- 1Wellcome-Medical Research Council (MRC) Cambridge Stem Cell Institute, University of Cambridge, Cambridge, United Kingdom
- 2Department of Hematology, University of Cambridge, Cambridge, United Kingdom
- 3National Health Service Blood and Transplant, Cambridge Biomedical Campus, Cambridge, United Kingdom
- 4Instituto de Biomedicina de Sevilla-IBiS, Hospitales Universitarios Virgen del Rocío y Macarena/Spanish National Research Council (CSIC)/Universidad de Sevilla, Seville, Spain
- 5Departamento de Fisiología Médica y Biofísica, Universidad de Sevilla, Seville, Spain
Hematopoietic stem cells (HSCs) rely on local interactions in the bone marrow (BM) microenvironment with stromal cells and other hematopoietic cells that facilitate their survival and proliferation, and also regulate their functions. HSCs and multipotent progenitor cells differentiate into lineage-specific progenitors that generate all blood and immune cells. Megakaryocytes (Mks) are hematopoietic cells responsible for producing blood platelets, which are essential for normal hemostasis and blood coagulation. Although the most prominent function of Mks is platelet production (thrombopoiesis), other increasingly recognized functions include HSC maintenance and host immune response. However, whether and how these diverse programs are executed by different Mk subpopulations remains poorly understood. This Perspective summarizes our current understanding of diversity in ontogeny, functions and cell-cell interactions. Cumulative evidence suggests that BM microenvironment dysfunction, partly caused by mutated Mks, can induce or alter the progression of a variety of hematologic malignancies, including myeloproliferative neoplasms (MPNs) and other disorders associated with tissue scarring (fibrosis). Therefore, as an example of the heterogeneous functions of Mks in malignant hematopoiesis, we will discuss the role of Mks in the onset and progression of BM fibrosis. In this regard, abnormal interactions between of Mks and other immune cells might directly contribute to fibrotic diseases. Overall, further understanding of megakaryopoiesis and how Mks interact with HSCs and immune cells has potential clinical implications for stem cell transplantation and other therapies for hematologic malignancies, as well as for treatments to stimulate platelet production and prevent thrombocytopenia.
Introduction
The BM hematopoietic niches are composed of non-hematopoietic and mature hematopoietic cells that support the survival, proliferation, and differentiation of HSCs and hematopoietic progenitor cells (HPCs). HSCs and multipotent progenitor cells differentiate into lineage-specific progenitors that generate all major lineages of hematopoietic and immune cells.
HSC niches can be defined based on their anatomical location in the BM and the type of blood vessel they contain [arterioles (1), sinusoids (2), or transition zone vessels (3)]. To date, there are two anatomically and functionally distinct niches that dictate HSC cell fate in mouse BM: 1) the central niche, which is located in the inner BM, and 2) the endosteal niche, which is close to the bone surface. The central niche contains the majority of venous sinusoids and arterioles and harbours 85% of the HSCs (2). While the endosteal niche is relatively enriched in HSCs (~15% of all HSCs) (4) and contains all transition zone vessels, it seems that both BM niches are functionally different. For instance, activated HSCs migrate through the sinusoidal niche and their migration is regulated by sympathetic nerve fibers (5, 6). By contrast, the endosteal niche seems to be necessary for hematopoietic regeneration (7, 8). In line with these findings, a recent study by the Lucas group reported that granulopoiesis is spatially organized, with the generation of granulocytes and of monocytes–dendritic cells taking place in central sinusoids (9). Therefore, local cues provided by distinct microenvironments could be responsible for the orchestration of hematopoiesis.
Megakaryopoiesis is the process giving rise to Mks within the myeloid branch of hematopoiesis. During steady-state hematopoiesis, all blood lineages are produced through a series of committed progenitors, the Mk being derived through the multipotent progenitor (MPP), common myeloid progenitor (CMP), and Mk erythroid progenitor (MEP) (10–12); however, recent studies have begun to redefine this hierarchy and shed new light on alternative routes by which HSCs can directly differentiate into Mks (13–15) (Figure 1).
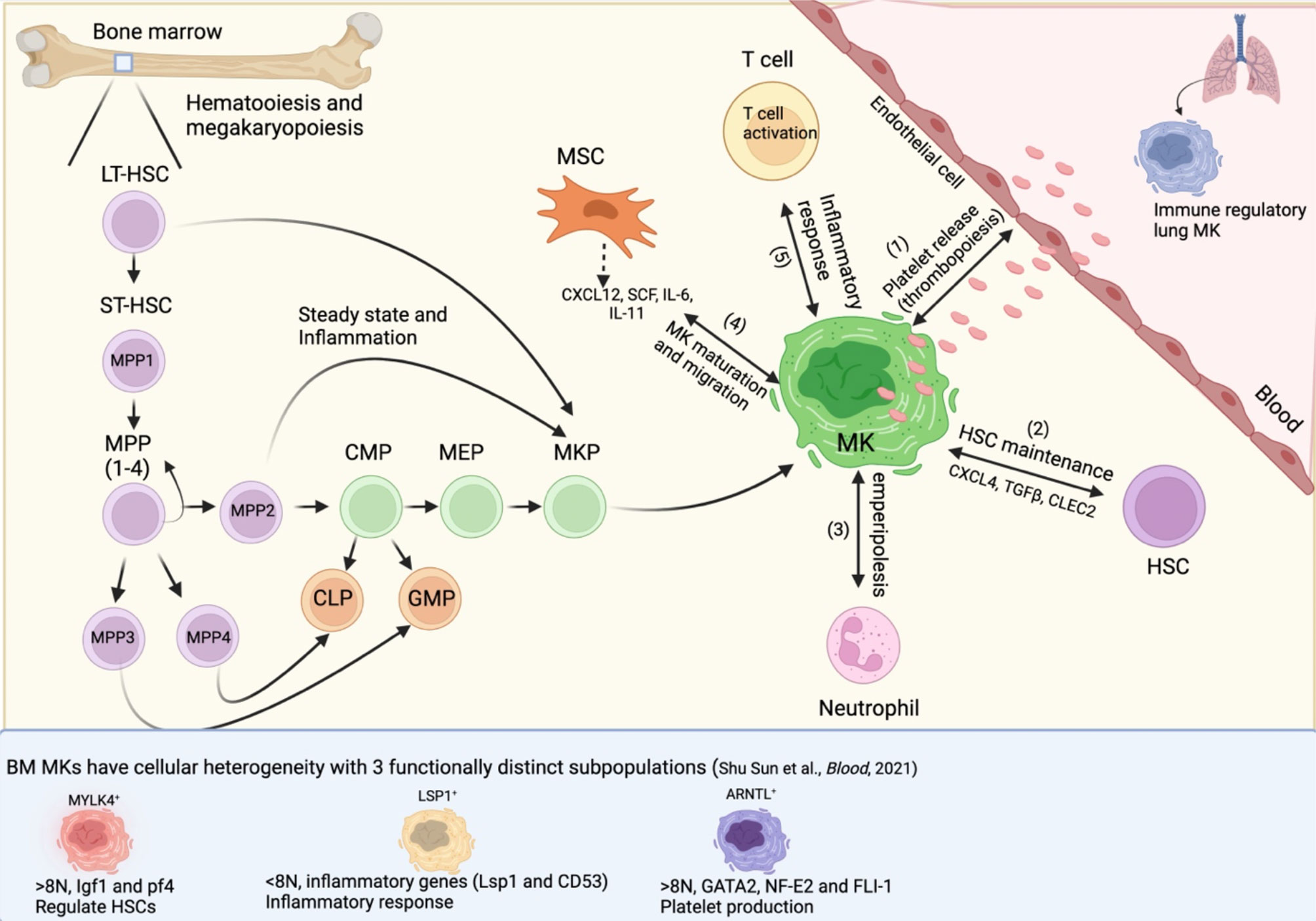
Figure 1 Heterogeneity in megakaryocyte development and functions. Megakaryopoiesis involves differentiation from long-term HSC (LT-HSC), short-term HSC (ST-HSC; or MPP1), multipotent progenitor (MPP2), common myeloid progenitor (CMP), Mk-erythroid progenitor (MEP), and Mk progenitor (MKP). Mks in the bone marrow undergo a maturation process involving an increase in size, extension of proplatelets and platelet release into circulation (thrombopoiesis) (1). Through divergent pathways, MPP3 differentiates into the granulocyte-macrophage progenitor (GMP), MPP4 gives rise to the common lymphoid progenitor (CLP), while MPP2 differentiates into MEP. The Mk-biased pathway is highlighted here, where MPP2 can differentiate directly into Mk bypassing intermediate progenitors, a phenomenon observed during steady-state but most frequently occurring after stress, such as inflammation. Besides platelet production and release, growing evidence supports other Mk functions, such as HSC niche cells (2). Additionally, Mks interact with other BM cells, such as neutrophils, through cellular engulfing (emperipolesis) or by regulating neutrophil migration and activation (3). In addition, Mks can interact directly with mesenchymal stem cells (MSCs) via adhesion molecules, while MSCs secrete cytokines, chemokines and soluble factors that affect Mk maturation and migration (4). New studies have shown that Mk can also act as inflammatory cells and enhance CD4+ T cell activation and function (both in BM and lung). BM Mks might comprise 3 functionally distinct subpopulations that can be identified using different markers: Myosin Light Chain Kinase Family Member 4 (MYLK4)+ Mks involved in regulating HSC maintenance; Lymphocyte Specific Protein 1 (LSP1)+ inflammatory response-associated Mks; and platelet-releasing Aryl hydrocarbon receptor nuclear translocator-like protein 1 (ARNTL)+ Mks (16).
Mks are one of the largest (50-100 μm) and rarest (0.05% to 0.1%) hematopoietic cells in the BM. Mk progenitors undergo multiple rounds of endomitosis to become polyploid cells (17, 18). Polyploid Mks further undergo terminal maturation and generate platelets, essential for normal hemostasis and blood coagulation. Although the most prominent function of Mks is the production and release of platelets (19), growing evidence attributes new functions to these cells in the generation and maintenance of HSCs, an effect mediated by cytokines and growth factors such as CXCL4 (PF4), TGF-β, FGF-1, and IGF-1 (20–24). In addition, recent studies have reported that Mks may participate in regulating the immune response during inflammation and infection because they express multiple inflammatory and immunologic surface markers. Thus, Mks can be considered immune cells, as well as hemostatic cells (25–27).
Furthermore, Mks are the primary source of pro- and anti-angiogenic proteins (e.g. VEGF, Thrombospondin-1 and Endostatin) (28) and the “profibrotic” protein TGF-β, which is involved in the onset and progression of MPNs (29, 30). All these findings raise the question of whether these distinct Mk functions are executed by the same cells or by different subsets of cells. This Perspective article focuses on recent studies that have expanded the functions of Mks beyond thrombopoiesis and shed light on Mk heterogeneity, Mk interactions with other BM cell types, and Mk functions under physiological and pathological conditions (particularly in MPN pathogenesis).
Megakaryocyte Development and Heterogeneity
BM long-term HSCs (LT-HSCs) are largely quiescent during steady-state hematopoiesis (31). However, during emergency hematopoiesis, HSCs lose quiescence, differentiate into mature hematopoietic and immune cells and are mobilized into circulation. Hematopoiesis is a stepwise differentiation process from LT-HSCs, short-term HSCs (which can reconstitute all mature lineages but have limited self-renewal capability) and MPPs, which are non-self-renewing lineage-biased progenitor cells. MPPs differentiate into committed progenitors, such as common lymphoid progenitors (CLP) and CMPs, which differentiate into MEPs and Mk progenitor (MKPs) (Figure 1).
