- Brain Research Institute, Jeffrey Cheah School of Medicine and Health Sciences, Monash University Malaysia, Bandar Sunway, Malaysia
Stress induces various neurobiological responses and causes psychiatric disorders, including depression. Monoamine oxidase A (MAO-A) plays an important role in various functions of the brain, such as regulation of mood, anxiety and aggression, and dysregulation of MAO-A is observed in stress-related psychiatric disorders. This study addressed the question whether acute social stress induces changes to transcriptional and/or post-transcriptional regulation of MAO-A expression in the brain. Using male Nile tilapia (Oreochromis niloticus), we investigated whether acute social stress, induced by the presence of a dominant male fish, changes the expression of MAO-A. We measured gene expression of MAO-A by quantitative PCR, enzymatic activity of MAO-A by the luminescent method, and 5-HT and 5-HIAA levels by liquid chromatography–mass spectrometry in the brain of socially stressed and control fish. Socially stressed males showed decreased MAO-A mRNA levels, consistent MAO-A enzymatic activity, increased 5-HT turnover in the brain, and elevated plasma cortisol levels, compared to controls. Our results suggest that acute social stress suppresses the transcription of MAO-A gene, enhances 5-HT metabolism but does not affect the production of MAO-A protein.
Introduction
Stress elicits various neurobiological responses and causes several psychiatric disorders, including depression, anxiety disorder, and post-traumatic stress disorder (Lucassen et al., 2014; Chiriţã et al., 2015). Dysregulation of monoamine oxidase A (MAO-A) is observed in stress-related psychiatric disorders. Elevated MAO-A activity and 5-hydroxytryptamine (5-HT) degradation in the brain are well-known features in depressed patients and animals exposed to chronic stress. Positron emission tomography shows higher density of MAO-A in the brain of patients with major depressive disorders, compared to healthy subjects (Meyer et al., 2006). Higher MAO-A density in the brain is associated with the recurrence of depressive symptoms (Meyer et al., 2009). In addition, chronically stressed animals show increased MAO-A levels, decreased 5-HT levels, and elevated 5-hydroxyindoleacetic acid (5-HIAA) levels (Winberg and Lepage, 1998; Grunewald et al., 2012).
Monoamine oxidase A is an enzyme that catalyzes the degradation of 5-HT to 5-HIAA. Increased MAO-A activity, therefore, reduces 5-HT levels in the brain. Degradation of 5-HT to 5-HIAA by MAO-A leads to the production of hydrogen peroxide, one of reactive oxygen species, which causes a decline of cellular functions (Maurel et al., 2003; Fitzgerald et al., 2014). In addition, the regulation of MAO-A is associated with the hypothalamus-pituitary-adrenal (HPA) axis. A selective glucocorticoid receptor agonist, dexamethasone, increases MAO-A expression by activating the transcription of MAO-A gene (Ou et al., 2006). Understanding the regulation of MAO-A during stress and finding an endogenous mechanism to reduce MAO-A levels would be important to develop a new approach to control MAO-A for treatment of stress-related psychiatric disorders.
Various species and stressors are used to test the influence of stress on animals. Chronic stress model contributes to the understanding of neurobiological differences between normal and depression-like profile; however, due to long exposure period, the endogenous mechanism to prevent further exaggeration of negative stress response might be missed (Musazzi et al., 2017). Therefore, this study uses acute stress to observe immediate responses of MAO-A regulation. Acute stress may induce physiological responses for the maintenance of normal biological functions of the brain (McEwen, 2004, 2008; Edwards et al., 2007; Sinyakov et al., 2017). Biological response to stress may vary depending on the duration of stress exposure (Øverli et al., 1999; Chen and Fernald, 2011; Nardocci et al., 2014). For example, stress responses in the 5-HT system and the HPA axis vary depending on the duration and frequency of stressors (Keeney et al., 2006; Moltesen et al., 2016).
In this study, using male Nile tilapia (Oreochromis niloticus), we studied whether acute social stress induces responses in the regulation of MAO-A by measuring the gene expression and enzymatic activity of MAO-A, and 5-HT turnover. Nile tilapia shows changes in body color (Corrêa et al., 2003), eye color (Volpato et al., 2003; Vera Cruz and Brown, 2007), and behavior (Corrêa et al., 2003) in response to stressors. Darkening of body color is an indicator of stress in Nile tilapia. Socially stressed fish shows darker body color, compared to the fish in higher hierarchical rank (Fernandes and Volpato, 1993; Corrêa et al., 2003). Nile tilapia are social animals, which show clear social hierarchy in the male conspecific group, as some other mammalian species do. It also has cortisol as a major endogenous glucocorticoid, as do humans. Therefore, we adopted this species as a model animal of acute social stress.
Materials and Methods
Experimental Animals
Male Nile tilapia used in this study were obtained from Inter Sea Fishery (Malaysia) Sdn Bhd or grown at the fish facility, Monash University Malaysia. Adult fish (4–8 months old) were group-housed in a home tank (90 cm × 45 cm × 45 cm) under 14 h light/10 h dark cycles. Male fish showing typical body color (silver) and behavioral pattern (aggression) of male Nile tilapia of high social status were used as fish going to play a dominant role in the social defeat stress procedure described below. The fish going to play a dominant role were kept in individual tanks (36 cm × 22 cm × 26 cm) before social defeat procedure. Fish were fed with commercial dry pellets (TP-1, Star Feedmills, Malaysia) three times per day. All the procedures for animal care and experiments in this study were approved by Monash University Animal Ethics Committee (MARP/2015/180).
Social Defeat Stress
In this study, socially stressed animals were defined as male fish which showed body color darkening, while control fish as male fish which showed neither aggressive/submissive behavior nor body color changes. In the social defeat stress experiment, the fish going to play a dominant role (15–16 cm standard length) was placed in an experimental tank (36 cm × 22 cm × 26 cm) for 1 h (9:00–10:00 h) for acclimatization. Test fish (11–12 cm standard length) was captured from the home tank, kept in the experimental tank from 10:00 to 12:30 or 15:00 h. Immediately after the exposure to social defeat stress, defeated fish were anesthetized in 0.02% benzocaine (Sigma-Aldrich, MO, United States) for approximately 1–2 min until the fish were immobilized. Blood samples were collected from a caudal vein of the fish, using 1 mL heparinized syringes and 25G syringe needles. The brain was removed and immediately dissected into three parts (Area I, telencephalon and preoptic area; Area II, optic tectum, midbrain, and hypothalamus; Area III, cerebellum and hindbrain; Figure 2A), frozen on dry ice, and stored at -80°C until analysis. Video recording during stress exposure was used to check the behavior of fish. Control fish were captured from the group-housed tank. Samples from control fish corresponding to 2.5 and 5.0 h stress groups were collected at 12:30 and 15:00 h, respectively, using the same sampling methods as stress groups.
