- 1Group of Brain Function and Development, Nagoya University Neuroscience Institute of the Graduate School of Science, Nagoya, Japan
- 2Research Unit for Developmental Disorders, Institute for Advanced Research, Nagoya University, Nagoya, Japan
- 3Department of Pediatrics, Asahikawa Medical University, Asahikawa, Japan
- 4Department of Pediatrics, Chiba University Hospital, Chiba, Japan
- 5Central Institute for Experimental Animals, Kawasaki, Japan
- 6Department of Physiology, Keio University School of Medicine, Tokyo, Japan
- 7Department of Radiology, Harvard Medical School, Boston, MA, United States
- 8Athinoula A. Martinos Center for Biomedical Imaging, Massachusetts General Hospital, Boston, MA, United States
- 9Department of Radiology, Juntendo University School of Medicine, Tokyo, Japan
- 10Department of Diagnostic Radiology, Tokyo Metropolitan Geriatric Hospital, Tokyo, Japan
- 11Department of Pediatrics, Nagoya University Graduate School of Medicine, Nagoya, Japan
- 12Department of Developmental Disability Medicine, Nagoya University Graduate School of Medicine, Nagoya, Japan
Rett syndrome (RTT) is a severe progressive neurodevelopmental disorder characterized by various neurological symptoms. Almost all RTT cases are caused by mutations in the X-linked methyl-CpG-binding protein 2 (MeCP2) gene, and several mouse models have been established to understand the disease. However, the neuroanatomical abnormalities in each brain region of RTT mouse models have not been fully understood. Here, we investigated the global and local neuroanatomy of the Mecp2 gene-deleted RTT model (Mecp2-KO) mouse brain using T2-weighted 3D magnetic resonance imaging with different morphometry to clarify the brain structural abnormalities that are involved in the pathophysiology of RTT. We found a significant reduction in global and almost all local volumes in the brain of Mecp2-KO mice. In addition, a detailed comparative analysis identified specific volume reductions in several brain regions in the Mecp2-deficient brain. Our analysis also revealed that the Mecp2-deficient brain shows changes in hemispheric asymmetry in several brain regions. These findings suggest that MeCP2 affects not only the whole-brain volume but also the region-specific brain structure. Our study provides a framework for neuroanatomical studies of a mouse model of RTT.
Introduction
Rett syndrome (RTT) is a severe and progressive neurodevelopmental disorder caused by mutations in the X-linked gene encoding methyl-CpG-binding protein 2 (MECP2) (Amir et al., 1999). With an incidence of approximately 1:10,000 female births, RTT is one of the most common causes of severe intellectual disability in adult female (Laurvick et al., 2006). Patients with RTT show normal development up to 18 months of age. This course is followed by the loss of acquired fine and gross motor skills and the ability to engage in social interaction. In many cases, patients develop seizures, cognitive impairment, autonomic dysfunction, and stereotypic hand movements (Chahrour and Zoghbi, 2007). Autopsy studies of patients with RTT revealed a 12–34% reduction in brain weight and volume, and the effect was most pronounced in the prefrontal, posterior frontal, and anterior temporal regions, with structural abnormalities at the cellular level, such as decreased dendritic length, reduced spine density, and cell body size (Belichenko et al., 1994; Bauman et al., 1995; Armstrong, 2005). In other studies, the RTT brain showed no obvious degeneration, atrophy, or inflammation, indicating that RTT is a postnatal neurodevelopmental disorder rather than a neurodegenerative disorder (Jellinger et al., 1988; Reiss et al., 1993; Chahrour and Zoghbi, 2007). In particular, anxiety is one of the prominent symptom of the behavioral phenotype of RTT (Barnes et al., 2015). A series of studies have reported a high prevalence of anxiety and anxiety-related disorders such as fear in RTT, such as sudden mood changes, screaming episodes, inability to stop crying, and self-abuse (Sansom et al., 1993; Barnes et al., 2015; Cianfaglione et al., 2015).
To further understand the pathophysiology of RTT, several mouse models with different Mecp2 mutations were generated in the past. Mecp2-knockout (Mecp2-KO) mice, lacking either exon 3 or both exons 3 and 4 (Chen et al., 2001; Guy et al., 2001) or carrying a truncated allele of Mecp2 at amino acid 308 (Shahbazian et al., 2002) undergo a period of normal development, followed by severe progressive neurological phenotypes such as motor impairments, seizures, stereotypic forepaw movements, hypoactivity, and microcephaly. Female Mecp2± mice also had behavioral abnormalities, but with a later age of onset. Conditional Mecp2 deletion in the brain, using the Nestin-Cre transgene, results in a phenotype similar to that observed in conventional Mecp2-KO mice, demonstrating that MeCP2 dysfunction in the brain is sufficient to cause the disease (Guy et al., 2001). In addition to mouse models, rat and zebrafish models that mimic Mecp2 loss have been developed (Pietri et al., 2013; Veeraragavan et al., 2016). While the rat model has been reported to show similar developmental and behavioral abnormalities, it has been shown that zebrafish model is viable and fertile, suggesting that Mecp2 might be more indispensable in higher organisms.
Although RTT patients and mouse models show profound neurological phenotypes, the major neuropathological changes in the brain are characterized by an overall decrease in brain size (Bauman et al., 1995; Kaufmann et al., 2000; Chen et al., 2001; Armstrong, 2005). Magnetic resonance imaging (MRI) has been used to identify specific pathological changes in the brain. MRI has the advantage of enabling non-invasive imaging, and it can be used to obtain functional and structural information of the whole brain. MRI completely covers brain volume without limiting depth penetration, and high-resolution three-dimensional (3D) imaging can be achieved accordingly (Wu and Lo, 2017). Therefore, a detailed analysis using MRI technology may reveal important insights into RTT pathology and the impacts of MeCP2 on brain structure development when conducted in mouse models with Mecp2 mutations. MRI studies of RTT patients have revealed volume reductions in frontal gray matter, basal ganglia, substantia nigra, midbrain, cerebellum, and brainstem area and preservation of the occipital cortex (Murakami et al., 1992; Reiss et al., 1993). Preferential reduction of the anterior frontal lobe area appears to correlate with clinical severity in patients (Carter et al., 2008). Another report of a study in which MRI was performed for children with RTT revealed decreased volumes of the cerebellum, whereas cerebral cortical volumes and subcortical gray matter volumes were preserved in the children (Shiohama et al., 2019). In addition, MRI studies using a mouse model for RTT such as Mecp2-null KO mice showed volume loss in many of the same areas as humans, suggesting that these models reproduce the human phenotypic gross anatomy (Saywell et al., 2006; Ward et al., 2009). However, these studies using RTT mouse models limited their analyses to certain areas and detailed volume changes throughout the Mecp2-deficient brain have not been fully investigated.
In this study, we performed a detailed whole-brain anatomical analysis of RTT mouse models using T2-weighted 3D MRI with different morphometric analysis processes. We identified Mecp2-deficient specific changes in brain structure and laterality, which are associated with the phenotypes of RTT patients and mouse models. Our study provides a framework for neuroanatomical studies of RTT mouse models.
Materials and Methods
Experimental Animals
All aspects of animal care and treatment were performed according to the guidelines of the Experimental Animal Care Committee of Nagoya University. Mecp2-KO mice (Mecp2tm1.1Jae) were generated by deleting exon 3, containing the methyl-DNA-binding domain of Mecp2 (Chen et al., 2001), and they were obtained from Jackson Laboratories. All mice used in this study, both mutant and wild-type (WT) littermates were bred from wild-type C57BL/6J males and Mecp2tm1.1Jae heterozygous females. All mice were housed as 2–5 animals per cage and maintained on a 12-h light/dark cycle with water and food available ad libitum. Mecp2-KO male mice at the age of 6 weeks and their WT littermates were used in this study (n = 4 WT males, 4 Mecp2-KO males).
Genotyping
After weaning, mouse genomic DNA was extracted from the tip of the tail using phenol-chloroform DNA extraction, and a polymerase chain reaction strategy was used to distinguish WT from mutant alleles using standard methodologies.
Tissue Preparation for Magnetic Resonance Imaging
The mice were initially anesthetized with an intraperitoneal injection of a mixture of medetomidine, midazolam, and butorphanol, and then intracardially perfused as described previously (Cahill et al., 2012). Briefly, following transcardial perfusion with phosphate-buffered saline and 4% paraformaldehyde, the heads of the mice were decapitated, and their skin and lower jaw were removed accordingly.
Magnetic Resonance Imaging Acquisition and Processing
Magnetic Resonance Imaging Acquisition
The MRI acquisition method has been described previously (Yano et al., 2018; Abe et al., 2019). The brains were immersed in 0.2 mM gadolinium containing PBS for 1 week. The brains were firmly fixed in an acrylic tube filled with Fluorinert (Sumitomo 3M Limited, Tokyo, Japan) to minimize the signal intensity attributable to the medium surrounding the specimen. Ex vivo MRI of mouse brains was performed with a 7 T Biospec 70/16 MRI scanner (Bruker Biospin GmbH, Ettlingen, Germany) equipped with actively shielded gradients at a maximum strength of 700 mT/m and a transmitting/receiving volume coil with an inner diameter of 22 mm. High-resolution anatomical images of the whole brain were acquired using a rapid acquisition with relaxation enhancement (RARE) sequence with the following parameters: effective echo time (eTE) = 20 ms, repetition time (TR) = 350 ms, RARE factor = 4, number of averages = 12, spatial resolution = 75 × 75 × 75 (μm)3, scan time 8 h 19 min 48 s.
Atlas Registration and Quantifying Anatomical Regions
Processing pipeline 1: The acquired structural T2-weighted images were registered to atlas coordinates (Lein et al., 2007; Oh et al., 2014; Hikishima et al., 2017) using the script ‘‘antsRegistrationSyN.sh’’ in Advanced Normalization Tools (ANTs) open-source software.1 Each brain label (575 regions in total) (Komaki et al., 2016) was obtained by applying an inverse transformation based on the registration information from the atlas coordinates to the native coordinates of the individual data (Uematsu et al., 2017; Takata et al., 2021). The individual label volume, which was automatically segmented by the ANT pipeline, was measured using the ITK-SNAP (Yushkevich et al., 2006; Seki et al., 2017).
