- 1Laboratory for Molecular Biodiscovery, Department of Pharmaceutical and Pharmacological Sciences, KU Leuven, Leuven, Belgium
- 2Research group Experimental Pharmacology
- 3Department of Development and Regeneration, Section Pediatric Neurology, University Hospital KU Leuven, Leuven, Belgium
Dravet syndrome (DS) is a genetic encephalopathy that is characterized by severe seizures and prominent co-morbidities (e.g., physical, intellectual disabilities). More than 85% of the DS patients carry an SCN1A mutation (sodium channel, voltage gated, type I alpha subunit). Although numerous anti-epileptic drugs have entered the market since 1990, these drugs often fail to adequately control seizures in DS patients. Nonetheless, current clinical data shows significant seizure reduction in DS patients treated with the serotonergic (5-hydroxytryptamine, 5-HT) drug fenfluramine (FA). Recent preclinical research confirmed the anti-epileptiform activity of FA in homozygous scn1a mutant zebrafish larvae that mimic DS well. Here we explored the anti-epileptiform mechanisms of FA by investigating whether selective agonists/antagonists of specific receptor subtypes were able to counteract the FA-induced inhibition of seizures and abnormal brain discharges observed in the scn1a mutants. We show that antagonists of 5-HT1D and 5-HT2C receptor subtypes were able to do so (LY 310762 and SB 242084, respectively), but notably, a 5-HT2A-antagonist (ketanserin) was not. In addition, exploring further the mechanism of action of FA beyond its serotonergic profile, we found that the anti-epileptiform brain activity of FA was significantly abolished when it was administered in combination with a σ1-agonist (PRE 084). Our study therefore provides the first evidence of an involvement of the σ1 receptor in the mechanism of FA. We further show that the level of some neurotransmitters [i.e., dopamine and noradrenaline (NAD)] in head homogenates was altered after FA treatment, whereas γ-aminobutyric acid (GABA) and glutamate levels were not. Of interest, NAD-decreasing drugs have been employed successfully in the treatment of neurological diseases; including epilepsy and this effect could contribute to the therapeutic effect of the compound. In summary, we hypothesize that the anti-epileptiform activity of FA not only originates from its 5-HT1D- and 5-HT2C-agonism, but likely also from its ability to block σ1 receptors. These findings will help in better understanding the pharmacological profile of compounds that is critical for their applicability in the treatment of DS and possibly also other drug-resistant epilepsies.
Introduction
Epilepsy is a severe neurological disease affecting 65 million people worldwide (Moshé et al., 2015). It is marked by abnormal electrical activity in the brain and is associated with precipitous recurrent episodes of involuntary movements and/or loss of consciousness. Various epilepsy syndromes have been described with different etiologies (e.g., genetic, structural, metabolic, infectious or unknown cause). In general, multiple genes and/or environmental factors are implicated in the pathogenesis of epilepsy but also monogenic epilepsy syndromes exist (Williams and Battaglia, 2013). Of interest, these monogenic pathologies can be mimicked rather easily by genetic animal models (Dinday and Baraban, 2015; Sourbron et al., 2016).
A de novo mutation in the SCN1A gene (sodium channel, voltage gated, type I alpha subunit) has been proven to be the cause in more than 85% of the DS patients (Mastrangelo and Leuzzi, 2012). This monogenic epilepsy syndrome, which was also known as severe myoclonic epilepsy of infancy (SMEI), is an uncommon but severe encephalopathy that starts in the first year of life (Dravet, 2011). Moreover it is one of the most drug-resistant epilepsy syndromes, highlighting the need for new innovative drugs with novel mechanisms of action.
Even though new AEDs have been developed over the past two decades, the treatment of drug-resistant epilepsy has not improved significantly (Ventola, 2014). Notably, some interesting progress has been made in the understanding of the mechanisms underlying epilepsy, revealing novel targets for future AEDs (Löscher et al., 2013). For instance, current evidence underscores the role of SER (5-HT) in epilepsy and it has been suggested that serotonergic drugs have great potential in the treatment of various epilepsy conditions (Gharedaghi et al., 2014).
One interesting example of a successful epilepsy therapy based on serotonergic modulation relates to the use of fenfluramine (FA) as an add-on drug in a small clinical trial of DS patients (Ceulemans et al., 2012). FA is a potent SER releaser and also directly acts on multiple 5-HT receptor subtypes, 14 of which have been described in humans. Prospective data also demonstrated the efficacy and safety of FA in treating DS patients (Schoonjans et al., 2015), which led to the initiation of clinical Phase III studies early in 20161.
Zebrafish (Danio rerio) are increasingly used in neurological research (Best and Alderton, 2008) and the high genetic homology between ZF and human (ca. 70%) makes them an attractive model to study genetic diseases (Howe et al., 2013). More than 80% of the known epilepsy-related genes are found in the ZF genome and different groups were able to generate ZF epilepsy models by specific gene manipulation, recapitulating the main characteristics of the human disease, e.g., fever-sensitive myoclonic epilepsy (CHD2 and SCN1A) (Suls et al., 2013; Zhang et al., 2015) and fever-associated epilepsy (STX1B) (Schubert et al., 2014). In addition, the phenotype of homozygous scn1Lab mutant ZF larvae (herein referred to as scn1a mutant larvae) has been studied and mirrors the clinical features of DS well. Significantly, these mutants did not respond to currently available AEDs and only valproic acid and FA were effective in treating seizures, as seen in DS patients (Dinday and Baraban, 2015; Sourbron et al., 2016).
Our pharmacological investigations using scn1a mutant larvae showed that stimulation of the 5-HT2B receptor (by BW 723C86, a selective 5-HT2B-agonist) did not generate an anti-epileptiform effect whereas the application of selective 5-HT1D- (GR 46611), 5-HT2A- (TCB 2) or 5-HT2C- (lorcaserin) agonists resulted in a significant decrease of epileptiform locomotor and brain activity (Sourbron et al., 2016). However, direct evidence that these 5-HT receptor subtypes are implicated in the pharmacological activity of FA is presently lacking. Moreover, in vitro assays interrogating potential targets suggested beta2-adrenergic- (β2) and sigma- (σ) antagonism as possibly involved in the mechanism of FA (Parthena et al., 2016). These receptors have relatively high protein similarity compared to their human counterparts, which amounts to 68.2 and 79.5 % for the β2 receptor and σ1 receptor, respectively [as calculated by EMBOSS stretcher, (Rice et al., 2000)]. In addition, they show a pronounced expression in the ZF brain (comparable to the human proteins) (Wang et al., 2009; Moritz et al., 2015). Structural data regarding the σ2 receptor subtype are more ill-defined, even though selective compounds with high affinity for the binding site are available (Rhoades et al., 2014).
In this study, the mechanistic profile of FA was explored in scn1a mutant larvae by using subtype selective agonists and antagonists of serotonergic, beta2-adrenergic- and sigma receptors. We demonstrate that the anti-epileptiform activity of FA originates both from its agonistic action at 5-HT1D and 5-HT2C receptors and its antagonistic action at σ1 receptors. Moreover, we found a significant decrease of certain monoamines in the head homogenates of ZF larvae after FA treatment. The results obtained allowed us to put forward a mechanistic hypothesis regarding the clinical efficacy of FA in the treatment of DS.
Materials and Methods
Compounds and Their Maximum Tolerable Concentration (MTC)
Compounds were purchased from Tocris Bioscience, except for the 5-HT2A-antagonist, ketanserin (Sigma-Aldrich) and (±)FA that was a gift from Prof. Berten Ceulemans (Child Neurology, University Hospital Antwerp, Belgium). Agonists and antagonists were selected by their high and selective affinity for a specific receptor (Tables 1, 2). In addition, all compounds have a cLogP value higher than one (cLogP > 1.0) ensuring a good bioavailability in ZF larvae (Milan, 2003; García-Pedraza et al., 2013; Watry and Lu, 2013) (Tables 1, 2). These LogP values were calculated by an interactive calculator after conversion of the chemical names to SMILES (cLogP range between 1.21 and 4.59). Compounds were dissolved in DMSO (99.9% spectroscopy grade, Acros Organics) (stock solution), and immediately before use diluted in embryo medium to achieve a final DMSO concentration of 0.1% w/v that also served as VHC control.
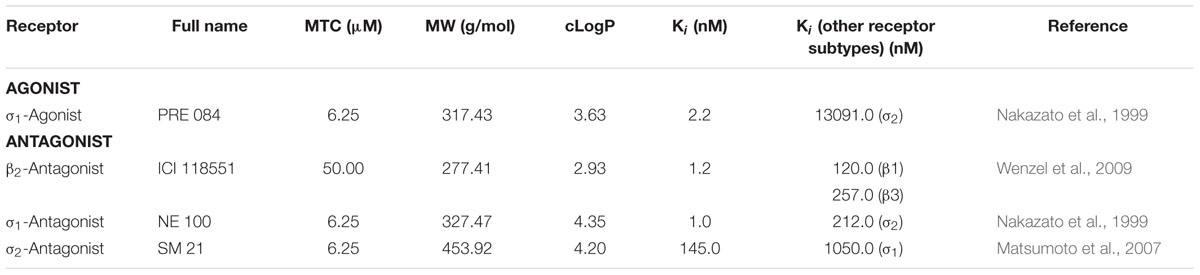
TABLE 1. Compounds with selective agonistic or antagonistic activity at the beta2-adrenergic (β2) or the sigma receptors (σ1 and σ2).
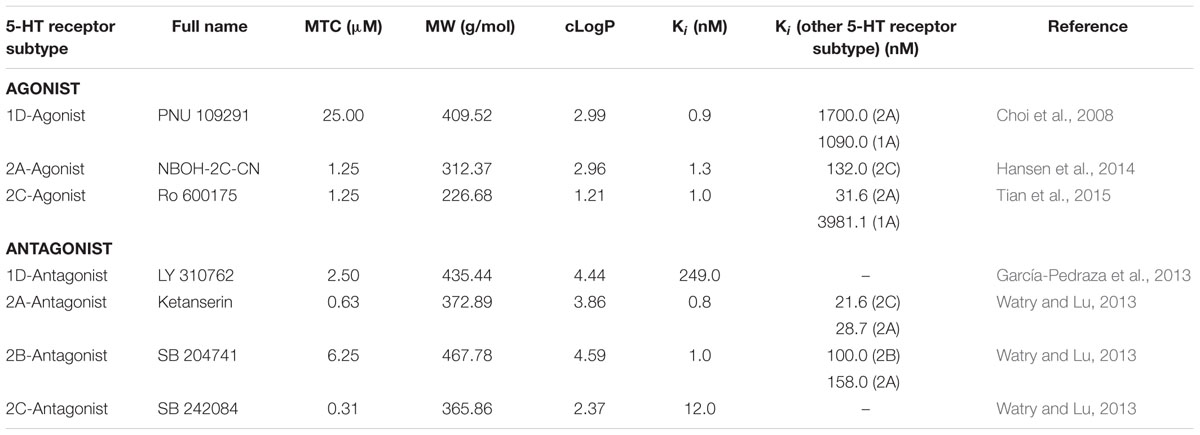
TABLE 2. Compounds with selective agonistic or antagonistic activity at specific SER (5-HT) receptor subtypes.