The traditional view of megakaryopoiesis describes the progressive commitment from hematopoietic stem cells, through a stepwise cascade of differentiation, into mature Mks. At the end stage, Mks undergo a terminal maturation process involving multiple steps of endomitosis and cytoplasmic restructuring to form platelets, which is the main function of Mks. However, recent studies have challenged this model of hematopoiesis and proposed alternative routes by which HSCs can directly differentiate into Mks (15).
As platelets play a role in inflammation, infection and vascular injury, it is expected that an alternative, faster megakaryopoiesis mechanism skipping intermediate steps is available. During stress megakaryopoiesis, rapid differentiation from HSCs might be required to replenish the platelet pool. It is important to note that the MPP population comprises subsets of lineage-biased MPPs (32), which have been designated MPP1–4 (33). Integrated quantitative proteome, transcriptome, and methylome analyses of these four MPP populations showed that MPP1 resemble short-term HSC, whereas MPP2 are biased towards Mks/erythroid cells, MPP3 are granulocyte/macrophage biased, and MPP4 are lymphoid biased (33) (Figure 1). Importantly, MPP2 seems to be the only MPP capable of replenishing platelets in a transplant model (32).
Along this line, a recent study has confirmed the existence of Mk-biased MPP2 and HSCs responsible for the generation of ~31% Mks during native hematopoiesis (15). Notably, mature Mks can differentiate directly from LT-HSCs by skipping the MPP2 intermediate (Figure 1). Taken together, these critical studies support the idea that Mk-biased HSCs are important to directly and rapidly differentiate into Mks, bypassing intermediate steps (like CMP or MEP). The above-mentioned studies also suggest that HSCs might originate as Mk-primed during development (13, 14, 34).
Mks have been found associated with vascular BM niches as they are frequently adjacent to BM sinusoids (35, 36). However, Mks can locate close to different types of BM vessels, such as venous sinusoids and arterioles (37), in the endosteal or central BM. Notably, it is known that positioning of Mk in close proximity of BM sinusoids relies on chemokines that are produced and released by endothelial cells and mesenchymal stromal cells (MSCs) associated with these vessels (38, 39). An investigation of the BM of mice two days after sub-lethal total body irradiation revealed that Mks can translocate from the epiphysis to the diaphysis in response to SDF1 (CXCL12) upregulation in these areas upon BM injury (39, 40).
What are the signaling pathways that regulate Mk development? Megakarypoiesis is regulated at multiple levels by different cytokines, with the most critical one being thrombopoietin (TPO). In 1994, TPO and its receptor, c-Mpl, were cloned and found to stimulate platelet production (41, 42). Several studies showed the same year that TPO signaling promotes both development and maturation of Mks from HSCs (41–46). Correspondingly, all Mk-competent or primed progenitor cells, including HSCs, CMPs and MEPs, express Mpl (47). Consequently, mutations in c-Mpl or TPO can cause congenital amegakaryocytic thrombocytopenia (CAMT), which is characterized by severely defective megakaryopoiesis (48–54).
TPO signaling results in the internalization of the Mpl-TPO complex and the initiation of signal transduction pathways. TPO induces phosphorylation of JAK2, which in turn phosphorylates and activates downstream targets, including the transcription factors STAT3 and STAT5 (55) (this specific pathway and its involvement in MPN pathogenesis is discussed in further detail below). Finally, signaling through these pathways causes downstream activation of Mk-specific transcription factors. To emphasize the role of TPO in HSC and Mk production, the group of Alexander used TPO and Mpl knock-out (KO) mice and found that these mice have decreased HSCs, leading to a significant reduction in Mks and platelets. Taken together, these data imply that TPO signaling through Mpl receptor plays a vital physiological role in the regulation of HSC production and function (56).
In 2007, the Suda group reported that TPO/Mpl signaling regulates HSC quiescence and mobilization by upregulating beta1-integrin and cyclin-dependent kinase inhibitors in HSCs (57).
Later, in 2015, the same group identified CLEC-2, a novel Mk-specific factor involved in TPO production in Mks. In this study, the authors demonstrated that Mk-specific deficiency of CLEC-2 disrupts HSC quiescence and that CLEC-2 crosstalks with other niche pathways, including TPO, in Mks (58). Indeed, TPO is essential for the rapid Mk commitment of platelet-biased HSCs. TPO-dependent changes in mitochondrial metabolism prime HSCs to undergo direct differentiation into Mks, while skipping intermediate progenitors (59).
Besides TPO, several other cytokines and chemokines have been identified as Mk-promoting factors. For example, a recent study showed that Insulin-like growth factor (IGF-1) can promote CD34+ cell differentiation toward Mks, as well as facilitate pro-platelet formation and platelet production from cultured Mks – a process mediated by AKT signaling, with the assistance of steroid receptor coactivator 3 (60). Additionally, the CCL5 chemokine has been shown to increase Mk ploidy and pro-platelet formation in a CCR5-dependent manner (61). In this study, using an in vivo murine acute colitis model, the authors found that platelet count significantly correlates with inflammation, whereas CCR5 antagonist treatment abolishes this correlation. Therefore, CCL5/CCR5 signaling may increase platelet counts during physiological stress.
In addition to IGF-1 and CCL5, several members of the IL-6 family, including IL-6, IL-11 and leukemia inhibitory factor (LIF), have a major role in Mk maturation (62, 63). However, genetic ablation of these cytokines or their specific receptor had no essential part in normal steady-state megakaryopoiesis and are not required for the residual Mk and platelet production found in the Mpl-/- mouse model (64, 65).
To conclude, excepting TPO, the cytokines discussed above, while efficiently stimulating megakaryopoiesis in-vitro or in-vivo, appear to be dispensable when ablated in genetically modified mice. These observations, combined with the fact that many of these cytokines are produced and released in high levels by different immune cells in response to viral/bacterial infection or inflammation, indicate that these cytokines might be important to drive emergency (rather than steady-state) thrombopoiesis.
As mentioned before, Mks have other functions different from platelet production. Mks can serve as HSC niche cells and regulate HSC function by secreting cytokines such as CXCL4 (PF4), TGF-β, FGF-1, and IGF-1 (20–23). Furthermore, Mks may participate in pathogen surveillance and antigen presentation because they express multiple inflammatory and immunologic surface markers, including members of the toll-like receptor (TLR) family (TLR1-6), FcγR, major histocompatibility complex (MHC) class I, and CD40L (25–27). In addition, Mks have anti-viral functions since they produce interferon (IFN)-induced transmembrane protein 3 (IFITM3) to limit viral infection (66). Mks also produce and release keratinocyte chemoattractant (KC or CXCL1), which promotes neutrophil motility and mobilization from the BM (67). However, it is not known whether these diverse programs are executed by a functionally homogeneous population or by distinct subsets of Mks. In other words, the functional heterogeneity among Mks remains unclear, partly due to the difficulty to isolate Mks due to their size and complexity.
However, a very recent study by Sun and colleagues (16) has combined improved isolation methods with scRNA-seq analysis, in situ 3D immunofluorescence and functional assays, to characterize the functional diversity and spatial distribution of Mks. The authors identified four subpopulations/clusters associated with unique functions, including platelet generation, maintenance of the HSC niche, and inflammatory responses (Figure 1). Importantly, these transcriptionally distinct groups of Mks were similarly identified in primary human BM Mks. The role of each subpopulation is discussed in further detail below.
Megakaryocyte Interaction With Different Bone Marrow Niches
Mks interact with different hematopoietic and stromal cellular components while migrating between endosteal and central niches within the BM. This section focuses on Mk-BM cell interactions and how these affect Mk development, maturation, and function.
Cumulative evidence indicates that MSCs are key regulators of Mk differentiation and function (68),. MSCs express and secrete different important regulators of Mk maturation, such as, IL-6, IL-11, and Stem Cell Factor (SCF) (Figure 1) (63, 69). In addition to secreting cytokines, MSCs directly interact with Mks through cell adhesion molecules like ICAM-1, VCAM-1 and E-Selectin (70). Furthermore, SDF-1 (CXCL12) secreted by MSCs can direct the migration of Mks, which express the CXCL12 receptor CXCR4 (Figure 1). CXCL12 is mainly produced in the BM by perivascular MSCs and, to a lesser extent, by endothelial cells (71, 72).
Apart from MSCs, endothelial cells can regulate Mk differentiation and thrombopoiesis (73, 74). BM endothelial cell monolayers can support long-term proliferation of HPCs, particularly megakaryocytic and myeloid progenitor cells, through the secretion of cytokines such as G-CSF, GM-CSF and IL-6. Direct cellular contact between HPCs, Mks and BM endothelial cells through specific adhesion molecules, including β1 and β2 integrins, plays a critical role in the migration and possibly in the proliferation of HSCs. However, dysfunction of endothelial cells within the hematopoietic microenvironment [e.g. during inflammation, hypoxia or exposure to damaging stimuli (75, 76)], which is characterized by higher levels of reactive oxygen species (ROS) and apoptosis, impaired migration and angiogenesis, may contribute to the pathogenesis of aplastic anemias and contribute to graft failure after BM transplantation (77–79).
Under pathological conditions (e.g. MPNs), the Zhan group used mice expressing the JAK2V617F mutation specifically in ECs (found in some MPN patients; using Tie2-Cre), to study the effect of the JAK2V617F-bearing vascular niche on MPN disease development in vivo (80). The authors observed thrombocytosis and clusters of Mks preferentially located near sinusoids, associated with reticulin fibrosis. Since Mks play an important role in the pathogenesis of marrow fibrosis (29), these findings suggest that the JAK2V617F mutation might have direct fibrotic effects on mutant Mks, but also niche-dependent effects, due to the impact of the mutation in ECs. The role of Mk-derived profibrotic cytokines is discussed in further detail below.
Bone turnover and remodeling is finely regulated by the concerted action of bone-resorbing osteoclasts and bone-forming osteoblasts. Increased bone resorption and/or decreased bone formation can reduce bone mass and quality, resulting in high fracture risk. Increasing in vitro and in vivo evidence suggest that Mks regulate both bone resorption and bone formation. A mouse model with increased Mks showed a high bone mass phenotype and decreased osteoclast number and bone resorption (81). In vitro experiments have demonstrated that Mks inhibit osteoclast precursors from differentiating into osteoclasts, suppressing bone resorption (82). In contrast, Mks promote osteoblast proliferation and bone formation (83). Thus, Mks play an osteoprotective role by inhibiting bone resorption and stimulating bone formation, making it an ideal therapeutic target.
To test the roles of Mk-secreted factors on bone formation and resorption, a recent study by Lee et al. found that Mk-conditioned media reduced in vitro bone resorption due to suppressed osteoclastic activity (84). Mk-conditioned media suppresses osteoblastic differentiation but stimulates osteoblast proliferation, prompting bone formation. While Mks have bone-anabolic effects, little is known about the regulation of megakaryopoiesis and platelet formation by osteoblasts. A recent study has suggested that osteoblasts support megakaryopoiesis by secreting interleukin-9 (IL-9), which stimulates IL-9 receptor (IL-9R)/STAT3 signaling to drive megakaryopoiesis (85).
