- 1Center for Neuroscience, Swammerdam Institute for Life Sciences, University of Amsterdam, Amsterdam, Netherlands
- 2Department of Basic and Clinical Neuroscience, Institute of Psychiatry, Psychology and Neuroscience, King’s College London, London, United Kingdom
There are sex differences in metabolic disease risk, and early-life stress (ES) increases the risk to develop such diseases, potentially in a sex-specific manner. It remains to be understood, however, how sex and ES affect such metabolic vulnerability. The hypothalamus regulates food intake and energy expenditure by sensing the organism’s energy state via metabolic hormones (leptin, insulin, ghrelin) and nutrients (glucose, fatty acids). Here, we investigated if and how sex and ES alter hypothalamic nutrient sensing short and long-term. ES was induced in mice by limiting the bedding and nesting material from postnatal day (P)2-P9, and the expression of genes critical for hypothalamic nutrient sensing were studied in male and female offspring, both at P9 and in adulthood (P180). At P9, we observed a sex difference in both Ppargc1a and Lepr expression, while the latter was also increased in ES-exposed animals relative to controls. In adulthood, we found sex differences in Acacb, Agrp, and Npy expression, whereas ES did not affect the expression of genes involved in hypothalamic nutrient sensing. Thus, we observe a pervasive sex difference in nutrient sensing pathways and a targeted modulation of this pathway by ES early in life. Future research is needed to address if the modulation of these pathways by sex and ES is involved in the differential vulnerability to metabolic diseases.
Introduction
The prevalence of obesity and the related chronic diseases rise, and postulate a global health concern (Malik et al., 2013). While both men and women are affected, there is a differential risk to develop metabolic diseases between sexes (Kanter and Caballero, 2012; Kautzky-Willer et al., 2016). Worldwide, more women than men are obese, although the prevalence of overweight and obesity among men and women vastly fluctuates between countries (Kanter and Caballero, 2012). Under healthy bodyweight conditions, men and women differ in fat quantity and distribution (Power and Schulkin, 2008; Fuente-Martín et al., 2013), and rodent studies show for example that males and females differently respond to high-fat diets (Grove et al., 2010; Estrany et al., 2011, 2013; Garg et al., 2011).
Next to sex as determinant of metabolic vulnerability, there is also evidence that the perinatal environment is critical for establishing a metabolic set point defining later-life metabolic health (Bouret and Simerly, 2006; Levin, 2006; Bouret, 2009; Yam et al., 2015, 2017a). Exposure to stress during this sensitive developmental period (e.g., neglect or abuse) increases the risk to develop metabolic diseases, including obesity and diabetes, later in life (Alciati et al., 2013; Danese and Tan, 2014; Basu et al., 2017). Indeed, we have previously shown in mice that early-life stress (ES)-exposed males and females have a leaner phenotype when fed a (healthy) standard chow diet, but that ES-exposed males accumulated more fat when later fed a western-style diet (WSD) with a moderate fat content, while how females respond to such a diet was not investigated in this study (Yam et al., 2017a). There is in fact increasing evidence that the effects of ES may be sexually dimorphic (Boynton-Jarrett et al., 2010; Kozyrskyj et al., 2011; Murphy et al., 2018; Park et al., 2018). However, the mechanisms contributing to the sex and ES-induced impacts on metabolic regulation are not well understood.
The arcuate nucleus of the hypothalamus (ARH) regulates energy homeostasis by controlling food intake, energy expenditure and glucose metabolism, and it has been proposed that its development is important for the establishment of the metabolic setpoint (Bouret and Simerly, 2006; Bouret, 2009, 2012; Dearden and Ozanne, 2015; Ramamoorthy et al., 2015). The ARH integrates a wide range of inputs, including those from metabolic hormones and peripheral nutrients (Lam et al., 2005; Timper and Brüning, 2017). The key neuropeptides regulating food intake in the ARH are agouti-related protein (AgRP) and neuropeptide Y (NPY), which stimulate food intake while inhibiting energy expenditure, and proopiomelanocortin (POMC), which has opposite regulatory effects (Barsh and Schwartz, 2002; Timper and Brüning, 2017). Metabolic hormones, including leptin, ghrelin and insulin, act on ARH neurons by binding to their respective receptors. Leptin is an adipokine secreted by the white adipose tissue, which, via binding to its receptor (LepR) inhibits food intake (Friedman, 2014). Insulin, via binding to the insulin receptor (InsR), has a comparable effect as leptin on food intake (Porte and Woods, 1981; Könner et al., 2009), whereas ghrelin (primarily secreted by the stomach) binds to the growth hormone secretagogue receptor (GHSR) (Gil-Campos et al., 2006), and stimulates food intake and bodyweight gain (Wren et al., 2001). Importantly, we have shown before that ES reduced circulating leptin levels and leptin expression in the adipose tissue, while increasing Lepr expression in the choroid plexus, which is an important side of leptin entry into the brain (Zlokovic et al., 2000), in both males and females when fed standard chow diet (Yam et al., 2017a). ES furthermore affected circulating ghrelin levels in an age-dependent manner specifically in females, while altering the fiber density of NPY and AgRP in both males and females at postnatal day (P)14, i.e., when the hypothalamus is fully developed (Yam et al., 2017b).
As mentioned above, next to the hormonal regulation of food intake, circulating nutrients [e.g., glucose, fatty acids (FA)] are sensed in the hypothalamus and provide information about the energy state (Matzinger et al., 2000; Lam et al., 2005; Migrenne et al., 2006; Rijnsburger et al., 2016; Le Foll, 2019). More specifically, FA metabolism in the hypothalamus is suggested to function as sensor for nutrient/energy availability integrating both nutritional and hormonal signals. The intracellular pool of long-chain FAs depends on both biosynthetic and oxidative pathways (breakdown of FAs) (Lam et al., 2005) and thought to signal energy abundance (Lam et al., 2005; Dragano et al., 2020). Indeed, FA administration has been shown to suppress Npy and Agrp expression in vitro (Ma et al., 2017), and in vivo experiments showed that intracerebroventricular FA administration inhibits food intake and Npy expression (Obici et al., 2002). Furthermore, manipulating key proteins involved in both FA synthesis and oxidation (e.g., FASN, CPT1, and ACC) centrally affects food intake and bodyweight (Loftus et al., 2000; Shimokawa et al., 2002; Obici et al., 2003; Cesquini et al., 2008; Jo et al., 2009), and central peroxisome proliferator–activated receptors (PPARs), a family of transcription factors regulating lipid and glucose metabolism, have also been shown to modulate food intake and fat mass (Lu et al., 2011; Ryan et al., 2011; Kocalis et al., 2012). Importantly, glucose, leptin, ghrelin and insulin also affect FA metabolism pathways in the hypothalamus (Lam et al., 2005; Gao et al., 2007; López et al., 2008; Lage et al., 2010; Diéguez et al., 2011; Taïb et al., 2013). Thus, hypothalamic FA metabolism integrates nutrient and hormonal signals and affects hypothalamic neuropeptide expression and food intake.
Yet, it remains to be determined if and how sex and ES impact hypothalamic neuropeptide systems and nutrient sensing pathways. Therefore, we here investigated the gene expression of hypothalamic neuropeptides, hormone receptors and fatty acid metabolism at P9 and P180 in male and female control (CTL) and ES-exposed mice. We studied this in the cohort of mice that we used to report on the metabolic effects of ES in males and females (Yam et al., 2017a), giving us the opportunity to link these gene expression profiles to their metabolic phenotype. We show pervasive sex differences in nutrient sensing pathways and, early in life, a targeted modulation of these pathways by ES.
Materials and Methods
Animals and Breeding
In total 17 male and 14 female pups, and 17 male and 13 female adult mice were used for this study. Animals were kept under standard housing conditions (temperature 20–22°C, 40–60% humidity, 12/12h light/dark schedule), and fed standard chow (CRM (P), 801722, Standard Diets Services, Essex, United Kingdom, 3.585 kcal/g, with 22% protein, 9% fat, and 69% carbohydrates) and water provided ad libitum. All experimental procedures were conducted under national law and European Union directives on animal experiments, and were approved by the animal welfare committee of the University of Amsterdam.
