- 1Department of Neurosurgery, The Third Affiliated Hospital of Soochow University, Changzhou, China
- 2Department of Neurosurgery, Huashan Hospital, Fudan University, Shanghai, China
Glioma, characterized by infiltrative growth and treatment resistance, is regarded as the most prevalent intracranial malignant tumor. Due to its poor prognosis, accumulating investigation has been performed for improvement of overall survival (OS) and progression-free survival (PFS) in glioma patients. Valproic acid (VPA), one of the most common histone deacetylase inhibitors (HDACIs), has been detected to directly or synergistically exert inhibitory effects on glioma in vitro and in vivo. In this review, we generalize the latest advances of VPA in treating glioma and its underlying mechanisms and clinical implications, providing a clearer profile for clinical application of VPA as a therapeutic agent for glioma.
1 Introduction
Glioma, originating from the neuroglial stem or progenitor cells, is the most prevalent and aggressive primary intracranial tumor (1, 2). Clinically, the standard therapy for glioma patients includes surgical intervention and adjuvant radiotherapy and chemotherapy (3). However, owing to its infiltrative growth and resistance to comprehensive treatment, the mortality and recurrence rate of glioma patients are still high, leading to poor prognosis (4). Therefore, it is crucial to summarize latest advances in glioma treatment and grope for promising investigational directions.
Valproic acid (VPA), one of the most common histone deacetylase inhibitors (HDACIs), is known as an anticonvulsant and mood-stabilizing drug clinically (5). Gathering evidence have manifested that VPA directly or synergistically exerted anti-tumor effects on various solid tumors (6, 7). For instance, VPA suppressed gastric cancer cell proliferation and induced autophagy through HDAC1/PTEN/Akt signaling (8). Similarly in breast cancer, the inhibitory role of VPA is mainly reflected in cellular proliferation, cell cycle, and apoptosis via Hsp70 acetylation (9), while in most cancers, VPA tended to serve as an adjuvant drug for chemotherapy, radiotherapy, and other therapies. In lung cancer, VPA and arsenic trioxide markedly potentiated cell death in vitro and in vivo (10). VPA also modulated invasion capability targeting MMP-1, MMP-3, and MMP-13 to enhance the radiotherapy effect of breast cancer cells (11). Furthermore, VPA sensitized pancreatic cancer cells to NK cell-mediated lysis by upregulating MICA and MICB via PI3K/Akt signaling pathway (12). Currently, various studies have ascertained that application of VPA is effectively involved in glioma treatment (13–15). In this review, we generalize the latest advances of VPA in treating glioma and its underlying mechanisms (Figures 1–3 and Tables 1, 2) and clinical implications (Table 3), providing a clearer profile for clinical application of VPA as a therapeutic agent for glioma.
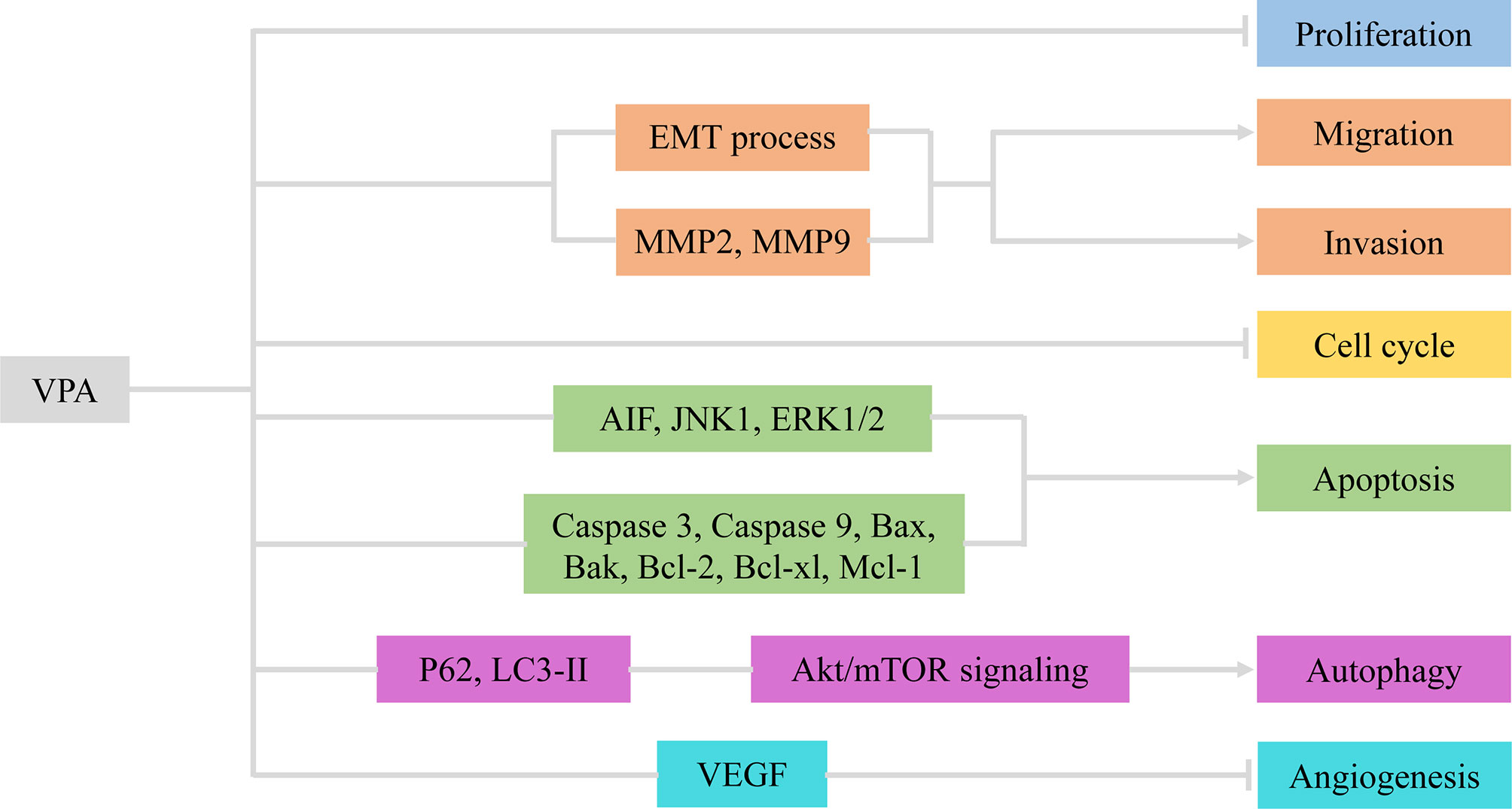
Figure 1 VPA exerted therapeutic effects via involvement with cellular activities of glioma. VPA, valproic acid; EMT, epithelial–mesenchymal transition; MMP2/9, matrix metalloproteinase 2/9; AIF, apoptosis-inducing factor; VEGF, vascular endothelial growth factor.
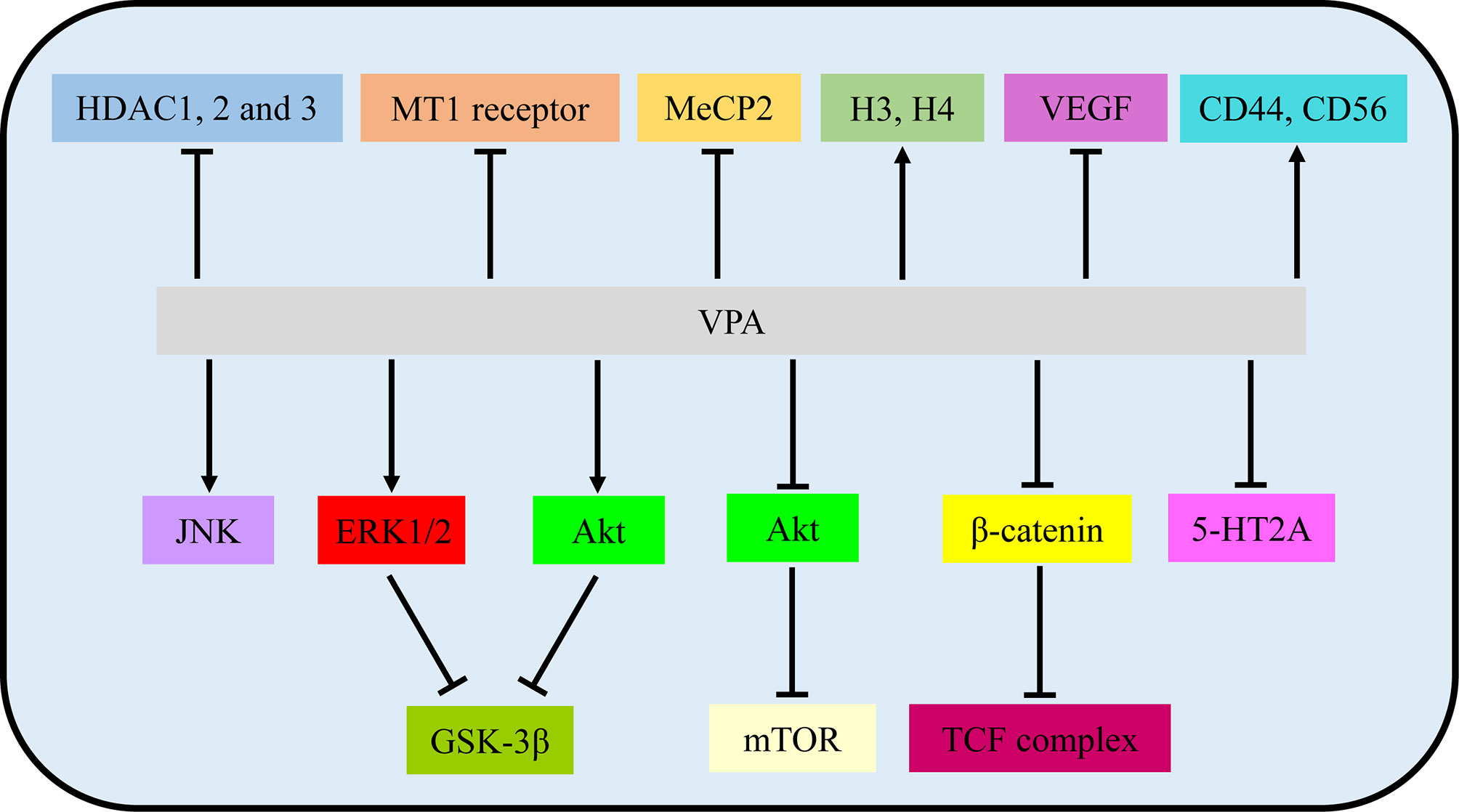
Figure 2 VPA exerted therapeutic effects via involvement with signaling pathways and molecular targets. VPA, valproic acid; HDAC, histone deacetylase; MT1, MT1: melatonin 1; MeCP2, methyl CpG binding protein 2; H3/4, histone 4; VEGF, vascular endothelial growth factor.
