- 1Department of Neurobiology, Systems Neuroscience Institute, University of Pittsburgh, Pittsburgh, PA, United States
- 2Center for Biologic Imaging, University of Pittsburgh, Pittsburgh, PA, United States
- 3Department of Cell Biology, University of Pittsburgh, Pittsburgh, PA, United States
- 4Department of Radiology, University of Pittsburgh, Pittsburgh, PA, United States
- 5Department of Bioengineering, University of Pittsburgh, Pittsburgh, PA, United States
- 6Department of Neurobiology, University of Pittsburgh, Pittsburgh, PA, United States
Objective: Reanimation of muscles paralyzed by disease states such as spinal cord injury remains a highly sought therapeutic goal of neuroprosthetic research. Optogenetic stimulation of peripheral motor nerves expressing light-sensitive opsins is a promising approach to muscle reanimation that may overcome several drawbacks of traditional methods such as functional electrical stimulation (FES). However, the utility of these methods has only been demonstrated in rodents to date, while translation to clinical practice will likely first require demonstration and refinement of these gene therapy techniques in non-human primates.
Approach: Three rhesus macaques were injected intramuscularly with either one or both of two optogenetic constructs (AAV6-hSyn-ChR2-eYFP and/or AAV6-hSyn-Chronos-eYFP) to transduce opsin expression in the corresponding nerves. Neuromuscular junctions were targeted for virus delivery using an electrical stimulating injection technique. Functional opsin expression was periodically evaluated up to 13 weeks post-injection by optically stimulating targeted nerves with a 472 nm fiber-coupled laser while recording electromyographic (EMG) responses.
Main Results: One monkey demonstrated functional expression of ChR2 at 8 weeks post-injection in each of two injected muscles, while the second monkey briefly exhibited contractions coupled to optical stimulation in a muscle injected with the Chronos construct at 10 weeks. A third monkey injected only in one muscle with the ChR2 construct showed strong optically coupled contractions at 5 ½ weeks which then disappeared by 9 weeks. EMG responses to optical stimulation of ChR2-transduced nerves demonstrated graded recruitment relative to both stimulus pulse-width and light intensity, and followed stimulus trains up to 16 Hz. In addition, the EMG response to prolonged stimulation showed delayed fatigue over several minutes.
Significance: These results demonstrate the feasibility of viral transduction of peripheral motor nerves for functional optical stimulation of motor activity in non-human primates, a variable timeline of opsin expression in a animal model closer to humans, and fundamental EMG response characteristics to optical nerve stimulation. Together, they represent an important step in translating these optogenetic techniques as a clinically viable gene therapy.
Introduction
Disease states such as severe spinal cord injuries (SCIs) are often accompanied by muscle paralysis and loss of motor function. In these cases, restoration of native muscle and motor function is the ultimate goal of therapeutic interventions. Brain-Machine Interfaces (BMIs), which attempt to reroute control signals from an intact brain to a motor effector and effectively bypass the site of injury, have made great strides toward achieving this goal over the last several decades. Motor BMIs have progressed from simple control of a computer cursor (Serruya et al., 2002; Taylor et al., 2002; Leuthardt et al., 2004; Revechkis et al., 2015) to high-dimensional control of a robotic arm (Hochberg et al., 2012; Collinger et al., 2013; Wodlinger et al., 2015). While these systems provide an excellent intermediate step and can restore a significant degree of independence to patients that they may not have experienced for many years, they do not address the desired goal of native limb reanimation.
The traditional approach to reanimating paralyzed limbs is to electrically stimulate muscles or their nerves. This approach, often referred to as Functional Electrical Stimulation (FES), was previously coupled to residual movements or muscle activity to control the electrical stimulation of paralyzed muscles (Peckham et al., 2002; Kilgore et al., 2008). More recently, intramuscular FES has been coupled with BMI control signals by several groups to produce brain-controlled modulation of native muscle activity and limb movements in both non-human primates (NHPs) (Moritz et al., 2008; Ethier et al., 2012) and human subjects (Bouton et al., 2016; Ajiboye et al., 2017; Biasiucci et al., 2018). While these studies demonstrate restoration of volitional control over previously paralyzed muscles and can convey a significant increase in independence to a patient, the control offered by these systems is far from ideal naturalistic control. For example, in the study by Ajiboye et al. (2017), a C4-level SCI patient implanted with intracortical microelectrode arrays regained some volitional control over paralyzed arm muscles after pairing FES of those muscles with intracortical control signals. This scheme allowed the subject to reclaim certain daily functions such as feeding himself, but these movements were quite slow, taking several tens of seconds for tasks that most people would complete in a second or two. FES-mediated movements in this study also required additional hardware to support the arm against gravity. These studies demonstrate that the current state-of-the art for muscle stimulation necessitates major technological advances to approach practical relevance or even approach the performance level of other BMI-driven effectors such as robotic arms.
The difficulties highlighted by that study may be due to several inherent drawbacks of FES. These potentially include a non-physiological, random or reverse recruitment order of muscle fibers (Henneman, 1957; Fang and Mortimer, 1991; Singh et al., 2000; Lertmanorat and Durand, 2004; Gregory and Bickel, 2005; Bickel et al., 2011), a poorly graded, steeply sigmoidal recruitment curve making controlled stimulation of intermediate force values difficult to achieve, and early fatigue of muscle contractions (Gregory and Bickel, 2005). These factors have limited the use of FES for reanimation of paralyzed muscle.
A potential alternative to FES that may circumvent some of these shortcomings is the use of peripheral optogenetic techniques to elicit muscle activity through Functional Optical Stimulation (FOS). In this approach, light sensitive ion channels, i.e., “opsins,” are inserted into the motor nerve axonal membrane, allowing the nerve to be depolarized using light stimulation. FOS experiments in rodents have suggested that this approach may hold several advantages over FES by overcoming the drawbacks of FES discussed above. In an initial study, Llewellyn et al. demonstrated in transgenic mice expressing the blue-light sensitive channelrhodopsin (ChR2) that optically stimulating motor nerves elicits a natural recruitment of muscle fibers; small diameter muscle fibers are recruited first with low-amplitude stimulation, and larger fibers are recruited with increasing stimulation intensities (Llewellyn et al., 2010). This combination of fiber activation produced a wide dynamic range of forces from fine to gross. This differs from the recruitment order of muscle fibers observed with FES. Related to these observations of natural recruitment order, Llewellyn et al. also found that muscle activation leads to decreased muscle fatigue, delaying the onset of muscle fatigue to repetitive optical stimulation for several minutes vs. only a few tens of seconds with electrical stimulation (Llewellyn et al., 2010). Additionally, viral transduction of opsins sensitive to different wavelengths of light makes it possible to selectively target only nerve fibers innervating a desired muscle. Conversely, FES is relatively non-selective in stimulating axons to individual muscles at proximal nerve sites. For example, electrically stimulating the sciatic nerve will activate contractions of the gastrocnemius, tibialis anterior, and other lower leg muscles non-specifically. However, Towne et al. have demonstrated selective activation of a single viral-targeted tibialis anterior muscle with optical stimulation at a common proximal sciatic nerve location (Towne et al., 2013). Although selective electrical stimulation can be accomplished to a degree, it typically requires complex spatial activation patterns or deforming the nerve (Tyler and Durand, 2002). Finally, although optical stimulation can cause photoelectric artifacts if shone directly on an electrode (Kozai and Vazquez, 2015), it does not cause electromyographic (EMG) artifacts to arise from distant optical stimulation, unlike the volume-conducted artifact associated with electrical stimulation of a muscle or nerve. This artifact-free stimulation could simplify signal processing in closed-loop stimulation schemes that rely on EMG or intraneural feedback (Yeom and Chang, 2010; Bruns et al., 2013). Overall, these potential advantages may make peripheral optogenetic stimulation a viable alternative to FES for muscle activation in neuroprosthetic applications.
To date, peripheral optogenetic activation of muscle activity displaying these potential benefits has only been demonstrated in rodents. Across these studies, several methods have been used to label motor nerves with stimulating opsins. Llewellyn et al. used a transgenic mouse line to express ChR2 in neurons in the peripheral nervous systems (PNS) under the Thy1 promoter, allowing the authors to elicit muscle activity through optical stimulation of peripheral motor nerve axons (Llewellyn et al., 2010). While use of transgenic mouse lines of this nature is useful for testing the neurophysiological characteristics of optogenetic stimulation, this type of germ-line manipulation is impractical for human applications. Bryson et al. demonstrated optical control of muscle in wild-type mice after transplanting motor neurons expressing ChR2 derived from embryonic stem cells into a nerve graft site (Bryson et al., 2014). A more common approach in line with genetic manipulation used in other systems and disease models (Asokan et al., 2012) is the utilization of adeno-associated virus (AAV) vectors to enable optical modulation of muscle activity. These include expression of ChR2 in rat peripheral motor nerves following muscle injection of an AAV vector (Towne et al., 2013; Maimon et al., 2017) or expression directly in mouse skeletal muscle tissue following systemic injection (Bruegmann et al., 2015). While direct optical modulation of muscle tissue is feasible, it would require individual light sources for each targeted muscle, and implanting optical stimulation hardware could be difficult in smaller or deep muscles such as intrinsic hand muscles. Conversely, viral transduction of opsins in motor nerve axons offers the potential to independently control multiple muscles from a single proximal nerve location more amenable to light source implantation, making it an appealing approach over muscle transduction.
While the rodent studies above are an important step in exploring the potential of peripheral optogenetic stimulation, translating these techniques to NHPs prior to human trials remains largely unexplored. Indeed, even the development of optogenetic techniques for NHPs in the central nervous system (CNS) has proven challenging with examples of successful viral transduction studies slowly beginning to accumulate. Multiple cortical studies have demonstrated optogenetic perturbation of local cortical network dynamics but did not report appreciable alteration of behavior (Han et al., 2009; Diester et al., 2011; Yazdan-Shahmorad et al., 2016). However, a small number of examples have demonstrated an effect of optogenetic modulation on behavior such as those related to reward (Stauffer et al., 2016), vision (Jazayeri et al., 2012), salience (Dai et al., 2014), eye movements (Cavanaugh et al., 2012; El-Shamayleh et al., 2017), and somatosensation (May et al., 2014). Notably, reports of optogenetic modulation of somatomotor behavior are still lacking. Outside of the brain, a handful of studies have demonstrated viral transduction of peripheral neuromuscular tissue with fluorescent proteins in NHP models (Towne et al., 2009; Okada et al., 2013), but optogenetic modulation of peripheral neural activity in primates similar to the aforementioned rodent studies has not been reported to date. With the difficulties in translating rodent-proven optogenetic techniques to primates, it is likely that similar challenges will be faced in translating peripheral motor optogenetic techniques due to the PNS's greater exposure to the immune system, the differences in rodent vs. primate immune responses, and sheer scale difference.