Processing pipeline 2: The acquired structural T2-weighted images were analyzed using the Atlas Normalization Toolbox using elastiX version 2 (ANTx2) (Lein et al., 2007; Hubner et al., 2017; Koch et al., 2019)2 running in MATLAB (MathWorks, Natick, MA, United States) toolbox for image registration of mouse MRI data. Through the ANTx2 pipeline, MR images were processed using SPM123 and non-linear warping of tissue probability maps in ELASTIX (Klein et al., 2010),4 and these were registered in the Allen Mouse Atlas 2017 (CCFv3) (Lein et al., 2007; Hikishima et al., 2017; Hubner et al., 2017). After checking the visual inspection of atlas registration, the estimated volumes for each anatomical region in the native space were individually calculated for each mouse.
Evaluation of Structural Hemispheric Asymmetry
The laterality index (LI) was calculated for each mouse as [VL − VR]/[VL + VR] × 100, as described previously (Springer et al., 1999). VL and VR were the volumes for the left and right hemispheres, respectively. LIs were subsequently classified as left hemisphere dominant (LI > 20), symmetric (−20 ≤ LI ≤ +20), or right hemisphere dominant (LI < −20).
Ethics Statement
All animal experiments were conducted under protocols approved by the Animal Experimental Committee and the recombinant DNA experiment committee of Nagoya University.
Statistical Analysis
Statistical analysis was performed using Prism 7 (GraphPad Software, San Diego, CA, United States) and IBM SPSS statistics (IBM, Armonk, NY, United States). All data are presented as mean ± standard error of the mean (SEM). Differences between groups were examined for statistical significance using the student’s t-test, Welch’s t-test or Mann-Whitney test, followed by Bonferroni correction or false discovery rate (FDR) adjustment for multiple comparisons. Statistical significance was set at a p-value of < 0.05.
Results
Mecp2-Null Mice Show Reduced Body Weight and Whole-Brain Volume
To investigate the effect of loss of the Mecp2 gene in the brain, we performed a T2-weighted MRI scan on hemizygous and matched WT controls (Figures 1A,B). There was a significant overall difference in body weight and whole-brain volume between Mecp2-KO mice and WT mice (Figures 1C,D and Table 1). Mecp2-KO mice weighted 49% as much as their WT littermates (WT 17.41 ± 0.32 g, Mecp2-KO 8.54 ± 1.30 g), and they had smaller whole-brain volumes (WT 338.62 ± 13.58 mm3, Mecp2-KO 260.82 ± 13.18 mm3).
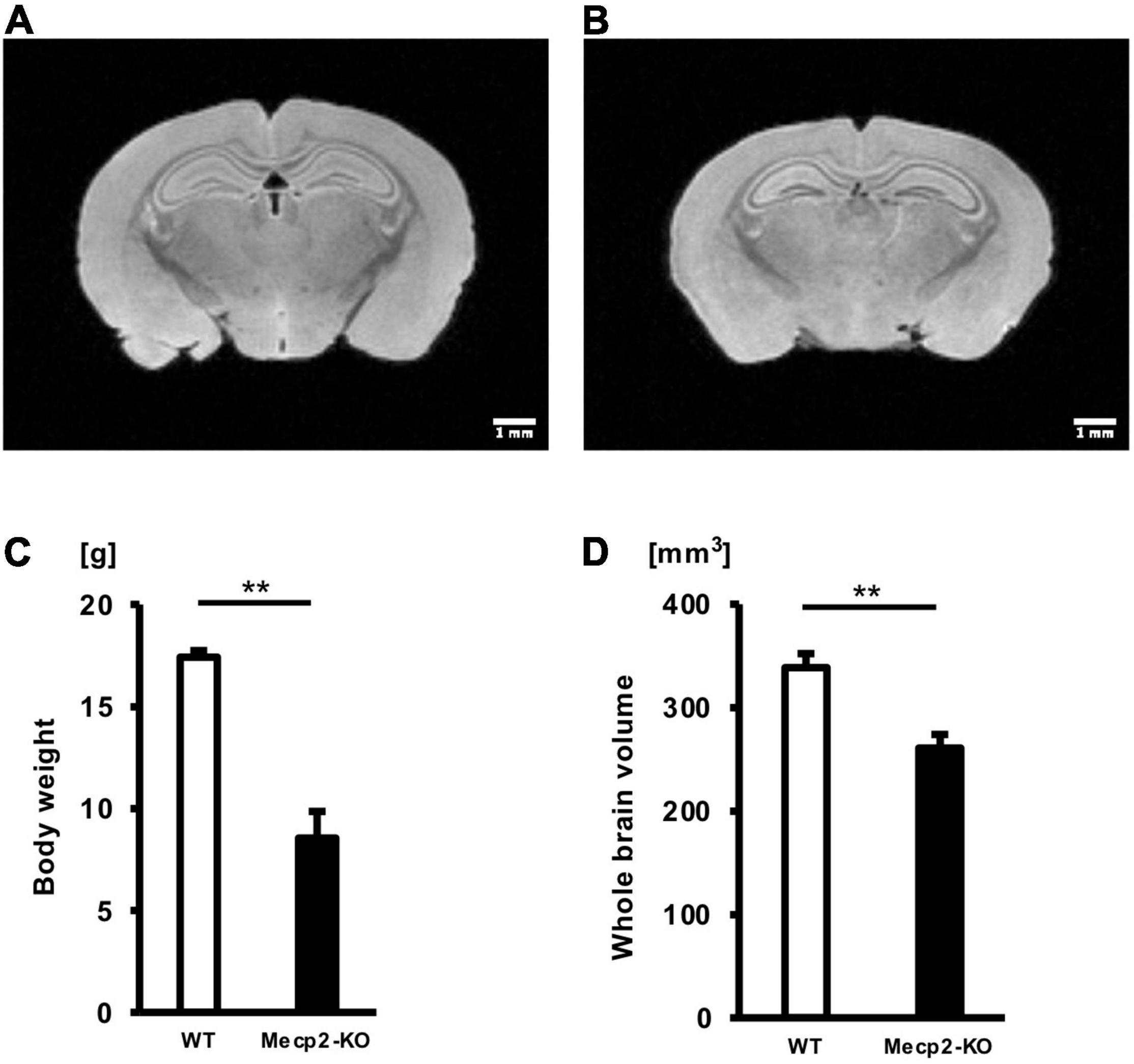
Figure 1. Mean body weights and whole-brain volumes for Mecp2-KO mice and WT mice. T2-weighted images of WT mice (A) and Mecp2-KO mice (B) are shown. Loss of Mecp2 leads to a drastic decrease in body weight (g) (C) and whole-brain volume (mm3) (D). Statistical test: Welch’s t-test. n = 4 mice per group. **p < 0.01.
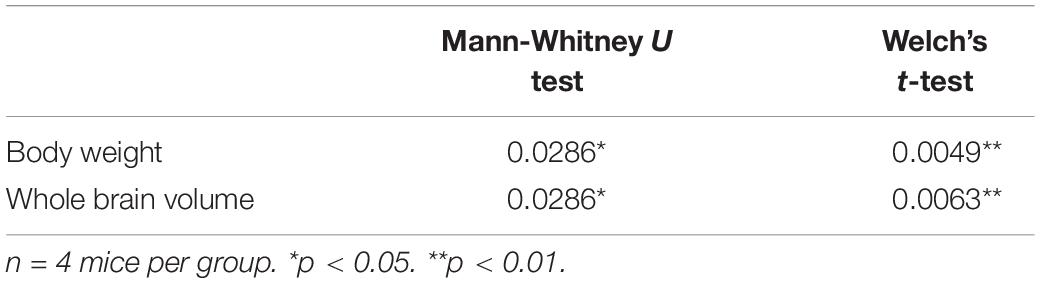
Table 1. Results of each statistical test of Figure 1.
Mecp2-Deficient Brain Exhibits Decreases in Regional Brain Volume
To evaluate potential neuroanatomical brain anomalies in Mecp2-KO mice, we performed an automated regional-based analysis using a mouse brain template (Processing pipeline 1). The analysis revealed that Mecp2-KO mice had volume reductions compared with their WT littermates across many regions of the brain, such as somatosensory area (WT 29.06 ± 0.77, Mecp2-KO 22.16 ± 0.71 mm3), visceral area (WT 1.97 ± 0.04, Mecp2-KO 1.54 ± 0.02 mm3), temporal association area (WT 2.47 ± 0.06, Mecp2-KO 1.84 ± 0.06 mm3), and ectorhinal area (WT 1.44 ± 0.03, Mecp2-KO 1.08 ± 0.01 mm3) (Figure 2, Tables 2, 3, and Supplementary Table 1). To further verify the neuroanatomical alterations in the Mecp2-KO brain, we conducted a more detailed analysis pipeline (Processing pipeline 2) focusing on four regions where significant volume changes were detected by processing pipeline 1. We then found volume reductions in several regions in the Mecp2-KO brain compared with the WT brain (Figures 3A,B, Table 4, and Supplementary Tables 2, 5). The detailed analysis revealed decreases of volume in several somatosensory areas (e.g., primary somatosensory area, lower limb (WT 2.87 ± 0.10, Mecp2-KO 2.09 ± 0.13 mm3), primary somatosensory area, upper limb (WT 4.83 ± 0.15, Mecp2-KO 3.51 ± 0.21 mm3), primary somatosensory area, trunk, layer 4 (WT 0.45 ± 0.02, Mecp2-KO 0.34 ± 0.01 mm3), and primary somatosensory area, unassigned (WT 2.61 ± 0.06, Mecp2-KO 1.89 ± 0.12 mm3) (Figures 3C–F). In addition, significant reductions were also found in ectorhinal area, layer 1 (WT 0.54 ± 0.01, Mecp2-KO 0.41 ± 0.01 mm3) (Figure 3G).
