- 1Department of Pathology, College of Korean Medicine, Kyung Hee University, Seoul, South Korea
- 2Korean Medicine-Based Drug Repositioning Cancer Research Center, College of Korean Medicine, Kyung Hee University, Seoul, South Korea
- 3School of Pharmacy, Sungkyunkwan University, Suwon, South Korea
Daemonorops draco Blume (DD), also called dragon’s blood, has been used as a traditional Korean medicine, especially for relieving pain caused by wound infection. Recently, it has been described that DD has antibacterial and analgesic effects. In this study, the underlying anticancer effect of DD associated with apoptosis was investigated in acute myeloid leukemia cell lines U937 and THP-1. DD exhibited cytotoxic effects and induced apoptosis in U937 and THP-1 cells. Moreover, DD treatment significantly reduced mitochondrial membrane potential (ΔΨ). The protein expression of cleaved poly(ADP-ribose) polymerase, cleaved caspase-3, p-H2A.X, CCAAT/enhancer-binding protein (CHOP), and activating transcription factor 4 was upregulated by DD treatment. Consistently, DD-treated cells had increased reactive oxygen species (ROS) level in a concentration-dependent manner via miR-216b activation in association with c-Jun inhibition. N-acetyl-L-cysteine pretreatment reversed the cytotoxic effect of DD treatment as well as prevented ROS accumulation. Collectively, the results of this study suggest that the anticancer effect of DD in AML was mediated by CHOP-dependent apoptosis along with ROS accumulation and included upregulation of miR-216b followed by a decrease in c-Jun.
Introduction
Acute myeloid leukemia (AML) is a heterogeneous malignant disease caused by uncontrolled proliferation of immature myeloid blast cells. The expansion of myeloid precursor cells in the bone marrow (BM) is a distinct pathological characteristic of AML, which disrupts hematopoiesis in the BM (1). More than half of patients with AML have chromosomal abnormalities, while the remaining 40%–50% had cytogenetically normal AML (CN-AML). Various genetic mutations or changes in gene phenotypes are detected in patients with CN-AML, which are important in determining prognosis and treatment (2). To date, 13 types of mutant genes have been discovered, including Nucleophosmin 1 (NPM1), DNA methyltransferase 3A (DNMT3A), FMS-like tyrosine kinase 3 (FLT3), Isocitrate dehydrogenase (IDH), and Ten–eleven-translocation 2 (TET2) (3). These chromosomal and gene mutations were used as an index for the four stages of risk stratification in the 2010 European Leukemia Net classification scheme (4).
The pathology and prognosis of AML are closely related to endoplasmic reticulum (ER) stress and the amount of reactive oxygen species (ROS). Doron et al. have reported that AML cells utilize ER stress to change the stromal composition in the BM. Therefore, the reduction of ER stress, change in ROS, and correlation between the two are important indicators for evaluating AML activity (5). Moreover, ROS was related to the lifespan of hematopoietic stem cells and alterations in leukemic oncogenes (6). The ER maintains cellular functions, including the synthesis and proper folding of proteins (7, 8). However, in undesirable conditions, such as hypoxia, ischemia, and turbulence in intracellular pH, ER stress occurs (9). Subsequently, unfolded protein response is induced by ER stress, which results in various symptoms, including neurodegenerative diseases and cancer (10). Thus, ER stress is one of the key mechanisms in the process of ROS-mediated apoptosis (11).
MicroRNAs (miRNA) play an essential role in maintaining homeostasis of cellular growth, differentiation, migration, and apoptosis, which are regulated by the development and differentiation of hematopoietic cells (12). In particular, alterations in miRNA genes have close relationships with the development of tumor and hematological diseases (13, 14). Impaired miRNA expression in AML that can stem from many causes, such as chromosome translocations, inversion, gene deletions, and mutations, is directly or indirectly controlled by post-transcriptional modification due to limitless clonal expansion of myeloid blast cells (15). Amanda et al. have reported that 33 types of miRNA were upregulated or downregulated in AML, suggesting the use of miRNAs in subclassifying the types of leukemia (16). Moreover, miR-15 and miR-16 were deleted or downregulated in chronic lymphocytic leukemia (17), whereas overexpression of the miR-181 family is associated with the high risk of cytogenetically normal AML along with CCAAT/enhancer-binding protein-alpha mutations (18). Modulation of miRNA genes is related to the mutation of different oncogenes, as miR-155 was regulated in patients with AML with FLT3-internal tandem duplication mutations, and miR-10a and miR-10b were capable predictors of AML with mutations (19, 20). Notably, miR-216b is downregulated in various types of cancer, including cervical cancer, non-small cell lung cancer (NSCLC), and colorectal cancer (21–23). The expression of miR-216b showed a higher frequency of U2AF1 and IDH1/2 mutations in patients with AML and was a valuable predictor of AML recurrence (24).
The proto-oncoprotein c-Jun is an initial transcription factor that regulates the expression of cellular mechanisms and carcinogen combination, which belongs to the Activation protein-1 (AP-1) family (25). The overexpression of c-Jun is superior to the mechanism caused by ER stress-related apoptosis, suppressing the death caused by the activation of cleaved caspase-3 and cleaved poly(ADP-ribose) polymerase (PARP) (26). Several studies have identified c-Jun as a target protein of miR-216b, which was effective in alleviating cancer-related symptoms. Xu et al. confirmed that miR-216b directly targeted c-Jun, consequently inhibiting AP-1-dependent transcription, and was susceptible to ER stress-related apoptosis (26). Overexpression of miR-216b improved cisplatin-induced apoptosis in NSCLC, which was mediated by inhibiting the expression of c-Jun (27). Hence, changes in c-Jun activity through the regulation of miR-216b will be a standard for observing changes in AML cell activity.
Daemonorops draco Blume (DD), a traditional medicine derived from a natural resin, is widely used for its analgesic effects in wound healing, ulcers, and diarrhea and has also hemostatic, anti-inflammatory effects and reduces genesis of osteoclasts (28, 29). DD is also referred to as dragon’s blood; however, this name collectively refers to plant extracts of various origins according to region (6). Although other types of dragon’s blood have shown antitumor effects, such as inhibiting liver cancer (30, 31), the effects of DD in treating cancer have yet to be explored. Flavone compounds derived from DD form a phenolic group, which has antioxidant and anti-inflammatory activities and properties that alleviate cancer toxicity (32, 33). Therefore, to determine various bioactive components derived from DD, liquid chromatography (LC)/mass spectrometry (MS)/ultraviolet detection (UV) was performed. In this study, the anticancer effect of DD was investigated; moreover, this study evaluated the relationship between DD and ER stress and ROS and attempted to verify the detailed mechanisms at a molecular level.
Materials and Methods
Materials
DD was cultivated in Kang Won province in Korea and was bought at Yak Won Herbal Pharmacy. DD was stored at the herbarium of the Department of Pathology, College of Korean Medicine, and Kyung Hee University. DD (200 g) was extracted using 99% ethyl alcohol (Duksan, Gyeonggi-do, South Korea) according to the procedure described in previous studies (34). Briefly, the solution was concentrated to 100-ml aqueous solution using an evaporator and kept at −80°C for 24 h. Then, DD was dissolved in dimethyl sulfoxide (Duksan, Gyeonggi-do, South Korea). DD stock was prepared to a concentration of 200 mg/ml and then stored at −20°C.
LC/MS/UV-Based Analysis for DD Extract
The extract of DD was prepared by dissolving the samples in methanol. The solutions were filtered through a 0.45-mm hydrophobic polytetrafluoroethylene filter and analyzed by LC/MS using an Agilent 1200 Series HPLC system (Agilent Technologies, Santa Clara, CA) equipped with a photodiode array detector combined with a 6130 Series electrospray ionization (ESI) mass spectrometer. The ESI conditions were set as follows: capillary voltage, 2.0 kV; convoltage, 50 V; source temperature, 120°C, desolvation temperature, 350°C; and desolvation gas flow rate, 800 L/h. High-purity nitrogen was used as the nebulizer and auxiliary gas. The collision energy for detecting the precursor ions was set to 3 eV. Analysis was performed by injecting 5 μl of the sample using Aglient Eclipse Plus C18 column (3.5 μm, 4.6 mm × 100 mm) set at 35°C. The mobile phase consisting of formic acid in H2O (0.1% [v/v]) (A) and methanol (B) was delivered at a flow rate of 0.3 ml/min by applying the following programmed gradient elution: 0%–100% (B) for 30 min, 100% (B) for 1 min, 100% (B) isocratic for 10 min, and then 0% (B) isocratic for 10 min, to perform post-run reconditioning of the column.
Cell Culture
The AML cell lines THP-1 and U937 were purchased from Korean Cell Line Bank (Seoul, South Korea). THP-1 and U937 were cultured in RPMI 1640 medium containing 10% fetal bovine serum, 10,000-U/ml penicillin/streptomycin, and 2-μM L-glutamine (Gibco, Grand Island, NY, USA). All cells were cultured in an incubator at 37°C in a humidified incubator containing 5% CO2.
Cytotoxicity Assay
A cytotoxicity assay was performed to examine THP-1 and U937 cells using EZ-Cytox Cell Viability Assay Kit (Daeil Lab Service, Seoul, South Korea) according to the manual. Cells were seeded and exposed to various concentrations of DD (i.e., 12.5, 25, 50, 100, and 200 μg/ml) for 24 h onto a 96-well plate. The cells were incubated with an EZ-Cytox solution until formazan was formed for 2 h. The absorbance values were measured at 450 nm using a microplate reader (Bio-Rad, Hercules, CA, USA).
Mitochondrial Membrane Potential Assay
JC1-MMP Assay Kit (ab113850, Abcam) was used. JC-1 Dye (Mitochondria Function Assay Kit, Thermo–Fisher Scientific, USA) for MMP can be detected using aggregated (excitation/emission = 535/595) and J-monomers (excitation/emission = 475/535). The signal ratio can be used to differentiate healthy mitochondria from depolarized ones in association with changes in mitochondrial calcium, superoxide, mitochondrial permeability transition, and membrane potential. THP-1 and U937 cells were seeded in a 96-well plate and pretreated with a density of 1 × 106 cells per well. After staining with 20-μM JC-1 Dye for 10 min at room temperature (RT) in the dark, the cells were treated with DD (15 and 30 μg/ml) for 4 h. Then, the 96-well plates were measured using an enzyme-linked immunosorbent assay (ELISA) reader (Bio-Rad, Hercules, CA, USA).