Given the potential benefits of FOS over current FES approaches for neuroprosthetic applications and the current gap regarding peripheral optogenetic modulation of motor activity in higher-order animal models, the current study examined the feasibility of virally mediated optogenetic modulation of motor activity in a macaque model. We explored the utility of an AAV vector, used successfully in prior rodent FOS studies, to transduce macaque motor nerves with commonly used opsins (ChR2 and Chronos) and drive PNS motor activity in an NHP model. To overcome some of the aforementioned translational challenges, we delivered the virus using a stimulating muscle injection technique to target and deliver virus locally near neuromuscular junctions. We then used optical stimulation of targeted nerves and EMG recordings to functionally assess opsin expression. We next examined the relationships between optical stimulation variables and elicited EMG activity for comparison with previous observations in rodents. Finally, we examined whole tissue imaging of opsin expression to correlate expression variability with observations of functional optical sensitivity in nerve samples. The results presented will not only help to address the feasibility of peripheral viral gene therapy and FOS in BMI applications, but may also help to identify further virus, opsin, and hardware development needed prior to clinical translation.
Methods
Subjects
For the main focus of this study, three male rhesus macaques (Macaca mulatta), Monkeys M, O, and P, weighing 7–9 kg were used in these experiments. All animal procedures were approved and conducted in accordance with the University of Pittsburgh's Institutional Animal Care and Use Committee.
Viral Constructs
Two high-titer AAV6-based viral vectors were obtained from Virovek, Inc. (Hayward, CA) for use in these experiments. The first vector, AAV6-hSyn-ChR2(H134R)-eYFP, was produced at a titer of 1.04 × 1014 vp/mL. This construct was previously used for AAV-mediated transduction of excitatory opsins in peripheral motor nerves (Towne et al., 2013; Maimon et al., 2017), and has been reported at a range of viral titers. The second construct tested in these experiments replaced the well-studied opsin, ChR2(H134R), with the more recently developed Chronos (Klapoetke et al., 2014). Chronos has faster kinetics and increased sensitivity over ChR2, but its utility has not been demonstrated in the periphery to date. The AAV6-hSyn-Chronos-eYFP construct was produced by Virovek at a titer of 1.00 × 1014 vp/mL.
Virus Injections
Aseptic techniques were used for all virus injection surgeries. Prior to virus injection, each monkey was sedated with a cocktail of ketamine (20 mg/kg) and xylazine (0.5 mg/kg). For each target muscle, a skin incision was made to expose the muscle while leaving the surrounding fascia intact. Following virus injection procedures as described below, all skin incisions were closed with subcuticular stitches. Injected animals received a 5 day course of antibiotics and were returned to their home cage to recover for at least 3 weeks before evaluation expression.
Monkeys M, O, and P received injections of AAV-based constructs in two, four, and one muscle(s), respectively, as shown in Figure 1 with injection parameters summarized in Table 1. Monkey M was injected in two muscles with the AAV6-hSyn-ChR2(H134R)-eYFP construct. The tibialis anterior (TA) muscle of each leg was injected with construct diluted to relatively low or high titer. The right TA (“high” titer leg) was injected with 160 μL of virus (1.66 × 1013 vp) diluted in hypertonic saline to a total volume of 2 mL (8.32 × 1012 vp/mL). The left TA (“low” titer) was injected with 20 μL of virus (2.08 × 1012 vp) diluted with hypertonic saline to a volume of 2 mL (1.04 × 1012 vp/mL). Ten individual injections were made per muscle with approximately 200 μL of virus solution injected per site.
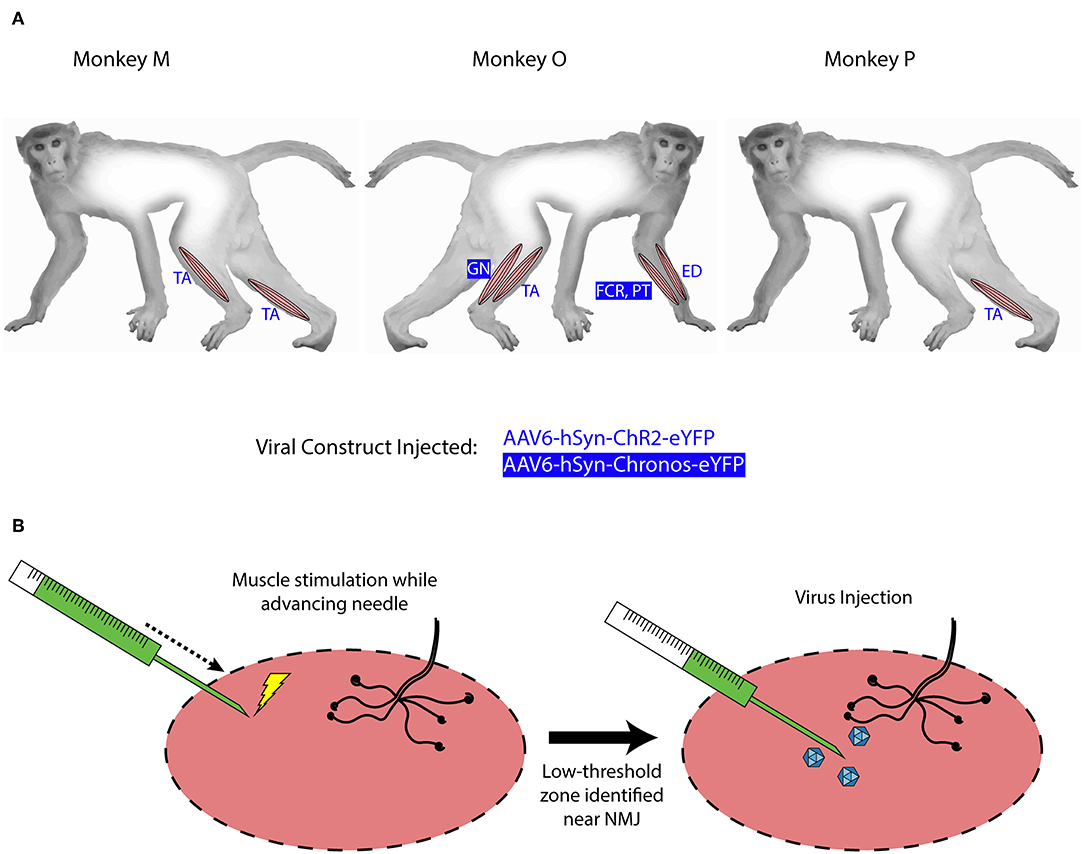
Figure 1. Intramuscular injections of viral optogenetic constructs. (A) Schematic of macaque subjects, viral constructs, and muscles injected. Blue/reverse blue lettering indicate whether a designated muscle was injected with the ChR2- or Chronos-based construct, respectively. Muscle abbreviations: TA, tibialis anterior; GN, lateral gastrocnemius; FCR, flexor carpi radialis; PT, pronator teres; ED, extensor digitorum. (B) Identification of injection zones. A stimulating injection needle was advanced slowly into the muscle while applying low-amplitude electrical stimulation. After observing near-maximal contractions, indicating a zone with higher density of neuromuscular junctions (NMJs), 200 μL of viral solution was injected slowly over 1 min. This process was repeated over 10 sites per muscle or muscle group.
At each injection site, low-threshold electrical stimulation was used to localize potential motor endplates to minimize the distance virus would have to diffuse before uptake at the neuromuscular junction. A 30 gauge monopolar injectable needle (Technomed, Netherlands) was attached to a tuberculin syringe filled with virus solution, while a metal hub needle attached to a ground lead was inserted through the skin edge. A biphasic waveform (200 μs at 0.25 mA, 400 μs at −0.125 mA) was applied between the needle tip and ground electrode via an analog stimulus isolator (A-M Systems, Model 2200). As electrical stimulation was applied, the needle was slowly advanced into the muscle by hand while monitoring muscle twitches. After finding a needle insertion position facilitating maximum contraction, stimulation was paused and 200 μL of virus was injected over ~1 min. The needle was held in place for an additional minute before slowly withdrawing it. This process was repeated for each injection site. Injections were aimed at the presumed line of neuromuscular junctions approximately 1/3 of the muscle length away from the proximal end of the muscle. Needle insertions were aimed in both proximal-to-distal and distal-to-proximal fashions toward this zone, and were spaced laterally across the muscle surface.
Monkey O received injections of both the ChR2 and Chronos viral constructs. Four muscles groups (two flexor/extensor pairs) were targeted. In the right leg, we injected the TA muscle with the AAV6-hSyn-ChR2-eYFP construct, and we injected the lateral gastrocnemius (GN) with AAV6-hSyn-Chronos-eYFP. In the left forearm, we injected the extensor digitorum (ED) with the ChR2 construct, and we injected both flexor carpi radialis (FCR) and pronator teres (PT) muscles with the Chronos solution. For each muscle, 100 μL of stock virus was diluted with hypertonic saline to 2 mL total volume (5.02 × 1012 vp/mL for ChR2, 5.0 × 1012 vp/mL for Chronos). The Chronos solution was split evenly between the FCR and PT muscles in the forearm (~1 mL per muscle over 4–5 sites). Targeting of the muscle endplates and muscle injections were performed in a similar fashion to those described for Monkey M.
Monkey P was injected with the AAV6-ChR2 construct in the right TA muscle. One-hundred microliters of stock virus (1.04 × 1013 vp) was diluted to a total volume of 1 mL with hypertonic saline at a slightly higher concentration (1.04 × 1013 vp/mL) than the highest used in Monkey M. Stimulating injections targeting neuromuscular junctions were used to deliver 900 μL of virus solution to the muscle over 5 sites. The deep peroneal (DP) nerve innervating the TA muscle was also exposed near its insertion into the TA via blunt separation of fibers of the overlying biceps femoris muscle. One-hundred microliters of virus solution was injected directly into the DP nerve over 3 sites.
Expression Evaluation
Each monkey was periodically evaluated for opsin expression over the course of 8–13 weeks. During an evaluation surgery, the monkey was anesthetized, and a previously injected muscle was re-exposed. Blunt dissection was used to separate fascia from the muscle and to expose the innervating nerve. Electrical stimulation of the nerve using a pair of bipolar hook electrodes (Cadwell Laboratories, Kennewick, WA) was used to confirm the identity of the desired nerve. Optical stimulation was delivered using a 400 μm diameter core multimode fiber (ThorLabs, Newton, NJ) connected to a 150 mW, 472 nm fiber-coupled laser (LaserGlow Technologies, Toronto, Ontario). Maximum laser output at the fiber tip was typically around 110 mW. While moving the fiber tip manually along the length of the nerve, optical stimulation trains of 15–20 ms pulses at 2.5 Hz and 100 mW were delivered to scan the nerve for areas sensitive to optical stimulation. A pair of the injectable electrode needles (same model as used for stimulation during muscle injection) was inserted into the muscle belly to measure EMG activity with a metal hub needle in the skin edge serving as electrical ground. EMG electrodes were connected to a low-impedance differential headstage with 20x gain (RA16LI-D, Tucker Davis Technologies (TDT), Alachua, FL). A TDT neurophysiology recording system (RZ-2) was used to coordinate optical stimulation waveforms with EMG recordings. All waveforms were sampled at 24 kHz.
Following periodic evaluations of nerve expression, any retracted muscle and fascia overlying target nerves were sutured in layers with absorbable suture. Skin incisions were closed with subcuticular stitches, and the animal was returned to its cage to recover.
