- 1Department of Physiology and Pharmacology, University of Rome “Sapienza”, Rome, Italy
- 2European Brain Research Institute, Rita Levi-Montalcini Foundation, Rome, Italy
- 3Department of Biology, University of Rome Tor Vergata, Rome, Italy
Ginkgo leaf is the most used form of supplement for cognitive ailments. The standardized extract formulation EGb 761 is a dietary supplement with proven benefit in several neurological and psychiatric conditions including memory decline in Alzheimer’s disease, schizophrenia and dementia. Ginkgolic acid (GA) is a component of this extract which shows pleiotropic effects including antitumoral and anti-HIV action; however, its effect on memory is still unknown. Here, we carried out an electrophysiological analysis to investigate the effects of GA on long term potentiation and synaptic transmission at CA1 hippocampal synapses. We also evaluated the potential rescuing effect of GA on the synaptic dysfunction following in vitro application of Aβ. Data obtained indicate that GA exerts neuroprotective effects against Aβ-induced impairment of neurotransmitter release and synaptic plasticity.
Introduction
Ginkgo biloba L. (Mantissa Plantarum Altera, 1771, Ginkgoaceae) leaves and the nuts have been in use for several centuries in traditional Chinese medicine. However, it was only in the last 20–30 years that the use of Ginkgo leaf and its standardized extract formulation (EGb 761) has been widely used as a form of supplement for cognitive ailments (Mahadevan and Park, 2008). Ginkgo leaf extract has shown beneficial effect in different pathologies such as Alzheimer’s disease (AD), memory loss, cardiovascular disease, cancer, age-related macular degeneration, and psychiatric disorders like schizophrenia (Gessner et al., 1985; Kennedy et al., 2007; Ramassamy et al., 2007; Scripnikov et al., 2007). Ginkgolic acid (GA) is a component of EGb 761, currently used as memory enhancer and as a dietary supplement with proven benefit in several neurological and psychiatric conditions (Le Bars et al., 1997; Hsu et al., 1999). Nonetheless, because EGb 761 is a complex combination of several potentially active components that may be acting synergistically, the exact mechanism of action remains difficult to elucidate. AD is a neurodegenerative disorder characterized by the progressive loss of neurons, deposition of insoluble aggregates of two proteins in the brain, amyloid-β (Aβ), and the microtubule associated protein tau (MAPT). Synaptic deterioration occurs early in the disease, well before the formation of amyloid plaques and neuron loss (Thompson et al., 2003; Arendt, 2009). This early synaptic impairment is evident in different brain areas, including the hippocampus, and entorhinal cortex, which are involved in cognitive process and memory formation (Thompson et al., 2003; Arendt, 2009).
Ginkgolic acid has controversial effects, which were characterized in several studies (Ude et al., 2013). Indeed, GA is able to induce neuronal death at high concentrations (Ahlemeyer et al., 2001); accordingly, today all commercial preparations of Ginkgo leaf contain low dose of GA in order to minimize side effects (McKenna et al., 2001). However, there is recent evidence that GA has different protective effects, as well as antitumoral and anti-HIV effects (Wang H. et al., 2010; Zhou et al., 2010; Lü et al., 2012).
To shed same light on the potential effects of GA on memory, we carried out an electrophysiological study analyzing long term potentiation (LTP), a molecular mechanism which underlies learning and memory (Bliss and Collingridge, 1993), and excitatory synaptic transmission in the in vitro hippocampus. Also, we investigated the protective effects of GA against Aβ-induced synaptic impairment (Klyubin et al., 2008; Olsen and Sheng, 2012; Yao et al., 2013; Varga et al., 2015).
Materials and Methods
Slices Preparation
All experiments followed international guidelines on the ethical use of animals from the European Communities (Santa Lucia Foundation, Rome, Italy) Council Directive 2010/64/EU. C57BL6 mice (30–40 days old) were deeply anesthetized with halothane and killed by decapitation. The brain was rapidly removed from the skull and parasagittal hippocampal slices (250 μm thick) were cut with a vibratome (VT 1200S, Leica) in cold (0°C) artificial cerebrospinal fluid (aCSF) containing (in mM): NaCl 124; KCl 3; MgSO4 1; CaCl2 2; NaH2PO4 1.25; NaHCO3 26; glucose 10; saturated with 95% O2, 5% CO2 (pH 7.4; Cold Spring Harbor Protocols), and left to recover for 1 h in ACSF at room temperature.
Whole-Cell Patch Clamp Recordings
Individual slices were placed in a recording chamber, on the stage of an upright microscope (Zeiss, Germany) and submerged in a continuously flowing (3 ml/min) solution at 30°C (±2°C). Individual neurons were visualized through a 40× water-immersion objective (Olympus, Japan) connected to infrared video microscopy (Hamamatsu, Japan). Borosilicate glass electrodes (5–7 MΩ), pulled with a PP 83 Narishige puller, were filled with a solution containing the following (in mM): CsCH3SO3 115; CsCl 10; KCl 10; CaCl2 0.45; EGTA 1; Hepes 10; QX-314 5; Na3-GTP 0.3; Mg-ATP 4.0; pH adjusted to 7.3 pH with CsOH. Whole-cell voltage clamp (at -70 mV holding potential) or current clamp experiments were carried out with a MultiClamp 700B amplifier (Axon Instruments, Foster City, CA, USA), filtered at 1 kHz and digitized (5 kHz).
Excitatory post-synaptic currents (EPSCs) were elicited by monopolar stimulating electrode placed in stratum radiatum in order to stimulate Schaffer collateral fibers. LTP was induced by 30 pulses (0 mV holding potential) as previously described (Peineau et al., 2007).
Some slices were incubated for 30 min with GA (1–30 μM) or Aβ1–42 (Aβ 200–500 nM) or both before recordings; other recordings (control condition) were performed from non-incubated slices. All experiments were made in the presence of the GABA antagonist picrotoxin in order to pharmacologically isolate the EPSC.
