- 1Department of Hepatobiliary and Pancreatic Surgery, The Second Hospital of Jilin University, Changchun, China
- 2Jilin Provincial Key Laboratory on Molecular and Chemical Genetic, The Second Hospital of Jilin University, Changchun, China
- 3Department of Radiotherapy, The Second Hospital of Jilin University, Changchun, China
Mitochondria participate in the progression of hepatocellular carcinoma (HCC) by modifying processes including but not limited to redox homeostasis, metabolism, and the cell death pathway. These processes depend on the health status of the mitochondria. Quality control processes in mitochondria can repair or eliminate “unhealthy mitochondria” at the molecular, organelle, or cellular level and form an efficient integrated network that plays an important role in HCC tumorigenesis, patient survival, and tumor progression. Here, we review the influence of mitochondria on the biological behavior of HCC. Based on this information, we further highlight the need for determining the role and mechanism of interaction between different levels of mitochondrial quality control in regulating HCC occurrence and progression as well as resistance development. This information may lead to the development of precision medicine approaches against targets involved in various mitochondrial quality control-related pathways.
1 Introduction
Hepatocellular carcinoma (HCC) accounts for approximately 75% of primary liver cancers Current therapies for HCC, including surgery, chemotherapy, and targeted therapy, are not effective for treating advanced HCC because they do not prevent the proliferation, metastasis, invasion, angiogenesis, and chemoresistance of this cancer (1–5); therefore, the 5-year relative survival rate of patients with HCC remains at only 18% (6). It has been reported that mitochondria can participate in the progression of HCC by modifying redox homeostasis (7), metabolism (8, 9), and the cell death pathway (10); these complex regulatory networks play important roles in tumorigenesis, patient survival, and tumor progression and depend on the mitochondrial health status. Cells can repair or eliminate “unhealthy mitochondria” via mitochondrial quality control to balance mitochondrial homeostasis. Several studies have focused on the influence of mitochondrial quality control on the maintenance of cell viability and chemoresistance by evaluating the metabolic pathways specific to liver cells. These studies identified key mitochondrial quality control molecules as new targets for liver cancer treatment. Here, we review the effects of mitochondrial quality control-related pathways and molecules that maintain cell survival on the efficacy of liver cancer therapy and provide new candidates for mitochondria-targeted liver cancer treatments.
2 Mitochondria Regulate the Progression and Death of Hepatocellular Carcinoma
The mitochondrial matrix is surrounded by two lipid membranes: the outer mitochondrial membrane and inner mitochondrial membrane (IMM). Various proteins and protein complexes are present on the mitochondrial membrane. Mitochondria function to supply energy to cells through oxidative phosphorylation, such as by producing ATP through the electron transport chain (ETC) and by using oxygen. This process is accompanied by the generation of reactive oxygen species (ROS) (11). Mitochondria are abundant in tumor cells, although aerobic glycolysis is the main mode of energy metabolism in these cells. This high-speed, low-efficiency mode of metabolism is known as the Warburg effect, and it allows cells to grow rapidly and promotes the tumor formation, proliferation, and progression (12, 13). Moreover, mitochondria in tumor cells maintain complete metabolic functions and play important roles in tumor metabolism. Studies have shown that phenformin inhibits the mitochondrial respiratory chain complex I to suppress the proliferation of liver cancer cells (14). Inhibiting the expression of mitochondrial ATP synthase subunit e restricts cell proliferation (15). These findings also indicate that mitochondria affect tumor cell metabolism and alter the biological behavior of a tumor. Additionally, when tumor cells respond to external stress stimulation, they maintain their survival through mitochondria-mediated metabolic reprogramming.
The liver is a metabolic factory in humans. Therefore, mitochondria are more abundant in liver cells than in other types of cells (16), suggesting that mitochondria play an important role in the occurrence and development of liver cancer. Excessive ROS production by mitochondria in hepatocytes can induce tumorigenesis. Rostkowska et al. found that N-nitrosodiethylamine induces excessive production of free radicals that disrupt protein folding and increase ATP demand in hepatocyte mitochondria. These effects indirectly increase mitochondrial oxidative phosphorylation and ROS accumulation, eventually leading to liver carcinogenesis (17–21). Preventive elimination of mitochondria-derived ROS (mtROS) has been suggested to inhibit the occurrence of HCC (22). In tumor cells, moderate levels of mtROS can stimulate the expression of nuclear genes involved in angiogenesis and oxygen supply, glycolysis, anti-oxidation, cell proliferation, epithelial-mesenchymal transition, anti-apoptosis, invasion, and metastasis through mitochondrial retrograde signaling pathways that help cells adapt to new metabolic requirements (23). For example, the production of low levels of mtROS can induce the proliferation of HCC cells through phosphoinositide 3-kinase-mediated phosphorylation of Akt (24). However, excessive levels of ROS can also have a tumor-killing effect. Several previous studies, including a study conducted by our group, have revealed that cisplatin-based chemotherapy drugs destroy liver cancer cells by inducing an increase in mtROS levels and inhibiting HCC proliferation. The increase in ROS levels induced by lenvatinib can promote apoptosis in liver cancer cells through the ATM and eIF2α signaling pathway (25, 26).
Mitochondria are also central organelles that regulate cell death such as apoptosis, necrosis, ferroptosis, pyroptosis, and other cell death processes. An increased expression of anti-apoptotic members of the Bcl-2 protein family (Bcl-XL and Mcl-1), which are localized to the mitochondria in HCC cells, can induce resistance to regorafenib and sorafenib (27, 28). Resistance to apoptosis also plays a role in the chemoresistance mechanisms of liver cancer cells. For example, an increased expression of mitochondrial phosphoglycerate mutase/protein phosphatase in liver cancer cells enhances chemoresistance to 5-fluorouracil by increasing the stability of Bcl-XL, and inhibiting the expression of Bax and cytochrome c, which eventually inhibit the apoptotic signaling pathway (29). A study suggested that HCC can resist apoptosis by overexpressing mitochondrial uncoupling protein to decrease mitochondrial membranes permeability (30). In addition to apoptosis, mitochondria are involved in ferroptosis and necrosis. Genes related to mitochondrial iron and fatty acid metabolism (RPL8, IREB2, ATP5G3, CS, TTC35, and ACSF35) can provide the required lipid precursors for mitochondrial ferroptosis and excess iron to induce ROS. Levels of ROS that exceed the antioxidant capacity of cells can cause oxidative stress, which directly or indirectly damages various macromolecules, such as proteins, nucleic acids, and lipids (31), and triggers the release of lipid peroxides from the mitochondria that leads to cell ferroptosis (32). Moreover, overexpression of mitochondrial CISD1 (CDGSH iron-sulfur domain 1) can inhibit ferroptosis in HCC cells by regulating membrane lipid peroxidation and mitochondrial iron uptake (33). These findings suggest that mitochondria play a role in regulating iron-induced death in HCC cells. To induce necroptosis, mitochondrial ROS activate autophosphorylation of receptor-interacting protein kinase 1, leading to the recruitment of RIPK3 to further promote the production of ROS. This forms a positive feedback loop that promotes necroptosis (11, 34–37). Recent studies have shown that in HepG2 cells with low levels of GSNOR, inhibition of the mitochondrial respiratory chain complex II expression leads to necroptosis of the PARP1/receptor-interacting protein kinase 1 pathway and suppresses cell growth (38). However, mitochondrial glutathione in HepG2 cells increases the tolerance of cells to azathioprine by protecting cytochrome C and inhibiting necroptosis via the JNK pathway (39). Pyroptosis is a process induced by inflammasomes, as an immune response to bacteria, pathogens, or their endotoxins (40). One of the main mechanisms is gasdermin E(GSDME)-dependent pyroptosis. A recent study has found that the mitochondrial apoptotic protein caspase3/7 can be activated by GSDME and then participate in cell apoptosis, additionally, mitochondrial endogenous apoptotic protein caspase3/9 can also induce GSDME-dependent pyroptosis (41), suggesting that mitochondria play an important role in the crosstalk between apoptosis and pyroptosis. NLRP3 as a kind of inflammasome was found to activate caspase-1, which results in pyroptosis activation. Yu et al. established a HCC cell model of Non-alcoholic steatohepatitis using double stimulation with palmitic acid and lipopolysaccharide, which caused mitochondrial dysfunction and ROS generation. ROS overproduction activated inflammasome by binding to NLRP3, and then initiate pyroptosis. But PINK/Parkin-dependent mitophagy induced by liraglutide could eliminate damaged mitochondria and inhibit pyropotosis (42).
In summary, stable energy and metabolism, redox homeostasis of mitochondria, and regulation of liver cancer cell death influence the tumorigenesis and progression of HCC (Figure 1). The maintenance of mitochondrial homeostasis is the basis of influence of development. Several studies have shown that HCC can maintain mitochondrial homeostasis through mitochondrial quality control, enabling the proliferation, invasion, chemotherapy resistance, and survival of HCC cells. Mitochondrial quality control changes occur at the molecular, organelle, and cellular levels to maintain mitochondrial function or eliminate damaged mitochondria to ensure mitochondrial and cellular homeostasis (21, 43).
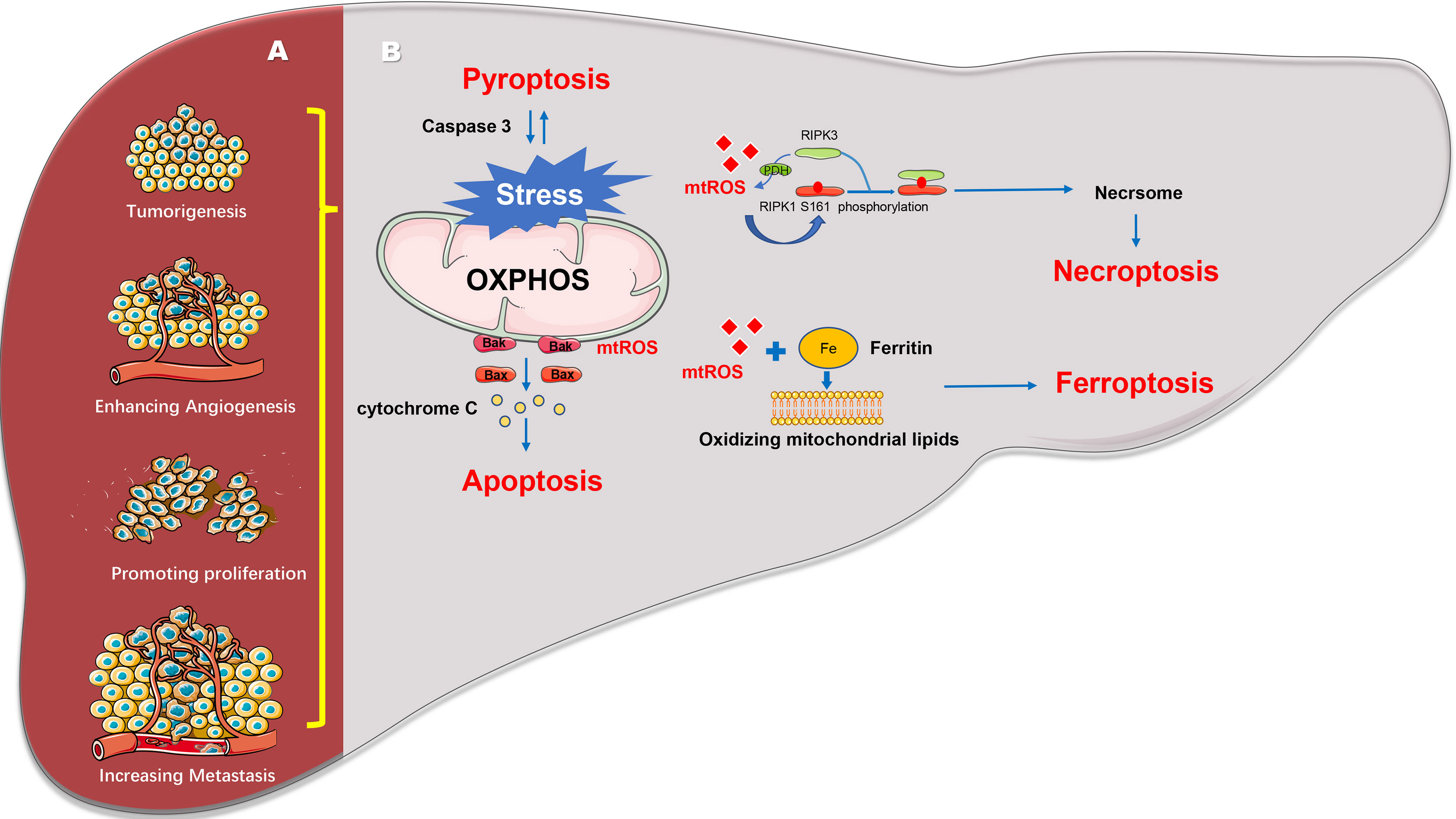
Figure 1 Mitochondrial regulates the progression and death of hepatocellular carcinoma. (A) Mitochondria can reverse regulate the expression of nuclear genes to induce cell tumorigenesis, angiogenesis, cell proliferation, invasion, and metastasis. (B) Mitochondria can regulate various cell death pathways including apoptosis, necroptosis, pyroptosis and ferroptosis and so on.