Measurement of Plasma Cortisol Levels
The experimental condition for the high performance liquid chromatography (HPLC) was adopted from a previous study (Thang et al., 2016) with minor modification. Plasma samples were separated from blood samples by centrifugation at 3000×g for 10 min. Cortisol was extracted from the plasma using the following procedure: dexamethasone (50 ng; internal standard; Nacalai Tesque, Kyoto, Japan) and dichloromethane (400 μL; Fisher Scientific UK Ltd., Loughborough, United Kingdom) were added to plasma (200 μL) in microcentrifuge tubes. The tubes were tightly capped, vortex mixed for 15 min and centrifuged at 15000 rpm for 10 min. The organic layer was transferred to a new centrifuge tube. Dichloromethane (400 μL) was added to the remaining aqueous phase and the extraction was repeated. After the organic phase was collected in the same centrifuge tube, the sample was washed with 0.1 M NaOH (100 μL). The organic layer was separated by centrifugation at 2000 ×g for 5 min and transferred into a new tube; then the solvent was evaporated at 50°C. The residue was reconstituted with 55% methanol in water (100 μL), filtered with Cosmospin filter G (hydrophilic PTFE membrane filter with 0.2 μm pores, Nacalai Tesque) and used for analysis. A stock solution of cortisol was prepared by dissolving 10 mg of cortisol powder (Nacalai Tesque) in 10 mL of methanol (Fisher Scientific UK Ltd.). Calibration solutions (5, 10, 50, 100, 200, 300, 400, and 500 ng/mL of cortisol) were prepared in 55% methanol with dexamethasone (500 ng/mL; internal standard). 20 μL of each sample was injected manually into HPLC-DAD system (1260 Infinity, Agilent Technologies, CA, United States). The separation was performed with Agilent Zorbax Eclipse Plus C18 Rapid Resolution (4.6 mm × 75 mm, 1.8 μm, Agilent Technologies) maintained at 30°C and mobile phase consisted of methanol-water (55/45; v/v). Flow rate of the mobile phase was 0.8 mL/min. Cortisol and dexamethasone were detected and quantified by UV absorbance at 245 nm. Peak areas of cortisol and dexamethasone on the chromatograms were calculated with ChemStation software (RRID:SCR_015742, Agilent Technologies).
Gene Expression Analysis
Total RNA was extracted from the brain samples with Favorprep Tri-RNA reagent (Favorgen Biotech Corporation, Taiwan), following the manufacturer’s protocol. The extracted total RNA (1000 ng for each brain sample) was transcribed into cDNA using High-Capacity cDNA Reverse Transcription Kit (Applied Biosystems, CA, United States). The cDNA samples were stored at -20°C until analysis. Quantitative real-time PCR (qPCR) was performed to measure the expression levels of MAO-A and β-actin (reference gene) mRNA using 7500 Fast Real-time PCR system (Applied Biosystems, CA, United States) with SensiFASTTM SYBR Hi-ROX Kit (Bioline Reagents, United Kingdom). The plasmid containing MAO-A or β-actin fragment was serially diluted to a concentration of 102, 103, 104, 105, 106, 107, and 108 copy/μL as a standard DNA for absolute quantification of mRNA. Reaction conditions were 95°C for 2 min, 40 cycles at 95°C for 5 s, and 60°C for 30 s followed by dissociation steps. Specific Primers for MAO-A and β-actin were used and designed as follows: MAO-A, 5′-AGCTGCTGGAGGGCTACTAA-3′ (forward) and 5′-TTCCATCCAACACGGTCTGC-3′ (reverse); β-actin, 5′-CACCGTGCTGTCTGGAGGTA-3′ (forward) and 5′-TTACGCTCAGGTGGGGCAA-3′ (reverse). The data was acquired and analyzed with 7500 Real-Time PCR Software (RRID:SCR_014596, Applied Biosystems), using ΔΔCt method.
Measurement of MAO-A Enzymatic Activity
Enzymatic activity of MAO-A in the brain was measured with an MAO-Glo Assay kit (Promega, WI, United States), following the manufacturer’s protocol. Brain tissue samples were homogenized in 10-fold volume of 100 mM HEPES-KOH buffer (pH 7.6) containing glycerol (5%) and protease inhibitor cocktail (Nacalai Tesque) and centrifuged at 600×g for 10 min. 3 μL of the supernatant was used for each MAO-A reaction. The luminescent signal was measured by a microplate reader (Infinite M200 PRO; Tecan, Switzerland).
Measurement of 5-HT and 5-HIAA
Measurement of 5-HT and 5-HIAA was performed with liquid chromatography–mass spectrometry (LC-MS). Brain tissues were homogenized in 350 μL of 50% acetonitrile (Fisher Scientific UK Ltd.) with 0.1% formic acid (Sigma-Aldrich) and 20 ng/mL of isoproterenol (internal standard; Nacalai Tesque) in microcentrifuge tubes, incubated for 5 min on ice and centrifuged at 15000 rpm for 15 min for protein precipitation. The supernatant was transferred into Cosmospin filter G (hydrophilic PTFE membrane filter with 0.2 μm pores, Nacalai Tesque), centrifuged at 5000 ×g for 10 min and used for analysis. Calibration solutions containing 1.25, 2.5, 5, 10, 20, 40, 80, 160 ng/mL of 5-HT (Sigma-Aldrich) and 5-HIAA (Sigma-Aldrich), and 20 ng/mL of isoproterenol (internal standard) were prepared in 50% acetonitrile with 0.1% formic acid. The measurement was performed with an Agilent Technologies 6410 Triple Quad LC/MS equipped with a Zorbax SB-C18 column (Narrow-Bore, 2.1 × 150 mm, 3.5 μm column; Agilent Technologies). The mobile phase was a mixture of water with 0.1% formic acid (A) and acetonitrile with 0.1% formic acid (B). The elution was as follows: 5% B from 0 to 0.3 min; 5% B to 50% B from 0.3 to 2.5 min; 50 to 100% B from 2.5 to 3.0 min. The flow rate was 0.5 mL/min. The mass spectrometer was operated in the positive-ion mode. The peaks of 5-HT, 5-HIAA, and isoproterenol were monitored by the product ion (m/z 177.1 → 160 for 5-HT, m/z 192.07 → 146 for 5-HIAA, and m/z 212.1 →194 for isoproterenol). Data acquisition and calculation were performed with Agilent Masshunter Quantitative Analysis software (RRID:SCR_015040, Agilent Technologies).
Statistics
Statistical differences were evaluated using Welch’s t-test for comparison of two groups. One-way ANOVA was followed by Tukey’s honest significant difference test for multiple comparisons. A value of p < 0.05 was considered statistically significant.
Results
Social Stress Changed Body Color of Fish and Increased Plasma Cortisol Levels
In social defeat stress experiment, the body color darkening was observed in all the socially stressed fish. An example of social stress-induced body color changes is shown in Figures 1A,B. Plasma cortisol levels were significantly higher in social stress group, compared to control groups [p < 0.01 for 2.5 h social stress (111.9 ng/mL) vs. control (28.9 ng/mL); p < 0.01 for 5 h social stress (139.3 ng/mL) vs. control (10.7 ng/mL); Figure 1C].
