- 1State Key Laboratory of Toxicology and Medical Countermeasures, Beijing Key Laboratory of Neuropsychopharmacology, Department of Biochemical Pharmacology, Beijing Institute of Pharmacology and Toxicology, Beijing, China
- 2Department of Neurobiology, Beijing Institute of Basic Medical Sciences, Beijing, China
The sigma-1 receptor is a 223 amino acids molecular chaperone with a single transmembrane domain. It is resident to eukaryotic mitochondrial-associated endoplasmic reticulum and plasma membranes. By chaperone-mediated interactions with ion channels, G-protein coupled receptors and cell-signaling molecules, the sigma-1 receptor performs broad physiological and pharmacological functions. Despite sigma-1 receptors have been confirmed to regulate various types of ion channels, the relationship between the sigma-1 receptor and N-type Ca2+ channel is still unclear. Considering both sigma-1 receptors and N-type Ca2+ channels are involved in intracellular calcium homeostasis and neurotransmission, we undertake studies to explore the possible interaction between these two proteins. In the experiment, we confirmed the expression of the sigma-1 receptors and the N-type calcium channels in the cholinergic interneurons (ChIs) in rat striatum by using single-cell reverse transcription-polymerase chain reaction (scRT-PCR) and immunofluorescence staining. N-type Ca2+ currents recorded from ChIs in the brain slice of rat striatum was depressed when sigma-1 receptor agonists (SKF-10047 and Pre-084) were administrated. The inhibition was completely abolished by sigma-1 receptor antagonist (BD-1063). Co-expression of the sigma-1 receptors and the N-type calcium channels in Xenopus oocytes presented a decrease of N-type Ca2+ current amplitude with an increase of sigma-1 receptor expression. SKF-10047 could further depress N-type Ca2+ currents recorded from oocytes. The fluorescence resonance energy transfer (FRET) assays and co-immunoprecipitation (Co-IP) demonstrated that sigma-1 receptors and N-type Ca2+ channels formed a protein complex when they were co-expressed in HEK-293T (Human Embryonic Kidney -293T) cells. Our results revealed that the sigma-1 receptors played a negative modulation on N-type Ca2+ channels. The mechanism for the inhibition of sigma-1 receptors on N-type Ca2+ channels probably involved a chaperone-mediated direct interaction and agonist-induced conformational changes in the receptor-channel complexes on the cell surface.
Introduction
Sigma receptors are originally discovered in the central nervous system of mammals in 1976 (Martin et al., 1976). Two subtypes of sigma receptors have been distinguished: sigma-1 and sigma-2 receptors, based on their different drug selectivity patterns and molecular weights (Quirion et al., 1992). Until now, sigma-2 receptors have not been cloned yet (Xu et al., 2011). On the contrary, the biological and physiological roles of the sigma-1 receptor have been examined more intensively, as it has been cloned in mice, rats and humans (Kekuda et al., 1996; Seth et al., 1998, 2001). The crystal structure of the human sigma-1 receptor is also available now (Schmidt et al., 2016). It is characterized as a 26 kDa single polypeptide containing 223 amino acids. The overall structure of the sigma-1 receptor reveals a trimeric organization. It contains only a single transmembrane domain for each promoter, and the domain includes a cupin-like β-barrel with the ligand-binding site buried at its center. Sigma-1 receptors are widely distributed in the brain and periphery organs, including lung, kidney, liver, pancreas, spleen, and adrenal gland (Hayashi and Su, 2007; Su et al., 2016). They are predominantly localized at the endoplasmic reticulum and mitochondrial-associated endoplasmic reticulum membrane (MAM, Langa et al., 2003; Marriott et al., 2012). As a molecular chaperone (Su et al., 2010), the sigma-1 receptor participates in many biological processes including nociception, cancer, stroke, memory, drug addiction, cardiac activity, and Alzheimer’s disease (Romieu et al., 2004; Renaudo et al., 2007; Tsai et al., 2009; Luty et al., 2010; Ruscher et al., 2011; Kourrich et al., 2012; David et al., 2013). Several lines of evidence reveal that sigma-1 receptors regulate a number of neurotransmitter systems, including the glutamatergic, dopaminergic, serotonergic, noradrenergic, and cholinergic systems (Cobos et al., 2008; Su et al., 2016), and many types of ion channels, including voltage-dependent Kv1.2 (Kourrich et al., 2013), Kv1.3 (Kinoshita et al., 2012), Kv1.4 (Lupardus et al., 2000; Aydar et al., 2002), voltage-gated calcium channels (Sabeti et al., 2007; Tchedre et al., 2008), Nav1.5 (Fontanilla et al., 2009; Johannessen et al., 2009), hERG (human Ether-à-go-go Related Gene) channel (Crottès et al., 2011) and acid-sensing ion channel (Carnally et al., 2010). It has been established that sigma-1 receptors maintain Ca2+ homeostasis and modulate Ca2+ signal through the inositol triphosphate (IP3) receptor (Hayashi and Su, 2001, 2003; Pal et al., 2008). The sigma-1 receptor has been considered as an important therapeutic target for treatment of many forms of neurodegenerative diseases in human (Guitart et al., 2004; Vidaltorres et al., 2014), even though the mechanism (Su and Hayashi, 2003) and structural basis for the regulation produced by sigma-1 receptor agonists remain poorly defined.
The N-type Ca2+ channel is a type of voltage-gated calcium channels (VGCCs). It mainly locates at presynaptic membrane and mediates rapid Ca2+ influx into the synaptic terminal that triggers synaptic vesicle exocytosis and neurotransmitter release (Llinás et al., 1981; Catterall, 2000; Momiyama and Koga, 2001). Inhibition of the N-type Ca2+ channels can regulate neuropsychiatric disorders in animals and humans and it has become a potential target for the treatment of certain types of pain, particularly neuropathic pain (Altier et al., 2006). It is obvious that both of sigma-1 receptors and N-type Ca2+ channels are involved in the same biological processes: calcium homeostasis regulation and neurotransmitter release. Sigma-1 receptor activation has been found to inhibit glutamate release from rat cortical nerve terminals by blocking N-type and P/Q-type Ca2+ channels (Lu et al., 2012). Despite many researches have revealed that sigma-1 receptors regulate various types of VGCCs probably by constitutive interaction (Chu and Ruoho, 2015), it is not convinced whether there is an interaction between sigma-1 receptors and N-type Ca2+ channels, and how the sigma-1 receptor regulates the neurotransmission.
Considering their important functions in human physiology and pharmacology, we conducted studies to clarify the hypothetical direct or indirect interaction between sigma-1 receptors and N-type Ca2+ channels. To address the possible interaction, at first, we confirmed the expression of sigma-1 receptors in the cholinergic interneurons (ChIs) in rat striatum. Then we used the method of electrophysiology in brain slices to observe the influence on N-type Ca2+ channels by sigma-1 receptors in rat striatal ChIs. To evaluate whether the effect attributes to chaperone-mediated regulation induced by sigma-1 receptors, we co-expressed sigma-1 receptors and N-type Ca2+ channels in Xenopus oocytes. At last, we investigated the protein-protein interaction between sigma-1 receptors and N-type Ca2+ channels with techniques of FRET assays and Co-IP in HEK-293T cells.
Materials and Methods
Brain Slice Preparation and Solution
All experiments were performed in accordance with the NIH guideline to the care and use of laboratory animals (Publication NO.85-23, revised 1985) and approved by the Animal Research Advisory Committee of Beijing Institute of Biological Science. The procedures were similar to those we described previously (Zhao et al., 2016). In brief, male Sprague Dawley rats (14–16 days old) were decapitated. The brain was rapidly removed and submerged in oxygenated sucrose solution at 4°C containing (in mM): 2.5 KCl, 1.25 NaH2PO4, 24 NaHCO3, 10 glucose, 10 MgSO4, 0.5 CaCl2, 2 sodium pyruvate, 230 sucrose (295–305 mOsm/l). The value of pH was adjusted to 7.4 with NaOH. Coronal striatal slices (300 μm) were prepared with a vibratome (MA 752, Campden instruments). Slices were then transferred into a chamber (BSC-PC, Warner instruments) continuously bubbled with 95% O2 and 5% CO2 gas mixture for 30 min at 32°C. The solution incubating the slices was the standard NaHCO3-buffered saline solution containing (in mM): 126 NaCl, 2.5 KCl, 1.25 NaH2PO4, 26 NaHCO3, 10 glucose, 2 sodium pyruvate, 2 CaCl2, 2 MgCl2 (295–305 mOsm/l). pH was adjusted to 7.4 with HCl. The chamber was then maintained at room temperature bubbled with O2/CO2 gas mixture. Under this condition, the slices could be stored for several hours.
Electrophysiology
Electrophysiological recording in the striatal ChIs was conducted as described previously (Zhao et al., 2016). Briefly, the pipettes had a resistance of 3–5 MΩ when filled with the internal solution consisted of (in mM): 80 CsOH, 80 gluconate acid, 30 CsCl, 40 HEPES, 10 tetraethylammonium chloride (TEA-Cl), 5 EGTA, 12 Na2phosphoceatine, 1 MgCl2, 2 Mg-ATP, 0.5 Na-GTP (265–270 mOsm/l), which was adjusted with CsOH to pH 7.3 (Miki et al., 2013). Slices were bathed in an external solution of (in mM): 105 NaCl, 20 TEA-Cl, 2 CaCl2, 6 MgCl2, 6 KCl, 26 NaHCO3, 10 glucose, 3 myo-inositol, 2 sodium pyruvate, 0.5 ascorbic acid, 1.25 NaH2PO4, 0.0005 tetrodotoxin (TTX, pH = 7.2 with TEA-OH, Miki et al., 2013). The slice in the recording chamber was visualized with a 40 × water-immersion objective (NIR Apo, Nikon, Japan) using standard infrared and differential interference contrast (IR-DIC) microscopy and a CCD camera. Cells in the striatum approximately 50 μm beneath the slice surface were patched. Electrophysiology was performed using an Axon 200B amplifier (Molecular devices, Foster city, CA, United States) and Clampex 10.1 software (Molecular devices) at room temperature 23 ∼ 25°C (Hawkins et al., 2015). Data were filtered at 2 kHz and digitized at 10 kHz online. Only those recordings with stable holding currents and access resistance were accepted.
Immunohistochemical Staining
The rats of the same age to those used for electrophysiological experiments were used for histochemical experiments. The rats were anesthetized with Nembutal. After perfused with phosphate buffered saline (PBS) and fixed in 4% paraformaldehyde, the brain was removed carefully, cut sagittally at the midline and post-fixed in the same fixative for 3 h at 4°C (Zhao et al., 2016). The brain was sectioned at 5 μm to form the sequential brain slices. The slices containing the striatum were mounted on polylysine-coated slides. The staining was performed by using commercially available antibodies: a rabbit anti-sigma-1 receptor antibody (Abcam, Cat#ab53852, RRID: AB_881796 using at 1:200) and a goat anti-ChAT antibody (Millipore, Cat#AB144P, RRID: AB_2079751 using at 1:500).