To standardize the perinatal environment, experimental animals were bred in house. For breeding, 8-week-old C57Bl/6J female and male mice were purchased from Envigo Laboratories B.V. (Venray, Netherlands), and allowed to habituate for 1–2 weeks. Next, two primiparous females were housed with one adult male to allow for mating. After 1 week, the male was removed and females were housed together for another week in a clean cage with nest material (square piece of cotton) to practice, and after another week, females were housed individually in a type II cage with nesting material and filtertop. Starting from 18 days after the start of the breeding, dams were checked for the birth of pups each morning before 09:00 a.m. When a litter was born, the previous day was designated as postnatal day 0.
ES Paradigm
The limited nesting and bedding material model was used to induce ES from P2 to P9, as described previously (Rice et al., 2008; Naninck et al., 2015). In short, litters were culled to 6 pups, including both males and females, and randomly allocated to the control (CTL) or ES condition at P2. Litters with less than 5 pups were excluded. CTL cages had a standard amount of sawdust and one square piece of cotton nesting material (5 × 5 cm, Tecnilab-BMI, Someren, The Netherlands), whereas ES cages had a thin layer of sawdust on the bottom, covered with a fine-gauge stainless steel mesh 1 cm above the bottom of the cage, and half a square piece of cotton nesting material (2.5 × 5 cm). All cages were covered with a filtertop. For the adult experiment, litters were moved to new cages containing standard amounts of sawdust at P9, and left undisturbed until weaning at P21, after which they were group-housed with same sex littermates.
Tissue Preparation
To study the direct effects of ES on nutrient sensing pathways in male and female offspring, pups were sacrificed in the morning of P9 by rapid decapitation. For adult studies, animals were fasted for 4 hours and sacrificed by rapid decapitation at P180. The hypothalamus was rapidly dissected, snap frozen and stored at −80°C. RNA was obtained using Trizol (Invitrogen, Carlsbad, CA, United States) according to manufacturer’s instructions and stored at −80°C until further use. Reverse transcription of RNA into cDNA was done with SuperScript II Reverse Transcriptase (Invitrogen, Carlsbad, CA, United States), and cDNA was stored at −20°C until further analysis.
Real-Time PCR
Relative gene expression was measured using a 7500 Real-time PCR system (Applied Biosystems, Foster City, CA, United States). In short, for all genes with exception of the leptin receptor (see below), hot FirePol EvaGreen Mastermix (Solis Biodyne, Tartu, Estonia) was used. Primers (Eurogentec, Liege, Belgium) of target and reference genes all had an efficiency between 90 and 110% (Supplementary Table 1). Gene specific forward and reverse primers together with cDNA template were added to the reaction mix according to manufacturer’s instructions. Cycling conditions were as follows: 15 min polymerase activation at 95°C, followed by 40 cycles of replication (15 s at 95°C, 20 s at 65°C, and 35 s at 72°C) and a dissociation program. For the quantification of the leptin receptor, Taqman® probes were used. TaqMan® Gene Expression Master Mix (4369016, Thermo Fisher Scientific, Waltham, MA, United States) was added to cDNA template and Lepr Taqman® probes (6749720_1, Thermo Fisher Scientific, Waltham, MA, United States), and the following cycling conditions were used: 2 min 50°C and 10 min 95°C for activation, followed by 40 cycles of replication (15 s at 95°C, 1 min at 60°C) and a dissociation program. Gene expression was calculated with the ΔΔCt method in Qbase+ (Biogazelle, Zwijnaarde, Belgium) and normalized for two reference genes (Rpl13a and Rplp0 for P9, Tbp and Tuba1a for P180) which were tested for stability.
Statistical Analysis
Data were analyzed with SPSS 25.0 (IBM software, Armonk, NY, United States), R studio 1.2.1335 (R Core Team, 2018) and Graphpad Prism 6 (Graphpad software, San Diego, CA, United States). All data are presented as mean ± standard error of the mean (SEM), and when p < 0.05, data was considered statistically significant. Gene expression data was scaled against the CTL male group, and log transformed for statistical analysis. Outliers were identified in SPSS and removed, and data was analyzed with a 2-way ANOVA with condition and sex as predictor variables. Multiple mice from the same litter were included in this study, and therefore data are nested. We verified if litter contributed to the outcome by performing mixed model analysis with litter as random factor. Litter did not affect any of the outcome variables. In addition, to test whether estrous cycle contributed to the variable outcomes, we performed mixed model with estrous cycle as random factor. Estrous cycle did not affect any of the outcomes. Total white adipose tissue levels (as percentage of bodyweight) and circulating leptin levels (previously published in Yam et al., 2017a) were correlated to hypothalamic gene expression levels with R studio software (version 1.2.1335) (R Core Team, 2018). Pearson correlations were calculated based on complete pairwise cases, and correlation coefficients were tested against critical values on a two-tailed distribution (alpha = 0.05).
Results
Effects of ES on Hypothalamic Gene Expression in Male and Female Offspring at P9
Figure 1A shows a full overview of the effects of ES and sex on hypothalamic gene expression. Among the genes that regulate fatty acid metabolism, Ppargc1a was higher in females compared to males (Figure 1B). Moreover, Lepr expression was higher in females, and lower in ES-exposed males and females compared to their respective controls (Figure 1C). The expression of other receptors and hypothalamic neuropeptides were not affected by sex or ES.
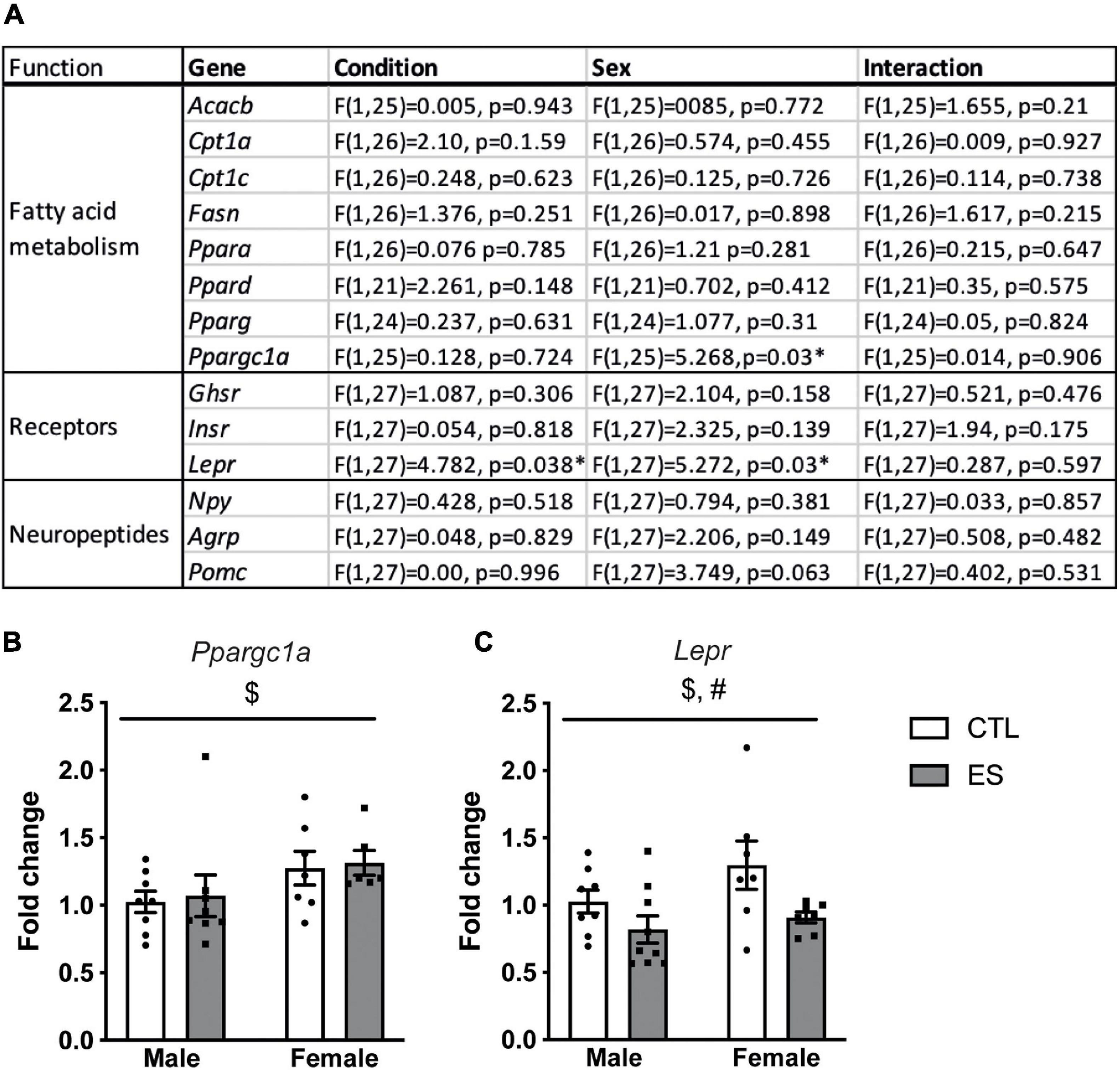
Figure 1. (A) Effects of ES and sex on hypothalamic gene expression at P9. (B) Hypothalamic Ppargc1a expression was higher in females. (C) Lepr expression was higher in females and lower in ES-exposed animals. Indicated is mean ± SEM, p < 0.05. # Main effect of condition; * = significant effect; $ main effect of sex.
