- 1Department of Veterinary Pharmacology, College of Veterinary Medicine, Huazhong Agricultural University, Wuhan, China
- 2National Reference Laboratory of Veterinary Drug Residues and MAO Key Laboratory for Detection of Veterinary Drug Residues, Huazhong Agriculture University, Wuhan, China
- 3State Key Laboratory of Agriculture Microbiology, College of Veterinary Medicine, Huazhong Agriculture University, Wuhan, China
A new, more palatable formulation of 10% enrofloxacin enteric-coated granules was investigated to evaluate the pharmacokinetic effect in plasma, the residue elimination in tissues and the clinical efficacy against Actinobacillus pleuropneumonia (APP) and Mycoplasam suis (MS) in pigs. In this study, the enrofloxacin concentrations in plasma and tissues were detected using high-performance liquid chromatography with phosphate buffer (pH = 3) and acetonitrile. The pharmacokinetics and elimination of enrofloxacin enteric-coated granules were performed after oral administration at a single dose of 10 mg/kg body weight (bw) and 5 mg/kg twice per day for 5 consecutive days, respectively. The in vivo antibacterial efficacy and clinical effectiveness of enrofloxacin enteric-coated granules against APP and MS were assayed at 2.5, 5, 10 mg/kg, compared with tiamulin (8 mg/kg) based on establishment of APP and MS infection models. 56 APP strains were selected and tested for in vitro antibacterial activity of enrofloxacin enteric-coated granules. The main parameters of elimination half-life (t1/2β), Tmax, and area under the curve (AUC) were 14.99 ± 4.19, 3.99 ± 0.10, and 38.93 ± 1.52 μg h/ml, respectively, revealing that the enrofloxacin concentration remained high and with a sustainable distribution in plasma. Moreover, the analysis on the evaluation of enrofloxacin and ciprofloxacin in muscle, fat, liver and kidney showed that the recovery were more than 84% recovery in accordance with the veterinary drug residue guidelines of United States pharmacopeia, and the withdrawal periods were 4.28, 3.81, 4.84, and 3.51 days, respectively, suggesting that the withdrawal period was 5 d after oral administration of 5 mg/kg twice per day. The optimal dosage of enrofloxacin enteric-coated granules against APP and MS was 5 mg/kg, with over 90% efficacy, which was significantly different (p < 0.05) to the 2.5 mg/kg group, but not to the 10 mg/kg group or the positive control group (tiamulin). In conclusion, 10% enrofloxacin enteric-coated granules had significant potential for treating APP and MS, and it provided an alternative enrofloxacin palatability formulation.
Introduction
Enrofloxacin (ENR), a classical fluoroquinolone antibiotic was developed for exclusive use in veterinary medicine for the treatment of respiratory and gastrointestinal infections. Its essential metabolite, ciprofloxacin (CP), is also widely active and effective against Gram-negative, positive aerobes, and mycoplasmas (Küng et al., 1993; Deveau, 2007; Reyesherrera et al., 2011). Although CP has not been approved for veterinary use, it is a metabolite of ENR in animals, which decreases animal mortality, promotes growth, and improves economic benefits (Sneeringer et al., 2015). With the indiscriminate use of ENR, the presence of its residues in animals may lead to adverse health effects on human, such as allergic reactions and ENR-resistant strains. In addition, sub-therapeutic metabolites of quinolones can persist in edible tissues and be ingested by human, and resistance genes may be transferred to endogenous or exogenous bacterium (Sa et al., 2008; Aliu and Sulaj, 2014; de Almeida et al., 2015; Wang et al., 2016). As a quinolone commonly used in poultry and mammalian production, ENR has a direct effect of inhibiting bacterial DNA-gyrase and topoisomerase IV enzyme activities (Ebrahimzadeh et al., 2014; Gouvêa et al., 2015). In Europe, ENR and CP are only approved for therapeutic use in animal production, and their residues could be detected in animal tissue caused by respective withdrawal periods before slaughter.
Actinobacillus pleuropneumonia (APP) is an obligate parasite of the porcine respiratory tract that can infect nasal cavities and tonsils of pigs (Dom et al., 1994a; Duff et al., 1996; Yoshimura et al., 2002; Jobert et al., 2010). There are 12 serotypes of biotype 1, and 6 serotypes of biotype 2, which are defined based on their surface polysaccharide antigens (Altman et al., 1990; Perry et al., 1990; Schaller et al., 2001; Bossé et al., 2002). All serotypes can be urease positive, and thus cause respiratory infection and serious economic loss. Mycoplasam suis (MS) is an uncultivable pathogen that can colonize the surface of porcine erythrocytes, inducing long-term hypocytosis anemia and mild fever. MS can also decrease reproductive efficiency in sows, and cause growth retardation in feeder pigs, causing economic reduction (Henry, 1979; Messick, 2004; Yuan et al., 2009, 2010).
Against APP and MS, ENR is widely used in veterinary clinics, but it has a bitter taste and causes irritation to the gastrointestinal tract, resulting in food refusal, vomiting, stomachache, and ventosity in animals when ENR is added to food. The application of coatings to the surface of pharmaceutical solid-dosage forms has been practiced for over 150 years. Previous reports have demonstrated enteric polymers to be safe and palatable, and have been widely accepted for use in drug products (Nykänen, 2003; Biju et al., 2004; Bushra et al., 2010). One type of enteric-coated pellet is 10% enrofloxacin enteric-coated granules (EEG), which can effectively cover the bitterness of ENR, and reduce adverse reactions in animals with sustained release. It was prepared by Shanghai Tongren Pharmaceutical Co., Ltd.
Most published studies have reported the pharmacokinetics (PK), residue and withdrawal period of ENR in broilers using HPLC, ELISA, or LC-MS/MS. The detection of ENR in edible tissues of broilers have also been evaluated in those reports (de Assis et al., 2016; Haag et al., 2016; Panzenhagen et al., 2016). Establishment of withdrawal periods are based on the depletion times of drugs, which allows the appropriate animal treatment and slaughter of treated animals, according to the requirement for the elimination and residues of drugs in animal tissues. However, there are few published reports on the residue and withdrawal period for ENR in swine.
In view of the physiological similarities of pigs with human and other animals, our study aimed to explore the metabolism, pharmacokinetic profiles, and clinical efficacy of EEG in pigs after oral administration. To ensure human food safety, this study has established compliance with withdrawal periods, maximum residue limits (MRLs), and evaluated the clinical treatment for a new formulation 10% EEG in pigs. Our results would be useful for the assessment of efficacy, safety, and effective dosage regimens and withdrawal periods of EEG for clinical use.
Materials and Methods
Chemicals and Reagents
The ENR reference standard (98.5% purity) and CP reference standard (95% purity) were purchased from Dr. Ehrenstorfer (Augsburg, Germany), 10% EEG test product, and 10% tiamulin fumarate were provided from Shanghai Tongren Pharmaceutical Co., Ltd (Shanghai, PR China). Acetonitrile and methanol were purchased from Fisher Chemicals Co., Ltd (New Jersey, USA). Phosphoric acid and triethylamine were purchased from Sinopharm Chemical Reagent Co., Ltd (Shanghai, PR China). Water was purified using the Milli-Q water purification system (Milli-Q Co., Ltd, France). All chemicals used in this study were analytical grade or higher and dissolved or diluted with deionized water.
