- 1Faculty of Engineering and Natural Sciences, Sabanci University, Orta Mahalle, Istanbul, Turkey
- 2Sabanci University Nanotechnology Research and Application Center (SUNUM), Istanbul, Turkey
- 3Department of Food Hygiene and Quality Control, Faculty of Veterinary Medicine, University of Tehran, Tehran, Iran
- 4Laboratory for Stem Cell Research, Shiraz University of Medical Sciences, Shiraz, Iran
- 5Department of Anatomical Sciences, School of Medicine, Student Research Committee, Shiraz University of Medical Sciences, Shiraz, Iran
- 6Islamic Azad University, Kazeroon, Iran
- 7Centre for Micro-BioRobotics, Istituto Italiano di Tecnologia, Pisa, Italy
- 8Department of Basic Medical Sciences, Neyshabur University of Medical Sciences, Neyshabur, Iran
- 9Department of Pharmacy, Abdul Wali Khan University, Mardan, Pakistan
- 10Student Research Committee, Department of Physiotherapy, Faculty of Rehabilitation, Ahvaz Jundishapur University of Medical Sciences, Ahvaz, Iran
- 11Medical Technology Research Center, Kermanshah University of Medical Sciences, Kermanshah, Iran
- 12Radiology and Nuclear Medicine Department, School of Paramedical Sciences, Kermanshah University of Medical Sciences, Kermanshah, Iran
- 13Research Center for Biochemistry and Nutrition in Metabolic Diseases, Institute for Basic Sciences, Kashan University of Medical Sciences, Kashan, Iran
Immune response, proliferation, migration and angiogenesis are juts a few of cellular events that are regulated by transforming growth factor-β (TGF-β) in cells. A number of studies have documented that TGF-β undergoes abnormal expression in different diseases, e.g., diabetes, cancer, fibrosis, asthma, arthritis, among others. This has led to great fascination into this signaling pathway and developing agents with modulatory impact on TGF-β. Curcumin, a natural-based compound, is obtained from rhizome and roots of turmeric plant. It has a number of pharmacological activities including antioxidant, anti-inflammatory, anti-tumor, anti-diabetes and so on. Noteworthy, it has been demonstrated that curcumin affects different molecular signaling pathways such as Wnt/β-catenin, Nrf2, AMPK, mitogen-activated protein kinase and so on. In the present review, we evaluate the potential of curcumin in regulation of TGF-β signaling pathway to corelate it with therapeutic impacts of curcumin. By modulation of TGF-β (both upregulation and down-regulation), curcumin ameliorates fibrosis, neurological disorders, liver disease, diabetes and asthma. Besides, curcumin targets TGF-β signaling pathway which is capable of suppressing proliferation of tumor cells and invading cancer cells.
Introduction
Thanks to the previously conducted research over past decades to assist scientists in comprehensive understanding of molecular signaling pathways and mechanisms, and how to deal with them in different diseases and disorders (Farooqi et al., 2020). Interdisciplinary research and the emergence of cutting-edge technologies have made it possible to look for major signaling pathways and their regulation (Farooqi et al., 2019a). There has been an explosion in the field of molecular biology and recently published articles have also confirmed this fact (Farooqi et al., 2019b; Fayyaz et al., 2019). Along with identification of molecular pathways, scientists have tried to develop synthetic drugs in their regulation. It is worth mentioning that plus to synthetic drugs, there has been a great trend toward plant derived-natural compounds in the regulation of molecular pathways and mechanisms (Najafi et al., 2019b; Mortezaee et al., 2020). Nowadays, researchers are more interested in naturally occurring compounds compared to synthetic therapeutics. This emanates from the fact that synthetic drugs are designed for just a purpose (for instance, treatment of a particular disease) and targeting a certain pathway and mechanism, while a large number of studies have revealed that naturally-occuring compounds are capable of affecting a wide variety of molecular signaling pathways (Guo et al., 2019; Mortezaee et al., 2019a; Mortezaee et al., 2019b; Mortezaee et al., 2019c). This multi-targeting nature of herbal compounds have attracted much fascination. In addition to multi-targeting property, it has been demonstrated that herbal compounds have minimal toxicity or even lack toxicity against normal cells (Farhood et al., 2019a; Farhood et al., 2019b), whereas synthetic drugs negatively affect organs of body. For instance, such story is obviously observed in chemotherapy. It seems that synthetic drugs applied in chemotherapy have a number of adverse effects against normal cells and may induce renotoxicity, hepatotoxicity and so on, while plant derived-natural compounds can be used as potential chemotherapeutic agents with negligible side effects (Najafi et al., 2019a). All of these statements advocate from the fact that herbal compounds are efficient agents in treatment of diseases and they can be applied to target various molecular pathways. In the current mechanistic review, we specifically discuss the potential of curcumin in targeting transforming growth factor-β (TGF-β) in disease therapy to direct further studies for research in this field.