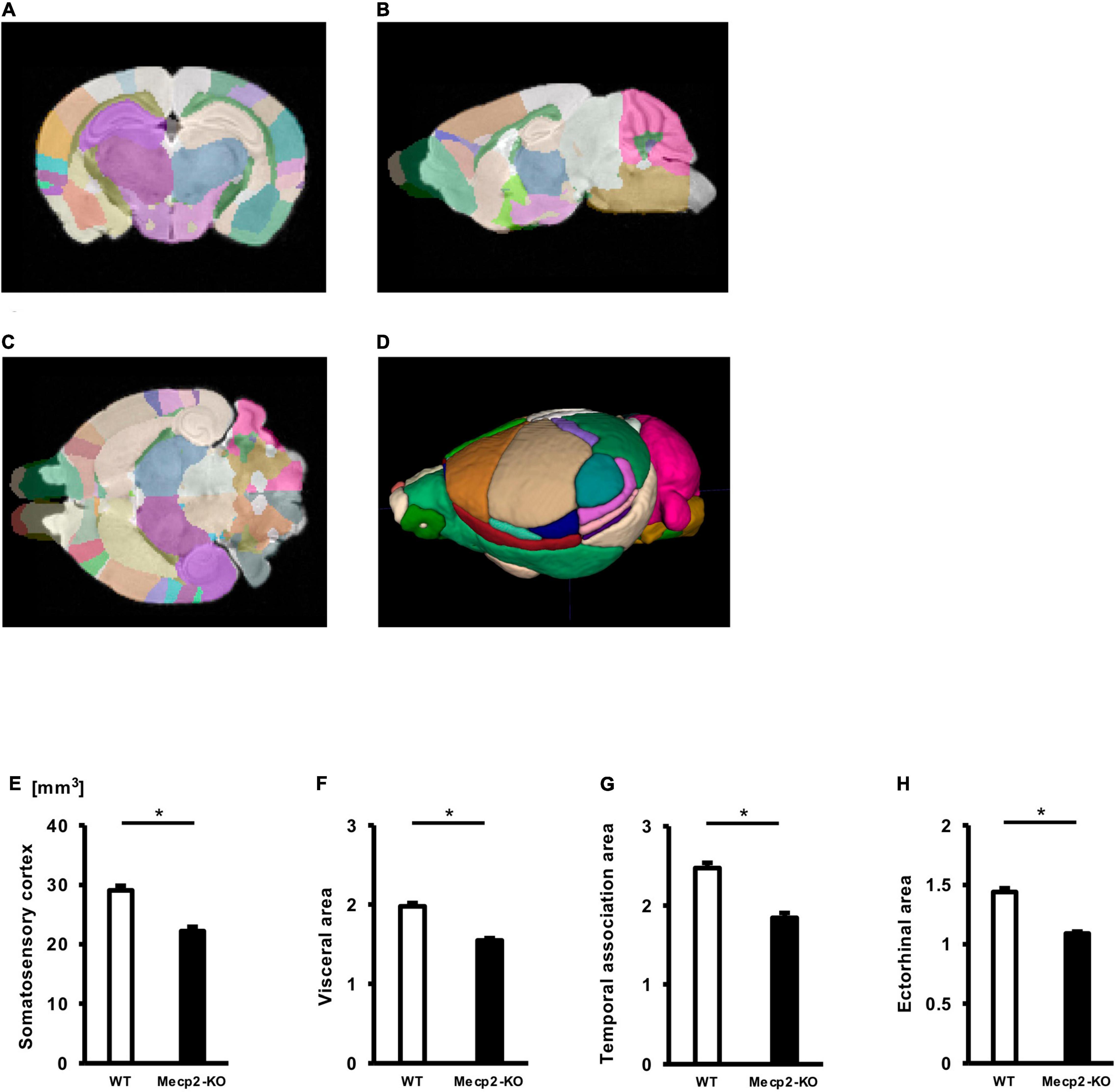
Figure 2. Comparison between brain region volumes of WT and Mecp2-KO mice obtained using volume-based morphometry. Atlas registration and quantification of anatomical regions in WT mice and Mecp2-KO mice were performed using volume-based morphometry (A–D). Several regional brain volumes in Mecp2-KO mice compared with WT mice were significantly decreased, including the somatosensory cortex (E), visceral area (F), temporal association area (G), and ectorhinal area (H). Statistical test: Welch’s t-test. n = 4 mice per group. *Significant difference.
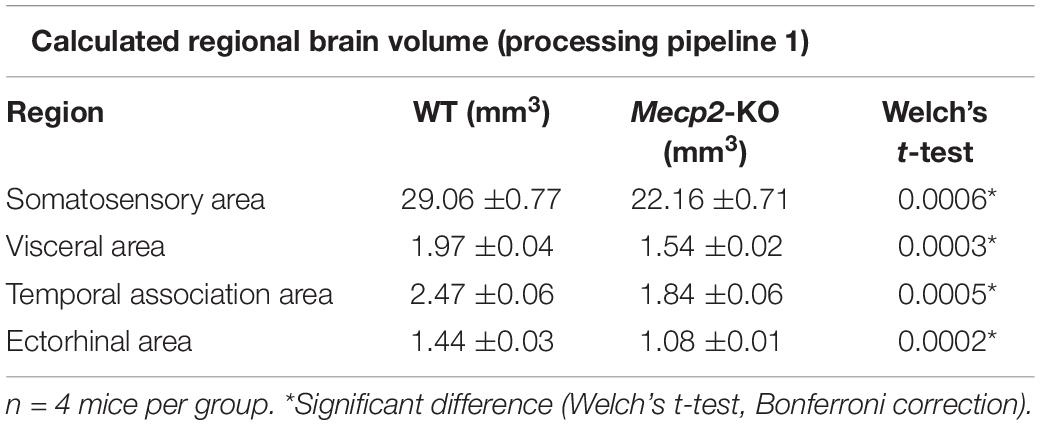
Table 2. List of significantly altered each regional brain volume in Welch’s t-test (Processing pipeline 1).
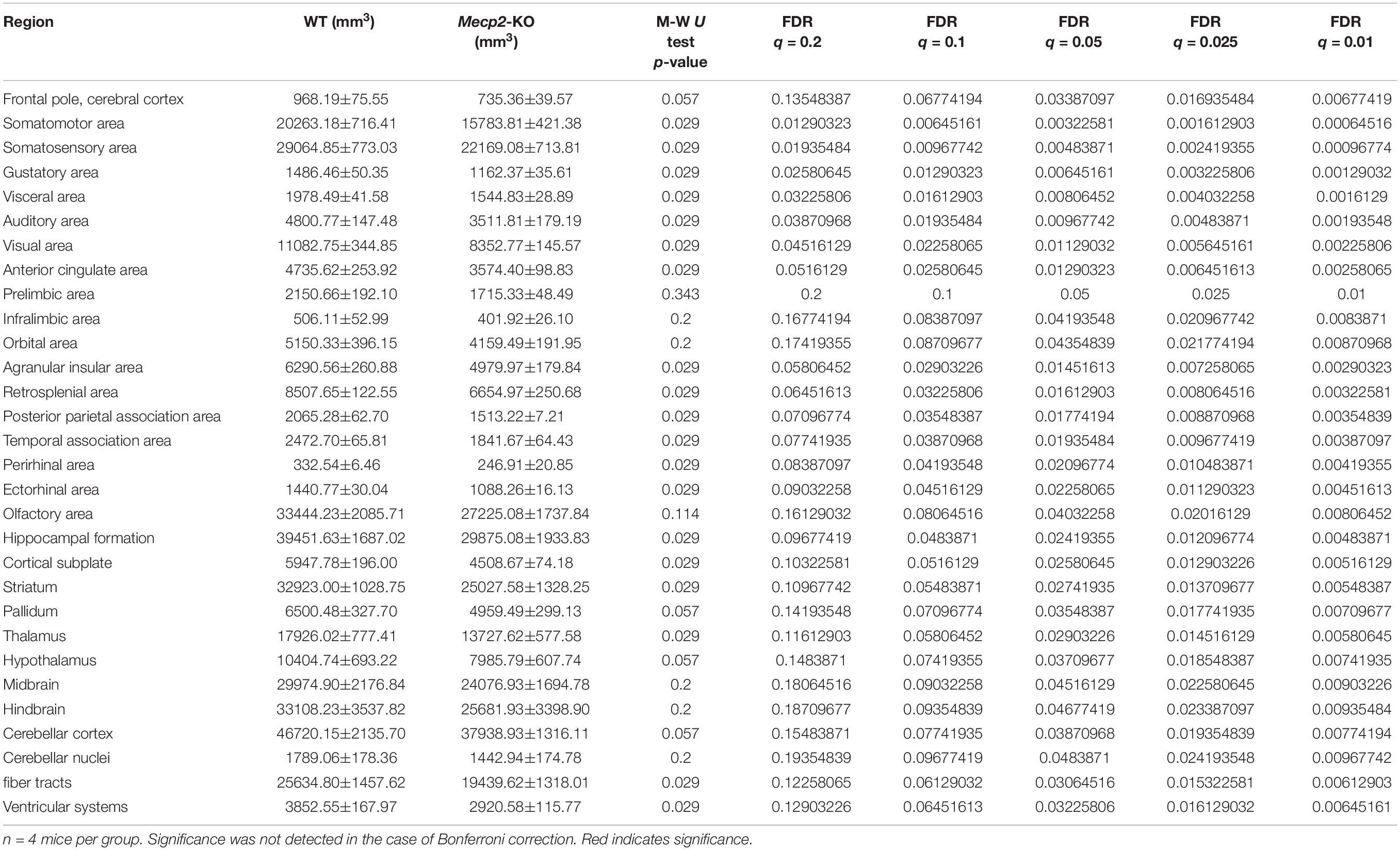
Table 3. Calculated regional brain volume (processing pipeline 1; Mann Whitney U test, FDR adjustment).
