- 1Division of Endocrinology, Diabetes and Bone Disease, Icahn School of Medicine at Mount Sinai, New York City, NY, United States
- 2Tisch Cancer Institute at Mount Sinai, Icahn School of Medicine at Mount Sinai, New York City, NY, United States
Obesity and type 2 diabetes have both been associated with increased cancer risk and are becoming increasingly prevalent. Metabolic abnormalities such as insulin resistance and dyslipidemia are associated with both obesity and type 2 diabetes and have been implicated in the obesity-cancer relationship. Multiple mechanisms have been proposed to link obesity and diabetes with cancer progression, including an increase in insulin/IGF-1 signaling, lipid and glucose uptake and metabolism, alterations in the profile of cytokines, chemokines, and adipokines, as well as changes in the adipose tissue directly adjacent to the cancer sites. This review aims to summarize and provide an update on the epidemiological and mechanistic evidence linking obesity and type 2 diabetes with cancer, focusing on the roles of insulin, lipids, and adipose tissue.
Introduction
An increase in obesity has been observed in children as well as adults, in both genders, and is prevalent in both developed and developing countries (1–3). Obesity is associated with an increased risk of overall mortality (4, 5) and constitutes a risk factor for diseases such as type 2 diabetes, dyslipidemia, hypertension, fatty liver disease and cardiovascular disease. In addition, obesity has been linked to increased cancer incidence and mortality (6–8). It has been estimated that 3.6% of all of new cancer cases diagnosed worldwide in adults aged 30 years and older could be attributed to high BMI (9). An assessment of temporal trends for cancer cases in the US suggests that development of some obesity-related cancers in younger generations is becoming increasingly common (10). Internationally, an increase in obesity-related cancers in adolescents and young adults has also been noted (11), highlighting the influence of obesity on cancer risk across ages. There is therefore, much interest in understanding how obesity-associated tumor growth is mediated and might be therapeutically targeted. This review aims to provide an update on some of the mechanisms proposed to underpin the relationship between obesity and cancer (Figure 1), with a focus on breast cancer.
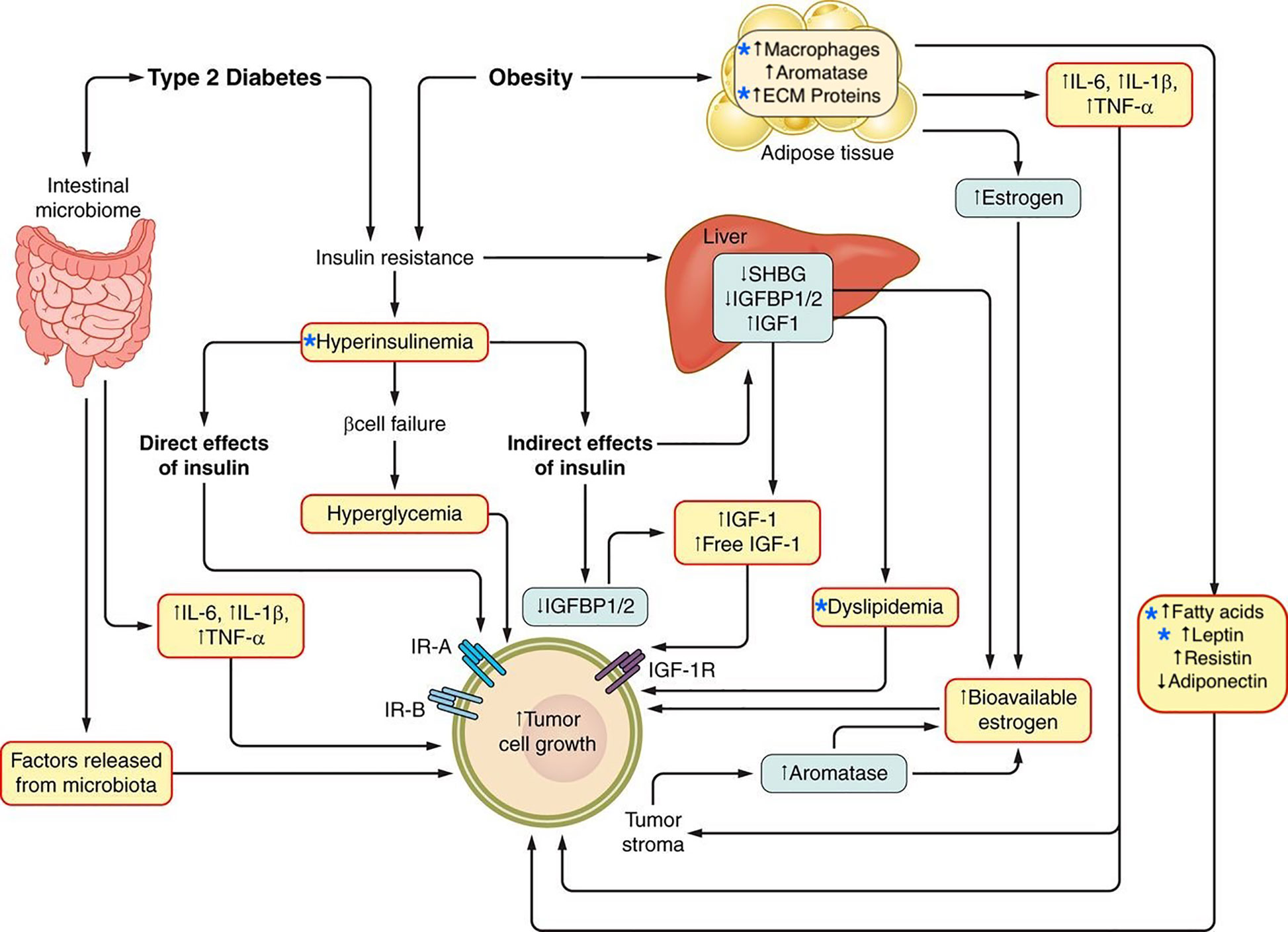
Figure 1 Potential mechanisms linking obesity and type 2 diabetes and cancer. The relationship between type 2 diabetes, obesity, and cancer is potentially mediated by multiple mechanisms, including metabolic conditions such as hyperinsulinemia and dyslipidemia as well as the alteration of adipose tissue which is characterized by inflammation and a tumor growth-promoting secretory profile. Stars indicate factors discussed in this review. Adapted from: Gallagher, E.J., and LeRoith, D (2015). Obesity and Diabetes: The Increased Risk of Cancer and Cancer-Related Mortality. Physiol. Rev. 95, 727–748.
Obesity and Type 2 Diabetes
Obesity
Obesity has been defined as an accumulation of fat mass at levels sufficiently high to adversely influence health (12). Body mass index (BMI) is one measurement used by the World Health Organization to define obesity, and is calculated as: body weight (kg)/height (m)2. Overweight is considered to be a BMI of 25–29.9 kg/m2 and obesity, a BMI of ≥30 kg/m2. Of note, lower BMI cut-off points have been applied to Asian populations, due to the increased percentage of body fat in these populations, compared to non-Asian populations, for a given BMI (13, 14).
A number of studies have examined the links between cancer and obesity, defined by BMI. Large cohort studies and meta-analyses, have reported the association between obesity and cancer to be gender-, site- and menopausal status-specific (6, 7). The International Agency for Research on Cancer (IARC) and World Cancer Research Fund/American Institute for Cancer Research (WCRF/AICR) have reviewed the strength of the evidence linking obesity with specific cancer types (15–17). The IARC assessed the cancer-preventative effect of the absence of excess adiposity. Both organizations found adequate evidence supporting the association of excess body fatness with increased risk of esophageal adenocarcinoma, colon and rectal, liver, pancreatic, postmenopausal breast, endometrial, and renal cell cancer (15–18). Additional cancers for which the WCRF/AICR identified a greater risk were gastric cardia, gallbladder, ovary, mouth, pharynx and larynx, and advanced prostate cancer (17, 18).
A study assessing the effect of adolescent obesity on cancer risk and mortality later in life found that BMI at age 17 was associated with an increased overall risk of cancer in men, but not in women. Inverse relationships were reported for BMI and both breast and cervical cancers in women (19). The cancer sites most strongly connected to adolescent obesity for men in this cohort were breast, pancreas and kidney, and in women, uterus, liver, bile duct, and pancreas. Increased BMI at adolescence was also linked with a greater risk of mortality in cancer-bearing individuals (19). Several studies suggest that accumulating adiposity throughout adulthood influences cancer risk (20–23). Weight gain (≥ 0.45 kg per year) over the course of 14 years increased cancer risk by 38% compared to the maintenance of constant weight (21).
The relationship between excess adiposity and breast cancer appears to be modulated by menopausal status and by breast cancer subtype, which is clinically based on the expression of the estrogen receptor (ER), progesterone receptor (PR), and human epidermal growth factor receptor-2 (HER2). Weight gain and elevated BMI have frequently been associated with an increased risk of postmenopausal breast cancer, particularly ER-positive and PR-positive invasive breast cancer (15, 17, 24). In contrast, BMI has been inversely associated with premenopausal breast cancer incidence. In a large pooled analysis of premenopausal women, the negative relationship between BMI and breast cancer risk was strongest in early adulthood (ages 18–24 years), and for hormone receptor-positive cancer (25). The negative relationship between breast cancer and premenopausal adiposity was not found with ER/PR-negative or triple negative (ER/PR negative, non-HER2 overexpressing) breast cancer in individuals over 24 years of age (25). It has been reported in a meta-analysis that a positive association exists between the presence of obesity in premenopausal women and the risk of triple negative breast cancer (TNBC) (26).
A large prospective cohort study following men and women over the course of 16 years found that being overweight or obese was associated with a higher risk of death from cancer (27). It has however, been noted that inconsistency exists regarding the relationship between obesity and increased cancer-specific mortality (15, 16, 28). The inconsistency between studies stems from multiple factors, including differences in study design and setting, timing of obesity measurements in relation to cancer diagnosis, cancer stage at diagnosis, presence of other risk factors such as smoking (29, 30), genetic variants, choice of treatment, and the effect of obesity on therapeutic dosing and adherence (15, 16, 28). Obesity has been consistently linked with breast cancer-specific mortality regardless of menopausal status or subtype across meta-analyses (31–33).
Although obesity has been defined using BMI in the majority of epidemiology studies, it does not always reflect metabolic health. High body fat levels correlated with fasting insulin, leptin, triglycerides and inflammatory markers (IL-6, C-reactive protein), and increased breast cancer risk in postmenopausal women with normal BMI (34). Waist circumference and waist-to-hip ratio are other measures that are used to define obesity (17, 35, 36). Waist circumference is one of the criteria that defines the metabolic syndrome. Abdominal obesity has a stronger correlation with insulin resistance than BMI or gluteofemoral (gynoid) obesity (37, 38). The metabolic syndrome, which is comprised of abdominal obesity, dyslipidemia, dysglycemia and hypertension, is a syndrome of insulin resistance (35). As discussed in subsequent sections, the metabolic dysfunction associated with insulin resistance may underlie the link between obesity and cancer.
The Metabolic Syndrome and Insulin Resistance
Insulin resistance and hyperinsulinemia have been noted in individuals for many years preceding the diagnosis of diabetes (39). Obesity, specifically abdominal adiposity has been correlated with insulin resistance (40, 41). Insulin resistance is also considered to underlie the development of the metabolic syndrome. Endogenous hyperinsulinemia occurs to compensate for insulin resistance in order to maintain euglycemia. In clinical studies fasting insulin levels, or the fasting concentrations of C-peptide (a cleavage product of the insulin precursor that is released at equal concentrations to insulin) have been used to examine the links between hyperinsulinemia and cancer.
Studies have largely reported that the metabolic syndrome increases the risk for developing cancers such as, breast, colorectal, liver, bladder, endometrial and pancreatic cancer (42–45). In particular, it was observed that women with insulin resistance in comparision to insulin-sensitive women were at greater risk of developing breast cancer, regardless of BMI-defined obesity status (46). Circulating C-peptide (47), and insulin levels have been associated with increased breast cancer risk and cancer-specific mortality (48, 49), even after adjustment for adiposity (46, 50). Likewise, increased C-peptide or insulin levels have been linked with a greater risk of colorectal cancer, independent of adiposity (51, 52). Studies examining the timing of cancer diagnoses in individuals with diabetes found that the risk of cancer was higher in the period before diabetes diagnosis (53, 54), when insulin resistance and hyperinsulinemia is likely to be present. Overall, these observations support the hypothesis that hyperinsulinemia promotes cancer development and progression.
In addition to cancer incidence, insulin resistance has been associated with both an increased risk of all-cause mortality and cancer-specific mortality in postmenopausal women (55). Insulin resistance has been further identified as a factor mediating the relationship between race and poor breast cancer prognosis (56). In a recent cross-sectional study, self-identified Black women showed greater insulin resistance and poorer prognosis for breast cancer compared to White women (56). It is however, important to note that multiple factors including socio-economic status, environmental exposures, access to healthcare, tumor biology, genetic susceptibility and systemic metabolism can all potentially contibute to the racial disparities in cancer mortality (57).
Type 2 Diabetes and Hyperglycemia
Obesity is commonly observed in individuals with type 2 diabetes and the rising cases of obesity have been proposed to explain the sharp increases in the prevalence and incidence of type 2 diabetes (58). Large cohort studies and meta-analyses have observed an increased risk of several types of cancer including breast (59), intrahepatic cholangiocarcinoma (60), colorectal cancer (59, 61) and pancreatic cancer (62) in individuals with type 2 diabetes. An umbrella review of meta-analyses, which also included an assessment of robustness and evidence of bias, showed that the incidence of breast, endometrial, colorectal cancers, and intrahepatic cholangiocarcinoma was greater in individuals with type 2 diabetes, compared to those without diabetes (63). A recent mendelian randomization study reported that a genetic predisposition to type 2 diabetes conveyed higher odds of cancer of the pancreas, kidney, uterus and cervix (64). For breast cancer, a meta-analysis across forty studies found that diabetes increased the risk of post-menopausal breast cancer by 16%, after adjustment for BMI (65). Subtypes of breast cancer that carry a poorer prognosis, including PR/HER2-negative breast cancers, TNBC, and the closely related basal-like breast cancer molecular subtype have also been reported to occur at greater frequency in women with diabetes than in those without diabetes (66, 67).
Diabetes has also been associated with increased cancer mortality (68, 69). One large cohort study followed individuals without a history of cancer at enrolment for 16 years, and found that diabetes was a significant predictor of mortality from liver, pancreatic, bladder and colon cancer in men, and pancreatic, colon, and breast cancer in women (68). The greater cancer mortality in individuals with diabetes was also reported in a large pooled analysis of 97 prospective studies with 820,900 participants and in many meta-analyses (61, 69–71). In particular, a meta-analysis examining survival outcomes for individuals with pre-existing diabetes and newly diagnosed cancer, found that those with diabetes had a 41% increased mortality compared to non-diabetic individuals (72). Although the evidence linking diabetes and all-cause mortality in individuals with cancer is strong, the evidence regarding cancer-specific mortality has been inconsistent, as individuals with diabetes have a greater mortality from non-cancer causes than those without diabetes (73). Diabetes may also have an impact on cancer treatment, as previously reported for breast cancer (74). However, a recent study which accounted for co-morbidities such as cardiovascular disease, found that cancer treatments were similar for patients with breast cancer regardless of diabetes status (75). Other possible factors relating to the increased cancer-specific mortality in individuals with diabetes, include presenting with advanced stage cancers at diagnosis (76), a higher risk of chemotherapy-related toxicity (77), as well as patient fragility resulting from chronic diabetes-associated complications (78).
Glycated hemoglobin (HbA1c) levels have been used as an indicator of glucose levels to examine possible associations between hyperglycemia and cancer in the UK biobank cohort, a large prospective population-based cohort study. Diabetes and HbA1c levels were observed to be positively linked with cancer risk across some organs, including liver and bladder (79). Another population-based cohort study, which also drew data from the UK biobank cohort, reported that with the exception of pancreatic cancer, HbA1c levels did not correlate with higher cancer risk, after adjustment for factors such as BMI, physical activity, alcohol consumption and ethnicity (80). A lack of association was also observed in a mendelian randomization study examining the relationship between breast and prostate cancer risk with glycemic traits (81).
Dyslipidemia
Dyslipidemia is frequently associated with obesity and type 2 diabetes. Elevated levels of triglycerides and decreased high-density lipoprotein (HDL) are components of the metabolic syndrome and are often observed in conjunction with high levels of low-density lipoprotein (LDL) cholesterol, and small dense LDL (82). Triglycerides are transported in the circulation in the form of chylomicrons and very low density lipoprotein (VLDL), which is synthesized and secreted by the liver along with apolipoproteins (ApoB-100, ApoC-I, ApoC-II, ApoC-III, ApoE), which bind the non-polar lipids and aqueous plasma, facilitating the transport of non-polar lipids through the circulation (83). Dyslipidemia has been associated with increased cancer risk in some studies (84–87). Meta-analyses have examined dietary cholesterol intake, and found that high dietary intake of cholesterol increased the risk of esophageal cancer (88), pancreatic cancer (8% increased risk per 100 mg cholesterol/day) (89) and ovarian cancer (1% increased risk per 15 mg cholesterol/day) (90). In breast cancer, a dose-response analysis found that a non-linear relationship existed between dietary cholesterol and breast cancer, and was statistically significant when cholesterol intake was greater than 370 mg/day (91).
In a cohort of 3,278 adults from the Framingham Offspring study, individuals with high VLDL and low HDL levels had a greater incidence of cancer (92). Higher incidence of prostate and colon cancer in men, and breast cancer in women were reported in individuals with high cholesterol (≥ 240mg/dL), compared with those with cholesterol <160mg/dL in a large prospective study from Korea (93). A lower incidence of lung, liver and stomach cancers were found in individuals with high total cholesterol in this cohort (93). A meta-analysis of twelve prospective studies examining cancer risk irrespective of site found an inverse relationship between total cholesterol and cancer risk (94). It is important to note the distinction between total and HDL cholesterol, where low HDL is a component of the metabolic syndrome. In the context of breast cancer, an inverse association with cancer risk has been observed for HDL cholesterol (95). Menopausal status may impact upon the influence of cholesterol on breast cancer. For example, low HDL has been associated with increased risk of postmenopausal breast cancer in some studies (96, 97), but has been linked with premenopausal breast cancer in other studies (98). A few studies have looked at cancer incidences and mortality rates in individuals with familial hypercholesterolemia (FH). Though no difference was found in total cancer mortality rate (99), a strong association was found with death rates from pancreatic cancer (100). In contrast, lower incidences of smoking-related cancers in individuals with FH have been observed, which may be attributed to decreased smoking as a lifestyle modification (101).
The presence of many variables may explain the conflicting results that have been reported in epidemiological studies. In the setting of hepatocellular carcinoma or hepatic metastasis, impaired liver function may result in aberrant lipid synthesis, decreasing cholesterol levels (102). Similarly, individuals with pancreatic cancers and other gastrointestinal cancers may have decreased dietary absorption of lipids that would affect systemic lipid levels (103, 104). A relationship between circulating lipid levels and cancer risk may also be obscured by a decrease in lipid levels arising from the low nutritional intake associated with advanced cancer and cachexia (105). Increased cholesterol uptake by cells in some hematological malignancies has been described, and may also account for low lipid levels, increasing the difficulty in establishing a direct relationship between cholesterol and cancer risk (106). Furthermore, dyslipidemic individuals are often treated with lipid-lowering medications (107) as cardiovascular disease constitutes a co-morbidity of dyslipidemia. This in turn, might mask a direct association between cholesterol levels and cancer risk. Individuals with untreated high cholesterol levels are also at an increased risk of pre-mature cardiovascular disease-related mortality (107, 108). Therefore, cardiovascular disease may be a competing risk factor for mortality independent of cancer.