Perfusion, Tissue Clearing, and Imaging
Following final evaluation of opsin expression, each animal was perfused transcardially with 1X phosphate buffer solution (PBS) followed by 4% paraformaldehyde (PFA). Sections of targeted nerves were harvested and post-fixed in 4% PFA overnight, after which they were stored in 0.02% sodium azide solution in PBS at 4°C while awaiting processing for tissue clearing. Several nerve samples from each animal were reserved for tissue clearing and whole sample imaging. 5–10 mm long sections of nerve were excised from the main nerve branch directly innervating virus targeted muscles. Nerve samples were cleared using the polyethylene glycol (PEG)-associated solvent system (PEGASOS) passive immersion protocol (Jing et al., 2018). Briefly, following tissue fixation in 4% PFA, tissues were first passively bathed in a 25% Quadrol to decolor the tissue step, followed by gradient solutions (30, 50, 70%) of tert-Butanol (tB) at 37° over 2 days for delipidation and dehydration. Samples were further dehydrated in a solution composed of 70% tB, 27% PEG methacrylate Mn 500 (PEGMMA500), and 3% Quadrol for 2 days. Finally tissues were cleared for at least 1 day in a solution of BB-PEG formed by mixing 75% benzyl benzoate (BB) and 25% PEGMMA500 supplemented with 3% Quadrol until tissues reached transparency. Following clearing, samples were preserved in the BB-PEG clearing medium at room temperature.
Following tissue clearing, whole nerve samples were mounted in BB-PEG and sealed between two rounded cover glass. Tissues were imaged using the RS-G4 ribbon scanning confocal microscope (Caliber I.D., Rochester, NY) (Watson et al., 2017) equipped with an iChrome MLE laser engine (Toptica Photonics, Munich Germany). Large-area mosaic images were captured using the Olympus XLPLN25XWMP2, 25x, 1.05NA, water immersion objective with a scan zoom of 1.7 and lateral resolution of 0.295 microns. Z-steps were acquired at 1.52 microns. Fluorescence from eYFP was detected by using 488 nm excitation and 520/44 nm emission filters. The tissue was imaged from bottom to top with the 488-laser power interpolated linearly through Z from 15% (top) to 30% (bottom). Mosaic images were stitched and assembled by the microscope software.
To facilitate analysis, mosaic images were processed in MATLAB R2017b by first flattening the image and then subtracting background. A unique background filter was calculated for each image by applying a gaussian filter with a standard deviation of 6 and then a morphologic opening with a disk structuring element of radius 200 pixels. A flattening filter was produced by taking the mean of the background filter divided by the background filter:
First the flattening filter was applied to both the RAW image and the background filter by multiplying the two images. The flattened background filter was subtracted from the resulting image:
Flattened and background-subtracted images were then assembled into volumes using the Imaris File Converter and analyzed using Imaris v9.2.1 (Bitplane). Models of eYFP expressing nerve axon segments were built using the Imaris Surpass surface tool. Manual cleaning of the surface rendering was performed to ensure that labeled regions represented eYFP expressing nerves. Volume and position data for eYFP model surface elements were exported from the surface tool to Matlab where expression volume was binned as a function of longitudinal (Y) position.
Results
Time Course of Expression
Each monkey was tested for expression in targeted nerves intermittently between the injection surgery and final terminal evaluation surgery. Injection results are summarized in Table 1. Monkey M was tested at 3 (right TA), 4 (left TA), and 8 weeks (both legs). During evaluation time points at weeks 3 and 4, neither leg showed visible contractions or EMG deflections upon laser stimulation at full power. The deep peroneal (DP) nerve innervating the TA muscle was exposed for stimulation but was not aggressively dissected to avoid permanently damaging the nerve. At week 8, both targeted nerves were re-exposed and tested. Initial optical stimulation along the length of the superficial (anterior) portion of the left DP nerve again did not suggest overt expression of ChR2. However, stimulation of the posteriolateral aspect of the nerve at a single proximal site demonstrated visible contraction of the TA muscle. Optical stimulation of the anterior aspect of the nerve at this location or proximal/distal to it did not elicit muscle contractions. However, following this initial display of sensitivity, the DP was dissected distally to its insertion into the TA muscle where it branches out. At this point, optical stimulation of the nerve and muscle activity became more consistent with several branches showing sensitivity. Evaluation of the DP nerve of the right leg proceeded in a similar fashion with dispersed sensitive spots along the nerve proximal to the muscle, and more consistent sensitivity where the nerve branched out close to the muscle.
Expression of optogenetic transduction in Monkey O was tested at 5, 10, and 13 weeks post-injection. We first tested expression in the nerves leading to the TA and lateral GN muscles of the right leg at 5 weeks. No visible muscle contractions were elicited with optical stimulation of either nerve. At 10 weeks, we tested all targeted nerves in the right leg and left forearm. Neither nerve branch in the leg nor in the nerve supplying the ED muscle of the forearm demonstrated optically sensitivity. A branch of the median nerve supplying the PT muscle in the left forearm (injected with the Chronos vector) did facilitate brisk contraction of the PT when stimulated optically with the fiber-coupled laser. Upon observing optical sensitivity, we further dissected the nerve to accommodate placement of an LED nerve cuff intended for chronic stimulation of the nerve. After initial placement of the cuff, the nerve no longer initiated PT contractions when stimulated with blue light from the cuff or optical fiber. We suspected the nerve may have become irritated by prolonged exposure or irritation during the LED cuff placement, so we removed the cuff, re-sutured all nerve and muscle exposures, and returned the monkey to its home cage. At 13 weeks, we re-tested each targeted nerve. During this experiment, no nerves (including the previously sensitive branch to the PT muscle) exhibited optical sensitivity. Electrical stimulation of the PT muscle's nerve elicited brisk contractions, suggesting that the nerve was healthy.
The DP nerve of Monkey P innervating the injected right TA muscle was tested at 5 ½ weeks and 9 weeks post-injection. At the first checkpoint, optical stimulation of the exposed nerve resulted in small contractions visible through the skin. Optical sensitivity was more consistent along the exposed portion of the nerve than in Monkeys M and O with no obvious insensitive portions of nerve. After returning to check the nerve at the 9 week time point, no visual or EMG evidence of sensitivity to optical stimulation of the target nerve was present.
Visually Observed Responses to Optical Stimulation
Visible contractions to optical stimulation of targeted nerves were observed in all three monkeys. In monkey M, contractions of the TA muscle were clearly visible in both legs (Supplementary Movie 1). Additionally, contractions of different portions of the muscle could be observed when different fascicles were stimulated at the branch-out location of the nerve near insertion into the muscle. However, even at full power stimulation (>100 mW, 30 ms pulse duration), optical stimulation along the nerve did not produce functional movement of the lower leg (i.e., dorsiflexion of the foot). For the short period of time that we observed optical sensitivity in monkey O, optical stimulation of a branch of the median nerve produced brisk contractions of the PT muscle similar to those observed in monkey M. Again, although clearly visible, these contractions did not result in pronation of the forearm. Finally, optical stimulation of the right DP nerve in Monkey P resulted in contractions of the TA that could be seen through the skin before further exposing the muscle belly.
As a set of visual checks that opsin expression was limited to nerve tissue innervating the target muscle, no muscle contractions were observed when the injected muscle was directly stimulated with blue light. In addition, optical stimulation of nearby non-injected muscles and their corresponding nerves did not induce visible contractions or EMG activity.
Individual Optical Pulses Elicit Graded EMG Responses to Pulse Duration and Intensity
After observing visual responses to optical stimulation in monkey M, we recorded the EMG response of each TA muscle to variations in several optical stimulation parameters. First, the pulse duration was varied from 1 to 30 ms (100 mW). The delay from the onset of the optical pulse to a deflection in EMG activity was consistent across muscles at approximately 12 ms. As shown in Figure 2A, the length of the evoked EMG waveform stays relatively constant while the peak amplitude and RMS of EMG activity (Figure 2B) increases gradually with pulse duration until plateauing at pulse durations above 10 ms. We then measured the evoked EMG activity as a function of the incident intensity of optical stimulation. Figure 2D shows that the magnitude of the EMG waveform changed with varying optical intensity, while Figure 2E depicts a near-linear monotonic increase of EMG activity with optical intensity within the range studied. Results from Monkey P showed similar trends with a plateau in elicited EMG activity near 10 ms and a linear increase in EMG with light intensity (Figures 2C,F). These trends are consistent with results from previous studies in rodents utilizing AAV6 and ChR2 (Llewellyn et al., 2010; Towne et al., 2013), and support the notion that optogenetic stimulation offers graded recruitment of muscle activity.
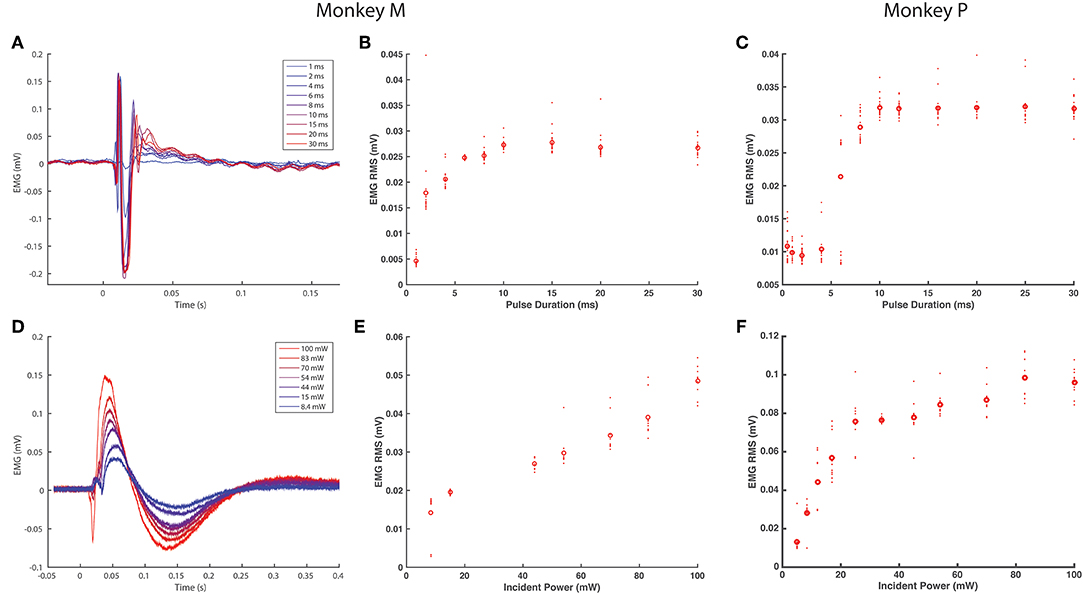
Figure 2. EMG response characteristics to optogenetic stimulation. (A–C) EMG response to varying optical pulse duration. (A) shows stimulus averaged EMG traces from the right TA muscle of monkey M, color-coded by optical pulse duration varying from 1 to 30 ms (20 pulses, 2.5 Hz trains), while (B) shows the corresponding distributions of RMS values. (C) shows the corresponding EMG vs. optical pulse duration curve from monkey P. (D–F) EMG response to varying optical power. (D) depicts stimulus-averaged EMG waveforms from the left TA muscle of monkey M, color-coded by optical power measured at the output of the optical fiber. Stimulus trains consisted of 20 pulses of 20 ms duration at 2.5 Hz. (E) illustrates the corresponding trend in EMG RMS vs. optical power, while (F) displays similar data from monkey P. Open circles in (B–E) and (D) indicate mean RMS values while dots indicate RMS responses for individual optical pulses.