Effects of ES on Hypothalamic Gene Expression in Male and Female Mice at P180
Figure 2A shows an overview of the effects of ES and sex on hypothalamic gene expression in adulthood (P180). Acacb expression was higher in females (Figure 2B). Moreover Agrp (Figure 2C) and Npy (Figure 2D) expression were higher in females compared to males. There were no effects of ES on the hypothalamic expression of hormone receptors, genes in fatty acid metabolism, or neuropeptides in adulthood.
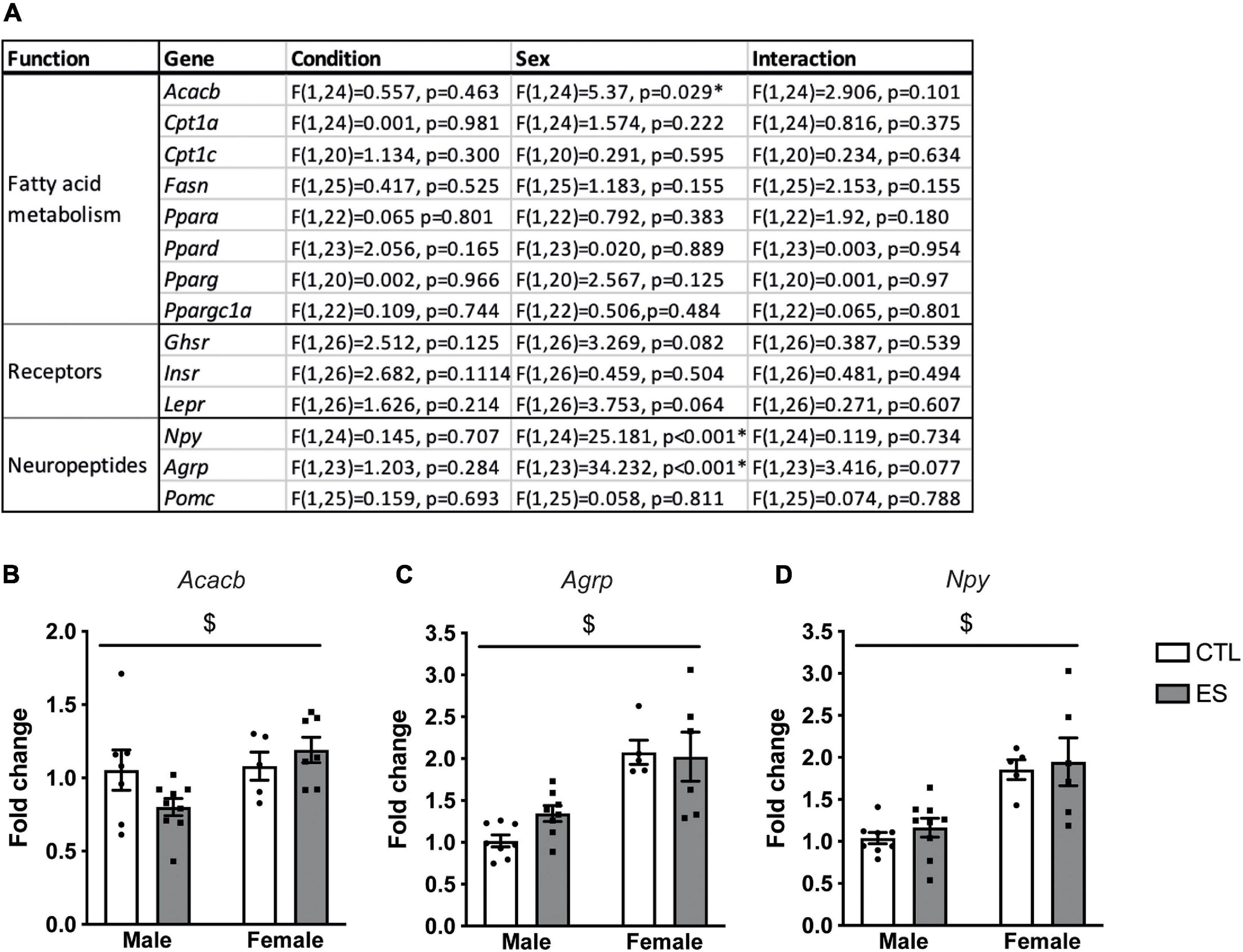
Figure 2. (A) Effects of ES and sex on hypothalamic gene expression in adulthood. (B) Acacb expression was higher in females. (C) Expression of Agrp was higher in females. (D) Npy expression was higher in females. Indicated is mean ± SEM, p < 0.05. * = significant effect; $ main effect of sex.
Hypothalamic Gene Expression Correlates With Metabolic Readouts in Adulthood
In the same cohort of mice as used in the current study, we previously reported that ES decreased white adipose tissue (WAT) levels at P9 and in adulthood, as well as reduced leptin expression and circulating leptin levels in both males and females (see Yam et al., 2017a). To explore whether hypothalamic gene expression is related to the metabolic profile, we correlated the genes that were significantly affected by either ES or sex with white adipose tissue (WAT) levels and circulating leptin levels (Figure 3A). At P9, Ppargc1a and LepR expression did not correlate with either WAT or leptin levels. In adulthood, Acacb expression correlated with leptin levels (Figure 3B), Agrp expression correlated with both WAT and leptin levels (Figures 3C,D), and Npy expression correlated with WAT levels (Figure 3E).