Bacterium
The serotype 1 of clinical APP pathogenic strain and MS, which were both isolated from Hubei Province were gifted by Huazhong Agriculture University State Key Laboratory of Microbiology. These strains were used to establish infection models for the clinical efficacy study. All strains were stored at −80°C until analysis. Prior to testing the MIC, each isolate was subcultured at least three times in tryptic soy broth (TSB) and tryptic soy agar (TSA; Qingdao Hai Bo Biological Technology Co., Ltd., Shangdong, China) containing 5% newborn calf serum (Zhejiang Tianhang Biotechnology Co., Ltd., Zhejiang, China).
Animals
Forty-three pigs used in the study were 2-month old (20 ± 5 kg) and 120 two-and-a-half-month old healthy landrace and large white cross pigs (30 ± 5 kg) were obtained from a commercial pig farm (Hubei Jianfeng Hubei province animal husbandry Co., Ltd). The pigs were placed in separate pens, had free access to water, and were fed antibiotic-free food twice daily. The pigs were allowed a 7-day acclimation period prior to the study. Animal housing was maintained at 25 ± 2°C and 45–65% relative humidity.
All animal experiments and experimental protocols were conducted in accordance with the Guide for the Care and Use of Laboratory Animals of Huibei Provincial Laboratory Animal Public Service Center (permit number SYXK 2013-0044) and approved by the Ethics Committee of Huazhong Agricultural Univeristy, Wuhan, China.
Susceptibility Determination In vitro
Susceptibility determination of ENR against APP was performed using the agar dilution method in accordance with the CLSI recommendations in a previously described report. Strains of APP (2–4 μl, ~108 CFU/ml) were inoculated onto TSA agar plates containing 5% newborn calf serum, with two-fold serial dilutions of ENR (0.0625–32 μg/ml). Plates of strains were incubated in the presence of CO2 for 48 h at 37°C. MICs were determined at the lowest drug concentrations that caused complete growth inhibition (100%). Escherichia coli (ATCC 25922) was used as the quality control (QC) strain to verify the results of the susceptibility testing.
Dose Administration and Experiment Design
Pharmacokinetic (PK) Experiment Design
Eight male pigs weighing 20 ± 5 kg were fed for 7 days before the PK experiment. All pigs received 10% EEG by oral administration at a dose of 10 mg/kg. Plasma samples (5 ml) were collected at 0.083, 0.25, 0.5, 1, 2, 3, 4, 6, 8, 12, 24, 36, 48, and 72 h after oral administering.
Distribution and Elimination of ENR
A total of 35 male pigs weighing 25 ± 5 kg were fed for 7 days before the distribution and elimination experiment. A 5 mg/kg dose of 10% EEG was orally gavage administered twice per day for 5 consecutive days to pigs. The pigs were slaughtered through exsanguination under diethyl ether inhalation of anesthesia 1, 3, 7, 9, 11, or 13 days after ending of administration. Tissues from the liver, fat, kidney, and muscle were collected. These tissues were thoroughly rinsed with deionized water to remove residual blood, blotted to dryness, and finally homogenization was performed. Control tissues containing no drugs were also collected in order to provide control matrices.
Sample Treatment
Blood
Blood samples collected with anticoagulant were centrifuged for 10 min at 3,000 rpm to obtain plasma. Plasma samples were transferred to a clean sterile tube and stored at −20°C until analysis. Then, 0.5 ml plasma samples and 1 ml methanol were added to tubes, and vortexed for 2 min and centrifuged at 5,000 rpm for 10 min. The clean aqueous phase was transferred to a clean tube and dried in nitrogen at 60°C. The samples were filtered with a 0.22 μm membrane and analyzed by HPLC.
Tissues
Tissues (2 g) were diluted with 10 ml phosphate buffer solution into 50 ml tubes and then the mixture was vortexed and sonicated for 1 and 5 min, respectively. The obtained mixture was centrifuged at 10,000 rpm for 10 min. The supernatant was aspirated and transferred to a new centrifuge tube. The lower pellet was again processed as above. The final supernatant was obtained through activated C18 solid phase extraction (SPE), and then the SPE was washed with 2 ml double distilled water. When the SPE was completely dry, 1 ml of the mobile phase was extracted through SPE to obtain the final samples. Samples were injected into the HPLC for identification and quantification of the potential metabolites after filtering with a 0.22 μm membrane.
APP and MS Infection Models
For infection models, 120 pigs were randomly divided into two groups: group A for the APP infection model (n = 60) and group B for the MS infection model (n = 60). In groups A and B, 60 pigs were randomly divided into six groups, which were orally administered twice per day for 5 consecutive days 24 h after inoculation: (a) 10 mg/kg; (b) 5 mg/kg; (c) 2.5 mg/kg EEG; (d) positive group 8 mg/kg t tiamulin fumarate; (e) negative group; and (f) blank no-infection group. Each pig in the infection groups was inoculated by intranasal administration with 5 × 107 to 108 CFU/kg of APP or MS culture, which was previously reported to be strongly virulent. Sterile tissue (lungs) samples were collected by necropsy from dead pigs and cultured to confirm that mortality was caused by the inoculated strain.
Clinical Effectiveness
Clinical symptoms were carefully recorded for each pig, especially temperature, coughing, breathing, vomiting, prostration, and anorexia status. When evaluation indicators (temperature, coughing, etc.) were all close to or surpassed the no-infection group, the EEG efficacy groups were assessed as cured. When indicators were significantly lower compared with the positive group, the drugs were assessed as having a significant effect. When indicators were eased more than the positive group, the drug would be assessed as effective. When indicators were not alleviatived compared with the positive group, the drug would be assessed as ineffective. The presence of pathological changes in lungs after MS infection were scored as follows in order to make a daily clinical score: when diseased areas in lungs were 0, 1–25, 26–50, 51–75, and above 75%, they were scored as 0, 1, 2, 3, 4, respectively (Mutlu et al., 2012; Sibila et al., 2014; Cheng et al., 2017).
HPLC Condition of ENR and CP and Pharmacokinetic Analysis
A C18 reverse-phase column (250 × 4.6 mm, i.d., 5 μm, Agilent, USA) was used for HPLC, which was performed with a 278 nm detection wavelength at 30°C. The mobile phase consisted of PBS mixed with triethylamine (phase A) and acetonitrile (phase B) (v:v, 86:14).
PK parameters for plasma and tissue ENR and CP concentrations were determined using WinNonlin software (version 5.2.1, Pharsight Corporation, Mountain View, CA, USA). Drug concentrations were plotted on semi-logarithmic graphs to choose appropriate PK models.
Statistical Analysis
MIC95 was calculated by using SPSS software, and statistical analysis was performed with Student's t-test and Bonferroni revision for comparing the clinical variables of before and after treatment. The p < 0.05 was considered to indicate statistically significant.
Results
MIC Distribution of APP
The minimal inhibitory concentration (MIC) distribution of the 56 APP strains to ENR are shown in Figure 1. The MIC values were 0.03125–0.5 μg/ml, and the MIC95 of ENR against APP was 0.125 μg/ml. This indicated that the APP strains were sensible to ENR according to the clinical and laboratory standards institute (CLSI) M100-S19 guide document. Generally MIC95 was the preference value if clinical extrapolations were to be made.
