- 1Department of Radiotherapy and Radiooncology, Klinikum rechts der Isar, Technical University of Munich, Munich, Germany
- 2Helmholtz Zentrum München (HMGU), CCG - Innate Immunity in Tumor Biology, Munich, Germany
The immature, chaotic microvasculature of most solid tumors can present a significant impediment to blood-borne delivery, uneven distribution, and compromised penetration of macromolecular anticancer drugs and diagnostic agents from tumor microvessels across the interstitial space to cancer cells. To reach viable tumor cells in relevant concentrations, macromolecular agents are confronted with several barriers to vascular, transvascular, and interstitial transport. Amongst those (1) heterogeneous and poor blood supply, (2) distinctly reduced or even abolished hydrostatic and oncotic pressure gradients across the microvessel wall abrogating the convective transport from the vessel lumen into the interstitial space (impairment of transvascular transport), and (3) impediment of convective transport within the interstitial compartment due to elevated interstitial fluid pressure (IFP) (resulting from hyperpermeable blood vessels coupled with non-functional lymphatics) and a dense structure of the interstitial matrix are the major mechanisms hindering drug delivery. Upon irradiation, changes in these barrier functions are inconclusive so far. Alterations in vascular transport properties following fractionated radiation up to 40 Gy are quite inconsistent in terms of direction, extent, and time course. Total doses above 45 Gy can damage tumor microvessels, additionally impeding vascular delivery. Vascular permeability for macromolecules might be enhanced up to a total dose of 45 Gy. However, this effect is counteracted/abolished by the elevated IFP in solid tumors. When assessing IFP during fractionated radiotherapy in patient tumors, inconsistent alterations have been observed, both in direction and extent. From these data it is concluded that modulations in vascular, transvascular, and interstitial transport by irradiation of solid tumors are rather unclear so far. Translation of experimental data into the clinical setting thus needs to be undertaken with especial care.
Introduction
The chaotic microvasculature of solid tumors leads to significant impediment of delivery, uneven distribution, and compromised penetration of macromolecules and nanotherapeutics from tumor microvessels across the interstitial compartment to cancer cells, especially to cells distant from microvessels. To reach viable tumor cells in relevant concentrations, diagnostic, and therapeutic agents are confronted with several obstacles: disturbed convective transport within the chaotic vascular compartment (vascular transport), spatio-temporally uneven distribution within the tissue, and significant shunt flow bypassing the exchange processes between the vascular bed and the extravascular space. Extravasation (transvascular transport) and extravascular convection (interstitial transport) of macromolecules and nanoparticles are mainly impaired by high interstitial fluid pressure (IFP). Furthermore, marked gradients in concentrations of macromolecules and nanoparticles exist within the extravascular space limiting anticancer therapies with increasing distance from tumor blood vessels (Jain, 1987, 1990; Vaupel, 2009b; Jain and Stylianopoulos, 2010; Vaupel and Multhoff, 2012).
Amongst the key pathophysiological abnormalities in solid tumors related to drug transport, chaotic vascular networks, abnormal blood flow, and elevated IFP (interstitial hypertension) seem to play the dominant roles (see Figure 1). Accumulated solid stress from the growing tumor (through unlimited proliferation of cancer cells and excessive production of collagen and hyaluran), a dense interstitial structure, and contractions of the interstitial matrix mediated by stromal fibroblasts add to the transport barrier to anticancer agents (Heldin et al., 2004; Chauhan et al., 2011; Wiig and Swartz, 2012).
