- Department of Radiotherapy, GROW School for Oncology Maastricht University Medical Centre, Maastricht, Netherlands
Radiation pneumonitis (RP) and radiation fibrosis (RF) are two dose-limiting toxicities of radiotherapy (RT), especially for lung, and esophageal cancer. It occurs in 5–20% of patients and limits the maximum dose that can be delivered, reducing tumor control probability (TCP) and may lead to dyspnea, lung fibrosis, and impaired quality of life. Both physical and biological factors determine the normal tissue complication probability (NTCP) by Radiotherapy. A better understanding of the pathophysiological sequence of radiation-induced lung injury (RILI) and the intrinsic, environmental and treatment-related factors may aid in the prevention, and better management of radiation-induced lung damage. In this review, we summarize our current understanding of the pathological and molecular consequences of lung exposure to ionizing radiation, and pharmaceutical interventions that may be beneficial in the prevention or curtailment of RILI, and therefore enable a more durable therapeutic tumor response.
Development of the RILI (Molecular and Clinical Response)
The lung is one of the most sensitive tissues to ionizing radiation, and its susceptibility to radiation damage limits the success of radiotherapy for lung cancer treatment. The effects of lung irradiation are typically divided into early radiation toxicity, occurring within hours to a few days after RT exposure, and late radiation toxicity, occurring months to years after the treatment, which includes tissue fibrosis, necrosis, atrophy, and vascular injury [Figure 1; (1, 2)].
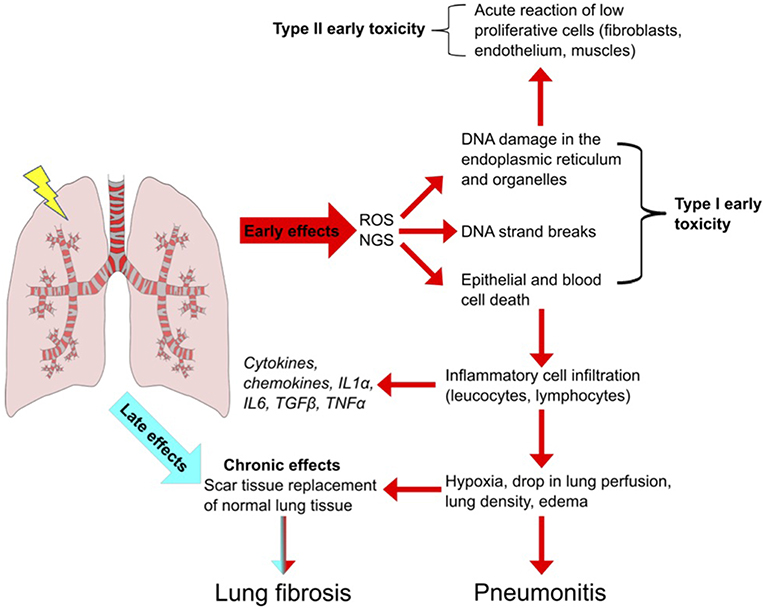
Figure 1. Radiation-Induced Lung Injury (RILI). Schematic overview of the important steps leading to pulmonary toxicity after radiotherapy. Radiation induces reactive oxygen and nitrogen species (ROS, NGS) which leads to DNA strand breaks and to epithelial cell death. Inflammatory cells infiltrate the affected region to remove death cells. Leucocytes and lymphocytes proliferate and produce cytokines and chemokines, leading to an inflammatory condition highly deregulated in duration and perpetuation. The persistence of the inflammatory state culminates in early reversible toxicity (pneumonitis) and can develop in to irreversible late toxicity (fibrosis).
Two primary mechanisms cause radiation-induced tissue injury: direct DNA damage and the generation of reactive oxygen species (3). Minutes after irradiation, the damage to DNA or cytoplasmic organelles triggers intracellular signaling, leading to altered gene expression and immediate release of growth factors such as transforming growth factor ß (TGF-ß), platelet-derived growth factor (PDGF), and interleukin 1 (IL-1) (4). Additionally, ionization of water molecules generates reactive oxygen species (ROS) such as superoxide, hydrogen peroxide, hydroxyl radicals and nitrogen species (NGS) (5) that account for 60% of the total damage inflicted (6, 7). ROS can directly modify proteins and organelles but in an iron dependent manner (Fenton reaction) can produce hydroxyl radicals that cause DNA damage (8, 9). ROS also induces DNA damage in mitochondrial DNA of which cells typically have thousands of variant copies and is more sensitive to damage than nuclear DNA because lack of repair. MtDNA damage acts as DNA damage associated molecular pattern that provoke inflammation and immune responses and apoptotic cell death and is strongly associated with immune related lung diseases (10, 11) Furthermore, ROS cause cell loss, edema of the alveolar walls, increased vascular permeability and exudation of proteins into the alveolar space which further reduces the alveolar septa, and vascular integrity leading to the apoptosis of alveolar type-I pneumocytes. The human alveolar epithelium is composed of type-I and II pneumocytes, which constitute 90 and 10% of cells in the alveolus, respectively. Type-II cells are the precursors of type-I cells and synthesize and secrete the pulmonary surfactant that regulates the alveolar-surface tension. The average turnover of the lung epithelium is 4 weeks but after radiation, the sensitive type-I pneumocytes are rapidly lost, and type-II pneumocytes drive re-epithelialization of the alveolus (12). Epithelial and endothelial cell loss, due to radiation-induced cell death leads to loss of barrier function and vessel integrity thereby reducing micro-vessel density and oxygen perfusion (1, 13). These effects of ROS are counteracted by direct activation of the hypoxia-inducible factors (HIF) 1α and 2α by ROS in cells, resulting in the activation of cytokines and growth factors including VEGF that promote endothelial cell proliferation. After tumor reoxygenation, nuclear accumulation of HIFα, and enhanced translation of HIF-1-regulated transcripts occur in response to ROS. The resulting increase in HIF-1-regulated cytokine expression enhances endothelial cell radio-resistance (14).
Following apoptotic death, damage associated molecular pattern molecules (DAMPs) are released from cells triggering the recruitment of immune effector cells from the innate immune system (neutrophils, macrophages, leukocytes, lymphocytes) that infiltrate into the damaged lung, and contribute to tissue remodeling (15, 16). Neutrophils are the first to arrive to the injured site. The increased endothelial expression of intercellular adhesion molecule 1 (ICAM-1) and platelet endothelial cell adhesion molecule 1 (PECAM-1) promotes neutrophil transmigration into the damaged lung followed by lymphocytic and macrophage transmigration. The inflammatory cells produce pro-inflammatory cytokines such as interleukins (IL) IL-3, IL-6, IL-7, TNF-α, TGF-β which results in the activation of fibroblasts, leading to the initiation of additional paracrine and autocrine loops between fibroblasts, endothelia, and macrophages (17, 18). The increased concentration of macrophages enhances the production of TNF-α, stimulating IL-6 secretion, and fibroblast proliferation (19, 20). The consumption of oxygen needed for the activation of the immune cells leads to tissue hypoxia. Hypoxia promotes the generation of ROS, upregulates TGF-β, and promotes collagen formation, which reduces the elasticity of the lung alveolus (13). Furthermore, hypoxia slows down the degradation of hypoxia-inducible factors (HIF) 1α and 2α in cells, resulting in the activation of genes encoding VEGF, erythropoietin and lactate dehydrogenase 5 (21). VEGF upregulation also occurs through TGF-β stimulation via SMAD3 signaling (22), which triggers an autocrine stimulus leading to late toxicity. All these events lead to radiation pneumonitis which is an acute a reversible event in RILI. Most patients develop only radiological signs of pneumonitis without symptoms.
Density changes of the lung parenchyma are a known effect of radiation therapy that can be monitored by CT. The dose dependent density change consists of 2 phases: a transient phase peaking (3–4 months) and a fibrotic phase (after 9 months). These coincide with the time points of pneumonitis and fibrosis. Significantly, pre-treatment (23). Thus, CT monitoring of density change is an important factor when symptoms are absent. In case of symptoms such as cough and dyspnea, other causes than RILI should be excluded since up to 45% of patients have these symptoms that are not due to RILI (24). More severe cases are treated with corticosteroids with mostly fast improvement and recovery. The optimal corticosteroid schedule has never been investigated prospectively and in view of the heterogeneity of RILI, it is conceivable that a one-fits-it-all approach is suboptimal.
The mechanism of how corticosteroids suppress, or reverse radiation pneumonitis has been mostly investigated in preclinical studies. Administration of dexamethasone 4 weeks after irradiation reduces inflammatory cell infiltration and cytokine expression of TNF-α, IL-6, IL-17A, and TGF-β1 in broncho-alveolar lavage fluid improving survival (p = 0.0323). In the RT only group, 13 mice (65.0%) died within 180 days after RT while in the dexamethasone group only 6 mice (30.0%) died (25). Similar results were obtained with a single dose administration of dexamethasone (5 mg/kg) after 20 Gy thoracic irradiation in C3H/HeN mice which suppressed expression of pro-inflammatory cytokines, TNF-α, IL-1α, and IL-1β mRNA within 6 h after irradiation (26). Expression profiling on the lungs of 20 Gy irradiated mice, showed that CTGF (connective tissue growth factor) a central mediator of tissue remodeling, was upregulated after irradiation. Administration of FG-3019, a human monoclonal antibody that binds human and rodent CTGF, extended median survival of irradiated mice from 161 days to 300 days. Pneumonitis was reduced within 2 weeks of FG-3019 treatment and almost completely reversed by 24 weeks. FG-3019, attenuated the lung density increase after RT, improved lung function, reduced lung wall erosion, collagen deposition, and leukocyte infiltration (27). In depth analysis of gene expression changes in mouse lungs treated with and without FG-3019 and irradiation showed amelioration of RT-associated expression pattern highly enriched in macrophage, dendritic cell, mast cell, and mesenchymal transcripts. Furthermore, the administration of FG-3019, 2 days prior RT for 8 weeks, reduced RT-induced radiologic, histologic, and functional lung deficits and attenuated growth factor and matrix remodeling genes which resulted in an improved lung function and a prolonged survival (28). In patients who cannot tolerate steroids or are unresponsive, other immunosuppressive agents such as azathioprine and cyclosporine can be considered; however, evidence for their efficacy is limited to case reports (29).
TGF-β produced by inflammatory cells is the primary driver of late lung toxicity. The increase in TGF-β levels after RT accompanies elevated collagen IV gene expression (30). This collagen is associated with basement membranes of endothelial and epithelial cells, leading to septal thickening, and indicative of microvascular injury. Pneumocytes and fibroblasts also contribute to TGF-β production in response to RT (31). TGF-β exerts its pro-fibrotic role by binding the transmembrane serine/threonine kinase, TGF-β receptors. Ligand binding induces activation of SMAD a transcriptional activator of collagen (32, 33). Through the stimulation of metalloproteinase inhibitors (TIMPs), TGF-β inhibits collagen catabolism which results in collagen accumulation and conversion of fibroblasts into myofibroblasts, leading to lung architecture remodeling. The differentiation of fibroblast into myofibroblasts as a consequence of TGF-β production results in increased expression of alpha-smooth muscle actin (α-SMA) (34). Myofibroblasts may also derive from circulating bone marrow-derived progenitor cells known as fibrocytes or from epithelial cells undergoing an epithelial-mesenchymal transition (EMT) (17). In response to TGF-β, myofibroblasts secrete excess collagen, fibronectin, and proteoglycans (35), resulting in increased stiffness, and thickening of the lung parenchyma. Furthermore, the increased activity of TIMPs and decreased matrix metalloproteinase (MMP) activity (MMP2-MMP9) leads to excessive ECM deposition (36), and excess collagen. These changes lead to pulmonary fibrosis resulting in fibrotic areas susceptible to physical trauma (i.e., rupture) and gradual ischemia, which further leads to loss of respiratory capacity, tissue atrophy, and necrosis (37, 38).
Risk Factors for RILI
The guidelines for measuring and reporting radiation toxicity in relation to dose/volume and clinical outcome have been described in the QUANTEC report: Quantitative Analysis of Normal Tissue Effects in the Clinic, that describe the development of Normal Tissue Complication Probability models (NTCP) (39). The likelihood of developing adverse lung effects after radiotherapy and the severity is strongly associated with patient characteristics and dosimetric parameters (40). Although the absolute risk of developing radiation-induced lung toxicity remains difficult to predict (41), the evaluation of the patient's clinical conditions and the potential risk factors to lung toxicity to upgrade the QUANTEC recommendations is setting the basis for a personalized treatment and setting dose-volume limits for personalized treatment [Table 1; (103)]. Radiologically abnormal Interstitial lung abnormalities are predictor for radiation pneumonitis (106). Clinical studies looking at RILI are often difficult to interpret because different endpoints are used (e.g., dyspnea score, corticoid use), and because only the maximum dyspnea is scored as an event after RT. Because approximately 50% of lung cancer patients have already some grade of dyspnea before radiotherapy, scoring dyspnea after RT, without considering baseline dyspnea, exaggerates the effect of RT on dyspnea, and proposed. Moreover, about 20% of patients have less dyspnea after RT, which is not taken into account in most models (107). Defraene et al. proposed a more accurate model for predicting radiation pneumonitis by combining dosimetric parameters with the ΔDyspnea score which is the maximum dyspnea score after 6 months corrected for baseline-dyspnea (108). Dyspnea scores are subjective, which hamper detailed quantitative analyses, including biomarker identification. The goal is the development of risk models to stratify patients according to their genetic risk for radiotherapy-induced damage and hence to more optimal personalized radiotherapy schedules (109). The single most important risk factor to develop severe radiation pneumonitis is interstitial lung disease (ILD) which can be quantified by the uptake of FDG in the lungs. In a retrospective study of 101 NSCLC patients a CT (4D-CT) scan directly followed by an [18F]FDG-PET scan was performed before radiotherapy treatment. Patients with high [18F]FDG uptake in 5 to 10% of the lungs before RT were more likely to develop radiation toxicity than patients with a low uptake. Furthermore, in patients with RILI the [18F]FDG uptake was higher in the lower lobe of the lung than in other regions. These results suggest that identifying patients at high risk for RILI on the basis of a pretreatment [18F]FDG-PET-CT can be used to individualize treatment (106, 110).