Western Blotting
Cells were lysed with a lysis buffer (pH = 7.4, 150-mM NaCl, 1% NP-40, 50-mM Tris-HCl, 0.25% sodium deoxycholic acid, 1-M ethylenediaminetetraacetic acid, 1-mM Na3VO4, and 1-mM NaF) containing a protease inhibitor cocktail (Amresco, Scolon, OH, USA). In the lysate sample, the protein concentration was quantified using Bio-Rad DC Protein Assay Kit II (Bio-Rad, Hercules, CA, USA) according to the manufacturer’s instructions. The proteins were separated using sodium dodecyl sulfate–polyacrylamide gel electrophoresis (8%–12%) by electrophoresis and transferred to polyvinylidene fluoride membranes (Millipore, USA). Then, 5% skim milk in Tris-buffered saline plus 0.1% Tween 20 (TBST) was used to block nonspecific protein binding sites. The following specific primary antibodies were used—c-PARP (1:1,000) (#9542) (Cell Signaling, Beverly, MA, USA), c-cas3 (1:1,000) (#9661), CCAAT/enhancer-binding protein (CHOP) (1:1,000) (#2895), p-H2A.X (1:1,000) (#2577), β-actin (1:1,000) (# 4967), p-ATF4 (1:1,000) (#PA5-105835) (Thermo–Fisher Scientific, Waltham, MA, USA), and p-c-Jun (1:1,000) (#822) (Santa Cruz Biotechnologies, Santa Cruz, CA, USA)—for 24 h at 4°C. After washing with TBST for 30 min, the membranes were incubated with rabbit horseradish peroxidase-conjugated immunoglobulin G (IgG) secondary anti-mouse or rabbit antibody (5% skim milk) (1:10,000, Santa Crus, Dallas, TX, USA) for 1 h at RT. Protein expression levels were identified using an enhanced chemiluminescence system (Amersham Pharmacia, Piscataway, NJ, USA).
Live and Dead Cell Assays
THP-1 (2 × 105 cells/ml) or U937 (2 × 105 cells/ml) cells were seeded into a 4-chamber slide (Nunc™ Lab-Tek™ II Chamber Slide™ System, Thermo–Fisher Scientific, USA) at 1 ml/well. After seeding, the culture medium was treated with 30-μg/ml DD for 24 h at 1 ml/well. The cells were washed with Dulbecco’s phosphate-buffered saline, then loaded with calcein-AM (LIVE/DEAD® Viability/Cytotoxicity Kit, Thermo–Fisher Scientific, USA) and ethidium homodimer-1 (LIVE/DEAD® Viability/Cytotoxicity Kit, Thermo–Fisher Scientific, USA) for 30 min, and added to each slide, according to the manufacturer’s protocol. Images were obtained using confocal microscopy FV10i (OLYMPUS Fluoview USA) (green: live cells; red: dead cells; scale bar = 100 μm).
Measurement of ROS
The Reactive Oxygen Species Detection Assay (Abcam, Cambridge, United Kingdom) using reagent 2’,7’-dichlorofluorescin diacetate (DCFDA) was used to identify hydroxyl, peroxyl, and other ROS of cellular cytosolic hydrogen peroxide (H2O2). THP-1 and U937 cells were seeded onto 96-well plates and pretreated with N-Acetyl-L-cysteine (NAC) (Sigma Aldrich Co., St. Louis, MO, USA) for 1 h, and the control group was not pretreated with NAC. Then, the cells were stained with 20-µM DCFDA for 30 min at RT in the dark. Consequently, both THP-1 and U937 cells were treated with 30-μg/ml DD for 4 h. Then, the 96-well plates were measured using an ELISA reader (Bio-Rad, Hercules, CA, USA) (Ex/Em = 450/570 nm)
Quantitative Real-Time Polymerase Chain Reaction
Total RNA was isolated using the RNeasy Mini Kit (EZ™ Total RNA Mini Prep Kit, Enzynomics, South Korea) according to the manufacturer’s protocol and reverse transcribed using the HB_I RT Reaction Kit. cDNAs were amplified by qRT-PCR using the synthesized specific HB_I Nucleic Mix II primers and RNU6B HB primers (HeimBiotek, South Korea). PCR was performed using the LightCycler instrument (Roche Applied Sciences, Indianapolis, IN, USA). PCR was started at 95°C for 15 min, followed by 40 cycles at 95°C for 10 s and 60°C for 40 s, and finished with 95°C for 60 s, 55°C for 30 s and 95°C for 30 s. The expression of RNU6B was used to normalize the expression of target genes. The specific primer Has-miR-216b was designed and synthesized by HeimBiotek Company (HeimBiotek, South Korea). Relative miRNA fold change was normalized using standard Ct values of RNU6B (U6) (HeimBiotek, South Korea). RT-PCR was performed using the LightCycler instrument (Roche Applied Science, Indianapolis, IN, USA).
Transfection miR-216b Inhibitor Study
THP-1 and U937 cells were transfected with a miR-216b inhibitor (HeimBiotek, South Korea) and ViaFect™ Transfection Reagent (Promega, Madison USA) and seeded onto 6-well plates with prewarmed serum-free medium. In this process, 10–50-nM miR-216b inhibitor and 3-μl ViaFect™ Transfection Reagent were added into 100-μl prewarmed serum-free medium at RT and mixed immediately. The cells were incubated with ViaFect™ Transfection Reagent: miR-216b inhibitor mixtures for 5 min transfected using ViaFect™ Transfection Reagent according to the manufacturer’s protocol. After the transfection of miR-216b inhibitor for 48 h, THP-1 and U937 cells were treated with 30-μg/ml DD for 24 h. MiR-216b inhibitor oligobase type with follow: 2’ O-Methyl RNA base was applied by HeimBiotek, South Korea.
Statistical Analysis
Data were presented as means ± standard deviation. Statistically significant differences between the control and MLT-treated groups were calculated using Student’s t-test using SigmaPlot 12 (SysTest Software Inc., San Jose, CA, USA). All experiments were performed in triplicate. Differences with p-values of less than 0.05 were considered statistically significant.
Results
Identification of Various Flavonoids in DD by HPLC-MS
LC/MS/UV-based analysis of the extract of DD revealed a major peak with molecular ions of m/z 257.1 [M+H]+ and m/z 255.1 [M-H]- at a retention time of 30.5 min, which also showed a unique UV spectrum (λmax 218, 236, 304, and 321 nm) (Figure 1). Based on the characteristic UV data and the molecular ions detected by LC/MS, as well as the chemical database of DD previously reported in available studies (29, 34–36), the major metabolite was determined to be (2S)-7-hydroxy-5-methoxyflavan (Figure 1).
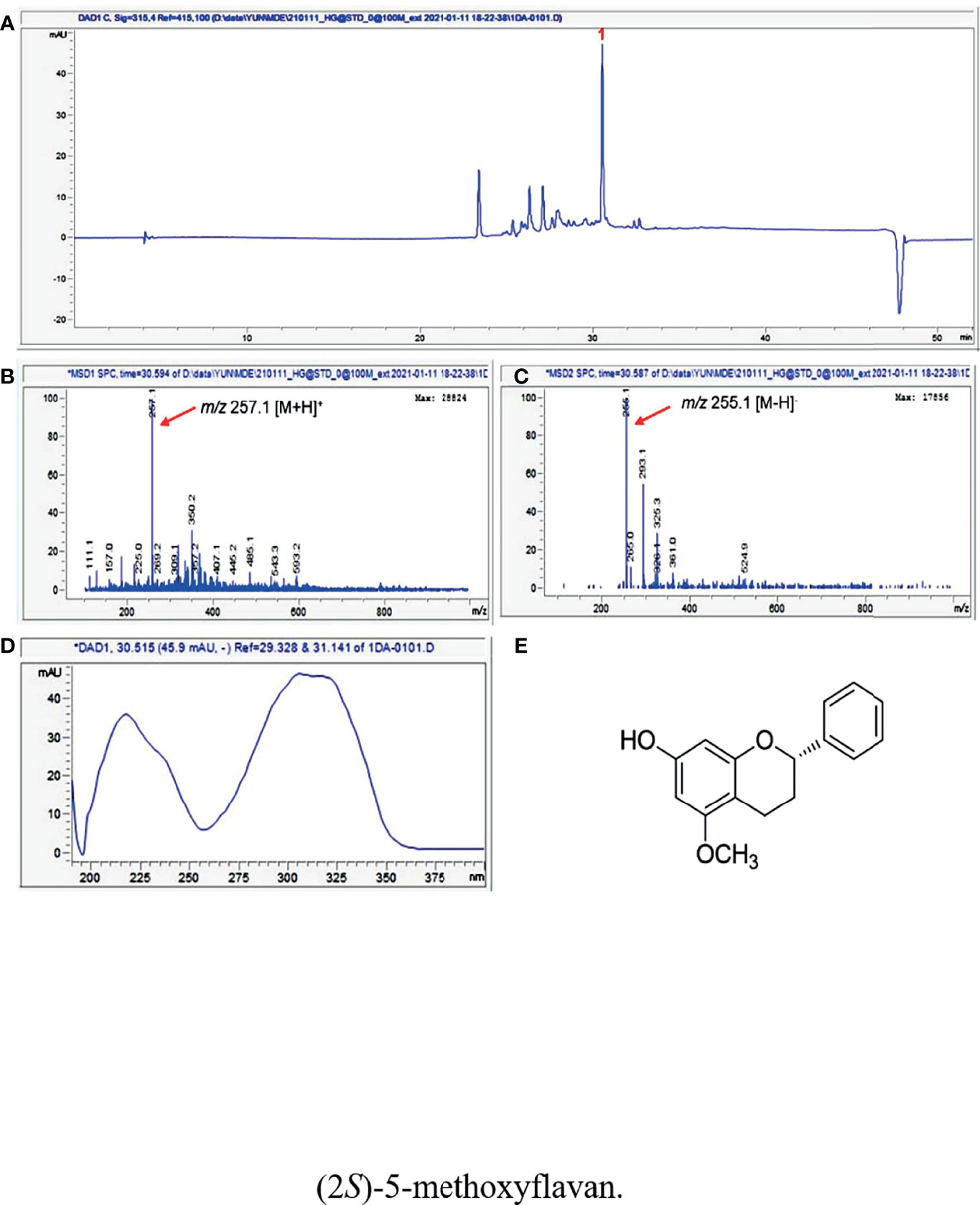
Figure 1 (A) UV chromatogram of LC/MS (detection wavelength was set at 315 nm) of the extract of DD. (B) Positive and (C) negative ion-mode ESI-MS data of the peak at retention time 30.5 min and (D) UV data of the peak. (E) The chemical structure of (2S)-7-hydroxy-5-methoxyflavan (1).
DD Had a Cytotoxic Effect on AML Cells
To investigate the cytotoxic effect of DD, EZ-Cytox was performed in AML cells, including U937 and THP-1 ells. In Figures 2A, B, the concentrations of 25 and 50 μg/ml showed a survival rate of approximately 55%–80%. Cell viability assay showed that the survival rate decreased in a concentration-dependent manner. This result was statistically significant.