Before performing pharmacological experiments, the MTC of each compound was determined. Six dpf WT scn1Lab+/+ ZF larvae (hereinafter referred to as WT larvae) were incubated in a 96-well plate, one larva per well (tissue culture plate, flat bottom, FALCON®, USA), with different concentrations of compound or VHC at 28°C on a 14/10 h light/dark cycle (medium was replenished daily). Each larva was checked under the microscope during a period of 48 h for the following signs of toxicity: decreased or no touch response upon a light touch of the tail, loss of posture, body abnormalities, edema, anomalies in heart rate or circulation and death. The MTC was determined using 12 WT ZF larvae (6 dpf) and defined as the highest concentration at which none of these larvae showed signs of toxicity within 48 h of exposure. Compounds were used at their MTC (Tables 1, 2), whereas FA was used at a final concentration of 25 μM as described before (Sourbron et al., 2016).
Zebrafish Research
Zebrafish Maintenance and Experimental Set-up
ZF (Danio rerio) heterozygous for the scn1Lab mutation (scn1Lab+/-), backcrossed with Tupfel longfin WT (WT scn1Lab+/+), were a generous gift of Dr. H. Baier (Freiburg, Germany). The adult ZF were kept at 28.0°C, on a 14/10 h light/dark cycle under standard aquaculture conditions. Fertilized eggs were collected via natural spawning. Genotyping of these ZF adults and embryos was performed as described previously (Sourbron et al., 2016). The embryos and larvae were kept in petridishes in embryo medium containing 1.5 mM HEPES, pH 7.6, 17.4 mM NaCl, 0.21 mM KCl, 0.12 mM MgSO4 and 0.18 mM Ca(NO3)2 in an incubator at 28.0°C, likewise on a 14/10 h light/dark cycle. Scn1a mutant larvae were selected by their darker appearance, lack of a swim bladder and slight curvature of the body (Schoonheim et al., 2010).
All ZF experiments were approved by the Ethics Committee of the University of Leuven (Ethische Commissie van de KU Leuven, approval number 154/2015) and by the Belgian Federal Department of Public Health, Food Safety & Environment (Federale Overheidsdienst Volksgezondheid, Veiligheid van de Voedselketen en Leefmileu, approval number LA1210199).
Locomotor Behavior
Scn1a mutant larvae and WT larvae were arrayed in a 96-well plate (one larva per well) and treated at 6 dpf with VHC, compound or co-treatment of compounds for a 22 h period as performed previously (Sourbron et al., 2016). The co-treatment of FA and subtype selective 5-HT-antagonists and/or the σ1-agonist was performed to investigate the mechanism of action of FA. After incubation at 28°C on a 14/10 h light/dark cycle and 30 min chamber habituation 7 dpf larvae were placed in an automated tracking device (ZebraBoxTM apparatus; Viewpoint, Lyon, France) and their locomotor behavior was evaluated during 10 min under dark conditions, as described before (Sourbron et al., 2016). Locomotor activity was quantified by the lardist parameter (total distance in large movements) and plotted in cm (ZebraLabTM software, Viewpoint, Lyon, France). Data were collected and pooled together from two or three independent experiments with at least eight larvae per treatment condition. The locomotor data were analyzed by normalizing the locomotor activity of treated ZF larvae against age-matched VHC-treated larvae (method A), and by calculating the percentage of scn1a mutant larvae with epileptiform activity below or above a 70% threshold value using the cumulative locomotor activity during 10 min of each larva separately (method B). Method A has been described previously (Sourbron et al., 2016) and is performed for both scn1a mutant larvae and WT larvae. The threshold value used in method B is based on sensitivity analyses with different cut-off values in ‘10%-steps’; i.e., 10, 20, 30, 40, 50, 60, 70, 80, and 90% of the average cumulative locomotor activity during 10 min of VHC-treated scn1a mutant larvae per experiment (data available in Supplementary Tables S1–S5).
Forebrain Local Field Potential Recordings
The epileptiform brain activity was measured by non-invasive surface recordings from the skin above the forebrain (Zdebik et al., 2013). Scn1a mutant and WT larvae were treated on 6 dpf as described above [see Locomotor Behavior (Single and Combined)] and recordings were performed at 7 dpf. ZF larvae were immobilized by 2% low-melting-point agarose (Invitrogen) (no paralytic was used).
The recording electrodes were made of borosilicate glass with microfilament (GC150TF-7.5, Harvard Apparatus, UK), pulled with DMZ Universal Puller (Zeitz, Germany) to an opening 15–20 microns, and filled with aCSF made from: 124 mM NaCl, 2 mM KCl, 2 mM MgSO4, 2 mM CaCl2, 1.25 mM KH2PO4, 26 mM NaHCO3 and 10 mM glucose. The differential signal was amplified 10,000 times using DAGAN 2400 amplifier (Minneapolis, MN, USA), band pass filtered at 0.3–300.0 Hz and digitized at 2.0 kHz via a PCI-6251 interface (National Instruments, UK) using WindEDR (John Dempster, University of Strathclyde, UK). Single recordings were performed for 10 min and epileptiform activity was quantified according to the duration of spiking paroxysms as described before (Sourbron et al., 2016). Electrograms were analyzed with Clampfit 10.2 software (Molecular devices corporation, USA). Spontaneous epileptiform activity was defined as events in which the amplitude exceeded three times the baseline and lasted longer than 50 ms. At least 10 (up to 50) ZF larvae were used per experimental condition.
Neurotransmitter Determination
The heads of 7 dpf ZF larvae were used to quantify the amount of the neurotransmitters 5-HT, NAD, DOP, GABA and GLUT. Both scn1a mutant larvae and WT larvae were treated with either VHC or FA (n = 48 for both treatment arms, leading to a total of 192 larvae). Six heads per tube were homogenized and data acquisition was carried out as described before (Sourbron et al., 2016). Thereafter, the amount of neurotransmitter (in nmol) was determined and expressed as nmol neurotransmitter per mass head homogenate (mg).
Statistical Analysis
GraphPad Prism 5 software (GraphPad Software, Inc.) was used for statistical analyses.
The locomotor activity was analyzed by the methods described above [see Locomotor Behavior (Single and Combined)]. The results obtained with method A were analyzed by One-way ANOVA and subsequent Dunnett’s multiple comparison tests. The results obtained with method B were performed for the individual experiments by contingency tables, followed by Fisher’s exact tests.
Electrographic brain activity was analyzed by a Student’s t-test [if data passed the normality test (D’Agostino & Pearson omnibus normality test)]. Mann–Whitney U tests were used if the data did not pass the normality test, as described before (Sourbron et al., 2016). To determine if the genotype (scn1a mutant vs. WT) and/or FA treatment affected the neurotransmitter content, data were analyzed by a two-way ANOVA for genotype and FA treatment as the inter-subject factors. Regarding the analyses of single vs. triple experiments [whether or not combined with FA; see Anti-epileptiform Activity Induced by Sigma1-Antagonism and 5-HT1D&2C-Agonism (Triple Treatment) and Counteraction of FA’s Anti-epileptiform Activity by Sigma1-Agonism and 5-HT1D&2C-Agonism (Triple Treatment), respectively] Mann–Whitney U tests were applied because the data did not pass the normality test.
For all analyses, differences between a treatment group and the equivalent control groups were considered statistically significant if the p-value was below 0.05 (p < 0.05).
Results
To explore the anti-epileptiform mechanism(s) of FA, we used an experimental workflow that is depicted in Figure 1.
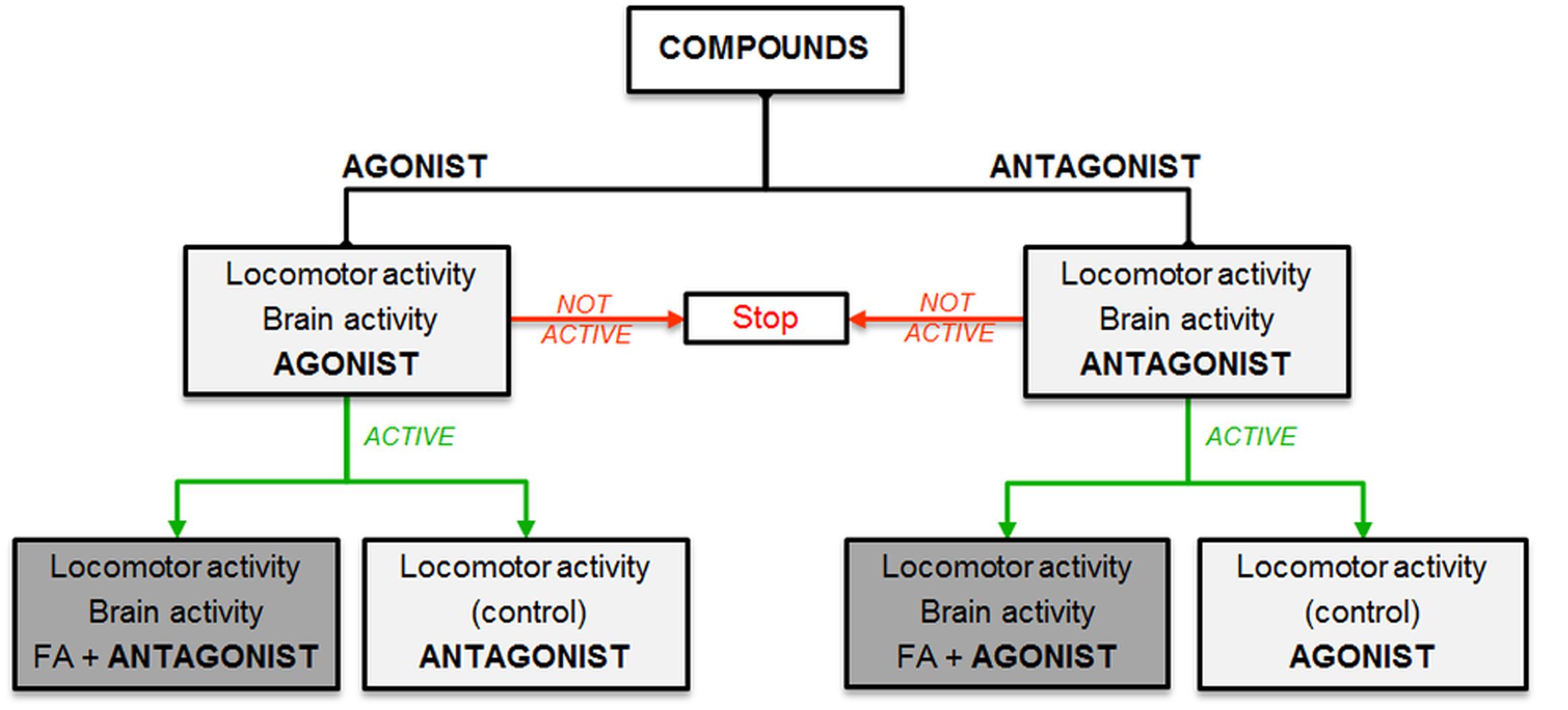
FIGURE 1. Experimental workflow. Selection of compounds based on hypothesized mechanisms of FA: stimulation (agonist, left side) or inhibition (antagonist, right side) of specific receptors. Light gray boxes show single treatment and dark gray boxes represent combinatorial treatment with FA.
