- 1Department of Oncology, Azienda Unità Sanitaria Locale (AUSL) Bologna, Bologna, Italy
- 2Nervous System Medical Oncology Department, Istituto di Ricovero e Cura a Carattere Scientifico (IRCCS) Istituto delle Scienze Neurologiche di Bologna, Bologna, Italy
The glioma-associated tumor microenvironment involves a multitude of different cells ranging from immune cells to endothelial, glial, and neuronal cells surrounding the primary tumor. The interactions between these cells and glioblastoma (GBM) have been deeply investigated while very little data are available on patients with lower-grade gliomas. In these tumors, it has been demonstrated that the composition of the microenvironment differs according to the isocitrate dehydrogenase status (mutated/wild type), the presence/absence of codeletion, and the expression of specific alterations including H3K27 and/or other gene mutations. In addition, mechanisms by which the tumor microenvironment sustains the growth and proliferation of glioma cells are still partially unknown. Nonetheless, a better knowledge of the tumor-associated microenvironment can be a key issue in the optic of novel therapeutic drug development.
Introduction
Gliomas are the most frequent primary tumors of the central nervous system (CNS) with an estimated incidence of 7.1/100,000 cases in the United States (1). Glioblastoma (GBM) encounters 55% of all glioma diagnoses while the remaining 45% of cases are represented by other glioma subtypes (1). Overall, gliomas are mainly divided into isocitrate dehydrogenase (IDH) mutant and IDH wild-type (wt) tumors (1–3). In the primary group, composed of IDH-mutated tumors, the World Health Organization (WHO) 2021 classification recognizes oligodendroglioma (presenting 1p19q codeletion) and astrocytoma (without 1p19q codeletion) (4). These tumors can be further divided into WHO grade 2 (oligodendroglioma and diffuse astrocytoma) and 3 (anaplastic oligodendroglioma and anaplastic astrocytoma) gliomas.
Gliomas without IDH mutations are defined as IDH-wt gliomas. According to the WHO 2021 classification, the presence of IDH-wt and other molecular alterations such as TERT (Telomerase Reverse Transcriptase), EGFR (Epidermal Growth Factor Receptor), or gain of chromosome 7/loss of chromosome 10 allows defining these tumors as a molecular GBM (4). Gliomas with H3K27 alterations (27th amino acid of Histone 3) is a new entity of IDH-wt gliomas diagnosed in pediatric patients but occasionally also in adults (4).
The prognosis of patients with gliomas (5) is extremely variable, ranging from decades in low-grade IDH-mutated gliomas to a few months in IDH-wt tumors (6). The standard clinical therapeutic approach is represented by maximal safe surgical resection followed by radiation therapy and chemotherapy (2, 3, 6).
In the last few years, important improvements toward a better understanding of genetic and epigenetic pathways regulating glioma development and growth have been done. These mechanisms could explain the different clinical courses and evolution of these malignancies. Indeed, genomic and epigenomic alterations differ in each glioma subtype explaining the different histology, clinical course, and biological behavior. The tumor-associated microenvironment appears to be another key element influencing the development, progression, and clinical evolution of gliomas.
Indeed, the tumor microenvironment (TME) composition is manipulated directly by cancer cells; therefore, TME composition changes according to the different alterations expressed by tumors. On the other hand, TME can sustain tumor growth and development in different ways (7).
Interactions between TME and GBM have been largely evaluated (7), while few data are available on patients with IDH-mutated/wt low-grade gliomas.
In this review, we examine current knowledge toward TME surrounding IDH-mutated and IDH-wt glioma (excluding GBM). To better understand TME composition, we analyzed the genomic landscape of each tumor subtype. Finally, we investigated possible therapeutic strategies aimed to target TME. Our focus is mainly oriented on adult gliomas; thus, we exclude pediatric malignancies and GBM from this review.
IDH-Mutated Gliomas
Microscopically IDH-mutated, 1p19q codeleted oligodendrogliomas appear as tumor cells with rounded nuclei, clear perinuclear halons, frail capillaries, and focal microcalcification (3, 4, 6). An increased number of mitoses, vascular proliferation, and necrosis is observed in CNS WHO grade 3 oligodendrogliomas (3, 4, 6).
IDH-mutated 1p19q non-codeleted astrocytomas spread with perineuronal, perivascular, or subpial patterns and present nuclear atypia and pleomorphism (Table 1). The higher tumor grade is associated with increased mitotic activity, angiogenesis, and necrosis (3, 4, 6).
Oligodendrogliomas and astrocytomas represent 40%–45% and 50%–55% of all glioma diagnoses with an estimated survival ranging from 6.5 to over 15 years (6, 11–13).
Maximal safe surgical resection is the standard of care for these tumors (2, 3). In WHO grade 2 astrocytomas and oligodendrogliomas, follow-up after surgery is considered in low-risk patients while adjuvant radiation therapy (RT) followed by chemotherapy [temozolomide/TMZ (9) or procarbazine plus lomustine plus vincristine/PCV (10, 15)] is commonly used in high-risk patients (8, 9).
Genomic Landscape of IDH-Mutated Gliomas
Oligodendroglioma and astrocytoma significantly differ in their genomic alterations (16).
In addition to IDH1 or IDH2 mutations and 1p19q codeletion, oligodendrogliomas frequently present mutations of TERT (96%), CIC (Capicua Transcriptional Repressor, 62%), FUBP1 (Far Upstream Element Binding Protein 1, 29%), and/or PI3K (phosphoinositide 3-kinase, 20%) with overexpression of NOTCH1 (Notch homolog 1, translocation-associated, 31%) genes (16). Notably, ATRX (X-linked nuclear protein) mutations are rare in oligodendrogliomas and are mutually exclusive with TERT since both these two genes target the lengthening telomeres (16).
Almost all astrocytomas present p53 (94%) alterations, ATRX (86%) loss, or CDKN2A/CDKN2B (cyclin-dependent kinase inhibitor 2A/2B, 10%) homozygous deletion (16, 17). Curiously, astrocytomas present often non-canonical IDH1 mutations that are associated with improved survival (18–20). Recently, a next-generation sequencing (NGS) analysis carried out on 432 patients with anaplastic astrocytomas enrolled in the CATNON trial revealed a prognostic role of some selected genes. In particular, amplification of PDGFR (platelet-derived growth factor receptor) genes, CDKN2A/CDKN2B homozygous deletion, and PI3K mutations were independently associated with worse prognosis in patients with anaplastic (WHO grade 3) astrocytomas (17).
Another improvement toward a better knowledge of glioma genomic assessment was carried out in a large study adopting single-cell RNA sequencing (21). Researchers were able to evaluate with high precision the single-cell expression silencing confounding factors related to intratumoral genetic heterogeneity and genomic analysis of TME that can pollute large NGS studies (16).
In this study, researchers were able to identify, within tumor specimens assessed, three specific cellular populations: two differentiated tumoral cells belonging to oligodendrogliomas or astrocytomas and an undifferentiated phenotype (21). Through this precise gene expression analysis, the authors highlighted a shared expression between undifferentiated cells from oligodendrogliomas and astrocytomas, suggesting a shared progenitor of these two entities. These progenitor cancer cells presented alterations of key transcription factors such as SOX4 (SRY-Box Transcription Factor 4), SOX11 (SRY-Box Transcription Factor 11), and TCF4 (Transcription Factor 4). The percentage of these alterations increased according to CNS grade and the number of recurrences (21).
IDH-mutated gliomas seem to originate from a shared progenitor stem cell. Early acquired alterations are IDH with or without 1p19q codeletion. These events drive subsequent genomic alterations and explain the differences in terms of gene expression and TME (see below) within these two subtypes (21).
The progenitor cell of oligodendrogliomas and astrocytomas has a transcriptional phenotype similar to progenitor neural cells. These neural lineages can differentiate toward astrocytic or oligodendrocyte-like tumoral cancer cells (21, 22). These findings overturned the previous belief that there were two distinct progenitor cells for oligodendrogliomas and astrocytomas (23).