Cholesterol lowering 3-hydroxy-3-methylglutaryl-coenzyme A (HMG CoA) reductase inhibitors (“statins”) may have therapeutic value as anticancer agents. Although the evidence regarding statin use and its effect on cancer risk and mortality is not unanimous, many studies have reported a strong inverse relationship between advanced prostate cancer and longer duration of statin use (109–111). A large Danish population study found lower incidences of prostate and breast cancer in statin users compared with those who did not use statins (112). However, meta-analyses did not find a link between statin use and the occurrence of lung (113) and breast cancer (114). The majority of the studies examining statin use and breast cancer recurrence and prognosis suggest statins improve recurrence-free and cancer-specific survival (115–117).
Mechanisms Underlying the Obesity–Cancer Relationship: Hyperglycemia and Insulin Signaling
Insulin/Insulin-Like Growth Factor Signaling and Cancer
At a cellular level, activation of insulin/IGF signaling pathway has been hypothesized to contribute to tumor initiation and/or progression through tumor cell-specific mechanisms including the promotion of cell division, glucose metabolism (118) and epithelial-to-mesenchymal transition (EMT) (119). In addition to activating mitogenic and pro-tumorigenc metabolic pathways via the induction of endogenous hyperinsulinemia, insulin resistance might also contribute to tumor growth by other mechanisms including: modulating sex hormone bioavailability by decreasing sex hormone binding globulin; reducing of levels of certain circulating IGF binding proteins (IGFBP-1) resulting in free IGFs that activate the cell surface receptors; raising circulating triglycerides by enhancing hepatic lipid synthesis and decreasing clearance; increasing circulating and tissue free fatty acids from adipose tissue lipolysis, and altering expression of adipokines (Figure 1) (82).
Insulin, insulin-like growth factor-1 (IGF-1), and insulin-like growth factor-2 (IGF-2) are ligands for the transmembrane tyrosine kinase receptors, insulin receptor (IR), and IGF-1 receptor (IGF-1R), which have important roles in growth, development, cancer, and metabolic disease (120, 121). The IR is the preferential receptor for insulin and is comprised of two heterodimeric hemi-receptors, of which there are two isoforms, IR-A and IR-B (120). IR-A, in comparison with IR-B, displays an increased affinity for IGF-2 and as such, also acts a receptor for circulating and locally produced IGF-2 (122). Activation of the receptors via ligand binding results in trans auto-phosphorylation within the intracellular subunits of the receptors. This leads to the activation and recruitment of various substrates including the IR substrates (IRS) 1-4 and adaptor proteins. Pathways that are activated as a result of IR/IGF-1R activation include the Phosphatidylinositol 3-kinase (PI3K)/Akt/mechanistic target of rapamycin (mTOR) and Ras/extracellular signal-regulated kinase (ERK)1/2 pathways (121, 123).
The circulating levels of IGF-1 are positively associated with both increasing BMI up to 27 kg/m2 (124, 125) and increased risk of pre- and post-menopausal breast cancer (126, 127). The contribution of IGF-1 to the obesity-cancer link is not simple, as the positive relationship between IGF-1 levels and BMI exists only up to 27 kg/m2 and becomes negative thereafter (124), secondary to hyperinsulinemia inhibiting growth hormone secretion (128). In contrast to total circulating IGF-1, most of which exists in complexes with IGFBPs, the actual bioavailability of IGF-1 at a tissue level in vivo is difficult to determine (129), which complicates the contribution of IGF-1 to the relationship between obesity and cancer (124).
Rodent studies found that reduced circulating IGF-1 levels led to decreased tumor development (130) and that increased signaling through the IGF-1R signaling pathway promoted tumor growth (131, 132). The stimulation of an ER-positive breast cancer cell line (MCF7) with IGF-1 led to the identification of an IGF-1 gene signature, which was enriched for signaling pathways that are involved in mitogenesis such as ER, Ras/ERK1/2, and PI3K/Akt/mTOR. This IGF-1 gene signature was, in turn, associated with poorer survival (133). Increased activation of the IGF-1R signaling pathway led to reduced E-cadherin expression, and the potentiation of response to IGF-1R inhibition in the context of invasive lobular breast cancer, which is largely ER-positive (134). In the context of TNBC, low IGF-1R expression has been found and linked to worse overall survival (135). In Wnt-driven tumors, the inhibition of IGF-1R signaling led to increased mammary tumor development (136), potentially through a loss of protection from cellular stress and the development of a pro-metastatic tumor microenvironment (135). These observations suggest that the effect of IGF-1R signaling on breast cancer progression may be context-dependent.
Insulin Signaling in Cancer
Relative to normal breast tissue, increased expression of the IR has been demonstrated in breast cancer tissue (137). The phosphorylation of the IR/IGF-1R has been noted across breast cancer sub-types, with 48.1% IR/IGF-1R phosphorylation in luminal, 64.3% in HER2-overexpressing, and 41.9% in TNBC cases examined (138). The IR has also been noted to be resistant to down-regulation in the setting of hyperinsulinemia (139). The stimulation of non-small cell lung, pancreatic and breast cancer cell lines, which express the IR, with insulin led to proliferation in vitro (140–142). Conversely proliferation was decreased with silencing of the IR (141–145). The tumor growth-promoting effect of endogenous hyperinsulinemia have also been shown in rodent models across several obesity-associated cancer types (118).
The stimulatory effect of endogenous hyperinsulinemia on breast cancer progression has been modeled through the use of a transgenic mouse model (MKR), in which a kinase-inactive form of the IGF1R is overexpressed in skeletal muscle under the muscle creatine kinase promoter (146). The female MKR mice display insulin resistance, as well as endogenous hyperinsulinemia in the absence of obesity (147). The stimulatory effect of hyperinsulinemia on both primary tumor growth and metastasis was demonstrated across a variety of breast cancer models employing different oncogenes (147–151) with tumors showing activation of the IR/IGF-1R and Akt (147). Tumors from the MKR mice, relative to wild-type mice, had increased levels of phosphorylated IR but not IGF-1R (152), thereby identifying the activation of the IR as a major contributor to hyperinsulinemia-associated tumor growth. The tumor promoting effects of hyperinsulinemia were ameliorated by either lowering circulating insulin levels with a β3-adrenergic agonist (153), or with inhibitors of the IR/IGF-1R, PI3K, and/or mTOR (147, 154, 155). These inhibitors while effective for reducing tumor growth, were shown to exacerbate the systemic metabolic abnormalities associated with insulin resistance: (hyperglycemia, hypertriglyceridemia, and hyperinsulinemia) in the MKR and wild-type mice (147, 154, 155). PI3K inhibitors contribute to hepatic glycogenolysis and reduced glucose disposal into adipose tissue, leading to greater secretion of insulin from the pancreas and hyperinsulinemia (123, 142). Insulin was observed to result in the re-activation of the PI3K signaling pathway and restoration of growth across a variey of cell lines including pancreatic and breast (142), ultimately reducing efficacy of PI3K inhibition in pre-clinical models. The use of PI3K inhibitors, in combination with insulin-lowering therapies, such as a ketogenic diet or sodium glucose co-transporter-2 (SGLT2) inhibition, resulted in sustained suppression of tumor growth in pre-clinical studies (142).
Insulin enhances glucose uptake in tissues such as muscle and adipose tissue by inducing the translocation of glucose transporter 4 (GLUT4) (82). The expression of the GLUT proteins, which comprise a family of 14 members, differs across tissues and tumor types, with the expression of GLUT1 and GLUT3 commonly being identified as elevated in cancer (156). Hyperglycemia is a defining feature of diabetes and has been postulated to mediate cancer progression through a variety of mechanisms including the promotion of DNA damage and accumulation of mutations, pro-tumorigenic post-translational protein modifications, acting as a metabolic substrate for cancer cells and by altering immune cell recruitment and activity (157–160). In particular, glucose is crucially involved in the Warburg effect which is commonly observed in cancer cells where the rate of glucose uptake is elevated and aerobic glycolysis occurs. The Warburg effect has been proposed to support cancer progression by allowing for the rapid generation of ATP and enhanced flux through biosynthetic pathways for cell proliferation and modulation of cell signaling and the tumor microenvironment, in part through acidification arising from lactate accumulation (161). Insulin has been suggested to influence tumor cell metabolism and anabolism by directing the utilization of glucose through PI3K-Akt signaling, leading to (1): enhanced glycolytic flux resulting in the generation of ATP (2); the promotion of aerobic glycolysis with the generation of lactate and the regeneration of NAD+ (3); increased production of ribose-5-phosphate, the precursor for purine and pyrimidine nucleotide synthesis, through the pentose phosphate pathway, and (4) increased lipid synthesis (162). In addition, insulin has also been shown to influence glucose metabolism through the regulation of cyclin D1-cyclin dependent kinase (Cdk) 4 activity (163), rendering hyperinsulinemia-associated tumor growth susceptible to CDK4 inhibitors in the context of liver cancer (164).
SGLT2 inhibitors are used to treat diabetes in clinical practice. SGLT2 inhibiton has also been examined in obesity-associated tumor studies in pre-clinical models of breast and colorectal cancer (165). The presence of hyperinsulinemia together with increased tumor proliferation and glucose uptake observed in obese rodents, was reduced with therapies with insulin-lowering effects, such as an SGLT2 inhibitor or a liver-specific mitochondrial uncoupler of oxidative phosphorylation. Treatment with insulin abrogated the tumor-suppressive effect associated with these therapies (165, 166). These observations taken together, suggest that insulin promotes tumor growth which is in turn, associated with increased glucose uptake, in some cancers. Interestingly, a positive association between glucose uptake into tumors and BMI has been found in the context of breast cancer, while glucose uptake in non-small cell lung cancer was inversely associated with BMI (167). In addition to increased tumor growth, the presence of elevated endogenous insulin levels and IR signaling have also been postulated to encourage metastasis through the promotion of EMT in both human (151) and mouse tumor models (149, 168) in the context of breast cancer and prostate cancer (169).
Mechanisms Underlying the Obesity–Cancer Relationship: The Contribution of Lipids
Cholesterol Uptake
In non-cancer cells, cholesterol and related sterols contribute to essential physiological functions and are crucial components in the membranes of eukaryotic cells, reducing permeability and influencing protein assembly (170). Cholesterol is also an essential molecule for the synthesis of other sterols, including steroid hormones and oxysterols (171). Cholesterol can be absorbed from extraneous sources, or synthesized intracellularly by the mevalonate pathway utilizing the rate-limiting enzyme, HMG CoA reductase. In addition to synthesizing cholesterol, the mevalonate pathway also gives rise to non-sterol isoprenoids, such as dolichol, coenzyme Q, farnesyl-pyrophosphate (FPP) and geranylgeranyl-pyrophosphate (GGPP) (172). Isoprenoids can prenylate many molecules important for carcinogenesis such as Ras GTPases that can lead to proliferation, migration, and metastasis (173).
Free cholesterol in cells is maintained at a constant level by homeostatic processes involving sensors like sterol regulatory element binding protein (SREBP) and liver X receptor (LXR). Nuclear translocation of SREBP leads to the enhanced expression of enzymes such as HMG CoA reductase, and the LDL receptor (LDLR), which contributes to increased exogenous lipid uptake (174). Enhanced activation of the SREBPs has been observed in cancers such as prostate (175), and breast cancer (176). Activation of the SREBP–mevalonate pathway sustained the proliferation and self-renewal of breast cancer cells in the setting of p53 mutations (177). The nuclear translocation of SREBP in the context of cancer is mediated by multiple factors such as the loss of the tumor suppressor p53, low pH of the tumor microenvironment, proinflammatory cytokines such as TNFα, and endoplasmic reticulum stress (178). LXRs are nuclear receptors that also modulate intracellular cholesterol levels by up-regulating the transcription of efflux protein such as ATP binding cassette subfamily A member 1 (ABCA1) and ATP binding cassette subfamily G member 1 (ABCG1). Upregulation of these efflux proteins by LXR agonists have induced apoptosis in prostate and breast cancer cell lines (179).
The influence of elevated circulating cholesterol on cancer growth has been modeled using rodents. Adiponectin knockout mice which have glucose intolerance, insulin resistance, and hyperlipidemia were observed to develop larger transgenic polyoma virus middle T antigen (PyMT) mammary tumors than control mice, and the effect was enhanced by high fat diet feeding (180). Similarly, syngeneic breast cancers in ApoE-/-, LDLR-/-, and APOE3+/+ mice, which are all models of hyperlipidemia, demonstrated increased growth compared to controls; ER-negative tumors in ApoE-/-and LDLR-/- mice, and ER-positive tumors in APOE3+/+ (181–183). The tumor growth-promoting effect of hyperlipidemia has also been recapitulated in human breast cancer xenografts in immunodeficient mice models of hyperlipidemia (182).
An increased uptake of cholesterol into cancer cells has been observed (184–186) with LDLR expression being upregulated in certain breast cancer cell lines (182, 187). The scavenger receptor, SR-B1, is another means by which tumor cells may take up cholesterol. An increased expression of SR-B1 in breast and prostate cancer cells has been associated with increased cell proliferation and tumor growth in vivo (188, 189). Higher LDL metabolism has been observed in gynecological cancer cell lines compared non-neoplastic cells (190). The tumor cell expression of LDLR plays a crucial in the uptake of circulating LDL and the growth of pancreatic adenocarcinoma and prostate cancer (184, 191). Silencing the LDLR in breast cancers reduced tumor growth, particularly in the setting of high circulating LDL (182). Furthermore, in human breast cancers a high expression of LDLR was associated with decreased recurrence-free survival in patients who have received systemic therapy (182). Interestingly, LDL uptake through the LDLR on cancer cells is being studied as a mechanism of targeted drug delivery to tumors, using lipidic emulsions. These molecules were reported to localize heavily in human breast cancer cells that were removed during surgery compared with normal cells (192). LDLR could therefore, be a potential drug target. Taken together these observations suggest that the increased cholesterol uptake and metabolism by cancer cells may support rapid cell division and growth.
Stored cholesterol in the form of cholesteryl esters (CE) may contribute to proliferation and aggressiveness of breast (193), prostate, and colon cancer (194), as well as leukemia (195). Increased activity of Acetyl-coenzyme acetyltransferase-1 (ACAT1), an enzyme that can catalyze cholesterol esterification (196) as well as lipase activity (197) has also been seen in cancer cells, suggesting that CE may allow cancer cells to store and quickly access energy when needed. Cholesterol is also located in lipid rafts, which are crucial for cell signaling, adhesion and migration of cancer cells. The depletion of cholesterol from lipid rafts using methyl-β-cyclodextrin (MβCD) resulted in the disruption of lipid rafts and increased apoptosis of breast cancer cells (198). Although high intracellular cholesterol levels appear to be conducive for cancer cell growth and survival, it has also been noted that low concentrations in some cases, facilitate metastasis by enhancing membrane fluidity and the consequent development of a migratory phenotype (199).
Cholesterol lowering statins may exert anti-cancer effects by lowering circulating cholesterol levels or targeting the mevalonate pathway in cancer cells leading to decreased cholesterol synthesis (200). Statins can either be hydrophilic, which are predominantly taken up by the liver, or lipophilic, which can be taken up by all cells by passive diffusion (201). Lipophilic statins have been found to reduce cancer risk suggesting that their beneficial effect may be by acting on tumor cells directly (202). Statins also confer pleiotropic benefits, such as improved endothelial function, antioxidant properties and anti-inflammatory properties (203). Their anti-inflammatory role is suggested by a reduction in C-reactive protein (204) and may occur in part, by lowering levels of pro-inflammatory cytokines such as TNFα and IFNγ (205). These effects are beneficial in treating cardiovascular disease. In breast cancer models, statins blocked tumor growth by: 1) inducing cell cycle arrest by cyclin D1-CDK4; 2) decreasing cell proliferation through inhibiting prenylation and activation Rho/Ras family of proteins; 3) modulating pro- and anti-apoptotic proteins such as Bcl2 and Bax, and by inducing reactive oxygen species (ROS) (202), and 4) contributing to oxidative stress by inhibiting coenzyme Q synthesis (206). Statins have also been shown to suppress angiogenesis, invasion and metastasis through reducing the expression of MMP2, MMP9 and VEGF (202).
Oxysterols: 27-Hydroxycholesterol
27-Hydroxycholesterol (27-HC) is the most abundant circulating oxysterol in humans (207). 27-HC is produced when cholesterol is hydroxylated by cytochrome P450 27A1 (CYP27A1) to yield 27-HC in both the liver and extrahepatic tissues. Levels of 27-HC are decreased by cytochrome P450 family 7 subfamily B member 1 (CYP7B1), which metabolizes it to produce an intermediate for bile acid synthesis in the liver (208, 209). 27-HC was the first discovered endogenous selective estrogen receptor modulator (SERM) (210). Though 27-HC binds to both ERα and ERβ, it binds to ERβ with >100 more affinity than ERα (211). In the breast it shows partial agonist activity to ERα, but has lower efficacy than 17β-estradiol (210, 212). However, in other tissues such as cardiovascular or bone, it acts as an antagonist and can attenuate the protective effects of estrogen (209, 213, 214). It is also a LXR agonist and has the ability to bind to both LXRα and LXRβ to modulate LXR dependent genes that are involved in lipid homeostasis such as ABCA1, ABCG1, and SREBP-1c. The expression of LXR-related genes can be modulated with cholesterol loading, suggesting that 27-HC may act as a cholesterol sensor (208).
Plasma levels of 27-HC have been shown to correlate with circulating cholesterol levels (215) and has been hypothesized to explain the link between hypercholesterolemia and cancer (216, 217). 27-HC content in normal breast tissue from individuals with cancer was increased compared with cancer-free controls, and was observed to be further elevated in tumors (218). 27-HC was also observed to be elevated in exosomes from ER-positive but not ER-negative breast cancer or other non-cancerous cell lines (219). Decreased expression of the enzyme, CYP7B1, has been linked to poorer cancer prognosis (218). Silencing of CYP7B1 can occur epigenetically by hypermethylation of the gene (220). In contrast, the relationship between the expression of CYP27A1 in tumors and cancer prognosis has been inconsistent. Intratumoral expression of CYP27A1 was linked to high grade cancer cells and ER-positive breast tumors (183). However, in three independent cohorts of breast cancer patients, elevated levels of CYP27A1 correlated with longer recurrence-free survival in women with ER-positive breast cancer who were less than 50 years of age (221). In a recent evaluation of a cohort from the European Investigation into Cancer and Nutrition (EPIC) study, no association between circulating 27-HC levels and premenopausal breast cancer risk was found, and an inverse correlation was reported in postmenopausal women (222, 223). These results suggest that the estrogen levels may influence the association between 27-HC and breast cancer risk and survival (224).
27-HC stimulates the proliferation of ER-positive breast cancer cells by increasing cyclin D activity (212) and by reducing p53 transcriptional activity by facilitating its interaction with MDM2 (225). The growth-promoting effect of 27-HC has also been re-capitulated in vivo, where ER-positive tumor xenografts displayed accelerated growth in the presence of 27-HC (183). Increased levels of 27-HC were also found to promote metastasis. Inhibition of Cyp27a1 with a small molecule inhibitor, or by genetic ablation resulted in reduced lung metastasis (226). 27-HC induced EMT in MCF7 cells by reducing the expression of E-cadherin and β-catenin and increasing the expression of MMP9 (227, 228). In both MCF7 and MDA-MB-231 breast cancer cell lines, 27-HC increased ROS production that led to increased migration of the cells via STAT3/VEGF signaling (229, 230). In addition to affecting tumor cells directly, 27-HC induced endothelial to mesenchymal transition in endothelial cell lines via STAT3 signaling and aided breast cancer cell migration (231). It also influenced the tumor immune microenvironment in the lung, where 27-HC increased recruitment of polymorphonuclear neutrophils (PMNs) and γδ T-cells, which suppresed the recruitment of anti-tumorigenic effector T-cells (226).
