- 1Department of Neuroscience and Behavioral Sciences, Ribeirão Preto Medical School, University of São Paulo, Ribeirão Preto, Brazil
- 2National Institute for Science and Technology-Translational Medicine, National Council for Scientific and Technological Development (CNPq), Ribeirão Preto, Brazil
Much of our knowledge of the endocannabinoid system in schizophrenia comes from behavioral measures in rodents, like prepulse inhibition of the acoustic startle and open-field locomotion, which are commonly used along with neurochemical approaches or drug challenge designs. Such methods continue to map fundamental mechanisms of sensorimotor gating, hyperlocomotion, social interaction, and underlying monoaminergic, glutamatergic, and GABAergic disturbances. These strategies will require, however, a greater use of neurophysiological tools to better inform clinical research. In this sense, electrophysiology and viral vector-based circuit dissection, like optogenetics, can further elucidate how exogenous cannabinoids worsen (e.g., tetrahydrocannabinol, THC) or ameliorate (e.g., cannabidiol, CBD) schizophrenia symptoms, like hallucinations, delusions, and cognitive deficits. Also, recent studies point to a complex endocannabinoid-endovanilloid interplay, including the influence of anandamide (endogenous CB1 and TRPV1 agonist) on cognitive variables, such as aversive memory extinction. In fact, growing interest has been devoted to TRPV1 receptors as promising therapeutic targets. Here, these issues are reviewed with an emphasis on the neurophysiological evidence. First, we contextualize imaging and electrographic findings in humans. Then, we present a comprehensive review on rodent electrophysiology. Finally, we discuss how basic research will benefit from further combining psychopharmacological and neurophysiological tools.
Introduction
Heavy cannabis use may precipitate or exacerbate schizophrenia symptoms. The substantial psychiatric documentation on this matter has been reviewed multiple times in the past two decades (Manseau and Goff, 2015). Concomitantly, a literature has emerged on the neurobiology underlying cannabis psychosis, including genetics, pathology, physiology, and imaging approaches in humans (Batalla et al., 2014; Bossong et al., 2014), as well as neurochemistry and behavioral pharmacology approaches in other animals, primarily rodents (Zamberletti et al., 2012; Zuardi et al., 2012). This literature has also been regularly reviewed by authors like Esteban and García-Sevilla (2012), El Khoury et al. (2012), and López-Moreno et al. (2008). Their hypotheses continuously help us to make sense of the relationship between cannabis effects and schizophrenia-spectrum symptoms. As a result of this exploration, our understanding of the endogenous cannabinoid and most recently the vanilloid system has rapidly evolved, creating therapeutic development opportunities (Robson et al., 2014).
Several approaches are still underutilized in the study of endocannabinoid/endovanilloid systems in schizophrenia, a field in which animal experimentation is relatively new (Giuffrida and Seillier, 2012). For instance, while drug challenge designs allow investigating the cannabinoid receptor type 1 (CB1) involvement in psychotic-like symptoms (Roser et al., 2010), not much is known about behavior-related neural activity patterns (Skosnik et al., 2016). Moreover, circuit dissection through optogenetics and chemogenetics, which have been productive in the study of, for example, spatial learning and reward (Deisseroth, 2015; Whissell et al., 2016), has not yet impacted the research on endocannabinoid/endovanilloid systems in schizophrenia. Our review seeks to identify methodological areas that might contribute to this research topic in the future.
We initially give an overview of endogenous cannabinoid and vanilloid systems in their overlap with the neurobiology of schizophrenia. For that, selected reviews and research articles are cited (for biochemically detailed reviews on the endocannabinoid system, we suggest; Ligresti et al., 2016; Lu and Mackie, 2016). We then move on to the methodological landscape of human studies, with an emphasis on functional imaging and electroencephalography (EEG). A final emphasis is given to electrophysiology in rodents, which has been increasingly used in the study of cannabinoids/vanilloids in schizophrenia, especially over the past 5 years. Some research directions in rodents are also proposed.
Endocannabinoid and Endovanilloid Systems
The endocannabinoid (eCB) system comprises lipid neuromodulatory pathways regulating multiple functions of the mammalian brain, such as neural development and synaptic plasticity (Chevaleyre et al., 2006; Elphick, 2012; Maccarrone et al., 2014). In both humans and rodents, the eCB system plays a fundamental role in sensory, cognitive and emotional processes (Piomelli, 2003), a topic that has been boosted by Cannabis sativa research (Di Marzo, 2006).
In the late 1960s, Mechoulam and colleagues were the first to isolate and identify Δ9-tetrahydrocannabinol (THC), the main psychoactive constituent of cannabis, as well as compounds devoid of typical cannabis effects, like cannabidiol (CBD), cannabinol, and cannabigerol. All of these compounds are collectively referred to as phytocannabinoids (Mechoulam and Gaoni, 1967; Mechoulam, 1970; Hanuš et al., 2016). There are at least 113 phytocannabinoids, each with a distinct pharmacological property (Izzo et al., 2009; Aizpurua-Olaizola et al., 2016), and their discovery stimulated the development of synthetic analogs: the exocannabinoids, e.g., WIN 55,212-2 (Pacher et al., 2006; Breuer et al., 2016). Today, phytocannabinoids and exocannabinoids comprise the large group of cannabinoids (Pertwee, 2010).
Although cannabinoids were previously thought to act via nonspecific membrane-associated mechanisms, their pharmacological actions have been demonstrated to be highly stereospecific (Mechoulam et al., 1988; Mechoulam and Parker, 2013). The first substantial evidence of binding site specificity was the finding that cannabinoids modulate the adenylyl cyclase, which is important to transduce signals from G protein-coupled receptors (Howlett and Fleming, 1984). Cannabinoid receptor binding sites were finally identified in neurons by the late 1980s (Devane et al., 1988; Matsuda et al., 1990). Nowadays, cannabinoid receptors are known to integrate the eCB system, along with eCB ligands, and enzymes for synthesis and degradation of eCBs (Lu and Mackie, 2016).
Endocannabinoid actions are primarily mediated by cannabinoid receptors of the subtypes 1 (CB1) and 2 (CB2) (Pertwee, 2008). CB1 receptors are widely expressed in central neurons, but are also found on peripheral terminals and non-neural tissues such as the vascular endothelium (Herkenham et al., 1990; Munro et al., 1993; Kendall and Yudowski, 2017). In fact, CB1 receptors are the most abundant Gi/Go-coupled receptors in the mammalian brain (Howlett et al., 2002; Aizpurua-Olaizola et al., 2017). CB2 receptors, in turn, were initially associated with microglia and the immune system, but recent works indicate that they are also expressed on central neurons, although at lower levels than CB1 (Xi et al., 2011; Ramirez et al., 2012; Stempel et al., 2016; Zhang et al., 2016; Chen et al., 2017). CB2 receptors are nowadays suggested to play functional and protective roles in the brain, as their expression has been demonstrated to increase upon brain injury and inflammation (Miller and Devi, 2011; Pacher and Mechoulam, 2011; Callén et al., 2012).
CB1 receptors are found in excitatory and inhibitory synapses across mesocorticolimbic circuits, including the prefrontal cortex (PFC), hippocampus, basolateral nucleus of the amygdala (BLA), ventral tegmental area (VTA), ventral pallidum (VP), and nucleus accumbens (NAc) (Mackie, 2005; Hu and Mackie, 2015). CB1 receptors ultimately inhibit adenylyl cyclase activity, thereby reducing the conversion of adenosine triphosphate (ATP) into cyclic adenosine monophosphate (cAMP) (Demuth and Molleman, 2006), and therefore lowering the concentration of several intracellular messengers related to gene transcription and synaptic function (Childers and Deadwyler, 1996; Waltereit and Weller, 2003). CB1 receptors also exert rapid actions, including the inhibition of voltage-dependent Ca2+ channels (mainly N- and P/Q-type) and the activation of K+ channels (mainly A-type) (Mackie and Hille, 1992; Deadwyler et al., 1995; Twitchell et al., 1997). As a consequence, CB1 receptors promote the reduction of presynaptic vesicle exocytosis, thus modulating the release of neurotransmitters such as glutamate and GABA (Katona et al., 1999, 2006; El Khoury et al., 2012). Important outcomes of such modulation are two forms of eCB-mediated synaptic plasticity: short- and long-term depression (respectively, eCB-STD and eCB-LTD) (Kano, 2014), which have implications for the therapeutic use of cannabinoids (Kano et al., 2009; Castillo et al., 2012).
The endogenous activation of presynaptic CB1 receptors occurs via post-synaptically synthesized ligands that are retrogradely released into the synaptic cleft, i.e., the eCBs. These ligands are small molecules derived from arachidonic acid (a plasma membrane fatty acid; Rodríguez de Fonseca et al., 2005), and are primarily represented by 2-arachidonoyl-glycerol (2-AG) and arachidonoyl ethanolamide (anandamide) (Fride and Mechoulam, 1993; Hanuš et al., 2001; Pertwee et al., 2010). The synthesis of 2-AG can be triggered by three mechanisms (reviewed by Kano, 2014; Ohno-Shosaku and Kano, 2014). (1) Postsynaptic depolarization mediated by Ca2+ influx. (2) Postsynaptic depolarization or hyperpolarization mediated by several metabotropic receptors, including M1/M3 muscarinic acetylcholine, group 1 metabotropic glutamate receptors (mGluRs), and D2 dopamine receptors (Melis et al., 2004a,b). (3) Combination of weak postsynaptic elevation of Ca2+ with also weak activation of Gq/11-coupled receptors (Hashimotodani et al., 2005). Once in the synaptic cleft, 2-AG interacts with presynaptic CB1 receptors, ultimately inhibiting the Ca2+ influx and promoting eCB-STD (Wilson and Nicoll, 2001; Brown et al., 2003). 2-AG can also promote eCB-LTD through several mechanisms, including inhibition of adenylyl cyclase and the cAMP/PKA pathway via activation of postsynaptic mGluR and AMPA receptors (Chevaleyre et al., 2006; Heifets and Castillo, 2009). Anandamide, in turn, can be synthesized via postsynaptic activation of group 5 mGluR (mGluR5) and consequent release of Ca2+ from intracellular stores (Liu et al., 2008; Castillo et al., 2012). Once in the synaptic cleft, anandamide preferentially participates in eCB-LTD (Ohno-Shosaku and Kano, 2014), which involves the activation of transient receptor potential vanilloid 1 (TRPV1) channels (Liu et al., 2008; Castillo et al., 2012).
The activation of TRPV1 receptors, as well as the orphan G protein-coupled receptor 55 (GPR55), has been recently brought into attention (Ligresti et al., 2016; Lu and Mackie, 2016). In particular, TRPV1 receptors—which are activated by anandamide both pre- and postsynaptically (Zygmunt et al., 1999; Smart et al., 2000)—are non-selective cation channels with a preferential permeability for Ca2+. They can be activated by physical stimuli, including high temperatures (>43°C), voltage changes, low pH, as well as cannabinoid and vanilloid ligands (Naziroğlu and Demirdaş, 2015). TRPV1 receptors were initially described as targets of capsaicin, the spicy active principle of red pepper, and other vanilloids, but are also widely present in the brain, including the already mentioned PFC, hippocampus, BLA, VTA, VP, and NAc (Caterina et al., 1997; Mezey et al., 2000; Roberts et al., 2004; Immke and Gavva, 2006; Szallasi et al., 2007; Aguiar et al., 2014). A range of responses is attributed to neuronal TRPV1 receptors. In presynaptic terminals, TRPV1 can facilitate glutamate release onto dopaminergic substantia nigra neurons (Marinelli et al., 2003, 2007). In postsynaptic terminals, the same receptors participate in eCB-LTD, and the inhibition of 2-AG synthesis (Maccarrone et al., 2008; Chávez et al., 2010; Grueter et al., 2010; Puente et al., 2011). Interestingly, TRPV1 receptors are preferentially activated by high concentrations of anandamide, while in low concentrations anandamide predominantly acts on CB1 receptors (Moreira et al., 2012). Since anandamide binds promiscuously to either TRPV1 or CB1 receptors, this particular eCB is also referred to as an endovanilloid (Malek and Starowicz, 2016).
Mechanisms by which 2-AG and anandamide are removed from the synaptic cleft include transport facilitation through the plasma membrane, concomitantly to diffusion across the lipid bilayer (Hermann et al., 2006; Nicolussi and Gertsch, 2015). Subsequently, 2-AG would be degraded by monoacylglycerol lipase (MAGL) in the presynaptic terminal (Dinh et al., 2002), and anandamide by fatty acid amide hydrolase (FAAH) mostly in the postsynaptic terminal (Cravatt et al., 1996; Egertová et al., 2003). These enzymes are widely distributed in the brain, and are considered to be the ending point of eCB signaling (Piomelli, 2003). Over the past decades, the inhibition of these enzymes emerged as therapeutic option for treating neuropsychiatric disorders, including major depression and anxiety (Batista et al., 2014; Ogawa and Kunugi, 2015). In fact, inhibiting MAGL or FAAH can prolong the homeostatic actions of released eCBs, thereby minimizing side effects from exogenous activation of CB1/CB2 receptors (Petrosino and Di Marzo, 2010; Tuo et al., 2017).
Cannabinoid and vanilloid drugs have been proven valuable tools for the neuropharmacological exploration of the eCB system. The phytocannabinoids THC (partial CB1/CB2 agonist), and CBD are among these tools (Izzo et al., 2009; Ibeas Bih et al., 2015). CBD can be described as a multi-target drug, whose pharmacological interactions vary with concentration and site of action (Ronan et al., 2016). Although CBD actions are not fully understood (Ibeas Bih et al., 2015; Pisanti et al., 2017), CBD has been demonstrated to combine: low-affinity CB1 and CB2 receptor binding (Pertwee, 2008), serotonergic 5-HT1A receptor agonism (Russo et al., 2005), and mu- and delta-opioid receptor allosteric modulation (Kathmann et al., 2006), as well as TRPV1 receptor agonism and FAAH inhibition (Bisogno et al., 2001).
Cannabinoid receptors can also be modulated with higher specificity using exocannabinoids, including CP-55940 and WIN 55,212-2 (CB1/CB2 agonists), AM-251 and SR-141716A (Rimonabant, CB1 inverse agonists), and resiniferatoxin (TRPV1 antagonist). Lastly, eCB upregulation can be induced by metabolic inhibitors, like URB-597 (FAAH inhibitor), URB-602 (2-AG degradation blocker), and AM404 (anandamide reuptake inhibitor/TRPV1 agonist) (Melis et al., 2004b; Tzavara et al., 2006; Lafourcade et al., 2007; Xing and Li, 2007; Dissanayake et al., 2008; Hajós et al., 2008; Aguilar et al., 2014; Raver and Keller, 2014).
It is evident, therefore, that the eCB and endovanilloid systems have intricate physiological roles. In general terms, they homeostatically regulate synaptic function “on demand,” meaning that postsynaptic activity triggers the release of eCBs, which in turn exert complex pre- and post-synaptic actions (Alger and Kim, 2011). As discussed below, these systems go awry in psychiatric disorders, such as schizophrenia (Skosnik et al., 2016). The fact is, however, that eCB and (especially) endovanilloid involvements in schizophrenia are still far from understood, which is increasingly motivating neurophysiological experiments using the aforementioned pharmacological tools.
The eCB System in Schizophrenia: Overview from Human Studies
Schizophrenia is a complex and heterogeneous psychiatric disorder, with a lifetime prevalence of 1% of the population. Symptoms usually appear during the late adolescence, i.e., 18–25 years, and are classified as positive (hallucinations, delusions, disorganized speech and behavior), negative (depression, blunted affection, social withdrawal, anhedonia), and cognitive deficits, such as in working and verbal memory, executive functions, and attention (Morris et al., 2005; Mesholam-Gately et al., 2009). The classical neurochemical concept of schizophrenia is the dopamine hypothesis (Carlsson, 1988), which derives from the fact that typical antipsychotics, such as haloperidol and chlorpromazine, are dopaminergic antagonists (Kapur and Remington, 2001). According to this hypothesis, positive symptoms would arise from an excessive dopaminergic function, especially across the striatum, along with dopaminergic deficits in frontal cortices (Davis et al., 1991; Laruelle, 1998). However, dopaminergic dysfunction is insufficient to explain the non-psychotic symptoms of schizophrenia, which required alternative conceptual models of schizophrenia. In this context, evidence has accumulated about glutamatergic mechanisms in schizophrenia, supporting the role of N-methyl-D-aspartate (NMDA) receptor hypofunction (Coyle, 1996; Olney et al., 1999). Blocking NMDA receptors in healthy subjects with psychotomimetic agents, like phencyclidine (PCP) and ketamine, can induce positive and negative symptoms, as well as cognitive alterations. These drugs can also exacerbate psychotic symptoms in schizophrenic individuals (Luby et al., 1962; Javitt and Zukin, 1991; Krystal et al., 1994).
In addition to dopaminergic and glutamatergic roles, compelling evidences point to abnormalities of the eCB system in schizophrenia. Patients with schizophrenia manifest elevated eCB levels in the blood and cerebrospinal fluid (Giuffrida et al., 2004; Leweke et al., 2007; Koethe et al., 2009; Leweke, 2012), which are normalized with both antipsychotics and clinical remission (Giuffrida et al., 2004; Koethe et al., 2009). Moreover, schizophrenia patients with a history of cannabis use show decreased gray matter density in the posterior cingulate cortex, when compared with non-using individuals (Bangalore et al., 2008). Also, schizophrenia patients who use cannabis show cortical thinning in areas known for the high density of CB1 receptors, such as the anterior cingulate cortex, and the dorsolateral PFC (Rais et al., 2010). Postmortem studies, on the other hand, have been conflicting. In vitro autoradiography studies report increased CB1 receptor binding in schizophrenic patients (Zavitsanou et al., 2004; Newell et al., 2006; Dalton et al., 2011; Jenko et al., 2012), while immunodetection methods resulted in diminished or unchanged CB1 expression (Koethe et al., 2007; Eggan et al., 2010; Volk et al., 2014). Results from positron emission tomography imaging have also been contradictory. Ceccarini et al. (2013) have reported an increase in CB1 receptor binding throughout mesocorticolimbic areas in schizophrenia patients (NAc, insula, cingulate cortex, inferior frontal cortex, and parietal and mediotemporal lobes). In contrast, Ranganathan et al. (2016) have found lower availability of CB1 receptors in male schizophrenic subjects compared with controls. Gender differences may partially account for these inconsistencies, as women have been shown to be more susceptible to THC than men during memory tasks (Craft et al., 2013; Rubino and Parolaro, 2015). Apart from these gender inconsistencies, an important implication from CB1 binding is its negative correlation with the depressive symptomatology in schizophrenia patients. Wong et al. (2010) have found that lower incidence of negative symptoms corresponds to elevated CB1 receptor binding in the frontal cortex and globus pallidus. This, together with the study of Ceccarini et al. (2013), implies the corticostriatal and mesocorticolimbic circuitry in the balance between positive and negative symptoms.
In addition to the eCB involvement in schizophrenia, heavy cannabis use is a risk factor for developing the disorder (Large et al., 2011; Skosnik et al., 2014). Chronic cannabis use, especially during adolescence, is associated with lasting impairments in cognitive and perceptual functions (Skosnik et al., 2012, 2014). THC itself can acutely elicit psychoses in healthy individuals, and precipitate relapse in abstinent schizophrenia patients (D'Souza et al., 2004, 2005). This effect is associated with reduced activation in the temporal cortex and cerebellum, implying brain-wide alterations in cannabis psychosis (Atakan et al., 2013). In fact, THC-induced psychotic symptoms have been associated with altered activity of the parahippocampal gyrus and ventral striatum during a verbal learning task (Seal and Fletcher, 2009). Furthermore, Bhattacharyya et al. (2015a) have found, in a visual stimulation task, that response inhibition errors are correlated with THC-induced psychotic symptoms, and diminished frontal activation. In another study (Bossong et al., 2013), THC has been linked with impaired performance in an executive task, which in turn has been correlated with reduced deactivation in brain regions related to the default mode network. Overall, these studies suggest that phytocannabinoid-induced cognitive deficits, which resemble those of schizophrenia, involve brain-wide alterations (Bossong et al., 2013). Interestingly, THC and CBD have opposite effects on the activity of the hippocampus, medial PFC (mPFC), striatum, and superior temporal and occipital cortices, depending on the cognitive task. Thus, different patterns of brain activation could underlie the opposing actions of THC and CBD on schizophrenia-related circuits (Bhattacharyya et al., 2010, 2012).
Cannabis effects are hypothesized to interfere in the relationship between the eCB and mesocorticolimbic systems (Voruganti et al., 2001). Initial studies have reported increased dopaminergic drive in the striatum after THC administration (Voruganti et al., 2001; Bossong et al., 2009). Recent studies have challenged this hypothesis, demonstrating modest, if existent, changes in dopamine release under THC (Stokes et al., 2009; Bossong et al., 2015), and absent alterations in striatal dopamine availability in volunteers with a history of cannabis use (Stokes et al., 2012). However, Kuepper et al. (2013) have shown that while THC does not affect dopamine release in healthy subjects, it promotes dopamine release in patients with psychosis and their relatives, demonstrating higher THC sensitivity in individuals at risk for psychosis. Therefore, phytocannabinoid sensitivity seems correlated with the propensity for developing schizophrenia.
There is evidence that genes related to the pathophysiology of schizophrenia also participate in cannabinoid effects (Silveira et al., 2016). For example, the CUB and Sushi multiple domains-1 gene (CSMD1) has been associated with increased risk for both schizophrenia and cannabis dependence (Sherva et al., 2016). Polymorphisms in the catechol-O-methyltransferase gene (COMT)—an enzyme involved in dopamine metabolism and some forms of psychosis (Silveira et al., 2016)—are linked with cannabis dependence, as well as THC-induced impairments in working memory (Tunbridge et al., 2015) and executive functions (Verdejo-Garcia et al., 2007). COMT knockout mice also present with a behavioral sensitivity to cannabinoid effects (O'Tuathaigh et al., 2014). Moreover, cannabis use is linked to a variety of epigenetic alterations, including methylation of the COMT gene (Szutorisz and Hurd, 2016). Finally, CB1 receptor expression is increased in blood lymphocytes of schizophrenia patients with a history of cannabis abuse, in addition to being inversely correlated to methylation of the promoter of the CB1 receptor gene (Liu et al., 2014). Thus, genetic and epigenetic studies further support the association between cannabinoid actions and schizophrenia.
The eCB System in Schizophrenia: Specific Functional Alterations in Humans
Although structural and functional abnormalities of schizophrenia have been identified in patients, understanding the pathophysiological substrates of this spectrum of disorders remains a challenge in neuropsychiatry (Uhlhaas and Singer, 2015). There are still no reliable biomarkers for early diagnosis, and pharmacological developments have been modest since typical antipsychotics were discovered (Lieberman et al., 2005; Uhlhaas and Singer, 2015). Furthermore, while positive symptoms can be treated with traditional pharmacological approaches, negative symptoms and cognitive deficits are harder to treat (Harrison, 1999). In fact, schizophrenia is currently proposed to emerge from dysfunctional dynamics of the brain as a whole, instead of alterations in specific brain regions (Uhlhaas and Singer, 2015).
We now review the functional abnormalities related to both schizophrenia and the eCB system in further detail. Subsections are organized according to the methods used for measuring the human brain function.
fMRI
Coordination of brain dynamics and regional connectivity are fundamental for perceptual and cognitive processes. In humans, functional connectivity between brain regions can be inferred from the blood-oxygen-level-dependent (BOLD) signal using functional magnetic resonance imaging (fMRI). In turn, electrophysiological oscillations measured non-invasively by electroencephalography (EEG) or magnetoencephalography (MEG) can inform about phase connectivity between brain regions and relationships between frequency bands.
One of the main findings in schizophrenia is the disrupted connectivity between the hippocampus and the dorsolateral PFC (Weinberger et al., 1992; Heckers et al., 1998), which has been shown to be affected during working memory demand (Meyer-Lindenberg et al., 2005; Rasetti, 2011). Furthermore, reduced resting state connectivity between the hippocampus, posterior cingulate cortex, extrastriate cortex, mPFC, and parahippocampal gyrus has been described in schizophrenia patients (Zhou et al., 2008). Interestingly, decreased connectivity between the hippocampus and PFC has also been observed in healthy subjects at risk for developing schizophrenia (Benetti et al., 2009; Rasetti, 2011).
Alterations of functional connectivity are also present during cannabinoid activation. Lee et al. (2013) have demonstrated that THC reduces the connectivity between the amygdala and primary sensorimotor areas during experimentally induced cutaneous pain. In a salience-processing task, fronto-striatal, and mediotemporal-prefrontal connectivity have been shown to be reduced and enhanced by THC, respectively (Bhattacharyya et al., 2015b). In the same study, CBD has been reported to exert opposite connectivity effects. Taken together, these data demonstrate that connectivity patterns react in different manners depending on the cannabinoid agent, brain regions, and sensory/cognitive stimulation.