Differently, the progenitor cells of IDH-wt gliomas assume high levels of cellular state plasticity. Indeed, these ancestral cells can differentiate toward different transcriptional subtypes named mesenchymal, classic, and/or proneural (24). Subsequent studies differentiated the transcriptional subtypes into four main groups named astrocyte (EGFR amplified), oligodendrocyte (PDGFRA amplified), neural progenitor (CDK mutated), and mesenchymal (NF1 mutated)-like lineages (25).
Another important issue is the absence of a defined hierarchy between tumoral subtypes within IDH-wt gliomas (25). Thus, cancer cells can proliferate and switch from one subtype to another with high plasticity and heterogeneity (25). This finding diverges from what was observed in IDH-mutated and IDH-wt H3K27 gliomas. Indeed, IDH-wt H3K27 altered gliomas present a specific progenitor cell that has an oligodendrocyte-like transcription lineage (26). During the evolution, these progenitor cells lose (due to the alterations of histone 3) oligodendrocyte lineage differentiating toward an astrocyte-like cancer cell phenotype (26).
Microenvironment of IDH-Mutated Gliomas
Interactions between tumor cells and surrounding cells are complex and depend on the genetic expression of a specific tumor subtype (Figure 1). In general, tumor cells interact with immune cells, endothelial cells, and neurons (Table 2).
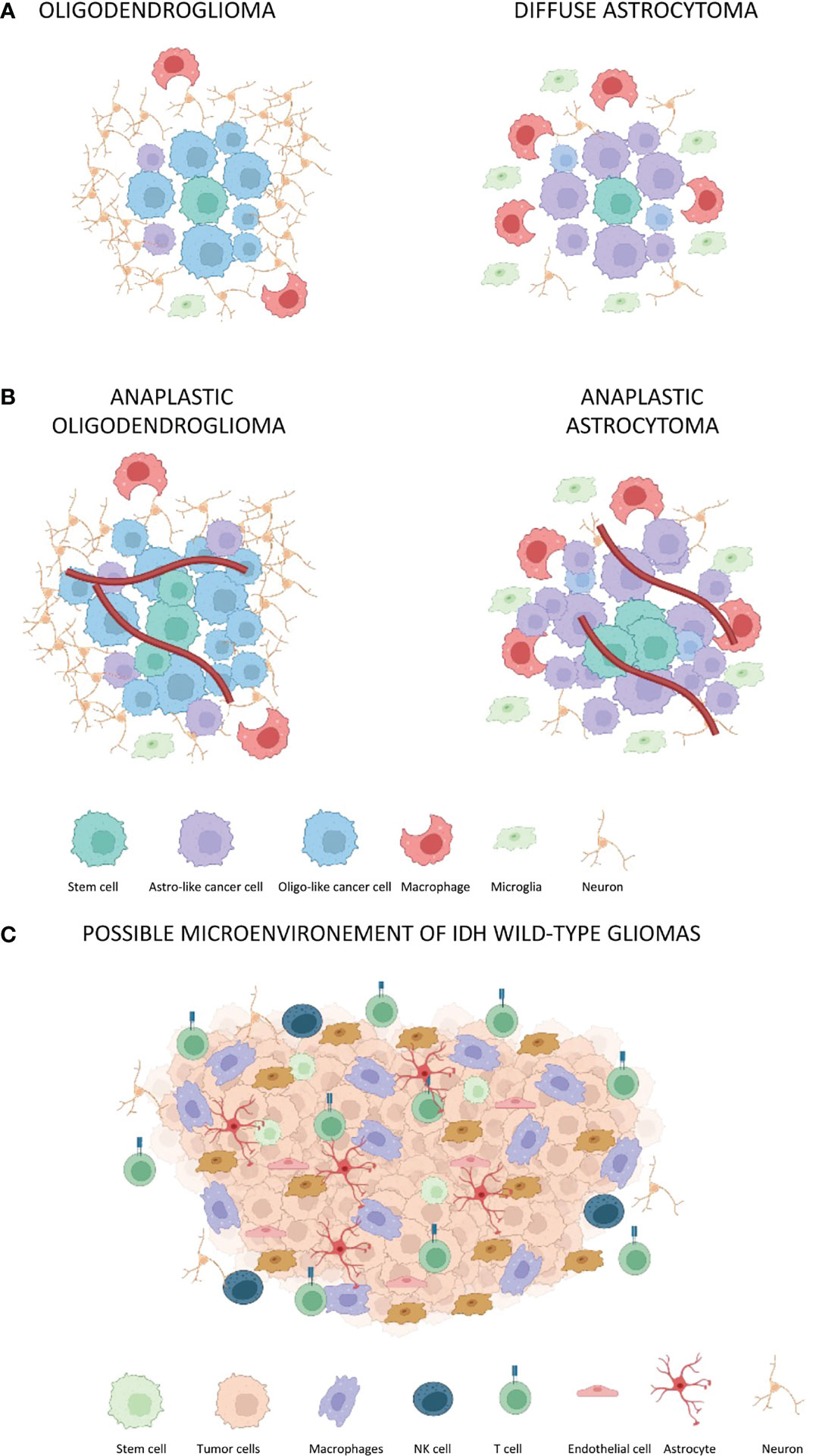
Figure 1 The tumor-associated microenvironment of IDH-mutated and IDH-wt gliomas. Red lines represent blood vessels. (A) Oligodendroglioma and diffuse astrocytoma without a significant blood vessel proliferation. The oligodendroglioma microenvironment presents a reduced percentage of macrophages, microglia, and astro-like cells as compared to diffuse astrocytoma. (B) The same composition of microenvironment associated with oligodendroglioma and astrocytoma is maintained in anaplastic gliomas. However, there is an increased tumor cell proliferation as well as increased angiogenesis. (C) Composition of microenvironment associated to GBM with CD4 immune regulatory and CD8 T-lymphocyte. Notably, CD8 lymphocytes assume the classical exhaustion phenotype expressing several inhibitory receptors including the PD-1, T-cell membrane protein 3 (TIM3), lymphocyte activation gene 3 protein (LAG3), and T-cell immunoreceptor with immunoglobulin and ITIM domains (TIGIT) (27).
Important insights into connections between IDH mutant gliomas and immune cells have been provided by the study carried out by Venteicher et al. investigating single-cell RNA expression (21).
The authors identified an inflammatory expression derived from two specific and different signatures (21). The first was a microglia signature characterized by specific markers such as CX3CR1 (C-X-C motif chemokine receptor 1), P2RY12 (purigenic receptor P2Y12), and P2RY13 (purigenic receptor P2Y13). The second signature was a macrophage signature identified by the expression of CD163, TGFβ1 (transforming growth factor β1), and F13A1 (coagulation factor XIII A chain) (21). Notably, the distribution of the signature markers of both microglia and macrophages followed a continuum more than a bimodal scheme. Therefore, a macrophage that has reached the TME can acquire a microglia-like expression according to the phenotype expressed by cancer cells (21). On the other hand, microglia cannot differentiate to a macrophage immune profile (21). Finally, a subtle but reported difference between resident and tissue-derived macrophages has been reported (21).
Other important findings of this study revealed that macrophage signature and expression were more frequently associated with astrocytoma as compared to oligodendroglioma (21). Moreover, the macrophage signature was significantly associated with angiogenesis and endothelial activities. The same association was not true in cells expressing microglia signature.
According to these results, the presence of macrophages seems to enhance angiogenesis, progression, and glioma development. However, the mechanisms behind these interactions are unknown (21).
In this optic, it is essential to observe that the role of macrophages on glioma proliferation and development has only been investigated with a single-cell approach; thus, no definitive conclusions can be deduced. This is mainly because mechanisms beyond macrophage and glioma proliferation stimulation are largely unknown.
A subsequent study investigated TME composition in patients with IDH-mutated gliomas. These studies adopted different approaches involving flow cytometry, RNA sequencing, protein arrays, culture assays, and spatial tissue characterization (42) or also high-dimensional single-cell profiling (43). These assessments revealed a disease-specific enrichment of immune cells with a significant difference in proportional abundance of microglia, macrophages, neutrophils, and T cells (42). In particular, macrophages showed a distinctive signature trajectory that differs according to the primary tumor subtype (43).
