- 1Department of Neurobiology, The Alexander Silberman Institute of Life Science, The Hebrew University of Jerusalem, Jerusalem, Israel
- 2The Charles E. Smith Family and Prof. Joel Elkes Laboratory for Collaborative Research in Psychobiology, The Hebrew University of Jerusalem, Jerusalem, Israel
- 3The Harvey M. Kruger Family Center for Nanoscience, The Hebrew University of Jerusalem, Jerusalem, Israel
- 4Edmond and Lily Safra Center for Brain Sciences, The Hebrew University of Jerusalem, Jerusalem, Israel
- 5Bio-Imaging Unit, The Alexander Silberman Institute of Life Science the Hebrew University of Jerusalem, Jerusalem, Israel
Despite increasing use of in vivo multielectrode array (MEA) implants for basic research and medical applications, the critical structural interfaces formed between the implants and the brain parenchyma, remain elusive. Prevailing view assumes that formation of multicellular inflammatory encapsulating-scar around the implants [the foreign body response (FBR)] degrades the implant electrophysiological functions. Using gold mushroom shaped microelectrodes (gMμEs) based perforated polyimide MEA platforms (PPMPs) that in contrast to standard probes can be thin sectioned along with the interfacing parenchyma; we examined here for the first time the interfaces formed between brains parenchyma and implanted 3D vertical microelectrode platforms at the ultrastructural level. Our study demonstrates remarkable regenerative processes including neuritogenesis, axon myelination, synapse formation and capillaries regrowth in contact and around the implant. In parallel, we document that individual microglia adhere tightly and engulf the gMμEs. Modeling of the formed microglia-electrode junctions suggest that this configuration suffice to account for the low and deteriorating recording qualities of in vivo MEA implants. These observations help define the anticipated hurdles to adapting the advantageous 3D in vitro vertical-electrode technologies to in vivo settings, and suggest that improving the recording qualities and durability of planar or 3D in vivo electrode implants will require developing approaches to eliminate the insulating microglia junctions.
Introduction
Basic and clinically oriented brain research and their applications rely on the use of sophisticated neuroimplants for long-term, simultaneous, multisite extracellular recordings of field potentials (FP) generated by neurons in freely behaving subjects. Despite significant technological progress, contemporary in vivo multielectrode array (MEA) technologies suffer from inherent limitations that include: (a) a low signal-to-noise ratio (S/N), (b) low source resolution, and (c) deterioration of the recording yield and FP amplitudes within days to weeks of implantation (Jackson and Fetz, 2007; Perge et al., 2013; Voigts et al., 2013; Harris et al., 2016; Lee et al., 2018, 2021). In addition, current in vivo brain implants are “blind” to sub-threshold synaptic potentials generated by individual neurons. This implies that critical elements of the brains signaling repertoire and computational components are ignored. The prevailing view relates these limitations to: (a) the gradual increase in the thickness of the inflammatory glia scar that displaces neurons from the implant surfaces (Edell et al., 1992; Biran et al., 2005; Polikov et al., 2005; Malaga et al., 2016; Salatino et al., 2017a; Michelson et al., 2018), (b) the glial scar encapsulating the implant (Szarowski et al., 2003; Johnson et al., 2005; Polikov et al., 2005; Otto et al., 2006; Williams et al., 2007; Prasad and Sanchez, 2012) and a biofouling layer assembled on the electrode surfaces insulate the electrodes from the current sources by their relatively high resistivity compared to the intact brain tissue (Sommakia et al., 2009, 2014; Malaga et al., 2016), (c) pro-inflammatory cytokines released from the glia and injured neurons lead to demyelination of the axons and thereby disrupt action potential propagation (Winslow and Tresco, 2010; Winslow et al., 2010), (d) released cytokines reduce the excitability and synaptic connectivity of neurons in the implant’s vicinity (Vezzani and Viviani, 2015; Salatino et al., 2017b, 2019; Hermann and Capadona, 2018; Thompson et al., 2020), (e) damage to blood capillaries by the implant leads to infiltration of neurotoxic factors and myeloid cells (Saxena et al., 2013) and reduces the blood supply to individual cells. Although objective experimental attempts to relate the thickness of the inflammatory foreign body response (FBR) to deterioration in recording qualities have failed, this concept has continued to dominate the field and still shapes extensive research efforts to mitigate or overcome this deterioration. Whereas ever-improving spike-detecting, spike-sorting and signal averaging techniques make it possible to extract significant information from monitoring extracellular FP (Quiroga et al., 2004; Einevoll et al., 2012; Carlson and Carin, 2019), the limited recording qualities of current multielectrode array-implants (MEA implants) and their deterioration in time considerably hinder the research progress.
The realization that the use of substrate integrated planar MEA technologies for extracellular recordings (Figure 1) inherently limits the qualities of in vitro and in vivo systems has prompted the development of new 3D in vitro technologies to enable parallel, multisite intracellular recordings and stimulation from many individual cultured cells (neurons, cardiomyocytes and striated muscles). In principle, this family of in vitro MEA technologies utilizes different forms of 3D vertical nano-structures (nano-pillars) that pierce the plasma membrane of cultured cells (by electroporation or spontaneously) in a way similar to classical sharp electrodes (Figure 1 and Tian et al., 2010; Angle and Schaefer, 2012; Duan et al., 2012; Gao et al., 2012; Robinson et al., 2012; Xie et al., 2012; Angle et al., 2014; Lin and Cui, 2014; Lin et al., 2014; Qing et al., 2014; Abbott et al., 2017, 2018, 2019; Dipalo et al., 2017; Liu et al., 2017; Mateus et al., 2019; Li et al., 2020; Teixeira et al., 2020; Yoo et al., 2020; Xu et al., 2021; Zhang et al., 2021).
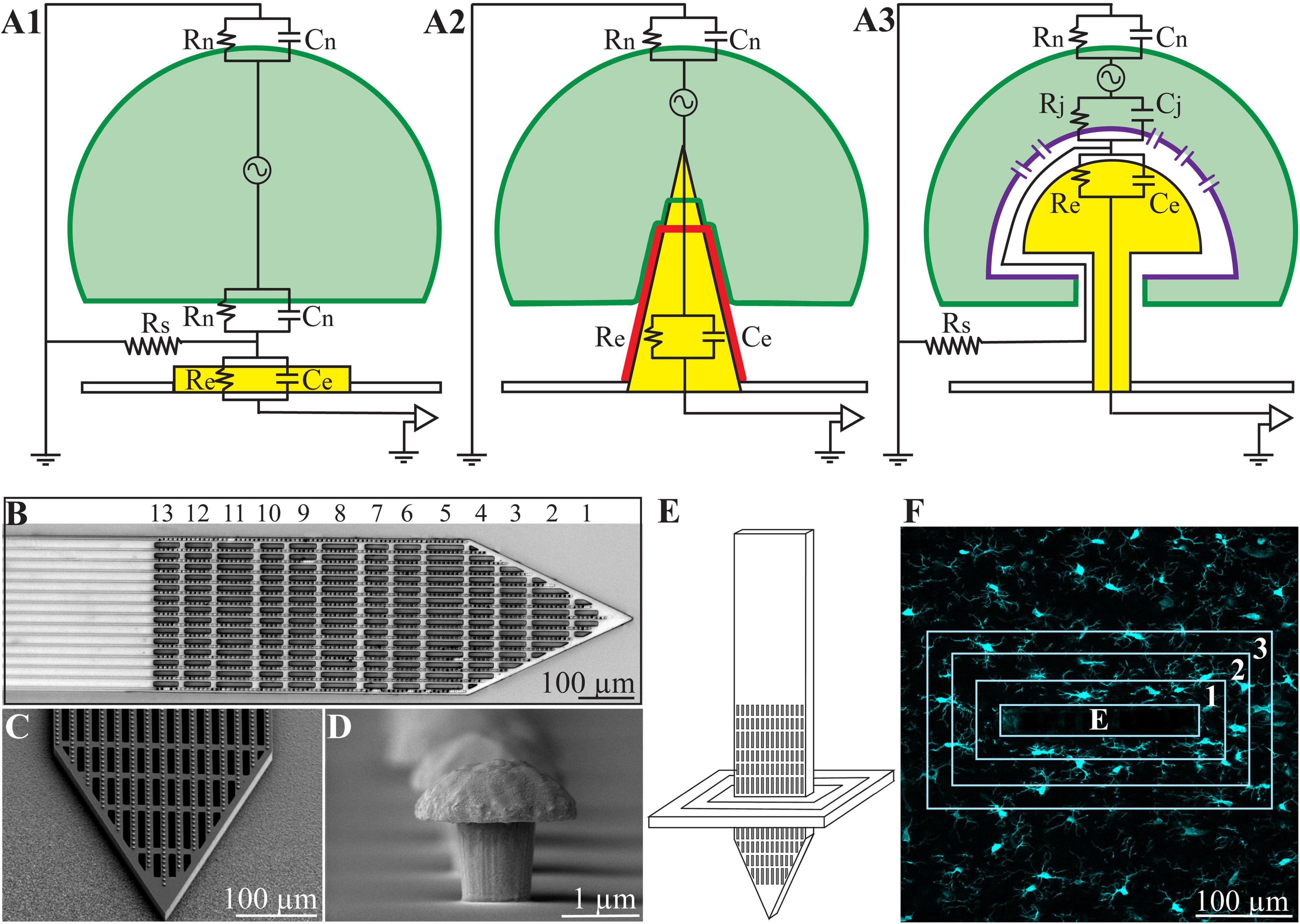
Figure 1. (A) Passive analog electrical circuit models depicting the structural interfaces formed between cells and recording microelectrodes under in vitro conditions. (A1) Substrate integrated planar electrode for extracellular field potential recordings, (A2) a vertical nano-pillar electrode that pierces the plasma membrane for intracellular recordings, and (A3) extracellular gold mushroom shaped vertical microelectrode (gMμE) for IN-CELL recordings. The three configurations differ mainly in terms of the nature and dimensions of the cleft formed between the cultured cells (neurons, cardiomyocytes, or striated muscle fibers) and the recording electrode. In panel (A1), the extracellular field potential generated by propagating action potentials is largely attenuated across the high resistance non-junctional membrane (Rn) and the low seal resistance (Rs). In panel (A2), the vertical nanoelectrode pierces the cell’s plasma membrane, gaining direct access to the cytosol (Rn is reduced to zero). A very high seal (∼GΩ) resistance (not drawn) formed between the vertical nanoelectrode’s (yellow electrode with a red insulating layer) surface and the plasma membrane (green). In panel (A3), the cell engulfs a mushroom shaped vertical electrode (yellow) to form relatively high Rs by the narrow cleft. Along with reduced junctional membrane resistance (Rj -purple) the configuration makes it possible to record attenuated intracellular potentials. (B) Low magnification image of the polyimide based perforated MEA platform (PPMP), the proximal solid part and distal perforated part are shown. For orientation, the rows of perforations are numbered. (C) SEM enlargement of a PPMP segment, showing the perforations of the polyimide platform and dense rows of gMμEs along the PI “ribs.” (D) A SEM image of a gMμE. (E) Schematic illustration of an implanted PPMP and the orientation of thick horizontal tissue slices. (F) The integrated immuno-fluorescent intensity within the electrode (central rectangle-E) and within 25 μm wide centripetal shells around it were measured and processed to establish the Normalized Fluorescent Intensity level (NFI) or the number of a given cell type at a given distance and time around the implant.
At the same time, a number of laboratories have developed the “IN-CELL” recording and stimulation configuration, in which micrometer-sized, extracellular gold mushroom-shaped microelectrodes (gMμEs) record attenuated synaptic and action potentials (Figure 1 and Spira et al., 2007; Hai et al., 2010a,b; Fendyur and Spira, 2012; Spira and Hai, 2013; Rabieh et al., 2016; Shmoel et al., 2016; Weidlich et al., 2017; McGuire et al., 2018; Spira et al., 2018, 2019; Mateus et al., 2019; Jones et al., 2020; Teixeira et al., 2020). Ultrastructural imaging complemented by electrophysiology and model system analysis of the cultured-neurons/gMμEs configuration have revealed that the biophysical principles of “IN-CELL” recordings are identical to those of the perforated patch electrode configuration (Horn and Marty, 1988; Akaike and Harata, 1994).
Successful adaptation of the vertical nano-pillar and gMμEs MEA approaches to in vivo brain research could effectively address the limitations of the currently used planar MEA technologies (low S/N, poor source resolution and deterioration), and importantly would make it possible to record the entire signaling repertoire from many individual neurons. It is thus expected that such adaptation will significantly improve the likelihood of understanding the codes of brain-circuit computations.
Ultrastructural examinations of the interfaces formed between cultured neurons and gMμEs or vertical nano-pillar based MEAs have played key roles in revealing that cultured neurons and other cell types tightly engulf vertical structures by evolutionarily conserved cell biological mechanisms (Hai et al., 2010b; Santoro et al., 2014, 2017b; McGuire et al., 2018). And, that the narrow cleft formed between the engulfing plasma membrane and the gMμEs form a high seal resistance (Rs). This, together with the increased conductance of the cell’s membrane that faces the gMμEs (the junctional membrane—Rj, Figure 1), make it possible to record attenuated action potentials and subthreshold synaptic potentials with features and biophysics similar to perforated patch recordings (Horn and Marty, 1988; Akaike and Harata, 1994; Spira et al., 2007; Hai et al., 2009a,b; Fendyur et al., 2011; Santoro et al., 2013, 2014, 2017a,b).
In contrast to meticulous ultrastructural studies of the interfaces formed between cultured cells and different types of vertical nanoelectrodes, structural studies of the interfaces formed between implanted neuroprobes and in vivo brain parenchyma were of very low resolution. Besides the inherent low spatial resolution of the immunohistological methods used, in the vast majority of light and electron microscope studies, the implants were pulled out (extracted) from the brain tissue prior to thin sectioning for histological examination. This unavoidably damages the parenchyma/implant interfaces, making it impossible to examine and understand the structural relationships between the abiotic implant and the tissue (for example Schultz and Willey, 1976; Moss et al., 2004; Grand et al., 2010; Marton et al., 2020).
