bWFpbC5jb20= Yihui Guan, Z3VhbnlpaHVpQGhvdG1haWwuY29t Fang Xie, ZmFuZ3hpZUBmdWRhbi5lZHUuY24=