A similar interaction has also been observed in GBM. This confirms that the macrophage signature is associated with angiogenesis and tumor progression (28–32). GBM cancer cells can attract monocyte, microglia, and macrophage by the production of several factors including CCL2 (C-C motif chemokine ligand 2), CCL7 (C-C motif chemokine ligand 7), GDNF (glial cell-derived neurotrophic factor), SDF1 (stromal cell-derived factor 1), TNF (tumor necrosis factor), VEGF (vascular endothelial growth factor), ATP (adenosine triphosphate), CSF-1 (colony-stimulating factor 1), GM-CSF (granulocyte-macrophage colony-stimulating factor), and expression of OLIG2 (oligodendrocyte transcription factor 2). Once attracted into the TME, macrophages can attract monocytes by the production of CCL2 and CCR2. Monocytes can be further oriented toward a macrophage signature by factors secreted by GBM (7, 28–32).
Macrophages contribute to the development of an immune-suppressive environment by the production of TGFβ1, ARG1 (arginase 1), and/or IL-10 (interleukin 10) (44). Macrophages can further stimulate angiogenesis through the production of VEGF (vascular endothelial growth factor) and metalloproteases (44).
It is important to remark that all these interactions have been demonstrated in GBM while no data are available on low-grade IDH-mutated gliomas.
A recent study investigating transcriptomic data of low-grade gliomas associated with TME identified three specific immune signatures (45). The first signature (Im1) identified a high number of T cells, Th17, and mast cells. The second signature (Im2) was composed of macrophages and exhausted CD8+ T cells. Tumors harboring the Im2 signature were associated with worst prognosis. Finally, the third signature (Im3) was composed of T-helper, antigen-presenting cells, and macrophages; 23.7% of tumor samples analyzed in this study were IDH-wt tumors; therefore, it is unknown which patterns are present in IDH mutant low-grade gliomas (45).
Another trial (37) assessed the single-cell expression of TME associated with 10 WHO grade 2 astrocytomas and 4 WHO grade 2 oligodendrogliomas. This study identified the presence of several immune cells like CD8+, CD4+, regulatory T cells, and natural killer cells (37). This is one of the first studies characterizing tumor-associated lymphocytes in low-grade gliomas. Notably, the authors identified that TME associated with astrocytoma presented a more inhibitory feature as compared with those observed in oligodendrogliomas. Finally, the increased percentage of CD8+ PD-1 (programmed death receptor 1) expressing T cell and CD4+ TIM3 (T-cell immunoglobulin and mucin-domaining containing-3) expressing T cell in association with regulatory T cells and macrophages was mainly responsible for an immune-suppressive contexture (37).
Interactions between IDH-mutated glioma cells and neurons and normal glial cells are largely unknown. It is well known that glioma is clinically associated with several neurological symptoms including cognitive or motility deficits, verbal fluency, headaches, and seizures (46–48). Notably, before diagnosis, low-grade gliomas often have a pre-symptomatic period. At this time, tumors may occupy a significant volume of the brain without manifesting significant symptoms. It has been proposed that neurons and glial cells can adapt to the tumor presence by activating a reactive response resulting in plasticity (49–51). There are several remarkable examples of this plasticity (50).
Brain tissue could adapt to the presence of tumors even if the mechanisms of this plasticity remain largely hidden. Furthermore, we ignore if low-grade IDH-mutated tumor cells can directly interact with neurons and altered glial cells. Tumor cells produce glutamate, which is released through the SLC7A11 (solute carrier family 7 member 11) glutamate–cysteine exchanger (38–40). The glutamate excess interacts with NMDA (N-methyl-D-aspartate receptor) receptors, inducing an influx of calcium on neurons and resulting in seizure onset and neuron death (38–40). Astrocytes activated after an injury can promote the secretion of cytokines and growth factors (52). Furthermore, astrocytes can also alter the permeability of the blood–brain barrier (BBB) (52). It has been demonstrated that GBM cells use astrocyte activation to sustain tumor growth and development while this has not been demonstrated in low-grade IDH-mutated tumors. Curiously, a recent study recognizes the importance of the glucose transporter GLUT1, which seems to mediate glioma cells’ perineuronal satellitosis in mice (53).
The study of the interactions between low-grade IDH-mutated tumor cells and surrounding neurons/glial cells is one of the most attractive and emerging issues. Future studies will probably offer novel insights into these important connections. Notably, it has been demonstrated that GBM interacting with neurons in a specific niche can differentiate toward an oligodendrocyte subtype (54), losing their infiltrative behaviors. Neuron stimulation could therefore modify tumor cell proliferation and development, making these interactions of particular interest.
IDH-wt Gliomas
The IDH-wt gliomas represent a heterogeneous family of tumors. In general, IDH-wt astrocytoma represent 5%–12% of all low-grade gliomas (6, 11–13). These tumors are diagnosed in older age (45–55 years) compared to IDH-mutated gliomas and are associated with shorter survival (from 15 to 36 months) (6, 11–13).
No randomized trials have been designed to assess the role of systemic treatments in IDH-wt WHO grade 2 or 3 tumors (2, 3). A second interim analysis of the CATNON trial showed in a subgroup analysis that neither concurrent nor adjuvant TMZ was associated with a survival improvement in this population (8). To date, the most appropriate clinical management after surgical resection of IDH-wt gliomas (excluding GBM) is unclear.
The diffuse midline H3K27M glioma is a novel entity recognized by the WHO 2021 classification (4). This tumor involves 80% of brain stem tumors in children and adolescents with an estimated median survival of 9–11 months. Occasionally, these tumors can also be diagnosed in adult patients and are associated with the same dismal prognosis observed in children (1). To date, no standard therapeutic approaches for midline gliomas have been approved, and inclusion in clinical trials should be encouraged (3).
Genomic Landscape of IDH-wt Gliomas
The TCGA assessed 282 low-grade gliomas, of which 56 (19.8%) were low-grade IDH-wt subtypes. In this study, the most frequent mutated genes were as follows: TERT (64%), EGFR (27%), PTEN (23%, phosphatase and tensin homolog), NF1 (20%, neurofibromin 1), TP53 (14%), and PIK3CA (9%) (16). Focal deletions of CDKN2A and RB1 (retinoblastoma-associated protein 1) occurred in 63% and 25% of cases, respectively. Gain of chromosome 7/loss of chromosome 10 was evident in 56% of cases (16).
As previously reported, IDH-mutated tumors could share a common progenitor that can differentiate into an astrocytic or oligodendroglioma phenotype (21). IDH-wt tumors are composed of cancer cells with the ability to modify their transcriptional profile assuming cellular plasticity (24, 25, 55, 56) and assuming all transcriptional subtypes (57) exchange within the same tumors (24, 25, 56). In other words, IDH-wt cancer cells can modify their phenotype ranging from the following subtypes:
1) Astrocytic-like cells characterized mainly by EGFR amplification,
2) Oligodendrocyte/neural progenitor phenotype frequently associated with PDGFRA/CDK4 amplification, and
3) Mesenchymal transcriptome associated with NF1 mutation.
The complexity of this scheme makes it clear how IDH-wt can be heterogeneous and therefore extremely similar to GBM (25).
It is important to remark that all these data have been provided by patients harboring an IDH-wt GBM while no studies focused on precursor cells within low-grade IDH-wt gliomas.
Genomic Landscape of IDH-wt H3K27 Midline Gliomas
In midline gliomas, the pathognomonic alterations are represented by the lysine-to-methionine substitution at position 27 of histones 3.1 and 3.3 (58–61).
