- 1Department of Biochemistry and Molecular Genetics, Stem Cell Institute, University of Alabama at Birmingham, Birmingham, AL, United States
- 2Genome Engineering and iPSC Center, Washington University, St. Louis, MO, United States
Friedreich’s ataxia (FRDA) is a neurodegenerative disorder caused by the expansion of guanine–adenine–adenine repeats within the first intron of the frataxin (FXN) gene. The location and nature of the expansion have been proven to contribute to transcriptional repression of FXN by decreasing the rate of polymerase II (RNA polymerase II) progression and increasing the presence of histone modifications associated with a heterochromatin-like state. Targeting impaired FXN transcription appears as a feasible option for therapeutic intervention, while no cure currently exists. We created a novel reporter cell line containing an FXN-Nanoluciferase (FXN-NLuc) fusion in induced pluripotent stem cells (iPSCs) reprogrammed from the fibroblasts of patients with FRDA, thus allowing quantification of endogenous FXN expression. The use of iPSCs provides the opportunity to differentiate these cells into disease-relevant neural progenitor cells (NPCs). NPCs derived from the FXN-NLuc line responded to treatments with a known FXN inducer, RG109. Results were validated by quantitative PCR and Western blot in multiple FRDA NPC lines. We then screened a commercially available library of compounds consisting of molecules targeting various enzymes and pathways critical for silencing or activation of gene expression. Only selected histone deacetylase inhibitors were capable of partial reactivation of FXN expression. This endogenous, FRDA iPSC-derived reporter can be utilized for high-throughput campaigns performed in cells most relevant to disease pathology in search of FXN transcription activators.
Introduction
Friedreich’s ataxia (FRDA; OMIM, #229300) is an autosomal recessive neurodegenerative disorder, affecting approximately 1:20,000–1:50,000 people of the Caucasian population (Bürk, 2017; Indelicato et al., 2020). It is the most common inherited ataxia (Marmolino, 2011; Cook and Giunti, 2017) with a typical onset at 10–15 years of age (Parkinson et al., 2013). FRDA is described as a multisystemic disease, manifesting in peripheral and central nervous systems, with a significant group of patients affected by cardiomyopathy, diabetes, and hearing and vision impairment (Koeppen, 2011; Delatycki and Corben, 2012). The molecular pathology of FRDA is driven by the large trinucleotide guanine–adenine–adenine (GAA) expansion in the first intron of the frataxin (FXN) gene, located on chromosome 9q13-q21.1 (Campuzano et al., 1996). For the majority of patients, an expansion is present on both FXN alleles (Dürr et al., 1996), with a smaller population harboring a single expanded GAA tract accompanied by a point mutation (Cossée et al., 1999; Clark et al., 2017). Healthy individuals carry up to 38 GAA repeats, whereas hyperexpansion in patients with FRDA can exceed 1,000 repeats (Pandolfo, 2009). FXN is a mitochondrial protein involved in iron–sulfur cluster (Fe–S) synthesis and overall iron metabolism (Martelli et al., 2007). Deficiency of FXN in FRDA leads to a broad spectrum of molecular aberrations including but not limited to mitochondrial iron accumulation, increased oxidative stress, and reactive oxygen species (ROS) formation (Clark et al., 2018).
The consequence of GAA repeat expansion is transcriptional silencing of the FXN gene, resulting in low levels of FXN (Bidichandani et al., 1998; Ohshima et al., 1998; Dion and Wilson, 2009). Two major, not mutually exclusive, mechanisms leading to FXN transcription inhibition have been proposed: formation of non-B DNA and/or DNA–RNA structures (Bidichandani et al., 1998) and localized chromatin changes (Saveliev et al., 2003). The formation of non-canonical DNA structures such as intramolecular triplexes or R-loops (Bergquist et al., 2016) leads to defects in transcription initiation (De Biase et al., 2009) and decreased rate of RNA polymerase II (RNAPII) elongation (Li et al., 2015a). At the same time, differences in histone modifications at the FXN locus, typically immediately upstream and downstream of the expanded repeats, between FRDA and unaffected controls have been recurrently observed (Herman et al., 2006). These include decreased acetylation of histones H3 and H4 (H3K9ac and H4K5ac) and increased histone H3 trimethylation at lysines 9 and 27 (H3K9me3 and H3K27me3) as well as elevated DNA cytosine methylation, all demonstrated in cellular and mouse FRDA models (Herman et al., 2006; Greene et al., 2007; Al-Mahdawi et al., 2008; Punga and Bühler, 2010; Yandim et al., 2013). Combined, data from numerous studies point toward DNA/DNA–RNA conformation(s)-induced chromatin changes as a trigger for FXN downregulation.
Targeting impaired FXN transcription in FRDA represents one of the main therapeutic strategies for this devastating disease (Gottesfeld, 2019; Zhang et al., 2019). Numerous small molecules aimed to reverse the repressive chromatin environment at the FXN locus have been tested (Sarsero et al., 2003; Herman et al., 2006; Sandi et al., 2014; Georges et al., 2019). FXN expression is upregulated by treatment with histone deacetylase inhibitors (HDACi) (Bürk, 2017), especially RG109 (also known as RG2833) (Soragni et al., 2014; Chutake et al., 2016; Codazzi et al., 2016), nicotinamide, and resveratrol (Chan et al., 2013; Li et al., 2013; Georges et al., 2019). In addition, treating FRDA cells with histone methyltransferase (HMT) inhibitors that reduce H3K9me2/3, H3K27me3 (Sherzai et al., 2020), and H4K20me2/3 (Vilema-Enríquez et al., 2020) demonstrated modest increases in FXN expression, indicating a role for histone methylation also in repressing FXN transcription.
FXN reactivation was also observed after targeting GAA tracts (or structures formed by these repeats) using chemically modified single- and double-stranded antisense oligonucleotides (ASOs) (Li L. et al., 2016; Li et al., 2018; Shen et al., 2018) or pyrrole-imidazole polyamides (PAs) (Burnett et al., 2006; Gottesfeld, 2007). Combining GAA-specific PAs with a small-molecule ligand engaging transcription elongation machinery resulted in a novel type of hybrid compound termed Synthetic Transcription Elongation Factor 1 (SynTef1), also capable of stimulating FXN transcription (Erwin et al., 2017). Lastly, several other compounds, including interferon gamma, antioxidants, and nuclear factor erythroid 2–related factor 2 (Nrf2) activators, were shown to increase FXN levels although their mechanisms of action have not been clearly defined (Gottesfeld, 2019).
Some of the described transcription activators have reached early phases of clinical trials (Gottesfeld, 2019); however, identification of novel lead compounds capable of stimulating FXN expression in FRDA cells is laborious and time consuming. To aid this process, several reporter cell lines designed to mimic the FXN transcription defect while allowing for robust evaluation of numerous compounds have been utilized (Grant et al., 2006; Soragni et al., 2008; Li et al., 2013; Lufino et al., 2013). Initial FRDA reporter cell lines were designed on the basis of engineered HeLa (Grant et al., 2006) or HEK293 FlpIn (Soragni et al., 2008) cells harboring a green fluorescent protein (GFP) gene with expanded GAA tracts. Neither of these reporter systems directly reported endogenous FXN levels. Next-generation reporters addressed this issue by using bacterial artificial chromosome-based constructs carrying the entire human FXN gene modified by an in-frame insertion of a reporter gene (enhanced GFP or luciferase) (Li et al., 2013; Lufino et al., 2013). These constructs were integrated in the genomes of HEK293 (Lufino et al., 2013) or HeLa (Li et al., 2013) cells (not at the FXN locus) and harbor short or moderately expanded GAA repeat tracts.
Recent studies indicate that both long-range interactions and locations of repeat-containing genes in the genome may be important for their functions (Sun et al., 2018; Ruiz Buendía et al., 2020). Thus, an FRDA reporter cell line, to better reflect physiological regulatory mechanisms, should be engineered at the endogenous FXN locus in its natural chromosomal/genomic context. It should also harbor expanded GAA repeats of lengths representative of the patient population, and it would be of benefit to create an FRDA reporter system using cells that represent tissues/organs primarily affected by the disease.
To fulfill all these criteria and to efficiently screen for compounds capable of reversing GAA-induced transcriptional silencing of FXN, we generated a novel reporter line using cells derived from a patient with FRDA, thus enabling the investigation of endogenous FXN levels impacted by long GAA repeats. We performed a knock-in of a Nanoluciferase (NLuc) reporter gene into the FXN locus in induced pluripotent stem cells (iPSCs) reprogrammed from patient fibroblasts. These iPSCs were further differentiated into neural progenitor cells (NPCs) and neurons. As a proof of concept, we screened a library containing 281 compounds targeting different pathways involved in chromatin maintenance and transcription regulation. Our luciferase-based screen validated the reporter as a reliable tool in search of novel inducers of FXN expression. Results of our analyses revealed HDACi as the only class of compounds represented in the library capable of reproducible reactivation of FXN expression.
Materials and Methods
Reprogramming Friedreich’s Ataxia Fibroblasts to Induced Pluripotent Stem Cells
FRDA fibroblasts containing expanded GAA repeats and control fibroblast cell lines were obtained from the Friedreich’s Ataxia Cell Line Repository (FACLR) established at the Napierala laboratory at the University of Alabama at Birmingham (UAB) in collaboration with Dr. David Lynch from Children’s Hospital of Philadelphia (CHOP) with appropriate approvals of the Institutional Review Boards (IRBs; CHOP IRB no. 10-007864; UAB IRB no. N131204003) (Li Y. et al., 2016). Skin biopsies were donated by patients with FRDA and unaffected individuals for derivation of primary fibroblast lines. All biopsy samples (and resulting cell lines) are de-identified. Fibroblasts were transduced with Sendai virus vectors, encoding the four reprogramming factors, namely, octamer-binding transcription factor 3/4 (Oct3/4), cellular myelocytomatosis oncogene (c-Myc), kruppel like factor 4 (Klf4), and sex determining region Y-Box 2 (Sox2), using the CytoTune iPS 2.0 Sendai Reprogramming Kit (Life Technologies) to generate iPSCs. Cells were cultured on Matrigel®-coated plates (Corning) with mTeSR medium (STEMCELL Technologies). Medium changes were performed daily with passages every 5–7 days with Dispase (STEMCELL Technologies) and 10 μM ROCK inhibitor (Stemgent) as described by Polak et al. (2016). Analyses of pluripotency, differentiation potential, and karyotype were performed as described by Li et al. (2015b). A description of iPSC lines used in this study can be found in Supplementary Table 1.