Curcumin: An Overview of the Pharmacological Impacts and Limitation
Curcumin, a phenolic compound, is also known as diferuloylmethane with chemical name of (1E, 6E)-1,7-bis(4-hydroxy-3-methoxyphenyl)hepta-1,6-diene-3,5-dione) (Figure 1) (Baldi et al., 2020; Chainoglou and Hadjipavlou-Litina, 2020; Salehi et al., 2020a; Stohs et al., 2020). This biologically active compound occurs in high amounts in rhizome and roots of turmeric plant (Curcuma longa) (Hesari et al., 2019). Apart from curcumin, there are also two other curcuminoids in this plant including demethoxycurcumin (DMC) and bis-demethoxycurcumin (BDMC). It has been demonstrated that curcuminoids comprise 2–4% of dry turmeric root powder (Ak and Gülçin, 2008; Prasad et al., 2014; Amalraj et al., 2017; Kocaadam and Şanlier, 2017; Kunnumakkara et al., 2017; Rahmani et al., 2018; Yeung et al., 2019). Curcumin has a yellow color and can be used in several applications, e.g., as a food flavoring and coloring agent, and herbal nutrition supplement (Aggarwal et al., 2006; Aggarwal et al., 2007). Curcumin was isolated for the first time at the impure form in 1815, but Lampe and colleagues characterized curcumin in 1910 in term of structure and chemically synthesized it (Lampe and Milobedzka, 1913; Gupta et al., 2012; Mehanny et al., 2016). As a bis-α,β-unsaturated β-diketone, curcumin exhibits keto-enol tautomerism. The enol form of curcumin is widely found in alkaline solutions, while its keto form is prevalent in acidic and natural pH (Sharma et al., 2005). It is worth mentioning that curcumin is used in food, pharmaceutical and textile industries (Aggarwal and Harikumar, 2009). It seems that curcumin has been common for treatment of diseases in Asia, particularly traditional Indian medicine and this returns back to 2,500 years ago (Unlu et al., 2016). The curcumin has been considered as an efficient agent in treatment of different ailments such as infection therapy in eye and skin diseases, rheumatism, dyspepsia, and irritable bowel disease, amonng others (Singh, 2007; Hatcher et al., 2008; Qin et al., 2009; Lucariello et al., 2015; Zhou et al., 2017a; Perna et al., 2018; Esposito et al., 2019; Hay et al., 2019).