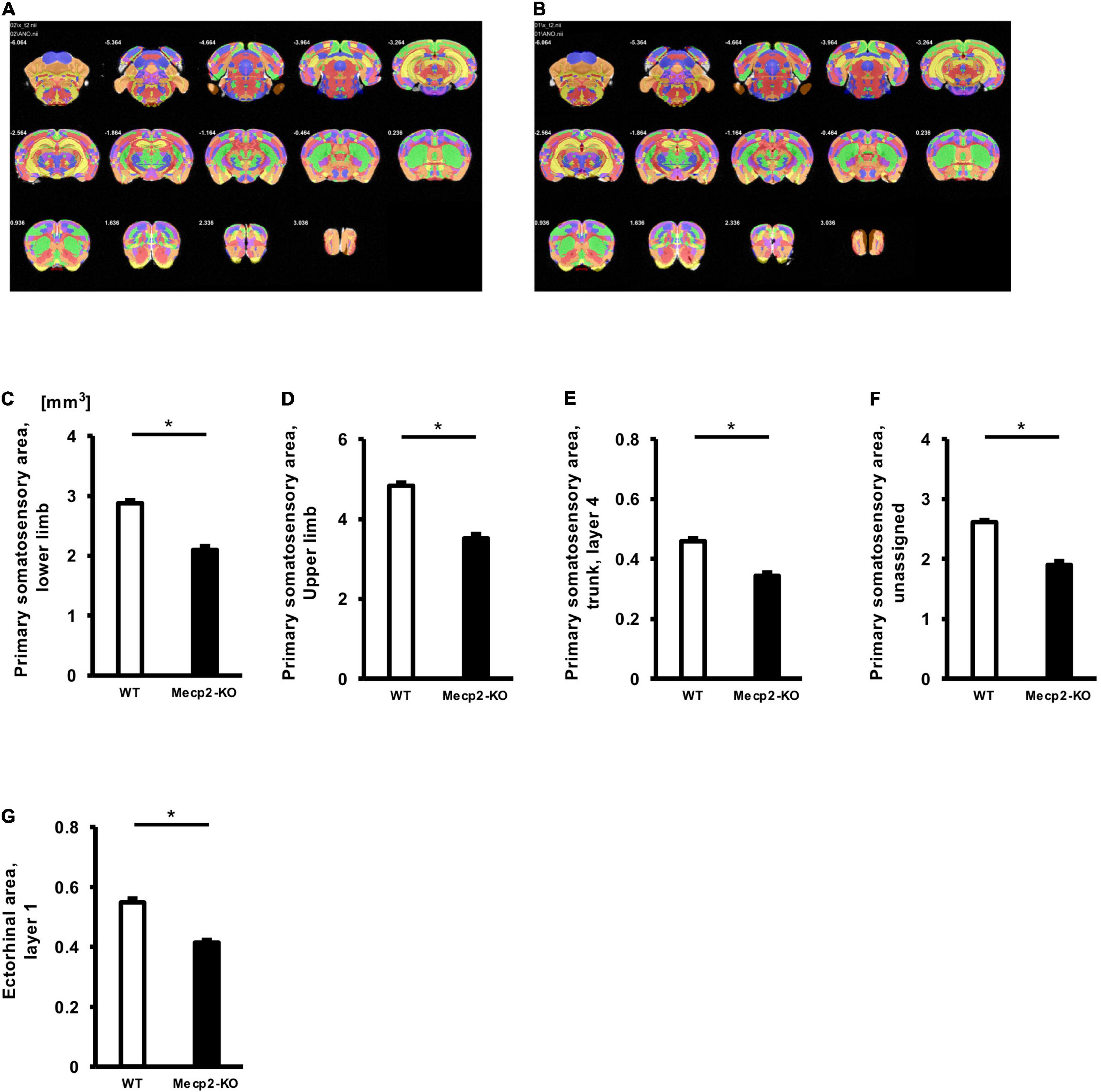
Figure 3. Comparison between brain region volumes of WT and Mecp2-KO mice obtained using detailed volume-based morphometry. Atlas registration and quantification of anatomical regions in WT mice (A) and Mecp2-KO mice (B) were performed using detailed volume-based morphometry. A decrease in volume was detected in brain regions such as the primary somatosensory area, lower limb (E), primary somatosensory area, mouth (C), primary somatosensory area, upper limb (D), primary somatosensory area, trunk, layer 4 (E), primary somatosensory area, unassigned (F), ectorhinal area, layer 1 (G) in Mecp2-KO mice. Statistical test: Welch’s t-test. n = 4 mice per group. *Significant difference.
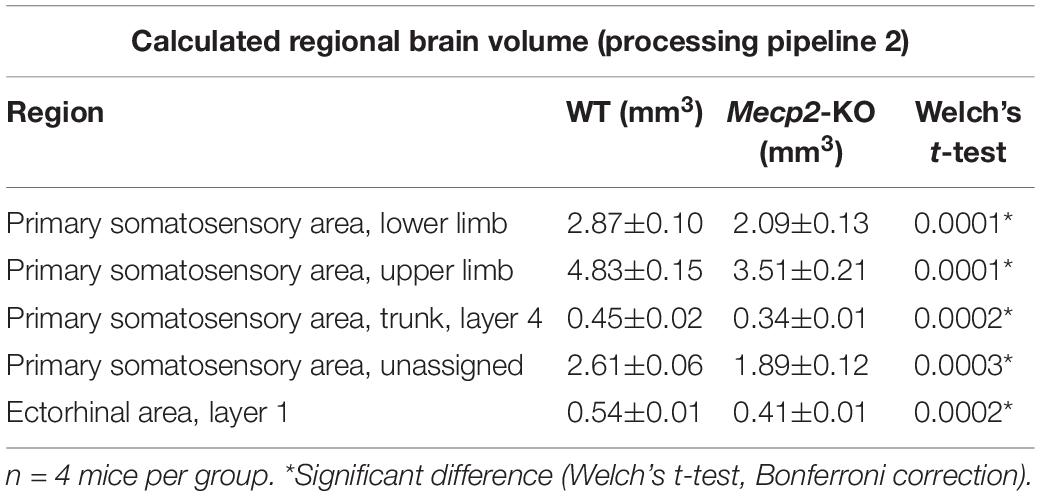
Table 4. List of significantly altered each regional brain volume in Welch’s t-test (Processing pipeline 2).
The Mecp2-Deficient Brain Exhibits Specific Decreases in Regional Brain Volume
To explore brain regions that are specifically altered in Mecp2-deficient brains, we evaluated the ratio of each regional brain volume to the whole-brain volume and focused on brain regions that are involved in the prominent symptom of the behavioral phenotype of RTT. Then we found specific alterations in several regions of the brain of Mecp2-KO mice, such as the bed nucleus of the anterior commissure (WT 0.00269 ± 0.000252, Mecp2-KO 0.00191 ± 0.0000913%), posteromedial visual area (layer 6b) (WT 0.00284 ± 0.000148, Mecp2-KO 0.00241 ± 0.000161%), retrosplenial area (dorsal part, layer 6b) (WT 0.00896 ± 0.000423, Mecp2-KO 0.00709 ± 0.000777%), and field CA2 stratum oriens (WT 0.0278 ± 0.00121, Mecp2-KO 0.0241 ± 0.00115%) (Figure 4 and Table 5). These results suggest that MeCP2 deficiency also contributes to specific changes in the brain structure.
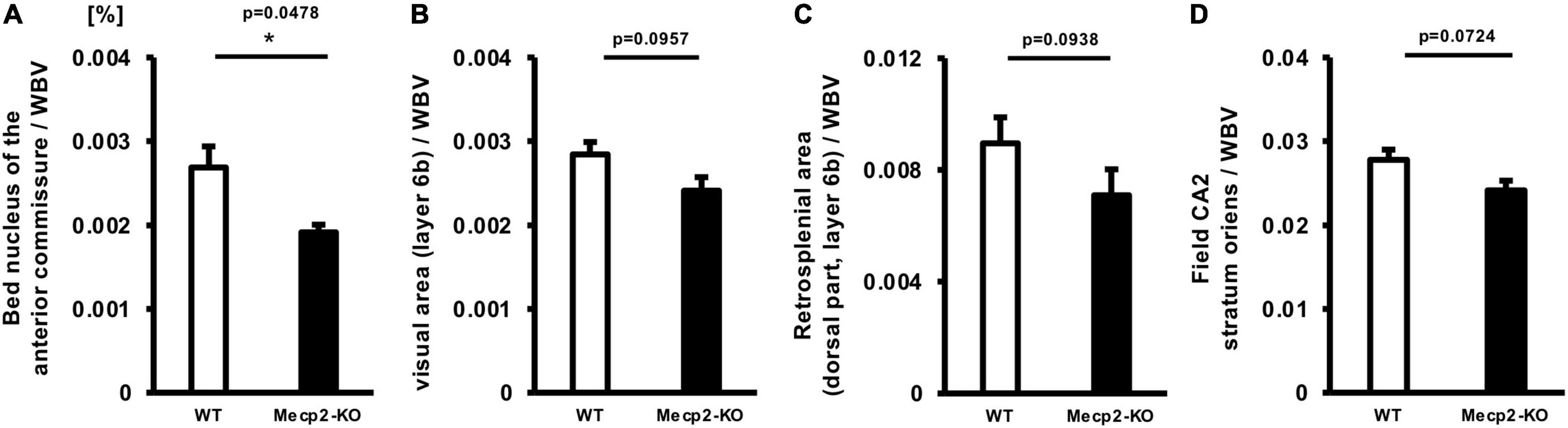
Figure 4. Comparison between normalized brain region volumes of WT and Mecp2-KO mice obtained using detailed volume-based morphometry. The ratio of each regional brain volume to the whole-brain volume was evaluated and specific regional changes, such as the bed nucleus of the anterior commissure (A), posteromedial visual area (layer 6b) (B), retrosplenial area (C), and field CA2 stratum oriens (D) of Mecp2-KO mice were detected. Statistical test: Welch’s t-test. n = 4 mice per group. *Significant difference.
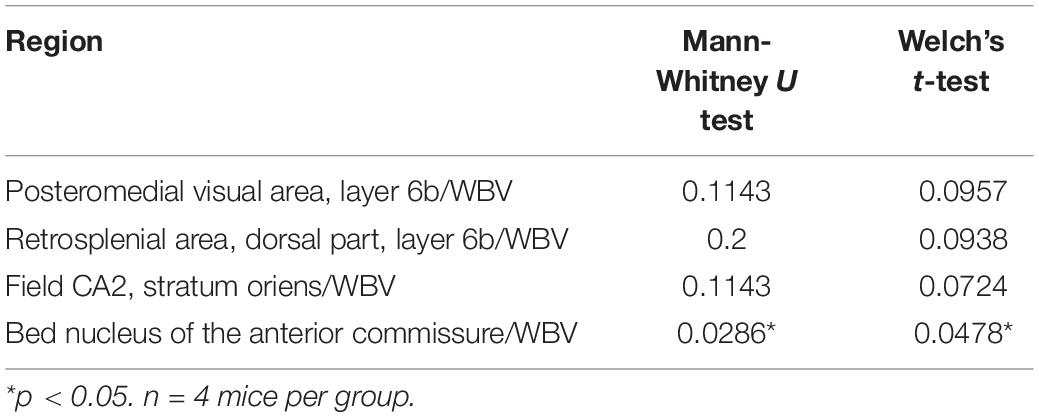
Table 5. Results of each statistical test of Figure 4.