Knock-in of Nanoluciferase Using CRISPR/Cas9
One million parental FRDA iPSCs were transfected with 2 μg of pX330-U6-Chimeric_BB-CBhhSpCas9 (Addgene, #42230) encoding SpCas9 and expressing a gRNA targeting FXN downstream of the stop codon using a Human Stem Cell Nucleofector Kit (Lonza). Three days after nucleofection, single cells were seeded at clonal density in mTeSR medium supplemented with CloneR (STEMCELL Technologies) and cultured for 4 days. Colonies were picked manually after 10–14 days. After expansion, genomic DNA was isolated from individual clones and screened for the presence of the correct 5′ and 3′ junctions using primers listed below. In addition, the locations of primers, gRNA binding sequence, and the exact amino acid sequence of the C-terminus of FXN fused with NLuc are shown in Figure 1 and Supplementary Figure 1. The polymerase chain reactions (PCRs) for 5′ and 3′ junctions and to detect the presence of the wild-type (WT), unedited allele were performed with 100 ng of DNA with the REDTaq® ReadyMix™ PCR Reaction Mix (Sigma-Aldrich) using the following cycling conditions: initial denaturation step at 94°C for 2 min, followed by 30 cycles of 30 s at 94°C, 30 s at 60°C, and 2 min at 72°C, and a final extension step of 5 min at 72°C. Expected sizes of the amplicons are described in the legend of Figure 1. Long GAA repeats were amplified using 100 ng of genomic DNA with the FailSafe™ PCR 2X PreMix D and FailSafe™ PCR Enzyme Mix (Epicenter) with primers spanning 498 base pairs (bp) of flanking GAA sequences (Li et al., 2015b) using the following cycling conditions: initial denaturation step for 3 min at 94°C, followed by 20 cycles of 20 s at 94°C, 30 s at 64°C, and 5 min at 68°C, followed by additional eight cycles of 20 s at 94°C and 5 min at 68°C, with 15-s increase of every elongation step and a final extension step of 7 min at 68°C. Sequences of primers (5′–3′) used for FXN-NLuc reporter DNA sequence validation: 5′ junction: 5′j forward: GTTTAGGTGATTGCTGGGGTGC3′; 5′j reverse: CCAACGAAATCTTCGAG TGTGA; 3′ junction: 3′j forward: GTTCCGAGTAACCATCAACGGAG; 3′j reverse: CCCTCATCTACCCAAT AGGGAAT; detection of unedited allele: WT_forward: TGGTTCATCTGAAGGGCTGTGCTGT GG; WT_reverse: TGGGGGCAAGGTAGGAGGCAACACA; GAA repeats: GAA_forward: GGCTTGAACTTCCCACACG TGTT; GAA_reverse: AGGACCATCATGGCCACACTT.
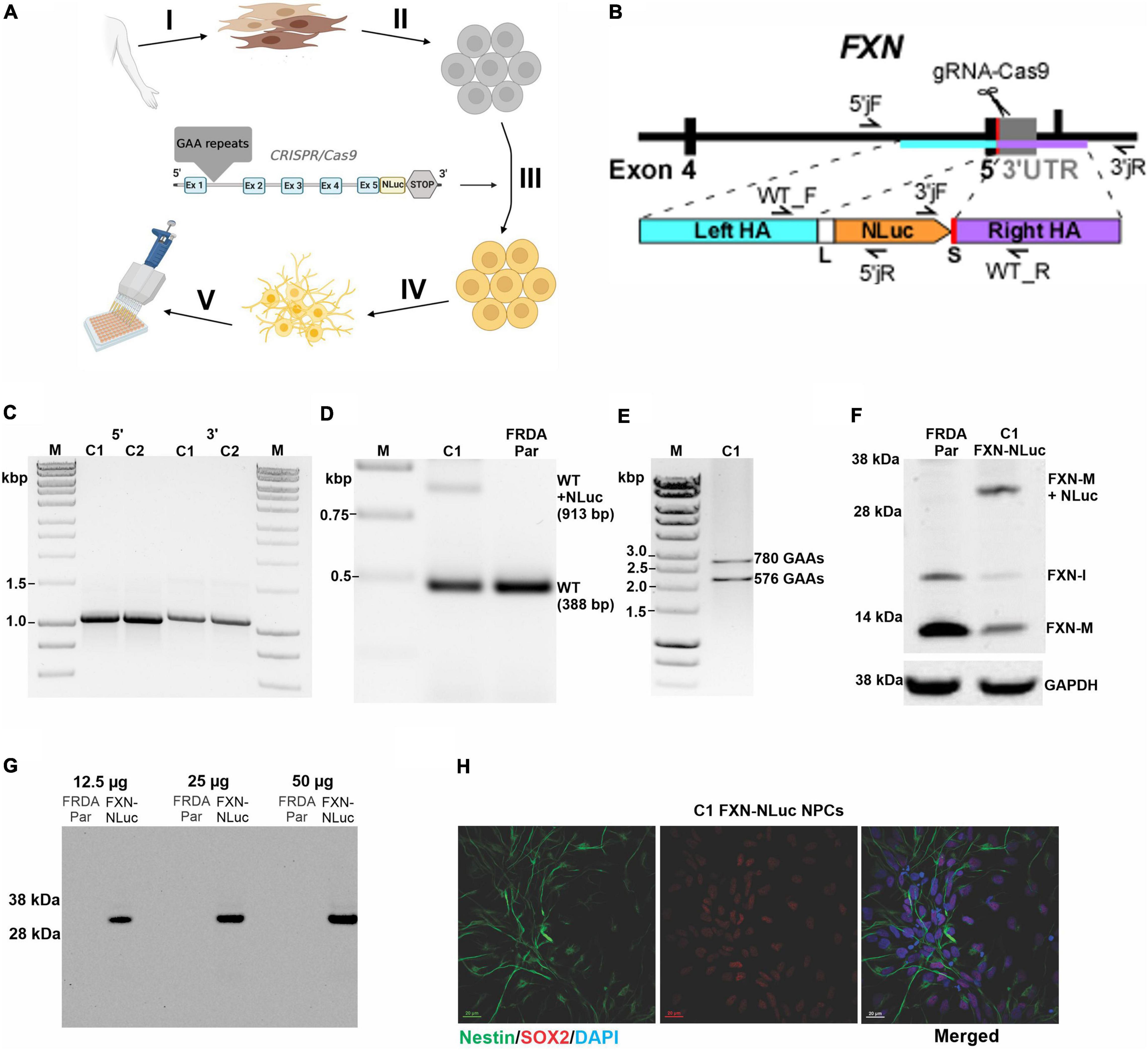
Figure 1. Generation and characterization of the FXN-NLuc reporter. (A) Schematic overview of FXN-NLuc reporter cell line generation. Primary fibroblasts of patients with FRDA were derived from a forearm skin biopsy (I) and reprogrammed to iPSCs (II). An in-frame fusion between the FXN gene and NLuc gene was generated via CRISPR/Cas9 genome editing (III). FXN-NLuc iPSCs were differentiated into NPCs (IV), generating a ready-to-use reporter cell line for compound screening (V). Image was created with BioRender.com. (B) Schematic presentation of the CRISPR/Cas9-mediated editing strategy used to generate the FXN-NLuc fusion. Approximate locations of the gRNA as well as primers used for confirmation of the editing are presented. (C) Validation of 5′ and 3′ junctions by PCR in two knock-in iPSC clones (C1 and C2). The 5′ junction was amplified using 5′jF and 5′jR (expected product size 1,068 bp); the 3′junction was amplified using 3′jF and 3′jR (expected product of 1,100 bp). M, size marker. (D) Confirmation of heterozygous knock-in of the NLuc gene in FRDA iPSCs. PCR was performed using primers WT_F and WT_R. C1 represents an edited clone [both unedited (WT) allele of 388 bp and edited WT + NLuc allele of 913 bp are amplified]; FRDA Par represents the unedited FRDA parental line. M, size marker. (E) Agarose gel electrophoresis of PCR products confirming the presence of expanded GAA repeats in edited FRDA iPSCs (clone C1 is shown). Primers amplify the GAA repeat tract and additional flanking sequences of 498 bp; M, size marker. (F) Western blot analysis of FXN and FXN-NLuc fusion expression in parental, unedited FRDA iPSCs (FRDA Par) and edited cells (C1, FXN-NLuc), FXN-M + NLuc (∼32 kDa)–fusion protein. GAPDH serves as a loading control. (G) In-gel detection of the luminescent FXN-NLuc fusion protein. Increasing amounts of cell lysates prepared from parental (FRDA Par) and edited (FXN-NLuc) iPSCs were separated by SDS-PAGE and the luminescent signal was detected using the Nano-Glo® In-Gel Detection System. (H) Differentiation of FXN-NLuc iPSCs into Nestin and Sox2 positive NPCs. Scale bar, 20 mm.
Differentiation of Neural Progenitor Cells
NPCs were differentiated using the STEMdiff™ SMADi Neural Induction Kit (STEMCELL Technologies) as monolayer cultures, according to the manufacturer’s recommendation. Two million iPSCs per well were plated on Matrigel®-coated six-well plates (Corning) in a single-cell suspension using STEMdiff™ Neural Induction Medium + SMADi (STEMCELL Technologies), supplemented with 10 μM ROCK inhibitor (Stemgent). Daily medium changes were performed with STEMdiff™ Neural Induction Medium + SMADi (STEMCELL Technologies). Cells were passaged after 7 days using Accutase (STEMCELL Technologies) and plated at a density of 2 × 106 cells per well. After 7 days of growth, cells were passaged for a total of two passages. Mature NPCs were then plated at a density of 1.2 × 106 cells per well on Matrigel®-coated six-well plates (Corning) and cultured in STEMdiff™ Neural Progenitor Medium (STEMCELL Technologies) with daily medium changes.
Generation of Terminally Differentiated Neurons
Neuronal differentiation was performed as described by Zhang et al. (2013), with modifications, using lentiviral vectors for Ngn2 (ys13-002 plasmid) and rtTA (ys13-003 plasmid) overexpression. On the initial day, 1 × 106 iPSC cells per well were plated on Matrigel®-coated six-well plates (Corning) in a single-cell suspension using mTeSR medium (STEMCELLTechnologies) with thiazovivin (BioVision). After 24 h, cells were infected with rtTA-Ub and Ngn2 viruses, in dulbecco’s modified eagle medium: nutrient mixture F-12 (DMEM/F12) medium (Gibco) supplemented with Polybrene (MilliporeSigma). Twenty-four hours after transfection, the culture medium was replaced with DMEM/F12 (Gibco), supplemented with N-2 (Life Technologies), non-essential amino acids (NEAA) (Invitrogen), human brain derived neurotrophin factor (BDNF) (PeproTech), human neurotrophin 3 (NT-3) (PeproTech), and mouse laminin (Invitrogen). To induce TetO expression, doxycycline (Clontech) was added to the medium. One day after induction of TetO expression, the medium was changed to Neurobasal (Gibco) supplemented with B-27 (Thermo Fisher Scientific), GlutaMAX (Invitrogen), human BDNF (PeproTech), human NT-3 (PeproTech), and doxycycline (Clontech). Puromycin (EMD Millipore) was added to the supplemented medium to start cell selection. Cells were cultured in Neurobasal supplemented medium for 12 days, with daily medium changes. After 2 weeks of differentiation, cells were treated with Accutase (STEMCELL Technologies) and plated onto poly-D-lysine (Sigma)/laminin (Invitrogen) coated plates, at a density of 2 × 106 cells per well. After re-plating, cortical neurons were cultured for 7 days in supplemented Neurobasal medium, with half of the medium changed every 2 days.