These alterations lead to the inactivation of the PRC2 (polycomb repressive complex-2 methyltransferases complex) hiding gene expression (58–61). This alteration is diagnosed in about 80% of cases of midline glioma. Nonetheless, other mechanisms converge to PRC2 altered function (4). Indeed, the EZH inhibitory protein (EZHIP, CXorf7) is overexpressed in some midline gliomas and posterior fossa type A ependymomas (62). The hyperexpression of EZHIP leads to an inhibitory activity on PCR2 similar to H3 mutations (62). These tumors often present p53 (42%), ACVR1 (activin A receptor type 1), PPM1D (9%–23%, phosphatase Mg2+/Mn2+-dependent 1D), and PI3K mutations (58–61, 63, 64). Notably, PPM1D mutations are mutually exclusive with p53 (activation of PPM1D leads to p53 inactivation) (65). Other amplified genes are PDGFB (platelet-derived growth factor B), CCND1 (cyclin D1), CCND2 (cyclin D2), CCND3 (cyclin D3), CDK4 (cyclin-dependent kinase 4), and CDK6 (cyclin-dependent kinase 6) (58–61, 63, 64).
Precursor cells of diffuse midline gliomas display an oligodendrocyte phenotype (26). Nonetheless, the alteration occurring in PRC2 blocks the differentiation toward oligodendrocyte subtype; thus, the cancer cells assume an astrocyte-like phenotype instead of an oligodendrocyte one (26).
Microenvironment of IDH-wt Gliomas
Before the 2021 WHO classification, which recognized the diagnosis of “molecular glioblastoma”, IDH-wt gliomas were considered distinct tumor subtypes (66). In a study published in 2015 by Reuss et al. evaluating the molecular assessment of 160 IDH-wt glioma specimens, almost all tumors were finally classified as GBM or midline glioma (66). Not surprisingly, studies assessing TME on IDH-wt gliomas present the same results observed in similar analyses carried out on GBM patients (7, 67). In 2020, a study assessed and validated a specific immune signature in cohorts of patients with low-grade IDH wild-type gliomas (68). The authors identified an immune signature associated with a worse prognosis and characterized by high expression of immune-exhaustion markers and immune-depressive cytokines released by macrophages (68). On the other hand, researchers also identified a second phenotype associated with an elevated expression of lymphocyte and plasma cell-related genes (68).
In conclusion, most studies assessing TME on IDH-wt gliomas assessed the TME composition of GBM (7, 67). The complex interactions between tumor cells and TME (42, 43, 69, 70) required a specific and detailed discussion and are outside the scope of the current paper.
There are few studies investigating the TME of patients with midline gliomas. Nonetheless, there are particular issues concerning the tumor immune-associated stroma of these malignancies. These are immunologically cold tumors with a low percentage of T/NK cells (14). The main factors expressed are CCL2, CCL5, CSF1, CXCL12, and PDGFA with mainly enhanced microglia surrounding tumor cells (14).
It has been demonstrated that microglia associated with midline glioma assume their morphology with enlarged cell bodies and shorter processes (33–35). Similar to IDH-mutated astrocytomas and GBM, the microenvironment of midline gliomas is also enriched with macrophages. The PDGFB seems to be mainly responsible for macrophage recruitment (33–35). Compared to other CNS primary tumors, macrophages associated with midline gliomas have a lower expression of IL6, IL1A, IL1B, CCL3, and CCL4. Of interest, a gene-expression study demonstrated a significant difference between macrophages associated with GBM and midline gliomas (35). In particular, GBM-associated macrophages express mainly genes related to monocyte/neutrophil chemotaxis and chemokine while midline glioma-associated macrophages express genes related to angiogenesis, extracellular matrix organization, and angiogenesis (33–35). Furthermore, these tumors present a non-inflammatory environment as demonstrated by a low concentration of natural killer cells and infiltrating lymphocytes. This inflammation-desert microenvironment can be partially attributed to the reduced presence of key cytokines including the IL2 resulting from high levels of TGFβ and IL8 (14, 34). The other two key factors commonly observed in midline gliomas are a high concentration of the chemokines CCL2, CCL5, and the receptor PDGFRA, which directly support proliferation and cell survival (71).
While the midline glioma-associated immune contexture has just begun to be investigated, there is no data regarding interactions of these cells with other elements such as neurons, white matter, and glial cells.
In brief, GBM TME has a large infiltration of immune cells; however, their immune activity is mainly shifted toward an immune-suppressive phenotype. Midline glioma TME presents unique macrophages and microglia with its characteristics and morphology. Midline glioma shows a reduced presence of inflammation cells rather than a high number of immune cells with an inhibited response as observed in GBM TME.
Therapeutic Implications and Future Developments
The TME offers novel targets for the treatment of malignant gliomas (72–74). The clinical importance of TME has been largely investigated in GBM (7), while only recently has it been assessed in patients with low-grade gliomas. Furthermore, the majority of studies concentrate their investigation on the immune cells’ composition of TME excluding other important elements such as neurons, stromal cells (such as fibroblasts), and other glial cells (75).
Among patients with IDH-mutated gliomas, the importance of tumor-associated cells is gaining increasing interest; however, no therapeutic drugs are acting directly on TME.
There are several systemic agents shown to modify the TME (76).
Immune checkpoint inhibitors (ICIs) are agents able to restore a suppressed immune response against tumors by targeting specific receptors named immune checkpoints. The programmed death receptor 1 (PD1) is the target of the monoclonal antibodies nivolumab and pembrolizumab, which have been tested in patients with GBM. In GBM, pembrolizumab has been investigated as a neoadjuvant treatment shown to induce a significant modification of the TME. These modifications consisted of an increased number of T cells, reduced monocytic population, activation of interferon γ-related gene expression, and downregulation of cell-cycle-related genes (77). Similarly, also the PD-1 inhibitor nivolumab can modulate the TME composition of GBM patients (78). Despite these positive results, no trials demonstrated that ICIs improved the clinical outcome of patients with GBM (79). To date, nivolumab is under evaluation in combination with the IDH inhibitor ivosidenib in patients with IDH-mutated tumors (NCT04056910).
Vaccines are other treatment strategies employed for the treatment of patients with gliomas (80). The NOA16 trial was a phase I study testing an IDH1-specific peptide vaccine among patients with IDH1-mutated gliomas. Notably, of the 33 patients receiving the vaccine, 93.3% presented an immune response (81). Patients responding to the vaccine more frequently had a pseudo-progression suggesting inflammation on the tumor site. Moreover, these same patients showed an increased T-cell response switching from an immune-inhibited to an immune-active environment (81). IDH vaccines are under evaluation in patients with gliomas (NCT03893903).
IDH inhibitors are a promising treatment approach (82), and a phase III trial (INDIGO, NCT04164901) is currently assessing the IDH pan-inhibitor vorasidenib. Modifications of TME during IDH inhibition are unknown and could be an interesting issue to investigate in case of a successful approval of these drugs.
Differently, manipulation of the TME is an emerging treatment strategy in patients with IDH-wt gliomas and especially H3K27 altered diffuse midline gliomas.
Peptide vaccines targeting the H3K27 mutation as well as dendritic cell vaccines have been investigated in the pre-clinical model (83, 84). In particular, peptide vaccine was shown to improve immune response against tumors shifting from an immune-suppressive microenvironment to an active one.
Phase I studies (NCT03396575 and NCT02960230) are currently investigating vaccines in this setting.
Other strategies that aimed to switch the immune microenvironment of midline gliomas consist of oncolytic virus (NCT02960230) and ICIs (NCT03330197 and NCT02359565). Engineered T lymphocytes (CAR T cells) are a new class of compounds consisting of lymphocytes obtained from patients, amplified and activated against tumor cells artificially, and then reinjected into the patients (85). This approach has been successfully tested on hematological malignancies and will also be evaluated in solid tumors. A phase I study employing HER 2 oriented CAR T cells showed promising activity in midline gliomas and a safety profile (86). There are some phase I trials evaluating this approach on patients with newly diagnosed (NCT04099797, NCT04196413, and NCT04185038) and recurrent (NCT04099797) midline glioma.
IDH-wt diffuse gliomas represent a heterogeneous class of tumors. It is reasonable to suppose that these tumors display a similar TME of GBM and thus could benefit from strategies aimed to target angiogenesis as well as an immune response against tumors (7). To date, there are no trials investigating agents modifying TME tailored for patients with IDH-wt diffuse gliomas.