Patient's Characteristics
In lung cancer patients, the incidence of adverse effects after radiotherapy is related to clinical factors and a variety of dosimetric parameters (111). Age is one of the main factors associated with radiation-induced lung toxicity (45). Older patients (>65 years old) have less tolerance to RT and a major risk of developing adverse effects (41, 77). A multivariate analysis of 369 patients with an age > 65 years, with stage III non-small cell lung cancer (NSCLC), revealed how age influence both grade 2 (OR = 1.99), and grade 3 radiation pneumonitis (OR = 8.90) (41). Similar results were confirmed by others, in a prospective study with 96 patients who received three-dimensional conformal radiotherapy (3D-CRT) for stage IA to IIIB NSCLC (44). Furthermore, grade 4 + toxicity occurred in 62% of NSCLC patients <70 years compared with 81% of elderly patients (P = 0.007) and Grade 4 + toxicity occurred in 1% of those younger than 70 years, compared with 6% of those older than 70 (P = 0.02) (112). This is primarily due to the fact that older patients have more comorbidities that are a risk factor for RP, than younger patients (46). Despite age being a strong risk factor, a threshold value has not been determined due to additional risk factors such as smoking status, and pulmonary function.
The effect of gender on RILI is still unclear (77). Women have smaller lung volumes and more often develop an autoimmune disease which increases their risk of RILI. A univariate analysis of 148 lung cancer patients with good performance status (ECOG 0–1) treated with chemo-radiation confirmed that the risk of severe pneumonitis was significantly higher in women vs. men (15% in women vs. 4% in men) (48). Among 214 consecutive patients with locally advanced NSCLC that received 3D CRT, gender was a predictor for the grade ≥2 group only (OR = 0.32, p = 0.028) (47). Other studies do not show a significant association between gender and RP risk, probably due to the contribution of different factors such as pre-existing diseases, or radiation schedule (46, 70, 113). Further studies are needed to clarify the role of gender as a clinical risk factor for RILI.
Pre-existing Disease
In contrast to the causative role of smoking and lung cancer, tobacco use seems to have a protective role against pneumonitis in lung cancer patients treated with chemo-radiation (50, 51). The frequency of Grade 3+ pneumonitis was higher in long time quitters in comparison to recent quitters or current smokers (72). In stage I–IV lung cancer patients (n = 182) treated with radical (chemo) radiotherapy the dyspnea grade was higher in patients who quit smoking compared to active smokers. A possible explanation may be that ex-smokers quit smoking because of the appearance of dyspnea. However, for a correct interpretation of the results the baseline dyspnea score has to be taken in to account in order to avoid false positive results. In 576 patients with stage III, NSCLC treated with radiotherapy the incidence of radiation-induced pneumonitis was higher (grade ≥3 pneumonitis) in non-smokers (37% at 1 year) in comparison to smokers (14% at 1 year). Patients who quit smoking before diagnosis showed an intermediate incidence of RP (23% at 1 year) although dosimetric parameters were not taken into account (52). The long-term exposure of the lung to chemicals in tobacco smoke destroys the lung tissue due to the replacement of the elastic walls with a fibrotic structure and the attraction of pro-inflammatory mediators. On the other hand, smoking leads to immunosuppression causing reduced antibody response against carcinogens compared to non-smokers (53, 114). The protective role of smoking is therefore probably related to reduced sensitivity of the damaged lung compared to a non-smokers healthy lung (48, 49, 53). Furthermore, a carbon monoxide–induced hypoxia caused by tobacco results in less ROS generation after radiolysis and therefore less lung DNA and tissue damage (53).
Significant differences in radio-sensitivity are associated with single nucleotide polymorphisms (SNP) that affect cell survival, DNA damage response, and DNA repair genes (71). SNPs in ATM, IL-1A, IL-8, TNF, TNFRSF1B, MIF rs2868371, rs1800469, TGF-β, TNF-α, VEGF, XRCC1, APEX1, IL-6 are associated with a 2.16-fold increased risk of lung injury upon irradiation (72). SNPs in IL-13 with 2 variant alleles rs20541 or rs180925 were approximately 3-times more likely to develop pneumonitis compared to those with wild-type genotypes. SNP rs10711, located in the 3′UTR region of CDK1 (encoding for cyclin-dependent kinase 1) was significantly associated with a higher risk of pneumonitis (OR = 2.67, 95% CI = 1.26–5.63, P = 0.010) (115). SNPs, in IL-1A, IL-8, TNFRSF1B, MIF, and NOS3 are also associated with 3.16-fold increased risk of radiation pneumonitis (72). Including SNPs in RP risk models, improves the discrimination accuracy especially when including SNPs with a high allele frequency or larger effect size. Therefore, stratification based on individual genetic differences in NTCP models could be an interesting approach to select patients at lowest risk for radiotherapy complications (74). However, despite numerous studies (SNP) associations have rarely been reproduced in independent validation studies. For example initial studies reported strong associations between TGF-β SNP and radiation pneumonitis but could not be validated in independent cohorts (55, 116, 117). Development of robust, standardized and quantitative endpoints integrating GWAS and gene-expression are needed before radiogenomics will be useful to predict radiation sensitivity (118).
Treatment Related Risk factors
Lung Function
Lung function is evaluated by assessing different parameters [forced expiratory volume (FEV), forced vital capacity (FVC), diffusing capacity of the lungs for carbon monoxide, total lung capacity (TLC), lung volume (LV)], and how they change before and after radiation. A decreased value of these parameters is an indicator of decreased pulmonary function and of capillary alveolar changes (DLCO), bronchial obstruction (FEV) (55), and lung stiffening (FVC, TLC) (45, 54, 57). Pre-existing lung disease strongly increases the risk for radiation pneumonitis after thoracic radiotherapy (58, 59, 106) by exacerbating inflammation and destruction of the connective tissue scaffold (60). Most lung cancer patients develop COPD, emphysema and therefore have poor pulmonary function (ECOG 3–4). In a multivariate analysis, Rancati et al. showed that lung cancer patients suffering from COPD receiving fractionated radiotherapy had 24.1% more lung toxicity compared to patients with no COPD (61). Similarly Kimura et al. found a strong correlation between the grade of pulmonary emphysema (PE) (grades 0–3) and radiation pneumonitis, ranging from 16.5% (grade 0) to 54.0% (grade 3) (62).
Dose
Dosimetric parameters (irradiated volume, mean lung dose (MLD), dose delivered, schedule, tumor location) are risk factors for RILI (119). Irradiating larger volumes of the lung causes compromises lung function (79, 113). Improvements in three-dimensional treatment planning systems describe the relationship between irradiated lung volume and the probability of tissue complications. Robnett et al. demonstrated that in a group of 540 patients, the risk for grade 2 pneumonitis strongly correlated with MLD (48). Other studies underline how V20 and V30 (lung volume receiving 20 or 30 Gy, respectively) are the only parameters significant in predicting RP (79, 113). One significant limitation is that most studies determine the DVH (Dose Volume Histogram) but do not consider lung movement and deformation resulting from breathing (43). Hernando and Guerrero et al. found that an MLD < 10 Gy is associated with a 10% radiation pneumonitis rate but this increases to 16% with an MLD of 11–20 Gy (43, 101). The NTCP model describes the probability of RP as a function of a sigmoidal dose relationship (39). To calculate the likelihood of lung injury this approach relates the tolerance dose for lung irradiation and the slope of the dose-response curve (120, 121). In a study of 42 lung cancer patients treated with fractionated radiotherapy, with a dose of 67 Gy, the NTCP average values were 73% for the patients with RP, and 25% for those without lung comorbidities (102). Thus, NTCP could be used for the optimization of the treatment plan (122).
While the mean dose to the lung is a key risk factor for RILI, the dose to the heart also influences pulmonary toxicity. Using high energy proton irradiation van Luijk et al. demonstrated that co-irradiation of the heart and lung significantly increased breathing rate as surrogate marker for lung function in rats compared to lung irradiation alone (123). Further work in preclinical models from the same group showed that that lung and heart irradiation through different mechanisms enhance lung toxicity by increasing hypertension and inducing vascular pulmonary damage and that both need to be avoided that to reduce lung toxicity after irradiation (124). Indeed using precision irradiation of the heart limiting dose- to small volumes in the lung induced lung pneumonitis in a dose-dependent manner in the absence of heart toxicity in mouse models (125). Also in patients reduced cardio-pulmonary function further exacerbates radiation induced lung toxicity (104). In large cohort studies no significant association between heart dose and RP was found (105). Quantitative parameters independent of dyspnea and cough that may result from pre-existing lung disease rather than a consequence of cardiac damage are needed.
Tumor Location
Tumor location is one of the main predictors for RP development in preclinical and clinical studies. In C3Hf/KAM mice where 70% of the lung was irradiated with a fixed dose (22 Gy), irradiation of the midlung was associated with less morbidity from RP than radiation of the base, the upper half of the lung, and the region adjacent to the pleura (75). In a retrospective study of 60 lung cancer patients that received chemo-radiation, RP was more frequent after irradiation of the base of the lobe (70%) rather than after irradiation of the upper lung lobes (20%) (51). In the study of Seppenwolde et al. the risk of RP has been evaluated in relation to the regional dose distribution in 106 lung cancer patients that received fractionated radiotherapy (2 Gy/fraction) was determined. Dividing the lung into different sub-volumes the incidence of RP was higher in posterior central and peripheral regions in comparison to anterior and contralateral zones (76). Another study of 324 lung cancer patients confirms the higher incidence of lung injury following the exposure of the lower region compared to the upper one, suggesting the importance of superior-to-inferior tumor position as a significant variable (70). Possible explanations for the different regional radio-sensitivity might be related to the better oxygenation, perfusion, and ventilation of the lower pulmonary region (77).
Systemic Treatment
There are conflicting results on the effects of concurrent, concomitant, or neoadjuvant chemotherapy on radiation toxicity. Neoadjuvant chemotherapy shrinks the tumor volume before RT and reduces the PTV (planned treatment volume), and the risk of RILI. A dosimetric analysis of 23 patients with stage IV small cell lung cancer receiving platinum-based chemotherapy as neoadjuvant showed that 30 had a 20% reduction in tumor volume after induction chemotherapy. This translated into a 5% reduction in risk for RILI (85), suggesting that neoadjuvant chemotherapy can reduce RILI (19, 79). In contrast, a retrospective study of 223 patients treated with concurrent chemoradiotherapy did not show a significant effect on RP (<3) among treatments (with and without RT) (126). The difference might be explained by different patient selection criteria, radiotherapy dose, chemotherapy doses, and clinical schedule (concurrent vs. neoadjuvant). Paclitaxel, taxol, dactinomycin, cyclophosphamide, doxorubicin, mitomycin C, gemcitabine, and irinotecan have all been reported to increase the risk of pulmonary toxicity when combined with fractionated radiation (56). In a phase I/II study of 24 patients with NSCLC treated with paclitaxel/cisplatin concurrent with radiotherapy, the incidence of pulmonary toxicity was higher in patients treated with chemoradiation compared to patients receiving both monotherapies (80). In a retrospective study of 84 lung cancer patients who received 3D-CRT, grade >2 RP was 3-fold higher in patients with mitomycin (31.2%) than those with RT only (61).
In NSCLC patients, radiation pneumonitis was a dose-limiting toxicity when gemcitabine was administered at a dose of 50 mg/m2 twice-weekly with concurrent radiotherapy (81) and the incidence of Grade >2 RP was several fold higher among patients who received irinotecan (56%) compared with those who did not (14%) (56, 82). RILI is highly dependent on the treatment schedule. Sequential chemotherapy is associated with an increased risk for RP compared with a concomitant regimen (46, 83). Fraction size is another critical parameter in RILI. Fractions >2.67 Gy enhance the risk of RILI compared to lower daily fractions (84).
Targeted Therapies
Tyrosine Kinase inhibitors (erlotinib and gefitinib) against EGFR are first-line treatment for patient with EGFR-mutated non-small cell lung cancer. A large meta-analysis with more than 15 thousand patients showed it increases the incidence of pneumonitis with ethnic differences in susceptibility (127). No differences were found in efficacy or toxicity between Erlotinib and Gefitinib in randomized phase trials of EGFR mutated NSCLC (95). Third generation TKI's such as Osimertinib are more potent than Erlotinib and Gefitinib and increase progression free survival and overall survival with comparable overall toxicity, although interstitial lung disease, and cough symptoms were elevated in the Osimertinib group (96). Acute side effects from TKI can be managed by cessation of treatment or with corticosteroids (128). There are a limited number of studies with low number of patiens and case reports describing increases in interstitial lung disease and pneumonitis with concurrent thoracic radiotherapy and erlotinib in the curative (73, 93), and palliative setting (94). Larger studies are needed to understand the understand the full scope of these adverse effects.