Sections were washed with PBS for 3 × 5 min and blocked with normal serum consistent with the host of secondary antibody for 1 h at RT. After that, the serum was removed and primary antibody (diluted in PBS containing 1% BSA) was then applied for overnight at 4°C. Thereafter, sections were rinsed with PBS for 3 min × 5 min and stained with the secondary antibody in blocking serum for 1 h at room temperature in dark. At last, after another three washes, the sections were mounted in medium fluoroshieldTM with DAPI (Sigma–Aldrich F6057, Saint Louis, MO, United States) for visualization. Images were acquired using an Olympus microscope under 40× lens.
Single-Cell Reverse Transcription-Polymerase Chain Reaction (scRT-PCR)
ScRT-PCR was performed using protocols previously described (Yan and Surmeier, 1996; Day et al., 2002; Zhao et al., 2016). Individual neuron was aspirated into micropipettes by applying negative pressure while being continuously perfused. After aspiration, the tip of the electrode was broken off and the content was expelled into a 0.5 ml Eppendorf (EP) tube containing 0.5 μl of random primer, 0.25 μl of RNasin (40 U/μl) and 4 μl of DEPC-treated water.
Single-stranded cDNA was acquired by reverse transcription. The neuron-containing mixture was heated to 70°C for 5 min to denature the nucleic acids, and then immediately chilled on ice for at least 5 min. The reverse transcription reaction mixture was added to the reaction tube. The reaction mixture contained primer mixture, GoScriptTM 5× Reaction buffer (4 μl), MgCl2 (2.4 μl), dNTP (1.0 μl), RNasin (0.25 μl), GoScriptTM Reverse Transcriptase (1.0 μl), nuclease-free water (6.35 μl), and was processed according to the following steps to synthesize the single-strand cDNA: annealing at 25°C for 5 min, extending at 42°C for 60 min, inactivating reverse transcriptase at 70°C for 15 min, and then icing. In the end, to eliminate any residual RNA, 1 μl of RNase H (2 U/μl) was added to the mixture that was incubated at 37°C for 20 min. All the reagents were from Promega Inc. (Madison, WI, United States).
The resulting cDNA from RT was amplified by using a modified protocol from Zhao’s report (Zhao et al., 2016). The amplification was carried out in a thermal cycler (Applied Biosystems). The PCR reaction mixture contained: 10 μl green GoTaq flexi buffer, 2.5 mM MgCl2, 0.2 mM each dNTP, 0.8 μM primers, 2 μl cDNA template, 125 U GoTaq G2 flexi DNA polymerase, and Nuclease-free water for a final volume of 50 μl. The thermal cycling protocol was set as: 94°C for 3min; and then 94°C for 1 min, 58°C for 1 min, 72°C for 1 min for 43 cycles; and followed by 72°C for 5 min.
The PCR primer sequences for β-actin, GAPDH, and ChAT are described in Yan and Surmeier (1996). The primer sequences of sigma-1 receptor and Ca2+ channels in scRT-PCR were reported by Zamanillo et al. (2000) and D’Ascenzo et al. (2004), respectively. The primers were synthesized by Beijing Biomed Inc. All the procedures were performed to minimize the cross-contamination (Mavournin et al., 1990). In each set of single-neuron reactions, negative controls were performed by omitting reverse transcriptase to ensure extraneous and genomic DNA did not contribute to the PCR product. To control for extraneous cDNA, the cellular template was replaced with Nuclease-free water. PCR products were then analyzed by the electrophoresis in 2% agarose gels with ethidium bromide staining. Both controls were consistently negative in these experiments.
Molecular Biology (Fusion Proteins and Expression Vectors)
Full-length cDNA for human sigma-1 receptor variant 1 was obtained from OriGene (Rockville, MD, United States). The sequence is available under GenBank accession number NM_005866.2. For oocyte expression, sigma-1 receptor was subcloned into the pGH-19 vector modified by BamH I and EcoR I restriction enzymes sites. The orientation of sigma-1 receptor-pGH19 was T7 and the linearizing site for RNA was Xhol I. The Cav2.2 (α1b) cDNA used in oocyte study was a gift from Dr. Diane Lipscombe (Lin et al., 1997). GenBank accession number of the α1b, β1b, and α2δ1 cDNA sequence is AF055477, L06110, and AF296488, respectively. The sigma-1 receptor cDNA was also subcloned into the CMV promotor-driven eukaryotic expression vector Dsred-N1 (Clontech) for expression in HEK-293T cells. The construct encoding the full-length GFP-tagged Cav2.2 subunit was a generous gift from Dr. Gerald W. Zamponi (Kisilevsky et al., 2008).
Oocyte Expression and Electrophysiology
Oocytes were harvested from anesthetized Xenopus laevis through a small abdominal incision. The follicular membranes were removed by digesting in OR2 solution (82.0 mM NaCl, 2.5 mM KCL, 1.0 mM MgCl2, 5.0 mM HEPES, pH 7.6 adjusted with NaOH) containing 1.5 mg/ml collagenase type I (Sigma–Aldrich, St. Louis, MO, United States) for total of 1 h at room temperature. Oocytes were then incubated in ND-96 solution (96.0 mM NaCl, 2.0 mM KCl, 1.0 mM MgCl2, 1.8 mM CaCl2, 5.0 mM HEPES, and 2.0 mM sodium pyruvate. pH was adjusted to 7.4 with NaOH) at 18°C for at least 12 h before injection. With a microinjection pipette, 46 nl cRNA was injected into oocytes. The injection was carried out using Drummond nano-injector (Drummond Scientific Co., Broomall, PA, United States). Oocytes were then cultured for 48–96 h before recording. cRNA was synthesized from a T7 promotor using the Ribo MAXTM Large Scale RNA Production System (Promega, Gaithersburg, MD, United States), which was diluted in nuclease-free water to different concentrations.
After 48 h, currents were recorded using an Axon 2B amplifier (Molecular devices, Foster city, CA, United States) and Clampex 10.1 software (Molecular devices) at room temperature. We recorded the N-type Ca2+ current using the following voltage protocol: the holding potential was held at -80 mV and the testing potential was stepped from -80 to +50 mV for 500 ms with an increment of 10 mV. Oocytes were impaled with two electrodes pulled from borosilicate glass pipettes and filled with 3 M KCl. Electrode resistance was 0.5–2.5 MΩ. Recording was performed with oocytes bathing in the external solution containing (in mM): 5 BaCl2, 50 N-methyl-d-glucamine, 5 KCl, 5 HEPES, and pH was adjusted with methanesulfonic acid to 7.4 (Zhang et al., 2015). Leak and capacitive currents were subtracted using the P/4 procedure.
Cell Culture and Transient Expression
HEK-293T cells were cultured in Dulecco’s modified Eagle’s medium (DMEM, Invitrogen) containing10% fetal bovine serum (v/v), 50 U/ml penicillin, and 50 μg/ml streptomycin at 37°C supplied with 5% CO2. Poly-l-lysine coated coverslips were put at the bottom of 35 mm dish. The cells were planted on the coverslips for transfection. The cells were then transfected with the transfection reagent PolyJet (SignaGen) in accordance with the manufacture’s protocol at a 3:1 reagent to DNA ratio (Cassidy et al., 2014). The DNA mixtures (1 μg) used in the transfection consisted of pEGFP-N1-Cacna1b, pcDNA3.1_Cacnb1b, and pcDNA3.1_Cacna2d1 at a ratio of 2:1:1. The medium was replaced 16 h after transfection. Forty-eight hours after transfection, the cells were washed three times in PBS for 5 min each time and mounted them with DAPI. Then fluorescence was observed using confocal microscopy (Olympus).
Fluorescence Resonance Energy Transfer (FRET) Assay
FRET was measured in HEK-293T cells that were co-transfected with sigma-1 receptor-Dsred and pEGFP-Cav2.2 (α1b + β1b + α2δ1) using PerkinElmer Ultra IEWVox. The pEGFP-Cav2.2 was the donor and sigma-1 receptor-Dsred was the acceptor. Band limited excitation (420–495 nm) was provided by a laser light and three filters. Cells were imaged using an inverted microscope (Zeiss LSM-880) and an oil-immersion 40× objective lens. Data analysis was done with the Volocity 6.0. The method of nFRET was as shown in Xia and Liu (2001). The equation was as following: NFRET = (FRET-(Acceptor × A)-(Donor × B))/sqrt (Acceptor × Donor).
Co-immunoprecipitation
All processes described below were performed on ice except noted specifically. Sigma-1 receptor-Dsred and pEGFP-Cav2.2 expressing cells were washed three times with cold PBS, then lysed with 500 μl RIPA Lysis buffer (CW Biotech) and incubated on ice for 30 min. Lysates were centrifuged at 12000 g for 20 min at 4°C, and the supernatants were removed to another EP tube. Protein concentration of cellular extracts was measured using a BCA assay kit (Thermo Scientific). After that, 500 μg of supernatants were mixed with 1 μg of the homolog antibody: the anti-sigma-1 receptor antibody (Abcam, Cat#ab53852, RRID: AB_881796), the anti-Cav2.2 antibody (Millipore, Cat#AB5154, RRID: AB_2069093), or 1 μg IgG (Sigma–Aldrich, Cat#I8140, RRID: AB_1163661), and rotated at 4°C for 4 h. Then, 20 μg agrose A beads (Vigorous Biotechnology) were added to each sample that was rotated continuously at 4°C for overnight. Thereafter, samples were centrifuged at 3000 rpm for 5 min at 4°C, and then the supernatants were removed. Immunoprecipitants were washed twice with 1 ml of 1× lysis buffer, then once with 1 ml of PBS for 5 min at 3000 rpm each time. Both washing buffers contained 10 μl of protease inhibitor. After wash, samples were boiled in 60 μl of 2 × SDS loading buffer at 95°C for 10 min. Proteins were probed with anti-sigma-1 receptor antibody (Proteintech, Cat#15168-1-AP, RRID: AB_2301712 using at 1:500) and anti-Cav2.2 antibody (Proteintech, Cat#19681-1-AP, RRID: AB_10638918 using at 1:500) separately. Bands were visualized with an ECL (Gene Co. Ltd) (Kourrich et al., 2013; Yan et al., 2014).
Chemicals and Data Analysis
Chemicals were obtained from Sigma–Aldrich (St. Louis, MO, United States), TCI (Shanghai, China), TOCRIS bioscience (Bristol, United Kingdom). SKF-10047, BD-1063, PRE-084, and NE-100 were dissolved in nuclease-free water to form a 10 mM stock solution and further diluted in the bathing solution for the final concentration that were perfused at a rate of 2 ml/min by a peristaltic pump. Data analysis was performed with softwares including Clampfit Version 10.2, Prism Version 6.0 and Origin Version 9.0. The significance of the fit parameters (Mean ± SEM) was tested using student’s t-test, one-way ANOVA, and two-way ANOVA. Difference of p < 0.05 was considered statistically significant.
