- 1Departamento de Patologia, Centro de Ciências Biológicas, Universidade Estadual de Londrina, Londrina, Brazil
- 2Departamento de Ciências Farmacêuticas, Centro de Ciências da Saúde, Universidade Estadual de Londrina, Londrina, Brazil
Despite the progress that has occurred in recent years in the development of therapies to treat painful and inflammatory diseases, there is still a need for effective and potent analgesics and anti-inflammatory drugs. It has long been known that several types of antioxidants also possess analgesic and anti-inflammatory properties, indicating a strong relationship between inflammation and oxidative stress. Understanding the underlying mechanisms of action of anti-inflammatory and analgesic drugs, as well as essential targets in disease physiopathology, is essential to the development of novel therapeutic strategies. The Nuclear factor-2 erythroid related factor-2 (Nrf2) is a transcription factor that regulates cellular redox status through endogenous antioxidant systems with simultaneous anti-inflammatory activity. This review summarizes the molecular mechanisms and pharmacological actions screened that link analgesic, anti-inflammatory, natural products, and other therapies to Nrf2 as a regulatory system based on emerging evidences from experimental disease models and new clinical trial data.
Introduction
A cross-talk between varied reactive oxygen species (ROS) and reactive nitrogen species (RNS) is a common feature of inflammatory and painful diseases. These oxidants can be generated by enzymes abundant in immune and non-immune cells (Mittal et al., 2014) as part of protective actions against infections and environmental threats, including microbial or any noxious insults (Janssen-Heininger et al., 2008). The agonist binding to cellular receptors dictates the actions of nicotinamide adenine dinucleotide phosphate (NADPH) oxidases (NOX) and nitric oxide synthases (NOS) to produce superoxide anion and nitric oxide, respectively. Besides the oxidative burst, ROS are also produced in mitochondrial compartments as a result of respiratory chain activity where oxygen consumption is high (Paiva and Bozza, 2014).
Compelling evidence also indicates that oxidative stress is not only related to tissue damage, but also cellular signaling pathways that tightly control cell division, migration and mediator production that ultimately regulate diverse cellular functions (Finkel, 2003; Janssen-Heininger et al., 2008; Pinho-Ribeiro et al., 2016b). Indeed, ROS/RNS sustain their own production and induce the release of cytokines, adhesion molecules, lipid mediators, inflammasome assembly and cyclooxygenase (COX)-2 expression by mechanisms involving the nuclear factor kappa B (NF-κB) activation (Verri et al., 2012; Wardyn et al., 2015; Hennig et al., 2018). NF-κB also induces mitochondrial activity and NADPH oxidase expression in the context of inflammation (Manea et al., 2007; Mauro et al., 2011; Wardyn et al., 2015). Activation of the nuclear factor erythroid 2 (NFE2)-related factor 2 (Nrf2) is central to the disruption of this circle. In fact, in vivo studies have shown that Nrf2 signaling has an essential role in limiting neuropathies (Arruri et al., 2017), arthritis (Ferrandiz et al., 2018), colitis (Xu et al., 2018), pneumonia (Athale et al., 2012), pulmonary fibrosis (Yan et al., 2017), skin diseases (Schafer and Werner, 2015), liver (Bae et al., 2013), and kidney damage (Shen et al., 2017), as well as affecting tumor development (Sporn and Liby, 2012). Importantly, the structural features and signaling of Nrf2 protein assign its activity to maintain cellular redox homeostasis (Hayes and Dinkova-Kostova, 2014).
It is well established that Nrf2 activity is controlled, in part, by the cytosolic protein Kelch-like ECH-associated protein 1 (Keap1), as portrayed in Figure 1. Under homeostatic conditions, Nrf2 levels and its activation are controlled essentially by Keap1. Two Keap1 molecules maintain Nrf2 attached to its DLG and ETH motifs, which favors CUL3-mediated ubiquitination of Nrf2 and subsequent proteasome degradation (Itoh et al., 1999). A small proportion of Nrf2 escapes the inhibitory complex and accumulates in the nucleus to mediate basal antioxidant responsive element (ARE)-dependent gene expression and maintains cellular homeostasis (Kansanen et al., 2013). Conversely, upon oxidative stress or in the presence of electrophilic or activating compounds, the modification of key Keap1 cysteine residues promotes the dissociation of the inhibitory complex and nuclear translocation of Nrf2. In the nucleus, Nrf2 forms a heterodimer with its partner sMAF (v-Maf avian musculoaponeurotic fibrosarcoma oncogene homolog) and binds to ARE, driving the expression of an array of Nrf2-target genes, for example NAD(P)H quinone-oxidoreductase 1 (NQO1), heme-oxygenase 1(HO-1), glutamate-cysteine ligase (GCL), glutathione S-transferases (GSTs), catalase (CAT), superoxide dismutase (SOD) and thioredoxin UDP-glucuronosyltransferase (Nguyen et al., 2009; Ruiz et al., 2013; Hayes and Dinkova-Kostova, 2014). This signaling is defined as the canonical mechanism of Nrf2 pathway (Silva-Islas and Maldonado, 2018). Importantly, this pathway can be modulated by protein kinases involved in signal transduction in the cytosol, such as protein kinase C (PKC), phosphoinositide 3-kinase (PI3K) and mitogen-activated protein kinase (MAPK) ERK1/2 (Bloom and Jaiswal, 2003; Nguyen et al., 2003; Manandhar et al., 2007).
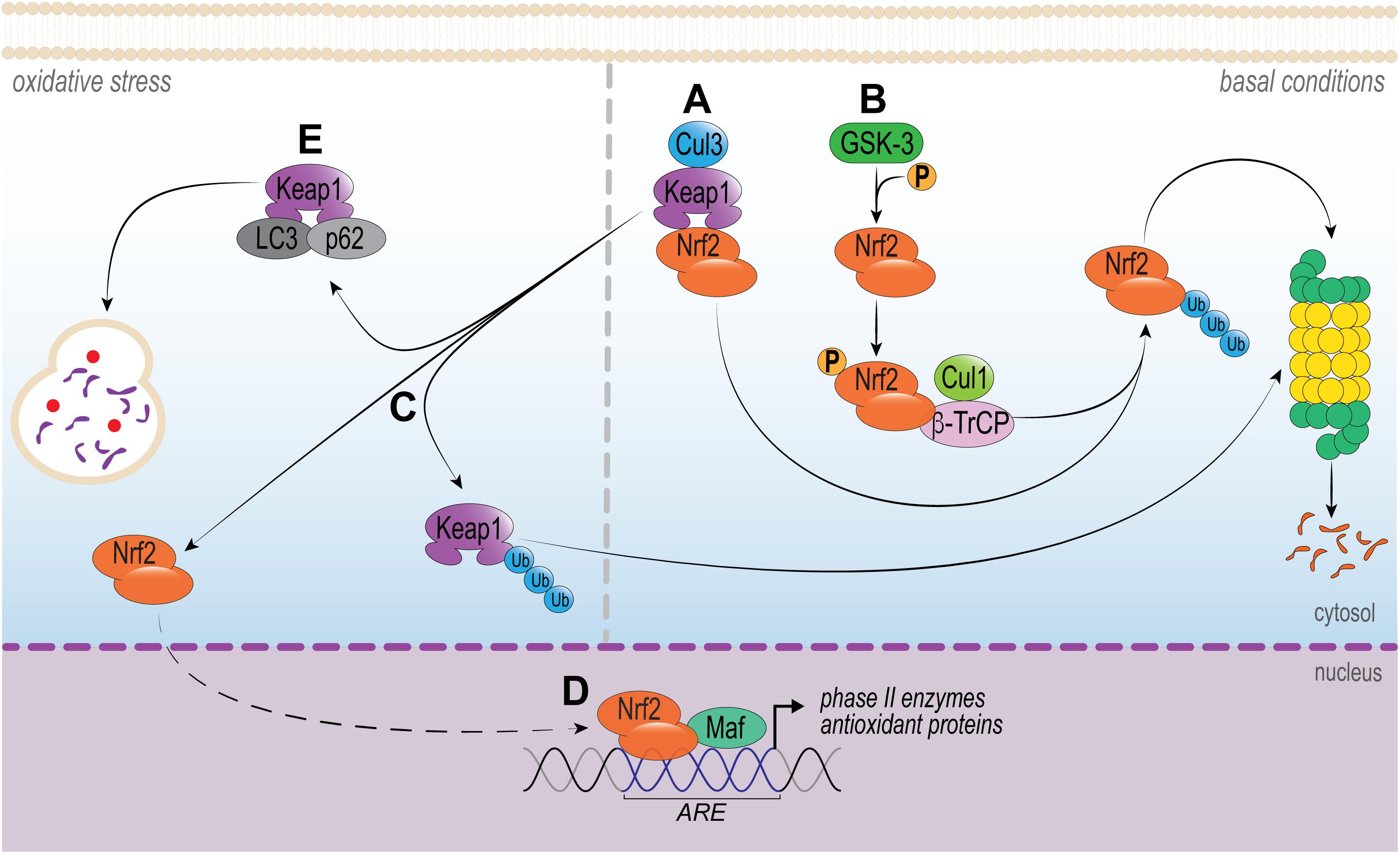
Figure 1. Intracellular signaling pathways that regulate Nrf2. In basal conditions, (A) Nrf2 is sequestered in the cytosol by Keap1 by two motifs (ETGE and DLG), which are essential to recruit Nrf2. Keap1 works as a dimeric redox sensitive substrate adaptor for cullin-based E3 ubiquitin ligase, which inhibits the transcriptional activity of Nrf2 via ubiquitination and proteasomal degradation. This signaling is known as canonical pathway. (B) Alternatively, Nrf2 is also regulated by a non-canonical pathway. The phosphorylation of Nrf2 by GSK-3 facilitates its recognition by β-TrCP, leading to Cul1-mediated ubiquitination, followed by Nrf2 proteasome degradation. Under oxidative stress or pathological conditions, (C,D) Keap1-CUL3 ubiquitin E3 ligase activity decreases and Nrf2 dissociates from Keap1. Nrf2 translocates to the nucleus and heterodimerizes with small musculoaponeurotic fibrosarcoma (Maf) protein and binds to DNA and other transcription partners to setting up a nuclear complex with the ubiquitin-conjugating enzyme UbcM2. These nuclear complexes formed with Nrf2 induce the expression of the ARE-gene battery, such as: NQO1, HMOX1, GCL, GSTs, CAT, SOD, and thioredoxin UDP-glucuronosyltransferase. (E) The multifunctional protein p62 and LC3 acts by sequestration of Keap1, which culminates in its autophagic degradation. As a consequence, Nrf2 can translocate to the nucleus and activate ARE.
Alternatively, the interaction of Keap1-Nrf2 can be disrupted by non-canonical mechanisms (Figure 1). These independent mechanisms involve the disruption of Keap1/Nrf2 interaction by competitive binding of disrupter proteins p62, p53-induced p21 (Toledano, 2009; Best and Sutherland, 2018); DPP3 (Hast et al., 2013), WTX (Karapetian et al., 2005), Prothymosin α (Karapetian et al., 2005), PALB2 (Ma et al., 2012), or BRCA1 (Gorrini et al., 2013) to Keap1 (Lau et al., 2010). Of note, p62 is particularly interesting, since it is the most studied non-canonical pathway of Nrf2 activation. The deficiency in autophagy upregulates p62, that binds to Keap1, thereby inhibiting the Keap1-Cul3-E3 ubiquitin ligase complex and stabilizing Nrf2 (Lau et al., 2010). In addition, PI3K/Akt signaling pathway can activate serine threonine kinase glycogen synthase kinase 3-beta (GSK-3β), which phosphorylates Nrf2 and, in turn, results in the induction of downstream HO-1, glutathione peroxidase, GST A1, NQO-1 and GCL expression (Salazar et al., 2006; Best and Sutherland, 2018). These recent discoveries on the mechanisms of Nrf2 regulation illustrate possible therapeutic strategies to modulate its activity.
Of particular interest is the notion that pharmacological activation of Nrf2 interferes in inflammation (Hayes and Dinkova-Kostova, 2014). It is also important to mention that Nrf2 is expressed in many tissues, mainly those exposed to the environment or associated with detoxification (Itoh et al., 2010). In other words, it is likely that Nrf2 can be exploited as a target in distinct organs. Thus, in this review we will discuss the involvement of Nrf2 in the mechanisms of action of classic analgesic and anti-inflammatory drugs, as well as natural products and other molecules that modulate Nrf2, in the context of experimental models (summarized in Table 1) and human diseases (summarized in Table 2).
Modulation of NRF2 by Analgesic Drugs and Synergic Effects
Despite their deleterious side effects, opioids are one of the most effective analgesic drugs employed in the clinic (Knezevic et al., 2017). Opioids produce their pain-relieving actions by interacting with μ, δ or κ opioid receptors (Bovill, 1997). Growing evidence has shown that Nrf2-activators can synergize with opioids to achieve better analgesic effects. Sulforaphane (SFN), for instance, is an activator of Nrf2 transcription factor that enhances the antiallodynic and antihyperalgesic effects produced by morphine. This effect was attributed to local increase in the expression of μ-opioid receptors, as observed in animals with peripheral inflammation. Notwithstanding, SFN enhances the production of HO-1 and NQO1 in the spinal cord and paw tissue, suggesting that the antiallodynic and antihyperalgesic effects of SFN and morphine are produced by a Nrf2 antioxidant-mediated mechanism (Redondo et al., 2017). Fentanyl, another opioid drug, when combined with butorphanol, activates Nrf2-ARE signaling via kappa-opioid receptor. The activation of this pathway increased the expression of downstream genes NQO1 and HO-1 and prevented oxidative stress in myocardial ischemia-reperfusion (I/R) injury model (Zhang et al., 2016). Corroborating these findings, the induction of Nrf2 can also enhance the antinociceptive effects of delta-opioid receptors in diabetic neuropathy associated to type 2 diabetes (McDonnell et al., 2017). Collectively, these data suggest that the use of Nrf2-activators may be beneficial, since lower doses of opioid could be used, thus likely reducing their side effects.
Cannabinoids are molecules that were originally isolated from Cannabis sativa and later discovered to be endogenously produced. Cannabinoids represent an alternative pain relief therapy group of drugs with varied origins (Elikkottil et al., 2009). Some molecular effects of cannabinoids depend on Nrf2. One postulated mechanism of action is that endogenous cannabinoids can enhance SOD synthesis and decrease ROS production via Nrf2. These effects were implicated in the neuroprotective effect of cannabinoids in a model of Parkinson’s disease, a progressive nervous system disorder with inflammatory features that affects movement (More and Choi, 2015). Anandamide, an endogenous cannabinoid, leads to Nrf2 activation and downstream HO-1 mRNA expression in MCF-7 and MDA-MB-231 breast cancer cell lines (Li H. et al., 2013). Cannabidiol, a natural cannabinoid constituent isolated from cannabis, controls LPS-induced oxidative stress and inflammation in BV2 cells by inducing Nrf2 and ATF4 transcription factors in a mechanism involving Nrf2-Hmox1 and the Nrf2/ATF4 pathways (Juknat et al., 2013).
Desipramine, a tricyclic antidepressant that displays pain-relief and anti-inflammatory effects, is commonly used in low doses to control neuropathic pain (Roumestan et al., 2007; Hearn et al., 2014). It has been demonstrated that desipramine elevates HO-1 expression through ERK and JNK pathways, leading to Nrf2 activation in Mes23.5 dopaminergic neurons. Desipramine-induced high HO-1 expression also protects dopaminergic neurons from cell death, supporting the notion that this drug could be a promising therapeutic approach to treat neurodegenerative disease (Lin et al., 2012).
In summary, the Keap1/Nrf2/HO-1 axis contributes to the analgesic effects of cannabinoids, anticonvulsant, antidepressant, and enhances the analgesic effects of opioids.
Modulation of NRF2 by Non-Steroidal Anti-Inflammatory Drugs
Non-steroidal anti-inflammatory drugs (NSAIDs) are a broad class of anti-inflammatory drugs commonly prescribed for pain and inflammation (Cashman, 1996). NSAIDs relieve pain by blocking COX subtypes 1 and 2 enzymes, which results in the inhibition of prostanoid production (Simmons et al., 2004) via peripheral and central actions (Cashman, 1996). Depending on the NSAID, its mechanism of action may also include the inhibition of NFκB activation and induction of pro-resolving lipid mediators (Serhan, 2017). The compounds in willow bark, for example salicylate, provide the basis for aspirin and many other traditional non-selective NSAIDs (Serhan, 2017). Accumulating evidence has shown that various NSAIDs have effects toward the Keap1/Nrf2/ARE pathway (Antman et al., 2007).
Aspirin, the most commonly used NSAID, has free radical scavenging property, specifically the removal of hydrogen peroxide. Aspirin has been reported to have protective effects against H2O2 in primary human melanocytes by activating Nrf2-ARE pathway and inducing HO-1 expression (Jian et al., 2016). Taken this into account, the authors suggested aspirin as a new antioxidant for the treatment to vitiligo. Aspirin also displayed neuroprotective effects in spinal cord injury model. This effect was attributed to a Nrf2/NQO1/HO-1 signaling pathway-dependent inhibition of neuronal apoptosis, astrocyte activation, oxidative stress and metabolic dysregulation (Wei et al., 2018).
The COX-2 transcription is activated by JNK2/c-jun pathway, which inhibits PI3-K activity and leads to suppression of Nrf2-ARE transcriptional activity (Healy et al., 2005). Corroborating these data, COX-2 selective NSAIDs activate the Nrf2/ARE pathway. Celecoxib is a selective COX-2 inhibitor (Gong et al., 2012) that has been reported to activate AMPK-CREB-Nrf2-dependent signaling and enhance vascular endothelium protection. Treatment of human endothelial cells with celecoxib leads to COX-2 independent signaling via phosphorylation of AMPK, resulting in the nuclear translocation of Nrf2. Together, CREB and Nrf2 pathways upregulate the expression of the antioxidant and anti-inflammatory genes such as HO-1 and H-Ferritin (FHC). In light of this, celecoxib improves endothelial function, minimizing cardiovascular risk in patients (Al-Rashed et al., 2018).
Another group later reported that Mosquito fish (Gambusia affinis) exposed to diclofenac for 7 days presented increased expression of Nrf2 mRNA and its downstream related genes. This response occurred as a protective mechanism due to large absorption and accumulation of diclofenac, which caused the buildup of ROS and induction of the antioxidant responses (Bao et al., 2017). In a model of choroidal neovascularization (CNV), indomethacin or bromfenac showed modulatory activity on Nrf2. In ARPE-19 [human diploid retinal pigment epithelium (RPE)] cells, indomethacin or bromfenac induced the translocation of Nrf2 into the nucleus and high levels of HO-1 in the perinuclear lesion and cytoplasm. In vivo, similar therapeutic effects were produced by these NSAIDs. In rat CNV model, indomethacin or bromfenac increased Nrf2 or HO-1 expression, inhibited macrophage infiltration and reduced VEGF levels. These data support NSAIDs treatment as a reasonable therapeutic approach for CNV due to their anti-angiogenic effect and that increasing Nrf2 signaling is a contributing underlying mechanism (Yoshinaga et al., 2011). Overall, these results demonstrate the potential protective effects of a NSAID in inflammatory conditions via the canonical Keap1/Nrf2 pathway.
