- 1Department of Physiology, University of Kentucky, Lexington, KY, United States
- 2Department of Biology, University of Kentucky, Lexington, KY, United States
- 3Department of Pharmacology and Nutritional Sciences, University of Kentucky, Lexington, KY, United States
- 4Research and Development, Lexington Veterans Affairs Medical Center, Lexington, KY, United States
People with diabetes are more likely to experience sleep disturbance than those without. Sleep disturbance can cause daytime sleepiness in diabetic patients, which may impair their daytime performance or even lead to workplace injuries. Therefore, restoring the normal sleep-wake cycle is critical for diabetic patients who experience daytime sleepiness. Previous data on a diabetic mouse model, the db/db mice, have demonstrated that the total sleep time and sleep fragmentation are increased and the daily rhythm of the sleep-wake cycle is attenuated. Accumulating evidence has shown that active time-restricted feeding (ATRF), in which the timing of food availability is restricted to the active-phase, is beneficial to metabolic health. However, it is unknown whether ATRF restores the normal sleep-wake cycle in diabetes. To test that, we used a non-invasive piezoelectric system to monitor the sleep-wake profile in the db/db mice with ad libitum feeding (ALF) as a baseline and then followed with ATRF. The results showed that at baseline, db/db mice exhibited abnormal sleep-wake patterns: the sleep time percent during the light-phase was decreased, while during the dark-phase it was increased with unusual cycling compared to control mice. In addition, the sleep bout length during both the light-phase and the full 24-h period was shortened in db/db mice. Analysis of the sleep-wake circadian rhythm showed that ATRF effectively restored the circadian but suppressed the ultradian oscillations of the sleep-wake cycle in the db/db mice. In conclusion, ATRF may serve as a novel strategy for treating diabetes-induced irregularity of the sleep-wake cycle.
Introduction
The prevalence of diabetes has been increasing over the past few decades. Over 400 million adults were estimated to have diabetes worldwide in 2017 (IDF Diabetes Atlas, 2017). It is well-documented that sleep disorders contribute to the development of diabetes (Cappuccio et al., 2010). Recent evidence has shown that the relationship between diabetes and sleep disorders is bidirectional (Tasali et al., 2008). Epidemiological studies have shown that insomnia, daytime sleepiness, prolonged sleep latency, and poor sleep maintenance is more frequent in diabetic patients than non-diabetic subjects (Lamond et al., 2000; Skomro et al., 2001; Nakanishi-Minami et al., 2012; Plantinga et al., 2012; Medeiros et al., 2013; Lecube et al., 2016). Daytime sleepiness such as that caused by sleep disturbance in diabetic patients may compromise daytime performance or even lead to workplace accidents (Horne and Reyner, 1995; Melamed and Oksenberg, 2002). Therefore, restoring the normal sleep-wake cycle and reducing daytime sleepiness is important for the life quality, daytime performance, and work safety of diabetic patients who experience daytime sleepiness.
The sleep-wake cycle is regulated by multiple factors, among which the circadian rhythm is one of the most important factors (Eban-Rothschild et al., 2018). A large body of evidence demonstrates that the timing of food intake plays a prominent role in entraining circadian clocks in peripheral tissues. When nocturnal animals are fed only during the inactive light-phase, the phase of clock gene oscillations in various tissues are reversed/shifted (Damiola et al., 2000; Stokkan et al., 2001). In contrast, when the feeding time is restricted to the active dark-phase in the nocturnal rodents, the disrupted clock gene oscillations are restored with high-fat diet- or genetic mutant-induced obese/diabetic rodents (Kudo et al., 2004; Hatori et al., 2012). In addition, active-phase time-restricted feeding (ATRF) also improves metabolic dysregulation in diabetes (Mistlberger et al., 1998; Arble et al., 2009; Hatori et al., 2012; Sherman et al., 2012; Tsai et al., 2013; Adamovich et al., 2014; Chaix et al., 2014; Yasumoto et al., 2016). However, it is unknown whether ATRF is able to improve the sleep-wake cycle in diabetic humans or other animal models of diabetes.
The leptin receptor mutant db/db mouse is a commonly used type 2 diabetic mouse model, which manifests syndromes that resemble maturity-onset diabetes in humans (Ktorza et al., 1997). The db/db mice exhibit a variety of alterations in sleep-wake architecture, including increased total sleep time in 24-h, mostly due to increased sleep time during the active dark-phase. In addition, sleep fragmentation is elevated (Laposky et al., 2008). To test whether ATRF improves sleep-wake cycles in diabetes, we monitored the sleep-wake states of the db/db mice with ad libitum feeding (ALF) and ATRF by using a non-invasive PiezoSleep system. Our results demonstrated that ATRF was able to improve the sleep-wake patterns in db/db mice to a state that is comparable to control mice.
Method
Animals
Male db/db and control (db/+) C57BL/KsJ mice (n = 8 in each strain) were purchased from the Jackson lab (Stock No. 000642). After arrival and throughout the experiment, the mice were housed in a 12L: 12D light: dark cycle with lights on from 9 pm to 9 am. Normal chow diet and water were provided ad libitum.
Experimental Procedure
The sleep-wake states were determined in 15-week-old control and db/db mice using a piezoelectric system. Prior to data collection, the mice were acclimated in the system for 4 weeks. The baseline sleep-wake states were recorded for 5 consecutive days in control and db/db mice with ALF. Then the mice were subjected to an ATRF regimen for a total of 18 days, during which sleep was monitored day 1–5 and day 15–17. The ATRF was administered by quietly manually adding food at ZT13 (10 am) and removing food at ZT21 (6 pm) by the same investigator. The disturbance caused by the food addition or removal was brief (<1 min for each mouse). Sham interaction between the investigator with the mice under ALF regimen was not performed because each mouse was fed ALF initially and then switched at the same time to ATRF. The water was given ad libitum. During the over 3 weeks of sleep recording, some data with artifacts were excluded: 2 control and 1 db/db mice at baseline and 2 db/db mice for day 1–5. The different numbers of the exclusion during different recording periods were due to the re-setup of the recording system when cleaning the cages. All animal procedures were approved by the Institutional Animal Care and Use Committee.
Body Weight and Blood Glucose Measurement
The body weight and non-fasting blood glucose were measured before starting the ATRF and after 18 days of ATRF, both at ZT20. To measure non-fasting blood glucose, the mouse tail was sniped to obtain a drop of blood. Then a disposable test strip (StatStrip XepressTM glucometer, NOVA Biomedical; Waltham, MA, United States) was used to measure the glucose level in the blood drop.
The Piezoelectric System
The piezoelectric system is a non-invasive, highly sensitive motion detector system, as described previously (Flores et al., 2007; Mang et al., 2014; Yaghouby et al., 2016) and at www.sigsoln.com (Signal Solutions LLC, Lexington, KY, United States). In brief, each cage unit housed a single mouse inside 18-cm × 18-cm walled compartments with attached food and water structures. The cages had open bottoms resting on polyvinylidene difluoride (PVDF) sensors serving as the cage floor. The PVDF sensors were coupled to an input differential amplifier, and pressure signals were generated and classified as motions consistent with either activity related to wake, or inactivity and regular breathing movements associated with sleep. Sleep was characterized primarily by periodic (2–3 Hz) and regular amplitude signals, which is typical of respiration from a sleeping mouse. In contrast, signals characteristic of wake were both the absence of characteristic sleep signals and higher amplitude, and irregular signals were associated with volitional movements, even subtle head movements during quiet wake. The piezoelectric signals in 2-s epochs were classified by a linear discriminant classifier algorithm based on multiple signal variables to assign a binary label of “sleep” or “wake.” Data collected from the cage system were binned over specified time periods (e.g., 1 h) using the average of percent sleep as well as by length of individual bouts of sleep.
