- 1The Cancer Center, The Fifth Affiliated Hospital of Sun Yat-Sen University, Zhuhai, China
- 2Department of Urology, The Fifth Affiliated Hospital of Sun Yat-Sen University, Zhuhai, China
- 3Department of Clinical Nutrition, The Fifth Affiliated Hospital of Sun Yat-Sen University, Zhuhai, China
- 4Department of Oncology, First Affiliated Hospital of Zhengzhou University, Zhengzhou, Henan, China
Head and neck cancer (HNC) is one of the most common cancers on the planet, with approximately 600,000 new cases diagnosed and 300,000 deaths every year. Research into the biological basis of HNC has advanced slowly over the past decades, which has made it difficult to develop new, more effective treatments. The patient-derived organoids (PDOs) are made from patient tumor cells, resembling the features of their tumors, which are high-fidelity models for studying cancer biology and designing new precision medicine therapies. In recent years, considerable effort has been focused on improving “organoids” technologies and identifying tumor-specific medicine using head and neck samples and a variety of organoids. A review of improved techniques and conclusions reported in publications describing the application of these techniques to HNC organoids is presented here. Additionally, we discuss the potential application of organoids in head and neck cancer research as well as the limitations associated with these models. As a result of the integration of organoid models into future precision medicine research and therapeutic profiling programs, the use of organoids will be extremely significant in the future.
Introduction
The phrase “head and neck cancer” (HNC) outlines a variety of cancers that affect the larynx, hypopharynx, nasopharynx, mouth, throat, thyroid, and salivary gland. These cancers are categorized based on the anatomical areas in which they develop, including lip, oral, oropharynx, larynx, hypopharynx, and nasopharynx cancers (1). In 2018, HNC was the 7th most prevalent cancer globally (2) with an incidence of more than half a million patients and 300,000 deaths every year (3, 4). Head and neck squamous cell carcinoma (HNSCC), the most prevalent cancer of the upper aerodigestive tract, accounts for over 90% of cases (5) and most of HNC. Other primary sites, such as salivary gland cancers (SGC), encompassing a rare type of HNCs. It is reported that SGC has a wide range of subtypes which preclinical research is lacking. The person-year incidence rate of SGC is less than 3 cases/per 100,000 people (6). In current clinical practice, treatment options include surgery, radiotherapy, chemotherapy, targeted therapy, and immunotherapy. However, HNC therapy is not effective, and various factors cause the high recurrence rates (7). Firstly, ascribing to their anatomic location, hidden lesions complicate surgery. On the other hand, chemotherapy is a crucial part of treatment, but unfortunately, the mechanisms underlying chemoresistance (to drugs like Cisplatin, for example) are still poorly understood (8). Furthermore, relatively high intra-tumor genetic heterogeneity is one of the important factors that lead to highly variable treatment responses. The comprehensive preclinical model which able to evaluate patient responses to therapy plans predictively and identify biomarkers with a high degree of sensitivity and specificity while taking into account interactions between the tumor microenvironment (TME). The preclinical model would make it easier for HNC patients to receive tailored treatment options (9), which link the molecular characteristics of a patient’s tumor with efficient therapeutic measures in a way that is saleable and compatible with systems biology techniques. Over the past few decades, organoid technology has improved the preclinical model and led to an ever-increasing number of breakthroughs toward the promise of precision cancer therapies.
Organoid and patient-derived organoid
In 2009, Hans Clevers and Toshiro Sato, created the first tiny gut organoids from adult stem cells derived from the gut of mice, setting off a frenzy of organoid research (10). Organoids are microscopic three-dimensional(3D) structures that are grown from stem cells in vitro. They recapitulate structural and functional traits of their in vivo corresponding organs and possess the capability of self-organize and self-renewal. This technology allows us to simulate complex organ structures and functions in vitro, greatly accelerating the development and application of genes and drugs. In the field of cancer research, for now, preclinical cancer research is regularly conducted by immortalized human cancer-derived cell lines (adhesion or suspension) (11). However, it cannot capture tumor architecture, as well as mimic the tumor microenvironment, which is crucial for understanding how cells respond to medications and for designing anti-cancer therapies. Patient-derived tumor xenograft (PDX)is a transplanted tumor model formed by using tumor tissues and primary cells of patients implanted into immune deficient mice. PDX model retains most of the features of primary tumor in histopathology, molecular biology and gene level, and has better clinical efficacy prediction. Therefore, PDX model has been increasingly widely used in many key nodes of new drug development. As PDX preserves the initial intra-tumor heterogeneity and tumor-stroma interactions, they are widely used (12–15). The process of generating a PDX, however, is time-consuming and costly (13).
Therefore, the clinical practice needs a rapid, low-cost, and individual 3D structures tumor model which derived from patients. In 2011, Sato et al. took the lead in establishing colorectal cancer organoids from patient samples with organoid technology (15). This advancing technology is named patient-derived organoid (PDO). PDO has several unique advantages over other model systems. In contrast to static sequencing data, it offers the opportunity to analyze specific tumors as a dynamic system and maintain the characteristics of the original tumor. Due to its biological features, PDO stand in the middle between cell lines and patient-derived xenografts (PDX) models. PDO can more closely resemble a tumor in vivo condition while also being simpler to set up and less expensive to maintain. Since the first PDO were created more than ten years ago, many tumor organoids have shown tremendous promise in both clinical and basic research, but head and neck malignancies have seen little use of this technique. Nevertheless, recent studies have revealed that there are several potential uses for head and neck tumor organoids in therapeutic settings.
Establishment of HNC PDOs
Construction
The advancement of organoid technology offers new opportunities for pre-clinical field testing of medicative solutions. Technically, the construction steps of most HNC PDOs are similar, with only a few differences, as shown in Table 1. For the creation of PDOs, a generic methodology might not be suitable for all types of tumors. Kijima and Karakasheva group described in detail the general establishment process of patient-derived head and neck cancer that most researchers used (18). In brief, after the specimens were washed, minced, and digested, the tissues are dissociated into single-cell suspension. And then mixed with Matrigel for further cultivated. The specific formula of the medium is different (16–18, 20, 22, 25).
Based on the work by Kondo et al (27), Noriaki Tanaka et al. constructed the PDOs for HNSCC by using cancer tissue-originated spheroids (CTOS) method in 2018 (16). Unlike traditional strategy, this method requires cultivation in advance in ultra-low culture dishes for 24-72h to form CTOSs. Following verification, CTOSs were moved into Matrigel to form solidoid and cultivated in a medium with added growth factors. The growth rate from original CTOS cultures to organoids is 37.2%. They claimed that the PDO exhibit histological features closely matched those of the donor tumor tissues and that the carcinoma stem cell marker (CD44) expression was similar to those of the original tumors. In order to optimize the digestive conditions, Xian-Wen Wang proposed a new two-step enzymatic strategy for nasopharyngeal carcinoma (23). And they claimed that the success rate is different between primary tumors and recurrence tumors. Zhaohui Wang has developed micro-organospheres technique to provide a more reliable platform for assessing drug response, based on the clinical need for rapid and batch establishment of a large number of organoids using smaller tissue blocks (24). In addition, the Clevers and Driehuis group also created organoids of normal oral mucosa and investigated how the herpes simplex virus infected these organoids (17).
Gerben Lassche et al. presented the first successful development and characterization of SGC PDO cultures. According to their findings, the 19% success rate is rather low when compared to PDOs culture attempts for other cancer types (21). Yoshihiro Aizawa not only established the patient-derived organoid and PDX model of SGC but also built a PDX-derived organoid (PDXO) by using specimens from SGC PDXs. Three subtypes of SGC were successfully generated, and they found these three models showed similar histological features as the original tumors (20). However, salivary gland tumor organoids should be used cautiously in clinical drug sensitivity screening. A case report of a patient with ETV6-NTRK3 gene fusion-positive secretory salivary gland carcinoma which was sensitive to selective TRK inhibitor larotrectinib showed that the drug response in PDOs differs from patient (28). The authors highlight that the growth factors in the medium may cause cellular defense mechanisms to develop that are independent of TRK signaling, which might potentially account for the absence of drug sensitivity.
In addition to using conventional surgical specimens or pathological biopsy specimens to construct PDOs, attempting to construct tumor organoids using circulating tumor cells (CTC) present in liquid biopsies in HNC patients reduces the risks associated with surgery for patients. Kuan-Chou Lin et al. developed the eSelect system to expand CTC ex vivo. Tumor organoids constructed in this way can mimic a patient’s response to clinical drug therapy (26). The success rate of organoid construction is 92.50%, which is much higher than traditional construction methods. This strategy could work for individuals with advanced HNC since many of them had metastatic or incurable conditions that precluded additional biopsies or operations. However, if there are few CTCs present in the early stages of the illness, it could not be effective.