Modulation of NRF2 by Steroidal Anti-Inflammatory Drugs (SADs)
Synthetic SADs resemble natural glucocorticoids with peculiar differences in both pharmacodynamics and pharmacokinetics features (Clark and Belvisi, 2012). They possess powerful immunosuppressive actions (Busillo and Cidlowski, 2013) and the most frequently prescribed SADs are prednisone/prednisolone, dexamethasone, and budesonide. Nevertheless, there are several other SADs that are used to treat numerous diseases (Clark and Belvisi, 2012). Synthetic glucocorticoids have been indispensable over the last half-century for treating several inflammatory and autoimmune diseases such as allergies, asthma, rheumatoid arthritis, Graves’ disease, psoriasis, sepsis, and transplanted organ rejections. It is noteworthy that the therapeutic benefits of glucocorticoids are limited by severe adverse effects that develop in patients subjected to long-term use. Adverse effects include osteoporosis, skin atrophy, diabetes, abdominal obesity, glaucoma, growth retardation in children, immunosuppression, inhibition of wound repair, and hypertension hypertension (Miner et al., 2005; Rhen and Cidlowski, 2005).
Regarding the mechanism to achieve the pharmacological effects, glucocorticoids transduce their actions by binding to the glucocorticoid receptor (GR) in the cell cytoplasm. Upon ligand binding, the complex glucocorticoid-GR undergoes conformational change that triggers its translocation to the nucleus. In the nucleus, the glucocorticoid-GR complex binds to glucocorticoid responsive elements, which recruit either coactivator or corepressor proteins. This biding results in the modification of chromatin structure, which can facilitate or inhibit the transcription machinery (Hebbar and Archer, 2003; Nagaich et al., 2004). Moreover, the complex can also interact with other transcription factors such as NF-κB (McKay and Cidlowski, 1999; De Bosscher et al., 2003) and Nrf2 (Ki et al., 2005; Kratschmar et al., 2012). Although glucocorticoids exert their actions mainly through genomic (transactivation and transrepression) mechanisms, non-genomic actions have also been described (Groeneweg et al., 2011).
Experimental approaches based on immunoprecipitation of Nrf2 and its interacting proteins identified GR as a novel Nrf2-binding partner (Alam et al., 2017). In agreement, numerous studies have shown that both natural and synthetic glucocorticoids can modulate Nrf2 activity via GR signaling (Alam et al., 2017; Choi et al., 2017). A microarray analysis of developing zebrafish larvae exposed to glucocorticoids (dexamethasone, prednisolone, and triamcinolone) revealed that Nrf2 was among the top perturbed canonical pathways in oxidative stress response. In agreement with these data, GR signaling can block Nrf2-mediated cytoprotection from oxidative stress (Kratschmar et al., 2012; Alam et al., 2017) and inhibition of GR nuclear translocation restores dexamethasone-induced inhibition of Nrf2/HO-1 expression (Singh and Haldar, 2016). Mechanistically, it was demonstrated that dexamethasone enhances GR recruitment to antioxidant response elements (AREs) without affecting chromatin binding of Nrf2, resulting in the inhibition of acetyltransferase CBP (CREB-binding protein) recruitment and histone acetylation at AREs. This repressive effect was inhibited by the addition of histone deacetylase inhibitors, suggesting that the reduction in Nrf2 transcriptional activation by GR signaling is dependent on the inhibition of histone acetylation (Alam et al., 2017).
In contrast to previous data showing that dexamethasone mitigates Nrf2-mediated response during oxidative stress, in Ataxia telangiectasia lymphoblastoid cells, dexamethasone increased GSH and NADPH levels, as well as improved the antioxidant capacity in a Nrf2-dependent manner. Dexamethasone was shown to induce the translocation of Nrf2 from the cytosol to the nucleus, where its accumulation continued up to 24-h after drug administration to sustain phase II antioxidant gene expression (Biagiotti et al., 2016). In experimental autoimmune encephalomyelitis (EAE), dexamethasone also presented antioxidant effect via up-regulation of Nrf2 and antioxidant enzymes and Nrf2-nuclear translocation (Li B. et al., 2013).
Glucocorticoid-mediated Nrf2 activation also plays an important role in various other physiological and pathological settings. In liver regeneration, Nrf2 has been shown to be a key player (Ishtiaq et al., 2018). A variety of factors regulate hepatic tissue regeneration, among them, augmenter of liver regeneration (ALR) (Dayoub et al., 2013). Chronic release of glucocorticoids increases intracellular ROS levels in hepatocytes, which induces Keap1-Nrf2 conformational changes. These events culminate in the release of the activated Nrf2, an important inducer of ALR expression (Adenuga et al., 2010; Dayoub et al., 2013). In the absence of Nrf2, decreased ALR levels and delayed liver regeneration are observed in mice subjected to partial hepatectomy (Zou et al., 2015). Therefore, glucocorticoid effect on liver regeneration is dependent on Nrf2 regulation of ALR expression. In airway epithelial cells, Nrf2 pathway was identified as essential to steroid (dexamethasone)-induced enhancement of airway epithelial barrier. The transfection of cells with specific siRNA reduced the enhancement of airway epithelial barrier integrity and the accumulation of tight junction and adherent junction proteins at sites of cell–cell contact. Moreover, transfecting cells with aldehyde oxidase 1 (AOX1)-specific siRNA, a downstream enzyme of Nrf2, also reduced steroid-induced enhancement of airway epithelial barrier integrity (Shintani et al., 2015). In cancer cells on the other hand, increased Nrf2 activation due to Keap1 or NRF2 mutations occurs frequently and this seems to be important in the maintenance of these cells. Therefore, Nrf2 inhibition could be a promising therapeutic strategy to target these cells (Ma et al., 2018; Rojo de la Vega et al., 2018). An initial screening of compounds with inhibitory effect on Nrf2 revealed the glucocorticoid clobetasol propionate (CP) as one of the most potent Nrf2 inhibitors. CP prevented nuclear accumulation and promoted Beta-transducin repeats-containing proteins (β-TrCP)-dependent degradation of Nrf2 in a GR- and GSK-3-dependent manner. As a result, CP induced oxidative stress in cancer cells, which strongly suppressed the growth of tumors with Keap1 mutation and high Nrf2 activity (Choi et al., 2017).
In addition to the various effects of GR-signaling via Nrf2, an inverse relation between Nrf2 and glucocorticoid activity has been reported. In a lung inflammation model, it was demonstrated that glucocorticoid activity is regulated by Nrf2. Compared to the WT, Nrf2-/- mice subjected to LPS-induced lung inflammation showed decreased HDAC2 levels and increased markers of inflammation, which was not reversed by the glucocorticoid budesonide (Adenuga et al., 2010). Abnormal lung inflammation and oxidant burden was associated with a significant reduction in HDAC2 abundance and glucocorticoid resistance, thus, glucocorticoid sensitivity during inflammatory response can be dependent on Nrf2-HDAC2 axis.
Natural Products-Derived Pharmacological Modulators of NRF2
Natural products have been described as extraordinarily rich sources of Nrf2 activators. Phytochemicals are biologically active compounds found in plants and considered to be the main representatives of Nrf2-activators (Kou et al., 2013; Zhu et al., 2016). In fact, analgesic and anti-inflammatory effects produced by many phytochemicals are considered to occur via Nrf2 pathway (Kumar et al., 2014), secondary to increased phase 2 enzymes, GSH production and turnover [GCL and glutathione reductase (GR)], increase of HO-1 and NQO1 levels, and reduced ROS levels (Ma, 2013; Figure 2). It is known that ROS such as superoxide anion induce inflammatory pain (Maioli et al., 2015) via NF-κB activation (Pinho-Ribeiro et al., 2016a) and increased TNFα, IL-1β (Fattori et al., 2015; Yamacita-Borin et al., 2015; Manchope et al., 2016) and endothelin levels (Serafim et al., 2015). These inflammatory peptides sensitize nociceptor sensory neuron terminals that transduce the nociceptive stimuli in peripheral tissue (Pinho-Ribeiro et al., 2017). Thus, the use of phytochemicals to induce Nrf2 pathway and reduce ROS production is a conceivable approach to treat inflammatory and painful conditions.
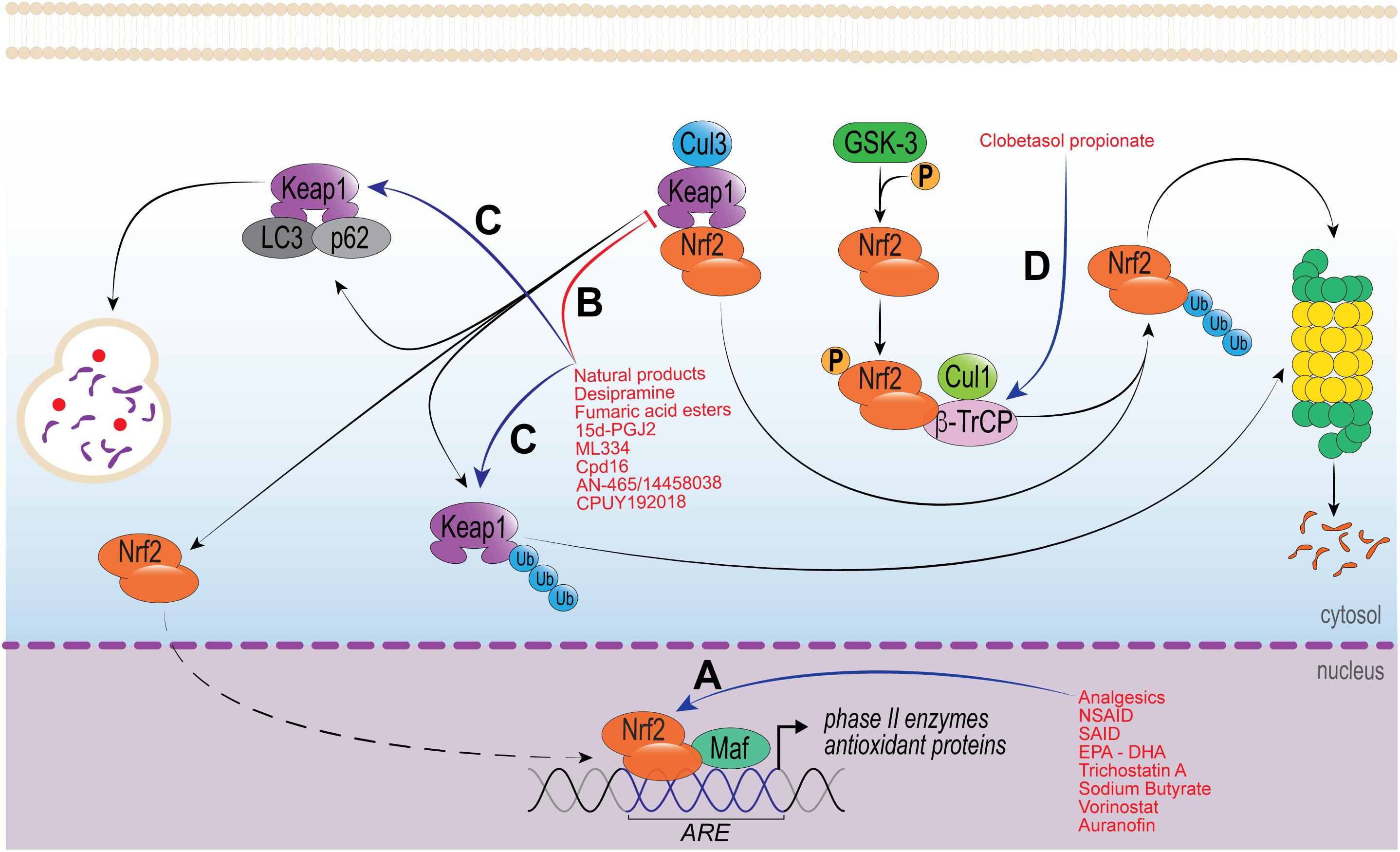
Figure 2. Molecular mechanisms of drugs that modulate Nrf2 activity. (A) Group of drugs that increase Nrf2 biding to DNA and/or, Nrf2-Maf affinity to ARE region and/or modulate histone acetyltransferase (HDAC). (B,C) Drugs modulating the disruption of Keap1/Nrf2 complex; either (B) by protein–protein interaction though Michael addition reaction and/or, (C) increasing Keap1 degradation through proteasome or autophagic pathways. (D) Drug targeting the degradation of Nrf2 via proteasome by increasing the GSK-3/β-TrCP signaling and reducing Nrf2 activity.
Nrf2 inducer phytochemicals can be classified into the following distinct classes: Michael acceptors, oxidizable phenols and quinones, isothiocyanates, dithiolethiones, polyenes or vicinal dimercaptans chemical types (Kumar et al., 2014). The common feature between them is the ability to react with sulfhydryl groups by alkylation, oxidation or reduction. Most phytochemicals are Michael acceptors (olefins or acetylenes-conjugated with electron-withdrawing groups) and undergo a conjugate addition with nucleophilic amino acids (cysteine, lysine, and serine) found in electrophile-sensitive proteins (Powers et al., 2002). Moreover, phytochemicals can react with sulfhydryl groups, acting as nucleophiles in Michael reaction signaling, culminating in the up-regulation of phase 2 enzymes (Dinkova-Kostova et al., 2001; Kumar et al., 2014).
Flavonoids are well known for their analgesic and anti-inflammatory properties. Hesperidin-methyl-chalcone (HMC), naringenin and quercetin, for example, exhibit analgesic and anti-inflammatory effects in acute and chronic inflammatory pain conditions. In fact, HMC, naringenin and quercetin inhibited carrageenan-, LPS-, and superoxide anion-induced inflammatory pain by mitigating leukocyte recruitment, oxidative stress, IL-33, TNFα, IL-1β, IL-6 production and NF-κB activation in mice (Maioli et al., 2015; Pinho-Ribeiro et al., 2015, 2016b,c; Manchope et al., 2016). Both the analgesic and anti-inflammatory actions of the aforementioned molecules have been attributed, at least in part, to Nrf2 pathway activation. The flavonoid HMC inhibited gout-induced inflammatory pain reducing synovitis, leukocyte recruitment, oxidative stress, inflammatory cytokines (TNFα, IL-1β, and IL-6) and inducing Nrf2 activation and HO-1 mRNA expression in mice (Ruiz-Miyazawa et al., 2018a). The flavanone naringenin inhibited titanium dioxide/prosthesis-like-induced chronic arthritis by mitigating leukocyte recruitment, cartilage degradation, bone resorption, oxidative stress, and inflammatory cytokine expression (IL-33, TNF-α, IL-1β, and IL-6), and NF-κB activation (Manchope et al., 2018). It is noteworthy that the chronic effect of naringenin in titanium dioxide/prosthesis-like model may be related to its inhibitory effect on superoxide anion-induced inflammatory pain through Nrf2 activation and increased HO-1 mRNA expression (Manchope et al., 2016). Mechanistically, naringenin increased Nrf2/HO-1 through PI3K/Akt signaling pathway in D-galactose-induced mice brain aging (Zhang et al., 2017). The flavonoid quercetin has also been reported to inhibit chronic inflammatory pain in titanium dioxide/prosthesis-like-induced chronic arthritis and Ehrlich tumor-induced pain in mice (Calixto-Campos et al., 2015b). The mechanisms attributed to this analgesic effect was reduced leukocyte recruitment, oxidative stress, and inflammatory cytokine production (TNF-α, IL-1β, and IL-6) secondary to increased Nrf2 and phase 2 enzymes HO-1, GPx1 and GR (Calixto-Campos et al., 2015b; Borghi et al., 2017). Quercetin also presents hepatoprotective effect in acetaminophen (APAP)-induced cytotoxicity in human liver L-02 cells by inducing Nrf2 activation and its downstream enzymes HO-1 and GCL. Mechanistically, quercetin-induced Nrf2 activation by Keap1-dependent and Keap1-independent mechanisms. Keap1-dependent mechanism was secondary to the interaction of H-benzene and H-bond of quercetin to Keap-1 Arg415 and Tyr527, and Gly364, respectively. The Keap1-independent mechanism, on the other hand, occurred through JNK MAP kinase signaling pathway. Interestingly, quercetin also induced hepatoprotection by increasing p62 protein via JNK signaling pathway (Ji et al., 2015). Collectively, the flavonoid induction of Nrf2 and its downstream enzymes may be Keap1-dependent and Keap1-independent, and by the non-canonical pathway via p62 (Figure 2). However, further investigations are needed to establish which pathways are being activated by each molecule.
Curcumin, a yellow pigment found in turmeric, inhibited superoxide anion-induced inflammatory pain via the inhibition of leukocyte recruitment, oxidative stress, TNF-α, and IL-1β production and induction of Nrf2 activation and HO-1 expression (Fattori et al., 2015). In addition, this polyphenol inhibited neurological impairment, brain edema and infarction volume, capillary leakage, and oxidative stress in middle cerebral artery occlusion-induced ischemic/reperfusion damage in rats by activating Nrf2 and inhibiting NF-κB (Li et al., 2016). The curcumin induction of Nrf2 expression and its downstream enzymes were dependent of PI3K/Akt signaling pathway activation in an in vitro model of ischemia/reperfusion (Wu et al., 2013). Studies show that curcumin may also exert therapeutic effect as a Michael acceptor by binding to COX-1, COX-2 (Selvam et al., 2005), and GSK-3β (Bustanji et al., 2009). Moreover, in a randomized double-blind placebo-controlled clinical trial, the positive effect of the dietary supplementation with curcumin on the redox status and Nrf2 activation was observed in patients with non-diabetic or diabetic proteinuria in chronic kidney disease (Jimenez-Osorio et al., 2016).
Cinnamic acid and its derivates are one of the simplest phenolic acids found in nature (Anantharaju et al., 2016). Caffeic acid, a cinnamic acid derivate found in thyme and oregano, possesses analgesic activity. This analgesic effect was observed in the formalin- (second phase) and acetic acid-induced writhing tests, as well as, LPS- and carrageenan-induced mechanical hyperalgesia in rats (Mehrotra et al., 2011). Later studies revealed that caffeic acid also has hepatoprotective and anti-inflammatory effects. In APAP-induced liver injury model, this molecule protected mice by reducing neutrophil recruitment and ROS levels and increasing GSH levels in the liver. Interestingly, caffeic acid alone, i.e., independent of APAP-induced injury, boosted hepatic GSH levels (Pang et al., 2016). These effects were attributed to the induction of Nrf2 and its downstream enzymes HO-1 and NQO1 by caffeic acid, as observed in human liver L-02 cells. Mechanistically, caffeic acid decreased Keap1 content through the H-benzene interaction with Arg415 of Keap1. In addition, the hydrogen atom of the hydroxyl at 3-position in caffeic acid formed water-mediated hydrogen bonds with Ser508, and the carbonyl group of CA could form H-bond with Gly603 and Ser363, enhancing the binding affinity between caffeic acid and Keap1 (Pang et al., 2016).
The flavoring agent vanillic acid is also an analgesic and anti-inflammatory. In carrageenan-induced inflammatory pain model, vanillic acid mitigated hyperalgesia, leukocyte recruitment, oxidative stress, IL-33, TNFα, and IL-1β production, and NF-κB activation in mice. Accordingly, the analgesic and anti-inflammatory effects of vanillic acid were related to Nrf2 activation (Calixto-Campos et al., 2015a). In agreement, vanillic acid also inhibited Aβ1-42-induced oxidative stress, neuroinflammation and cognitive impairment in mice through Nrf2 activation and HO-1 expression (Amin et al., 2017). Although the effects were described to be secondary to Nrf-2 pathway activation, these studies did not show how this occurred. Therefore, further investigations demonstrating the precise molecular mechanism involved in vanillic acid activity are necessary.