For paired-pulse ratio (PPR) experiments, paired-pulse stimuli (50 ms inter-pulse interval) were elicited with stimulating electrode placed close to the recording neuron. Spontaneous excitatory post-synaptic currents (sEPSCs) were recorded in the presence of picrotoxin (100 μM).
Statistical Analysis
Excitatory post-synaptic currents peak amplitude was normalized to baseline; statistical significance was evaluated by paired or unpaired Student’s t-test, according to the groups compared, between 50 and 60 min following delivery of conditioning trains. The PPR was calculated as the ratio of the second EPSC amplitude to the first. Amplitude and frequency of sEPSC was evaluated on 3 min recordings. All values were described as mean ± SEM. Statistical significance was set at p < 0.05. For all statistical comparisons, the n used reflected the number of neurons recorded.
Drugs
Picrotoxin and GA was purchased from Abcam (Milan, Italy); Aβ1–42 was purchased from Sigma-Aldrich. When drugs were dissolved in DMSO, the final concentration of DMSO did not exceed 0.5%.
Results
Ginkgolic Acid Enhanced Hippocampal LTP
Whole-cell recordings were obtained from hippocampal CA1 pyramidal neurons and synaptic responses were evoked by electrical stimulation of Schaffer collateral afferents using a monopolar electrode. To induce LTP, the stimulating train was applied 5 min after the whole cell configuration, as previously described (Peineau et al., 2007).
In our experimental condition, in nine control neurons the LTP elicited was 164 ± 11%. In neurons from slices incubated with GA, we found a dose-dependent increase in LTP magnitude compared to control condition. Indeed, GA, at the concentration of 1 μM, did not affect LTP magnitude (155 ± 13%, n = 6, p > 0.05, Figures 1A,B). On the other hand, treatment with GA between 3 and 30 μM was able to increase significantly LTP compared to vehicle (3 μM, 237 ± 14%, n = 6; 10 μM, 270 ± 15%, n = 7; 30 μM, 275 ± 11%, n = 8, p < 0.001, Figures 1A,B).
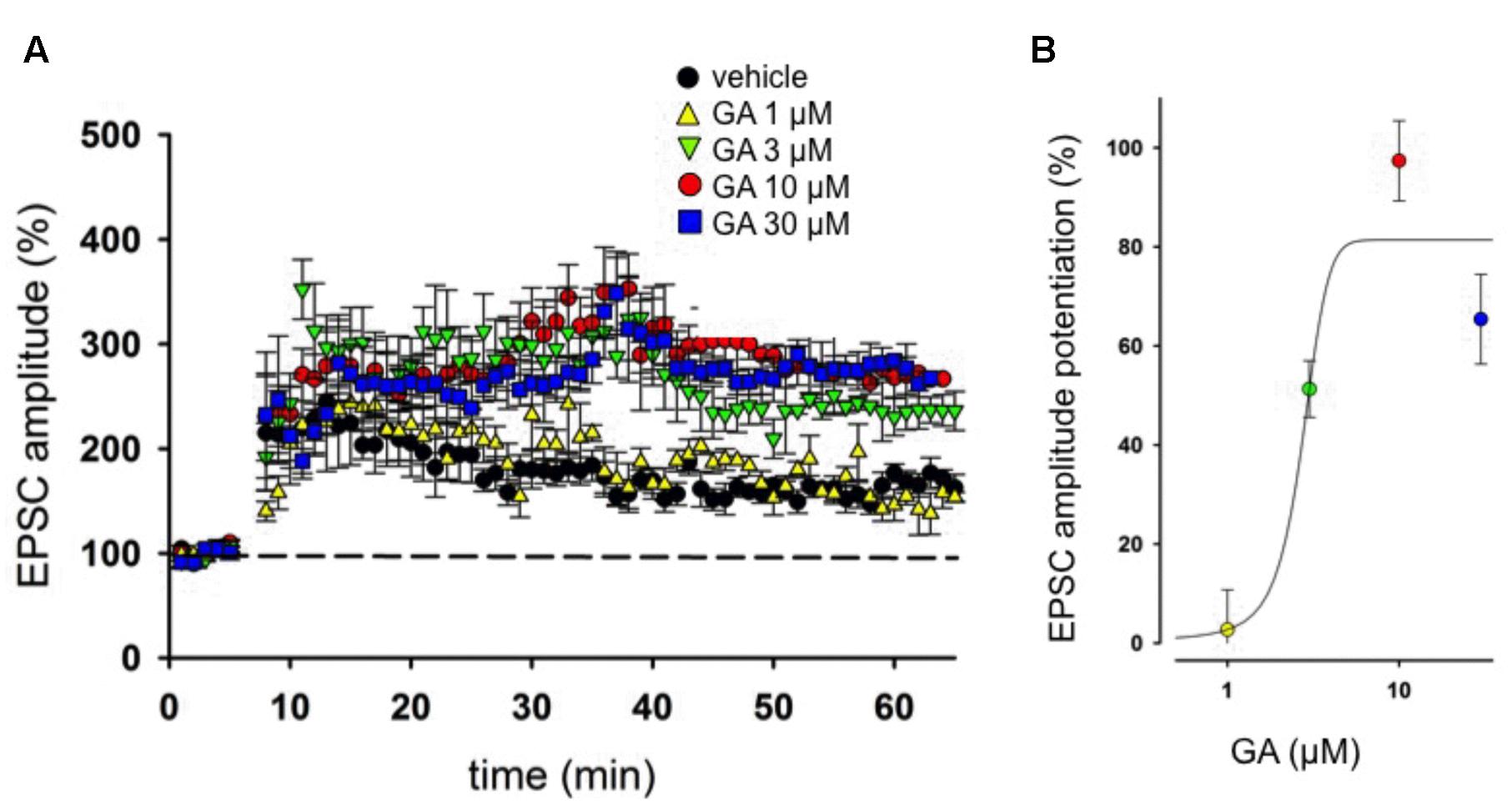
FIGURE 1. Ginkgolic acid (GA) modulates long term potentiation (LTP) in hippocampus. (A) Superimposed pooled data showing the effect of GA (1–30 μM) on LTP compared to control. (B) Concentration-response curve showing the effects of GA on the Excitatory post-synaptic currents (EPSC; measured as % of control) 1 h after induction of LTP. Each plot represents the recording of at least six separate neurons.
