- 1Brain Research Center, National Yang Ming Chiao Tung University, Taipei, Taiwan
- 2School of Medicine, National Defense Medical Center, Taipei, Taiwan
- 3Department of Nursing, Hsin-Sheng College of Medical Care and Management, Taoyuan, Taiwan
- 4Department of Nuclear Medicine, Cheng-Hsin General Hospital, Taipei, Taiwan
- 5Department of Nuclear Medicine, Taipei Medical University Hospital, Taipei, Taiwan
- 6Department of Nuclear Medicine, Tri-Service General Hospital, Taipei, Taiwan
- 7Radiomedix, Inc., Houston, TX, United States
- 8Department of Biology and Anatomy, National Defense Medical Center, Taipei, Taiwan
Alterations to the serotonergic system due to 3,4-methylenedioxymethamphetamine (MDMA) (ecstasy) consumption have been extensively documented. However, knowledge of the reversibility of these neurotoxic effects based on in vivo evaluations of serotonin transport (SERT) availability remains limited. This study aimed to evaluate the long-term neurotoxicity of MDMA after 66 months abstinence and explored whether Dextromethorphan, a non-competitive N-methyl-D-aspartate (NMDA) receptor, could attenuate MDMA-induced neurotoxicity using 4-[18F]-ADAM, an imaging ligand that selectively targets SERT, with positron emission tomography technology (PET). Nine monkeys (Macaca cyclopis) were used in this study: control, MDMA, and DM + MDMA. Static 4-[18F]-ADAM PET was performed at 60 and 66 months after drug treatment. Serotonin transport (SERT) availability was presented as the specific uptake ratios (SURs) of 4-[18F]-ADAM in brain regions. Voxel-based region-specific SERT availability was calculated to generate 3D PET/MR images. Structural Magnetic Resonance Imaging (MRI) volumetric analysis was also conducted at 60 months. Significantly decreased 4-[18F]-ADAM SURs were observed in the striatum and thalamus of the MDMA group at 60 and 66 months compared to controls; the midbrain and frontal cortex SURs were similar at 60 and 66 months in the MDMA and control groups. All eleven brain regions showed significantly lower (∼13%) self-recovery rates over time; the occipital cortex and cingulate recovered to baseline by 66 months. DM attenuated MDMA-induced SERT deficiency on average, by ∼8 and ∼1% at 60 and 66 months, respectively; whereas significant differences were observed between the thalamus and amygdala of the MDMA and DM + MDMA groups at 66 months. Compared to controls, the MDMA group exhibited significantly increased (∼6.6%) gray matter volumes in the frontal cortex, occipital cortex, caudate nucleus, hippocampus, midbrain, and amygdala. Moreover, the gray matter volumes of the occipital cortex, hippocampus and amygdala correlated negatively with the 4-[18F]-ADAM SURs of the same regions. DM (n = 2) did not appear to affect MDMA-induced volumetric changes. The 4-[18F]-ADAM SURs, lower self-recovery rate and increased volumetric values indicate the occipital cortex, hippocampus and amygdala still exhibit MDMA-induced neurotoxicity after 66 months’ abstinence. Moreover, DM may prevent MDMA-induced serotonergic deficiency, as indicated by increased 4-[18F]-ADAM SURs and SERT availability, but not volumetric changes.
Introduction
3,4-Methylenedioxymethamphetamine (MDMA), commonly known as ecstasy or molly, is a synthetic drug that acts as a stimulant, hallucinogen, and entactogen. MDMA is used recreationally for its mild hallucinogenic and stimulant properties, as well as its ability to increase emotional closeness (Lyles and Cadet, 2003). However, studies have also demonstrated the effectiveness of MDMA might serve a potential treatment for anxiety in people with post-traumatic stress disorder (PTSD) and terminal illnesses (Morgan, 2020).
3,4-Methylenedioxymethamphetamine boosts the activity of three neurotransmitters: dopamine, serotonin (Schmidt et al., 1987; Gough et al., 1991), and norepinephrine (Rothman et al., 2001). The drug enhances synaptic release of these neurotransmitters (Sabol and Seiden, 1998) and/or blocks their reuptake (Berger et al., 1992; Verrico et al., 2008), resulting in increased levels of the neurotransmitters within the synaptic cleft, which in turn affects mood, energy levels, appetite, trust, sexual activity, emotions, and sleep. MDMA also carries serious risks, including hyperthermia, cardiovascular effects, mental impairment, risky behavior, and fatal overdose (Bolla et al., 1998).
While the recreational effects of MDMA usually last for about 3–6 h, the half-life of MDMA is 8–9 h (Freye, 2009). De La Torre et al. (2000) found the peak effects of MDMA are observed within the first 1 and 2 h, and decrease around 4–6 h after taking the drug (De La Torre et al., 2000). Research in rodents has shown that repeated administration of moderate to high doses of MDMA induces long-lasting sensitization of noradrenergic and serotonergic neurons, which correlates with behavioral sensitization (Kirilly, 2010) and selective ablation of serotonergic axon terminals in the forebrain (O’hearn et al., 1988). MDMA-induced 5-HT neural injury in non-human primates lasted for at least 7 years, and may well be permanent (Hatzidimitriou et al., 1999). Several factors are known to influence the recovery of 5-HT axons after administration of MDMA, including the distance between the affected axon terminal field and the rostral raphe nuclei, the degree of initial 5-HT axonal injury, and possibly the proximity of the damaged 5-HT axons to myelinated fiber tracts (Hatzidimitriou et al., 1999; Chiu et al., 2015; Vegting et al., 2016).
The SERT functional imaging have been employed for in vivo using single photon emission computed tomography (SPECT) or positron emission tomography (PET) to further explore the effects of MDMA on the serotonergic system (Skye and Hwang, 2016). Using [11C]-(+)-McN5652 PET, Mccann et al. (2005) observed decreased global and local binding to SERT in 23 previous ecstasy users compared to 19 non-MDMA controls (Mccann et al., 2005). Semple et al. (1999) used [123I]-2β-carbomethoxy-3β-(4-fluorophenyl)tropane ([123I]-β-CIT) SPECT and detected a cortical reduction in cerebral SERTs in long-term MDMA users compared with MDMA-naive subjects who use other drugs (Semple et al., 1999). Buchert et al. (2003) demonstrated that the distribution volume ratios (DVR) in the mesencephalon and thalamus were significantly lower in current ecstasy users compared to drug-naive control subjects, and concluded that ecstasy-induced protracted alterations to the brain serotonergic system last several weeks (Buchert et al., 2003).
Dextromethorphan (DM, 3-methoxy-17-methylmorphinan), a non-competitive N-methyl-D-aspartate (NMDA) receptor antagonist, has been widely used as an antitussive agent. DM has been suggested to reduce neuronal damage and modulate pain sensations via non-competitive antagonism of excitatory amino acids (EAAs) (Siu and Drachtman, 2007). Excitotoxicity is a phenomenon that describes the toxic actions of excessive excitatory neurotransmitters, primarily the EAA glutamate, that lead to exacerbated or prolonged activation of receptors, which starts a cascade of neurotoxicity that ultimately leads to the loss of neuronal function and cell death (Armada-Moreira et al., 2020; Suzuki et al., 2021). In addition to its therapeutic effects in terms of antitussive activity (Craviso and Musacchio, 1983), cancer pain relief (Weinbroum et al., 2000), and methotrexate toxicity (Kishi et al., 2000), DM has also been used as neuroprotective agent for seizures (Schmitt et al., 1994), cerebral ischemia (Lo and Steinberg, 1991) and Parkinson’s disease (Liu et al., 2019).
As described above, in the last two decades, a number of SERT imaging agents have become available for human SPECT or PET studies, and some of these agents labeled with 11C or 123I have been used for MDMA-related neuroimaging. These include [123I]I-β-CIT (Laruelle et al., 1994) and [123I]I-ADAM (Newberg et al., 2004) for SPECT, and [11C]-(+) McN5652 (Buck et al., 2000), [11C]DASB (Ginovart et al., 2001) and [11C]AFM (Naganawa et al., 2013) for PET.
However, 18F has some advantages over 11C, notably that the 18F-labeled radioligands can be transported offsite if a cyclotron is not available. The most important advantages of PET imaging are its much higher sensitivity and better contrast and spatial resolution compared to SPECT (Rahmim and Zaidi, 2008). Therefore, a number of 18F-labeled SERT imaging agents such as S-[18F]fluoroethyl)-(+)-McN5652 (Suehiro et al., 1996), S-[18F]fluoromethyl)-(+)-McN5652 (Brust et al., 2003), and [18F]F-ACF (Oya et al., 2002) have been reported. Based on its structure and our experience with [123I]I-ADAM, our group and others made efforts to develop 18F-labeled SERT imaging agents, including 4-[18F]F-ADAM (Shiue et al., 2003; Peng et al., 2008; Huang et al., 2009), 5-[18F]F-ADAM (Fang et al., 2004), and [18F]F-AFM (Huang et al., 2005). The structures of [123I]I-ADAM and 4-[18F]F-ADAM are shown in Supplementary Figure 1.
Among these 18F-labeled SERT imaging agents, S-[18F]fluoromethyl)-(+)-McN5652 has been studied in humans and shown to be suitable for in vivo quantification of SERT with PET (Hesse et al., 2012), and 4-[18F]F-ADAM developed by our group has been synthesized and evaluated in translational studies of its specificity for SERT in rodents (Ma et al., 2009) and primates (Huang et al., 2010). Moreover, the safety and high specificity of 4-[18F]-ADAM for SERT have also been demonstrated in human studies (Huang et al., 2013).
With 4-[18F]-ADAM PET, we also reported that MDMA induced neurite damage and neuron death in serotonergic neurons in vitro (Li et al., 2016). MDMA-treated rats exhibited reduced SERT availability in the midbrain and thalamus at 2 weeks, and these changes were associated with depressive-like behaviors (Shih et al., 2016).
In parallel to developing 4-[18F]-ADAM, in order to investigate the topic of MDMA-induced SERT deficiency and explore whether DM exerts a neuroprotective effect, we performed SERT imaging using [123I]-ADAM SPECT in the primate brain (Ma et al., 2009). We observed the MDMA-induced decrease in central SERT levels persisted for over 4 years. The [123I]-ADAM signals were significantly lower in the brains of the MDMA group than the control group, indicating the MDMA-treated monkeys had lower brain SERT levels. We also reported that DM exerts a protective effect against MDMA-induced serotonergic aberrations (Ma et al., 2016).
In the present study, by using [18F]-4-ADAM PET/CT/MR, we further evaluated SERT availability, self-recovery rate, and volumetric changes in various brain regions of MDMA-exposed primates 6.5 years after administration of the drug using and also assessed the neuroprotective effects of DM. To extend our previous [123I]-ADAM SPECT results, the motivation of this study was to (1) to gain a better understanding of the extended long-term SERT deficiency post-MDMA up to 66 months; (2) to emphasize that certain specific regions; i.e., 3D detailed regional-specific SERT PET imaging post-MDMA, is superior to SPECT images; and (3) to highlight that current state-of-the-art PET and MRI scanners can greatly benefit from improvements in innovative image reconstruction algorithms; i.e., MDMA-induced alterations of structural MRI volumetric analysis vs. functional PET SERT activity.