First, we selected compounds with specific agonistic or antagonistic activity at distinct receptor subtypes (i.e., 5-HT1D, 5-HT2A, 5-HT2C receptors, and β2, σ1, σ2 receptors, respectively) and studied their effects on the locomotor and brain activity of scn1a mutant larvae.
The locomotor data were analyzed by normalizing the locomotor activity of treated ZF larvae against age-matched VHC-treated larvae (method A), and by calculating the percentage of scn1a mutant larvae with epileptiform activity below or above a 70% threshold value (method B). Method A was used to evaluate the results of both scn1a mutant larvae and WT larvae and has the advantage to demonstrate non-specific effects, e.g., a decrease in both mutant and WT larvae by sedative effects. Method B was applied to the data of the scn1a mutant larvae and has the advantage to account for the inherent variability of locomotor assays (i.e., different larvae at different days) since each larva is analyzed separately for each individual experiment.
In case of a significant decrease of epileptiform activity for a specific agonist/antagonist [as shown by at least one method of locomotor analysis (A and/or B) and confirmed by forebrain LFP recordings], we continued by treating larvae in separate experiments with a combination of FA and a compound (combinatorial treatment) with a counteractive profile of the agonist/antagonist previously used (in other words: in case of an active agonist, we combined FA with an antagonist and vice versa). Thereafter, we also tested the activity of the agonist/antagonist used in the combinatorial treatment as a single compound (control experiment). Finally, we performed triple treatment experiments to examine additional effects when combining all anti-epileptiform compounds. Similarly, we explored FA’s pharmacological profile by combining FA with triple treatment of compounds with the counteractive profile of the agonists/antagonists previously used.
Anti-epileptiform Activity Induced by Sigma1-Antagonism, 5-HT1D&2C-Agonism
Blocking the β2-adrenergic receptor (by ICI 118551) or the σ1 receptor (by NE 100) resulted in a significant decrease of epileptiform locomotor activity of the scn1a mutant larvae, as identified by one of the two methods assessing locomotor activity (i.e., method A and method B, respectively) (Figures 2A,B). The β2-adrenergic receptor antagonist, however, also reduced the locomotor activity of WT larvae, whereas the σ1-antagonist did not (Figure 2A1). Subsequently, treated scn1a mutant larvae were subjected to brain activity recordings, showing that only the σ1-antagonist (NE 100) was able to significantly reduce epileptiform brain activity (Figures 3A,B). Representative traces of brain activity during 5 min recordings are depicted in Figure 3C. σ2-antagonism (by SM 21) did not decrease epileptiform locomotor or brain activity in scn1a mutant larvae but lowered the locomotor activity of WT larvae (Figures 2, 3).
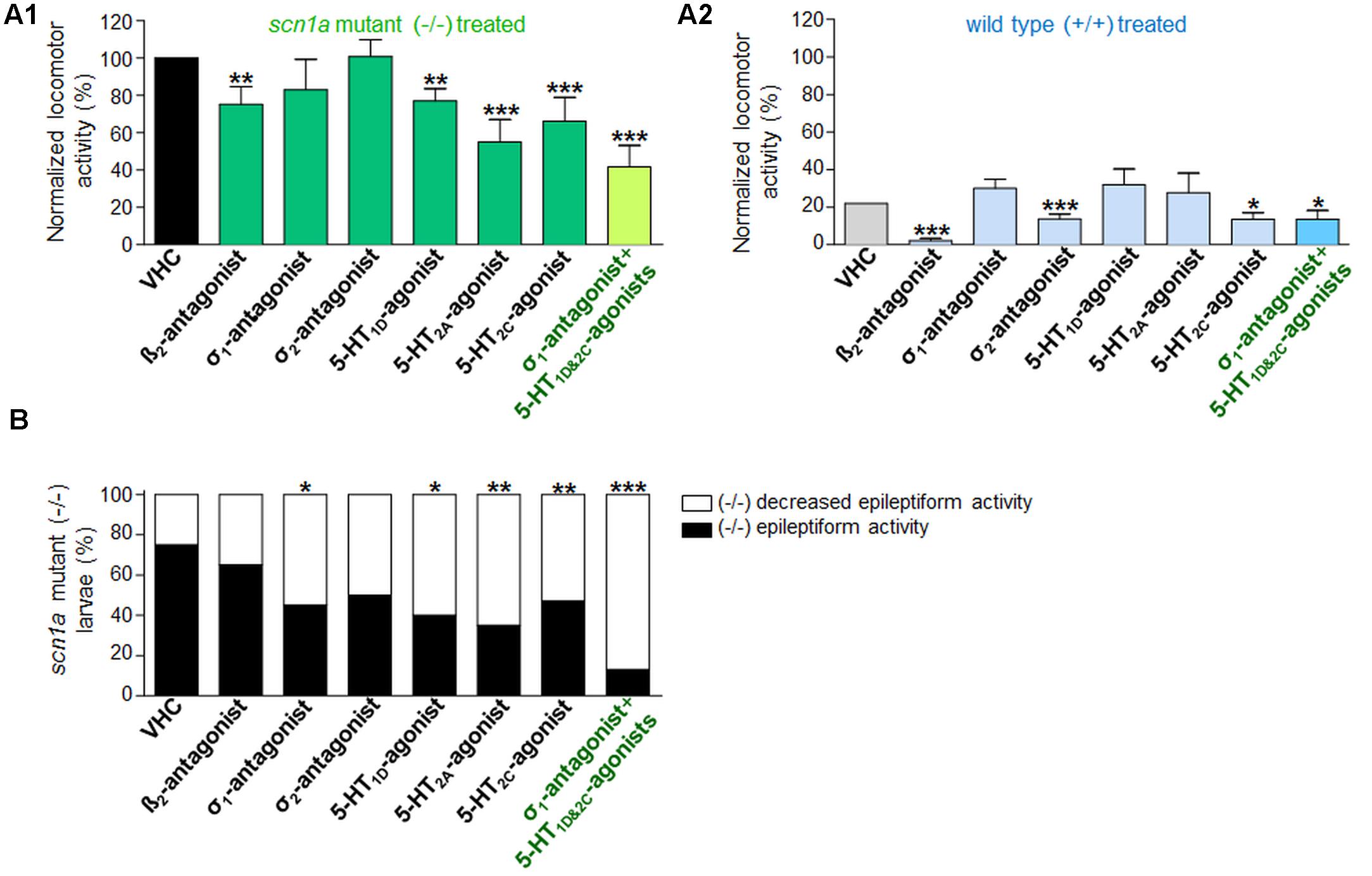
FIGURE 2. Activity profile of the selective beta2-antagonist (β2), sigma1-antagonist (σ1), sigma2-antagonist (σ2), subtype selective 5-HT-agonists (1D, 2A, 2C) and triple treatment with anti-epileptiform compounds [σ1-antagonist and subtype selective 5-HT-agonists (1D and 2C)] (locomotor behavioral assays). Locomotor activity was normalized against VHC-treated scn1a mutant larvae and displayed as a percentage ± SD. (A1) Normalized locomotor activity of treated scn1a mutant larvae (-/-). (A2) Normalized locomotor activity of treated WT larvae (+/+). (B) Percentage of scn1a mutant larvae with epileptiform activity below (white area) or above the threshold value (black area). Treatment with the selective β2-antagonist, σ1-antagonist, the subtype selective 5-HT-agonists (1D, 2A, 2C) and triple treatment with anti-epileptiform compounds [σ1-antagonist and subtype selective 5-HT-agonists (1D and 2C)] induced anti-epileptiform locomotor activity. A statistical difference is indicated by: ∗p < 0.05, ∗∗p < 0.01 and ∗∗∗p < 0.001 vs. VHC-treated controls. n = 16–30 ZF larvae for all experimental conditions.
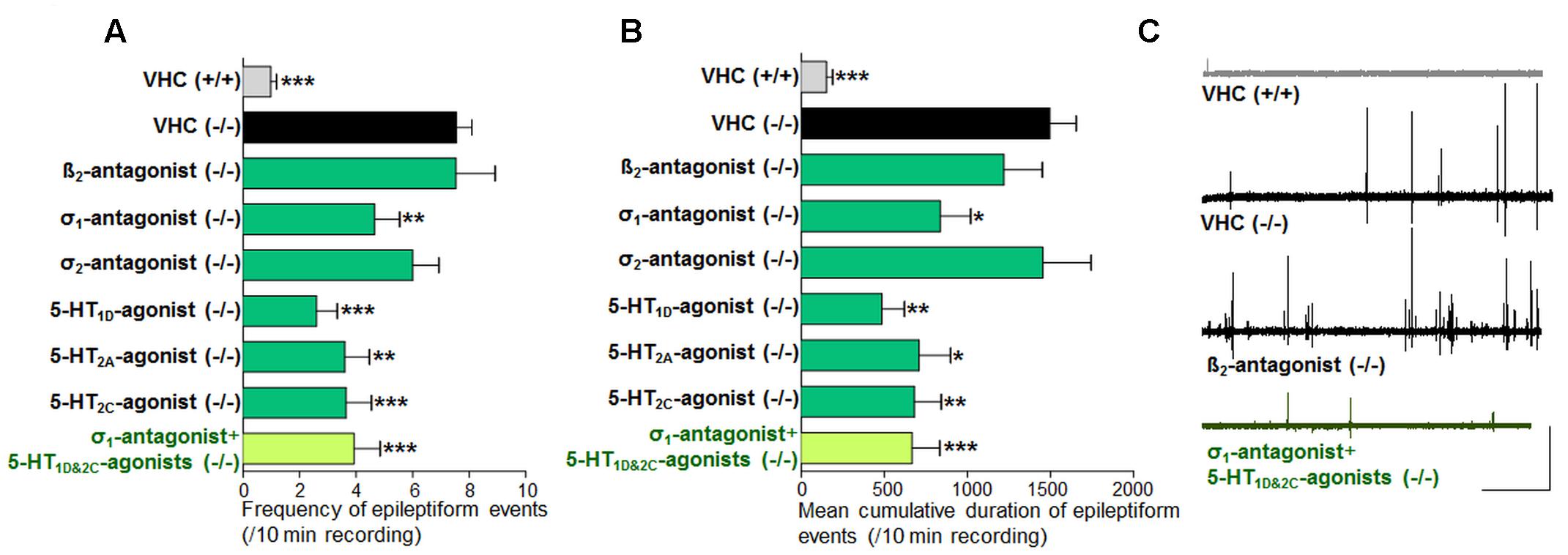
FIGURE 3. Activity profile of the selective beta2-antagonist (β2), sigma1-antagonist (σ1), sigma2-antagonist (σ2), subtype selective 5-HT-agonists (1D, 2A, 2C) and triple treatment with anti-epileptiform compounds [σ1-antagonist and subtype selective 5-HT-agonists (1D and 2C)] (forebrain LFP recordings). Quantification of brain recordings of VHC-treated WT larvae [VHC(+/+), n = 42], VHC-treated scn1a mutant larvae [VHC(-/-), n = 41] and compound-treated scn1a mutant larvae [compound(-/-)]. (A) Frequency of epileptiform events during 10 min recording. (B) Mean cumulative duration of epileptiform events during 10 min recording. Treatment with the selective β2-antagonist (n = 19) or the σ2-antagonist (n = 14) did not affect epileptiform brain activity in scn1a mutant larvae. In contrast, the σ1-antagonist (n = 20), 5-HT1D-agonist (n = 10), 5-HT2A-agonist (n = 10), the 5-HT2C-agonist (n = 14) and triple treatment with anti-epileptiform compounds [σ1-antagonist and subtype selective 5-HT-agonists (1D and 2C); n = 11] significantly reduced the frequency and the mean cumulative duration of epileptiform brain activity in scn1a mutant larvae. A statistical difference is indicated by: ∗p < 0.05, ∗∗p < 0.01 and ∗∗∗p < 0.001 vs. VHC-treated scn1a mutant larvae. (C) Visualization of representative electrograms of 7 dpf ZF larvae: VHC-treated WT larva [VHC (+/+)], VHC-treated scn1a mutant larva [VHC (-/-)]; β2-antagonist-treated scn1a mutant larva [β2-antagonist (-/-)]; triple treatment of scn1a mutant larva with anti-epileptiform compounds [σ1-antagonist+5-HT1D&2C-agonists (-/-)]; scale bars: 1 mV, 30 s.