Ginkgolic Acid Restores LTP Impairment Following Aβ Application
It is known that Aβ oligomers are able to perturb hippocampal LTP (Chapman et al., 1999; Chen et al., 2000; Varga et al., 2015) and were also reported to alter synaptic glutamate (Glu)-recycling and transmission (Yao et al., 2013; Varga et al., 2015). Here we wanted to test whether GA exerts a neuroprotective action against Aβ-induced LTP deficiency. In our experimental system, pretreatment of slices for 30 min with 200 nM of Aβ was able to fully block LTP in hippocampal CA1 pyramidal neurons (109 ± 10%, n = 7, p > 0.05, Figures 2A,B). This effect did not occur when slices were pretreated with scrambled form of Aβ (157 ± 8%, n = 5, p < 0.001, Figures 2A,B). Notably, co-application of GA (1 μM) was able to reverse LTP impairment induced by Aβ (199 ± 14%, n = 5, p < 0.001, Figures 2C,D).
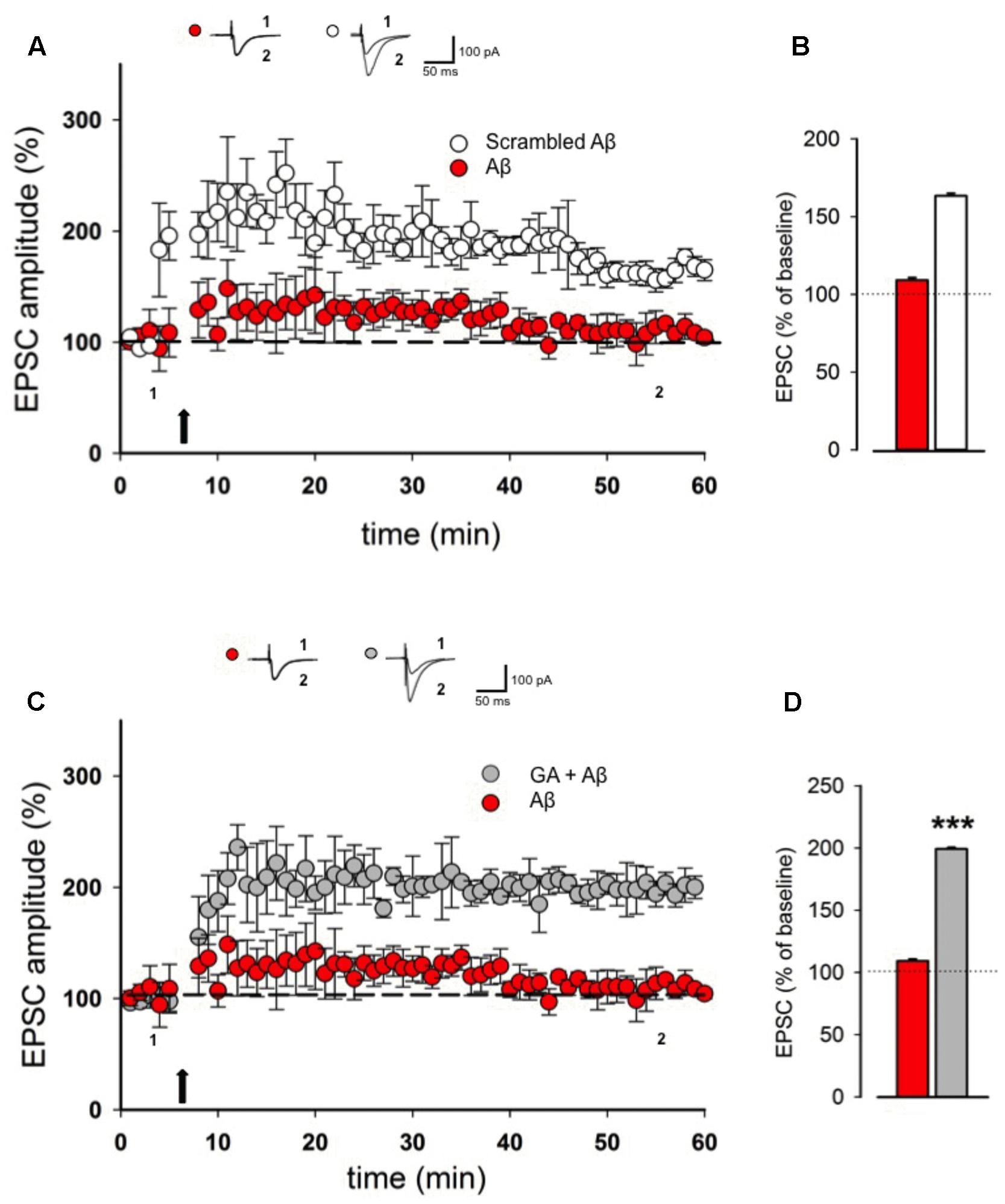
FIGURE 2. Ginkgolic acid reverses Aβ-mediated LTP impairment. (A) Superimposed pooled data showing LTP in slices treated with Aβ or scrambled Aβ. On top, representative traces for both conditions are shown. (B) Histograms illustrating the magnitude of LTP (% of baseline) in the two experimental conditions. (C) Superimposed pooled data showing LTP in slices treated with Aβ or GA + Aβ. On top, representative traces for both conditions are shown. (D) Histograms illustrating the magnitude of LTP (% of baseline) in the two experimental conditions. ∗∗∗p < 0.001.