As we injected each TA muscle in monkey M with different viral loads approximately an order of magnitude apart (1.66 × 1013 vp in the right TA vs. 2.08 × 1012 vp in the left TA), we examined whether viral load impacted viral transduction and optically elicited muscle activity. We compared the EMG RMS activity in each leg elicited by similar trains (20 ms pulses, 100 mW, 2.5 Hz). Although visual observation did not suggest distinct differences in the magnitude of muscle contractions, the EMG recorded from each leg showed appreciable differences in the shape and duration of the stimulus-averaged waveform. The right TA demonstrated a sharp, transient spike lasting <100 ms (Figure 2A) while the left TA demonstrated a waveform lasting 250 ms (Figure 2C). Counterintuitive to the trend expected with respect to viral load, these waveforms correspond to EMG RMS values of 0.027 and 0.051 mV, respectively. As we observed above that optical sensitivity was not consistent along a nerve, these differences could arise due to the accessibility of labeled fibers at a given location as opposed to the total number of transduced nerve fibers. In general, however, the range of viral loads injected in this study did not appear to directly correlate with differences in optically stimulated EMG activity.
EMG Response to Optical Pulse Trains
After measuring basic EMG responses of optogenetically labeled nerves to single pulses of varying duration and intensity, we then examined the response to longer trains. EMG activity was measured over 10 s blocks of continuous stimulation (20 ms, 100 mW) at increasing pulse frequencies from 2 to 30 Hz. The train of responses within a frequency block (RMS value of 600 sample/24.6 ms window following the onset of each light pulse) was then normalized to the response of the block's first stimulus pulse to assess how well the nerve and corresponding muscle activation could track the optical stimulus. As shown in Figures 3A,B, EMG responses in Monkey M tracked optical stimulation relatively well for pulse frequencies below 16 Hz, retaining EMG responses near 50% of their maximum initial response. Between 16 and 20 Hz, however, stimulus tracking appears to suffer as the normalized response drops precipitously. At 20 Hz, occasional EMG responses near 50% are interspersed throughout the train from 2 to 10 s, but these are dominated by weak EMG spikes as the nerve/muscle fail to recover. Monkey P demonstrated a similar frequency response with a noticeable dropoff in EMG-optical stimulus coupling between 12 and 16 Hz (see Figures 3C,D). These results suggest a functional maximum stimulation frequency below 20 Hz, similar to reports of the frequency response of ChR2 in neuronal culture (Nagel et al., 2003; Boyden et al., 2005; Mattis et al., 2012).
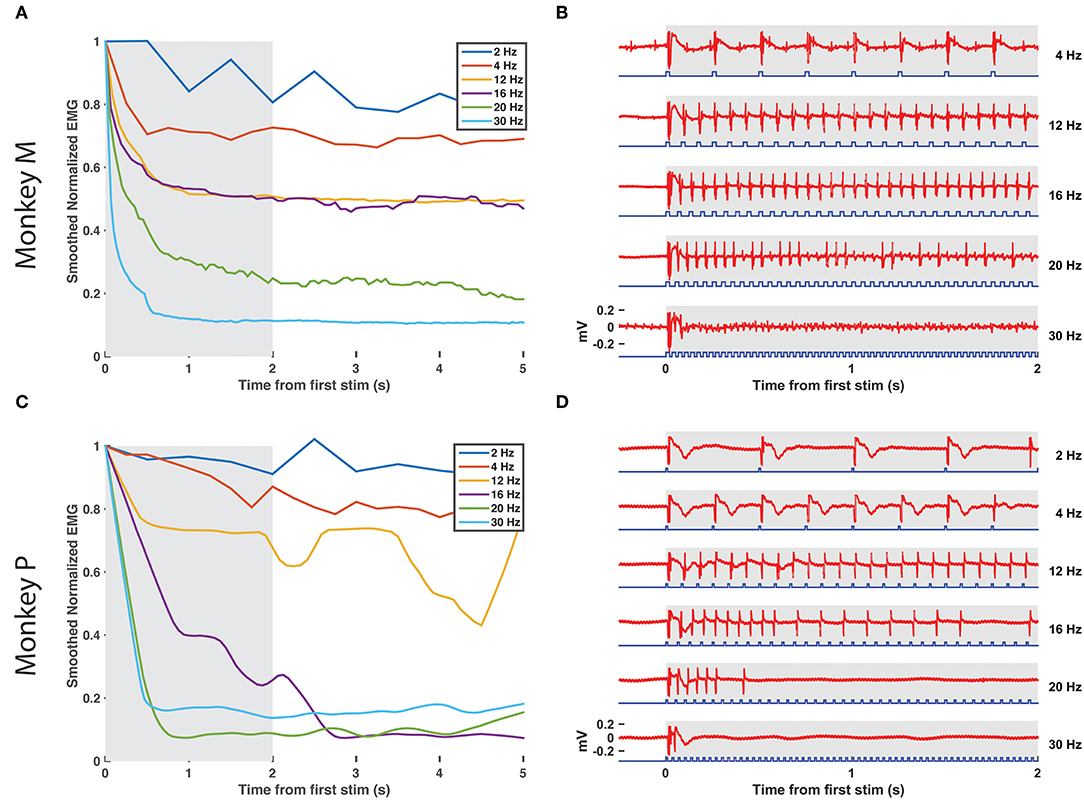
Figure 3. EMG tracking of optical stimulation trains. (A,B) The right DP nerve of monkey M was stimulated with trains of varying frequency (2–30 Hz, 20 ms pulse width, 100 mW) for 10 s while recording EMG from the corresponding TA muscle. The RMS value of the EMG activity elicited by each stimulus pulse (24.6 ms window following pulse onset) was calculated and normalized by the RMS elicited by the first pulse in the train. (A) depicts the smoothed EMG response to prolonged optical stimulation at various frequencies, while (B) depicts the first 2 s of raw EMG responses from the shaded window in (A). Although a significant drop in elicited EMG activity within the first 1–2 s is present at each frequency, stimulus trains below 16 Hz are able to maintain normalized EMG activity at or above 50% of first stimulus magnitude. Between 16 and 20 Hz, however, elicited EMG waveforms become more erratic as some spikes are missed. EMG responses to stimulus trains above 20 Hz drop off precipitously to below 20% of first stimulus response magnitude. (C,D) Similar experimental analysis and results as in (A,B) from the right DP nerve and TA muscle of monkey P.
EMG Shows Delayed Decay With Prolonged Optical Stimulation
Finally, we assessed for any decay in optical sensitivity following optical stimulation in a transduced nerve in Monkey M. The right DP nerve was stimulated continuously via the blue laser at maximum power with a 10 Hz, 20 ms optical pulse train for 2 min. Figures 4A,B depicts the raw EMG trace from monkey M's right TA muscle as well as the normalized RMS response to stimulation over time. The normalized response falls to 70% of maximum within a few seconds and then levels off similar to traces above 12 Hz in Figure 3. However, after 40 s, the muscle response again trends gradually downward over the next 80 s before approaching 40% of the initial EMG RMS response at the end of stimulation. Optical stimulation of the right DP nerve of Monkey P showed a similar profile with an initial drop in EMG rms after the initial few pulses followed by a sustained, consistent EMG activity for the rest of the 2 min (see Figures 4C,D). The slow decline observed in this study is again consistent with the delayed time course of muscle fatigue with optical stimulation observed in rodent studies (Llewellyn et al., 2010).
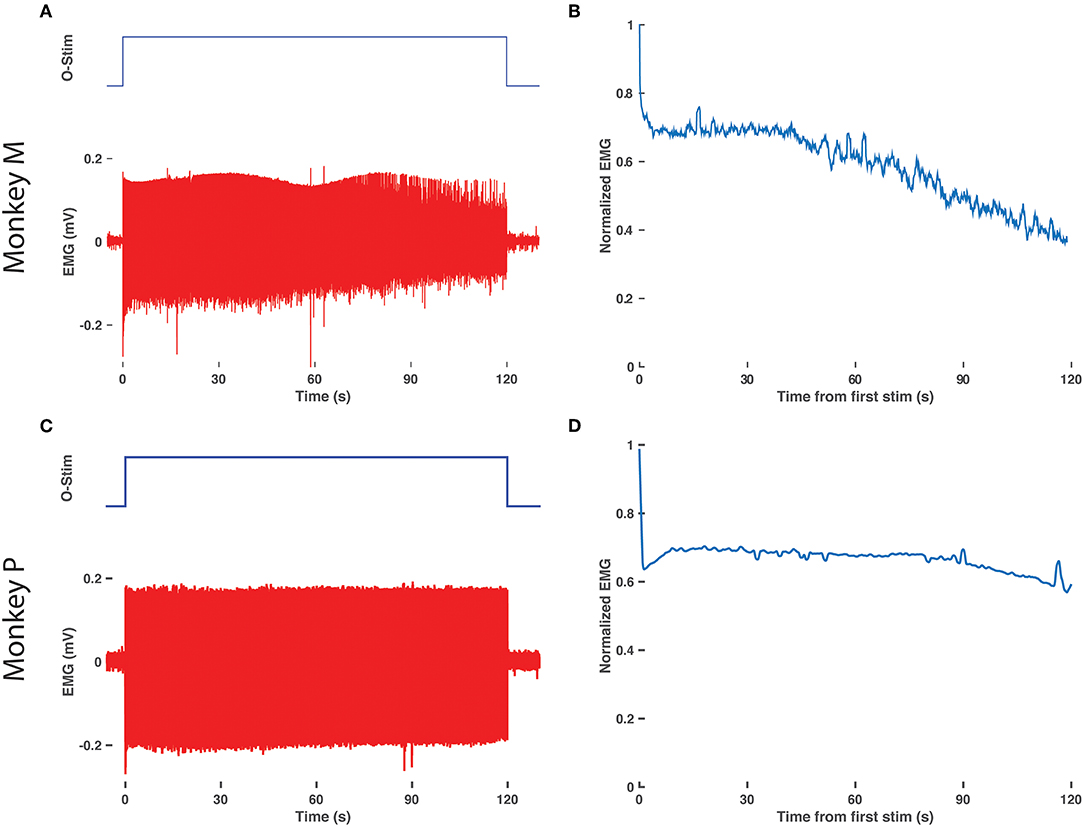
Figure 4. Delay in EMG decay with prolonged optical stimulation. (A) Raw EMG traces (bottom, red) from the right TA muscle of monkey M in response to 2 min of optical stimulation (top blue trace, duration of train of 20 ms pulses at 10 Hz). (B) Normalized EMG response over time. The RMS response to each optical stimulation pulse was calculated over a 600 sample/24.6 ms window following the onset of each pulse and then normalized to the response to the first pulse of the 2 min train. (C,D) Same as in (A,B), repeated for the right TA muscle of monkey P.
