- 1Neural Stem Cell Research Lab, Research Department, National Neuroscience Institute, Singapore, Singapore
- 2Department of Diagnostic Radiology, Singapore General Hospital, Singapore, Singapore
- 3Neuroscience & Behavioral Disorders Program, Duke University and National University of Singapore (DUKE-NUS), Graduate Medical School, Singapore, Singapore
- 4Department of Neurology, National Neuroscience Institute, Singapore General Hospital Campus, Singapore, Singapore
- 5Lee Kong Chian School of Medicine, Nanyang Technological University, Novena Campus, Singapore, Singapore
Curative therapies or treatments reversing the progression of Parkinson’s disease (PD) have attracted considerable interest in the last few decades. PD is characterized by the gradual loss of dopaminergic (DA) neurons and decreased striatal dopamine levels. Current challenges include optimizing neuroprotective strategies, developing personalized drug therapy, and minimizing side effects from the long-term prescription of pharmacological drugs used to relieve short-term motor symptoms. Transplantation of DA cells into PD patients’ brains to replace degenerated DA has the potential to change the treatment paradigm. Herein, we provide updates on current progress in stem cell-derived DA neuron transplantation as a therapeutic alternative for PD. We briefly highlight cell sources for transplantation and focus on cell assessment methods such as identification of genetic markers, single-cell sequencing, and imaging modalities used to access cell survival and function. More importantly, we summarize clinical reports of patients who have undergone cell-derived transplantation in PD to better perceive lessons that can be drawn from past and present clinical outcomes. Modifying factors include (1) source of the stem cells, (2) quality of the stem cells, (3) age of the patient, (4) stage of disease progression at the time of cell therapy, (5) surgical technique/practices, and (6) the use of immunosuppression. We await the outcomes of joint efforts in clinical trials around the world such as NYSTEM and CiRA to further guide us in the selection of the most suitable parameters for cell-based neurotransplantation in PD.
Introduction
Parkinson’s disease (PD) is one of the most prevalent chronic neurodegenerative disorder characterized by the selective, progressive loss of nigrostriatal dopaminergic (DA) neurons in the substantia nigra pars compacta. The main hallmarks of PD include the presence of α-synuclein positive Lewy bodies and neuroinflammation (MacGeer and McGeer, 2008; More et al., 2013) that extends across many areas of the central nervous system (CNS), affecting the enteric and autonomic systems, in particular (Goedert et al., 2013), causing impairments in motor movements such as bradykinesia (slowed movements), tremors, postural instability, and muscle rigidity. Furthermore, PD patients have shown non-motor disease manifestations such as rapid eye movement (REM), sleep behavior disorders, depression, hyposmia, and constipation (Olanow et al., 2009). Unfortunately, there are no curative therapies available to modify or reverse the progression of the underlying disease processes to date.
The current gold standard for PD treatment is through the ingestion of levodopa, which has been approved by the US Food and Drug Administration in the 1970s and has continuously shown positive results in temporal amelioration of PD symptoms (Fahn, 2003, 2006). However, long-term exposure to levodopa results in a gradual decrease in drug effectiveness and shorter periods of benefit, leading to levodopa-induced dyskinesias (motor fluctuations), as well as psychiatric and cognitive problems. Alternatively, surgical strategies, such as deep brain stimulation (DBS), have shown to alleviate PD motor symptoms (Siegfried and Lippitz, 1994; Limousin et al., 1995) and offer symptomatic relief that cannot be controlled with medications (Alamri et al., 2015). However, its application is not only limited to early-to-mid PD stages but also loses efficacy after a few years (deSouza et al., 2013).
In the last few decades, cell-based therapy using human stem cells has made large strides in overcoming the abovementioned limitations in PD treatment. Also known as regenerative medicine, stem cell therapy is believed to replace diseased, dysfunctional, or damaged tissue in hopes to restore lost neuronal circulatory caused by focal degeneration of mesencephalic dopaminergic (mDA) neurons. Specifically, neural progenies from pluripotent stem cells (PSCs) are known to hold great potential as a succeeding treatment for neurodegenerative diseases (Hu et al., 2010; Kriks et al., 2011; Ma et al., 2012). Today, DA neurons differentiated from stem cells are paving the way as a new, alternative approach in the treatment of PD. In this review article, we briefly highlight the major sources of stem cells used in preclinical and clinical PD observations (have been thoroughly reviewed in various articles, refer to Stoker, 2018). We focus on key methodologies currently applied in cell assessment, imaging modalities, and also further discuss ongoing stem cell-based clinical trials in PD. This also includes key challenges that the field is encountering and the prospects of stem cell therapy in PD.
Cell Sources
First, we briefly discuss the various types of stem cells currently being used as a source for cell-based therapy in PD. We also include the pros and cons of each cell line (Table 1), followed by the characterization of graft quality through various cell assessment methods (Cell Assessment of Differentiated DA Neurons section).
Fetal Ventral Mesencephalic Cells
In the early 1970s, Olson and colleagues successfully transplanted adrenal chromaffin cells and embryonic DA neurons into the anterior chamber of the eye in rats and showed that the viability of grafted neurons was best achieved using developing embryonic neurons (Olson and Malmfors, 1970; Olson and Seiger, 1972). Parkinsonism rat and monkey models grafted with early gestational age dopamine-rich mesencephalic neurons formed neurite protrusions and synthesize dopamine (Dunnett et al., 1983; Brundin et al., 1986; Redmond et al., 1986; Stromberg et al., 1986; Bakay et al., 1987). Furthermore, successful integration of transplanted cells into the host brain neuronal network was demonstrated through synaptic integration using a rabies-based monosynaptic tracing method (Cardoso et al., 2018). Behavioral studies in PD rodents and primates with human fetal DA neuron transplantation showed higher efficacy in improvement of behavioral deficits as compared to conventional adrenal medullary tissue transplants (Bjorklund and Stenevi, 1979, Perlow et al., 1979; Freed et al., 1981; Morihisa et al., 1984). Also, pioneering clinical studies in human fetal ventral mesencephalic (fVM) transplantation into the caudate and putamen of PD patients in Sweden, United Kingdom, and United States reported moderate amelioration of PD symptoms (Lindvall et al., 1988; Madrazo et al., 1988; Freed et al., 1990; Freed et al., 2001; Olanow et al., 2003). Moreover, normal striatal F-DOPA uptake was 3–5 years post-surgery, including gradual motor improvements that sustained up to 18 years post-transplantation (Kefalopoulou et al., 2014). However, the majority of successful cases were performed in PD patients under the age of 60 (Ma et al., 2010). Whether graft-induced dyskinesias are characteristics of neural transplantation has to be better studied and analyzed (Freed et al., 2001; Hagell et al., 2002; Olanow et al., 2003; Ma et al., 2010). Nonetheless, obtaining as many as up to seven human fetal donors (aged 6–9 weeks after conception) for each host raises many ethical concerns and logistical challenges for a disease affecting millions of people worldwide (Steinbeck and Studer, 2015; Barker and Consortium, 2019). Furthermore, the difficulty in preaccessing a cell type before transplantation is a major challenge in standardization as the heterogenicity of cell population within the graft is inevitable, contributing to high variability in the degree of symptomatic recovery. All in all, the additional risk in cell contamination of unwanted cell types during tissue extraction hampered the downstream translation of fVM transplantation as an alternative therapeutic option.
Human Embryonic Stem Cells
Due to the abovementioned ethical controversies in utilizing hfVM tissues for cell-based therapy (and other limitations), human embryonic stem cells (hESCs) were identified as a prospective substitute (Thomson et al., 1998; Reubinoff et al., 2000; Barker, 2014). These subsets of pluripotent cells are located in the inner cell mass of early embryonic blastocyst commonly derived from in vitro fertilization (Evans and Kaufman, 1981; Thomson et al., 1998) and hold the capability to generate into a plethora of cell lines through a spontaneous differentiation protocol in vitro (Itskovitz-Eldor et al., 2000; Lee et al., 2000; Reubinoff et al., 2001; Zhang et al., 2001). In the case of neuroepithelial cell-derived DA neuron differentiation, cells showed an increase in a multitude of cellular marker expression for midbrain DA neurons with fiber outgrowth (Thomson et al., 1998; Kawasaki et al., 2000; Kim et al., 2002) and electrophysiologically active neurons that produced DA in an activity-dependent manner (Yan et al., 2005). In later years, it was identified that DA neurons unlike all other neurons are generated from the midbrain floor plate. With newly improvised DA neuron differentiation protocol (Fasano et al., 2010; Kriks et al., 2011; Kirkeby et al., 2012), a significant upregulation of midbrain DA neuronal markers was observed along with recovery in motor defects in preclinical studies (Kirkeby et al., 2012, 2017a; Grealish et al., 2014). Unfortunately, key limitations lie in the difficulty in controlling the maturation stage of embryonic cultures and cellular heterogeneity, which may lead to negative outcomes in therapeutic applications (Stewart et al., 2006; Roy et al., 2006; Cho et al., 2008; Koch et al., 2009). Other caveats include the associated risk in tumor generation and teratoma due to their high pluripotent phenotype (Ben-Hur et al., 2004; Roy et al., 2006; Brederlau et al., 2006; Sonntag et al., 2007; Yang et al., 2008). In 2001, ethical concerns in hESC research resulted in a restriction on federal fundings in the United States. Fortunately, this legislation has been revoked by President Barack Obama in 2007. With this advantage, New York Stem Cell Science Consortia at Memorial Sloan Kettering Cancer Center conducted ongoing projects such as the development of good manufacturing practice (GMP) clinical-grade hESC-derived DA neurons for FDA approval in future transplantation studies (refer to section “GMP cryopreservation of cells”), optimization of cell purification to enrich A9 type DA neurons, and also, active involvement in strategical planning for clinical trial of hESCs in Parkinson’s disease.1
Human-Induced Pluripotent Stem Cells (hiPSCs)
The field of stem cell research and regenerative medicine was revolutionized in 2006 when human fibroblast cells were successfully reprogrammed into pluripotent cell lines using four transcription factors: c-Myc (or Nanog, Lin28), Oct3/4, Klf4, and Sox2 (Takahashi and Yamanaka, 2006; Takahashi et al., 2007; Yu et al., 2007). Reprogrammed iPSCs have been a highly attractive cell source as they have the characteristics of hESCs (in terms of morphology and genetic profile) (Fairchild, 2010; Phanstiel et al., 2011), and they have a relatively simpler extraction process. Tissue collection is non-invasive as host cells from skin fibroblast (Pulecio et al., 2014), peripheral blood mononuclear cells, and umbilical cord mesenchymal cells (Park et al., 2008; Senju et al., 2011; Biju et al., 2013; Qin et al., 2013) could be used to differentiate into patient-specific neurons in vitro (Soldner et al., 2009; Beevers et al., 2013; Eigentler et al., 2013; Sison et al., 2018). This would also avoid allogenic recognition and ethical concerns (Takahashi and Yamanaka, 2016). In PD studies, the quality of iPSC-derived DA neurons was highly similar to that of hESCs (Cooper et al., 2010; Doi et al., 2014; Kikuchi et al., 2017; Lehnen et al., 2017), and human leukocyte antigen (HLA)-matched allogeneic neural transplantation into monkeys increased the efficacy of cell survival and function (Morizane et al., 2017). Animal studies demonstrated successful amelioration of PD symptoms resulting from iPSC-derived DA neuron transplantation (Wakeman et al., 2017). Further refinement and characterization are necessary to achieve precise cell fate conversion of reprogrammed cells. Similar to ESCs, it is important that minimal manipulation is made during reprogramming prior to cell delivery.
GMP Cryopreservation of Cells
The generation of good manufacturing practice (GMP)-compliant, deliverable midbrain DA (mDA) progenitors/neurons optimized for cell-based therapy for PD is a major challenge. Currently, a diverse collection of clinical-grade hESC lines are available as starting material to generate GMP-compliant mDA progenitors/neurons. In fact, GMP compliant differentiation protocols and reagents have been successfully applied to generate GMP mDA neurons (Liu et al., 2013; Peng et al., 2014).
In comparison, the availability of clinical-grade iPSCs is relatively lesser due to the lack of technology that involves complex reprogramming methodologies. Major hurdles of the clinical translation of mDA cells therapy include (i) quality control of the identity, safety, and efficacy of cell product in a consistent and real-time manner, (ii) determination of the precise time points at which DA precursors/neurons can be cryopreserved and banked without affecting its’ quality, (iii) good postthaw viability of mDA cells, and (iv) characteristics and functionality of the population of cells should have minimal to no alterations after thawing. XCell Science has generated GMP-compatible authentic DA neurons, which are functional when transplanted into PD animal model (Peng et al., 2014) where cells were cryopreserved at day 14 after neuronal stem cell (NSC) stage. Similar studies were also reported by Cellular Dynamics International using more mature mDA cells in postmitotic stage (Wakeman et al., 2017). Successful generation of GMP-grade cryopreserved cells would allow for storage of a large batch of DA neurons and also increase the flexibility in operational schedule organization without the dependence on GMP-manufacturing site.