Non-parametric Circadian Rhythm Analysis
The daily rhythm of wake percent was analyzed by a non-parametric circadian rhythm analysis method using the Clocklab analysis software (van Someren et al., 1996). The non-parametric circadian rhythm method measures several parameters, including interdaily stability (IS), intradaily variability (IV), L5 average and L5 start time, M10 average and M10 start time, and relative amplitude (RA). IS quantifies the strength of resemblance from 1 day to the next; IV measures the fragmentation between rest and activity; L5 average and L5 start are the average wake time and the start time of the 5-h period with the least wake time; M10 average and M10 start are the average wake time and the start time of the 10-h period with the most wake time; and RA compares the periods of lowest and highest wake time: RA = (M10 Avg – L5 Avg)/(M10 Avg + L5 Avg).
Statistical Analysis
The statistical analysis was performed using GraphPad Prism (version 8). Two-way ANOVA with repeated measurement with Geisser-Greenhouse correction and Sidak post hoc test was used. A mixed-effects model was performed if there are missing data. The data were expressed as mean ± SEM. A p value less than 0.05 was considered statistically significant.
Results
The Sleep-Wake Pattern Was Altered in the db/db Mice With ad libitum Feeding (ALF)
We monitored the sleep-wake profile in groups of db/db and control mice with ALF as baseline and then switched to ATRF to determine the effects of ATRF on the sleep-wake profile of the diabetic db/db mice. As shown in the representative recordings (Figure 1) and the mean with 95% confidence interval plots (Supplementary Figure S1), at baseline with ALF, the daily sleep profile in the db/db mice (Figure 1B and Supplementary Figure S1B) was significantly different from the control mice (Figure 1A and Supplementary Figure S1A). In contrast to the clear and large difference in the wake percent between the light- and dark-phase, the percent waking time of the db/db mice in either the light- or dark-phase fluctuated with a sawtooth-like pattern (Figure 1B) and the difference between the light- and dark-phase seems to be smaller compared to the controls.
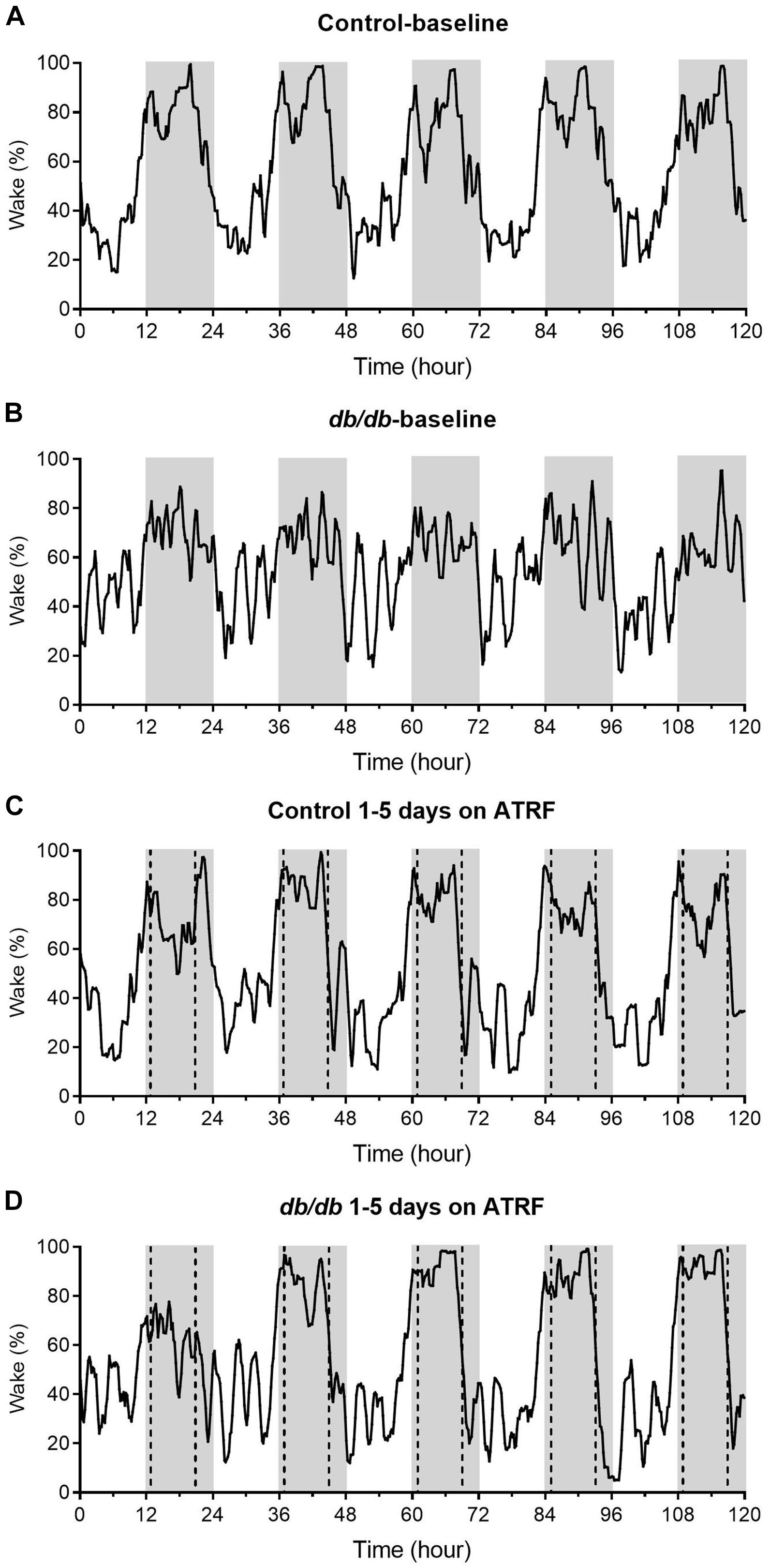
Figure 1. Representative sleep-wake profiles of the control and db/db mice with ALF and the 1–5 days of ATRF. The sleep-wake profiles in the control mice with ALF (A) or ATRF (C) and in the db/db mice with ALF (B) or ATRF (D). The gray box indicates the dark-phase. The dotted vertical line in C,D indicates the time when food was added or withdrawn during the ATRF feeding paradigm.
Quantification of the sleep time showed that the sleep percent during the light-phase was significantly decreased from 64.9 ± 3.33% in the control to 54.8 ± 2.56% in the db/db mice (p < 0.01, Figure 2A and Supplementary Table S1). In contrast, the sleep percent during the dark-phase was significantly increased from 23.7 ± 2.39% in the control to 37.5 ± 2.77% in the db/db mice (p < 0.001, Figure 2B and Supplementary Table S1). With a decrease in the light-phase and an increase in the dark-phase sleep percent, the 24-h sleep percent showed no difference between the control and db/db mice (p > 0.05, Figure 2C and Supplementary Table S1). For the sleep bout length, there was a significant decrease during the light-phase from 701.5 ± 68.57 s in the control to 455.2 ± 113.5 s in the db/db mice (p < 0.01, Figure 2D and Supplementary Table S2). There was no change during the dark-phase (p > 0.05, Figure 2E and Supplementary Table S2), which resulted in a significant decrease in the 24-h sleep bout length from 495.7 ± 69.43 s in the control to 337.0 ± 97.26 s in the db/db mice (p < 0.05, Figure 2F and Supplementary Table S2).