On the other hand, THC effects on emotional processing are controversial. For example, THC has been shown to increase amygdala-PFC functional coupling (Gorka et al., 2015a), while attenuating amygdala activation during presentation of emotionally negative images (Phan et al., 2005). Other studies have demonstrated that THC increases amygdala activation (Bhattacharyya et al., 2010) while having no impact on amygdala-PFC connectivity in subjects exposed to fearful faces (Fusar-Poli, 2009). THC has also been shown to increase amygdala activation, while reducing the functional coupling between the amygdala and dorsolateral PFC during cognitive reappraisal of emotionally negative pictures (Gorka et al., 2015b). Conversely, CBD has been associated with decreased anxiety and attenuated BOLD signal in the amygdala (Fusar-Poli, 2009). Although inconsistent, these findings indicate that the eCB system somehow modulates fronto-limbic substrates, and therefore the emotional processing (Gorka et al., 2015b).
Field Oscillations
Field oscillations are essential for coordinating the brain activity. Low-frequency oscillatory patterns are known to functionally connect distant regions, while high-frequency oscillations enable local network synchronization (Uhlhaas and Singer, 2010). These activity patterns have been related with a variety of cognitive processes such as working memory, attention and perception (Uhlhaas and Singer, 2010). Field oscillations in human studies are usually classified as induced, resting-state, steady-state, or evoked (Bertrand and Tallon-Baudry, 2000; Uhlhaas and Singer, 2010). Induced oscillations are observed during cognitive tasks, and can occur at different phase and latencies in relation to stimulus presentation (Skosnik et al., 2014). They are self-sustained rather than directly evoked by stimuli, and are associated with stimulus-triggered cognitive processes (Uhlhaas and Singer, 2006). Resting-state oscillations, on the other hand, are spontaneous task-unrelated patterns (Lang et al., 2014). They reflect the excitatory/inhibitory balance, as well as the connectivity between brain regions without behavior-related biases (Leuchter et al., 2012). Steady-state oscillations are produced by entraining the EEG activity to a particular frequency of sensory stimulation, allowing to test the ability of neural networks to engage in that frequency (O'Donnell et al., 2013). Finally, evoked oscillations are phase-locked to sensory stimuli, typically a few hundred milliseconds after each stimulus, thus allowing to probe sensory processes (Bertrand and Tallon-Baudry, 2000).
Induced and Resting-State Field Oscillations
Studies on induced oscillations have reported that cognition-related gamma-band oscillations (30–80 Hz) are reduced in schizophrenia patients (Haenschel et al., 2009; Minzenberg et al., 2010). These patients also present with reduced theta (3–7 Hz) and gamma activity in frontal regions during executive and working memory tasks (Schmiedt et al., 2005; Cho et al., 2006; Haenschel et al., 2009). Deficits in gamma oscillations (60–120 Hz) have also been observed during a perceptual organization task (Grützner et al., 2013). These results indicate that dysfunctions in local circuit-driven high-frequency oscillations may be involved in the cognitive deficits of schizophrenia. Concerning long-range synchronization, several studies have shown a decrease in phase synchrony in the beta and gamma frequency bands during visual perceptual organization, and auditory processing (Spencer et al., 2003; Symond et al., 2005; Uhlhaas et al., 2006). Ford et al. (2002) have observed reduced fronto-temporal coherence in the delta (1–3 Hz) and theta bands during speech. Resting-state recordings from schizophrenia patients also indicate a reduction in high-frequency activity (Rutter et al., 2009), an increase in low-frequency activity (Boutros et al., 2008), and a decrease in theta coherence (Koenig et al., 2001). Therefore, multiple oscillatory patterns, either induced or spontaneous, seem involved in the cognitive deficits of schizophrenia.
Exogenous cannabinoid effects on induced theta and gamma synchrony have also been described in human studies. For example, a reduction in induced gamma oscillations during a coherent motion task has been observed in chronic cannabis users (Skosnik et al., 2014). Another study has shown that acute THC increases low-gamma band oscillations (27–45 Hz) during resting state, while enhancing high-gamma power (85–130 Hz) during a motor task (Nottage et al., 2014). The authors suggest that this gamma over-activity may lead to neuronal noise, producing erroneous processing of the environmental information. Indeed, at psychosis-inducing doses, THC has been shown to increase neural noise in the EEG (Cortes-Briones et al., 2015a). This effect has also been correlated with psychosis-like symptoms induced by THC (Cortes-Briones et al., 2015a).
In a study by Morrison et al. (2011), the effects of intravenous THC on EEG power and coherence have been tested during a working memory test. Results show that THC impairs working memory performance, and precipitates positive and negative symptoms. The authors have also shown a reduction in theta power and coherence between bi-frontal EEG electrodes. Coherence reduction has been associated with positive psychotic symptoms, suggesting that the psychotic effects of THC can be partially due to impaired dynamics between the frontal lobes. Other studies have also found a decrease in both theta power and working memory performance after smoking marijuana (Ilan et al., 2004; Böcker et al., 2010). Furthermore, a specific polymorphism within the CB1 receptor gene has been associated with a reduction in theta power recorded from frontal, central, and parietal electrodes during resting state in humans (Heitland et al., 2014). Given the importance of theta and gamma oscillations for cognition, CB1-mediated deficits have been suggested to contribute to the pathophysiology of schizophrenia (Heitland et al., 2014).
Steady State and Evoked Field Oscillations
Additional evidence from abnormalities in gamma frequency in schizophrenia comes from studies using steady state or evoked oscillations. Studies on auditory steady-state responses have shown reduced 40 and 80 Hz power in both schizophrenia patients (Kwon et al., 1999; Tsuchimoto et al., 2011) and their relatives (Hong et al., 2004). In this same sense, Light et al. (2006) have observed reduced power and phase synchronization upon 30 and 40 Hz. Several studies on sensory stimulus-evoked oscillations have also demonstrated abnormalities in schizophrenic patients (Uhlhaas and Singer, 2010). In fact, decreased amplitude and phase locking in these oscillations have been observed during visual processing (Spencer et al., 2004, 2008). Auditory processing studies have in turn shown reduced amplitude and phase locking of evoked beta (15–30 Hz) and gamma frequencies (Hirano et al., 2008; Johannesen et al., 2008; Roach and Mathalon, 2008). These results suggest an impaired ability to coordinate oscillatory activity and sensory responsivity, which may underlie the perceptual and cognitive deficits of schizophrenia (Uhlhaas and Singer, 2010).
Reduction in evoked gamma synchrony is also evident under cannabinoid manipulation. In fact, presynaptic CB1 receptors throughout the hippocampus and neocortex inhibit GABA release from cholecystokinin (CCK)-containing interneurons (Bacci et al., 2004; Eggan and Lewis, 2007; Ali and Todorova, 2010). These interneurons are fundamental for generating gamma oscillations (30–80 Hz) (Buzsaki and Draguhn, 2004; Gonzalez-Burgos and Lewis, 2008; Uhlhaas and Singer, 2010). Using an auditory sensory gating paradigm, Edwards et al. (2009) have shown reduced evoked gamma power in heavy cannabis users. Also in chronic cannabis users, Skosnik et al. (2006, 2012) have observed a decrease in 40-Hz steady-state entrainment. Interestingly, the earlier the subject started using cannabis during adolescence, the weaker their 40-Hz steady-state entrainment (Skosnik et al., 2012). Also, acute THC administration in humans has been shown to reduce 40 Hz-peaked gamma oscillations after auditory steady-state responses (Cortes-Briones et al., 2015b). These studies indicate that exposure to cannabinoids modify the neocortical ability to undergo evoked, steady-state, and induced field oscillations, especially within the gamma range. Because decreased gamma band activity is also present in schizophrenia patients and their relatives, it is possible that these deficits are mediated by a disruption in eCB and GABA transmission (Skosnik et al., 2012).
Event-Related Field Responses
Event-related responses (ERP) are time-locked voltage deflections observed in the EEG upon sensory stimulation, e.g., a sequence of sound pulses (Korostenskaja and Kähkönen, 2009). Infrequent deviant stimuli among this sequence provoke changes in ERP, i.e., mismatch negativity (MMN) components, which are thought to reflect change detection and sensory memory (Onitsuka et al., 2013). Reduced MMN is a common feature in schizophrenia patients (Salisbury et al., 2007; Näätänen and Kähkönen, 2009), as well as their healthy first-degree relatives (Michie et al., 2002), and is therefore suggested as an endophenotype of schizophrenia vulnerability. THC administration also reduces MMN amplitude, while the use of a cannabis extract containing both THC and CBD enhances the MMN amplitude (Juckel et al., 2007). Acute subanesthetic ketamine, which is known to produce psychotic symptoms, does not reduce MMN by itself but does so when the CB1 inverse agonist rimonabant is co-administered. These findings suggest that exogenous CB1 agonism is implied in the cognitive impairments of schizophrenia, and that this disruption seems to involve both the eCB system and the glutamatergic neurotransmission (Roser et al., 2011).
Another ERP feature associated with change detection—for example during the oddball stimulation paradigm—is P300: a positive component peaking at ~300 ms post-stimulus latency (Onitsuka et al., 2013). P300 is thought to reflect working memory and attention (Polich, 2007). Alterations in auditory evoked P300 have been frequently reported in patients with schizophrenia (Bramon et al., 2004). Auditory P300 amplitude is negatively correlated with age in schizophrenia patients (Wang et al., 2003), and is also seen as a trait marker for schizophrenia, as P300 amplitude is reduced even when the patients are less symptomatic (Mathalon et al., 2000). THC administration in healthy subjects weakens the P300 response recorded from midline frontal, central, and parietal electrodes during a choice reaction task, indicating the involvement of the eCB system in attention and working memory (Roser et al., 2008). Weak P300 has also been shown in chronic cannabis users (Rentzsch et al., 2016) which however manifest increased P300 amplitude when exposed to unpleasant trait words, and decreased negative symptoms such as affective blunting (Skosnik et al., 2008). These findings suggest that the eCB system may be particularly relevant for the positive symptoms of schizophrenia.
Therefore, similar EEG observations arise from schizophrenic patients and cannabinoid effects, including a reduction in gamma band reactivity, reduction of theta coherence, and disruption of ERP components, suggesting common alterations in cognitive and perceptual processing.
Endovanilloid System in Schizophrenia: Indirect Electrophysiological Implications from Humans
The neurophysiological study of the endovanilloid system in mental disorders is still at an early stage. In one study (Mori et al., 2012), motor-evoked potentials induced by transcranial magnetic stimulation (TMS) were examined in patients with two TRPV1 genetic polymorphisms. Depending on the polymorphism, subjects presented with weaker or stronger motor-evoked potentials upon paired-pulse TMS. In addition, TRPV1 has been linked to pain perception and cognition deficits in schizophrenia (Madasu et al., 2015). Given that abnormal motor-evoked potentials and pain sensitivity are observed in schizophrenia patients (Pascual-Leone et al., 2002; Bonnot et al., 2009; Lakatos et al., 2013; Zhou et al., 2016), TRPV1 channels—and therefore the endovanilloid system—could be altered in schizophrenia, which deserves neurophysiological investigation.
Animal Models of Schizophrenia
Animal models allow neuronal circuits to be examined in more detail than in humans. In this sense, relatively modern techniques, such as large-scale electrophysiological recordings and optogenetics, have been increasingly used in animal models of schizophrenia (Sigurdsson, 2016). These animal models will be outlined below.
Behavioral Assessment
Reproducing the etiology of schizophrenia, or even its specific symptoms in non-human animals remains a challenge. However, it is still conceivable to use animal models that reproduce some of the disease “endophenotypes,” i.e., abnormalities consistently observed in schizophrenia patients, even though they do not constitute the core symptoms for diagnosis (Sigurdsson, 2016). For example, patients with schizophrenia show reduced prepulse inhibition of the startle reflex (PPI) (Braff et al., 1978), which is the ability to attenuate reflex responses (e.g., eye blinks evoked by intense sound pulses) when they are preceded by weak stimuli (Swerdlow and Geyer, 1998). PPI is associated with schizophrenia symptoms (Weinberger et al., 1992), particularly thought disorders and distractibility (Turetsky et al., 2007). In the rodent PPI procedure, sound-evoked startle responses (sudden movements detected by a load-cell platform) can be attenuated by a weak stimulus (i.e., prepulse), allowing the assessment of sensorimotor gating (Swerdlow and Geyer, 1998). This response is disrupted in genetic models of schizophrenia (Powell et al., 2009).
Assessing behavioral alterations that resemble positive and negative symptoms has been important to evaluate the effects of novel antipsychotics. Hyperlocomotion is frequently assessed in animal models of schizophrenia as it resembles positive symptoms such as psychotic agitation (Powell et al., 2009), and is associated with hyperdopaminergic states (van den Buuse, 2010). Hyperlocomotion can be measured by monitoring rodents while they roam in a novel space, like an open field. In turn, social interaction deficits represent negative symptoms, and can be tested by monitoring subjects while they interact with unfamiliar congeners (Sams-Dodd, 1995, 1996).
Schizophrenia patients also manifest a range of cognitive deficits, especially working memory impairments (Park and Holzman, 1992). Deficits in specific types of memory are identified as distinct schizophrenia symptoms, which in turn can be assessed in rodents using different tasks (Saperstein et al., 2006). Testing the novel object recognition (NOR) evaluates the ability to distinguish a new object from a familiar one (non-spatial learning), or the ability to remember when objects are moved (spatial learning), thus indirectly measuring memory. Associative learning, which is also deranged in schizophrenia (Rushe et al., 1999), can be tested through contextual fear conditioning, measuring the animal's capacity to associate non-aversive contexts with aversive stimuli (Fanselow, 1980). Other paradigms that assess spatial learning, like the Morris water maze, T-maze, and radial maze, are also commonly used in schizophrenia-oriented studies (Jentsch et al., 1997; Beraki et al., 2009; Enomoto and Floresco, 2009).
Induction Strategies
Experimental research has developed strategies to model different aspects of human schizophrenia, each of them reflecting genetic and environmental factors, as well as pathophysiological mechanisms related with the disease (Sigurdsson, 2016). A number of genetic risk factors have been identified in schizophrenia (Moran et al., 2016), and many of them have been reproduced in mouse models. Microdeletions in the region q11.2 of chromosome 22 and mutations in the Disrupted in Schizophrenia 1 (DISC1) gene, which are both related to the human schizophrenia (Clair et al., 1990; Jonas et al., 2014), are associated with schizophrenia-relevant abnormalities in mice, like reduced PPI (Paylor et al., 2001; Long et al., 2006; Stark et al., 2008), impaired fear conditioning (Paylor et al., 2001; Stark et al., 2008; Fenelon et al., 2013), working memory deficits (Koike et al., 2006; Kvajo et al., 2008; Stark et al., 2008; Sigurdsson et al., 2010; Juan et al., 2014) and depressive-like behaviors (Shen et al., 2008; Sauer et al., 2015).
Environmental factors can also favor schizophrenia. Epidemiological studies have demonstrated that viral infections during human pregnancy (e.g., influenza) put children at increased risk of developing the disorder (Canetta and Brown, 2012). Since these infections do not directly affect fetal development, the activation of the mother's immune system is believed to be a causal factor. Thus, maternal immune activation (MIA) through gestational viral-like infection has been frequently used as an animal model of schizophrenia, in which the offspring shows behavioral abnormalities, including deficits in PPI and latent inhibition (Shi et al., 2003; Dickerson and Bilkey, 2013).
A different approach is to directly model the pathophysiological mechanisms of schizophrenia. Acute pharmacological models are based on the dopaminergic and glutamatergic hypotheses of schizophrenia, and they include NMDA hypofunction (induced by NMDA receptor antagonists, such as ketamine, MK-801, and PCP), and dopaminergic activation (induced by psychostimulants, such as amphetamine and methamphetamine). In rodents, NMDA antagonists induce hyperlocomotion, PPI deficits, and decreased social interest, which can be reversed by antipsychotics (Kitaichi et al., 1994; Bakshi and Geyer, 1995; Sams-Dodd, 1995, 1996; Geyer et al., 2001). PCP, MK-801, and methamphetamine are also known to induce NOR deficits in mice (Karasawa et al., 2008; Mizoguchi et al., 2008; Vigano et al., 2009). In addition, rodents chronically treated with PCP display long-lasting impairments in associative learning, which can be reversed by olanzapine (Enomoto et al., 2005).
Evidences also indicate that schizophrenia is a neurodevelopmental disorder that may culminate in dysfunctional brain circuits in adulthood (Lewis and Levitt, 2002). Directly disturbing neural development during pregnancy or early life can generate adults that display schizophrenia-like abnormalities. This is what proposes the neonatal ventral hippocampal lesion (NVHL) model (Lipska et al., 2002; Tseng et al., 2009), in which the ventral hippocampus (vHipp) is lesioned by ibotenic acid at postnatal day 7. NVHL-lesioned animals present with a number of behavioral abnormalities, like hypersensitivity to psychostimulants, reduced PPI, reduced latent inhibition, and deficits in social interaction, spatial learning, working memory, attention set-shifting, and reversal learning (Tseng and O'Donnell, 2007; O'Donnell, 2012). Abnormally behaving adults can also be generated by injecting methylazoxymethanol acetate (MAM, a mitotoxin) in pregnant rats during gestational day 17. Once in adulthood, the MAM-exposed offspring shows reduced PPI and latent inhibition, hypersensitivity to psychostimulants, and working memory deficits (Lodge et al., 2009).
Electrophysiological Measurements
Based on the outline above, we can now mention representative electrophysiological findings from animal models of schizophrenia. This will contextualize the following section, which reviews electrophysiological findings on the eCB and endovanilloid systems in schizophrenia (see Sigurdsson, 2016 for an extensive review).
Synaptic Plasticity
Synaptic plasticity is increasingly implicated in the pathological alterations of schizophrenia (Crabtree and Gogos, 2014). Synaptic plasticity data from genetic models of schizophrenia are primarily from in vitro experiments. The 22q11.2 mouse model present with impaired long-term potentiation (LTP) and increased short-term depression in the mPFC (Fenelon et al., 2013). These mice display schizophrenia-relevant alterations in sensorimotor gating, fear conditioning, and working memory (Sigurdsson, 2016). Also, hippocampal CA3-CA1 synaptic plasticity, but not basal synaptic transmission, is altered in 22q11.2 mice (Earls et al., 2010; Drew et al., 2011). Alterations on hippocampal synaptic plasticity have also been observed in the DISC1 mouse model of genetic risk for schizophrenia, including abnormal LTP in CA3-CA1 synapses (Kvajo et al., 2008; Booth et al., 2014), and reduced short-term plasticity in the DG-CA3 pathway (Kvajo et al., 2011). Thus, short and long-term forms of synaptic plasticity are differentially impaired in genetic models of schizophrenia. Of note, synaptic plasticity—which is generally associated with sensory/cognitive processes and memory consolidation—is hypothesized to participate in the connectivity abnormalities of the disease (Sigurdsson, 2016), as further outlined below.
Local Synchrony
Abnormalities in beta and gamma oscillations are described both in schizophrenia patients and animal models. Increased gamma power during the awake state (Del Pino et al., 2013) and reduced evoked gamma oscillations (Barz et al., 2016) have been reported in genetic models. Also, a sub-anesthetic dose of ketamine strengthens gamma power both in awake and anesthetized rodents (Ma and Leung, 2000; Pinault, 2008; Ehrlichman et al., 2009; Hakami et al., 2009; Lazarewicz et al., 2009; Kulikova et al., 2012), while stimulus-evoked gamma oscillations are reduced after ketamine injection (Lazarewicz et al., 2009; Kulikova et al., 2012). Likewise, evoked (but not spontaneous) beta/gamma oscillations in vHipp and mPFC are reduced in the gestational MAM model (Lodge et al., 2009). Evidences indicate that interneurons expressing the calcium-binding protein parvalbumin (PV) are related to gamma oscillation abnormalities in schizophrenia models. Particularly in DISC1 mice, reduced theta and gamma power have been observed during the awake state, concomitantly with a loss of PV interneurons (Sauer et al., 2015). Mice lacking NMDA receptors on interneurons (including PV-expressing ones) also show a higher propensity for gamma potentiation in the hippocampus, somatosensory cortex (SCx), and auditory cortex (Korotkova et al., 2010; Carlén et al., 2011; Nakao and Nakazawa, 2014).
Long-Range Synchrony
Long-range synchrony deficits are observed in a variety of animal models of schizophrenia. In DISC1 mice, an impaired hippocampal-mPFC coordination has been observed after MIA (Hartung et al., 2016). Other genetic risk models also manifest impaired synchrony in the hippocampus-mPFC (Del Pino et al., 2013) and hippocampus-NAc pathways (Nason et al., 2011). Hippocampal-mPFC synchrony is also impaired in the MIA model, including lower LFP coherence between these regions, and reduced phase locking of prefrontal neurons to hippocampal theta oscillations (Dickerson et al., 2010). Interestingly, the antipsychotic clozapine has been shown to enhance theta coherence between the two regions (Dickerson et al., 2012). Moreover, hippocampal-mPFC synchrony is impaired in NVHL animals (Lee et al., 2014), and both hippocampal-mPFC high-frequency synchrony and spike cross-correlation are diminished in MAM-exposed rats (Phillips et al., 2012).
In addition, through recording from the hippocampus and mPFC of 22q11.2 mice, Sigurdsson et al. (2010) have found reduced phase locking between mPFC neural activity and hippocampal theta oscillations during a spatial working memory task. However, hippocampal and mPFC local field potentials (LFP) were intact. This suggests that, in 22q11.2 mice, the ability to synchronize between remote regions is affected, whereas the capacity to generate local synchrony is not. The authors suggest that long-range synchrony impairments could reflect long-term plasticity dysfunctions in mPFC afferents (Sigurdsson, 2016), which is in agreement with the prefrontal LTP deficits observed in the 22q11.2 model (Fenelon et al., 2013).
Single Unit Activity
Excitatory/inhibitory balance is critical for neuronal ensemble function. Convergent lines of evidence indicate a reduction of inhibitory function in schizophrenia patients and animal models (Lodge et al., 2009; Lewis et al., 2012; Sauer et al., 2015), which could reflect increased firing and therefore impaired neuronal processing. Indeed, lower signal-to-noise ratio in the SCx is observed in genetic mouse models of schizophrenia, in addition to increased baseline firing, and disrupted firing responses to sensory stimulation (Barz et al., 2016). An increase in PFC firing rate is observed in the MIA model (Dickerson et al., 2010), and under subanesthetic ketamine (Jackson et al., 2004; Wood et al., 2012). It has also been demonstrated that pyramidal cells increase, while interneurons decrease their firing rates, suggesting that NMDA hypofunction in interneurons can be responsible for the behavioral and neural activity observations in these models (Homayoun and Moghaddam, 2007). A similar effect has been observed in the SCx of mice lacking NMDA receptors (Carlén et al., 2011), although reduced pyramidal firing has been reported in the hippocampus (Korotkova et al., 2010).
Finally, disruptions in the excitatory/inhibitory balance can derive from alterations in neuromodulatory systems (Sigurdsson, 2016). NVHL alters the response of mPFC pyramidal neurons to VTA stimulation: in control animals, VTA stimulation transiently inhibits the mPFC through feedforward interneuronal processing (Tseng et al., 2006), while the opposite is observed in vHipp-lesioned animals (O'Donnell et al., 2002). A similar effect is observed in the MAM model (Goto and Grace, 2006), in which mPFC responses to reward-predictive stimuli are enhanced (Gruber et al., 2010). A higher number of spontaneously active VTA dopamine neurons is also observed in the MAM model (Lodge and Grace, 2007; Gomes et al., 2015), which seems to be associated with stronger vHipp influence (Lodge and Grace, 2007), and altered interneuronal activity (Perez and Logde, 2013). It is possible, therefore, that increased firing in these animal models could disturb sensory encoding, ultimately affecting cognitive performance.
The eCB System in Schizophrenia: Electrophysiological Findings from Rodents
In this section (see Figure 1 for a graphical summary), we first review in vivo single-neuron recordings in anesthetized or chronically implanted rodents, either accompanied or not by behavioral testing. Secondly, we move on to in vivo field potential recordings, either after repeated drug administration, or during acute drug effects. Then, we review in vitro studies, which primarily include synaptic transmission and plasticity experiments. Finally, we map the available electrophysiological evidence to speculate on research trends in the following section.
