- 1Department of Physiology and Biophysics, University of Illinois at Chicago, Chicago, IL, United States
- 2Department of Neuroscience, Washington University School of Medicine, St. Louis, MO, United States
Despite the fact that sleep deprivation substantially affects the way animals regulate their body temperature, the specific mechanisms behind this phenomenon are not well understood. In both mammals and flies, neural circuits regulating sleep and thermoregulation overlap, suggesting an interdependence that may be relevant for sleep function. To investigate this relationship further, we exposed flies to 12 h of sleep deprivation, or 48 h of sleep fragmentation and evaluated temperature preference in a thermal gradient. Flies exposed to 12 h of sleep deprivation chose warmer temperatures after sleep deprivation. Importantly, sleep fragmentation, which prevents flies from entering deeper stages of sleep, but does not activate sleep homeostatic mechanisms nor induce impairments in short-term memory also resulted in flies choosing warmer temperatures. To identify the underlying neuronal circuits, we used RNAi to knock down the receptor for Pigment dispersing factor, a peptide that influences circadian rhythms, temperature preference and sleep. Expressing UAS-PdfrRNAi in subsets of clock neurons prevented sleep fragmentation from increasing temperature preference. Finally, we evaluated temperature preference after flies had undergone a social jet lag protocol which is known to disrupt clock neurons. In this protocol, flies experience a 3 h light phase delay on Friday followed by a 3 h light advance on Sunday evening. Flies exposed to social jet lag exhibited an increase in temperature preference which persisted for several days. Our findings identify specific clock neurons that are modulated by sleep disruption to increase temperature preference. Moreover, our data indicate that temperature preference may be a more sensitive indicator of sleep disruption than learning and memory.
Introduction
Although the precise function of sleep remains unknown, there is little question that sleep plays an essential role in maintaining the integrity of a large and diverse set of biological systems. In recent years, a main focus of sleep research has been on the relationship between sleep and synaptic plasticity (Stickgold and Walker, 2013; Dissel et al., 2015a; Dissel and Shaw, 2017; Seibt and Frank, 2019; Tononi and Cirelli, 2020; Frank, 2021). Nevertheless, it is important to note that thermoregulation has been implicated in sleep regulation and function from the earliest days of research in the field (De Manaceine, 1894; Kleitman and Doktorsky, 1933; Bentivoglio and Grassi-Zucconi, 1997). Indeed, decades of research have firmly established that sleep and thermoregulation are inextricably intertwined on many levels (Glotzbach and Heller, 1976; Parmeggiani et al., 1983; Szymusiak and McGinty, 1990). Given this intimate interrelationship, it is exciting that recent studies have established that subsets of neural circuits regulating sleep also overlap with circuits regulating thermoregulation (Head et al., 2015; Harding et al., 2018; Szymusiak, 2018; Yadlapalli et al., 2018). However, it is unclear why the same neurons regulate sleep and thermoregulation and whether such overlap is relevant for sleep function.
The interaction between sleep and thermoregulation has clear adaptive value. That is, by coordinating the timing of sleep with daily changes in ambient temperature, animals can avoid extreme conditions and confine waking behaviors to the most optimal times of day (Dillon et al., 2009). Not surprisingly then, circadian mechanisms synergize with sleep and thermoregulatory circuits to regulate behavior. In Drosophila, the clock is comprised of 150 neurons that can be divided into two major groups: (1) Lateral neurons (LNd, sLNvs, lLNvs) and (2) Dorsal neurons (DN1, DN2, DN3; Helfrich-Forster, 2003; Taghert and Shafer, 2006; Ma et al., 2021). These neurons have been the topic of intensive investigation and are known to regulate both sleep and temperature regulation (Hamada et al., 2008; Goda and Hamada, 2019; Shafer and Keene, 2021; Schlichting et al., 2022). For example, DN1s are sleep-promoting, coordinate a temperature preference rhythm and can respond to changes in ambient temperature to control the timing of sleep (Kunst et al., 2014; Head et al., 2015; Guo et al., 2016; Tang et al., 2017; Yadlapalli et al., 2018; Alpert et al., 2020). Although clock neurons are known to regulate temperature preference across the biological day, their precise role in mediating the effects of sleep loss remains unclear.
Historically, sleep deprivation has been used as a powerful tool to evaluate sleep regulation and function (Kleitman, 1963; Rechtschaffen et al., 1989a; Bentivoglio and Grassi-Zucconi, 1997). As mentioned, sleep deprivation was used in the earliest of sleep studies where it was found that sleep loss changed temperature regulation. Importantly, sleep deprivation profoundly effects thermoregulation in rodents and humans (Kleitman and Doktorsky, 1933; Rechtschaffen et al., 1989b; Savourey and Bittel, 1994; Shaw et al., 1997). Indeed, feeling cold is an extremely common experience that people report during sleep deprivation (Romeijn et al., 2012). Rats, like humans, also behave as if they feel cold following sleep loss. In fact, rats immediately increase operant responses for heat during sleep deprivation, indicating that changes in thermoregulation are among the earliest detectable changes induced by sleep loss (Shaw et al., 1997). To determine whether the effects of sleep deprivation on temperature regulation are evolutionarily conserved, we evaluated temperature preference in flies following sleep deprivation and sleep fragmentation.
Methods
Flies
Flies were cultured at 25°C with 50–60% relative humidity and kept on a diet of yeast, dark corn syrup and agar under a 12-h light:12-h dark cycle. Tim-GAL4 (BL #7126); Clk.856-GAL4(BL #93198); Clk4.1 M-GAL4 (BL #36316); Clk4.5F-GAL4 (BL #37526) and UAS-Pdfr-RNAi (BL #42508) were obtained from the Bloomington stock center. per01, Pdfr503, C929-GAL4; and R6-GAL4 were a kind gift from Paul Taghert (Washington University in St. Louis).
Thermal preference
A 30 cm X 2.5 cm aluminum runway was positioned between a hot plate and ice to generate a gradient ranging between 30°C and 17.9°C (Figures 1A,B). Clear Plexiglas walls and ceiling were coated with Fluon (Fisher Scientific #NC0533515) to prevent flies from avoiding contact with the runway by walking on the walls. The chamber was allowed to reach equilibrium for 10 min and the temperature range was verified using an infrared thermometer (Fisher Scientific, 15–077-968; Figure 1C).Thermal preference was assessed in a room at approximately 25°C, a relative humidity of ~50–65% using stable light illumination. Individual flies were then introduced into the chamber for the specified time and their precise temperature was recorded using the infrared thermometer. The temperature range of the thermal gradient was validated between flies. Unless stated otherwise, all experiments were conducted between 2:30–4:30 pm. All experiments were replicated by at least two independent investigators.
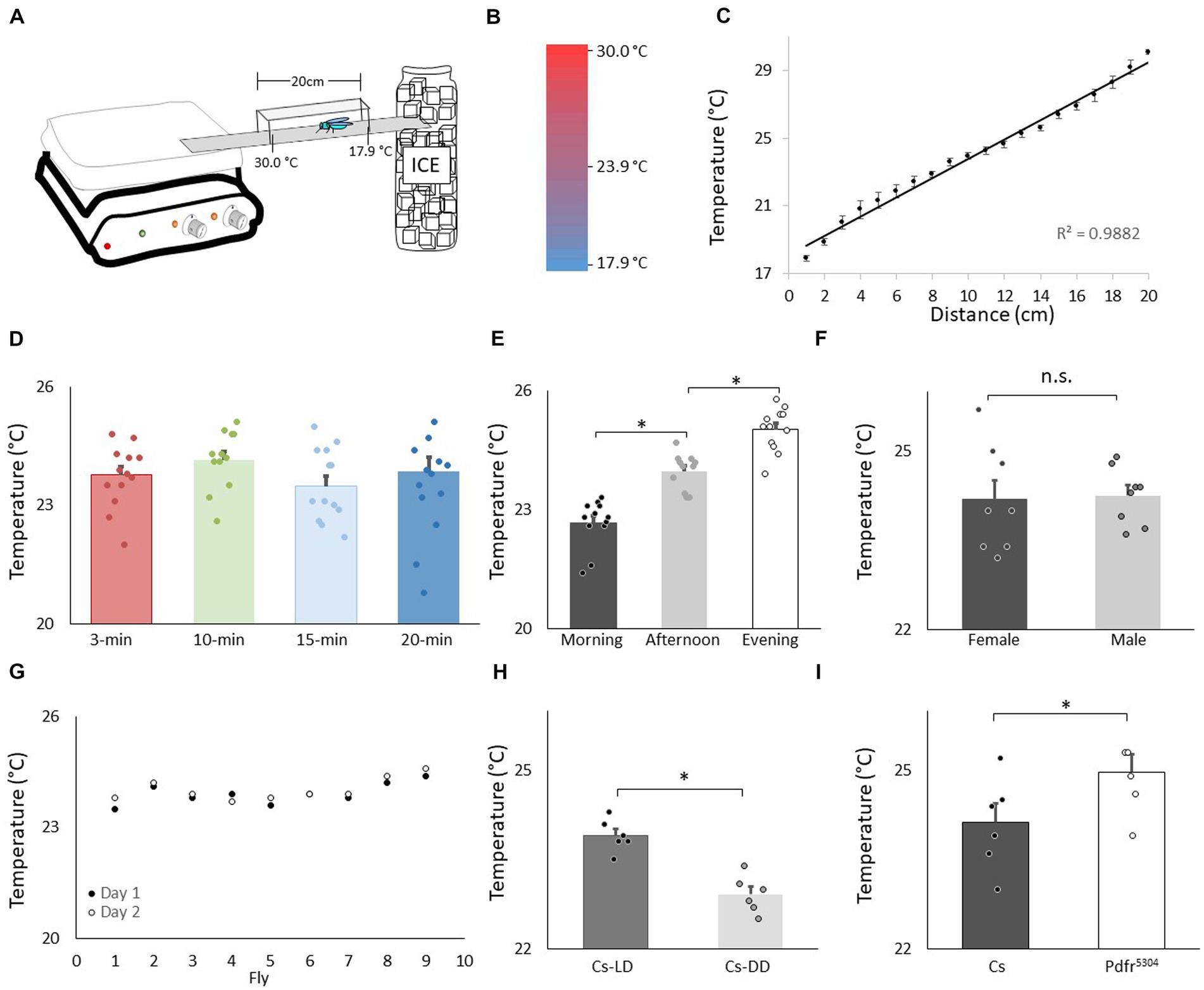
Figure 1. Validation of thermal preference assay. (A) Schematic for thermal preference apparatus. (B) Heat map of the floor surface. (C) Temperature readings in 1 cm increments measured using an infrared Thermometer (4 independent samples were taken at each location). (D) Temperature preference in Canton-S (Cs) flies after being placed into the apparatus for different time intervals (n = 11 flies/interval); One way ANOVA: F(3,43) = 1.36; p = 0.26. (E) Temperature preference for Cs flies tested in the morning 8:30–10:30 am, n = 12; afternoon 2-4 pm, n = 11; evening 6-8 pm; One way ANOVA for condition: F(2,34) = 57.4; p = 2.6E-11 *p < 0.05, corrected Bonferroni test. Power analysis calculates of Cohen’s D of 8.28 between morning and afternoon. (F) Thermal preference in male and female flies (n = 8 flies/condition; p = 0.86, t-test). (G) Temperature preference in individual flies test on two consecutive days. (H) Temperature preference in Cs flies tested in flies maintained on a 12:12 Light: dark schedule (LD) and constant darkness (DD) (n = 6/condition, p = 9.72E-05, t-test). Power analysis calculates of Cohen’s D of 8.08. (I) Temperature preference in Cs and Pdfr5304mutants (n = 6/condition p = 0.03, t-test).
Sleep
Sleep was assessed as previously described (Shaw et al., 2000). Briefly, flies were placed into individual 65 mm tubes containing the same food as they were reared on. All activity was continuously measured through the Trikinetics Drosophila Activity Monitoring System (1Waltham, MA). Locomotor activity was measured in 1-min bins and sleep was defined as periods of quiescence lasting at least 5 min. All sleep experiments were replicated a minimum of two times.