Terpenoids are naturally occurring organic chemicals derived from five-carbon isoprene unit comprising mono-, di-, and tri-terpenoids. Kaurenoic acid is a diterpene found in Sphagneticola trilobata with analgesic and anti-inflammatory properties in vivo. Both carrageenan-induced inflammatory pain and TNF-α and IL-1β production were inhibited by kaurenoic acid in mice (Mizokami et al., 2012). It is postulated that the analgesic effect of this compound could be related to Nrf2 activation, since kaurenoic acid attenuated LPS-induced acute lung injury, neutrophil recruitment and inflammatory cytokine gene expression (TNFα and IL-1β) by activating Nrf2 and regulating the expression of phase 2 enzymes NQO-1, HO-1 and glutamate-cysteine ligase catalytic subunit (GCLC) (Kim et al., 2016). Glycyrrhizin a pentacyclic triterpenoid found in licorice root inhibited CFA-induced mechanical and thermal hyperalgesia in mice paw and increased IL-6, TNFα, and IL-1β levels, microglia activation, HMGB1, and NF-κB activation in spinal cord (Sun et al., 2018). Mechanistically, glycyrrhizin inhibited LPS-induced HMGB1 release through p38/Nrf2-dependent induction of HO-1 in Raw 264.7 cells (Kim et al., 2015). In silico molecular docking assay showed that glycyrrhizin interacted directly with 16-mer Nrf2 peptide binding site on Keap1, suggesting that the interference in Keap1/Nrf2 binding was involved (Kamble et al., 2017). These findings suggest that Nrf2 activation by glycyrrhizin may be both Keap1 dependent and independent.
Oxidizable phenols and quinones were one of the first classes described as phase 2 inducers before the discovery of Nrf2/ARE pathway (Prochaska et al., 1985; Kumar et al., 2014). Catechol (1,2-diphenol) and hydroquinone (1,4-diphenol) are Nrf2 inducers that undergo oxidation by cytochrome p450 in vivo. This reaction results in the formation of its quinone derivate, which contains a Michael acceptor. This quinone can react with critical cysteine residues in Keap1, resulting in Nrf2 activation and increased phase 2 enzyme expression (Bensasson et al., 2008). Rosmarinic acid is a polyphenol-containing a catechol moiety found in rosemary and peppermint. It has been demonstrated that pre- or post-treatment with this compound mitigates chronic constriction injury (CCI)-induced neuropathic pain by inhibiting mechanical and thermal allodynia, oxidative stress, glial cell activation, and TNFα production (Anantharaju et al., 2016). These effects were related to Nrf2 activation. Rosmarinic acid also inhibited carbon tetrachloride-induced liver intoxication in mice by reducing oxidative and nitrosative stress, TNFα, and COX-2 protein expression, and NF-κB activation, as well as inducing Nrf2 activation and HO-1 in the liver (Domitrovic et al., 2013). Importantly, it was demonstrated that Nrf2 activation ocurred through Akt/GSK-3β/Fyn pathway, because the inhibition of β-amyloid-induced oxidative stress by rosmarinic acid was abrogated by Akt inhibitor LY294002, GSK-3β inhibitor LiCl, Nrf2 shRNA, or Fyn shRNA in PC12 cells (Rong et al., 2018). Nevertheless, it remains to be determined if Nrf2 activation by rosmarinic acid is also dependent on Keap1 interaction. In contrast, the interaction with Keap1 was demonstrated for carsonic acid. This molecule interacts with Keap1 via alkylation of critical cysteine residues. This interaction was central to the inhibition of oxidative stress in immature cortical neurons (Satoh et al., 2008). Moreover, the importance of Nrf2 activation in carsonic acid activity was highlighted by the abrogation of this effect in dominant negative Nrf2 cortical neurons (Satoh et al., 2008). In vivo, carsonic acid translocates into the brain, increases the level of reduced glutathione in vivo, and protects the brain against middle cerebral artery ischemia/reperfusion (Satoh et al., 2008).
Epigallocatechin gallate (EGCG), a catechin polyphenol found in green tea, inhibited bone cancer-induced pain and neuroinflammation by decreasing TNFα in mice spinal cord (Li and Zhang, 2015). ECCG is protective in fluoride-induced intoxication kidney damage in rats. The mechanism attributed to this effect was decreasing oxidative stress, NFκB activation, and inflammatory cytokine (TNF-α, and IL-6) levels, secondary to Nrf2 activation and upregulation of phase 2 enzymes HO-1, GCL, and GST (Thangapandiyan and Miltonprabu, 2014). Collectively, these data indicate that the analgesic and anti-inflammatory effects described for EGCG could be related to Nrf2 activation. Mechanistically EGCG effects involve the activation of ERK and p38 MAPK signaling pathways. In human umbilical vein endothelial cells, PD98059 (a selective inhibitor of extracellular signal regulated kinase [ERK]-1/2) and SB203580 (a selective inhibitor of p38 MAPK), but not SP600125 [a selective inhibitor of c-jun N-terminal kinase (JNK)], attenuated the EGCG-induced Nrf2 and HO-1 expression. Moreover, silencing Nrf2 abolished EGCG-induced enhancement of cell viability and the upregulation of Nrf2 and HO-1 (Yang et al., 2015). Collectively, the studies discussed above show that oxidizable phenols and quinones also induce Nrf2 and its downstream enzymes through Keap1 dependent and independent mechanisms.
Isothiocyanates are derived from their glucosinolate precursors, which are found in cruciferous plants. Glucosinolates are hydrolyzed by plant enzyme myrosinase or by mammalian gastrointestinal microflora (Fahey et al., 2001; Shapiro et al., 2001) and important biological activity has been attributed to these compounds. Sulforaphane, an isothiocyanate found in broccoli, brussels sprout and cabbage, inhibits CCI-induced neuropathic pain and the expression of inflammatory cytokines, COX-2 and iNOS proteins in the spinal cord. Interestingly, the opioid pathway seemed to be involved in sulforaphane analgesic effect, since naloxone inhibited its anti-allodynic action (Wang and Wang, 2017). Anhedonia (loss of pleasure) is a common feature in patients with neuropathic pain and spared nerve ligation (SNI) in rats, which can induce a depression-like phenotype in some of the animals. This phenotype is related with reduction in Nrf2 levels in medial prefrontal cortex (mPFC), hippocampus, spinal cord and skeletal muscle, but not nucleus accumbens in anhedonia-susceptible compared to anhedonia-resistant and sham rats (Li S. et al., 2018). Sulforaphane pretreatment inhibited SNI-induced mechanical hyperalgesia in anhedonia susceptible and resistant rats and normalized Nrf2 levels in mPFC, hippocampus, spinal cord and skeletal muscle in anhedonia-susceptible rats (Yao et al., 2016). In agreement, sulforaphane also inhibited nitroglycerin-induced hyperalgesia and neuronal activation (c-fos and nNOS immunoreactivity) in trigeminal nucleus caudalis in mice by increasing nuclear Nrf2 and phase 2 proteins HO-1 and NQO-1 in neurons (Di et al., 2016). In other contexts, sulforaphane controls inflammation and improves bacterial clearance in chronic obstructive pulmonary disease (COPD). Mice exposed to cigarette smoke for 6 months and challenged with Haemophilus influenza or Pseudomonas aeruginosa presented pulmonary inflammation, increased in leukocyte recruitment, and impairment in bacterial clearance by alveolar macrophages. The treatment with sulforaphane ameliorated these events through Nrf2 activation and upregulation of macrophage receptor with collagenous structure (MARCO), its downstream target. In agreement, sulforaphane restored the capacity of bacteria recognition and phagocytosis by alveolar macrophages from COPD patients (Harvey et al., 2011). Mechanistically, sulforaphane seems to induce Nrf2 activation through Keap1 cysteine residue 151 interaction (Hu et al., 2011). Regarding the effects of sulforaphane in humans (Table 2), the oral consumption of sulforaphane doses contained in standardized broccoli sprout homogenate increased phase II enzymes in nasal lavage cells in a placebo-controlled dose escalation trial (Riedl et al., 2009). On the other hand, in a randomized double-blind clinical trial, patients with COPD receiving oral sulforaphane treatment did not present significant changes in Nrf2 target genes or markers of inflammation in alveolar macrophages or bronchial epithelial cells (Wise et al., 2016). Interestingly, although similar doses of sulforaphane were tested, these studies reported different outcomes. Differences in the study subjects may account for this. The first trial was conducted with healthy non-smokers, whereas in the second, the subjects were either active or former smokers with COPD. It is plausible that higher doses of sulforaphane may be necessary to produce significant changes in Nrf2 target genes and disease outcome as the disease severity increases. In this case, inhalable formulation of sulforaphane may be more effective in guaranteeing higher delivery in the lungs. Nevertheless, further studies should be conducted to test this hypothesis.
Capsaicin, a transient receptor potential cation channel subfamily V member 1 (TRPV1) agonist, is a compound found in the red pepper. Acutely, capsaicin induces pain, but chronically, it displays analgesic effects when administrated centrally or peripherally by depleting neuropeptides at supraspinal level (Fattori et al., 2016). Unexpectedly, capsaicin induces the production of ROS, which can interact with NQO1. Subsequently, Nrf2-ARE binding can occur; followed by Nrf2 activation and induction of HO-1 expression via the PI3K/Akt signaling pathways in HepG2 cells (Joung et al., 2007). However, it is unknown whether the analgesic effect of chronic capsaicin treatment depends on Nrf2 induction.
In summary, this section of the review brought data from the key phytochemical classes that have analgesic and anti-inflammatory activity through Nrf2 pathway (Figure 2). It is noteworthy that natural products are good candidates to associate with conventional analgesic and anti-inflammatory therapeutic protocols. The association of these molecules could reduce the dose of drugs such as opioids, non-steroidal and steroidal anti-inflammatory, that have several known adverse reactions. Seeking for novel analgesics and anti-inflammatory drugs with lessened side effects is also important and may produce substitute drugs for cases in which the side effects of current drugs do not allow their therapeutic use.
Other Therapies Modulating NRF2
In addition to the previously discussed classes of drugs, many other compounds have shown important biological activity via Nrf2. There are a variety of Nrf2 inducers, most of which are electrophilic molecules. These molecules react with cysteine thiols of Keap1, being Cys151, Cys273, and Cys288 residues the most prone to electrophile reaction. Electrophile adducts can inhibit Keap1 in two different ways. First, by the induction of a conformational change in Keap1, which results in the loss of its binding capacity to Nrf2. Second, by blocking the interaction between Keap1 and CUL3/RBX1, resulting in sequestration of Keap1 with Nrf2 and further stabilization of newly synthetized Nrf2 (reviewed in Cuadrado et al., 2018; Figure 2).
Fumaric acid esters, including dimethyl fumarate (DMF) and the monoester form monomethyl fumarate (MMF), are the most prominent examples of Keap1 cysteine residue modifiers (Lin et al., 2011). DMF [Tecfidera® by Biogen] is to date the only Food and Drug Administration approved drug registered as NRF2 activator. DMF and other fumaric acid esters have been used to treat psoriasis for over 50 years, when the role of Nrf2 in disease was still unknown. Nevertheless, clinical trials have demonstrated the effectiveness of these compounds in reducing psoriasis area and severity index (Altmeyer et al., 1994), and treating cases of moderate to severe chronic plaque psoriasis (Mrowietz et al., 2017; Tzaneva et al., 2018; Table 2). The mechanisms of action related to these effects include a shift from a T helper (Th)1 toward a Th2 immune response, in addition to the overall decrease in the number of peripheral T cells (Ghoreschi et al., 2011; Tahvili et al., 2015). Considering the immune modulatory actions of fumaric acid esters, it is not surprising that these compounds have also therapeutic effects in other auto-immune diseases such as cutaneous lupus erythematosus (Kuhn et al., 2016; Saracino and Orteu, 2017) and multiple sclerosis (MS) Table 2. DMF was approved in 2013 for the treatment of MS (Xu et al., 2015) and is currently used as the first line treatment of relapsing-remitting MS that does not respond to traditional therapies (Bar-Or et al., 2013). Positive results in EAE, a mouse model for MS, were early indications that fumaric acid esters may have beneficial effects in this disease (Schilling et al., 2006). Schilling et al. (2006) reported important therapeutic effects on the course of disease and histology attributed to the reduction in macrophage-mediated inflammation in the spinal cord and increase in systemic IL-10 levels (Schilling et al., 2006). Accordingly, in this same model of MS, DMF-mediated beneficial effects on clinical course and preservation of myelin, axons, and neurons were observed in WT, but not in Nrf2-/- mice (Ellrichmann et al., 2011; Linker et al., 2011). In line with the potent anti-inflammatory effects described, DMF also modulates the immune response in dendritic cells and T cells by reducing the release of inflammatory cytokines (Ockenfels et al., 1998). Moreover, DMF prevents endothelial dysfunction and cardiovascular pathologic ROS formation and inflammation, as well as decrease atherosclerosis and kidney dysfunction in diabetic mice (Tan et al., 2014; Sharma et al., 2017). Overall, the anti-inflammatory and immune modulatory activities of fumaric acid esters indicate that these compounds may also be successful in treating other disease in which chronic inflammation and ROS production are important pathological mechanisms.
As previously discussed in this review, Nrf2 acetylation mediated by histone acetyltransferase/HDAC enhances its transcriptional ability and the expression of downstream targets. This is the mechanism of action of the pan-HDAC inhibitor trichostatin A, which protects against cartilage degradation via the reduction in matrix metalloproteinase (MMP)s and proinflammatory cytokines TNF-α, IL-1β, and IL-6 in osteoarthritis (Cai et al., 2015). Moreover, protective effects via Nrf2 in inflammatory cystic fibrosis lung disease and cerebral ischemic damage have also been observed for trichostatin A (Wang et al., 2012; Bodas et al., 2018). Other HDAC inhibitors, for example sodium butyrate and vorinostat, also mitigate inflammation and the up regulation of MMPs and aggrecanase 2 in human osteoarthritis chondrocytes. As a result, HDAC inhibitors have protective effects against cartilage degradation through mechanisms such as Nrf2 activation and the inhibition of NF-κB and MAPK (Khan and Haqqi, 2018). In addition to the drugs discussed in this section, numerous other Nrf2 inducers acting as electrophilic Keap1 modifiers have been or are currently being tested in clinical trials for the treatment of diseases/conditions such as kidney disease, diabetes, liver diseases (non-alcoholic fatty liver disease, and liver fibrosis and cirrhosis), and cancer. Some examples of these molecules are Bardoxolone methyl (Pergola et al., 2011; Hong et al., 2012; de Zeeuw et al., 2013) and Oltipraz (Kim S.G. et al., 2010; Kim et al., 2017a), which are shown in Table 2.
Auranofin (2,3,4,6-Tetra-O-acetyl-1-thio-beta-D-glucopyranosato-S [triethylphosphine] gold) is a gold(I)-containing antirheumatic drug that possesses anti-inflammatory properties mainly via HO-1 induction. The antirheumatic gold(I)-containing compound selectively activates the DNA binding of the heterodimer Nrf2 and small Maf. Once bound to the ARE or Maf-recognition element, Nrf2/small Maf induces a range of antioxidative stress genes, including HO-1 and γ-glutamylcysteine synthetase, which contribute to the scavenging of ROS and exert anti-inflammatory effects (Kataoka et al., 2001). Moreover, auranofin can elevate cellular levels of Nrf2 by increasing protein stability. Co-immunoprecipitation and Western blot analysis indicated that auranofin inhibits Nrf2 degradation by inducing the dissociation of the Nrf2-Keap1 complex, resulting in nuclear accumulation of Nrf2. Additionally, mechanistic studies revealed that upregulation of Keap1/Nrf2 signaling and downstream HO-1 by auranofin is dependent on Rac1/iNOS induction and MAPK activation (Kim N.H et al., 2010).
The role of lipid mediators in promoting the resolution of inflammation has been widely demonstrated (Serhan et al., 2008). Strikingly, studies are now showing that these effects might be induced via Nrf2 activation. The pro-resolving lipid mediator 15d-PGJ2, for example, has been reported to interact with Nrf2. 15d-PGJ2 forms an adduct to Keap1 and disrupts Nrf2 ubiquitination, leading to the accumulation of Nrf2 in the nucleus (Joo et al., 2017). Numerous studies have demonstrated the anti-inflammatory activity of 15d-PGJ2 via Nrf2 activation (Kudoh et al., 2014; Li et al., 2015; Ruiz-Miyazawa et al., 2018b). In a model of gout arthritis in mice, for example, 15d-PGJ2-loaded nanocapsules reduced monosodium urate (MSU)-induced pain, inflammatory cytokine production, and NLRP3 inflammasome and NF-κB activation (Ruiz-Miyazawa et al., 2018b). Treatment with 15d-PGJ2-loaded NC mitigated oxidative stress and increased both Nrf2 and HO1 mRNA expression, which were reverted by the PPAR-γ inhibitor GW9662. These observations suggest that these effects of 15d-PGJ2-loaded NC are PPAR-γ dependent, which is in line with previous studies showing that PPAR-γ activation results in increased Nrf2/HO-1 signaling and antioxidant defenses (Hsu et al., 2013). Further substantiating the central role of Nrf2 in the anti-inflammatory activity of 15d-PGJ2, Bretscher et al. (2015) demonstrated that the reduction in IL-6 and IL-12 expression by 15d-PGJ2 was not present in Nrf2-deficient myeloid cells (Bretscher et al., 2015). Interestingly, 15d-PGJ2 also potentiates macrophage efferocytosis through Nrf2-mediated upregulation of CD36 and HO-1. Macrophage efferocytosis is central to the clearance of apoptotic neutrophils during the resolution of inflammation, thus 15d-PGJ2 also promotes the resolution of inflammation (Kim et al., 2017b).
Other lipid mediators that also seem to mediate anti-inflammatory effects via Nrf2 are lipoxin A4 (LXA4) and Resolvin D1 (RvD1) (Martinez et al., 2018; Saito et al., 2018). In ultraviolet (UV) radiation-induced skin inflammation in mice, LXA4 and RvD1 greatly diminished inflammation, matrix metalloproteinase 9 expression, and sunburn cell counts (Martinez et al., 2018; Saito et al., 2018). These lipids also induced Nrf2 and Nqo1 expression, as well as mitigated oxidative stress. Skin damage induced by UVB irradiation is highly dependent on ROS; and Nrf2 has an important role in the restorative adaptive response to UV radiation-induced inflammation and sunburn reaction (Patwardhan and Bhatt, 2015). Therefore, it is likely that increased Nrf2 expression is important in the protective effect of LXA4 and RvD1 against UV radiation-induced skin inflammation. In the same rationale, polyunsaturated fatty acids have also been shown to activate Nrf2 in inflammation. The omega-3 fatty acids docosahexaenoic acid (DHA) and eicosapentaenoic acid (EPA), are sources for the production of Protectin and Resolvin D-series, and Resolvin-E series, respectively (Serhan et al., 2008). Therefore it is conceivable that they also may modulate Nrf2 activity. Both DHA and EPA were shown to be cytoprotective against oxidative insults in endothelial cells in a Nrf2-dependent manner (Lee et al., 2015; Sakai et al., 2017). DHA also significantly mitigated the toxicity and increase in MCP-1 levels by organic pollutants such as polychlorinated biphenyls (PCBs) in vascular endothelial cells; and this effect was attributed to increased Nrf2 DNA binding and downstream expression of antioxidants (Majkova et al., 2011). Although several of these studies have reported Nrf2 pathway as being central to the anti-inflammatory and antioxidant effects of the aforementioned lipid mediators and polyunsaturated fatty acids, the precise molecular mechanism involved in the activation of Nrf2 was not demonstrated. In this sense, the interaction between Nrf2 and these molecules is still unclear.