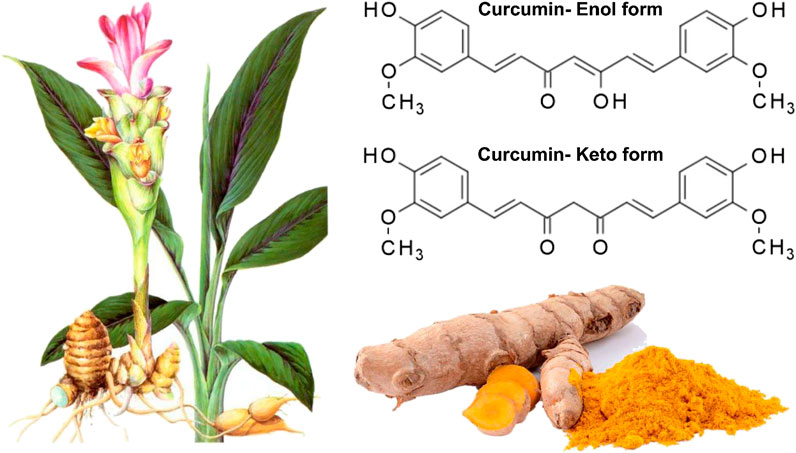
FIGURE 1. The Structure of curcumin in which its enolic exsits organic solvents whereas its keto form presence in aquoues mwedia (Zheng et al., 2018). Reprinted with permission from the publishers.
The first experiment related to therapeutic impact of curcumin was published in 1937 (Zhou et al., 2011), and since then, much attention has been directed toward revealing the extraordinary pharmacological activities of curcumin. It has been reported that curcumin has valuable therapeutic and biological activities such as antioxidant (Kharat et al., 2020), anti-inflammatory (Sneharani, 2019), anti-diabetic (Xia et al., 2020), hepatoprotective (Dogaru et al., 2020), cardioprotective (Hadi et al., 2019; Hallajzadeh et al., 2019; Kuszewski et al., 2019), neuroprotective (Sturzu et al., 2019), anti-microbial (Rai et al., 2020), anti-tumor (Bahrami et al., 2019; Shabaninejad et al., 2020; Weng and Goel, 2020) and improving dyslipidemia (Roxo et al., 2019) and ischemic-reperfusion (Ahmed et al., 2019). Curcumin possesses great solubility in oil-based solutions. Besides, being insoluble in water at acidic and neutral pH, curcumin is soluble at alkaline pH. As mentioned earlier, in spite of excellent therapeutic activities, a variety of issues have remarkably restricted the effectiveness of curcumin. The most important hurdle is its poor solubility in aqoues media (11 ng/ml) as well as its rapid metabolism into an inactive metabolite (Valencia et al., 2019; Moeini et al., 2020). . In light of this, a number of research have been devoted on the solubility enhancements or encapsulation of curcumin targeted drug delivery platforms for biomedical applications (Deljoo et al., 2019; Zare et al., 2019a; Zare et al., 2019b). For instance, a number of nanoscale carriers (e.g., micelles, liposomes, polymeric nanocarriers, lipid nanoparticles, and carbon nanotubes) have been developed to encapsulate hydrophobic active compounds such as curcumin (Bian and Guo, 2020; Li et al., 2020b; Hu et al., 2020; Varshosaz et al., 2020; Zhao et al., 2020). Such platforms enhanced the therapeutic efficacy of curcumin along with prolonged delivery. In the following section, the influence of curcumin on molecular pathways in exerting its pharmacological activities is highlighted.