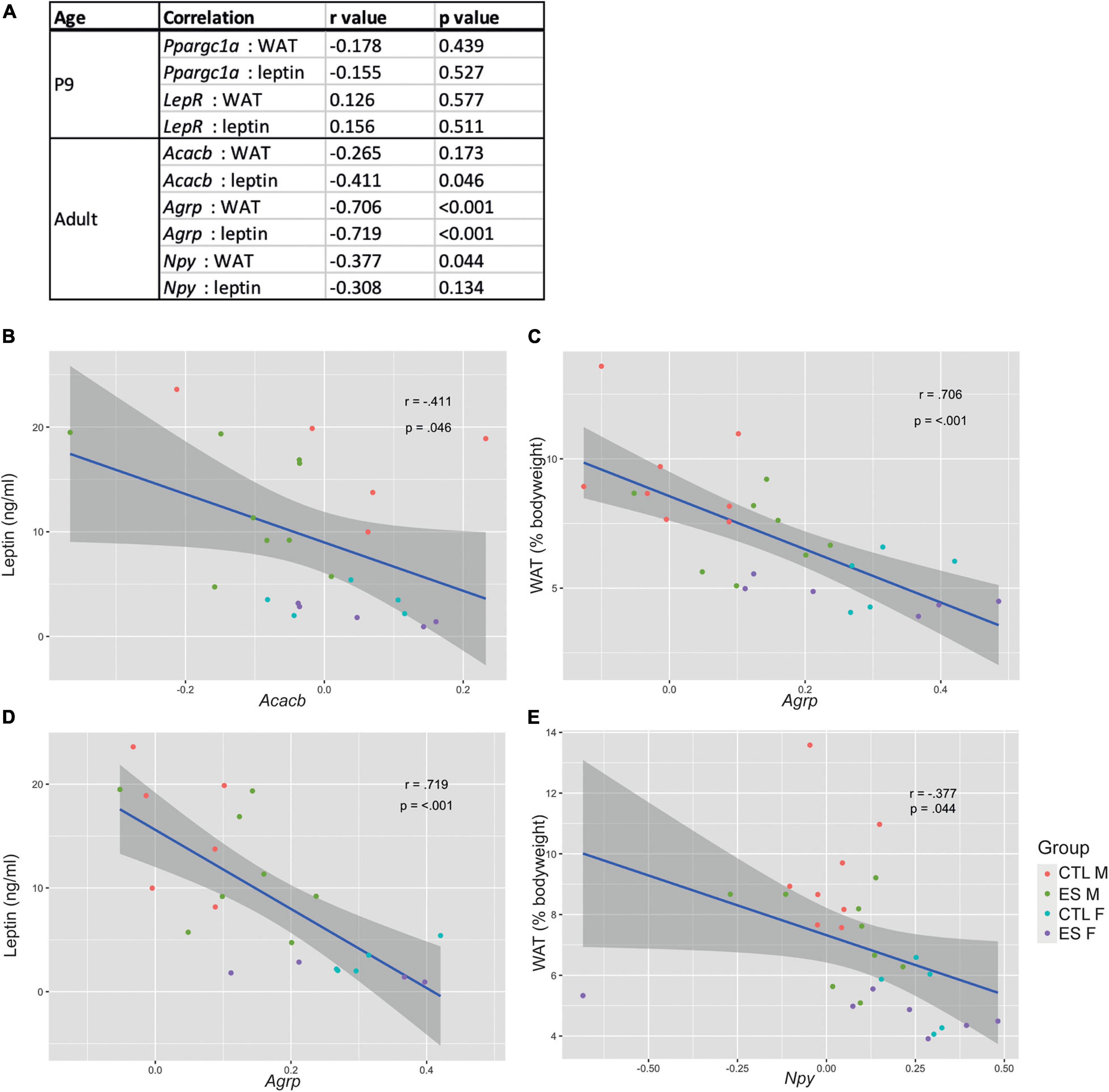
Figure 3. (A) Correlations between significantly affected genes and metabolic readouts at P9 and in adulthood. (B) Acacb expression correlated with leptin levels. (C) Agrp expression correlated with white adipose tissue (WAT) levels. (D) Agrp expression correlated with leptin levels. (E) Npy expression correlated with WAT levels.
Discussion
We presented here for the first time the effect of sex and ES on hypothalamic fatty acid metabolism, hormone receptors and neuropeptides at P9 and in adulthood. Pervasive sex differences emerge at both ages with a targeted impact of ES at P9, which was independent of sex. In particular, at P9, females had higher Ppargc1a expression compared to males. Moreover, while Lepr expression was higher in females compared to males, ES decreased Lepr expression independent of sex. In adulthood, females, compared to males, had higher Acacb, Npy, and Agrp expression. We will first discuss the sex differences in hypothalamic gene expression at P9 and adulthood, followed by the effects of ES, and will relate this to the previously described metabolic phenotype (Yam et al., 2017a,b).
Sex Differences in Hypothalamic Nutrient Sensing
At P9, females had higher Ppargc1a and Lepr expression compared to males. At this age, females also have a lower bodyweight compared to males, whereas their adiposity (as percentage of bodyweight) is similar (Yam et al., 2017a). PGC-1α (Ppargc1a) is known as transcription factor that responds to environmental and energy signals and targets the PPAR family (Sugden et al., 2010). As such, it is involved in the regulation of mitochondrial oxidative metabolism, glucose and lipid homeostasis, and was implicated in metabolic diseases (Lin et al., 2005). Whole body PGC-1α null mice are lean, resistant to diet-induced obesity and show hyperactivity (Lin et al., 2004), and also a selective inactivation of hypothalamic PGC-1α protected mice against diet-induced obesity (Ma et al., 2010). A high-fat diet furthermore downregulated hypothalamic PGC-1α in male but not female mice (Morselli et al., 2014). Since we did not find significant correlations between Ppargc1a expression and adiposity or leptin levels, it remains to be determined what is the functional implication of the observed sex differences in Ppargc1a expression early in life.
The increased Lepr expression in females at P9, despite similar leptin levels in male and female pups (Yam et al., 2017a), suggests there are sex-differences in leptin signaling in the hypothalamus. Sex differences in Lepr expression seem to be brain region specific, as hippocampal LepR expression was similar in males and females (Yam et al., 2017a). Leptin signaling early in life, rather than modulating food intake, is crucial for the development of the hypothalamic circuitry (Ahima et al., 1998; Mistry et al., 1999; Bouret et al., 2004; Granado et al., 2012). Although in the current study we did not observe sex differences in whole hypothalamic neuropeptide expression levels at this age, with a more anatomically precise approach we previously described higher ARH NPY fiber density in females compared to males at P14 (Yam et al., 2017b).
In adulthood, females had higher Acacb, Agrp, and Npy levels in the hypothalamus compared to males. Female mice at this age weigh less, and have lower adiposity independent of bodyweight, as well as lower circulating leptin levels when fed a standard chow diet (Yam et al., 2017a). Acetyl-CoA carboxylases (ACCs) occur in two isozymes [ACC1 (Acaca) and ACC2 (Acacb)] that are activated in the fed state. While ACC1 plays a key role in fatty acid synthases, ACC2 inhibits β-oxidation (Tong and Harwood, 2006), and higher Acacb expression thus indicates more inhibition of β-oxidation. The higher hypothalamic Acacb expression in female hypothalamus is in line with a previous report showing higher hepatic Acacb levels in female mice (Tuazon et al., 2015). To get a better understanding of what could be the functional implication of such sex-specific change in expression, is notable that polymorphisms in the Acacb gene influence the risk on developing metabolic syndrome in humans (Phillips et al., 2010). Whether ACCs are involved in sex differences in metabolism remains to be determined.
Increased Acacb expression in females suggests lower Npy and Agrp gene expression (Rijnsburger et al., 2016). In contrast, we observed higher hypothalamic expression of Npy and Agrp mRNA in females compared to males. A previous study found lower numbers of NPY expressing cells in the ARH of females (in proestrus) compared to males (Urban et al., 1993), and for Agrp, both lower (Viveros et al., 2010) as well as higher (Lensing et al., 2016) expression levels have been described in females. In fact, Npy and Agrp expression are affected by sex steroids. Testosterone increases Npy expression and release (Sahu et al., 1989; Urban et al., 1993), and in females, Npy and Agrp expression fluctuates throughout the estrous cycle, parallel to the cyclic changes in food intake and bodyweight (Olofsson et al., 2009; Fontana et al., 2014). Although estrous cycle was not a contributing factor in our statistical models, our study was not designed to specifically investigate the effect of estrous cycle in females. Another difference between our study and others is whether the animals were fasted before sacrificing. We fasted the mice for 4 h, while other studies describe no fasting period (Urban et al., 1993; Viveros et al., 2010; Lensing et al., 2016). Indeed, female rats show an increase in food intake after fasting, while male rats do not show such rebound feeding (Funabashi et al., 2009). This was accompanied by an increased number of activated hypothalamic orexin neurons in females (independent of estrous cycle) but not males. Sex differences in Agrp and Npy expression dynamics thus require further investigation.