Sleep deprivation/restriction
Sleep deprivation was performed as previously described (Shaw et al., 2002; Seugnet et al., 2008). Briefly, flies were placed into individual 65 mm tubes and the sleep-nullifying apparatus (SNAP) was used to sleep deprive or sleep restrict flies Sleep deprivation was performed for 12 h during the dark phase (lights out to lights on). For sleep deprivation, the SNAP was activated once every 20 s for the duration of the experiment. Sleep restriction was performed for 48 h. The SNAP was activated for 18 s once every 15 min for 48 h, yielding a total of 192 stimuli lasting ~60 min; this regime both reduced and fragmented sleep as previously described (Klose and Shaw, 2019). To determine whether the stimulus induced by the SNAP was able to alter temperature preference flies were continually exposed to the SNAP for an equal number of exposures (Bang-Control). Sleep homeostasis was calculated for each individual as a ratio of the minutes of sleep gained above baseline during the 48 h of recovery divided by the total min of sleep lost during 12 h of sleep deprivation.
Short-term memory
Short-term memory (STM) was assessed by Aversive Phototaxic Suppression (APS) as previously described (Seugnet et al., 2008, 2009). The experimenters were blinded to conditions. In the APS, flies are individually placed in a T-maze and allowed to choose between a lighted and darkened chamber over 16 trials. Flies that do not display phototaxis during the first block of 4 trials are excluded from further analysis (Le Bourg and Buecher, 2002; Seugnet et al., 2009). During 16 trials, flies learn to avoid the lighted chamber that is paired with an aversive stimulus (Filter paper is wetted with 10-1M quinine hydrochloride). The performance index is calculated as the percentage of times the fly chooses the dark vial during the last 4 trials of the 16-trial test. In the absence of quinine, where no learning is possible, it is common to observe flies choosing the dark vial once during the last 4 trials in Block 4 (Seugnet et al., 2009). In contrast, flies never choose the dark vial 2 or more times during Block 4 in the absence of quinine (Seugnet et al., 2009). Thus, short term memory is defined as two or more photonegative choices in Block 4. For short term memory experiments following a 12 h sleep deprivation, the deprivation continued until evaluation by the APS. Power analysis using G*Power calculates a Cohen’s d of 1.8 and indicates that 8 flies/group are needed to obtain statistical differences (Seugnet et al., 2009).
Statistics
All comparisons were done using a Student’s T-test or, if appropriate, ANOVA and subsequent planned comparisons using modified Bonferroni test unless otherwise stated. Note that a significant omnibus-F is not a requirement for conducting planned comparisons (Keppel, 1982). All statistically different groups are defined as *p < 0.05.
Results
Validation of thermal preference
We evaluated temperature preference in adult Canton-S (Cs) flies using a slightly modified protocol from earlier studies (Sayeed and Benzer, 1996; Hamada et al., 2008). A schematic of the apparatus is shown in Figure 1A. A heat map of the runway floor is shown in Figure 1B. The temperature along the gradient, was measured using an infrared thermometer and is shown in Figure 1C. Importantly, the temperature, was stable, reproducible, and linear, with a slope of 0.6°C/cm, as previously described (Sayeed and Benzer, 1996). Previous studies have evaluated thermal preference in groups of 10–30 flies that are monitored for 20–30 min (Tang et al., 2017; Hague et al., 2020; Ito and Awasaki, 2022). However, we prefer studying individual flies in which (1) the sleep history of that individual has been well characterized, and (2) the individual fly can be retrieved at the end of the assay and their behavior can be further evaluated (Seugnet et al., 2009; Dissel et al., 2015b). Interestingly, when we evaluated individual flies, it appeared as if they settled down and chose a preferred temperature much sooner than 20–30 min. Thus, we examined temperature preference in individual flies in 3 min, 10 min, 15 min and 20 min intervals. As seen in Figure 1D, temperature preference did not change across intervals ranging from 3 to 20 min when tested individually. It is worth noting that the temperature preference of individual flies defined using our protocol closely matches that reported by Sayeed and Benzer (1996).
Evaluating temperature preference in individual flies for 3 min differs from previous studies. Thus, we asked whether we could replicate published findings with our approach. Previous studies indicate that flies display a daily temperature preference rhythm in which they select warmer temperatures across the light period (Kaneko et al., 2012). As seen in Figure 1E, when assessed after 3 min, individual flies select progressively warmer temperatures as the day progresses. Interestingly, previous studies indicate that temperature preference is similar between male and female flies run in groups. As seen in Figure 1F, no sexual dimorphisms were observed in our temperature preference assay when flies were assessed individually. To determine whether the temperature preference of an individual fly was stable, we examined temperature preference in individual flies over two successive day. As seen in Figure 1G, temperature preference is stable in an individual fly across days. A previous report indicates that flies select cooler temperature in the dark compared to siblings maintained in the light (Head et al., 2015). We find similar results using individual flies (Figure 1H). Finally, mutants for the receptor for the Pigment dispersing factor receptor (Pdfr5304) select warmer temperatures than controls; we find similar results when temperature preference is evaluated for 3 min in individual flies (Figure 1I). Together these data indicate that monitoring thermal preference in individual flies for 3 min accurately identifies thermal preferences and replicates published studies even when using a slightly different protocol.
Sleep deprivation increase temperature preference
To determine the effects of sleep loss on temperature preference, Cs flies were exposed to 12 h of sleep deprivation. As can be seen in Figures 2A,B, sleep deprivation was effective in keeping flies awake and Cs flies showed a typical sleep rebound (Shaw et al., 2002). Importantly, sleep deprived flies selected warmer temperatures in the thermal gradient compared to their untreated controls when tested between in the morning (8:30–10:30 am; Figure 2C). Flies also selected warmer temperatures than controls when sleep deprivation was extended into the afternoon and tested for thermal preference between 2:30–4:30 pm (25.4 ± 0.12°C vs. 23.8 ± 0.08°C, respectively, p < 0.05). We have previously shown that sleep deprivation disrupts short-term memory and that these memory deficits are completely restored after only 2 h of recovery sleep (Seugnet et al., 2008). Thus, we asked how long it would take for fly’s temperature preference to return to baseline. An independent cohort of flies was sleep deprived for 12 h during their primary sleep period. The effectiveness of the sleep deprivation is shown in Figure 2D. Importantly, siblings that were allowed to recover showed sleep rebound (Figure 2E). As seen in Figure 2F, sleep deprivation resulted in flies selecting warmer temperatures replicating the data shown in Figure 2C. Similarly to what we have observed for short-term memory, 2 h of recovery sleep was sufficient to return temperature preference to baseline levels (Figure 2F). Thus, sleep deprivation increases temperature preference in flies as it does in mammals.
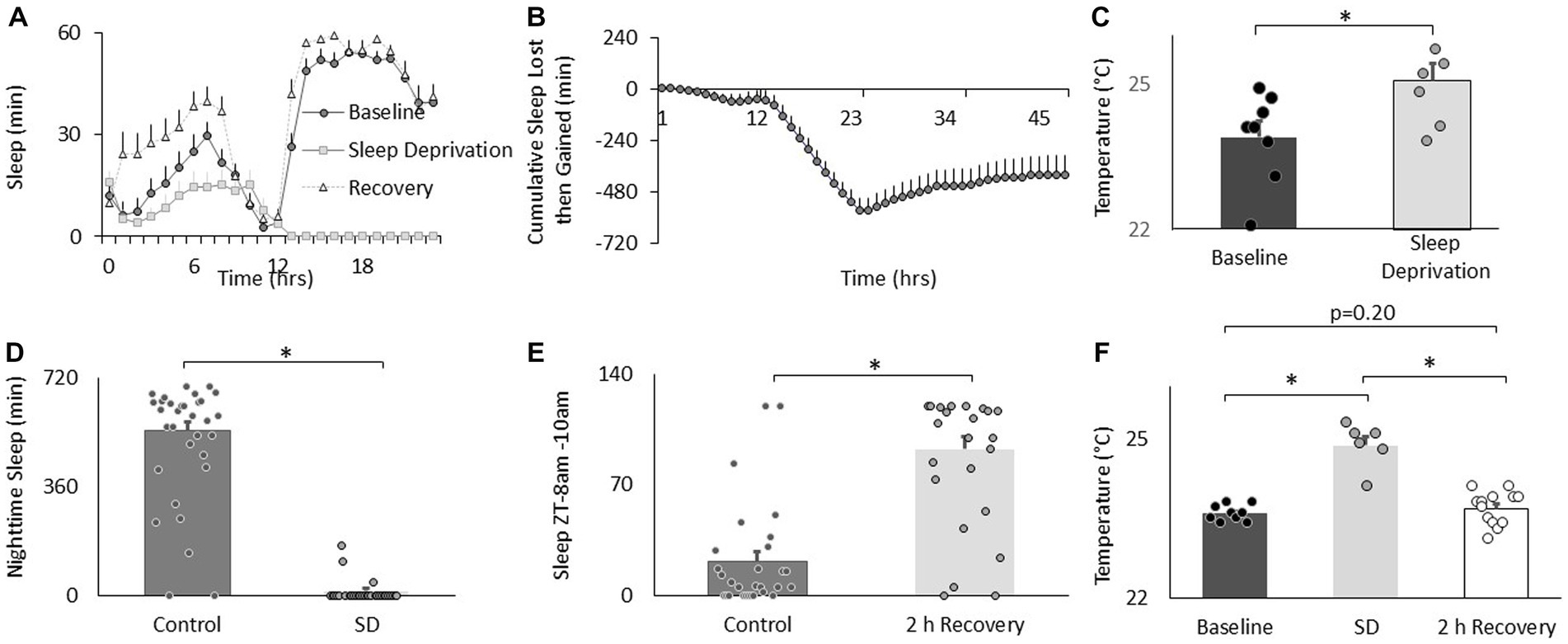
Figure 2. Sleep deprivation increases thermal preference. (A) Sleep in min/h in Cs flies during baseline, sleep deprivation and recovery (n = 16 flies). (B) Cumulative sleep lost then gained plot. (C) Temperature preference is increased following 12 h of sleep deprivation (n = 7) compared to baseline (n = 8) (p = 0.01, t-test). (D) Nighttime sleep in untreated controls (n = 30) compared to sleep deprived siblings (n = 20; p = 2.40E-22, t-test). (E) Sleep in untreated controls (n = 29) compared to sleep deprived flies during 8-10 am 2 h sleep recovery period (n = 20) and (p = 6.88E-10, t-test). (F) Thermal preference in baseline (n = 9), sleep deprivation (n = 7), and sleep deprivation after 2 h of recovery sleep flies (One way ANOVA:F(2,27) = 39.9; p = 1.62E-08).
Sleep fragmentation increase temperature preference
We have validated an ethologically relevant sleep fragmentation protocol in flies that can disrupt sleep for extended periods (Klose and Shaw, 2019). The protocol is based upon observations that conditions that support brain plasticity increase daytime sleep-bout duration from ~10 min to ~25 min (Seugnet et al., 2008, 2011; Donlea et al., 2011, 2014; Dissel et al., 2015a). These data suggest that there is a minimum amount of sleep consolidation that is required for sleep to fulfil its functions. Thus, in our sleep fragmentation protocol, flies are kept awake for 1 min every 15 min to limit their ability to obtain restorative sleep. Sleep fragmentation is achieved using the sleep nullifying apparatus as previously described(Klose and Shaw, 2019). As seen in Figures 3A–C, sleep fragmentation modestly disrupts sleep, is effective in preventing long sleep-bouts and does not robustly activate sleep homeostasis. Importantly, sleep fragmentation did not disrupt short-term memory as assessed using Aversive Phototaxic Suppression (APS; Figure 3D). In the APS, flies are individually placed in a T-maze and must learn to avoid a lighted chamber that is paired with an aversive stimulus (quinine/ humidity; Seugnet et al., 2008). The performance index is calculated as the percentage of times the fly chooses the dark vial during the last 4 trials of the 16 trial test and short-term memory is defined as selecting the dark vial on 2 or more occasions during Block 4 (Dissel et al., 2015a,b,c). Despite having a normal short-term memory, flies exposed to 48 h of sleep fragmentation selected warmer temperatures in the thermal gradient compared to untreated siblings when tested in the afternoon (2:30–4:30 pm; Figure 3E). Temperature preference was also increased when flies were exposed to 48 h of sleep fragmentation and tested in the morning (8:30–10:30 am; 25.06 ± 0.06°C vs. 23.4 ± 0.12°C, p < 0.05). To exclude the possibility that the stimulus used to keep the animal awake altered temperature preference independently from sleep fragmentation, we exposed flies to the same number of stimuli that accrued during sleep fragmentation but in a consolidated block of 60 min during the light period and immediately evaluated temperature preference. As seen in Figure 3F, no changes in temperature preference were observed. Finally, we examined temperature preference during 2 h, 6 h and 24 h recovery from 48 h of sleep fragmentation. To facilitate comparisons across groups, the timing of recovery was staggered such that temperature preference was evaluated in all groups between 2:30 pm and 4:30 pm. Thus, sleep fragmentation ended at 12:30 pm and 8:30 am for the 2 h and 6 h recovery groups, respectively. The 2:30–4:30 pm time window is ideal since flies can choose either lower or higher temperatures at this time and thereby minimizes the impact of ceiling and floor effects present during the morning and evening time points (Figure 1E; Head et al., 2015). As seen in Figure 3G, temperature preference remained elevated even after 6 h of recovery. Thus, sleep fragmentation increases temperature preference in a thermal gradient and these effects persist for several hours.