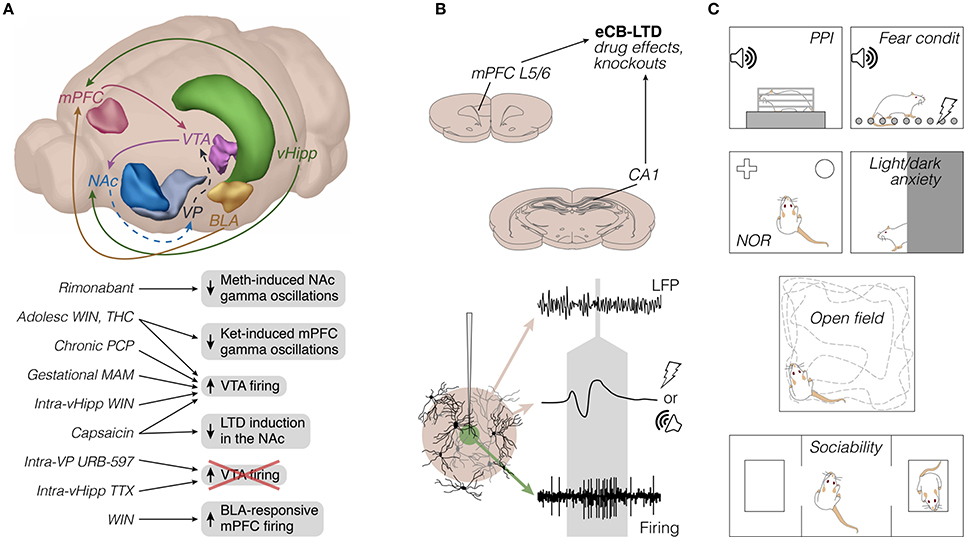
Figure 1. Rodent electrophysiology literature on cannabinoids and vanilloids in schizophrenia-relevant circuits: emphasis on methods. (A) Top: Frequently studied brain sites and axonal pathways. To our knowledge, projections like VTA-mPFC, mPFC-NAc, and mPFC-BLA have not yet been directly examined, and are therefore omitted for simplicity. Dashed lines represent GABAergic pathways. Only the left hemisphere is represented (brain sites adapted from the Brain Explorer, Allen Institute). Bottom: Main electrophysiological findings, mostly from in vivo experiments (see also Figures 2, 3). (B) Top: representative brain sites and manipulations of in vitro studies (coronal sections adapted from Paxinos and Watson, 2007; see also Figure 3). Bottom: illustrative recording probe, e.g., glass or steel microelectrode, from which LFP (beige area) and single-unit firing (green area) can be recorded upon adequate filtering, amplification, and digitization. The middle voltage trace represents a field potential response to afferent electrical or auditory stimulation, both of which present in the reviewed literature. The gray area roughly indicates the timescale between types of signal. (C) Prevalent behavioral tests in the reviewed literature, most of them performed separately from electrophysiological experiments. Adolesc, adolescent; BLA, basolateral amygdala; condit, conditioning; eCB-LTD, endocannabinoid long-term depression; Ket, ketamine; L5/6, layers 5/6; LFP, local field potentials; MAM, methylazoxymethanol acetate; Meth, methamphetamine; mPFC, medial prefrontal cortex; NAc, nucleus accumbens; NOR, novel object recognition; PCP, phencyclidine; PPI, prepulse inhibition of the acoustic startle; THC, delta-9-tetrahydrocannabinol; TTX, tetrodotoxin; vHipp, ventral hippocampus; VP, ventral pallidum; VTA, ventral tegmental area; WIN, WIN 55,212-2.
Unit Activity In vivo
Melis et al. (2004a) and Laviolette and Grace (2006) are among the initial electrophysiological studies assessing the cannabinoid transmission in schizophrenia-relevant substrates: mPFC, VTA, and BLA. Using urethane-anesthetized rats, Melis et al. (2004a) have shown that intravenous SR-141716A (SRA, CB1 inverse agonist) dose-dependently potentiates monosynaptic spiking responses of VTA dopamine cells to mPFC electrical stimulation. The opposite was observed under WIN 55,212-2 (or simply WIN: CB1/CB2 receptor agonist), implying the eCB participation in the top-down control of dopamine signaling. Using chloral hydrate-anesthetized rats, Laviolette and Grace (2006) have identified mPFC neurons responsive to both BLA orthodromic electrical stimulation and footshock-paired odors. Specifically, in these neurons, the authors have found that intravenous WIN before conditioning increases the frequency of odor-elicited spikes, which is suppressed by AM-251 (CB1 inverse agonist). Therefore, each axonal pathway, BLA-mPFC or mPFC-VTA, react differently to CB1 agonism, which seems associated with Pavlovian fear conditioning (Figure 2A).
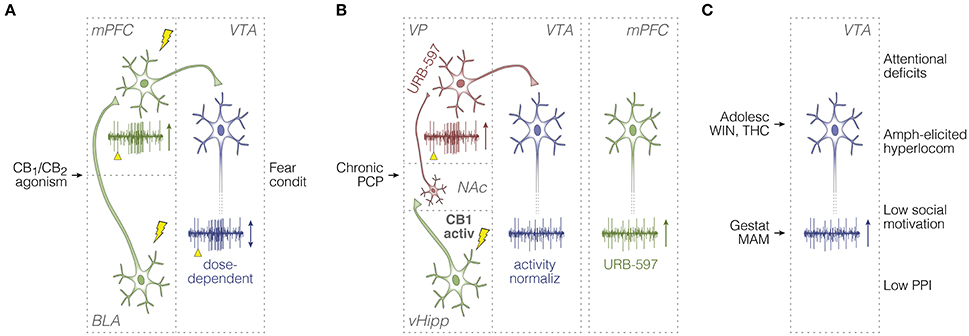
Figure 2. Summary of in vivo unit activity studies in rodents. (A) Afferent stimulation experiments on BLA-responsive mPFC cells (Laviolette and Grace, 2006), and the top-down control of dopamine signaling (Draycott et al., 2014; Melis et al., 2004a). Electrical pulses (lightning icons) and their timestamps (yellow arrowheads) are illustrated along with recording sweeps, and overall effects of cannabinoid manipulations on unit activity responses (vertical arrows). Green and blue neurons are glutamatergic and dopaminergic, respectively. (B) Studies on: (1) CB1 receptor activation in vHipp (Loureiro et al., 2015, 2016); (2) downstream consequences of vHipp hyperactivity (i.e., abnormal NAc-VP-VTA disinhibition) induced by the chronic PCP model of schizophrenia, and ameliorating effects of anandamide upregulation through FAAH inhibition (URB-597) (Aguilar et al., 2014); and (3) URB-597 effects on mPFC firing in PCP-treated rats (Aguilar et al., 2016). Red neurons are GABAergic. (C) Studies on behavioral phenotypes and VTA spontaneous activity, either after pubertal cannabinoid exposure, or in the gestational MAM model (Gomes et al., 2015; Renard et al., 2016). Activ, activation; Adolesc, adolescent; amph, amphetamine; condit, conditioning; BLA, basolateral amygdala; gestat, gestational; hyperlocom, hyperlocomotion; MAM, methylazoxymethanol acetate; mPFC, medial prefrontal cortex; NAc, nucleus accumbens; normaliz, normalization; PCP, phencyclidine; PPI, prepulse inhibition of the acoustic startle; THC, delta-9-tetrahydrocannabinol; vHipp, ventral hippocampus; VP, ventral pallidum; VTA, ventral tegmental area; WIN, WIN 55,212-2.
More recently, Draycott et al. (2014) have brought about the mPFC-VTA projections in further detail. In urethane-anesthetized rats, they have shown that intra-mPFC injection of WIN modulates the spontaneous activity of VTA dopamine cells, but in a biphasic dose-dependent manner: a lower dose of WIN increased the firing rate and the incidence of bursts, while a ten-fold higher dose inhibited both patterns. Using a separate cohort of chronically cannulated rats, Draycott et al. (2014) have observed a similar dose-dependent effect on fear conditioning: lower, but not higher, intra-mPFC dose of WIN, during odor-footshock pairing, promoted freezing responses during the test session. In addition, co-administration of WIN and a dopamine receptor antagonist (cis-α-flupenthixol, or simply α-flu) into the mPFC blocked this behavioral effect, which could be restored by GABA receptor antagonists into the VTA. These findings suggest that the degree of CB1 receptor activation—and possibly the endogenous fluctuation in eCB transmission—can exert different effects on the mPFC-VTA loop, feedforward interneuronal processing within the VTA, and related behaviors (Figure 2A).
Using the sub-chronic PCP model, Aguilar et al. (2014) have provided a more direct link between VTA dopamine neuron activity and schizophrenia. Using chloral hydrate-anesthetized rats, Aguilar et al. (2014) have shown that PCP-induced VTA hyperactivity could be normalized by up-regulating anandamide through URB-597 (FAAH inhibitor) into the VP. Moreover, the authors have demonstrated that vHipp electrical stimulation evokes an inhibitory spiking response in VP (<60 ms latency), which is converted to post-stimulus excitation upon systemic URB-597. Also, reduction of PCP-induced aberrant activity in the VTA could be achieved through tetrodotoxin inactivation of the vHipp. Because increased VTA activity in the PCP model might partially derive from downstream effects of higher vHipp influence (i.e., abnormal disinhibition from the NAc-VP system), augmenting the cannabinoid drive onto VP GABAergic neurons could be a therapeutic strategy against vHipp-related hyperdopaminergia, and therefore schizophrenia (Lodge and Grace, 2007; Aguilar et al., 2014). These results are consistent with Loureiro et al. (2015, 2016), according to which vHipp CB1 agonism during urethane anesthesia increases the average neural activity in VTA and NAc shell. Thus, both the PCP model and intra-hippocampal CB1 receptor activation have been shown to disarrange the NAc-VP-VTA processing, which seems to be treatable with anandamide up-regulation in the VP (Figure 2B).
These brain site-specific evidences are consistent with systemic observations. In fact, a relationship is known between cerebrospinal fluid levels of anandamide and the severity of schizophrenia symptoms (Giuffrida et al., 2004; Leweke et al., 2007; Koethe et al., 2009; Morgan et al., 2013; Aguilar et al., 2016). This relationship reinforces how elusive are the actions of anandamide and exogenous cannabinoids in either protecting against schizophrenia symptoms, or exacerbating them. Disparate effects of anandamide up-regulation and THC have indeed been demonstrated in the mPFC of non-anesthetized animals using the PCP model (Aguilar et al., 2016). According to the authors, systemic URB-597 potentiates the mPFC firing rate in PCP-treated rats, but not their controls, whereas systemic THC reduces the mPFC firing rate in control rats, but not PCP-treated ones. A possible interpretation resides in the fact that URB-597 interacts with an enzyme (FAAH), while THC binds to receptors (CB1). Differently from the direct THC actions on CB1, the indirect influence of URB-597 on these receptors would be contingent upon the FAAH dynamics. This would balance the anandamide up-regulation, making it more similar to endogenous increases in anandamide transmission. Such possibility would explain the symptom-relieving outcomes of anandamide up-regulation, manifested as prefrontal net excitation, and VTA activity normalization in the PCP model (Aguilar et al., 2014, 2016; Figure 2B).
Besides anandamide up-regulation, exogenous cannabinoid agonism per se can affect schizophrenia-like symptoms in complex manners, depending on the experimental design. Repeated administration of WIN throughout rat puberty has been reported to potentiate attentional set-shifting deficits, amphetamine-elicited hyperlocomotion, and the number of spontaneously active dopaminergic neurons in VTA, as recorded during chloral hydrate anesthesia in adults (Gomes et al., 2015). The authors have observed the same in the MAM developmental disruption model, implying that both gestational MAM and pubertal WIN end up promoting schizophrenia-like signs in adulthood. However, pubertal WIN treatment was not able to exacerbate MAM-induced alterations in attentional set shifting or VTA neural activity; actually, WIN attenuated the amphetamine-elicited hyperlocomotion in MAM-exposed rats (Gomes et al., 2015; Figure 2C). As discussed by the authors, chronic administration of exogenous cannabinoid agonists during puberty could trigger plastic mechanisms in hyperlocomotion-related structures, especially NAc, which could compensate for schizophrenia-relevant upstream abnormalities in the ventral hippocampal formation and VTA. These findings provide a neurophysiological-behavioral link between chronic cannabinoid exposure during adolescence and cannabinoid-unrelated propensity for developing schizophrenia, with implications for the hypothesis of cannabis self-medication (Sherif et al., 2016). Most importantly, however, these findings underscore that the intermingled relationship between the eCB system and schizophrenia requires multidisciplinary exploration. In this sense, chronic treatment with WIN during adolescence has been shown to cause gene transcription alterations that are potentially related with memory impairments in adulthood (Tomas-Roig et al., 2016). Furthermore, histone acetylation—related to neural development—is known to be altered in the hippocampus in the MAM model, and such alteration can be reverted by AM-251 (Večeřa et al., 2017). Therefore, epigenetic processes may contribute to the developmental disruptions from chronic cannabinoid exposure.
Laviolette and colleagues have recently linked a variety of schizophrenia-like behavioral phenotypes with THC-induced dopaminergic hyperactivity, and mPFC molecular alterations (Renard et al., 2016). Specifically, chronic injections of THC in adolescent, but not in adult, rats were associated with lower social motivation, lower basal locomotion (i.e., without hyperlocomotion-inducing drugs, like amphetamine), higher light/dark box anxiety, and lower PPI of the acoustic startle. After behavioral tests, single-unit recordings under urethane anesthesia replicated the VTA hyperactivity of the PCP and MAM models, but only in rats treated with THC during adolescence (Figure 2C). In addition, western blotting from mPFC micro-punches revealed diminished levels of mTOR-related synaptic proteins (e.g., GSK-3, β-catenin, AKT) in rats treated with THC during adolescence, but not adulthood. Actually, many of these synaptic markers were increased by adult THC exposure (Renard et al., 2016). The authors discuss the opposing molecular results between adolescent and adult THC treatments in terms of synaptic plasticity, neuropsychiatric disorders, and dopamine transmission. They speculate that adult, but not adolescent, prefrontal cells would be more able to adapt their molecular machinery in response to THC-induced alterations in the dopaminergic drive (Renard et al., 2016).
Field Potentials In vivo
Before Renard et al. (2016) the mPFC participation in adolescent exposure to exocannabinoids had been investigated in two field electrophysiology reports (Raver et al., 2013; Cass et al., 2014). In the Cass et al. (2014) study, adult rats repeatedly treated with systemic WIN (or vehicle) during early adolescence (P35-40) were chloral hydrate-anesthetized for implantation of a stimulating electrode into vHipp and a recording electrode into mPFC. Different trains of pulses (10, 20, or 40 Hz) were then delivered into vHipp while recording voltage deflections from prefrontal LFP. Through analyzing stimulation-disrupted LFP epochs, the authors claim that adolescent WIN treatment facilitates LFP responses to 20-Hz trains, while attenuating LFP inhibition triggered by 40-Hz trains (Cass et al., 2014; Figure 3A). In the same work, three subsequent experiments were performed: early adolescent co-treatment with WIN and AM-251, WIN treatment during late adolescence (postnatal days 50–55), and intra-mPFC microinfusion of indiplon (a GABA-A positive allosteric modulator) before recording from early adolescence-treated rats. All manipulations reproduced the results from vehicle-treated rats of the first experiment. These converging results point to early adolescence as the actual window of vulnerability to exocannabinoids, during which the maturation of mPFC local GABAergic transmission would be sensitive to exogenous disturbances.
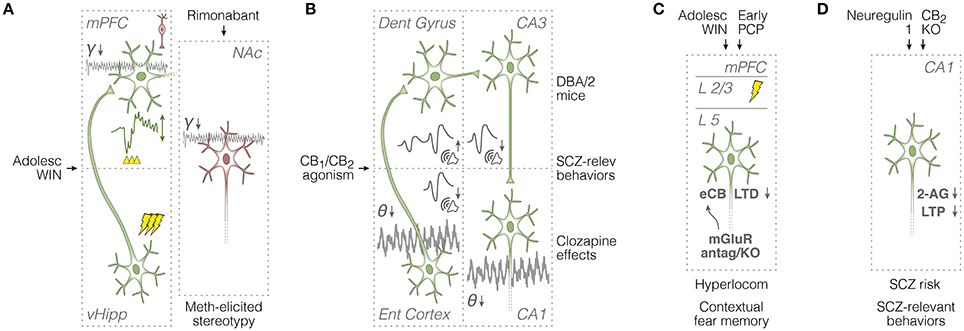
Figure 3. Summary of in vivo field potential and in vitro synaptic plasticity studies in rodents. (A) Left: evaluation of mPFC responses (see voltage deflection) to vHipp train stimulation (see lightning icons), and the mPFC capacity to engage in ketamine-potentiated gamma oscillations (see spontaneous field potentials) after adolescent WIN treatment (Raver et al., 2013; Cass et al., 2014). Right: attenuating effects of rimonabant on methamphetamine-potentiated stereotypy and accumbal gamma oscillations (Morra et al., 2012). Green and red neurons are glutamatergic and GABAergic, respectively. (B) Overall effects of cannabinoid agonists on spontaneous theta oscillations and auditory evoked potentials across entorhinal cortical and hippocampal circuits (Dissanayake et al., 2008; Hajós et al., 2008), and relationships with schizophrenia-relevant mouse models and manipulations (Smucny et al., 2014). (C) In vitro assessment of mPFC synaptic plasticity and glutamatergic neurotransmission after adolescent WIN or early-life PCP exposure: association with schizophrenia-like symptoms (Lafourcade et al., 2007; Jew et al., 2013; Lovelace et al., 2014, 2015). (D) In vitro assessment of CA1 synaptic plasticity and eCB neurotransmission: relationships with schizophrenia risk factors and cannabinoid receptor activation (Du et al., 2013; Kim and Li, 2015; Li and Kim, 2016). 2-AG, 2-arachidonoyl-glycerol; adolesc, adolescent; antag, antagonism; dent, dentate; eCB-LTD, endocannabinoid long-term depression; ent, entorhinal; γ, gamma oscillations; hyperlocom, hyperlocomotion; KO, knockout; L, layer; LTP, long-term potentiation; meth, methamphetamine; mGluR, metabotropic glutamate receptors; mPFC, medial prefrontal cortex; NAc, nucleus accumbens; PCP, phencyclidine; relev, relevant; SCZ, schizophrenia; θ, theta oscillations; vHipp, ventral hippocampus; WIN, WIN 55,212-2.
Prefrontal GABAergic interneurons are considered to be critical for entraining pyramidal neuron activity into cognition-relevant gamma oscillations (Bartos et al., 2007), which can be transiently potentiated by a sub-anesthetic dose of ketamine (Kocsis et al., 2013). This brings us to the in vivo electrocorticogram experiment of Raver et al. (2013). Using adult mice treated with WIN (or vehicle) during adolescence, the authors have found that psychotic-like effects of ketamine on frontal gamma oscillations are much weaker in WIN-exposed mice. Indeed, gamma synchrony is known to be impaired in regular cannabis users, and exogenous cannabinoid agonists are known to reduce the firing precision of fast-spiking interneurons (Skosnik et al., 2016). A combined scenario from Raver et al. (2013) and Cass et al. (2014) is that chronic exogenous CB1 agonism during early adolescence alters both the vHipp-mPFC communication, and the mPFC capacity to engage in interneuron-dependent fast oscillations (Figure 3A). Intriguingly, Morra et al. (2012) had previously shown, in adult rats, that a single intravenous dose of rimonabant (CB1 inverse agonist) reduces both methamphetamine-induced stereotypy, and potentiation of NAc gamma activity, particularly its fast 60-100 Hz band. Since Morra et al. (2012) have also been able to associate accumbal fast-spiking interneurons, but not medium spiny neurons, with methamphetamine effects on LFP, they provide evidence for exogenous CB1 antagonism as a suppressor of NAc gamma oscillations (Figure 3A).
Methodological distinctions between Morra et al. (2012) and Raver et al. (2013) including the recording site (NAc or mPFC) and the cannabinoid treatment (chronic adolescent regime, or single adult dose), probably account for the apparent contradictions on CB1 agonism and antagonism. Apart from discussing this issue based on the non-electrophysiological literature, one important conclusion is that mesocorticolimbic activity patterns in schizophrenia and cannabinoid modulation are just emerging, especially over the past five years. Worthy of mention are three other reports with a less explicit relationship with the mesocorticolimbic system (Dissanayake et al., 2008; Hajós et al., 2008; Smucny et al., 2014). Hajós et al. (2008) have shown that CB1/CB2 agonism (CP-55940) during chloral hydrate anesthesia reduces LFP theta power from entorhinal cortex and hippocampal CA1, weakens theta activity of medial septal neurons without altering their average firing rate, and reduces the amplitude of auditory evoked potentials from entorhinal cortex and CA3 (Figure 3B). The authors have also demonstrated that CP-55940 attenuates gamma band oscillations in the entorhinal cortex while enhancing them in CA3, and all these effects could be reverted by AM-251 (Hajós et al., 2008). These results indicate that sensory gating disruption by exogenous cannabinoid activation is partially due to a functional disorganization of the septo-hippocampal system.
In turn, Dissanayake et al. (2008) have examined systemic WIN effects on paired auditory responses from the dentate gyrus, CA3, and mPFC during isoflurane anesthesia. Results show that WIN potentiates the amplitude ratio between responses to test and conditioning stimuli in all three sites. Paired auditory responses from CA3 under anesthesia (chloral hydrate) have also been investigated by Smucny et al. (2014). Using DBA/2 mice, which are known to display schizophrenia-like symptoms (Singer et al., 2009), Smucny et al. (2014) have found that auditory gating improvement by systemic clozapine (atypical antipsychotic) is indifferent to THC co-administration. As different species, anesthetics, and drugs have been used in these three studies (Dissanayake et al., 2008; Hajós et al., 2008; Smucny et al., 2014), it is difficult to compare them. For instance, according to Smucny et al. (2014) THC alone is innocuous for CA3 response amplitudes, whereas according to Dissanayake et al. (2008) WIN is able to change these amplitudes, at least in a proportion of subjects. It seems anyway clear that cannabinoid and psychosis-relevant manipulations can effectively modulate auditory gating within the temporal lobe and connected areas (Figure 3B).
In vitro Studies
Two of the references above (Raver et al., 2013; Cass et al., 2014) have included in vivo and in vitro experiments after adolescent exocannabinoid exposure. Raver et al. (2013), who have reported that adolescent WIN treatment precludes frontal gamma-potentiating effects of ketamine, have made converging observations from LFP in vitro. This time, they have analyzed prefrontal and somatosensory cortical gamma oscillations potentiated by perfusion of kainic acid + carbachol, a method known to shift the LFP spectrum toward fast frequencies, thanks to higher excitatory drive and cholinergic activation of interneurons (Raver et al., 2013). Slices from adults treated with WIN during adolescence presented weaker gamma power reactivity in both brain sites. Intriguingly, an equivalent adolescent treatment with THC replicated these results in mPFC, but not SCx. This finding might represent an electrophysiological correlate of the WIN vs. THC pharmacological distinction, which has been further examined by the same group using AM-251 co-treatment (Raver and Keller, 2014). Complementarily, Raver et al. (2013) have also described NOR deficits in a separate cohort of adolescent WIN-treated adults, suggesting that the exocannabinoid-exposed neocortex has lower sensitivity to any gamma-potentiating event: either psychotomimetic drug administration or cognitive effort.
In turn, Cass et al. (2014), who have found a GABAergic involvement in WIN-induced alterations of the vHipp-mPFC communication in vivo, have reinforced their conclusions through an in vitro experiment. Whole-cell patch-clamp recordings from the adult mPFC—specifically its deep-layer pyramidal neurons—provided a link between repeated adolescent WIN exposure and lower incidence of spontaneous inhibitory postsynaptic currents. WIN treatment during adulthood failed to reproduce this effect. We again interpret the Raver et al. (2013) and Cass et al. (2014) studies together: chronic exposure to exocannabinoids during adolescence seems to impair interneuronal activity within the mPFC, as well as its responsivity to hippocampal afferent inputs, which can both account for adult susceptibility to schizophrenia symptoms, including cognitive deficits. Adolescent exposure to WIN has also been demonstrated to affect mPFC intracortical synaptic plasticity in vitro. Lovelace et al. (2015) have shown that eCB-LTD at layer 2/3 → layer 5 synapses of the mPFC is suppressed in brain slices from adult female mice exposed to WIN during adolescence (Figure 3C). Neither input-output curves nor short-term forms of synaptic plasticity were affected by the WIN treatment. These findings indicate that the underpinnings of cannabinoid tolerance, i.e., CB1 receptor down-regulation or desensitization, can affect the prefrontal capacity to undergo long-term presynaptic plasticity without altering its basal intra-cortical transmission. Lovelace et al. (2015) have also shown that JZL-184 (inhibitor of 2-AG hydrolysis) can rescue the eCB-LTD deficit caused by adolescent WIN exposure. As discussed by the authors, these results are in line with the post-mortem evidence of abnormal CB1 expression in schizophrenia (Curran et al., 2016).
