- 1Centre for Clinical Diagnostics, Royal Brisbane and Women's Hospital, University of Queensland Centre for Clinical Research, Brisbane, QLD, Australia
- 2Department of Obstetric and Gynaecology, Faculty of Medicine, Universidad de los Andes, Santiago, Chile
Background: Vascular smooth muscle cells (VSMCs) migration is a critical process during human uterine spiral artery (SpA) remodeling and a successful pregnancy. Extravillous trophoblast cells (EVT) interact with VSMC and enhance their migration, however, the mechanisms by which EVT remodel SpA remain to be fully elucidated. We hypothesize that exosomes released from EVT promote VSMC migration.
Methods: JEG-3 and HTR-8/SVneo cell lines were used as models for EVT. Cells were cultured at 37°C and humidified under an atmosphere of 5% CO2-balanced N2 to obtain 8% O2. Cell-conditioned media were collected, and exosomes (exo-JEG-3 and exo- HTR-8/SVneo) isolated by differential and buoyant density centrifugation. The effects of exo-EVT on VSMC migration were established using a real-time, live-cell imaging system (Incucyte™). Exosomal proteins where identified by mass spectrometry and submitted to bioinformatic pathway analysis (Ingenuity software).
Results: HTR-8/SVneo cells were significantly more (~30%) invasive than JEG-3 cells. HTR-8/SVneo cells released 2.6-fold more exosomes (6.39 × 108 ± 2.5 × 108 particles/106 cells) compared to JEG-3 (2.86 × 108 ± 0.78 × 108 particles/106 cells). VSMC migration was significantly increased in the presence of exo-JEG-3 and exo-HTR-8/SVneo compared to control (−exosomes) (21.83 ± 0.49 h and 15.57 ± 0.32, respectively, vs. control 25.09 ± 0.58 h, p < 0.05). Sonication completely abolished the effect of exosomes on VSMC migration. Finally, mass spectrometry analysis identified unique exosomal proteins for each EVT cell line-derived exosomes.
Conclusion: The data obtained in this study are consistent with the hypothesis that the release, content, and bioactivity of exosomes derived from EVT-like cell lines is cell origin-dependent and differentially regulates VSMC migration. Thus, an EVT exosomal signaling pathway may contribute to SpA remodeling by promoting the migration of VSMC out of the vessel walls.
Introduction
Remodeling of the uterine spiral arteries (SpA) into low resistance, high capacity vessel begins as extravillous trophoblasts (EVT) invade the decidua during first trimester and is essential for successful pregnancy (Kam et al., 1999). When EVT “plugs” are lost during early second trimester, maternal blood flows through the modified vessels to deliver nutrients and oxygen to support fetal growth and development (Pijnenborg et al., 2006a). EVT continue to invade into the myometrium and remodel the SpA until mid-second trimester (Hamilton and Boyd, 1970; Pijnenborg et al., 1983; Blackburn et al., 2003; American Diabetes Association, 2012). The initial steps of uterine spiral artery remodeling consists of vessel dilatation, vascular smooth muscle cells (VSMC) separation, endothelial cell swelling, EVT infiltration, and fibrinoid deposition (Pijnenborg et al., 2006b). VSMC migrate or undergo apoptosis and are replaced by fibriniod material, in which EVT cells embed. Recently Bulmer et al. showed that during SpA remodeling, VSMC migrate outside of the artery, and this phenomenon is enhanced in the presence of EVT (Bulmer et al., 2012). While the mechanisms by which EVTs remodel SpA remain to be fully elucidated, available data are consistent with the hypothesis that EVT directly interact with VSMC of the uterine spiral arteries. We propose that the releases of nanoparticles (i.e., exosomes) that contain specific effector molecules (e.g., proteins and miRNAs) are released from EVT and affect the loss of VSMC.
Exosomes (30–100 nm) are nanovesicles released when late endosomes fuse with the cell membrane (Thery, 2011; Salomon et al., 2013a). Exosomes interact with target cells via multiple pathways including: by directly activating target cell membrane receptors; by modifying the extracellular milieu of the target cell; and by fusing with the cell membrane and releasing the molecular cargo into the target cell (Pegtel et al., 2010). Their molecular cargo is: cell-specific (Kobayashi et al., 2014); regulated by tissue physiology and cellular function; and fundamental to their bioactivity.
Exosomes are identified in cell-conditioned media and body fluids indicate that they can be released from different types of cells (Vlassov et al., 2012). Recently, the role of exosomes isolated from placental cells (Salomon et al., 2013a,b) and other cell types (Chen et al., 2014; Lee et al., 2014; Yoon et al., 2014) on cell migration has been established. Exosomes released from first trimester placental mesenchymal stem cells (pMSC) increase endothelial cell migration and vascular tube formation in vitro (Salomon et al., 2013a). Similarly, cytotrophoblast-derived exosomes increase EVT migration in vitro (Salomon et al., 2013b).
Consistent with the proposal that exosomal signaling regulates cell migration and invasion, proteins associated with actin cytoskeleton, growth hormone, and VEGF signaling have been identified within exosomes. The effect of EVT-derived exosomes on VSMC migration, however, remains to be established. We, therefore, hypothesize that exosomes released by EVT act paracellularly to promote VSMC migration and thus contributing to SpA remodeling. The aims of this study were: (1) to compare the exosome release and exosomal protein composition derived from EVT cell lines from different origin (JEG-3 and HTR-8/SVneo); and (2) to establish the effect of exosomes from both JEG-3 and HTR-8/SVneo cells on human VSMC migration.
Numerous human trophoblastic cell lines have been established, which basically originated from normal tissues or from pathological tissues. JEG3 is a choriocarcinoma cell line cloned from primary choriocarcinoma (Kohler and Bridson, 1971), and HTR8/SVneo is a transformed extravillous trophoblast cell line established by immortalizing primary EVT cells via transfection with simian virus 40 large T antigen (SV40) (Graham et al., 1993); both cell lines are frequently used as models of physiologically invasive extravillous trophoblast. EVT invasion into the myometrium is a critical process for remodeling the uterine spiral artery (in this stage EVT interact with VSMC), however, the invasiveness capacity between these two cells lines are different. HTR-8/SVneo have significantly higher invasion capacity than JEG-3 (Suman and Gupta, 2012). Moreover, differences between these two cells lines are not just in the invasion capacity, but also in their miRNA profiles (Morales-Prieto et al., 2012) as well as their protease (e.g., metalloproteases-9) expressions (Suman and Gupta, 2012), however, functional differences between exosome vesicles derived from JEG-3 and HTR-8/SVneo remain to be established. Previously, these cells lines have been validated and used routinely as models of EVT function (Suman and Gupta, 2012; Weber et al., 2013).
The aim of this study was to test the hypotheses that: (1) exosomes from EVT act paracellularly to promote VSMC migration; and (2) The release, protein content and bioactivity of exosomes is cell origin-dependent (i.e., EVT cell lines from choriocarcinoma and chorionic villi). The effects of exosomes isolated from the EVT-like cell lines, JEG-3 and HTR-8/SVneo cells on VSMC migration were assessed.