Using gMμEs based perforated polyimide MEA platforms that can be thin sectioned along with the interfacing parenchyma; we examined here for the first time the interfaces formed between brains parenchyma and implanted 3D vertical microelectrode (gMμEs) platforms at the ultrastructural level. Our study demonstrates remarkable structural parenchyma regenerative processes including neuritogenesis, axon myelination and synapse formation in contact and around the implant. In parallel, we documented that individual microglia adhere tightly and engulf the gMμE electrodes. The extracellular cleft formed between the implant and the adhering microglia in parallel to the microglia’s input resistance suggest that high resistance barriers are formed in contact with the electrodes. We posit that these microglia-electrode-junctions, rather than the thick multicellular inflammatory encapsulation that is thought to displace neuronal cell bodies and induce axon demyelination or structural synapse degeneration, are the underlying mechanisms governing the deterioration of the electrical coupling between neurons and the in vivo implanted electrodes. In addition, our ultrastructural observations objectively highlight the expected hurdles to applying arrays of vertical nano-pillars in general and gMμEs in particular to record intracellular potentials from cortical neurons in freely behaving rats. Approaches to mitigate or selectively eliminate the adhering microglia are thus needed to advance the application of 3D microelectrode arrays for intracellular recording of the entire signaling repertoire of the in vivo brain.
Materials and Methods
Animals
All the procedures in the present study were approved by the Committee for Animal Experimentation at the Institute of Life Sciences of the Hebrew University of Jerusalem. This study was conducted using female Sprague Dawley rats (215–340 g).
Neuroimplants
To address the technical features required to prepare thin sections of implanted gMμE-platforms along with the parenchyma around it, we fabricated non-functional implants constructed of a Perforated Polyimide (PI)-based MEA Platform (PPMP) that carries a dense array of gold mushroom shaped microelectrodes (gMμE, Figure 1). The 1.7 mm long, 280 μm wide, and 16 μm thick non-functional gMμE-PPMPs were divided to a 0.9 mm long solid proximal part and a 0.8 mm perforated distal part (Figure 1). The perforated segment tapered to form a sharp tip. The width of all the rectangular perforations was 7–8 μm and the lengths of the different perforations were 65, 47, and 44 μm (Figure 1). gMμEs were electroplated at a pitch of 8 μm in rows along the 15 gold conducting lines that run along the platform (Figure 1 and Supplementary Figure 1).
Implant Fabrication
The gMμE-PPMPs were constructed using standard photolithography fabrication methods as follows (Supplementary Figure 1). First, an aluminum releasing layer was sputtered on a 3-inch silicon wafer (University Wafer, United States), followed by a spin-coated 15 μm thick polyimide layer (PI 2610, HD Microsystems, Germany) that served as an insulating layer and the main mechanical backbone of the platform. A triple metal layer of Cr/Au/Cr (20/120/20 nm) was then patterned and e-beam evaporated as interconnects, pads and scribe-lines. Next, a second 1 μm thick insulating layer of polyimide was spin-coated, followed by the deposition of a 1 μm SiO2 with Plasma Enhanced Chemical Vapor Deposition (PECVD). A 1.5 μm photoresist layer was then patterned. Dry etching by RIE was used to define 1.5 μm molds for electroplating the gMμEs and pads through the SiO2 and the one micrometer thick insulating PI layer. After removal of the top Cr layer by wet etch, gMμEs with cap diameters of ∼2 μm were electrodeposited at a pitch of 8 μm along the tip and a perforated section of the platform (Figure 1 and Supplementary Figure 1). An additional 300 nm of SiO2 was deposited with PECVD and a photoresist layer were used to define the perforated pattern of the platform. The photoresist and SiO2 layers were then removed and the platforms were then released from the wafer by anodic metal dissolution and thoroughly rinsed in distilled deionized water.
Platform Implantations
A 1–1.5 cm longitudinal cut of the skin on the head was made and the anterior, dorsal surfaces of the skull were exposed. Two craniotomies, one in the left and the other in the right frontal bones, were performed at the desired reference points (coordinates: AP: +3.5 mm; ML: ±2.5 mm from the Bregma) and the dura was gently resected (0.3–0.5 mm long incision). The 1.7 mm long platforms held by forceps mounted on a micromanipulator were slowly inserted into the motor cortex to a depth of 1.8 mm. The electrodes were gently released from the holder and the craniotomy was sealed with melted bone wax (W810, Ethicon, Belgium). The wound was treated in situ with antibiotic ointment (Synthomycine, chloramphenicol 5%) and sutured with nylon sutures. Then the rats received an intraperitoneal injection of the antibiotic Enrofloxacin 50 mg/ml (5% W/V) at a dose of 15 mg/kg diluted with saline to 1 ml (Baytril, Bayer Animal Health GmbH, Leverkusen, Germany). In line with standard protocols to prevent postoperative pain, the rats received for three consecutive days after gMμE-PPMP implantation non-steroidal anti-inflammatory/analgesic drugs. A subcutaneous injection of Carprofen 50 mg/ml (5% W/V) in a dose of about 12 mg/kg (Norocarp, Norbrook Laboratories Limited, Newry, Co. Down, Northern Ireland) during surgery. Then, to further reduce the stress and pain caused by injections and prevent mechanical stress to the skin around the implantation site, the rats were fed on days 2 and 3 post PPMP implantation by Meloxicam (Rheumocam, oral suspension 1.5 mg/ml, Chanelle pharma) dissolved in palatable Jelly. To that end, Meloxicam dissolved in agar (Meloxicam-jelly) prepared in a small Petri dish (diameter of 35 mm) was placed in the rat cages. The Petri dishes were removed at the end of days 2 and 3. Visual checks confirmed that the rats consumed the entire volume of the Meloxicam-jelly. After surgery, the animals were housed individually to prevent them from chewing the implants. Actually, this is relevant only for transcuteneous implants. In case of dummy probes it is important to keep rats separately only for the first 10–14 days after the surgery. I overlooked it because automatically, i keep all my transcutaneously implanted animals separately.
Tissue Processing for Immunohistology and Transmission Electron Microscopy
For brain tissue fixation, individual rats were deeply anesthetized with isoflurane (Piramal, United States) followed by an IP overdose injection of pentobarbital (4.5 ml per 250 g rat, CTS Group, Israel). When breathing had stopped, the rats were transcardially perfused with phosphate buffer saline (PBS). This was followed by a 4% paraformaldehyde in PBS (PFA, Sigma-Aldrich) perfusion for immunohistology and 1–2.5% glutaraldehyde/2% paraformaldehyde (Agar Scientific) for transmission electron microscopy (TEM). In both cases, the perfusion rate was 10 ml/min and lasted for 40 min. Next, the skulls were removed and the implanted brains were post-fixed at 4°C for an additional 12–24 h. in either PFA (for immunohistology), or glutaraldehyde/paraformaldehyde (for TEM). Thereafter, the fixed and exposed brains destined for immunohistology were washed in PBS and incubated for 1–3 days in a 30% sucrose solution in PBS at 4°C.
To prepare the brain tissue for cryosectioning (immunohistology), cubic shaped portions of tissue (approximately 1 × 1 × 1 cm) with the PPMP in their center were isolated. The isolated piece was placed in a freezing medium (Tissue- Plus O.C.T. Compound, Scigen) and frozen at −80°C. The frozen tissues along with the implanted platform were then horizontally sectioned into 40 μm thick slices using a Leica CM1850 Cryostat. Individual slices were collected and placed in 24 well plates containing PBS. The tissue slices were then incubated in blocking solution (1xPBS, 1% heat-inactivated horse serum (Biological Industries), 0.1% Triton X-100 (Sigma Aldrich)) for 1 h at room temperature (RT) under gentle shaking. Next, the slices were incubated with a diluted primary antibody for 3 h at room temperature (RT) and washed three times with the blocking solution. This was followed by 1-h incubation at RT with the diluted secondary antibody after which the slices were washed with the blocking solution three times and stained with the nuclear marker DAPI (Sigma–Aldrich, 1 mg/ml 1:1,000) for 15 min at RT. After washing with the blocking solution and PBS, the slices were mounted on Superfrost Plus Slides (Thermo Fisher Scientific) and sealed by a Vectashield (VE-H-1000 -Vector Labs) mounting medium.
Electron Microscopy
For TEM imaging, glutaraldehyde/paraformaldehyde fixed tissue along with the PI, MEA platform implants were washed with PBS and sliced by a Leica VT1000S Vibrotome using a ceramic blade (Campden Instruments Ltd.) into 200 μm thick horizontal sections. The slices were deposited in 24 well plates with PBS.
After eight washes with 0.1 M cacodylate buffer at pH 7.4 (SigmaAldrich) the tissue was post fixed by 1% osmium tetroxide (Electron Microscopy Sciences) and 0.6% K3Fe(CN)6 in a 0.1 M cacodylate buffer for 1 hr. at room temperature. The slices were then washed again in a 0.1 M cacodylate buffer and dehydrated by a series of increasing concentrations of ethanol solutions of 10, 25, 50, 75, 90, 96, 100, and 100%. Finally, the slices were embedded in Agar 100 (Agar Scientific). The embedded preparation was then thin-sectioned and observed using a TEM Tecnai 12 microscope at 100 kV. The shown TEM images were taken from sections prepared across the perforated part of the implant (rows 2–6 as marked in Figure 1). Efforts were made to orientate the thin sections perpendicular to the long axis of the polyimide platform and along the gMμEs. As the diameter of the stalks and caps of the gMμEs are in the range of 1–3 μm, a slight deviation from a perfect sectioning angle, resulted in imperfect sections that did not pass through the entire length of the gMμE cap and stalk. Thus, in some cases, the sections went through the entire length of the mushrooms cap, stalks and the contact between the stalk and the conducting line (for example Figures 6, 7A). In others, the thin sections were slightly tilted in respect to the long axis of the gMμE stalks. In these cases the stalk of the mushroom appears to taper toward the polyimide platform. The observed TEM images and conclusions represent transmission electron microscope imaging of over 250 thin sections prepared from 25 different PPMPs implants.
Immunolabeling, Confocal Imaging, Image Processing, Analysis, and Statistics
Immunolabeling, imaging, image processing, and analysis were conducted as detailed in previous studies from our laboratory (Huang et al., 2020; Sharon et al., 2021). Briefly, neurons were concomitantly labeled with two antibodies: one for neurite labeling [mouse anti-Neurofilament 160/200 monoclonal antibody (Sigma-Aldrich N2912, 1:10,000–1:20,000)] and the other for neuronal nuclei [mouse anti-NeuN monoclonal antibody (Merck MAB377, 1:200)]. Astrocytes were labeled with chicken anti-glial fibrillary acidic protein (GFAP) polyclonal antibodies (Thermo Fisher PA1-10004, 1:500–1,000). Microglia were labeled using rabbit anti-Iba-1 monoclonal antibody (Abcam ab178846, 1:1,000). For the secondary antibodies we used goat anti-mouse Alexa 488, goat anti-chicken Alexa 647 (Thermo Fisher A-11001 and A21449, respectively, 1:100) and sheep anti-rabbit Cy3 (Sigma–Aldrich C2306, 1:100).
Confocal image stacks of the immunolabeled slices were acquired with an Olympus FLUOVIEW FV3000 confocal scan head coupled to an IX83 inverted microscope, using a 20X air objective (NA = 0.75). Typically, 15–30 confocal slices were acquired, with a vertical spacing of 1 μm. Image processing of the immunolabeled sections was conducted using the Fiji distribution of ImageJ (Schindelin et al., 2012; Schneider et al., 2012).
Two methods of analysis and representation of the cell densities in contact and around the PPMPs were used: (1) The densities of the astrocytes and neurons, including their cell bodies and neurites, were analyzed and displayed as the relative fluorescent intensities with respect to the normal background. These are referred to in Figures 2E2,E3 as the Normalized Fluorescent Intensity (NFI) values (Huang et al., 2020). (2) The density of the microglia and neuronal cell bodies per 100 μm2, at a given shell around the implant, and within the pores were calculated by manual counting (Figures 2E1,E4). The counting of these cell bodies was done by merging Iba1 labeled microglia or NeuN labeled neurons with the nuclear marker DAPI.