Cell Assessment of Differentiated DA Neurons
Understanding the key type of DA neurons required to achieve downstream restoration of PD pathology is essential. The mesotelencephalic DA system in the midbrain contains two main groups: the A9 neuronal clusters of the nigrostriatal DA pathway located in the zona compacta, the substantia nigra involved in the control of posture, and the A10 neurons located in the ventromedial mesencephalic tegmentum that regulates the locomotor activity and emotional behavior (Dahlstroem and Fuxe, 1964; Anden et al., 1966; Ungerstedt, 1971; Lindvall and Bjorklund, 1974; Pijnenburg et al., 1976; Papp and Bal, 1986). Dysfunction of the nigrostriatal system has been linked to Parkinsonism and later to schizophrenia, drug addiction, and depression (Robinson and Berridge, 1993; Meyer-Lindenberg et al., 2002). Differences between the two DA cell populations have been observed in neurochemistry and in spontaneous neuronal firing (Grenhoff et al., 1988; Wolfart et al., 2001; Neuhoff et al., 2002). More importantly, A9 neurons display significantly enhanced levels of neuromelanin pigmentation as compared to other dopamine-producing neurons (Mann and Yates, 1983; Hirsch et al., 1988; Gibb, 1992; Kastner et al., 1992). This could account for the association of early loss of A9 DA neurons in Parkinson’s disease with increased vulnerability upon disease progression with the relative preservation of A10 DA neurons (Hirsch et al., 1988; German et al., 1989; German et al., 1992; Damier et al., 1999; Halliday et al., 2005; Alavian et al., 2008).
Generally, stem cells are differentiated into specific nigra A9 DA neurons in large quantities prior to PD transplantation. This step has been thoroughly reviewed by many articles such as in Fan et al. (2020) and, thus, will not be further discussed here. However, we focus on developments in technology in cell assessment of differentiated DA neurons.
Assessment of the Efficacy of Cell Transplants With Immunostaining Characterization
Prior to stem cell transplantation, it is important to be able to fully characterize differentiated cell types to avoid heterogenicity of cell population (also known as cellular contamination). Previous studies have shown that transplantation of fetal SN-A9 DA neurons suffices the requirement for striatal reinnervation and recovery of PD-like behavioral observations (Grealish et al., 2010). However, tumor formation (Roy et al., 2006; Brederlau et al., 2006; Elkabetz et al., 2008; Doi et al., 2012) and development of graft-induced dyskinesia could arise from the high heterogenicity of serotonergic neurons (Carlsson et al., 2007; Politis et al., 2010). As cells are normally transplanted as immature progenitor cells, developing methods that can characterize and predict its functional maturation and therapeutic efficacy is crucial. Hence, to circumvent these limitations prior to proceeding into clinical trials, methods to isolate homogenous population of DA progenitor cells have been closely evaluated (Fukuda et al., 2006; Pruszak et al., 2009; Jonsson et al., 2009; Ganat et al., 2012; Sundberg et al., 2013). This includes developing meaningful quality control assays to assess cell type to avoid having heterogeneous mixtures of cells (includes phenotypes and degree of maturity) and batch-to-batch variation. The quality of differentiated mesencephalic A9 DA neurons that represent those in the substantia nigral para compacta or into immature progenitor cells is vital to determine the therapeutic efficacy of cell transplantation in the Parkinsonian brain. It is well understood that the orchestration of specific gene expression patterns is highly correlated to DA cell differentiation and survival. Therefore, the establishment and determination of specific gene expression markers have been used to positively characterize differentiated cells in vitro.
In the case of mDA progenitor neuron specifications, positive gene expression of common transcription factors FOXA2, LMX1A, and OTX2 and negative markers (non-neural) such as Afp, Gata4, and Brachyury have been quantitatively analyzed (Chung et al., 2009; Lin et al., 2009; Jaeger et al., 2011; Kriks et al., 2011; Kirkeby et al., 2012; Salti et al., 2013; Doi et al., 2014). More importantly, the upregulation and downregulation of these markers at a given stage in vitro governs the efficiency of cell fate determination. Unfortunately, these markers have been shown to coexpress in the diencephalic progenitor cells of the subthalamic nucleus (STN) (Kee et al., 2017). Furthermore, the expression of the positive genetic marker for DA neurons, tyrosine hydroxylase (TH), a rate-limiting enzyme in dopamine synthesis (Daadi and Weiss, 1999; Sonntag et al., 2004; Kirkeby et al., 2017a), and the levels of GIRK2 have also been observed in many cell types in vitro (Thompson et al., 2005; Kirkeby et al., 2012; Reyes et al., 2012; Grow et al., 2016). Moreover, common positive markers used to isolate high-quality DA progenitor cells include EN1 and SPRY1 (Simon et al., 2001; Alberi et al., 2004; Kirkeby et al., 2017a); Nurr1 (Le et al., 1999); FOXA2, LMX1B, and MSX1 (Andersson et al., 2006; Chung et al., 2011), and the bicoid-related homeodomain factor Ptx3/Pitx3 (Hargus et al., 2010). It is noteworthy that some discrepancies have been found with the requirement for the presence of floor plate-specific cell surface marker CORIN expression (Ono et al., 2007; Chung et al., 2011; Kriks et al., 2011; Kirkeby et al., 2012, 2017a; Doi et al., 2014; Arenas et al., 2015; Fan et al., 2020). A more recent study has identified a cell surface marker integrin-associated protein (IAP, CD47) as a positive marker for FOXA2-positive DA progenitor cells (Lehnen et al., 2017).
While these positive markers are required to narrow down the search for pure DA progenitor cells, negative markers such as Oct3/4, PAX6, and SOX1 for other midbrain neurons act as good controls to prevent introducing contamination with other neuronal subtypes during sorting. Last, terminal differentiation of DA neurons post-transplantation can be identified by the expression of neurotransmitter phenotype markers, namely, TH, dopamine transporter (DAT), Vmat2, Girk2, and Calbindin (Di Porzio et al., 1990; Sgado et al., 2006). It is crucial to take into consideration the wide genetic variation of iPSCs, which may harbor a large spectrum of genetic variation and even retain donor-specific gene expression pattern depending on multiple factors, such as the number of passages of the lineage or transcriptional factors introduced to induce cell differentiation (Rouhani et al., 2014; Thomas et al., 2015; Burrows et al., 2016; Carcamo-Orive et al., 2017). Nonetheless, growing evidence strongly suggests the need for heightened stringency in cell type evaluation. This is particularly important to avoid incomplete differentiation of cells, which could result in undesired reprogrammed cell lineages affecting functional deficits when transplanted into PD models (Park et al., 2005; Grow et al., 2016; Kirkeby et al., 2017a).
Single-Cell RNA-Seq to Evaluate the Quality of Cells
More recently, high-resolution analyses of cell type specificity such as single-cell transcriptomic analyses of neuronal populations of induced stem cells have pathed its way to become a new tool to increase the specificity during DA neuron extraction. This method would allow gene expression profiling of individual cells to better understand population heterogeneity and to distinguish between distinct cell subpopulations to increase the purity of desired cell lines (Poulin et al., 2014; La Manno et al., 2016; Reid and Wernisch, 2016; Lang et al., 2019; Tiklova et al., 2019). However, to achieve this, a specific set of cellular and gene regulatory network contexts have to be determined (as mentioned in the Assessment of the Efficacy of Cell Transplants With Immunostaining Characterization section). Although the presence of the PITX3 gene expression in adult mDA neurons suffices the criteria (Smidt et al., 1997), PITX3 was later shown to be present in both TH-positive and TH-negative cells (Tiklova et al., 2019). In the same study, single-cell RNA sequencing (scRNAseq) analyses were used to distinguish between several mDA subtypes with gene targeting. Moving forward, providing key proof-of-concept in utilizing scRNAseq as a tool for quality control would be the future for cell replacement therapies.
Assessment of the Efficacy of Cell Transplants With Imaging
Last, concurrent with the high demand for the optimization of cell graft visualization in PD, growing emphasis has been placed on enhancing the sensitivity and precision of the spatiotemporal resolution of functional neuroimaging. En route to successful cell transplantation as a therapeutic regenerative method for Parkinson’s disease, neuroimaging techniques have to be employed for better patient care. Some key features required to elucidate the therapeutic efficacy of transplanted cells for clinical diagnostics are (1) innervation, (2) survival, (3) differentiation, and (4) functional biochemistry composition. Furthermore, it is crucial that these imaging techniques are time efficient, safe, non-invasive, and allow repeated measures in an individual to determine longitudinal post-operative progression in patients with cell transplantation (Barrow et al., 2015; Ramos-Gomez et al., 2015). In this section, we summarize the pros and cons of current imaging modalities used in tracking cell grafts in PD and their respective biomarkers (Table 2).
Magnetic resonance imaging (MRI) is a popular method for examining brain tissue morphology that uses strong magnetic fields coupled with contrast agents such as paramagnetic contrast agent (Gadolinium [III] [Gd3+], Manganese [Mn2+]), perfluorocarbons, or superparamagnetic iron oxide (SPIO) despite its challenges in differentiating tissues with structures that naturally emits low MRI signals like bones. Its biggest advantage is its superior spatial resolution, non-invasiveness, and relatively cost efficiency compared to other neuroimaging methods discussed below. Various lines of evidence strongly suggest the reliability of MRI in visualizing prelabeled transplanted cells such as ESCs (Sykova and Jendelova, 2007), fetal rat cortical cells (Hawrylak et al., 1993), and fetal striatal tissues (Norman et al., 1992) in rats. Furthermore, MRI has been used to evaluate edema and inflammation in tissues surrounding cell-transplanted sites in mice and primates (Anderson et al., 2005; Iwanami et al., 2005). It is important to note that false MRI signals may result from the residual build-up of SPIO nanoparticles released from dead transplanted cells and engulfed by macrophages and activated microglia (Amsalem et al., 2007; Liu and Frank, 2009; Cupaioli et al., 2014; Ramos-Gomez and Martinez-Serrano, 2016). Additionally, cells prelabeled with contrast agents prior to transplantation may show diluted and faded contrast over time as cells proliferate within the transplanted site, which may lead to a reduction in signal. Finally, MRI technology is predominately used in multimodality neuroimaging of cell transplantation by combining both structural and functional readouts for the improved refinement of clinical diagnostics. To this end, it could be coupled with the high sensitivity but low-resolution bioluminescence imaging (Tennstaedt et al., 2013), an economical and non-invasive technique using enzymatic chemiluminescence that allows full temporal live tracking of viable transplanted grafts.
Single-photon emission computed tomography (SPECT) is a type of nuclear imaging technique that utilizes specific gamma-emitting isotopes (compounds derived from cocaine that bind to the dopamine transporter) to analyze the integrity of the nigrostriatal DA pathway in PD (Son et al., 2016). SPECT biomarkers allow for the detection of presynaptic neuronal degeneration (Marshall and Grosset, 2003) and D2-type post-synaptic receptor density (Thobois et al., 2001). The clinical utility of such metabolic and neurochemical changes in PD is reviewed by Wang et al. (2012). To improve the diagnostic accuracy of SPECT imaging, current studies have employed the combined evaluation of both pre- and post-synaptic measurements through striatal dopamine transporters (DAT) and dopamine D2 receptor analysis, respectively (Koch et al., 2007). Further refinements must be made for SPECT imaging modality to be able to differentiate diseases with impairments in presynaptic DA neuronal survival such as PD, progressive supranuclear palsy, multiple system atrophy, and others (Bajaj et al., 2013). Also, potential leakage of radiotracers into adjacent cells resulting in diluted signals during cell proliferation has to be rectified. More importantly, the optimal concentration of tracers must be determined to avoid tissue damage due to exposure to toxic radioactive reagents. One disadvantage of this technique is its inability to examine cell survival and function.
Positron emission tomography (PET) is also a common imaging tool that employs specific radionuclides to elucidate the functional consequences of transplantation on the DA system in the brain, such as receptor distribution, metabolic activity, and inflammation (Visnyei et al., 2006). The measurement of aromatic L-amino acid decarboxylase activity using [18F]FDOPA is regarded as the gold standard to examine DA function and disease severity in Parkinson’s disease (Morrish et al., 1996; Punal-Rioboo et al., 2009), also shown in PD non-human primate model (Muramatsu et al., 2009; Emborg et al., 2013; Hallett et al., 2015) and clinical reports (Lindvall et al., 1990; Peschanski et al., 1994; Piccini et al., 2000, 2005; Ma et al., 2010) (refer to citation in Table 2). PET images can also be used in conjunction with SPECT data to further evaluate the negative association between striatal DAT and motor severity (Shih et al., 2006; Wu et al., 2014). Interestingly, recent clinical studies have shown that [11C]PE2I has higher predictive value and sensitivity toward the differential detection of motor impairments than [18F]DOPA imaging; hence, [11C]PE2I could be a prospective biomarker to investigate novel interventions (Fazio et al., 2015; Li et al., 2018). PET would be advantageous for studying the early maturation of cells transplanted in vivo and for follow-up examinations months after cell transplantation. A comprehensive and concise review on the development of functional neuroimaging is discussed in the cited works (Zheng et al., 2017; Helmich et al., 2018).
With no doubt, one of the most understudied limitations in neuroimaging is in deciphering the complexity of neuropathological overlap and clinical heterogeneity in the progression of individual neurological diseases. Improvements in bioimaging tools, such as the identification of specialized biomarkers for specific cell types to evaluate differential functional signatures, are important to circumvent the high level of variation in the prognosis of PD and its management by patients. In addition, the paucity of imaging modalities available for quantitation and of their respective analytical tools continues to hinder the further development of cell-based therapeutics toward clinically competitive treatments for PD. As discussed above (also refer to Table 2), we cannot rely on a single imaging technique for clinical diagnosis especially post-transplantation; thus, researchers are actively searching for the development of multimodality imaging (Waerzeggers et al., 2008) along with the identification of novel biomarkers and tracers to escalate the accuracy of post-operative care. A better understanding of neuroanatomical and pathophysiological processes would be highly advantageous for cell-derived therapeutics.