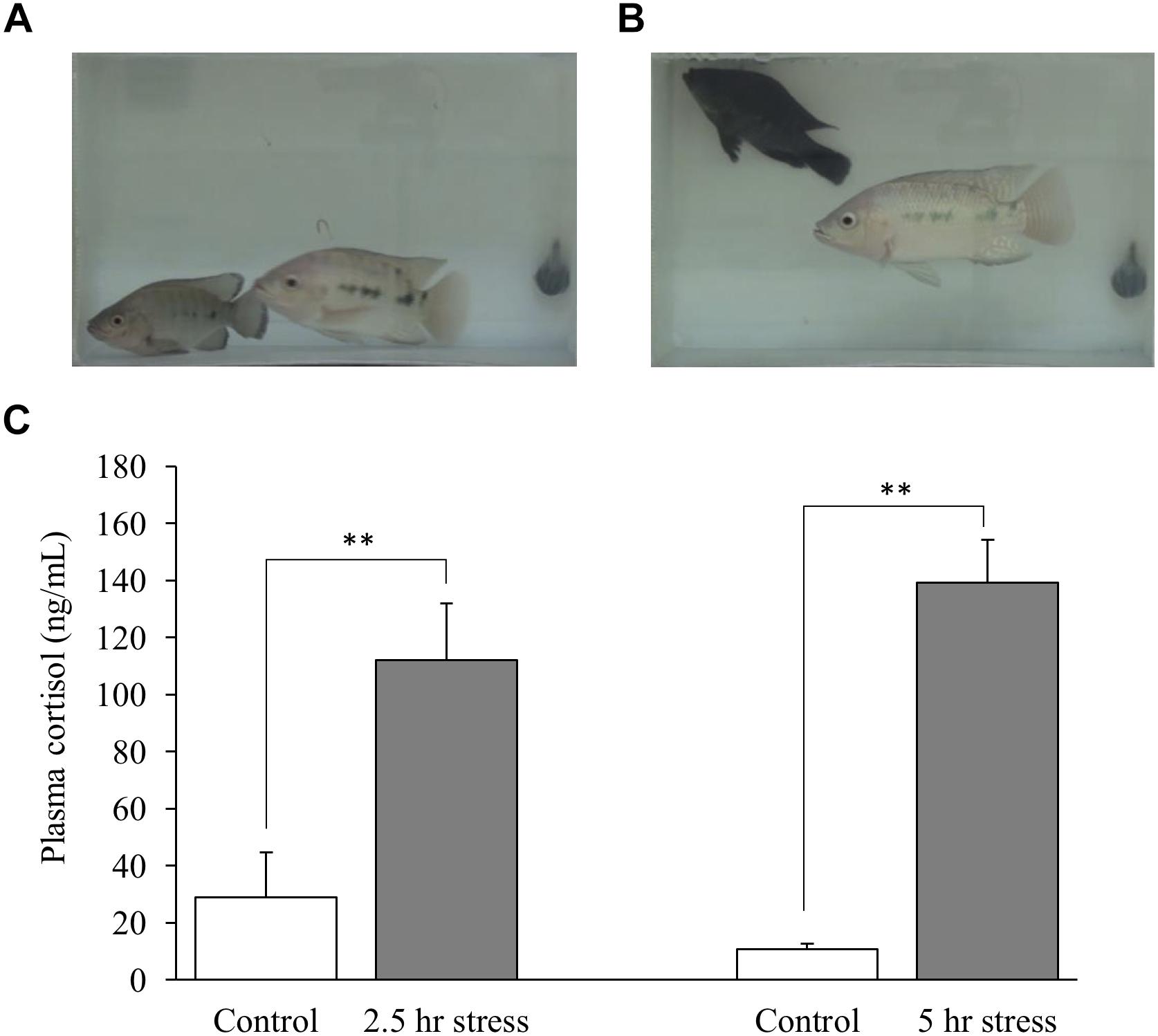
Figure 1. Changes in body color of fish (A,B) and plasma cortisol levels (C). (A) Focal fish (bottom left) showed normal body color at the beginning of stress exposure. (B) Focal fish (top left) showed darker body color after stress exposure. (C) Plasma cortisol levels were significantly elevated by social stress. Fish in 2.5 and 5 h stress groups were exposed to stress from 10:00–12:30 to10:00–15:00 h, respectively. Blood sampling from fish in the control group corresponding to each stress group was conducted at 12:30 or 15:00 h, respectively. n = 6 for control (12:30 h); n = 8 for 2.5 h stress; n = 9 for control (15:00 h); n = 4 for 5 h stress. ∗∗p < 0.01.
Social Stress Downregulates MAO-A Gene Expression
Gene expression of MAO-A was measured by qPCR. Gene expression levels of MAO-A in the brain were significantly decreased by social stress both in 2.5 and 5.0 h stress groups, compared to control groups, except for brain area I after 2.5 h social stress [0.49, 0.46, 0.42-fold (2.5 h stress vs. control); 0.42, 0.47, 0.37-fold (5.0 h stress vs. control) in the brain area I, II, and III, respectively, (Figures 2B,C)]. In addition, significant differences in MAO-A mRNA levels were detected between brain areas [one-way ANOVA, F(2,27) = 10.88, p < 0.001]. MAO-A mRNA is mainly localized in brain area II and III [1.19 × 103± 1.8 × 102, 6.78 × 103± 8.0 × 102, and 6.54 × 103± 1.4 × 103 copies/μg total RNA in Area I, II, and III, respectively. p < 0.001 for Area I vs. II; p < 0.01 for Area I vs. III; p = 0.98 for Area II vs. III (Figure 2D)].
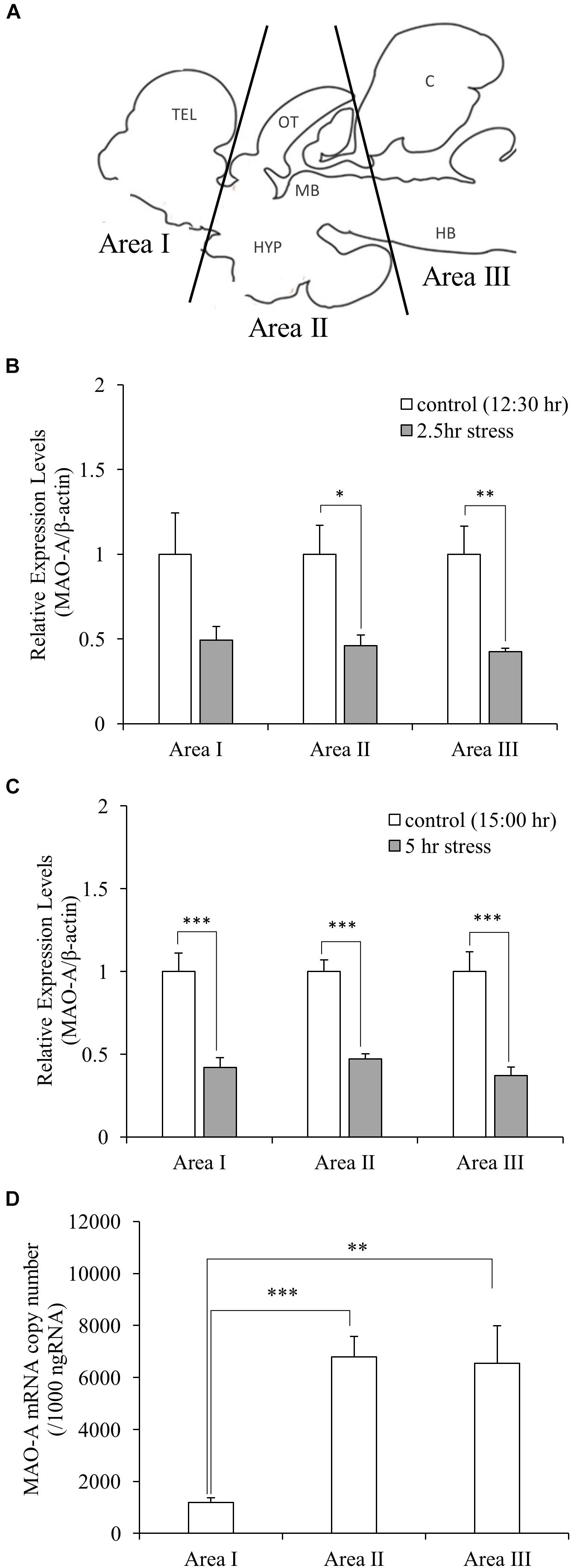
Figure 2. (A) Dissection of the brain samples. TEL, telencephalon; OT, optic tectum; MB, midbrain; HYP, hypothalamus; C, cerebellum; HB, hindbrain. ((B,C) Relative gene expression levels of MAO-A. MAO-A expression levels in the brain were decreased by social stress, except for brain area I after 2.5 h stress. (D) Copy number of MAO-A mRNA in the brain. MAO-A gene was expressed mainly in Area II and III. n = 10/group ∗p < 0.05, ∗∗p < 0.01, ∗∗∗p < 0.001.)