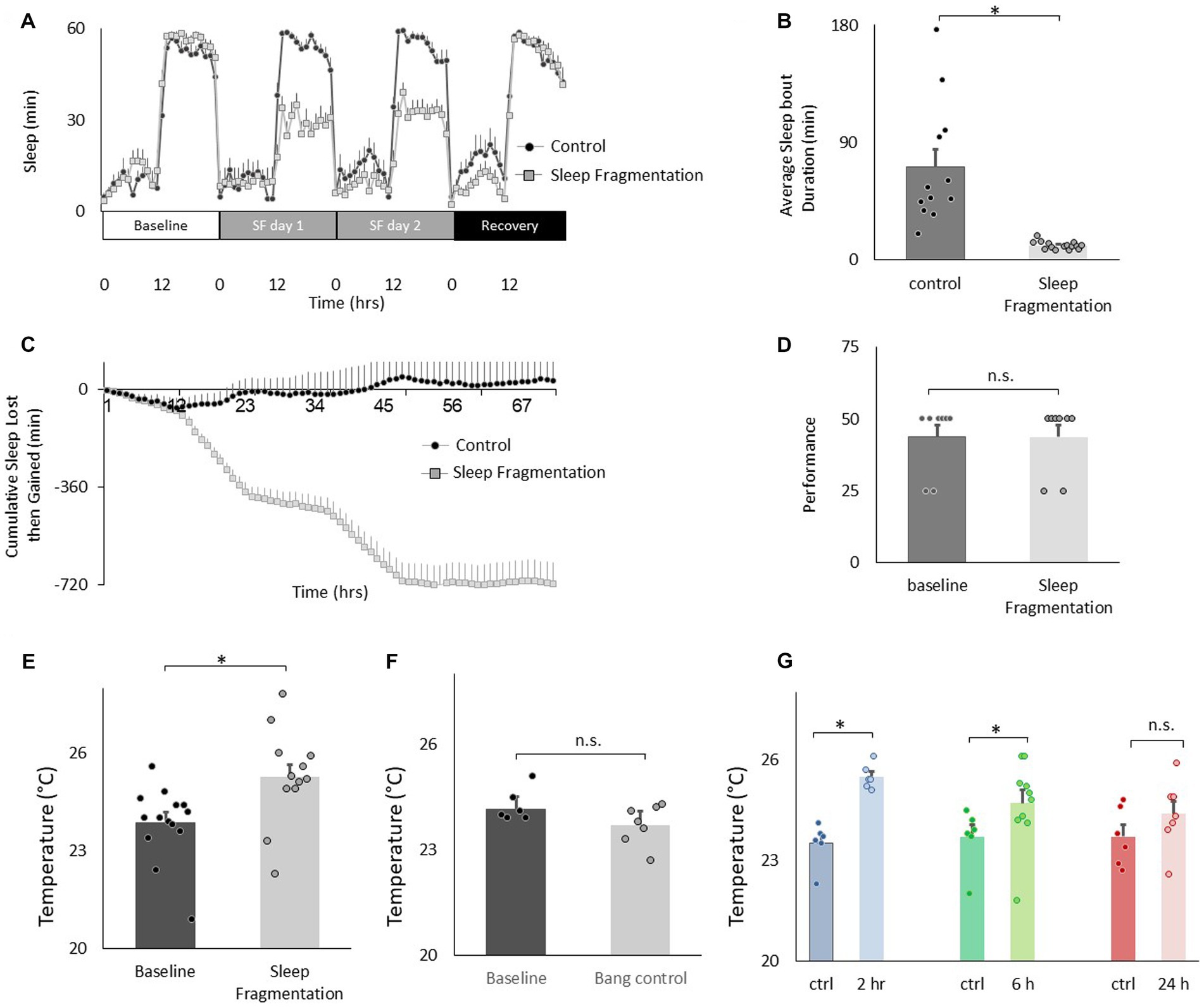
Figure 3. Sleep fragmentation increases thermal preference. (A) Sleep (min/h) in untreated controls and sleep fragmented siblings (n = 12 and16 flies/condition). (B) Average sleep bout duration during the night in untreated controls and sleep fragmented siblings (n = 12 and 16 flies/condition, p = 1.89E-05, t-test). (C) Cumulative sleep lost then gained plot in untreated controls and their sleep fragmented siblings. (D) Short-term memory, as assessed using Aversive Phototaxic Suppression, is not impaired by sleep fragmentation (n = 8 flies/condition, p = 0.5, t-test). (E). Sleep fragmentation increases thermal preference (n = 12 and 14 flies/condition, p = 0.004, t-test). (F) Temperature preference was not changed when flies were exposed to the same number of stimuli as sleep fragmented siblings but did not lose sleep (n = 6 and 7 flies/condition, p = 0.065 t-test). (G) Following sleep fragmentation, flies were allowed to recover for 2 h, 6 h and 24 h. Thermal preference remained elevated for 2 h and 6 h compared to untreated siblings. One way ANOVA F(3,40) = 7.3; p = 0.0005,*p < 0.05, corrected Bonferroni test.
Pigment dispersing factor receptor modulates temperature preference following sleep fragmentation
Recent studies highlight the importance of the clock circuitry in regulating light dependent temperature preference (Head et al., 2015). Specifically, light dependent temperature preference was not dependent upon the canonical clock gene period (per01) but was modulated in Pigment dispersing factor receptor (Pdfr5403) mutants. To evaluate whether these genes also play a role in the changes in temperature preference seen after sleep fragmentation, per01 and Pdfr5403 mutants were exposed to 48 h of sleep fragmentation and evaluated in the thermal gradient between 2:30 and 4:30 pm as described above. As seen in Figures 4A,B, sleep fragmentation modestly disrupted sleep in per01 mutants without altering sleep homeostasis. Importantly, per01 mutants selected warmer temperature in the thermal gradient indicating that the sleep-fragmentation induced changes in temperature preference do not require the molecular clock (Figure 4C). As seen in Figures 4D,E, sleep fragmentation modestly disrupted sleep in Pdfr5403 mutants without altering sleep homeostasis. However, Pdfr5403 mutants did not select warmer temperatures in the thermal gradient (Figure 4F), suggesting that Pdfr5403 may play a role in mediating the effects of sleep fragmentation on temperature preference.
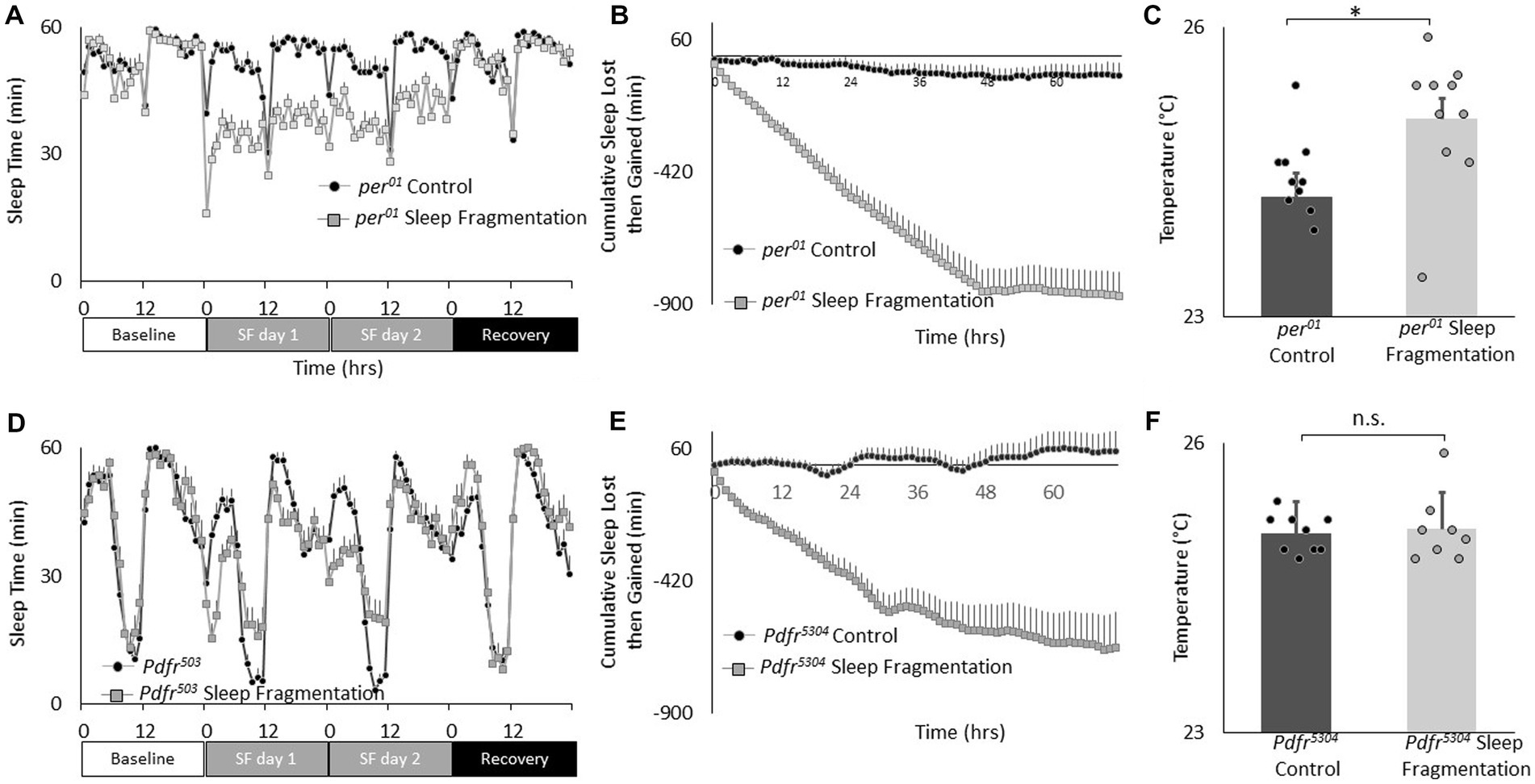
Figure 4. Sleep fragmentation alters thermal preference in Pdfr5304 mutants. (A) Sleep (min/h) in per01 mutants during baseline, sleep fragmentation and recovery (n = 16 flies/condition). (B) Cumulative sleep lost then gained plot in sleep fragmented per01 mutants. (C) Temperature preference is increased in per01 following sleep fragmentation (n = 11 and 10 flies/condition p = 0. 0.01, t-test). (D) Sleep in min/h in Pdfr5304 mutants during baseline, sleep fragmentation and recovery (n = 16 flies/condition). (E) Cumulative sleep lost then gained plot in sleep fragmented Pdfr5304 mutants. (F) Temperature preference is unchanged in Pdfr5304 following sleep fragmentation (n = 7 flies/condition p = 0.47, t-test).
Clock neurons play a role in temperature preference following sleep fragmentation
The clock is comprised of 150 neurons that can be divided into two major groups. (1) Lateral neurons (LNd, sLNvs, lLNvs) and (2) Dorsal neurons (DN1, DN2, DN3; Helfrich-Forster, 2003; Taghert and Shafer, 2006; Ma et al., 2021). Given the role that clock neurons play in regulating temperature preference (Head et al., 2015; Goda et al., 2016), we evaluated their role in mediating the effects of sleep fragmentation. To begin we used RNAi to knock down Pdfr using the pan-clock drivers, tim-GAL4 and Clk856-GAL4. As seen in Figure 5A, sleep fragmentation produced modest changes in sleep time in all genotypes. Importantly, sleep fragmentation increased temperature preference in the thermal gradient for tim-GAL4/+, UAS-PdfrRNAi/+, Clk856-GAL4/+ parental controls. However, sleep fragmentation did not alter temperature preference in the thermal gradient in either tim-GAL4/+ > UAS-PdfrRNAi/+ or Clk856-GAL4/+ > UAS-PdfrRNAi/+ experimental lines (Figure 5B). Together these data indicate that the clock circuitry can influence the impact of sleep fragmentation on temperature preference via the Pdfr.