Luciferase Assays
Luciferase assays were performed using Nano-Glo® Luciferase Assay System (Promega) according to the manufacturer’s recommendation. The FXN-NLuc NPCs were plated on 96-well Matrigel-coated (Corning) white plates in triplicate, at a density of 2.5 × 104 cells per well. Cells were treated with compounds in different concentrations (as indicated in the “Results” section) and cultured in STEMdiff™ Neural Progenitor Medium (STEMCELL Technologies) for 24, 48, or 72 h depending on experimental design. Nano-Glo® Luciferase Assay Substrate was mixed with Nano-Glo Luciferase Assay Buffer in a proportion of 1:50. Subsequently, Nano-Glo® Luciferase Assay Reagent was added to FXN-NLuc NPCs at a volume equal to the culture media and mixed. Luminescence was measured using FLUOstar Omega plate reader (BMG Labtech) with blank media and Nano-Glo® Luciferase Assay Reagent as a background control.
In-Gel Luciferase Assays
To verify the size of the FXN-NLuc fusion protein and identify any potential non-specific NLuc expression, in-gel luciferase assays were performed. Cell lysates were prepared using Passive Lysis Buffer (Promega) supplemented with protease inhibitor cocktail (Sigma-Aldrich). Protein concentration was determined using a Bradford Protein Assay kit (Bio-Rad). Whole-cell extracts (12.5, 25, and 50 μg) along with the SeeBlue Plus2 Pre-stained Protein Standard (Fisher Scientific) were electrophoresed on NuPAGE 4–12% Bis-Tris gels (Life Technologies). Gels were washed with 25% isopropanol, rinsed with double-distilled water, then covered in Nano-Glo® In-Gel Detection Reagent (Promega), and incubated for 10 min. The luminescent signal was detected with Nano-Glo® In-Gel Detection System (Promega) and visualized using a ChemiDoc MP Imaging System (Bio-Rad).
Epigenetics Compound Library Description and Screening
The luciferase-based screening was performed using DiscoveryProbe™ Epigenetics Compound Library (APExBIO). The compounds were diluted to 10 μM concentration in dimethyl sulfoxide (DMSO) (Sigma-Aldrich). FXN-NLuc NPCs were plated on 96-well white plates at a density of 2.5 × 104 cells per well. Treatments were performed for 24 h. Screening was performed in duplicate using two independently cultured batches of FXN-NLuc NPCs with DMSO as a vehicle control. Luminescence detection was conducted as described above.
Half maximal effective concentration values for selected compounds were calculated using GraphPad Prism 9 software.
RNA Isolation and Quantitative Real-Time PCR
All RNA samples were isolated using the RNeasy Plus Kit (QIAGEN), followed by DNase treatment with Turbo DNase (Thermo Fisher Scientific). Quantitative real-time PCR (qRT-PCR) reactions were performed using 50 ng of RNA with the iTaq™ Universal SYBR® Green One-Step Kit (Bio-Rad) on a CFX Opus 96 Real-Time PCR Instrument (Bio-Rad). FXN transcript levels were normalized to glyceraldehyde 3-phosphate dehydrogenase (GAPDH) or hypoxanthine phosphoribosyltransferase 1 (HPRT1) and quantified relative to the respective control sample using the ΔΔCt method. The following primers (shown as 5′–3′) were used in qRT-PCR analyses: FXN forward: CAGAGGAAACGCTGGACTCT; FXN reverse: AGCCAGATTTGCTTGTTTGG; GAPDH forward: GAAGGTGAAGGTCGGAGTC; GAPDH reverse: GAAGATGG TGATGGGATTTC; HPRT1 forward: TGACACTGGCAAAAC AATGCA, HPRT1 reverse: GGTCCTTTTCACCAGCAAGCT.
Protein Isolation and Western Blot
Protein isolation and Western blot were performed as described by Li et al. (2019). Briefly, cell lysates were prepared in whole-cell extraction buffer containing 0.1% IGEPAL CA-630 (IGEPAL) CA-630 (NP-40), 0.25 M sodium chloride (NaCl), 5 mM edetic acid (EDTA), and 50 mM 4-(2-hydroxyethyl)piperazine-1-ethanesulfonic acid (pH 7.5) supplemented with protease inhibitor cocktail (Sigma-Aldrich). Protein concentration was determined using a Bradford Protein Assay kit (Bio-Rad). Fifty micrograms of protein extracts were electrophoresed on NuPAGE 4–12% Bis-Tris gel (Life Technologies) followed by protein transfer onto 0.2 mM nitrocellulose membrane (Bio-Rad). Human FXN protein was detected using anti-FXN antibody diluted to 1:1,000 (ProteinTech Group) incubated overnight at 4°C. Membranes were then incubated with anti-rabbit Immunoglobulin G (IgG) conjugated to horseradish peroxidase (HRP) (GE Healthcare) diluted to 1:7,500 for 1 h at room temperature. Human GAPDH protein was detected using anti-GAPDH antibody (Sigma-Aldrich) diluted to 1:10,000 and incubated for 1 h at room temperature. Human HPRT1 protein was detected using anti-HPRT1 antibody (Cell Signaling Technology) diluted to 1:1,000 and incubated for 1 h at room temperature. Membranes were then incubated with anti-mouse IgG conjugated to HRP (GE Healthcare) diluted to 1:7,500 for 1 h at room temperature. Signals were visualized using Thermo Scientific™ SuperSignal™ West Femto Maximum Sensitivity Substrate (Life Technologies) using a ChemiDoc MP Imaging System (Bio-Rad) and quantified using ImageLab software (Bio-Rad).
Immunocytochemistry
NPCs markers SOX2 and Nestin were detected by imaged-based immunocytochemistry analyses. Cells were plated onto Matrigel-coated (Corning) 12-well plates on cover slips at a density of 0.4 × 106 cells per well. After 2 days, cells were fixed using 4% paraformaldehyde, permeabilized with 0.1% Triton-X in phosphate-buffered saline (PBS), and blocked with 2.5% normal donkey serum (Abcam) in PBS/Tween 20. SOX2 protein was detected using anti-SOX2 antibody (ProteinTech Group) diluted to 1:500, and Nestin protein was detected using anti-Nestin antibody (Sigma-Aldrich) diluted to 1:200, following 1-h incubation at room temperature. Cells were incubated with Alexa Fluor 488 antibody (Thermo Fisher Scientific) diluted to 1:2,000 for Nestin detection and with Alexa Fluor 568 antibody (Thermo Fisher Scientific) diluted to 1:1,000 for SOX2 detection at room temperature for 45 min. Prepared coverslips were mounted onto slides using ProLong™ Gold Antifade Mountant with 4′,6-diamidino-2-phenylindole (DAPI) (Invitrogen) and imaged using a Nikon A1R confocal microscope (UAB High Resolution Imaging Facility).
Lactate Dehydrogenase Cytotoxicity Assay
Cytotoxicity assays were performed using the CyQUANT™ Lactate Dehydrogenase (LDH) Cytotoxicity Assay (Invitrogen) according to the manufacturer’s recommendation. Cells were seeded in 96-well tissue culture plates in triplicate in STEMdiffperformed using the Neural Progenitor Medium (STEMCELL Technologies) at a density of 2.5 × 104 cells per well. Besides compound-treated samples, two assay controls were added: untreated cells (spontaneous LDH activity) and lysed cells (maximum LDH activity). Fifty microliters of medium from each sample was transferred to a flat bottom 96-well plate and supplemented with 50 μl of LDH Cytotoxicity assay reaction mixture (containing assay substrate in assay buffer prepared according to the manufacturer’s recommendations), followed by 30 min incubation in the dark. Subsequently, 50 μl of Stop Solution was added before absorbance measurements at 490 and 680 nm were taken using a FLUOstar Omega plate reader (BMG Labtech). The A680 nm was subtracted from A490 nm as background before the calculations. Relative cytotoxicity was expressed as follows:
Statistical Analyses
Statistical analyses and graphs were performed using unpaired two-tailed Student’s t-tests and one-way ANOVA in GraphPad Prism 9 software. The significance of the differences between groups of datasets was set to the following p-values: *p < 0.05, **p < 0.01, and ***p < 0.001.
Results
Generation of Endogenous Frataxin-Nanoluciferase Fusion as a Novel Friedreich’s Ataxia Reporter Cell Line
A schematic overview of generation of the novel FXN-NLuc reporter cell line is shown in Figure 1A. FRDA iPSCs reprogrammed from patient fibroblasts were edited using CRISPR/Cas9 to site-specifically knock-in the NLuc gene at the 3′ end of exon 5 of endogenous FXN (Figure 1B and Supplementary Figure 1). A five-amino acid-long linker was inserted between the last FXN amino acid (Ala) and the first amino acid of NLuc (Val). Technical details are provided in section “Materials and Methods” and Supplementary Figure 1. Using three sets of primers, we confirmed both 5′ and 3′ junctions (Figure 1C and Supplementary Figure 1) as well as heterozygosity of the knock-in (Figure 1D). The sequence of the junction between FXN and NLuc was also confirmed by DNA sequencing (data not shown). We did not identify homozygous knock-in of NLuc, indicating that the FXN-NLuc fusion protein is likely not functional. Two independent clones (C1 and C2) were obtained, and all subsequent studies described herein were performed using clone C1. This line harbored two expanded GAA tracts of ∼780 and 580 repeats (Figure 1E) and Western blot analysis demonstrated expression of both unmodified FXN (∼13 kDa) and FXN-NLuc fusion (∼32 kDa) (Figure 1F). Although the possibility of random integration and expression of the promoterless NLuc DNA construct is low, we analyzed total protein extracts from the FXN-NLuc line for the presence of a non-specific NLuc signal using an in-gel luciferase assay. We detected only a single band at ∼32 kDa corresponding to the molecular weight of the FXN-NLuc fusion protein (Figure 1G). As iPSCs do not represent a disease-relevant cell type, we differentiated them to NPCs. These dividing cells demonstrate neuronal characteristics and can be easily passaged, cryopreserved, and expanded to large numbers necessary for HTS campaigns. Differentiated FXN-NLuc NPCs expressed Nestin and Sox2 as determined by immunostaining (Figure 1H).