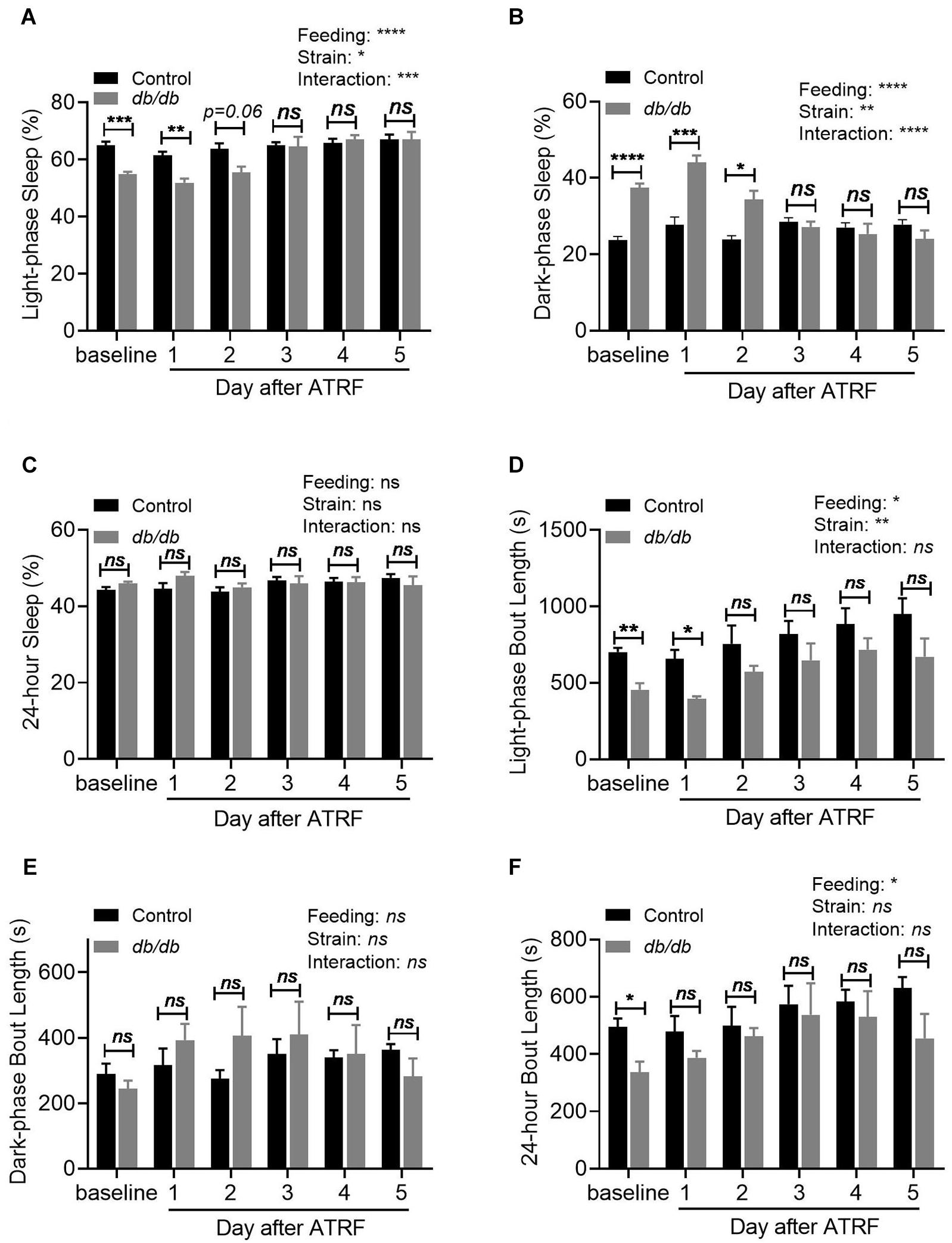
Figure 2. Quantification of the sleep-wake profile of the control and db/db mice with ALF and the 1–5 days of ATRF. (A–C) Average sleep time percent during the light-phase (A), dark-phase (B), and over 24-h (C). (D–F) Average sleep bout length during the light-phase (D), dark-phase (E), and over 24-h (F). Control, n = 6–8; db/db, n = 6–7. ∗p < 0.05; ∗∗p < 0.01; ∗∗∗p < 0.001; ****p < 0.0001; ns, no significant.
Active Time-Restricted Feeding (ATRF) Improved the Sleep-Wake Pattern in the db/db Mice
The sleep-wake profile showed that ATRF did not alter the sleep-wake pattern in the control mice (Figure 1C vs. Figure 1A and Supplementary Figure S1A). However, ATRF gradually improved the pattern in the db/db mice to resemble the control mice (Figure 1D vs. Figure 1B and Supplementary Figure S1B). By the third day of ATRF, the sleep-wake pattern in the db/db mice was similar to that in the control mice (Figure 1D and Supplementary Figure S1B).
Quantification of the sleep time showed that the db/db mice still exhibited lower light-phase sleep percent than the control mice on the first and second days of ATRF but increased to the same level as that of the control mice by the third day of ATRF (Figure 2A and Supplementary Table S1). ATRF significantly increased the light-phase sleep percent in the db/db mice (baseline vs. fifth day of ATRF: p < 0.001), but did not affect the light-phase sleep percent in the control mice. The dark-phase sleep percent in the db/db mice remained higher than in controls on the first two days of ATRF but was equivalent to that of control mice by the third day of ATRF (Figure 2B and Supplementary Table S1). The dark-phase sleep percent was not significantly different in the control mice between baseline vs. the fifth day of ATRF; however, it was significantly decreased in the db/db mice (p < 0.0001). The 24-h sleep percent was not significantly different between db/db and control mice or between baseline vs. ATRF (Figure 2C and Supplementary Table S1). The decrease of the sleep bout length during the light-phase (Figure 2D and Supplementary Table S2) or the 24 h (Figure 2F and Supplementary Table S2) in the db/db mice was abolished by the ATRF on the 2nd and 1st day, respectively.
ATRF Significantly Improved the Daily, but Suppressed the Ultradian Sleep-Wake Oscillations in the db/db Mice
To further characterize the daily oscillation of the sleep-wake cycle, we analyzed its pattern using a non-parametric method. The results showed that the IS was significantly decreased (Figure 3A and Supplementary Table S3) whereas the IV was significantly increased (Figure 3B and Supplementary Table S3) in the db/db mice compared to control mice under baseline with ALF feeding. The ATRF effectively restored both the IS and IV in the db/db mice to the control level (Figures 3A,B and Supplementary Tables S3, S4). During the least awake 5-h period in 1 day, the db/db mice had a higher mean wake percent at the baseline (Figure 3C and Supplementary Table S3). ATRF effectively suppressed it to the control level (Figure 3C and Supplementary Tables S3, S4) without changing the delayed starting time of the least awake 5-h (Figure 3D and Supplementary Table S4). For the most awake 10-h period in 1 day, the db/db mice had a decreased mean wake percent (Figure 3E and Supplementary Table S3). ATRF increased it to a level even higher than in the control mice (Figure 3E and Supplementary Tables S3, S4). The delayed starting time of the most awake 10-h period in 1 day in the db/db mice was restored to the controls (Figure 3F and Supplementary Tables S3, S4). The RA of the daily oscillation (Figure 3G and Supplementary Tables S3, S4) and the ratio of dark/light-phase wake time (Figure 3H and Supplementary Tables S3, S4) were suppressed in the db/db under ALF and restored to control level with ATRF.