Whole Tissue Imaging Demonstrates Variable Opsin Expression
After final evaluation of functional expression, nerve samples were harvested, cleared, and imaged as whole samples using ribbon confocal microscopy to examine opsin expression patterns. Figure 5A depicts native eYFP fluorescence of an intact whole nerve sample from the right DP nerve of Monkey M, while no similar fluorescence of fiber tracts was observed in a control nerve from an uninjected muscle as seen in Figure 5B. Imaris software was used to trace the eYFP expression in Figure 5A and approximate a longitudinal profile of expression. 3D surfaces corresponding to positive eYFP expression were first computed using a built-in local background signal subtraction algorithm and manual removal of noisy features, with the resulting surfaces highlighted in Figure 5C. The volume of these surfaces was then binned as a function of distance along the length of the nerve (200 μm bins), and the resulting longitudinal profile of opsin/eYFP expression was plotted in Figure 5D. As seen from Figures 5C,D, expression of the viral gene product was not uniform along the nerve as patches of expression would emerge and disappear along the nerve. This finding corroborated the previously described variability in the nerve's sensitivity to optical stimulation (section Time Course of Expression) as well as similar observations in some of our parallel rodent pilot experiments (Williams et al., 2016). Nerves that had demonstrated optical sensitivity at some point during an animal's experimental timeline but were insensitive by the terminal checkpoint (i.e., right median nerve branch to the PT muscle of Monkey O, right DP nerve to the right TA muscle of Monkey P) were cleared and imaged similarly to the nerves in Figure 5. Reporter expression in these nerves at the time of perfusion was grossly absent or indistinguishable from background suggesting that loss of optical sensitivity was related to loss of opsin expression along the nerve (see Supplementary Figure S1).
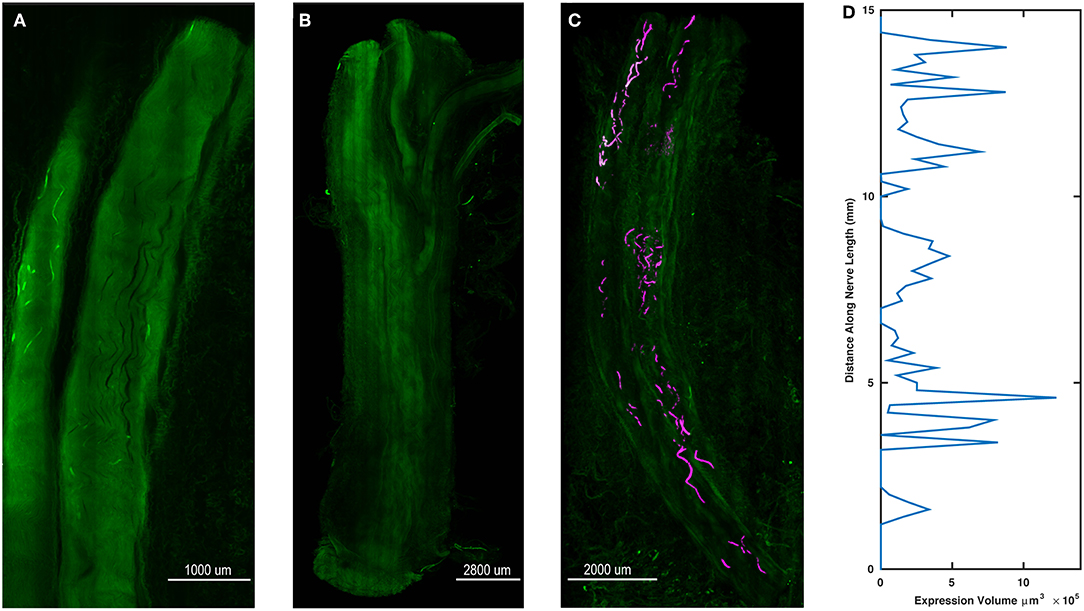
Figure 5. Spatial variability of transgene expression in cleared whole nerve sections. (A) Native eYFP fluorescence observed in a cleared nerve section from the right DP nerve of Monkey M using ribbon confocal microscopy. Fluorescent labeling of individual axonal fibers appears to fade in and out within the displayed segment. (B) Control nerve from non-injected muscle. In contrast to (A), no distinct patterns of increased fluorescence along individual axons are discernable. (C) Maximum intensity projection zoomed-out view of the cleared nerve segment in (A) with expression sites marked. eYFP positive axon segments were labeled in software as 3D surfaces (purple) to highlight the variable labeling of axons along the length of the nerve segment. (D) Volume of eYFP expression (horizontal axis) as a function of longitudinal distance (vertical axis) along the nerve in (A,C). The dependent variable axis (distance along nerve length) has been rotated to the vertical axis to roughly align with the nerve in (C) and displays how viral expression varies along the length of the nerve.
Discussion
This study represents a critical step in translating the potential of virally mediated peripheral optogenetics to a clinical therapy capable of alleviating a number of motor diseases or injuries. We have demonstrated that the AAV6-hSyn-ChR2 vector previously shown to be efficacious in transducing peripheral motor axons in rodents following muscle injection (Towne et al., 2013; Maimon et al., 2017) is also a viable vector for peripheral expression of light-sensitive opsins in non-human primates. Our results also exhibit several of the suggested benefits of peripheral optogenetic stimulation over electrical stimulation of muscle activity including graded muscle activation and delayed muscle fatigue. Finally, the correlation of EMG responses to basic optical stimulation parameters lays a foundation from which to approach the design of functional optical stimulation paradigms. Although this study is an important proof-of-concept demonstration, our results also highlight several of the necessary hurdles to be addressed prior to clinical viability as well as new potential avenues of investigation.
Time Course of Opsin Expression
Because we employed a novel longitudinal study of nerve expression in Rhesus monkeys with periodic checks of optical sensitivity, we were able to construct a gross timeline of expression in each animal for comparison with other studies and species. Towne et al. utilized a 4–6 week incubation period prior to assessing expression of ChR2 following intramuscular virus injection in rats (Towne et al., 2013). Similarly, a 4 week incubation period was utilized prior to evaluating the expression of eGFP in the spinal cord following intramuscular injection of AAV6-CMV-eGFP in African green monkeys (Towne et al., 2009). However, a recent study utilizing transdermal stimulation of ChR2-labeled nerves mediated by AAV6 (Maimon et al., 2017) suggests that peak transgene expression may occur later in rats, between 5 and 8 weeks, although even these gross time points of peak sensitivity showed considerable variability. Our findings agree with this variable and potentially extended time course of expression as optical sensitivity was observed initially at 5 ½, 8, and 10 weeks post-injection. In the case of monkey P, although expression was evident relatively early at 5 ½ weeks, optical sensitivity had disappeared by the next check at 9 weeks. As we did not test each injected muscle during earlier evaluations in the first two monkeys in order to minimize surgical manipulations at a given site, we cannot rule out that some sites may have demonstrated optical sensitivity at earlier time points similar to Monkey P. Additionally, the focal sensitivity observed along the left DP nerve of monkey M raises the possibility we did not fully expose or probe one of these focal “hotspots” of sensitivity during our earlier assessments while attempting to leave the surrounding tissue grossly intact. Once we more aggressively exposed the DP nerve and its insertion into the TA muscle, stimulation of one of these hotspots likely became more probable. In any case, the time course of expression, as well as differences between species, remains a critical yet poorly understood process.
One potential confound in our experimental design is the multiple surgical procedures utilized to test nerves for optical sensitivity. The variable timeline of functional expression observed in this study supports testing at several time points so as not to miss a window of expression as opposed to using a single checkpoint and perfusion at 6 or 8 weeks as is often employed in similar studies (Towne et al., 2009). However, it is possible that each surgical manipulation of the muscle and nerve could cause an inflammatory or immune response that could interfere with future expression and optical sensitivity. Whereas transdermal illumination of targeted nerves offers a non-invasive approach to probe functional expression in rodents (Maimon et al., 2017), the scattering of blue light caused by additional tissue thickness between skin and nerve in a macaque makes this approach ill-suited to evaluating expression in large primates. Chronically implanted light sources (e.g., LED cuff or fiber) would offer the possibility of evaluating sensitivity over time without additional surgical events, but it is very likely that a device implanted around the nerve could induce a foreign body response that might also compromise expression or efficient light delivery. In the current study, the nerve, muscle, and surrounding fascia did not typically display significant scar tissue buildup between evaluation surgeries, and functional expression was observed in nerves that had already been exposed in a prior evaluation surgery. Thus, we hypothesize that our surgical protocol had minimal impact on opsin expression and offered a reasonable approach to grossly evaluate optical sensitivity over an uncertain timeline compared to unproven alternatives.
Considerations for Chronic Optical Stimulation
Our results also bring forth several considerations for chronic FOS. As one potential application of this gene therapy is to restore volitional control of paralyzed muscle activity through a hybrid optogenetic-BMI, optical nerve stimulation hardware such as chronic LED or fiber optic nerve cuffs must be able to consistently stimulate opsin-labeled axons over a period of years. A potential benefit of using chronically implanted optical nerve cuffs on virally targeted nerves would be the ability to assess the time course of expression without additional surgical procedures. However, the variable expression and sensitivity pattern of ChR2 observed in monkey M in Figure 5 and some of our parallel rat studies (Williams et al., 2016) suggests that proper placement of stimulation hardware for either of these applications may be more challenging than initially anticipated. Correct temporal assessment of opsin expression patterns would require blind, accurate placement of nerve cuffs soon after injection over high expression zones on the nerve. Similarly, to provide consistent chronic optical stimulation capabilities in a rehabilitation setting would require (1) an additional evaluation surgery following the virus incubation period to properly place optical cuffs, (2) securing the cuff such that it does not move relative to the hotspot of expression on the nerve, and (3) stability of expression/low turnover at the hotspot. It is possible that the variable optical sensitivity and fluorescent expression observed in this study is due in part to poor expression and trafficking of opsins to the axonal membrane, although another likely contributor may be the immune system through a piecemeal recognition and degradation of opsins by an immune response. Elucidating the underlying cause of this problem could then direct further development of opsins or promoters (Chaffiol et al., 2017) with better expression and trafficking characteristics vs. development of injection techniques (Favre et al., 2000; Burger et al., 2005; Harris et al., 2012; Tosolini and Morris, 2016; Williams et al., 2016), recombinant viruses (Bartel, 2011; Tervo et al., 2016) to increase the efficiency and total number of axons transduced within a nerve, or immunosuppressive approaches to maintain expressed opsins.
Transduction as a Function of Viral Load
As we contemplate potential approaches to improve opsin expression along the nerve, we must also examine the results of this study with respect to viral load delivered to the muscle. As a first pass study, we used a range of viral loads grossly spanning approximately an order of magnitude (~1012-1013 vp per muscle) between the three monkeys with no obvious trend in expression. The lower end of this range is consistent with a previous study by Towne et al. in African green monkeys (Towne et al., 2009). However, the range of viral loads per kilogram of bodyweight used in this study (9.24 × 1011-1.84 × 1012 vp/kg) is an order of magnitude lower than the high titer intramuscular injections used by Maimon et al. in rats (1.5–2.5 × 1013 vp/kg) (Maimon et al., 2017). Assuming that the mass and volume of the muscles targeted in these studies scale approximately with total body weight, a lack of functional limb movement from optical stimulation in this study could be explained by an insufficient dose of viral particles delivered to muscles with much greater volume compared to prior mouse and rat models. The limited sample size afforded by early NHP studies such as this often precludes the systematic examination of factors such as viral load, but based on rodent studies, it is possible that doses on the order of 1014 vp per muscle might be necessary to yield consistent opsin expression that is functional for eliciting limb movements in primates.