Ginkgolic Acid Restores PPR Alteration Following Aβ Application
To test whether Aβ influences basal synaptic transmission, we recorded evoked EPSCs of hippocampal CA1 pyramidal neurons. After a stable baseline was obtained, we perfused Aβ (200 nM, 30 min) and found it had no effect on basal synaptic transmission (98 ± 6%, n = 6, p > 0.05). However, when applied at 500 nM, Aβ caused a marked depression in EPSC amplitude when compared to baseline (53 ± 7%, n = 7, p < 0.01). We then studied paired pulse facilitation (PPF) paradigm, which represents a presynaptic form of synaptic plasticity. Perfusion of 200 nM Aβ did not alter the PPR, whereas 500 nM Aβ induced a significant increase in the PPR (4 ± 0.8, n = 7, p < 0.05, Figure 3A) compared to baseline (2 ± 0.9, Figure 3B), which is associated with a reduced release of neurotransmitter. Pretreatment with GA (1 μM) before Aβ application (500 nM) normalized the PPF alteration to the baseline level (n = 7, p > 0.05, Figure 3B), while GA per se did not affect PPR (n = 5, p > 0.05, Figure 3C).
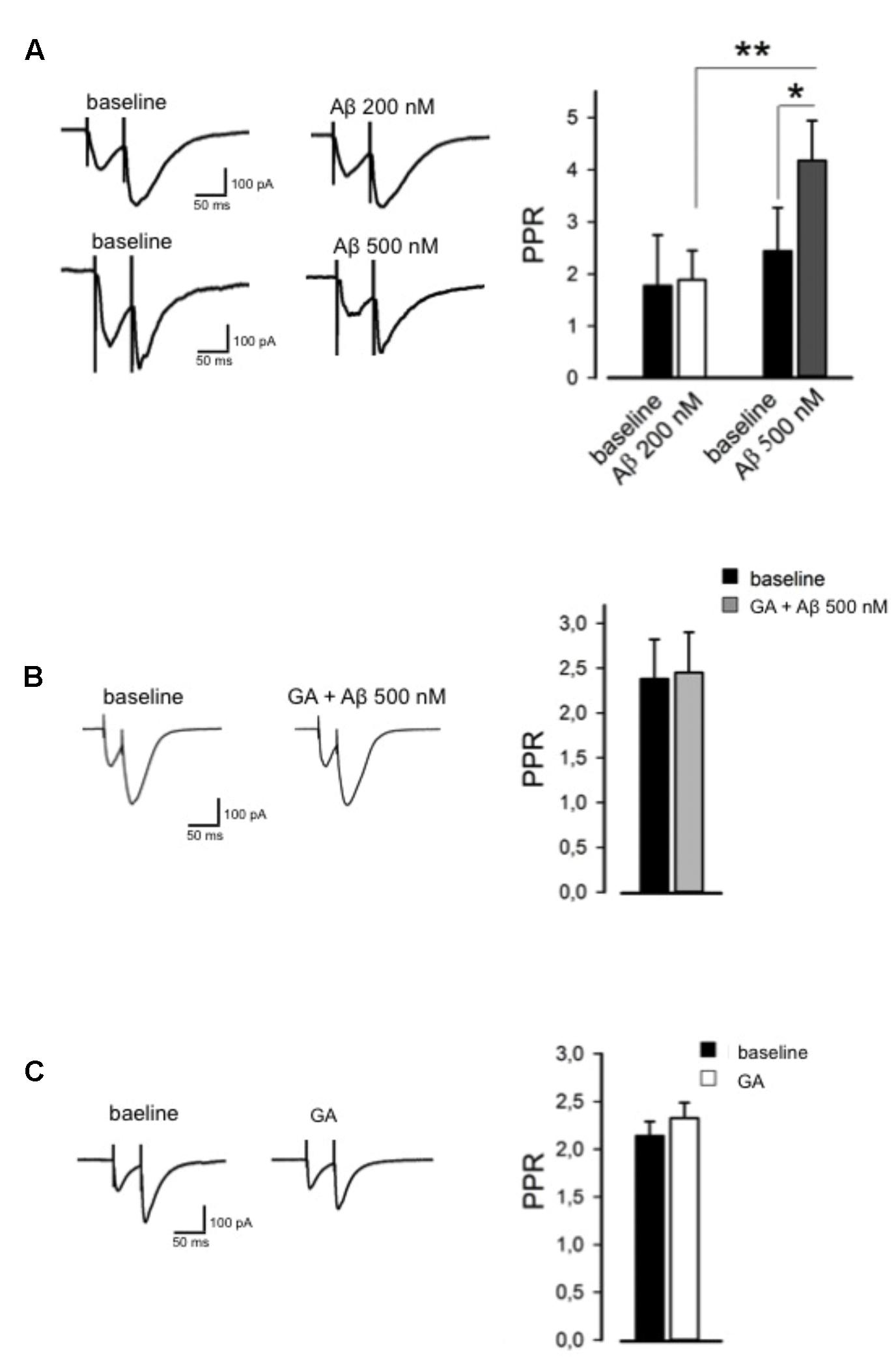
FIGURE 3. Ginkgolic acid reverses Aβ-mediated paired-pulse ratio (PPR) alteration. (A) On the left, representative traces are shown; on the right, histograms show change in PPR. (B) GA reverses Aβ-induced change in PPR. On the left, representative traces in baseline and GA+Aβ conditions are shown. On the right, histograms show PPR in both conditions. (C) GA alone did not alter PPR. On the left, representative traces are shown; on the right, histograms show no change in PPR. ∗p < 0.05; ∗∗p < 0.01.