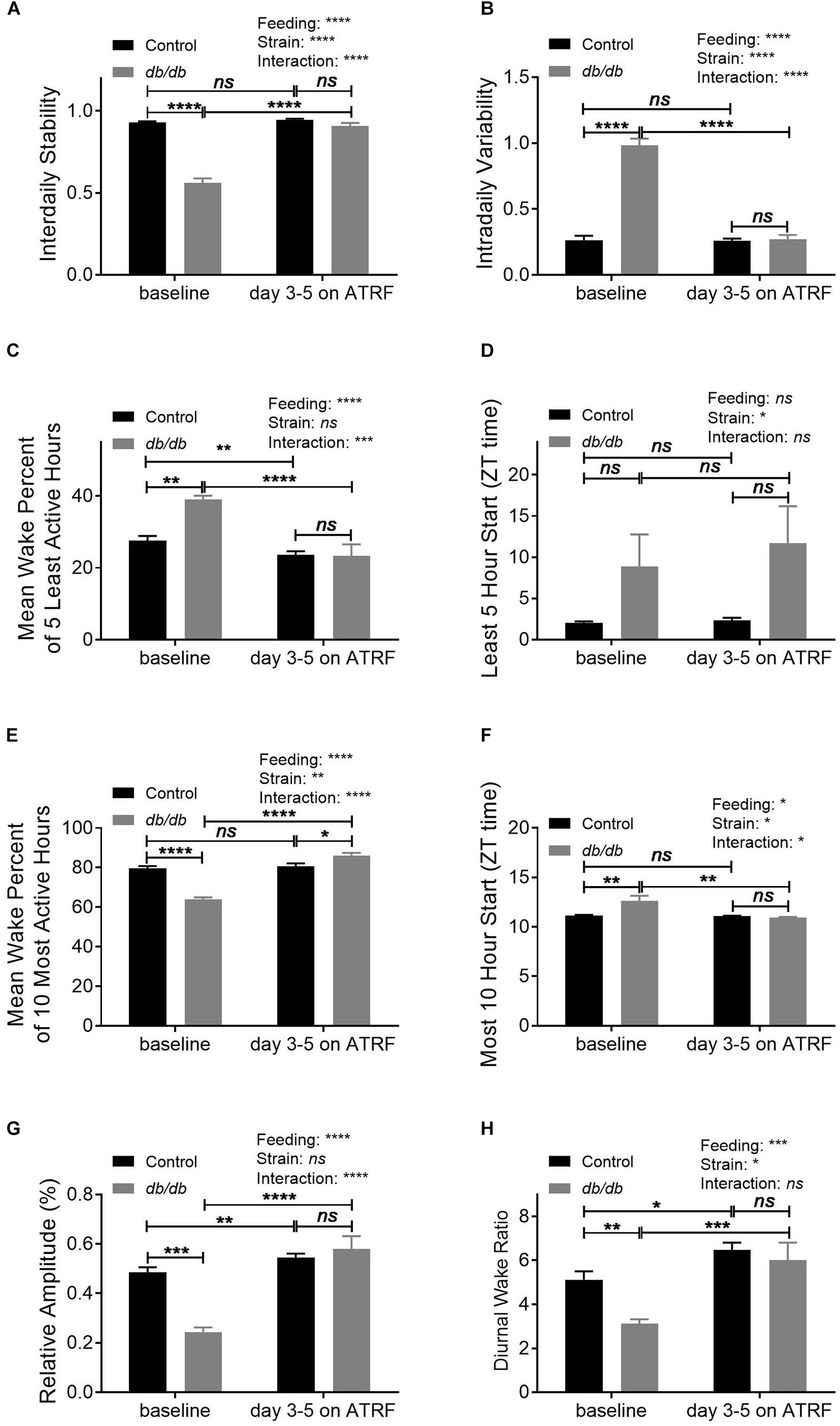
Figure 3. Non-parametric circadian rhythm analysis of the sleep-wake cycles of the control and db/db mice with ALF and the 1–5 days of ATRF. The non-parametric circadian rhythm analysis was performed using Clocklab analysis software. (A) Interdaily stability, (B) intradaily variability, (C,D) mean wake percent of the 5 least wake hours (C) and start time (D), (E,F) mean wake percent of 10 most wake hours (E) and start time (F), (G) relative amplitude, and (H) diurnal wake ratio calculated by the Piezoelectric system. Control, n = 6–8; db/db, n = 6–7. ∗p < 0.05; ∗∗p < 0.01; ∗∗∗p < 0.001; ****p < 0.0001; ns, no significant.
While the near 24-h daily oscillation was the dominant rhythm in both the control and db/db mice, the amplitude of the daily oscillation was compromised in the db/db mice (Supplementary Figure S2A vs. Supplementary Figures S2B,E and Supplementary Table S3) and restored by ATRF (Supplementary Figure S2C vs. Supplementary Figures S2D,E and Supplementary Tables S3, S4). In contrast, there was an increase in the ultradian oscillations in the db/db mice at baseline, especially oscillations with period length shorter than 6 h (Supplementary Figure S2B vs. Supplementary Figures S2A,F and Supplementary Table S3). Impressively, ATRF suppressed the ultradian oscillations of db/db mice to the control level (Supplementary Figure S2D vs. Supplementary Figures S2C,F and Supplementary Tables S3, S4). Taken together, ATRF was effective in restoring the daily and suppressing the ultradian oscillations in the db/db mice to the control level.
The Improvement of the Sleep-Wake Cycle in the db/db Mice Induced by ATRF Persisted Through 17 Days and Was Associated With Body Weight Loss
To investigate further whether the improvement of the sleep-wake oscillation in the db/db mice would be maintained after the initial 5 days, we monitored the sleep-wake cycle in the two groups of mice on ATRF for the 15th through 17th days. As shown in Figures 4A,B, the sleep-wake cycle remained very similar between the control and db/db mice after the 17 days on ATRF, indicating the improvement was maintained at least for 17 days, the longest time monitored. The light-phase sleep percent remained at similar low levels on day 3–5 and on day 15–17 (Figure 4C and Supplementary Tables S5, S7), the dark-phase sleep percent remained at similar high levels on day 3–5 and on day 15–17 (Figure 4D and Supplementary Tables S5, S7), and the 24-h sleep percent remain unchanged (Figure 4E and Supplementary Tables S5, S7). Interestingly, while the dark-phase sleep bout length remained unchanged (Figure 4G and Supplementary Tables S6, S8), the increased sleep bout length during the light-phase, and 24-h observed during the initial 5 days seemed to be lost by day 15 to 17 (Figures 4F,H and Supplementary Tables S6, S8).