Immunotherapy
Recently, by combining immune checkpoint inhibitors with chemoradiotherapy significant responses and prolonged survival are seen in treatment-refractory advanced Stage III NSCLC (129). There is mounting evidence that radiation may induce expression of checkpoint receptors on normal tissues as well (130, 131). NSCLC cancer patients often have pre-existing conditions such as COPD, asthma, and emphysema that are associated with inflammation and hypoxia that may upregulate PDL-1 in normal tissue resident cells or in infiltrating immune cells (132). Autoimmune-related response are strong predictors for outcome in patients treated with checkpoint inhibitors (133). However, they are also associated with treatment related side-effects. In a meta-analysis of serious adverse events of PD-1 and PD-L1 inhibitors in clinical trials, pneumonitis was among the most frequent common causes of death (134, 135). Stereotactic high dose radiotherapy is standard of care in inoperable node-negative NSCLC with manageable lung toxicity (136). Hypofractionated radiation has been shown to convert “cold' tumors to” hot 'inflammatory tumors by activating damage associated pathways and releasing pro-inflammatory factors that boost an anti-tumor T-cell response (137, 138). Indeed, pre-treatment immunological status predicts response to stereotactic hypofractionated RT in patients (139). With the promise of combining immunotherapy and radiotherapy we may see an increase of stereotactic radiation in the thorax. Thus, it will be important to monitor for increases in immune-related side effect as well. To date, immune-checkpoint inhibitors show objective responses in up to 30% of patients.
Intervention and Prevention
Approaches are sought to prevent, dampen, or delay late normal tissue complication. Conformal radiotherapy, as well as intensity-modulated radiotherapy (IMRT) and particle (e.g., carbon, proton) therapy, are used to improve irradiation of tumor volumes while sparing the healthy tissue. While these approaches substantially contribute to more precise tumor irradiation dose, exposure of the healthy tissue is inevitable. The study of a cohort of 188 NSCLC patients that underwent (chemo-)radiotherapy with IMRT or VMAT show grade four and grade five late toxicity only in VMAT (Volumetric Arc Therapy) treated patients when compared to IMRT. Grade >3 lung pulmonary toxicity nearly doubled in the VMAT group compared to the IMRT treated patients. The differences may be related to the different dose distribution characteristics which may result in differences in radiation exposure (140). In the study of Jegadesh et al. IMRT was associated with an improvement in median overall survival and 5-year survival rate (17.2 vs. 14.6 months; 19.9% vs. 13.4%, p = 0.021). Different studies demonstrate the ability of IMRT to reduce mean lung dose, lung V20, maximum dos to the spinal cord and multiple heart dosimetric parameters and improves quality of life, dosimetry, and toxicity in comparison to 3D CRT in the treatment of locally advanced NSCLC (141). Furthermore, IMRT may improve dosimetric parameters via increased dose conformity to the target volume. A more detailed description of technological advances in precision irradiation falls outside of the scope of this review, and the reader is referred to other studies (142, 143).
Biological approaches exploit differences in radiation- sensitivity between tumor and healthy tissue. These include the use of radiation protectors, modifiers, or mitigators. These drugs are specific for acute or late responding normal tissues, ideally without any protective effect on the tumor cells. There are three types of pharmaceuticals that can be administered at different stages of treatment. Firstly, radio-protectors are given before radiation exposure; secondly, radio-mitigators are given during or immediately after radiotherapy but before the appearance of toxicity; and thirdly treatments can be administered after the appearance of toxicity to attenuate progression or reverse the damage (144, 145). However, multiple approaches are often used to obtain successful results, due to different factors that contribute to RP (lung sensitivity, cellular turnover, dosimetric, and clinical parameters) (40).
Radioprotectors
Amifostine is a thiol prodrug whose damage repair mechanism acts through a hydrogen atom donation and to date, the only thiol-drug approved for clinical use (146). It is administered as an inactive prodrug that after de-phosphorylation by Alkaline Phosphatase is activated (147). It is relatively specific for normal cells due to the relatively high expression of Alkaline Phosphatases in normal tissue vs. tumor cells. Furthermore, the low pH of the tumor microenvironment inactivates amifostine contributing to its normal tissue specificity (148). The thiol group of amifostine plays a key role in activating redox-sensitive transcription nuclear factor kappa-light-chain-enhancer of activated B cells (NFκB), resulting in an increased expression of Superoxide Dismutase 2 (SOD2) (149). Increased SOD enzyme expression further contributes to ROS neutralization into less reactive products (hydrogen peroxide and oxygen) (149). Once oxidized amifostine induces oxygen depletion, by increasing oxygen consumption in normal cells making them more resistant to permanent radiation-induced DNA damage. Antonodau et al. reported a significant reduction in esophagitis > grade 2 and RP (grade 3) (P <0.001) in lung cancer patients receiving thoracic radiation post amifostine (340 mg/m2 IV before daily RT) (150). Hildebrandt et al. showed that both esophagitis > grade 3 (16 vs. 35%), and radiation pneumonitis (0 vs. 16%; P < 0.02) was significantly lower in 62 patients receiving neoadjuvant amifostine, concurrent chemotherapy (cisplatin and oral etoposide), and hypofractionated radiotherapy (1.2 Gy bid to 69.6 Gy) (72). Although amifostine is generally well-tolerated, side-effects include nausea, fever, hypotension and allergic reactions in a dose-dependent manner. The treatment is rarely interrupted however, suggesting a safe and tolerable profile (148, 151).
ROS can also be neutralized by engineered nanoparticles which have shown radioprotective effects in preclinical models. For example, the administration of Manganese Superoxide Dismutase-Plasmid Liposomes (MnSOD-PL) in a single dose, before RT has been shown to decrease the magnitude and duration of cytokine production, and thiol and lipid peroxidation in vivo studies. Intratracheal administration of MnSOD-PL in C57Bl/6 mice with orthotopic Lewis Lung carcinoma 24 h before whole thorax irradiation (18Gy) resulted in tumor radiosensitization but spared the surrounding normal tissue, prolonging mice survival (152). Similar results were shown by Carpenter et al. who showed that inhalation of MnSOD-PL 24 h before radiotherapy increased survival in 20 Gy irradiated mice compared to controls. Furthermore, a decrease in alveolitis, lung fibrosis, and weight loss was observed (153). Together with SOD nanoparticles, porphyrins also showed a reduction in radiation damage. Vujaskovic et al. showed a reduction of RT lung injury after the supplementation of AEOL 10113 [manganese (III) mesotetrakis (N-ethylpyridinium-2-yl) porphyrin (MnTE-2-PyP(5+)] in 28 Gy irradiated rats. AEOL 10113 significantly reduces the severity of RT-induced lung injury by reducing collagen deposition, pro-fibrogenic cytokines and TGF-β in irradiated rats (154). Inactivation of ROS can be accomplished by administration of cerium oxide nanoparticles (CNPs) (155). Intraperitoneal injection of high dose (10 μM) CNP-18, and CNP-ME, 2 h post irradiation for 4 weeks improves 15 Gy whole-thorax irradiated CBA/J mice survival (survival rates of 40%) compared to irradiation alone (10%). Additionally, a reduction of vascular damage, collagen deposition, and inflammatory response was recorded (156).
Genistein is a soy-derivative and isoflavone, which acts as radical scavenger and protein kinase inhibitor (157). In irradiated murine lungs, it exerts anti-inflammatory and antioxidant properties by limiting macrophages infiltration, reducing micronuclei formation, lowering ROS levels, and preventing DNA damage (158). Genistein supplementation before and immediately after radiotherapy protects from the onset of pneumonitis and reduces fibrosis in C57Bl/6 mice (159). Humanetics Corporation is developing BIO300SOD/ a nanoparticle formulation of Genistein to prevent pneumonitis and fibrogenesis. The efficacy and the toxicological profile of BIO300 has been tested in vivo models while its pharmacokinetic and safety has been evaluated in a phase I safety and pharmacokinetic trial (NCT 00504335).
Targeting DNA damage caused by ROS is another promising strategy to reduce radiation side effects. Intraperitoneal administration of the catalase mimetic Eukarion (2–30 mg/kg) post irradiation (10 to 20 Gy 60Co γ-rays) reduced micronuclei induction, when given after irradiation (160). Berberine and other ROS scavenger at 20 mg/kg once a day for 6 weeks reduces the incidence of lung injury in NSCLC patients treated with 2 Gy to a total of 60–70 Gy. Improved pulmonary function and decreased intercellular adhesion molecule 1 (ICAM-1), and TGF-β levels were observed (161). Nitroxides are potent free radical scavengers and Tempol (4-hydroxy-2,2,6,6-tetramethylpiperidine-1-oxyl), has shown a protective role against ROS in in vivo models (148). Preclinical studies showed a radioprotective effect of systemic administration of Tempol (275 mg/kg) in C3H mice exposed to whole body irradiation without any effect on tumor growth (162). The paramagnetic property of the oxidized Tempol permits non-invasive Magnetic Resonance Imaging (MRI) resulting in image contrast enhancement (40). Thus, the concentration of its radioprotective state and site of accumulation in tissue provides essential information about the compound and the radiation treatment.
Pentoxifylline is a xanthine derivative agent which down-regulates the production of pro-inflammatory cytokines, particularly TNF-α, and inhibits platelet aggregation. Pentoxifylline (400 mg/3 times daily), in patients with lung and breast cancer receiving a daily dose of 2 Gy, 5 days/week, protects against both early and late lung toxicity (163). In preclinical studies administration of pentoxifylline (500 mg/l), 1 week before irradiation in C57Bl/6 mice receiving whole thorax irradiation (12 Gy) reduces the RP phase (164). The radioprotective effects are related to the increase in locoregional blood flow. Pentoxifylline inhibits cAMP phosphodiesterase causing a cascade of events reducing leukocyte adherence to endothelial cells, minimized platelet aggregation and dilatation of capillaries through enhanced prostacyclin synthesis. Consequently, blood viscosity, and systemic vascular resistance are reduced (165). High levels of cAMP reduce the release of bioactive TNF-α by downregulating the expression of the TNF-α gene.
Radiomitigators
Targeting inflammation represents an alternative approach to reduce adverse effects during radiotherapy. In 18 Gy irradiated C57Bl/6 mice, intraperitoneal injection of methyl prednisone, an immune suppressor (40 mg/kg a day), for 7 days reduces TGF-β1 and TNF-α in lung tissue at 9 weeks after radiation exposure delaying the development of fibrosis at 12 weeks after irradiation (166). Statins (HMG-co-reductase inhibitors) ameliorate endothelial function by increasing the concentration of endothelial nitric oxide synthase (eNOS), which promotes anti-inflammatory signaling, prevents apoptosis, increases vasodilatation, and reduces platelet adhesion (157). Treatment with Ulinastatin for 3 days pre- and 4 days post-irradiation in 20 Gy irradiated Sprague Dawley rats attenuated pulmonary injury compared to the control group which developed severe fibrosis (167). Although the mechanism of action of Ulinastatin is not fully understood, it caused the downregulation of pro-inflammatory chemokines and alleviated pulmonary edema and inflammation of the alveoli (168). Lovastatin shows similar results. Statins have also been shown to reduce O2 consumption, which could counteract the protective effect of statins by reducing inflammation. In C57Bl/6 mice exposed to a single dose, 15 Gy whole thorax irradiation Lovastatin reduced radiation-induced pneumonitis by exerting anti-inflammatory, anti-apoptotic and antifibrotic effects (169). A recent study shows how Ethyl Pyruvate (EP), an effective inflammatory injury ameliorator, alleviates radiation injury in C57Bl/6 mice exposed to whole lung irradiation (16 Gy). Intraperitoneal injection of EP once every day for 1 month after RT was associated with a lower histological grade of inflammation and reduced pro-inflammatory cytokines, IL-1β, IL-6, and GM-CSF in irradiated mice. Furthermore, EP suppresses the production of TGF-β1 showing anti-fibrotic effects (54).
ACE (angiotensin-converting enzyme) inhibitors and angiotensin-2 antagonists, normally used to regulate blood pressure and prevent cardiovascular diseases, have been shown to diminish radiation-induced tissue damage in preclinical models. Robbins reviewed the use of the renin-angiotensin-system agents (RAS agents) for mitigating late radiation effects (144, 170). Angiotensin II Receptor Antagonist (AT2RA 158, 809) and the ACE inhibitors (Captopril and Enalapril) attenuate the effects of radiation damage by targeting the oxidant, inflammatory and fibrogenic pathways. Angiotensin II regulates TGF-β and α-smooth muscle actin (SMA), two proteins with a critical role in the pathogenesis of pulmonary fibrosis (171, 172). Enalapril reduces vascular remodeling and decreases levels of TGF-β (173). Supplementing RAS agents (ACEIs Captopril, CL 24817, Enalapril, and CGS 13945) in irradiated Sprague Dawley rats has been shown to reduce expression of endothelial dysfunction markers (chemokine secretion and leukocyte adherence, cell permeability, enhanced low-density lipoprotein oxidation, platelet activation). The AT2RA 158,809, Captopril and Enalapril, significantly delay radiation-induced lung injury. The incidence of Grade > 2 pneumonitis was significantly lower in 62 patients with stage I or III treated with ACEIs during thoracic irradiation compared to 100 non-users (2 vs. 11%) (174).
Curcumin (diferuloylmethane), a natural compound extracted from Curcuma longa, inhibits NSCLC metastasis by blocking the Adiponectin/NF-κB/MMPs Signaling Pathway (175), and reducing cell viability, invasion, and migration. This was shown in the metastatic 95D NSCLC cell line (176). Curcumin attenuates radiation-induced lung inflammation and fibrosis in 18 Gy irradiated rats by exerting anti-inflammatory effects, reducing macrophage infiltration and attenuating the increase of alveolar septal thickness after radiation. Furthermore, collagen accumulation, TGF-β content, and CTGF and NF-κB expression levels were significant reduced in curcumin treated rats (177).