Although Keap1 oxidation by electrophilic compounds (classic inducers) is essential for Nrf2 activation and its downstream effects (canonical pathway), the lack of selectivity of electrophilic Keap1 inhibitors is frequently overlooked and may account for some of the off-target and undesired side effects. Bardoxolone methyl, for example, can interact with over 500 different proteins, including many different transcription factors (Yore et al., 2011; Zhang, 2013). To overcome the lack of selectivity and off-target effects, a new class of NRF2 inducers that prevent the docking of NRF2 to KEAP1 has emerged (Richardson et al., 2015). The Kelch-DC domain of Keap1 binds to Nrf2 via either its DLG or ETGE motif; both of which are thought to be the major targets of small peptides capable of disrupting Keap1-Nrf2 protein–protein interaction (PPI) (Xu et al., 2015; Gazaryan and Thomas, 2016; Jiang et al., 2016; Saito et al., 2016; Dinkova-Kostova et al., 2017). To date, five families of Keap1-Nrf2 PPI inhibitors have been described: tetrahydroisoquinoline, thiopyrimidine, naphthalene, carbazone, and urea derivatives (reviewed recently in Cuadrado et al., 2018) and studies show that these peptides are excellent candidates to activate Nrf2 due to their potent activity and specificity (Hancock et al., 2012, 2013).
The discovery that the DEETGE sequence in Nrf2 is critical for the Keap1-Nrf2 interaction led to the development of DEETGE-CAL-Tat synthetic peptides. These peptides containing the DEETGE sequence, a caplain cleavage site, and a HIV-Tat cell transduction domain, were shown to disrupt Keap1-Nrf2 interaction and induce Nrf2 genes in vitro and in vivo in brain injured mice (Zhao et al., 2011). A subsequent study revealed that this peptide also had neuroprotective and cognitive-preserving effects in rats subjected to global cerebral ischemia. The administration of the DEETGE-CAL-Tat peptide strongly enhanced nuclear translocation and DNA binding of Nrf2, as well as expression of known Nrf2-regulated target antioxidant/cell-defense proteins in the hippocampal CA1 region. Intracerebroventricular pre-treatment or peripheral post-treatment also induced robust neuroprotection in the hippocampal CA1 region and strongly preserved cognitive function in this model (Tu et al., 2015). In a study by Lu et al. (2018), on the other hand, the head-to-tail cyclic strategy was applied in the development of novel peptide inhibitors (Lu et al., 2018). A novel cyclic peptide 3 showed high binding affinity with Keap1 and potency in Nrf2 activation at cellular level. This peptide exhibited effective anti-inflammatory effects in mouse RAW 264.7 cells by activating the Nrf2-regulated defense system and enhancing the antioxidant capacity (Lu et al., 2018). Importantly, from the large number of compounds indexed, LH601, benzenesulfonyl- pyrimidone 2, N-phenyl-benzenesulfonamide, and a series of 1,4-diphenyl-1,2,3-triazoles seem to be more promising candidates to inhibit the PPI with KEAP1, due to favorable atomic interaction with KEAP1, affinity, and thermodynamic parameters of binding (Cuadrado et al., 2018). Nevertheless, the effect of these molecules has yet to be tested. Moreover, more studies are necessary to test the effects, potency, and safety and better elucidate the mechanisms of the newly developed small peptides discussed herein.
Role of Drugs Acting Via NRF2 in Cancer
Chronic inflammation, pain and oxidative stress often accompanies cancer. Nrf2 has been conventionally considered as a tumor suppressor, especially in the early stages of cancer. The definition that Nrf2 is a tumor suppressor comes from its cytoprotective effect against exogenous and endogenous insults such as xenobiotics (Sporn and Liby, 2012). More recently, molecular analysis have stablished that Nrf2 is a oncogenic factor and its activation leads to chemotherapy resistance (Ganan-Gomez et al., 2013). In this context, it is controversial whether the activation of Nrf2 by pharmacological agents with anti-inflammatory and antioxidant properties are useful for the prevention or treatment of cancer.
In experimental studies, the chemopreventive role of Nrf2 inducers was mainly addressed by using the naturally occurring isothiocyanate, sulforaphane. Sulforaphane has received audience because of its ability to simultaneously modulate early stages of carcinogenetic events (initiation) or hamper steps involved in cancer development (Fimognari and Hrelia, 2007; Jiang et al., 2018). Mechanistically, sulforaphane, via Nrf2, promotes DNA protection by inducing phase II enzymes (Morimitsu et al., 2002). This complex association between sulforaphane-Nrf2 is defined by the reversible modification of Keap1 cysteine residues (Hu et al., 2011), interaction with MAPK, phosphatidylinositol 3-kinase (PI3K), PKC pathways, NF-κB, or epigenetic modifications (Su et al., 2018). Therefore, the modulation of kinases or DNA methyltransferases results in phosphorylation, nuclear accumulation, and increased transcription and stability of Nrf2. In addition, all effects were observed in nanomolar range (Guan et al., 1990; Su et al., 2018).
Besides sulforaphane, others molecules with distinct mechanisms, including phenethyl isothiocyanate, oltipraz, curcumin, resveratrol, fumaric acid and its esters, and synthetic oleanane triterpenoids also have therapeutic effects in cancer by targeting Nrf2 (Gupta et al., 2004). Of note, although all these chemopreventive molecules can interact with proteins other than Nrf2, indicating that Nrf2-independent mechanisms were also present, their beneficial effects were abrogated or decreased in Nrf2 knockouts (Ramos-Gomez et al., 2001). Additionally, ARE reporter mice and measurements of NAD(P)H:quinone oxidoreductase (NQO1) and GST transcripts levels were also used to confirm the involvement of Nrf2 (Lewis et al., 2006; Sporn and Liby, 2012). Importantly, all these chemopreventive drugs reduce at low doses the uncontrollable oxidative stress generated by carcinogens that would damage DNA and induce persistent inflammation (Hayes et al., 2010; Hu et al., 2010; Sporn and Liby, 2012). So far, the beneficial role of Nrf2 induction has been widely explored at multiple organ sites including skin (Ben Yehuda Greenwald et al., 2017; Alyoussef and Taha, 2018), lungs (Kumar et al., 2011; To et al., 2015; Creelan et al., 2017; Lin et al., 2017), bladder (Iida et al., 2004; Leone et al., 2017), breast (Pledgie-Tracy et al., 2007; Soto-Balbuena et al., 2018; Soundararajan and Kim, 2018), colon (Rajendran et al., 2015; Guo et al., 2018), pancreas (Kallifatidis et al., 2009), stomach (Fahey et al., 2002), and oral cancer (Bauman et al., 2016; Soundararajan and Kim, 2018).
Because Nrf2 promotes cell survival under stress, it is coherent to assume that an increase in Nrf2 could be protective for cancer cells. In this context, the hyperactivation or unbalanced regulation of Nrf2 may participate in tumor growth or be involved in chemoresistance (Ganan-Gomez et al., 2013). However, only a few studies reporting a cancer-promoting role of Nrf2 have been published.
The frequencies of Nrf2 and Keap1 mutation in tumors are often low (Taguchi et al., 2011). Following the discoveries and characterization, it was observed that common oncogenes, such as KRAS, BRAF, and MYC, increase the transcription and activity of Nrf2, resulting in an increase in cytoprotection and, most notably, a decrease in free radical generation (DeNicola et al., 2011; Sutcliffe et al., 2011). Thus, oncogenes may promote tumorigenesis, in part, in a Nrf2-dependent manner by enhancing the survival of tumor cells (DeNicola et al., 2011). Therefore, considering that cells do not appear to become refractory to repeated activation of the NRF2 pathway by drugs, it is possible that cancer cells utilize a non-mutated pathway to support tumor growth in early stages (Perera and Bardeesy, 2011). Despite these effects, studies point out that increased levels of fumarate, due to chronic exposition to DMF, can become carcinogenic to cells (Sporn and Liby, 2012; To et al., 2015). However, additional studies are required to determine whether phytochemicals, synthetic chemopreventive agents or others drugs targeting Nrf2 increase or decrease cancer risk.
Persistent activation of Nrf2 also enhances resistance to etoposide, doxorubicin, tamoxifen and cisplatin. Alongside with this, many Nrf2 downstream genes, in particular, heme oxygenase-1 (HO-1) have been shown to contribute to the observed Nrf2-dependent chemoresistance (Jozkowicz et al., 2007; Jeddi et al., 2017). In this sense, the abrogation of drug-induced ROS by Nrf2 can confer chemoresistance. Moreover, there is no clear understanding between pharmacological agents acting via Nrf2 and direct resistance, since the persistent activation of Nrf2 is usually a result of a genetic mutation (Homma et al., 2009; Kensler and Wakabayashi, 2010).
Overall, the timing of Nrf2 activation is important in the context of cancer, as summarized in Figure 3. Enhancing Nrf2 is essential for the prevention of cancer, especially at low doses by drugs that enhance this pathway. On the other hand, in fully malignant cells and in advanced stages of cancer, the enhancement of Nrf2 caused by mutations can protect the tumor microenvironment. At this point, modulating unique redox regulatory mechanism or avoiding use of Nrf2 inducers from general source that greatly accumulate in cells might be effective. However, the direct effects of Nrf2 inducers or even palliative drugs acting via Nrf2, for instance, morphine on cells at intermediate stage of cancer, need further investigation.
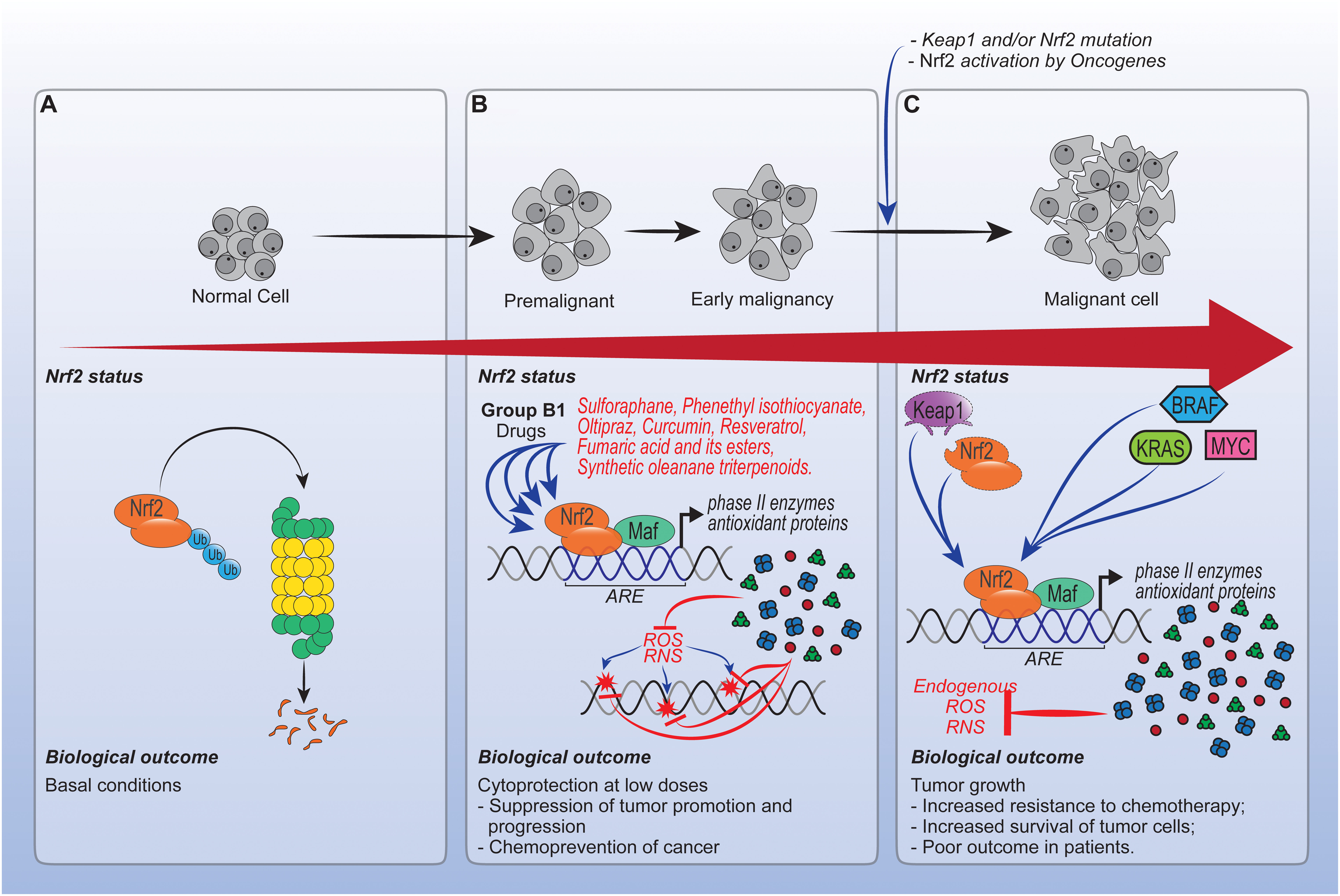
Figure 3. Role of drugs acting via Nrf2 in cancer. (A) In normal cells, Nrf2 activity is regulated by canonical and non-canonical pathways, which in the absence of oxidative stress, culminate in Nrf2 proteossomal degradation. (B) Enhancing Nrf2 in premalignant and early malignant cells is important to prevent cancer development, specially by low doses of drugs capable of inducing phase II enzymes and antioxidant proteins expression. (C) Otherwise, in malignant cells the enhancement of Nrf2 activity caused by mutations such as Kras, Bras and Myc, can protect tumors from the cytotoxic effects of reactive oxygen species (ROS) induced by chemotherapy. However, the effects of drugs that act via Nrf2 at intermediate and chemotherapy stages still need investigation. Overall, the effects of Nrf2 in cancer depend on the biological development stage of tumor cells.
Non-Pharmacological Approaches Targeting KEAP1-NRF2 Pathway
In this section we will discuss data from preclinical studies on non-pharmacological approaches of targeting Keap1-Nrf2 signaling pathway in varied inflammatory disorders. Among the non-pharmacological approaches, the use of interference RNA (iRNA) and genetic constructions targeting the knockdown (KD) of keap1 gene are widely applied in a variety of in vivo and in vitro studies. In fact, a keap1 KD with background on scurfy mice was developed (Suzuki et al., 2017). Scurfy mice are deficient in Treg cells; therefore, they succumb to severe multi-organ inflammation by 4 weeks of age. The systemic activation of Nrf2, by KD of Keap1, considerably ameliorated inflammation and lethality. In addition, increases in Nrf2 activation reduced the number of activated T cells and the amount of pro-inflammatory cytokines (Suzuki et al., 2017).
Similarly, in vivo KD of Keap1 decreased fasting-induced steatosis (Xu et al., 2013). The overt-activation of Nrf2 in this particular case decreased the levels of lipid accumulation in the liver and reduced the expression of lipogenic genes and genes related to fatty acid transport. In addition to these findings, the authors propose that Nrf2 plays a role in insulin signaling regulation and enhances insulin sensitivity in skeletal muscle (Xu et al., 2013). The Keap1 KD was also investigated in glomerulosclerosis (Miyazaki et al., 2014). The increase in Nrf2 activity ameliorates podocyte injury caused by an immunotoxin. These findings suggest that the Keap1-Nrf2 system is a promising target in the treatment of chronic liver and kidney diseases.
Although great efforts have been made to study the role of Nrf2 in various diseases, the in vivo study of Keap1 is limited to its knockdown, as previously described. Interestingly, Keap1-null mice present postnatal lethality (Wakabayashi et al., 2003). In fact, constitutive activation of Nrf2 culminate in morphological alterations on esophagus and forestomach, manifesting as hyperkeratosis lesions. Two major hypotheses were postulated to explain the lethality of Keap1-null mice. First, a subset of genes for squamous cell differentiation is within the ARE region, therefore, Nrf2 activation induces their expression. Second, the process of desquamation and keratin degradation is dependent on oxidative stress. In this sense, the high amounts of antioxidant proteins prevent keratin oxidation, avoiding desquamation (Wakabayashi et al., 2003). In spite of the protective role Nrf2 plays in many scenarios of diseases, driving its uncontrolled activation may be a dangerous path, as will be discussed further in this section.
The increase o Nrf2 activity was also investigated on human renal tubular HK-2 cell line by KD keap1 gene with a short hairpin iRNA. Cells with decreased expression of keap1 showed up-regulation in a set of antioxidant and detoxifying proteins, which increased the resistance to cisplatin and doxorubicin cytotoxicity (Jeong et al., 2015). This work demonstrated the potential of Nrf2 to induce the expression of four renal transporters involved in the excretion of drugs, which in a translational manner suggest the importance of Nrf2 in human xenobiotic-induced nephrotoxicity. In a similar way, the KD of keap1 in Hep2 cancer cells line increased the expression of antioxidant agents and reduced the apoptosis rate when cells were challenged with H2O2 (Li C. et al., 2018). As discussed in this review, the up-regulation of Nrf2 may favor tumor growth by reducing apoptosis and the relation between Nrf2 and cancer cells is contradictory, therefore, genetic interventions that increase Nrf2 activity may not be a suitable therapeutic approach for cancer.
Despite effective, KD methodologies listed above are limited to the basic research level and obviously not reliable to be considered as therapy for humans today. Nevertheless, the effort of these alternative approaches placed Keap1 as an important target to elucidate the function of genes as well as finding new therapeutic interventions.
Concluding Remarks
Considering that Nrf2 signaling pathway can regulate at least 600 genes, of which 200 encode cytoprotective proteins that are involved in diseases and the dynamic connections between diseases and drugs, modulating Nrf2 activity is a promising pharmacological approach in inflammatory and painful diseases (Ahmed et al., 2017). Nrf2 activators are commonly naturally occurring and plant-derived, but many others are synthetic compounds as represented by pharmacological classes of analgesics, glucocorticoids, NSAIDs, pro-resolution lipid mediators, electrophilic compounds and others as discussed above. To improve comprehension, we highlighted some examples of drugs with analgesic or anti-inflammatory actions that act via Nrf2 by canonical or non-canonical pathways in Figure 2. Moreover, for a comprehensive overview of the current evidence on molecules that mediate analgesic and anti-inflammatory actions via Nrf2 using in vivo and in vitro approaches, we include in Table 1 examples of compounds that belong to the aforementioned pharmacological classes and the main mechanisms demonstrated in experimental models. In Table 2, we provide an overview of molecules tested in completed clinical trials that were discussed in this review. Additionally, we have included molecules that were tested, but no outcome was reported following the completion of the study, for example CXA-10, cytarabine, daunorubicin hydrochloride, fluvastatin, grape powder, idarubicin, L-carnosine, melatonin, resveratrol, RTA 408, valsartan. Data from Table 2 and other clinical trials that were not included herein can be found at: www.clinicaltrials.gov/ct2/results?term=nrf2&Search=Apply&age_v=&gndr=&type=&rslt=.