Validation of the Frataxin-Nanoluciferase Neural Progenitor Cell Reporter Using a Known Frataxin Expression Inducer
To test the reporter cell line utility, the FXN-NLuc cells were subjected to treatments with a known inducer of FXN expression, HDACi RG109. RG109 increased expression of FXN-NLuc in a concentration-dependent manner; however, the activation was modest and never reached statistical significance (Figure 2A). At the same time, 10 μM RG109 significantly increased FXN mRNA by ∼1.5-fold as determined by qRT-PCR (Figure 2B). Similar to luminescence, the assessment of the effect of RG109 treatment on FXN and FXN-NLuc protein expression using Western blot revealed slight but not significant increases (Figures 2C,D).
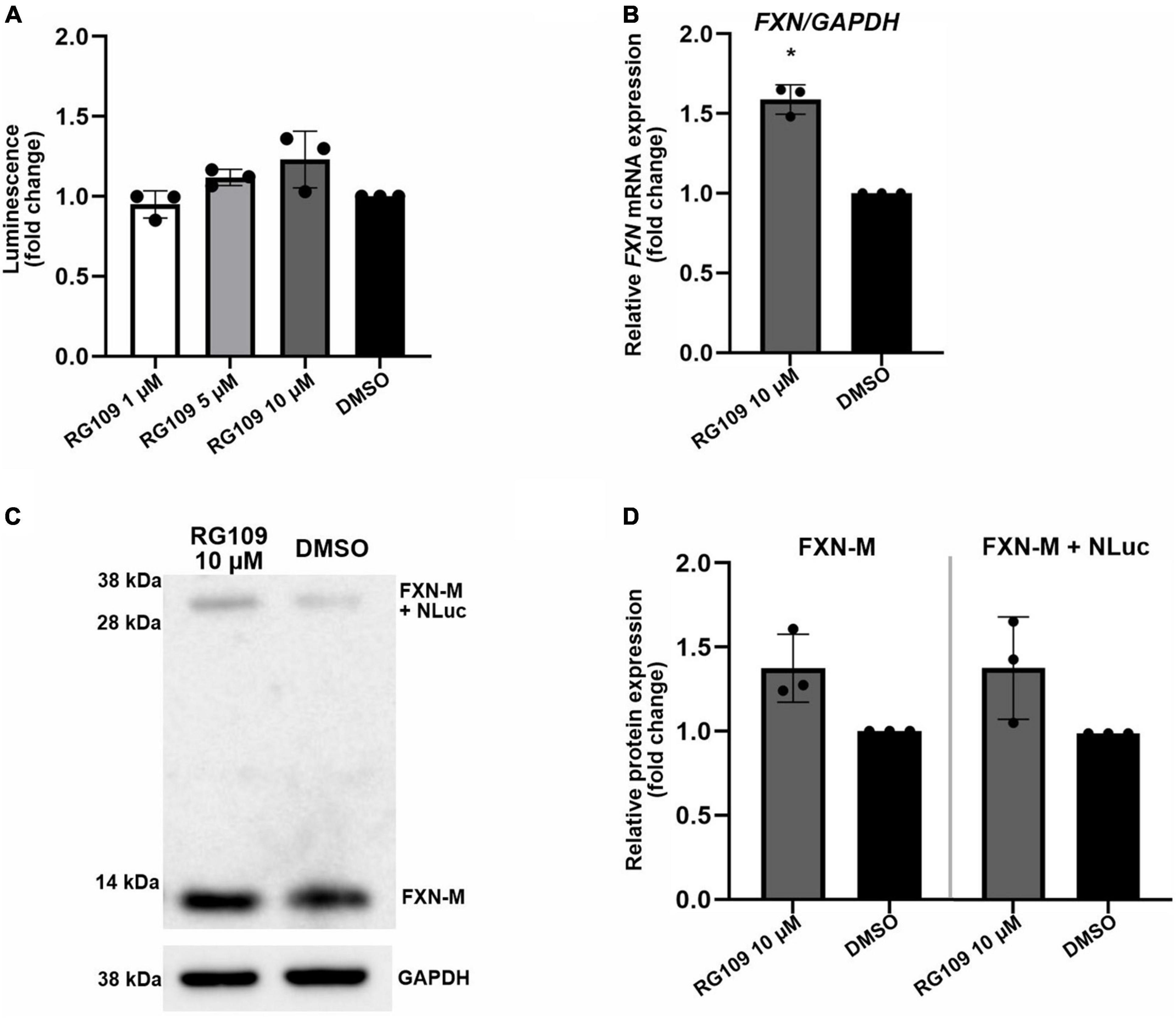
Figure 2. Validation of FXN-NLuc reporter NPCs with known FXN inducer RG109. (A) Increase of FXN-NLuc expression (luminescence) by HDACi RG109 treatment of NPCs for 24 h at 1, 5, and 10 μM. Results are shown relative to DMSO control. (B) qRT-PCR determination of FXN mRNA and FXN-NLuc mRNA expression upon 24-h treatment of NPCs with 10 μM HDACi RG109. Primers used for amplification are located in exons 3 and 4 of FXN. DMSO treatment served as a vehicle control. (C) Representative results of Western blot analyses of FXN-NLuc NPCs treated with 10 μM RG109 for 24 h. DMSO was used as a control. Endogenous mature FXN (FXN-M, ∼13 kDa) and FXN-NLuc fusion (FXN-M + NLuc, ∼32 kDa) are indicated. GAPDH served as a loading control. (D) Western blot quantitation. FXN-M and FXN-M + NLuc were quantified and plotted independently. Statistical calculations were performed using one-way ANOVA (non-parametric Kruskal–Wallis test) for data in (A) or Student’s t-test for data in (B,D); *p < 0.05. Unless otherwise indicated, results represent mean ± SD from three independent experiments.
These results indicate that the FXN-NLuc reporter responds to a known FXN expression activator and can be used as a reliable model to identify new compounds capable of reactivating FXN in FRDA cells. Importantly, the response level of the reporter signal (luminescence) is comparable to the FXN and FXN-NLuc increase measured at the protein level using Western blot (Figure 2). Moreover, when measured separately, the response of the unmodified FXN allele is identical to the signal increase observed from the FXN-NLuc allele, indicating preservation of the FXN regulatory mechanisms at the stages of transcription, translation, and protein stability.
Screening of Epigenetic Compound Library Identified Frataxin Expression Activator Candidates
Next, as a proof of concept, we performed a luciferase-based screening of the Discovery Probe Epigenetic Compound Library (APExBIO) that includes 281 compounds predominantly targeting various epigenetic and chromatin pathways (Supplementary Table 2). Treatment of FXN-NLuc NPCs was conducted at 10 μM concentration of the compounds for 24 h in a 96-well format (Figure 3A and Supplementary Figure 2), with DMSO-treated cells used as the vehicle control. Two independent screening rounds were performed. A total of 16 compounds were identified as candidates for further evaluation based on the following criteria: (i) luminescence signal greater than the average of all samples on a plate (library was screened on four separate plates) plus one standard deviation of the mean and (ii) consistent increase of the luminescence signal in two independent screening rounds (Table 1).
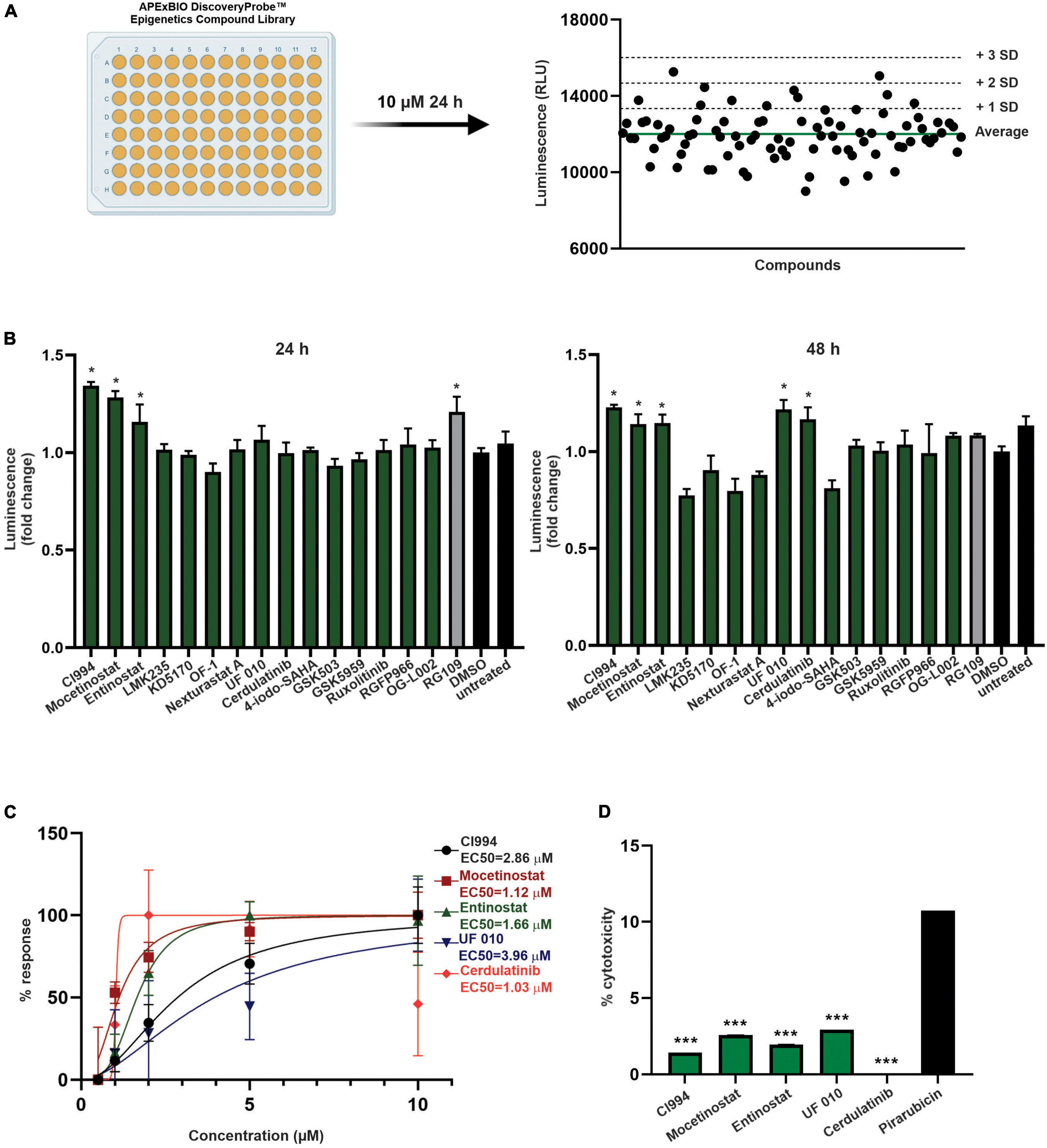
Figure 3. Screening of epigenetic compound library to identify novel inducers of FXN expression. (A) An overview of the screening process. All compounds of the APExBIO DiscoveryProbe™ Epigenetics Compound Library were tested at 10 μM concentration. Luminescence signal was plotted independently per each plate. The green line corresponds to plate average signal; dashed lines indicate average signal plus 1, 2, and 3 standard deviations of the mean. All data from two rounds of screening are included as Supplementary Figure 2. (B) Secondary validation of the efficacy of 16 compounds selected by initial screens. Luminescence detection was performed after 24 and 48 h of treatment. DMSO- and RG109-treated FXN-NLuc NPCs were used as controls. Five compounds were selected for further studies: CI994, Mocetinostat, Entinostat, UF 010, and Cerdulatinib. Results are mean ± SD from three technical replicates; * indicates p < 0.05 by one-way ANOVA. (C) Dose–response analyses for the selected compounds. Luminescence analyses were performed after 48 h of treatment with compounds at 0.5, 1, 2, 5, and 10 μM. EC50 is indicated for each compound. (D) Determination of cytotoxicity in FXN-NLuc NPCs using an LDH assay. Cytotoxicity is calculated relative to the spontaneous LDH release detected in DMSO-treated cells. Cells were treated with 10 μM of each compound for 48 h. Pirarubicin served as a positive control for cytotoxicity. Results are mean ± SD from three independent experiments; *** indicates p < 0.001 by one-way ANOVA.