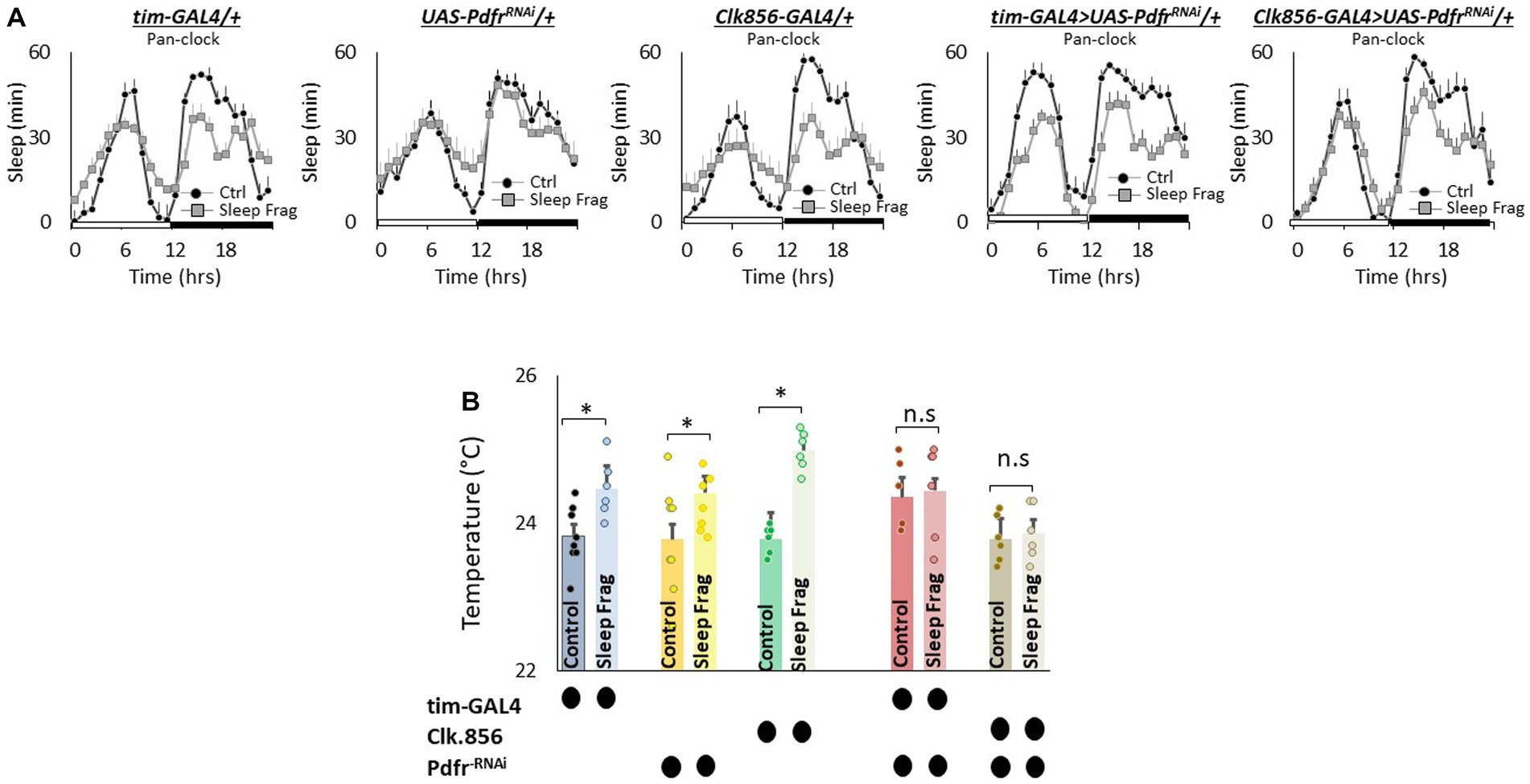
Figure 5. Knocking Down Pdfr in Clock Neurons Prevents Changes in Thermal Preference Induced by Sleep Fragmentation. (A) Sleep in min/h in tim-GAL4/+, UAS-PdfrRNAi/+, Clk856-GAL4/+, tim-GAL4/+ > UAS-PdfrRNAi/+and Clk856-GAL4/+ > UAS-PdfrRNAi/+during baseline (Ctrl) and sleep fragmentation (n = 16 flies/condition). (B) Sleep fragmentation increases temperature preference tim-GAL4/+, UAS-PdfrRNAi/+, Clk856-GAL4/+ parental controls. Temperature preference in tim-GAL4/+ > UAS-PdfrRNAi/+and Clk856-GAL4/+ > UAS-PdfrRNAi/+ is not altered by sleep fragmentation; A 5(genotype) X 2(condition)ANOVA F(4,57) = 2.92; p = 0.029,*p < 0.05, corrected Bonferroni test. Temperature preference in untreated experimental lines (Ctrl) do not differ from parental controls, One-way ANOVA for genotype F(4,34) = 1.68; p = 0.17; corrected Bonferroni test for tim-GAL4/+ > UAS-PdfrRNAi/+ p = 0.17 and p = 0.24 and Clk856-GAL4/+ > UAS-PdfrRNAi/+ p = 1 and p = 0.80.
We next evaluated the role of Pdfr using GAL4 lines that express in DN1 neurons. DN1 neurons play a role in sleep regulation and modulate light-dependent temperature preference (Kunst et al., 2014; Guo et al., 2016; Lamaze et al., 2018; Schlichting et al., 2022). We used RNAi to knock down Pdfr using Clk4.1 M-GAL4 and Clk4.5F-GAL4. As seen in Figure 6A, sleep fragmentation produced modest changes in sleep time in all genotypes. Interestingly, sleep fragmentation increased temperature preference in the thermal gradient for Clk4.1 M-GAL4/+, UAS-PdfrRNAi/+ and Clk4.5F-GAL4/+ parental controls. However, sleep fragmentation did not alter temperature preference in the thermal gradient in either Clk4.1 M-GAL4/+ > UAS-PdfrRNAi/+ or Clk4.5F-GAL4/+ > UAS-PdfrRNAi/+ experimental lines (Figure 6B). These data indicate that the DN1s neurons can influence the impact of sleep fragmentation on temperature preference via the Pdfr.
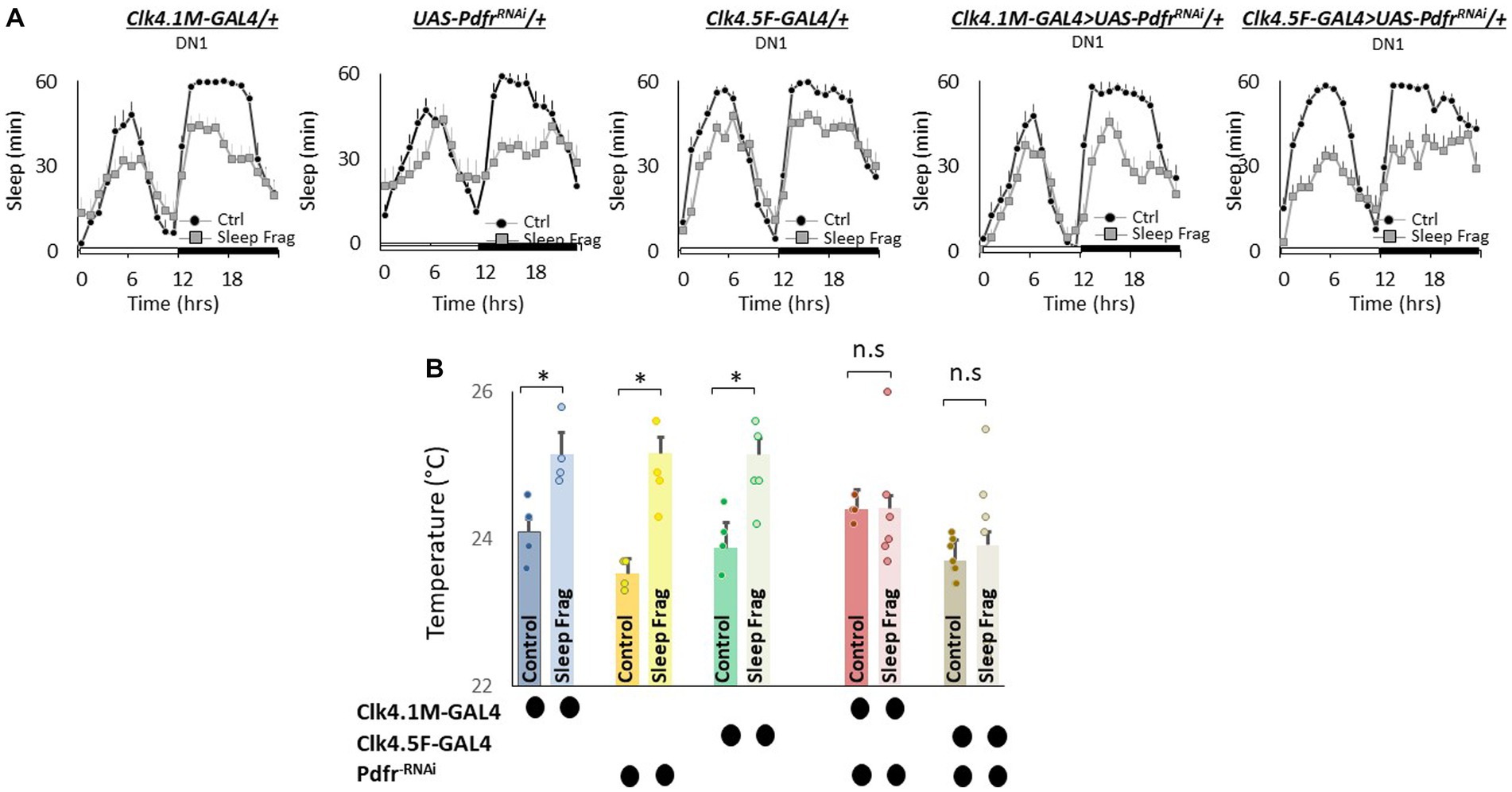
Figure 6. Knocking Down Pdfr in dorsal Clock Neurons (DN1s) neurons Prevents Changes in Thermal Preference induced by Sleep Fragmentation. (A) Sleep in min/h in Clk4.1 M-GAL4/+, UAS-PdfrRNAi/+, Clk4.F5-GAL4/+, Clk4.1 M/+ > UAS-PdfrRNAi/+and Clk4.5F-GAL4/+ > UAS-PdfrRNAi/+during baseline (Ctrl) and sleep fragmentation (n = 16 flies/condition). (B) Sleep fragmentation increases temperature preference in Clk4.1-GAL4/+, UAS-PdfrRNAi/+, Clk4.F5-GAL4/+ parental controls. Temperature preference in Clk4.1-GAL4/+ > UAS-PdfrRNAi/+and Clk4.F5-GAL4/+ > UAS-PdfrRNAi/+ is not altered by sleep fragmentation; A 5(genotype) X 2(condition) ANOVA F(4,45) = 3.02; p = 0.021,*p < 0.05, corrected Bonferroni test. Temperature preference in untreated experimental lines (Ctrl) do not differ from both parental controls, One-way ANOVA for genotype F(4,27) = 5.2; p = 0.003; corrected Bonferroni test for Clk4.1-GAL4/+ > UAS-PdfrRNAi/+; p = 0.24 and p = 0.24 and Clk4.F5-GAL4/+ > UAS-PdfrRNAi/+; p = 0.24 and p = 0.08.