Materials and Methods
Radiosynthesis of 4-[18F]-ADAM
4-[18F]-ADAM, a specific radioligand for SERT, was synthesized using an automated synthesis device, as previously reported (Peng et al., 2008). In brief, the precursor 4-[18F]-ADAM in 0.5 mL of dimethyl sulfoxide was added to dried potassium [18F]-fluoride/Kryptofix 2.2.2 residue and reduced with NaBH4/Cu(OAc)2. Purification by high-performance liquid chromatography produced the desired compound with a radiochemical yield of approximately 3% at the end of synthesis (EOS) in a synthesis period of 120 min. The EOS of 4-[18F]-ADAM can be increased to approximately 15% by using a different precursor and manual synthesis (Huang et al., 2009). The chemical and radiochemical purities were >95%, and the specific activity was >3 Ci μmol–1 (111 GBq μmol–1).
Experimental Animals
Nine Formosan rock monkeys (Macaca cyclopis) aged 4–6-years-old were assessed in this study (Table 1). The animals were housed separately in a facility accredited by the Association for Assessment and Accreditation of Laboratory Animal Care International (AAALAC International), at National Defense Medical Center. All experiments were performed following National Defense Medical Center’s guidelines for conducting experiments in non-human primates under an Institutional Animal Care and Use Committee approved research protocol (IAUIC No 12-188).
Study Design and Drug Treatment
The experimental design is illustrated in Figure 1. Nine monkeys (Macaca cyclopis) were used in this study: control, MDMA (5 mg/kg, twice a day for four consecutive days), and DM co-administered with MDMA (MDMA 5 mg/kg with DM 5 mg/kg, twice a day for four consecutive days). The animals used in this study were the same animals used in our previous work, in which we monitored MDMA-induced serotonergic aberrations up to 54 months using [123I]ADAM SPECT (Ma et al., 2016); the current study was a follow-up extension up to 66 months based on 4-[18F]ADAM PET/CT/MRI imaging.
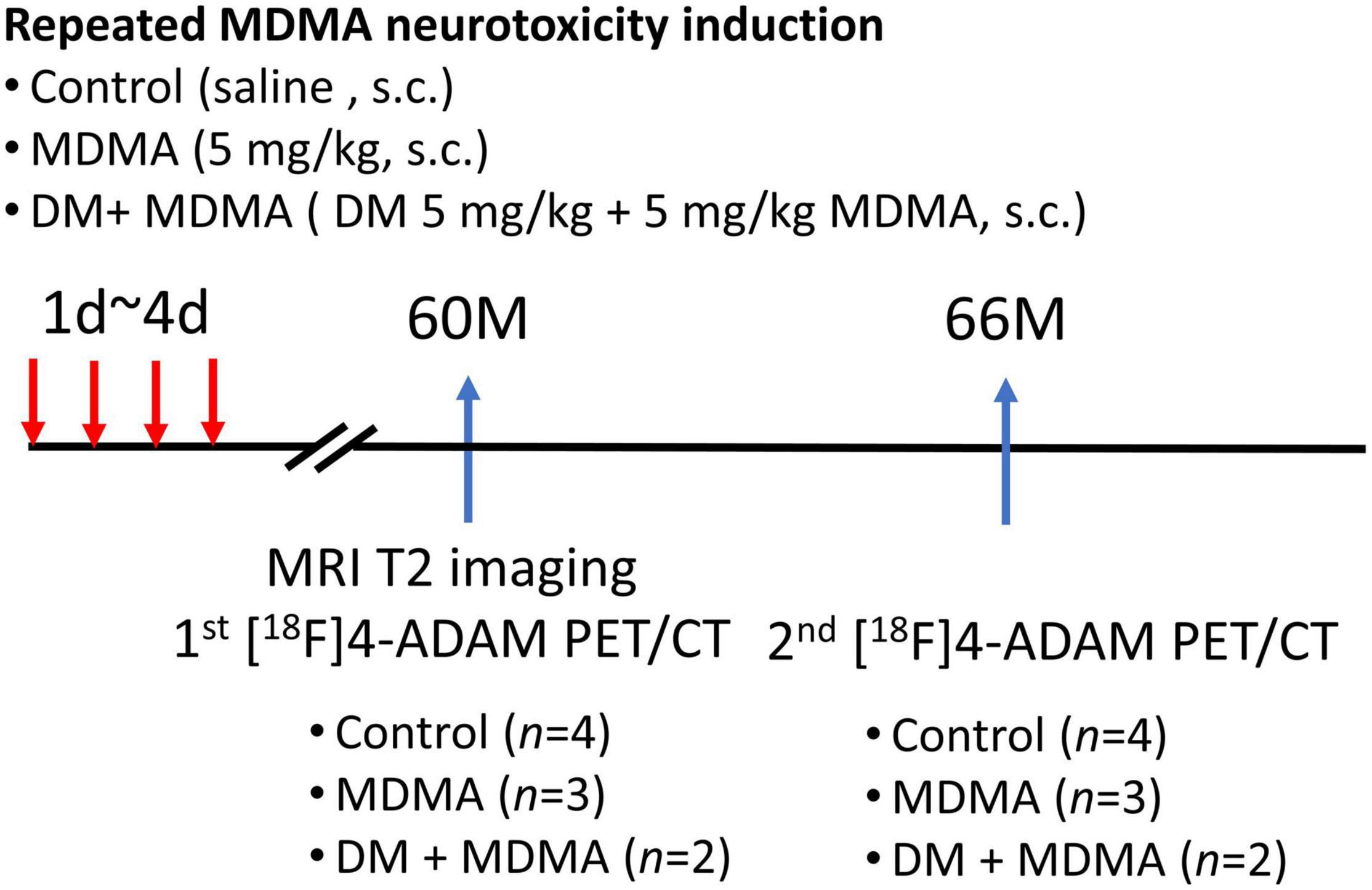
Figure 1. Flowchart of the study design. A total of nine monkeys were randomized into three groups: control, MDMA, and DM + MDMA. The drugs were administered between day 1 and day 4 (twice a day for four consecutive days). MRI imaging was performed at 60 months and PET/CT imaging was performed at 60 and 66 months.
3,4-Methylenedioxymethamphetamine was provided by the Investigation Bureau of Taiwan and DM (Sigma-Aldrich) challenge studies were used to explore the protective effects of DM against MDMA neurotoxicity. In the MDMA challenge studies, the dose regimen of MDMA was modified from the dose used in a previous study (Szabo et al., 2002). The monkeys were subcutaneously injected with 5 mg/kg MDMA twice a day for four consecutive days to damage the serotonergic system. This particular dosage regimen has been proven to lead to moderate to severe damage in the serotonergic system (Ricaurte et al., 1988; Hatzidimitriou et al., 1999).
Brain 4-[18F]-ADAM PET studies were performed 60 and 66 months after the neurotoxin treatment in the MDMA-treated group, MDMA + DM co-administration group, and the control group. All animals in each group were scanned two times to access SERT availability and the progression and recovery of neurotoxicity of the serotonergic systems. The interval between each PET imaging session was 6 months. The average body weight of animal over study period is shown in Table 2.
Positron Emission Tomography Imaging and Data Acquisition
Prior to imaging, the animals were fasted overnight with free access to water. The animals were immobilized with Zoletil™ (4 mg/kg, I.M. injection), and anesthesia was maintained with passive inhalation of oxygen containing 1.8% isoflurane at a rate of 2 L/min (to maintain >95% oxygen saturation). If necessary, Atropine (0.05 mg/kg I.M. injection) was administered to reduce salivary and bronchial secretions during Zoletil™ anesthesia to avoid excessive swallowing, reflexes, and accumulation of saliva during the experiment, which may cause vomiting and nausea. Intravenous infusion of 0.9% NaCl at a rate of 5 mL/kg per hour via the cephalic vein was used to maintain hydration and for radiotracer administration.
Positron emission tomography/CT measurements were performed in 3D mode on a Biogragh 2 scanner (Biograph Duo, Siemens), which includes a dual slice detector CT scanner (Somatom Emotion; Siemens Medical Systems). This scanner has a transverse FOV of 58.5 cm, an axial FOV of 15.5 cm and a spatial resolution of 4.8 mm. Each subject was carefully placed on the motorized adjustable bed of the scanner and their head was immobilized using a cradle. Before the emission scan, a scout scan was performed to define the imaging field, followed by a low-dose CT scan (130 kVp, 50 mAs, 0.8 s tube rotation, 4-mm slice collimation, pitch 3), followed by a static PET scan in 3D mode at 120–150 min after intravenous bolus injection of 192.48 ± 23.93 MBq (5.13 ± 0.59 mCi) of 4-[18F]-ADAM. Emission images were reconstructed in a 512 × 512 × 64 matrix with a pixel size of 0.519 mm × 0.519 mm × 2.4 mm using OSEM (six iterations and 16 subsets) using a Gaussian filter with a FWHM of 3 mm. All reconstructed PET images were corrected for attenuation using the CT images. The CT images also served as anatomic references for registering the PET data when defining ROIs.
Magnetic Resonance Imaging Protocol
T2-weighted Magnetic Resonance (MR) imaging was performed using a 3.0 Tesla GE SIGNA 450 system. After three-plane tri-pilot imaging, 22 contiguous coronal, sagittal and horizontal T2WIs were acquired using a fast spin echo sequence with a TR/TE of 3,000/100 ms, echo train length of 8, NEX of 4, matrix size of 256 × 256, FOV of 20 × 20 mm2, slice thickness (SLTH) of 4 mm, flip angle of 90°, bandwidth of 50.0 kHz, and acquisition time of 6 min 44 s. All animals were imaged using the same MR parameters. After the image acquisition was complete, the images were transferred to a stand-alone personal computer and analyzed using N.I.H. Image 1.52 software. The MR images were resliced, resized, and co-registered to all corresponding PET images in the plane parallel to the canthomeatal line (CML).
Positron Emission Tomography/Magnetic Resonance Data Analyses
We delineated the region of interest (ROIs) using an automated anatomical labeling template (monkey atlas) in PNEURO (PMOD version 4.0, PMOD Technologies, Zurich, Switzerland) in order to prevent bias from inter- or intra-rater reliability issues arising from manual delineation. Then, the mean standardized uptake value (SUV) in frontal cortex (FCX), midbrain (MB), thalamus (TH), striatum (STR), and cerebellum (CB) were extracted from unsmoothed SUV images in the standard stereotactic space. The CB expresses very low concentrations of SERT and was used as the reference region (Kish et al., 2005). The cerebellar vermis was excluded and only the posterior half of the cerebellar cortex was delineated.
Specific Uptake Ratio of 4-[18F]-ADAM
The specific binding of 4-[18F]-ADAM in SERT-rich regions was semi-quantitatively analyzed and expressed as the specific uptake ratio (SUR). The CB was chosen as the reference region for free and non-specific binding in the brain. The SUR of various ROIs, including the MB, TH, STR, and FCX, were calculated and expressed using Equation (1):
where CROI and CCB are the mean radioactivity at “pseudo” equilibrium in the ROIs and CB, respectively.
