- 1Institut de Biologie de l’École Normale Supérieure (IBENS), École Normale Supérieure, CNRS, INSERM, Université PSL, Paris, France
- 2Center for Interdisciplinary Research in Biology, Collège de France, CNRS, INSERM, Université PSL, Paris, France
- 3Collège Doctoral, Sorbonne Université, Paris, France
Microglia, the brain resident macrophages, are multifaceted glial cells that belong to the central nervous and immune systems. As part of the immune system, they mediate innate immune responses, regulate brain homeostasis and protect the brain in response to inflammation or injury. At the same time, they can perform a wide array of cellular functions that relate to the normal functioning of the brain. Importantly, microglia are key actors of brain development. Indeed, these early brain invaders originate outside of the central nervous system from yolk sac myeloid progenitors, and migrate into the neural folds during early embryogenesis. Before the generation of oligodendrocytes and astrocytes, microglia thus occupy a unique position, constituting the main glial population during early development and participating in a wide array of embryonic and postnatal processes. During this developmental time window, microglia display remarkable features, being highly heterogeneous in time, space, morphology and transcriptional states. Although tremendous progress has been made in our understanding of their ontogeny and roles, there are several limitations for the investigation of specific microglial functions as well as their heterogeneity during development. This review summarizes the current murine tools and models used in the field to study the development of these peculiar cells. In particular, we focus on the methodologies used to label and deplete microglia, monitor their behavior through live-imaging and also discuss the progress currently being made by the community to unravel microglial functions in brain development and disorders.
Introduction
Microglia, the central nervous system (CNS) resident macrophages remained poorly studied until an exponential growth in interest during the last two decades led to fascinating insights into the origin of microglia, their functions, as well as dysfunctions in pathological conditions (Mosser et al., 2017; Hammond et al., 2018; Hoeffel and Ginhoux, 2018; Li and Barres, 2018; Thion et al., 2018a; Prinz et al., 2019). Contrary to most brain cells, microglia were shown to originate from mesodermal yolk sac (YS) macrophage progenitors that travel to reach the CNS during early embryonic development, around Embryonic day (E)9 in mice and gestational week 4/5 in humans (Monier et al., 2007; Ginhoux et al., 2010; Verney et al., 2010; Menassa et al., 2022). As such, these brain invaders constitute the main glial population before the emergence of other glial cells such as oligodendrocytes and astrocytes. After closure of the blood brain barrier around E14 in mice, microglia are believed to be enclosed in the brain under steady-state conditions. These pioneer microglia thus proliferate, seed the entire parenchyma and progressively mature in symbiosis with the neural tissue microenvironment (Matcovitch-Natan et al., 2016; Thion et al., 2018b; Kracht et al., 2020) before self-renewing throughout life. This situation is different in zebrafish where microglia are fully replaced by another source of microglia by adulthood (Xu et al., 2015; Ferrero et al., 2018). A key aspect of their development is the high heterogeneity in their colonization patterns, morphologies and molecular properties. In particular, microglial colonization of the brain parenchyma is a long-lasting process that spans embryogenesis until the end of the second postnatal week, following a very stereotypical and uneven spatiotemporal pattern (Swinnen et al., 2013; Squarzoni et al., 2014; Menassa et al., 2022). These cells transiently accumulate at specific hotspots such as the cortico-striatal-amygdalar boundary and are excluded from others regions such as the cortical plate. In addition, they display a variety of morphologies (ameboid, poorly ramified, and elongated) associated with different brain localizations. Finally, owing to high throughput approaches, microglia have been shown to exhibit different transcriptomic states, specifically during development, in both mice and humans (Hammond et al., 2019; Li et al., 2019; Sankowski et al., 2019; Kracht et al., 2020). This high developmental heterogeneity contrasts with a relatively uniform distribution in the whole parenchyma alongside homogeneously ramified morphologies and molecular signatures at adult stages. Finally, sex-specific microglial features have been highlighted in postnatal steady-state conditions but also in response to environmental challenges (Schwarz et al., 2012; Lenz et al., 2013; Rebuli et al., 2016; Hanamsagar et al., 2017; Guneykaya et al., 2018; Thion et al., 2018b; Villa et al., 2018; VanRyzin et al., 2019).
Several seminal studies have demonstrated that, beyond their immune functions, microglia also perform a wide array of cellular functions that relate to the normal functioning of the brain and importantly to its development. In particular, they interact with synapses to mediate remodeling, pruning and transmission but have also been involved in synaptogenesis (Andoh and Koyama, 2021). They further participate to neurogenesis and oligodendrogenesis, partly through their regulation of cell death and survival (Sierra et al., 2010; Cunningham et al., 2013; Hagemeyer et al., 2017; Wlodarczyk et al., 2017; Nemes-Baran et al., 2020; Sherafat et al., 2021; Cserep et al., 2022). They also contribute to the refinement of axonal tracts (Pont-Lezica et al., 2014; Squarzoni et al., 2014) and to the development of cortical inhibitory circuits (Squarzoni et al., 2014; Thion et al., 2019; Favuzzi et al., 2021; Yu et al., 2022). Besides, microglia express various pattern recognition, purinergic, chemokine and cytokine receptors, collectively described as the sensome (Hickman et al., 2013), enabling them to detect and integrate environmental changes. Importantly and consistently with their wide array of cellular functions, microglial dysfunction has been associated with the etiology of neurodevelopmental disorders, including autism spectrum disorders and schizophrenia in humans and mouse models (Lukens and Eyo, 2022). Therefore, during this crucial period of development, it is key to better grasp their functions, the regulatory mechanisms underpinning their heterogeneity and how their molecular states may regulate their roles. Furthermore, elucidating how external signals can impact on these fundamental processes is a major challenge. This will be crucial to illuminate specific and diverse microglial contributions to brain wiring as well as shed light on pathological mechanisms of neurodevelopmental disorders.
Beside microglia, other non-parenchymal macrophages called Border-Associated Macrophages (BAMs) are present at the interfaces of the brain: the meninges, choroid plexus and perivascular space (Lee et al., 2021; Figure 1). Although microglia and BAMs originate from yolk-sac derived progenitors and seed the brain during embryogenesis, some of them are further replaced by monocyte-derived cells, arising from hematopoietic stem cells (Goldmann et al., 2016; Mrdjen et al., 2018; Van Hove et al., 2019; Utz et al., 2020; Masuda et al., 2022). Moreover, while generally referred to as BAMs, they display age- and tissue-specific signatures (Kierdorf et al., 2019; Mildenberger et al., 2022). Most of the well-recognized microglial markers, reporter mouse lines and models that currently exist to label and deplete microglia can also target a large part of macrophages such as the BAMs in the CNS but also populations of peripheral macrophages (Green et al., 2020). Consequently, despite intense research efforts, these limitations prevent the identification and characterization of specific microglia functions, especially during development. Herein, we discuss about the current murine tools and models available to label or deplete microglia and subsequently assess their developmental functions in physiological and disease conditions.
