- 1Department Pharmaceutical Sciences, Northeastern University, Boston, MA, United States
- 2Center for Translational Neuroimaging, Northeastern University, Boston, MA, United States
- 3Department of Chemistry & Biochemistry, New Mexico State University, Las Cruces, NM, United States
- 4Department of Psychology, Northern Illinois University, DeKalb, IL, United States
- 5Department of Mathematics, College of Science & Humanity Studies, Riyadh, Saudi Arabia
- 6Psychological & Brain Sciences, Program in Neuroscience, Indiana University, Bloomington, IN, United States
- 7Department Psychology & Pharmaceutical Sciences, Northeastern University, Boston, MA, United States
Psilocybin is a hallucinogen with complex neurobiological and behavioral effects. This is the first study to use MRI to follow functional changes in brain activity in response to different doses of psilocybin in fully awake, drug naive rats. We hypothesized that psilocybin would show a dose-dependent increase in activity in the prefrontal cortex and thalamus, while decreasing hippocampal activity. Female and male rats were given IP injections of vehicle or psilocybin in doses of 0.03 mg/kg, 0.3 mg/kg, and 3.0 mg/kg while fully awake during the imaging session. These levels were validated by measuring psilocybin and its metabolite, psilocin. Changes in BOLD signal were recorded over a 20 min window. Data for resting state functional connectivity were collected approximately 35 min post injection. All data were registered to rat 3D MRI atlas with 169 brain areas providing site-specific changes in global brain activity and changes in functional connectivity. Treatment with psilocybin resulted in a significant dose-dependent increase in positive BOLD signal. The areas most affected by the acute presentation of psilocybin were the somatosensory cortex, basal ganglia and thalamus. Males and females showed different sensitivity to psilocybin dose, with females exhibiting greater activation than males at 0.3 mg/kg, especially in thalamic and basal ganglia regions. There was a significant dose-dependent global increase in functional connectivity, highlighted by hyperconnectivity to the cerebellum. Brain areas hypothesized to be involved in loss of sensory filtering and organization of sensory motor stimuli, such as the cortico-striato-thalamo-cortical circuit and the claustrum, showed increased activation at higher doses of psilocybin. Indeed, the general neuroanatomical circuitry associated with the psychedelic experience was affected but the direction of the BOLD signal and pattern of activity between neural networks was inconsistent with the human literature.
Introduction
Psilocybin (PSI) has gained interest in psychological research in recent years for its potential therapeutic use in treatment-resistant mood and anxiety disorders. Though it has been used by humans for centuries, research on PSI was curtailed for approximately 30 years following the US DEA’s assignment of psychedelic substances as Schedule I drugs in 1970, despite thousands of studies that showed promising results in the treatment of anxiety, obsessive-compulsive disorders, addiction, and sexual dysfunction (Vollenweider and Preller, 2020). The advancement of magnetic resonance imaging (MRI) and positron emission tomography (PET) imaging in the 1990s improved our understanding of the mechanisms within the central nervous system, reigniting interest in psychedelics research, and leading to pilot studies and eventual FDA approvals for the therapeutic use of psychedelics in the treatment of various mental health conditions (Banushi and Polito, 2023). Recent studies show efficacy in treating substance dependence (de Veen et al., 2017), depression (Vollenweider and Preller, 2020; Goodwin et al., 2022), and anxiety (Griffiths et al., 2016).
Several clinical studies have been conducted with healthy human volunteers. de Veen et al. (2017) concluded that PSI may exert anti-addictive properties by relieving negative emotional states and stress or by lessening cognitive inflexibility and compulsivity. In a recent study, PSI has been suggested to have some use in the treatment of acquired brain injury by way of modulating excessive neuroinflammation and promoting neuroplasticity (Allen et al., 2024). It has also been shown to modulate self-focus, reduce negative emotional processing, and improve social functioning in healthy patients, as well as patients with psychiatric illness (Vollenweider and Preller, 2020). Human studies are limited, however, in the range of experiments that can be performed using PSI, due to the lack of knowledge about the exact nature of the neurological mechanisms at play as well as ethical concerns about inducing a psychologically vulnerable state (Allen et al., 2024). This is an issue that has been partially addressed with research in mammals.
Using rodents, researchers have been able to identify the dose-dependent effects of PSI more precisely on the brain on a much shorter timescale. Jefsen et al. (2021) showed the drug’s dose-dependent facilitation of plasticity-related gene expression in the rat prefrontal cortex and hippocampus. Similarly, it was shown to induce long-lasting increases in spine density and spine head width in the mouse medial frontal cortex as a result of a higher rate of dendritic formation (Shao et al., 2021). Increased neuroplasticity, or the structural and functional adaptation of neurons over time, has been hypothesized to alleviate symptoms of depression, a condition associated with the loss of synapses in the frontal cortex (Shao et al., 2021). This is one of the major mechanisms by which PSI is proposed to be of therapeutic use in the treatment of mental illnesses. Though a significant amount of information has been drawn from animal histology and behavioral studies, very few studies have specifically examined PSI’s functional effects in rodents; hence, there is a lack of a localized functional understanding of substances like PSI on the brain. Within that smaller amount of literature, there is an even smaller amount of imaging that has been done on awake as opposed to anesthetized rodents.
One such recent study, though not using MRI, examined neural activity in awake mice. Golden and Chadderton (2022) found that PSI desynchronized neural oscillations in the anterior cingulate cortex by increasing the power of gamma oscillations and decreasing the power of low frequency oscillations. The resulting change in cortical activity and disruption of top-down processing is suggested to be the potential mechanism of action of psychedelic compounds. Although the studies were performed in anesthetized rats, Liu et al. (2023) reported an increase in BOLD (Blood Oxygen Level Dependent) signal across multiple cortical areas, hippocampus, and striatum following IP injection of PSI. In the present study we used BOLD imaging to record changes in brain function in response to PSI given to awake rats during the imaging session. We found dose-dependent global increases in positive BOLD volume of activation and hyperconnectivity with PSI treatment.
Methods and materials
Animals
Adult male (n = 24) and female (n = 24) Sprague Dawley rats were purchased from Charles River Laboratories (Wilmington, MA, United States). Animals were housed in Plexiglas cages (two per cage) and maintained in ambient temperature (22–24°C). Animals were maintained on a reverse L-D cycle with lights off at 0900 h and studied during the dark phase when they are normally active. All experiments were conducted between 1000 and 1800 h to avoid the transitions between the L-D dark cycles. Food and water were provided ad libitum. All animals were acquired and cared for in accordance with the guidelines published in the NIH Guide for the Care and Use of Laboratory Animals. All methods and procedures described below were pre-approved by the Northeastern University Institutional Animal Care and Use Committee under protocol number 23-0407R. Northeastern University’s animal care and use program and housing facilities are fully accredited by AAALAC, International. The protocols used in this study followed the ARRIVE guidelines for reporting in vivo experiments in animal research (Kilkenny et al., 2010). Animals were monitored daily over the duration of the study for general health, food and water consumption. A 15% loss in body weight was set as a humane endpoint.
Psilocybin and ketanserin preparation and administration
Psilocybin was acquired through the National Institute on Drug Abuse (NIDA) Drug Supply Program and distributed by the Research Triangle Institute. On the day of imaging PSI was prepared in sterile saline (0.9% NaCl). To deliver drug remotely during the imaging session, a poly-ethylene tube (PE-20), approximately 30 cm in length, was positioned in the peritoneal cavity. The range of doses of PSI were taken from the literature (Higgins et al., 2021; Wojtas et al., 2022; Spain et al., 2015). Rats were randomly assigned to one of four dose groups (vehicle, 0.03, 0.3, 3.0 mg/kg PSI), ensuring each group had a similar distribution of body weights. Each group consisted of 12 rats divided equally between males and females. Due to motion artifact five rats were excluded from the study. Two male rats from the vehicle treatment, one male from the low dose treatment and one male and one female from the high dose group. Following the original study with 48 rats, we ran a pilot study with seven male Sprague Dawley rats to evaluate the effect of the 5-HT2A receptor antagonist ketanserin on changes in brain activity in response to the 3.0 mg/kg dose of PSI. Rats were housed and acclimated as described above. One hour prior to imaging, rats were given an I.P injection of ketanserin (2.0 mg/kg in saline).
Acclimation for awake imaging
To mitigate the stress associated with head restraint, rats underwent an acclimation protocol to familiarize them with the restraining system, which consisted of a head holder and body tube. This system was designed to exclude surgery and included a cushioned head support, eliminating the need for ear bars and, in turn, reducing discomfort to the animals while minimizing any unintended motion artifacts. These acclimation sessions were conducted daily for five consecutive days. During these sessions, rats were briefly anesthetized with 1–2% isoflurane for placement into the restraining system. Their forepaws were fastened using surgical tape.
Once fully conscious, the rats were positioned within an opaque black box, a “mock scanner,” for 60 min. Inside the mock scanner, a tape recording of the MRI pulse sequence was played to simulate the environment of the magnet bore and the imaging protocol. Under these conditions there is a significant decreases in respiration, heart rate, motor activity, and plasma corticosterone levels when comparing the first and last acclimation sessions, as reported by King et al. (2005). This reduction in autonomic and somatic signs of arousal and stress improved signal resolution and image quality.
Image acquisition
Five to six rats were imaged in a day. Each day had a mix of the different experimental groups known by all the investigators. Rats were scanned at 300 MHz using a quadrature transmit/receive volume coil built into the rat head holder and restraining system for awake animal imaging (Ekam Imaging, Boston, MA, United States). A video of the rat preparation for imaging is available at www.youtube.com/watch?v=JQX1wgOV3K4. The design of the coil provided complete coverage of the brain from olfactory bulbs to brain stem. Radio frequency signals were sent and received with a quadrature volume coil built into the animal restrainer (Ekam Imaging, Boston MA, United States) (Ferris, 2022). Imaging sessions were conducted using a Bruker Biospec 7.0 T/20-cm USR horizontal magnet (Bruker, Billerica, MA, USA) and a 2 T/m magnetic field gradient insert (ID = 12 cm) capable of a 120-μs rise time. At the beginning of each imaging session, a high-resolution anatomical data set was collected RARE factor 8; 25 slices, 1 mm; FOV 3.0 cm2; data matrix 256 × 256; TR 3 s; TE 12 ms; effective TE 48 ms; NEX 3; 4.48 min acquisition time; in-plane resolution 117.2 μm2. Functional images were captured using HASTE pulse sequence: 22 slices, 1.1 mm, FOV of 3.0 cm2; data matrix 96 × 96, TR 6 s, TE 3.75 ms, effective TE 22.5 ms, 25 min acquisition time, in-plane resolution of 312.5 μm2. It should also be emphasized that high neuroanatomical fidelity and spatial resolution are critical in identifying distributed neural circuits in any animal imaging study. Many brain areas in the segmented rat atlas have in-plane boundaries of less than 400 μm2 and may extend for over 1,000 μm in the rostral/caudal plane. With the development of a segmented, annotated 3D Rat Brain Atlas© for rats (Ekam Solutions, Boston, MA, United States), it was possible to localize functional imaging data to precise 3D “volumes of interest” in clearly delineated brain areas. This spatial resolution was sufficient to identify the bilateral habenula, with approximately 4–5 voxels on each side, but not to differentiate between the lateral and medial habenula.
Data analysis
The fMRI data analysis consisted of three main steps: pre-processing, processing, and post-processing. All these steps were executed using SPM-12 (available at https://www.fil.ion.ucl.ac.uk/spm/) and in house Matlab software. In the pre-processing stage, several operations were performed, including co-registration, motion correction, smoothing, and detrending. Co-registration was carried out with specific parameters: Quality set at 0.97, Smoothing at 0.6 mm Full Width at Half Maximum (FWHM), and Separation at 0.4 mm. Additionally, Gaussian smoothing was applied with a FWHM of 0.8 mm.
The processing step involved aligning the data to a rat atlas, followed by segmentation and statistical analysis. To achieve registration and segmentation, all images were initially aligned and registered to the 3D Rat Brain Atlas©, which included 169 segmented and annotated brain areas. This alignment was performed using the GUI-based EVA software developed by Ekam Solutions (Boston, MA). The image registration process encompassed translation, rotation, and scaling adjustments, performed independently in all three dimensions (see Supplementary Data Sheet 1). All spatial transformations applied were compiled into a matrix [Tj] for each subject. Each transformed anatomical pixel location was tagged with its corresponding brain area, resulting in fully segmented representations of individual subjects within the atlas.