In our study, despite increased Npy and Agrp expression in females, which stimulate food intake and reduce energy expenditure (Timper and Brüning, 2017), females were leaner and did not eat more of the standard chow than males (food intake after fasting was not investigated) (Yam et al., 2017a). In fact, male rodents are more vulnerable to develop adiposity and have more greatly impaired glucose tolerance upon long-term high-fat diet feeding (Grove et al., 2010; Estrany et al., 2011, 2013). Sex differences in the quantity and functioning of fat depots (Fuente-Martín et al., 2013) likely contribute to this increased metabolic vulnerability in males, and the same applies to the sympathetic innervation and projections from the hypothalamus to the adipose tissue (Adler et al., 2012). We here show that Acacb, Agrp, and Npy, genes that were differentially expressed between males and females, correlated with metabolic readouts (adiposity and/or leptin levels). Although these correlation analyses cannot imply causality nor directionality, our data suggest that sex-differences in nutrient sensing pathways could also contribute to this sex-bias in metabolic vulnerability, an avenue worth investigating in the future.
Effects of ES on Nutrient Sensing Pathways
ES exposure affected hypothalamic nutrient sensing pathways early in life, in a targeted manner. More specifically, at P9, Lepr expression was lower in ES-exposed mice. At this age, ES also led to a reduction in circulating leptin levels, that was notably similar in males and females, and did not affect hippocampal Lepr expression (Yam et al., 2017a), indicative of brain-region specific effects of ES on Lepr expression. Moreover, the reduction in leptin levels together with the lower hypothalamic Lepr expression suggests reductions in leptin signaling during hypothalamic development. In line with the altered hypothalamic leptin signaling at P9, we had previously found that at P14, ES also affected NPY and AgRP fiber density in the ARH and paraventricular nucleus, respectively (Yam et al., 2017b). In our current study, however, ES did not affect the gene expression Npy and Agrp in whole hypothalamic lysates. This could be due to the different method of assessment (i.e., gene expression of the whole hypothalamus versus fiber density in specific regions), and/or depend on the specific developmental stage due to the rapid development of the hypothalamus during the first two postnatal weeks (Bouret and Simerly, 2006; Bouret, 2012). Despite ES-induced changes in ghrelin levels in females at P9 (Yam et al., 2017b), hypothalamic Ghsr levels were not affected by ES, nor did ES affect the expression of genes involved in fatty acid metabolism. Thus, ES alters the leptin system at P9, whereas hypothalamic fatty acid metabolism seems unaffected. Interestingly, the leptin signaling pathway has been suggested to be a top upstream regulator of the effects of chronic stress in the prefrontal cortex, indicating leptin could be one of the key mediators by which stress exerts its effect on the brain (Musaelyan et al., 2020). Whether this might be the case also in the context of ES remains to be addressed.
In adulthood, ES did no longer impact the expression of genes involved in hypothalamic nutrient sensing. While we and others have previously shown lasting effects of ES on adipose tissue levels under basal circumstances and/or in the response to unhealthy diets (Murphy et al., 2017, 2018; Yam et al., 2017a), it remains unclear what leads to these ES-induced metabolic alterations. Investigating the expression of genes involved in nutrient sensing in (whole) hypothalamic samples provides a first indication of potential effects of ES on these pathways. However, it would be important in future studies to investigate these pathways with better spatial resolution and on the protein level, and next to under basal circumstances, also in response to an unhealthy diet. For example, we have previously shown that ES alters hypothalamic microglia in response to a western-style diet, but not under standard dietary circumstances (Ruigrok et al., 2021). Next to the hypothalamus, also other brain circuits and/or peripheral organs could be involved in the ES-induced metabolic risk. For example, we have previously shown ES-induced changes in the adipose tissue gene expression (Yam et al., 2017a; Ruigrok et al., 2021). Adipose tissue has a crucial role in regulating energy homeostasis, and its dysfunction has been related to the negative health consequences associated with obesity (Longo et al., 2019). In addition, the microbiome is implicated in obesity development (Davis, 2016), and has been shown to be affected by ES exposure (O’Mahony et al., 2009). ES has furthermore been shown to affect the reward circuitry (Spyrka et al., 2020), which is involved in the hedonic aspects of food intake, an important aspect of human obesity development, and there is initial evidence that food preference indeed is affected by ES exposure (Lussana et al., 2008; Jackson and Vaughn, 2019). In addition to the above described sex differences in the hypothalamus and adipose tissue, sex differences in the microbiome and reward circuitry have also been reported (Fuente-Martín et al., 2013; Becker and Chartoff, 2019; Kim et al., 2020), but it remains to be determined whether ES affects these systems in a sex-dependent manner. More research is thus needed to further elucidate how ES increases the risk for developing obesity, potentially in a sex-specific manner.
Conclusion
To the best of our knowledge, this is the first description of effects of ES and sex on hypothalamic nutrient sensing pathways. Clear sex differences were present in these pathways, while ES induced a minor modulation early in life that was similar in both sexes. Our data contribute to a further understanding of sex differences in the circuits that regulate metabolism and the impact of ES on these pathways.
Data Availability Statement
The raw data supporting the conclusions of this article will be made available by the authors, without undue reservation.
Ethics Statement
All experimental procedures were conducted under national law and European Union directives on animal experiments and were approved by the animal welfare committee [dierexperimentencommissie (DEC)] of the University of Amsterdam.
Author Contributions
SR and AK: conceptualization, writing—original draft, project administration. SR: formal analysis and visualization. AK: funding acquisition and supervision. SR, NS, and KYY: investigation. SR, AK, CL, PL, and ST: writing—review and editing. All authors contributed to the article and approved the submitted version.
Funding
AK and SR were funded by JPI HDHL. AK was supported by Alzheimer Nederland and NWO-Food Cognition and Behavior. PL was supported by Alzheimer Nederland and the Center for Urban Mental Health of the University of Amsterdam.
Conflict of Interest
The authors declare that the research was conducted in the absence of any commercial or financial relationships that could be construed as a potential conflict of interest.
Publisher’s Note
All claims expressed in this article are solely those of the authors and do not necessarily represent those of their affiliated organizations, or those of the publisher, the editors and the reviewers. Any product that may be evaluated in this article, or claim that may be made by its manufacturer, is not guaranteed or endorsed by the publisher.