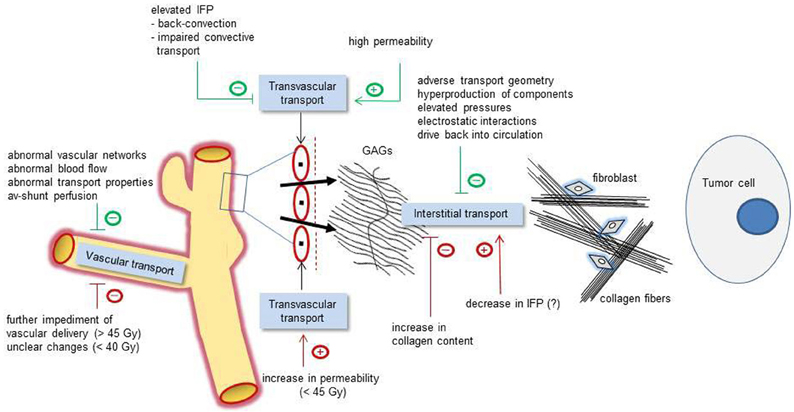
Figure 1. Schematic representation of relevant pathophysiological mechanisms affecting the vascular (left), transvascular (center), and interstitial transport (right) of macromolecular compounds (e.g., anti-tumor and diagnostic large-size molecules). Green tags: basic pathophysiological obstacles (negative signs) or facilitating mechanisms (positive signs). Red tags: irradiation-induced modulations affecting the transport properties in a positive or negative direction. Interstitial transport of macromolecules is hindered by an adverse transport geometry (including enlarged interstitial volumes and transport distances), by a hyperproduction of interstitial components (e.g., stromal cells, collagen fibers, interstitial matrix), by elevated pressures (IFP, OP, and accumulated solid stress), electrostatic interactions, and drive back into the circulation. IFP, interstitial fluid pressure; OP, oncotic pressure; GAGs, negatively charged glycosaminoglycans.
While some data suggest that interstitial hypertension might not be a significant barrier to therapy as has generally been proposed (Wiig and Swartz, 2012), in the following sections the impact of irradiation on the key pathophysiological characteristics mentioned above will be discussed with regard to their effect on the delivery of macromolecules and nanotherapeutics to primary and metastatic tumors.
Vascular Transport
Vascular transport, i.e., the delivery of anticancer and diagnostic agents via the blood stream, includes the convective transport to the tumor and the subsequent distribution within the tumor (“blood-borne delivery,” Vaupel and Multhoff, 2012). The development of a disorganized microvasculature and significant arterio-venous shunt perfusion leads to an inefficient delivery of (macromolecular) agents and nutrients (e.g., oxygen, glucose) through the vascular system of the tumor (see Table 1). The situation is further aggravated by flow-dependent spatio-temporal heterogeneities in the distribution of plasma-borne agents (and their metabolites). These “4D-heterogeneities” are not static, but instead are quite dynamic, and therefore more complex than has been previously assumed (for reviews see Vaupel et al., 1989; Vaupel, 2006, 2009a,b, 2012).
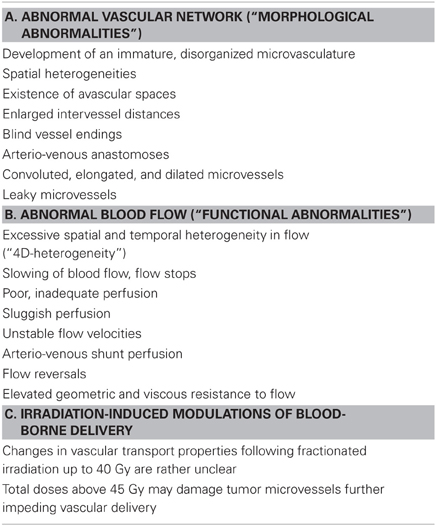
Table 1. Obstacles in blood-borne delivery of macromolecular anticancer and diagnostic agents and modulations following irradiation (selection; Vaupel, 2006, 2009a).
The status of the tumor microvasculature and blood flow (direction, extent, and time course of changes) upon irradiation remains largely unclear, both for single large doses (12–50 Gy) and fractionated radiation (25 fractions, 5 weeks, up to a total dose of 75 Gy), but also appears to depend on the tumor type studied, the radiation dose, the time interval between exposures, and irradiation stage (during vs. post). The literature provides quite conflicting data on whether or not radiation-related biologically or clinically relevant changes in microvascular structures and functions occur.