Magnetic Resonance Imaging-Based Volumetric Quantification
In addition to the PET data, the MRI images obtained at the 60-month imaging timepoint were reconstructed; brain segment images representing a labeled atlas of the brain structures identified by the segmentation algorithm were created in PNEURO (PMOD version 4.0). Volumetric quantification was performed for the stratum, midbrain, thalamus, frontal cortex, and cerebellum of each subject.
Statistical Analysis
Data are expressed as mean ± SEM. One-way ANOVA with the post-hoc Bonferroni or unpaired Student’s t-test were used for statistical evaluation; p < 0.05 was defined as statistically significant. Statistical analyses were performed using GraphPad Prism 8 (GraphPad software, La Jolla, CA, United States).
Results
Repeated 3,4-Methylenedioxymethamphetamine Administration Induces Long-Lasting Serotonergic Neurotoxicity in the Striatum
Figure 2 is the illustration of the location of the brain regions was used to estimate the SERT binding of 4-[18F]-ADAM. The 3D PET/MRI brain images shown in Figure 3 present evaluation of the 4-[18F]-ADAM uptakes in the control group and at 60 and 66 months after administration of MDMA with or without DM. On average, the midbrain showed the highest uptake of [18F]-ADAM during the study period, followed by the thalamus, putamen, caudate nucleus, amygdala, and the other regions. The reference region, the cerebellum, had relatively lower SURs compared to other regions.
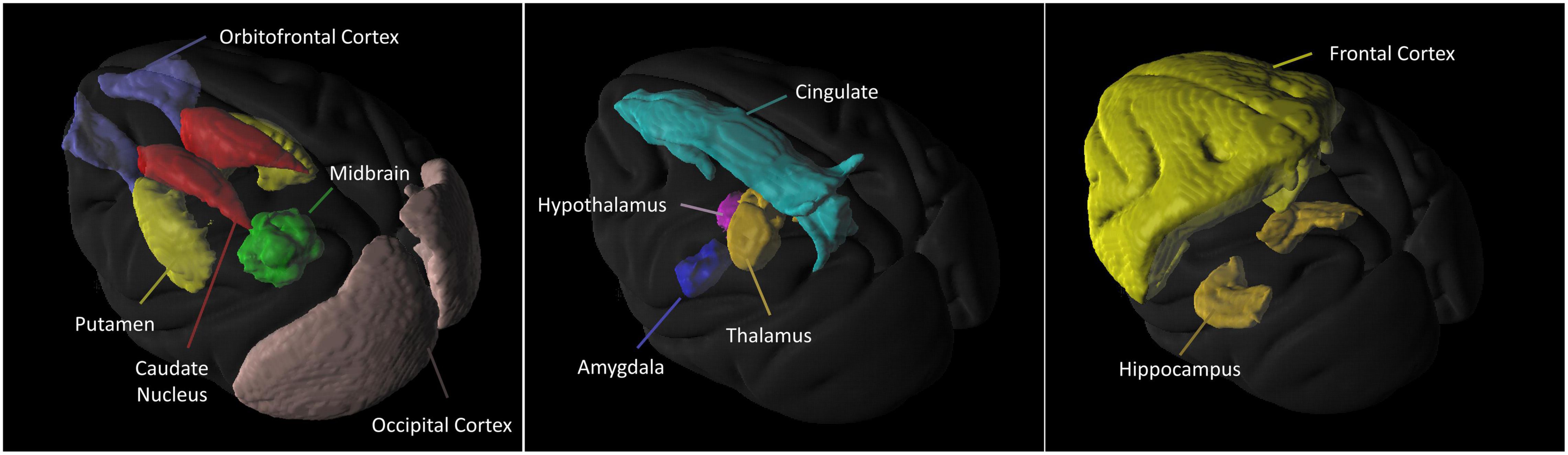
Figure 2. 3D 4-[18F]-ADAM PET images. Illustration of the location of the brain regions was used to estimate the SERT binding of 4-[18F]-ADAM.
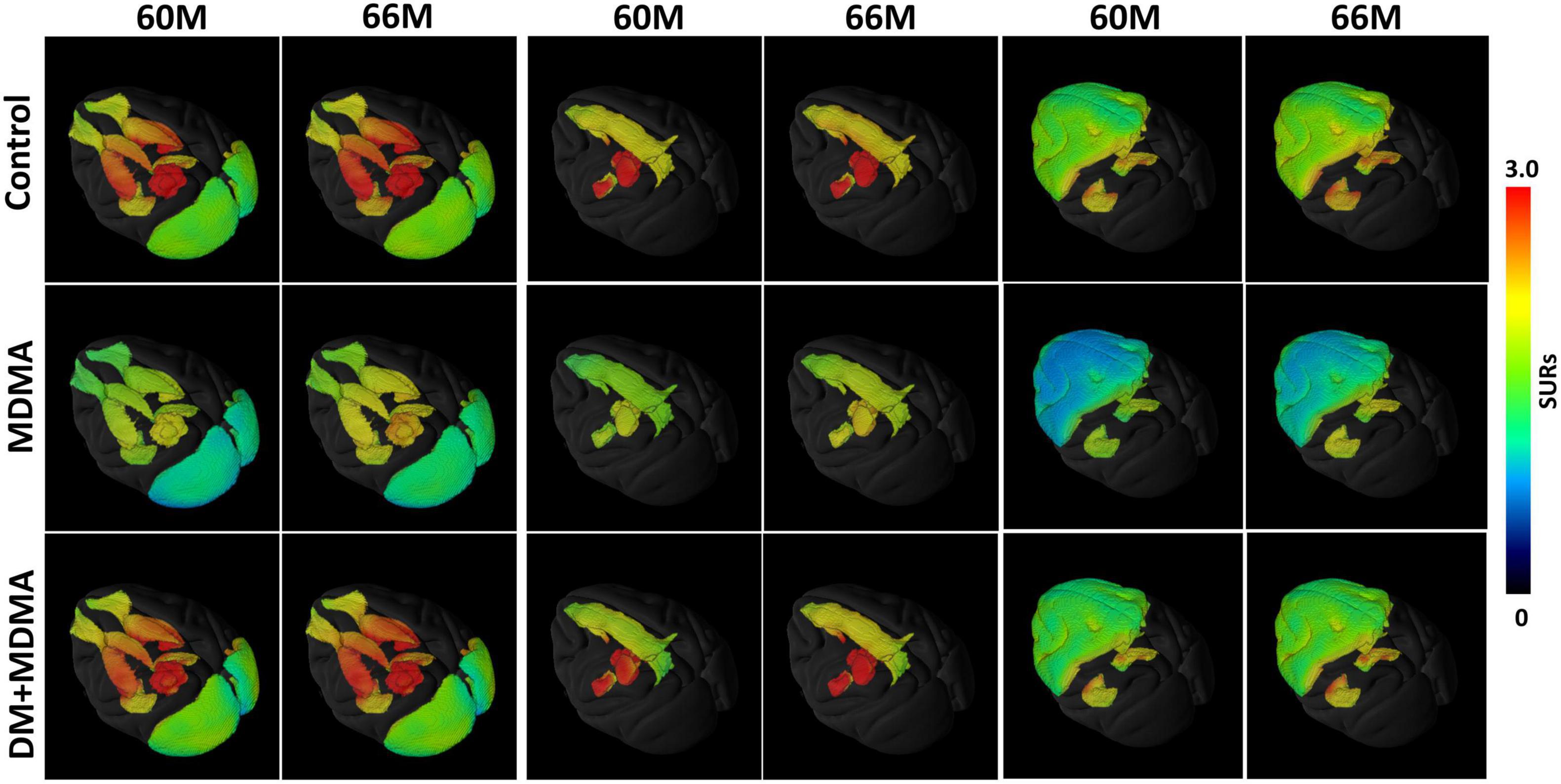
Figure 3. 3D PET parametric SUR imaging obtained 120–150 min after intravenous injection of [18F]-4-ADAM in different regions. Multiple-assembly brain region (top row); control group (second row); MDMA group (third row); and DM + MDMA group (bottom row). PET images were co-registered to MRI-based atlas to generate 3D PET/MR images (PMOD version 4.0, PMOD Technologies, Zurich, Switzerland).
As shown in Figure 4, lower 4-[18F]-ADAM SURs were observed in all regions in the MDMA groups at 60 and 66 months compared to the controls. Significant reductions were detected in the frontal cortex, occipital cortex, hippocampus, midbrain and amygdala (p < 0.05). Detailed results of the specific uptake ratio (SURs) in vivo are shown in Supplementary Table 1.
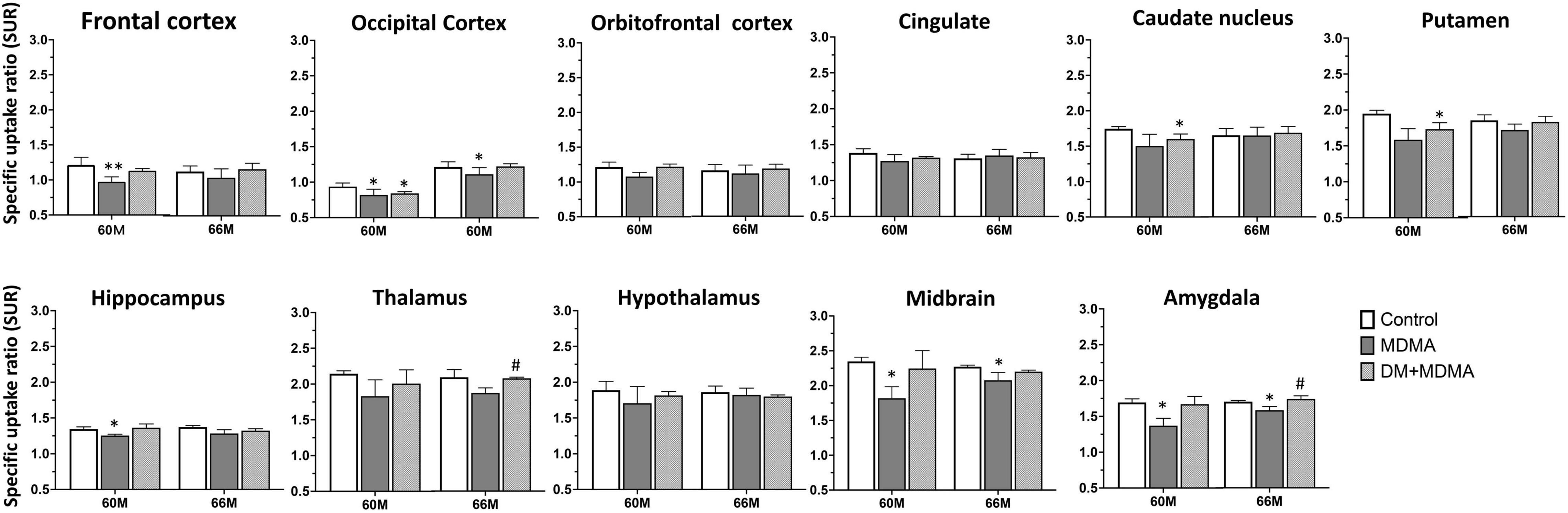
Figure 4. Specific uptake ratios of [18F]-4-ADAM PET obtained 120–150 min post-injection for various brain regions in the control, MDMA, and DM + MDMA groups at 60 and 66 months. Data is presented as mean ± SEM; *p < 0.05 compared to control group. #p < 0.05 compared to the MDMA group. **p < 0.01.