In conclusion, modulating Nrf2 activity is a promising approach to achieve homeostasis during inflammatory responses where oxidative stress is an essential player. Nevertheless, a better understanding of how the activation or inhibition of Nrf2 can modulate the course and/or outcome of inflammatory diseases is an important strategy for the discovery of new drugs or the repurposing of drugs that target NRF2. Importantly, recent studies focusing on the development of more specific and potent peptides that target Keap1-Nrf2 PPI are promising. However, the effect of many of these molecules have not been tested in vivo yet. Therefore, investigations on their effect, mechanisms of action, and safety will be of great value. Further, translational investigations on the therapeutic effect and safety of new or repurposed modulators of Nrf2 pathway in humans is warranted.
Author Contributions
LS-F, SB-G, MH, MM, TZ, RC, and WV wrote the manuscript, read and approved the final version of the manuscript. All authors contributed equally.
Funding
This work was supported by grants from National Council for Scientific and Technological Development (CNPq, Brazil), Coordination for the Improvement of Higher Education Personnel (CAPES, Brazil), Programa de Pesquisa para o SUS (PPSUS) from Department of Science and Technology of Science, Technology and Strategic Inputs Secretariat (Decit/SCTIE/MS, Brazil) intermediated by CNPq (Brazil) with support of Araucária Foundation and State Health Secretariat, Paraná (SESA-PR, Brazil) (agreement 041/2017, protocol 48095.501.37488.12042017), and Programa de Apoio a Grupos de Excelência (PRONEX) grant supported by SETI/ Araucária Foundation, MCTI/CNPq, and Parana State Government (agreement 014/2017, protocol 46.843).
Conflict of Interest Statement
The authors declare that the research was conducted in the absence of any commercial or financial relationships that could be construed as a potential conflict of interest.
Acknowledgments
We thank the support of Multiuser Center of Research Laboratories from Londrina State University (CMLP-UEL).
References
Adenuga, D., Caito, S., Yao, H., Sundar, I. K., Hwang, J. W., Chung, S., et al. (2010). Nrf2 deficiency influences susceptibility to steroid resistance via HDAC2 reduction. Biochem. Biophys. Res. Commun. 403, 452–456. doi: 10.1016/j.bbrc.2010.11.054
Ahmed, S. M., Luo, L., Namani, A., Wang, X. J., and Tang, X. (2017). Nrf2 signaling pathway: pivotal roles in inflammation. Biochim. Biophys. Acta 1863, 585–597. doi: 10.1016/j.bbadis.2016.11.005
Alam, M. M., Okazaki, K., Nguyen, L. T. T., Ota, N., Kitamura, H., Murakami, S., et al. (2017). Glucocorticoid receptor signaling represses the antioxidant response by inhibiting histone acetylation mediated by the transcriptional activator NRF2. J. Biol. Chem. 292, 7519–7530. doi: 10.1074/jbc.M116.773960
Al-Rashed, F., Calay, D., Lang, M., Thornton, C. C., Bauer, A., Kiprianos, A., et al. (2018). Celecoxib exerts protective effects in the vascular endothelium via COX-2-independent activation of AMPK-CREB-Nrf2 signalling. Sci. Rep. 8:6271. doi: 10.1038/s41598-018-24548-z
Altmeyer, P. J., Matthes, U., Pawlak, F., Hoffmann, K., Frosch, P. J., Ruppert, P., et al. (1994). Antipsoriatic effect of fumaric acid derivatives, Results of a multicenter double-blind study in 100 patients. J. Am. Acad. Dermatol. 30, 977–981. doi: 10.1016/S0190-9622(94)70121-0
Alyoussef, A., and Taha, M. (2018). Antitumor activity of sulforaphane in mice model of skin cancer via blocking sulfatase-2. Exp. Dermatol. doi: 10.1111/exd.13802 [Epub ahead of print].
Amin, F. U., Shah, S. A., and Kim, M. O. (2017). Vanillic acid attenuates Abeta1-42-induced oxidative stress and cognitive impairment in mice. Sci. Rep. 7:40753. doi: 10.1038/srep40753
Antman, E. M., Bennett, J. S., Daugherty, A., Furberg, C., Roberts, H., and Taubert, K. A. (2007). Use of nonsteroidal antiinflammatory drugs: an update for clinicians: a scientific statement from the American Heart Association. Circulation 115, 1634–1642. doi: 10.1161/CIRCULATIONAHA.106.181424
Anantharaju, P. G., Gowda, P. C., Vimalambike, M. G., and Madhunapantula, S. V. (2016). An overview on the role of dietary phenolics for the treatment of cancers. Nutr. J. 15:99. doi: 10.1186/s12937-016-0217-2
Arruri, V., Komirishetty, P., Areti, A., Dungavath, S. K. N., and Kumar, A. (2017). Nrf2 and NF-kappaB modulation by Plumbagin attenuates functional, behavioural and biochemical deficits in rat model of neuropathic pain. Pharmacol. Rep. 69, 625–632. doi: 10.1016/j.pharep.2017.02.006
Athale, J., Ulrich, A., Macgarvey, N. C., Bartz, R. R., Welty-Wolf, K. E., Suliman, H. B., et al. (2012). Nrf2 promotes alveolar mitochondrial biogenesis and resolution of lung injury in Staphylococcus aureus pneumonia in mice. Free Radic. Biol. Med. 53, 1584–1594. doi: 10.1016/j.freeradbiomed.2012.08.009
Bae, S. H., Sung, S. H., Oh, S. Y., Lim, J. M., Lee, S. K., Park, Y. N., et al. (2013). Sestrins activate Nrf2 by promoting p62-dependent autophagic degradation of Keap1 and prevent oxidative liver damage. Cell Metab. 17, 73–84. doi: 10.1016/j.cmet.2012.12.002
Bao, S., Nie, X., Ou, R., Wang, C., Ku, P., and Li, K. (2017). Effects of diclofenac on the expression of Nrf2 and its downstream target genes in mosquito fish (Gambusia affinis). Aquat. Toxicol. 188, 43–53. doi: 10.1016/j.aquatox.2017.04.008
Bar-Or, A., Gold, R., Kappos, L., Arnold, D. L., Giovannoni, G., Selmaj, K., et al. (2013). Clinical efficacy of BG-12 (dimethyl fumarate) in patients with relapsing-remitting multiple sclerosis: subgroup analyses of the DEFINE study. J. Neurol. 260, 2297–2305. doi: 10.1007/s00415-013-6954-7
Bauman, J. E., Zang, Y., Sen, M., Li, C., Wang, L., Egner, P. A., et al. (2016). Prevention of carcinogen-induced oral cancer by sulforaphane. Cancer Preven. Res. (Phila) 9, 547–557. doi: 10.1158/1940-6207.CAPR-15-0290
Yehuda Greenwald, B. M., Frusic-Zlotkin, M., Soroka, Y., Ben Sasson, S., Bitton, R., Bianco-Peled, H., et al. (2017). Curcumin protects skin against UVB-induced cytotoxicity via the keap1-Nrf2 pathway: the use of a microemulsion delivery system. Oxid. Med. Cell Longev. 2017:5205471. doi: 10.1155/2017/5205471
Bensasson, R. V., Zoete, V., Dinkova-Kostova, A. T., and Talalay, P. (2008). Two-step mechanism of induction of the gene expression of a prototypic cancer-protective enzyme by diphenols. Chem. Res. Toxicol. 21, 805–812. doi: 10.1021/tx7002883
Best, S. A., and Sutherland, K. D. (2018). “Keaping” a lid on lung cancer: the Keap1-Nrf2 pathway. Cell Cycle 17, 1696–1707. doi: 10.1080/15384101.2018.1496756
Biagiotti, S., Menotta, M., Orazi, S., Spapperi, C., Brundu, S., Fraternale, A., et al. (2016). Dexamethasone improves redox state in ataxia telangiectasia cells by promoting an NRF2-mediated antioxidant response. FEBS J. 283, 3962–3978. doi: 10.1111/febs.13901
Bloom, D. A., and Jaiswal, A. K. (2003). Phosphorylation of Nrf2 at Ser40 by protein kinase C in response to antioxidants leads to the release of Nrf2 from INrf2, but is not required for Nrf2 stabilization/accumulation in the nucleus and transcriptional activation of antioxidant response element-mediated NAD(P)H:quinone oxidoreductase-1 gene expression. J. Biol. Chem. 278, 44675–44682. doi: 10.1074/jbc.M307633200
Bodas, M., Mazur, S., Min, T., and Vij, N. (2018). Inhibition of histone-deacetylase activity rescues inflammatory cystic fibrosis lung disease by modulating innate and adaptive immune responses. Respir. Res. 19:2. doi: 10.1186/s12931-017-0705-8
Borghi, S. M., Mizokami, S. S., Pinho-Ribeiro, F. A., Fattori, V., Crespigio, J., Clemente-Napimoga, J. T., et al. (2017). The flavonoid quercetin inhibits titanium dioxide (TiO2)-induced chronic arthritis in mice. J. Nutr. Biochem. 53, 81–95. doi: 10.1016/j.jnutbio.2017.10.010
Bovill, J. G. (1997). Mechanisms of actions of opioids and non-steroidal anti-inflammatory drugs. Eur. J. Anaesthesiol. Suppl. 15, 9–15. doi: 10.1097/00003643-199705001-00003
Bretscher, P., Egger, J., Shamshiev, A., Trotzmuller, M., Kofeler, H., Carreira, E. M., et al. (2015). Phospholipid oxidation generates potent anti-inflammatory lipid mediators that mimic structurally related pro-resolving eicosanoids by activating Nrf2. EMBO Mol. Med. 7, 593–607. doi: 10.15252/emmm.201404702
Busillo, J. M., and Cidlowski, J. A. (2013). The five Rs of glucocorticoid action during inflammation: ready, reinforce, repress, resolve, and restore. Trends Endocrinol. Metab. 24, 109–119. doi: 10.1016/j.tem.2012.11.005
Bustanji, Y., Taha, M. O., Almasri, I. M., Al-Ghussein, M. A., Mohammad, M. K., and Alkhatib, H. S. (2009). Inhibition of glycogen synthase kinase by curcumin: investigation by simulated molecular docking and subsequent in vitro/in vivo evaluation. J. Enzyme Inhib. Med. Chem. 24, 771–778. doi: 10.1080/14756360802364377
Cai, D., Yin, S., Yang, J., Jiang, Q., and Cao, W. (2015). Histone deacetylase inhibition activates Nrf2 and protects against osteoarthritis. Arthritis Res. Ther. 17:269. doi: 10.1186/s13075-015-0774-3
Calixto-Campos, C., Carvalho, T. T., Hohmann, M. S. N., Pinho-Ribeiro, F. A., Fattori, V., Manchope, M. F., et al. (2015a). Vanillic acid inhibits inflammatory pain by inhibiting neutrophil recruitment, oxidative stress, cytokine production, and NFκB activation in mice. J. Nat. Prod. 78, 1799–1808. doi: 10.1021/acs.jnatprod.5b00246
Calixto-Campos, C., Corrêa, M. P., Carvalho, T. T., Zarpelon, A. C., Hohmann, M. S. N., Rossaneis, A. C., et al. (2015b). Quercetin reduces ehrlich tumor-induced cancer pain in mice. Anal. Cell. Pathol. (Amsterdam) 2015, 285708–285708. doi: 10.1155/2015/285708
Cashman, J. N. (1996). The mechanisms of action of NSAIDs in analgesia. Drugs 52(Suppl. 5), 13–23. doi: 10.2165/00003495-199600525-00004
Chen, Q., Li, C., Gong, Z., Chan, E. C. Y., Snyder, S. A., and Lam, S. H. (2017). Common deregulated gene expression profiles and morphological changes in developing zebrafish larvae exposed to environmental-relevant high to low concentrations of glucocorticoids. Chemosphere 172, 429–439. doi: 10.1016/j.chemosphere.2017.01.036
Choi, E. J., Jung, B. J., Lee, S. H., Yoo, H. S., Shin, E. A., Ko, H. J., et al. (2017). A clinical drug library screen identifies clobetasol propionate as an NRF2 inhibitor with potential therapeutic efficacy in KEAP1 mutant lung cancer. Oncogene 36, 5285–5295. doi: 10.1038/onc.2017.153
Clark, A. R., and Belvisi, M. G. (2012). Maps and legends: the quest for dissociated ligands of the glucocorticoid receptor. Pharmacol. Ther. 134, 54–67. doi: 10.1016/j.pharmthera.2011.12.004
Creelan, B. C., Gabrilovich, D. I., Gray, J. E., Williams, C. C., Tanvetyanon, T., Haura, E. B., et al. (2017). Safety, pharmacokinetics, and pharmacodynamics of oral omaveloxolone (RTA 408), a synthetic triterpenoid, in a first-in-human trial of patients with advanced solid tumors. Onco Targets Ther. 10, 4239–4250. doi: 10.2147/OTT.S136992
Cuadrado, A., Manda, G., Hassan, A., Alcaraz, M. J., Barbas, C., Daiber, A., et al. (2018). Transcription factor NRF2 as a therapeutic target for chronic diseases: a systems medicine approach. Pharmacol. Rev. 70, 348–383. doi: 10.1124/pr.117.014753
Dayoub, R., Vogel, A., Schuett, J., Lupke, M., Spieker, S. M., Kettern, N., et al. (2013). Nrf2 activates augmenter of liver regeneration (ALR) via antioxidant response element and links oxidative stress to liver regeneration. Mol. Med. 19, 237–244. doi: 10.2119/molmed.2013.00027
De Bosscher, K., Vanden Berghe, W., and Haegeman, G. (2003). The interplay between the glucocorticoid receptor and nuclear factor-kappaB or activator protein-1: molecular mechanisms for gene repression. Endocr. Rev. 24, 488–522. doi: 10.1210/er.2002-0006
de Zeeuw, D., Akizawa, T., Audhya, P., Bakris, G. L., Chin, M., Christ-Schmidt, H., et al. (2013). Bardoxolone methyl in type 2 diabetes and stage 4 chronic kidney disease. N. Engl. J. Med. 369, 2492–2503. doi: 10.1056/NEJMoa1306033
DeNicola, G. M., Karreth, F. A., Humpton, T. J., Gopinathan, A., Wei, C., Frese, K., et al. (2011). Oncogene-induced Nrf2 transcription promotes ROS detoxification and tumorigenesis. Nature 475, 106–109. doi: 10.1038/nature10189
Di, W., Shi, X., Lv, H., Liu, J., Zhang, H., Li, Z., et al. (2016). Activation of the nuclear factor E2-related factor 2/anitioxidant response element alleviates the nitroglycerin-induced hyperalgesia in rats. J. Headache Pain 17:99. doi: 10.1186/s10194-016-0694-x
Dinkova-Kostova, A. T., Kostov, R. V., and Canning, P. (2017). Keap1, the cysteine-based mammalian intracellular sensor for electrophiles and oxidants. Arch. Biochem. Biophys. 617, 84–93. doi: 10.1016/j.abb.2016.08.005
Dinkova-Kostova, A. T., Massiah, M. A., Bozak, R. E., Hicks, R. J., and Talalay, P. (2001). Potency of michael reaction acceptors as inducers of enzymes that protect against carcinogenesis depends on their reactivity with sulfhydryl groups. Proc. Natl. Acad. Sci. U.S.A. 98, 3404–3409. doi: 10.1073/pnas.051632198
Domitrovic, R., Skoda, M., Vasiljev Marchesi, V., Cvijanovic, O., Pernjak Pugel, E., and Stefan, M. B. (2013). Rosmarinic acid ameliorates acute liver damage and fibrogenesis in carbon tetrachloride-intoxicated mice. Food Chem. Toxicol. 51, 370–378. doi: 10.1016/j.fct.2012.10.021
Elikkottil, J., Gupta, P., and Gupta, K. (2009). The analgesic potential of cannabinoids. J. Opioid. Manage. 5, 341–357. doi: 10.5055/jom.2009.0034
Ellrichmann, G., Petrasch-Parwez, E., Lee, D. H., Reick, C., Arning, L., Saft, C., et al. (2011). Efficacy of fumaric acid esters in the R6/2 and YAC128 models of Huntington’s disease. PLoS One 6:e16172. doi: 10.1371/journal.pone.0016172
Fahey, J. W., Haristoy, X., Dolan, P. M., Kensler, T. W., Scholtus, I., Stephenson, K. K., et al. (2002). Sulforaphane inhibits extracellular, intracellular, and antibiotic-resistant strains of Helicobacter pylori and prevents benzo[a]pyrene-induced stomach tumors. Proc. Natl. Acad. Sci. U.S.A. 99, 7610–7615. doi: 10.1073/pnas.112203099
Fahey, J. W., Zalcmann, A. T., and Talalay, P. (2001). The chemical diversity and distribution of glucosinolates and isothiocyanates among plants. Phytochemistry 56, 5–51. doi: 10.1016/S0031-9422(00)00316-2
Fattori, V., Hohmann, M. S., Rossaneis, A. C., Pinho-Ribeiro, F. A., and Verri, W. A. (2016). Capsaicin: current understanding of its mechanisms and therapy of pain and other pre-clinical and clinical uses. Molecules 21:E844. doi: 10.3390/molecules21070844
Fattori, V., Pinho-Ribeiro, F. A., Borghi, S. M., Alves-Filho, J. C., Cunha, T. M., Cunha, F. Q., et al. (2015). Curcumin inhibits superoxide anion-induced pain-like behavior and leukocyte recruitment by increasing Nrf2 expression and reducing NF-κB activation. Inflamm. Res. 64, 993–1003. doi: 10.1007/s00011-015-0885-y
Ferrandiz, M. L., Nacher-Juan, J., and Alcaraz, M. J. (2018). Nrf2 as a therapeutic target for rheumatic diseases. Biochem. Pharmacol. 152, 338–346. doi: 10.1016/j.bcp.2018.04.010
Fimognari, C., and Hrelia, P. (2007). Sulforaphane as a promising molecule for fighting cancer. Mutat. Res. 635, 90–104. doi: 10.1016/j.mrrev.2006.10.004
Finkel, T. (2003). Oxidant signals and oxidative stress. Curr. Opin. Cell Biol. 15, 247–254. doi: 10.1016/S0955-0674(03)00002-4
Ganan-Gomez, I., Wei, Y., Yang, H., Boyano-Adanez, M. C., and Garcia-Manero, G. (2013). Oncogenic functions of the transcription factor Nrf2. Free Radic. Biol. Med. 65, 750–764. doi: 10.1016/j.freeradbiomed.2013.06.041
Gazaryan, I. G., and Thomas, B. (2016). The status of Nrf2-based therapeutics: current perspectives and future prospects. Neural Regen. Res. 11, 1708–1711. doi: 10.4103/1673-5374.194706