Descriptive and morphometric studies performed between 1927 and 1977 using experimental tumors suggested that fractionated doses commonly led to an increase in vascular density, while single large doses often destroyed the vasculature and shut down blood flow (for details see Narayan and Cliff, 1982; Fajardo and Berthrong, 1988; Baker and Krochak, 1989; Dewhirst, 1991). However, experiments using single large dose irradiation are quite inconclusive since changes in tumor blood flow were both dose-and time-dependent (Vaupel et al., 1984). In a recent review, a very contradictory data set for single large dose local irradiation in the experimental setting has been presented (Kozin et al., 2012).
In conventional fractionation schedules, tumor microvessels are distinctly damaged above doses of 40–45 Gy (Zywietz et al., 1994). Above this “critical cumulated dose” tumor oxygenation and ATP levels progressively decreased (Thews et al., 1999), clearly showing that these parameters are critically determined by the efficacy of tumor blood flow. Continuous hyperfractionation (2 daily fractions of 2.5 Gy, up to 60 Gy), however, induced only relatively discreet alterations of the tumor microvasculature (Lorke et al., 1999).
Published data on changes in tumor blood flow and oxygenation upon radiation therapy in the clinical setting showed no clear direction in observed alterations (Feldmann et al., 2000; Molls et al., 2000). From this compilation of data, there is evidence that changes in tumor microcirculation (i.e., vascular transport properties) following γ-irradiation (fractionated doses, up to 40 Gy) are rather unclear so far due to obvious variabilities in the direction, extent, and time course of changes observed. There is at least some consensus that upon conventional fractionation with total doses above 45 Gy microvessels are damaged, further impeding vascular delivery of blood-borne anticancer (macro-) molecules.
Transvascular Transport
Therapeutic (and diagnostic) molecules and nanomedicines cross the leaky vessel walls by two major mechanisms: diffusion and convection. Large pore sizes of tumor microvessels facilitate these transport processes. Diffusion is the prevailing molecular transport modality of small-size molecules driven by concentration gradients. Convection is driven by hydrostatic pressure gradients and is the dominant mode of transport for large molecules, liposomes, and other nanoparticles (Kuszyk et al., 2001). Due to the elevated interstitial fluid pressure (IFP, interstitial hypertension, see section below), transvascular pressure gradients are approaching zero. As a result of this “equilibration” of hydraulic pressures, significant hindering of the transport of macromolecules and nanoparticles into the extravascular space by convection has to be considered (see Table 2). For this reason, the main mechanism of mass transport across vessel walls is diffusion (for a review see Vaupel and Multhoff, 2012). This process is significantly slower than convection, especially for macromolecules and nanoparticles (Jain and Stylianopoulos, 2010). Vessel wall hyperpermeability (enhanced porosity) is thus counteracted by elevated IFP in tumors (and by the large size of nanoparticles).
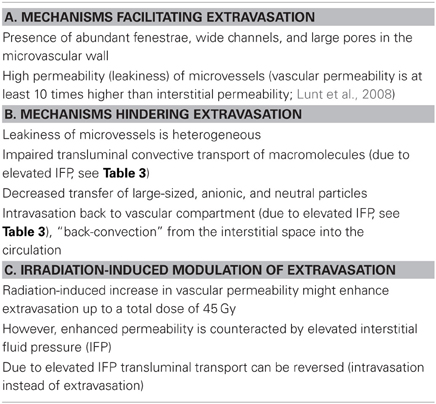
Table 2. Obstacles to transvascular transport (extravasation) of macromolecular therapeutic and diagnostic agents in solid tumors and modulations upon irradiation (selection, Vaupel and Multhoff, 2012).
