- 1Department of Physiology, Harbin Medical University, Harbin, China
- 2Exercise Medicine Research Institute, Edith Cowan University, Joondalup, WA, Australia
- 3School of Medical and Health Sciences, Edith Cowan University, Joondalup, WA, Australia
- 4Department of Pathology, Harbin Medical University, Harbin, China
- 5School of Human Movement and Nutrition Sciences, University of Queensland, Brisbane, QLD, Australia
- 6UQ Centre for Clinical Research, University of Queensland, Brisbane, QLD, Australia
- 7Department of Urology, Royal Brisbane and Women’s Hospital, Brisbane, QLD, Australia
- 8QIMR Berghofer Medical Research Institute, Brisbane, QLD, Australia
Physical exercise is increasingly recognized as a valuable treatment strategy in managing prostate cancer, not only enhancing supportive care but potentially influencing disease outcomes. However, there are limited studies investigating mechanisms of the tumor-suppressive effect of exercise. Recently, extracellular vesicles (EVs) have been recognized as a therapeutic target for cancer as tumor-derived EVs have the potential to promote metastatic capacity by transferring oncogenic proteins, integrins, and microRNAs to other cells and EVs are also involved in developing drug resistance. Skeletal muscle has been identified as an endocrine organ, releasing EVs into the circulation, and levels of EV-containing factors have been shown to increase in response to exercise. Moreover, preclinical studies have demonstrated the tumor-suppressive effect of protein and microRNA contents in skeletal muscle-derived EVs in various cancers, including prostate cancer. Here we review current knowledge of the tumor-derived EVs in prostate cancer progression and metastasis, the role of exercise in skeletal muscle-derived EVs circulating levels and the alteration of their contents, and the potential tumor-suppressive effect of skeletal muscle-derived EV contents in prostate cancer. In addition, we review the proposed mechanism of exercise in the uptake of skeletal muscle-derived EVs in prostate cancer.
Introduction
Prostate cancer (PCa) is the most frequently diagnosed cancer in 112 countries with over 1.4 million new cases estimated in 2020, which is 14.1% of all new cancer diagnoses (1). Moreover, 370,000 men were estimated to die from prostate cancer in 2020 (6.8% of deaths caused by all cancer) (1). Early detection and advancement in treatments have improved survival for patients with PCa (1). However, these treatments can also have enduring adverse effects, such as the loss of lean mass and bone mass, fat mass gain, post-surgery incontinence, metabolic imbalance, and reduced quality of life (2–4).
Exercise or physical activity has been receiving attention in patient care in the oncology setting (5) due to the increasing body of research in the field of exercise oncology. Multiple epidemiological studies (6, 7) and clinical trials (8–10) consistently report improvements in health-related outcomes for PCa patients. In addition, preclinical murine model studies have also demonstrated a reduced PCa tumor volume and delayed tumor growth with an exercise stimulus (11, 12), and provide a strong mechanistic case for clinical trials to be tested on cancer outcomes. However, while numerous hypotheses exist, the mechanisms by which exercise influences tumor biology are not fully understood (13).
As such, multiple studies have been conducted to reveal the mechanisms of exercise-induced benefits for cancer patients. Alteration in circulating factors, epigenetic modulation, gene expression modulation, immune function improvement, and systemic inflammation reduction have been suggested as potential mechanisms for exercise-induced tumor suppression (13, 14). For instance, serum levels of myokines, skeletal muscle secreted cytokines and peptides, are known to be altered with skeletal muscle stimulation, and multiple preclinical studies have shown the tumor-suppressive role of myokines with direct application in different cancer cell lines, including PCa (13). Although the beneficial role of exercise in reduced disease progression, increased survival, and patient care is promising, the mechanisms underlying how exercise-induced physiological changes provide tumor-suppressive effects are not clearly understood.
One potential mechanism proposed is the involvement of extracellular vesicles (EVs) (15, 16), as exercise-induced skeletal muscle-derived EVs may reduce cancer cell proliferation and metastasis (17). EVs are small membrane-surrounded structures released from various cells (18) that transfer bioactive molecules (including DNA, RNA, and proteins) from donor to acceptor cells (19). Two main types of EVs are defined based on their cellular route of release, exosomes and microvesicles or microparticles (18). The term ‘exosome’ refers to vesicles of the endosomal system that are released through the fusion of the multivesicular body delimiting membrane with the plasma membrane, while ‘microvesicles’ or ‘microparticles’ refer to vesicles that directly pinch off the cell surface (18).
During exercise, the release of EVs packaging cytokines and myokines plays a crucial role in the communication between muscle and other tissues (20). For instance, the skeletal muscle-derived EVs have been shown to increase in response to exercise, and increased uptake of skeletal muscle-derived EVs in the liver has been shown in animal models (21). Thus, the potential role of skeletal muscle-derived EVs in reducing cancer proliferation and migration by transporting anti-oncogenic proteins and microRNAs (miRNAs) has been proposed (20). This review will provide the current evidence for the role of exercise in skeletal muscle-derived EVs concentration in the circulatory system, the alteration of EV contents, and the potential role of exercise-induced skeletal muscle-derived EVs content in PCa. In addition, we propose a potential mechanism whereby exercise enhances skeletal muscle-derived EV uptake and delivery in PCa.
Extracellular Vesicles in Prostate Cancer
EV cargoes are considered to be biologically influential in cancer progression, metastasis, and development of drug resistance (22–24). Furthermore, particular miRNAs in EV cargoes have been considered as potential diagnostic, prognostic, and predictive markers for PCa (24–26) (Figure 1). For instance, miR-107, miR-130b, miR-141, miR-2110, miR-301a, miR-326, miR-331-3p, miR-432, miR-484, miR-574-3p, and miR-625 were shown to be substantially increased in circulating EVs from prostate cancer patients (n=78) compared to healthy individuals (n=28) (P<0.05) (27). Out of 11 miRNAs shown to increase in prostate cancer patients, miR-141 and miR-375 were significantly increased in patients with metastatic prostate cancer (n=16) compared to patients with localized prostate cancer (n=55) (P<0.05) (27), suggesting potential of circulating exosomes as prognostic markers for PCa. Furthermore, exosomal RNA analysis of plasma from 100 castration-resistant prostate cancer (CRPC) patients showed significantly shorter overall survival in patients with higher miR-375 and miR-1290 levels compared to those with lower miR-375 and miR-1290 levels (P=0.0045) (28). Although further validation will be required, this study also showed improved performance of predictive models for overall survival by incorporating miR-375 and miR-1290 levels with clinical prognostic factors (time to ADT failure and PSA level at the time of CRPC diagnosis) (28).
