- 1Faculty of Biology, University of Warsaw, Warsaw, Poland
- 2Dominick P. Purpura Department of Neuroscience, Albert Einstein College of Medicine, New York, NY, United States
- 3Nencki Institute of Experimental Biology of Polish Academy of Sciences, Warsaw, Poland
- 4College of Inter-Faculty Individual Studies in Mathematics and Natural Sciences, University of Warsaw, Warsaw, Poland
Despite the fact that there is a growing awareness to the callosal connections between hemispheres the two hemispheres of the brain are commonly treated as independent structures when peripheral or cortical manipulations are applied to one of them. The contralateral hemisphere is often used as a within-animal control of plastic changes induced onto the other side of the brain. This ensures uniform conditions for producing experimental and control data, but it may overlook possible interhemispheric interactions. In this paper we provide, for the first time, direct proof that cortical, experience-dependent plasticity is not a unilateral, independent process. We mapped metabolic brain activity in rats with 2-[14C] deoxyglucose (2DG) following experience-dependent plasticity induction after a month of unilateral (left), partial whiskers deprivation (only row B was left). This resulted in ∼45% widening of the cortical sensory representation of the spared whiskers in the right, contralateral barrel field (BF). We show that the width of 2DG visualized representation is less than 20% when only contralateral stimulation of the spared row of whiskers is applied in immobilized animals. This means that cortical map remodeling, which is induced by experience-dependent plasticity mechanisms, depends partially on the contralateral hemisphere. The response, which is observed by 2DG brain mapping in the partially deprived BF after standard synchronous bilateral whiskers stimulation, is therefore the outcome of at least two separately activated plasticity mechanisms. A focus on the integrated nature of cortical plasticity, which is the outcome of the emergent interactions between deprived and non-deprived areas in both hemispheres may have important implications for learning and rehabilitation. There is also a clear implication that there is nothing like “control hemisphere” since any plastic changes in one hemisphere have to have influence on functioning of the opposite one.
Introduction
Rodents whiskers’ representation in the somatosensory cortex is widely used as a model of cortical plasticity due to its highly somatotopic organization. Each whisker has its sensory representation in the cortical area of the barrel field (BF) in the contralateral hemisphere (Figure 1A). Each barrel is a representation of one whisker. Barrels are easily visualized in layer IV and are constructed by a dense composition of cells around the middle part of columnar representation of the whisker. The barrels are arranged in the cortex like the whiskers on the snout (Fox and Woolsey, 2008), and this makes them a good model for cortical map remodeling. The whisker sensory information passes through the ventroposteromedial (VPM) and the medial division of the posterior nucleus (POm) in the contralateral thalamus, reaching, respectively, the barrel center and the septas between barrels (Kim and Ebner, 1999). These two distinct thalamocortical systems are supposed to be separate channels for processing sensory information, since signals from the two systems are combined in SII (Alloway, 2008; Feldmeyer, 2012). The sensory information from the ipsi- and contralateral cortices is supposed to be fused for the very first time when transferred calossally between SI areas (Fabri et al., 2005) and SII (Carvell and Simons, 1987). The cortical sensory information input from the whiskers in rodents is supposed to be completely crossed (White and DeAmicis, 1977; Simons and Woolsey, 1979; Olavarria et al., 1984; Koralek et al., 1990; Cauller et al., 1998; Renier et al., 2017) and at the same time requires representations in both hemispheres for the animal to perform bilateral tactile discrimination (Shuler et al., 2001, unilateral tasks are not supposed to engage the ipsilateral SI; Hutson and Masterton, 1986).
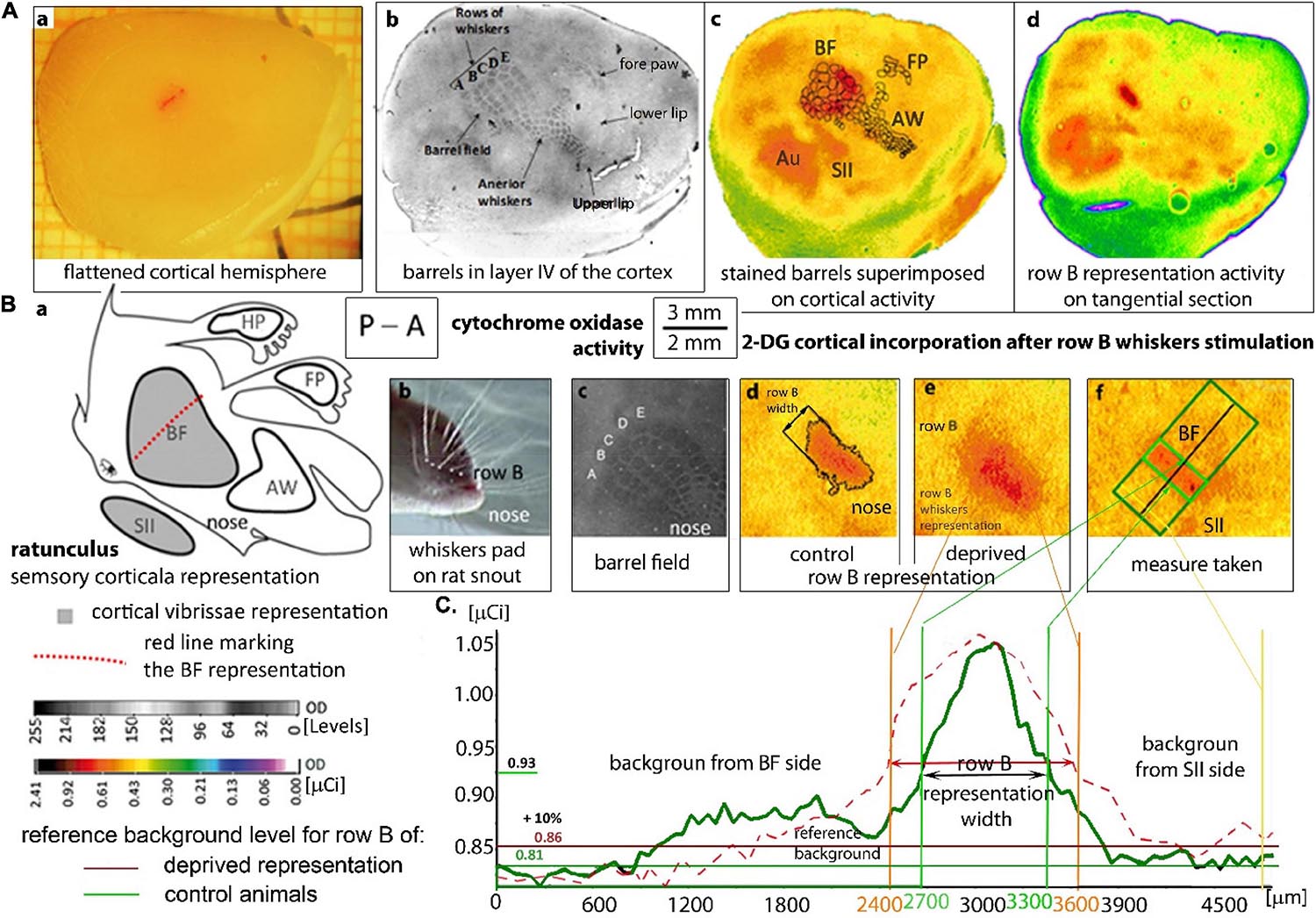
Figure 1. Localization and measurement of the row B cortical representation: (A) A flatted hemisphere and tangential sections of the cortex after 2DG brain activity mapping. (A,a) Isolated hemisphere with a marked line perpendicular to the representation in row B. Localization of the rats’ cortical whiskers somatosensory representations on a tangential section of layer IV (A,b–d) stained by cytochrome oxidase activity (A,b) and 2DG-incorporation on autoradiograms visualizing the brain activity (A,c,d). (A,c) Superimposed barrels from the histological staining to the suitable autoradiogram image which is shown in pseudocolors. (A,d): the autoradiogram presenting stimulated row B activity. (AW) anterior whiskers representation; (FP) front paw representation; (SII) secondary somatosensory cortex, (Au) auditory cortex which is always activated by the white noise. The left whiskers stimulation in intact rats activates, the right BF and SII (A,c) in the contralateral hemisphere and do not activate AW, hind paw and FP cortical representations (A,c,d). (B) The cortical vibrissal representation in SI and SII. (B,a) “Rattunculus” with areas of increased activation during all whiskers stimulations marked in gray. (B,b) Photograph of the whiskers pad on the rat snout showing row B. (B,c) Magnification of the barrel field on the cortical tangential section stained for cytochrome oxidase activity. (B,d,e) A magnification of row B cortical representation on digitized autoradiograms of tangential sections in undeprived (B,d) and deprived (B,e) animals. (B,f) Row B representation with the marked area of the width measured with the rotatable box. (C) Comparison of the OD graphs showing 2DG incorporation from the animals with non-deprived (green line) and deprived BF (dashed red line). OD analysis by the MCID program, where the area exceeding the background over 10% were treated as the activated representation of row B. The mean value of OD from the neighborhood of the BF (right side of the graph, from the SII side of the stimulated row B) was treated as a reference area. The subscribed “background from the BF side” was altered by the whiskers stimulation. OD—optical density in arbitrary units with a direct set of 255 gray scale levels or calibrated to μCi radiation units of [14 C]-2DG; P–A—posterior to anterior.