Curcumin and Molecular Pathways and Mechanisms
Notably, curcumin is suggested to affect various molecular signaling pathways and mechanisms (Ghasemi et al., 2019; Bagherian et al., 2020; Mardani et al., 2020; Salehi et al., 2020b). Until now, no naturally occurring compound has been investigated similar to curcumin. Herein, the potential therapeutic impacts of curcumin mediated by its effect on molecular pathways and mechanisms are discussed. The nuclear factor erythroid 2-related factor 2 (Nrf2) is well-known due to its capability in improving antioxidant defense system via targeting down-stream mediators including heme oxygenease-1 (HO-1), superoxide dismutase (SOD) and NADPH quinone reductase 1 (NQO1) (Song et al., 2020). It is said that antioxidant activity of curcumin is mainly mediated by stimulation of Nrf2 signaling pathway (Zhang et al., 2020a). Multiple studies have investigated the potential of curcumin in diabetes mellitus (DM) treatment, as a chronic metabolic disorder (Funamoto et al., 2019). Mechanistically, curcumin improves insulin resistance and dyslipidemia, and remarkably diminishes levels of glucose via upregulation of GLUT1 and GLUT4 (Al-Saud, 2020). The calcified aortic valve disease (CAVD) is a primary valve disease that negatively affects a high number of people worldwide (Kirchhof et al., 2016). A variety of factors are involved in CAVD development, but it appears that inflammatory factors play a pivotal role (Lee and Choi, 2018). The nuclear factor-kappaB (NF-κB) is suggested to induce inflammation (Sinjari et al., 2019). The administration of curcumin effectively suppresses the progression and development of CAVD via down-regulation of NF-κB and inhibiting its nuclear translocation (Zhou et al., 2020). Two factors are vital in amelioration of damages on cells and improving a disease that include reducing stress and inhibition of apoptotic cell death. In attenuation of diabetic nephropathy, curcumin diminishes apoptosis via down-regulation of pro-apoptotic factors Bax and caspase-3, while it induces autophagy through upregulation of Beclin-1 and ATG5, resulting in reduced cell stress (Zhang et al., 2020c). It is worth mentioning that curcumin induces apoptotic- and autophagic-cell death in cancer therapy. However, it is held that autophagy can determine the number of cancer cells undergoing apoptosis, so that cancer cells with high autophagy influx demonstrate a relative resistance into apoptosis (Lee et al., 2020a). Apoptosis can be triggered by endoplasmic reticulum (ER) stress in which glucose-regulated protein 78 (GRP78), CCAAT-enhancer-binding protein homologous protein (CHOP) and activating transcription factor 4 (ATF4) are induced by unfold protein response (UPR) to ameliorate ER stress by stimulation of apoptosis (Di Conza and Ho, 2020). Curcumin stimulates neuroprotective effects by down-regulation of GRP78 and ATF4 to attenuate ER stress-mediated apoptosis in neuronal cells (Keshk et al., 2020). Noteworthy, curcumin-mediated Notch upregulation protects neuronal cells against cytotoxic agents such as bisphenol A (BPA) (Tandon et al., 2020). Taking everything into account, based on the recently published articles, it can be said that curcumin is a potential naturally occurring compound in treatment of various disorders and diseases. This is due to capability of curcumin in affecting a variety of molecular signaling pathways and mechanisms that are discussed in this section (Liczbiński et al., 2020; Mohajeri et al., 2020; Sharifi et al., 2020).
Transforming Growth Factor-Beta Signaling Pathway: From Basics to Role in Pathological Events
The TGF-β is a dynamic and sophisticated molecular signaling pathway with pleiotropic impacts that modulate various biological mechanisms such as cell proliferation, cell differentiation, angiogenesis, motility, invasion, and immune response (Bai et al., 2019; Boguslawska et al., 2019; Finnson et al., 2019; Soleimani et al., 2019; Lai et al., 2020; Li and Wu, 2020; Lin and Wu, 2020; Tzavlaki and Moustakas, 2020). The TGF-β family possesses 33 genes that are capable of encoding homodimeric or heterodimeric secreted cytokines (Heldin and Moustakas, 2016; Derynck and Budi, 2019). Then, these proteins are cleaved via secretory pathway to produce mature dimeric ligands (Ten Dijke and Arthur, 2007; Heldin and Moustakas, 2016). The ribosomes present on the rough ER participate in synthesis of TGF-β and then, other processes including eliminating N-terminal signal peptide, protein folding and glycosylation occur in their route of ER into Golgi apparatus (Manning et al., 2002; Ten Dijke and Arthur, 2007). The TGF-β protein folding relies on formation of intermolecular disulfide bonds in N- and C-terminal region (Ten Dijke and Arthur, 2007). The glycosidation of N-terminal segment of TGF-β leads to the inactivation of TGF-β (Miyazono and Heldin, 1989), showing that further process is needed to activate TGF-β. Next, proteolytic cleavage of disulfide bonds by furin family proteins result in generation of two characteristic proteins including N-terminal long dimeric and disulfide-linked propeptide, known as latency-associated peptide (LAP) and C-terminal short dimeric disulfide-linked polypeptide, known as mature TGF-β (Ten Dijke and Arthur, 2007; Derynck and Budi, 2019). The signaling pathway of TGF-β is of interest and includes canonical and non-canonical pathways.