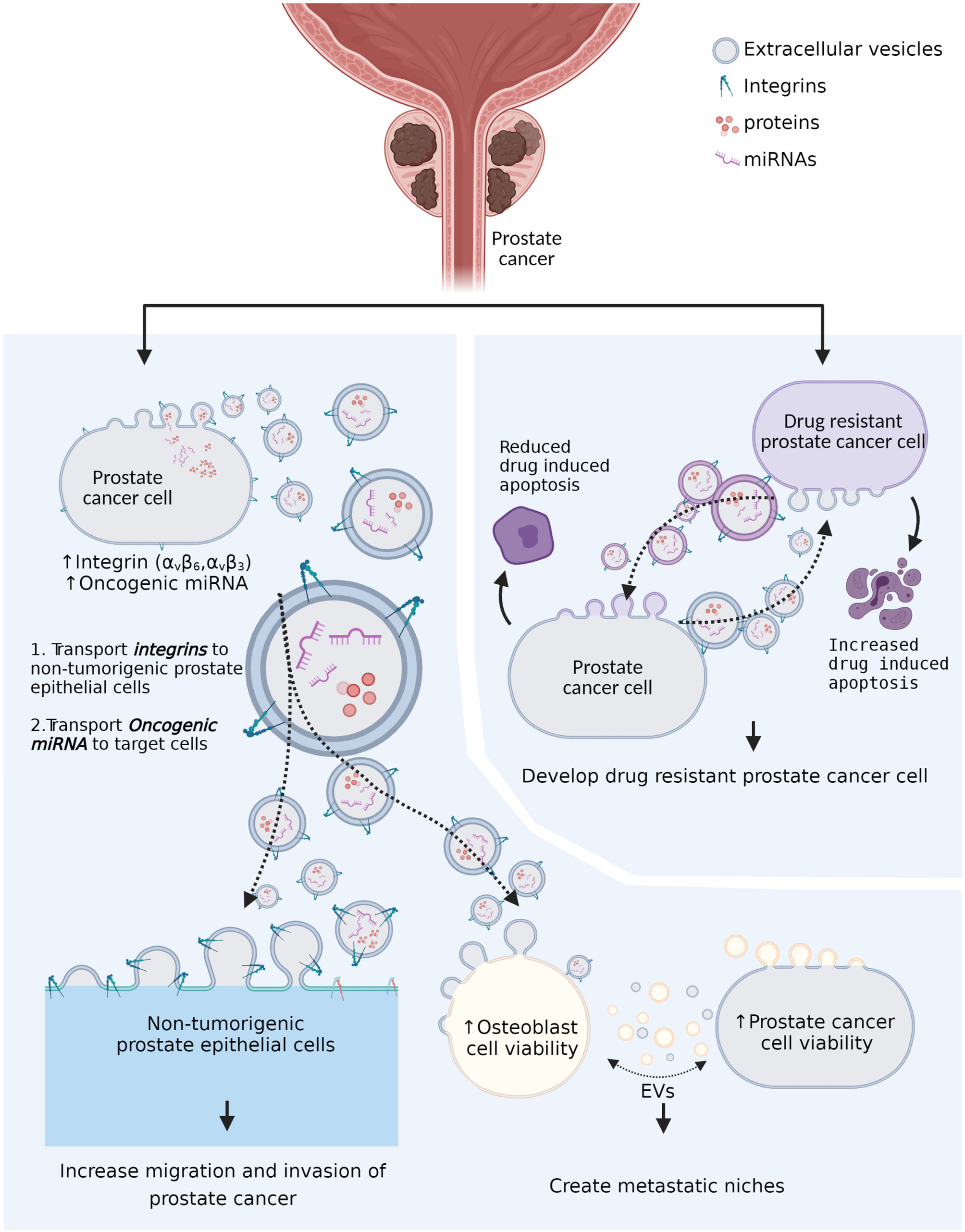
Figure 1 The role of tumor-derived extracellular vesicles in prostate cancer progression and drug resistance. Tumor-derived extracellular vesicles (EVs) transport the integrins (αV family integrins) to non-αV family integrin presenting cells and promote cell-extracellular matrix communication to promote cancer growth. Tumor-derived extracellular vesicles also transport oncogenic microRNAs (miRNAs) to recipient cells and promote remodeling of metastatic niches. In addition, drug resistant prostate cancer cell-derived extracellular vesicles can reduce drug-induced apoptosis in non-drug resistant prostate cancer cells. Moreover, non-drug-resistant prostate cancer cell-derived extracellular vesicles can increase drug-induced apoptosis in drug-resistant prostate cancer cells.
The study by Albino and colleagues (29) investigating the role of circulating miR-424 positive EVs in PCa patients demonstrated that metastatic PCa patients (metastatic castration-sensitive (mCSPC), n=16; metastatic castration-resistance (mCRPC), n=17) showed a higher frequency (P<0.05) of circulating miR-424 positive EVs compared to patients with primary tumors (n=25) and benign prostatic hyperplasia (BPH, n=6). Moreover, the application of EVs isolated from plasma of patients (n=17) in the in vitro environment showed increased tumor-sphere formation in the application of EVs from patients with mCSPC and mCRPC compared to primary or BPH patients (P<0.02) (29). In addition, the level of miR-424 containing EVs was positively associated with tumor growth in the 3D cell culture environment (tumor-sphere formation) (P=0.003) (29), suggesting a potential role of EV-contained miRNAs in PCa progression.
The study by Albino et al. (29) also generated a castration-resistant cell model using the LNCaP cell line by culturing in an androgen-depleted condition and showed significantly increased miR-424 in castration-resistant LNCaP-derived EVs compared to EVs derived from normal LNCaP cells (P<0.005), confirming the elevation in circulating miR-424 positive EVs in patients with advanced PCa (29). Furthermore, the application of EVs isolated from the castrate-resistant LNCaP-derived EVs to another PCa cell line, RWPE-1, showed increased tumor formation in a 3D cell culture model and cell migration compared to the application of normal LNCaP cell-derived EVs (P<0.005) (29), confirming the result by applying human plasma isolated EVs to cancer cell lines.
Preclinical studies involving PCa cell line-derived EVs and osteoblast cells also suggest a role for cancer cell-derived EVs in creating favorable niches for metastasis (30–32). In an in vitro study investigating the role of prostate cancer cell-induced EVs in osteoclastogensis and osteoblast proliferation, Inder and colleagues (30) demonstrated an increase in osteoclastogenesis of murine RAW264.7 pre-osteoclast cells (37 fold) and human primary-osteoblast proliferation (1.43 fold) with the presence of EVs isolated from the human prostate cancer cell line PC3 compared with no PC3- induced EVs (P<0.005). However, secreted soluble factors from PC3 cells were not able to increase osteoclastogensis of RAW264.7 cells, indicating requirement for PCa-induced EVs in osteoclastogenesis (30).
Probert and colleagues (31) reported that culture of osteoblast cells with PC-3 (high metastasis capacity to bone), C4-2 (moderate metastasis capacity to bone), and C4-2-4B (a bone metastatic lineage of C4-2) PCa cell line-derived EVs resulted in a significant increase of osteoblast cell viability (P=0.004, P=0.032, and P=0.001, respectively). In addition, co-culture of the osteoblast cells preincubated with PCa derived-EVs with PC-3 and C4-2 cells showed a significant increase (P<0.001) in PCa cell line viability compared to PCa cell lines co-cultured with osteoblast cells precultured with non- PCa cell lines (31). This study also showed a significant increase of PCa abundant miRNA in osteoblast and induced functional changes of osteoblast via EVs transported miRNA, suggesting a role of PCa cell line-derived EVs in generating metastatic niches (31).
Similarly, there is also preclinical evidence for the role of EVs in transferring integrins to different subsets of PCa cell lines (33, 34). The integrins are a diverse family of glycoproteins that allows cells to interact with extracellular matrix (ECM) molecules and, in cancer, overexpression of the integrins in cancer cells contributes to migration and invasion by disrupting the ECM molecules (35). Studies have showed increased integrins (such as αVβ6 and αVβ3) in PCa cell lines (PC-3 and RWPE) and PCa cell line-derived exosomes (33, 34). Incubation of αVβ6 negative PCa cell line (DU145) or non-tumorigenic prostate epithelial cells with PCa cell line-derived exosomes results in de novo expression of these integrins in αVβ6 negative PCa cell and non-tumorigenic prostate epithelial cells (33, 34). These results suggest that tumor-derived EVs can transfer surface proteins, especially integrins, and enhance migration and invasion of tumor cells.
EVs have also been reported to be involved in PCa drug resistance (36). Panagopoulos and co-workers (37) showed increased camptothecin (CPT, chemotherapy drug) resistance in CPT sensitive DU145 cells when EVs isolated from CPT resistant RC1 PCa cell line conditioned growth media was applied. However, when RC1 cells were cultured with EVs isolated from DU145, CPT-induced apoptosis was increased in the RC1 cells. In addition, when PCa cell lines DU-145 and 22Rv1 cells were cultured with the presence of EVs isolated from docetaxel resistance, PCa cell lines demonstrated docetaxel resistance (38). Furthermore, application of exosomes isolated from docetaxel responding PCa patients (n=6) and non-responding PCa patients (n=2) to the DU145 PCa cell line showed increased docetaxel-resistance in DU145 cells cultured with exosomes isolated from docetaxel non-responders, suggesting the potential role of tumor-cell derived EVs in drug resistance (38).