Results
N-type Ca2+ Channels and Sigma-1 Receptors Expression on ChIs
To observe a possible interaction between sigma-1 receptors and N-type Ca2+ channels, we had to use a specific cell that expressed both of these proteins. It was reported that N-type Ca2+ channel was the dominant one among all the voltage-gated Ca2+ channels expressed in ChIs (Yan and Surmeier, 1996). However, the expression of sigma-1 receptor in ChIs is not reported until now. Therefore, the expression of sigma-1 receptors and N-type Ca2+ channels in ChIs should be clarified firstly.
Cholinergic interneurons were picked out by their large and specific shape in rat striatal slices (Figure 1A). scRT-PCR was performed by sucking the cellular content without nucleus into the recording pipette. PCR products were separated by the electrophoreses in 2% agarose gels, stained with ethidium bromide and visualized by UV light. In Figure 1B, the positive ChAT lane revealed that the tested cell was ChI neuron, and sigma-1 receptors, Cavα1A and α1B were observed in the same neuron. For analysis of calcium channel expression, the same experiment was performed on 38 ChIs and the summarized result was plotted in Figure 1C. Briefly, three types of voltage-gated Ca2+ channels were found with different detection rates of 78.9% (α1A, P/Q-type), 92.1% (α1B, N-type), and 28.9% (α1C, L-type), respectively. It was obvious that N-type Ca2+ channel was the prominent isoform in all the high-voltage gated Ca2+ channels in ChIs.
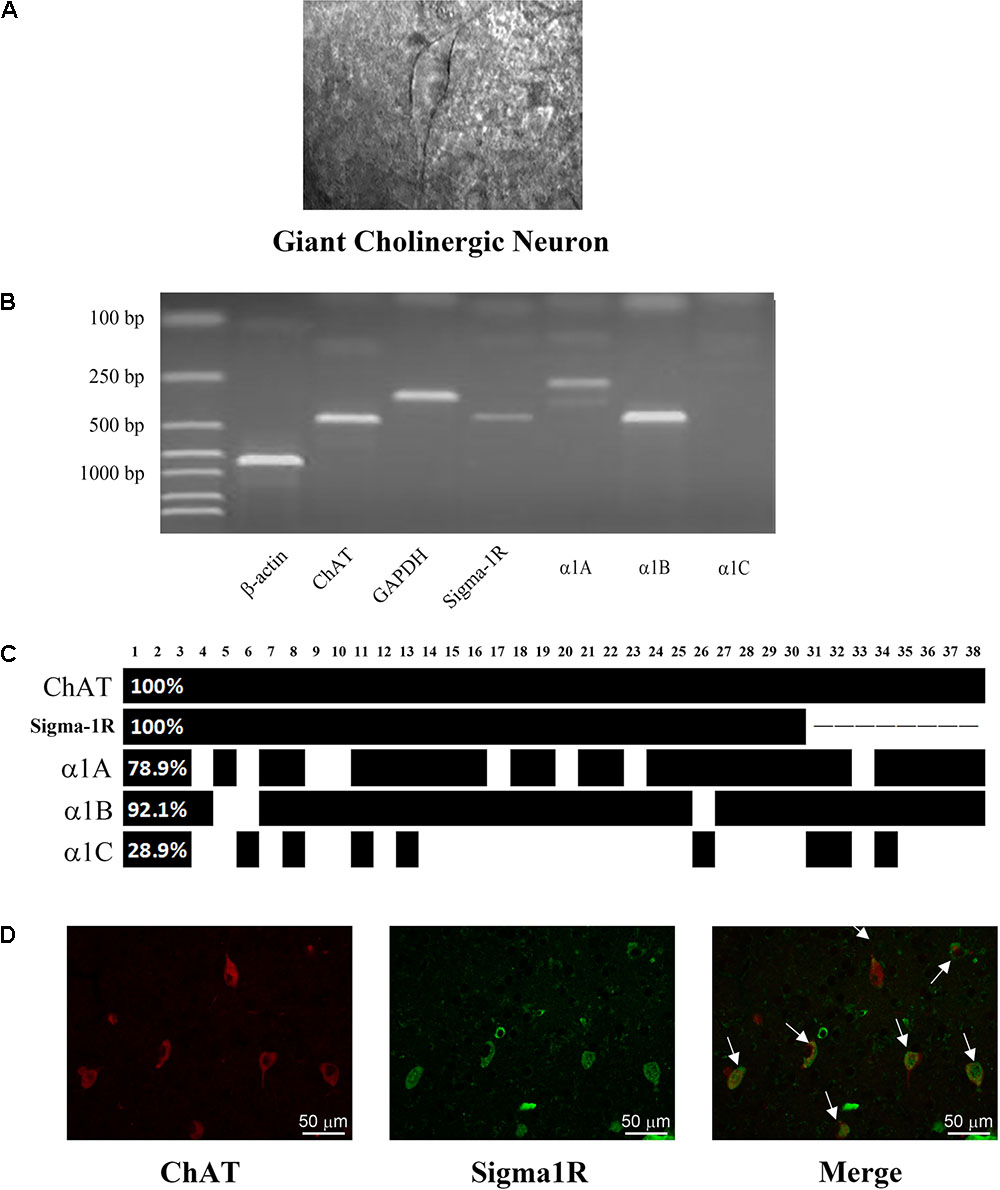
FIGURE 1. Identification of the expression of N-type Ca2+ channel and sigma-1 receptor in ChIs. (A) An IR-DIC image of a striatal slice illustrating the characteristic appearance of giant cholinergic interneurons (ChIs). (B) Representative photograph of single-cell reverse transcription polymerase chain reaction (scRT-PCR). Experiment products were stained in a 2% agarose gel. Molecular mass markers were shown in the first lane. β-actin and GAPDH were introduced as positive control. The presence of ChAT indicated the aspirated cell was a ChI. (C) Bar plot indicated the co-expression of ChAT, sigma-1 receptor and α1A-C in ChIs by scRT-PCR. ChAT and sigma-1 receptor were in every detected neuron. The α1B subunit of N-type Ca2+ channel was found in 92.1% detected cells. (D) Immunofluorescence of successive paraffin-embedded rat striatum slice observed under 40× objective lens. Left: using the anti-sigma-1 receptor antibody at a dilution of 1:20 and FITC-conjugated affinipure goat anti-rabbit IgG. Middle: Using the anti-ChAT antibody at a dilution of 1:200 and TRITC-conjugated affinipure rabbit anti-goat IgG. Right: the merged picture of the sigma-1 receptor and ChAT.
Meanwhile, we detected the expression of sigma-1 receptor on 30 neurons which are included in the 38 neurons. The mRNA of sigma-1 receptor was found in every tested ChI neuron (Figure 1C). There were about 90% (27/30) ChIs that co-expressed the sigma-1 receptors and N-type Ca2+ channels. The protein of sigma-1 receptor in ChIs was further verified by using immunofluorescence staining on sequential brain slices (5 μm). Two adjacent slices were separately incubated with goat anti-ChAT and rabbit anti-Sigma-1 receptor antibodies. Red immunofluorescence of ChAT staining, as illustrated in Figure 1D, showed the morphology and the localization of ChIs, while the green staining represented sigma-1 receptor. When two slices were merged, colocalization of both ChAT and sigma-1 receptor was clearly observed in several ChIs (as indicated by the arrows). In combination with scRT-PCR, these results proved that sigma-1 receptors were also expressed in ChIs.
Inhibition of Ca2+ Current by Sigma-1 Receptor Agonist in Rat Striatum ChIs
To investigate whether the sigma-1 receptor can regulate the N-type Ca2+ channel, the Ca2+ current of ChIs in the striatal slice was first observed using the whole-cell recording technology. Ca2+ currents were evoked by a stimulating protocol as following: the holding potential was -80 mV and the testing potential was stepped from -80 mV to +20 mV for 500 ms with an increment of 10 mV. Recorded Ca2+ current was shown in Figure 2A and its related I–V curve was plotted in Figure 2B. When a specific N-type Ca2+ channel blocker, ω–conotoxin-GVIA (CTX, 0.5 μM), was applied, the current was inhibited by 72.3% (n = 3, p < 0.05; Figures 2C,F), which further confirmed that N-type Ca2+ channels were predominant voltage-gated Ca2+ channels in rat striatal ChIs.
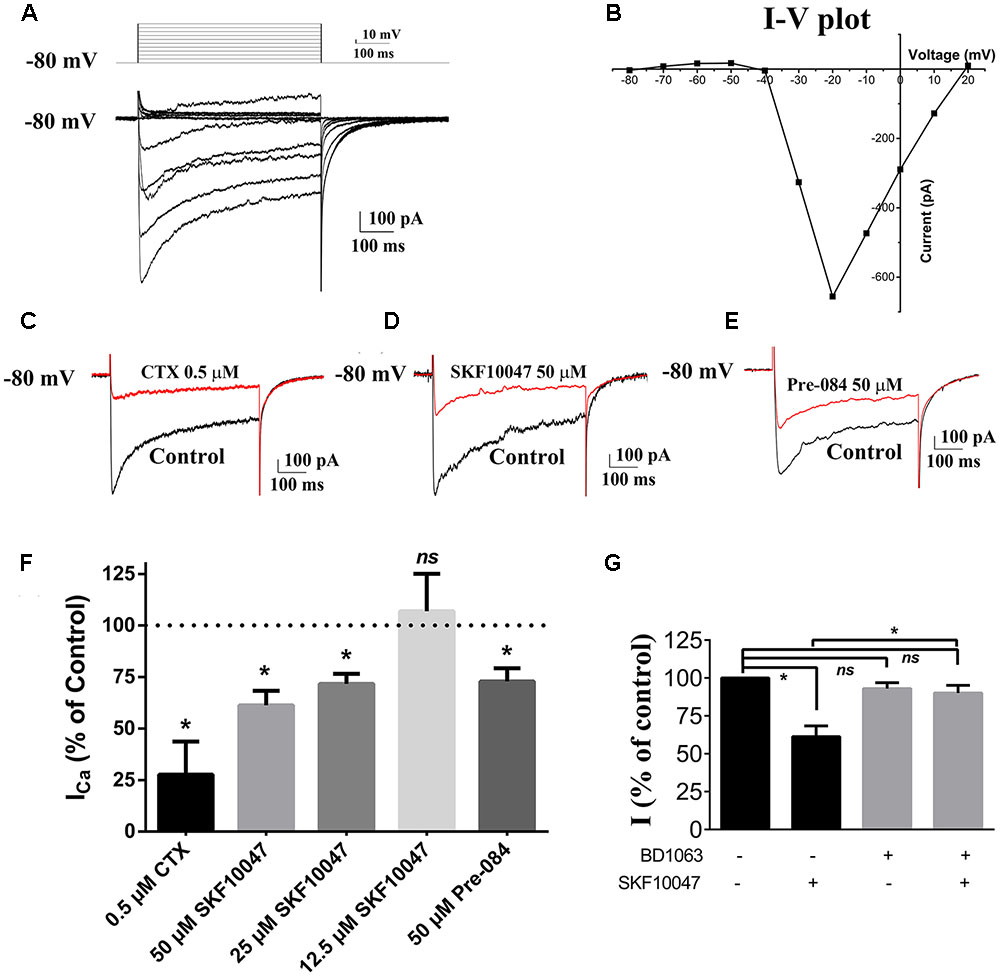
FIGURE 2. Sigma-1 receptor agonist reduced currents of Ca2+ channel in ChIs. (A) Representative trace of Ca2+ channel current obtained from the rat striatum ChIs in the brain slice. (B) I–V plot of the recorded Ca2+ channel current trace in (A). (C–E) Representative traces of current evoked before and after the bath application of 0.5 μM CTX, 50 μM SKF-10047, and 50 μM Pre-084, respectively. (F) Summarized bar graph to present the effect of CTX (N-type Ca2+ channel blocker), different concentrations of SKF-10047 and Pre-084 (sigma-1 receptor agonists) on Ca2+ currents. (G) Summarized bar graph to present the effect of BD-1063 (sigma-1 receptor antagonist) on the Ca2+ current inhibition induced by SKF-10047. ∗p < 0.05.