Ghoreschi, K., Bruck, J., Kellerer, C., Deng, C., Peng, H., Rothfuss, O., et al. (2011). Fumarates improve psoriasis and multiple sclerosis by inducing type II dendritic cells. J. Exp. Med. 208, 2291–2303. doi: 10.1084/jem.20100977
Gong, L., Thorn, C. F., Bertagnolli, M. M., Grosser, T., Altman, R. B., and Klein, T. E. (2012). Celecoxib pathways: pharmacokinetics and pharmacodynamics. Pharmacogenet. Genomics 22, 310–318. doi: 10.1097/FPC.0b013e32834f94cb
Gorrini, C., Baniasadi, P. S., Harris, I. S., Silvester, J., Inoue, S., Snow, B., et al. (2013). BRCA1 interacts with Nrf2 to regulate antioxidant signaling and cell survival. J. Exp. Med. 210, 1529–1544. doi: 10.1084/jem.20121337
Groeneweg, F. L., Karst, H., De Kloet, E. R., and Joels, M. (2011). Rapid non-genomic effects of corticosteroids and their role in the central stress response. J. Endocrinol. 209, 153–167. doi: 10.1530/JOE-10-0472
Guan, D., Ohta, H., Tawil, T., Liddle, R. A., and Green, G. M. (1990). CCK-releasing activity of rat intestinal secretion: effect of atropine and comparison with monitor peptide. Pancreas 5, 677–684. doi: 10.1097/00006676-199011000-00007
Guo, Y., Wu, R., Gaspar, J. M., Sargsyan, D., Su, Z. Y., Zhang, C., et al. (2018). DNA methylome and transcriptome alterations and cancer prevention by curcumin in colitis-accelerated colon cancer in mice. Carcinogenesis 39, 669–680. doi: 10.1093/carcin/bgy043
Gupta, S., Adhami, V. M., Subbarayan, M., Maclennan, G. T., Lewin, J. S., Hafeli, U. O., et al. (2004). Suppression of prostate carcinogenesis by dietary supplementation of celecoxib in transgenic adenocarcinoma of the mouse prostate model. Cancer Res. 64, 3334–3343. doi: 10.1158/0008-5472.CAN-03-2422
Hancock, R., Bertrand, H. C., Tsujita, T., Naz, S., El-Bakry, A., Laoruchupong, J., et al. (2012). Peptide inhibitors of the Keap1-Nrf2 protein-protein interaction. Free Radic. Biol. Med. 52, 444–451. doi: 10.1016/j.freeradbiomed.2011.10.486
Hancock, R., Schaap, M., Pfister, H., and Wells, G. (2013). Peptide inhibitors of the Keap1-Nrf2 protein-protein interaction with improved binding and cellular activity. Org. Biomol. Chem. 11, 3553–3557. doi: 10.1039/c3ob40249e
Harvey, C. J., Thimmulappa, R. K., Sethi, S., Kong, X., Yarmus, L., Brown, R. H., et al. (2011). Targeting Nrf2 signaling improves bacterial clearance by alveolar macrophages in patients with COPD and in a mouse model. Sci. Transl. Med. 3:78ra32. doi: 10.1126/scitranslmed.3002042
Hast, B. E., Goldfarb, D., Mulvaney, K. M., Hast, M. A., Siesser, P. F., Yan, F., et al. (2013). Proteomic analysis of ubiquitin ligase KEAP1 reveals associated proteins that inhibit NRF2 ubiquitination. Cancer Res. 73, 2199–2210. doi: 10.1158/0008-5472.CAN-12-4400
Hayes, J. D., and Dinkova-Kostova, A. T. (2014). The Nrf2 regulatory network provides an interface between redox and intermediary metabolism. Trends Biochem. Sci. 39, 199–218. doi: 10.1016/j.tibs.2014.02.002
Hayes, J. D., Mcmahon, M., Chowdhry, S., and Dinkova-Kostova, A. T. (2010). Cancer chemoprevention mechanisms mediated through the Keap1-Nrf2 pathway. Antioxid. Redox. Signal. 13, 1713–1748. doi: 10.1089/ars.2010.3221
Healy, Z. R., Lee, N. H., Gao, X., Goldring, M. B., Talalay, P., Kensler, T. W., et al. (2005). Divergent responses of chondrocytes and endothelial cells to shear stress: cross-talk among COX-2, the phase 2 response, and apoptosis. Proc. Natl. Acad. Sci. U.S.A. 102, 14010–14015. doi: 10.1073/pnas.0506620102
Hearn, L., Moore, R. A., Derry, S., Wiffen, P. J., and Phillips, T. (2014). Desipramine for neuropathic pain in adults. Cochrane Database Syst. Rev. 9:CD011003. doi: 10.1002/14651858.CD011003
Hebbar, P. B., and Archer, T. K. (2003). Chromatin remodeling by nuclear receptors. Chromosoma 111, 495–504. doi: 10.1007/s00412-003-0232-x
Hennig, P., Garstkiewicz, M., Grossi, S., Di Filippo, M., French, L. E., and Beer, H. D. (2018). The crosstalk between Nrf2 and inflammasomes. Int. J. Mol. Sci. 19:E562. doi: 10.3390/ijms19020562
Homma, S., Ishii, Y., Morishima, Y., Yamadori, T., Matsuno, Y., Haraguchi, N., et al. (2009). Nrf2 enhances cell proliferation and resistance to anticancer drugs in human lung cancer. Clin. Cancer Res. 15, 3423–3432. doi: 10.1158/1078-0432.CCR-08-2822
Hong, D. S., Kurzrock, R., Supko, J. G., He, X., Naing, A., Wheler, J., et al. (2012). A phase I first-in-human trial of bardoxolone methyl in patients with advanced solid tumors and lymphomas. Clin. Cancer Res. 18, 3396–3406. doi: 10.1158/1078-0432.CCR-11-2703
Hsu, W. H., Lee, B. H., Chang, Y. Y., Hsu, Y. W., and Pan, T. M. (2013). A novel natural Nrf2 activator with PPARgamma-agonist (monascin) attenuates the toxicity of methylglyoxal and hyperglycemia. Toxicol. Appl. Pharmacol. 272, 842–851. doi: 10.1016/j.taap.2013.07.004
Hu, C., Eggler, A. L., Mesecar, A. D., and Van Breemen, R. B. (2011). Modification of keap1 cysteine residues by sulforaphane. Chem. Res. Toxicol. 24, 515–521. doi: 10.1021/tx100389r
Hu, R., Saw, C. L., Yu, R., and Kong, A. N. (2010). Regulation of NF-E2-related factor 2 signaling for cancer chemoprevention: antioxidant coupled with antiinflammatory. Antioxid. Redox. Signal. 13, 1679–1698. doi: 10.1089/ars.2010.3276
Iida, K., Itoh, K., Kumagai, Y., Oyasu, R., Hattori, K., Kawai, K., et al. (2004). Nrf2 is essential for the chemopreventive efficacy of oltipraz against urinary bladder carcinogenesis. Cancer Res. 64, 6424–6431. doi: 10.1158/0008-5472.CAN-04-1906
Ishtiaq, S. M., Khan, J. A., and Arshad, M. I. (2018). Psychosocial-stress, liver regeneration and weight gain: a conspicuous pathophysiological triad. Cell Physiol. Biochem. 46, 1–8. doi: 10.1159/000488378
Itoh, K., Mimura, J., and Yamamoto, M. (2010). Discovery of the negative regulator of Nrf2. Keap1: a historical overview. Antioxid. Redox. Signal. 13, 1665–1678. doi: 10.1089/ars.2010.3222
Itoh, K., Wakabayashi, N., Katoh, Y., Ishii, T., Igarashi, K., Engel, J. D., et al. (1999). Keap1 represses nuclear activation of antioxidant responsive elements by Nrf2 through binding to the amino-terminal Neh2 domain. Genes Dev. 13, 76–86. doi: 10.1101/gad.13.1.76
Janssen-Heininger, Y. M., Mossman, B. T., Heintz, N. H., Forman, H. J., Kalyanaraman, B., Finkel, T., et al. (2008). Redox-based regulation of signal transduction: principles, pitfalls, and promises. Free Radic. Biol. Med. 45, 1–17. doi: 10.1016/j.freeradbiomed.2008.03.011
Jeddi, F., Soozangar, N., Sadeghi, M. R., Somi, M. H., and Samadi, N. (2017). Contradictory roles of Nrf2/Keap1 signaling pathway in cancer prevention/promotion and chemoresistance. DNA Repair (Amst.) 54, 13–21. doi: 10.1016/j.dnarep.2017.03.008
Jeong, H. S., Ryoo, I. G., and Kwak, M. K. (2015). Regulation of the expression of renal drug transporters in KEAP1-knockdown human tubular cells. Toxicol. In Vitro 29, 884–892. doi: 10.1016/j.tiv.2015.03.013
Ji, L. L., Sheng, Y. C., Zheng, Z. Y., Shi, L., and Wang, Z. T. (2015). The involvement of p62-Keap1-Nrf2 antioxidative signaling pathway and JNK in the protection of natural flavonoid quercetin against hepatotoxicity. Free Radic. Biol. Med. 85, 12–23. doi: 10.1016/j.freeradbiomed.2015.03.035
Jian, Z., Tang, L., Yi, X., Liu, B., Zhang, Q., Zhu, G., et al. (2016). Aspirin induces Nrf2-mediated transcriptional activation of haem oxygenase-1 in protection of human melanocytes from H2 O2 -induced oxidative stress. J. Cell Mol. Med. 20, 1307–1318. doi: 10.1111/jcmm.12812
Jiang, X., Liu, Y., Ma, L., Ji, R., Qu, Y., Xin, Y., et al. (2018). Chemopreventive activity of sulforaphane. Drug Des. Dev. Ther. 12, 2905–2913. doi: 10.2147/DDDT.S100534
Jiang, Z. Y., Lu, M. C., and You, Q. D. (2016). Discovery and development of Kelch-like ECH-associated protein 1. nuclear factor erythroid 2-related factor 2 (KEAP1:NRF2) protein-protein interaction inhibitors: achievements, challenges, and future directions. J. Med. Chem. 59, 10837–10858. doi: 10.1021/acs.jmedchem.6b00586
Jimenez-Osorio, A. S., Garcia-Nino, W. R., Gonzalez-Reyes, S., Alvarez-Mejia, A. E., Guerra-Leon, S., Salazar-Segovia, J., et al. (2016). The effect of dietary supplementation with curcumin on redox status and Nrf2 activation in patients with nondiabetic or diabetic proteinuric chronic kidney disease: a pilot study. J. Ren. Nutr. 26, 237–244. doi: 10.1053/j.jrn.2016.01.013
Joo, M., Lee, J. Y., and Kim, K. H. (2017). 15-deoxy-Δ12,14-prostaglandin J2 enhances the interaction between Keap1 and p62/SQSTM1. J. Immunol. 198:222.222.
Joung, E. J., Li, M. H., Lee, H. G., Somparn, N., Jung, Y. S., Na, H. K., et al. (2007). Capsaicin induces heme oxygenase-1 expression in HepG2 cells via activation of PI3K-Nrf2 signaling: NAD(P)H:quinone oxidoreductase as a potential target. Antioxid. Redox. Signal. 9, 2087–2098. doi: 10.1089/ars.2007.1827
Jozkowicz, A., Was, H., and Dulak, J. (2007). Heme oxygenase-1 in tumors: is it a false friend? Antioxid. Redox Signal. 9, 2099–2117. doi: 10.1089/ars.2007.1659
Juknat, A., Pietr, M., Kozela, E., Rimmerman, N., Levy, R., Gao, F., et al. (2013). Microarray and pathway analysis reveal distinct mechanisms underlying cannabinoid-mediated modulation of LPS-induced activation of BV-2 microglial cells. PLoS One 8:e61462. doi: 10.1371/journal.pone.0061462
Kallifatidis, G., Rausch, V., Baumann, B., Apel, A., Beckermann, B. M., Groth, A., et al. (2009). Sulforaphane targets pancreatic tumour-initiating cells by NF-kappaB-induced antiapoptotic signalling. Gut 58, 949–963. doi: 10.1136/gut.2008.149039
Kamble, S. M., Patel, H. M., Goyal, S. N., Noolvi, M. N., Mahajan, U. B., Ojha, S., et al. (2017). In silico evidence for binding of pentacyclic triterpenoids to Keap1-Nrf2 protein-protein binding site. Comb. Chem. High Throughput Screen 20, 215–234. doi: 10.2174/1386207319666161214111822
Kansanen, E., Kuosmanen, S. M., Leinonen, H., and Levonen, A. L. (2013). The Keap1-Nrf2 pathway: mechanisms of activation and dysregulation in cancer. Redox Biol. 1, 45–49. doi: 10.1016/j.redox.2012.10.001
Karapetian, R. N., Evstafieva, A. G., Abaeva, I. S., Chichkova, N. V., Filonov, G. S., Rubtsov, Y. P., et al. (2005). Nuclear oncoprotein prothymosin alpha is a partner of Keap1: implications for expression of oxidative stress-protecting genes. Mol. Cell. Biol. 25, 1089–1099. doi: 10.1128/MCB.25.3.1089-1099.2005
Kataoka, K., Handa, H., and Nishizawa, M. (2001). Induction of cellular antioxidative stress genes through heterodimeric transcription factor Nrf2/small Maf by antirheumatic gold(I) compounds. J. Biol. Chem. 276, 34074–34081. doi: 10.1074/jbc.M105383200
Kensler, T. W., and Wakabayashi, N. (2010). Nrf2: friend or foe for chemoprevention? Carcinogenesis 31, 90–99. doi: 10.1093/carcin/bgp231
Khan, N. M., and Haqqi, T. M. (2018). Epigenetics in osteoarthritis: potential of HDAC inhibitors as therapeutics. Pharmacol. Res. 128, 73–79. doi: 10.1016/j.phrs.2017.08.007
Ki, S. H., Cho, I. J., Choi, D. W., and Kim, S. G. (2005). Glucocorticoid receptor (GR)-associated SMRT binding to C/EBPbeta TAD and Nrf2 Neh4/5: role of SMRT recruited to GR in GSTA2 gene repression. Mol. Cell. Biol. 25, 4150–4165. doi: 10.1128/MCB.25.10.4150-4165.2005
Kim, K. H., Sadikot, R. T., and Joo, M. (2016). Therapeutic effect of ent-kaur-16-en-19-oic acid on neutrophilic lung inflammation and sepsis is mediated by Nrf2. Biochem. Biophys. Res. Commun. 474, 534–540. doi: 10.1016/j.bbrc.2016.04.122
Kim, N. H., Oh, M. K., Park, H. J., and Kim, I. S. (2010). Auranofin, a gold(I)-containing antirheumatic compound, activates Keap1/Nrf2 signaling via Rac1/iNOS signal and mitogen-activated protein kinase activation. J. Pharmacol. Sci. 113, 246–254. doi: 10.1254/jphs.09330FP
Kim, S. G., Kim, Y. M., Choi, Y. H., Lee, M. G., Choi, J. Y., Han, J. Y., et al. (2010). Pharmacokinetics of oltipraz and its major metabolite (RM) in patients with liver fibrosis or cirrhosis: relationship with suppression of circulating TGF-beta1. Clin. Pharmacol. Ther. 88, 360–368. doi: 10.1038/clpt.2010.89
Kim, W., Kim, B. G., Lee, J. S., Lee, C. K., Yeon, J. E., Chang, M. S., et al. (2017a). Randomised clinical trial: the efficacy and safety of oltipraz, a liver X receptor alpha-inhibitory dithiolethione in patients with non-alcoholic fatty liver disease. Aliment. Pharmacol. Ther. 45, 1073–1083. doi: 10.1111/apt.13981
Kim, W., Lee, H. N., Jang, J. H., Kim, S. H., Lee, Y. H., Hahn, Y. I., et al. (2017b). 15-deoxy-delta(12,14)-prostaglandin J2 exerts proresolving effects through nuclear factor E2-related factor 2-induced expression of CD36 and heme oxygenase-1. Antioxid. Redox Signal. 27, 1412–1431. doi: 10.1089/ars.2016.6754
Kim, Y. M., Kim, H. J., and Chang, K. C. (2015). Glycyrrhizin reduces HMGB1 secretion in lipopolysaccharide-activated RAW 264.7 cells and endotoxemic mice by p38/Nrf2-dependent induction of HO-1. Int. Immunopharmacol. 26, 112–118. doi: 10.1016/j.intimp.2015.03.014
Knezevic, N. N., Yekkirala, A., and Yaksh, T. L. (2017). Basic/translational development of forthcoming opioid- and nonopioid-targeted pain therapeutics. Anesth. Analg. 125, 1714–1732. doi: 10.1213/ANE.0000000000002442
Kou, X., Kirbergerb, M., and Chen, Y. N. (2013). Natural products for cancer prevention associated with Nrf2–ARE pathway. Food Sci. Hum. Wellness 2, 22–28. doi: 10.1016/j.fshw.2013.01.001
Kratschmar, D. V., Calabrese, D., Walsh, J., Lister, A., Birk, J., Appenzeller-Herzog, C., et al. (2012). Suppression of the Nrf2-dependent antioxidant response by glucocorticoids and 11beta-HSD1-mediated glucocorticoid activation in hepatic cells. PLoS One 7:e36774. doi: 10.1371/journal.pone.0036774
Kudoh, K., Uchinami, H., Yoshioka, M., Seki, E., and Yamamoto, Y. (2014). Nrf2 activation protects the liver from ischemia/reperfusion injury in mice. Ann. Surg. 260, 118–127. doi: 10.1097/SLA.0000000000000287
Kuhn, A., Landmann, A., Patsinakidis, N., Ruland, V., Nozinic, S., Perusquia Ortiz, A. M., et al. (2016). Fumaric acid ester treatment in cutaneous lupus erythematosus (CLE): a prospective, open-label, phase II pilot study. Lupus 25, 1357–1364. doi: 10.1177/0961203316644335
Kumar, H., Kim, I. S., More, S. V., Kim, B. W., and Choi, D. K. (2014). Natural product-derived pharmacological modulators of Nrf2/ARE pathway for chronic diseases. Nat. Prod. Rep. 31, 109–139. doi: 10.1039/C3NP70065H
Kumar, V., Kumar, S., Hassan, M., Wu, H., Thimmulappa, R. K., Kumar, A., et al. (2011). Novel chalcone derivatives as potent Nrf2 activators in mice and human lung epithelial cells. J. Med. Chem. 54, 4147–4159. doi: 10.1021/jm2002348
Lau, A., Wang, X. J., Zhao, F., Villeneuve, N. F., Wu, T., Jiang, T., et al. (2010). A noncanonical mechanism of Nrf2 activation by autophagy deficiency: direct interaction between Keap1 and p62. Mol. Cell. Biol. 30, 3275–3285. doi: 10.1128/MCB.00248-10
Lee, S. E., Kim, G. D., Yang, H., Son, G. W., Park, H. R., Cho, J. J., et al. (2015). Effects of eicosapentaenoic acid on the cytoprotection through Nrf2-mediated heme oxygenase-1 in human endothelial cells. J. Cardiovasc. Pharmacol. 66, 108–117. doi: 10.1097/FJC.0000000000000251
Leone, A., Diorio, G., Sexton, W., Schell, M., Alexandrow, M., Fahey, J. W., et al. (2017). Sulforaphane for the chemoprevention of bladder cancer: molecular mechanism targeted approach. Oncotarget 8, 35412–35424. doi: 10.18632/oncotarget.16015
Lewis, P. A., Mullany, D. V., Courtney, M., and Coyer, F. (2006). Australasian trends in intra-aortic balloon counterpulsation weaning: results of a postal survey. Crit. Care Resuscitation 8, 361–367.
Li, B., Li, R., Xie, X., Song, X., Zhang, J., Wang, Y., et al. (2013). [Anti-oxidative effects of dexamethasone on mice with experimental autoimmune encephalomyelitis]. Xi Bao Yu Fen Zi Mian Yi Xue Za Zhi 29, 1155–1158.