Each scanning session consisted of 250 data acquisitions with a period of 6 s each for a total lapse time of 25 min. The first 50 scans (5 min) were the control window while the stimulation window was 200–240 (for ca 20 min post-injection) scans. Statistical t-tests were performed on each voxel (~ 36,000 voxels in the whole brain) of each subject within their original coordinate system. Before statistical testing, a baseline signal change threshold of 1% was applied to identify voxels with potentially meaningful signal changes. This excluded voxels that never exceeded ±1% BOLD signal change from baseline during the post-injection period. This 1% cutoff was chosen as a conservative floor to eliminate very small fluctuations that likely reflect noise or minor physiological drift, ensuring that only voxels with at least a minimal response were considered for significance testing. Importantly, this thresholding did not automatically deem 1% as significant; it was simply a preprocessing step. All voxels meeting the threshold were then subjected to statistical analysis using a one sample t-test against zero change. The reference for these t-tests was a null hypothesis of zero change from baseline. The t test statistics used a 95% confidence level (p < 0.05), two-tailed distributions, and heteroscedastic variance assumptions. As a result of the multiple t-test analyses performed, a false-positive detection controlling mechanism was introduced. We set the acceptable False Discovery Rate (FDR) at Q = 0.05 (5%). After performing voxel-wise tests, an FDR correction across all voxels in the brain was applied to control for multiple comparisons using the Benjamini–Hochberg procedure. This subsequent filter guaranteed that the FDR rate was below our cutoff of 0.05. The formulation of the filter satisfied the following expression:
In the equation, Pi represents the p-value derived from the t-test conducted at the i-th pixel within the region of interest, comprising V pixels, with each pixel ranked according to its probability value. For our analysis, we set the false-positive filter value q at 0.2, and we fixed the predetermined constant c(V) at unity, following a conservative approach for assessing significance (Genovese et al., 2002).
To create composite maps displaying the percent changes in the BOLD signal for each experimental group, we mapped each composite pixel location (in terms of rows, columns, and slices) to a voxel within the j-th subject using the inverse transformation matrix [Tj]-1. A trilinear interpolation method was used to determine the contribution of subject-specific voxel values to the composite representation. The use of inverse matrices ensured that the entire composite volume was populated with subject inputs. The average of all contributions was assigned as the percentage change in the BOLD signal at each voxel within the composite representation of the brain for the respective experimental group.
In the post-processing phase, we compared the number of activated voxels in each of the 169 brain areas between the control and PSI doses using a Kruskal–Wallis test statistic. The data were ranked in order of significance, as detailed in Tables 1–4. We generated activation maps, depicted in Figure 1, showing brain areas with significant differences when comparing two groups, e.g., veh vs. 3.0 mg/kg PSI. For Figures 2, 3 we limited statistical comparisons to either global metrics or a priori regions of interest (e.g., somatosensory cortex) rather than every individual brain area.
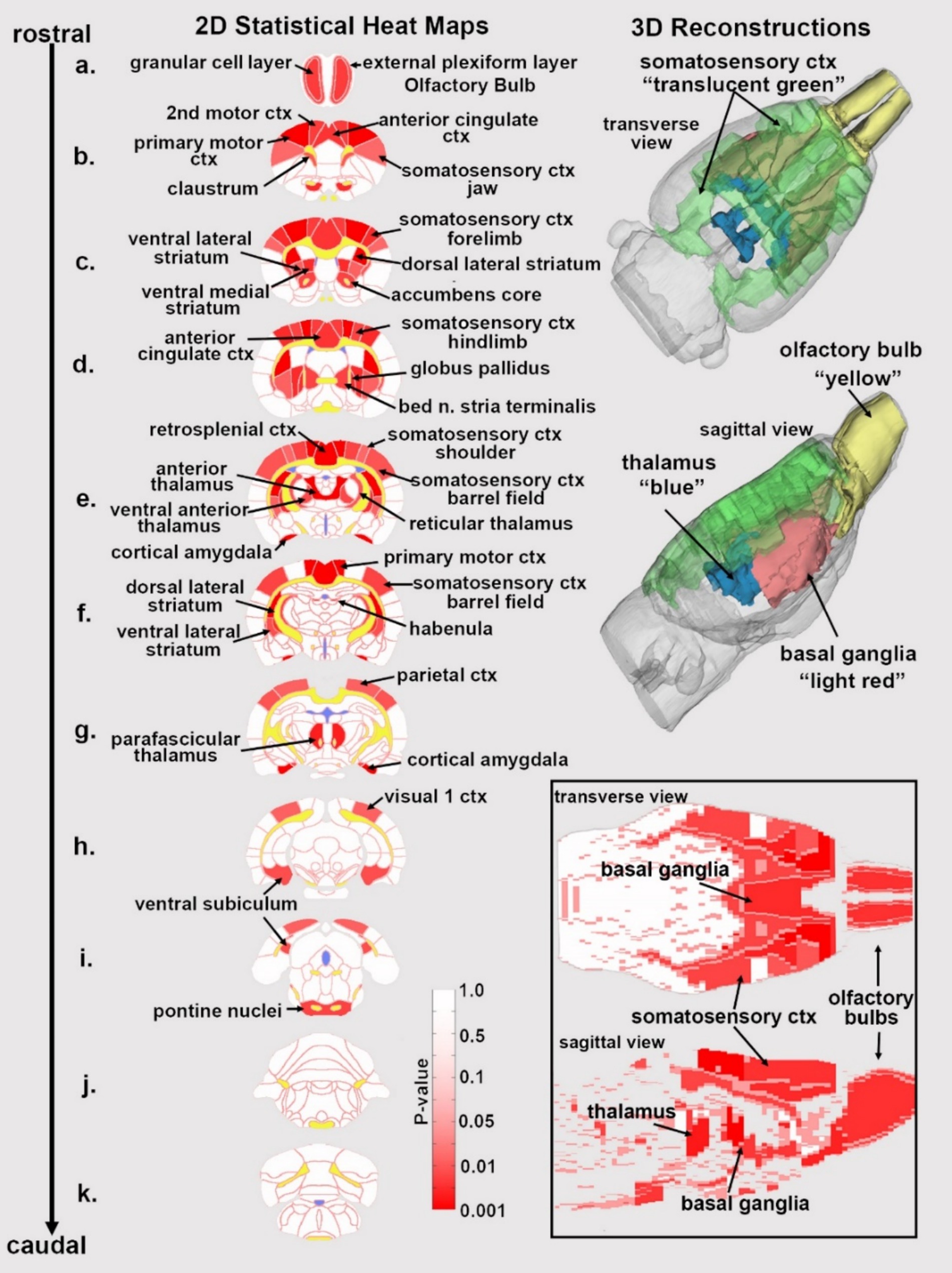
Figure 1. Statistical heat maps of positive BOLD activation. Depicted are 2D coronal sections (a-k) showing the location of brain areas that were significantly different in positive BOLD VoA (red highlight) between vehicle and high dose (3.0 mg/kg) PSI. White matter tracts are highlighted in yellow. The insert in the lower right shows statistical heat maps in transverse and sagittal views. The 3D color-coded reconstructions summarize the major brain areas that were significantly different.
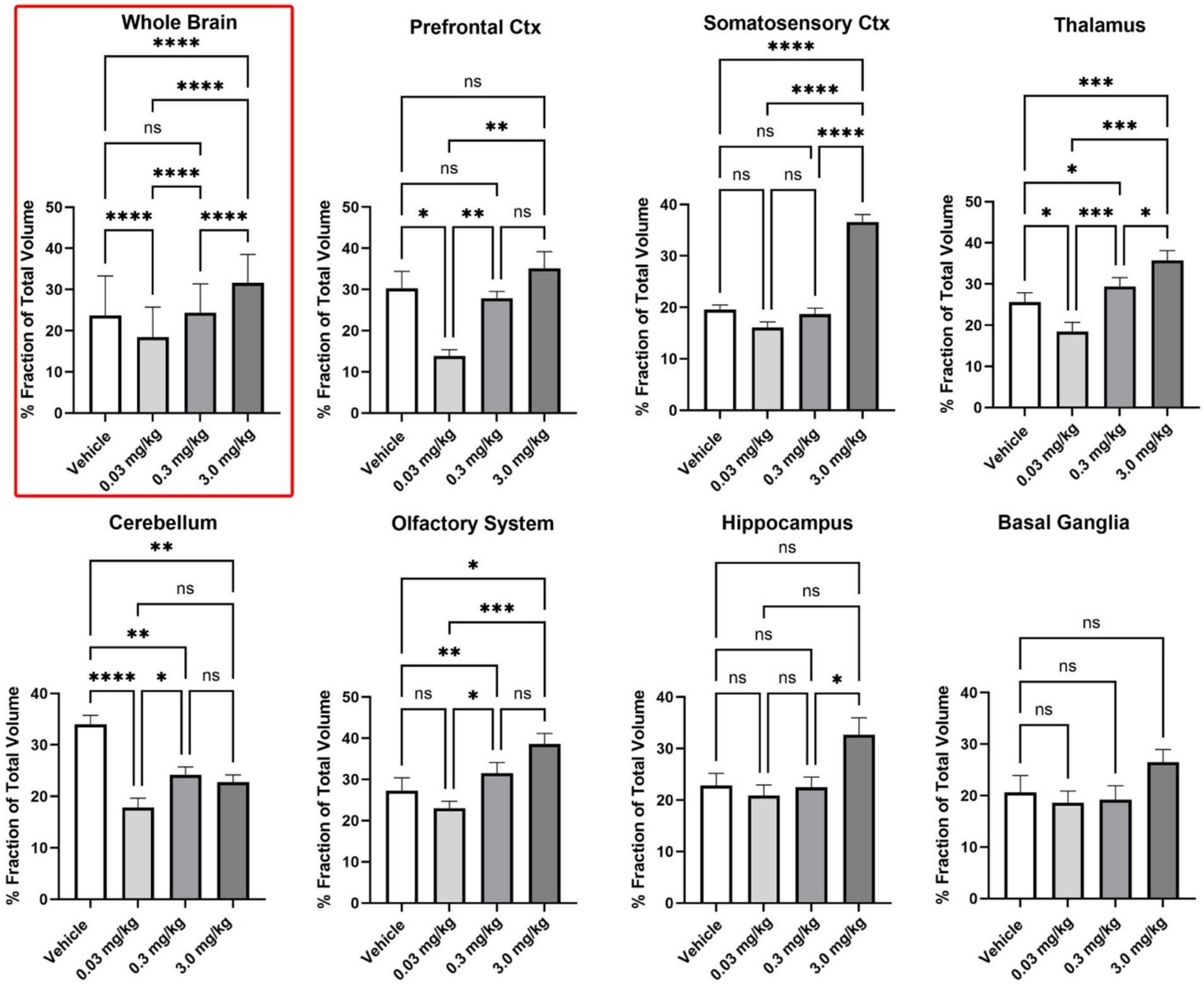
Figure 2. Regional differences in BOLD volume of activation. Shown are bar graphs (mean ± SD) for the dose-dependent change in positive VoA for different brain regions. Data was compared using a matched one-way ANOVA followed by Tukey’s multiple comparison post hoc test. * p < 0.05; ** p < 0.01; *** p < 0.001; **** p < 0.0001.
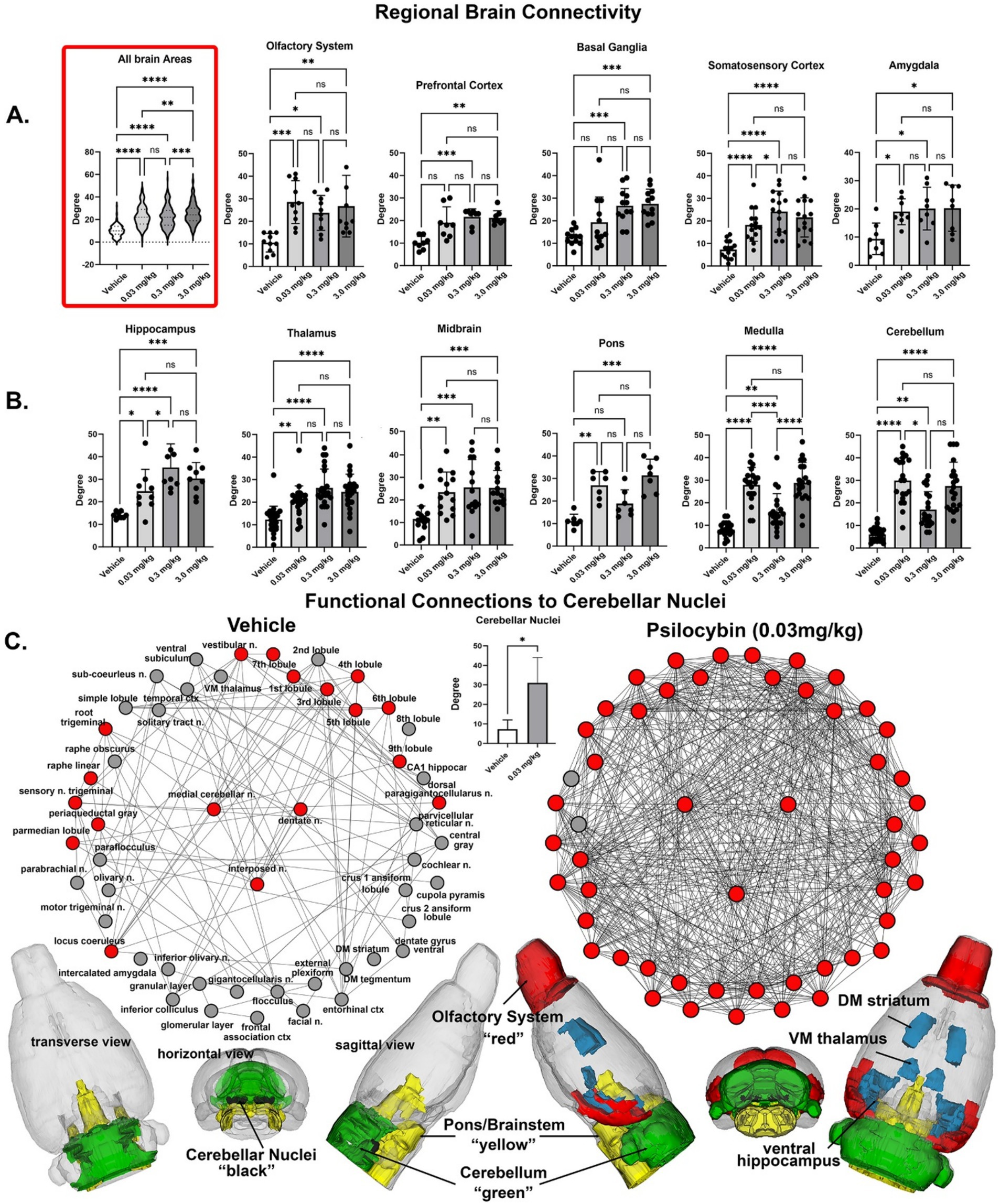
Figure 3. Regional differences in functional connectivity. Rows (a,b) shown are dot plots and bar graphs (mean ± SD) for the number of degrees or connections to other brain areas for veh 0.03 mg/kg, 0.3 mg/kg and 3.0 m/kg PSI. The violin plot show as an insert at the beginning of row (a) is the total of all degrees between the veh, 0.03 mg/kg 169 different brain areas. Shown in row (c) re functional connections to the cerebellum. Shown are radial representations of the connections or degrees (lines) to the three cerebellar nuclei (center red), e.g., fastigial, dentate and interposed, following vehicle or 3.0 mg/kg PSI. The total network is represented by 52 brain areas as the union of both treatments. All brain areas highlighted in red have direct connections to the cerebellar nuclei under each experimental condition. The significant difference in connectivity (student t-test, *p < 0.05) is shown in the inserted bar graph at the center of the figure. The 52 brain areas comprising the network can be organized in three major brain regions—olfactory bulbs (red), cerebellum (green), and brainstem/pons (yellow), with connections to the thalamus, striatum and hippocampus (all in blue). The data are presented for different brain regions. *p < 0.05; **p < 0.01; ***p < 0.001; ****p < 0.0001.