Region-Specific Recovery of Serotonin Transport Availability After 3,4-Methylenedioxymethamphetamine Induction
We next evaluated whether SERT availability could recover over time. All regions showed significantly lower self-recovery rates over time after administration of MDMA (p < 0.05∼p < 0.005), whereas the occipital cortex and cingulate recovered to baseline levels (control group) within 66 months (Figure 5).
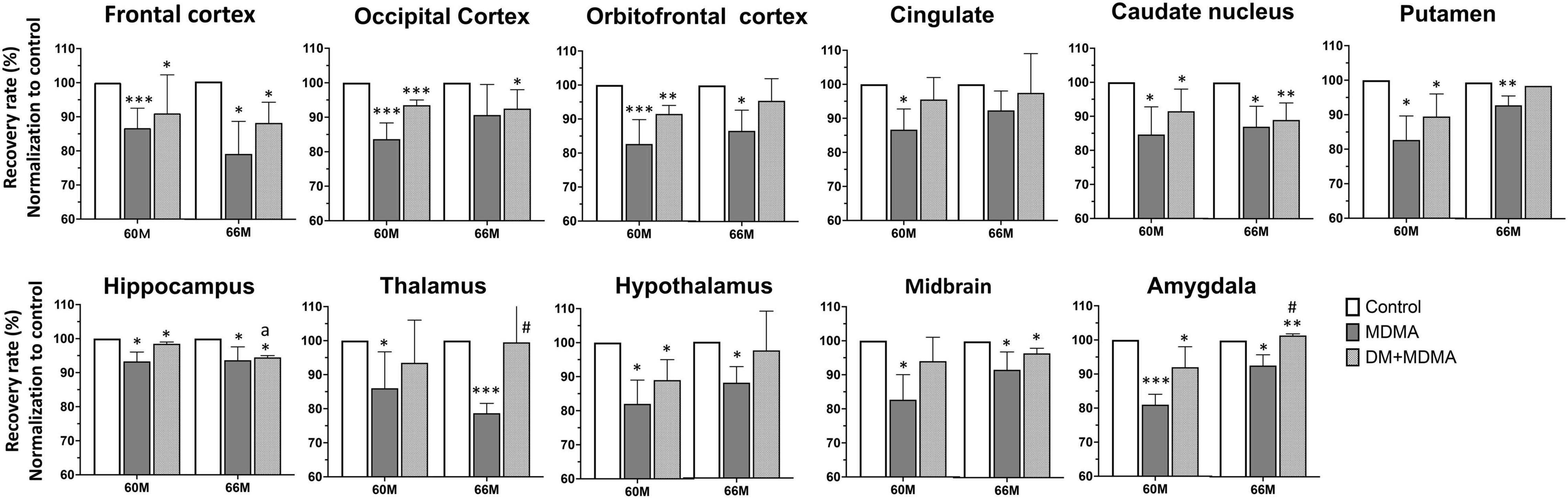
Figure 5. Recovery rate of SERT availability in various brain regions in the control, MDMA, and DM + MDMA groups at 60 and 66 months. Data is presented as mean ± SEM; *p < 0.05, **p < 0.01, ***p < 0.005 compared to the control group; #p < 0.05 compared to the MDMA group. ap < 0.01 when compared to DM+MDMA at 60 months.
Dextromethorphan Attenuates 3,4-Methylenedioxymethamphetamine-Induced Serotonin Transport Deficiency
Next, we tested the hypothesis that treatment with DM attenuates MDMA-induced SERT deficiency by accelerating the recovery of SERT availability. Compared to the MDMA group, DM attenuated MDMA-induced SERT deficiency from 85.55 ± 5.72% to 93.23 ± 3.48% and 88.91 ± 6.17% to 89.91 ± 7.41% at 60 and 66 months, respectively. There were significant differences between the thalamus and amygdala of the MDMA and DM + MDMA groups at 66 months (#p < 0.05, Figure 4). Detailed results of the recovery rate of SERT availability in vivo are shown in Supplementary Table 2.
3,4-Methylenedioxymethamphetamine Affects Brain Volume, but Dextromethorphan Does Not Attenuate These Changes
The volumes of the frontal cortex, occipital cortex, caudate nucleus, hippocampus, midbrain and amygdala were significantly increased in the MDMA group compared to controls (P < 0.01∼0.005, Figure 6). On average, MDMA increased the brain volume in the regions mentioned above by 6.06 ± 2.18% compared to controls. However, DM had no effect on the changes in volume induced by MDMA (DM + MDMA vs. MDMA; Figure 7). Detailed results of the MRI volumetric differences in vivo are shown in Table 3.
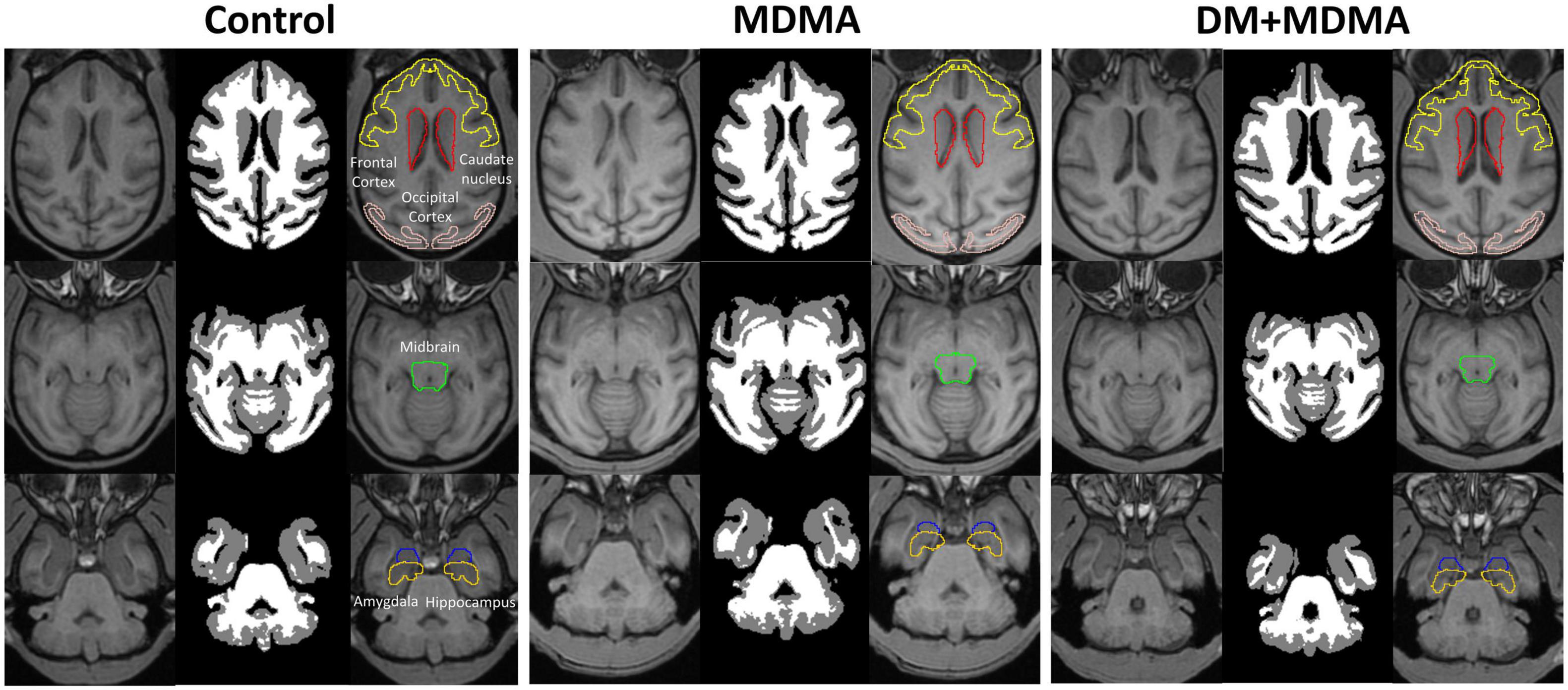
Figure 6. Volumetric differences between the control (Left), MDMA (middle), and DM + MDMA (right) groups. MDMA led to higher gray matter volumes in young primates; DM + MDMA had no effect on the MDMA-induced changes in brain volume.
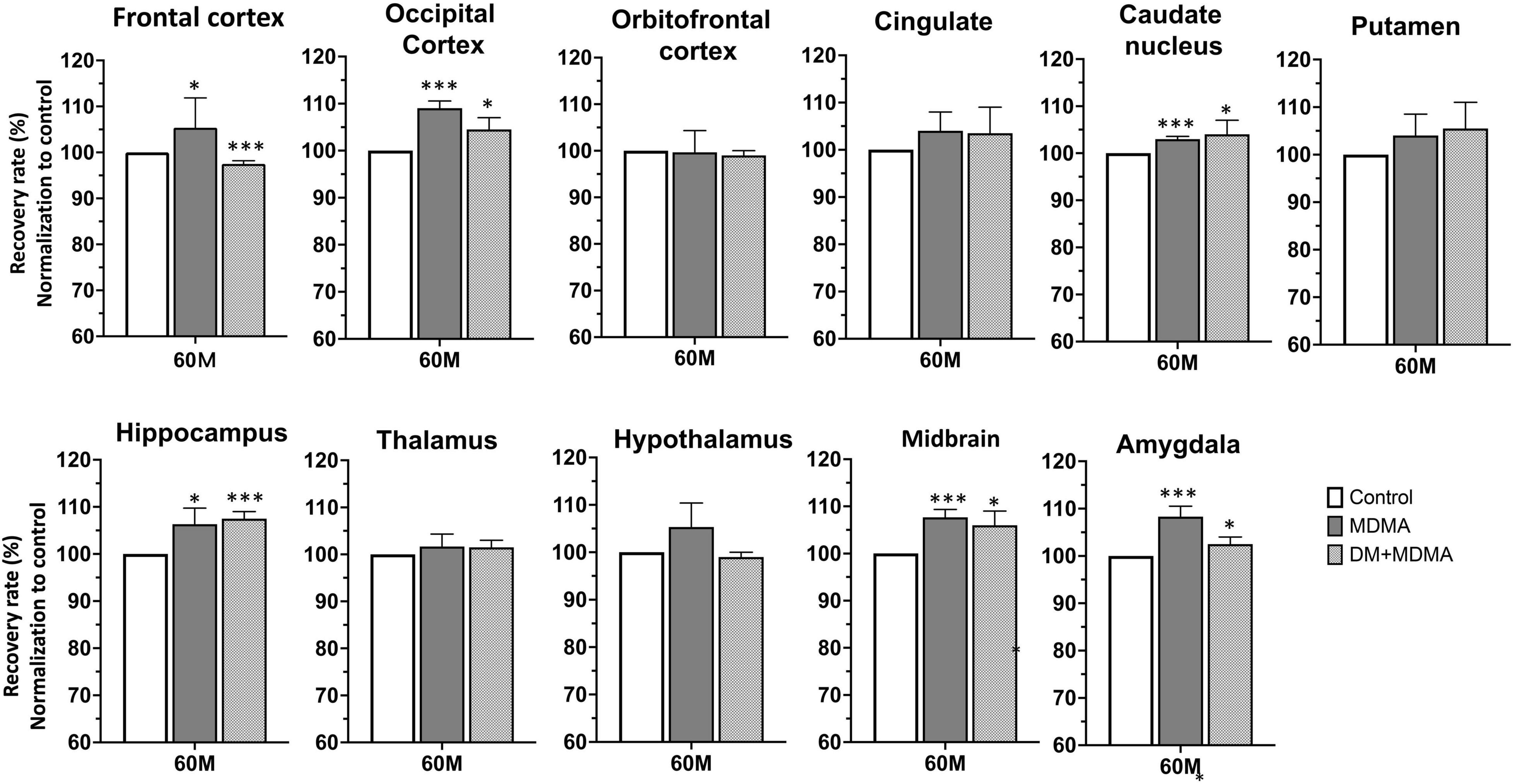
Figure 7. Volumetric analysis of brain MR images. Comparison showing the volumes of the striatum, midbrain, thalamus and frontal cortex in MDMA group were significantly larger (p < 0.01∼0.005) compared to controls. Co-administration of DM with MDMA had no significant neuroprotective effect on volumetric values. Data are presented as mean ± SEM; *p < 0.05, ** p < 0.01, ***p < 0.005 compared to the control group.