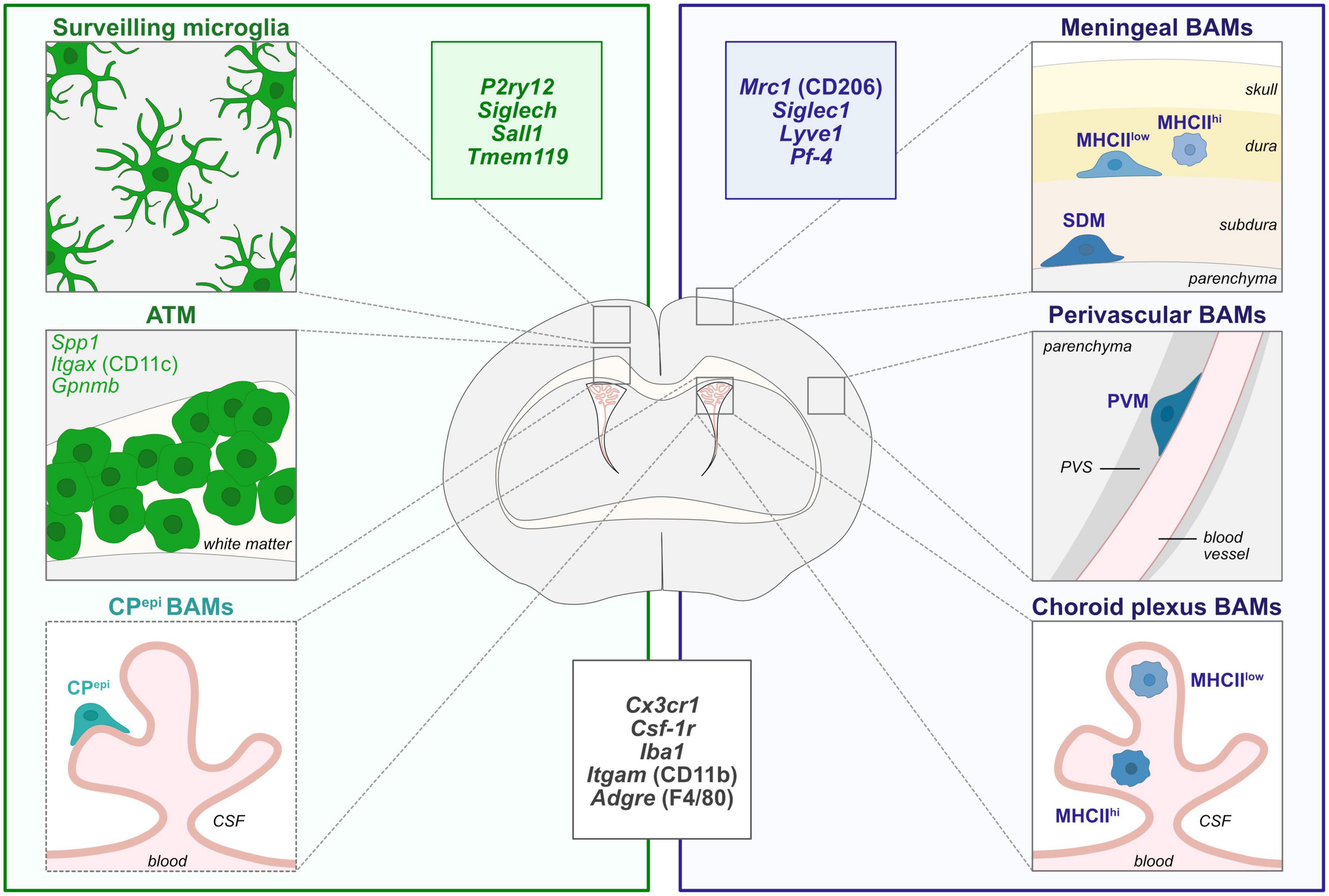
Figure 1. Diversity of CNS macrophages in steady-state conditions. During steady-state, microglia are localized within the brain parenchyma and are highly heterogeneous in morphologies, localization and molecular signatures throughout brain development. In particular, axon tract-associated microglia (ATM)/proliferative-region-associated microglia (PAM)/Cd11c-positive microglia have been described during early postnatal development in the corpus callosum and other white matter regions. Border-associated macrophages (BAMs) reside at distinct interfaces of the CNS such as the meninges, choroid plexus and perivascular space (Goldmann et al., 2016; Mrdjen et al., 2018; Van Hove et al., 2019; Utz et al., 2020; Masuda et al., 2022). Non-parenchymal microglia-like Kolmer’s epiplexus BAMs (CPepi) reside in the apical surface of the choroid plexus facing the cerebrospinal fluid (CSF) and share some transcriptional features with microglia and ATM signature (Van Hove et al., 2019). In particular, meningeal MHCIIlow and MHCIIhigh macrophages are localized in the dura matter while subdural macrophages (SDM) are restrained to the subdural area. Finally, while perivascular macrophages (PVM) are found in the perivascular space, between the vascular basement membrane and the glia limitans of the parenchyma, choroid plexus contains MHCIIlow and MHCIIhigh BAMs. The insets depict the most commonly used markers for microglia (green), BAMs (blue), and common markers to both populations (black). ATM, axon tract-associated microglia; BAMs, border-associated macrophages; CPepi, Kolmer’s epiplexus; CSF, cerebrospinal fluid; MHCII, major histocompatibility complex class II; PVS, perivascular space; PVM, perivascular macrophage; SDM, subdural macrophage.
From broad microglial targeting to specific states labeling
Catch me if you can: Microglia and other brain macrophages
Historically, microglia were mainly identified either through Iba1 immunostaining or using the Cx3cr1GFP/+ (Jung et al., 2000) that labels many macrophages, including microglia and BAMs. Similarly, the well-established Cx3cr1creERT2 mouse lines have been very useful to inactivate genes in these cells (Parkhurst et al., 2013; Yona et al., 2013). Nevertheless, they are not specific to microglia and may trigger microglial reactivity in neonates upon tamoxifen administration (Sahasrabuddhe and Ghosh, 2022). Recent studies, including single cell RNA sequencing analyses, highlighted more specific homeostatic microglia markers including p2ry12, Sall1, Tmem119, Hexb, Siglech (Gautier et al., 2012; Buttgereit et al., 2016; Satoh et al., 2016; Cserep et al., 2020; Masuda et al., 2020) allowing the use of specific antibodies to label either microglia (P2ry12, Tmem119, and SiglecH) or BAMs (CD206, Lyve1, and Siglec1) (Mrdjen et al., 2018; Figure 1). Fluorescent In Situ Hybridization (FISH) has also been used to circumvent the absence of specific antibodies, particularly by taking advantage of the RNAscope technique (Matcovitch-Natan et al., 2016; Hammond et al., 2019). To specifically visualize, manipulate and assess microglia functions, reporter and creERT2-expressing lines were generated with minor recombination in BAMs: Tmem119eGFP and Tmem119creERT2 (Kaiser and Feng, 2019), Tmem119tdTomato (Ruan et al., 2020), HexbtdTomato and HexbcreERT2 (Masuda et al., 2020), p2ry12creERT2 (McKinsey et al., 2020), Sall1GFP, and Sall1creERT2 (Buttgereit et al., 2016), the latter recombining in neurons and other glia (Chappell-Maor et al., 2020; Table 1). Nonetheless, since most of these genes start to be expressed as microglia mature (Bennett et al., 2016), it is important to stress that there are so far no alternatives to the Cx3cr1GFP/+ or Cx3cr1creERT2 lines to efficiently target early embryonic microglia.
Targeting microglia more specifically is crucial, since studies often assign a variety of roles to microglia using depletion models that target both microglia and BAMs. Kim et al. (2021) took advantage of an elegant “split cre” binary genetic construct to generate the Cx3cr1ccre:Sall1ncre and Cx3cr1ccre:Lyve1ncre, to selectively target microglia and BAMs, respectively. Furthermore, several mouse lines including the Siglec1cre (Utz et al., 2020), Pf4-Cre (McKinsey et al., 2020), Lyve1creERT2, and Mrc1CreERT2 lines (Masuda et al., 2022) have been shown to specifically label BAMs during development and thereby enable to follow their trajectory (Table 1). In addition, while no circulating cells are thought to enter in the brain parenchyma during steady-state after BBB closure, monocyte infiltration can occur in disease, aging and injury, and can be monitored using bone marrow chimeras (Mills et al., 2022). These new tools will be important to decipher the relative contributions of microglia, BAMs or infiltrating myeloid cells, but still require thorough characterization with regards to efficiency and rate of spontaneous recombination at different timepoints along development.
Finally, cell-specific viral gene delivery has been extensively used to target different CNS population but robust transduction in microglia remained quite inefficient until recently (Maes et al., 2019). In a ground-breaking study, Lin et al. (2022) successfully targeted 80% of microglia in vivo using adeno-associated viruses without inducing microglia reactivity or changes in gene expression, although it remains elusive to what extend BAMs were also affected. Such approach opens new avenues to study microglia but also have tremendous translational potential.