Resting state functional connectivity
Image acquisition
Scans were collected using a spin-echo triple-shot EPI sequence: matrix size 96 × 96 × 20 (H x W x D), TR/TE = 1000/15 msec, voxel size 0.312 × 0.312 × 1.2 mm, with 200 repetitions, time of acquisition 15 min. For preprocessing, we utilized a combination of various software tools, including Analysis of Functional NeuroImages (AFNI_17.1.12), the FMRIB Software Library (FSL, v5.0.9), Deformable Registration via Attribute Matching and Mutual-Saliency Weighting (DRAMMS 1.4.1), and MATLAB. Brain tissue masks for resting-state functional images were manually delineated using 3DSlicer and applied for skull-stripping. We identified motion outliers, which are data segments affected by substantial motion, and recorded the corresponding time points for later regression. Large motion spikes were also detected and removed from the time-course signals. Following this step, slice timing correction was applied to account for interleaved slice acquisition order. We performed head motion correction using the six motion parameters, with the first volume serving as the reference image. Normalization involved registering functional data to the 3D MRI Rat Brain Atlas© using affine registration through DRAMMS. After quality control, a band-pass filter (0.01 Hz to 0.1 Hz) was applied to reduce low-frequency drift effects and high-frequency physiological noise for each subject. The resulting images underwent detrending and spatial smoothing, with a full width at half maximum of 0.8 mm. Additionally, regressors, including motion outliers, the six motion parameters, the mean white matter, and cerebrospinal fluid time series, were incorporated into general linear models for nuisance regression to eliminate unwanted effects.
The region-to-region functional connectivity analysis was conducted to measure the correlations in spontaneous BOLD fluctuations. In this analysis, a network consists of nodes (brain areas of interest) and edges (connections between brain areas). We averaged the voxel time series data within each node based on the residual images obtained through the nuisance regression procedure. Pearson’s correlation coefficients were computed across all pairs of nodes (14,196 pairs) for each subject within all four experimental groups to assess interbrain area temporal correlations. The resulting r-values, ranging from −1 to 1, were z-transformed using Fisher’s Z transform to improve their normality. We constructed 169 × 169 symmetric connectivity matrices, with each entry representing the strength of an edge. Group-level analysis was then conducted to examine functional connectivity in the experimental groups. The Z-score matrices obtained from one-group t-tests were clustered using the K-nearest neighbors clustering method to identify how nodes cluster together and form resting-state networks. A Z-score threshold of |Z| = 2.3 was applied to eliminate spurious or weak node connections for visualization purposes.
Functional connectivity analysis
Degree centrality
We conducted all network analysis using Gephi, which is an open-source software for network analysis and visualization (Bastian et al., 2009). We imported the absolute values of the symmetric connectivity matrices for both PSI and vehicle data, treating the edges as undirected networks. Degree centrality analysis measures the number of connections that a particular node has within the entire network. Degree centrality is defined as:
Here, “n” represents the total number of rows in the adjacency matrix denoted as “A,” and the individual elements of the matrix are indicated as “Aij,” which signifies the count of edges connecting nodes i and j.
Statistics
We conducted all statistical analysis for the graph theory assessment using GraphPad Prism. To decide whether parametric or non-parametric assumptions were appropriate for different group subregions, we performed normality tests. We used Shapiro–Wilk’s tests to assess the normality assumption. Subregion degree centrality p-values exceeding 0.05 were considered to exhibit a normal distribution. Once the normality assumptions were confirmed, we employed one-way ANOVA to compare the degree centrality between the experimental groups in various subregions.
Sex differences
In an attempt to determine if there were sex differences in the sensitivity to PSI we divided the each of the experimental groups into their male and female cohorts. The final distributions were Vehicle (4 males, 6 females), 0.03 mg/kg (5 males, 6 females), 0.3 mg/kg (6 males, 6 females), and 3.0 mg/kg (5 males, 5 females) PSI. While underpowered a two-way ANOVA comparing the sexes across all doses for whole brain activation was significant (p < 0.0001) as reported in the Results. Dividing each experimental group into male and female cohorts for rsFC analysis was unsuccessful as the analysis requires a minimum of 7–8 subjects as determined from previous studies (Harris-Blum et al., 2024; Ghaw et al., 2024).
Psilocybin assay and eCB lipidomics measurements
Sample preparation
75 μL of plasma were added to 500 μL methanol (high performance liquid chromography-HPLC grade) in a centrifuge tube and spiked with 5 μL of 1 μM d4-PSI (Cayman). The 1 μM d4-PSI spike was prepared in a 20:80 methanol/water solution to match optimal elution conditions. Samples were briefly vortexed, after which they were incubated in the dark on ice for 30 min. After incubation, samples were centrifuged for 20 min at 19000 x g at 20o C. Supernatant was added to 9.5 mL of HPLC grade water to form a 2.5% aqueous load. 1.5 mL of this was passed through a solid phase extraction (SPE) column (Agilent Bond Elut) and collected in a 2 mL autosampler vial. The remaining 8.5 mL of the load was passed through the SPE column and emptied to waste. 1 mL elutions were subsequently passed through the SPE column and collected in autosampler vials in the following concentrations: (water/methanol) 100:0, 80:20, 35:65: 25:75.
HPLC properties
Mobile phase A consisted of 0.1% formic acid in 100% water (LCMS grade), and mobile phase B consisted of 0.1% formic acid in 100% methanol (LCMS grade). Mobile phase percentages were adjusted during the 10-min run according adapted from Kolaczynska et al. (2021). In brief, mobile phase A was started at 100% for 30 s. A linear ramp up to 100% B occurred from 0.5 min to 3 min. 100% B was maintained for one more min (min 3–4), followed by a return to 100% A for the remaining six min.
Autosampler conditions
The autosampler (Shimadzu SIL-40C) was kept at room temperature (25o C). Shimadzu pumps (SIL-40D) provided control of mobile phase percentages. The column oven, which housed the mixer and analytical column, was maintained at 40o C. A Phenomenex Luna C18 (Banushi and Polito, 2023) analytical column was used for chromatographic separation (50 mm length, 2 mm internal diameter, 3 μm particle size, 100 angstrom pore size). The analytical column was preceded by a Zorbax Eclipse XDB-C8 guard column.
Mass spectrometric analysis
Mass spectrometry was performed with a SCIEX 7500 triple quadrupole in positive Multiple reactions monitoring (MRM) mode with voltage set to 2,300. Analysis of unknown peaks from plasma were quantified using standard curves as previously described (Bradshaw and Johnson, 2023). A mass spec method for PSI, d4-PSI, and psilocin was developed and parent/fragment pairs were optimized. Supplementary Table 1 lists all mass spectrometric parameters used for analysis in the API 7500. Each compound was validated with more than one parent/daughter pair combination. Chromatograms for psilocybin standards and an example plasma samples are shown in Supplementary Figure 1. The overall lipidomic analysis was validated by evaluating 8 additional endogenous plasma lipids in each sample as previously described (Kolaczynska et al., 2021).
Results
Shown in Figures 4A,B are dose-dependent plasma levels of PSI and its active dephosphorylated metabolite psilocin and measured 30 min after IP administration. There were four blood samples drawn for each dose and the levels detected at lowest dose of 0.03 mg/kg was at the limit of sensitivity with only one sample providing a measure, therefore, we were able to determine statistical significance between 0.3 mg and 3 mg/kg. In brief, at 0.03 mg/kg, psilocin levels were at or below the detection limit (~5 nM); at 0.3 mg/kg, psilocin was around 70 nM (mean 0.07 μM); and at 3.0 mg/kg, psilocin reached roughly 5 μM (mean 1.14 μM). The medium and high dose of PSI yielded significantly higher plasma psilocin than the low dose (which was near zero), confirming dose-proportional pharmacokinetics. To validate that the plasma lipid extraction and qualifications were within normal ranges for these samples, levels of endocannabinoids were evaluated. Figures 4C–J show these levels. All N-acyl ethanolamines were equivalent across all treatment groups, which demonstrates that the extractions were consistent and, also that acute PSI treatment did not affect this class of lipids. Interestingly, the levels of 2-arachidonyl glycerol (2-AG) and 2-linoleoyl glycerol (2-LG) were significantly lowered in all treatment groups and not in a dose-dependent manner suggesting that that PSI has an acute effect on these lipid-signaling molecules even at the lowest dose.
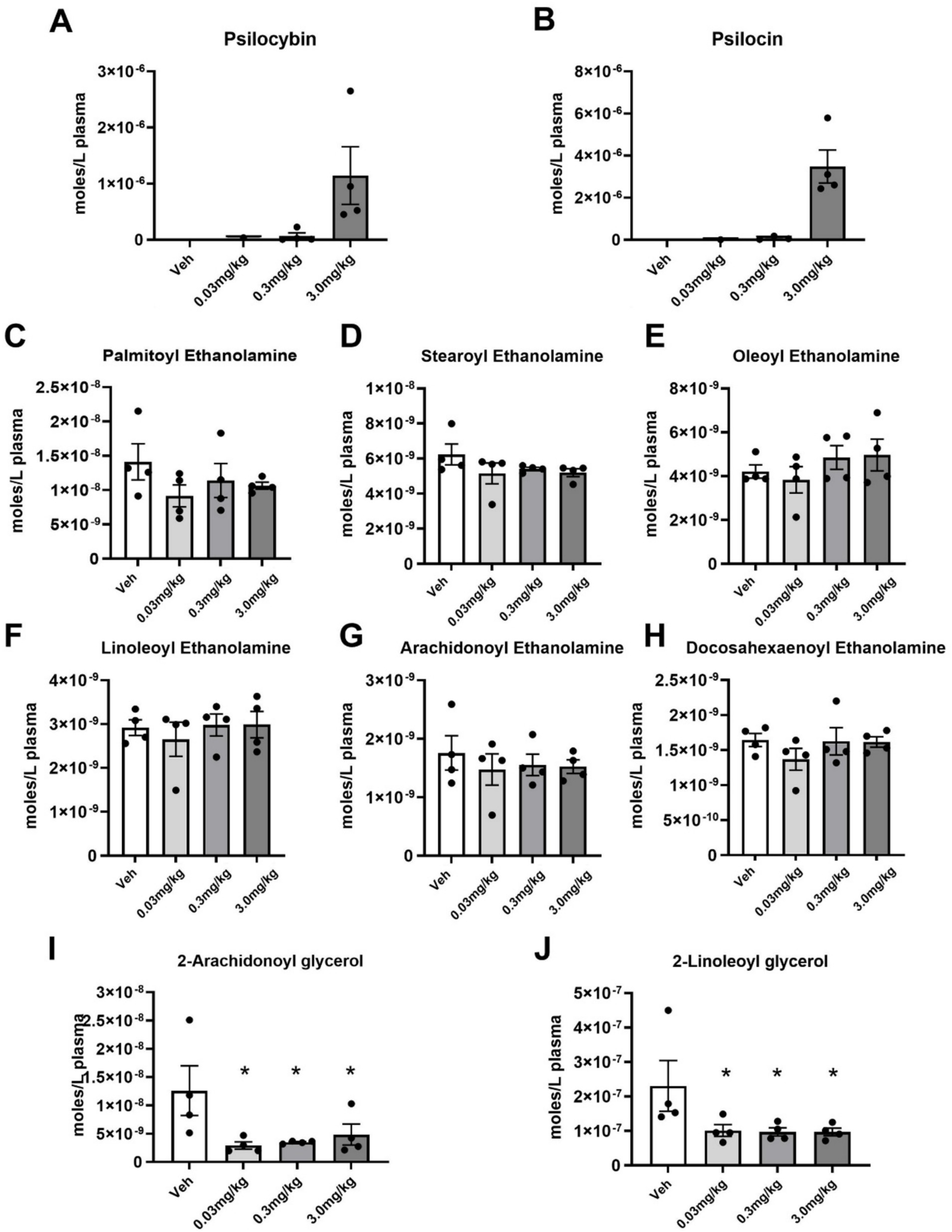
Figure 4. Plasma levels of psilocybin, psilocin, and endogenous lipids measured 30 min after PSI injection. (A,B) Average (Mean ± SEM) levels of psilocybin and its metabolite, psilocin. (C–H) Average levels of N-acyl ethanolamine endogenous lipids, including the endocannabinoid (eCB), Anandamide (G). (I,J) Average levels of 2-acyl glycerol endolipids, including the eCB, 2-AG. * p ≤ 0.05.