MAO-A Enzymatic Activity
Monoamine oxidase A enzymatic activity was measured to understand whether stress-induced decreased MAO-A gene expression caused changes in MAO-A enzymatic activity. MAO-A enzymatic activity did not change following social stress in the brain areas tested (Figures 3A,B). Significant differences in MAO-A enzymatic activity were found between different brain regions (one-way ANOVA, F(2,27) = 19.98, p < 0.001). Area I and II showed significantly higher MAO-A enzymatic activity, compared to Area III (p < 0.001 for Area I vs. III; p < 0.01 for Area II vs. III; Figure 3C).
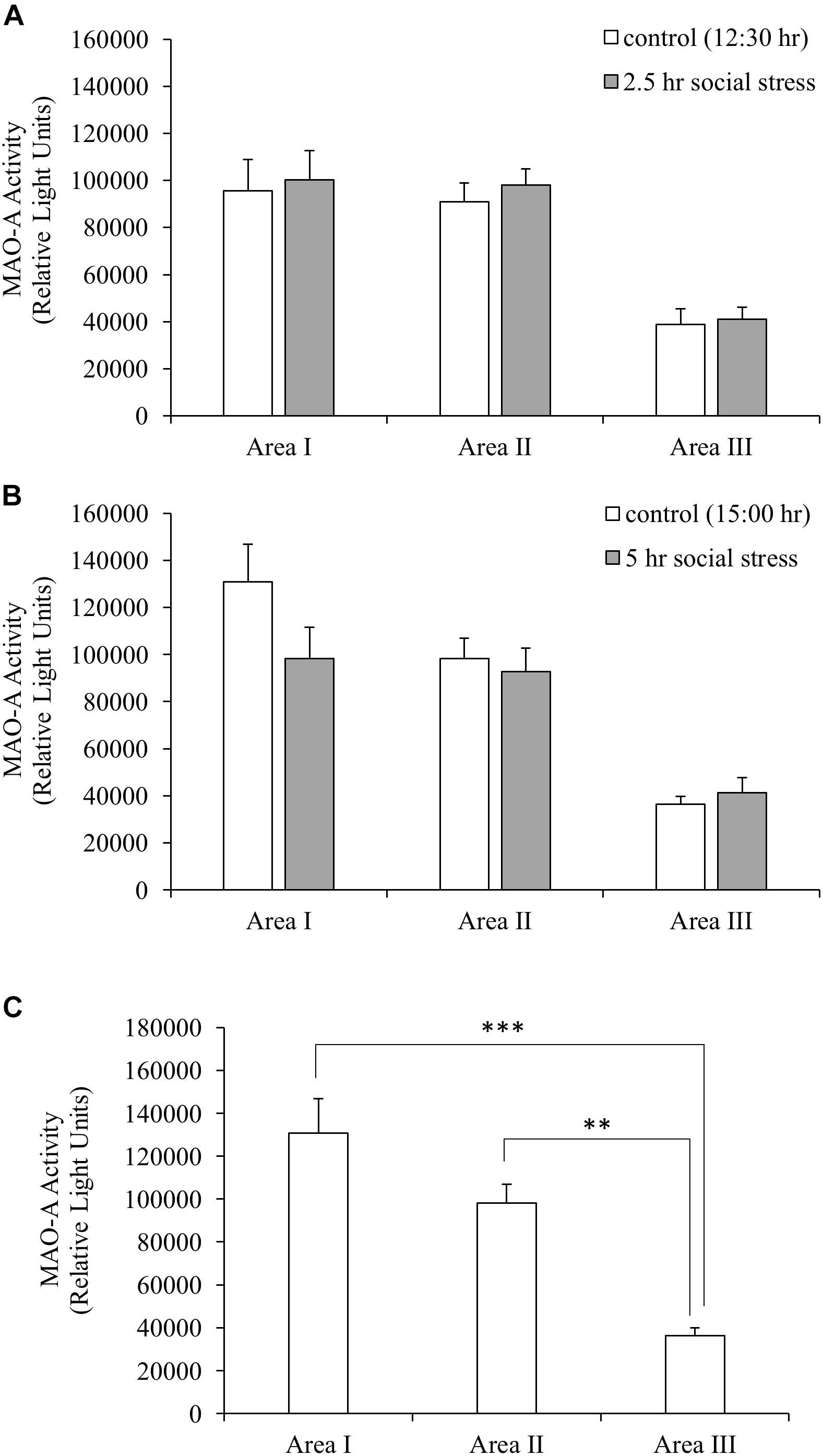
Figure 3. (A,B) MAO-A enzymatic activity. MAO-A enzymatic activity in the brain was not changed by social stress. (C) MAO-A enzymatic activity in the brain (control group sampled at 15:00 h). MAO-A enzymatic activity in Area I and II were significantly higher than that of Area III. n = 10/group ∗∗p < 0.01, ∗∗∗p < 0.001.
Social Stress Increased 5-HT Turnover in the Brain
The influences of social stress on 5-HT, 5-HIAA, and 5-HT turnover were tested in three brain areas (Figure 2A). 5-HIAA levels were significantly increased (1.56, 1.63, and 2.17-fold in the brain area I, II, and III, respectively) by 5 h social stress exposure, whereas 5-HT levels remained unchanged (Table 1). In parallel with the increases in 5-HIAA levels, 5-HIAA/5-HT ratios were significantly increased [1.88, 1.82, and 2.27-fold in area I, II, and III, respectively, (Table 1)].
Discussion
Social Stress Model of Male Nile Tilapia
In social defeat stress experiment, the test fish was introduced into a tank where fish with a larger body size was placed in advance, and the test fish was forced to experience stress by the presence of the dominant fish. Therefore, this model is similar to learned helplessness model. Socially stressed fish showed clear changes in body color. Previous studies showed stress-induced changes in body color in teleost (Höglund et al., 2000; Barreto and Volpato, 2006; Carpenter et al., 2014), which is consistent with our results in social stress model of male Nile tilapia. In addition, socially stressed fish showed higher cortisol levels in plasma, compared to control fish, indicating that social stress acutely activates the hypothalamus-pituitary-interrenal (HPI) axis, a teleost homolog of mammalian HPA axis. This result is also consistent with previous studies that show high plasma cortisol levels in teleost after exposure to stress (Øverli et al., 2004; Carpenter et al., 2014) and human subject with depression (Carroll et al., 2012).