The construction of PDOs has always been the biggest obstacle to its application. Different methods can result in inconsistencies between the produced organoids and the primary tissue. At present, the variation of non-standardized schemes mainly comes from: 1. Different tissue sources (representing only a subset of tumors); 2. Different mediums, growth factors, and poorly defined culture components will unpredictably change organoid phenotypes, leading to differences in growth and biological behavior; 3. The Matrigel varies from batch to batch and contamination will lead to different results. In addition, there are still great differences between in vitro culture matrix and actual tumor micro-environment.
Evaluation of PDOs in HNC
The main difference between tumor and normal tissue is due to genetic heterogeneity. As early as 1953, researchers identified the multifocal origin of squamous epithelial tumors (29), which is the main pathological pattern in HNC. Thus, one of the criteria for evaluating PDOs is whether the genetic characteristics of genes are preserved. The map of genetic mutations in head and neck tumors is extremely complex. Numerous studies have documented frequent chromosomal instability and somatic genomic changes based on data from sequencing technology-based genetic profiling of HNC tissues (30, 31). The inactivation of the tumor suppressor genes TP53, CDKN2A, and PTEN, as well as the amplification of the CCND1 gene, are the primary genetic modifications that turn dysplasia into invasive HNSCC (32). In addition, following bulk sequencing investigations, it was discovered that HNSCC frequently has mutations in TP53, FAT1, CDKN2A, PI3KA, and NOTCH (31, 33). Also, genetic alteration profiles may differently depend on HPV status. While CDKN2A and TP53 mutations are the most frequent in HPV-negative cancers, HPV-positive tumors frequently include TP63, TRAF3, and E2F1 mutations (31, 33, 34). Based on multiple types of mutations, De Cecco et al. proposed six distinct subtypes (immunoreactive, inflammatory, HPV-like, classical, hypoxia-associated, and mesenchymal) in HNSCCs (35). There is also a strong link between genetic mutation and treatment response. More recently, study showed HRAS mutations in HNC make tumors more vulnerable to farnesyltransferase inhibitor tipifarnib (36). Another high-frequency mutation of PI3K leads to a wide range of applications of PI3K inhibitors in HNC (37–39). Heterogeneity is difficult to reproduce in 2D culture, and the PDX model may also have gene changes due to the doping of the mouse tumor microenvironment. Due to their ability to mimic the genetic changes associated with a primary tumor, organoids have many advantages.
In the case of HNC, an ideal marker is required to describe molecular changes in tumor cells and predict therapeutic targets. PDOs provide a way to describe the genetic map of tumors at different stages of the different expression patterns of tumor heterogeneity. Almost all studies on HNC PDOs have used immunological methods to assess the genetic similarity to primary tumors (16–18, 27, 40), and unanimously described that organoids retain intra-tumoral heterogeneity of the original tumor. As shown in Table 2, most studies used immunostaining to detect the expression of some markers, for example, TP53, Ki67, KRT5, and CD44 (16–18). Whole-exome sequencing (WES) detected common mutations in HNC PDOs, such as TP53, PIK3CA, BRAF, and CDKN2A (17). Single-nucleotide variants (SNV) and small insertions or deletions (Indel) throughout the genome were compared between organoids and primary tumors (19). And simultaneously it was found that organoids retained the chromosome missegregation of the primary tumor well. Genetic uniformity of salivary gland carcinoma PDOs also have been evaluated by sequencing (20). Therefore, PDOs largely recapitulated the genetic alterations that were detected in the tumor. In addition, it was shown that PDOs retained their tumorigenic potential upon xenotransplantation (20).
Next, the drug response of PDOs is also an important aspect of evaluation, which is also the premise of whether these models can be personalized and applied to patients’ treatment prediction. More than 60% of patients diagnosed with head and neck cancer tumors are in the local advanced stage and therefore require multi-mode treatment such as surgery combined with radiotherapy and chemotherapy (44). Even so, 65% of patients had a relapse (45), which need more effective therapy. In recent years, the rise of targeted therapy and immunotherapy offers hope for patients. Cetuximab has been established as first-line standard-of-care therapy for recurrent or metastatic head and neck cancer (46). Additionally, the approval of programmed death 1(PD-1) immune-checkpoint inhibitors makes the treatment of HNC is more promising. Unfortunately, the objective response rate remains low (46–48). Moreover, the treatment of patients with advanced head and neck tumors is highly heterogeneous, and the prediction based on protein marker expression is controversial (2). Therefore, in vitro models that can simulate patients are needed to test drug sensitivity. Current studies have shown that in vitro testing of HNC PDOs reveals that they respond to chemotherapeutics, targeted treatments, and immunological agents (16, 17). Most studies of organoids in head and neck tumors have tested susceptibility to chemotherapy, for example, cisplatin. There have also been individual studies that have tested the sensitivity of targeted EGFR therapy (See Table 2). However, due to the limitations of organoid culture, no PDOs studies have been conducted to probe the sensitivity of immunotherapy in HNC. Advances in culture technology allow us to create PDOs that are smaller with a larger surface area (24), which opens up the possibility of immunotherapy tests in the future.
It is worth remembering that conventional colony-forming assays, which are commonly used to assess 2D cell proliferation conditions, were inapplicable for PDOs because the size changed so inapparently after treatment, even though there was significant cell death in particular. There have been some ambiguous results for cell viability tests due to 3D growth and the existence of complex cell clusters, such as Celltiter-Glo (16, 41, 49). Simple and straightforward methods are needed to detect organoid drug responses. ATP-based end-point luminescence assays(Celltiter-Glo) used to consider to be a better option to assess viability of 3D cultures (50). When the organoid whole volume is observed at the single-cell level, A novel method that evaluates cell metabolism utilizing intrinsic fluorescence from NAD(P)H and FAD on a single cell level for a 3-D in vitro model was tested in HNC PDOs (51).
Technological improvement
Organoid culture technology advancements have both sped up mass production culture and improved simulations of the microenvironment in the human body. Here, we summarized the present state of organoid culture technologies (Figure 1) and discussed the potential applications for them in PDOs of head and neck tumors (Table 3).
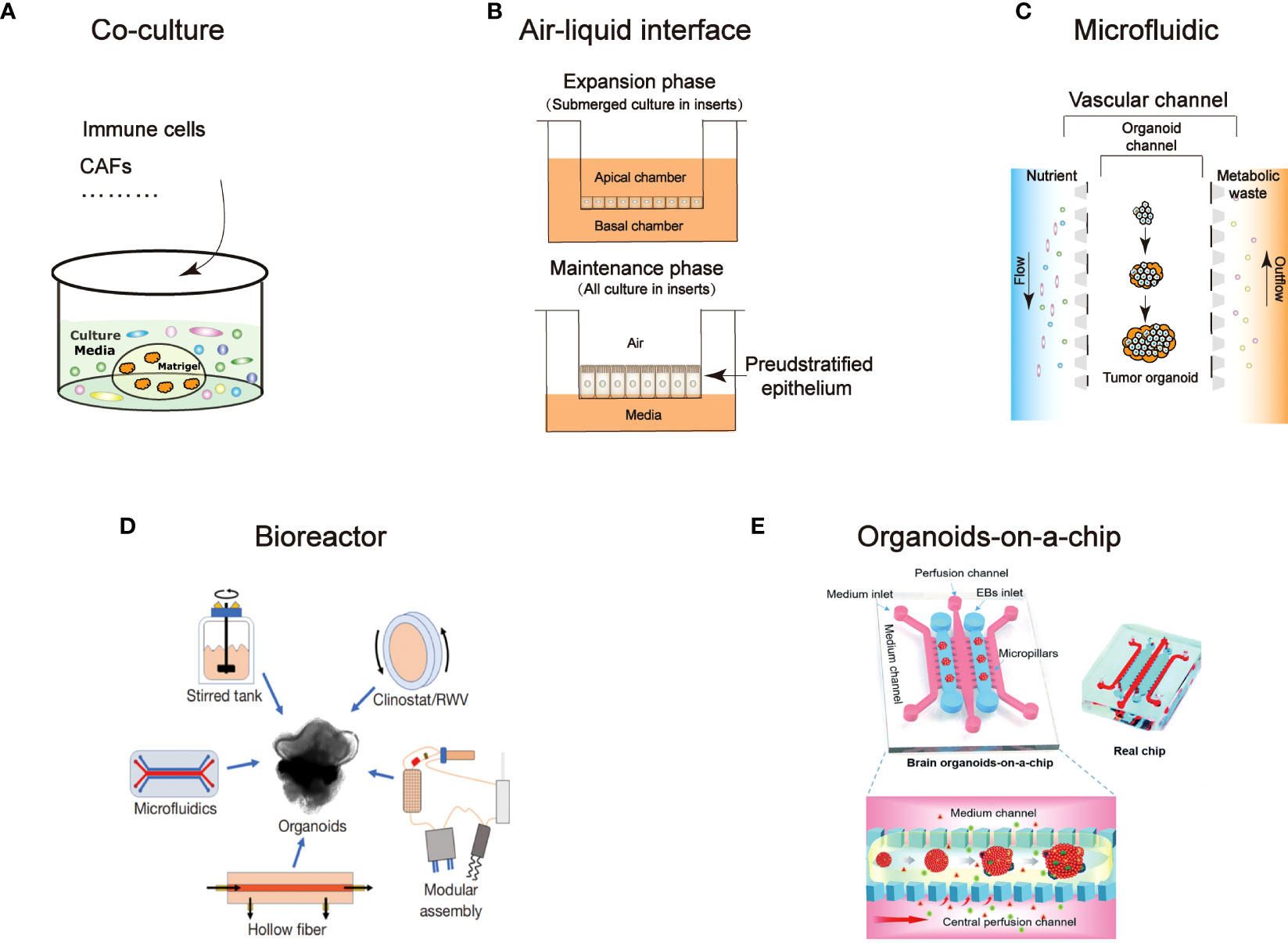
Figure 1 An overview of technological developments in organoids. Characterize the different technical features: (A) Co-culture (B) Air-liquid interface (C) Microfluidic (D) Bioreactor (94) (E) Organoids-on-a-chip (95).