Loss of Mecp2 Affects Hemispheric Asymmetry of Brain Structure
Recent studies have reported that patients with neurodevelopmental and neuropsychiatric diseases show abnormal laterality of brain structures (Ribolsi et al., 2014; Okada et al., 2016; Postema et al., 2019). Since mutations in the MeCP2 gene have been associated with a wide range of neurological disorders, we reasoned that MeCP2 affects structural asymmetry of the brain. We then investigated the laterality of the Mecp2-null brain. We found 69 regions of the left hemisphere dominant in volume in Mecp2-KO mice compared with WT mice, including the dorsal tegmental nucleus (WT LI = −8.75 ± 3.31, Mecp2-KO LI = 39.0 ± 13.12), area postrema (WT LI = 16.8 ± 2.62, Mecp2-KO LI = 61.1 ± 12.81), spinal nucleus of the trigeminal (caudal part) (WT LI = −1.08 ± 0.27, Mecp2-KO LI = 30.6 ± 9.99), and nucleus ambiguous (WT LI = 4.58 ± 0.79, Mecp2-KO LI = 24.0 ± 3.90) (Figures 5A–D and Supplementary Table 3). The analysis also revealed that the right hemisphere was dominant in 20 regions, including the frontal pole (WT LI = 1.18 ± 1.08, Mecp2-KO LI = −22.5 ± 2.01), anterior cingulate area (ventral part, layer 1) (WT LI = −10.7 ± 7.08, Mecp2-KO LI = −28.9 ± 8.80), main olfactory bulb (WT LI = −6.44 ± 5.34, Mecp2-KO LI = −29.2 ± 8.53), and subfornical organ (WT LI = −14.9 ± 3.99, Mecp2-KO LI = −33.4 ± 10.12) (Figures 5E–H and Supplementary Table 4). Together, these findings suggest that MeCP2 dysfunction influences the structural laterality of the brain.
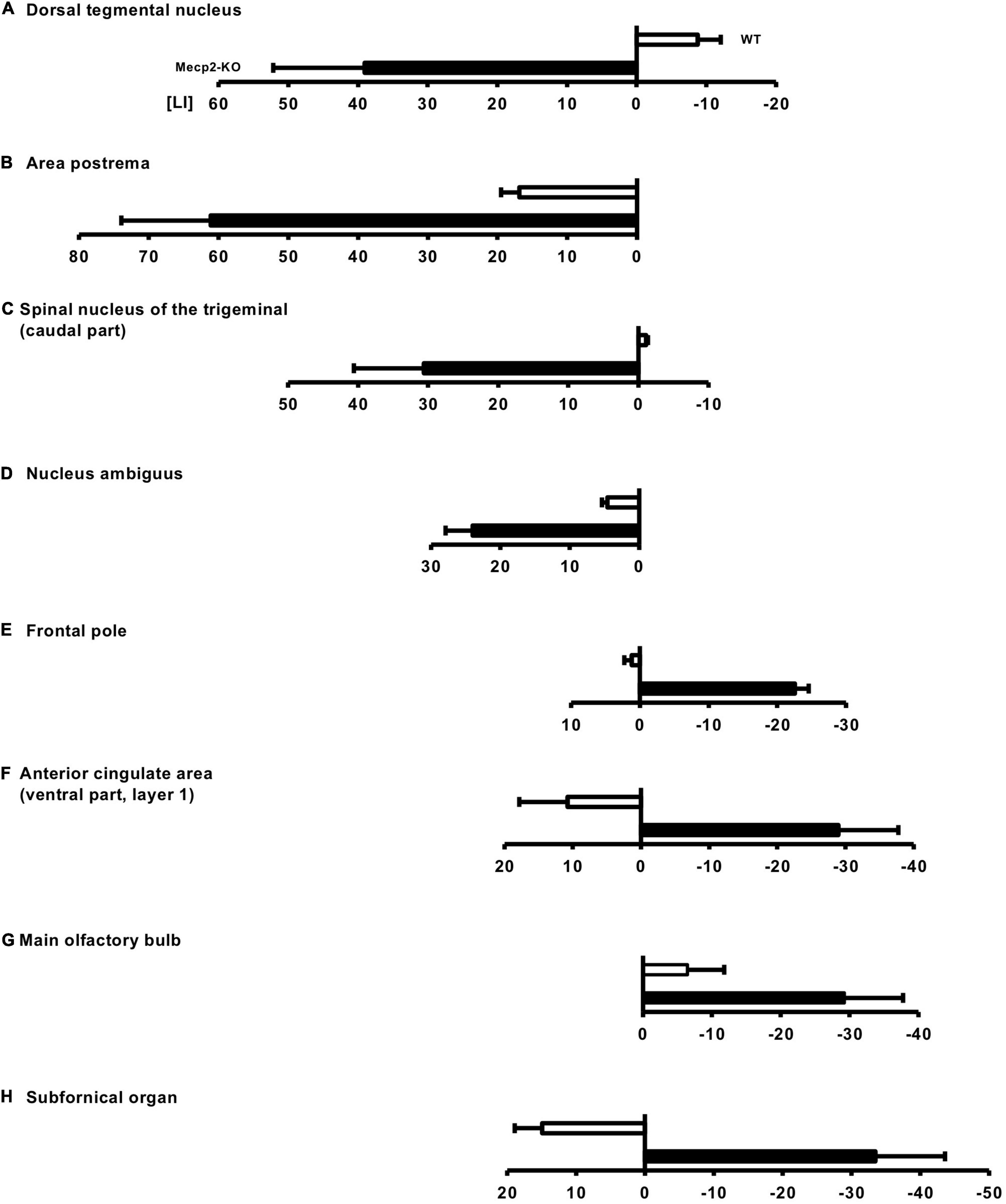
Figure 5. Altered laterality of the regional brain volume in Mecp2-KO mice. LI was calculated to evaluate the laterality of the regional brain volume in Mecp2-KO mice. In total, 69 regions of the left hemisphere dominant were detected in Mecp2-KO mice, including the dorsal tegmental nucleus (A), area postrema (B), spinal nucleus of the trigeminal (caudal part) (C), and nucleus ambiguous (D). Twenty regions of right hemisphere dominance were also detected in Mecp2-KO mice, including the frontal pole (E), anterior cingulate area (ventral part, layer 1) (F), main olfactory bulb (G), and (subfornical organ) (H). n = 4 mice per group.
Discussion
In this study, we analyzed neuroanatomical measurements in T2-weighted 3D MRI of the brain of Mecp2-KO mice using different morphometry methods to identify the structural abnormalities that are associated with the pathophysiology of RTT. Our data showed the global and local volume reduction in the brain of Mecp2-KO mice compared with WT mice, and further analysis revealed the specific volume changes and laterality in several brain regions.
The overall volume reduction of the brain has consistently been found in RTT patients (Murakami et al., 1992; Reiss et al., 1993; Carter et al., 2008) and in mouse models (Saywell et al., 2006; Ward et al., 2009). These gross reductions in the RTT brain may correlate with cellular phenotypes, such as generalized reductions in neuronal soma size and dendritic arborizations (Armstrong et al., 1995). Recent studies suggest that loss of Mecp2 induces abnormal neural stem cell (NSC)/neural progenitor cell (NPC) differentiation (Tsujimura et al., 2009, 2015; Nakashima et al., 2021) and abnormal fate specification of NSC/NPC may affect changes in brain volume. Also, since body weight of Mecp2-KO mice is decrease to about 50% of that of WT, alterations of brain volume are expected to be dependent on their body weight. However, further research on younger stage would be needed to investigate this assumption.
Furthermore, region-specific structural abnormalities that are involved in each diverse RTT phenotype including anxiety and fear have not been clarified till date. In the present study, we revealed specific changes in volume in several RTT brain regions, the bed nucleus of the anterior commissure, the posteromedial visual area (layer 6b), the retrosplenial area (dorsal part, layer 6b), and field CA2 stratum oriens, of Mecp2-KO mice. The ratio of the bed nucleus of the anterior commissure volume to the whole-brain volume was significantly decreased in Mecp2-KO mice brain, and the other three regions also showed a trend toward a decrease when compared with that of the WT brain. The bed nucleus of the anterior commissure is predominantly linked with the medial habenula and it has been implicated in the control of fear responses (Yamaguchi et al., 2013). In a previous study, ablation of the bed nucleus of the anterior commissure projection neurons selectively enhanced fear responses (Yamaguchi et al., 2013). Anxiety/fear behavior is a prominent component of the behavioral phenotype of RTT patients and it has functional consequences (Barnes et al., 2015). Fear behaviors such as “fear in unfamiliar situations” in RTT patients were reported in 46/63 cases (Coleman et al., 1988). The pathophysiology underlying anxiety/fear behavior in RTT patients is unclear and it is expected to be associated with autonomic dysfunction, as well (Robertson et al., 2006). Our data suggest that the volume reduction of bed nucleus of the anterior commissure may be directly involved in these behavioral abnormalities. The posteromedial visual area mediates visual information between the primary visual cortex and the retrosplenial cortex, which further projects to the hippocampus (Wang and Burkhalter, 2007). In humans, the retrosplenial cortex is engaged in spatial navigation and representation of familiar visual environments (Harvey et al., 2009). RTT patients have been reported to show altered visual evoked potentials (LeBlanc et al., 2015). This result indicates that MeCP2-deficiency causes abnormality in visual cortical processing, and alteration of the posteromedial visual area may contribute to visual processing impairment. The field CA2 of the hippocampus, particularly the vasopressin 1b receptor expressed in the hippocampus, is necessary for the regulation of social memory (Smith et al., 2016), and it promotes social aggression depending on CA2 output to the lateral septum, which is selectively enhanced immediately prior to attack (Leroy et al., 2018). RTT mouse models have deficits in long-term social learning and memory in a paradigm that has been shown to be dependent on hippocampal function of the hippocampus (Moretti et al., 2006). Externalizing behaviors, such as impulsivity, hyperactivity, aggression, self-abuse, inconsolable crying, and screaming are less frequently reported in RTT patients (Buchanan et al., 2019). A recent study showed low levels of overactivity, impulsivity, and self-abuse in RTT patients compared with a control group matched for age, sex, language, self-help skills, and intellectual ability (Cianfaglione et al., 2015). Although these facts suggest the involvement of the field CA2 in the hippocampus, Mecp2 conditional knockout mice have also been reported to be aggressive (Fyffe et al., 2008), and several different pathophysiologies may be involved in this behavior. Since almost all of the above brain regions have not been reported as phenotype-associating regions, these findings provide novel insight into the fundamental mechanisms of pathophysiology of RTT and an interesting future study would be to evaluate the changes in the region identified in the present study in RTT patients.