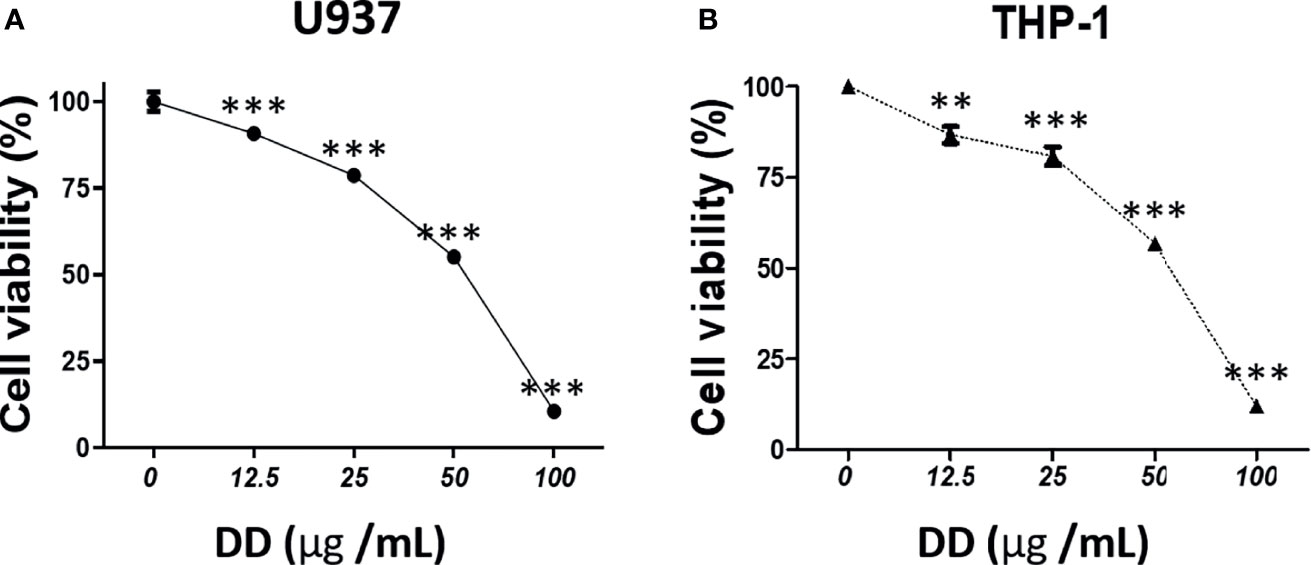
Figure 2 Daemonorops draco Blume (DD) exerted a cytotoxic effect on acute myeloid leukemia cells. The cytotoxicity of DD in (A) U937 and (B) THP-1 cells. The cells were treated with DD (i.e., 12.5, 25, 50, 100, or 200 μg/ml) for 24 h. Cell viability assay was performed using EZ-Cytox. Values above represent the means of three experiments. Means ± standard deviation; **p < 0.01 and ***p < 0.001 compared with the untreated groups.
DD Reduced MMP and Induced Apoptosis in AML Cells
To establish the mechanism of apoptosis controlled by DD, JC-1 staining and Western blotting were conducted. As shown in Figures 3A, B, DD reduced MMP (ΔΨ) in a concentration-dependent manner in AML cells. Furthermore, caspase-3 is a critical executioner of mitochondria-mediated apoptosis (37). Western blotting showed that DD significantly induced the activation of caspase-3 along with the expression of cleaved PARP via the inhibitory regulation of c-Jun. Furthermore, CHOP is a major mediator of ER stress-related pathways closely related to caspase-3 activation (38, 39). CHOP alone does not exert sufficient effect to cause cell destruction but enhances the effect of activating transcription factor 4 (ATF4) to decrease cell viability through ER stress. Furthermore, ATF4 and CHOP were found to act on the same target gene to increase protein synthesis related to stress-induced transcription, inducing apoptosis (40). Nevertheless, the underlying anticancer mechanism of DD related to ER stress-related apoptotic proteins and caspase-mediated apoptosis has not been identified so far. Furthermore, DD significantly reduced MMP (ΔΨ), cleaved caspase 3, and cleaved PARP; increased CHOP and p-ATF4; and attenuated p-c-Jun in a concentration-dependent manner in AML cells (Figures 3C–F). These results demonstrated that DD is involved in apoptosis via the mitochondria-mediated caspase and ER stress-related apoptosis activation pathways.
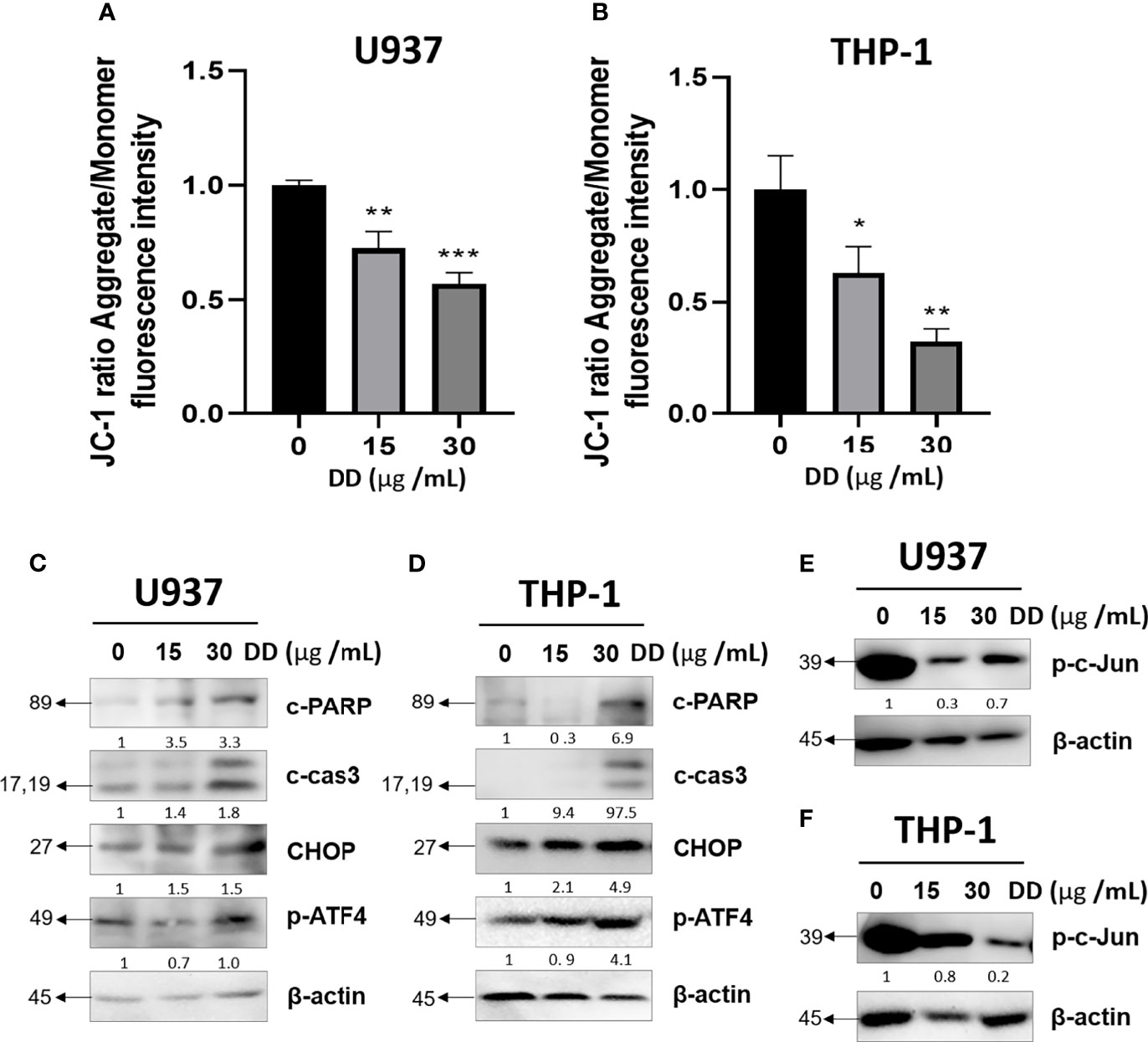
Figure 3 Daemonorops draco Blume (DD) reduced mitochondrial membrane potential and induced apoptosis in U937 and THP-1 cells treated with DD. (A, B) Cells were pretreated at JC-1 (20 μM) and DD (15 and 30 μg/ml) for 4 h. Green monomeric fluorescence form changed to red fluorescent aggregates in a concentration-dependent manner, which was measured using a microplate reader. (C–F) After treatment with DD (15 and 30 μg/ml) for 24 h, the cells were subjected to Western blotting due to the expression of apoptosis-related proteins, such as cleaved caspase-3, cleaved poly(ADP-ribose)polymerase, CCAAT/enhancer-binding protein, p-ATF4, p-c-Jun, and β-actin, in (C, E) U937 and (D, F) THP-1 cells. Fluorescein isothiocyanate (excitation/emission = 540/570) and rhodamine (excitation/emission = 540/570). Values above represent the means of three experiments. Means ± standard deviation; *p < 0.05, **p < 0.01, and ***p < 0.001 compared with the untreated groups.
DD Increased DNA Damage in AML Cells
To evaluate the cytotoxic effects of DD, Western blotting and live/dead staining were adopted in U937 and THP-1 cells. DD significantly increased p-H2A.X by Western blotting in a concentration-dependent manner compared with the untreated groups (Figures 4A, B). Similarly, DD-treated cells emitted significantly more red fluorescence due to dead cells compared with the control group (Figures 4C, D). Consistently, DD effectively induced apoptosis by causing DNA damage.