Looking with new eyes
Along with new markers, mouse lines and viral approaches to target microglia, technical advances in diverse fields shed new light on ways to study microglia, in particular going from fixed immobile imaging in brain slices to dynamic and global approaches. First, tissue clearing methods are constantly improving, with some of them perfectly preserving the signal from reporter lines and antibodies [reviewed in Eme-Scolan and Dando (2020)]. This allows visualization of microglia in whole intact brains. However, while these techniques are becoming well-established and easier to use routinely, the difficulty lies in the analysis of the generated data. Annotated 3D atlases of the developing brain will offer many exciting possibilities toward a more comprehensive study of microglia development.
Another revolution in the field of microglia came with two-photon live-imaging experiments, which revealed never resting microglia with their processes constantly surveilling their environment as well as rapidly reacting in case of injury (Davalos et al., 2005; Nimmerjahn et al., 2005). In combination with other markers, it is thus possible to monitor microglial interaction with blood vessels (Csaszar et al., 2022), radial glia (Rosin et al., 2021), neuronal populations as well as track microglial processes and their specific contact with synapses (Wake et al., 2009) or nodes of Ranvier (Ronzano et al., 2021). While some experiments are performed on brain slices, inducing tissue damage and possibly altering microglial behavior, most studies use cranial windows or skull thinning that allows microglial observation in their homeostatic environment. For pups, adapted approaches are being developed taking advantage of the thinness of the embryonic skull to perform ex utero live-imaging of microglia and macrophages in the brain of intact embryos (Hattori et al., 2020, 2022; Munz et al., 2022), that will probably be critical to better characterize key aspects of microglia development such as their entry in the brain parenchyma (Stremmel et al., 2018).
As a self-renewing population arising from a restricted pool of pioneer cells, the questions of microglial expansion during development, their migration and turnover were raised–and still retain some mystery. The “Microfetti” mice developed by Tay et al. (2017) in which microglia express randomly one out of four fluorophores after tamoxifen induction, highlighted clonal expansion of adult microglia in pathology. In addition, Ratz et al. (2022) developed the new TREX technique, which combines single-cell and spatial transcriptomic coupled to early (E9.5) in vivo barcoding, in order to analyze the lineage relationships between mature cells and progenitors. Thereby, they highlighted drastic microglial expansion from a limited pool of progenitors. Though not extensively used yet, these tools for clonal analysis and lineage tracing will be valuable in the context of development to better understand microglial expansion, migration and final distribution in the brain.
Targeting the different flavors of microglia
Historically, microglia were classified as displaying either a neurotoxic pro-inflammatory M1 or neuroprotective anti-inflammatory M2 phenotype but this binary perspective has been largely revisited (Paolicelli et al., 2022). The development of high throughput technologies such as single cell and single nucleus RNA sequencing (sc/snRNA-seq), cytometry by time-of-flight (CyTOF), or multiplex error-robust fluorescent in situ hybridization (MERFISH) revealed a richer heterogeneity in microglial profiles. While microglia constitute a relatively homogeneous population in adulthood, they display a striking heterogeneity during prenatal/early postnatal development, aging and neurodegeneration (Deczkowska et al., 2018; Hammond et al., 2019; Li et al., 2019; Masuda et al., 2019; Sankowski et al., 2019; Kracht et al., 2020; Marschallinger et al., 2020; Safaiyan et al., 2021; Stogsdill et al., 2022). Nevertheless, we should not underestimate the experimental bias introduced by cell dissociation and sorting-strategies, sequencing technologies and subsequent analyzes selected to characterize microglial heterogeneity (Marsh et al., 2022; Paolicelli et al., 2022; Sankowski et al., 2022). To avoid extensive confusion in the field, it remains crucial to be cautious about the semantic implication of microglial heterogeneity and the subsequent functional diversity associated with it (Paolicelli et al., 2022).
Specific developmental microglial states are thus starting to be described such as the Axon Tract-associated Microglia (ATM), also known as Proliferative-region-Associated Microglia (PAM), Cd11c-positive microglia, and Youth-Associated Microglia (YAM). They are characterized by the expression of several genes such as Spp1, Itgax, Gpnmb and were initially identified in the postnatal white matter as regulators of the development of oligodendrocyte precursors and of subsequent myelinogenesis (Hagemeyer et al., 2017; Wlodarczyk et al., 2017; Hammond et al., 2019; Li et al., 2019; Silvin et al., 2022). Interestingly, there are remarkable similarities in transcriptomic signatures between ATM and Disease Associated Microglia (DAM), initially characterized in mouse models of Alzheimer’s disease (Keren-Shaul et al., 2017; Krasemann et al., 2017), raising the question of their potential similarities, differences–and relationship. Apart from the use of specific antibodies or in situ hybridization probes, the field lacks genetic tools to specifically label and target microglia states. So far, only a CD11ccre line has been used to target ATM (Wlodarczyk et al., 2017), but was unsuccessful in depleting ATM in combination with Diphtheria Toxin strategies. In addition, a novel Spp1tdTomato mouse line has been generated and used to monitor SPP1 from perivascular macrophages (De Schepper et al., 2022), and could constitute an interesting tool for ATM-lineage studies. Based on their emerging molecular characterization, novel mouse lines or viral approaches will enable specific depletion or inactivation of microglial states to further assess their fates and functions. Moreover, in-depth characterization of transcriptomic, epigenomic, and metabolomic landscapes in microglia would enable to better understand their regulatory mechanisms, the transientness of these states and to which extent they can be induced by the microenvironment at different stages of life. Along these lines, the progress of spatial transcriptomics toward increased structural resolution should lead to a tremendous breakthrough to characterize microglial heterogeneity (Stogsdill et al., 2022), in particular at hotspots of accumulating microglia during development. Altogether, the field is moving onto a specific targeting of developmental microglial states which should shed new light on their regulatory mechanisms, their plasticity and functions in steady-state and disease conditions.
En route for specific depletion approaches
Microglial functions in brain development, homeostasis and diseases were historically assessed using in vivo depletion strategies summarized in Table 1. Though a lot of techniques have been developed, their diversity illustrates the difficulty to obtain a specific, efficient and long-lasting depletion of microglia while limiting its off-target effects.
Killing by numbers
The first strategies employed were aiming to directly trigger microglial apoptosis by administration of clodronate liposomes, that are specifically phagocytosed by microglia and BAMs and induce cell death upon cytoplasmic release (Green et al., 2020). This approach is efficient in the early post-natal brain and in adults, but the inability of the clodronate liposomes to cross the blood brain barrier requires an intracerebral injection, inducing an injury and possible release of inflammatory cytokines (Han et al., 2019). Another approach uses the diphtheria-toxin (DT) based systems, either with direct expression of the DTA or with DT receptor, the latter needing administration of DT. The first studies were performed in Cx3cr1CreER:R26iDTR and Iba1-tTA:DTAtetO/tetO targeting both microglia and BAMs (Parkhurst et al., 2013; Miyamoto et al., 2016; Takeda et al., 2018). On the contrary, the use of the Siglechdtr/+ mice led to specific and transient depletion of embryonic microglia, without affecting BAMs (Li et al., 2021). Thus, the constitutive or inducible DTR/DTA expression, combined with specific microglial lines provides a better temporal control as well as selective effect, though their efficiency remains variable.
CSF-1R inhibitors
The CSF-1 receptor (CSF-1R) is expressed by microglia, macrophages and their progenitors and its signaling is essential for their survival and proliferation. It thus became a preferential target in the quest for microglial depletion methods. Injections of an anti-CSF-1R antibody performed at E6.5 and E7.5 lead to a drastic depletion of myeloid progenitors, macrophages and microglia, while repopulation spans the first postnatal week (Squarzoni et al., 2014; Hoeffel and Ginhoux, 2015; Thion et al., 2019). On the other hand, embryonic targeting of CSF-1, one of the two CSF-1R ligands, through anti-CSF-1 antibody, leads to a drastic depletion of forebrain microglia (Easley-Neal et al., 2019), with possible off-target effects of the circulating antibodies. Pharmacological inhibitors of the CSF-1R injected or delivered non-invasively via food pellets have been broadly developed to deplete adult microglia, such as PLX3397 and PLX5622, the latter having a higher specificity and improved brain penetrance (Elmore et al., 2014; Green et al., 2020) while it efficiently depletes embryonic microglia, enabling a temporal control of the depletion during gestation (Rosin et al., 2018; Marsters et al., 2020). Nevertheless, as this treatment can affect lactation by depleting maternal macrophages essential for mammary gland development (O’Brien et al., 2012), it can impact pup survival following birth. For early postnatal depletion, direct subcutaneous injections of PLX3397 or PLX5622 during the first post-natal week allows for an efficient depletion of microglia (Li et al., 2020; Favuzzi et al., 2021; Gesuita et al., 2022). Although beyond the scope of this review, CSF-1R inhibitors are also used in other species including humans, bringing novel therapeutic approaches [reviewed in Han et al. (2022)]. Overall, targeting the CSF-1R pathway has proven to be efficient and convenient, but also affects peripheral macrophages and BAMs, preventing the identification of specific microglial functions.