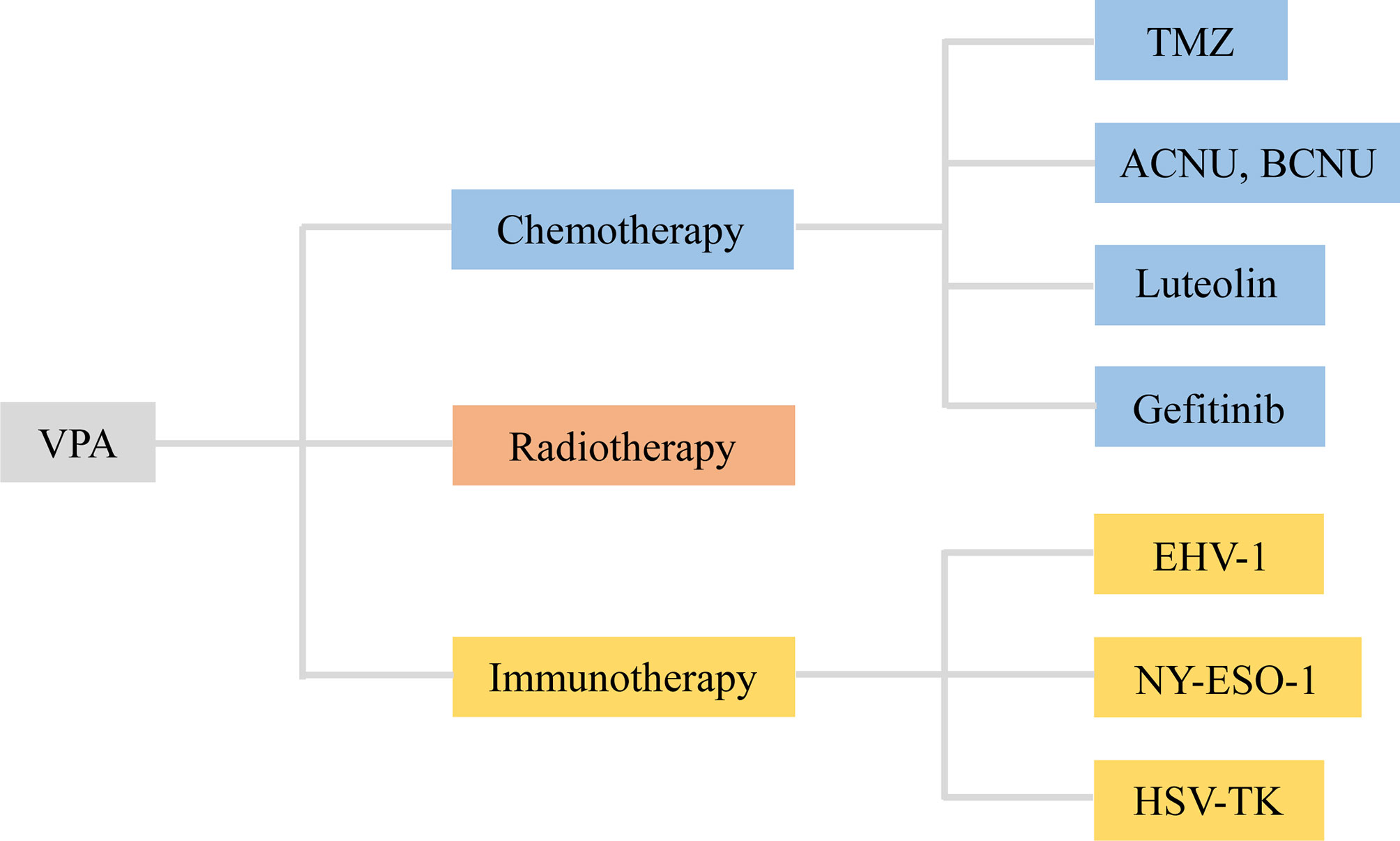
Figure 3 VPA served as an adjuvant agent in chemotherapy, radiotherapy, and immunotherapy of glioma. VPA, valproic acid; ACNU, nimustine; BCNU, 1, 3-bis (2-chloroethyl)-1-nitrosourea; EHV-1, equine herpesvirus type 1; HSV-TK, herpes simplex virus type I thymidine kinase.
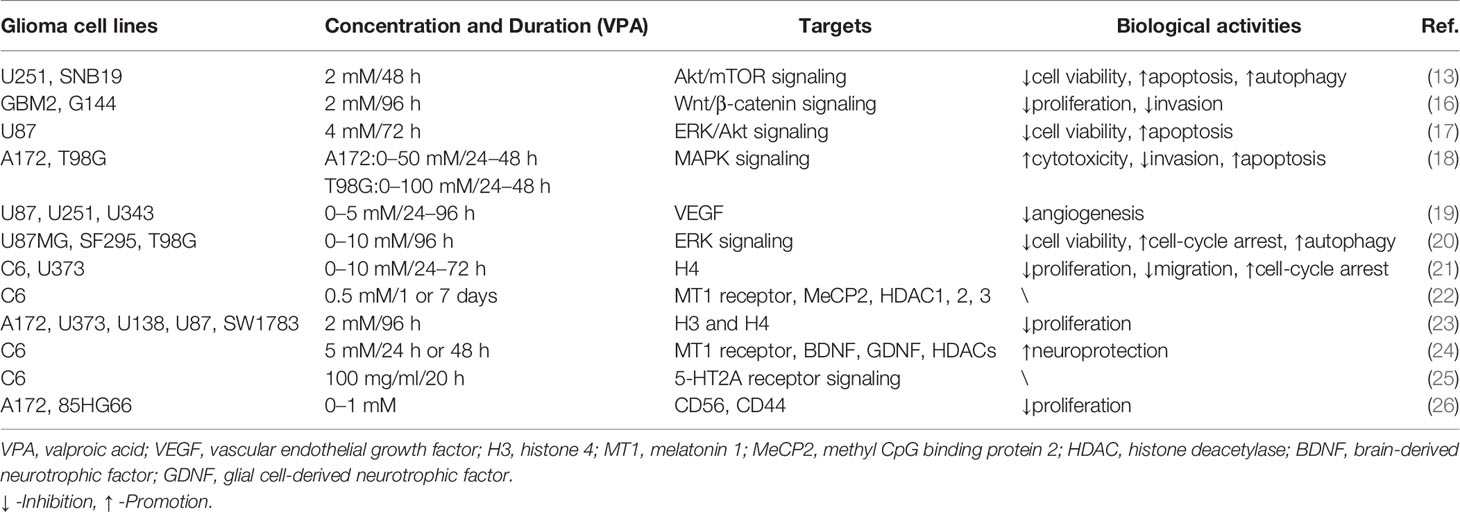
Table 1 VPA was involved in cellular activities of glioma through signaling pathways and molecular targets.