Decreased Expression of MAO-A Gene During Social Stress
This study was designed to investigate how acute social stress influences transcriptional and post-transcriptional regulation of MAO-A and 5-HT metabolism in the brain. The expression of MAO-A gene was downregulated in the brain during social stress. This result indicates that MAO-A expression in our social stress model is regulated differently from that of chronic stress models where MAO-A mRNA is increased by chronic social stress (Grunewald et al., 2012; Harris et al., 2015). This result also supports the advantage of the acute stress model in exploring the endogenous mechanism which downregulates the gene expression of MAO-A, which may lead to identification of novel approach to control MAO-A expression. Upregulation of MAO-A gene expression, protein levels and enzymatic activity during depression and chronic stress have been observed in previous studies (Filipenko et al., 2002a,b; Meyer et al., 2006, 2009; Chiuccariello et al., 2014; Sacher et al., 2015). Glucocorticoid is an activator of MAO-A gene expression (Ou et al., 2006). In this study, however, gene expression levels of MAO-A were decreased, while plasma cortisol levels were elevated by social stress, indicating that circulating cortisol is not a determinating factor for MAO-A gene expression during acute stress exposure in our social stress model. The discrepancy between these previous studies and this study might result from the difference in duration of stress. Social stress in this study increased plasma cortisol levels in relatively short period of time (2.5 or 5.0 h), compared to previous studies using chronic stress models (Filipenko et al., 2002a; Grunewald et al., 2012; Harris et al., 2015). The downregulation observed in this study might be a result of stress coping responses of the brain to get the increased 5-HT turnover back to normal levels. In addition to glucocorticoids, expression of MAO-A gene is regulated by transcription factors, such as Krüppel-like factor 11 (Grunewald et al., 2012) and R1 (Chen et al., 2005). Changes in these transcription factors were found in the post-mortem brains of patients with depression (Johnson et al., 2011; Harris et al., 2015). The role of these transcription factors in the acute phase of stress response needs to be investigated to explore the molecular mechanism which decreases MAO-A mRNA during acute stress.
MAO-A Enzymatic Activity and 5-HT Turnover in the Brain
In this study social stress upregulated 5-HT turnover in the brain. This result is consistent with previous studies which tested the influences of chronic social stress on 5-HT turnover (Beitia et al., 2005). However, MAO-A enzymatic activity did not show significant changes, suggesting that increased 5-HIAA levels and 5-HT turnover were not caused by changes in the production of MAO-A protein at the post-transcriptional levels. Enzymatic activity measured in our experiment reflects the total amount of MAO-A protein in the brain tissues. The gap between the increased 5-HT turnover and the unchanged MAO-A enzymatic activity might be explained by changes in the bioavailability of 5-HT (substrate) for MAO-A (enzyme) in the brain. 5-HT needs to be in MAO-A expressing cell to be degraded by MAO-A protein localized on the outer membrane of mitochondria. Several possible explanations can describe the elevated 5-HT turnover in our study. An increase in the release of 5-HT would explain the elevated 5-HIAA and 5-HIAA/5-HT ratios. If 5-HT release and reuptake are increased, the amount of cytosolic 5-HT would be increased; thus 5-HT turnover would also be increased. An increase in 5-HT release in response to stress is strongly supported by previous studies (Keeney et al., 2006; Mo et al., 2008; Mongeau et al., 2010). Reuptake transporters of monoamines, such as serotonin transporter (SERT), organic cation transporters (Gasser, 2006, Gasser et al., 2009; Bacq et al., 2012; Matsui et al., 2016), and plasma membrane monoamine transporter (Engel et al., 2004; Engel and Wang, 2005; Duan and Wang, 2010), may change the availability of 5-HT for MAO-A in the cells and thus affect 5-HT turnover in the brain. Dexamethasone (glucocorticoid receptor agonist) acutely enhances the cell surface localization of SERT in serotonergic neurons derived from mouse embryonic stem cells (Lau et al., 2013), supporting the possibility that SERT might increase the availability of 5-HT for MAO-A and thus increase 5-HT turnover during stress. It is also possible to hypothesize that a decrease in 5-HT recycling into synaptic vesicles could increase the supply of 5-HT to MAO-A, which can lead to an increase in 5-HT turnover. Finally, it is possible to postulate that increases in 5-HT synthesis and/or 5-HT receptors could increases the supply of 5-HT to MAO-A and enhance the 5-HT turnover (Figure 4).
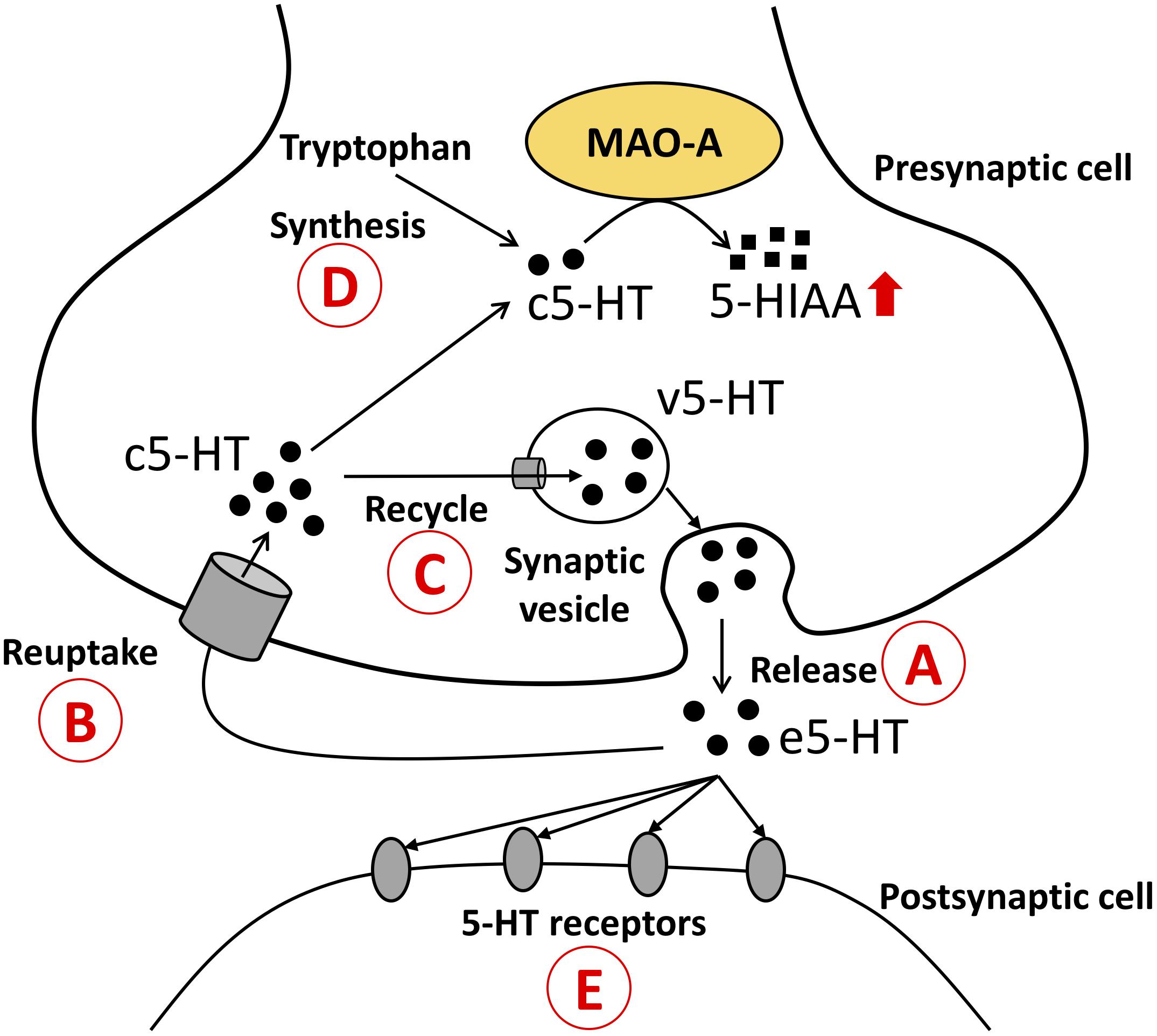
Figure 4. Possible mechanisms that explain the increased 5-HT turnover (5-HIAA/5-HT ratios). The amount of 5-HT available for MAO-A can be changed by release of 5-HT;
reuptake of 5-HT;
recycle of 5-HT into synaptic vesicles;
5-HT synthesis in the cells; and
binding of 5-HT with receptors. Possibility 1: Increases in 5-HT release
and 5-HT reuptake
lead to an increase in the supply of c5-HT to MAO-A; thus 5-HT turnover is increased. Possibility 2: Decreased 5-HT recycling into synaptic vesicles
leads to an increase in the supply of c5-HT to MAO-A; thus 5-HT turnover is increased. Possibility 3: Increased 5-HT synthesis
leads to an increase in c5-HT available for MAO-A; thus 5-HT turnover is increased. Possibility 4: Decreased 5-HT receptor
causes an increase in e5-HT, which can be taken up into 5-HT cells, and leads to an increase in the supply of c5-HT to MAO-A; thus 5-HT turnover is increased. 5-HT, 5-hydroxytryptamine; 5-HIAA, 5-hydroxyindoleacetic acid; c5-HT, cytosolic 5-HT; v5-HT, vesicular 5-HT; e5-HT, extracellular 5-HT.