Ginkgolic Acid Rescues Aβ-mediated Effects on Excitatory Transmission
Next, we studied synaptic transmission by recording sEPSC from CA1 pyramidal neurons. We found that 500 nM Aβ significantly suppressed both sEPSCs amplitude (80 ± 4%, n = 8, p < 0.05, Figure 4A) and frequency (74 ± 4%, n = 8, p < 0.05, Figure 4A) compared to baseline, suggesting that Aβ-induced inhibition of synaptic transmission relies on both presynaptic and postsynaptic mechanisms. Next, we investigated whether GA was able to reverse the Aβ-mediated effect on excitatory synaptic transmission. In the same conditions, GA was able to reverse the changes induced by Aβ on both the amplitude and frequency of sEPSC (n = 6, p > 0.05, Figure 4B). Notably, GA alone neither influenced the amplitude nor the frequency of sEPSC (n = 6, p > 0.05, Figure 4C).
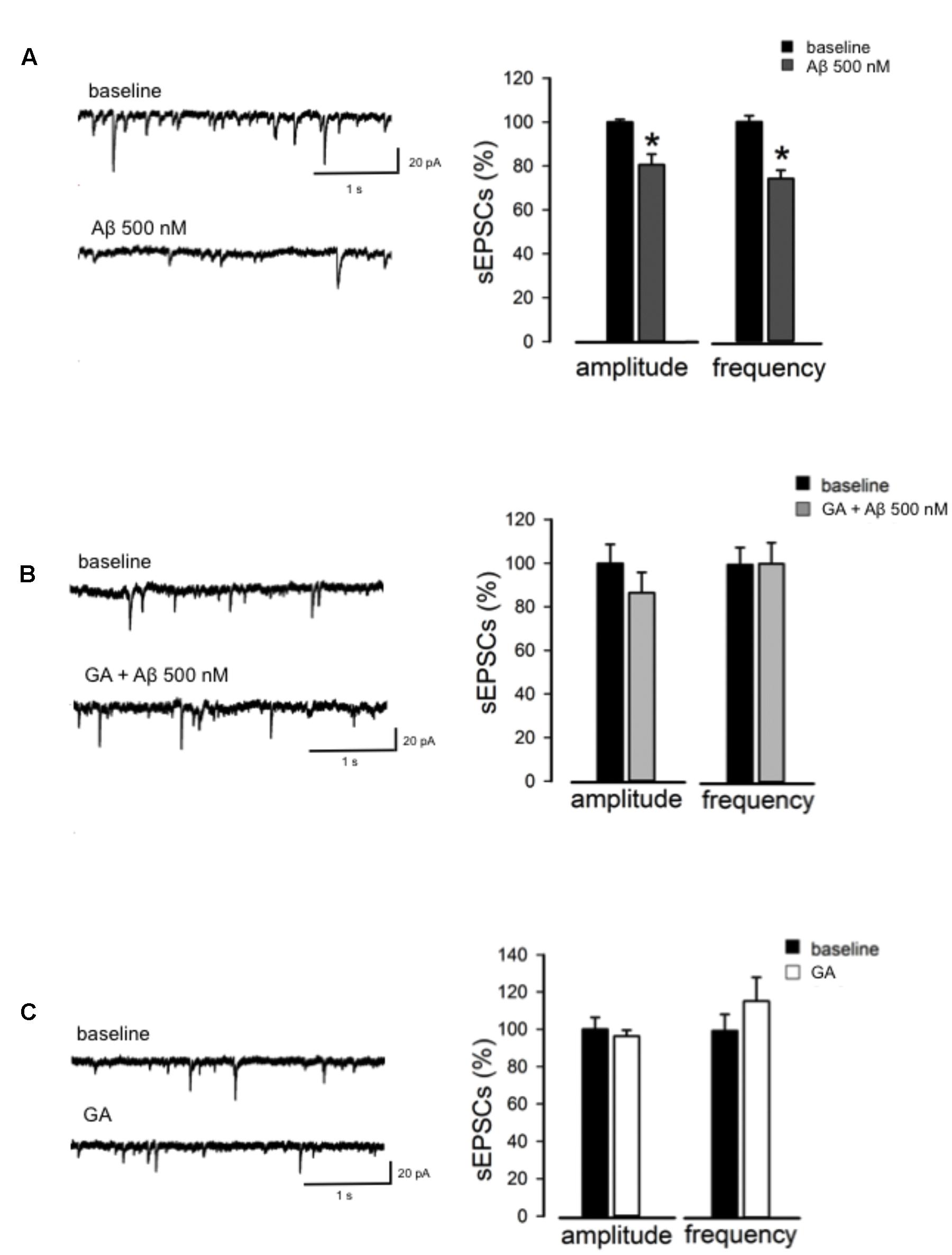
FIGURE 4. GA normalizes Aβ-mediated change in excitatory neurotransmission. (A) Reduction in amplitude and frequency of Spontaneous excitatory post-synaptic currents (sEPSC) following Aβ perfusion. On the left, representative traces are shown; on the right, histograms show the effect of Aβ on amplitude and frequency of sEPSC (as % of baseline). (B) GA prevented Aβ-triggered impairment of sEPSC. On the left, traces of sEPSC are shown. On the right, histograms show effect of GA+Aβ on amplitude and frequency of sEPSC (as % of baseline). (C) GA alone did not alter sEPSC amplitude and frequency. On the left, representative traces in baseline conditions and following perfusion of GA are shown. On the right, histograms show effect of GA on amplitude and frequency of sEPSC (as % of baseline). ∗p < 0.05.