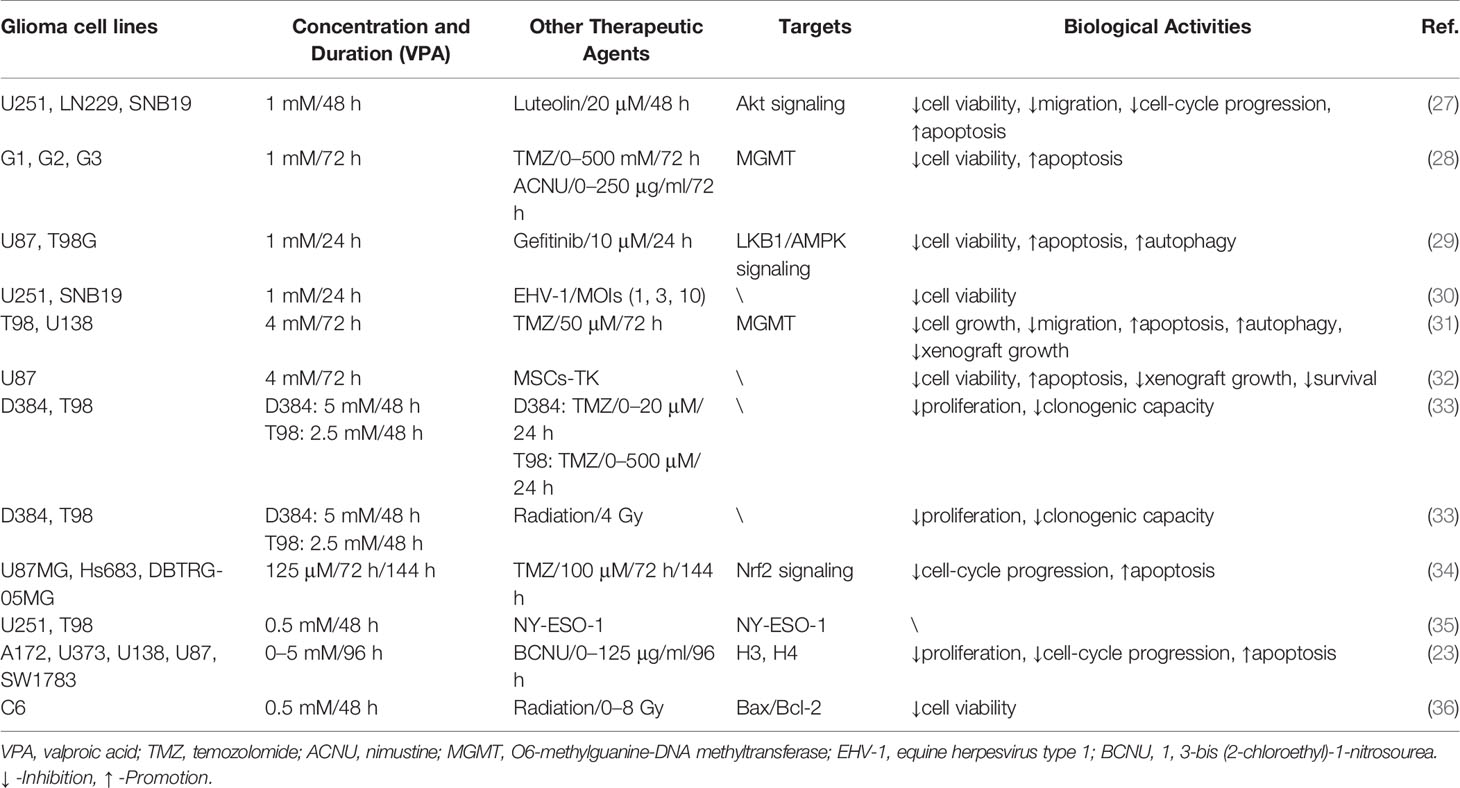
Table 2 VPA served as an adjuvant agent in glioma treatment via involvement with cellular activities and biological targets.
2 Individual Anti-Tumor Effects of VPA in Glioma
2.1 Involvement With Cellular Activities
2.1.1 VPA Inhibited Glioma Cell Proliferation, Migration, and Invasion
Cell proliferation, one of the important physiological functions of tumor cells, is the basis of growth, development, reproduction, and heredity (43). Moreover, cellular migration and invasion are also the most important features of malignant tumors, mainly involved in cancer metastasis (44). In most glioma cells and glioma stem cells (GSCs), VPA repressed cell viability in a dose- and time-dependent manner (2-10 mM for 48, 72, and 96 h) at varying degrees due to tumor heterogeneity (13, 17, 20). Subsequently, Trypan Blue dye exclusion assay was utilized to detect proliferative rates. Treatment with VPA induced a statistically significant reduction of the cell growth, ranging from 10% to 40%, with an increase in the LDH release (16, 17). Benítez et al. further validated the particular correlation between the decreased cell proliferation and increased cell death (21).
Indeed, VPA was verified to impair the migratory process of glioma cells according to Boyden chamber findings (16, 21). Meanwhile, the invasive capability of glioma cells was also influenced by VPA treatment (18, 45). As is well-known, the epithelial–mesenchymal transition (EMT) process and matrix metalloproteinases (MMPs) are vital for migration and invasion (46, 47). Concluding from results of Western blot and immunofluorescence, VPA treatment potentiated downregulation of Snail1 and Twist1 levels and relocalization of E-Cadherin, thus inactivating the EMT process (16). Ryu et al. also found that MMP-2 and MMP-9 were knocked down by VPA (18). Therefore, VPA exerted inhibitory effects on cellular proliferation, migration, and invasion in glioma via the EMT process and MMPs.
2.1.2 VPA-Induced Glioma Cell-Cycle Arrest and Apoptosis
Cell cycle refers to the whole process that the genetic material of a cell is duplicated and equally distributed to the two daughter cells (48). It could be modulated by gene mutations and physical and chemical factors, which is indicative of apoptosis (49). Cell apoptosis is an active process of the orderly death of cells controlled by genes to maintain the stability of the internal environment for better adaptation to the living environment (50). To explore whether VPA had an inhibitory effect on cell cycle, DNA flow cytometric analyses and immunocytochemistry were carried out (20, 21). Obviously, VPA treatment could increase the population at the G0/G1 phase and decrease the population at the S phase, indicating that VPA induces G0/G1 arrest in malignant glioma cell lines (20). A growth arrest process is also involved in the decrease of cell proliferation, together with cell death and inhibition of cell migration (21). However, the cell-cycle-related proteins and mechanisms have not been detected, which appeals to additional experiments.
The apoptosis induced by VPA was measured by flow cytometry Annexin V-FITC/PI and TUNEL staining. Zhang et al. found that apoptotic rates of glioma cells were induced by VPA in a dose-dependent manner (17, 18). Further functional investigation illustrated that VPA upregulated expression of cleaved caspase 3 and cleaved caspase 9. Moreover, the expression of Bcl-2 family protein Bax and Bak was increased, whereas Bcl-2, Bcl-xl, and Mcl-1, the anti-apoptotic members, were decreased by VPA treatment (13, 17). Additionally, the release of cytochrome c from the mitochondria under VPA treatment has also been increased. The expression of apoptosis-inducing factor (AIF) and poly ADP-ribose polymerase (PARP) was also upregulated (17). Moreover, Chen et al. found that VPA treatment mildly suppressed the expression of JNK1 and increased the expression of phospho-JNK1 and phospho-ERK1/2, but had no effect on the expression of ERK1/2 (18). However, inhibition of JNK1 and/or ERK1/2 reversed the VPA-induced cytotoxicity and changes in apoptosis (18). Similarly, ERK and Akt proteins were phosphorylated, indicating that their activities were induced but GSK3β activity was inhibited, because of increased GSK3β phosphorylation (17). In summary, VPA promoted cell-cycle arrest at the G0/G1 phase, thus inducing apoptosis via activation of the mitochondria-mediated apoptosis and ERK/Akt pathway.
2.1.3 VPA Was Involved in Cellular Autophagy and Angiogenesis
Autophagy is a highly conserved process that is essential for cell survival, host defense, and energy consumption (51). Autophagy in cancer is often described as a “double-edged sword”, which either promotes tumor survival under microenvironmental stress and increases growth and aggressiveness or suppresses tumorigenesis via its quality control function (52). Results of electron microscopy (EM), MDC staining, and GFP-LC3-labeling analyses revealed that autophagic vacuoles increased under treatment with VPA (13, 20). Since LC3-II is closely associated with the membrane of autophagosomes and p62 is a selective autophagy adaptor/receptor, binding ubiquitinated proteins and LC3 for engulfment, the expression of LC3-II and p62 was examined by Western blot analysis (53). Han et al. found that p62 expression was downregulated, while LC3-II expression was obviously upregulated in glioma cells treated with VPA (13). Similar promotion of autophagy could also be seen for Beclin-1 (20). Subsequently, p-Akt/Akt and p-mTOR/mTOR expression was apparently downregulated, thus inhibiting the Akt/mTOR pathway to promote autophagy (13). All these data suggested that VPA facilitated the induction of autophagy via the Akt/mTOR signaling pathway.
Angiogenesis refers to the formation of new blood vessels from existing capillaries or veins behind capillaries, which plays an important role in the development and metastasis of tumors (19). VPA reduced vascular endothelial growth factor (VEGF) secretion of glioma cells in a dose-dependent manner under both normoxic and hypoxic conditions. VPA was also found to inhibit tube formation in the angiogenesis assay. In vivo, treatment with VPA combined with irinotecan reduced the number of vessels expressing factor VIII in the brain tumor model. Therefore, VPA inhibited glioma angiogenesis by direct inhibition of endothelial cell proliferation and tube formation and indirectly decreased secretion of VEGF by glioma cells (14). Above all, VPA inhibited angiogenesis in vitro and in vivo targeting VEGF (Figure 1 and Table 1).
2.2 Signaling Pathways and Molecular Targets
2.2.1 Signaling Pathways
Several signaling pathways have been verified to participate in the initiation and progress of malignant tumors (54, 55). In addition, all these pathways have been involved in proliferation, migration, invasion, cell-cycle progression, apoptosis, autophagy, and angiogenesis, which could also be therapeutic targets for multiple therapies, especially for chemotherapy (56, 57).
In VPA treatment, VPA activated the Akt/mTOR signaling by decreased expression of p-Akt/Akt and p-mTOR/mTOR in U251 and SNB19 (13). While Zhang et al. reported that incubation with VPA increased phosphorylation of ERK and Akt in ERK/Akt signaling in U87, thus in turn inhibiting GKS3β activation by the induction of GKS3β phosphorylation (17). Further loss-of-function experiments illustrated that inhibitors of MAPK and PI3K pathways abolished apoptotic induction of VPA, but GSK3β inhibitor mimicked effects of VPA (17). Furthermore, p-JNK1 and p-ERK1/2 were also increased by VPA, while inhibition of JNK1 and/or ERK1/2 partially reversed the VPA effects, involved in MAPK signaling (18, 20). Moreover, DNA methylation changes of Wnt pathway-related genes and transcriptional activity of the β-catenin/TCF complex were obviously induced by VPA via a TOP/FOP flash reporter assay (16). Additionally, VPA treatment resulted in an enhancement of 5-HT2A receptor-stimulated PI hydrolysis (25). Hence, Akt/mTOR signaling, ERK/Akt signaling, MAPK signaling, Wnt/β-catenin signaling, and 5-HT2A signaling play vital roles in the functional activities of VPA.