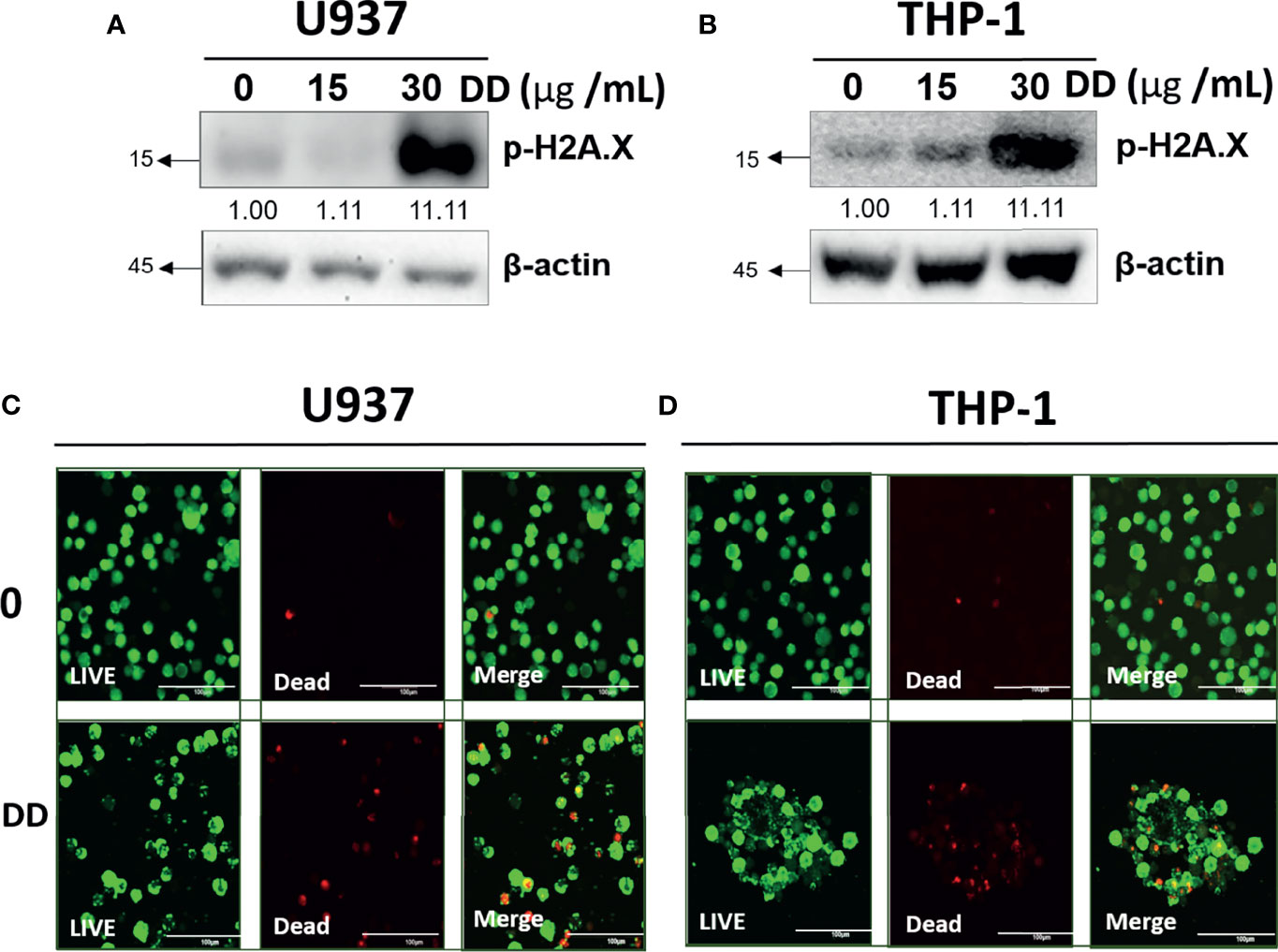
Figure 4 Daemonorops draco Blume (DD) increased DNA damage in U937 and THP-1 cells. (A, B) The effect of DD on H2A.X, which was treated with various concentrations of DD (i.e., 15 and 30 μg/ml for 24 h), in U937 and THP-1 cells, which were subjected to Western blotting with the antibodies of p-H2A.X and β-actin. (C, D) Confocal images of AML cells represented live cells (left panel), dead cells (middle panel), and the combination of both (right panel). AML cells were treated with DD (30 μg/ml) for 24 h, and double dyes were incubated at 37°C for 30 min. AML cells were stained with calcium AM (excitation/emission = 494/517) and ethidium homodimer-1 (excitation/emission = 528/617). Scale bar = 100 μm.
DD Increased ROS and NAC Reversed DD-Induced Cytotoxicity in AML Cells
Furthermore, chemotherapy increases the consumption of glutathione and sulfhydryl in cells, followed by an increase in ROS, which eventually leads to DNA damage (41). To determine whether DD induces ROS accumulation, a DCFDA staining assay kit was used. As shown in Figures 5A, B, the amount of intracellular ROS was significantly increased compared with that in the untreated control group in AML cells. To determine the role of ROS in DD-induced apoptosis, ROS was measured in AML cells with and without NAC pretreatment. NAC is an antioxidant and a safe and inexpensive drug that induces glutathione production and inhibits the depletion of MMP (ΔΨ) as an ROS scavenger (42). As shown in Figures 5C, D, ROS accumulation was effectively attenuated by NAC pretreatment compared with that in the untreated group in U937 and THP-1 cells. Consistently, the reduced cell viability caused by DD was significantly recovered by NAC pretreatment in both cells (Figures 5E, F). These findings showed that the DD-induced apoptosis in AML cells may depend on the regulation of ROS accumulation.
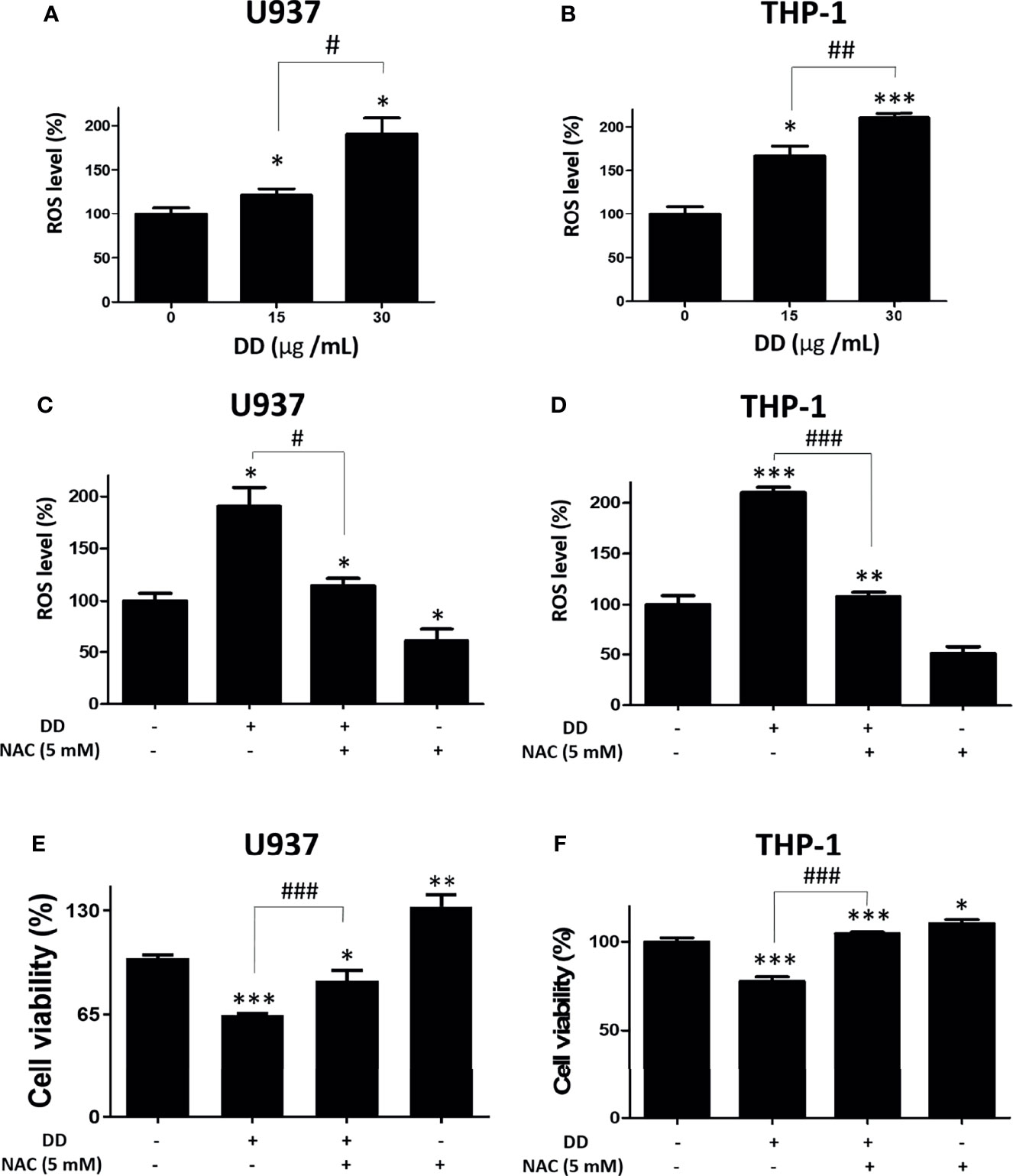
Figure 5 Daemonorops draco Blume (DD) increased reactive oxygen species (ROS) accumulation and N-Acetyl-L-cysteine (NAC) pretreatment reversed the cytotoxic effect of DD in U937 and THP-1 cells. (A, B) Both cells were incubated with 20-μM 2’,7’-dichlorofluorescin diacetate (DCFDA) for 30 min at 37°C in the dark and subjected to ROS assay. The cells were exposed to 30-μg/ml DD for 4 h. DCFDA fluorescence was determined using a dual microplate reader. (C, D) Both cells were exposed to NAC (5 mM) pretreatment for 60 min and subjected to ROS measurement. (E, F) A cell viability assay was conducted with absorbance measurement using an optical spectrometer. (Ex/Em = 450/570). Values represent the means of three experiments. Means ± standard deviation; *p < 0.05, **p < 0.01, and ***p < 0.001 compared to untreated control group. #p < 0.05 and ###p < 0.001 between the two groups.
DD Regulated the Expression Level of miR-216b Along and Inhibited p-c-Jun in AML Cells
Several studies have identified c-Jun as a target protein of miR-216b, which was effective in alleviating cancer-related symptoms (26). Since DD significantly reduced c-Jun, its upstream miRNA, miR-216b, was measured. To measure the expression of miR-216b, qRT-PCR was performed in U936 and THP-1 cell lines. The treatment of DD significantly upregulated the expression of miR216b in a concentration-dependent manner (Figures 6A, B). To measure the role of miR-216b in DD-induced apoptosis, qRT-PCR and cell viability assay were performed. MiR-216b inhibitor transfection reversed the increased miR-216b by DD treatment (Figures 6C, D). Consistently, reduced cell viability by DD treatment was increased by miR-216 inhibitor transfection (Figures 6E, F). These results indicate that miR-216b is involved in the anticancer effects of DD. Additionally, to examine the involvement of miR-216b in the anticancer effect of DD and apoptosis, we performed qRT-PCR of miR-216b, together with Western blotting of p-c-Jun in AML cells, and we observed that miR-216b level was highly increased in THP-1 and moderately in U937 cells (Figures 6A–D). Transfection of miR-216b inhibitor in the presence of DD significantly upregulated p-c-Jun level and reduced CHOP, an ER stress-related apoptosis marker, compared to the DD-only treated cells (Figures 6G, H). Collectively, these results document that miR-216b-mediated c-Jun and CHOP are closely related to DD-induced apoptosis of AML cells.