Emperipolesis: An Abnormal Megakaryocyte -Neutrophil Interaction
Mks have long been known to internalize other intact hematopoietic cells, but the pathophysiological implications of these observations have remained unclear. Already in 1970, while studying fresh BM samples from thrombocytopenic and anemic patients, Larsen et al. noted neutrophils invading and moving inside the demarcation membrane system of Mks – the cytoplasmic network that forms the plasma membrane of future platelets – enabling the transfer of membrane from the neutrophil to the Mks and vice versa, and later exiting, without compromised cell viability (86). This phenomenon was termed “emperipolesis”. Since then, BM Mk emperipolesis has been observed in a number of pathological conditions, where neutrophils are usually the most frequently internalized cells. More than 50 years since its initial observation and description, the cell biology, regulation, and function of Mk emperipolesis remain largely unknown.
Emperipolesis occurs at low frequency in the healthy BM and may increase across a range of pathological conditions. Both in vitro modeling and in vivo studies have established that emperipolesis is a distinctive form of cell-cell interaction different from phagocytosis, entosis, or transcellular migration (87). Studies focused on the interaction between Mks and neutrophils underscore the need of active cytoskeletal engagement by both cell types for emperipolesis. Ultrastructure (electron microscopy) and immunofluorescence studies have suggested a multi-step process: First, neutrophil enter Mks in vesicles termed “emperisomes”, which are larger than the engulfed cells, but contract over time. Next, the emperisome membrane disappears, leaving the intact neutrophil in the demarcation membrane system of Mks. After a period of residence, neutrophils exit the host Mk without evident damage to either cell type (87, 88).
Emperipolesis is likely mediated by specific receptor-ligand interactions. Tanaka M et al. found enhanced intracellular adhesion molecule 1 (ICAM-1) staining in Mks engaged in emperipolesis (89). At the same time, internalized neutrophils expressed the lymphocyte function-associated antigen1 (LFA-1, or CD18/CD11a), which is the receptor for ICAM-1. Consequently, LFA-1 blockade reduces lipopolysaccharide (LPS)-induced emperipolesis in vivo (89).
In 2000 Cramer and colleagues made the observation that Mks from primary myelofibrosis (PMF) patients and mouse models highly express the adhesion molecule P-selectin on their demarcation membrane system, associated with very frequent emperipolesis (90). Studies by the Miggliacio group supported this concept by demonstrating that impaired Mk maturation in the GATA1low animal model of myelofibrosis leads to abnormal P-Selectin distribution and emperipolesis (91), raising the possibility that neutrophil proteases might facilitate the pathological release/activation of Mk α-granule proteins and growth factors, such as TGF-β, which are critical in the pathogenesis of myelofibrosis (92). However, Mks from PMF patients and mouse models exhibit numerous maturation abnormalities. Consequently, whether abnormal P-selectin in the DMS-mediated neutrophil emperipolesis within Mk is solely responsible for TGF-β-induced myelofibrosis remains to be demonstrated.
These abnormal Mk-neutrophil interactions raise the question whether Mks regulate neutrophil activation, migration or function. Mk cytoplasm and α-granules are rich in soluble mediators that can impact immune cells, and particularly neutrophils [reviewed in (93)]. For instance, platelet factor 4 (PF4), TGF-β, and CXCL1 are produced by Mks and promote neutrophil motility and mobilization from the BM (67, 94, 95). Mks and platelets also produce pro-inflammatory cytokines considered as potent neutrophil activators, including IL-1α, IL-1β and IL-6 (93, 96, 97). Thus, emperipolesis likely modulates the function of neutrophils transiting inside Mks, which in turn affect the function of Mks and platelets. However, further investigations are warranted to elucidate the molecular underpinning and functional implications of Mk-neutrophil emperipolesis in myelofibrosis.
Mk-neutrophil emperipolesis is frequently observed in other types of myelofibrosis independent from MPN, such as in those associated to Mk genetic disorders, such as Gray Platelet Syndrome (GPS). GPS is a rare inherited bleeding disorder characterized by a deficiency of platelet α-granules, macro-thrombocytopenia and BM fibrosis. The autosomal recessive form of GPS is linked to loss of function mutations in NBEAL2, which is predicted to regulate granule trafficking in Mks. Unexpectedly, the Guerrero and Ouwehand group observed that GPS platelets are enriched in proteins normally found within neutrophil granules (98). Due to the lack of concordant increase in expression of the genes encoding these proteins, two potential mechanisms are: 1) endocytosis by circulating platelets or 2) emperipolesis by Mks. Although we cannot discard the former, we and others hypothesize that emperipolesis likely affects the cargo of platelets. A transfer of membranes between Mks and neutrophils has been observed in emperipolesis (87), and a functional genomic study reported an association of myeloperoxidase (MPO, normally contained within neutrophil-specific granules) with mean platelet volume, highlighting the crosstalk between neutrophils and platelets (99). Hence, the release of neutrophil granule proteins (such proteolytic enzymes, elastase and MPO) inside Mks during emperipolesis could provide a plausible explanation for the higher level of these proteins in GPS platelets (98).
Megakaryocytes as HSC Niche Cells
In both central and endosteal BM, HSCs reside in perivascular niches, where endothelial cells and MSCs critically maintain and regulate HSC function. Pioneering studies by the Nilsson group showed that transplanted HSCs preferentially lodge close to mature Mks in the mouse BM and that HSCs isolated from the endosteal BM proliferate more when co-cultured with mature Mks (22). However, in vivo studies suggest that Mks preserve HSC quiescence; whole-mount 3D BM imaging revealed that a subset of quiescent HSCs specifically associates with Mks (20). In addition, Mks, which are mainly adjacent to sinusoids, regulate HSC quiescence by several mechanisms under homeostatic conditions, including secretion of the chemokine CXCL4/PF4 (20) or TGF-β (23, 100). Furthermore, Mks can also regulate HSC quiescence through C-type lectin-like receptor-2 (CLEC-2) signaling, since Clec2MkΔ/Δ mice exhibit reduced BM HSC quiescence and repopulation potential, along with extramedullary hematopoiesis. The low level of TPO production may account for the decline in HSC potential in Clec2MkΔ/Δ mice, as administration of recombinant TPO to Clec2MkΔ/Δ mice restores HSC potential. Overall, this study shows that CLEC-2 signaling is involved in various molecular pathways to produce niche factors, including TPO (58). Taken together, these studies reinforce the idea that Mks function as a niche to maintain HSC quiescence. However, under stressed conditions, when rapid expansion of HSCs is required, Mks produce fibroblast growth factor-1 (FGF-1), promoting HSC expansion after injury (23, 24). In addition to the direct release of proteins, recent attention has also turned to the release of microparticles within the BM. Mk-derived microparticles bind to HSPCs and induce them to differentiate into functional Mks (101), providing a possible mechanism of direct communication between the Mk and the HSC.
The spatial localization of HSCs in the BM remains controversial, with some studies suggesting that they are maintained in homogeneously distributed niches, while others have suggested the contributions of distinct niche structures. HSC quiescence may be differently regulated between steady-state and emergency and/or malignant hematopoiesis. However, lineage commitment appears to be influenced by the location of HSCs in the BM. Growing evidence suggests that lymphopoiesis preferentially occurs in the endosteal BM, while myelopoiesis/erythropoiesis/megakaryopoiesis mainly occurs in central BM regions. A study by the Frenette group supported this concept by using von Willebrand factor (vWF)-eGFP mice model to label platelet-biased HSCs (102). In this study, the authors demonstrated that platelet and myeloid-biased HSCs, marked by vWF expression (vWF+ HSCs), are highly enriched near MKs. Moreover, they found that depletion of Mk selectively expands vWF+ HSCs, whereas the depletion of NG2+ arteriolar niche cells selectively depletes vWF- lymphoid-biased/balanced HSCs (102). In other words, vWF+ platelet/myeloid-biased HSCs are associated with Mks, whereas vWF- lymphoid-biased/balanced HSCs are located close to arterioles. Therefore, alterations in specialized niches might directly affect myeloid/lymphoid output, and the imbalanced production of mature hematopoietic cells at specific niches might in turn, remodel the local microenvironment for these cells.
As described above, Mks are known to play critical roles in supporting HSCs in the BM niche. Recently, Sun et al. identified a transcriptionally unique Mk subpopulation that expresses high levels of Mylk4 (cluster 2; HSC-niche MKs) (16). These Mks highly express other HSC regulators, such as CXCL4, IGF1, WNT signaling and cell adhesion molecules. The unique spatial distributions of cluster 2 (HSC niche Mks) and cluster 4 (platelet generation Mks) populations were consistent with the distinct function inferred from their respective transcriptomic signatures and support the concept that platelet generation and HSC maintenance are properties of 2 different Mk subpopulations. Additionally, these observations provide the foundation for future studies to investigate the interaction between Mks and HSCs.
Mks promote the quiescence of neighboring HSCs. Nonetheless, whether Mk-HSC interactions change during pathological conditions or during aging is unclear. Sympathetic adrenergic signals regulate stromal MSC proliferation (71) and are affected during age-related myeloproliferative neoplasms (103). Cumulative evidence suggests that different BM microenvironments regulate myeloid differentiation and megakaryopoiesis during aging (104). One study suggests that the BM microenvironment regulates HSPC lineage commitment through β2-adrenergic-receptor (β2-AR) and β3-AR. Interestingly, β2-AR and β3-AR exhibit opposite roles on myeloid differentiation: increased BM noradrenergic innervation promotes β2-AR-IL-6-dependent megakaryopoiesis and becomes predominant during aging, while reduced β3-AR activity correlates with decreased endosteal niches and Mk apposition to sinusoids (104). Additionally, increased sympathetic adrenergic activity has been previously described during aging (105) and might increase osteoporosis and fracture risk by restraining bone formation (106).
Megakaryocytes and Their Important Roles in Immunity
Emerging evidence demonstrates that Mks sense and respond to inflammatory stress and participate in host immune responses. For instance, infections are associated with extensive platelet consumption, representing a high risk to health. However, the mechanism coordinating the rapid regeneration of the platelet pool during such stress conditions is not entirely understood. Haas S et al. reported that the phenotypic HSC compartment contains stem-like Mk-committed progenitors (SL-MkPs) (107). This cell population shares many features with multipotent HSCs and serves as a lineage-restricted emergency pool. Inflammatory signaling associated with infections directly triggers cell cycle activation of quiescent SL-MkPs, resulting in rapid maturation of SL-MkPs and other Mk progenitors, thereby efficiently replenishing platelets lost during acute inflammation (107).
Under stress conditions, such as inflammation and infection, murine Mks express MHC class II genes, which are predominant markers of professional antigen-presenting cells, such as dendritic cells, macrophages, and B cells, and co-stimulatory molecule CD40L – the ligand for the CD40 on T cells and B cells. However, whether the expression of these immune-related genes is ubiquitous in the Mk lineage or restricted to a subset of Mk populations in vivo is unknown. Using freshly isolated mouse cells, Sun et al. found that inflammatory CD53+ Mks are capable of engulfing and ingesting bacteria particles, whereas CD53– Mks lack phagocytic capability (16). Moreover, when co-cultured with T cells, inflammatory CD53+ Mks, but not CD53– Mks, can stimulate T-cell expansion. Together, these observations indicate that CD53+ Mks can respond to pathogen-derived agonists and inflammatory stimulation. Therefore, the CD53+ Mk subset appears to have specialized functions in innate and adaptive immunity.