Clinical Trials for Stem Cell-Derived DA Neuron Transplantation in Parkinson’s Disease
Historically, fVM cell transplantation showed varied outcomes in human clinical trials (Table 3) (Freed et al., 2001; Olanow et al., 2001, Olanow et al., 2003, Redmond et al., 2001; Barker et al., 2013). A double-blind study of bilateral injection of fVM transplantation and sham surgery into the putamen was first performed in 19 PD patients by Freed and colleagues in 2001 (Freed et al., 2001). Interestingly, only younger age groups showed clinical improvements compared to the sham control (Freed et al., 2001). Using available data extracted from individual clinical papers cited in Table 3, we have performed systematic statistical analysis of the clinical outcomes of PD patients with fVM transplantation against various parameters, namely, age of onset (old, > 40 years vs. young, ≤ 40 years), disease stage (severe vs. mild), and disease duration (long, > 10 years vs. short, ≤ 10 years). The fold change of PET readings post-transplantation from the baseline reading of individual patients was used to access graft survival. We show that graft survival is independent of the age of disease onset (Figure 1A) but is dependent on variations in disease stage (Figure 1B) and the length of disease duration (Figure 1C), where better graft survival was observed in mild stage PD and patients with shorter disease duration (≤ 10 years). Moreover, we used the Unified Parkinson Disease Rating Scale (UPDRS) motor scores to examine clinical improvements post-transplantation of PD patients in various factors (Figure 2). We have demonstrated that in all three parameters (as mentioned above), PD patients with fVM transplantation have shown significant clinical improvements (correlated to the decrease in UPDRS motor scores) post-transplantation. Also, comparison between post-transplantation within each parameter (i.e., old vs. young or severe vs. mild or long vs. short) showed no significant differences. In summary, although clinical improvements can be observed throughout the wide spectrum of PD patients with fVM transplantation (Figure 2), the optimal condition with the most potential could be seen in mild stage PD patients with short disease duration (Figure 1).
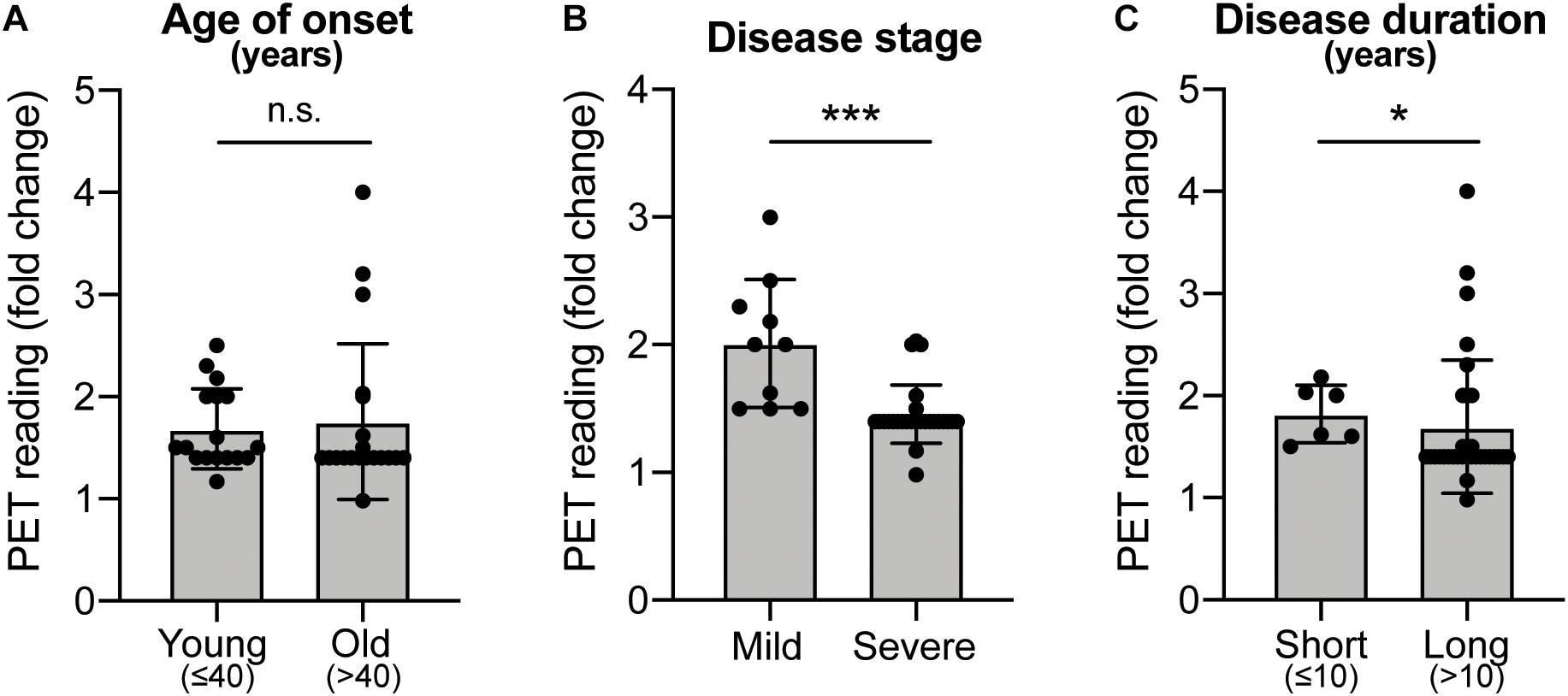
Figure 1. Systematic analysis of various factors associated with clinical outcomes using positron emission tomography (PET) readings of Parkinson’s disease (PD) patients with fetal ventral mesencephalic (fVM) cell transplantation. Statistical comparison was performed on various parameters against fold change of PET readings pre- and post-transplantation. (A) Age on onset: old (> 40 years) vs. young (≤ 40 years) PD patients. (B) Disease stage in mild and severe conditions. (C) Disease duration: long (> 10 years) vs. short (≤ 10 years). Student t-test, *p < 0.05, ***p < 0.001.
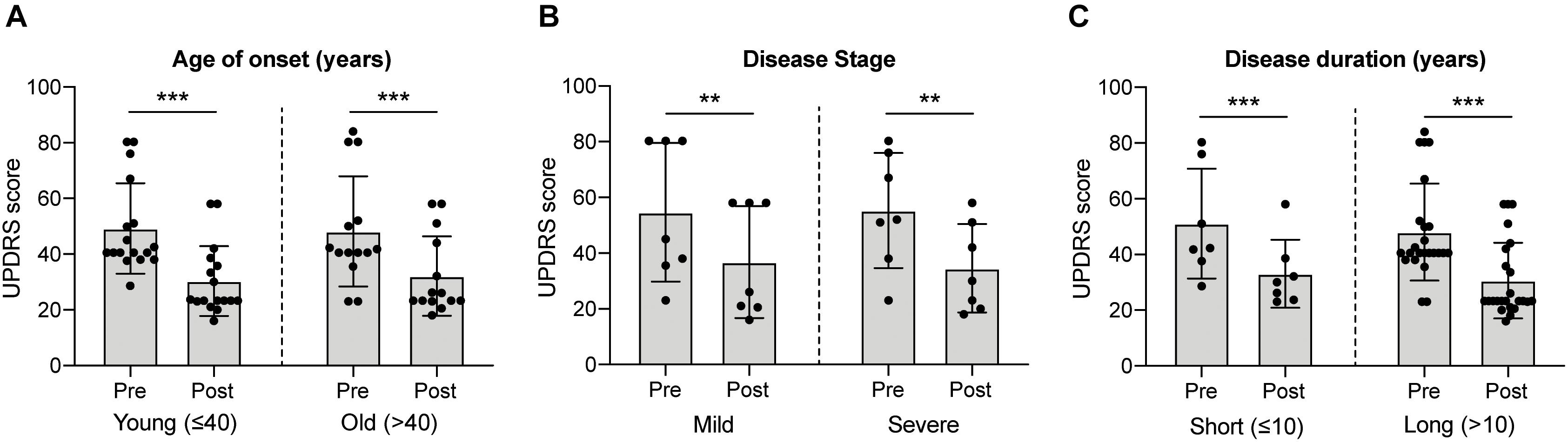
Figure 2. Systematic analysis of various factors associated with clinical outcome in fVM cell transplantation in PD patients using UPDRS motor scores. Statistical comparison was performed on varying parameters against the Unified Parkinson Disease Rating Scale (UPDRS) motor scores of pre- and post-transplantation. (A) Age on onset: old (> 40 years) vs. young (≤ 40 years) PD patients. (B) Disease stage in severe vs. mild condition. (C) Disease duration: long (> 10 years) vs. short (≤ 10 years). Two-way ANOVA, Sidak’s multiple comparisons test, **p < 0.005, ***p < 0.001.
In line with our data, the high prevalence of long-term graft survival with low to no immune response in the majority of fVM recipients could be represented for future/ongoing stem cell-based clinical trials as a basis for host tissue innervation and reconnection to host DA circuitry. It is to note that occasional appearance of graft-induced dyskinesia cannot be attributed to cell transplantation as of date, as there are very limited follow-up studies. Upcoming clinical studies must include detailed surgical procedures, characterization of PD hallmarks such as α-synuclein-positive Lewy bodies, ubiquitin expression, and imaging analysis for F-DOPA uptake in graft region in addition to clinical observations. It is believed that the differences in quality and heterogeneity in the transplanted cells, patient selection, and surgical methodologies could have been the reason for failures in some trials. The current status of the TRANSEURO trial (NCT01898390), a large collaboration between the European Union multicenters of fetal nigral cell transplantation, which started in 2012, has grafted 11 young patients with early-stage PD in Cambridge, 2019, and will be subjected to clinical observations for 36 months post-surgery, which is estimated to be completed in early 2021.
With the improvement in the human DA neuron differentiation protocol (Nolbrant et al., 2017), more authentic midbrain DA neurons can now be derived from ESCs or iPSCs in vitro. These more defined ESC/iPSC-derived DA neurons show satisfactory therapeutic effectiveness in PD animal models (Studer, 2017), which has led to new waves of initiatives for cell transplantation in PD patients. Furthermore, with ES-derived DA neuronal transplantation being equipotent (Grealish et al., 2014) to that of the current gold standard for PD cell therapy (Li et al., 2016), stem cells rather than fetal neurons hold high expectation in the near future. However, we must bear in mind that animal models cannot fully reproduce human PD. Confounders, including aging, disease duration, disease severity, diabetes, and depression, should be taken into account when cell therapy is translated from preclinical models to clinical trials (Aarsland et al., 2011; Athauda et al., 2017; Henchcliffe and Parmar, 2018). Currently, ongoing clinical trials of the GForce-PD Consortium include European-based STEM-PD trial, NYSTEM trial, CiRA trial;2 Cyto Therapeutics Pty Limited founded trial (NCT02452723), and the Chinese Academy of Sciences founded trial (NCT03119636) lead by Qi Zhou. STEM-PD trial was designed to use GMP-grade hESCs as the clinical cell source, employing full GMP-grade production procedure (Kirkeby et al., 2017b), and transplanting 100,000 TH+ D16 mDA progenitors per graft as a target dose. In contrast, CiRA was designed to develop clinical-grade DA cell therapy from autologous iPSCs taken from PD patients (Barker et al., 2017). More recently, iPSC-derived dopamine progenitor cells have been bilaterally injected into a 69 year old PD patient and have demonstrated signs of improvements in motor assessment 24 months post-surgery (Schweitzer et al., 2020). It is interesting to note that clinical improvements were significantly associated with the right (second) surgical procedure than the left. One explanation would be the improved procedural efficiency including shorter time taken from cell harvest to implantation. Further double-blind studies will be essential to better understand the full potential of iPSC-derived dopamine cells in PD.
Notably, neural progenitor stem cells (NPCs) are an alternative cell source for cell replacement strategy. These multipotent cells can self-renew and differentiate into all mature neural cells in the CNS in large quantities (Ribeiro et al., 2013). The first autologous differentiated neural stem cell clinical trial was conducted using tissue samples collected in the prefrontal cortical and subcortical region along the trajectory of the electrode implant prior to further expansion and differentiation. The patient showed clinical improvements during the first 3 years post-transplantation with subsequent decline back to baseline by the fifth year (Levesque et al., 2009). Ongoing clinical trials sponsored by NeuroGenerations involves 12–20 PD patients at the age of 35–85 years (Hoehn and Yahr stage III or IV) with an estimated completion date by 2021 (NCT03309514, NCT01329926).
Another factor we need to consider for PD cell transplantation clinical trials is the patient stratification. Since aging-induced BBB leakage can lead to infection and inflammation, age can greatly compromise the survival of DA neurons. Moreover, cell transplantation is usually less effective in patients with a longer and more severe disease progression (Barker et al., 2017). Discrepancies were also found in some patients with substantial survival of grafted DA neurons but no beneficial behavioral improvements (Barker et al., 2013, 2015a, 2017; Barker, 2014). These observations possibly indicate the degeneration of other brain systems, especially the post-synaptic component of the DA system. Thus, DA neuron transplantation clinical trials initiated by various organizations only include patients who 1) are younger than 65 years old, 2) have a disease duration of less than 10 years, and 3) are in the early stage of the disease (Kirkeby et al., 2017b; Studer, 2017; Takahashi, 2017). Moving forward, the identification of these confounders would be helpful for clinicians to be able to better stratify PD patients and suggest the most suitable treatment strategy for each patient.