The modulation of sigma-1 receptors on N-type Ca2+ channels was observed by using sigma-1 receptor agonists of SKF-10047 and Pre-084. When SKF-10047 was added to the bath solution, the amplitude of Ca2+ current was inhibited by 28.4% ± 4.9% (n = 9, p < 0.05) and 38.7% ± 7.1% (n = 11, p < 0.05) at the concentration of 25 and 50 μM, respectively (Figures 2D,F). Pre-084 (50 μM) also depressed the currents by 27.1% ± 6.4% (n = 7, p < 0.05) (Figures 2E,F). BD-1063, a selective antagonist of sigma-1 receptor, did not display any visible effect on Ca2+ currents. However, the inhibition of SKF-10047 on Ca2+ currents was abolished in the presence of BD-1063 (Figure 2G).
Direct Interaction between Sigma-1 Receptors and N-type Ca2+ Channels
In order to investigate the possible constitutive interaction between sigma-1 receptors and N-type Ca2+ channels, the two proteins were co-expressed in Xenopus oocytes. N-type Ca2+ channel cRNA contained α1b, β1b, and α2δ1 in a 2:1:1 ratio. No visible currents were evoked in native oocytes. However, big inward currents were recorded after cRNA of N-type Ca2+ channel was injected into Xenopus oocytes after 48 h and CTX (0.2 μM) blocked the currents completely (Figures 3A,B), which indicated that functional N-type Ca2+ channels were successfully expressed in oocytes. After sigma-1 receptor cRNA was injected into oocytes for 48 h, Western Blot was performed. The schematic Western blot strap in the left lane showed the sigma-1 receptor expressed successfully in oocytes (Figure 3C).
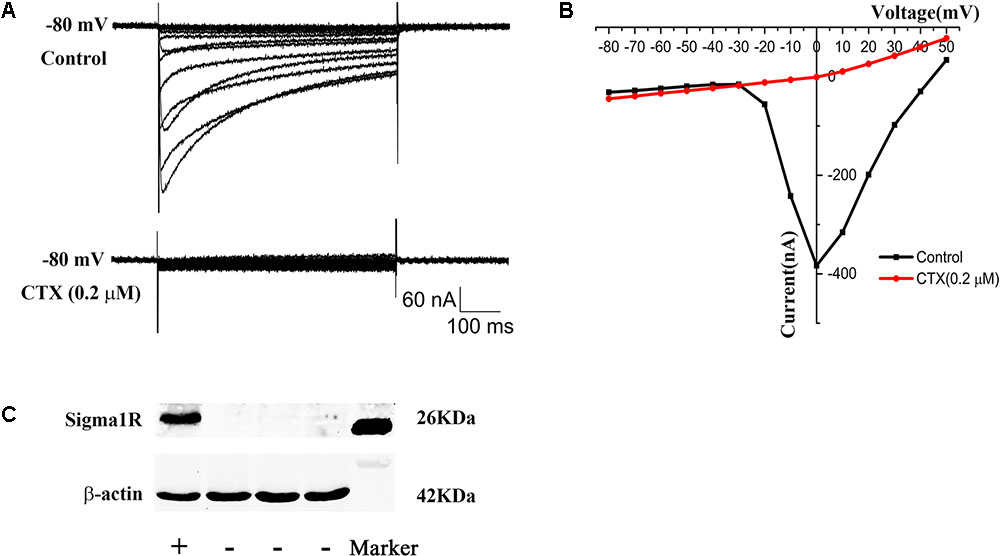
FIGURE 3. Expression of N-type Ca2+ channel and sigma-1 receptor in xenopus oocytes. (A) Upper panel: the current of N-type Ca2+ channel recorded in oocytes after microinjection with N-type Ca2+ channel cRNA. Lower panel: the current was blocked by N-type Ca2+ channel blocker CTX (0.2 μM). (B) I–V plot of the recorded N-type Ca2+ channel current in (A). (C) Schematic Western blot strap of oocytes injected with sigma-1 receptor cRNA (the first lane) and control group (the middle three lanes), which indicated the sigma-1 receptor expressed successfully. The last lane showed the Marker.
To observe the possible interaction between sigma-1 receptors and N-type Ca2+ channels, a mixture of their cRNA was injected into oocytes with a ratio of 1:1. Surprisingly, no visible currents were induced by a depolarized stimulation. To clarify a possible chaperone-mediated interaction between sigma-1 receptors and N-type Ca2+ channels, we decreased the concentration of sigma-1 receptor cRNA and mix it with the constant concentration of N-type Ca2+ channel cRNA at the ratio of 0.5:1, 0.25:1, 0.125:1, 0.0625:1, and 0.03125:1, respectively. The results showed that the amplitude of N-type Ca2+ channel current was increased obviously with decreased sigma-1 receptor cRNA (Figures 4A,B). Compared to the currents recorded in oocytes with only expression of N-type Ca2+ channels, the current amplitudes were 29.9% ± 3.9% (n = 18, p < 0.001), 52.6% ± 3.8% (n = 17, p < 0.001), 72.1% ± 3.7% (n = 26, p < 0.001), 85.0% ± 5.8% (n = 17, p < 0.05), and 89.9% ± 7.5% (n = 10, p > 0.05) with the cRNA ratio of 0.5:1, 0.25:1, 0.125:1, 0.0625:1, and 0.03125:1, respectively. However, the expression levels of the two proteins was positively correlated with the amount of cRNA injected, indicating that the expression of each protein was not affected by each other (Figure 4C). These results suggested that sigma-1 receptors probably regulated N-type Ca2+ channels constitutively by direct interaction. As a negative control, we studied sigma-1 receptor and another ion channel, HCN1, in the same way as with N-type Ca2+ channel in oocytes. We found HCN1 current was not affected by sigma-1 receptor. This experiment indicated us the effect on N-type Ca2+ channels was specific (Data was not shown).
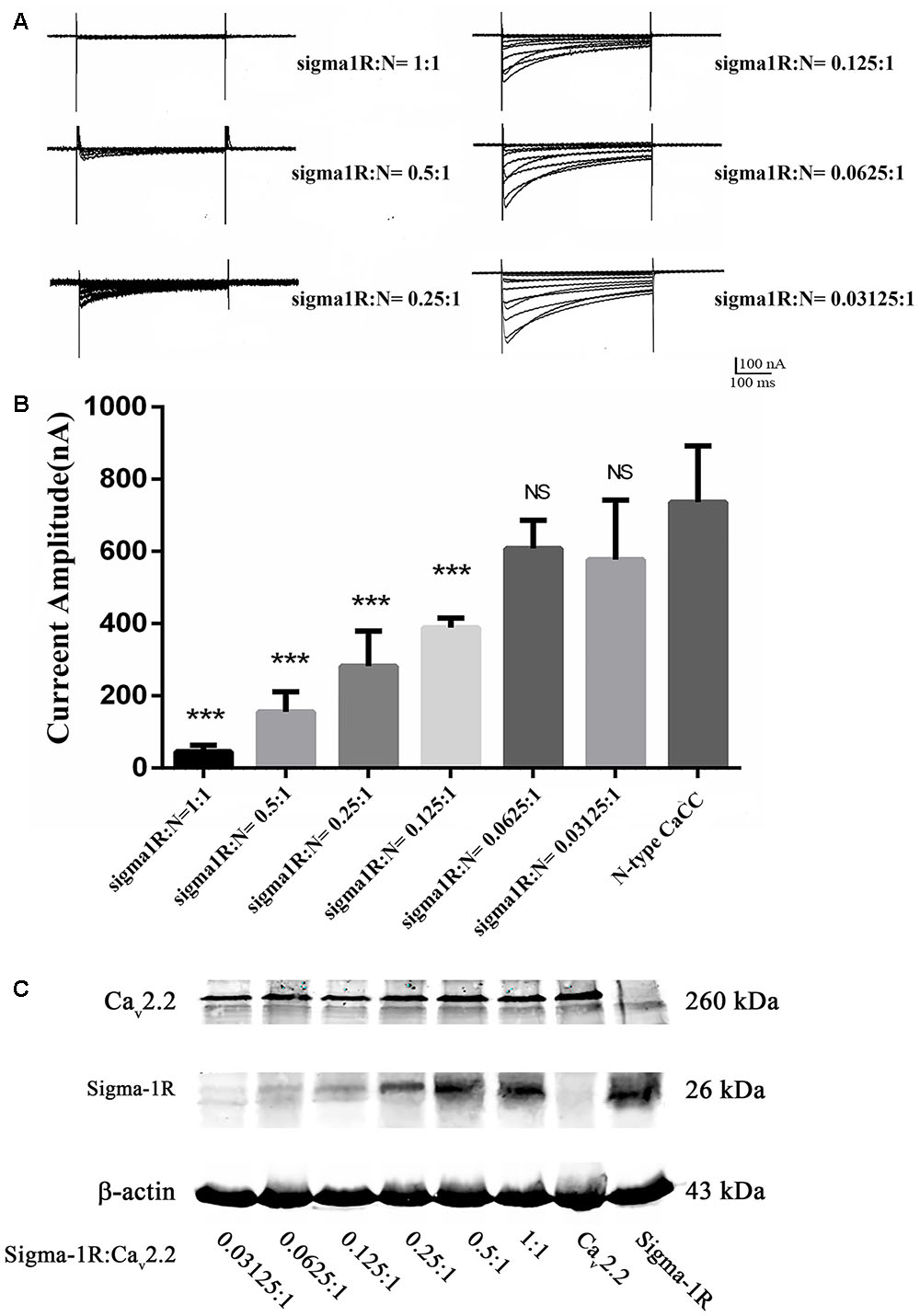
FIGURE 4. Currents recorded at different cRNA ratio of sigma-1 receptor to N-type Ca2+ channel. (A) Currents recorded from the oocytes injected with different cRNA ratio. The cRNA concentration ratio of Sigma-1 receptor: N-type Ca2+ channel = 1:1, 0.5:1, 0.25:1, 0.125:1, 0.0625:1, and 0.03125:1, respectively. (B) Summarized bar graph to present the N-type Ca2+ currents decreased with the increase of sigma-1 receptor cRNA injected in oocytes. The current amplitude recorded from oocytes with injection of N-type Ca2+ channel cRNA only was normalized as 100%. Values are Mean ± SEM (n = 18 for 1:1, n = 18 for 0.5:1, n = 17 for 0.25:1, n = 26 for 0.125:1, n = 17 for 0.0625:1, and n = 10 for 0.03125:1 group, respectively. ∗∗∗p < 0.001, ∗p < 0.05). (C) The expression levels of the two proteins from oocytes injected with the cRNA ratio of sigma-1 receptor: N-type Ca2+ channel at 0.03125:1, 0.0625:1, 0.125:1, 0.25:1, 0.5:1, and 1:1 from left to right. The blots in the right two lanes showed the protein expression in oocytes of control groups, which were injected with the N-type Ca2+ channel cRNA or the sigma-1 receptor cRNA alone.