Interestingly, in a systemic lupus erythematosus mouse model, Lin–c-Kit+CD41+ Mk progenitors express MHC class II molecules and can promote Th17 cell development in vitro (108). In line with this result, Mks and platelets are also involved in induction of immune tolerance as they can control the differentiation of Th17 and the expression of Foxp3+ Treg cells (109, 110). Taken together, these findings suggest that Mks have dual functions, as they may serve as antigen-presenting cells through MHC class II molecules for platelet-mediated immunoregulation while they might also undergo active thrombopoiesis to replenish the pool of circulating platelets during emergency thrombopoiesis, such as after bleeding (108, 111). However, it is currently unclear whether Mks with immune functions undergo thrombopoiesis, or whether the resulting platelets are functionally different from those derived from other Mk populations.
Platelet-derived CD40L was shown to induce monocyte differentiation into dendritic cell (DC), DC maturation and upregulation of co-stimulatory molecules (112). This function of CD40L derived by platelets may be highly relevant to autoimmune diseases, such as systemic lupus erythematosus, in which platelets induce DC differentiation and type-I interferon release, thereby promoting B-cell secretion of antibodies (113).
In addition to CD40L, Mks also express CD80 and CD86 lymphocyte co-stimulation molecules (114). This study demonstrates that Mks can endocytose ovalbumin and proteolytically generate its immunogenic peptide ligand, which is cross-presented on their surface in association with MHC class I. The authors also found that MKs can present Mk-associated (CD61) peptides to activate CD61-specific CD8+ T cells and mediate immune thrombocytopenia in vivo. Together, these results suggest that, in addition to their hemostatic role, mature Mks can significantly affect CD8+ T-cell responses via antigen presentation and are able to spread this immunogenic information through platelets. Interestingly, a recent study by the Ziebuhr group identified an expansion of interferon-activated circulating Mks and increased erythropoiesis in patients with COVID-19 (115). Mk- and erythroid-cell-derived co-expression modules were predictive of fatal disease outcome. The study demonstrates broad cellular effects of SARS-CoV-2 infection beyond adaptive immune cells.
In summary, Mks can play vital roles in adaptive immunity and during infections. In addition to their support of professional antigen-presenting cells and lymphocyte functions, Mks can process and present antigens.
Megakaryocytes Outside the Bone Marrow
Mks have long-been considered BM-resident cells. However, platelet-producing Mks can be found in other tissues, such as lung interstitial tissue (116), although these cells could be derived from the BM and their contribution to physiological thrombopoiesis is unclear. Compared with BM, lung interstitial Mks are enriched with mRNAs associated with immune regulatory functions, such as immunoreceptors, chemokines, and cytokines (116). The role of Mks as immune regulatory cells is poorly understood in general, particularly regarding lung interstitial Mks. Since these cells increase during pulmonary and cardiovascular diseases (117), lung interstitial Mks may be dynamically responsive to inflammatory states.
Mks have been mainly studied in the immune-quiescent BM environment, and it is unknown whether Mks in other tissues have a different immune phenotype and functions. Recently, Pariser et al. have demonstrated that lung and BM Mks have distinct immune phenotypes and functions: lung Mks secrete inflammatory cytokines and express molecules that are similar to many tissue-resident leukocytes and antigen-presenting cells (118, 119). Moreover, lung Mks process live intact bacteria and present bacteria-derived antigen to CD4+ T cells both in vitro and in vivo. This study suggests that lung Mks have important roles in the early activation of T cell responses to pulmonary pathogens, supporting an immune regulatory function of lung interstitial Mks (Figure 1) (118, 119). Sun et al. compared the identified four BM MK clusters with lung Mks (16). BM immune Mks are preferentially enriched in the expression of antigen presentation-related genes. In contrast, lung immune Mks exhibit stronger expression signatures of phagocytosis and bacteria clearance, which is consistent with the notion that lungs face more constant challenges from pathogens, compared with BM.
Megakaryocyte Dysfunction in Hematological Malignancies, Including MPNs
The past few years have seen extraordinary support for the dysfunction of the BM microenvironment, affecting BM Mks, in the progression of a variety of hematologic malignancies, including MPNs (120). MPNs are clonal hematopoietic disorders characterized by excessive production of mature blood cells. The Philadelphia-negative MPNs include three major diseases: polycythemia vera (PV), essential thrombocythemia (ET), and primary myelofibrosis (PMF) (121). Several mutations affecting the genes encoding JAK2, CALR, or MPL, referred to herein as the “oncogenic drivers”, are considered to drive the myeloproliferative phenotype. These mutations lead to constitutive activation of cytokine-regulated intracellular signaling pathways (122, 123). The last two decades have spotted dramatic advances in understanding of the molecular and cellular basis of excessive myeloproliferation, leading to the development and therapeutic use of novel targeted treatments, such as JAK inhibitors. In this section, the role of Mks in the onset and progression of MPNs and MPN-related myelofibrosis, will be discussed.
Myelofibrosis can occur as a primary disorder (PMF) or develop secondary to the other MPN classifications (PV or ET). PMF is the prototypic example of progressive development of BM fibrosis and is associated with poor prognosis and morbidity. In addition, myelofibrosis is characterized by increased numbers of abnormal BM Mks, and progressive BM fibrosis that destroys the hematopoietic microenvironment, resulting in the cardinal disease features of cytopenias, mobilization of HSPCs to peripheral blood, extramedullary hematopoiesis, splenomegaly, and a high propensity for leukemic transformation. Survival is typically 5–10 years from diagnosis and is not substantially improved by currently available drug therapies (124).
Mks are dramatically increased in number in myelofibrosis and are one of the critical cellular drivers of the destructive BM remodelling by releasing excess pro-fibrotic growth factors, cytokines including TGF-β, cross-linking enzymes and extracellular matrix proteins (28, 125–128). All these proteins are known to directly cause BM fibrosis by stimulating MSCs to become fibroblastic cells or produce collagen, leading to ECM remodeling and BM scarring. An alternative source of collagen- and fibronectin-producing cells in the BM and spleens of JAK2V617F-induced myelofibrosis mice are fibrocytes derived from neoplastic hematopoietic cells (mainly from neoplastic monocytes) upon TGF-β stimulation (129).
TGF-β is a member of a large family of growth factors involved in tissue development and repair, as well as in cancer progression and myelofibrosis. Using transmission electron-microscopy, the Migliaccio group identified Mks with abnormally high levels of TGF-β and collagen fibres embedded in their cytoplasm in the spleen of PMF patients and Gata1low mouse model of PMF (130). Importantly, TGF-β inhibition or loss of P-selectin (involved in Mk-neutrophil interaction) could prevent fibrosis in this study.
The cellular and molecular pathways that give rise to the dramatically increased Mk numbers and Mk dysfunction leading to tissue fibrosis are unclear. Recently, the Mead group investigated the cellular and molecular basis for aberrant megakaryopoiesis in myelofibrosis (131). In this study, the authors captured peripheral blood HSPCs and performed single-cell analysis of abnormal Mk differentiation and function in patients with myelofibrosis. Using multi-parameter immunophenotyping, functional studies, single-cell RNA sequencing, TARGET-seq (132), and single-cell proteomics, they found a fibrosis signature already at the Mk progenitor stage in myelofibrosis (PMF, post ET myelofibrosis, post PV myelofibrosis), identifying potential targets for future studies (131). Among these was G6B, an immunoreceptor tyrosine-based inhibition motif (ITIM), a Mk marker over-expressed in mutant clone-derived HSPCs from myelofibrosis patients (131). These investigators propose the combinatorial targeting of HSCs and Mks with bi-specific antibody therapies as a potential future strategy for selective ablation of the myelofibrosis clone.
Although the molecular and cellular mutations involved in the pathogenesis of MPN and PMF have been extensively investigated (133), reactive cellular alterations in the non-hematopoietic compartment (mainly in stromal cells) remain comparatively less clear. For instance, a recent study by Baryawno et al. found that different stromal subtypes have distinct roles in normal BM hematopoiesis and leukemia (134). However, the sequential events that drive stromal cell activation by hematopoietic–stromal crosstalk remain elusive. Recently, through an unbiased approach and validation in patients with MPN, the Schneider group found that the differential spatial expression of the chemokine CXCL4 might trigger the progression to fibrosis (135). In this study, the authors show that the absence of hematopoietic CXCL4 ameliorates the MPN phenotype, reduces stromal cell activation and BM fibrosis, which in turn decreases the activation of profibrotic pathways in Mks, inflammation in fibrosis-driving cells, and JAK activation in both Mks and stromal cells in three murine PMF models (TPOhigh, JAK2V617F, MPLW515L). These data indicate that excessive CXCL4 levels, associated with the characteristically high inflammation in MPN, have pro-fibrotic effects. Therefore, targeting CXCL4 might be a promising strategy to reduce inflammation and fibrosis in PMF.
Patients with BM fibrosis have increased levels of extracellular matrix (ECM) proteins, particularly reticulin, fibronectin fibers and, in some cases, collagen fibers. Mks are presumed to be the neoplastic cell subtype that predominantly forces fibroblasts to produce ECMs in the disease, through an uncontrolled production and release of several cytokines, such as TGF-β1, platelet-derived growth factor, or basic fibroblast growth factor [as reviewed in (136)]. Among the ECM proteins that fill the BM cavity, fibronectin is a glycoprotein whose mRNA has three alternative splicing sites (termed extra domain A [EDA], extra domain B [EDB], and IIICS or EIIIA, EIIIB, and V), that allow several forms of fibronectin, whose expression has been proven to be altered in tumors (137). Different studies have described the involvement of EDA fibronectin isoforms in critical pathological processes, such as atherosclerosis (138), lung fibrosis (139), and liver fibrosis (140). On the contrary, its expression and function in BM fibrosis and its effect on the hematopoietic tissue has been less studied (141). A recent study by the Balduini group (142) showed that mice constitutively expressing the EDA domain, but not EDA knockout mice, are more prone to develop BM fibrosis upon treatment with TPO. Mechanistically, EDA fibronectin binds to TLR4 and sustains progenitor cell proliferation and Mk differentiation through activation of STAT5 and ERK 1/2 signaling pathways, inducing LPS-like responses, such as release of profibrotic IL-6. Moreover, pharmacological inhibition of TLR4 or TLR4 deletion in TPOhigh mice abolishes BM fibrosis, IL-6 release, and splenomegaly. Supporting a clinical correlate, EDA fibronectin is similarly increased in plasma and BM biopsies of PMF patients, compared with healthy controls, correlating with fibrotic phase.
Together, these studies suggest that abnormal Mk differentiation and emperipolesis are two major drivers of the release of cytokines with fibrogenic potential. However, the mechanisms underlying cytokine secretion, Mk interactions with other BM cells (e.g. neutrophils and mesenchymal stromal cells) and their functional activities in physiological conditions, as well as during myelofibrosis progression, are not yet well understood and will be an exciting subject of future studies. These could lead to new therapies aimed at disrupting abnormal Mk-BM cell interactions as a way to interfere with myelofibrosis progression.
Concluding Remarks
Research in recent years has made substantial progress in understanding Mk development and their heterogeneous specialized functions. The changing dogma of megakaryopoiesis prompts new research areas intersecting with different fields, including immune response, cancer and stem cell biology. The ontogenic and functional relationships among Mk subpopulations are interesting areas for future studies harnessing the regenerative potential of these cells.
Emerging research suggests that Mk subpopulations exhibit specific functions beyond their roles in platelet production and hemostasis. Therefore, Mks should be studied not only as a platelet-producing cells, but also as important regulators of immune responses, HSCs and their niches. Although much has been learned recently about Mk heterogeneity, many unknowns remain regarding the signals that regulate these events through dynamic interactions of Mks with different cell types of the BM microenvironment.