2.2.2 Molecular Targets
In addition to main signaling pathways, molecular targets also participated in functional activities of VPA. As one of the most common HDACIs, VPA caused significant time-dependent changes in histone deacetylase (HDAC) 1, 2, and 3 (22). The mRNA expression of the melatonin 1 (MT1) receptor and methyl CpG binding protein 2 (MeCP2) was also decreased by VPA (22, 24). At the same time, histone 3 (H3) and H4 acetylation were induced by VPA (21, 23). Rincón Castro et al. also detected the upregulation of brain-derived neurotrophic factor (BDNF) and glial cell line-derived neurotrophic factor (GDNF) in VPA activities (24). Apart from these, VPA reduced VEGF secretion of glioma cells in a dose-dependent manner under both normoxic and hypoxic conditions (14). Moreover, incubation with VPA markedly increased the expression level of CD44 and CD56 (26). All these findings manifested that VPA mainly exerted its biological activities via signalings and molecular targets mentioned above, providing evidence for pre-clinical experiments (Figure 2 and Table 1).
3 Synergistic Effects of VPA With Therapeutic Agents in Glioma
3.1 VPA-Adjuvant Chemotherapy
3.1.1 VPA and Temozolomide (TMZ)
TMZ, a 3-methyl derivative of mitozolomide, is the first-line chemotherapy drug of patients with gliomas, which easily pass through the blood–brain barrier (58). Despite its primary efficiency in glioma treatment, drug resistance is inevitable in patients with high O6-methylguanine-DNA methyltransferase (MGMT) (59). Gathering experiments have suggested that the combination of VPA with TMZ has combined or enhanced antitumor effects in glioma. For cytotoxic response, the combination of VPA and TMZ suppressed the survival rate and migration of glioma cells compared with that of the TMZ alone, which verified the sensitivity of VPA (28, 31, 33). More importantly, the combination induced apoptotic cell death, accompanied by enhanced DNA damage, intracellular GSH depletion, ROS production, and mitochondrial transmembrane potential disruption, via upregulation of Bax/Bcl-2 and cleaved caspase-3/caspase-3 (31, 34). Additionally, autophagic effects could also be activated by the combination of VPA and TMZ (31).
3.1.2 VPA and ACNU/BCNU
ACNU and BCNU, referred to as common nitrosourea alkylating agents, have affinity to the blood–brain barrier. Researchers have verified their direct or indirect inhibitory effects on several tumors, including brain tumors, lung cancer, and gastric cancer (60, 61). Li et al. found that VPA enhanced the inhibitory effects of ACNU on the growth and apoptosis of MGMT-negative/positive cells, particularly in the MGMT-positive cells. Further mechanical investigation illustrated that VPA downregulated the expression of MGMT protein and promoted the methylation of MGMT promoter (28). As for BCNU, detailed analysis of combination of VPA and BCNU revealed that the synergistic effect was mainly reflected in cell growth inhibition and cell-cycle arrest rather than an increased programmed cell death (23).
3.1.3 VPA and Luteolin
Luteolin is a natural flavonoid that could be extracted from traditional Chinese medical herbs (62). Indeed, it has been well established that luteolin has a variety of pharmacological effects, including anti-tumor, anti-inflammation, anti-oxidation, and immune regulation (63). Luteolin was verified to exert limited inhibitory effects on glioma cells, which contributes to application of VPA and luteolin. In this study, VPA enhanced the anticancer effects of luteolin in cell viability, colony formation, and migration. More importantly, VPA treatment induced cell-cycle arrest and cellular apoptosis via upregulation of p-PARP/PARP, cleaved caspase 3/caspase 3, and Bax/bcl-2. Moreover, VPA activated Akt signaling to promote autophagic response via accumulation of LC3-II and decrease of p62 (27).
3.1.4 VPA and Gefitinib
Gefitinib, an oral tyrosine kinase inhibitor, selectively targeted the epidermal growth factor receptor (EGFR) (64). Due to its application in cancer treatment, its inhibitory effects, especially anti-angiogenesis, have been extensively acknowledged (65). However, a nontoxic concentration of VPA sensitized U87 and T98G glioma cells to gefitinib by inhibiting cell growth and long-term clonogenic survival via increased intracellular reactive oxygen species generation. In addition, the combination therapy induced autophagic response, accompanied by conversion of microtubule-associated protein-1 light chain 3-II (LC3-II), and degradation of p62 through activation of liver kinase-B1 (LKB1)/AMP-activated protein kinase (AMPK)/ULK1. Subsequent loss-of-function assay ascertained that knockdown of AMPK and ULK1 reversed the biological effect of the combination therapy-induced autophagy and growth inhibition (29). Therefore, VPA served as an adjuvant therapeutic agent for chemotherapy of glioma, contributing to subsequent exploration for novel chemotherapy drugs.
3.2 VPA-Adjuvant Radiotherapy
Radiotherapy, used in over 50% of cancer patients, mainly targets tumor tissues by using ionizing radiation with a little damage on normal tissues (66). However, emerging resistance to radiotherapy is the main obstacle in the clinical application (67). Therefore, it is an urgent need to combine an effective sensitizer with radiotherapy to obtain better outcome of tumor patients. Accumulating evidence showed that VPA enhanced radiation-induced cell death and the clonogenic formation at varying radiation doses from 0 to 6 Gy (33). Zhou et al. also explored that VPA induced apoptotic responses to irradiation by inhibiting Bcl-2 and increasing Bax at the mRNA and protein levels (36). However, the amount of trials focusing on VPA-adjuvant radiotherapy is too rare to verify its sensitization role in glioma.
3.3 VPA-Adjuvant Immunotherapy
Immunotherapy has made much progress with the cropping up of the immune checkpoint inhibitors (ICIs) (68). Apart from this, several immunotherapies, concerning dendritic cell, T lymphocytes, and oncolytic viruses, have been applied in the treatment of glioma (69). White et al. firstly evaluated the combination therapy of the lytic animal virus equine herpesvirus type 1 (EHV-1) with VPA. Surprisingly, VPA pretreatment promoted the infection and the yield of EHV-1, thus strengthening the ability spread laterally among cells (30). NY-ESO-1, an immunogenic cancer antigen, has been a specific target for immunotherapy. Sachie Oi et al. explored that VPA enhanced the induction of NY-ESO-1 by DNA-methyltransferase inhibitors (DNMTi). Further chromatin assays illustrated that the combination induced DNA demethylation, H3 Lys9 demethylation, and acetylation (35). Additionally, MSCs with high expression level of herpes simplex virus type I thymidine kinase (HSV-TK) were applied into glioma treatment. The results demonstrated that VPA and MSCs-TK synergistically induced cellular apoptosis of glioma cells via caspase activation, which was evaluated by TUNEL staining assay. Subsequent in vivo treatment also contributed to the same effects, including the suppression of tumor growth and survival time (32). Thus, the effects of VPA-adjuvant immunotherapy in glioma were so satisfactory that investigation should be carried out for more immunotherapy agents (Figure 3 and Table 2).
4 Clinical Trials of Gliomas With VPA
A few clinical trials have illustrated that the clinical application of VPA showed ambiguous significance in glioma patients. For 44 glioma patients, only 3 patients developed somnolence, and average trough blood levels of VPA were below the safe standard, which verified that it had no severe toxicity (40). Further investigation performed by Francisco et al. revealed that compared to the non-treated group of six patients, median event-free survival and median survival of VPA group were much longer (15). Meanwhile, VPA seemed to serve as an adjuvant drug in glioma patients. Watanabe et al. explored that VPA contributed to survival improvement, including delayed hair loss and prolonged survival time (38). Similar improvements in prognosis of 165 GBM patients were also detected (39). In 38 children with DIPGs or HGGs, treatment of radiation and VPA could prolong event-free survival and overall survival, while only three patients developed thrombocytopenia, weight gain, and pancreatitis, respectively (37). Due to the different pathological grades and types of gliomas, more detailed research has been carried out. For GBM patients, VPA could reduce hazard ratio and improve overall survival (41, 42). For grade II/III glioma patients, prolonged PFS and decreased histological progression were correlated with positive VPA treatment (42). However, clinical significance of VPA treatment for recurrent diffuse intrinsic pontine gliomas (DIPGs) have not been testified (70). Therefore, though its safety has been initially confirmed, the clinical efficacy of VPA is still uncertain, appealing to a certain number of clinical studies (Table 3).
Additionally, application of VPA has been equipped with auxiliary benefits. Aiming at irreversible damage of radiotherapy, including apoptotic response of normal neuronal cells and neurocognitive deficits, VPA specifically protected hippocampal neurons from radiation-induced damage in vivo and in vitro (71). More concretely, VPA improved radiation-related hair loss in 112 glioma patients (39). Castro et al. also attributed the neuroprotective properties of VPA to modulation of BDNF, GDNF, and melatonin receptors (24), while there were still a few adverse effects, mainly in psychiatric, neurological, gastrointestinal, hematopoietic, and metabolic disorders (72). Firstly, hyperammonemia induced by VPA treatment would lead to unpredictable damage of nervous system, contributing to psychiatric disorders and neurological disorders, including cognitive dysfunction, Parkinsonism, emotional instability, insomnia, and neurasthenia (73–76). The defects of neural tube and axial skeleton further verified the role of VPA as a teratogen (77). Secondly, VPA would induce nausea, vomiting, indigestion, diarrhea, and constipation in a certain proportion of patients receiving VPA treatment (78). Thirdly, VPA might alter hematopoietic homeostasis for occurrence of thrombocytopenia and megakaryocyte dysplasia (38, 79). Fourthly, endocrine disturbances and subsequent weight gain were also main side effects of VPA (80). In addition, allergic symptoms, fever, hearing loss, menstrual disorders, and damage of liver and kidney function could also be observed (72, 81). Of course, stopping oral VPA and applying symptomatic drugs would be a great option for these related clinical symptoms (37).