Genetic models
Different genetic models, including constitutive knock-outs of fundamental transcription or survival factors, like Pu.1–/– (McKercher et al., 1996) and Csf1r–/– (Erblich et al., 2011), fail to develop microglia as well as most macrophages (Green et al., 2020). This results in many off-target effects and early death in Pu.1–/– and Csf1r–/–. On the other hand, the Csf1rfl/fl allows for a more specific targeting of microglia, thanks to the microglia specific mouse lines now available and their possible temporal induction. This strategy has been used with Sall1CreER;Csf1rfl/fl (Buttgereit et al., 2016) and HexbCreERT2;Csf1rfl/fl mice (Masuda et al., 2020), with a respective efficiency varying across brain regions from 70% to 90% and of 60%.
An additional important model is the Csf1rΔFIRE/ΔFIRE mice, in which a Csf1r enhancer is deleted (Rojo et al., 2019; Munro et al., 2020). This leads to the absence of microglia from the brain parenchyma, and of resident macrophages of the skin, kidney, heart and peritoneum; while other macrophages and monocytes are unaffected. Importantly, these mice are healthy and fertile in a mixed B6CBAF1/C57BL/6J background, and do not display strong developmental defects described in the Csf1r–/–. So far, they were used to investigate the role of resident microglia in Alzheimer disease mice (Kiani Shabestari et al., 2022) and they will provide a more specific and long-lasting model to explore microglial functions.
Finally, full inactivation of CSF-1R ligands, Csf-1 (Chang et al., 1994; Kondo and Duncan, 2009) or Il34 (Greter et al., 2012; Wang et al., 2012), expressed by the neural tissue, results in time- and region-dependent partial depletion of microglia albeit there are still some controversies amongst studies and major impact on peripheral macrophages (Green et al., 2020; Table 1). Nevertheless, under the pan neuronal driver Nestincre that restricts depletion to the nervous system, thus mainly affecting microglia, the Nestincre;Csf1fl/fl and Nestincre;Il34fl/fl are respectively deprived of microglia in the white matter and cerebellum or in the gray matter highlighting region-specific dependency (Kana et al., 2019; Badimon et al., 2020). On the other hand, macrophages are unaffected in the yolk sac, fetal liver and fetal limbs. Last, because the CSF-1R ligands are differentially expressed and required from microglia through development, these lines provide promising models to investigate local microglial functions.
Concluding remarks
Microglia now appear as key actors of brain development, interacting with most brain cells and regulating several crucial developmental processes. Nevertheless, these exciting advances were done in models affecting both microglia and BAMs. Thus, dissecting their respective contributions thanks to new specific tools will be key to the field. In addition, further understanding microglial diversity during development and the functions played by specific states should bring new light on their importance in neurodevelopmental pathologies and open new avenues for therapeutic intervention.
Author contributions
CB and MST wrote the review. Both authors contributed to the article and approved the submitted version.
Funding
The laboratories acknowledge support from the INSERM, CNRS, École Normale Supérieure, Collège de France and Fondation du Collège de France. CB was supported by an AMX doctoral fellowship and an ARC fellowship. MST is a CNRS investigator supported by the Foundation for Brain Research (FRC).
Acknowledgments
We are grateful to members of the Thion and Garel laboratories for stimulating discussions and comments on the manuscript.
Conflict of interest
The authors declare that the research was conducted in the absence of any commercial or financial relationships that could be construed as a potential conflict of interest.
Publisher’s note
All claims expressed in this article are solely those of the authors and do not necessarily represent those of their affiliated organizations, or those of the publisher, the editors and the reviewers. Any product that may be evaluated in this article, or claim that may be made by its manufacturer, is not guaranteed or endorsed by the publisher.
References
Andoh, M., and Koyama, R. (2021). Microglia regulate synaptic development and plasticity. Dev. Neurobiol. 81, 568–590. doi: 10.1002/dneu.22814
Badimon, A., Strasburger, H. J., Ayata, P., Chen, X., Nair, A., Ikegami, A., et al. (2020). Negative feedback control of neuronal activity by microglia. Nature 586, 417–423. doi: 10.1038/s41586-020-2777-8
Bennett, M. L., Bennett, F. C., Liddelow, S. A., Ajami, B., Zamanian, J. L., Fernhoff, N. B., et al. (2016). New tools for studying microglia in the mouse and human CNS. Proc. Natl. Acad. Sci. U.S.A. 113, E1738–E1746. doi: 10.1073/pnas.1525528113
Buttgereit, A., Lelios, I., Yu, X., Vrohlings, M., Krakoski, N. R., Gautier, E. L., et al. (2016). Sall1 is a transcriptional regulator defining microglia identity and function. Nat. Immunol. 17, 1397–1406. doi: 10.1038/ni.3585
Chang, Y., Albright, S., and Lee, F. (1994). Cytokines in the central nervous system: Expression of macrophage colony stimulating factor and its receptor during development. J. Neuroimmunol. 52, 9–17. doi: 10.1016/0165-5728(94)90156-2
Chappell-Maor, L., Kolesnikov, M., Kim, J. S., Shemer, A., Haimon, Z., Grozovski, J., et al. (2020). Comparative analysis of CreER transgenic mice for the study of brain macrophages: A case study. Eur. J. Immunol. 50, 353–362. doi: 10.1002/eji.201948342
Csaszar, E., Lenart, N., Cserep, C., Kornyei, Z., Fekete, R., Posfai, B., et al. (2022). Microglia modulate blood flow, neurovascular coupling, and hypoperfusion via purinergic actions. J. Exp. Med. 219:e20211071. doi: 10.1084/jem.20211071
Cserep, C., Posfai, B., Lenart, N., Fekete, R., Laszlo, Z. I., Lele, Z., et al. (2020). Microglia monitor and protect neuronal function through specialized somatic purinergic junctions. Science 367, 528–537. doi: 10.1126/science.aax6752
Cserep, C., Schwarcz, A. D., Posfai, B., Laszlo, Z. I., Kellermayer, A., Kornyei, Z., et al. (2022). Microglial control of neuronal development via somatic purinergic junctions. Cell Rep. 40:111369. doi: 10.1016/j.celrep.2022.111369
Cunningham, C. L., Martinez-Cerdeno, V., and Noctor, S. C. (2013). Microglia regulate the number of neural precursor cells in the developing cerebral cortex. J. Neurosci. 33, 4216–4233. doi: 10.1523/JNEUROSCI.3441-12.2013
Davalos, D., Grutzendler, J., Yang, G., Kim, J. V., Zuo, Y., Jung, S., et al. (2005). ATP mediates rapid microglial response to local brain injury in vivo. Nat. Neurosci. 8, 752–758. doi: 10.1038/nn1472