Co-culture
Studies on cancer therapy are limited as a result of the inability of in vitro models to analyze the connection between the vasculature and the stroma (52). Co-culture method could retain natural stromal elements, such as different immune cells, or by incorporating foreign immune cells, cancer-associated fibroblasts (CAFs), vasculature, and other elements, which could closely mirror the tumor microenvironment (65). The expression of traditional mesenchymal markers and EMT-associated genes in partial HNSCC cells supports the idea that cancer cells and CAFs interact with one another in a regulatory manner (66). On this point, Xu Chen reported the method for the model of fibroblast-attached organoid to investigate the contact-dependent mechanisms (67). The mix cluster of single fibroblasts and oral squamous cell carcinoma cells were resuspended in medium in an ultra-low attached (ULA) plate before co-culture in Matrigel. In this model, they revealed that the stimulation of fibroblasts associated to the oral squamous cell carcinoma PDOs is mediated by Notch pathway. Coincidentally, another study reported that co-culture of CAFs with oral squamous cell carcinoma PDOs promotes stem-like properties of tumor (43). Hui Zhao et al. used co-culture method to demonstrated nicotinamide N-methyltransferase in cancer-associated fibroblasts is corelated with tumor growth and extracellular matrix remodeling is a potential therapeutic target for patients with oral squamous cell carcinoma (65). Moreover, co-culturing PDOs with immune cells or PBMCs can simulate features of the tumor immune regulation, such as T cell activation, infiltration into tumors, T cells recognition, and cancer cell eradication (52). Consequently, co-culture technique is a good platform for CAR-T (chimeric antigen receptor T-cell immunotherapy) evaluation (68, 69). Tsai et al. created pancreatic cancer PDOs using co-culture method with patient-matched peripheral blood lymphocytes and CAFs to study relevant between the immunotherapy and tumor-immune cell interaction (70). In colon cancer, Theresa E et al. provided the evidence that CAR-engineered NK-92 cells exhibit tumor antigen-specific cytotoxicity in organoid (71). However, the cytotoxic effects of T cells in PDOs have not been studied in the field of head and neck cancer, adoptive cell therapy advancement recently may provide opportunity for new perspective (72). It will be appealing to employ co-culture systems in the future to anticipate the efficiency of immunotherapy since several studies have shown that it is beneficial for HNCs (73–75).
Air-liquid interface
To explore patterns in how tumors interact with the microenvironment, the ALI technique is designed for the evaluation of the interaction between the epithelium and stromal microenvironment. Two dished (inner and outer) were used to separate collagen gel matrix and culture media in which the growth of organoid tissue retain native tissue architecture without reconstitution (53). And the organoid constructed in this way does not need to add additional growth factors because the endogenous factors secreted by stromal cells are sufficient (76). ALI technique produces organoids that include immune cells like T cells and B cells adjacent to the tumor epithelium (52) and they successfully mimic immune checkpoint inhibition by inhibiting PD-1/PD-L1, activating tumor antigen-specific tumor-infiltrating cells, and generating tumor cytotoxicity (52). It has so far been applied to a number of cancers, such as kidney (77) and colorectal cancer (77, 78). Despite the fact that the anticancer effect of tumor-infiltrating lymphocytes offers immune treatment strategies for the prevention of HNCs progression to improve patients’ overall survival (79, 80), ALI technology has not been tested in HNC PDOs. As the effectiveness of immune medicines in HNC PDOs has not yet been established, the ALI technology offers a promising avenue of investigation.
Microfluidic
Photolithography and 3D printing technology allows us to accurately build frameworks that simulate microenvironments in organism (58). The scientific progress of devices that control a submillimeter volume of fluid is known as microfluidics. Organoids and cultural medium are distributed in different channels, continuously feeding the organoids through inflow and outflow by microfluidic device (81). It can be used to investigate stromal cell cross-talk and T cell penetration into cancer organoid by adding exogenous T cells into the media channels (82). Recently, custom designed 3D printed microfluidic chip co-culture technique provide possibility to de novo generated vasculature with cerebral organoids (55, 83). It is a simple, extremely efficient method of vascularizing organoids that may be used with any organoid system. This microfluidic technique has been used to successfully culture up to two types of organoids(liver and islet) and detect metabolism-related pathway activity (84). Well-designed microfluidic devices that maintain autologous myeloid and lymphoid cell populations similar to the original donor can be used to detect dynamic response and resistance to immune checkpoint inhibitors (85). However, the materials used in the microfluidic devices are different from those used in microscopic cultures, making generalizations difficult (56). In fact, cells cultivated on polystyrene or glass still form the basis of the bulk of newly published research on in vitro cell biology. Zhaohui Wang recently designed a microfluidic device to generate nanoliter-sized droplets contains organospheres, and in this way they were able to rapidly and massively produce organoids for head and neck tumors (24).
Bioreactor
Bioreactor is a device that allows cells or tissues to grow in a controlled environment. Through a series of mechanical regulations, the cells are provided with sufficient nutrients and a suitable environment in which cells or tissues can grow rapidly. Specific and detailed techniques can be referred to Xuyu Qian et al.’s work (59). In many studies, bioreactors have been shown to significantly enhance the efficiency of 3D culture (86, 87). The rapid cultivation of large numbers of organoids in small samples is a widely faced problem. The HNC biopsy specimens are frequently too small and inadequate to establish direct organoids. Additionally, restrictions in the transport of oxygen and nutrients may impede the formation of organoids in static cultures. It has been reported that rotating-wall vessel bioreactors (88) and bioreactor SpinΩ (58) can rapidly promote retinal organoid and brain organoid production respectively. The development of materials technology has provided a great impetus for the translation of basic research into the clinic, new-type bioreactor may be possible to solve the problem in expanding the culture of organoids from small biopsy tissue.
Organoids-on-a-chip
Organoids-on-a-chip is an integration which combines the advantages of organoids and organ-on-a-chip technology. This is a microfabricated cell culture devices designed to imitate the functional units of human organs in vitro (89, 90). This model can mimic integrated organ-level functions necessary for physiological homeostasis, as well as complex disease processes (90, 91) by integrating living human cells with synthetically generated yet physiologically relevant micro-environments, and furthermore, simulate multiorgan interactions and physiological responses at the systemic level (62). The precise micro-environment control in this technology opens up a new idea for organoid and provides solutions to several problems. The precise control of micro-environment is the main barrier in organoid development, in which contains biochemical signaling, mechanical forces and nutrient supply. Yuli Wang et al., utilized a cross-linked collagen hydrogel to form a biomimetic scaffold, in which small intestinal epithelium cells were guided to form a crypt-villus architecture. Cells polarized by application of gradients of soluble biochemical reagents along the crypt-villus axis (92). Homan et al. used a 3D printed chamber to form a kidney organoid-on-chip model. He and his co-worker found out that high fluid flow stress (FFS) resulted in acceleration of hPSCs-derived kidney organoid vascularization and maturation of glomerular and tubular compartments. Additionally, concurrent morphogenesis of podocytes and tubular epithelial cells was accelerated in a shear stress-dependent manner, indicating that the flow-generated microenvironment was a key factor in the structural and functional development of the kidney organoid (93). Despite micro-environmental control, organoids-on-a-chip technology also achieve modeling biological interactions and reducing variability (63). Although there is no definitive organoids-on-a-chip study in head and neck tumor research, but Zhaohui Wang et al. have developed a chip to produces head and neck tumor organoids rapidly (24).