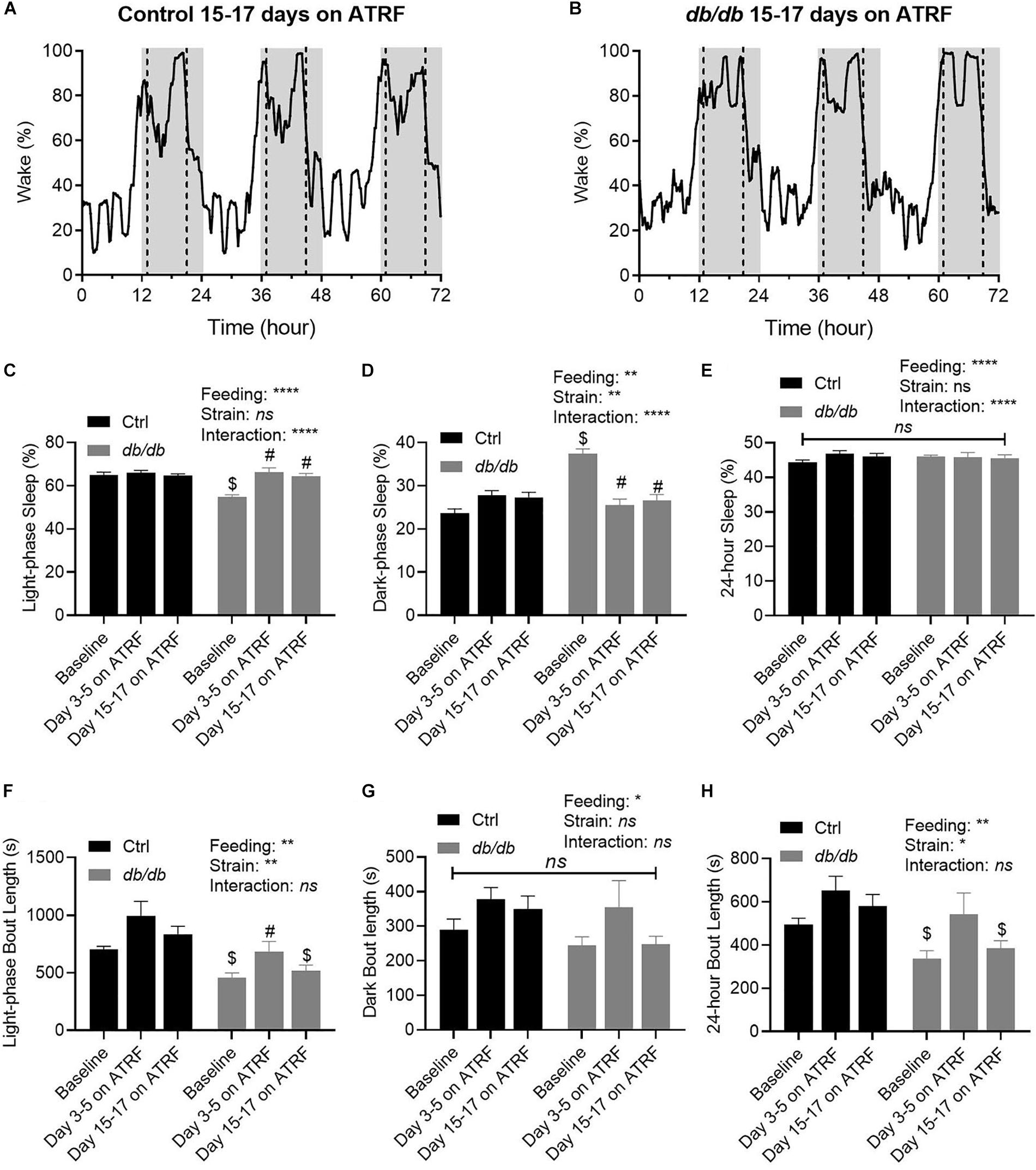
Figure 4. Time-dependent improvements of sleep-wake profile by ATRF in the control and db/db mice. Representative sleep-wake profiles in the control (A) and db/db mice (B) with 15–17 days of ATRF. The gray box indicates the dark-phase. The dotted vertical line indicates the time when food was added or withdrawn. (C–E) Average sleep time percent at baseline (ALF), 3–5 days and 15–17 days of ATRF during the light-phase (C), dark-phase (D), and over 24-h (E). (F–H) Average bout length at baseline (ALF), 3–5 days, and 15–17 days of ATRF during the light-phase (F), dark-phase (G), and over 24-h (H). Control, n = 6–8; db/db, n = 6–7. $p < 0.05 when compares with control. #p < 0.05 when compares with baseline. ∗p < 0.05; ∗∗p < 0.01; ****p < 0.0001; ns, no significant.
To determine whether the ATRF-induced sleep-wake improvement is associated with metabolic improvements in the db/db mice, we measured the body weight in db/db and control mice at baseline and after 18 days of ATRF: db/db mice body weight was decreased from 41.7 ± 0.9 g to 34.9 ± 1.1 g (p < 0.0001); however, the body weight of the control mice remained unchanged (28.1 ± 0.5 g vs. 29.1 ± 0.8 g, p > 0.05). The non-fasting blood glucose level measured at ZT20 was not significantly altered by ATRF in the db/db or control mice (mg/dl): 625.8 ± 27.0 vs. 665.3 ± 34.1, p > 0.05 in db/db mice and 136.5 ± 6.2 vs. 118.5 ± 3.4, p > 0.05 in the control mice.
Discussion
In the present study, we used a non-invasive piezoelectric system to monitor the daily sleep-wake rhythm in the type 2 diabetic db/db mice and control mice under ALF and then under the active time-restricted feeding (ATRF) paradigm. The findings are (1) Compared with the controls, the db/db mice exhibited altered daily sleep-wake rhythms: during the light-phase, the sleep percent and sleep bout length were reduced, while during the active dark-phase, the sleep percentage but not bout length were increased, and (2) ATRF restored the sleep-wake pattern in the db/db mice to become similar to that of the control strain.
Previous human and animal studies have provided a great deal of evidence to demonstrate the effects of meal timing on metabolic health. However, very few studies have examined the effects of ATRF on sleep. Interestingly, previous investigations of time-restricted feeding on sleep were mostly focused on whether dissociation between feeding and circadian time would affect sleep-wake states. Animal studies showed that when the feeding time is restricted to the normal inactive-phase, the diurnal distribution of REM and NREM sleep is attenuated (Mouret and Bobillier, 1971; Roky et al., 1999; Mieda et al., 2004; Szentirmai et al., 2009). In contrast, experimental investigations of ATRF on sleep-wake rhythms are rare. One previous study in Drosophila demonstrated that ATRF decreases daytime sleep and increases nighttime sleep (Gill et al., 2015). Another study showed that ATRF improves sleep-wake timing in a Huntington’s Disease mouse model (Wang et al., 2018). In the present study, we first reported that ATRF is able to improve the sleep-wake cycle in diabetic db/db mice by increasing daytime sleep and decreasing nighttime sleep. However, due to the limitation of the piezoelectric system, it is unknown whether ATRF improves the rhythm of NREM EEG delta power, a marker of sleep homeostasis. However, the improved diurnal sleep-wake ratio (more sleep in the light period, less sleep in the dark period) as is typical in most mice, along with the reduced ultradian sleep-wake cycle at night in ad lib db/db mice, suggest improved sleep homeostatic function.