Like other primary representations, the cortical columns in the BF are involved in the first step of cortical information processing. The sensory input arrives to layer IV in the BF, goes through circuits of columnar loops of cellular connections and is combined with the thalamocortical and cortico-cortical loops that modify the contextual response (Alloway, 2008). Prolonged changes in the sensory input from the whisker pad can be observed as connectivity remodeling in the well-defined structure of BF. It was shown (in rats) that a month of unilateral, partial whiskers deprivation results in the widening of the spared whiskers representation at the expense of surrounding area of the deprived whiskers representations (Kossut et al., 1988; Diamond et al., 1993; Fox, 1994). The widening can be visualized by [14C]-2-deoxyglucose (2DG) mapping of the metabolic correlates of brain activity (McCasland and Woolsey, 1988).
The enlargement of one representation in response to surrounding whiskers deprivation that is visualized by 2DG was already shown by Dietrich et al., 1985. Since that time it has been widely used in experiments exploring plasticity mechanisms in the healthy and injured cortex. However, the difference in response to uni- vs. bilateral stimulation was hardly considered and studied. Simultaneous bilateral stimulation of corresponding rows of whiskers resulted in a similar area of 2DG incorporation in layer IV and more superficial layers in both hemispheres (Siucinska and Kossut, 2004). A unilateral stimulation led to 2DG incorporation only in the contralateral hemisphere, a result that is in accordance with the theory of full crossing of this sensory pathways (Debowska et al., 2011). However, the two hemispheres were usually treated as independent even in experiments involving unilateral, peripheral and/or cortical manipulations (Fusco et al., 2003; Sun et al., 2003; Jablonka et al., 2007, 2012; Chung et al., 2013). Bilateral whiskers’ stimulation was often used to visualize the cortical plasticity changes in one hemisphere and was compared to the opposite hemisphere, which was treated as a control (Jablonka et al., 2007, 2012; Kaliszewska et al., 2012). In this experimental model, the unilateral partial whiskers deprivation induces plastic changes in the contralateral hemisphere, increasing it by almost 50% when visualized by 2DG incorporation.
It has been shown that the transfer of the effect of learning between BFs in both hemispheres is topographically arranged and is more closely related to strongly connected homotopic whiskers (Harris and Diamond, 2000). Although interhemispheric cortical interactions were reported (Iwamura, 2000; Innocenti, 2009; Ragert et al., 2011) and the inhibitory and excitatory influences of the interactions were shown for a few modalities (Bloom and Hynd, 2005), the possibility that opposite homotopic regions may participate in the remodeling of any representations was rarely considered. Wiest et al. (2005) suggested a revision to the concept of the highly segregated hemispheric processing in BFs and presented data supporting the conjecture that callosal connections constitutively mediate the activity of BF. Glazewski et al. (2007) demonstrated that ipsilateral whiskers can constrain the experience-dependent enlargement of the cortical representation during chronic, unilateral, partial whiskers deprivation. Since it was shown that the information processed in SII may interfere with the contralateral plasticity mechanisms in the BF (Debowska et al., 2011), we hypothesized that interhemispheric interactions may be engaged in the cortical circuits’ remodeling following experience-dependent plasticity in SI as well. We therefore compared metabolic activity during bilateral and unilateral whiskers stimulation for the areas newly engaged in information processing following 1 month of deprivation. We also checked if the changes observed in one hemisphere are somehow reflected in the contralateral homotopic area the undeprived one. To ensure whether the changes observed in one hemisphere are somehow reflected in the contralateral homotopic area the undeprived one row B unilateral whiskers stimulation was performed.
Specifically, we wanted to see if the response to an acute unilateral whiskers stimulation after chronic experience-dependent plasticity induction results in the same pattern of plastic rearrangement of the spared whiskers cortical representations as that resulting from bilateral stimulation.
Materials and Methods
Animals
Male Wistar rats weighing about 300 g from the Bialystok colony were used. Animals were housed separately in plastic cages in a 12 h light-dark cycle at approximately 20°C, and had a free access to food and water. There were six groups of rats: three experimental groups with whiskers deprivation (D) of which two groups received unilateral whiskers stimulation (n = 6) and one group received bilateral stimulation (n = 8) during brain activity mapping; there were also three naïve control groups (n = 6) where individuals did not receive sensory deprivation (C) but received the same type of whiskers stimulation as the experimental groups. Animals were handled and habituated to the restraining procedure for 1 week before deprivation. All the procedures were accepted by the First Regional Ethical Commission in Warsaw (270/2012) and were in accordance with the European Communities Directive (86/609/EEC).
Unilateral Partial Whiskers Deprivation
Sensory deprivation was performed by trimming all the left whiskers except for row B. The trimming was repeated every second day for 1 month.
Brain Mapping
After 4 weeks of vibrissae deprivation, 2DG functional brain mapping was performed (Figures 1Ac,d,Bd–f,C; Kennedy et al., 1975). Animals were put in a restrainer and the whiskers of the undeprived right side were cut close to the skin apart from row B. [14C] – 2DG (7 μCi/100 g b.m., American Radiolabeled Chemical, spec. act. 55 mCi/mmol; St. Louis, MO, United States) was injected intramuscularly. During brain mapping the whiskers of the both rows B were stroked manually uni- or bilaterally in experimental and control groups, in rostro-caudal direction with frequency of 2 Hz. After 30 min of stimulation the animal was deeply anesthetized by intraperitoneal injection of Morbital (0.1 ml/100 g).
Tissue Histological Preparation
Rats were killed by i.p., Morbital injection (0.1 ml/100 g; Biovet, Pulawy, Poland) and intracardially perfused with 4% paraformaldehyde (Sigma-Aldrich; St. Louis, MO, United States). Brains were removed and cortices of separated hemispheres were flattened between glass slides to 3 mm, snap frozen in heptane at −70°C and stored at −80°C. Hemispheres were cut tangentially (Figure 1Aa) on a cryostat at −16°C into 20 μm tangential sections, which were collected alternately on specimen slides and cover slips. The sections, which were on cover slips were immediately dried and exposed on an X-ray film (MIN-R 2000; Kodak) for 3 weeks with a set of [14C] standards (American Radiolabeled Chemicals; St. Louis, MO, United States). The remaining sections were stained for cytochrome oxidase (CO) activity to identify the BF (Figures 1Ab,Bc), and the BF marked perpendicularly to row B, with DiI (Figure 1Aa).
The histological staining images of tangential sections with the barrels present for HP, BF and anterior whiskers were superimposed on corresponding autoradiogram images which mark the regions of the sensory representations.
Data Analysis and Statistics
The autoradiograms were analyzed by a computer image analysis program (MCID; InterFocus Ltd., Cambridge, United Kingdom). The optical density (OD) was measured by the program with 8 bits accuracy with 255 gray scale levels and OD and distance calibration were performed. The software allowed us to display an image of the section stained for CO activity next to the section from which the autoradiogram was obtained and to superimpose these images on each other so that the position of the barrel field, hind limb, forepaw, anterior whiskers and the relation of the other areas can be precisely defined.
After establishing the OD of the autoradiograms within the range of [14C] standards and the increase of OD in a linear standard manner, autoradiograms were compared with stained brain sections (Figure 1Ab,c). Assuming that the OD in autoradiograms is proportional to the concentration of the [14C] isotope (that in turn reflects local glucose consumption; Sokoloff et al., 1977), we used the μCi values as a direct measure of cortical activity. We converted the OD values from digitized images into μCi based on the ODs of the [14C] standards that were co-exposed with the cortex sections. The software measured OD values in labeled regions. The geometric properties of the marked areas were measured automatically by the software or with the software tools. The width of the labeled cortical representation of row B whiskers was measured in layer IV. The pixels with 2-DG-uptake intensity that were consistently above 15% of the mean surrounding cortex incorporation were considered as a labeled representation (Chmielowska et al., 1986). The width was taken by rotatable box tool crossing perpendicularly to the middle of the delineated region. The results were averaged for all sections from layer IV, II/III, and V/VI of one hemisphere.