Since the discovery of the role of 27-HC in breast cancer progression, new research shows that its effect is not restricted to breast cancer. 27-HC increased the proliferation of lung, endometrial and melanoma cancer cell lines (232–234). In contrast, 27-HC suppressed the proliferation of gastric, and colon cancer cell lines. (236–237). In DU145 prostate cancer cells, 27HC inhibited proliferation by disrupting lipid rafts (235), but increased the proliferation of LNCaP and PC3 cells via an ERβ-mediated pathway (238). However, lower CYP27A1 transcript levels were associated with shorter disease-free survival and higher tumor grade in patient samples (239). Therefore, 27-HC may mediate some of the pro-tumorigenic effects of cholesterol through direct effects on tumor cells, and indirectly by its effects on the tumor microenvironment (Figure 2). However, a greater understanding of its tumor promoting and inhibiting properties is needed, in addition to understanding whether circulating or locally produced 27-HC has a greater influence on tumor growth and metastasis.
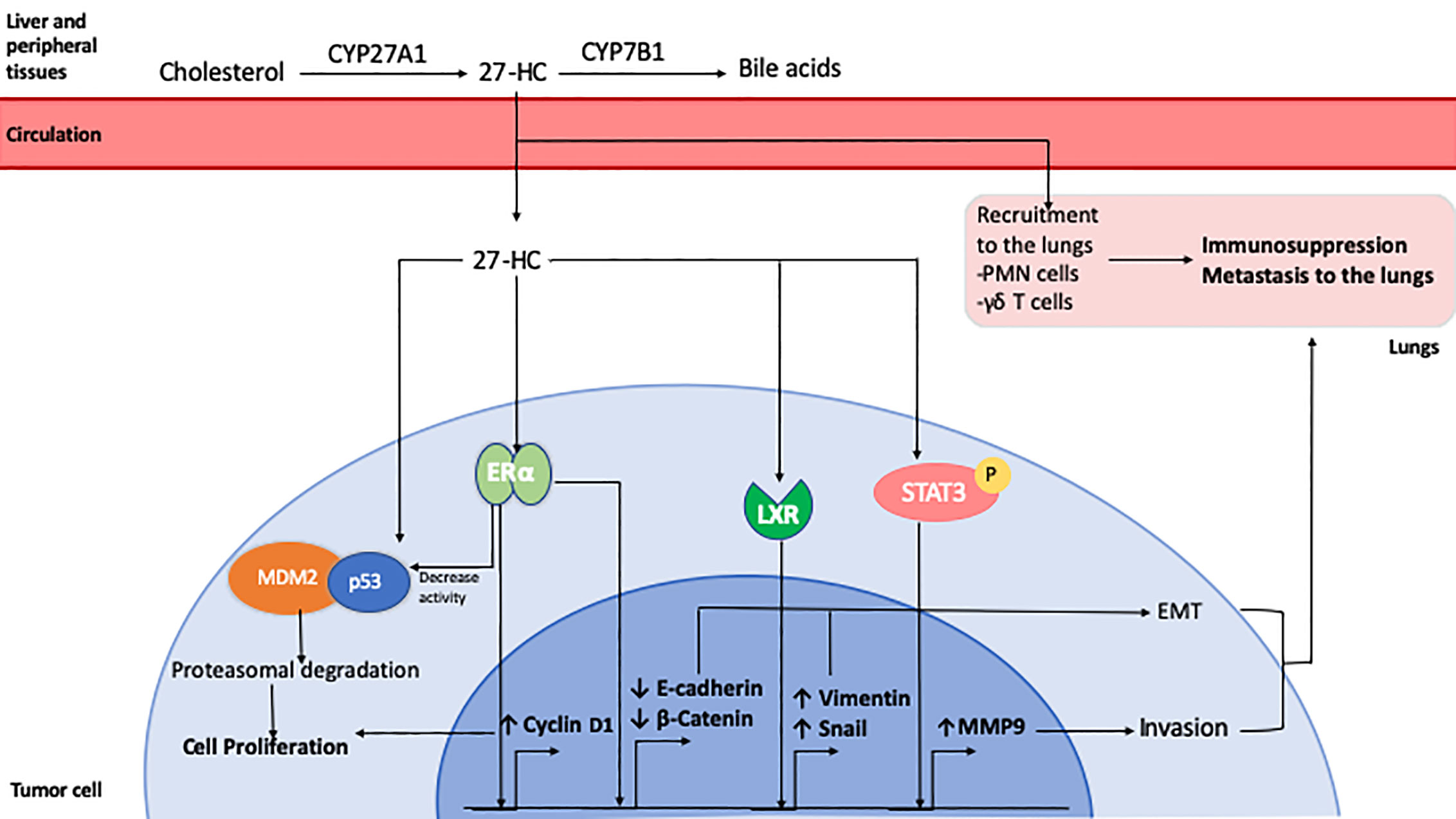
Figure 2 Role of 27-Hydroxycholesterol in breast cancer. 27-HC is synthesized from cholesterol by CYP27A1 in the liver and other peripheral tissues. It can be further metabolized for bile acid synthesis by CYP7B1. 27-HC can be taken up by tumor cells from the circulation where it exerts ERα agonistic activity, inducing the expression of Cyclin D1 which leads to cell cycle progression and proliferation. It can also enhance the association of tumor suppressor p53 protein with MDM2 leading to its degradation. 27-HC has also been shown to promote EMT by reducing E-Cadherin and β-Catenin expression and by inducing an LXR-mediated increase in Snail and Vimentin expression. It can also activate Stat3 signaling, resulting in increased expression of MMP9 and cell invasion. 27-HC in the lungs can promote the recruitment of polymorphonuclear cells and T cells which favors the development of an immunosuppressive microenvironment that facilitates metastatic seeding and growth of breast cancer cells.
Fatty Acid Transfer
Insulin resistance in adipose tissue leads to lipolysis and the release of fatty acids may also support tumor growth (240). Fatty acid uptake and metabolism has been reported to promote tumor cell proliferation, survival, invasion, and tumor-initiating capacity (241–247). The expression of fatty acid translocase, CD36, was identified as a characteristic of metastasis-initiating cells (243). CD36 expression was induced in cancer cells co-cultured with adipocytes in the context of ovarian cancer, leading to increased tumor cell fatty acid uptake, invasion and proliferation (248). Fatty acid transfer and the expression of genes associated with lipid metabolism and inflammation were enhanced when tumor cells were cultured with hypertrophied adipocytes isolated under obesity-associated conditions compared to adipocytes from non-obese conditions (249, 250).
The enhanced provision of fatty acids in the context of adipose tissue lipolysis may also influence immune cells. In natural killer (NK) cells, obesity was associated with enhanced expression of lipid transport genes, such as Ldlr, Cd36, and fatty acid binding proteins (Fabp), along with decreased mTOR-associated glycolysis, which was related to activation of PPARα/δ signaling. These metabolic changes in NK cells resulted in reduced cytotoxic activity (251). While not studied directly in the context of obesity, macrophages, myeloid-derived suppressor cells (MDSCs) and regulatory T-cells (Treg), have also been shown to up-regulate lipid transport receptors and increase lipid uptake in lipid-enriched tumor microenvironments, leading to pro-tumorigenic effects (252–254). In particular, CD36 was shown to mediate lipid accumulation and fatty acid oxidation in tumor-associated macrophages, resulting in a pro-tumorigenic gene expression profile (253). In MDSCs, the expression of lipid transporters including Cd36, Vldlr, Ldlr, and Fabp were induced by cytokines, through STAT3/5 signaling, leading to enhanced immunosuppressive capacity (252). Tregs are associated with the suppression of anti-tumor immune responses (255). Tregs were shown to utilize fatty acid oxidation during their development and possess an enhanced capacity for fatty acid oxidation through the expression of the Treg-specific transcription factor, Foxp3, which has been proposed to afford them protection from fatty acid toxicity (256, 257). Tregs situated in tumors increased both the expression of the fatty acid receptor, CD36, and lipid uptake, leading to a PPARβ-driven increase in capacity to survive in lactic acid-rich tumor microenvironments (254). Ablation or neutralization of CD36 led to the loss of suppressive function and reduced tumor growth in mice, demonstrating the importance of fatty acid uptake by Tregs (254).
Effector T-cell numbers were observed to be reduced in tumors of mice with diet-induced obesity. Inhibiting fatty acid oxidation or the ablation of STAT3, increased effector T-cell glycolysis and T-cell numbers, resulting in decreased tumor growth (258). However, fatty acid oxidation has been found to be important for effector T-cell function under hypoxic and hypoglycemic conditions in melanoma models (259). Enhancing fatty acid oxidation concurrently with glycolysis with a PGC-1α/PPAR agonist improved responses to immunotherapy in models of colon cancer and skin sarcoma (260).
A lipid-enriched environment may therefore, promote tumor growth by reducing NK cell cytotoxic function and effector T cell numbers, and enhancing the accumulation and immunosuppressive function of macrophages, MDSCs, and Tregs.
Mechanisms Underlying the Obesity–Cancer Relationship: Obesity-Associated Adipose Tissue Expansion
Adipose Stromal Cells
Adipose tissue is composed of a variety of cell types in addition to adipocytes, such as adipocyte progenitor cells, fibroblasts, endothelial cells, and immune cells, that contribute to its behavior and diverse adipocytokine secretion profile (261, 262). The expansion of adipose tissue with the development of obesity occurs due to a combination of adipogenesis and lipogenesis, processes that are regulated by insulin/IR signaling (263–265).
The obese phenotype has been found to promote breast cancer growth through an enhanced provision of adipose tissue progenitor cells (also known as adipose stromal cells), which express CD34 and can be differentiated into multiple cell types such as chondrocytes, osteocytes and adipocytes (266). These adipose tissue progenitor cells are detectable in the circulation of both rodents (267) and humans and are present at increased levels with obesity (268, 269). Co-injection experiments involving adipose tissue-derived CD34+ progenitor cells and tumor cells resulted in increased tumor growth and metastasis in mice compared to injections of tumor cells alone, demonstrating the potential for adipose tissue progenitor cells to contribute to the tumor vasculature and promote tumor growth (270). In rodent models, diet-induced obesity resulted in both an elevation in the levels of adipose stromal cells in the circulation and increased engraftment of these stromal cells in tumors, with an increased number of actively proliferating tumor cells positioned closer in proximity to the adipose tissue-derived structures (267). C-X-C motif chemokine ligand 1 (CXCL1) signaling has been shown to recruit C-X-C motif chemokine receptor (CXCR)1/2 expressing progenitor cells into prostate cancers (266, 267, 271). The recruitment of adipose stromal cells has also been associated with increased levels of GM-CSF and MMP9 levels in tumors, which promote the development of a tumor-supportive microenvironment (272). In addition to contributing to the development of the tumor microenvironment and vasculature, the recruitment of adipose stromal cells has also been linked to the promotion of EMT and chemo-resistance of prostate cancer cells, where the ablation of adipose stromal cells was shown to augment the efficacy of chemotherapy (273).
Obesity-Associated Extracellular Matrix Deposition
The expansion of adipose tissue also involves the synthesis and deposition of extracellular matrix proteins (fibrosis) (274), which has been proposed to contribute to the development of dysfunctional adipose tissue in obesity and cancer (275). Compared to lean individuals, breast tissue from obese individuals contain a greater number of myofibroblasts, a cell type implicated in fibrotic remodeling, and stiffer extracellular matrices (276). These changes resulted in increased tumor cell growth in vitro (276).
Extracellular matrix (ECM) proteins such as collagen VI have been implicated in the link between obesity and cancer. Collagen VI expression in human subcutaneous adipose tissue was observed to be elevated in obese individuals compared to lean individuals, and has been associated with decreased insulin sensitivity and adipose tissue inflammation (277). Collagen VI, which is secreted by adipocytes, has been shown to stimulate cancer growth, while collagen VI-null mice had reduced tumor growth in the context of breast cancer (278, 279).
The expression of endotrophin (ETP), a collagen VI fragment, is higher in the adipose tissue of obese mice and has been implicated in both obesity-associated inflammation and metabolic dysfunction (280). ETP was also present at greater levels in the circulation of women with breast cancer relative to cancer-free women (281). ETP promoted EMT and chemo-resistance in human breast cancer cell lines (281) and enhanced primary and metastatic tumor growth in mice (279). Neutralization of endotrophin with a humanized anti-ETP antibody reduced tumor growth in a preclinical model (281). Taken together, these observations illustrate how excess ECM protein deposition, which is linked to obesity-associated adipose tissue inflammation and metabolic health, promotes tumor growth.
Adipose Tissue Macrophages
Macrophages are an essential part of the tumor microenvironment with the ability to influence various processes that are involved in cancer development and progression, such as the promotion of tumor cell migration, angiogenesis, and regulation of the tumor immune response (282–284). Increased levels of the cytokines, C-C motif chemokine ligand 2 (CCL2) and Interleukin (IL)-1β, in adipose tissue from obese mice induced macrophage secretion of CXCL12, a cytokine that promotes angiogenesis (283). Accumulation of tumor-infiltrating macrophages with activated NOD-like receptor C4 (NLRC4) inflammasomes in the obese tumor microenvironment has been linked to increased IL-1β activation (284). IL-1β has been shown to promote tumor angiogenesis by stimulating adipocyte secretion of the proangiogenic cytokines, VEGF-A and ANGPTL4 (284, 285). Changes in adipose tissue ECM proteins associated with obesity skewed macrophage function towards a tumor-associated macrophage-like phenotype (286). The role of macrophages in obesity-associated cancer growth is however, complex. In a pre-neoplastic model, the depletion of macrophages in diet-induced obese mice led to greater numbers of mammary epithelial cells exhibiting DNA damage and increased mammary epithelial cell progenitor activity, suggesting that macrophages potentially play a modulatory role in the early stages of cancer development (287).
The accumulation of crown-like structures (CLS), which are dying (necrotic-like) adipocytes surrounded by macrophages, has been used as an indicator of inflammation and macrophage infiltration in adipose tissue (288). Breast adipose tissues from women with the metabolic syndrome have increased numbers of CLS. This association has been observed in both obese and non-obese women (BMI < 25 kg/m2), suggesting a link between insulin resistance and breast adipose tissue inflammation (289, 290). Breast adipose tissue CLS have been correlated with increased breast cancer risk (291–294), and decreased relapse-free survival (289). The relationship between the increased CLS formation and BMI (295) has been reported in African American, Hispanic/Latina, Asian, and White populations (290, 296). In prostate cancer, inflammation in periprostatic white adipose tissue was associated with higher grade, suggesting adipose tissue inflammation may have tumor-promoting effects in a number of cancers (297).
The accumulation of CLS in breast adipose tissue has been linked to increased ER-positive breast cancer risk through the increased secretion of aromatase inducers (TNF α, IL-1β and prostaglandin E2) by macrophages (298), and higher circulating concentrations of IL-6, leading to greater aromatase expression in pre-adipocytes (299). Aromatase is the enzyme that converts androgens to estrogens (292). In postmenopausal women, greater levels of breast adipose tissue inflammation and aromatase expression have been observed, potentially explaining the high incidence of ER-positive breast cancer compared to other breast cancer subtypes after menopause (300, 301).
The Role of Leptin
In addition to the obesity-associated influence on macrophage behavior, the altered anti-tumor immune response observed in obesity has been proposed to be mediated by other mechanisms, some of which are linked to leptin.
Leptin is an adipokine that affects various physiological processes, including metabolism, reproduction and body weight regulation (302). Due to the development of leptin resistance, the circulating levels of leptin increase with BMI in both rodents and humans (303). Leptin has largely been reported to have a tumor growth-promoting influence in rodent models of breast cancer (304). Leptin-deficient obese mice had decreased mammary tumor growth relative to wild type in syngeneic models, and leptin receptor (ObR)-deficient mice (with high leptin levels), showed greater mammary tumor growth with syngeneic cancer models (305). Of note, tumor growth in the A-ZIP/F-1 mice, which are fatless and do not have detectable levels of leptin, was enhanced compared to wild-type mice. The A-ZIP/F-1 mice had increased levels of pro-inflammatory cytokines, and were also hyperinsulinemic and hyperglycemic, highlighting the pro-tumorigenic influence of these conditions even in the absence of leptin (306). In addition to stimulating tumor cell proliferation and invasion (307–309), leptin signaling was also reported to promote EMT and the generation of cancer stem cells (310, 311). Leptin can also modulate the behavior of immune cells.
The ObR is present in multiple types of leukocytes such as bone marrow CD34+ hematopoietic precursor cells, monocytes, macrophages, T- and B-cells (312), as well as a sub-population of NK cells (313). Leptin stimulation has been reported to affect the function of these immune cells, although the duration of exposure to leptin results in different effects. In human NK cells, short-term exposure to leptin led to increased anti-tumor activity and long-term exposure resulted in impaired anti-tumor function (313), suggesting that NK cell activity in the context of obesity might be impaired in the setting of chronic hyperleptinemia. Blocking the ObR decreased the number of circulating MDSCs (314).
Studies have found that the response to immunotherapies such as anti-CTLA-4 monoclonal antibodies (mAb), or recombinant adenoviral-encoded TNF-related apoptosis-inducing ligand Ad(TRAIL) combined with the TLR agonist, CpG, was impaired in obese mice compared to lean mice (315). The neutralization of leptin in obese mice potentiated the response to AdTrail/CpG immunotherapy leading to increased dendritic cell function and intratumoral T-cell accumulation, resulting in decreased tumor growth (315). In contrast to the response to immunotherapies involving AdTrail/CpG or anti-CTLA-4 mAb, the response to anti-PD-1 mAb was augmented in the context of obesity preclinical models (316). The favorable response was shown to be leptin-mediated. Exposure to chronically elevated levels of leptin in the context of obesity led to the development of an exhausted phenotype in T-cells, concomitant with an increased expression of PD-1. In contrast, PD-1 expression was not increased in T-cells from leptin-deficient or ObR-deficient mice, providing a mechanistic explanation for how response to this form of immunotherapy is potentially enhanced in the setting of obesity (316).
Consistent with the pre-clinical studies, an obesity paradox has been described for treatment responses to immune check-point inhibitors where high BMI has been associated with greater treatment efficacy (316–319). This effect has been described for cancers such as melanoma (319) and non-small cell lung cancer (318). It has also been noted that obesity was associated with the development of immune-related adverse effects (320–322), which in turn, was associated with better response to therapy (323, 324).
Discussion
The obesity-cancer relationship is mediated by multiple mechanisms which are inter-related. Dysfunctional adipose tissue, characterized by increased inflammation and fibrosis plays a major role in driving obesity-associated cancer progression. Evidence from both preclinical studies and cross-sectional human clinical studies (261, 262, 325, 326) suggest that there is substantial interaction between adipose tissue and tumor cells, contributing to the obesity-associated promotion of tumor growth. In addition to a direct influence on tumor cells, the secretion of cytokines and fatty acids from adipose tissue may also promote tumor progression by modulating the accumulation and function of tumor-infiltrating leukocytes. As discussed, factors comprising metabolic health, such as dyslipidemia, insulin resistance and its corollary, hyperinsulinemia, promote cancer progression, largely through their effects on tumor cell growth and invasiveness. Further understanding how dyslipidemia and hyperinsulinemia might influence the development of the tumor microenvironment is an important area requiring futher investigation.
Therapeutic approaches to target different aspects of the obesity-cancer link have been reviewed recently (119, 261). These include targeting adipose tissue inflammation, reducing circulating insulin levels through dietary, pharmacological or surgical means (119, 261, 327), and decreasing circulating and tumor cell lipid synthesis, uptake and metabolism (244, 328). Given the complex relationship between systemic metabolic disease and the tumor immune microenvironment, it seems important that we not only consider how the anti-tumor immune response can be augmented with therapies targeting systemic metabolism, but also consider systemic metabolic conditions as variables in determining response to immune-based therapies in clinical trials.