Several growth factors act as radiation damage mitigators when supplemented close to the time of irradiation. Among these compounds Keratinocyte Growth Factor (KGF) is the most common. KGF is a paracrine growth factor which stimulates the proliferation and differentiation of alveolar type 2 cell and protects the lung from radiation injury. Liu et al. showed, in Sprague–Dawley rat models (20 Gy), how radiation-induced fibrosis is delayed after KGF administration, stimulating cell proliferation, inhibiting inflammatory response and decreasing lipid peroxidation as a direct result of ROS. KGF also promotes alveolar fluid clearance, decreases lung edema after pulmonary damage and reduces the reactive oxygen species (161). KGF protects against increases in endothelium permeability, decreasing lung edema driven by hydrogen peroxide in human airway epithelial cells (178). Despite its safety profile and efficacy in treating RILI, its instability, cost and inability to enter the distal lung have limited its use. Better results are reported with Palifermin a recombinant human KGF which showed promising results in clinical trials where it decreased the incidence and duration of dysphagia in patients with an unresectable stage III NSCLC treated with concurrent CT/RT (179).
Cell Based Therapies
Stem cell therapy has the potential to repair and restore tissue function from the adverse effects of radiotherapy in multiple tissues (180). However, there are limited preclinical studies and no clinical experience yet that guide the optimal timing of transplantation of stem cells and application to early or late radiation toxicity in lung.
Bone marrow derived mesenchymal stem cells (MSCs) have demonstrated great promise in regenerative medicine including in the lung. Due to their anti-inflammatory properties and enhanced repair capacity, MSCs are used for the treatment of inflammatory diseases and COPD, resulting in improved lung architecture, decreased apoptosis and increased cell proliferation and could be a promising therapeutic approach to mitigate radiation-induced pneumonitis. MSCs have been shown to successfully migrate toward the injury site in the lung after irradiation and adopt lung cell phenotypes (181, 182). In C57Bl/6 at 25 weeks after 15 Gy whole thorax irradiation, MSC infusion at 24 h and 14 days after RT protected the irradiated lung from severe radiation-induced vascular endothelial cell (EC) damage and delayed EC loss. Radiation-induced increase in infiltration of myeloid cells was also significantly reduced in MSC-treated animals at 25 weeks after whole thorax irradiation, which might be due to the protection of lung EC. Furthermore, MSC-derived cell culture efficiently rescued cultured lung EC from the radiation-induced side effects in short-term and long-term survival assays, indicating the protective contribution of MSC-secreted factors. Administration of MSCs after RT restores SOD1 levels in irradiated murine lung tissue, likely contributing to their protective effect. Improved vascular function and normalization of immune cell infiltration, favors both prevention and recovery from radiation injury to vascular and other resident lung cells. However, further studies are required to better understand the effects of MSCs on bronchial-alveolar and epithelial cells in order to develop MSC based therapeutic strategies (183). Adipose-derived mesenchymal stromal cells (Ad-MSCs) also have significant potential for clinical application. The delivery of Ad-MSC through the tail veins 2 h after irradiation (15 Gy) in Sprague-Dawley rats attenuates radiation-induced lung toxicity by blocking inflammatory, apoptotic, and fibrotic responses. Ad-MSc were associated with decreased serum IL-1, IL-6 and TNF-α expression within the first 28 days after irradiation and decreased fibrotic markers (TGF-β, CTGF, α-SMA, and Col1a1). Hydroxyproline content was a direct index reflecting lung fibrosis, suggesting that stem cell therapy delayed fibrosis. Thus, Ad-MSCs have therapeutic potential in RILI management as well (184).
Induced pluripotent stem cells (iPS) are a useful tool in regenerative medicine (185). The pathways that play a leading role during lung embryogenesis and morphogenesis may also be involved in the regeneration of lung tissue after injury (186). The murine lung developmental process has been elucidated through technologically advanced techniques such as whole transcriptome analysis (187, 188). Recapitulating the sequence of the developmental stages during lung development represents a promising approach to differentiate pluripotent stem cells into lung lineages. The differentiation of induced pluripotent stem cells into endodermal progenitors occurs throughout the stimulation of the Nodal signaling pathway (189). Consequently, the dual inhibition of TGF-β and BMP4 pathways and the supplementation of WNT, RA, and BMP leads to NKX2+progenitors, which further differentiate toward bronchial (Sox2+) and alveolar (Sox9+) lung lineages (185, 190). This process is known as “directed differentiation” and culminates with NKX2+ progenitors, from which all lung epithelia derived. These derived progenitors can re-populate decellularized whole lung scaffolds (186). Furthermore, the generation of ESC from patients with cystic fibrosis allows their use for lung pathologies (191) although their clinical use has not yet been documented. An understanding of the iPS mechanism of action and the molecular mechanisms of lung repair may promote their use in the irradiated lung to facilitate stem cells spreading over the damaged tissue.
Although several cell biology studies are based on submerged culture procedure, they are far from mimicking the in vivo lung microenvironment. 3D models such as lung organoids are a useful tool to answer several questions about lung regeneration. Broncosphere culture assays have been performed using both iPS and lung basal cells (192). Fluorescence-activated cell sorting allows the isolation of P63, NGFR, K5 positive stem, and progenitor lung epithelial cells that can be cultured in Matrigel which provides a three-dimensional structure to study the mechanisms involved in basal cell renewal. The same model can be used to investigate the mechanism of lung regeneration after irradiation in an experimental condition closer to reality (193). Three-dimensional scaffolds have been generated from synthetic or biomimetic materials to develop ex vivo lung parenchyma and vascular system and could be exploited for transplantation to produce functioning lung tissue in animal models (194).
Translation From Preclinical Models
With the diversity of preclinical models and differential sensitivity, breadth and penetrance of radiation-induced lung toxicity, there is a need for a consensus on preclinical models that best resemble aspects of human disease phenotypes (195). Most models use single dose irradiation rather than clinically relevant fractionated irradiation, and there are significant strain-differences in the sensitivity to pneumonitis and fibrosis (196, 197). Treatment toxicity should also be measured in immune-tolerant orthotopic tumor bearing models using clinically relevant combination-treatment schedules and (surrogate) endpoints. Furthermore, the dose to other organs at risk in the irradiation field such as the heart influence pulmonary function and indirectly contribute to adverse effects and should be appropriately modeled as well (198). In this respect dose calculations on human tissues irradiation studies with MeV beams cannot be directly translated to mouse preclinical irradiation studies using KeV beams (199). The use of preclinical micro-irradiators with integrated CT imaging are a new tool to evaluate radiation-induced toxicity closer to clinical irradiation set-up. These small animal iGRT systems enable precision irradiation to lung subvolumes and demonstrate that CT density correlates with histopathological factors of lung remodeling (200, 201) although this may not be sensitive enough to measure small reductions in lung remodeling when using anti-fibrotics (202). These studies are important in parallel to the development of treatment planning and delivery for orthotopic lung tumor models (203) Standardization of these preclinical irradiation platforms is crucial and guidelines have recently been proposed (204).
Concluding Remarks
Modern precision radiotherapy techniques may reduce normal tissue exposure to radiation, but many patients still experience adverse effects. Radiation-induced toxicity is dependent on many parameters, such as the tissue location, the functional status of an organ, the dose and irradiated volume and other factors. In most cancers, radiotherapy is combined with systemic treatment concurrently or concomitantly, and in many cases, they sensitize to radiation-induced lung injury or worsen pre-existing co-morbidities. There is a high need for biomarkers that can predict responders and select those patients that will only suffer from side effects. In patients individual radiation sensitivity is a quantitative and tissue-specific trait and large patient numbers and standardized outcome measures are essential as well as independent validation cohorts to identify QT-loci and the inclusion of ‘real world' data (205). While such studies provide hypothesis for future mechanistic investigation it is not yet evident how this can be translated into radiotherapy practice. One potential application is that such ‘sensitive' signatures may be used for patient selection to receive more precise but also more expensive proton therapy while keeping the maximum dose to the tumor (206). More research is therefore needed to understand which preclinical models should be exploited to identify crucial parameters that contribute to radiation toxicity and how these can be used to inform the design of clinical studies were radiation therapy is combined with other treatments to minimize radiotherapy induced toxicity at optimal tumor control.
Author Contributions
LG, DD, and MV wrote the manuscript. LG and JI prepared the figures and tables. MV supervised the work.
Funding
Marie Sklodowska-Curie ITN Actions of the EU H2020 Programme grant 642623.
Conflict of Interest Statement
The authors declare that the research was conducted in the absence of any commercial or financial relationships that could be construed as a potential conflict of interest.
References
1. Trott KR, Herrmann T, Kasper M. Target cells in radiation pneumopathy. Int J Radiat Oncol Biol Phys. (2004) 58:463–9. doi: 10.1016/j.ijrobp.2003.09.045
2. Wirsdorfer F, Jendrossek V. The role of lymphocytes in radiotherapy-induced adverse late effects in the lung. Front Immunol. (2016) 7:591. doi: 10.3389/fimmu.2016.00591
3. Azzam EI, Jay-Gerin JP, Pain D. Ionizing radiation-induced metabolic oxidative stress and prolonged cell injury. Cancer Lett. (2012) 327:48–60. doi: 10.1016/j.canlet.2011.12.012
4. Jack CI, Cottier B, Jackson MJ, Cassapi L, Fraser WD, Hind CR. Indicators of free radical activity in patients developing radiation pneumonitis. Int J Radiat Oncol Biol Phys. (1996) 34:149–54. doi: 10.1016/0360-3016(95)00209-X
5. Tak JK, Park J-W. The use of ebselen for radioprotection in cultured cells and mice. Free Rad Biol Med. (2009) 46:1177–85. doi: 10.1016/j.freeradbiomed.2009.01.023
6. Terasaki Y, Ohsawa I, Terasaki M, Takahashi M, Kunugi S, Dedong K, et al. Hydrogen therapy attenuates irradiation-induced lung damage by reducing oxidative stress. Am J Physiol Lung Cell Mol Physiol. (2011) 301:L415–426. doi: 10.1152/ajplung.00008.2011
7. Zhao W, Robbins ME. Inflammation and chronic oxidative stress in radiation-induced late normal tissue injury: therapeutic implications. Curr Med Chem. (2009) 16:130–43. doi: 10.2174/092986709787002790
8. Imlay JA. The molecular mechanisms and physiological consequences of oxidative stress: lessons from a model bacterium. Nat Rev Microbiol. (2013) 11:443–54. doi: 10.1038/nrmicro3032
9. Schieber M, Chandel NS. ROS function in redox signaling and oxidative stress. Curr Biol. (2014) 24:R453–62. doi: 10.1016/j.cub.2014.03.034
10. Kim SR, Kim DI, Kim SH, Lee H, Lee KS, Cho SH, et al. NLRP3 inflammasome activation by mitochondrial ROS in bronchial epithelial cells is required for allergic inflammation. Cell Death Dis. (2014) 5:e1498. doi: 10.1038/cddis.2014.460
11. Liu X, Chen Z. The pathophysiological role of mitochondrial oxidative stress in lung diseases. J Transl Med. (2017) 15:207. doi: 10.1186/s12967-017-1306-5
12. Kasper M, Haroske G. Alterations in the alveolar epithelium after injury leading to pulmonary fibrosis. Histol Histopathol. (1996) 11:463–83.