This study also revealed that brain Mecp2-null mice exhibit alterations in structural laterality in several brain regions, such as the anterior cingulate area, subfornical organ, and dorsal tegmental nucleus (DTN). To the best of our knowledge, no studies on the alteration of structural laterality in the RTT brain have been reported so far. It is possible that these changes in laterality are associated with the pathophysiology of RTT and mouse models. A recent study showed that manipulation of the function of DTN neurons by the brainstem-acting drug cloperastine (CPS) improves breathing disorders in Mecp2-disrupted mice (Johnson et al., 2020). It is possible that functional alterations induced by abnormal laterality of DTN may contribute to breathing disorders in Mecp2-disrupted mice. The nucleus ambiguous comprises the motor neurons of the branchiogenic muscles of the 3rd–6th pharyngeal arch (CN IX, X, medullary section of XI). These form a large longitudinal rostrocaudal pars compacta of the original nucleus ambiguus. In addition, preganglionic fibers for parasympathetic innervation of the heart originate from the external part of the nucleus ambiguus and dorsal motor nucleus of the vagus nerve (dmnX) (Zandstra et al., 2021). As mentioned above, patients with RTT encounter breathing disorders. These breathing disturbances may be mechanically related to other disturbances, such as dysphagia (Ramirez et al., 2020). Dysphagia mostly arises from factors influencing chewing, such as abnormal tongue movements, usually caused by lack of movement in the central and frontal areas of the tongue, hypotonia, or hypertonia of the tongue muscles, poor posture of the cervical and thoracic spine, muscular rigidity of the shoulder girdle, hyperextension of the neck, and tongue protrusion (Mezzedimi et al., 2017). RTT patients are also associated with cardiovascular autonomic disturbances that predispose patients to cardiac arrhythmias and sudden death (Kumar et al., 2017). Immaturity of the brainstem and lack of integrative inhibition due to poor parasympathetic development have been suggested as possible explanations for autonomic dysfunction (Julu et al., 2001; Kumar et al., 2017). However, the mechanism of autonomic dysfunction in RTT is not clearly known, and altered neurotrophin signaling, serotonergic dysfunction, and substance P deficiency have been suggested as possible causes for the same (Kumar et al., 2017). We believe that abnormal laterality of the nucleus ambiguus might influence problems related to speech, shortness of breath, swallowing, and autonomic function of the heart. Only a limited number of studies have reported oral findings of Rett syndrome (Bianco and Rota, 2018). Although Rett syndrome is mostly associated with bruxism (Mahdi et al., 2021) and oral manifestations such as mouth breathing, tongue thrusting, digit/thumb sucking, high arch palate, and drooling (Ribeiro et al., 1997; Bianco and Rota, 2018; Mahdi et al., 2021), there are no reports of sensory findings or abnormalities in this area. Our findings showed a marked abnormal laterality in the caudal part (nucleus caudalis) of the spinal trigeminal nucleus, which is associated with orofacial thermal and noxious stimuli. Previously, deficits in pain sensitivity were reported in patients (Zhang et al., 2015). Zhang et al. (2014) reported that MeCP2 plays a crucial role in persistent pain sensation in mice. MeCP2 has been implicated in the early cascade of molecular steps for the initiation of pain states (Downs et al., 2010). This has also been linked to altered responses to noxious stimulation in RTT syndrome (Geranton et al., 2008; Downs et al., 2010). In addition, Zhang et al. (2015) showed that MeCP2 plays an analgesic role in both acute pain transduction and chronic pain formation in mice models by regulating the CREB-miR-132 pathway. In this light, future studies evaluating the structural laterality of the brain of patients would provide novel insights into RTT pathophysiology.
RTT was originally thought to occur exclusively in females, however, MECP2 mutations have been identified in males presenting with classic RTT. MECP2 mutations that cause classic RTT in females typically lead to neonatal encephalopathy and death in the first year of life in male and some of these mutations have been reported in males with classic RTT and a normal karyotype (Chahrour and Zoghbi, 2007), suggesting that MECP2 mutations in males lead to more severe effects due to absence of normal MECP2 and MECP2 mutations in females exhibit moderate phenotypes compared with the case of males. Although the present our study using male hemizygote has limitations to elucidate pathology of RTT in females, the findings of this study examining the effects of MECP2 loss on brain structure would contribute to our understanding of the brain structural pathology of female RTT. Moreover, it is very important to investigate the correlation between the severity of the symptoms and the changes in morphology for deeper understanding of the impact of MECP2 mutation. Therefore, the future study addressing this important the issue using heterozygote female mice and other mice lines that show more severe phenotype such as a mice line generated by Bird is needed.
The mechanisms of RTT pathophysiology are still unclear, and the downstream factors of MeCP2 that are involved in the changes in brain volume have not been identified. Recent studies have shown that MeCP2 specifically promotes the processing of a subset of microRNAs (miRNAs), and miR-199a has been identified as a MeCP2-target miRNA associated with RTT pathophysiology (Tsujimura et al., 2015; Nakashima et al., 2021). These studies have also reported that genetic reduction of miR-199a in mice reproduces the phenotypes of RTT mouse models, such as microcephaly, short life, and abnormal differentiation of neural stem cells. Therefore, further research examining the global and local neuroanatomy of miR-199a-deficient mice using MRI would be an important and interesting work.
In addition, although Mecp2-KO mice used in this study are considered to bear a complete null mutation, they actually express a small segment of MeCP2 protein. Therefore, the possibility that the small segment of MeCP2 may affect brain structural phenotype cannot be fully ruled out, and it is needed to be addressed in future study.
In conclusion, our study performed a comprehensive volumetric analysis of Mecp2-null mice brain by T2-weighted 3D MRI and revealed the detailed neuroanatomical alterations and changes in structural laterality of Mecp2-deficient brains. These findings provide novel insights into regional alterations for RTT.
Data Availability Statement
The original contributions presented in the study are included in the article/Supplementary Material, further inquiries can be directed to the corresponding author.
Ethics Statement
The animal study was reviewed and approved by the Experimental Animal Care Committee of Nagoya University.
Author Contributions
YA and KT performed the experiments and sample preparation for the MRI. YA, TSh, YK, FS, ET, and KT analyzed the data. YA, TSh, YK, DS, WU, KK, KS, SA, ST, TSu, JN, AO, ET, and KT wrote the manuscript. KT designed and supervised the entire project. All authors contributed to the article and approved the submitted version.
Funding
This research was funded by the Japan Agency for Medical Research and Development (AMED) (JP21ek0109411 and JP21ek0109498), Japan Society for the Promotion of Science (JSPS) KAKENHI (JP21K06834, JP19H05211, and JP18K06484), a grant from Kawano Masanori Memorial Public Interest Incorporated Foundation for Promotion of Pediatrics (29-11), and NPO Rett syndrome support organization grant (2019-01-09, 2020-01-27). This work was supported by the “MRI platform” as a Program for Promoting Public Utilization of Advanced Research Infrastructure of the Ministry of Education, Culture, Sports, Science, and Technology (MEXT), Japan. This work was also supported by JSPS KAKENHI Grant No. JP16H06280, Grant-in-Aid for Scientific Research on Innovative Areas – Platforms for Advanced Technologies and Research Resources “Advanced Bioimaging Support.”
Conflict of Interest
The authors declare that the research was conducted in the absence of any commercial or financial relationships that could be construed as a potential conflict of interest.
Publisher’s Note
All claims expressed in this article are solely those of the authors and do not necessarily represent those of their affiliated organizations, or those of the publisher, the editors and the reviewers. Any product that may be evaluated in this article, or claim that may be made by its manufacturer, is not guaranteed or endorsed by the publisher.
Acknowledgments
We thank all members of the Group of Brain Function and Development, Nagoya University Neuroscience Institute Graduate School of Science. We appreciate the technical assistance provided by the Animal Center at Nagoya University.