In conclusion, characterizing and understanding the cross-talk between adipose tissue and tumors, the effect of obesity on immune cell function, and the effect of metabolic health on cancer progression will be key to improving responses to current therapies, and developing novel therapies that target systemic metabolic disease and cancer.
Author Contributions
TS and AE drafted sections of the manuscript and edited it for cohesion. DL and EG conceived the project, reviewed and edited the manuscript. All authors contributed to the article and approved the submitted version.
Funding
EG received research support from the National Institutes of Health/National Cancer Institute K08CA190770, Alkeon Capital, the Department of Medicine and the Tisch Cancer Institute at Mount Sinai. DL received research support from National Institutes of Health/National Cancer Institute R01CA200553 and R01CA128799.
Conflict of Interest
TS and AE declare no conflicts of interest. EG declares the following potential conflicting interests: EG has served on an advisory board for Novartis Pharmaceuticals and as consultant for Seattle Genetics. DR declares the following conflicting interests: DR has served on advisory boards for Mannkind and AstraZeneca.
References
1. Ng M, Fleming T, Robinson M, Thomson B, Graetz N, Margono C, et al. Global, regional, and national prevalence of overweight and obesity in children and adults during 1980-2013: a systematic analysis for the Global Burden of Disease Study 2013. Lancet Lond Engl (2014) 384(9945):766–81. doi: 10.1016/S0140-6736(14)60460-8
2. NCD Risk Factor Collaboration (NCD-RisC). Worldwide trends in body-mass index, underweight, overweight, and obesity from 1975 to 2016: a pooled analysis of 2416 population-based measurement studies in 128·9 million children, adolescents, and adults. Lancet Lond Engl (2017) 390(10113):2627–42 doi: 10.1016/S0140-6736(17)32129-3
3. NCD Risk Factor Collaboration (NCD-RisC). Trends in adult body-mass index in 200 countries from 1975 to 2014: a pooled analysis of 1698 population-based measurement studies with 19·2 million participants. Lancet (2016) 387(10026):1377–96. doi: 10.1016/S0140-6736(16)30054-X
4. Xu H, Cupples LA, Stokes A, Liu C-T. Association of Obesity With Mortality Over 24 Years of Weight History: Findings From the Framingham Heart Study. JAMA Netw Open (2018) 21(7):e184587–e184587. doi: 10.1001/jamanetworkopen.2018.4587
5. Bhaskaran K, dos-Santos-Silva I, Leon DA, Douglas IJ, Smeeth L. Association of BMI with overall and cause-specific mortality: a population-based cohort study of 3·6 million adults in the UK. Lancet Diabetes Endocrinol (2018) 16(12):944–53. doi: 10.1016/S2213-8587(18)30288-2
6. Bhaskaran K, Douglas I, Forbes H, dos-Santos-Silva I, Leon DA, Smeeth L. Body-mass index and risk of 22 specific cancers: a population-based cohort study of 5·24 million UK adults. Lancet Lond Engl (2014) 384(9945):755–65. doi: 10.1016/S0140-6736(14)60892-8
7. Renehan AG, Tyson M, Egger M, Heller RF, Zwahlen M. Body-mass index and incidence of cancer: a systematic review and meta-analysis of prospective observational studies. Lancet Lond Engl (2008) 371(9612):569–78. doi: 10.1016/S0140-6736(08)60269-X
8. Parr CL, Batty GD, Lam TH, Barzi F, Fang X, Ho SC, et al. Body-mass index and cancer mortality in the Asia-Pacific Cohort Studies Collaboration: pooled analyses of 424,519 participants. Lancet Oncol (2010) 11(8):741–52. doi: 10.1016/S1470-2045(10)70141-8
9. Arnold M, Pandeya N, Byrnes G, Renehan PAG, Stevens GA, Ezzati PM, et al. Global burden of cancer attributable to high body-mass index in 2012: a population-based study. Lancet Oncol (2015) 16(1):36–46. doi: 10.1016/S1470-2045(14)71123-4
10. Sung H, Siegel RL, Rosenberg PS, Jemal A. Emerging cancer trends among young adults in the USA: analysis of a population-based cancer registry. Lancet Public Health (2019) 14(3):e137–47. doi: 10.1016/S2468-2667(18)30267-6
11. Gupta S, Harper A, Ruan Y, Barr R, Frazier AL, Ferlay J, et al. International trends in the incidence of cancer among adolescents and young adults. J Natl Cancer Inst (2020) 112(11):1105–17. doi: 10.1093/jnci/djaa007
12. van der Klaauw AA, Farooqi IS. The hunger genes: pathways to obesity. Cell (2015) 26161(1):119–32. doi: 10.1016/j.cell.2015.03.008
13. Seo MH, Lee W-Y, Kim SS, Kang J-H, Kang J-H, Kim KK, et al. Korean Society for the Study of Obesity Guideline for the Management of Obesity in Korea. J Obes Metab Syndr (2019) 28(1):40–5. doi: 10.7570/jomes.2019.28.1.40
14. WHO Expert Consultation. Appropriate body-mass index for Asian populations and its implications for policy and intervention strategies. Lancet Lond Engl (2004) 363(9403):157–63. doi: 10.1016/S0140-6736(03)15268-3
15. IARC. Absence of excess body fatness. IARC Handb Cancer Prev (2018) 16:1–646. Available at: http://publications.iarc. fr/570 .
16. Lauby-Secretan B, Scoccianti C, Loomis D, Grosse Y, Bianchini F, Straif K. Body Fatness and Cancer — Viewpoint of the IARC Working Group. N Engl J Med (2016) 375(8):794–8. doi: 10.1056/NEJMsr1606602
17. World Cancer Research Fund/American Institute for Cancer Research. Diet, Nutrition, Physical Activity and Cancer: a Global Perspective. World Cancer Res Fund (2018). [cited 2020 Sep 5]. Available from: dietandcancerreport.org.
18. López-Suárez A. Burden of cancer attributable to obesity, type 2 diabetes and associated risk factors. Metabolism (2019) 92:136–46. doi: 10.1016/j.metabol.2018.10.013
19. Furer A, Afek A, Sommer A, Keinan-Boker L, Derazne E, Levi Z, et al. Adolescent obesity and midlife cancer risk: a population-based cohort study of 2·3 million adolescents in Israel. Lancet Diabetes Endocrinol (2020) 8(3):216–25. doi: 10.1016/S2213-8587(20)30019-X
20. Keum N, Greenwood DC, Lee DH, Kim R, Aune D, Ju W, et al. Adult weight gain and adiposity-related cancers: a dose-response meta-analysis of prospective observational studies. J Natl Cancer Inst (2015) 107(2):djv088. doi: 10.1093/jnci/djv088
21. Chadid S, Singer MR, Kreger BE, Bradlee ML, Moore LL. Midlife weight gain is a risk factor for obesity-related cancer. Br J Cancer (2018) 118(12):1665–71. doi: 10.1038/s41416-018-0106-x
22. Zheng Y, Manson JE, Yuan C, Liang MH, Grodstein F, Stampfer MJ, et al. Associations of Weight Gain From Early to Middle Adulthood With Major Health Outcomes Later in Life. JAMA (2017) 318(3):255–69. doi: 10.1001/jama.2017.7092
23. Azrad M, Blair CK, Rock CL, Sedjo RL, Wolin KY, Demark-Wahnefried W. Adult weight gain accelerates the onset of breast cancer. Breast Cancer Res Treat (2019) Aug176(3):649–56. doi: 10.1007/s10549-019-05268-y
24. Neuhouser ML, Aragaki AK, Prentice RL, Manson JE, Chlebowski R, Carty CL, et al. Overweight, Obesity, and Postmenopausal Invasive Breast Cancer Risk: A Secondary Analysis of the Women’s Health Initiative Randomized Clinical Trials. JAMA Oncol (2015) 1(5):611–21. doi: 10.1001/jamaoncol.2015.1546
25. Premenopausal Breast Cancer Collaborative Group, MJ S, HB N, LB W, MN B, ME J, et al. Association of Body Mass Index and Age With Subsequent Breast Cancer Risk in Premenopausal Women. JAMA Oncol (2018) 014(11):e181771. doi: 10.1001/jamaoncol.2018.1771
26. Pierobon M, Frankenfeld CL. Obesity as a risk factor for triple-negative breast cancers: a systematic review and meta-analysis. Breast Cancer Res Treat (2013) 37(1):307–14. doi: 10.1007/s10549-012-2339-3
27. Calle EE, Rodriguez C, Walker-Thurmond K, Thun MJ. Overweight, obesity, and mortality from cancer in a prospectively studied cohort of U.S. Adults N Engl J Med (2003) Apr 24348(17):1625–38. doi: 10.1056/NEJMoa021423
28. Slawinski CGV, Barriuso J, Guo H, Renehan AG. Obesity and Cancer Treatment Outcomes: Interpreting the Complex Evidence. Clin Oncol R Coll Radiol G B (2020) Sep32(9):591–608. doi: 10.1016/j.clon.2020.05.004
29. GBD 2015 Risk Factors Collaborators. Global, regional, and national comparative risk assessment of 79 behavioural, environmental and occupational, and metabolic risks or clusters of risks, 1990-2015: a systematic analysis for the Global Burden of Disease Study 2015. Lancet Lond Engl (2016) Oct 8;388(10053):1659–724. doi: 10.1016/S0140-6736(16)31679-8
30. Piirtola M, Jelenkovic A, Latvala A, Sund R, Honda C, Inui F, et al. Association of current and former smoking with body mass index: A study of smoking discordant twin pairs from 21 twin cohorts. PloS One (2018) 13(7):e0200140. doi: 10.1371/journal.pone.0200140
31. Chan DSM, Vieira AR, Aune D, Bandera EV, Greenwood DC, McTiernan A, et al. Body mass index and survival in women with breast cancer-systematic literature review and meta-analysis of 82 follow-up studies. Ann Oncol Off J Eur Soc Med Oncol (2014) 25(10):1901–14. doi: 10.1093/annonc/mdu042
32. Niraula S, Ocana A, Ennis M, Goodwin PJ. Body size and breast cancer prognosis in relation to hormone receptor and menopausal status: a meta-analysis. Breast Cancer Res Treat (2012) Jul134(2):769–81. doi: 10.1007/s10549-012-2073-x
33. Protani M, Coory M, Martin JH. Effect of obesity on survival of women with breast cancer: systematic review and meta-analysis. Breast Cancer Res Treat (2010) 123(3):627–35. doi: 10.1007/s10549-010-0990-0
34. Iyengar NM, Arthur R, Manson JE, Chlebowski RT, Kroenke CH, Peterson L, et al. Association of Body Fat and Risk of Breast Cancer in Postmenopausal Women With Normal Body Mass Index: A Secondary Analysis of a Randomized Clinical Trial and Observational Study. JAMA Oncol (2019) 5(2):155–63. doi: 10.1001/jamaoncol.2018.5327
35. Alberti KGMM, Eckel RH, Grundy SM, Zimmet PZ, Cleeman JI, Donato KA, et al. Harmonizing the metabolic syndrome: a joint interim statement of the International Diabetes Federation Task Force on Epidemiology and Prevention; National Heart, Lung, and Blood Institute; American Heart Association; World Heart Federation; International Atherosclerosis Society; and International Association for the Study of Obesity. Circulation (2009) 120(16):1640–5. doi: 10.1161/CIRCULATIONAHA.109.192644
36. O’Neill S, O’Driscoll L. Metabolic syndrome: a closer look at the growing epidemic and its associated pathologies. Obes Rev Off J Int Assoc Study Obes (2015) 16(1):1–12. doi: 10.1111/obr.12229
37. Smith U. Abdominal obesity: a marker of ectopic fat accumulation. J Clin Invest (2015) May125(5):1790–2. doi: 10.1172/JCI81507
38. Wiklund P, Toss F, Weinehall L, Hallmans G, Franks PW, Nordström A, et al. Abdominal and gynoid fat mass are associated with cardiovascular risk factors in men and women. J Clin Endocrinol Metab (2008) Nov93(11):4360–6. doi: 10.1210/jc.2008-0804
39. Tabák AG, Jokela M, Akbaraly TN, Brunner EJ, Kivimäki M, Witte DR. Trajectories of glycaemia, insulin sensitivity, and insulin secretion before diagnosis of type 2 diabetes: an analysis from the Whitehall II study. Lancet Lond Engl (2009) 27373(9682):2215–21. doi: 10.1016/S0140-6736(09)60619-X
40. Hotamisligil GS. Inflammation and metabolic disorders. Nature (2006) Dec 14444(7121):860–7. doi: 10.1038/nature05485
41. Lalia AZ, Dasari S, Johnson ML, Robinson MM, Konopka AR, Distelmaier K, et al. Predictors of Whole-Body Insulin Sensitivity Across Ages and Adiposity in Adult Humans. J Clin Endocrinol Metab (2016) 101(2):626–34. doi: 10.1210/jc.2015-2892
42. Dibaba DT, Braithwaite D, Akinyemiju T. Metabolic Syndrome and the Risk of Breast Cancer and Subtypes by Race, Menopause and BMI. Cancers (2018) 10(9):299. doi: 10.3390/cancers10090299
43. Esposito K, Chiodini P, Colao A, Lenzi A, Giugliano D. Metabolic syndrome and risk of cancer: a systematic review and meta-analysis. Diabetes Care (2012) 35(11):2402–11. doi: 10.2337/dc12-0336
44. Liang X, Margolis KL, Hendryx M, Rohan T, Groessl EJ, Thomson CA, et al. Metabolic phenotype and risk of colorectal cancer in normal-weight postmenopausal women. Cancer Epidemiol Biomark Prev Publ Am Assoc Cancer Res Cosponsored Am Soc Prev Oncol (2017) 26(2):155–61. doi: 10.1158/1055-9965.EPI-16-0761
45. Xia B, He Q, Pan Y, Gao F, Liu A, Tang Y, et al. Metabolic syndrome and risk of pancreatic cancer: A population-based prospective cohort study. Int J Cancer (2020) 147(12):3384–93. doi: 10.1002/ijc.33172
46. Gunter MJ, Xie X, Xue X, Kabat GC, Rohan TE, Wassertheil-Smoller S, et al. Breast cancer risk in metabolically healthy but overweight postmenopausal women. Cancer Res (2015) Jan 1575(2):270–4. doi: 10.1158/0008-5472.CAN-14-2317
47. Hills CE, Brunskill NJ. Cellular and physiological effects of C-peptide. Clin Sci Lond Engl 1979 (2009) Apr116(7):565–74. doi: 10.1042/CS20080441
48. Gunter MJ, Hoover DR, Yu H, Wassertheil-Smoller S, Rohan TE, Manson JE, et al. Insulin, insulin-like growth factor-I, and risk of breast cancer in postmenopausal women. J Natl Cancer Inst (2009) 101(1):48–60. doi: 10.1093/jnci/djn415
49. Irwin ML, Duggan C, Wang C-Y, Smith AW, McTiernan A, Baumgartner RN, et al. Fasting C-peptide levels and death resulting from all causes and breast cancer: the health, eating, activity, and lifestyle study. J Clin Oncol Off J Am Soc Clin Oncol (2011) 29(1):47–53. doi: 10.1200/JCO.2010.28.4752
50. Ahern TP, Hankinson SE, Willett WC, Pollak MN, Eliassen AH, Tamimi RM. Plasma C-peptide, mammographic breast density, and risk of invasive breast cancer. Cancer Epidemiol Biomark Prev Publ Am Assoc Cancer Res Cosponsored Am Soc Prev Oncol (2013) 22(10):1786–96. doi: 10.1158/1055-9965.EPI-13-0375
51. Yoon YS, Keum N, Zhang X, Cho E, Giovannucci EL. Hyperinsulinemia, insulin resistance and colorectal adenomas: A meta-analysis. Metabolism (2015) 64(10):1324–33. doi: 10.1016/j.metabol.2015.06.013
52. Murphy N, Cross AJ, Abubakar M, Jenab M, Aleksandrova K, Boutron-Ruault M-C, et al. A Nested Case-Control Study of Metabolically Defined Body Size Phenotypes and Risk of Colorectal Cancer in the European Prospective Investigation into Cancer and Nutrition (EPIC). PloS Med (2016) 13(4):e1001988. doi: 10.1371/journal.pmed.1001988
53. Lega IC, Wilton AS, Austin PC, Fischer HD, Johnson JA, Lipscombe LL. The temporal relationship between diabetes and cancer: A population-based study. Cancer (2016) 01122(17):2731–8. doi: 10.1002/cncr.30095
54. Onitilo AA, Stankowski RV, Berg RL, Engel JM, Glurich I, Williams GM, et al. Breast cancer incidence before and after diagnosis of type 2 diabetes mellitus in women: increased risk in the prediabetes phase. Eur J Cancer Prev Off J Eur Cancer Prev Organ ECP (2014) 23(2):76–83. doi: 10.1097/CEJ.0b013e32836162aa
55. Pan K, Nelson RA, Wactawski-Wende J, Lee DJ, Manson JE, Aragaki AK, et al. Insulin Resistance and Cancer-Specific and All-Cause Mortality in Postmenopausal Women: The Women’s Health Initiative. J Natl Cancer Inst (2020) 01112(2):170–8. doi: 10.1093/jnci/djz069
56. Gallagher EJ, Fei K, Feldman SM, Port E, Friedman NB, Boolbol SK, et al. Insulin resistance contributes to racial disparities in breast cancer prognosis in US women. Breast Cancer Res (2020) May 1222(1):40. doi: 10.1186/s13058-020-01281-y
57. Zavala VA, Bracci PM, Carethers JM, Carvajal-Carmona L, Coggins NB, Cruz-Correa MR, et al. Cancer health disparities in racial/ethnic minorities in the United States. Br J Cancer (2020) Sep 9. doi: 10.1038/s41416-020-01038-6 Online ahead of print.