Furthermore, Mk subtypes might exhibit disparate roles in steady state versus emergency hematopoiesis. The studies discussed in this Perspective demonstrate essential roles in steady state and emergency hematopoiesis, as well as in the development of malignancies, such as MPNs. However, it’s unclear whether these myriad functions are explained by distinct Mk subpopulations dynamically interacting with other cell types in the BM. These are exciting areas for future multidisciplinary research.
Data Availability Statement
The original contributions presented in the study are included in the article/supplementary material. Further inquiries can be directed to the corresponding authors.
Author Contributions
Both authors conceived and wrote the manuscript. EK-M prepared the figure. All authors contributed to the article and approved the submitted version.
Funding
EK-M received outstanding postdoctoral Arab students Scholarship (from the Israeli council for higher education) and National Postdoctoral Award Program for Women in Science (from Weizmann institute of science). Original work discussed in this article was supported by core support grants from the Wellcome Trust [203151/Z/16/Z] and the MRC to the Cambridge Stem Cell Institute, National Health Service Blood and Transplant (United Kingdom), European Union’s Horizon 2020 research (ERC-2014-CoG-648765), MRC-AMED grant MR/V005421/1, a Programme Foundation Award (C61367/A26670) from Cancer Research UK to SM-F. For the purpose of Open Access, the authors have applied a CC BY public copyright license to any Author Accepted Manuscript version arising from this submission. The authors regret that some relevant literature could not be discussed because of space limitation.
Conflict of Interest
The authors declare that the research was conducted in the absence of any commercial or financial relationships that could be construed as a potential conflict of interest.
Publisher’s Note
All claims expressed in this article are solely those of the authors and do not necessarily represent those of their affiliated organizations, or those of the publisher, the editors and the reviewers. Any product that may be evaluated in this article, or claim that may be made by its manufacturer, is not guaranteed or endorsed by the publisher.
References
1. Kunisaki Y, Bruns I, Scheiermann C, Ahmed J, Pinho S, Zhang D, et al. Arteriolar Niches Maintain Haematopoietic Stem Cell Quiescence. Nature (2013) 502:637–43. doi: 10.1038/nature12612
2. Acar M, Kocherlakota KS, Murphy MM, Peyer JG, Oguro H, Inra CN, et al. Deep Imaging of Bone Marrow Shows Non-Dividing Stem Cells Are Mainly Perisinusoidal. Nature (2015) 526:126–30. doi: 10.1038/nature15250
3. Kusumbe AP, Ramasamy SK, Adams RH. Coupling of Angiogenesis and Osteogenesis by a Specific Vessel Subtype in Bone. Nature (2014) 507:323–8. doi: 10.1038/NATURE13145
4. Beerman I, Luis TC, Singbrant S, lo Celso C, Méndez-Ferrer S. The Evolving View of the Hematopoietic Stem Cell Niche. Exp Hematol (2017) 50:22–6. doi: 10.1016/J.EXPHEM.2017.01.008
5. Méndez-Ferrer S, Lucas D, Battista M, Frenette PS. Haematopoietic Stem Cell Release Is Regulated by Circadian Oscillations. Nature (2008) 452:442–7. doi: 10.1038/nature06685
6. García-García A, Korn C, García-Fernández M, Domingues O, Villadiego J, Martín-Pérez D, et al. Dual Cholinergic Signals Regulate Daily Migration of Hematopoietic Stem Cells and Leukocytes. Blood (2019) 133:224–36. doi: 10.1182/BLOOD-2018-08-867648
7. Zhao M, Tao F, Venkatraman A, Li Z, Smith SE, Unruh J, et al. N-Cadherin-Expressing Bone and Marrow Stromal Progenitor Cells Maintain Reserve Hematopoietic Stem Cells. Cell Rep (2019) 26:652–69.e6. doi: 10.1016/J.CELREP.2018.12.093
8. Méndez-Ferrer S, Bonnet D, Steensma DP, Hasserjian RP, Ghobrial IM, Gribben JG, et al. Bone Marrow Niches in Haematological Malignancies. Nat Rev Cancer (2020) 20:285–98. doi: 10.1038/s41568-020-0245-2
9. Zhang J, Wu Q, Johnson CB, Pham G, Kinder JM, Olsson A, et al. In Situ Mapping Identifies Distinct Vascular Niches for Myelopoiesis. Nature (2021) 590:457–62. doi: 10.1038/s41586-021-03201-2
10. Akashi K, Traver D, Miyamoto T, Weissman IL. A Clonogenic Common Myeloid Progenitor That Gives Rise to All Myeloid Lineages. Nature (2000) 404:193–7. doi: 10.1038/35004599
11. Debili N, Coulombel L, Croisille L, Katz A, Guichard J, Breton-Gorius J, et al. Characterization of a Bipotent Erythro-Megakaryocytic Progenitor in Human Bone Marrow. Blood (1996) 88:1284–96. doi: 10.1182/BLOOD.V88.4.1284.BLOODJOURNAL8841284
12. Carrelha J, Meng Y, Kettyle LM, Luis TC, Norfo R, Alcolea V, et al. Hierarchically Related Lineage-Restricted Fates of Multipotent Haematopoietic Stem Cells. Nature (2018) 554:106–11. doi: 10.1038/nature25455
13. Sanjuan-Pla A, Macaulay IC, Jensen CT, Woll PS, Luis TC, Mead A, et al. Platelet-Biased Stem Cells Reside at the Apex of the Haematopoietic Stem-Cell Hierarchy. Nature (2013) 502:232–6. doi: 10.1038/NATURE12495
14. Yamamoto R, Morita Y, Ooehara J, Hamanaka S, Onodera M, Rudolph KL, et al. Clonal Analysis Unveils Self-Renewing Lineage-Restricted Progenitors Generated Directly From Hematopoietic Stem Cells. Cell (2013) 154:1112–26. doi: 10.1016/J.CELL.2013.08.007
15. Rodriguez-Fraticelli AE, Wolock SL, Weinreb CS, Panero R, Patel SH, Jankovic M, et al. Clonal Analysis of Lineage Fate in Native Haematopoiesis. Nature (2018) 553:212–6. doi: 10.1038/nature25168
16. Sun S, Jin C, Si J, Lei Y, Chen K, Cui Y, et al. Single-Cell Analysis of Ploidy and the Transcriptome Reveals Functional and Spatial Divergency in Murine Megakaryopoiesis. Blood (2021) 39:1288–300. doi: 10.1182/blood.2021010697
17. Noetzli LJ, French SL, Machlus KR. New Insights Into the Differentiation of Megakaryocytes From Hematopoietic Progenitors. Arteriosclerosis Thrombosis Vasc Biol (2019) 39:1288–300. doi: 10.1161/ATVBAHA.119.312129
18. Ebaugh FG, Bird RM. The Normal Megakaryocyte Concentration in Aspirated Human Bone Marrow. Blood (1951) 6:75–80. doi: 10.1182/BLOOD.V6.1.75.75
19. Tavassoli M. Megakaryocyte-Platelet Axis and the Process of Platelet Formation and Release. Blood (1980) 55:537–45. doi: 10.1182/BLOOD.V55.4.537.537
20. Bruns I, Lucas D, Pinho S, Ahmed J, Lambert MP, Kunisaki Y, et al. Megakaryocytes Regulate Hematopoietic Stem Cell Quiescence Through CXCL4 Secretion. Nat Med (2014) 20:1315–20. doi: 10.1038/nm.3707
21. Gong Y, Zhao M, Yang W, Gao A, Yin X, Hu L, et al. Megakaryocyte-Derived Excessive Transforming Growth Factor β1 Inhibits Proliferation of Normal Hematopoietic Stem Cells in Acute Myeloid Leukemia. Exp Hematol (2018) 60:40–6.e2. doi: 10.1016/J.EXPHEM.2017.12.010
22. Heazlewood SY, Neaves RJ, Williams B, Haylock DN, Adams TE, Nilsson SK. Megakaryocytes Co-Localise With Hemopoietic Stem Cells and Release Cytokines That Up-Regulate Stem Cell Proliferation. Stem Cell Res (2013) 11:782–92. doi: 10.1016/J.SCR.2013.05.007
23. Zhao M, Perry JM, Marshall H, Venkatraman A, Qian P, He XC, et al. Megakaryocytes Maintain Homeostatic Quiescence and Promote Post-Injury Regeneration of Hematopoietic Stem Cells. Nat Med (2014) 20:1233–4. doi: 10.1038/nm.3706
24. Day RB, Link DC. Megakaryocytes in the Hematopoietic Stem Cell Niche. Nat Med (2014) 20:1233–4. doi: 10.1038/nm.3745
25. Beaulieu LM, Lin E, Morin KM, Tanriverdi K, Freedman JE. Regulatory Effects of TLR2 on Megakaryocytic Cell Function. Blood (2011) 117:5963. doi: 10.1182/BLOOD-2010-09-304949
26. Shiraki R, Inoue N, Kawasaki S, Takei A, Kadotani M, Ohnishi Y, et al. Expression of Toll-Like Receptors on Human Platelets. Thromb Res (2004) 113:379–85. doi: 10.1016/J.THROMRES.2004.03.023
27. Crist SA, Elzey BD, Ahmann MT, Ratliff TL. Early Growth Response-1 (EGR-1) and Nuclear Factor of Activated T Cells (NFAT) Cooperate to Mediate CD40L Expression in Megakaryocytes and Platelets. J Biol Chem (2013) 288:33985–96. doi: 10.1074/JBC.M113.511881
28. Ciurea SO, Merchant D, Mahmud N, Ishii T, Zhao Y, Hu W, et al. Pivotal Contributions of Megakaryocytes to the Biology of Idiopathic Myelofibrosis. Blood (2007) 110:986–93. doi: 10.1182/BLOOD-2006-12-064626
29. Badalucco S, di Buduo CA, Campanelli R, Pallotta I, Catarsi P, Rosti V, et al. Involvement of Tgfβ1 in Autocrine Regulation of Proplatelet Formation in Healthy Subjects and Patients With Primary Myelofibrosis. Haematologica (2013) 98:514–7. doi: 10.3324/HAEMATOL.2012.076752
30. Malara A, Gruppi C, Rebuzzini P, Visai L, Perotti C, Moratti R, et al. Megakaryocyte-Matrix Interaction Within Bone Marrow: New Roles for Fibronectin and Factor XIII-A. Blood (2011) 117:2476–83. doi: 10.1182/BLOOD-2010-06-288795
31. Hirao A, Arai F, Suda T. Regulation of Cell Cycle in Hematopoietic Stem Cells by the Niche. Cell Cycle (2004) 3:1481–3. doi: 10.4161/cc.3.12.1281