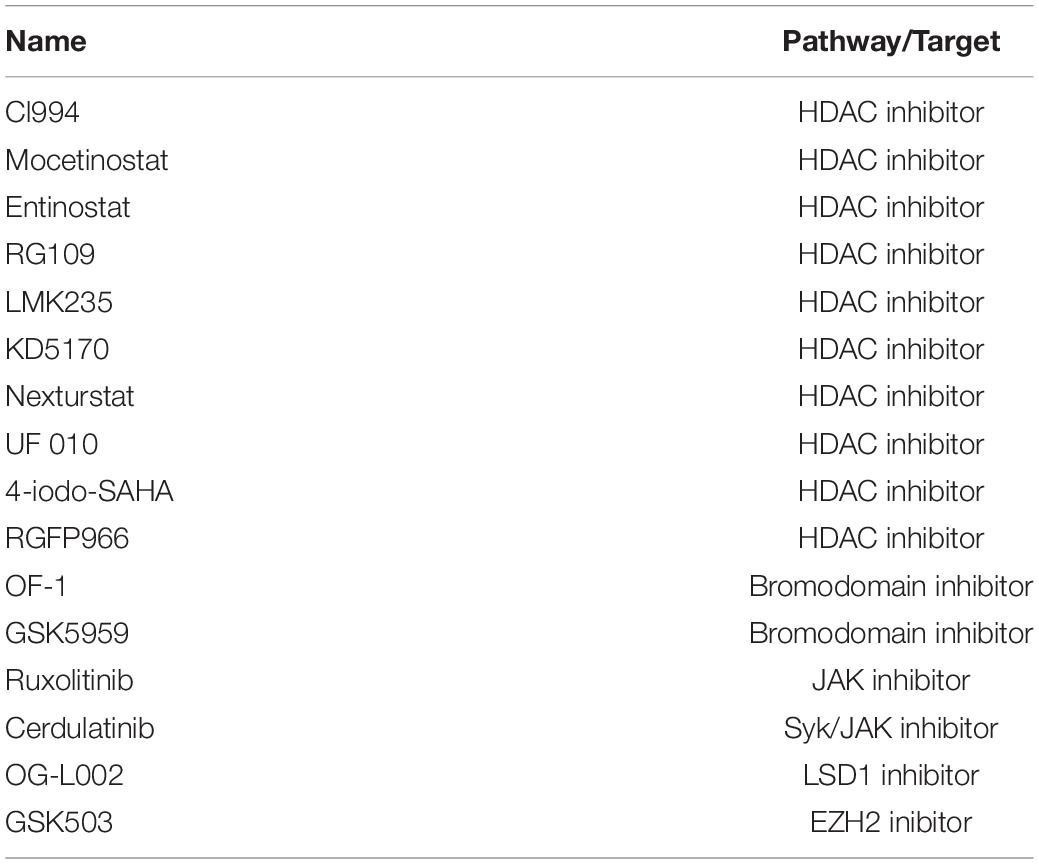
Table 1. Compounds identified in the screen of the APExBIO DiscoveryProbe™ Epigenetics Compound Library to increase expression of FXN-NLuc in FRDA NPCs.
One of the initial 16 upregulators of FXN-NLuc expression was RG109, confirming previously reported results and further validating the reporter line. The remaining hits represented HDACi (9), bromodomain inhibitors (2), janus kinase (JAK) and Syk/JAK inhibitors (2), and unique LSD1 as well as EZH2 inhibitors (Table 1). To confirm whether selected compounds indeed increase FXN-NLuc expression, additional assays were conducted with 24- and 48-h treatments (Figure 3B). Five compounds increased FXN-NLuc luminescence signal following either 24- or 48-h treatments: CI994, Entinostat, Mocetinostat, UF 010, and Cerdulatinib. Three of them (CI994, Entinostat, and Mocetinostat) stimulated reporter expression at both time points. We focused further studies on these five compounds.
Efficacy and Toxicity of Candidate Compounds
To determine the most effective concentration of each compound, we performed dose–response analyses within the range of 0.5–10 μM. Cerdulatinib, Mocetinostat, and Entinostat demonstrated the lowest half maximal effective concentration (EC50) values (∼1.0, 1.1, and 1.7 μM, respectively), while CI994 and UF 010 required higher concentrations (∼2.9 and 4.0 μM, respectively) to increase FXN-NLuc expression (Figure 3C). Next, to determine whether the selected compounds exert cytotoxic effects, we treated FXN-NLuc NPCs for 48 h at 10 μM concentration and then performed cytotoxicity assays by measuring LDH release to the media. No overt toxicity of the compounds was detected relative to vehicle-treated control (Figure 3D).
Histone Deacetylase Inhibitors Are Capable of Increasing Frataxin mRNA and Protein Expression in Friedreich’s Ataxia Neural Progenitor Cells
Next, we performed secondary validation of the five hits identified by the screen by qRT-PCR and Western blot using FXN-NLuc NPCs treated with 10 μM of the compounds. FXN transcript levels were increased following Mocetinostat and Entinostat treatment and, to a lesser extent, upon treatment with CI994 and UF 010 when compared to DMSO control (Figure 4A). Similarly, we detected ∼2–2.5-fold increase of the mature FXN protein and FXN-NLuc fusion (Figures 4B,C). Cerdulatinib failed to upregulate FXN expression at the transcript or protein levels. These results were confirmed in two additional FRDA NPC lines derived from different patient iPSCs (Figure 5). Although variable upregulation of FXN mRNA by CI994, Mocetinostat, and Entinostat treatments was determined, a consistent ∼2-fold increase in FXN protein was observed (Figures 5B,C). In addition, treatment with HDACi UF 010 resulted in ∼1.5-fold increase of FXN protein. In summary, testing in three independent FRDA NPC lines confirmed the efficacy of three HDACi identified by our reporter screen.
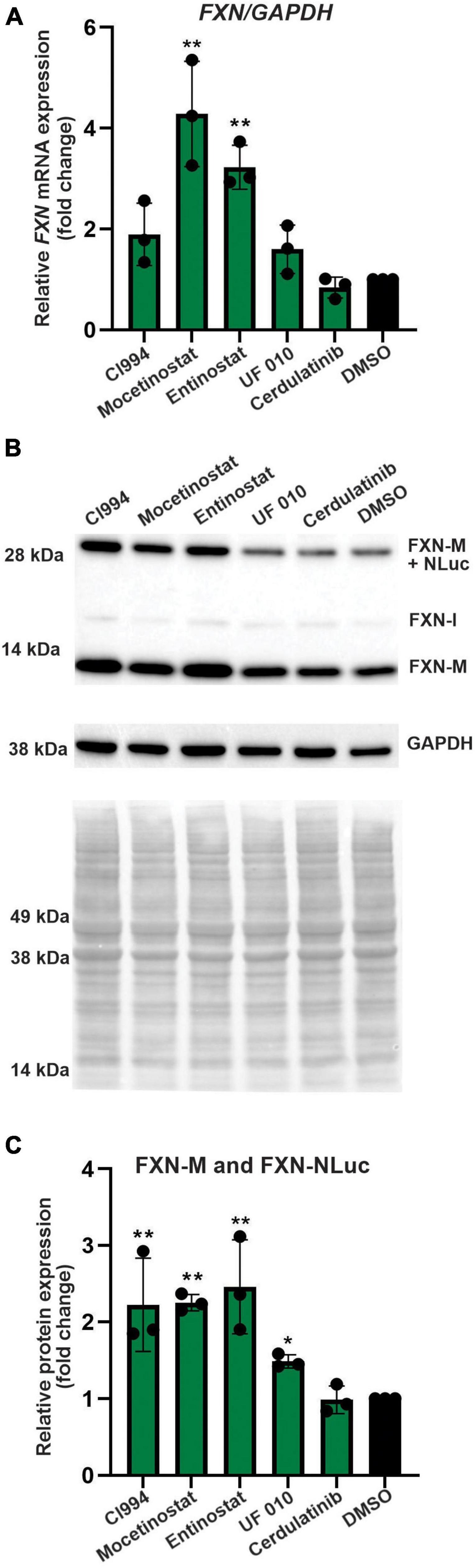
Figure 4. Selected HDACi increase FXN transcript and protein in FXN-NLuc NPCs. (A) Results of qRT-PCR analysis of FXN mRNA expression upon 48-h treatment with 10 μM CI994, Mocetinostat, Entinostat, UF 010, and Cerdulatinib. Results represent mean ± SD from three independent experiments; one-way ANOVA, **p < 0.01. DMSO treatment served as a control. (B) FXN and FXN-NLuc protein levels determined after treatment with indicated compounds for 48 h at 10 μM. Signals corresponding to mature FXN (FXN-M), intermediate FXN isoform (FXN-I), and FXN-NLuc fusion (FXN-M + NLuc) are indicated. GAPDH and Ponceau S staining served as loading controls. (C) Quantification of FXN and FXN-NLuc protein expression relative to Ponceau S staining. Cumulative signal from FXN and FXN-NLuc is shown. Results represent mean ± SD from three independent experiments; *p < 0.05 and **p < 0.01 determined by one-way ANOVA.