Pharmacokinetic Analysis of ENR in Plasma by HPLC
The proposed method of high performance liquid chromatography (HPLC) was suitable for ENR quantification in plasma. It showed specificity and a recovery of over 82% in accordance with the veterinary drug residue guidelines of Agriculture department and United States Pharmacopeia (Gad, 2014; Millipore, 2015), and a good linear relationship from 0.05 to 10 μg/ml. The chromatogram in Figures 2A–C showed the blank Figure 2A, the lower limit of quantification (LLOQ) Figure 2B, and measured samples 1 h after oral administration in plasma Figure 2C, which indicated that the proposed method for ENR detection was specific and accurate. The typical regression equation was y = 0.008X–0.0036, R2 = 0.9999. The lower limit of determination (LLOD) was 0.025 μg/ml, and the LLOQ was 0.05 μg/ml in plasma.
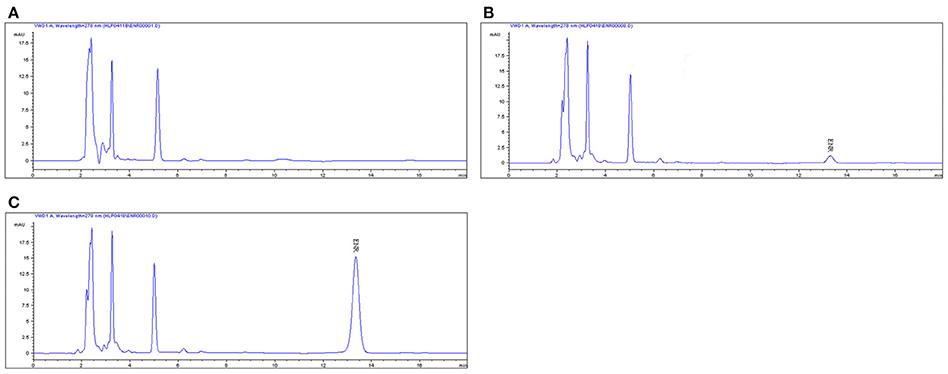
Figure 2. The HPLC method for ENR quantification in plasma. The representative HPLC chromatograms of plasma were shown: (A) blank plasma sample; (B) plasma sample at the LLOQ of 0.05 μg/ml; (C) plasma sample after oral administration of EEG at the point of 1 h; ENR, ENR at the peak time of 13.5 min.
The mean ± SD of ENR concentration-time profile was presented in Figure 3 after oral gavage administration, and the main PK parameters were shown in Table 1 using an absorbing two-compartment open model with a lower Akaike's Information Criterion (AIC) value (−2.43) compared other models by WinNonlin software. The results in Table 1 showed that Cmax, AUC, Tmax, and t1/2β, t1/2ka were 3.38 ± 0.06 μg/ml, 38.93 ± 1.52 μg·h/ml, 3.99 ± 0.1, 14.99 ± 4.19, and 1.76 ± 0.29 h, respectively.
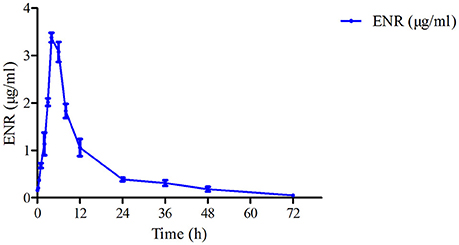
Figure 3. The curve of ENR concentration–time in plasma of pigs at a dose of 10 mg/kg after oral administering. ENR in the plasma was determined at 0.083, 0.25, 0.5, 1, 2, 3, 4, 6, 8, 12, 24, 36, 48, and 72 h.
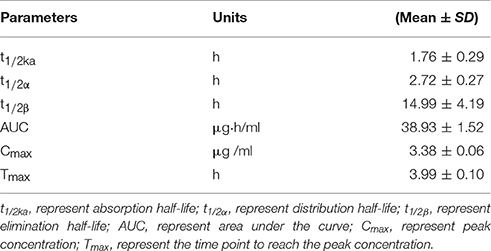
Table 1. The main pharmacokinetic parameters in pigs after a single oral dose of 10% enrofloxacin enteric-coated granules (10 mg/kg.bw).
Distribution and Elimination of ENR and CP in Tissues
The chromatogram in Figures 4A–H showed the blank, LLOQs of ENR and CP in muscle, fat, liver, and kidney, respectively, which indicated the proposed methods for ENR and CP detection in tissues were specific and accurate. The lower limit of determination (LLOD) was 0.02 μg/ml, and LLOQ was 0.05 μg/ml for tissues. The coefficient of determination (R2) in 0.05–10 μg/ml of standard curves were 0.9995–0.9999 for tissues (muscle, fat, liver, and kidney). The inter-day variation was determined to be 3.6–8.0% in tissues, and the intra-day variation was 1.62–4.94%. The recovery ratios were 84.92 ± 4.72–93.83 ± 3.18% in tissues. After oral administration of 10% EEG 5 mg/kg twice per day for 5 consecutive days, distribution profiles of ENR and CP in liver and fat were similar to those in kidney and muscle, respectively (Figure 5). ENR and CP underwent a rapid and wide distribution, and reached the highest concentration in various tissues within 1 day (Figure 5). The highest concentrations were 242.4–409.4 and 188.4–270.9 μg/kg for ENR and CP, respectively, in muscle, fat, liver, kidney (Table 2). The observed drug concentrations in liver and kidney were higher than those in fat and muscle. In fat and muscle, the drug rapidly declined for the first 3 days and then decreased slowly, and was detectable after 5 days, while in liver and kidney, the drug declined rapidly for 7 days after the dose administration (Figure 5). These data in tissues represented elimination of first order kinetics, which were in accordance with the equation C = C0·e−kt, where C is the concentration at time t, C0 is the pre-exponential term, and k is the elimination rate constant. According to the equation (t1/2ke = 0.693/k) t1/2kevalues in each tissue were evaluated 1.67, 1.19, 2.11, 2.20 days in muscle, fat, liver, kidney, respectively (Table 3). At the same time, withdrawal periods (WDTs) were evaluated based on the provided maximum residue limit (MRL) in each tissue and the equation MRL = C0·e−k(WDT), which were 4.28, 3.81, 4.84, 3.51 days in muscle, fat, liver, kidney, respectively (Table 3). As a result, the ENR and CP elimination rate of tissues from fast to slow were kidney, liver, muscle, and fat. Moreover, the WDT of tissues from short to long were kidney, fat, muscle, and liver, which were all below 5 days. Thus, the suggested WDT was 5 days after oral administration of 10% EEG 5 mg/kg twice per day.
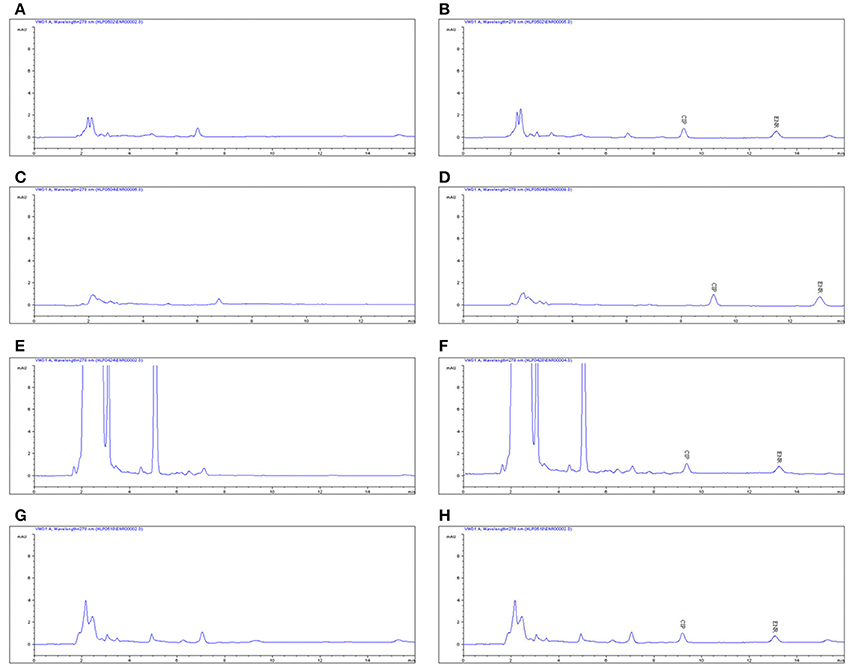
Figure 4. The HPLC methods for ENR quantification in tissues. The representative HPLC chromatograms of tissues were shown: (A,C,E,G) represent the blank samples in muscle, fat, liver, and kidney, respectively. (B,D,F,H) represent the LLOQ of 0.05 μg/ml in muscle, fat, liver, and kidney, respectively.