Exercise and Prostate Cancer
Multiple epidemiological studies in clinical oncology have consistently reported the positive impact of exercise in reducing PCa progression and enhancing survival. For instance, Kenfield and co-workers reported a 61% reduced risk (P=0.03) of PCa-related death (6) while Richman and co-workers reported a 57% reduction in disease progression (P=0.03) (7) in those with higher physical activity levels (≥3 hours/week) compared to those with lower physical activity levels. Furthermore, in preclinical studies, direct application of human serum obtained after exercise from healthy individuals to PCa cell line LNCaP showed a significant reduction in LNCaP cell growth (12). In addition, reduced tumor volume and delayed tumor growth was evident in a murine model injected with LNCaP cells exposed to human serum obtained after a bout of exercise compared with cells exposed to human serum acquired before exercise, suggesting a potential role for exercise in reducing tumor progression in vivo (12). Similar results were shown in the report by Hwang et al. (11), where human serum obtained after exercise from healthy older individuals (age > 60) was directly applied to PC-3 PCa cell lines. In addition, exercise increased blood delivery at the tumor site in a PCa animal model (R-3327 MatLyLu tumor cell orthotopically injected mice model) and reduced aggressiveness of PCa cells via reduced hypoxia at the tumor site (39) suggesting that exercise-induced physiological changes might have positive effects on cancer progression.
In addition, increased lean mass in PCa patients might also positively impact patient outcomes (10). In our recent systemic review and meta-analysis of the efficacy of exercise in improving supportive care outcomes in PCa patients with a range of treatments, improvements (P<0.001) in whole-body fat mass (-0.6 kg), lean mass (+0.5 kg), and appendicular lean mass (+0.4 kg) after exercise compared to usual care were noted (9). Furthermore, in our randomized controlled trial involving 57 PCa patients undergoing androgen deprivation therapy (ADT), 3 months of exercise significantly increased muscle strength, physical function, lean mass, and a number of patient-reported outcomes (8). Moreover, a recent retrospective report also showed that men with PCa exhibited increased prostate-specific antigen (PSA) progression-free survival and radiological progression-free survival in those with higher lean mass (P=0.03 and P<0.001, respectively) (10), providing a strong case for PCa patients to engage in exercise, especially of an anabolic nature to enhance or preserve lean mass.
Skeletal Muscle Derived EVs During Exercise and Prostate Cancer
Over the past decade, skeletal muscle has been identified as an important secretory organ producing a range of cytokines and peptides called myokines (40). In addition, in response to exercise training, skeletal muscle releases miRNAs into the circulation (41), and miRNAs, messenger RNAs (mRNAs), DNA, piwi-interacting RNAs (piRNAs), transfer RNAs (tRNAs), and myokines loaded into EVs for intercellular communication (20, 21). Moreover, clinical and preclinical studies have shown alteration of skeletal muscle-derived EVs concentration in the circulatory system and their contained protein levels are also altered by exercise (21, 42–48) (Table 1 and Figure 2).
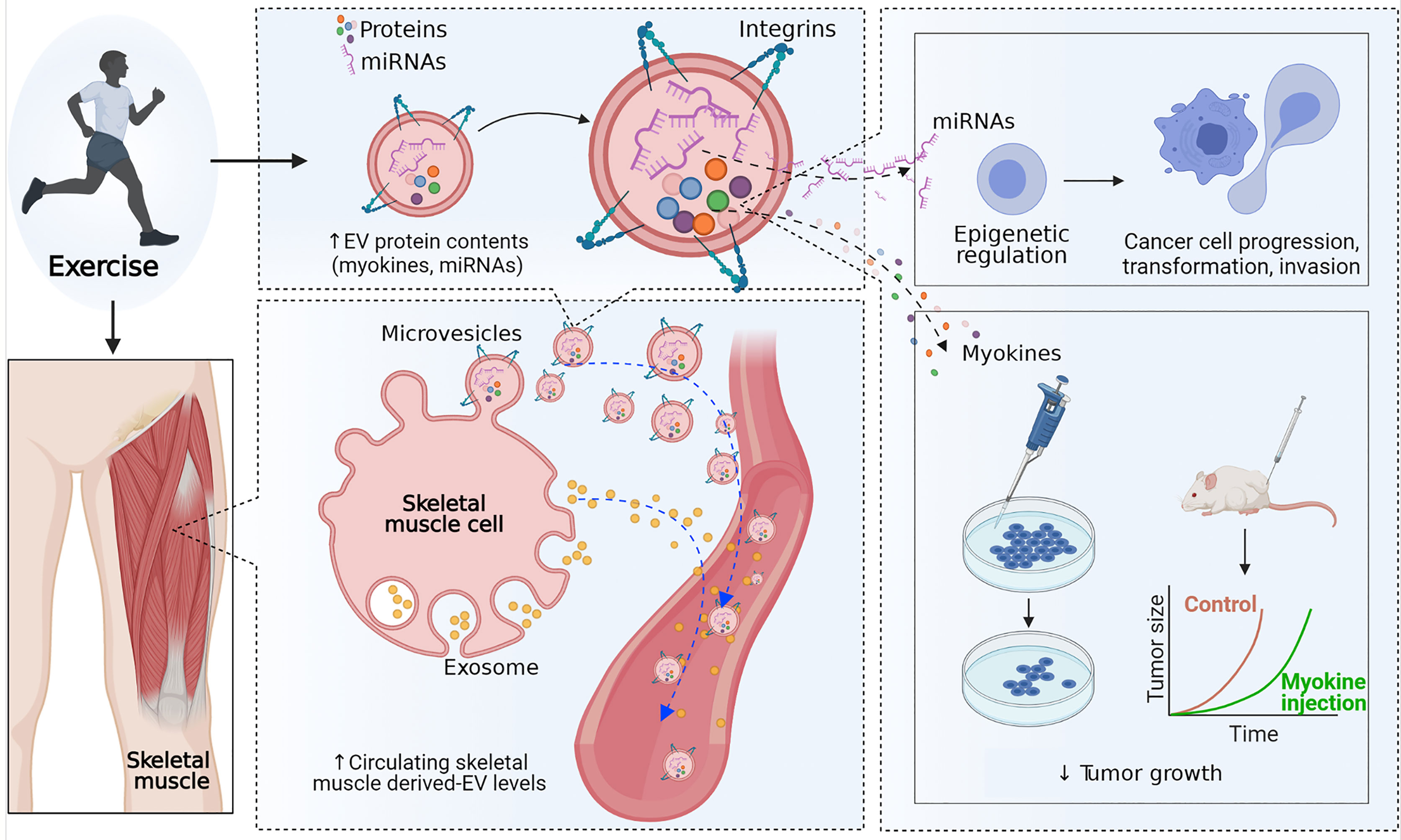
Figure 2 Effect of exercise on extracellular vesicles. Exercise may increase the secretion of extracellular vesicles (EVs) into the circulatory system and alter the concentration of proteins and microRNAs (miRNAs) in skeletal muscle-derived extracellular vesicles. MicroRNAs in skeletal muscle-derived extracellular vesicles may induce epigenetic regulation in prostate cancer cells and reduce cell progression, transformation, and invasion. In addition, skeletal muscle-derived extracellular vesicles containing proteins, including myokines, have a direct tumor-suppressive effect.