The study of microenvironment composition is an emerging issue in patients with low-grade gliomas. In the majority of cases, we have only limited data about its composition according to tumor subtype. On the other hand, improved knowledge of this key element would improve the clinical management of these tumors in different possible ways. For example, microenvironment composition could be an element associated with prognosis and/or different responses to treatment provided. In this optic, the microenvironment could be assessed as a prognostic or predictive factor independently associated with clinical outcomes. A deeper knowledge of microenvironment composition would also improve the selection of patients to enroll in clinical trials (which is essential considering the rarity of the diseases and the long follow-up required for final data). Targeting microenvironment composition could also be a promising therapeutic option. Due to the approval of ICIs, the composition of immune cells surrounding tumors is the most assessed issue. Nonetheless, interactions between neurons, glial cells, endothelial cells, and stromal cells could hide important potential targets for therapy.
Conclusion
Few studies investigated the TME of patients with glioma (excluding GBM). The composition of TME differs according to the genomic expression and mutations exhibited by cancer cells and can be modified by systemic treatments. Vaccines built with IDH1 and H3K27 peptides could be an interesting approach as these agents showed to modify microenvironment composition and improve immune response against tumors. A combination of different agents can further amplify the effect on TME resulting in improved anti-tumor activity.
Author Contributions
VN and LG: writing and draft. EF, AB, AT, and SB: project conception and reviewing. All authors contributed to the article and approved the submitted version.
Conflict of Interest
The authors declare that the research was conducted in the absence of any commercial or financial relationships that could be construed as a potential conflict of interest.
Publisher’s Note
All claims expressed in this article are solely those of the authors and do not necessarily represent those of their affiliated organizations, or those of the publisher, the editors and the reviewers. Any product that may be evaluated in this article, or claim that may be made by its manufacturer, is not guaranteed or endorsed by the publisher.
Abbreviations
ACVR1, Activin A receptor type 1; ARG1, Arginase 1; ATP, Adenosine triphosphate; ATRX, X-linked nuclear protein; CCL2, C-C Motif Chemokine Ligand 2; CCL3, C-C Motif Chemokine Ligand 3; CCL4, C-C Motif Chemokine Ligand 4; CCL7, C-C Motif Chemokine Ligand 7; CCND1, Cyclin D1; CCND2, Cyclin D2; CCND3, Cyclin D3; CDK4, Cyclin-dependent kinase 4; CDK6, Cyclin-dependent kinase 6; CDKN2A/CDKN2B, cyclin-dependent kinase inhibitor 2A/2B; CIC, Capicua Transcriptional Repressor; CNS, Central nervous system; CX3CR1, C-X-C Motif Chemokine Receptor 1; CSF-1, Colony-stimulating factor 1; EZHIP, EZH inhibitory protein; F13A1, Coagulation Factor XIII A Chain; FUBP1, Far Upstream Element Binding Protein 1; EGFR, Epidermal Growth Factor Receptor; GBM, Glioblastoma; GDNF, Glial Cell-Derived Neurotrophic Factor; GM-CSF, Granulocyte-Macrophage Colony-Stimulating Factor; H3K27, 27th amino acid of Histone 3; H3G34, 34th amino acid of Histone 3; IDH, Isocitrate Dehydrogenase; IL-1A/B, Interleukin 1A/1B; IL-6, Interleukin 6; IL-10, Interleukin 10; NF1, Neurofibromin 1; NGS, Next-Generation Sequencing; NMDA, N-methyl-D-aspartate receptor; NOTCH1, Notch homolog 1, translocation-associated; OLIG2, Oligodendrocyte transcription factor 2; RT, Radiation Therapy; P2RY12, Purigenic Receptor P2Y12; P2RY13, Purigenic Receptor P2Y13; P53, Tumor suppressor protein 53; PCV, Procarbazine, lomunstine, vincristine; PD-1, Programmed Death Receptor 1; PDGFA, Platelet-derived growth factor subunit A; PDGFB, Platelet-derived growth factor subunit B; PDGFR, Platelet-Derived Growth Factor Receptor; PI3K, Phosphoinositide 3-kinase; PPM1D, Phosphatase Mg2+/Mn2+ dependent 1D; PRC2, Polycomb repressive complex-2 methyltransferases complex; PTEN, Phosphatase and tensin homolog; RB1, Retinoblastoma-associated protein; SLC7A11, Solute Carrier Family 7 Member 11; SDF1, Stromal cell-derived factor 1; SOX4, SRY-Box Transcription Factor 4; SOX11, SRY-Box Transcription Factor 11; TCF4, Transcription Factor 4; TERT, Telomerase Reverse Transcriptase; TGCA, The Cancer Genome Atlas; TGFβ1, Transforming growth factor β1; TIM3, T-cell immunoglobulin and mucin-domaining containing-3; TME, Tumor microenvironment; TMZ, Temozolomide; TNF, Tumor necrosis factor; VEGF, Vascular Endothelial Growth Factor; WHO, World Health Organization;
References
1. Miller KD, Ostrom QT, Kruchko C, Patil N, Tihan T, Cioffi G, et al. Brain, and Other Central Nervous System Tumor Statistics, 2021. CA Cancer J Clin (2021) 71(5):381–406. doi: 10.3322/caac.21693
2. Weller M, van den Bent M, Preusser M, Le Rhun E, Tonn JC, Minniti G, et al. EANO Guidelines on the Diagnosis and Treatment of Diffuse Gliomas of Adulthood. Nat Rev Clin Oncol (2021) 18(3):170–86. doi: 10.1038/s41571-020-00447-z
3. Mohile NA, Messersmith H, Gatson NT, Hottinger AF, Lassman A, Morton J, et al. Therapy for Diffuse Astrocytic and Oligodendroglial Tumors in Adults: ASCO-SNO Guideline. J Clin Oncol (2022) 40(4):403–26. doi: 10.1200/JCO.21.02036
4. Louis DN, Perry A, Wesseling P, Cree IA, Figarella-Branger D, Hawkins C, et al. The 2021 WHO Classification of Tumors of the Central Nervous System: A Summary. Neuro Oncol (2021) 23(8):1231–51. doi: 10.1093/neuonc/noab106
5. Compostella A, Tosoni A, Blatt V, Franceschi E, Brandes AA. Prognostic Factors for Anaplastic Astrocytomas. J Neurooncol (2007) 81(3):295–303. doi: 10.1007/s11060-006-9232-z
6. Mair MJ, Geurts M, van den Bent MJ, Berghoff AS. A Basic Review on Systemic Treatment Options in WHO Grade II-III Gliomas. Cancer Treat Rev (2021) 92:102124. doi: 10.1016/j.ctrv.2020.102124
7. Broekman ML, Maas SLN, Abels ER, Mempel TR, Krichevsky AM, Breakefield XO. Multidimensional Communication in the Microenvirons of Glioblastoma. Nat Rev Neurol (2018) 14(8):482–95. doi: 10.1038/s41582-018-0025-8