Effect of Sigma-1 Receptor Agonist on N-type Ca2+ Channels
To prove if sigma-1 receptor agonists could modulate the Ca2+ channel in oocytes the same way as in ChIs, we observed the effect of SKF-10047 on the currents recorded in oocytes in which sigma-1 receptor to N-type Ca2+ channel was injected at 0.25:1 ratio (Figure 5A). When SKF-10047 (50 μM) was added into the bath solution, the current was inhibited by 29.7% ± 6.7% comparing with control (Figures 5A–C, n = 6, p < 0.05). BD-1063 (50 μM) alone did not present remarkable inhibition on N-type Ca2+ currents (93.2 ± 2.1% of control, Figure 5C, n = 6, p > 0.05), but could block the inhibition of SFK-10047 on N-type Ca2+ current (87.2 ± 2.2% of control, Figure 5C, n = 6, p > 0.05). This result indicated that SKF-10047 inhibited N-type Ca2+ channel through sigma-1 receptor activation.
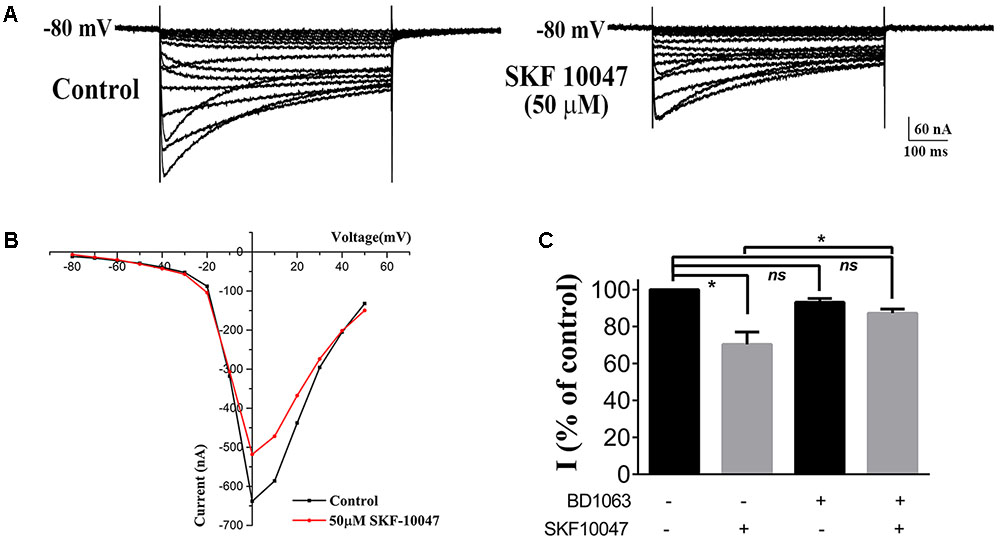
FIGURE 5. Effect of sigma-1 receptor agonist and antagonist on N-type Ca2+ channel. (A) Example trace of the inhibition on N-type Ca2+ current induced by 50 μM SKF-10047. The oocyte was injected with a cRNA ratio of sigma 1R: N-type Ca2+ channel = 0.25:1. (B) I–V plot of the recorded N-type Ca2+ current from the oocyte in (A). (C) Summarized bar graph to present the effect of SFK-10047 (sigma-1 receptor agonist) and BD-1063 (sigma-1 receptor antagonist) on N-type Ca2+ currents. Two-way ANOVA analysis, Mean ± SEM, ∗p < 0.05.
Expression of Sigma-1 Receptors and N-type Ca2+ Channels in HEK-293T Cells
As reviewed in Su et al. (2016), we speculated that the inhibition of sigma-1 receptor on N-type Ca2+ channels may be mediated by protein–protein interaction. To investigate if there is a protein–protein interaction between receptor and channel, we took HEK-293T cells as an exogenous expressing model. Cultured HEK-293T cells were transiently transfected with the GFP-Cav2.2 channels (α1b+β1b+α2δ1subunits) in the absence and presence of sigma-1-Dsred receptor. The cells were observed using confocal microscopy. As illustrated in Figures 6A,B, the red and green fluorescence indicated sigma-1 receptors and N-type Ca2+ channels were successfully expressed, respectively. When cells were co-transfected with sigma-1 receptor and N-type Ca2+ channel, there is yellow fluorescence in merged channel. This indicated that receptors and channels were co-expressed in the same cell, as shown in Figure 6C. These results allowed us to further study the interaction of the receptors and channels.
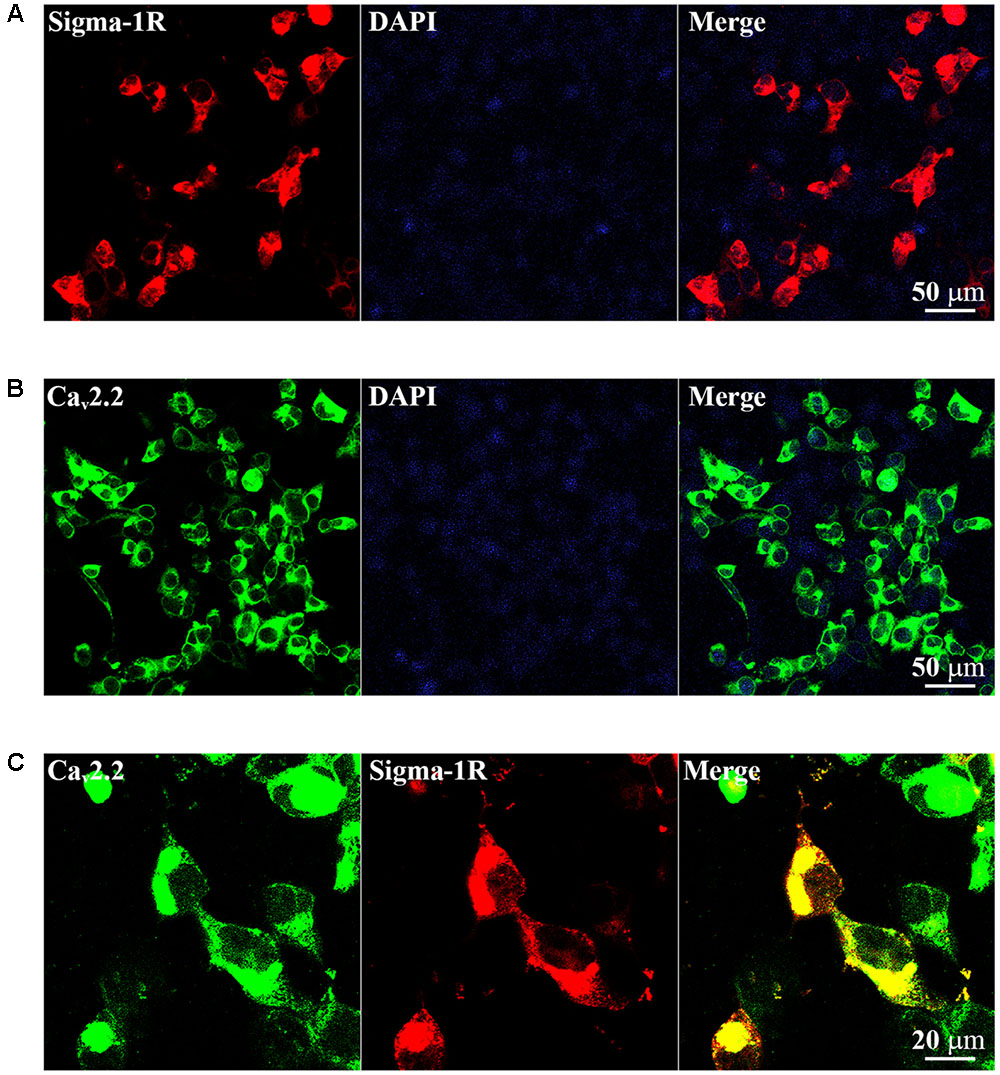
FIGURE 6. Exogenous expression of Sigma-1-Dsred receptor and Cav2.2-GFP in HEK-293T cell line. (A) Fluorescence images of HEK-293T cells transfected with 0.5 μg Sigma-1-Dsred receptor cDNA. (B) Fluorescence images of HEK-293T cells transfected with 0.5 μg Cav2.2-GFP (α1b+β1b+α2δ1subunits) cDNA. (C) Confocal microscopy images of HEK-293T cells with both 0.5 μg Cav2.2-GFP and 0.5 μg Sigma-1-Dsred receptor cDNA. Co-localization was shown in yellow.
Sigma-1 Receptor Physically Associates with Cav2.2 Channels in HEK-293T Cells
To examine a direct interaction between sigma-1 receptors and N-type Ca2+ channels, the technology of FRET was performed. Using the co-transfected HEK-293T cells, we chose GFP-Cav2.2 as the FRET donor and sigma-1 receptor-Dsred as the acceptor. In the experimental group, we used 488 nm laser-light to stimulate the donor (GFP), which then excites the red fluorescence from FRET channel when both are in close proximity as shown in Figure 7A. Using the Volocity 6.0, we measured the intensity of the signals from nFRET channel. We set the area of signals 0.02 μm2 and the intensity ≥0.5 as valid standards. Then we counted the number of signals under this condition. In experimental group, we obtained 203 signals from 11 fields subjected to the condition (Figure 7C). An example including the signals in this field was shown in Figure 7B, where the nFRET value of each signal was 0.81 ± 0.06 (Mean ± SD). However, in the control experiment, where pEGFP vector alone was co-transfected with sigma-1 receptor-Dsred, the number of signals matched the condition was 0 (zero) (also see Figure 7C). This indicated the existence of the receptor-channel complex.