Virus Delivery Approaches
The differences in viral load as a function of body weight across animal models highlights another difficulty in scaling this gene therapy approach up to humans. Because the volume of muscle and corresponding zone of neuromuscular junctions targeted for viral uptake increases dramatically from rodent to primate, efficient delivery of viral particles to the entire motor end plate may become both expensive and technically challenging. Our first attempt to address this challenge was to simply increase the volume of viral solution injected with hypertonic saline to be on the same order of magnitude used in rodent studies (~200 μL/kg bodyweight vs. 100 μL/kg bodyweight in Maimon et al., 2017) with the potential ramifications discussed above. Our second approach was to attempt to localize zones of high neuromuscular junction density near the motor end plate using electrical stimulation. Previous rodent studies have demonstrated that targeting muscle injections along motor endplates greatly enhances motor neuron transduction (Tosolini et al., 2013; Tosolini and Morris, 2016). Targeting of the motor end plate as in these studies requires prior histological mapping of the motor end plate in a given muscle in situ followed by visual alignment of anatomical landmarks in the subject to be injected. Conversely, our approach uses electrophysiological responses to map the end plate and potentially account for anatomical variability between animals. A third injection approach that we employed in Monkey P that may be promising for scaling up injections with animal size was to inject virus directly into the nerve branch of interest. Our experience with this technique has shown that intraneural injections near the insertion of the nerve into the muscle may effectively utilize the nerve sheath to contain and funnel the virus toward the motor end plate as the nerve branches out within the muscle (Williams et al., 2016). Therefore, virus that does not directly enter nerve axons upon injection but instead resides in the connective tissue perineurium may still be guided back down to the muscle where it may have a greater probability of uptake at neuromuscular junctions. Utilization of nerve injections in this manner could significantly reduce the volume of virus needed for effective motor neuron transduction in larger animals such as the macaque. Intraneural injections at sites more proximal to the spinal cord such as the sciatic nerve do hold the possibility of transducing unwanted sensory neurons. However, injecting the nerve closer to the target muscle would likely minimize unrelated sensory transduction or limit it to proprioceptive fibers that could be utilized for feedback. Alternatively, future muscle injection approaches may seek to incorporate advancements utilized in convection enhanced delivery (CED) currently used to deliver drugs and gene therapy in the brain (Yazdan-Shahmorad et al., 2016).
Viral Vector Design
In addition to the load and route of viral particles delivered to motor nerves, the composition of the viral construct itself holds great potential for improvement of motor nerve transduction. The mechanisms by which AAV vectors undergo uptake at the neuromuscular junction and traffic to the spinal cord are not completely understood, but presumably it is a receptor-mediated process facilitated by domains on the viral capsid which confer tissue tropism to various serotypes. A better fundamental understanding of these uptake and transport processes could inform the design of viral vectors for peripheral motor gene therapies. An alternative approach recently taken by several groups is “directed evolution” or high-throughput screening and selection of recombinant AAV variants for a desired trait (Dalkara et al., 2013; Choudhury et al., 2016; Tervo et al., 2016). Similarly, the hSyn promoter has been commonly used for peripheral nerve transduction due to its specificity for neural tissues yet relatively strong expression. Using a promoter restricting expression to specific nerve fiber types (e.g., slow/fast fatiguable motor units, proprioceptive fibers, etc.) would enable selective modulation of efferent or afferent activity as well as an approach to artificially specify the recruitment order of motor unit types. However, in general, more specific promoters result in weaker expression in the target tissues, so this tradeoff of nerve optical sensitivity vs. fiber type specificity would have to be addressed.
Immune Response to AAV and Gene Products
AAV6 was chosen as the gene delivery vehicle in this study due to AAV's safety profile and low immunogenicity (Calcedo and Wilson, 2013) as well as its previously demonstrated success in transducing peripheral motor nerves in non-human primates (Towne et al., 2009). However, a considerable proportion of both humans and macaques naturally exhibit pre-existing neutralizing antibodies (NAbs) to a variety of AAV serotypes (Boutin et al., 2010; Hurlbut et al., 2010; Calcedo and Wilson, 2013). Even at low NAb levels, transgene expression may be significantly inhibited in non-human primates (Jiang et al., 2006; Hurlbut et al., 2010). We did not assess the status of preexisting NAbs to AAV6 in our subjects, so its role regarding differences in expression between monkeys in this study is unclear. Nevertheless, several transient immunosuppression strategies such as those used for organ transplants have shown efficacy in maintaining AAV6-mediated transgene expression in canine models (Shin et al., 2012; Wang et al., 2012) as well as non-human primate models examining AAV8 (Jiang et al., 2006). Employing such regimens may not only increase transduction efficiency and prolong transgene expression, it could also enable separate viral injections of multiple muscle groups over several surgeries without decreased efficacy after an initial viral exposure (Riviere et al., 2006).
In addition to the viral vector, a recent rodent study has strongly suggested that opsins expressed along a peripheral nerve may also elicit a strong immune response and is likely to be a primary factor for the decay of optical sensitivity over time (Maimon et al., 2018). Indeed, results from the aforementioned rodent study and a preliminary study from our group in non-human primates (Williams et al., 2019) have demonstrated that opsin expression and functional sensitivity can be prolonged with the use of chronic immunosuppression similar to regimens used for organ transplants. However, an alternative approach to this problem would be to engineer the opsin itself, screening opsin variants for those that might be minimally immunogenic. Use of such an opsin for expression through a viral vector might preclude the need for subjects to endure long or potentially lifelong courses of immunosuppression.
Optical Stimulation Parameters and Opsin Selection
The results from this study provide baseline practical guidelines for optical stimulation parameters. EMG responses were modulated with pulse widths up to 10 ms, above which responses appeared to plateau. From a frequency response perspective, EMG responses tracked optical stimulation trains up 16 Hz, suggesting an upper bound for use in FOS stimulation schemes. This limit is relatively low compared to the frequency of stimulus trains commonly used for FES, often ranging from 20 to 50 Hz for clinical applications (Doucet et al., 2012). However, due to the previously discussed differences in recruitment order between optical and electrical stimulation, further study is required to elucidate how these optical stimulation parameters translate to functional force production and how to optimize modulation strategies for neuroprosthetic driven movements.
The EMG relation to optical stimulation parameters explored here was only characterized for the opsin ChR2. Although its use in this and similar prior studies in rodents as a first line of investigation is warranted by ChR2's well-characterized behavior and consistent expression patterns in a wide array of neural systems, other recently developed opsins may hold properties beneficial to peripheral motor stimulation. Indeed, we injected several muscles in our second monkey with a construct using the opsin Chronos to exploit its increased sensitivity and faster kinetics to (1) lower the light intensity and consequently power requirements for implantable optical stimulation hardware, and (2) increase the frequency range of pulsed stimulation trains to at least comparable levels used for FES. Recent studies have supported the fast temporal advantages of Chronos over ChR2 in the central auditory pathway (Guo et al., 2015; Hight et al., 2015). Although this study demonstrated a novel use of Chronos in the motor periphery, the brief period during which we were able to observe its response left us unable to fully examine whether these purported benefits extend to the peripheral motor system. However, preliminary data from our parallel rat studies (Williams et al., 2016) suggest that Chronos does have a better frequency response for light stimulus-EMG coupling in the periphery than ChR2. Other opsins which may prove beneficial for peripheral applications include red-shifted variants such as Chrimson (Klapoetke et al., 2014). The use of longer stimulation wavelengths would allow greater tissue penetration that could prove highly desirable when scaling stimulation hardware up to target primate nerves several millimeters in diameter.
Finally, the stimulus response characteristics referenced above may be influenced by both the level of expression within a given axon as well as the number of axons transduced within a given nerve in addition to the basic channel properties of the opsin. As there was a disconnect between the window of functional expression and the time of perfusion for histological evaluation in two of the three monkeys, we were unable to evaluate any such trends in the current study. However, based on our own experience with stimulation of transgenic mice expressing ChR2 in peripheral nerves and virally transduced rats similar to previous rodent studies (Llewellyn et al., 2010; Towne et al., 2013), the EMG frequency response characteristics are unlikely to appreciably change with expression levels while the magnitude of EMG responses will likely show a stronger correlation with expression. How these relationships scale with animal and nerve size is a question worthy of further investigation.
Comparison With Spinal Electrical Stimulation Approaches
Our main goal in this study was to introduce peripheral optogenetic stimulation as an alternative to FES in BMI applications. FES is typically associated with electrical stimulation of muscles directly or through nerve stimulation. However, several groups have used brain-controlled electrical intraspinal (Zimmermann and Jackson, 2014) or epidural spinal (Capogrosso et al., 2016) stimulation in NHPs for grasping and hindlimb locomotion, respectively. This mode of stimulation elicits muscle activation patterns either directly by stimulating pools of alpha motoneurons or indirectly by inducing motor patterns through interneurons following stimulation of dorsal roots. Thus, our peripheral FOS approach may not be directly comparable to electrical stimulation approaches at the spinal level. Nonetheless, it is possible that the potential benefits of FOS observed in the periphery may also extend to analogous optical stimulation of the spinal cord as has been recently investigated (Mondello et al., 2018).
Peripheral Optogenetics Relevance to Motor Behavior
As discussed in the introduction, success in using viral optogenetics to alter behavior has been difficult to achieve in non-human primates, especially regarding direct modulation of somatomotor activity. The current study represents an early success toward this goal. Although the muscle activity elicited by optical stimulation in this study was likely small compared to those typically elicited during normal locomotion, it did produce an easily observable change in the activity of the target effector. Even small optically induced contractions could potentially be used in perturbation studies of natural movements, while larger scales of virus expression and induced muscle contractions will likely be necessary to be therapeutically useful. A primary difference between the success achieved in this study and past unsuccessful attempts at optogenetic modulation of somatomotor behavior in the brain (Diester et al., 2011) may lie in the proximity of targeted opsin expression to the desired output. While even a small cortical volume transduced by a single virus injection may result in transfection of multiple downstream effector pathways, injection of a vector such as AAV into a single desired end effector (e.g., muscle) virtually guarantees that only the desired effector will be optically activated without upstream transmission. This simplicity in targeting and stimulating desired effector pathways further supports the role of this approach in motor disease therapy.
Conclusions
In summary, the viral transduction and functional expression of opsins for peripheral optical modulation of muscle activity in non-human primates is a step toward effective reanimation of movement in paralyzed subjects. The introduction of neuromuscular junction targeting for virus injection is a useful technique for increasing the likelihood of virus uptake. In addition, the EMG response characteristics to optical stimulation parameters described here serve as an important base upon which to build future primate studies and FOS algorithms.
While the jump from rodent to primate is important in itself, this study also highlights problems due to differences in scale and species that may not have been as pronounced in prior rodent studies. Potential variability in both the timeline and spatial profile of expression, the immune system's probable role in this variability, and effectiveness of the virus as well as light delivery in much larger target muscles/nerves are all challenges that must be addressed before FOS may become a clinically viable approach to restoring lost motor function.
Ethics Statement
This study was carried out in accordance with the recommendations of the University of Pittsburgh's Institutional Animal Care and Use Committee. The protocol was approved by the University of Pittsburgh's Institutional Animal Care and Use Committee.
Author Contributions
JW, AV, and AS contributed to study conception and experimental design. JW performed all surgeries and experiments. AW performed tissue clearing and imaging while JW and AW analyzed imaging data. JW wrote the first draft of the manuscript. All authors contributed to manuscript revision, read, and approved the submitted version.
Funding
This work was funded in part by the DARPA Grant W911NF1420107, Brain Control Optical Stimulation of Muscles, as well as Chair in Systems Neuroscience funds from the University of Pittsburgh.