8. van den Bent MJ, Tesileanu CMS, Wick W, Sanson M, Brandes AA, Clement PM, et al. Adjuvant and Concurrent Temozolomide for 1p/19q non-Co-Deleted Anaplastic Glioma (CATNON; EORTC Study 26053-22054): Second Interim Analysis of a Randomised, Open-Label, Phase 3 Study. Lancet Oncol (2021) 22(6):813–23. doi: 10.1016/S1470-2045(21)00090-5
9. van den Bent MJ, Baumert B, Erridge SC, Vogelbaum MA, Nowak AK, Sanson M, et al. Interim Results From the CATNON Trial (EORTC Study 26053-22054) of Treatment With Concurrent and Adjuvant Temozolomide for 1p/19q Non-Co-Deleted Anaplastic Glioma: A Phase 3, Randomised, Open-Label Intergroup Study. Lancet (2017) 390(10103):1645–53. doi: 10.1016/S0140-6736(17)31442-3
10. Shaw EG, Wang M, Coons SW, Brachman DG, Buckner JC, Stelzer KJ, et al. Randomized Trial of Radiation Therapy Plus Procarbazine, Lomustine, and Vincristine Chemotherapy for Supratentorial Adult Low-Grade Glioma: Initial Results of RTOG 9802. J Clin Oncol (2012) 30(25):3065–70. doi: 10.1200/JCO.2011.35.8598
11. Reuss DE, Mamatjan Y, Schrimpf D, Capper D, Hovestadt V, Kratz A, et al. IDH Mutant Diffuse and Anaplastic Astrocytomas Have Similar Age at Presentation and Little Difference in Survival: A Grading Problem for WHO. Acta Neuropathol (2015) 129(6):867–73. doi: 10.1007/s00401-015-1438-8
12. Aoki K, Nakamura H, Suzuki H, Matsuo K, Kataoka K, Shimamura T, et al. Prognostic Relevance of Genetic Alterations in Diffuse Lower-Grade Gliomas. Neuro Oncol (2018) 20(1):66–77. doi: 10.1093/neuonc/nox132
13. Pekmezci M, Rice T, Molinaro AM, Walsh KM, Decker PA, Hansen H, et al. Adult Infiltrating Gliomas With WHO 2016 Integrated Diagnosis: Additional Prognostic Roles of ATRX and TERT. Acta Neuropathol (2017) 133(6):1001–16. doi: 10.1007/s00401-017-1690-1
14. Price G, Bouras A, Hambardzumyan D, Hadjipanayis CG. Current Knowledge on the Immune Microenvironment and Emerging Immunotherapies in Diffuse Midline Glioma. EBioMedicine (2021) 69:103453. doi: 10.1016/j.ebiom.2021.103453
15. van den Bent MJ, Carpentier AF, Brandes AA, Sanson M, Taphoorn MJ, Bernsen HJ, et al. Adjuvant Procarbazine, Lomustine, and Vincristine Improves Progression-Free Survival But Not Overall Survival in Newly Diagnosed Anaplastic Oligodendrogliomas and Oligoastrocytomas: A Randomized European Organisation for Research and Treatment of Cancer Phase III Trial. J Clin Oncol (2006) 24(18):2715–22. doi: 10.1200/JCO.2005.04.6078
16. Brat DJ, Verhaak RG, Aldape KD, Yung WK, Salama SR, Cooper LA, et al. Comprehensive, Integrative Genomic Analysis of Diffuse Lower-Grade Gliomas. N Engl J Med (2015) 372(26):2481–98.doi: 10.1056/NEJMoa1402121
17. Tesileanu CMS, van den Bent MJ, Sanson M, Wick W, Brandes AA, Clement PM, et al. Prognostic Significance of Genome-Wide DNA Methylation Profiles Within the Randomized, Phase 3, EORTC CATNON Trial on non-1p/19q Deleted Anaplastic Glioma. Neuro Oncol (2021) 23(9):1547–59. doi: 10.1093/neuonc/noab088
18. Di Nunno V, Franceschi E, Tosoni A, Gatto L, Maggio I, Lodi R, et al. Clinical and Molecular Features of Patients With Gliomas Harboring IDH1 Non-Canonical Mutations: A Systematic Review and Meta-Analysis. Adv Ther (2022) 39(1):165–77. doi: 10.1007/s12325-021-01977-3
19. Franceschi E, Biase D, Di Nunno V, Pession A, Tosoni A, Gatto L, et al. IDH1(105GGT) Single Nucleotide Polymorphism Improves Progression Free Survival in Patients With IDH Mutated Grade II and III Gliomas. Pathol Res Pract (2021) 221:153445. doi: 10.1016/j.prp.2021.153445
20. Franceschi E, De Biase D, Di Nunno V, Pession A, Tosoni A, Gatto L, et al. IDH1 Non-Canonical Mutations and Survival in Patients With Glioma. Diagnostics (Basel) (2021) 11(2):342. doi: 10.3390/diagnostics11020342
21. Venteicher AS, Tirosh I, Hebert C, Yizhak K, Neftel C, Filbin MG, et al. Decoupling Genetics, Lineages, and Microenvironment in IDH-Mutant Gliomas by Single-Cell RNA-Seq. Science (2017) 355(6332):eaai8478. doi: 10.1126/science.aai8478
22. Tirosh I, Venteicher AS, Hebert C, Escalante LE, Patel AP, Yizhak K, et al. Single-Cell RNA-Seq Supports a Developmental Hierarchy in Human Oligodendroglioma. Nature (2016) 539(7628):309–13. doi: 10.1038/nature20123
23. Pei Y, Wechsler-Reya RJ. A Malignant Oligarchy: Progenitors Govern the Behavior of Oligodendrogliomas. Cancer Cell (2010) 18(6):546–7. doi: 10.1016/j.ccr.2010.11.031
24. Patel AP, Tirosh I, Trombetta JJ, Shalek AK, Gillespie SM, Wakimoto H, et al. Single-Cell RNA-Seq Highlights Intratumoral Heterogeneity in Primary Glioblastoma. Science (2014) 344(6190):1396–401. doi: 10.1126/science.1254257
25. Neftel C, Laffy J, Filbin MG, Hara T, Shore ME, Rahme GJ, et al. An Integrative Model of Cellular States, Plasticity, and Genetics for Glioblastoma. Cell (2019) 178(4):835–849.e21. doi: 10.1016/j.cell.2019.06.024
26. Filbin MG, Tirosh I, Hovestadt V, Shaw ML, Escalante LE, Mathewson ND, et al. Developmental and Oncogenic Programs in H3K27M Gliomas Dissected by Single-Cell RNA-Seq. Science (2018) 360(6386):331–5. doi: 10.1126/science.aao4750
27. Wherry EJ, Kurachi M. Molecular and Cellular Insights Into T Cell Exhaustion. Nat Rev Immunol (2015) 15(8):486–99. doi: 10.1038/nri3862
28. Li W, Graeber MB. The Molecular Profile of Microglia Under the Influence of Glioma. Neuro Oncol (2012) 14(8):958–78. doi: 10.1093/neuonc/nos116
29. Hambardzumyan D, Gutmann DH, Kettenmann H. The Role of Microglia and Macrophages in Glioma Maintenance and Progression. Nat Neurosci (2016) 19(1):20–7. doi: 10.1038/nn.4185
30. Chang AL, Miska J, Wainwright DA, Dey M, Rivetta CV, Yu D, et al. CCL2 Produced by the Glioma Microenvironment Is Essential for the Recruitment of Regulatory T Cells and Myeloid-Derived Suppressor Cells. Cancer Res (2016) 76(19):5671–82. doi: 10.1158/0008-5472.CAN-16-0144
31. Brandenburg S, Müller A, Turkowski K, Radev YT, Rot S, Schmidt C, et al. Resident Microglia Rather Than Peripheral Macrophages Promote Vascularization in Brain Tumors and are Source of Alternative Pro-Angiogenic Factors. Acta Neuropathol (2016) 131(3):365–78. doi: 10.1007/s00401-015-1529-6
32. Bayik D, Zhou Y, Park C, Hong C, Vail D, Silver DJ, et al. Myeloid-Derived Suppressor Cell Subsets Drive Glioblastoma Growth in a Sex-Specific Manner. Cancer Discov (2020) 10(8):1210–25. doi: 10.1158/2159-8290.CD-19-1355