The differences between the groups were examined using a multi-factor ANOVA and a post hoc Tukey test (for unequal N). The data were also compared by using bootstrap analysis in 1000 iterations with replacement (Efron, 1979). The significance of the differences between hemispheres of bilaterally stimulated rats was calculated with a two-tailed paired Student’s T-test.
Results
2DG Cortical Activity Mapping
Uni- and bilateral whiskers stimulations lead to a different pattern of experience-dependent plasticity effects. In these two cases the visualization of the experience dependent plasticity by 2DG incorporation differed significantly (p ≤ 0.05). Both types of stimulation generated a band of the representation of sensory row B in the BF that is contralateral to the stimulated rows and more laterally in the neighboring SII (Figures 1Ac,d). However, there were differences in rows B representations visualized in uni- and bilaterally stimulated rats in the deprived animals.
Row B Representation Width
Autoradiograms analysis showed differences in rows B representations width between groups (Figures 2, 3; F(4,24) = 17, p < 0.0001). In the control naïve animals rows B representations’ width in both hemispheres after uni- and bilateral stimulations (Supplementary Table 1) were similar to that in undeprived hemisphere of deprived animals, i.e., around 800 μm (Figure 3; 796 ± 47 vs. 833 ± 71 μm).
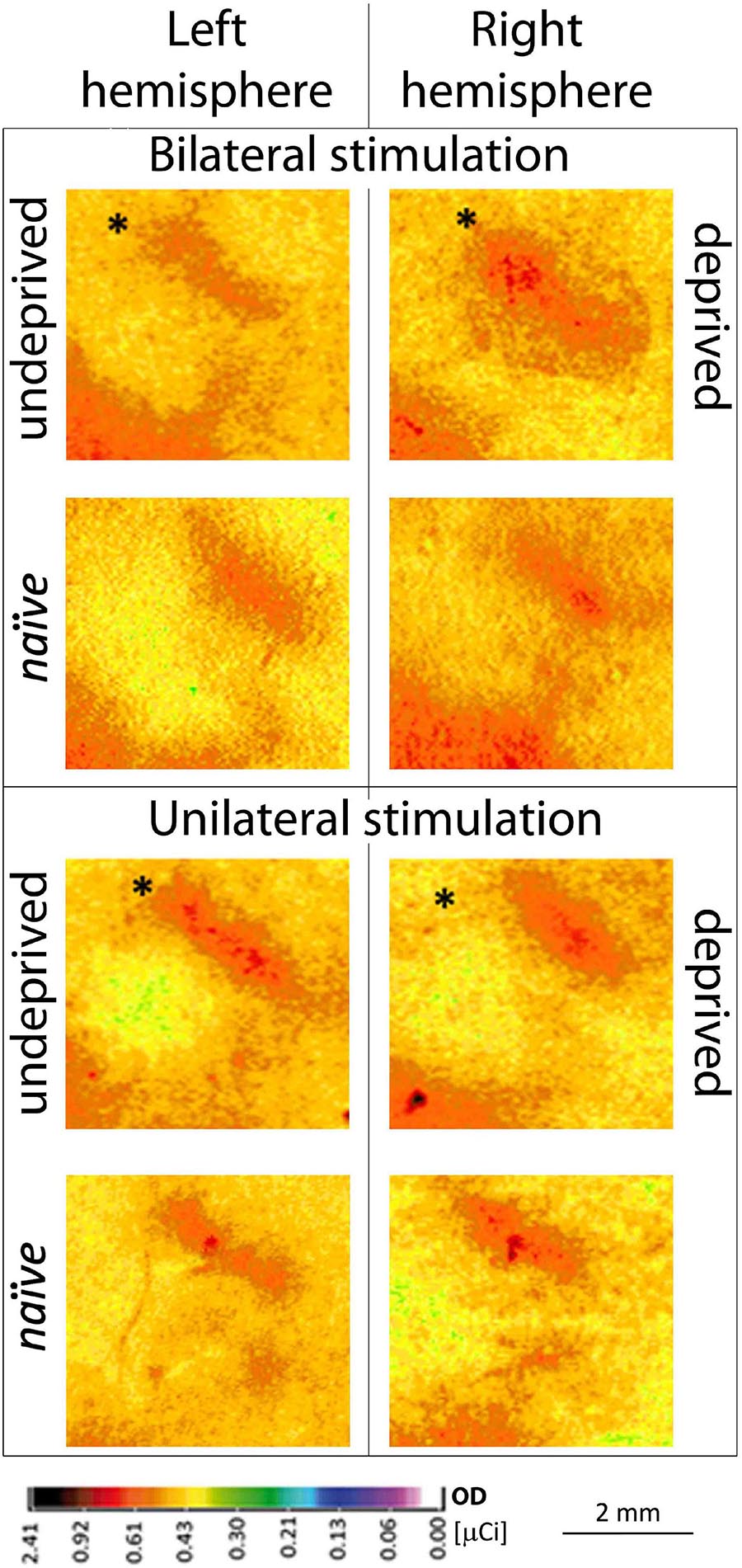
Figure 2. Contralateral 2DG incorporation of row B representations in the two hemispheres during uni- vs. bilateral whiskers stimulation in deprived experimental groups. Individuals with unilateral, partial whiskers cut and bilateral stimulation (n = 8) or individuals with unilateral stimulation (n = 6) of whiskers’ row B during brain activity mapping are shown; bottom row: naïve animal; ∗Mean ± SD; ∗P < 0.05.
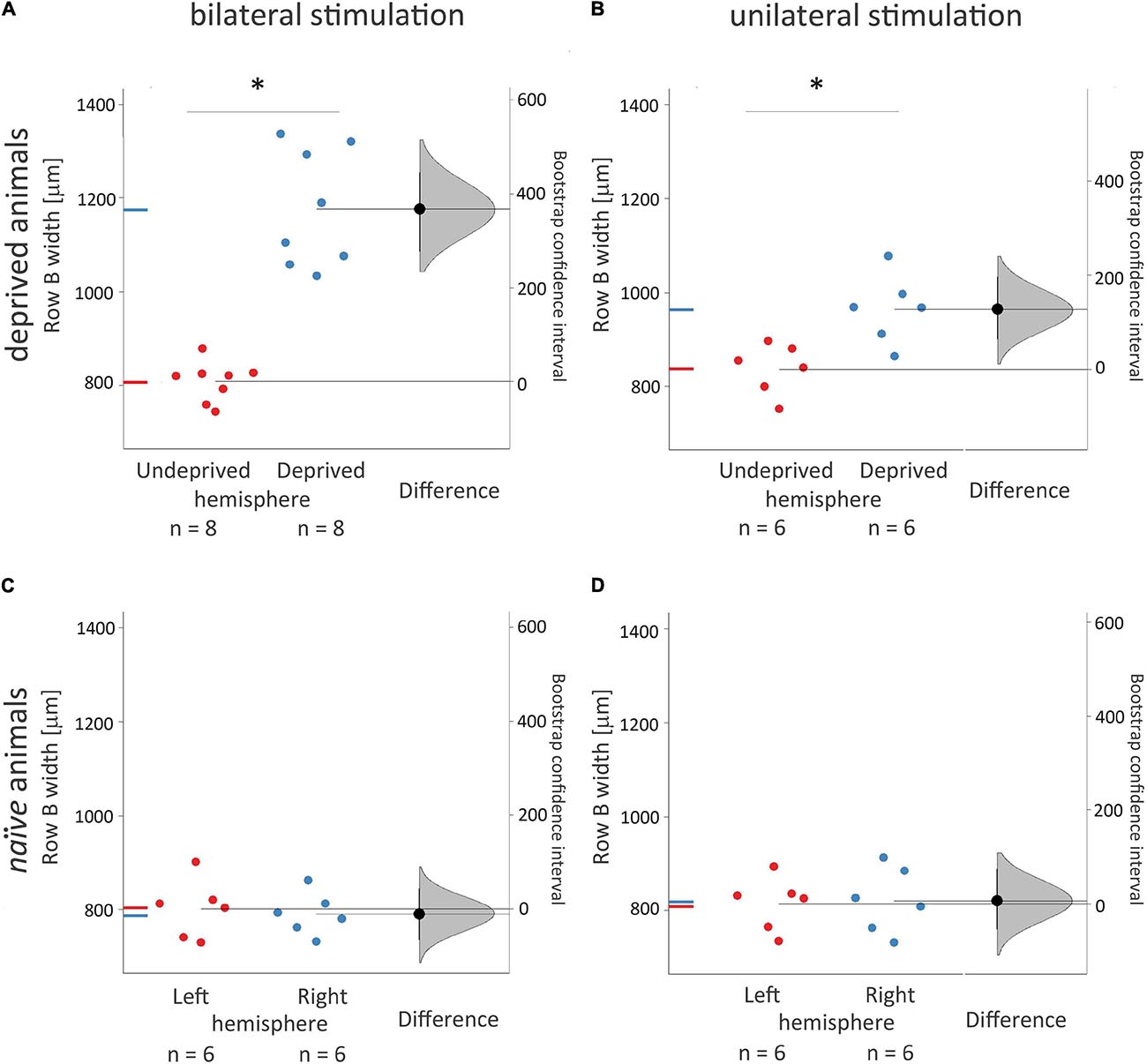
Figure 3. Width of 2DG incorporation in rows B representations in the contralateral, deprived, and non-deprived hemispheres and its dependence on uni- vs. bilateral whiskers stimulation. Experimental groups were deprived and bilaterally (A. n = 8) or unilaterally (B. n = 6) stimulated during brain activity mapping; naïve rats (C.,D.) received just whiskers stimulation during brain activity mapping. ANOVA with post hoc Tuckey test and bootstrap analysis were used. The right curve shows an estimation plot emphasizing the effect size – the difference between the group means of the left and right hemispheres. The 0 point of the “difference axis” (on the right) is based on the mean of the reference group (the naive control group, presented below). The filled triangles show the difference between left and right hemispheres, with 95% confidence intervals. The curves illustrate the range of expected sampling error in estimating the mean difference. *Mean ± SD; *P < 0.05.