58. Eckel RH, Kahn SE, Ferrannini E, Goldfine AB, Nathan DM, Schwartz MW, et al. Obesity and type 2 diabetes: what can be unified and what needs to be individualized? J Clin Endocrinol Metab (2011) Jun;96(6):1654–63. doi: 10.1210/jc.2011-0585
59. De Bruijn KMJ, Arends LR, Hansen BE, Leeflang S, Ruiter R, van Eijck CHJ. Systematic review and meta-analysis of the association between diabetes mellitus and incidence and mortality in breast and colorectal cancer. Br J Surg (2013) Oct100(11):1421–9. doi: 10.1002/bjs.9229
60. Petrick JL, Thistle JE, Zeleniuch-Jacquotte A, Zhang X, Wactawski-Wende J, Van Dyke AL, et al. Body Mass Index, Diabetes and Intrahepatic Cholangiocarcinoma Risk: The Liver Cancer Pooling Project and Meta-analysis. Am J Gastroenterol (2018) 113(10):1494–505. doi: 10.1038/s41395-018-0207-4
61. Larsson SC, Orsini N, Wolk A. Diabetes mellitus and risk of colorectal cancer: a meta-analysis. J Natl Cancer Inst (2005) Nov 1697(22):1679–87. doi: 10.1093/jnci/dji375
62. Pang Y, Kartsonaki C, Guo Y, Bragg F, Yang L, Bian Z, et al. Diabetes, plasma glucose and incidence of pancreatic cancer: A prospective study of 0.5 million Chinese adults and a meta-analysis of 22 cohort studies. Int J Cancer (2017) Apr 15140(8):1781–8. doi: 10.1002/ijc.30599
63. Tsilidis KK, Kasimis JC, Lopez DS, Ntzani EE, Ioannidis JPA. Type 2 diabetes and cancer: umbrella review of meta-analyses of observational studies. BMJ (2015) 350:g7607. doi: 10.1136/bmj.g7607
64. Yuan S, Kar S, Carter P, Vithayathil M, Mason AM, Burgess S, et al. Is Type 2 Diabetes Causally Associated With Cancer Risk? Evidence From a Two-Sample Mendelian Randomization Study. Diabetes (2020) Jul69(7):1588–96. doi: 10.2337/db20-0084
65. Boyle P, Boniol M, Koechlin A, Robertson C, Valentini F, Coppens K, et al. Diabetes and breast cancer risk: a meta-analysis. Br J Cancer (2012) 107(9):1608–17. doi: 10.1038/bjc.2012.414
66. Bronsveld HK, Jensen V, Vahl P, De Bruin ML, Cornelissen S, Sanders J, et al. Diabetes and Breast Cancer Subtypes. PloS One (2017) 12(1):e0170084. doi: 10.1371/journal.pone.0170084
67. Chen H, Cook LS, Tang M-TC, Hill DA, Wiggins CL, Li CI. Relationship between diabetes and diabetes medications and risk of different molecular subtypes of breast cancer. Cancer Epidemiol Biomark Prev Publ Am Assoc Cancer Res Cosponsored Am Soc Prev Oncol (2019) Nov28(11):1802–8. doi: 10.1158/1055-9965.EPI-19-0291
68. Coughlin SS, Calle EE, Teras LR, Petrelli J, Thun MJ. Diabetes mellitus as a predictor of cancer mortality in a large cohort of US adults. Am J Epidemiol (2004) Jun 15159(12):1160–7. doi: 10.1093/aje/kwh161
69. Rao Kondapally Seshasai S, Kaptoge S, Thompson A, Di Angelantonio E, Gao P, Sarwar N, et al. Diabetes mellitus, fasting glucose, and risk of cause-specific death. N Engl J Med (2011) Mar 3364(9):829–41. doi: 10.1056/NEJMoa1008862
70. Mao Y, Tao M, Jia X, Xu H, Chen K, Tang H, et al. Effect of Diabetes Mellitus on Survival in Patients with Pancreatic Cancer: A Systematic Review and Meta-analysis. Sci Rep (2015) Nov 24;5:17102. doi: 10.1038/srep17102
71. Mills KT, Bellows CF, Hoffman AE, Kelly TN, Gagliardi G. Diabetes mellitus and colorectal cancer prognosis: a meta-analysis. Dis Colon Rectum (2013) Nov56(11):1304–19. doi: 10.1097/DCR.0b013e3182a479f9
72. Barone BB, Yeh H-C, Snyder CF, Peairs KS, Stein KB, Derr RL, et al. Long-term All-Cause Mortality in Cancer Patients With Preexisting Diabetes Mellitus: A Systematic Review and Meta-analysis. JAMA (2008) 300(23):2754–64. doi: 10.1001/jama.2008.824
73. Lega IC, Lipscombe LL. Review: Diabetes, Obesity, and Cancer—Pathophysiology and Clinical Implications. Endocr Rev (2020) 41(1):33–52. doi: 10.1210/endrev/bnz014
74. Peairs KS, Barone BB, Snyder CF, Yeh H-C, Stein KB, Derr RL, et al. Diabetes mellitus and breast cancer outcomes: a systematic review and meta-analysis. J Clin Oncol Off J Am Soc Clin Oncol (2011) 129(1):40–6. doi: 10.1200/JCO.2009.27.3011
75. Lega IC, Austin PC, Fischer HD, Fung K, Krzyzanowska MK, Amir E, et al. The Impact of Diabetes on Breast Cancer Treatments and Outcomes: A Population-Based Study. Diabetes Care (2018) 41(4):755–61. doi: 10.2337/dc17-2012
76. Lipscombe LL, Fischer HD, Austin PC, Fu L, Jaakkimainen RL, Ginsburg O, et al. The association between diabetes and breast cancer stage at diagnosis: a population-based study. Breast Cancer Res Treat (2015) 150(3):613–20. doi: 10.1007/s10549-015-3323-5
77. Srokowski TP, Fang S, Hortobagyi GN, Giordano SH. Impact of diabetes mellitus on complications and outcomes of adjuvant chemotherapy in older patients with breast cancer. J Clin Oncol Off J Am Soc Clin Oncol (2009) May 127(13):2170–6. doi: 10.1200/JCO.2008.17.5935
78. Zylla D, Gilmore G, Eklund J, Richter S, Carlson A. Impact of diabetes and hyperglycemia on health care utilization, infection risk, and survival in patients with cancer receiving glucocorticoids with chemotherapy. J Diabetes Complications (2019) 33(4):335–9. doi: 10.1016/j.jdiacomp.2018.12.012
79. Peila R, Rohan TE. Diabetes, Glycated Hemoglobin, and Risk of Cancer in the UK Biobank Study. Cancer Epidemiol Biomark Prev Publ Am Assoc Cancer Res Cosponsored Am Soc Prev Oncol (2020) 29(6):1107–19. doi: 10.1158/1055-9965.EPI-19-1623
80. Rentsch CT, Farmer RE, Eastwood SV, Mathur R, Garfield V, Farmaki A-E, et al. Risk of 16 cancers across the full glycemic spectrum: a population-based cohort study using the UK Biobank. BMJ Open Diabetes Res Care (2020) 8(1):e001600. doi: 10.1136/bmjdrc-2020-001600
81. Au Yeung SL, Schooling CM. Impact of glycemic traits, type 2 diabetes and metformin use on breast and prostate cancer risk: a Mendelian randomization study. BMJ Open Diabetes Res Care (2019) 7(1):e000872. doi: 10.1136/bmjdrc-2019-000872
82. Gallagher EJ, LeRoith D. Obesity and Diabetes: The Increased Risk of Cancer and Cancer-Related Mortality. Physiol Rev (2015) 95(3):727–48. doi: 10.1152/physrev.00030.2014
83. Rader DJ. Lipoprotein Metabolism. In: Offermanns S, Rosenthal W, editors. Encyclopedia of Molecular Pharmacology. Berlin, Heidelberg: Springer Berlin Heidelberg (2008). 696–700. doi: 10.1007/978-3-540-38918-7_190
84. Jamnagerwalla J, Howard LE, Allott EH, Vidal AC, Moreira DM, Castro-Santamaria R, et al. Serum cholesterol and risk of high-grade prostate cancer: results from the REDUCE study. Prostate Cancer Prostatic Dis (2018) 21(2):252–9. doi: 10.1038/s41391-017-0030-9
85. Pelucchi C, Serraino D, Negri E, Montella M, Dellanoce C, Talamini R, et al. The metabolic syndrome and risk of prostate cancer in Italy. Ann Epidemiol (2011) 21(11):835–41. doi: 10.1016/j.annepidem.2011.07.007
86. Tian Y, Wang K, Li J, Wang J, Wang Z, Fan Y, et al. The association between serum lipids and colorectal neoplasm: a systemic review and meta-analysis. Public Health Nutr (2015) 18(18):3355–70. doi: 10.1017/S1368980015000646
87. Wulaningsih W, Garmo H, Holmberg L, Hammar N, Jungner I, Walldius G, et al. Serum Lipids and the Risk of Gastrointestinal Malignancies in the Swedish AMORIS Study. J Cancer Epidemiol (2012) 2012:792034. doi: 10.1155/2012/792034
88. Jin Y, Yang T, Li D, Ding W. Effect of dietary cholesterol intake on the risk of esophageal cancer: a meta-analysis. J Int Med Res (2019) 47(9):4059–68. doi: 10.1177/0300060519865632
89. Chen H, Qin S, Wang M, Zhang T, Zhang S. Association between cholesterol intake and pancreatic cancer risk: evidence from a meta-analysis. Sci Rep (2015) 45:8243. doi: 10.1038/srep08243
90. Sadeghi A, Shab-Bidar S, Parohan M, Djafarian K. Dietary Fat Intake and Risk of Ovarian Cancer: A Systematic Review and Dose-Response Meta-Analysis of Observational Studies. Nutr Cancer (2019) 71(6):939–53. doi: 10.1080/01635581.2019.1595049
91. Li C, Yang L, Zhang D, Jiang W. Systematic review and meta-analysis suggest that dietary cholesterol intake increases risk of breast cancer. Nutr Res N Y N (2016) 36(7):627–35. doi: 10.1016/j.nutres.2016.04.009
92. Mainous AG, Wells BJ, Koopman RJ, Everett CJ, Gill JM. Iron, lipids, and risk of cancer in the Framingham Offspring cohort. Am J Epidemiol (2005) Jun 15161(12):1115–22. doi: 10.1093/aje/kwi131
93. Kitahara CM, Berrington de González A, Freedman ND, Huxley R, Mok Y, Jee SH, et al. Total cholesterol and cancer risk in a large prospective study in Korea. J Clin Oncol Off J Am Soc Clin Oncol (2011) Apr 2029(12):1592–8. doi: 10.1200/JCO.2010.31.5200
94. Wu B, Teng L, He D, Yu D-D, Jiang F. Dose-response relation between serum total cholesterol levels and overall cancer risk: evidence from 12 prospective studies involving 1,926,275 participants. Int J Food Sci Nutr (2019) Jun70(4):432–41. doi: 10.1080/09637486.2018.1529147
95. Touvier M, Fassier P, His M, Norat T, Chan DSM, Blacher J, et al. Cholesterol and breast cancer risk: a systematic review and meta-analysis of prospective studies. Br J Nutr (2015) 14114(3):347–57. doi: 10.1017/S000711451500183X
96. Furberg A-S, Veierød MB, Wilsgaard T, Bernstein L, Thune I. Serum high-density lipoprotein cholesterol, metabolic profile, and breast cancer risk. J Natl Cancer Inst (2004) Aug 496(15):1152–60. doi: 10.1093/jnci/djh216
97. Ni H, Liu H, Gao R. Serum Lipids and Breast Cancer Risk: A Meta-Analysis of Prospective Cohort Studies. PloS One (2015) 10(11):e0142669. doi: 10.1371/journal.pone.0142669
98. Kucharska-Newton AM, Rosamond WD, Mink PJ, Alberg AJ, Shahar E, Folsom AR. HDL-cholesterol and incidence of breast cancer in the ARIC cohort study. Ann Epidemiol (2008) 18(9):671–7. doi: 10.1016/j.annepidem.2008.06.006
99. Mundal L, Sarancic M, Ose L, Iversen PO, Borgan J-K, Veierød MB, et al. Mortality among patients with familial hypercholesterolemia: a registry-based study in Norway, 1992-2010. J Am Heart Assoc (2014) 23(6):e001236. doi: 10.1161/JAHA.114.001236
100. Mabuchi H, Miyamoto S, Ueda K, Oota M, Takegoshi T, Wakasugi T, et al. Causes of death in patients with familial hypercholesterolemia. Atherosclerosis (1986) 61(1):1–6. doi: 10.1016/0021-9150(86)90107-3
101. Krogh HW, Svendsen K, Igland J, Mundal LJ, Holven KB, Bogsrud MP, et al. Lower risk of smoking-related cancer in individuals with familial hypercholesterolemia compared with controls: a prospective matched cohort study. Sci Rep (2019) 179(1):19273. doi: 10.1038/s41598-019-55682-x
102. Jiang J, Nilsson-Ehle P, Xu N. Influence of liver cancer on lipid and lipoprotein metabolism. Lipids Health Dis (2006) 5:4. doi: 10.1186/1476-511X-5-4
103. Tomiki Y, Suda S, Tanaka M, Okuzawa A, Matsuda M, Ishibiki Y, et al. Reduced low-density-lipoprotein cholesterol causing low serum cholesterol levels in gastrointestinal cancer: a case control study. J Exp Clin Cancer Res CR (2004) Jun23(2):233–40.
104. Vujasinovic M, Valente R, Del Chiaro M, Permert J, Löhr J-M. Pancreatic Exocrine Insufficiency in Pancreatic Cancer. Nutrients (2017) 9(3):183. doi: 10.3390/nu9030183
105. Zwickl H, Hackner K, Köfeler H, Krzizek E-C, Muqaku B, Pils D, et al. Reduced LDL-Cholesterol and Reduced Total Cholesterol as Potential Indicators of Early Cancer in Male Treatment-Naïve Cancer Patients With Pre-cachexia and Cachexia. Front Oncol (2020) 10:1262. doi: 10.3389/fonc.2020.01262
106. Kuliszkiewicz-Janus M, Małecki R, Mohamed AS. Lipid changes occuring in the course of hematological cancers. Cell Mol Biol Lett (2008) 13(3):465–74. doi: 10.2478/s11658-008-0014-9
107. Authors/Task Force Members, ESC Committee for Practice Guidelines (CPG), ESC National Cardiac Societies. ESC/EAS guidelines for the management of dyslipidaemias: Lipid modification to reduce cardiovascular risk. Atherosclerosis (2019) 290:140–205. doi: 10.1016/j.atherosclerosis.2019.08.014
108. Koene RJ, Prizment AE, Blaes A, Konety SH. Shared Risk Factors in Cardiovascular Disease and Cancer. Circulation (2016) 133(11):1104–14. doi: 10.1161/CIRCULATIONAHA.115.020406
109. Bonovas S, Filioussi K, Sitaras NM. Statin use and the risk of prostate cancer: A metaanalysis of 6 randomized clinical trials and 13 observational studies. Int J Cancer (2008) 123(4):899–904. doi: 10.1002/ijc.23550
110. Murtola TJ, Tammela TLJ, Lahtela J, Auvinen A. Cholesterol-lowering drugs and prostate cancer risk: a population-based case-control study. Cancer Epidemiol Biomark Prev Publ Am Assoc Cancer Res Cosponsored Am Soc Prev Oncol (2007) 16(11):2226–32. doi: 10.1158/1055-9965.EPI-07-0599
111. Tan P, Wei S, Tang Z, Gao L, Zhang C, Nie P, et al. LDL-lowering therapy and the risk of prostate cancer: a meta-analysis of 6 randomized controlled trials and 36 observational studies. Sci Rep (2016) 146:24521. doi: 10.1038/srep24521
112. Nielsen SF, Nordestgaard BG, Bojesen SE. Statin use and reduced cancer-related mortality. N Engl J Med (2012) 8367(19):1792–802. doi: 10.1056/NEJMoa1201735
113. Wang J, Li C, Tao H, Cheng Y, Han L, Li X, et al. Statin use and risk of lung cancer: a meta-analysis of observational studies and randomized controlled trials. PloS One (2013) 8(10):e77950. doi: 10.1371/journal.pone.0077950
114. Undela K, Srikanth V, Bansal D. Statin use and risk of breast cancer: a meta-analysis of observational studies. Breast Cancer Res Treat (2012) Aug135(1):261–9. doi: 10.1007/s10549-012-2154-x
115. Ahern TP, Pedersen L, Tarp M, Cronin-Fenton DP, Garne JP, Silliman RA, et al. Statin prescriptions and breast cancer recurrence risk: a Danish nationwide prospective cohort study. J Natl Cancer Inst (2011) Oct 5103(19):1461–8. doi: 10.1093/jnci/djr291
116. Manthravadi S, Shrestha A, Madhusudhana S. Impact of statin use on cancer recurrence and mortality in breast cancer: A systematic review and meta-analysis. Int J Cancer (2016) Sep 15139(6):1281–8. doi: 10.1002/ijc.30185
117. Zhong S, Zhang X, Chen L, Ma T, Tang J, Zhao J. Statin use and mortality in cancer patients: Systematic review and meta-analysis of observational studies. Cancer Treat Rev (2015) 41(6):554–67. doi: 10.1016/j.ctrv.2015.04.005
118. Perry RJ, Shulman GI. Mechanistic Links between Obesity, Insulin, and Cancer. Trends Cancer (2020) 6(2):75–8. doi: 10.1016/j.trecan.2019.12.003
119. Gallagher EJ, LeRoith D. Hyperinsulinaemia in cancer. Nat Rev Cancer (2020) 20(11):629–44. doi: 10.1038/s41568-020-0295-5
120. Belfiore A, Frasca F, Pandini G, Sciacca L, Vigneri R. Insulin receptor isoforms and insulin receptor/insulin-like growth factor receptor hybrids in physiology and disease. Endocr Rev (2009) 30(6):586–623. doi: 10.1210/er.2008-0047
121. Gallagher EJ, LeRoith D. The proliferating role of insulin and insulin-like growth factors in cancer. Trends Endocrinol Metab TEM (2010) Oct21(10):610–8. doi: 10.1016/j.tem.2010.06.007
122. Vella V, Milluzzo A, Scalisi NM, Vigneri P, Sciacca L. Insulin Receptor Isoforms in Cancer. Int J Mol Sci (2018) 19(11):3615. doi: 10.3390/ijms19113615
123. Hopkins BD, Goncalves MD, Cantley LC. Insulin–PI3K signalling: an evolutionarily insulated metabolic driver of cancer. Nat Rev Endocrinol (2020) May16(5):276–83. doi: 10.1038/s41574-020-0329-9
124. Renehan AG, Zwahlen M, Egger M. Adiposity and cancer risk: new mechanistic insights from epidemiology. Nat Rev Cancer (2015) Aug15(8):484–98. doi: 10.1038/nrc3967
125. Crowe FL, Key TJ, Allen NE, Appleby PN, Overvad K, Grønbæk H, et al. A cross-sectional analysis of the associations between adult height, BMI and serum concentrations of IGF-I and IGFBP-1 -2 and -3 in the European Prospective Investigation into Cancer and Nutrition (EPIC). Ann Hum Biol (2011) Mar38(2):194–202. doi: 10.3109/03014460.2010.507221
126. Endogenous Hormones and Breast Cancer Collaborative Group, TJ K, PN A, GK R, Roddam AW. Insulin-like growth factor 1 (IGF1), IGF binding protein 3 (IGFBP3), and breast cancer risk: pooled individual data analysis of 17 prospective studies. Lancet Oncol (2010) Jun11(6):530–42. doi: 10.1016/S1470-2045(10)70095-4
127. Clayton PE, Banerjee I, Murray PG, Renehan AG. Growth hormone, the insulin-like growth factor axis, insulin and cancer risk. Nat Rev Endocrinol (2011) Jan7(1):11–24. doi: 10.1038/nrendo.2010.171
128. Gahete MD, Córdoba-Chacón J, Lin Q, Brüning JC, Kahn CR, Castaño JP, et al. Insulin and IGF-I inhibit GH synthesis and release in vitro and in vivo by separate mechanisms. Endocrinology (2013) 54(7):2410–20. doi: 10.1210/en.2013-1261
129. Baxter RC. IGF binding proteins in cancer: mechanistic and clinical insights. Nat Rev Cancer (2014) 14(5):329–41. doi: 10.1038/nrc3720
130. Wu Y, Cui K, Miyoshi K, Hennighausen L, Green JE, Setser J, et al. Reduced circulating insulin-like growth factor I levels delay the onset of chemically and genetically induced mammary tumors. Cancer Res (2003) Aug 163(15):4384–8.