Li, H., Wood, J. T., Whitten, K. M., Vadivel, S. K., Seng, S., Makriyannis, A., et al. (2013). Inhibition of fatty acid amide hydrolase activates Nrf2 signalling and induces heme oxygenase 1 transcription in breast cancer cells. Br. J. Pharmacol. 170, 489–505. doi: 10.1111/bph.12111
Li, C., Cheng, L., Wu, H., He, P., Zhang, Y., Yang, Y., et al. (2018). Activation of the KEAP1NRF2ARE signaling pathway reduces oxidative stress in Hep2 cells. Mol. Med. Rep. 18, 2541–2550.
Li, S., Yang, C., Fang, X., Zhan, G., Huang, N., Gao, J., et al. (2018). Role of Keap1-Nrf2 signaling in anhedonia symptoms in a rat model of chronic neuropathic pain: improvement with sulforaphane. Front. Pharmacol. 9:887. doi: 10.3389/fphar.2018.00887
Li, Q., and Zhang, X. (2015). Epigallocatechin-3-gallate attenuates bone cancer pain involving decreasing spinal tumor necrosis factor-alpha expression in a mouse model. Int. Immunopharmacol. 29, 818–823. doi: 10.1016/j.intimp.2015.08.037
Li, W., Suwanwela, N. C., and Patumraj, S. (2016). Curcumin by down-regulating NF-kB and elevating Nrf2, reduces brain edema and neurological dysfunction after cerebral I/R. Microvasc. Res. 106, 117–127. doi: 10.1016/j.mvr.2015.12.008
Li, X. Y., Luo, B. L., Wang, L. J., Zhang, W. D., and Liu, Z. G. (2015). 15-Deoxy-prostaglandin J2 anti-inflammation in a rat model of chronic obstructive pulmonary disease and human bronchial epithelial cells via Nrf2 activation. Genet. Mol. Res. 14, 14037–14042. doi: 10.4238/2015.October.29.22
Lin, H. Y., Yeh, W. L., Huang, B. R., Lin, C., Lai, C. H., Lin, H., et al. (2012). Desipramine protects neuronal cell death and induces heme oxygenase-1 expression in Mes23.5 dopaminergic neurons. PLoS One 7:e50138. doi: 10.1371/journal.pone.0050138
Lin, K., Yang, R., Zheng, Z., Zhou, Y., Geng, Y., Hu, Y., et al. (2017). Sulforaphane-cysteine-induced apoptosis via phosphorylated ERK1/2-mediated maspin pathway in human non-small cell lung cancer cells. Cell Death Discov. 3:17025. doi: 10.1038/cddiscovery.2017.25
Lin, S. X., Lisi, L., Dello Russo, C., Polak, P. E., Sharp, A., Weinberg, G., et al. (2011). The anti-inflammatory effects of dimethyl fumarate in astrocytes involve glutathione and haem oxygenase-1. ASN Neuro 3:e00055. doi: 10.1042/AN20100033
Linker, R. A., Lee, D. H., Ryan, S., Van Dam, A. M., Conrad, R., Bista, P., et al. (2011). Fumaric acid esters exert neuroprotective effects in neuroinflammation via activation of the Nrf2 antioxidant pathway. Brain 134, 678–692. doi: 10.1093/brain/awq386
Lu, M. C., Jiao, Q., Liu, T., Tan, S. J., Zhou, H. S., You, Q. D., et al. (2018). Discovery of a head-to-tail cyclic peptide as the Keap1-Nrf2 protein-protein interaction inhibitor with high cell potency. Eur. J. Med. Chem. 143, 1578–1589. doi: 10.1016/j.ejmech.2017.10.052
Ma, J., Cai, H., Wu, T., Sobhian, B., Huo, Y., Alcivar, A., et al. (2012). PALB2 interacts with KEAP1 to promote NRF2 nuclear accumulation and function. Mol. Cell. Biol. 32, 1506–1517. doi: 10.1128/MCB.06271-11
Ma, Q. (2013). Role of nrf2 in oxidative stress and toxicity. Annu. Rev. Pharmacol. Toxicol. 53, 401–426. doi: 10.1146/annurev-pharmtox-011112-140320
Ma, S., Paiboonrungruan, C., Yan, T., Williams, K. P., Major, M. B., and Chen, X. L. (2018). Targeted therapy of esophageal squamous cell carcinoma: the NRF2 signaling pathway as target. Ann. N. Y. Acad. Sci. 1434, 164–172. doi: 10.1111/nyas.13681
Maioli, N. A., Zarpelon, A. C., Mizokami, S. S., Calixto-Campos, C., Guazelli, C. F. S., Hohmann, M. S. N., et al. (2015). The superoxide anion donor, potassium superoxide, induces pain and inflammation in mice through production of reactive oxygen species and cyclooxygenase-2. Braz. J. Med. Biol. Res. 48, 321–331. doi: 10.1590/1414-431X20144187
Majkova, Z., Layne, J., Sunkara, M., Morris, A. J., Toborek, M., and Hennig, B. (2011). Omega-3 fatty acid oxidation products prevent vascular endothelial cell activation by coplanar polychlorinated biphenyls. Toxicol. Appl. Pharmacol. 251, 41–49. doi: 10.1016/j.taap.2010.11.013
Manandhar, S., Cho, J. M., Kim, J. A., Kensler, T. W., and Kwak, M. K. (2007). Induction of Nrf2-regulated genes by 3H-1, 2-dithiole-3-thione through the ERK signaling pathway in murine keratinocytes. Eur. J. Pharmacol. 577, 17–27. doi: 10.1016/j.ejphar.2007.08.018
Manchope, M. F., Artero, N. A., Fattori, V., Mizokami, S. S., Pitol, D. L., Issa, J. P. M., et al. (2018). Naringenin mitigates titanium dioxide (TiO2)-induced chronic arthritis in mice: role of oxidative stress, cytokines, and NFkappaB. Inflamm. Res. 67, 997–1012. doi: 10.1007/s00011-018-1195-y
Manchope, M. F., Calixto-Campos, C., Coelho-Silva, L., Zarpelon, A. C., Pinho-Ribeiro, F. A., Georgetti, S. R., et al. (2016). Naringenin inhibits superoxide anion-induced inflammatory pain: role of oxidative stress, cytokines, Nrf-2 and the NO-cGMP-PKG-KATPChannel signaling pathway. PLoS One 11:e0153015. doi: 10.1371/journal.pone.0153015
Manea, A., Manea, S. A., Gafencu, A. V., and Raicu, M. (2007). Regulation of NADPH oxidase subunit p22(phox) by NF-kB in human aortic smooth muscle cells. Arch. Physiol. Biochem. 113, 163–172. doi: 10.1080/13813450701531235
Martinez, R. M., Fattori, V., Saito, P., Melo, C. B. P., Borghi, S. M., Pinto, I. C., et al. (2018). Lipoxin A4 inhibits UV radiation-induced skin inflammation and oxidative stress in mice. J. Dermatol. Sci. doi: 10.1016/j.jdermsci.2018.04.014 [Epub ahead of print].
Mauro, C., Leow, S. C., Anso, E., Rocha, S., Thotakura, A. K., Tornatore, L., et al. (2011). NF-kappaB controls energy homeostasis and metabolic adaptation by upregulating mitochondrial respiration. Nat. Cell Biol. 13, 1272–1279. doi: 10.1038/ncb2324
McDonnell, C., Leanez, S., and Pol, O. (2017). The induction of the transcription factor Nrf2 enhances the antinociceptive effects of delta-opioid receptors in diabetic mice. PLoS One 12:e0180998. doi: 10.1371/journal.pone.0180998
McKay, L. I., and Cidlowski, J. A. (1999). Molecular control of immune/inflammatory responses: interactions between nuclear factor-kappa B and steroid receptor-signaling pathways. Endocr. Rev. 20, 435–459.
Mehrotra, A., Shanbhag, R., Chamallamudi, M. R., Singh, V. P., and Mudgal, J. (2011). Ameliorative effect of caffeic acid against inflammatory pain in rodents. Eur. J. Pharmacol. 666, 80–86. doi: 10.1016/j.ejphar.2011.05.039
Miner, J. N., Hong, M. H., and Negro-Vilar, A. (2005). New and improved glucocorticoid receptor ligands. Expert Opin. Investig. Drugs 14, 1527–1545. doi: 10.1517/13543784.14.12.1527
Mittal, M., Siddiqui, M. R., Tran, K., Reddy, S. P., and Malik, A. B. (2014). Reactive oxygen species in inflammation and tissue injury. Antioxid. Redox. Signal. 20, 1126–1167. doi: 10.1089/ars.2012.5149
Miyazaki, Y., Shimizu, A., Pastan, I., Taguchi, K., Naganuma, E., Suzuki, T., et al. (2014). Keap1 inhibition attenuates glomerulosclerosis. Nephrol. Dial. Transplant. 29, 783–791. doi: 10.1093/ndt/gfu002
Mizokami, S. S., Arakawa, N. S., Ambrosio, S. R., Zarpelon, A. C., Casagrande, R., Cunha, T. M., et al. (2012). Kaurenoic acid from Sphagneticola trilobata inhibits inflammatory pain: effect on cytokine production and activation of the NO-cyclic GMP-protein kinase G-ATP-sensitive potassium channel signaling pathway. J. Nat. Prod. 75, 896–904. doi: 10.1021/np200989t
More, S. V., and Choi, D. K. (2015). Promising cannabinoid-based therapies for Parkinson’s disease: motor symptoms to neuroprotection. Mol. Neurodegener. 10:17. doi: 10.1186/s13024-015-0012-0
Morimitsu, Y., Nakagawa, Y., Hayashi, K., Fujii, H., Kumagai, T., Nakamura, Y., et al. (2002). A sulforaphane analogue that potently activates the Nrf2-dependent detoxification pathway. J. Biol. Chem. 277, 3456–3463. doi: 10.1074/jbc.M110244200
Mrowietz, U., Szepietowski, J. C., Loewe, R., Van De Kerkhof, P., Lamarca, R., Ocker, W. G., et al. (2017). Efficacy and safety of LAS41008 (dimethyl fumarate) in adults with moderate-to-severe chronic plaque psoriasis: a randomized, double-blind, Fumaderm((R)) – And placebo-controlled trial (BRIDGE). Br. J. Dermatol. 176, 615–623. doi: 10.1111/bjd.14947
Nagaich, A. K., Rayasam, G. V., Martinez, E. D., Becker, M., Qiu, Y., Johnson, T. A., et al. (2004). Subnuclear trafficking and gene targeting by steroid receptors. Ann. N. Y. Acad. Sci. 1024, 213–220. doi: 10.1196/annals.1321.002
Nguyen, T., Nioi, P., and Pickett, C. B. (2009). The Nrf2-antioxidant response element signaling pathway and its activation by oxidative stress. J. Biol. Chem. 284, 13291–13295. doi: 10.1074/jbc.R900010200
Nguyen, T., Sherratt, P. J., Huang, H. C., Yang, C. S., and Pickett, C. B. (2003). Increased protein stability as a mechanism that enhances Nrf2-mediated transcriptional activation of the antioxidant response element. Degradation of Nrf2 by the 26 S proteasome. J. Biol. Chem. 278, 4536–4541. doi: 10.1074/jbc.M207293200
Ockenfels, H. M., Schultewolter, T., Ockenfels, G., Funk, R., and Goos, M. (1998). The antipsoriatic agent dimethylfumarate immunomodulates T-cell cytokine secretion and inhibits cytokines of the psoriatic cytokine network. Br. J. Dermatol. 139, 390–395. doi: 10.1046/j.1365-2133.1998.02400.x
Paiva, C. N., and Bozza, M. T. (2014). Are reactive oxygen species always detrimental to pathogens? Antioxid. Redox Signal. 20, 1000–1037. doi: 10.1089/ars.2013.5447
Pang, C., Zheng, Z., Shi, L., Sheng, Y., Wei, H., Wang, Z., et al. (2016). Caffeic acid prevents acetaminophen-induced liver injury by activating the Keap1-Nrf2 antioxidative defense system. Free Radic. Biol. Med. 91, 236–246. doi: 10.1016/j.freeradbiomed.2015.12.024
Patwardhan, J., and Bhatt, P. (2015). Ultraviolet-B protective effect of flavonoids from Eugenia caryophylata on human dermal fibroblast cells. Pharmacogn. Mag. 11, S397–S406. doi: 10.4103/0973-1296.168979
Perera, R. M., and Bardeesy, N. (2011). Cancer: when antioxidants are bad. Nature 475, 43–44. doi: 10.1038/475043a
Pergola, P. E., Raskin, P., Toto, R. D., Meyer, C. J., Huff, J. W., Grossman, E. B., et al. (2011). Bardoxolone methyl and kidney function in CKD with type 2 diabetes. N. Engl. J. Med. 365, 327–336. doi: 10.1056/NEJMoa1105351
Pinho-Ribeiro, F. A., Fattori, V., Zarpelon, A. C., Borghi, S. M., Staurengo-Ferrari, L., Carvalho, T. T., et al. (2016a). Pyrrolidine dithiocarbamate inhibits superoxide anion-induced pain and inflammation in the paw skin and spinal cord by targeting NF-(B and oxidative stress. Inflammopharmacology 24, 97–107. doi: 10.1007/s10787-016-0266-3
Pinho-Ribeiro, F. A., Zarpelon, A. C., Fattori, V., Manchope, M. F., Mizokami, S. S., Casagrande, R., et al. (2016b). Naringenin reduces inflammatory pain in mice. Neuropharmacology 105, 508–519. doi: 10.1016/j.neuropharm.2016.02.019
Pinho-Ribeiro, F. A., Zarpelon, A. C., Mizokami, S. S., Borghi, S. M., Bordignon, J., Silva, R. L., et al. (2016c). The citrus flavonone naringenin reduces lipopolysaccharide-induced inflammatory pain and leukocyte recruitment by inhibiting NF-(B activation. J. Nutr. Biochem. 33, 8–14. doi: 10.1016/j.jnutbio.2016.03.013
Pinho-Ribeiro, F. A., Hohmann, M. S. N., Borghi, S. M., Zarpelon, A. C., Guazelli, C. F. S., Manchope, M. F., et al. (2015). Protective effects of the flavonoid hesperidin methyl chalcone in inflammation and pain in mice: role of TRPV1, oxidative stress, cytokines and NF-κB. Chem. Biol. Interact 228, 88–99. doi: 10.1016/j.cbi.2015.01.011
Pinho-Ribeiro, F. A., Verri, W. A., and Chiu, I. M. (2017). Nociceptor sensory neuron–immune interactions in pain and inflammation. Trends Immunol. 38, 5–19. doi: 10.1016/j.it.2016.10.001
Pledgie-Tracy, A., Sobolewski, M. D., and Davidson, N. E. (2007). Sulforaphane induces cell type-specific apoptosis in human breast cancer cell lines. Mol. Cancer Ther. 6, 1013–1021. doi: 10.1158/1535-7163.MCT-06-0494
Powers, J. C., Asgian, J. L., Ekici, O. D., and James, K. E. (2002). Irreversible inhibitors of serine, cysteine, and threonine proteases. Chem. Rev. 102, 4639–4750. doi: 10.1021/cr010182v
Prochaska, H. J., De Long, M. J., and Talalay, P. (1985). On the mechanisms of induction of cancer-protective enzymes: a unifying proposal. Proc. Natl. Acad. Sci. U.S.A. 82, 8232–8236. doi: 10.1073/pnas.82.23.8232
Rajendran, P., Dashwood, W. M., Li, L., Kang, Y., Kim, E., Johnson, G., et al. (2015). Nrf2 status affects tumor growth, HDAC3 gene promoter associations, and the response to sulforaphane in the colon. Clin. Epigenet. 7:102. doi: 10.1186/s13148-015-0132-y
Ramos-Gomez, M., Kwak, M. K., Dolan, P. M., Itoh, K., Yamamoto, M., Talalay, P., et al. (2001). Sensitivity to carcinogenesis is increased and chemoprotective efficacy of enzyme inducers is lost in nrf2 transcription factor-deficient mice. Proc. Natl. Acad. Sci. U.S.A. 98, 3410–3415. doi: 10.1073/pnas.051618798
Redondo, A., Chamorro, P. A. F., Riego, G., Leanez, S., and Pol, O. (2017). Treatment with sulforaphane produces antinociception and improves morphine effects during inflammatory pain in mice. J. Pharmacol. Exp. Ther. 363, 293–302. doi: 10.1124/jpet.117.244376
Rhen, T., and Cidlowski, J. A. (2005). Antiinflammatory action of glucocorticoids–new mechanisms for old drugs. N. Engl. J. Med. 353, 1711–1723. doi: 10.1056/NEJMra050541
Richardson, B. G., Jain, A. D., Speltz, T. E., and Moore, T. W. (2015). Non-electrophilic modulators of the canonical Keap1/Nrf2 pathway. Bioorg. Med. Chem. Lett. 25, 2261–2268. doi: 10.1016/j.bmcl.2015.04.019
Riedl, M. A., Saxon, A., and Diaz-Sanchez, D. (2009). Oral sulforaphane increases Phase II antioxidant enzymes in the human upper airway. Clin. Immunol. 130, 244–251. doi: 10.1016/j.clim.2008.10.007
Rojo de la Vega, M., Chapman, E., and Zhang, D. D. (2018). NRF2 and the Hallmarks of Cancer. Cancer Cell 34, 21–43. doi: 10.1016/j.ccell.2018.03.022
Rong, H., Liang, Y., and Niu, Y. (2018). Rosmarinic acid attenuates beta-amyloid-induced oxidative stress via Akt/GSK-3beta/Fyn-mediated Nrf2 activation in PC12 cells. Free Radic. Biol. Med. 120, 114–123. doi: 10.1016/j.freeradbiomed.2018.03.028
Roumestan, C., Michel, A., Bichon, F., Portet, K., Detoc, M., Henriquet, C., et al. (2007). Anti-inflammatory properties of desipramine and fluoxetine. Respir Res. 8:35. doi: 10.1186/1465-9921-8-35
Ruiz, S., Pergola, P. E., Zager, R. A., and Vaziri, N. D. (2013). Targeting the transcription factor Nrf2 to ameliorate oxidative stress and inflammation in chronic kidney disease. Kidney Int. 83, 1029–1041. doi: 10.1038/ki.2012.439
Ruiz-Miyazawa, K. W., Pinho-Ribeiro, F. A., Borghi, S. M., Staurengo-Ferrari, L., Fattori, V., Amaral, F. A., et al. (2018a). Hesperidin methylchalcone suppresses experimental gout arthritis in mice by inhibiting NF-kappaB activation. J. Agric. Food Chem. 66, 6269–6280. doi: 10.1021/acs.jafc.8b00959
Ruiz-Miyazawa, K. W., Staurengo-Ferrari, L., Pinho-Ribeiro, F. A., Fattori, V., Zaninelli, T. H., Badaro-Garcia, S., et al. (2018b). 15d-PGJ2-loaded nanocapsules ameliorate experimental gout arthritis by reducing pain and inflammation in a PPAR-gamma-sensitive manner in mice. Sci. Rep. 8:13979. doi: 10.1038/s41598-018-32334-0
Saito, P., Melo, C. P. B., Martinez, R. M., Fattori, V., Cezar, T. L. C., Pinto, I. C., et al. (2018). The lipid mediator resolvin D1 reduces the skin inflammation and oxidative stress induced by UV irradiation in hairless mice. Front. Pharmacol. 9:1242. doi: 10.3389/fphar.2018.01242
Saito, R., Suzuki, T., Hiramoto, K., Asami, S., Naganuma, E., Suda, H., et al. (2016). Characterizations of three major cysteine sensors of keap1 in stress response. Mol. Cell. Biol. 36, 271–284.