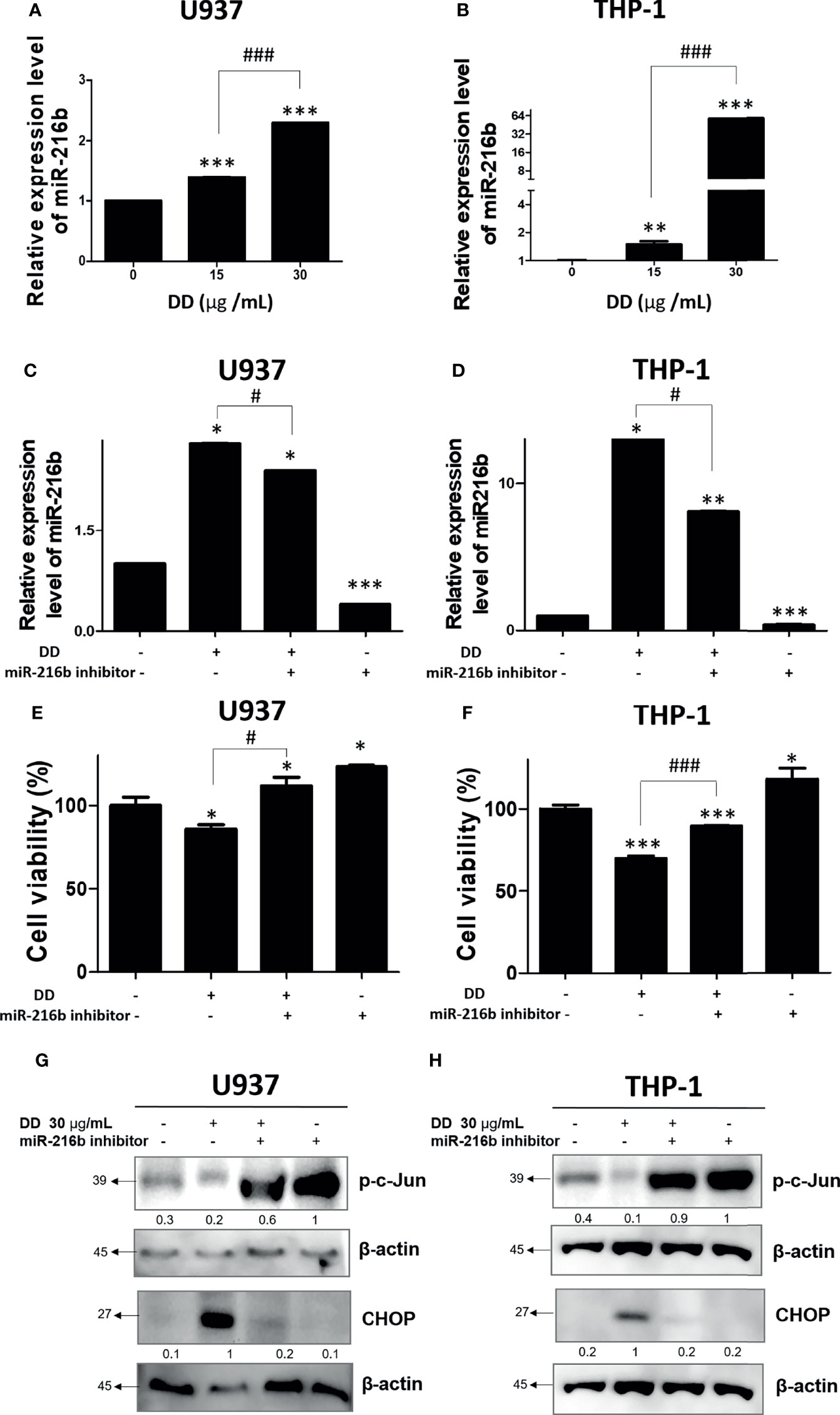
Figure 6 Anticancer effect of Daemonorops draco Blume (DD) and reactivation of c-Jun and CCAAT/enhancer-binding protein-mediated apoptosis in acute myeloid leukemia cells. DD elevated the expression of miR-216b in (A) U937 and (B) THP-1 cells. AML cells were transfected with miR-216b inhibitor. (C–H) A total of 1 × 105 cells/ml were seeded into 6-well plates and allowed to reach approximately 50% density of transfection. The cells were transfected with miR-216b inhibitor for 48 h and exposed to the indicated doses of DD (i.e., 15 and 30 μg/ml) for 24 h. Following transfection for 48 h, miRNA was isolated and adopted to quantitative analysis of miRNA expression level or cell viability (E, F), and Western blotting (G, H) was performed. Values represent the means of three experiments. Means ± standard deviation; *p < 0.05, **p < 0.01, and ***p < 0.001 compared with untreated control group. #p < 0.05, and ###p < 0.001 between the two groups.
Discussion
AML is one of the most aggressive types of cancer with a low treatment success rate (43, 44). The survival rate of AML is estimated to be less than 10% when a relapse occurs (43, 45). Symptoms and prognosis of AML are associated with numerous gene mutations, which leads to the difficulty of making clear diagnosis and treatment decision. The mutation in hematopoietic stem cell that has the multipotent ability of self-renewal could be related to clonal expansion, making it the distinct characteristic responsible for the variability of AML (46). In a recent cohort study, 86% of patients showed two or more gene mutations, and co-mutated gene increased the mortality of AML (47). Induction therapy is the main treatment for patients with AML, using chemotactic agents, such as anthracyclines and cytarabine (48). Consolidation therapy is used in AML relapse or minimal residual leukemia, in which chemotherapy and hematopoietic stem cell transplantation are used alone or in combination. Recently, new treatment strategies, such as FLT3 inhibitors, IDH inhibitors, nuclear exporter inhibitors, and immune therapies, are introduced to regulate genetic expression and immunological responses in AML (48). However, complete remission of chemotherapy in older patients is relatively low, while specific treatment methods for relapsed/refractory AML have yet to be identified (49). Furthermore, short-term and long-term side effects of chemotherapy were identified, accompanied by significant impacts on quality of life in patients with AML (50). Diverse variations in AML and the limitations of existing anticancer drugs suggest the need for research on alternative treatments for AML.
Meanwhile, the efficacy of DD in AML has not been explored, and the underlying mechanisms of DD were identified in U937 and THP-1 cells, including apoptosis, ROS, and miRNA regulation. U937 is a pro-monocytic, human myeloid leukemia cell line, which is commonly used to elucidate mechanisms of monocyte and macrophage differentiation. THP-1 is a human monocytic leukemia cell line, characterized by the expression of Fc and C3b receptors, with the lack of surface immunoglobulins (51). The classification of membrane receptors, such as IgG or C3b, is thought to be consistent with the AML model because they are detected in the blast cells of patients with AML. Blast cells are classified into myeloblasts, myelomonocytes, and monocytes (52).
Recently, clinical case studies have reported that the apoptosis pathway involving caspase 3 and cleaved PARP are major mediators that enhance chemotherapy effectiveness (53, 54). Moreover, cleaved caspase 3 triggers various pathways involved in apoptosis signaling (53). The mitochondrion is a sensor of apoptosis-promoted caspase activation in response to the apoptotic signaling pathway caused by DNA damage or various cellular stresses (55). Notably, the reduction in MMP (ΔΨ) is characterized by inevitable apoptosis resulting in the cleaved form of executioner caspase 3, causing the proteolysis of PARP (56). Here, the ER stress-related factor CHOP induces the activation of caspase 3 due to DNA damage caused by drug treatment, leading to apoptosis (56, 57). CHOP induced by numerous cellular stresses is a pro-apoptotic factor that promotes the activation of apoptotic genes and the hyper-oxidation of the ER lumen (58). Meanwhile, ATF4 plays a dual role in maintaining protein homeostasis while inducing apoptosis and cell cycle arrest. ATF4 is related to the reduction of stress in cancer cells due to lipid accumulation and malnutrition, as well as angiogenesis and metastasis, and conversely, when the situation changes, cancer cells are vulnerable to apoptosis through chemotherapy (59). Notably, ATF4 and CHOP prefer binding to similar motifs (GCATCAT/G) that share target gene sets (26). The forced expression of ATF4 and CHOP induced ATP depletion and oxidative stress protein synthesis that could result in cell death (52). Conversely, c-Jun N-terminal kinase (JNK), referred to as a serine/threonine (Ser/Thr) protein kinase, is included in the mitogen-activated protein kinase family. JNK mediates various cellular responses, such as proliferation, differentiation, survival, migration, invasion, and apoptosis, and stimulates inflammation, fibrosis, cancer progression, and metabolic diseases (60–62).
Thus, to determine the anticancer effect of DD on AML, in this study, the underlying apoptotic signaling of DD was studied in connection with the regulation of c-Jun or ER stress-mediated apoptosis signaling. Here, the viability of AML cells treated with DD was inhibited in a dose-dependent manner, indicating the anticancer effect of DD on AML cells (Figure 2). Consistently, DD altered MMP (ΔΨ) and increased the expression of cleaved PARP, cleaved caspase 3, p-ATF4, and CHOP due to the activation of the apoptotic pathway in a dose-dependent manner, implying the potent involvement of ER stress-related pathway and mitochondrial-mediated caspase activation signaling in the anticancer effect of DD (Figure 3).
Several studies have reported that excessive ROS can cause DNA damage, such as DNA double-strand break or DNA protein cross-linking break generation, illustrating their genotoxic nature (63–66). p-H2A.X is a marker of DNA damage due to DNA double-strand break (67, 68). The underlying anticancer effect of DD was associated with DNA damage followed by apoptosis induction at the living. Consistently, DD significantly increased p-H2A.X due to DNA damage, which was confirmed using DNA-binding polar fluorescent probe through confocal microscopy. The red fluorescent probe could not penetrate live cell membranes and selectively binds to the DNA of dead cells (69), indicating increased DNA damage due to DD treatment (Figure 4).
Furthermore, to confirm whether the DD-induced apoptosis signaling is regulated by ROS, NAC pretreatment was performed. Importantly, NAC pretreatment alone or NAC with DD treatment increased cell viability compared with untreated controls, indicating that ROS accumulation plays a critical role in DD-induced activation of the apoptotic pathway in AML cells (Figure 5). Accumulating evidence showed that ER stress-related and mitochondria-mediated pathways are closely involved in ROS accmulation, thereby exerting potential anticancer effects of DD. The Human Genome Project has provided the genetic blueprint of humans and helps to uncover the genetic causes of human diseases including cancer. As human genome sequences have begun to be solved using algorithms, cancer progression has been found to be caused by miRNA, which controls tumor suppressor genes and oncogenes. Therefore, numerous studies on miRNA related to human diseases and cancer have been conducted (70). Of note, the importance of natural products in regulating miRNA has only recently begun to focus on determining therapeutic targets for cancer (71). Consistently, several studies have reported that herbal extracts are extensively related to modulating miRNA in association with the inhibition of epithelial–mesenchymal transition, chemoresistance, and metastasis (72, 73). Interestingly, miRNA is easy to acquire from patient blood or tissue samples and is used as a diagnostic and prognostic marker as it provides crucial information concerning gene expression profiling (74), suggesting that cancer-specific characterization of AML contributes to the advantage of targeting of miRNA-based therapy. Therefore, to assess whether DD has a miRNA-based therapeutic effect on AML, the underlying anticancer effect of DD was investigated in association with the miR-216b-mediated pathway. Here, DD significantly induced the expression of miR-216b in both U937 and THP-1 cells, revealing that DD is a regulator of miR-216b. Notably, it has been recently identified that plants significantly inhibit miRNA, which is required for controlling processes by the introduction of a sponge RNA involved in fine-tuning targeting miRNA (75, 76). MiR-216b directly inhibited c-Jun in response to ER stress, which led to CHOP-dependent apoptosis (26). Additionally, c-Jun is involved in cell survival in various cancers by the dysregulation of the PI3K/AKT axis, including NSCLC (77) and gastric cancer (61).