After screening for acceptable motion artifact (See Supplementary Figure 2) rats were analyzed for volume of activation (VoA). See Supplementary Table 2 for complete tables of VoA PSI dose response together with vehicle. Table 1 is a truncated list of brain areas (mean ± SE) that show a significant dose-dependent change in positive VoA, i.e., number of positive BOLD voxels, in response to increasing doses of PSI. Several areas are associated with the sensorimotor cortices, e.g., somatosensory cortex barrel field and forelimb, motor cortices and visual cortex. Significant positive activation was observed in 25/169 brain areas. The brain areas are ranked in order of their significance using a critical value of p < 0.05. Shown are the p values and effect size given as omega square (ω Sq). The false discovery rate (FDR) was p = 0.03. Note the effect size for many of the brain areas exceeds 0.14 and indicates a large effect where much of the variance in the dependent variable, i.e., VoA, is attributed to the experimental doses of PSI (Olejnik and Algina, 2003). In Table 2, only 7/169 areas displayed a significant change in negative VoA, an inconsequential effect when considering an FDR p = 0.008.
To determine if there were regional differences in VoA all 169 brain areas were parsed into 11 different brain regions. The organization of these brain regions is shown in Supplementary Table 3. Shown in Figure 2 are bar graphs (mean ± SD) for the dose-dependent change in positive VoA for different brain regions. The number of voxels for each subject in each individual brain area were normalized to the whole volume of the brain region and presented as percent fraction of total volume. The mean ± SD for all subjects in a brain area for that brain region were compared across treatments using a matched ANOVA followed by Tukey’s multiple comparison test for post-hoc differences. The differences across treatments for the whole brain (non-parametric data) were compared using a Friedman test (p < 0.0001). Differences across treatments for the cerebellum (F = 18.06, p < 0.0001), somatosensory cortex (F = 68.57, p < 0.0001), thalamus (F = 19.08, p < 0.0001) and basal ganglia (F = 3.907, p = 0.050) were compared with a one-way ANOVA followed by Tukey’s multiple comparison test. Note for whole brain and each brain region the low 0.03 mg/kg dose of PSI has the lowest VoA significantly less than vehicle for the whole brain, prefrontal cortex, thalamus, and cerebellum. The high 3.0 mg/kg dose of PSI was significantly greater than vehicle for whole brain, somatosensory cortex, thalamus, cerebellum and olfactory system. In several cases there was a staircase rise in VoA across the different PSI doses, e.g., whole brain prefrontal cortex, and thalamus. The dose–response pattern appears non-linear. Low-dose PSI does not produce significant activation above the vehicle baseline and may even blunt some of the spontaneous activation seen with vehicle, while the high dose produces robust activation.
Due to an outsized effect of the high dose, the 3.0 mg/kg group was used for further comparison with the vehicle group. Tables 3, 4 compare positive and negative VoA between the vehicle and high dose treatment, with the same reported metrics as Table 1. As such, results are also ordered by significance. An FDR analyses resulted in a p-value of 0.039. A total of 33 brain areas were significantly activated in the high dose group out of 169 brain areas observed. The pilot study pretreating rats with ketanserin and subsequently challenging them with the 3.0 mg/kg dose of PSI in the magnet showed a complete reduction in positive BOLD signal (Supplementary Table 4). Indeed, rather than an increase in BOLD signal there was a decrease in positive BOLD and an increase in negative BOLD to 13 different brain areas. When 5-HT2A receptors are blocked the PSI effect is inhibiting to a small number of brain areas.
Figure 1 shows the anatomical location of the brain areas listed in Table 3 for positive BOLD volume of activation presented as 2D statistical heat maps. The coronal sections are labeled (a.) through (k.) and arranged from rostral (top) to caudal (bottom). Areas in red are areas of significant activation following 3.0 mg/kg dose of PSI. Areas in yellow denote the location of white matter tracts. Brain section (a.) shows significant activity in the olfactory bulb. Sections (c., d.) highlight afferent connections of the dopamine system (e.g., striatum, globus pallidus, anterior cingulate cortex), all producing significant activation. Sections (c., f.) denote the somatosensory cortex, of significant interest due to its role in producing psychedelic hallucinations. Section (e.) highlights the thalamus, thought to play a role in psychedelic hallucinations. Note that sections (j., k.) are not significantly affected by PSI. The essential findings taken from the 2D images, sections (a.–f.) are summarized in color-coded 3D reconstructions to the right. A transverse view of the brain shows the somatosensory cortex as a green translucent cover over all of the brain minus the olfactory bulb (yellow). A sagittal view of the brain displays the thalamus (blue) and basal ganglia (red).
Figure 5 shows the change in BOLD signal over time for the somatosensory cortex in response to vehicle (black line) and 3.0 mg/kg of PSI (red line). 250 images were acquired over the 25 min imaging session. Each acquisition is the mean ± SE of vehicle and high dose PSI rats combining the data from the somatosensory cortex (e.g., barrel filed, hindlimb, forelimb, trunk etc.) for all subjects for each experimental condition. With a two-way repeated measures ANOVA there was a significant interaction between time and treatment [F(2.49, 202.42) = 4.424, p < 0.0001] with a main effect for treatment [F(1,117) = 10.55, p = 0.0015] Note the immediate increase in BOLD signal (2%) following injection (image acquisition # 51) of PSI. There was a continuous increase in BOLD signal that remained above threshold (blue line) for almost the entire imaging session, peaking at 4% positive BOLD signal. In contrast, vehicle injection was characterized by a slow and steady increase in positive BOLD signal that did not breach the threshold until image acquisition #71, peaking at 2.5% positive BOLD signal. Shown in See Supplementary Figure 3 is the time course of BOLD signal change in the somatosensory cortices in response to 3.0 mg/kg PSI but in the presence of ketanserin. There is no significant difference between vehicle and PSI with the blockade of 5-HT2A receptors.
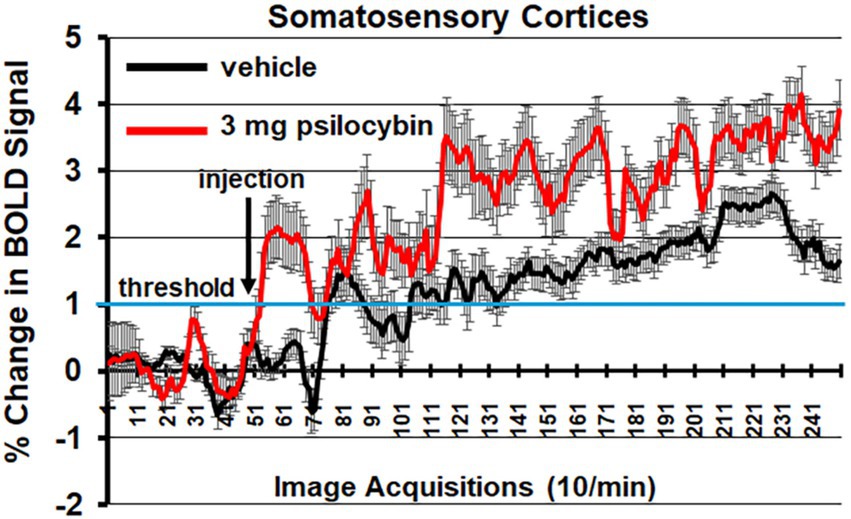
Figure 5. Somatosensory cortex time course. Shown is the change in BOLD signal over time for the somatosensory cortices in response to vehicle (black line) and 3.0 mg/kg PSI (red line). 250 images were acquired over the 25 min imaging session. Each acquisition is the mean ± SE of vehicle and high dose PSI rats combining the data from the different somatosensory cortices, e.g., barrel field, hindlimb, forelimb, trunk, and upper lip. The 1% threshold is highlighted by the blue lines to account for the normal fluctuations in BOLD signal in the awake rat brain. A two-way ANOVA showed a significant Time x Treatment interaction F(2.49, 202.42) = 4.424, p < 0.0001.
When the mean volume of activation for each of the brain areas for male and female rats was compared for all experimental groups (veh, 0.03 mg/kg, 0.3 mg/kg, and 3.0 mg/kg PSI) there was a significant sex by dose interaction [two-way ANOVA F(3,672) = 72.39, p < 0.0001]. Subsequent analysis (two-way ANOVA) looking at the sex difference in brain areas for each of the experimental groups showed no significant differences for vehicle [F(169,1,352) = 0,849, p = 0.912)] or the 3.0 mg/kg dose of PSI [F(170, 1,360) = 0.9420, p = 0.686]. However, male were significantly more sensitive to the low 0.03 mg/kg dose of PSI as compared to females [F(169,1,352) = 1.271, p = 0.0148]. A Wilcoxon signed-ranked test showed only six brain regions to be significantly activated. Females, on the other hand were far more sensitive to the 0.3 mg/kg dose of PSI as compared to males [two-way ANOVA, F(169, 1,690) = 3.273, p < 0.0001]. A Wilcoxon post hoc test showed 45 brain areas were significantly activated. See Supplementary Table 5 for a table of female/male differences for the 0.3 mg/kg dose of PSI.
Shown in Figure 6 are the location of many of the brain areas in female rats that were more sensitive to the 0.3 mg/kg dose of PSI than males. Brain section (b.) shows a sex difference in the prefrontal cortex, e.g., prelimbic, ventral orbital and lateral orbital cortices. Section (c.) highlights the ventral striatum comprised of the ventral medial and ventral lateral striatum, accumbens core and shell, and ventral pallidum. Sections (d., e.) note the sensitivity of the thalamus. Section (f.) shows the ventral tegmental area. Sections (f.–i.) highlight numerous areas comprising the ascending reticular activating system (e.g., midbrain reticular n., parabrachial n., paragigantocellularis and gigantocellularis reticular nuclei) together with the raphe nuclei and the periaqueductal gray, areas involved in arousal, sleep/wake cycles and consciousness. Sections (h., i.) highlight a sex difference in the sensitivity of the cerebellum.