Previous work showed evidence for a role of 5-HT1D, 5-HT2A, and 5-HT2C receptors in seizure modulation (Sourbron et al., 2016). Here we reconfirmed the anti-epileptiform effect of a distinct set of 5-HT-agonists (Figures 2A,B and Table 1). Agonism at the 5-HT1D (by PNU 109291) and 5-HT2A receptor (by NBOH-2C-CN) also decreased epileptiform brain activity of scn1a mutant larvae (Figures 3A,B) and did not affect the locomotor activity of WT larvae (Figure 2A1). Similarly, the 5-HT2C-agonist (Ro 600175) decreased locomotor activity of scn1a mutant but also of the WT larvae (Figure 2A1). However, consecutive brain recordings proved the selective anti-epileptiform activity of this compound in scn1a mutant larvae (Figures 3A,B).
Anti-epileptiform Activity of FA and the Counteraction by Sigma1-Agonism or 5-HT1D&2C-Antagonism (Combinatorial Treatment)
In order to explore the mechanisms of action of FA, we combined FA with the σ1-agonist (PRE 084) or 5-HT subtype selective antagonists (Figure 1 and Table 2). First, we reconfirmed the anti-epileptiform activity of FA in both assays (locomotor and brain recordings) (Figures 4, 5) as shown before (Dinday and Baraban, 2015; Sourbron et al., 2016). The σ1-agonist was unable to counteract the anti-epileptiform activity of FA in the locomotor assay (Figures 4A,B), although brain recordings revealed a statistically significant counteraction (Figures 5A,B). Treatment with the 5-HT1D-antagonist (LY 310762) or the 5-HT2C-antagonist (SB 242084) significantly counteracted the decrease in locomotor activity as elicited by FA in scn1a mutant larvae (Figures 4A,B). This was not the case for the 5-HT2A-antagonist (ketanserin). The locomotor behavior was not significantly altered in age-matched WT larvae by combinatorial treatments (Figure 4A2), pointing to a selective effect on scn1a mutant larvae. Interestingly, quantification of the corresponding electrograms confirmed the significant counteraction of the 5-HT1D-antagonist and 5-HT2C-antagonist (Figures 5A,B). Representative traces of brain activity during 5 min recording are depicted in Figure 5C. Finally, we performed experiments with a selective 5-HT2B-antagonist (SB 204741, negative control). This antagonist was unable to counteract the anti-epileptiform activity of FA in the locomotor (Figures 4A,B) and the brain recording assays (Figures 5A,B).
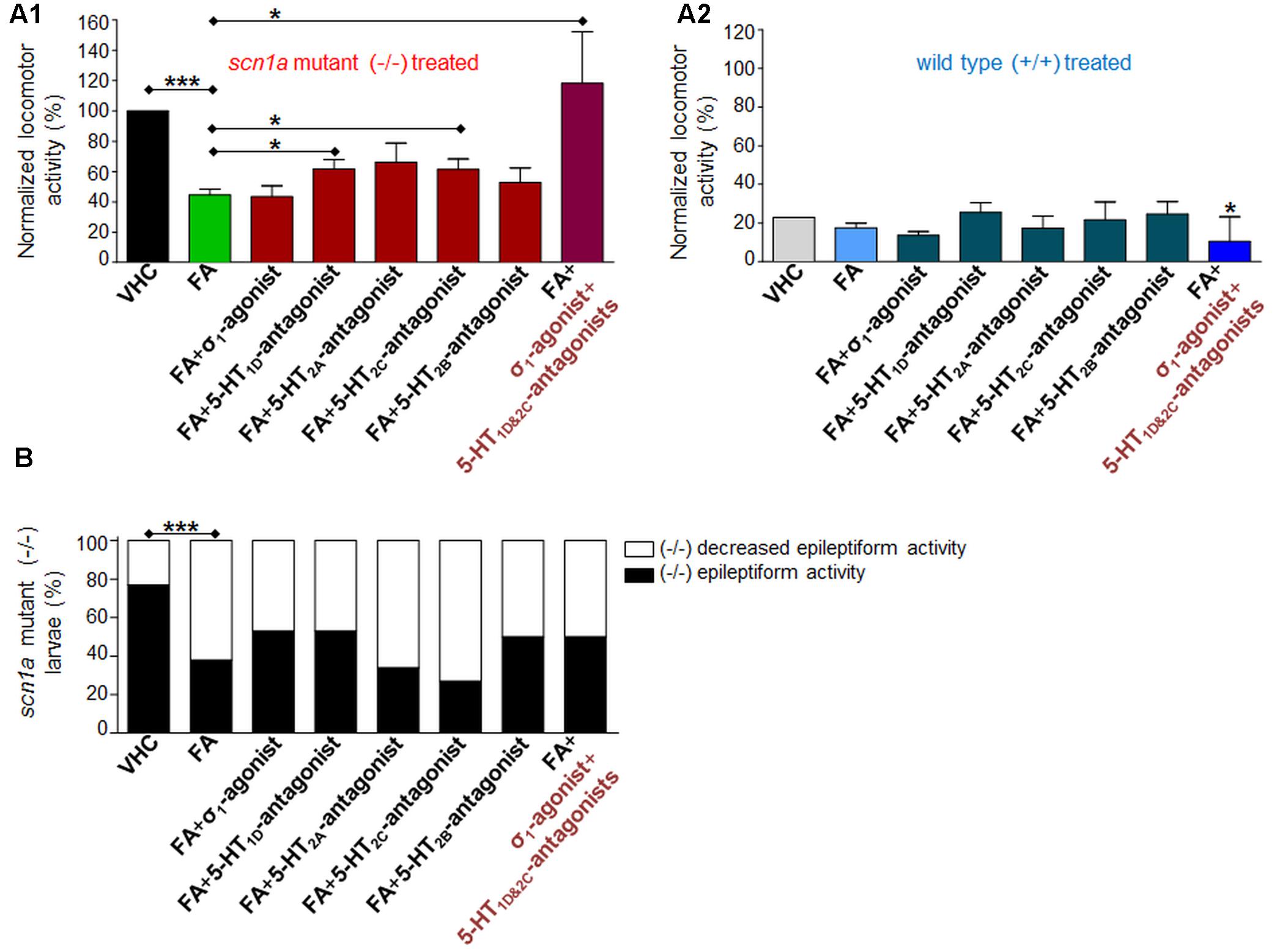
FIGURE 4. Activity profile of FA [single treatment with FA and combinatorial treatment of FA with the sigma1-agonist (σ1) or subtype selective 5-HT-antagonists (1D, 2A, 2C, 2B) or triple treatment of epileptiform compounds [σ1-agonist and subtype selective 5-HT-antagonists (1D and 2C)] (locomotor behavioral assays)]. Locomotor activity was normalized against VHC-treated scn1a mutant larvae (-/-) and displayed as a percentage ± SD. (A1) Normalized locomotor activity of treated scn1a mutant larvae (-/-). (A2) Normalized locomotor activity of treated WT larvae (+/+). (B) Percentage of scn1a mutant larvae with epileptiform activity below (white area) or above the threshold value (black area). Single treatment with FA induced anti-epileptiform locomotor activity (FA), which was counteracted by combinatorial treatment with the 5-HT1D-antagonist or the 5-HT2C-antagonist or triple treatment of epileptiform compounds [σ1-agonist and subtype selective 5-HT-antagonists (1D and 2C)]. A statistical difference is indicated by: ∗p < 0.05 and ∗∗∗p < 0.001 vs. VHC-treated controls (or FA-treated larvae). n = 16–30 ZF larvae for all experimental conditions.