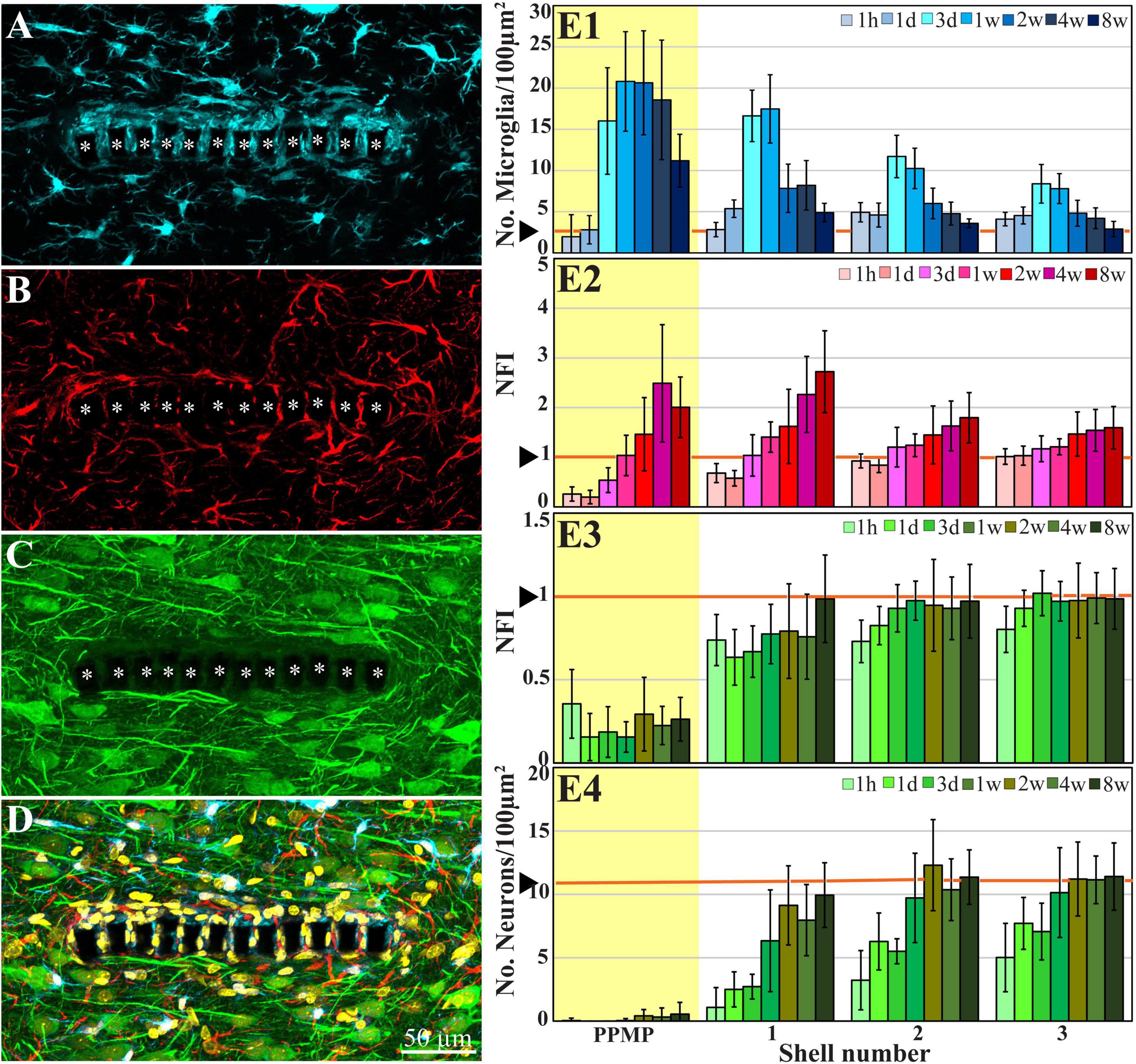
Figure 2. Confocal microscope images showing horizontal-sections of immuno-labeled cortical brain tissue along with cross sections of the perforated segment of an implanted PPMP, 2 weeks post implantation. Shown are: microglia (cyan) within and around the implant marked by asterisks (A), astrocytes (B, red), neurons and neurites (C, green), and a merged image of (A–C) which also includes the nuclei of the cells labeled in yellow (D). (E) Histograms depicting the average Normalized Fluorescent Intensity (NFI) or number of cells/100 μm2. Microglia (E1, cyan), astrocytes (E2, red), neurites and cell bodies (E3, green), and neuronal cell bodies (E4, green) within and around the platform’s perforated segments. The time post-platform implantation is coded by the darkening of the column color as indicated by the legend on the right hand side of the histograms. The average NFI values or the cells/100 μm2 within the platforms (PPMP) are highlighted in yellow. The distance of the average NFI from the MEA platform is given by shell number. Each shell is 25 μm wide (as illustrated in Figure 1F). Vertical lines correspond to one standard deviation. The orange lines indicated by the arrowheads depict the normal NFI values or the number of cell/100 μm2 in the control cortices. An enlarged image of (D) is presented as Supplementary Figure 2.
Average fluorescent values and cell counting characterizing the FBR in space and time were measured and calculated from cortical brain slices prepared from sections across rows 4–8 of the perforated part of the implant (Figures 1B, 2). We used 2–10 hemispheres/experimental points in time (Supplementary Table 1). Each brain hemisphere was used to prepare 1–6 tissue slices. Each slice was used to prepare a single maximal projected image generated by 10 consecutive optical sections. For more data on the numerical values and statistical tests, see Supplementary Table 2.
Results
Probe Design Principles
To address the technical features required to prepare thin sections of implanted gMμE platforms along with the parenchyma around it, we fabricated non-functional implants constructed of a Perforated Polyimide (PI)-based MEA Platform (PPMP) that carries a dense array of gold mushroom shaped microelectrodes (gMμE-PPMP, Figure 1). PI was selected because it is a biocompatible polymer with a Young’s modulus of 2.5 GP. Importantly, based on studies demonstrating that PI implants can be thin-sectioned for histological examinations (Mercanzini et al., 2007, 2008; Richter et al., 2013; Xie et al., 2014; Boehler et al., 2017; Huang et al., 2020) our laboratory has developed procedures to section implanted gMμE-PPMPs along with the surrounding brain parenchyma for light and transmission electron-microscope (TEM) studies (Huang et al., 2020; Sharon et al., 2021). In the present study, we fabricated 1.7 mm long, 280 μm wide and 16 μm thick non-functional gMμE-PPMPs. The proximal 0.9 mm of the implant was constructed of solid PI, and the remaining distal part was perforated (Figure 1). The perforated segment tapered to form a sharp tip. The width of all the rectangular perforations was 7–8 μm and the lengths of the different perforations were 65, 47, and 44 μm (Figure 1). gMμEs were electroplated in rows along the conducing gold lines which run in between the perforations (Supplementary Figure 1). The high density of the gMμEs served to increase the probability of successfully preparing thin sections (80 nm) for TEM imaging through gMμEs and PI along with the interfacing brain parenchyma. The perforated microarchitecture of the platform reduced the projected solid surface area of the perforated part by 35% and allowed cells to extend branches or migrate through the perforations. Each pore in the PI platform approximately doubled the PI surface to which the cells could adhere.
Ultrastructure of the Implant and Parenchyma
To examine the interfaces formed between the brain parenchyma and implanted gMμE-PPMPs, cross-sections for transmission electron microscopy of gMμE-PPMPs along with the surrounding tissue were prepared. We selected to examine the ultrastructure of the inflammatory scar at 2, 4, and 8 weeks after electrode implantation, since our earlier immunohistological studies showed characteristic alterations in the distribution and densities of the microglia, astrocytes and neurons at these points in time (Figure 2 and Huang et al., 2020; Sharon et al., 2021). For the reader’s convenience, the overall spatiotemporal relationships between microglia, astrocytes, neurons, and PPMP implants is briefly presented in Figure 2 using conventional immunohistological imaging.
For the ultrastructural analysis, gMμE-PPMP implanted brains were chemically fixed by standard transcardial perfusion of glutaraldehyde/paraformaldehyde fixative. Since MEA platform implantation unavoidably damages blood capillaries along the insertion path, concern was raised whether the quality of tissue fixation around the implant will suffice to preserve the tissue ultrastructure. In retrospect, based on the preservation qualities of the cell membranes and subcellular organelles including the mitochondria, the smooth and rough endoplasmic reticulum, synaptic vesicles, post-synaptic densities and myelin, we concluded that the perfusion of the fixative was not impaired in the surroundings of the implant. It is important to note, however, that as in other ultrastructural studies of the CNS, the extracellular spaces between the various cell types is reduced by approximately 20% (Korogod et al., 2015; Hrabetova et al., 2018; Soria et al., 2020). Since the volume of the implanted PPMPs is not altered by the fixatives, transcardial fixation led to the generation of mechanical tension around the implant. This often tears the tissue around the implant. Importantly, tissue growing into the PPMP pores and adhering to the platform surfaces remained tightly attached to the platforms, and the break in the tissue took place between cells or even across cells a few micrometers away from the implant surface.
Since the relative positioning of the PPMP and the cells around it are not altered by the classical method of transcardial fixative perfusion, the TEM analysis presented here suffices to provide the essential and missing information on implant brain-tissue interfaces. In addition, because the range of the shrinkage factor is known, the genuine extracellular clefts can be estimated. It is important to note that TEM examination of hundreds of thin sections representing over 25 gMμE-PPMP implants revealed that the gMμEs maintain stable contact with the conducting lines used for their electroplating. That is, the gMμEs are not striped off during the platforms insertion or during the thin sectioning of the tissue along with the implant for TEM imaging.
Insulation of the Perforated Polyimide MEA Platform Implants by Microglia at 2 Weeks Post-perforated Polyimide MEA Platform Implantation
Typically, at 2 weeks post gMμE-PPMPs implantation, tightly adhering dark cytoplasmic microglia processes (dark as compared to other cell profiles in their surroundings) encapsulated individual PI “ribs” (Figures 3, 4). The cell bodies from which the dark cytoplasm emanated contained characteristic microglia nuclei with clumps of heterochromatin beneath the nuclear envelope and throughout their nucleoplasm (Figure 3 and see Tremblay et al., 2012; Garcia-Cabezas et al., 2016; Savage et al., 2018; Nahirney and Tremblay, 2021). The electron-dense cytoplasm of these microglia was bordered by a clear plasma membrane and contained rough endoplasmic reticulum, mitochondria and dark inclusions which may plausibly be lysosomes and lipofuscin granules (Figures 3, 4).
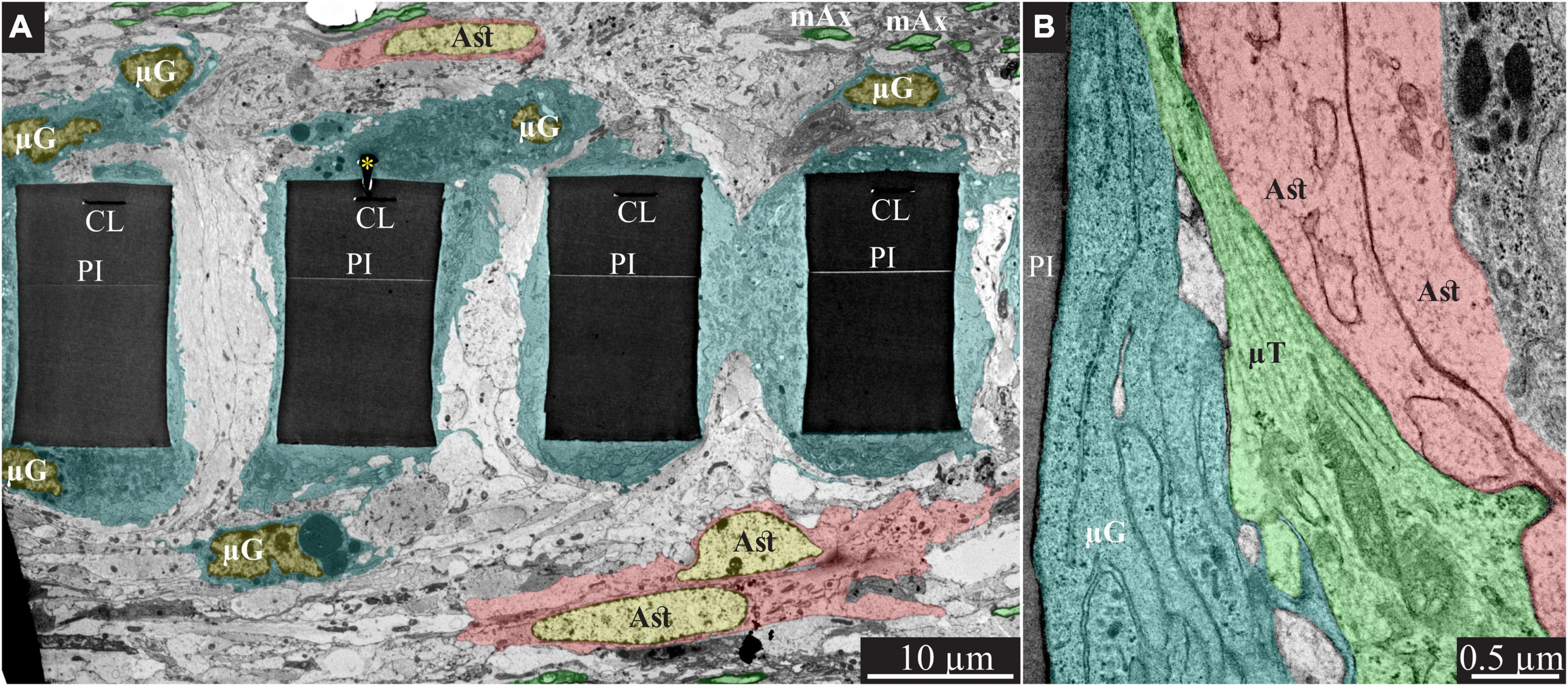
Figure 3. Encapsulation of individual PPMPs “ribs” by adhering microglia. (A) A low magnification transmission electron microscope cross-section of an implanted PPMP along with the parenchyma around it 2 weeks after platform implantation. The PI “ribs” are encompassed by microglia (cytoplasm marked in cyan and typical microglia nuclei μG -yellow) and astrocytes (cytoplasm marked pink, and astrocyte nuclei Ast- yellow). (B) Microglia adhering to the PI surface interposed between the implant and non-myelinated neurites containing microtubules and astrocyte branches that invaded the platform pores. No myelinated axons were seen in the immediate vicinity of the implant at this point in time after PPMP implantation. PI, polyimide ribs; gMμE, yellow asterisks, mAx- myelinated axons (green); CL, conducting line; μG, microglia; μT, microtubule; Ast, astrocyte. Note that an unmarked copy of this figure is presented as Supplementary Figure 3.