A previous work from the same group (Lovelace et al., 2014) had indeed reported abnormal CB1 expression in a rodent model of schizophrenia. Using confocal microscopy, they report reduced CB1 fluorescent signal in mPFC and dorsal hippocampus (dentate gyrus and CA1) from adult mice treated with PCP during early development (postnatal days 7-11). As Lovelace et al. (2014) had also shown impaired eCB-LTD and deficient contextual fear memory in PCP-treated mice (Figure 3C), it can be concluded that NMDA receptor hypofunction, during mPFC maturation, might result in both eCB and cognitive dysfunctions later in life. Using naïve adult mice, Lafourcade et al. (2007) and Jew et al. (2013) have also explored the interplay between glutamatergic and eCB transmission in the mPFC. Lafourcade et al. (2007) have found, in mPFC deep layers, a co-localization of presynaptic CB1 receptors, mGluR5, and diacylglycerol lipase α, which is key in the synthesis of 2-AG. eCB-LTD in layers 5/6 of the mPFC was suppressed by the mGluR antagonist MPEP, and a sub-threshold tetanic stimulation required URB-602 (2-AG degradation blocker), but not URB-597, to induce LTD (Lafourcade et al., 2007). Consistently, Jew et al. (2013) have observed impaired eCB-LTD in knockout mice lacking mGluR5 in principal cortical neurons. Behaviorally, the same mice manifested higher novelty-induced locomotion, higher open-field locomotion after injection of methylphenidate (a psychostimulant), but unaffected anxiety, fear conditioning, and PPI (Jew et al., 2013). These findings suggest that specific schizophrenia-like symptoms may depend on specific dysfunctions of the mGluR/eCB-LTD cooperation (Figure 3C).
Lastly, we mention three studies on the intra-hippocampal synaptic transmission (Du et al., 2013; Kim and Li, 2015; Li and Kim, 2016). According to Du et al. (2013), chronic exposure to hippocampal organotypic cultures to neuregulin-1—whose over-expression is a risk factor for schizophrenia—increases the enzymatic degradation of 2-AG, resulting in weaker mGluR agonist-induced LTD in CA1. Using a similar preparation, the same research group (Kim and Li, 2015) has demonstrated that chronic CB2 receptor agonism elevates the frequency of quantal glutamate release in CA1, which does not occur in slices from schizophrenia-like CB2 knockout mice. Slices from the same transgenic mice have also been reported to undergo weaker LTP in CA1 (Li and Kim, 2016; Figure 3D). Therefore, the hippocampus itself is prone to alterations in the mGluR/eCB interplay, which could contribute to downstream dysfunctions in mPFC, VTA, and other schizophrenia-relevant brain sites.
Methodological Overview
For delimitating the literature of this section via PubMed, we searched for all possible combinations between keywords related to schizophrenia, cannabinoids, vanilloids, and the endocannabinoid and endovanilloid systems, provided that they belonged to electrophysiological recording studies from non-human animals. No articles were found using non-human primates, or more recent neurophysiological techniques, like optogenetics or chemogenetics. Hence, whether directly involving models of schizophrenia, or at least discussing schizophrenia, the main citations above (total of 23) reflect an exhaustive review.
We can draw the following methodological outline from such review (see also Table 1). (1) Most of the electrophysiological recordings (13 of 23) have been performed in anesthetized rodents, either accompanied (Laviolette and Grace, 2006; Draycott et al., 2014; Gomes et al., 2015; Loureiro et al., 2015, 2016; Renard et al., 2016) or not (Melis et al., 2004a; Dissanayake et al., 2008; Hajós et al., 2008; Raver et al., 2013; Aguilar et al., 2014; Cass et al., 2014; Smucny et al., 2014) by behavioral testing. (2) Some in vitro studies have also included separate behavioral experiments (Jew et al., 2013; Raver et al., 2013; Lovelace et al., 2014, 2015), but most of them have been purely electrophysiological, either accompanied or not by anesthetized recordings (Melis et al., 2004a; Lafourcade et al., 2007; Du et al., 2013; Cass et al., 2014; Raver and Keller, 2014; Kim and Li, 2015; Li and Kim, 2016). (3) Only two studies have performed chronic recordings (Morra et al., 2012; Aguilar et al., 2016) one of them with simultaneous behavioral monitoring (Morra et al., 2012).
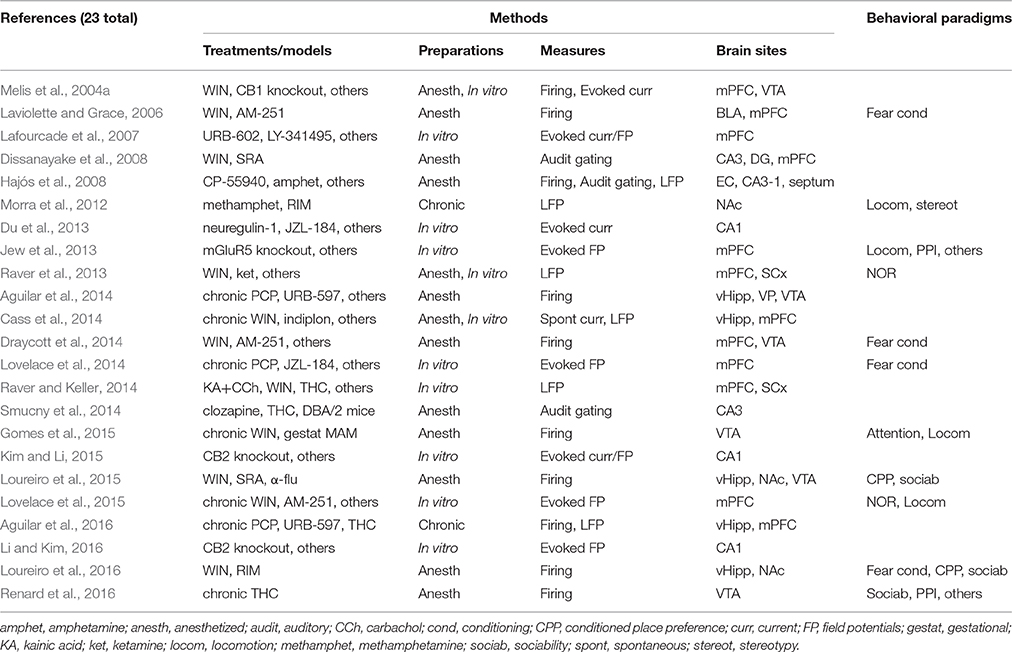
Table 1. Rodent electrophysiology studies involving the eCB system and schizophrenia-relevant treatments/models.
In general, cannabinoid interactions with three other neurotransmitter systems (dopamine, glutamate, and GABA) across mesocorticolimbic circuits (vHipp, NAc, VP, VTA, mPFC, and BLA) have been prioritized. Relationships between cannabinoid transmission and schizophrenia have been investigated using animal models (sub-chronic PCP, gestational MAM, subanesthetic ketamine, amphetamine, and methamphetamine), and behavioral measures (open-field locomotion/stereotypy, sociability, PPI of the acoustic startle, fear conditioning, attentional set shifting, light/dark box anxiety, and NOR). Chronic cannabinoid exposure during adolescence and its impacts on mPFC interneurons, oscillatory activity, and protein expression during adulthood have also received attention. A minor proportion of articles reports cannabinoid effects on auditory evoked potentials from the hippocampus. Finally, in vitro studies have provided all available information on eCB-mediated synaptic plasticity in the mPFC or hippocampus in schizophrenia-relevant assays (e.g., adolescent cannabinoid exposure, mGluR and CB2 knockouts, and acute psychostimulant effects).
Endovanilloid System in Schizophrenia: Indirect Electrophysiological Implications from Rodents
We found six electrophysiological studies on the non-human endovanilloid system with potential implications for schizophrenia (see also Table 2). They report findings on schizophrenia-relevant neurochemical and behavioral alterations without explicitly focusing on this disorder. Marinelli et al. (2005) performed in vitro and in vivo experiments on the TRPV1 participation in dopamine release. In VTA slices, the TRPV1 agonist capsaicin has been shown to increase the firing rate of dopamine neurons. This response could be blocked by antagonists of ionotropic glutamate receptors (CNQX and AP5), providing a link between the endovanilloid, dopaminergic, and glutamatergic systems. Using in vivo experiments, the same studies have found that both capsaicin microinjection into the VTA and noxious tail stimulation increases dopamine release in the NAc. Therefore, this finding is an indirect evidence of the endovanilloid involvement in schizophrenia, since pain sensitivity is disarranged in this disorder (Stubbs et al., 2015). Grueter et al. (2010) have also studied the endovanilloid modulation of the NAc. According to the authors, mGluR-mediated release of eCB from medium spiny neurons activated both postsynaptic TRPV1 and presynaptic CB1 receptors. Noteworthy, whereas this CB1 recruitment induced eCB-LTD, TRPV1 activation triggered the endocytosis of AMPA receptors, thus inducing a postsynaptic form of LTD (Grueter et al., 2010). Therefore, these two electrophysiological studies point to an interplay between the eCB and endovanilloid systems in schizophrenia, at least regarding plasticity mechanisms within the NAc.
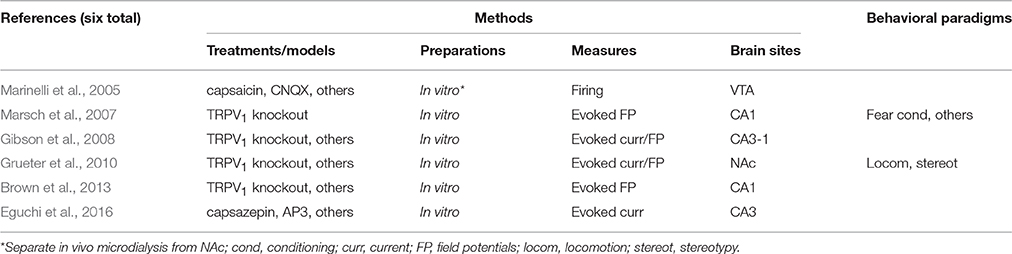
Table 2. Rodent electrophysiology studies involving the endovanilloid system and schizophrenia-relevant treatments/models.
TRPV1 receptors have also been shown to play a role in the inhibitory neurotransmission within the hippocampus, which is usually associated with disrupted field oscillations in schizophrenia. Using hippocampal slices from TRPV1-knockout mice, Marsch et al. (2007) have found a lower ability of CA1 pyramidal neurons to undergo LTP, an effect that has been later demonstrated to be reverted by GABAA antagonism (Brown et al., 2013). Also using TRPV1-knockout mice, Gibson et al., (2008) have reported a lower ability of CA1 interneurons to undergo LTD. Therefore, TRPV1 receptors seem to modulate hippocampal synaptic plasticity and interneuronal activity in complex manners. Also in the hippocampus, Eguchi et al. (2016) have found that TPRV1 antagonism suppresses mGluR-dependent excitatory postsynaptic currents in voltage-clamped CA3 interneurons. These findings, together with those from Marinelli et al. (2005) and Grueter et al. (2010), suggest that complex interactions exist between the eCB and endovanilloid systems across hippocampal and mesolimbic circuits.
Psychopharmacology studies had already observed that TRPV1 receptors modulate behavioral changes in schizophrenia models (Tzavara et al., 2006; Almeida et al., 2014). In addition, systemic capsaicin in hyperdopaminergic animals has been reported to suppress the hyperlocomotion associated with decreased nigrostriatal activity (De Lago et al., 2004; Lee et al., 2006; Tzavara et al., 2006). It seems clear, therefore, that exploring these same psychopharmacological issues using neurophysiological tools tends to detail the relationship between the endovanilloid system and schizophrenia.
Implications from Rodent Electrophysiology and Research Directions
What emerges from the animal model literature is that cannabinoid and vanilloid drugs affect the mesocorticolimbic system depending on their dose and pharmacological action. As a result, cannabinoid and vanilloid treatments can exacerbate or reverse schizophrenia-like symptoms. In fact, there is a myriad of hippocampal and prefrontal cortical changes in receptor expression, synaptic plasticity, and oscillatory activity that might result from aberrant eCB and endovanilloid drive. Of note, altered VTA single-unit activity appears to be the consistent outcome of both cannabinoid and TRPV1 manipulations, implicating the vHipp-NAc-VP disinhibition system and related symptoms.
Such a scenario is expectedly elusive, given the low number of studies within this review's scope. The majority of rodent electrophysiological reports were published in the past 5 years, indicating this is a rapidly expanding subfield. In almost all of in vivo works, behavioral and electrophysiological data come from separate experiments, or even separate groups of subjects, thus offering disconnected results and less analytical opportunities. Moreover, combined analyses of LFP and unit activity are still neglected in the subfield. The remaining text reflects the authors' opinion on how these limitations may be overcome by recent (but already established) neurophysiological approaches. Directions take into account functional data from humans (reviewed above), and the paucity of electrophysiological studies explicitly interested in the endovanilloid-schizophrenia relationship.
The endovanilloid role in schizophrenia is clearly underexplored, making its neurophysiological study a promising research avenue. Rodent studies so far have only provided indirect relationships between TRPV1 and schizophrenia symptoms (Chahl, 2007). Therefore, in vivo approaches tend to provide meaningful information, even from simple behavioral pharmacology experiments. Chronic electrophysiological recordings during such experiments will additionally reveal how behavioral abnormalities relate to functional markers, e.g., local synchrony within theta and gamma bands, as well as long-range synchrony between hippocampus and PFC during cognitive tasks.
CBD, in turn, has been shown to be a promising antipsychotic (Crippa et al., 2010; Zuardi et al., 2012). Despite controversies, CBD is known to ameliorate hyperlocomotion and PPI deficits in acute models of schizophrenia (Zuardi et al., 2012). From the mechanistic perspective, however, CBD's actions on psychosis-relevant brain regions is still unclear (Gururajan and Malone, 2016), thus making electrophysiological experiments on this matter highly desirable. For example, interrogating whether CBD attenuates the effects of PCP, ketamine, or amphetamine on gamma oscillations and single-unit activity will begin to clarify CBD's antipsychotic properties.
In this same sense, electrophysiological measures may shed light on the still elusive interactions among CBD, psychosis, and serotonin receptors (Russo et al., 2005). Similarly to the antipsychotic aripiprazole, CBD is known to facilitate 5-HT1A receptor-mediated neurotransmission in schizophrenia-related areas, including the rodent mPFC (Campos et al., 2012). Comparing the systemic effects of these two drugs while assessing behavior (e.g., locomotion), and selectively manipulating 5-HT1A receptors (e.g., intra-mPFC WAY100635) is an opportunity for chemotrode LFP recordings, either separately or simultaneously to behavioral monitoring. Employing these approaches in animal models of schizophrenia is a feasible way to further explore the serotonergic character of CBD, and its neuropsychiatric relevance.
In addition to psychopharmacology-oriented designs, circuit-level studies are necessary. Assessing synaptic plasticity and brain connectivity disruptions under both schizophrenia symptoms and eCB/endovanilloid manipulations is likely to yield relevant mechanistic information. For example, CB1 and TRPV1 receptors contribute to LTD induction across the mPFC, hippocampus, and NAc (Gibson et al., 2008; Grueter et al., 2010; Lovelace et al., 2014). Thus, monitoring these plasticity processes in schizophrenia-like phenotypes (e.g., adult rats previously exposed to MAM, or chronic THC) is a promising approach, both in anesthetized and behaving subjects.
Plasticity dysfunctions can also be reflected in long-range connectivity impairments (Sigurdsson, 2016). The hippocampal-PFC synchrony is disrupted in schizophrenia patients, animal models, and under CB1 agonism (Cass et al., 2014; Sigurdsson, 2016). In fact, this is an ideal substrate for studying how eCB interacts with glutamatergic, cholinergic, and monoaminergic systems in modulating inter-areal communication (Schlicker and Kathmann, 2001; Nagode et al., 2014). Chronic multi-site recording experiments might, therefore, unveil these interactions, thus pushing the research topic into a systems-level perspective. Addressing cognitive and perceptual alterations in this context would also be relevant for providing neuropsychiatric implications.
Oscillatory activity abnormalities are well documented in schizophrenia, especially in the gamma range (McNally and McCarley, 2016). Complex interactions between interneuron subtypes are supposedly responsible for generating gamma oscillations (Sohal et al., 2009; Sohal, 2012). Interestingly, hippocampal and neocortical CB1 receptors are preferentially found on certain interneurons, i.e., CCK-containing non-fast-spiking cells (Bacci et al., 2004; Eggan and Lewis, 2007; Ali and Todorova, 2010), which in turn provide inputs to both pyramidal cells, and PV-containing fast-spiking interneurons (Karson et al., 2009; Keimpema et al., 2012). Skosnik et al. (2016) proposed that fine tuning within this CCK-PV-pyramidal arrangement depends on the eCB modulation, which would be disrupted by exogenous cannabinoid agents. Considering that gamma oscillations rely on this fine tuning, the hypotheses of Skosnik et al. (2016) could be explored using electrophysiology and optogenetics. More specifically, each cell type involved in gamma entrainment could be optically tagged (Kim et al., 2016; Nomoto et al., 2016), and subsequently monitored before and after CB1 activation (e.g., a psychotomimetic dose of THC). Such an experiment would gain additional relevance if performed in chronically treated animals (e.g., adolescent THC treatment), and/or animal models of schizophrenia.
Oscillatory patterns outside the gamma range can be linked with other schizophrenia-related neurotransmitter systems, especially dopamine (Lisman, 2012). The VTA-hippocampus-mPFC activity is known to coordinate 4 Hz, theta, and low-gamma oscillations in intricate manners (Fujisawa and Buzsáki, 2011). Furthermore, dopamine into the mPFC is known to promote hippocampal-mPFC theta coherence (Benchenane et al., 2010). Given that both power (Ducharme et al., 2012) and coherence (Sigurdsson et al., 2010) in the theta band have been found to be diminished in schizophrenia models, this reduction could be potentially attributed to abnormal dopaminergic signaling. In this sense, the firing rate of VTA dopaminergic cells has been shown to increase upon local TRPV1 agonism (Marinelli et al., 2005; Ali and Todorova, 2010). Thus, an interesting approach would be to electrophysiologically probe the VTA-mPFC communication under TRPV1 agonists (e.g., capsaicin) in schizophrenia models.
In general, the proposed directions suggest potential lines of research. While the above scenario does not cover experimental possibilities in an exhaustive fashion, it may be helpful for experiment designing in the near future.
Concluding Remarks
Dysfunctions of eCB and endovanilloid signaling across mesocorticolimbic circuits may contribute to schizophrenia-like symptoms. Identifying neural activity patterns under these dysfunctions tends to motivate further electrophysiological experimentation, which (according to this review) is in need of denser analyses, as well as brain stimulation and chronic recording approaches. Potential therapeutic targets or procedures will likely arise from such exploration.
Author Contributions
Wrote the manuscript: RR, LB, MR, and JD. Made figure/tables: LB and MR. Revised the manuscript: LB, JL, and JH.
Conflict of Interest Statement
The authors declare that the research was conducted in the absence of any commercial or financial relationships that could be construed as a potential conflict of interest.
Acknowledgments
This work was funded by the National Council for Scientific and Technological Development, CNPq (142451/2014-2, 465458/2014-9), the São Paulo Research Foundation, FAPESP (2012/23918-0, 2016/17882-4), and the Coordination for Improvement of Higher Education Personnel, CAPES (PNPD 1645824).