Conflict of Interest Statement
The authors declare that the research was conducted in the absence of any commercial or financial relationships that could be construed as a potential conflict of interest.
Acknowledgments
We would like to thank Chris Towne for his consultation regarding peripheral optogenetic techniques. We thank Richard Dum, Jean-Alban Rathelot, and Peter Strick for their guidance and expertise with primate virus injections, Doug Weber for his aid with EMG recordings, and Bistra Iordanova for her help with histology. Finally, we would like to thank Kathy Hansell-Prigg, Scott Kennedy, Hongwei Mao, Steve Suway, Rex Tien, and Sally Zheng for their assistance with surgeries and experiments.
Note: A version of this manuscript was previously released as a pre-print (Williams et al., 2018) to the bioRxiv server hosted by the Cold Spring Harbor Laboratory.
Supplementary Material
The Supplementary Material for this article can be found online at: https://www.frontiersin.org/articles/10.3389/fnins.2019.00759/full#supplementary-material
References
Ajiboye, A. B., Willett, F. R., Young, D. R., Memberg, W. D., Murphy, B. A., Miller, J. P., et al. (2017). Restoration of reaching and grasping movements through brain-controlled muscle stimulation in a person with tetraplegia: a proof-of-concept demonstration. Lancet 389, 1821–1830. doi: 10.1016/S0140-6736(17)30601-3
Asokan, A., Schaffer, D. V., and Jude Samulski, R. (2012). The AAV vector toolkit: poised at the clinical crossroads. Mol. Ther. 20, 699–708. doi: 10.1038/mt.2011.287
Bartel, M. (2011). Enhancing the clinical potential of AAV vectors by capsid engineering to evade pre-existing immunity. Front. Microbiol. 2:204. doi: 10.3389/fmicb.2011.00204
Biasiucci, A., Leeb, R., Iturrate, I., Perdikis, S., Al-Khodairy, A., Corbet, T., et al. (2018). Brain-actuated functional electrical stimulation elicits lasting arm motor recovery after stroke. Nat. Commun. 9:2421. doi: 10.1038/s41467-018-04673-z
Bickel, C. S., Gregory, C. M., and Dean, J. C. (2011). Motor unit recruitment during neuromuscular electrical stimulation: a critical appraisal. Eur. J. Appl. Physiol. 111, 2399–2407. doi: 10.1007/s00421-011-2128-4
Boutin, S., Monteilhet, V., Veron, P., Leborgne, C., Benveniste, O., Montus, M. F., et al. (2010). Prevalence of serum IgG and neutralizing factors against adeno-associated virus (AAV) types 1, 2, 5, 6, 8, and 9 in the healthy population: implications for gene therapy using AAV vectors. Hum. Gene Ther. 21, 704–712. doi: 10.1089/hum.2009.182
Bouton, C. E., Shaikhouni, A., Annetta, N. V., Bockbrader, M. A., Friedenberg, D. A., Nielson, D. M., et al. (2016). Restoring cortical control of functional movement in a human with quadriplegia. Nature 533, 247–250. doi: 10.1038/nature17435
Boyden, E. S., Zhang, F., Bamberg, E., Nagel, G., and Deisseroth, K. (2005). Millisecond-timescale, genetically targeted optical control of neural activity. Nat. Neurosci. 8, 1263–1268. doi: 10.1038/nn1525
Bruegmann, T., van Bremen, T., Vogt, C. C., Send, T., Fleischmann, B. K., and Sasse, P. (2015). Optogenetic control of contractile function in skeletal muscle. Nat. Commun. 6:7153. doi: 10.1038/ncomms8153
Bruns, T. M., Wagenaar, J. B., Bauman, M. J., Gaunt, R. A., and Weber, D. J. (2013). Real-time control of hind limb functional electrical stimulation using feedback from dorsal root ganglia recordings. J. Neural Eng. 10:026020. doi: 10.1088/1741-2560/10/2/026020
Bryson, J. B., Machado, C. B., Crossley, M., Stevenson, D., Bros-Facer, V., Burrone, J., et al. (2014). Optical control of muscle function by transplantation of stem cell–derived motor neurons in mice. Science 344, 94–97. doi: 10.1126/science.1248523
Burger, C., Nguyen, F. N., Deng, J., and Mandel, R. J. (2005). Systemic mannitol-induced hyperosmolality amplifies rAAV2-mediated striatal transduction to a greater extent than local co-infusion. Mol. Ther. 11, 327–331. doi: 10.1016/j.ymthe.2004.08.031
Calcedo, R., and Wilson, J. M. (2013). Humoral immune response to AAV. Front. Immunol. 4:341. doi: 10.3389/fimmu.2013.00341
Capogrosso, M., Milekovic, T., Borton, D., Wagner, F., Moraud, E. M., Mignardot, J.-B., et al. (2016). A brain–spine interface alleviating gait deficits after spinal cord injury in primates. Nature 539, 284–288. doi: 10.1038/nature20118
Cavanaugh, J., Monosov, I. E., McAlonan, K., Berman, R., Smith, M. K., Cao, V., et al. (2012). Optogenetic inactivation modifies monkey visuomotor behavior. Neuron 76, 901–907. doi: 10.1016/j.neuron.2012.10.016
Chaffiol, A., Caplette, R., Jaillard, C., Brazhnikova, E., Desrosiers, M., Dubus, E., et al. (2017). A new promoter allows optogenetic vision restoration with enhanced sensitivity in macaque retina. Mol. Ther. 25, 2546–2560. doi: 10.1016/j.ymthe.2017.07.011
Choudhury, S. R., Fitzpatrick, Z., Harris, A. F., Maitland, S. A., Ferreira, J. S., Zhang, Y., et al. (2016). In vivo selection yields AAV-B1 capsid for central nervous system and muscle gene therapy. Mol. Ther. 24, 1247–1257. doi: 10.1038/mt.2016.84
Collinger, J. L., Wodlinger, B., Downey, J. E., Wang, W., Tyler-Kabara, E. C., Weber, D. J., et al. (2013). High-performance neuroprosthetic control by an individual with tetraplegia. Lancet 381, 557–564. doi: 10.1016/S0140-6736(12)61816-9
Dai, J., Brooks, D. I., and Sheinberg, D. L. (2014). Optogenetic and electrical microstimulation systematically bias visuospatial choice in primates. Curr. Biol. 24, 63–69. doi: 10.1016/j.cub.2013.11.011
Dalkara, D., Byrne, L. C., Klimczak, R. R., Visel, M., Yin, L., Merigan, W. H., et al. (2013). In vivo–directed evolution of a new adeno-associated virus for therapeutic outer retinal gene delivery from the vitreous. Sci. Transl. Med. 5:189ra76. doi: 10.1126/scitranslmed.3005708
Diester, I., Kaufman, M. T., Mogri, M., Pashaie, R., Goo, W., Yizhar, O., et al. (2011). An optogenetic toolbox designed for primates. Nat. Neurosci. 14, 387–397. doi: 10.1038/nn.2749
Doucet, B. M., Lam, A., and Griffin, L. (2012). Neuromuscular electrical stimulation for skeletal muscle function. Yale J. Biol. Med. 85, 201–215.
El-Shamayleh, Y., Kojima, Y., Soetedjo, R., and Horwitz, G. D. (2017). Selective optogenetic control of purkinje cells in monkey cerebellum. Neuron 95, 51–62.e4. doi: 10.1016/j.neuron.2017.06.002
Ethier, C., Oby, E. R., Bauman, M. J., and Miller, L. E. (2012). Restoration of grasp following paralysis through brain-controlled stimulation of muscles. Nature 485, 368–371. doi: 10.1038/nature10987
Fang, Z.-P., and Mortimer, J. T. (1991). Selective activation of small motor axons by quasitrapezoidal current pulses. IEEE Trans. Biomed. Eng. 38, 168–174. doi: 10.1109/10.76383
Favre, D., Cherel, Y., Provost, N., Blouin, V., Ferry, N., Moullier, P., et al. (2000). Hyaluronidase enhances recombinant adeno-associated virus (rAAV)-mediated gene transfer in the rat skeletal muscle. Gene Ther. 7, 1417–1420. doi: 10.1038/sj.gt.3301256
Gregory, C. M., and Bickel, C. S. (2005). Recruitment patterns in human skeletal muscle during electrical stimulation. Phys. Ther. 85, 358–364. doi: 10.1093/ptj/85.4.358
Guo, W., Hight, A. E., Chen, J. X., Klapoetke, N. C., Hancock, K. E., Shinn-Cunningham, B. G., et al. (2015). Hearing the light: neural and perceptual encoding of optogenetic stimulation in the central auditory pathway. Sci. Rep. 5:10319. doi: 10.1038/srep10319
Han, X., Qian, X., Bernstein, J. G., Zhou, H., Franzesi, G. T., Stern, P., et al. (2009). Millisecond-timescale optical control of neural dynamics in the nonhuman primate brain. Neuron 62, 191–198. doi: 10.1016/j.neuron.2009.03.011
Harris, J. A., Wook Oh, S., and Zeng, H. (2012). Adeno-associated viral vectors for anterograde axonal tracing with fluorescent proteins in nontransgenic and cre driver mice. Curr. Protoc. Neurosci. 59, 20.1–1.20.18. doi: 10.1002/0471142301.ns0120s59
Henneman, E. (1957). Relation between size of neurons and their susceptibility to discharge. Science 126, 1345–1347.