The data obtained are consistent with the hypothesis that the function of EVT-derived exosomes is cell origin specific and showed differences in the release, content and effects on VSMC migration. EVT may communicate with VSMC during SpA remodeling, stimulating their migration through these specific nanovesicles (i.e., exosomes).
Materials and Methods
Materials
Medium RPMI 1640, Medium 231, Smooth Muscle Growth Supplement (SMGS), glutamine, antibiotics, HEPES, and phosphate buffered saline (PBS) were obtained from Life Technologies Corporation (Mulgrave, Victoria, Australia). CD63 ELISA kits were obtained from SBI (ExoELISA™, System Biosciences, Mountain View, CA).
Cell Culture
All experimental procedures were conducted within an ISO17025 accredited (National Association of Testing Authorities, Australia) research facility. All data were recorded within a 21 Code of Federal Regulation (CFR) part 11 compliant electronic laboratory notebook (Irisnote, Redwood City, CA, USA). JEG-3 human choriocarcinoma cell line was purchased from the European Collection of Cell Cultures (Porton Down, Salisbury, UK). The HTR-8/SVneo cell line was kindly donated by Dr. Charles H. Graham (Queen's University, Ontario, Canada). HTR-8/SVneo was established by the transfection of trophoblast cells isolated from first trimester villous explants, with a gene encoding simian virus 40 large T antigen to immortalize them (Graham et al., 1993). JEG-3 and HTR-8/SVneo cells were maintained in phenol red-free RPMI 1640 medium supplemented with 10% heat-inactivated fetal bovine serum, 1% non-essential amino acids, 1 mM sodium pyruvate and 100 U/mL penicillin, and 100 mg/mL streptomycin. Cultures were maintained at 37°C and humidified under an atmosphere of 5% CO2-balanced N2 to obtain 8% O2 (pO2 ~54 mmHg) in an automated PROOX 110-scaled hypoxia chamber (BioSphericsacona, NY, USA). Cells were subcultured with dissociation media, TrypLE™ Express (Life technologies, USA) and cellular viability was determined by Trypan Blue exclusion and Countess® Automated cell counter (Life Technologies, USA).
Human Vascular Smooth Muscle cells (hVSMC) were purchased from LONZA (Lonza Group Ltd.). VSMC were cultured in 231 media (Life Technologies Corporation) supplemented with SMGS, 100 U/ml penicillin, and 100 μg/ml streptomycin, at 37°C and humidified under an atmosphere of 5% CO2-balanced N2 to obtain 8% O2 (pO2 ~54 mmHg) in an automated PROOX 110-scaled hypoxia chamber (BioSpherics™, Lacona, NY, USA).
The in vitro cell migration rates of JEG-3 and HTR-8/SVneo we established using real-time cell imaging system (IncuCyte™ live-cell ESSEN BioScience Inc., Ann Arbor, MI, USA). Using a scratch assay format, cell were imaged every 3 h to monitor cell migration as previously described (Salomon et al., 2013b).
Exosome Isolation
Exosomes were isolated from cell-free JEG-3 and HTR-8/SVneo- conditioned media as previously described (Salomon et al., 2013a; Kobayashi et al., 2014). In brief, cell-conditioned media was centrifuged at 300 × g for 15 min, 2000 × g for 30 min, and 12,000 × g for 45 min to remove whole cells and debris. The resultant supernatant were passed through a 0.22 μm filter sterilize Steritop™ (Millipore, Billerica, MA, USA) and then centrifuged at 120,000 × g for 70 min (Thermo Fisher Scientific Ins., Asheville, NC, USA, Sorvall, SureSpin™ 630/36, fixed angle rotor). The pellet was resuspended in PBS, washed and re-centrifuged (120,000 × g, 75 min). The pellet was resuspended in PBS, layered on a cushion of 30% (w/v) sucrose and centrifuged at 110,000 g for 75 min. The fraction containing exosomes [~3.5 ml, 1.127 density using OPTi digital refractometer (Bellingham+Stanley Inc., Lawrenceville, GA, USA)] was recovered using a Pulse-Free Flow Peristaltic Pump with a flow rate range of 1 ml per min (GILSON Miniplus® model 3) and Fraction Collector (GILSON FC 203B model) and diluted in PBS, and then ultracentrifuged at 110,000 × g of 70 min. Recovered exosomes were resuspended in 50 μl PBS and their protein contents were determined using the Bradford assay (Bio-Rad DC). Exosome samples (5 μl) were prepared by adding RIPA buffer (50 mM Tris, 1% Triton × 100, 0.1% SDS, 0.5% DOC, 1 mM EDTA, 150 mM NaCl, protease inhibitor) directly to exosomes suspended in PBS and sonicated at 37°C for 15 s three times to disrupt exosomes and solubilise the proteins. Bovine serum albumin (BSA) diluted in RIPA buffer and PBS mixture (1:1) were prepared as protein standards (0, 200, 400, 600, 800, 1000, 1500 μg/mL). Standards and samples (exosomes) were transferred to 96-well plates and procedures outlined by the manufacture were followed. In brief, alkaline copper tartrate solution (Bio-Rab Laboratories, Hercules, CA, USA) and dilute Folin Reagent (Bio-Rab Laboratories,) were added to the samples and incubated for 15 min. The absorbance was read at 750 nm with Paradigm Detection Platform (Beckman Coulter, USA).
Nanoparticle Tracking Analysis (NTA)
NTA measurements were performed using a NanoSight NS500 instrument (NanoSight NTA 2.3 Nanoparticle Tracking and Analysis Release Version Build 0033) following the manufacturer's instructions. The NanoSight NS500 instrument measured the rate of Brownian motion of nanoparticles and consists in a light scattering system that provides a reproducible platform for specific and general nanoparticle characterization (NanoSight Ltd., Amesbury, UK). Samples were processed in duplicate and diluted with PBS over a range of concentration to obtain between 10 and 100 particles per image (optimal ~50 particles × image) before the analysis with the NTA system. The samples were mixed before introducting into the chamber (temperature: 25°C and viscosity: 0.89 cP) and the camera level set to obtain an image that had sufficient contrast to clearly identify particles while minimizing background noise with video recording (camera level: 10 and capture duration: 60 s). Afterwards, the capture videos (2 videos per sample) were processed and analyzed. A combination of high shutter speed (450) and gain (250) followed by manual focusing enables optimum visualization of the maximum number of vesicles. A minimum of 200 completed tracks per video were collected in duplicate for each sample analyzed. NTA post acquisition settings were optimized and kept constant between samples (Frames Processed: 1496 of 1496, Frames per Second: 30, camera shutter: 20 ms; Calibration: 139 nm/pixel, Blur: 3 × 3; Detection Threshold: 10; Min Track Length: Auto; Min Expected Size: Auto), and each video was then analyzed to give the mean, mode, and median particle size together with an estimated number of particles. An Excel spreadsheet (Microsoft Corp., Redmond, Washington) was also automatically generated, recording the concentration at each particle size.