The stimulated deprived, left row B representation width in the right hemisphere after unilateral the left whiskers stimulation was 22% smaller than after the bilateral one (Figure 2; 964 ± 73 vs. 1176 ± 125 μm, p = 0.02). In unilaterally stimulated rats, the width of the cortical representation of the spared row B in the right hemisphere contralateral to the deprived whiskers pad was 15% wider than that seen in the ipsilateral to the deprived whiskers, the left hemisphere after contralateral (the right) row B whiskers’ stimulation (Figures 2, 3.; 835 ± 65 vs. 964 ± 73 μm, p = 0.03), while in bilaterally stimulated rats it was 45% wider (Figures 2, 3; 808 ± 42 vs. 1176 ± 125 μm, p < 0.001; similar to the magnitude of the response previously reported by Jablonka et al., 2007, 2012). The relations were similar for all the layers (Supplementary Table 1 and Supplementary Figure 1). To ensure whether the changes in one hemisphere are somehow reflected in the contralateral homotopic area unilateral whiskers stimulation of the undeprived row B was performed and results compared to those of naïve animals. There were no significant differences.
Ipsilateral Response
When rats were stimulated unilaterally, also ipsilateral activity appeared in the supragranular layers (Figure 4, >1000 μm) of the entire BF in the hemisphere ipsilateral to the whiskers stimulation (mean area 1340 ± 200 μm2). Activity was observed in both deprived and undeprived animals and had a uniform 2DG incorporation (1.24 ± 0.03 μCi) just below 1000 μm. In deeper layers activity was smaller and more focused (549 ± 240 mm2; p < 0.001): it was restricted to the area homotopic to the stimulated row B representation (Figure 4). No statistically significant differences were observed between groups that were unilaterally stimulated. However, the bilateral stimulation gave more focused response in the area restricted to the row’s representation in the supragranular layers (Figure 4; 1.18 ± t0.08 uCi, 229 ± 68 mm2, p < 0.0001).
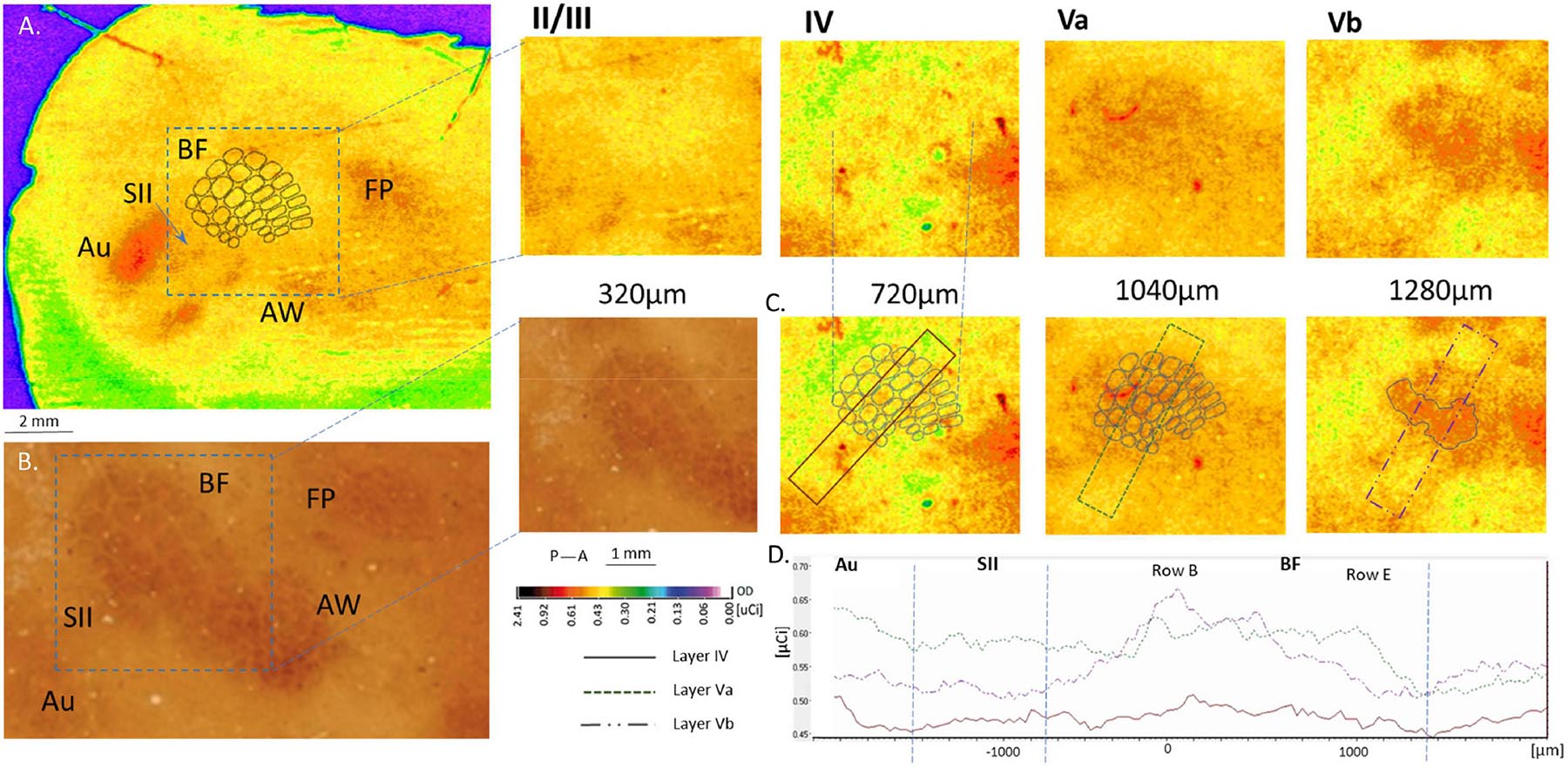
Figure 4. Cortical 2DG incorporation following the ipsilateral row B whiskers stimulation shown in four layers of the barrel field cortex. (A) no response is seen in layers II/III and IV (slightly higher incorporation might be observed in deep layer IV). Layer Va shows evident excitation in the entire surface of the BF with stronger activation of row B representation in layer Vb; (B) BF in layer IV stained for cytochrome oxidase activity. (C) OD measured across the BF in layer IV, Va and Vb. (D) 2DG incorporation in the above chosen areas in the tree layers.
