- 1Department of Physiology, Cell Physiology Research Group, University of Extremadura, Cáceres, Spain
- 2Department of Medical Physiology and Biophysic, Institute of Biomedicine of Seville, Virgen del Rocio University Hospital, Consejo Superior de Investigaciones Científicas, University of Seville, Sevilla, Spain
Store-operated Ca2+ entry (SOCE) is an ubiquitous mechanism for Ca2+ entry in eukaryotic cells. This route for Ca2+ influx is regulated by the filling state of the intracellular Ca2+ stores communicated to the plasma membrane channels by the proteins of the Stromal Interaction Molecule (STIM) family, STIM1, and STIM2. Store-dependent, STIM1-modulated, channels include the Ca2+ release-activated Ca2+ channels, comprised of subunits of Orai proteins, as well as the store-operated Ca2+ (SOC) channels, involving Orai1, and members of the canonical transient receptor potential family of proteins. Recent studies have revealed the expression of splice variants of STIM1, STIM2, and Orai1 in different cell types. While certain variants are ubiquitously expressed, others, such as STIM1L, show a more restricted expression. The splice variants for STIM and Orai1 proteins exhibit significant functional differences and reveal that alternative splicing enhance the functional diversity of STIM1, STIM2, and Orai1 genes to modulate the dynamics of Ca2+ signals.
Introduction
Eukaryotic cells finely modulate cytosolic calcium concentration ([Ca2+]c) to trigger a myriad of physiological events, from short term responses, such muscle contraction, impulse transmission, secretion, and aggregation, to long term events, including activation of transcription factors, growth and in the last instance, apoptosis, and cellular death. Evolution has provided the cells with a highly complex machinery, which finely tunes, orchestrates and coordinates intracellular Ca2+ homeostasis. Physiological agonists modulates [Ca2+]c by the activation of more or less selective Ca2+ channels and transporters, both in the intracellular Ca2+ compartments [endoplasmic reticulum (ER), mitochondria or acidic vesicles] and in the plasma membrane (PM). Once the stimulus ends, [Ca2+]c returns to basal level and the cell is ready for a new stimulation (Berridge et al., 2003; Redondo and Rosado, 2015). Cells have a number of mechanisms to induce Ca2+ entry and although all these events were studied in the past as independent pathways, mostly due to technical challenges to address Ca2+ signal as a whole, new studies and a deeper comprehension of Ca2+ entry support a sophisticated relation encompassed by all these pathways (Mignen et al., 2007; Wang et al., 2010; Rodriguez-Moyano et al., 2013; Zhang et al., 2014).
Store-Operated Calcium Entry (SOCE), a major mechanism for Ca2+ influx, is regulated by the filling state of the intracellular Ca2+ reservoirs, mainly the ER. A reduction in the intraluminal ER Ca2+ concentration ([Ca2+]ER), evokes the opening of channels in the PM leading to Ca2+ entry from the extracellular medium (Putney, 1986). After intense investigation, the mechanism that communicate the filling state of the intracellular Ca2+ stores to the PM channels was found to be mediated by the Stromal Interaction Molecule 1 (STIM1), a protein discovered in Oritani and Kincade (1996) and known as a cell–cell interaction mediator. STIM1 is the ER Ca2+ sensor that stimulate Ca2+ entry, triggering the activation of store-operated channels located in the PM (Roos et al., 2005; Zhang et al., 2005). Concerning the Ca2+-permeable channels that conduct SOCE, soon after the identification of STIM1 as the ER Ca2+ sensor Orai1 was proposed as the pore-forming subunit of the Ca2+ release-activated Ca2+ (CRAC) channels (Feske et al., 2005, 2006; Mercer et al., 2006; Peinelt et al., 2006; Prakriya et al., 2006). In addition, STIM1 might activate the less Ca2+ selective store-operated Ca2+ (SOC) channels, which require the interaction of Orai1 with the canonical transient receptor potential (TRP) family member TRPC1 (Rosado and Sage, 2000; Singh et al., 2000; Huang et al., 2006; Yuan et al., 2007; Jardin et al., 2008; Cheng et al., 2011, 2013; Choi et al., 2014; Desai et al., 2015). STIM1 activates Orai1 through a cytosolic STIM1-Orai1 activation region (SOAR; aa 344–442; Yuan et al., 2009) also identified as the CRAC activating domain (CAD; aa 342–448; Park et al., 2009); the Orai-activating small fragment (OASF; aa 233–450/474; Muik et al., 2009) and the Ccb9 (aa 339–44; Kawasaki et al., 2009). SOAR dimerization is essential for the activation of Orai1 and the polybasic region (aa 382–387) within the SOAR structure is required for Orai1 binding (Yang et al., 2012). The activation of TRPC1 by STIM1 has been reported to require both, the SOAR region, which is important for the STIM1–TRPC1 interaction (Lee et al., 2014), and the last 14 amino acids of STIM1, which constitute a polybasic lysine-rich domain required for the activation of TRPC channels by STIM1 upon store depletion (Zeng et al., 2008).
Furthermore, Orai1, together with Orai3, and the PM-resident STIM1 have also been reported to participate in a store-independent mechanism for Ca2+ entry activated by arachidonate (Mignen et al., 2008a,b, 2009), which reveals the diversity and complexity of the regulation of Ca2+ entry in eukaryotic cells.
STIM Proteins
Members of the STIM family, STIM1 (Figure 1) and STIM2 (Figure 2), have highly conserved structure and present slightly divergences giving them different functions. Upon store depletion, STIM1 oligomerizes and redistributes into discrete puncture nearby the PM (Luik et al., 2008; Cahalan, 2009; Park et al., 2009; Covington et al., 2010).
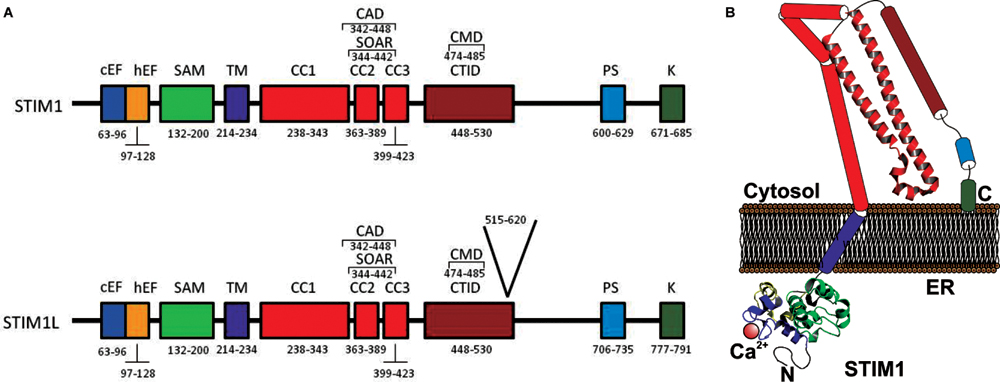
FIGURE 1. (A) Molecular structure of STIM1 and STIM1L. (A) The ER luminal N-terminal region includes a Ca2+-binding canonical EF-hand motif (cEF), a hidden EF-hand (hEF) motif, and a sterile α-motif (SAM). STIM1 has a single transmembrane domainTM. The cytosolic C-terminal region includes three coiled-coil (CC) regions (CC1, CC2, and CC3), which contain the SOAR (STIM–Orai activating region) or CAD (CRAC activation domain), the minimal sequence required for the activation of Orai1 channels. Downstream of CC3 there is a C-terminal inhibitory domain (CTID), which overlaps the CRAC modulatory domain. The C-terminal region also contains a Pro/Ser-rich domain (PS) and a Lys-rich domain (K) (Soboloff et al., 2012). STIM1L also includes 106 amino acids located a position 515–620 (Darbellay et al., 2011). (B) Cartoon depicting a possible model of STIM1 monomer in the coalescent state, where STIM1 solved crystal structures are shown. CC1 is divided in CC1α1, CC1α2, and CC1α3 (Fahrner et al., 2014).