Vascular permeability decreases with increasing size of the transported nanoparticles (according to the Organization for Standardization, nanomedical approaches use particles from 1 to 100 nm; e.g., gold nanoparticles 2.5 nm, monoclonal antibodies 10–15 nm, oncolytic viruses 30–40 nm, magnetic nanoparticles for drug targeting 15–100 nm, liposome-encapsulated doxorubicin 80–130 nm, gadolinium-based nanoparticles 115 nm, and albumin-paclitaxel nanoparticles 130 nm). Furthermore, permeability is higher for cationic compounds than for their anionic or neutral counterparts (Jain and Stylianopoulos, 2010).
Upon fractionated γ-irradiation, time- and dose-dependent changes in vascular permeability have been described in the experimental setting due to direct vessel wall damage and the action of indirect inflammatory stimuli (Lorke et al., 1999). A discrete increase in leakiness (associated with interstitial edema) has been observed already after a total dose of 15 Gy, with more pronounced leakiness at higher radiation doses. Upon radiation with a total dose of 30 Gy, hyperpermeability was further increased. Prolonged irradiation was eventually associated with progressive destruction of the vascular wall and disruption of the basal lamina.
In principle, radiation-triggered increases in vascular permeability may enhance extravasation of anti-cancer macromolecules up to a total dose of approximately 45 Gy. However, this facilitation is severely counteracted or totally abolished by mechanisms occurring in the interstitial compartment as outlined in the following section.
Interstitial Transport
The interstitial compartment of tumors differs significantly from that of normal tissue (Vaupel and Multhoff, 2012). As a result of (1) vessel leakiness, (2) lack of functional lymphatics, (3) interstitial fibrosis, (4) contraction of the interstitial matrix mediated by stromal fibroblasts, and (5) cell proliferation in a confined space, most solid tumors develop an elevated interstitial/hydrostatic fluid pressure (IFP), which is in contrast to normal tissues where IFP is close to atmospheric pressure (Jain, 1987, 1990; Heldin et al., 2004; Milosevic et al., 2004; Cairns et al., 2006; Wiig and Swartz, 2012).
As already mentioned above, increased IFP within solid tumors decreases extravasation. In addition, high IFP severely inhibits interstitial transport of larger molecules (e.g., antibodies, antibody drug conjugates, and liposomes) by convection (see Table 3). Macromolecules rely more heavily on convection as opposed to simple diffusional transport of low-molecular weight drugs. Compounds larger than 60 nm in diameter are not able to effectively diffuse through the extracellular matrix of highly fibrotic tumors. Interstitial transport of macromolecules is further impaired by a much denser network of interconnected collagen fibers in the extracellular matrix of tumors (as compared to normal tissues) leaving them in higher concentrations in perivascular areas only (Jain and Stylianopoulos, 2010). The transport of compounds with sizes of up to 1000 nm is further hindered by highly negatively charged heparan sulfate in the matrix.
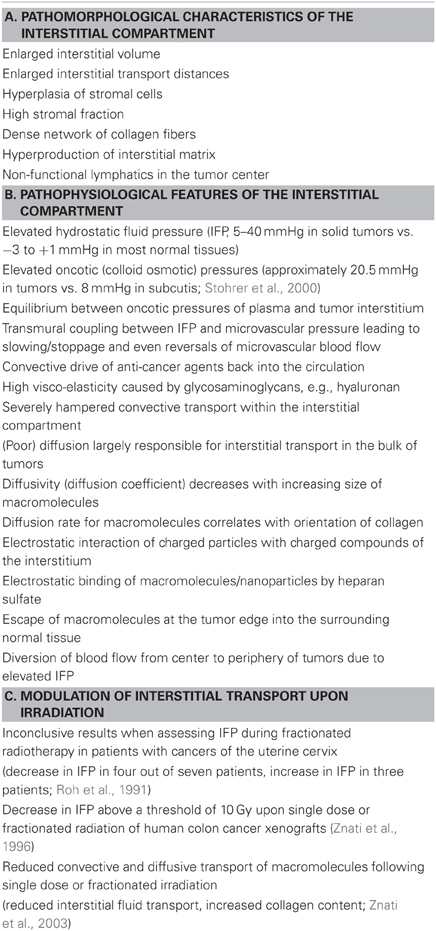
Table 3. Obstacles in interstitial transport of macromolecular anti-cancer agents and nanomedicines and modulations following irradiation (selection, Vaupel and Multhoff, 2012).