Application
Prediction of treatment effectiveness
40% of patients with HNSCC relapse despite conventional therapeutic approaches, in part because they lack the effective strategy to choose the sensitive treatment. To forecast patients’ response to therapy in vitro is one of the most crucial uses of PDOs. The outcomes offer specific directions for clinical patient care. In HNC PDOs, several studies have explored the sensitivity of cisplatin (16–18) and found that this sensitivity could simulate patient response to treatment partially. One HNC subtype, nasopharyngeal cancer, maintains unique molecular characteristics, medication response, and graded radiation sensitivity (19). Potential subtype-specific therapy regimens are identified through the use of PDO-based pharmacological tests. It is reported that the epithelial subtype is more sensitive to EGFR inhibitor and sarcomatoid subtype and mixed sarcomatoid-epithelial is more sensitive to microtubule inhibitors (19). In addition to chemotherapeutic drugs, targeted drugs is also used to test organoids for drug sensitivity. Epidermal Growth Factor Receptor (EGFR) protein level is much higher in head and neck tumors than in normal tissues, making it an effective therapeutic target. EGFR-targeted photodynamic therapy (PDT) could successfully kill head and neck tumor organoids and does not affect organoids of normal tissue formation due to low EGFR expression level in the latter (41). Radiotherapy is an effective treatment for head and neck tumors, so it is of great significance to predict the sensitivity of radiotherapy for clinical treatment. Researchers Driehuis and colleagues found a connection between the clinical outcome of the relevant donor patient and the HNSCC tumoroid’s radiation sensitivity. In patients who relapsed after radiotherapy, the corresponding organoids showed the same resistance to radiotherapy (17). Furthermore, the HNC PDOs can also be used to establish radiobiological parameters (42). On this detail, A technique for medium-throughput drug screening using HNSCC and colorectal PDOs was reported by Putker and his colleagues in 2021. They stated that any tissue-derived organoid model with in vitro exposure to chemotherapy and/or radiation can use this platform (96).
However, the use of PDOs to predict therapeutic outcomes is still in its infancy. These results need to be interpreted with caution, although many researchers have produced organoid drug responses similar to those in patients. Appropriate gene-targeting mutations do not necessarily mean that specific drug therapy is effective. For instance, on one occasion an organoid responded favorably to a PI3K inhibitor despite the absence of a PI3K gene-activating mutation in the organoid (17). As a result, functional research may occasionally be more insightful than genetic research.
Biobank construction and biomarker prediction
The Cancer Genome Atlas (TCGA) and the International Cancer Genome Consortium used a large number of tumor gene expression profiles to create a comprehensive “atlas” that provides a powerful tool for understanding tumorigenesis, progression, and therapeutic response (97, 98). Theoretically, PDOs provide a faster and more convenient way to obtain tumor expression information and to obtain expression profiles at any stage and location, especially in patients with advanced tumors. Patients with HNCs are characterized by numerous subtypes and obvious heterogeneity. In some small sample studies, PDOs recapitulate genetic alterations found in HNSCC patients (16, 17, 19, 28). For instance, PIK3CA mutations and the lack of EGFR amplifications, two characteristics of HPV-positive HNSCC that are lost in established cell lines, are retained in PDOs (99). In addition, biobank established using PDOs can also be used as a biomarker prediction for sophisticated tumor subtypes. Bo Wang et al. established a patient-derived organoid biobank with salivary gland tumors (benign and malignant) to explore the subtypes of tumors. The biobank replicated the transcriptional and anatomical characteristics of the original malignancies, which uncover PTP4A1 as a mucoepidermoid carcinoma diagnostic biomarker (22). As a result of the use of PDOs in the future, it is expected that more “atlases” will be established for the guidance of clinical treatment.
Studying the risk factor of HNC using PDOs
Research on humans and animals has been hampered by questions of access to samples and ethics. Fortunately, organoids are a powerful alternative, preserving genetic and phenotypic information, and simulating the progression of tumors and metastasis (100). The ability to be expanded in vitro rapidly distinguishes tumoroids from PDX models and 2D cell cultures, allowing for the quick production of a large number of clones for further research on clinically orphan disease (101). And organoid system serves as a physiologically relevant experimental platform to determine the effects of epithelial exposure to harmful environmental chemicals such as alcohol (102) and acetaldehyde (103). Shimonosono et al. studied the mechanism of ethanol exposure by HNC organoids in 2021 since alcohol use is one of the major risk factors for HNC (104). The results demonstrated in non-CD44H cells, mitochondrial damage is induced by oxidative stress and apoptosis. Meanwhile, ethanol exposure improved intratumorally CD44H cells growth in an autophagy-dependent way. At present, there are very few basic studies applied to head and neck tumor organoids. It is expected that more studies will follow with the progress of modeling methods.
Limitation and perspective
Limitation
In recent years, tumor organoids have developed rapidly, but deficiencies and challenges still exist. First and foremost, the success rate of PDOs construction varies across tumor pathological types, sometimes, rather low (105). Underlying factors contribute to the low success rate, such as non-standard culture medium, pathological type of tumor, cancer heterogeneity, and lack of standardized protocols. Therefore, a standard tumor organoid procedure for HNC is required, in which the sample processing, digestion, and culture conditions of different tumor types should be specified. Of course, this will consume a lot of time cost and labor cost to build. Notably, a study has shown that optimization of the culture medium composition could partially improve organoid growth in breast cancer (106).
Secondly, whether the PDOs are truly representative of the primary tumor and whether they introduce unexpected bias while cultured in vitro remains unknown. Under some circumstances, the dominant growth of epithelial cells contamination could be detrimental for cancer cells in the organoid cultures and is a problem in some cancers (107). ALK inhibitor A83-01 (108) and p38 inhibitor SB202190 (109) are two typical additives to organoid culture media that may interact with medications that target the same signaling pathway. Consequently, like the CCK-8 assay in 2D cell culture, the development and validation of a universal and reliable protocol with predetermined threshold values for medication response will be necessary for the therapeutic application of PDOs. In addition to technical obstacles, PDOs do not comprise the tumor microenvironment which is consist of fibroblasts or immune cells. The emergence of co-cultures, which are detailed in more detail above, has partially addressed this.
Last but not least, tumoroid lack of vasculatures and neuronal networks restrains their capability to be used as precise models to investigate the effects of personalized therapeutic strategies. Technically, some of the technologies mentioned above are attempting to address this shortcoming, such as co-culture method and air-liquid interface, but there is still much work to be done. Furthermore, before adoption in clinical cancer treatment can be taken into consideration, there are still numerous challenges to be solved, and large cohort investigations are urgently required.
Perspective
In the past, it took at least 10 years for a drug to be developed into a clinical application. The use of organoids has greatly accelerated the transfer of drugs from the bench to the bedside. An ongoing multicenter observational study (NCT04261192) is going to assess the feasibility of using PDO from HNSCC as tools for predicting response to treatments. The study plans to verify the sensitivity and clinical predictive value of organoids to chemotherapy, radiotherapy, RAPR inhibitors, and immunotherapy. And new targets are predicted based on molecular signatures and therapeutic response (110). Of note, this study will coculture of PDO with immune cells. Another clinical trial (NCT05400239), which has not yet begun recruiting, is planned to assess whether patient derived organoids can be used to predict treatment sensitivity in HNC patients. The completion of clinical trials will promote the further application of head and neck tumor organoids in precision therapy. Narmal tissue organoids can be used to better understand and estimate treatment-related adverse effects, which are frequently seen with targeted therapy. This use is less well known. For instance, E Driehuis et al. studied the effect of MTX on oral mucosa organoid (111). Another noteworthy example is the investigation of nephrotoxic medicines using kidney organoids (112).