32. Pietras EM, Reynaud D, Kang Y-A, Carlin D, Calero-Nieto FJ, Leavitt AD, et al. Functionally Distinct Subsets of Lineage-Biased Multipotent Progenitors Control Blood Production in Normal and Regenerative Conditions. Cell Stem Cell (2015) 17:35–46. doi: 10.1016/j.stem.2015.05.003
33. Cabezas-Wallscheid N, Klimmeck D, Hansson J, Lipka DB, Reyes A, Wang Q, et al. Identification of Regulatory Networks in HSCs and Their Immediate Progeny via Integrated Proteome, Transcriptome, and DNA Methylome Analysis. Cell Stem Cell (2014) 15:507–22. doi: 10.1016/j.stem.2014.07.005
34. Gekas C, Graf T. CD41 Expression Marks Myeloid-Biased Adult Hematopoietic Stem Cells and Increases With Age. Blood (2013) 121:4463–72. doi: 10.1182/BLOOD-2012-09-457929
35. Junt T, Schulze H, Chen Z, Massberg S, Goerge T, Krueger A, et al. Dynamic Visualization of Thrombopoiesis Within Bone Marrow. Science (2007) 317:1767–70. doi: 10.1126/science.1146304
36. Becker RP, De Bruyn PPH. The Transmural Passage of Blood Cells Into Myeloid Sinusoids and the Entry of Platelets Into the Sinusoidal Circulation; a Scanning Electron Microscopic Investigation. Am J Anat (1976) 145:183–205. doi: 10.1002/aja.1001450204
37. Tamura S, Suzuki-Lnoue K, Tsukiji N, Shirai T, Sasaki T, Osada M, et al. Podoplanin-Positive Periarteriolar Stromal Cells Promote Megakaryocyte Growth and Proplatelet Formation in Mice by CLEC-2. Blood (2016) 127:1701–10. doi: 10.1182/BLOOD-2015-08-663708
38. Wang J-F, Liu Z-Y, Groopman JE. The α-Chemokine Receptor CXCR4 Is Expressed on the Megakaryocytic Lineage From Progenitor to Platelets and Modulates Migration and Adhesion. Blood (1998) 92:756–64. doi: 10.1182/BLOOD.V92.3.756
39. Hamada T, Möhle R, Hesselgesser J, Hoxie J, Nachman RL, Moore MAS, et al. Transendothelial Migration of Megakaryocytes in Response to Stromal Cell-Derived Factor 1 (SDF-1) Enhances Platelet Formation. J Exp Med (1998) 188:539–48. doi: 10.1084/jem.188.3.539
40. Dominici M, Rasini V, Bussolari R, Chen X, Hofmann TJ, Spano C, et al. Restoration and Reversible Expansion of the Osteoblastic Hematopoietic Stem Cell Niche After Marrow Radioablation. Blood (2009) 114:2333–43. doi: 10.1182/blood-2008-10-183459
41. Bartley TD, Bogenberger J, Hunt P, Li YS, Lu HS, Martin F, et al. Identification and Cloning of a Megakaryocyte Growth and Development Factor That Is a Ligand for the Cytokine Receptor Mpl. Cell (1994) 77:1117–24. doi: 10.1016/0092-8674(94)90450-2
42. Lok S, Kaushansky K, Holly RD, Kuijper JL, Lofton-Day CE, Oort PJ, et al. Cloning and Expression of Murine Thrombopoietin cDNA and Stimulation of Platelet Production In Vivo. Nature (1994) 369:565–8. doi: 10.1038/369565a0
43. de Sauvage FJ, Hass PE, Spencer SD, Malloy BE, Gurney AL, Spencer SA, et al. Stimulation of Megakaryocytopoiesis and Thrombopoiesis by the C-Mpl Ligand. Nature (1994) 369:533–8. doi: 10.1038/369533A0
44. Kaushansky K, Lok S, Holly RD, Broudy VC, Lin N, Bailey MC, et al. Promotion of Megakaryocyte Progenitor Expansion and Differentiation by the C-Mpl Ligand Thrombopoietin. Nature (1994) 369:568–71. doi: 10.1038/369568A0
45. Wendling F, Maraskovsky E, Debili N, Florido C, Teepe M, Titeux M, et al. Cmpl Ligand Is a Humoral Regulator of Megakaryocytopoiesis. Nature (1994) 369:571–4. doi: 10.1038/369571A0
46. Kaushansky K. The Mpl Ligand: Molecular and Cellular Biology of the Critical Regulator of Megakaryocyte Development. Stem Cells (Dayton Ohio) (1994) 12(Suppl 1):91–6; discussion 96–7. doi: 10.3324/HAEMATOL.2019.241406
47. O’Neill A, Chin D, Tan D, Abdul Majeed ABB, Nakamura-Ishizu A, Suda T. Thrombopoietin Maintains Cell Numbers of Hematopoietic Stem and Progenitor Cells With Megakaryopoietic Potential. Haematologica (2021) 106:1883–91. doi: 10.3324/HAEMATOL.2019.241406
48. van den Oudenrijn S, Bruin M, Folman CC, Peters M, Faulkner LB, de Haas M, et al. Mutations in the Thrombopoietin Receptor, Mpl, in Children With Congenital Amegakaryocytic Thrombocytopenia. Br J Haematol (2000) 110:441–8. doi: 10.1046/J.1365-2141.2000.02175.X
49. Tonelli R, Scardovi AL, Pession A, Strippoli P, Bonsi L, Vitale L, et al. Compound Heterozygosity for Two Different Amino-Acid Substitution Mutations in the Thrombopoietin Receptor (C-Mpl Gene) in Congenital Amegakaryocytic Thrombocytopenia (CAMT). Hum Genet (2000) 107:225–33. doi: 10.1007/S004390000357
50. Ballmaier M, Germeshausen M, Schulze H, Cherkaoui K, Lang S, Gaudig A, et al. C-Mpl Mutations Are the Cause of Congenital Amegakaryocytic Thrombocytopenia. Blood (2001) 97:139–46. doi: 10.1182/BLOOD.V97.1.139
51. Dasouki MJ, Rafi SK, Olm-Shipman AJ, Wilson NR, Abhyankar S, Ganter B, et al. Exome Sequencing Reveals a Thrombopoietin Ligand Mutation in a Micronesian Family With Autosomal Recessive Aplastic Anemia. Blood (2013) 122:3440–9. doi: 10.1182/BLOOD-2012-12-473538
52. Seo A, Ben-Harosh M, Sirin M, Stein J, Dgany O, Kaplelushnik J, et al. Bone Marrow Failure Unresponsive to Bone Marrow Transplant Is Caused by Mutations in Thrombopoietin. Blood (2017) 130:875–80. doi: 10.1182/BLOOD-2017-02-768036
53. Pecci A, Ragab I, Bozzi V, de Rocco D, Barozzi S, Giangregorio T, et al. Thrombopoietin Mutation in Congenital Amegakaryocytic Thrombocytopenia Treatable With Romiplostim. EMBO Mol Med (2018) 10:63–75. doi: 10.15252/EMMM.201708168
54. Ihara K, Ishii E, Eguchi M, Takada H, Suminoe A, Good RA, et al. Identification of Mutations in the C-Mpl Gene in Congenital Amegakaryocytic Thrombocytopenia. Proc Natl Acad Sci (1999) 96:3132–6. doi: 10.1073/PNAS.96.6.3132
55. Miyakawa Y, Oda A, Druker BJ, Miyazaki H, Handa M, Ohashi H, et al. Thrombopoietin Induces Tyrosine Phosphorylation of Stat3 and Stat5 in Human Blood Platelets. Blood (1996) 87:439–46. doi: 10.1182/BLOOD.V87.2.439.BLOODJOURNAL872439
56. Kimura S, Roberts AW, Metcalf D, Alexander WS. Hematopoietic Stem Cell Deficiencies in Mice Lacking C-Mpl, the Receptor for Thrombopoietin. Proc Natl Acad Sci USA (1998) 95:1195. doi: 10.1073/PNAS.95.3.1195
57. Yoshihara H, Arai F, Hosokawa K, Hagiwara T, Takubo K, Nakamura Y, et al. Thrombopoietin/MPL Signaling Regulates Hematopoietic Stem Cell Quiescence and Interaction With the Osteoblastic Niche. Cell Stem Cell (2007) 1:685–97. doi: 10.1016/J.STEM.2007.10.020
58. Nakamura-Ishizu A, Takubo K, Kobayashi H, Suzuki-Inoue K, Suda T. CLEC-2 in Megakaryocytes Is Critical for Maintenance of Hematopoietic Stem Cells in the Bone Marrow. J Exp Med (2015) 25:1772–85.e6. doi: 10.1084/jem.20150057
59. Nakamura-Ishizu A, Matsumura T, Stumpf PS, Umemoto T, Takizawa H, Takihara Y, et al. Thrombopoietin Metabolically Primes Hematopoietic Stem Cells to Megakaryocyte-Lineage Differentiation. Cell Rep (2018) 25:1772–85.e6. doi: 10.1016/J.CELREP.2018.10.059
60. Chen S, Hu M, Shen M, Wang S, Wang C, Chen F, et al. IGF-1 Facilitates Thrombopoiesis Primarily Through Akt Activation. Blood (2018) 132:210–22. doi: 10.1182/BLOOD-2018-01-825927
61. Machlus KR, Johnson KE, Kulenthirarajan R, Forward JA, Tippy MD, Soussou TS, et al. CCL5 Derived From Platelets Increases Megakaryocyte Proplatelet Formation. Blood (2016) 127:921–6. doi: 10.1182/BLOOD-2015-05-644583
62. Burstein SA, Mei R -L, Henthorn J, Friese P, Turner K. Leukemia Inhibitory Factor and Interleukin-11 Promote Maturation of Murine and Human Megakaryocytes In Vitro. J Cell Physiol (1992) 153:305–12. doi: 10.1002/JCP.1041530210
63. Ishibashi T, Kimura H, Uchida T, Kariyone S, Friese P, Burstein SA. Human Interleukin 6 Is a Direct Promoter of Maturation of Megakaryocytes In Vitro. Proc Natl Acad Sci (1989) 86:5953–7. doi: 10.1073/PNAS.86.15.5953
64. Nandurkar HH, Robb L, Tarlinton D, Barnett L, Köntgen F, Begley CG. Adult Mice With Targeted Mutation of the Interleukin-11 Receptor (IL11Ra) Display Normal Hematopoiesis. Blood (1997) 90:2148–59. doi: 10.1182/BLOOD.V90.6.2148
65. Gainsford T, Nandurkar M, Metcalf D, Robb L, Glenn Begley C, Alexander WS. The Residual Megakaryocyte and Platelet Production in C-Mpl–deficient Mice Is Not Dependent on the Actions of Interleukin-6, Interleukin-11, or Leukemia Inhibitory Factor. Blood (2000) 95:528–34. doi: 10.1182/BLOOD.V95.2.528
66. Campbell RA, Schwertz H, Hottz ED, Rowley JW, Manne BK, Washington AV, et al. Human Megakaryocytes Possess Intrinsic Antiviral Immunity Through Regulated Induction of IFITM3. Blood (2019) 133:2013–26. doi: 10.1182/blood-2018-09-873984
67. Köhler A, de Filippo K, Hasenberg M, van den Brandt C, Nye E, Hosking MP, et al. G-CSF-Mediated Thrombopoietin Release Triggers Neutrophil Motility and Mobilization From Bone Marrow via Induction of Cxcr2 Ligands. Blood (2011) 117:4349–57. doi: 10.1182/BLOOD-2010-09-308387
68. Cheng L, Qasba P, Vanguri P, Thiede MA. Human Mesenchymal Stem Cells Support Megakaryocyte and Pro-Platelet Formation From CD34+ Hematopoietic Progenitor Cells. J Cell Physiol (2000) 184:58–69. doi: 10.1002/(SICI)1097-4652(200007)184:1<58::AID-JCP6>3.0.CO;2-B