131. Carboni JM, Lee AV, Hadsell DL, Rowley BR, Lee FY, Bol DK, et al. Tumor development by transgenic expression of a constitutively active insulin-like growth factor I receptor. Cancer Res (2005) 165(9):3781–7. doi: 10.1158/0008-5472.CAN-04-4602
132. Jones RA, Campbell CI, Gunther EJ, Chodosh LA, Petrik JJ, Khokha R, et al. Transgenic overexpression of IGF-IR disrupts mammary ductal morphogenesis and induces tumor formation. Oncogene (2007) 826(11):1636–44. doi: 10.1038/sj.onc.1209955
133. Creighton CJ, Casa A, Lazard Z, Huang S, Tsimelzon A, Hilsenbeck SG, et al. Insulin-like growth factor-I activates gene transcription programs strongly associated with poor breast cancer prognosis. J Clin Oncol Off J Am Soc Clin Oncol (2008) 126(25):4078–85. doi: 10.1200/JCO.2007.13.4429
134. Nagle AM, Levine KM, Tasdemir N, Scott JA, Burlbaugh K, Kehm J, et al. Loss of E-cadherin Enhances IGF1-IGF1R Pathway Activation and Sensitizes Breast Cancers to Anti-IGF1R/InsR Inhibitors. Clin Cancer Res Off J Am Assoc Cancer Res (2018) 1524(20):5165–77. doi: 10.1158/1078-0432.CCR-18-0279
135. Obr AE, Kumar S, Chang Y-J, Bulatowicz JJ, Barnes BJ, Birge RB, et al. Insulin-like growth factor receptor signaling in breast tumor epithelium protects cells from endoplasmic reticulum stress and regulates the tumor microenvironment. Breast Cancer Res BCR (2018) 2020(1):138. doi: 10.1186/s13058-018-1063-2
136. Rota LM, Albanito L, Shin ME, Goyeneche CL, Shushanov S, Gallagher EJ, et al. IGF1R inhibition in mammary epithelia promotes canonical Wnt signaling and Wnt1-driven tumors. Cancer Res (2014) 174(19):5668–79. doi: 10.1158/0008-5472.CAN-14-0970
137. Papa V, Pezzino V, Costantino A, Belfiore A, Giuffrida D, Frittitta L, et al. Elevated insulin receptor content in human breast cancer. J Clin Invest (1990) Nov86(5):1503–10. doi: 10.1172/JCI114868
138. Law JH, Habibi G, Hu K, Masoudi H, Wang MYC, Stratford AL, et al. Phosphorylated insulin-like growth factor-i/insulin receptor is present in all breast cancer subtypes and is related to poor survival. Cancer Res (2008) Dec 1568(24):10238–46. doi: 10.1158/0008-5472.CAN-08-2755
139. Mulligan AM, O’Malley FP, Ennis M, Fantus IG, Goodwin PJ. Insulin receptor is an independent predictor of a favorable outcome in early stage breast cancer. Breast Cancer Res Treat (2007) Nov106(1):39–47. doi: 10.1007/s10549-006-9471-x
140. Milazzo G, Giorgino F, Damante G, Sung C, Stampfer MR, Vigneri R, et al. Insulin receptor expression and function in human breast cancer cell lines. Cancer Res (1992) Jul 1552(14):3924–30.
141. Frisch CM, Zimmermann K, Zilleßen P, Pfeifer A, Racké K, Mayer P. Non-small cell lung cancer cell survival crucially depends on functional insulin receptors. Endocr Relat Cancer (2015) 22(4):609–21. doi: 10.1530/ERC-14-0581
142. Hopkins BD, Pauli C, Du X, Wang DG, Li X, Wu D, et al. Suppression of insulin feedback enhances the efficacy of PI3K inhibitors. Nature (2018) Aug560(7719):499–503. doi: 10.1038/s41586-018-0343-4
143. Zhang H, Fagan DH, Zeng X, Freeman KT, Sachdev D, Yee D. Inhibition of cancer cell proliferation and metastasis by insulin receptor downregulation. Oncogene (2010) Apr 2929(17):2517–27. doi: 10.1038/onc.2010.17
144. Chan JY, LaPara K, Yee D. Disruption of insulin receptor function inhibits proliferation in endocrine-resistant breast cancer cells. Oncogene (2016) 1135(32):4235–43. doi: 10.1038/onc.2015.488
145. ostoker R, Abelson S, Bitton-Worms K, Genkin I, Ben-Shmuel S, Dakwar M, et al. Highly specific role of the insulin receptor in breast cancer progression. Endocr Relat Cancer (2015) Apr22(2):145–57. doi: 10.1530/ERC-14-0490
146. Fernández AM, Kim JK, Yakar S, Dupont J, Hernandez-Sanchez C, Castle AL, et al. Functional inactivation of the IGF-I and insulin receptors in skeletal muscle causes type 2 diabetes. Genes Dev (2001) Aug 115(15):1926–34. doi: 10.1101/gad.908001
147. Novosyadlyy R, Lann DE, Vijayakumar A, Rowzee A, Lazzarino DA, Fierz Y, et al. Insulin-mediated acceleration of breast cancer development and progression in a non-obese model of type 2 diabetes. Cancer Res (2010) Jan 1570(2):741–51. doi: 10.1158/0008-5472.CAN-09-2141
148. Ferguson RD, Novosyadlyy R, Fierz Y, Alikhani N, Sun H, Yakar S, et al. Hyperinsulinemia enhances c-Myc-mediated mammary tumor development and advances metastatic progression to the lung in a mouse model of type 2 diabetes. Breast Cancer Res BCR (2012) Jan 7;14(1):R8. doi: 10.1186/bcr3089
149. Ferguson RD, Gallagher EJ, Cohen D, Tobin-Hess A, Alikhani N, Novosyadlyy R, et al. Hyperinsulinemia promotes metastasis to the lung in a mouse model of Her2-mediated breast cancer. Endocr Relat Cancer (2013) 20(3):391–401. doi: 10.1530/ERC-12-0333
150. Shlomai G, Zelenko Z, Antoniou IM, Stasinopoulos M, Tobin-Hess A, Vitek MP, et al. OP449 inhibits breast cancer growth without adverse metabolic effects. Endocr Relat Cancer (2017) 24(10):519–29. doi: 10.1530/ERC-17-0077
151. Zelenko Z, Gallagher EJ, Antoniou IM, Sachdev D, Nayak A, Yee D, et al. EMT reversal in human cancer cells after IR knockdown in hyperinsulinemic mice. Endocr Relat Cancer (2016) 23(9):747–58. doi: 10.1530/ERC-16-0142
152. Gallagher EJ, Alikhani N, Tobin-Hess A, Blank J, Buffin NJ, Zelenko Z, et al. Insulin receptor phosphorylation by endogenous insulin or the insulin analog AspB10 promotes mammary tumor growth independent of the IGF-I receptor. Diabetes (2013) Oct62(10):3553–60. doi: 10.2337/db13-0249
153. Fierz Y, Novosyadlyy R, Vijayakumar A, Yakar S, LeRoith D. Insulin-sensitizing therapy attenuates type 2 diabetes-mediated mammary tumor progression. Diabetes (2010) 59(3):686–93. doi: 10.2337/db09-1291
154. Fierz Y, Novosyadlyy R, Vijayakumar A, Yakar S, LeRoith D. Mammalian target of rapamycin inhibition abrogates insulin-mediated mammary tumor progression in type 2 diabetes. Endocr Relat Cancer (2010) 17(4):941–51. doi: 10.1677/ERC-10-0091
155. Gallagher EJ, Fierz Y, Vijayakumar A, Haddad N, Yakar S, LeRoith D. Inhibiting PI3K reduces mammary tumor growth and induces hyperglycemia in a mouse model of insulin resistance and hyperinsulinemia. Oncogene (2012) 531(27):3213–22. doi: 10.1038/onc.2011.495
156. Ancey P-B, Contat C, Meylan E. Glucose transporters in cancer – from tumor cells to the tumor microenvironment. FEBS J (2018) 285(16):2926–43. doi: 10.1111/febs.14577
157. Fainsod-Levi T, Gershkovitz M, Völs S, Kumar S, Khawaled S, Sagiv JY, et al. Hyperglycemia Impairs Neutrophil Mobilization Leading to Enhanced Metastatic Seeding. Cell Rep (2017) 2821(9):2384–92. doi: 10.1016/j.celrep.2017.11.010
158. Ramteke P, Deb A, Shepal V, Bhat MK. Hyperglycemia Associated Metabolic and Molecular Alterations in Cancer Risk, Progression, Treatment, and Mortality. Cancers (2019) 11(9):1402. doi: 10.3390/cancers11091402
159. Rodrigues Mantuano N, Stanczak MA, Oliveira I de A, Kirchhammer N, Filardy AA, Monaco G, et al. Hyperglycemia Enhances Cancer Immune Evasion by Inducing Alternative Macrophage Polarization through Increased O-GlcNAcylation. Cancer Immunol Res (2020) 8(10):1262–72. doi: 10.1158/2326-6066.CIR-19-0904
160. Zhang IY, Zhou H, Liu H, Zhang L, Gao H, Liu S, et al. Local and Systemic Immune Dysregulation Alters Glioma Growth in Hyperglycemic Mice. Clin Cancer Res Off J Am Assoc Cancer Res (2020) 126(11):2740–53. doi: 10.1158/1078-0432.CCR-19-2520
161. Liberti MV, Locasale JW. The Warburg Effect: How Does it Benefit Cancer Cells? Trends Biochem Sci (2016) 41(3):211–8. doi: 10.1016/j.tibs.2015.12.001
162. Hoxhaj G, Manning BD. The PI3K-AKT network at the interface of oncogenic signalling and cancer metabolism. Nat Rev Cancer (2020) 20(2):74–88. doi: 10.1038/s41568-019-0216-7
163. Lee Y, Dominy JE, Choi YJ, Jurczak M, Tolliday N, Camporez JP, et al. Cyclin D1-Cdk4 controls glucose metabolism independently of cell cycle progression. Nature (2014) 510(7506):547–51. doi: 10.1038/nature13267
164. Luo C, Liang J, Sharabi K, Hatting M, Perry EA, Tavares CDJ, et al. Obesity/Type 2 Diabetes-Associated Liver Tumors Are Sensitive to Cyclin D1 Deficiency. Cancer Res (2020) 580(16):3215–21. doi: 10.1158/0008-5472.CAN-20-0106
165. Nasiri AR, Rodrigues MR, Li Z, Leitner BP, Perry RJ. SGLT2 inhibition slows tumor growth in mice by reversing hyperinsulinemia. Cancer Metab (2019) 11:7. doi: 10.1186/s40170-019-0203-1
166. Wang Y, Nasiri AR, Damsky WE, Perry CJ, Zhang X-M, Rabin-Court A, et al. Uncoupling Hepatic Oxidative Phosphorylation Reduces Tumor Growth in Two Murine Models of Colon Cancer. Cell Rep (2018) 0324(1):47–55. doi: 10.1016/j.celrep.2018.06.008
167. Leitner BP, Perry RJ. The Impact of Obesity on Tumor Glucose Uptake in Breast and Lung Cancer. JNCI Cancer Spectr (2020) 4(2):pkaa007. doi: 10.1093/jncics/pkaa007
168. Zelenko Z, Gallagher EJ, Tobin-Hess A, Belardi V, Rostoker R, Blank J, et al. Silencing vimentin expression decreases pulmonary metastases in a pre-diabetic mouse model of mammary tumor progression. Oncogene (2017) 36(10):1394–403. doi: 10.1038/onc.2016.305
169. Sarkar PL, Lee W, Williams ED, Lubik AA, Stylianou N, Shokoohmand A, et al. Insulin Enhances Migration and Invasion in Prostate Cancer Cells by Up-Regulation of FOXC2. Front Endocrinol (2019) 10:481. doi: 10.3389/fendo.2019.00481
170. Simons K, Ikonen E. How cells handle cholesterol. Science (2000) Dec 1290(5497):1721–6. doi: 10.1126/science.290.5497.1721
171. Ikonen E. Cellular cholesterol trafficking and compartmentalization. Nat Rev Mol Cell Biol (2008) Feb9(2):125–38. doi: 10.1038/nrm2336
172. Mullen PJ, Yu R, Longo J, Archer MC, Penn LZ. The interplay between cell signalling and the mevalonate pathway in cancer. Nat Rev Cancer (2016) 16(11):718–31. doi: 10.1038/nrc.2016.76
173. Berndt N, Hamilton AD, Sebti SM. Targeting protein prenylation for cancer therapy. Nat Rev Cancer (2011) 2411(11):775–91. doi: 10.1038/nrc3151
174. Brown MS, Goldstein JL. A proteolytic pathway that controls the cholesterol content of membranes, cells, and blood. Proc Natl Acad Sci U S A (1999) 896(20):11041–8. doi: 10.1073/pnas.96.20.11041
175. Krycer JR, Phan L, Brown AJ. A key regulator of cholesterol homoeostasis, SREBP-2, can be targeted in prostate cancer cells with natural products. Biochem J (2012) Sep 1446(2):191–201. doi: 10.1042/BJ20120545
176. Freed-Pastor WA, Mizuno H, Zhao X, Langerød A, Moon S-H, Rodriguez-Barrueco R, et al. Mutant p53 disrupts mammary tissue architecture via the mevalonate pathway. Cell (2012) 148(1–2):244–58. doi: 10.1016/j.cell.2011.12.017
177. Sorrentino G, Ruggeri N, Specchia V, Cordenonsi M, Mano M, Dupont S, et al. Metabolic control of YAP and TAZ by the mevalonate pathway. Nat Cell Biol (2014) Apr16(4):357–66. doi: 10.1038/ncb2936
178. Huang B, Song B-L, Xu C. Cholesterol metabolism in cancer: mechanisms and therapeutic opportunities. Nat Metab (2020) 2(2):132–41. doi: 10.1038/s42255-020-0174-0
179. Lin C-Y, Gustafsson J-Å. Targeting liver X receptors in cancer therapeutics. Nat Rev Cancer (2015) 15(4):216–24. doi: 10.1038/nrc3912
180. Liu J, Xu A, Lam KS-L, Wong N-S, Chen J, Shepherd PR, et al. Cholesterol-induced mammary tumorigenesis is enhanced by adiponectin deficiency: role of LDL receptor upregulation. Oncotarget (2013) 4(10):1804–18. doi: 10.18632/oncotarget.1364
181. Alikhani N, Ferguson RD, Novosyadlyy R, Gallagher EJ, Scheinman EJ, Yakar S, et al. Mammary tumor growth and pulmonary metastasis are enhanced in a hyperlipidemic mouse model. Oncogene (2013) 132(8):961–7. doi: 10.1038/onc.2012.113
182. Gallagher EJ, Zelenko Z, Neel BA, Antoniou IM, Rajan L, Kase N, et al. Elevated tumor LDLR expression accelerates LDL cholesterol-mediated breast cancer growth in mouse models of hyperlipidemia. Oncogene (2017) 1636(46):6462–71. doi: 10.1038/onc.2017.247
183. Nelson ER, Wardell SE, Jasper JS, Park S, Suchindran S, Howe MK, et al. 27-Hydroxycholesterol links hypercholesterolemia and breast cancer pathophysiology. Science (2013) 342(6162):1094–8. doi: 10.1126/science.1241908
184. Guillaumond F, Bidaut G, Ouaissi M, Servais S, Gouirand V, Olivares O, et al. Cholesterol uptake disruption, in association with chemotherapy, is a promising combined metabolic therapy for pancreatic adenocarcinoma. Proc Natl Acad Sci U S A (2015) 112(8):2473–8. doi: 10.1073/pnas.1421601112
185. Ho YK, Smith RG, Brown MS, Goldstein JL. Low-density lipoprotein (LDL) receptor activity in human acute myelogenous leukemia cells. Blood (1978) 52(6):1099–114. doi: 10.1182/blood.V52.6.1099.bloodjournal5261099
186. Murtola TJ, Syvälä H, Pennanen P, Bläuer M, Solakivi T, Ylikomi T, et al. The importance of LDL and cholesterol metabolism for prostate epithelial cell growth. PloS One (2012) 7(6):e39445. doi: 10.1371/journal.pone.0039445
187. Stranzl A, Schmidt H, Winkler R, Kostner GM. Low-density lipoprotein receptor mRNA in human breast cancer cells: influence by PKC modulators. Breast Cancer Res Treat (1997) 42(3):195–205. doi: 10.1023/A:1005754026205
188. Danilo C, Gutierrez-Pajares JL, Mainieri MA, Mercier I, Lisanti MP, Frank PG. Scavenger receptor class B type I regulates cellular cholesterol metabolism and cell signaling associated with breast cancer development. Breast Cancer Res BCR (2013) 15(5):R87. doi: 10.1186/bcr3483
189. Gordon JA, Noble JW, Midha A, Derakhshan F, Wang G, Adomat HH, et al. Upregulation of Scavenger Receptor B1 Is Required for Steroidogenic and Nonsteroidogenic Cholesterol Metabolism in Prostate Cancer. Cancer Res (2019) 0179(13):3320–31. doi: 10.1158/0008-5472.CAN-18-2529
190. Gal D, MacDonald PC, Porter JC, Simpson ER. Cholesterol metabolism in cancer cells in monolayer culture. III. Low-density Lipoprotein Metab Int J Cancer (1981) 28(3):315–9. doi: 10.1002/ijc.2910280310
191. Yue S, Li J, Lee S-Y, Lee HJ, Shao T, Song B, et al. Cholesteryl ester accumulation induced by PTEN loss and PI3K/AKT activation underlies human prostate cancer aggressiveness. Cell Metab (2014) Mar 419(3):393–406. doi: 10.1016/j.cmet.2014.01.019
192. Graziani SR, Igreja FAF, Hegg R, Meneghetti C, Brandizzi LI, Barboza R, et al. Uptake of a cholesterol-rich emulsion by breast cancer. Gynecol Oncol (2002) 85(3):493–7. doi: 10.1006/gyno.2002.6654
193. de Gonzalo-Calvo D, López-Vilaró L, Nasarre L, Perez-Olabarria M, Vázquez T, Escuin D, et al. Intratumor cholesteryl ester accumulation is associated with human breast cancer proliferation and aggressive potential: a molecular and clinicopathological study. BMC Cancer (2015) 915:460. doi: 10.1186/s12885-015-1469-5
194. Accioly MT, Pacheco P, Maya-Monteiro CM, Carrossini N, Robbs BK, Oliveira SS, et al. Lipid bodies are reservoirs of cyclooxygenase-2 and sites of prostaglandin-E2 synthesis in colon cancer cells. Cancer Res (2008) Mar 1568(6):1732–40. doi: 10.1158/0008-5472.CAN-07-1999
195. Mulas MF, Abete C, Pulisci D, Pani A, Massidda B, Dessì S, et al. Cholesterol esters as growth regulators of lymphocytic leukaemia cells. Cell Prolif (2011) Aug44(4):360–71. doi: 10.1111/j.1365-2184.2011.00758.x
196. Antalis CJ, Uchida A, Buhman KK, Siddiqui RA. Migration of MDA-MB-231 breast cancer cells depends on the availability of exogenous lipids and cholesterol esterification. Clin Exp Metastasis (2011) 28(8):733–41. doi: 10.1007/s10585-011-9405-9
197. Wang J, Tan M, Ge J, Zhang P, Zhong J, Tao L, et al. Lysosomal acid lipase promotes cholesterol ester metabolism and drives clear cell renal cell carcinoma progression. Cell Prolif (2018) 51(4):e12452. doi: 10.1111/cpr.12452
198. Badana A, Chintala M, Varikuti G, Pudi N, Kumari S, Kappala VR, et al. Lipid Raft Integrity Is Required for Survival of Triple Negative Breast Cancer Cells. J Breast Cancer (2016) 19(4):372–84. doi: 10.4048/jbc.2016.19.4.372
199. Szlasa W, Zendran I, Zalesińska A, Tarek M, Kulbacka J. Lipid composition of the cancer cell membrane. J Bioenerg Biomembr (2020) 52(5):321–42. doi: 10.1007/s10863-020-09846-4
200. Stancu C, Sima A. Statins: mechanism of action and effects. J Cell Mol Med (2001) 5(4):378–87. doi: 10.1111/j.1582-4934.2001.tb00172.x
201. Schachter M. Chemical, pharmacokinetic and pharmacodynamic properties of statins: an update. Fundam Clin Pharmacol (2005) 19(1):117–25. doi: 10.1111/j.1472-8206.2004.00299.x
202. Beckwitt CH, Brufsky A, Oltvai ZN, Wells A. Statin drugs to reduce breast cancer recurrence and mortality. Breast Cancer Res BCR (2018) 2020(1):144. doi: 10.1186/s13058-018-1066-z
203. Davignon J. Beneficial cardiovascular pleiotropic effects of statins. Circulation (2004) 109(23 Suppl 1):III39–43. doi: 10.1161/01.CIR.0000131517.20177.5a
204. Albert MA, Danielson E, Rifai N, Ridker PM, Investigators PRINCE. Effect of statin therapy on C-reactive protein levels: the pravastatin inflammation/CRP evaluation (PRINCE): a randomized trial and cohort study. JAMA (2001) 286(1):64–70. doi: 10.1001/jama.286.1.64
205. Link A, Ayadhi T, Böhm M, Nickenig G. Rapid immunomodulation by rosuvastatin in patients with acute coronary syndrome. Eur Heart J (2006) 27(24):2945–55. doi: 10.1093/eurheartj/ehl277
206. McGregor GH, Campbell AD, Fey SK, Tumanov S, Sumpton D, Blanco GR, et al. Targeting the Metabolic Response to Statin-Mediated Oxidative Stress Produces a Synergistic Antitumor Response. Cancer Res (2019) 80(2):175–88. doi: 10.1158/0008-5472.CAN-19-0644
207. Björkhem I, Meaney S, Diczfalusy U. Oxysterols in human circulation: which role do they have? Curr Opin Lipidol (2002) Jun;13(3):247–53. doi: 10.1097/00041433-200206000-00003
208. Fu X, Menke JG, Chen Y, Zhou G, MacNaul KL, Wright SD, et al. 27-hydroxycholesterol is an endogenous ligand for liver X receptor in cholesterol-loaded cells. J Biol Chem (2001) 276(42):38378–87. doi: 10.1074/jbc.M105805200
209. Umetani M, Ghosh P, Ishikawa T, Umetani J, Ahmed M, Mineo C, et al. The cholesterol metabolite 27-hydroxycholesterol promotes atherosclerosis via proinflammatory processes mediated by estrogen receptor alpha. Cell Metab (2014) 120(1):172–82. doi: 10.1016/j.cmet.2014.05.013
210. DuSell CD, McDonnell DP. 27-Hydroxycholesterol: a potential endogenous regulator of estrogen receptor signaling. Trends Pharmacol Sci (2008) Oct29(10):510–4. doi: 10.1016/j.tips.2008.07.003
211. Starkey NJE, Li Y, Drenkhahn-Weinaug SK, Liu J, Lubahn DB. 27-Hydroxycholesterol Is an Estrogen Receptor β-Selective Negative Allosteric Modifier of 17β-Estradiol Binding. Endocrinology (2018) 01159(5):1972–81. doi: 10.1210/en.2018-00081
212. DuSell CD, Umetani M, Shaul PW, Mangelsdorf DJ, McDonnell DP. 27-hydroxycholesterol is an endogenous selective estrogen receptor modulator. Mol Endocrinol Baltim Md (2008) 22(1):65–77. doi: 10.1210/me.2007-0383
213. DuSell CD, Nelson ER, Wang X, Abdo J, Mödder UI, Umetani M, et al. The endogenous selective estrogen receptor modulator 27-hydroxycholesterol is a negative regulator of bone homeostasis. Endocrinology (2010) Aug151(8):3675–85. doi: 10.1210/en.2010-0080
214. Umetani M, Domoto H, Gormley AK, Yuhanna IS, Cummins CL, Javitt NB, et al. 27-Hydroxycholesterol is an endogenous SERM that inhibits the cardiovascular effects of estrogen. Nat Med (2007) Oct13(10):1185–92. doi: 10.1038/nm1641
215. Hirayama T, Mizokami Y, Honda A, Homma Y, Ikegami T, Saito Y, et al. Serum concentration of 27-hydroxycholesterol predicts the effects of high-cholesterol diet on plasma LDL cholesterol level. Hepatol Res Off J Jpn Soc Hepatol (2009) 39(2):149–56. doi: 10.1111/j.1872-034X.2008.00450.x
216. Kloudova A, Guengerich FP, Soucek P. The Role of Oxysterols in Human Cancer. Trends Endocrinol Metab TEM (2017) 28(7):485–96. doi: 10.1016/j.tem.2017.03.002
217. Munir MT, Ponce C, Powell CA, Tarafdar K, Yanagita T, Choudhury M, et al. The contribution of cholesterol and epigenetic changes to the pathophysiology of breast cancer. J Steroid Biochem Mol Biol (2018) 183:1–9. doi: 10.1016/j.jsbmb.2018.05.001
218. Wu Q, Ishikawa T, Sirianni R, Tang H, McDonald JG, Yuhanna IS, et al. 27-Hydroxycholesterol promotes cell-autonomous, ER-positive breast cancer growth. Cell Rep (2013) 145(3):637–45. doi: 10.1016/j.celrep.2013.10.006
219. Roberg-Larsen H, Lund K, Seterdal KE, Solheim S, Vehus T, Solberg N, et al. Mass spectrometric detection of 27-hydroxycholesterol in breast cancer exosomes. J Steroid Biochem Mol Biol (2017) 169:22–8. doi: 10.1016/j.jsbmb.2016.02.006
220. Shi S-Z, Lee E-J, Lin Y-J, Chen L, Zheng H-Y, He X-Q, et al. Recruitment of monocytes and epigenetic silencing of intratumoral CYP7B1 primarily contribute to the accumulation of 27-hydroxycholesterol in breast cancer. Am J Cancer Res (2019) 9(10):2194–208.