13. Fleckenstein K, Zgonjanin L, Chen L, Rabbani Z, Jackson IL, Thrasher B, et al. Temporal onset of hypoxia and oxidative stress after pulmonary irradiation. Int J Radiat Oncol Biol Phys. (2007) 68:196–204. doi: 10.1016/j.ijrobp.2006.12.056
14. Moeller BJ, Cao Y, Li CY, Dewhirst MW. Radiation activates HIF-1 to regulate vascular radiosensitivity in tumors: role of reoxygenation, free radicals, and stress granules. Cancer Cell. (2004) 5:429–41. doi: 10.1016/S1535-6108(04)00115-1
15. Ryter SW, Kim HP, Hoetzel A, Park JW, Nakahira K, Wang X, et al. Mechanisms of cell death in oxidative stress. Antioxid Redox Signal. (2007) 9:49–89. doi: 10.1089/ars.2007.9.49
16. Zhang B, Wang Y, Pang X, Su Y, Ai G, Wang T. ER stress induced by ionising radiation in IEC-6 cells. Int J Radiat Biol. (2010) 86:429–35. doi: 10.3109/09553001003668014
17. Darby IA, Hewitson TD. Fibroblast differentiation in wound healing and fibrosis. Int Rev Cytol. (2007) 257:143–79. doi: 10.1016/S0074-7696(07)57004-X
18. Barker HE, Paget JT, Khan AA, Harrington KJ. The tumour microenvironment after radiotherapy: mechanisms of resistance and recurrence. Nat Rev Cancer. (2015) 15:409–25. doi: 10.1038/nrc3958
19. Tsoutsou PG, Belkacemi Y, Gligorov J, Kuten A, Boussen H, Bese N, et al. Optimal sequence of implied modalities in the adjuvant setting of breast cancer treatment: an update on issues to consider. Oncologist. (2010) 15:1169–78. doi: 10.1634/theoncologist.2010-0187
20. Barthelemy-Brichant N, Bosquee L, Cataldo D, Corhay JL, Gustin M, Seidel L, et al. Increased IL-6 and TGF-beta1 concentrations in bronchoalveolar lavage fluid associated with thoracic radiotherapy. Int J Radiat Oncol Biol Phys. (2004) 58:758–67. doi: 10.1016/S0360-3016(03)01614-6
21. Semenza GL. Signal transduction to hypoxia-inducible factor 1. Biochem Pharmacol. (2002) 64:993–8. doi: 10.1016/S0006-2952(02)01168-1
22. Kobayashi T, Liu X, Wen F-Q, Fang Q, Abe S, Wang XQ, et al. Smad3 mediates TGF-β1 induction of VEGF production in lung fibroblasts. Biochem Biophys Res Commun. (2005) 327:393–8. doi: 10.1016/j.bbrc.2004.12.032
23. Defraene G, Van Elmpt W, Crijns W, Slagmolen P, De Ruysscher D. CT characteristics allow identification of patient-specific susceptibility for radiation-induced lung damage. Radiother Oncol. (2015) 117:29–35. doi: 10.1016/j.radonc.2015.07.033
24. De Ruysscher D, Faivre-Finn C, Moeller D, Nestle U, Hurkmans CW, Le Pechoux C, et al. European organization for research and treatment of cancer (EORTC) recommendations for planning and delivery of high-dose, high precision radiotherapy for lung cancer. Radiother Oncol. (2017) 124:1–10. doi: 10.1016/j.radonc.2017.06.003
25. Wang LP, Wang YW, Wang BZ, Sun GM, Wang XY, Xu JL. Expression of interleukin-17A in lung tissues of irradiated mice and the influence of dexamethasone. Sci World J. (2014) 2014:251067. doi: 10.1155/2014/251067
26. Hong JH, Chiang CS, Tsao CY, Lin PY, Wu CJ, McBride WH. Can short-term administration of dexamethasone abrogate radiation-induced acute cytokine gene response in lung and modify subsequent molecular responses? Int J Radiat Oncol Biol Phys. (2001) 51:296–303. doi: 10.1016/S0360-3016(01)01702-3
27. Bickelhaupt S, Erbel C, Timke C, Wirkner U, Dadrich M, Flechsig P, et al. Effects of CTGF blockade on attenuation and reversal of radiation-induced pulmonary fibrosis. J Natl Cancer Inst. (2017) 109:djw339. doi: 10.1093/jnci/djw339
28. Sternlicht MD, Wirkner U, Bickelhaupt S, Lopez Perez R, Tietz A, Lipson KE, et al. Radiation-induced pulmonary gene expression changes are attenuated by the CTGF antibody Pamrevlumab. Respir Res. (2018) 19:14. doi: 10.1186/s12931-018-0720-4
29. Muraoka T, Bandoh S, Fujita J, Horiike A, Ishii T, Tojo Y, et al. Corticosteroid refractory radiation pneumonitis that remarkably responded to cyclosporin A. Intern Med. (2002) 41:730–3. doi: 10.2169/internalmedicine.41.730
30. Rubin P, Johnston CJ, Williams JP, Mcdonald S, Finkelstein JN. A perpetual cascade of cytokines postirradiation leads to pulmonary fibrosis. Int J Radiat Oncol Biol Phys. (1995) 33:99–109. doi: 10.1016/0360-3016(95)00095-G
31. Barcellos-Hoff M, Dix TA. Redox-mediated activation of latent transforming growth factor-beta 1. Mol Endocrinol. (1996) 10:1077–83. doi: 10.1210/me.10.9.1077
32. Anscher MS, Chen L, Rabbani Z, Kang S, Larrier N, Huang H, et al. Recent progress in defining mechanisms and potential targets for prevention of normal tissue injury after radiation therapy. Int J Radiat Oncol Biol Phys. (2005) 62:255–9. doi: 10.1016/j.ijrobp.2005.01.040
33. Verrecchia F, Mauviel A. Transforming growth factor-beta and fibrosis. World J Gastroenterol. (2007) 13:3056–62. doi: 10.3748/wjg.v13.i22.3056
34. Tomasek JJ, Gabbiani G, Hinz B, Chaponnier C, Brown RA. Myofibroblasts and mechano-regulation of connective tissue remodelling. Nat Rev Mol Cell Biol. (2002) 3:349. doi: 10.1038/nrm809
35. Kendall RT, Feghali-Bostwick CA. Fibroblasts in fibrosis: novel roles and mediators. Front Pharmacol. (2014) 5:123. doi: 10.3389/fphar.2014.00123
36. Pardo A, Selman M. Matrix metalloproteases in aberrant fibrotic tissue remodeling. Proc Am Thorac Soc. (2006) 3:383–8. doi: 10.1513/pats.200601-012TK
37. Denham JW, Hauer-Jensen M. The radiotherapeutic injury–a complex ‘wound'. Radiother Oncol. (2002) 63:129–45. doi: 10.1016/S0167-8140(02)00060-9
38. Toussaint O, Remacle J, Dierick J-F, Pascal T, Frippiat C, Royer V, et al. Approach of evolutionary theories of ageing, stress, senescence-like phenotypes, calorie restriction and hormesis from the view point of far-from-equilibrium thermodynamics. Mech Ageing Dev. (2002) 123:937–46. doi: 10.1016/S0047-6374(02)00031-3
39. Bentzen SM, Constine LS, Deasy JO, Eisbruch A, Jackson A, Marks LB, et al. Quantitative analyses of normal tissue effects in the clinic (QUANTEC): an introduction to the scientific issues. Int J Radiat Oncol Biol Phys. (2010) 76:S3–S9. doi: 10.1016/j.ijrobp.2009.09.040
40. Citrin D, Cotrim AP, Hyodo F, Baum BJ, Krishna MC, Mitchell JB. Radioprotectors and mitigators of radiation-induced normal tissue injury. Oncologist. (2010) 15:360–71. doi: 10.1634/theoncologist.2009-S104
41. Dang J, Li G, Zang S, Zhang S, Yao L. Risk and predictors for early radiation pneumonitis in patients with stage III non-small cell lung cancer treated with concurrent or sequential chemoradiotherapy. Radiation oncology. (2014) 9:172. doi: 10.1186/1748-717X-9-172
42. Quon H, Shepherd FA, Payne DG, Coy P, Murray N, Feld R, et al. The influence of age on the delivery, tolerance, and efficacy of thoracic irradiation in the combined modality treatment of limited stage small cell lung cancer. Int J Radiat Oncol Biol Phys. (1999) 43:39–45. doi: 10.1016/S0360-3016(98)00373-3
43. Hernando ML, Marks LB, Bentel GC, Zhou SM, Hollis D, Das SK, et al. Radiation-induced pulmonary toxicity: a dose-volume histogram analysis in 201 patients with lung cancer. Int J Radiat Oncol Biol Phys. (2001) 51:650–9. doi: 10.1016/S0360-3016(01)01685-6
44. Claude L, Pérol D, Ginestet C, Falchero L, Arpin D, Vincent M, et al. A prospective study on radiation pneumonitis following conformal radiation therapy in non-small-cell lung cancer: clinical and dosimetric factors analysis. Radiother Oncol. (2004) 71:175–81. doi: 10.1016/j.radonc.2004.02.005
45. Dehing-Oberije C, De Ruysscher D, Van Baardwijk A, Yu S, Rao B, Lambin P. The importance of patient characteristics for the prediction of radiation-induced lung toxicity. Radiother Oncol. (2009) 91:421–6. doi: 10.1016/j.radonc.2008.12.002
46. Vogelius IR, Bentzen SM. A literature-based meta-analysis of clinical risk factors for development of radiation induced pneumonitis. Acta Oncol. (2012) 51:975–83. doi: 10.3109/0284186X.2012.718093
47. Movsas B, Swann S, Curran W, Coyne J, Konski A, Komaki R, et al. 109: sociodemographic factors are significant predictors of toxicity in RTOG non-operative NSCLC Trials. Int J Rad Oncol Biol Phys. (2006) 66:S62. doi: 10.1016/j.ijrobp.2006.07.139
48. Robnett TJ, Machtay M, Vines EF, Mckenna MG, Algazy KM, Mckenna WG. Factors predicting severe radiation pneumonitis in patients receiving definitive chemoradiation for lung cancer. Int J Radiat Oncol Biol Phys. (2000) 48:89–94. doi: 10.1016/S0360-3016(00)00648-9
49. Monson JM, Stark P, Reilly JJ, Sugarbaker DJ, Strauss GM, Swanson SJ, et al. Clinical radiation pneumonitis and radiographic changes after thoracic radiation therapy for lung carcinoma. Cancer. (1998) 82:842–50. doi: 10.1002/(SICI)1097-0142(19980301)82:5<842::AID-CNCR7>3.0.CO;2-L
50. Senzer N. A phase III randomized evaluation of amifostine in stage IIIA/IIIB non-small cell lung cancer patients receiving concurrent carboplatin, paclitaxel, and radiation therapy followed by gemcitabine and cisplatin intensification: preliminary findings. Semin Oncol. (2002) 29:38–41. doi: 10.1016/S0093-7754(02)70008-0
51. Mehta V. Radiation pneumonitis and pulmonary fibrosis in non-small-cell lung cancer: pulmonary function, prediction, and prevention. Int J Radiat Oncol Biol Phys. (2005) 63:5–24. doi: 10.1016/j.ijrobp.2005.03.047
52. Jin H, Tucker SL, Liu HH, Wei X, Yom SS, Wang S, et al. Dose-volume thresholds and smoking status for the risk of treatment-related pneumonitis in inoperable non-small cell lung cancer treated with definitive radiotherapy. Radiother Oncol. (2009) 91:427–32. doi: 10.1016/j.radonc.2008.09.009
53. Gokula K, Earnest A, Wong LC. Meta-analysis of incidence of early lung toxicity in 3-dimensional conformal irradiation of breast carcinomas. Radiat Oncol. (2013) 8:268. doi: 10.1186/1748-717X-8-268
54. Chen S, Zhou S, Zhang J, Yin FF, Marks LB, Das SK. A neural network model to predict lung radiation-induced pneumonitis. Med Phys. (2007) 34:3420–7. doi: 10.1118/1.2759601
55. Yuan X, Liao Z, Liu Z, Wang LE, Tucker SL, Mao L, et al. Single nucleotide polymorphism at rs1982073:T869C of the TGFbeta 1 gene is associated with the risk of radiation pneumonitis in patients with non-small-cell lung cancer treated with definitive radiotherapy. J Clin Oncol. (2009) 27:3370–8. doi: 10.1200/JCO.2008.20.6763
56. Graves PR, Siddiqui F, Anscher MS, Movsas B. Radiation pulmonary toxicity: from mechanisms to management. Semin Radiat Oncol. (2010) 20:201–7. doi: 10.1016/j.semradonc.2010.01.010
57. Wang D, Zhu J, Sun J, Li B, Wang Z, Wei L, et al. Functional and biologic metrics for predicting radiation pneumonitis in locally advanced non-small cell lung cancer patients treated with chemoradiotherapy. Clin Trans Oncol. (2012) 14:943–52. doi: 10.1007/s12094-012-0890-3
58. Li C, Wu W, Chen N, Song H, Lu T, Yang Z, et al. Clinical characteristics and outcomes of lung cancer patients with combined pulmonary fibrosis and emphysema: a systematic review and meta-analysis of 13 studies. J Thorac Dis. (2017) 9:5322–34. doi: 10.21037/jtd.2017.12.72
59. Zhou Z, Song X, Wu A, Liu H, Wu H, Wu Q, et al. Pulmonary emphysema is a risk factor for radiation pneumonitis in NSCLC patients with squamous cell carcinoma after thoracic radiation therapy. Sci Rep. (2017) 7:2748. doi: 10.1038/s41598-017-02739-4
60. Houghton AM. Mechanistic links between COPD and lung cancer. Nat Rev Cancer. (2013) 13:233–45. doi: 10.1038/nrc3477
61. Rancati T, Ceresoli GL, Gagliardi G, Schipani S, Cattaneo GM. Factors predicting radiation pneumonitis in lung cancer patients: a retrospective study. Radiother Oncol. (2003) 67:275–83. doi: 10.1016/S0167-8140(03)00119-1
62. Kimura T, Togami T, Takashima H, Nishiyama Y, Ohkawa M, Nagata Y. Radiation pneumonitis in patients with lung and mediastinal tumours: a retrospective study of risk factors focused on pulmonary emphysema. Br J Radiol. (2012) 85:135–41. doi: 10.1259/bjr/32629867
63. Wurm R, Burnet NG, Duggal N, Yarnold JR, Peacock JH. Cellular radiosensitivity and DNA damage in primary human fibroblasts. Int J Radiat Oncol Biol Phys. (1994) 30:625–33. doi: 10.1016/0360-3016(92)90949-I
64. Scott D, Hu Q, Roberts SA. Dose-rate sparing for micronucleus induction in lymphocytes of controls and ataxia-telangiectasia heterozygotes exposed to 60Co gamma-irradiation in vitro. Int J Radiat Biol. (1996) 70:521–7. doi: 10.1080/095530096144725
65. Slonina D, Klimek M, Szpytma T, Gasinska A. Comparison of the radiosensitivity of normal-tissue cells with normal-tissue reactions after radiotherapy. Int J Radiat Biol. (2000) 76:1255–64. doi: 10.1080/09553000050134483
66. Hill RP, Rodemann HP, Hendry JH, Roberts SA, Anscher MS. Normal tissue radiobiology: from the laboratory to the clinic. Int J Radiat Oncol Biol Phys. (2001) 49:353–65. doi: 10.1016/S0360-3016(00)01484-X
67. Zhang X, Li Y, Pan X, Xiaoqiang L, Mohan R, Komaki R, et al. Intensity-modulated proton therapy reduces the dose to normal tissue compared with intensity-modulated radiation therapy or passive scattering proton therapy and enables individualized radical radiotherapy for extensive stage IIIB non-small-cell lung cancer: a virtual clinical study. Int J Radiat Oncol Biol Phys. (2010) 77:357–66. doi: 10.1016/j.ijrobp.2009.04.028
68. Sinitsky MY, Larionov AV, Asanov MA, Druzhinin VG. Associations of DNA-repair gene polymorphisms with a genetic susceptibility to ionizing radiation in residents of areas with high radon (222Rn) concentration. Int J Radiat Biol. (2015) 91:486–94. doi: 10.3109/09553002.2015.1012306
69. Rosenstein BS. Identification of SNPs associated with susceptibility for development of adverse reactions to radiotherapy. Pharmacogenomics. (2011) 12:267–75. doi: 10.2217/pgs.10.186
70. Bradley JD, Hope A, El Naqa I, Apte A, Lindsay PE, Bosch W, et al. A nomogram to predict radiation pneumonitis, derived from a combined analysis of RTOG 9311 and institutional data. Int J Radiat Oncol Biol Phys. (2007) 69:985–92. doi: 10.1016/j.ijrobp.2007.04.077
71. Akulevich NM, Saenko VA, Rogounovitch TI, Drozd VM, Lushnikov EF, Ivanov VK, et al. Polymorphisms of DNA damage response genes in radiation-related and sporadic papillary thyroid carcinoma. Endocr Relat Cancer. (2009) 16:491–503. doi: 10.1677/ERC-08-0336
72. Hildebrandt MA, Komaki R, Liao Z, Gu J, Chang JY, Ye Y, et al. Genetic variants in inflammation-related genes are associated with radiation-induced toxicity following treatment for non-small cell lung cancer. PLoS ONE. (2010) 5:e12402. doi: 10.1371/journal.pone.0012402
73. Zhuang H, Yuan Z, Chang JY, Wang J, Pang Q, Zhao L, et al. Radiation pneumonitis in patients with non–small-cell lung cancer treated with erlotinib concurrent with thoracic radiotherapy. J Thor Oncol. (2014) 9:882–5. doi: 10.1097/JTO.0000000000000126
74. Kerns SL, Kundu S, Oh JH, Singhal SK, Janelsins M, Travis LB, et al. The prediction of radiotherapy toxicity using single nucleotide polymorphism-based models: a step toward prevention. Semin Radiat Oncol. (2015) 25:281–91. doi: 10.1016/j.semradonc.2015.05.006
75. Tucker SL, Liao ZX, Travis EL. Estimation of the spatial distribution of target cells for radiation pneumonitis in mouse lung. Int J Radiat Oncol Biol Phys. (1997) 38:1055–66. doi: 10.1016/S0360-3016(97)00131-4
76. Seppenwoolde Y, De Jaeger K, Boersma LJ, Belderbos JS, Lebesque JV. Regional differences in lung radiosensitivity after radiotherapy for non-small-cell lung cancer. Int J Radiat Oncol Biol Phys. (2004) 60:748–58. doi: 10.1016/j.ijrobp.2004.04.037
77. Kong FM, Wang S. Nondosimetric risk factors for radiation-induced lung toxicity. Semin Radiat Oncol. (2015) 25:100–9. doi: 10.1016/j.semradonc.2014.12.003
78. Kong FM, Hayman JA, Griffith KA, Kalemkerian GP, Arenberg D, Lyons S, et al. Final toxicity results of a radiation-dose escalation study in patients with non-small-cell lung cancer (NSCLC): predictors for radiation pneumonitis and fibrosis. Int J Radiat Oncol Biol Phys. (2006) 65:1075–86. doi: 10.1016/j.ijrobp.2006.01.051
79. Fay M, Tan A, Fisher R, Mac Manus M, Wirth A, Ball D. Dose-volume histogram analysis as predictor of radiation pneumonitis in primary lung cancer patients treated with radiotherapy. Int J Radiat Oncol Biol Phys. (2005) 61:1355–63. doi: 10.1016/j.ijrobp.2004.08.025
80. Robert F, Childs H, Spencer S, Redden D, Hawkins M. Phase I/IIa study of concurrent paclitaxel and cisplatin with radiation therapy in locally advanced non-small cell lung cancer: analysis of early and late pulmonary morbidity. Sem Radiat Oncol. (1999) 9:136–47.