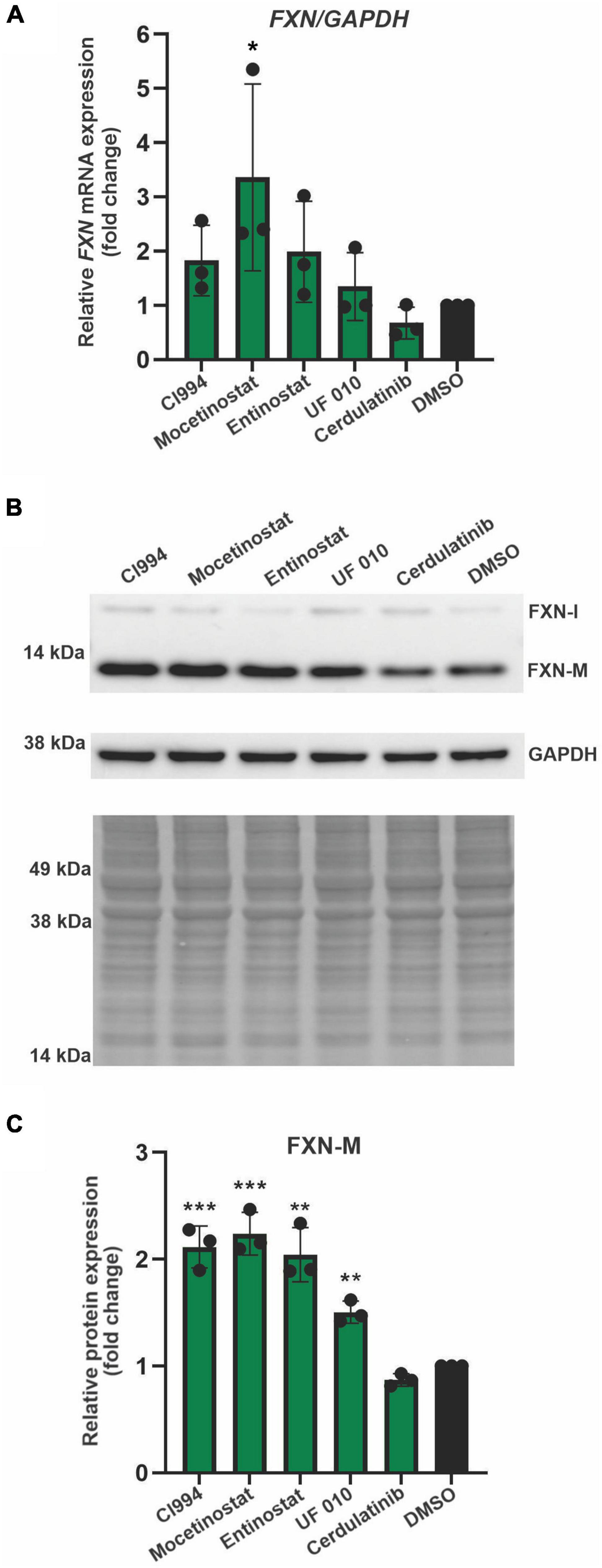
Figure 5. Validation of efficacy of identified HDACi in additional FRDA NPC lines. (A) Results of qRT-PCR analysis of FXN mRNA expression upon 48-h treatment with 10 μM CI994, Mocetinostat, Entinostat, UF 010, and Cerdulatinib. Results represent mean ± SD from three independent experiments conducted using three different NPC lines; *p < 0.05 determined by one-way ANOVA. DMSO treatment served as a control. (B) FXN protein expression determined after treatment with indicated compounds for 48 h at 10 μM. Signals corresponding to mature FXN (FXN-M) and intermediate FXN (FXN-I) are indicated. GAPDH and Ponceau S staining served as loading controls. A representative Western blot for the FRDA NPC lines tested is shown. (C) Quantification of FXN-M levels relative to Ponceau S staining. Results represent mean ± SD from three independent experiments obtained using three different FRDA NPC lines; **p < 0.01 and ***p < 0.001 determined by one-way ANOVA.
To determine whether the effect of the compounds is specific to FRDA cells, we also conducted analyses of FXN expression in control NPCs derived from an unaffected individual. CI994 and Mocetinostat treatment demonstrated ∼1.5-fold upregulation of FXN mRNA (Supplementary Figure 3A). A moderate (∼1.5-fold) upregulation of the FXN protein level was also detected upon treatment with CI994 and Entinostat when compared to DMSO control (Supplementary Figures 3B–D). Overall, tested compounds increased FXN levels in control cells to a lesser extent than in FRDA NPCs likely due to already higher basal FXN expression in the unaffected cells.
Histone Deacetylase Inhibitors Reactivate Frataxin Expression in Terminally Differentiated Neurons
Thus far, all results were obtained in proliferating NPCs. To determine whether the HDACi identified in our screen are capable of FXN upregulation in non-dividing cells, we tested the efficacy of CI994, Entinostat, and Mocetinostat in terminally differentiated neurons. The FRDA iPSC lines used for NPC differentiation were differentiated to cortical neurons (Zhang et al., 2013) and then treated with HDACi. The qRT-PCR results demonstrated, similarly to NPCs, that all three HDACi can upregulate FXN mRNA by ∼ 2–3-fold compared to the DMSO control (Figure 6A). We were also able to detect ∼1.5–1.75-fold increase of the mature FXN protein, confirming the efficacy of the three selected HDACi (Figures 6B,C). This concordance of results suggests that NPCs, which are significantly more amenable to large screening campaigns, represent a good surrogate for mature neuronal cells.
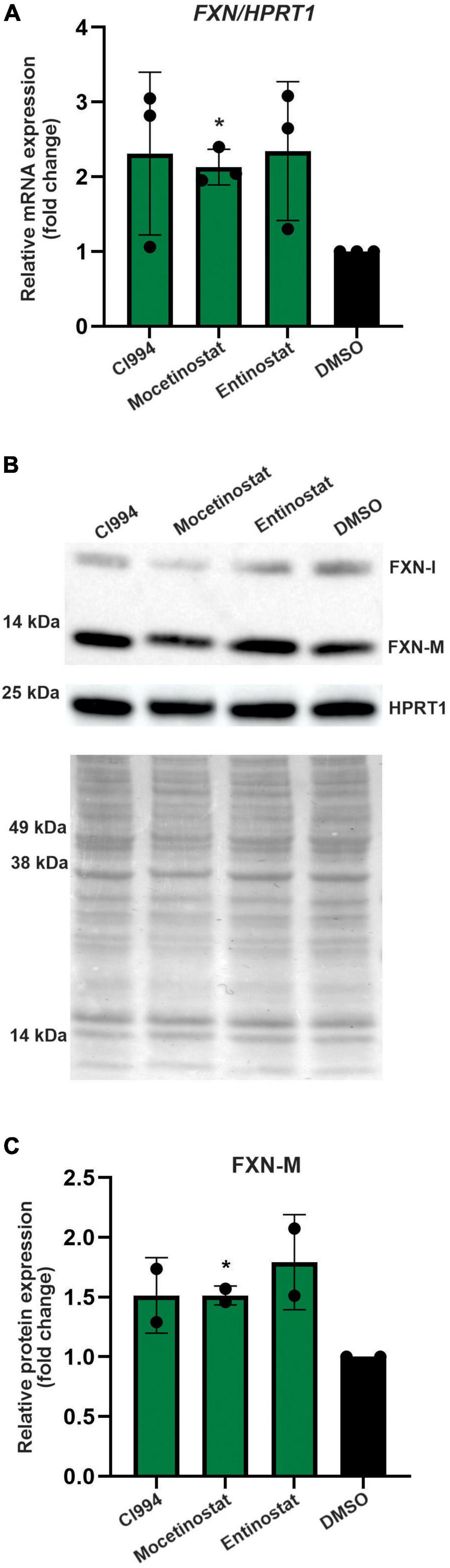
Figure 6. Validation of the most potent identified HDACi in terminally differentiated FRDA neurons. (A) Results of qRT-PCR analysis of FXN mRNA expression upon 48-h treatment with 10 μM CI994, Mocetinostat, and Entinostat. Results represent mean ± SD from three independent experiments conducted using three different FRDA neuronal lines; *p < 0.05 determined by independent Student’s t-test. DMSO treatment served as a control. (B) FXN protein expression determined after treatment with indicated compounds for 48 h at 10 μM concentration. The signals corresponding to mature FXN-M and FXN-I are indicated. HPRT1 and Ponceau S staining served as loading controls. A representative Western blot is shown. (C) Quantification of FXN-M levels relative to Ponceau S staining. Results represent mean ± SD from two independent experiments obtained using two different FRDA neuronal lines; *p < 0.01 determined by independent Student’s t-test.
Discussion
In this work, we designed, generated, and validated a novel FRDA reporter cell line derived from patient iPSCs that were modified by CRISPR/Cas9 to incorporate the NLuc gene fused to the terminal exon 5 of endogenous FXN. The reporter contains expanded GAA repeats within their natural genomic context of the FXN gene and enables luminescence-based detection of the FXN-NLuc fusion protein. FXN-NLuc reporter upregulation correlated well with both qRT-PCR and Western blot measurements of FXN mRNA and protein. The FXN-NLuc cell line is, to our knowledge, the first FRDA reporter based on patient-derived cells harboring long GAA repeats. Previously utilized reporter systems were predominantly derived from HeLa (Grant et al., 2006; Li et al., 2013) or HEK293 cells (Soragni et al., 2008; Lufino et al., 2013). Differentiation of iPSCs into NPCs and neurons (and potentially any other cell type of interest) presents an opportunity for testing the efficacy of FXN upregulation in disease-relevant cells or organoids. This flexibility represents an important advantage of iPSC-based reporters over systems created in fully committed cells from the perspective of variable efficacy of candidate compounds in different cell types. Recent studies on nicotinamide and resveratrol demonstrated their lack of efficacy toward FXN upregulation in terminally differentiated neurons (Georges et al., 2019) despite prior demonstration of FXN stimulation in fibroblasts and lymphocytes (Chan et al., 2013; Li et al., 2013), thus emphasizing the importance of conducting drug discovery research in disease-relevant cell types.
The molecular cause of FRDA has been known for 25 years and a role for chromatin involvement for almost 20 years. However, despite numerous efforts and screening campaigns, only a few small molecules capable of modest re-activation of FXN expression have been identified. A clear efficacy of specific HDACi (e.g., RG109) has been recurrently demonstrated in various model systems (Sandi et al., 2014; Soragni et al., 2014; Chutake et al., 2016; Codazzi et al., 2016). Targeting the HMT G9a affected histone methylation at FXN but did not increase its expression in FRDA lymphoblasts and fibroblasts (Punga and Bühler, 2010; Sherzai et al., 2020). On the other hand, a recent study targeting G9a and EZH2 HMTs reported, in addition to changes in H3K9me2/me3 and H3K27me3 levels, an increase of FXN mRNA expression in FRDA fibroblasts but without noticeable effects on FXN protein levels (Sherzai et al., 2020). This may be a result of an FRDA-specific patient cell response, as the length of the GAAs may influence the efficacy of some small molecules (Punga and Bühler, 2010). Inhibition of SUV420H1 (lysine methyltransferase 5B, KMT5B) by the small-molecule A-196 resulted in an FRDA-specific ∼1.5- to 2-fold upregulation of FXN levels via targeting H4K20me2/me3 (Vilema-Enríquez et al., 2020). Thus, despite numerous efforts, efficacious small-molecule-mediated reactivation of FXN expression in FRDA cells proves to be a challenging task.