De Schepper, S., Ge, J. G., Crowley, G., Ferreira, L. S. S., Garceau, D., Toomey, C. E., et al. (2022). Perivascular SPP1 mediates microglial engulfment of synapses in Alzheimer’s disease models. bioRxiv [Preprint]. doi: 10.1101/2022.04.04.486547
Deczkowska, A., Keren-Shaul, H., Weiner, A., Colonna, M., Schwartz, M., and Amit, I. (2018). Disease-associated microglia: A universal immune sensor of neurodegeneration. Cell 173, 1073–1081. doi: 10.1016/j.cell.2018.05.003
Easley-Neal, C., Foreman, O., Sharma, N., Zarrin, A. A., and Weimer, R. M. (2019). CSF1R ligands IL-34 and CSF1 are differentially required for microglia development and maintenance in white and gray matter brain regions. Front. Immunol. 10:2199. doi: 10.3389/fimmu.2019.02199
Elmore, M. R., Najafi, A. R., Koike, M. A., Dagher, N. N., Spangenberg, E. E., Rice, R. A., et al. (2014). Colony-stimulating factor 1 receptor signaling is necessary for microglia viability, unmasking a microglia progenitor cell in the adult brain. Neuron 82, 380–397. doi: 10.1016/j.neuron.2014.02.040
Eme-Scolan, E., and Dando, S. J. (2020). Tools and approaches for studying microglia in vivo. Front. Immunol. 11:583647. doi: 10.3389/fimmu.2020.583647
Erblich, B., Zhu, L., Etgen, A. M., Dobrenis, K., and Pollard, J. W. (2011). Absence of colony stimulation factor-1 receptor results in loss of microglia, disrupted brain development and olfactory deficits. PLoS One 6:e26317. doi: 10.1371/journal.pone.0026317
Favuzzi, E., Huang, S., Saldi, G. A., Binan, L., Ibrahim, L. A., Fernandez-Otero, M., et al. (2021). GABA-receptive microglia selectively sculpt developing inhibitory circuits. Cell 184, 4048–4063.e32. doi: 10.1016/j.cell.2021.06.018
Ferrero, G., Mahony, C. B., Dupuis, E., Yvernogeau, L., Di Ruggiero, E., Miserocchi, M., et al. (2018). Embryonic microglia derive from primitive macrophages and are replaced by cmyb-dependent definitive microglia in zebrafish. Cell Rep. 24, 130–141. doi: 10.1016/j.celrep.2018.05.066
Gautier, E. L., Shay, T., Miller, J., Greter, M., Jakubzick, C., Ivanov, S., et al. (2012). Gene-expression profiles and transcriptional regulatory pathways that underlie the identity and diversity of mouse tissue macrophages. Nat. Immunol. 13, 1118–1128. doi: 10.1038/ni.2419
Gesuita, L., Cavaccini, A., Argunsah, A. O., Favuzzi, E., Ibrahim, L. A., Stachniak, T. J., et al. (2022). Microglia contribute to the postnatal development of cortical somatostatin-positive inhibitory cells and to whisker-evoked cortical activity. Cell Rep. 40:111209. doi: 10.1016/j.celrep.2022.111209
Ginhoux, F., Greter, M., Leboeuf, M., Nandi, S., See, P., Gokhan, S., et al. (2010). Fate mapping analysis reveals that adult microglia derive from primitive macrophages. Science 330, 841–845. doi: 10.1126/science.1194637
Goldmann, T., Wieghofer, P., Jordao, M. J., Prutek, F., Hagemeyer, N., Frenzel, K., et al. (2016). Origin, fate and dynamics of macrophages at central nervous system interfaces. Nat. Immunol. 17, 797–805. doi: 10.1038/ni.3423
Green, K. N., Crapser, J. D., and Hohsfield, L. A. (2020). To kill a microglia: A case for CSF1R Inhibitors. Trends Immunol. 41, 771–784. doi: 10.1016/j.it.2020.07.001
Greter, M., Lelios, I., Pelczar, P., Hoeffel, G., Price, J., Leboeuf, M., et al. (2012). Stroma-derived interleukin-34 controls the development and maintenance of langerhans cells and the maintenance of microglia. Immunity 37, 1050–1060. doi: 10.1016/j.immuni.2012.11.001
Guneykaya, D., Ivanov, A., Hernandez, D. P., Haage, V., Wojtas, B., Meyer, N., et al. (2018). Transcriptional and translational differences of microglia from male and female brains. Cell Rep. 24, 2773–2783.e6. doi: 10.1016/j.celrep.2018.08.001
Hagemeyer, N., Hanft, K. M., Akriditou, M. A., Unger, N., Park, E. S., Stanley, E. R., et al. (2017). Microglia contribute to normal myelinogenesis and to oligodendrocyte progenitor maintenance during adulthood. Acta Neuropathol. 134, 441–458. doi: 10.1007/s00401-017-1747-1
Hammond, T. R., Dufort, C., Dissing-Olesen, L., Giera, S., Young, A., Wysoker, A., et al. (2019). Single-Cell RNA sequencing of microglia throughout the mouse lifespan and in the injured brain reveals complex cell-state changes. Immunity 50, 253–271.e6. doi: 10.1016/j.immuni.2018.11.004
Hammond, T. R., Robinton, D., and Stevens, B. (2018). Microglia and the brain: Complementary partners in development and disease. Annu. Rev. Cell Dev. Biol. 34, 523–544. doi: 10.1146/annurev-cellbio-100616-060509
Han, J., Chitu, V., Stanley, E. R., Wszolek, Z. K., Karrenbauer, V. D., and Harris, R. A. (2022). Inhibition of colony stimulating factor-1 receptor (CSF-1R) as a potential therapeutic strategy for neurodegenerative diseases: Opportunities and challenges. Cell Mol. Life Sci. 79, 219. doi: 10.1007/s00018-022-04225-1
Han, X., Li, Q., Lan, X., El-Mufti, L., Ren, H., and Wang, J. (2019). Microglial depletion with clodronate liposomes increases proinflammatory cytokine levels, induces astrocyte activation, and damages blood vessel integrity. Mol. Neurobiol. 56, 6184–6196. doi: 10.1007/s12035-019-1502-9
Hanamsagar, R., Alter, M. D., Block, C. S., Sullivan, H., Bolton, J. L., and Bilbo, S. D. (2017). Generation of a microglial developmental index in mice and in humans reveals a sex difference in maturation and immune reactivity. Glia 65, 1504–1520. doi: 10.1002/glia.23176
Hattori, Y., Kato, D., Murayama, F., Koike, S., Naito, Y., Kawaguchi, A., et al. (2022). Border-associated macrophages transventricularly infiltrate the early embryonic cerebral wall to differentiate into microglia. bioRxiv [Preprint]. doi: 10.1101/2022.07.27.501563
Hattori, Y., Naito, Y., Tsugawa, Y., Nonaka, S., Wake, H., Nagasawa, T., et al. (2020). Transient microglial absence assists postmigratory cortical neurons in proper differentiation. Nat. Commun. 11:1631. doi: 10.1038/s41467-020-15409-3
Hickman, S. E., Kingery, N. D., Ohsumi, T. K., Borowsky, M. L., Wang, L. C., Means, T. K., et al. (2013). The microglial sensome revealed by direct RNA sequencing. Nat. Neurosci. 16, 1896–1905. doi: 10.1038/nn.3554
Hoeffel, G., and Ginhoux, F. (2015). Ontogeny of tissue-resident macrophages. Front Immunol. 6:486. doi: 10.3389/fimmu.2015.00486
Hoeffel, G., and Ginhoux, F. (2018). Fetal monocytes and the origins of tissue-resident macrophages. Cell Immunol. 330, 5–15. doi: 10.1016/j.cellimm.2018.01.001
Huang, Y., Xu, Z., Xiong, S., Sun, F., Qin, G., Hu, G., et al. (2018). Repopulated microglia are solely derived from the proliferation of residual microglia after acute depletion. Nat Neurosci. 21, 530–540. doi: 10.1038/s41593-018-0090-8
