- 1The First Clinical College, Gannan Medical University, Ganzhou, Jiangxi, China
- 2Department of Urology, The First Affiliated hospital of Gannan Medical University, Ganzhou, Jiangxi, China
Angiogenesis is an essential process in the growth and metastasis of cancer cells, which can be hampered by an anti-angiogenesis mechanism, thereby delaying the progression of tumors. However, the benefit of this treatment modality could be restricted, as most patients tend to develop acquired resistance during treatment. Vasculogenic mimicry (VM) is regarded as a critical alternative mechanism of tumor angiogenesis, where studies have demonstrated that patients with tumors supplemented with VM generally have a shorter survival period and a poorer prognosis. Inhibiting VM may be an effective therapeutic strategy to prevent cancer progression, which could prove helpful in impeding the limitations of lone use of anti-angiogenic therapy when performed concurrently with other anti-tumor therapies. This review summarizes the mechanism of VM signaling pathways in urological tumors, i.e., prostate cancer, clear cell renal cell carcinoma, and bladder cancer. Furthermore, it also summarizes the potential of VM as a therapeutic strategy for urological tumors.
1 Introduction
Urological cancers include cancers of the urinary system, including kidneys, urinary tract epithelium (including bladder, ureter, and urethra), prostate, testes, and penis. The American Cancer Society predicted in 2022 that there would be 1,918,030 new cases of cancer diagnosed with estimated 31,990 death from urological cancers in the United States. Prostate cancer is the most common malignancy and the third leading cause of cancer-related deaths in men, while kidney and bladder cancer are regarded among the top ten most common malignancies in the United States (1). The growth, invasion, and metastasis of malignant tumors are closely related to angiogenesis, which has become one of the hot topics in cancer research (2). Over the past few decades, it has been thought that the growth of tumors is dependent on angiogenesis. Tumors larger than 2mm in diameter cannot rely solely on diffusion for oxygen supply, and without the intervention of neovascularization, tumors cannot continue to grow (3). Based on this hypothesis, it has been proposed that antiangiogenic drugs should be able to inhibit the growth of all solid tumors (4). However, anti-angiogenic therapy has so far shown only limited efficacy in patients. As early as before, researchers have proposed the idea of non-angiogenic tumors. But only recently has the special biological status of non-angiogenic tumors been formally described (5). Non-angiogenic tumors grow through two main mechanisms in the absence of angiogenesis. One way is to utilize pre-existing blood vessels by infiltrating cancer cells and occupying normal tissue, this is called vessel co-option. The second is through the formation of channels that provide blood flow through the cancer cells themselves, known as vasculogenic mimicry (6). To ensure sufficient nutrient supply, tumors release angiogenic factors that promote neovascularization. The coexistence of angiogenesis and VM is common in invasive tumors, and anti-angiogenic agents have been found to have little to no effect on VM (7). The VM can replace the angiogenesis role, providing tumors with oxygen and nutrients. Further, Qu et al. reported that anti-angiogenic therapy might even facilitate the formation of VM (8). As research progresses, more and more scientists are paying attention to the potential of vasculogenic mimicry (VM) in cancer treatment, and it has been found that a treatment plan using a combination of anti-angiogenic and anti-VM drugs is imperative. Currently, VM is of particular interest in the three types of cancers mentioned above. This review will focus on the research progress made regarding VM in prostate, kidney, and bladder tumors.
2 Forms of tumor angiogenesis
2.1 Angiogenesis and vasculogenesis
Angiogenesis is the process of forming new blood vessels from pre-existing ones through sprouting, which is stimulated by endothelial growth factors promoting paracrine signaling, leading to the proliferation and migration of endothelial cells, in addition to recruitment of other cell types such as smooth muscle cells (9). Angiogenesis occurs under physiological conditions, such as during embryonic development or adult wound healing. However, half a century ago, Dr. Judah Folkman proposed that pathological angiogenesis is mandatory for the growth of solid tumors (10). Cancer is characterized by the dysregulated function of angiogenesis, where the newly formed blood vessels no longer regulated by the body will promote tumor growth, metastasis, and invasion (11). The classical angiogenesis theory believes that there are two modes of tumor angiogenesis: angiogenesis based on the original blood vessel (12). The other is vasculogenesis (13). At present, more research is angiogenesis, that is, host mature vascular endothelial cells are exposed to pro-angiogenic factors in the tumor microenvironment, such as vascular endothelial growth factor (VEGF), basic fibroblast growth factor (bFGF), platelet-derived growth factor (PDGF). Under the action of these angiogenic factors and chemokines, a new collateral blood vessel of the tumor tissue is formed to provide nutrients to the tumor tissue (14). Another form of tumor angiogenesis is vasculogenesis, which refers to endothelial precursors derived from bone marrow and hematopoietic cells, called endothelial progenitor cells (EPCs) (15). And through growth factors, cytokines and hypoxia-related signaling pathways recruited to the tumor site, where they differentiate into mature endothelial cells, and under the stimulation of angiogrowth factor, division, proliferation, endothelial cells clump together to form a vascular channel to supply nutrients to tumor cells (16, 17). Targeting tumor angiogenesis has become an important target in cancer treatment, as angiogenesis plays a significant role in tumor progression. Targeted drugs such as bevacizumab, Sorafenib, and Sunitinib have been successfully used in clinical practice, advocating the success of anti-angiogenic therapy in cancer treatment (18). However, some studies have reported that these drugs have poor therapeutic effects on certain patients and could even promote tumor progression (19, 20). Therefore, some researchers speculated that new microcirculation patterns might exist for tumor blood nutrition supply.
2.2 Vessel co-option
Vessel co-option(VCO) is a phenomenon associated with tumor growth and progression, which differs from the traditional tumor angiogenic process. In tumor co-selection, tumor cells do not induce new angiogenesis, but choose to “borrow” existing blood vessels from surrounding normal tissues to supply their own nutritional and oxygen needs (6). In some cases, cells from malignant tumors move along existing vascular pathways, invading and occupying the blood vessels of normal tissue, thereby obtaining nutrients and oxygen from the blood (21). VCO causes less prominent vascular structures in morphology, making tumors more difficult to detect (22). VCO has been observed in a variety of cancers, such as liver, brain, skin, lymph node, and many others (23–25). Several studies have shown that many solid tumors can progress through vascular co-selection, and blocking co-selection and anti-angiogenic therapy can more effectively inhibit tumor growth (26–28). And the effect of inhibiting VCO can be achieved by targeting the signaling pathways related to VCO, such as targeting the Ang-2 pathway, VEGF pathway and YAP-TAZ pathway (29). Therefore, understanding tumor co-selection is essential to develop more precise treatment strategies and predict tumor growth patterns (Figure 1).
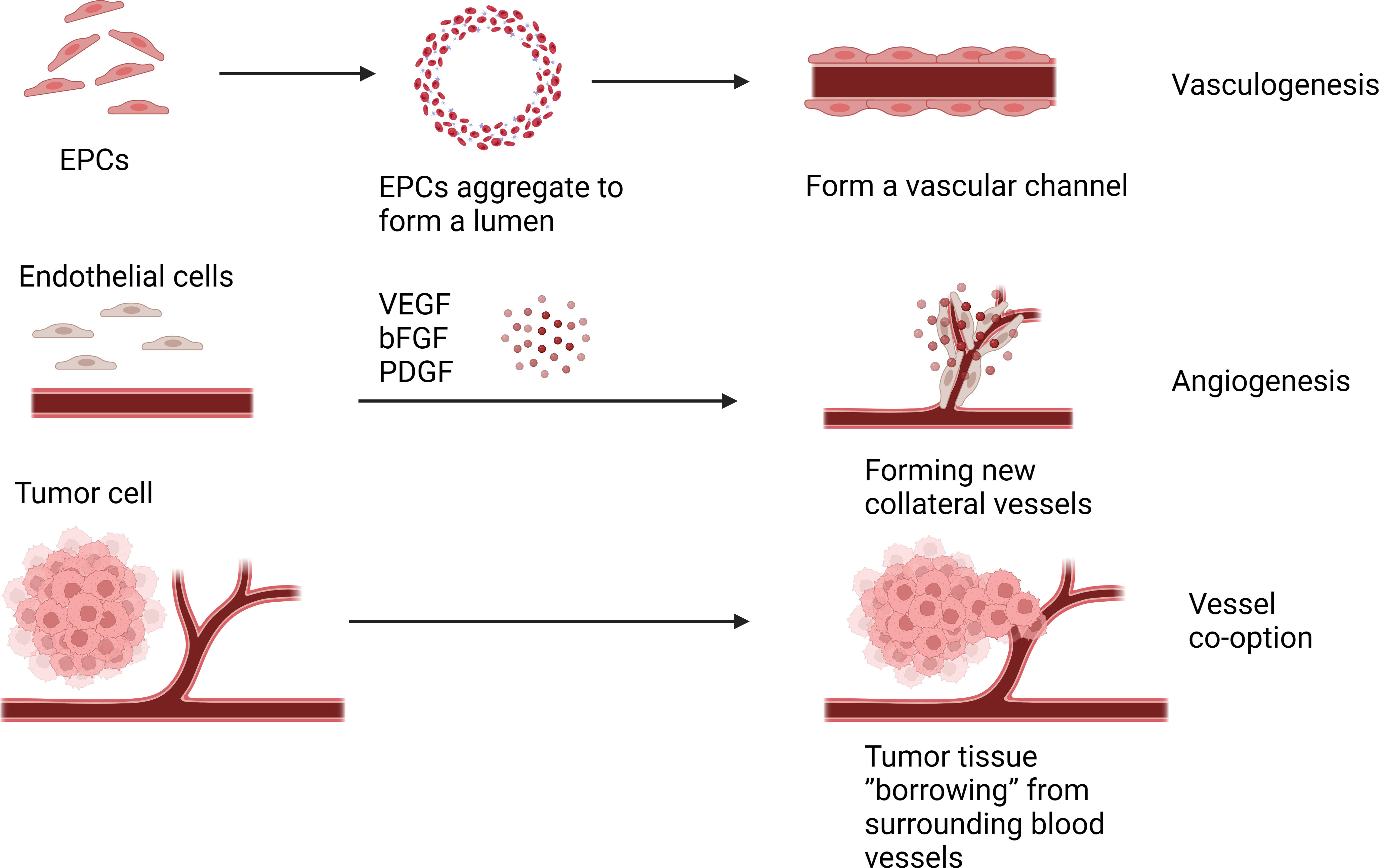
Figure 1 Molecular mechanisms related to VM in urological tumors. This figure summarizes the signal pathways involved in VM regulation in urological tumors. Red represents VM related signaling pathway in prostate cancer, yellow represents VM related signaling pathway in renal cell carcinoma, and the green represents VM related signaling pathway in bladder cancer.
2.3 Vasculogenic mimicry
In 1999, Maniotis et al. discovered the formation of tumor blood vessels lacking endothelial cells in highly invasive melanoma tissue samples. These vessels were positive for PAS staining, but staining of endothelial cell markers (Factor VIII-related antigen and CD31) failed to stain the luminal contents of the vessels (30). Similarly, culturing highly invasive cell lines in a 3D extracellular matrix (ECM) demonstrated that tumor cells cultured in vitro produce patterned vascular channels and are PAS-positive, indicating its functionality as a blood vessel. This phenomenon was named VM (30). With further research, VM was also reported in other invasive tumors such as breast, ovarian and liver cancer and some urological tumors (31–35). VM is closely related to tumor growth, invasion, metastasis, and patient prognosis, and patients with VM generally had a shorter survival time and poorer prognosis (36).