221. Kimbung S, Chang C-Y, Bendahl P-O, Dubois L, Thompson JW, McDonnell DP, et al. Impact of 27-hydroxylase (CYP27A1) and 27-hydroxycholesterol in breast cancer. Endocr Relat Cancer (2017) 24(7):339–49. doi: 10.1530/ERC-16-0533
222. Le Cornet C, Walter B, Sookthai D, Johnson TS, Kühn T, Herpel E, et al. Circulating 27-hydroxycholesterol and breast cancer tissue expression of CYP27A1, CYP7B1, LXR-β, and ERβ: results from the EPIC-Heidelberg cohort. Breast Cancer Res BCR (2020) 1922(1):23. doi: 10.1186/s13058-020-1253-6
223. Lu D-L, Le Cornet C, Sookthai D, Johnson TS, Kaaks R, Fortner RT. Circulating 27-Hydroxycholesterol and Breast Cancer Risk: Results From the EPIC-Heidelberg Cohort. J Natl Cancer Inst (2019) 01111(4):365–71. doi: 10.1093/jnci/djy115
224. Asghari A, Umetani M. Obesity and Cancer: 27-Hydroxycholesterol, the Missing Link. Int J Mol Sci (2020) 21(14):4822. doi: 10.3390/ijms21144822
225. Raza S, Ohm JE, Dhasarathy A, Schommer J, Roche C, Hammer KDP, et al. The cholesterol metabolite 27-hydroxycholesterol regulates p53 activity and increases cell proliferation via MDM2 in breast cancer cells. Mol Cell Biochem (2015) Dec410(1–2):187–95. doi: 10.1007/s11010-015-2551-7
226. Baek AE, Yu Y-RA, He S, Wardell SE, Chang C-Y, Kwon S, et al. The cholesterol metabolite 27 hydroxycholesterol facilitates breast cancer metastasis through its actions on immune cells. Nat Commun (2017) 118(1):864. doi: 10.1038/s41467-017-00910-z
227. Shen Z, Zhu D, Liu J, Chen J, Liu Y, Hu C, et al. 27-Hydroxycholesterol induces invasion and migration of breast cancer cells by increasing MMP9 and generating EMT through activation of STAT-3. Environ Toxicol Pharmacol (2017) 51:1–8. doi: 10.1016/j.etap.2017.02.001
228. Torres CG, Ramírez ME, Cruz P, Epuñan MJ, Valladares LE, Sierralta WD. 27-hydroxycholesterol induces the transition of MCF7 cells into a mesenchymal phenotype. Oncol Rep (2011) 26(2):389–97. doi: 10.3892/or.2011.1284
229. Shen Z, Jiao K, Teng M, Li Z. Activation of STAT-3 signalling by RECK downregulation via ROS is involved in the 27-hydroxycholesterol-induced invasion in breast cancer cells. Free Radic Res (2020) Mar54(2–3):126–36. doi: 10.1080/10715762.2020.1715965
230. Zhu D, Shen Z, Liu J, Chen J, Liu Y, Hu C, et al. The ROS-mediated activation of STAT-3/VEGF signaling is involved in the 27-hydroxycholesterol-induced angiogenesis in human breast cancer cells. Toxicol Lett (2016) Dec 15264:79–86. doi: 10.1016/j.toxlet.2016.11.006
231. Jiao K, Zhen J, Wu M, Teng M, Yang K, Zhou Q, et al. 27-Hydroxycholesterol-induced EndMT acts via STAT3 signaling to promote breast cancer cell migration by altering the tumor microenvironment. Cancer Biol Med (2020) Feb 1517(1):88–100. doi: 10.20892/j.issn.2095-3941.2019.0262
232. Gibson DA, Collins F, Cousins FL, Esnal Zufiaurre A, Saunders PTK. The impact of 27-hydroxycholesterol on endometrial cancer proliferation. Endocr Relat Cancer (2018) 25(4):381–91. doi: 10.1530/ERC-17-0449
233. Hiramitsu S, Ishikawa T, Lee W-R, Khan T, Crumbley C, Khwaja N, et al. Estrogen Receptor Beta-Mediated Modulation of Lung Cancer Cell Proliferation by 27-Hydroxycholesterol. Front Endocrinol (2018) 9:470. doi: 10.3389/fendo.2018.00470
234. Tian W, Pang W, Ge Y, He X, Wang D, Li X, et al. Hepatocyte-generated 27-hydroxycholesterol promotes the growth of melanoma by activation of estrogen receptor alpha. J Cell Biochem (2018) 119(3):2929–38. doi: 10.1002/jcb.26498
235. Dambal S, Alfaqih M, Sanders S, Maravilla E, Ramirez-Torres A, Galvan GC, et al. 27-Hydroxycholesterol Impairs Plasma Membrane Lipid Raft Signaling as Evidenced by Inhibition of IL6-JAK-STAT3 Signaling in Prostate Cancer Cells. Mol Cancer Res MCR (2020) 18(5):671–84. doi: 10.1158/1541-7786.MCR-19-0974
236. Guo F, Hong W, Yang M, Xu D, Bai Q, Li X, et al. Upregulation of 24(R/S),25-epoxycholesterol and 27-hydroxycholesterol suppresses the proliferation and migration of gastric cancer cells. Biochem Biophys Res Commun (2018) 12504(4):892–8. doi: 10.1016/j.bbrc.2018.09.058
237. Warns J, Marwarha G, Freking N, Ghribi O. 27-hydroxycholesterol decreases cell proliferation in colon cancer cell lines. Biochimie (2018) 153:171–80. doi: 10.1016/j.biochi.2018.07.006
238. Raza S, Meyer M, Goodyear C, Hammer KDP, Guo B, Ghribi O. The cholesterol metabolite 27-hydroxycholesterol stimulates cell proliferation via ERβ in prostate cancer cells. Cancer Cell Int (2017) 17:52. doi: 10.1186/s12935-017-0422-x
239. Alfaqih MA, Nelson ER, Liu W, Safi R, Jasper JS, Macias E, et al. CYP27A1 Loss Dysregulates Cholesterol Homeostasis in Prostate Cancer. Cancer Res (2017) 0177(7):1662–73. doi: 10.1158/0008-5472.CAN-16-2738
240. Hoy AJ, Balaban S, Saunders DN. Adipocyte-Tumor Cell Metabolic Crosstalk in Breast Cancer. Trends Mol Med (2017) 23(5):381–92. doi: 10.1016/j.molmed.2017.02.009
241. Corn KC, Windham MA, Rafat M. Lipids in the tumor microenvironment: From cancer progression to treatment. Prog Lipid Res (2020) Aug 11:101055. doi: 10.1016/j.plipres.2020.101055
242. Nieman KM, Kenny HA, Penicka CV, Ladanyi A, Buell-Gutbrod R, Zillhardt MR, et al. Adipocytes promote ovarian cancer metastasis and provide energy for rapid tumor growth. Nat Med (2011) Oct 3017(11):1498–503. doi: 10.1038/nm.2492
243. Pascual G, Avgustinova A, Mejetta S, Martín M, Castellanos A, Attolini CS-O, et al. Targeting metastasis-initiating cells through the fatty acid receptor CD36. Nature (2017) 05541(7635):41–5. doi: 10.1038/nature20791
244. Snaebjornsson MT, Janaki-Raman S, Schulze A. Greasing the Wheels of the Cancer Machine: The Role of Lipid Metabolism in Cancer. Cell Metab (2020) Jan 731(1):62–76. doi: 10.1016/j.cmet.2019.11.010
245. Wang T, Fahrmann JF, Lee H, Li Y-J, Tripathi SC, Yue C, et al. JAK/STAT3-Regulated Fatty Acid β-Oxidation Is Critical for Breast Cancer Stem Cell Self-Renewal and Chemoresistance. Cell Metab (2018) 27(1):136–150.e5. doi: 10.1016/j.cmet.2017.11.001
246. Wang YY, Attané C, Milhas D, Dirat B, Dauvillier S, Guerard A, et al. Mammary adipocytes stimulate breast cancer invasion through metabolic remodeling of tumor cells. JCI Insight (2017) 2(4):e87489. doi: 10.1172/jci.insight.87489
247. Zhang M, Di Martino JS, Bowman RL, Campbell NR, Baksh SC, Simon-Vermot T, et al. Adipocyte-Derived Lipids Mediate Melanoma Progression via FATP Proteins. Cancer Discovery (2018) 8(8):1006–25. doi: 10.1158/2159-8290.CD-17-1371
248. Ladanyi A, Mukherjee A, Kenny HA, Johnson A, Mitra AK, Sundaresan S, et al. Adipocyte-induced CD36 expression drives ovarian cancer progression and metastasis. Oncogene (2018) 37(17):2285–301. doi: 10.1038/s41388-017-0093-z
249. Balaban S, Shearer RF, Lee LS, van Geldermalsen M, Schreuder M, Shtein HC, et al. Adipocyte lipolysis links obesity to breast cancer growth: adipocyte-derived fatty acids drive breast cancer cell proliferation and migration. Cancer Metab (2017) 5:1. doi: 10.1186/s40170-016-0163-7
250. Blucher C, Iberl S, Schwagarus N, Muller S, Liebisch G, Horing M, et al. Secreted factors from adipose tissue reprogram tumor lipid metabolism and induce motility by modulating PPARα/ANGPTL4 and FAK. Mol Cancer Res (2020) 18(12):1849–62. doi: 10.1158/1541-7786.MCR-19-1223
251. Michelet X, Dyck L, Hogan A, Loftus RM, Duquette D, Wei K, et al. Metabolic reprogramming of natural killer cells in obesity limits antitumor responses. Nat Immunol (2018) 19(12):1330–40. doi: 10.1038/s41590-018-0251-7
252. Al-Khami AA, Zheng L, Del Valle L, Hossain F, Wyczechowska D, Zabaleta J, et al. Exogenous lipid uptake induces metabolic and functional reprogramming of tumor-associated myeloid-derived suppressor cells. OncoImmunology (2017) 36(10):e1344804. doi: 10.1080/2162402X.2017.1344804
253. Su P, Wang Q, Bi E, Ma X, Liu L, Yang M, et al. Enhanced Lipid Accumulation and Metabolism Are Required for the Differentiation and Activation of Tumor-Associated Macrophages. Cancer Res (2020) 0180(7):1438–50. doi: 10.1158/0008-5472.CAN-19-2994
254. Wang H, Franco F, Tsui Y-C, Xie X, Trefny MP, Zappasodi R, et al. CD36-mediated metabolic adaptation supports regulatory T cell survival and function in tumors. Nat Immunol (2020) 21(3):298–308. doi: 10.1038/s41590-019-0589-5
255. Plitas G, Rudensky AY. Regulatory T Cells in Cancer. Annu Rev Cancer Biol (2020) 4(1):459–77. doi: 10.1146/annurev-cancerbio-030419-033428
256. Howie D, Cobbold SP, Adams E, Ten Bokum A, Necula AS, Zhang W, et al. Foxp3 drives oxidative phosphorylation and protection from lipotoxicity. JCI Insight (2017) Feb 92(3):e89160. doi: 10.1172/jci.insight.89160
257. Lochner M, Berod L, Sparwasser T. Fatty acid metabolism in the regulation of T cell function. Trends Immunol (2015) 136(2):81–91. doi: 10.1016/j.it.2014.12.005
258. Zhang C, Yue C, Herrmann A, Song J, Egelston C, Wang T, et al. STAT3 Activation-Induced Fatty Acid Oxidation in CD8+ T Effector Cells Is Critical for Obesity-Promoted Breast Tumor Growth. Cell Metab (2020) 731(1):148–61.e5. doi: 10.1016/j.cmet.2019.10.013
259. Zhang Y, Kurupati R, Liu L, Zhou XY, Zhang G, Hudaihed A, et al. Enhancing CD8+ T Cell Fatty Acid Catabolism within a Metabolically Challenging Tumor Microenvironment Increases the Efficacy of Melanoma Immunotherapy. Cancer Cell (2017) 32(3):377–91.e9. doi: 10.1016/j.ccell.2017.08.004
260. Chowdhury PS, Chamoto K, Kumar A, Honjo T. PPAR-Induced Fatty Acid Oxidation in T Cells Increases the Number of Tumor-Reactive CD8+ T Cells and Facilitates Anti-PD-1 Therapy. Cancer Immunol Res (2018) 6(11):1375–87. doi: 10.1158/2326-6066.CIR-18-0095
261. Quail DF, Dannenberg AJ. The obese adipose tissue microenvironment in cancer development and progression. Nat Rev Endocrinol (2019) Mar15(3):139–54. doi: 10.1038/s41574-018-0126-x
262. Lengyel E, Makowski L, DiGiovanni J, Kolonin MG. Cancer as a Matter of Fat: The Crosstalk between Adipose Tissue and Tumors. Trends Cancer (2018) 4(5):374–84. doi: 10.1016/j.trecan.2018.03.004
263. Klöting N, Blüher M. Adipocyte dysfunction, inflammation and metabolic syndrome. Rev Endocr Metab Disord (2014) Dec15(4):277–87. doi: 10.1007/s11154-014-9301-0
264. Arner E, Westermark PO, Spalding KL, Britton T, Rydén M, Frisén J, et al. Adipocyte turnover: relevance to human adipose tissue morphology. Diabetes (2010) Jan59(1):105–9. doi: 10.2337/db09-0942
265. Cignarelli A, Genchi VA, Perrini S, Natalicchio A, Laviola L, Giorgino F. Insulin and Insulin Receptors in Adipose Tissue Development. Int J Mol Sci (2019) 20(3):759. doi: 10.3390/ijms20030759
266. Zhang T, Tseng C, Zhang Y, Sirin O, Corn PG, Li-Ning-Tapia EM, et al. CXCL1 mediates obesity-associated adipose stromal cell trafficking and function in the tumour microenvironment. Nat Commun (2016) 317:11674. doi: 10.1038/ncomms11674
267. Zhang Y, Daquinag AC, Amaya-Manzanares F, Sirin O, Tseng C, Kolonin MG. Stromal progenitor cells from endogenous adipose tissue contribute to pericytes and adipocytes that populate the tumor microenvironment. Cancer Res (2012) 72(20):5198–208. doi: 10.1158/0008-5472.CAN-12-0294
268. Bellows CF, Zhang Y, Simmons PJ, Khalsa AS, Kolonin MG. Influence of BMI on level of circulating progenitor cells. Obes Silver Spring Md (2011) Aug19(8):1722–6. doi: 10.1038/oby.2010.347
269. Bellows CF, Zhang Y, Chen J, Frazier ML, Kolonin MG. Circulation of progenitor cells in obese and lean colorectal cancer patients. Cancer Epidemiol Biomark Prev Publ Am Assoc Cancer Res Cosponsored Am Soc Prev Oncol (2011) 20(11):2461–8. doi: 10.1158/1055-9965.EPI-11-0556
270. Martin-Padura I, Gregato G, Marighetti P, Mancuso P, Calleri A, Corsini C, et al. The white adipose tissue used in lipotransfer procedures is a rich reservoir of CD34+ progenitors able to promote cancer progression. Cancer Res (2012) 172(1):325–34. doi: 10.1158/0008-5472.CAN-11-1739
271. Zhang Y, Daquinag A, Traktuev DO, Amaya-Manzanares F, Simmons PJ, March KL, et al. White adipose tissue cells are recruited by experimental tumors and promote cancer progression in mouse models. Cancer Res (2009) 69(12):5259–66. doi: 10.1158/0008-5472.CAN-08-3444
272. Reggiani F, Labanca V, Mancuso P, Rabascio C, Talarico G, Orecchioni S, et al. Adipose Progenitor Cell Secretion of GM-CSF and MMP9 Promotes a Stromal and Immunological Microenvironment That Supports Breast Cancer Progression. Cancer Res (2017) 77(18):5169–82. doi: 10.1158/0008-5472.CAN-17-0914
273. Su F, Ahn S, Saha A, DiGiovanni J, Kolonin MG. Adipose stromal cell targeting suppresses prostate cancer epithelial-mesenchymal transition and chemoresistance. Oncogene (2019) 38(11):1979–88. doi: 10.1038/s41388-018-0558-8
274. Strissel KJ, Stancheva Z, Miyoshi H, Perfield JW, DeFuria J, Jick Z, et al. Adipocyte death, adipose tissue remodeling, and obesity complications. Diabetes (2007) 56(12):2910–8. doi: 10.2337/db07-0767
275. Crewe C, An YA, Scherer PE. The ominous triad of adipose tissue dysfunction: inflammation, fibrosis, and impaired angiogenesis. J Clin Invest (2017) 03127(1):74–82. doi: 10.1172/JCI88883
276. Seo BR, Bhardwaj P, Choi S, Gonzalez J, Andresen Eguiluz RC, Wang K, et al. Obesity-dependent changes in interstitial ECM mechanics promote breast tumorigenesis. Sci Transl Med (2015) 7(301):301ra130. doi: 10.1126/scitranslmed.3010467
277. Spencer M, Yao-Borengasser A, Unal R, Rasouli N, Gurley CM, Zhu B, et al. Adipose tissue macrophages in insulin-resistant subjects are associated with collagen VI and fibrosis and demonstrate alternative activation. Am J Physiol Endocrinol Metab (2010) 299(6):E1016–1027. doi: 10.1152/ajpendo.00329.2010
278. Iyengar P, Espina V, Williams TW, Lin Y, Berry D, Jelicks LA, et al. Adipocyte-derived collagen VI affects early mammary tumor progression in vivo, demonstrating a critical interaction in the tumor/stroma microenvironment. J Clin Invest (2005) 115(5):1163–76. doi: 10.1172/JCI23424
279. Park J, Scherer PE. Adipocyte-derived endotrophin promotes malignant tumor progression. J Clin Invest (2012) 122(11):4243–56. doi: 10.1172/JCI63930
280. Sun K, Park J, Gupta OT, Holland WL, Auerbach P, Zhang N, et al. Endotrophin triggers adipose tissue fibrosis and metabolic dysfunction. Nat Commun (2014) 195:3485. doi: 10.1038/ncomms4485
281. Bu D, Crewe C, Kusminski CM, Gordillo R, Ghaben AL, Kim M, et al. Human endotrophin as a driver of malignant tumor growth. JCI Insight (2019) 21:5. doi: 10.1172/jci.insight.125094
282. Qian B, Pollard JW. Macrophage Diversity Enhances Tumor Progression and Metastasis. Cell (2010) 141(1):39–51. doi: 10.1016/j.cell.2010.03.014
283. Arendt LM, McCready J, Keller PJ, Baker DD, Naber SP, Seewaldt V, et al. Obesity promotes breast cancer by CCL2-mediated macrophage recruitment and angiogenesis. Cancer Res (2013) 73(19):6080–93. doi: 10.1158/0008-5472.CAN-13-0926
284. Kolb R, Phan L, Borcherding N, Liu Y, Yuan F, Janowski AM, et al. Obesity-associated NLRC4 inflammasome activation drives breast cancer progression. Nat Commun (2016) 067:13007. doi: 10.1038/ncomms13007
285. Kolb R, Kluz P, Tan ZW, Borcherding N, Bormann N, Vishwakarma A, et al. Obesity-associated inflammation promotes angiogenesis and breast cancer via angiopoietin-like 4. Oncogene (2019) 38(13):2351–63. doi: 10.1038/s41388-018-0592-6
286. Springer NL, Iyengar NM, Bareja R, Verma A, Jochelson MS, Giri DD, et al. Obesity-Associated Extracellular Matrix Remodeling Promotes a Macrophage Phenotype Similar to Tumor-Associated Macrophages. Am J Pathol (2019) 189(10):2019–35. doi: 10.1016/j.ajpath.2019.06.005
287. Chamberlin T, Clack M, Silvers C, Kuziel G, Thompson V, Johnson H, et al. Targeting obesity-induced macrophages during preneoplastic growth promotes mammary epithelial stem/progenitor activity, DNA damage and tumor formation. Cancer Res (2020) 80(20):4465–75. doi: 10.1158/0008-5472.CAN-20-0789. https://cancerres.aacrjournals.org/content/early/2020/08/29/0008-5472.CAN-20-0789. Jan 1 [cited 2020 Sep 6].