5 Discussion
The reviewed data have provided supporting evidence for application of VPA as a therapeutic agent in glioma treatment. VPA was involved in several cellular activities, including cell proliferation, migration, invasion, cell-cycle arrest, apoptosis, autophagy, and angiogenesis. These biological actions focused on several signaling pathways, including Akt/mTOR, ERK/Akt, JNK1, ERK1/2, Wnt pathway, and 5-HT2A signalings. Apart from these targets, molecules like HDAC 1, 2, and 3, MeCP2, H3, H4, VEGF, CD44, and CD56 also played vital roles in treating glioma. Meanwhile, VPA was also verified to be an adjunctive agent in the treatment of chemotherapy, radiotherapy, and immunotherapy. Of course, a few clinical trials demonstrated that VPA improved survival of glioma patients. Surprisingly, knockdown of SEL1L, a crucial protein involved in homeostatic pathways, cancer aggressiveness, and stem cell state maintenance, increased VPA sensitivity to glioma (82). All these evidence have confirmed the therapeutic role of VPA in glioma treatment.
However, results of a few experiments were contrary to findings above. For example, Riva et al. reported that VPA induced the genome-wide DNA methylation profile and the differentiation behavior to elevate the sensitivity of VPA, while not TMZ (83). However, incubation of VPA promoted secretion of amphiregulin (AR), facilitating TMZ resistance (84). In addition to this, Han et al. found that VPA inhibited the Akt/mTOR pathway by reducing the expression of p-Akt/AKT and p-mTOR/mTOR in glioma cell lines U251 and SNB19 (13), while in the study conducted by Zhang et al., VPA could increase the phosphorylation of ERK and Akt in the ERK/Akt pathway in U87 (17). The reason for these differential expressions might be attributed to tumor heterogeneity, origin, gene drift, and experimental conditions. Therefore, more experimental and clinical investigation should be carried out for qualification of the inhibitory role of VPA.
Furthermore, many other signaling pathways and molecular targets have been detected in the functional processes of VPA in other tumors. For example, the STAT3/Bmi1 pathway could be modulated by VPA to increase the sensitivity of gemcitabine to pancreatic cancer cells (10). VPA also triggered the EMT process of colorectal cancer cells targeting Snail via the Akt/GSK-3β pathway (85). In addition, HIF-1α and Survivin played significant roles in the activities of VPA (86, 87). Gathering evidence demonstrated that synergistic antitumor effects of VPA and other therapeutic agents have been explored. The combination therapy of VPA and simvastatin sensitized prostate cancer cells via YAP inhibition (6). VPA and Arsenic Trioxide were verified to induce cell-cycle arrest at the G2/M phase and apoptotic cell death in lung cancer (88). Moreover, VPA could also enhance anti-PD-L1 tumor immunotherapy in blocking myeloid-derived suppressor cell function (89). To our surprise, VPA was also involved in gene therapy and antiblastic therapy (90, 91), which might be directions for subsequent research. Hence, it is an urgent demand to explore more reliable signaling pathways and adjuvant therapeutic agents in biological activities of VPA.
Currently, more and more anti-epilepsy drugs (AEDs), including VPA, phenobarbital, carbamazepine, clonazepam, levetiracetam, lamotrigine, topiramate, and oxcarbazepine, have been concerned in studying tumors of CNS. Among these AEDs, VPA and levetiracetam exhibits superiority in the clinical application of brain tumor-related epilepsy (BTE), due to their sensitization of TMZ through MGMT-dependent or MGMT-independent mechanisms (92, 93). In addition, levetiracetam and VPA would be beneficial to verbal memory and cognitive function via downregulating excitatory amino acid transporter-2 expression (94, 95). However, further evidence-based guidelines demonstrated that levetiracetam is the best first-line agent for BTE patients due to its efficacy and tolerability, especially in patients undergoing 5-ALA-mediated fluorescence-guided resection (FGR) (96). Different from levetiracetam, VPA contributed to prolonged survival of glioma patients, particularly in glioblastomas (97). Therefore, prospective evaluation of VPA and levetiracetam treatment for glioma patients is warranted to confirm these findings.
Despite these deficiencies and prospects of VPA treatment in glioma, accumulating evidence demonstrated that VPA exerted inhibitory effects on glioma targeting several signaling pathways or molecules individually or with chemotherapy, radiotherapy, and immunotherapy, contributing to further exploration.
Author Contributions
WH designed and wrote the manuscript, and drafted the schematic figures and tables. WG contributed to the overall design, supervision of the work, and essential reading. All authors contributed to the article and approved the submitted version.
Conflict of Interest
The authors declare that the research was conducted in the absence of any commercial or financial relationships that could be construed as a potential conflict of interest.
Publisher’s Note
All claims expressed in this article are solely those of the authors and do not necessarily represent those of their affiliated organizations, or those of the publisher, the editors and the reviewers. Any product that may be evaluated in this article, or claim that may be made by its manufacturer, is not guaranteed or endorsed by the publisher.
Abbreviations
VPA, valproic acid; OS, overall survival; PFS, progression-free survival; HDACIs, histone deacetylase inhibitors; GSCs, glioma stem cells; EMT, epithelial–mesenchymal transition; MMPs, matrix metalloproteinases; AIF, apoptosis-inducing factor; PARP, poly ADP-ribose polymerase; EM, electron microscopy; VEGF, vascular endothelial growth factor; HDAC, histone deacetylase; MT1, melatonin 1; MeCP2, methyl CpG binding protein 2; H3, histone 3; BDNF, brain-derived neurotrophic factor; GDNF, glial cell line-derived neurotrophic factor; TMZ, temozolomide; MGMT, O6-methylguanine-DNA methyltransferase; EGFR, epidermal growth factor receptor; LC3-II, light chain 3-II; LKB1, liver kinase-B1; AMPK, AMP-activated protein kinase; ICIs, immune checkpoint inhibitors; EHV-1, equine herpesvirus type 1; DNMTi, DNA-methyltransferase inhibitors; HSV-TK, herpes simplex virus type I thymidine kinase; DIPG, diffuse intrinsic pontine glioma; AED, anti-epilepsy drug; BTE, brain tumor-related epilepsy; FGR, fluorescence-guided resection; AR, amphiregulin.