Jung, S., Aliberti, J., Graemmel, P., Sunshine, M. J., Kreutzberg, G. W., Sher, A., et al. (2000). Analysis of fractalkine receptor CX(3)CR1 function by targeted deletion and green fluorescent protein reporter gene insertion. Mol. Cell Biol. 20, 4106–4114. doi: 10.1128/MCB.20.11.4106-4114.2000
Kaiser, T., and Feng, G. (2019). Tmem119-EGFP and tmem119-CreERT2 transgenic mice for labeling and manipulating microglia. eNeuro 6, ENEURO.0448–18.2019. doi: 10.1523/ENEURO.0448-18.2019
Kana, V., Desland, F. A., Casanova-Acebes, M., Ayata, P., Badimon, A., Nabel, E., et al. (2019). CSF-1 controls cerebellar microglia and is required for motor function and social interaction. J. Exp. Med. 216, 2265–2281. doi: 10.1084/jem.20182037
Keren-Shaul, H., Spinrad, A., Weiner, A., Matcovitch-Natan, O., Dvir-Szternfeld, R., Ulland, T. K., et al. (2017). A unique microglia type associated with restricting development of Alzheimer’s disease. Cell 169, 1276–1290.e17. doi: 10.1016/j.cell.2017.05.018
Kiani Shabestari, S., Morabito, S., Danhash, E. P., McQuade, A., Sanchez, J. R., Miyoshi, E., et al. (2022). Absence of microglia promotes diverse pathologies and early lethality in Alzheimer’s disease mice. Cell Rep. 39:110961. doi: 10.1016/j.celrep.2022.110961
Kierdorf, K., Masuda, T., Jordao, M. J. C., and Prinz, M. (2019). Macrophages at CNS interfaces: Ontogeny and function in health and disease. Nat. Rev. Neurosci. 20, 547–562. doi: 10.1038/s41583-019-0201-x
Kim, J. S., Kolesnikov, M., Peled-Hajaj, S., Scheyltjens, I., Xia, Y., Trzebanski, S., et al. (2021). A binary Cre transgenic approach dissects microglia and CNS border-associated macrophages. Immunity 54, 176–190.e7. doi: 10.1016/j.immuni.2020.11.007
Kondo, Y., and Duncan, I. D. (2009). Selective reduction in microglia density and function in the white matter of colony-stimulating factor-1-deficient mice. J. Neurosci. Res. 87, 2686–2695. doi: 10.1002/jnr.22096
Konishi, H., Kobayashi, M., Kunisawa, T., Imai, K., Sayo, A., Malissen, B., et al. (2017). Siglec-H is a microglia-specific marker that discriminates microglia from CNS-associated macrophages and CNS-infiltrating monocytes. Glia 65, 1927–1943. doi: 10.1002/glia.23204
Kracht, L., Borggrewe, M., Eskandar, S., Brouwer, N., Chuva de Sousa Lopes, S. M., Laman, J. D., et al. (2020). Human fetal microglia acquire homeostatic immune-sensing properties early in development. Science 369, 530–537. doi: 10.1126/science.aba5906
Krasemann, S., Madore, C., Cialic, R., Baufeld, C., Calcagno, N., El Fatimy, R., et al. (2017). The TREM2-APOE pathway drives the transcriptional phenotype of dysfunctional microglia in neurodegenerative diseases. Immunity 47, 566–581.e9. doi: 10.1016/j.immuni.2017.08.008
Lee, E., Eo, J. C., Lee, C., and Yu, J. W. (2021). Distinct features of brain-resident macrophages: Microglia and non-parenchymal brain macrophages. Mol. Cells 44, 281–291. doi: 10.14348/molcells.2021.0060
Lenz, K. M., Nugent, B. M., Haliyur, R., and McCarthy, M. M. (2013). Microglia are essential to masculinization of brain and behavior. J. Neurosci. 33, 2761–2772. doi: 10.1523/JNEUROSCI.1268-12.2013
Li, C., Konishi, H., Nishiwaki, K., Sato, K., Miyata, T., and Kiyama, H. (2021). A mouse model of microglia-specific ablation in the embryonic central nervous system. Neurosci. Res. 173, 54–61. doi: 10.1016/j.neures.2021.06.002
Li, Q., and Barres, B. A. (2018). Microglia and macrophages in brain homeostasis and disease. Nat. Rev. Immunol. 18, 225–242. doi: 10.1038/nri.2017.125
Li, Q., Cheng, Z., Zhou, L., Darmanis, S., Neff, N. F., Okamoto, J., et al. (2019). Developmental heterogeneity of microglia and brain myeloid cells revealed by deep single-cell RNA sequencing. Neuron 101, 207–223.e10. doi: 10.1016/j.neuron.2018.12.006
Li, Y., He, X., Kawaguchi, R., Zhang, Y., Wang, Q., Monavarfeshani, A., et al. (2020). Microglia-organized scar-free spinal cord repair in neonatal mice. Nature 587, 613–618. doi: 10.1038/s41586-020-2795-6
Lin, R., Zhou, Y., Yan, T., Wang, R., Li, H., Wu, Z., et al. (2022). Directed evolution of adeno-associated virus for efficient gene delivery to microglia. Nat. Methods 19, 976–985. doi: 10.1038/s41592-022-01547-7
Lukens, J. R., and Eyo, U. B. (2022). Microglia and neurodevelopmental disorders. Annu. Rev. Neurosci. 45, 425–445. doi: 10.1146/annurev-neuro-110920-023056
MacDonald, K. P., Palmer, J. S., Cronau, S., Seppanen, E., Olver, S., Raffelt, N. C., et al. (2010). An antibody against the colony-stimulating factor 1 receptor depletes the resident subset of monocytes and tissue- and tumor-associated macrophages but does not inhibit inflammation. Blood 116, 3955–3963. doi: 10.1182/blood-2010-02-266296
Maes, M. E., Colombo, G., Schulz, R., and Siegert, S. (2019). Targeting microglia with lentivirus and AAV: Recent advances and remaining challenges. Neurosci. Lett. 707:134310. doi: 10.1016/j.neulet.2019.134310
Marschallinger, J., Iram, T., Zardeneta, M., Lee, S. E., Lehallier, B., Haney, M. S., et al. (2020). Lipid-droplet-accumulating microglia represent a dysfunctional and proinflammatory state in the aging brain. Nat. Neurosci. 23, 194–208. doi: 10.1038/s41593-019-0566-1
Marsh, S. E., Walker, A. J., Kamath, T., Dissing-Olesen, L., Hammond, T. R., de Soysa, T. Y., et al. (2022). Dissection of artifactual and confounding glial signatures by single-cell sequencing of mouse and human brain. Nat. Neurosci. 25, 306–316. doi: 10.1038/s41593-022-01022-8
Marsters, C. M., Nesan, D., Far, R., Klenin, N., Pittman, Q. J., and Kurrasch, D. M. (2020). Embryonic microglia influence developing hypothalamic glial populations. J. Neuroinflammation 17:146. doi: 10.1186/s12974-020-01811-7
Masuda, T., Amann, L., Monaco, G., Sankowski, R., Staszewski, O., Krueger, M., et al. (2022). Specification of CNS macrophage subsets occurs postnatally in defined niches. Nature 604, 740–748. doi: 10.1038/s41586-022-04596-2
Masuda, T., Amann, L., Sankowski, R., Staszewski, O., Lenz, M., d’Errico, P., et al. (2020). Novel Hexb-based tools for studying microglia in the CNS. Nat. Immunol. 21, 802–815. doi: 10.1038/s41590-020-0707-4
Masuda, T., Sankowski, R., Staszewski, O., Bottcher, C., Amann, L., and Sagar, et al. (2019). Spatial and temporal heterogeneity of mouse and human microglia at single-cell resolution. Nature 566, 388–392. doi: 10.1038/s41586-019-0924-x
Matcovitch-Natan, O., Winter, D. R., Giladi, A., Vargas Aguilar, S., Spinrad, A., Sarrazin, S., et al. (2016). Microglia development follows a stepwise program to regulate brain homeostasis. Science 353:aad8670. doi: 10.1126/science.aad8670
McKercher, S. R., Torbett, B. E., Anderson, K. L., Henkel, G. W., Vestal, D. J., Baribault, H., et al. (1996). Targeted disruption of the PU.1 gene results in multiple hematopoietic abnormalities. EMBO J. 15, 5647–5658.