Compared to the controls (white circle), MRI brain volume increased post-administration of MDMA, but PET SERT recovery values decreased in all regions (red dot in pink block in Figure 8). In animals co-administered DM, the PET SERT recovery values tended to increase (x-axis), but DM had no effect on the brain volume (y-axis; blue dot in blue block in Figure 8). Furthermore, after fitting all data sets (black line in Figure 7), the increased brain volumes correlated negatively and significantly with the PET SERT recovery values in the occipital cortex (R2 = 0.757), hippocampus (R2 = 0.601; all P < 0.05). Detailed results of the in vivo PET vs. MRI correlations are shown in Table 4.
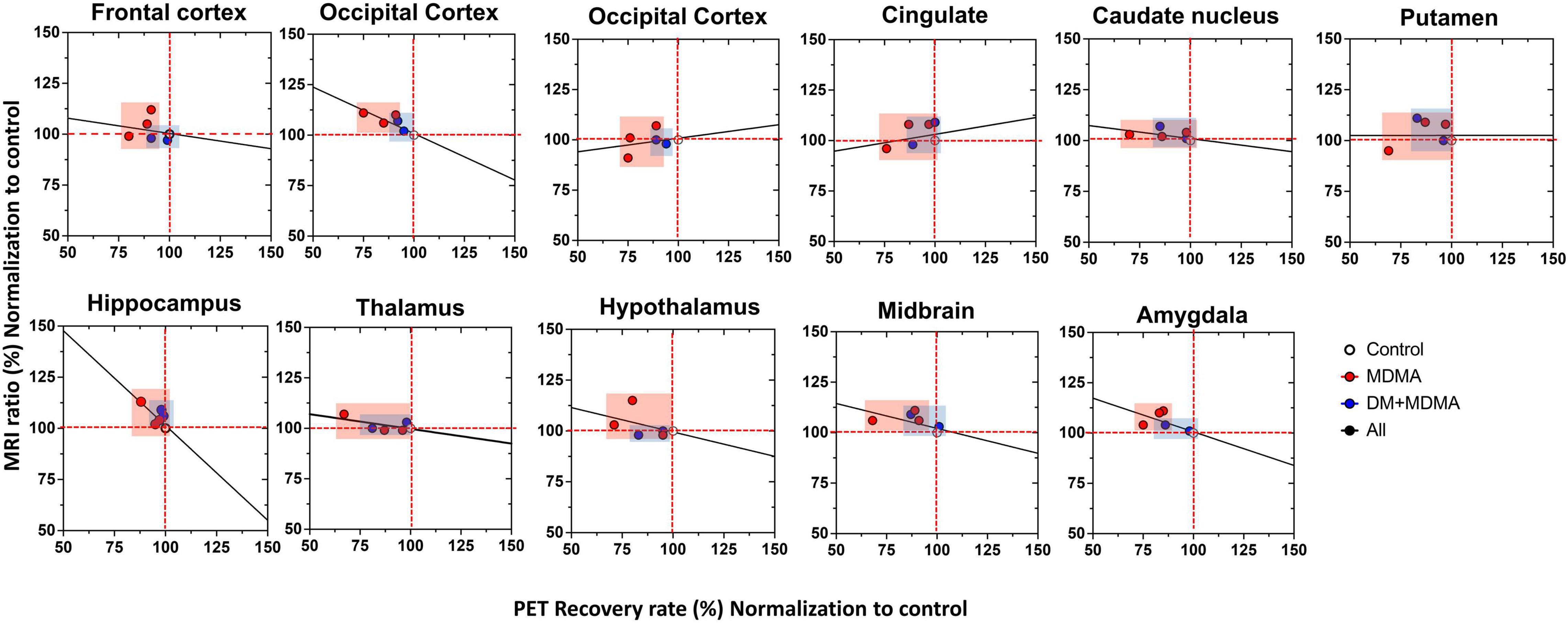
Figure 8. Relationship between the volumetric ratio determined by MRI (y-axis) and SERT recovery rate determined by [18F]-4-ADAM PET (x-axis). Increased brain volume negatively correlated with reduced uptake of [18F]-4-ADAM after MDMA administration. Linear correlation coefficients between X and Y are shown when the two variables ranges; MDMA group-pink block, DM-MDMA-blue block, black line-all grange/group (R2-values for each region are shown in Table 4).
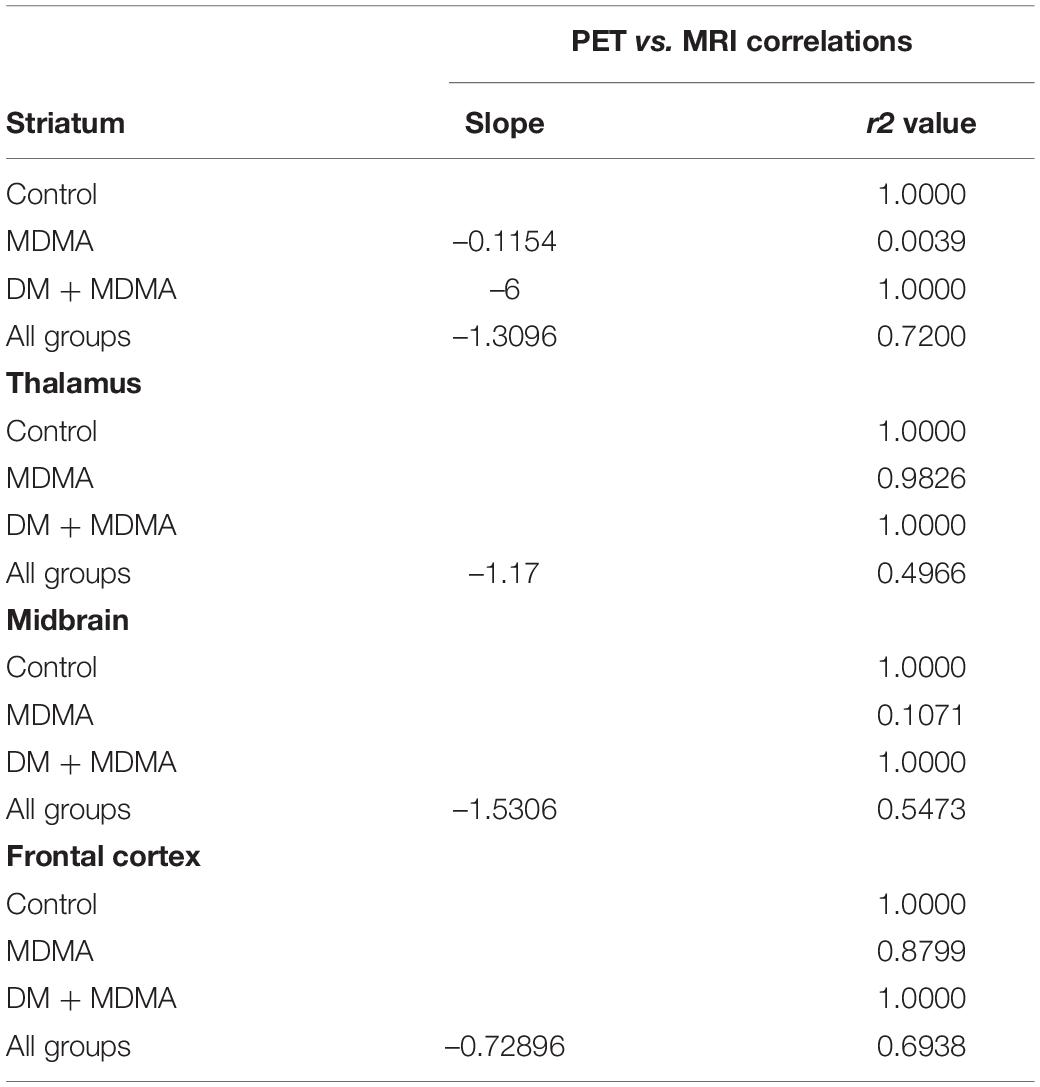
Table 4. Simple linear regression model of MRI ratio and PET 4-[18F]ADAM recovery rate (R-squared) for the data set of each group or all groups.
Discussion
This in vivo functional 4-[18F]-ADAM PET/CT/MR imaging study demonstrated that MDMA could induce long-lasting SERT over 66 months in the central nervous system (CNS) of primates. Moreover, the recovery rate of SERT availability and neuroprotective effects of DM were region-specific. These results, particularly after administration of high dosages, are in good agreement with our previous study (Ma et al., 2016) and recent findings (Vegting et al., 2016).
Compared to the aforementioned studies of SERT reversibility in humans after MDMA exposure, we observed some interesting findings in primates. In 2016, we reported that the MDMA-induced decrease in brain SERT levels could persist for over 4 years (48 months) based on the SURs of [123I]ADAM SPECT (Ma et al., 2016); we also reported that DM abolished MDMA-induced aberrations in SERT density in several brain regions.
In this study, we extended the follow-up to 66 months. More accurate and detailed 3D PET/MR images revealed the 4-[18F]-ADAM PET uptake ratio varied in different brain regions, and we further analyzed the recovery rate of SERT availability to determine self-recovery over time. We also evaluated whether DM attenuates the MDMA-induced reduction in SERT availability or accelerates the recovery rate.
At 60 months post-MDMA exposure (without DM), the mean self-recovery rate of the 11 tested brain regions had reached >88%. At 66 months, the self-recovery rate remained unchanged (89%), suggesting self-recovery had stopped occurring. Significantly lower self-recovery rates for the occipital cortex, hippocampus and amygdala relative to other brain regions tested indicate these regions still exhibit MDMA-induced neurotoxicity after 66 months after last MDMA exposure. These results indicate region-specific recovery of SERT availability after MDMA induction occurs.