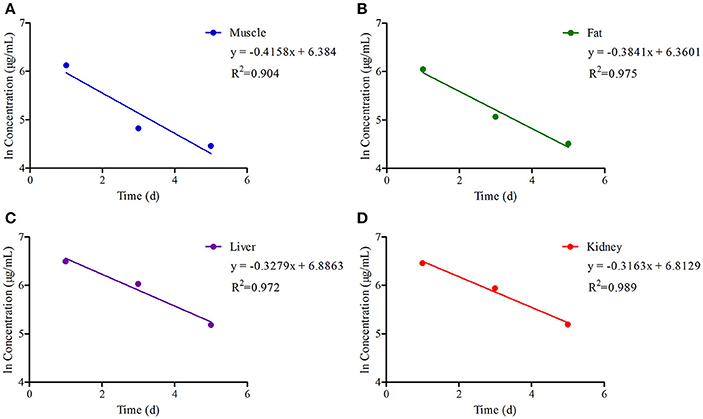
Figure 5. The ln concentration-time curves of (ENR+CP) in tissues of pigs with regression lines and correlation coefficients at a dose of 5 mg/kg EEG after orally administered twice per day for 5 consecutive days.
Efficacy of 10% EEG in the Treatment of APP and MS
There were no clinical symptoms of APP and MS in any pigs during the acclimation period prior to the infection challenge. After 24 h of the APP infection, the group not treated with drugs (negative group) exhibited dyspnea, cough, and fever. Swabs from the center of the lung lesions of dead pigs from the group treated with drugs (positive group) showed the satellite phenomenon around Staphylococcus aureus (Supplementary Figure 1) and were pink in the urease test (Supplementary Figure 2). In addition, the isolate was verified as APP content by PCR. These results demonstrated that the isolate from swabs was positive for APP, and the APP infection models were established successfully. After 15 days of the MS infection, most pigs presented rapid breathing, droopy appearance and abdominal breathing. When dead pigs from the negative group were dissected, carnification in lungs was observed (Supplementary Figure 3). The strain isolated from the lung was verified as obtaining MS by PCR (Supplementary Figure 4). These results demonstrated that the isolated strain from lung was positive for MS, and that the MS infection models were established successfully.
All pigs in infected groups showed lethargy and loss of appetite before treatment. The symptoms of most pigs in the treatment groups disappeared after 2 days in high-dose (10 mg/kg) and middle-dose (5 mg/kg) groups, 3 days in the positive group (tiamulin fumarate), and 4 days in the low-dose (2.5 mg/kg) group. As shown in Table 4, the ineffective ratios were 10% in high-, middle-dose, and positive groups, while the ratios in the low-dose and the negative groups were 40 and 90%, which were higher than the former groups. Moreover, the results in Table 5 showed there were no deaths in high-, middle-dose groups and positive groups, whereas the mortality rates of the low-dose groups and negative were 20 and 50%, respectively. The high-, middle-, low-dose groups displayed markedly higher effectiveness and higher average daily gain (ADG), and significantly lower detox rates than the negative group; while the high- and middle-dose groups displayed no significant difference compared with the positive group, and the low-dose group displayed lower than the positive group, in effectiveness, detox rates and ADG.
As shown in Tables 6, 7, the ineffective ratios were 10% in high-, middle-dose, and positive groups, while the ratios in the low-dose and negative groups were 50 and 90%, which were higher than the former groups. The results in Table 6 showed there were no deaths in high-, middle-dose and positive groups, whereas the mortality rates of the negative and low-dose groups were 30 and 10%, respectively. The high-, middle-, low-dose groups displayed markedly higher effective rate and higher ADG, and significantly lower lung lesion score (LLS) than the negative group; while the high- and middle-dose groups displayed no significant difference compared with the positive group, and the low-dose group displayed lower than the positive group, in effective rate, LLS, and ADG.
These revealed that the efficacy of 10% EEG against APP and MS had a 90% effective ratio, which was equal to tiamulin fumarate and the dosage of 5 mg/kg twice per day for 5 consecutive days was the optimum dose.
Discussion
The PK and residue of ENR in the serum of goats, pigs, calves, horses, sheep, and broilers have already been investigated in previous reports (Kaartinen et al., 1995; Giguère et al., 1996; Mckellar et al., 1997, 1999; Anadón et al., 1999; Elmas et al., 2001; Sang et al., 2015; Haag et al., 2016). However, most ENR products were difficult to feed in forage or water because of poor palatability. In this study, ENR coated with enteric particles was studied to evaluate PK, residue and clinical efficacy in pigs comprehensively.
For PK study in plasma, the parameters obtained in this study were compared with previous reports. Cmax, Tmax, and t1/2β were 3.38 μg /ml, 3.99, 14.99 h, respectively, by oral administration of 10% EEG at a signal dose of 10 mg/kg (1 mg/kg ENR) (Table 1), while these parameter values in published studies were 0.63–1.17 μg/ml, 0.92–1.81, and 1.96–6.69 h after intramuscular administration 2.5 mg/kg of ENR (Wiuff et al., 2002, 2003; Bimazubute et al., 2010; Peng-Peng et al., 2013; Wang et al., 2016). The value of t1/2β in plasma (14.99 h) in this study was double compared with previous reports (6.69 h) by Wang et al. (2016), which showed 10% EEG had a remarkably sustained release action in pigs. Moreover, Cmax was higher than MIC95 for APP (0.125 μg/ml), and remained above 1 μg/ml for 12 h in plasma, which was higher than the MIC of most pathogenic bacteria. Thus, these results revealed that 10% EEG had high concentration, wide distribution and long-acting properties in plasma.
Regarding the accumulation and elimination kinetics of ENR and CP in pigs, total concentrations of 661.5, 638.0, 457.0, and 422.9 μg/kg were observed 1 day after drug administration in liver, kidney, muscle, and fat, respectively, and these were above MRL values for 3–4 days (Table 2). After 5 days of decontamination, the concentration of ENR and CP in each tissue were lower compared with MRL values regulated by the European Union1. Similar results were obtained in a previous study at a daily dose of 5 mg/kg ENR (5%) for 5 consecutive days (Garcia et al., 2005). However, in this study the administration dosage was 10 mg/kg EEG (10% ENR), which doubled that in previous work by Garcia (Garcia et al., 2005), but the ENR and CP concentrations were lower than the results by Garcia for the duration of the experiment. This result showed that 10% EEG had a faster elimination ratio in each tissue than Garcia's work. In our study, after 6 days post-administration, the drug was only detected in fat at a level below the LLOD, and not detected in any other tissues. The t1/2ke values of kidney and liver were 2.20, 2.11 days, and these were higher than those of muscle and fat, which were 1.67, 1.19 days, respectively.