Transmission Electron Microscopy
Exosome pellets (as described above, 30 μg protein) were fixed in 3% (w/v) glutaraldehyde and 2% paraformaldehyde in cacodylate buffer, pH 7.3. Exosome samples were then applied to a continuous carbon grid and negatively stained with 2% uranyl acetate. The samples were examined in an FEI Tecnai 12 transmission electron microscope (FEI™, Hillsboro, OR, USA).
Quantification of Cell-Derived Exosome
The concentration of exosomes from EVT cells lines (JEG-3 and HTR-8/SVneo) in maternal circulation was expressed as total immunoreactive exosomal CD63 (ExoELISA™, System Biosciences, Mountain View, CA). Briefly, 10 μg of exosomal protein was immobilized in micro-titer plate wells and incubated overnight (binding step). Plates were washed three times for 5 min using a wash buffer solution and then incubated with exosome specific primary antibody (CD63) at room temperature (RT) for 1 h on a shaker. Plates were washed and incubated with secondary antibody (1:5000) at RT 1 h on a shaker. Plates were washed and incubated with Super-sensitive TMB ELISA substrate at RT for 45 min while shaking. The reaction was terminated using Stop Buffer solution. Absorbance was measured at 450 nm. The number of exosomes/ml, (ExoELISA™ kit) was obtained using an exosomal CD63 standard curve calibrated against nanoparticle tracking data (i.e., number of exosomes, NanoSight™). The coefficients of intra- and inter-assay variations were less than 8%.
Effect of Exosomes on Vascular Smooth Muscle Cells (VSMC) Migration
VSMC were cultured in 231 media supplemented with 0.2% FBS-exosome free in 96-well culture plate (Corning Life Science, Tewksbury, MA, USA) according to the manufacturer's instructions for 18–24 h. Firstly, we analyzed the effect of cell proliferation on cell migration assay in our experimental conditions using an anti-proliferative drug Mitomycin C (SIGMA-ALDRICH). Cells were plated (1 × 105 cells per well) onto 24-well plate, and after 24 h Mitomycin C (50 and 100 ng/ml) was added to the cell for 48 h. Cell proliferation was quantified (time-curve) by measuring the cell confluence using a real time imagining system IncuCyte™. Simultaneously, VSMC migration assay in the presence of Mitomycin C (50 and 100 ng/ml) for 48 h was performed.
During experiments, VSMC cells were incubated in the presence (treatment: 100 μg exosomal protein/ml) or absence (control) of exosomes for up to 48 h under 8% O2 (n = 12). The concentration used in this study was based upon exosome dose-response curves from our previously published studies (Salomon et al., 2013a,b, 2014). Exosomes were subjected to heat inactivation (30 min at 65°C) or sonication for 30 min (sonicator bath) before the incubation on VSMC. Cell migration was assessed using a scratch assay format. A scratch was made on confluent monolayers using a 96-pin WoundMaker™ (BioScience Inc, Ann Arbor, MI, USA). Wound images were automatically acquired and registered by the IncuCyte™ software system. CellPlayer™ 96-Well Invasion Assay software was use to fully automate data collection. Data were processed and analyzed using IncuCyte™ 96-Well Cell Invasion Software Application Module. Data are presented as the Relative Wound Density (RWD, Eizen, v1.0 algorithm). RWD is a representation of the spatial cell density in the wound area relative to the spatial cell density outside of the wound area at every time point (time-curve). Migration assays were performed in the presence of Mitomycin C (100 ng/ml) to minimize any confounding effects of cell proliferation. The rate of wound closure was compared using the half-maximal stimulatory time (ST50) and area under the time course curve (AUC).
Exosome Internalization
For exosome uptake analysis, the exosomes pellet (before purification using 30% sucrose cushion) isolated from JEG-3 and HTR-8/SVneo cells was resuspended in PBS and stained with PKH67 green fluorescent cell linker kit follow the manufacturer's instructions (Sigma-Aldrich). The staining reaction was stopped after 5 min with exosome-free FBS. Exosomes were then purified using 30% sucrose cushion as described above. Exosomes-PKH67 (100 μg/ml from JEG-3 and HTR-8/svneo cells) were incubated on VSMC for 24 h and exosome uptake was quantified using IncuCyte™ (fluorescent mode). Simultaneously, VSMC were grown in glass bottom chamber slides, and labeled exosomes (100 μg/ml) were added to subconfluent cells. After 24 h, VSMC were washed and fixed using 2% paraformaldehyde for 5 min at RT. Cells were immediately analyzed using a Zeiss fluorescence microscope (Axio Imager M1, Zeiss EC Plan-NEOFLUAR 40×/0.75, the Carl Zeiss Group, Germany) with FITC and DAPI absorbance setting.
Proteomic Analysis of Cytotrophoblast Derived-Exosomes by Mass Spectrometry (MS)
Isolated exosomes from JEG-3 and HTR-8/SVneo were solubilized in 8 M urea in 50 mM ammonium bicarbonate, pH 8.5, and reduced with DTT for 1 h. Proteins were then alkylated in 10 mM iodoacetic acid (IAA) for 1 h in the dark. The sample was diluted to 1:10 with 50 mM ammonium bicarbonate and digested with trypsin (20 μg) at 37°C for 18 h. The samples were desalted by solid phase extraction using a STAGE tip protocol (Stop and go extraction tips for matrix-assisted laser desorption/ionization, nano-electrospray, and LC/MS sample pre-treatment in proteomics). The eluted peptides were dried by centrifugal evaporation to remove acetonitrile and redissolved in Solvent A. The resulting peptide mixture was analyzed by liquid chromatography (LC)/mass spectrometry (MS) LC-MS/MS on a 5600 Triple TOF mass spectrometer (AB Sciex, Framingham, USA) equipped with an Eksigent Nanoflow binary gradient HPLC system and a nanospray III ion source. Solvent A was 0.1% formic acid in water and solvent B was 0.1% formic acid in acetonitrile. MS/MS spectra were collected using Information Dependent Acquisition (IDA) using a survey scan (m/z 350–1500) followed by 25 data-dependent product ion scans of the 25 most intense precursor ions. All mass spectra were analyzed using the Mascot and Protein Pilot search engines against the Swissprot-database with the species set as human. Positive identifications were ascribed where Mascot scores were greater than 30. False discovery rate (FDR) was estimated using a reversed sequence database. Finally, proteins identified were submitted to bioinformatic pathway analysis (Ingenuity Pathway Analysis [IPA]; Ingenuity Systems, Mountain View, CA; www.ingenuity.com).
Statistical Analysis
Data are represented as mean ± s.e.m. Comparisons between two group means were performed by unpaired Student's t-tests. Multiple groups were compared using the analysis of variance (ANOVA). Post-hoc analyses were used for pairwise comparisons (Bonferroni correction test). Statistical significance was defined as at least p < 0.05. Statistical analyses were preformed using commercially available packages (Stata 11, StatCorp, College Station, TX, USA and Prism 6, GraphPad Inc, La Jolla, CA 92037 USA).