References
1. Deng Y, Zhu H, Xiao L, Liu C, Meng X. Circ_0005198 Enhances Temozolomide Resistance of Glioma Cells Through miR-198/TRIM14 Axis. Aging (Albany NY) (2020) 13(2):2198–211. doi: 10.18632/aging.202234
2. Mu M, Niu W, Zhang X, Hu S, Niu C. LncRNA BCYRN1 Inhibits Glioma Tumorigenesis by Competitively Binding With miR-619-5p to Regulate CUEDC2 Expression and the PTEN/AKT/p21 Pathway. Oncogene (2020) 39(45):6879–92. doi: 10.1038/s41388-020-01466-x
3. Lin C, Lin L, Mao S, Yang L, Yi L, Lin X, et al. Reconstituting Glioma Perivascular Niches on a Chip for Insights Into Chemoresistance of Glioma. Anal Chem (2018) 90(17):10326–33. doi: 10.1021/acs.analchem.8b02133
4. D’Amico RS, Englander ZK, Canoll P, Bruce JN. Extent of Resection in Glioma-A Review of the Cutting Edge. World Neurosurg (2017) 103:538–49. doi: 10.1016/j.wneu.2017.04.041
5. Kawashima N, Nishimiya Y, Takahata S, Nakayama KI. Induction of Glycosphingolipid GM3 Expression by Valproic Acid Suppresses Cancer Cell Growth. J Biol Chem (2016) 291(41):21424–33. doi: 10.1074/jbc.M116.751503
6. Iannelli F, Roca MS, Lombardi R, Ciardiello C, Grumetti L, Rienzo S, et al. Synergistic Antitumor Interaction of Valproic Acid and Simvastatin Sensitizes Prostate Cancer to Docetaxel by Targeting CSCs Compartment via YAP Inhibition. J Exp Clin Cancer Res (2020) 39(1):213. doi: 10.1186/s13046-020-01723-7
7. Makarevic J, Rutz J, Juengel E, Maxeiner S, Tsaur I, Chun FK, et al. Influence of the HDAC Inhibitor Valproic Acid On the Growth and Proliferation of Temsirolimus-Resistant Prostate Cancer Cells In Vitro. Cancers (Basel) (2019) 11(4):566. doi: 10.3390/cancers11040566
8. Sun J, Piao J, Li N, Yang Y, Kim KY, Lin Z. Valproic Acid Targets HDAC1/2 and HDAC1/PTEN/Akt Signalling to Inhibit Cell Proliferation via the Induction of Autophagy in Gastric Cancer. FEBS J (2020) 287(10):2118–33. doi: 10.1111/febs.15122
9. Mawatari T, Ninomiya I, Inokuchi M, Harada S, Hayashi H, Oyama K, et al. Valproic Acid Inhibits Proliferation of HER2-Expressing Breast Cancer Cells by Inducing Cell Cycle Arrest and Apoptosis Through Hsp70 Acetylation. Int J Oncol (2015) 47(6):2073–81. doi: 10.3892/ijo.2015.3213
10. Li H, Zhang Z, Gao C, Wu S, Duan Q, Wu H, et al. Combination Chemotherapy of Valproic Acid (VPA) and Gemcitabine Regulates STAT3/Bmi1 Pathway to Differentially Potentiate the Motility of Pancreatic Cancer Cells. Cell Biosci (2019) 9:50. doi: 10.1186/s13578-019-0312-0
11. Artacho-Cordon F, Rios-Arrabal S, Olivares-Urbano MA, Storch K, Dickreuter E, Munoz-Gamez JA, et al. Valproic Acid Modulates Radiation-Enhanced Matrix Metalloproteinase Activity and Invasion of Breast Cancer Cells. Int J Radiat Biol (2015) 91(12):946–56. doi: 10.3109/09553002.2015.1087067
12. Shi P, Yin T, Zhou F, Cui P, Gou S, Wang C. Valproic Acid Sensitizes Pancreatic Cancer Cells to Natural Killer Cell-Mediated Lysis by Upregulating MICA and MICB via the PI3K/Akt Signaling Pathway. BMC Cancer (2014) 14:370. doi: 10.1186/1471-2407-14-370
13. Han W, Yu F, Cao J, Dong B, Guan W, Shi J. Valproic Acid Enhanced Apoptosis by Promoting Autophagy via Akt/mTOR Signaling in Glioma. Cell Transplant (2020) 29:963689720981878. doi: 10.1177/0963689720981878
14. Osuka S, Takano S, Watanabe S, Ishikawa E, Yamamoto T, Matsumura A. Valproic Acid Inhibits Angiogenesis In Vitro and Glioma Angiogenesis In Vivo in the Brain. Neurol Med Chir (Tokyo) (2012) 52(4):186–93. doi: 10.2176/nmc.52.186
15. Felix FH, de Araujo OL, da Trindade KM, Trompieri NM, Fontenele JB. Retrospective Evaluation of the Outcomes of Children With Diffuse Intrinsic Pontine Glioma Treated With Radiochemotherapy and Valproic Acid in a Single Center. J Neurooncol (2014) 116(2):261–6. doi: 10.1007/s11060-013-1280-6
16. Riva G, Cilibrasi C, Bazzoni R, Cadamuro M, Negroni C, Butta V, et al. Valproic Acid Inhibits Proliferation and Reduces Invasiveness in Glioma Stem Cells Through Wnt/beta Catenin Signalling Activation. Genes (Basel) (2018) 9(11):522. doi: 10.3390/genes9110522
17. Zhang C, Liu S, Yuan X, Hu Z, Li H, Wu M, et al. Valproic Acid Promotes Human Glioma U87 Cells Apoptosis and Inhibits Glycogen Synthase Kinase-3beta Through ERK/Akt Signaling. Cell Physiol Biochem (2016) 39(6):2173–85. doi: 10.1159/000447912
18. Chen Y, Tsai YH, Tseng SH. Valproic Acid Affected the Survival and Invasiveness of Human Glioma Cells Through Diverse Mechanisms. J Neurooncol (2012) 109(1):23–33. doi: 10.1007/s11060-012-0871-y
19. Liang W, Guo B, Ye J, Liu H, Deng W, Lin C, et al. Vasorin Stimulates Malignant Progression and Angiogenesis in Glioma. Cancer Sci (2019) 110(8):2558–72. doi: 10.1111/cas.14103
20. Fu J, Shao CJ, Chen FR, Ng HK, Chen ZP. Autophagy Induced by Valproic Acid Is Associated With Oxidative Stress in Glioma Cell Lines. Neuro Oncol (2010) 12(4):328–40. doi: 10.1093/neuonc/nop005
21. Benitez JA, Arregui L, Cabrera G, Segovia J. Valproic Acid Induces Polarization, Neuronal-Like Differentiation of a Subpopulation of C6 Glioma Cells and Selectively Regulates Transgene Expression. Neuroscience (2008) 156(4):911–20. doi: 10.1016/j.neuroscience.2008.07.065
22. Kim B, Rincon Castro LM, Jawed S, Niles LP. Clinically Relevant Concentrations of Valproic Acid Modulate Melatonin MT(1) Receptor, HDAC and MeCP2 mRNA Expression in C6 Glioma Cells. Eur J Pharmacol (2008) 589(1-3):45–8. doi: 10.1016/j.ejphar.2008.04.058
23. Ciusani E, Balzarotti M, Calatozzolo C, de Grazia U, Boiardi A, Salmaggi A, et al. Valproic Acid Increases the In Vitro Effects of Nitrosureas On Human Glioma Cell Lines. Oncol Res (2007) 16(10):453–63. doi: 10.3727/096504007783338340
24. Castro LM, Gallant M, Niles LP. Novel Targets for Valproic Acid: Up-Regulation of Melatonin Receptors and Neurotrophic Factors in C6 Glioma Cells. J Neurochem (2005) 95(5):1227–36. doi: 10.1111/j.1471-4159.2005.03457.x
25. Sullivan NR, Burke T, Siafaka-Kapadai A, Javors M, Hensler JG. Effect of Valproic Acid On Serotonin-2a Receptor Signaling in C6 Glioma Cells. J Neurochem (2004) 90(5):1269–75. doi: 10.1111/j.1471-4159.2004.02690.x
26. Knupfer MM, Hernaiz-Driever P, Poppenborg H, Wolff JE, Cinatl J. Valproic Acid Inhibits Proliferation and Changes Expression of CD44 and CD56 of Malignant Glioma Cells In Vitro. Anticancer Res (1998) 18(5A):3585–9.
27. Han W, Yu F, Wang R, Guan W, Zhi F. Valproic Acid Sensitizes Glioma Cells to Luteolin Through Induction of Apoptosis and Autophagy via Akt Signaling. Cell Mol Neurobiol (2020). doi: 10.1007/s10571-020-00930-2
28. Li Z, Xia Y, Bu X, Yang D, Yuan Y, Guo X, et al. Effects of Valproic Acid on the Susceptibility of Human Glioma Stem Cells for TMZ and ACNU. Oncol Lett (2018) 15(6):9877–83. doi: 10.3892/ol.2018.8551
29. Chang CY, Li JR, Wu CC, Ou YC, Chen WY, Kuan YH, et al. Valproic Acid Sensitizes Human Glioma Cells to Gefitinib-Induced Autophagy. IUBMB Life (2015) 67(11):869–79. doi: 10.1002/iub.1445
30. White MC, Frampton AR. The Histone Deacetylase Inhibitor Valproic Acid Enhances Equine Herpesvirus Type 1 (EHV-1)-Mediated Oncolysis of Human Glioma Cells. Cancer Gene Ther (2013) 20(2):88–93. doi: 10.1038/cgt.2012.89
31. Ryu CH, Yoon WS, Park KY, Kim SM, Lim JY, Woo JS, et al. Valproic Acid Downregulates the Expression of MGMT and Sensitizes Temozolomide-Resistant Glioma Cells. J BioMed Biotechnol (2012) 2012:987495. doi: 10.1155/2012/987495
32. Ryu CH, Park KY, Kim SM, Jeong CH, Woo JS, Hou Y, et al. Valproic Acid Enhances Anti-Tumor Effect of Mesenchymal Stem Cell Mediated HSV-TK Gene Therapy in Intracranial Glioma. Biochem Biophys Res Commun (2012) 421(3):585–90. doi: 10.1016/j.bbrc.2012.04.050
33. Van Nifterik KA, Van den Berg J, Slotman BJ, Lafleur MV, Sminia P, Stalpers LJ. Valproic Acid Sensitizes Human Glioma Cells for Temozolomide and Gamma-Radiation. J Neurooncol (2012) 107(1):61–7. doi: 10.1007/s11060-011-0725-z
34. Chen CH, Chang YJ, Ku MS, Chung KT, Yang JT. Enhancement of Temozolomide-Induced Apoptosis by Valproic Acid in Human Glioma Cell Lines Through Redox Regulation. J Mol Med (Berl) (2011) 89(3):303–15. doi: 10.1007/s00109-010-0707-1
35. Oi S, Natsume A, Ito M, Kondo Y, Shimato S, Maeda Y, et al. Synergistic Induction of NY-ESO-1 Antigen Expression by a Novel Histone Deacetylase Inhibitor, Valproic Acid, With 5-Aza-2’-Deoxycytidine in Glioma Cells. J Neurooncol (2009) 92(1):15–22. doi: 10.1007/s11060-008-9732-0
36. Zhou Y, Xu Y, Wang H, Niu J, Hou H, Jiang Y. Histone Deacetylase Inhibitor, Valproic Acid, Radiosensitizes the C6 Glioma Cell Line In Vitro. Oncol Lett (2014) 7(1):203–8. doi: 10.3892/ol.2013.1666
37. Su JM, Murray JC, McNall-Knapp RY, Bowers DC, Shah S, Adesina AM, et al. A Phase 2 Study of Valproic Acid and Radiation, Followed by Maintenance Valproic Acid and Bevacizumab in Children With Newly Diagnosed Diffuse Intrinsic Pontine Glioma or High-Grade Glioma. Pediatr Blood Cancer (2020) 67(6):e28283. doi: 10.1002/pbc.28283
38. Watanabe S, Kuwabara Y, Suehiro S, Yamashita D, Tanaka M, Tanaka A, et al. Valproic Acid Reduces Hair Loss and Improves Survival in Patients Receiving Temozolomide-Based Radiation Therapy for High-Grade Glioma. Eur J Clin Pharmacol (2017) 73(3):357–63. doi: 10.1007/s00228-016-2167-1
39. Li C, Chen H, Tan Q, Xie C, Zhan W, Sharma A, et al. The Therapeutic and Neuroprotective Effects of An Antiepileptic Drug Valproic Acid in Glioma Patients. Prog Brain Res (2020) 258:369–79. doi: 10.1016/bs.pbr.2020.09.008
40. Wolff JE, Kramm C, Kortmann RD, Pietsch T, Rutkowski S, Jorch N, et al. Valproic Acid Was Well Tolerated in Heavily Pretreated Pediatric Patients With High-Grade Glioma. J Neurooncol (2008) 90(3):309–14. doi: 10.1007/s11060-008-9662-x
41. Kuo YJ, Yang YH, Lee IY, Chen PC, Yang JT, Wang TC, et al. Effect of Valproic Acid On Overall Survival in Patients With High-Grade Gliomas Undergoing Temozolomide: A Nationwide Population-Based Cohort Study in Taiwan. Med (Baltimore) (2020) 99(28):e21147. doi: 10.1097/MD.0000000000021147
42. Redjal N, Reinshagen C, Le A, Walcott BP, McDonnell E, Dietrich J, et al. Valproic Acid, Compared to Other Antiepileptic Drugs, Is Associated With Improved Overall and Progression-Free Survival in Glioblastoma But Worse Outcome in Grade II/III Gliomas Treated With Temozolomide. J Neurooncol (2016) 127(3):505–14. doi: 10.1007/s11060-016-2054-8
43. Yamazaki H, Kasai S, Mimura J, Ye P, Inose-Maruyama A, Tanji K, et al. Ribosome Binding Protein GCN1 Regulates the Cell Cycle and Cell Proliferation and Is Essential for the Embryonic Development of Mice. PloS Genet (2020) 16(4):e1008693. doi: 10.1371/journal.pgen.1008693
44. Lambert AW, Pattabiraman DR, Weinberg RA. Emerging Biological Principles of Metastasis. Cell (2017) 168(4):670–91. doi: 10.1016/j.cell.2016.11.037
45. Knupfer MM, Pulzer F, Schindler I, Hernaiz Driever P, Knupfer H, Keller E. Different Effects of Valproic Acid on Proliferation and Migration of Malignant Glioma Cells In Vitro. Anticancer Res (2001) 21(1A):347–51.