Conclusion and Future Directions
We have highlighted four different types of cell sources and have addressed their pros and cons to better understand the characteristics of individual cell types and have also provided detailed analysis of the discrepancies observed in clinical outcomes of PD patients. This also includes methodologies in cell type specification and various imaging modalities. The emphasis on cell line availability, quality, and ability to innervate into host tissues, develop into functional A9 DA neurons, which would efficiently repair the host DA system is of topmost importance. Furthermore, long-term survivability for years after surgery without graft-induced dyskinesia or immune rejection by the host are some safety requirements and is the key to successful translation into large-scale therapeutic application and also in biomedical research to better recapitulate in vitro disease models. To avoid further inconsistencies in clinical results, we need to ensure standardization across several aspects of transplantations, including tissue preparation for engrafts, surgical technique, patient selection, immune therapy, synaptic integration capacity of cells transplanted into the human brain, and ways to bypass ethical issues revolving around cell transplantation in humans and the usage of ESCs (more details can be found in Barker et al., 2015b). The need to develop minimal, but precise, surgical techniques and achieving a better understanding of striatal functions are necessary, and these improvements could be accomplished by acquiring higher-resolution diagnostic imaging with heightened specificity for targeted graft placement and post-op observational study. Clinical endpoint observations from aforementioned ongoing clinical trials would pave a way to develop a coherent and systematic blueprint for therapeutic strategies such as improved surgical methodologies, optimized and standardized protocols, development of appropriate safeguards, and objective long-term outcome measures. The field of stem cell-based therapies for PD has entered into an exciting era, and we believe there is greater optimism that neurotransplantation may provide a viable option for treatment of PD in the future.
Author Contributions
SEJ, LQ, and LZ have drafted the manuscript with inputs from LLC and E-KT. All authors contributed to the article and approved the submitted version.
Funding
This research was supported by the Open Fund-Large Collaborative Grant, SPARK II Program, STaR Award, and CSA awards.
Conflict of Interest
The authors declare that the research was conducted in the absence of any commercial or financial relationships that could be construed as a potential conflict of interest.
Footnotes
- ^ https://www.mskcc.org
- ^ https://upload.umin.ac.jp/cgi-open-bin/ctr_e/ctr_view.cgi?recptno=R000038278
References
Aarsland, D., Pahlhagen, S., Ballard, C. G., Ehrt, U., and Svenningsson, P. (2011). Depression in Parkinson disease–epidemiology, mechanisms and management. Nat. Rev. Neurol. 8, 35–47. doi: 10.1038/nrneurol.2011.189
Alamri, A., Ughratdar, I., Samuel, M., and Ashkan, K. (2015). Deep brain stimulation of the subthalamic nucleus in Parkinson’s disease 2003-2013: where are we another 10 years on? Br. J. Neurosurg. 29, 319–328. doi: 10.3109/02688697.2014.997669
Alavian, K. N., Scholz, C., and Simon, H. H. (2008). Transcriptional regulation of mesencephalic dopaminergic neurons: the full circle of life and death. Mov. Disord. 23, 319–328. doi: 10.1002/mds.21640
Alberi, L., Sgado, P., and Simon, H. H. (2004). Engrailed genes are cell-autonomously required to prevent apoptosis in mesencephalic dopaminergic neurons. Development 131, 3229–3236. doi: 10.1242/dev.01128
Amsalem, Y., Mardor, Y., Feinberg, M. S., Landa, N., Miller, L., Daniels, D., et al. (2007). Iron-oxide labeling and outcome of transplanted mesenchymal stem cells in the infarcted myocardium. Circulation 116, I38–I45. doi: 10.1161/CIRCULATIONAHA.106.680231
Anden, N. E., Dahlstrom, A., Fuxe, K., and Larsson, K. (1966). Functional role of the nigro-neostriatal dopamine neurons. Acta Pharmacol. Toxicol. 24, 263–274. doi: 10.1111/j.1600-0773.1966.tb00389.x
Anderson, S. A., Glod, J., Arbab, A. S., Noel, M., Ashari, P., Fine, H. A., et al. (2005). Noninvasive Mr imaging of magnetically labeled stem cells to directly identify neovasculature in a glioma model. Blood 105, 420–425. doi: 10.1182/blood-2004-06-2222
Andersson, E., Jensen, J. B., Parmar, M., Guillemot, F., and Bjorklund, A. (2006). Development of the mesencephalic dopaminergic neuron system is compromised in the absence of neurogenin 2. Development 133, 507–516. doi: 10.1242/dev.02224
Arenas, E., Denham, M., and Villaescusa, J. C. (2015). How to make a midbrain dopaminergic neuron. Development 142, 1918–1936. doi: 10.1242/dev.097394
Athauda, D., Maclagan, K., Skene, S. S., Bajwa-Joseph, M., Letchford, D., Chowdhury, K., et al. (2017). Exenatide once weekly versus placebo in Parkinson’s disease: a randomised, double-blind, placebo-controlled trial. Lancet 390, 1664–1675. doi: 10.1016/S0140-6736(17)31585-4
Bajaj, N., Hauser, R. A., and Grachev, I. D. (2013). Clinical utility of dopamine transporter single photon emission Ct (DaT-Spect) with (123I) ioflupane in diagnosis of parkinsonian syndromes. J. Neurol. Neurosurg. Psychiatry 84, 1288–1295. doi: 10.1136/jnnp-2012-304436
Bakay, R. A., Barrow, D. L., Fiandaca, M. S., Iuvone, P. M., Schiff, A., and Collins, D. C. (1987). Biochemical and behavioral correction of Mptp Parkinson-like syndrome by fetal cell transplantation. Ann. N. Y. Acad. Sci. 495, 623–640. doi: 10.1111/j.1749-6632.1987.tb23705.x
Barker, R. A. (2014). Developing stem cell therapies for Parkinson’s disease: waiting until the time is right. Cell Stem Cell 15, 539–542. doi: 10.1016/j.stem.2014.09.016
Barker, R. A., Barrett, J., Mason, S. L., and Bjorklund, A. (2013). Fetal dopaminergic transplantation trials and the future of neural grafting in Parkinson’s disease. Lancet Neurol. 12, 84–91. doi: 10.1016/s1474-4422(12)70295-8
Barker, R. A., and Consortium, T. (2019). Designing stem-cell-based dopamine cell replacement trials for Parkinson’s disease. Nat. Med. 25, 1045–1053. doi: 10.1038/s41591-019-0507-2
Barker, R. A., Drouin-Ouellet, J., and Parmar, M. (2015a). Cell-based therapies for Parkinson disease-past insights and future potential. Nat. Rev. Neurol. 11, 492–503. doi: 10.1038/nrneurol.2015.123
Barker, R. A., Parmar, M., Studer, L., and Takahashi, J. (2017). Human trials of stem cell-derived dopamine neurons for parkinson’s disease: dawn of a new era. Cell Stem Cell 21, 569–573. doi: 10.1016/j.stem.2017.09.014
Barker, R. A., Studer, L., Cattaneo, E., Takahashi, J., and Consortium, G. F. P. (2015b). G-Force Pd: a global initiative in coordinating stem cell-based dopamine treatments for Parkinson’s disease. NPJ Parkinsons Dis. 1:15017. doi: 10.1038/npjparkd.2015.17
Barrow, M., Taylor, A., Murray, P., Rosseinsky, M. J., and Adams, D. J. (2015). Design considerations for the synthesis of polymer coated iron oxide nanoparticles for stem cell labelling and tracking using Mri. Chem. Soc. Rev. 44, 6733–6748. doi: 10.1039/c5cs00331h
Beevers, J. E., Caffrey, T. M., and Wade-Martins, R. (2013). Induced pluripotent stem cell (ipsc)-derived dopaminergic models of Parkinson’s disease. Biochem. Soc. Trans. 41, 1503–1508. doi: 10.1042/bst20130194
Ben-Hur, T., Idelson, M., Khaner, H., Pera, M., Reinhartz, E., Itzik, A., et al. (2004). Transplantation of human embryonic stem cell-derived neural progenitors improves behavioral deficit in Parkinsonian rats. Stem Cells 22, 1246–1255. doi: 10.1634/stemcells.2004-0094
Biju, K. C., Santacruz, R. A., Chen, C., Zhou, Q., Yao, J., Rohrabaugh, S. L., et al. (2013). Bone marrow-derived microglia-based neurturin delivery protects against dopaminergic neurodegeneration in a mouse model of Parkinson’s disease. Neurosci. Lett. 535, 24–29. doi: 10.1016/j.neulet.2012.12.034
Bjorklund, A., and Stenevi, U. (1979). Reconstruction of the nigrostriatal dopamine pathway by intracerebral nigral transplants. Brain Res. 177, 555–560. doi: 10.1016/0006-8993(79)90472-4
Brederlau, A., Correia, A. S., Anisimov, S. V., Elmi, M., Paul, G., Roybon, L., et al. (2006). Transplantation of human embryonic stem cell-derived cells to a rat model of Parkinson’s disease: effect of in vitro differentiation on graft survival and teratoma formation. Stem Cells 24, 1433–1440. doi: 10.1634/stemcells.2005-0393
Brundin, P., Nilsson, O. G., Strecker, R. E., Lindvall, O., Astedt, B., and Bjorklund, A. (1986). Behavioural effects of human fetal dopamine neurons grafted in a rat model of Parkinson’s disease. Exp. Brain Res. 65, 235–240. doi: 10.1007/BF00243848
Brundin, P., Pogarell, O., Hagell, P., Piccini, P., Widner, H., Schrag, A., et al. (2000). Bilateral caudate and putamen grafts of embryonic mesencephalic tissue treated with lazaroids in Parkinson’s disease. Brain 123(Pt 7), 1380–1390. doi: 10.1093/brain/123.7.1380
Burrows, C. K., Banovich, N. E., Pavlovic, B. J., Patterson, K., Gallego Romero, I., Pritchard, J. K., et al. (2016). Genetic variation, not cell type of origin, underlies the majority of identifiable regulatory differences in ipscs. PLoS Genet. 12:e1005793. doi: 10.1371/journal.pgen.1005793
Carcamo-Orive, I., Hoffman, G. E., Cundiff, P., Beckmann, N. D., D’souza, S. L., Knowles, J. W., et al. (2017). Analysis of transcriptional variability in a large human ipsc library reveals genetic and non-genetic determinants of heterogeneity. Cell Stem Cell 20, 518.e9–532.e9. doi: 10.1016/j.stem.2016.11.005
Cardoso, T., Adler, A. F., Mattsson, B., Hoban, D. B., Nolbrant, S., Wahlestedt, J. N., et al. (2018). Target-specific forebrain projections and appropriate synaptic inputs of hesc-derived dopamine neurons grafted to the midbrain of parkinsonian rats. J. Comp. Neurol. 526, 2133–2146. doi: 10.1002/cne.24500
Carlsson, T., Carta, M., Winkler, C., Bjorklund, A., and Kirik, D. (2007). Serotonin neuron transplants exacerbate L-Dopa-induced dyskinesias in a rat model of Parkinson’s disease. J. Neurosci. 27, 8011–8022. doi: 10.1523/jneurosci.2079-07.2007
Cho, M. S., Lee, Y. E., Kim, J. Y., Chung, S., Cho, Y. H., Kim, D. S., et al. (2008). Highly efficient and large-scale generation of functional dopamine neurons from human embryonic stem cells. Proc. Natl. Acad. Sci. U.S.A. 105, 3392–3397. doi: 10.1073/pnas.0712359105
Chung, S., Leung, A., Han, B. S., Chang, M. Y., Moon, J. I., Kim, C. H., et al. (2009). Wnt1-lmx1a forms a novel autoregulatory loop and controls midbrain dopaminergic differentiation synergistically with the Shh-FoxA2 pathway. Cell Stem Cell 5, 646–658. doi: 10.1016/j.stem.2009.09.015
Chung, S., Moon, J. I., Leung, A., Aldrich, D., Lukianov, S., Kitayama, Y., et al. (2011). Es cell-derived renewable and functional midbrain dopaminergic progenitors. Proc. Natl. Acad. Sci. U.S.A. 108, 9703–9708. doi: 10.1073/pnas.1016443108
Cooper, O., Hargus, G., Deleidi, M., Blak, A., Osborn, T., Marlow, E., et al. (2010). Differentiation of human Es and Parkinson’s disease ips cells into ventral midbrain dopaminergic neurons requires a high activity form of Shh, Fgf8a and specific regionalization by retinoic acid. Mol. Cell. Neurosci. 45, 258–266. doi: 10.1016/j.mcn.2010.06.017
Cupaioli, F. A., Zucca, F. A., Boraschi, D., and Zecca, L. (2014). Engineered nanoparticles. How brain friendly is this new guest? Prog. Neurobiol. 119-120, 20–38. doi: 10.1016/j.pneurobio.2014.05.002
Daadi, M. M., and Weiss, S. (1999). Generation of tyrosine hydroxylase-producing neurons from precursors of the embryonic and adult forebrain. J. Neurosci. 19, 4484–4497. doi: 10.1523/jneurosci.19-11-04484.1999
Dahlstroem, A., and Fuxe, K. (1964). Evidence for the existence of monoamine-containing neurons in the central nervous system. I. demonstration of monoamines in the cell bodies of brain stem neurons. Acta Physiol. Scand. Suppl. Suppl. 232, 1–55.
Damier, P., Hirsch, E. C., Agid, Y., and Graybiel, A. M. (1999). The substantia nigra of the human brain. Ii. Patterns of loss of dopamine-containing neurons in Parkinson’s disease. Brain 122(Pt 8), 1437–1448. doi: 10.1093/brain/122.8.1437
deSouza, R. M., Moro, E., Lang, A. E., and Schapira, A. H. (2013). Timing of deep brain stimulation in Parkinson disease: a need for reappraisal? Ann. Neurol. 73, 565–575. doi: 10.1002/ana.23890
Di Porzio, U., Zuddas, A., Cosenza-Murphy, D. B., and Barker, J. L. (1990). Early appearance of tyrosine hydroxylase immunoreactive cells in the mesencephalon of mouse embryos. Int. J. Dev. Neurosci. 8, 523–532. doi: 10.1016/0736-5748(90)90044-3
Doi, D., Morizane, A., Kikuchi, T., Onoe, H., Hayashi, T., Kawasaki, T., et al. (2012). Prolonged maturation culture favors a reduction in the tumorigenicity and the dopaminergic function of human Esc-derived neural cells in a primate model of Parkinson’s disease. Stem Cells 30, 935–945. doi: 10.1002/stem.1060
Doi, D., Samata, B., Katsukawa, M., Kikuchi, T., Morizane, A., Ono, Y., et al. (2014). Isolation of human induced pluripotent stem cell-derived dopaminergic progenitors by cell sorting for successful transplantation. Stem Cell Rep. 2, 337–350. doi: 10.1016/j.stemcr.2014.01.013
Dunnett, S. B., Bjorklund, A., Schmidt, R. H., Stenevi, U., and Iversen, S. D. (1983). Intracerebral grafting of neuronal cell suspensions. V. Behavioural recovery in rats with bilateral 6-Ohda lesions following implantation of nigral cell suspensions. Acta Physiol. Scand. Suppl. 522, 39–47.