Canonical Pathway
The transmembrane serine/threonine kinases such as TGF-β type II (TβRII) and type I (ALK5) are involved in canonical pathway of TGF-β signaling (Wrighton et al., 2009; Liu and Feng, 2010). The TGF-β induces phosphorylation of ALK5 by binding into TβRII (Massagué, 1998; Wrighton et al., 2009; Ahmadi et al., 2019). Then, Smad cascade is activated, so that ALK5 stimulates phosphorylation of Smad2 and Smad3 proteins to form a complex with Smad4. This complex translocates and accumulates in nucleus to regulate gene expression by cooperation with co-activators such as CBP/p300 and co-repressors such as tumor growth-interacting factor (TGIF), Ski and SnoN (Schmierer and Hill, 2007; Hill, 2009). Notably, there are a number of Smads, known as inhibitor Smads (I-Smads) that include Smad6 and Smad7. They are able to inhibit TGF-β signaling pathway by acting as an antagonist. The Smad6 is suggested to suppress Smad1 by competing with Smad4 for binding into phosphorylated Smad1 (Hata et al., 1998). The Smad7 forms a negative feedback loop with TGF-β signaling (Yan et al., 2009). The Smad7 suppresses TGF-β signaling by competing with Smad2/3 for binding into ALK5 (Yan et al., 2009).
Non-Canonical Pathway
The most important axis in non-canonical pathway of TGF-β is ALK1-Smad1/5. In fact, ALK1 as a TGF-β type I receptor, plays a key role in non-canonical pathway of TGF-β and is expressed in chondrocytes, endothelial cells and so on. Upon TGF-β attachment, ALK1 is induced to form a complex with ALK5, resulting in Smad1/5 activation (Finnson et al., 2008; Finnson et al., 2010; Pardali et al., 2010; van der Kraan et al., 2010; Farhood et al., 2020), and suppressing ALK5-Smad2/3 signaling (Finnson et al., 2008). The mitogen-activated protein kinase (MAPK), phosphatidylinositol 3-kinase (PI3K) and Rho-like GTPase contribute to non-canonical pathway of TGF-β (Figure 2) (Derynck and Zhang, 2003; Moustakas and Heldin, 2005; Zhang, 2009).