Discussion
The present study demonstrates, for the first time, that GA, the major component of Gingko biloba standardized extract formulation EGb 761, rescues Aβ-mediated alteration of LTP, PPF, and spontaneous excitatory transmission in CA1 hippocampal pyramidal neurons. In line with this, previous studies have demonstrated that EGb 761 component acts as memory enhancer thus improving cognitive decline in AD patients (Amieva et al., 2013), as well as LTP and hippocampal-dependent working memory following acute perfusion or chronic treatment, respectively (Williams et al., 2004; Wang et al., 2006).
It has been established that nanomolar concentrations of Aβ cause inhibition of LTP mainly through postsynaptic mechanisms, whereas keeping basal synaptic transmission intact (Vitolo et al., 2002; Puzzo et al., 2005; Monfort and Felipo, 2010; Wang X.H. et al., 2010). On the other hand, presynaptic dysfunction has only been observed following the perfusion of high nanomolar to low micromolar concentrations of Aβ (Santos-Torres et al., 2007; Yao et al., 2013). To test neuroprotective action of GA against Aβ, we chose the concentration at which GA per se does not affect synaptic plasticity.
Interestingly, here we show that GA was able to rescue Aβ-mediated LTP impairment at a concentration ineffective to affect basal neurotransmission and LTP. In addition, our results demonstrate that GA could reverse Aβ-induced alterations of either PPR or sEPSC, which measure both pre- and post-synaptic function, respectively. We have not investigated so far the mechanism underlying GA effect. Our results might suggest that both pre- and post-synaptic mechanisms might be involved in its neuroprotective action.
Based on the current literature, putative molecular targets might involve SUMOylation, a post-translational modification that is known to control many aspects of cell function also at the neuronal level (Martin et al., 2007; Henley et al., 2014). In particular, it has been demonstrated that SUMOylation is among the mechanisms that links Aβ to synaptic dysfunction (Fukuda et al., 2009; Lee et al., 2013, 2014, Nisticò et al., 2014). Indeed, GA directly binds E1 and inhibits the formation of E1-SUMO intermediate at the same range of concentrations here used to prevent LTP inhibition by Aβ (Fukuda et al., 2009). In addition, GA can also inhibit the SUMOylation of p53 (Fukuda et al., 2009), which is known to play a crucial role in the early phase of LTP (Pustylnyak et al., 2015) and has been shown to be involved in aging and AD (LaFerla et al., 1996; Lanni et al., 2012).
Another possible mechanism might rely on the Bcl-2/Bax pathway, which contributes to the antitumoral and anti-HIV effects of GA (Wang H. et al., 2010; Zhou et al., 2010; Lü et al., 2012). Inasmuch, as Bcl-2/Bax is also involved in Aβ-mediated impairment of LTP (Olsen and Sheng, 2012), it can be hypothesized that the protective effect of GA here observed might be mediated by Bcl-2/Bax inhibition.
In addition, a recent work suggested that GA inactivates PI3K/Akt/mTOR in lung cancer cells (Baek et al., 2016). The PI3K/Akt/mTOR is a crucial pathway regulating autophagy and synaptic plasticity in the brain (Heras-Sandoval et al., 2014). Dysregulation of this pathway is commonly reported in brains from AD patients and in AD model mice and was demonstrated to occur by means of Aβ-promoted autophagy (Heras-Sandoval et al., 2014). Thus, inactivation of PI3K/Akt/mTOR pathway by GA might be a protective mechanism against Aβ-promoted autophagy.
To conclude, our work investigated the effect of GA on hippocampal plasticity, neurotransmitter release, and excitatory neurotransmission. In particular, we demonstrate for the first time that GA acts at the synaptic level affording neuroprotection against Aβ-mediated impairment, possibly representing a novel approach to AD prevention and cure.
Author Contributions
DM designed the research, performed experiments, analyzed data, and wrote the paper, FW analyzed data, RN designed the research, interpreted data, and wrote the paper.
Conflict of Interest Statement
The authors declare that the research was conducted in the absence of any commercial or financial relationships that could be construed as a potential conflict of interest.
Acknowledgments
DM was supported by SIF-MSD research fellowship. This research was partly supported by from the Italian Ministry of Health to IRCCS National Neurological Institute “C. Mondino” (Ricerca Corrente).
References
Ahlemeyer, B., Selke, D., Schaper, C., Klumpp, S., and Krieglstein, J. (2001). Ginkgolic acids induce neuronal death and activate protein phosphatase type-2C. Eur. J. Pharmacol. 430, 1–7. doi: 10.1016/S0014-2999(01)01237-7
Amieva, H., Meillon, C., Helmer, C., Barberger-Gateau, P., and Dartigues, J. F. (2013). Ginkgo biloba extract and long-term cognitive decline: a 20-year follow-up population-based study. PLoS ONE 8:e52755. doi: 10.1371/journal.pone.0052755
Arendt, T. (2009). Synaptic degeneration in Alzheimer’s disease. Acta Neuropathol. 118, 167–179. doi: 10.1007/s00401-009-0536-x
Baek, S. H., Ko, J. H., Lee, J. H., Kim, C., Lee, H., Nam, D., et al. (2016). Ginkgolic acid inhibits invasion and migration and TGF-β-induced EMT of lung cancer cells through PI3K/Akt/mTOR inactivation. J. Cell Physiol. doi: 10.1002/jcp.25426 [Epub ahead of print],
Bliss, T. V., and Collingridge, G. L. (1993). A synaptic model of memory: long-term potentiation in the hippocampus. Nature 361, 31–39. doi: 10.1038/361031a0
Chapman, P. F., White, G. L., Jones, M. W., Cooper-Blacketer, D., Marshall, V. J., Irizarry, M., et al. (1999). Impaired synaptic plasticity and learning in aged amyloid precursor protein transgenic mice. Nat. Neurosci. 2, 271–276. doi: 10.1038/6374
Chen, Q. S., Kagan, B. L., Hirakura, Y., and Xie, C. W. (2000). Impairment of hippocampal long-term potentiation by Alzheimer amyloid beta-peptides. J. Neurosci. Res. 60, 65–72. doi: 10.1002/(SICI)1097-4547(20000401)60:1<65::AID-JNR7>3.0.CO;2-Q
Fukuda, I., Ito, A., Hirai, G., Nishimura, S., Kawasaki, H., Saitoh, H., et al. (2009). Ginkgolic acid inhibits protein SUMOylation by blocking formation of the E1-SUMO intermediate. Chem. Biol. 16, 133–140. doi: 10.1016/j.chembiol.2009.01.009
Gessner, B., Voelp, A., and Klasser, M. (1985). Study of the long-term action of a Ginkgo biloba extract on vigilance and mental performance as determined by means of quantitative pharmaco- EEG and psy- chometric measurements. Arzneimittelforschung 35, 1459–1465.