Eigentler, A., Boesch, S., Schneider, R., Dechant, G., and Nat, R. (2013). Induced pluripotent stem cells from friedreich ataxia patients fail to upregulate frataxin during in vitro differentiation to peripheral sensory neurons. Stem Cells Dev. 22, 3271–3282. doi: 10.1089/scd.2013.0126
Elkabetz, Y., Panagiotakos, G., Al Shamy, G., Socci, N. D., Tabar, V., and Studer, L. (2008). Human Es cell-derived neural rosettes reveal a functionally distinct early neural stem cell stage. Genes Dev. 22, 152–165. doi: 10.1101/gad.1616208
Emborg, M. E., Liu, Y., Xi, J., Zhang, X., Yin, Y., Lu, J., et al. (2013). Induced pluripotent stem cell-derived neural cells survive and mature in the nonhuman primate brain. Cell Rep. 3, 646–650. doi: 10.1016/j.celrep.2013.02.016
Evans, M. J., and Kaufman, M. H. (1981). Establishment in culture of pluripotential cells from mouse embryos. Nature 292, 154–156. doi: 10.1038/292154a0
Fahn, S. (2003). Description of Parkinson’s disease as a clinical syndrome. Ann. N. Y. Acad. Sci. 991, 1–14. doi: 10.1016/b978-0-12-374028-1.00001-4
Fahn, S. (2006). Levodopa in the treatment of Parkinson’s disease. J. Neural Transm. Suppl. 71, 1–15. doi: 10.2147/ce.s7031
Fairchild, P. J. (2010). The challenge of immunogenicity in the quest for induced pluripotency. Nat. Rev. Immunol. 10, 868–875. doi: 10.1038/nri2878
Fan, Y., Winanto, and Ng, S. Y. (2020). Replacing what’s lost: a new era of stem cell therapy for Parkinson’s disease. Transl. Neurodegener. 9:2. doi: 10.1186/s40035-019-0180-x
Fasano, C. A., Chambers, S. M., Lee, G., Tomishima, M. J., and Studer, L. (2010). Efficient derivation of functional floor plate tissue from human embryonic stem cells. Cell Stem Cell 6, 336–347. doi: 10.1016/j.stem.2010.03.001
Fazio, P., Svenningsson, P., Forsberg, A., Jonsson, E. G., Amini, N., Nakao, R., et al. (2015). Quantitative analysis of (1)(8)F-(E)-N-(3-Iodoprop-2-Enyl)-2beta-carbofluoroethoxy-3beta-(4’-Methyl-Phenyl) nortropane binding to the dopamine transporter in parkinson disease. J. Nucl. Med. 56, 714–720. doi: 10.2967/jnumed.114.152421
Freed, C. R., Breeze, R. E., Rosenberg, N. L., Schneck, S. A., Wells, T. H., Barrett, J. N., et al. (1990). Transplantation of human fetal dopamine cells for Parkinson’s disease. Results at 1 year. Arch. Neurol. 47, 505–512. doi: 10.1001/archneur.1990.00530050021007
Freed, C. R., Greene, P. E., Breeze, R. E., Tsai, W. Y., Dumouchel, W., Kao, R., et al. (2001). Transplantation of embryonic dopamine neurons for severe Parkinson’s disease. N. Engl. J. Med. 344, 710–719. doi: 10.1056/nejm200103083441002
Freed, W. J., Morihisa, J. M., Spoor, E., Hoffer, B. J., Olson, L., Seiger, A., et al. (1981). Transplanted adrenal chromaffin cells in rat brain reduce lesion-induced rotational behaviour. Nature 292, 351–352. doi: 10.1038/292351a0
Freeman, T. B., Olanow, C. W., Hauser, R. A., Nauert, G. M., Smith, D. A., Borlongan, C. V., et al. (1995). Bilateral fetal nigral transplantation into the postcommissural putamen in Parkinson’s disease. Ann. Neurol. 38, 379–388. doi: 10.1002/ana.410380307
Fukuda, H., Takahashi, J., Watanabe, K., Hayashi, H., Morizane, A., Koyanagi, M., et al. (2006). Fluorescence-activated cell sorting-based purification of embryonic stem cell-derived neural precursors averts tumor formation after transplantation. Stem Cells 24, 763–771. doi: 10.1634/stemcells.2005-0137
Ganat, Y. M., Calder, E. L., Kriks, S., Nelander, J., Tu, E. Y., Jia, F., et al. (2012). Identification of embryonic stem cell-derived midbrain dopaminergic neurons for engraftment. J. Clin. Invest. 122, 2928–2939. doi: 10.1172/jci58767
German, D. C., Manaye, K., Smith, W. K., Woodward, D. J., and Saper, C. B. (1989). Midbrain dopaminergic cell loss in Parkinson’s disease: computer visualization. Ann. Neurol. 26, 507–514. doi: 10.1002/ana.410260403
German, D. C., Manaye, K. F., Sonsalla, P. K., and Brooks, B. A. (1992). Midbrain dopaminergic cell loss in Parkinson’s disease and Mptp-induced parkinsonism: sparing of calbindin-D28k-containing cells. Ann. N. Y. Acad. Sci. 648, 42–62. doi: 10.1111/j.1749-6632.1992.tb24523.x
Gibb, W. R. (1992). Melanin, tyrosine hydroxylase, calbindin and substance P in the human midbrain and substantia nigra in relation to nigrostriatal projections and differential neuronal susceptibility in Parkinson’s disease. Brain Res. 581, 283–291. doi: 10.1016/0006-8993(92)90719-p
Goedert, M., Spillantini, M. G., Del Tredici, K., and Braak, H. (2013). 100 years of Lewy pathology. Nat. Rev. Neurol. 9, 13–24. doi: 10.1038/nrneurol.2012.242
Goggi, J. L., Qiu, L., Liao, M. C., Khanapur, S., Jiang, L., Boominathan, R., et al. (2020). Dopamine transporter neuroimaging accurately assesses the maturation of dopamine neurons in a preclinical model of Parkinson’s disease. Stem Cell Res. Ther. 11:347. doi: 10.1186/s13287-020-01868-4
Grealish, S., Diguet, E., Kirkeby, A., Mattsson, B., Heuer, A., Bramoulle, Y., et al. (2014). Human Esc-derived dopamine neurons show similar preclinical efficacy and potency to fetal neurons when grafted in a rat model of Parkinson’s disease. Cell Stem Cell 15, 653–665. doi: 10.1016/j.stem.2014.09.017
Grealish, S., Jonsson, M. E., Li, M., Kirik, D., Bjorklund, A., and Thompson, L. H. (2010). The A9 dopamine neuron component in grafts of ventral mesencephalon is an important determinant for recovery of motor function in a rat model of Parkinson’s disease. Brain 133, 482–495. doi: 10.1093/brain/awp328
Grenhoff, J., Ugedo, L., and Svensson, T. H. (1988). Firing patterns of midbrain dopamine neurons: differences between A9 and A10 cells. Acta Physiol. Scand. 134, 127–132. doi: 10.1111/j.1748-1716.1988.tb08468.x
Grow, D. A., Simmons, D. V., Gomez, J. A., Wanat, M. J., Mccarrey, J. R., Paladini, C. A., et al. (2016). Differentiation and characterization of dopaminergic neurons from baboon induced pluripotent stem cells. Stem Cells Transl. Med. 5, 1133–1144. doi: 10.5966/sctm.2015-0073
Hagell, P., Piccini, P., Bjorklund, A., Brundin, P., Rehncrona, S., Widner, H., et al. (2002). Dyskinesias following neural transplantation in Parkinson’s disease. Nat. Neurosci. 5, 627–628. doi: 10.1038/nn863
Hallett, P. J., Deleidi, M., Astradsson, A., Smith, G. A., Cooper, O., Osborn, T. M., et al. (2015). Successful function of autologous ipsc-derived dopamine neurons following transplantation in a non-human primate model of Parkinson’s disease. Cell Stem Cell 16, 269–274. doi: 10.1016/j.stem.2015.01.018
Halliday, G. M., Ophof, A., Broe, M., Jensen, P. H., Kettle, E., Fedorow, H., et al. (2005). Alpha-synuclein redistributes to neuromelanin lipid in the substantia nigra early in Parkinson’s disease. Brain 128, 2654–2664. doi: 10.1093/brain/awh584
Hargus, G., Cooper, O., Deleidi, M., Levy, A., Lee, K., Marlow, E., et al. (2010). Differentiated Parkinson patient-derived induced pluripotent stem cells grow in the adult rodent brain and reduce motor asymmetry in Parkinsonian rats. Proc. Natl. Acad. Sci. U.S.A. 107, 15921–15926. doi: 10.1073/pnas.1010209107
Hawrylak, N., Ghosh, P., Broadus, J., Schlueter, C., Greenough, W. T., and Lauterbur, P. C. (1993). Nuclear magnetic resonance (Nmr) imaging of iron oxide-labeled neural transplants. Exp. Neurol. 121, 181–192. doi: 10.1006/exnr.1993.1085
Helmich, R. C., Vaillancourt, D. E., and Brooks, D. J. (2018). The future of brain imaging in Parkinson’s disease. J. Parkinsons Dis. 8, S47–S51. doi: 10.3233/JPD-181482
Henchcliffe, C., and Parmar, M. (2018). Repairing the brain: cell replacement using stem cell-based technologies. J. Parkinsons Dis. 8, S131–S137. doi: 10.3233/JPD-181488
Hirsch, E., Graybiel, A. M., and Agid, Y. A. (1988). Melanized dopaminergic neurons are differentially susceptible to degeneration in Parkinson’s disease. Nature 334, 345–348. doi: 10.1038/334345a0
Hu, B. Y., Weick, J. P., Yu, J., Ma, L. X., Zhang, X. Q., Thomson, J. A., et al. (2010). Neural differentiation of human induced pluripotent stem cells follows developmental principles but with variable potency. Proc. Natl. Acad. Sci. U.S.A. 107, 4335–4340. doi: 10.1073/pnas.0910012107
Itskovitz-Eldor, J., Schuldiner, M., Karsenti, D., Eden, A., Yanuka, O., Amit, M., et al. (2000). Differentiation of human embryonic stem cells into embryoid bodies compromising the three embryonic germ layers. Mol. Med. 6, 88–95. doi: 10.1007/bf03401776
Iwanami, A., Kaneko, S., Nakamura, M., Kanemura, Y., Mori, H., Kobayashi, S., et al. (2005). Transplantation of human neural stem cells for spinal cord injury in primates. J. Neurosci. Res. 80, 182–190. doi: 10.1002/jnr.20436
Jaeger, I., Arber, C., Risner-Janiczek, J. R., Kuechler, J., Pritzsche, D., Chen, I. C., et al. (2011). Temporally controlled modulation of Fgf/Erk signaling directs midbrain dopaminergic neural progenitor fate in mouse and human pluripotent stem cells. Development 138, 4363–4374. doi: 10.1242/dev.066746
Jonsson, M. E., Ono, Y., Bjorklund, A., and Thompson, L. H. (2009). Identification of transplantable dopamine neuron precursors at different stages of midbrain neurogenesis. Exp. Neurol. 219, 341–354. doi: 10.1016/j.expneurol.2009.06.006
Kastner, A., Hirsch, E. C., Lejeune, O., Javoy-Agid, F., Rascol, O., and Agid, Y. (1992). Is the vulnerability of neurons in the substantia nigra of patients with Parkinson’s disease related to their neuromelanin content? J. Neurochem. 59, 1080–1089. doi: 10.1111/j.1471-4159.1992.tb08350.x
Kawasaki, H., Mizuseki, K., Nishikawa, S., Kaneko, S., Kuwana, Y., Nakanishi, S., et al. (2000). Induction of midbrain dopaminergic neurons from Es cells by stromal cell-derived inducing activity. Neuron 28, 31–40. doi: 10.1016/s0896-6273(00)00083-0
Kee, N., Volakakis, N., Kirkeby, A., Dahl, L., Storvall, H., Nolbrant, S., et al. (2017). Single-cell analysis reveals a close relationship between differentiating dopamine and subthalamic nucleus neuronal lineages. Cell Stem Cell 20, 29–40. doi: 10.1016/j.stem.2016.10.003
Kefalopoulou, Z., Politis, M., Piccini, P., Mencacci, N., Bhatia, K., Jahanshahi, M., et al. (2014). Long-term clinical outcome of fetal cell transplantation for Parkinson disease: two case reports. JAMA Neurol. 71, 83–87. doi: 10.1001/jamaneurol.2013.4749
Kikuchi, T., Morizane, A., Doi, D., Magotani, H., Onoe, H., Hayashi, T., et al. (2017). Human ips cell-derived dopaminergic neurons function in a primate Parkinson’s disease model. Nature 548, 592–596. doi: 10.1038/nature23664
Kim, J. H., Auerbach, J. M., Rodriguez-Gomez, J. A., Velasco, I., Gavin, D., Lumelsky, N., et al. (2002). Dopamine neurons derived from embryonic stem cells function in an animal model of Parkinson’s disease. Nature 418, 50–56. doi: 10.1038/nature00900
Kirkeby, A., Grealish, S., Wolf, D. A., Nelander, J., Wood, J., Lundblad, M., et al. (2012). Generation of regionally specified neural progenitors and functional neurons from human embryonic stem cells under defined conditions. Cell. Rep. 1, 703–714. doi: 10.1016/j.celrep.2012.04.009
Kirkeby, A., Nolbrant, S., Tiklova, K., Heuer, A., Kee, N., Cardoso, T., et al. (2017a). Predictive markers guide differentiation to improve graft outcome in clinical translation of hesc-based therapy for Parkinson’s disease. Cell Stem Cell 20, 135–148. doi: 10.1016/j.stem.2016.09.004
Kirkeby, A., Parmar, M., and Barker, R. A. (2017b). Strategies for bringing stem cell-derived dopamine neurons to the clinic: a european approach (Stem-Pd). Prog. Brain Res. 230, 165–190. doi: 10.1016/bs.pbr.2016.11.011