Discussion
Our results show that the cortical areas engaged in experience-dependent plasticity cortical map remodeling are differently activated during uni- and bilateral stimulation, thus suggesting contralateral hemisphere participation in cortical plasticity. We assume that the undeprived hemisphere, which is active all the time, influences the deprived areas of the deprived hemisphere. The result is, as we have shown in this paper, that the neighborhood of the active non-deprived row of whiskers is activated by contralateral homotopic cortical area stimulation in the undeprived hemisphere. It is possible, however, that what we actually observe is a plasticity effects of the inhibitory loop from the contralateral hemisphere, activating the inhibitory interneurons surrounding the only active area in the deprived hemisphere. The control groups showed no such effect. 2DG incorporation, which visualizes the overall effect of inhibitory and excitatory activity as well as the neuronal and astrocyte metabolic response to neuronal activity (Kennedy et al., 1975; Sokoloff et al., 1977), showed deprivation-induced enlargement of the area around the spared + depending on whether unilateral or bilateral whiskers’ stimulation was applied within the area engaged in experience-dependent plasticity.
The 2DG visualized emanation of experience-dependent plasticity cortical map remodeling after partial whiskers deprivation differed following the two treatments: the 2DG incorporation area of cortical representation of the spared whiskers was smaller by 22% after contralateral whiskers stimulation than that manifest after bilateral stimulation, although both were enlarged in comparison to the representations in intact animals (by 45 and 15%, respectively). Since it is assumed that information transferred from the vibrissae to the cortex crosses between hemispheres through the corpus callosum (Smith, 1973; White and DeAmicis, 1977; Simons and Woolsey, 1979; Olavarria et al., 1984; Koralek et al., 1990; Cauller et al., 1998; Renier et al., 2017) any additional response presented after bilateral stimulation (in comparison to unilateral stimulation) must be due to transfer through callosal connections.
The corpus callosum is the biggest connective pathway between the brain hemispheres. In the sensory areas the callosal connections are mainly between layers II/III and layer V (Huang et al., 2013; Decosta-Fortune et al., 2015). Neurons responsible for callosal connections can also innervate other associative areas like SII (Krubitzer and Kaas, 1990), and callosal connections were suggested to transmit both inhibitory and excitatory stimulations (Kobayashi and Pascual-Leone, 2003; Bloom and Hynd, 2005). The conclusion that the corpus callosum is an active structure, constitutively establishing interhemispheric integration (Hellige, 1993; Banich, 1995; Tame et al., 2016) is inescapable.
The diversity of the signals communicated through the corpus callosum may depend on the context in which excitatory and inhibitory interhemispheric interactions occur (Banich, 1995; Cooke and Bear, 2013; Reed et al., 2010, 2011). This means that there are grounds to believe that both the inhibitory and excitatory signals transferred through callosal fibers influence innervated regions in the cortex (Hlushchuk and Hari, 2006). Previous studies demonstrated the direct influence of contralateral homotopic regions on the activity patterns of sensory representations (Meissirel et al., 1991; Clarke and Zaidel, 1994; Iwamura, 2000) and it was shown that sensory information from the whiskers can be modulated by incoming information from the contralateral BF, probably via direct connections through callosal afferenciation of both BFs (Li et al., 2005).
Celikel et al., 2004 demonstrated the immediate changes after deprivation in connectivity of layer IV and II/III, and Li et al. (2005) also showed that the unilateral suppression of BF activity modifies responses to incoming information in the contralateral BF with no impact on spontaneous activity. However, as far as we know, there were as yet no studies (except one, Glazewski et al., 2007) that supported the idea that the constitutive cooperation between the hemispheres may contain cortical remodeling through neuronal plasticity mechanisms in SI. The results presented in this paper further support the idea that the rewiring of the deprived hemisphere has some influence on the contralateral one and that there are on-going re-entrant interactions between the two hemispheres. They also suggests that incoming information processing in the “intact” hemisphere of deprived animals is likely to differ from information processing in naïve (intact) animals.
The response of the cortical neurons in ipsilateral layer V was described by Shuler et al. (2001), who showed that ipsilateral input from multiple whiskers increases the probability of spike recording in direct proportion to the amount of whiskers’ stimulation. In the experiments described in this paper, continuous stroking for half an hour of one row of 5 whiskers gave a uniform 2DG incorporation in the entire BF of the ipsilateral hemisphere in layer Va. This illustrates the active connectivity of the transcallosal axons. The transcallosal connections may trigger the induction of background activity, changing the sensitivity of the whole BF to the processing of signals entering layer IV, which becomes integrated with the current activity induced by the stimulation of the ipsilateral whiskers. The pattern of 2-DG incorporation does not, however, provide information regarding the electrophysiological properties of the stimulus-related activity. Nevertheless, analysis of the post-stimulus latency and time histograms for multi-unit activity showed responses in layer V of the principal and neighboring barrels as well as in the surrounding septal regions. Contralateral whisker stimulation resulted in a greater receptive field of the cells in layer V than that of the cells in layer IV (Wright and Fox, 2010).
Our results clearly show that 2DG incorporation in layer Vb and VI was targeted onto the area homotopic to the stimulated contralateral cortical representation of the stroked ipsilateral whiskers’ row B (Figure 3). This result may seem contrary to the cellular recordings reported by Wright and Fox (2010), who showed lower septum/barrel response differentiation in layer Vb than in Va; however, since they focused on contralateral responses while we applied ipsilateral whiskers stimulation, there is no contradiction. Moreover, Wright and Fox (2010) recordings showed a lower range of differences than the 2DG-incorporation detection threshold.
The homotopic characteristic of the transcallosal connections in the subgranular layers of the BF was described by Chovsepian et al. (2017). This characteristic corresponds in our study to the hyperactivity of layer Vb of row B representation, which changed smoothly to encompass the entire BF activation in layer Va (Figures 4, 5). It is also compatible with the time points of responses recorded by Wright and Fox (2010), who showed 0.7 ms earlier activation in layer Vb than in Va. This suggests that incoming transcallosal information was first transmitted to the homotopic area of layer Vb, and was later distributed to the entire BF in the Va layer via one or two synapses. Since most sensory areas propagate their callosal connections to layer V (Decosta-Fortune et al., 2015) this could reflect the transfer of incoming information from the stimulated hemisphere to the contralateral one, which influence the propensity of the contralateral homotopic areas to respond to any other incoming activation. A hypothetical reconstruction of this processes in presented in Figure 5.
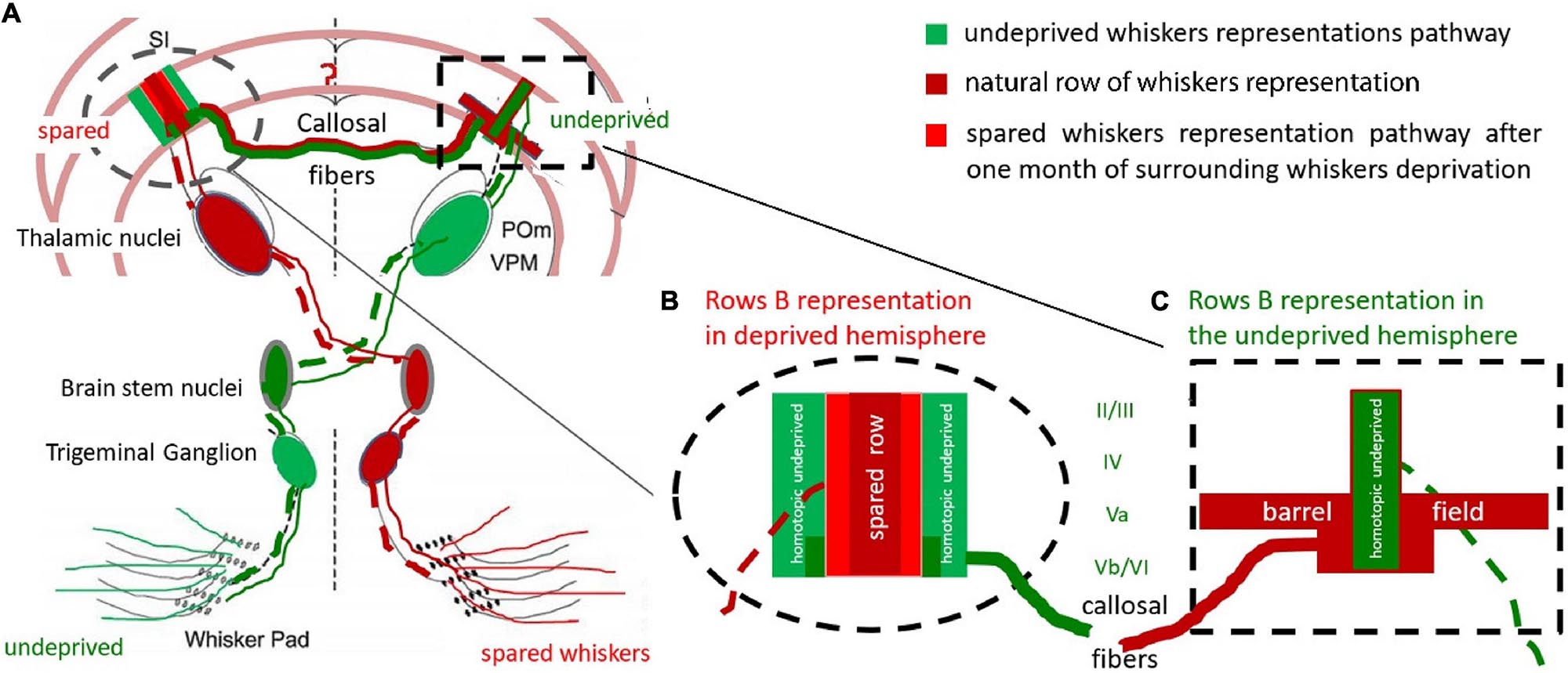
Figure 5. Hypothetical reconstruction of the ExDP in the cortex of BF. (A) Whiskers-BF pathway. The cortical row of whiskers’ representations shows the profile of the activation perpendicular to the row during bilateral whiskers stimulation of spared and homotopic contralateral row of whiskers; (B) cortical representation of a spared row of whiskers. (C) BF with contralateral, homotopic to the spared row of whiskers, row B cortical representation (green), and spread activation in response to ipsilateral spared whiskers stimulation (red); Red: response to unilateral, spared whiskers’ stimulation, from the deprived whisker pad; Dark red: response typical to the stimulation of undeprived row of whiskers (when the whisker pad is intact); Green: undeprived whiskers activity effects in deprived animals during bilateral homotopic rows stimulation (the undeprived and spared one). The homotopic undeprived row B stimulation effect on (the green on Figure 5B) is supposedly the transcallosal inhibition from activated during the deprivation period homotopic to the spared one row of whiskers. dashed line – contralateral whiskers-BF pathway; solid line – ipsilateral pathway; POm – Posteromedial nucleus; VPM – ventroposteromedial nucleus. Adapted from Shuler et al., 2001.