69. Broudy VC, Lin NL, Kaushansky K. Thrombopoietin (C-Mpl Ligand) Acts Synergistically With Erythropoietin, Stem Cell Factor, and Interleukin-11 to Enhance Murine Megakaryocyte Colony Growth and Increases Megakaryocyte Ploidy In Vitro. Blood (1995) 85:1719–26. doi: 10.1182/blood.v85.7.1719.bloodjournal8571719
70. Pallotta I, Lovett M, Rice W, Kaplan DL, Balduini A. Bone Marrow Osteoblastic Niche: A New Model to Study Physiological Regulation of Megakaryopoiesis. PloS One (2009) 4. doi: 10.1371/JOURNAL.PONE.0008359
71. Méndez-Ferrer S, Michurina TV, Ferraro F, Mazloom AR, MacArthur BD, Lira SA, et al. Mesenchymal and Haematopoietic Stem Cells Form a Unique Bone Marrow Niche. Nature (2010) 466:829–34. doi: 10.1038/nature09262
72. Sugiyama T, Kohara H, Noda M, Nagasawa T. Maintenance of the Hematopoietic Stem Cell Pool by CXCL12-CXCR4 Chemokine Signaling in Bone Marrow Stromal Cell Niches. Immunity (2006) 25:977–88. doi: 10.1016/j.immuni.2006.10.016
73. Rafii S, Shapiro F, Pettengell R, Ferris B, Nachman RL, Moore MA, et al. Human Bone Marrow Microvascular Endothelial Cells Support Long-Term Proliferation and Differentiation of Myeloid and Megakaryocytic Progenitors. Blood (1995) 86:3353–63. doi: 10.1182/blood.V86.9.3353.bloodjournal8693353
74. Rafii S, Mohle R, Shapiro F, Frey BM, Moore MAS. Regulation of Hematopoiesis by Microvascular Endothelium. Leukemia Lymphoma (1997) 27:375–86. doi: 10.3109/10428199709058305
75. Pober JS, Sessa WC. Evolving Functions of Endothelial Cells in Inflammation. Nat Rev Immunol (2007) 7:803–15. doi: 10.1038/nri2171
76. Yoshizumi M, Perrella MA, Burnett JC, Lee ME. Tumor Necrosis Factor Downregulates an Endothelial Nitric Oxide Synthase mRNA by Shortening Its Half-Life. Circ Res (1993) 73:205–9. doi: 10.1161/01.RES.73.1.205
77. Galasso G, Schiekofer S, Sato K, Shibata R, Handy DE, Ouchi N, et al. Impaired Angiogenesis in Glutathione Peroxidase-1– Deficient Mice Is Associated With Endothelial Progenitor Cell Dysfunction. Circ Res (2006) 98:254. doi: 10.1161/01.RES.0000200740.57764.52
78. Füreder W, Krauth MT, Sperr WR, Sonneck K, Simonitsch-Klupp I, Müllauer L, et al. Evaluation of Angiogenesis and Vascular Endothelial Growth Factor Expression in the Bone Marrow of Patients With Aplastic Anemia. Am J Pathol (2006) 168:123. doi: 10.2353/AJPATH.2006.050034
79. Hooper AT, Butler JM, Nolan DJ, Kranz A, Iida K, Kobayashi M, et al. Engraftment and Reconstitution of Hematopoiesis Is Dependent on VEGFR2 Mediated Regeneration of Sinusoidal Endothelial Cells. Cell Stem Cell (2009) 4:263. doi: 10.1016/J.STEM.2009.01.006
80. Zhan H, Lin CHS, Segal Y, Kaushansky K. The JAK2V617F-Bearing Vascular Niche Promotes Clonal Expansion in Myeloproliferative Neoplasms. Leukemia (2018) 32:462–9. doi: 10.1038/leu.2017.233
81. Suva LJ, Hartman E, Dilley JD, Russell S, Akel NS, Skinner RA, et al. Platelet Dysfunction and a High Bone Mass Phenotype in a Murine Model of Platelet-Type Von Willebrand Disease. Am J Pathol (2008) 172:430. doi: 10.2353/AJPATH.2008.070417
82. Ciovacco WA, Cheng YH, Horowitz MC, Kacena MA. Immature and Mature Megakaryocytes Enhance Osteoblast Proliferation and Inhibit Osteoclast Formation. J Cell Biochem (2010) 109:774–81. doi: 10.1002/JCB.22456
83. Kacena MA, Shivdasani RA, Wilson K, Xi Y, Troiano N, Nazarian A, et al. Megakaryocyte-Osteoblast Interaction Revealed in Mice Deficient in Transcription Factors GATA-1 and NF-E2. J Bone Mineral Res: Off J Am Soc Bone Mineral Res (2004) 19:652–60. doi: 10.1359/JBMR.0301254
84. Lee YS, Kwak MK, Moon SA, Choi YJ, Baek JE, Park SY, et al. Regulation of Bone Metabolism by Megakaryocytes in a Paracrine Manner. Sci Rep (2020) 10:1–12. doi: 10.1038/s41598-020-59250-6
85. Xiao M, Wang Y, Tao C, Wang Z, Yang J, Chen Z, et al. Osteoblasts Support Megakaryopoiesis Through Production of Interleukin-9. Blood (2017) 129:3196–209. doi: 10.1182/BLOOD-2016-11-749838
86. Larsen TE. Emperipolesis of Granular Leukocytes Within Megakaryocytes in Human Hemopoietic Bone Marrow. Am J Clin Pathol (1970) 53:485–9. doi: 10.1093/AJCP/53.4.485
87. Cunin P, Bouslama R, Machlus KR, Bonet MM, Lee PY, Wactor A, et al. Megakaryocyte Emperipolesis Mediates Membrane Transfer From Intracytoplasmic Neutrophils to Platelets. eLife (2019) 8. doi: 10.7554/ELIFE.44031
88. Cunin P, Nigrovic PA. Megakaryocyte Emperipolesis: A New Frontier in Cell-in-Cell Interaction. Platelets (2020) 31:700–6. doi: 10.1080/09537104.2019.1693035
89. Tanaka M, Aze Y, Fujita T. Adhesion Molecule LFA-1/ICAM-1 Influences on LPS-Induced Megakaryocytic Emperipolesis in the Rat Bone Marrow. Veterinary Pathol (1997) 34:463–6. doi: 10.1177/030098589703400511
90. Schmitt A, Drouin A, Massé J-M, Guichard J, Shagraoui H, Cramer EM. Polymorphonuclear Neutrophil and Megakaryocyte Mutual Involvement in Myelofibrosis Pathogenesis. Leukemia Lymphoma (2002) 43:719–24. doi: 10.1080/10428190290016809
91. Centurione L, di Baldassarre A, Zingariello M, Bosco D, Gatta V, Rana RA, et al. Increased and Pathologic Emperipolesis of Neutrophils Within Megakaryocytes Associated With Marrow Fibrosis in GATA-1(Low) Mice. Blood (2004) 104:3573–80. doi: 10.1182/blood-2004-01-0193
92. Spangrude GJ, Lewandowski D, Martelli F, Marra M, Zingariello M, Sancillo L, et al. P-Selectin Sustains Extramedullary Hematopoiesis in the Gata1 Low Model of Myelofibrosis. Stem Cells (Dayton Ohio) (2016) 34:67–82. doi: 10.1002/stem.2229
93. Cunin P, Nigrovic PA. Megakaryocytes as Immune Cells. J Leukocyte Biol (2019) 105:1111. doi: 10.1002/JLB.MR0718-261RR
94. Brandes ME, Mai UE, Ohura K, Wahl SM. Type I Transforming Growth Factor-Beta Receptors on Neutrophils Mediate Chemotaxis to Transforming Growth Factor-Beta. J Immunol (Baltimore Md: 1950) (1991) 147:1600–6. doi: 10.1002/JLB.MR0718-261RR
95. Deuel TF, Senior RM, Chang D, Griffin GL, Heinrikson RL, Kaiser ET. Platelet Factor 4 Is Chemotactic for Neutrophils and Monocytes. Proc Natl Acad Sci USA (1981) 78:4584. doi: 10.1073/PNAS.78.7.4584
96. Boilard E, Nigrovic PA, Larabee K, Watts GFM, Coblyn JS, Weinblatt ME, et al. Platelets Amplify Inflammation in Arthritis via Collagen-Dependent Microparticle Production. Science (New York NY) (2010) 327:580–3. doi: 10.1126/SCIENCE.1181928
97. Boilard E. Platelet-Derived Interleukin-1β Fuels the Fire in Blood Vessels in Systemic Lupus Erythematosus. Arteriosclerosis Thrombosis Vasc Biol (2017) 37:607–8. doi: 10.1161/ATVBAHA.117.309108
98. Sims MC, Mayer L, Collins JH, Bariana TK, Megy K, Lavenu-Bombled C, et al. Novel Manifestations of Immune Dysregulation and Granule Defects in Gray Platelet Syndrome. Blood (2020) 136:1956. doi: 10.1182/BLOOD.2019004776
99. Lee DH, Yao C, Bhan A, Schlaeger T, Keefe J, Rodriguez BAT, et al. Integrative Genomic Analysis Reveals Four Protein Biomarkers for Platelet Traits. Circ Res (2020) 127:1182–94. doi: 10.1161/CIRCRESAHA.119.316447
100. Jiang L, Han X, Wang J, Wang C, Sun X, Xie J, et al. SHP-1 Regulates Hematopoietic Stem Cell Quiescence by Coordinating TGF-ß Signaling. J Exp Med (2018) 215:1337–47. doi: 10.1084/jem.20171477
101. Jiang J, Kao CY, Papoutsakis ET. How do Megakaryocytic Microparticles Target and Deliver Cargo to Alter the Fate of Hematopoietic Stem Cells? J Controlled Release: Off J Control Release Soc (2017) 247:1. doi: 10.1016/J.JCONREL.2016.12.021
102. Pinho S, Marchand T, Yang E, Wei Q, Nerlov C, Frenette PS. Lineage-Biased Hematopoietic Stem Cells Are Regulated by Distinct Niches. Dev Cell (2018) 44:634–41. doi: 10.1016/j.devcel.2018.01.016
103. Arranz L, Sánchez-Aguilera A, Martín-Pérez D, Isern J, Langa X, Tzankov A, et al. Neuropathy of Haematopoietic Stem Cell Niche Is Essential for Myeloproliferative Neoplasms. Nature (2014) 512:78–81. doi: 10.1038/nature13383
104. Ho YH, del Toro R, Rivera-Torres J, Rak J, Korn C, García-García A, et al. Remodeling of Bone Marrow Hematopoietic Stem Cell Niches Promotes Myeloid Cell Expansion During Premature or Physiological Aging. Cell Stem Cell (2019) 25:407. doi: 10.1016/J.STEM.2019.06.007
105. Hart ECJ, Charkoudian N. Sympathetic Neural Regulation of Blood Pressure: Influences of Sex and Aging. Physiology (2014) 29:8–15. doi: 10.1152/PHYSIOL.00031.2013
106. Elefteriou F, Ahn JD, Takeda S, Starbuck M, Yang X, Liu X, et al. Leptin Regulation of Bone Resorption by the Sympathetic Nervous System and CART. Nature (2005) 434:514–20. doi: 10.1038/NATURE03398
107. Haas S, Hansson J, Klimmeck D, Loeffler D, Velten L, Uckelmann H, et al. Inflammation-Induced Emergency Megakaryopoiesis Driven by Hematopoietic Stem Cell-Like Megakaryocyte Progenitors. Cell Stem Cell (2015) 17:422–34. doi: 10.1016/J.STEM.2015.07.007
108. Morrell CN, Aggrey AA, Chapman LM, Modjeski KL. Emerging Roles for Platelets as Immune and Inflammatory Cells. Blood (2014) 123:2759–67. doi: 10.1182/blood-2013-11-462432