Effect of an Exercise Bout on Skeletal Muscle-Derived EVs
Emerging clinical exercise trials are building our understanding of the effect of exercise bouts on exercise-derived EVs in both human and animal models (Table 1 and Figure 2). Frühbeis and colleagues (44) showed that the level of small EVs of 100-130 nm that carry proteins characteristic of exosomes in plasma increased by an average of 5.2 times (Flot1, P=0.0021; Hsp/Hsc70, P=0.0021) in 12 healthy individuals in response to cycling or running until exhaustion. Similarly, another acute exhaustive exercise trial involving 16 healthy subjects also demonstrated a significant increase of EVs in serum at the peak exercise workload compared to at rest (P<0.05) (42), indicating an effect of exhaustive exercise in increasing circulating EVs concentration.
Elevation of serum EV concentrations and protein contents have also been shown after moderate-intensity aerobic exercise. Nielsen and colleagues demonstrated elevation of CD36+ and FATP4+ skeletal muscle-derived EVs in serum after 60 minutes of moderate-intensity aerobic exercise (70% VO2max) in both healthy (n=14; CD36+, 52%, P=0.019) and patients with metabolic disease (n=13; CD36+, 55%, P=0.016; FATP4+, 53%, P=0.007) compared to at rest (46). Moreover, Rigamonti and colleagues (48) showed a significant elevation of skeletal muscle-derived EVs (sarcoglycan-α+EVs) in serum obtained immediately after 30 minutes of aerobic exercise (60% VO2max) compared to at rest (P=0.016), whereas monocyte/macrophage (CD14+EVs), endothelium (CD62E+EVs), and adipose tissue (FABP+EVs) derived EVs were unchanged. In addition, a study investigating the effect of 60 minutes of cycling exercise on circulating small vesicle concentrations and contents in 11 healthy subjects showed elevated circulating small vesicle levels with a significant alteration of 322 proteins after the exercise bout compared to pre-exercise (21).
Not only moderate-intensity aerobic exercise but high and low-intensity exercise also have been shown to increase serum EVs concentration and EVs protein content (45, 47). A clinical study involving a 40-minute bout of vigorous-intensity aerobic exercise (80%VO2 max) in 22 healthy subjects reported the presence of skeletal muscle-derived EVs (sarcoglycan-α+EV) in a cytofluorimetric analysis and level of muscle-specific mRNAs, such as miR-181a-5p and mi-133b, in sarcoglycan-α+EVs to be significantly increased after exercise (P<0.05) (45). Furthermore, in a preclinical study, 18 mice were divided into 4 groups, non-exercise, low-intensity exercise, moderate-intensity exercise, and high-intensity exercise, and undertook 40 minutes of treadmill exercise at a speed of 14-16 m/min (20% below maximum lactate steady state (MLSS), 20-22 m/min (at MLSS), and 24-26 m/min (20% above MLSS) (47). Although there were no significant differences among exercise groups in serum EVs concentration, a significant difference in EVs concentration was shown in all exercise groups compared to the non-exercise group (low-ex vs. non-ex, P=0.014; mod-ex vs. non-ex, P=0.021; high-ex vs. non-ex, P=0.02) (47), whereas the size of EVs in the exercise groups was not changed compared to the non-exercise group. Similarly, EV protein concentrations were also significantly increased in all exercise groups compared to the non-exercise group (P=0.014) (47). In addition, 12 miRNAs in serum EVs (rno-miR-128-3p, 1033p, 330-5p, 148a-3p, 191a-5p, 10b-5p, 93-5p, 25-3p, 142-5p, 3068-3p, 142-3p, and 410-3p), predicted to target genes involved in the MAPK signal transduction pathway, were found to be differentially expressed after exercise in the animal model (47). These results suggest that low- and high-intensity exercise may also increase EV concentrations and protein content levels.
Effect of Chronic Exercise Training on Skeletal Muscle-Derived EVs
Due to the lack of clinical studies investigating the effect of chronic exercise training on circulating EVs concentration, insight into the effect of exercise training on resting circulating EVs concentration can only be derived from animal studies. However, positive associations between aerobic capacity (VO2max) and EVs containing miRNAs (miR-1, R=0.58, P=0.01; miR-133b, R=0.54, P=0.02; miR-181a-5p, R=0.63, P=0.006; miR-206, R=0.5, P=0.003; miR-499, R=0.54, P=0.02) were found in the study by Guescini and colleagues (45) involving 18 healthy subjects suggesting that improvements in aerobic capacity due to chronic exercise might have a role in altering EV contents. Furthermore, a study by Bei and co-workers showed a 1.85-fold increase of circulating EVs after 3 weeks of swimming exercise in a mice model (42), and Bertoldi and colleagues demonstrated elevation of CD36 (exosome marker) in serum after 2 weeks of daily moderate-intensity exercise in a rat model at different ages (43). Although these studies suggest the elevation of circulating EVs concentration, the exercise period was short, and substantial exercise adaptation may not have occurred in the animals. Longer duration studies for exercise adaptation and investigation of the origin of these EV responses to chronic training are required to enhance our understanding of the effect of chronic exercise on circulating skeletal muscle-derived EV resting concentrations.
Potential Role of Skeletal Muscle-Derived EV Cargoes in Prostate Cancer
Exercise can modify the biology of PCa via its effects on muscle hypertrophy, adipose tissue oxidation, increased insulin sensitivity, increased osteogenesis, reduced inflammation, and increased antitumor activity (49). Among the physiological alterations induced by exercise, the tumor-suppressive role of skeletal muscle secreted proteins (myokines) and miRNAs in PCa suggests a regulatory role of skeletal muscle-derived EV-containing proteins in PCa (Figure 2).
Myokines, such as IL-6, irisin/FNDC5, decorin, oncostatin M (OSM), and secreted protein acidic and rich in cysteine (SPARC), have shown the potential of a direct tumor-suppressive effect in different cancer cell lines, including PCa (13). For example, in vitro administration of IL-6 resulted in a reduction of hormone-sensitive PCa cell line proliferation by reducing androgen receptor expression (50), and application of irisin to PCa cell lines significantly reduced cell viability (P<0.05) (51). Moreover, direct application of SPARC and decorin significantly reduced PCa cell line growth by reduced Cyclin D1 and epidermal growth factor receptor (EGFR) activation, respectively (52, 53). Levels of myokines have also been shown to be altered in intercellular muscle protein and mRNA levels (13), supporting the analytic results of protein contents in skeletal muscle-derived EV contents by Whitham and co-workers, which showed alteration of protein contents in skeletal muscle-derived EVs (21).
The importance of epigenetics (including DNA methylation, histone modification, and miRNA and long non-coding RNA (lncRNA) regulation) in cellular transformation, tissue invasion, induction of angiogenesis, escape from immune surveillance, and metastasis is increasingly being recognized in cancer development and progression. In the pathogenesis of human PCa, somatic epigenetic alterations appear earlier than genetic changes, as well as more commonly and more consistently (54). Recent research has implicated EVs in epigenetic regulation of the cancer microenvironment to affect cancer progression (55). Bioinformatic analysis has indicated that many mRNAs and proteins contained in EVs are involved in epigenetic modulation (56). Proteins, mRNAs, microRNAs, and non-coding RNAs in EVs alter the phenotype of target cells by transferring mRNA, a transcriptional modulator, or degrading mRNA rapidly (57). Recently, it has been shown that aerobic exercise is a potential epigenetic modifier. Aerobic exercise induces epigenetic changes through several mechanisms, including chromatin methylation, histone acetylation, DNA methylation, and miR expression. MiRs secreted into the extracellular microenvironment via EVs may play an important role in epigenetic modulation (58).
Uptake of Skeletal Muscle-Derived EVs by Prostate Cancer Cells: Potential Myokine Involvement
Uptake of EVs by targeted cells is an important process to elicit the functional effects by initiating signaling events at the surface of recipient cells or transferring EV contents into recipient cells (59). Although the uptake of EVs by recipient cells is a critical process in cell-to-cell or ECM-to-cell communication via EVs, this process is poorly understood because the uptake of EVs depends on the specific properties of the recipient cells (60). However, the potential role of skeletal muscle-induced myokines, especially irisin, in skeletal muscle-derived EVs and cancer cell communication has been proposed in a recent review by Darkwah et al. (17) (Figure 3).