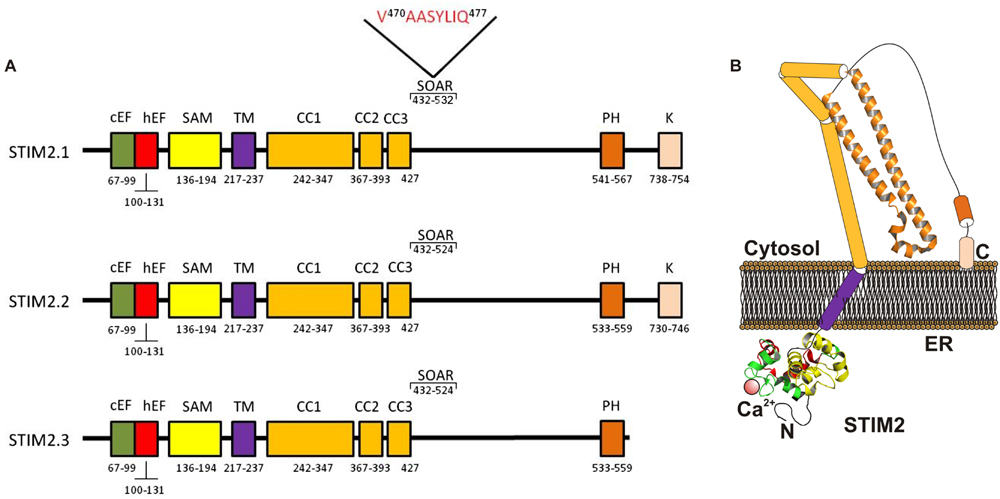
FIGURE 2. Molecular structure of STIM2 variants. (A) The ER luminal N-terminal region includes a Ca2+-binding canonical EF-hand motif (cEF), a non-Ca2+ -binding hidden EF-hand hEF motif, and a sterile α-motif (SAM). The cytosolic C-terminal region includes the CC regions (CC1 and CC2), the SOAR region a Pro/His-rich (PH) and a Lys-rich domain (K). The STIM2.1 variant contains an eight-residue insert (VAASYLIQ) in its SOAR region (Miederer et al., 2015; Rana et al., 2015). (B) Cartoon depicting a possible model of STIM2.2 monomer in the coalescent state, where STIM1 solved crystal structures conserved within STIM2 are shown. CC1 is divided in CC1α1, CC1α2, and CC1α3 (Fahrner et al., 2014).
Both STIMs are single spanning transmembrane (TM) proteins that are located mainly in the ER (Roos et al., 2005; Zhang et al., 2005; Baba et al., 2006), but also in acidic stores (Zbidi et al., 2011) and in the PM (Sabbioni et al., 1999; Spassova et al., 2006; Jardin et al., 2013). The STIM N-terminal region is located in the intraluminal compartment (or the extracellular medium when located in the PM), harboring the canonical and hidden EF-hand (hEF) motives (for STIM1 aa 63–128; Liou et al., 2005; Roos et al., 2005). The Ca2+ binding canonical EF-hand is the Ca2+ sensor. Mutations within this region incapacitate Ca2+ association, thus, inducing constitutive Ca2+ entry (Spassova et al., 2006). Next, STIM presents the steril-α-motif (SAM) domain (aa 132–200) exhibiting distinct properties in STIM1 and STIM2 (Stathopulos et al., 2009; Zheng et al., 2011). SAM is followed by the TM domain (aa 214–343), which has been recently shown to undergo structural changes from the resting state, where Ca2+ is bond to the EF-hand, to the activated one, when store depletion occurs (Ma et al., 2015). Located in the cytosol, STIM C-terminus comprises 3 conserved coiled-coil (CC) domains, CC1 (aa 238–343), CC2 (aa 363–389), and CC3 (aa 363–389), the CRAC modulatory domain (CMD; aa 448–530), which includes the STIM1 homomerization domain SHD (aa 420–450), followed by a serine/proline rich region (aa 600–629) and a lysine-rich region (aa 671–685) at the very end of the C-terminus, that binds to membrane phospholipids, thus anchoring STIM1 toward its target (Liou et al., 2005). Furthermore, the polybasic lysine-rich region regulates Ca2+ entry by PM-resident STIM1 (Jardin et al., 2009, 2013). The long CC1 domain might be separated into CC1α1, CC1α2, and CC1α3 (Soboloff et al., 2012; Yang et al., 2012; Stathopulos et al., 2013). Furthermore, CC2 and CC3 domains, which comprise the SOAR domain, could be divided in four regions, Sα1, Sα2, Sα3, and Sα4 (Yang et al., 2012; Wang et al., 2014). Beside the differences between STIM1 and STIM2, the CC regions are highly conserved, presenting, however, noticeable disparity in their functions (Wang et al., 2014).
Orai Proteins
The Orai channels family is composed by three remarkably conserved homologs: Orai1, Orai2, and Orai3 (Feske et al., 2005; Mercer et al., 2006; Zhang et al., 2006; Gwack et al., 2007; Rothberg et al., 2013). A single Orai1 monomer spams four times the PM, exposing two loops (1 and 3) to the extracellular medium and with the N- and C-terminus domains and one loop (2) facing the cytoplasm (Figure 3). Both, N- and C- termini are required for STIM1 interaction and regulation (Muik et al., 2009; Park et al., 2009; Yuan et al., 2009; Derler et al., 2013; Palty et al., 2015; Palty and Isacoff, 2016).
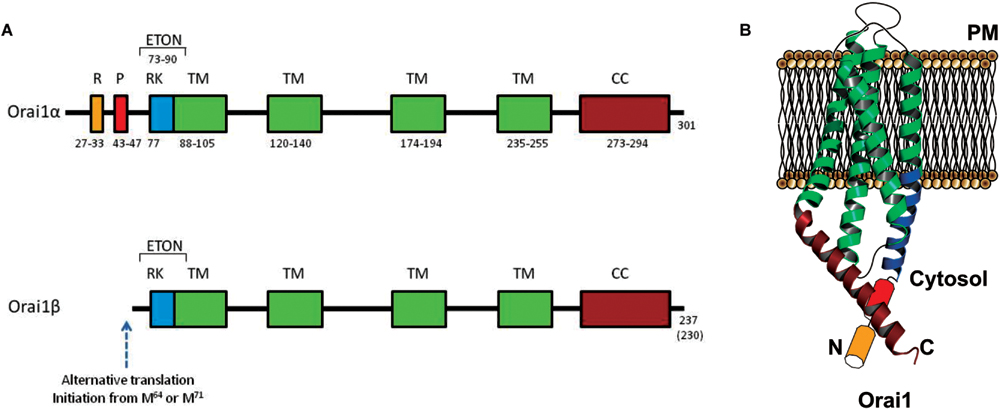
FIGURE 3. Molecular structure of Orai1α and Orai1β variants. (A) Orai1α and Orai1β architecture. R, arginin-rich region; P, proline-rich region; RK, arginine-lysine-rich region; TM, transmembrane domain; CC, coiled-coil domain (Yuan et al., 2009; Derler et al., 2012). Orai1β has an alternative translation initiation from methionine at position 64 or 71 (Fukushima et al., 2012). (B) Cartoon depicting Orai1α monomer crystal structure in the resting state.
Prior to its crystal structure determination in Drosophila, the human Orai1 channel was thought to form a tetramer with high selectivity to Ca2+ (Mignen et al., 2008b; Penna et al., 2008; Maruyama et al., 2009). Crystallization of Drosophila Orai1 showed a hexameric molecule permeable to Ca2+ as well as to monovalent ions in the presence of divalent cations (Hou et al., 2012; Thompson and Shuttleworth, 2013a). Furthermore, the Orai channels may form heteropentamers (3 Orai1 and 2 Orai3) to function as arachidonate-regulated Ca2+ (ARC) channels, a store-independent channel regulated by the STIM1 population located in the PM (Thompson and Shuttleworth, 2013b; Zhang et al., 2014).