109. Mudd JC, Panigrahi S, Kyi B, Moon SH, Manion MM, Younes SA, et al. Inflammatory Function of CX3CR1+ CD8+ T Cells in Treated HIV Infection Is Modulated by Platelet Interactions. J Infect Dis (2016) 214:1808–16. doi: 10.1093/INFDIS/JIW463
110. Shi G, Field DJ, Ko KA, Ture S, Srivastava K, Levy S, et al. Platelet Factor 4 Limits Th17 Differentiation and Cardiac Allograft Rejection. J Clin Invest (2014) 124:543–52. doi: 10.1172/JCI71858
111. Finkielsztein A, Schlinker AC, Zhang L, Miller WM, Datta SK. Human Megakaryocyte Progenitors Derived From Hematopoietic Stem Cells of Normal Individuals Are MHC Class II-Expressing Professional APC That Enhance Th17 and Th1/Th17 Responses. Immunol Lett (2015) 163:84–95. doi: 10.1016/j.imlet.2014.11.013
112. Kaneider NC, Kaser A, Tilg H, Ricevuti G, Wiedermann CJ. CD40 Ligand-Dependent Maturation of Human Monocyte-Derived Dendritic Cells by Activated Platelets. Int J Immunopathol Pharmacol (2003) 16:225–31. doi: 10.1177/039463200301600307
113. Duffau P, Seneschal J, Nicco C, Richez C, Lazaro E, Douchet I, et al. Platelet CD154 Potentiates Interferon-α Secretion by Plasmacytoid Dendritic Cells in Systemic Lupus Erythematosus. Sci Trans Med (2010) 2. doi: 10.1126/SCITRANSLMED.3001001
114. Zufferey A, Speck ER, Machlus KR, Aslam R, Guo L, McVey MJ, et al. Mature Murine Megakaryocytes Present Antigen-MHC Class I Molecules to T Cells and Transfer Them to Platelets. Blood Adv (2017) 1:1773–85. doi: 10.1182/BLOODADVANCES.2017007021
115. Bernardes JP, Mishra N, Tran F, Bahmer T, Best L, Blase JI, et al. Longitudinal Multi-Omics Analyses Identify Responses of Megakaryocytes, Erythroid Cells, and Plasmablasts as Hallmarks of Severe COVID-19. Immunity (2020) 53:1296–314.e9. doi: 10.1016/J.IMMUNI.2020.11.017
116. Lefrançais E, Ortiz-Muñoz G, Caudrillier A, Mallavia B, Liu F, Sayah DM, et al. The Lung Is a Site of Platelet Biogenesis and a Reservoir for Haematopoietic Progenitors. Nature (2017) 544:105–9. doi: 10.1038/nature21706
117. Sharma GK, Talbot IC. Pulmonary Megakaryocytes: “Missing Link” Between Cardiovascular and Respiratory Disease? J Clin Pathol (1986) 39:969–76. doi: 10.1136/JCP.39.9.969
118. Pariser DN, Hilt ZT, Ture SK, Blick-Nitko SK, Looney MR, Cleary SJ, et al. Lung Megakaryocytes Are Immune Modulatory Cells. J Clin Invest (2021) 131. doi: 10.1172/JCI137377
119. Boilard E, Machlus KR. Location Is Everything When It Comes to Megakaryocyte Function. J Clin Invest (2021) 131. doi: 10.1172/JCI144964
120. Kaushansky K, Zhan H. The Marrow Stem Cell Niche in Normal and Malignant Hematopoiesis. Ann New York Acad Sci (2020) 1466:17. doi: 10.1111/NYAS.14028
121. Arber DA, Orazi A, Hasserjian R, Thiele J, Borowitz MJ, le Beau MM, et al. The 2016 Revision to the World Health Organization Classification of Myeloid Neoplasms and Acute Leukemia. Blood (2016) 127:2391–405. doi: 10.1182/BLOOD-2016-03-643544
122. Beer PA, Erber WN, Campbell PJ, Green AR. How I Treat Essential Thrombocythemia. Blood (2011) 117:1472–82. doi: 10.1182/BLOOD-2010-08-270033
123. Nangalia J, Green AR. Myeloproliferative Neoplasms: From Origins to Outcomes. Blood (2017) 130:2475–83. doi: 10.1182/BLOOD-2017-06-782037
124. O’Sullivan JM, Harrison CN. Myelofibrosis: Clinicopathologic Features, Prognosis, and Management. Clin Adv Hematol Oncol: H&O (2018) 16:121–31.
125. Jeremy Wen Q, Yang Q, Goldenson B, Malinge S, Lasho T, Schneider RK, et al. Targeting Megakaryocytic-Induced Fibrosis in Myeloproliferative Neoplasms by AURKA Inhibition. Nat Med (2015) 21:1473–80. doi: 10.1038/NM.3995
126. Martyré MC, le Bousse-Kerdiles MC, Romquin N, Chevillard S, Praloran V, Demory JL, et al. Elevated Levels of Basic Fibroblast Growth Factor in Megakaryocytes and Platelets From Patients With Idiopathic Myelofibrosis. Br J Haematol (1997) 97:441–8. doi: 10.1046/j.1365-2141.1997.292671.x
127. Cecchetti L, Tolley ND, Michetti N, Bury L, Weyrich AS, Gresele P. Megakaryocytes Differentially Sort mRNAs for Matrix Metalloproteinases and Their Inhibitors Into Platelets: A Mechanism for Regulating Synthetic Events. Blood (2011) 118:1903–11. doi: 10.1182/blood-2010-12-324517
128. Malara A, Abbonante V, Zingariello M, Migliaccio A, Balduini A. Megakaryocyte Contribution to Bone Marrow Fibrosis: Many Arrows in the Quiver. Mediterranean J Hematol Infect Dis (2018) 10:e2018068. doi: 10.4084/MJHID.2018.068
129. Ozono Y, Shide K, Kameda T, Kamiunten A, Tahira Y, Sekine M, et al. Neoplastic Fibrocytes Play an Essential Role in Bone Marrow Fibrosis in Jak2V617F-Induced Primary Myelofibrosis Mice. Leukemia (2021) 35:454–67. doi: 10.1038/s41375-020-0880-3
130. Zingariello M, Ruggeri A, Martelli F, Marra M, Sancillo L, Ceglia I, et al. A Novel Interaction Between Megakaryocytes and Activated Fibrocytes Increases TGF-β Bioavailability in the Gata1low Mouse Model of Myelofibrosis. Am J Blood Res (2015) 5:34.
131. Psaila B, Wang G, Rodriguez-Meira A, Li R, Heuston EF, Murphy L, et al. Single-Cell Analyses Reveal Megakaryocyte-Biased Hematopoiesis in Myelofibrosis and Identify Mutant Clone-Specific Targets. Mol Cell (2020) 78:477–92.e8. doi: 10.1016/J.MOLCEL.2020.04.008/ATTACHMENT/2A661996-558A-46B0-AF9F-F7F3E6E42411/MMC5.XLSX
132. Rodriguez-Meira A, Buck G, Clark S-A, Povinelli BJ, Alcolea V, Louka E, et al. Unravelling Intratumoral Heterogeneity Through High-Sensitivity Single-Cell Mutational Analysis and Parallel RNA Sequencing. Mol Cell (2019) 73:1292–305.e8. doi: 10.1016/j.molcel.2019.01.009
133. Rampal R, Al-Shahrour F, Abdel-Wahab O, Patel JP, Brunel JP, Mermel CH, et al. Integrated Genomic Analysis Illustrates the Central Role of JAK-STAT Pathway Activation in Myeloproliferative Neoplasm Pathogenesis. Blood (2014) 123:e123–33. doi: 10.1182/BLOOD-2014-02-554634
134. Baryawno N, Przybylski D, Kowalczyk MS, Kfoury Y, Severe N, Gustafsson K, et al. A Cellular Taxonomy of the Bone Marrow Stroma in Homeostasis and Leukemia. Cell (2019) 177:1915–32.e16. doi: 10.1016/J.CELL.2019.04.040
135. Gleitz HFE, Dugourd AJF, Leimkühler NB, Snoeren IAM, Fuchs SNR, Menzel S, et al. Increased CXCL4 Expression in Hematopoietic Cells Links Inflammation and Progression of Bone Marrow Fibrosis in MPN. Blood (2020) 136:2051–64. doi: 10.1182/blood.2019004095
136. Malara A, Abbonante V, di Buduo CA, Tozzi L, Currao M, Balduini A. The Secret Life of a Megakaryocyte: Emerging Roles in Bone Marrow Homeostasis Control. Cell Mol Life Sci: CMLS (2015) 72:1517. doi: 10.1007/S00018-014-1813-Y
137. Oyama F, Hirohashi S, Shimosato Y, Titani K, Sekiguchi K. Oncodevelopmental Regulation of the Alternative Splicing of Fibronectin Pre-Messenger RNA in Human Lung Tissues. Cancer Res (1990) 50:1075–8.
138. Doddapattar P, Gandhi C, Prakash P, Dhanesha N, Grumbach IM, Dailey ME, et al. Fibronectin Splicing Variants Containing Extra Domain A Promote Atherosclerosis in Mice Through Toll-Like Receptor 4. Arteriosclerosis Thrombosis Vasc Biol (2015) 35:2391–400. doi: 10.1161/ATVBAHA.115.306474
139. Muro AF, Moretti FA, Moore BB, Yan M, Atrasz RG, Wilke CA, et al. An Essential Role for Fibronectin Extra Type III Domain A in Pulmonary Fibrosis. Am J Respir Crit Care Med (2008) 177:638–45. doi: 10.1164/RCCM.200708-1291OC
140. Jarnagin WR, Rockey DC, Koteliansky VE, Wang SS, Montgomery Bissell D. Expression of Variant Fibronectins in Wound Healing: Cellular Source and Biological Activity of the EIIIA Segment in Rat Hepatic Fibrogenesis. J Cell Biol (1994) 127:2037–48. doi: 10.1083/JCB.127.6.2037
141. Malara A, Gruppi C, Celesti G, Abbonante V, Viarengo G, Laghi L, et al. Alternatively Spliced Fibronectin Extra Domain A Is Required for Hemangiogenic Recovery Upon Bone Marrow Chemotherapy. Haematologica (2018) 103:e42–5. doi: 10.3324/HAEMATOL.2017.173070
Keywords: megakaryocyte (MK), niche, bone marrow, heterogeneity, hematopoietic stem and progenitor cell (HSPC), emperipolesis, immune
Citation: Khatib-Massalha E and Méndez-Ferrer S (2022) Megakaryocyte Diversity in Ontogeny, Functions and Cell-Cell Interactions. Front. Oncol. 12:840044. doi: 10.3389/fonc.2022.840044
Received: 20 December 2021; Accepted: 17 January 2022;
Published: 04 February 2022.
Edited by:
Anna Rita Migliaccio, Icahn School of Medicine at Mount Sinai, United StatesReviewed by:
Mortimer Poncz, Children’s Hospital of Philadelphia, United StatesJames Palis, University of Rochester, United States
Copyright © 2022 Khatib-Massalha and Méndez-Ferrer. This is an open-access article distributed under the terms of the Creative Commons Attribution License (CC BY). The use, distribution or reproduction in other forums is permitted, provided the original author(s) and the copyright owner(s) are credited and that the original publication in this journal is cited, in accordance with accepted academic practice. No use, distribution or reproduction is permitted which does not comply with these terms.
*Correspondence: Eman Khatib-Massalha, ZWs1NTBAY2FtLmFjLnVr; Simón Méndez-Ferrer, c20yMTE2QGNhbS5hYy51aw==