The experience-dependent plasticity we observed is probably the consequence of the lack of counterbalancing equilibria of the circuits in the deprived hemisphere (Barnes et al., 2015; Iwamura et al., 2001). This may explain why only the areas included in the spared row whiskers’ representation were susceptible to type of the stimulation in deprived animals. The ipsilateral response observed in layer V provides additional support to the suggestion that there is ongoing interhemispheric cooperation during unilateral whiskers stimulation (Figure 5). Although we did not observe any differences in the undeprived hemisphere, SII may participate in the interhemispheric integration of plasticity changes (Debowska et al., 2011). We demonstrated that the effect of experience-dependent plasticity in SI, which is visualized by 2DG might be mainly the result of modified interhemispheric interactions. The profile of the 2DG incorporation shows that layer V participates in the integration of incoming sensory information, although our results cannot prove that the ipsilateral responses were modified by experience-dependent plasticity.
Although the effects of the feedback relations between the hemispheres require additional exploration, our results clearly show that the responses of the stimulated ipsilateral whiskers participate in experience-dependent rearrangement of cortical map representations most probably via the contralateral hemisphere inhibitory feedback loops (see Palmer et al., 2013; Kokinovic and Medini, 2018). Part of the enlargement would be a result of the direct enlargement of the area processing the incoming stimulation – the area which belongs to the cortical representation of the spared whiskers – and the rest could be due the inhibitory activities around it (Sachdev et al., 2012), which result from the feadback of ipsilateral homotopic to the deprived cortex activity (Figure 5). It seems that both this parts undergow rearangemant after partial whiskers deprivation.
In the visual cortex monocular deprivation results in increase of GABAB inhibition (Kokinovic and Medini, 2018) and since the inhibition is greater and imbalanced only in binocular cortical areas (Iurilli et al., 2012) it has been suggested that a switch from interocular to interhemispheric suppression is crucial in the ocular dominance changes induced by MD (Pietrasanta et al., 2014). The neighboring rows of barrels may represent a similar condition for the layer four neurons and therefore we conclude the observed widening of increased activation in response to the spared row stimulation may be accompanied by the interhemispheric inhibition increase, observed only when bilateral stimulation is applied. Additional studies of the electrophysiological properties of the responses are required to determine the nature and location of the inhibitory or excitatory activity involved and the time-scale of the response. Neverthelss, our results support the idea that cortical plasticity is sensitive to bilateral interactions, and is probably the outcome of the counterbalancing effects of interhemispheric activity. Therefore there is nothing like “control hemisphere” since any plastic changes in one hemisphere have to have implications for the functioning of the opposite one. The invovlement of hemispheric interactions in cortical map rearragement has far reaching implications for rehabiliation therapies following unilateral injuries like strokes (Dietrich et al., 1986; Fernández et al., 2003; Hlushchuk and Hari, 2006; Turco et al., 2019).
It is already well known that ipsilateral stimulation in patients after stroke inhibits the injured cortex rehabilitation due to callosal inhibition from the overactivated intact hemisphere (Wang et al., 2012). However, the fact that the contralateral hemisphere participates in intact cortex plasticity in the context of the barrel field is a new phenomenon. On humans so far it was shown that unilateral application of cathodal direct current improved visual acuity in amblyopic patients (Bocci et al., 2018). Probably this effect was caused by cortical plasticity modifying ocular dominance via transcallosal disinhibition. It may be also connected to another similar characteristic of brain functioning - the interhemispheric integration during the cortical map reorganization. If it were confirmed on humans that both hemispheres participate in unilaterally induced plasticity changes and that the interhemispheric interactions are crucial for its arrangement, this would have an important theaupathic implications.
Data Availability Statement
The raw data supporting the conclusions of this article will be made available by the authors, without undue reservation.
Ethics Statement
The animal study was reviewed and approved by the local Ethical Committee for Animal Experiments in Warsaw (The I Lokalna Komisja Etyczna ds. Doświadczeń na Zwierzętach w Warszawie) with the agreement number 270/2012; 15/03/2012.
Author Contributions
JJ: experimental project, 2DG brain mapping and histologial staining performance, data acquisition and analysis, manuscript preparation. RB: optical density measurement analysis. MK: methodological set up of the 2DG brain mapping. MS: histological staining, data acquisition, data analysis and preparation for the manuscript. WS: statistics and data analysis. AS: experimental methodological set up and data acquisition and preparation for the manuscript. PU: data analysis and statistic. All authors contributed to the article and approved the submitted version.
Funding
JJ and collaborators were funded by grant: MNiSW IP2011 018471.
Conflict of Interest
The authors declare that the research was conducted in the absence of any commercial or financial relationships that could be construed as a potential conflict of interest.
Acknowledgments
We would like to thank Prof. Piotr Bembas for supporting our experimental work at the University, and Prof. Malgorzata Kossut for supporting the scientific development, and Dr. Katarzyna Kisiel with animal house staff for excellent care for our rats from this project.
Supplementary Material
The Supplementary Material for this article can be found online at: https://www.frontiersin.org/articles/10.3389/fnins.2021.631328/full#supplementary-material
References
Alloway, K. D. (2008). Information processing streams in rodent barrel cortex : the differential functions of barrel and septal circuits. Cereb Cortex 18, 979–989. doi: 10.1093/cercor/bhm138
Banich, M. (1995). “Interhemispheric processing: theoretical considerations and empirical approaches,” in Brain Asymmetry, eds R. J. Davidson and K. Hugdahl (Cambridge, MA: The MIT Press), 427–450.
Barnes, S. J., Cheetham, C. E., Liu, Y., Bennett, S. H., Albieri, G., Jorstad, A. A., et al. (2015). Delayed and temporally imprecise neurotransmission in reorganizing cortical microcircuits. J. Neurosci. 35, 9024–9037. doi: 10.1523/JNEUROSCI.4583-14.2015
Bloom, J. S., and Hynd, G. W. (2005). The role of the corpus callosum in interhemispheric transfer of information: excitation or inhibition? Neuropsychol. Rev. 15, 59–71. doi: 10.1007/s11065-005-6252-y
Bocci, T., Nasini, F., Caleo, M., Restani, L., Barloscio, D., Ardolino, G., et al. (2018). Unilateral application of cathodal tDCS reduces transcallosal inhibition and improves visual acuity in amblyopic patients. Front. Behav. Neurosci. 12:109. doi: 10.3389/fnbeh.2018.00109
Carvell, G. E., and Simons, D. J. (1987). Thalamic and corticocortical connections of the second somatic sensory area of the mouse. The Journal of comparative neurology, 265, 409–427. doi: 10.1002/cne.902650309
Cauller, L. J., Clancy, B., and Connors, B. W. (1998). Backward cortical projections to primary somatosensory cortex in rats extend long horizontal axons in layer I. J. Comp. Neurol. 390, 297–310.