Hight, A. E., Kozin, E. D., Darrow, K., Lehmann, A., Boyden, E., Brown, M. C., et al. (2015). Superior temporal resolution of Chronos versus channelrhodopsin-2 in an optogenetic model of the auditory brainstem implant. Hear. Res. 322, 235–241. doi: 10.1016/j.heares.2015.01.004
Hochberg, L. R., Bacher, D., Jarosiewicz, B., Masse, N. Y., Simeral, J. D., Vogel, J., et al. (2012). Reach and grasp by people with tetraplegia using a neurally controlled robotic arm. Nature 485, 372–375. doi: 10.1038/nature11076
Hurlbut, G. D., Ziegler, R. J., Nietupski, J. B., Foley, J. W., Woodworth, L. A., Meyers, E., et al. (2010). Preexisting immunity and low expression in primates highlight translational challenges for liver-directed AAV8-mediated gene therapy. Mol. Ther. 18, 1983–1994. doi: 10.1038/mt.2010.175
Jazayeri, M., Lindbloom-Brown, Z., and Horwitz, G. D. (2012). Saccadic eye movements evoked by optogenetic activation of primate V1. Nat. Neurosci. 15, 1368–1370. doi: 10.1038/nn.3210
Jiang, H., Couto, L. B., Patarroyo-White, S., Liu, T., Nagy, D., Vargas, J. A., et al. (2006). Effects of transient immunosuppression on adenoassociated, virus-mediated, liver-directed gene transfer in rhesus macaques and implications for human gene therapy. Blood 108, 3321–3328. doi: 10.1182/blood-2006-04-017913
Jing, D., Zhang, S., Luo, W., Gao, X., Men, Y., Ma, C., et al. (2018). Tissue clearing of both hard and soft tissue organs with the PEGASOS method. Cell Res. 28, 803–818. doi: 10.1038/s41422-018-0049-z
Kilgore, K. L., Hoyen, H. A., Bryden, A. M., Hart, R. L., Keith, M. W., and Peckham, P. H. (2008). An implanted upper-extremity neuroprosthesis using myoelectric control. J. Hand Surg. 33, 539–550. doi: 10.1016/j.jhsa.2008.01.007
Klapoetke, N. C., Murata, Y., Kim, S. S., Pulver, S. R., Birdsey-Benson, A., Cho, Y. K., et al. (2014). Independent optical excitation of distinct neural populations. Nat. Methods 11, 338–346. doi: 10.1038/nmeth.2836
Kozai, T. D. Y., and Vazquez, A. L. (2015). Photoelectric artefact from optogenetics and imaging on microelectrodes and bioelectronics: new challenges and opportunities. J. Mater. Chem. B 3, 4965–4978. doi: 10.1039/C5TB00108K
Lertmanorat, Z., and Durand, D. M. (2004). Extracellular voltage profile for reversing the recruitment order of peripheral nerve stimulation: a simulation study. J. Neural Eng. 1, 202–211. doi: 10.1088/1741-2560/1/4/003
Leuthardt, E. C., Schalk, G., Wolpaw, J. R., Ojemann, J. G., and Moran, D. W. (2004). A brain-computer interface using electrocorticographic signals in humans. J. Neural Eng. 1, 63–71. doi: 10.1088/1741-2560/1/2/001
Llewellyn, M. E., Thompson, K. R., Deisseroth, K., and Delp, S. L. (2010). Orderly recruitment of motor units under optical control in vivo. Nat. Med. 16, 1161–1165. doi: 10.1038/nm.2228
Maimon, B. E., Diaz, M., Revol, E. C. M., Schneider, A. M., Leaker, B., Varela, C. E., et al. (2018). Optogenetic peripheral nerve immunogenicity. Sci. Rep. 8:14076. doi: 10.1038/s41598-018-32075-0
Maimon, B. E., Zorzos, A. N., Bendell, R., Harding, A., Fahmi, M., Srinivasan, S., et al. (2017). Transdermal optogenetic peripheral nerve stimulation. J. Neural Eng. 14:034002. doi: 10.1088/1741-2552/aa5e20
Mattis, J., Tye, K. M., Ferenczi, E. A., Ramakrishnan, C., O'Shea, D. J., Prakash, R., et al. (2012). Principles for applying optogenetic tools derived from direct comparative analysis of microbial opsins. Nat. Methods 9, 159–172. doi: 10.1038/nmeth.1808
May, T., Ozden, I., Brush, B., Borton, D., Wagner, F., Agha, N., et al. (2014). Detection of optogenetic stimulation in somatosensory cortex by non-human primates - towards artificial tactile sensation. PLoS ONE 9:e114529. doi: 10.1371/journal.pone.0114529
Mondello, S. E., Sunshine, M. D., Fischedick, A. E., Dreyer, S. J., Horwitz, G. D., Anikeeva, P., et al. (2018). Optogenetic surface stimulation of the rat cervical spinal cord. J. Neurophysiol. 120, 795–811. doi: 10.1152/jn.00461.2017
Moritz, C. T., Perlmutter, S. I., and Fetz, E. E. (2008). Direct control of paralysed muscles by cortical neurons. Nature 456, 639–642. doi: 10.1038/nature07418
Nagel, G., Szellas, T., Huhn, W., Kateriya, S., Adeishvili, N., Berthold, P., et al. (2003). Channelrhodopsin-2, a directly light-gated cation-selective membrane channel. Proc. Natl. Acad. Sci. U. S. A. 100, 13940–13945. doi: 10.1073/pnas.1936192100
Okada, H., Ishibashi, H., Hayashita-Kinoh, H., Chiyo, T., Nitahara-Kasahara, Y., Baba, Y., et al. (2013). Robust long-term transduction of common marmoset neuromuscular tissue with rAAV1 and rAAV9. Mol. Ther. Nucleic Acids 2:e95. doi: 10.1038/mtna.2013.21
Peckham, P. H., Kilgore, K. L., Keith, M. W., Bryden, A. M., Bhadra, N., and Montague, F. W. (2002). An advanced neuroprosthesis for restoration of hand and upper arm control using an implantable controller. J. Hand Surg. 27, 265–276. doi: 10.1053/jhsu.2002.30919
Revechkis, B., Aflalo, T. N. S., Kellis, S., Pouratian, N., and Andersen, R. A. (2015). Parietal neural prosthetic control of a computer cursor in a graphical-user-interface task. J. Neural Eng. 12:019601. doi: 10.1088/1741-2560/12/1/019601
Riviere, C., Danos, O., and Douar, A. M. (2006). Long-term expression and repeated administration of AAV type 1, 2 and 5 vectors in skeletal muscle of immunocompetent adult mice. Gene Ther. 13, 1300–1308. doi: 10.1038/sj.gt.3302766
Serruya, M. D., Hatsopoulos, N. G., Paninski, L., Fellows, M. R., and Donoghue, J. P. (2002). Instant neural control of a movement signal. Nature 416, 141–142. doi: 10.1038/416141a
Shin, J.-H., Yue, Y., Srivastava, A., Smith, B., Lai, Y., and Duan, D. (2012). A simplified immune suppression scheme leads to persistent micro-dystrophin expression in duchenne muscular dystrophy dogs. Hum. Gene Ther. 23, 202–209. doi: 10.1089/hum.2011.147
Singh, K., Richmond, F. J., and Loeb, G. E. (2000). Recruitment properties of intramuscular and nerve-trunk stimulating electrodes. IEEE Trans. Rehabil. Eng. 8, 276–285. doi: 10.1109/86.867869
Stauffer, W. R., Lak, A., Yang, A., Borel, M., Paulsen, O., Boyden, E. S., et al. (2016). Dopamine neuron-specific optogenetic stimulation in rhesus macaques. Cell 166, 1564–1571.e6. doi: 10.1016/j.cell.2016.08.024
Taylor, D. M., Tillery, S. I., and Schwartz, A. B. (2002). Direct cortical control of 3D neuroprosthetic devices. Science 296, 1829–1832. doi: 10.1126/science.1070291
Tervo, D. G. R., Hwang, B.-Y., Viswanathan, S., Gaj, T., Lavzin, M., Ritola, K. D., et al. (2016). A Designer AAV variant permits efficient retrograde access to projection neurons. Neuron 92, 372–382. doi: 10.1016/j.neuron.2016.09.021
Tosolini, A. P., Mohan, R., and Morris, R. (2013). Targeting the full length of the motor end plate regions in the mouse forelimb increases the uptake of fluoro-gold into corresponding spinal cord motor neurons. Front. Neurol. 4:58. doi: 10.3389/fneur.2013.00058
Tosolini, A. P., and Morris, R. (2016). Targeting motor end plates for delivery of adenoviruses: an approach to maximize uptake and transduction of spinal cord motor neurons. Sci. Rep. 6:33058. doi: 10.1038/srep33058
Towne, C., Montgomery, K. L., Iyer, S. M., Deisseroth, K., and Delp, S. L. (2013). Optogenetic control of targeted peripheral axons in freely moving animals. PLoS ONE 8:e72691. doi: 10.1371/journal.pone.0072691
Towne, C., Schneider, B. L., Kieran, D., Redmond, D. E., and Aebischer, P. (2009). Efficient transduction of non-human primate motor neurons after intramuscular delivery of recombinant AAV serotype 6. Gene Ther. 17, 141–146. doi: 10.1038/gt.2009.119
Tyler, D. J., and Durand, D. M. (2002). Functionally selective peripheral nerve stimulation with a flat interface nerve electrode. IEEE Trans. Neural Syst. Rehabil. Eng. 10, 294–303. doi: 10.1109/TNSRE.2002.806840
Wang, Z., Storb, R., Halbert, C. L., Banks, G. B., Butts, T. M., Finn, E. E., et al. (2012). Successful regional delivery and long-term expression of a dystrophin gene in canine muscular dystrophy: a preclinical model for human therapies. Mol. Ther. 20, 1501–1507. doi: 10.1038/mt.2012.111
Watson, A. M., Rose, A. H., Gibson, G. A., Gardner, C. L., Sun, C., Reed, D. S., et al. (2017). Ribbon scanning confocal for high-speed high-resolution volume imaging of brain. PloS ONE 12:e0180486. doi: 10.1371/journal.pone.0180486
Williams, J. J., Vazquez, A. L., and Schwartz, A. B. (2019). “Prolonged functional optical sensitivity in non-human primate motor nerves following Cyclosporine-based immunosuppression and rAAV2-retro mediated expression of ChR2,” in Proceedings of the 9th International IEEE EMBS Conference on Neural Engineering (San Francisco, CA).
Williams, J. J., Vazquez, A. L., Wirblich, C., Schnell, M. J., and Schwartz, A. B. (2016). “Virus, opsin, and immunomodulation selection for optogenetic control of peripheral motor function,” in 2016 Neuroscience Meeting Planner (San Diego, CA). Available online at: http://www.abstractsonline.com/pp8/#!/4071/presentation/15933 (accessed September 27, 2017).
Williams, J. J., Watson, A. M., Vazquez, A. L., and Schwartz, A. B. (2018). Viral-mediated optogenetic stimulation of peripheral motor nerves in non-human primates. bioRxiv 261925. doi: 10.1101/261925
Wodlinger, B., Downey, J. E., Tyler-Kabara, E. C., Schwartz, A. B., Boninger, M. L., and Collinger, J. L. (2015). Ten-dimensional anthropomorphic arm control in a human brain-machine interface: difficulties, solutions, and limitations. J. Neural Eng. 12:016011. doi: 10.1088/1741-2560/12/1/016011
Yazdan-Shahmorad, A., Diaz-Botia, C., Hanson, T. L., Kharazia, V., Ledochowitsch, P., Maharbiz, M. M., et al. (2016). A large-scale interface for optogenetic stimulation and recording in nonhuman primates. Neuron 89, 927–939. doi: 10.1016/j.neuron.2016.01.013
Yeom, H., and Chang, Y.-H. (2010). Autogenic EMG-controlled functional electrical stimulation for ankle dorsiflexion control. J. Neurosci. Methods 193, 118–125. doi: 10.1016/j.jneumeth.2010.08.011
Keywords: optogenetics, neural prosthetics, peripheral nerve stimulation, functional optical stimulation, neurostimulation, muscle control, tissue clearing
Citation: Williams JJ, Watson AM, Vazquez AL and Schwartz AB (2019) Viral-Mediated Optogenetic Stimulation of Peripheral Motor Nerves in Non-human Primates. Front. Neurosci. 13:759. doi: 10.3389/fnins.2019.00759
Received: 30 November 2018; Accepted: 08 July 2019;
Published: 31 July 2019.
Edited by:
Jeffrey R. Capadona, Case Western Reserve University, United StatesReviewed by:
Amy L. Orsborn, University of Washington, United StatesAndres Canales, Massachusetts Institute of Technology, United States
Copyright © 2019 Williams, Watson, Vazquez and Schwartz. This is an open-access article distributed under the terms of the Creative Commons Attribution License (CC BY). The use, distribution or reproduction in other forums is permitted, provided the original author(s) and the copyright owner(s) are credited and that the original publication in this journal is cited, in accordance with accepted academic practice. No use, distribution or reproduction is permitted which does not comply with these terms.
*Correspondence: Andrew B. Schwartz, YWJzMjFAcGl0dC5lZHU=