Henley, J. M., Craig, T. J., and Wilkinson, K. A. (2014). Neuronal SUMOylation: mechanisms, physiology, and roles in neuronal dysfunction. Physiol. Rev. 94, 1249–1285. doi: 10.1152/physrev.00008.2014
Heras-Sandoval, D., Pérez-Rojas, J. M., Hernández-Damián, J., and Pedraza-Chaverri, J. (2014). The role of PI3K/AKT/mTOR pathway in the modulation of autophagy and the clearance of protein aggregates in neurodegeneration. Cell. Signal. 26, 2694–2701. doi: 10.1016/j.cellsig.2014.08.019
Hsu, K. S., Ho, W. C., Huang, C. C., and Tsai, J. J. (1999). Prior short-term synaptic disinhibition facilitates long-term potentiation and suppresses long-term depression at CA1 hippocampal synapses. Eur. J. Neurosci. 11, 4059–4069. doi: 10.1046/j.1460-9568.1999.00819.x
Kennedy, D. O., Jackson, P. A., Haskell, C. F., and Scholey, A. B. (2007). Modulation of cognitive per- formance following single doses of 120 mg Ginkgo biloba extract administered to healthy young volunteers. Hum. Psychopharmacol. doi: 10.1002/hup.885 [Epub ahead of print],
Klyubin, I., Betts, V., Welzel, A. T., Blennow, K., Zetterberg, H., Wallin, A., et al. (2008). Amyloid beta protein dimer-containing human CSF disrupts synap- tic plasticity: prevention by systemic passive immunization. J. Neurosci. 28, 4231–4237. doi: 10.1523/JNEUROSCI.5161-07.2008
LaFerla, F. M., Hall, C. K., Ngo, L., and Jay, G. (1996). Extracellular deposition of beta-amyloid upon p53-dependent neuronal cell death in transgenic mice. J. Clin. Invest. 98, 1626–1632. doi: 10.1172/JCI118957
Lanni, C., Racchi, M., Memo, M., Govoni, S., and Uberti, D. (2012). p53 at the crossroads between cancer and neurodegeneration. Free Radic. Biol. Med. 52, 1727–1733. doi: 10.1016/j.freeradbiomed.2012.02.034
Le Bars, P. L., Katz, M. M., Berman, N., Itil, T. M., Freedman, A. M., and Schatzberg, A. F. (1997). A placebo-controlled, double-blind, randomized trial of an extract of Ginkgo biloba for dementia. North American EGb Study Group. JAMA 278, 1327–1332.
Lee, L., Dale, E., Staniszewski, A., Zhang, H., Saeed, F., Sakurai, M., et al. (2014). Regulation of synaptic plasticity and cognition by SUMO in normal physiology and Alzheimer’s disease. Sci. Rep. 4:7190. doi: 10.1038/srep07190
Lee, L., Sakurai, M., Matsuzaki, S., Arancio, O., and Fraser, P. (2013). SUMO and Alzheimer’s disease. Neuromolecular Med. 15, 720–736. doi: 10.1007/s12017-013-8257-7
Lü, J. M., Yan, S., Jamaluddin, S., Weakley, S. M., Liang, Z., Siwak, E. B., et al. (2012). Ginkgolic acid inhibits HIV protease activity and HIV infection in vitro. Med. Sci. Monit. 18, 293–298.
Mahadevan, S., and Park, Y. (2008). Multifaceted therapeutic benefits of Ginkgo biloba L.: chemistry, efficacy, safety, and uses. J. Food Sci. 73, 14–19. doi: 10.1111/j.1750-3841.2007.00597.x
Martin, S., Wilkinson, K. A., Nishimune, A., and Henley, J. M. (2007). Emerging extranuclear roles of protein SUMOylation in neuronal function and dysfunction. Nat. Rev. Neurosci. 8, 948–959. doi: 10.1038/nrn2276
McKenna, D. J., Jones, K., and Hughes, K. (2001). Efficacy, safety, and use of Ginkgo biloba in clinical and preclinical applications. Altern. Ther. Health Med. 86, 88–90.