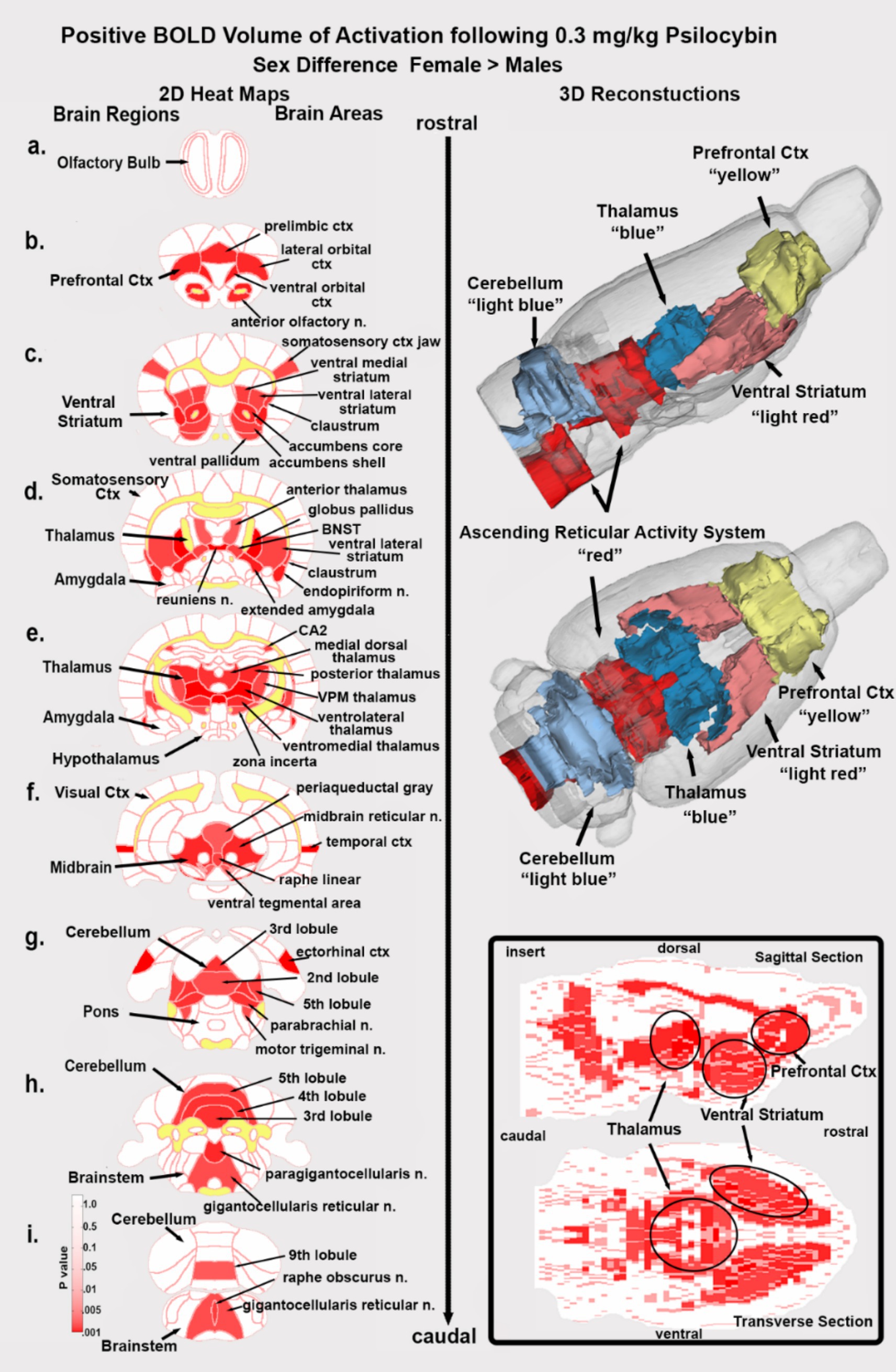
Figure 6. Sex differences in brain activation. Depicted are 2D coronal sections (a-i) showing the location of brain areas that were significantly different in positive BOLD VoA (red highlight) between males and females for the 0.3 mg/kg dose of PSI. Females were greater than males. White matter tracts are highlighted in yellow. The insert in the lower right shows statistical heat maps in transverse and sagittal views the 3D color-coded reconstructions summarize the major brain areas that were significantly different.
Shown in Figure 3 are the dose-dependent differences in degrees (connections to other brain areas) for 11 major brain regions that make up the whole brain. The highlighted box in row A. shows the connections between all 169 brain areas as violin plots, i.e., the probability distribution of the numerical data for each dose and vehicle. There was a significant treatment effect using a matched one-way ANOVA [F(2.60, 442.1) = 72.46, p < 0.0001] followed by Tukey’s multiple comparison post hoc test **p < 0.01, *** p < 0.001; **** p < 0.0001. For many brain regions, e.g., olfactory system, somatosensory cortex, amygdala, thalamus and midbrain all doses were significantly greater than vehicle, but not between themselves. In contrast in row B. the medulla [F(2.76, 52.45) = 76.49, p < 0.0001] and cerebellum [F(2.76,52.52) = 72.46, p < 0.0001] showed a significant difference between PSI doses and displayed a U-shaped profile with the medium 0.3 mg/kg dose having the lowest numbers of degrees. Note the high level of degrees (29.95 ± 10.1, mean and SD) for the low 0.03 mg/kg dose of PSI as compared to vehicle (6.05 ± 3.08). Row C. looks at these differences from the perspective of the medial cerebellar, dentate and interposed deep cerebellar nuclei. All efferent functional connections leaving the cerebellum and projecting to extra cerebellar sites go through these three nuclei. The significant difference (two-tailed t-test p = 0.039) between vehicle and 0.03 mg/kg dose of PSI for the cerebellar nuclei is shown in the bar graph in row C. Based on weighted degree analysis, a metric that analyzes total strength of node connections, the cerebellar nuclei of the psilocybin group exhibited significantly greater influence than the control group (t-test, p = 0.045). The individual connections between brain areas for vehicle and low dose PSI are shown as wheels with the three cerebellar nuclei in the middle. The cerebellar nuclei with vehicle treatment have only 15 connections between them show in red. With PSI treatment these connections are increase to 46 with the exception of the raphe linear and periaqueductal gray. The connections with PSI expand to include all of the cerebellum, areas of the hippocampus (dentate, subiculum, CA1), all of the olfactory bulb (glomerular, external plexiform and granular layers), temporal ctx, ventral medial thalamus, dorsal medial striatum and many areas in the brainstem. The differences between vehicle and low dose PSI are shown in the color-coded 3D reconstruction below. The most dramatic change occurs between vehicle and the lowest dose, with more moderate increases at higher doses, suggesting even small amounts of psilocybin significantly reorganize brain network dynamics.
The global statistics using graph theory analysis are shown in Table 5. Both average degree and network density show substantial increases from vehicle to all PSI doses suggesting PSI promotes more connections between brain areas. There is a general trend where connectivity metrics increase with dose, particularly notable in the jump from vehicle to the lowest dose (0.03 mg/kg). The average path length decreases as dose increases, indicating information can travel more efficiently across the brain network under the influence of PSI; while the average weighted degree shows a consistent dose-dependent increase, suggesting not just more connections but stronger ones at higher doses. The most dramatic change occurs between vehicle and the lowest dose, with more moderate increases at higher doses, suggesting even small amounts of PSI significantly reorganize brain network dynamics.
Discussion
To the best of our knowledge this is one of the few pharmacological functional MRI (phMRI) study in animals or humans to show a dose-dependent change in BOLD signal in response to PSI. To make these data more relevant to the human condition, imaging was performed while male and female rats were fully awake without the confound of anesthesia and during the dark phase of L-D cycle when rats are normally active. There was a dose-dependent increase in BOLD VoA in much of the forebrain, basal ganglia and somatosensory cortex and a global dose-dependent increase in functional connectivity. These findings are discussed with respect to imaging studies in healthy human volunteers exposed to PSI and their relevance, if any, to understanding the complex hallucinogen experience.
Psilocybin is in the chemical class of indoleamine hallucinogens that also includes DMT and LSD (Banushi and Polito, 2023). PSI is relatively non-selective for serotonin receptors having a high affinity for 5-HT1A, 5-HT2A, and 5-HT2C receptor subtypes (Halberstadt et al., 2011; Halberstadt and Geyer, 2011). The CNS effects of PSI are primarily attributed to activation of the 5-HT2A receptor localized to pyramidal neurons in the somatosensory and prefrontal cortices (Vollenweider et al., 1998). There is evidence that PSI activation of 5-HT2A receptor in the cortex leads to a rise in extracellular levels of glutamate (Mason et al., 2020). PSI can also elevate levels of dopamine in the basal ganglia (Vollenweider et al., 1999) and prefrontal cortex (Wojtas et al., 2022). The involvement of glutamate and dopamine signaling are two examples of down-stream effects of PSI that could contribute to the complex changes in brain activity and functional circuitry associated with the psychedelic experience. To that end, phMRI is agnostic and not wedded to any particular mechanism, providing a global map of site-specific changes in brain activity and connectivity.
Acute BOLD response
Areas comprising the different body-specific parts of the primary somatosensory cortex (e.g., barrel field, hindlimb, truck, forelimb) were activated. Indeed, the primary somatosensory cortices showed both a significant increase in volume of activation as well as percent change in BOLD signal over time, two separate ways to assess functional activity. Liu et al. (2023) using anesthetized male rats also reported that 2.0 mg/kg PSI given IP during the scanning session causes widespread positive BOLD activation and increased functional connectivity. Spain et al. (2015) also imaged male rats under anesthesia given a low (0.03 mg/kg) or high (2.0 mg/kg) dose of psilocin during the scanning session. The low dose of psilocn had little or no effect. However, the high dose of psilocn caused a significant increase in BOLD signal in the olfactory system, limbic and visual cortices but a decrease in the somatosensory cortex, thalamus, and hippocampus. Using a laser scanning technique, these same rats were also studied for changes in localized blood flow to the barrel field of the somatosensory cortex in response to whisker stimulation in the absence and presence of psilocn while also recording local field potentials from implanted electrodes in the same site. The 2.0 mg/kg dose of psilocn enhanced blood flow during whisker stimulation, a response that was augmented by psilocin. Paradoxically, the drug-evoked increase in blood flow occurred during a decrease in neural activity raising questions about neurovascular coupling in the presence of PSI. However, vascular reactivity in response to a hypercapnic challenge was normal when comparing vehicle and psilocin treatments. Golden and Chadderton (2022) reported recordings from the anterior cingulate cortex on awake mice showing an increase in neuronal firing in response to a dose of 2.0 mg/kg of PSI. In addition there was a decrease in low frequency band power but an increase in gamma band power evidence of cortical desynchronization. These studies by Liu, Spain and Golden showing regional increases in BOLD signal and electrical activity and ours showing a global increase to PSI are in sharp contrast to the initial human BOLD imaging study performed by Carhart-Harris et al. (2012a). In healthy volunteers with a history of hallucinogen exposure there was a sharp decrease in cerebral blood flow (CBF) measured with arterial spin labeling in all areas of the brain. The same study was repeated with BOLD imaging again finding a decrease particularly in the prefrontal cortex and anterior cingulate. The magnitude of the decrease in CBF and BOLD was inversely correlated with the subjective effects of PSI. In a subsequent publication on data collected from the same subjects, Carhart-Harris reported PSI enhanced BOLD signal in the visual cortex and somatosensory areas in response to memory recall enacted during the scanning session showing a difference between resting and task driven effects of PSI (Carhart-Harris et al., 2012b). However, the global decrease in hemodynamic measures of brain activity in humans under resting conditions is contrary to PET imaging studies using [F-18] FDG to follow glucose metabolism. Vollenweider et al. (1997) reported a global increase in glucose metabolism following PSI treatment with the greatest changes occurring in the prefrontal and anterior cortical areas. The increase in metabolic hyperfrontality was also reported by Gouzoulis-Mayfrank et al. (1999) in healthy volunteers in response to PSI exposure. The Vollenweider group followed up their PET imaging study with another using arterial spin labeling to follow changes in CBF in response to PSI (Lewis et al., 2017). Correcting for global CBF in each subject, they reported regional differences in blood flow highlighted by an increase in the frontal cortex but a decrease in other brain areas. N,N-dimethyltryptamine (DMT) a hallucinogen with comparable chemistry and activity to PSI, when tested in human volunteers also increases CBF to the frontal, limbic, and anterior cingulate cortices (Riba et al., 2006).
Pharmacokinetics
5-HT2A receptors typically have high affinity in the low nanomolar range, so a plasma psilocin concentration of ~5 nM at the 0.03 mg/kg suggests minimal occupancy/activation of 5-HT2A and likely below the threshold for measurable effect (Nichols, 2004). In contrast, ~5 μM at 3.0 mg/kg is orders of magnitude higher – enough to occupy nearly all 5-HT2A receptors and even engage lower-affinity sites like 5-HT1B and 5-HT1D receptors. The pharmacological breadth at the high PSI dose could explain why we see widespread activation as the drug is hitting multiple receptor systems, possibly including vasoconstrictive 5-HT1 receptors, as discussed by Spain et al. (2015). This dose-dependent transition from no effect to hyperactivation might reflect the steep occupancy-response curve of 5-HT2A and recruitment of additional targets at high dose. In addition to the direct effects on 5-HT2A receptors, we show here that even the lowest dose of 0.03 mg/kg had a profound effect on plasma levels of the endocannabinoid, 2-AG. There is a growing literature on the relationship of 2-AG and serotonin regulation (Oakes et al., 2019; Parrish and Nichols, 2006; Granberg et al., 2001) that may underly some of the functional changes observed here at the 0.03 mg/kg dose.
Sex differences
Dividing each of the experimental groups into male and female cohorts enabled us to test for sex differences to the sensitivity of different doses of PSI. Neither the vehicle nor the high 3.0 mg/kg dose of PSI presented with a significant sex difference. However, the low 0.03 mg/kg dose of PSI revealed a modest (only six areas activated) but significant male sensitivity to this hallucinogen. More compelling was the sensitivity of females to the medium 0.3 mg/kg dose of PSI with 45/169 brain areas showing a sex difference. The anatomical organization of the female sensitivity was most interesting with clearly defined activation of the ventral tegmental area and its dopaminergic efferent connections to the ventral striatum, accumbens and ventral pallidum (see Figure 6). Almost all of the thalamus was similarly activated together with key areas of the ascending reticular activating system. What was missing from this pattern of global brain activation was almost the entire somatosensory cortex and pons and all the hippocampus, amygdala and olfactory system. Notably, male rats exhibited far less activation in these areas compared to females. Behavioral studies showing a sex differences in response to PSI or psilocin are sparse. Tyls and coworkers treated male and female rats SQ with 0.25 mg/kg, 1.0 mg/kg or 4.0 mg/kg of psilocin and 15 min later measured locomotor activity in an open field (Tyls et al., 2016). There was a dose-dependent decrease in locomotion for both males and diestrus females but not estrus females. However, when tested for acoustic startle for prepulse inhibition there were no sex differences. Adult male mice but not female mice treated IP with PSI in doses ranging from 0.1 mg/kg to 2.0 mg/kg show reduced voluntary ethanol consumption (Alper et al., 2022). In a recent study Effinger et al. (2023) reported the central amygdala of female rats is more sensitive to a 2.0 mg/kg dose of psilocin as compared to males in measures of c-fos immunostaining and photometry in response to an aversive stimulus. There is no clear evidence in the clinical literature of sex difference in sensitivity to PSI (Shadani et al., 2024).