33. Ross JL, Chen Z, Herting CJ, Grabovska Y, Szulzewsky F, Puigdelloses M, et al. Platelet-Derived Growth Factor Beta Is a Potent Inflammatory Driver in Paediatric High-Grade Glioma. Brain (2021) 144(1):53–69. doi: 10.1093/brain/awaa382
34. Lin GL, Nagaraja S, Filbin MG, Suvà ML, Vogel H, Monje M, et al. Non-Inflammatory Tumor Microenvironment of Diffuse Intrinsic Pontine Glioma. Acta Neuropathol Commun (2018) 6(1):51. doi: 10.1186/s40478-018-0553-x
35. Gieryng A, Pszczolkowska D, Walentynowicz KA, Rajan WD, Kaminska B. Immune Microenvironment of Gliomas. Lab Invest (2017) 97(5):498–518. doi: 10.1038/labinvest.2017.19
36. Di Nunno V, Franceschi E, Tosoni A, atto L, Bartolini S, Brandes AA. Glioblastoma Microenvironment: From an Inviolable Defense to a Therapeutic Chance. Front Oncol (2022) 12:852950. doi: 10.3389/fonc.2022.852950
37. Fu W, Wang W, Li H, Jiao Y, Weng J, Huo R, et al. High Dimensional Mass Cytometry Analysis Reveals Characteristics of the Immunosuppressive Microenvironment in Diffuse Astrocytomas. Front Oncol (2020) 10:78. doi: 10.3389/fonc.2020.00078
38. Buckingham SC, Campbell SL, Haas BR, Montana V, Robel S, Ogunrinu T, et al. Glutamate Release by Primary Brain Tumors Induces Epileptic Activity. Nat Med (2011) 17(10):1269–74. doi: 10.1038/nm.2453
39. Campbell SL, Buckingham SC, Sontheimer H. Human Glioma Cells Induce Hyperexcitability in Cortical Networks. Epilepsia (2012) 53(8):1360–70. doi: 10.1111/j.1528-1167.2012.03557.x
40. Sontheimer H. A Role for Glutamate in Growth and Invasion of Primary Brain Tumors. J Neurochem (2008) 105(2):287–95. doi: 10.1111/j.1471-4159.2008.05301.x
41. Nakanishi H, Ni J, Nonaka S, Hayashi Y. Microglial Circadian Clock Regulation of Microglial Structural Complexity, Dendritic Spine Density and Inflammatory Response. Neurochem Int (2021) 142:104905. doi: 10.1016/j.neuint.2020.104905
42. Klemm F, Maas RR, Bowman RL, Kornete M, Soukup K, Nassiri S, et al. Interrogation of the Microenvironmental Landscape in Brain Tumors Reveals Disease-Specific Alterations of Immune Cells. Cell (2020) 181(7):1643–1660.e17. doi: 10.1016/j.cell.2020.05.007
43. Friebel E, Kapolou K, Unger S, Núñez NG, Utz S, Rushing EJ, et al. Single-Cell Mapping of Human Brain Cancer Reveals Tumor-Specific Instruction of Tissue-Invading Leukocytes. Cell (2020) 181(7):1626–1642.e20. doi: 10.1016/j.cell.2020.04.055
44. Ma T, Hu C, Lal B, Zhou W, Ma Y, Ying M, et al. Reprogramming Transcription Factors Oct4 and Sox2 Induce a BRD-Dependent Immunosuppressive Transcriptome in GBM-Propagating Cells. Cancer Res (2021) 81(9):2457–69. doi: 10.1158/0008-5472.CAN-20-2489
45. Wu F, Wang ZL, Wang KY, Li GZ, Chai RC, Liu YQ, et al. Classification of Diffuse Lower-Grade Glioma Based on Immunological Profiling. Mol Oncol (2020) 14(9):2081–95. doi: 10.1002/1878-0261.12707
46. Bosma I, Vos MJ, Heimans JJ, Taphoorn MJ, Aaronson NK, Postma TJ, et al. The Course of Neurocognitive Functioning in High-Grade Glioma Patients. Neuro Oncol (2007) 9(1):53–62. doi: 10.1215/15228517-2006-012
47. Miotto EC, Junior AS, Silva CC, Cabrera HN, Machado MA, Benute GR, et al. Cognitive Impairments in Patients With Low Grade Gliomas and High Grade Gliomas. Arq Neuropsiquiatr (2011) 69(4):596–601. doi: 10.1590/S0004-282X2011000500005
48. Taphoorn MJ, Klein M. Cognitive Deficits in Adult Patients With Brain Tumours. Lancet Neurol (2004) 3(3):159–68. doi: 10.1016/S1474-4422(04)00680-5
49. Duffau H. Diffuse Low-Grade Gliomas and Neuroplasticity. Diagn Interv Imaging (2014) 95(10):945–55. doi: 10.1016/j.diii.2014.08.001
50. Kong NW, Gibb WR, Tate MC. Neuroplasticity: Insights From Patients Harboring Gliomas. Neural Plast (2016) 2016:2365063. doi: 10.1155/2016/2365063
51. Torres D, Canoll P. Alterations in the Brain Microenvironment in Diffusely Infiltrating Low-Grade Glioma. Neurosurg Clin N Am (2019) 30(1):27–34. doi: 10.1016/j.nec.2018.08.001
52. Liddelow SA, Barres BA. Reactive Astrocytes: Production, Function, and Therapeutic Potential. Immunity (2017) 46(6):957–67. doi: 10.1016/j.immuni.2017.06.006
53. Miyai M, Kanayama T, Hyodo F, Kinoshita T, Ishihara T, Okada H, et al. Glucose Transporter Glut1 Controls Diffuse Invasion Phenotype With Perineuronal Satellitosis in Diffuse Glioma Microenvironment. Neurooncol Adv (2021) 3(1):vdaa150. doi: 10.1093/noajnl/vdaa150
54. Brooks LJ, Clements MP, Burden JJ, Kocher D, Richards L, Devesa SC, et al. The White Matter Is a Pro-Differentiative Niche for Glioblastoma. Nat Commun (2021) 12(1):2184. doi: 10.1038/s41467-021-22225-w
55. Bhaduri A, Di Lullo E, Jung D, Müller S, Crouch EE, Espinosa CS, et al. Outer Radial Glia-Like Cancer Stem Cells Contribute to Heterogeneity of Glioblastoma. Cell Stem Cell (2020) 26(1):48–63.e6. doi: 10.1016/j.stem.2019.11.015
56. Weng Q, Wang J, Wang J, He D, Cheng Z, Zhang F, et al. Single-Cell Transcriptomics Uncovers Glial Progenitor Diversity and Cell Fate Determinants During Development and Gliomagenesis. Cell Stem Cell (2019) 24(5):707–723.e8. doi: 10.1016/j.stem.2019.03.006
57. Nicholson JG, Fine HA. Diffuse Glioma Heterogeneity and Its Therapeutic Implications. Cancer Discov (2021) 11(3):575–90. doi: 10.1158/2159-8290.CD-20-1474
58. Khuong-Quang DA, Buczkowicz P, Rakopoulos P, Liu XY, Fontebasso AM, Bouffet E, et al. K27M Mutation in Histone H3.3 Defines Clinically and Biologically Distinct Subgroups of Pediatric Diffuse Intrinsic Pontine Gliomas. Acta Neuropathol (2012) 124(3):439–47. doi: 10.1007/s00401-012-0998-0
59. Lewis PW, Müller MM, Koletsky MS, Cordero F, Lin S, Banaszynski LA, et al. Inhibition of PRC2 Activity by a Gain-of-Function H3 Mutation Found in Pediatric Glioblastoma. Science (2013) 340(6134):857–61. doi: 10.1126/science.1232245
60. Schwartzentruber J, Korshunov A, Liu XY, Jones DT, Pfaff E, Jacob K, et al. Driver Mutations in Histone H3.3 and Chromatin Remodelling Genes in Paediatric Glioblastoma. Nature (2012) 482(7384):226–31. doi: 10.1038/nature10833
61. Wu G, Broniscer A, McEachron TA, Lu C, Paugh BS, Becksfort J, et al. Somatic Histone H3 Alterations in Pediatric Diffuse Intrinsic Pontine Gliomas and non-Brainstem Glioblastomas. Nat Genet (2012) 44(3):251–3. doi: 10.1038/ng.1102
62. Jain SU, Rashoff AQ, Krabbenhoft SD, Hoelper D, Do TJ, Gibson TJ, et al. H3 K27M and EZHIP Impede H3K27-Methylation Spreading by Inhibiting Allosterically Stimulated Prc2. Mol Cell (2020) 80(4):726–735.e7. doi: 10.1016/j.molcel.2020.09.028