Heterogeneous mobility and distribution of large-sized molecules is additionally caused by two phases in the matrix: a more aqueous phase is found in regions with low fiber content (“fast” compartment with relatively high diffusivity), and a more viscous phase is due to a high concentration of collagen fibers in a dense matrix (“slow” compartment with high retention of compounds). Collagen content in tumors is much higher and collagen fibers are much thicker than in normal tissue leading to an increased mechanical stiffness of the tissue (Netti et al., 2000; Heldin et al., 2004). The interstitium also contains abundant stromal cells and enzymes that can affect the activity and delivery of agents to the tumor cells (Kuszyk et al., 2001).
It is assumed that IFP is almost uniform throughout a tumor and that relevant gradients of IFP do not exist. However, IFP drops precipitously at the tumor/normal tissue interface. For this reason, the interstitial fluid oozes out of the tumor into the surrounding normal tissue, carrying away anticancer agents, growth factors or released heat shock proteins, and cancer cells with it (Fukumura and Jain, 2007). Shedded cancer cells may mediate metastasis. As another consequence of this peripheral drop in IFP, blood flow may be diverted away from the tumor center toward the periphery where anticancer agents may be lost from larger vessels.
Transmural coupling between IFP and microvascular pressure can critically reduce perfusion pressure between up- and downstream tumor blood vessels leading to flow stasis and thus, inadequate delivery of anticancer agents, in addition to the mechanisms impairing blood flow already mentioned above.
In the experimental setting, radiocurability of human tumor xenografts decreases with increasing IFP (Rofstad et al., 2009, 2010). In these experiments, IFP showed a strong positive correlation to the extent of acute hypoxia in the tumors investigated (Rofstad et al., 2009), an increased number of clonogenic cells (Rofstad et al., 2010), stimulation of proliferation, occurring presumably via modulation of signaling pathways (Nathan et al., 2005), and upregulation of VEGF-A expression (Nathan et al., 2008).
Studies in patients with cervix cancers have explored the relationship between IFP and outcome following radiotherapy (Milosevic et al., 2001; Yeo et al., 2009). In these studies, IFP was found to be a strong, negative, and independent prognostic factor for local control and distant metastasis. Several compounds have been shown to decrease tumor IFP in patients (for a review see Heldin et al., 2004). This reduction in IFP has been attributed to a substantial decrease in vascular permeability, lowered microvascular pressure and changes in the extracellular matrix.
Assessing interstitial hypertension during fractionated radiotherapy in patients with cervix cancers showed inconclusive results, since only 4 out of 7 patients experienced a drop in IFP during treatment, whereas in 3 patients IFP distinctly increased (Roh et al., 1991). Measurements after single dose or fractionated radiation in human colon cancer xenografts yielded a reduction in IFP above a threshold of 10 Gy. Below this threshold there was no significant change in IFP (Znati et al., 1996). A decrease in microvascular pressure has been discussed as a plausible explanation for the radiation-induced reduction in IFP by these authors. Furthermore, the authors argued that this radiation-related decrease in IFP may have been responsible for an improved uptake of monoclonal antibodies following single dose or fractionated irradiation as reported earlier by others. In contrast to these data, in a later publication by this group a reduced interstitial fluid transport and increased collagen content in tumors has been communicated (Znati et al., 2003), implicating a reduced transport of macromolecular agents in tumors upon radiation.