Current research suggests that VM formation may be primarily related to cancer stem cells (CSCs) and epithelial-mesenchymal transition (EMT). CSCs are a subset of tumor cells with self-renewal and differentiation capabilities and are considered the main drivers of tumor growth, metastasis, and recurrence (37). Among them, the VEGF (vascular endothelial growth factor) pathway is considered the most important, promoting the generation of new blood vessels through signal transduction mediated by VEGFR. Mirshahi et al. found that CD133+/CD34+ stem cells derived from acute leukemia (AL) patients could secrete more IGF-1 and SDF-1, leading to the formation of VM in Matrigel (38). In melanoma, Lai et al. found that a population of cells with stem cell-like characteristics, marked by CD133, drove tumor growth by promoting VM formation and the morphogenesis of a specialized perivascular niche (39).
EMT is the process by which epithelial cells transform into mesenchymal cells and plays a vital role in embryonic development, inflammation, fibrosis, and cancer progression (40). It has been reported that EMT activation triggers cancer cell invasion and metastasis and contributes to VM (41). During EMT, epithelial cells lose polarity and epithelial characteristics and acquire mesenchymal cell features (13). These cells can attract endothelial cells by releasing various cytokines and signaling molecules, promoting endothelial cell migration and invasion, thereby promoting angiogenesis and expansion of the vascular network. In addition, EMT also releases some matrix-degrading enzymes, such as MMPs, which can degrade the matrix and provide more space for new blood vessels (42). On the other hand, tumor vasculature can also inversely affect the process of EMT. Newly formed tumor vessels can release various factors such as VEGF and TGF-β (transforming growth factor-β), promoting EMT of tumor cells, thereby enhancing their invasive and metastatic ability (43–45). In addition, the lack of tumor vasculature can lead to tumor cell hypoxia, thereby promoting the occurrence and progression of EMT (Figure 2).
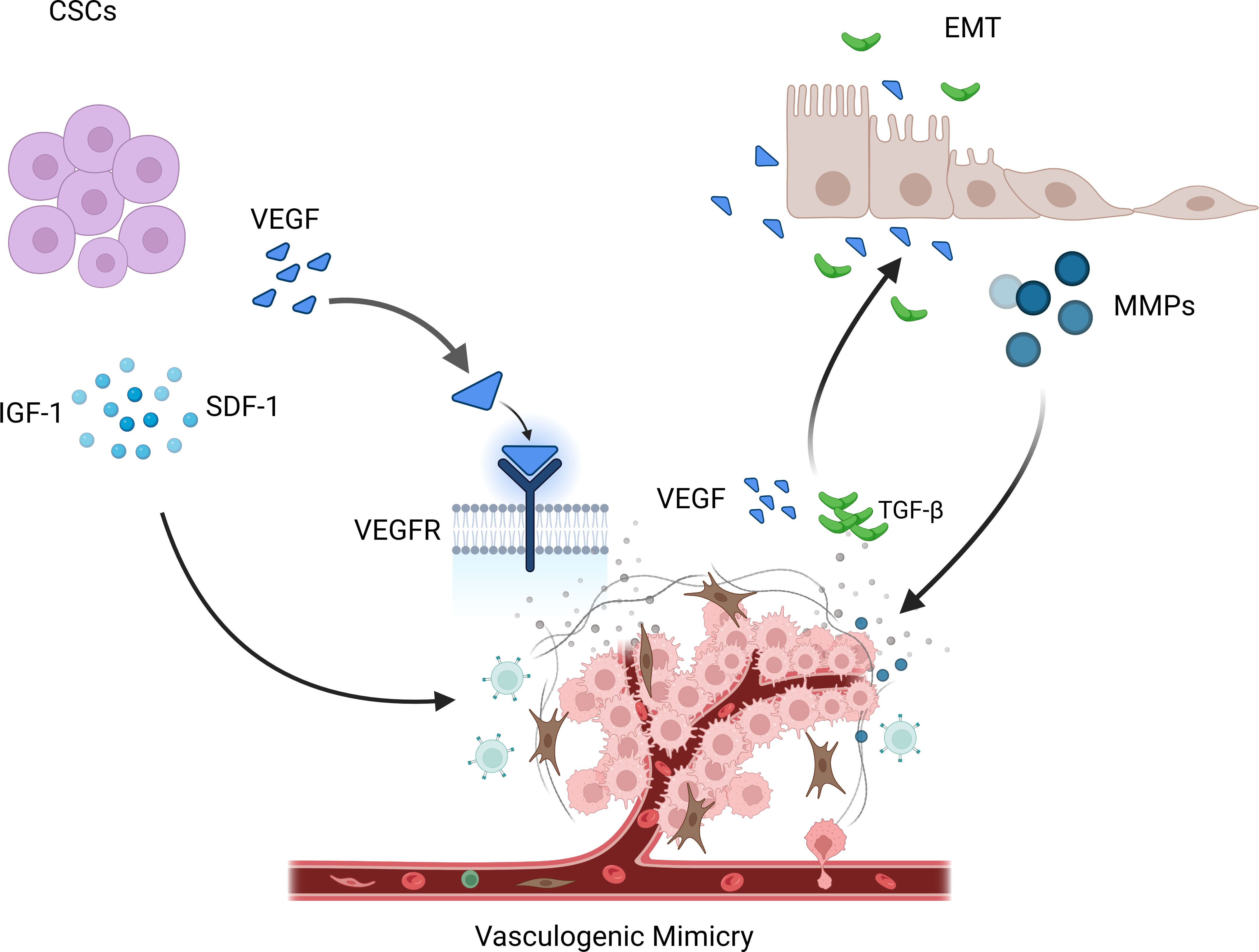
Figure 2 Angiogenesis is under the action of these angiogenic factors and chemokines, a new collateral blood vessel of the tumor tissue is formed to provide nutrients to the tumor tissue. Vasculogenesis is endothelial cells clump together to form a vascular channel to supply nutrients to tumor cells. Vessel co-option(VCO) is the tumor cell choose to “borrow” existing blood vessels from surrounding normal tissues to supply their own nutritional and oxygen needs.
Moreover, some studies have suggested an interaction between tumor angiogenesis and immune evasion. Vasculogenic mimicry can weaken the body’s immune system’s attack on tumors by forming immune escape areas on the vascular wall (46). Therefore, simultaneously inhibiting tumor vasculogenic mimicry and enhancing the immune system’s ability to attack tumors may be an important therapeutic strategy in treating tumors.
3 Factors involved in urological tumor vasculogenic mimicry
3.1 Vasculogenic mimicry in prostate cancer
Prostate cancer (PCa) is one of the most common solid malignancies in the male urogenital system, second only to lung cancer in incidence, and ranked second among male malignant tumors (47). The treatment for localized PCa includes radical surgery and radiation therapy, while androgen deprivation therapy (ADT) is used for metastatic PCa. However, after 18 months, most metastatic PCa eventually progresses to ADT-resistant PCa (48). In patients with PCa, the incidence of VM is higher in those with higher Gleason scores, TNM staging, more lymph nodes, and distant metastases (32).
The presence of VM in prostate cancer is associated with higher expression of certain related factors, like HIF1α, EphA2, ZEB1, and Sp1. Luo et al. found that MCT1 can stabilize HIF1α through lactylation by introducing MCT1 into PCa cells, thereby promoting the transcriptional activity of KIAA1199 (49). KIAA1199 further reduces the expression of Sema3A, increases the expression of VE-cadherin and phosphorylated EphA2, and enhances angiogenesis and vasculogenic mimicry in prostate cancer by enhancing hyaluronic acid-mediated VEGFA signaling (49). EphA2 is a receptor tyrosine kinase expressed in most epithelial cells (50). In gastric cancer cells, cancer-associated fibroblasts overexpressing EphA2 promote VM formation by activating the EphA2-PI3K pathway (51). While PI3K is a heterodimeric protein composed of a catalytic subunit (p110α/β/γ/δ) and a regulatory subunit (p85α/β) (52). Wang et al. demonstrated that higher levels of EphA2 expression and PI3K activity were associated with VM in more invasive prostate cancer cell lines PC3 and DU-145, but with no significant correlation between EphA2 and PI3K expression levels (53), Luo et al. suggested that PI3K is necessary for VM in PCa and may function by regulating the phosphorylation of EphA2 (53).
ZEB1 is a critical activator of EMT, which upregulates tumor cell plasticity and EMT to acquire cancer stem cell properties (54). In a previous study, Peng et al. found that ZEB1 promotes EMT in lung cancer cells by activating Fak/Src signaling (55). Wang et al. found that ZEB1 is required for VM formation in vitro, mediating the expression of EMT-related and CSC-related proteins in PCa cells.The study data showed that ZEB1 knockdown reduced the inhibition of Src phosphorylation at the p-Src527 site in PCa cells while reducing the formation of VMs. They further confirmed that treating PCa cells with the Src inhibitor PP2 resulted in a decrease in VM formation, while Src overexpression in stable ZEB1 knockdown cells restored VM formation (56). Where similar results were also observed in in vivo studies, indicating depletion of ZEB1 protein in PC3 cells inhibited the growth of xenograft tumors in mice (56).
The transcription factor Sp1 is overexpressed in many types of cancer cells, including PCa, and is associated with various fundamental biological processes. It has been shown to play an important role in cell growth, differentiation, apoptosis, and carcinogenesis (57). Han et al. found that Sp1 controls the nuclear expression of the transcription factor twist to regulate the expression of VE-cadherin in PC3 cells. Sp1 induces the upregulation of twist/VE-cadherin, activating the AKT pathway, activated AKT enhances the expression of matrix metalloproteinases (MMPs) such as MMP-2 and -14, leading to VM formation by remodeling the extracellular matrix including LAMC2, leading to VM occurrence and development (58). Sp1, Twist, VE-cadherin, and AKT form a loop, and targeting Sp1 expression may provide a new therapeutic strategy for PCa patients with VM.
As mentioned earlier, VM formation involves proliferation, migration, and invasive changes. Therefore, the treatment for inhibiting VM can target any of these processes. Kaempferol is a natural flavonol found in many fruits and vegetables, which was reported to significantly inhibit the proliferation of AR-positive prostate cancer cell line LNCaP and promote apoptosis (59). Resveratrol (3,5,4’-trihydroxy-trans-stilbene, RES) is one of the most well-known phytochemicals found in red wine, grapes, berries, and peanuts with potent antioxidant and anticancerous properties (60). Han et al. found that RES inhibited the VM structure formation at non-cytotoxic concentrations by inactivating EphA2 and reducing twist-mediated VE-cadherin expression when co-cultured with prostate cancer cell line PC-3 cells (61). Chrysin, also known as 5,7-dihydroxyflavone, is another natural compound with anti-tumor properties, chrysin inhibits the growth and VM formation of prostate cancer cell line PC-3 by inhibiting HIF-1α, SPHK-1, and phosphorylation of the AKT/GSK-3β signaling pathway (62).