Finally, we evaluated the role of the small (sLNvs) and large ventrolateral neurons (lLNvs) in modulating temperature preference after sleep fragmentation. The sLNvs and lLNvs play a role in regulating sleep and waking (Nitabach et al., 2006; Parisky et al., 2008; Sheeba et al., 2008; Chung et al., 2009; Shang et al., 2013). However, the relationship between the sLNvs and lLNvs and temperature preference is more complicated. Initial studies indicated that neither the sLNvs nor the lLNvs influence daytime temperature preference rhythms or light-dependent temperature preference (Kaneko et al., 2012; Head et al., 2015). However, Tang and colleagues report that the sLNvs play a role in setting preferred temperature before dawn (Tang et al., 2017). Interestingly, while pigment dispersing factor (pdf) is expressed in both the sLNvs and lLNvs; the Pdfr is only expressed in the sLNvs in unperturbed adult flies (Shafer et al., 2008; Im and Taghert, 2010). However, the Pdfr is re-expressed in the lLNvs of adult flies after sleep deprivation, sleep fragmentation and starvation (Klose and Shaw, 2019). Since Pdfr is only expressed in the lLNvs of adult flies after a perturbation, its role in regulating temperature preference remains unexplored. Thus, we used UAS-PdfrRNAi to knock down the Pdfr in sLNvs (R6-GAL4) and lLNvs (c929-GAL4). As seen in Figure 7A, sleep fragmentation produced modest changes in sleep time in all genotypes. Interestingly, sleep fragmentation increased temperature preference in the thermal gradient for R6-GAL4/+, UAS-PdfrRNAi/+ and c929-GAL4/+ parental controls (Figure 7B). However, sleep fragmentation did not alter temperature preference in the thermal gradient in either R6-GAL4/+ > UAS-PdfrRNAi/+ or c929-GAL4/+ > UAS-PdfrRNAi/+ experimental lines. These data indicate that both the sLNvs and the lLNvs can influence the impact of sleep fragmentation on temperature preference via the Pdfr.
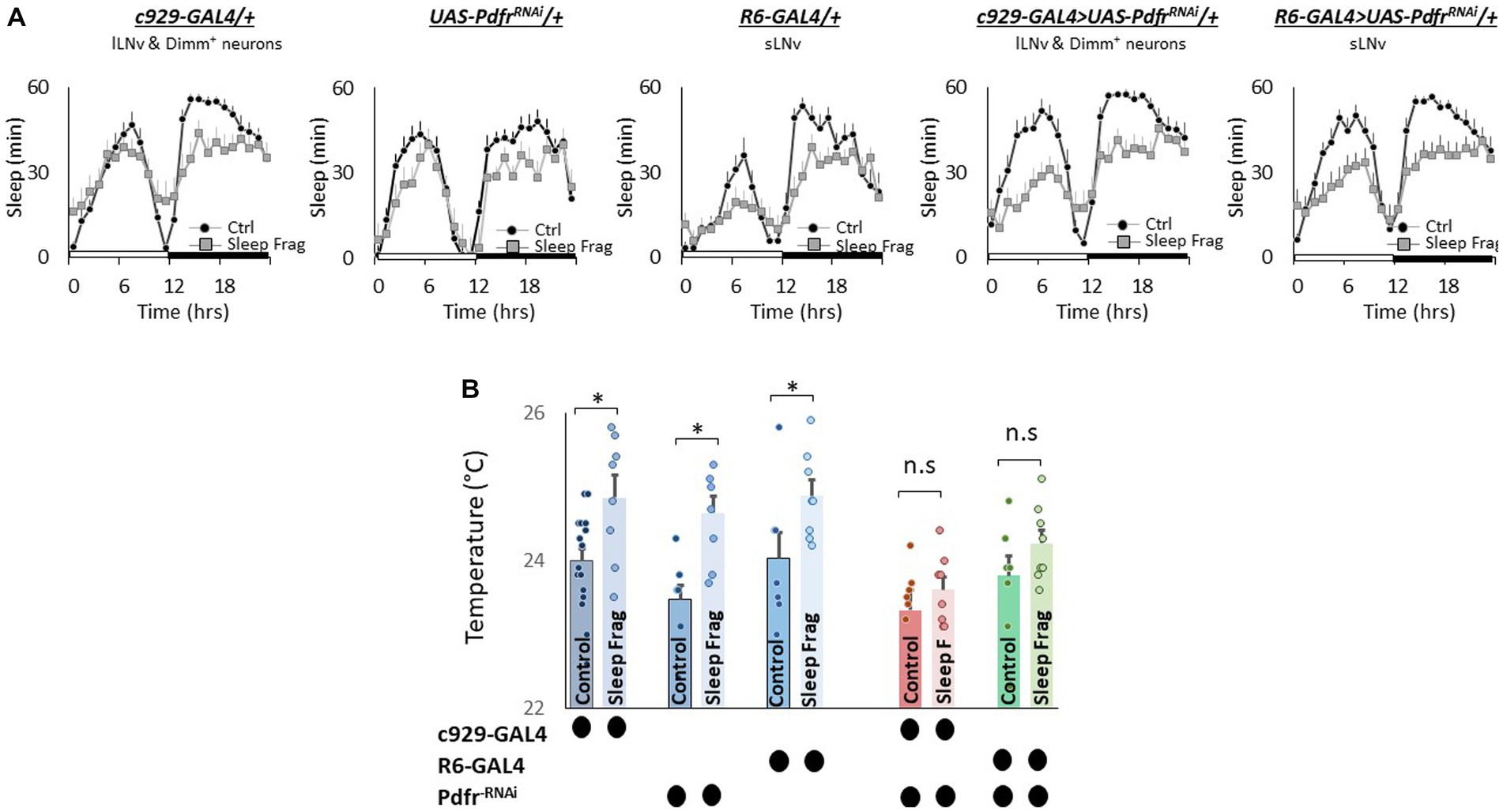
Figure 7. Knocking Down Pdfr in ventral Lateral (LNvs) neurons Prevents Changes in Thermal Preference Induced by Sleep Fragmentation. (A) Sleep in min/h in c929-GAL4/+, UAS-PdfrRNAi/+, R6-GAL4/+, c929/+ > UAS-PdfrRNAi/+and R6-GAL4/+ > UAS-PdfrRNAi/+during baseline (Ctrl) and sleep fragmentation (n = 16 flies/condition). (B) Sleep fragmentation increasses temperature preference c929-GAL4/+, UAS-PdfrRNAi/+, R6-GAL4/+ parental controls. Temperature preference in c929-GAL4/+ > UAS-PdfrRNAi/+ and R6-GAL4/+ > UAS-PdfrRNAi/+ is not altered by sleep fragmentation; A 5(genotype) X 2(condition)ANOVA F(4,77) = 1.12; p = 0.35,*p < 0.05, corrected Bonferroni test. Temperature preference in untreated experimental lines (Ctrl) do not differ from both parental controls, One-way ANOVA for genotype F(4,46) = 1.67; p = 0.17; corrected Bonferroni test for c929-GAL4/+ > UAS-PdfrRNAi/+; p = 0.03 and p = 0.68 and R6-GAL4/+ > UAS-PdfrRNAi/+; p = 0.36 and p = 0.59.
Social jet lag increases temperature preference
Social jet lag is a misalignment between an individual’s internal biological clock and their social schedule. Social jet lag can occur when people wake up early for work during the week and then stay up later and sleep in on the weekends to make up for lost sleep (Juda et al., 2013; Pilz et al., 2018; Reis et al., 2020; Fischer et al., 2021). Social Jet lag can be modeled in Drosophila where it has been shown to dampen rhythmicity in most circadian neurons (Nave et al., 2020). Thus, we explored the effects of social jet lag on temperature preference in Cs flies. To induce social jet lag, the timing of lights-off is delayed by 3 h on Friday night and then shifted back 3 h on Sunday night to mimic a weekend schedule (Figure 8A, arrows). As can be seen in Figure 8A, sleep in flies exposed to Social Jet Lag closely resembles that seen in their age-matched untreated controls beginning on Monday after the light schedule has returned to normal to mimic a typical work week schedule. Indeed, quantification of sleep parameters, including total sleep time and sleep consolidation (average sleep bout duration during the day and average sleep bout duration at night) do not differ between flies exposed to social jet lag and their controls after being placed on a work-week schedule (Figure 8B). To further explore how social jet lag alters sleep, we evaluated metrics designed to measure sleep pressure PDoze and sleep depth PWake (Wiggin et al., 2020). As seen in Figure 8C, social jet lag did not alter either metric compared to untreated siblings. Social Jet lag has been reported to disrupt short-term memory (Nave et al., 2020). We replicate that finding here (Figure 8D). In addition, to memory impairment, we show that flies exposed to social jet lag choose warmer temperatures in a thermal gradient when tested in the afternoon (2:30–4:30 pm; Figure 8D). Flies exposed to social jet lag also select warmer temperatures when tested in the morning (8:30–10:30 am; 23.4 ± 0.24°C vs. 24.2 ± 0.08°C, p < 0.05). Together, these data indicate that social jet lag disrupts both learning and memory and temperature preference.
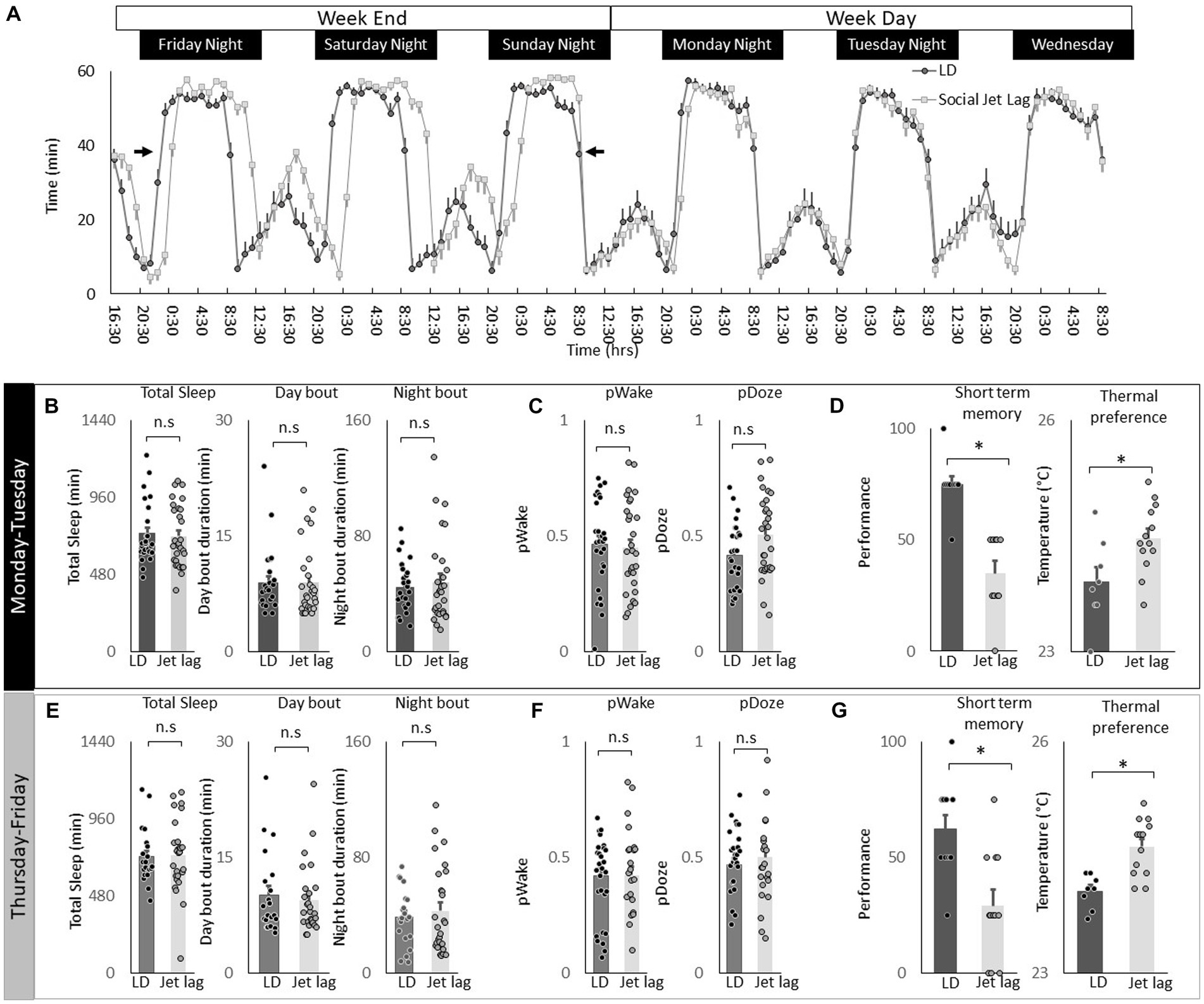
Figure 8. Social Jet Lag increases Thermal Preference. (A) Sleep in min/h in Cs flies maintained on a 12:12 LD schedule and siblings that have been exposed to social jet lag. For social jet lag, the timing of lights out was delayed 3 h on Friday night and then advanced back 3 h on Sunday night (arrows). (B) Social Jet lag did not disrupt total sleep time, or sleep bout duration measured during the day or night compared to untreated siblings (n = 28 and 30 flies/condition, p > 0.05, t-test). Data are presented from Monday morning at lights-on to Tuesday morning at lights-on. (C) Social Jet lag did not disrupt PWake or PDoze (n = 28,30 flies/condition, p > 0.05, t-test). (D) Social jet lag impaired short-term memory compared to untreated siblings (n = 10 flies/condition) *p = 5.63E-06. Flies exposed to social jet lag selected warmer temperatures in the thermal gradient compared to controls (n = 8 and13 flies/condition, *p = 0.01, t-test). (E) Social Jet lag did not disrupt total sleep time, or sleep bout duration measured during the day or night compared to untreated siblings (n = 28 and 30 flies/condition, p > 0.05, t-test). Data are presented from Thursday morning at lights-on to Friday morning at lights-on. (F) Social Jet lag did not disrupt PWake or PDoze (n = 28 and 30 flies/condition, p > 0.05, t-test). (G) Social jet lag impaired short-term memory compared to untreated siblings (n = 12 flies/condition; *p = 0.001, t-test). Flies exposed to social jet lag selected warmer temperatures in the thermal gradient compared to controls (n = 13 and 16 flies. Condition, *p = 0.0007, t-test).