81. Blackstock AW, Lesser GJ, Fletcher-Steede J, Case LD, Tucker RW, Russo SM, et al. Phase I study of twice-weekly gemcitabine and concurrent thoracic radiation for patients with locally advanced non–small-cell lung cancer. Int J Radiat Oncol Biol Phys. (2001) 51:1281–9. doi: 10.1016/S0360-3016(01)01732-1
82. Yamada M, Kudoh S, Hirata K, Nakajima T, Yoshikawa J. Risk factors of pneumonitis following chemoradiotherapy for lung cancer. Eur J Cancer. (1998) 34:71–5. doi: 10.1016/S0959-8049(97)00377-8
83. Shahidi M, Mozdarani H, Mueller WU. Radiosensitivity and repair kinetics of gamma-irradiated leukocytes from sporadic prostate cancer patients and healthy individuals assessed by alkaline comet assay. Iran Biomed J. (2010) 14:67–75.
84. Roach M III, Gandara DR, Yuo H-S, Swift PS, Kroll S, Shrieve DC, et al. Radiation pneumonitis following combined modality therapy for lung cancer: analysis of prognostic factors. J Clin Oncol. (1995) 13:2606–12. doi: 10.1200/JCO.1995.13.10.2606
85. Amin NP, Miften M, Thornton D, Ryan N, Kavanagh B, Gaspar LE. Effect of induction chemotherapy on estimated risk of radiation pneumonitis in bulky non–small cell lung cancer. Med Dosimetry. (2013) 38:320–6. doi: 10.1016/j.meddos.2013.03.003
86. Tsoutsou PG, Koukourakis MI. Radiation pneumonitis and fibrosis: mechanisms underlying its pathogenesis and implications for future research. Int J Radiat Oncol Biol Phys. (2006) 66:1281–93. doi: 10.1016/j.ijrobp.2006.08.058
87. Palma DA, Senan S, Tsujino K, Barriger RB, Rengan R, Moreno M, et al. Predicting radiation pneumonitis after chemoradiation therapy for lung cancer: an international individual patient data meta-analysis. Int J Radiat Oncol Biol Phys. (2013) 85:444–50. doi: 10.1016/j.ijrobp.2012.04.043
88. Kharofa J, Choong N, Wang D, Firat S, Schultz C, Sadasiwan C, et al. Continuous-course reirradiation with concurrent carboplatin and paclitaxel for locally recurrent, nonmetastatic squamous cell carcinoma of the head-and-neck. Int J Radiat Oncol Biol Phys. (2012) 83:690–5. doi: 10.1016/j.ijrobp.2011.06.2010
89. Gore E. Celecoxib and radiation therapy in non-small-cell lung cancer. Oncology. (2004) 18:10–4. doi: 10.1016/j.semradonc.2004.06.001
90. Kirkbride P, Hatton M, Lorigan P, Joyce P, Fisher P. Fatal pulmonary fibrosis associated with induction chemotherapy with carboplatin and vinorelbine followed by CHART radiotherapy for locally advanced non-small cell lung cancer. Clin Oncol. (2002) 14:361–6. doi: 10.1053/clon.2002.0119
91. Leprieur EG, Fernandez D, Chatellier G, Klotz S, Giraud P, Durdux C. Acute radiation pneumonitis after conformational radiotherapy for nonsmall cell lung cancer: clinical, dosimetric, and associated-treatment risk factors. J Cancer Res Ther. (2013) 9:447–51. doi: 10.4103/0973-1482.119339
92. Inoue A, Saijo Y, Maemondo M, Gomi K, Tokue Y, Kimura Y, et al. Severe acute interstitial pneumonia and gefitinib. Lancet. (2003) 361:137–9. doi: 10.1016/S0140-6736(03)12190-3
93. Chang CC, Chi KH, Kao SJ, Hsu PS, Tsang YW, Chang HJ, et al. Upfront gefitinib/erlotinib treatment followed by concomitant radiotherapy for advanced lung cancer: a mono-institutional experience. Lung Cancer. (2011) 73:189–94. doi: 10.1016/j.lungcan.2010.12.007
94. Awad R, Nott L. Radiation recall pneumonitis induced by erlotinib after palliative thoracic radiotherapy for lung cancer: case report and literature review. Asia Pac J Clin Oncol. (2016) 12:91–5. doi: 10.1111/ajco.12447
95. Yang JJ, Zhou Q, Yan HH, Zhang XC, Chen HJ, Tu HY, et al. A phase III randomised controlled trial of erlotinib vs gefitinib in advanced non-small cell lung cancer with EGFR mutations. Br J Cancer. (2017) 116:568–74. doi: 10.1038/bjc.2016.456
96. Soria JC, Ohe Y, Vansteenkiste J, Reungwetwattana T, Chewaskulyong B, Lee KH, et al. Osimertinib in untreated EGFR-mutated advanced non-small-cell lung cancer. N Engl J Med. (2018) 378:113–25. doi: 10.1056/NEJMoa1713137
97. Oh YT, Noh OK, Jang H, Chun M, Park KJ, Kim MH, et al. The features of radiation induced lung fibrosis related with dosimetric parameters. Radiother Oncol. (2012) 102:343–6. doi: 10.1016/j.radonc.2012.02.003
98. Fowler JF. Review: total doses in fractionated radiotherapy–implications of new radiobiological data. Int J Radiat Biol Relat Stud Phys Chem Med. (1984) 46:103–20. doi: 10.1080/09553008414551181
99. Oetzel D, Schraube P, Hensley F, Sroka-Perez G, Menke M, Flentje M. Estimation of pneumonitis risk in three-dimensional treatment planning using dose-volume histogram analysis. Int J Radiat Oncol Biol Phys. (1995) 33:455–60. doi: 10.1016/0360-3016(95)00009-N
100. Kwa SL, Lebesque JV, Theuws JC, Marks LB, Munley MT, Bentel G, et al. Radiation pneumonitis as a function of mean lung dose: an analysis of pooled data of 540 patients. Int J Radiat Oncol Biol Phys. (1998) 42:1–9. doi: 10.1016/S0360-3016(98)00196-5
101. Guerrero T, Johnson V, Hart J, Pan T, Khan M, Luo D, et al. Radiation pneumonitis: local dose versus [18F]-fluorodeoxyglucose uptake response in irradiated lung. Int J Radiat Oncol Biol Phys. (2007) 68:1030–5. doi: 10.1016/j.ijrobp.2007.01.031
102. Martel MK, Ten Haken RK, Hazuka MB, Turrisi AT, Fraass BA, Lichter AS. Dose-volume histogram and 3-D treatment planning evaluation of patients with pneumonitis. Int J Radiat Oncol Biol Phys. (1994) 28:575–81. doi: 10.1016/0360-3016(94)90181-3
103. Appelt AL, Vogelius IR, Farr KP, Khalil AA, Bentzen SM. Towards individualized dose constraints: adjusting the QUANTEC radiation pneumonitis model for clinical risk factors. Acta Oncol. (2014) 53:605–12. doi: 10.3109/0284186X.2013.820341
104. Huang EX, Hope AJ, Lindsay PE, Trovo M, El Naqa I, Deasy JO, et al. Heart irradiation as a risk factor for radiation pneumonitis. Acta Oncol. (2011) 50:51–60. doi: 10.3109/0284186X.2010.521192
105. Tucker SL, Liao Z, Dinh J, Bian SX, Mohan R, Martel MK, et al. Is there an impact of heart exposure on the incidence of radiation pneumonitis? Anal Data Large Clin Cohort Acta Oncol. (2014) 53:590–6. doi: 10.3109/0284186X.2013.831185
106. Li F, Zhou Z, Wu A, Cai Y, Wu H, Chen M, et al. Preexisting radiological interstitial lung abnormalities are a risk factor for severe radiation pneumonitis in patients with small-cell lung cancer after thoracic radiation therapy. Radiat Oncol. (2018) 13:82. doi: 10.1186/s13014-018-1030-1
107. De Ruysscher D, Dehing C, Yu S, Wanders R, Ollers M, Dingemans AM, et al. Dyspnea evolution after high-dose radiotherapy in patients with non-small cell lung cancer. Radiother Oncol. (2009) 91:353–9. doi: 10.1016/j.radonc.2008.10.006
108. Defraene G, Schuit E, De Ruysscher D. Development and internal validation of a multinomial NTCP model for the severity of acute dyspnea after radiotherapy for lung cancer. Radiother Oncol. (2019) 136:176–84. doi: 10.1016/j.radonc.2019.03.034
109. De Ruysscher D, Defraene G, Ramaekers BLT, Lambin P, Briers E, Stobart H, et al. Optimal design and patient selection for interventional trials using radiogenomic biomarkers: A REQUITE and radiogenomics consortium statement. Radiother Oncol. (2016) 121:440–6. doi: 10.1016/j.radonc.2016.11.003
110. Petit SF, Van Elmpt WJ, Oberije CJ, Vegt E, Dingemans AM, Lambin P, et al. [(1)(8)F]fluorodeoxyglucose uptake patterns in lung before radiotherapy identify areas more susceptible to radiation-induced lung toxicity in non-small-cell lung cancer patients. Int J Radiat Oncol Biol Phys. (2011) 81:698–705. doi: 10.1016/j.ijrobp.2010.06.016
111. Shi A, Zhu G, Wu H, Yu R, Li F, Xu B. Analysis of clinical and dosimetric factors associated with severe acute radiation pneumonitis in patients with locally advanced non-small cell lung cancer treated with concurrent chemotherapy and intensity-modulated radiotherapy. Radiat Oncol. (2010) 5:35. doi: 10.1186/1748-717X-5-35
112. Schild SE, Bonner JA, Hillman S, Kozelsky TF, Vigliotti AP, Marks RS, et al. Results of a phase II study of high-dose thoracic radiation therapy with concurrent cisplatin and etoposide in limited-stage small-cell lung cancer (NCCTG 95-20-53). J Clin Oncol. (2007) 25:3124–9. doi: 10.1200/JCO.2006.09.9606
113. Wang D, Shi J, Liang S, Lu S, Qi X, Wang Q, et al. Dose-volume histogram parameters for predicting radiation pneumonitis using receiver operating characteristic curve. Clin Trans Oncol. (2013) 15:364–9. doi: 10.1007/s12094-012-0931-y
114. Qiu F, Liang CL, Liu H, Zeng YQ, Hou S, Huang S, et al. Impacts of cigarette smoking on immune responsiveness: up and down or upside down? Oncotarget. (2017) 8:268–84. doi: 10.18632/oncotarget.13613
115. Pu X, Wang L, Chang JY, Hildebrandt MA, Ye Y, Lu C, et al. Inflammation-related genetic variants predict toxicity following definitive radiotherapy for lung cancer. Clin Pharmacol Ther. (2014) 96:609–15. doi: 10.1038/clpt.2014.154
116. Wang L, Bi N. TGF-beta1 gene polymorphisms for anticipating radiation-induced pneumonitis in non-small-cell lung cancer: different ethnic association. J Clin Oncol. (2010) 28:e621–2. doi: 10.1200/JCO.2010.31.0458
117. Voets AM, Oberije C, Struijk RB, Reymen B, De Ruyck K, Thierens H, et al. No association between TGF-beta1 polymorphisms and radiation-induced lung toxicity in a European cohort of lung cancer patients. Radiother Oncol. (2012) 105:296–8. doi: 10.1016/j.radonc.2012.09.016
118. Andreassen CN, Schack LM, Laursen LV, Alsner J. Radiogenomics - current status, challenges and future directions. Cancer Lett. (2016) 382:127–36. doi: 10.1016/j.canlet.2016.01.035
119. Han S, Gu F, Lin G, Sun X, Wang Y, Wang Z, et al. Analysis of clinical and dosimetric factors influencing radiation-induced lung injury in patients with lung cancer. J Cancer. (2015) 6:1172–8. doi: 10.7150/jca.12314
120. Lyman JT, Wolbarst AB. Optimization of radiation therapy, III: a method of assessing complication probabilities from dose-volume histograms. Int J Radiat Oncol Biol Phys. (1987) 13:103–9. doi: 10.1016/0360-3016(87)90266-5
121. Kutcher GJ, Burman C. Calculation of complication probability factors for non-uniform normal tissue irradiation: the effective volume method. Int J Radiat Oncol Biol Phys. (1989) 16:1623–30. doi: 10.1016/0360-3016(89)90972-3