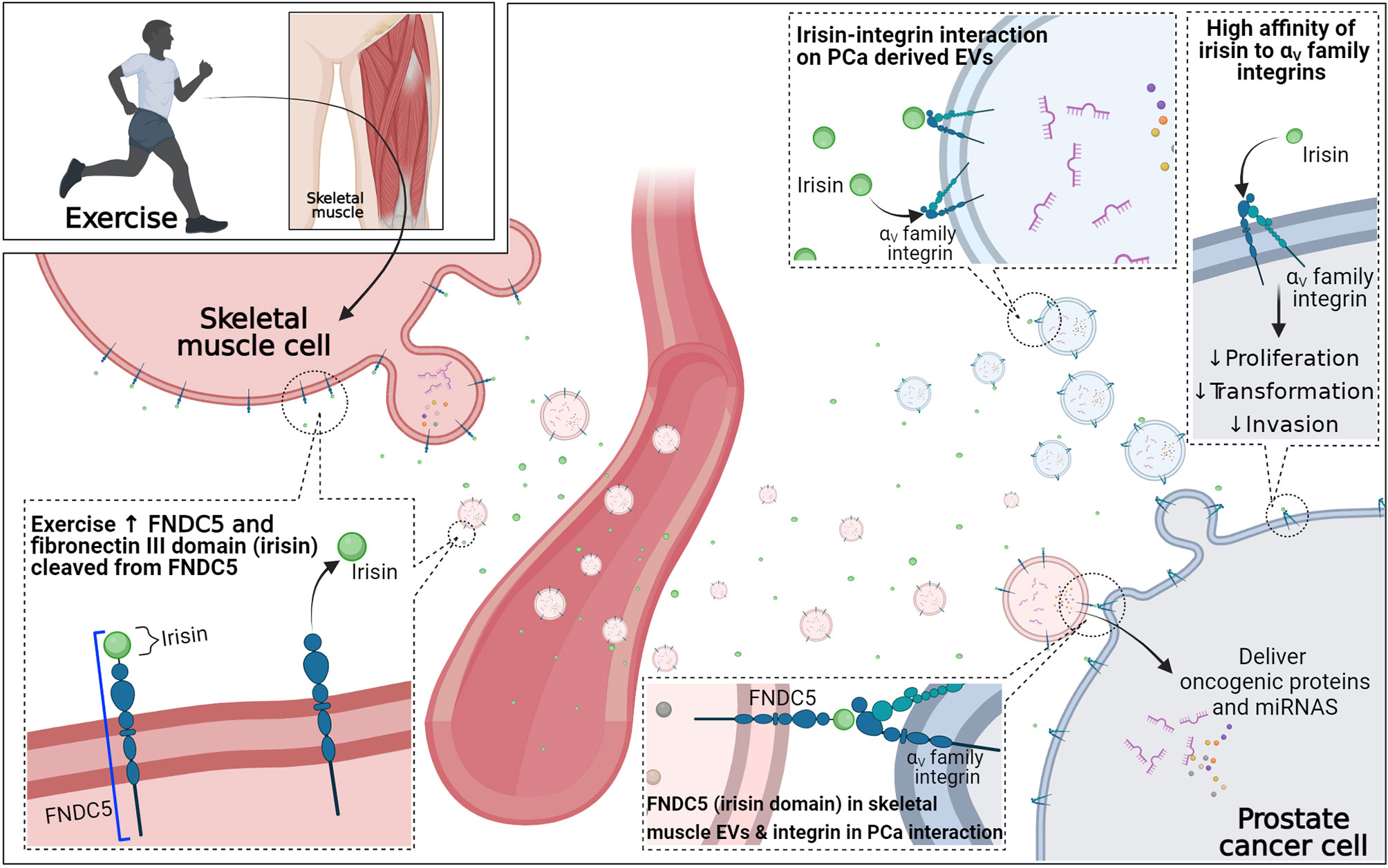
Figure 3 Potential role of exercise-induced myokines in extracellular uptake in prostate cancer cells. Irisin has a high affinity to αV family integrins, which is highly expressed in prostate cancer. FNDC5 is a precursor of irisin and with exercise stimulation FNDC5 is increased on the membrane, as is the secretion of irisin from skeletal muscle. Free irisin and skeletal muscle-derived extracellular vesicles (EVs) containing FNDC5 may travel to the prostate cancer site through the circulatory system. The high affinity of free irisin to αV family integrins on the prostate cancer cell may directly induce irisin-integrin interaction to elicit the direct tumor-suppressive effect. Moreover, free irisin may also interact with αV family integrins on tumor-derived extracellular vesicles to interfere with the remodeling of metastatic niches. Lastly, the irisin domain of FNDC5 on the membrane of skeletal muscle-derived extracellular vesicles may increase internalization of skeletal muscle-derived extracellular vesicles to prostate cancer cells through FNDC5 (irisin domain)- αV family integrin interaction.
Fibronectin type III domain containing 5 (FNDC5), a type1 transmembrane glycoprotein embedded in the skeletal muscle cell membrane, is a precursor of irisin and with exercise stimulus not only does FNDC5 expression increase on the membrane of skeletal muscle cells but the fibronectin III domain is cleaved and released to the extracellular site as irisin (61, 62). Irisin has been shown to increase energy expenditure by inducing white adipocyte browning and helps to maintain metabolic homeostasis, reducing body weight, improving glucose metabolism, and improving insulin sensitivity (62–64). Furthermore, various preclinical studies have demonstrated a direct reduction of growth of various cancer cell lines after applying exogenous irisin including PCa (13).
The receptors of irisin were not identified until recently. However, irisin has been shown to have a high affinity to specific integrin families, αV family, in bone and fat cells (65). Furthermore, previous studies have demonstrated high αV family integrins (such as αVβ6 and αVβ3) expression in PCa cell lines, and these transmembrane proteins can be transferred to other recipient cells (33, 34), suggesting the potential role of irisin in increasing skeletal muscle-derived EVs and PCa cell communication. Although there is no research investigating the expression of FNDC5 in the membrane of skeletal muscle-derived EVs, the process of microvesicle biogenesis suggests the surface protein of the cell might be transferred to EVs (17). Taken together, it could be proposed that irisin-αV family integrins interaction can directly induce a tumor-suppressive effect on PCa cells and possibly increase skeletal muscle-derived EVs-PCa cell communication through an extracellular domain of FNDC5 (irisin)-integrin interaction. This may increase the internalization of skeletal muscle-derived EVs into PCa cells to elicit the functional role of EV contents in PCa (17). Furthermore, circulating irisin may also interact with PCa cell-derived EV containing integrins and interfere with the delivery of PCa cell-derived EVs to the cells near PCa cells and contribute to remodeling the pre-metastatic environment (17). However, further investigation examining the role of irisin-integrin interaction in PCa is required to fully elucidate skeletal muscle-derived EVs uptake in PCa and involvement of myokines.
Discussion
The importance of exercise oncology, the application of exercise medicine in cancer, has been well recognized in clinical oncology (5). Epidemiological (6, 7, 10) and clinical (8, 9) studies examining the effect of exercise in PCa patients have further established the role of exercise in patient care for men with PCa. Moreover, exercise-induced circulating factor alteration, epigenetic modulation, and gene expression have been suggested as potential mechanisms whereby exercise may impact disease progression in men with PCa (13, 14).