3.2 Vasculogenic mimicry in renal cell carcinoma
Renal cell carcinoma (RCC) accounts for 3.8% of all cancers and 2.5% of all cancer deaths worldwide (63). Early-stage RCC is commonly treated with partial or radical nephrectomy, with a 5-year survival rate of 92.6%. However, about 25% of RCC patients are diagnosed at the metastatic stage and one-third of patients undergoing local tumor resection experience recurrence (64). Angiogenesis is a key aspect of pathogenesis, and anti-angiogenic drugs such as Sunitinib have been shown to significantly reduce tumor blood flow. However, most Sunitinib-treated patients eventually experience tumor progression after several months of treatment (65). More than a decade ago, Amalia A Vartanian et al. found through retrospective studies that RCC patients who tested positive for VM had a significantly lower disease-free survival rate and a significantly increased risk of recurrence (66). Therefore, VM as a novel neovascularization pathway cannot be ignored in treating RCC.
The MMP family plays a role in promoting VM progression in various cancers. Lin et al. found that MMP9 was overexpressed in RCC patient tissues, which was positively correlated with clinical stage, pathological grade, RCC metastasis, and VM formation (67). Targeted inhibition of MMP9 prevented the formation of VM in RCC cell lines 786-O and 769-P that were originally able to form VM (67). Vimentin (VIM) is a major component of the intermediate filament (IF) protein family and a hallmark of EMT (68). In RCC, VIM overexpression is one of the independent predictors of poor clinical outcomes (69). Many studies have also shown that VIM plays an important role in the formation of VM (67, 69–71). Bai et al. found that TR4 downregulates the expression of miR490-3p, which upregulates VIM expression, thereby promoting RCC VM formation and metastasis (72). Lin et al. further validated the role of VIM in promoting VM in RCC by inducing EMT through hypoxia, upregulating VIM and AXL, and downregulating E-cadherin expression to promote RCC cell VM formation (71). He et al. found Sunitinib regulates ERβ signaling to increase cancer stem cell and angiogenic mimicry formation (73), However, first-line anti-angiogenesis drugs such as Sunitinib or Bevacizumab cannot inhibit VM and may even induce VM, Ding et al. found that targeting ERβ/circDGKD by downregulating VE-cadherin reduced RCC growth and proliferation and significantly weakened the VM formation, which is envisaged to enhance the efficacy of Sunitinib providing a new combinational therapy strategy to prevent RCC progression (74).
Paired-related homeobox 1 (PRRX1) is a novel inducer of EMT, and its expression is associated with metastasis and prognosis in multiple tumors (75, 76). Protein phosphatase 2A (PP2A) is an effective tumor suppressor that acts on various oncogenic transcription factors (77). CIP2A is an important oncogene that inhibits the activity of PP2A, thereby maintaining the malignant phenotype of tumor cells and playing an important role in the occurrence, development, and biological behavior of tumor cells (78). Both PRRX1 and CIP2A are major inducers of EMT. Wang et al. investigated the roles of PRRX1, CIP2A, and VM in RCC and found that PRRX1 expression was negatively correlated with VM and CIP2A, whereas CIP2A expression was positively correlated with VM development. Low PRRX1 expression combined with high VM and CIP2A was associated with poor prognosis and metastasis in RCC (79). However, Wang et al. only observed this correlation in RCC patient specimens and did not perform animal or cell line investigations to explore the underlying mechanisms. Further research is needed to clarify the mechanisms involved.
Androgen receptor (AR) has an oncogenic function in RCC, promoting progression and hematogenous metastasis (80). You et al. showed that a long non-coding RNA, lncRNA-TANAR, regulated by AR transcription, increased the stability of TWIST1 mRNA by directly binding to its 5′UTR, disrupting UPF1-mediated nonsense-mediated TWIST1 mRNA decay, thereby leading to a decrease in VM formation (81).
Tumor-associated macrophages (TAMs) play a crucial role in reshaping the tumor microenvironment (TME) to promote tumor development (82). Numerous studies have shown that TAMs can promote tumor cell proliferation, invasion, and migration (83, 84). Polarized macrophages commonly exist as either M1 or M2 macrophages. Unlike M1 macrophages, which have pro-inflammatory and immune-stimulatory effects, M2 polarized macrophages are similar to TAMs and have pro-tumor functions (85). It has been found that macrophages can affect cancer progression through miRNAs carried by extracellular vesicles (86). Liu et al. evaluated ten VM-related genes in RCC cells co-cultured with or without TAMs using protein imprinting and found that TIMP2, which was restrained by TAMs, might be a key VM regulatory factor in RCC (87). Subsequently, through bioinformatics analysis and experimental validation, Liu et al. found that miR-193a-5p derived from macrophage-derived extracellular vesicles targeted TIMP2 in RCC cells, enhancing VM and cell invasion capability (87).
Metabolic reprogramming is a hallmark of cancer and is critical in tumor progression. Accumulation of the tumor metabolite L-2-hydroxyglutarate (L-2HG) occurs in cancer due to hypoxia (88), which is also an important factor in VM formation. Wang et al. found that tumors with high levels of L-2HG exhibited more VM structures than tumors with low levels of L-2HG. They also compared RNA sequencing analysis of RCC cell lines with and without L-2HG treatment and found that PHLDB2 was downregulated by L-2H (89). PHLDB2 (also known as LL5β) is a protein containing a PH domain that plays an important role in mediating cell migration by forming complexes with partners such as CLASPs and Prickle 1 (90). Wang et al. found that inhibiting PHLDB2 reduced VM formation while restoring PHLDB2 expression levels reversed this phenomenon. They also found that decreasing PHLDB2 expression increased ERK1/2 phosphorylation, but there was no statistically significant difference in ERK1/2 phosphorylation due to limited replicates. However, the trend of changes in ERK1/2 phosphorylation was consistent in each group (89). Therefore, the L-2HG/PHLDB2 pathway may be a potential signaling pathway for treating VM in RCC.
3.3 Vasculogenic mimicry in bladder cancer
It is estimated that there are reported 500,000 new cases and 200,000 deaths from bladder cancer (BCa) globally. Over 80,000 new cases are reported in the United States alone, with 17,000 deaths from BCa annually (91). Despite various treatments such as surgery, bladder infusion, and immunotherapy being used in clinical practice, the rate of tumor progression within 5 years is still very high (92). In particular, the treatment options for advanced BCa are minimal (93). Early on, Fujimoto et al. found that ECV304, derived initially from BCa epithelial cells and now known as the T24/83 BCa epithelial cell line, can connect with blood vessels around the normal endothelial source, forming tumor tissues with vascular characteristics and is typically found in highly invasive tumors with poor prognosis (94).
A protein TG2, which has been demonstrated to be associated with endothelial cell-derived angiogenesis (95), can be overexpressed under pathological and stress conditions, leading to increased cell surface externalization and deposition into the extracellular matrix (ECM), thereby exerting crosslinking effects with various ECM proteins such as fibronectin and laminin, etc. (96). Moreover, previous studies have suggested that exogenous TG2 added to a rat dorsal skin flap wound healing model can enhance angiogenesis (97). Jones et al. found that TG2 was not detected in normal human fibroblast C378, while there was an abundant expression of TG2 in ECV304 cells. Targeted inhibition of TG2 expression in ECV304 cells could block cell migration, thereby preventing the formation of the actin cytoskeleton and focal adhesion (98).
MicroRNAs (miRNAs) are evolutionarily conserved small non-coding RNAs that function as endogenous regulators of gene expression (99). Dysregulation of certain miRNAs has been associated with numerous tumorigenic changes, including growth, apoptosis, metastasis, and tumor angiogenesis (100). It has been reported that miR-124 inhibits the malignant potential, proliferation, and invasiveness of malignant tumor cells by targeting multiple proteins (101–103). Studies have shown that UHRF1 is an oncogene promoting cancer cell development (104). In different types of cancers, the expression of UHRF1 incurs many changes getting out of control. The expression or activity of this protein is often modified, leading to transformation and increased proliferation, motility, and invasiveness, as well as providing tumor cells with resistance to chemotherapy (105). Wang et al. found that miR-124 and UHRF1 are negatively correlated in BCa tissue, where miR-124 inhibited BCa invasiveness by reducing UHRF1 expression (106).
Many studies have proposed that EMT is crucial for VM formation and tumor progression, with ZEB1 as an essential EMT inducer that is elevated in colorectal cancer specimens showing EMT features both in vivo and in vitro (107). Li et al. found that ZEB1 is also highly expressed in BCa, and to further elucidate the relationship between VM and ZEB1 in BCa, they performed 3D culture assays after transfection with specific siRNA to reduce ZEB1 expression in bladder transitional cell carcinoma cell lines. Moreover, after ZEB1 restoration, VM formation was inhibited in UM-UC-3 and J82 cell lines (35). However, Li et al. did not observe changes in EMT markers after suppressing ZEB1 expression in BCa, suggesting that ZEB1 is an intermediate step in BCa VM formation, regulated or influenced by some unknown upstream molecules and downstream genes, and it may not have a direct relationship with epithelial phenotype (35).
It is well known that the tumor microenvironment exists in a hypoxic state (108). In the hypoxic microenvironment, tumor cells form new blood vessels to obtain the oxygen and nutrients they need to support their continued proliferation. Numerous studies have shown that hypoxia is closely related to the development of VM. For example, in the melanoma mouse model, mice in the ischemic model group were found to exhibit higher VMs compared to the control group, which was positively correlated with HIF-1α and HIF-2α expression, indicating that hypoxia promoted VM (109). Liu et al. developed and validated a novel hypoxia risk score that can predict clinical outcomes and TME characteristics of BLCA, and for patients in the high-risk score group, they may benefit from immunotherapy, chemotherapy, and radiotherapy, and patients in the low-risk score group may benefit from targeted therapy with VM-associated signaling pathways (WNT-β-catenin network, PPARG network, and FGFR3 network), contributing to the development of BCa precision medicine (108).
In recent years, there has been increasing evidence that the status and formation of angiogenic mimetics (VMs) in the tumor microenvironment is regulated by various factors, especially the immune factors present in the tumor microenvironment (110). BCATRANSFERASE 2 (BCAT2) is a core enzyme in sulfur amino acid metabolism (111). Cai et al. found that BCAT2 has the effect of modulating TME immune status, and patients with high BCAT2 expression may have better efficacy in anti-VM therapy (112). Siglec15, a member of the sialic acid-bound immunoglobulin-like lectin family, is an emerging broad-spectrum target for normalizing cancer immunotherapy (113). Jiao et al. found that BCa patients in the high Siglec15 group were more sensitive to targeting vascular mimicry-related signaling pathways (β-catenin, PPAR-γ, and FGFR3 pathways) (114, 115). Therefore, Siglec15 may be used as an indicator of targeted therapy for VM.
In BCa, DNA methylation plays a key role in early diagnosis, predicting prognosis, predicting therapeutic opportunities, and serving as a potential therapeutic target (116), 5-Methylcytosine (5mC) in DNA is the most important epigenetic modification that shapes TME by influencing genomic stability, determining cancer cell differentiation status, and selecting cell identity (117, 118). Jiao et al. found that the high 5 mC score group may not be sensitive to neoadjuvant chemotherapy, but there are several immunosuppressive oncogenic pathways significantly enriched in the heterogeneous high 5 mC score group associated with VM, including WNT-β-catenin network, FGFR3 network, and VEGFA (114, 119–121). These VM pathways may provide promising therapeutic opportunities for BLCA patients in the high 5mC scoring group.