Celikel, T., Szostak, V. A., and Feldman, D. E. (2004). Modulation of spike timing by sensory deprivation during induction of cortical map plasticity. Nat. Neurosci. 7, 534–542. doi: 10.1038/nn1222
Chmielowska, J., Kossut, M., and Chmielowski, M. (1986). Single vibrissal column in a mouse labeled with 2-deoxyglucose. Exp. Brain Res. 63, 607–619.
Chovsepian, A., Empl, L., Correa, D., and Bareyre, F. M. (2017). Heterotopic transcallosal projections are present throughout the mouse cortex. Front. Cell Neurosci. 11:36. doi: 10.3389/fncel.2017.00036
Chung, J.-Y., Kim, M.-W., Bang, M.-S., and Kim, M. (2013). Increased expression of neurotrophin 4 following focal cerebral ischemia in adult rat brain with treadmill exercise. PLoS One 8:e52461. doi: 10.1371/journal.pone.0052461
Clarke, J. M., and Zaidel, E. (1994). Anatomical-behavioral relationships: corpus callosum morphometry and hemispheric specialization. Behav. Brain Res. 64, 185–202. doi: 10.1016/0166-4328(94)90131-7
Cooke, S. F., and Bear, M. F. (2013). How the mechanisms of long-term synaptic potentiation and depression serve experience-dependent plasticity in primary visual cortex. Philos. Trans. R. Soc. Lond. B Biol. Sci. 369:20130284. doi: 10.1098/rstb.2013.0284
Debowska, W., Liguz-Lecznar, M., and Kossut, M. (2011). Bilateral plasticity of vibrissae SII representation induced. J. Neurosci. 31, 5447–5453. doi: 10.1523/JNEUROSCI.5989-10.2011
Decosta-Fortune, T. M., Li, C. X., de Jongh Curry, A. L., and Waters, R. S. (2015). Differential pattern of interhemispheric connections between homotopic layer V regions in the forelimb representation in rat barrel field cortex. Anat. Rec. 298, 1885–1902. doi: 10.1002/ar.23262
Diamond, M. E., Armstrong-James, M., and Ebner, F. F. (1993). Experience-dependent plasticity in adult rat barrel cortex. Proc. Natl. Acad. Sci. U.S.A. 90, 2082–2086. doi: 10.1073/pnas.90.5.2082
Dietrich, W. D., Ginsberg, M. D., Busto, R., and Smith, D. W. (1985). Metabolic alterations in rat somatosensory cortex following unilateral vibrissal removal. J. Neurosci. 5, 874–880. doi: 10.1523/JNEUROSCI.05-04-00874.1985
Dietrich, W. D., Ginsberg, M. D., Busto, R., and Watson, B. D. (1986). Photochemically induced cortical infarction in the rat. 2. acute and subacute alterations in local glucose utilization. J. Cereb. Blood Flow Metab. 6, 195–202. doi: 10.1038/jcbfm.1986.32
Efron, B. (1979). Bootstrap methods: another look at the jackknife. Ann. Statist. 7, 1–26. doi: 10.1214/aos/1176344552
Fabri, M., Polonara, G., Salvolini, U., and Manzoni, T. (2005). Bilateral cortical representation of the trunk midline in human first somatic sensory area. Human brain mapping, 25, 287–296. doi: 10.1002/hbm.20099
Feldmeyer, D. (2012). Excitatory neuronal connectivity in the barrel cortex. Front. Neuroanat. 6:24. doi: 10.3389/fnana.2012.00024
Fernández, G., Weis, S., Stoffel-Wagner, B., Tendolkar, I., Reuber, M., Beyenburg, S., et al. (2003). Menstrual cycle-dependent neural plasticity in the adult human brain is hormone, task, and region specific. J. Neurosci. 23, 3790–3795. doi: 10.1523/JNEUROSCI.23-09-03790.2003
Fox, K. (1994). The cortical component of experience-dependent synaptic plasticity in the rat barrel cortex. J. Neurosci. 14, 7665–7679. doi: 10.1523/jneurosci.14-12-07665.1994
Fusco, F. R., Zuccato, C., Tartari, M., Martorana, A., De March, Z., Giampà, C., et al. (2003). Co-localization of brain-derived neurotrophic factor (BDNF) and wild-type huntingtin in normal and quinolinic acid-lesioned rat brain. Eur. J. Neurosci. 18, 1093–1102. doi: 10.1046/j.1460-9568.2003.02844.x
Glazewski, S., Benedetti, B. L., and Barth, A. L. (2007). Ipsilateral whiskers suppress experience-dependent plasticity in the barrel cortex. J. Neurosci. 27, 3910–3920. doi: 10.1523/JNEUROSCI.0181-07.2007
Harris, J. A., and Diamond, M. E. (2000). Ipsilateral and contralateral transfer of tactile learning. Neuroreport, 11, 263–266. doi: 10.1097/00001756-200002070-00008
Hellige, J. B. (1993). Hemispheric Asymmetry: What’s Right and What ‘s Left. Cambridge, MA: Harvard University Press.
Hlushchuk, Y., and Hari, R. (2006). Transient suppression of ipsilateral primary somatosensory cortex during tactile finger stimulation. J. Neurosci. 26, 5819–5824. doi: 10.1523/JNEUROSCI.5536-05.2006
Huang, Y., Song, N. N., Lan, W., Zhang, Q., Zhang, L., Zhang, L., et al. (2013). Sensory input is required for callosal axon targeting in the somatosensory cortex. Mol. Brain 6:53. doi: 10.1186/1756-6606-6-53
Hutson, K. A., and Masterton, R. B. (1986). The sensory contribution of a single vibrissa’s cortical barrel. Journal of neurophysiology, 56, 1196–1223. doi: 10.1152/jn.1986.56.4.1196
Innocenti, G. M. (2009). Dynamic interactions between the cerebral hemispheres. Exp. Brain Res. 192, 417–423. doi: 10.1007/s00221-008-1484-8
Iurilli, G., Benfenati, F., and Medini, P. (2012). Loss of visually driven synaptic responses in layer 4 regular-spiking neurons of rat visual cortex in absence of competing inputs. Cerebral cortex 22, 2171–2181. doi: 10.1093/cercor/bhr304
Iwamura, Y. (2000). Bilateral receptive field neurons and callosal connections in the somatosensory cortex. Philosophical transactions of the Royal Society of London. Series B, Biological sciences, 355, 267–273. doi: 10.1098/rstb.2000.0563
Iwamura, Y., Taoka, M., and Iriki, A. (2001). Bilateral activity and callosal connections in the somatosensory cortex. Neuroscientist 7, 419–429. doi: 10.1177/107385840100700511
Jablonka, J. A., Kossut, M., Witte, O. W., and Liguz-Lecznar, M. (2012). Experience-dependent brain plasticity after stroke: effect of ibuprofen and poststroke delay. Eur. J. Neurosci. 36, 2632–2639. doi: 10.1111/j.1460-9568.2012.08174.x
Jablonka, J. A., Witte, O. W., and Kossut, M. (2007). Photothrombotic infarct impairs experience-dependent plasticity in neighboring cortex. Neuroreport 18, 165–169. doi: 10.1097/WNR.0b013e328010feff
Kaliszewska, A., Bijata, M., Kaczmarek, L., and Kossut, M. (2012). Experience-dependent plasticity of the barrel cortex in mice observed with 2-DG brain mapping and c-Fos: effects of MMP-9 KO. Cerebral cortex (New York, NY: 1991), 22, 2160–2170. doi: 10.1093/cercor/bhr303
Kennedy, C., Des Rosiers, M. H., Jehle, J. W., Reivich, M., Sharpe, F., and Sokoloff, L. (1975). Mapping of functional neural pathways by autoradiographic survey of local metabolic rate with (14C)deoxyglucose. Science 187, 850–853. doi: 10.1126/science.1114332
Kim, U., and Ebner, F. F. (1999). Barrels and septa: separate circuits in rat barrels field cortex. J. Comp. Neurol. 408, 489–505. doi: 10.1002/(SICI)1096-9861(19990614)408:4<489::AID-CNE4>3.0.CO;2-E
Kobayashi, M., and Pascual-Leone, A. (2003). Transcranial magnetic stimulation in neurology. Lancet Neurol. 2, 145–156. doi: 10.1016/s1474-4422(03)00321-1
Kokinovic, B., and Medini, P. (2018). Loss of GABAB -mediated interhemispheric synaptic inhibition in stroke periphery. J. Physiol. 596, 1949–1964. doi: 10.1113/JP275690