46. Cui J, Yin Z, Liu G, Chen X, Gao X, Lu H, et al. Activating Transcription Factor 1 Promoted Migration and Invasion in Lung Cancer Cells Through Regulating EGFR and MMP-2. Mol Carcinog (2019) 58(10):1919–24. doi: 10.1002/mc.23086
47. Li S, Cong X, Gao H, Lan X, Li Z, Wang W, et al. Tumor-Associated Neutrophils Induce EMT by IL-17a to Promote Migration and Invasion in Gastric Cancer Cells. J Exp Clin Cancer Res (2019) 38(1):6. doi: 10.1186/s13046-018-1003-0
48. McInerny CJ. Cell Cycle-Regulated Transcription in Fission Yeast. Biochem Soc Trans (2004) 32(Pt 6):967–72. doi: 10.1042/BST0320972
49. Aiello P, Sharghi M, Mansourkhani SM, Ardekan AP, Jouybari L, Daraei N, et al. Medicinal Plants in the Prevention and Treatment of Colon Cancer. Oxid Med Cell Longev (2019) 2019:2075614. doi: 10.1155/2019/2075614
50. Burke PJ. Mitochondria, Bioenergetics and Apoptosis in Cancer. Trends Cancer (2017) 3(12):857–70. doi: 10.1016/j.trecan.2017.10.006
51. Liu X, Zhao P, Wang X, Wang L, Zhu Y, Gao W. Triptolide Induces Glioma Cell Autophagy and Apoptosis via Upregulating the ROS/JNK and Downregulating the Akt/mTOR Signaling Pathways. Front Oncol (2019) 9:387. doi: 10.3389/fonc.2019.00387
52. Li H, Li J, Chen L, Qi S, Yu S, Weng Z, et al. HERC3-Mediated SMAD7 Ubiquitination Degradation Promotes Autophagy-Induced EMT and Chemoresistance in Glioblastoma. Clin Cancer Res (2019) 25(12):3602–16. doi: 10.1158/1078-0432.CCR-18-3791
53. Martens S. A Division of Labor in mTORC1 Signaling and Autophagy. Sci Signal (2018) 11(559):eaav3530. doi: 10.1126/scisignal.aav3530
54. Zhan T, Rindtorff N, Boutros M. Wnt Signaling in Cancer. Oncogene (2017) 36(11):1461–73. doi: 10.1038/onc.2016.304
55. Du Y, Xin Z, Liu T, Xu P, Mao F, Yao J. Overexpressed CA12 Has Prognostic Value in Pancreatic Cancer and Promotes Tumor Cell Apoptosis via NF-kappaB Signaling. J Cancer Res Clin Oncol (2021) 147(5):1557–64. doi: 10.1007/s00432-020-03447-9
56. Zhang J, Wu L, Lian C, Lian S, Bao S, Zhang J, et al. Nitidine Chloride Possesses Anticancer Property in Lung Cancer Cells Through Activating Hippo Signaling Pathway. Cell Death Discov (2020) 6:91. doi: 10.1038/s41420-020-00326-7
57. Zhu M, Yu K, Wang L, Yu S. Contribution of Drugs Acting On the TLRs/MyD88 Signaling Pathway On Colitis-Associated Cancer. Pharmazie (2018) 73(7):363–8. doi: 10.1691/ph.2018.8388
58. Xu X, Wang Z, Liu N, Cheng Y, Jin W, Zhang P, et al. Association Between SOX9 and CA9 in Glioma, and Its Effects On Chemosensitivity to TMZ. Int J Oncol (2018) 53(1):189–202. doi: 10.3892/ijo.2018.4382
59. Han B, Meng X, Wu P, Li Z, Li S, Zhang Y, et al. ATRX/EZH2 Complex Epigenetically Regulates FADD/PARP1 Axis, Contributing to TMZ Resistance in Glioma. Theranostics (2020) 10(7):3351–65. doi: 10.7150/thno.41219
60. Zhao J, Zhu J, Lv X, Xing J, Liu S, Chen C, et al. Curcumin Potentiates the Potent Antitumor Activity of ACNU Against Glioblastoma by Suppressing the PI3K/AKT and NF-Kappab/COX-2 Signaling Pathways. Onco Targets Ther (2017) 10:5471–82. doi: 10.2147/OTT.S149708
61. Sun X, Sun G, Huang Y, Hao Y, Tang X, Zhang N, et al. 3-Bromopyruvate Regulates the Status of Glycolysis and BCNU Sensitivity in Human Hepatocellular Carcinoma Cells. Biochem Pharmacol (2020) 177:113988. doi: 10.1016/j.bcp.2020.113988
62. Wu HT, Lin J, Liu YE, Chen HF, Hsu KW, Lin SH, et al. Luteolin Suppresses Androgen Receptor-Positive Triple-Negative Breast Cancer Cell Proliferation and Metastasis by Epigenetic Regulation of MMP9 Expression via the AKT/mTOR Signaling Pathway. Phytomedicine (2021) 81:153437. doi: 10.1016/j.phymed.2020.153437
63. Oyagbemi AA, Adejumobi OA, Ajibade TO, Asenuga ER, Afolabi JM, Ogunpolu BS, et al. Luteolin Attenuates Glycerol-Induced Acute Renal Failure and Cardiac Complications Through Modulation of Kim-1/NF-KappaB/Nrf2 Signaling Pathways. J Diet Suppl (2020) 18(5):543–65. doi: 10.1080/19390211.2020.1811442
64. Hu H, Hao L, Yan B, Li X, Zhu Y, Yao J, et al. Gefitinib Inhibits Retina Angiogenesis by Affecting VEGF Signaling Pathway. BioMed Pharmacother (2018) 102:115–9. doi: 10.1016/j.biopha.2018.02.110
65. Tryfonidis K, Basaran G, Bogaerts J, Debled M, Dirix L, Thery JC, et al. A European Organisation for Research and Treatment of Cancer Randomized, Double-Blind, Placebo-Controlled, Multicentre Phase II Trial of Anastrozole in Combination With Gefitinib or Placebo in Hormone Receptor-Positive Advanced Breast Cancer (NCT00066378). Eur J Cancer (2016) 53:144–54. doi: 10.1016/j.ejca.2015.10.012
66. De Ruysscher D, Niedermann G, Burnet NG, Siva S, Lee AWM, Hegi-Johnson F. Radiotherapy Toxicity. Nat Rev Dis Primers (2019) 5(1):13. doi: 10.1038/s41572-019-0064-5
67. Guo Z, Wang YH, Xu H, Yuan CS, Zhou HH, Huang WH, et al. LncRNA Linc00312 Suppresses Radiotherapy Resistance by Targeting DNA-PKcs and Impairing DNA Damage Repair in Nasopharyngeal Carcinoma. Cell Death Dis (2021) 12(1):69. doi: 10.1038/s41419-020-03302-2
68. Wang B, Zhao Q, Zhang Y, Liu Z, Zheng Z, Liu S, et al. Targeting Hypoxia in the Tumor Microenvironment: A Potential Atrategy to Improve Cancer Immunotherapy. J Exp Clin Cancer Res (2021) 40(1):24. doi: 10.1186/s13046-020-01820-7
69. Wilcox JA, Ramakrishna R, Magge R. Immunotherapy in Glioblastoma. World Neurosurg (2018) 116:518–28. doi: 10.1016/j.wneu.2018.04.020
70. Wolff JE, Rytting ME, Vats TS, Zage PE, Ater JL, Woo S, et al. Treatment of Recurrent Diffuse Intrinsic Pontine Glioma: The MD Anderson Cancer Center Experience. J Neurooncol (2012) 106(2):391–7. doi: 10.1007/s11060-011-0677-3
71. Thotala D, Karvas RM, Engelbach JA, Garbow JR, Hallahan AN, DeWees TA, et al. Valproic Acid Enhances the Efficacy of Radiation Therapy by Protecting Normal Hippocampal Neurons and Sensitizing Malignant Glioblastoma Cells. Oncotarget (2015) 6(33):35004–22. doi: 10.18632/oncotarget.5253
72. Du Y, Lin J, Shen J, Ding S, Ye M, Wang L, et al. Adverse Drug Reactions Associated With Six Commonly Used Antiepileptic Drugs in Southern China From 2003 to 2015. BMC Pharmacol Toxicol (2019) 20(1):7. doi: 10.1186/s40360-019-0285-y
73. Baddour E, Tewksbury A, Stauner N. Valproic Acid-Induced Hyperammonemia: Incidence, Clinical Significance, and Treatment Management. Ment Health Clin (2018) 8(2):73–7. doi: 10.9740/mhc.2018.03.073
74. Muralidharan A, Rahman J, Banerjee D, Hakim Mohammed AR, Malik BH. Parkinsonism: A Rare Adverse Effect of Valproic Acid. Cureus (2020) 12(6):e8782. doi: 10.7759/cureus.8782
75. Mahmoud F, Tampi RR. Valproic Acid-Induced Parkinsonism in the Elderly: A Comprehensive Review of the Literature. Am J Geriatr Pharmacother (2011) 9(6):405–12. doi: 10.1016/j.amjopharm.2011.09.002
76. Krishnan P, Sinha S, Taly AB, Ramachandraiah CT, Rao S, Satishchandra P. Sleep Disturbances in Juvenile Myoclonic Epilepsy: A Sleep Questionnaire-Based Study. Epilepsy Behav (2012) 23(3):305–9. doi: 10.1016/j.yebeh.2011.12.018
77. Li AS, Marikawa Y. Adverse Effect of Valproic Acid On An In Vitro Gastrulation Model Entails Activation of Retinoic Acid Signaling. Reprod Toxicol (2016) 66:68–83. doi: 10.1016/j.reprotox.2016.09.015
78. Hassamal S, Waller S, Reese K, Testa C. Reversible Valproic Acid-Induced Parkinsonism and Cognitive Impairment in An Elderly Patient With Bipolar Disorder I. Turk Psikiyatri Derg (2016) 27(3):213–7.