This revealed that ENR and CP were more prevalent and metabolized in kidney and liver, a result similar to published reports (Feng et al., 2005; San et al., 2007; San Martin et al., 2010; de Assis et al., 2016). This result also revealed that the liver is an appropriate target tissue for residue monitoring. For WDT evaluation of pharmacological compounds in target tissues, it was necessary to consider the administered dosage, therapy time and species. CP, as the main and crucial metabolite generated from ENR biotransformation, also had antibacterial activity for the treatment of bacterial infections (Aydemir et al., 2006; Hickerson and Carson, 2006; San et al., 2007; Schilt, 2012). According to European Medicines Agency (EMA) regulation, the target tissues for ENR and CP residue were liver, muscle and kidney2. However, the FDA had stated that edible tissues which eliminated slowly should also be considered as target tissue (San et al., 2007). WDTs in liver, kidney, muscle and fat were 4.84, 3.51, 4.28, and 3.81 days, respectively. Moreover, ENR was also detected after 6 days in fat, and this coincided with its slow WDT of about 3.81 days, suggesting the fat, together with liver and kidney, should also be considered as a key tissue for food safety control and toxicology concern. For the WDT of ENR, our findings in pigs were 5 days, which was similar to 5 or 6 days in poultry in previous reports (San Martin et al., 2010; Terrado-Campos et al., 2017). The previous studies revealed that after exposure to ENR at a dose of 10 mg/kg, the WDT was 5, 7, and 10 days in pigs (Delsol et al., 2004; San et al., 2007; Liu et al., 2011), and 23 days in piaractus mesopotamicus (Luo et al., 2010), most of which was far longer than that in our study (Wiuff et al., 2002; San et al., 2007; Godoy et al., 2011; Paschoal et al., 2013). Therefore, EEG had an advantage with a short WDT. And the final recommended WDT for 10% EEG was 5 days in pigs.
This study evaluated the efficacy of 10% EEG against APP and MS at high-, middle-, and low-doses compared with tiamulin fumarate. Previous reports had established a close link between the respiratory disease risk and the productivity decrease in animals (Godoy et al., 2011; Paschoal et al., 2013; Hassanpouraghdam et al., 2015; Sala et al., 2015). Generally, the strain with MIC95 or MIC90 was the preference for establishment of infection model and indicators for clinical treatment. However, correlating MIC and MIC breakpoints with clinical efficacy could not be assumed in the case of APP infections. To alleviate the uncertainty, we isolated the strain from the lung lesions of the dead pigs and the strain was verified to be APP, thus indicating the successful establishment of the infection model (Seah et al., 2003). A previous study had been conducted to detect the susceptibility (MIC) of 12 alternative antibiotics including ENR against 12 serotypes of 138 APP and acquired resistance of APP was found in oxytetracycline, ampicillin, and chloramphenicol while not in ENR (Reeve-Johnson, 2000). The MIC range of ENR against APP was from 0.06 to 0.12 μg/ml, and the MIC90 in the previous report was equal to MIC95 in this study (Dom et al., 1994b; Reeve-Johnson, 2000; Seah et al., 2003). There had been a suggestion in published study for APP infections in pigs that clinical demeanor and respiratory scores did not give a absolutely reliable indication of the pathology occurring in the animal (Smith et al., 1991). Although, all serotypes could cause disease, but a few reports had provided evidence that biotype 2 strains were less virulent than biotype 1 strains, and it had been reported that biotype 1 serotypes 1, 5a, 5b, 9, and 10 strains were more virulent than other biotype 1 serotypes (Smith et al., 1991; Reeve-Johnson, 1999, 2000). Thus, this study selected APP serotype 1 with MIC95 and MS, were selected for infection models and evaluated the in vivo antibacterial activity of EEG in pigs.
For evaluating the therapeutic effect, plasma concentrations should be higher or equal to the MIC (Reeve-Johnson, 1998; Cheng et al., 2017). The plasma concentrations of ENR were higher than MICs of APP based on the calculated pharmacokinetic values (Table 1). According to the results of MIC and pharmacokinetics in pigs, the 10, 5, 2.5 mg/kg administration dosages were set as the high-, middle-, and low-doses, respectively. For treatment efficacy of EEG against APP and MS, the efficacies of the high- and middle-dose groups were greater than that of the low-dose treatment group, while the efficacy of the middle-dose group was equal to the positive and high-dose groups, as indicators of the major efficacy parameters were not significantly different (p > 0.05). Therefore, the suggested therapeutic dose of 10% EEG for treatment of APP and MS was 5–10 mg/kg; the low-dose of 2.5 mg/kg could be considered as a preventive dose. In other published reports for therapeutic doses, most were i.m. administration at a higher dosage compared with that in this study (Sutter et al., 2010). Several reports gave oral administration at 5–10 mg/kg twice daily, a dosage equal to that of this study (Breitschwerdt et al., 1991; Heins et al., 2014). Previous study suggested that after treatment of ENR to serotype 1 APP by oral administration for 7 days, lung lesions were observed in five pigs (35.71%) with an average damage of 1.16%, and four pigs (28.57%) with 1.24% after at a dosage 40 and 150 mg/kg, respectively (Robb et al., 2007). In another study on the treatment of serotype 3 APP infection in the pigs, it revealed that only 150 mg/kg ENR could produce marked control of the infection in terms of reduced average severity of thoracic lesions (Dom et al., 1994b). However, the dose of 5 mg/kg of EEG produced 90% effective and 30% detox ratios in fighting APP infection (Tables 4, 5), and this dose was lower than those reported in the previously published studies (Herradora and Martínezgamba, 2003). Although the MS infection has caused more and more attention, few studies had been conducted to investigate the treatment of ENR to MS. Our data demonstrated an dosage of 5 mg/kg EEG produced the highest efficacy against MS, which was much lower dosage than 50 mg/kg ENR in drinking water for 3 days in one published research on the treatment of Mycoplasma gallisepticum (Hinz and Rottmann, 1990; Wallgren et al., 1999).
Conclusions
In this study, PK in plasma, residue elimination in edible tissues, MICs and clinical efficacy of 10% EEG to APP and MS in pigs were assessed. The results showed that EEG had a high antibacterial activity, was fast absorbed, widely distributed, had a high concentration in plasma, and short WDT in tissues. Moreover, it presented high efficacy for APP and MS, which could provide reasonable theoretical foundation for clinical application. And EEG, as a more palatable formulation, could be used for veterinary medicine conveniently and widely.
Author Contributions
JC and QH conceived the study, JC and ZL designed the experiments. ZL, KL, and BY performed the experiments. ZL and QL wrote the manuscript. JX, LH, SA, and PC improved the language. All authors reviewed the manuscript.
Conflict of Interest Statement
The authors declare that the research was conducted in the absence of any commercial or financial relationships that could be construed as a potential conflict of interest.
Acknowledgments
This work was supported by grants from the National Key Research and Development Program of China (2016YFD0500702) and the China Agricultural Research System (CARS-36).
Supplementary Material
The Supplementary Material for this article can be found online at: http://journal.frontiersin.org/article/10.3389/fphar.2017.00294/full#supplementary-material
Footnotes
1. ^Committee for veterinary medicinal products Committee for veterinary medicinal products Enrofloxacin summary report for MRL in tissues (http://www.ema.europa.eu/docs/en_GB/document_library/Maximum_Residue_Limits_-_Report/2009/11/WC500014142.pdf.