Koralek, K. A., Olavarria, J., and Killackey, H. P. (1990). Areal and laminar organization of corticocortical projections in the rat somatosensory cortex. J. Comp. Neurol. 299, 133–150. doi: 10.1002/cne.902990202
Kossut, M., Hand, P. J., Greenberg, J., and Hand, C. L. (1988). Single vibrissal cortical column in SI cortex of rat and its alterations in neonatal and adult vibrissa-deafferented animals: a quantitative 2DG study. J. Neurophysiol. 60, 829–852. doi: 10.1152/jn.1988.60.2.829
Krubitzer, L. A., and Kaas, J. H. (1990). The organization and connections of somatosensory cortex in marmosets. J. Neurosci. 10, 952–974. doi: 10.1523/JNEUROSCI.10-03-00952.1990
Li, L., Rema, V., and Ebner, F. F. (2005). Chronic suppression of activity in barrel field cortex downregulates sensory responses in contralateral barrel field cortex. J. Neurophysiol. 94, 3342–3356. doi: 10.1152/jn.00357.2005
McCasland, J. S., and Woolsey, T. A. (1988). High-resolution 2-deoxyglucose mapping of functional cortical columns in mouse barrel cortex. J. Comp. Neurol. 278, 555–569. doi: 10.1002/cne.902780407
Meissirel, C., Dehay, C., Berland, M., and Kennedy, H. (1991). Segregation of callosal and association pathways during development in the visual cortex of the primate. The Journal of neuroscience: the official journal of the Society for Neuroscience, 11, 3297–3316. doi: 10.1523/JNEUROSCI.11-11-03297.1991
Olavarria, J., Van Sluyters, R. C., and Killackey, H. P. (1984). Evidence for the complementary organization of callosal and thalamic connections within rat somatosensory cortex. Brain Res. 291, 364–368. doi: 10.1016/0006-8993(84)91270-8
Palmer, L. M., Schulz, J. M., and Larkum, M. E. (2013). Layer-specific regulation of cortical neurons by interhemispheric inhibition. Commun. Integr. Biol. 6:e23545. doi: 10.4161/cib.23545
Pietrasanta, M., Restani, L., Cerri, C., Olcese, U., Medini, P., and Caleo, M. (2014). A switch from inter-ocular to inter-hemispheric suppression following monocular deprivation in the rat visual cortex. Eur. J. Neurosci. 40, 2283–2292. doi: 10.1111/ejn.12573
Ragert, P., Nierhaus, T., Cohen, L. G., and Villringer, A. (2011). Interhemispheric interactions between the human primary somatosensory cortices. PLoS One 6:e16150. doi: 10.1371/journal.pone.0016150
Reed, J. L., Qi, H. X., and Kaas, J. H. (2011). Spatiotemporal properties of neuron response suppression in owl monkey primary somatosensory cortex when stimuli are presented to both hands. J. Neurosci. 31, 3589–3601.
Reed, J. L., Qi, H. X., Zhou, Z., Bernard, M. R., Burish, M. J., Bonds, A. B., et al. (2010). Response properties of neurons in primary somatosensory cortex of owl monkeys reflect widespread spatiotemporal integration. J. Neurophysiol. 103, 2139–2157. doi: 10.1152/jn.00709.2009
Renier, N., Dominici, C., Erzurumlu, R. S., Kratochwil, C. F., Rijli, F. M., Gaspar, P., et al. (2017). A mutant with bilateral whisker to barrel inputs unveils somatosensory mapping rules in the cerebral cortex. ELife 6:e23494. doi: 10.7554/eLife.23494
Sachdev, R. N., Krause, M. R., and Mazer, J. A. (2012). Surround suppression and sparse coding in visual and barrel cortices. Front. Neural Circuits 6:43. doi: 10.3389/fncir.2012.00043
Shuler, M. G., Krupa, D. J., and Nicolelis, M. A. (2001). Bilateral integration of whisker information in the primary somatosensory cortex of rats. J. Neurosci. 21, 5251–5261. doi: 10.1523/JNEUROSCI.21-14-05251.2001
Simons, D. J., and Woolsey, T. A. (1979). Functional organization in mouse barrel cortex. Brain research, 165, 327–332. doi: 10.1016/0006-8993(79)90564-x
Siucinska, E., and Kossut, M. (2004). Experience-dependent changes in cortical whisker representation in the adult mouse: a 2-deoxyglucose study. Neuroscience 127, 961–971. doi: 10.1016/j.neuroscience.2004.06.004
Smith, R. L. (1973). The ascending fiber projections from the principal sensory trigeminal nucleus in the rat. J. Comp. Neurol. 148, 423–445. doi: 10.1002/cne.901480403
Sokoloff, L., Reivich, M., Kennedy, C., Des Rosiers, M. H., Patlak, C. S., Pettigrew, K. D., et al. (1977). The [14C] deoxyglucose method for the measurement of local cerebral glucose utilization: theory, procedure, and normal values in the conscious and anesthetized albino rat. J. Neurochem. 28, 897–916. doi: 10.1111/j.1471-4159.1977.tb10649.x
Sun, M. C., Honey, C. R., Berk, C., Wong, N. L., and Tsui, J. K. (2003). Regulation of aquaporin-4 in a traumatic brain injury model in rats. J. Neurosurg. 98, 565–569. doi: 10.3171/jns.2003.98.3.0565
Tame, L., Braun, C., Holmes, N. P., Farnè, A., and Pavani, F. (2016). Bilateral representations of touch in the primary somatosensory cortex. Cogn. Neuropsychol. 33, 48–66. doi: 10.1080/02643294.2016.1159547
Turco, C. V., Fassett, H. J., Locke, M. B., El-Sayes, J., and Nelson, A. J. (2019). Parallel modulation of interhemispheric inhibition and the size of a cortical hand muscle representation during active contraction. J. Neurophysiol. 122, 368–377. doi: 10.1152/jn.00030.2019
Wang, L. E., Tittgemeyer, M., Imperati, D., Diekhoff, S., Ameli, M., Fink, G. R., et al. (2012). Degeneration of corpus callosum and recovery of motor function after stroke: a multimodal magnetic resonance imaging study. Hum. Brain Mapp. 33, 2941–2956. doi: 10.1002/hbm.21417
White, E. L., and DeAmicis, R. A. (1977). Afferent and efferent projections of the region in mouse SmL cortex which contains the posteromedial barrel subfield. J. Comp. Neurol. 175, 455–482. doi: 10.1002/cne.901750405
Wiest, M. C., Bentley, N., and Nicolelis, M. A. (2005). Heterogeneous integration of bilateral whisker signals by neurons in primary somatosensory cortex of awake rats. J. Neurophysiol. 93, 2966–2973. doi: 10.1152/jn.00556.2004
Keywords: cortical plasticity, barrel field, 2DG, 2-deoxy-D-glucose, interhemispheric
Citation: Jablonka JA, Binkowski R, Kazmierczak M, Sadowska M, Sredniawa W, Szlachcic A and Urban P (2021) The Role of Interhemispheric Interactions in Cortical Plasticity. Front. Neurosci. 15:631328. doi: 10.3389/fnins.2021.631328
Received: 19 November 2020; Accepted: 18 May 2021;
Published: 09 July 2021.
Edited by:
Claudia Lunghi, UMR 8248 Laboratoire des Systèmes Perceptifs, FranceReviewed by:
Laura Restani, Institute of Neuroscience, National Research Council (CNR), ItalyTommaso Bocci, University of Milan, Italy
Copyright © 2021 Jablonka, Binkowski, Kazmierczak, Sadowska, Sredniawa, Szlachcic and Urban. This is an open-access article distributed under the terms of the Creative Commons Attribution License (CC BY). The use, distribution or reproduction in other forums is permitted, provided the original author(s) and the copyright owner(s) are credited and that the original publication in this journal is cited, in accordance with accepted academic practice. No use, distribution or reproduction is permitted which does not comply with these terms.
*Correspondence: Jan Antoni Jablonka, ja.jablonka@uw.edu.pl