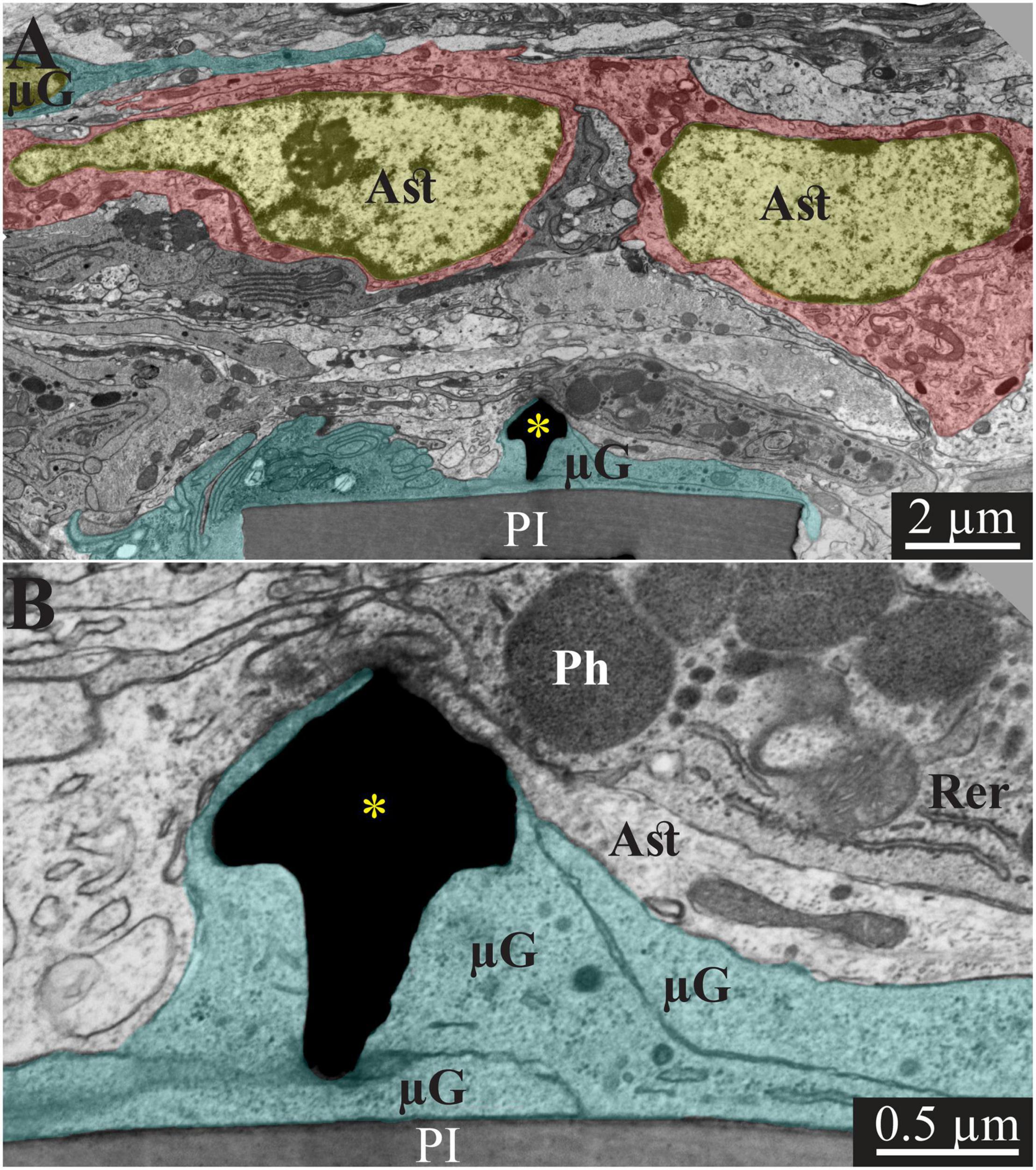
Figure 4. Transmission electron microscope images of a gMμE (black mushroom shaped profiles A,B) tightly engulfed by microglia, 2 weeks after PPMP implantation. Note the thin 0.5–1 μm layers of microglia branches that adhered tightly to the PI surface and the gold mushroom microelectrode (cyan). Additional microglia layers characterized by dark cytoplasm (not labeled in color) contained a rough endoplasmic reticulum and dark inclusions which plausibly were lysosomes and lipofuscine granules. Astrocyte branches characterized by sparse electron dense material containing intermediate filaments invaded in between the microglia branches but did not form direct contact with the implant. Note that the TEM section is slightly tilted in respect to the long axis of the gMμE. For that reason the mushroom’s stalk appears to taper toward the polyimide platform and looks like it end in the tissue rather than attached to polyimide. Astrocyte cell bodies resided micrometers away from the implant. PI, polyimide ribs; gMμE, yellow asterisks; μG, microglia; Rer, rough endoplasmic reticulum; Ast, astrocyte; Ph, phagocytosed materials. An unmarked copy of this figure is presented as Supplementary Figure 4.
In line with Figure 2 and our earlier immunohistological studies (Huang et al., 2020; Sharon et al., 2021), 2 weeks post-PPMP implantation, TEM images revealed the presence of astrocyte cell bodies and branches in close proximity to the platform surface and within the platform pores (Figures 3B, 4A). Astrocyte cell bodies could be identified by their pale nuclei that had a thin rim of heterochromatin and pale cytoplasm (Figures 3, 4A). Typically, the cytoplasm of astrocyte branches are characterized by sparse electron- dense material containing intermediate filaments (glial fibrillary acidic protein, GFAP, Garcia-Cabezas et al., 2016; Nahirney and Tremblay, 2021).
Two weeks after implantation, the dark microglia cytoplasm that adheres tightly to the gMμE-PPMPs surfaces are often interposed between the PPMP surfaces and the astrocytic branches, thus mechanically preventing direct contact between the neurite and astrocytic branches and the platform surfaces (Figure 3B).
Immunohistological imaging of the neurite revealed that at 2 weeks post-implantation, neurites extended into the PI platform pores (Figures 2C,E3). Based on the presence of microtubules in axons and GFAP in astrocytes (Figure 3B) it was possible to differentiate between the branches of the astrocytes and the unmyelinated neurites (axons and dendrites). At 2 weeks post-PPMP implantation, no myelinated axonal profiles were observed in the immediate vicinity (<10 μm) to the gMμE-PPMPs. Further away from the implants (>10 μm) myelinated axons were observed (Figure 3).
Neuronal cell bodies characterized by typical round euchromatic nuclei, the presence of electron dense nucleoli and nuclear membrane invaginations were observed as close as ∼20 μm from the PI platform and onwards (see also Figure 2E4). Chemical presynaptic terminals identified by the presence of profiles containing clusters of synaptic vesicles or chemical synapses identified by presynaptic fibers in association with typical post-synaptic densities were imaged at a distances of approximately 10 μm from the platform.
Regenerative Processes in Contact and Around Perforated Polyimide MEA Platform Implants at 4 Weeks Post-perforated Polyimide MEA Platform Implantation
Immunohistological examination of the changes in cell composition and distribution within and around the implanted PPMPs 4 weeks after PPMP implantation (Figure 2) suggested that the parenchyma around the implant had undergone regenerative processes. These included: (a) a reduction in the average microglia density in the first shells around the implant, but not within the PPMP pores (Figure 2E1 and Huang et al., 2020); (b) a significant increase in the average density of the neuronal cell bodies in the first shell around the implant, preceded by the extension of neurites toward the implant and into the PPMP pores (Figures 2E3,E4 and Huang et al., 2020). In contrast to these regenerative processes, the astrocyte branches and cell bodies continued to increase during the fourth week post- implantation both within the PPMPs and in the first shell around it (Figure 2E2).
The overall regenerative processes observed at the confocal microscope resolution were reflected and more finely delineated at the ultrastructural levels. TEM images revealed myelinated axons extending toward and in the vicinity of the implant surface (Figure 5). A considerable increase in the density of structurally mature chemical synapses was seen. At 4 weeks post-implantation, the PPMP’s “ribs” were no longer enwrapped by dark protoplasmic protrusions emanating from microglia cell bodies. Rather, relatively thin layers of electron opaque cytoplasm adhered to the surface of the PPMPs and the gMμEs (Figure 5). Relevant to the electrophysiological recording functions of the implant (see discussion), it is noted that the extracellular cleft formed between the microglia membranes that enwrapped the gMμE remained in the range of 10–20 nm (Figure 5B). The microglia clearly interposed between the myelinated axons extending in the electrodes’ vicinity and between pale cytoplasmic profiles of astrocytic branches and unmyelinated axons. Astrocyte branches and cell bodies, microglia and unmyelinated axonal profiles were seen occupy the pores between the PI “ribs” and directly adhere to the PI surfaces.
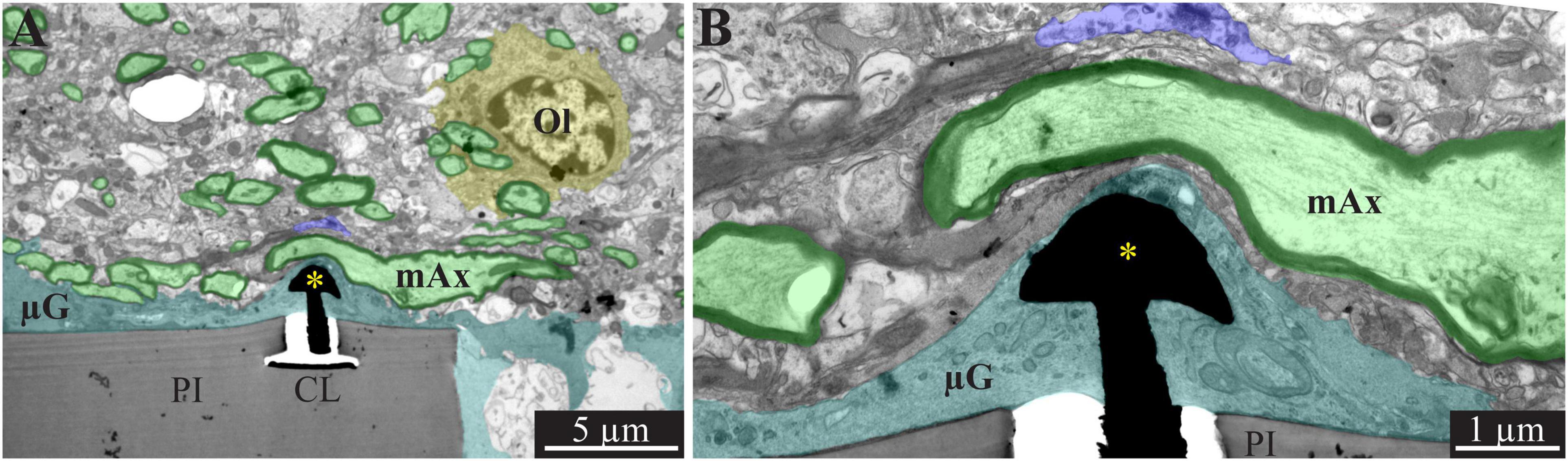
Figure 5. A low and high magnification, transmission electron microscope image of the interfaces formed between a gold mushroom shaped microelectrode extending from a polyimide platform implanted for 4 weeks and the surrounding cortical tissue. The mushroom shaped microelectrode (black) emerges from the PI substrate (gray). Note the thin layer of dark microglia (cyan in A,B, and unmarked dark gray branches in B) adheres tightly to the gMμE and PI substrate. The parenchyma around the implant underwent regenerative processes as indicated by the large number of myelinated axons (green and black envelope) and the presence of oligodendrocytes in the immediate vicinity of the implant (yellow). Whereas, axonal branches with a relatively large diameter (∼3 μm) extended close to the gMμE, it is conceivable that the adhering microglia (and in this instance the myelin as well) insulated the electrode from the surrounding excitable tissue. PI, polyimide ribs; CL, conducting line; gMμE, yellow asterisks; Ol, oligodendrocyte; μG, microglia; mAx, myelinated axons. Note an unmarked copy of this figure is presented as Supplementary Figure 6.
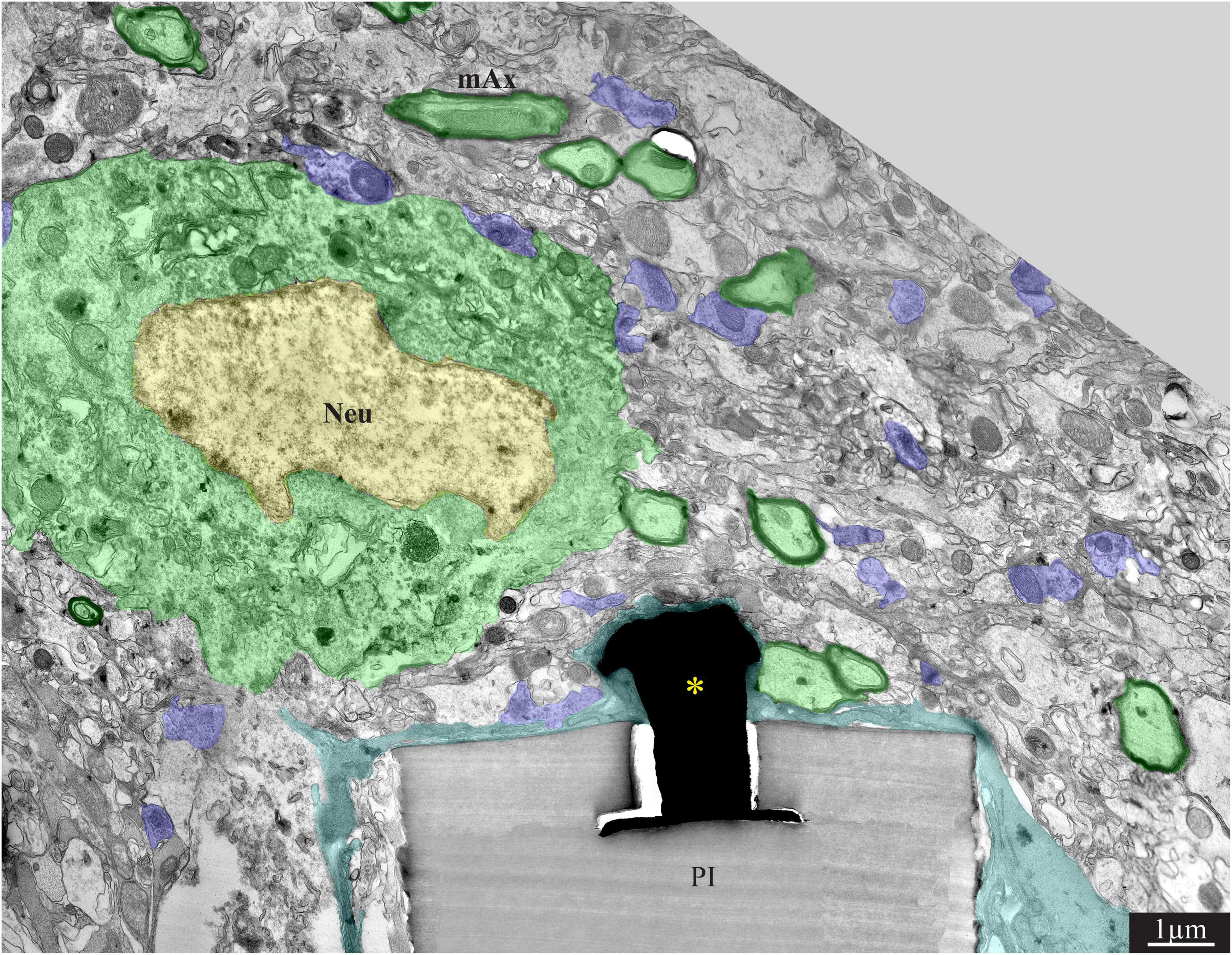
Figure 6. A low magnification, transmission electron microscope image of the interfaces formed between a gold mushroom shaped microelectrode extending from a polyimide platform implanted for 8 weeks and the surrounding cortical tissue. The mushroom shaped microelectrode (black) immerges from the PI substrate (gray). A thin layer of dark microglia (cyan) adheres tightly to the gMμE and the PI substrate. A neuronal cell body with a typical nuclear structure (yellow) and cytoplasm (green) resides approximately a micrometer away from the gMμE and the PI platform’s surface. Myelinated axons (green surrounded by a black sheath) are distributed in the parenchyma in contact with the microglia that adheres to the platform. Unmyelinated neurites and synaptic structures (labeled purple) were identified (using large magnification of the image) by the presence of presynaptic vesicles. The remainder of the unmarked profiles are astrocyte branches and non-myelinated neurites. PI, polyimide ribs; gMμE, yellow asterisks; Neu, neuron; mAx, myelinated axons. Note that an unmarked copy of this figure is presented as Supplementary Figure 7.