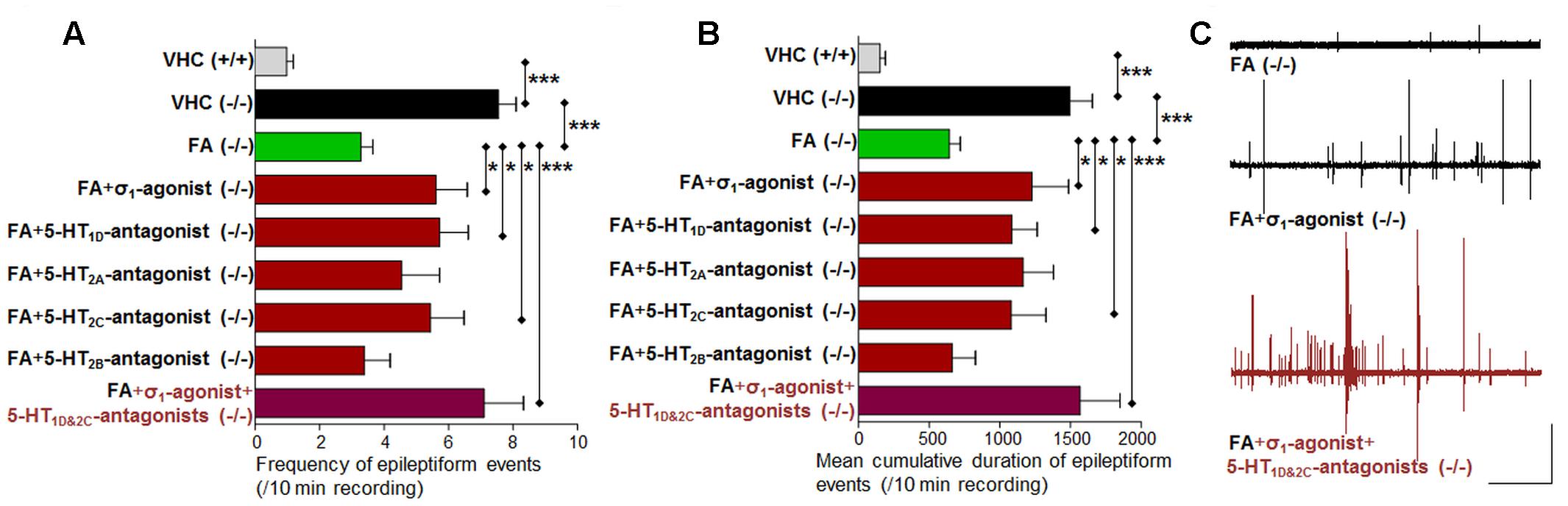
FIGURE 5. Activity profile of FA [single treatment with FA and combinatorial treatment of FA with the sigma1-agonist (σ1) or subtype selective 5-HT-antagonists (1D, 2A, 2C, 2B) or triple treatment of epileptiform compounds [σ1-agonist and subtype selective 5-HT-antagonists (1D and 2C)] (forebrain LFP recordings)]. Quantification of brain recordings of VHC-treated WT larvae [VHC(+/+), n = 42], VHC-treated scn1a mutant larvae [VHC(-/-), n = 41] and compound-treated scn1a mutant larvae [compound(-/-)]. (A) Frequency of epileptiform events during 10 minutes recording. (B) Mean cumulative duration of epileptiform events during 10 min recording. Single treatment with FA reduced epileptiform brain activity (n = 50), which was counteracted by combinatorial treatment with the σ1-agonist (n = 13), the 5-HT1D-antagonist (n = 18) or the 5-HT2C-antagonist (n = 16). A statistical difference is indicated by ∗p < 0.05 and ∗∗∗p < 0.001. (C) Visualization of representative electrograms of 7 dpf ZF larvae: FA-treated scn1a mutant larva [FA(-/-)]; FA-treated scn1a mutant larva in combination with the σ1-agonist [FA+ σ1-agonist(-/-)] or triple treatment with epileptiform compounds [σ1-agonist+5-HT1D&2C-antagonists (-/-)]; scale bars: 1 mV, 30 s.
No Anti-epileptiform Activity of Sigma1-Agonism or 5-HT-Antagonism
Subsequently, we performed single treatment experiments to investigate whether the compounds, used in combinatorial treatment with FA [see Anti-epileptiform Activity of FA and the Counteraction by Sigma1-Agonism or 5-HT1D&2C-Antagonism (Combinatorial Treatment)], affected the locomotor activity of ZF larvae in the absence of FA (Figure 6). Treatment with the σ1-agonist did not significantly influence the locomotor activity of ZF larvae, although a slight statistically insignificant increase in the epileptiform behavior of scn1a mutant larvae was present (p > 0.05, Figure 6A). The pharmacological inhibition of the 5-HT1D, 5-HT2A, and the 5-HT2C receptor did not alter epileptiform locomotor activity in scn1a mutant larvae (Figures 6A,B). The 5-HT1D-antagonist and the 5-HT2B-antagonist significantly affected locomotor activity of age-matched WT larvae (Figure 6A1).
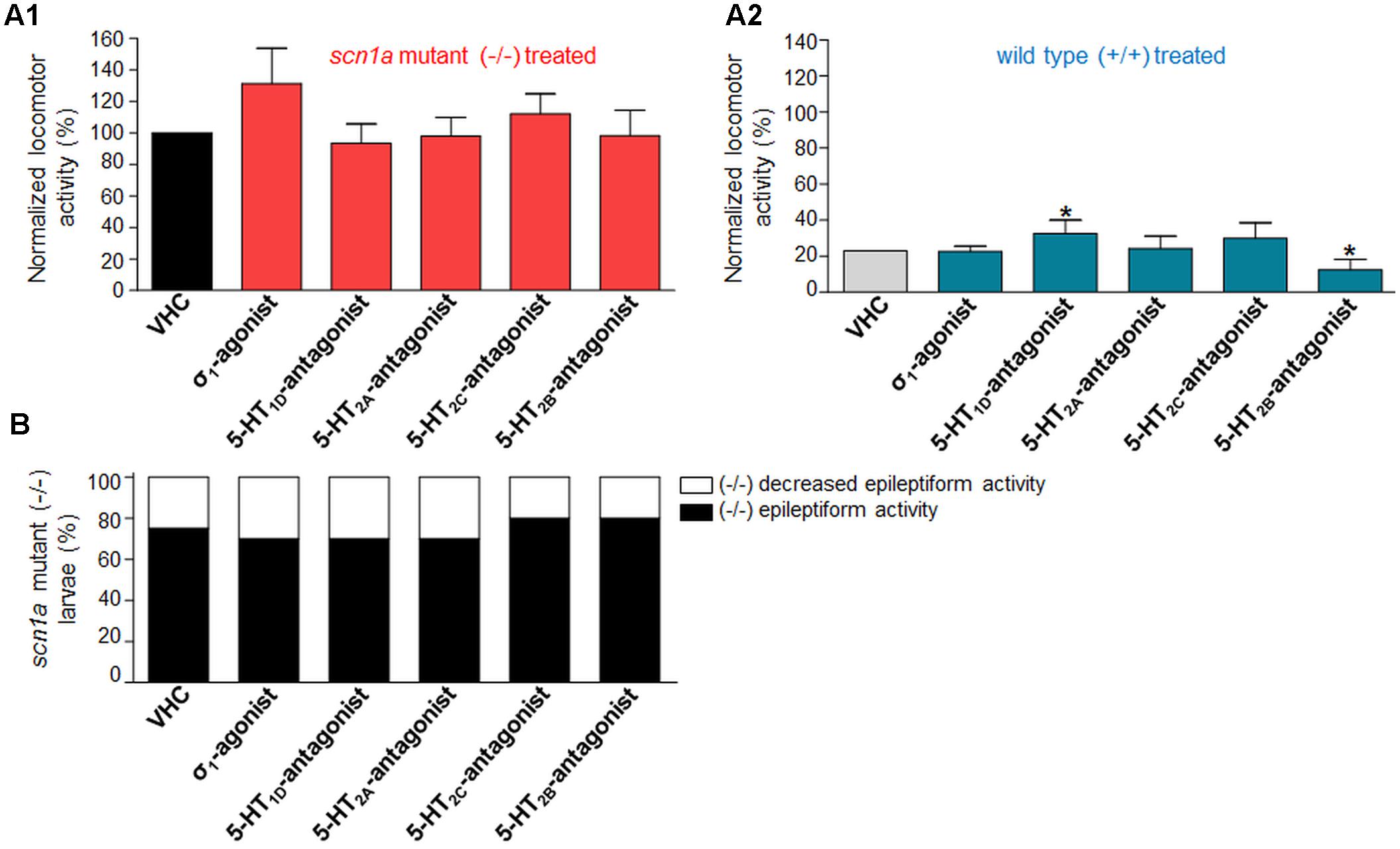
FIGURE 6. Activity profile of the sigma1-agonist (σ1) and subtype selective 5-HT-antagonists (1D, 2A, 2C, 2B) (locomotor behavioral assays). Locomotor activity was normalized against VHC-treated scn1a mutant larvae (-/-) and displayed as a percentage ± SD. (A1) Normalized locomotor activity of treated scn1a mutant larvae (-/-). (A2) Normalized locomotor activity of treated WT larvae (+/+). (B) Percentage of scn1a mutant larvae with epileptiform activity below (white area) or above the threshold value (black area). Single treatment with the σ1-agonist (σ1) and the subtype selective 5-HT-antagonists (1D, 2A, 2C, 2B) did not significantly affect the epileptiform locomotor behavior. A statistical difference is indicated by: ∗p < 0.05 vs. VHC-treated controls. n = 20 ZF larvae for all experimental conditions.
Anti-epileptiform Activity Induced by Sigma1-Antagonism and 5-HT1D&2C-Agonism (Triple Treatment)
Single treatment (σ1-antagonist, 5-HT1D- or 5-HT2C-agonist) decreased the abnormal (epileptiform) locomotor activity (range 17–34% decrease compared to VHC; Figure 2A1). Triple treatment (combination of the aforementioned three compounds) decreased the epileptiform locomotor activity to a greater extent (58% decrease compared to VHC, Figure 2A1), which is similar to the decrease elicited by FA (>55% decrease compared to VHC; Figure 4A1). Subsequent brain recordings confirmed these anti-epileptiform effects, though no major differences were observed between single and triple treatment (Figures 3, 5). Consistently, statistical analyses did not show any significant differences between triple treatment and single treatments (p < 0.05).
Counteraction of FA’s Anti-epileptiform Activity by Sigma1-Agonism and 5-HT1D&2C-Antagonism (Triple Treatment)
Combinatorial treatment of FA with single treatment of the σ1-agonist, 5-HT1D- or 5-HT2C-antagonist counteracted FA’s decrease in locomotor activity (range 0–41% increase relative to FA; Figure 4A1). Triple treatment (combination of the aforementioned three compounds) counteracted FA’s decrease in locomotor activity more profoundly (89% increase relative to FA; Figure 4A1). Consistently, brain recordings confirmed the counteraction of FA’s anti-epileptiform effect and showed that triple treatment counteracted FA’s effect more profoundly (116–144% increase relative to FA for the frequency and cumulative duration of epileptiform events, respectively; Figure 5), in comparison to the single treatment counteraction experiments (74–91% relative to FA for the frequency and cumulative duration of epileptiform events, respectively; Figure 5). Overall, these more pronounced counteractive effects by triple treatment compared to single treatments were not statistically significant (p > 0.05).
Neurotransmitter Changes after FA Treatment
Our data indicate an overall reduction of two out of three monoamines after FA treatment, i.e., DOP and NAD (Figure 7). The most pronounced and significant decrease was shown for NAD (Figure 7 and Supplementary Table S6).