To determine how long the effects of social jet lag might persist, we evaluated sleep, short-term memory and temperature preference on Friday. Recall that the light schedule had been returned to baseline on Sunday evening. As seen in Figures 8E,F measures of sleep time, sleep architecture and sleep depth are not altered by social jet lag compared to untreated controls. Despite normal sleep metrics, social jet lag treated flies shown memory impairments and increases in temperature preference several days after being placed on their typical weekday schedule (Figure 8G). These data indicate that social jet lag results in long-lasting changes to short-term memory as well as temperature preference.
Discussion
Our data indicate that the effects of sleep deprivation on thermoregulation are evolutionarily conserved. In addition, we show that even small disruptions in sleep induced by sleep fragmentation are able to alter thermoregulatory centers. Furthermore, we localize the effects of sleep disruption to subsets of clock neurons that play dual roles in sleep and thermoregulation. Finally, we show that while clock circuits play a role in mediating the effects of sleep disruption on temperature preference, changes in temperature preference do not require a functioning molecular clock.
The success of sleep deprivation as a tool to study sleep function depends upon the ability to monitor relevant outcome variables and their underlying neuronal circuitry. However, sleep deprivation alters a variety of physiological processes including learning and memory, metabolic, immune, digestive, cardiovascular, respiratory, and endocrine systems, to name a few (Rechtschaffen et al., 1989b; Spiegel et al., 1999; Imeri and Opp, 2009). Each of these systems are under complex regulatory control and require specialized equipment and training to evaluate properly. In contrast, temperature preference is an evolutionarily relevant outcome-variable that can be quantified using equipment found in most labs. Importantly, the temperature preference assay is exceedingly robust and the effect sizes that are seen following sleep disruption are notably high. For example, calculating the effects size for observing a difference between control and sleep deprived siblings using short-term memory reveals a Cohen’s D of 1.8, a result seen for other performance metrics in humans (Frey et al., 2004; Seugnet et al., 2009). However, when examining the impact of sleep deprivation or sleep restriction, on temperature preference the Cohen’s D is substantially higher (Cohen’s D > 3). Thus temperature preference is a unique outcome variable that can be evaluated following sleep disruption to address sleep function.
Historically, temperature preference is conducted on groups of 20–30 flies tested together as a group for 30 min. To our surprise, individual flies quickly settled down in the thermal gradient after ~3 min. The temperatures individual flies chose are remarkably similar to the values obtained when flies were evaluated in groups (Sayeed and Benzer, 1996; Hamada et al., 2008). Importantly, we were able to replicate key findings from previously published manuscripts. Furthermore the temperature chosen by a fly was stable across days. The data presented here were collected by two independent investigators indicating that this assay produces consistent, reliable outcomes that are easily reproduced. Together, our results indicate that temperature preference in flies is a robust phenotype that can return similar results even when the protocol is changed modestly between labs.
In contrast to sleep deprivation, sleep fragmentation disrupts sleep consolidation without inducing a strong homeostatic response or deficits in short-term memory (Klose and Shaw, 2019). Nonetheless, sleep fragmentation is not without its consequences. Indeed, we have previously shown that sleep fragmentation can alter the expression of the Pdfr within the clock circuitry of adult flies. In the current study, we show that sleep fragmentation does not alter temperature preference in Pdfr5403 mutants or when Pdfr is knocked down in clock neurons. Within the clock circuitry, subsets of neurons regulate different aspects of temperature preference (Goda and Hamada, 2019). For example, light dependent temperature preference is mediated by DN1s, while body temperature rhythms are modulated by DN2s in concert with the sLNvs (Head et al., 2015; Goda et al., 2016). Surprisingly, expressing PDFR in DN1s restores light dependent temperature preference in Pdfr5304 mutants even though mutants for the neuropeptide, Pigment dispersing factor (pdf01), display normal temperature preference (Head et al., 2015). Flies mutant for Diuretic hormone 31, a putative ligand for the PDFR, also display normal light dependent temperature preference leaving the mechanisms leading to the activation of the PDFR unknown in this context (Head et al., 2015). Given these observations, it is possible that the changes in temperature preference we observe following sleep fragmentation may not be due exclusively to PDF. However, sleep fragmentation impacts additional sets of clock neurons compared to those utilized during light dependent temperature preference. The clock neurons modulated by sleep fragmentation include the lLNvs that only express the PDFR following challenges that increase sleep drive (Klose and Shaw, 2019) as well as the sLNvs. Nonetheless, the finding that pdf01 mutants maintain their ability to modulate light dependent temperature preference indicates that light, and perhaps sleep fragmentation, can alter clock circuitry, and thus temperature preference via recruiting additional neurotransmitters (e.g., glutamate, dopamine) or peptides (e.g., sNPF). Consistent with this hypothesis, ambient temperature feeds back on specific clock neurons to sculpt the timing of sleep and waking across the biological day (Yadlapalli et al., 2018; Alpert et al., 2020; Fan et al., 2022). Together, these observations highlight the interconnectedness of clock neurons and suggest that sleep fragmentation may disrupt temperature preference by altering the balance of activity within the clock circuit via a variety of peptides/ transmitters (Chen et al., 2022; Reinhard et al., 2022; Shafer et al., 2022; Sun et al., 2022).
In humans, social jet lag disrupts circadian rhythms and results in a variety of adverse health outcomes (Foster et al., 2013; Chellappa et al., 2019; Reis et al., 2020). The social jet lag protocol we used was designed to mimic sleep/wake schedules commonly seen in humans (Nave et al., 2020). This protocol dampens rhythmicity in most clock neurons and alters sleep regulation (Nave et al., 2020). We show that social jet lag disrupts short-term memory and increases temperature preference. In addition, we show that the impact of social jet lag are long lasting. That is, while the light schedule is restored on Sunday evening, flies display alterations to short-term memory and temperature preference that persist at least until the following Friday. If flies were maintained on this schedule through the subsequent weekend, as is the case for many humans, they might not fully recover.
We chose to focus on Pdfr as a mediator of sleep loss induced changes to temperature preference given its ability to impact such a diverse set of neurons. Ongoing studies are underway to determine whether the effects of social jet lag are mediated by Pdfr and to identify the underlying circuitry. As noted above, PDF can modulate the timing of behavior by staggering when, during the biological day, neurons display peak activity (Liang et al. 2017). PDF modulates the timing of peak activity both within subsets of neurons in the clock circuit and on their downstream output targets (Liang et al., 2016, 2023). Thus social jet lag has the opportunity to disrupt short-term memory and temperature preference by disrupting a large set of diverse neurons in flies. Social jet lag has a seemingly large reach in humans as well, adversely effecting cardiovascular disease, metabolic disorders and mood. Identifying the mechanisms used by social jet lag to disrupt temperature preference in flies may provide new clues into how social jet lag adversely impacts a variety of physiological systems that are relevant for human health.
It is important to note that mechanisms regulating sleep and waking are plastic such that sleep and wake promoting neurons modulate their response properties to match sleep need with environmental demands (Rattenborg et al., 2004; Lyamin et al., 2005; Keene et al., 2010; Thimgan et al., 2010; Lesku et al., 2012; Gravett et al., 2017; Machado et al., 2017). For example, the wake-promoting lLNvs do not typically express the Pdfr in healthy adults (Shafer et al., 2008). However, the Pdfr is re-expressed in the lLNvs during conditions of high sleep drive, and the presence of the Pdfr in the lLNvs plays an important for maintaining adaptive behavior (Klose and Shaw, 2019). Similarly, the Dopamine 1-like receptor 1 (Dop1R1) is not expressed in the sleep-promoting dorsal Fan Shaped Body neurons in healthy adults (Pimentel et al., 2016). However, following starvation or time restricted feeding the Dop1R1 is recruited to the dorsal Fan Shaped body to provide additional inhibitory tone to sleep promoting neurons (Dissel et al., 2022). Finally, a sleep circuit that is primarily active during a narrow developmental time-window can be reactivated in adults when flight is impaired (Melnattur et al., 2020). These findings imply that it might be important to re-examine signaling pathways that have been previously ruled out as relevant to sleep regulation in order to better understand the effects of sleep disturbance on temperature preference. Nonetheless, understanding sleep plasticity will be important for fully understanding sleep regulation and function, and may provide crucial insight into elucidating the molecular mechanisms induced by sleep deprivation and sleep fragmentation.
The function of sleep has puzzled scientists for decades. We propose that the evolutionary origins of sleep may be better understood by determining how sleep deprivation affects neurons controlling thermoregulation in the poikilothermic fly. Both euthermic and poikilothermic animals use behavioral thermoregulation to accomplish similar goals. For example, both poikilothermic and euthermic animals can use behavior to adjust body temperature and thus conserve energy. Similarly, both poikilothermic and euthermic animals can use behavioral thermoregulation to optimize their ability to avoid predators, engage in reproductive behavior, or select the best environment for sleep (Goda and Hamada, 2019; Harding et al., 2019). Indeed, ambient temperature has a profound effect on sleep in natural environments (Yetish et al., 2015; Gravett et al., 2017). Moreover, animals are known to engage in a number of behavioral adaptations that allow them to sleep in suboptimal thermal environments (Rakotomalala et al., 2017; Campbell et al., 2018; Harding et al., 2019; Reinhardt et al., 2019; Mills et al., 2021). Thus, the interaction between sleep and behavioral thermoregulation is evolutionarily conserved. We expect that understanding the molecular mechanism linking sleep with temperature regulation in flies will provide insight into sleep regulation and function in mammals.
Data availability statement
The original contributions presented in the study are included in the article/Supplementary material, further inquiries can be directed to the corresponding author.
Author contributions
SR, MF, VS, VL, HF, ZL EP, DA, IH, AK, and PS performed experiments. SR, MF, and PS oversaw experiments, analysis, and project direction. PS designed the experiments. SR, MF, EP, and PS wrote the paper. All authors contributed to the article and approved the submitted version.
Funding
This work was supported by NIH grants 5R01NS051305-14 and 5R01NS076980-08 to PS. The confocal facility is supported by NIH shared instrument grant S1OD21629-01A1.
Acknowledgments
The authors thank Matthew Thimgan and Stephane Dissel for useful comments. They also thank Paul Taghert for sharing reagents and flies.
Conflict of interest
The authors declare that the research was conducted in the absence of any commercial or financial relationships that could be construed as a potential conflict of interest.
Publisher’s note
All claims expressed in this article are solely those of the authors and do not necessarily represent those of their affiliated organizations, or those of the publisher, the editors and the reviewers. Any product that may be evaluated in this article, or claim that may be made by its manufacturer, is not guaranteed or endorsed by the publisher.