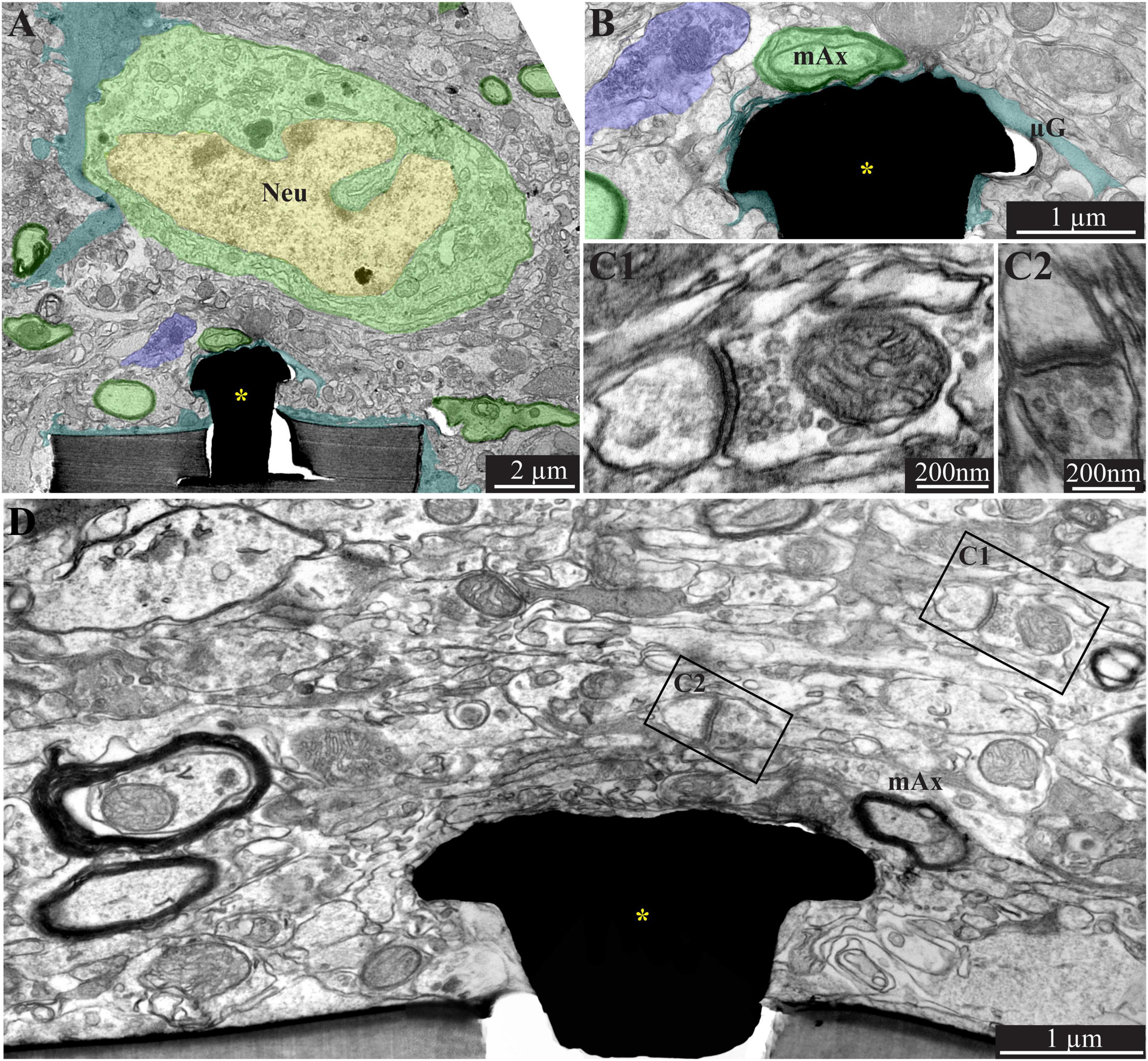
Figure 7. A low (A) and high magnification (B), transmission electron microscope image of the interfaces formed between a gold mushroom shaped microelectrode extending from a polyimide platform implanted for 8 weeks and the surrounding cortical tissue. As the regenerative processes of the brain parenchyma proceed with time, the dark microglia adhering layer becomes thinner (A,B, cyan). It is conceivable that even a thin microglia layer might insulate the electrodes from the surrounding parenchyma. (D) The regenerative processes of the parenchyma are also evidenced by the presence of a chemical synaptic profile as close as a few micrometers from the implant (C1,C2, see D for the location of the synapses with respect to the electrode). Note that the TEM section (in D) is slightly tilted in respect to the long axis of the gMμE. For that reason the base of the mushrooms stalk appears to taper toward the polyimide platform. Interestingly, 8 weeks after implantation we also observed gMμE that were not enwrapped by microglia and formed a direct contact with the small profile of astrocyte and possibly neurons (D). gMμE, yellow asterisks; Neu, neuron; mAx, myelinated axons; μG, microglia. An unmarked copy of this figure is presented as Supplementary Figure 8.
In addition, large profiles of dark cytoplasm containing phagocytosed materials were occasionally observed to reside within the pores (Supplementary Figure 5). In a few cases, the section went through the nucleus of these large cells. Based on the heterochromatin distribution of the nucleus, these cells were likely to be microglia. These cell types were never observed outside the PI implant pores.
In summary, whereas clear regeneration of the neuron cell body densities, axons, dendrites and synapses took place within the first shell around the implant, dark microglia branches adhering to the gMμEs and PPMPs were still present. These adhering microglia can be assumed to electrically insulate the electrodes from the surrounding neurons (see section “Discussion”).
Increased Density of Neurons Near the Implant Surface 8 Weeks Post-perforated Polyimide MEA Platform Implantation
Confocal microscope imaging of the cortical parenchyma interfaced 8 weeks after PPMP implantation revealed that the overall regenerative processes that were observed 2–4 weeks after implantation persisted. (a) The microglia density within the implant was further reduced to half of its peak value and to a third in the first shell around the implant (Figure 2E1 and Huang et al., 2020). (b) Whereas, the neurite density (NFI values, Figure 2E3) did not change, the average neuronal cell body density in the first shell around the implant further increased to 86% with respect to the control level (Figure 2E4). (c) On the other hand, the astrocyte (branches and cell bodies together) continued to increase mainly in the first shell around the implant (Figure 2E2). These regenerative trends were reflected at the TEM level, in particular in that neuronal cell bodies were imaged to reside as close as ∼2 μm from the gMμE caps (Figures 6, 7A). The narrow space between the cell bodies membrane and the gMμE caps were occupied by small profiles (with a diameter in the range of <1 μm) of either astrocytes or neurites. Parts of the gMμE stalk and caps were enwrapped by narrow (∼100 nm) dark cytoplasmic protrusions probably corresponding to microglia branches, while other parts appeared to be free of microglia. Myelinated axonal profiles were observed to form a direct contact with gMμE caps (Figure 7B). Chemical synaptic profiles were observed as close as ∼0.5 μm to the PI platform and the gMμEs, and within the parenchyma surrounding the implant (Figures 6, 7). The pores within the PI platform were mainly occupied by astrocytic branches and unmyelinated axonal profiles. No synaptic structures were observed within the pores.
TEM observations conducted 8 weeks after the PPMP implantation, occasionally revealed gMμE that were not insulated by microglia. Under these conditions, the gMμEs formed a direct contact with many small (∼100 nm) axonal or astrocytic profiles (Figure 7D). It is conceivable that the small surface area of these tentatively identified unmyelinated axonal profiles were too small to generate sufficient current to be measured by the gMμE system.
Discussion
Despite significant progress, contemporary in vivo MEA technologies suffer from inherent limitations that include a low signal-to-noise ratio, low source resolution and deterioration of the recording yield and FP amplitudes within days to weeks of implantation. Whereas, these drawbacks constitute a critical impediment to the progress of basic and clinically oriented brain research, the mechanisms that generate these limitations remain elusive. For that reason, attempts to develop effective methods to overcome these drawbacks have only been marginally successful.
To achieve a better understanding of the mechanisms that limit the functions of implanted electrophysiological neuroprobes, for the first time, the present study examined the intact ultrastructural interfaces formed between the cortical parenchyma and a large footprint implanted neuroprobes. The findings reveal remarkable tissue regeneration around and in contact with the large-footprint implanted MEA platform. This include the regrowth of neurites toward the implant, myelination of the newly grown axons, the formation of structurally mature chemical synapses, the recovery of neuronal cell body densities in the vicinity of the electrodes (at a distance of ∼1 μm from the electrodes’ surfaces) and cortical capillaries (Figure 8). Along with this remarkable tissue regeneration, we documented that individual microglia adhering to the gMμEs-PPMP surfaces formed a micrometer-thin barrier in contact with the PI backbone and the gMμEs which we dub the “microglia-insulating-junction.” For a period of approximately 8 weeks post-PPMP implantation (the longest observation period made here), the adhering microglia prevented the formation of a direct contact between the axons or neuronal cell bodies and the gMμE. Thus, engulfment of gMμEs and most likely other 3D or planar microelectrodes by neurons is likely to be impeded. Because the microglia insulating junctions are formed at the electrode surfaces, this configuration offers an explanation to the enigma as to why no correlation has been found between the dimensions and density of the FBR and recording qualities (Kozai et al., 2014, 2015; McCreery et al., 2016; Du et al., 2017; Salatino et al., 2017a; Michelson et al., 2018).
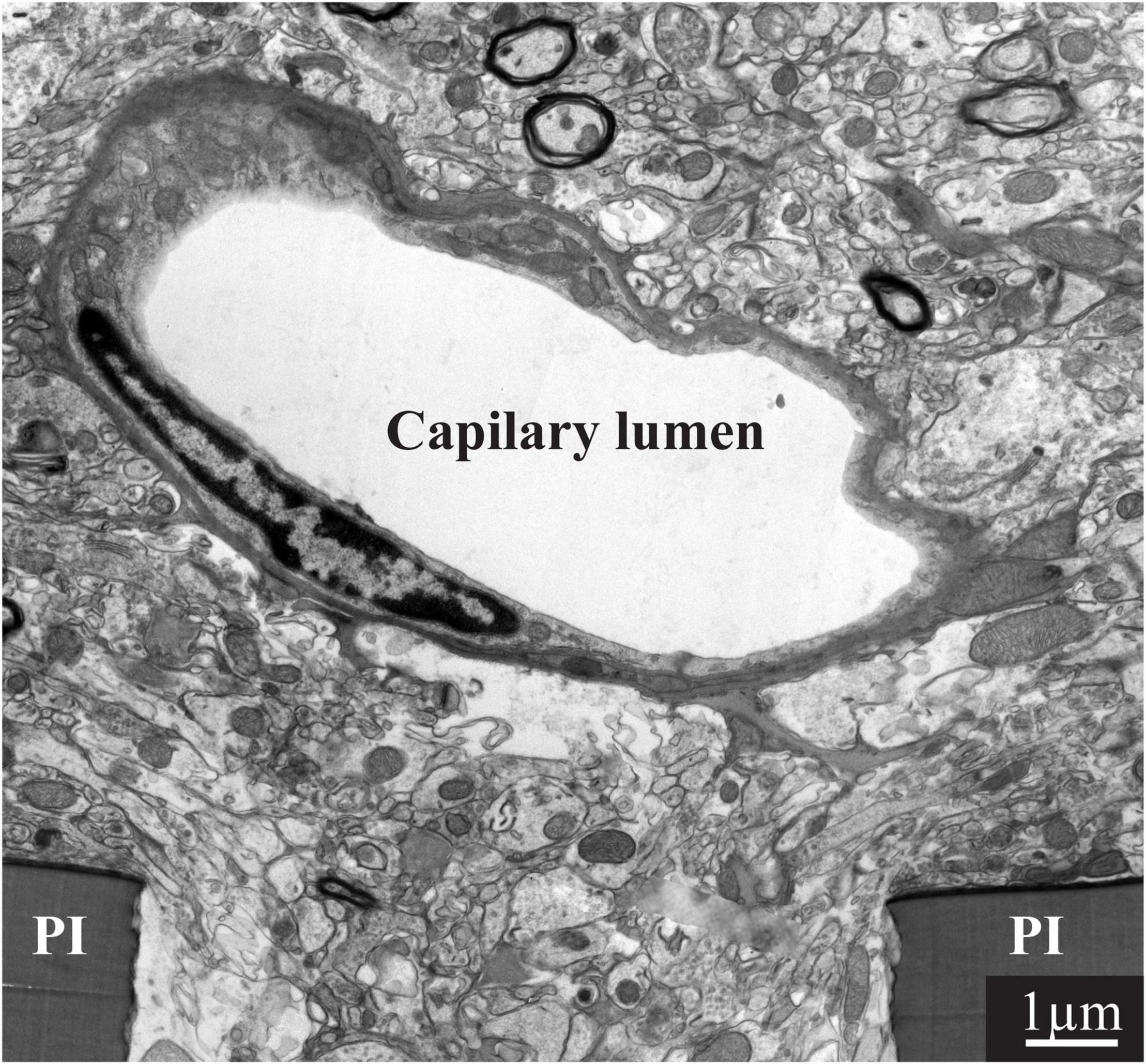
Figure 8. Regeneration of capillaries close to implanted PPMPs. A capillary located micrometers away from the surface of the polyimide (PI) “ribs” of an implanted PPMP for 8 weeks.
Ultrastructural analysis of the junctions formed between different cell types and planar or 3D microelectrodes under in vitro conditions have served a pivotal role in deciphering the biophysics and potential applications of the junctions formed. An order of magnitude estimate of microglia- gMμE-junction impedance can be derived using a passive electrical circuit model composed of two parallel resistors: the seal resistance (Rs) formed by the cleft between the plasma membrane of the microglia and the surface of the gMμEs, and the input resistance (Rμg) of the adhering microglia.