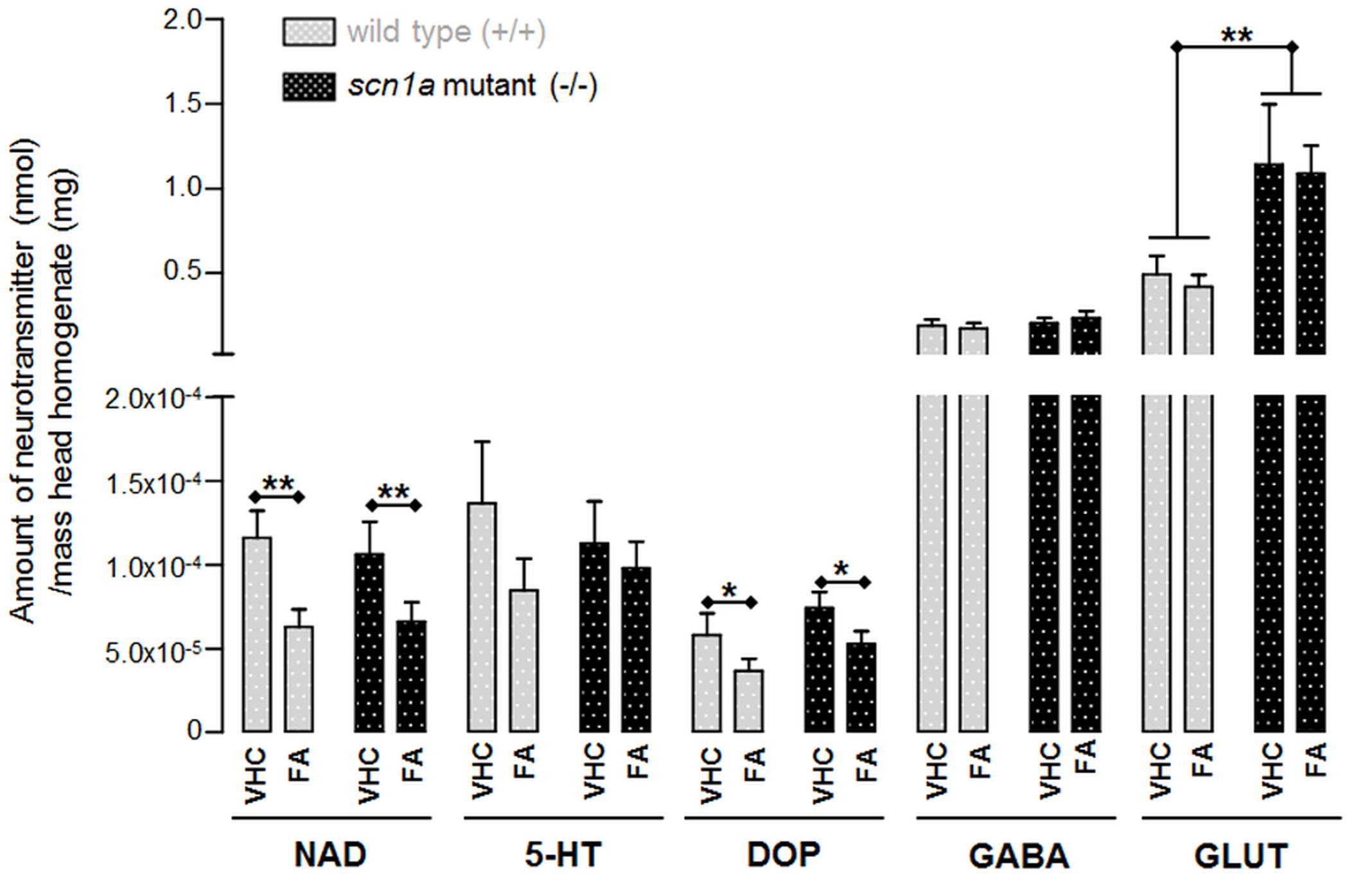
FIGURE 7. Neurotransmitter content in head homogenates of ZF larvae (nmol/mg). Data for each condition were collected from eight samples with each six heads (n = 48). ∗p < 0.05 and ∗∗p < 0.01 indicate a statistically significant alteration of some neurotransmitters (Supplementary Table S6; Two-way ANOVA).
The only statistical significant difference between both genotypes was the GLUT content which was significantly higher in scn1a mutant larvae (-/-) when compared to WT larvae (+/+). However, GLUT content was not significantly altered after FA treatment (Figure 7 and Supplementary Table S6).
Discussion
Dravet syndrome is one of the most drug-resistant forms of epilepsy that typically starts in the first year of life (Nolan et al., 2011). Recently, it was shown that the serotonergic drug FA is a potent add-on AED in DS patients (Ceulemans et al., 2012). Although this compound shows great promise for future therapeutic regimens in the treatment of DS and possibly other drug-resistant epileptic syndromes, it is presently unknown which 5-HT receptor subtypes are involved in its action and whether other mechanisms beyond its serotonergic profile play a part. This situation hampered a full mechanistic appreciation of the clinical performance of the compound.
Serotonergic Activity (5-HT)
In previous experimental work using scn1a mutant larvae, which mimic well the epileptiform features of DS in ZF, we have shown that selective pharmacological agonists of the 5-HT1D, 5-HT2A and 5-HT2C receptor subtypes (GR 46611, TCB 2 and lorcaserin, respectively) substantially decreased the epileptiform activity. Of importance, stimulation of other 5-HT receptor subtypes was not met with success. Using an identical approach here, we explored whether selective antagonists of 5-HT1D, 5-HT2A and 5-HT2C receptor subtypes were able to counteract the FA-induced inhibition of epileptiform locomotor and brain activity observed in scn1a mutant larvae. We show that antagonists of 5-HT1D and the 5-HT2C receptor subtypes were able to do so (LY 310762 and SB 242084, respectively), but notably, the 5-HT2A-antagonist (ketanserin) was not.
Of importance, and in line with our previous findings (Sourbron et al., 2016), a 5-HT2B-antagonist (SB 204741) did not counteract the anti-epileptiform activity of FA. Consequently, we can conclude that the 5-HT2B receptor subtype, that is proven to cause cardiotoxic effects after prolonged use of FA (Rothman et al., 2000), is not involved in the anti-epileptiform effect of FA.
We also examined the anti-epileptiform activity of the subtype selective 5-HT1D-, 5-HT2A- and 5-HT2C-antagonists without combinatorial FA treatment (i.e., single treatment) as other studies have shown that blocking these receptor subtypes can protect against seizures in other seizure/epilepsy models. For example, the 5-HT2A-antagonist used in this study, ketanserin, protected against seizures induced by hippocampal kindling in cats (Wada et al., 1992). The same antagonist was also involved in increasing the latency to audiogenic seizures in DBA/2 mice (Semenova and Tiku, 1997). Consistently, the 5-HT2C-antagonist, mesulergine, inhibited myoclonic jerks induced by 5-HTP in guinea pigs. This latter antagonist primarily blocks the 5-HT2C receptor and displays affinity for the 5-HT2A receptor subtype (Pappert et al., 1998). Selective pharmacological blockade of the 5-HT1D receptor also inhibited myoclonic jerks in the same model of generalized myoclonus (Hagan et al., 1995). However, our data clearly show that antagonists for these 5-HT receptor subtypes did not show any anti-epileptiform activity in our ZF model of DS.
Beta2-Adrenergic Activity (β2)
The activity of FA has always been labeled as 5-HT related, as confirmed in this study. Nonetheless, recent in vitro data suggested antagonism of sigma (σ) and beta2-adrenergic (β2) receptors as part of its pharmacological spectrum (Parthena et al., 2016). Hence, in this study we used the scn1a mutant larvae to explore the anti-epileptiform activity of σ- and β2- antagonism in in vivo conditions.
A selective antagonist of the β2-adrenergic receptor (ICI 118551) was able to significantly reduce the locomotor activity of scn1a mutant larvae. This is in agreement with the findings of Luchowska et al. (2001), that showed an anti-epileptiform effect against maximal electroshock-induced seizures in mice by antagonizing the β-adrenergic receptor. Additionally, β-adrenergic receptors have been implicated in audiogenic seizures and pindolol, a potent β-antagonist, was found to attenuate audiogenic seizures in DBA/2 mice (Lints and Nyquist-Battie, 1985). Nevertheless, in this work we show that the percentage of scn1a mutant larvae with decreased epileptiform activity was not significantly changed. In addition, the locomotor activity of WT larvae was decreased, suggesting a non-specific alteration of locomotor activity (i.e., potential sedative or muscle-relaxant effect). In line with these latter findings, the β2-antagonist did not decrease epileptiform brain activity. Thus, our in vivo data imply that the β2-adrenergic receptor is not involved in modulating epileptiform activity in the ZF DS model.
Sigma Activity (σ1 and σ2)
As far as the sigma receptors are concerned, the σ1-antagonist (NE 100) decreased both epileptiform behavior and especially brain activity in scn1a mutant larvae. On the other hand, the σ1-agonist (PRE 084) did not counteract FA’s decrease in abnormal locomotor activity (behavioral assays, Figure 4), whereas brain activity recordings clearly demonstrated a statistically significant counteraction (Figure 5). Locomotion is regulated in a complex way in different parts of the brain (Dunn et al., 2016) and it is possible that these neuronal activities are not always consistent with the brain activity alterations recorded by a single electrode positioned at the forebrain. Alternatively, it is also conceivable that the σ1-agonist (PRE 084) does not uniformly distribute over the brain, and that especially the peripheral areas probed by the electrode accumulated more compound resulting in a more pronounced effect.
Altogether, the data therefore suggest an involvement of the σ1 receptor in the mechanism of FA, beyond its serotonergic profile. In contrast, treatment with the σ2-antagonist (SM 21) did not reduce epileptiform activity in scn1a mutant larvae. This result was somewhat anticipated as most studies underline the role of the σ2 subtype receptor in cancer-related diseases, rather than in brain-related disorders (Rousseaux and Greene, 2015).
Of interest, a number of studies demonstrate the role of σ1 receptors in neurological diseases like epilepsy (Rousseaux and Greene, 2015) and overstimulation of σ1 receptors has been associated with GLUT-related excitatory effects (via NMDA receptors) (Borlot et al., 2014; Nicita et al., 2014; Rousseaux and Greene, 2015). In addition, blockade of σ1 receptors was associated directly with anti-epileptiform activity (Matsumoto et al., 2002). In contrast, also σ1-agonists have also been shown to exert clear anti-seizure effects (Meurs et al., 2007; Guo et al., 2015), and possibly σ1-agonist/antagonists might exert different activities depending on the seizure/epilepsy model. Anyhow, our data show that σ1-agonism was ineffective in reducing epileptiform events, whereas σ1-antagonism likely plays a role in the mechanism of FA in the ZF DS model.
Neurotransmitter Content
The neurotransmitter content analysis of scn1a mutants compared to WT only demonstrated a statistically significant increase in GLUT (p = 0.0036). This finding is in line with the neuro-excitatory properties related to GLUT and its role in epilepsy (Meldrum, 1994). Hence it is not surprising that decreasing glutamatergic effects is one of the main targets for currently available AEDs (Landmark, 2007). Nonetheless, the anti-epileptic compound FA did not alter GLUT levels. Interestingly, the concentration of other neurotransmitters (i.e., NAD and DOP) in head homogenates was altered after FA treatment. Our data indicate a significant DOP decrease by almost one third compared to the VHC treated group (p = 0.0335). The NAD content was even nearly halved when comparing the FA vs. the VHC treated group (p = 0.0035). The latter result is therefore in line with previous work that demonstrated a FA-related decrease of the NAD content in rat brain (Calderini et al., 1975).
As 5-HT-agonism (Astorne Figari et al., 2014) but also σ-antagonism (Monnet et al., 1992) can result in decreased NAD signaling, we hypothesize that the reduction of NAD content after FA treatment as observed in this study is due the effects of FA at 5-HT2C and σ1 receptors. Of interest, elevated NAD transmission has been associated with some cases of epilepsy (Fitzgerald, 2010) and although some studies show contradictory results (Svob Strac et al., 2016), also evidence exists supporting the use of NAD-decreasing drugs in the treatment of neurological diseases, including epilepsy (Fitzgerald, 2015).
Of importance, the concentrations measured encompass the total intra- and extracellular levels, so inter-synaptic level alterations were not assessed.