References
Aguiar, D. C., Moreira, F. A., Terzian, A. L., Fogaça, M. V., Lisboa, S. F., Wotjak, C. T., et al. (2014). Modulation of defensive behavior by Transient Receptor Potential Vanilloid Type-1 (TRPV1) Channels. Neurosci. Biobehav. Rev. 46, 418–428. doi: 10.1016/j.neubiorev.2014.03.026
Aguilar, D. D., Chen, L., and Lodge, D. J. (2014). Increasing endocannabinoid levels in the ventral pallidum restore aberrant dopamine neuron activity in the subchronic PCP rodent model of schizophrenia. Int. J. Neuropsychopharmacol. 18, 1–9. doi: 10.1093/ijnp/pyu035
Aguilar, D. D., Giuffrida, A., and Lodge, D. J. (2016). THC and endocannabinoids differentially regulate neuronal activity in the prefrontal cortex and hippocampus in the subchronic PCP model of schizophrenia. J. Psychopharmacol. 30, 169–181. doi: 10.1177/0269881115612239
Aizpurua-Olaizola, O., Elezgarai, I., Rico-Barrio, I., Zarandona, I., Etxebarria, N., and Usobiaga, A. (2017). Targeting the endocannabinoid system: future therapeutic strategies. Drug Discov. Today 22, 105–110. doi: 10.1016/j.drudis.2016.08.005
Aizpurua-Olaizola, O., Soydaner, U., Ekin, O., Schibano, D., Simsir, Y., Navarro, P., et al. (2016). Evolution of the cannabinoid and terpene content during the growth of Cannabis sativa plants from different chemotypes. J. Nat. Prod. 79, 324–331. doi: 10.1021/acs.jnatprod.5b00949
Alger, B. E., and Kim, J. (2011). Supply and demand for endocannabinoids. Trends Neurosci. 34, 304–315. doi: 10.1016/j.tins.2011.03.003
Ali, A. B., and Todorova, M. (2010). Asynchronous release of GABA via tonic cannabinoid receptor activation at identified interneuron synapses in rat CA1. Eur. J. Neurosci. 31, 1196–1207. doi: 10.1111/j.1460-9568.2010.07165.x
Almeida, V., Peres, F. F., Levin, R., Suiama, M. A., Calzavara, M. B., Zuardi, A. W., et al. (2014). Effects of cannabinoid and vanilloid drugs on positive and negative-like symptoms on an animal model of schizophrenia: the SHR strain. Schizophr. Res. 153, 150–159. doi: 10.1016/j.schres.2014.01.039
Atakan, Z., Bhattacharyya, S., Allen, P., Martín-Santos, R., Crippa, J. A., Borgwardt, S. J., et al. (2013). Cannabis affects people differently: inter-subject variation in the psychotogenic effects of Δ9-tetrahydrocannabinol: a functional magnetic resonance imaging study with healthy volunteers. Psychol. Med. 43, 1255–1267. doi: 10.1017/S0033291712001924
Bacci, A., Huguenard, J. R., and Prince, D. A. (2004). Long-lasting self-inhibition of neocortical interneurons mediated by endocannabinoids. Nature 431, 312–316. doi: 10.1038/nature02913
Bakshi, V. P., and Geyer, M. A. (1995). Antagonism of phencyclidine induced deficits in prepulse inhibition by the putative atypical antipsychotic olanzapine. Psychopharmacology (Berl). 122, 198–201. doi: 10.1007/BF02246096
Bangalore, S. S., Prasad, K. M. R., Montrose, D. M., Goradia, D. D., Diwadkar, V. A., and Keshavan, M. S. (2008). Cannabis use and brain structural alterations in first episode schizophrenia - A region of interest, voxel based morphometric study. Schizophr. Res. 99, 1–6. doi: 10.1016/j.schres.2007.11.029
Bartos, M., Vida, I., and Jonas, P. (2007). Synaptic mechanisms of synchronized gamma oscillations in inhibitory interneuron networks. Nat. Rev. Neurosci. 8, 45–56. doi: 10.1038/nrn2044
Barz, C. S., Bessaih, T., Abel, T., Feldmeyer, D., and Contreras, D. (2016). Sensory encoding in Neuregulin 1 mutants. Brain Struct. Funct. 221, 1067–1081. doi: 10.1007/s00429-014-0955-x
Batalla, A., Crippa, J. A., Busatt, G. F., Guimarães, F. S., Zuardi, A. W., Valverde, O., et al. (2014). Neuroimaging studies of acute effects of THC and CBD in humans and animals: a systematic review. Curr. Pharm. Des. 20, 2168–2185. doi: 10.2174/13816128113199990432
Batista, L. A., Gobira, P. H., Viana, T. G., Aguiar, D. C., and Moreira, F. A. (2014). Inhibition of endocannabinoid neuronal uptake and hydrolysis as strategies for developing anxiolytic drugs. Behav. Pharmacol. 25, 425–433. doi: 10.1097/FBP.0000000000000073
Benchenane, K., Peyrache, A., Khamassi, M., Tierney, P. L., Gioanni, Y., Battaglia, F. P., et al. (2010). Coherent theta oscillations and reorganization of spike timing in the hippocampal- prefrontal network upon learning. Neuron 66, 921–936. doi: 10.1016/j.neuron.2010.05.013
Benetti, S., Mechelli, A., Picchioni, M., Broome, M., Williams, S., and McGuire, P. (2009). Functional integration between the posterior hippocampus and prefrontal cortex is impaired in both first episode schizophrenia and the at risk mental state. Brain 132, 2426–2436. doi: 10.1093/brain/awp098
Beraki, S., Diaz-Heijtz, R., Tai, F., and Ogren, S. O. (2009). Effects of repeated treatment of phencyclidine on cognition and gene expression in C57BL/6 mice. Int. J. Neuropsychopharmacol. 12, 243–255. doi: 10.1017/S1461145708009152
Bertrand, O., and Tallon-Baudry, C. (2000). Oscillatory gamma activity in humans: a possible role for object representation. Int. J. Psychophysiol. 38, 211–223. doi: 10.1016/S0167-8760(00)00166-5
Bhattacharyya, S., Atakan, Z., Martin-Santos, R., Crippa, J. A., Kambeitz, J., Malhi, S., et al. (2015a). Impairment of inhibitory control processing related to acute psychotomimetic effects of cannabis. Eur. Neuropsychopharmacol. 25, 26–37. doi: 10.1016/j.euroneuro.2014.11.018
Bhattacharyya, S., Crippa, J. A., Allen, P., Martin-Santos, R., Borgwardt, S., Fusar-Poli, P., et al. (2012). Induction of psychosis by Δ9-tetrahydrocannabinol reflects modulation of prefrontal and striatal function during attentional salience processing. Arch. Gen. Psychiatric 69, 27–36. doi: 10.1001/archgenpsychiatry.2011.161
Bhattacharyya, S., Falkenberg, I., Martin-Santos, R., Atakan, Z., Crippa, J. A., Giampietro, V., et al. (2015b). Cannabinoid modulation of functional connectivity within regions processing attentional salience. Neuropsychopharmacology 40, 1343–1352. doi: 10.1038/npp.2014.258
Bhattacharyya, S., Morrison, P. D., Fusar-Poli, P., Martin-Santos, R., Borgwardt, S., Winton-Brown, T., et al. (2010). Opposite effects of delta-9-tetrahydrocannabinol and cannabidiol on human brain function and psychopathology. Neuropsychopharmacology 35, 764–774. doi: 10.1038/npp.2009.184
Bisogno, T., Hanus, L., De Petrocellis, L., Tchilibon, S., Ponde, D. E., Brandi, I., et al. (2001). Molecular targets for cannabidiol and its synthetic analogues: effects on vanilloid VR1 receptors and on the cellular uptake and enzymatic hydrolysis of anandamide. Br. J. Pharmacol. 134, 845–852. doi: 10.1038/sj.bjp.0704327
Böcker, K. B. E., Hunault, C. C., Gerritsen, J., Kruidenier, M., Mensinga, T. T., and Kenemans, J. L. (2010). Cannabinoid modulations of resting state EEG theta power and working memory are correlated in humans. J. Cogn. Neurosci. 22, 1906–1916. doi: 10.1162/jocn.2009.21355
Bonnot, O., Anderson, G. M., Cohen, D., Willer, J. C., and Tordjman, S. (2009). Are patients with schizophrenia insensitive to pain? A reconsideration of the question. Clin. J. Pain 25, 244–252. doi: 10.1097/AJP.0b013e318192be97
Booth, C. A., Brown, J. T., and Randall, A. D. (2014). Neurophysiological modification of CA1 pyramidal neurons in a transgenic mouse expressing a truncated form of disrupted-in-schizophrenia 1. Eur. J. Neurosci. 39, 1074–1090. doi: 10.1111/ejn.12549
Bossong, M. G., Jansma, J. M., Bhattacharyya, S., and Ramsey, N. F. (2014). Role of the endocannabinoid system in brain functions relevant for schizophrenia: an overview of human challenge studies with cannabis or Δ9-tetrahydrocannabinol (THC). Prog. Neuropsychopharmacol. Biol. Psychiatry 52, 53–69. doi: 10.1016/j.pnpbp.2013.11.017
Bossong, M. G., Jansma, J. M., van Hell, H. H., Jager, G., Kahn, R. S., and Ramsey, N. F. (2013). Default mode network in the effects of delta9-tetrahydrocannabinol (THC) on human executive function. PLoS ONE 8:e70074. doi: 10.1371/journal.pone.0070074
Bossong, M. G., Mehta, M. A., Van Berckel, B. N. M., Howes, O. D., Kahn, R. S., and Stokes, P. R. A. (2015). Further human evidence for striatal dopamine release induced by administration of Δ9-tetrahydrocannabinol (THC): selectivity to limbic striatum. Psychopharmacology Berl 232, 2723–2729. doi: 10.1007/s00213-015-3915-0
Bossong, M. G., van Berckel, B. N., Boellaard, R., Zuurman, L., Schuit, R. C., Windhorst, A. D., et al. (2009). Delta9-tetrahydrocannabinol induces dopamine release in the human striatum. Neuropsychopharmacology 34, 759–766. doi: 10.1038/npp.2008.138
Boutros, N. N., Arfken, C., Galderisi, S., Warrick, J., Pratt, G., and Iacono, W. (2008). The status of spectral EEG abnormality as a diagnostic test for schizophrenia. Schizophr. Res. 99, 225–237. doi: 10.1016/j.schres.2007.11.020
Braff, D., Stone, C., Callaway, E., Geyer, M., Glick, I., and Bali, L. (1978). Prestimulus effects on human startle reflex in normals and schizophrenics. Psychophysiology 15, 339–343. doi: 10.1111/j.1469-8986.1978.tb01390.x
Bramon, E., Rabe-Hesketh, S., Sham, P., Murray, R. M., and Frangou, S. (2004). Meta-analysis of the P300 and P50 waveforms in schizophrenia. Schizophr. Res. 70, 315–329. doi: 10.1016/j.schres.2004.01.004
Breuer, A., Haj, C. G., Fogaça, M. V., Gomes, F. V., Silva, N. R., Pedrazzi, J. F., et al. (2016). Fluorinated cannabidiol derivatives: enhancement of activity in mice models predictive of anxiolytic, antidepressant and antipsychotic effects. PLoS ONE 11:e0158779. doi: 10.1371/journal.pone.0158779
Brown, T. E., Chirila, A. M., Schrank, B. R., and Kauer, J. A. (2013). Loss of interneuron ltd and attenuated pyramidal cell LTP in TRPV1 and Trpv3 KO mice. Hippocampus 23, 662–671. doi: 10.1002/hipo.22125
Brown, T. M., Brotchie, J. M., and Fitzjohn, S. M. (2003). Cannabinoids decrease corticostrialtal synaptic transmission via an effect on glutamate uptake. J. Neurosci. 23, 11073–11077.
Buzsaki, G., and Draguhn, A. (2004). Neuronal oscillations in cortical networks. Science 304, 1926–1929. doi: 10.1126/science.1099745
Callén, L., Moreno, E., Barroso-Chinea, P., Moreno-Delgado, D., Cortés, A., Mallol, J., et al. (2012). Cannabinoid receptors CB1 and CB2 form functional heteromers in the brain. J. Biol. Chem. 287, 20851–20865. doi: 10.1074/jbc.M111.335273
Campos, A. C., Moreira, F. A., Gomes, F. V., Del Bel, E. A., and Guimarães, F. S. (2012). Multiple mechanisms involved in the large-spectrum therapeutic potential of cannabidiol in psychiatric disorders. Phil. Trans. R. Soc. B. 367, 3364–3378. doi: 10.1098/rstb.2011.0389
Canetta, S. E., and Brown, A. S. (2012). Prenatal infection, maternal immune activation, and risk for schizophrenia. Transl. Neurosci. 3, 320–327. doi: 10.2478/s13380-012-0045-6
Carlén, M., Meletis, K., Siegle, J., Cardin, J., Futai, K., Vierling-Claassen, D., et al. (2011). A critical role for NMDA receptors in parvalbumin interneurons for gamma rhythm induction and behavior. Mol. Psychiatry 17, 537–548. doi: 10.1038/mp.2011.31
Carlsson, A. (1988). The current status of the dopamine hypothesis of schizophrenia. Neuropsychopharmacology 1, 179–186.
Cass, D. K., Flores-Barrera, E., Thomases, D. R., Vital, W. F., Caballero, A., and Tseng, K. Y. (2014). CB1 cannabinoid receptor stimulation during adolescence impairs the maturation of GABA function in the adult rat prefrontal cortex. Mol. Psychiatry 19, 536–543. doi: 10.1038/mp.2014.14.CB1
Castillo, P. E., Younts, T. J., Chávez, A. E., and Hashimotodani, Y. (2012). Endocannabinoid signaling and synaptic function. Neuron 76, 70–81. doi: 10.1016/j.neuron.2012.09.020
Caterina, M. J., Schumacher, M. A., Tominaga, M., Rosen, T. A., Levine, J. D., and Julius, D. (1997). The capsaicin receptor: a heat-activated ion channel in the pain pathway. Nature 389, 816–824. doi: 10.1038/39807
Ceccarini, J., De Hert, M., Van Winkel, R., Peuskens, J., Bormans, G., Kranaster, L., et al. (2013). Increased ventral striatal CB1 receptor binding is related to negative symptoms in drug-free patients with schizophrenia. Neuroimage 79, 304–312. doi: 10.1016/j.neuroimage.2013.04.052
Chahl, L. A. (2007). TRP's: links to schizophrenia? Biochim. Biophys. Acta 1772, 968–977. doi: 10.1016/j.bbadis.2007.05.003
Chávez, A. E., Chiu, C. Q., and Castillo, P. E. (2010). TRPV1 activation by endogenous anandamide triggers postsynaptic long-term depression in dentate gyrus. Nat. Neurosci. 13, 1511–1518. doi: 10.1038/nn.2684
Chen, D. J., Gao, M., Gao, F. F., Su, Q. X., and Wu, J. (2017). Brain cannabinoid receptor 2: expression, function and modulation. Acta Pharmacol. Sin. 38, 312–316. doi: 10.1038/aps.2016.149
Chevaleyre, V., Takahashi, K. A., and Castillo, P. E. (2006). Endocannabinoid-mediated synaptic plasticity in the CNS. Annu. Rev. Neurosci. 29, 37–76. doi: 10.1146/annurev.neuro.29.051605.112834
Childers, S. R., and Deadwyler, S. A. (1996). Role of cyclic AMP in the actions of cannabinoid receptors. Biochem. Pharmacol. 52, 819–827. doi: 10.1016/0006-2952(96)00419-4
Cho, R. Y., Konecky, R. O., and Carter, C. S. (2006). Impairments in frontal cortical gamma synchrony and cognitive control in schizophrenia. Proc. Natl. Acad. Sci. U.S.A. 103, 19878–19883. doi: 10.1073/pnas.0609440103
Clair, D. S., Blackwood, D., Muir, W., Carothers, A., Walkers, M., Spowart, G., et al. (1990). Association within a family of a balanced autosomal translocation with major mental illness. Lancet 336, 13–16.
Cortes-Briones, J. A., Cahill, J. D., Skosnik, P. D., Mathalon, D. H., Williams, A., Sewell, R. A., et al. (2015a). The psychosis-like effects of delta9-tetrahydrocannabinol are associated with increased cortical noise in healthy humans. Biol. Psychiatry 78, 805–813. doi: 10.1016/j.biopsych.2015.03.023
Cortes-Briones, J., Skosnik, P. D., Mathalon, D., Cahill, J., Pittman, B., Williams, A., et al. (2015b). Δ9-THC disrupts gamma (γ)–band neural oscillations in humans. Neuropsychopharmacology 40, 2124–2134. doi: 10.1038/npp.2015.53
Coyle, J. T. (1996). The glutamatergic dysfunction hypothesis for schizophrenia. Harv. Rev. Psychiatry 3, 241–253.
Crabtree, G. W., and Gogos, J. A. (2014). Synaptic plasticity, neural circuits, and the emerging role of altered short-term information processing in schizophrenia. Front. Synaptic Neurosci. 6:28. doi: 10.3389/fnsyn.2014.00028
Craft, M. R., Marusich, J. A., and Wiley, J. L. (2013). Sex differences in cannabinoid pharmacology: a reflection of differences in the endocannabinoid system? Life Sci. 92, 476–481. doi: 10.1016/j.lfs.2012.06.009
Cravatt, B. F., Giang, D. K., Mayfield, S. P., Boger, D. L., Lerner, R. A., and Gilula, N. B. (1996). Molecular characterization of an enzyme that degrades neuromodulatory fatty-acid amides. Nature 384, 83–87. doi: 10.1038/384083a0
Crippa, J. A. S., Zuardi, A. W., and Hallak, J. E. C. (2010). Therapeutical use of the cannabinoids in psychiatry. Rev. Bras. Psiquiatr. 32, S56–S66. doi: 10.1590/S1516-44462010000500009
Curran, H. V., Freeman, T. P., Mokrysz, C., Lewis, D. A., Morgan, C., and Parsons, L. H. (2016). Keep off the grass? Cannabis, cognition and addiction. Nat. Rev. Neurosci. 17, 293–306. doi: 10.1038/nrn.2016.28
D'Souza, D. C., Abi-Saab, W. M., Madonick, S., Forselius-Bielen, K., Doersch, A., Braley, G., et al. (2005). Delta-9-tetrahydrocannabinol effects in schizophrenia: implications for cognition, psychosis, and addiction. Biol. Psychiatry 57, 594–608. doi: 10.1016/j.biopsych.2004.12.006
D'Souza, D. C., Perry, E., MacDougall, L., Ammerman, Y., Cooper, T., Wu, Y., et al. (2004). The psychotomimetic effects of intravenous delta-9-tetrahydrocannabinol in healthy individuals: implications for psychosis. Neuropsychopharmacology 29, 1558–1572. doi: 10.1038/sj.npp.1300496
Dalton, V. S., Long, L. E., Weickert, C. S., and Zavitsanou, K. (2011). Paranoid schizophrenia is characterized by increased CB1 receptor binding in the dorsolateral prefrontal cortex. Neuropsychopharmacology 36, 1620–1630. doi: 10.1038/npp.2011.43
Davis, K. L., Kahn, R. S., Ko, G., and Davidson, M. (1991). Dopamine in schizophrenia: a review and reconceptualization. Am. J. Psychiatry 148, 1474–1486. doi: 10.1176/ajp.148.11.1474
De Lago, E., De Miguel, R., Lastres-Becker, I., Ramos, J. A., and Fernández-Ruiz, J. (2004). Involvement of vanilloid-like receptors in the effects of anandamide on motor behavior and nigrostriatal dopaminergic activity: in vivo and in vitro evidence. Brain Res. 1007, 152–159. doi: 10.1016/j.brainres.2004.02.016
Deadwyler, S. A., Hampson, R. E., Mu, J., Whyte, A., and Childers, S. (1995). Cannabinoids modulate voltage sensitive potassium A-current in hippocampal neurons via a cAMP-dependent process. J. Pharmacol. Exp. Ther. 273, 734–743.
Deisseroth, K. (2015). Optogenetics: 10 years of microbial opsins in neuroscience. Nat. Neurosci. 18, 1213–1225. doi: 10.1038/nn.4091
Del Pino, I., García-Frigola, C., Dehorter, N., Brotons-Mas, J. R., Alvarez-Salvado, E., Martínez de Lagrán, M., et al. (2013). Erbb4 deletion from Fast-Spiking interneurons causes Schizophrenia-like Phenotypes. Neuron 79, 1152–1168. doi: 10.1016/j.neuron.2013.07.010
Demuth, D. G., and Molleman, A. (2006). Cannabinoid signalling. Life Sci. 78, 549–563. doi: 10.1016/j.lfs.2005.05.055
Devane, W. A., Dysarz, F. A. III., Johnson, M. R., Melvin, L. S., and Howlett, A. C. (1988). Determination and characterization of a cannabinoid receptor in rat brain. Mol. Pharmacol. 34, 605–613.
Di Marzo, V. (2006). A brief history of cannabinoid and endocannabinoid pharmacology as inspired by the work of British scientists. Trends Pharmacol. Sci. 27, 134–140. doi: 10.1016/j.tips.2006.01.010
Dickerson, D. D., and Bilkey, D. K. (2013). Aberrant neural synchrony in the maternal immune activation model: using translatable measures to explore targeted interventions. Front. Behav. Neurosci. 7:217. doi: 10.3389/fnbeh.2013.00217
Dickerson, D. D., Restieaux, A. M., and Bilkey, D. K. (2012). Clozapine administration ameliorates disrupted long-range synchrony in a neurodevelopmental animal model of schizophrenia. Schizophr. Res. 135, 112–115. doi: 10.1016/j.schres.2011.12.016
Dickerson, D. D., Wolff, A. R., and Bilkey, D. K. (2010). Abnormal long-range neural synchrony in a maternal immune activation animal model of schizophrenia. J. Neurosci. 30, 12424–12431. doi: 10.1523/JNEUROSCI.3046-10.2010
Dinh, T. P., Carpenter, D., Leslie, F. M., Freund, T. F., Katona, I., Sensi, S. L., et al. (2002). Brain monoglyceride lipase participating in endocannabinoid inactivation. Proc. Natl. Acad. Sci. U.S.A. 99, 10819–10824. doi: 10.1073/pnas.152334899
Dissanayake, D. W. N., Zachariou, M., Marsden, C. A., and Mason, R. (2008). Auditory gating in rat hippocampus and medial prefrontal cortex: effect of the cannabinoid agonist WIN55,212-2. Neuropharmacology 55, 1397–1404. doi: 10.1016/j.neuropharm.2008.08.039
Draycott, B., Loureiro, M., Ahmad, T., Tan, H., Zunder, J., and Laviolette, S. R. (2014). Cannabinoid transmission in the prefrontal cortex bi-phasically controls emotional memory formation via functional interactions with the ventral tegmental area. J. Neurosci. 34, 13096–13109. doi: 10.1523/JNEUROSCI.1297-14.2014
Drew, L. J., Stark, K. L., Fénelon, K., Karayiorgou, M., Macdermott, A. B., and Gogos, J. A. (2011). Evidence for altered hippocampal function in a mouse model of the human 22q11.2 microdeletion. Mol. Cell. Neurosci. 47, 293–305. doi: 10.1016/j.mcn.2011.05.008
Du, H., Kwon, I. K., and Kim, J. (2013). Neuregulin-1 impairs the long-term depression of hippocampal inhibitory synapses by facilitating the degradation of endocannabinoid 2-AG. J. Neurosci. 33, 15022–15031. doi: 10.1523/JNEUROSCI.5833-12.2013
Ducharme, G., Lowe, G. C., Goutagny, R., and Williams, S. (2012). Early alterations in hippocampal circuitry and theta rhythm generation in a mouse model of prenatal infection: implications for schizophrenia. PLoS ONE 7:e29754. doi: 10.1371/journal.pone.0029754
Earls, L. R., Bayazitov, I. T., Fricke, R. G., Berry, R. B., Illingworth, E., Mittleman, G., et al. (2010). Dysregulation of presynaptic calcium and synaptic plasticity in a mouse model of 22q11 deletion syndrome. J. Neurosci. 30, 15843–15855. doi: 10.1523/JNEUROSCI.1425-10.2010
Edwards, C. R., Skosnik, P. D., Steinmetz, A. B., O'Donnell, B. F., and Hetrick, W. P. (2009). Sensory gating impairments in heavy cannabis users are associated with altered neural oscillations. Behav. Neurosci. 123, 894–904. doi: 10.1037/a0016328
Egertová, M., Cravatt, B. F., and Elphick, M. R. (2003). Comparative analysis of fatty acid amide hydrolase and cb(1) cannabinoid receptor expression in the mouse brain: evidence of a widespread role for fatty acid amide hydrolase in regulation of endocannabinoid signaling. Neuroscience 119, 481–496. doi: 10.1016/S0306-4522(03)00145-3
Eggan, S. M., and Lewis, D. A. (2007). Immunocytochemical distribution of the cannabinoid CB1 receptor in the primate neocortex: a regional and laminar analysis. Cereb. Cortex 17, 175–191. doi: 10.1093/cercor/bhj136
Eggan, S. M., Stoyak, S. R., Verrico, C. D., and Lewis, D. A. (2010). Cannabinoid CB1 receptor immunoreactivity in the prefrontal cortex: comparison of schizophrenia and major depressive disorder. Neuropsychopharmacology 35, 2060–2071. doi: 10.1038/npp.2010.75
Eguchi, N., Hishimoto, A., Sora, I., and Mori, M. (2016). Slow synaptic transmission mediated by TRPV1 channels in CA3 interneurons of the hippocampus. Neurosci. Lett. 616, 170–176. doi: 10.1016/j.neulet.2015.12.065
Ehrlichman, R. S., Gandal, M. J., Maxwell, C. R., Lazarewicz, M. T., Finkel, L. H., Contreras, D., et al. (2009). N-methyl-d-aspartic acid receptor antagonist-induced frequency oscillations in mice recreate pattern of electrophysiological deficits in schizophrenia. Neuroscience 158, 705–712. doi: 10.1016/j.neuroscience.2008.10.031
El Khoury, M., Gorgievski, V., Moutsimilli, L., Giros, B., and Tzavara, E. T. (2012). Interactions between the cannabinoid and dopaminergic systems: evidence from animal studies. Prog. Neuropsychopharmacol. Biol. Psychiatry 38, 36–50. doi: 10.1016/j.pnpbp.2011.12.005
Elphick, M. R. (2012). The evolution and comparative neurobiology of endocannabinoid signalling. Philos. Trans. R. Soc. Lond. B. Biol. Sci. 367, 3201–3215. doi: 10.1098/rstb.2011.0394
Enomoto, T., and Floresco, S. B. (2009). Disruptions in spatial working memory, but not short-term memory, induced by repeated ketamine exposure. Prog. Neuropsychopharmacol. Biol. Psychiatry 33, 668–675. doi: 10.1016/j.pnpbp.2009.03.013
Enomoto, T., Noda, Y., Mouri, A., Shin, E. J., Wang, D., Murai, R., et al. (2005). Long-lasting impairment of associative learning is correlated with a dysfunction of N-methyl-D-aspartate-extracellular signaling-regulated kinase signaling in mice after withdrawal from repeated administration of phencyclidine. Mol. Pharmacol. 68, 1765–1774. doi: 10.1124/mol.105.011304
Esteban, S., and García-Sevilla, J. A. (2012). Effects induced by cannabinoids on monoaminergic systems in the brain and their implications for psychiatric disorders. Prog. Neuropsychopharmacol. Biol. Psychiatry 38, 78–87. doi: 10.1016/j.pnpbp.2011.11.007
Fanselow, M. S. (1980). Conditional and unconditional components of post-shock freezing. Pavlov. J. Biol. Sci. Off. J. Pavlov. 15, 177–182. doi: 10.1007/BF03001163
Fenelon, K., Xu, B., Lai, C. S., Mukai, J., Markx, S., Stark, K. L., et al. (2013). The pattern of cortical dysfunction in a mouse model of a schizophrenia-related microdeletion. J. Neurosci. 33, 14825–14839. doi: 10.1523/JNEUROSCI.1611-13.2013
Ford, J. M., Mathalon, D. H., Whitfield, S., Faustman, W. O., and Roth, W. T. (2002). Reduced communication between frontal and temporal lobes during talking in schizophrenia. Biol. Psychiatry 51, 485–492. doi: 10.1016/S0006-3223(01)01335-X
Fride, E., and Mechoulam, R. (1993). Pharmacological activity of the cannabinoid receptor agonist, anandamide, a brain constituent. Eur. J. Pharmacol. 231, 313–314. doi: 10.1016/0014-2999(93)90468-W
Fujisawa, S., and Buzsáki, G. (2011). A 4 Hz oscillation adaptively synchronizes prefrontal, vta, and hippocampal activities. Neuron 72, 153–165. doi: 10.1016/j.neuron.2011.08.018
Fusar-Poli, P. (2009). Distinct effects of delta9-tetrahydrocannabinol and cannabidiol on neural activation during emotional processing. Arch. Gen. Psychiatry 66, 95–105. doi: 10.1001/archgenpsychiatry.2008.519
Geyer, M. A., Krebs-Thomson, K., Braff, D. L., and Swerdlow, N. R. (2001). Pharmacological studies of prepulse inhibition models of sensorimotor gating deficits in schizophrenia: a decade in review. Psychopharmacology Berl 156, 117–154. doi: 10.1007/s002130100811
Gibson, H. E., Edwards, J. G., Page, R. S., Van Hook, M. J., and Kauer, J. A. (2008). TRPV1 channels mediate long-term depression at synapses on hippocampal interneurons. Neuron 57, 746–759. doi: 10.1016/j.neuron.2007.12.027
Giuffrida, A., and Seillier, A. (2012). New insights on endocannabinoid transmission in psychomotor disorders. Prog. Neuropsychopharmacol. Biol. Psychiatry 38, 51–58. doi: 10.1016/j.pnpbp.2012.04.002
Giuffrida, A., Leweke, F. M., Gerth, C. W., Schreiber, D., Koethe, D., Faulhaber, J., et al. (2004). Cerebrospinal anandamide levels are elevated in acute schizophrenia and are inversely correlated with psychotic symptoms. Neuropsychopharmacology 29, 2108–2114. doi: 10.1038/sj.npp.1300558
Gomes, F. V., Guimarães, F. S., and Grace, A. A. (2015). Effects of pubertal cannabinoid administration on attentional set-shifting and dopaminergic hyper-responsivity in a developmental disruption model of schizophrenia. Int. J. Neuropsychopharmacol. 18, 1–10. doi: 10.1093/ijnp/pyu018
Gonzalez-Burgos, G., and Lewis, D. A. (2008). GABA neurons and the mechanisms of network oscillations: implications for understanding cortical dysfunction in schizophrenia. Schizophr. Bull. 34, 944–961. doi: 10.1093/schbul/sbn070
Gorka, S. M., Fitzgerald, D. A., De Wit, H., and Phan, K. L. (2015a). Cannabinoid modulation of amygdala subregion functional connectivity to social signals of threat. Int. J. Neuropsychopharmacol. 18, 1–6. doi: 10.1093/ijnp/pyu104
Gorka, S. M., Phan, K. L., Lyons, M., Mori, S., Angstadt, M., and Rabinak, C. A. (2015b). Cannabinoid modulation of frontolimbic activation and connectivity during volitional regulation of negative affect. Neuropsychopharmacology 41, 1–9. doi: 10.1038/npp.2015.359
Goto, Y., and Grace, A. A. (2006). Alterations in medial prefrontal cortical activity and plasticity in rats with disruption of cortical development. Biol. Psychiatry 60, 1259–1267. doi: 10.1016/j.biopsych.2006.05.046
Gruber, A. J., Calhoon, G. G., Shusterman, I., Schoenbaum, G., Roesch, M. R., and O'Donnell, P. (2010). More is less: a disinhibited prefrontal cortex impairs cognitive flexibility. J. Neurosci. 30, 17102–17110. doi: 10.1523/JNEUROSCI.4623-10.2010
Grueter, B. A., Brasnjo, G., and Malenka, R. C. (2010). Postsynaptic TRPV1 triggers cell type-specific long-term depression in the nucleus accumbens. Nat. Neurosci. 13, 1519–1525. doi: 10.1038/nn.2685
Grützner, C., Wibral, M., Sun, L., Rivolta, D., Singer, W., Maurer, K., et al. (2013). Deficits in high- (>60 Hz) gamma-band oscillations during visual processing in schizophrenia. Front. Hum. Neurosci. 7:88. doi: 10.3389/fnhum.2013.00088
Gururajan, A., and Malone, D. T. (2016). Does cannabidiol have a role in the treatment of schizophrenia? Schizophr. Res. 176, 281–290. doi: 10.1016/j.schres.2016.06.022
Haenschel, C., Bittner, R. A., Waltz, J., Haertling, F., Wibral, M., Singer, W., et al. (2009). Cortical oscillatory activity is critical for working memory as revealed by deficits in early-onset schizophrenia. J. Neurosci. 29, 9481–9489. doi: 10.1523/JNEUROSCI.1428-09.2009
Hajós, M., Hoffmann, W. E., and Kocsis, B. (2008). Activation of Cannabinoid-1 receptors disrupts sensory gating and neuronal oscillation: relevance to Schizophrenia. Biol. Psychiatry 63, 1075–1083. doi: 10.1016/j.biopsych.2007.12.005
Hakami, T., Jones, N. C., Tolmacheva, E. A., Gaudias, J., Chaumont, J., Salzberg, M., et al. (2009). NMDA receptor hypofunction leads to generalized and persistent aberrant gamma oscillations independent of hyperlocomotion and the state of consciousness. PLoS ONE 4:e6755. doi: 10.1371/journal.pone.0006755
Hanuš, L. O., Abu-Lafi, S., Fride, E., Breuer, A., Vogel, Z., Shalev, D. E., et al. (2001). 2-arachidonyl glyceryl ether, an endogenous agonist of the cannabinoid CB1 receptor. Proc. Natl. Acad. Sci. U.S.A. 98, 3662–3665. doi: 10.1073/pnas.061029898
Hanuš, L. O., Meyer, S. M., Muñoz, E., Taglialatela-Scafati, O., and Appendino, G. (2016). Phytocannabinoids: a unified critical inventory. Nat. Prod. Rep. 33, 1357–1392. doi: 10.1039/c6np00074f
Harrison, P. J. (1999). The neuropathology of schizophrenia: a critical review of the data and their interpretation. Brain 122, 593–624. doi: 10.1093/brain/122.4.593
Hartung, H., Cichon, N., De Feo, V., Riemann, S., Schildt, S., Lindemann, C., et al. (2016). From shortage to surge: a developmental switch in hippocampal–prefrontal coupling in a gene–environment model of neuropsychiatric disorders. Cereb. Cortex. 26, 4265–4281. doi: 10.1093/cercor/bhw274
Hashimotodani, Y., Ohno-Shosaku, T., Tsubokawa, H., Ogata, H., Emoto, K., Maeijma, T., et al. (2005). Phospholipase Cbeta serves as a coincidence detector through its Ca2+ dependency for triggering retrograde endocannabinoid signal. Neuron 45, 257–268. doi: 10.1016/j.neuron.2005.01.004
Heckers, S., Rauch, S. L., Goff, D., Savage, C. R., Schacter, D. L., Fischman, A. J., et al. (1998). Impaired recruitment of the hippocampus during conscious recollection in schizophrenia. Nat. Neurosci. 1, 318–323. doi: 10.1038/1137
Heifets, B. D., and Castillo, P. E. (2009). Endocannabinoid signaling and long-term synaptic plasticity. Annu. Rev. Physiol. 71, 283–306. doi: 10.1146/annurev.physiol.010908.163149
Heitland, I., Kenemans, J. L., Böcker, K. B. E., and Baas, J. M. P. (2014). Genetic variability in the human cannabinoid receptor 1 is associated with resting state EEG theta power in humans. Behav. Brain Res. 274, 344–348. doi: 10.1016/j.bbr.2014.08.003
Herkenham, M., Lynn, A. B., Litrle, M. D., Johnsont, M. R., Melvin, L. S., De Costa, B. R., et al. (1990). Cannabinoid receptor localization in brain. Prog. Natl. Acad. Sci. U. S. A. 87, 1932–1936. doi: 10.1073/pnas.87.5.1932
Hermann, A., Kaczocha, M., and Deutsch, D. G. (2006). 2-Arachidonoylglycerol (2-AG) membrane transport: history and outlook. AAPS J. 8, E409–E412. doi: 10.1208/aapsj080247
Hirano, S., Hirano, Y., Maekawa, T., Obayashi, C., Oribe, N., Kuroki, T., et al. (2008). Abnormal neural oscillatory activity to speech sounds in schizophrenia: a magnetoencephalography study. J. Neurosci. 28, 4897–4903. doi: 10.1523/JNEUROSCI.5031-07.2008
Homayoun, H., and Moghaddam, B. (2007). NMDA receptor hypofunction produces opposite effects on prefrontal cortex interneurons and pyramidal neurons. J. Neurosci. 27, 11496–11500. doi: 10.1523/JNEUROSCI.2213-07.2007
Hong, L. E., Summerfelt, A., McMahon, R., Adami, H., Francis, G., Elliott, A., et al. (2004). Evoked gamma band synchronization and the liability for schizophrenia. Schizophr. Res. 70, 293–302. doi: 10.1016/j.schres.2003.12.011
Howlett, A. C., and Fleming, R. M. (1984). Cannabinoid inhibition of adenylate cyclase. Pharmacology of the response in neuroblastoma cell membranes. Mol. Pharmacol. 26, 532–538.