Supplementary Material
The Supplementary Material for this article can be found online at: https://www.frontiersin.org/articles/10.3389/fnins.2022.885335/full#supplementary-material
Footnotes
- ^ http://stnava.github.io/ANTs/
- ^ https://github.com/ChariteExpMri/antx2
- ^ https://www.fil.ion.ucl.ac.uk/spm/software/spm12
- ^ https://elastix.lumc.nl
References
Abe, Y., Komaki, Y., Seki, F., Shibata, S., Okano, H., and Tanaka, K. F. (2019). Correlative study using structural MRI and super-resolution microscopy to detect structural alterations induced by long-term optogenetic stimulation of striatal medium spiny neurons. Neurochem. Int. 125, 163–174. doi: 10.1016/j.neuint.2019.02.017
Amir, R. E., Van den Veyver, I. B., Wan, M., Tran, C. Q., Francke, U., and Zoghbi, H. Y. (1999). Rett syndrome is caused by mutations in X-linked MECP2, encoding methyl-CpG-binding protein 2. Nat. Genet. 23, 185–188. doi: 10.1038/13810
Armstrong, D., Dunn, J. K., Antalffy, B., and Trivedi, R. (1995). Selective dendritic alterations in the cortex of Rett syndrome. J. Neuropathol. Exp. Neurol. 54, 195–201. doi: 10.1097/00005072-199503000-00006
Armstrong, D. D. (2005). Neuropathology of Rett syndrome. J. Child Neurol. 20, 747–753. doi: 10.1177/08830738050200090901
Barnes, K. V., Coughlin, F. R., O’Leary, H. M., Bruck, N., Bazin, G. A., Beinecke, E. B., et al. (2015). Anxiety-like behavior in Rett syndrome: characteristics and assessment by anxiety scales. J. Neurodev. Disord. 7:30. doi: 10.1186/s11689-015-9127-4
Bauman, M. L., Kemper, T. L., and Arin, D. M. (1995). Pervasive neuroanatomic abnormalities of the brain in three cases of Rett’s syndrome. Neurology 45, 1581–1586. doi: 10.1212/wnl.45.8.1581
Belichenko, P. V., Oldfors, A., Hagberg, B., and Dahlstrom, A. (1994). Rett syndrome: 3-D confocal microscopy of cortical pyramidal dendrites and afferents. Neuroreport 5, 1509–1513. doi: 10.1097/00001756-199407000-00025
Bianco, E., and Rota, D. (2018). Oral findings in Rett syndrome: an update and review of the literature. Dent. Med. Probl. 55, 441–445. doi: 10.17219/dmp/99203
Buchanan, C. B., Stallworth, J. L., Scott, A. E., Glaze, D. G., Lane, J. B., Skinner, S. A., et al. (2019). Behavioral profiles in Rett syndrome: data from the natural history study. Brain Dev. 41, 123–134. doi: 10.1016/j.braindev.2018.08.008
Cahill, L. S., Laliberte, C. L., Ellegood, J., Spring, S., Gleave, J. A., Eede, M. C., et al. (2012). Preparation of fixed mouse brains for MRI. Neuroimage 60, 933–939. doi: 10.1016/j.neuroimage.2012.01.100
Carter, J. C., Lanham, D. C., Pham, D., Bibat, G., Naidu, S., and Kaufmann, W. E. (2008). Selective cerebral volume reduction in Rett syndrome: a multiple-approach MR imaging study. AJNR Am. J. Neuroradiol. 29, 436–441. doi: 10.3174/ajnr.A0857
Chahrour, M., and Zoghbi, H. Y. (2007). The story of Rett syndrome: from clinic to neurobiology. Neuron 56, 422–437. doi: 10.1016/j.neuron.2007.10.001
Chen, R. Z., Akbarian, S., Tudor, M., and Jaenisch, R. (2001). Deficiency of methyl-CpG binding protein-2 in CNS neurons results in a Rett-like phenotype in mice. Nat. Genet. 27, 327–331. doi: 10.1038/85906
Cianfaglione, R., Clarke, A., Kerr, M., Hastings, R. P., Oliver, C., Moss, J., et al. (2015). A national survey of Rett syndrome: behavioural characteristics. J. Neurodev. Disord. 7:11. doi: 10.1186/s11689-015-9104-y
Coleman, M., Brubaker, J., Hunter, K., and Smith, G. (1988). Rett syndrome: a survey of North American patients. J. Ment. Defic. Res. 32(Pt 2), 117–124. doi: 10.1111/j.1365-2788.1988.tb01397.x
Downs, J., Geranton, S. M., Bebbington, A., Jacoby, P., Bahi-Buisson, N., Ravine, D., et al. (2010). Linking MECP2 and pain sensitivity: the example of Rett syndrome. Am. J. Med. Genet. A 152A, 1197–1205. doi: 10.1002/ajmg.a.33314
Fyffe, S. L., Neul, J. L., Samaco, R. C., Chao, H. T., Ben-Shachar, S., Moretti, P., et al. (2008). Deletion of Mecp2 in Sim1-expressing neurons reveals a critical role for MeCP2 in feeding behavior, aggression, and the response to stress. Neuron 59, 947–958. doi: 10.1016/j.neuron.2008.07.030
Geranton, S. M., Fratto, V., Tochiki, K. K., and Hunt, S. P. (2008). Descending serotonergic controls regulate inflammation-induced mechanical sensitivity and methyl-CpG-binding protein 2 phosphorylation in the rat superficial dorsal horn. Mol. Pain 4:35. doi: 10.1186/1744-8069-4-35
Guy, J., Hendrich, B., Holmes, M., Martin, J. E., and Bird, A. (2001). A mouse Mecp2-null mutation causes neurological symptoms that mimic Rett syndrome. Na. Genet. 27, 322–326. doi: 10.1038/85899
Harvey, C. D., Collman, F., Dombeck, D. A., and Tank, D. W. (2009). Intracellular dynamics of hippocampal place cells during virtual navigation. Nature 461, 941–946. doi: 10.1038/nature08499
Hikishima, K., Komaki, Y., Seki, F., Ohnishi, Y., Okano, H. J., and Okano, H. (2017). In vivo microscopic voxel-based morphometry with a brain template to characterize strain-specific structures in the mouse brain. Sci. Rep. 7:85. doi: 10.1038/s41598-017-00148-1
Hubner, N. S., Mechling, A. E., Lee, H. L., Reisert, M., Bienert, T., Hennig, J., et al. (2017). The connectomics of brain demyelination: functional and structural patterns in the cuprizone mouse model. Neuroimage 146, 1–18. doi: 10.1016/j.neuroimage.2016.11.008
Jellinger, K., Armstrong, D., Zoghbi, H. Y., and Percy, A. K. (1988). Neuropathology of Rett syndrome. Acta Neuropathol. 76, 142–158. doi: 10.1007/BF00688098
Johnson, C. M., Cui, N., Xing, H., Wu, Y., and Jiang, C. (2020). The antitussive cloperastine improves breathing abnormalities in a Rett Syndrome mouse model by blocking presynaptic GIRK channels and enhancing GABA release. Neuropharmacology 176:108214. doi: 10.1016/j.neuropharm.2020.108214
Julu, P. O., Kerr, A. M., Apartopoulos, F., Al-Rawas, S., Engerstrom, I. W., Engerstrom, L., et al. (2001). Characterisation of breathing and associated central autonomic dysfunction in the Rett disorder. Arch. Dis. Child 85, 29–37. doi: 10.1136/adc.85.1.29
Kaufmann, W. E., MacDonald, S. M., and Altamura, C. R. (2000). Dendritic cytoskeletal protein expression in mental retardation: an immunohistochemical study of the neocortex in Rett syndrome. Cereb. Cortex 10, 992–1004. doi: 10.1093/cercor/10.10.992
Klein, S., Staring, M., Murphy, K., Viergever, M. A., and Pluim, J. P. (2010). elastix: a toolbox for intensity-based medical image registration. IEEE Trans. Med. Imaging 29, 196–205. doi: 10.1109/TMI.2009.2035616
Koch, S., Mueller, S., Foddis, M., Bienert, T., von Elverfeldt, D., Knab, F., et al. (2019). Atlas registration for edema-corrected MRI lesion volume in mouse stroke models. J. Cereb. Blood Flow Metab. 39, 313–323. doi: 10.1177/0271678X17726635
Komaki, Y., Hikishima, K., Shibata, S., Konomi, T., Seki, F., Yamada, M., et al. (2016). Functional brain mapping using specific sensory-circuit stimulation and a theoretical graph network analysis in mice with neuropathic allodynia. Sci. Rep. 6:37802. doi: 10.1038/srep37802
Kumar, A., Jaryal, A., Gulati, S., Chakrabarty, B., Singh, A., Deepak, K. K., et al. (2017). Cardiovascular autonomic dysfunction in children and adolescents with Rett Syndrome. Pediatr. Neurol. 70, 61–66. doi: 10.1016/j.pediatrneurol.2017.01.010
Laurvick, C. L., de Klerk, N., Bower, C., Christodoulou, J., Ravine, D., Ellaway, C., et al. (2006). Rett syndrome in Australia: a review of the epidemiology. J. Pediatr. 148, 347–352. doi: 10.1016/j.jpeds.2005.10.037
LeBlanc, J. J., DeGregorio, G., Centofante, E., Vogel-Farley, V. K., Barnes, K., Kaufmann, W. E., et al. (2015). Visual evoked potentials detect cortical processing deficits in Rett syndrome. Ann. Neurol. 78, 775–786. doi: 10.1002/ana.24513
Lein, E. S., Hawrylycz, M. J., Ao, N., Ayres, M., Bensinger, A., Bernard, A., et al. (2007). Genome-wide atlas of gene expression in the adult mouse brain. Nature 445, 168–176. doi: 10.1038/nature05453
Leroy, F., Park, J., Asok, A., Brann, D. H., Meira, T., Boyle, L. M., et al. (2018). A circuit from hippocampal CA2 to lateral septum disinhibits social aggression. Nature 564, 213–218. doi: 10.1038/s41586-018-0772-0
Mahdi, S. S., Jafri, H. A., Allana, R., Amenta, F., Khawaja, M., and Qasim, S. S. B. (2021). Oral manifestations of Rett syndrome-A systematic review. Int. J. Environ. Res. Public Health 18:1162. doi: 10.3390/ijerph18031162
Mezzedimi, C., Livi, W., De Felice, C., and Cocca, S. (2017). Dysphagia in Rett syndrome: a descriptive study. Ann. Otol. Rhinol. Laryngol. 126, 640–645. doi: 10.1177/0003489417723033
Moretti, P., Levenson, J. M., Battaglia, F., Atkinson, R., Teague, R., Antalffy, B., et al. (2006). Learning and memory and synaptic plasticity are impaired in a mouse model of Rett syndrome. J. Neurosci. 26, 319–327. doi: 10.1523/JNEUROSCI.2623-05.2006
Murakami, J. W., Courchesne, E., Haas, R. H., Press, G. A., and Yeung-Courchesne, R. (1992). Cerebellar and cerebral abnormalities in Rett syndrome: a quantitative MR analysis. AJR Am. J. Roentgenol. 159, 177–183. doi: 10.2214/ajr.159.1.1609693
Nakashima, H., Tsujimura, K., Irie, K., Imamura, T., Trujillo, C. A., Ishizu, M., et al. (2021). MeCP2 controls neural stem cell fate specification through miR-199a-mediated inhibition of BMP-Smad signaling. Cell Rep. 35:109124. doi: 10.1016/j.celrep.2021.109124
Oh, S. W., Harris, J. A., Ng, L., Winslow, B., Cain, N., Mihalas, S., et al. (2014). A mesoscale connectome of the mouse brain. Nature 508, 207–214. doi: 10.1038/nature13186
Okada, N., Fukunaga, M., Yamashita, F., Koshiyama, D., Yamamori, H., Ohi, K., et al. (2016). Abnormal asymmetries in subcortical brain volume in schizophrenia. Mol. Psychiatry 21, 1460–1466. doi: 10.1038/mp.2015.209
Pietri, T., Roman, A. C., Guyon, N., Romano, S. A., Washbourne, P., Moens, C. B., et al. (2013). The first mecp2-null zebrafish model shows altered motor behaviors. Front. Neural Circuits 7:118. doi: 10.3389/fncir.2013.00118
Postema, M. C., van Rooij, D., Anagnostou, E., Arango, C., Auzias, G., Behrmann, M., et al. (2019). Altered structural brain asymmetry in autism spectrum disorder in a study of 54 datasets. Nat. Commun. 10:4958. doi: 10.1038/s41467-019-13005-8
Ramirez, J. M., Karlen-Amarante, M., Wang, J. J., Bush, N. E., Carroll, M. S., Weese-Mayer, D. E., et al. (2020). The pathophysiology of Rett syndrome with a focus on breathing dysfunctions. Physiology 35, 375–390. doi: 10.1152/physiol.00008.2020
Reiss, A. L., Faruque, F., Naidu, S., Abrams, M., Beaty, T., Bryan, R. N., et al. (1993). Neuroanatomy of Rett syndrome: a volumetric imaging study. Ann. Neurol. 34, 227–234. doi: 10.1002/ana.410340220
Ribeiro, R. A., Romano, A. R., Birman, E. G., and Mayer, M. P. (1997). Oral manifestations in Rett syndrome: a study of 17 cases. Pediatr. Dent. 19, 349–352.