3 Molecular Mechanisms Involved in the Quality Control of Mitochondria
In response to external nutrient deficiency, hypoxia, oxidative stress, abnormal metabolite accumulation, viruses, and other pathophysiological factors, mitochondria can stimulate the mitochondrial quality control system to ensure that a sufficient number of functional mitochondria are present to meet the cellular needs in case of cell damage (43, 44). Proteins in the mitochondria are encoded by nuclear and mitochondrial genes. Moreover, mitochondrial quality control is an extremely complicated system involving coordinated protein synthesis and mitochondrial import of nuclear gene-encoded proteins.
Most HCC patients are accompanied by viral hepatitis, alcoholic liver disease, or non-alcoholic fatty liver disease (NAFLD), which causes the microenvironment of HCC to be different from that of other tumors. These factors also affect the mitochondrial status and mitochondrial quality control. Studies have shown that HCC cells can induce the down-regulation of MFN2 and the recruitment of DRP1 to mitochondria after HCV infection, causing mitochondrial fission and subsequent mitophagy. These changes in mitochondrial quality control can help reduce HCC cell apoptosis and may lead to persistent HCV infection (45). A similar phenomenon was also found in HBV-infected HCC cells. HBV can induce mitochondrial translocation of Drp1. In addition, HBV upregulates the expression of genes Parkin, PINK1 and LC3B and induces Parkin recruitment to mitochondria and MFN2 degradation by ubiquitination. Mitochondrial fission and mitophagy also promote the survival of HCC cells and may promote the continued existence of HBV (46). In an experiment using oleic acid to treat HCC cells to build NAFLD model, it was found that oleic acid induced NAFLD can induce mitophagy in HCC cells (47). In addition, alcohol can also damage the mitochondria of HCC cells and induce mitochondrial quality control (48). Above all, compared with other tumor cells, HCC cells may need to adapt to the unique tumor microenvironment through mitochondrial quality control for maintaining its own survival.
3.1 Transport of Mitochondrial Proteins and Mitochondrial Unfolded Protein Response in HCC
The nucleus encodes most mitochondrial proteins that undergo unfolding and refolding to enter the mitochondria (43, 49). Mitochondria produce ROS during energy metabolism, leading to damage to the mitochondrial DNA (mtDNA). These factors eventually interfere with protein folding. Therefore, to ensure mitochondrial homeostasis, cells must degrade abnormal (misfolded or unfolded) proteins via the mitochondrial unfolded protein response (mtUPR) (Figure 2) (43). This method of degradation or refoldation separately depends on mitochondrial proteases and chaperone proteins, such as mitochondrial heat shock protein 60, TNF receptor-associated protein 1 (TRAP1), and mortalin (HSPA9) as well as the ubiquitin-proteasome system for mitochondrial quality control at the molecular level (50). In the mitochondrial matrix, misfolded or unfolded proteins are degraded by mitochondrial proteases (LON and mtClpP) or refold by mitochondrial protein chaperones (Hsp60/Hsp70/Hsp100). Misfolded or unfolded proteins in the inner mitochondrial membrane, particularly unassembled subunits of the respiratory chain complex, are mainly degraded by two membrane-integrated protease systems (i-AAA/m-AAA) and two AAAs (ATPases associated with diverse cellular activities) that belong to the AAA+ enzyme family (51, 52). In the outer mitochondrial membrane, misfolded or unfolded proteins are ubiquitinated by the ubiquitin-proteasome system in the cytosol and then degraded (53, 54).
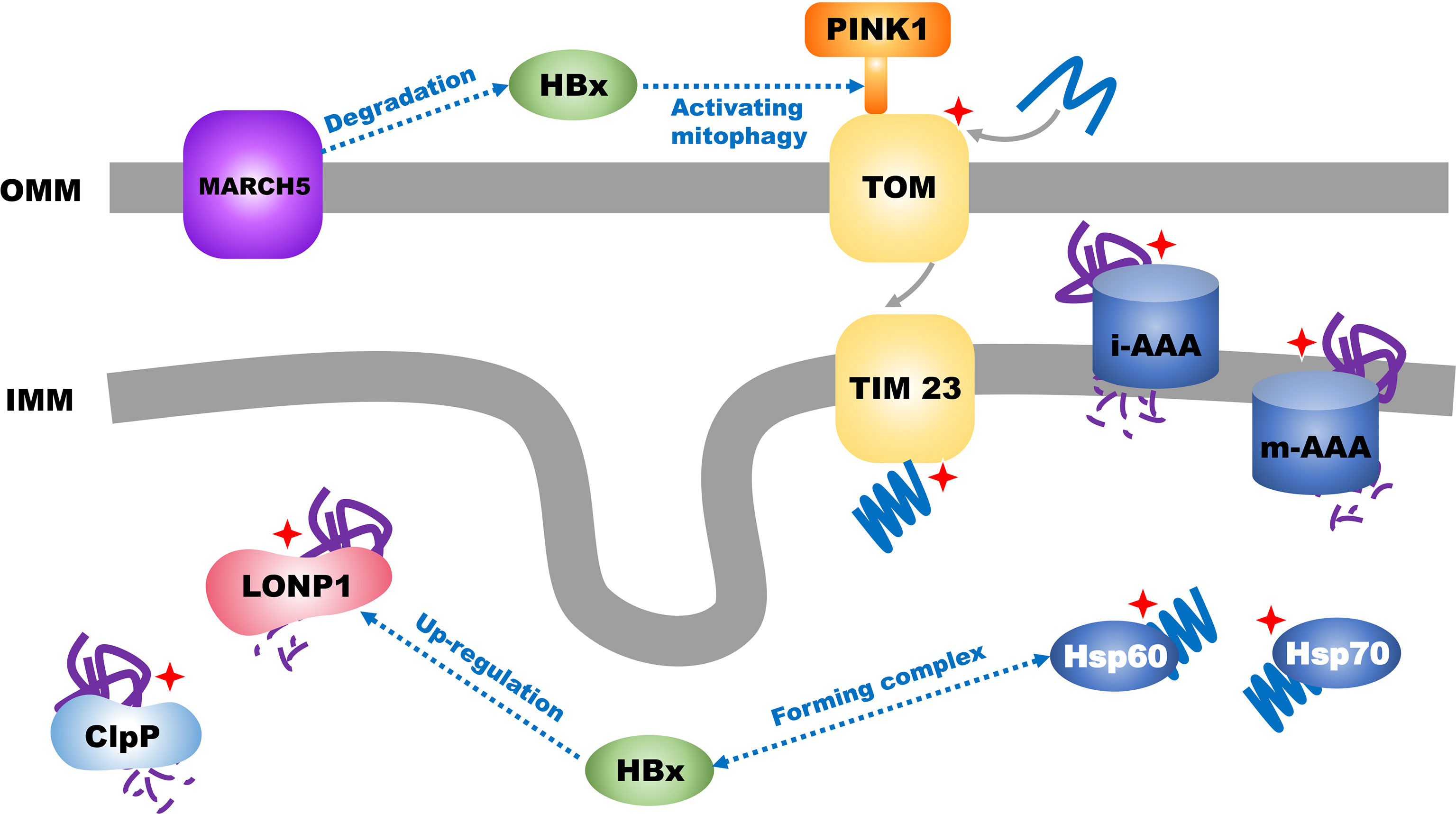
Figure 2 Transport of mitochondrial proteins and mitochondrial unfolded protein response in HCC. Mitochondrial unfolded protein response is labelled with red star.
In mammals, the mtUPR is triggered by an integrated stress response caused by misfolded proteins in the mitochondria. The integrated stress response promotes overexpression of ATF5 by phosphorylating the translation initiation factor eIF2α (55, 56). Under normal conditions, ATF5 is introduced into the mitochondria by its own mitochondrial targeting sequence for degradation; however, under mitochondrial-nucleoprotein imbalance conditions, the nuclear localization signal of ATF5 binds to the nuclear mtUPR element. This initiates the transcription of genes related to the mtUPR, including HSP60, HSP10, and LONP1. These transcripts are translated and introduced into the mitochondria (anterograde signals). Inside the mitochondria, molecular chaperones, such as Hsp60 and Hsp10, co-fold damaged proteins, and mitochondrial proteases, such as LONP1, cut and degrade the irreparable proteins to maintain mitochondrial protein homeostasis (57).
Approximately 40% of HCCs are caused by hepatitis B virus (HBV) infection (58). HBV can promote the progression and worsen the prognosis of HCC (59) through the expression of the open reading frame of HBV X protein (HBx). Xu et al. found that HBx could promote an increase in the expression of ATF5 through the HPIP/AKT/ERK/FOXO4 pathway (60); another study showed that HBx could form a complex with the mtUPR molecular chaperones HSP60 and HSP70 (61) which facilitate HBx-induced cell apoptosis (62). Furthermore, HBx can promote the mtUPR. Studies have found that HBx can induce the translocation of LONP1 from the cytoplasm to the mitochondria and activate the LONP1/PINK1 pathway to promote mitochondrial autophagy and eventually inhibit the mitochondrial apoptotic pathway (63). However, HBx in the mitochondria is also regulated by the ubiquitination system of the outer mitochondrial membrane in the mtUPR; the outer mitochondrial membrane mitochondrial ubiquitin ligase (MARCH5) mediates the degradation of HBx via the ubiquitin proteasome and inhibits ROS generation, stabilizes the quality of mitochondria, reduces inflammation caused by HBx, and inhibits the initiation of HCC. Western blotting and mRNA expression analyses of tumor tissues from patients with HCC have suggested that the expression of MARCH5 gradually decreases with increasing tumor grades (G1–G4), and Kaplan-Meier analysis has shown that high MARCH5 expression is associated with prolonged survival in patients with HCC (64). Efavirenz (EFV) is the most widely used non-nucleoside reverse transcriptase inhibitor and has been shown to cause liver damage by affecting mitochondrial homeostasis in hepatocytes. Nadezda et al. found that in Hep3B cells, EFV could induce endoplasmic reticulum stress, which is mediated by the mitochondria. However, the harmful effects of EFV are markedly reduced in cells lacking functional mitochondria (65). Another study has shown that although EFV can reduce the content of LON in the mitochondria in Hep3B cells, it can increase its content in the outer membrane of mitochondria and mitochondria-associated endoplasmic reticulum membranes, and thus, it may lead to mitochondrial/endoplasmic reticulum stress in HCC cells (66).
In addition to maintaining the homeostasis of mitochondrial proteins, studies have found that the degradation of the mitochondrial chaperone, TRAP1, induced by S-nitrosylation, leads to increased succinate dehydrogenase activity and inhibition of mitochondrial complex IV expression. Increased succinate dehydrogenase activity sensitizes HepG2 cells to α-tocopheryl succinate (α-TOS), which is a succinate dehydrogenase inhibitor, and oxidative stress caused by the mitochondrial targeting agent mitocans. Additionally, α-TOS can inhibit the proliferation of HCC cells by degrading TRAP1 and inducing necroptosis (38).
Proper transport of mitochondrial proteins ensures both mitochondrial protein and nucleic acid homeostasis. For example, the mitochondrial transport protein mia40 is essential for transporting purine/pyrimidine endonuclease 1, which repairs mtDNA. Moreover, as cell proliferation is accelerated in the early stage of HCC, high expression of mia40 promotes purine/pyrimidine endonuclease 1 mitochondrial translocation, which is beneficial for maintaining mitochondrial homeostasis during the rapid cell proliferation stage (67).
Few studies have focused on the mitochondrial proteases involved in the mtUPR in the mitochondrial matrix and intima in HCC cells, with most studies investigating the diagnosis, evaluation of the therapeutic effect, and prognosis of HCC (68–71). These limited studies of the mtUPR molecular chaperones and proteases have shown that mtUPR has a promotes HCC progression. Therefore, further research is needed to clarify whether these molecules affect the biological behavior of tumor cells and are related to the regulation of mitochondrial quality control.
3.2 Mitochondrial Quality Control at the Organelle Level in HCC
Mitochondria are highly motile and are transported along the cytoskeleton. As the degree of stress increases, mitochondria and cells initiate mitochondrial quality control at the organelle level, which involves mitochondrial fusion, fission, mitophagy, and mitochondrial biogenesis (Figure 3).