Results
Characterization of EVT Cell Lines
Figure 1A presents photomicrographs of wound closure over 40 h incubation for both cell lines and the percent change in relative wound density over time. The migration rate of HTR-8/SVneo was significantly greater than that observed for JEG-3 cells. Half-maximal stimulatory time (ST50) was 31.29 ± 0.14 h and 15.26 ± 0.15 h for JEG-3 and HTR-8SV/neo, respectively (p < 0.05, Figure 1A, bottom). Area under curves analysis showed that the migration of HTR-8SV/neo was ~2.0-fold greater than that obtained for JEG-3 cells (Figure 1B).
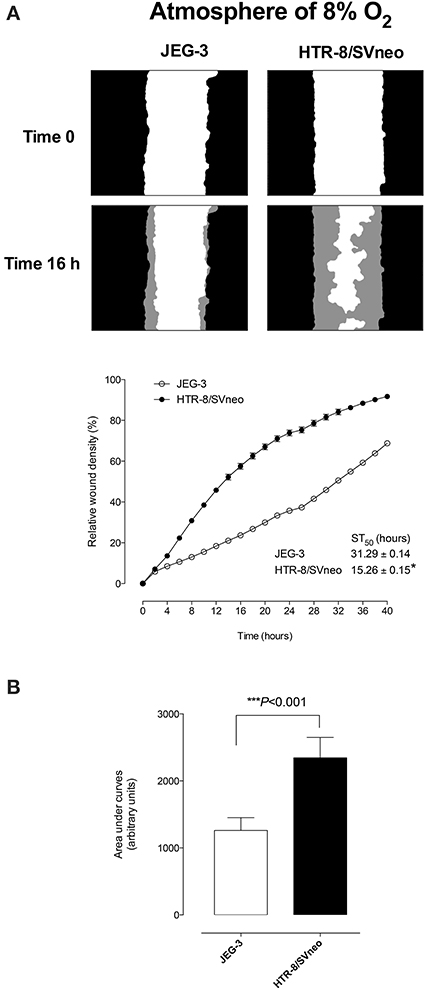
Figure 1. Extravillous Trophoblast invasion. JEG-3 and HTR-8/SVneo cells were grown to confluence in complete media. A wound was made using 96 well WoundMaker and cells were imaged with IncuCyte™ (Essen BioSciences, USA) every 4 h for 40 h (see Materials and Methods). (A) Top: Representative images of JEG-3 and HTR-8/SVneo cell migration at 16 h. The gray region denotes the area of the initial wound covered by advancing cells. Bottom: The time course of JEG-3 and HTR-8/SVneo migration. Insert: half-maximal stimulatory time (ST50). (B) Area under curves analysis from (A). Data represented as mean ± s.e.m. (n = 12). In (B) ***p < 0.0001 vs. HTR-8/SVneo.
Characterization of EVT Cell Line-Derived Exosomes
Defining vesicle size range is one of the standard metrics used to confirm that the vesicle preparation in not contaminated with non-exosome vesicles. After differential centrifugation (i.e., 300 × g, 2000 × g, and 12,000 × g), the supernatant was analyzed using a nanoparticles tracking analysis (NTA). Particle size distribution ranged from 30 to 500 nm, with an average of 180 ± 100 nm (Figure 2A). Supernatant was filtered and ultracentrifuged to obtain a particulate fraction (including exosomes particles) with a particle size ranged of 30–200 nm (with a mean diameter of 119 ± 65 nm, Figure 2B). The exosomes were purified by ultracentrifugation over a sucrose cushion and analyzed using a NTA. The exosome fractions displayed a particle size ranged from 30 to 150 nm in diameter (with mean of 79 ± 68 nm, Figure 2C). The presence of exosomal marker CD63 in exosomes isolated from both cell lines was confirmed by Western blot (Figure 2D, top). Finally, exosomes isolated from JEG-3 and HTR-8/SVneo were visualized by transmission electron microscopy (Figure 2D, bottom). Exosomes were identified as small vesicles between 40 and 100 nm. No significant differences in NTA data, CD63 expression and electron microscopy were identified between cell lines.
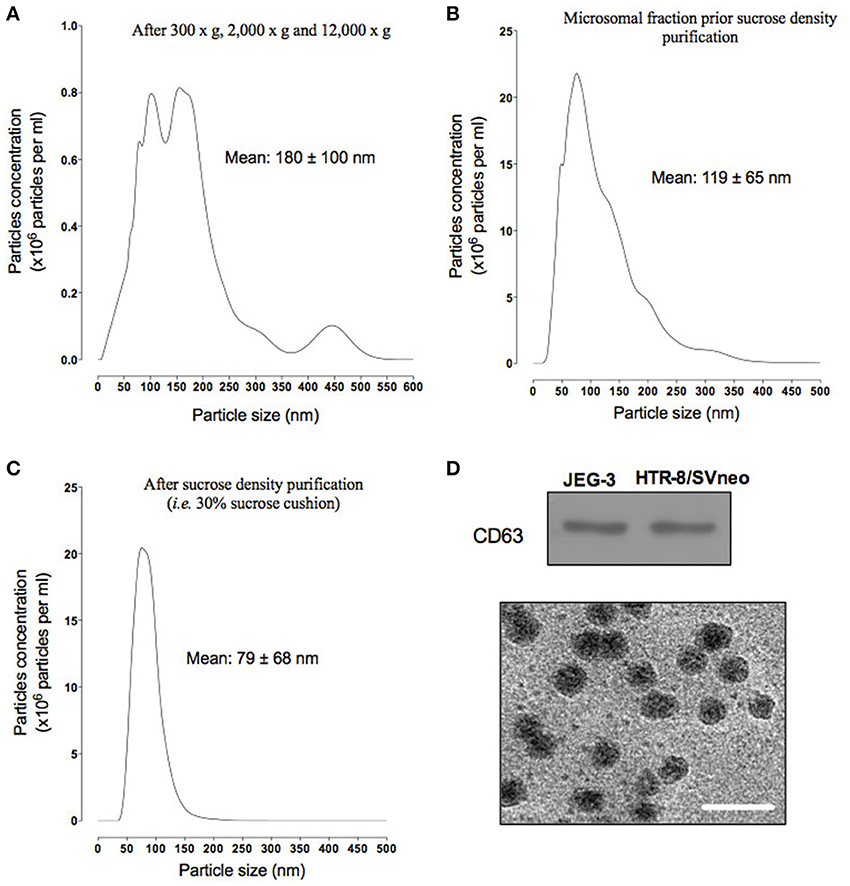
Figure 2. Characterization of exosome from EVT cells. Exosomes were isolated from JEG-3 and HTR-8/SV cells by differential and buoyant density centrifugation (see Materials and Methods). (A–D) shown representative particles size distribution of exosomes isolation steps from JEG-3 and HTR-8/SV cells. (A) After differential centrifugation (i.e., 300 × g, 2000 × g, and 12,000 × g). (B) Microsomal fraction prior sucrose density purification. (C) After sucrose density purification (30% sucrose cushion). (D) Top: representative Western blot for exosome markers: CD63. Bottom: representative electron micrograph exosome fractions, Scale bar 200 nm.
Exosome Release from EVT Cell Lines
Exosomal protein release was expressed as total exosomal protein (i.e., particulate material with a buoyant density of 1.127 g/ml) per million cells/24 h. JEG-3 cells released 46 ± 16 ng exosomal protein per million cells per 24 h (n = 4; i.e., 4 different isolations from ~200 × 106 cells each) whereas HTR-8/SVneo cells released 120 ± 35 ng exosomal protein per million cells per 24 h (n = 4, Figure 3A). HTR-8/SVneo cells released significantly more exosomes (~2.6-folds) in 24 h compared to JEG-3 cells (p < 0.0001). These results were confirmed by quantifying immunoreactive exosomal CD63. The number of exosomes (NEP) released from HTR-8/SVneo cells (6.39 × 108 ± 2.5 × 108/24 h) was ~2.3-fold greater (p < 0.0001) than that observed for JEG-3 cells (2.86 × 108 ± 0.78 × 108/24 h, Figure 3B).
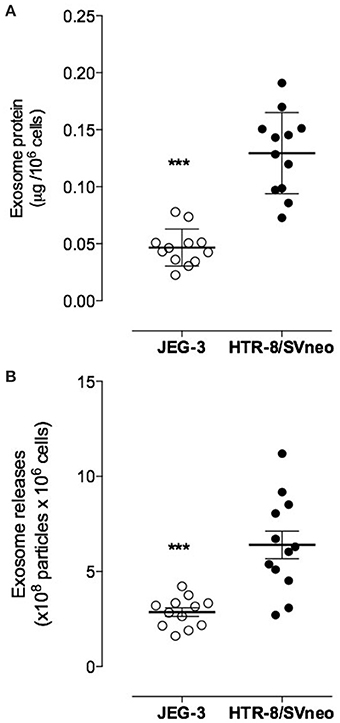
Figure 3. Exosome releases from EVT cell lines. Exosomal protein and number of exosomes were quantified cell conditioned media from JEG-3 and HTR-8/SVneo cells using a colorimetric assay and ELISA kit, respectively. (A) exosomes concentration presented as μg exosomal protein per 106 cells and (B) Number of exosomes particles. Data are presented as scatter dot plot and values are mean ± s.e.m. In (A,B) ***p < 0.001 vs. HTR-8/SVneo.
Effect of EVT-Derived Exosomes on Cell Migration
A VSMC was used to establish the effects of EVT-derived exosomes on cell migration. An anti-proliferative drug Mitomycin C (50 and 100 ng/ml) reduces significantly (p < 0.01) VSMC proliferation (Figure 4A). A dose of 100 ng/ml decreased VSMC migration compared to 50 ng/ml of Mitomycin C and control (Figure 4B). The effects of exosomes (100 μg protein/ml) isolated from JEG-3 and HTR-8/SVneo cultured under 8% O2 on VSMC migration under 8% O2 are presented in Figures 4C,D. All experiments were done in the presence of Mitomycin C (100 ng/ml). The rate of wound closure was significantly increased in the presence of HTR-8/SVneo-derived exosome compared to control (-exosomes) as measured by ST50 (15.57 ± 0.32 vs. 25.09 ± 0.58, p < 0.01) (Table 1). Area under curves analysis showed that HTR-8/SVneo-derived exosome increased ~35 ± 0.2% VSMC migration compared to control. Similarly, exosomes from JEG-3 cells increased VSMC migration ~12 ± 0.1% compared to values in the absence of exosomes (control), however, the effect was smaller compared to exosomes from HTR-8/SVneo. Exosomes were exposed to heat inactivation before incubation on VSMC; however, heat inactivation did not affect the effect of exosomes on VSMC migration. In contrast, sonication completely abolished the HTR-8/SVneo and JEG-3-derived exosomes effect on VSMC migration.
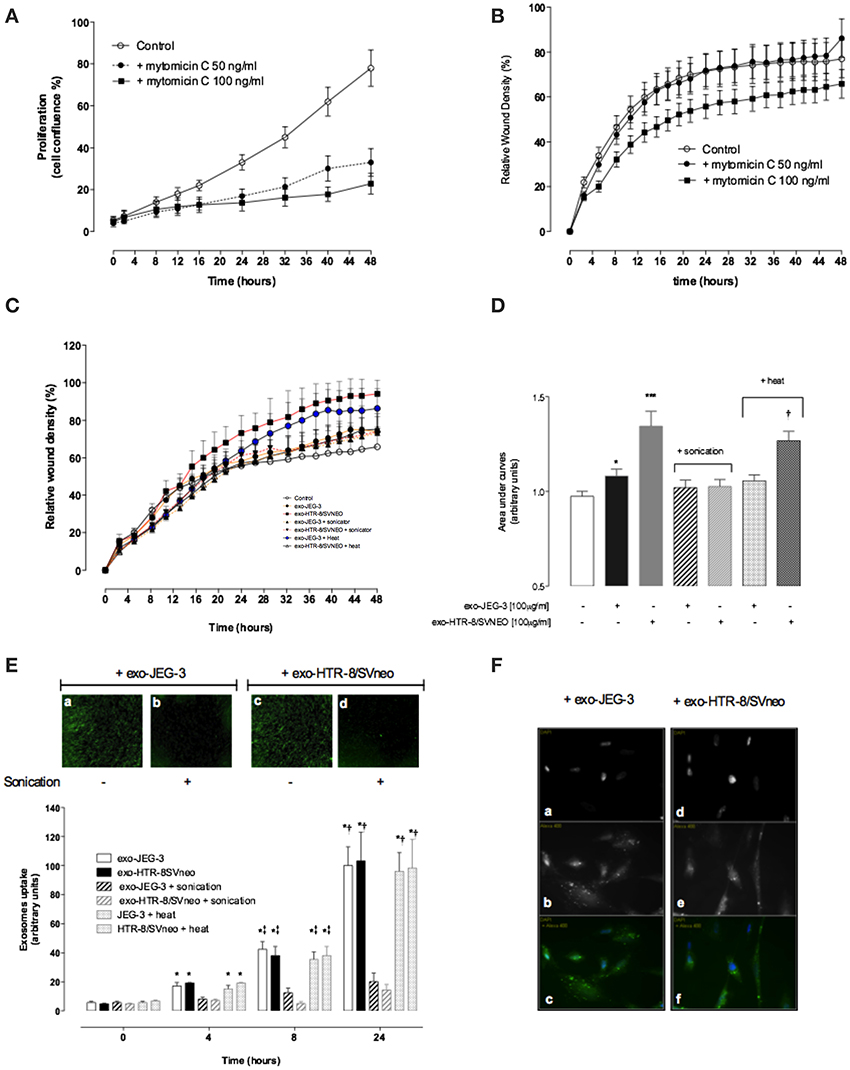
Figure 4. EVT-derived exosomes effects on hVSMC migration. hVSMC were grown to confluence in 231 media and a wound was made using 96 well WoundMaker (see Materials and Methods). hVSMC Migration was measured in absence or presence of 100 ug/ml of exosomes from JEG-3 and HTR-8/SVneo cells and mitomycin C (100 ng/ml) for 48 h. Exosome particles were subjected to sonication (+sonication) or heat inactivation (+heat) before exposure to hVSMC cells (A,B) hVSMC proliferation and migration in the presence of Mitomycin C, respectively. (C) Time course of wound closure for hVSMC expressed as relative wound density (%). (D) Area under curves from data in (C). (E) Time-dependent uptake of exosomes using a real time imaging system analysis. Top: images after 24 h; Bottom: Graphical representation of exosome uptake. (F) Fluorescent microscopy analysis of exosome uptake (40X). Data represent an n = 12 well each point with 3 different cells culture. Values are mean ± SD. In (D) ***p < 0.001 vs. all condition except exo-HTR-8/SVneo + heat; and †p < 0.05 vs. control. In (E) *p < 0.001 vs. corresponding values at 0 h; ‡p < 0.05 vs. corresponding values control at 4 h; †p < 0.05 vs. corresponding values control at 8 and 4 h.
The internalization of exosomes labeled with PKH67 (green) in VSMC was quantified and visualized using The IncuCyte and a fluorescence microscope, respectively (Figures 4E,F). Exosome uptake by VSMC was observed in a time-dependent manner with the maximum at 24 h (Figure 4E, top panel a and c). Sonication abolished the uptake of fluorescent exosomes (Figure 4E, top panel b and d) compared to exosomes without sonication. Exosome uptake is presented as fluorescent per cell confluence normalized to maximum uptake of 100%. Heat inactivation did not affect the exosomes uptake by VSMC (Figure 4, lower panel). Finally, fluorescence microscope analysis showed an intracellular fluorescent in VSMC cells exposed to intact vesicles from JEG-3 and HTR-8/SVneo (Figure 4F).
Proteomic Analysis of EVT-Derived Exosome
Mass spectrometry analysis identified over 140 exosomal proteins, including cell-line specific exosomal proteins (Table 2 and Figure 5A). Exosomal proteins isolated from JEG-3 and HTR-8/SVneo cells were associated with cellular movement and morphology, immune cell trafficking and cellular assembly and organization in accordance with Ingenuity Pathway Analysis (IPA) analysis. The canonical pathways associated with our exosomal proteins isolated from JEG-3 and HTR-8/SVneo cells and defined by IPA Core comparison analysis showed that the score (−log [p-value]) for proteins associated with cell movement and migration was significantly higher (~1.2-fold, p < 0.05) in HTR-8/SVneo-derived exosomes to compare to exosomes from JEG-3 cells (Figure 5B).
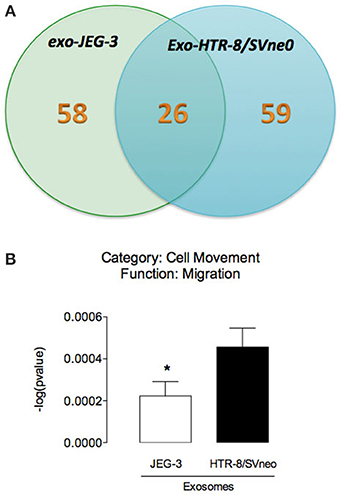
Figure 5. Analysis of EVT cell-derived exosomes proteins. (A) The Venn diagram represents the distribution of common and unique proteins identified by nanospray LC-MS/MS (ABSciex 5600) in exosomes released from JEG-3 and HTR-8/SVneo cells. (B) Comparison of biological function (cell movement and migration) identified by IPA core analysis. In (B) values are mean ± s.e.m. In (B) *p < 0.05 vs. HTR-8/SVneo cells.
Discussion
A tenet of contemporary obstetrics is that events that compromise placentation and the development of the materno-fetal exchange increase the risk of complications of pregnancy and contribute to poor pregnancy outcome. In particular, conditions that affect the differentiation and invasion of placental cells compromise placental perfusion and function (Jauniaux et al., 2006, 2010; Burton et al., 2009) and the subsequent growth and development of the fetus (Khong et al., 1987). EVT migrate into maternal decidua and myometrium and interact with VSMC in uterine spiral arteries. Conversion of these arteries is associated with the loss of VSMC from the vessel wall by migration out of the vessel. In this study we established that: (1) exosome isolated from EVT-like cell lines (JEG-3 and HTR-8/SVneo) with different cellular origins (choriocarcinoma and EVT) exhibit differences in their rate of release, protein content and bioactivity; and (2) both exosomes from JEG-3 and HTR-8/SVneo cells increase VSMC migration. However, the effect of exosomes from HTR-8/SVneo was significantly higher. Exosomes released from first trimester trophoblast cells (EVT), therefore, may regulate processes that play a key role in placentation and remodeling of the uterine spiral arteries.
The interactions between EVT and VSMC are not fully understood, in part due to difficulties of accessing first trimester samples and the lack of suitable animal models (Carter, 2007; Ackerman et al., 2013). We obtained nanovesicles with high purity. The data presented establish that the final preparation used display a size distribution and buoyant consistent with exosomes and the lack of significant contamination by microsomes. In this study, we quantified the release of exosomes from JEG-3 and HTR-8/SVneo cells (as indicated by immunoreactive exosomal CD63). The data obtained establish that exosome release is cell type specific and correlated with cell migration capacity. Consistent with these data, a correlation between exosome release and cell invasiveness has been described previously in ovarian cancer cells (Kobayashi et al., 2014).
The initial stages of uterine SpA remodeling involves a loss of the VSMC component by apoptosis, migration or a combination of both processes. In this study, we identify a novel exosomal signaling pathway by which EVT (HTR-8/SVneo cells) promote the migration of VSMC. Consistent with these data, Bulmer et al. (2012) observed that VSMC migration plays a major role in remodeling of the artery by migration into the decidua and vessel lumen and this phenomenon is enhanced in the presence of EVT cells (Bulmer et al., 2012). Based upon the available data, we propose that perivascular EVT release exosomes that interaction with VSMC and promote their migration out of SpAs and alter the vasoreactive of these vessels. This effect of exosomes on VSMC migration was cell-type specific (i.e., only exosomes from HTR-8/SVneo cells promote cell migration) and may reflect the different cellular origin of the two cell lines compared in this study. HTR-8/SVneo cells are derived by transfection of first trimester trophoblast cells (Graham et al., 1993). JEG-3 cells are derived from a choriocarcinoma (Kohler and Bridson, 1971). Difference in the molecular cargo carried by exosomes released from HTR-8/SVneo and JEG-3 cell may account for their different effects on target cell migration. Consistent with this suggestion, proteins involved in cell migration were differentially represented in exosomes isolated from the two-cell lines.
The exosomal content is highly dependent on the cell origin and on pre-conditioning of the cell. Exosomes function as a carrier of specific molecules such as, proteins, lipids, mRNA, and miRNA and can interact with neighboring cells or travel long distances in the bloodstream to reprogram the phenotype and regulate their function (Denzer et al., 2000). In this study, we identified unique proteins (58 and 59 proteins in exosome from JEG-3 and HTR-8/SVneo cells) and common proteins (26) between these EVT cell lines. Ingenuity Pathway Analysis (IPA) of exosomal proteins identified cell-dependent changes in cell movement and migration signaling pathways. Exosomes have been reported to express a diverse range of cell surface receptors, proteins (including, heat shock proteins, cytoskeletal proteins, adhesion molecules, membrane transport, and fusion proteins), mRNA and miRNA with the potential to affect the acute and long-term function of the cells with which they interact (Ambros, 2004). Recent data demonstrate that trophoblast-derived exosomes induce proinflammatory cytokines such IL-1 β in human macrophages cells (Atay et al., 2011). Furthermore, in vitro exposure of PBMC and dendritic cells to exosomal proteins induce differentiation of stem cells; suppression of activation of natural killer cells and macrophages; and stimulation of cell migration (Mincheva-Nilsson et al., 2006; Knight, 2008; Soo et al., 2012). Interestingly, protein analysis revealed that exosome release from cytrophoblast cells increases with low oxygen tension and their exosome promotes cell migration in extravillous cytotrophoblast (HTR-8/SVneo) (Salomon et al., 2013b).
The observed effects of HTR-8/SVneo-derived exosomes on VSCM migration were also dependent upon exosome structural integrity. Disruption of exosomes by sonication (Delorme-Axford et al., 2013) completely abolished their effect on VSMC migration. While the precise mechanisms by which sonication abolishes the effects of exosomes remains to be established, preventing exosomal fusion with VSMC cell membrane and the intracellular delivery of signaling molecules and/or the loss of capacity to appropriately present exosomal surface moieties to target cell receptors represent possible pathways and warrant further investigation. We establish in this study that exosome integrity is critical to mediating their effects on VSMC migration. It is not possible from the data obtained in this study to differentiate between sonication–induced disruption of exosome: uptake; fusion; activation of VSMC cell surface receptors, or a combination of these (and other) mechanisms. The elucidation of contribution of these mechanisms will require additional extensive studies to establish.
In conclusion, using in vitro EVT-like cell lines, we have demonstrated difference in release, composition and bioactivity between exosomes from JEG-3 and HTR-8/SVneo cells. Exosomes released from EVT may play a role in remodeling SpA by promoting migration of VSMC. Exosomal-induced VSCM migration is associated with increased representation of proteins involved in cell migration processes within their molecular cargo and dependent upon exosomal structural integrity. The identification of this EVT-VSMC exosomal communication pathway not only affords opportunity for developing biomarkers of placentation but also the assessment of exosome targeted therapeutic innervation strategies.
Author Contributions
Carlos Salomon, Sarah Yee, Katherin Scholz-Romero, Kanchan Vaswani, Miharu Kobayashi and David Kvaskoff contributed in generating experimental data. Carlos Salomon, Sebastian E. Illanes, Murray D. Mitchell and Gregory E. Rice contributed in discussion and reviewed/edited manuscript. Carlos Salomon and Gregory E. Rice wrote the manuscript and drew the figures.
Conflict of Interest Statement
The authors declare that the research was conducted in the absence of any commercial or financial relationships that could be construed as a potential conflict of interest.
Acknowledgments
We acknowledge the assistance of Dr. Jamie Riches and Dr. Rachel Hancock of the Central Analytical Research Facility, Institute for Future Environments, Queensland University of Technology (QUT) for the electron microscope analyses.
References
American Diabetes Association. (2012). Diagnosis and classification of diabetes mellitus. Diabetes Care 35(Suppl. 1), S64–S71. doi: 10.2337/dc12-s064
Ackerman, W. E. 4th., Carter, A. M., De Mestre, A. M., Golos, T. G., Jeschke, U., Kusakabe, K., et al. (2013). IFPA Meeting 2012 Workshop Report I: comparative placentation and animal models, advanced techniques in placental histopathology, human pluripotent stem cells as a model for trophoblast differentiation. Placenta 34(Suppl.), S3–S5. doi: 10.1016/j.placenta.2012.11.006
Atay, S., Gercel-Taylor, C., Suttles, J., Mor, G., and Taylor, D. D. (2011). Trophoblast-derived exosomes mediate monocyte recruitment and differentiation. Am. J. Reprod. Immunol. 65, 65–77. doi: 10.1111/j.1600-0897.2010.00880.x
Blackburn, C. A., Keelan, J. A., Taylor, R. S., and North, R. A. (2003). Maternal serum activin A is not elevated before preeclampsia in women who are at high risk. Am. J. Obstet. Gynecol. 188, 807–811. doi: 10.1067/mob.2003.173
Bulmer, J. N., Innes, B. A., Levey, J., Robson, S. C., and Lash, G. E. (2012). The role of vascular smooth muscle cell apoptosis and migration during uterine spiral artery remodeling in normal human pregnancy. FASEB J. 26, 2975–2985. doi: 10.1096/fj.12-203679
Burton, G. J., Charnock-Jones, D. S., and Jauniaux, E. (2009). Regulation of vascular growth and function in the human placenta. Reproduction 138, 895–902. doi: 10.1530/REP-09-0092
Carter, A. M. (2007). Animal models of human placentation–a review. Placenta 28(Suppl. A), S41–S47. doi: 10.1016/j.placenta.2006.11.002
Chen, G., Zhang, Y., and Wu, X. (2014). 786-0 Renal cancer cell line-derived exosomes promote 786-0 cell migration and invasion. Oncol. Lett. 7, 1576–1580. doi: 10.3892/ol.2014.1962
Delorme-Axford, E., Donker, R. B., Mouillet, J. F., Chu, T., Bayer, A., Ouyang, Y., et al. (2013). Human placental trophoblasts confer viral resistance to recipient cells. Proc. Natl. Acad. Sci. U.S.A. 110, 12048–12053. doi: 10.1073/pnas.1304718110
Denzer, K., Kleijmeer, M. J., Heijnen, H. F., Stoorvogel, W., and Geuze, H. J. (2000). Exosome: from internal vesicle of the multivesicular body to intercellular signaling device. J. Cell Sci. 113, 3365–3374.