Consistent with these conclusions in animals, human studies also revealed significantly lower SERT binding in specific brain areas, predominantly the occipital cortex (p < 0.05∼0.0001) (Mccann et al., 1998; Semple et al., 1999; Mccann et al., 2005; De Win et al., 2008; Mccann et al., 2008; Frokjaer et al., 2014), hippocampus (p < 0.05∼0.0001) (Mccann et al., 2005, 2008; De Win et al., 2008; Kish et al., 2010; Frokjaer et al., 2014), and thalamus (p < 0.05∼ < 0.003) (Mccann et al., 2005; Buchert et al., 2007; De Win et al., 2008).
Moreover, studies that included cognitive performance measures support the imaging findings described above. Reneman et al. (2000) reported higher binding ratios for the post-synaptic 5-HT2A receptors observed using [123I]R91150 SPECT were associated with poor memory function, as indicated by a verbal memory test, in MDMA users compared to controls (Reneman et al., 2000). In addition to cortical regions, Bosch et al. (2013) demonstrated significantly decreased regional cerebral brain glucose metabolism (rMRGlu) correlated with poorer verbal learning and delayed recall performance (Bosch et al., 2013). Taken together, this evidence indicates MDMA-induced serotonergic deficiency may be brain region-specific.
In this study, DM attenuated MDMA-induced serotonergic deficiency, and was associated with a reduced density of cerebral SERT; this protective effect was observed up to 66 months later. The protective mechanism of DM may include serotonin reuptake inhibition (Henderson and Fuller, 1992; Gillman, 2005) through its proposed high-affinity binding to the serotonin transporter (Meoni and Bowery, 1997) or inhibition of serotonin (Narita et al., 1995). DM only had a significant neuroprotective effect in the thalamus and amygdala at 66 months after MDMA exposure, but not in the other brain regions tested. This could be due to individual variations and the small sample size (n = 2), which resulted in high standard deviations for the MDMA or DM + MDMA group and may have reduced the power to detect significant differences. The variations in the regional distribution of SERT may also help to explain why the protective effects of DM were less pronounced in the tested regions, as larger doses of DM may be required to block the increased number of SERT.
However, studies have also reported that if DM replaces codeine in cough medicines and the approved doses are exceeded, DM produces dissociative effects similar to ketamine and phencyclidine (PCP) (Roy et al., 2015). Therefore, further experiments employing higher doses of DM would help to clarify the relationship between increased SERT availability and the regional alterations in MDMA-induced serotonergic deficiency.
Additionally, it is interesting to note that the brain volumes of the frontal cortex, occipital cortex, caudate nucleus, hippocampus, midbrain, and amygdala increased in the MDMA group compared to controls. Similarly, global reductions in the volumes of gray matter and left orbitofrontal and right occipital regions have been reported in long-term users (Daumann et al., 2011; Koester et al., 2012). Unlike the findings on chronic use (Cowan, 2007; Mueller et al., 2016), Mackey et al. (2014) reported that the gray matter volumes in the vmPFC and insula increased in a population of younger college-aged occasional users of amphetamine-type stimulants (ATS) and cocaine compared to controls (Mackey et al., 2014). They concluded that the increased gray matter volumes in the vmPFC and insula contrasted with the age-related decline in gray matter volume in the healthy developing brain, suggesting that gray matter development may be delayed in young occasional ATS and cocaine users (Mackey et al., 2014).
The orbito- and middle frontal cortex and striatum are important neural structures involved in decision-making. These regions are associated with the reward system and also play a role in addictive disorders (Betzler et al., 2017). One possible explanation for the higher volumes of the frontal cortex, occipital cortex, caudate nucleus, hippocampus, midbrain, and amygdala in the MDMA group could be that acute, repeated low-dose MDMA (5 mg/kg MDMA twice a day for four consecutive days) may lead to selective synaptic reorganization in the ventral striatum and medial frontal cortex (Robinson and Kolb, 2004). Diaz Heijtz et al. (2003) demonstrated that low doses of amphetamine increased spine density and dendritic branching in young rats (Diaz Heijtz et al., 2003). It is possible that the relationship observed between MDMA exposure and increased frontal cortex, occipital cortex, caudate nucleus, hippocampus, midbrain, and amygdala volumes in young primates may be related to excessive proliferation of neuronal processes, associated with structural plasticity, and reflect reorganization of patterns of synaptic connectivity in these neural systems that alters their operation, which may thus contribute to some of the persistent sequela associated with drug use (Robinson and Kolb, 2004).
Taken together, the present study demonstrates that the brain volume values of the occipital cortex, hippocampus and amygdala correlate negatively with the rate of recovery of PET 4-[18F]ADAM SERT activity in the same regions. Our findings suggest that MDMA exposure leads to functional abnormalities in a network of brain regions that are important in decision-making processes. In addition, the increased brain volume may compensate for the decreased brain SERT activity in the same regions, and reflect compensatory or neural plasticity in the MDMA-exposed group.
Limitations
The animals used in this study were the same animals used in our previous work reported in 2016 (Ma et al., 2016); thus, the major drawback is the lack of pre-MDMA baseline scans, although non-MDMA animals were used as controls. Moreover, we did not collect enough data to investigate the links between functional PET SERT activity and structural MRI measures of volumetric values. In addition, most statistical analyses of the DM + MDMA group did not reach statistical significance, although strong decreasing trends were observed. This may be due to the small sample size of this study; further studies with larger sample sizes and additional data are needed to validate our findings.
Conclusion
The present study examined MDMA-induced serotonergic deficiency in primates at 60 and 66 months after drug administration. 4-[18F]-ADAM PET/CT/MRI revealed MDMA-induced serotonergic deficiency may be region-specific, as indicated by the significantly lower 4-[18F]-ADAM binding ratios and recovery rates in most brain regions. Unlike findings on chronic use, the increased occipital cortex, hippocampus and amygdala volumes in younger primates exposed to MDMA tended to negatively correlate with the 4-[18F]-ADAM PET SURs in the same regions. This may be a compensatory effect for the decreased brain SERT activity in the reward regions, and reflect compensatory or neural plasticity in the MDMA-exposed group. DM appears to exert a neuroprotective effect on SERT activity in the hippocampus and amygdala at 66 months after MDMA exposure, but not against MDMA-induced changes in brain volume. Additional research based on molecular imaging paired with functional neuroimaging, genetics, or pharmacological challenge of the serotonin system is necessary to decipher the link between the serotonergic and cognitive changes induced by MDMA.
Data Availability Statement
The raw data supporting the conclusions of this article will be made available by the authors, without undue reservation.
Ethics Statement
This study involving animals was reviewed and approved by the Institutional Animal Care and Use Committee approved research protocol (IAUIC No 12-188). All experiments were performed following National Defense Medical Center’s guidelines for conducting experiments in nonhuman primates.
Author Contributions
K-HM, W-SH, and C-YC: conceptualization. C-YC: radiosynthesis. Y-YK, C-HC, T-HY, and LF: investigation. SY and K-HM: writing and editing. K-HM, C-YC, and C-JT: funding acquisition and study resources.
Funding
Funding for this study was provided by the Ministry of Science and Technology R.O.C. (MOST), Development Center for Biotechnology, and The Featured Areas Research Center Program within the framework of the Higher Education Sprout Project by the Ministry of Education (MOE) in Taiwan (MOST-110-2314-B-038-167, MOST-110-2314-B-A49A-528, MOST 109-2314-B-016-014-MY2, and 110M0156).
Conflict of Interest
LF was employed by Radiomedix, Inc.
The remaining authors declare that the research was conducted in the absence of any commercial or financial relationships that could be construed as a potential conflict of interest.
Publisher’s Note
All claims expressed in this article are solely those of the authors and do not necessarily represent those of their affiliated organizations, or those of the publisher, the editors and the reviewers. Any product that may be evaluated in this article, or claim that may be made by its manufacturer, is not guaranteed or endorsed by the publisher.
Acknowledgments
We thank the Molecular Imaging Facility Small Animal 7T PET/MR and Brain Research Center at National Yang Ming Chaio Tung University for technical support.
Supplementary Material
The Supplementary Material for this article can be found online at: https://www.frontiersin.org/articles/10.3389/fnins.2022.837194/full#supplementary-material
Supplementary Figure 1 | Structures of [123I]I-ADAM and 4-[18F]ADAM.
Supplementary Table 1 | Regional specific uptake ratios (SUR) of 4-[18F]ADAM in the brain. Data are presented as mean ± SEM; *p < 0.05, **p < 0.01 compared to the control group.
Supplementary Table 2 | Recovery of serotonin transport (SERT) availability during the study period. Data are presented as mean ± SEM; *p < 0.05, **p < 0.01, ***p < 0.005 compared to the control group; #p < 0.05 compared to the MDMA group.
References
Armada-Moreira, A., Gomes, J. I., Pina, C. C., Savchak, O. K., Goncalves-Ribeiro, J., Rei, N., et al. (2020). Going the Extra (Synaptic) Mile: excitotoxicity as the Road Toward Neurodegenerative Diseases. Front. Cell Neurosci. 14:90. doi: 10.3389/fncel.2020.00090
Berger, U. V., Gu, X. F., and Azmitia, E. C. (1992). The substituted amphetamines 3,4-methylenedioxymethamphetamine, methamphetamine, p-chloroamphetamine and fenfluramine induce 5-hydroxytryptamine release via a common mechanism blocked by fluoxetine and cocaine. Eur. J. Pharmacol. 215, 153–160. doi: 10.1016/0014-2999(92)90023-w
Betzler, F., Viohl, L., and Romanczuk-Seiferth, N. (2017). Decision-making in chronic ecstasy users: a systematic review. Eur. J. Neurosci. 45, 34–44. doi: 10.1111/ejn.13480
Bolla, K. I., Mccann, U. D., and Ricaurte, G. A. (1998). Memory impairment in abstinent MDMA (“Ecstasy”) users. Neurology 51, 1532–1537. doi: 10.1212/wnl.51.6.1532
Bosch, O. G., Wagner, M., Jessen, F., Kuhn, K. U., Joe, A., Seifritz, E., et al. (2013). Verbal memory deficits are correlated with prefrontal hypometabolism in (18)FDG PET of recreational MDMA users. PLoS One 8:e61234. doi: 10.1371/journal.pone.0061234
Brust, P., Hinz, R., Kuwabara, H., Hesse, S., Zessin, J., Pawelke, B., et al. (2003). In vivo measurement of the serotonin transporter with (S)-([18F]fluoromethyl)-(+)-McN5652. Neuropsychopharmacology 28, 2010–2019. doi: 10.1038/sj.npp.1300281
Buchert, R., Thiele, F., Thomasius, R., Wilke, F., Petersen, K., Brenner, W., et al. (2007). Ecstasy-induced reduction of the availability of the brain serotonin transporter as revealed by [11C](+)McN5652-PET and the multi-linear reference tissue model: loss of transporters or artifact of tracer kinetic modelling? J. Psychopharmacol. 21, 628–634. doi: 10.1177/0269881106071975
Buchert, R., Thomasius, R., Nebeling, B., Petersen, K., Obrocki, J., Jenicke, L., et al. (2003). Long-term effects of “ecstasy” use on serotonin transporters of the brain investigated by PET. J. Nucl. Med. 44, 375–384.