Ribolsi, M., Daskalakis, Z. J., Siracusano, A., and Koch, G. (2014). Abnormal asymmetry of brain connectivity in schizophrenia. Front. Hum. Neurosci. 8:1010. doi: 10.3389/fnhum.2014.01010
Robertson, L., Hall, S. E., Jacoby, P., Ellaway, C., de Klerk, N., and Leonard, H. (2006). The association between behavior and genotype in Rett syndrome using the Australian Rett syndrome database. Am. J. Med. Genet. B Neuropsychiatr. Genet. 141B, 177–183. doi: 10.1002/ajmg.b.30270
Sansom, D., Krishnan, V. H., Corbett, J., and Kerr, A. (1993). Emotional and behavioural aspects of Rett syndrome. Dev. Med. Child Neurol. 35, 340–345. doi: 10.1111/j.1469-8749.1993.tb11646.x
Saywell, V., Viola, A., Confort-Gouny, S., Le Fur, Y., Villard, L., and Cozzone, P. J. (2006). Brain magnetic resonance study of Mecp2 deletion effects on anatomy and metabolism. Biochem. Biophys. Res. Commun. 340, 776–783. doi: 10.1016/j.bbrc.2005.12.080
Seki, F., Hikishima, K., Komaki, Y., Hata, J., Uematsu, A., Okahara, N., et al. (2017). Developmental trajectories of macroanatomical structures in common marmoset brain. Neuroscience 364, 143–156. doi: 10.1016/j.neuroscience.2017.09.021
Shahbazian, M., Young, J., Yuva-Paylor, L., Spencer, C., Antalffy, B., Noebels, J., et al. (2002). Mice with truncated MeCP2 recapitulate many Rett syndrome features and display hyperacetylation of histone H3. Neuron 35, 243–254. doi: 10.1016/s0896-6273(02)00768-7
Shiohama, T., Levman, J., and Takahashi, E. (2019). Surface- and voxel-based brain morphologic study in Rett and Rett-like syndrome with MECP2 mutation. Int. J. Dev. Neurosci. 73, 83–88. doi: 10.1016/j.ijdevneu.2019.01.005
Smith, A. S., Williams Avram, S. K., Cymerblit-Sabba, A., Song, J., and Young, W. S. (2016). Targeted activation of the hippocampal CA2 area strongly enhances social memory. Mol. Psychiatry 21, 1137–1144. doi: 10.1038/mp.2015.189
Springer, J. A., Binder, J. R., Hammeke, T. A., Swanson, S. J., Frost, J. A., Bellgowan, P. S., et al. (1999). Language dominance in neurologically normal and epilepsy subjects: a functional MRI study. Brain 122(Pt 11), 2033–2046. doi: 10.1093/brain/122.11.2033
Takata, N., Sato, N., Komaki, Y., Okano, H., and Tanaka, K. F. (2021). Flexible annotation atlas of the mouse brain: combining and dividing brain structures of the Allen Brain Atlas while maintaining anatomical hierarchy. Sci. Rep. 11:6234. doi: 10.1038/s41598-021-85807-0
Tsujimura, K., Abematsu, M., Kohyama, J., Namihira, M., and Nakashima, K. (2009). Neuronal differentiation of neural precursor cells is promoted by the methyl-CpG-binding protein MeCP2. Exp. Neurol. 219, 104–111. doi: 10.1016/j.expneurol.2009.05.001
Tsujimura, K., Irie, K., Nakashima, H., Egashira, Y., Fukao, Y., Fujiwara, M., et al. (2015). miR-199a links MeCP2 with mTOR signaling and its dysregulation leads to Rett syndrome phenotypes. Cell Rep. 12, 1887–1901. doi: 10.1016/j.celrep.2015.08.028
Uematsu, A., Hata, J., Komaki, Y., Seki, F., Yamada, C., Okahara, N., et al. (2017). Mapping orbitofrontal-limbic maturation in non-human primates: a longitudinal magnetic resonance imaging study. Neuroimage 163, 55–67. doi: 10.1016/j.neuroimage.2017.09.028
Veeraragavan, S., Wan, Y. W., Connolly, D. R., Hamilton, S. M., Ward, C. S., Soriano, S., et al. (2016). Loss of MeCP2 in the rat models regression, impaired sociability and transcriptional deficits of Rett syndrome. Hum. Mol. Genet. 25, 3284–3302. doi: 10.1093/hmg/ddw178
Wang, Q., and Burkhalter, A. (2007). Area map of mouse visual cortex. J. Comp. Neurol. 502, 339–357. doi: 10.1002/cne.21286
Ward, B. C., Kolodny, N. H., Nag, N., and Berger-Sweeney, J. E. (2009). Neurochemical changes in a mouse model of Rett syndrome: changes over time and in response to perinatal choline nutritional supplementation. J. Neurochem. 108, 361–371. doi: 10.1111/j.1471-4159.2008.05768.x
Wu, Y. L., and Lo, C. W. (2017). Diverse application of MRI for mouse phenotyping. Birth Defects Res. 109, 758–770. doi: 10.1002/bdr2.1051
Yamaguchi, T., Danjo, T., Pastan, I., Hikida, T., and Nakanishi, S. (2013). Distinct roles of segregated transmission of the septo-habenular pathway in anxiety and fear. Neuron 78, 537–544. doi: 10.1016/j.neuron.2013.02.035
Yano, R., Hata, J., Abe, Y., Seki, F., Yoshida, K., Komaki, Y., et al. (2018). Quantitative temporal changes in DTI values coupled with histological properties in cuprizone-induced demyelination and remyelination. Neurochem. Int. 119, 151–158. doi: 10.1016/j.neuint.2017.10.004
Yushkevich, P. A., Piven, J., Hazlett, H. C., Smith, R. G., Ho, S., Gee, J. C., et al. (2006). User-guided 3D active contour segmentation of anatomical structures: significantly improved efficiency and reliability. Neuroimage 31, 1116–1128. doi: 10.1016/j.neuroimage.2006.01.015
Zandstra, T. E., Notenboom, R. G. E., Wink, J., Kies, P., Vliegen, H. W., Egorova, A. D., et al. (2021). Asymmetry and heterogeneity: part and parcel in cardiac autonomic innervation and function. Front. Physiol. 12:665298. doi: 10.3389/fphys.2021.665298
Zhang, R., Huang, M., Cao, Z., Qi, J., Qiu, Z., and Chiang, L. Y. (2015). MeCP2 plays an analgesic role in pain transmission through regulating CREB / miR-132 pathway. Mol. Pain 11:19. doi: 10.1186/s12990-015-0015-4
Keywords: Rett syndrome, methyl-CpG-binding protein 2, magnetic resonance imaging, brain structure, volumetric analysis, neurodevelopmental disorder
Citation: Akaba Y, Shiohama T, Komaki Y, Seki F, Ortug A, Sawada D, Uchida W, Kamagata K, Shimoji K, Aoki S, Takahashi S, Suzuki T, Natsume J, Takahashi E and Tsujimura K (2022) Comprehensive Volumetric Analysis of Mecp2-Null Mouse Model for Rett Syndrome by T2-Weighted 3D Magnetic Resonance Imaging. Front. Neurosci. 16:885335. doi: 10.3389/fnins.2022.885335
Received: 28 February 2022; Accepted: 20 April 2022;
Published: 10 May 2022.
Edited by:
Simona Lodato, Humanitas University, ItalyReviewed by:
Manxiu Ma, Virginia Tech Carilion, United StatesSteven Andrew Baker, The University of Utah, United States
Daniela Tropea, Trinity College Dublin, Ireland
Copyright © 2022 Akaba, Shiohama, Komaki, Seki, Ortug, Sawada, Uchida, Kamagata, Shimoji, Aoki, Takahashi, Suzuki, Natsume, Takahashi and Tsujimura. This is an open-access article distributed under the terms of the Creative Commons Attribution License (CC BY). The use, distribution or reproduction in other forums is permitted, provided the original author(s) and the copyright owner(s) are credited and that the original publication in this journal is cited, in accordance with accepted academic practice. No use, distribution or reproduction is permitted which does not comply with these terms.
*Correspondence: Keita Tsujimura, dHN1amltdXJhLmtlaXRhLmszQGYubWFpbC5uYWdveWEtdS5hYy5qcA==
†These authors have contributed equally to this work