Interestingly, the excessive depletion of miR-216b and the activation of c-Jun by miR-216b inhibitor were observed compared with those in groups treated with DD alone, indicating that DD induces apoptosis in sensitized AML cells via miR-216b-dependent signaling (Figure 6). Additionally, the biological effects of natural products have been studied for decades, and recent analysis methods related to HPLC-MS have enabled gathering scientific data on effective compounds (78). Furthermore, it is well documented that effective compounds of natural products, such as alkaloids, phenolics, and carotenoids, have apoptotic effects on AML (79). Salvia miltiorrhiza, another traditional herbal medicine, classified similarly with DD in terms of blood circulatory effects, induces apoptosis and necrosis in a ROS-independent and caspase-independent manner in acute lymphoblastic leukemia cells (80). Additionally, Spatholobi caulis, an effective Chinese medicine for relieving blood stasis, was proved to exert caspase-dependent apoptotic activity on U937, a human monocyte leukemia cell line (81). In previous studies, compared with the efficacy of natural products targeting a single mechanism for leukemia caused by complex mutations, DD significantly activated mitochondria-mediated caspase activation, ER stress-related regulation of ROS, c-Jun, and miR-216b, indicating the multiple anticancer mechanisms of DD (Figure 7). However, the prepared DD solution is highly concentrated and bioavailability and inability could be the issues to achieve the therapeutic dose in vivo. Further investigation is needed for future studies about the anticancer effect of DD. The antitumor properties of DD should be examined further in in-depth studies on the specific therapeutic application methods of DD in treating AML. One of the constituents of DD, dracorhodin has been reported to have an anticancer effect in melanoma (82), esophageal squamous cell carcinoma (83), lung cancer (84), breast cancer (85), etc. Other compounds from DD including abietic acid (86) and nordracorubin (87) showed anticancer activities. DD could be a potent candidate for in vivo and clinical studies. This study is limited in that the effects of DD on AML are confined to in vitro studies. In future studies, the practical effects of DD should be explored through in vivo experiments. The determination of the specific interrelationships by which ROS lowered the viability of AML cells and regulated ER stress should be further addressed. Furthermore, to confirm the efficacy of DD in patients with AML, further studies are required for the identification of proper dosage of DD through animal experiments; moreover, studies on the subtypes of various AML cell lines and miRNA genes should be conducted.
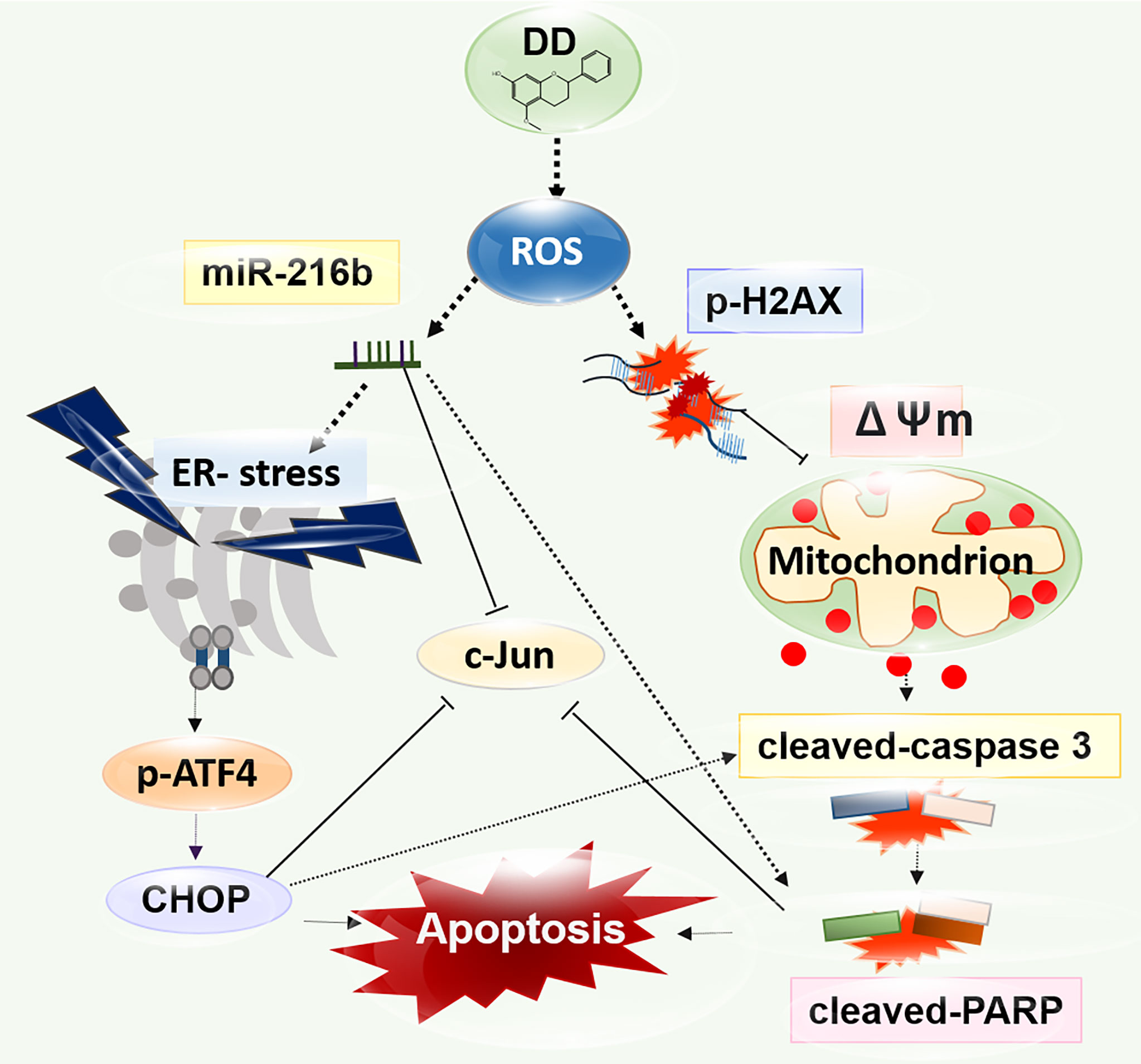
Figure 7 A diagram of the anticancer effect of Daemonorops draco Blume (DD) research strategy. DD significantly promoted mitochondria-mediated caspase activation and endoplasmic reticulum stress-related apoptosis pathway involved in reactive oxygen species generation, leading to apoptosis due to DNA damage in AML cells. DD treatment significantly showed anticancer effects on AML cells by significantly inhibiting c-Jun, leading to apoptosis via the regulation of miR-216b.
Conclusions
DD has a significant cytotoxic effect on AML cells. Notably, DD treatment efficiently induced ROS-mediated ER-associated degradation, including CHOP and p-ATF4 along with cleaved caspase 3 and cleaved PARP, and attenuated c-Jun, activating p-γH2A.X. Moreover, DD treatment regulated miR-216b-dependent ER stress-related apoptosis in AML cells. Overall, this study opens up the possibility of therapeutic application of DD against AML, involving upregulation of miR-216b.
Data Availability Statement
The original contributions presented in the study are included in the article/supplementary material. Further inquiries can be directed to the corresponding authors.
Author Contributions
Conceptualization and writing—original draft preparation: MP and HJ. Formal analysis: SJ, SP, and KK. Data curation: SJ and SP. Writing—review and editing: MR, S-HK, and BK. Visualization: MP, SJ, and KK. Supervision: S-HK, WK, and BK. Project administration: BK. Funding acquisition: BK. All authors contributed to the article and approved the submitted version.
Funding
This research was supported by Basic Science Research Program through the National Research Foundation of Korea (NRF) funded by the Ministry of Education (NRF-2020R1I1A2066868), the National Research Foundation of Korea (NRF) grant funded by the Korea government (MSIT) (No. 2020R1A5A2019413), a grant of the Korea Health Technology R&D Project through the Korea Health Industry Development Institute (KHIDI), funded by the Ministry of Health & Welfare, South Korea (grant number: HF20C0116), and a grant of the Korea Health Technology R&D Project through the Korea Health Industry Development Institute (KHIDI), funded by the Ministry of Health & Welfare, South Korea (grant number: HF20C0038).
Conflict of Interest
The authors declare that the research was conducted in the absence of any commercial or financial relationships that could be construed as a potential conflict of interest.
Publisher’s Note
All claims expressed in this article are solely those of the authors and do not necessarily represent those of their affiliated organizations, or those of the publisher, the editors and the reviewers. Any product that may be evaluated in this article, or claim that may be made by its manufacturer, is not guaranteed or endorsed by the publisher.