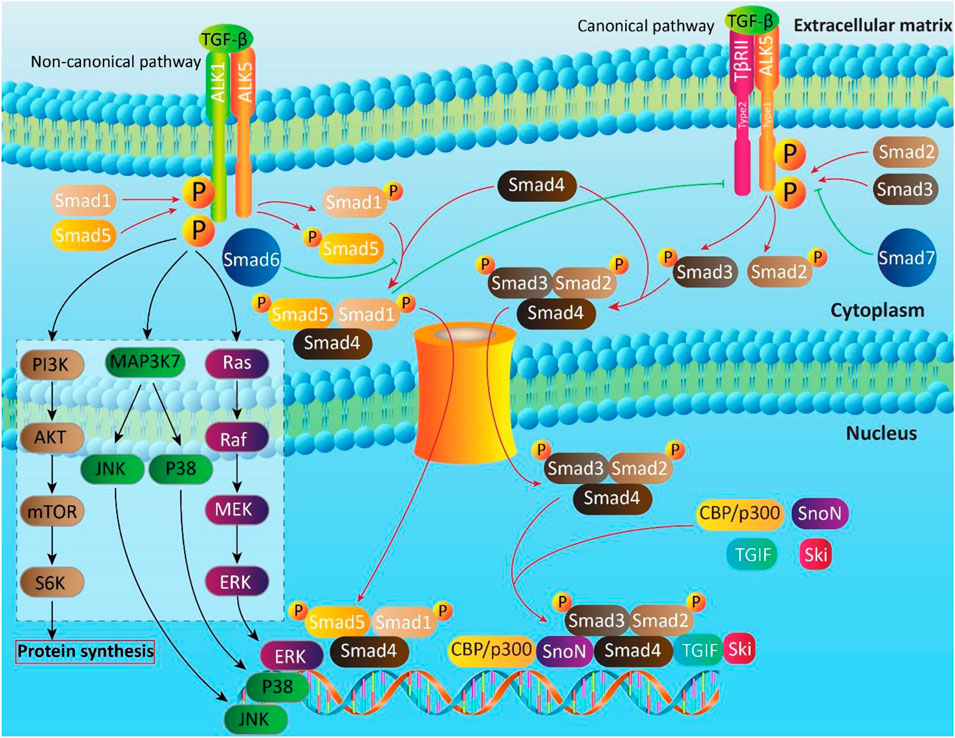
FIGURE 2. The canonical and non-canonical pathways of TGF-β signaling. Smads are key executers in TGF-b signaling that regulate expression of target genes by translocating into nucleus. In non-canonical pathway, in addition to Smads, other moleculra pathways such as PI3K, MAPK and Ras are involved.
The Role of Transforming Growth Factor-Beta in Pathological Events
Multiple studies have evaluated the role of TGF-β signaling pathway in different malignancies and disorders. It is not possible to comprehensively discuss the role of TGF-β signaling in diseases in this article (limitation of space) and we cite some of the great reviews for further information (Fionda et al., 2020; Munoz et al., 2020; Regis et al., 2020). However, we briefly describe the role of TGF-β signaling pathway in pathological events to pave the road for discussing the relationship between curcumin and TGF-β in different diseases.
Based on the recently published articles, it seems that enhanced activity of TGF-β predisposes muscle into damage. The TGF-β elevates the levels of fibroadipogenic progenitors (FAPs) to induce fibro-calcification of muscle, resulting in muscle degeneration and inhibition of regenerative myogenesis (Mazala et al., 2020). In respect to the role of TGF-β in degeneration, studies have focused on regulation of TGF-β signaling in disease therapy. It appears that administration of ginsenoside Rg1 remarkably diminishes airway collagen volume fraction, decreases the levels of inflammatory cytokines, and ameliorates pulmonary fraction. The examination of molecular pathways demonstrates that down-regulation of TGF-β1/Smad3 axis mediates antifibrotic impact of this compound (Guan et al., 2020). In fact, these studies confirm the pro-inflammatory role of TGF-β, and its association with organ damage. Compounds similar to ginsenoside Rg1 with inhibitory effects on TGF-β can be beneficial in preventing TGF-β-mediated organ damage. As pulmonary fibrosis is going to be an increasing concern, researchers have focused on finding both pharmacological and genetic interventions for this disorder. It is suggested that tripartite motif-containing 33 (TRIM33) alleviates pulmonary fibrosis via inhibition of TGF-β signaling pathway (Boutanquoi et al., 2020). This study demonstrates that upstream molecular pathways of TGF-β can be targeted in disease therapy.