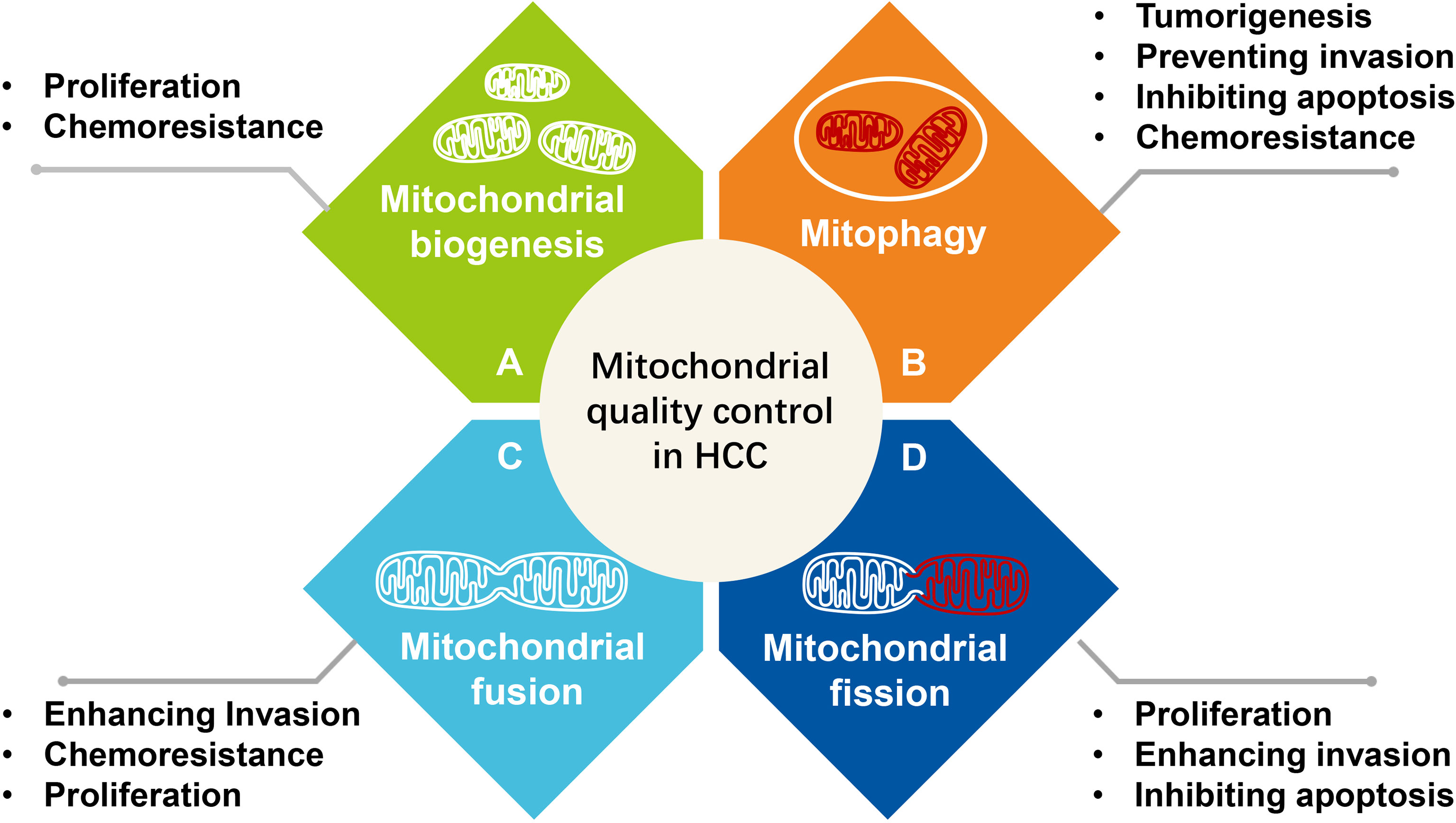
Figure 3 The effect of mitochondrial quality control at the organelle level on the survival and progression of hepatocellular carcinoma. (A) Mitochondrial biogenesis, (B) Mitophagy, (C) Mitochondrial fusion, (D) Mitochondrial fission.
Mitochondrial fission is the division of a mitochondrion into two smaller mitochondria. This division starts with the recruitment of cytoplasmic Drp1 (also known as DNM1L) by mitochondrial outer membrane proteins—mitochondrial fission 1 protein (FIS1) and mitochondrial fission factor (MFF)—following which Drp1 oligomerizes and drives scission (72, 73). The recruited Drp1 combines with mitochondrial outer membrane proteins (FIS1, MFF, MID49, and MID51) and then oligomerizes to form a band, compressing the site at which mitochondrial division occurs (74). The hydrolysis of GTP in the MID49/MID51–DRP1–GTP complex assists in shrinking of the complex, which curls into a closed loop with an inner diameter of 16 nm until the outer mitochondrial membrane shrinks (75). The cleaved mitochondria can be further transported by autophagosomes to lysosomes for degradation, a process known as mitophagy (44), making mitochondrial fission an important mode of mitophagy sorting. Under hypoxic conditions, HCC cells increase mitochondrial fission by overexpressing Drp1 and increasing expression of the mitophagy-related protein, BNIP3, to promote the mitophagy and survival of HCC cells. However, after Midiv-1 inhibits fission, hypoxia induces apoptosis via the mitochondrial pathway in HCC cells. This suggests that HCC cells can use mitochondrial fission as a stress-responsive mechanism to maintain cell survival (76). Other studies have shown that mitochondrial division plays a role in the separation of mitochondria with damaged proteins, membrane instability, and mutations or damaged mtDNA (77–81). Additionally, mitochondrial division markedly affects the production of ROS (82), regulation of cell proliferation (83–86), and apoptosis (87).
Mitochondrial fusion is a mechanism through which mitochondria combine. This process requires two mitochondria to be in close contact (88). Once a close connection is established, two MFN1 molecules dock in the trans position, possibly via the HR2 or GTPase domain; this association induces conformational changes that drive GTP hydrolysis by MFN1 molecules, leading to the fusion of the two outer mitochondrial membranes (73). Further, IMM fusion mediated by OPA1 depends on the inner membrane potential. However, fusion helps balance the matrix metabolite levels, regulate the distribution of mtDNA in the mitochondrial network, or help balance the levels of membrane components, such as electron transport chain complex I (77, 89–92). These components enable mitochondria to resist metabolic damage, maintain cell integrity and prevent autophagy, and improve the aerobic metabolism of cells to respond to stimuli, such as starvation and low protein synthesis (93, 94).
Mitophagy is an important mechanism of mitochondrial quality control at the organelle level. Normally PINK1 is imported into the IMM, and a lack of import induces mitophagy by stabilizing PINK1 to recruit Parkin1 in mitochondrial outer membrane (44, 95). Mitophagy involves five steps: formation of isolation membrane, elongation, closure of the isolation membrane and autophagosome formation, autophagosome–lysosome fusion, and lysosomal degradation (96). PINK1 is constitutively imported, likely via the TIM/TOM complex, to the inner membrane, where it is cleaved by several proteases and then proteolytically degraded (97–100). Accumulation of PINK1 on the mitochondrial surface recruits Parkin from the cytosol to the damaged mitochondria. After mitochondrial translocation, the E3 ubiquitin ligase activity of Parkin increases (101), which mediates the formation of two types of polyubiquitin chains: lysine (K) 48 linkage associated with proteasomal degradation of the substrate and lysine (K) 63 linkage associated with autophagic degradation (98). K48-mediated degradation of substrates, such as Miro and Mitofusin, inhibits mitochondrial fusion and transport, whereas K63 is recognized by ubiquitin-binding adaptors, such as p62, HDAC6, and other unknown ubiquitin-binding adaptors, that recruit damaged mitochondria to the isolation membrane by interacting with the autophagosomal protein LC3 (44). This pathway is known as PINK1/Parkin-dependent mitochondrial autophagy.
Furthermore, the mitochondrial outer membrane proteins—BNIP3, NIX, and FUNDC1—combine with the isolation membrane LC3 to participate in recruiting and extending the isolation membrane, eventually leading to the formation of autophagosomes (44, 50). BNIP3 is a Bcl-2 homology 3only protein with an LC3-binding motif known as the LC3 interaction region and binds to the isolation membrane LC3/GABARAP to promote mitophagy (102). Nix (BNIP3L), a mitochondrial outer membrane protein related to Bcl2, has an atypical BH3 domain containing a conserved LC3 interaction region; thus, it may be similar to the yeast recruiting autophagy ATG32/ATG11 receptors that target autophagosomes. FUNDC1 is present on the outer mitochondrial membrane. Under normal conditions, FUNDC1 is phosphorylated by Src kinase and CK2 at Tyr18 and Ser13, respectively, to reduce its affinity for LC3. Under hypoxic conditions or following the loss of mitochondrial potential, FUNDC1 is dephosphorylated by phosphoglycerate mutase/protein phosphatase and accumulates through the mitochondrial-endoplasmic reticulum junction, binds to the isolation membrane LC3 through the LC3 interaction region sequence, and recruits Drp1 to promote mitochondrial phagocytosis by autophagosomes (103). Autophagosome encapsulation triggers lysis in lysosomes (104–106) and maintains a healthy mitochondrial population (21). When mitophagy eliminates damaged mitochondria before caspase-dependent apoptosis is activated, mitochondrial autophagy functions as a cell survival mechanism.
An excessive reduction in the mitochondrial mass caused by persistent mitochondrial fission or mitophagy is not conducive to cell survival; these processes induce the cell to undergo apoptosis ensure mitochondrial quality. Mitochondrial biogenesis refers to the coordinated expression of genes in the nuclear and mitochondrial genome that help generate new mitochondria from existing mitochondria, which includes the synthesis of internal and external mitochondrial membranes and mitochondrial gene-encoded proteins, synthesis and entry of nuclear gene-encoded mitochondrial proteins, and mtDNA replication. Of these, peroxisome proliferation-activated receptor-γ co-activator-1α (PGC-1a) is considered the main regulator of mitochondrial biogenesis. It interacts with two key nuclear transcription factors, nuclear respiratory factor 1 and 2 (NRF1 and NRF2), and increases the expression and activity of NRF1 and NRF2 through protein interactions. NRF1 and NRF2 activate mitochondrial transcription factor A (TFAM) and bind to the promoter regions of nuclear genes encoding the five subunits of the ETC complex, thereby increasing the assembly of respiratory complexes and regulating participation in heme biosynthesis, transcription of nuclear genes encoding mitochondrial proteins, and mtDNA replication and transcription (107). Mitochondrial quality control at the organelle level ensures flexibility at the metabolic level, thus enabling tumor cells to adapt to targeted therapy and the tumor microenvironment and ultimately support their growth, survival, and migration (21). Studies have shown that the overexpression of c-Myc in hepatocytes promotes mitochondrial production of MAMs (endoplasmic reticulum-associated mitochondrial membrane), which induce the release of Ca2+ from the endoplasmic reticulum into mitochondria and oxidative stress and inhibit oxidative phosphorylation in hepatocytes in organoid containers. These events promote the initiation of hepatocellular carcinogenesis (108).
3.2.1 Mitochondrial Fission
3.2.1.1 Effect of Mitochondrial Fission on Proliferation of HCC Cells
Studies of the effects of mitochondrial division on the regulation of cell proliferation have (86, 87, 109) suggested that tumor cell mitochondrial dynamics affect proliferation by influencing the cell cycle. Imaging studies of cultured cells have indicated that mitochondrial morphology undergoes stereotypical changes during progression through the cell cycle (109–112). The two most apparent features are tubulation of the mitochondrial network at the G1/S transition and extensive fragmentation during mitosis. The elongated, “hyperfused” network at G1/S is associated with increased ATP production and affects the entry of cells into S phase by controlling the levels of cyclin E (111), whereas extensive fragmentation ensures an equal distribution of mitochondria to the daughter cells. Moreover, the CDK1/cyclin B-mediated phosphorylation of Drp1 at Ser616 facilitates daughter cells to accept part of the mitochondrial network, which is essential for proper progression of cell division (109).
Furthermore, Drp1 is involved in the G1/S transition in HCC cells. Overexpression of Drp1 induces mitochondrial fission, which can be inhibited by p53 (by activating the ROS-dependent Akt/MDM2 pathway) and increased activity of NF-κB (which induces cyclin D1 and E1 expression) to promote the proliferation of HCC cells (113). Inhibition of Drp1 phosphorylation suppresses mitochondrial fission, thus inhibiting cell proliferation, migration, invasion, and epithelial-mesenchymal transition (114).
Mitochondrial fission process protein 1 (MTP18) participates in mitochondrial fission by regulating the division of mitochondrial membranes. However, there limited studies have explored the function of MTP18 in mitochondrial division. Studies have suggested that the overexpression of MTP18 increases mitochondrial fragmentation and promotes HCC cell growth both in vitro and in vivo by participating in G1/S transition and inhibiting cellular apoptosis. Midiv1, an inhibitor of mitochondrial division, inhibits the growth of HCC cells (115).
3.2.1.2 Impact of Mitochondrial Fission on Invasion and Metastasis in HCC
Mitochondrial fission affects the invasiveness of HCC, and increased expression of Drp1 at the mRNA and protein levels in distant metastatic tissues does not improve disease-free survival in patients with HCC. Moreover, overexpression and knockdown of Drp1 in an orthotopic liver cancer model suggested that Drp1 increases intrahepatic lung metastasis and that Drp1-induced mitochondrial fission regulates reprogramming of the adhesion complex and lamellar pseudopodia and promotes invasion in HCC by Ca2+/calmodulin-dependent protein kinase II. Phosphorylation of downstream extracellular signal-regulated kinase 1/2 and focal-adhesion kinase eventually promotes liver cancer invasion. Additionally, increased ATP production (by promoting Ca2+ influx) can repress the inhibitory effect of mitochondrial fission on cell migration, suggesting that mitochondrial division can affect invasion and metastasis in HCC by regulating mitochondrial energy production (116).
Studies of other mitochondrial fission factors have revealed high FIS1 expression in HCC metastases and showed that this protein promotes HCC invasion and migration by inducing epithelial-mesenchymal transition (117). Increased expression of MTP18 also increases the invasion of HCC cells, whereas its decreased expression inhibits invasion by suppressing epithelial-mesenchymal transition and downregulating the expression of matrix metallopeptidase 9. Furthermore, knockout of FIS1 inhibited the migration of HCC cells, although MTP18 promoted this migration via mitochondrial fission (115).
3.2.1.3 Impact of Mitochondrial Fission on Apoptosis in HCC
Studies suggest that Drp1-induced increased mitochondrial division enhances the survival of HCC cells by promoting mitophagy and inhibiting mitochondria-dependent apoptosis. Specifically, Drp1-mediated mitochondrial fission increases mtROS levels, which can increase NF-κB activity and inhibit TP53. Therefore, mitochondrial fission increases the viability of HCC via crosstalk between the two pathways (118).
However, as a regulator of mitochondrial morphology, FIS1 plays a major role in the response to stress. For example, under high-energy fructose-palmitate culture conditions, high expression of FIS1 leads to steatosis in HepG2 cells as a link in the mitochondrial stress response and induces mitochondrial fission. The mitochondria can then produce sufficient ROS to damage themselves. Other changes in the mitochondria include dissipation of the membrane potential, low expression of ETC molecules, decreased mitochondrial biosynthesis (PGC-1α), and increased caspase 3-mediated apoptosis (119). Furthermore, the addition of silver nanoparticles reduces the expression of FIS1, fragments the mitochondrial morphology (by increasing p-Drp1 levels), decreases mitochondrial biogenesis, and transiently enhances antioxidant capacity and improves mitochondrial pathway-mediated apoptosis (120).
However, some studies have shown that treatment with suberoylanilide hydroxamic acid (SAHA), an acetylase inhibitor, can significantly induce apoptosis in HCC cells through mechanisms other than the overexpression of Bcl-2. Moreover, SAHA significantly reduces the expression of FIS1 and translocation of Drp1 to the mitochondria, which inhibits mitochondrial fission. However, downregulation of the expression of proteins involved in mitochondrial division and changes in the mitochondrial morphology are not involved in SAHA-induced apoptosis in HCC cells (121).
3.2.1.4 Impact of Mitochondrial Fission on Immune Regulation of HCC
Few studies focus on the impact of mitochondrial quality control of immune regulation of HCC. Bao et al. have shown that Drp1-mediated mitochondrial fission in HCC cells can induce cytoplasmic mtDNA, and promote the secretion of CCL2 which augment tumor-associated macrophages recruitment and M2 polarization eventually promote HCC cell proliferation (122). Mitochondrial quality control changes in tumor microenvironment of hepatocellular carcinoma can also affect the progression of hepatocellular carcinoma. Zheng et al. showed that Drp1-mediated mitochondrial fragmentation promoted the increase of caspase3 levels in NK cells, which augmented the apoptosis of NK cells, and objectively caused the immune escape of HCC (123).
3.2.2 Mitochondrial Fusion
3.2.2.1 Effect of Mitochondrial Fusion on HCC Cell Growth and Proliferation
Wang et al. found that the overexpression of MFN2 inhibits proliferation of hepatoma cells by arresting cells in S phase, and mitochondrial calcium overload caused by mitochondrial fusion mediates an increase in the expression of proteins related to the BAX apoptotic pathway (124, 125). Analysis of HCC and the surrounding tissues showed that reduced expression of MFN2 is negatively associated with the overall survival of patients with HCC (126, 127). Moreover, in HBV-related HCC, high expression of HBX induces ubiquitination and degradation of MFN2 (46), and low expression of MFN2 negatively affects the prognosis of patients with HBV-related liver cancer (128).
Tissue-specific deletion of Opa1 suggests the importance of Opa1-mediated mitochondrial fusion in the expression of ETC and cell oxidative phosphorylation metabolism (129). Compared with its expression in normal tissues, Opa1 shows low expression in HCC tissues. By reducing the expression of Opa1, Meng et al. found that this protein mediates mitochondrial fusion, promotes cell oxidative phosphorylation, and functionally supports the growth of subcutaneous HCC in mice (130).
3.2.2.2 Impact of Mitochondrial Fusion on Invasion and Metastasis of HCC
Zhang et al. (131) found that MFN1 promotes mitochondrial fusion but inhibits the proliferation, invasion, and migration of HCC cells both in vivo and in vitro and that patients with HCC with high levels of MFN1 have prolonged disease-free and overall survival. Furthermore, MFN1-induced mitochondrial fusion inhibits aerobic glycolysis and a shift to oxidative phosphorylation, which induces an increase in E-cadherin expression and decrease in N-cadherin, vimentin, and SNAIL expression to eventually suppress the invasion of HCC cells.
3.2.2.3 Impact of Mitochondrial Fusion on Chemoresistance in HCC
A study suggested that downregulation of Opa1 expression during treatment with sorafenib induced mitochondrial fragmentation and the release of cytochrome C to mediate apoptosis. In contrast, inhibition of Opa1 expression sensitized HCC cells to sorafenib (132).
3.2.2.4 Impact of Mitochondrial Fusion on Metabolism in HCC
Most studies found that mitochondrial fusion could promote the conversion of cells metabolism from aerobic glycolysis to oxidative phosphorylation. Zhang et al. found that overexpression of MFN1 induced mitochondrial fusion in HCC can inhibit the expression of enzymes involved in aerobic glycolysis, further inhibiting cell proliferation and invasion (131). While Li et al. pointed out that MFN1 and Opa1 cooperate for prolonging mitochondria fusion, promoting oxygen consumption rate and ATP content, augment HCC proliferation (130). Li et al. found that, after the mitochondrial fusion induced by DRP1 S637 phosphorylation, the amount and width of the mitochondrial cristae were increased, assembly of the respiratory chain complex I-IV were increased, and glycolysis mediated by the NAD/SIRT1 pathway was inhibited, ultimately promoting HCC cell survival under starvation (133).
3.2.3 Mitochondrial Biogenesis
3.2.3.1 Effect of Mitochondrial Biogenesis on Proliferation of HCC Cells
Under hypoxic conditions, HCC and other liver cancer cell lines show increased mitochondrial biogenesis and proliferation by upregulating the expression of PGC-1α. To achieve this effect, HMGB1 translocated from the nucleus to the cytoplasm binds to cytoplasmic Toll-like receptor-9; this complex activates p38 to subsequently phosphorylate PGC-1α (134). Additionally, the p53 pathway can reduce the expression of PGC-1α, which suppresses mitochondrial biogenesis and oxidative phosphorylation by inhibiting the expression of downstream TFAM and p53R2, thus eventually decreasing the proliferation of liver cancer cells (135).
Studies of hepatocytes suggested that increased expression of Myc promotes the transcription of genes involved in biosynthesis of the mitochondrial membrane, matrix, and ribosomes; carboxylic acid metabolism; electron transporter activity; oxidative phosphorylation; and mtDNA replication by directly upregulating the expression of transcription factors, such as TFAM. This enhanced transcriptional activity increases the mitochondrial mass, which indirectly promotes the growth and proliferation of hepatocytes (21, 136).
3.2.3.2 Impact of Mitochondrial Biogenesis on Invasion and Metastasis of HCC
The influence of PGC-1α-induced mitochondrial biogenesis on the invasiveness of HCC cells remains controversial. A clinical study showed that low expression of PGC-1α in HCC is related to more frequent vascular invasion. In vitro experiments and analysis of in vivo mouse models showed that PGC-1α inhibits the migration and invasion of HCC cells. Specifically, PGC-1α inhibits aerobic glycolysis by regulating the peroxisome proliferation-activated receptor-γ-dependent WNT/β-catenin/PDK1 axis, thereby inhibiting metastasis of HCC (137). NPAS2 inhibits mitochondrial oxidative phosphorylation and mitochondrial biogenesis by inhibiting the expression of PGC-1α. This inhibitory effect is synergistic with hypoxia-inducible factor-1α–mediated aerobic glycolysis in tumor cells to promote the proliferation and metastasis of HCC cells through the energy supply (138). However, another study showed that SIRT1, as part of the SIRT1/PGC-1α axis, promotes the metastasis of HCC by increasing PGC-1α-mediated mitochondrial biogenesis; a corresponding reduction in the mitochondrial mass and oxidized phosphoric acid caused by low expression of SIRT1 in liver cancer tissues inhibits invasion and metastasis (139). Therefore, a higher level of energy metabolism increases cell migration, and the mitochondrial mass and level of cellular glycolysis together affect invasion by HCC.
3.2.3.3 Impact of Mitochondria Biogenesis on Chemoresistance in HCC
Further, tigecycline has been shown to inhibit the translation of mitochondrial respiratory chain complexes, enhance mitochondrial oxidative stress, and reduce resistance to cisplatin in liver cancer (140). Moreover, in cells resistant to doxorubicin and sorafenib, inhibition of TFAM, an essential factor for mitochondrial synthesis, reduces drug resistance (141).
3.2.3.4 Impact of Mitochondria Biogenesis on Metabolism in HCC
PGC-1α can enhance oxidative phosphorylation, mitochondrial biogenesis and oxygen consumption rate in cancer cells (142). Under hypoxia conditions, HCC undergo changes in their bioenergetic profile to favor mitochondrial respiration by activating the PGC-1α (134). The mitochondrial respiratory capacity and proliferation was diminished when PGC-1α was suppressed in hypoxic HCC cells (143). Activation of PGC-1α was also found to promote cell growth by facilitating mitochondrial and fatty acid metabolism in liver cancer cells (144).
3.2.4 Mitophagy
3.2.4.1 Impact of Mitochondrial Autophagy on the Occurrence of HCC
A dysfunction in mitophagy causes tumorigenesis (145). For example, loss of mitophagy can lead to tumor formation in the liver through oxidative stress and DNA damage (146). In contrast, FUNDC1 enhances mitophagy to reduce hepatocyte inflammation and the inflammatory response to inhibit the occurrence of HCC in diethylnitrosamine-induced mouse models (147). The thyroid hormone T3 mediates ubiquitination of mitochondrial HBX in HBV-infected cells through PINK1 to simultaneously promote mitophagy and mitochondrial biogenesis, inhibit the production of mitochondrial ROS, and reduce the tumor-promoting effect of HBV (82).
3.2.4.2 Impact of Mitophagy on Invasion and Metastasis in HCC
The regulation of mitophagy, via mechanisms other than PINK1/Parkin, can inhibit the migration of tumor cells. For example, ubiquitination of Yap, but not Parkin, reduces mitophagy by inhibiting Bnip3 to ensure the supply of ATP required for cellular maintenance. Intracellular SERCA activity and calcium homeostasis can effectively block the activation of calmodulin-dependent protein kinase II, prevent the calmodulin-dependent protein kinase II/cofilin pathway from degrading F-actin, and maintain the lamellipodium to promote the metastasis of HCC (148).
3.2.4.3 Effect of Mitophagy on Chemoresistance and Resistance to Apoptosis in HCC
Studies of chemoresistance revealed that chemotherapy-induced activation of Drp1 induces mitochondrial autophagy. However, the mitochondrial division inhibitor Mdivi-1 promotes the release of cytochrome C from the mitochondria into the cytoplasm by reducing the mitochondrial membrane potential and increasing the sensitivity of HCC cells to cisplatin. These findings indicate that mitophagy increases chemoresistance in HCC. Further, inhibition of Drp1 and mitophagy can participate in downregulating the expression of Bcl-XL and upregulating the expression of Bax to enhance apoptosis, which ultimately increases the lethality of platinum-based drugs (149).
The regulation of mitophagy by the PINK1/Parkin pathway is observed in HBV-related HCC. HBV promotes cell survival and virus persistence by disrupting mitochondrial fission. Kim et al. found that HBV could induce translocation of Drp1 and Parkin to the mitochondria to mediate the degradation of MFN-2, thereby promoting mitophagy, inhibiting the mitochondrial apoptotic pathway, and maintaining tumor cell survival (46). Ketoconazole inhibits the growth of HCC cells by inhibiting prostaglandin-endoperoxide synthase 2, which activates the accumulation of PINK1 on the mitochondrial membrane and induces HCC cell apoptosis (150, 151).
A lack of mitophagy not only causes HCC but also reduces chemoresistance in HCC. It has been reported that α-TOS, a vitamin E derivative, can cause mitochondrial damage and trigger mitophagy. However, defects in the denitrosylating enzyme S-nitrosoglutathione reductase or Parkin reduce the ability of cells to specifically eliminate mitochondria damaged by α-TOS and decrease chemoresistance in HCC cells (152). In contrast, Wang et al. found that Cepharanthine hydrochloride increased mitochondrial fission and autophagy through the AMPK pathway, thereby increasing the chemoresistance of liver cancer cells. However, inhibition of mitophagy by 3-methyladenine in vivo and in vitro reduced the tumor volume, indicating improvement in the antitumor activity of Cepharanthine hydrochloride; thus, the inhibition of mitophagy suppresses chemoresistance (153). Increased autophagy also enhances tumor chemoresistance. For example, in HCC cells induced by melatonin, the sensitivity to sorafenib is related to the production of ROS and mitophagy. Melatonin may promote the expression of Parkin and its translocation to the mitochondrial membrane to promote mitophagy (154). A previous study by our group also showed that mitophagy induced by PINK1/Parkin influences the resistance to cisplatin, as it effectively degrades mitochondria damaged by cisplatin in HCC cells and maintains cell survival (26).
4 Summary and Prospects
The therapy strategy targeting mitochondrial quality control in HCC is still in the basic research stage. Mdivi-1 (mitochondrial division inhibitor 1) is a kind of selective Drp1 inhibitor. Ma et al. found that Mdivi-1 could inhibit mitophagy induced by cisplatin and initiate mitochondria pathway apoptosis, and then enhance the sensitivity of HCC to cisplatin (149). Mdivi-1 can also reduce the levels of oxidative phosphorylation and weaken the stemness in liver cancer stem cells by inhibiting mitochondrial fission (155). Lin et al. found that under hypoxic conditions, Mdivi-1 inhibits mitophagy by inhibiting the expression of BNIP3 and LC3B, up-regulating p62 and promote mitochondrial Bax accumulation, eventually stimulating mitochondrial-dependent apoptosis in HCC (76). Several other drugs like Dynasore and P110, which can target mitochondrial dynamin have been found, but few of them have been reported in the treatment of HCC, these drugs are likely to be promising therapy strategies in HCC.
In summary, mitochondrial quality control plays an important role in the occurrence, progression, and treatment of HCC. Studies have mainly focused on mitochondrial quality control at the organelle level. Mitochondrial quality control at the molecular and organelle levels can regulate bioenergy metabolism in mitochondria, control cell death, and alter mitochondrial retrograde signaling to maintain homeostasis in HCC cells under stress conditions (hypoxia and chemotherapy). However, research on mitochondrial quality control at the molecular level is primarily limited to the diagnosis, curative effect, and prognosis of patients with HCC. Studies of the impact of mitochondrial quality control at the molecular and cellular levels on the biological behavior of HCC are required.
Future research should focus on the role and mechanism of interaction between different levels of mitochondrial quality control in the regulation of the occurrence, progression, and resistance of HCC. Continuous improvements in precision medicine will clarify the process of mitochondrial quality control and role of key molecules in the precision treatment of HCC. Individual evaluation and regulation of mitochondrial quality control in HCC tissues in patients undergoing different treatments will aid in individualized treatment.
Author Contributions
JB and DZ wrote the first draft. JS, YW, WY, and HQ provided the organization and framework of the article. JS and RC provided critical revisions. All authors contributed to the article and approved the submitted version.
Funding
This work was supported by grants from the National Key R&D Program of China (Grant #2018YFC1311600), Natural Science Foundation of China (81902484), China Postdoctoral Science Foundation (2020M670864), Medical and Health Talents Project of Jilin Province (2019SCZT003, 2019SCZT035, 2020SCZT039), and Youth Support Project of Jilin Association for Science and Technology (202028).
Conflict of Interest
The authors declare that the research was conducted in the absence of any commercial or financial relationships that could be construed as a potential conflict of interest.
Publisher’s Note
All claims expressed in this article are solely those of the authors and do not necessarily represent those of their affiliated organizations, or those of the publisher, the editors and the reviewers. Any product that may be evaluated in this article, or claim that may be made by its manufacturer, is not guaranteed or endorsed by the publisher.
References
1. Ng KKC, Chok KSH, Chan ACY, Cheung TT, Wong TCL, Fung JYY, et al. Randomized Clinical Trial of Hepatic Resection Versus Radiofrequency Ablation for Early-Stage Hepatocellular Carcinoma. Br J Surg (2017) 104(13):1775–84. doi: 10.1002/bjs.10677
2. Varghese J, Kedarisetty C, Venkataraman J, Srinivasan V, Deepashree T, Uthappa M, et al. Combination of TACE and Sorafenib Improves Outcomes in BCLC Stages B/C of Hepatocellular Carcinoma: A Single Centre Experience. Ann Hepatol (2017) 16(2):247–54. doi: 10.5604/16652681.1231583
3. Kuczynski EA, Lee CR, Man S, Chen E, Kerbel RS. Effects of Sorafenib Dose on Acquired Reversible Resistance and Toxicity in Hepatocellular Carcinoma. Cancer Res (2015) 75(12):2510–9. doi: 10.1158/0008-5472.Can-14-3687
4. Wong MC, Jiang JY, Goggins WB, Liang M, Fang Y, Fung FD, et al. International Incidence and Mortality Trends of Liver Cancer: A Global Profile. Sci Rep (2017) 7:45846. doi: 10.1038/srep45846
5. McGlynn KA, Petrick JL, El-Serag HB. Epidemiology of Hepatocellular Carcinoma. Hepatol (Baltimore Md) (2021) 73(Suppl 1):4–13. doi: 10.1002/hep.31288
6. Siegel RL, Miller KD, Jemal A. Cancer Statistics, 2020. CA: Cancer J Clin (2020) 70(1):7–30. doi: 10.3322/caac.21590
7. Ciccarone F, Castelli S, Ciriolo MR. Oxidative Stress-Driven Autophagy acROSs Onset and Therapeutic Outcome in Hepatocellular Carcinoma. Oxid Med Cell Longev (2019) 2019:6050123. doi: 10.1155/2019/6050123
8. Guri Y, Colombi M, Dazert E, Hindupur SK, Roszik J, Moes S, et al. Mtorc2 Promotes Tumorigenesis via Lipid Synthesis. Cancer Cell (2017) 32(6):807–23.e12. doi: 10.1016/j.ccell.2017.11.011
9. Xu Z, Chen L, Leung L, Yen TS, Lee C, Chan JY. Liver-Specific Inactivation of the Nrf1 Gene in Adult Mouse Leads to Nonalcoholic Steatohepatitis and Hepatic Neoplasia. Proc Natl Acad Sci USA (2005) 102(11):4120–5. doi: 10.1073/pnas.0500660102
10. Mello T, Simeone I, Galli A. Mito-Nuclear Communication in Hepatocellular Carcinoma Metabolic Rewiring. Cells (2019) 8(5):517. doi: 10.3390/cells8050417
11. Bock FJ, Tait SWG. Mitochondria as Multifaceted Regulators of Cell Death. Nat Rev Mol Cell Biol (2020) 21(2):85–100. doi: 10.1038/s41580-019-0173-8
12. Zunft HJ, Lehninger A, Nelson DL, Cox MM. Principles of Biochemistry. 2. Auflage, 1090 Seiten, Zahlreiche Abb. Und Tab. New York: Worth Publishers (1994) p. 223. 1993. Preis: 65,95 US. Weinheim: WILEY-VCH Verlag GmbH.
13. Sonveaux P, Végran F, Schroeder T, Wergin MC, Verrax J, Rabbani ZN, et al. Targeting Lactate-Fueled Respiration Selectively Kills Hypoxic Tumor Cells in Mice. J Clin Invest (2008) 118(12):3930–42. doi: 10.1172/jci36843
14. Veiga SR, Ge X, Mercer CA, Hernández-Álvarez MI, Thomas HE, Hernandez-Losa J, et al. Phenformin-Induced Mitochondrial Dysfunction Sensitizes Hepatocellular Carcinoma for Dual Inhibition of mTOR. Clin Cancer Res an Off J Am Assoc Cancer Res (2018) 24(15):3767–80. doi: 10.1158/1078-0432.Ccr-18-0177
15. Ying H, Yu Y, Xu Y. Antisense of ATP Synthase Subunit E Inhibits the Growth of Human Hepatocellular Carcinoma Cells. Oncol Res (2001) 12(11-12):485–90. doi: 10.3727/096504001108747495
16. Auger C, Alhasawi A, Contavadoo M, Appanna VD. Dysfunctional Mitochondrial Bioenergetics and the Pathogenesis of Hepatic Disorders. Front Cell Dev Biol (2015) 3:40. doi: 10.3389/fcell.2015.00040
17. Rostkowska K, Zwierz K, Rózański A, Moniuszko-Jakoniuk J, Roszczenko A. Formation and Metabolism of N-Nitrosamines. Polish J Environ Stud (1998) 7(6):321–5.
18. Haynes CM, Titus EA, Cooper AA. Degradation of Misfolded Proteins Prevents ER-Derived Oxidative Stress and Cell Death. Mol Cell (2004) 15(5):767–76. doi: 10.1016/j.molcel.2004.08.025
19. Santos CX, Tanaka LY, Wosniak J, Laurindo FR. Mechanisms and Implications of Reactive Oxygen Species Generation During the Unfolded Protein Response: Roles of Endoplasmic Reticulum Oxidoreductases, Mitochondrial Electron Transport, and NADPH Oxidase. Antioxid Redox Signal (2009) 11(10):2409–27. doi: 10.1089/ars.2009.2625
20. Jin T, Wang C, Tian Y, Dai C, Zhu Y, Xu F. Mitochondrial Metabolic Reprogramming: An Important Player in Liver Cancer Progression. Cancer Lett (2020) 470:197–203. doi: 10.1016/j.canlet.2019.11.029
21. Zong WX, Rabinowitz JD, White E. Mitochondria and Cancer. Mol Cell (2016) 61(5):667–76. doi: 10.1016/j.molcel.2016.02.011
22. Shetty S, Kumar R, Bharati S. Mito-TEMPO, a Mitochondria-Targeted Antioxidant, Prevents N-Nitrosodiethylamine-Induced Hepatocarcinogenesis in Mice. Free Radical Biol Med (2019) 136:76–86. doi: 10.1016/j.freeradbiomed.2019.03.037
23. Dengler VL, Galbraith M, Espinosa JM. Transcriptional Regulation by Hypoxia Inducible Factors. Crit Rev Biochem Mol Biol (2014) 49(1):1–15. doi: 10.3109/10409238.2013.838205
24. Dong-Yun S, Yu-Ru D, Shan-Lin L, Ya-Dong Z, Lian W. Redox Stress Regulates Cell Proliferation and Apoptosis of Human Hepatoma Through Akt Protein Phosphorylation. FEBS Lett (2003) 542(1-3):60–4. doi: 10.1016/s0014-5793(03)00338-7
25. Roberts JL, Poklepovic A, Booth L, Dent P. The Multi-Kinase Inhibitor Lenvatinib Interacts With the HDAC Inhibitor Entinostat to Kill Liver Cancer Cells. Cell Signal (2020) 70:109573. doi: 10.1016/j.cellsig.2020.109573
26. Sheng J, Shen L, Sun L, Zhang X, Cui R, Wang L. Inhibition of PI3K/mTOR Increased the Sensitivity of Hepatocellular Carcinoma Cells to Cisplatin via Interference With Mitochondrial-Lysosomal Crosstalk. Cell Prolif (2019) 52(3):e12609. doi: 10.1111/cpr.12609
27. Cucarull B, Tutusaus A, Subías M, Stefanovic M, Hernáez-Alsina T, Boix L, et al. Regorafenib Alteration of the BCL-Xl/MCL-1 Ratio Provides a Therapeutic Opportunity for BH3-Mimetics in Hepatocellular Carcinoma Models. Cancers (2020) 12(2):332. doi: 10.3390/cancers12020332
28. Zheng JF, He S, Zeng Z, Gu X, Cai L, Qi G. PMPCB Silencing Sensitizes HCC Tumor Cells to Sorafenib Therapy. Mol Ther J Am Soc Gene Ther (2019) 27(10):1784–95. doi: 10.1016/j.ymthe.2019.06.014
29. Cheng J, Qian D, Ding X, Song T, Cai M, Dan X, et al. High PGAM5 Expression Induces Chemoresistance by Enhancing Bcl-xL-Mediated Anti-Apoptotic Signaling and Predicts Poor Prognosis in Hepatocellular Carcinoma Patients. Cell Death Dis (2018) 9(10):991. doi: 10.1038/s41419-018-1017-8
30. Li J, Jiang R, Cong X, Zhao Y. UCP2 Gene Polymorphisms in Obesity and Diabetes, and the Role of UCP2 in Cancer. FEBS Lett (2019) 593(18):2525–34. doi: 10.1002/1873-3468.13546
31. Ray PD, Huang BW, Tsuji Y. Reactive Oxygen Species (ROS) Homeostasis and Redox Regulation in Cellular Signaling. Cell Signal (2012) 24(5):981–90. doi: 10.1016/j.cellsig.2012.01.008
32. Yu H, Guo P, Xie X, Wang Y, Chen G. Ferroptosis, A New Form of Cell Death, and Its Relationships With Tumourous Diseases. J Cell Mol Med (2017) 21(4):648–57. doi: 10.1111/jcmm.13008
33. Yuan H, Li X, Zhang X, Kang R, Tang D. CISD1 Inhibits Ferroptosis by Protection Against Mitochondrial Lipid Peroxidation. Biochem Biophys Res Commun (2016) 478(2):838–44. doi: 10.1016/j.bbrc.2016.08.034
34. Wang YQ, Chang SY, Wu Q, Gou YJ, Jia L, Cui YM, et al. The Protective Role of Mitochondrial Ferritin on Erastin-Induced Ferroptosis. Front Aging Neurosci (2016) 8:308. doi: 10.3389/fnagi.2016.00308
35. Schenk B, Fulda S. Reactive Oxygen Species Regulate Smac Mimetic/Tnfα-Induced Necroptotic Signaling and Cell Death. Oncogene (2015) 34(47):5796–806. doi: 10.1038/onc.2015.35
36. Zhang Y, Su SS, Zhao S, Yang Z, Zhong CQ, Chen X, et al. RIP1 Autophosphorylation Is Promoted by Mitochondrial ROS and Is Essential for RIP3 Recruitment Into Necrosome. Nat Commun (2017) 8:14329. doi: 10.1038/ncomms14329
37. Fang X, Wang H, Han D, Xie E, Yang X, Wei J, et al. Ferroptosis as a Target for Protection Against Cardiomyopathy. Proc Natl Acad Sci USA (2019) 116(7):2672–80. doi: 10.1073/pnas.1821022116
38. Rizza S, Montagna C, Cardaci S, Maiani E, Di Giacomo G, Sanchez-Quiles V, et al. S-Nitrosylation of the Mitochondrial Chaperone TRAP1 Sensitizes Hepatocellular Carcinoma Cells to Inhibitors of Succinate Dehydrogenase. Cancer Res (2016) 76(14):4170–82. doi: 10.1158/0008-5472.Can-15-2637
39. Hernández-Breijo B, Monserrat J, Ramírez-Rubio S, Cuevas EP, Vara D, Díaz-Laviada I, et al. Preclinical Evaluation of Azathioprine Plus Buthionine Sulfoximine in the Treatment of Human Hepatocarcinoma and Colon Carcinoma. World J Gastroenterol (2011) 17(34):3899–911. doi: 10.3748/wjg.v17.i34.3899
40. Miller DR, Cramer SD, Thorburn A. The Interplay of Autophagy and non-Apoptotic Cell Death Pathways. Int Rev Cell Mol Biol (2020) 352:159–87. doi: 10.1016/bs.ircmb.2019.12.004
41. Zhang X, Zhang P, An L, Sun N, Peng L, Tang W, et al. Miltirone Induces Cell Death in Hepatocellular Carcinoma Cell Through GSDME-Dependent Pyroptosis. Acta Pharm Sin B (2020) 10(8):1397–413. doi: 10.1016/j.apsb.2020.06.015
42. Yu X, Hao M, Liu Y, Ma X, Lin W, Xu Q, et al. Liraglutide Ameliorates non-Alcoholic Steatohepatitis by Inhibiting NLRP3 Inflammasome and Pyroptosis Activation via Mitophagy. Eur J Pharmacol (2019) 864:172715. doi: 10.1016/j.ejphar.2019.172715
43. Pickles S, Vigié P, Youle RJ. Mitophagy and Quality Control Mechanisms in Mitochondrial Maintenance. Curr Biol CB (2018) 28(4):R170–85. doi: 10.1016/j.cub.2018.01.004
44. Ashrafi G, Schwarz TL. The Pathways of Mitophagy for Quality Control and Clearance of Mitochondria. Cell Death Differ (2013) 20(1):31–42. doi: 10.1038/cdd.2012.81
45. Kim S-J, Syed GH, Khan M, Chiu W-W, Sohail MA, Gish RG, et al. Hepatitis C Virus Triggers Mitochondrial Fission and Attenuates Apoptosis to Promote Viral Persistence. Proc Natl Acad Sci (2014) 111(17):6413. doi: 10.1073/pnas.1321114111
46. Kim S-J, Khan M, Quan J, Till A, Subramani S, Siddiqui A. Hepatitis B Virus Disrupts Mitochondrial Dynamics: Induces Fission and Mitophagy to Attenuate Apoptosis. PloS Pathog (2013) 9(12):e1003722–e. doi: 10.1371/journal.ppat.1003722
47. Pang L, Liu K, Liu D, Lv F, Zang Y, Xie F, et al. Differential Effects of Reticulophagy and Mitophagy on Nonalcoholic Fatty Liver Disease. Cell Death Dis (2018) 9(2):90. doi: 10.1038/s41419-017-0136-y
48. Castañeda F, Kinne RKH. Apoptosis Induced in HepG2 Cells by Short Exposure to Millimolar Concentrations of Ethanol Involves the Fas-Receptor Pathway. J Cancer Res Clin Oncol (2001) 127(7):418–24. doi: 10.1007/s004320000227
49. Burbulla LF, Krüger R. Converging Environmental and Genetic Pathways in the Pathogenesis of Parkinson's Disease. J Neurol Sci (2011) 306(1-2):1–8. doi: 10.1016/j.jns.2011.04.005
50. Vigié P, Camougrand N. Role of Mitophagy in the Mitochondrial Quality Control. Med Sci M/S (2017) 33(3):231–7. doi: 10.1051/medsci/20173303008
51. Voos W, Jaworek W, Wilkening A, Bruderek M. Protein Quality Control at the Mitochondrion. Essays Biochem (2016) 60(2):213–25. doi: 10.1042/ebc20160009
52. Baker MJ, Tatsuta T, Langer T. Quality Control of Mitochondrial Proteostasis. Cold Spring Harb Perspect Biol (2011) 3(7):a007559. doi: 10.1101/cshperspect.a007559
53. Livnat-Levanon N, Glickman MH. Ubiquitin-Proteasome System and Mitochondria - Reciprocity. Biochim Biophys Acta (2011) 1809(2):80–7. doi: 10.1016/j.bbagrm.2010.07.005
54. Haynes CM, Petrova K, Benedetti C, Yang Y, Ron D. ClpP Mediates Activation of a Mitochondrial Unfolded Protein Response in C. Elegans. Dev Cell (2007) 13(4):467–80. doi: 10.1016/j.devcel.2007.07.016
55. Zhao Q, Wang J, Levichkin IV, Stasinopoulos S, Ryan MT, Hoogenraad NJ. A Mitochondrial Specific Stress Response in Mammalian Cells. EMBO J (2002) 21(17):4411–9. doi: 10.1093/emboj/cdf445
56. Deng P, Haynes CM. Mitochondrial Dysfunction in Cancer: Potential Roles of ATF5 and the Mitochondrial UPR. Semin Cancer Biol (2017) 47:43–9. doi: 10.1016/j.semcancer.2017.05.002
57. Melber A, Haynes CM. UPR(mt) Regulation and Output: A Stress Response Mediated by Mitochondrial-Nuclear Communication. Cell Res (2018) 28(3):281–95. doi: 10.1038/cr.2018.16
58. James SL, Abate D, Abate KH, Abay SM, Abbafati C, Abbasi N, et al. Global, Regional, and National Incidence, Prevalence, and Years Lived With Disability for 354 Diseases and Injuries for 195 Countries and Territories, 1990-2017: A Systematic Analysis for the Global Burden of Disease Study 2017. Lancet (2018) 392(10159):1789–858. doi: 10.1016/s0140-6736(18)32279-7
59. Stevens M. HBV May Lead to More Aggressive Form of HCC Than HCV. Infect Dis News (2015) 28(7):45.
60. Xu X, Fan Z, Kang L, Han J, Jiang C, Zheng X, et al. Hepatitis B Virus X Protein Represses miRNA-148a to Enhance Tumorigenesis. J Clin Invest (2013) 123(2):630–45. doi: 10.1172/jci64265
61. Zhang SM, Sun DC, Lou S, Bo XC, Lu Z, Qian XH, et al. HBx Protein of Hepatitis B Virus (HBV) can Form Complex With Mitochondrial HSP60 and HSP70. Arch Virol (2005) 150(8):1579–90. doi: 10.1007/s00705-005-0521-1
62. Tanaka Y, Kanai F, Kawakami T, Tateishi K, Ijichi H, Kawabe T, et al. Interaction of the Hepatitis B Virus X Protein (HBx) With Heat Shock Protein 60 Enhances HBx-Mediated Apoptosis. Biochem Biophys Res Commun (2004) 318(2):461–9. doi: 10.1016/j.bbrc.2004.04.046
63. Huang XY, Li D, Chen ZX, Huang YH, Gao WY, Zheng BY, et al. Hepatitis B Virus X Protein Elevates Parkin-Mediated Mitophagy Through Lon Peptidase in Starvation. Exp Cell Res (2018) 368(1):75–83. doi: 10.1016/j.yexcr.2018.04.016
64. Yoo YS, Park YJ, Lee HS, Oanh NTK, Cho MY, Heo J, et al. Mitochondria Ubiquitin Ligase, MARCH5 Resolves Hepatitis B Virus X Protein Aggregates in the Liver Pathogenesis. Cell Death Dis (2019) 10(12):938. doi: 10.1038/s41419-019-2175-z
65. Apostolova N, Gomez-Sucerquia LJ, Alegre F, Funes HA, Victor VM, Barrachina MD, et al. ER Stress in Human Hepatic Cells Treated With Efavirenz: Mitochondria Again. J Hepatol (2013) 59(4):780–9. doi: 10.1016/j.jhep.2013.06.005
66. Polo M, Alegre F, Moragrega AB, Gibellini L, Marti-Rodrigo A, Blas-Garcia A, et al. Lon Protease: A Novel Mitochondrial Matrix Protein in the Interconnection Between Drug-Induced Mitochondrial Dysfunction and Endoplasmic Reticulum Stress. Br J Pharmacol (2017) 174(23):4409–29. doi: 10.1111/bph.14045
67. Bazzani V, Barchiesi A, Radecka D, Pravisani R, Guadagno A, Di Loreto C, et al. Mitochondrial Apurinic/Apyrimidinic Endonuclease 1 Enhances mtDNA Repair Contributing to Cell Proliferation and Mitochondrial Integrity in Early Stages of Hepatocellular Carcinoma. BMC Cancer (2020) 20(1):969. doi: 10.1186/s12885-020-07258-6
68. Yi X, Luk JM, Lee NP, Peng J, Leng X, Guan XY, et al. Association of Mortalin (HSPA9) With Liver Cancer Metastasis and Prediction for Early Tumor Recurrence. Mol Cell Proteomics MCP (2008) 7(2):315–25. doi: 10.1074/mcp.M700116-MCP200
69. Xu Z, Chen X, Peng C, Liu E, Li Y, Li C, et al. The Expression and Clinical Significance of Omi/Htra2 in Hepatocellular Carcinoma. Hepato-Gastroenterology (2013) 60(121):6–13.
70. Xu Z, Chen Y, Xu G, Peng C, Liu E, Li Y, et al. Omi/HtrA2 Pro-Apoptotic Marker Differs in Various Hepatocellular Carcinoma Cell Lines Owing to Ped/Pea-15 Expression Level. Oncol Rep (2015) 33(2):905–12. doi: 10.3892/or.2014.3656
71. Zhang X, Lin C, Song J, Chen H, Chen X, Ren L, et al. Parkin Facilitates Proteasome Inhibitor-Induced Apoptosis via Suppression of NF-κb Activity in Hepatocellular Carcinoma. Cell Death Dis (2019) 10(10):719. doi: 10.1038/s41419-019-1881-x
72. Chan DC. Mitochondrial Dynamics and Its Involvement in Disease. Annu Rev Pathol (2020) 15:235–59. doi: 10.1146/annurev-pathmechdis-012419-032711
73. Giacomello M, Pyakurel A, Glytsou C, Scorrano L. The Cell Biology of Mitochondrial Membrane Dynamics. Nat Rev Mol Cell Biol (2020) 21(4):204–24. doi: 10.1038/s41580-020-0210-7
74. Cho B, Cho HM, Jo Y, Kim HD, Song M, Moon C, et al. Constriction of the Mitochondrial Inner Compartment Is a Priming Event for Mitochondrial Division. Nat Commun (2017) 8:15754. doi: 10.1038/ncomms15754
75. Kalia R, Wang RY, Yusuf A, Thomas PV, Agard DA, Shaw JM, et al. Structural Basis of Mitochondrial Receptor Binding and Constriction by DRP1. Nature (2018) 558(7710):401–5. doi: 10.1038/s41586-018-0211-2
76. Lin XH, Qiu BQ, Ma M, Zhang R, Hsu SJ, Liu HH, et al. Suppressing DRP1-Mediated Mitochondrial Fission and Mitophagy Increases Mitochondrial Apoptosis of Hepatocellular Carcinoma Cells in the Setting of Hypoxia. Oncogenesis (2020) 9(7):67. doi: 10.1038/s41389-020-00251-5
77. Hoppins S, Lackner L, Nunnari J. The Machines That Divide and Fuse Mitochondria. Annu Rev Biochem (2007) 76:751–80. doi: 10.1146/annurev.biochem.76.071905.090048
78. Twig G, Hyde B, Shirihai OS. Mitochondrial Fusion, Fission and Autophagy as a Quality Control Axis: The Bioenergetic View. Biochim Biophys Acta (2008) 1777(9):1092–7. doi: 10.1016/j.bbabio.2008.05.001
79. Gomes LC, Scorrano L. High Levels of Fis1, a Pro-Fission Mitochondrial Protein, Trigger Autophagy. Biochim Biophys Acta (2008) 1777(7-8):860–6. doi: 10.1016/j.bbabio.2008.05.442
80. Malena A, Loro E, Di Re M, Holt IJ, Vergani L. Inhibition of Mitochondrial Fission Favours Mutant Over Wild-Type Mitochondrial DNA. Hum Mol Genet (2009) 18(18):3407–16. doi: 10.1093/hmg/ddp281
81. Suen DF, Narendra DP, Tanaka A, Manfredi G, Youle RJ. Parkin Overexpression Selects Against a Deleterious mtDNA Mutation in Heteroplasmic Cybrid Cells. Proc Natl Acad Sci USA (2010) 107(26):11835–40. doi: 10.1073/pnas.0914569107
82. Chi HC, Chen SL, Lin SL, Tsai CY, Chuang WY, Lin YH, et al. Thyroid Hormone Protects Hepatocytes From HBx-Induced Carcinogenesis by Enhancing Mitochondrial Turnover. Oncogene (2017) 36(37):5274–84. doi: 10.1038/onc.2017.136
83. Pang R, Lee TK, Poon RT, Fan ST, Wong KB, Kwong YL, et al. Pin1 Interacts With a Specific Serine-Proline Motif of Hepatitis B Virus X-Protein to Enhance Hepatocarcinogenesis. Gastroenterology (2007) 132(3):1088–103. doi: 10.1053/j.gastro.2006.12.030
84. Yoo YG, Oh SH, Park ES, Cho H, Lee N, Park H, et al. Hepatitis B Virus X Protein Enhances Transcriptional Activity of Hypoxia-Inducible Factor-1alpha Through Activation of Mitogen-Activated Protein Kinase Pathway. J Biol Chem (2003) 278(40):39076–84. doi: 10.1074/jbc.M305101200
85. Wang Y, Lu Y, Toh ST, Sung WK, Tan P, Chow P, et al. Lethal-7 is Down-Regulated by the Hepatitis B Virus X Protein and Targets Signal Transducer and Activator of Transcription 3. J Hepatol (2010) 53(1):57–66. doi: 10.1016/j.jhep.2009.12.043
86. Mitra K. Mitochondrial Fission-Fusion as an Emerging Key Regulator of Cell Proliferation and Differentiation. BioEssays News Rev Mol Cell Dev Biol (2013) 35(11):955–64. doi: 10.1002/bies.201300011
87. Qian W, Wang J, Roginskaya V, McDermott LA, Edwards RP, Stolz DB, et al. Novel Combination of Mitochondrial Division Inhibitor 1 (Mdivi-1) and Platinum Agents Produces Synergistic Pro-Apoptotic Effect in Drug Resistant Tumor Cells. Oncotarget (2014) 5(12):4180–94. doi: 10.18632/oncotarget.1944
88. Meeusen S, McCaffery JM, Nunnari J. Mitochondrial Fusion Intermediates Revealed In Vitro. Sci (New York NY) (2004) 305(5691):1747–52. doi: 10.1126/science.1100612
89. Partikian A, Olveczky B, Swaminathan R, Li Y, Verkman AS. Rapid Diffusion of Green Fluorescent Protein in the Mitochondrial Matrix. J Cell Biol (1998) 140(4):821–9. doi: 10.1083/jcb.140.4.821
90. Ono T, Isobe K, Nakada K, Hayashi JI. Human Cells are Protected From Mitochondrial Dysfunction by Complementation of DNA Products in Fused Mitochondria. Nat Genet (2001) 28(3):272–5. doi: 10.1038/90116
91. Busch KB, Bereiter-Hahn J, Wittig I, Schagger H, Jendrach M. Mitochondrial Dynamics Generate Equal Distribution But Patchwork Localization of Respiratory Complex I. Mol Membr Biol (2006) 23(6):509–20. doi: 10.1080/09687860600877292
92. Dimmer KS, Scorrano L. (De)constructing Mitochondria: What for? Physiol (Bethesda Md) (2006) 21:233–41. doi: 10.1152/physiol.00010.2006
93. Maycotte P, Marín-Hernández A, Goyri-Aguirre M, Anaya-Ruiz M, Reyes-Leyva J, Cortés-Hernández P. Mitochondrial Dynamics and Cancer. Tumour Biol J Int Soc Oncodevelopmental Biol Med (2017) 39(5):1010428317698391. doi: 10.1177/1010428317698391
94. Wai T, Langer T. Mitochondrial Dynamics and Metabolic Regulation. Trends Endocrinol Metab: TEM (2016) 27(2):105–17. doi: 10.1016/j.tem.2015.12.001
95. Bernardini JP, Lazarou M, Dewson G. Parkin and Mitophagy in Cancer. Oncogene (2017) 36(10):1315–27. doi: 10.1038/onc.2016.302
96. Tanida I. Autophagy Basics. Microbiol Immunol (2011) 55(1):1–11. doi: 10.1111/j.1348-0421.2010.00271.x
97. Meissner C, Lorenz H, Weihofen A, Selkoe DJ, Lemberg MK. The Mitochondrial Intramembrane Protease PARL Cleaves Human Pink1 to Regulate Pink1 Trafficking. J Neurochem (2011) 117(5):856–67. doi: 10.1111/j.1471-4159.2011.07253.x
98. Narendra DP, Jin SM, Tanaka A, Suen DF, Gautier CA, Shen J, et al. PINK1 Is Selectively Stabilized on Impaired Mitochondria to Activate Parkin. PloS Biol (2010) 8(1):e1000298. doi: 10.1371/journal.pbio.1000298
99. Deas E, Plun-Favreau H, Gandhi S, Desmond H, Kjaer S, Loh SH, et al. PINK1 Cleavage at Position A103 by the Mitochondrial Protease PARL. Hum Mol Genet (2011) 20(5):867–79. doi: 10.1093/hmg/ddq526
100. Greene AW, Grenier K, Aguileta MA, Muise S, Farazifard R, Haque ME, et al. Mitochondrial Processing Peptidase Regulates PINK1 Processing, Import and Parkin Recruitment. EMBO Rep (2012) 13(4):378–85. doi: 10.1038/embor.2012.14
101. Matsuda N, Sato S, Shiba K, Okatsu K, Saisho K, Gautier CA, et al. PINK1 Stabilized by Mitochondrial Depolarization Recruits Parkin to Damaged Mitochondria and Activates Latent Parkin for Mitophagy. J Cell Biol (2010) 189(2):211–21. doi: 10.1083/jcb.200910140
102. Liu L, Sakakibara K, Chen Q, Okamoto K. Receptor-Mediated Mitophagy in Yeast and Mammalian Systems. Cell Res (2014) 24(7):787–95. doi: 10.1038/cr.2014.75
103. Drake LE, Springer MZ, Poole LP, Kim CJ, Macleod KF. Expanding Perspectives on the Significance of Mitophagy in Cancer. Semin Cancer Biol (2017) 47:110–24. doi: 10.1016/j.semcancer.2017.04.008
104. Ding WX, Yin XM. Mitophagy: Mechanisms, Pathophysiological Roles, and Analysis. Biol Chem (2012) 393(7):547–64. doi: 10.1515/hsz-2012-0119
105. Youle RJ, Narendra DP. Mechanisms of Mitophagy. Nat Rev Mol Cell Biol (2011) 12(1):9–14. doi: 10.1038/nrm3028
106. Lemasters JJ. Variants of Mitochondrial Autophagy: Types 1 and 2 Mitophagy and Micromitophagy (Type 3). Redox Biol (2014) 2:749–54. doi: 10.1016/j.redox.2014.06.004
107. Li PA, Hou X, Hao S. Mitochondrial Biogenesis in Neurodegeneration. J Neurosci Res (2017) 95(10):2025–9. doi: 10.1002/jnr.24042
108. Sun L, Wang Y, Cen J, Ma X, Cui L, Qiu Z, et al. Modelling Liver Cancer Initiation With Organoids Derived From Directly Reprogrammed Human Hepatocytes. Nat Cell Biol (2019) 21(8):1015–26. doi: 10.1038/s41556-019-0359-5
109. Taguchi N, Ishihara N, Jofuku A, Oka T, Mihara K. Mitotic Phosphorylation of Dynamin-Related GTPase Drp1 Participates in Mitochondrial Fission. J Biol Chem (2007) 282(15):11521–9. doi: 10.1074/jbc.M607279200
110. Margineantu DH, Gregory Cox W, Sundell L, Sherwood SW, Beechem JM, Capaldi RA. Cell Cycle Dependent Morphology Changes and Associated Mitochondrial DNA Redistribution in Mitochondria of Human Cell Lines. Mitochondrion (2002) 1(5):425–35. doi: 10.1016/s1567-7249(02)00006-5
111. Mitra K, Wunder C, Roysam B, Lin G, Lippincott-Schwartz J. A Hyperfused Mitochondrial State Achieved at G1-S Regulates Cyclin E Buildup and Entry Into S Phase. Proc Natl Acad Sci USA (2009) 106(29):11960–5. doi: 10.1073/pnas.0904875106
112. Chen H, Chan DC. Mitochondrial Dynamics in Regulating the Unique Phenotypes of Cancer and Stem Cells. Cell Metab (2017) 26(1):39–48. doi: 10.1016/j.cmet.2017.05.016
113. Zhan L, Cao H, Wang G, Lyu Y, Sun X, An J, et al. Drp1-Mediated Mitochondrial Fission Promotes Cell Proliferation Through Crosstalk of P53 and NF-κb Pathways in Hepatocellular Carcinoma. Oncotarget (2016) 7(40):65001–11. doi: 10.18632/oncotarget.11339
114. Yi T, Luo H, Qin F, Jiang Q, He S, Wang T, et al. LncRNA LL22NC03-N14H11.1 Promoted Hepatocellular Carcinoma Progression Through Activating MAPK Pathway to Induce Mitochondrial Fission. Cell Death Dis (2020) 11(10):832. doi: 10.1038/s41419-020-2584-z
115. Zhang Y, Li H, Chang H, Du L, Hai J, Geng X, et al. MTP18 Overexpression Contributes to Tumor Growth and Metastasis and Associates With Poor Survival in Hepatocellular Carcinoma. Cell Death Dis (2018) 9(10):956. doi: 10.1038/s41419-018-0987-x
116. Sun X, Cao H, Zhan L, Yin C, Wang G, Liang P, et al. Mitochondrial Fission Promotes Cell Migration by Ca(2+) /CaMKII/ERK/FAK Pathway in Hepatocellular Carcinoma. Liver Int Off J Int Assoc Study Liver (2018) 38(7):1263–72. doi: 10.1111/liv.13660
117. Geng X, Xing G, Zhang Y, Hai J, Zhang Z, Du L. Mitochondrial Fission 1 Protein(FIS1) Promotes the Migration and Invasion of Hepatocellular Carcinoma by Inducing Epithelial-Mesenchymal Transition. Progress in Modern Biomedicine (2017) 17(32):6242–46. doi: 10.13241/j.cnki.pmb.2017.32.008
118. Huang Q, Zhan L, Cao H, Li J, Lyu Y, Guo X, et al. Increased Mitochondrial Fission Promotes Autophagy and Hepatocellular Carcinoma Cell Survival Through the ROS-Modulated Coordinated Regulation of the NFKB and TP53 Pathways. Autophagy (2016) 12(6):999–1014. doi: 10.1080/15548627.2016.1166318
119. Swapna Sasi US, Sindhu G, Raghu KG. Fructose-Palmitate Based High Calorie Induce Steatosis in HepG2 Cells via Mitochondrial Dysfunction: An In Vitro Approach. Toxicol Vitro an Int J Published Assoc BIBRA (2020) 68:104952. doi: 10.1016/j.tiv.2020.104952
120. Li J, Zhang B, Chang X, Gan J, Li W, Niu S, et al. Silver Nanoparticles Modulate Mitochondrial Dynamics and Biogenesis in HepG2 Cells. Environ pollut (Barking Essex 1987) (2020) 256:113430. doi: 10.1016/j.envpol.2019.113430
121. Lee JS, Yoon YG, Yoo SH, Jeong NY, Jeong SH, Lee SY, et al. Histone Deacetylase Inhibitors Induce Mitochondrial Elongation. J Cell Physiol (2012) 227(7):2856–69. doi: 10.1002/jcp.23027
122. Bao D, Zhao J, Zhou X, Yang Q, Chen Y, Zhu J, et al. Mitochondrial Fission-Induced mtDNA Stress Promotes Tumor-Associated Macrophage Infiltration and HCC Progression. Oncogene (2019) 38(25):5007–20. doi: 10.1038/s41388-019-0772-z
123. Zheng X, Qian Y, Fu B, Jiao D, Jiang Y, Chen P, et al. Mitochondrial Fragmentation Limits NK Cell-Based Tumor Immunosurveillance. Nat Immunol (2019) 20(12):1656–67. doi: 10.1038/s41590-019-0511-1
124. Wang W, Lu J, Zhu F, Wei J, Jia C, Zhang Y, et al. Pro-Apoptotic and Anti-Proliferative Effects of Mitofusin-2 via Bax Signaling in Hepatocellular Carcinoma Cells. Med Oncol (2012) 29(1):70–6. doi: 10.1007/s12032-010-9779-6
125. Wang W, Xie Q, Zhou X, Yao J, Zhu X, Huang P, et al. Mitofusin-2 Triggers Mitochondria Ca2+ Influx From the Endoplasmic Reticulum to Induce Apoptosis in Hepatocellular Carcinoma Cells. Cancer Lett (2015) 358(1):47–58. doi: 10.1016/j.canlet.2014.12.025
126. Wu Y, Zhou D, Xu X, Zhao X, Huang P, Zhou X, et al. Clinical Significance of Mitofusin-2 and Its Signaling Pathways in Hepatocellular Carcinoma. World J Surg Oncol (2016) 14(1):179. doi: 10.1186/s12957-016-0922-5
127. Feng X, Zhu K, Liu J, Chen J, Tang J, Liang Y, et al. The Evaluative Value of Sema3C and MFN2 Co-Expression Detected by Immunohistochemistry for Prognosis in Hepatocellular Carcinoma Patients After Hepatectomy. OncoTargets Ther (2016) 9:3213–21. doi: 10.2147/ott.S98322
128. Wang X, Liu Y, Sun J, Gong W, Sun P, Kong X, et al. Mitofusin-2 Acts as Biomarker for Predicting Poor Prognosis in Hepatitis B Virus Related Hepatocellular Carcinoma. Infect Agents Cancer (2018) 13:36. doi: 10.1186/s13027-018-0212-7
129. Zhang Z, Wakabayashi N, Wakabayashi J, Tamura Y, Song WJ, Sereda S, et al. The Dynamin-Related GTPase Opa1 Is Required for Glucose-Stimulated ATP Production in Pancreatic Beta Cells. Mol Biol Cell (2011) 22(13):2235–45. doi: 10.1091/mbc.E10-12-0933
130. Li M, Wang L, Wang Y, Zhang S, Zhou G, Lieshout R, et al. Mitochondrial Fusion Via OPA1 and MFN1 Supports Liver Tumor Cell Metabolism and Growth. Cells (2020) 9(1):121. doi: 10.3390/cells9010121
131. Zhang Z, Li TE, Chen M, Xu D, Zhu Y, Hu BY, et al. MFN1-Dependent Alteration of Mitochondrial Dynamics Drives Hepatocellular Carcinoma Metastasis by Glucose Metabolic Reprogramming. Br J Cancer (2020) 122(2):209–20. doi: 10.1038/s41416-019-0658-4
132. Zhao X, Tian C, Puszyk WM, Ogunwobi OO, Cao M, Wang T, et al. OPA1 Downregulation Is Involved in Sorafenib-Induced Apoptosis in Hepatocellular Carcinoma. Lab Invest; J Tech Methods Pathol (2013) 93(1):8–19. doi: 10.1038/labinvest.2012.144
133. Li J, Huang Q, Long X, Guo X, Sun X, Jin X, et al. Mitochondrial Elongation-Mediated Glucose Metabolism Reprogramming is Essential for Tumour Cell Survival During Energy Stress. Oncogene (2017) 36(34):4901–12. doi: 10.1038/onc.2017.98
134. Tohme S, Yazdani HO, Liu Y, Loughran P, van der Windt DJ, Huang H, et al. Hypoxia Mediates Mitochondrial Biogenesis in Hepatocellular Carcinoma to Promote Tumor Growth Through HMGB1 and TLR9 Interaction. Hepatol (Baltimore Md) (2017) 66(1):182–97. doi: 10.1002/hep.29184
135. Huang Q, Li J, Xing J, Li W, Li H, Ke X, et al. CD147 Promotes Reprogramming of Glucose Metabolism and Cell Proliferation in HCC Cells by Inhibiting the P53-Dependent Signaling Pathway. J Hepatol (2014) 61(4):859–66. doi: 10.1016/j.jhep.2014.04.035
136. Li F, Wang Y, Zeller KI, Potter JJ, Wonsey DR, O'Donnell KA, et al. Myc Stimulates Nuclearly Encoded Mitochondrial Genes and Mitochondrial Biogenesis. Mol Cell Biol (2005) 25(14):6225–34. doi: 10.1128/mcb.25.14.6225-6234.2005
137. Zuo Q, He J, Zhang S, Wang H, Jin G, Jin H, et al. PPAR Gamma Coactivator-1 Alpha Suppresses Metastasis of Hepatocellular Carcinoma by Inhibiting Warburg Effect by PPAR Gamma-Dependent WNT/beta-Catenin/Pyruvate Dehydrogenase Kinase Isozyme 1 Axis. Hepatol (Baltimore Md) (2021) 73(2):644–60. doi: 10.1002/hep.31280
138. Yuan P, Yang T, Mu J, Zhao J, Yang Y, Yan Z, et al. Circadian Clock Gene NPAS2 Promotes Reprogramming of Glucose Metabolism in Hepatocellular Carcinoma Cells. Cancer Lett (2020) 469:498–509. doi: 10.1016/j.canlet.2019.11.024
139. Li Y, Xu S, Li J, Zheng L, Feng M, Wang X, et al. SIRT1 Facilitates Hepatocellular Carcinoma Metastasis by Promoting PGC-1α-Mediated Mitochondrial Biogenesis. Oncotarget (2016) 7(20):29255–74. doi: 10.18632/oncotarget.8711
140. Tan J, Song M, Zhou M, Hu Y. Antibiotic Tigecycline Enhances Cisplatin Activity Against Human Hepatocellular Carcinoma Through Inducing Mitochondrial Dysfunction and Oxidative Damage. Biochem Biophys Res Commun (2017) 483(1):17–23. doi: 10.1016/j.bbrc.2017.01.021
141. Zhu Y, Xu J, Hu W, Wang F, Zhou Y, Xu W, et al. TFAM Depletion Overcomes Hepatocellular Carcinoma Resistance to Doxorubicin and Sorafenib Through AMPK Activation and Mitochondrial Dysfunction. Gene (2020) 753:144807. doi: 10.1016/j.gene.2020.144807
142. LeBleu VS, O'Connell JT, Gonzalez Herrera KN, Wikman H, Pantel K, Haigis MC, et al. PGC-1α Mediates Mitochondrial Biogenesis and Oxidative Phosphorylation in Cancer Cells to Promote Metastasis. Nat Cell Biol (2014) 16(10):992–15. doi: 10.1038/ncb3039
143. Chang H, Li J, Luo Y, Wu B, Yuan C, Geng X. TFB2M Activates Aerobic Glycolysis in Hepatocellular Carcinoma Cells Through the NAD(+) /SIRT3/HIF-1α Signaling. J Gastroenterol Hepatol (2021). doi: 10.1111/jgh.15548
144. Bhalla K, Hwang BJ, Dewi RE, Ou L, Twaddel W, Fang H-B, et al. Pgc1α Promotes Tumor Growth by Inducing Gene Expression Programs Supporting Lipogenesis. Cancer Res (2011) 71(21):6888. doi: 10.1158/0008-5472.CAN-11-1011
145. Chang JY, Yi HS, Kim HW, Shong M. Dysregulation of Mitophagy in Carcinogenesis and Tumor Progression. Biochim Biophys Acta Bioenerg (2017) 1858(8):633–40. doi: 10.1016/j.bbabio.2016.12.008
146. Tian Y, Wang L, Ou JJ. New Insight Into the Role of Autophagy in Tumorigenesis. Cell Stress (2017) 1(3):136–8. doi: 10.15698/cst2017.12.116
147. Li W, Li Y, Siraj S, Jin H, Fan Y, Yang X, et al. FUN14 Domain-Containing 1-Mediated Mitophagy Suppresses Hepatocarcinogenesis by Inhibition of Inflammasome Activation in Mice. Hepatol (Baltimore Md) (2019) 69(2):604–21. doi: 10.1002/hep.30191
148. Shi C, Cai Y, Li Y, Li Y, Hu N, Ma S, et al. Yap Promotes Hepatocellular Carcinoma Metastasis and Mobilization via Governing Cofilin/F-Actin/Lamellipodium Axis by Regulation of JNK/Bnip3/SERCA/CaMKII Pathways. Redox Biol (2018) 14:59–71. doi: 10.1016/j.redox.2017.08.013
149. Ma M, Lin XH, Liu HH, Zhang R, Chen RX. Suppression of DRP1−mediated Mitophagy Increases the Apoptosis of Hepatocellular Carcinoma Cells in the Setting of Chemotherapy. Oncol Rep (2020) 43(3):1010–8. doi: 10.3892/or.2020.7476
150. Chen HN, Chen Y, Zhou ZG, Wei Y, Huang C. A Novel Role for Ketoconazole in Hepatocellular Carcinoma Treatment: Linking PTGS2 to Mitophagy Machinery. Autophagy (2019) 15(4):733–4. doi: 10.1080/15548627.2019.1569934
151. Chen Y, Chen HN, Wang K, Zhang L, Huang Z, Liu J, et al. Ketoconazole Exacerbates Mitophagy to Induce Apoptosis by Downregulating Cyclooxygenase-2 in Hepatocellular Carcinoma. J Hepatol (2019) 70(1):66–77. doi: 10.1016/j.jhep.2018.09.022
152. Rizza S, Di Leo L, Mandatori S, De Zio D, Filomeni G. Mitophagy Contributes to Alpha-Tocopheryl Succinate Toxicity in GSNOR-Deficient Hepatocellular Carcinoma. Biochem Pharmacol (2020) 176:113885. doi: 10.1016/j.bcp.2020.113885
153. Wang Y, Su GF, Huang ZX, Wang ZG, Zhou PJ, Fan JL, et al. Cepharanthine Hydrochloride Induces Mitophagy Targeting GPR30 in Hepatocellular Carcinoma (HCC). Expert Opin Ther Targets (2020) 24(4):389–402. doi: 10.1080/14728222.2020.1737013
154. Prieto-Domínguez N, Ordóñez R, Fernández A, Méndez-Blanco C, Baulies A, Garcia-Ruiz C, et al. Melatonin-Induced Increase in Sensitivity of Human Hepatocellular Carcinoma Cells to Sorafenib Is Associated With Reactive Oxygen Species Production and Mitophagy. J Pineal Res (2016) 61(3):396–407. doi: 10.1111/jpi.12358
Keywords: mitochondrial quality control, hepatocellular carcinoma, tumorigenesis, tumor progression, chemoresistance
Citation: Bian J, Zhang D, Wang Y, Qin H, Yang W, Cui R and Sheng J (2021) Mitochondrial Quality Control in Hepatocellular Carcinoma. Front. Oncol. 11:713721. doi: 10.3389/fonc.2021.713721
Received: 24 May 2021; Accepted: 27 August 2021;
Published: 13 September 2021.
Edited by:
Xing-Xing He, Huazhong University of Science and Technology, ChinaReviewed by:
Wei Liu, Sun Yat-sen University, ChinaKe Chen, Huazhong University of Science and Technology, China
Copyright © 2021 Bian, Zhang, Wang, Qin, Yang, Cui and Sheng. This is an open-access article distributed under the terms of the Creative Commons Attribution License (CC BY). The use, distribution or reproduction in other forums is permitted, provided the original author(s) and the copyright owner(s) are credited and that the original publication in this journal is cited, in accordance with accepted academic practice. No use, distribution or reproduction is permitted which does not comply with these terms.
*Correspondence: Jiyao Sheng, c2hlbmdqaXlhb0BqbHUuZWR1LmNu
†These authors have contributed equally to this work and share first authorship