The seal resistance (Rs) is given by Rseal = ρs⋅δ/d, where ρs is the resistivity of the electrolyte solution (ρs = 0.7 ΩCm), d is the average cleft width between the neuron’s plasma membrane and the electrodes’ surface, and δ is the overlapping surface coefficient that takes into account the percentage of the electrodes’ sensitive area in contact with the microglia (Massobrio et al., 2016). Because of the unavoidable ∼20% shrinking artifact of the extracellular spaces due to the chemical fixation of the tissue for TEM imaging (Korogod et al., 2015; Hrabetova et al., 2018; Soria et al., 2020) the actual width (d) of the clefts formed between the microglia and the implanted gMμEs-PPMPs surfaces cannot be extracted with precision from the ultrastructural images. In addition, the fraction of the surface area of the contact between a gMμE or planar electrode and the adhering microglia (δ) cannot be obtained from classical TEM images. Nonetheless, an order of magnitude estimate of Rs formed by different cell types can be obtained by using parameters published previously in the literature. A large number of in vitro studies have revealed that the cleft width formed between different cultured cell types and artificial substrates ranges from 20 to 100 nm (Braun and Fromherz, 1998; Iwanaga et al., 2001; Straub et al., 2001; Lambacher and Fromherz, 2002; Brittinger and Fromherz, 2005; Gleixner and Fromherz, 2006; Wrobel et al., 2008) and the contact surface area of these junctions has been estimated. The estimated seal resistance derived in these studies ranged from ∼1 MΩ in the case of planar electrodes (Weis and Fromherz, 1997; Buitenweg et al., 1998, 2002) to ∼40–100 MΩs for gMμEs (Hai et al., 2009a; Fendyur et al., 2011; Spira and Hai, 2013; Ojovan et al., 2015; Shmoel et al., 2016; Massobrio et al., 2018; Spira et al., 2019).
The input resistance of mice microglia (Rμg) was reported to be 2–5 GΩ (Avignone et al., 2008; Schilling and Eder, 2015). Since the morphology and physiology of microglia are known to change under different functional states and in response to different substrates (Eder, 1998, 2005, 2010; Kettenmann et al., 2011), it is conceivable that the input resistance of microglia adhering to the gMμEs is <2–5 GΩ. Assuming that the input resistance of adhering microglia is reduced to the range of 10–100 MΩ, the resistance formed by an adhering “microglia insulating junction” is in the range of ∼1 MΩ for a planar electrode and ∼50 MΩs for a gMμE or a vertical nano-pillar engulfed by a microglia (Rμg⋅Rs/Rμg + Rs.).
Given that the estimated resistance of intact brain parenchyma is in the range of 1–4 Ω (Logothetis et al., 2007), and 300–6,000 Ω, across an encapsulation glial scar (Turner et al., 1999; Szarowski et al., 2003; Moffitt and McIntyre, 2005; Grill and Mortimer, 2014), the current generated by neurons positioned very close or in contact with microglia adhering to a sensing electrode is expected to be attenuated by 1–3 orders of magnitude. Thus, the FPs generated by neurons positioned in the immediate vicinity of a microglia-insulating-junction might be below the level of detection.
It is worth noting that recent progress in bioengineering has led to the implementation of ultra-small and ultra-flexible platforms, with dimensions comparable to those of a single neuron (Xiang et al., 2014; Fu et al., 2016; Luan et al., 2017; Zhao et al., 2017; Wei et al., 2018; Guan et al., 2019; Yang et al., 2019; Zhang et al., 2021). Immunohistological observations have shown that these ultra-small, flexible implants integrate seamlessly with brain tissue, and that under these conditions neuronal cell bodies are seen to reside in close proximity to the implant (Fu et al., 2016; Luan et al., 2017; Zhou et al., 2017; Hong et al., 2018; Yang et al., 2019). Despite the fact that the impedances of these ultra- small and ultra-flexible electrodes are similar to those of conventional implants (0.5–1 MΩ) and despite the seamless integration of these platforms with brain tissue, the recorded amplitudes of the FP have been within the range of those recorded by implants that trigger FBR. These observations are inconsistent with the prevailing hypothesis that in the absence of a histological FBR the FPs amplitudes should be larger. This apparent paradox may be resolved by assuming that even if a “classical” FBR is not imaged as having been formed by these implants, microglia insulating-junctions that were not detected by standard immunohistology nevertheless formed and insulated the electrodes.
Overall, the present study resolves two critical questions: (1) what are the cellular mechanisms that underlie the limited electrophysiological functions of implanted in vivo neuroprobes and (2), can the successfully developed and advantageous gMμE or other 3D vertical nano-pillars be applied to in vivo settings? We posited that the insulation formed by individual microglia that tightly adhere to or engulf in vivo implanted electrodes rather than multicellular FBR deteriorate the electrical coupling coefficient between the neurons and the implanted electrodes. The microglia electrode junctions structurally isolate and electrically insulate the electrodes from the neurons and hence limit the electrophysiological functions of the electrodes. Overcoming the challenging microglia insulating-junction requires developing new protocols to specifically and temporally target the adhering microglia. This should be complemented by methods to increase the density of neuronal cell bodies to enable the formation of direct contact with the electrodes.
It is conceivable that the effective structural regeneration of the parenchyma in the immediate vicinity of the gMμE-PPMP implants and the implant-parenchyma integration documented here reflect compound abiotic and biotic factors. For that reason, it is premature to extrapolate the observation made here to implants composed of different materials, with different microarchitecture, sizes and shapes, and implanted in different brain regions in different organisms.
Data Availability Statement
The raw data supporting the conclusions of this article will be made available by the authors, without undue reservation.
Ethics Statement
The animal study was reviewed and approved by the Committee for Animal Experimentation at the Institute of Life Sciences of the Hebrew University of Jerusalem.
Author Contributions
AS implanted the platforms and processed the tissues for both immunohistological and transmission electron microscope sectioning together with HE. AS and HE analyzed the images. NS headed the fabrication of the perforated polyimide MEA platforms. MJ supervised the PPMP implantations. YF thin sectioned the tissues for TEM imaging and helped with the analysis. MS conceived, designed, and supervised the project. All authors contributed to the article and approved the submitted version.
Funding
This work was supported by the Israel Science Foundation grant number 1808/19. Part of this work was conducted at the Charles E. Smith and Prof. Joel Elkes Laboratory for Collaborative Research in Psychobiology. This study is based on an earlier research project supported by the National Institute of Neurological Disorders and Stroke of the National Institutes of Health under Award Number U01NS099687.
Author Disclaimer
The content is solely the responsibility of the authors and does not necessarily represent any official views of the granting agencies.
Conflict of Interest
The authors declare that the research was conducted in the absence of any commercial or financial relationships that could be construed as a potential conflict of interest.
Publisher’s Note
All claims expressed in this article are solely those of the authors and do not necessarily represent those of their affiliated organizations, or those of the publisher, the editors and the reviewers. Any product that may be evaluated in this article, or claim that may be made by its manufacturer, is not guaranteed or endorsed by the publisher.
Acknowledgments
We thank Shimon Eliav, Galina Chechelinsky, Maurice Saidian, and Evgenia Blayvas from the Harvey M. Kruger Family Center for Nanoscience for taking part in the fabrication of the perforated polyimide-based MEA platforms.
Supplementary Material
The Supplementary Material for this article can be found online at: https://www.frontiersin.org/articles/10.3389/fnins.2021.764448/full#supplementary-material
References
Abbott, J., Ye, T., Ham, D., and Park, H. (2018). Optimizing nanoelectrode arrays for scalable intracellular electrophysiology. Acc. Chem. Res. 51, 600–608. doi: 10.1021/acs.accounts.7b00519
Abbott, J., Ye, T., Krenek, K., Gertner, R. S., Ban, S., Kim, Y., et al. (2019). A nanoelectrode array for obtaining intracellular recordings from thousands of connected neurons. Nat. Biomed. Eng. 4, 232–241. doi: 10.1038/s41551-019-0455-7
Abbott, J., Ye, T., Qin, L., Jorgolli, M., Gertner, R. S., Ham, D., et al. (2017). CMOS nanoelectrode array for all-electrical intracellular electrophysiological imaging. Nat. Nanotechnol. 12, 460–466. doi: 10.1038/nnano.2017.3
Akaike, N., and Harata, N. (1994). Nystatin perforated patch recording and its applications to analyses of intracellular mechanisms. Jpn. J. Physiol. 44, 433–473. doi: 10.2170/jjphysiol.44.433
Angle, M. R., and Schaefer, A. T. (2012). Neuronal recordings with solid-conductor intracellular nanoelectrodes (SCINEs). PLoS One 7:e43194. doi: 10.1371/journal.pone.0043194
Angle, M. R., Wang, A., Thomas, A., Schaefer, A. T., and Melosh, N. A. (2014). Penetration of cell membranes and synthetic lipid bilayers by nanoprobes. Biophys. J. 107, 2091–2100. doi: 10.1016/j.bpj.2014.09.023
Avignone, E., Ulmann, L., Levavasseur, F., Rassendren, F., and Audinat, E. (2008). Status epilepticus induces a particular microglial activation state characterized by enhanced purinergic signaling. J. Neurosci. 28, 9133–9144. doi: 10.1523/JNEUROSCI.1820-08.2008
Biran, R., Martin, D. C., and Tresco, P. A. (2005). Neuronal cell loss accompanies the brain tissue response to chronically implanted silicon microelectrode arrays. Exp. Neurol. 195, 115–126. doi: 10.1016/j.expneurol.2005.04.020
Boehler, C., Kleber, C., Martini, N., Xie, Y., Dryg, I., Stieglitz, T., et al. (2017). Actively controlled release of Dexamethasone from neural microelectrodes in a chronic in vivo study. Biomaterials 129, 176–187. doi: 10.1016/j.biomaterials.2017.03.019
Braun, D., and Fromherz, P. (1998). Fluorescence interferometry of neuronal cell adhesion on microstructured silicon. Phys. Rev. Lett. 81, 5241–5244. doi: 10.1103/physrevlett.81.5241
Brittinger, M., and Fromherz, P. (2005). Field-effect transistor with recombinant potassium channels: fast and slow response by electrical and chemical interactions. Appl. Phys. Mat. Sci. Process. 81, 439–447. doi: 10.1007/s00339-005-3272-7
Buitenweg, J. R., Rutten, W. L., Marani, E., Polman, S. K., and Ursum, J. (2002). Extracellular detection of active membrane currents in the neuron-electrode interface. J. Neurosci. Methods 115, 211–221. doi: 10.1016/s0165-0270(02)00021-3
Buitenweg, J. R., Rutten, W. L., Willems, W. P., and Van Nieuwkasteele, J. W. (1998). Measurement of sealing resistance of cell-electrode interfaces in neuronal cultures using impedance spectroscopy. Med. Biol. Eng. Comput. 36, 630–637. doi: 10.1007/BF02524436
Carlson, D., and Carin, L. (2019). Continuing progress of spike sorting in the era of big data. Curr. Opin. Neurobiol. 55, 90–96. doi: 10.1016/j.conb.2019.02.007
Dipalo, M., Amin, H., Lovato, L., Moia, F., Caprettini, V., Messina, G. C., et al. (2017). Intracellular and extracellular recording of spontaneous action potentials in mammalian neurons and cardiac cells with 3D plasmonic nanoelectrodes. Nano. Lett. 17, 3932–3939. doi: 10.1021/acs.nanolett.7b01523
Du, Z. J., Kolarcik, C. L., Kozai, T. D. Y., Luebben, S. D., Sapp, S. A., Zheng, X. S., et al. (2017). Ultrasoft microwire neural electrodes improve chronic tissue integration. Acta Biomater. 53, 46–58. doi: 10.1016/j.actbio.2017.02.010
Duan, X., Gao, R., Xie, P., Cohen-Karni, T., Qing, Q., Choe, H. S., et al. (2012). Intracellular recordings of action potentials by an extracellular nanoscale field-effect transistor. Nat. Nanotechnol. 7, 174–179. doi: 10.1038/nnano.2011.223
Edell, D. J., Toi, V. V., Mcneil, V. M., and Clark, L. D. (1992). Factors influencing the biocompatibility of insertable silicon microshafts in cerebral cortex. IEEE Trans. Biomed. Eng. 39, 635–643. doi: 10.1109/10.141202
Eder, C. (1998). Ion channels in microglia (brain macrophages). Am. J. Physiol. 275, C327–C342. doi: 10.1007/978-1-4899-5031-4_17
Eder, C. (2005). Regulation of microglial behavior by ion channel activity. J. Neurosci. Res. 81, 314–321.
Eder, C. (2010). Ion channels in monocytes and microglia/brain macrophages: promising therapeutic targets for neurological diseases. J. Neuroimmunol. 224, 51–55. doi: 10.1016/j.jneuroim.2010.05.008
Einevoll, G. T., Franke, F., Hagen, E., Pouzat, C., and Harris, K. D. (2012). Towards reliable spike-train recordings from thousands of neurons with multielectrodes. Curr. Opin. Neurobiol. 22, 11–17. doi: 10.1016/j.conb.2011.10.001
Fendyur, A., Mazurski, N., Shappir, J., and Spira, M. E. (2011). Formation of essential ultrastructural interface between cultured hippocampal cells and gold mushroom-shaped MEA- Toward “IN-CELL” recordings from vertebrate neurons. Front. Neuroeng. 4:14. doi: 10.3389/fneng.2011.00014
Fendyur, A., and Spira, M. E. (2012). Toward on-chip, in-cell recordings from cultured cardiomyocytes by arrays of gold mushroom-shaped microelectrodes. Front. Neuroeng. 5:21. doi: 10.3389/fneng.2012.00021
Fu, T. M., Hong, G., Zhou, T., Schuhmann, T. G., Viveros, R. D., and Lieber, C. M. (2016). Stable long-term chronic brain mapping at the single-neuron level. Nat. Methods 13, 875–882. doi: 10.1038/nmeth.3969
Gao, R., Strehle, S., Tian, B., Cohen-Karni, T., Xie, P., Duan, X., et al. (2012). Outside looking in: nanotube transistor intracellular sensors. Nano Lett. 12, 3329–3333. doi: 10.1021/nl301623p
Garcia-Cabezas, M. A., John, Y. J., Barbas, H., and Zikopoulos, B. (2016). Distinction of neurons, glia and endothelial cells in the cerebral cortex: an algorithm based on cytological features. Front. Neuroanat. 10:107. doi: 10.3389/fnana.2016.00107
Gleixner, R., and Fromherz, P. (2006). The extracellular electrical resistivity in cell adhesion. Biophys. J. 90, 2600–2611.