Supplementary Material
The Supplementary Material for this article can be found online at: https://www.frontiersin.org/articles/10.3389/fnins.2021.695367/full#supplementary-material
References
Adler, E. S., Hollis, J. H., Clarke, I. J., Grattan, D. R., and Oldfield, B. J. (2012). Neurochemical characterization and sexual dimorphism of projections from the brain to abdominal and subcutaneous white adipose tissue in the rat. J. Neurosci. 32, 15913–15921. doi: 10.1523/JNEUROSCI.2591-12.2012
Ahima, R. S., Prabakaran, D., and Flier, J. S. (1998). Postnatal leptin surge and regulation of circadian rhythm of leptin by feeding. Implications for energy homeostasis and neuroendocrine function. J. Clin. Invest. 101, 1020–1027. doi: 10.1172/JCI1176
Alciati, A., Gesuele, F., Casazza, G., and Foschi, D. (2013). The relationship between childhood parental loss and metabolic syndrome in obese subjects. Stress Health 29, 5–13. doi: 10.1002/smi.1435
Barsh, G. S., and Schwartz, M. W. (2002). Genetic approaches to studying energy balance: perception and integration. Nat. Rev. Genet. 3, 589–600. doi: 10.1038/nrg862
Basu, A., McLaughlin, K. A., Misra, S., and Koenen, K. C. (2017). Childhood maltreatment and health impact: the examples of cardiovascular disease and type 2 diabetes mellitus in adults. Clin. Psychol. Sci. Pract. 24, 125–139. doi: 10.1111/cpsp.12191
Becker, J. B., and Chartoff, E. (2019). Sex differences in neural mechanisms mediating reward and addiction. Neuropsychopharmacology 44, 166–183. doi: 10.1038/s41386-018-0125-6
Bouret, S. G. (2009). Early life origins of obesity: role of hypothalamic programming. J. Pediatr. Gastroenterol. Nutr. 48(Suppl. 1), S31–S38. doi: 10.1097/MPG.0b013e3181977375
Bouret, S. G. (2012). Nutritional programming of hypothalamic development: critical periods and windows of opportunity. Int. J. Obes. Supp. 2, S19–S24. doi: 10.1038/ijosup.2012.17
Bouret, S. G., Draper, S. J., and Simerly, R. B. (2004). Trophic action of leptin on hypothalamic neurons that regulate feeding. Science 304, 108–110. doi: 10.1126/science.1095004
Bouret, S. G., and Simerly, R. B. (2006). Developmental programming of hypothalamic feeding circuits. Clin. Genet. 70, 295–301. doi: 10.1111/j.1399-0004.2006.00684.x
Boynton-Jarrett, R., Fargnoli, J., Suglia, S. F., Zuckerman, B., and Wright, R. J. (2010). Association between maternal intimate partner violence and incident obesity in preschool-aged children: results from the fragile families and child well-being study. Arch. Pediatr. Adolesc. Med. 164, 540–546. doi: 10.1001/archpediatrics.2010.94
Cesquini, M., Stoppa, G. R., Prada, P. O., Torsoni, A. S., Romanatto, T., Souza, A., et al. (2008). Citrate diminishes hypothalamic acetyl-CoA carboxylase phosphorylation and modulates satiety signals and hepatic mechanisms involved in glucose homeostasis in rats. Life Sci. 82, 1262–1271. doi: 10.1016/j.lfs.2008.04.015
Danese, A., and Tan, M. (2014). Childhood maltreatment and obesity: systematic review and meta-analysis. Mol. Psychiatry 19, 544–554. doi: 10.1038/mp.2013.54
Davis, C. D. (2016). The gut microbiome and its role in obesity. Nutr. Today 51, 167–174. doi: 10.1097/NT.0000000000000167
Dearden, L., and Ozanne, S. E. (2015). Early life origins of metabolic disease: developmental programming of hypothalamic pathways controlling energy homeostasis. Front. Neuroendocrinol. 39:3–16. doi: 10.1016/j.yfrne.2015.08.001
Diéguez, C., Vazquez, M. J., Romero, A., López, M., and Nogueiras, R. (2011). Hypothalamic control of lipid metabolism: focus on leptin, ghrelin and melanocortins. Neuroendocrinology 94, 1–11. doi: 10.1159/000328122
Dragano, N. R., Monfort-Pires, M., and Velloso, L. A. (2020). Mechanisms mediating the actions of fatty acids in the hypothalamus. Neuroscience 447, 15–27. doi: 10.1016/j.neuroscience.2019.10.012
Estrany, M. E., Proenza, A. M., Gianotti, M., and Lladó, I. (2013). High-fat diet feeding induces sex-dependent changes in inflammatory and insulin sensitivity profiles of rat adipose tissue. Cell Biochem. Funct. 31, 504–510. doi: 10.1002/cbf.2927
Estrany, M. E., Proenza, A. M., Lladó, I., and Gianotti, M. (2011). Isocaloric intake of a high-fat diet modifies adiposity and lipid handling in a sex dependent manner in rats. Lipids Health Dis. 10:52. doi: 10.1186/1476-511X-10-52
Fontana, R., Della Torre, S., Meda, C., Longo, A., Eva, C., and Maggi, A. C. (2014). Estrogen replacement therapy regulation of energy metabolism in female mouse hypothalamus. Endocrinology 155, 2213–2221. doi: 10.1210/en.2013-1731
Fuente-Martín, E., Argente-Arizón, P., Ros, P., Argente, J., and Chowen, J. A. (2013). Sex differences in adipose tissue: it is not only a question of quantity and distribution. Adipocyte 2, 128–134. doi: 10.4161/adip.24075
Funabashi, T., Hagiwara, H., Mogi, K., Mitsushima, D., Shinohara, K., and Kimura, F. (2009). Sex differences in the responses of orexin neurons in the lateral hypothalamic area and feeding behavior to fasting. Neurosci. Lett. 463, 31–34. doi: 10.1016/j.neulet.2009.07.035
Gao, S., Kinzig, K. P., Aja, S., Scott, K. A., Keung, W., Kelly, S., et al. (2007). Leptin activates hypothalamic acetyl-CoA carboxylase to inhibit food intake. Proc. Natl. Acad. Sci. U.S.A. 104, 17358–17363. doi: 10.1073/pnas.0708385104
Garg, N., Thakur, S., McMahan, C. A., and Adamo, M. L. (2011). High fat diet induced insulin resistance and glucose intolerance are gender-specific in IGF-1R heterozygous mice. Biochem. Biophys. Res. Commun. 413, 476–480. doi: 10.1016/j.bbrc.2011.08.123
Gil-Campos, M., Aguilera, C. M., Cañete, R., and Gil, A. (2006). Ghrelin: a hormone regulating food intake and energy homeostasis. Br. J. Nutr. 96, 201–226. doi: 10.1079/bjn20061787
Granado, M., Fuente-Martín, E., García-Cáceres, C., Argente, J., and Chowen, J. A. (2012). Leptin in early life: a key factor for the development of the adult metabolic profile. Obes. Facts 5, 138–150. doi: 10.1159/000336967
Grove, K. L., Fried, S. K., Greenberg, A. S., Xiao, X. Q., and Clegg, D. J. (2010). A microarray analysis of sexual dimorphism of adipose tissues in high-fat-diet-induced obese mice. Int. J. Obes. 34, 989–1000. doi: 10.1038/ijo.2010.12
Jackson, D. B., and Vaughn, M. G. (2019). Obesogenic food consumption among young children: the role of maltreatment. Public Health Nutr. 22, 1840–1849. doi: 10.1017/S1368980019000065
Jo, Y. H., Su, Y., Gutierrez-Juarez, R., and Chua, S. (2009). Oleic acid directly regulates POMC neuron excitability in the hypothalamus. J. Neurophysiol. 101, 2305–2316. doi: 10.1152/jn.91294.2008
Kanter, R., and Caballero, B. (2012). Global gender disparities in obesity: a review. Adv. Nutr. 3, 491–498. doi: 10.3945/an.112.002063
Kautzky-Willer, A., Harreiter, J., and Pacini, G. (2016). Sex and gender differences in risk, pathophysiology and complications of type 2 diabetes mellitus. Endocr. Rev. 37, 278–316. doi: 10.1210/er.2015-1137
Kim, Y. S., Unno, T., Kim, B. Y., and Park, M. S. (2020). Sex differences in gut microbiota. World J. Mens Health 8, 48–60. doi: 10.5534/wjmh.190009
Kocalis, H. E., Turney, M. K., Printz, R. L., Laryea, G. N., Muglia, L. J., Davies, S. S., et al. (2012). Neuron-specific deletion of peroxisome proliferator-activated receptor delta (PPARδ) in mice leads to increased susceptibility to diet-induced obesity. PLoS One 7:e42981. doi: 10.1371/journal.pone.0042981
Könner, A. C., Klöckener, T., and Brüning, J. C. (2009). Control of energy homeostasis by insulin and leptin: targeting the arcuate nucleus and beyond. Physiol. Behav. 97, 632–638. doi: 10.1016/j.physbeh.2009.03.027
Kozyrskyj, A. L., Zeng, Y., Colman, I., Hayglass, K. T., Sellers, E. A. C., Becker, A. B., et al. (2011). Maternal distress in early life predicts the waist-to-hip ratio in schoolchildren. J. Dev. Orig. Health Dis. 2, 72–80. doi: 10.1017/S2040174410000723