Concluding Remarks
Preceding cellular pharmacodynamics, three important pharmacokinetic steps govern the delivery of anti-cancer drugs and diagnostic agents to tumor cells: vascular, transvascular, and interstitial transport. Barriers to delivery of macromolecular drugs mainly arise from immature, chaotic vascular networks and abnormal tumor blood flow, hyperpermeability of leaky microvessels, and elevated fluid pressure within the interstitial compartment abrogating convective transport. Upon tumor irradiation, changes in these barriers and thus in transport properties are inconsistent so far, so that definite conclusions for the clinical (and experimental) setting cannot be drawn. Therefore, transport mechanisms for (macro-) molecules should increasingly receive attention. One of the goals of translational cancer research is to obtain a better understanding of the compromised delivery and distribution of anti-cancer compounds in solid tumors (i.e., intratumor pharmacokinetics) in order to improve patients' outcomes.
Conflict of Interest Statement
The authors declare that the research was conducted in the absence of any commercial or financial relationships that could be construed as a potential conflict of interest.
Acknowledgments
The authors want to thank Mrs. Anett Lange (Munich) and Dr. Debra K. Kelleher (Mainz) for excellent editorial assistance. This review is partly based on a previous article (Vaupel and Multhoff, 2012).
References
Baker, D. G., and Krochak, R. J. (1989). The response of the microvascular system to radiation: a review. Cancer Invest. 7, 287–294.
Cairns, R., Papandreou, I., and Denko, N. (2006). Overcoming physiologic barriers to cancer treatment by molecularly targeting the tumor microenvironment. Mol. Cancer Res. 4, 61–70.
Chauhan, V. P., Stylianopoulos, T., Boucher, Y., and Jain, R. K. (2011). Delivery of molecular and nanoscale medicine to tumors: transport barriers and strategies. Annu. Rev. Chem. Biomol. Eng. 2, 281–298.
Dewhirst, M. W. (1991). Microvasular changes induced by radiation exposure: implications for therapy. Funktionsanalyse Biolog. Systeme 20, 109–122.
Fajardo, L. F., and Berthrong, M. (1988). Vascular lesions following radiation. Pathol. Annu. 23(Pt 1), 297–330.
Feldmann, H. J., Molls, M., and Vaupel, P. (2000). “Clinical Investigations on blood perfusion in human tumors,” in Blood Perfusion and Microenvironment of Human Tumors, eds M. Molls and P. Vaupel (Berlin/Heidelberg/New York, NY: Springer), 47–62.
Fukumura, D., and Jain, R. K. (2007). Tumor microenvironment abnormalities: causes, consequences, and strategies to normalize. J. Cell. Biochem. 101, 937–949.
Heldin, C. H., Rubin, K., Pietras, K., and Ostman, A. (2004). High interstitial fluid pressure – an obstacle in cancer therapy. Nat. Rev. Cancer 4, 806–813.
Jain, R. K. (1987). Transport of molecules across tumor vasculature. Cancer Metastasis Rev. 6, 559–593.
Jain, R. K. (1990). Physiological barriers to delivery of monoclonal antibodies and other macromolecules in tumors. Cancer Res. 50, 814s–819s.
Jain, R. K., and Stylianopoulos, T. (2010). Delivering nanomedicine to solid tumors. Nat. Rev. Clin. Oncol. 7, 653–664.
Kozin, S. V., Duda, D. G., Munn, L. L., and Jain, R. K. (2012). Neovascularization after irradiation: what is the source of newly formed vessels in recurring tumors? J. Natl. Cancer Inst. 104, 899–905.
Kuszyk, B. S., Corl, F. M., Franano, F. N., Bluemke, D. A., Hofmann, L. V., Fortman, B. J., et al. (2001). Tumor transport physiology: implications for imaging and imaging-guided therapy. Am. J. Roentgenol. 177, 747–753.
Lorke, D. E., Wenzel, S., Siebert, K., and Zywietz, F. (1999). Microvascular and tumor cell alterations during continuous hyperfractionated irradiation: an electron microscopic investigation on the rat R1H rhabdomyosarcoma. Int. J. Radiat. Oncol. Biol. Phys. 44, 895–904.