Recently, extracellular vesicles have been highlighted in cell-to-cell and cell-to-extracellular matrix communications, and in PCa tumor-derived EVs have been suggested as a therapeutic target (24–26). Tumor-derived EVs have potential in delivering oncogenic proteins, surface proteins, and miRNAs to non-tumor cells and contribute to progression of PCa by initiating growth-promoting signal cascades or creating metastatic niches among non-tumoral cells near cancerous cells (29, 31, 33, 34). As such, multiple proteins, miRNAs, and surface proteins in tumor-derived EVs have been identified as potential predictable markers for PCa progression (22, 23, 26). However, a few clinical exercise trials demonstrating elevation of skeletal muscle-induced EVs after exercise and preclinical studies demonstrating a potential tumor-suppressive effect of skeletal muscle-derived factors (such as myokines and miRNAs) suggest EVs as a potential delivery mechanism for skeletal muscle induced proteins and miRNAs in PCa (13, 21, 42–48, 58). Furthermore, the potential role of myokines in the facilitation of skeletal muscle-derived EV uptake in cancer has been recently proposed in a review article by Darkwah and co-workers (17).
It is important to note that research into skeletal muscle-derived EVs in cancer is at an early stage; more research is required to fully elucidate the role of exercise in PCa. For instance, the clinical exercise trials investigating the alteration of skeletal muscle-derived EVs after exercise are limited to healthy populations, limiting the generalizability of these outcomes to cancer patients. Moreover, as common adverse effects of ADT, a widspread treatment in men with PCa, are a significant loss of skeletal muscle mass and gain of fat mass (4), physiology of skeletal muscle biogenesis may differ from that of healthy subjects (13). The lack of preclinical studies investigating the direct effect of skeletal muscle-derived EVs on PCa cells also prevents a clear understanding of the role of exercise-induced skeletal muscle-derived EVs. Although skeletal muscle secretomes, such as myokines and miRNAs, have been shown to have potential in suppressing tumor growth (13, 49), the direct communication between skeletal muscle cells and PCa cells via EVs is yet to be reported.
Conclusions
Exercise-derived EVs have received increased attention as they provide an opportunity to understand the mechanistic benefit of exercise in cancer patients. Emerging evidence indicates that aerobic exercise affects circulating EV dynamics, including the size, morphology, and composition. Proteins, miRNAs, mRNAs, and DNAs packed in exercise-specific EVs may potentially play a role in preventing PCa development and disease progression. This review has summarized the preliminary evidence for an effect of exercise on circulatory levels of skeletal muscle-derived EV secretion, EV-containing protein or miRNA contents and the role of EV-containing factors in PCa progression, as well as the potential involvement of myokines in EV uptake in PCa. Given the speculative nature of the role of exercise-derived EVs to date, in the coming decade research will likely clarify the role of EVs with a focus on the dynamics of EVs in response to specific exercise modes and dosages, providing opportunities to enhance our understanding of the tailoring of exercise prescription on mediating possible cancer outcomes.
Author Contributions
YZ and J-SK contributed equality to this work. YZ and T-ZW drafted the initial manuscript, and J-SK developed the manuscript. J-SK, RN, DG, RG, MH, and DT performed the revision. All authors contributed to the article and approved the submitted version.
Funding
J-SK is supported by National Health and Research Council Centre of Research Excellence (NHMRC-CRE) in Prostate Cancer Survivorship Scholarship. DG and RN are funded by an NHMRC-CRE in Prostate Cancer Survivorship (APP1116334).
Conflict of Interest
The authors declare that the research was conducted in the absence of any commercial or financial relationships that could be construed as a potential conflict of interest.
Publisher’s Note
All claims expressed in this article are solely those of the authors and do not necessarily represent those of their affiliated organizations, or those of the publisher, the editors and the reviewers. Any product that may be evaluated in this article, or claim that may be made by its manufacturer, is not guaranteed or endorsed by the publisher.
References
1. Sung H, Ferlay J, Siegel RL, Laversanne M, Soerjomataram I, Jemal A, et al. Global Cancer Statistics 2020: GLOBOCAN Estimates of Incidence and Mortality Worldwide for 36 Cancers in 185 Countries. CA Cancer J Clin (2021) 71:209–49. doi: 10.3322/caac.21660
2. Boorjian SA, Eastham JA, Graefen M, Guillonneau B, Karnes RJ, Moul JW, et al. A Critical Analysis of the Long-Term Impact of Radical Prostatectomy on Cancer Control and Function Outcomes. Eur Urol (2012) 61:664–75. doi: 10.1016/j.eururo.2011.11.053
3. Parsons BA, Evans S, Wright MP. Prostate Cancer and Urinary Incontinence. Maturitas (2009) 63:323–8. doi: 10.1016/j.maturitas.2009.06.005
4. Smith MR, Finkelstein JS, McGovern FJ, Zietman AL, Fallon MA, Schoenfeld DA, et al. Changes in Body Composition During Androgen Deprivation Therapy for Prostate Cancer. J Clin Endocrinol Metab (2002) 87:599–603. doi: 10.1210/jcem.87.2.8299
5. Schmitz KH, Campbell AM, Stuiver MM, Pinto BM, Schwartz AL, Morris GS, et al. Exercise is Medicine in Oncology: Engaging Clinicians to Help Patients Move Through Cancer. CA Cancer J Clin (2019) 69:468–84. doi: 10.3322/caac.21579
6. Kenfield SA, Stampfer MJ, Giovannucci E, Chan JM. Physical Activity and Survival After Prostate Cancer Diagnosis in the Health Professionals Follow-Up Study. J Clin Oncol (2011) 29:726–32. doi: 10.1200/JCO.2010.31.5226
7. Richman EL, Kenfield SA, Stampfer MJ, Paciorek A, Carroll PR, Chan JM. Physical Activity After Diagnosis and Risk of Prostate Cancer Progression: Data From the Cancer of the Prostate Strategic Urologic Research Endeavor. Cancer Res (2011) 71:3889–95. doi: 10.1158/0008-5472.CAN-10-3932
8. Galvão DA, Taaffe DR, Spry N, Joseph D, Newton RU. Combined Resistance and Aerobic Exercise Program Reverses Muscle Loss in Men Undergoing Androgen Suppression Therapy for Prostate Cancer Without Bone Metastases: A Randomized Controlled Trial. J Clin Oncol (2010) 28:340–7. doi: 10.1200/JCO.2009.23.2488
9. Lopez P, Taaffe DR, Newton RU, Galvao DA. Resistance Exercise Dosage in Men With Prostate Cancer: Systematic Review, Meta-Analysis, and Meta-Regression. Med Sci Sports Exerc (2020) 53:459–69. doi: 10.1249/MSS.0000000000002503
10. Pak S, Kim MS, Park EY, Kim SH, Lee KH, Joung JY. Association of Body Composition With Survival and Treatment Efficacy in Castration-Resistant Prostate Cancer. Front Oncol (2020) 10:558. doi: 10.3389/fonc.2020.00558
11. Hwang JH, McGovern J, Minett GM, Della Gatta PA, Roberts L, Harris JM, et al. Mobilizing Serum Factors and Immune Cells Through Exercise to Counteract Age-Related Changes in Cancer Risk. Exerc Immunol Rev (2020) 26:80–99.