Sleep disturbance is common in diabetes. People with diabetes are more likely to experience sleep disturbance than those without (Lamond et al., 2000; Skomro et al., 2001; Nakanishi-Minami et al., 2012; Plantinga et al., 2012; Medeiros et al., 2013; Lecube et al., 2016). Compared to the large number of human studies, investigations of sleep-wake states in animals have been limited, and probably because of the difficulties in monitoring sleep in small animals. The present study used a non-invasive piezoelectric system to monitor sleep-wake states in the db/db mice. Since db/db mice are overweight and move less than smaller mice, it is possible that the PiezoSleep system might mis-score db/db mice more than normal mice. However, the db/db mice still display postural adjustments and subtle head movements for olfactory sampling and other needs that provide the same disruption of the breath signal to score quiet wake vs. sleep with good accuracy. Importantly, the db/db sleep data in this paper matches well with previous publications using the “gold standard” of EEG/EMG in db/db or ob/ob mice (Laposky et al., 2008; Silvani et al., 2009) showing similar dysregulation of sleep-wake cycles. The previous studies showed that the total sleep time, the dark-phase NREM, and REM sleep were all increased, whereas the light-phase REM sleep was decreased in db/db and ob/ob mice compared to wildtype mice (Laposky et al., 2008; Silvani et al., 2009). Although the piezoelectric system used in the current study cannot distinguish between NREM and REM sleep, we observed increased night-phase sleep and decreased light-phase sleep in db/db mice compared to the heterozygous control db/ + mice. However, the total sleep time was not significantly different between db/db and db/+ mice. The discrepancy may be due to the different control mice used. The db/+ mice, which carry one allele of leptin receptor mutation, have normal body weight and blood glucose, but higher fat mass and plasma leptin levels relative to wildtype mice (Chung et al., 1998), and develop gestational diabetes during pregnancy (Kaufmann et al., 1981; Ishizuka et al., 1999). Therefore, it is possible that the one allele of leptin receptor mutation eliminates the total sleep time difference between db/db and db/+ mice. For the sleep bout length, the previous study showed that the light-phase and 24-h sleep bout length of NREM sleep were shortened in db/db mice compared to wildtype mice whereas no difference was found in REM sleep, which indicates the summed sleep bout length was shortened during light-phase and 24-h sleep. Consistent with that, we also detected similar alternations of sleep bout length in db/db mice. We also observed the unusual fragmentation of sleep and wake during the dark period (“spike” pattern), which demonstrated consistency between our study and prior EEG/EMG research.
The mechanisms underlying diabetes-induced disruption of sleep-wake states in db/db mice remain elusive. One possibility is diabetes-associated metabolic problems, such as obesity and impaired glucose control, contribute to the irregularity of sleep-wake states. Beside metabolic factors, another possibility is that diabetes disrupts clock gene circadian rhythms and thus the sleep-wake cycle. Interestingly, we and others have demonstrated that the mRNA and protein expressions of clock and clock-controlled genes are altered in db/db mice (Kudo et al., 2004; Caton et al., 2011; Su et al., 2012; Nernpermpisooth et al., 2015; Hou et al., 2019). In addition, alterations of clock genes are linked not only to the expected circadian disruptions but also to fundamental aspects of sleep homeostasis, suggesting clock genes may provide a previously uncharacterized “bridge” between process c and process s (Curie et al., 2013; Striz and O’Hara, 2013), the two process models of sleep regulation. Hence, it is possible that diabetes-associated clock gene alternations may contribute to sleep disturbances in db/db mice.
The precise mechanisms underlying the beneficial effects of ATRF on the db/db mouse’s sleep-wake cycle remain to be elucidated. The decrease in body weight likely contributes, however, we noted that the body weight of the db/db mice remained significantly higher than that of control mice after 18 days of ATRF. A decrease in blood glucose does not seem to contribute, as the non-fasting blood glucose was not significantly altered by ATRF in the db/db mice. In addition, ATRF-associated changes in the body temperature oscillation (Szentirmai et al., 2009) may also contribute to the improvement of sleep-wake cycle as body temperature modifies the sleep behavior (Liao, 2002). However, future studies are required to test this possibility. Evidence showed that the timing of food intake is a strong zeitgeber of peripheral clocks (Mendoza, 2007), and a previous study has demonstrated ATRF restores clock gene oscillation in db/db mouse liver (Kudo et al., 2004). We also demonstrated that ATRF-induced restoration of clock gene oscillation not only occurs in the liver but also in various other tissues (unpublished data). Hence it is possible that the restored rhythms of clock gene expression contribute to the improved sleep-wake patterns. In addition, we found that ATRF completely restored the sleep-wake cycle within 3 days suggesting the possibility of direct effects on sleep- and wake-promoting neuronal pathways or fundamental aspects of individual neurons (e.g., resting membrane potential). Further examinations on the activity of sleep- and wake-promoting neurons with ATRF are needed.
One limitation of the current study is that only the db/db mouse model was used. Although the phenotype of disrupted sleep in the obese diabetic db/db mice resembles that of human patients, the etiology of diabetes is different. Interestingly, an increase of sleep during the active dark-phase has also been reported in the high-fat diet-induced obese mouse model (Jenkins et al., 2006). It will be valuable to determine whether ATRF would be effective in improving the sleep-wake pattern in this additional mouse model in the future. In addition, an added ALF session after the ATRF would have provided data to verify that the ATRF-induced sleep-wake cycle improvement is not a time-dependent effect and to determine whether the ATRF-induced improvement would sustain for some time or not after the feeding regimen returns to ALF.
Conclusion
In conclusion, the current study demonstrated an attenuated rhythm of the sleep-wake cycle and shortened sleep bout length in db/db mice and that ATRF is able to restore a more normal sleep-wake cycle (and tended to lengthen the sleep bout length), indicating ATRF may serve as a therapeutic strategy for improving the disrupted sleep-wake patterns that are characteristic of diabetes.
Data Availability
All datasets generated for this study are included in the manuscript and/or the Supplementary Files.
Ethics Statement
The animal study was reviewed and approved by the University of Kentucky Animal Care and Use Committee.
Author Contributions
TH performed the experiments. CW, SJ, and TH analyzed the data. TH, ZG, and MG contributed to the idea, experimental design, and writing and editing of the manuscript. BO’H contributed to the interpretation of the sleep–wake data and editing of the manuscript.
Funding
This work was supported by the NIH grants HL 106843 and HL 141103 (MG and ZG).
Conflict of Interest Statement
BO’H is a co-founder and owner of Signal Solutions LLC that manufactures and sells the PiezoSleep system. However, BO’H was not involved in any of the data collection or production of the graphs and results of this manuscript.
The remaining authors declare that the research was conducted in the absence of any commercial or financial relationships that could be construed as a potential conflict of interest.
Acknowledgments
The authors thank Dr. Marilyn Duncan for editing the manuscript.
Supplementary Material
The Supplementary Material for this article can be found online at: https://www.frontiersin.org/articles/10.3389/fnins.2019.00969/full#supplementary-material
FIGURE S1 | The sleep-wake profiles in the control and db/db mice with ALF and 1–5 days of ATRF. The sleep-wake profiles with 95% confidence interval in the control (A) and db/db (B) mice. The gray box indicates the dark-phase.
FIGURE S2 | Lomb-Scargle Periodogram of the control and db/db mice with ALF and 3–5 days of ATRF. (A–D) Individual mouse periodogram of the control mice with ALF (A) or ATRF (C) and db/db mice with ALF (B) or ATRF (D). (E) The amplitude of the peak period length. (F) The sum amplitude of period length less than 6 h. ****p < 0.0001; ns, no significant.
TABLE S1 | The light-, dark-phase, and 24-h sleep percent in control and db/db mice with ALF (baseline), and 1–5 days of ATRF.
TABLE S2 | The light-, dark-phase, and 24-h sleep bout length in control and db/db mice with ALF (baseline), and 1–5 days of ATRF.
TABLE S3 | Non-parametric circadian rhythm analysis of ALF (baseline) and 3–5 days of ATRF in control and db/db mice.
TABLE S4 | Within-subject comparisons of non-parametric circadian rhythm analysis between ALF (baseline) and 3–5 days of ATRF.