McKinsey, G. L., Lizama, C. O., Keown-Lang, A. E., Niu, A., Santander, N., Larpthaveesarp, A., et al. (2020). A new genetic strategy for targeting microglia in development and disease. Elife 9:e54590. doi: 10.7554/eLife.54590
Menassa, D. A., Muntslag, T. A. O., Martin-Estebane, M., Barry-Carroll, L., Chapman, M. A., Adorjan, I., et al. (2022). The spatiotemporal dynamics of microglia across the human lifespan. Dev. Cell 57, 2127–2139.e6. doi: 10.1016/j.devcel.2022.07.015
Michaelson, M. D., Bieri, P. L., Mehler, M. F., Xu, H., Arezzo, J. C., Pollard, J. W., et al. (1996). CSF-1 deficiency in mice results in abnormal brain development. Development 122, 2661–2672. doi: 10.1242/dev.122.9.2661
Mildenberger, W., Stifter, S. A., and Greter, M. (2022). Diversity and function of brain-associated macrophages. Curr. Opin. Immunol. 76:102181. doi: 10.1016/j.coi.2022.102181
Mills, J., Ladner, L., Soliman, E., Leonard, J., Morton, P. D., and Theus, M. H. (2022). Cross-talk and subset control of microglia and associated myeloid cells in neurological disorders. Cells 11:3364. doi: 10.3390/cells11213364
Miyamoto, A., Wake, H., Ishikawa, A. W., Eto, K., Shibata, K., Murakoshi, H., et al. (2016). Microglia contact induces synapse formation in developing somatosensory cortex. Nat. Commun. 7:12540. doi: 10.1038/ncomms12540
Monier, A., Adle-Biassette, H., Delezoide, A. L., Evrard, P., Gressens, P., and Verney, C. (2007). Entry and distribution of microglial cells in human embryonic and fetal cerebral cortex. J. Neuropathol. Exp. Neurol. 66, 372–382. doi: 10.1097/nen.0b013e3180517b46
Mosser, C. A., Baptista, S., Arnoux, I., and Audinat, E. (2017). Microglia in CNS development: Shaping the brain for the future. Prog. Neurobiol. 14, 1–20. doi: 10.1016/j.pneurobio.2017.01.002
Mrdjen, D., Pavlovic, A., Hartmann, F. J., Schreiner, B., Utz, S. G., Leung, B. P., et al. (2018). High-dimensional single-cell mapping of central nervous system immune cells reveals distinct myeloid subsets in health, aging, and disease. Immunity 48:599. doi: 10.1016/j.immuni.2018.02.014
Munro, D. A. D., Bradford, B. M., Mariani, S. A., Hampton, D. W., Vink, C. S., Chandran, S., et al. (2020). CNS macrophages differentially rely on an intronic Csf1r enhancer for their development. Development 147:dev194449. doi: 10.1242/dev.194449
Munz, M., Bharioke, A., Kosche, G., Moreno-Juan, V., Brignall, A., Graff-Meyer, A., et al. (2022). Embryonic cortical layer 5 pyramidal neurons form an active, transient circuit motif perturbed by autism-associated mutations. bioRxiv [Preprint]. doi: 10.1101/2022.08.31.506080
Nemes-Baran, A. D., White, D. R., and DeSilva, T. M. (2020). Fractalkine-dependent microglial pruning of viable oligodendrocyte progenitor cells regulates myelination. Cell Rep. 32:108047. doi: 10.1016/j.celrep.2020.108047
Nimmerjahn, A., Kirchhoff, F., and Helmchen, F. (2005). Resting microglial cells are highly dynamic surveillants of brain parenchyma in vivo. Science 308, 1314–1318. doi: 10.1126/science.1110647
O’Brien, J., Martinson, H., Durand-Rougely, C., and Schedin, P. (2012). Macrophages are crucial for epithelial cell death and adipocyte repopulation during mammary gland involution. Development 139, 269–275. doi: 10.1242/dev.071696
Paolicelli, R. C., Sierra, A., Stevens, B., Tremblay, M. E., Aguzzi, A., Ajami, B., et al. (2022). Microglia states and nomenclature: A field at its crossroads. Neuron 110, 3458–3483. doi: 10.1016/j.neuron.2022.10.020
Parkhurst, C. N., Yang, G., Ninan, I., Savas, J. N., Yates, J. R. III, Lafaille, J. J., et al. (2013). Microglia promote learning-dependent synapse formation through brain-derived neurotrophic factor. Cell 155, 1596–1609. doi: 10.1016/j.cell.2013.11.030
Pont-Lezica, L., Beumer, W., Colasse, S., Drexhage, H., Versnel, M., and Bessis, A. (2014). Microglia shape corpus callosum axon tract fasciculation: Functional impact of prenatal inflammation. Eur. J. Neurosci. 39, 1551–1557. doi: 10.1111/ejn.12508
Prinz, M., Jung, S., and Priller, J. (2019). Microglia biology: One century of evolving concepts. Cell 179, 292–311. doi: 10.1016/j.cell.2019.08.053
Ratz, M., von Berlin, L., Larsson, L., Martin, M., Westholm, J. O., La Manno, G., et al. (2022). Clonal relations in the mouse brain revealed by single-cell and spatial transcriptomics. Nat. Neurosci. 25, 285–294. doi: 10.1038/s41593-022-01011-x
Rebuli, M. E., Gibson, P., Rhodes, C. L., Cushing, B. S., and Patisaul, H. B. (2016). Sex differences in microglial colonization and vulnerabilities to endocrine disruption in the social brain. Gen. Comp. Endocrinol. 238, 39–46. doi: 10.1016/j.ygcen.2016.04.018
Rojo, R., Raper, A., Ozdemir, D. D., Lefevre, L., Grabert, K., Wollscheid-Lengeling, E., et al. (2019). Deletion of a Csf1r enhancer selectively impacts CSF1R expression and development of tissue macrophage populations. Nat. Commun. 10:3215. doi: 10.1038/s41467-019-11053-8
Ronzano, R., Roux, T., Thetiot, M., Aigrot, M. S., Richard, L., Lejeune, F. X., et al. (2021). Microglia-neuron interaction at nodes of Ranvier depends on neuronal activity through potassium release and contributes to remyelination. Nat. Commun. 12:5219. doi: 10.1038/s41467-021-25486-7
Rosin, J. M., Marsters, C. M., Malik, F., Far, R., Adnani, L., Schuurmans, C., et al. (2021). Embryonic microglia interact with hypothalamic radial glia during development and upregulate the TAM receptors MERTK and AXL following an insult. Cell Rep. 34:108587. doi: 10.1016/j.celrep.2020.108587
Rosin, J. M., Vora, S. R., and Kurrasch, D. M. (2018). Depletion of embryonic microglia using the CSF1R inhibitor PLX5622 has adverse sex-specific effects on mice, including accelerated weight gain, hyperactivity and anxiolytic-like behaviour. Brain Behav. Immun. 73, 682–697. doi: 10.1016/j.bbi.2018.07.023
Ruan, C., Sun, L., Kroshilina, A., Beckers, L., De Jager, P., Bradshaw, E. M., et al. (2020). A novel Tmem119-tdTomato reporter mouse model for studying microglia in the central nervous system. Brain Behav. Immun. 83, 180–191. doi: 10.1016/j.bbi.2019.10.009
Safaiyan, S., Besson-Girard, S., Kaya, T., Cantuti-Castelvetri, L., Liu, L., Ji, H., et al. (2021). White matter aging drives microglial diversity. Neuron 109, 1100–1117.e10. doi: 10.1016/j.neuron.2021.01.027
Sahasrabuddhe, V., and Ghosh, H. S. (2022). Cx3Cr1-Cre induction leads to microglial activation and IFN-1 signaling caused by DNA damage in early postnatal brain. Cell Rep. 38:110252. doi: 10.1016/j.celrep.2021.110252
Sankowski, R., Bottcher, C., Masuda, T., Geirsdottir, L., Sagar Sindram, E., et al. (2019). Mapping microglia states in the human brain through the integration of high-dimensional techniques. Nat. Neurosci. 22, 2098–2110. doi: 10.1038/s41593-019-0532-y
Sankowski, R., Monaco, G., and Prinz, M. (2022). Evaluating microglial phenotypes using single-cell technologies. Trends Neurosci. 45, 133–144. doi: 10.1016/j.tins.2021.11.001