12. Rundqvist H, Augsten M, Stromberg A, Rullman E, Mijwel S, Kharaziha P, et al. Effect of Acute Exercise on Prostate Cancer Cell Growth. PloS One (2013) 8:e67579. doi: 10.1371/journal.pone.0067579
13. Kim J-S, Galvão DA, Newton RU, Gray E, Taaffe DR. Exercise-Induced Myokines and Their Effect on Prostate Cancer. Nat Rev Urol (2021) 18:519–42. doi: 10.1038/s41585-021-00476-y
14. Galvao DA, Taaffe DR, Spry N, Gardiner RA, Taylor R, Risbridger GP, et al. Enhancing Active Surveillance of Prostate Cancer: The Potential of Exercise Medicine. Nat Rev Urol (2016) 13:258–65. doi: 10.1038/nrurol.2016.46
15. Becker A, Thakur BK, Weiss JM, Kim HS, Peinado H, Lyden D. Extracellular Vesicles in Cancer: Cell-to-Cell Mediators of Metastasis. Cancer Cell (2016) 30:836–48. doi: 10.1016/j.ccell.2016.10.009
16. Suchorska WM, Lach MS. The Role of Exosomes in Tumor Progression and Metastasis (Review). Oncol Rep (2016) 35:1237–44. doi: 10.3892/or.2015.4507
17. Darkwah S, Park EJ, Myint PK, Ito A, Appiah MG, Obeng G, et al. Potential Roles of Muscle-Derived Extracellular Vesicles in Remodeling Cellular Microenvironment: Proposed Implications of the Exercise-Induced Myokine, Irisin. Front Cell Dev Biol (2021) 9:634853. doi: 10.3389/fcell.2021.634853
18. Gyorgy B, Szabo TG, Pasztoi M, Pal Z, Misjak P, Aradi B, et al. Membrane Vesicles, Current State-of-the-Art: Emerging Role of Extracellular Vesicles. Cell Mol Life Sci (2011) 68:2667–88. doi: 10.1007/s00018-011-0689-3
19. Mathieu M, Martin-Jaular L, Lavieu G, Théry C. Specificities of Secretion and Uptake of Exosomes and Other Extracellular Vesicles for Cell-to-Cell Communication. Nat Cell Biol (2019) 21:9–17. doi: 10.1038/s41556-018-0250-9
20. Trovato E, Di Felice V, Barone R. Extracellular Vesicles: Delivery Vehicles of Myokines. Front Physiol (2019) 10:522. doi: 10.3389/fphys.2019.00522
21. Whitham M, Parker BL, Friedrichsen M, Hingst JR, Hjorth M, Hughes WE, et al. Extracellular Vesicles Provide a Means for Tissue Crosstalk During Exercise. Cell Metab (2018) 27:237–51. doi: 10.1016/j.cmet.2017.12.001
22. Fujita Y, Yoshioka Y, Ochiya T. Extracellular Vesicle Transfer of Cancer Pathogenic Components. Cancer Sci (2016) 107:385–90. doi: 10.1111/cas.12896
23. Jabalee J, Towle R, Garnis C. The Role of Extracellular Vesicles in Cancer: Cargo, Function, and Therapeutic Implications. Cells (2018) 7:93. doi: 10.3390/cells7080093
24. Ludwig M, Rajvansh R, Drake JM. Emerging Role of Extracellular Vesicles in Prostate Cancer. Endocrinology (2021) 162:bqab139. doi: 10.1210/endocr/bqab139
25. Linxweiler J, Junker K. Extracellular Vesicles in Urological Malignancies: An Update. Nat Rev Urol (2020) 17:11–27. doi: 10.1038/s41585-019-0261-8
26. Sita-Lumsden A, Dart DA, Waxman J, Bevan CL. Circulating microRNAs as Potential New Biomarkers for Prostate Cancer. Br J Cancer (2013) 108:1925–30. doi: 10.1038/bjc.2013.192
27. Bryant RJ, Pawlowski T, Catto JW, Marsden G, Vessella RL, Rhees B, et al. Changes in Circulating microRNA Levels Associated With Prostate Cancer. Br J Cancer (2012) 106:768–74. doi: 10.1038/bjc.2011.595
28. Huang X, Yuan T, Liang M, Du M, Xia S, Dittmar R, et al. Exosomal miR-1290 and miR-375 as Prognostic Markers in Castration-Resistant Prostate Cancer. Eur Urol (2015) 67:33–41. doi: 10.1016/j.eururo.2014.07.035
29. Albino D, Falcione M, Uboldi V, Temilola DO, Sandrini G, Merulla J, et al. Circulating Extracellular Vesicles Release Oncogenic miR-424 in Experimental Models and Patients With Aggressive Prostate Cancer. Commun Biol (2021) 4:119. doi: 10.1038/s42003-020-01642-5
30. Inder KL, Ruelcke JE, Petelin L, Moon H, Choi E, Rae J, et al. Cavin-1/PTRF Alters Prostate Cancer Cell-Derived Extracellular Vesicle Content and Internalization to Attenuate Extracellular Vesicle-Mediated Osteoclastogenesis and Osteoblast Proliferation. J Extracell Vesicles (2014) 3:23784. doi: 10.3402/jev.v3.23784
31. Probert C, Dottorini T, Speakman A, Hunt S, Nafee T, Fazeli A, et al. Communication of Prostate Cancer Cells With Bone Cells via Extracellular Vesicle RNA; A Potential Mechanism of Metastasis. Oncogene (2019) 38:1751–63. doi: 10.1038/s41388-018-0540-5
32. Robinson H, Ruelcke JE, Lewis A, Bond CS, Fox AH, Bharti V, et al. Caveolin-1-Driven Membrane Remodelling Regulates hnRNPK-Mediated Exosomal microRNA Sorting in Cancer. Clin Transl Med (2021) 11:e381. doi: 10.1002/ctm2.381
33. Fedele C, Singh A, Zerlanko BJ, Iozzo RV, Languino LR. The Alphavbeta6 Integrin Is Transferred Intercellularly via Exosomes. J Biol Chem (2015) 290:4545–51. doi: 10.1074/jbc.C114.617662
34. Singh A, Fedele C, Lu H, Nevalainen MT, Keen JH, Languino LR. Exosome-Mediated Transfer of Alphavbeta3 Integrin From Tumorigenic to Nontumorigenic Cells Promotes a Migratory Phenotype. Mol Cancer Res (2016) 14:1136–46. doi: 10.1158/1541-7786.MCR-16-0058
35. Hood JD, Cheresh DA. Role of Integrins in Cell Invasion and Migration. Nat Rev Cancer (2002) 2:91–100. doi: 10.1038/nrc727
36. Wang JQ, DeChalus A, Chatterjee DN, Keller ET, Mizokami A, Camussi G, et al. Extracellular Vesicle-Mediated Reversal of Paclitaxel Resistance in Prostate Cancer. Crit Rev Oncog (2015) 20:407–17. doi: 10.1615/CritRevOncog.v20.i5-6.120
37. Panagopoulos K, Cross-Knorr S, Dillard C, Pantazatos D, Del Tatto M, Mills D, et al. Reversal of Chemosensitivity and Induction of Cell Malignancy of a Non-Malignant Prostate Cancer Cell Line Upon Extracellular Vesicle Exposure. Mol Cancer (2013) 12:118. doi: 10.1186/1476-4598-12-118
38. Corcoran C, Rani S, O’Brien K, O’Neill A, Prencipe M, Sheikh R, et al. Docetaxel-Resistance in Prostate Cancer: Evaluating Associated Phenotypic Changes and Potential for Resistance Transfer via Exosomes. PloS One (2012) 7:e50999. doi: 10.1371/journal.pone.0050999
39. McCullough DJ, Stabley JN, Siemann DW, Behnke BJ. Modulation of Blood Flow, Hypoxia, and Vascular Function in Orthotopic Prostate Tumors During Exercise. J Natl Cancer Inst (2014) 106:dju036. doi: 10.1093/jnci/dju036
40. Pedersen BK, Febbraio MA. Muscles, Exercise and Obesity: Skeletal Muscle as a Secretory Organ. Nat Rev Endocrinol (2012) 8:457–65. doi: 10.1038/nrendo.2012.49
41. Baggish AL, Hale A, Weiner RB, Lewis GD, Systrom D, Wang F, et al. Dynamic Regulation of Circulating microRNA During Acute Exhaustive Exercise and Sustained Aerobic Exercise Training. J Physiol (2011) 589:3983–94. doi: 10.1113/jphysiol.2011.213363