A previous study which tested the impact of confinement stress on MAO in the brain of rainbow trout showed that MAO activity in the telencephalon is initially decreased after 1 h stress exposure but increased again back to normal levels after 3 h stress exposure (Schjolden et al., 2006), indicating that the response of the enzymatic activity can be different depending on the duration of stress. This previous study also reported increases in MAO activity in the optic tectum and hypothalamus, which is different from our results, suggesting that the impact of stress on MAO activity differs depending on the types of stressors or species. The increases in 5-HT turnover observed in this study was similar to those reported in Schjolden et al. (2006), suggesting that the increased 5-HT turnover is a common response to social defeat stress and confinement stress.
Distribution of MAO-A mRNA and Protein in the Brain
Our data suggest that MAO-A mRNA is mainly distributed in the brain areas including the midbrain, raphe, and hindbrain (Area II and III in Figure 2A) rather than the forebrain (Area I). However, 5-HT turnover was observed in the forebrain as well as other brain areas. In addition, brain area I and II showed higher MAO-A enzymatic activity, compared to area III. These results indicate that MAO-A protein is transported from the posterior regions to the telencephalon. MAO-A protein is localized on the outer membrane of mitochondria. Therefore, the localization of MAO-A is likely to be dependent on mitochondria, which can be transported from soma to terminal through axons (Saxton and Hollenbeck, 2012). Further study will be required to elucidate the mechanism by which the telencephalon recruits MAO-A from other brain regions. The teleost brain has three populations of 5-HT neurons: the raphe, hypothalamus, and pretectal populations (Lillesaar, 2011; Loveland et al., 2014). In zebrafish, MAO is localized mainly in serotonergic and noradrenergic neurons (Anichtchik et al., 2006). The raphe population of 5-HT neurons have projections to the forebrain (Gaspar and Lillesaar, 2012). Therefore, the high MAO-A enzymatic activity in the forebrain observed in this study can be dependent on MAO-A enzyme transported from the raphe to the forebrain region through the axons of the raphe population of serotonergic neurons.
In conclusion, this study found that acute social stress downregulates the expression of MAO-A at the transcriptional level and increases 5-HT metabolism. In addition, our data suggested the possibility of axonal transport of MAO-A enzyme. The acute social stress model using Nile tilapia would be useful to explore the mechanism underlying stress-induced changes in MAO-A transcription and 5-HT turnover rates.
Author Contributions
IP, TS, and YH designed the research. YH performed the experiments and wrote the manuscript. IP and TS edited the manuscript.
Funding
This work was supported by research grant from Monash University Malaysia and Fundamental Research Grant Scheme (FRGS), FRGS/2/2014/STO3/MUSM/02/01 (TS and IP) from the Ministry of Higher Education, Malaysia.
Conflict of Interest Statement
The authors declare that the research was conducted in the absence of any commercial or financial relationships that could be construed as a potential conflict of interest.
Acknowledgments
We would like to thank Ms. Nurziana Sharmilla Nawawi for her technical assistance. This study was conducted using the Drug Discovery platform of Brain Research Institute Monash Sunway, Monash University Malaysia. YH is the recipient of Graduate Research Merit Scholarship of Monash University Malaysia.
References
Anichtchik, O., Sallinen, V., Peitsaro, N., and Panula, P. (2006). Distinct structure and activity of monoamine oxidase in the brain of zebrafish (Danio rerio). J. Comp. Neurol. 498, 593–610. doi: 10.1002/cne.21057
Bacq, A., Balasse, L., Biala, G., Guiard, B., Gardier, A. M., Schinkel, A., et al. (2012). Organic cation transporter 2 controls brain norepinephrine and serotonin clearance and antidepressant response. Mol. Psychiatry 17, 926–939. doi: 10.1038/mp.2011.87
Barreto, R. E., and Volpato, G. L. (2006). Stress responses of the fish Nile tilapia subjected to electroshock and social stressors. Braz. J. Med. Biol. Res. 39, 1605–1612. doi: 10.1590/S0100-879X2006001200012
Beitia, G., Garmendia, L., Azpiroz, A., Vegas, O., Brain, P. F., and Arregi, A. (2005). Time-dependent behavioral, neurochemical, and immune consequences of repeated experiences of social defeat stress in male mice and the ameliorative effects of fluoxetine. Brain Behav. Immun. 19, 530–539. doi: 10.1016/j.bbi.2004.11.002
Carpenter, R. E., Maruska, K. P., Becker, L., and Fernald, R. D. (2014). Social opportunity rapidly regulates expression of CRF and CRF receptors in the brain during social ascent of a teleost fish, Astatotilapia burtoni. PLoS One 9:e96632. doi: 10.1371/journal.pone.0096632
Carroll, B. J., Iranmanesh, A., Keenan, D. M., Cassidy, F., Wilson, W. H., and Veldhuis, J. D. (2012). Pathophysiology of hypercortisolism in depression: pituitary and adrenal responses to low glucocorticoid feedback. Acta Psychiatr. Scand. 125, 478–491. doi: 10.1111/j.1600-0447.2011.01821.x
Chen, C.-C., and Fernald, R. D. (2011). Visual information alone changes behavior and physiology during social interactions in a cichlid fish (Astatotilapia burtoni). PLoS One 6:e20313. doi: 10.1371/journal.pone.0020313
Chen, K., Ou, X.-M., Chen, G., Choi, S. H., and Shih, J. C. (2005). R1, a novel repressor of the human monoamine oxidase A. J. Biol. Chem. 280, 11552–11559. doi: 10.1074/jbc.M410033200
Chiriţã, A. L., Gheorman, V., Bondari, D., and Rogoveanu, I. (2015). Current understanding of the neurobiology of major depressive disorder. Rom. J. Morphol. Embryol. 56, 651–658.