The following, yet incomplete, hypothesis emerges regarding the pharmacological profile of FA that underlies the treatment of DS and possibly other SCN1A-related epilepsies. Of importance, the present study used only one agonist or antagonist at their respective MTCs for the exploration of the involvement of some type of receptors, and further experiments using alternative methods (e.g., a genetic approach using receptor gene knock-down or overexpression) are needed to confirm the present results.
SCN1A mutations, as a major monogenic cause of DS, lead to a malfunction of the encoded voltage-gated sodium channels 1.1 (Nav1.1). In mammalian (rodent) DS models these channels are mainly affected in inhibitory (GABAergic) interneurons and to a lesser extent in excitatory pyramidal neurons (Dutton et al., 2013; Mistry et al., 2014). As a result, neuronal inhibition is impaired, which is assumed to cause seizures (Wong et al., 2016). Our data suggest that FA reduces epileptiform activity by stimulating 5-HT2C and 5-HT1D receptors, leading to enhanced GABAergic neurotransmission (as proven for 5-HT2C receptors) (Shen and Andrade, 1998; Higgins et al., 2014), and by a σ1-antagonistic action that can reduce the excitatory neurotransmission by modulating NMDA responses (Rousseaux and Greene, 2015). In addition, FA also significantly decreases the NAD brain content that might contribute to its anti-epileptic effects.
Conclusion
This work extends our current understanding of the anti-epileptic mechanisms of FA, beyond its serotonergic activity. Using an in vivo scn1a mutant ZF model we demonstrated that the anti-epileptiform activity of FA not only originates from its 5-HT1D- and 5-HT2C-agonism, but likely also from its ability to block σ1 receptors. These findings will help to understand the pharmacological profile of compounds that is critical for their applicability in the treatment of DS and possibly also other drug-resistant epilepsies.
Author Contributions
All authors took part in the outlines of the research. JS carried out all the zebrafish experiments and data-analysis. Neurotransmitter analyses were done by IS. JS and PdW were involved in the manuscript and figure preparation. All authors approved the final manuscript version.
Funding
This project was carried out with the support of Zogenix and the Agency for Innovation by Science and Technology (project nr 131179, IWT, Flanders).
Conflict of Interest Statement
LL obtains consultancy honoraria from Zogenix. No conflict of interest is declared regarding materials, methods or results in this research.
The other authors declare that the research was conducted in the absence of any commercial or financial relationships that could be construed as a potential conflict of interest.
Acknowledgments
We sincerely thank Aleksandra Siekierska and Borbála Hunyadi for advice on the analysis of the brain activity recordings, Michèle Partoens for the excellent assistance during experimental work, and Ria Berckmans for the neurotransmitter determinations [Center for Neurosciences, C4N, Vrije Universiteit Brussel (VUB), Brussels, Belgium].
Abbreviations
5-HT, 5-hydroxytryptamine (serotonin); 5-HTP, 5-Hydroxy tryptophan; aCSF, artificial cerebrospinal fluid; AED, anti-epileptic drug; cLogP, calculated LogP; DMSO, dimethylsulfoxide; DOP, dopamine; dpf, days post fertilization; DS, Dravet syndrome; EEG, electroencephalogram; GABA, γ-aminobutyric acid; GLUT, glutamate; lardist, distance in large movements (cm); LFP, local field potential; ms, milliseconds; MTC, maximum tolerable concentration; mV, millivolt; NAD, noradrenaline; Nav1.1, sodium channel protein type 1 subunit alpha isoform 1; Nav1.5, sodium channel protein type 1 subunit alpha isoform 5; NMDA, N-methyl-D-aspartate; SCN1A, sodium channel, voltage gated, type I alpha subunit (human); scn1Lab, sodium channel, voltage-gated, type I like, alpha b (zebrafish); SER, serotonin; VHC, vehicle; WT, wild type; ZF, zebrafish.
Supplementary Material
The Supplementary Material for this article can be found online at: http://journal.frontiersin.org/article/10.3389/fphar.2017.00191/full#supplementary-material
Footnotes
References
Astorne Figari, W. J., Herrmann, S., Akogyeram, C., and Qian, Q. (2014). New onset hypertension following abrupt discontinuation of citalopram. Clin. Nephrol. 82, 202–204. doi: 10.5414/CN107731
Best, J. D., and Alderton, W. K. (2008). Zebrafish: an in vivo model for the study of neurological diseases. Neuropsychiatr. Dis. Treat. 4, 567–576. doi: 10.2147/NDT.S2056
Borlot, F., Wither, R. G., Ali, A., Wu, N., Verocai, F., and Andrade, D. M. (2014). A pilot double-blind trial using verapamil as adjuvant therapy for refractory seizures. Epilepsy Res. 108, 1642–1651. doi: 10.1016/j.eplepsyres.2014.08.009
Calderini, G., Morselli, P. L., and Garattini, S. (1975). Effect of amphetamine and fenfluramine on brain noradrenaline and MOPEG-SO4. Eur. J. Pharmacol. 34, 345–350. doi: 10.1016/0014-2999(75)90261-7
Ceulemans, B., Boel, M., Leyssens, K., Van Rossem, C., Neels, P., Jorens, P. G., et al. (2012). Successful use of fenfluramine as an add-on treatment for Dravet syndrome. Epilepsia 53, 1131–1139. doi: 10.1111/j.1528-1167.2012.03495.x
Choi, S.-K., Green, D., Ho, A., Klein, U., Marquess, D., Taylor, R., et al. (2008). Designing selective, high affinity ligands of 5-HT1D receptor by covalent dimerization of 5-HT1F ligands derived from 4-Fluoro-N-[3-(1-methyl-4-piperidinyl)-1H-indol-5-yl]benzamide. J. Med. Chem. 51, 3609–3616. doi: 10.1021/jm7011722
Dinday, M. T., and Baraban, S. C. (2015). Large-scale phenotype-based antiepileptic drug screening in a zebrafish model of dravet syndrome. eNeuro 2, ENEURO.0068–15. doi: 10.1523/ENEURO.0068-15.2015
Dravet, C. (2011). The core Dravet syndrome phenotype. Epilepsia 52(Suppl. 2), 3–9. doi: 10.1111/j.1528-1167.2011.02994.x
Dunn, T. W., Mu, Y., Narayan, S., Randlett, O., Naumann, E. A., Yang, C.-T., et al. (2016). Brain-wide mapping of neural activity controlling zebrafish exploratory locomotion. eLife 5:e12741. doi: 10.7554/eLife.12741
Dutton, S. B., Makinson, C. D., Papale, L. A., Shankar, A., Balakrishnan, B., Nakazawa, K., et al. (2013). Preferential inactivation of Scn1a in parvalbumin interneurons increases seizure susceptibility. Neurobiol. Dis. 49, 211–220. doi: 10.1016/j.nbd.2012.08.012
Fitzgerald, P. J. (2010). Is elevated norepinephrine an etiological factor in some cases of epilepsy? Seizure 19, 311–318. doi: 10.1016/j.seizure.2010.04.011
Fitzgerald, P. J. (2015). Noradrenaline transmission reducing drugs may protect against a broad range of diseases. Auton. Autacoid Pharmacol. 34, 15–26. doi: 10.1111/aap.12019
García-Pedraza, J. Á., García, M., Martín, M. L., Gómez-Escudero, J., Rodríguez-Barbero, A., Román, L. S., et al. (2013). Peripheral 5-HT1D and 5-HT7 serotonergic receptors modulate sympathetic neurotransmission in chronic sarpogrelate treated rats. Eur. J. Pharmacol. 714, 65–73. doi: 10.1016/j.ejphar.2013.05.045
Gharedaghi, M. H., Seyedabadi, M., Ghia, J.-E., Dehpour, A. R., and Rahimian, R. (2014). The role of different serotonin receptor subtypes in seizure susceptibility. Exp. Brain Res. 232, 347–367. doi: 10.1007/s00221-013-3757-0
Guo, L., Chen, Y., Zhao, R., Wang, G., Friedman, E., Zhang, A., et al. (2015). Allosteric modulation of sigma-1 receptors elicits anti-seizure activities. Br. J. Pharmacol. 172, 4052–4065. doi: 10.1111/bph.13195
Hagan, J. J., Hatcher, J. P., and Slade, P. (1995). The role of 5-HT1D and 5-HT1A receptors in mediating 5-hydroxytryptophan induced myoclonic jerks in guinea pigs. Eur. J. Pharmacol. 294, 743–751. doi: 10.1016/0014-2999(95)00627-3
Hansen, M., Phonekeo, K., Paine, J. S., Leth-Petersen, S., Begtrup, M., Brauner-Osborne, H., et al. (2014). Synthesis and structure-activity relationships of N-benzyl phenethylamines as 5-HT2A/2C agonists. ACS Chem. Neurosci. 5, 243–249. doi: 10.1021/cn400216u
Higgins, G. A., Desnoyer, J., Niekerk, A., Van Silenieks, L. B., Lau, W., Thevarkunnel, S., et al. (2014). Characterization of the 5-HT 2C receptor agonist lorcaserin on efficacy and safety measures in a rat model of diet-induced obesity. Pharmacol. Res. Perspect. 3:e00084. doi: 10.1002/prp2.84
Howe, K., Clark, M. D., Torroja, C. F., Torrance, J., Berthelot, C., Muffato, M., et al. (2013). The zebrafish reference genome sequence and its relationship to the human genome. Nature 496, 498–503. doi: 10.1038/nature12111
Landmark, C. J. (2007). Targets for antiepileptic drugs in the synapse. Medical Sci. Monit. 13, RA1–RA7. doi: 10.12659/MSM.897626
Lints, C. E., and Nyquist-Battie, C. (1985). A possible role for beta-adrenergic receptors in the expression of audiogenic seizures. Pharmacol. Biochem. Behav. 22, 711–716. doi: 10.1016/0091-3057(85)90518-0
Löscher, W., Klitgaard, H., Twyman, R. E., and Schmidt, D. (2013). New avenues for anti-epileptic drug discovery and development. Nat. Rev. Drug Discov. 12, 757–776. doi: 10.1038/nrd4126
Luchowska, E., Luchowski, P., Wielosz, M., Kleinrok, Z., and Urbanska, E. M. (2001). beta-Adrenoceptor blockade enhances the anticonvulsant effect of glutamate receptor antagonists against maximal electroshock. Eur. J. Pharmacol. 431, 209–214.
Mastrangelo, M., and Leuzzi, V. (2012). Genes of early-onset epileptic encephalopathies: from genotype to phenotype. Pediatr. Neurol. 46, 24–31. doi: 10.1016/j.pediatrneurol.2011.11.003
Matsumoto, R. R., McCracken, K. A., Pouw, B., Zhang, Y., and Bowen, W. D. (2002). Involvement of sigma receptors in the behavioral effects of cocaine: evidence from novel ligands and antisense oligodeoxynucleotides. Neuropharmacology 42, 1043–1055. doi: 10.1016/S0028-3908(02)00056-4
Matsumoto, R. R., Pouw, B., Mack, A. L., Daniels, A., and Coop, A. (2007). Effects of UMB24 and ( ± )-SM 21, putative σ(2)-preferring antagonists, on behavioral toxic and stimulant effects of cocaine in mice. Pharmacol. Biochem. Behav. 86, 86–91. doi: 10.1016/j.pbb.2006.12.011
Meldrum, B. S. (1994). The role of glutamate in epilepsy and other CNS disorders. Neurology 44, S14–S23.