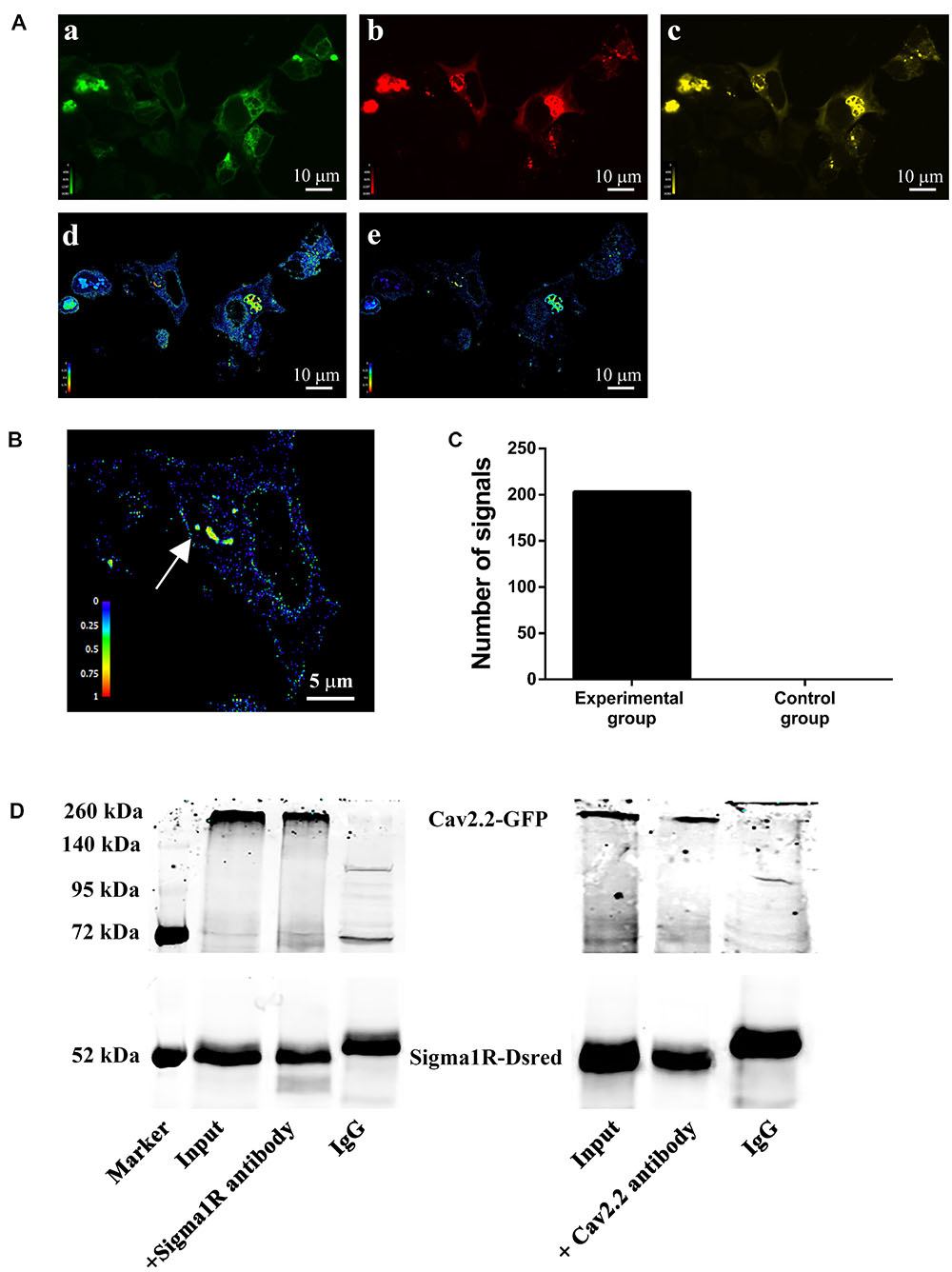
FIGURE 7. Protein–protein interaction between sigma-1 receptors and N-type Ca2+ channels. (A) HEK-293T cells were transfected with both Cav2.2-GFP (α1b+β1b+α2δ1) and sigma-1-Dsred receptor. Pseudo-color images were obtained under the three filter sets: GFP (a), Dsred (b), and FRET (c). After subtraction of background and bleed-through signals, net FRET (d) was acquired. Normalized FRET (e) values were got by using equation described in section “Materials and Methods.” Color bars represented relative degree of net FRET and normalized FRET within the cells. (B) Representative cell enlarged from (e). The net FRET value of the point with the arrow was 0.81 ± 0.06 (Mean ± SD). (C) Bar graph of the statistical numbers in 11 experimental fields (203) and 5 negative control fields (0). (D) Co-immunoprecipitation of GFP-Cav2.2 channels and sigma-1-Dsred receptors. Total lysates were prepared from HEK-293T cells. Immunoprecipitated samples were run on the gels and the blots probed with either anti-Cav2.2 antibody or anti-sigma-1 receptor antibody.
To investigate the receptor-channel physical interaction further, we performed the co-immunoprecipitation. Samples were processed with anti-Cav2.2 antibody or anti-sigma-1 receptor antibody. Probing the gel with antibody against N-type Ca2+ channel in the sample immunoprecipitated with anti-sigma-1 antibody revealed a band at 260 kDa shown in Figure 7D left panel. On the other hand, probing with antibodies against sigma-1 receptor in the sample immunoprecipitated with anti-N-type Ca2+ channel antibody revealed a band at 52 kDa, as shown in Figure 7D right panel. The input of the cell lysates indicated that the receptor and the channel were co-expressed, IgG was used as negative control, as shown in Figure 7D. These results demonstrated the physical association of sigma-1 receptors with N-type Ca2+ channels.
Discussion
Since the sigma-1 receptor was discovered decades ago (Martin et al., 1976; Su, 1982), it has been investigated broadly in many aspects. It is now recognized as an ligand-regulated chaperone protein that resides at MAM and some plasma membrane compartments (Hayashi and Su, 2007). The human crystal structure of the sigma-1 receptor reveals a trimeric organization and each promoter contains only a single transmembrane domain, which includes a cupin-like β-barrel with the ligand-binding site buried at its center (Schmidt et al., 2016). The unique structural features of sigma-1 receptors probably underwrite its multitasking functions. The receptor has been demonstrated to be involved in various biological and pathological processes, and has been considered as an important therapeutic target in many central nervous system diseases in human (Maurice and Lockhart, 1997; Maurice et al., 2002; Maurice and Su, 2009). The N-type Ca2+ channel is well established as a critical factor to control neurotransmitter release. The N-type Ca2+ channel mainly locates at presynaptic membrane and mediate rapid Ca2+ influx into the synaptic terminal that triggers synaptic vesicle exocytosis and neurotransmitter release (Llinás et al., 1981; Catterall, 2000; Momiyama and Koga, 2001). Accumulative evidence reveals that sigma-1 receptors are also involved in the regulation of neurotransmitter release. Sigma-1 receptor activation inhibits glutamate release from rat cortical nerve terminals, which is prevented by blocking N-type and P/Q-type Ca2+ channels, but not by blocking the ryanodine receptors or the mitochondrial Na+/Ca2+ exchange (Lu et al., 2012). In rat primary retinal ganglion cells (RGCs), sigma-1 receptor agonist inhibits calcium currents and an association between L-type calcium channels and the sigma-1 receptors is demonstrated (Tchedre et al., 2008). Despite many kinds of VGCCs appear to be constitutively regulated by direct interaction with sigma-1 receptors (Chu and Ruoho, 2015), it still remains unclear that if there is an interaction between sigma-1 receptors and N-type Ca2+ channels.
To identify the possible interaction between sigma-1 receptors and N-type Ca2+ channels, we need to find a specific cell that has both proteins co-expressed. ChIs in rat striatum has been reported to express several subtypes of VGCCs, and the N-type Ca2+ channel is predominant one (Yan and Surmeier, 1996). However, it is unclear if sigma-1 receptors are also expressed in ChIs. In the experiment, we first identify that sigma-1 receptors are expressed in ChIs by using scRT-PCR and immunofluorescence staining techniques. Three subtypes of VGCCs (N-type, P/Q-type, and L-type) present a different detection rate and N-type Ca2+ channels are found nearly in every ChI. A large portion (72.3%) of VGCC currents induced in ChIs is inhibited by CTX, a specific N-type Ca2+ channel blocker, which further confirms that N-type Ca2+ channels are predominant voltage-gated Ca2+ channels, and the result is identical with Yan’s reports (Yan and Surmeier, 1996). When agonists of sigma-1 receptors (SKF-10047, Pre-084) are applied into the bath solution, the VGCC currents are depressed remarkably. The sigma-1 receptor antagonist (BD-1063) alone is not able to block the Ca2+ currents, but it completely abolishes the blockage induced by sigma-1 receptor agonist SKF-10047, which suggests the receptor activation underlies the mechanism of the inhibition. Agonist of sigma-1 receptors has also been reported to block VGCC currents in neurons and other tissues and it is considered as a directly activation of the receptors at the level of the plasma lemma (Zhang and Cuevas, 2002; Tchedre et al., 2008; Lu et al., 2012; Pan et al., 2014). However, the evidence for a direct interaction between sigma-1 receptors and N-type VGCC is lacking.
To observe the possible interaction between sigma-1 receptors and N-type Ca2+ channels, Xenopus laevis oocyte is used to co-express both of the two proteins. The results show that no currents are evoked when cRNAs of sigma-1 receptors and N-type Ca2+ channels are injected into oocytes at a concentration ratio of 1:1. The possible explanation may be a chaperon-mediated interaction induced by sigma-1 receptors. To verify the consideration, we reduce the concentration of sigma-1 receptor cRNA and mix it with a fixed concentration of N-type Ca2+ channel cRNA. Then N-type Ca2+ channel currents appear and their amplitudes increase with decreased sigma-1 receptor cRNA. In this situation, sigma-1 receptor agonist can still display its blockage on N-type Ca2+ currents. Even though the relationship between ligand binding to sigma-1 receptor and the subsequent biological response is not well addressed, evidence for ligand-mediated changes in sigma-1 receptor oligomerization state is confirmed (Mishra et al., 2014). Sigma-1 receptor agonists alter oligomeric structures and favor dissociation of the complexes of the receptor. Therefore, we consider that sigma-1 receptors inhibit N-type Ca2+ channels probably by two mechanisms: a direct physical interaction constitutively and a conformational change induced by receptor activation at the surface of cell membrane. To clarify the molecular basis of interactions between sigma-1 receptors and N-type Ca2+ channels, HEK-293T cells are used to express both proteins. The fluorescence indicates that sigma-1 receptors and N-type Ca2+ channels are successfully co-expressed in HEK-293T cells. FRET experiments reveal that sigma-1 receptors and N-type Ca2+ channels locate in a close proximity. However, the three-filter system is affected by a number of factors, e.g., photobleaching/quenching, quantum yield of fluorophores, and molecular position/orientation, and critical in collecting data (Xia and Liu, 2001). To derive energy transfer efficiencies accurately, we should perform the experiments combining the three-filter system with acceptor photobleaching. Co-IP experiment displays a protein complex formed by sigma-1 receptors and N-type Ca2+ channels. These results establish the molecular basis for the protein–protein interaction between sigma-1 receptors and N-type Ca2+ channels.