References
1. Döhner H, Weisdorf DJ, Bloomfield CD. Acute Myeloid Leukemia. N Engl J Med (2015) 373:1136–52. doi: 10.1056/NEJMra1406184
2. Saultz JN, Garzon R. Acute Myeloid Leukemia: A Concise Review. J Clin Med (2016) 5. doi: 10.3390/jcm5030033
3. Takahashi S. Current Findings for Recurring Mutations in Acute Myeloid Leukemia. J Hematol Oncol (2011) 4:36. doi: 10.1186/1756-8722-4-36
4. Ley TJ, Miller C, Ding L, Raphael BJ, Mungall AJ, Robertson A, et al. Genomic and Epigenomic Landscapes of Adult De Novo Acute Myeloid Leukemia. N Engl J Med (2013) 368:2059–74. doi: 10.1056/NEJMoa1301689
5. Doron B, Abdelhamed S, Butler JT, Hashmi SK, Horton TM, Kurre P. Transmissible ER Stress Reconfigures the AML Bone Marrow Compartment. Leukemia (2019) 33:918–30. doi: 10.1038/s41375-018-0254-2
6. Irwin ME, Rivera-Del Valle N, Chandra J. Redox Control of Leukemia: From Molecular Mechanisms to Therapeutic Opportunities. Antioxid Redox Signal (2013) 18:1349–83. doi: 10.1089/ars.2011.4258
7. Wu J, Kaufman RJ. From Acute ER Stress to Physiological Roles of the Unfolded Protein Response. Cell Death Differ (2006) 13:374–84. doi: 10.1038/sj.cdd.4401840
8. Kim C, Kim B. Anti-Cancer Natural Products and Their Bioactive Compounds Inducing ER Stress-Mediated Apoptosis: A Review. Nutrients (2018) 10. doi: 10.3390/nu10081021
9. Shore GC, Papa FR, Oakes SA. Signaling Cell Death From the Endoplasmic Reticulum Stress Response. Curr Opin Cell Biol (2011) 23:143–9. doi: 10.1016/j.ceb.2010.11.003
10. Yadav RK, Chae SW, Kim HR, Chae HJ. Endoplasmic Reticulum Stress and Cancer. J Cancer Prev (2014) 19:75–88. doi: 10.15430/JCP.2014.19.2.75
11. Malhotra JD, Kaufman RJ. Endoplasmic Reticulum Stress and Oxidative Stress: A Vicious Cycle or a Double-Edged Sword? Antioxid Redox Signal (2007) 9:2277–93. doi: 10.1089/ars.2007.1782
12. Arif KMT, Elliott EK, Haupt LM, Griffiths LR. Regulatory Mechanisms of Epigenetic miRNA Relationships in Human Cancer and Potential as Therapeutic Targets. Cancers (Basel) (2020) 12. doi: 10.3390/cancers12102922
13. Weiss CN, Ito K. A Macro View of MicroRNAs: The Discovery of MicroRNAs and Their Role in Hematopoiesis and Hematologic Disease. Int Rev Cell Mol Biol (2017) 334:99–175. doi: 10.1016/bs.ircmb.2017.03.007
14. Khan AQ, Ahmed EI, Elareer NR, Junejo K, Steinhoff M, Uddin S. Role of miRNA-Regulated Cancer Stem Cells in the Pathogenesis of Human Malignancies. Cells (2019) 8. doi: 10.3390/cells8080840
15. Liao Q, Wang B, Li X, Jiang G. miRNAs in Acute Myeloid Leukemia. Oncotarget (2017) 8:3666–82. doi: 10.18632/oncotarget.12343
16. Dixon-Mciver A, East P, Mein CA, Cazier JB, Molloy G, Chaplin T, et al. Distinctive Patterns of microRNA Expression Associated With Karyotype in Acute Myeloid Leukaemia. PloS One (2008) 3:e2141. doi: 10.1371/journal.pone.0002141
17. Calin GA, Dumitru CD, Shimizu M, Bichi R, Zupo S, Noch E, et al. Frequent Deletions and Down-Regulation of Micro- RNA Genes Mir15 and Mir16 at 13q14 in Chronic Lymphocytic Leukemia. Proc Natl Acad Sci USA (2002) 99:15524–9. doi: 10.1073/pnas.242606799
18. Marcucci G, Maharry K, Radmacher MD, Mrózek K, Vukosavljevic T, Paschka P, et al. Prognostic Significance of, and Gene and microRNA Expression Signatures Associated With, CEBPA Mutations in Cytogenetically Normal Acute Myeloid Leukemia With High-Risk Molecular Features: A Cancer and Leukemia Group B Study. J Clin Oncol (2008) 26:5078–87. doi: 10.1200/JCO.2008.17.5554
19. Garzon R, Volinia S, Liu CG, Fernandez-Cymering C, Palumbo T, Pichiorri F, et al. MicroRNA Signatures Associated With Cytogenetics and Prognosis in Acute Myeloid Leukemia. Blood (2008) 111:3183–9. doi: 10.1182/blood-2007-07-098749
20. Jongen-Lavrencic M, Sun SM, Dijkstra MK, Valk PJ, Löwenberg B. MicroRNA Expression Profiling in Relation to the Genetic Heterogeneity of Acute Myeloid Leukemia. Blood (2008) 111:5078–85. doi: 10.1182/blood-2008-01-133355
21. He S, Liao B, Deng Y, Su C, Tuo J, Liu J, et al. MiR-216b Inhibits Cell Proliferation by Targeting FOXM1 in Cervical Cancer Cells and is Associated With Better Prognosis. BMC Cancer (2017) 17:673. doi: 10.1186/s12885-017-3650-5
22. Yao Y, Li Q, Wang H. MiR-216b Suppresses Colorectal Cancer Proliferation, Migration, and Invasion by Targeting SRPK1. Onco Targets Ther (2018) 11:1671–81. doi: 10.2147/OTT.S161835
23. Wang L, Wang Y, Du X, Yao Y, Wang L, Jia Y. MiR-216b Suppresses Cell Proliferation, Migration, Invasion, and Epithelial-Mesenchymal Transition by Regulating FOXM1 Expression in Human non-Small Cell Lung Cancer. Onco Targets Ther (2019) 12:2999–3009. doi: 10.2147/OTT.S202523
24. Zhang TJ, Wu DH, Zhou JD, Li XX, Zhang W, Guo H, et al. Overexpression of miR-216b: Prognostic and Predictive Value in Acute Myeloid Leukemia. J Cell Physiol (2018) 233:3274–81. doi: 10.1002/jcp.26171
25. Vogt PK. Fortuitous Convergences: The Beginnings of JUN. Nat Rev Cancer (2002) 2:465–9. doi: 10.1038/nrc818
26. Xu Z, Bu Y, Chitnis N, Koumenis C, Fuchs SY, Diehl JA. miR-216b Regulation of C-Jun Mediates GADD153/CHOP-Dependent Apoptosis. Nat Commun (2016) 7:11422. doi: 10.1038/ncomms11422
27. Huang G, Pan J, Ye Z, Fang B, Cheng W, Cao Z. Overexpression of miR-216b Sensitizes NSCLC Cells to Cisplatin-Induced Apoptosis by Targeting C-Jun. Oncotarget (2017) 8:104206–15. doi: 10.18632/oncotarget.22171
28. Gupta D, Bleakley B, Gupta RK. Dragon's Blood: Botany, Chemistry and Therapeutic Uses. J Ethnopharmacol (2008) 115:361–80. doi: 10.1016/j.jep.2007.10.018
29. Wang X, Batubara I, Yamauchi K, Mitsunaga T. Identification and Structure-Activity Relationship (SAR) of Chemical Constituents From Daemonorops Draco (Willd.) Blume and Selected Commercial Flavonoids on Anti-Osteoclastogenesis Activity. Fitoterapia (2019) 138:104280. doi: 10.1016/j.fitote.2019.104280
30. Fan JY, Yi T, Sze-To CM, Zhu L, Peng WL, Zhang YZ, et al. A Systematic Review of the Botanical, Phytochemical and Pharmacological Profile of Dracaena Cochinchinensis, a Plant Source of the Ethnomedicine "Dragon's Blood". Molecules (2014) 19:10650–69. doi: 10.3390/molecules190710650
31. Chen X, Zhao Y, Yang A, Tian Y, Pang D, Sun J, et al. Chinese Dragon's Blood EtOAc Extract Inhibits Liver Cancer Growth Through Downregulation of Smad3. Front Pharmacol (2020) 11:669. doi: 10.3389/fphar.2020.00669
32. Lopes MI, Saffi J, Echeverrigaray S, Henriques JA, Salvador M. Mutagenic and Antioxidant Activities of Croton Lechleri Sap in Biological Systems. J Ethnopharmacol (2004) 95:437–45. doi: 10.1016/j.jep.2004.08.025
33. Natsume M. Polyphenols: Inflammation. Curr Pharm Des (2018) 24:191–202. doi: 10.2174/1381612823666171109104141
34. Shi J, Hu R, Lu Y, Sun C, Wu T. Single-Step Purification of Dracorhodin From Dragon's Blood Resin of Daemonorops Draco Using High-Speed Counter-Current Chromatography Combined With pH Modulation. J Sep Sci (2009) 32:4040–7. doi: 10.1002/jssc.200900392
35. Apaza Ticona L, Rumbero Sánchez Ã, Sánchez Sánchez-Corral J, Iglesias Moreno P, Ortega Domenech M. Anti-Inflammatory, Pro-Proliferative and Antimicrobial Potential of the Compounds Isolated From Daemonorops Draco (Willd.) Blume. J Ethnopharmacol (2021) 268:113668. doi: 10.1016/j.jep.2020.113668
36. Pham-Huy LA, He H, Pham-Huy C. Free Radicals, Antioxidants in Disease and Health. Int J BioMed Sci (2008) 4:89–96.
37. Puig B, Tortosa A, Ferrer I. Cleaved Caspase-3, Caspase-7 and Poly (ADP-Ribose) Polymerase are Complementarily But Differentially Expressed in Human Medulloblastomas. Neurosci Lett (2001) 306:85–8. doi: 10.1016/S0304-3940(01)01873-0
38. Oyadomari S, Mori M. Roles of CHOP/GADD153 in Endoplasmic Reticulum Stress. Cell Death Differ (2004) 11:381–9. doi: 10.1038/sj.cdd.4401373
39. Kim C, Song HS, Park H, Kim B. Activation of ER Stress-Dependent miR-216b Has a Critical Role in Salviamiltiorrhiza Ethanol-Extract-Induced Apoptosis in U266 and U937 Cells. Int J Mol Sci (2018) 19. doi: 10.3390/ijms19041240
40. Han J, Back SH, Hur J, Lin YH, Gildersleeve R, Shan J, et al. ER-Stress-Induced Transcriptional Regulation Increases Protein Synthesis Leading to Cell Death. Nat Cell Biol (2013) 15:481–90. doi: 10.1038/ncb2738
41. Zheng J, Zhuo L, Ran D, Ma Y, Luo T, Zhao H, et al. Cadmium Induces Apoptosis via Generating Reactive Oxygen Species to Activate Mitochondrial P53 Pathway in Primary Rat Osteoblasts. Toxicology (2020) 446:152611. doi: 10.1016/j.tox.2020.152611
42. Yamada M, Tsukimura N, Ikeda T, Sugita Y, Att W, Kojima N, et al. N-Acetyl Cysteine as an Osteogenesis-Enhancing Molecule for Bone Regeneration. Biomaterials (2013) 34:6147–56. doi: 10.1016/j.biomaterials.2013.04.064
43. Steffen B, Müller-Tidow C, Schwäble J, Berdel WE, Serve H. The Molecular Pathogenesis of Acute Myeloid Leukemia. Crit Rev Oncol Hematol (2005) 56:195–221. doi: 10.1016/j.critrevonc.2004.10.012
44. Liu J, Huang B, Xiao Y, Xiong HM, Li J, Feng DQ, et al. Aberrant Expression of Splicing Factors in Newly Diagnosed Acute Myeloid Leukemia. Onkologie (2012) 35:335–40. doi: 10.1159/000338941
45. Christ O, Feuring-Buske M, Hiddemann W, Buske C. Pathobiology of Acute Myeloid Leukemia. Med Klin (Munich) (2007) 102:290–5. doi: 10.1007/s00063-007-1035-2
46. Gibson CJ, Steensma DP. New Insights From Studies of Clonal Hematopoiesis. Clin Cancer Res (2018) 24:4633–42. doi: 10.1158/1078-0432.CCR-17-3044
47. Papaemmanuil E, Gerstung M, Bullinger L, Gaidzik VI, Paschka P, Roberts ND, et al. Genomic Classification and Prognosis in Acute Myeloid Leukemia. N Engl J Med (2016) 374:2209–21. doi: 10.1056/NEJMoa1516192
48. Dombret H, Gardin C. An Update of Current Treatments for Adult Acute Myeloid Leukemia. Blood (2016) 127:53–61. doi: 10.1182/blood-2015-08-604520
49. Bose P, Vachhani P, Cortes JE. Treatment of Relapsed/Refractory Acute Myeloid Leukemia. Curr Treat Options Oncol (2017) 18:17. doi: 10.1007/s11864-017-0456-2
50. Crossnohere NL, Richardson DR, Reinhart C, O'donoghue B, Love SM, Smith BD, et al. Side Effects From Acute Myeloid Leukemia Treatment: Results From a National Survey. Curr Med Res Opin (2019) 35:1965–70. doi: 10.1080/03007995.2019.1631149
51. Chanput W, Peters V, Wichers H. “THP-1 and U937 Cells”. In: Verhoeckx K, Cotter P, López-Expósito I, Kleiveland C, Lea T, Mackie A, et al, editors. The Impact of Food Bioactives on Health: In Vitro and Ex Vivo Models. Cham, CH: Springer. Copyright 2015, The Authors (2015). p. 147–59.