TABLE S5 | The light-, dark-phase, and 24-h sleep percent in control and db/db mice with ALF (baseline), 3–5 days, and 15–17 days of ATRF.
TABLE S6 | The light-, dark-phase, and 24-h sleep bout length in control and db/db mice with ALF (baseline), 3–5 days, and 15–17 days of ATRF.
TABLE S7 | Within-subject comparisons of sleep percent between ALF (baseline), 3–5 days, and 15–17 days of ATRF.
TABLE S8 | Within-subject comparisons of sleep bout length between ALF (baseline), 3–5 days, and 15–17 days of ATRF.
References
Adamovich, Y., Rousso-Noori, L., Zwighaft, Z., Neufeld-Cohen, A., Golik, M., Kraut-Cohen, J., et al. (2014). Circadian clocks and feeding time regulate the oscillations and levels of hepatic triglycerides. Cell Metab. 19, 319–330. doi: 10.1016/j.cmet.2013.12.016
Arble, D. M., Bass, J., Laposky, A. D., Vitaterna, M. H., and Turek, F. W. (2009). Circadian timing of food intake contributes to weight gain. Obesity 17, 2100–2102. doi: 10.1038/oby.2009.264
Cappuccio, F. P., D’Elia, L., Strazzullo, P., and Miller, M. A. (2010). Quantity and quality of sleep and incidence of type 2 diabetes: a systematic review and meta-analysis. Diabetes Care 33, 414–420. doi: 10.2337/dc09-1124
Caton, P. W., Kieswich, J., Yaqoob, M. M., Holness, M. J., and Sugden, M. C. (2011). Metformin opposes impaired AMPK and SIRT1 function and deleterious changes in core clock protein expression in white adipose tissue of genetically-obese db/db mice. Diabetes Obes. Metab. 13, 1097–1104. doi: 10.1111/j.1463-1326.2011.01466.x
Chaix, A., Zarrinpar, A., Miu, P., and Panda, S. (2014). Time-restricted feeding is a preventative and therapeutic intervention against diverse nutritional challenges. Cell Metab. 20, 991–1005. doi: 10.1016/j.cmet.2014.11.001
Chung, W. K., Belfi, K., Chua, M., Wiley, J., Mackintosh, R., Nicolson, M., et al. (1998). Heterozygosity for Lepob or Leprdb affects body composition and leptin homeostasis in adult mice. Am. J. Physiol. 274, R985–R990.
Curie, T., Mongrain, V., Dorsaz, S., Mang, G. M., Emmenegger, Y., and Franken, P. (2013). Homeostatic and circadian contribution to EEG and molecular state variables of sleep regulation. Sleep 36, 311–323. doi: 10.5665/sleep.2440
Damiola, F., Le Minh, N., Preitner, N., Kornmann, B., Fleury-Olela, F., and Schibler, U. (2000). Restricted feeding uncouples circadian oscillators in peripheral tissues from the central pacemaker in the suprachiasmatic nucleus. Genes Dev. 14, 2950–2961. doi: 10.1101/gad.183500
Eban-Rothschild, A., Appelbaum, L., and de Lecea, L. (2018). Neuronal Mechanisms for Sleep/Wake regulation and modulatory drive. Neuropsychopharmacology 43, 937–952. doi: 10.1038/npp.2017.294
Flores, A. E., Flores, J. E., Deshpande, H., Picazo, J. A., Xie, X., Franken, P., et al. (2007). Pattern recognition of sleep in rodents using piezoelectric signals generated by gross body movements. IEEE Trans. Biomed. Eng. 54, 225–233. doi: 10.1109/tbme.2006.886938
Gill, S., Le, H. D., Melkani, G. C., and Panda, S. (2015). Time-restricted feeding attenuates age-related cardiac decline in Drosophila. Science 347, 1265–1269. doi: 10.1126/science.1256682
Hatori, M., Vollmers, C., Zarrinpar, A., DiTacchio, L., Bushong, E. A., Gill, S., et al. (2012). Time-restricted feeding without reducing caloric intake prevents metabolic diseases in mice fed a high-fat diet. Cell Metab. 15, 848–860. doi: 10.1016/j.cmet.2012.04.019
Horne, J. A., and Reyner, L. A. (1995). Sleep related vehicle accidents. BMJ 310, 565–567. doi: 10.1136/bmj.310.6979.565
Hou, T., Su, W., Guo, Z., and Gong, M. C. (2019). A novel diabetic mouse model for real-time monitoring of clock gene oscillation and blood pressure circadian rhythm. J. Biol. Rhythms 34, 51–68. doi: 10.1177/0748730418803719
Ishizuka, T., Klepcyk, P., Liu, S., Panko, L., Gibbs, E., and Friedman, J. (1999). Effects of overexpression of human GLUT4 gene on maternal diabetes and fetal growth in spontaneous gestational diabetic C57BLKS/J Lepr (db/+) mice. Diabetes Metab. Res. Rev. 48, 1061–1069. doi: 10.2337/diabetes.48.5.1061
Jenkins, J. B., Omori, T., Guan, Z., Vgontzas, A. N., Bixler, E. O., and Fang, J. (2006). Sleep is increased in mice with obesity induced by high-fat food. Physiol. Behav. 87, 255–262. doi: 10.1016/j.physbeh.2005.10.010
Kaufmann, R. C., Amankwah, K. S., Dunaway, G., Maroun, L., Arbuthnot, J., and Roddick, J. Jr. (1981). An animal model of gestational diabetes. Am. J. Obstet. Gynecol. 141, 479–482.
Ktorza, A., Bernard, C., Parent, V., Penicaud, L., Froguel, P., Lathrop, M., et al. (1997). Are animal models of diabetes relevant to the study of the genetics of non-insulin-dependent diabetes in humans? Diabetes Metab. 23(Suppl. 2), 38–46.
Kudo, T., Akiyama, M., Kuriyama, K., Sudo, M., Moriya, T., and Shibata, S. (2004). Night-time restricted feeding normalises clock genes and Pai-1 gene expression in the db/db mouse liver. Diabetologia 47, 1425–1436.
Lamond, N., Tiggemann, M., and Dawson, D. (2000). Factors predicting sleep disruption in Type II diabetes. Sleep 23, 415–416.