The sigma-1 receptor agonists have recently attracted much attention as potential therapeutic drugs for cognitive and affective disorders. However, it is still unclear whether they act via modulation of neurotransmitter release (Antonini et al., 2011). As our data reveal that agonists of sigma-1 receptor block N-type Ca2+ channels, neurotransmitter release is probably also inhibited while the agonists are administrated. The negative modulation of sigma-1 receptor and its agonists on N-type Ca2+ channels might partially contribute to their therapeutic effect on nervous system disorders.
Conclusion
Our data first identify that the sigma-1 receptor is expressed in rat striatal ChIs and agonists of sigma-1 receptors can depress VGCC currents by activating the receptors. Heterogeneous express experiment demonstrates that sigma-1 receptors and N-type Ca2+ channels form a complex on the plasma lemma by Co-IP and FRET assays. Via the protein-protein interaction, sigma-1 receptors exercise negative modulation on N-type Ca2+ channels by a direct physical contact and an agonist-induced conformational change. Considering the critical regulation of N-type Ca2+ channels on neurotransmission, our data should be benefit to better understand the multitasking functions of sigma-1 receptors.
Author Contributions
Study concept design: KZ, ZZ, and JZ. Collection of data: KZ and ZZ. Analysis and interpretation of data: KZ, ZZ, and JZ. Drafting of the manuscript: KZ and ZZ. Critical revision of the manuscript: KZ and JZ. Study supervision and help: LL, XL, XW, LW, and HY. All authors approved the final version of the manuscript. All experiments were performed in State Key Laboratory of Toxicology and Medical Countermeasures in China.
Funding
This research was supported by grants from the National Key Technology R&D Program of China (No. 2012BAI01B07), the National Integrated Drug Discovery Technology Platform Foundation of China (No. 2012ZX09301003), the Beijing Nature Science Foundation (No. 7132146), and the General Program of National Natural Science Foundation of China (No. 81472817 and No. 81573404).
Conflict of Interest Statement
The authors declare that the research was conducted in the absence of any commercial or financial relationships that could be construed as a potential conflict of interest.
Acknowledgment
We are extremely grateful to Dr. Weifeng Yu for the critical revision of the manuscript.
References
Altier, C., Khosravani, H., Evans, R. M., Hameed, S., Peloquin, J. B., Vartian, B. A., et al. (2006). ORL1 receptor-mediated internalization of N-type Ca2+ channels. Nat. Neurosci. 9, 31–40. doi: 10.1038/nn1605
Antonini, V., Marrazzo, A., Kleiner, G., Coradazzi, M., Ronsisvalle, S., Prezzavento, O., et al. (2011). Anti-amnesic and neuroprotective actions of the sigma-1 receptor agonist (-)-MR22 in rats with selective cholinergic lesion and amyloid infusion. J. Alzheimers Dis. 24, 569–586. doi: 10.3233/JAD-2011-101794
Aydar, E., Palmer, C. P., Klyachko, V. A., and Jackson, M. B. (2002). The sigma receptor as a ligand-regulated auxiliary potassium channel subunit. Neuron 34, 399–410. doi: 10.1016/S0896-6273(02)00677-3
Carnally, S. M., Johannessen, M., Henderson, R. M., Jackson, M. B., and Edwardson, J. M. (2010). Demonstration of a direct interaction between σ-1 receptors and acid-sensing ion channels. Biophys. J. 98, 1182–1191. doi: 10.1016/j.bpj.2009.12.4293
Cassidy, J. S., Ferron, L., Kadurin, I., Pratt, W. S., and Dolphin, A. C. (2014). Functional exofacially tagged N-type Ca2+ channels elucidate the interaction with auxiliary α2δ-1 subunits. Proc. Natl. Acad. Sci. U.S.A. 111, 8979–8984. doi: 10.1073/pnas.1403731111
Catterall, W. A. (2000). Structure and regulation of voltage-gated Ca2+ channels. Annu. Rev. Cell Dev. Biol. 16, 521–555. doi: 10.1146/annurev.cellbio.16.1.521
Chu, U. B., and Ruoho, A. E. (2015). Biochemical pharmacology of the sigma-1 receptor. Mol. Pharmacol. 89, 142–153. doi: 10.1124/mol.115.101170
Cobos, E. J., Entrena, J. M., Nieto, F. R., Cendán, C. M., and Del, P. E. (2008). Pharmacology and therapeutic potential of sigma1 receptor ligands. Curr. Neuropharmacol. 6, 344–366. doi: 10.2174/157015908787386113
Crottès, D., Martial, S., Rapetti-Mauss, R., Pisani, D. F., Loriol, C., Pellissier, B., et al. (2011). Sig1R protein regulates hERG channel expression through a post- translational mechanism in leukemic cells. J. Biol. Chem. 286, 27947–27958. doi: 10.1074/jbc.M111.226738
D’Ascenzo, M., Vairano, M., Andreassi, C., Navarra, P., Azzena, G. B., and Grassi, C. (2004). Electrophysiological and molecular evidence of L-(Cav1), N- (Cav2.2), and R-(Cav2.3) type Ca2+ channels in rat cortical astrocytes. Glia 45, 354–363. doi: 10.1002/glia.10336
David, C., Hélène, G., Patrick, M., Franck, B., and Olivier, S. (2013). The sigma-1 receptor: a regulator of cancer cell electrical plasticity? Front. Physiol. 4:175. doi: 10.3389/fphys.2013.00175
Day, M., Olson, P. A., Platzer, J., Striessnig, J., and Surmeier, D. J. (2002). Stimulation of 5-HT2 receptors in prefrontal pyramidal neurons inhibits Cav1.2 L-type Ca2+ Currents Via a PLCβ/IP3/calcineurin signaling cascade. J. Neurophysiol. 87, 2490–2504. doi: 10.1152/jn.00843.2001
Fontanilla, D., Johannessen, M., Hajipour, A. R., Cozzi, N. V., Jackson, M. B., and Ruoho, A. E. (2009). The hallucinogen N,N-dimethyltryptamine (DMT) is an endogenous sigma-1 receptor regulator. Science 323, 934–937. doi: 10.1126/science.1166127
Guitart, X., Codony, X., and Monroy, X. (2004). Sigma receptors: biology and therapeutic potential. Psychopharmacology 174, 301–319. doi: 10.1007/s00213-004-1920-9
Hawkins, V. E., Hawryluk, J. M., Takakura, A. C., Tzingounis, A. V., Moreira, T. S., and Mulkey, D. K. (2015). HCN channels contribute to serotonergic modulation of ventral surface chemosensitive neurons and respiratory activity. J. Neurophysiol. 113, 1195–1205. doi: 10.1152/jn.00487.2014
Hayashi, T., and Su, T. P. (2001). Regulating ankyrin dynamics: roles of sigma-1 receptors. Proc. Natl. Acad. Sci. U.S.A. 98, 491–496. doi: 10.1073/pnas.98.2.491
Hayashi, T., and Su, T. P. (2003). Intracellular dynamics of σ-1 receptors (s-1binding sites) in NG108-15 cells. J. Pharmacol. Exp. Ther. 306, 726–733. doi: 10.1124/jpet.103.051292
Hayashi, T., and Su, T. P. (2007). Sigma-1 receptor chaperones at the ER- mitochondrion interface regulate Ca2+ signaling and cell survival. Cell 131, 596–610. doi: 10.1016/j.cell.2007.08.036
Johannessen, M., Ramachandran, S., Riemer, L., Ramos-Serrano, A., Ruoho, A. E., and Jackson, M. B. (2009). Voltage-gated sodium channel modulation by sigma-receptors in cardiac myocytes and heterologous systems. Am. J. Physiol. Cell Physiol. 296, C1049–C1057. doi: 10.1152/ajpcell.00431.2008
Kekuda, R., Prasad, P. D., Fei, Y. J., Leibach, F. H., and Ganapathy, V. (1996). Cloning and functional expression of the human type 1 sigma receptor (hSigmaR1). Biochem. Biophys. Res. Commun. 229, 553–558. doi: 10.1006/bbrc.1996.1842
Kinoshita, M., Matsuoka, Y., Suzuki, T., Mirrielees, J., and Yang, J. (2012). Sigma-1 receptor alters the kinetics of Kv1.3 voltage gated potassium channels but not the sensitivity to receptor ligands. Brain Res. 1452, 1–9. doi: 10.1016/.brainres.2012.02.070
Kisilevsky, A., Mulligan, S. C., Iftinca, M., Varela, D., Tai, C., Chen, L., et al. (2008). D1 receptors physically interact with N-type Ca2+ channels to regulate channel distribution and dendritic Ca2+ entry. Neuron 58, 557–570. doi: 10.1016/j.neuron.2008.03.002
Kourrich, S., Hayashi, T., Chuang, J. Y., Tsai, S. Y., Su, T. P., and Bonci, A. (2013). Dynamic interaction between sigma-1 receptor and Kv1.2 shapes neuronal and behavioral responses to cocaine. Cell 152, 236–247. doi: 10.1016/j.cell.2012.12.004
Kourrich, S., Su, T. P., Fujimoto, M., and Bonci, A. (2012). The sigma-1 receptor: roles in neuronal plasticity and disease. Trends. Neurosci. 35, 762–771. doi: 10.1016/j.tins.2012.09.007
Langa, F., Codony, X., Tovar, V., Lavado, A., Giménez, E., Cozar, P., et al. (2003). Generation and phenotypic analysis of sigma receptor type I (σ1) knockout mice. Eur. J. Neurosci. 18, 2188–2196. doi: 10.1046/j.1460-9568.2003.02950.x
Lin, Z., Haus, S., Edgerton, J., and Lipscombe, D. (1997). Identification of functionally distinct isoforms of the N-type Ca2+ channel in rat sympathetic ganglia and brain. Neuron 18, 153–166. doi: 10.1016/S0896-6273(01)80054-4
Llinás, R., Steinberg, I. Z., and Walton, K. (1981). Relationship between presynaptic Ca2+ current and postsynaptic potential in squid giant synapse. Biophys. J. 33, 323–351. doi: 10.1016/S0006-3495(81)84899-0
Lu, C. W., Lin, T. Y., Wang, C. C., and Wang, S. J. (2012). σ-1 receptor agonist SKF10047 inhibits glutamate release in rat cerebral cortex nerve endings. J. Pharmacol. Exp. Ther. 341, 532–542. doi: 10.1124/jpet.111.191189
Lupardus, P. J., Wilke, R. A., Aydar, E., Palmer, C. P., Chen, Y., Ruoho, A. E., et al. (2000). Membrane-delimited coupling between sigma receptors and K+ channels in rat neurohypophysial terminals requires neither G-protein nor ATP. J. Physiol. 526, 527–539. doi: 10.1111/j.1469-7793.2000.00527.x
Luty, A. A., Kwok, J. B., Dobson-Stone, C., Loy, C. T., Coupland, K. G., Karlström, H., et al. (2010). Sigma nonopioid intracellular receptor 1 mutations cause frontotemporal lobar degeneration-motor neuron disease. Ann. Neurol. 68, 639–649. doi: 10.1002/ana.22274
Marriott, K. S. C., Prasad, M., Thapliyal, V., and Bose, H. S. (2012). σ-1 receptor at the mitochondrial-associated endoplasmic reticulum membrane is responsible for mitochondrial metabolic regulation. J. Pharmacol. Exp. Ther. 343, 578–586. doi: 10.1124/jpet.112.198168
Martin, W. R., Eades, C. G., Thompson, J. A., Huppler, R. E., and Gilbert, P. E. (1976). The effects of morphine- and nalorphine-like drugs in the nondependent and morphine-dependent chronic spinal dog. J. Pharmacol. Exp. Ther. 197, 517–532.