Howlett, A. C., Barth, F., Bonner, T. I., Cabral, G., Casellas, P., Devane, W. A., et al. (2002). International union of pharmacology. XXVII. Classification of cannabinoid receptors. Pharmacol. Rev. 54, 161–202. doi: 10.1124/pr.54.2.161
Hu, S. S., and Mackie, K. (2015). Distribution of the endocannabinoid system in the central nervous system. Handb. Exp. Pharmacol. 231, 59–93. doi: 10.1007/978-319-20825-1_3
Ibeas Bih, C., Chen, T., Nunn, A. V. W., Bazelot, M., Dallas, M., and Whalley, B. J. (2015). Molecular targets of cannabidiol in neurological disorders. Neurotherapeutics 12, 699–730. doi: 10.1007/s13311-015-0377-3
Ilan, A. B., Smith, M. E., and Gevins, A. (2004). Effects of marijuana on neurophysiological signals of working and episodic memory. Psychopharmacology Berl. 176, 214–222. doi: 10.1007/s00213-004-1868-9
Immke, D. C., and Gavva, N. R. (2006). The TRPV1 receptor and nociception. Semin. Cell Dev. Biol. 17, 582–591. doi: 10.1016/j.semcdb.2006.09.004
Izzo, A. A., Borrelli, F., Capasso, R., Di Marzo, V., and Mechoulam, R. (2009). Non-psychotropic plant cannabinoids: new therapeutic opportunities from an ancient herb. Trends Pharmacol. Sci. 30, 515–527. doi: 10.1016/j.tips.2009.07.006
Jackson, M. E., Homayoun, H., and Moghaddam, B. (2004). NMDA receptor hypofunction produces concomitant firing rate potentiation and burst activity reduction in the prefrontal cortex. Proc. Natl. Acad. Sci. U.S.A. 101, 8467–8472. doi: 10.1073/pnas.0308455101
Javitt, D. C., and Zukin, S. R. (1991). Recent advance in the phencyclidine model of schizophrenia. Am. J. Psychiatry 148, 1301–1308.
Jenko, K. J., Hirvonen, J., Henter, I. D., Anderson, K. B., Zoghbi, S. S., Hyde, T. M., et al. (2012). Binding of a tritiated inverse agonist to cannabinoid CB1 receptors is increased in patients with schizophrenia. Schizophr. Res. 141, 185–188. doi: 10.1016/j.schres.2012.07.021
Jentsch, J. D., Tran, A., Le, D., Youngren, K. D., and Roth, R. H. (1997). Subchronic phencyclidine administration reduces mesoprefrontal dopamine utilization and impairs prefrontal cortical-dependent cognition in the rat. Neuropsychopharmacology 17, 92–99. doi: 10.1016/S0893-133X(97)00034-1
Jew, C. P., Wu, C. S., Sun, H., Zhu, J., Huang, J. Y., Yu, D., et al. (2013). mGluR5 ablation in cortical glutamatergic neurons increases novelty-induced locomotion. PLoS ONE 8:e70415. doi: 10.1371/journal.pone.0070415
Johannesen, J. K., Bodkins, M., O'Donnell, B. F., Shekhar, A., and Hetrick, W. P. (2008). Perceptual anomalies in schizophrenia co-occur with selective impairments in the gamma frequency component of midlatency auditory ERPs. J. Abnorm. Psychol. 117, 106–118. doi: 10.1037/0021-843X.117.1.106
Jonas, R. K., Montojo, C. A., and Bearden, C. E. (2014). The 22q11.2 deletion syndrome as a window into complex neuropsychiatric disorders over the lifespan. Biol. Psychiatry 75, 351–360. doi: 10.1016/j.biopsych.2013.07.019
Juan, L. W., Liao, C. C., Lai, W. S., Chang, C. Y., Pei, J. C., Wong, W. R., et al. (2014). Phenotypic characterization of C57BL/6J mice carrying the Disc1 gene from the 129S6/SvEv strain. Brain Struct. Funct. 219, 1417–1431. doi: 10.1007/s00429-013-0577-8
Juckel, G., Roser, P., Nadulski, T., Stadelmann, A. M., and Gallinat, J. (2007). Acute effects of Δ9-tetrahydrocannabinol and standardized cannabis extract on the auditory evoked mismatch negativity. Schizophr. Res. 97, 109–117. doi: 10.1016/j.schres.2007.08.015
Kano, M. (2014). Control of synaptic function by endocannabinoid-mediated retrograde signaling. Proc. Jpn. Acad. Ser. B. Phys. Biol. Sci. 90, 235–250. doi: 10.2183/pjab.90.235
Kano, M., Ohno-Shosaku, T., Hashimotodani, Y., Uchigashima, M., and Watanabe, M. (2009). Endocannabinoid-mediated control of synaptic transmission. Physiol. Rev. 89, 309–380. doi: 10.1152/physrev.00019.2008
Kapur, S., and Remington, G. (2001). Dopamine D(2) receptors and their role in atypical antipsychotic action: still necessary and may even be sufficient. Biol Psychiatry 50, 873–883.
Karasawa, J., Hashimoto, K., and Chaki, S. (2008). d-Serine and a glycine transporter inhibitor improve MK-801-induced cognitive deficits in a novel object recognition test in rats. Behav. Brain Res. 186, 78–83. doi: 10.1016/j.bbr.2007.07.033
Karson, M. A., Tang, A. H., Milner, T. A., and Alger, B. E. (2009). Synaptic cross talk between perisomatic-targeting interneuron classes expressing cholecystokinin and parvalbumin in hippocampus. J. Neurosci. 29, 4140–4154. doi: 10.1523/JNEUROSCI.5264-08.2009
Kathmann, M., Flau, K., Redmer, A., Tränkle, C., and Schlicker, E. (2006). Cannabidiol is an allosteric modulator at mu- and delta-opioid receptors. Naunyn. Schmeideb. Arch. Pharmacol. 372, 354–361. doi: 10.1007/s00210-006-0033-x
Katona, I., Sperlágh, B., Sík, A., Käfalvi, A., Vizi, E. S., and Mackie, K. (1999). Presynaptically Located CB1 Cannabinoid Receptors Regulate GABA Release from Axon Terminals of Specific. J. Neurosci. 19, 4544–4558.
Katona, I., Urba, G. M., Wallace, M., Ledent, C., Jung, K., Piomelli, D., et al. (2006). Molecular composition of the endocannabinoid system at glutamatergic synapses. J. Neurosci. 26, 5628–5637. doi: 10.1523/JNEUROSCI.0309-06.2006
Keimpema, E., Straiker, A., Mackie, K., Harkany, T., and Hjerling-Leffler, J. (2012). Sticking out of the crowd: the molecular identity and development of cholecystokinin-containing basket cells. J. Physiol. 590, 703–714. doi: 10.1113/jphysiol.2011.224386
Kendall, D. A., and Yudowski, G. A. (2017). Cannabinoid receptors in the central nervous system: their signaling and roles in disease. Front. Cell Neurosci. 10:294. doi: 10.3389/fncel.2016.00294
Kim, J., and Li, Y. (2015). Chronic activation of CB2 cannabinoid receptors in the hippocampus increases excitatory synaptic transmission. J. Physiol. 593, 871–886. doi: 10.1113/jphysiol.2014.286633
Kim, Y. C., Han, S. W., Alberico, S. L., Ruggiero, R. N., De Corte, B., Chen, K. H., et al. (2016). Optogenetic stimulation of frontal D1 neurons compensates for impaired temporal control of action in dopamine-depleted mice. Curr. Biol. 27, 39–47. doi: 10.1016/j.cub.2016.11.029
Kitaichi, K., Yamada, K., Hasegawa, T., Furukawa, H., and Nabeshima, T. (1994). Effects of risperidone on phencyclidine-induced behaviors: comparison with haloperidol and ritanserin. Jpn. J. Pharmacol 66, 181–189.
Kocsis, B., Brown, R. E., Mccarley, R. W., and Hajos, M. (2013). Impact of ketamine on neuronal network dynamics: translational modeling of schizophrenia-relevant deficits. CNS Neurosci. Ther. 19, 437–447. doi: 10.1111/cns.12081
Koenig, T., Lehmann, D., Saito, N., Kuginuki, T., Kinoshita, T., and Koukkou, M. (2001). Decreased functional connectivity of EEG theta-frequency activity in first-episode, neuroleptic-naïve patients with schizophrenia: preliminary results. Schizophr. Res. 50, 55–60. doi: 10.1016/S0920-9964(00)00154-7
Koethe, D., Giuffrida, A., Schreiber, D., Hellmich, M., Schultze-Lutter, F., Ruhrmann, S., et al. (2009). Anandamide elevation in cerebrospinal fluid in initial prodromal states of psychosis. Br. J. Psychiatry 194, 371–372. doi: 10.1192/bjp.bp.108.053843
Koethe, D., Llenos, I. C., Dulay, J. R., Hoyer, C., Torrey, E. F., Leweke, F. M., et al. (2007). Expression of CB1 cannabinoid receptor in the anterior cingulate cortex in schizophrenia, bipolar disorder, and major depression. J. Neural Transm. 114, 1055–1063. doi: 10.1007/s00702-007-0660-5
Koike, H., Arguello, P. A., Kvajo, M., Karayiorgou, M., and Gogos, J. A. (2006). Disc1 is mutated in the 129S6/SvEv strain and modulates working memory in mice. Proc. Natl. Acad. Sci. U.S.A. 103, 3693–3697. doi: 10.1073/pnas.0511189103
Korostenskaja, M., and Kähkönen, S. (2009). What do ERPs and ERFs reveal about the effect of antipsychotic treatment on cognition in schizophrenia? Curr. Pharm. Des. 15, 2573–2593. doi: 10.2174/138161209788957474
Korotkova, T., Fuchs, E. C., Ponomarenko, A., von Engelhardt, J., and Monyer, H. (2010). NMDA Receptor Ablation on Parvalbumin-Positive interneurons impairs hippocampal synchrony, spatial representations, and working memory. Neuron 68, 557–569. doi: 10.1016/j.neuron.2010.09.017
Krystal, J. H., Karper, L. P., Seibyl, J. P., Freeman, G. K., Delaney, R., Bremner, J. D., et al. (1994). Subanesthetic effects of the noncompetitive NMDA antagonist, ketamine, in humans. Psychotomimetic, perceptual, cognitive and neuroendocrine responses. Arch. Gen. Psychiatry 51, 199–214. doi: 10.1001/archpsyc.1994.03950030035004
Kuepper, R., Ceccarini, J., Lataster, J., van Os, J., van Kroonenburgh, M., van Gerven, J. M. A., et al. (2013). Delta-9-tetrahydrocannabinol-induced dopamine release as a function of psychosis risk: 18f-fallypride positron emission tomography study. PLoS ONE 8:e70378. doi: 10.1371/journal.pone.0070378
Kulikova, S. P., Tolmacheva, E. A., Anderson, P., Gaudias, J., Adams, B. E., Zheng, T., et al. (2012). Opposite effects of ketamine and deep brain stimulation on rat thalamocortical information processing. Eur. J. Neurosci. 36, 3407–3419. doi: 10.1111/j.1460-9568.2012.08263.x
Kvajo, M., McKellar, H., Arguello, P. A., Drew, L. J., Moore, H., MacDermott, A. B., et al. (2008). A mutation in mouse Disc1 that models a schizophrenia risk allele leads to specific alterations in neuronal architecture and cognition. Proc. Natl. Acad. Sci. U.S.A. 105, 7076–7081. doi: 10.1073/pnas.0802615105
Kvajo, M., McKellar, H., Drew, L. J., Lepagnol-Bestel, A. M., Xiao, L., Levy, R. J., et al. (2011). Altered axonal targeting and short-term plasticity in the hippocampus of Disc1 mutant mice. Proc. Natl. Acad. Sci. U.S.A. 108, E1349–E1358. doi: 10.1073/pnas.1114113108
Kwon, J. S., O'Donnell, B. F., Wallenstein, G. V., Greene, R. W., Hirayasu, Y., Nestor, P. G., et al. (1999). Gamma frequency-range abnormalities to auditory stimulation in schizophrenia. Arch. Gen. Psychiatry 56, 1001–1005. doi: 10.1007/s11920-010-0124-8
Lafourcade, M., Elezgarai, I., Mato, S., Bakiri, Y., Grandes, P., and Manzoni, O. J. (2007). Molecular components and functions of the endocannabinoid system in mouse prefrontal cortex. PLoS ONE 2:e709 doi: 10.1371/journal.pone.0000709
Lakatos, P., Schroeder, C. E., Leitman, D. I., and Javitt, D. C. (2013). Predictive suppression of cortical excitability and its deficit in schizophrenia. J. Neurosci. 33, 11692–11702. doi: 10.1523/JNEUROSCI.0010-13.2013
Lang, S., Duncan, N., and Northoff, G. (2014). Resting-state functional magnetic resonance imaging: review of neurosurgical applications. Neurosurgery 74, 453–464. doi: 10.1227/NEU.0000000000000307
Large, M., Sharma, S., Compton, M. T., Slade, T., and Nielssen, O. (2011). Cannabis use and earlier onset of psychosis: a systematic meta-analysis. Arch. Gen. Psychiatry 68, 555–561. doi: 10.1016/j.ypsy.2011.09.029
Laruelle, M. (1998). Imaging dopamine transmission in schizophrenia. A review and meta-analysis Q. J. Nucl. Med. 42, 211–221.
Laviolette, S. R., and Grace, A. A. (2006). Cannabinoids potentiate emotional learning plasticity in neurons of the medial prefrontal cortex through basolateral amygdala inputs. J. Neurosci. 26, 6458–6468. doi: 10.1523/JNEUROSCI.0707-06.2006
Lazarewicz, M. T., Ehrlichman, R. S., Maxwell, C. R., Gandal, M. J., Finkel, L. H., and Siegel, S. J. (2009). Ketamine modulates theta and gamma oscillations. J. Cogn. Neurosci. 22, 1452–1464. doi: 10.1162/jocn.2009.21305
Lee, H., Dvorak, D., and Fenton, A. A. (2014). Targeting neural synchrony deficits is sufficient to improve cognition in a schizophrenia-related neurodevelopmental model. Front. Psychiatry 5:e15. doi: 10.3389/fpsyt.2014.00015
Lee, J., Di Marzo, V., and Brotchie, J. M. (2006). A role for vanilloid receptor 1 (TRPV1) and endocannabinnoid signalling in the regulation of spontaneous and L-DOPA induced locomotion in normal and reserpine-treated rats. Neuropharmacology 51, 557–565. doi: 10.1016/j.neuropharm.2006.04.016
Lee, M. C., Ploner, M., Wiech, K., Bingel, U., Wanigasekera, V., Brooks, J., et al. (2013). Amygdala activity contributes to the dissociative effect of cannabis on pain perception. Pain 154, 124–134. doi: 10.1016/j.pain.2012.09.017
Leuchter, A. F., Cook, I. A., Hunter, A. M., Cai, C., and Horvath, S. (2012). Resting-state quantitative electroencephalography reveals increased neurophysiologic connectivity in depression. PLoS ONE 7:e32508. doi: 10.1371/journal.pone.0032508
Leweke, F. M. (2012). Anandamide dysfunction in prodromal and established psychosis. Curr. Pharm. Des. 18, 5188–5193.