2. ^Committee for veterinary medicinal products Enrofloxacin summary report for target tissues.http://www.ema.europa.eu/docs/en_GB/document_library/Maximum_Residue_Limits_-_Report/2009/11/WC500014139.pdf
References
Aliu, H., and Sulaj, K. (2014). Screening of quinolone antibiotic residues in beef sold in Kosovo. Albanian J. Agric. Sci. 13, 541–544.
Altman, E., Brisson, J. R., and Perry, M. B. (1990). Structural characterization of the capsular polysaccharide and O-chain of the lipopolysaccharide of Actinobacillus pleuropneumoniae serotype 8 (strain 405). Can. J. Chem. 68, 329–333. doi: 10.1139/v90-047
Anadón, A., Martínez-Larrañaga, M. R., Díaz, M. J., Fernández-Cruz, M. L., Martínez, M. A., Frejo, M. T., et al. (1999). Pharmacokinetic variables and tissue residues of enrofloxacin and ciprofloxacin in healthy pigs. Am. J. Vet. Res. 60, 1377–1382.
Aydemir, S., Tunger, A., and Cilli, F. (2006). In vitro activity of fluoroquinolones against common respiratory pathogens. West Indian Med. J. 55, 9–12. doi: 10.1590/S0043-31442006000100003
Biju, S. S., Saisivam, S., Rajan, N. S., and Mishra, P. R. (2004). Dual coated erodible microcapsules for modified release of diclofenac sodium. Eur. J. Pharmaceut. Biopharmaceut. 58, 61–67. doi: 10.1016/j.ejpb.2004.03.021
Bimazubute, M., Cambier, C., Baert, K., Vanbelle, S., Chiap, P., Albert, A., et al. (2010). Penetration of enrofloxacin into the nasal secretions and relationship between nasal secretions and plasma enrofloxacin concentrations after intramuscular administration in healthy pigs. J. Vet. Pharmacol. Ther. 33, 183–188. doi: 10.1111/j.1365-2885.2009.01123.x
Bossé, J. T., Janson, H., Sheehan, B. J., Beddek, A. J., Rycroft, A. N., Kroll, J. S., et al. (2002). Actinobacillus pleuropneumoniae: pathobiology and pathogenesis of infection. Microbes Infect. 4, 225–235. doi: 10.1016/S1286-4579(01)01534-9
Breitschwerdt, E. B., Davidson, M. G., Aucoin, D. P., Levy, M. G., Szabados, N. S., Hegarty, B. C., et al. (1991). Efficacy of chloramphenicol, enrofloxacin, and tetracycline for treatment of experimental Rocky Mountain spotted fever in dogs. Antimicrob. Agents Chemother. 35, 2375–2381. doi: 10.1128/AAC.35.11.2375
Bushra, A., Shoaib, M. H., Aslam, N., and Mehmood, Z. A. (2010). Enteric coating of ibuprofen tablets (200 mg) using an aqueous dispersion system. Braz. J. Pharmaceut. Sci. 46, 99–107. doi: 10.1590/S1984-82502010000100011
Cheng, G., Xu, Y., Zhu, X., Xie, S., Wang, L., Huang, L., et al. (2017). The antibacterial activities of aditoprim and its efficacy in the treatment of swine streptococcosis. Sci. Rep. 7:41370. doi: 10.1038/srep41370
de Almeida, M. P., Rezende, C. P., Ferreira, F. D., de Souza, L. F., de Assis, D. C., de Figueiredo, T. C., et al. (2015). Optimization and validation method to evaluate the residues of β-lactams and tetracyclines in kidney tissue by UPLC–MS/MS. Talanta 144, 922–932. doi: 10.1016/j.talanta.2015.07.048
de Assis, D. C. S. D., da Silva, G. R., Lanza, I. P., Ribeiro, A. C., Lana, M., Lara, L. J., et al. (2016). Evaluation of the presence and levels of enrofloxacin, ciprofloxacin, sulfaquinoxaline and oxytetracycline in broiler chickens after drug administration. PLoS ONE 11:e0166402. doi: 10.1371/journal.pone.0166402
Delsol, A. A., Woodward, M. J., and Roe, J. M. (2004). Effect of a 5 day enrofloxacin treatment on Salmonella enterica serotype Typhimurium DT104 in the pig. J. Antimicrob. Chemother. 53, 396–398. doi: 10.1093/jac/dkh038
Deveau, I. (2007). United States Pharmacopeia convention standards and clinical information for compounded medications used in veterinary medicine. Int. J. Pharm. Compd. 11, 357–362.
Dom, P., Haesebrouck, F., Ducatelle, R., and Charlier, G. (1994a). In vivo association of Actinobacillus pleuropneumoniae serotype 2 with the respiratory epithelium of pigs. Infect. Immun. 62, 1262–1267.
Dom, P., Hommez, J., Castryck, F., Devriese, L. A., and Haesebrouck, F. (1994b). Serotyping and quantitative determination of in vitro antibiotic susceptibility of Actinobacillus pleuropneumoniae strains isolated in Belgium (July 1991-August 1992). Vet. Q. 16:10. doi: 10.1080/01652176.1994.9694407
Duff, J. P., Scott, W. A., Wilkes, M. K., and Hunt, B. (1996). Otitis in a weaned pig: a new pathological role for Actinobacillus (Haemophilus) pleuropneumoniae. Vet. Rec. 139, 561–563.
Ebrahimzadeh, A. V., Mesgari, A. M., Abedimanesh, N., Ostadrahimi, A., and Gorbani, A. (2014). Investigation of enrofloxacin and chloramphenicol residues in broiler chickens carcasses collected from local markets of tabriz, northwestern iran. Health Promot. Perspect. 4:151. doi: 10.5681/hpp.2014.020
Elmas, M., Tras, B., Kaya, S., Bas, A. L., Yazar, E., and Yarsan, E. (2001). Pharmacokinetics of enrofloxacin after intravenous and intramuscular administration in Angora goats. Can. J. Vet. Res. 65, 64–67.
Feng, Z., Xiaohua, G., Qiyi, H., Linlin, D., Yanhua, L., et al. (2005). Studies on the deletion of enrofloxacin residues in the procine tissues. Chinese J. Vet. Drug 39, 6–9.
Gad, S. C. (2014). “United States Pharmacopoeia (USP),” in Encyclopedia of Toxicology, 881–882. doi: 10.1016/b978-0-12-386454-3.00959-3
Garcia, M. A., Solans, C., Calvo, A., Hernandez, E., Rey, R., Bregante, M. A., et al. (2005). Determination of enrofloxacin and its primary metabolite, ciprofloxacin, in pig tissues. Application to residue studies. Biomed. Chromatogr. 19, 27–31. doi: 10.1002/bmc.411
Giguère, S., Sweeney, R. W., and Bélanger, M. (1996). Pharmacokinetics of enrofloxacin in adult horses and concentration of the drug in serum, body fluids, and endometrial tissues after repeated intragastrically administered doses. Am. J. Vet. Res. 57, 1025–1030.