Regarding CRAC channels, and despite that further studies are needed to determine human Orai1 structure, it is mostly accepted that each Orai1 channel comprises 6 Orai1 monomers, accurately arranged, forming the highly Ca2+ selective ion channel in the PM. The pore is located amid the hexamer, involving the six TM1 domains and including the residues 74–90 (ETON region) within the N-terminus (Derler et al., 2013) which contributes to STIM1 binding. Briefly, the pore acts as a funnel formed by the external vestibule, negatively charged (aa D110, D112, and D114) and supposed to attract Ca2+ to the immediacies to the pore; next the selectivity filter (aa E106); the hydrophobic cavity (aa V102, F99 and L95) and a basic region (aa R91, K87, and R83). It is surrounded by three rings, subsequently comprised by TM2, TM3, and TM4 (Hou et al., 2012). Even though TM2–4 do not form the pore themselves, it is known that several residues within those, are key regulators of the closed stated of Orai1. For instance, the Orai1 mutations L138F and P245L, located in TM2 and TM4, trigger constitutive currents and have been found to be causing tubular aggregate myopathy (TAM; Endo et al., 2015) and the Stormorken disease (Nesin et al., 2014), respectively. Included into the C-terminus, and in addition to the STIM1-binding region, Orai1 monomers exhibit a highly conserved hinge region, which allows the Orai1 subunits to pair with its neighbor in an antiparallel manner, and to coexist as dimers (Hou et al., 2012).
STIM1-Orai1 Coupling
STIM1-mediated Orai1 activation has been studied since their partnership was disclosed in 2006. ER Ca2+ depletion prompted by a stimulus leads to Ca2+ displacement from the STIM1 EF-hand and a number of rearrangements within the N-terminus domain, transferred through the TM domain to the C-termini, which culminates in Orai1 activation and Ca2+ entry. Nowadays and despite to the fact that a resting STIM1 crystal structure has proved elusive, it is common consensus that in its coalescent state, STIM1 forms dimers, its EF-hand domain is occupied by Ca2+, and that the Orai1 triggering regions are hidden from the channel (Fahrner et al., 2013). While STIM1 response obeys solely to large variations in [Ca2+]ER, STIM2 shows faster reaction to smaller changes in intraluminal Ca2+ (Stathopulos et al., 2009). As stated above, Ca2+ dissociation from the EF-hand changes both the later and SAM conformation exposing hydrophobic domains and habilitating the formation of STIM1 dimers and oligomers (Stathopulos et al., 2006, 2009). Next, the TM region, which has been recently shown to interact in an angled manner within the ER membrane, provides support to the control of the active/inactive state of STIM1 dimers. Upon ER depletion the angle lessened bringing the C-termini together (Ma et al., 2015). Ultimately, the signal travels through the molecule to the cytosolic domain where CC1, which is clamping the rest of C-terminal portion in a tight state, releases it in order to reinforce the oligomerizated conformation, via the SHD region, and to grant STIM1 activating regions access to Orai1 C- and N-terminus (Derler et al., 2013; Stathopulos et al., 2013; Fahrner et al., 2014). The interaction between STIM1 C-terminus and Orai1 C-terminus has been recently solved by NMR. In Stathopulos et al. (2013) have demonstrated that the critical positively charged residues mentioned above, K382, K284, K385, and K386, two aromatic ones Y361 and Y362; and finally four hydrophobic amino acids L347, L351, L373, and A376 are the key players within STIM1 to interact with Orai1; meanwhile the channel includes the residues L273, L276, R281, L286, R289 from its C-terminus, forming what the authors have named the STIM1-Orai1 Association Pocket (SOAP; Stathopulos et al., 2013). However, the association between STIM1 and Orai1 N-terminus is still yet completely unsolved and further approaches are required to fully understand the STIM1-mediated gating mechanism of Orai1.
Recent studies have revealed the expression of splice variants of STIM1, STIM2, and Orai1 in different cell types. It has been reported that over 95% multiexonal proteins in vertebrates undergo alternative splicing (Kornblihtt et al., 2013), which expands the functional diversity of a number of genes. Here we present the most prominent STIM1 and Orai1 variants and the differences among them.
STIM Splicing Variants
STIM1
As mentioned before, upon cell stimulation, the ER Ca2+ concentration decreases and Ca2+ unbinds from the canonical EF-hand, leading to an oligomerization of STIM1 molecules followed by a translocation toward the PM and aggregation as punctae structures (Lewis, 2007). Subsequently, STIM1 C-terminal region unfolds, exposing the SOAR domain and locating the polybasic lysine-rich region close to the SOC channels.
Darbellay et al. (2011) an alternatively spliced long variant of STIM1 (STIM1L, L for long to differentiate it from the conventional STIM1 isofom of 90 kDa) was identified in adult human muscle fibers and in in vitro–differentiated myotubes. STIM1L was described to be the product of an alternative splicing on exon 11, and contains an extra 106 residues (aa 515–620) inserted in the cytosolic region (Figure 1), an actin-binding domain, that allows STIM1L to interact with Orai1 Ca2+ channels to form permanent clusters (Darbellay et al., 2011).
While STIM1 is ubiquitously expressed, STIM1L is expressed in human skeletal muscle (Horinouchi et al., 2012), in skeletal muscle, as well as in heart and brain of mice (Darbellay et al., 2011) and in neonatal rat cardiomyocytes (Luo et al., 2012). The expression of STIM1L, as well as that of the conventional STIM1 variant, decreased in adult rat cardiomyocytes, where their expression levels have been found to be upregulated under pathological cardiac hypertrophy (Luo et al., 2012).
The function of STIM1L has been associated to the particularly rapid maximal activation of SOCE in skeletal muscle cells (<1 s) in comparison with other cells where full SOCE activation requires several seconds (>5 s in human platelets (Redondo et al., 2006), and up to 260 s in other cell types (Parekh and Putney, 2005; Liou et al., 2007). STIM1L was initially found to allow rapid activation of SOCE and is required to trigger repetitive cytosolic Ca2+ signals (Darbellay et al., 2011). The rapid activation of SOCE in STIM1L expressing cells was proposed to rely on the interaction between STIM1L and Orai1 at rest even when Ca2+ stores were full. This interaction was suggested to be stabilized by STIM1L-actin filament association as actin depolymerization has been found to disrupt STIM1L–Orai1 complexes at rest, which, subsequently delays SOCE activation (Darbellay et al., 2011). More recent studies have revealed that both STIM1 and STIM1L are distributed throughout the cortical ER vesicles, while Orai1 channels are localized in the PM. Following agonist stimulation and reduction in ER Ca2+ concentration STIM1 induces cortical ER expansion by a mechanism that requires the lysine-rich motif thus recruiting Orai1 channels in large ER–PM clusters. By contrast, STIM1L is unable to enlarge cortical ER structures and recruits Orai1 channels in reduced ER–PM clusters (Sauc et al., 2015). The greater efficiency of STIM1L mediating Ca2+ entry through SOCE has been hypothesized to occur due to the slower diffusion of Ca2+ in the cytoplasm in large STIM1–Orai1 clusters which might trap Ca2+ in the proximity of Orai1 channels promoting Ca2+-dependent inactivation of the channel (Sauc et al., 2015).
Furthermore, STIM1L has been reported to modulate store-independent Ca2+ entry through the TRPC channels TRPC3 and TRPC6. In HEK-293 cells stably expressing endothelin type A receptor STIM1L expression was found to attenuate receptor-operated Ca2+ entry via TRPC3 and TRPC6 more strongly that STIM1 by interaction with both channels (Horinouchi et al., 2012). Overexpression of STIM1 and STIM1L did not modify the expression level of TRPC3 and TRPC6. Although STIM1L exhibits a greater capacity to bind TRPC3 and TRPC6 than STIM1 (Horinouchi et al., 2012), which might suggest the recruitment of these channels into the store-dependent signalplex, the precise mechanism involved in the suppression of store-independent, receptor-operated, Ca2+ entry by STIM1 and STIM1L in this cell model has not been further clarified.