Buck, A., Gucker, P. M., Schonbachler, R. D., Arigoni, M., Kneifel, S., Vollenweider, F. X., et al. (2000). Evaluation of serotonergic transporters using PET and [11C](+)McN-5652: assessment of methods. J. Cereb. Blood. Flow Metab. 20, 253–262. doi: 10.1097/00004647-200002000-00005
Chiu, C. H., Siow, T. Y., Weng, S. J., Hsu, Y. H., Huang, Y. S., Chang, K. W., et al. (2015). Effect of MDMA-Induced Axotomy on the Dorsal Raphe Forebrain Tract in Rats: an In Vivo Manganese-Enhanced Magnetic Resonance Imaging Study. PLoS One 10:e0138431. doi: 10.1371/journal.pone.0138431
Cowan, R. L. (2007). Neuroimaging research in human MDMA users: a review. Psychopharmacology (Berl) 189, 539–556. doi: 10.1007/s00213-006-0467-3
Craviso, G. L., and Musacchio, J. M. (1983). High-affinity dextromethorphan binding sites in guinea pig brain. II. Competition experiments. Mol. Pharmacol. 23, 629–640.
Daumann, J., Koester, P., Becker, B., Wagner, D., Imperati, D., Gouzoulis-Mayfrank, E., et al. (2011). Medial prefrontal gray matter volume reductions in users of amphetamine-type stimulants revealed by combined tract-based spatial statistics and voxel-based morphometry. Neuroimage 54, 794–801. doi: 10.1016/j.neuroimage.2010.08.065
De La Torre, R., Farre, M., Roset, P. N., Lopez, C. H., Mas, M., Ortuno, J., et al. (2000). Pharmacology of MDMA in humans. Ann. N. Y. Acad. Sci. 914, 225–237.
De Win, M. M., Jager, G., Booij, J., Reneman, L., Schilt, T., Lavini, C., et al. (2008). Neurotoxic effects of ecstasy on the thalamus. Br. J. Psychiatr. 193, 289–296. doi: 10.1192/bjp.bp.106.035089
Diaz Heijtz, R., Kolb, B., and Forssberg, H. (2003). Can a therapeutic dose of amphetamine during pre-adolescence modify the pattern of synaptic organization in the brain? Eur. J. Neurosci. 18, 3394–3399. doi: 10.1046/j.0953-816x.2003.03067.x
Fang, P., Shiue, G. G., Shimazu, T., Greenberg, J. H., and Shiue, C. Y. (2004). Synthesis and evaluation of N,N-dimethyl-2-(2-amino-5-[18F]fluorophenylthio)benzylamine (5-[18F]-ADAM) as a serotonin transporter imaging agent. Appl. Radiat. Isot. 61, 1247–1254. doi: 10.1016/j.apradiso.2004.03.003
Frokjaer, V. G., Erritzoe, D., Holst, K. K., Madsen, K. S., Fisher, P. M., Madsen, J., et al. (2014). In abstinent MDMA users the cortisol awakening response is off-set but associated with prefrontal serotonin transporter binding as in non-users. Int. J. Neuropsychopharmacol. 17, 1119–1128. doi: 10.1017/S1461145714000066
Gillman, P. K. (2005). Monoamine oxidase inhibitors, opioid analgesics and serotonin toxicity. Br. J. Anaesth. 95, 434–441. doi: 10.1093/bja/aei210
Ginovart, N., Wilson, A. A., Meyer, J. H., Hussey, D., and Houle, S. (2001). Positron emission tomography quantification of [(11)C]-DASB binding to the human serotonin transporter: modeling strategies. J. Cereb. Blood Flow Metab. 21, 1342–1353. doi: 10.1097/00004647-200111000-00010
Gough, B., Ali, S. F., Slikker, W. Jr., and Holson, R. R. (1991). Acute effects of 3,4-methylenedioxymethamphetamine (MDMA) on monoamines in rat caudate. Pharmacol. Biochem. Behav. 39, 619–623. doi: 10.1016/0091-3057(91)90137-q
Hatzidimitriou, G., Mccann, U. D., and Ricaurte, G. A. (1999). Altered serotonin innervation patterns in the forebrain of monkeys treated with (+/-)3,4-methylenedioxymethamphetamine seven years previously: factors influencing abnormal recovery. J. Neurosci. 19, 5096–5107. doi: 10.1523/JNEUROSCI.19-12-05096.1999
Henderson, M. G., and Fuller, R. W. (1992). Dextromethorphan antagonizes the acute depletion of brain serotonin by p-chloroamphetamine and H75/12 in rats. Brain Res. 594, 323–326. doi: 10.1016/0006-8993(92)91144-4
Hesse, S., Brust, P., Mading, P., Becker, G. A., Patt, M., Seese, A., et al. (2012). Imaging of the brain serotonin transporters (SERT) with 18F-labelled fluoromethyl-McN5652 and PET in humans. Eur. J. Nucl. Med. Mol. Imaging 39, 1001–1011. doi: 10.1007/s00259-012-2078-z
Huang, W. S., Huang, S. Y., Ho, P. S., Ma, K. H., Huang, Y. Y., Yeh, C. B., et al. (2013). PET imaging of the brain serotonin transporters (SERT) with N,N-dimethyl-2-(2-amino-4-[18F]fluorophenylthio)benzylamine (4-[18F]-ADAM) in humans: a preliminary study. Eur. J. Nucl. Med. Mol. Imaging 40, 115–124. doi: 10.1007/s00259-012-2250-5
Huang, Y., Bae, S. A., Zhu, Z., Guo, N., Roth, B. L., and Laruelle, M. (2005). Fluorinated diaryl sulfides as serotonin transporter ligands: synthesis, structure-activity relationship study, and in vivo evaluation of fluorine-18-labeled compounds as PET imaging agents. J. Med. Chem. 48, 2559–2570. doi: 10.1021/jm0400808
Huang, Y. Y., Huang, W. S., Chu, T. C., and Shiue, C. Y. (2009). An improved synthesis of 4-[18F]-ADAM, a potent serotonin transporter imaging agent. Appl. Radiat. Isot. 67, 1063–1067. doi: 10.1016/j.apradiso.2009.02.090
Huang, Y. Y., Ma, K. H., Tseng, T. W., Chou, T. K., Ng, H., Mirsalis, J. C., et al. (2010). Biodistribution, toxicity and radiation dosimetry studies of the serotonin transporter radioligand 4-[18F]-ADAM in rats and monkeys. Eur. J. Nucl. Med. Mol. Imaging 37, 545–555. doi: 10.1007/s00259-009-1281-z
Kirilly, E. (2010). Long-term neuronal damage and recovery after a single dose of MDMA: expression and distribution of serotonin transporter in the rat brain. Neuropsychopharmacol. Hung. 12, 413–423.
Kish, S. J., Furukawa, Y., Chang, L. J., Tong, J., Ginovart, N., and Wilson, A. (2005). Regional distribution of serotonin transporter protein in postmortem human brain: is the cerebellum a SERT-free brain region? Nucl. Med. Biol. 32, 123–128. doi: 10.1016/j.nucmedbio.2004.10.001
Kish, S. J., Lerch, J., Furukawa, Y., Tong, J., Mccluskey, T., Wilkins, D., et al. (2010). Decreased cerebral cortical serotonin transporter binding in ecstasy users: a positron emission tomography/[(11)C]DASB and structural brain imaging study. Brain 133, 1779–1797. doi: 10.1093/brain/awq103
Kishi, T., Tanaka, Y., and Ueda, K. (2000). Evidence for hypomethylation in two children with acute lymphoblastic leukemia and leukoencephalopathy. Cancer 89, 925–931. doi: 10.1002/1097-0142(20000815)89:4<925::aid-cncr28>3.0.co;2-w
Koester, P., Tittgemeyer, M., Wagner, D., Becker, B., Gouzoulis-Mayfrank, E., and Daumann, J. (2012). Cortical thinning in amphetamine-type stimulant users. Neuroscience 221, 182–192. doi: 10.1016/j.neuroscience.2012.06.049
Laruelle, M., Wallace, E., Seibyl, J. P., Baldwin, R. M., Zea-Ponce, Y., Zoghbi, S. S., et al. (1994). Graphical, kinetic, and equilibrium analyses of in vivo [123I] beta-CIT binding to dopamine transporters in healthy human subjects. J. Cereb. Blood Flow Metab. 14, 982–994. doi: 10.1038/jcbfm.1994.131
Li, I. H., Ma, K. H., Kao, T. J., Lin, Y. Y., Weng, S. J., Yen, T. Y., et al. (2016). Involvement of autophagy upregulation in 3,4-methylenedioxymethamphetamine (’ecstasy’)-induced serotonergic neurotoxicity. Neurotoxicology 52, 114–126. doi: 10.1016/j.neuro.2015.11.009
Liu, C. T., Kao, L. T., Shih, J. H., Chien, W. C., Chiu, C. H., Ma, K. H., et al. (2019). The effect of dextromethorphan use in Parkinson’s disease: a 6-hydroxydopamine rat model and population-based study. Eur. J. Pharmacol. 862:172639. doi: 10.1016/j.ejphar.2019.172639
Lo, E. H., and Steinberg, G. K. (1991). Effects of dextromethorphan on regional cerebral blood flow in focal cerebral ischemia. J. Cereb. Blood Flow Metab. 11, 803–809. doi: 10.1038/jcbfm.1991.138
Lyles, J., and Cadet, J. L. (2003). Methylenedioxymethamphetamine (MDMA, Ecstasy) neurotoxicity: cellular and molecular mechanisms. Brain Res. Brain Res. Rev. 42, 155–168. doi: 10.1016/s0165-0173(03)00173-5
Ma, K. H., Huang, W. S., Kuo, Y. Y., Peng, C. J., Liou, N. H., Liu, R. S., et al. (2009). Validation of 4-[18F]-ADAM as a SERT imaging agent using micro-PET and autoradiography. Neuroimage 45, 687–693. doi: 10.1016/j.neuroimage.2008.12.060
Ma, K. H., Liu, T. T., Weng, S. J., Chen, C. F., Huang, Y. S., Chueh, S. H., et al. (2016). Effects of dextromethorphan on MDMA-induced serotonergic aberration in the brains of non-human primates using [(123)I]-ADAM/SPECT. Sci. Rep. 6:38695. doi: 10.1038/srep38695
Mackey, S., Stewart, J. L., Connolly, C. G., Tapert, S. F., and Paulus, M. P. (2014). A voxel-based morphometry study of young occasional users of amphetamine-type stimulants and cocaine. Drug Alcohol Dep. 135, 104–111. doi: 10.1016/j.drugalcdep.2013.11.018
Mccann, U. D., Szabo, Z., Scheffel, U., Dannals, R. F., and Ricaurte, G. A. (1998). Positron emission tomographic evidence of toxic effect of MDMA (“Ecstasy”) on brain serotonin neurons in human beings. Lancet 352, 1433–1437. doi: 10.1016/s0140-6736(98)04329-3
Mccann, U. D., Szabo, Z., Seckin, E., Rosenblatt, P., Mathews, W. B., Ravert, H. T., et al. (2005). Quantitative PET studies of the serotonin transporter in MDMA users and controls using [11C]McN5652 and [11C]DASB. Neuropsychopharmacology 30, 1741–1750. doi: 10.1038/sj.npp.1300736
Mccann, U. D., Szabo, Z., Vranesic, M., Palermo, M., Mathews, W. B., Ravert, H. T., et al. (2008). Positron emission tomographic studies of brain dopamine and serotonin transporters in abstinent (+/-)3,4-methylenedioxymethamphetamine (“ecstasy”) users: relationship to cognitive performance. Psychopharmacology (Berl) 200, 439–450. doi: 10.1007/s00213-008-1218-4
Meoni, T. F., and Bowery, Ng (1997). An autoradiographic study of dextromethorphan high-affinity binding sites in rat brain: sodium- dependency and colocalization with paroxetine. Br. J. Pharmacol. 120, 1255–1262. doi: 10.1038/sj.bjp.0701043
Morgan, L. (2020). MDMA-assisted psychotherapy for people diagnosed with treatment-resistant PTSD: what it is and what it isn’t. Ann. Gen. Psychiatry 19:33. doi: 10.1186/s12991-020-00283-6
Mueller, F., Lenz, C., Steiner, M., Dolder, P. C., Walter, M., Lang, U. E., et al. (2016). Neuroimaging in moderate MDMA use: a systematic review. Neurosci Biobehav Rev 62, 21–34. doi: 10.1016/j.neubiorev.2015.12.010
Naganawa, M., Nabulsi, N., Planeta, B., Gallezot, J. D., Lin, S. F., Najafzadeh, S., et al. (2013). Tracer kinetic modeling of [(11)C]AFM, a new PET imaging agent for the serotonin transporter. J. Cereb. Blood Flow Metab. 33, 1886–1896. doi: 10.1038/jcbfm.2013.134
Narita, N., Hashimoto, K., Iyo, M., Minabe, Y., and Yamazaki, K. (1995). Lack of neuroprotective effect of σ receptor ligands in the neurotoxicity of p-chloroamphetamine in rat brain. Eur. J. Pharmacol. 293, 277–280. doi: 10.1016/0926-6917(95)00018-6
Newberg, A. B., Plossl, K., Mozley, P. D., Stubbs, J. B., Wintering, N., Udeshi, M., et al. (2004). Biodistribution and imaging with (123)I-ADAM: a serotonin transporter imaging agent. J. Nucl. Med. 45, 834–841.