Lage, R., Vázquez, M. J., Varela, L., Saha, A. K., Vidal−Puig, A., Nogueiras, R., et al. (2010). Ghrelin effects on neuropeptides in the rat hypothalamus depend on fatty acid metabolism actions on BSX but not on gender. FASEB J. 24, 2670–2679. doi: 10.1096/fj.09-150672
Lam, T. K. T., Schwartz, G. J., and Rossetti, L. (2005). Hypothalamic sensing of fatty acids. Nat. Neurosci. 8, 579–584. doi: 10.1038/nn1456
Le Foll, C. (2019). Hypothalamic fatty acids and ketone bodies sensing and role of FAT/CD36 in the regulation of food intake. Front. Physiol. 10:1036. doi: 10.3389/fphys.2019.01036
Lensing, C. J., Adank, D. N., Doering, S. R., Wilber, S. L., Andreasen, A., Schaub, J. W., et al. (2016). Ac-Trp-DPhe(p-I)-Arg-Trp-NH2, a 250-fold selective melanocortin-4 receptor (MC4R) antagonist over the melanocortin-3 receptor (MC3R), affects energy homeostasis in male and female mice differently. ACS Chem. Neurosci. 7, 1283–1291. doi: 10.1021/acschemneuro.6b00156
Levin, B. E. (2006). Metabolic imprinting: critical impact of the perinatal environment on the regulation of energy homeostasis. Philos. Trans. R. Soc. B 361, 1107–1121. doi: 10.1098/rstb.2006.1851
Lin, J., Handschin, C., and Spiegelman, B. M. (2005). Metabolic control through the PGC-1 family of transcription coactivators. Cell Metab. 1, 361–370. doi: 10.1016/j.cmet.2005.05.004
Lin, J., Wu, P. H., Tarr, P. T., Lindenberg, K. S., St-Pierre, J., Zhang, C. Y., et al. (2004). Defects in adaptive energy metabolism with CNS-linked hyperactivity in PGC-1α null mice. Cell 119, 121–135. doi: 10.1016/j.cell.2004.09.013
Loftus, T. M., Jaworsky, D. E., Frehywot, C. L., Townsend, C. A., Ronnett, G. V., Daniel Lane, M., et al. (2000). Reduced food intake and body weight in mice treated with fatty acid synthase inhibitors. Science 288, 2379–2381. doi: 10.1126/science.288.5475.2379
Longo, M., Zatterale, F., Naderi, J., Parrillo, L., Formisano, P., Raciti, G. A., et al. (2019). Adipose tissue dysfunction as determinant of obesity-associated metabolic complications. Int. J. Mol. Sci. 20:2358. doi: 10.3390/ijms20092358
López, M., Lage, R., Saha, A. K., Pérez-Tilve, D., Vázquez, M. J., Varela, L., et al. (2008). Hypothalamic fatty acid metabolism mediates the orexigenic action of ghrelin. Cell Metab. 7, 389–399. doi: 10.1016/j.cmet.2008.03.006
Lu, M., Sarruf, D. A., Talukdar, S., Sharma, S., Li, P., Bandyopadhyay, G., et al. (2011). Brain PPAR-γ promotes obesity and is required for the insuling-sensitizing effect of thiazolidinediones. Nat. Med. 17, 618–622. doi: 10.1038/nm.2332
Lussana, F., Painter, R. C., Ocke, M. C., Buller, H. R., Bossuyt, P. M., and Roseboom, T. J. (2008). Prenatal exposure to the Dutch famine is associated with a preference for fatty foods and a more atherogenic lipid profile. Am. J. Clin. Nutr. 88, 1648–1652. doi: 10.3945/ajcn.2008.26140
Ma, D., Li, S., Lucas, E. K., Cowell, R. M., and Lin, J. D. (2010). Neuronal inactivation of peroxisome proliferator-activated receptor γ coactivator 1α(PGC-1α) protects mice from diet-induced obesity and leads to degenerative lesions. J. Biol. Chem. 285, 39087–39095. doi: 10.1074/jbc.M110.151688
Ma, Y., Wang, X., Yang, H., Zhang, X., and Yang, N. (2017). Involvement of CD36 in modulating the decrease of NPY and AgRP induced by acute palmitic acid stimulation in N1E-115 cells. Nutrients 9:626. doi: 10.3390/nu9060626
Malik, V. S., Willett, W. C., and Hu, F. B. (2013). Global obesity: trends, risk factors and policy implications. Nat. Rev. Endocrinol. 9, 13–27. doi: 10.1038/nrendo.2012.199
Matzinger, D., Degen, L., Drewe, J., Meuli, J., Duebendorfen, R., Ruckstuhl, N., et al. (2000). The role of long chain fatty acids in regulating food intake and cholecystokinin release in humans. Gut 46, 688–693. doi: 10.1136/gut.46.5.689
Migrenne, S., Cruciani-Guglielmacci, C., Kang, L., Wang, R., Rouch, C., Lefèvre, A. L., et al. (2006). Fatty acid signaling in the hypothalamus and the neural control of insulin secretion. Diabetes 55, S139–S144. doi: 10.2337/db06-S017
Mistry, A. M., Swick, A., and Romsos, D. R. (1999). Leptin alters metabolic rates before acquisition of its anorectic effect in developing neonatal mice. Am. J. Physiol. Integr. Comp. Physiol. 277, R742–R747. doi: 10.1152/ajpregu.1999.277.3.R742
Morselli, E., Fuente-Martin, E., Finan, B., Kim, M., Frank, A., Garcia-Caceres, C., et al. (2014). Hypothalamic PGC-1α protects against high-fat diet exposure by regulating ERα. Cell Rep. 9, 633–645. doi: 10.1016/j.celrep.2014.09.025
Murphy, M. O., Herald, J. B., Leachman, J., Villasante Tezanos, A., Cohn, D. M., and Loria, A. S. (2018). A model of neglect during postnatal life heightens obesity-induced hypertension and is linked to a greater metabolic compromise in female mice. Int. J. Obes. 42, 1354–1365. doi: 10.1038/s41366-018-0035-z
Murphy, M. O., Herald, J. B., Wills, C. T., Unfried, S. G., Cohn, D. M., and Loria, A. S. (2017). Postnatal treatment with metyrapone attenuates the effects of diet-induced obesity in female rats exposed to early-life stress. Am. J. Physiol. Endocrinol. Metab. 312, E98–E108. doi: 10.1152/ajpendo.00308.2016
Musaelyan, K., Yildizoglu, S., Bozeman, J., Du Preez, A., Egeland, M., Zunszain, P. A., et al. (2020). Chronic stress induces significant gene expression changes in the prefrontal cortex alongside alterations in adult hippocampal neurogenesis. Brain Commun. 2:fcaa153. doi: 10.1093/braincomms/fcaa153
Naninck, E. F. G. G., Hoeijmakers, L., Kakava-Georgiadou, N., Meesters, A., Lazic, S. E., Lucassen, P. J., et al. (2015). Chronic early life stress alters developmental and adult neurogenesis and impairs cognitive function in mice. Hippocampus 25, 309–328. doi: 10.1002/hipo.22374
O’Mahony, S. M., Marchesi, J. R., Scully, P., Codling, C., Ceolho, A. M., Quigley, E. M. M., et al. (2009). Early life stress alters behavior, immunity, and microbiota in rats: implications for irritable bowel syndrome and psychiatric illnesses. Biol. Psychiatry 65, 263–267. doi: 10.1016/j.biopsych.2008.06.026
Obici, S., Feng, Z., Arduini, A., Conti, R., and Rossetti, L. (2003). Inhibition of hypothalamic carnitine palmitoyltransferase-1 decreases food intake and glucose production. Nat. Med. 9, 756–761. doi: 10.1038/nm873
Obici, S., Feng, Z., Morgan, K., Stein, D., Karkanias, G., and Rossetti, L. (2002). Central administration of oleic acid inhibits glucose production and food intake. Diabetes 51, 271–275. doi: 10.2337/diabetes.51.2.271
Olofsson, L. E., Pierce, A. A., and Xu, A. W. (2009). Functional requirement of AgRP and NPY neurons in ovarian cycle-dependent regulation of food intake. Proc. Natl. Acad. Sci. U.S.A. 106, 15932–15937. doi: 10.1073/pnas.0904747106
Park, H., Sundaram, R., Gilman, S. E., Bell, G., Louis, G. M. B., and Yeung, E. H. (2018). Timing of maternal depression and sex-specific child growth, the upstate KIDS study. Obesity 26, 160–166. doi: 10.1002/oby.22039
Phillips, C. M., Goumidi, L., Bertrais, S., Field, M. R., Cupples, L. A., Ordovas, J. M., et al. (2010). ACC2 gene polymorphisms, metabolic syndrome, and gene-nutrient interactions with dietary fat. J. Lipid Res. 51, 3500–3507. doi: 10.1194/jlr.M008474
Porte, D., and Woods, S. C. (1981). Regulation of food intake and body weight by insulin. Diabetologia 20, 274–280. doi: 10.1007/BF00254493
Power, M. L., and Schulkin, J. (2008). Sex differences in fat storage, fat metabolism, and the health risks from obesity: possible evolutionary origins. Br. J. Nutr. 99, 931–940. doi: 10.1017/S0007114507853347
R Core Team (2018). R: A Language and Environment for Statistical Computing [WWW Document]. Available online at: https://scholar.google.com/scholar?cluster=1636566325440065690&hl=en&oi=scholarr (accessed March 17, 2021).