Godoy, C., Castells, G., Martí, G., Capece, B. P., Pérez, F., Colom, H., et al. (2011). Influence of a pig respiratory disease on the pharmacokinetic behaviour of amoxicillin after oral ad libitum administration in medicated feed. J. Vet. Pharmacol. Ther. 34, 265–276. doi: 10.1111/j.1365-2885.2010.01220.x
Gouvêa, R., Santos, F., Machado, L. S., and de Almeida Pereira, V. L. (2015). Fluoroquinolones in industrial poultry production, bacterial resistance and food residues:a review. Revista Brasileira De Ciência Avícola 17, 1–10. doi: 10.1590/1516-635x17011-10
Haag, G., Marin, G. H., and Errecalde, J. (2016). Quantification of residual enrofloxacin and ciprofloxacin in feathers of broiler chickens by high-performance liquid chromatography-fluorescence after oral administration of the drugs. J. Adv. Pharmaceut. Technol. Res. 7, 2–5. doi: 10.4103/2231-4040.173265
Hassanpouraghdam, M. B., Elamery, E. M. S. M., and Mazid, M. (2015). Note on the enzyme activities, productivity and quality parameters of chickpea cultivars under influence of diverse synthetic plant growth promoters. Indian J. Agric. Res. 49:327. doi: 10.5958/0976-058X.2015.00059.1
Heins, B. D., Nydam, D. V., Woolums, A. R., Berghaus, R. D., and Overton, M. W. (2014). Comparative efficacy of enrofloxacin and tulathromycin for treatment of preweaning respiratory disease in dairy heifers. J. Dairy Sci. 97, 372–382. doi: 10.3168/jds.2013-6696
Herradora, L. M., and Martínezgamba, R. (2003). Effect of oral enrofloxacin and florfenicol on pigs experimentally infected with Actinobacillus pleuropneumoniae serotype 1. J. Vet. Med. A Physiol. Pathol. Clin. Med. 50, 259–263. doi: 10.1046/j.1439-0442.2003.00529.x
Hickerson, A. D., and Carson, C. C. (2006). The treatment of urinary tract infections and use of ciprofloxacin extended release. Expert Opin. Investig. Drugs 15:519. doi: 10.1517/13543784.15.5.519
Hinz, K. H., and Rottmann, S. (1990). Studies in vivo on the efficacy of enrofloxacin against Mycoplasma gallisepticum. Avian Pathology Pathol. 19, 511–522. doi: 10.1080/03079459008418704
Jobert, J. L., Savoye, C., Cariolet, R., Kobisch, M., and Madec, F. (2010). Diffusion aandeacute;rienne d'Actinobacillus pleuropneumoniae dans des conditions expandeacute;rimentales et dandeacute;veloppement de pleuropneumonie chez les porcs. J. Rech. Porcine France 33, 263–267.
Kaartinen, L., Salonen, M., Alli, L., and Pyörälä, S. (1995). Pharmacokinetics of enrofloxacin after single intravenous, intramuscular and subcutaneous injections in lactating cows. J. Vet. Pharmacol. Ther. 18:357. doi: 10.1111/j.1365-2885.1995.tb00604.x
Küng, K., Riond, J. L., and Wanner, M. (1993). Pharmacokinetics of enrofloxacin and its metabolite ciprofloxacin after intravenous and oral administration of enrofloxacin in dogs. J. Vet. Pharmacol. Ther. 16:462. doi: 10.1111/j.1365-2885.1993.tb00212.x
Liu, X. Y., Hai-Kun, W. U., Fang, B. H., Yuan, Z. H., and Yang, L. J. (2011). Study on residue elimination of enrofloxacin suspension in pigs. Prog. Vet. Med. 32, 89–93. doi: 10.16437/j.cnki.1007-5038.2011.05.023
Luo, Y. J., Sun, Y. X., Xiao-Na, L. I., and Zeng, D. P. (2010). Studies on residues depletion of enrofloxacin microcapsules in pigs. China Anim. Husb. Vet. Med. 37, 129–131.
Mckellar, Q. A., Gibson, I., and Monteiro, A. (1997). Pharmacokinetic study comparing enrofloxacin and danofloxacin in calves. J. Vet. Pharmacol. Therapeut. 20, 23–24.
Mckellar, Q., Gibson, I., Monteiro, A., and Bregante, M. (1999). Pharmacokinetics of enrofloxacin and danofloxacin in plasma, inflammatory exudate, and bronchial secretions of calves following subcutaneous administration. Antimicrob. Agents Chemother. 43, 1988–1992.
Messick, J. B. (2004). Hemotrophic mycoplasmas (hemoplasmas): a review and new insights into pathogenic potential. Vet. Clin. Pathol. 33, 2–13. doi: 10.1111/j.1939-165X.2004.tb00342.x
Millipore, E. (2015). New possibilities when adapting the HPLC method in United States pharmacopoeia monograph for atovaquone oral suspension. Cell 136, 62–74.
Mutlu, Y. E., Sunbul, M., Aksoy, A., Yilmaz, H., Guney, A. K., and Guvenc, T. (2012). Efficacy of tigecycline/colistin combination in a pneumonia model caused by extensively drug-resistant Acinetobacter baumannii. Int. J. Antimicrob. Agents 40, 332–336. doi: 10.1016/j.ijantimicag.2012.06.003
Nykänen, P. (2003). Development of Multiple-Unit Oral Formulations for Colon-Specific Drug Delivery using Enteric Polymers and Organic Acids as Excipients. Dissertation, Faculty of Science, University of Helsinki.
Panzenhagen, P. H. N., Aguiar, W. S., Gouvêa, R., de Oliveira, A. M., Barreto, F., Pereira, V. L., et al. (2016). Investigation of enrofloxacin residues in broiler tissues using ELISA and LC-MS/MS. Food Addit. Contam. A Chem. Anal. Control Expo. Risk Assess. 33, 639–643. doi: 10.1080/19440049.2016.1143566
Paschoal, J. A., Quesada, S. P., Gonçalves, L. U., Cyrino, J. E., and Reyes, F. G. (2013). Depletion study and estimation of the withdrawal period for enrofloxacin in pacu (Piaractus mesopotamicus). J. Vet. Pharmacol. Ther. 36, 594–602. doi: 10.1111/jvp.12043
Peng-Peng, L. I., Zhou, D. G., Xu-Dong, F. U., and Zhang, X. H. (2013). The research of pharmacokinetics of Enrofloxacin injection in the body of Swine. Chinese J. Vet. Med. 49, 81–83.
Perry, M. B., Altman, E., Brisson, J. R., Beynon, L. M., and Richards, J. C. (1990). Structural characteristics of the antigenic capsular polysaccharides and lipopolysaccharides involved in the serological classification of Actinobacillus (Haemophilus) pleuropneumoniae strains. Serodiagn. Immunother. Infect. Dis. 4, 299–308. doi: 10.1016/0888-0786(90)90018-J
Reeve-Johnson, L. (1998). Use of disease models in the development and evaluation of therapeutic agents. Vet. Rec. 142:638. doi: 10.1136/vr.142.23.638
Reeve-Johnson, L. (1999). The Use of Experimental Infection Models to Investigate the Correlation between Clinical and Pathological Measures of the Severity of Respiratory Disease in Three Species. University of Edinburgh.
Reeve-Johnson, L. (2000). The Pitfalls of Using Minimum Inhibitory Concentrations (MIC's) as Indicators of Clinical Efficacy. Using the Macrolide antibiotics as a comparative case study.
Reyesherrera, I., Schneider, M. J., Blore, P. J., and Donoghue, D. J. (2011). The relationship between blood and muscle samples to monitor for residues of the antibiotic enrofloxacin in chickens. Poult. Sci. 90:481. doi: 10.3382/ps.2010-01057
Robb, E. J., Tucker, C. M., Corley, L., Bryson, W. L., Rogers, K. C., Sturgess, K., et al. (2007). Efficacy of tulathromycin or enrofloxacin for initial treatment of naturally occurring bovine respiratory disease in feeder calves. Vet. Ther. 8, 127–135.