O’hearn, E., Battaglia, G., De Souza, E. B., Kuhar, M. J., and Molliver, M. E. (1988). Methylenedioxyamphetamine (MDA) and methylenedioxymethamphetamine (MDMA) cause selective ablation of serotonergic axon terminals in forebrain: immunocytochemical evidence for neurotoxicity. J. Neurosci. 8, 2788–2803. doi: 10.1523/JNEUROSCI.08-08-02788.1988
Oya, S., Choi, S. R., Coenen, H., and Kung, H. F. (2002). New PET imaging agent for the serotonin transporter: [(18)F]ACF (2-[(2-amino-4-chloro-5-fluorophenyl)thio]-N,N-dimethyl-benzenmethanamine). J. Med. Chem. 45, 4716–4723. doi: 10.1021/jm020167y
Peng, C. J., Huang, Y. Y., Huang, W. S., and Shiue, C. Y. (2008). An automated synthesis of N,N-dimethyl-2-(2-amino-4-[18F]fluorophenylthio)benzylamine (4-[18F]-ADAM) for imaging serotonin transporters. Appl. Radiat. Isot. 66, 625–631. doi: 10.1016/j.apradiso.2007.11.009
Rahmim, A., and Zaidi, H. (2008). PET versus SPECT: strengths, limitations and challenges. Nucl. Med. Commun. 29, 193–207. doi: 10.1097/MNM.0b013e3282f3a515
Reneman, L., Booij, J., Schmand, B., Van Den Brink, W., and Gunning, B. (2000). Memory disturbances in “Ecstasy” users are correlated with an altered brain serotonin neurotransmission. Psychopharmacology (Berl) 148, 322–324. doi: 10.1007/s002130050057
Ricaurte, G. A., Delanney, L. E., Irwin, I., and Langston, J. W. (1988). Toxic effects of MDMA on central serotonergic neurons in the primate: importance of route and frequency of drug administration. Brain Res. 446, 165–168. doi: 10.1016/0006-8993(88)91309-1
Robinson, T. E., and Kolb, B. (2004). Structural plasticity associated with exposure to drugs of abuse. Neuropharmacology 47, (Suppl. 1), 33–46. doi: 10.1016/j.neuropharm.2004.06.025
Rothman, R. B., Baumann, M. H., Dersch, C. M., Romero, D. V., Rice, K. C., Carroll, F. I., et al. (2001). Amphetamine-type central nervous system stimulants release norepinephrine more potently than they release dopamine and serotonin. Synapse 39, 32–41. doi: 10.1002/1098-2396(20010101)39:1<32::AID-SYN5>3.0.CO;2-3
Roy, A. K. III, Hsieh, C., and Crapanzano, K. (2015). Dextromethorphan Addiction Mediated Through the NMDA System: common Pathways With Alcohol? J. Addict. Med. 9, 499–501. doi: 10.1097/ADM.0000000000000152
Sabol, K. E., and Seiden, L. S. (1998). Reserpine attenuates D-amphetamine and MDMA-induced transmitter release in vivo: a consideration of dose, core temperature and dopamine synthesis. Brain Res. 806, 69–78. doi: 10.1016/s0006-8993(98)00720-3
Schmidt, C. J., Levin, J. A., and Lovenberg, W. (1987). In vitro and in vivo neurochemical effects of methylenedioxymethamphetamine on striatal monoaminergic systems in the rat brain. Biochem. Pharmacol. 36, 747–755. doi: 10.1016/0006-2952(87)90729-5
Schmitt, B., Netzer, R., Fanconi, S., Baumann, P., and Boltshauser, E. (1994). Drug refractory epilepsy in brain damage: effect of dextromethorphan on EEG in four patients. J. Neurol. Neurosurg. Psychiatr. 57, 333–339. doi: 10.1136/jnnp.57.3.333
Semple, D. M., Ebmeier, K. P., Glabus, M. F., O’carroll, R. E., and Johnstone, E. C. (1999). Reduced in vivo binding to the serotonin transporter in the cerebral cortex of MDMA (’ecstasy’) users. Br. J. Psychiatr. 175, 63–69. doi: 10.1192/bjp.175.1.63
Shih, J. H., Ma, K. H., Chen, C. F., Cheng, C. Y., Pao, L. H., Weng, S. J., et al. (2016). Evaluation of brain SERT occupancy by resveratrol against MDMA-induced neurobiological and behavioral changes in rats: a 4-[(1)(8)F]-ADAM/small-animal PET study. Eur. Neuropsychopharmacol. 26, 92–104. doi: 10.1016/j.euroneuro.2015.11.001
Shiue, G. G., Fang, P., and Shiue, C. Y. (2003). Synthesis of N,N-dimethyl-2-(2-amino-4-[18F]fluorophenylthio)benzylamine as a serotonin transporter imaging agent. Appl. Radiat. Isot. 58, 183–191. doi: 10.1016/s0969-8043(02)00271-3
Siu, A., and Drachtman, R. (2007). Dextromethorphan: a review of N-methyl-d-aspartate receptor antagonist in the management of pain. CNS Drug Rev. 13, 96–106. doi: 10.1111/j.1527-3458.2007.00006.x
Skye, F. C., and Hwang, Jj (2016). “Effects of Club Drugs on Dopaminergic and Serotonergic Systems and Diagnosis with [18F]FDOPA, [99mTc]TRODAT-1 and [I*]ADAM,” in Neuropathology of Drug addictions and Substance Misuse Part II. Club Drugs, ed. V. R. Preedy (Cambridge: Academic Press), 391–405.
Suehiro, M., Greenberg, J. H., Shiue, C. Y., Gonzalez, C., Dembowski, B., and Reivich, M. (1996). Radiosynthesis and biodistribution of the S-[18F]fluoroethyl analog of McN5652. Nucl. Med. Biol. 23, 407–412. doi: 10.1016/0969-8051(96)00013-3
Suzuki, H., Kawakita, F., Asada, R., Nakano, F., Nishikawa, H., and Fujimoto, M. (2021). Old but Still Hot Target, Glutamate-Mediated Neurotoxicity in Stroke. Transl. Stroke Res. 13, 216–217. doi: 10.1007/s12975-021-00958-6
Szabo, Z., Mccann, U. D., Wilson, A. A., Scheffel, U., Owonikoko, T., Mathews, W. B., et al. (2002). Comparison of (+)-(11)C-McN5652 and (11)C-DASB as serotonin transporter radioligands under various experimental conditions. J. Nucl. Med. 43, 678–692.
Vegting, Y., Reneman, L., and Booij, J. (2016). The effects of ecstasy on neurotransmitter systems: a review on the findings of molecular imaging studies. Psychopharmacology (Berl) 233, 3473–3501. doi: 10.1007/s00213-016-4396-5
Verrico, C. D., Lynch, L., Fahey, M. A., Fryer, A. K., Miller, G. M., and Madras, B. K. (2008). MDMA-induced impairment in primates: antagonism by a selective norepinephrine or serotonin, but not by a dopamine/norepinephrine transport inhibitor. J. Psychopharmacol. 22, 187–202. doi: 10.1177/0269881107083639
Keywords: 4-[18F]-ADAM PET, MDMA-induced serotonergic deficiency, SERT reversibility, volumetric changes, neuroprotective effects of dextromethorphan
Citation: Yeh SH-H, Kuo Y-Y, Huang W-S, Chiu C-H, Yu T-H, Flores LG II, Tsai C-J, Cheng C-Y and Ma K-H (2022) Preliminary Results on the Long-Term Effects of Dextromethorphan on MDMA-Mediated Serotonergic Deficiency and Volumetric Changes in Primates Based on 4-[18F]-ADAM PET/MRI. Front. Neurosci. 16:837194. doi: 10.3389/fnins.2022.837194
Received: 16 December 2021; Accepted: 26 April 2022;
Published: 19 May 2022.
Edited by:
Patrick Arthur Randall, The Pennsylvania State University, United StatesReviewed by:
Lakshmi Rajagopal, Northwestern University, United StatesPaul Meyer, University at Buffalo, United States
Copyright © 2022 Yeh, Kuo, Huang, Chiu, Yu, Flores, Tsai, Cheng and Ma. This is an open-access article distributed under the terms of the Creative Commons Attribution License (CC BY). The use, distribution or reproduction in other forums is permitted, provided the original author(s) and the copyright owner(s) are credited and that the original publication in this journal is cited, in accordance with accepted academic practice. No use, distribution or reproduction is permitted which does not comply with these terms.
*Correspondence: Kuo-Hsing Ma, a3VvaHNpbmc5MUB5YWhvby5jb20udHc=; Chi-Jung Tsai, Z2lyb25nZ2lybEBob3RtYWlsLmNvbQ==; Cheng-Yi Cheng, Y2hlbmdjeTYwQGdtYWlsLmNvbQ==
†These authors have contributed equally to this work and share first authorship