Supplementary material
The Supplementary material for this article can be found online at: https://www.frontiersin.org/articles/10.3389/fnins.2023.1175478/full#supplementary-material
Footnotes
References
Alpert, M. H., Frank, D. D., Kaspi, E., Flourakis, M., Zaharieva, E. E., Allada, R., et al. (2020). A circuit encoding absolute cold temperature in Drosophila. Curr. Biol. 30, 2275–2288.e5. doi: 10.1016/j.cub.2020.04.038
Bentivoglio, M., and Grassi-Zucconi, G. (1997). The pioneering experimental studies on sleep deprivation. Sleep 20, 570–576. doi: 10.1093/sleep/20.7.570
Campbell, L. A. D., Tkaczynski, P. J., Mouna, M., Derrou, A., Oukannou, L., Majolo, B., et al. (2018). Behavioural thermoregulation via microhabitat selection of winter sleeping areas in an endangered primate: implications for habitat conservation. R. Soc. Open Sci. 5:181113. doi: 10.1098/rsos.181113
Chellappa, S. L., Vujovic, N., Williams, J. S., and Scheer, F. (2019). Impact of circadian disruption on cardiovascular function and disease. Trends Endocrinol Metab 30, 767–779. doi: 10.1016/j.tem.2019.07.008
Chen, S. C., Tang, X., Goda, T., Umezaki, Y., Riley, A. C., Sekiguchi, M., et al. (2022). Dorsal clock networks drive temperature preference rhythms in Drosophila. Cell Rep. 39:110668. doi: 10.1016/j.celrep.2022.110668
Chung, B. Y., Kilman, V. L., Keath, J. R., Pitman, J. L., and Allada, R. (2009). The GABA(a) receptor RDL acts in peptidergic PDF neurons to promote sleep in Drosophila. Curr. Biol. 19, 386–390. doi: 10.1016/j.cub.2009.01.040
De Manaceine, M. (1894). Quelques observations experimentales sur I'influence de I'insomnie absolue. Arch. Ital. Biol. 21, 322–325.
Dillon, M. E., Wang, G., Garrity, P. A., and Huey, R. B. (2009). Review: thermal preference in Drosophila. J. Therm. Biol. 34, 109–119. doi: 10.1016/j.jtherbio.2008.11.007
Dissel, S., Angadi, V., Kirszenblat, L., Suzuki, Y., Donlea, J., Klose, M., et al. (2015a). Sleep restores behavioral plasticity to Drosophila mutants. Curr. Biol. 25, 1270–1281. doi: 10.1016/j.cub.2015.03.027
Dissel, S., Klose, M. K., van Swinderen, B., Cao, L., Ford, M., Periandri, E. M., et al. (2022). Sleep-promoting neurons remodel their response properties to calibrate sleep drive with environmental demands. PLoS Biol. 20:e3001797. doi: 10.1371/journal.pbio.3001797
Dissel, S., Melnattur, K., and Shaw, P. J. (2015c). Sleep, performance, and memory in flies. Curr. Sleep Med. Rep. 1, 47–54. doi: 10.1007/s40675-014-0006-4
Dissel, S., Seugnet, L., Thimgan, M. S., Silverman, N., Angadi, V., Thacher, P. V., et al. (2015b). Differential activation of immune factors in neurons and glia contribute to individual differences in resilience/vulnerability to sleep disruption. Brain Behav. Immun. 47, 75–85. doi: 10.1016/j.bbi.2014.09.019
Dissel, S., and Shaw, P. J. (2017). “Sleep and memory formation in Drosophila” in Learning and memory: A comprehensive reference. ed. J. H. Byrne (Cambridge, Massachusetts: Academic Press)
Donlea, J. M., Ramanan, N., Silverman, N., and Shaw, P. J. (2014). Genetic rescue of functional senescence in synaptic and behavioral plasticity. Sleep 37, 1427–1437. doi: 10.5665/sleep.3988
Donlea, J. M., Thimgan, M. S., Suzuki, Y., Gottschalk, L., and Shaw, P. J. (2011). Inducing sleep by remote control facilitates memory consolidation in Drosophila. Science 332, 1571–1576. doi: 10.1126/science.1202249
Fan, Y., Wang, Y., Gu, P., Han, J., and Tian, Y. (2022). How temperature influences sleep. Int. J. Mol. Sci. 23:12191. doi: 10.3390/ijms232012191
Fischer, D., Roenneberg, T., and Vetter, C. (2021). Chronotype-specific sleep in two versus four consecutive shifts. J. Biol. Rhythm. 36, 395–409. doi: 10.1177/07487304211006073
Foster, R. G., Peirson, S. N., Wulff, K., Winnebeck, E., Vetter, C., and Roenneberg, T. (2013). Sleep and circadian rhythm disruption in social jetlag and mental illness. Prog. Mol. Biol. Transl. Sci. 119, 325–346. doi: 10.1016/B978-0-12-396971-2.00011-7
Frank, M. G. (2021). Renormalizing synapses in sleep: the clock is ticking. Biochem. Pharmacol. 191:114533. doi: 10.1016/j.bcp.2021.114533
Frey, D. J., Badia, P., and Wright, K. P. (2004). Inter–and intra-individual variability in performance near the circadian nadir during sleep deprivation. J. Sleep Res. 13, 305–315. doi: 10.1111/j.1365-2869.2004.00429.x
Glotzbach, S. F., and Heller, H. C. (1976). Central nervous regulation of body temperature during sleep. Science 194, 537–539. doi: 10.1126/science.973138
Goda, T., and Hamada, F. N. (2019). Drosophila temperature preference rhythms: an innovative model to understand body temperature rhythms. Int. J. Mol. Sci. 20:1988. doi: 10.3390/ijms20081988
Goda, T., Tang, X., Umezaki, Y., Chu, M. L., Kunst, M., Nitabach, M. N. N., et al. (2016). Drosophila DH31 neuropeptide and PDF receptor regulate night-onset temperature preference. J. Neurosci. 36, 11739–11754. doi: 10.1523/JNEUROSCI.0964-16.2016
Gravett, N., Bhagwandin, A., Sutcliffe, R., Landen, K., Chase, M. J., Lyamin, O. I., et al. (2017). Inactivity/sleep in two wild free-roaming African elephant matriarchs–does large body size make elephants the shortest mammalian sleepers? PLoS One 12:e0171903. doi: 10.1371/journal.pone.0171903
Guo, F., Yu, J., Jung, H. J., Abruzzi, K. C., Luo, W., Griffith, L. C., et al. (2016). Circadian neuron feedback controls the Drosophila sleep--activity profile. Nature 536, 292–297. doi: 10.1038/nature19097
Hague, M. T. J., Caldwell, C. N., and Cooper, B. S. (2020). Pervasive effects of Wolbachia on host temperature preference. MBio 11:e01768. doi: 10.1128/mBio.01768-20
Hamada, F. N., Rosenzweig, M., Kang, K., Pulver, S. R., Ghezzi, A., Jegla, T. J., et al. (2008). An internal thermal sensor controlling temperature preference in Drosophila. Nature 454, 217–220. doi: 10.1038/nature07001
Harding, E. C., Franks, N. P., and Wisden, W. (2019). The temperature dependence of sleep. Front. Neurosci. 13:336. doi: 10.3389/fnins.2019.00336
Harding, E. C., Yu, X., Miao, A., Andrews, N., Ma, Y., Ye, Z., et al. (2018). A neuronal hub binding sleep initiation and body cooling in response to a warm external stimulus. Curr. Biol. 28, 2263–2273.e4. doi: 10.1016/j.cub.2018.05.054
Head, L. M., Tang, X., Hayley, S. E., Goda, T., Umezaki, Y., Chang, E. C., et al. (2015). The influence of light on temperature preference in Drosophila. Curr. Biol. 25, 1063–1068. doi: 10.1016/j.cub.2015.02.038
Helfrich-Forster, C. (2003). The neuroarchitecture of the circadian clock in the brain of Drosophila melanogaster. Microsc. Res. Tech. 62, 94–102. doi: 10.1002/jemt.10357
Im, S. H., and Taghert, P. H. (2010). PDF receptor expression reveals direct interactions between circadian oscillators in Drosophila. J. Comp. Neurol. 518, 1925–1945. doi: 10.1002/cne.22311
Imeri, L., and Opp, M. R. (2009). How (and why) the immune system makes us sleep. Nat. Rev. Neurosci. 10, 199–210. doi: 10.1038/nrn2576
Ito, F., and Awasaki, T. (2022). Comparative analysis of temperature preference behavior and effects of temperature on daily behavior in 11 Drosophila species. Sci. Rep. 12:12692. doi: 10.1038/s41598-022-16897-7
Juda, M., Vetter, C., and Roenneberg, T. (2013). Chronotype modulates sleep duration, sleep quality, and social jet lag in shift-workers. J. Biol. Rhythm. 28, 141–151. doi: 10.1177/0748730412475042
Kaneko, H., Head, L. M., Ling, J., Tang, X., Liu, Y., Hardin, P. E., et al. (2012). Circadian rhythm of temperature preference and its neural control in Drosophila. Curr. Biol. 22, 1851–1857. doi: 10.1016/j.cub.2012.08.006
Keene, A. C., Duboue, E. R., McDonald, D. M., Dus, M., Suh, G. S., Waddell, S., et al. (2010). Clock and cycle limit starvation-induced sleep loss in Drosophila. Curr. Biol. 20, 1209–1215. doi: 10.1016/j.cub.2010.05.029
Keppel, G.. (1982). Design and analysis a researcher's handbook. Englewood Cliffs, NJ: Prentice-Hall.