Grand, L., Wittner, L., Herwik, S., Gothelid, E., Ruther, P., Oscarsson, S., et al. (2010). Short and long term biocompatibility of NeuroProbes silicon probes. J. Neurosci. Methods 189, 216–229. doi: 10.1016/j.jneumeth.2010.04.009
Grill, W. M., and Mortimer, J. T. (2014). Electrical Properties of Implant Encapsulation Tissue. Ann. Biomed. Eng. 22, 23–33. doi: 10.1007/bf02368219
Guan, S., Wang, J., Gu, X., Zhao, Y., Hou, R., Fan, H., et al. (2019). Elastocapillary self-assembled neurotassels for stable neural activity recordings. Sci. Adv. 5:eaav2842. doi: 10.1126/sciadv.aav2842
Hai, A., Dormann, A., Shappir, J., Yitzchaik, S., Bartic, C., Borghs, G., et al. (2009a). Spine-shaped gold protrusions improve the adherence and electrical coupling of neurons with the surface of micro-electronic devices. J. R. Soc. Interface 6, 1153–1165. doi: 10.1098/rsif.2009.0087
Hai, A., Kamber, D., Malkinson, G., Erez, H., Mazurski, N., Shappir, J., et al. (2009b). Changing gears from chemical adhesion of cells to flat substrata toward engulfment of micro-protrusions by active mechanisms. J. Neural. Eng. 6:066009. doi: 10.1088/1741-2560/6/6/066009
Hai, A., Shappir, J., and Spira, M. E. (2010a). In-cell recordings by extracellular microelectrodes. Nat. Methods 7, 200–202. doi: 10.1038/nmeth.1420
Hai, A., Shappir, J., and Spira, M. E. (2010b). Long-term, multisite, parallel, in-cell recording and stimulation by an array of extracellular microelectrodes. J. Neurophysiol. 104, 559–568. doi: 10.1152/jn.00265.2010
Harris, K. D., Quiroga, R. Q., Freeman, J., and Smith, S. L. (2016). Improving data quality in neuronal population recordings. Nat. Neurosci. 19, 1165–1174. doi: 10.1038/nn.4365
Hermann, J. K., and Capadona, J. R. (2018). Understanding the Role of Innate Immunity in the Response to Intracortical Microelectrodes. Crit. Rev. Biomed. Eng. 46, 341–367. doi: 10.1615/critrevbiomedeng.2018027166
Hong, G., Yang, X., Zhou, T., and Lieber, C. M. (2018). Mesh electronics: a new paradigm for tissue-like brain probes. Curr. Opin. Neurobiol. 50, 33–41.
Horn, R., and Marty, A. (1988). Muscarinic activation of ionic currents measured by a new whole-cell recording method. J. Gen. Physiol. 92, 145–159. doi: 10.1085/jgp.92.2.145
Hrabetova, S., Cognet, L., Rusakov, D. A., and Nagerl, U. V. (2018). Unveiling the extracellular space of the brain: from super-resolved microstructure to in vivo function. J. Neurosci. 38, 9355–9363. doi: 10.1523/JNEUROSCI.1664-18.2018
Huang, S. H., Shmoel, N., Jankowski, M. M., Erez, H., Sharon, A., Abu-Salah, W., et al. (2020). Immunohistological and ultrastructural study of the inflammatory response to perforated polyimide cortical implants: mechanisms underlying deterioration of electrophysiological recording quality. Front. Neurosci. 14:926. doi: 10.3389/fnins.2020.00926
Iwanaga, Y., Braun, D., and Fromherz, P. (2001). No correlation of focal contacts and close adhesion by comparing GFP-vinculin and fluorescence interference of Dil. Eur. Biophys. J. 30, 17–26. doi: 10.1007/s002490000119
Jackson, A., and Fetz, E. E. (2007). Compact movable microwire array for long-term chronic unit recording in cerebral cortex of primates. J. Neurophysiol. 98, 3109–3118. doi: 10.1152/jn.00569.2007
Johnson, M. D., Otto, K. J., and Kipke, D. R. (2005). Repeated voltage biasing improves unit recordings by reducing resistive tissue impedances. IEEE Trans. Neural. Syst. Rehabil. Eng. 13, 160–165. doi: 10.1109/TNSRE.2005.847373
Jones, P. D., Moskalyuk, A., Barthold, C., Gutohrlein, K., Heusel, G., Schroppel, B., et al. (2020). Low-Impedance 3D PEDOT:PSS Ultramicroelectrodes. Front. Neurosci. 14:405. doi: 10.3389/fnins.2020.00405
Kettenmann, H., Hanisch, U. K., Noda, M., and Verkhratsky, A. (2011). Physiology of microglia. Physiol. Rev. 91, 461–553.
Korogod, N., Petersen, C. C., and Knott, G. W. (2015). Ultrastructural analysis of adult mouse neocortex comparing aldehyde perfusion with cryo fixation. Elife 4:e05793. doi: 10.7554/eLife.05793
Kozai, T. D., Jaquins-Gerstl, A. S., Vazquez, A. L., Michael, A. C., and Cui, X. T. (2015). Brain tissue responses to neural implants impact signal sensitivity and intervention strategies. ACS Chem. Neurosci. 6, 48–67. doi: 10.1021/cn500256e
Kozai, T. D., Li, X., Bodily, L. M., Caparosa, E. M., Zenonos, G. A., Carlisle, D. L., et al. (2014). Effects of caspase-1 knockout on chronic neural recording quality and longevity: insight into cellular and molecular mechanisms of the reactive tissue response. Biomaterials 35, 9620–9634. doi: 10.1016/j.biomaterials.2014.08.006
Lambacher, A., and Fromherz, P. (2002). Luminescence of dye molecules on oxidized silicon and fluorescence interference contrast microscopy of biomembranes. J. Opt. Soc. Am. Opt. Phys. 19, 1435–1453. doi: 10.1364/josab.19.001435
Lee, H. C., Gaire, J., Roysam, B., and Otto, K. J. (2018). Placing Sites on the Edge of Planar Silicon Microelectrodes Enhances Chronic Recording Functionality. IEEE Trans. Biomed. Eng. 65, 1245–1255. doi: 10.1109/TBME.2017.2715811
Lee, Y., Shin, H., Lee, D., Choi, S., Cho, I. J., and Seo, J. (2021). A Lubricated Nonimmunogenic Neural Probe for Acute Insertion Trauma Minimization and Long-Term Signal Recording. Adv. Sci. (Weinh) 8:e2100231. doi: 10.1002/advs.202100231
Li, X. L., Mo, J. S., Fang, J. R., Xu, D. X., Yang, C., Zhang, M., et al. (2020). Vertical nanowire array-based biosensors: device design strategies and biomedical applications. J. Mater. Chem. B 8, 7609–7632. doi: 10.1039/d0tb00990c
Lin, Z. C., and Cui, B. (2014). Nanowire transistors: room for manoeuvre. Nat. Nanotechnol. 9, 94–96. doi: 10.1038/nnano.2014.10
Lin, Z. C., Xie, C., Osakada, Y., Cui, Y., and Cui, B. (2014). Iridium oxide nanotube electrodes for sensitive and prolonged intracellular measurement of action potentials. Nat. Commun. 5:3206. doi: 10.1038/ncomms4206
Liu, R., Chen, R., Elthakeb, A. T., Lee, S. H., Hinckley, S., Khraiche, M. L., et al. (2017). High density individually addressable nanowire arrays record intracellular activity from primary rodent and human stem cell derived neurons. Nano Lett. 17, 2757–2764. doi: 10.1021/acs.nanolett.6b04752
Logothetis, N. K., Kayser, C., and Oeltermann, A. (2007). In vivo measurement of cortical impedance spectrum in monkeys: implications for signal propagation. Neuron 55, 809–823. doi: 10.1016/j.neuron.2007.07.027
Luan, L., Wei, X., Zhao, Z., Siegel, J. J., Potnis, O., Tuppen, C. A., et al. (2017). Ultraflexible nanoelectronic probes form reliable, glial scar-free neural integration. Sci. Adv. 3:e1601966. doi: 10.1126/sciadv.1601966
Malaga, K. A., Schroeder, K. E., Patel, P. R., Irwin, Z. T., Thompson, D. E., Nicole Bentley, J., et al. (2016). Data-driven model comparing the effects of glial scarring and interface interactions on chronic neural recordings in non-human primates. J. Neural. Eng. 13:016010. doi: 10.1088/1741-2560/13/1/016010
Marton, G., Toth, E. Z., Wittner, L., Fiath, R., Pinke, D., Orban, G., et al. (2020). The neural tissue around SU-8 implants: a quantitative in vivo biocompatibility study. Mater. Sci. Eng. C Mater. Biol. Appl. 112:110870. doi: 10.1016/j.msec.2020.110870
Massobrio, G., Martinoia, S., and Massobrio, P. (2018). Equivalent circuit of the neuro-electronic junction for signal recordings from planar and engulfed micro-nano-electrodes. IEEE Trans. Biomed. Circ. Syst. 12, 3–12. doi: 10.1109/TBCAS.2017.2749451
Massobrio, P., Massobrio, G., and Martinoia, S. (2016). Interfacing cultured neurons to microtransducers arrays: a review of the neuro-electronic junction models. Front. Neurosci. 10:282. doi: 10.3389/fnins.2016.00282
Mateus, J. C., Lopes, C. D. F., Cerquido, M., Leitao, L., Leitao, D., Cardoso, S., et al. (2019). Improved in vitro electrophysiology using 3D-structured microelectrode arrays with a micro-mushrooms islets architecture capable of promoting topotaxis. J. Neural. Eng. 16:036012. doi: 10.1088/1741-2552/ab0b86
McCreery, D., Cogan, S., Kane, S., and Pikov, V. (2016). Correlations between histology and neuronal activity recorded by microelectrodes implanted chronically in the cerebral cortex. J. Neural. Eng. 13:036012.
McGuire, A. F., Santoro, F., and Cui, B. (2018). Interfacing cells with vertical nanoscale devices: applications and characterization. Annu. Rev. Anal. Chem. 11, 101–126. doi: 10.1146/annurev-anchem-061417-125705
Mercanzini, A., Cheung, K., Buhl, D., Boers, M., Maillard, A., Colin, P., et al. (2007). Demonstration of cortical recording and reduced inflammatory response using flexible polymer neural probes. Proc. IEEE Twentieth Annu. Int. Conf. Micro Electro Mech. Syst. 1, 573–576.
Mercanzini, A., Cheung, K., Buhl, D. L., Boers, M., Maillard, A., Colin, P., et al. (2008). Demonstration of cortical recording using novel flexible polymer neural probes. Sens. Actuat. Phys. 143, 90–96. doi: 10.1016/j.sna.2007.07.027
Michelson, N. J., Vazquez, A. L., Eles, J. R., Salatino, J. W., Purcell, E. K., Williams, J. J., et al. (2018). Multi-scale, multi-modal analysis uncovers complex relationship at the brain tissue-implant neural interface: new emphasis on the biological interface. J. Neural. Eng. 15:033001. doi: 10.1088/1741-2552/aa9dae
Moffitt, M. A., and McIntyre, C. C. (2005). Model-based analysis of cortical recording with silicon microelectrodes. Clin. Neurophysiol. 116, 2240–2250. doi: 10.1016/j.clinph.2005.05.018
Moss, J., Ryder, T., Aziz, T. Z., Graeber, M. B., and Bain, P. G. (2004). Electron microscopy of tissue adherent to explanted electrodes in dystonia and Parkinson’s disease. Brain 127, 2755–2763. doi: 10.1093/brain/awh292
Nahirney, P. C., and Tremblay, M. E. (2021). Brain Ultrastructure: putting the pieces together. Front. Cell Dev. Biol. 9:629503. doi: 10.3389/fcell.2021.629503
Ojovan, S. M., Rabieh, N., Shmoel, N., Erez, H., Maydan, E., Cohen, A., et al. (2015). A feasibility study of multi-site, intracellular recordings from mammalian neurons by extracellular gold mushroom-shaped microelectrodes. Sci. Rep. 5:14100. doi: 10.1038/srep14100
Otto, K. J., Johnson, M. D., and Kipke, D. R. (2006). Voltage pulses change neural interface properties and improve unit recordings with chronically implanted microelectrodes. IEEE Trans. Biomed. Eng. 53, 333–340. doi: 10.1109/TBME.2005.862530
Perge, J. A., Homer, M. L., Malik, W. Q., Cash, S., Eskandar, E., Friehs, G., et al. (2013). Intra-day signal instabilities affect decoding performance in an intracortical neural interface system. J. Neural. Eng. 10:036004. doi: 10.1088/1741-2560/10/3/036004
Polikov, V. S., Tresco, P. A., and Reichert, W. M. (2005). Response of brain tissue to chronically implanted neural electrodes. J. Neurosci. Methods 148, 1–18. doi: 10.1016/j.jneumeth.2005.08.015
Prasad, A., and Sanchez, J. C. (2012). Quantifying long-term microelectrode array functionality using chronic in vivo impedance testing. J. Neural. Eng. 9:026028. doi: 10.1088/1741-2560/9/2/026028
Qing, Q., Jiang, Z., Xu, L., Gao, R. X., Mai, L. Q., and Lieber, C. M. (2014). Free-standing kinked nanowire transistor probes for targeted intracellular recording in three dimensions. Nat. Nanotechnol. 9, 142–147. doi: 10.1038/nnano.2013.273
Quiroga, R. Q., Nadasdy, Z., and Ben-Shaul, Y. (2004). Unsupervised spike detection and sorting with wavelets and superparamagnetic clustering. Neural. Comput. 16, 1661–1687. doi: 10.1162/089976604774201631
Rabieh, N., Ojovan, S. M., Shmoel, N., Erez, H., Maydan, E., and Spira, M. E. (2016). On-chip, multisite extracellular and intracellular recordings from primary cultured skeletal myotubes. Sci. Rep. 6:36498. doi: 10.1038/srep36498
Richter, A., Xie, Y., Schumacher, A., Loffler, S., Kirch, R. D., Al-Hasani, J., et al. (2013). A simple implantation method for flexible, multisite microelectrodes into rat brains. Front. Neuroeng. 6:6. doi: 10.3389/fneng.2013.00006
Robinson, J. T., Jorgolli, M., Shalek, A. K., Yoon, M. H., Gertner, R. S., and Park, H. (2012). Vertical nanowire electrode arrays as a scalable platform for intracellular interfacing to neuronal circuits. Nat. Nanotechnol. 7, 180–184. doi: 10.1038/nnano.2011.249
Salatino, J. W., Kale, A. P., and Purcell, E. K. (2019). Alterations in ion channel expression surrounding implanted microelectrode arrays in the brain. bioRxiv [Preprint]. doi: 10.1101/518811
Salatino, J. W., Ludwig, K. A., Kozai, T. D. Y., and Purcell, E. K. (2017a). Glial responses to implanted electrodes in the brain. Nat. Biomed. Eng. 1, 862–877. doi: 10.1038/s41551-017-0154-1
Salatino, J. W., Winter, B. M., Drazin, M. H., and Purcell, E. K. (2017b). Functional remodeling of subtype-specific markers surrounding implanted neuroprostheses. J. Neurophysiol. 118, 194–202. doi: 10.1152/jn.00162.2017
Santoro, F., Dasgupta, S., Schnitker, J., Auth, T., Neumann, E., Panaitov, G., et al. (2014). Interfacing electrogenic cells with 3D nanoelectrodes: position, shape, and size matter. ACS Nano 8, 6713–6723. doi: 10.1021/nn500393p
Santoro, F., Schnitker, J., Panaitov, G., and Offenhäusser, A. (2013). On chip guidance and recording of cardiomyocytes with 3D mushroom-shaped electrodes. Nano Lett. 13, 5379–5384. doi: 10.1021/nl402901y
Santoro, F., Zhao, W., Joubert, L. M., Duan, L., Schnitker, J., Van De Burgt, Y., et al. (2017b). Revealing the cell-material interface with nanometer resolution by focused ion beam/scanning electron microscopy. ACS Nano 11, 8320–8328. doi: 10.1021/acsnano.7b03494
Santoro, F., Van De Burgt, Y., Keene, S. T., Cui, B. X., and Salleo, A. (2017a). Enhanced Cell-Chip Coupling by Rapid Femtosecond Laser Patterning of Soft PEDOT:PSS Biointerfaces. ACS Appl. Mater. Interfaces 9, 39116–39121. doi: 10.1021/acsami.7b12308
Savage, J. C., Picard, K., Gonzalez-Ibanez, F., and Tremblay, M. E. (2018). A brief history of microglial ultrastructure: distinctive features, phenotypes, and functions discovered over the past 60 years by electron microscopy. Front. Immunol. 9:803. doi: 10.3389/fimmu.2018.00803
Saxena, T., Karumbaiah, L., Gaupp, E. A., Patkar, R., Patil, K., Betancur, M., et al. (2013). The impact of chronic blood-brain barrier breach on intracortical electrode function. Biomaterials 34, 4703–4713. doi: 10.1016/j.biomaterials.2013.03.007
Schilling, T., and Eder, C. (2015). Microglial K(+) channel expression in young adult and aged mice. Glia 63, 664–672.