Lunt, S. J., Fyles, A., Hill, R. P., and Milosevic, M. (2008). Interstitial fluid pressure in tumors: therapeutic barrier and biomarker of angiogenesis. Future Oncol. 4, 793–802.
Milosevic, M., Fyles, A., Hedley, D., and Hill, R. (2004). The human tumor microenvironment: invasive (needle) measurement of oxygen and interstitial fluid pressure. Semin. Radiat. Oncol. 14, 249–258.
Milosevic, M., Fyles, A., Hedley, D., Pintilie, M., Levin, W., Manchul, L., et al. (2001). Interstitial fluid pressure predicts survival in patients with cervix cancer independent of clinical prognostic factors and tumor oxygen measurements. Cancer Res. 61, 6400–6405.
Molls, M., Feldmann, H. J., Stadler, P., and Jund, R. (2000). “Changes in tumor oxygenation during radiation therapy,” in Blood Perfusion and Microenvironment of Human Tumors, eds M. Molls and P. Vaupel (Berlin/Heidelberg/New York, NY: Springer), 81–87.
Narayan, K., and Cliff, W. J. (1982). Morphology of irradiated microvasculature: a combined in vivo and electron-microscopic study. Am. J. Pathol. 106, 47–62.
Nathan, S. S., Di Resta, G. R., Casas-Ganem, J. E., Hoang, B. H., Sowers, R., Yang, R., et al. (2005). Elevated physiologic tumor pressure promotes proliferation and chemosensitivity in human osteosarcoma. Clin. Cancer Res. 11, 2389–2397.
Nathan, S. S., Huvos, A. G., Casas-Ganem, J. E., Yang, R., Linkov, I., Sowers, R., et al. (2008). Tumor interstitial fluid pressure may regulate angiogenic factors in osteosarcoma. J. Orthop. Res. 26, 1520–1525.
Netti, P. A., Berk, D. A., Swartz, M. A., Grodzinsky, A. J., and Jain, R. K. (2000). Role of extracellular matrix assembly in interstitial transport in solid tumors. Cancer Res. 60, 2497–2503.
Rofstad, E. K., Gaustad, J. V., Brurberg, K. G., Mathiesen, B., Galappathi, K., and Simonsen, T. G. (2009). Radiocurability is associated with interstitial fluid pressure in human tumor xenografts. Neoplasia 11, 1243–1251.
Rofstad, E. K., Ruud, E. B., Mathiesen, B., and Galappathi, K. (2010). Associations between radiocurability and interstitial fluid pressure in human tumor xenografts without hypoxic tissue. Clin. Cancer Res. 16, 936–945.
Roh, H. D., Boucher, Y., Kalnicki, S., Buchsbaum, R., Bloomer, W. D., and Jain, R. K. (1991). Interstitial hypertension in carcinoma of uterine cervix in patients: possible correlation with tumor oxygenation and radiation response. Cancer Res. 51, 6695–6698.
Stohrer, M., Boucher, Y., Stangassinger, M., and Jain, R. K. (2000). Oncotic pressure in solid tumors is elevated. Cancer Res. 60, 4251–4255.
Thews, O., Zywietz, F., Lecher, B., and Vaupel, P. (1999). Quantitative changes of metabolic and bioenergetic parameters in experimental tumors during fractionated irradiation. Int. J. Radiat. Oncol. Biol. Phys. 45, 1281–1288.
Vaupel, P. (2006). “Abnormal microvasculature and defective microcirculatory function in solid tumors,” in Vascular-targeted Therapies in Oncology, ed D. W. Siemann (Chichester: John Wiley and Sons, Ltd.), 9–29.