Leweke, F. M., Giuffrida, A., Koethe, D., Schreiber, D., Nolden, B. M., Kranaster, L., et al. (2007). Anandamide levels in cerebrospinal fluid of first-episode schizophrenic patients: impact of cannabis use. Schizophr. Res. 94, 29–36. doi: 10.1016/j.schres.2007.04.025
Lewis, D. A., and Levitt, P. (2002). Schizophrenia as a disorder of neurodevelopment. Annu. Rev. Neurosci. 25, 409–432. doi: 10.1146/annurev.neuro.25.112701.142754
Lewis, D. A., Curley, A. A., Glausier, J. R., and Volk, D. W. (2012). Cortical parvalbumin interneurons and cognitive dysfunction in schizophrenia. Trends Neurosci. 35, 57–67. doi: 10.1016/j.tins.2011.10.004
Li, Y., and Kim, J. (2016). Deletion of CB2 cannabinoid receptors reduces synaptic transmission and long-term potentiation in the mouse hippocampus. Hippocampus 26, 275–281. doi: 10.1002/hipo.22558
Lieberman, J. A., Stroup, T. S., McEvoy, J., Swartz, M. S., Rosenheck, R., Perkins, D. O., et al. (2005). Effectiveness of antipsychotic drugs in patients with chronic schizophrenia. N. Engl. J. Med. 353, 1209–1223. doi: 10.1056/NEJMp1415160
Light, G. A., Hsu, J. L., Hsieh, M. H., Meyer-Gomes, K., Sprock, J., Swerdlow, N. R., et al. (2006). Gamma band oscillations reveal neural network cortical coherence dysfunction in schizophrenia patients. Biol. Psychiatry 60, 1231–1240. doi: 10.1016/j.biopsych.2006.03.055
Ligresti, A., De Petrocellis, L., and Di Marzo, V. (2016). From phytocannabinoids to cannabinoid receptors and endocannabinoids: pleiotropic physiological and pathological roles through complex pharmacology. Physiol. Rev. 96, 1593–1659. doi: 10.1152/physrev.00002.2016
Lipska, B. K., Aultman, J. M., Verma, A., Weinberger, D. R., and Moghaddam, B. (2002). Neonatal damage of the rat ventral hippocampus impairs acquisition of a working memory task. Neuropsychopharmacology 27, 47–54. doi: 10.1016/S0893-133X(02)00282-8
Lisman, J. (2012). Excitation, inhibition, local oscillations, or large-scale loops: what causes the symptoms of schizophrenia? Curr. Opin. Neurobiol. 22, 537–544. doi: 10.1016/j.conb.2011.10.018
Liu, J., Chen, J., Ehrlich, S., Walton, E., White, T., Perrone-Bizzozero, N., et al. (2014). Methylation patterns in whole blood correlate with symptoms in schizophrenia patients. Schizophr. Bull. 40, 769–776. doi: 10.1093/schbul/sbt080
Liu, J., Wang, L., Harvey-White, J., Huang, B. X., Kim, H. Y., Luquet, S., et al. (2008). Multiple pathways involved in the biosynthesis of anandamide. Neuropharmacology 54, 1–7. doi: 10.1016/j.neuropharm.2007.05.020
Lodge, D. J., and Grace, A. A. (2007). Aberrant hippocampal activity underlies the dopamine dysregulation in an animal model of schizophrenia. J. Neurosci. 27, 11424–11430. doi: 10.1523/JNEUROSCI.2847-07.2007
Lodge, D. J., Behrens, M. M., and Grace, A. A. (2009). A loss of parvalbumin-containing interneurons is associated with diminished oscillatory activity in an animal model of schizophrenia. J. Neurosci. 29, 2344–2354. doi: 10.1523/JNEUROSCI.5419-08.2009
Long, J. M., LaPorte, P., Merscher, S., Funke, B., Saint-Jore, B., Puech, A., et al. (2006). Behavior of mice with mutations in the conserved region deleted in velocardiofacial/DiGeorge syndrome. Neurogenetics 7, 247–257. doi: 10.1007/s10048-006-0054-0
López-Moreno, J. A., González-Cuevas, G., Moreno, G., and Navarro, M. (2008). The pharmacology of the endocannabinoid system: functional and structural interactions with other neurotransmitter systems and their repercussions in behavioral addiction. Addict. Biol. 13, 160–187. doi: 10.1111/j.1369-1600.2008.00105.x
Loureiro, M., Kramar, C., Renard, J., Rosen, L. G., and Laviolette, S. R. (2016). Cannabinoid transmission in the hippocampus activates nucleus accumbens neurons and modulates reward and aversion-related emotional salience. Biol. Psychiatry 80, 216–225. doi: 10.1016/j.biopsych.2015.10.016
Loureiro, M., Renard, J., Zunder, J., and Laviolette, S. R. (2015). Hippocampal cannabinoid transmission modulates dopamine neuron activity: impact on rewarding memory formation and social interaction. Neuropsychopharmacology 40, 1436–1447. doi: 10.1038/npp.2014.329
Lovelace, J. W., Corches, A., Vieira, P. A., Hiroto, A. S., Mackie, K., and Korzus, E. (2015). An animal model of female adolescent cannabinoid exposure elicits a long-lasting deficit in presynaptic long-term plasticity. Neuropharmacology 99, 242–255. doi: 10.1016/j.neuropharm.2015.04.034
Lovelace, J. W., Vieira, P. A., Corches, A., Mackie, K., and Korzus, E. (2014). Impaired fear memory specificity associated with deficient endocannabinoid-dependent long-term plasticity. Neuropsychopharmacology 39, 1685–1693. doi: 10.1038/npp.2014.15
Lu, H. C., and Mackie, K. (2016). An introduction to the endogenous cannabinoid system. Biol. Psychiatry 79, 516–525. doi: 10.1016/j.biopsych.2015.07.028
Luby, E. D., Gottlieb, J. S., Cohen, B. D., Rosenbaum, G., and Domino, E. F. (1962). Model psychoses and schizophrenia. Am. J. Psychiatry 119, 61–67. doi: 10.1176/ajp.119.1.61
Ma, J., and Leung, L. W. (2000). Relation between hippocampal gamma waves and behavioral disturbances induced by phencyclidine and methamphetamine. Behav. Brain Res. 111, 1–11. doi: 10.1016/S0166-4328(00)00138-8
Maccarrone, M., Guzmán, M., Mackie, K., Doherty, P., and Harkany, T. (2014). Programming of neural cells by (endo)cannabinoids: from physiological rules to emerging therapies. Nat. Rev. Neurosci. 15, 786–801. doi: 10.1038/nrn3846
Maccarrone, M., Rossi, S., Bari, M., De Chiara, V., Fezza, F., Musella, A., et al. (2008). Anandamide inhibits metabolism and physiological actions of 2-arachidonoylglycerol in the striatum. Nat. Neurosci. 11, 152–159. doi: 10.1038/nn2042
Mackie, K. (2005). Distribution of cannabinoid receptors in the central and peripheral nervous system. Handb. Exp. Pharmacol. 168, 299–325. doi: 10.1007/3-540-26573-2_10
Mackie, K., and Hille, B. (1992). Cannabinoids inhibit N-type calcium channels in neuroblastoma-glioma cells. Proc. Natl. Acad. Sci. U.S.A. 89, 3825–3829.
Madasu, M. K., Roche, M., and Finn, D. P. (2015). Supraspinal transient receptor potential subfamily V member 1 (TRPV1) in pain and psychiatric disorders. Mod. Trends Pharmacopsychiatry 30, 80–93. doi: 10.1159/000435934
Malek, N., and Starowicz, K. (2016). Dual-acting compounds targeting endocannabinoid and endovanilloid systems-a novel treatment option for chronic pain management. Front. Pharmacol. 7:257. doi: 10.3389/fphar.2016.00257
Manseau, M. W., and Goff, D. C. (2015). Cannabinoids and schizophrenia: risks and therapeutic potential. Neurotherapeutics 12, 816–824. doi: 10.1007/s13311-015-0382-6
Marinelli, S., Di Marzo, V., Berretta, N., Matias, I., Maccarrone, M., Bernardi, G., et al. (2003). Presynaptic facilitation of glutamatergic synapses to dopaminergic neurons of the rat substantia nigra by endogenous stimulation of vanilloid receptors. J. Neurosci. 23, 3136–3144.
Marinelli, S., Di Marzo, V., Florenzano, F., Fezza, F., Viscomi, M. T., van der Stelt, M., et al. (2007). N-arachidonoyl-dopamine tunes synaptic transmission onto dopaminergic neurons by activating both cannabinoid and vanilloid receptors. Neuropsychopharmacology 32, 298–308. doi: 10.1038/sj.npp.1301118
Marinelli, S., Pascucci, T., Bernardi, G., Puglisi-Allegra, S., and Mercuri, N. B. (2005). Activation of TRPV1 in the VTA excites dopaminergic neurons and increases chemical- and noxious-induced dopamine release in the nucleus accumbens. Neuropsychopharmacology 30, 864–870. doi: 10.1038/sj.npp.1300615
Marsch, R., Foeller, E., Rammes, G., Bunck, M., Kössl, M., Holsboer, F., et al. (2007). Reduced anxiety, conditioned fear, and hippocampal long-term potentiation in transient receptor potential vanilloid type 1 receptor-deficient mice. J. Neurosci. 27, 832–839. doi: 10.1523/JNEUROSCI.3303-06.2007
Mathalon, D. H., Ford, J. M., and Pfefferbaum, A. (2000). Trait and state aspects of p300 amplitude reduction in schizophrenia: a retrospective longitudinal study. Biol. Psychiatry 47, 434–449. doi: 10.1016/S0006-3223(99)00277-2
Matsuda, L. A., Lolait, S. J., Brownstein, M. J., Young, A. C., and Bonner, T. I. (1990). Structure of a cannabinoid receptor and functional expression of the cloned cDNA. Nature 346, 561–564. doi: 10.1038/346561a0
McNally, J. M., and McCarley, R. W. (2016). Gamma band oscillations: a key to understanding schizophrenia symptoms and neural circuit abnormalities. Curr. Opin. Psychiatry 29, 202–210. doi: 10.1097/YCO.0000000000000244
Mechoulam, B. R., and Gaoni, Y. (1967). Recent advances in the chemistry of hashish. Fortschr. Chem. Org. Naturst. 25, 175–213.
Mechoulam, R., and Parker, L. A. (2013). The endocannabinoid system and the brain. Annu. Rev. Psychol. 64, 21–47. doi: 10.1146/annurev-psych-113011-143739
Mechoulam, R., Feigenbaum, J. J., Lander, N., Segal, M., Jarbe, T. U. C., Consroe, P., et al. (1988). Enantiomeric cannabinoids: stereospecificity of psychotropic activity. Experientia 44, 762–764.
Melis, M., Perra, S., Muntoni, A. L., Pillolla, G., Lutz, B., Marsicano, G., et al. (2004a). Prefrontal cortex stimulation induces 2-arachidonoyl-glycerol-mediated suppression of excitation in dopamine neurons. J. Neurosci. 24, 10707–10715. doi: 10.1523/JNEUROSCI.3502-04.2004
Melis, M., Pistis, M., Perra, S., Muntoni, A. L., Pillolla, G., and Gessa, G. L. (2004b). Endocannabinoids mediate presynaptic inhibition of glutamatergic transmission in rat ventral tegmental area dopamine neurons through activation of CB1 receptors. J. Neurosci. 24, 53–62. doi: 10.1523/JNEUROSCI.4503-03.2004
Mesholam-Gately, R. I., Giuliano, A. J., Goff, K. P., Faraone, S. V., and Seidman, L. J. (2009). Neurocognition in first-episode schizophrenia: a meta-analytic review. Neuropsychology 23, 315–336. doi: 10.1037/a0014708
Meyer-Lindenberg, A. S., Olsen, R. K., Kohn, P. D., Brown, T., Egan, M. F., Weinberger, D. R., et al. (2005). Regionally specific disturbance of dorsolateral prefrontal-hippocampal functional connectivity in schizophrenia. Arch. Gen. Psychiatry 62, 379–386. doi: 10.1001/archpsyc.62.4.379
Mezey, E., Toth, Z. E., Cortright, D. N., Arzubi, M. K., Krause, J. E., Elde, R., et al. (2000). Distribution of mRNA for vanilloid receptor subtype 1 (VR1), and VR1-like immunoreactivity, in the central nervous system of the rat and human. Proc. Natl. Acad. Sci. U.S.A. 97, 3655–3660. doi: 10.1073/pnas.97.7.3655
Michie, P. T., Innes-Brown, H., Todd, J., and Jablensky, A. V. (2002). Duration mismatch negativity in biological relatives of patients with schizophrenia spectrum disorders. Biol. Psychiatry 52, 749–758. doi: 10.1016/S0006-3223(02)01379-3
Miller, L. K., and Devi, L. A. (2011). The highs and lows of cannabinoid receptor expression in disease: mechanisms and their therapeutic implications. Pharmacol. Rev. 63, 461–470. doi: 10.1124/pr.110.003491
Minzenberg, M. J., Firl, A. J., Yoon, J. H., Gomes, G. C., Reinking, C., and Carter, C. S. (2010). Gamma oscillatory power is impaired during cognitive control independent of medication status in first-episode schizophrenia. Neuropsychopharmacology 35, 2590–2599. doi: 10.1038/npp.2010.150
Mizoguchi, H., Takuma, K., Fukakusa, A., Ito, Y., Nakatani, A., Ibi, D., et al. (2008). Improvement by minocycline of methamphetamine-induced impairment of recognition memory in mice. Psychopharmacology (Berl). 196, 233–241. doi: 10.1007/s00213-007-0955-0
Moran, P., Stokes, J., Marr, J., Bock, G., Desbonnet, L., Waddington, J., et al. (2016). Gene x environment interactions in schizophrenia: evidence from genetic mouse models. Neural Plast. 2016:2173748. doi: 10.1155/2016/2173748
Moreira, F. A., Aguiar, D. C., Terzian, A. L. B., Guimarães, F. S., and Wotjak, C. T. (2012). Cannabinoid type 1 receptors and transient receptor potential vanilloid type 1 channels in fear and anxiety-two sides of one coin? Neuroscience 204, 186–192. doi: 10.1016/j.neuroscience.2011.08.046
Morgan, C. J. A., Page, E., Schaefer, C., Chatten, K., Manocha, A., Gulati, S., et al. (2013). Cerebrospinal fluid anandamide levels, cannabis use and psychotic-like symptoms. Br. J. Psychiatry 202, 381–382. doi: 10.1192/bjp.bp.112.121178
Mori, F., Ribolsi, M., Kusayanagi, H., Monteleone, F., Mantovani, V., Buttari, F., et al. (2012). TRPV1 channels regulate cortical excitability in humans. J. Neurosci. 32, 873–879. doi: 10.1523/JNEUROSCI.2531-11.2012
Morra, J. T., Glick, S. D., and Cheer, J. F. (2012). Cannabinoid receptors mediate methamphetamine induction of high frequency gamma oscillations in the nucleus accumbens. Neuropharmacology 63, 565–574. doi: 10.1016/j.neuropharm.2012.04.036
Morris, B. J., Cochran, S. M., and Pratt, J. A. (2005). PCP: from pharmacology to modelling schizophrenia. Curr. Opin. Pharmacol. 5, 101–106. doi: 10.1016/j.coph.2004.08.008
Morrison, P. D., Nottage, J., Stone, J. M., Bhattacharyya, S., Tunstall, N., Brenneisen, R., et al. (2011). Disruption of frontal theta coherence by delta(9)-tetrahydrocannabinol is associated with positive psychotic symptoms. Neuropsychopharmacology 36, 827–836. doi: 10.1038/npp.2010.222
Munro, S., Thomas, K. L., and Abu-Shaar, M. (1993). Molecular characterization of a peripheral receptor of cannabinoids. Nature 365, 61–65.
Näätänen, R., and Kähkönen, S. (2009). Central auditory dysfunction in schizophrenia as revealed by the mismatch negativity (MMN) and its magnetic equivalent MMNm: a review. Int. J. Neuropsychopharmacol. 12, 125–135. doi: 10.1017/S1461145708009322
Nagode, D.A., Tang, A.-H., Yang, K., and Alger, B. E. (2014). Optogenetic identification of an intrinsic cholinergically driven inhibitory oscillator sensitive to cannabinoids and opioids in hippocampal CA1. J. Physiol. 592, 103–123. doi: 10.1113/jphysiol.2013.257428
Nakao, K., and Nakazawa, K. (2014). Brain state-dependent abnormal LFP activity in the auditory cortex of a schizophrenia mouse model. Front. Neurosci. 8:168. doi: 10.3389/fnins.2014.00168
Nason, M. W., Adhikari, A., Bozinoski, M., Gordon, J. A., and Role, L. W. (2011). Disrupted activity in the hippocampal-accumbens circuit of type III neuregulin 1 mutant mice. Neuropsychopharmacology 36, 488–496. doi: 10.1038/npp.2010.180
Naziroğlu, M., and Demirdaş, A. (2015). Psychiatric disorders and TRP channels: focus on psychotropic drugs. Curr. Neuropharmacol. 13, 248–257. doi: 10.2174/1570159X13666150304001606
Newell, K. A., Deng, C., and Huang, X. F. (2006). Increased cannabinoid receptor density in the posterior cingulate cortex in schizophrenia. Exp. Brain Res. 172, 556–560. doi: 10.1007/s00221-006-0503-x
Nicolussi, S., and Gertsch, J. (2015). Endocannabinoid transport revisited. Vitam. Horm. 98, 441–485. doi: 10.1016/bs.vh.2014.12.011
Nomoto, M., Ohkawa, N., Nishizono, H., Yokose, J., Suzuki, A., Matsuo, M., et al. (2016). Cellular tagging as a neural network mechanism for behavioural tagging. Nat. Commun. 7, 10–12. doi: 10.1038/ncomms12319
Nottage, J. F., Stone, J., Murray, R. M., Sumich, A., Bramon-Bosch, E., Ffytche, D., et al. (2014). Delta-9-tetrahydrocannabinol, neural oscillations above 20 Hz and induced acute psychosis. Psychopharmacology Berl. 232, 519–528. doi: 10.1007/s00213-014-3684-1
O'Donnell, B. F., Vohs, J. L., Krishnan, G. P., Rass, O., Hetrick, W. P., and Morzorati, S. L. (2013). The auditory steady-state response (ASSR): a translational biomarker for schizophrenia. Suppl. Clin. Neurophysiol. 62, 101–112. doi: 10.1016/B978-0-7020-5307-8.00006-5
O'Donnell, P. (2012). Cortical disinhibition in the neonatal ventral hippocampal lesion model of schizophrenia: new vistas on possible therapeutic approaches. Pharmacol. Ther. 133, 19–25. doi: 10.1016/j.pharmthera.2011.07.005
O'Donnell, P., Lewis, B. L., Weinberger, D. R., and Lipska, B. K. (2002). Neonatal hippocampal damage alters electrophysiological properties of prefrontal cortical neurons in adult rats. Cereb. Cortex 12, 975–982. doi: 10.1093/cercor/12.9.975
Ogawa, S., and Kunugi, H. (2015). Inhibitors of fatty acid amide hydrolase and monoacylglycerol lipase: new targets for future antidepressants. Curr. Neuropharmacol. 13, 760–775. doi: 10.2174/1570159X13666150612225212
Ohno-Shosaku, T., and Kano, M. (2014). Endocannabinoid-mediated retrograde modulation of synaptic transmission. Curr. Opin. Neurobiol. 29, 1–8. doi: 10.1016/j.conb.2014.03.017
Olney, J. W., Newcomer, J. W., and Farber, N. B. (1999). NMDA receptor hypofunction model of schizophrenia. J. Psychiatr. Res. 33, 523–533. doi: 10.1016/S0022-3956(99)00029-1
Onitsuka, T., Oribe, N., Nakamura, I., and Kanba, S. (2013). Review of neurophysiological findings in patients with schizophrenia. Psychiatry Clin. Neurosci. 67, 461–470. doi: 10.1111/pcn.12090
O'Tuathaigh, C. M., Gantois, I., and Waddington, J. L. (2014). Genetic dissection of the psychotomimetic effects of cannabinoid exposure. Prog. Neuropsychopharmacol. Biol. Psychiatry 52, 33–40. doi: 10.1016/j.pnpbp.2013.11.002
Pacher, P., and Mechoulam, R. (2011). Is lipid signaling throught cannabinoid 2 receptors part of a protective system? Prog. Lipid. Res. 50, 193–211. doi: 10.1016/j.plipres.2011.01.001
Pacher, P., Batkai, S., and Kunos, G. (2006). The endocannabinoid system as an emerging target of pharmacotherapy. Pharmacol. Rev. 58, 389–462. doi: 10.1124/pr.58.3.2
Park, S., and Holzman, P. S. (1992). Schizophrenics show spatial working memory deficits. Arch. Gen. Psychiatry. 49, 975–982.
Pascual-Leone, A., Manoach, D. S., Birnbaum, R., and Goff, D. C. (2002). Motor cortical excitability in schizophrenia. Biol. Psychiatry 52, 24–31. doi: 10.1016/S0006-3223(02)01317-3
Paxinos, G., and Watson, C. (2007). The Rat Brain in Stereotaxic Coordinates. Amsterdam: Academic Press/Elsevier.
Paylor, R., McIlwain, K. L., McAninch, R., Nellis, A., Yuva-Paylor, L. A., Baldini, A., et al. (2001). Mice deleted for the DiGeorge/velocardiofacial syndrome region show abnormal sensorimotor gating and learning and memory impairments. Hum. Mol. Genet. 10, 2645–2650. doi: 10.1093/hmg/10.23.2645
Perez, S. M., and Logde, D. J. (2013). Hippocampal interneuron transplants reverse aberrant dopamine system function and behavior in a rodent model of schizophrenia. Mol. Psychiatry 18, 1193–1198. doi: 10.1038/mp.2013.111
Pertwee, R. G. (2008). The diverse CB1 and CB2 receptor pharmacology of three plant cannabinoids: delta9-tetrahydrocannabinol, cannabidiol and delta9-tetrahydrocannabivarin. Br. J. Pharmacol. 153, 199–215. doi: 10.1038/sj.bjp.0707442
Pertwee, R. G. (2010). Receptors and channels targeted by synthetic cannabinoid receptor agonists and antagonists. Curr. Med. Chem. 17, 1360–1381. doi: 10.2174/092986710790980050
Pertwee, R. G., Howlett, A. C., Abood, M. E., Alexander, S. P. H., Di Marzo, V., Elphick, M. R., et al. (2010). International union ofbasic and clinical pharmacology. LXXIX. Cannabinoid receptors and their ligands: beyond CB1 and CB2. Pharmacol. Rev. 62, 588–631. doi: 10.1124/pr.110.003004
Petrosino, S., and Di Marzo, V. (2010). FAAH and MAGL inhibitors: therapeutic opportunities from regulating endocannabinoid levels. Curr. Opin. Investig. Drugs 11, 51–62.
Phan, K. L., Fitzgerald, D. A., Nathan, P. J., Moore, G. J., Uhde, T. W., and Tancer, M. E. (2005). Neural substrates for voluntary suppression of negative affect: a functional magnetic resonance imaging study. Biol. Psychiatry 57, 210–219. doi: 10.1016/j.biopsych.2004.10.030
Phillips, K. G., Bartsch, U., McCarthy, A. P., Edgar, D. M., Tricklebank, M. D., Wafford, K. A., et al. (2012). Decoupling of sleep-dependent cortical and hippocampal interactions in a neurodevelopmental model of schizophrenia. Neuron 76, 526–533. doi: 10.1016/j.neuron.2012.09.016
Pinault, D. (2008). N-methyl D-aspartate receptor antagonists ketamine and MK-801 induce wake-related aberrant gamma oscillations in the rat neocortex. Biol. Psychiatry 63, 730–735. doi: 10.1016/j.biopsych.2007.10.006
Piomelli, D. (2003). The molecular logic of endocannabinoid signalling. Nat. Rev. Neurosci. 4, 873–884. doi: 10.1038/nrn1247
Pisanti, S., Malfitano, A. M., Ciaglia, E., Lamberti, A., Ranieri, R., Cuomo, G., et al. (2017). Cannabidiol: state of the art and new challenges for therapeutic applications. Pharmacol. Ther. 175, 133–150. doi: 10.1016/j.pharmthera.2017.02.041
Polich, J. (2007). Updating P300: an integrative theory of P3a and P3b. Clin. Neurophysiol. 118, 2128–2148. doi: 10.1016/j.clinph.2007.04.019
Powell, S. B., Zhou, X., and Geyer, M. A. (2009). Prepulse inhibition and genetic mouse models of schizophrenia. Behav. Brain Res. 204, 282–294. doi: 10.1016/j.bbr.2009.04.021
Puente, N., Cui, Y., Lassalle, O., Lafourcade, M., Georges, F., Venance, L., et al. (2011). Polymodal activation of the endocannabinoid system in the extended amygdala. Nat. Neurosci. 14, 1542–1547. doi: 10.1038/nn.2974
Rais, M., van Haren, N. E. M., Cahn, W., Schnack, H. G., Lepage, C., Collins, L., et al. (2010). Cannabis use and progressive cortical thickness loss in areas rich in CB1 receptors during the first five years of schizophrenia. Eur. Neuropsychopharmacol. 20, 855–865. doi: 10.1016/j.euroneuro.2010.08.008
Ramirez, S. H., Skuba, A., Fan, S., Dykstra, H., McCormick, R., Reichenbach, N., et al. (2012). Activation of cannabinoid receptor 2 attenuates leukocyte-endothelial cell interactions and blood-brain barrier dysfunction under inflammatory conditions. J. Neurosci. 32, 4004–4016. doi: 10.1523/JNEUROSCI.4628-11.2012
Ranganathan, M., Cortes-Briones, J., Radhakrishnan, R., Thurnauer, H., Planeta, B., Skosnik, P., et al. (2016). Reduced brain cannabinoid receptor availability in schizophrenia. Biol. Psychiatry 79, 997–1005. doi: 10.1016/j.biopsych.2015.08.021
Rasetti, R. (2011). Altered cortical network dynamics. Arch. Gen. Psychiatry 68, 1207–1217. doi: 10.1001/archgenpsychiatry.2011.103
Raver, S. M., and Keller, A. (2014). Permanent suppression of cortical oscillations in mice after adolescent exposure to cannabinoids: receptor mechanisms. Neuropharmacology 86, 161–173. doi: 10.1016/j.neuropharm.2014.07.006
Raver, S. M., Haughwout, S. P., and Keller, A. (2013). Adolescent cannabinoid exposure permanently suppresses cortical oscillations in adult mice. Neuropsychopharmacology 38, 2338–2347. doi: 10.1038/npp.2013.164
Renard, J., Rosen, L. G., Loureiro, M., De Oliveira, C., Schmid, S., Rushlow, W. J., et al. (2016). Adolescent cannabinoid exposure induces a persistent sub-cortical hyper-dopaminergic state and associated molecular adaptations in the prefrontal cortex. Cereb. Cortex 27, 1297–1310. doi: 10.1093/cercor/bhv335
Rentzsch, J., Stadtmann, A., Montag, C., Kunte, H., Plöckl, D., Hellweg, R., et al. (2016). Attentional dysfunction in abstinent long-term cannabis users with and without schizophrenia. Eur. Arch. Psychiatry Clin. Neurosci. 266, 409–421. doi: 10.1007/s00406-015-0616-y
Roach, B. J., and Mathalon, D. H. (2008). Event-related EEG time-frequency analysis: an overview of measures and an analysis of early gamma band phase locking in schizophrenia. Schizophr. Bull. 34, 907–926. doi: 10.1093/schbul/sbn093
Roberts, J. C., Davis, J. B., and Benham, C. D. (2004). [3H]Resiniferatoxin autoradiography in the CNS of wild-type and TRPV1 null mice defines TRPV1 (VR-1) protein distribution. Brain Res. 995, 176–183. doi: 10.1016/j.brainres.2003.10.001
Robson, P. J., Guy, G. W., and Di Marzo, V. (2014). Cannabinoids and schizophrenia: therapeutic prospects. Curr. Pharm. Des. 20, 2194–2204. doi: 10.2174/13816128113199990427
Rodríguez de Fonseca, F., Del Arco, I., Bermudez-Silva, F. J., Bilbao, A., Cippitelli, A., and Navarro, M. (2005). The endocannabinoid system: physiology and pharmacology. Alcohol Alcohol. 40, 2–14. doi: 10.1093/alcalc/agh110
Ronan, P. J., Wongngamnit, N., and Beresford, T. P. (2016). Molecular mechanisms of cannabis signaling in the brain. Prog. Mol. Biol. Transl. Sci. 137, 123–147. doi: 10.1016/bs.pmbts.2015.10.002
Roser, P., Haussleiter, I. S., Chong, H. J., Maier, C., Kawohl, W., Norra, C., et al. (2011). Inhibition of cerebral type 1 cannabinoid receptors is associated with impaired auditory mismatch negativity generation in the ketamine model of schizophrenia. Psychopharmacology Berl. 218, 611–620. doi: 10.1007/s00213-011-2352-y
Roser, P., Juckel, G., Rentzsch, J., Nadulski, T., Gallinat, J., and Stadelmann, A. M. (2008). Effects of acute oral Δ9-tetrahydrocannabinol and standardized cannabis extract on the auditory P300 event-related potential in healthy volunteers. Eur. Neuropsychopharmacol. 18, 569–577. doi: 10.1016/j.euroneuro.2008.04.008
Roser, P., Vollenweider, F. X., and Kawohl, W. (2010). Potential antipsychotic properties of central cannabinoid (CB1) receptor antagonists. World J. Biol. Psychiatry 11, 208–219. doi: 10.3109/15622970801908047
Rubino, T., and Parolaro, D. (2015). Sex-dependent vulnerability to cannabis abuse in adolescence. Front. Psychiatry 6:56. doi: 10.3389/fpsyt.2015.00056
Rushe, T. M., Woodruff, P. W., Murray, R. M., and Morris, R. G. (1999). Episodic memory and learning in patients with chronic schizophrenia. Schizophr. Res. 35, 85–96. doi: 10.1016/S0920-9964(98)00117-0
Russo, E. B., Burnett, A., Hall, B., and Parker, K. K. (2005). Agnostic properties of cannabidiol at 5-HT1a receptors. Neurochem. Res. 30, 1037–1043. doi: 10.1007/s11064-005-6978-1
Rutter, L., Carver, F. W., Holroyd, T., Nadar, S. R., Mitchell-Francis, J., Apud, J., et al. (2009). Magnetoencephalographic gamma power reduction in patients with schizophrenia during resting condition. Hum. Brain Mapp. 30, 3254–3264. doi: 10.1002/hbm.20746
Salisbury, D. F., Kuroki, N., Kasai, K., Shenton, M. E., and McCarley, R. W. (2007). Progressive and interrelated functional and structural evidence of post-onset brain reduction in schizophrenia. Arch. Gen. Psychiatry 64, 521–529. doi: 10.1001/archpsyc.64.5.521
Sams-Dodd, F. (1995). Distinct effects of d-amphetamine and phencyclidine on the social behaviour of rats. Behav. Pharmacol. 6, 55–65. doi: 10.1097/00008877-199501000-00009
Sams-Dodd, F. (1996). Phencyclidine-induced stereotyped behaviour and social isolation in rats: a possible animal model of schizophrenia. Behav. Pharmacol. 7, 3–23.