Recently, the application of genetic engineering can enable us to better understand the mechanism of tumor occurrence and progression at the genetic level. As a powerful genome engineering tool, CRISPR/Cas9 may be used to modulate genes in organoids to deliver various target gene mutations for further underlying mechanism research (105). In other words, this technique can introduce oncogenic mutations for simulating tumorigenesis. Due to the urgent need for a thorough understanding of the various mutational drivers of the specific cancer subtype, which may not be available, there are significant challenges in the implementation of this technique. There have already been reports of successful use of CRISPR/Cas9 in tumor organoids, such as colorectal, breast, glioma, and ovarian (113–115). It might represent a promising approach to modeling oral carcinogenesis. Additionally, organoid technology can be used to evaluate people who have a family history of head and neck cancer to rule out major risk factors in their lifestyle, such as smoking, drinking, and being susceptible to HPV infection, thereby reducing the likelihood of potentially disastrous events like invasion. Regeneration of surgical tissues by organoids can give HNSCC patients with autologous transplantation and improve the quality of their lives in addition to early detection. PDOs may provide a platform to facilitate investigation into the utility of novel therapeutic strategies, including CAR-T cell therapies (71) and oncolytic virotherapy,
As PDOs research expands significantly, there is an increased potential for understanding aspects of cancer, stratification of patients, and the development of personalized therapeutic strategies based on these findings. However, to fully exploit its potential, it is necessary to further adapt the state-of-the-art methods and technologies related to organoids. It is imperative to continue the quest for biologically relevant inquiries and technical progress, designing studies crucial to patients. The expansion of collaborations and bio-bank sharing is also critical to increase the sample size of our cohorts and apply the appropriate expertise. To conclude, the validation of organoid-derived data may help develop precision medicine approaches that can optimize health outcomes in patients with head and neck cancer by providing meaningful results that can potentially be used in diagnostics and therapeutics.
Author contributions
HQ, XT, WZ, YZ, and DZ wrote the manuscript. SC and SW contributed to the limitation and perspective. JW revised the manuscript and responsible for the overall content. All authors contributed to the article and approved the submitted version.
Conflict of interest
The authors declare that the research was conducted in the absence of any commercial or financial relationships that could be construed as a potential conflict of interest.
Publisher’s note
All claims expressed in this article are solely those of the authors and do not necessarily represent those of their affiliated organizations, or those of the publisher, the editors and the reviewers. Any product that may be evaluated in this article, or claim that may be made by its manufacturer, is not guaranteed or endorsed by the publisher.
References
1. Huang SH, O’Sullivan B. Overview of the 8th edition TNM classification for head and neck cancer. Curr Treat Options Oncol (2017) 18(7):40. doi: 10.1007/s11864-017-0484-y
3. Ferlay J, Colombet M, Soerjomataram I, Mathers C, Parkin DM, Pieros M, et al. Estimating the global cancer incidence and mortality in 2018: GLOBOCAN sources and methods. Int J Cancer (2019) 144(8):1941–53. doi: 10.1002/ijc.31937
4. Bray F, Ferlay J, Soerjomataram I, Siegel RL, Torre LA, Jemal A. Global cancer statistics 2018: GLOBOCAN estimates of incidence and mortality worldwide for 36 cancers in 185 countries. CA: Cancer J For Clin (2018) 68(6):394–424. doi: 10.3322/caac.21492
5. Vigneswaran N, Williams MD. Epidemiologic trends in head and neck cancer and aids in diagnosis. Oral Maxillofac Surg Clin North Am (2014) 26(2):123–41. doi: 10.1016/j.coms.2014.01.001
6. Skálová A, Hyrcza MD, Leivo I. Update from the 5th edition of the world health organization classification of head and neck tumors: salivary glands. Head Neck Pathol (2022) 16(1):40–53. doi: 10.1007/s12105-022-01420-1
7. Argiris A, Karamouzis MV, Raben D, Ferris RL. Head and neck cancer. Lancet (2008) 371(9625):1695–709. doi: 10.1016/S0140-6736(08)60728-X
8. Kaidar-Person O, Gil Z, Billan S. Precision medicine in head and neck cancer. Drug Resist Updat (2018) 40:13–6. doi: 10.1016/j.drup.2018.09.001
9. Rothenberg SM, Ellisen LW. The molecular pathogenesis of head and neck squamous cell carcinoma. J Clin Invest (2012) 122(6):1951–7. doi: 10.1172/JCI59889
10. Sato T, Vries RG, Snippert HJ, van de Wetering M, Barker N, Stange DE, et al. Single Lgr5 stem cells build crypt-villus structures in vitro without a mesenchymal niche. Nature (2009) 459(7244):262–5. doi: 10.1038/nature07935
11. Boehm JS, Hahn WC. Immortalized cells as experimental models to study cancer. Cytotechnology (2004) 45(1-2):47–59. doi: 10.1007/s10616-004-5125-1
12. Haney MG, Moore LH, Blackburn JS. Drug screening of primary patient derived tumor xenografts in zebrafish. J Visualized Experiments: JoVE (2020) 158. doi: 10.3791/60996
13. Hidalgo M, Amant F, Biankin AV, Budinsk E, Byrne AT, Caldas C, et al. Patient-derived xenograft models: an emerging platform for translational cancer research. Cancer Discovery (2014) 4(9):998–1013. doi: 10.1158/2159-8290.CD-14-0001
14. Bleijs M, van de Wetering M, Clevers H, Drost J. Xenograft and organoid model systems in cancer research. EMBO J (2019) 38(15):e101654. doi: 10.15252/embj.2019101654
15. Sato T, Stange DE, Ferrante M, Vries RGJ, Van Es JH, Van den Brink S, et al. Long-term expansion of epithelial organoids from human colon, adenoma, adenocarcinoma, and barrett’s epithelium. Gastroenterology (2011) 141(5):1762–72. doi: 10.1053/j.gastro.2011.07.050
16. Tanaka N, Osman AA, Takahashi Y, Lindemann A, Patel AA, Zhao M, et al. Head and neck cancer organoids established by modification of the CTOS method can be used to predict in vivo drug sensitivity. Oral Oncol (2018) 87:49–57. doi: 10.1016/j.oraloncology.2018.10.018
17. Driehuis E, Kolders S, Spelier S, Lohmussaar K, Willems SM, Devriese LA, et al. Oral mucosal organoids as a potential platform for personalized cancer therapy. Cancer Discovery (2019) 9(7):852–71. doi: 10.1158/2159-8290.CD-18-1522
18. Karakasheva TA, Kijima T, Shimonosono M, Maekawa H, Sahu V, Gabre JT, et al. Generation and characterization of patient-derived head and neck, oral, and esophageal cancer organoids. Curr Protoc Stem Cell Biol (2020) 53(1):e109. doi: 10.1002/cpsc.109
19. Ding RB, Chen P, Rajendran BK, Lyu X, Wang H, Bao J, et al. Molecular landscape and subtype-specific therapeutic response of nasopharyngeal carcinoma revealed by integrative pharmacogenomics. Nat Commun (2021) 12(1):3046. doi: 10.1038/s41467-021-23379-3
20. Aizawa Y, Takada K, Aoyama J, Sano D, Yamanaka S, Seki M, et al. Establishment of experimental salivary gland cancer models using organoid culture and patient-derived xenografting. Cell Oncol (Dordr) (2022) 46(2)409–421. doi: 10.21203/rs.3.rs-1621957/v1