42. Bei Y, Xu T, Lv D, Yu P, Xu J, Che L, et al. Exercise-Induced Circulating Extracellular Vesicles Protect Against Cardiac Ischemia-Reperfusion Injury. Basic Res Cardiol (2017) 112:38. doi: 10.1007/s00395-017-0628-z
43. Bertoldi K, Cechinel LR, Schallenberger B, Corssac GB, Davies S, Guerreiro ICK, et al. Circulating Extracellular Vesicles in the Aging Process: Impact of Aerobic Exercise. Mol Cell Biochem (2018) 440:115–25. doi: 10.1007/s11010-017-3160-4
44. Fruhbeis C, Helmig S, Tug S, Simon P, Kramer-Albers EM. Physical Exercise Induces Rapid Release of Small Extracellular Vesicles Into the Circulation. J Extracell Vesicles (2015) 4:28239. doi: 10.3402/jev.v4.28239
45. Guescini M, Canonico B, Lucertini F, Maggio S, Annibalini G, Barbieri E, et al. Muscle Releases Alpha-Sarcoglycan Positive Extracellular Vesicles Carrying miRNAs in the Bloodstream. PloS One (2015) 10:e0125094. doi: 10.1371/journal.pone.0125094
46. Nielsen MH, Sabaratnam R, Pedersen AJT, Hojlund K, Handberg A. Acute Exercise Increases Plasma Levels of Muscle-Derived Microvesicles Carrying Fatty Acid Transport Proteins. J Clin Endocrinol Metab (2019) 104:4804–14. doi: 10.1210/jc.2018-02547
47. Oliveira GP Jr., Porto WF, Palu CC, Pereira LM, Petriz B, Almeida JA, et al. Effects of Acute Aerobic Exercise on Rats Serum Extracellular Vesicles Diameter, Concentration and Small RNAs Content. Front Physiol (2018) 9:532. doi: 10.3389/fphys.2018.00532
48. Rigamonti AE, Bollati V, Pergoli L, Iodice S, De Col A, Tamini S, et al. Effects of an Acute Bout of Exercise on Circulating Extracellular Vesicles: Tissue-, Sex-, and BMI-Related Differences. Int J Obes (Lond) (2020) 44:1108–18. doi: 10.1038/s41366-019-0460-7
49. Hayes BD, Brady L, Pollak M, Finn SP. Exercise and Prostate Cancer: Evidence and Proposed Mechanisms for Disease Modification. Cancer Epidemiol Biomarkers Prev (2016) 25:1281–8. doi: 10.1158/1055-9965.EPI-16-0223
50. Lee SO, Chun JY, Nadiminty N, Lou W, Gao AC. Interleukin-6 Undergoes Transition From Growth Inhibitor Associated With Neuroendocrine Differentiation to Stimulator Accompanied by Androgen Receptor Activation During LNCaP Prostate Cancer Cell Progression. Prostate (2007) 67:764–73. doi: 10.1002/pros.20553
51. Tekin S, Erden Y, Sandal S, Yilmaz B. Is Irisin an Anticarcinogenic Peptide? Med-Science (2015) 4:2172–80. doi: 10.5455/medscience.2014.03.8210
52. Hu Y, Sun H, Owens RT, Wu J, Chen YQ, Berquin IM, et al. Decorin Suppresses Prostate Tumor Growth Through Inhibition of Epidermal Growth Factor and Androgen Receptor Pathways. Neoplasia (2009) 11:1042–53. doi: 10.1593/neo.09760
53. Said N, Frierson HF Jr, Chernauskas D, Conaway M, Motamed K, Theodorescu D. The Role of SPARC in the TRAMP Model of Prostate Carcinogenesis and Progression. Oncogene (2009) 28:3487–98. doi: 10.1038/onc.2009.205
54. Nelson WG, Yegnasubramanian S, Agoston AT, Bastian PJ, Lee BH, Nakayama M, et al. Abnormal DNA Methylation, Epigenetics, and Prostate Cancer. Front Biosci (2007) 12:4254–66. doi: 10.2741/2385
55. Agnati LF, Fuxe K. Extracellular-Vesicle Type of Volume Transmission and Tunnelling-Nanotube Type of Wiring Transmission Add a New Dimension to Brain Neuro-Glial Networks. Philos Trans R Soc Lond B Biol Sci (2014) 369:20130505. doi: 10.1098/rstb.2013.0505
56. Sharma A. Bioinformatic Analysis Revealing Association of Exosomal mRNAs and Proteins in Epigenetic Inheritance. J Theor Biol (2014) 357:143–9. doi: 10.1016/j.jtbi.2014.05.019
57. Quesenberry PJ, Goldberg LR, Aliotta JM, Dooner MS, Pereira MG, Wen S, et al. Cellular Phenotype and Extracellular Vesicles: Basic and Clinical Considerations. Stem Cells Dev (2014) 23:1429–36. doi: 10.1089/scd.2013.0594
58. Masi LN, Serdan TD, Levada-Pires AC, Hatanaka E, Silveira LD, Cury-Boaventura MF, et al. Regulation of Gene Expression by Exercise-Related Micrornas. Cell Physiol Biochem (2016) 39:2381–97. doi: 10.1159/000452507
59. French KC, Antonyak MA, Cerione RA. Extracellular Vesicle Docking at the Cellular Port: Extracellular Vesicle Binding and Uptake. Semin Cell Dev Biol (2017) 67:48–55. doi: 10.1016/j.semcdb.2017.01.002
60. Lee TH, Chennakrishnaiah S, Meehan B, Montermini L, Garnier D, D’Asti E, et al. Barriers to Horizontal Cell Transformation by Extracellular Vesicles Containing Oncogenic H-Ras. Oncotarget (2016) 7:51991–2002. doi: 10.18632/oncotarget.10627
61. Huh JY, Panagiotou G, Mougios V, Brinkoetter M, Vamvini MT, Schneider BE, et al. FNDC5 and Irisin in Humans: I. Predictors of Circulating Concentrations in Serum and Plasma and II. mRNA Expression and Circulating Concentrations in Response to Weight Loss and Exercise. Metabolism (2012) 61:1725–38. doi: 10.1016/j.metabol.2012.09.002
62. Novelle MG, Contreras C, Romero-Pico A, Lopez M, Dieguez C. Irisin, Two Years Later. Int J Endocrinol (2013) 2013:746281. doi: 10.1155/2013/746281
63. Arhire LI, Mihalache L, Covasa M. Irisin: A Hope in Understanding and Managing Obesity and Metabolic Syndrome. Front Endocrinol (Lausanne) (2019) 10:524. doi: 10.3389/fendo.2019.00524
64. Reddy NL, Tan BK, Barber TM, Randeva HS. Brown Adipose Tissue: Endocrine Determinants of Function and Therapeutic Manipulation as a Novel Treatment Strategy for Obesity. BMC Obes (2014) 1:13. doi: 10.1186/s40608-014-0013-5
Keywords: prostate cancer, exercise oncology, extracellular vesicles, cancer physiology, exercise physiology
Citation: Zhang Y, Kim J-S, Wang T-Z, Newton RU, Galvão DA, Gardiner RA, Hill MM and Taaffe DR (2021) Potential Role of Exercise Induced Extracellular Vesicles in Prostate Cancer Suppression. Front. Oncol. 11:746040. doi: 10.3389/fonc.2021.746040
Received: 23 July 2021; Accepted: 27 August 2021;
Published: 14 September 2021.
Edited by:
Tanya I Stoyanova, Stanford University, United StatesReviewed by:
Lucas Delmonico, Federal University of Rio de Janeiro, BrazilAvishay Sella, Yitzhak Shamir Medical Center, Israel
Copyright © 2021 Zhang, Kim, Wang, Newton, Galvão, Gardiner, Hill and Taaffe. This is an open-access article distributed under the terms of the Creative Commons Attribution License (CC BY). The use, distribution or reproduction in other forums is permitted, provided the original author(s) and the copyright owner(s) are credited and that the original publication in this journal is cited, in accordance with accepted academic practice. No use, distribution or reproduction is permitted which does not comply with these terms.
*Correspondence: Dennis R. Taaffe, ZC50YWFmZmVAZWN1LmVkdS5hdQ==
†These authors have contributed equally to work and share first authorship