Saperstein, A. M., Fuller, R. L., Avila, M. T., Adami, H., McMahon, R. P., Thaker, G. K., et al. (2006). Spatial working memory as a cognitive endophenotype of schizophrenia: assessing risk for pathophysiological dysfunction. Schizophr. Bull. 32, 498–506. doi: 10.1093/schbul/sbj072
Sauer, J. F., Strüber, M., and Bartos, M. (2015). Impaired fast-spiking interneuron function in a genetic mouse model of depression. Elife 4:e04979. doi: 10.7554/eLife.04979
Schlicker, E., and Kathmann, M. (2001). Modulation of transmitter release via presynaptic cannabinoid receptors. Trends Pharmacol. Sci. 22, 565–572. doi: 10.1016/S0165-6147(00)01805-8
Schmiedt, C., Brand, A., Hildebrandt, H., and Basar-Eroglu, C. (2005). Event-related theta oscillations during working memory tasks in patients with schizophrenia and healthy controls. Brain Res. Cogn. Brain Res. 25, 936–947. doi: 10.1016/j.cogbrainres.2005.09.015
Seal, M. L., and Fletcher, P. C. (2009). Modulation of mediotemporal and ventrostriatal function in humans by Delta9-tetrahydrocannabinol. Arch. Gen. Psychiatry 66, 442–451. doi: 10.1001/archgenpsychiatry.2009.17
Shen, S., Lang, B., Nakamoto, C., Zhang, F., Pu, J., Kuan, S. L., et al. (2008). Schizophrenia-related neural and behavioral phenotypes in transgenic mice expressing truncated Disc1. J. Neurosci. 28, 10893–10904. doi: 10.1523/JNEUROSCI.3299-08.2008
Sherif, M., Radhakrishnan, R., D'Souza, D. C., and Ranganathan, M. (2016). Human laboratory studies on cannabinoids and psychosis. Biol. Psychiatry 79, 526–538. doi: 10.1016/j.biopsych.2016.01.011
Sherva, R., Wang, Q., Kranzler, H., Zhao, H., Koesterer, R., Herman, A., et al. (2016). Genome-wide association study of cannabis dependence severity, novel risk variants, and shared genetic risks. JAMA Psychiatry 73, 472–480. doi: 10.1001/jamapsychiatry.2016.0036
Shi, L., Fatemi, S. H., Sidwell, R. W., and Patterson, P. H. (2003). Maternal influenza infection causes marked behavioral and pharmacological changes in the offspring. J. Neurosci. 23, 297–302.
Sigurdsson, T. (2016). Neural circuit dysfunction in schizophrenia: insights from animal models. Neuroscience 321, 42–65. doi: 10.1016/j.neuroscience.2015.06.059
Sigurdsson, T., Stark, K. L., Karayiorgou, M., Gogos, J. A., and Gordon, J. A. (2010). Impaired hippocampal–prefrontal synchrony in a genetic mouse model of schizophrenia. Nature 464, 763–767. doi: 10.1038/nature08855
Silveira, M. M., Arnold, J. C., Laviolette, S. R., Hillard, C. J., Celorrio, M., Aymerich, M. S., et al. (2016). Seeing through the smoke: human and animal studies of cannabis use and endocannabinoid signalling in corticolimbic networks. Neurosci. Biobehav. Rev. 76, 380–395. doi: 10.1016/j.neubiorev.2016.09.007
Singer, P., Feldon, J., and Yee, B. K. (2009). Are DBA/2 mice associated with schizophrenia-like endophenotypes? A behavioural contrastwith C57BL/6 mice. Psychopharmacology Berl. 206, 677–698. doi: 10.1007/s00213-009-1568-6
Skosnik, P. D., Cortes-Briones, J. A., and Hajós, M. (2016). It's all in the rhythm: the role of cannabinoids in neural oscillations and psychosis. Biol. Psychiatry 79, 568–577. doi: 10.1016/j.biopsych.2015.12.011
Skosnik, P. D., D'Souza, D. C., Steinmetz, A. B., Edwards, C. R., Vollmer, J. M., Hetrick, W. P., et al. (2012). The effect of chronic cannabinoids on broadband EEG neural oscillations in humans. Neuropsychopharmacology 37, 2184–2193. doi: 10.1038/npp.2012.65
Skosnik, P. D., Krishnan, G. P., Aydt, E. E., Kuhlenshmidt, H. A., and O'Donnell, B. F. (2006). Psychophysiological evidence of altered neural synchronization in cannabis use: relationship to schizotypy. Am. J. Psychiatry 163, 1798–1805. doi: 10.1176/appi.ajp.163.10.1798
Skosnik, P. D., Krishnan, G. P., D'Souza, D. C., Hetrick, W. P., and O'Donnell, B. F. (2014). Disrupted gamma-band neural oscillations during coherent motion perception in heavy cannabis users. Neuropsychopharmacology 39, 3087–3099. doi: 10.1038/npp.2014.166
Skosnik, P. D., Park, S., Dobbs, L., and Gardner, W. L. (2008). Affect processing and positive syndrome schizotypy in cannabis users. Psychiatry Res. 157, 279–282. doi: 10.1016/j.psychres.2007.02.010
Smart, D., Gunthorpe, M. J., Jerman, J. C., Nasir, S., Gray, J., Muir, A. I., et al. (2000). The endogenous lipid anandamide is a full agonist at the human vanilloid receptor (hVR1). Br. J. Pharmacol. 129, 227–230. doi: 10.1038/sj.bjp.0703050
Smucny, J., Stevens, K. E., and Tregellas, J. R. (2014). Acute administration of Δ9 tetrahydrocannabinol does not prevent enhancement of sensory gating by clozapine in DBA/2 mice. Pharmacol. Biochem. Behav. 118, 22–29. doi: 10.1016/j.pbb.2014.01.001
Sohal, V. S. (2012). Insights into cortical oscillations arising from optogenetic studies. Biol. Psychiatry 71, 1039–1045. doi: 10.1016/j.biopsych.2012.01.024
Sohal, V. S., Zhang, F., Yizhar, O., and Deisseroth, K. (2009). Parvalbumin neurons and gamma rhythms enhance cortical circuit performance. Nature 459, 698–702. doi: 10.1038/nature07991
Spencer, K. M., Nestor, P. G., Niznikiewicz, M. A., Salisbury, D. F., Shenton, M. E., and McCarley, R. W. (2003). Abnormal neural synchrony in schizophrenia. J. Neurosci. 23, 7407–7411.
Spencer, K. M., Nestor, P. G., Perlmutter, R., Niznikiewicz, M. A., Klump, M. C., Frumin, M., et al. (2004). Neural synchrony indexes disordered perception and cognition in schizophrenia. Proc. Natl. Acad. Sci. U.S.A. 101, 17288–17293. doi: 10.1073/pnas.0406074101
Spencer, K. M., Niznikiewicz, M. A., Shenton, M. E., and McCarley, R. W. (2008). Sensory-evoked gamma oscillations in chronic schizophrenia. Biol. Psychiatry 63, 744–747. doi: 10.1016/j.biopsych.2007.10.017
Stark, K. L., Xu, B., Bagchi, A., Lai, W. S., Liu, H., Hsu, R., et al. (2008). Altered brain microRNA biogenesis contributes to phenotypic deficits in a 22q11-deletion mouse model. Nat. Genet. 40, 751–760. doi: 10.1038/ng.138
Stempel, A. V., Stumpf, A., Zhang, H. Y., Özdoğan, T., Pannasch, U., Theis, A. K., et al. (2016). Cannabinoid type 2 receptors mediate a cell type-specific plasticity in the hippocampus. Neuron 90, 795–809. doi: 10.1016/j.neuron.2016.03.034
Stokes, P. R. A., Mehta, M. A., Curran, H. V., Breen, G., and Grasby, P. M. (2009). Can recreational doses of THC produce significant dopamine release in the human striatum? Neuroimage 48, 186–190. doi: 10.1016/j.neuroimage.2009.06.029
Stokes, P. R., Egerton, A., Watson, B., Reid, A., Lappin, J., Howes, O. D., et al. (2012). History of cannabis use is not associated with alterations in striatal dopamine D2/D3 receptor availability. J. Psychopharmacol. 26, 144–149. doi: 10.1177/0269881111414090
Stubbs, B., Thompson, T., Acaster, S., Vancampfort, D., Gaughran, F., and Correll, C. U. (2015). Decreased pain sensitivity among people with schizophrenia: a meta-analysis of experimental pain induction studies. Pain 156, 2121–2131. doi: 10.1097/j.pain.0000000000000304
Swerdlow, N. R., and Geyer, M. A. (1998). Using an animal model of deficient sensorimotor gating to study the pathophysiology and new treatments of schizophrenia. Schizophr. Bull. 24, 285–301.
Symond, M. B., Harris, A. W. F., Gordon, E., and Williams, L. M. (2005). “Gamma synchrony” in first-episode schizophrenia: a disorder of temporal connectivity? Am. J. Psychiatry 162, 459–465. doi: 10.1176/appi.ajp.162.3.459
Szallasi, A., Cortright, D. N., Blum, C. A., and Eid, S. R. (2007). The vanilloid receptor TRPV1: 10 years from channel cloning to antagonist proof-of-concept. Nat. Rev. Drug Discov. 6, 357–372. doi: 10.1038/nrd2280
Szutorisz, H., and Hurd, Y. L. (2016). Epigenetic effects of cannabis exposure. Biol. Psychiatry 79, 586–594. doi: 10.1016/j.biopsych.2015.09.014.
Tomas-Roig, J., Benito, E., Agis-Balboa, R. C., Piscitelli, F., Hoyer-Fender, S., Di Marzo, V., et al. (2016). Chronic exposure to cannabinoids during adolescence causes long-lasting behavioral deficits in adult mice. Addict. Biol. doi: 10.1111/adb.12446. [Epub ahead of print].
Tseng, K. Y., and O'Donnell, P. (2007). Dopamine modulation of prefrontal cortical interneurons changes during adolescence. Cereb. Cortex 17, 1235–1240. doi: 10.1093/cercor/bhl034
Tseng, K. Y., Chambers, R. A., and Lipska, B. K. (2009). The neonatal ventral hippocampal lesion as a heuristic neurodevelopmental model of schizophrenia. Behav. Brain Res. 204, 295–305. doi: 10.1016/j.bbr.2008.11.039
Tseng, K. Y., Mallet, N., Toreson, K. L., Le Moine, C., Gonon, F., and O'Donnell, P. (2006). Excitatory response of prefrontal cortical fast-spiking interneurons to ventral tegmental area stimulation in vivo. Synapse 59, 412–417. doi: 10.1002/syn.20255
Tsuchimoto, R., Kanba, S., Hirano, S., Oribe, N., Ueno, T., Hirano, Y., et al. (2011). Reduced high and low frequency gamma synchronization in patients with chronic schizophrenia. Schizophr. Res. 133, 99–105. doi: 10.1016/j.schres.2011.07.020
Tunbridge, E. M., Dunn, G., Murray, R. M., Evans, N., Lister, R., Stumpenhorst, K., et al. (2015). Genetic moderation of the effects of cannabis: catechol-O-methyltransferase (COMT) affects the impact of Δ9-tetrahydrocannabinol (THC) on working memory performance but not on the occurrence of psychotic experiences. J. Psychopharmacol. 29, 1146–1151. doi: 10.1177/0269881115609073
Tuo, W., Leleu-Chavain, N., Spencer, J., Sansook, S., Millet, R., and Chavatte, P. (2017). Therapeutic potential of fatty acid amide hydrolase, monoacylglycerol lipase, and N-acylethanolamine acid amidase inhibitors. J. Med. Chem. 60, 4–46. doi: 10.1021/acs.jmedchem.6b00538
Turetsky, B. I., Calkins, M. E., Light, G. A., Olincy, A., Radant, A. D., and Swerdlow, N. R. (2007). Neurophysiological endophenotypes of schizophrenia: the viability of selected candidate measures. Schizophr. Bull. 33, 69–94. doi: 10.1093/schbul/sbl060
Twitchell, W., Brown, S., and Mackie, K. (1997). Cannabinoids inhibit N- and P/Q-type calcium channels in cultured rat hippocampal neurons. J. Neurophysiol. 78, 43–50.
Tzavara, E. T., Li, D. L., Moutsimilli, L., Bisogno, T., Di Marzo, V., Phebus, L. A., et al. (2006). Endocannabinoids activate transient receptor potential vanilloid 1 receptors to reduce hyperdopaminergia-related hyperactivity: therapeutic implications. Biol. Psychiatry 59, 508–515. doi: 10.1016/j.biopsych.2005.08.019
Uhlhaas, P. J., and Singer, W. (2006). Neural synchrony in brain disorders: relevance for cognitive dysfunctions and pathophysiology. Neuron 52, 155–168. doi: 10.1016/j.neuron.2006.09.020
Uhlhaas, P. J., and Singer, W. (2010). Abnormal neural oscillations and synchrony in schizophrenia. Nat. Rev. Neurosci. 11, 100–113. doi: 10.1038/nrn2774
Uhlhaas, P. J., and Singer, W. (2015). Oscillations and neuronal dynamics in schizophrenia: the search for basic symptoms and translational opportunities. Biol. Psychiatry 77, 1001–1009. doi: 10.1016/j.biopsych.2014.11.019
Uhlhaas, P. J., Linden, D. E. J., Singer, W., Haenschel, C., Lindner, M., Maurer, K., et al. (2006). Dysfunctional long-range coordination of neural activity during gestalt perception in schizophrenia. J. Neurosci. 26, 8168–8175. doi: 10.1523/JNEUROSCI.2002-06.2006
van den Buuse, M. (2010). Modeling the positive symptoms of schizophrenia in genetically modified mice: pharmacology and methodology aspects. Schizophr. Bull. 36, 246–270. doi: 10.1093/schbul/sbp132
Večeřa, J., Bártová, E., Krejčí, J., Legartová, S., Komrková, D., Rudá-Kučerová, J., et al. (2017). HDAC1 and HDAC3 underlie dynamic H3K9 acetylation during embryonic neurogenesis and in schizophrenia-like animals. J. Cell Physiol. doi: 10.1002/jcp.25914. [Epub ahead of print].
Verdejo-Garcia, A., Benbrook, A., Funderburk, F., David, P., Cadet, J. L., and Bolla, K. I. (2007). The differential relationship between cocaine use and marijuana use on decision-making performance over repeat testing with the Iowa Gambling Task. Drug Alcohol Depend. 90, 2–11. doi: 10.1016/j.drugalcdep.2007.02.004
Vigano, D., Guidali, C., Petrosino, S., Realini, N., Rubino, T., Di Marzo, V., et al. (2009). Involvement of the endocannabinoid system in phencyclidine-induced cognitive deficits modelling schizophrenia. Int. J. Neuropsychopharmacol. 12, 599–614. doi: 10.1017/S1461145708009371
Volk, D. W., Eggan, S. M., Horti, A. G., Wong, D. F., and Lewis, D. A. (2014). Reciprocal alterations in cortical cannabinoid receptor 1 binding relative to protein immunoreactivity and transcript levels in schizophrenia. Schizophr. Res. 159, 124–129. doi: 10.1016/j.schres.2014.07.017
Voruganti, L. N. P., Slomka, P., Zabel, P., Mattar, A., and Awad, A. G. (2001). Cannabis induced dopamine release: an in-vivo SPECT study. Psychiatry Res. 107, 173–177. doi: 10.1016/S0925-4927(01)00104-4
Waltereit, R., and Weller, M. (2003). Signaling from cAMP/PKA to MAPK and synaptic plasticity. Mol. Neurobiol. 27, 99–106. doi: 10.1385/MN:27:1:99
Wang, J., Hirayasu, Y., Hiramatsu, K. I., Hokama, H., Miyazato, H., and Ogura, C. (2003). Increased rate of P300 latency prolongation with age in drug-naive and first episode schizophrenia. Clin. Neurophysiol. 114, 2029–2035. doi: 10.1016/S1388-2457(03)00207-4
Weinberger, D. R., Berman, K. F., Suddath, R., and Fuller Torrey, E. (1992). Evidence of dysfunction of a prefrontal-limbic network in schizophrenia: a magnetic resonance imaging and regional cerebral blood flow study of discordant monozygotic twins. Am. J. Psychiatry 149, 890–897. doi: 10.1176/ajp.149.7.890
Whissell, P. D., Tohyama, S., and Martin, L. J. (2016). The use of DREADDs to deconstruct behavior. Front. Genet. 7:70. doi: 10.3389/fgene.2016.00070
Wilson, R. I., and Nicoll, R. A. (2001). Endogenous cannabinoids mediate retrograde signalling at hippocampal synapses. Nature 410, 588–592. doi: 10.1038/35069076
Wong, D. F., Kuwabara, H., Horti, A. G., Raymont, V., Brasic, J., Guevara, M., et al. (2010). Quantification of cerebral cannabinoid receptor subtype 1 (CB1) in healthy subjects and schizophrenia by the novel PET radioligand [11C]OMAR. Neuroimage 52, 1505–1305. doi: 10.1016/j.neuroimage.2010.04.034
Wood, J., Kim, Y., and Moghaddam, B. (2012). Disruption of prefrontal cortex large scale neuronal activity by different classes of psychotomimetic drugs. J. Neurosci. 32, 3022–3031. doi: 10.1523/JNEUROSCI.6377-11.2012
Xi, Z. X., Peng, X. Q., Li, X., Song, R., Zhang, H. Y., Liu, Q. R., et al. (2011). Brain cannabinoid CB2 receptors modulate cocaine's actions in mice. Nat. Neurosci. 14, 1160–1166. doi: 10.1038/nm.2874
Xing, J., and Li, J. (2007). TRPV1 receptor mediates glutamatergic synaptic input to dorsolateral periaqueductal gray (dl-PAG) neurons. J. Neurophysiol. 97, 503–511. doi: 10.1152/jn.01023.2006
Zamberletti, E., Rubino, T., and Parolaro, D. (2012). The endocannabinoid system and schizophrenia: integration of evidence. Curr. Pharm. Des. 18, 4980–4990. doi: 10.2174/138161212802884744
Zavitsanou, K., Garrick, T., and Huang, X. F. (2004). Selective antagonist [3H]SR141716A binding to cannabinoid CB1 receptors is increased in the anterior cingulate cortex in schizophrenia. Prog. Neuropsychopharmacol. Biol. Psychiatry 28, 355–360. doi: 10.1016/j.pnpbp.2003.11.005
Zhang, H. Y., Gao, M., Shen, H., Bi, G. H., Yang, H. J., Liu, Q. R., et al. (2016). Expression of functional cannabinoid CB2 receptor in VTA dopamine neurons in rats. Addict. Biol. 22, 752–765. doi: 10.1111/adb.12367
Zhou, D., Pang, F., Liu, S., Shen, Y., Liu, L., Fang, Z., et al. (2016). Altered motor-striatal plasticity and cortical functioning in patients with schizophrenia. Neurosci. Bull. 33, 307–311. doi: 10.1007/s12264-016-0079-9
Zhou, Y., Shu, N., Liu, Y., Song, M., Hao, Y., Liu, H., et al. (2008). Altered resting-state functional connectivity and anatomical connectivity of hippocampus in schizophrenia. Schizophr. Res. 100, 120–132. doi: 10.1016/j.schres.2007.11.039
Zuardi, A. W., Crippa, J. A., Hallak, J. E., Bhattacharyya, S., Atakan, Z., Martin-Santos, R., et al. (2012). A critical review of the antipsychotic effects of cannabidiol: 30 years of a translational investigation. Curr. Pharm. Des. 18, 5131–5140. doi: 10.2174/138161212802884681
Keywords: cannabinoids, vanilloids, schizophrenia, functional imaging, electrophysiology, animal models
Citation: Ruggiero RN, Rossignoli MT, De Ross JB, Hallak JEC, Leite JP and Bueno-Junior LS (2017) Cannabinoids and Vanilloids in Schizophrenia: Neurophysiological Evidence and Directions for Basic Research. Front. Pharmacol. 8:399. doi: 10.3389/fphar.2017.00399
Received: 23 February 2017; Accepted: 06 June 2017;
Published: 21 June 2017.
Edited by:
Andrew C. McCreary, Janssen Prevention Center, NetherlandsReviewed by:
Marco Pistis, Università degli studi di Cagliari, ItalyFrancis Bambico, Centre for Addiction and Mental Health, Canada
Copyright © 2017 Ruggiero, Rossignoli, De Ross, Hallak, Leite and Bueno-Junior. This is an open-access article distributed under the terms of the Creative Commons Attribution License (CC BY). The use, distribution or reproduction in other forums is permitted, provided the original author(s) or licensor are credited and that the original publication in this journal is cited, in accordance with accepted academic practice. No use, distribution or reproduction is permitted which does not comply with these terms.
*Correspondence: Matheus T. Rossignoli, cm9zc2lnbm9saS5tdEBnbWFpbC5jb20=