Sakai, C., Ishida, M., Ohba, H., Yamashita, H., Uchida, H., Yoshizumi, M., et al. (2017). Fish oil omega-3 polyunsaturated fatty acids attenuate oxidative stress-induced DNA damage in vascular endothelial cells. PLoS One 12:e0187934. doi: 10.1371/journal.pone.0187934
Salazar, M., Rojo, A. I., Velasco, D., De Sagarra, R. M., and Cuadrado, A. (2006). Glycogen synthase kinase-3beta inhibits the xenobiotic and antioxidant cell response by direct phosphorylation and nuclear exclusion of the transcription factor Nrf2. J. Biol. Chem. 281, 14841–14851. doi: 10.1074/jbc.M513737200
Saracino, A. M., and Orteu, C. H. (2017). Severe recalcitrant cutaneous manifestations in systemic lupus erythematosus successfully treated with fumaric acid esters. Br. J. Dermatol. 176, 472–480. doi: 10.1111/bjd.14698
Satoh, T., Kosaka, K., Itoh, K., Kobayashi, A., Yamamoto, M., Shimojo, Y., et al. (2008). Carnosic acid, a catechol-type electrophilic compound, protects neurons both in vitro and in vivo through activation of the Keap1/Nrf2 pathway via S-alkylation of targeted cysteines on Keap1. J. Neurochem. 104, 1116–1131. doi: 10.1111/j.1471-4159.2007.05039.x
Schafer, M., and Werner, S. (2015). Nrf2–A regulator of keratinocyte redox signaling. Free Radic. Biol. Med. 88, 243–252. doi: 10.1016/j.freeradbiomed.2015.04.018
Schilling, S., Goelz, S., Linker, R., Luehder, F., and Gold, R. (2006). Fumaric acid esters are effective in chronic experimental autoimmune encephalomyelitis and suppress macrophage infiltration. Clin. Exp. Immunol. 145, 101–107. doi: 10.1111/j.1365-2249.2006.03094.x
Selvam, C., Jachak, S. M., Thilagavathi, R., and Chakraborti, A. K. (2005). Design, synthesis, biological evaluation and molecular docking of curcumin analogues as antioxidant, cyclooxygenase inhibitory and anti-inflammatory agents. Bioorg. Med. Chem. Lett. 15, 1793–1797. doi: 10.1016/j.bmcl.2005.02.039
Serafim, K. G. G., Navarro, S. A., Zarpelon, A. C., Pinho-Ribeiro, F. A., Fattori, V., Cunha, T. M., et al. (2015). Bosentan, a mixed endothelin receptor antagonist, inhibits superoxide anion-induced pain and inflammation in mice. Naunyn-Schmiedeberg’s Arch. Pharmacol. 388, 1211–1221. doi: 10.1007/s00210-015-1160-z
Serhan, C. N. (2017). Treating inflammation and infection in the 21st century: new hints from decoding resolution mediators and mechanisms. FASEB J. 31, 1273–1288. doi: 10.1096/fj.201601222R
Serhan, C. N., Yacoubian, S., and Yang, R. (2008). Anti-inflammatory and proresolving lipid mediators. Annu. Rev. Pathol. 3, 279–312. doi: 10.1146/annurev.pathmechdis.3.121806.151409
Shapiro, T. A., Fahey, J. W., Wade, K. L., Stephenson, K. K., and Talalay, P. (2001). Chemoprotective glucosinolates and isothiocyanates of broccoli sprouts: metabolism and excretion in humans. Cancer Epidemiol. Biomarkers. Preven. 10, 501–508.
Sharma, A., Rizky, L., Stefanovic, N., Tate, M., Ritchie, R. H., Ward, K. W., et al. (2017). The nuclear factor (erythroid-derived 2)-like 2 (Nrf2) activator dh404 protects against diabetes-induced endothelial dysfunction. Cardiovasc. Diabetol. 16:33. doi: 10.1186/s12933-017-0513-y
Shen, X., Hu, B., Xu, G., Chen, F., Ma, R., Zhang, N., et al. (2017). Activation of Nrf2/HO-1 pathway by glycogen synthase kinase-3beta inhibition attenuates renal ischemia/reperfusion injury in diabetic rats. Kid. Blood Press. Res. 42, 369–378. doi: 10.1159/000477947
Shintani, Y., Maruoka, S., Gon, Y., Koyama, D., Yoshida, A., Kozu, Y., et al. (2015). Nuclear factor erythroid 2-related factor 2 (Nrf2) regulates airway epithelial barrier integrity. Allergol. Int. 64(Suppl.), S54–S63. doi: 10.1016/j.alit.2015.06.004
Silva-Islas, C. A., and Maldonado, P. D. (2018). Canonical and non-canonical mechanisms of Nrf2 activation. Pharmacol. Res. 134, 92–99. doi: 10.1016/j.phrs.2018.06.013
Simmons, D. L., Botting, R. M., and Hla, T. (2004). Cyclooxygenase isozymes: the biology of prostaglandin synthesis and inhibition. Pharmacol. Rev. 56, 387–437. doi: 10.1124/pr.56.3.3
Singh, A. K., and Haldar, C. (2016). Melatonin modulates glucocorticoid receptor mediated inhibition of antioxidant response and apoptosis in peripheral blood mononuclear cells. Mol. Cell. Endocrinol. 436, 59–67. doi: 10.1016/j.mce.2016.07.024
Soto-Balbuena, C., Rodriguez, M. F., Escudero Gomis, A. I., Ferrer Barriendos, F. J., Le, H. N., and Pmb-Huca, G. (2018). Incidence, prevalence and risk factors related to anxiety symptoms during pregnancy. Psicothema 30, 257–263.
Soundararajan, P., and Kim, J. S. (2018). Anti-carcinogenic glucosinolates in cruciferous vegetables and their antagonistic effects on prevention of cancers. Molecules 23:E2983. doi: 10.3390/molecules23112983
Sporn, M. B., and Liby, K. T. (2012). NRF2 and cancer: the good, the bad and the importance of context. Nat. Rev. Cancer 12, 564–571. doi: 10.1038/nrc3278
Su, X., Jiang, X., Meng, L., Dong, X., Shen, Y., and Xin, Y. (2018). Anticancer activity of sulforaphane: the epigenetic mechanisms and the Nrf2 signaling pathway. Oxid. Med. Cell Longev. 2018:5438179. doi: 10.1155/2018/5438179
Sun, X., Zeng, H., Wang, Q., Yu, Q., Wu, J., Feng, Y., et al. (2018). Glycyrrhizin ameliorates inflammatory pain by inhibiting microglial activation-mediated inflammatory response via blockage of the HMGB1-TLR4-NF-kB pathway. Exp. Cell Res. 369, 112–119. doi: 10.1016/j.yexcr.2018.05.012
Sutcliffe, J. G., Hedlund, P. B., Thomas, E. A., Bloom, F. E., and Hilbush, B. S. (2011). Peripheral reduction of beta-amyloid is sufficient to reduce brain beta-amyloid: implications for Alzheimer’s disease. J. Neurosci. Res. 89, 808–814. doi: 10.1002/jnr.22603
Suzuki, T., Murakami, S., Biswal, S. S., Sakaguchi, S., Harigae, H., Yamamoto, M., et al. (2017). Systemic activation of NRF2 alleviates lethal autoimmune inflammation in scurfy mice. Mol. Cell. Biol. 37:e00063-17. doi: 10.1128/MCB.00063-17
Taguchi, K., Motohashi, H., and Yamamoto, M. (2011). Molecular mechanisms of the Keap1-Nrf2 pathway in stress response and cancer evolution. Genes Cells 16, 123–140. doi: 10.1111/j.1365-2443.2010.01473.x
Tahvili, S., Zandieh, B., and Amirghofran, Z. (2015). The effect of dimethyl fumarate on gene expression and the level of cytokines related to different T helper cell subsets in peripheral blood mononuclear cells of patients with psoriasis. Int. J. Dermatol. 54, e254–e260. doi: 10.1111/ijd.12834
Tan, S. M., Sharma, A., Stefanovic, N., Yuen, D. Y., Karagiannis, T. C., Meyer, C., et al. (2014). Derivative of bardoxolone methyl, dh404, in an inverse dose-dependent manner lessens diabetes-associated atherosclerosis and improves diabetic kidney disease. Diabetes Metab. Res. Rev. 63, 3091–3103. doi: 10.2337/db13-1743
Thangapandiyan, S., and Miltonprabu, S. (2014). Epigallocatechin gallate supplementation protects against renal injury induced by fluoride intoxication in rats: role of Nrf2/HO-1 signaling. Toxicol. Rep. 1, 12–30. doi: 10.1016/j.toxrep.2014.01.002
To, C., Ringelberg, C. S., Royce, D. B., Williams, C. R., Risingsong, R., Sporn, M. B., et al. (2015). Dimethyl fumarate and the oleanane triterpenoids, CDDO-imidazolide and CDDO-methyl ester, both activate the Nrf2 pathway but have opposite effects in the A/J model of lung carcinogenesis. Carcinogenesis 36, 769–781. doi: 10.1093/carcin/bgv061
Toledano, M. B. (2009). The guardian recruits cops: the p53-p21 axis delegates prosurvival duties to the Keap1-Nrf2 stress pathway. Mol. Cell. 34, 637–639. doi: 10.1016/j.molcel.2009.06.005
Tu, J., Zhang, X., Zhu, Y., Dai, Y., Li, N., Yang, F., et al. (2015). Cell-permeable peptide targeting the Nrf2-keap1 interaction: a potential novel therapy for global cerebral ischemia. J. Neurosci. 35, 14727–14739. doi: 10.1523/JNEUROSCI.1304-15.2015
Tzaneva, S., Geroldinger, A., Trattner, H., and Tanew, A. (2018). Fumaric acid esters in combination with a 6-week course of narrowband ultraviolet B provides an accelerated response compared with fumaric acid esters monotherapy in patients with moderate-to-severe plaque psoriasis: a randomized prospective clinical study. Br. J. Dermatol. 178, 682–688. doi: 10.1111/bjd.16106
Verri, W. A., Vicentini, F. T. M. C., Baracat, M. M., Georgetti, S. R., Cardoso, R. D., Cunha, T. M., et al. (2012). “Flavonoids as anti-inflammatory and analgesic drugs: mechanisms of action and perspectives in the development of pharmaceutical forms,” in Studies in Natural Products Chemistry, ed. Atta-U-Rahman (Amsterdam: Elsevier). doi: 10.1016/B978-0-444-53836-9.00026-8
Wakabayashi, N., Itoh, K., Wakabayashi, J., Motohashi, H., Noda, S., Takahashi, S., et al. (2003). Keap1-null mutation leads to postnatal lethality due to constitutive Nrf2 activation. Nat. Genet. 35, 238–245. doi: 10.1038/ng1248
Wang, B., Zhu, X., Kim, Y., Li, J., Huang, S., Saleem, S., et al. (2012). Histone deacetylase inhibition activates transcription factor Nrf2 and protects against cerebral ischemic damage. Free Radic. Biol. Med. 52, 928–936. doi: 10.1016/j.freeradbiomed.2011.12.006
Wang, C., and Wang, C. (2017). Anti-nociceptive and anti-inflammatory actions of sulforaphane in chronic constriction injury-induced neuropathic pain mice. Inflammopharmacology 25, 99–106. doi: 10.1007/s10787-016-0307-y
Wardyn, J. D., Ponsford, A. H., and Sanderson, C. M. (2015). Dissecting molecular cross-talk between Nrf2 and NF-kappaB response pathways. Biochem. Soc. Trans. 43, 621–626. doi: 10.1042/BST20150014
Wei, J., Zhu, H., Lord, G., Bhattachayya, M., Jones, B. M., Allaway, G., et al. (2017). Nrf2 exerts cell-autonomous antifibrotic effects: compromised function in systemic sclerosis and therapeutic rescue with a novel heterocyclic chalcone derivative. Transl. Res. 183:e71. doi: 10.1016/j.trsl.2016.12.002
Wei, W., Shurui, C., Zipeng, Z., Hongliang, D., Hongyu, W., Yuanlong, L., et al. (2018). Aspirin suppresses neuronal apoptosis, reduces tissue inflammation, and restrains astrocyte activation by activating the Nrf2/HO-1 signaling pathway. Neuroreport 29, 524–531. doi: 10.1097/WNR.0000000000000969
Wise, R. A., Holbrook, J. T., Criner, G., Sethi, S., Rayapudi, S., Sudini, K. R., et al. (2016). Lack of effect of oral sulforaphane administration on Nrf2 expression in COPD: a randomized, double-blind, placebo controlled trial. PLoS One 11:e0163716. doi: 10.1371/journal.pone.0175077
Wu, J., Li, Q., Wang, X., Yu, S., Li, L., Wu, X., et al. (2013). Neuroprotection by curcumin in ischemic brain injury involves the Akt/Nrf2 pathway. PLoS One 8:e59843. doi: 10.1371/journal.pone.0059843
Xu, J., Donepudi, A. C., Moscovitz, J. E., and Slitt, A. L. (2013). Keap1-knockdown decreases fasting-induced fatty liver via altered lipid metabolism and decreased fatty acid mobilization from adipose tissue. PLoS One 8:e79841. doi: 10.1371/journal.pone.0079841
Xu, L. L., Liu, T., Wang, L., Li, L., Wu, Y. F., Li, C. C., et al. (2018). 3-(1H-Benzo[d]imidazol-6-yl)-5-(4-fluorophenyl)-1,2,4-oxadiazole (DDO7232), a Novel Potent Nrf2/ARE inducer, ameliorates DSS-induced murine colitis and protects NCM460 cells against oxidative stress via ERK1/2 phosphorylation. Oxid. Med. Cell. Longev. 2018:3271617. doi: 10.1155/2018/3271617
Xu, Z., Zhang, F., Sun, F., Gu, K., Dong, S., and He, D. (2015). Dimethyl fumarate for multiple sclerosis. Cochrane Database Syst. Rev. 4:CD11076. doi: 10.1002/14651858.CD011076.pub2
Yamacita-Borin, F. Y., Zarpelon, A. C., Pinho-Ribeiro, F. A., Fattori, V., Alves-Filho, J. C., Cunha, F. Q., et al. (2015). Superoxide anion-induced pain and inflammation depends on TNF(/TNFR1 signaling in mice. Neurosci. Lett. 605, 53–58. doi: 10.1016/j.neulet.2015.08.015
Yan, B., Ma, Z., Shi, S., Hu, Y., Ma, T., Rong, G., et al. (2017). Sulforaphane prevents bleomycininduced pulmonary fibrosis in mice by inhibiting oxidative stress via nuclear factor erythroid 2related factor2 activation. Mol. Med. Rep. 15, 4005–4014. doi: 10.3892/mmr.2017.6546
Yang, G. Z., Wang, Z. J., Bai, F., Qin, X. J., Cao, J., Lv, J. Y., et al. (2015). Epigallocatechin-3-gallate protects HUVECs from PM2.5-induced oxidative stress injury by activating critical antioxidant pathways. Molecules 20, 6626–6639. doi: 10.3390/molecules20046626
Yao, W., Zhang, J. C., Ishima, T., Dong, C., Yang, C., Ren, Q., et al. (2016). Role of Keap1-Nrf2 signaling in depression and dietary intake of glucoraphanin confers stress resilience in mice. Sci. Rep. 6:30659. doi: 10.1038/srep30659
Yore, M. M., Kettenbach, A. N., Sporn, M. B., Gerber, S. A., and Liby, K. T. (2011). Proteomic analysis shows synthetic oleanane triterpenoid binds to mTOR. PLoS One 6:e22862. doi: 10.1371/journal.pone.0022862
Yoshinaga, N., Arimura, N., Otsuka, H., Kawahara, K., Hashiguchi, T., Maruyama, I., et al. (2011). NSAIDs inhibit neovascularization of choroid through HO-1-dependent pathway. Lab. Invest. 91, 1277–1290. doi: 10.1038/labinvest.2011.101
Zhang, D. D. (2013). Bardoxolone brings Nrf2-based therapies to light. Antioxid. Redox Signal. 19, 517–518. doi: 10.1089/ars.2012.5118
Zhang, X., Li, J., Yang, J., and Huang, Z. (2016). Fentanyl combined with butorphanol protects myocardial ischemia/reperfusion injury via κ-opioid receptor-mediated Nrf2-ARE signaling. Int. J. Clin. Exp. Med. 9, 2500–2506.
Zhang, Y., Liu, B., Chen, X., Zhang, N., Li, G., Zhang, L. H., et al. (2017). Naringenin ameliorates behavioral dysfunction and neurological deficits in a d-galactose-induced aging mouse model through activation of PI3K/Akt/Nrf2 pathway. Rejuvenation Res. 20, 462–472. doi: 10.1089/rej.2017.1960
Zhao, J., Redell, J. B., Moore, A. N., and Dash, P. K. (2011). A novel strategy to activate cytoprotective genes in the injured brain. Biochem. Biophys. Res. Commun. 407, 501–506. doi: 10.1016/j.bbrc.2011.03.046
Zhu, J., Wang, H., Chen, F., Fu, J., Xu, Y., Hou, Y., et al. (2016). An overview of chemical inhibitors of the Nrf2-ARE signaling pathway and their potential applications in cancer therapy. Free Radic. Biol. Med. 99, 544–556. doi: 10.1016/j.freeradbiomed.2016.09.010
Keywords: Nrf2, Keap1, pain, inflammation, oxidative stress, analgesic, anti-inflammatory, antioxidant
Citation: Staurengo-Ferrari L, Badaro-Garcia S, Hohmann MSN, Manchope MF, Zaninelli TH, Casagrande R and Verri WA Jr (2019) Contribution of Nrf2 Modulation to the Mechanism of Action of Analgesic and Anti-inflammatory Drugs in Pre-clinical and Clinical Stages. Front. Pharmacol. 9:1536. doi: 10.3389/fphar.2018.01536
Received: 24 August 2018; Accepted: 17 December 2018;
Published: 11 January 2019.
Edited by:
Carmen Gomez-Guerrero, Universidad Autónoma de Madrid, SpainReviewed by:
Khalid El Bairi, Mohamed Premier University, MoroccoElena Ossipova, Karolinska Institute (KI), Sweden
Copyright © 2019 Staurengo-Ferrari, Badaro-Garcia, Hohmann, Manchope, Zaninelli, Casagrande and Verri. This is an open-access article distributed under the terms of the Creative Commons Attribution License (CC BY). The use, distribution or reproduction in other forums is permitted, provided the original author(s) and the copyright owner(s) are credited and that the original publication in this journal is cited, in accordance with accepted academic practice. No use, distribution or reproduction is permitted which does not comply with these terms.
*Correspondence: Waldiceu A. Verri Jr., d2FsZGljZXVqckB5YWhvby5jb20uYnI=; d2F2ZXJyaUB1ZWwuYnI= orcid.org/0000-0003-2756-9283
†Present address: Waldiceu A. Verri Jr., Departamento de Ciências Patológicas, Centro de Ciências Biológicas, Universidade Estadual de Londrina, Londrina, Brazil