Ramamoorthy, T. G., Begum, G., Harno, E., and White, A. (2015). Developmental programming of hypothalamic neuronal circuits: impact on energy balance control. Front. Neurosci. 9:126. doi: 10.3389/fnins.2015.00126
Rice, C. J., Sandman, C. A., Lenjavi, M. R., and Baram, T. Z. (2008). A novel mouse model for acute and long-lasting consequences of early life stress. Endocrinology 149, 4892–4900. doi: 10.1210/en.2008-0633
Rijnsburger, M., Belegri, E., Eggels, L., Unmehopa, U. A., Boelen, A., Serlie, M. J., et al. (2016). The effect of diet interventions on hypothalamic nutrient sensing pathways in rodents. Physiol. Behav. 162, 61–68. doi: 10.1016/j.physbeh.2016.04.011
Ruigrok, S. R., Abbink, M. R., Geertsema, J., Kuindersma, J. E., Stöberl, N., van der Beek, E. M., et al. (2021). Effects of early-life stress, postnatal diet modulation and long-term western-style diet on peripheral and central inflammatory markers. Nutrients 13:288. doi: 10.3390/nu13020288
Ryan, K. K., Li, B., Grayson, B. E., Matter, E. K., Woods, S. C., and Seeley, R. J. (2011). A role for central nervous system PPAR-γ in the regulation of energy balance. Nat. Med. 17, 623–626. doi: 10.1038/nm.2349
Sahu, A., Kalra, S. P., Crowley, W. R., and Kalra, P. S. (1989). Testosterone raises neuropeptide-Y concentration in selected hypothalamic sites and in vitro release from the medial basal hypothalamus of castrated male rats∗. Endocrinology 124, 410–414. doi: 10.1210/endo-124-1-410
Shimokawa, T., Kumar, M. V., and Lane, M. D. (2002). Effect of a fatty acid synthase inhibitor on food intake and expression of hypothalamic neuropeptides. Proc. Natl. Acad. Sci. U.S.A. 99, 66–71. doi: 10.1073/pnas.012606199
Spyrka, J., Gugula, A., Rak, A., Tylko, G., Hess, G., and Blasiak, A. (2020). Early life stress-induced alterations in the activity and morphology of ventral tegmental area neurons in female rats. Neurobiol. Stress 13:100250. doi: 10.1016/j.ynstr.2020.100250
Sugden, M. C., Caton, P. W., and Holness, M. J. (2010). PPAR control: it’s SIRTainly as easy as PGC. J. Endocrinol. 204, 93–104. doi: 10.1677/JOE-09-0359
Taïb, B., Bouyakdan, K., Hryhorczuk, C., Rodaros, D., Fulton, S., and Alquier, T. (2013). Glucose regulates hypothalamic long-chain fatty acid metabolism via AMP-activated kinase (AMPK) in neurons and astrocytes. J. Biol. Chem. 288, 37216–37229. doi: 10.1074/jbc.M113.506238
Timper, K., and Brüning, J. C. (2017). Hypothalamic circuits regulating appetite and energy homeostasis: pathways to obesity. DMM Dis. Models Mech. 10, 679–689. doi: 10.1242/dmm.026609
Tong, L., and Harwood, H. J. (2006). Acetyl-coenzyme a carboxylases: versatile targets for drug discovery. J. Cell Biochem. 99, 1476–1488. doi: 10.1002/jcb.21077
Tuazon, M. A., McConnell, T. R., Wilson, G. J., Anthony, T. G., and Henderson, G. C. (2015). Intensity-dependent and sex-specific alterations in hepatic triglyceride metabolism in mice following acute exercise. J. Appl. Physiol. 118, 61–70. doi: 10.1152/japplphysiol.00440.2014
Urban, J. H., Bauer-Dantoin, A. C., and Levine, J. E. (1993). Neuropeptide Y gene expression in the arcuate nucleus: sexual dimorphism and modulation by testosterone. Endocrinology 132, 139–145. doi: 10.1210/endo.132.1.8419120
Viveros, M. P., Llorente, R., Díaz, F., Romero-Zerbo, S. Y., Bermudez-Silva, F. J., Rodríguez de Fonseca, F., et al. (2010). Maternal deprivation has sexually dimorphic long-term effects on hypothalamic cell-turnover, body weight and circulating hormone levels. Horm. Behav. 58, 808–819. doi: 10.1016/j.yhbeh.2010.08.003
Wren, A. M., Small, C. J., Abbott, C. R., Dhillo, W. S., Seal, L. J., Cohen, M. A., et al. (2001). Ghrelin causes hyperphagia and obesity in rats. Diabetes 50, 2540–2547. doi: 10.2337/diabetes.50.11.2540
Yam, K. Y., Naninck, E. F. G. G., Abbink, M. R., la Fleur, S. E., Schipper, L., van den Beukel, J. C., et al. (2017a). Exposure to chronic early-life stress lastingly alters the adipose tissue, the leptin system and changes the vulnerability to western-style diet later in life in mice. Psychoneuroendocrinology 77, 186–195. doi: 10.1016/j.psyneuen.2016.12.012
Yam, K. Y., Naninck, E. F. G. G., Schmidt, M. V., Lucassen, P. J., and Korosi, A. (2015). Early-life adversity programs emotional functions and the neuroendocrine stress system: the contribution of nutrition, metabolic hormones and epigenetic mechanisms. Stress 18, 328–342. doi: 10.3109/10253890.2015.1064890
Yam, K. Y., Ruigrok, S. R., Ziko, I., De Luca, S. N., Lucassen, P. J., Spencer, S. J., et al. (2017b). Ghrelin and hypothalamic NPY/AgRP expression in mice are affected by chronic early-life stress exposure in a sex-specific manner. Psychoneuroendocrinology 86, 73–77. doi: 10.1016/j.psyneuen.2017.09.006
Zlokovic, B. V., Jovanovic, S., Miao, W., Samara, S., Verma, S., and Farrell, C. L. (2000). Differential regulation of leptin transport by the choroid plexus and blood-brain barrier and high affinity transport systems for entry into hypothalamus and across the blood-cerebrospinal fluid barrier. Endocrinology 141, 1434–1441. doi: 10.1210/endo.141.4.7435
Keywords: nutrient sensing, hypothalamus, sex differences, early-life stress, metabolism
Citation: Ruigrok SR, Stöberl N, Yam KY, de Lucia C, Lucassen PJ, Thuret S and Korosi A (2021) Modulation of the Hypothalamic Nutrient Sensing Pathways by Sex and Early-Life Stress. Front. Neurosci. 15:695367. doi: 10.3389/fnins.2021.695367
Received: 14 April 2021; Accepted: 28 June 2021;
Published: 23 July 2021.
Edited by:
Julie A. Chowen, Niño Jesús University Children’s Hospital, SpainReviewed by:
Rim Hassouna, Danone, FranceEmmanuel N. Pothos, Tufts University School of Medicine, United States
Copyright © 2021 Ruigrok, Stöberl, Yam, de Lucia, Lucassen, Thuret and Korosi. This is an open-access article distributed under the terms of the Creative Commons Attribution License (CC BY). The use, distribution or reproduction in other forums is permitted, provided the original author(s) and the copyright owner(s) are credited and that the original publication in this journal is cited, in accordance with accepted academic practice. No use, distribution or reproduction is permitted which does not comply with these terms.
*Correspondence: Aniko Korosi, YS5rb3Jvc2lAdXZhLm5s