The broad spectrum of chromatin and epigenetic processes targeted by the compounds utilized in this study as well as prior works by numerous laboratories raises a question regarding the role of chromatin changes in the molecular pathology FRDA. The small molecules tested in our study represent compounds targeting HDACs, HMTs, histone demethylases, bromodomains, and DNA methyltransferases, to name a few major classes (Supplementary Table 2). Out of this range of targets, predominantly, class 1, benzamide HDACi: CI994 (HDAC1 specific), Mocetinostat (pan HDACi), and Entinostat (class 1 HDACi) were capable of reproducible FXN upregulation in NPCs and terminally differentiated neurons. All three of them are potent HDAC inhibitors, developed and tested in clinical trials for various malignancies (Bondarev et al., 2021).
One possible explanation of relative inefficiency of chromatin manipulation in restoring FXN expression is that epigenome changes are secondary consequences of a physical barrier imposed by the expanded GAAs. Hence, without correcting the underlying issue at the DNA level, correcting the chromatin landscape is rather transient and inefficient. The relatively high efficacy of HDACi, demonstrated in this work and by numerous prior studies, may rely on the proximity of the GAA region to the promoter. Increased histone acetylation may facilitate the recruitment of RNAPII or promote an efficient transition from a paused state to productive elongation. In either of these scenarios, increased transcription of FXN would result from a higher rate of transcription through the gene rather than more efficient traversing of the expanded GAA repeats by the transcription machinery. That would also imply that a single compound targeting chromatin may not be completely efficacious in restoring FXN levels to asymptomatic carrier levels, especially in severe FXN deficiency cases caused by the longest GAA repeats. Although any upregulation of FXN levels may be of therapeutic significance (e.g., to delay disease progression), curative effects are likely to be achieved by ∼3- to 10-fold increase, depending on the length of the GAA tracts and correlated FXN repression observed in a given patient. Therefore, a combinational approach by targeting expanded GAA repeat tracts (e.g., with ASOs) and a small molecule targeting repressive chromatin may be required. In addition, hybrid molecules (e.g., SynTef1) combining GAA targeting with transcriptional modulation may represent an alternative as they could help reduce risks of non-specific effects of targeting enzymes involved in global control of gene expression.
Conclusion
In conclusion, despite challenges associated with developing a small-molecule therapy to increase FXN expression, a patient cell-based reporter system utilizing endogenous FXN expression as a readout represents an excellent model to evaluate any pre-clinical approach aimed at increasing FXN mRNA or protein levels.
Data Availability Statement
The original contributions presented in the study are included in the article/Supplementary Material, further inquiries can be directed to the corresponding author/s.
Author Contributions
AS, YL, and Y-HC performed all experiments and analyzed the data. AS, YL, Y-HC, JN, and MN designed the experiments. AS, JN, and MN wrote the article. JN and MN acquired funding. All authors read the article, contributed comments and suggestions, and approved the final version of the article.
Funding
This study was supported by the research grants from Friedreich’s Ataxia Research Alliance (to MN and independently to JN). Support was also provided by National Institutes of Health R01NS081366 and R01NS121038 from the National Institute of Neurological Disorders and Stroke (to MN).
Conflict of Interest
The authors declare that the research was conducted in the absence of any commercial or financial relationships that could be construed as a potential conflict of interest.
The handling editor declared a past co-authorship with several of the authors AS, YL, JN, and MN.
Publisher’s Note
All claims expressed in this article are solely those of the authors and do not necessarily represent those of their affiliated organizations, or those of the publisher, the editors and the reviewers. Any product that may be evaluated in this article, or claim that may be made by its manufacturer, is not guaranteed or endorsed by the publisher.
Acknowledgments
We would like to thank the UAB High Resolution Imaging Facility for their expert assistance in acquiring microscopy images.
Supplementary Material
The Supplementary Material for this article can be found online at: https://www.frontiersin.org/articles/10.3389/fnins.2022.836476/full#supplementary-material
References
Al-Mahdawi, S., Pinto, R. M., Ismail, O., Varshney, D., Lymperi, S., Sandi, C., et al. (2008). The Friedreich Ataxia GAA Repeat Expansion Mutation Induces Comparable Epigenetic Changes in Human and Transgenic Mouse Brain and Heart Tissues. Hum. Mol. Genet. 17, 735–746. doi: 10.1093/hmg/ddm346
Bergquist, H., Rocha, C. S. J., Álvarez-Asencio, R., Nguyen, C.-H., Rutland, M. W., Edvard Smith, C. I., et al. (2016). Disruption of Higher Order DNA Structures in Friedreich’s Ataxia (GAA)n Repeats by PNA or LNA Targeting. PLoS One 11:e0165788. doi: 10.1371/journal.pone.0165788
Bidichandani, S. I., Ashizawa, T., and Patel, P. I. (1998). The GAA triplet-repeat expansion in Friedreich ataxia interferes with transcription and may be associated with an unusual DNA structure. Am. J. Hum. Genet. 62, 111–121. doi: 10.1086/301680
Bondarev, A. D., Attwood, M. M., Jonsson, J., Chubarev, V. N., Tarasov, V. V., and Schiöth, H. B. (2021). Recent developments of HDAC inhibitors: emerging indications and novel molecules. Br. J. Clin. Pharmacol. 87, 4577–4597. doi: 10.1111/bcp.14889
Bürk, K. (2017). Friedreich Ataxia: current status and future prospects. Cerbellum Cerbellum Ataxias 4:4. doi: 10.1186/s40673-017-0062-x
Burnett, R., Melander, C., Puckett, J. W., Son, L. S., Wells, R. D., Dervan, P. B., et al. (2006). DNA sequence-specific polyamides alleviate transcription inhibition associated with long GAA⋅TTC repeats in Friedreich’s ataxia. PNAS 103, 11497–11502. doi: 10.1073/pnas.0604939103
Campuzano, V., Montermini, L., Moltò, M. D., Pianese, L., Cossée, M., Cavalcanti, F., et al. (1996). Friedreich’s ataxia: autosomal recessive disease caused by an intronic GAA triplet repeat expansion. Science 271, 1423–1427. doi: 10.1126/science.271.5254.1423
Chan, P. K., Torres, R., Yandim, C., Law, P. P., Khadayate, S., Mauri, M., et al. (2013). Heterochromatinization induced by GAA-repeat hyperexpansion in Friedreich’s ataxia can be reduced upon HDAC inhibition by vitamin B3. Hum. Mol. Genet. 22, 2662–2675. doi: 10.1093/hmg/ddt115
Chutake, Y. K., Lam, C. C., Costello, W. N., Anderson, M. P., and Bidichandani, S. I. (2016). Reversal of epigenetic promoter silencing in Friedreich ataxia by a class I histone deacetylase inhibitor. Nucleid Acid Res. 44, 5095–5104. doi: 10.1093/nar/gkw107
Clark, E., Butler, J. S., Isaacs, C. J., Napierala, M., and Lynch, D. R. (2017). Selected Missense Mutations Impair Frataxin Processing in Friedreich Ataxia. Ann. Clin. Transl. Neurol. 4, 575–584. doi: 10.1002/acn3.433
Clark, E., Johnson, J., Dong, Y. N., Mercado-Ayon, E., Warren, N., Zhai, M., et al. (2018). Role of frataxin protein deficiency and metabolic dysfunction in Friedreich ataxia, an autosomal recessive mitochondrial disease. Neuronal Signal. 2:NS20180060. doi: 10.1042/NS20180060
Codazzi, F., Hu, A., Rai, M., Donatello, S., Salerno Scarzella, F., Mangiameli, E., et al. (2016). Friedreich ataxia-induced pluripotent stem cell-derived neurons show a cellular phenotype that is corrected by a benzamide HDAC inhibitor. Hum. Mol. Genet. 25, 4847–4855. doi: 10.1093/hmg/ddw308
Cook, A., and Giunti, P. (2017). Friedreich’s ataxia: Clinical features, pathogenesis and management. Br. Med. Bull. 124, 19–30. doi: 10.1093/bmb/ldx034
Cossée, M., Dürr, M., Schmitt, M., Dahl, N., Trouillas, P., Allinson, P., et al. (1999). Friedreich’s ataxia: point mutations and clinical presentation of compound heterozygotes. Ann. Neurol. 45, 200–206. doi: 10.1002/1531-8249(199902)45:2<200::AID-ANA10<3.0.CO;2-U
De Biase, I., Chutake, Y. K., Rindler, P. M., and Bidichandani, S. I. (2009). Epigenetic Silencing in Friedreich Ataxia Is Associated with Depletion of CTCF (CCCTC-Binding Factor) and Antisense Transcription. PLoS One 4:e7914. doi: 10.1371/journal.pone.0007914
Delatycki, M. B., and Corben, L. A. (2012). Clinical features of Friedreich ataxia. J. Child Neurol. 27, 1133–1137. doi: 10.1177/0883073812448230
Dion, V., and Wilson, J. H. (2009). Instability and Chromatin Structure of Expanded Trinucleotide Repeats. Trends Genet. 25, 288–297. doi: 10.1016/j.tig.2009.04.007
Dürr, A., Cossee, M., Agid, Y., Campuzano, V., Mignard, C., Penet, C., et al. (1996). Clinical and Genetic Abnormalities in Patients With Friedreich’s Ataxia. New England J. Med. 335, 1169–1175. doi: 10.1056/NEJM199610173351601