Monfort, P., and Felipo, V. (2010). Amyloid-beta impairs, and ibuprofen restores, the cGMP pathway, synaptic expression of AMPA receptors and long-term potentiation in the hippocampus. J. Alzheimers Dis. 22, 795–809. doi: 10.3233/JAD-2010-101092
Nisticò, R., Ferraina, C., Marconi, V., Blandini, F., Negri, L., Egebjerg, J., et al. (2014). Age-related changes of protein SUMOylation balance in the AβPP Tg2576 mouse model of Alzheimer’s disease. Front. Pharmacol. 5:63. doi: 10.3389/fphar.2014.00063
Olsen, K. M., and Sheng, M. (2012). NMDA receptors and BAX are essential for Abeta impairment of LTP. Sci. Rep. 2:225. doi: 10.1038/srep00225
Peineau, S., Taghibiglou, C., Bradley, C., Wong, T. P., Liu, L., Lu, J., et al. (2007). LTP inhibits LTD in the hippocampus via regulation of GSK3beta. Neuron 53, 703–717. doi: 10.1016/j.neuron.2007.01.029
Pustylnyak, V. O., Lisachev, P. D., and Shtark, M. B. (2015). Expression of p53 target genes in the early phase of long-term potentiation in the rat hippocampal CA1 area. Neural Plast. 2015:242158. doi: 10.1155/2015/242158
Puzzo, D., Vitolo, O., Trinchese, F., Jacob, J. P., Palmeri, A., and Arancio, O. (2005). Amyloid-beta peptide inhibits activation of the nitric oxide/cGMP/cAMP-responsive element-binding protein pathway during hippocampal synaptic plasticity. J. Neurosci. 25, 6887–6897. doi: 10.1523/JNEUROSCI.5291-04.2005
Ramassamy, C., Longpre, F., and Christen, Y. (2007). Ginkgo biloba extract (EGb 761) in Alzheimer’s disease: is there any evidence? Curr. Alzheimer Res. 4, 253–262. doi: 10.2174/156720507781077304
Santos-Torres, J., Fuente, A., Criado, J. M., Riolobos, A. S., Heredia, M., and Yajeya, J. (2007). Glutamatergic synaptic depression by synthetic amyloid beta-peptide in the medial septum. J. Neurosci. Res. 85, 634–648. doi: 10.1002/jnr.21150
Scripnikov, A., Khomenko, A., and Napryeyenko, O. (2007). GINDEM-NP Study Group. Effects of Ginkgo biloba extract EGb 761 on neuropsychiatric symptoms of demen- tia: findings from a randomised controlled trial. Wien. Med. Wochenschr. 157, 295–300. doi: 10.1007/s10354-007-0427-5
Thompson, P. M., Hayashi, K. M., de Zubicaray, G., Janke, A. L., Rose, S. E., Semple, J., et al. (2003). Dynamics of gray matter loss in Alzheimer’s disease. J. Neurosci. 23, 994–1005.
Ude, C., Schubert-Zsilavecz, M., and Wurglics, M. (2013). Ginkgo biloba extracts: a review of the pharmacokinetics of the active ingredients. Clin. Pharmacokinet. 52, 727–749. doi: 10.1007/s40262-013-0074-5
Varga, E., Juhász, G., Bozsó, Z., Penke, B., Fülöp, L., and Szegedi, V. (2015). Amyloid-β1-42 disrupts synaptic plasticity by altering glutamate recycling at the synapse. J. Alzheimers Dis. 45, 449–456. doi: 10.3233/JAD-142367
Vitolo, O. V., Sant’Angelo, A., Costanzo, V., Battaglia, F., Arancio, O., and Shelanski, M. (2002). Amyloid beta -peptide inhibition of the PKA/CREB pathway and long-term potentiation: reversibility by drugs that enhance cAMP signaling. Proc. Nat. Acad. Sci. U.S.A. 99, 13217–13221. doi: 10.1073/pnas.172504199
Wang, H., Zhou, C. C., Feng, Y., Dai, L. N., Chen, J., Chen, S. X., et al. (2010). The effect of ginkgolic acids on multidrug resistance in oral squamous cell carcinoma. Hua Xi Kou Qiang Yi Xue Za Zhi. 28, 668–671.
Wang, X. H., Li, L., Hölscher, C., Pan, Y. F., Chen, X. R., and Qi, J. S. (2010). Val8-glucagon-like peptide-1 protects against Abeta1-40- induced impairment of hippocampal late-phase long-term potentiation and spatial learning in rats. Neuroscience 170, 1239–1248. doi: 10.1016/j.neuroscience.2010.08.028
Wang, Y., Wang, L., Wu, J., and Cai, J. (2006). The in vivo synaptic plasticity mechanism of EGb 761-induced enhancement of spatial learning and memory in aged rats. Br. J. Pharmacol. 148, 147–153. doi: 10.1038/sj.bjp.0706720
Williams, B., Watanabe, C. M., Schultz, P. G., Rimbach, G., and Krucker, T. (2004). Age-related effects of Ginkgo biloba extract on synaptic plasticity and excitability. Neurobiol. Aging 25, 955–962. doi: 10.1016/j.neurobiolaging.2003.10.008
Yao, W., Zou, H. J., Sun, D., and Ren, S. Q. (2013). Aβ induces acute depression of excitatory glutamatergic synaptic transmission through distinct phosphatase-dependent mechanisms in rat CA1 pyramidal neurons. Brain Res. 1515, 88–97. doi: 10.1016/j.brainres.2013.03.049
Keywords: ginkgolic acid, hippocampus, LTP, Aβ, electrophysiology
Citation: Mango D, Weisz F and Nisticò R (2016) Ginkgolic Acid Protects against Aβ-Induced Synaptic Dysfunction in the Hippocampus. Front. Pharmacol. 7:401. doi: 10.3389/fphar.2016.00401
Received: 13 September 2016; Accepted: 11 October 2016;
Published: 26 October 2016.
Edited by:
Cesare Mancuso, Catholic University of the Sacred Heart, ItalyReviewed by:
Simone Lista, Pitié-Salpêtrière University Hospital, FranceCarla Petrella, National Research Council, Italy
Copyright © 2016 Mango, Weisz and Nisticò. This is an open-access article distributed under the terms of the Creative Commons Attribution License (CC BY). The use, distribution or reproduction in other forums is permitted, provided the original author(s) or licensor are credited and that the original publication in this journal is cited, in accordance with accepted academic practice. No use, distribution or reproduction is permitted which does not comply with these terms.
*Correspondence: Dalila Mango, ZC5tYW5nb0BlYnJpLml0 Robert Nisticò, ci5uaXN0aWNvQGVicmkuaXQ=