Satoh, J., Kino, Y., Asahina, N., Takitani, M., Miyoshi, J., Ishida, T., et al. (2016). TMEM119 marks a subset of microglia in the human brain. Neuropathology 36, 39–49. doi: 10.1111/neup.12235
Schwarz, J. M., Sholar, P. W., and Bilbo, S. D. (2012). Sex differences in microglial colonization of the developing rat brain. J. Neurochem. 120, 948–963. doi: 10.1111/j.1471-4159.2011.07630.x
Sherafat, A., Pfeiffer, F., Reiss, A. M., Wood, W. M., and Nishiyama, A. (2021). Microglial neuropilin-1 promotes oligodendrocyte expansion during development and remyelination by trans-activating platelet-derived growth factor receptor. Nat. Commun. 12:2265. doi: 10.1038/s41467-021-22532-2
Sierra, A., Encinas, J. M., Deudero, J. J., Chancey, J. H., Enikolopov, G., Overstreet-Wadiche, L. S., et al. (2010). Microglia shape adult hippocampal neurogenesis through apoptosis-coupled phagocytosis. Cell Stem Cell 7, 483–495. doi: 10.1016/j.stem.2010.08.014
Silvin, A., Uderhardt, S., Piot, C., Da Mesquita, S., Yang, K., Geirsdottir, L., et al. (2022). Dual ontogeny of disease-associated microglia and disease inflammatory macrophages in aging and neurodegeneration. Immunity 55, 1448–1465.e6. doi: 10.1016/j.immuni.2022.07.004
Squarzoni, P., Oller, G., Hoeffel, G., Pont-Lezica, L., Rostaing, P., Low, D., et al. (2014). Microglia modulate wiring of the embryonic forebrain. Cell Rep. 8, 1271–1279. doi: 10.1016/j.celrep.2014.07.042
Stogsdill, J. A., Kim, K., Binan, L., Farhi, S. L., Levin, J. Z., and Arlotta, P. (2022). Pyramidal neuron subtype diversity governs microglia states in the neocortex. Nature 608, 750–756. doi: 10.1038/s41586-022-05056-7
Stremmel, C., Schuchert, R., Wagner, F., Thaler, R., Weinberger, T., Pick, R., et al. (2018). Yolk sac macrophage progenitors traffic to the embryo during defined stages of development. Nat. Commun. 9:75. doi: 10.1038/s41467-017-02492-2
Swinnen, N., Smolders, S., Avila, A., Notelaers, K., Paesen, R., Ameloot, M., et al. (2013). Complex invasion pattern of the cerebral cortex bymicroglial cells during development of the mouse embryo. Glia 61, 150–163. doi: 10.1002/glia.22421
Takeda, A., Shinozaki, Y., Kashiwagi, K., Ohno, N., Eto, K., Wake, H., et al. (2018). Microglia mediate non-cell-autonomous cell death of retinal ganglion cells. Glia 66, 2366–2384. doi: 10.1002/glia.23475
Tay, T. L., Mai, D., Dautzenberg, J., Fernandez-Klett, F., Lin, G., and Sagar, et al. (2017). A new fate mapping system reveals context-dependent random or clonal expansion of microglia. Nat. Neurosci. 20, 793–803. doi: 10.1038/nn.4547
Thion, M. S., Ginhoux, F., and Garel, S. (2018a). Microglia and early brain development: An intimate journey. Science 362, 185–189. doi: 10.1126/science.aat0474
Thion, M. S., Low, D., Silvin, A., Chen, J., Grisel, P., Schulte-Schrepping, J., et al. (2018b). Microbiome influences prenatal and adult microglia in a sex-specific manner. Cell 172, 500–516.e16. doi: 10.1016/j.cell.2017.11.042
Thion, M. S., Mosser, C. A., Ferezou, I., Grisel, P., Baptista, S., Low, D., et al. (2019). Biphasic impact of prenatal inflammation and macrophage depletion on the wiring of neocortical inhibitory circuits. Cell Rep. 28, 1119–1126.e14. doi: 10.1016/j.celrep.2019.06.086
Utz, S. G., See, P., Mildenberger, W., Thion, M. S., Silvin, A., Lutz, M., et al. (2020). Early fate defines microglia and non-parenchymal brain macrophage development. Cell 181, 557–573.e18. doi: 10.1016/j.cell.2020.03.021
Van Hove, H., Martens, L., Scheyltjens, I., De Vlaminck, K., Pombo Antunes, A. R., De Prijck, S., et al. (2019). A single-cell atlas of mouse brain macrophages reveals unique transcriptional identities shaped by ontogeny and tissue environment. Nat. Neurosci. 22, 1021–1035. doi: 10.1038/s41593-019-0393-4
VanRyzin, J. W., Marquardt, A. E., Argue, K. J., Vecchiarelli, H. A., Ashton, S. E., Arambula, S. E., et al. (2019). Microglial phagocytosis of newborn cells is induced by endocannabinoids and sculpts sex differences in juvenile rat social play. Neuron 102, 435–449.e6. doi: 10.1016/j.neuron.2019.02.006
Verney, C., Monier, A., Fallet-Bianco, C., and Gressens, P. (2010). Early microglial colonization of the human forebrain and possible involvement in periventricular white-matter injury of preterm infants. J. Anat. 217, 436–448. doi: 10.1111/j.1469-7580.2010.01245.x
Villa, A., Gelosa, P., Castiglioni, L., Cimino, M., Rizzi, N., Pepe, G., et al. (2018). Sex-specific features of microglia from adult mice. Cell Rep. 23, 3501–3511. doi: 10.1016/j.celrep.2018.05.048
Wake, H., Moorhouse, A. J., Jinno, S., Kohsaka, S., and Nabekura, J. (2009). Resting microglia directly monitor the functional state of synapses in vivo and determine the fate of ischemic terminals. J. Neurosci. 29, 3974–3980. doi: 10.1523/JNEUROSCI.4363-08.2009
Wang, Y., Szretter, K. J., Vermi, W., Gilfillan, S., Rossini, C., Cella, M., et al. (2012). IL-34 is a tissue-restricted ligand of CSF1R required for the development of Langerhans cells and microglia. Nat. Immunol. 13, 753–760. doi: 10.1038/ni.2360
Wlodarczyk, A., Holtman, I. R., Krueger, M., Yogev, N., Bruttger, J., Khorooshi, R., et al. (2017). A novel microglial subset plays a key role in myelinogenesis in developing brain. EMBO J. 36, 3292–3308. doi: 10.15252/embj.201696056
Xu, J., Zhu, L., He, S., Wu, Y., Jin, W., Yu, T., et al. (2015). Temporal-spatial resolution fate mapping reveals distinct origins for embryonic and adult microglia in zebrafish. Dev. Cell 34, 632–641. doi: 10.1016/j.devcel.2015.08.018
Yona, S., Kim, K. W., Wolf, Y., Mildner, A., Varol, D., Breker, M., et al. (2013). Fate mapping reveals origins and dynamics of monocytes and tissue macrophages under homeostasis. Immunity 38, 79–91. doi: 10.1016/j.immuni.2012.12.001
Keywords: microglia, brain, development, models, tools
Citation: Bridlance C and Thion MS (2023) Multifaceted microglia during brain development: Models and tools. Front. Neurosci. 17:1125729. doi: 10.3389/fnins.2023.1125729
Received: 16 December 2022; Accepted: 24 February 2023;
Published: 23 March 2023.
Edited by:
Subashika Govindan, DBT/Wellcome Trust India Alliance, IndiaReviewed by:
Deborah R. Winter, Northwestern University, United StatesKathryn M. Lenz, The Ohio State University, United States
Copyright © 2023 Bridlance and Thion. This is an open-access article distributed under the terms of the Creative Commons Attribution License (CC BY). The use, distribution or reproduction in other forums is permitted, provided the original author(s) and the copyright owner(s) are credited and that the original publication in this journal is cited, in accordance with accepted academic practice. No use, distribution or reproduction is permitted which does not comply with these terms.
*Correspondence: Morgane Sonia Thion, dGhpb25AYmlvbG9naWUuZW5zLmZy