122. Zhou Z-R, Han Q, Liang S-X, He X-D, Cao N-Y, Zi Y-J. Dosimetric factors and Lyman normal-tissue complication modelling analysis for predicting radiation-induced lung injury in postoperative breast cancer radiotherapy: a prospective study. Oncotarget. (2017) 8:33855. doi: 10.18632/oncotarget.12979
123. Van Luijk P, Novakova-Jiresova A, Faber H, Schippers JM, Kampinga HH, Meertens H, et al. Radiation damage to the heart enhances early radiation-induced lung function loss. Cancer Res. (2005) 65:6509–11. doi: 10.1158/0008-5472.CAN-05-0786
124. Ghobadi G, Van Der Veen S, Bartelds B, De Boer RA, Dickinson MG, De Jong JR, et al. Physiological interaction of heart and lung in thoracic irradiation. Int J Radiat Oncol Biol Phys. (2012) 84:e639–46. doi: 10.1016/j.ijrobp.2012.07.2362
125. Sievert W, Stangl S, Steiger K, Multhoff G. Improved overall survival of mice by reducing lung side effects after high-precision heart irradiation using a small animal radiation research platform. Int J Radiat Oncol Biol Phys. (2018) 101:671–9. doi: 10.1016/j.ijrobp.2018.02.017
126. Wang S, Liao Z, Wei X, Liu HH, Tucker SL, Hu CS, et al. Analysis of clinical and dosimetric factors associated with treatment-related pneumonitis (TRP) in patients with non-small-cell lung cancer (NSCLC) treated with concurrent chemotherapy and three-dimensional conformal radiotherapy (3D-CRT). Int J Radiat Oncol Biol Phys. (2006) 66:1399–407. doi: 10.1016/j.ijrobp.2006.07.1337
127. Suh CH, Park HS, Kim KW, Pyo J, Hatabu H, Nishino M. Pneumonitis in advanced non-small-cell lung cancer patients treated with EGFR tyrosine kinase inhibitor: meta-analysis of 153 cohorts with 15,713 patients: Meta-analysis of incidence and risk factors of EGFR-TKI pneumonitis in NSCLC. Lung Cancer. (2018) 123:60–9. doi: 10.1016/j.lungcan.2018.06.032
128. Sun TY, Sung AW, Neal JW. Steroid-sparing therapy for tyrosine kinase inhibitor-induced pneumonitis. J Thorac Oncol. (2019) 14:e75–7. doi: 10.1016/j.jtho.2018.11.030
129. Antonia SJ, Villegas A, Daniel D, Vicente D, Murakami S, Hui R, et al. Overall survival with durvalumab after chemoradiotherapy in stage III NSCLC. N Engl J Med. (2018) 379:2342–50. doi: 10.1056/NEJMoa1809697
130. De Ruysscher D, Dingemans AM, Vooijs M, Heymans S. PD-(L)1 inhibition and cardiac damage: a relevant toxicity? J Thorac Oncol. (2018) 13:478–9. doi: 10.1016/j.jtho.2018.02.008
131. Wirsdorfer F, De Leve S, Jendrossek V. Combining radiotherapy and immunotherapy in lung cancer: can we expect limitations due to altered normal tissue toxicity? Int J Mol Sci. (2018) 20:24. doi: 10.3390/ijms20010024
132. Noman MZ, Desantis G, Janji B, Hasmim M, Karray S, Dessen P, et al. PD-L1 is a novel direct target of HIF-1alpha, and its blockade under hypoxia enhanced MDSC-mediated T cell activation. J Exp Med. (2014) 211:781–90. doi: 10.1084/jem.20131916
133. Toi Y, Sugawara S, Sugisaka J, Ono H, Kawashima Y, Aiba T, et al., Profiling preexisting antibodies in patients treated with anti-PD-1 therapy for advanced non-small cell lung cancer. JAMA Oncol. (2018) 5:376–83. doi: 10.1001/jamaoncol.2018.5860
134. Abdel-Rahman O, Fouad M. Risk of pneumonitis in cancer patients treated with immune checkpoint inhibitors: a meta-analysis. Ther Adv Respir Dis. (2016) 10:183–93. doi: 10.1177/1753465816636557
135. Wang Y, Zhou S, Yang F, Qi X, Wang X, Guan X, et al., Treatment-related adverse events of PD-1 and PD-L1 inhibitors in clinical trials: a systematic review and meta-analysis. JAMA Oncol. (2019) 5:1008–19. doi: 10.1001/jamaoncol.2019.0393
136. Bezjak A, Paulus R, Gaspar LE, Timmerman RD, Straube WL, Ryan WF, et al. (2019). Safety and efficacy of a five-fraction stereotactic body radiotherapy schedule for centrally located non-small-cell lung cancer: NRG oncology/RTOG 0813 Trial. J Clin Oncol. 37:1316–25. doi: 10.1200/JCO.18.00622
137. Formenti SC, Rudqvist NP, Golden E, Cooper B, Wennerberg E, Lhuillier C, et al. Radiotherapy induces responses of lung cancer to CTLA-4 blockade. Nat Med. (2018) 24:1845–51. doi: 10.1038/s41591-018-0232-2
138. Marciscano AE, Haimovitz-Friedman A, Lee P, Tran PT, Tome WA, Guha C, et al. Immunomodulatory effects of stereotactic body radiation therapy: preclinical insights and clinical opportunities. Int J Radiat Oncol Biol Phys. (2019). doi: 10.1016/j.ijrobp.2019.02.046. [Epub ahead of print].
139. Shaverdian N, Veruttipong D, Wang J, Schaue D, Kupelian P, Lee P. Pretreatment immune parameters predict for overall survival and toxicity in early-stage non-small-cell lung cancer patients treated with stereotactic body radiation therapy. Clin Lung Cancer. (2016) 17:39–46. doi: 10.1016/j.cllc.2015.07.007
140. Wijsman R, Dankers F, Troost EGC, Hoffmann AL, Van Der Heijden E, De Geus-Oei LF, et al. Comparison of toxicity and outcome in advanced stage non-small cell lung cancer patients treated with intensity-modulated (chemo-)radiotherapy using IMRT or VMAT. Radiother Oncol. (2017) 122:295–9. doi: 10.1016/j.radonc.2016.11.015
141. Jegadeesh N, Liu Y, Gillespie T, Fernandez F, Ramalingam S, Mikell J, et al. Evaluating Intensity-modulated radiation therapy in locally advanced non-small-cell lung cancer: results from the national cancer data base. Clin Lung Cancer. (2016) 17:398–405. doi: 10.1016/j.cllc.2016.01.007
142. Horinouch H. Precision radiotherapy for patients with locally advanced non-small cell lung cancer in the era of immunotherapy and precision medicine. Trans Lung Cancer Res. (2018) 7:S146–8. doi: 10.21037/tlcr.2018.03.13
143. Constanzo J, Wei L, Tseng HH, El Naqa I. Radiomics in precision medicine for lung cancer. Trans Lung Cancer Res. (2017) 6:635–47. doi: 10.21037/tlcr.2017.09.07
144. Prasanna PG, Stone HB, Wong RS, Capala J, Bernhard EJ, Vikram B, et al. Normal tissue protection for improving radiotherapy: where are the Gaps? Transl Cancer Res. (2012) 1:35–48. doi: 10.3978/j.issn.2218-676X.2012.05.05
145. Akita S. Treatment of radiation injury. Adv Wound Care. (2014) 3:1–11. doi: 10.1089/wound.2012.0403
146. Dziegielewski J, Baulch JE, Goetz W, Coleman MC, Spitz DR, Murley JS, et al. WR-1065, the active metabolite of amifostine, mitigates radiation-induced delayed genomic instability. Free Radic Biol Med. (2008) 45:1674–81. doi: 10.1016/j.freeradbiomed.2008.09.004
147. Kouvaris JR, Kouloulias VE, Vlahos LJ. Amifostine: the first selective-target and broad-spectrum radioprotector. Oncologist. (2007) 12:738–47. doi: 10.1634/theoncologist.12-6-738
148. Koukourakis MI. Radiation damage and radioprotectants: new concepts in the era of molecular medicine. Br J Radiol. (2012) 85:313–30. doi: 10.1259/bjr/16386034
149. Murley JS, Kataoka Y, Baker KL, Diamond AM, Morgan WF, Grdina DJ. Manganese superoxide dismutase (SOD2)-mediated delayed radioprotection induced by the free thiol form of amifostine and tumor necrosis factor alpha. Radiat Res. (2007) 167:465–74. doi: 10.1667/RR0758.1
150. Antonadou D, Coliarakis N, Synodinou M, Athanassiou H, Kouveli A, Verigos C, et al. Randomized phase III trial of radiation treatment +/- amifostine in patients with advanced-stage lung cancer. Int J Radiat Oncol Biol Phys. (2001) 51:915–22. doi: 10.1016/S0360-3016(01)01713-8
151. Bourhis J, Blanchard P, Maillard E, Brizel DM, Movsas B, Buentzel J, et al. Effect of amifostine on survival among patients treated with radiotherapy: a meta-analysis of individual patient data. J Clin Oncol. (2011) 29:2590–7. doi: 10.1200/JCO.2010.33.1454
152. Greenberger JS, Epperly MW. Review. Antioxidant gene therapeutic approaches to normal tissue radioprotection and tumor radiosensitization. In Vivo. (2007) 21:141–6.
153. Carpenter M, Epperly M, Agarwal A, Nie S, Hricisak L, Niu Y, et al. Inhalation delivery of manganese superoxide dismutase-plasmid/liposomes protects the murine lung from irradiation damage. Gene Ther. (2005) 12:685. doi: 10.1038/sj.gt.3302468
154. Vujaskovic Z, Batinic-Haberle I, Rabbani ZN, Feng QF, Kang SK, Spasojevic I, et al. A small molecular weight catalytic metalloporphyrin antioxidant with superoxide dismutase (SOD) mimetic properties protects lungs from radiation-induced injury. Free Rad Biol Med. (2002) 33:857–63. doi: 10.1016/S0891-5849(02)00980-2
155. Colon J, Herrera L, Smith J, Patil S, Komanski C, Kupelian P, et al. Protection from radiation-induced pneumonitis using cerium oxide nanoparticles. Nanomedicine. (2009) 5:225–31. doi: 10.1016/j.nano.2008.10.003
156. Xu P, Maidment B 3rd, Antonic V, Jackson I, Das S, Zodda A, et al. Cerium oxide nanoparticles: a potential medical countermeasure to mitigate radiation-induced lung injury in CBA/J mice. Radiat. Res. (2016) 185:516–26. doi: 10.1667/RR14261.1
157. Williams JP, Johnston CJ, Finkelstein JN. Treatment for radiation-induced pulmonary late effects: spoiled for choice or looking in the wrong direction? Curr Drug Targets. (2010) 11:1386–94. doi: 10.2174/1389450111009011386
158. Para AE, Bezjak A, Yeung IW, Van Dyk J, Hill RP. Effects of genistein following fractionated lung irradiation in mice. Radiother Oncol. (2009) 92:500–10. doi: 10.1016/j.radonc.2009.04.005
159. Lee JC, Krochak R, Blouin A, Kanterakis S, Chatterjee S, Arguiri E, et al. Dietary flaxseed prevents radiation-induced oxidative lung damage, inflammation and fibrosis in a mouse model of thoracic radiation injury. Cancer Biol Ther. (2009) 8:47–53. doi: 10.4161/cbt.8.1.7092
160. Langan AR, Khan MA, Yeung IW, Van Dyk J, Hill RP. Partial volume rat lung irradiation: the protective/mitigating effects of Eukarion-189, a superoxide dismutase-catalase mimetic. Radiother Oncol. (2006) 79:231–8. doi: 10.1016/j.radonc.2006.03.017
161. Liu CJ, Ha XQ, Jiang JJ, Lv TD, Wu C. Keratinocyte growth factor (KGF) gene therapy mediated by an attenuated form of Salmonella typhimurium ameliorates radiation induced pulmonary injury in rats. J Radiat Res. (2011) 52:176–84. doi: 10.1269/jrr.10148
162. Hahn SM, Tochner Z, Krishna CM, Glass J, Wilson L, Samuni A, et al. Tempol, a stable free radical, is a novel murine radiation protector. Cancer Res. (1992) 52:1750–3.