The utilization of glucose in tumor cells significantly increases, producing a large number of intermediate metabolites through glycolysis to meet the needs of tumor cell proliferation. Increasing evidence indicated that accumulated lactate, as the final product of glycolysis, is a key regulatory factor in tumor development, immune escape, metastasis, and angiogenesis (122, 123). Hepatitis B X-interacting protein (HBXIP), also known as LAMTOR5, is a conserved protein that is often expressed in various tissues in mammals (124). HBXIP is highly expressed in several types of cancers and is associated with a series of clinical pathological features and poor prognosis (125). Overexpression of HBXIP in BCa tissues is related to clinical staging, lymph node metastasis, tumor recurrence, and patient survival. In addition, silencing HBXIP reduces the proliferation, migration, and invasion of BCa cells in vitro and tumor formation in vivo (126). Moreover, the high expression of HBXIP in high-grade tissues suggests that HBXIP may be an important indicator for judging the prognosis of BCa patients. Some studies have shown that the PI3K/AKT/mTOR pathway is a central signaling pathway that coordinates aerobic glycolysis and cell biosynthesis in malignant tumor cells (127). Liu et al. provided evidence that HBXIP as an oncogene regulates glycolysis of BC cells through the AKT/mTOR pathway, thereby promoting VM in BCa cells (128). They found that reducing HBXIP in BCa cells affected the migration and angiogenesis of HUVECs and decreased the expression of VEGF and EPO. Both glucose and lactate stimulation reversed the cell viability, migration, and tubular formation of HUVECs co-cultured with HBXIP-silenced BCa cells. Glucose stimulation demonstrated that HBXIP further promotes glycolysis by regulating glucose uptake by tumor cells, while lactate stimulation demonstrated that glycolysis further promotes VM, suggesting HBXIP plays a key role in VM (Figure 3) (128) (Table 1).
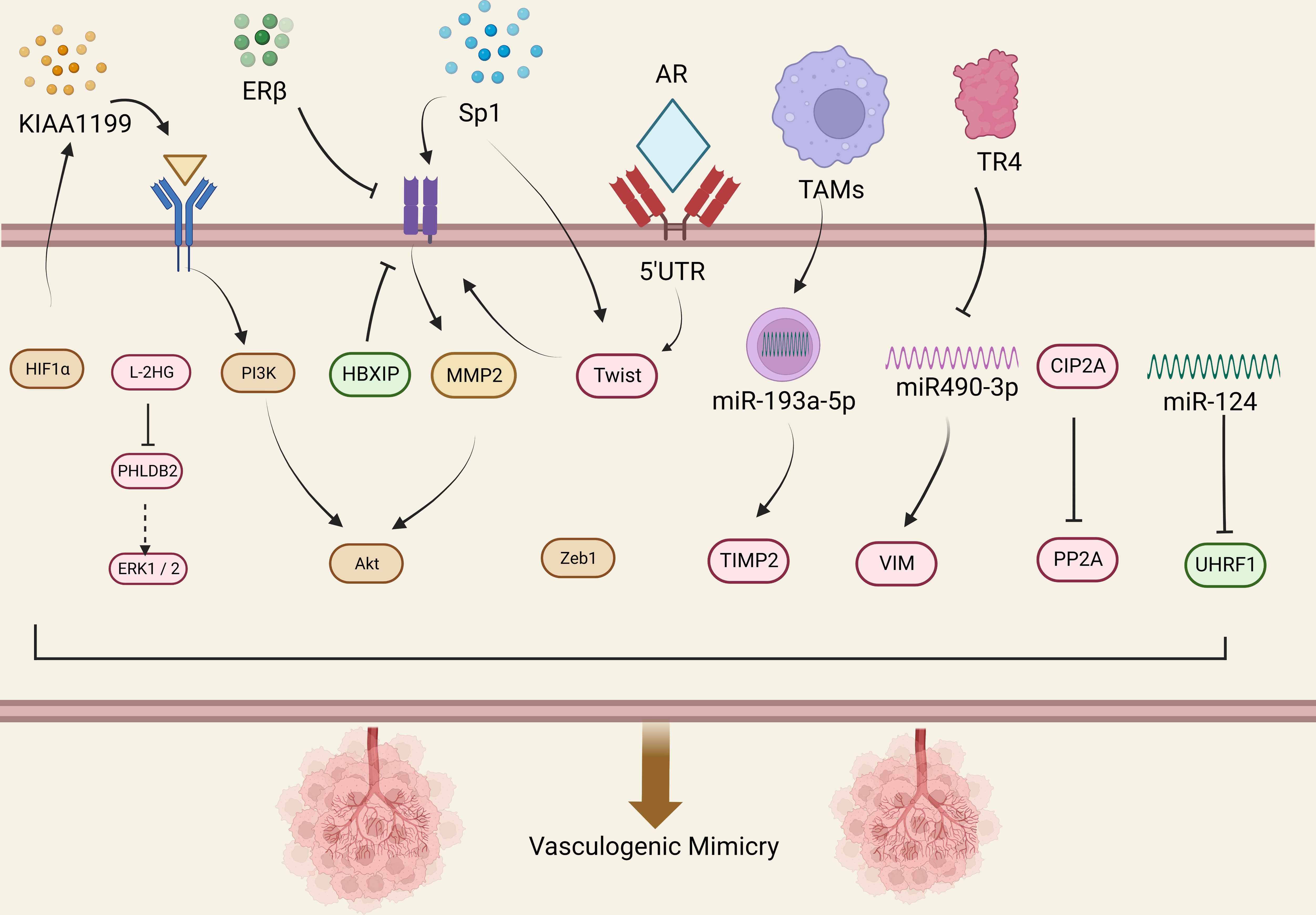
Figure 3 VM is regulated by various mechanisms. CSCs promote the occurrence of VM phenomenon by releasing IGF-1, SDF-1, and VEGF. During EMT, some matrix degrading enzymes are released, such as MMPs, which can promote the occurrence of the VM phenomenon. At the same time, new tumor blood vessels release various factors, such as VEGF and TGF- β, thereby promoting the occurrence and progress of EMT.
4 Potential targeted drugs for vasculogenic mimicry
4.1 Targeting VEGF and VEGFR
It is now widely believed that VM plays a crucial role in tumor growth, proliferation, and metastasis formation. VEGF is one of the important promoting factors for VM, and the VEGF and its receptor are one of the main inducers of tumor angiogenesis (129). Inhibitors targeting the VEGF/VEGFR system have been used clinically. Clinical trials using VEGF molecules can induce moderate improvement in overall survival, measured in weeks to just a few months, and tumors respond differently to these drugs (130). Antibodies targeting VEGF, such as Bevacizumab, can effectively inhibit tumor angiogenesis and growth and have been widely used to treat various cancers (130). In addition to directly inhibiting VEGF, tumor angiogenesis can also be inhibited by hindering the activity of VEGF receptors. Sorafenib and Sunitinib are tyrosine kinase inhibitors that block VEGFR-2, which are currently approved for treating cancers such as hepatocellular carcinoma, neuroendocrine pancreatic tumors, and metastatic renal cell carcinoma (130, 131). However, tumor cells are prone to develop resistance to these drugs, resulting in poor clinical efficacy (132).
Studies have shown that when VEGF is blocked, other angiogenic factors modulate sensitivity against VEGF therapy and allow regeneration of the tumor-associated vasculature (133). A phase III trial by Rini et al. on bevacizumab plus interferon α in patients with metastatic renal cell carcinoma showed that bevacizumab blocked the VEGF pathway and that patients with bevacizumab plus IFN-α had significantly improved PFS and OS compared with IFN-α alone (134). Although the data emphasize the importance of VEGF signaling, there are many intracellular pathways in tumorigenesis VM, and inhibitors targeting individual signaling pathways have limited inhibitory effect on VMs because other signaling pathways immediately compensate and eventually resume the process of switching to the VM phenotype (135). Therefore, exploring multi-target combination therapies to limit VM-mediated tumor resistance is expected to maximize anti-tumor efficacy in the future.
4.2 Targeting extracellular matrix
An extracellular matrix (ECM) is a complex extracellular biological macromolecular network involved in angiogenesis. Drugs targeting ECM can inhibit angiogenesis by interfering with the biological functions of ECM. MMPs is particularly important in ECM degradation. Under hypoxic conditions, high expression of MMP-9 molecules increases tumor invasiveness and promotes VM (136). Therefore, MMP inhibitors can inhibit MMP activity, thus blocking ECM degradation and remodeling and reducing VM. In addition, ECM is an important structure for cell adhesion, and inhibiting the binding of ECM to cells can inhibit the proliferation and migration of endothelial cells. For example, using RGD peptide sequences can block the binding of ECM to cells, thereby inhibiting VM (137).
4.3 Targeting PI3K/Akt/mTOR
The PI3K/Akt/mTOR signaling pathway is a crucial pathway in regulating cell proliferation, survival, and metabolism and is considered an important regulatory mechanism for tumor angiogenesis and development (138). Drugs targeting this pathway, such as Rapamycin, have been extensively studied to inhibit tumor angiogenesis and growth, thereby suppressing tumor progression and metastasis (139). Huang et al. found that under normal oxygen or hypoxic conditions, with the increase of rapamycin concentration, the duct-forming structure of glioma cell line U87-MG in stromal gum decreased, demonstrating that inhibition of mTOR can eliminate glioma VM formation (139). Therefore, Huang et al. deduced that the mTOR signaling pathway is related to VM formation and that mTOR is an upstream molecule of HIF-1α. In the final stage of the VM signaling pathway, express and activate MMP-14 to activate MMP-2. MMP-2 combined with MMP-14 cuts Ln-5-γ2 chains into migration fragments. The release of fragments of these into the tumor microenvironment can increase the migration of tumor cells, invasion, and eventually lead to VM (139). It is worth mentioning that since PI3K/Akt/mTOR signaling pathway also plays an important physiological role in normal cells, these drugs lack selective activity on cancerous cells and thus may adversely affect the normal cells (140). The results of a recent study have shown a delicate balance between the growth-promoting activity of AKT and the growth-promoting activity of p53, which is essential for preventing cellular aging and cancer (141). PI3K/AKT/mTOR inhibition also has associated clinical adverse effects, including hyperglycemia, hyperlipidemia, myelosuppression, and severe hepatotoxicity (142). Further research and optimization of targeted therapy for the PI3K/Akt/mTOR signaling pathway are imperative to improve treatment efficacy and reduce the incidence of adverse reactions.
4.4 Targeting perivascular cells
The tumor microenvironment contains a series of non-cancer cells around the tumor, such as fibroblasts, macrophages, and endothelial cells, as well as some ECM and molecular signaling substances. These non-cancerous cells and molecules play an important role in tumorigenesis, growth, invasion, metastasis, and tumor angiogenesis (143). In tumor angiogenesis, tumor cells release some promoting factors, such as VEGF, PDGF, etc., to stimulate tumor cells to generate VM. At the same time, tumor cells induce surrounding fibroblasts and macrophages to transform into tumor-related cell types, releasing various factors that promote tumor growth and invasion (144). Therefore, targeting non-cancer cells and molecules in the tumor microenvironment has become a strategy for treating tumor angiogenesis. Among them, drugs targeting fibroblasts and macrophages, such as Imatinib and Dasatinib.Studies have shown that dasatinib can effectively inhibit the growth of fibroblasts by inhibiting PDGF receptor signaling at biologically relevant concentrations (145, 146). PDGF is normally expressed in a variety of cell types, including fibroblasts, neuronal cells, macrophages, smooth muscle cells, platelets, and preosteoclastic cells. They typically use autocrine or paracrine mechanisms to perform their biological functions, and Imatinib can target inhibition of PDGFR (147), can inhibit the proliferation and function of these cells, thereby reducing their promotion of VM (148). In addition, drugs targeting endothelial cells, such as Bevacizumab and Ramucirumab, can inhibit the migration and proliferation of endothelial cells(EC), thereby reducing tumor vascular density and tumor growth rate (149). Bevacizumab is the first humanized anti-VEGF neutralizing antibody approved by the FDA for the treatment of metastatic colon cancer (150), Bevacizumab treatment blocked extracellular VEGF-induced apoptosis, inhibiting EC proliferation (149). In short, targeting non-cancer cells and molecules in the tumor microenvironment can effectively inhibit tumor angiogenesis and growth, a potential tumor treatment strategy (Table 2).
5 Conclusion and future directions
The review on VM in urinary tumors provides in-depth new ideas and solutions for treating urinary tumors. Tumor growth and metastasis can be slowed down by targeted inhibition of tumor VM, and VM inhibitors can also be used in combination with other therapeutic methods to increase anti-cancer effects.
However, the current study faces some challenges. First, different VM targeted inhibitors may have different efficacy for different urinary tumors. Secondly, the dose and timing of VM inhibitors in clinical application and the combination regimen and sequence of combination therapy strategies require further research.
With the in-depth study of tumor VM, the efficacy and safety of treatments targeting to inhibit VM will continue to improve. Combinational use of VM targeted inhibitors with other antitumor methods is envisaged to improve the therapeutic effect further and reduce the side effects. Therefore, we can expect targeted therapy for tumor VM to play an increasingly important role in the future.
Author contributions
XL: validation, writing, formal analysis and visualization. SL and CY reviewed the manuscript and polished the grammar. SH, SL, XZ and GZ: searched related publications. GW and JZ: supervision. All authors contributed to the article and approved the submitted version.
Funding
This project was supported by the Graduate Innovation Special Fund Project of Gannan Medical University (YC2022-S955).
Acknowledgments
We thank Home for Researchers editorial team (www.home-for-researchers.com) for language editing service.
Conflict of interest
The authors declare that the research was conducted in the absence of any commercial or financial relationships that could be construed as a potential conflict of interest.
Publisher’s note
All claims expressed in this article are solely those of the authors and do not necessarily represent those of their affiliated organizations, or those of the publisher, the editors and the reviewers. Any product that may be evaluated in this article, or claim that may be made by its manufacturer, is not guaranteed or endorsed by the publisher.
References
1. Siegel RL, Miller KD, Fuchs HE, Jemal A. Cancer statistics, 2022. CA: Cancer J Clin (2022) 72:7–33. doi: 10.3322/caac.21708
2. Janic B, Arbab AS. The role and therapeutic potential of endothelial progenitor cells in tumor neovascularization. TheScientificWorldJournal (2010) 10:1088–99. doi: 10.1100/tsw.2010.100
3. Hillen F, Griffioen AW. Tumour vascularization: sprouting angiogenesis and beyond. Cancer metastasis Rev (2007) 26:489–502. doi: 10.1007/s10555-007-9094-7
4. Folkman J. Tumor angiogenesis: therapeutic implications. New Engl J Med (1971) 285:1182–6. doi: 10.1056/nejm197111182852108
5. Pezzella F, Ribatti D. Vascular co-option and vasculogenic mimicry mediate resistance to antiangiogenic strategies. Cancer Rep (Hoboken N.J.) (2022) 5:e1318. doi: 10.1002/cnr2.1318
6. Donnem T, Reynolds AR, Kuczynski EA, Gatter K, Vermeulen PB, Kerbel RS, et al. Non-angiogenic tumours and their influence on cancer biology. Nat Rev Cancer (2018) 18:323–36. doi: 10.1038/nrc.2018.14
7. van der Schaft DW, Seftor RE, Seftor EA, Hess AR, Gruman LM, Kirschmann DA, et al. Effects of angiogenesis inhibitors on vascular network formation by human endothelial and melanoma cells. J Natl Cancer Institute (2004) 96:1473–7. doi: 10.1093/jnci/djh267
8. Qu B, Guo L, Ma J, Lv Y. Antiangiogenesis therapy might have the unintended effect of promoting tumor metastasis by increasing an alternative circulatory system. Med Hypotheses (2010) 74:360–1. doi: 10.1016/j.mehy.2009.08.020
9. Aldebasi YH, Rahmani AH, Khan AA, Aly SM. The effect of vascular endothelial growth factor in the progression of bladder cancer and diabetic retinopathy. Int J Clin Exp Med (2013) 6:239–51.
10. Folkman J, Merler E, Abernathy C, Williams G. Isolation of a tumor factor responsible for angiogenesis. J Exp Med (1971) 133:275–88. doi: 10.1084/jem.133.2.275
11. Bernardini S, Fauconnet S, Chabannes E, Henry PC, Adessi G, Bittard H. Serum levels of vascular endothelial growth factor as a prognostic factor in bladder cancer. J Urol (2001) 166:1275–9. doi: 10.1016/S0022-5347(05)65752-7
12. Yang J, Weinberg RA. Epithelial-mesenchymal transition: at the crossroads of development and tumor metastasis. Dev Cell (2008) 14:818–29. doi: 10.1016/j.devcel.2008.05.009
13. Hugo H, Ackland ML, Blick T, Lawrence MG, Clements JA, Williams ED, et al. Epithelial–mesenchymal and mesenchymal–epithelial transitions in carcinoma progression. J Cell Physiol (2007) 213:374–83. doi: 10.1002/jcp.21223
14. Lugano R, Ramachandran M, Dimberg A. Tumor angiogenesis: causes, consequences, challenges and opportunities. Cell Mol Life Sci CMLS (2020) 77:1745–70. doi: 10.1007/s00018-019-03351-7
15. Chopra H, Hung MK, Kwong DL, Zhang CF, Pow EHN. Insights into endothelial progenitor cells: origin, classification, potentials, and prospects. Stem Cells Int (2018) 2018:9847015. doi: 10.1155/2018/9847015
16. Asahara T, Murohara T, Sullivan A, Silver M, van der Zee R, Li T, et al. Isolation of putative progenitor endothelial cells for angiogenesis. Sci (New York N.Y.) (1997) 275:964–7. doi: 10.1126/science.275.5302.964
17. Reale A, Melaccio A, Lamanuzzi A, Saltarella I, Dammacco F, Vacca A, et al. Functional and biological role of endothelial precursor cells in tumour progression: A new potential therapeutic target in haematological malignancies. Stem Cells Int (2016) 2016:7954580. doi: 10.1155/2016/7954580
18. Li T, Kang G, Wang T, Huang H. Tumor angiogenesis and anti-angiogenic gene therapy for cancer. Oncol Lett (2018) 16:687–702. doi: 10.3892/ol.2018.8733
19. Carbone C, Moccia T, Zhu C, Paradiso G, Budillon A, Chiao PJ, et al. Anti-VEGF treatment-resistant pancreatic cancers secrete proinflammatory factors that contribute to Malignant progression by inducing an EMT cell phenotype. Clin Cancer Res (2011) 17:5822–32. doi: 10.1158/1078-0432.Ccr-11-1185
20. Pàez-Ribes M, Allen E, Hudock J, Takeda T, Okuyama H, Viñals F, et al. Antiangiogenic therapy elicits Malignant progression of tumors to increased local invasion and distant metastasis. Cancer Cell (2009) 15:220–31. doi: 10.1016/j.ccr.2009.01.027
21. Kuczynski EA, Vermeulen PB, Pezzella F, Kerbel RS, Reynolds AR. Vessel co-option in cancer. Nat Rev Clin Oncol (2019) 16:469–93. doi: 10.1038/s41571-019-0181-9
22. Pezzella F, Pastorino U, Tagliabue E, Andreola S, Sozzi G, Gasparini G, et al. Non-small-cell lung carcinoma tumor growth without morphological evidence of neo-angiogenesis. Am J Pathol (1997) 151:1417–23.
23. Valiente M, Obenauf AC, Jin X, Chen Q, Zhang XH, Lee DJ, et al. Serpins promote cancer cell survival and vascular co-option in brain metastasis. Cell (2014) 156:1002–16. doi: 10.1016/j.cell.2014.01.040
24. Mandelcorn ED, Palestine AG, Dubovy S, Davis JL. Vascular co-option in lung cancer metastatic to the eye after treatment with bevacizumab. J ophthalmic Inflammation infection (2010) 1:35–8. doi: 10.1007/s12348-010-0013-7
25. Kuczynski EA, Yin M, Bar-Zion A, Lee CR, Butz H, Man S, et al. Co-option of liver vessels and not sprouting angiogenesis drives acquired sorafenib resistance in hepatocellular carcinoma. J Natl Cancer Institute (2016) 108. doi: 10.1093/jnci/djw030
26. Ribatti D, Pezzella F. Vascular co-option and other alternative modalities of growth of tumor vasculature in glioblastoma. Front Oncol (2022) 12:874554. doi: 10.3389/fonc.2022.874554
27. Billaud M, Santoro M. Is Co-option a prevailing mechanism during cancer progression? Cancer Res (2011) 71:6572–5. doi: 10.1158/0008-5472.Can-11-2158
28. Frentzas S, Simoneau E, Bridgeman VL, Vermeulen PB, Foo S, Kostaras E, et al. Vessel co-option mediates resistance to anti-angiogenic therapy in liver metastases. Nat Med (2016) 22:1294–302. doi: 10.1038/nm.4197
29. Cuypers A, Truong AK, Becker LM, Saavedra-García P, Carmeliet P. Tumor vessel co-option: The past & the future. Front Oncol (2022) 12:965277. doi: 10.3389/fonc.2022.965277
30. Maniotis AJ, Folberg R, Hess A, Seftor EA, Gardner LM, Pe'er J, et al. Vascular channel formation by human melanoma cells in vivo and in vitro: vasculogenic mimicry. Am J Pathol (1999) 155:739–52. doi: 10.1016/s0002-9440(10)65173-5
31. Lim D, Do Y, Kwon BS, Chang W, Lee MS, Kim J, et al. Angiogenesis and vasculogenic mimicry as therapeutic targets in ovarian cancer. BMB Rep (2020) 53:291–8. doi: 10.5483/BMBRep.2020.53.6.060
32. Liu R, Yang K, Meng C, Zhang Z, Xu Y. Vasculogenic mimicry is a marker of poor prognosis in prostate cancer. Cancer Biol Ther (2012) 13:527–33. doi: 10.4161/cbt.19602
33. Morales-Guadarrama G, García-Becerra R, Méndez-Pérez EA, García-Quiroz J, Avila E, Díaz L. Vasculogenic mimicry in breast cancer: clinical relevance and drivers. Cells (2021) 10. doi: 10.3390/cells10071758
34. Zheng N, Zhang S, Wu W, Zhang N, Wang J. Regulatory mechanisms and therapeutic targeting of vasculogenic mimicry in hepatocellular carcinoma. Pharmacol Res (2021) 166:105507. doi: 10.1016/j.phrs.2021.105507
35. Li B, Mao X, Wang H, Su G, Mo C, Cao K, et al. Vasculogenic mimicry in bladder cancer and its association with the aberrant expression of ZEB1. Oncol Lett (2018) 15:5193–200. doi: 10.3892/ol.2018.7975
36. Cao Z, Bao M, Miele L, Sarkar FH, Wang Z, Zhou Q. Tumour vasculogenic mimicry is associated with poor prognosis of human cancer patients: a systemic review and meta-analysis. Eur J Cancer (Oxford Engl 1990) (2013) 49:3914–23. doi: 10.1016/j.ejca.2013.07.148
37. Nassar D, Blanpain C. Cancer stem cells: basic concepts and therapeutic implications. Annu Rev Pathol (2016) 11:47–76. doi: 10.1146/annurev-pathol-012615-044438
38. Mirshahi P, Rafii A, Vincent L, Berthaut A, Varin R, Kalantar G, et al. Vasculogenic mimicry of acute leukemic bone marrow stromal cells. Leukemia (2009) 23:1039–48. doi: 10.1038/leu.2009.10
39. Lai CY, Schwartz BE, Hsu MY. CD133+ melanoma subpopulations contribute to perivascular niche morphogenesis and tumorigenicity through vasculogenic mimicry. Cancer Res (2012) 72:5111–8. doi: 10.1158/0008-5472.Can-12-0624
40. Chen T, You Y, Jiang H, Wang ZZ. Epithelial-mesenchymal transition (EMT): A biological process in the development, stem cell differentiation, and tumorigenesis. J Cell Physiol (2017) 232:3261–72. doi: 10.1002/jcp.25797
41. Fan YL, Zheng M, Tang YL, Liang XH. A new perspective of vasculogenic mimicry: EMT and cancer stem cells (Review). Oncol Lett (2013) 6:1174–80. doi: 10.3892/ol.2013.1555
42. Scheau C, Badarau IA, Costache R, Caruntu C, Mihai GL, Didilescu AC, et al. The role of matrix metalloproteinases in the epithelial-mesenchymal transition of hepatocellular carcinoma. Analytical Cell Pathol (Amsterdam) (2019) 2019:9423907. doi: 10.1155/2019/9423907
43. Chen L, Lin G, Chen K, Liang R, Wan F, Zhang C, et al. VEGF promotes migration and invasion by regulating EMT and MMPs in nasopharyngeal carcinoma. J Cancer (2020) 11:7291–301. doi: 10.7150/jca.46429
44. Bowman T, Broome MA, Sinibaldi D, Wharton W, Pledger WJ, Sedivy JM, et al. Stat3-mediated Myc expression is required for Src transformation and PDGF-induced mitogenesis. Proc Natl Acad Sci United States America (2001) 98:7319–24. doi: 10.1073/pnas.131568898
45. Butler JM, Kobayashi H, Rafii S. Instructive role of the vascular niche in promoting tumour growth and tissue repair by angiocrine factors. Nat Rev Cancer (2010) 10:138–46. doi: 10.1038/nrc2791
46. Reiman JM, Knutson KL, Radisky DC. Immune promotion of epithelial-mesenchymal transition and generation of breast cancer stem cells. Cancer Res (2010) 70:3005–8. doi: 10.1158/0008-5472.Can-09-4041
47. Wang H, Huang B, Li BM, Cao KY, Mo CQ, Jiang SJ. Erratum: Global cancer statistics 2018: GLOBOCAN estimates of incidence and mortality worldwide for 36 cancers in 185 countries. CA: Cancer J Clin (2020) 70:313. doi: 10.3322/caac.21609
48. Chang AJ, Autio KA, Roach M. 3rd; Scher, H.I. High-risk prostate cancer-classification and therapy. Nat Rev Clin Oncol (2014) 11:308–23. doi: 10.1038/nrclinonc.2014.68
49. Luo Y, Yang Z, Yu Y, Zhang P. HIF1α lactylation enhances KIAA1199 transcription to promote angiogenesis and vasculogenic mimicry in prostate cancer. Int J Biol macromolecules (2022) 222:2225–43. doi: 10.1016/j.ijbiomac.2022.10.014
50. Lindberg RA, Hunter T. cDNA cloning and characterization of eck, an epithelial cell receptor protein-tyrosine kinase in the eph/elk family of protein kinases. Mol Cell Biol (1990) 10:6316–24. doi: 10.1128/mcb.10.12.6316-6324.1990
51. Kim HS, Won YJ, Shim JH, Kim HJ, Kim BS, Hong HN. Role of EphA2-PI3K signaling in vasculogenic mimicry induced by cancer-associated fibroblasts in gastric cancer cells. Oncol Lett (2019) 18:3031–8. doi: 10.3892/ol.2019.10677
52. Shukla S, Maclennan GT, Hartman DJ, Fu P, Resnick MI, Gupta S. Activation of PI3K-Akt signaling pathway promotes prostate cancer cell invasion. Int J Cancer (2007) 121:1424–32. doi: 10.1002/ijc.22862
53. Wang H, Lin H, Pan J, Mo C, Zhang F, Huang B, et al. Vasculogenic mimicry in prostate cancer: the roles of ephA2 and PI3K. J Cancer (2016) 7:1114–24. doi: 10.7150/jca.14120
54. Zhang P, Sun Y, Ma L. ZEB1: at the crossroads of epithelial-mesenchymal transition, metastasis and therapy resistance. Cell Cycle (Georgetown Tex.) (2015) 14:481–7. doi: 10.1080/15384101.2015.1006048
55. Peng DH, Ungewiss C, Tong P, Byers LA, Wang J, Canales JR, et al. ZEB1 induces LOXL2-mediated collagen stabilization and deposition in the extracellular matrix to drive lung cancer invasion and metastasis. Oncogene (2017) 36:1925–38. doi: 10.1038/onc.2016.358
56. Wang H, Huang B, Li BM, Cao KY, Mo CQ, Jiang SJ, et al. ZEB1-mediated vasculogenic mimicry formation associates with epithelial-mesenchymal transition and cancer stem cell phenotypes in prostate cancer. J Cell Mol Med (2018) 22:3768–81. doi: 10.1111/jcmm.13637
57. Vizcaíno C, Mansilla S, Portugal J. Sp1 transcription factor: A long-standing target in cancer chemotherapy. Pharmacol Ther (2015) 152:111–24. doi: 10.1016/j.pharmthera.2015.05.008
58. Han DS, Lee EO. Sp1 plays a key role in vasculogenic mimicry of human prostate cancer cells. Int J Mol Sci (2022) 23. doi: 10.3390/ijms23031321
59. Da J, Xu M, Wang Y, Li W, Lu M, Wang Z. Kaempferol promotes apoptosis while inhibiting cell proliferation via androgen-dependent pathway and suppressing vasculogenic mimicry and invasion in prostate cancer. Analytical Cell Pathol (Amsterdam) (2019) 2019:1907698. doi: 10.1155/2019/1907698
60. Aggarwal BB, Bhardwaj A, Aggarwal RS, Seeram NP, Shishodia S, Takada Y. Role of resveratrol in prevention and therapy of cancer: preclinical and clinical studies. Anticancer Res (2004) 24:2783–840.
61. Han DS, Lee HJ, Lee EO. Resveratrol suppresses serum-induced vasculogenic mimicry through impairing the EphA2/twist-VE-cadherin/AKT pathway in human prostate cancer PC-3 cells. Sci Rep (2022) 12:20125. doi: 10.1038/s41598-022-24414-z
62. Han H, Lee SO, Xu Y, Kim JE, Lee HJ. SPHK/HIF-1α Signaling pathway has a critical role in chrysin-induced anticancer activity in hypoxia-induced PC-3 cells. Cells (2022) 11. doi: 10.3390/cells11182787
63. Capitanio U, Montorsi F. Renal cancer. Lancet (London England) (2016) 387:894–906. doi: 10.1016/s0140-6736(15)00046-x
64. Lam JS, Shvarts O, Pantuck AJ. Changing concepts in the surgical management of renal cell carcinoma. Eur Urol (2004) 45:692–705. doi: 10.1016/j.eururo.2004.02.002
65. Nerich V, Hugues M, Paillard MJ, Borowski L, Nai T, Stein U, et al. Clinical impact of targeted therapies in patients with metastatic clear-cell renal cell carcinoma. OncoTargets Ther (2014) 7:365–74. doi: 10.2147/ott.S56370
66. Vartanian AA, Stepanova EV, Gutorov SL, Solomko E, Grigorieva IN, Sokolova IN, et al. Prognostic significance of periodic acid-Schiff-positive patterns in clear cell renal cell carcinoma. Can J Urol (2009) 16:4726–32.
67. Lin H, Pan JC, Zhang FM, Huang B, Chen X, Zhuang JT, et al. Matrix metalloproteinase-9 is required for vasculogenic mimicry by clear cell renal carcinoma cells. Urologic Oncol 2015 33 (2015) 168:e169–116. doi: 10.1016/j.urolonc.2014.12.007
68. Thiery JP. Epithelial-mesenchymal transitions in tumour progression. Nat Rev Cancer (2002) 2:442–54. doi: 10.1038/nrc822
69. Ingels A, Hew M, Algaba F, de Boer OJ, van Moorselaar RJ, Horenblas S, et al. Vimentin over-expression and carbonic anhydrase IX under-expression are independent predictors of recurrence, specific and overall survival in non-metastatic clear-cell renal carcinoma: a validation study. World J Urol (2017) 35:81–7. doi: 10.1007/s00345-016-1854-y
70. Du J, Sun B, Zhao X, Gu Q, Dong X, Mo J, et al. Hypoxia promotes vasculogenic mimicry formation by inducing epithelial-mesenchymal transition in ovarian carcinoma. Gynecologic Oncol (2014) 133:575–83. doi: 10.1016/j.ygyno.2014.02.034
71. Lin H, Hong Y, Huang B, Liu X, Zheng J, Qiu S. Vimentin overexpressions induced by cell hypoxia promote vasculogenic mimicry by renal cell carcinoma cells. BioMed Res Int (2019) 2019:7259691. doi: 10.1155/2019/7259691
72. Bai J, Yeh S, Qiu X, Hu L, Zeng J, Cai Y, et al. TR4 nuclear receptor promotes clear cell renal cell carcinoma (ccRCC) vasculogenic mimicry (VM) formation and metastasis via altering the miR490-3p/vimentin signals. . Oncogene (2018) 37:5901–12. doi: 10.1038/s41388-018-0269-1
73. He M, Yang H, Shi H, Hu Y, Chang C, Liu S, et al. Sunitinib increases the cancer stem cells and vasculogenic mimicry formation via modulating the lncRNA-ECVSR/ERβ/Hif2-α signaling. Cancer Lett (2022) 524:15–28. doi: 10.1016/j.canlet.2021.08.028
74. Ding J, Cui XG, Chen HJ, Sun Y, Yu WW, Luo J, et al. Targeting circDGKD intercepts TKI's effects on up-regulation of estrogen receptor β and vasculogenic mimicry in renal cell carcinoma. Cancers (2022) 14. doi: 10.3390/cancers14071639
75. Takahashi Y, Sawada G, Kurashige J, Uchi R, Matsumura T, Ueo H, et al. Paired related homoeobox 1, a new EMT inducer, is involved in metastasis and poor prognosis in colorectal cancer. Br J Cancer (2013) 109:307–11. doi: 10.1038/bjc.2013.339
76. Hirata H, Sugimachi K, Takahashi Y, Ueda M, Sakimura S, Uchi R, et al. Downregulation of PRRX1 confers cancer stem cell-like properties and predicts poor prognosis in hepatocellular carcinoma. Ann Surg Oncol (2015) 22 Suppl 3:S1402–1409. doi: 10.1245/s10434-014-4242-0
77. Westermarck J, Hahn WC. Multiple pathways regulated by the tumor suppressor PP2A in transformation. Trends Mol Med (2008) 14:152–60. doi: 10.1016/j.molmed.2008.02.001
78. Tang Q, Wang Q, Zeng G, Li Q, Jiang T, Zhang Z, et al. Overexpression of CIP2A in clear cell renal cell carcinoma promotes cellular epithelial-mesenchymal transition and is associated with poor prognosis. Oncol Rep (2015) 34:2515–22. doi: 10.3892/or.2015.4217
79. Wang X, Yang R, Wang Q, Wang Y, Ci H, Wu S. Aberrant expression of vasculogenic mimicry, PRRX1, and CIP2A in clear cell renal cell carcinoma and its clinicopathological significance. Medicine (2019) 98:e17028. doi: 10.1097/md.0000000000017028
80. Zhao H, Leppert JT, Peehl DMA. Protective role for androgen receptor in clear cell renal cell carcinoma based on mining TCGA data. PloS One (2016) 11:e0146505. doi: 10.1371/journal.pone.0146505
81. You B, Sun Y, Luo J, Wang K, Liu Q, Fang R, et al. Androgen receptor promotes renal cell carcinoma (RCC) vasculogenic mimicry (VM) via altering TWIST1 nonsense-mediated decay through lncRNA-TANAR. Oncogene (2021) 40:1674–89. doi: 10.1038/s41388-020-01616-1
82. Pollard JW. Tumour-educated macrophages promote tumour progression and metastasis. Nat Rev Cancer (2004) 4:71–8. doi: 10.1038/nrc1256
83. Komohara Y, Hasita H, Ohnishi K, Fujiwara Y, Suzu S, Eto M, et al. Macrophage infiltration and its prognostic relevance in clear cell renal cell carcinoma. Cancer Sci (2011) 102:1424–31. doi: 10.1111/j.1349-7006.2011.01945.x
84. Chen XW, Yu TJ, Zhang J, Li Y, Chen HL, Yang GF, et al. CYP4A in tumor-associated macrophages promotes pre-metastatic niche formation and metastasis. Oncogene (2017) 36:5045–57. doi: 10.1038/onc.2017.118
85. Sica A, Erreni M, Allavena P, Porta C. Macrophage polarization in pathology. Cell Mol Life Sci CMLS (2015) 72:4111–26. doi: 10.1007/s00018-015-1995-y
86. Lan J, Sun L, Xu F, Liu L, Hu F, Song D, et al. M2 macrophage-derived exosomes promote cell migration and invasion in colon cancer. Cancer Res (2019) 79:146–58. doi: 10.1158/0008-5472.Can-18-0014
87. Liu Q, Zhao E, Geng B, Gao S, Yu H, He X, et al. Tumor-associated macrophage-derived exosomes transmitting miR-193a-5p promote the progression of renal cell carcinoma via TIMP2-dependent vasculogenic mimicry. Cell Death Dis (2022) 13:382. doi: 10.1038/s41419-022-04814-9
88. Intlekofer AM, Dematteo RG, Venneti S, Finley LW, Lu C, Judkins AR, et al. Hypoxia induces production of L-2-hydroxyglutarate. Cell Metab (2015) 22:304–11. doi: 10.1016/j.cmet.2015.06.023
89. Wang H, Wang L, Zheng Q, Lu Z, Chen Y, Shen D, et al. Oncometabolite L-2-hydroxyglurate directly induces vasculogenic mimicry through PHLDB2 in renal cell carcinoma. Int J Cancer (2021) 148:1743–55. doi: 10.1002/ijc.33435
90. Lim BC, Matsumoto S, Yamamoto H, Mizuno H, Kikuta J, Ishii M, et al. Prickle1 promotes focal adhesion disassembly in cooperation with the CLASP-LL5β complex in migrating cells. J Cell Sci (2016) 129:3115–29. doi: 10.1242/jcs.185439
91. Richters A, Aben KKH, Kiemeney L. The global burden of urinary bladder cancer: an update. World J Urol (2020) 38:1895–904. doi: 10.1007/s00345-019-02984-4
92. Dy GW, Gore JL, Forouzanfar MH, Naghavi M, Fitzmaurice C. Global burden of urologic cancers, 1990-2013. Eur Urol (2017) 71:437–46. doi: 10.1016/j.eururo.2016.10.008
93. Mayr R, Fritsche HM, Pycha A, Pycha A. Radical cystectomy and the implications of comorbidity. Expert Rev Anticancer Ther (2014) 14:289–95. doi: 10.1586/14737140.2014.868775
94. Fujimoto A, Onodera H, Mori A, Nagayama S, Yonenaga Y, Tachibana T. Tumour plasticity and extravascular circulation in ECV304 human bladder carcinoma cells. Anticancer Res (2006) 26:59–69. doi: 10.1586/14737140.6.1.59
95. Griffin M, Casadio R, Bergamini CM. Transglutaminases: nature's biological glues. Biochem J (2002) 368:377–96. doi: 10.1042/bj20021234
96. Collighan RJ, Griffin M. Transglutaminase 2 cross-linking of matrix proteins: biological significance and medical applications. Amino Acids (2009) 36:659–70. doi: 10.1007/s00726-008-0190-y
97. Haroon ZA, Hettasch JM, Lai TS, Dewhirst MW, Greenberg CS. Tissue transglutaminase is expressed, active, and directly involved in rat dermal wound healing and angiogenesis. FASEB J (1999) 13:1787–95. doi: 10.1096/fasebj.13.13.1787
98. Jones RA, Wang Z, Dookie S, Griffin M. The role of TG2 in ECV304-related vasculogenic mimicry. Amino Acids (2013) 44:89–101. doi: 10.1007/s00726-011-1214-6
99. Yang L, Zou X, Zou J, Zhang G. Functions of circular RNAs in bladder, prostate and renal cell cancer (Review). Mol Med Rep (2021) 23. doi: 10.3892/mmr.2021.11946
100. Sato F, Tsuchiya S, Meltzer SJ, Shimizu K. MicroRNAs and epigenetics. FEBS J (2011) 278:1598–609. doi: 10.1111/j.1742-4658.2011.08089.x
101. Zhang T, Wang J, Zhai X, Li H, Li C, Chang J. MiR-124 retards bladder cancer growth by directly targeting CDK4. Acta Biochim Biophys Sin (2014) 46:1072–9. doi: 10.1093/abbs/gmu105
102. Qian C, Wang Y, Ji Y, Chen D, Wang C, Zhang G, et al. Neural stem cell−derived exosomes transfer miR−124−3p into cells to inhibit glioma growth by targeting FLOT2. Int J Oncol (2022) 61. doi: 10.3892/ijo.2022.5405
103. Ren X, Fan Y, Shi D, Xu E, Liu Y. MicroRNA-124 inhibits canine mammary carcinoma cell proliferation, migration and invasion by targeting CDH2. Res veterinary Sci (2022) 146:5–14. doi: 10.1016/j.rvsc.2022.03.004
104. Reardon ES, Shukla V, Xi S, Gara SK, Liu Y, Straughan D, et al. UHRF1 is a novel druggable epigenetic target in Malignant pleural mesothelioma. J Thorac Oncol (2021) 16:89–103. doi: 10.1016/j.jtho.2020.08.024
105. Bronner C, Krifa M, Mousli M. Increasing role of UHRF1 in the reading and inheritance of the epigenetic code as well as in tumorogenesis. Biochem Pharmacol (2013) 86:1643–9. doi: 10.1016/j.bcp.2013.10.002
106. Wang X, Wu Q, Xu B, Wang P, Fan W, Cai Y, et al. MiR-124 exerts tumor suppressive functions on the cell proliferation, motility and angiogenesis of bladder cancer by fine-tuning UHRF1. FEBS J (2015) 282:4376–88. doi: 10.1111/febs.13502
107. Liu Z, Sun B, Qi L, Li H, Gao J, Leng X. Zinc finger E-box binding homeobox 1 promotes vasculogenic mimicry in colorectal cancer through induction of epithelial-to-mesenchymal transition. Cancer Sci (2012) 103:813–20. doi: 10.1111/j.1349-7006.2011.02199.x
108. Liu Z, Tang Q, Qi T, Othmane B, Yang Z, Chen J, et al. Robust hypoxia risk score predicts the clinical outcomes and tumor microenvironment immune characters in bladder cancer. Front Immunol (2021) 12:725223. doi: 10.3389/fimmu.2021.725223
109. Sun B, Zhang D, Zhang S, Zhang W, Guo H, Zhao X. Hypoxia influences vasculogenic mimicry channel formation and tumor invasion-related protein expression in melanoma. Cancer Lett (2007) 249:188–97. doi: 10.1016/j.canlet.2006.08.016
110. Wei X, Chen Y, Jiang X, Peng M, Liu Y, Mo Y, et al. Mechanisms of vasculogenic mimicry in hypoxic tumor microenvironments. Mol Cancer (2021) 20:7. doi: 10.1186/s12943-020-01288-1
111. Mayers JR, Torrence ME, Danai LV, Papagiannakopoulos T, Davidson SM, Bauer MR, et al. Tissue of origin dictates branched-chain amino acid metabolism in mutant Kras-driven cancers. Sci (New York N.Y.) (2016) 353:1161–5. doi: 10.1126/science.aaf5171
112. Cai Z, Chen J, Yu Z, Li H, Liu Z, Deng D, et al. BCAT2 shapes a noninflamed tumor microenvironment and induces resistance to anti-PD-1/PD-L1 immunotherapy by negatively regulating proinflammatory chemokines and anticancer immunity. Advanced Sci (Weinheim Baden-Wurttemberg Germany) (2023) 10:e2207155. doi: 10.1002/advs.202207155
113. Wang J, Sun J, Liu LN, Flies DB, Nie X, Toki M, et al. Siglec-15 as an immune suppressor and potential target for normalization cancer immunotherapy. Nat Med (2019) 25:656–66. doi: 10.1038/s41591-019-0374-x
114. Zhang C, Xiao J, Yuan T, He Y, Deng D, Xiao Z, et al. Molecular vasculogenic mimicry-Related signatures predict clinical outcomes and therapeutic responses in bladder cancer: Results from real-world cohorts. Front Pharmacol (2023) 14:1163115. doi: 10.3389/fphar.2023.1163115
115. Hu J, Yu A, Othmane B, Qiu D, Li H, Li C, et al. Siglec15 shapes a non-inflamed tumor microenvironment and predicts the molecular subtype in bladder cancer. Theranostics (2021) 11:3089–108. doi: 10.7150/thno.53649
116. Nunes SP, Henrique R, Jerónimo C, Paramio JM. DNA methylation as a therapeutic target for bladder cancer. Cells (2020) 9. doi: 10.3390/cells9081850
117. Kelly AD, Issa JJ. The promise of epigenetic therapy: reprogramming the cancer epigenome. Curr Opin Genet Dev (2017) 42:68–77. doi: 10.1016/j.gde.2017.03.015
118. Biswas S, Rao CM. Epigenetic tools (The Writers, The Readers and The Erasers) and their implications in cancer therapy. Eur J Pharmacol (2018) 837:8–24. doi: 10.1016/j.ejphar.2018.08.021
119. Hu J, Othmane B, Yu A, Li H, Cai Z, Chen X, et al. 5mC regulator-mediated molecular subtypes depict the hallmarks of the tumor microenvironment and guide precision medicine in bladder cancer. BMC Med (2021) 19:289. doi: 10.1186/s12916-021-02163-6
120. Qi L, Song W, Liu Z, Zhao X, Cao W, Sun B. Wnt3a promotes the vasculogenic mimicry formation of colon cancer via Wnt/β-catenin signaling. Int J Mol Sci (2015) 16:18564–79. doi: 10.3390/ijms160818564
121. Liu X, He H, Zhang F, Hu X, Bi F, Li K, et al. m6A methylated EphA2 and VEGFA through IGF2BP2/3 regulation promotes vasculogenic mimicry in colorectal cancer via PI3K/AKT and ERK1/2 signaling. Cell Death Dis (2022) 13:483. doi: 10.1038/s41419-022-04950-2
122. Deng F, Zhou R, Lin C, Yang S, Wang H, Li W, et al. Tumor-secreted dickkopf2 accelerates aerobic glycolysis and promotes angiogenesis in colorectal cancer. Theranostics (2019) 9:1001–14. doi: 10.7150/thno.30056
123. Mashreghi M, Azarpara H, Bazaz MR, Jafari A, Masoudifar A, Mirzaei H, et al. Angiogenesis biomarkers and their targeting ligands as potential targets for tumor angiogenesis. J Cell Physiol (2018) 233:2949–65. doi: 10.1002/jcp.26049
124. Liu B, Wang T, Wang H, Zhang L, Xu F, Fang R, et al. Oncoprotein HBXIP enhances HOXB13 acetylation and co-activates HOXB13 to confer tamoxifen resistance in breast cancer. J Hematol Oncol (2018) 11:26. doi: 10.1186/s13045-018-0577-5
125. Xiu M, Zeng X, Shan R, Wen W, Li J, Wan R. The oncogenic role of HBXIP. Biomedicine pharmacotherapy = Biomedecine pharmacotherapie (2021) 133:111045. doi: 10.1016/j.biopha.2020.111045
126. Li X, Liu S. Suppression of HBXIP reduces cell proliferation, migration and invasion in vitro, and tumorigenesis in vivo in human urothelial carcinoma of the bladder. Cancer biotherapy radiopharmaceuticals (2016) 31:311–6. doi: 10.1089/cbr.2016.2038
127. Zheng YL, Li L, Jia YX, Zhang BZ, Li JC, Zhu YH, et al. LINC01554-mediated glucose metabolism reprogramming suppresses tumorigenicity in hepatocellular carcinoma via downregulating PKM2 expression and inhibiting AKT/mTOR signaling pathway. Theranostics (2019) 9:796–810. doi: 10.7150/thno.28992
128. Liu X, Li H, Che N, Zheng Y, Fan W, Li M, et al. HBXIP accelerates glycolysis and promotes cancer angiogenesis via AKT/mTOR pathway in bladder cancer. Exp Mol Pathol (2021) 121:104665. doi: 10.1016/j.yexmp.2021.104665
129. Prager GW, Poettler M, Unseld M, Zielinski CC. Angiogenesis in cancer: Anti-VEGF escape mechanisms. Trans Lung Cancer Res (2012) 1:14–25. doi: 10.3978/j.issn.2218-6751.2011.11.02
130. Aguilar-Cazares D, Chavez-Dominguez R, Carlos-Reyes A, Lopez-Camarillo C, Hernadez de la Cruz ON, Lopez-Gonzalez JS. Contribution of angiogenesis to inflammation and cancer. Front Oncol (2019) 9:1399. doi: 10.3389/fonc.2019.01399
131. Escudier B, Eisen T, Stadler WM, Szczylik C, Oudard S, Siebels M, et al. Sorafenib in advanced clear-cell renal-cell carcinoma. New Engl J Med (2007) 356:125–34. doi: 10.1056/NEJMoa060655
132. Lacal PM, Graziani G. Therapeutic implication of vascular endothelial growth factor receptor-1 (VEGFR-1) targeting in cancer cells and tumor microenvironment by competitive and non-competitive inhibitors. Pharmacol Res (2018) 136:97–107. doi: 10.1016/j.phrs.2018.08.023
133. Ellis LM, Hicklin DJ. Pathways mediating resistance to vascular endothelial growth factor-targeted therapy. Clin Cancer Res (2008) 14:6371–5. doi: 10.1158/1078-0432.Ccr-07-5287
134. Rini BI, Halabi S, Rosenberg JE, Stadler WM, Vaena DA, Archer L, et al. Phase III trial of bevacizumab plus interferon alfa versus interferon alfa monotherapy in patients with metastatic renal cell carcinoma: final results of CALGB 90206. J Clin Oncol (2010) 28:2137–43. doi: 10.1200/jco.2009.26.5561
135. Hori A, Shimoda M, Naoi Y, Kagara N, Tanei T, Miyake T, et al. Vasculogenic mimicry is associated with trastuzumab resistance of HER2-positive breast cancer. Breast Cancer Res BCR (2019) 21:88. doi: 10.1186/s13058-019-1167-3
136. Sun L, Diamond ME, Ottaviano AJ, Joseph MJ, Ananthanarayan V, Munshi HG. Transforming growth factor-beta 1 promotes matrix metalloproteinase-9-mediated oral cancer invasion through snail expression. Mol Cancer Res MCR (2008) 6:10–20. doi: 10.1158/1541-7786.Mcr-07-0208
137. Munger JS, Sheppard D. Cross talk among TGF-β signaling pathways, integrins, and the extracellular matrix. Cold Spring Harbor Perspect Biol (2011) 3:a005017. doi: 10.1101/cshperspect.a005017
138. Jiang L, Shi S, Li F, Shi Q, Zhong T, Zhang H, et al. miR−519d−3p/HIF−2α axis increases the chemosensitivity of human cervical cancer cells to cisplatin via inactivation of PI3K/AKT signaling. Mol Med Rep (2021) 23. doi: 10.3892/mmr.2021.11992
139. Huang M, Ke Y, Sun X, Yu L, Yang Z, Zhang Y, et al. Mammalian target of rapamycin signaling is involved in the vasculogenic mimicry of glioma via hypoxia-inducible factor-1α. . Oncol Rep (2014) 32:1973–80. doi: 10.3892/or.2014.3454
140. Metcalfe SM, Canman CE, Milner J, Morris RE, Goldman S, Kastan MB. Rapamycin and p53 act on different pathways to induce G1 arrest in mammalian cells. Oncogene (1997) 15:1635–42. doi: 10.1038/sj.onc.1201341
141. Jung SH, Hwang HJ, Kang D, Park HA, Lee HC, Jeong D, et al. mTOR kinase leads to PTEN-loss-induced cellular senescence by phosphorylating p53. Oncogene (2019) 38:1639–50. doi: 10.1038/s41388-018-0521-8
142. Zhang Y, Yan H, Xu Z, Yang B, Luo P, He Q. Molecular basis for class side effects associated with PI3K/AKT/mTOR pathway inhibitors. Expert Opin Drug Metab Toxicol (2019) 15:767–74. doi: 10.1080/17425255.2019.1663169
143. Cannone S, Greco MR, Carvalho TMA, Guizouarn H, Soriani O, Di Molfetta D, et al. Cancer associated fibroblast (CAF) regulation of PDAC parenchymal (CPC) and CSC phenotypes is modulated by ECM composition. Cancers (2022) 14. doi: 10.3390/cancers14153737
144. Li F, Xu J, Liu S. Cancer stem cells and neovascularization. Cells (2021) 10. doi: 10.3390/cells10051070
145. Akhmetshina A, Dees C, Pileckyte M, Maurer B, Axmann R, Jüngel A, et al. Dual inhibition of c-abl and PDGF receptor signaling by dasatinib and nilotinib for the treatment of dermal fibrosis. FASEB J (2008) 22:2214–22. doi: 10.1096/fj.07-105627
146. Ferician AM, Ferician OC, Cumpanas AD, Berzava PL, Nesiu A, Barmayoun A, et al. Heterogeneity of platelet derived growth factor pathway gene expression profile defines three distinct subgroups of renal cell carcinomas. Cancer Genomics Proteomics (2022) 19:477–89. doi: 10.21873/cgp.20334
147. Mundhenke C, Weigel MT, Sturner KH, Roesel F, Meinhold-Heerlein I, Bauerschlag DO, et al. Novel treatment of ovarian cancer cell lines with Imatinib mesylate combined with Paclitaxel and Carboplatin leads to receptor-mediated antiproliferative effects. J Cancer Res Clin Oncol (2008) 134:1397–405. doi: 10.1007/s00432-008-0408-0
148. Kwon YC, Bose SK, Steele R, Meyer K, Di Bisceglie AM, Ray RB, et al. Promotion of cancer stem-like cell properties in hepatitis C virus-infected hepatocytes. J Virol (2015) 89:11549–56. doi: 10.1128/jvi.01946-15
149. Carneiro A, Falcão M, Azevedo I, Falcão Reis F, Soares R. Multiple effects of bevacizumab in angiogenesis: implications for its use in age-related macular degeneration. Acta ophthalmologica (2009) 87:517–23. doi: 10.1111/j.1755-3768.2008.01257.x
150. Ferrara N, Hillan KJ, Gerber HP, Novotny W. Discovery and development of bevacizumab, an anti-VEGF antibody for treating cancer. Nat Rev Drug Discovery (2004) 3:391–400. doi: 10.1038/nrd1381
151. Motzer RJ, Hutson TE, Tomczak P, Michaelson MD, Bukowski RM, Rixe O, et al. Sunitinib versus interferon alfa in metastatic renal-cell carcinoma. New Engl J Med (2007) 356:115–24. doi: 10.1056/NEJMoa065044
152. Kopetz S, Hoff PM, Morris JS, Wolff RA, Eng C, Glover KY, et al. Phase II trial of infusional fluorouracil, irinotecan, and bevacizumab for metastatic colorectal cancer: efficacy and circulating angiogenic biomarkers associated with therapeutic resistance. J Clin Oncol (2010) 28:453–9. doi: 10.1200/jco.2009.24.8252
Keywords: vasculogenic mimicry, urological, tumors, therapeutic potential, angiogenesis
Citation: Lin X, Long S, Yan C, Zou X, Zhang G, Zou J and Wu G (2023) Therapeutic potential of vasculogenic mimicry in urological tumors. Front. Oncol. 13:1202656. doi: 10.3389/fonc.2023.1202656
Received: 09 April 2023; Accepted: 06 September 2023;
Published: 21 September 2023.
Edited by:
Wen-Hao Xu, Fudan University, ChinaReviewed by:
Jiao Hu, Central South University, ChinaFrancesco Pezzella, University of Oxford, United Kingdom
Copyright © 2023 Lin, Long, Yan, Zou, Zhang, Zou and Wu. This is an open-access article distributed under the terms of the Creative Commons Attribution License (CC BY). The use, distribution or reproduction in other forums is permitted, provided the original author(s) and the copyright owner(s) are credited and that the original publication in this journal is cited, in accordance with accepted academic practice. No use, distribution or reproduction is permitted which does not comply with these terms.
*Correspondence: Gengqing Wu, gengqing169@126.com