Chiuccariello, L., Houle, S., Miler, L., Cooke, R. G., Rusjan, P. M., Rajkowska, G., et al. (2014). Elevated monoamine oxidase a binding during major depressive episodes is associated with greater severity and reversed neurovegetative symptoms. Neuropsychopharmacology 39, 973–980. doi: 10.1038/npp.2013.297
Corrêa, S. A., Fernandes, M. O., Iseki, K. K., and Negrão, J. A. (2003). Effect of the establishment of dominance relationships on cortisol and other metabolic parameters in Nile tilapia (Oreochromis niloticus). Brazilian J. Med. Biol. Res. 36, 1725–1731. doi: 10.1590/S0100-879X2003001200015
Duan, H., and Wang, J. (2010). Selective transport of monoamine neurotransmitters by human plasma membrane monoamine transporter and organic cation transporter 3. J. Pharmacol. Exp. Ther. 335, 743–753. doi: 10.1124/jpet.110.170142
Edwards, K. M., Burns, V. E., Carroll, D., Drayson, M., and Ring, C. (2007). The acute stress-induced immunoenhancement hypothesis. Exerc. Sport Sci. Rev. 35, 150–155. doi: 10.1097/JES.0b013e3180a031bd
Engel, K., and Wang, J. (2005). Interaction of organic cations with a newly identified plasma membrane monoamine transporter. Mol. Pharmacol. 68, 1397–1407. doi: 10.1124/mol.105.016832
Engel, K., Zhou, M., and Wang, J. (2004). Identification and characterization of a novel monoamine transporter in the human brain. J. Biol. Chem. 279, 50042–50049. doi: 10.1074/jbc.M407913200
Fernandes, M. O., and Volpato, G. L. (1993). Heterogeneous growth in the Nile tilapia: social stress and carbohydrate metabolism. Physiol. Behav. 54, 319–323. doi: 10.1016/0031-9384(93)90117-X
Filipenko, M. L., Beilina, A. G., Alekseyenko, O. V., Dolgov, V. V., and Kudryavtseva, N. N. (2002a). Increase in expression of brain serotonin transporter and monoamine oxidase a genes induced by repeated experience of social defeats in male mice. Biochemistry 67, 451–455.
Filipenko, M. L., Beilina, A. G., Alekseyenko, O. V., Dolgov, V. V., and Kudryavtseva, N. N. (2002b). Repeated experience of social defeats increases serotonin transporter and monoamine oxidase A mRNA levels in raphe nuclei of male mice. Neurosci. Lett. 321, 25–28. doi: 10.1016/S0304-3940(01)02495-2498
Fitzgerald, J. C., Ugun-Klusek, A., Allen, G., De Girolamo, L. A., Hargreaves, I., Ufer, C., et al. (2014). Monoamine oxidase-A knockdown in human neuroblastoma cells reveals protection against mitochondrial toxins. FASEB J. 28, 218–229. doi: 10.1096/fj.13-235481
Gaspar, P., and Lillesaar, C. (2012). Probing the diversity of serotonin neurons. Philos. Trans. R. Soc. B Biol. Sci. 367, 2382–2394. doi: 10.1098/rstb.2011.0378
Gasser, P. J. (2006). Corticosterone-sensitive monoamine transport in the rat dorsomedial hypothalamus: potential role for organic cation transporter 3 in stress-induced modulation of monoaminergic neurotransmission. J. Neurosci. 26, 8758–8766. doi: 10.1523/JNEUROSCI.0570-06.2006
Gasser, P. J., Orchinik, M., Raju, I., and Lowry, C. A. (2009). Distribution of organic cation transporter 3, a corticosterone-sensitive monoamine transporter, in the rat brain. J. Comp. Neurol. 512, 529–555. doi: 10.1002/cne.21921
Grunewald, M., Johnson, S., Lu, D., Wang, Z., Lomberk, G., Albert, P. R., et al. (2012). Mechanistic role for a novel glucocorticoid-KLF11 (TIEG2) protein pathway in stress-induced monoamine oxidase A expression. J. Biol. Chem. 287, 24195–24206. doi: 10.1074/jbc.M112.373936
Harris, S., Johnson, S., Duncan, J. W., Udemgba, C., Meyer, J. H., Albert, P. R., et al. (2015). Evidence revealing deregulation of The KLF11-Mao A pathway in association with chronic stress and depressive disorders. Neuropsychopharmacology 40, 1373–1382. doi: 10.1038/npp.2014.321
Höglund, E., Balm, P. H., and Winberg, S. (2000). Skin darkening, a potential social signal in subordinate arctic charr (Salvelinus alpinus): the regulatory role of brain monoamines and pro-opiomelanocortin-derived peptides. J. Exp. Biol. 203, 1711–1721.
Johnson, S., Stockmeier, C. A., Meyer, J. H., Austin, M. C., Albert, P. R., Wang, J., et al. (2011). The reduction of R1, a novel repressor protein for monoamine oxidase A, in major depressive disorder. Neuropsychopharmacology 36, 2139–2148. doi: 10.1038/npp.2011.105
Keeney, A., Jessop, D. S., Harbuz, M. S., Marsden, C. A., Hogg, S., and Blackburn-Munro, R. E. (2006). Differential effects of acute and chronic social defeat stress on hypothalamic-pituitary-adrenal axis function and hippocampal serotonin release in mice. J. Neuroendocrinol. 18, 330–338. doi: 10.1111/j.1365-2826.2006.01422.x
Lau, T., Heimann, F., Bartsch, D., Schloss, P., and Weber, T. (2013). Nongenomic, glucocorticoid receptor-mediated regulation of serotonin transporter cell surface expression in embryonic stem cell derived serotonergic neurons. Neurosci. Lett. 554, 115–120. doi: 10.1016/j.neulet.2013.08.070
Lillesaar, C. (2011). The serotonergic system in fish. J. Chem. Neuroanat. 41, 294–308. doi: 10.1016/j.jchemneu.2011.05.009
Loveland, J. L., Uy, N., Maruska, K. P., Carpenter, R. E., and Fernald, R. D. (2014). Social status differences regulate the serotonergic system of a cichlid fish, Astatotilapia burtoni. J. Exp. Biol. 217, 2680–2690. doi: 10.1242/jeb.100685
Lucassen, P. J., Pruessner, J., Sousa, N., Almeida, O. F. X., Van Dam, A. M., Rajkowska, G., et al. (2014). Neuropathology of stress. Acta Neuropathol. 127, 109–135. doi: 10.1007/s00401-013-1223-1225
Matsui, T., Nakata, T., and Kobayashi, Y. (2016). Localization of organic cation transporter 2 (OCT2) in monoaminergic and cholinergic axon terminals of the mouse brain. Neurosci. Lett. 633, 118–124. doi: 10.1016/j.neulet.2016.09.025
Maurel, A., Hernandez, C., Kunduzova, O., Bompart, G., Cambon, C., Parini, A., et al. (2003). Age-dependent increase in hydrogen peroxide production by cardiac monoamine oxidase A in rats. Am. J. Physiol. Heart Circ. Physiol. 284, H1460–H1467. doi: 10.1152/ajpheart.00700.2002
McEwen, B. S. (2004). Protection and damage from acute and chronic stress: allostasis and allostatic overload and relevance to the pathophysiology of psychiatric disorders. Ann. N. Y. Acad. Sci. 1032, 1–7. doi: 10.1196/annals.1314.001
McEwen, B. S. (2008). Central effects of stress hormones in health and disease: understanding the protective and damaging effects of stress and stress mediators. Eur. J. Pharmacol. 583, 174–185. doi: 10.1016/j.ejphar.2007.11.071
Meyer, J. H., Ginovart, N., Boovariwala, A., Sagrati, S., Hussey, D., Garcia, A., et al. (2006). Elevated monoamine oxidase a levels in the brain. Arch. Gen. Psychiatry 63, 1209–1216. doi: 10.1001/archpsyc.63.11.1209
Meyer, J. H., Wilson, A. A., Sagrati, S., Miler, L., Rusjan, P., Bloomfield, P. M., et al. (2009). Brain monoamine oxidase a binding in major depressive disorder. Arch. Gen. Psychiatry 66, 1304–1312. doi: 10.1001/archgenpsychiatry.2009.156
Mo, B., Feng, N., Renner, K., and Forster, G. (2008). Restraint stress increases serotonin release in the central nucleus of the amygdala via activation of corticotropin-releasing factor receptors. Brain Res. Bull. 76, 493–498. doi: 10.1016/j.brainresbull.2008.02.011
Moltesen, M., Laursen, D. C., Thörnqvist, P.-O., Andersson, M. Å., Winberg, S., and Höglund, E. (2016). Effects of acute and chronic stress on telencephalic neurochemistry and gene expression in rainbow trout ( Oncorhynchus mykiss ). J. Exp. Biol. 219, 3907–3914. doi: 10.1242/jeb.139857
Mongeau, R., Martin, C. B. P., Chevarin, C., Maldonado, R., Hamon, M., Robledo, P., et al. (2010). 5-HT2C receptor activation prevents stress-induced enhancement of brain 5-HT turnover and extracellular levels in the mouse brain: modulation by chronic paroxetine treatment. J. Neurochem. 115, 438–449. doi: 10.1111/j.1471-4159.2010.06932.x
Musazzi, L., Tornese, P., Sala, N., and Popoli, M. (2017). Acute or chronic? A stressful question. Trends Neurosci. 40, 525–535. doi: 10.1016/j.tins.2017.07.002
Nardocci, G., Navarro, C., Cortés, P. P., Imarai, M., Montoya, M., Valenzuela, B., et al. (2014). Neuroendocrine mechanisms for immune system regulation during stress in fish. Fish Shellfish Immunol. 40, 531–538. doi: 10.1016/j.fsi.2014.08.001
Ou, X.-M., Chen, K., and Shih, J. C. (2006). Glucocorticoid and androgen activation of monoamine oxidase A is regulated differently by R1 and Sp1. J. Biol. Chem. 281, 21512–21525. doi: 10.1074/jbc.M600250200
Øverli,Ø., Harris, C. A., and Winberg, S. (1999). Short-term effects of fights for social dominance and the establishment of dominant-subordinate relationships on brain monoamines and cortisol in rainbow trout. Brain Behav. Evol. 54, 263–275. doi: 10.1159/000006627
Øverli,Ø., Korzan, W. J., Höglund, E., Winberg, S., Bollig, H., Watt, M., et al. (2004). Stress coping style predicts aggression and social dominance in rainbow trout. Horm. Behav. 45, 235–241. doi: 10.1016/j.yhbeh.2003.12.002
Sacher, J., Rekkas, P. V., Wilson, A. A., Houle, S., Romano, L., Hamidi, J., et al. (2015). Relationship of monoamine oxidase-A distribution volume to postpartum depression and postpartum crying. Neuropsychopharmacology 40, 429–435. doi: 10.1038/npp.2014.190
Saxton, W. M., and Hollenbeck, P. J. (2012). The axonal transport of mitochondria. J. Cell Sci. 125, 2095–2104. doi: 10.1242/jcs.053850
Schjolden, J., Pulman, K. G. T., Pottinger, T. G., Tottmar, O., and Winberg, S. (2006). Serotonergic characteristics of rainbow trout divergent in stress responsiveness. Physiol. Behav. 87, 938–947. doi: 10.1016/j.physbeh.2006.02.009
Sinyakov, M. S., Haimovich, A., and Avtalion, R. R. (2017). Acute stress promotes post-injury brain regeneration in fish. Brain Res. 1676, 28–37. doi: 10.1016/j.brainres.2017.09.009
Thang, N. Q., Phuong, N. T. K., and Van Tan, L. (2016). Endocrine stress response in Oreochromis sp. from exposure to waterborne cadmium: the plasma cortisol analysis. Toxicol. Environ. Chem. 2248, 1–9. doi: 10.1080/02772248.2016.1172583
Vera Cruz, E. M., and Brown, C. L. (2007). The influence of social status on the rate of growth, eye color pattern and insulin-like growth factor-I gene expression in Nile tilapia, Oreochromis niloticus. Horm. Behav. 51, 611–619. doi: 10.1016/j.yhbeh.2007.02.010
Volpato, G. L., Luchiari, A. C., Duarte, C. R. A., Barreto, R. E., and Ramanzini, G. C. (2003). Eye color as an indicator of social rank in the fish Nile tilapia. Braz. J. Med. Biol. Res. 36, 1659–1663. doi: 10.1590/S0100-879X2003001200007
Keywords: stress, serotonin, 5-HIAA, MAO, depression, tilapia, neurotransmitter
Citation: Higuchi Y, Soga T and Parhar IS (2019) Social Defeat Stress Decreases mRNA for Monoamine Oxidase A and Increases 5-HT Turnover in the Brain of Male Nile Tilapia (Oreochromis niloticus). Front. Pharmacol. 9:1549. doi: 10.3389/fphar.2018.01549
Received: 28 August 2018; Accepted: 19 December 2018;
Published: 09 January 2019.
Edited by:
Luis F. Callado, Universidad del País Vasco, SpainReviewed by:
Svante Winberg, Uppsala University, SwedenAllyson K. Friedman, The City University of New York, United States
Copyright © 2019 Higuchi, Soga and Parhar. This is an open-access article distributed under the terms of the Creative Commons Attribution License (CC BY). The use, distribution or reproduction in other forums is permitted, provided the original author(s) and the copyright owner(s) are credited and that the original publication in this journal is cited, in accordance with accepted academic practice. No use, distribution or reproduction is permitted which does not comply with these terms.
*Correspondence: Tomoko Soga, dG9tb2tvLnNvZ2FAbW9uYXNoLmVkdQ==