Koch, P., Opitz, T., Steinbeck, J. A., Ladewig, J., and Brustle, O. (2009). A rosette-type, self-renewing human Es cell-derived neural stem cell with potential for in vitro instruction and synaptic integration. Proc. Natl. Acad. Sci. U.S.A. 106, 3225–3230. doi: 10.1073/pnas.0808387106
Koch, W., Hamann, C., Radau, P. E., and Tatsch, K. (2007). Does combined imaging of the pre- and postsynaptic dopaminergic system increase the diagnostic accuracy in the differential diagnosis of parkinsonism? Eur. J. Nucl. Med. Mol. Imaging 34, 1265–1273. doi: 10.1007/s00259-007-0375-8
Kordower, J. H., Chu, Y., Hauser, R. A., Freeman, T. B., and Olanow, C. W. (2008). Lewy body-like pathology in long-term embryonic nigral transplants in Parkinson’s disease. Nat. Med. 14, 504–506. doi: 10.1038/nm1747
Kordower, J. H., Freeman, T. B., Snow, B. J., Vingerhoets, F. J., Mufson, E. J., Sanberg, P. R., et al. (1995). Neuropathological evidence of graft survival and striatal reinnervation after the transplantation of fetal mesencephalic tissue in a patient with Parkinson’s disease. N. Engl. J. Med. 332, 1118–1124. doi: 10.1056/nejm199504273321702
Kordower, J. H., Goetz, C. G., Chu, Y., Halliday, G. M., Nicholson, D. A., Musial, T. F., et al. (2017). Robust graft survival and normalized dopaminergic innervation do not obligate recovery in a Parkinson disease patient. Ann. Neurol. 81, 46–57. doi: 10.1002/ana.24820
Kriks, S., Shim, J. W., Piao, J., Ganat, Y. M., Wakeman, D. R., Xie, Z., et al. (2011). Dopamine neurons derived from human Es cells efficiently engraft in animal models of Parkinson’s disease. Nature 480, 547–551. doi: 10.1038/nature10648
Kurowska, Z., Englund, E., Windner, H., Lindvall, O., Li, J. Y., and Brundin, P. (2011). Signs of degeneration in 12-22 year old grafts of mesencephalic dopamine neurons in patients with Parkinson’s disease. J. Parkinsons Dis. 1, 83–92. doi: 10.3233/jpd-2011-11004
La Manno, G., Gyllborg, D., Codeluppi, S., Nishimura, K., Salto, C., Zeisel, A., et al. (2016). Molecular diversity of midbrain development in mouse, human, and stem cells. Cell 167, 566.e19–580.e19. doi: 10.1016/j.cell.2016.09.027
Lang, C., Campbell, K. R., Ryan, B. J., Carling, P., Attar, M., Vowles, J., et al. (2019). Single-cell sequencing of ipsc-dopamine neurons reconstructs disease progression and identifies Hdac4 as a regulator of parkinson cell phenotypes. Cell Stem Cell 24, 93.e6–106.e6. doi: 10.1016/j.stem.2018.10.023
Le, W., Conneely, O. M., He, Y., Jankovic, J., and Appel, S. H. (1999). Reduced Nurr1 expression increases the vulnerability of mesencephalic dopamine neurons to Mptp-induced injury. J. Neurochem. 73, 2218–2221. doi: 10.1046/j.1471-4159.1999.02218.x
Lee, S. H., Lumelsky, N., Studer, L., Auerbach, J. M., and Mckay, R. D. (2000). Efficient generation of midbrain and hindbrain neurons from mouse embryonic stem cells. Nat. Biotechnol. 18, 675–679. doi: 10.1038/76536
Lehnen, D., Barral, S., Cardoso, T., Grealish, S., Heuer, A., Smiyakin, A., et al. (2017). Iap-based cell sorting results in homogeneous transplantable dopaminergic precursor cells derived from human pluripotent stem cells. Stem Cell Rep. 9, 1207–1220. doi: 10.1016/j.stemcr.2017.08.016
Levesque, M. F., Neuman, T., and Rezak, M. (2009). Therapeutic microinjection of autologous adult human neural stem cells and differentiated neurons for Parkinson’s Disease: five year post-operative outcome. Open Stem Cell J. 1, 20–29. doi: 10.2174/1876893800901010020
Li, J. Y., Englund, E., Widner, H., Rehncrona, S., Bjorklund, A., Lindvall, O., et al. (2010). Characterization of Lewy body pathology in 12- and 16-year-old intrastriatal mesencephalic grafts surviving in a patient with Parkinson’s disease. Mov. Disord. 25, 1091–1096. doi: 10.1002/mds.23012
Li, W., Englund, E., Widner, H., Mattsson, B., Van Westen, D., Latt, J., et al. (2016). Extensive graft-derived dopaminergic innervation is maintained 24 years after transplantation in the degenerating parkinsonian brain. Proc. Natl. Acad. Sci. U.S.A. 113, 6544–6549. doi: 10.1073/pnas.1605245113
Li, W., Lao-Kaim, N. P., Roussakis, A. A., Martin-Bastida, A., Valle-Guzman, N., Paul, G., et al. (2018). (11) C-Pe2I and (18) F-dopa pet for assessing progression rate in Parkinson’s: a longitudinal study. Mov. Disord. 33, 117–127. doi: 10.1002/mds.27183
Limousin, P., Pollak, P., Benazzouz, A., Hoffmann, D., Le Bas, J. F., Broussolle, E., et al. (1995). Effect of parkinsonian signs and symptoms of bilateral subthalamic nucleus stimulation. Lancet 345, 91–95. doi: 10.1016/s0140-6736(95)90062-4
Lin, W., Metzakopian, E., Mavromatakis, Y. E., Gao, N., Balaskas, N., Sasaki, H., et al. (2009). Foxa1 and Foxa2 function both upstream of and cooperatively with Lmx1a and Lmx1b in a feedforward loop promoting mesodiencephalic dopaminergic neuron development. Dev. Biol. 333, 386–396. doi: 10.1016/j.ydbio.2009.07.006
Lindvall, O., and Bjorklund, A. (1974). The organization of the ascending catecholamine neuron systems in the rat brain as revealed by the glyoxylic acid fluorescence method. Acta Physiol. Scand. Suppl. 412, 1–48.
Lindvall, O., Brundin, P., Widner, H., Rehncrona, S., Gustavii, B., Frackowiak, R., et al. (1990). Grafts of fetal dopamine neurons survive and improve motor function in Parkinson’s disease. Science 247, 574–577. doi: 10.1126/science.2105529
Lindvall, O., Rehncrona, S., Brundin, P., Gustavii, B., Astedt, B., Windner, H., et al. (1989). Human fetal dopamine neurons grafted into the striatum in two patients with severe Parkinson’s disease. A detailed account of methodology and a 6-month follow-up. Arch. Neurol. 46, 615–631. doi: 10.1001/archneur.1989.00520420033021
Lindvall, O., Rehncrona, S., Gustavii, B., Brundin, P., Astedt, B., Widner, H., et al. (1988). Fetal dopamine-rich mesencephalic grafts in Parkinson’s disease. Lancet 2, 1483–1484. doi: 10.1016/s0140-6736(88)90950-6
Lindvall, O., Sawle, G., Widner, H., Rothwell, J. C., Bjorklund, A., Brooks, D., et al. (1994). Evidence for long-term survival and function of dopaminergic grafts in progressive Parkinson’s disease. Ann. Neurol. 35, 172–180. doi: 10.1002/ana.410350208
Liu, Q., Pedersen, O. Z., Peng, J., Couture, L. A., Rao, M. S., and Zeng, X. (2013). Optimizing dopaminergic differentiation of pluripotent stem cells for the manufacture of dopaminergic neurons for transplantation. Cytotherapy 15, 999–1010. doi: 10.1016/j.jcyt.2013.03.006
Liu, W., and Frank, J. A. (2009). Detection and quantification of magnetically labeled cells by cellular Mri. Eur. J. Radiol. 70, 258–264. doi: 10.1016/j.ejrad.2008.09.021
Ma, L., Hu, B., Liu, Y., Vermilyea, S. C., Liu, H., Gao, L., et al. (2012). Human embryonic stem cell-derived Gaba neurons correct locomotion deficits in quinolinic acid-lesioned mice. Cell Stem Cell 10, 455–464. doi: 10.1016/j.stem.2012.01.021
Ma, Y., Tang, C., Chaly, T., Greene, P., Breeze, R., Fahn, S., et al. (2010). Dopamine cell implantation in Parkinson’s disease: long-term clinical and (18)F-Fdopa Pet outcomes. J. Nucl. Med. 51, 7–15. doi: 10.2967/jnumed.109.066811
MacGeer, P. L., and McGeer, E. G. (2008). Glial reactions in Parkinson’s disease. Mov. Disord. 23, 474–483. doi: 10.1002/mds.21751
Madrazo, I., Leon, V., Torres, C., Aguilera, M. C., Varela, G., Alvarez, F., et al. (1988). Transplantation of fetal substantia nigra and adrenal medulla to the caudate nucleus in two patients with Parkinson’s disease. N. Engl. J. Med. 318:51. doi: 10.1056/nejm198801073180115
Malloy, K. E., Li, J., Choudhury, G. R., Torres, A., Gupta, S., Kantorak, C., et al. (2017). Magnetic resonance imaging-guided delivery of neural stem cells into the basal ganglia of nonhuman primates reveals a pulsatile mode of cell dispersion. Stem Cells Transl. Med. 6, 877–885. doi: 10.5966/sctm.2016-0269
Mann, D. M., and Yates, P. O. (1983). Possible role of neuromelanin in the pathogenesis of Parkinson’s disease. Mech. Ageing Dev. 21, 193–203. doi: 10.1016/0047-6374(83)90074-x
Marshall, V., and Grosset, D. (2003). Role of dopamine transporter imaging in routine clinical practice. Mov. Disord. 18, 1415–1423. doi: 10.1002/mds.10592
Mendez, I., Sanchez-Pernaute, R., Cooper, O., Vinuela, A., Ferrari, D., Bjorklund, L., et al. (2005). Cell type analysis of functional fetal dopamine cell suspension transplants in the striatum and substantia nigra of patients with Parkinson’s disease. Brain 128, 1498–1510. doi: 10.1093/brain/awh510
Mendez, I., Vinuela, A., Astradsson, A., Mukhida, K., Hallet, P., Robertson, H., et al. (2008). Dopamine neurons implanted into people with Parkinson’s disease survive without pathology for 14 years. Nat. Med. 14, 507–509. doi: 10.1038/nm1752
Meyer-Lindenberg, A., Miletich, R. S., Kohn, P. D., Esposito, G., Carson, R. E., Quarantelli, M., et al. (2002). Reduced prefrontal activity predicts exaggerated striatal dopaminergic function in schizophrenia. Nat. Neurosci. 5, 267–271. doi: 10.1038/nn804
More, S. V., Kumar, H., Kim, I. S., Song, S. Y., and Choi, D. K. (2013). Cellular and molecular mediators of neuroinflammation in the pathogenesis of Parkinson’s disease. Mediators Inflamm. 2013:952375. doi: 10.1155/2013/952375
Morihisa, J. M., Nakamura, R. K., Freed, W. J., Mishkin, M., and Wyatt, R. J. (1984). Adrenal medulla grafts survive and exhibit catecholamine-specific fluorescence in the primate brain. Exp. Neurol. 84, 643–653. doi: 10.1016/0014-4886(84)90211-5
Morizane, A., Doi, D., Kikuchi, T., Okita, K., Hotta, A., Kawasaki, T., et al. (2013). Direct comparison of autologous and allogeneic transplantation of ipsc-derived neural cells in the brain of a non-human primate. Stem Cell Rep. 1, 283–292. doi: 10.1016/j.stemcr.2013.08.007
Morizane, A., Kikuchi, T., Hayashi, T., Mizuma, H., Takara, S., Doi, H., et al. (2017). Mhc matching improves engraftment of ipsc-derived neurons in non-human primates. Nat. Commun. 8:385. doi: 10.1038/s41467-017-00926-5
Morrish, P. K., Sawle, G. V., and Brooks, D. J. (1996). An [18F]dopa-Pet and clinical study of the rate of progression in Parkinson’s disease. Brain 119(Pt 2), 585–591. doi: 10.1093/brain/119.2.585
Muramatsu, S., Okuno, T., Suzuki, Y., Nakayama, T., Kakiuchi, T., Takino, N., et al. (2009). Multitracer assessment of dopamine function after transplantation of embryonic stem cell-derived neural stem cells in a primate model of Parkinson’s disease. Synapse 63, 541–548. doi: 10.1002/syn.20634
Neuhoff, H., Neu, A., Liss, B., and Roeper, J. (2002). I(h) channels contribute to the different functional properties of identified dopaminergic subpopulations in the midbrain. J. Neurosci. 22, 1290–1302. doi: 10.1523/jneurosci.22-04-01290.2002
Nolbrant, S., Heuer, A., Parmar, M., and Kirkeby, A. (2017). Generation of high-purity human ventral midbrain dopaminergic progenitors for in vitro maturation and intracerebral transplantation. Nat. Protoc. 12, 1962–1979. doi: 10.1038/nprot.2017.078
Norman, A. B., Thomas, S. R., Pratt, R. G., Lu, S. Y., and Norgren, R. B. (1992). Magnetic resonance imaging of neural transplants in rat brain using a superparamagnetic contrast agent. Brain Res. 594, 279–283. doi: 10.1016/0006-8993(92)91135-2
Olanow, C. W., Freeman, T., and Kordower, J. (2001). Transplantation of embryonic dopamine neurons for severe Parkinson’s disease. N. Engl. J. Med. 345:146.
Olanow, C. W., Goetz, C. G., Kordower, J. H., Stoessl, A. J., Sossi, V., Brin, M. F., et al. (2003). A double-blind controlled trial of bilateral fetal nigral transplantation in Parkinson’s disease. Ann. Neurol. 54, 403–414. doi: 10.1002/ana.10720
Olanow, C. W., Stern, M. B., and Sethi, K. (2009). The scientific and clinical basis for the treatment of Parkinson disease. Neurology 72, S1–S136. doi: 10.1212/WNL.0b013e3181a1d44c
Olson, L., and Malmfors, T. (1970). Growth characteristics of adrenergic nerves in the adult rat. Fluorescence histochemical and 3H-noradrenaline uptake studies using tissue transplantations to the anterior chamber of the eye. Acta Physiol. Scand. Suppl. 348, 1–112.
Olson, L., and Seiger, A. (1972). Brain tissue transplanted to the anterior chamber of the eye. 1. Fluorescence histochemistry of immature catecholamine and 5-hydroxytryptamine neurons reinnervating the rat iris. Z. Zellforsch. Mikrosk. Anat. 135, 175–194. doi: 10.1007/bf00315125
Ono, Y., Nakatani, T., Sakamoto, Y., Mizuhara, E., Minaki, Y., Kumai, M., et al. (2007). Differences in neurogenic potential in floor plate cells along an anteroposterior location: midbrain dopaminergic neurons originate from mesencephalic floor plate cells. Development 134, 3213–3225. doi: 10.1242/dev.02879
Papp, M., and Bal, A. (1986). Motivational versus motor impairment after haloperidol injection or 6-Ohda lesions in the ventral tegmental area or substantia nigra in rats. Physiol. Behav. 38, 773–779. doi: 10.1016/0031-9384(86)90042-9
Park, C. H., Minn, Y. K., Lee, J. Y., Choi, D. H., Chang, M. Y., Shim, J. W., et al. (2005). In vitro and in vivo analyses of human embryonic stem cell-derived dopamine neurons. J. Neurochem. 92, 1265–1276. doi: 10.1111/j.1471-4159.2004.03006.x
Park, H. J., Lee, P. H., Bang, O. Y., Lee, G., and Ahn, Y. H. (2008). Mesenchymal stem cells therapy exerts neuroprotection in a progressive animal model of Parkinson’s disease. J. Neurochem. 107, 141–151. doi: 10.1111/j.1471-4159.2008.05589.x
Peng, J., Liu, Q., Rao, M. S., and Zeng, X. (2014). Survival and engraftment of dopaminergic neurons manufactured by a good manufacturing practice-compatible process. Cytotherapy 16, 1305–1312. doi: 10.1016/j.jcyt.2014.06.002
Perez-Bouza, A., Di Santo, S., Seiler, S., Meyer, M., Andereggen, L., Huber, A., et al. (2017). Simultaneous transplantation of fetal ventral mesencephalic tissue and encapsulated genetically modified cells releasing gdnf in a hemi-parkinsonian rat model of Parkinson’s disease. Cell Transplant. 26, 1572–1581. doi: 10.1177/0963689717721202
Perlow, M. J., Freed, W. J., Hoffer, B. J., Seiger, A., Olson, L., and Wyatt, R. J. (1979). Brain grafts reduce motor abnormalities produced by destruction of nigrostriatal dopamine system. Science 204, 643–647. doi: 10.1126/science.571147
Peschanski, M., Defer, G., N’guyen, J. P., Ricolfi, F., Monfort, J. C., Remy, P., et al. (1994). Bilateral motor improvement and alteration of L-dopa effect in two patients with Parkinson’s disease following intrastriatal transplantation of foetal ventral mesencephalon. Brain 117(Pt 3), 487–499. doi: 10.1093/brain/117.3.487
Phanstiel, D. H., Brumbaugh, J., Wenger, C. D., Tian, S., Probasco, M. D., Bailey, D. J., et al. (2011). Proteomic and phosphoproteomic comparison of human Es and ips cells. Nat. Methods 8, 821–827. doi: 10.1038/nmeth.1699
Piccini, P., Brooks, D. J., Bjorklund, A., Gunn, R. N., Grasby, P. M., Rimoldi, O., et al. (1999). Dopamine release from nigral transplants visualized in vivo in a Parkinson’s patient. Nat. Neurosci. 2, 1137–1140. doi: 10.1038/16060
Piccini, P., Lindvall, O., Bjorklund, A., Brundin, P., Hagell, P., Ceravolo, R., et al. (2000). Delayed recovery of movement-related cortical function in Parkinson’s disease after striatal dopaminergic grafts. Ann. Neurol. 48, 689–695. doi: 10.1002/1531-8249(200011)48:5<689::aid-ana1>3.0.co;2-n
Piccini, P., Pavese, N., Hagell, P., Reimer, J., Bjorklund, A., Oertel, W. H., et al. (2005). Factors affecting the clinical outcome after neural transplantation in Parkinson’s disease. Brain 128, 2977–2986. doi: 10.1093/brain/awh649
Pijnenburg, A. J., Honig, W. M., Van Der Heyden, J. A., and Van Rossum, J. M. (1976). Effects of chemical stimulation of the mesolimbic dopamine system upon locomotor activity. Eur. J. Pharmacol 35, 45–58. doi: 10.1016/0014-2999(76)90299-5
Pogarell, O., Koch, W., Gildehaus, F. J., Kupsch, A., Lindvall, O., Oertel, W. H., et al. (2006). Long-term assessment of striatal dopamine transporters in Parkinsonian patients with intrastriatal embryonic mesencephalic grafts. Eur. J. Nucl. Med. Mol. Imaging 33, 407–411. doi: 10.1007/s00259-005-0032-z
Politis, M., Oertel, W. H., Wu, K., Quinn, N. P., Pogarell, O., Brooks, D. J., et al. (2011). Graft-induced dyskinesias in Parkinson’s disease: high striatal serotonin/dopamine transporter ratio. Mov. Disord. 26, 1997–2003. doi: 10.1002/mds.23743
Politis, M., Wu, K., Loane, C., Quinn, N. P., Brooks, D. J., Rehncrona, S., et al. (2010). Serotonergic neurons mediate dyskinesia side effects in Parkinson’s patients with neural transplants. Sci. Transl. Med. 2:38ra46. doi: 10.1126/scitranslmed.3000976
Poulin, J. F., Zou, J., Drouin-Ouellet, J., Kim, K. Y., Cicchetti, F., and Awatramani, R. B. (2014). Defining midbrain dopaminergic neuron diversity by single-cell gene expression profiling. Cell. Rep. 9, 930–943. doi: 10.1016/j.celrep.2014.10.008
Pruszak, J., Ludwig, W., Blak, A., Alavian, K., and Isacson, O. (2009). Cd15, Cd24, and Cd29 define a surface biomarker code for neural lineage differentiation of stem cells. Stem Cells 27, 2928–2940. doi: 10.1002/stem.211
Pulecio, J., Nivet, E., Sancho-Martinez, I., Vitaloni, M., Guenechea, G., Xia, Y., et al. (2014). Conversion of human fibroblasts into monocyte-like progenitor cells. Stem Cells 32, 2923–2938. doi: 10.1002/stem.1800
Punal-Rioboo, J., Serena-Puig, A., Varela-Lema, L., Alvarez-Paez, A. M., and Ruano-Ravina, A. (2009). [Clinical utility of (18)F-Dopa-Pet in movement disorders. A systematic review]. Rev. Esp. Med. Nucl. 28, 106–113. doi: 10.1016/s1578-200x(09)70018-x
Qin, J., Song, B., Zhang, H., Wang, Y., Wang, N., Ji, Y., et al. (2013). Transplantation of human neuro-epithelial-like stem cells derived from induced pluripotent stem cells improves neurological function in rats with experimental intracerebral hemorrhage. Neurosci. Lett. 548, 95–100. doi: 10.1016/j.neulet.2013.05.007
Ramos-Gomez, M., and Martinez-Serrano, A. (2016). Tracking of iron-labeled human neural stem cells by magnetic resonance imaging in cell replacement therapy for Parkinson’s disease. Neural Regen. Res. 11, 49–52. doi: 10.4103/1673-5374.169628
Ramos-Gomez, M., Seiz, E. G., and Martinez-Serrano, A. (2015). Optimization of the magnetic labeling of human neural stem cells and Mri visualization in the hemiparkinsonian rat brain. J. Nanobiotechnol. 13:20. doi: 10.1186/s12951-015-0078-4
Redmond, D. E. Jr., Sladek, J. R., and Spencer, D. D. (2001). Transplantation of embryonic dopamine neurons for severe Parkinson’s disease. N. Engl. J. Med. 345, 146–147. doi: 10.1056/nejm200107123450214
Redmond, D. E., Sladek, J. R. Jr., Roth, R. H., Collier, T. J., Elsworth, J. D., Deutch, A. Y., et al. (1986). Fetal neuronal grafts in monkeys given methylphenyltetrahydropyridine. Lancet 1, 1125–1127. doi: 10.1016/s0140-6736(86)91839-8
Reid, J. E., and Wernisch, L. (2016). Pseudotime estimation: deconfounding single cell time series. Bioinformatics 32, 2973–2980. doi: 10.1093/bioinformatics/btw372
Reubinoff, B. E., Itsykson, P., Turetsky, T., Pera, M. F., Reinhartz, E., Itzik, A., et al. (2001). Neural progenitors from human embryonic stem cells. Nat. Biotechnol. 19, 1134–1140. doi: 10.1038/nbt1201-1134
Reubinoff, B. E., Pera, M. F., Fong, C. Y., Trounson, A., and Bongso, A. (2000). Embryonic stem cell lines from human blastocysts: somatic differentiation in vitro. Nat. Biotechnol. 18, 399–404. doi: 10.1038/74447
Reyes, S., Fu, Y., Double, K., Thompson, L., Kirik, D., Paxinos, G., et al. (2012). Girk2 expression in dopamine neurons of the substantia nigra and ventral tegmental area. J. Comp. Neurol. 520, 2591–2607. doi: 10.1002/cne.23051
Ribeiro, D., Laguna Goya, R., Ravindran, G., Vuono, R., Parish, C. L., Foldi, C., et al. (2013). Efficient expansion and dopaminergic differentiation of human fetal ventral midbrain neural stem cells by midbrain morphogens. Neurobiol. Dis. 49, 118–127. doi: 10.1016/j.nbd.2012.08.006
Robinson, T. E., and Berridge, K. C. (1993). The neural basis of drug craving: an incentive-sensitization theory of addiction. Brain Res. Brain Res. Rev. 18, 247–291. doi: 10.1016/0165-0173(93)90013-p
Rouhani, F., Kumasaka, N., De Brito, M. C., Bradley, A., Vallier, L., and Gaffney, D. (2014). Genetic background drives transcriptional variation in human induced pluripotent stem cells. PLoS Genet. 10:e1004432. doi: 10.1371/journal.pgen.1004432
Roy, N. S., Cleren, C., Singh, S. K., Yang, L., Beal, M. F., and Goldman, S. A. (2006). Functional engraftment of human Es cell-derived dopaminergic neurons enriched by coculture with telomerase-immortalized midbrain astrocytes. Nat. Med. 12, 1259–1268. doi: 10.1038/nm1495
Salti, A., Nat, R., Neto, S., Puschban, Z., Wenning, G., and Dechant, G. (2013). Expression of early developmental markers predicts the efficiency of embryonic stem cell differentiation into midbrain dopaminergic neurons. Stem Cells Dev 22, 397–411. doi: 10.1089/scd.2012.0238
Sawle, G. V., Bloomfield, P. M., Bjorklund, A., Brooks, D. J., Brundin, P., Leenders, K. L., et al. (1992). Transplantation of fetal dopamine neurons in Parkinson’s disease: pet [18F]6-L-fluorodopa studies in two patients with putaminal implants. Ann. Neurol. 31, 166–173. doi: 10.1002/ana.410310207
Schweitzer, J. S., Song, B., Herrington, T. M., Park, T. Y., Lee, N., Ko, S., et al. (2020). Personalized ipsc-derived dopamine progenitor cells for Parkinson’s disease. N. Engl. J. Med. 382, 1926–1932. doi: 10.1056/NEJMoa1915872
Senju, S., Matsunaga, Y., Fukushima, S., Hirata, S., Motomura, Y., Fukuma, D., et al. (2011). Immunotherapy with pluripotent stem cell-derived dendritic cells. Semin. Immunopathol. 33, 603–612. doi: 10.1007/s00281-011-0263-y
Sgado, P., Alberi, L., Gherbassi, D., Galasso, S. L., Ramakers, G. M., Alavian, K. N., et al. (2006). Slow progressive degeneration of nigral dopaminergic neurons in postnatal Engrailed mutant mice. Proc. Natl. Acad. Sci. U.S.A. 103, 15242–15247. doi: 10.1073/pnas.0602116103
Shih, M. C., Amaro, E. Jr., Ferraz, H. B., Hoexter, M. Q., Goulart, F. O., Wagner, J., et al. (2006). [Neuroimaging of the dopamine transporter in Parkinsons disease: first study using [99mTc]-Trodat-1 and Spect in Brazil]. Arq. Neuropsiquiatr. 64, 628–634. doi: 10.1590/S0004-282X2006000400021
Siegfried, J., and Lippitz, B. (1994). Bilateral chronic electrostimulation of ventroposterolateral pallidum: a new therapeutic approach for alleviating all parkinsonian symptoms. Neurosurgery 35, 1126–1130. doi: 10.1227/00006123-199412000-00016
Simon, H. H., Saueressig, H., Wurst, W., Goulding, M. D., and O’leary, D. D. (2001). Fate of midbrain dopaminergic neurons controlled by the engrailed genes. J. Neurosci. 21, 3126–3134. doi: 10.1523/jneurosci.21-09-03126.2001
Sison, S. L., Vermilyea, S. C., Emborg, M. E., and Ebert, A. D. (2018). Using patient-derived induced pluripotent stem cells to identify parkinson’s disease-relevant phenotypes. Curr. Neurol. Neurosci. Rep. 18:84. doi: 10.1007/s11910-018-0893-8
Smidt, M. P., Van Schaick, H. S., Lanctot, C., Tremblay, J. J., Cox, J. J., Van Der Kleij, A. A., et al. (1997). A homeodomain gene Ptx3 has highly restricted brain expression in mesencephalic dopaminergic neurons. Proc. Natl. Acad. Sci. U.S.A. 94, 13305–13310. doi: 10.1073/pnas.94.24.13305
Soldner, F., Hockemeyer, D., Beard, C., Gao, Q., Bell, G. W., Cook, E. G., et al. (2009). Parkinson’s disease patient-derived induced pluripotent stem cells free of viral reprogramming factors. Cell 136, 964–977.