21. Lassche G, van Boxtel W, Aalders TW, van Hooij O, van Engen-van Grunsven ACH, Verhaegh GW, et al. Development and characterization of patient-derived salivary gland cancer organoid cultures. Oral Oncol (2022) 135:106186. doi: 10.1016/j.oraloncology.2022.106186
22. Wang B, Gan J, Liu Z, Hui Z, Wei J, Gu X, et al. An organoid library of salivary gland tumors reveals subtype-specific characteristics and biomarkers. J Exp Clin Cancer Res: CR (2022) 41(1):350. doi: 10.1186/s13046-022-02561-5
23. Wang XW, Xia TL, Tang HC, Liu X, Han R, Zou X, et al. Establishment of a patient-derived organoid model and living biobank for nasopharyngeal carcinoma. Ann Transl Med (2022) 10(9):526. doi: 10.21037/atm-22-1076
24. Wang Z, Boretto M, Millen R, Natesh N, Reckzeh ES, Hsu C, et al. Rapid tissue prototyping with micro-organospheres. Stem Cell Rep (2022) 17(9):1959–75. doi: 10.1016/j.stemcr.2022.07.016
25. Yang H, Liang Q, Zhang J, Liu J, Wei H, Chen H, et al. Establishment of papillary thyroid cancer organoid lines from clinical specimens. Front Endocrinol (Lausanne) (2023) 14:1140888. doi: 10.3389/fendo.2023.1140888
26. Lin KC, Ting LL, Chang CL, Lu LS, Lee HL, Hsu FC, et al. Ex vivo expanded circulating tumor cells for clinical anti-cancer drug prediction in patients with head and neck cancer. Cancers (Basel) (2021) 13(23):6076. doi: 10.3390/cancers13236076
27. Kondo J, Endo H, Okuyama H, Ishikawa O, Iishi H, Tsujii M, et al. Retaining cell-cell contact enables preparation and culture of spheroids composed of pure primary cancer cells from colorectal cancer. Proc Natl Acad Sci U S A (2011) 108(15):6235–40. doi: 10.1073/pnas.1015938108
28. Lassche G, van Engen-van Grunsven ACH, van Hooij O, Aalders TW, Am Weijers J, Cocco E, et al. Precision oncology using organoids of a secretory carcinoma of the salivary gland treated with TRK-inhibitors. Oral Oncol (2023) 137:106297. doi: 10.1016/j.oraloncology.2022.106297
29. Slaughter DP, Southwick HW, Smejkal W. Field cancerization in oral stratified squamous epithelium; clinical implications of multicentric origin. Cancer (1953) 6(5):963–8. doi: 10.1002/1097-0142(195309)6:5<963::AID-CNCR2820060515>3.0.CO;2-Q
30. Mroz EA, Tward AD, Hammon RJ, Ren Y, Rocco JW. Intra-tumor genetic heterogeneity and mortality in head and neck cancer: analysis of data from the cancer genome atlas. PloS Med (2015) 12(2):e1001786. doi: 10.1371/journal.pmed.1001786
31. Cancer Genome Atlas N. Comprehensive genomic characterization of head and neck squamous cell carcinomas. Nature (2015) 517(7536):576–82. doi: 10.1038/nature14129
32. Califano J, van der Riet P, Westra W, Nawroz H, Clayman G, Piantadosi S, et al. Genetic progression model for head and neck cancer: implications for field cancerization. Cancer Res (1996) 56(11):2488–92. doi: 10.1016/S0194-5998(96)80631-0
33. Stransky N, Egloff AM, Tward AD, Kostic AD, Cibulskis K, Sivachenko A, et al. The mutational landscape of head and neck squamous cell carcinoma. Sci (New York NY) (2011) 333(6046):1157–60. doi: 10.1126/science.1208130
34. Johnson DE, Burtness B, Leemans CR, Lui VWY, Bauman JE, Grandis JR. Head and neck squamous cell carcinoma. Nat Rev Dis Primers (2020) 6(1):92. doi: 10.1038/s41572-020-00224-3
35. De Cecco L, Nicolau M, Giannoccaro M, Daidone MG, Bossi P, Locati L, et al. Head and neck cancer subtypes with biological and clinical relevance: meta-analysis of gene-expression data. Oncotarget (2015) 6(11):9627–42. doi: 10.18632/oncotarget.3301
36. Gilardi M, Wang Z, Proietto M, Chillà A, Calleja-Valera JL, Goto Y, et al. Tipifarnib as a precision therapy for HRAS-mutant head and neck squamous cell carcinomas. Mol Cancer Ther (2020) 19(9):1784–96. doi: 10.1158/1535-7163.MCT-19-0958
37. Sambandam V, Frederick MJ, Shen L, Tong P, Rao X, Peng S, et al. PDK1 mediates NOTCH1-mutated head and neck squamous carcinoma vulnerability to therapeutic PI3K/mTOR inhibition. Clin Cancer Res (2019) 25(11):3329–40. doi: 10.1158/1078-0432.CCR-18-3276
38. Juric D, Rodon J, Tabernero J, Janku F, Burris HA, Schellens JHM, et al. Phosphatidylinositol 3-Kinase-Selective inhibition with alpelisib (BYL719) in PIK3CA-altered solid tumors: results from the first-in-Human study. J Clin Oncol (2018) 36(13):1291–9. doi: 10.1200/JCO.2017.72.7107
39. Keam B, Kim S, Ahn Y-O, Kim TM, Lee S-H, Kim D-W, et al. In vitro anticancer activity of PI3K alpha selective inhibitor BYL719 in head and neck cancer. Anticancer Res (2015) 35(1):175–82.
40. Melissaridou S, Wiechec E, Magan M, Jain MV, Chung MK, Farnebo L, et al. The effect of 2D and 3D cell cultures on treatment response, EMT profile and stem cell features in head and neck cancer. Cancer Cell Int (2019) 19:16. doi: 10.1186/s12935-019-0733-1
41. Driehuis E, Spelier S, Beltran Hernandez I, de Bree R, MW S, Clevers H, et al. Patient-derived head and neck cancer organoids recapitulate EGFR expression levels of respective tissues and are responsive to EGFR-targeted photodynamic therapy. J Clin Med (2019) 8(11):1880. doi: 10.3390/jcm8111880
42. Lucky SS, Law M, Lui MH, Mong J, Shi J, Yu S, et al. Patient-derived nasopharyngeal cancer organoids for disease modeling and radiation dose optimization. Front Oncol (2021) 11:622244. doi: 10.3389/fonc.2021.622244
43. Zhao H, Jiang E, Shang Z. 3D Co-culture of cancer-associated fibroblast with oral cancer organoids. J Dent Res (2021) 100(2):201–8. doi: 10.1177/0022034520956614
44. Braakhuis BJM, Brakenhoff RH, Leemans CR. Treatment choice for locally advanced head and neck cancers on the basis of risk factors: biological risk factors. Ann Oncol (2012) 23(Suppl 10):x173–x7. doi: 10.1093/annonc/mds299
45. Lefebvre JL. Current clinical outcomes demand new treatment options for SCCHN. Ann Oncol (2005) 16(Suppl 6):vi7–vi12. doi: 10.1093/annonc/mdi452
46. Vermorken JB, Mesia R, Rivera F, Remenar E, Kawecki A, Rottey S, et al. Platinum-based chemotherapy plus cetuximab in head and neck cancer. N Engl J Med (2008) 359(11):1116–27. doi: 10.1056/NEJMoa0802656
47. Seiwert TY, Burtness B, Mehra R, Weiss J, Berger R, Eder JP, et al. Safety and clinical activity of pembrolizumab for treatment of recurrent or metastatic squamous cell carcinoma of the head and neck (KEYNOTE-012): an open-label, multicentre, phase 1b trial. Lancet Oncol (2016) 17(7):956–65. doi: 10.1016/S1470-2045(16)30066-3
48. Mehra R, Seiwert TY, Gupta S, Weiss J, Gluck I, Eder JP, et al. Efficacy and safety of pembrolizumab in recurrent/metastatic head and neck squamous cell carcinoma: pooled analyses after long-term follow-up in KEYNOTE-012. Br J Cancer (2018) 119(2):153–9. doi: 10.1038/s41416-018-0131-9
49. Driehuis E, Kretzschmar K, Clevers H. Establishment of patient-derived cancer organoids for drug-screening applications. Nat Protoc (2020) 15(10):3380–409. doi: 10.1038/s41596-020-0379-4
50. Zanoni M, Piccinini F, Arienti C, Zamagni A, Santi S, Polico R, et al. 3D tumor spheroid models for in vitro therapeutic screening: a systematic approach to enhance the biological relevance of data obtained. Sci Rep (2016) 6:19103. doi: 10.1038/srep19103
51. Shah AT, Heaster TM, Skala MC. Metabolic imaging of head and neck cancer organoids. PloS One (2017) 12(1):e0170415. doi: 10.1371/journal.pone.0170415
52. Neal JT, Li X, Zhu J, Giangarra V, Grzeskowiak CL, Ju J, et al. Organoid modeling of the tumor immune microenvironment. Cell (2018) 175(7):1972–88.e16. doi: 10.1016/j.cell.2018.11.021
53. Li X, Ootani A, Kuo C. An air-liquid interface culture system for 3D organoid culture of diverse primary gastrointestinal tissues. Methods In Mol Biol (Clifton NJ) (2016) 1422:33–40. doi: 10.1007/978-1-4939-3603-8_4
54. Giandomenico SL, Mierau SB, Gibbons GM, Wenger LMD, Masullo L, Sit T, et al. Cerebral organoids at the air-liquid interface generate diverse nerve tracts with functional output. Nat Neurosci (2019) 22(4):669–79. doi: 10.1038/s41593-019-0350-2
55. Salmon I, Grebenyuk S, Abdel Fattah AR, Rustandi G, Pilkington T, Verfaillie C, et al. Engineering neurovascular organoids with 3D printed microfluidic chips. Lab Chip (2022) 22(8):1615–29. doi: 10.1039/D1LC00535A
56. Halldorsson S, Lucumi E, Gómez-Sjöberg R, Fleming RMT. Advantages and challenges of microfluidic cell culture in polydimethylsiloxane devices. Biosens Bioelectron (2015) 63:218–31. doi: 10.1016/j.bios.2014.07.029
57. Sackmann EK, Fulton AL, Beebe DJ. The present and future role of microfluidics in biomedical research. Nature (2014) 507(7491):181–9. doi: 10.1038/nature13118
58. Qian X, Jacob F, Song MM, Nguyen HN, Song H, Ming G-L. Generation of human brain region-specific organoids using a miniaturized spinning bioreactor. Nat Protoc (2018) 13(3):565–80. doi: 10.1038/nprot.2017.152
59. Qian X, Nguyen Ha N, Song Mingxi M, Hadiono C, Ogden Sarah C, Hammack C, et al. Brain-Region-Specific organoids using mini-bioreactors for modeling ZIKV exposure. Cell (2016) 165(5):1238–54. doi: 10.1016/j.cell.2016.04.032
60. Ovando-Roche P, West EL, Branch MJ, Sampson RD, Fernando M, Munro P, et al. Use of bioreactors for culturing human retinal organoids improves photoreceptor yields. Stem Cell Res Ther (2018) 9(1):156. doi: 10.1186/s13287-018-0907-0
61. Ellis MA, Dalwadi MP, Ellis MJ, Byrne HM, Waters SL. A systematically reduced mathematical model for organoid expansion. Front Bioeng Biotechnol (2021) 9:670186. doi: 10.3389/fbioe.2021.670186
62. Sung JH, Wang YI, Narasimhan N Sriram, Jackson M, Long C, Hickman JJ, et al. Recent advances in body-on-a-Chip systems. Anal Chem (2019) 91(1):330–51. doi: 10.1021/acs.analchem.8b05293
63. Park SE, Georgescu A, Huh D. Organoids-on-a-chip. Sci (New York NY) (2019) 364(6444):960–5. doi: 10.1126/science.aaw7894
64. Unagolla JM, Jayasuriya AC. Recent advances in organoid engineering: a comprehensive review. Appl Mater Today (2022) 29:101582. doi: 10.1016/j.apmt.2022.101582
65. Zhao H, Li R, Chen Y, Yang X, Shang Z. Stromal nicotinamide n-methyltransferase orchestrates the crosstalk between fibroblasts and tumour cells in oral squamous cell carcinoma: evidence from patient-derived assembled organoids. Oncogene (2023) 42(15):1166–1180. doi: 10.1038/s41388-023-02642-5
66. Patel AK, Vipparthi K, Thatikonda V, Arun I, Bhattacharjee S, Sharan R, et al. A subtype of cancer-associated fibroblasts with lower expression of alpha-smooth muscle actin suppresses stemness through BMP4 in oral carcinoma. Oncogenesis (2018) 7(10):78. doi: 10.1038/s41389-018-0087-x
67. Chen X, Li R, Zhao H, Wang X, Shao Z, Shang Z. Phenotype transition of fibroblasts incorporated into patient-derived oral carcinoma organoids. Oral Dis (2021) 29(3):913–922. doi: 10.1111/odi.14071
68. Dijkstra KK, Cattaneo CM, Weeber F, Chalabi M, van de Haar J, Fanchi LF, et al. Generation of tumor-reactive T cells by Co-culture of peripheral blood lymphocytes and tumor organoids. Cell (2018) 174(6):1586–1598.e12. doi: 10.1016/j.cell.2018.07.009
69. Michie J, Beavis PA, Freeman AJ, Vervoort SJ, Ramsbottom KM, Narasimhan V, et al. Antagonism of IAPs enhances CAR T-cell efficacy. Cancer Immunol Res (2019) 7(2):183–92. doi: 10.1158/2326-6066.CIR-18-0428
70. Tsai S, McOlash L, Palen K, Johnson B, Duris C, Yang Q, et al. Development of primary human pancreatic cancer organoids, matched stromal and immune cells and 3D tumor microenvironment models. BMC Cancer (2018) 18(1):335. doi: 10.1186/s12885-018-4238-4
71. Schnalzger TE, de Groot MH, Zhang C, Mosa MH, Michels BE, Rder J, et al. 3D model for CAR-mediated cytotoxicity using patient-derived colorectal cancer organoids. EMBO J (2019) 38(12):e100928. doi: 10.15252/embj.2018100928
72. Qureshi HA, Lee SM. Immunotherapy approaches beyond PD-1 inhibition: the future of cellular therapy for head and neck squamous cell carcinoma. Curr Treat Options Oncol (2019) 20(4):31. doi: 10.1007/s11864-019-0630-9
73. Cohen EEW, Bell RB, Bifulco CB, Burtness B, Gillison ML, Harrington KJ, et al. The society for immunotherapy of cancer consensus statement on immunotherapy for the treatment of squamous cell carcinoma of the head and neck (HNSCC). J Immunother Cancer (2019) 7(1):184. doi: 10.1186/s40425-019-0662-5
74. Cramer JD, Burtness B, Ferris RL. Immunotherapy for head and neck cancer: recent advances and future directions. Oral Oncol (2019) 99:104460. doi: 10.1016/j.oraloncology.2019.104460
75. Obradovic A, Graves D, Korrer M, Wang Y, Roy S, Naveed A, et al. Immunostimulatory cancer-associated fibroblast subpopulations can predict immunotherapy response in head and neck cancer. Clin Cancer Res (2022) 28(10):2094–109. doi: 10.1158/1078-0432.CCR-21-3570
76. Ootani A, Li X, Sangiorgi E, Ho QT, Ueno H, Toda S, et al. Sustained in vitro intestinal epithelial culture within a wnt-dependent stem cell niche. Nat Med (2009) 15(6):701–6. doi: 10.1038/nm.1951
77. Esser LK, Branchi V, Leonardelli S, Pelusi N, Simon AG, Klmper N, et al. Cultivation of clear cell renal cell carcinoma patient-derived organoids in an air-liquid interface system as a tool for studying individualized therapy. Front In Oncol (2020) 10:1775. doi: 10.3389/fonc.2020.01775
78. Zhao Y, Zhang B, Ma Y, Zhao F, Chen J, Wang B, et al. Colorectal cancer patient-derived 2D and 3D models efficiently recapitulate inter- and intratumoral heterogeneity. Advanced Sci (Weinheim Baden-Wurttemberg Germany) (2022) 9(22):e2201539. doi: 10.1002/advs.202201539
79. von Witzleben A, Wang C, Laban S, Savelyeva N, Ottensmeier CH. HNSCC: tumour antigens and their targeting by immunotherapy. Cells (2020) 9(9):2103. doi: 10.3390/cells9092103
80. Almangush A, Leivo I, Mäkitie AA. Overall assessment of tumor-infiltrating lymphocytes in head and neck squamous cell carcinoma: time to take notice. Acta Otolaryngol (2020) 140(3):246–8. doi: 10.1080/00016489.2020.1720284
81. Hou X, Zhang YS, Santiago G-d, Alvarez MM, Ribas J, Jonas SJ, et al. Interplay between materials and microfluidics. Nat Rev Mater (2017) 2(5):17016. doi: 10.1038/natrevmats.2017.16
82. Kitajima S, Ivanova E, Guo S, Yoshida R, Campisi M, Sundararaman SK, et al. Suppression of STING associated with LKB1 loss in KRAS-driven lung cancer. Cancer Discovery (2019) 9(1):34–45. doi: 10.1158/2159-8290.CD-18-0689
83. Rajasekar S, Lin DSY, Abdul L, Liu A, Sotra A, Zhang F, et al. IFlowPlate-a customized 384-well plate for the culture of perfusable vascularized colon organoids. Advanced mater (Deerfield Beach Fla) (2020) 32(46):e2002974. doi: 10.1002/adma.202002974
84. Tao T, Deng P, Wang Y, Zhang X, Guo Y, Chen W, et al. Microengineered multi-organoid system from hiPSCs to recapitulate human liver-islet axis in normal and type 2 diabetes. Advanced Sci (Weinheim Baden-Wurttemberg Germany) (2022) 9(5):e2103495. doi: 10.1002/advs.202103495
85. Jenkins RW, Aref AR, Lizotte PH, Ivanova E, Stinson S, Zhou CW, et al. Ex vivo profiling of PD-1 blockade using organotypic tumor spheroids. Cancer Discovery (2018) 8(2):196–215. doi: 10.1158/2159-8290.CD-17-0833
86. Gerecht-Nir S, Cohen S, Itskovitz-Eldor J. Bioreactor cultivation enhances the efficiency of human embryoid body (hEB) formation and differentiation. Biotechnol Bioeng (2004) 86(5):493–502. doi: 10.1002/bit.20045