BOLD functional connectivity
One of the more compelling findings in this study was the dose-dependent increase in global functional connectivity. Reinwald et al. (2023) in a cross-over experimental design, imaged females rats treated with 1.0 mg/kg PSI and vehicle during the MRI scanning session. The studies were done under anesthesia and during the light phase of the L-D cycle. Unfortunately, the cerebellum was not included in the study. There was an overall global decrease in connectivity with PSI treatment, in stark contrast to our findings. The most plausible explanation for this disparity is the confound of anesthesia and impact of circadian biology. The importance of circadian timing and brain function cannot be underestimated. Perivascular clearance of an MRI contrast agent from the brain of awake rats is regulated by the circadian clock being highest during the light phase and lowest during the dark phase of the L-D cycle (Cai et al., 2020). Brain temperature is a circadian rhythm entrained by the L-D cycle (Refinetti and Menaker, 1992; Scheer et al., 2005). These concerns notwithstanding, the data from the Reinwald study was consistent with many human imaging studies that report a general decrease in intra-network connectivity, particularly in association areas like the default mode and salience networks in healthy volunteers treated with PSI (Carhart-Harris et al., 2013; Lebedev et al., 2015; Muthukumaraswamy et al., 2013). Preller et al. (2020) reported a time-dependent change in global connectivity in healthy volunteers following PSI treatment. At 20 min post treatment these was hyperconnectivity in the occipital cortex. At later time points, 40 and 70 min post treatment, the pattern of brain activity evolved into hypoconnectivity in association areas and hyperconnectivity in sensory networks. Preller et al. further demonstrated that the altered brain connectivity was positively correlated with brain areas high in 5-HT2A gene expression and negatively correlated with the localization of 5-HT1A gene expression. Preller’s findings align with ours, demonstrating that PSI increases global brain connectivity across sensory networks. In a just published study Siegel and coworkers followed healthy volunteers prior to, during, and three weeks following a high 25 mg/kg dose of PSI (Siegel et al., 2024). PSI had a dramatic effect on disrupting rsFC particularly in the default mode network. The data from global graph theory analysis presented in Table 5 align with the “entropic brain hypothesis” proposed by Carhart-Harris et al. (2014) where psychedelics like PSI increase brain network integration and reduce segregation between normally distinct networks. The PSI induced increased entropy (disorder/uncertainty) in brain activity, particularly in high-level networks may explain the altered states of consciousness associated with classical hallucinogens.
One brain area highlighted in this study and previously overlooked in both preclinical and clinical literature is the cerebellum. Its involvement was anticipated, given the numerous awake animal imaging studies demonstrating alterations in cerebellar activity following the administration of cannabinoids, psychostimulants, ketamine, opioids, and neuropeptides (Demaree et al., 2021; Sadaka et al., 2021; Coleman et al., 2022; Kawazoe et al., 2022; Moore et al., 2016) and most recently the hallucinogen LSD (Ghaw et al., 2024). Traditionally, the cerebellum has been considered primarily responsible for motor coordination. However, it also plays a role in autonomic physiological functions such as heart rate, blood pressure, and respiration (Krohn et al., 2023; Cavdar et al., 2001; Haines et al., 1984; Snider et al., 1976). Additionally, the cerebellum is recognized for its significant involvement in emotional and cognitive functions (Zhang et al., 2023; Schmahmann, 2019; Adamaszek et al., 2017), feeding (Low et al., 2021), and addiction (Miquel et al., 2016). The cerebellum’s connectivity is unique, with all efferent information passing through the fastigial, interposed, and dentate nuclei. These nuclei have extensive bidirectional connections with various brain regions, including the thalamus, hypothalamus, limbic cortex, amygdala, hippocampus, and brainstem (Kebschull et al., 2023). The cerebellum also receives a substantial portion of its nerve connections from the vestibular complex, which relays auditory information from the ear to the cortex. In this study, cerebellar connections extended to the olfactory bulb, frontal association cortex, and hippocampus. This extensive network of connections and its involvement in various behavioral and sensory functions raise the question—could the cerebellum play a role in hallucinogenic effects that mimic psychosis? Notably, given the cerebellum’s involvement in cognitive, emotional, and sensory processes, it may contribute to the wide range of symptoms and cognitive impairments observed in schizophrenia (Andreasen and Pierson, 2008).
Limitations
This study would have benefited from a suite of behavioral assays measuring cognition, locomotion and emotionality for each dose of PSI. These measures would have been particularly relevant to understanding the region specific pattern of brain activation shown in females to the medium 0.3 mg/kg dose of PSI. The involvement of the ascending reticular activating system and dopaminergic neural circuitry would suggest an increase in motivation.
This study did not include pharmacokinetics. While we analyzed plasma for PSI and its metabolite psilocin these samples were collected at single time point 30 min following IP injection. The drug-induced changes in BOLD signal were recorded 20–24 min after IP injection while the rsFC data were collected ca 35 min post injection. Would the data have differed if we chose later time points, e.g., 45–60 min post injection of PSI? In a recent studying looking at the pharmacokinetics of 1.0 and 3.0 mg/kg PSI in mice injected IP, the levels of plasma psilocin peak at 15 with a half-life of ca 30 min (Passie et al., 2002). Hence, waiting a longer period of time for the acute drug-induced effect of PSI may have been limiting. The rapid rise of psilocin in plasma may account for the early rise in the BOLD signal observed in the time course series shown in Figure 6 for the somatosensory ctx.
Data interpretation
This phMRI study testing the effects of PSI on brain activity is similar to many others used to characterize a drug’s effect on the brain (Kawazoe et al., 2022; Sathe et al., 2023; Brems et al., 2023), yet the robust dose-dependent increase in global BOLD signal and connectivity are unprecedented and at odds with much of the preclinical and clinical literature. Spain and coworkers raised concerns around the potential confounding effects of 5-HT agonists and vascular tone with respective to the hemodynamic changes that contribute to the BOLD signal. Psilocin, psilocybin’s active metabolite, primarily acts through the 5-HT2A receptor. Psilocin also binds with lower, but potentially relevant, affinity for the 5-HT1B and 5-HT1D receptors. Serotonin receptors lining the vasculature may modulate vascular tone and causes subsequent changes to BOLD signal independent of metabolism. Spain and colleagues also noted that 5-HT2A receptors on cortical inhibitory interneurons may induce vasoconstriction and vasodilation, constituting a scenario where increased neuronal activity may lead to either positive or negative BOLD. Conscious, unrestrained rats treated with the hallucinogen DOI (2,5-dimethoxy-4-iodoamphetamine), a 5-HT2A receptor agonist, reduces blood flow to the cutaneous circulation of the tail through vasoconstriction, an effect blocked by ketanserin pretreatment (Blessing and Seaman, 2003). Whether this vasoconstriction in the peripheral cutaneous circulation generalizes to the cerebral vasculature is unknown. The dose-dependent increase in BOLD signal in this study is not consistent with a decrease in cerebral blood flow; however, it aligns with the decreases in cerebral blood flow and BOLD signal reported in the Carhart-Harris study (Carhart-Harris et al., 2012a).
How do we reconcile these differences? Imaging done in awake animals and during the circadian L-D cycle when they are normally active could account for the discrepancies in the acute BOLD response between our study and that of Spain and Reinwald. When comparing our results to the human literature many of the differences could be explained by the stress of the imaging protocol. Psilocybin and other 5-HT2A agonists are known to acutely activate the hypothalamic–pituitary–adrenal (HPA) axis. For example, administration of serotonergic psychedelics in rodents triggers robust increases in corticosterone via 5-HT2A receptor activation (Romanova et al., 2022; Van de Kar et al., 2001). Notably, this effect can be blocked by a 5-HT2A antagonist (Van de Kar et al., 2001), indicating it is mediated by the same receptor involved in psychedelic effects. In humans, PSI similarly elevates cortisol levels for an hour or more post-administration (Constantino et al., 2025). While rats have been acclimated to the imaging procedure there still maybe some restraint stress and corticosterone that, in combination with PSI, may lead to hypermetabolism or different network engagement than a relatively calm human during the scanning session.
Differences in neurobiology may also account for the discrepancies. There are inherent species differences in serotonin receptor distribution and neural circuitry (Nichols, 2004). Rodents have differences in cortical architecture and potentially in the baseline oscillatory activity compared to humans. For example, the rat default mode network (DMN) is not as pronounced as in humans (Liang et al., 2011), which might affect how PSI modulates “global connectivity.” Differences in 5-HT2A receptor density (e.g., rats have high densities in certain retrosplenial and frontal areas) could lead to different activation patterns. Additionally, dosing relative to metabolism differs as our rats were scanned during the peak drug effect (within 30 min of injection), whereas human fMRI studies often capture a slightly later timeframe of PSI effects when subjective effects plateau. Comparisons between rodents and humans must be made cautiously and with these confounds in mind. Our results in rats – robust hyperactivation and hyperconnectivity – might reflect an interaction between PSI’s direct pharmacological effects and the stress/arousal state of the animal.
Drawing comparisons between awake rats and awake humans when trying to explain the complex psychedelic effect of psilocybin or any hallucinogen by the pattern of brain activity may be a difficult endeavor. Commercially bred rats are, by most measures, genetically identical. All share a common restricted environment minimizing cognitive, emotional and sensory experience. This neurobiological portrait of their environment is limited to a short window of life—only 3–4 months. The food they eat is identical. They are drug naïve. Hence the variance between experimental doses may be small enough to observe dose-dependent changes across subjects as shown here. All of these controlled conditions are ideal for preclinical discovery but impossible in the world of human neuroscience. The psychedelic experience, by all accounts, is a personalized, untethered, and unfiltered emergence of experience and perception leading to “oceanic boundlessness” and “ego dissolution” (Vollenweider and Preller, 2020). This complex subjective experience cannot be modeled on a rodent. It is unlikely that the head twitch in a rat is a proxy for a hallucinogenic experience (Robbins, 2017; Corne et al., 1963). At face value it is a peripheral measure of a motor response to the CNS effect of 5-HT2A receptor activation (Gonzalez-Maeso et al., 2007). Nonetheless PSI activation of 5-HT2A receptor in this study did favor enhanced BOLD signal in the sensorimotor cortex and other brain regions with a high density of 5-HT2A receptors. Indeed, ketanserin treatment in this study blocked the activation of the somatosensory cortices by PSI. Brain areas hypothesized to be involved in loss of sensory filtering and organization of sensory motor stimuli such as the claustrum (Barrett et al., 2020) and the cortico-basal ganglia-thalamic-cortical loop are all affected by PSI in a dose-dependent manner (Banushi and Polito, 2023). So much of the neuroanatomical circuitry described in the human literature is affected in this study but not in the direction or pattern reported in the clinic.
Summary
To the best of our knowledge there has never been a published study—preclinical or clinical—showing a dose-dependent change in resting state functional connectivity using PSI. Furthermore we show a dose and sex-dependent difference in BOLD activity. We observed a sex-dependent pattern at the intermediate dose (0.3 mg/kg) with females showed significantly greater brain activation than males, whereas at the highest dose (3.0 mg/kg), male and female activation levels were more similar. This suggests that females may reach peak activation at a lower dose than males – in that sense showing greater sensitivity at 0.3 mg/kg – but both sexes ultimately exhibit large-scale activation at the high dose. The enhanced female sensitivity at the intermediate dose presents with a very region-specific pattern of activation around the thalamus and dopaminergic neural circuitry. These data were registered a rat 3D MRI atlas with 169 different bilateral brain areas providing global site-specific changes in male and female rats. All imaging was done in fully awake rats without the confound of anesthesia and during the dark phase of the L-D cycle when rodents are normally active. These experimental conditions were done to mimic the experience of a human imaging study. The neural circuitry associated with hallucinations in humans. e.g., the cortico-striato-thalamo-cortical circuit and the claustrum, showed increased activation at higher doses. Moreover, the cerebellum showed a dose-dependent increase in hyperconnectivity to numerous brain areas.
Data availability statement
The original contributions presented in the study are included in the article/Supplementary material, further inquiries can be directed to the corresponding author.
Ethics statement
All methods and procedures described below were pre-approved by the Northeastern University Institutional Animal Care and Use Committee under protocol number 23-0407R. The study was conducted in accordance with the local legislation and institutional requirements.