STIM2
The homologue of the STIM1 protein, STIM2 was identified in Williams et al. (2001) as a type I TM protein located in the ER and also identified in acidic organelles (Liou et al., 2005; Zbidi et al., 2011). The human STIM2 gene comprises 13 exons located at 4p15.1 (Williams et al., 2001), which lead to a variety of splice isoforms with different properties.
The best characterized STIM2 isoform is STIM2.2 (Miederer et al., 2015), also known as STIM2α (Rana et al., 2015). Human STIM2.2 consists of 833 amino acid with a molecular weight of 105 (115 kDa for the phosphorylated form), which shares amino acid sequence as well as domain architecture with STIM1 (Lopez et al., 2012). STIM2.2 mRNA is encoded by 12 exons (exons 1–8 and 10–13).
As previously mentioned for STIM1, the N-terminal region of STIM2 is located in the lumen of the ER and comprises a canonical EF-hand motif, a “hidden” EF-hand motif, and a SAM, (Soboloff et al., 2006). The canonical EF-hand motif has the ability to bind Ca2+ and exhibits an affinity for Ca2+ greater than that of STIM1 (STIM2 EF-hand motif Kd∼0.5 mM, STIM1 EF-hand motif Kd∼0.6 mM; Stathopulos et al., 2006; Zheng et al., 2008), therefore, STIM2 protein shows a greater sensitivity to minor changes in ER Ca2+ concentration as compared to STIM1. As a result, STIM2 has been reported to be partially active at resting ER Ca2+ concentrations and further actives by small reductions in ER Ca2+ concentrations, while STIM1 requires much larger reductions in ER Ca2+ concentration, such as those induced by physiological agonists, to become active (Brandman et al., 2007). Such greater sensitivity for free Ca2+ confers STIM2 the ability to sense ER Ca2+ concentration fluctuations as well as to activate earlier than STIM1 upon agonist-induced ER Ca2+ store discharge (Brandman et al., 2007).
The hEF domain, which is unable to bind Ca2+, plays an important role in the stability of the canonical EF-hand motif and SAM domains (Stathopulos et al., 2006, 2008; Zheng et al., 2011). On the other hand, the SAM domain plays an essential role in STIM oligomerization (Zheng et al., 2008, 2011).
The C-terminal region of STIM2 is located in the cytosol and comprises an ezrin/radixin/moesin (ERM) domain that contains three CC domains (CC1-3) including the including the SOAR/CAD, which has been reported to bind to Orai and TRPC channels (Yuan et al., 2009; Lee et al., 2014; Prakriya and Lewis, 2015). STIM2 structure differs from STIM1 in the adjacent proline- and histidine-rich (P/H) motif (PHAPHPSHPRHPHHPQHTPHSLPSPDP) located in a position that resembles that of the serine- and proline-rich (S/P) region present in STIM1 (SPSAPPGGSPHLDSSRSHSPSSPDPDTPSP), whose function is still unclear. From this point the sequences of STIM1 and STIM2 are significantly different, except for the distal lysine-rich (K) motif which consists of 14 amino acids in STIM1 (five lysines) and 17 residues in STIM2 (nine lysines; Williams et al., 2001).
The function of STIM2 differs from that of STIM1. While STIM1 is the main activator of SOCE, STIM2 mainly controls the resting cytosolic and ER Ca2+ concentrations and modulates prolonged Ca2+ entry and response to low concentrations of physiological agonists (Brandman et al., 2007; Oh-Hora et al., 2008). STIM2 is also involved in the regulation of the store-operated Imin Ca2+ channels in HEK-293 cells (Shalygin et al., 2015). In addition, the greater sensitivity of STIM2 to changes in ER Ca2+ concentration mentioned above leads to a role for STIM2 in the regulation of Ca2+ oscillations that differs from that attributed to STIM1. Thus, silencing of STIM2 expression has been reported to impair agonist-mediated Ca2+ oscillations at low levels of store depletion, without interfering with STIM1-mediated Ca2+ responses induced by full store discharge (Thiel et al., 2013).
Although the role of STIM2 in the activation of SOCE and its interaction with store-operated channels has been less investigated than that of STIM1, it has been reported that STIM2 can interact functionally with overexpressed as well as endogenously expressed Orai1, -2, and -3 and TRPC1 (Brandman et al., 2007; Parvez et al., 2008; Bandyopadhyay et al., 2011; Zbidi et al., 2011; Berna-Erro et al., 2012; Kar et al., 2012; Stanisz et al., 2014); however, whether STIM2 is relevant for the activation of store-independent Ca2+ entry remains unclear.
Two recent studies have identified three STIM2 splice variants: STIM2.1, STIM2.2, and STIM2.3 (Miederer et al., 2015; Rana et al., 2015). The structure and function described above for STIM2 concerns the STIM2.2 variant (Figure 2), the conventional isoform of STIM2, which will be named STIM2.2 from now on.
STIM2.1, also known as STIM2β, has been reported to contain an eight-residue insert (VAASYLIQ) in its SOAR/CAD region, encoded by an additional exon 9, that disrupts binding to Orai (Miederer et al., 2015; Rana et al., 2015; Figure 2). The expression of the STIM2.1 variant has been reported to be ubiquitous and its abundance relative to STIM2.2 depends upon the cell type but is significantly high in naive T cells, where the expression of both variants is similar (Miederer et al., 2015). In contrast to the role of STIM2.2 as an activator of SOCE, STIM2.1 has been shown to play an inhibitory role. STIM2.1 knockdown increases SOCE in CD4+ T cells, while overexpression of STIM2.1 decreases SOCE (Miederer et al., 2015).
The mechanism underlying the inhibitory role of the STIM2.1 variant in SOCE remains unclear. STIM2.1 by itself has been reported to interact poorly with Orai1 as detected by FRET or puncta formation assays (Rana et al., 2015); however, it has been indicated that STIM2.1 might heterodimerize with STIM1 or STIM2.2, which might recruit it to Orai1 channels, increasing the possibility to inhibit SOCE despite its low affinity for the channel. When STIM2.1 is recruited to the Orai1 channel signalplex, it might inhibit SOCE passively by direct interaction with STIM1 or STIM2.2, thus reducing the number of SOAR/CAD domains available for channel activation. However, this passive inhibition is unlikely to play a significant role in the modulation of SOCE under physiological conditions, since STIM2.1 is generally not as highly expressed as STIM1, and, therefore, a stronger active inhibitory role has also been hypothesized (Rana et al., 2015).
The transcript of the third STIM2 variant, STIM2.3, contains an alternative exon 13 that leads to an upstream end of translation and a transcript shortened by 444 bp, which results in a protein with approximately 17 kDa smaller (Miederer et al., 2015; Figure 2). The function of the STIM2.3 variant is unknown at present and its expression seems to be quite limited and has not been detected in lymphocytes, where the other two variants are significantly expressed (Miederer et al., 2015).
Orai1 Splicing Variants
Two variants of Orai1, Orai1α, and Orai1β, have been found to be expressed. Orai1α is the conventional variant of 301 amino acids (∼33 kDa, although the predicted molecular weight might be significantly modified by post-translational modifications, such as glycosylation on the asparagine residue at position 223 (Gwack et al., 2007) or phosphorylation on serine residues at positions 27 and 30 (Kawasaki et al., 2010). The short Orai1 variant, Orai1β, is generated by alternative translation initiation from a methionine at position 64, and possibly also 71, leading to a protein of approximately 23 kDa. Both Orai1 variants have been found to be ubiquitously expressed in human cell lines from a number of tissues, including HEK293 cells, Jurkat T cells, HeLa cells, epidermal HaCaT cells or the T84 lung carcinoma cell line, and show similar cellular localization (Fukushima et al., 2012).