Sa, K., Moltmann, J. F., and Knacker, T. (2008). Estimating the use of veterinary medicines in the European union. Regul. Toxicol. Pharmacol. 50, 59–65. doi: 10.1016/j.yrtph.2007.06.003
Sala, V., Faveri, E. D., Gusmara, C., and Costa, A. (2015). Comparative evaluation of two quinolones in the treatment of bacterial acute respiratory disease of pig during growing-fattening phase. Large Anim. Rev. 21, 129–134.
San Martin, B. S., Cornejo, J., Lapierre, L., Iragüen, D., Pérez, F., Hidalgo, H., et al. (2010). Withdrawal time of four pharmaceutical formulations of enrofloxacin in poultry according to different maximum residues limits. J. Vet. Pharmacol. Ther. 33:246. doi: 10.1111/j.1365-2885.2009.01127.x
San, M. B., Cornejo, J., Iragüen, D., Hidalgo, H., and Anadón, A. (2007). Depletion study of enrofloxacin and its metabolite ciprofloxacin in edible tissues and feathers of white leghorn hens by liquid chromatography coupled with tandem mass spectrometry. J. Food Prot. 70:1952. doi: 10.4315/0362-028X-70.8.1952
Sang, K. N., Hao, H. H., Huang, L. L., Wang, X., and Yuan, Z. H. (2015). Pharmacokinetic–Pharmacodynamic Modeling of Enrofloxacin Against Escherichia coli in Broilers. Front. Vet. Sci. 2:80. doi: 10.3389/fvets.2015.00080
Schaller, A., Djordjevic, S. P., Eamens, G. J., Forbes, W. A., Kuhn, R., Kuhnert, P., et al. (2001). Identification and detection of Actinobacillus pleuropneumoniae by PCR based on the gene apxIVA. Vet. Microbiol. 79, 47–62. doi: 10.1016/S0378-1135(00)00345-X
Schilt, R. (2012). “Residues of veterinary drugs in food,” in Proceedings of the Euroresidue Vii Conference, Vols. 1-3 (Egmond Aan Zee).
Seah, W. L., Raymond, C., and Hon, S. W. (2003). Evaluation of the efficacy of herbal gutmotil and animon in the presention of post-weaning diarrhoea and respiratory disease complex in piglents. Tertium Comparationis 9, 101–104.
Sibila, M., Aragón, V., Fraile, L., and Segalés, J. (2014). Comparison of four lung scoring systems for the assessment of the pathological outcomes derived from Actinobacillus pleuropneumoniae experimental infections. BMC Vet. Res. 10:165. doi: 10.1186/1746-6148-10-165
Smith, I. M., Mackie, A., and Lida, J. (1991). Effect of giving enrofloxacin in the diet to pigs experimentally infected with Actinobacillus pleuropneumoniae. Vet. Rec. 129:25. doi: 10.1136/vr.129.2.25
Sneeringer, S., Macdonald, J., Key, N., Mcbride, W., and Mathews, K. (2015). Economics of antibiotic use in U.S. livestock production. Indus. Eng. Chem. 51, 4424–4432. Available online at: http://purl.umn.edu/229202
Sutter, H. M., Ludwig, B., Bremer, K. D., Jordan, J.-C., Rehm, W. F., Wanner, M., et al. (2010). Pharmacokinetics of aditoprim in dogs after intravenous and oral administration: a preliminary study. J. Small Anim. Pract. 32, 517–520. doi: 10.1111/j.1748-5827.1991.tb00870.x
Terrado-Campos, D., Tayeb-Cherif, K., Peris-Vicente, J., Carda-Broch, S., and Esteve-Romero, J. (2017). Determination of oxolinic acid, danofloxacin, ciprofloxacin, and enrofloxacin in porcine and bovine meat by micellar liquid chromatography with fluorescence detection. Food Chem. 221, 1277–1284. doi: 10.1016/j.foodchem.2016.11.029
Wallgren, P., Segall, T., Pedersen, M. A., and Gunnarsson, A. (1999). Experimental infections with Actinobacillus pleuropneumoniae in pigs–I. Comparison of five different parenteral antibiotic treatments. J. Vet. Med. 46, 249–260.
Wang, J., Hao, H., Huang, L., Liu, Z., Chen, D., and Yuan, Z. (2016). Pharmacokinetic and Pharmacodynamic Integration and Modeling of Enrofloxacin in Swine for Escherichia coli. Front. Microbiol. 7:36. doi: 10.3389/fmicb.2016.00036
Wiuff, C., Lykkesfeldt, J., Aarestrup, F. M., and Svendsen, O. (2002). Distribution of enrofloxacin in intestinal tissue and contents of healthy pigs after oral and intramuscular administrations. J. Vet. Pharmacol. Ther. 25, 335–342. doi: 10.1046/j.1365-2885.2002.00430.x
Wiuff, C., Lykkesfeldt, J., Svendsen, O., and Aarestrup, F. M. (2003). The effects of oral and intramuscular administration and dose escalation of enrofloxacin on the selection of quinolone resistance among Salmonella and coliforms in pigs. Res. Vet. Sci. 75, 185–193. doi: 10.1016/S0034-5288(03)00112-7
Yoshimura, H., Takagi, M., Ishimura, M., and Endoh, Y. S. (2002). Comparative in vitro activity of 16 antimicrobial agents against Actinobacillus Pleuropneumoniae. Vet. Res. Commun. 26, 11–19. doi: 10.1023/A:1013397419995
Yuan, C., Ningyu, Z., Zhibiao, Y., and JiaFu, W. (2010). “A novel hemoplasma species confirmed in swine reveals the possibility of interspecies transmission of Mycoplasma wenyonii,” in 4th International Conference on Bioinformatics and Biomedical Engineering, 1–4. doi: 10.1109/icbbe.2010.5515331
Keywords: enrofloxacin, enteric-coated granules, elimination, APP, MS, withdrawal
Citation: Lei Z, Liu Q, Yang B, Xiong J, Li K, Ahmed S, Hong L, Chen P, He Q and Cao J (2017) Clinical Efficacy and Residue Depletion of 10% Enrofloxacin Enteric-Coated Granules in Pigs. Front. Pharmacol. 8:294. doi: 10.3389/fphar.2017.00294
Received: 29 March 2017; Accepted: 08 May 2017;
Published: 23 May 2017.
Edited by:
Thomas Dorlo, Netherlands Cancer Institute and Antoni van Leeuwenhoek Hospital, NetherlandsReviewed by:
Lloyd Reeve-Johnson, University of the Sunshine Coast, AustraliaConstantin Ion Mircioiu, Carol Davila University of Medicine and Pharmacy, Romania
Taewon Kim, Chungnam National University, South Korea
Copyright © 2017 Lei, Liu, Yang, Xiong, Li, Ahmed, Hong, Chen, He and Cao. This is an open-access article distributed under the terms of the Creative Commons Attribution License (CC BY). The use, distribution or reproduction in other forums is permitted, provided the original author(s) or licensor are credited and that the original publication in this journal is cited, in accordance with accepted academic practice. No use, distribution or reproduction is permitted which does not comply with these terms.
*Correspondence: Qigai He, aGU2MjhAbWFpbC5oemF1LmVkdS5jbg==
Jiyue Cao, Y2Fvaml5dWUyQDE2My5jb20=