Erwin, G. S., Grieshop, M. P., Ali, A., Qi, J., Lawlor, M., Kumar, D., et al. (2017). Synthetic Transcription Elongation Factors License Transcription Across Repressive Chromatin. Science 358, 1617–1622. doi: 10.1126/science.aan6414
Georges, P., Boza-Moran, M.-G., Gide, J., Pêche, G. E., Forêt, B., Bayot, A., et al. (2019). Induced pluripotent stem cells-derived neurons from patients with Friedreich ataxia exhibit differential sensitivity to resveratrol and nicotinamide. Sci. Rep. 9:14568. doi: 10.1038/s41598-019-49870-y
Gottesfeld, J. M. (2007). Small molecules affecting transcription in Friedreich ataxia. Pharmacol. Ther. 116, 236–248. doi: 10.1016/j.pharmthera.2007.06.014
Gottesfeld, J. M. (2019). Molecular Mechanisms and Therapeutics for the GAA⋅TTC Expansion Disease Friedreich Ataxia. Neurotherapeutics 16, 1032–1049. doi: 10.1007/s13311-019-00764-x
Grant, K., Sun, J., Xu, H., Subramony, S. H., Chaires, J. B., and Hebert, M. D. (2006). Rational Selection of Small Molecules That Increase Transcription Through the GAA Repeats Found in Friedreich’s Ataxia. FEBS Lett. 580, 5399–5405. doi: 10.1016/j.febslet.2006.09.006
Greene, E., Mahishi, L., Entezam, A., Kumari, D., and Usdin, K. (2007). Repeat-induced epigenetic changes in intron 1 of the frataxin gene and its consequences in Friedreich ataxia. Nucl. Acid Res. 35, 3383–3390. doi: 10.1093/nar/gkm271
Herman, D., Jenssen, K., Burnett, R., Soragni, E., Perlman, S. L., and Gottesfeld, J. M. (2006). Histone Deacetylase Inhibitors Reverse Gene Silencing in Friedreich’s Ataxia. Nat. Chem. Biol. 2, 551–558. doi: 10.1038/nchembio815
Indelicato, E., Nachbauer, W., Eigentler, A., Amprosi, M., Matteucci Gothe, R., Giunti, P., et al. (2020). Onset features and time to diagnosis in Friedreich’s Ataxia. Orphanet J. Rare Dis. 15:198. doi: 10.1186/s13023-020-01475-9
Koeppen, A. H. (2011). Friedreich’s ataxia: pathology, pathogenesis, and molecular genetics. J. Neurol. Sci. 303, 1–12. doi: 10.1016/j.jns.2011.01.010
Li, J., Rozwadowska, N., Clark, A., Fil, D., Napierala, J. S., and Napierala, M. (2019). Excision of the expanded GAA repeats corrects cardiomyopathy phenotypes of iPSC-derived Friedreich’s ataxia cardiomyocytes. Stem Cell Res. 4:101529. doi: 10.1016/j.scr.2019.101529
Li, L., Matsui, M., and Corey, D. R. (2016). Activating frataxin expression by repeat-targeted nucleic acids. Nat. Commun. 7:10606. doi: 10.1038/ncomms10606
Li, L., Shen, X., Liu, Z., Norrbom, M., Prakash, T. P., O’reilly, D., et al. (2018). Activation of Frataxin Protein Expression by Antisense Oligonucleotides Targeting the Mutant Expanded Repeat. Nucl. Acid Ther. 28, 23–33. doi: 10.1089/nat.2017.0703
Li, L., Voullaire, L., Sandi, C., Pook, M., Ioannou, P. A., Delatycki, M. B., et al. (2013). Pharmacological Screening Using an FXN-EGFP Cellular Genomic Reporter Assay for the Therapy of Friedreich Ataxia. PLoS One 8:e55940. doi: 10.1371/journal.pone.0055940
Li, Y., Lu, Y., Polak, U., Lin, K., Shen, J., Farmer, J., et al. (2015a). Expanded GAA repeats impede transcription elongation through the FXN gene and induce transcriptional silencing that is restricted to the FXN locus. Hum. Mol. Genet. 24, 6932–6943. doi: 10.1093/hmg/ddv397
Li, Y., Polak, U., Bhalla, A. D., Rozwadowska, N., Butler, J. S., Lynch, D. R., et al. (2015b). Excision of Expanded GAA Repeats Alleviates the Molecular Phenotype of Friedreich’s Ataxia. Mol. Ther. 23, 1055–1065. doi: 10.1038/mt.2015.41
Li, Y., Polak, U., Clark, A. D., Bhalla, A. D., Chen, Y., Li, J., et al. (2016). Establishment and Maintenance of Primary Fibroblast Repositories for Rare Diseases-Friedreich’s Ataxia Example. Biopreserv. Biobank. 14, 324–329. doi: 10.1089/bio.2015.0117
Lufino, M. M. P., Silva, A. M., Németh, A. H., Alegre-Abarrategui, J., Russell, A. J., and Wade-Martins, R. (2013). A GAA repeat expansion reporter model of Friedreich’s ataxia recapitulates the genomic context and allows rapid screening of therapeutic compounds. Hum. Mol. Genet. 22, 5173–5187. doi: 10.1093/hmg/ddt370
Marmolino, D. (2011). Friedreich’s Ataxia: past. Brain Res. Rev. 24, 311–330. doi: 10.1016/j.brainresrev.2011.04.001
Martelli, A., Wattenhofer-Donzé, M., Schmucker, S., Bouvet, S., Reutenauer, L., and Puccio, H. (2007). Frataxin is essential for extramitochondrial Fe-S cluster proteins in mammalian tissues. Hum. Mol. Genet. 16, 2651–2658. doi: 10.1093/hmg/ddm163
Ohshima, K., Montermini, L., Wells, R. D., and Pandolfo, M. (1998). Inhibitory effects of expanded GAA.TTC triplet repeats from intron I of the Friedreich ataxia gene on transcription and replication in vivo. J. Biol. Chem. 273, 14588–14595. doi: 10.1074/jbc.273.23.14588
Pandolfo, M. (2009). Friedreich ataxia: the clinical picture. J. Neurol. 256, 3–8. doi: 10.1007/s00415-009-1002-3
Parkinson, M. H., Boesch, S., Nachbauer, W., Mariotti, C., and Giunti, P. (2013). Clinical features of Friedreich’s ataxia: classical and atypical phenotypes. J. Neurochem. 126, 103–117. doi: 10.1111/jnc.12317
Polak, U., Li, Y., Butler, J. S., and Napierala, M. (2016). Alleviating GAA Repeat Induced Transcriptional Silencing of the Friedreich’s Ataxia Gene During Somatic Cell Reprogramming. Stem Cells Develop. 25, 1788–1800. doi: 10.1089/scd.2016.0147
Punga, T., and Bühler, M. (2010). Long intronic GAA repeats causing Friedreich ataxia impede transcription elongation. EMBO Mol. Med. 2, 120–129. doi: 10.1002/emmm.201000064
Ruiz Buendía, G. A., Leleu, M., Marzetta, F., Vanzan, L., Tan, J. Y., Ythier, V., et al. (2020). Three-dimensional chromatin interactions remain stable upon CAG/CTG repeat expansion. Sci. Adv. 6:eaaz4012. doi: 10.1126/sciadv.aaz4012
Sandi, C., Sandi, M., Anjomani Virmouni, S., Al-Mahdawi, S., and Pook, M. (2014). Epigenetic-based therapies for Friedreich ataxia. Front. Genet. 5:165. doi: 10.3389/fgene.2014.00165
Sarsero, J. P., Li, L., Wardan, H., Sitte, K., Williamson, R., and Ioannou, P. A. (2003). Upregulation of expression from the FRDA genomic locus for the therapy of Friedreich ataxia. J. Gene Med. 5, 72–81. doi: 10.1002/jgm.320
Saveliev, A., Everett, C., Sharpe, T., Webster, Z., and Festenstein, R. (2003). DNA triplet repeats mediate heterochromatin-protein-1-sensitive variegated gene silencing. Nature 422, 909–913. doi: 10.1038/nature01596
Shen, X., Kilikevicius, A., O’reilly, D., Prakash, T. P., Damha, M. J., Rigo, F., et al. (2018). Activating frataxin expression by single-stranded siRNAs targeting the GAA repeat expansion. Bioorg. Med. Chem. Lett. 28, 2850–2855. doi: 10.1016/j.bmcl.2018.07.033
Sherzai, M., Valle, A., Perry, N., Kalef-Ezra, E., Al-Mahdawi, S., Pook, M., et al. (2020). HMTase Inhibitors as a Potential Epigenetic-Based Therapeutic Approach for Friedreich’s Ataxia. Front. Genet. 11:584. doi: 10.3389/fgene.2020.00584
Soragni, E., Herman, D., Dent, S. Y. R., Gottesfeld, J. M., Wells, R. D., and Napierala, M. (2008). Long Intronic GAA*TTC Repeats Induce Epigenetic Changes and Reporter Gene Silencing in a Molecular Model of Friedreich Ataxia. Nucl. Acid Res. 36, 6056–6065. doi: 10.1093/nar/gkn604
Soragni, E., Miao, W., Iudicello, M., Jacoby, D., De Mercanti, S., Clerico, M., et al. (2014). Epigenetic Therapy for Friedreich Ataxia. Ann. Neurol. 76, 489–508. doi: 10.1002/ana.24260
Sun, J. H., Zhou, L., Emerson, D. J., Phyo, S. A., Titus, K. R., Gong, W., et al. (2018). Disease-Associated Short Tandem Repeats Co-localize with Chromatin Domain Boundaries. Cell 175:224–238.e15. doi: 10.1016/j.cell.2018.08.005
Vilema-Enríquez, G., Quinlan, R., Kilfeather, P., Mazzone, R., Saqlain, S., Del Molino Del Barrio I. et al. (2020). Inhibition of the SUV4-20 H1 histone methyltransferase increases frataxin expression in Friedreich’s ataxia patient cells. J. Biol. Chem. 295, 17973–17985.
Yandim, C., Natisvili, T., and Festenstein, R. (2013). Gene regulation and epigenetics in Friedreich’s ataxia. J. Neurochem. 126, 21–42. doi: 10.1111/jnc.12254
Zhang, S., Napierala, M., and Napierala, J. S. (2019). Therapeutic prospects for Friedreich’s ataxia. Trends Pharmacol. Sci. 40, 229–233. doi: 10.1016/j.tips.2019.02.001
Keywords: Friedreich’s ataxia (FRDA), reporter cell line, induced pluripotent stem cells, neural progenitor cells (NPCs), Nanoluciferase, screening
Citation: Schreiber AM, Li Y, Chen Y-H, Napierala JS and Napierala M (2022) Selected Histone Deacetylase Inhibitors Reverse the Frataxin Transcriptional Defect in a Novel Friedreich’s Ataxia Induced Pluripotent Stem Cell-Derived Neuronal Reporter System. Front. Neurosci. 16:836476. doi: 10.3389/fnins.2022.836476
Received: 15 December 2021; Accepted: 12 January 2022;
Published: 23 February 2022.
Edited by:
David Lynch, University of Pennsylvania, United StatesCopyright © 2022 Schreiber, Li, Chen, Napierala and Napierala. This is an open-access article distributed under the terms of the Creative Commons Attribution License (CC BY). The use, distribution or reproduction in other forums is permitted, provided the original author(s) and the copyright owner(s) are credited and that the original publication in this journal is cited, in accordance with accepted academic practice. No use, distribution or reproduction is permitted which does not comply with these terms.
*Correspondence: Marek Napierala, bW5hcGllcmFAdWFiLmVkdQ==