The sequence upstream of the translation initiation of Orai1β includes a proline-rich motif previously suggested to be important for Orai1 gating by STIM1 (Takahashi et al., 2007), and an arginine-rich sequence that has been found to be involved in the interaction of Orai1 with PM phosphatidylinositol-4,5-bisphosphate that might be important for the mobility of Orai1 in the PM (Calloway et al., 2011). In agreement with the latter, Fukushima and coworkers have reported that Orai1β has faster mobility in the PM (Fukushima et al., 2012).
A recent report by Desai et al. (2015) has revealed that both Orai1 variants might be subunits of the store-operated CRAC and SOC channels, with some biophysical differences that includes a stronger Ca2+-dependent inactivation of Orai1α. However, the most significant functional difference between these variants lies in the participation of Orai1α, but not Orai1β, in the ARC channels (Desai et al., 2015), although the molecular mechanisms underlying the different biological significance of both variants remain unclear.
Summarizing, different STIM1, STIM2, and Orai1 variants have been reported to be expressed as a result of alternative splicing in a number of cell types. The expression of certain variants, such as STIM1L, is quite restricted while that of other variants is ubiquitous. As it has been hypothesized (Kornblihtt et al., 2013), alternative splicing might expands the functional diversity of multiexonal genes. Consistent with this, significant functional differences have been reported between STIM1 and STIM1L, STIM2.1 and STIM2.2 and between the α and β variants of Orai1, which lead to distinct mechanisms of regulation of Ca2+ entry through store-operated (CRAC and SOC) as well as store-independent (ARC) channels. The analysis of the expression ratios of the different variants in a particular cellular model might be of great interest to understand the fine modulation of Ca2+ entry.
Author Contributions
JR designed the manuscript, contribute to write it and performed the final edition. RD, TS, and IJ contributed to write the manuscript and discussion.
Conflict of Interest Statement
The authors declare that the research was conducted in the absence of any commercial or financial relationships that could be construed as a potential conflict of interest.
Acknowledgments
This work was supported by MINECO (Grants BFU2013-45564-C2-1-P and BFU2013-45564-C2-2-P) and Junta de Extremadura-FEDER (GR15029).
References
Baba, Y., Hayashi, K., Fujii, Y., Mizushima, A., Watarai, H., Wakamori, M., et al. (2006). Coupling of STIM1 to store-operated Ca2+ entry through its constitutive and inducible movement in the endoplasmic reticulum. Proc. Natl. Acad. Sci. U.S.A. 103, 16704–16709. doi: 10.1073/pnas.0608358103
Bandyopadhyay, B. C., Pingle, S. C., and Ahern, G. P. (2011). Store-operated Ca(2)+ signaling in dendritic cells occurs independently of STIM1. J. Leukoc. Biol. 89, 57–62. doi: 10.1189/jlb.0610381
Berna-Erro, A., Galan, C., Dionisio, N., Gomez, L. J., Salido, G. M., and Rosado, J. A. (2012). Capacitative and non-capacitative signaling complexes in human platelets. Biochim. Biophys. Acta 1823, 1242–1251. doi: 10.1016/j.bbamcr.2012.05.023
Berridge, M. J., Bootman, M. D., and Roderick, H. L. (2003). Calcium signalling: dynamics, homeostasis and remodelling. Nat. Rev. Mol. Cell Biol. 4, 517–529. doi: 10.1038/nrm1155
Brandman, O., Liou, J., Park, W. S., and Meyer, T. (2007). STIM2 is a feedback regulator that stabilizes basal cytosolic and endoplasmic reticulum Ca2+ levels. Cell 131, 1327–1339. doi: 10.1016/j.cell.2007.11.039
Cahalan, M. D. (2009). STIMulating store-operated Ca(2+) entry. Nat. Cell Biol. 11, 669–677. doi: 10.1038/ncb0609-669
Calloway, N., Owens, T., Corwith, K., Rodgers, W., Holowka, D., and Baird, B. (2011). Stimulated association of STIM1 and Orai1 is regulated by the balance of PtdIns(4,5)P(2) between distinct membrane pools. J. Cell Sci. 124, 2602–2610. doi: 10.1242/jcs.084178
Cheng, K. T., Ong, H. L., Liu, X., and Ambudkar, I. S. (2011). Contribution of TRPC1 and Orai1 to Ca(2+) entry activated by store depletion. Adv. Exp. Med. Biol. 704, 435–449. doi: 10.1007/978-94-007-0265-3_24
Cheng, K. T., Ong, H. L., Liu, X., and Ambudkar, I. S. (2013). Contribution and regulation of TRPC channels in store-operated Ca2+ entry. Curr. Top. Membr. 71, 149–179. doi: 10.1016/B978-0-12-407870-3.00007-X
Choi, S., Maleth, J., Jha, A., Lee, K. P., Kim, M. S., So, I., et al. (2014). The TRPCs-STIM1-orai interaction. Handb. Exp. Pharmacol. 223, 1035–1054. doi: 10.1007/978-3-319-05161-1_13
Covington, E. D., Wu, M. M., and Lewis, R. S. (2010). Essential role for the CRAC activation domain in store-dependent oligomerization of STIM1. Mol. Biol. Cell 21, 1897–1907. doi: 10.1091/mbc.E10-02-0145
Darbellay, B., Arnaudeau, S., Bader, C. R., Konig, S., and Bernheim, L. (2011). STIM1L is a new actin-binding splice variant involved in fast repetitive Ca2+ release. J. Cell Biol. 194, 335–346. doi: 10.1083/jcb.201012157
Derler, I., Madl, J., Schutz, G., and Romanin, C. (2012). Structure, regulation and biophysics of I(CRAC), STIM/Orai1. Adv. Exp. Med. Biol. 740, 383–410. doi: 10.1007/978-94-007-2888-2_16
Derler, I., Plenk, P., Fahrner, M., Muik, M., Jardin, I., Schindl, R., et al. (2013). The extended transmembrane Orai1 N-terminal (ETON) region combines binding interface and gate for Orai1 activation by STIM1. J. Biol. Chem. 288, 29025–29034. doi: 10.1074/jbc.M113.501510
Desai, P. N., Zhang, X., Wu, S., Janoshazi, A., Bolimuntha, S., Putney, J. W., et al. (2015). Multiple types of calcium channels arising from alternative translation initiation of the Orai1 message. Sci. Signal. 8, ra74. doi: 10.1126/scisignal.aaa8323
Endo, Y., Noguchi, S., Hara, Y., Hayashi, Y. K., Motomura, K., Miyatake, S., et al. (2015). Dominant mutations in ORAI1 cause tubular aggregate myopathy with hypocalcemia via constitutive activation of store-operated Ca(2)(+) channels. Hum. Mol. Genet. 24, 637–648. doi: 10.1093/hmg/ddu477
Fahrner, M., Derler, I., Jardin, I., and Romanin, C. (2013). The STIM1/Orai signaling machinery. Channels (Austin) 7, 330–343. doi: 10.4161/chan.26742
Fahrner, M., Muik, M., Schindl, R., Butorac, C., Stathopulos, P., Zheng, L., et al. (2014). A coiled-coil clamp controls both conformation and clustering of stromal interaction molecule 1 (STIM1). J. Biol. Chem. 289, 33231–33244. doi: 10.1074/jbc.M114.610022