288. Cinti S, Mitchell G, Barbatelli G, Murano I, Ceresi E, Faloia E, et al. Adipocyte death defines macrophage localization and function in adipose tissue of obese mice and humans. J Lipid Res (2005) 46(11):2347–55. doi: 10.1194/jlr.M500294-JLR200
289. Iyengar NM, Zhou XK, Gucalp A, Morris PG, Howe LR, Giri DD, et al. Systemic Correlates of White Adipose Tissue Inflammation in Early-Stage Breast Cancer. Clin Cancer Res Off J Am Assoc Cancer Res (2016) 0122(9):2283–9. doi: 10.1158/1078-0432.CCR-15-2239
290. Iyengar NM, Brown KA, Zhou XK, Gucalp A, Subbaramaiah K, Giri DD, et al. Metabolic Obesity, Adipose Inflammation and Elevated Breast Aromatase in Women with Normal Body Mass Index. Cancer Prev Res Phila Pa (2017) 10(4):235–43. doi: 10.1158/1940-6207.CAPR-16-0314
291. Carter JM, Hoskin TL, Pena MA, Brahmbhatt R, Winham SJ, Frost MH, et al. Macrophagic “Crown-like Structures” Are Associated with an Increased Risk of Breast Cancer in Benign Breast Disease. Cancer Prev Res Phila Pa (2018) 11(2):113–9. doi: 10.1158/1940-6207.CAPR-17-0245
292. Morris PG, Hudis CA, Giri D, Morrow M, Falcone DJ, Zhou XK, et al. Inflammation and increased aromatase expression occur in the breast tissue of obese women with breast cancer. Cancer Prev Res Phila Pa (2011) 4(7):1021–9. doi: 10.1158/1940-6207.CAPR-11-0110
293. Sun X, Casbas-Hernandez P, Bigelow C, Makowski L, Joseph Jerry D, Smith Schneider S, et al. Normal breast tissue of obese women is enriched for macrophage markers and macrophage-associated gene expression. Breast Cancer Res Treat (2012) 131(3):1003–12. doi: 10.1007/s10549-011-1789-3
294. Vaysse C, Lømo J, Garred Ø, Fjeldheim F, Lofteroed T, Schlichting E, et al. Inflammation of mammary adipose tissue occurs in overweight and obese patients exhibiting early-stage breast cancer. NPJ Breast Cancer (2017) 3:19. doi: 10.1038/s41523-017-0030-x
295. Maliniak ML, Cheriyan AM, Sherman ME, Liu Y, Gogineni K, Liu J, et al. Detection of crown-like structures in breast adipose tissue and clinical outcomes among African-American and White women with breast cancer. Breast Cancer Res (2020) 22(1):65. doi: 10.1186/s13058-020-01308-4
296. Greenlee H, Shi Z, Hibshoosh H, Giri DD, Ahmed A, Williams S, et al. Obesity-associated Breast Inflammation among Hispanic/Latina Breast Cancer Patients. Cancer Prev Res Phila Pa (2019) 12(1):21–30. doi: 10.1158/1940-6207.CAPR-18-0207
297. Gucalp A, Iyengar NM, Zhou XK, Giri DD, Falcone DJ, Wang H, et al. Periprostatic adipose inflammation is associated with high-grade prostate cancer. Prostate Cancer Prostatic Dis (2017) 20(4):418–23. doi: 10.1038/pcan.2017.31
298. Subbaramaiah K, Howe LR, Bhardwaj P, Du B, Gravaghi C, Yantiss RK, et al. Obesity is associated with inflammation and elevated aromatase expression in the mouse mammary gland. Cancer Prev Res Phila Pa (2011) 4(3):329–46. doi: 10.1158/1940-6207.CAPR-10-0381
299. Bowers LW, Brenner AJ, Hursting SD, Tekmal RR, deGraffenried LA. Obesity-associated systemic interleukin-6 promotes pre-adipocyte aromatase expression via increased breast cancer cell prostaglandin E2 production. Breast Cancer Res Treat (2015) 149(1):49–57. doi: 10.1007/s10549-014-3223-0
300. Iyengar NM, Morris PG, Zhou XK, Gucalp A, Giri D, Harbus MD, et al. Menopause is a determinant of breast adipose inflammation. Cancer Prev Res Phila Pa (2015) May8(5):349–58. doi: 10.1158/1940-6207.CAPR-14-0243
301. Brown KA, Iyengar NM, Zhou XK, Gucalp A, Subbaramaiah K, Wang H, et al. Menopause Is a Determinant of Breast Aromatase Expression and Its Associations With BMI, Inflammation, and Systemic Markers. J Clin Endocrinol Metab (2017) 01;102(5):1692–701. doi: 10.1210/jc.2016-3606
302. Ahima RS, Flier JS. Leptin. Annu Rev Physiol (2000) 62:413–37. doi: 10.1146/annurev.physiol.62.1.413
303. Maffei M, Halaas J, Ravussin E, Pratley RE, Lee GH, Zhang Y, et al. Leptin levels in human and rodent: measurement of plasma leptin and ob RNA in obese and weight-reduced subjects. Nat Med (1995) 1(11):1155–61. doi: 10.1038/nm1195-1155
304. Andò S, Gelsomino L, Panza S, Giordano C, Bonofiglio D, Barone I, et al. Obesity, Leptin and Breast Cancer: Epidemiological Evidence and Proposed Mechanisms. Cancers (2019) 11(1):62. doi: 10.3390/cancers11010062
305. Zheng Q, Dunlap SM, Zhu J, Downs-Kelly E, Rich J, Hursting SD, et al. Leptin deficiency suppresses MMTV-Wnt-1 mammary tumor growth in obese mice and abrogates tumor initiating cell survival. Endocr Relat Cancer (2011) 18(4):491–503. doi: 10.1530/ERC-11-0102
306. Nunez NP, Oh W-J, Rozenberg J, Perella C, Anver M, Barrett JC, et al. Accelerated tumor formation in a fatless mouse with type 2 diabetes and inflammation. Cancer Res (2006) 1566(10):5469–76. doi: 10.1158/0008-5472.CAN-05-4102
307. Yin N, Wang D, Zhang H, Yi X, Sun X, Shi B, et al. Molecular mechanisms involved in the growth stimulation of breast cancer cells by leptin. Cancer Res (2004) 64(16):5870–5. doi: 10.1158/0008-5472.CAN-04-0655
308. Strong AL, Ohlstein JF, Biagas BA, Rhodes LV, Pei DT, Tucker HA, et al. Leptin produced by obese adipose stromal/stem cells enhances proliferation and metastasis of estrogen receptor positive breast cancers. Breast Cancer Res BCR (2015) 17:112. doi: 10.1186/s13058-015-0622-z
309. Yan D, Avtanski D, Saxena NK, Sharma D. Leptin-induced epithelial-mesenchymal transition in breast cancer cells requires β-catenin activation via Akt/GSK3- and MTA1/Wnt1 protein-dependent pathways. J Biol Chem (2012) 287(11):8598–612. doi: 10.1074/jbc.M111.322800
310. Chang C-C, Wu M-J, Yang J-Y, Camarillo IG, Chang C-J. Leptin-STAT3-G9a Signaling Promotes Obesity-Mediated Breast Cancer Progression. Cancer Res (2015) 75(11):2375–86. doi: 10.1158/0008-5472.CAN-14-3076
311. Bowers LW, Rossi EL, McDonell SB, Doerstling SS, Khatib SA, Lineberger CG, et al. Leptin Signaling Mediates Obesity-Associated CSC Enrichment and EMT in Preclinical TNBC Models. Mol Cancer Res MCR (2018) 16(5):869–79. doi: 10.1158/1541-7786.MCR-17-0508
312. La Cava A, Matarese G. The weight of leptin in immunity. Nat Rev Immunol (2004) 4(5):371–9. doi: 10.1038/nri1350
313. Wrann CD, Laue T, Hübner L, Kuhlmann S, Jacobs R, Goudeva L, et al. Short-term and long-term leptin exposure differentially affect human natural killer cell immune functions. Am J Physiol-Endocrinol Metab (2011) 302(1):E108–16. doi: 10.1152/ajpendo.00057.2011
314. Clements VK, Long T, Long R, Figley C, Smith DMC, Ostrand-Rosenberg S. Frontline Science: High fat diet and leptin promote tumor progression by inducing myeloid-derived suppressor cells. J Leukoc Biol (2018) 103(3):395–407. doi: 10.1002/JLB.4HI0517-210R
315. Murphy KA, James BR, Sjaastad FV, Kucaba TA, Kim H, Brincks EL, et al. Cutting Edge: Elevated Leptin during Diet-Induced Obesity Reduces the Efficacy of Tumor Immunotherapy. J Immunol Baltim Md (1950) 2018 01201(7):1837–41. doi: 10.4049/jimmunol.1701738
316. Wang Z, Aguilar EG, Luna JI, Dunai C, Khuat LT, Le CT, et al. Paradoxical effects of obesity on T cell function during tumor progression and PD-1 checkpoint blockade. Nat Med (2019) 25(1):141–51. doi: 10.1038/s41591-018-0221-5
317. Cortellini A, Bersanelli M, Buti S, Cannita K, Santini D, Perrone F, et al. A multicenter study of body mass index in cancer patients treated with anti-PD-1/PD-L1 immune checkpoint inhibitors: when overweight becomes favorable. J Immunother Cancer (2019) 277(1):57. doi: 10.1186/s40425-019-0527-y
318. Kichenadasse G, Miners JO, Mangoni AA, Rowland A, Hopkins AM, Sorich MJ. Association Between Body Mass Index and Overall Survival With Immune Checkpoint Inhibitor Therapy for Advanced Non-Small Cell Lung Cancer. JAMA Oncol (2020) 6(4):512–8. doi: 10.1001/jamaoncol.2019.5241
319. McQuade JL, Daniel CR, Hess KR, Mak C, Wang DY, Rai RR, et al. Association of body-mass index and outcomes in patients with metastatic melanoma treated with targeted therapy, immunotherapy, or chemotherapy: a retrospective, multicohort analysis. Lancet Oncol (2018) 19(3):310–22. doi: 10.1016/S1470-2045(18)30078-0
320. Cortellini A, Bersanelli M, Santini D, Buti S, Tiseo M, Cannita K, et al. Another side of the association between body mass index (BMI) and clinical outcomes of cancer patients receiving programmed cell death protein-1 (PD-1)/ Programmed cell death-ligand 1 (PD-L1) checkpoint inhibitors: A multicentre analysis of immune-related adverse events. Eur J Cancer Oxf Engl 1990 (2020) 128:17–26. doi: 10.1016/j.ejca.2019.12.031
321. Mirsoian A, Bouchlaka MN, Sckisel GD, Chen M, Pai C-CS, Maverakis E, et al. Adiposity induces lethal cytokine storm after systemic administration of stimulatory immunotherapy regimens in aged mice. J Exp Med (2014) Nov 17211(12):2373–83. doi: 10.1084/jem.20140116
322. Pollack R, Ashash A, Cahn A, Rottenberg Y, Stern H, Dresner-Pollak R. Immune Checkpoint Inhibitor-induced Thyroid Dysfunction Is Associated with Higher Body Mass Index. J Clin Endocrinol Metab (2020) 105(10):dgaa458. doi: 10.1210/clinem/dgaa458
323. Cortellini A, Chiari R, Ricciuti B, Metro G, Perrone F, Tiseo M, et al. Correlations Between the Immune-related Adverse Events Spectrum and Efficacy of Anti-PD1 Immunotherapy in NSCLC Patients. Clin Lung Cancer (2019) 20(4):237–247.e1. doi: 10.1016/j.cllc.2019.02.006
324. Rogado J, Sánchez-Torres JM, Romero-Laorden N, Ballesteros AI, Pacheco-Barcia V, Ramos-Leví A, et al. Immune-related adverse events predict the therapeutic efficacy of anti-PD-1 antibodies in cancer patients. Eur J Cancer Oxf Engl (1990) 2019109:21–7. doi: 10.1016/j.ejca.2018.10.014
325. Holowatyj AN, Haffa M, Lin T, Scherer D, Gigic B, Ose J, et al. Multi-omics analysis reveals adipose-tumor crosstalk in colorectal cancer patients. Cancer Prev Res Phila Pa (2020) 13(10):817–28. doi: 10.1158/1940-6207.CAPR-19-0538
326. Himbert C, Delphan M, Scherer D, Bowers LW, Hursting S, Ulrich CM. Signals from the Adipose Microenvironment and the Obesity-Cancer Link-A Systematic Review. Cancer Prev Res Phila Pa (2017) 10(9):494–506. doi: 10.1158/1940-6207.CAPR-16-0322
327. Faria SS, Corrêa LH, Heyn GS, de Sant’Ana LP, Almeida R das N, Magalhães KG. Obesity and Breast Cancer: The Role of Crown-Like Structures in Breast Adipose Tissue in Tumor Progression, Prognosis, and Therapy. J Breast Cancer (2020) 23(3):233–45. doi: 10.4048/jbc.2020.23.e35
Keywords: obesity, Type 2 diabetes, cancer, insulin, IGF-1, lipids, leptin
Citation: Scully T, Ettela A, LeRoith D and Gallagher EJ (2021) Obesity, Type 2 Diabetes, and Cancer Risk. Front. Oncol. 10:615375. doi: 10.3389/fonc.2020.615375
Received: 09 October 2020; Accepted: 09 December 2020;
Published: 02 February 2021.
Edited by:
Riccarda Granata, University of Turin, ItalyReviewed by:
Justyna Magdalena Hermanowicz, Medical University of Bialystok, PolandSteven A. Rosenzweig, Medical University of South Carolina, United States
Riccardo Vigneri, University of Catania, Italy
Copyright © 2021 Scully, Ettela, LeRoith and Gallagher. This is an open-access article distributed under the terms of the Creative Commons Attribution License (CC BY). The use, distribution or reproduction in other forums is permitted, provided the original author(s) and the copyright owner(s) are credited and that the original publication in this journal is cited, in accordance with accepted academic practice. No use, distribution or reproduction is permitted which does not comply with these terms.
*Correspondence: Derek LeRoith, ZGVyZWsubGVyb2l0aEBtc3NtLmVkdQ==