Kleitman, N., and Doktorsky, A. (1933). Studies on the physiology of sleep: VII. The effect of the position of the body and of sleep on rectal temperature in man. Am. J. Physiol. Leg. Content 104, 340–343. doi: 10.1152/ajplegacy.1933.104.2.340
Klose, M., and Shaw, P. (2019). Sleep-drive reprograms clock neuronal identity through CREB-binding protein induced PDFR expression. bioRxiv 2019:874628. doi: 10.1101/2019.12.12.874628
Kunst, M., Hughes, M. E., Raccuglia, D., Felix, M., Li, M., Barnett, G., et al. (2014). Calcitonin gene-related peptide neurons mediate sleep-specific circadian output in Drosophila. Curr. Biol. 24, 2652–2664. doi: 10.1016/j.cub.2014.09.077
Lamaze, A., Krätschmer, P., Chen, K. F., Lowe, S., and Jepson, J. E. C. (2018). A wake-promoting circadian output circuit in Drosophila. Curr. Biol. 28, 3098–3105.e3. doi: 10.1016/j.cub.2018.07.024
Le Bourg, E., and Buecher, C. (2002). Learned suppression of photopositive tendencies in Drosophila melanogaster. Anim. Learn. Behav. 30, 330–341. doi: 10.3758/BF03195958
Lesku, J. A., Rattenborg, N. C., Valcu, M., Vyssotski, A. L., Kuhn, S., Kuemmeth, F., et al. (2012). Adaptive sleep loss in polygynous pectoral sandpipers. Science 337, 1654–1658. doi: 10.1126/science.1220939
Liang, X., Holy, T. E., and Taghert, P. H. (2016). Synchronous Drosophila circadian pacemakers display nonsynchronous ca(2)(+) rhythms in vivo. Science 351, 976–981. doi: 10.1126/science.aad3997
Liang, X., Holy, T. E., and Taghert, P. H. (2017). A series of suppressive signals within the Drosophila circadian neural circuit generates sequential daily outputs. Neuron 94, 1173–1189.e4. doi: 10.1016/j.neuron.2017.05.007
Liang, X., Holy, T. E., and Taghert, P. H. (2023). Polyphasic circadian neural circuits drive differential activities in multiple downstream rhythmic centers. Curr. Biol. 33, 351–363.e3. doi: 10.1016/j.cub.2022.12.025
Lyamin, O., Pryaslova, J., Lance, V., and Siegel, J. (2005). Animal behaviour: continuous activity in cetaceans after birth. Nature 435:1177. doi: 10.1038/4351177a
Ma, D., Przybylski, D., Abruzzi, K. C., Schlichting, M., Li, Q., Long, X., et al. (2021). A transcriptomic taxonomy of Drosophila circadian neurons around the clock. Elife 10:e63056. doi: 10.7554/eLife.63056
Machado, D. R., Afonso, D. J., Kenny, A. R., Öztu Rk-Çolak, A., Moscato, E. H., Mainwaring, B., et al. (2017). Identification of octopaminergic neurons that modulate sleep suppression by male sex drive. Elife 6:e23130. doi: 10.7554/eLife.23130
Melnattur, K., Zhang, B., and Shaw, P. J. (2020). Disrupting flight increases sleep and identifies a novel sleep-promoting pathway in Drosophila. Sci. Adv. 6:eaaz2166. doi: 10.1126/sciadv.aaz2166
Mills, C. J., Nekaris, K. A. I., Campera, M., and Patel, E. (2021). Silky Sifakas (Propithecus candidus) use sleep sites for thermoregulation, food access and predator avoidance. Folia Primatol. 92, 315–326. doi: 10.1159/000520710
Nave, C., Roberts, L., Hwu, P., Estrella, J. D., Vo, T. C., Nguyen, T. H., et al. (2020). Weekend light shifts evoke persistent Drosophila circadian neural network desynchrony. J. Neurosci. 41, 5173–5189. doi: 10.1523/JNEUROSCI.3074-19.2021
Nitabach, M. N., Wu, Y., Sheeba, V., Lemon, W. C., Strumbos, J., Zelensky, P. K., et al. (2006). Electrical hyperexcitation of lateral ventral pacemaker neurons desynchronizes downstream circadian oscillators in the fly circadian circuit and induces multiple behavioral periods. J. Neurosci. 26, 479–489. doi: 10.1523/JNEUROSCI.3915-05.2006
Parisky, K. M., Agosto, J., Pulver, S. R., Shang, Y., Kuklin, E., Hodge, J. J., et al. (2008). PDF cells are a GABA-responsive wake-promoting component of the Drosophila sleep circuit. Neuron 60, 672–682. doi: 10.1016/j.neuron.2008.10.042
Parmeggiani, P. L., Azzaroni, A., Cevolani, D., and Ferrari, G. (1983). Responses of anterior hypothalamic-preoptic neurons to direct thermal stimulation during wakefulness and sleep. Brain Res. 269, 382–385. doi: 10.1016/0006-8993(83)90152-X
Pilz, L. K., Keller, L. K., Lenssen, D., and Roenneberg, T. (2018). Time to rethink sleep quality: PSQI scores reflect sleep quality on workdays. Sleep 41:29. doi: 10.1093/sleep/zsy029
Pimentel, D., Donlea, J. M., Talbot, C. B., Song, S. M., Thurston, A. J., and Miesenbock, G. (2016). Operation of a homeostatic sleep switch. Nature 536, 333–337. doi: 10.1038/nature19055
Rakotomalala, E. J., Rakotondraparany, F., Perofsky, A. C., and Lewis, R. J. (2017). Characterization of the tree holes used by Lepilemur ruficaudatus in the dry, deciduous Forest of Kirindy Mitea National Park. Folia Primatol. 88, 28–41. doi: 10.1159/000464406
Rattenborg, N. C., Mandt, B. H., Obermeyer, W. H., Winsauer, P. J., Huber, R., Wikelski, M., et al. (2004). Migratory sleeplessness in the white-crowned sparrow (Zonotrichia leucophrys gambelii). PLoS Biol. 2:E212. doi: 10.1371/journal.pbio.0020212
Rechtschaffen, A., Bergmann, B. M., Everson, C. A., Kushida, C. A., and Carol, A. E. (1989a). Sleep deprivation in the rat: I. conceptual issues. Sleep 12, 1–4. doi: 10.1093/sleep/12.1.1
Rechtschaffen, A., Bergmann, B. M., Everson, C. A., Kushida, C. A., and Gilliland, M. A. (1989b). Sleep deprivation in the rat: X. integration and discussion of the findings. Sleep 12, 68–87.
Reinhard, N., Schubert, F. K., Bertolini, E., Hagedorn, N., Manoli, G., Sekiguchi, M., et al. (2022). The neuronal circuit of the dorsal circadian clock neurons in Drosophila melanogaster. Front. Physiol. 13:886432. doi: 10.3389/fphys.2022.886432
Reinhardt, K. D., Vyazovskiy, V. V., Hernandez-Aguilar, R. A., Imron, M. A., and Nekaris, K. A. (2019). Environment shapes sleep patterns in a wild nocturnal primate. Sci. Rep. 9:9939. doi: 10.1038/s41598-019-45852-2
Reis, C., Pilz, L. K., Keller, L. K., Paiva, T., and Roenneberg, T. (2020). Social timing influences sleep quality in patients with sleep disorders. Sleep Med. 71, 8–17. doi: 10.1016/j.sleep.2020.02.019
Romeijn, N., Verweij, I. M., Koeleman, A., Mooij, A., Steimke, R., Virkkala, J., et al. (2012). Cold hands, warm feet: sleep deprivation disrupts thermoregulation and its association with vigilance. Sleep 35, 1673–1683. doi: 10.5665/sleep.2242
Savourey, G., and Bittel, J. (1994). Cold thermoregulatory changes induced by sleep deprivation in men. Eur. J. Appl. Physiol. Occup. Physiol. 69, 216–220. doi: 10.1007/BF01094791
Sayeed, O., and Benzer, S. (1996). Behavioral genetics of thermosensation and hygrosensation in Drosophila. Proc. Natl. Acad. Sci. U. S. A. 93, 6079–6084. doi: 10.1073/pnas.93.12.6079
Schlichting, M., Richhariya, S., Herndon, N., Ma, D., Xin, J., Lenh, W., et al. (2022). Dopamine and GPCR-mediated modulation of DN1 clock neurons gates the circadian timing of sleep. Proc. Natl. Acad. Sci. U. S. A. 119:e2206066119. doi: 10.1073/pnas.2206066119
Seibt, J., and Frank, M. G. (2019). Primed to sleep: the dynamics of synaptic plasticity across brain states. Front. Syst. Neurosci. 13:2. doi: 10.3389/fnsys.2019.00002
Seugnet, L., Suzuki, Y., Donlea, J. M., Gottschalk, L., and Shaw, P. J. (2011). Sleep deprivation during early-adult development results in long-lasting learning deficits in adult Drosophila. Sleep 34, 137–146. doi: 10.1093/sleep/34.2.137
Seugnet, L., Suzuki, Y., Stidd, R., and Shaw, P. J. (2009). Aversive phototaxic suppression: evaluation of a short-term memory assay in Drosophila melanogaster. Genes Brain Behav. 8, 377–389. doi: 10.1111/j.1601-183X.2009.00483.x
Seugnet, L., Suzuki, Y., Vine, L., Gottschalk, L., and Shaw, P. J. (2008). D1 receptor activation in the mushroom bodies rescues sleep-loss-induced learning impairments in Drosophila. Curr. Biol. 18, 1110–1117. doi: 10.1016/j.cub.2008.07.028
Shafer, O. T., Gutierrez, G. J., Li, K., Mildenhall, A., Spira, D., Marty, J., et al. (2022). Connectomic analysis of the Drosophila lateral neuron clock cells reveals the synaptic basis of functional pacemaker classes. Elife 11:e79139. doi: 10.7554/eLife.79139
Shafer, O. T., and Keene, A. C. (2021). The regulation of Drosophila sleep. Curr. Biol. 31, R38–r49. doi: 10.1016/j.cub.2020.10.082
Shafer, O. T., Kim, D. J., Dunbar-Yaffe, R., Nikolaev, V. O., Lohse, M. J., and Taghert, P. H. (2008). Widespread receptivity to neuropeptide PDF throughout the neuronal circadian clock network of Drosophila revealed by real-time cyclic AMP imaging. Neuron 58, 223–237. doi: 10.1016/j.neuron.2008.02.018
Shang, Y., Donelson, N. C., Vecsey, C. G., Guo, F., Rosbash, M., and Griffith, L. C. (2013). Short neuropeptide F is a sleep-promoting inhibitory modulator. Neuron 80, 171–183. doi: 10.1016/j.neuron.2013.07.029
Shaw, P. J., Bergmann, B. M., and Rechtschaffen, A. (1997). Operant control of ambient temperature during sleep deprivation. Am. J. Phys. 272, R682–R690. doi: 10.1152/ajpregu.1997.272.2.R682
Shaw, P. J., Cirelli, C., Greenspan, R. J., and Tononi, G. (2000). Correlates of sleep and waking in Drosophila melanogaster. Science 287, 1834–1837. doi: 10.1126/science.287.5459.1834
Shaw, P. J., Tononi, G., Greenspan, R. J., and Robinson, D. F. (2002). Stress response genes protect against lethal effects of sleep deprivation in Drosophila. Nature 417, 287–291. doi: 10.1038/417287a
Sheeba, V., Fogle, K. J., Kaneko, M., Rashid, S., Chou, Y. T., Sharma, V. K., et al. (2008). Large ventral lateral neurons modulate arousal and sleep in Drosophila. Curr. Biol. 18, 1537–1545. doi: 10.1016/j.cub.2008.08.033
Spiegel, K., Leproult, R., and Van Cauter, E. (1999). Impact of sleep debt on metabolic and endocrine function. Lancet 354, 1435–1439. doi: 10.1016/S0140-6736(99)01376-8
Stickgold, R., and Walker, M. P. (2013). Sleep-dependent memory triage: evolving generalization through selective processing. Nat. Neurosci. 16, 139–145. doi: 10.1038/nn.3303
Sun, L., Jiang, R. H., Ye, W. J., Rosbash, M., and Guo, F. (2022). Recurrent circadian circuitry regulates central brain activity to maintain sleep. Neuron 110, 2139–2154.e5. doi: 10.1016/j.neuron.2022.04.010
Szymusiak, R. (2018). Body temperature and sleep. Handb. Clin. Neurol. 156, 341–351. doi: 10.1016/B978-0-444-63912-7.00020-5
Szymusiak, R., and McGinty, D. (1990). Control of slow wave sleep by thermoregulatory mechanisms. Prog. Clin. Biol. Res. 345, 53–64.
Taghert, P. H., and Shafer, O. T. (2006). Mechanisms of clock output in the Drosophila circadian pacemaker system. J. Biol. Rhythm. 21, 445–457. doi: 10.1177/0748730406293910
Tang, X., Roessingh, S., Hayley, S. E., Chu, M. L., Tanaka, N. K., Wolfgang, W., et al. (2017). The role of PDF neurons in setting the preferred temperature before dawn in Drosophila. Elife 6:23206. doi: 10.7554/eLife.23206
Thimgan, M. S., Suzuki, Y., Seugnet, L., Gottschalk, L., and Shaw, P. J. (2010). The perilipin homologue, lipid storage droplet 2, regulates sleep homeostasis and prevents learning impairments following sleep loss. PLoS Biol. 8:e1000466. doi: 10.1371/journal.pbio.1000466
Tononi, G., and Cirelli, C. (2020). Sleep and synaptic down-selection. Eur. J. Neurosci. 51, 413–421. doi: 10.1111/ejn.14335
Wiggin, T. D., Goodwin, P. R., Donelson, N. C., Liu, C., Trinh, K., Sanyal, S., et al. (2020). Covert sleep-related biological processes are revealed by probabilistic analysis in Drosophila. Proc. Natl. Acad. Sci. U. S. A. 117, 10024–10034. doi: 10.1073/pnas.1917573117
Yadlapalli, S., Jiang, C., Bahle, A., Reddy, P., Meyhofer, E., and Shafer, O. T. (2018). Circadian clock neurons constantly monitor environmental temperature to set sleep timing. Nature 555, 98–102. doi: 10.1038/nature25740
Keywords: sleep, sleep deprivation, Drosophila, temperature preference behavior, sleep fragmentation
Citation: Roach ST, Ford MC, Simhambhatla V, Loutrianakis V, Farah H, Li Z, Periandri EM, Abdalla D, Huang I, Kalra A and Shaw PJ (2023) Sleep deprivation, sleep fragmentation, and social jet lag increase temperature preference in Drosophila. Front. Neurosci. 17:1175478. doi: 10.3389/fnins.2023.1175478
Edited by:
Ritchie Edward Brown, United States Department of Veterans Affairs, United StatesReviewed by:
Shuancheng Ren, Army Medical University, ChinaSergio I. Hidalgo, University of California, Davis, United States
Copyright © 2023 Roach, Ford, Simhambhatla, Loutrianakis, Farah, Li, Periandri, Abdalla, Huang, Kalra and Shaw. This is an open-access article distributed under the terms of the Creative Commons Attribution License (CC BY). The use, distribution or reproduction in other forums is permitted, provided the original author(s) and the copyright owner(s) are credited and that the original publication in this journal is cited, in accordance with accepted academic practice. No use, distribution or reproduction is permitted which does not comply with these terms.
*Correspondence: Paul J. Shaw, c2hhd3BAd3VzdGwuZWR1
†These authors have contributed equally to this work