Vaupel, P. (2009a). “Pathophysiology of solid tumors,” in The Impact of Tumor Biology on Cancer Treatment and Multidisciplinary Strategies, eds M. Molls, P. Vaupel, C. Nieder, and M. S. Anscher (Berlin/Heidelberg/New York, NY: Springer), 51–92.
Vaupel, P. (2009b). “Physiological mechanisms of treatment resistance,” in The Impact of Tumor Biology on Cancer Treatment and Multidisciplinary Strategies, eds M. Molls, P. Vaupel, C. Nieder, and M. S. Anscher (Berlin/Heidelberg/New York, NY: Springer), 273–290.
Vaupel, P. (2012). “Pathophysiological and vascular characteristics of solid tumors in relation to drug delivery,” in Drug Delivery in Oncology. Vol. 1, eds F. Kratz, P. Senter, and H. Steinhagen (Weinheim: Wiley-VCH), 33–64.
Vaupel, P., Frinak, S., and O'Hara, M. (1984). Direct measurement of reoxygenation in malignant mammary tumors after a single large dose of irradiation. Adv. Exp. Med. Biol. 180, 773–782.
Vaupel, P., Kallinowski, F., and Okunieff, P. (1989). Blood flow, oxygen and nutrient supply, and metabolic microenvironment of human tumors: a review. Cancer Res. 49, 6449–6465.
Vaupel, P., and Multhoff, G. (2012). “Pathophysiological barriers impeding the delivery of heat shock protein (HSP)-based macromolecules, and nanotherapeutics to solid tumors,” in Cellular Trafficking of Cell Stress Proteins in Health and Disease, eds B. Henderson and A. G. Pockley (Dordrecht: Springer), 185–199.
Wiig, H., and Swartz, M. A. (2012). Interstitial fluid and lymph formation and transport: physiological regulation and roles in inflammation and cancer. Physiol. Rev. 92, 1005–1060.
Yeo, S. G., Kim, J. S., Cho, M. J., Kim, K. H., and Kim, J. S. (2009). Interstitial fluid pressure as a prognostic factor in cervical cancer following radiation therapy. Clin. Cancer Res. 15, 6201–6207.
Znati, C. A., Rosenstein, M., Boucher, Y., Epperly, M. W., Bloomer, W. D., and Jain, R. K. (1996). Effect of radiation on interstitial fluid pressure and oxygenation in a human tumor xenograft. Cancer Res. 56, 964–968.
Znati, C. A., Rosenstein, M., McKee, T. D., Brown, E., Turner, D., Bloomer, W. D., et al. (2003). Irradiation reduces interstitial fluid transport and increases the collagen content in tumors. Clin. Cancer Res. 9, 5508–5513.
Keywords: irradiation, tumor microcirculation, transport barriers, tumor interstitial fluid pressure, macromolecular agents, intratumor pharmacokinetics
Citation: Multhoff G and Vaupel P (2012) Radiation-induced changes in microcirculation and interstitial fluid pressure affecting the delivery of macromolecules and nanotherapeutics to tumors. Front. Oncol. 2:165.doi: 10.3389/fonc.2012.00165
Received: 17 October 2012; Paper pending published: 24 October 2012;
Accepted: 25 October 2012; Published online: 15 November 2012.
Edited by:
Udo S. Gaipl, University Hosptial Erlangen, GermanyReviewed by:
Alan G. Pockley, Nottingham Trent University, UKFranz Rödel, Johann Wolfgang Goethe-University Frankfurt am Main, Germany
Copyright © 2012 Multhoff and Vaupel. This is an open-access article distributed under the terms of the Creative Commons Attribution License, which permits use, distribution and reproduction in other forums, provided the original authors and source are credited and subject to any copyright notices concerning any third-party graphics etc.
*Correspondence: Peter Vaupel, Department of Radiotherapy and Radiooncology, Klinikum rechts der Isar, Technical University of Munich, Ismaninger Strasse 22, 81675 Munich, Germany. e-mail:dmF1cGVsQHVuaS1tYWluei5kZQ==