79. Gesundheit B, Kirby M, Lau W, Koren G, Abdelhaleem M. Thrombocytopenia and Megakaryocyte Dysplasia: An Adverse Effect of Valproic Acid Treatment. J Pediatr Hematol Oncol (2002) 24(7):589–90. doi: 10.1097/00043426-200210000-00022
80. Li H, Wang X, Zhou Y, Ni G, Su Q, Chen Z, et al. Association of LEPR and ANKK1 Gene Polymorphisms With Weight Gain in Epilepsy Patients Receiving Valproic Acid. Int J Neuropsychopharmacol (2015) 18(7):pyv021. doi: 10.1093/ijnp/pyv021
81. Bota RG, Ligasan AP, Najdowski TG, Novac A. Acute Hypersensitivity Syndrome Caused by Valproic Acid: A Review of the Literature and a Case Report. Perm J (2011) 15(2):80–4. doi: 10.7812/tpp/10-140
82. Cattaneo M, Baronchelli S, Schiffer D, Mellai M, Caldera V, Saccani GJ, et al. Down-Modulation of SEL1L, An Unfolded Protein Response and Endoplasmic Reticulum-Associated Degradation Protein, Sensitizes Glioma Stem Cells to the Cytotoxic Effect of Valproic Acid. J Biol Chem (2014) 289(5):2826–38. doi: 10.1074/jbc.M113.527754
83. Riva G, Butta V, Cilibrasi C, Baronchelli S, Redaelli S, Dalpra L, et al. Epigenetic Targeting of Glioma Stem Cells: Short-Term and Long-Term Treatments With Valproic Acid Modulate DNA Methylation and Differentiation Behavior, But Not Temozolomide Sensitivity. Oncol Rep (2016) 35(5):2811–24. doi: 10.3892/or.2016.4665
84. Chen JC, Lee IN, Huang C, Wu YP, Chung CY, Lee MH, et al. Valproic Acid-Induced Amphiregulin Secretion Confers Resistance to Temozolomide Treatment in Human Glioma Cells. BMC Cancer (2019) 19(1):756. doi: 10.1186/s12885-019-5843-6
85. Feng J, Cen J, Li J, Zhao R, Zhu C, Wang Z, et al. Histone Deacetylase Inhibitor Valproic Acid (VPA) Promotes the Epithelial Mesenchymal Transition of Colorectal Cancer Cells via Upregulation of Snail. Cell Adh Migr (2015) 9(6):495–501. doi: 10.1080/19336918.2015.1112486
86. Yang FQ, Liu M, Yang FP, Che J, Li W, Zhai W, et al. VPA Inhibits Renal Cancer Cell Migration by Targeting HDAC2 and Down-Regulating HIF-1alpha. Mol Biol Rep (2014) 41(3):1511–8. doi: 10.1007/s11033-013-2996-2
87. Zhang L, Wang G, Wang L, Song C, Leng Y, Wang X, et al. VPA Inhibits Breast Cancer Cell Migration by Specifically Targeting HDAC2 and Down-Regulating Survivin. Mol Cell Biochem (2012) 361(1-2):39–45. doi: 10.1007/s11010-011-1085-x
88. Park HK, Han BR, Park WH. Combination of Arsenic Trioxide and Valproic Acid Efficiently Inhibits Growth of Lung Cancer Cells via G2/M-Phase Arrest and Apoptotic Cell Death. Int J Mol Sci (2020) 21(7):2649. doi: 10.3390/ijms21072649
89. Adeshakin AO, Yan D, Zhang M, Wang L, Adeshakin FO, Liu W, et al. Blockade of Myeloid-Derived Suppressor Cell Function by Valproic Acid Enhanced Anti-PD-L1 Tumor Immunotherapy. Biochem Biophys Res Commun (2020) 522(3):604–11. doi: 10.1016/j.bbrc.2019.11.155
90. Pan Z, Oh J, Huang L, Zeng Z, Duan P, Li Z, et al. The Combination of Forskolin and VPA Increases Gene Expression Efficiency to the Hypoxia/Neuron-Specific System. Ann Transl Med (2020) 8(15):933. doi: 10.21037/atm-20-3871
91. Angelucci A, Muzi P, Cristiano L, Millimaggi D, Cimini A, Dolo V, et al. Neuroendocrine Transdifferentiation Induced by VPA Is Mediated by PPAR Gamma Activation and Confers Resistance to Antiblastic Therapy in Prostate Carcinoma. Prostate (2008) 68(6):588–98. doi: 10.1002/pros.20708
92. Kerkhof M, Benit C, Duran-Pena A, Vecht CJ. Seizures in Oligodendroglial Tumors. CNS Oncol (2015) 4(5):347–56. doi: 10.2217/cns.15.29
93. Nakada M, Furuta T, Hayashi Y, Minamoto T, Hamada J. The Strategy for Enhancing Temozolomide Against Malignant Glioma. Front Oncol (2012) 2:98. doi: 10.3389/fonc.2012.00098
94. Groot M, Douw L, Sizoo EM, Bosma I, Froklage FE, Heimans JJ, et al. Levetiracetam Improves Verbal Memory in High-Grade Glioma Patients. Neuro Oncol (2013) 15(2):216–23. doi: 10.1093/neuonc/nos288
95. Lange F, Wesslau K, Porath K, Hornschemeyer J, Bergner C, Krause BJ, et al. AMPA Receptor Antagonist Perampanel Affects Glioblastoma Cell Growth and Glutamate Release In Vitro. PloS One (2019) 14(2):e0211644. doi: 10.1371/journal.pone.0211644
96. Piotrowski AF, Blakeley J. Clinical Management of Seizures in Patients With Low-Grade Glioma. Semin Radiat Oncol (2015) 25(3):219–24. doi: 10.1016/j.semradonc.2015.02.009
Keywords: valproic acid (VPA), glioma, anti-tumor effects, synergistic effects, clinical trials
Citation: Han W and Guan W (2021) Valproic Acid: A Promising Therapeutic Agent in Glioma Treatment. Front. Oncol. 11:687362. doi: 10.3389/fonc.2021.687362
Received: 29 March 2021; Accepted: 20 August 2021;
Published: 10 September 2021.
Edited by:
Gerardo Caruso, University Hospital of Policlinico G. Martino, ItalyReviewed by:
Hongtao Qu, University of South China, ChinaMonica Malik, Nizam’s Institute of Medical Sciences, India
Copyright © 2021 Han and Guan. This is an open-access article distributed under the terms of the Creative Commons Attribution License (CC BY). The use, distribution or reproduction in other forums is permitted, provided the original author(s) and the copyright owner(s) are credited and that the original publication in this journal is cited, in accordance with accepted academic practice. No use, distribution or reproduction is permitted which does not comply with these terms.
*Correspondence: Wei Guan, Z3VhbndlaTE0MDJAMTYzLmNvbQ==