Meurs, A., Clinckers, R., Ebinger, G., Michotte, Y., and Smolders, I. (2007). Sigma 1 receptor-mediated increase in hippocampal extracellular dopamine contributes to the mechanism of the anticonvulsant action of neuropeptide Y. Eur. J. Neurosci. 26, 3079–3092. doi: 10.1111/j.1460-9568.2007.05911.x
Milan, D. J. (2003). Drugs that induce repolarization abnormalities cause bradycardia in zebrafish. Circulation 107, 1355–1358. doi: 10.1161/01.CIR.0000061912.88753.87
Mistry, A. M., Thompson, C. H., Miller, A. R., Vanoye, C. G., George, A. L. J., and Kearney, J. A. (2014). Strain- and age-dependent hippocampal neuron sodium currents correlate with epilepsy severity in Dravet syndrome mice. Neurobiol. Dis. 65, 1–11. doi: 10.1016/j.nbd.2014.01.006
Monnet, F. P., Blier, P., Debonnel, G., and de Montigny, C. (1992). Modulation by sigma ligands of N-methyl-D-aspartate-induced [3H]noradrenaline release in the rat hippocampus: G-protein dependency. Naunyn Schmiedebergs Arch. Pharmacol. 346, 32–39. doi: 10.1007/BF00167567
Moritz, C., Berardi, F., Abate, C., and Peri, F. (2015). Live imaging reveals a new role for the sigma-1 (sigma1) receptor in allowing microglia to leave brain injuries. Neurosci. Lett. 591, 13–18. doi: 10.1016/j.neulet.2015.02.004
Moshé, S. L., Perucca, E., Ryvlin, P., and Tomson, T. (2015). Epilepsy: new advances. Lancet 385, 884–898. doi: 10.1016/S0140-6736(14)60456-6
Nakazato, A., Ohta, K., Sekiguchi, Y., Okuyama, S., Chaki, S., Kawashima, Y., et al. (1999). Design, synthesis, structure-activity relationships, and biological characterization of novel arylalkoxyphenylalkylamine sigma ligands as potential antipsychotic drugs. J. Med. Chem. 42, 1076–1087. doi: 10.1021/jm980212v
Nicita, F., Spalice, A., Papetti, L., Nikanorova, M., Iannetti, P., and Parisi, P. (2014). Efficacy of verapamil as an adjunctive treatment in children with drug-resistant epilepsy: a pilot study. Seizure 23, 36–40. doi: 10.1016/j.seizure.2013.09.009
Nolan, K. J., Kay, E., Camfield, C. S., and Camfield, P. R. (2011). Does Dravet syndrome have a recognizable face? Pediatr. Neurol. 45, 392–394. doi: 10.1016/j.pediatrneurol.2011.09.008
Pappert, E. J., Goetz, C. G., Stebbins, G. T., Belden, M., and Carvey, P. M. (1998). 5-Hydroxytryptophan-induced myoclonus in guinea pigs: mediation through 5-HT1/2 receptor subtypes. Eur. J. Pharmacol. 347, 51–56. doi: 10.1016/S0014-2999(98)00086-7
Parthena, M., Boyd, B., Gail, F., Arnold, G., Galer, B., and Ca, U. Z. I. E. (2016). An Examination of the Mechanism of Action of Fenfluramine in Dravet Syndrome: A Look Beyond Serotonin. Available at: http://www.zogenix.com/c/newsroom/publications-presentations.php
Rhoades, D. J., Kinder, D. H., and Mahfouz, T. M. (2014). A comprehensive ligand based mapping of the σ2 receptor binding pocket. Med. Chem. 10, 98–121. doi: 10.2174/1573406409999131119103621
Rice, P., Longden, I., and Bleasby, A. (2000). EMBOSS: the European Molecular Biology Open Software Suite. Trends Genet. 16, 276–277. doi: 10.1016/S0168-9525(00)02024-2
Rothman, R. B., Baumann, M. H., Savage, J. E., Rauser, L., McBride, A., Hufeisen, S. J., et al. (2000). Evidence for possible involvement of 5-HT(2B) receptors in the cardiac valvulopathy associated with fenfluramine and other serotonergic medications. Circulation 102, 2836–2841. doi: 10.1161/01.CIR.102.23.2836
Rousseaux, C. G., and Greene, S. F. (2015). Sigma receptors [sigmaRs]: biology in normal and diseased states. J. Recept. Signal Transduct. Res. 36, 327–388. doi: 10.3109/10799893.2015.1015737
Schoonheim, P. J., Arrenberg, A. B., Del Bene, F., and Baier, H. (2010). Optogenetic localization and genetic perturbation of saccade-generating neurons in zebrafish. J. Neurosci. 30, 7111–7120. doi: 10.1523/JNEUROSCI.5193-09.2010
Schoonjans, A.-S., Lagae, L., and Ceulemans, B. (2015). Low-dose fenfluramine in the treatment of neurologic disorders: experience in Dravet syndrome. Ther. Adv. Neurol. Disord. 8, 328–338. doi: 10.1177/1756285615607726
Schubert, J., Siekierska, A., Langlois, M., May, P., Huneau, C., Becker, F., et al. (2014). Mutations in STX1B, encoding a presynaptic protein, cause fever-associated epilepsy syndromes. Nat. Genet. 46, 1327–1332. doi: 10.1038/ng.3130
Semenova, T. P., and Tiku, M. K. (1997). Interactions between the serotoninergic and noradrenergic systems of the brain and their role in the regulation of animal behavior. Neurosci. Behav. Physiol. 27, 280–282. doi: 10.1007/BF02462895
Shen, R., and Andrade, R. (1998). 5-Hydroxytryptamine 2 receptor facilitates GABAergic neurotransmission in rat hippocampus 1. J. Pharmacol. Exp. Ther. 285, 805–812.
Sourbron, J., Schneider, H., Kecskes, A., Liu, Y., Buening, E. M., Lagae, L., et al. (2016). Serotonergic modulation as effective treatment for dravet syndrome in a zebrafish mutant model. ACS Chem. Neurosci. 7, 588–598. doi: 10.1021/acschemneuro.5b00342
Suls, A., Jaehn, J. A., Kecskés, A., Weber, Y., Weckhuysen, S., Craiu, D. C., et al. (2013). De novo loss-of-function mutations in CHD2 cause a fever-sensitive myoclonic epileptic encephalopathy sharing features with Dravet syndrome. Am. J. Hum. Genet. 93, 967–975. doi: 10.1016/j.ajhg.2013.09.017
Svob Strac, D., Pivac, N., Smolders, I. J., Fogel, W. A., De Deurwaerdere, P., and Di Giovanni, G. (2016). Monoaminergic mechanisms in epilepsy may offer innovative therapeutic opportunity for monoaminergic multi-target drugs. Front. Neurosci. 10:492. doi: 10.3389/fnins.2016.00492
Tian, X.-L., Yu, L.-H., Li, W.-Q., Hu, Y., Yin, M., and Wang, Z.-J. (2015). Activation of 5-HT(2C) receptor promotes the expression of neprilysin in U251 human glioma cells. Cell Mol. Neurobiol. 35, 425–432. doi: 10.1007/s10571-014-0138-6
Ventola, C. L. (2014). Epilepsy management: newer agents, unmet needs, and future treatment strategies. Pharm. Ther. 39, 776–792.
Wada, Y., Nakamura, M., Hasegawa, H., and Yamaguchi, N. (1992). Role of serotonin receptor subtype in seizures kindled from the feline hippocampus. Neurosci. Lett. 141, 21–24. doi: 10.1016/0304-3940(92)90325-2
Wang, Z., Nishimura, Y., Shimada, Y., Umemoto, N., Hirano, M., Zang, L., et al. (2009). Zebrafish beta-adrenergic receptor mRNA expression and control of pigmentation. Gene 446, 18–27. doi: 10.1016/j.gene.2009.06.005
Watry, S., and Lu, J. (2013). A brief summary for 5-HT receptors. J. Genet. Syndr. Gene Ther. 4, 4–9. doi: 10.4172/2157-7412.1000129
Wenzel, D., Knies, R., Matthey, M., Klein, A. M., Welschoff, J., Stolle, V., et al. (2009). beta(2)-adrenoceptor antagonist ICI 118,551 decreases pulmonary vascular tone in mice via a G(i/o) protein/nitric oxide-coupled pathway. Hypertension 54, 157–163. doi: 10.1161/HYPERTENSIONAHA.109.130468
Williams, C. A., and Battaglia, A. (2013). Molecular biology of epilepsy genes. Exp. Neurol. 244, 51–58. doi: 10.1016/j.expneurol.2011.12.001
Wong, J. C., Dutton, S. B. B., Collins, S. D., Schachter, S., and Escayg, A. (2016). Huperzine a provides robust and sustained protection against induced seizures in Scn1a mutant mice. Fronti. Pharmacol. 7:357. doi: 10.3389/fphar.2016.00357
Zdebik, A. A., Mahmood, F., Stanescu, H. C., Kleta, R., Bockenhauer, D., and Russell, C. (2013). Epilepsy in kcnj10 morphant zebrafish assessed with a novel method for long-term EEG recordings. PLoS ONE 8:e79765. doi: 10.1371/journal.pone.0079765
Zhang, Y., Kecskes, A., Copmans, D., Langlois, M., Crawford, A. D., Ceulemans, B., et al. (2015). Pharmacological characterization of an antisense knockdown zebrafish model of dravet syndrome: inhibition of epileptic seizures by the serotonin agonist fenfluramine. PLoS ONE 10:e0125898. doi: 10.1371/journal.pone.0125898
Keywords: epilepsy, Dravet syndrome, Zebrafish, pharmacological modulation, GABA, glutamate, monoamines, sigma
Citation: Sourbron J, Smolders I, de Witte P and Lagae L (2017) Pharmacological Analysis of the Anti-epileptic Mechanisms of Fenfluramine in scn1a Mutant Zebrafish. Front. Pharmacol. 8:191. doi: 10.3389/fphar.2017.00191
Received: 21 December 2016; Accepted: 23 March 2017;
Published: 06 April 2017.
Edited by:
Yukihiro Ohno, Osaka University of Pharmaceutical Sciences, JapanReviewed by:
Seok-Yong Choi, Chonnam National University, South KoreaYukio Ago, Osaka University, Japan
Copyright © 2017 Sourbron, Smolders, de Witte and Lagae. This is an open-access article distributed under the terms of the Creative Commons Attribution License (CC BY). The use, distribution or reproduction in other forums is permitted, provided the original author(s) or licensor are credited and that the original publication in this journal is cited, in accordance with accepted academic practice. No use, distribution or reproduction is permitted which does not comply with these terms.
*Correspondence: Peter de Witte, cGV0ZXIuZGV3aXR0ZUBwaGFybS5rdWxldXZlbi5iZQ==