Schindelin, J., Arganda-Carreras, I., Frise, E., Kaynig, V., Longair, M., Pietzsch, T., et al. (2012). Fiji: an open-source platform for biological-image analysis. Nat. Methods 9, 676–682. doi: 10.1038/nmeth.2019
Schneider, C. A., Rasband, W. S., and Eliceiri, K. W. (2012). NIH Image to ImageJ: 25 years of image analysis. Nat. Methods 9, 671–675. doi: 10.1038/nmeth.2089
Schultz, R. L., and Willey, T. J. (1976). Ultrastructure of Sheath around Chronically Implanted Electrodes in Brain. J. Neurocytol. 5, 621–642. doi: 10.1007/BF01181577
Sharon, A., Jankowski, M. M., Shmoel, N., Erez, H., and Spira, M. E. (2021). Inflammatory Foreign Body Response Induced by Neuro-Implants in Rat Cortices Depleted of Resident Microglia by a CSF1R Inhibitor and Its Implications. Front. Neurosci. 15:646914. doi: 10.3389/fnins.2021.646914
Shmoel, N., Rabieh, N., Ojovan, S. M., Erez, H., Maydan, E., and Spira, M. E. (2016). Multisite electrophysiological recordings by self-assembled loose-patch-like junctions between cultured hippocampal neurons and mushroom-shaped microelectrodes. Sci. Rep. 6:27110. doi: 10.1038/srep27110
Sommakia, S., Gaire, J., Rickus, J. L., and Otto, K. J. (2014). Resistive and reactive changes to the impedance of intracortical microelectrodes can be mitigated with polyethylene glycol under acute in vitro and in vivo settings. Front. Neuroeng. 7:33. doi: 10.3389/fneng.2014.00033
Sommakia, S., Rickus, J. L., and Otto, K. J. (2009). Effects of adsorbed proteins, an antifouling agent and long-duration DC voltage pulses on the impedance of silicon-based neural microelectrodes. Conf. Proc. IEEE Eng. Med. Biol. Soc. 2009, 7139–7142. doi: 10.1109/IEMBS.2009.5332456
Soria, F. N., Miguelez, C., Penagarikano, O., and Tonnesen, J. (2020). Current techniques for investigating the brain extracellular space. Front. Neurosci. 14:570750. doi: 10.3389/fnins.2020.570750
Spira, M. E., and Hai, A. (2013). Multi-electrode array technologies for neuroscience and cardiology. Nat. Nanotechnol. 8, 83–94. doi: 10.1038/nnano.2012.265
Spira, M. E., Huang, S. H., Shmoel, N., and Erez, H. (2019). “In vitro neuronal networks -from culturing methods toneuro-technological applications,” in Advance Neurobiology, eds M. Chiappalone, V. Pasquale, and M. Frega (Berlin: Springer), 125–153. doi: 10.1007/978-3-030-11135-9_5
Spira, M. E., Kamber, D., Dormann, A., Cohen, A., Bartic, C., Borghs, G., et al. (2007). Improved neuronal adhesion to the surface of electronic device by engulfment of protruding micro-nails fabricated on the chip surface. Trans. Eurosens. 1, 1247–1250.
Spira, M. E., Shmoel, N., Huang, S. M., and Erez, H. (2018). Multisite attenuated intracellular recordings by extracellular multielectrode arrays, a perspective. Front. Neurosci. 12:212. doi: 10.3389/fnins.2018.00212
Straub, B., Meyer, E., and Fromherz, P. (2001). Recombinant maxi-K channels on transistor, a prototype of iono-electronic interfacing. Nat. Biotechnol. 19, 121–124. doi: 10.1038/84369
Szarowski, D. H., Andersen, M. D., Retterer, S., Spence, A. J., Isaacson, M., Craighead, H. G., et al. (2003). Brain responses to micro-machined silicon devices. Brain Res. 983, 23–35. doi: 10.1016/s0006-8993(03)03023-3
Teixeira, H., Dias, C., Aguiar, P., and Ventura, J. (2020). Gold-mushroom microelectrode arrays and the quest for intracellular-like recordings: perspectives and outlooks. Adv. Mater. Technol. 6:e2000770.
Thompson, C. H., Riggins, T. E., Patel, P. R., Chestek, C. A., Li, W., and Purcell, E. (2020). Toward guiding principles for the design of biologically-integrated electrodes for the central nervous system. J. Neural. Eng. 17:021001. doi: 10.1088/1741-2552/ab7030
Tian, B., Cohen-Karni, T., Qing, Q., Duan, X., Xie, P., and Lieber, C. M. (2010). Three-dimensional, flexible nanoscale field-effect transistors as localized bioprobes. Science 329, 830–834. doi: 10.1126/science.1192033
Tremblay, M. E., Zettel, M. L., Ison, J. R., Allen, P. D., and Majewska, A. K. (2012). Effects of aging and sensory loss on glial cells in mouse visual and auditory cortices. Glia 60, 541–558. doi: 10.1002/glia.22287
Turner, J. N., Shain, W., Szarowski, D. H., Andersen, M., Martins, S., Isaacson, M., et al. (1999). Cerebral astrocyte response to micromachined silicon implants. Exp. Neurol. 156, 33–49. doi: 10.1006/exnr.1998.6983
Vezzani, A., and Viviani, B. (2015). Neuromodulatory properties of inflammatory cytokines and their impact on neuronal excitability. Neuropharmacology 96, 70–82. doi: 10.1016/j.neuropharm.2014.10.027
Voigts, J., Siegle, J. H., Pritchett, D. L., and Moore, C. I. (2013). The flexDrive: an ultra-light implant for optical control and highly parallel chronic recording of neuronal ensembles in freely moving mice. Front. Syst. Neurosci. 7:8. doi: 10.3389/fnsys.2013.00008
Wei, X., Luan, L., Zhao, Z., Li, X., Zhu, H., Potnis, O., et al. (2018). Nanofabricated Ultraflexible Electrode Arrays for High-Density Intracortical Recording. Adv. Sci. 5:1700625. doi: 10.1002/advs.201700625
Weidlich, S., Krause, K. J., Schnitker, J., Wolfrum, B., and Offenhausser, A. (2017). MEAs and 3D nanoelectrodes: electrodeposition as tool for a precisely controlled nanofabrication. Nanotechnology 28:095302. doi: 10.1088/1361-6528/aa57b5
Weis, R., and Fromherz, P. (1997). Frequency dependent signal transfer in neuron transistors. Phys. Rev. 55, 877–889. doi: 10.1002/smll.201906640
Williams, J. C., Hippensteel, J. A., Dilgen, J., Shain, W., and Kipke, D. R. (2007). Complex impedance spectroscopy for monitoring tissue responses to inserted neural implants. J. Neural. Eng. 4, 410–423. doi: 10.1088/1741-2560/4/4/007
Winslow, B. D., Christensen, M. B., Yang, W. K., Solzbacher, F., and Tresco, P. A. (2010). A comparison of the tissue response to chronically implanted Parylene-C-coated and uncoated planar silicon microelectrode arrays in rat cortex. Biomaterials 31, 9163–9172. doi: 10.1016/j.biomaterials.2010.05.050
Winslow, B. D., and Tresco, P. A. (2010). Quantitative analysis of the tissue response to chronically implanted microwire electrodes in rat cortex. Biomaterials 31, 1558–1567. doi: 10.1016/j.biomaterials.2009.11.049
Wrobel, G., Holler, M., Ingebrandt, S., Dieluweit, S., Sommerhage, F., Bochem, H. P., et al. (2008). Transmission electron microscopy study of the cell-sensor interface. J. R. Soc. Interface 5, 213–222. doi: 10.1098/rsif.2007.1094
Xiang, Z. L., Yen, S. C., Xue, N., Sun, T., Tsang, W. M., Zhang, S. S., et al. (2014). Ultra-thin flexible polyimide neural probe embedded in a dissolvable maltose-coated microneedle. J. Micromech. Microeng. 24:065015. doi: 10.1088/0960-1317/24/6/065015
Xie, C., Lin, Z., Hanson, L., Cui, Y., and Cui, B. (2012). Intracellular recording of action potentials by nanopillar electroporation. Nat. Nanotechnol. 7, 185–190. doi: 10.1038/nnano.2012.8
Xie, Y., Martini, N., Hassler, C., Kirch, R. D., Stieglitz, T., Seifert, A., et al. (2014). In vivo monitoring of glial scar proliferation on chronically implanted neural electrodes by fiber optical coherence tomography. Front. Neuroeng. 7:34. doi: 10.3389/fneng.2014.00034
Xu, D., Mo, J., Xie, X., and Hu, N. (2021). In-Cell Nanoelectronics: opening the door to intracellular electrophysiology. Nanomicro. Lett. 13:127. doi: 10.1007/s40820-021-00655-x
Yang, X., Zhou, T., Zwang, T. J., Hong, G., Zhao, Y., Viveros, R. D., et al. (2019). Bioinspired neuron-like electronics. Nat. Mater. 18, 510–517. doi: 10.1038/s41563-019-0292-9
Yoo, J., Kwak, H., Kwon, J., Ha, G. E., Lee, E. H., Song, S., et al. (2020). Long-term intracellular recording of optogenetically-induced electrical activities using vertical nanowire multi electrode array. Sci. Rep. 10:4279. doi: 10.1038/s41598-020-61325-3
Zhang, A., Lee, J. H., and Lieber, C. M. (2021). Nanowire-enabled bioelectronics. Nano Today 38, 1–17.
Zhao, W., Hanson, L., Lou, H. Y., Akamatsu, M., Chowdary, P. D., Santoro, F., et al. (2017). Nanoscale manipulation of membrane curvature for probing endocytosis in live cells. Nat. Nanotechnol. 12, 750–756. doi: 10.1038/nnano.2017.98
Keywords: neural-engineering, neuroelectronics, neuroimplant, interfacing, microelectrodes, ultrastructure (electron microscopy), immunohistology, polyimide
Citation: Sharon A, Shmoel N, Erez H, Jankowski MM, Friedmann Y and Spira ME (2021) Ultrastructural Analysis of Neuroimplant-Parenchyma Interfaces Uncover Remarkable Neuroregeneration Along-With Barriers That Limit the Implant Electrophysiological Functions. Front. Neurosci. 15:764448. doi: 10.3389/fnins.2021.764448
Received: 25 August 2021; Accepted: 21 October 2021;
Published: 22 November 2021.
Edited by:
Ulrich G. Hofmann, University of Freiburg Medical Center, GermanyReviewed by:
Paolo Massobrio, University of Genoa, ItalyEllis Meng, University of Southern California, United States
Copyright © 2021 Sharon, Shmoel, Erez, Jankowski, Friedmann and Spira. This is an open-access article distributed under the terms of the Creative Commons Attribution License (CC BY). The use, distribution or reproduction in other forums is permitted, provided the original author(s) and the copyright owner(s) are credited and that the original publication in this journal is cited, in accordance with accepted academic practice. No use, distribution or reproduction is permitted which does not comply with these terms.
*Correspondence: Micha E. Spira, spira@cc.huji.ac.il