Graham, C. H., Hawley, T. S., Hawley, R. G., MacDougall, J. R., Kerbel, R. S., Khoo, N., et al. (1993). Establishment and characterization of first trimester human trophoblast cells with extended lifespan. Exp. Cell Res. 206, 204–211. doi: 10.1006/excr.1993.1139
Jauniaux, E., Poston, L., and Burton, G. J. (2006). Placental-related diseases of pregnancy: involvement of oxidative stress and implications in human evolution. Hum. Reprod. Update 12, 747–755. doi: 10.1093/humupd/dml016
Jauniaux, E., Van Oppenraaij, R. H. F., and Burton, G. J. (2010). Obstetric outcome after early placental complications. Curr. Opin. Obstetr. Gynecol. 22, 452–457. doi: 10.1097/GCO.0b013e3283404e44
Kam, E. P., Gardner, L., Loke, Y. W., and King, A. (1999). The regulation of trophoblast differentiation by oxygen in the physiological change in decidual spiral arteries. Hum. Reprod. 14, 2131–2138. doi: 10.1093/humrep/14.8.2131
Khong, T. Y., Liddell, H. S., and Robertson, W. B. (1987). Defective hemochorial placentation as a cause of miscarriage - a preliminary-study. Br. J. Obstetr. Gynaecol. 94, 649–655. doi: 10.1111/j.1471-0528.1987.tb03169.x
Knight, A. M. (2008). Regulated release of B cell-derived exosomes: do differences in exosome release provide insight into different APC function for B cells and DC? Eur. J. Immunol. 38, 1186–1189. doi: 10.1002/eji.200838374
Kobayashi, M., Salomon, C., Tapia, J., Illanes, S. E., Mitchell, M. D., and Rice, G. E. (2014). Ovarian cancer cell invasiveness is associated with discordant exosomal sequestration of Let-7 miRNA and miR-200. J. Transl. Med. 12, 4. doi: 10.1186/1479-5876-12-4
Kohler, P. O., and Bridson, W. E. (1971). Isolation of hormone-producing clonal lines of human choriocarcinoma. J. Clin. Endocrinol. Metab. 32, 683–687. doi: 10.1210/jcem-32-5-683
Lee, H. D., Kim, Y. H., and Kim, D. S. (2014). Exosomes derived from human macrophages suppress endothelial cell migration by controlling integrin trafficking. Eur. J. Immunol. 44, 1156–1169. doi: 10.1002/eji.201343660
Mincheva-Nilsson, L., Nagaeva, O., Chen, T., Stendahl, U., Antsiferova, J., Mogren, I., et al. (2006). Placenta-derived soluble MHC class I chain-related molecules down-regulate NKG2D receptor on peripheral blood mononuclear cells during human pregnancy: a possible novel immune escape mechanism for fetal survival. J. Immunol. 176, 3585–3592. doi: 10.4049/jimmunol.176.6.3585
Morales-Prieto, D. M., Chaiwangyen, W., Ospina-Prieto, S., Schneider, U., Herrmann, J., Gruhn, B., et al. (2012). MicroRNA expression profiles of trophoblastic cells. Placenta 33, 725–734. doi: 10.1016/j.placenta.2012.05.009
Pegtel, D. M., Cosmopoulos, K., Thorley-Lawson, D. A., van Eijndhoven, M. A. J., Hopmans, E. S., Lindenberg, J. L., et al. (2010). Functional delivery of viral miRNAs via exosomes. Proc. Natl. Acad. Sci. U.S.A. 107, 6328–6333. doi: 10.1073/pnas.0914843107
Pijnenborg, R., Ball, E., Bulmer, J. N., Hanssens, M., Robson, S. C., and Vercruysse, L. (2006a). In vivo analysis of trophoblast cell invasion in the human. Methods Mol. Med. 122, 11–44. doi: 10.1385/1-59259-989-3:9
Pijnenborg, R., Vercruysse, L., and Hanssens, M. (2006b). The uterine spiral arteries in human pregnancy: facts and controversies. Placenta 27, 939–958. doi: 10.1016/j.placenta.2005.12.006
Pijnenborg, R., Bland, J. M., Robertson, W. B., and Brosens, I. (1983). Uteroplacental arterial changes related to interstitial trophoblast migration in early human pregnancy. Placenta 4, 397–413. doi: 10.1016/S0143-4004(83)80043-5
Salomon, C., Ryan, J., Sobrevia, L., Kobayashi, M., Ashman, K., Mitchell, M., et al. (2013a). Exosomal signaling during hypoxia mediates microvascular endothelial cell migration and vasculogenesis. PLoS ONE 8:e68451. doi: 10.1371/journal.pone.0068451
Salomon, C., Kobayashi, M., Ashman, K., Sobrevia, L., Mitchell, M. D., and Rice, G. E. (2013b). Hypoxia-induced changes in the bioactivity of cytotrophoblast-derived exosomes. PLoS ONE 8:e79636. doi: 10.1371/journal.pone.0079636
Salomon, C., Torres, M., Kobayashi, M., Scholz-Romero, K., Sobrevia, L., Dobierzewska, A., et al. (2014). A gestational profile of placental exosomes in maternal plasma and their effects on endothelial cell migration. PLoS ONE. 9:e98667. doi: 10.1371/journal.pone.0098667
Soo, C. Y., Song, Y., Zheng, Y., Campbell, E. C., Riches, A. C., Gunn-Moore, F., et al. (2012). Nanoparticle tracking analysis monitors microvesicle and exosome secretion from immune cells. Immunology 136, 192–197. doi: 10.1111/j.1365-2567.2012.03569.x
Suman, P., and Gupta, S. K. (2012). Comparative analysis of the invasion-associated genes expression pattern in first trimester trophoblastic (HTR-8/SVneo) and JEG-3 choriocarcinoma cells. Placenta 33, 874–877. doi: 10.1016/j.placenta.2012.06.017
Thery, C. (2011). Exosomes: secreted vesicles and intercellular communications. F1000 Biol. Rep. 3, 15. doi: 10.3410/B3-15
Vlassov, A. V., Magdaleno, S., Setterquist, R., and Conrad, R. (2012). Exosomes: current knowledge of their composition, biological functions, and diagnostic and therapeutic potentials. Bba-Gen. Subjects 1820, 940–948. doi: 10.1016/j.bbagen.2012.03.017
Weber, M., Knoefler, I., Schleussner, E., Markert, U. R., and Fitzgerald, J. S. (2013). HTR8/SVneo cells display trophoblast progenitor cell-like characteristics indicative of self-renewal, repopulation activity, and expression of stemness- associated transcription factors. BioMed. Res. Int. 2013, 243649. doi: 10.1155/2013/243649
Keywords: exosomes, cell migration, placenta, pregnancy, proteomics
Citation: Salomon C, Yee S, Scholz-Romero K, Kobayashi M, Vaswani K, Kvaskoff D, Illanes SE, Mitchell MD and Rice GE (2014) Extravillous trophoblast cells-derived exosomes promote vascular smooth muscle cell migration. Front. Pharmacol. 5:175. doi: 10.3389/fphar.2014.00175
Received: 01 May 2014; Accepted: 07 July 2014;
Published online: 11 August 2014.
Edited by:
Carlos Alonso Escudero, Universidad del Bio Bio, ChileReviewed by:
Siu-Lung Chan, University of Vermont, USACarlos Alonso Escudero, Universidad del Bio Bio, Chile
Motohiro Komaki, Tokyo Medical and Dental University Graduate School, Japan
Copyright © 2014 Salomon, Yee, Scholz-Romero, Kobayashi, Vaswani, Kvaskoff, Illanes, Mitchell and Rice. This is an open-access article distributed under the terms of the Creative Commons Attribution License (CC BY). The use, distribution or reproduction in other forums is permitted, provided the original author(s) or licensor are credited and that the original publication in this journal is cited, in accordance with accepted academic practice. No use, distribution or reproduction is permitted which does not comply with these terms.
*Correspondence: Carlos Salomon, Faculty of Health Sciences, Royal Brisbane Hospital, University of Queensland Centre for Clinical Research, University of Queensland, Building 71/918, Herston, Brisbane, QLD 4029, Australia e-mail:Yy5zYWxvbW9uZ2FsbG9AdXEuZWR1LmF1Web: www.uqccr.uq.edu.au/