As it was mentioned earlier, DM is a chronic metabolic disorder with high incidence rate, demanding novel strategies in its treatment and management. The β-cell dysfunction is a risk factor of DM. So, protection of β-cells is of considerable importance in DM therapy. It has been reported that TGF-β/Smad3 contributes to apoptotic cell death in β-cells, leading to their dysfunction and glucose tolerance (Lee et al., 2020b). Although upstream mediators are able to affect TGF-β in pathological events, increasing evidence exhibits that TGF-β signaling pathway can induce fibrosis and tracheal stenosis by stimulation of down-stream fibrotic mediators PI3K/Akt (Xiao et al., 2020). By inhibition of TGF-β, a decrease occurs in levels of inflammatory factors such as ILs and TNF-α to attenuate inflammatory diseases (Liu et al., 2020b). The TGF-β induces impairments in airway via enhancing cell migration and extracellular matrix (ECM) production, and by inhibition of TGF-β, the aforementioned mechanisms undergo down-regulation (Kim et al., 2020). It is worth mentioning that microRNAs (miRs) can function as upstream regulators of TGF-β. In stimulation of cardiac fibrosis, miR-21 activates TGF-β/Smad3 axis, while it decreases expression of Smad7, as an I-Smad (Yang et al., 2020).
The epithelial-to-mesenchymal transition (EMT) is a vital mechanism for inflammation, metastasis of cancer cells and fibrosis (Gui et al., 2020; Lee et al., 2020d). The TGF-β is able to stimulate EMT via upregulation of Smad2/3 (Fang et al., 2020). So, suppressing TGF-β can ameliorate inflammation and fibrosis in lung epithelial cells. It has been reported that TGF-β alleviates development of ovarian hyperstimulation syndrome via VEGF overexpression (Wan et al., 2020). These studies obviously demonstrate the role of TGF-β in diseases. Noteworthy, TGF-β plays a significant role in cancer progression. In enhancing migration and metastasis of cancer cells, TGF-β induces EMT through Smad4 upregulation (Xiong et al., 2020). Upstream oncogenic factors such as HOXD9 stimulate expression of TGF-β, leading to enhanced proliferation and growth of tumor cells (Wardhani et al., 2020). Notably, TGF-β overexpression induces resistance of cancer cells into chemotherapy (Qin et al., 2020). Consequently, studies have focused on inhibition of TGF-β signaling pathway in cancer therapy. It is held that down-regulation of TGF-β sensitizes cancer cells into anti-tumor immunity and remarkably diminishes their growth and proliferation (Horn et al., 2020).
These studies highlight the ponteitla contribution of TGF-β signaling in disease development. Notworthy, a variety of down-stream and upstream mediators of TGF-β exist that mediate its role in pathological events. Even molecular mechanisms are down-stream targets of TGF-β. For instance, DM treatment and preventing apoptosis in β-cells are performed via down-regulation of TGF-β. In fact, TGF-β induces Smad3 to trigger apoptosis in β-cells, providing condition for DM emergence (Lee et al., 2020c). Autophagy is another type of programmed cell death that can be affected by TGF-β. This molecular pathway is able to dually down-regulate/upregulate autophagy in normal and cancerous cells (Mao et al., 2019; Jin et al., 2020). So, revealing interaction between TGF-β and autophagy can be of importance for developing therapeutics. The interesting point is that TGF-β can affect various molecular pathways in disease development including mTOR (Chen et al., 2020c), PI3K/Akt (Wang et al., 2020a), Wnt (Liu et al., 2019), and STAT3 (Dees et al., 2020). There are also molecular pathways that can function as upstream mediators of TGF-β in pathological events. MiRs (Ge et al., 2019), lncRNAs (Tang et al., 2019), circRNAs (Bai et al., 2020), TRPM2 (Wang et al., 2019b) and so on can regulate TGF-β signaling in different disorders. Pharmacological or genetic interventions of aforementioned signaling networks can pave the road into effective treatment of diseases.
Most of the experiments are in line with pro-inflammatory role of TGF-β in diseases. This pro-inflammatory role is in favor of disease development and progression. So, down-regulation of TGF-β is advantageous in suppressing inflammation-mediated disease development. For instance, TGF-β induces inflammation to enhance progression and aggressive behavior of hepatocellular carcinoma cells. