- Department of Cardiology, University Medical Center Göttingen, Göttingen, Germany
Cardiac K channels are critical determinants of cardiac excitability. In hypertrophied and failing myocardium, alterations in the expression and activity of voltage-gated K channels are frequently observed and contribute to the increased propensity for life-threatening arrhythmias. Thus, understanding the mechanisms of disturbed K channel regulation in heart failure (HF) is of critical importance. Amongst others, Ca/calmodulin-dependent protein kinase II (CaMKII) has been identified as an important regulator of K channel activity. In human HF but also various animal models, increased CaMKII expression and activity has been linked to deteriorated contractile function and arrhythmias. This review will discuss the current knowledge about CaMKII regulation of several K channels, its influence on action potential properties, dispersion of repolarization, and arrhythmias with special focus on HF.
Introduction
Heart failure (HF) is a leading cause of death in western countries (United States and Europe), (Neumann et al., 2009; Go et al., 2012; Nichols et al., 2013) but also in developing countries like China (Hu et al., 2012). Morbidity in HF is characterized by contractile dysfunction and an increased propensity for arrhythmias (Luo and Anderson, 2013). Both are known consequences of the electro-mechanical remodeling of the cardiomyocyte. It is well established that reduced expression of K channels in hypertrophied and failing myocardium (Kääb et al., 1996) can lead to action potential (AP) prolongation, which is known to be pro-arrhythmogenic. Moreover, AP prolongation also leads to greater systolic Ca entry through voltage-gated L-type Ca channels (CaV1.2) and impairs the Ca export function of cardiac Na/Ca exchange (NCX, Bers, 2002a), which results in cytosolic Ca overload and dramatically impairs diastolic contractile function (Figure 1).
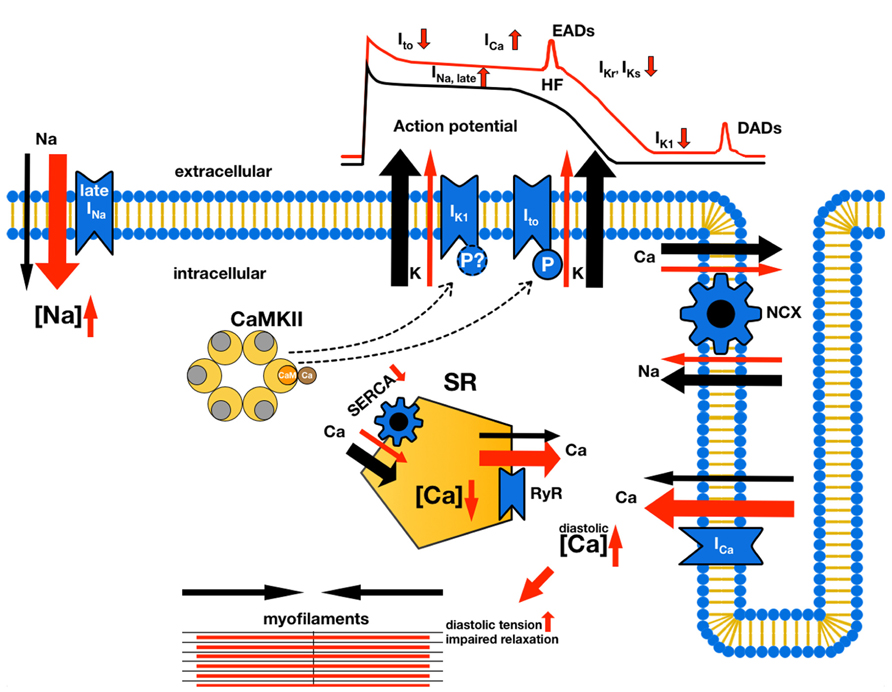
FIGURE 1. Electro-mechanical remodeling in ventricular myocytes in HF. Normal currents are indicated by black arrows, while changes in HF are indicated by red arrows and changes in size to indicate an increase or decrease in current density. CaMKII effects on potassium currents are indicated by bar-headed lines (in this figure only CaMKII effects on K currents are shown, for a detailed review of CaMKII effects refer to Maier and Bers, 2007). Decreased expression and function of repolarizing K currents (Ito, IKr, IKs, IK1), for instance due CaMKII-mediated effects leads to prolongation of the AP duration. This can result in greater systolic Ca entry through voltage-gated Ca channels, but also Na entry through increased late Na current (via voltage-gated Na channels, Shryock et al., 2013). Increased cytosolic Na concentrations, a feature also observed in HF (Pieske et al., 2002), together with prolonged AP duration also impairs the Ca export function of cardiac Na/Ca exchanger (Bers, 2002a), which further aggravates the net gain in cytosolic Ca. In the face of a reduced function of the sarcoplasmic reticulum (SR) Ca ATPase in HF (SERCA; ), this Ca remains in the cytosol thereby dramatically impairing diastolic function. Moreover, increased depolarizing currents (Na and Ca currents) during the plateau phase of the AP could lead to early afterdepolarizations (EADs), while increased diastolic SR Ca leak through ryanodine-receptor 2 (RyR) facilitates delayed afterdepolarizations (DADs).
Thus, understanding the mechanisms that are involved in the regulation of cardiac K channel expression and function in HF could greatly improve patient treatment.
Ca/calmodulin-dependent protein kinase II (CaMKII) has been identified as an important regulator of ion channels and transporters involved in cardiac excitation–contraction coupling under physiological but also pathophysiological conditions (Maier and Bers, 2007). Increased CaMKII expression and function was found in HF and is linked to contractile dysfunction and arrhythmias. Interestingly, there is substantial evidence that CaMKII is also involved in K channel regulation (Nerbonne, 2011). This review will discuss CaMKII-dependent regulation of several cardiac potassium channels and its significance for arrhythmogenesis and contractile function in HF.
K Channels are Important Regulators of Cardiac Excitability
The cardiac AP is initiated by activation of voltage-gated Na channels (NaV1.5). The resulting Na current (INa) leads to a rapid depolarization, i.e., the AP upstroke (phase 0; Bers, 2002b). The upstroke is limited by inactivation of INa and voltage-dependent activation of transient outward K channels (KV4.2, KV4.3, and KV1.4 generating Ito). Ito activation results in an early repolarization (notch, phase 1), thus setting the voltage plateau of the AP. Activation of L-type Ca channels generates a depolarizing Ca current (ICa) that stabilizes the membrane potential during the plateau phase (phase 2). Repolarization in phase 3 is mainly caused by activation of delayed rectifying K channels [hERG (KCNH2), Kv7.1 (KCNQ1), and Kv1.5 (KCNA5) responsible for IKr, IKs, and IKur, respectively]. Additionally, activation of inward rectifying K channels (Kir2.x, generating IK1) contributes to late phase repolarization. The resting membrane potential (phase 4) is stabilized by IK1, but ion conductance in phase 4 is also influenced by the Na/K-ATPase and NCX.
In pacemaker cells, the absence of a stabilizing IK1 is responsible for a more positive resting membrane potential (Cho et al., 2003). The non-specific cation current If (channel protein HCN) can thus generate diastolic depolarization leading to the generation of APs (Bers, 2002b).
Several mechanisms of arrhythmogenesis involving K channels have been described. Reduced function of Kv7.1 and hERG are the hallmark of congential long QT syndrome 1 and 2, respectively (Brenyo et al., 2012). A smaller IKs and IKr results in prolonged repolarization that is associated with torsade de pointes and sudden cardiac death (Roden, 2008). The underlying arrhythmic mechanisms involve increased triggered activity due to early afterdepolarizations (EADs) or reentry due to increased spatial heterogeneities in repolarization (see below). Recently, a mutation of an ATP-sensitive K channel (Medeiros-Domingo et al., 2010) has been identified in a patient with early repolarization syndrome, which is characterized by a prominent J wave on the ECG (see below) and is associated with an increased risk of ventricular fibrillation (VF) and cardiac death (Tikkanen et al., 2009). It was shown that this mutation results in gain of function in KATP (Medeiros-Domingo et al., 2010), consequently resulting in increased transmural heterogeneity of repolarization (see below).
Interestingly, besides rare congenital disease, altered K channel function has also been described for HF. It was shown that decreased IK1 and Ito density could lead to AP prolongation (Kaab et al., 1998).
Increased triggered activity is an important consequence of prolonged repolarization. The longer phase 2 of the AP results in reactivation of Ca channels that generate a depolarizing current possibly resulting in an EAD and ultimately leading to a triggered AP (Weiss et al., 2010). On the other hand, K channels have been also been shown to be involved in the generation of delayed afterdepolarizations (DADs) that are a consequence of cytosolic and sarcoplasmic reticulum (SR) Ca overload. The latter causes an increased propensity of spontaneous ryanodine-receptor (RyR) activation leading to a depolarizing inward NCX current (Kääb et al., 1996). Interestingly, this inward NCX current is more likely to induce DADs if IK1 is functionally downregulated, causing an unstable resting membrane potential (Dhamoon and Jalife, 2005).
Differential K channel expression across the ventricular wall is the basis for transmural dispersion of repolarization (TDR, Antzelevitch and Fish, 2001). Physiologically, the endocardial myocyte has a smaller Ito amplitude compared to the epicardial myocyte. This, together with increased depolarizing currents, contributes to a more positive AP plateau and a longer AP duration in the endocardial compared to the epicardial myocyte. The result is a physiological TDR that also determines the positive T wave on the surface ECG. However, under pathophysiological conditions this fine balanced regional difference in K channel function can be substantially altered. A preferential shortening of the epicardial AP by enhanced Ito, for instance, together with a preferential prolongation of the endocardial AP by enhanced late INa and minor changes in the small Ito would increase the TDR. While a TDR increase in phase 1 and 2 of the AP results in the occurance of a J wave (positive deflection at the QRS-ST junction; Yan and Antzelevitch, 1996), increased TDR in phase 3 and 4 can cause abnormal T waves. If the increase in TDR in phase 3 and 4 reached a threshold, abnormal electrical activity would find excitable myocytes, resulting in reentry and leading to torsade de pointes (Yan and Antzelevitch, 1996). Computational modeling of a rabbit ventricular myocyte overexpressing CaMKII was used to investigate the importance of the expression level of Ito for AP duration (Grandi et al., 2007). If 100% Ito expression was used ( = epicardial myocytes), CaMKII overexpression resulted in a shortening of the AP duration mainly due to a CaMKII-dependent enhancement of Ito. With 10% Ito expression ( = endocardial myocytes), however, AP duration increased because CaMKII-enhanced late INa and L-type Ca current outweighed the effect on the smaller Ito. The mechanisms by which CaMKII alters potassium channel expression and function will be discussed in this review.
CaMKII and HF
Calcium-Calmodulin-dependent kinase II is a serine/threonine kinase that can regulate multiple ion channels and transporters including K channels (see below). Currently, four isoforms and up to 30 splice-variants of the serine/threonine CaMKII have been identified, with CaMKIIδ as the predominant cardiac isoform (Maier and Bers, 2007). CaMKII contains an N-terminal catalytic kinase-domain with an ATP-binding site as well as substrate binding sites. Adjacent to the catalytic subunit, an autoregulatory domain with a calmodulin (CaM)-binding site and important regulatory threonine (T287, T306, T307) and methionine residues (M281/282) precedes the C-terminal association-domain, which is critical for the assembly of the holoenzyme. In vivo, self-association of CaMKII holoenzymes forms two ring-like CaMKII-hexamers which are stacked on top of each other (dodecameric configuration; Rellos et al., 2010). CaMKII is activated by binding of a Ca/CaM complex to its autoregulatory domain, resulting in conformational changes which expose the catalytic subunit, enabling ATP and substrate binding. An important substrate is the autoregulatory domain of an adjacent subunit, resulting in inter-subunit phosphorylation at T287 (auto-phosphorylation). The latter enables CaM-independent activity after the dissociation of Ca/CaM (Maier and Bers, 2007). Novel alternative activation pathways have also been described involving oxidation or glycosylation at M281/282, both of which result in Ca-independent activity similar to auto-phosphorylation (Erickson et al., 2008, 2013).
CaMKII has been associated with HF development. In human HF, expression and activity of CaMKII is increased (Hoch et al., 1999; Kirchhefer et al., 1999; Ai et al., 2005). Moreover, CaMKIIδ-transgenic mice develop HF with increased AP duration, disturbed Ca handling, and are prone to ventricular arrhythmias (Maier, 2003; Wagner et al., 2011). In contrast, transgenic CaMKII inhibition or CaMKII knockout prevents cardiac remodeling and HF development after myocardial infarction or increased afterload (Zhang et al., 2005; Backs et al., 2009; Ling et al., 2009).
Transient Outward K Current
Ito is generated by a pore-forming α-subunit with six transmembrane segments (S1–S6). Accessory β-subunits can associate with this α-subunit (Figure 2A, Niwa and Nerbonne, 2010). In their either homologous or heterologous tetrameric assembly, the subunits’ S5 and S6 segments face each other to form the pore, while segment S4 senses voltage (Snyders, 1999; Niwa and Nerbonne, 2010). Ito is critical for the early repolarization (“notch”) immediately following the upstroke in phase 0 of the cardiac AP. There are at least two components of Ito generated by different channel isoforms, that can be distinguished according to their recovery and inactivation kinetics (Figure 2B; Brahmajothi et al., 1999). The fast component (Ito,fast) inactivates and recovers with time constants (τ ) of less than 100 ms, whereas the slow component (Ito,slow) inactivates with τ of about 200 ms and recovers with τ ranging from hundreds of milliseconds up to several seconds (Brahmajothi et al., 1999; Xu et al., 1999).
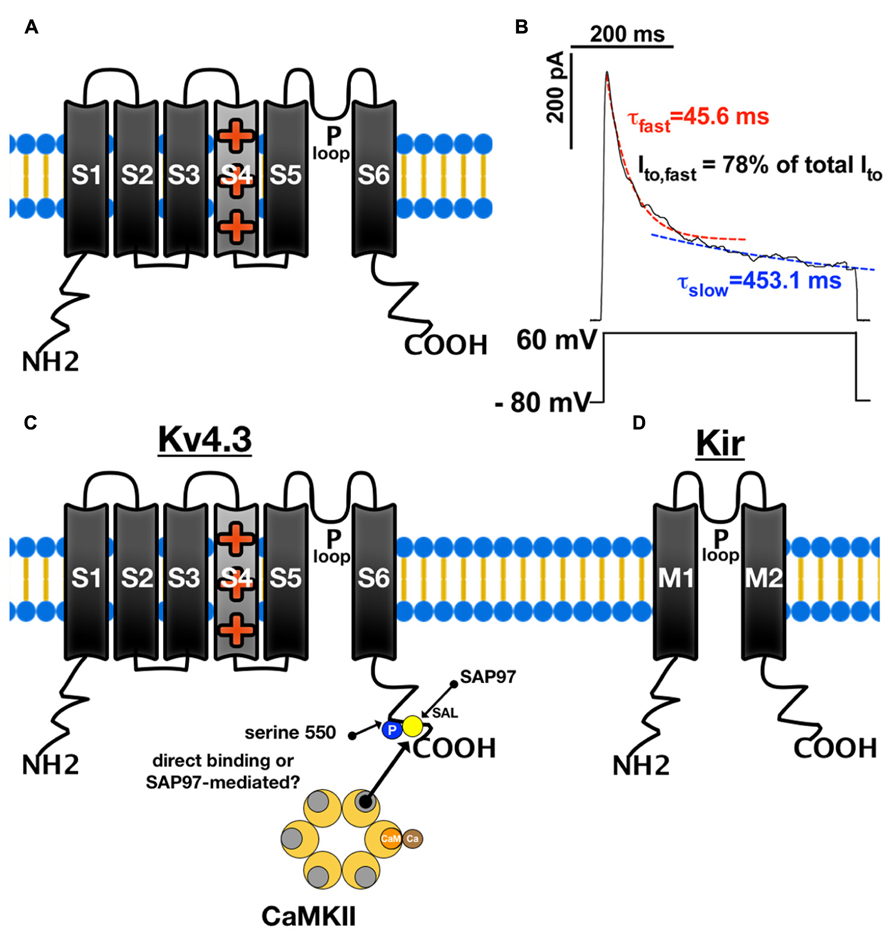
FIGURE 2. Structure and function of K channels. (A) Structure of voltage-gated K channel α-sunbunit (Kv) with six transmembrane segments (S1–S6). The S5–S6 segments face each other to form the central pore. The P-loop between the S5 and S6 segments acts as an ion conductance pathway and its signature motif G(Y/F)G functions as a K ion selectivity filter. Segment S4 senses voltage and moves outward during cell membrane depolarization resulting in conformational changes which open the pore. (B) There are two current components of Ito generated that can be distinguished according to their inactivation kinetics. Ito,fast inactivates with time constants (τ) of less than 100 ms, whereas the Ito,slow inactivates with τ of about 200 ms. (C) CaMKII can bind to Kv4.3 and phosphorylate serine 550 of its C terminus, which leads to altered current kinetics. SAP97 can also bind to Kv4.3 [at its Ser-Ala-Leu (SAL) segment] and possibly mediates the CaMKII-Kv4.3 interaction. (D) Inward rectifying potassium channels are formed by four α-subunits containing only two transmembrane segments (M1–M2) with a central P-loop as ion conductance pathway.
In human, rat, and canine tissue, Ito is generated mostly by the rapidly recovering channel population Kv4.3 (KCND3, Dixon et al., 1996). In a tachycardia-induced canine model of HF, a reduced ventricular Kv4.3 protein expression has been reported along with decreased Ito density (Zicha et al., 2004). It has also been shown that Kv4.3 expression is significantly reduced in human HF and that this is associated with a significant decrease in Ito density (Kaab et al., 1998; Zicha et al., 2004). Reduced Ito density is known to contribute to AP prolongation and prolonged QT intervals (Barry et al., 1998).
Despite this important role of Kv4.3 for Ito in human cardiac tissue, many animals species show a rather heterogeneous channel population comprised of Kv4.3, Kv1.4 (KCNA4), Kv4.2 (KCND2), and accessory KChIP subunits. In these species, Ito can be separated into the fast and slow component with varying relative contributions to total Ito. In rabbit and mouse cardiac myocytes, for instance, Kv1.4 has been shown to be responsible for the slow component while a complex of Kv4.2, Kv4.3, and KChIPs is responsible for the fast component (Niwa and Nerbonne, 2010). Similar to the dog model of HF, tachycardia-induced HF in rabbits showed reduced total Ito density while AP duration was prolonged (Rose, 2005). Interestingly, mRNA levels of Kv1.4 and Kv4.2, as well as KChIP2, were significantly reduced, while Kv4.3 mRNA was unchanged. Protein expression of Kv4.2 and KChIP2 was significantly reduced, though Kv4.3 and Kv1.4 were unchanged (Rose, 2005). In a TNF-α-overexpressing mouse model of HF, Ito,fast density and Kv4.2 protein expression was significantly reduced (Petkova-Kirova, 2006). Other mouse models of HF exhibited similar reductions in Ito density and increased AP duration (Knollmann et al., 2000; Mitarai, 2000).
The differential regulation in the expression and function of the various channel isoforms underlying Ito suggests that the two components Ito,fast and Ito,slow are functionally and structurally independent ion currents.
CaMKII-Dependent Regulation of Ito Expression
CaMKII has been shown to influence the expression of channel isoforms underlying Ito. In mice overexpressing CaMKIIδ, it was shown that total Ito density is significantly reduced (Wagner et al., 2009). This reduction was secondary to a reduced expression of Kv4.2 with reduced Ito,fast and accompanied by a prolongation of the cardiac AP (Wagner et al., 2009). In contrast, expression of Kv1.4 and Ito,slow were increased but this increase could not fully compensate the reduction in Ito,fast (Wagner et al., 2009). Interestingly, chronic CaMKII inhibition in mice by transgenic expression of the specific CaMKII inhibitory peptide AC3-I, a derivative of CaMKII substrate autocamtide-3, resulted in an increase in Ito,fast and shorter AP duration. On the other hand, the increase in Ito density was absent in crossbred mice expressing AC3-I but lacking phospholamban (PLN; Li et al., 2006). Since mice overexpressing CaMKIIδ also develop HF and chronic CaMKII inhibition may also affect the SR, it is not clear whether these changes are CaMKII-specific or secondary to remodeling or interference with other pathways. This is supported by the fact that short-term overexpression of CaMKIIδ in rabbit myocytes increases Ito (Wagner et al., 2009). Similary, in silico experiments with simulated CaMKII overexpression in rabbit myocytes also led to an increase in Ito along with faster Ito, slow recovery from inactivation (Grandi et al., 2007). Furthermore, Li et al. (2006) found no change in the expression of pore-forming subunits Kv4.2/Kv4.3 underlying increased Ito but only a downregulation of the accessory subunit KChIP2, suggesting that the regulation of Ito is complex, involving many interacting partners. In this respect it is not surprising that Kv4.3 and Kv4.2 form large macromolecular complexes with other proteins such as diaminopeptidyl transferase-like protein 6 (DPP6) and Eps15 homology domain-containing protein 4 (EHD4) (Marionneau et al., 2011). Recent evidence suggests that these proteins are important for endocytosis, vesicular recycling and trafficking (Cai et al., 2013). Perhaps more importantly, it has been shown that KChIP1 clamps two adjacent Kv4.3 α-subunits together via two contact interfaces that interact with the N-termini of Kv4.3 (Pioletti et al., 2006; Wang et al., 2006b). This stabilizes KV4.3 tetramers and also exerts an influence on current kinetics with current density being increased, inactivation slowed, and recovery from inactivation enhanced (Wang et al., 2006b). In addition, KChIP1 has been shown to be essential for proper Kv4 trafficking to the membrane (Cui et al., 2008).
More evidence that the downregulation of Kv4 in HF after CaMKII overexpression may be secondary and not directly mediated by CaMKII is derived from experiments investigating the interaction of the MAGUK (membrane-associated guanylate kinase) protein SAP97 with Kv4.
In neurons, the interaction of the C-terminal Ser-Ala-Leu (SAL)-sequence of Kv4.2 with SAP97 has been shown to be crucial for trafficking of Kv4.2 to the synaptic membrane (Gardoni et al., 2007). Interestingly, this trafficking has been shown to be enhanced by CaMKII phosphorylation of SAP97 at Serin-39 (Gardoni et al., 2007). Furthermore, it was shown in cardiac myocytes that Kv4.2/Kv4.3 channels form complexes with SAP97 and CaMKII (El-Haou et al., 2009). In the same publication, suppression of SAP97 in rat atrial myocytes via shRNA led to a decrease in Ito, whereas SAP97 overexpression resulted in enhanced Ito. Moreover, expression of Kv4.3 lacking the C-terminal SAL-sequence or SAP97 silencing via shRNA abolished the co-precipitation with CaMKII (El-Haou et al., 2009). Also, inhibition of CaMKII with autocamtide-2 related inhibitory peptide (AIP) resulted in reduced Ito and the inhibition was more pronounced after SAP97 overexpression (El-Haou et al., 2009). Interestingly, recent evidence suggests that SAP97 is downregulated in patients with dilated cardiomyopathy (Szuts et al., 2013).
CaMKII-Dependent Regulation of Ito Gating
The first evidence for a CaMKII-dependent regulation of cardiac potassium channel gating came from a study investigating human atrial myocytes (Tessier et al., 1999). The myocytes were isolated from donors with either chronic atrial dilation or chronic atrial fibrillation (AF). Patch–clamp experiments showed that inactivation of Ito was accelerated by CaMKII inhibition with either KN-93 or AIP (Tessier et al., 1999). Moreover, Tessier et al. (1999) also showed an increased expression level of CaMKII in the atrium of donors with chronic atrial dilation or chronic AF. More evidence for CaMKII regulating Ito came from experiments using transgenic mice overexpressing CaMKIIδc and also short-term CaMKIIδc overexpression in rabbit myocytes. It was shown that the recovery from inactivation of Ito,fast and Ito,slow was enhanced by CaMKII overexpression (Wagner et al., 2009). This enhancement could be blocked by acute CaMKII inhibition suggesting that this was not secondary to HF development (Wagner et al., 2009). The underlying mechanism of CaMKII-dependent regulation of Ito may involve direct CaMKII-dependent phosphorylation of Kv4.2 at serine 438/459, and of Kv1.4 at serine 123 (Roeper, 1997; Varga, 2004). Patch-clamp experiments in HEK-293 cells transfected with Kv4.3 showed that addition of autothiophosphorylated (pre-activated) CaMKII slowed Ito inactivation consistent with the results obtained by Tessier et al. (1999) and enhanced recovery from inactivation (Sergeant, 2005). Conversely, HEK cells treated with CaMKII-inhibitor KN-93 or CaMKII-inhibitory-peptide displayed significantly accelerated current inactivation and slowed recovery from inactivation (Sergeant, 2005). Moreover, if the C-terminal Kv4.3 mutant (serine 550 to alanine) was expressed, Ito inactivation was enhanced and Ito recovery was slowed (Sergeant, 2005). Neither addition of autothiophosphorylated CaMKII nor dialysis of CaMKII inhibitors could affect Ito recovery in HEK cells expressing this Kv4.3 S550A mutant (Sergeant, 2005), supporting the concept that the C-terminus of Kv4.3 is a hotspot for CaMKII-dependent association and regulation of Ito,fast (Figure 2C). Further evidence for a direct regulation of Ito,fast by CaMKII comes from studies in rat ventricular myocyte lysates showing that CaMKII co-immunoprecipitates with both Kv4.3 and Kv4.2 (Colinas, 2006), and inhibition of CaMKII with KN-93 resulted in a significant acceleration of Ito inactivation even through recovery from inactivation was unaffected (Colinas, 2006).
Interestingly, this CaMKII-dependent enhancement of Ito may also be important for reactive oxygen-species (ROS) induced arrhythmogenesis. ROS are known to oxidize and activate CaMKII (Erickson et al., 2008; Wagner et al., 2011) and ROS-induced arrhythmias are known to be CaMKII-dependent (Wagner et al., 2011). Recently, it was proposed that ROS-dependent activation of Ito favors EADs by facilitating ICa reactivation (Zhao et al., 2012).
Thus, CaMKIIδc appears to regulate both channel expression and/or trafficking, but also acutely regulates channel gating properties. In both cases, acute regulation results in an enhancement of Ito. In contrast to this, chronic CaMKII overexpression that leads to HF development results in a reduction of Ito but this appears to be a secondary effect.
Kv4.3 as an Important Regulator of CaMKII Activity
While Kv4.3 is an important target for CaMKII, it may also influence CaMKII localization and activity. Recently, in HEK-293 cells transfected with Kv4.3 and His-tagged CaMKII, it was shown that Kv4.3 binds to CaM-dissociated CaMKII competitively at its CaM binding site (residues 301 and 307; Keskanokwong et al., 2010). This binding was independent from the auto-phosphorylation status of CaMKII, since both constitutively active (T-287D) or inactive (T-287A) CaMKII-mutants could also bind to Kv4.3 (Keskanokwong et al., 2010).
Since the CaMKII inhibitor KN93 also binds CaMKII at the CaM binding site (Sumi, 1991), it is conceivable that KN-93 disturbs the interaction of CaMKII and Kv4.3. Consistent with this idea, Keskanokwong et al. showed that co-purification of Kv4.3 and CaMKII is abolished upon addition of KN-93. Furthermore, it was shown that application of the Kv4.3 blocker 4-aminopyridine (4-AP) disturbes the co-purification of CaMKII and Kv4.3 in HEK-293 cells, while CaMKII auto-phosphorylation is increased (Keskanokwong et al., 2010). Similarly, increased CaMKII activity was found in guinea pig ventricular myocytes treated with 4-AP (Wang et al., 2006a). Moreover, 4-AP-induced blockade of Kv4.3 in HEK-293 cells has been shown to result in increased apoptosis and enhanced CaMKII-auto-phosphorylation, while the authors were able to prevent apoptosis by inhibition of CaMKII with KN-93 (Zhang et al., 2012).
This suggests that Kv4.3 may function as a reservoir for inactive CaMKII-units and exert an influence on CaMKII activation levels (Figure 2C). In accordance with this hypothesis, in vivo overexpression of Kv4.3 in mouse ventricular myocardium via multiple-site virus injection decreased the level of phosphorylated CaMKII, while CaMKII expression was not affected. CaMKII bound to Kv4.3 was also shown to be protected from activation by systolic Ca transients (Keskanokwong et al., 2010). The Kv4.3-CaMKII interaction may also be important for the regulation of other CaMKII target proteins. For instance, it was shown that blockade of Kv4.3 with 4-AP results in increased ICa that could be blocked by buffering cytosolic Ca with BAPTA or application of AIP (Wang et al., 2006a).
As previously mentioned, Kv4.3 is downregulated in HF (Kaab et al., 1998) while CaMKII is upregulated (Hoch et al., 1999). The CaMKII-Kv4.3 interaction may thus be severely altered in HF, contributing to higher CaMKII activity. In this context, the previously mentioned role for SAP97 in the regulation of Kv4.3 expression and Kv4.3-CaMKII interaction may be important. Downregulation of SAP97 in HF (Szuts et al., 2013) may underlie reduced Kv4.3 and may contribute to increased CaMKII activity.
Inwardly Rectifying Current IK1
In contrast to the voltage-gated K channels, inwardly rectifying potassium channels [Kir2.1 (KCNJ2), Kir2.2 (KCNJ12), Kir2.3 (KCNJ4), and Kir2.4 (KCNJ14)] are formed by four α-subunits containing only two transmembrane segments (M1–M2) with a central P-loop but without a voltage-sensor (Figure 2D; Hibino et al., 2010). The main characteristic of this class of potassium channels is inward rectification, which features a strong potassium conductance during hyperpolarization, but a decrease in ion conductance upon depolarization due to blockade of the pore by Mg, Ca and cell membrane polyamines (Matsuda et al., 1987; Matsuda and Cruz, 1993; Hibino et al., 2010).
This peculiar inward rectifying property of the Kir2.x channels that generate IK1 renders these channels important stabilizers of the resting membrane potential by neutralizing resting influx of positive ions (Fauconnier et al., 2005). In addition, Kir2.x channels also contribute to late-phase (phase 4) repolarization (Dhamoon, 2004; Dhamoon and Jalife, 2005; Fauconnier et al., 2005).
In sinoatrial myocytes, the expression of channels forming IK1 is notably reduced, which allows for an unstable resting membrane potential that can be depolarized by If, thus inducing diastolic depolarization (Bers, 2002b).
There is evidence that Kir2.x isoforms can assemble as homo- or heterotetrameres (Zobel et al., 2003). The functional characteristics of IK1 depend very much on the Kir isoforms that comprise IK1 (Panama et al., 2010), since rectification of current at depolarized membrane potentials ( > -30 mV) is complete for Kir2.1 and Kir2.2, but incomplete for Kir2.3 (Dhamoon, 2004). There is great variability in the expression of these isoforms between left and right ventricle (Warren et al., 2003) but also atrium and ventricle (Gaborit et al., 2007). Similar to channel subunits generating Ito, expression of the Kir isoforms appears to be strongly species-dependent (Jost et al., 2013).
Ca or CaMKII-Dependent Regulation of IK1
IK1 functional expression also seems to be regulated differently under pathophysiological conditions. It was shown that IK1 density is reduced in failing rat ventricular myocytes (Fauconnier et al., 2005). Interestingly, this reduction was attenuated in the presence of high EGTA (10 mmol/L) and abolished if intracellular Ca was buffered with BAPTA (20 mM; Fauconnier et al., 2005). Moreover, activation of RyR by application of ryanodine or FK506 led to a similar reduction of IK1 density in non-failing wild-type rat ventricular cells and this effect could be blocked by Ca-buffering with BAPTA (Fauconnier et al., 2005). Whether this reduction occurs via direct Ca-dependent blockade of IK1 via the mechanism described (Matsuda and Cruz, 1993) or mechanisms involving altered expression/trafficking of the underlying Kir isoforms is, however, completely unknown. Fauconnier et al. (2005) also suggested the involvement of protein kinase C (PKC), since the PKC inhibitor staurosporine antagonized the effect of ryanodine on IK1. PKC has been shown to phosphorylate Kir2.1 at serine 64 and threonine 353, leading to reduced IK1 in human atrial myocytes (Karle, 2002). On the other hand, opposite results have recently been shown in canine ventricular myocytes. Addition of 900 nmol/L Ca in the patch pipette significantly increased IK1 current compared to measurements with 160 nM Ca (Nagy et al., 2013). Therefore, the effect of Ca on IK1 may be species-dependent. Supporting evidence comes from intact field-stimulated (1 Hz) canine right ventricular papillary muscle. Increasing extracellular Ca from 2 to 4 mmol/L, resulted in increased Ca transient amplitude and significantly shortened AP duration. This Ca-dependent AP shortening could be prevented by inhibition of IK1 using BaCl2. Moreover, BaCl2 preferentially prolonged AP duration at 4 mM [Ca]o vs. 2 mM [Ca]o. The authors conclude that Ca-dependent enhancement of IK1, at least in canine myocytes, may be an important contributor to repolarization reserve and an endogenous negative feedback mechanism inhibiting the generation of DADs due to high Ca levels (Nagy et al., 2013). Moreover, Nagy et al. (2013) also showed that CaMKII inhibition with KN-93 abolished the Ca-induced activation of IK1, suggesting that CaMKII is also involved in IK1 regulation. Supporting evidence for a CaMKII-dependent activation of IK1 also comes from rabbit ventricular myocytes. Acute overexpression of CaMKIIδ by adenovirus-mediated gene transfer resulted in a significant increase in IK1 density that could be blocked by addition of CaMKII-inhibitory peptide AIP to the pipette (Wagner et al., 2009). In the same, study, transgenic CaMKIIδ overexpression in mice that develop HF, however, resulted in a reduced IK1 density and reduced expression of Kir2.1 (Wagner et al., 2009). Thus, the discrepancy between the studies showing either increased or decreased IK1 may be due to species-differences but this remains speculative. In accordance, mouse ventricular myocytes with transgenic inhibition of CaMKII showed an increased IK1 and a shorter AP duration without a significant change in Kir2.1 and Kir2.2 expression levels (Li et al., 2006).
Aside from the species, it could also be relevant if the studied model results in HF. It was shown that SAP97 co-immunoprecipitates with Kir2.2 in rat hearts (Leonoudakis et al., 2004). Interestingly, in human dilated cardiomyopathy, the co-localization of SAP97 with Kir2.x was shown to be disturbed (Szuts et al., 2013). This suggests that a mechanism similar to the above mentioned Kv4.x-SAP97 interaction may be present. Therefore, further studies are greatly needed to clarify the importance of Ca and CaMKII for the regulation of IK1 in different animal models and in human disease (Table 1).
IK1 and Arrhythmias
IK1 is generally regarded as anti-arrhythmic by stabilizing resting membrane potential. In a canine model of tachycardia-induced HF, reduced IK1 has been shown to increase the propensity for sudden cardiac death and ventricular tachycardia (Kääb et al., 1996). Also, loss of function mutations in KCNJ2 have been associated with long QT syndrome (LQT7), in which increased AP duration and increased propensity for arrhythmias can be observed (Tsuboi and Antzelevitch, 2006).
On the other hand, contrasting results have been shown for wild-type Kir2.1 overexpressing mice that have an increased propensity for ventricular arrhythmias (Noujaim et al., 2006; Piao et al., 2007) or AF (Li, 2004). Kir2.1 knock-down in mice was associated with longer AP duration and a reduced incidence of premature ventricular contractions before and after AV node ablation, reduced arrhythmias due to extracellular hypokalemia, and a reduced incidence of halothane-induced ventricular tachycardia (Piao et al., 2007).
This discrepancy may be solved by the fact that both increase or decrease of IK1 can be pro-arrhythmic if there is a substantial spatial heterogeneity in the functional expression profile (Sekar et al., 2009). In accordance with this, gain-of-function mutations in KCNJ2 can result in short QT syndrome (SQT3), which is also pro-arrhythmogenic (Brenyo et al., 2012).
Delayed Rectifying K Channels
The three channels Kv1.5 (KCNA5), hERG (KCNH2), and Kv7.1 (KCNQ1) comprise the group of the delayed rectifying K channels. They generate IKur (ultra rapid), IKr (rapid), and IKs (slow), respectively. Together, they are important currents for phase 3 repolarization.
IKur is only present in atrial myocardium. In chronic human AF, it was shown that AP duration is reduced, possibly contributing to the arrhythmogenic mechanisms (Wettwer, 2004). Evidence for a role of Kv1.5 in AF came from a study investigating pharmacological Kv1.5 inhibition in a canine model of AF (Regan et al., 2007). They could show that AF terminates if Kv1.5 is inhibited. Furthermore, SAP97 was reported to co-immunoprecipitate with Kv1.5 (Murata et al., 2001) resulting in increased IKur (Godreau et al., 2002; Eldstrom et al., 2003).
Since SAP97 and CaMKII have been shown to interact (El-Haou et al., 2009), CaMKII expression is increased in AF (Tessier et al., 1999; Neef et al., 2010), and given the similarities between SAP97-dependent Kv4.3 and Kv1.5 regulation (Tessier et al., 1999; Godreau et al., 2002; El-Haou et al., 2009), it seems tempting to speculate that CaMKII could also regulate Kv1.5. Interestingly, in human atrial myocytes it was shown that CaMKIIδ is especially localized at intercalated disks, the region where Kv1.5 is also located (Tessier et al., 1999). Furthermore, Tessier et al. (1999) showed that selective inhibition of CaMKII with KN-93 or AIP reduced the amplitude of the sustained component of outward K current (Isus), whereas inhibition of phosphatases with okadaic acid increased Isus (Tessier et al., 1999; Tessier, 2001). This Isus is regarded as mainly generated by Kv1.5 (Fedida et al., 1993), suggesting that CaMKII, possibly by phosphorylation, regulates Kv1.5 (Tessier et al., 1999).
Besides IKur, other K currents may also be involved in AF. IK1, for instance, has been shown to be upregulated in AF possibly contributing to shortening of AP duration (Dobrev and Ravens, 2003).
IKs is comprised of the pore-forming α-subunit Kv7.1, but also the auxiliary β-subunit KCNE1 (Ruscic et al., 2013). Loss-of-function mutations in KCNQ1 are linked to an increase in AP duration associated with long QT-syndrome type I, whereas gain-of-function mutations in KCNQ1 are associated with short QT-syndrome (SQT2) (Brenyo et al., 2012) and familial AF (Chen et al., 2003). Additionally, loss-of-function mutations in KCNE1 have been associated with long QT-syndrome 5 (Splawski et al., 1997), which points out the important role of KCNE1 for the generation of IKs. Indeed it has been shown that KCNE1 is important in slowing down the movement of the voltage-sensor S4 of Kv7.1 upon depolarization, thus explaining the slow activation kinetics of IKs (Ruscic et al., 2013). Similar mechanisms may very well regulate other voltage-gated channels and underlie their distinct activation kinetics.
Interestingly, co-immunoprecipitation experiments in yeast cells expressing wild-type Kv7.1 or mutated Kv7.1 with truncated α-helices showed that calmodulin can bind to the C-terminus of Kv7.1 (Shamgar, 2006). This IQ-motif appears to be a hot spot for mutations: yeast 2-hybrid experiments indicated that KCNQ1 mutations A371T and S373P, which are associated with LQTS, lose their calmodulin-Kv7.1 interaction.
Moreover, agarose-pulldown assays in HEK-293 cells revealed that LQTS-associated Kv7.1 mutants W392R, S373P, and A371T bound significantly less calmodulin than wild-type Kv7.1 (Shamgar, 2006). This disturbed calmodulin- Kv7.1 interaction may be important for channel expression. Cell surface expression experiments with biotinylated channel proteins showed that mutants with impaired CaM-binding are significantly less expressed than wild-type Kv7.1 (Shamgar, 2006). Interestingly, overexpression of calmodulin in HEK-293 cells either expressing wild-type Kv7.1 or mutant S373P showed significant increases in Kv7.1 (5x) as well as S373P (100x) cell surface and protein expression, which highlights the important role of calmodulin for IKs assembly and cell surface expression (Shamgar, 2006).
In addition, the CaM- Kv7.1 interaction may also be relevant for the regulation of IKs gating. Patch-clamp experiments of inside-out membrane from Xenopus oocytes showed that application of calmodulin antagonist W7 significantly reduced current density of Kv7.1/KCNE1, while an increase in Ca significantly shifted voltage-dependence of channel activation toward more hyperpolarized membrane potentials (Shamgar, 2006).
Thus, the interaction of calmodulin and Kv7.1 appears to be critical for expression and function of IKs, with the intriguing possibility that regulatory mechanisms could also involve some form of CaMKII interaction with calmodulin and Kv7.1 or KCNE1.
ATP-Sensitive Potassium Current KATP
The ATP-sensitive potassium current KATP, comprising of Kir6.1 (KCNJ8) and Kir6.2 (KCNJ11) α-subunits, plays an important role in ischemic preconditioning (Li et al., 2007). KATP can be a substrate for CaMKII: in mice expressing CaMKII-inhibitory peptide AC3-I, an increased KATP current density has been shown along with an increase in the sarcolemmal Kir6.2 membrane surface expression (Li et al., 2007). Also, recent evidence from pancreatic β-cells suggests that Kir6.2 can be phosphorylated by CaMKII at threonine 224 (Kline et al., 2013). Co-expression of CaMKII and Kir6.2 in COS-cells resulted in a decreased KATP current.
The significance of KATP in HF and arrhythmogenesis is still largely unknown. There is evidence suggesting that KATP-channel opening with cromakalim produces more stable ventricular arrhythmias (Quintanilla et al., 2013). In addition, Langendorff-perfused canine failing hearts with induced VF showed an increased rate of spontaneous VF termination, if KATP was blocked with glibenclamide (Taylor et al., 2012). Also, recently, a mutation in cardiac Kir6.1 that is associated with gain of function has been identified in a patient with early repolarization syndrome (see above; Medeiros-Domingo et al., 2010).
On the other hand, KATP-blockade with glibenclamide in non-failing canine hearts with induced VF delayed the termination of VF (Taylor et al., 2012). Thus, the role of cardiac KATP and its regulation by CaMKII has yet to be evaluated.
Summary
While there is increasing evidence for an involvement of CaMKII in the regulation of K channels, many discrepancies are not yet understood. These discrepancies result from the great variability in the expression profile of K channels in different species and disease models. The greatest evidence so far exists for CaMKII-dependent regulation of Kv4.x expression, trafficking and function. Most intriguingly, the Kv4.x macromolecular complex appears to serve as a hotspot and reservoir for CaMKII, which may have profound impact on the regulation of various other CaMKII targets like Ca channels. CaMKII expression and activity has been shown to be increased in many animal models of HF, but also in human HF. Increased CaMKII activity has been shown to induce contractile dysfunction and arrhythmias. Therefore, a more detailed understanding of the mechanisms of K channel regulation by CaMKII is warranted.
Conflict of Interest Statement
The authors declare that the research was conducted in the absence of any commercial or financial relationships that could be construed as a potential conflict of interest.
Acknowledgments
Stefan Wagner and Lars S. Maier are funded by Deutsche Forschungsgemeinschaft (DFG) through an International Research Training Group GRK 1816. Lars S. Maier is funded by DFG grant MA 1982/4-2 and TPA03 SFB 1002. Lars S. Maier is also funded by the Fondation Leducq Transatlantic Network on “Redox and Nitrosative Regulation of Cardiac Remodeling.” Lars S. Maier, and Stefan Wagner, are funded by the DZHK (Deutsches Zentrum für Herz-Kreislauf-Forschung – German Centre for Cardiovascular Research). We acknowledge support by the German Research Foundation and the Open Access Publication Funds of the Göttingen University.
References
Ai, X., Curran, J. W., Shannon, T. R., Bers, D. M., and Pogwizd, S. M. (2005). Ca2+/calmodulin–dependent protein kinase modulates cardiac ryanodine receptor phosphorylation and sarcoplasmic reticulum Ca2+ leak in heart failure. Circ. Res. 97, 1314–1322. doi: 10.1161/01.RES.0000194329.41863.89
Antzelevitch, C., and Fish, J. (2001). Electrical heterogeneity within the ventricular wall. Basic Res. Cardiol. 96, 517–527. doi: 10.1007/s003950170002
Backs, J., Backs, T., Neef, S., Kreusser, M. M., Lehmann, L. H., Patrick, D. M., et al. (2009). The δ isoform of CaM kinase II is required for pathological cardiac hypertrophy and remodeling after pressure overload. Proc. Natl. Acad. Sci. U.S.A. 106, 2342–2347. doi: 10.1073/pnas.0813013106
Barry, D. M., Xu, H., Schuessler, R. B., and Nerbonne, J. M. (1998). Functional knockout of the transient outward current, long-QT syndrome, and cardiac remodeling in mice expressing a dominant-negative Kv4 subunit. Circ. Res. 83, 560–567. doi: 10.1161/01.RES.83.5.560
Bers, D. M. (2002a). Cardiac excitation-contraction coupling. Nature 415, 198–205. doi: 10.1038/415198a
Bers, D. M. (2002b). Excitation-Contraction Coupling and Cardiac Contractile Force, ed. D. M. Bers (Boston, MA: Kluwer Academic Publishers), 39–62.
Beuckelmann, D. J., Nabauer, M., and Erdmann, E. (1993). Alterations of K+ currents in isolated human ventricular myocytes from patients with terminal heart failure. Circ. Res. 73, 379–385. doi: 10.1161/01.RES.73.2.379
Brahmajothi, M. V., Campbell, D. L., Rasmusson, R. L., Morales, M. J., Trimmer, J. S., Nerbonne, J. M., et al. (1999). Distinct transient outward potassium current (Ito) phenotypes and distribution of fast-inactivating potassium channel alpha subunits in ferret left ventricular myocytes. J. Gen. Physiol. 113, 581–600. doi: 10.1085/jgp.113.4.581
Brenyo, A. J., Huang, D. T., and Aktas, M. K. (2012). Congenital long and short QT syndromes. Cardiology 122, 237–247. doi: 10.1159/000339537
Cai, B., Giridharan, S. S. P., Zhang, J., Saxena, S., Bahl, K., Schmidt, J. A., et al. (2013). Differential roles of C-terminal Eps15 homology domain proteins as vesiculators and tubulators of recycling endosomes. J. Biol. Chem. 288, 30172–30180. doi: 10.1074/jbc.M113.488627
Chen, Y.-H., Xu, S.-J., Bendahhou, S., Wang, X.-L., Wang, Y., Xu, W.-Y., et al. (2003). KCNQ1 gain-of-function mutation in familial atrial fibrillation. Science 299, 251–254. doi: 10.1126/science.1077771
Cho, H.-S., Takano, M., and Noma, A. (2003). The electrophysiological properties of spontaneously beating pacemaker cells isolated from mouse sinoatrial node. J. Physiol. 550, 169–180. doi: 10.1113/jphysiol.2003.040501
Colinas, O. (2006). Differential modulation of Kv4.2 and Kv4.3 channels by calmodulin-dependent protein kinase II in rat cardiac myocytes. Am. J. Physiol. Heart Circ. Physiol. 291, H1978–H1987. doi: 10.1152/ajpheart.01373.2005
Cui, Y. Y., Liang, P., and Wang, K. W. (2008). Enhanced trafficking of tetrameric Kv4.3 channels by KChIP1 clamping. Neurochem. Res. 33, 2078–2084. doi: 10.1007/s11064-008-9688-7
Dhamoon, A. S. (2004). Unique Kir2.x properties determine regional and species differences in the cardiac inward rectifier K+ current. Circ. Res. 94, 1332–1339. doi: 10.1161/01.RES.0000128408.66946.67
Dhamoon, A. S., and Jalife, J. (2005). The inward rectifier current (IK1) controls cardiac excitability and is involved in arrhythmogenesis. Heart Rhythm 2, 316–324. doi: 10.1016/j.hrthm.2004.11.012
Dixon, J. E., Shi, W., Wang, H.-S., McDonald, C., Yu, H., Wymore, R. S., et al. (1996). Role of the Kv4.3 K+ channel in ventricular muscle: a molecular correlate for the transient outward current. Circ. Res. 79, 659–668. doi: 10.1161/01.RES.79.4.659
Dobrev, D., and Ravens, U. (2003). Remodeling of cardiomyocyte ion channels in human atrial fibrillation. Basic Res. Cardiol. 98, 137–148. doi: 10.1007/s00395-003-0409-8
Eldstrom, J., Choi, W. S., Steele, D. F., and Fedida, D. (2003). SAP97 increases Kv1.5 currents through an indirect N-terminal mechanism. FEBS Lett. 547, 205–211. doi: 10.1016/S0014-5793(03)00668-9
El-Haou, S., Balse, E., Neyroud, N., Dilanian, G., Gavillet, B., Abriel, H., et al. (2009). Kv4 potassium channels form a tripartite complex with the anchoring protein SAP97 and CaMKII in cardiac myocytes. Circ. Res. 104, 758–769. doi: 10.1161/CIRCRESAHA.108.191007
Erickson, J. R., Joiner, M. A., Guan, X., Kutschke, W., Yang, J., Oddis, C. V., et al. (2008). A dynamic pathway for calcium-independent activation of CaMKII by methionine oxidation. Cell 133, 462–474. doi: 10.1016/j.cell.2008.02.048
Erickson, J. R., Pereira, L., Wang, L., Han, G., Ferguson, A., Dao, K., et al. (2013). Diabetic hyperglycemia activates CaMKII and arrhythmias by O-linked glycosylation. Nature 502, 372–376. doi: 10.1038/nature12537
Fauconnier, J., Lacampagne, A., Rauzier, J., Vassort, G., and Richard, S. (2005). Ca-dependent reduction of I in rat ventricular cells: a novel paradigm for arrhythmia in heart failure? Cardiovasc. Res. 68, 204–212. doi: 10.1016/j.cardiores.2005.05.024
Fedida, D., Wible, B., Wang, Z., Fermini, B., Faust, F., Nattel, S., et al. (1993). Identity of a novel delayed rectifier current from human heart with a cloned K+ channel current. Circ. Res. 73, 210–216. doi: 10.1161/01.RES.73.1.210
Gaborit, N., Le Bouter, S., Szuts, V., Varro, A., Escande, D., Nattel, S., et al. (2007). Regional and tissue specific transcript signatures of ion channel genes in the non-diseased human heart. J. Physiol. 582, 675–693. doi: 10.1113/jphysiol.2006.126714
Gardoni, F., Mauceri, D., Marcello, E., Sala, C., Di Luca, M., and Jeromin, A. (2007). SAP97 directs the localization of Kv4.2 to spines in hippocampal neurons: regulation by CaMKII. J. Biol. Chem. 282, 28691–28699. doi: 10.1074/jbc.M701899200
Go, A. S., Mozaffarian, D., Roger, V. L., Benjamin, E. J., Berry, J. D., Borden, W. B., et al. (2012). Heart disease and stroke statistics–2013 update: a report from the american heart association. Circulation 127, e6–e245. doi: 10.1161/CIR.0b013e31828124ad
Godreau, D., Vranckx, R., Maguy, A., Rücker-Martin, C., Goyenvalle, C., Abdelshafy, S., et al. (2002). Expression, regulation and role of the MAGUK protein SAP-97 in human atrial myocardium. Cardiovasc. Res. 56, 433–442. doi: 10.1016/S0008-6363(02)00602-8
Grandi, E., Puglisi, J. L., Wagner, S., Maier, L. S., Severi, S., and Bers, D. M. (2007). Simulation of Ca-calmodulin-dependent protein kinase II on rabbit ventricular myocyte Ion currents and action potentials. Biophys. J. 93, 3835–3847. doi: 10.1529/biophysj.107.114868
Hasenfuss, G. (1998). Alterations of calcium-regulatory proteins in heart failure. Cardiovasc. Res. 37, 279–289. doi: 10.1016/S0008-6363(97)00277-0
Hibino, H., Inanobe, A., Furutani, K., Murakami, S., Findlay, I., and Kurachi, Y. (2010). Inwardly rectifying potassium channels: their structure, function, and physiological roles. Physiol. Rev. 90, 291–366. doi: 10.1152/physrev.00021.2009
Hoch, B., Meyer, R., Hetzer, R., Krause, E.-G., and Karczewski, P. (1999). Identification and expression of -isoforms of the multifunctional Ca2+/calmodulin-dependent protein kinase in failing and nonfailing human myocardium. Circ. Res. 84, 713–721. doi: 10.1161/01.RES.84.6.713
Hu, S. S., Kong, L. Z., Gao, R. L., Zhu, M. L., Wang, W., Wang, Y. J., et al. (2012). Outline of the report on cardiovascular disease in China, 2010. Biomed. Environ. Sci. 25, 251–256.
Jost, N., Virag, L., Comtois, P., Ordög, B., Szuts, V., Seprenyi, G., et al. (2013). Ionic mechanisms limiting cardiac repolarization reserve in humans compared to dogs. J. Physiol. 591, 4189–4206. doi: 10.1113/jphysiol.2013.261198
Kaab, S., Dixon, J., Duc, J., Ashen, D., Nabauer, M., Beuckelmann, D. J., et al. (1998). Molecular basis of transient outward potassium current downregulation in human heart failure: a decrease in Kv4.3 mRNA correlates with a reduction in current density. Circulation 98, 1383–1393. doi: 10.1161/01.CIR.98.14.1383
Kääb, S., Nuss, H. B., Chiamvimonvat, N., O’Rourke, B., Pak, P. H., Kass, D. A., et al. (1996). Ionic mechanism of action potential prolongation in ventricular myocytes from dogs with pacing-induced heart failure. Circ. Res. 78, 262–273. doi: 10.1161/01.RES.78.2.262
Karle, C. A. (2002). Human cardiac inwardly-rectifying K+ channel Kir2.1b is inhibited by direct protein kinase C-dependent regulation in human isolated cardiomyocytes and in an expression system. Circulation 106, 1493–1499. doi: 10.1161/01.CIR.0000029747.53262.5C
Keskanokwong, T., Lim, H. J., Zhang, P., Cheng, J., Xu, L., Lai, D., et al. (2010). Dynamic Kv4.3-CaMKII unit in heart: an intrinsic negative regulator for CaMKII activation. Eur. Heart J. 32, 305–315. doi: 10.1093/eurheartj/ehq469
Kirchhefer, U., Schmitz, W., Scholz, H., and Neumann, J. (1999). Activity of cAMP-dependent protein kinase and Ca2+/calmodulin-dependent protein kinase in failing and nonfailing human hearts. Cardiovasc. Res. 42, 254–261. doi: 10.1016/S0008-6363(98)00296-X
Kline, C. F., Wright, P. J., Koval, O. M., Zmuda, E. J., Johnson, B. L., Anderson, M. E., et al. (2013). IV-Spectrin and CaMKII facilitate Kir6.2 regulation in pancreatic beta cells. Proc. Natl. Acad. Sci. U.S.A. 110, 17576–17581. doi: 10.1073/pnas.1314195110
Knollmann, B. C., Knollmann-Ritschel, B. E., Weissman, N. J., Jones, L. R., and Morad, M. (2000). Remodelling of ionic currents in hypertrophied and failing hearts of transgenic mice overexpressing calsequestrin. J. Physiol. 525, 483–498. doi: 10.1111/j.1469-7793.2000.t01-1-00483.x
Leonoudakis, D., Conti, L. R., Radeke, C. M., McGuire, L. M. M., and Vandenberg, C. A. (2004). A multiprotein trafficking complex composed of SAP97, CASK, Veli, and Mint1 is associated with inward rectifier Kir2 potassium channels. J. Biol. Chem. 279, 19051–19063. doi: 10.1074/jbc.M400284200
Li, J. (2004). Transgenic upregulation of IK1 in the mouse heart leads to multiple abnormalities of cardiac excitability. Am. J. Physiol. Heart Circ. Physiol. 287, H2790–H2802. doi: 10.1152/ajpheart.00114.2004
Li, J., Marionneau, C., Koval, O., Zingman, L., Mohler, P. J., Nerbonne, J. M., et al. (2007). Calmodulin kinase II inhibition enhances ischemic preconditioning by augmenting ATP-sensitive K+ current. Channels 1, 387–394.