63. Wu G, Diaz AK, Paugh BS, Rankin SL, Ju B, Li Y, et al. The Genomic Landscape of Diffuse Intrinsic Pontine Glioma and Pediatric Non-Brainstem High-Grade Glioma. Nat Genet (2014) 46(5):444–50. doi: 10.1038/ng.2938
64. Zarghooni M, Bartels U, Lee E, Buczkowicz P, Morrison A, Huang A, et al. Whole-Genome Profiling of Pediatric Diffuse Intrinsic Pontine Gliomas Highlights Platelet-Derived Growth Factor Receptor Alpha and Poly (ADP-Ribose) Polymerase as Potential Therapeutic Targets. J Clin Oncol (2010) 28(8):1337–44. doi: 10.1200/JCO.2009.25.5463
65. Zhang L, Chen LH, Wan H, Yang R, Wang Z, Feng J, et al. Exome Sequencing Identifies Somatic Gain-of-Function PPM1D Mutations in Brainstem Gliomas. Nat Genet (2014) 46(7):726–30. doi: 10.1038/ng.2995
66. Reuss DE, Kratz A, Sahm F, Capper Schrimpf D, Koelsche D, C, et al. Adult IDH Wild Type Astrocytomas Biologically and Clinically Resolve Into Other Tumor Entities. Acta Neuropathol (2015) 130(3):407–17. doi: 10.1007/s00401-015-1454-8
67. Chen Z, Hambardzumyan D. Immune Microenvironment in Glioblastoma Subtypes. Front Immunol (2018) 9. doi: 10.3389/fimmu.2018.01004
68. Wu F, Li GZ, Liu HJ, Zhao Z, Chai RC, Liu YQ, et al. Molecular Subtyping Reveals Immune Alterations in IDH Wild-Type Lower-Grade Diffuse Glioma. J Pathol (2020) 251(3):272–83. doi: 10.1002/path.5468
69. Abdelfattah N, Kumar P, Wang C, Leu JS, Flynn WF, Gao R, et al. Single-Cell Analysis of Human Glioma and Immune Cells Identifies S100A4 as an Immunotherapy Target. Nat Commun (2022) 13(1):767. doi: 10.1038/s41467-022-28372-y
70. Liu F, Peng B, Li M, Ma J, Deng G, Zhang S, et al. Targeted Disruption of Tumor Vasculature via Polyphenol Nanoparticles to Improve Brain Cancer Treatment. Cell Rep Phys Sci (2022) 3(1):100691. doi: 10.1016/j.xcrp.2021.100691
71. Paugh BS, Zhu X, Qu C, Endersby R, Diaz AK, Zhang J, et al. Novel Oncogenic PDGFRA Mutations in Pediatric High-Grade Gliomas. Cancer Res (2013) 73(20):6219–29. doi: 10.1158/0008-5472.CAN-13-1491
72. Di Nunno V, Franceschi E, Tosoni A, Di Battista M, Gatto L, Lamperini C, et al. Treatment of Recurrent Glioblastoma: State-of-the-Art and Future Perspectives. Expert Rev Anticancer Ther (2020) 20(9):785–95. doi: 10.1080/14737140.2020.1807949
73. Di Nunno V, Franceschi E, Tosoni A, Gatto L, Lodi R, Bartolini S, et al. Glioblastoma: Emerging Treatments and Novel Trial Designs. Cancers (Basel) (2021) 13(15):3750. doi: 10.3390/cancers13153750
74. Gatto L, Di Nunno V, Franceschi E, Tosoni A, Bartolini S, Brandes AA. Pharmacotherapeutic Treatment of Glioblastoma: Where Are We to Date? Drugs (2022) 82(5):491–510. doi: 10.1007/s40265-022-01702-6
75. Busek P, Mateu R, Zubal M, Kotackova L, Sedo A. Targeting Fibroblast Activation Protein in Cancer - Prospects and Caveats. Front Biosci (Landmark Ed) (2018) 23(10):1933–68. doi: 10.2741/4682
76. Tosoni A, Franceschi E, Poggi R, Brandes AA. Relapsed Glioblastoma: Treatment Strategies for Initial and Subsequent Recurrences. Curr Treat Options Oncol (2016) 17(9):49. doi: 10.1007/s11864-016-0422-4
77. Cloughesy TF, Mochizuki AY, Orpilla JR, Hugo W, Lee AH, Davidson TB, et al. Neoadjuvant Anti-PD-1 Immunotherapy Promotes a Survival Benefit With Intratumoral and Systemic Immune Responses in Recurrent Glioblastoma. Nat Med (2019) 25(3):477–86. doi: 10.1038/s41591-018-0337-7
78. Schalper KA, Rodriguez-Ruiz ME, Diez-Valle R, López-Janeiro A, Porciuncula A, Idoate MA, et al. Neoadjuvant Nivolumab Modifies the Tumor Immune Microenvironment in Resectable Glioblastoma. Nat Med (2019) 25(3):470–6. doi: 10.1038/s41591-018-0339-5
79. Reardon DA, Brandes AA, Omuro A, Mulholland P, Lim M, Wick A, et al. Effect of Nivolumab vs Bevacizumab in Patients With Recurrent Glioblastoma: The CheckMate 143 Phase 3 Randomized Clinical Trial. JAMA Oncol (2020) 6(7):1003–10. doi: 10.1001/jamaoncol.2020.1024
80. Crunkhorn S. Vaccine for IDH Mutant Glioma. Nat Rev Drug Discov (2021) 20(5):344. doi: 10.1038/d41573-021-00058-y
81. Platten M, Bunse L, Wick A, Bunse T, Le Cornet L, Harting I, et al. A Vaccine Targeting Mutant IDH1 in Newly Diagnosed Glioma. Nature (2021) 592(7854):463–8. doi: 10.1038/s41586-021-03363-z
82. Gatto L, Franceschi E, Tosoni A, Di Nunno V, Maggio I, Lodi R, et al. IDH Inhibitors and Beyond: The Cornerstone of Targeted Glioma Treatment. Mol Diagn Ther (2021) 25(4):457–73. doi: 10.1007/s40291-021-00537-3
83. Benitez-Ribas D, Cabezón R, Flórez-Grau G, Molero MC, Puerta P, Guillen A, et al. Immune Response Generated With the Administration of Autologous Dendritic Cells Pulsed With an Allogenic Tumoral Cell-Lines Lysate in Patients With Newly Diagnosed Diffuse Intrinsic Pontine Glioma. Front Oncol (2018) 8:127. doi: 10.3389/fonc.2018.00127
84. Ochs K, Ott M, Bunse T, Sahm F, Bunse L, Deumelandt K, et al. K27M-Mutant Histone-3 as a Novel Target for Glioma Immunotherapy. Oncoimmunology (2017) 6(7):e1328340. doi: 10.1080/2162402X.2017.1328340
85. Gatto L, Nunno VD, Franceschi E, Brandes AA. Chimeric Antigen Receptor Macrophage for Glioblastoma Immunotherapy: The Way Forward. Immunotherapy (2021) 13(11):879–83. doi: 10.2217/imt-2021-0054
Keywords: microenvironment, glioma, oligodendroglioma, astrocytoma, H3K27 altered glioma, midline glioma, IDH wild type glioma
Citation: Di Nunno V, Franceschi E, Tosoni A, Gatto L, Bartolini S and Brandes AA (2022) Tumor-Associated Microenvironment of Adult Gliomas: A Review. Front. Oncol. 12:891543. doi: 10.3389/fonc.2022.891543
Received: 07 March 2022; Accepted: 06 June 2022;
Published: 07 July 2022.
Edited by:
Aleksi Sedo, Charles University, CzechiaReviewed by:
Pravesh Gupta, University of Texas MD Anderson Cancer Center, United StatesTomas Kazda, Masaryk Memorial Cancer Institute (MMCI), Czechia
Copyright © 2022 Di Nunno, Franceschi, Tosoni, Gatto, Bartolini and Brandes. This is an open-access article distributed under the terms of the Creative Commons Attribution License (CC BY). The use, distribution or reproduction in other forums is permitted, provided the original author(s) and the copyright owner(s) are credited and that the original publication in this journal is cited, in accordance with accepted academic practice. No use, distribution or reproduction is permitted which does not comply with these terms.
*Correspondence: Enrico Franceschi, ZW5yaWNvZnJhQHlhaG9vLml0