Son, S. J., Kim, M., and Park, H. (2016). Imaging analysis of Parkinson’s disease patients using Spect and tractography. Sci. Rep. 6:38070. doi: 10.1038/srep38070
Sonntag, K. C., Pruszak, J., Yoshizaki, T., Van Arensbergen, J., Sanchez-Pernaute, R., and Isacson, O. (2007). Enhanced yield of neuroepithelial precursors and midbrain-like dopaminergic neurons from human embryonic stem cells using the bone morphogenic protein antagonist noggin. Stem Cells 25, 411–418. doi: 10.1634/stemcells.2006-0380
Sonntag, K. C., Simantov, R., Kim, K. S., and Isacson, O. (2004). Temporally induced Nurr1 can induce a non-neuronal dopaminergic cell type in embryonic stem cell differentiation. Eur. J. Neurosci. 19, 1141–1152. doi: 10.1111/j.1460-9568.2004.03204.x
Spencer, D. D., Robbins, R. J., Naftolin, F., Marek, K. L., Vollmer, T., Leranth, C., et al. (1992). Unilateral transplantation of human fetal mesencephalic tissue into the caudate nucleus of patients with Parkinson’s disease. N. Engl. J. Med. 327, 1541–1548. doi: 10.1056/nejm199211263272201
Steinbeck, J. A., and Studer, L. (2015). Moving stem cells to the clinic: potential and limitations for brain repair. Neuron 86, 187–206. doi: 10.1016/j.neuron.2015.03.002
Stewart, M. H., Bosse, M., Chadwick, K., Menendez, P., Bendall, S. C., and Bhatia, M. (2006). Clonal isolation of hescs reveals heterogeneity within the pluripotent stem cell compartment. Nat. Methods 3, 807–815. doi: 10.1038/nmeth939
Stoker, T. B. (2018). “Stem cell treatments for Parkinson’s disease,” in Parkinson’s Disease: Pathogenesis and Clinical Aspects, eds T. B. Stoker and J. C. Greenland (Brisbane: Codon Publications). doi: 10.15586/codonpublications.parkinsonsdisease.2018.ch9
Stroh, A., Boltze, J., Sieland, K., Hild, K., Gutzeit, C., Jung, T., et al. (2009). Impact of magnetic labeling on human and mouse stem cells and their long-term magnetic resonance tracking in a rat model of Parkinson disease. Mol. Imaging 8, 166–178. doi: 10.2310/7290.2009.00017
Stromberg, I., Bygdeman, M., Goldstein, M., Seiger, A., and Olson, L. (1986). Human fetal substantia nigra grafted to the dopamine-denervated striatum of immunosuppressed rats: evidence for functional reinnervation. Neurosci. Lett. 71, 271–276. doi: 10.1016/0304-3940(86)90632-4
Studer, L. (2017). Strategies for bringing stem cell-derived dopamine neurons to the clinic-The Nystem trial. Prog. Brain Res. 230, 191–212. doi: 10.1016/bs.pbr.2017.02.008
Sundberg, M., Bogetofte, H., Lawson, T., Jansson, J., Smith, G., Astradsson, A., et al. (2013). Improved cell therapy protocols for Parkinson’s disease based on differentiation efficiency and safety of hesc-, hipsc-, and non-human primate ipsc-derived dopaminergic neurons. Stem Cells 31, 1548–1562. doi: 10.1002/stem.1415
Sykova, E., and Jendelova, P. (2007). In vivo tracking of stem cells in brain and spinal cord injury. Prog. Brain Res. 161, 367–383. doi: 10.1016/s0079-6123(06)61026-1
Takahashi, J. (2017). Strategies for bringing stem cell-derived dopamine neurons to the clinic: the Kyoto trial. Prog. Brain Res. 230, 213–226. doi: 10.1016/bs.pbr.2016.11.004
Takahashi, K., Okita, K., Nakagawa, M., and Yamanaka, S. (2007). Induction of pluripotent stem cells from fibroblast cultures. Nat. Protoc. 2, 3081–3089. doi: 10.1038/nprot.2007.418
Takahashi, K., and Yamanaka, S. (2006). Induction of pluripotent stem cells from mouse embryonic and adult fibroblast cultures by defined factors. Cell 126, 663–676. doi: 10.1016/j.cell.2006.07.024
Takahashi, K., and Yamanaka, S. (2016). A decade of transcription factor-mediated reprogramming to pluripotency. Nat. Rev. Mol. Cell Biol. 17, 183–193. doi: 10.1038/nrm.2016.8
Tennstaedt, A., Aswendt, M., Adamczak, J., and Hoehn, M. (2013). Noninvasive multimodal imaging of stem cell transplants in the brain using bioluminescence imaging, and magnetic resonance imaging. Methods Mol Biol. 1052, 153–166. doi: 10.1007/7651_2013_14
Thobois, S., Guillouet, S., and Broussolle, E. (2001). Contributions of Pet and Spect to the understanding of the pathophysiology of Parkinson’s disease. Neurophysiol. Clin. 31, 321–340. doi: 10.1016/s0987-7053(01)00273-8
Thomas, S. M., Kagan, C., Pavlovic, B. J., Burnett, J., Patterson, K., Pritchard, J. K., et al. (2015). Reprogramming Lcls to ipscs results in recovery of donor-specific gene expression signature. PLoS Genet. 11:e1005216. doi: 10.1371/journal.pgen.1005216
Thompson, L., Barraud, P., Andersson, E., Kirik, D., and Bjorklund, A. (2005). Identification of dopaminergic neurons of nigral and ventral tegmental area subtypes in grafts of fetal ventral mesencephalon based on cell morphology, protein expression, and efferent projections. J. Neurosci. 25, 6467–6477. doi: 10.1523/jneurosci.1676-05.2005
Thomson, J. A., Itskovitz-Eldor, J., Shapiro, S. S., Waknitz, M. A., Swiergiel, J. J., Marshall, V. S., et al. (1998). Embryonic stem cell lines derived from human blastocysts. Science 282, 1145–1147. doi: 10.1126/science.282.5391.1145
Tiklova, K., Bjorklund, A. K., Lahti, L., Fiorenzano, A., Nolbrant, S., Gillberg, L., et al. (2019). Single-cell Rna sequencing reveals midbrain dopamine neuron diversity emerging during mouse brain development. Nat. Commun. 10:581. doi: 10.1038/s41467-019-08453-1
Ungerstedt, U. (1971). Stereotaxic mapping of the monoamine pathways in the rat brain. Acta Physiol. Scand. Suppl. 367, 1–48. doi: 10.1111/j.1365-201x.1971.tb10998.x
Visnyei, K., Tatsukawa, K. J., Erickson, R. I., Simonian, S., Oknaian, N., Carmichael, S. T., et al. (2006). Neural progenitor implantation restores metabolic deficits in the brain following striatal quinolinic acid lesion. Exp. Neurol. 197, 465–474. doi: 10.1016/j.expneurol.2005.10.023
Waerzeggers, Y., Klein, M., Miletic, H., Himmelreich, U., Li, H., Monfared, P., et al. (2008). Multimodal imaging of neural progenitor cell fate in rodents. Mol. Imaging 7, 77–91. doi: 10.2310/7290.2008.0010
Wakeman, D. R., Hiller, B. M., Marmion, D. J., Mcmahon, C. W., Corbett, G. T., Mangan, K. P., et al. (2017). Cryopreservation maintains functionality of human ipsc dopamine neurons and rescues parkinsonian phenotypes in vivo. Stem Cell Rep. 9, 149–161. doi: 10.1016/j.stemcr.2017.04.033
Wang, L., Zhang, Q., Li, H., and Zhang, H. (2012). Spect molecular imaging in Parkinson’s disease. J. Biomed. Biotechnol. 2012:412486. doi: 10.1155/2012/412486
Wang, S., Zou, C., Fu, L., Wang, B., An, J., Song, G., et al. (2015). Autologous ipsc-derived dopamine neuron transplantation in a nonhuman primate Parkinson’s disease model. Cell Discov. 1:15012. doi: 10.1038/celldisc.2015.12
Wenning, G. K., Odin, P., Morrish, P., Rehncrona, S., Widner, H., Brundin, P., et al. (1997). Short- and long-term survival and function of unilateral intrastriatal dopaminergic grafts in Parkinson’s disease. Ann. Neurol. 42, 95–107. doi: 10.1002/ana.410420115
Widner, H., Tetrud, J., Rehncrona, S., Snow, B., Brundin, P., Gustavii, B., et al. (1992). Bilateral fetal mesencephalic grafting in two patients with parkinsonism induced by 1-methyl-4-phenyl-1,2,3,6-tetrahydropyridine (Mptp). N. Engl. J. Med. 327, 1556–1563. doi: 10.1056/nejm199211263272203
Wolfart, J., Neuhoff, H., Franz, O., and Roeper, J. (2001). Differential expression of the small-conductance, calcium-activated potassium channel Sk3 is critical for pacemaker control in dopaminergic midbrain neurons. J. Neurosci. 21, 3443–3456. doi: 10.1523/jneurosci.21-10-03443.2001
Wu, X., Cai, H., Ge, R., Li, L., and Jia, Z. (2014). Recent progress of imaging agents for Parkinson’s disease. Curr. Neuropharmacol. 12, 551–563. doi: 10.2174/1570159x13666141204221238
Yan, Y., Yang, D., Zarnowska, E. D., Du, Z., Werbel, B., Valliere, C., et al. (2005). Directed differentiation of dopaminergic neuronal subtypes from human embryonic stem cells. Stem Cells 23, 781–790. doi: 10.1634/stemcells.2004-0365
Yang, D., Zhang, Z. J., Oldenburg, M., Ayala, M., and Zhang, S. C. (2008). Human embryonic stem cell-derived dopaminergic neurons reverse functional deficit in parkinsonian rats. Stem Cells 26, 55–63. doi: 10.1634/stemcells.2007-0494
Yu, J., Vodyanik, M. A., Smuga-Otto, K., Antosiewicz-Bourget, J., Frane, J. L., Tian, S., et al. (2007). Induced pluripotent stem cell lines derived from human somatic cells. Science 318, 1917–1920. doi: 10.1126/science.1151526
Zhang, S. C., Wernig, M., Duncan, I. D., Brustle, O., and Thomson, J. A. (2001). In vitro differentiation of transplantable neural precursors from human embryonic stem cells. Nat. Biotechnol. 19, 1129–1133. doi: 10.1038/nbt1201-1129
Keywords: Parkinson’s disease, dopaminergic neurons, transplantation, stem cells, imaging modalities, neuroimaging, clinical trials
Citation: Jang SE, Qiu L, Chan LL, Tan EK and Zeng L (2020) Current Status of Stem Cell-Derived Therapies for Parkinson’s Disease: From Cell Assessment and Imaging Modalities to Clinical Trials. Front. Neurosci. 14:558532. doi: 10.3389/fnins.2020.558532
Received: 03 May 2020; Accepted: 17 September 2020;
Published: 16 October 2020.
Edited by:
Woon-Man Kung, Chinese Culture University, TaiwanReviewed by:
Darius Widera, University of Reading, United KingdomPaolo Solla, Azienda Ospedaliero-Universitaria Cagliari, Italy
Copyright © 2020 Jang, Qiu, Chan, Tan and Zeng. This is an open-access article distributed under the terms of the Creative Commons Attribution License (CC BY). The use, distribution or reproduction in other forums is permitted, provided the original author(s) and the copyright owner(s) are credited and that the original publication in this journal is cited, in accordance with accepted academic practice. No use, distribution or reproduction is permitted which does not comply with these terms.
*Correspondence: Eng-King Tan, dGFuLmVuZy5raW5nQHNpbmdoZWFsdGguY29tLnNn; Li Zeng, bGlfemVuZ0BubmkuY29tLnNn