52. Scott CS, Bynoe AG, Linch DC, Allen C, Hough D, Roberts BE. Membrane Fc-IgG and C3b Receptors on Myeloid Leukaemia Cells: A Comparison With Cytoplasmic Acid Naphthyl Acetate Esterase Cytochemistry. J Clin Pathol (1983) 36:555–8. doi: 10.1136/jcp.36.5.555
53. Porter AG, Jänicke RU. Emerging Roles of Caspase-3 in Apoptosis. Cell Death Differ (1999) 6:99–104. doi: 10.1038/sj.cdd.4400476
54. Hsu HY, Lin TY, Hu CH, Shu DTF, Lu MK. Fucoidan Upregulates TLR4/CHOP-Mediated Caspase-3 and PARP Activation to Enhance Cisplatin-Induced Cytotoxicity in Human Lung Cancer Cells. Cancer Lett (2018) 432:112–20. doi: 10.1016/j.canlet.2018.05.006
55. Bock FJ, Tait SWG. Mitochondria as Multifaceted Regulators of Cell Death. Nat Rev Mol Cell Biol (2020) 21:85–100. doi: 10.1038/s41580-019-0173-8
56. Zhuang J, Dinsdale D, Cohen GM. Apoptosis, in Human Monocytic THP.1 Cells, Results in the Release of Cytochrome C From Mitochondria Prior to Their Ultracondensation, Formation of Outer Membrane Discontinuities and Reduction in Inner Membrane Potential. Cell Death Differ (1998) 5:953–62. doi: 10.1038/sj.cdd.4400440
57. Hayes MA, Tompkins SF, Herndon WA, Gruel CR, Kopta JA, Howard TC. Clinical and Radiological Evaluation of Lumbosacral Motion Below Fusion Levels in Idiopathic Scoliosis. Spine (Phila Pa 1976) (1988) 13:1161–7. doi: 10.1097/00007632-198810000-00019
58. Darling NJ, Cook SJ. The Role of MAPK Signalling Pathways in the Response to Endoplasmic Reticulum Stress. Biochim Biophys Acta (2014) 1843:2150–63. doi: 10.1016/j.bbamcr.2014.01.009
59. Wortel IMN, van der Meer LT, Kilberg MS, Van Leeuwen FN. Surviving Stress: Modulation of ATF4-Mediated Stress Responses in Normal and Malignant Cells. Trends Endocrinol Metab (2017) 28:794–806. doi: 10.1016/j.tem.2017.07.003
60. Wang J, Tai G. Role of C-Jun N-Terminal Kinase in Hepatocellular Carcinoma Development. Target Oncol (2016) 11:723–38. doi: 10.1007/s11523-016-0446-5
61. Zhang F, Li K, Pan M, Li W, Wu J, Li M, et al. miR-589 Promotes Gastric Cancer Aggressiveness by a LIFR-PI3K/AKT-C-Jun Regulatory Feedback Loop. J Exp Clin Cancer Res (2018) 37:152. doi: 10.1186/s13046-018-0821-4
62. Wu Q, Wu W, Fu B, Shi L, Wang X, Kuca K. JNK Signaling in Cancer Cell Survival. Med Res Rev (2019) 39:2082–104. doi: 10.1002/med.21574
63. Oh S-H, Lim S-C. A Rapid and Transient ROS Generation by Cadmium Triggers Apoptosis via Caspase-Dependent Pathway in HepG2 Cells and This Is Inhibited Through N-Acetylcysteine-Mediated Catalase Upregulation. Toxicol Appl Pharmacol (2006) 212:212–23. doi: 10.1016/j.taap.2005.07.018
64. Wang S-H, Shih Y-L, Kuo T-C, Ko W-C, Shih C-M. Cadmium Toxicity Toward Autophagy Through ROS-Activated GSK-3β in Mesangial Cells. Toxicol Sci (2009) 108:124–31. doi: 10.1093/toxsci/kfn266
65. Cuypers A, Plusquin M, Remans T, Jozefczak M, Keunen E, Gielen H, et al. Cadmium Stress: An Oxidative Challenge. BioMetals (2010) 23:927–40. doi: 10.1007/s10534-010-9329-x
66. Nemmiche S. Oxidative Signaling Response to Cadmium Exposure. Toxicol Sci (2016) 156:4–10. doi: 10.1093/toxsci/kfw222
67. Sharma A, Singh K, Almasan A. Histone H2AX Phosphorylation: A Marker for DNA Damage. Methods Mol Biol (2012) 920:613–26. doi: 10.1007/978-1-61779-998-3_40
68. Sharma A, Singh K, Almasan A. “Histone H2AX Phosphorylation: A Marker for DNA Damage”. In: Bjergbæk L, editor. DNA Repair Protocols. Totowa, NJ: Humana Press (2012). p. 613–26.
69. Jiménez-Hernández ME, Orellana G, Montero F, Portolés MT. A Ruthenium Probe for Cell Viability Measurement Using Flow Cytometry, Confocal Microscopy and Time-Resolved Luminescence. Photochem Photobiol (2000) 72:28–34. doi: 10.1562/0031-8655(2000)072<0028:ARPFCV>2.0.CO;2
70. Tutar Y. miRNA and Cancer; Computational and Experimental Approaches. Curr Pharm Biotechnol (2014) 15:429. doi: 10.2174/138920101505140828161335
71. Mohammadi A, Mansoori B, Baradaran B. Regulation of miRNAs by Herbal Medicine: An Emerging Field in Cancer Therapies. BioMed Pharmacother (2017) 86:262–70. doi: 10.1016/j.biopha.2016.12.023
72. Li Y, Kong D, Wang Z, Sarkar FH. Regulation of microRNAs by Natural Agents: An Emerging Field in Chemoprevention and Chemotherapy Research. Pharm Res (2010) 27:1027–41. doi: 10.1007/s11095-010-0105-y
73. Melkamu T, Zhang X, Tan J, Zeng Y, Kassie F. Alteration of microRNA Expression in Vinyl Carbamate-Induced Mouse Lung Tumors and Modulation by the Chemopreventive Agent Indole-3-Carbinol. Carcinogenesis (2010) 31:252–8. doi: 10.1093/carcin/bgp208
74. Pereira DM, Rodrigues PM, Borralho PM, Rodrigues CM. Delivering the Promise of miRNA Cancer Therapeutics. Drug Discov Today (2013) 18:282–9. doi: 10.1016/j.drudis.2012.10.002
75. Franco-Zorrilla JM, Valli A, Todesco M, Mateos I, Puga MI, Rubio-Somoza I, et al. Target Mimicry Provides a New Mechanism for Regulation of microRNA Activity. Nat Genet (2007) 39:1033–7. doi: 10.1038/ng2079
76. Ebert MS, Sharp PA. Emerging Roles for Natural microRNA Sponges. Curr Biol (2010) 20:R858–861. doi: 10.1016/j.cub.2010.08.052
77. Zhang E, Feng X, Liu F, Zhang P, Liang J, Tang X. Roles of PI3K/Akt and C-Jun Signaling Pathways in Human Papillomavirus Type 16 Oncoprotein-Induced HIF-1α, VEGF, and IL-8 Expression and In Vitro Angiogenesis in Non-Small Cell Lung Cancer Cells. PloS One (2014) 9:e103440. doi: 10.1371/journal.pone.0103440
78. Bezverkhniaia EA, Ermilova EV, Kadyrova TV, Krasnov EA, Brazovskii KS, Ponkratova AO, et al. Phytochemistry, Ethnopharmacology and Pharmacology of the Genus Empetrum: A Review. Adv Tradit Med (2021). doi: 10.1007/s13596-021-00612-4
79. Hwang D, Kim M, Park H, Jeong MI, Jung W, Kim B. Natural Products and Acute Myeloid Leukemia: A Review Highlighting Mechanisms of Action. Nutrients (2019) 11. doi: 10.3390/nu11051010
80. Wu CF, Hong C, Klauck SM, Lin YL, Efferth T. Molecular Mechanisms of Rosmarinic Acid From Salvia Miltiorrhiza in Acute Lymphoblastic Leukemia Cells. J Ethnopharmacol (2015) 176:55–68. doi: 10.1016/j.jep.2015.10.020
81. Ha ES, Lee EO, Yoon TJ, Kim JH, Park JO, Lim NC, et al. Methylene Chloride Fraction of Spatholobi Caulis Induces Apoptosis via Caspase Dependent Pathway in U937 Cells. Biol Pharm Bull (2004) 27:1348–52. doi: 10.1248/bpb.27.1348
82. Xia M, Wang M, Tashiro S-I, Onodera S, Minami M, Ikejima T. Dracorhodin Perchlorate Induces A375-S2 Cell Apoptosis via Accumulation of P53 and Activation of Caspases. Biol Pharm Bull (2005) 28:226–32. doi: 10.1248/bpb.28.226
83. Lu Z, Lu C, Li C, Jiao Y, Li Y, Zhang G. Dracorhodin Perchlorate Induces Apoptosis and G2/M Cell Cycle Arrest in Human Esophageal Squamous Cell Carcinoma Through Inhibition of the JAK2/STAT3 and AKT/FOXO3a Pathways. Mol Med Rep (2019) 20:2091–100. doi: 10.3892/mmr.2019.10474
84. Zhang G, Sun M, Zhang Y, Hua P, Li X, Cui R, et al. Dracorhodin Perchlorate Induces G(1)/G(0) Phase Arrest and Mitochondria-Mediated Apoptosis in SK-MES-1 Human Lung Squamous Carcinoma Cells. Oncol Lett (2015) 10:240–6. doi: 10.3892/ol.2015.3212
85. Yu JH, Zheng GB, Liu CY, Zhang LY, Gao HM, Zhang YH, et al. Dracorhodin Perchlorate Induced Human Breast Cancer MCF-7 Apoptosis Through Mitochondrial Pathways. Int J Med Sci (2013) 10:1149–56. doi: 10.7150/ijms.6275
86. Liu X, Chen W, Liu Q, Dai J. Abietic Acid Suppresses non-Small-Cell Lung Cancer Cell Growth via Blocking IKKβ/NF-κB Signaling. Onco Targets Ther (2019) 12:4825–37. doi: 10.2147/OTT.S199161
Keywords: Daemonorops draco Blume, acute myeloid leukemia, apoptosis, miR-216b, c-Jun, ER stress, reactive oxygen species
Citation: Park MN, Jeon HW, Rahman MA, Park SS, Jeong SY, Kim KH, Kim S-H, Kim W and Kim B (2022) Daemonorops draco Blume Induces Apoptosis Against Acute Myeloid Leukemia Cells via Regulation of the miR-216b/c-Jun. Front. Oncol. 12:808174. doi: 10.3389/fonc.2022.808174
Received: 03 November 2021; Accepted: 07 February 2022;
Published: 09 March 2022.
Edited by:
Suman Kumar Samanta, Ministry of Science and Technology, IndiaReviewed by:
Suchandra Chowdhury, Bethune College, IndiaJaroslav Truksa, Institute of Biotechnology (ASCR), Czechia
Copyright © 2022 Park, Jeon, Rahman, Park, Jeong, Kim, Kim, Kim and Kim. This is an open-access article distributed under the terms of the Creative Commons Attribution License (CC BY). The use, distribution or reproduction in other forums is permitted, provided the original author(s) and the copyright owner(s) are credited and that the original publication in this journal is cited, in accordance with accepted academic practice. No use, distribution or reproduction is permitted which does not comply with these terms.
*Correspondence: Bonglee Kim, bongleekim@khu.ac.kr
†These authors have contributed equally to this work