Maurice, T., and Lockhart, B. P. (1997). Neuroprotective and anti-amnesic potentials of sigma (sigma) receptor ligands. Prog. Neuropsychopharmacol. Biol. Psychiatry 21, 69–102. doi: 10.1016/S0278-5846(96)00160-1
Maurice, T., Martin-Fardon, R., Romieu, P., and Matsumoto, R. R. (2002). Sigma1 (σ1) antagonists represent a new strategy against cocaine addiction and toxicity. Neurosci. Biobehav. Rev. 26, 499–527. doi: 10.1016/S0149-7634(02)00017-9
Maurice, T., and Su, T. P. (2009). The pharmacology of sigma-1 receptors. Pharmacol. Ther. 124, 195–206. doi: 10.1016/j.pharmthera.2009.07.001
Mavournin, K. H., Blakey, D. H., Cimino, M. C., Salamone, M. F., and Heddle, J. A. (1990). The in vivo micronucleus assay in mammalian bone marrow and peripheral blood. A report of the U.S. Environmental Protection Agency Gene-Tox Program. Mutat. Res. 239, 29–80. doi: 10.1016/0165-1110(90)90030-F
Miki, T., Hirai, H., and Takahashi, T. (2013). Activity-dependent neurotrophin signaling underlies developmental switch of Ca2+ channel subtypes mediating neurotransmitter release. J. Neurosci. 33, 18755–18763. doi: 10.1523/JNEUROSCI.3161-13.2013
Mishra, A. K., Mavlyutov, T., Singh, D. R., Biener, G., Yang, J., Oliver, J. A., et al. (2014). The sigma-1 receptors are present in monomeric and oligomeric forms in living cells in the presence and absence of ligands. Biochem. J. 466, 263–271. doi: 10.1042/BJ20141321
Momiyama, T., and Koga, E. (2001). Dopamine D2-like receptors selectively block N-type Ca2+ channels to reduce GABA release onto rat striatal cholinergic interneurones. J. Physiol. 533(Pt 2), 479–492. doi: 10.1111/j.1469-7793.2001.0479a.x
Pal, A., Chu, U. B., Ramachandran, S., Grawoig, D., Guo, L. W., Hajipour, A. R., et al. (2008). Juxtaposition of the steroid binding domain-like I and II regions constitutes a ligand binding site in the sigma-1 receptor. J. Biol. Chem. 283, 19646–19656. doi: 10.1074/jbc.M802192200
Pan, B., Guo, Y., Kwok, W. M., Hogan, Q., and Wu, H. E. (2014). Sigma-1 receptor antagonism restores injury-induced decrease of voltage-gated Ca2+ current in sensory neurons. J. Pharmacol. Exp. Ther. 350, 290–300. doi: 10.1124/jpet.114.214320
Quirion, R., Bowen, W. D., Itzhak, Y., Junien, J. L., Musacchio, J. M., Rothman, R. B., et al. (1992). A proposal for the classification of sigma binding sites. Trends. Pharmacol. Sci. 13, 85–86. doi: 10.1016/0165-6147(92)90030-A
Renaudo, A., L’Hoste, S., Guizouarn, H. F., and Soriani, O. (2007). Cancer cell cycle modulated by a functional coupling between sigma-1 receptors and Cl- channels. J. Biol. Chem. 282, 2259–2267. doi: 10.1074/jbc.M607915200
Romieu, P., Meunier, J., Garcia, D., Zozime, N., Martin-Fardon, R., Bowen, W. D., et al. (2004). The sigma 1 (σ 1) receptor activation is a key step for the reactivation of cocaine conditioned place preference by drug priming. Psychopharmacology 175, 154–162. doi: 10.1007/s00213-004-1814-x
Ruscher, K., Shamloo, M., Rickhag, M., Ladunga, I., Soriano, L., Gisselsson, L., et al. (2011). The sigma-1 receptor enhances brain plasticity and functional recovery after experimental stroke. Brain 134, 732–746. doi: 10.1093/brain/awq367
Sabeti, J., Nelson, T. E., Purdy, R. H., and Gruol, D. L. (2007). Steroid pregnenolone sulfate enhances NMDA-receptor independent long-term potentiation at hippocampal CA1 synapses: role for L-type Ca2+ channels and sigma-receptors. Hippocampus 17, 349–369. doi: 10.1002/hipo.20273
Schmidt, H. R., Zheng, S., Gurpinar, E., Koehl, A., Manglik, A., and Kruse, A. C. (2016). Crystal structure of the human s1 receptor. Nature 532, 527–530. doi: 10.1038/nature17391
Seth, P., Ganapathy, M. E., Conway, S. J., Bridges, C. D., Smith, S. B., Casellas, P., et al. (2001). Expression pattern of the type 1 sigma receptor in the brain and identity of critical anionic amino acid residues in the ligand-binding domain of the receptor. Biochim. Biophys. Acta 1540, 59–67. doi: 10.1016/S0167-4889(01)117-113
Seth, P., Leibach, F. H., and Ganapathy, V. (1998). Cloning and Structural analysis of the cDNA and the gene encoding the murine type 1 sigma receptor. Biochem. Biophys. Res. Commun. 241, 535–540. doi: 10.1006/bbrc.1997.7840
Su, T. P. (1982). Evidence for sigma opioid receptor: binding of [3H]SKF-10047 to etorphine-inaccessible sites in guinea-pig brain. J. Pharmacol. Exp. Ther. 223, 284–290.
Su, T. P., and Hayashi, T. (2003). Understanding the molecular mechanism of sigma-1 receptors: towards a hypothesis that sigma-1 receptors are intracellular amplifiers for signal transduction. Curr. Med. Chem. 10, 2073–2080. doi: 10.2174/0929867033456783
Su, T. P., Hayashi, T., Maurice, T., Buch, S., and Ruoho, A. E. (2010). The sigma-1 receptor chaperone as an inter-organelle signaling modulator. Trends Pharmacol. Sci. 31, 557–566. doi: 10.1016/j.tips.2010.08.007
Su, T. P., Su, T. C., Nakamura, Y., and Tsai, S. Y. (2016). The sigma-1 receptor as a pluripotent modulator in living systems. Trends Pharmacol. Sci. 37, 262–278. doi: 10.1016/j.tips.2016.01.003
Tchedre, K. T., Huang, R. Q., Dibas, A., Krishnamoorthy, R. R., Dillon, G. H., and Yorio, T. (2008). Sigma-1 receptor regulation of voltage-gated Ca2+ channels involves a direct interaction. Invest. Ophthalmol. Vis. Sci. 49, 4993–5002. doi: 10.1167/iovs.08-1867
Tsai, S. Y., Hayashi, T., Mori, T., and Su, T. P. (2009). Sigma-1 receptor chaperones and diseases. Cent. Nerv. Syst. Agents Med. Chem. 9, 184–189. doi: 10.2174/1871524910909030184
Vidaltorres, A., Fernándezpastor, B., Carceller, A., Vela, J. M., Merlos, M., and Zamanillo, D. (2014). Effects of the selective sigma-1 receptor antagonist S1RA on formalin-induced pain behavior and neurotransmitter release in the spinal cord in rats. J. Neurochem. 129, 484–494. doi: 10.1111/jnc.12648
Xia, Z., and Liu, Y. (2001). Reliable and global measurement of fluorescence resonance energy transfer using fluorescence microscopes. Biophys. J. 81, 2395–2402. doi: 10.1016/S0006-3495(01)75886-9
Xu, J., Zeng, C., Chu, W., Pan, F., Rothfuss, J. M., Zhang, F., et al. (2011). Identification of the PGRMC1 protein complex as the putative sigma-2 receptor binding site. Nat. Commun. 2:380. doi: 10.1038/ncomms1386
Yan, J. Q., Yuan, Y. H., Gao, Y. N., Huang, J. Y., Ma, K. L., Gao, Y., et al. (2014). Overexpression of human E46K mutant α-synuclein impairs macroautophagy via inactivation of JNK1-Bcl-2 pathway. Mol. Neurobiol. 50, 702–703. doi: 10.1007/s12035-014-8738-1
Yan, Z., and Surmeier, D. J. (1996). Muscarinic (m2/m4) receptors reduce N- and P-type Ca2+ currents in rat neostriatal cholinergic interneurons through a fast, membrane-delimited, G-protein pathway. J. Neurosci. 16, 2592–2604.
Zamanillo, D., Andreu, F., Ovalle, S., Pérez, M. P., Romero, G., Farré, A. J., et al. (2000). Up-regulation of sigma1 receptor mRNA in rat brain by a putative atypical antipsychotic and sigma receptor ligand. Neurosci. Lett. 282, 169–172. doi: 10.1016/S0304-3940(00)00884-3
Zhang, H., and Cuevas, J. (2002). Sigma receptors inhibit high-voltage-activated calcium channels in rat sympathetic and parasympathetic neurons. J. Neurophysiol. 87, 2867–2879.
Zhang, S., Yang, L., Zhang, K., Liu, X., Dai, W., Zhang, C., et al. (2015). ZC88, a novel N-type calcium channel blocker from 4-amino-piperidine derivatives state-dependent inhibits Cav2.2 calcium channels. Brain Res. 1605, 12–21. doi: 10.1016/j.brainres.2015.01.054
Keywords: sigma-1 receptor, N-type Ca2+ channel, electrophysiology, ion channels modulation, protein–protein interaction
Citation: Zhang K, Zhao Z, Lan L, Wei X, Wang L, Liu X, Yan H and Zheng J (2017) Sigma-1 Receptor Plays a Negative Modulation on N-type Calcium Channel. Front. Pharmacol. 8:302. doi: 10.3389/fphar.2017.00302
Received: 20 December 2016; Accepted: 10 May 2017;
Published: 26 May 2017.
Edited by:
Hanting Zhang, West Virginia University, United StatesReviewed by:
Víctor Fernández-Dueñas, University of Barcelona, SpainSuying Cui, University at Buffalo, United States
Copyright © 2017 Zhang, Zhao, Lan, Wei, Wang, Liu, Yan and Zheng. This is an open-access article distributed under the terms of the Creative Commons Attribution License (CC BY). The use, distribution or reproduction in other forums is permitted, provided the original author(s) or licensor are credited and that the original publication in this journal is cited, in accordance with accepted academic practice. No use, distribution or reproduction is permitted which does not comply with these terms.
*Correspondence: Haitao Yan, eWFuaHQ3ODA5QGFsaXl1bi5jb20= Jianquan Zheng, anF6aGVuZzIwMTJAMTYzLmNvbQ==