Author contributions
EF: Data curation, Formal analysis, Writing – original draft. AC: Formal analysis, Writing – review & editing. RO: Data curation, Writing – original draft. TN: Formal analysis, Writing – review & editing. JE: Data curation, Formal analysis, Writing – original draft. ML: Formal analysis, Methodology, Writing – original draft. EG: Formal analysis, Methodology, Writing – original draft. TW: Formal analysis, Writing – original draft. BA: Formal analysis, Writing – review & editing. AM: Formal analysis, Writing – review & editing. NC: Data curation, Formal analysis, Writing – original draft. HB: Formal analysis, Writing – original draft, Writing – review & editing. PK: Formal analysis, Project administration, Writing – review & editing. CF: Conceptualization, Writing – original draft, Writing – review & editing, Formal analysis.
Funding
The author(s) declare that financial support was received for the research and/or publication of this article. This work was supported by Ekam Imaging, Inc.
Acknowledgments
We thank the National Institute on Drug Abuse, Drug Supply Program and Research Triangle Institute for providing the psilocybin used in these studies. We also thank the Center for Translational NeuroImaging and the Department Pharmaceutical Sciences for financial support.
Conflict of interest
CF and PK have a financial interest in Ekam Imaging.
The remaining authors declare that the research was conducted in the absence of any commercial or financial relationships that could be construed as a potential conflict of interest.
Generative AI statement
The author(s) declare that no Gen AI was used in the creation of this manuscript.
Publisher’s note
All claims expressed in this article are solely those of the authors and do not necessarily represent those of their affiliated organizations, or those of the publisher, the editors and the reviewers. Any product that may be evaluated in this article, or claim that may be made by its manufacturer, is not guaranteed or endorsed by the publisher.
Supplementary material
The Supplementary material for this article can be found online at: https://www.frontiersin.org/articles/10.3389/fnins.2025.1554049/full#supplementary-material
References
Adamaszek, M., D’Agata, F., Ferrucci, R., Habas, C., Keulen, S., Kirkby, K. C., et al. (2017). Consensus paper: cerebellum and emotion. Cerebellum 16, 552–576. doi: 10.1007/s12311-016-0815-8
Allen, J., Dames, S. S., Foldi, C. J., and Shultz, S. R. (2024). Psychedelics for acquired brain injury: a review of molecular mechanisms and therapeutic potential. Mol. Psychiatry 29, 671–685. doi: 10.1038/s41380-023-02360-0
Alper, K., Cange, J., Sah, R., Schreiber-Gregory, D., Sershen, H., and Vinod, K. Y. (2022). Psilocybin sex-dependently reduces alcohol consumption in C57BL/6J mice. Front. Pharmacol. 13:1074633. doi: 10.3389/fphar.2022.1074633
Andreasen, N. C., and Pierson, R. (2008). The role of the cerebellum in schizophrenia. Biol. Psychiatry 64, 81–88. doi: 10.1016/j.biopsych.2008.01.003
Banushi, B., and Polito, V. (2023). A comprehensive review of the current status of the cellular neurobiology of psychedelics. Biology (Basel) 12, 1–26. doi: 10.3390/biology12111380
Barrett, F. S., Krimmel, S. R., Griffiths, R. R., Seminowicz, D. A., and Mathur, B. N. (2020). Psilocybin acutely alters the functional connectivity of the claustrum with brain networks that support perception, memory, and attention. NeuroImage 218:116980. doi: 10.1016/j.neuroimage.2020.116980
Bastian, M, Heymann, S, and Jacomy, M (2009) Gephi: an open source software for exploring and manipulating networks. in International AAAI Conference on Weblogs and Social Media.
Blessing, W. W., and Seaman, B. (2003). 5-hydroxytryptamine(2A) receptors regulate sympathetic nerves constricting the cutaneous vascular bed in rabbits and rats. Neuroscience 117, 939–948. doi: 10.1016/S0306-4522(02)00810-2
Bradshaw, H. B., and Johnson, C. T. (2023). Measuring the content of endocannabinoid-like compounds in biological fluids: a critical overview of sample preparation methodologies. Methods Mol. Biol. 2576, 21–40. doi: 10.1007/978-1-0716-2728-0_3
Brems, B. M., Sullivan, E. E., Connolly, J. G., Zhang, J., Chang, A., Ortiz, R., et al. (2023). Dose-dependent effects of GAT107, a novel allosteric agonist-positive allosteric modulator (ago-PAM) for the alpha7 nicotinic cholinergic receptor: a BOLD phMRI and connectivity study on awake rats. Front. Neurosci. 17:1196786. doi: 10.3389/fnins.2023.1196786
Cai, X., Qiao, J., Kulkarni, P., Harding, I. C., Ebong, E., and Ferris, C. F. (2020). Imaging the effect of the circadian light-dark cycle on the glymphatic system in awake rats. Proc. Natl. Acad. Sci. USA 117, 668–676. doi: 10.1073/pnas.1914017117
Carhart-Harris, R. L., Erritzoe, D., Williams, T., Stone, J. M., Reed, L. J., Colasanti, A., et al. (2012a). Neural correlates of the psychedelic state as determined by fMRI studies with psilocybin. Proc. Natl. Acad. Sci. USA 109, 2138–2143. doi: 10.1073/pnas.1119598109
Carhart-Harris, R. L., Leech, R., Erritzoe, D., Williams, T. M., Stone, J. M., Evans, J., et al. (2013). Functional connectivity measures after psilocybin inform a novel hypothesis of early psychosis. Schizophr. Bull. 39, 1343–1351. doi: 10.1093/schbul/sbs117
Carhart-Harris, R. L., Leech, R., Hellyer, P. J., Shanahan, M., Feilding, A., Tagliazucchi, E., et al. (2014). The entropic brain: a theory of conscious states informed by neuroimaging research with psychedelic drugs. Front. Hum. Neurosci. 8:20. doi: 10.3389/fnhum.2014.00020
Carhart-Harris, R. L., Leech, R., Williams, T. M., Erritzoe, D., Abbasi, N., Bargiotas, T., et al. (2012b). Implications for psychedelic-assisted psychotherapy: functional magnetic resonance imaging study with psilocybin. Br. J. Psychiatry 200, 238–244. doi: 10.1192/bjp.bp.111.103309
Cavdar, S., San, T., Aker, R., Sehirli, U., and Onat, F. (2001). Cerebellar connections to the dorsomedial and posterior nuclei of the hypothalamus in the rat. J. Anat. 198, 37–45. doi: 10.1046/j.1469-7580.2001.19810037.x
Coleman, J. R., Madularu, D., Ortiz, R. J., Athanassiou, M., Knudsen, A., Alkislar, I., et al. (2022). Changes in brain structure and function following chronic exposure to inhaled vaporised cannabis during periadolescence in female and male mice: a multimodal MRI study. Addict. Biol. 27:e13169. doi: 10.1111/adb.13169
Constantino, J. L., van Dalfsen, J. H., Massetti, S., Kamphuis, J., and Schoevers, R. A. (2025). Neurobiological mechanisms of antidepressant properties of psilocybin: a systematic review of blood biomarkers. Prog. Neuro-Psychopharmacol. Biol. Psychiatry 136:111251. doi: 10.1016/j.pnpbp.2025.111251
Corne, S. J., Pickering, R. W., and Warner, B. T. (1963). A method for assessing the effects of drugs on the central actions of 5-hydroxytryptamine. Br. J. Pharmacol. Chemother. 20, 106–120. doi: 10.1111/j.1476-5381.1963.tb01302.x
de Veen, B. T., Schellekens, A. F., Verheij, M. M., and Homberg, J. R. (2017). Psilocybin for treating substance use disorders? Expert. Rev. Neurother. 17, 203–212. doi: 10.1080/14737175.2016.1220834
Demaree, J. L., Ortiz, R. J., Cai, X., Aggarwal, D., Senthilkumar, I., Lawson, C., et al. (2021). Exposure to methylphenidate during peri-adolescence decouples the prefrontal cortex: a multimodal MRI study. Am. J. Transl. Res. 13, 8480–8495
Effinger, D. P., Quadir, S. G., Ramage, M. C., Cone, M. G., and Herman, M. A. (2023). Sex-specific effects of psychedelic drug exposure on central amygdala reactivity and behavioral responding. Transl. Psychiatry 13:119. doi: 10.1038/s41398-023-02414-5
Ferris, C. F. (2022). Applications in awake animal magnetic resonance imaging. Front. Neurosci. 16:854377. doi: 10.3389/fnins.2022.854377
Genovese, C. R., Lazar, N. A., and Nichols, T. (2002). Thresholding of statistical maps in functional neuroimaging using the false discovery rate. NeuroImage 15, 870–878. doi: 10.1006/nimg.2001.1037
Ghaw, A., Chunduri, A., Chang, A., Ortiz, R. J., Kozlowska, M., Kulkarni, P. P., et al. (2024). Dose-dependent LSD effects on cortical/thalamic and cerebellar activity: brain oxygen level-dependent fMRI study in awake rats. Brain Commun 6:fcae194. doi: 10.1093/braincomms/fcae194
Golden, C. T., and Chadderton, P. (2022). Psilocybin reduces low frequency oscillatory power and neuronal phase-locking in the anterior cingulate cortex of awake rodents. Sci. Rep. 12:12702. doi: 10.1038/s41598-022-16325-w
Gonzalez-Maeso, J., Weisstaub, N. V., Zhou, M., Chan, P., Ivic, L., Ang, R., et al. (2007). Hallucinogens recruit specific cortical 5-HT(2A) receptor-mediated signaling pathways to affect behavior. Neuron 53, 439–452. doi: 10.1016/j.neuron.2007.01.008
Goodwin, G. M., Aaronson, S. T., Alvarez, O., Arden, P. C., Baker, A., Bennett, J. C., et al. (2022). Single-dose psilocybin for a treatment-resistant episode of major depression. N. Engl. J. Med. 387, 1637–1648. doi: 10.1056/NEJMoa2206443
Gouzoulis-Mayfrank, E., Schreckenberger, M., Sabri, O., Arning, C., Thelen, B., Spitzer, M., et al. (1999). Neurometabolic effects of psilocybin, 3,4-methylenedioxyethylamphetamine (MDE) and d-methamphetamine in healthy volunteers. A double-blind, placebo-controlled PET study with [18F]FDG. Neuropsychopharmacology 20, 565–581. doi: 10.1016/S0893-133X(98)00089-X
Granberg, M., Fowler, C. J., and Jacobsson, S. O. (2001). Effects of the cannabimimetic fatty acid derivatives 2-arachidonoylglycerol, anandamide, palmitoylethanolamide and methanandamide upon IgE-dependent antigen-induced beta-hexosaminidase, serotonin and TNF alpha release from rat RBL-2H3 basophilic leukaemic cells. Naunyn Schmiedeberg's Arch. Pharmacol. 364, 66–73. doi: 10.1007/s002100100424
Griffiths, R. R., Johnson, M. W., Carducci, M. A., Umbricht, A., Richards, W. A., Richards, B. D., et al. (2016). Psilocybin produces substantial and sustained decreases in depression and anxiety in patients with life-threatening cancer: a randomized double-blind trial. J. Psychopharmacol. 30, 1181–1197. doi: 10.1177/0269881116675513
Haines, D. E., Dietrichs, E., and Sowa, T. E. (1984). Hypothalamo-cerebellar and cerebello-hypothalamic pathways: a review and hypothesis concerning cerebellar circuits which may influence autonomic centers affective behavior. Brain Behav. Evol. 24, 198–220. doi: 10.1159/000121317
Halberstadt, A. L., and Geyer, M. A. (2011). Multiple receptors contribute to the behavioral effects of indoleamine hallucinogens. Neuropharmacology 61, 364–381. doi: 10.1016/j.neuropharm.2011.01.017
Halberstadt, A. L., Koedood, L., Powell, S. B., and Geyer, M. A. (2011). Differential contributions of serotonin receptors to the behavioral effects of indoleamine hallucinogens in mice. J. Psychopharmacol. 25, 1548–1561. doi: 10.1177/0269881110388326
Harris-Blum, L., Smith, Z., Ortiz, R. J., Athreya, D., Chang, A., Kulkarni, P. P., et al. (2024). Developmental changes in brain structure and function following exposure to oral LSD during adolescence. Sci. Rep. 14:18632. doi: 10.1038/s41598-024-69597-9
Higgins, G. A., Carroll, N. K., Brown, M., MacMillan, C., Silenieks, L. B., Thevarkunnel, S., et al. (2021). Low doses of psilocybin and ketamine enhance motivation and attention in poor performing rats: evidence for an antidepressant property. Front. Pharmacol. 12:640241. doi: 10.3389/fphar.2021.640241
Jefsen, O. H., Elfving, B., Wegener, G., and Muller, H. K. (2021). Transcriptional regulation in the rat prefrontal cortex and hippocampus after a single administration of psilocybin. J. Psychopharmacol. 35, 483–493. doi: 10.1177/0269881120959614
Kawazoe, K., McGlynn, R., Felix, W., Sevilla, R., Liao, S., Kulkarni, P., et al. (2022). Dose-dependent effects of esketamine on brain activity in awake mice: a BOLD phMRI study. Pharmacol. Res. Perspect. 10:e01035. doi: 10.1002/prp2.1035
Kebschull, J. M., Casoni, F., Consalez, G. G., Goldowitz, D., Hawkes, R., Ruigrok, T. J. H., et al. (2023). Cerebellum lecture: the cerebellar nuclei-Core of the cerebellum. Cerebellum 23, 620–677. doi: 10.1007/s12311-022-01506-0
Kilkenny, C., Browne, W., Cuthill, I. C., Emerson, M., and Altman, D. G.NC3Rs Reporting Guidelines Working Group (2010). Animal research: reporting in vivo experiments: the ARRIVE guidelines. Br. J. Pharmacol. 160, 1577–1579. doi: 10.1111/j.1476-5381.2010.00872.x
King, J. A., Garelick, T. S., Brevard, M. E., Chen, W., Messenger, T. L., Duong, T. Q., et al. (2005). Procedure for minimizing stress for fMRI studies in conscious rats. J. Neurosci. Methods 148, 154–160. doi: 10.1016/j.jneumeth.2005.04.011
Kolaczynska, K. E., Liechti, M. E., and Duthaler, U. (2021). Development and validation of an LC-MS/MS method for the bioanalysis of psilocybin's main metabolites, psilocin and 4-hydroxyindole-3-acetic acid, in human plasma. J. Chromatogr. B Analyt. Technol. Biomed. Life Sci. 1164:122486. doi: 10.1016/j.jchromb.2020.122486
Krohn, F., Novello, M., van der Giessen, R. S., de Zeeuw, C. I., Pel, J. J. M., and Bosman, L. W. J. (2023). The integrated brain network that controls respiration. eLife 12, 1–67. doi: 10.7554/eLife.83654
Lebedev, A. V., Lövdén, M., Rosenthal, G., Feilding, A., Nutt, D. J., and Carhart-Harris, R. L. (2015). Finding the self by losing the self: neural correlates of ego-dissolution under psilocybin. Hum. Brain Mapp. 36, 3137–3153. doi: 10.1002/hbm.22833
Lewis, C. R., Preller, K. H., Kraehenmann, R., Michels, L., Staempfli, P., and Vollenweider, F. X. (2017). Two dose investigation of the 5-HT-agonist psilocybin on relative and global cerebral blood flow. NeuroImage 159, 70–78. doi: 10.1016/j.neuroimage.2017.07.020
Liang, Z., King, J., and Zhang, N. (2011). Uncovering intrinsic connectional architecture of functional networks in awake rat brain. J. Neurosci. 31, 3776–3783. doi: 10.1523/JNEUROSCI.4557-10.2011
Liu, J., Wang, Y., Xia, K., Wu, J., Zheng, D., Cai, A., et al. (2023). Acute psilocybin increased cortical activities in rats. Front. Neurosci. 17:1168911. doi: 10.3389/fnins.2023.1168911
Low, A. Y. T., Goldstein, N., Gaunt, J. R., Huang, K. P., Zainolabidin, N., Yip, A. K. K., et al. (2021). Reverse-translational identification of a cerebellar satiation network. Nature 600, 269–273. doi: 10.1038/s41586-021-04143-5
Mason, N. L., Kuypers, K. P. C., Müller, F., Reckweg, J., Tse, D. H. Y., Toennes, S. W., et al. (2020). Me, myself, bye: regional alterations in glutamate and the experience of ego dissolution with psilocybin. Neuropsychopharmacology 45, 2003–2011. doi: 10.1038/s41386-020-0718-8
Miquel, M., Vazquez-Sanroman, D., Carbo-Gas, M., Gil-Miravet, I., Sanchis-Segura, C., Carulli, D., et al. (2016). Have we been ignoring the elephant in the room? Seven arguments for considering the cerebellum as part of addiction circuitry. Neurosci. Biobehav. Rev. 60, 1–11. doi: 10.1016/j.neubiorev.2015.11.005
Moore, K., Madularu, D., Iriah, S., Yee, J. R., Kulkarni, P., Darcq, E., et al. (2016). BOLD imaging in awake wild-type and mu-opioid receptor Knock-out mice reveals on-target activation maps in response to oxycodone. Front. Neurosci. 10:471. doi: 10.3389/fnins.2016.00471
Muthukumaraswamy, S. D., Carhart-Harris, R. L., Moran, R. J., Brookes, M. J., Williams, T. M., Errtizoe, D., et al. (2013). Broadband cortical desynchronization underlies the human psychedelic state. J. Neurosci. 33, 15171–15183. doi: 10.1523/JNEUROSCI.2063-13.2013
Nichols, D. E. (2004). Hallucinogens. Pharmacol. Ther. 101, 131–181. doi: 10.1016/j.pharmthera.2003.11.002
Oakes, M., Law, W. J., and Komuniecki, R. (2019). Cannabinoids stimulate the TRP Channel-dependent release of both serotonin and dopamine to modulate behavior in C. elegans. J. Neurosci. 39, 4142–4152. doi: 10.1523/JNEUROSCI.2371-18.2019
Olejnik, S., and Algina, J. (2003). Generalized eta and omega squared statistics: measures of effect size for some common research designs. Psychol. Methods 8, 434–447. doi: 10.1037/1082-989X.8.4.434
Parrish, J. C., and Nichols, D. E. (2006). Serotonin 5-HT(2A) receptor activation induces 2-arachidonoylglycerol release through a phospholipase c-dependent mechanism. J. Neurochem. 99, 1164–1175. doi: 10.1111/j.1471-4159.2006.04173.x
Passie, T., Seifert, J., Schneider, U., and Emrich, H. M. (2002). The pharmacology of psilocybin. Addict. Biol. 7, 357–364. doi: 10.1080/1355621021000005937
Preller, K. H., Duerler, P., Burt, J. B., Ji, J. L., Adkinson, B., Stämpfli, P., et al. (2020). Psilocybin induces time-dependent changes in global functional connectivity. Biol. Psychiatry 88, 197–207. doi: 10.1016/j.biopsych.2019.12.027
Refinetti, R., and Menaker, M. (1992). The circadian rhythm of body temperature. Physiol. Behav. 51, 613–637. doi: 10.1016/0031-9384(92)90188-8
Reinwald, J. R., Schmitz, C. N., Skorodumov, I., Kuchar, M., Weber-Fahr, W., Spanagel, R., et al. (2023). Psilocybin-induced default mode network hypoconnectivity is blunted in alcohol-dependent rats. Transl. Psychiatry 13:392. doi: 10.1038/s41398-023-02690-1
Riba, J., Romero, S., Grasa, E., Mena, E., Carrió, I., and Barbanoj, M. J. (2006). Increased frontal and paralimbic activation following ayahuasca, the pan-Amazonian inebriant. Psychopharmacology 186, 93–98. doi: 10.1007/s00213-006-0358-7
Robbins, T. W. (2017). Animal models of hallucinations observed through the modern Lens. Schizophr. Bull. 43, 24–26. doi: 10.1093/schbul/sbw134
Romanova, Z., Hlavacova, N., and Jezova, D. (2022). Psychotropic drug effects on steroid stress hormone release and possible mechanisms involved. Int. J. Mol. Sci. 23, 1–5. doi: 10.3390/ijms23020908
Sadaka, A. H., Ozuna, A. G., Ortiz, R. J., Kulkarni, P., Johnson, C. T., Bradshaw, H. B., et al. (2021). Cannabidiol has a unique effect on global brain activity: a pharmacological, functional MRI study in awake mice. J. Transl. Med. 19:220. doi: 10.1186/s12967-021-02891-6
Sathe, P. K., Ramdasi, G. R., Giammatteo, K., Beauzile, H., Wang, S., Zhang, H., et al. (2023). Effects of (−)-MBP, a novel 5-HT(2C) agonist and 5-HT(2A/2B) antagonist/inverse agonist on brain activity: a phMRI study on awake mice. Pharmacol. Res. Perspect. 11:e01144. doi: 10.1002/prp2.1144
Scheer, F. A., Pirovano, C., Van Someren, E. J., and Buijs, R. M. (2005). Environmental light and suprachiasmatic nucleus interact in the regulation of body temperature. Neuroscience 132, 465–477. doi: 10.1016/j.neuroscience.2004.12.012
Schmahmann, J. D. (2019). The cerebellum and cognition. Neurosci. Lett. 688, 62–75. doi: 10.1016/j.neulet.2018.07.005
Shadani, S., Conn, K., Andrews, Z. B., and Foldi, C. J. (2024). Potential differences in psychedelic actions based on biological sex. Endocrinology 165, 1–11. doi: 10.1210/endocr/bqae083
Shao, L. X., Liao, C., Gregg, I., Davoudian, P. A., Savalia, N. K., Delagarza, K., et al. (2021). Psilocybin induces rapid and persistent growth of dendritic spines in frontal cortex in vivo. Neuron 109, 2535–2544.e4. doi: 10.1016/j.neuron.2021.06.008
Siegel, J. S., Subramanian, S., Perry, D., Kay, B. P., Gordon, E. M., Laumann, T. O., et al. (2024). Psilocybin desynchronizes the human brain. Nature 632, 131–138. doi: 10.1038/s41586-024-07624-5
Snider, R. S., Maiti, A., and Snider, S. R. (1976). Cerebellar connections to catecholamine systems: anatomical and biochemical studies. Trans. Am. Neurol. Assoc. 101, 295–297
Spain, A., Howarth, C., Khrapitchev, A. A., Sharp, T., Sibson, N. R., and Martin, C. (2015). Neurovascular and neuroimaging effects of the hallucinogenic serotonin receptor agonist psilocin in the rat brain. Neuropharmacology 99, 210–220. doi: 10.1016/j.neuropharm.2015.07.018
Tyls, F., Palenicek, T., Kaderabek, L., Lipski, M., Kubesova, A., and Horacek, J. (2016). Sex differences and serotonergic mechanisms in the behavioural effects of psilocin. Behav. Pharmacol. 27, 309–320. doi: 10.1097/FBP.0000000000000198
Van de Kar, L. D., Javed, A., Zhang, Y., Serres, F., Raap, D. K., and Gray, T. S. (2001). 5-HT2A receptors stimulate ACTH, corticosterone, oxytocin, renin, and prolactin release and activate hypothalamic CRF and oxytocin-expressing cells. J. Neurosci. 21, 3572–3579. doi: 10.1523/JNEUROSCI.21-10-03572.2001
Vollenweider, F. X., Leenders, K. L., Scharfetter, C., Maguire, P., Stadelmann, O., and Angst, J. (1997). Positron emission tomography and fluorodeoxyglucose studies of metabolic hyperfrontality and psychopathology in the psilocybin model of psychosis. Neuropsychopharmacology 16, 357–372. doi: 10.1016/S0893-133X(96)00246-1
Vollenweider, F. X., and Preller, K. H. (2020). Psychedelic drugs: neurobiology and potential for treatment of psychiatric disorders. Nat. Rev. Neurosci. 21, 611–624. doi: 10.1038/s41583-020-0367-2
Vollenweider, F. X., Vollenweider-Scherpenhuyzen, M. F., Babler, A., Vogel, H., and Hell, D. (1998). Psilocybin induces schizophrenia-like psychosis in humans via a serotonin-2 agonist action. Neuroreport 9, 3897–3902. doi: 10.1097/00001756-199812010-00024
Vollenweider, F. X., Vontobel, P., Hell, D., and Leenders, K. L. (1999). 5-HT modulation of dopamine release in basal ganglia in psilocybin-induced psychosis in man—a PET study with [11C]raclopride. Neuropsychopharmacology 20, 424–433. doi: 10.1016/S0893-133X(98)00108-0
Wojtas, A., Bysiek, A., Wawrzczak-Bargiela, A., Szych, Z., Majcher-Maślanka, I., Herian, M., et al. (2022). Effect of psilocybin and ketamine on brain neurotransmitters, glutamate receptors, DNA and rat behavior. Int. J. Mol. Sci. 23, 1–20. doi: 10.3390/ijms23126713
Keywords: psilocin, cerebellar nuclei, BOLD resting state functional connectivity, 5-HT2A receptor, hyperconnectivity
Citation: Fuini E, Chang A, Ortiz RJ, Nasseef T, Edwards J, Latta M, Gonzalez E, Woodward TJ, Axe B, Maheswari A, Cavallaro N, Bradshaw HB, Kulkarni PP and Ferris CF (2025) Dose-dependent changes in global brain activity and functional connectivity following exposure to psilocybin: a BOLD MRI study in awake rats. Front. Neurosci. 19:1554049. doi: 10.3389/fnins.2025.1554049
Edited by:
Robert H. Lipsky, Uniformed Services University of the Health Sciences, United StatesReviewed by:
Eilidh MacNicol, King’s College London, United KingdomAndreas Brydenfelt Wulff, University of Maryland, United States
Copyright © 2025 Fuini, Chang, Ortiz, Nasseef, Edwards, Latta, Gonzalez, Woodward, Axe, Maheswari, Cavallaro, Bradshaw, Kulkarni and Ferris. This is an open-access article distributed under the terms of the Creative Commons Attribution License (CC BY). The use, distribution or reproduction in other forums is permitted, provided the original author(s) and the copyright owner(s) are credited and that the original publication in this journal is cited, in accordance with accepted academic practice. No use, distribution or reproduction is permitted which does not comply with these terms.
*Correspondence: Craig F. Ferris, Yy5mZXJyaXNAbm9ydGhlYXN0ZXJuLmVkdQ==