Feske, S., Gwack, Y., Prakriya, M., Srikanth, S., Puppel, S. H., Tanasa, B., et al. (2006). A mutation in Orai1 causes immune deficiency by abrogating CRAC channel function. Nature 441, 179–185. doi: 10.1038/nature04702
Feske, S., Prakriya, M., Rao, A., and Lewis, R. S. (2005). A severe defect in CRAC Ca2+ channel activation and altered K+ channel gating in T cells from immunodeficient patients. J. Exp. Med. 202, 651–662. doi: 10.1084/jem.20050687
Fukushima, M., Tomita, T., Janoshazi, A., and Putney, J. W. (2012). Alternative translation initiation gives rise to two isoforms of Orai1 with distinct plasma membrane mobilities. J. Cell Sci. 125, 4354–4361. doi: 10.1242/jcs.104919
Gwack, Y., Srikanth, S., Feske, S., Cruz-Guilloty, F., Oh-Hora, M., Neems, D. S., et al. (2007). Biochemical and functional characterization of Orai proteins. J. Biol. Chem. 282, 16232–16243. doi: 10.1074/jbc.M609630200
Horinouchi, T., Higashi, T., Higa, T., Terada, K., Mai, Y., Aoyagi, H., et al. (2012). Different binding property of STIM1 and its novel splice variant STIM1L to Orai1, TRPC3, and TRPC6 channels. Biochem. Biophys. Res. Commun. 428, 252–258. doi: 10.1016/j.bbrc.2012.10.034
Hou, X., Pedi, L., Diver, M. M., and Long, S. B. (2012). Crystal structure of the calcium release-activated calcium channel Orai. Science 338, 1308–1313. doi: 10.1126/science.1228757
Huang, G. N., Zeng, W., Kim, J. Y., Yuan, J. P., Han, L., Muallem, S., et al. (2006). STIM1 carboxyl-terminus activates native SOC, I(crac) and TRPC1 channels. Nat. Cell Biol. 8, 1003–1010. doi: 10.1038/ncb1454
Jardin, I., Dionisio, N., Frischauf, I., Berna-Erro, A., Woodard, G. E., Lopez, J. J., et al. (2013). The polybasic lysine-rich domain of plasma membrane-resident STIM1 is essential for the modulation of store-operated divalent cation entry by extracellular calcium. Cell. Signal. 25, 1328–1337. doi: 10.1016/j.cellsig.2013.01.025
Jardin, I., Lopez, J. J., Redondo, P. C., Salido, G. M., and Rosado, J. A. (2009). Store-operated Ca2+ entry is sensitive to the extracellular Ca2+ concentration through plasma membrane STIM1. Biochim. Biophys. Acta 1793, 1614–1622. doi: 10.1016/j.bbamcr.2009.07.003
Jardin, I., Lopez, J. J., Salido, G. M., and Rosado, J. A. (2008). Orai1 mediates the interaction between STIM1 and hTRPC1 and regulates the mode of activation of hTRPC1-forming Ca2+ channels. J. Biol. Chem. 283, 25296–25304. doi: 10.1074/jbc.M802904200
Kar, P., Bakowski, D., Di Capite, J., Nelson, C., and Parekh, A. B. (2012). Different agonists recruit different stromal interaction molecule proteins to support cytoplasmic Ca2+ oscillations and gene expression. Proc. Natl. Acad. Sci. U.S.A. 109, 6969–6974. doi: 10.1073/pnas.1201204109
Kawasaki, T., Lange, I., and Feske, S. (2009). A minimal regulatory domain in the C terminus of STIM1 binds to and activates ORAI1 CRAC channels. Biochem. Biophys. Res. Commun. 385, 49–54. doi: 10.1016/j.bbrc.2009.05.020
Kawasaki, T., Ueyama, T., Lange, I., Feske, S., and Saito, N. (2010). Protein kinase C-induced phosphorylation of Orai1 regulates the intracellular Ca2+ level via the store-operated Ca2+ channel. J. Biol. Chem. 285, 25720–25730. doi: 10.1074/jbc.M109.022996
Kornblihtt, A. R., Schor, I. E., Allo, M., Dujardin, G., Petrillo, E., and Munoz, M. J. (2013). Alternative splicing: a pivotal step between eukaryotic transcription and translation. Nat. Rev. Mol. Cell Biol. 14, 153–165. doi: 10.1038/nrm3525
Lee, K. P., Choi, S., Hong, J. H., Ahuja, M., Graham, S., Ma, R., et al. (2014). Molecular determinants mediating gating of Transient Receptor Potential Canonical (TRPC) channels by stromal interaction molecule 1 (STIM1). J. Biol. Chem. 289, 6372–6382. doi: 10.1074/jbc.M113.546556
Lewis, R. S. (2007). The molecular choreography of a store-operated calcium channel. Nature 446, 284–287. doi: 10.1038/nature05637
Liou, J., Fivaz, M., Inoue, T., and Meyer, T. (2007). Live-cell imaging reveals sequential oligomerization and local plasma membrane targeting of stromal interaction molecule 1 after Ca2+ store depletion. Proc. Natl. Acad. Sci. U.S.A. 104, 9301–9306. doi: 10.1073/pnas.0702866104
Liou, J., Kim, M. L., Heo, W. D., Jones, J. T., Myers, J. W., Ferrell, J. E., et al. (2005). STIM is a Ca2+ sensor essential for Ca2+-store-depletion-triggered Ca2+ influx. Curr. Biol. 15, 1235–1241. doi: 10.1016/j.cub.2005.05.055
Lopez, E., Salido, G. M., Rosado, J. A., and Berna-Erro, A. (2012). Unraveling STIM2 function. J. Physiol. Biochem. 68, 619–633. doi: 10.1007/s13105-012-0163-1
Luik, R. M., Wang, B., Prakriya, M., Wu, M. M., and Lewis, R. S. (2008). Oligomerization of STIM1 couples ER calcium depletion to CRAC channel activation. Nature 454, 538–542. doi: 10.1038/nature07065
Luo, X., Hojayev, B., Jiang, N., Wang, Z. V., Tandan, S., Rakalin, A., et al. (2012). STIM1-dependent store-operated Ca(2)(+) entry is required for pathological cardiac hypertrophy. J. Mol. Cell. Cardiol. 52, 136–147. doi: 10.1016/j.yjmcc.2011.11.003
Ma, G., Wei, M., He, L., Liu, C., Wu, B., Zhang, S. L., et al. (2015). Inside-out Ca(2+) signalling prompted by STIM1 conformational switch. Nat. Commun. 6, 7826. doi: 10.1038/ncomms8826
Maruyama, Y., Ogura, T., Mio, K., Kato, K., Kaneko, T., Kiyonaka, S., et al. (2009). Tetrameric Orai1 is a teardrop-shaped molecule with a long, tapered cytoplasmic domain. J. Biol. Chem. 284, 13676–13685. doi: 10.1074/jbc.M900812200
Mercer, J. C., Dehaven, W. I., Smyth, J. T., Wedel, B., Boyles, R. R., Bird, G. S., et al. (2006). Large store-operated calcium selective currents due to co-expression of Orai1 or Orai2 with the intracellular calcium sensor, Stim1. J. Biol. Chem. 281, 24979–24990. doi: 10.1074/jbc.M604589200
Miederer, A. M., Alansary, D., Schwar, G., Lee, P. H., Jung, M., Helms, V., et al. (2015). A STIM2 splice variant negatively regulates store-operated calcium entry. Nat. Commun. 6, 6899. doi: 10.1038/ncomms7899
Mignen, O., Thompson, J. L., and Shuttleworth, T. J. (2007). STIM1 regulates Ca2+ entry via arachidonate-regulated Ca2+-selective (ARC) channels without store-depletion or translocation to the plasma membrane. J. Physiol. (Lond.) 579(Pt 3), 703–715. doi: 10.1113/jphysiol.2006.122432
Mignen, O., Thompson, J. L., and Shuttleworth, T. J. (2008a). Both Orai1 and Orai3 are essential components of the arachidonate-regulated Ca2--selective (ARC) channels. J. Physiol. 586, 185–195. doi: 10.1113/jphysiol.2007.146258