163. Ozturk B, Egehan I, Atavci S, Kitapci M. Pentoxifylline in prevention of radiation-induced lung toxicity in patients with breast and lung cancer: a double-blind randomized trial. Int J Radiat Oncol Biol Phys. (2004) 58:213–9. doi: 10.1016/S0360-3016(03)01444-5
164. Rube CE, Wilfert F, Uthe D, Schmid KW, Knoop R, Willich N, et al. Modulation of radiation-induced tumour necrosis factor alpha (TNF-alpha) expression in the lung tissue by pentoxifylline. Radiother Oncol. (2002) 64:177–87. doi: 10.1016/S0167-8140(02)00077-4
165. Okunieff P, Augustine E, Hicks JE, Cornelison TL, Altemus RM, Naydich BG, et al. Pentoxifylline in the treatment of radiation-induced fibrosis. J Clin Oncol. (2004) 22:2207–13. doi: 10.1200/JCO.2004.09.101
166. Sun Y, Du Y-J, Zhao H, Zhang G-X, Sun N, Li X-J. Protective effects of ulinastatin and methylprednisolone against radiation-induced lung injury in mice. J Radiat Res. (2016) 57:505–11. doi: 10.1093/jrr/rrw036
167. Bao P, Gao W, Li S, Zhang L, Qu S, Wu C, et al. Effect of pretreatment with high-dose ulinastatin in preventing radiation-induced pulmonary injury in rats. Eur J Pharmacol. (2009) 603:114–9. doi: 10.1016/j.ejphar.2008.12.007
168. Sun ZJ, Yu T, Chen JS, Sun X, Gao F, Zhao XL, et al. Effects of ulinastatin and cyclophosphamide on the growth of xenograft breast cancer and expression of CXC chemokine receptor 4 and matrix metalloproteinase-9 in cancers. J Int Med Res. (2010) 38:967–76. doi: 10.1177/147323001003800323
169. Williams JP, Hernady E, Johnston CJ, Reed CM, Fenton B, Okunieff P, et al. Effect of administration of lovastatin on the development of late pulmonary effects after whole-lung irradiation in a murine model. Radiat Res. (2004) 161:560–7. doi: 10.1667/RR3168
170. Robbins ME, Diz DI. Pathogenic role of the renin-angiotensin system in modulating radiation-induced late effects. Int J Radiat Oncol Biol Phys. (2006) 64:6–12. doi: 10.1016/j.ijrobp.2005.08.033
171. Yuhas JM, Storer JB. Differential chemoprotection of normal and malignant tissues. J Natl Cancer Inst. (1969) 42:331–5.
172. Moding EJ, Kastan MB, Kirsch DG. Strategies for optimizing the response of cancer and normal tissues to radiation. Nat Rev Drug Discovery. (2013) 12:526–42. doi: 10.1038/nrd4003
173. Medhora M, Gao F, Jacobs ER, Moulder JE. Radiation damage to the lung: mitigation by angiotensin-converting enzyme (ACE) inhibitors. Respirology. (2012) 17:66–71. doi: 10.1111/j.1440-1843.2011.02092.x
174. Buentzel J, Micke O, Adamietz IA, Monnier A, Glatzel M, De Vries A. Intravenous amifostine during chemoradiotherapy for head-and-neck cancer: a randomized placebo-controlled phase III study. Int J Radiat Oncol Biol Phys. (2006) 64:684–91. doi: 10.1016/j.ijrobp.2005.08.005
175. Tsai J-R, Liu P-L, Chen Y-H, Chou S-H, Cheng Y-J, Hwang J-J, et al. Curcumin inhibits non-small cell lung cancer cells metastasis through the adiponectin/NF-κb/MMPs signaling pathway. PLoS ONE. (2015) 10:e0144462. doi: 10.1371/journal.pone.0144462
176. Jiao D-M, Yan L, Wang L-S, Hu H-Z, Tang X-L, Chen J, et al. Exploration of inhibitory mechanisms of curcumin in lung cancer metastasis using a miRNA-transcription factor-target gene network. PLoS ONE. (2017) 12:e0172470. doi: 10.1371/journal.pone.0189070
177. Cho YJ, Yi CO, Jeon BT, Jeong YY, Kang GM, Lee JE, et al. Curcumin attenuates radiation-induced inflammation and fibrosis in rat lungs. Korean J Physiol Pharmacol. (2013) 17:267–74. doi: 10.4196/kjpp.2013.17.4.267
178. Savla U, Waters CM. Barrier function of airway epithelium: effects of radiation and protection by keratinocyte growth factor. Radiat Res. (1998) 150:195–203. doi: 10.2307/3579855
179. Schuette W, Krzakowski MJ, Massuti B, Otterson GA, Lizambri R, Wei H, et al. Randomized phase II study of palifermin for reducing dysphagia in patients receiving concurrent chemoradiotherapy for locally advanced unresectable non-small cell lung cancer. J Thorac Oncol. (2012) 7:157–64. doi: 10.1097/JTO.0b013e31822f6526
180. Benderitter M, Caviggioli F, Chapel A, Coppes RP, Guha C, Klinger M, et al. Stem cell therapies for the treatment of radiation-induced normal tissue side effects. Antioxid Redox Signal. (2014) 21:338–55. doi: 10.1089/ars.2013.5652
181. Maria OM, Maria AM, Ybarra N, Jeyaseelan K, Lee S, Perez J, et al. Mesenchymal stem cells adopt lung cell phenotype in normal and radiation-induced lung injury conditions. Appl Immunohistochem Mol Morphol. (2016) 24:283–95. doi: 10.1097/PAI.0000000000000180
182. Perez JR, Ybarra N, Chagnon F, Serban M, Lee S, Seuntjens J, et al. Tracking of mesenchymal stem cells with fluorescence endomicroscopy imaging in radiotherapy-induced lung injury. Sci Rep. (2017) 7:40748. doi: 10.1038/srep40748
183. Klein D, Steens J, Wiesemann A, Schulz F, Kaschani F, Röck K, et al. Mesenchymal stem cell therapy protects lungs from radiation-induced endothelial cell loss by restoring superoxide dismutase 1 expression. Antioxid Redox Signal. (2017) 26:563–82. doi: 10.1089/ars.2016.6748
184. Jiang X, Jiang X, Qu C, Chang P, Zhang C, Qu Y, et al. Intravenous delivery of adipose-derived mesenchymal stromal cells attenuates acute radiation-induced lung injury in rats. Cytotherapy. (2015) 17:560–70. doi: 10.1016/j.jcyt.2015.02.011
185. Hawkins F, Kotton DN. Embryonic and induced pluripotent stem cells for lung regeneration. Ann Am Thorac Soc. (2015) 12:S50–3. doi: 10.1513/AnnalsATS.201410-457MG
186. Kotton DN, Morrisey EE. Lung regeneration: mechanisms, applications and emerging stem cell populations. Nat Med. (2014) 20:822. doi: 10.1038/nm.3642
187. Peng T, Tian Y, Boogerd CJ, Lu MM, Kadzik RS, Stewart KM, et al. Coordination of heart and lung co-development by a multipotent cardiopulmonary progenitor. Nature. (2013) 500:589. doi: 10.1038/nature12358
188. Cardoso WV, Lü J. Regulation of early lung morphogenesis: questions, facts and controversies. Development. (2006) 133:1611–24. doi: 10.1242/dev.02310
189. Kubo A, Shinozaki K, Shannon JM, Kouskoff V, Kennedy M, Woo S, et al. Development of definitive endoderm from embryonic stem cells in culture. Development. (2004) 131:1651–62. doi: 10.1242/dev.01044
190. Huang SX, Islam MN, O'neill J, Hu Z, Yang YG, Chen YW, et al. Efficient generation of lung and airway epithelial cells from human pluripotent stem cells. Nat. Biotechnol. (2014) 32:84–91. doi: 10.1038/nbt.2754
191. Pickering SJ, Minger SL, Patel M, Taylor H, Black C, Burns CJ, et al. Generation of a human embryonic stem cell line encoding the cystic fibrosis mutation deltaF508, using preimplantation genetic diagnosis. Reprod Biomed Online. (2005) 10:390–7. doi: 10.1016/S1472-6483(10)61801-9
192. Tadokoro T, Wang Y, Barak LS, Bai Y, Randell SH, Hogan BL. IL-6/STAT3 promotes regeneration of airway ciliated cells from basal stem cells. Proc Natl Acad Sci USA. (2014) 111:E3641–9. doi: 10.1073/pnas.1409781111
193. Fishman JM, De Coppi P, Elliott MJ, Atala A, Birchall MA, Macchiarini P. Airway tissue engineering. Expert Opin Biol Ther. (2011) 11:1623–35. doi: 10.1517/14712598.2011.623696
194. Nichols JE, Cortiella J. Engineering of a complex organ: progress toward development of a tissue-engineered lung. Proc Am Thorac Soc. (2008) 5:723–30. doi: 10.1513/pats.200802-022AW
195. Dabjan MB, Buck CM, Jackson IL, Vujaskovic Z, Marples B, Down JD. A survey of changing trends in modelling radiation lung injury in mice: bringing out the good, the bad, and the uncertain. Lab Invest. (2016) 96:936–49. doi: 10.1038/labinvest.2016.76
196. Paun A, Haston CK. Genomic and genome-wide association of susceptibility to radiation-induced fibrotic lung disease in mice. Radiother Oncol. (2012) 105:350–7. doi: 10.1016/j.radonc.2012.08.004
197. Walkin L, Herrick SE, Summers A, Brenchley PE, Hoff CM, Korstanje R, et al. The role of mouse strain differences in the susceptibility to fibrosis: a systematic review. Fibrogenesis Tissue Repair. (2013) 6:18. doi: 10.1186/1755-1536-6-18
198. Van Der Veen SJ, Ghobadi G, De Boer RA, Faber H, Cannon MV, Nagle PW, et al. ACE inhibition attenuates radiation-induced cardiopulmonary damage. Radiother Oncol. (2015) 114:96–103. doi: 10.1016/j.radonc.2014.11.017
199. Schyns LE, Eekers DB, Van Der Heyden B, Almeida IP, Vaniqui A, Verhaegen F. Murine vs human tissue compositions: implications of using human tissue compositions for photon energy absorption in mice. Br J Radiol. (2019) 92:20180454. doi: 10.1259/bjr.20180454
200. Granton PV, Dubois L, Van Elmpt W, Van Hoof SJ, Lieuwes NG, De Ruysscher D, et al. A longitudinal evaluation of partial lung irradiation in mice by using a dedicated image-guided small animal irradiator. Int J Radiat Oncol Biol Phys. (2014) 90:696–704. doi: 10.1016/j.ijrobp.2014.07.004
201. Van Berlo D, Khmelinskii A, Gasparini A, Salguero FJ, Floot B, De Wit N, et al. Micro cone beam computed tomography for sensitive assessment of radiation-induced late lung toxicity in preclinical models. Radiother Oncol. (2019) 138:17–24. doi: 10.1016/j.radonc.2019.05.007
202. De Ruysscher D, Granton PV, Lieuwes NG, Van Hoof S, Wollin L, Weynand B, et al. Nintedanib reduces radiation-induced microscopic lung fibrosis but this cannot be monitored by CT imaging: a preclinical study with a high precision image-guided irradiator. Radiother Oncol. (2017) 124:482–7. doi: 10.1016/j.radonc.2017.07.014
203. Sosa Iglesias V, Van Hoof SJ, Vaniqui A, Schyns LE, Lieuwes N, Yaromina A, et al. An orthotopic non-small cell lung cancer model for image-guided small animal radiotherapy platforms. Br J Radiol. (2019) 92:20180476. doi: 10.1259/bjr.20180476
204. Verhaegen F, Dubois L, Gianolini S, Hill MA, Karger CP, Lauber K, et al. ESTRO ACROP: technology for precision small animal radiotherapy research: optimal use and challenges. Radiother Oncol. (2018) 126:471–8. doi: 10.1016/j.radonc.2017.11.016
205. Brothwell MRS, West CM, Dunning AM, Burnet NG, Barnett GC. Radiogenomics in the era of advanced radiotherapy. Clin Oncol. (2019) 31:319–25. doi: 10.1016/j.clon.2019.02.006
Keywords: radiotherapy, adverse effects, RILI, RILT, pneumonitis, fibrosis, lung
Citation: Giuranno L, Ient J, De Ruysscher D and Vooijs MA (2019) Radiation-Induced Lung Injury (RILI). Front. Oncol. 9:877. doi: 10.3389/fonc.2019.00877
Received: 07 July 2019; Accepted: 23 August 2019;
Published: 06 September 2019.
Edited by:
Minesh P. Mehta, Baptist Health South Florida, United StatesReviewed by:
Zhongxing Liao, University of Texas MD Anderson Cancer Center, United StatesYevgeniy Vinogradskiy, University of Colorado Denver, United States
Copyright © 2019 Giuranno, Ient, De Ruysscher and Vooijs. This is an open-access article distributed under the terms of the Creative Commons Attribution License (CC BY). The use, distribution or reproduction in other forums is permitted, provided the original author(s) and the copyright owner(s) are credited and that the original publication in this journal is cited, in accordance with accepted academic practice. No use, distribution or reproduction is permitted which does not comply with these terms.
*Correspondence: Marc A. Vooijs, bWFyYy52b29panNAbWFhc3RyaWNodHVuaXZlcnNpdHkubmw=