Li, J., Marionneau, C., Zhang, R., Shah, V., Hell, J. W., Nerbonne, J. M., et al. (2006). Calmodulin kinase II Inhibition shortens action potential duration by upregulation of K+ currents. Circ. Res. 99, 1092–1099. doi: 10.1161/01.RES.0000249369.71709.5c
Li, Y., Kranias, E. G., Mignery, G. A., and Bers, D. M. (2002). Protein kinase A phosphorylation of the ryanodine receptor does not affect calcium sparks in mouse ventricular myocytes. Circ. Res. 90, 309–316. doi: 10.1161/hh0302.105660
Liao, S.-Y., Tse, H.-F., Chan, Y.-C., Mei-Chu Yip, P., Zhang, Y., Liu, Y., et al. (2013). Overexpression of Kir2.1 channel in embryonic stem cell-derived cardiomyocytes attenuates posttransplantation proarrhythmic risk in myocardial infarction. Heart Rhythm 10, 273–282. doi: 10.1016/j.hrthm.2012.10.008
Ling, H., Zhang, T., Pereira, L., Means, C. K., Cheng, H., Gu, Y., et al. (2009). Requirement for Ca2+/calmodulin–dependent kinase II in the transition from pressure overload–induced cardiac hypertrophy to heart failure in mice. J. Clin. Invest. 119, 1230–1240. doi: 10.1172/JCI38022
Luo, M., and Anderson, M. E. (2013). Mechanisms of altered Ca2+ handling in heart failure. Circ. Res. 113, 690–708. doi: 10.1161/CIRCRESAHA.113.301651
Maier, L., and Bers, D. (2007). Role of Ca2+/calmodulin-dependent protein kinase (CaMK) in excitation–contraction coupling in the heart. Cardiovasc. Res. 73, 631–640. doi: 10.1016/j.cardiores.2006.11.005
Maier, L. S. (2003). Transgenic CaMKIIdeltaC overexpression uniquely alters cardiac myocyte Ca2+ handling: reduced SR Ca2+ load and activated SR Ca2+ release. Circ. Res. 92, 904–911. doi: 10.1161/01.RES.0000069685.20258.F1
Marionneau, C., Townsend, R. R., and Nerbonne, J. M. (2011). Proteomic analysis highlights the molecular complexities of native Kv4 channel macromolecular complexes. Semin. Cell Dev. Biol. 22, 145–152. doi: 10.1016/j.semcdb.2010.10.004
Matsuda, H., and Cruz, J. D. S. (1993). Voltage-dependent block by internal Ca2+ ions of inwardly rectifying K+ channels in guinea-pig ventricular cells. J. Physiol. 470, 295–311.
Matsuda, H., Saigusa, A., and Irisawa, H. (1987). Ohmic conductance through the inwardly rectifying K channel and blocking by internal Mg2+. Nature 325, 156–159. doi: 10.1038/325156a0
McLerie, M. (2003). Dominant-negative suppression of I(K1) in the mouse heart leads to altered cardiac excitability. J. Mol. Cell. Cardiol. 35, 367–378. doi: 10.1016/S0022-2828(03)00014-2
Medeiros-Domingo, A., Tan, B.-H., Crotti, L., Tester, D. J., Eckhardt, L., Cuoretti, A., et al. (2010). Gain-of-function mutation S422L in the KCNJ8-encoded cardiac KATP channel Kir6.1 as a pathogenic substrate for J-wave syndromes. Heart Rhythm 7, 1466–1471. doi: 10.1016/j.hrthm.2010.06.016
Miake, J., Marbán, E., and Nuss, H. B. (2003). Functional role of inward rectifier current in heart probed by Kir2.1 overexpression and dominant-negative suppression. J. Clin. Invest. 111, 1529–1536. doi: 10.1172/JCI200317959
Mitarai, S. (2000). Changes in ionic currents and beta-adrenergic receptor signaling in hypertrophied myocytes overexpressing Gaq. Am. J. Physiol. Heart Circ. Physiol. H139–H148. doi: 10.1016/S0008-6363(02)00403-0
Murata, M., Buckett, P. D., Zhou, J., Brunner, M., Folco, E., and Koren, G. (2001). SAP97 interacts with Kv1. 5 in heterologous expression systems. Am. J. Physiol. Heart Circ. Physiol. 281, H2575–H2584.
Nagy, N., Acsai, K., Kormos, A., Sebõk, Z., Farkas, A. S., Jost, N., et al. (2013). [Ca2+]i-induced augmentation of the inward rectifier potassium current (IK1) in canine and human ventricular myocardium. Pflügers Arch. Eur. J. Physiol. Available at: http://link.springer.com/10.1007/s00424-013-1309-x (accessed August 29, 2013).
Neef, S., Dybkova, N., Sossalla, S., Ort, K. R., Fluschnik, N., Neumann, K., et al. (2010). CaMKII-dependent diastolic SR Ca2+ leak and elevated diastolic Ca2+ levels in right atrial myocardium of patients with atrial fibrillation. Circ. Res. 106, 1134–1144. doi: 10.1161/CIRCRESAHA.109.203836
Nerbonne, J. M. (2011). Repolarizing cardiac potassium channels: multiple sites and mechanisms for CaMKII-mediated regulation. Heart Rhythm 8, 938–941. doi: 10.1016/j.hrthm.2011.01.018
Neumann, T., Biermann, J., Neumann, A., Wasem, J., Ertl, G., Dietz, R., et al. (2009). Herzinsuffizienz: Häufigster Grund für Krankenhausaufenthalte. Dtsch. Ärztebl. 106, 269–275.
Nichols, M., Townsend, N., Scarborough, P., and Rayner, M. (2013). Cardiovascular disease in Europe: epidemiological update. Eur. Heart J. 34, 3028–3034. doi: 10.1093/eurheartj/eht356
Niwa, N., and Nerbonne, J. M. (2010). Molecular determinants of cardiac transient outward potassium current (Ito) expression and regulation. J. Mol. Cell. Cardiol. 48, 12–25. doi: 10.1016/j.yjmcc.2009.07.013
Noujaim, S. F., Pandit, S. V., Berenfeld, O., Vikstrom, K., Cerrone, M., Mironov, S., et al. (2006). Up-regulation of the inward rectifier K+ current (IK1) in the mouse heart accelerates and stabilizes rotors. J. Physiol. 578, 315–326. doi: 10.1113/jphysiol.2006.121475
Pak, P. H., Nuss, H. B., Tunin, R. S., Kääb, S., Tomaselli, G. F., Marban, E., et al. (1997). Repolarization abnormalities, arrhythmia and sudden death in canine tachycardia-induced cardiomyopathy. J. Am. Coll. Cardiol. 30, 576–584. doi: 10.1016/S0735-1097(97)00193-9
Panama, B. K., McLerie, M., and Lopatin, A. N. (2010). Functional consequences of Kir2.1/Kir2.2 subunit heteromerization. Pflügers Arch. Eur. J. Physiol. 460, 839–849. doi: 10.1007/s00424-010-0864-7
Petkova-Kirova, P. S. (2006). Electrical remodeling of cardiac myocytes from mice with heart failure due to the overexpression of tumor necrosis factor-alpha. Am. J. Physiol. Heart Circ. Physiol. 290, H2098–H2107. doi: 10.1152/ajpheart.00097.2005
Petrashevskaya, N. N., Bodi, I., Rubio, M., Molkentin, J. D., and Schwartz, A. (2002). Cardiac function and electrical remodeling of the calcineurin-overexpressed transgenic mouse. Cardiovasc. Res. 54, 117–132. doi: 10.1016/S0008-6363(02)00241-9
Piao, L., Li, J., McLerie, M., and Lopatin, A. N. (2007). Transgenic upregulation of IK1 in the mouse heart is proarrhythmic. Basic Res. Cardiol. 102, 416–428. doi: 10.1007/s00395-007-0659-y
Pieske, B., Maier, L. S., Piacentino, V., Weisser, J., Hasenfuss, G., and Houser, S. (2002). Rate Dependence of [Na+]i and contractility in nonfailing and failing human myocardium. Circulation 106, 447–453. doi: 10.1161/01.CIR.0000023042.50192.F4
Pioletti, M., Findeisen, F., Hura, G. L., and Minor., D. L. (2006). Three-dimensional structure of the KChIP1–Kv4.3 T1 complex reveals a cross-shaped octamer. Nat. Struct. Mol. Biol. 13, 987–995. doi: 10.1038/nsmb1164
Quintanilla, J. G., Moreno, J., Archondo, T., Chin, A., Pérez-Castellano, N., Usandizaga, E., et al. (2013). KATP channel opening accelerates and stabilizes rotors in a swine heart model of ventricular fibrillation. Cardiovasc. Res. 99, 576–585. doi: 10.1093/cvr/cvt093
Regan, C. P., Kiss, L., Stump, G. L., McIntyre, C. J., Beshore, D. C., Liverton, N. J., et al. (2007). Atrial antifibrillatory effects of structurally distinct IKur blockers 3-[(dimethylamino)methyl]-6-methoxy-2-methyl-4-phenylisoquinolin-1(2H)-one and 2-Phenyl-1,1-dipyridin-3-yl-2-pyrrolidin-1-yl-ethanol in dogs with underlying heart failure. J. Pharmacol. Exp. Ther. 324, 322–330. doi: 10.1124/jpet.107.127654
Rellos, P., Pike, A. C. W., Niesen, F. H., Salah, E., Lee, W. H., Von Delft, F., et al. (2010). Structure of the CaMKII delta calmodulin complex reveals the molecular mechanism of CaMKII kinase activation. PLoS Biol. 8:e1000426. doi: 10.1371/journal.pbio.1000426
Roeper, J. (1997). Frequency-dependent inactivation of mammalian A-type K channel KV1.4 regulated by Ca2/calmodulin- dependent protein kinase. J. Neurosci. 3379–3391.
Rose, J. (2005). Molecular correlates of altered expression of potassium currents in failing rabbit myocardium. Am. J. Physiol. Heart Circ. Physiol. 288, H2077–H2087. doi: 10.1152/ajpheart.00526.2003
Ruscic, K. J., Miceli, F., Villalba-Galea, C. A., Dai, H., Mishina, Y., Bezanilla, F., et al. (2013). PNAS Plus: IKs channels open slowly because KCNE1 accessory subunits slow the movement of S4 voltage sensors in KCNQ1 pore-forming subunits. Proc. Natl. Acad. Sci. U.S.A. 110, E559–E566. doi: 10.1073/pnas.1222616110
Sekar, R. B., Kizana, E., Cho, H. C., Molitoris, J. M., Hesketh, G. G., Eaton, B. P., et al. (2009). IK1 heterogeneity affects genesis and stability of spiral waves in cardiac myocyte monolayers. Circ. Res. 104, 355–364. doi: 10.1161/CIRCRESAHA.108.178335
Sergeant, G. P. (2005). Regulation of Kv4.3 currents by Ca2+/calmodulin-dependent protein kinase II. Am. J. Physiol. Cell Physiol. 288, C304–C313. doi: 10.1152/ajpcell.00293.2004
Shamgar, L. (2006). Calmodulin is essential for cardiac IKS channel gating and assembly: impaired function in long-QT mutations. Circ. Res. 98, 1055–1063. doi: 10.1161/01.RES.0000218979.40770.69
Shryock, J. C., Song, Y., Rajamani, S., Antzelevitch, C., and Belardinelli, L. (2013). The arrhythmogenic consequences of increasing late INa in the cardiomyocyte. Cardiovasc. Res. Available at: http://cardiovascres.oxfordjournals.org/content/early/2013/07/01/cvr.cvt145.abstract (accessed December 12, 2013).
Snyders, D. J. (1999). Structure and function of cardiac potassium channels. Cardiovasc. Res. 42, 377–390. doi: 10.1016/S0008-6363(99)00071-1
Splawski, I., Tristani-Firouzi, M., Lehmann, M. H., Sanguinetti, M. C., and Keating, M. T. (1997). Mutations in the hminK gene cause long QT syndrome and suppress lKs function. Nat. Genet. 17, 338–340. doi: 10.1038/ng1197-338
Sumi. (1991). The newly synthesized selective Ca2+/calmodulin dependent protein kinase II inhibitor KN-93 reduces dopamine contents in PC12h cells. Biochem. Biophys. Res. Commun. 181, 968–975. doi: 10.1016/0006-291X(91)92031-E
Szuts, V., Ménesi, D., Varga-Orvos, Z., Zvara, Á., Houshmand, N., Bitay, M., et al. (2013). Altered expression of genes for Kir ion channels in dilated cardiomyopathy1. Can. J. Physiol. Pharmacol. 91, 648–656. doi: 10.1139/cjpp-2012-0413
Taylor, T. G., Venable, P. W., Shibayama, J., Warren, M., and Zaitsev, A. V. (2012). Role of KATP channel in electrical depression and asystole during long-duration ventricular fibrillation in ex vivo canine heart. Am. J. Physiol. Heart Circ. Physiol. 302, H2396–H2409. doi: 10.1152/ajpheart.00752.2011
Tessier, S. (2001). Cumulative inactivation of the outward potassium current: a likely mechanism underlying electrical memory in human atrial myocytes. J. Mol. Cell. Cardiol. 33, 755–767. doi: 10.1006/jmcc.2001.1345
Tessier, S., Karczewski, P., Krause, E.-G., Pansard, Y., Acar, C., Lang-Lazdunski, M., et al. (1999). Regulation of the transient outward K+ current by Ca2+/calmodulin-dependent protein kinases II in human atrial myocytes. Circ. Res. 85, 810–819. doi: 10.1161/01.RES.85.9.810
Tikkanen, J. T., Anttonen, O., Junttila, M. J., Aro, A. L., Kerola, T., Rissanen, H. A., et al. (2009). Long-term outcome associated with early repolarization on electrocardiography. N. Engl. J. Med. 361, 2529–2537. doi: 10.1056/NEJMoa0907589
Tsuboi, M., and Antzelevitch, C. (2006). Cellular basis for electrocardiographic and arrhythmic manifestations of Andersen-Tawil syndrome (LQT7). Heart Rhythm 3, 328–335. doi: 10.1016/j.hrthm.2005.11.026
Varga, A. W. (2004). Calcium-calmodulin-dependent kinase II modulates Kv4.2 channel expression and upregulates neuronal A-type potassium currents. J. Neurosci. 24, 3643–3654. doi: 10.1523/JNEUROSCI.0154-04.2004
Wagner, S., Dybkova, N., Rasenack, E. C., Jacobshagen, C., Fabritz, L., Kirchhof, P., et al. (2006). Ca/Calmodulin-dependent protein kinase II regulates cardiac Na+ channels. J. Clin. Invest. 116, 3127–3138. doi: 10.1172/JCI26620
Wagner, S., Hacker, E., Grandi, E., Weber, S. L., Dybkova, N., Sossalla, S., et al. (2009). Ca/calmodulin kinase II differentially modulates potassium currents. Circ. Arrhythm. Electrophysiol. 2, 285–294. doi: 10.1161/CIRCEP.108.842799
Wagner, S., Ruff, H. M., Weber, S. L., Bellmann, S., Sowa, T., Schulte, T., et al. (2011). Reactive oxygen species–activated Ca/calmodulin kinase IIδ is required for late INa augmentation leading to cellular Na and Ca overload. Circ. Res. 108, 555–565. doi: 10.1161/CIRCRESAHA.110.221911
Wang, Y., Cheng, J., Tandan, S., Jiang, M., McClosey, D. T., and Hill, J. A. (2006a). Transient-outward K+ channel inhibition facilitates L-type Ca2+ current in heart. J. Cardiovasc. Electrophysiol. 17, 298–304. doi: 10.1111/j.1540-8167.2006.00362.x
Wang, H., Yan, Y., Liu, Q., Huang, Y., Shen, Y., Chen, L., et al. (2006b). Structural basis for modulation of Kv4 K+ channels by auxiliary KChIP subunits. Nat. Neurosci. 10, 32–39. doi: 10.1038/nn1822
Warren, M., Guha, P. K., Berenfeld, O., Zaitsev, A., Anumonwo, J. M. B., Dhamoon, A. S., et al. (2003). Blockade of the inward rectifying potassium current terminates ventricular fibrillation in the guinea pig heart. J. Cardiovasc. Electrophysiol. 14, 621–631. doi: 10.1046/j.1540-8167.2003.03006.x
Weiss, J. N., Garfinkel, A., Karagueuzian, H. S., Chen, P.-S., and Qu, Z. (2010). Early afterdepolarizations and cardiac arrhythmias. Heart Rhythm 7, 1891–1899. doi: 10.1016/j.hrthm.2010.09.017
Wettwer, E. (2004). Role of IKur in controlling action potential shape and contractility in the human atrium: influence of chronic atrial fibrillation. Circulation 110, 2299–2306. doi: 10.1161/01.CIR.0000145155.60288.71
Wickenden, A. D., Lee, P., Sah, R., Huang, Q., Fishman, G. I., and Backx, P. H. (1999). Targeted expression of a dominant-negative Kv4.2 K+ channel subunit in the mouse heart. Circ. Res. 85, 1067–1076. doi: 10.1161/01.RES.85.11.1067
Xu, H., Guo, W., and Nerbonne, J. M. (1999). Four kinetically distinct depolarization-activated K+ currents in adult mouse ventricular myocytes. J. Gen. Physiol. 113, 661–678. doi: 10.1085/jgp.113.5.661
Yan, G.-X., and Antzelevitch, C. (1996). Cellular basis for the electrocardiographic J wave. Circulation 93, 372–379. doi: 10.1161/01.CIR.93.2.372
Zhang, R., Khoo, M. S. C., Wu, Y., Yang, Y., Grueter, C. E., Ni, G., et al. (2005). Calmodulin kinase II inhibition protects against structural heart disease. Nat. Med. 11, 409–417. doi: 10.1038/nm1215
Zhang, X., Szeto, C., Gao, E., Tang, M., Jin, J., Fu, Q., et al. (2012). Cardiotoxic and cardioprotective features of chronic -adrenergic signaling. Circ. Res. 112, 498–509. doi: 10.1161/CIRCRESAHA.112.273896
Zhao, Z., Xie, Y., Wen, H., Xiao, D., Allen, C., Fefelova, N., et al. (2012). Role of the transient outward potassium current in the genesis of early afterdepolarizations in cardiac cells. Cardiovasc. Res. 95, 308–316. doi: 10.1093/cvr/cvs183
Zicha, S., Xiao, L., Stafford, S., Cha, T. J., Han, W., Varro, A., et al. (2004). Transmural expression of transient outward potassium current subunits in normal and failing canine and human hearts. J. Physiol. 561, 735–748. doi: 10.1113/jphysiol.2004.075861
Zobel, C., Cho, H. C., Nguyen, T.-T., Pekhletski, R., Diaz, R. J., Wilson, G. J., et al. (2003). Molecular dissection of the inward rectifier potassium current (IK1) in rabbit cardiomyocytes: evidence for heteromeric co-assembly of Kir2.1 and Kir2.2. J. Physiol. 550, 365–372. doi: 10.1113/jphysiol.2002.036400
Keywords: CaMKII, K channel, heart failure, action potential, arrhythmias
Citation: Mustroph J, Maier LS and Wagner S (2014) CaMKII regulation of cardiac K channels. Front. Pharmacol. 5:20. doi: 10.3389/fphar.2014.00020
Received: 13 December 2013; Accepted: 31 January 2014;
Published online: 21 February 2014.
Edited by:
Eleonora Grandi, University of California at Davis, USAReviewed by:
Stephane Hatem, Pierre-and-Marie-Curie University, FranceDaniel C. Bartos, University of California at Davis, USA
Copyright © 2014 Mustroph, Maier and Wagner. This is an open-access article distributed under the terms of the Creative Commons Attribution License (CC BY). The use, distribution or reproduction in other forums is permitted, provided the original author(s) or licensor are credited and that the original publication in this journal is cited, in accordance with accepted academic practice. No use, distribution or reproduction is permitted which does not comply with these terms.
*Correspondence: Stefan Wagner, Department of Cardiology, University Medical Center Göttingen, Robert-Koch-Strasse 40, 37075 Göttingen, Germany e-mail:c3dhZ25lckBtZWQudW5pLWdvZXR0aW5nZW4uZGU=