Mignen, O., Thompson, J. L., and Shuttleworth, T. J. (2008b). Orai1 subunit stoichiometry of the mammalian CRAC channel pore. J. Physiol. 586, 419–425. doi: 10.1113/jphysiol.2007.147249
Mignen, O., Thompson, J. L., and Shuttleworth, T. J. (2009). The molecular architecture of the arachidonate-regulated Ca2+-selective ARC channel is a pentameric assembly of Orai1 and Orai3 subunits. J. Physiol. 587, 4181–4197. doi: 10.1113/jphysiol.2009.174193
Muik, M., Fahrner, M., Derler, I., Schindl, R., Bergsmann, J., Frischauf, I., et al. (2009). A cytosolic homomerization and a modulatory domain within STIM1 C terminus determine coupling to ORAI1 channels. J. Biol. Chem. 284, 8421–8426. doi: 10.1074/jbc.C800229200
Nesin, V., Wiley, G., Kousi, M., Ong, E. C., Lehmann, T., Nicholl, D. J., et al. (2014). Activating mutations in STIM1 and ORAI1 cause overlapping syndromes of tubular myopathy and congenital miosis. Proc. Natl. Acad. Sci. U.S.A. 111, 4197–4202. doi: 10.1073/pnas.1312520111
Oh-Hora, M., Yamashita, M., Hogan, P. G., Sharma, S., Lamperti, E., Chung, W., et al. (2008). Dual functions for the endoplasmic reticulum calcium sensors STIM1 and STIM2 in T cell activation and tolerance. Nat. Immunol. 9, 432–443. doi: 10.1038/ni1574
Oritani, K., and Kincade, P. W. (1996). Identification of stromal cell products that interact with pre-B cells. J. Cell Biol. 134, 771–782. doi: 10.1083/jcb.134.3.771
Palty, R., and Isacoff, E. Y. (2016). Cooperative binding of stromal interaction molecule 1 (STIM1) to the N and C termini of calcium release-activated calcium modulator 1 (Orai1). J. Biol. Chem. 291, 334–341. doi: 10.1074/jbc.M115.685289
Palty, R., Stanley, C., and Isacoff, E. Y. (2015). Critical role for Orai1 C-terminal domain and TM4 in CRAC channel gating. Cell Res. 25, 963–980. doi: 10.1038/cr.2015.80
Parekh, A. B., and Putney, J. W. Jr (2005). Store-operated calcium channels. Physiol. Rev. 85, 757–810. doi: 10.1152/physrev.00057.2003
Park, C. Y., Hoover, P. J., Mullins, F. M., Bachhawat, P., Covington, E. D., Raunser, S., et al. (2009). STIM1 clusters and activates CRAC channels via direct binding of a cytosolic domain to Orai1. Cell 136, 876–890. doi: 10.1016/j.cell.2009.02.014
Parvez, S., Beck, A., Peinelt, C., Soboloff, J., Lis, A., Monteilh-Zoller, M., et al. (2008). STIM2 protein mediates distinct store-dependent and store-independent modes of CRAC channel activation. FASEB J. 22, 752–761. doi: 10.1096/fj.07-9449com
Peinelt, C., Vig, M., Koomoa, D. L., Beck, A., Nadler, M. J., Koblan-Huberson, M., et al. (2006). Amplification of CRAC current by STIM1 and CRACM1 (Orai1). Nat. Cell Biol. 8, 771–773. doi: 10.1038/ncb1435
Penna, A., Demuro, A., Yeromin, A. V., Zhang, S. L., Safrina, O., Parker, I., et al. (2008). The CRAC channel consists of a tetramer formed by Stim-induced dimerization of Orai dimers. Nature 456, 116–120. doi: 10.1038/nature07338
Prakriya, M., Feske, S., Gwack, Y., Srikanth, S., Rao, A., and Hogan, P. G. (2006). Orai1 is an essential pore subunit of the CRAC channel. Nature 443, 230–233. doi: 10.1038/nature05122
Prakriya, M., and Lewis, R. S. (2015). Store-operated calcium channels. Physiol. Rev. 95, 1383–1436. doi: 10.1152/physrev.00020.2014
Putney, J. W. Jr. (1986). A model for receptor-regulated calcium entry. Cell Calcium 7, 1–12. doi: 10.1016/0143-4160(86)90026-6
Rana, A., Yen, M., Sadaghiani, A. M., Malmersjo, S., Park, C. Y., Dolmetsch, R. E., et al. (2015). Alternative splicing converts STIM2 from an activator to an inhibitor of store-operated calcium channels. J. Cell Biol. 209, 653–669. doi: 10.1083/jcb.201412060
Redondo, P. C., Harper, M. T., Rosado, J. A., and Sage, S. O. (2006). A role for cofilin in the activation of store-operated calcium entry by de novo conformational coupling in human platelets. Blood 107, 973–979. doi: 10.1182/blood-2005-05-2015
Redondo, P. C., and Rosado, J. A. (2015). Store-operated calcium entry: unveiling the calcium handling signalplex. Int. Rev. Cell Mol. Biol. 316, 183–226. doi: 10.1016/bs.ircmb.2015.01.007
Rodriguez-Moyano, M., Diaz, I., Dionisio, N., Zhang, X., Avila-Medina, J., Calderon-Sanchez, E., et al. (2013). Urotensin-II promotes vascular smooth muscle cell proliferation through store operated calcium entry and EGFR transactivation. Cardiovasc. Res. 100, 297–306. doi: 10.1093/cvr/cvt196
Roos, J., Digregorio, P. J., Yeromin, A. V., Ohlsen, K., Lioudyno, M., Zhang, S., et al. (2005). STIM1, an essential and conserved component of store-operated Ca2+ channel function. J. Cell Biol. 169, 435–445. doi: 10.1083/jcb.200502019
Rosado, J. A., and Sage, S. O. (2000). Coupling between inositol 1,4,5-trisphosphate receptors and human transient receptor potential channel 1 when intracellular Ca2+ stores are depleted. Biochem. J. 350(Pt 3), 631–635. doi: 10.1042/bj3500631
Rothberg, B. S., Wang, Y., and Gill, D. L. (2013). Orai channel pore properties and gating by STIM: implications from the Orai crystal structure. Sci. Signal. 6, e9. doi: 10.1126/scisignal.2003971
Sabbioni, S., Veronese, A., Trubia, M., Taramelli, R., Barbanti-Brodano, G., Croce, C. M., et al. (1999). Exon structure and promoter identification of STIM1 (alias GOK), a human gene causing growth arrest of the human tumor cell lines G401 and RD. Cytogenet. Cell Genet. 86, 214–218. doi: 10.1159/000015341
Sauc, S., Bulla, M., Nunes, P., Orci, L., Marchetti, A., Antigny, F., et al. (2015). STIM1L traps and gates Orai1 channels without remodeling the cortical ER. J. Cell Sci. 128, 1568–1579. doi: 10.1242/jcs.164228
Shalygin, A., Skopin, A., Kalinina, V., Zimina, O., Glushankova, L., Mozhayeva, G. N., et al. (2015). STIM1 and STIM2 proteins differently regulate endogenous store-operated channels in HEK293 cells. J. Biol. Chem. 290, 4717–4727. doi: 10.1074/jbc.M114.601856
Singh, B. B., Liu, X., and Ambudkar, I. S. (2000). Expression of truncated transient receptor potential protein 1alpha (Trp1alpha ): evidence that the Trp1 C terminus modulates store-operated Ca2+ entry. J. Biol. Chem. 275, 36483–36486. doi: 10.1074/jbc.C000529200
Soboloff, J., Rothberg, B. S., Madesh, M., and Gill, D. L. (2012). STIM proteins: dynamic calcium signal transducers. Nat. Rev. Mol. Cell Biol. 13, 549–565. doi: 10.1038/nrm3414
Soboloff, J., Spassova, M. A., Hewavitharana, T., He, L. P., Xu, W., Johnstone, L. S., et al. (2006). STIM2 is an inhibitor of STIM1-mediated store-operated Ca2+ Entry. Curr. Biol. 16, 1465–1470. doi: 10.1016/j.cub.2006.05.051
Spassova, M. A., Soboloff, J., He, L. P., Xu, W., Dziadek, M. A., and Gill, D. L. (2006). STIM1 has a plasma membrane role in the activation of store-operated Ca(2+) channels. Proc. Natl. Acad. Sci. U.S.A. 103, 4040–4045. doi: 10.1073/pnas.0510050103
Stanisz, H., Saul, S., Muller, C. S., Kappl, R., Niemeyer, B. A., Vogt, T., et al. (2014). Inverse regulation of melanoma growth and migration by Orai1/STIM2-dependent calcium entry. Pigment Cell Melanoma Res. 27, 442–453. doi: 10.1111/pcmr.12222
Stathopulos, P. B., Li, G. Y., Plevin, M. J., Ames, J. B., and Ikura, M. (2006). Stored Ca2+ depletion-induced oligomerization of stromal interaction molecule 1 (STIM1) via the EF-SAM region: an initiation mechanism for capacitive Ca2+ entry. J. Biol. Chem. 281, 35855–35862. doi: 10.1074/jbc.M608247200
Stathopulos, P. B., Schindl, R., Fahrner, M., Zheng, L., Gasmi-Seabrook, G. M., Muik, M., et al. (2013). STIM1/Orai1 coiled-coil interplay in the regulation of store-operated calcium entry. Nat. Commun. 4, 2963. doi: 10.1038/ncomms3963
Stathopulos, P. B., Zheng, L., and Ikura, M. (2009). Stromal interaction molecule (STIM) 1 and STIM2 calcium sensing regions exhibit distinct unfolding and oligomerization kinetics. J. Biol. Chem. 284, 728–732. doi: 10.1074/jbc.C800178200
Stathopulos, P. B., Zheng, L., Li, G. Y., Plevin, M. J., and Ikura, M. (2008). Structural and mechanistic insights into STIM1-mediated initiation of store-operated calcium entry. Cell 135, 110–122. doi: 10.1016/j.cell.2008.08.006
Takahashi, Y., Murakami, M., Watanabe, H., Hasegawa, H., Ohba, T., Munehisa, Y., et al. (2007). Essential role of the N-terminus of murine Orai1 in store-operated Ca2+ entry. Biochem. Biophys. Res. Commun. 356, 45–52. doi: 10.1016/j.bbrc.2007.02.107
Thiel, M., Lis, A., and Penner, R. (2013). STIM2 drives Ca2+ oscillations through store-operated Ca2+ entry caused by mild store depletion. J. Physiol. 591, 1433–1445. doi: 10.1113/jphysiol.2012.245399
Thompson, J. L., and Shuttleworth, T. J. (2013a). How many Orai’s does it take to make a CRAC channel? Nat. Sci. Rep. 3, 1961. doi: 10.1038/srep01961
Thompson, J. L., and Shuttleworth, T. J. (2013b). Molecular basis of activation of the arachidonate-regulated Ca2+ (ARC) channel, a store-independent Orai channel, by plasma membrane STIM1. J. Physiol. 591, 3507–3523. doi: 10.1113/jphysiol.2013.256784
Wang, X., Wang, Y., Zhou, Y., Hendron, E., Mancarella, S., Andrake, M. D., et al. (2014). Distinct Orai-coupling domains in STIM1 and STIM2 define the Orai-activating site. Nat. Commun. 5, 3183. doi: 10.1038/ncomms4183
Wang, Y., Deng, X., Mancarella, S., Hendron, E., Eguchi, S., Soboloff, J., et al. (2010). The calcium store sensor, STIM1, reciprocally controls Orai and CaV1.2 channels. Science 330, 105–109. doi: 10.1126/science.1191086
Williams, R. T., Manji, S. S., Parker, N. J., Hancock, M. S., Van Stekelenburg, L., Eid, J. P., et al. (2001). Identification and characterization of the STIM (stromal interaction molecule) gene family: coding for a novel class of transmembrane proteins. Biochem. J. 357, 673–685. doi: 10.1042/0264-6021:3570673
Yang, X., Jin, H., Cai, X., Li, S., and Shen, Y. (2012). Structural and mechanistic insights into the activation of Stromal interaction molecule 1 (STIM1). Proc. Natl. Acad. Sci. U.S.A. 109, 5657–5662. doi: 10.1073/pnas.1118947109
Yuan, J. P., Zeng, W., Dorwart, M. R., Choi, Y. J., Worley, P. F., and Muallem, S. (2009). SOAR and the polybasic STIM1 domains gate and regulate Orai channels. Nat. Cell Biol. 11, 337–343. doi: 10.1038/ncb1842
Yuan, J. P., Zeng, W., Huang, G. N., Worley, P. F., and Muallem, S. (2007). STIM1 heteromultimerizes TRPC channels to determine their function as store-operated channels. Nat. Cell Biol. 9, 636–645. doi: 10.1038/ncb1590
Zbidi, H., Jardin, I., Woodard, G. E., Lopez, J. J., Berna-Erro, A., Salido, G. M., et al. (2011). STIM1 and STIM2 are located in the acidic Ca2+ stores and associates with Orai1 upon depletion of the acidic stores in human platelets. J. Biol. Chem. 286, 12257–12270. doi: 10.1074/jbc.M110.190694
Zeng, W., Yuan, J. P., Kim, M. S., Choi, Y. J., Huang, G. N., Worley, P. F., et al. (2008). STIM1 gates TRPC channels, but not Orai1, by electrostatic interaction. Mol. Cell 32, 439–448. doi: 10.1016/j.molcel.2008.09.020
Zhang, S. L., Yeromin, A. V., Zhang, X. H. F., Yu, Y., Safrina, O., Penna, A., et al. (2006). Genome-wide RNAi screen of Ca2+ influx identifies genes that regulate Ca2+ release-activated Ca2+ channel activity. Proc. Natl. Acad. Sci. U.S.A. 103, 9357–9362. doi: 10.1073/pnas.0603161103
Zhang, S. L., Yu, Y., Roos, J., Kozak, J. A., Deerinck, T. J., Ellisman, M. H., et al. (2005). STIM1 is a Ca2+ sensor that activates CRAC channels and migrates from the Ca2+ store to the plasma membrane. Nature 437, 902–905. doi: 10.1038/nature04147
Zhang, X., Zhang, W., Gonzalez-Cobos, J. C., Jardin, I., Romanin, C., Matrougui, K., et al. (2014). Complex role of STIM1 in the activation of store-independent Orai1/3 channels. J. Gen. Physiol. 143, 345–359. doi: 10.1085/jgp.201311084
Zheng, L., Stathopulos, P. B., Li, G. Y., and Ikura, M. (2008). Biophysical characterization of the EF-hand and SAM domain containing Ca2+ sensory region of STIM1 and STIM2. Biochem. Biophys. Res. Commun. 369, 240–246. doi: 10.1016/j.bbrc.2007.12.129
Keywords: calcium entry, STIM1, STIM2, orai1, splice variants
Citation: Rosado JA, Diez R, Smani T and Jardín I (2016) STIM and Orai1 Variants in Store-Operated Calcium Entry. Front. Pharmacol. 6:325. doi: 10.3389/fphar.2015.00325
Received: 01 December 2015; Accepted: 30 December 2015;
Published: 13 January 2016.
Edited by:
Antonio Ferrer-Montiel, Universitas Miguel Hernández, SpainReviewed by:
Hiroshi Hibino, Niigata University, JapanCarlos Villalobos, Consejo Superior de Investigaciones Científicas, Spain
Copyright © 2016 Rosado, Diez, Smani and Jardín. This is an open-access article distributed under the terms of the Creative Commons Attribution License (CC BY). The use, distribution or reproduction in other forums is permitted, provided the original author(s) or licensor are credited and that the original publication in this journal is cited, in accordance with accepted academic practice. No use, distribution or reproduction is permitted which does not comply with these terms.
*Correspondence: Juan A. Rosado, amFyb3NhZG9AdW5leC5lcw==