Laposky, A. D., Bradley, M. A., Williams, D. L., Bass, J., and Turek, F. W. (2008). Sleep-wake regulation is altered in leptin-resistant (db/db) genetically obese and diabetic mice. Am. J. Physiol. 295, R2059–R2066. doi: 10.1152/ajpregu.00026.2008
Lecube, A., Sanchez, E., Gomez-Peralta, F., Abreu, C., Valls, J., Mestre, O., et al. (2016). Global assessment of the impact of type 2 diabetes on sleep through specific questionnaires. A case-control study. PLoS One 11:e0157579. doi: 10.1371/journal.pone.0157579
Liao, W.-C. (2002). Effects of passive body heating on body temperature and sleep regulation in the elderly: a systematic review. Int. J. Nurs. Stud. 39, 803–810. doi: 10.1016/s0020-7489(02)00023-8
Mang, G. M., Nicod, J., Emmenegger, Y., Donohue, K. D., O’Hara, B. F., and Franken, P. (2014). Evaluation of a piezoelectric system as an alternative to electroencephalogram/electromyogram recordings in mouse sleep studies. Sleep 37, 1383–1392. doi: 10.5665/sleep.3936
Medeiros, C., Bruin, V., Férrer, D., Paiva, T., Montenegro Júnior, R., Forti, A., et al. (2013). Excessive daytime sleepiness in type 2 diabetes. Arq. Bras. Endocrinol. Metabol. 57, 425–430. doi: 10.1590/s0004-27302013000600003
Melamed, S., and Oksenberg, A. (2002). Excessive daytime sleepiness and risk of occupational injuries in non-shift daytime workers. Sleep 25, 315–322. doi: 10.1093/sleep/25.3.315
Mendoza, J. (2007). Circadian clocks: setting time by food. J. Neuroendocrinol. 19, 127–137. doi: 10.1111/j.1365-2826.2006.01510.x
Mieda, M., Williams, S. C., Sinton, C. M., Richardson, J. A., Sakurai, T., and Yanagisawa, M. (2004). Orexin neurons function in an efferent pathway of a food-entrainable circadian oscillator in eliciting food-anticipatory activity and wakefulness. J. Neurosci. 24, 10493–10501. doi: 10.1523/jneurosci.3171-04.2004
Mistlberger, R. E., Lukman, H., and Nadeau, B. G. (1998). Circadian rhythms in the Zucker obese rat: assessment and intervention. Appetite 30, 255–267. doi: 10.1006/appe.1997.0134
Mouret, J., and Bobillier, P. (1971). Diurnal rhythms of sleep in the rat: augmentation of paradoxical sleep following alterations of the feeding schedule. Int. J. Neurosci. 2, 265–269. doi: 10.3109/00207457109147009
Nakanishi-Minami, T., Kishida, K., Funahashi, T., and Shimomura, I. (2012). Sleep-wake cycle irregularities in type 2 diabetics. Diabetol. Metab. Syndr. 4:18. doi: 10.1186/1758-5996-4-18
Nernpermpisooth, N., Qiu, S., Mintz, J. D., Suvitayavat, W., Thirawarapan, S., Rudic, D. R., et al. (2015). Obesity alters the peripheral circadian clock in the aorta and microcirculation. Microcirculation 22, 257–266. doi: 10.1111/micc.12192
Plantinga, L., Rao, M. N., and Schillinger, D. (2012). Prevalence of self-reported sleep problems among people with diabetes in the United States, 2005-2008. Prev. Chronic Dis. 9:E76.
Roky, R., Kapás, L., Taishi, P., Fang, J., and Krueger, J. M. (1999). Food restriction alters the diurnal distribution of sleep in rats. Physiol. Behav. 67, 697–703. doi: 10.1016/s0031-9384(99)00137-7
Sherman, H., Genzer, Y., Cohen, R., Chapnik, N., Madar, Z., and Froy, O. (2012). Timed high-fat diet resets circadian metabolism and prevents obesity. FASEB J. 26, 3493–3502. doi: 10.1096/fj.12-208868
Silvani, A., Bastianini, S., Berteotti, C., Franzini, C., Lenzi, P., Lo Martire, V., et al. (2009). Sleep modulates hypertension in leptin-deficient obese mice. Hypertension 53, 251–255. doi: 10.1161/HYPERTENSIONAHA.108.125542
Skomro, R. P., Ludwig, S., Salamon, E., and Kryger, M. H. (2001). Sleep complaints and restless legs syndrome in adult type 2 diabetics. Sleep Med. 2, 417–422. doi: 10.1016/s1389-9457(01)00110-1
Stokkan, K.-A., Yamazaki, S., Tei, H., Sakaki, Y., and Menaker, M. (2001). Entrainment of the circadian clock in the liver by feeding. Science 291, 490–493. doi: 10.1126/science.291.5503.490
Striz, M., and O’Hara, B. F. (2013). Clock genes and sleep homeostasis: a fundamental link within the two-process model? Sleep 36, 301–302. doi: 10.5665/sleep.2430
Su, W., Xie, Z., Guo, Z., Duncan, M. J., Lutshumba, J., and Gong, M. C. (2012). Altered clock gene expression and vascular smooth muscle diurnal contractile variations in type 2 diabetic db/db mice. Am. J. Physiol. Heart Circ. Physiol. 302, H621–H633. doi: 10.1152/ajpheart.00825.2011
Szentirmai, É., Kapás, L., Sun, Y., Smith, R. G., and Krueger, J. M. (2009). Restricted feeding-induced sleep, activity, and body temperature changes in normal and preproghrelin-deficient mice. Am. J. Physiol. 298, R467–R477. doi: 10.1152/ajpregu.00557.2009
Tasali, E., Mokhlesi, B., and Van Cauter, E. (2008). Obstructive sleep apnea and type 2 diabetes: interacting epidemics. Chest 133, 496–506. doi: 10.1378/chest.07-0828
Tsai, J. Y., Villegas-Montoya, C., Boland, B. B., Blasier, Z., Egbejimi, O., Gonzalez, R., et al. (2013). Influence of dark phase restricted high fat feeding on myocardial adaptation in mice. J. Mol. Cell Cardiol. 55, 147–155. doi: 10.1016/j.yjmcc.2012.09.010
van Someren, E. J., Hagebeuk, E. E., Lijzenga, C., Scheltens, P., de Rooij, S. E., Jonker, C., et al. (1996). Circadian rest-activity rhythm disturbances in Alzheimer’s disease. Biol. Psychiatry 40, 259–270.
Wang, H.-B., Loh, D. H., Whittaker, D. S., Cutler, T., Howland, D., and Colwell, C. S. (2018). Time-restricted feeding improves circadian dysfunction as well as motor symptoms in the Q175 mouse model of Huntington’s disease. eNeuro 5:ENEURO.0431-17.2017.
Yaghouby, F., Donohue, K. D., O’Hara, B. F., and Sunderam, S. (2016). Noninvasive dissection of mouse sleep using a piezoelectric motion sensor. J. Neurosci. Methods 259, 90–100. doi: 10.1016/j.jneumeth.2015.11.004
Yasumoto, Y., Hashimoto, C., Nakao, R., Yamazaki, H., Hiroyama, H., Nemoto, T., et al. (2016). Short-term feeding at the wrong time is sufficient to desynchronize peripheral clocks and induce obesity with hyperphagia, physical inactivity and metabolic disorders in mice. Metabolism 65, 714–727. doi: 10.1016/j.metabol.2016.02.003
Keywords: active time-restricted feeding, sleep, circadian rhythm, diabetes, db/db mice
Citation: Hou T, Wang C, Joshi S, O’Hara BF, Gong MC and Guo Z (2019) Active Time-Restricted Feeding Improved Sleep-Wake Cycle in db/db Mice. Front. Neurosci. 13:969. doi: 10.3389/fnins.2019.00969
Received: 14 June 2019; Accepted: 29 August 2019;
Published: 20 September 2019.
Edited by:
Marcos G. Frank, Washington State University Health Sciences Spokane, United StatesReviewed by:
Ralph E. Mistlberger, Simon Fraser University, CanadaAlessandro Silvani, University of Bologna, Italy
Sigrid Veasey, University of Pennsylvania, United States
Copyright © 2019 Hou, Wang, Joshi, O’Hara, Gong and Guo. This is an open-access article distributed under the terms of the Creative Commons Attribution License (CC BY). The use, distribution or reproduction in other forums is permitted, provided the original author(s) and the copyright owner(s) are credited and that the original publication in this journal is cited, in accordance with accepted academic practice. No use, distribution or reproduction is permitted which does not comply with these terms.
*Correspondence: Zhenheng Guo, emd1bzJAdWt5LmVkdQ==