87. Lancaster MA, Knoblich JA. Organogenesis in a dish: modeling development and disease using organoid technologies. Sci (New York NY) (2014) 345(6194):1247125. doi: 10.1126/science.1247125
88. DiStefano T, Chen HY, Panebianco C, Kaya KD, Brooks MJ, Gieser L, et al. Accelerated and improved differentiation of retinal organoids from pluripotent stem cells in rotating-wall vessel bioreactors. Stem Cell Rep (2018) 10(1):300–13. doi: 10.1016/j.stemcr.2017.11.001
89. Huh D, Hamilton GA, Ingber DE. From 3D cell culture to organs-on-chips. Trends Cell Biol (2011) 21(12):745–54. doi: 10.1016/j.tcb.2011.09.005
90. Esch EW, Bahinski A, Huh D. Organs-on-chips at the frontiers of drug discovery. Nat Rev Drug discovery (2015) 14(4):248–60. doi: 10.1038/nrd4539
91. Benam KH, Dauth S, Hassell B, Herland A, Jain A, Jang K-J, et al. Engineered in vitro disease models. Annu Rev Pathol (2015) 10:195–262. doi: 10.1146/annurev-pathol-012414-040418
92. Wang Y, Gunasekara DB, Reed MI, DiSalvo M, Bultman SJ, Sims CE, et al. A microengineered collagen scaffold for generating a polarized crypt-villus architecture of human small intestinal epithelium. Biomaterials (2017) 128:44–55. doi: 10.1016/j.biomaterials.2017.03.005
93. Homan KA, Gupta N, Kroll KT, Kolesky DB, Skylar-Scott M, Miyoshi T, et al. Flow-enhanced vascularization and maturation of kidney organoids in vitro. Nat Methods (2019) 16(3):255–62. doi: 10.1038/s41592-019-0325-y
94. Phelan MA, Lelkes PI, Swaroop A. Mini and customized low-cost bioreactors for optimized high-throughput generation of tissue organoids. Stem Cell Investig (2018) 5:33. doi: 10.21037/sci.2018.09.06
95. Wang Y, Wang L, Guo Y, Zhu Y, Qin J. Engineering stem cell-derived 3D brain organoids in a perfusable organ-on-a-chip system. RSC Adv (2018) 8(3):1677–85. doi: 10.1039/C7RA11714K
96. Putker M, Millen R, Overmeer R, Driehuis E, Zandvliet MMJM, Clevers H, et al. Medium-throughput drug- and radiotherapy screening assay using patient-derived organoids. J Visualized Experiments: JoVE (2021) 170. doi: 10.3791/62495
97. Cooper LA, Demicco EG, Saltz JH, Powell RT, Rao A, Lazar AJ. PanCancer insights from the cancer genome atlas: the pathologist’s perspective. J Pathol (2018) 244(5):512–24. doi: 10.1002/path.5028
98. Hudson TJ, Anderson W, Artez A, Barker AD, Bell C, Bernabé RR, et al. International network of cancer genome projects. Nature (2010) 464(7291):993–8. doi: 10.1038/nature08987
99. Facompre ND, Rajagopalan P, Sahu V, Pearson AT, Montone KT, James CD, et al. Identifying predictors of HPV-related head and neck squamous cell carcinoma progression and survival through patient-derived models. Int J Cancer (2020) 147(11):3236–49. doi: 10.1002/ijc.33125
100. Lau HCH, Kranenburg O, Xiao H, Yu J. Organoid models of gastrointestinal cancers in basic and translational research. Nat Rev Gastroenterol Hepatol (2020) 17(4):203–22. doi: 10.1038/s41575-019-0255-2
101. Xu H, Lyu X, Yi M, Zhao W, Song Y, Wu K. Organoid technology and applications in cancer research. J Hematol Oncol (2018) 11(1):116. doi: 10.1186/s13045-018-0662-9
102. Chandramouleeswaran PM, Guha M, Shimonosono M, Whelan KA, Maekawa H, Sachdeva UM, et al. Autophagy mitigates ethanol-induced mitochondrial dysfunction and oxidative stress in esophageal keratinocytes. PloS One (2020) 15(9):e0239625. doi: 10.1371/journal.pone.0239625
103. Tanaka K, Whelan KA, Chandramouleeswaran PM, Kagawa S, Rustgi SL, Noguchi C, et al. ALDH2 modulates autophagy flux to regulate acetaldehyde-mediated toxicity thresholds. Am J Cancer Res (2016) 6(4):781–96.
104. Shimonosono M, Tanaka K, Flashner S, Takada S, Matsuura N, Tomita Y, et al. Alcohol metabolism enriches squamous cell carcinoma cancer stem cells that survive oxidative stress via autophagy. Biomolecules (2021) 11(10):1479. doi: 10.3390/biom11101479
105. LeSavage BL, Suhar RA, Broguiere N, Lutolf MP, Heilshorn SC. Next-generation cancer organoids. Nat Mater (2022) 21(2):143–59. doi: 10.1038/s41563-021-01057-5
106. Sachs N, de Ligt J, Kopper O, Gogola E, Bounova G, Weeber F, et al. A living biobank of breast cancer organoids captures disease heterogeneity. Cell (2018) 172(1-2):373–386.e10. doi: 10.1016/j.cell.2017.11.010
107. Karthaus WR, Iaquinta PJ, Drost J, Gracanin A, van Boxtel R, Wongvipat J, et al. Identification of multipotent luminal progenitor cells in human prostate organoid cultures. Cell (2014) 159(1):163–75. doi: 10.1016/j.cell.2014.08.017
108. Zhang R-R, Koido M, Tadokoro T, Ouchi R, Matsuno T, Ueno Y, et al. Human iPSC-derived posterior gut progenitors are expandable and capable of forming gut and liver organoids. Stem Cell Rep (2018) 10(3):780–93. doi: 10.1016/j.stemcr.2018.01.006
109. Wang Y, Hou Q, Wu Y, Xu Y, Liu Y, Chen J, et al. Methionine deficiency and its hydroxy analogue influence chicken intestinal 3-dimensional organoid development. Anim Nutr (2022) 8(1):38–51. doi: 10.1016/j.aninu.2021.06.001
110. Perreard M, Florent R, Divoux J, Grellard JM, Lequesne J, Briand M, et al. ORGAVADS: establishment of tumor organoids from head and neck squamous cell carcinoma to assess their response to innovative therapies. BMC Cancer (2023) 23(1):223. doi: 10.1186/s12885-023-10692-x
111. Driehuis E, Oosterom N, Heil SG, Muller IB, Lin M, Kolders S, et al. Patient-derived oral mucosa organoids as an in vitro model for methotrexate induced toxicity in pediatric acute lymphoblastic leukemia. PloS One (2020) 15(5):e0231588. doi: 10.1371/journal.pone.0231588
112. Lawlor KT, Vanslambrouck JM, Higgins JW, Chambon A, Bishard K, Arndt D, et al. Cellular extrusion bioprinting improves kidney organoid reproducibility and conformation. Nat Mater (2021) 20(2):260–71. doi: 10.1038/s41563-020-00853-9
113. Dekkers JF, Whittle JR, Vaillant F, Chen H-R, Dawson C, Liu K, et al. Modeling breast cancer using CRISPR-Cas9-Mediated engineering of human breast organoids. J Natl Cancer Inst (2020) 112(5):540–4. doi: 10.1093/jnci/djz196
114. Bian S, Repic M, Guo Z, Kavirayani A, Burkard T, Bagley JA, et al. Genetically engineered cerebral organoids model brain tumor formation. Nat Methods (2018) 15(8):631–9. doi: 10.1038/s41592-018-0070-7
Keywords: head and neck cancer, organoids, techniques, precision medicine, drug testing
Citation: Qi H, Tan X, Zhang W, Zhou Y, Chen S, Zha D, Wang S and Wen J (2023) The applications and techniques of organoids in head and neck cancer therapy. Front. Oncol. 13:1191614. doi: 10.3389/fonc.2023.1191614
Received: 22 March 2023; Accepted: 09 June 2023;
Published: 23 June 2023.
Edited by:
Peter Polverini, University of Michigan, United StatesReviewed by:
Steven Donald Forsythe, National Cancer Institute (NIH), United StatesXiaohui Shen, Shanghai Jiao Tong University, China
Copyright © 2023 Qi, Tan, Zhang, Zhou, Chen, Zha, Wang and Wen. This is an open-access article distributed under the terms of the Creative Commons Attribution License (CC BY). The use, distribution or reproduction in other forums is permitted, provided the original author(s) and the copyright owner(s) are credited and that the original publication in this journal is cited, in accordance with accepted academic practice. No use, distribution or reproduction is permitted which does not comply with these terms.
*Correspondence: Jinming Wen, d2VuamptaW5nQDE2My5jb20=
†These authors have contributed equally to this work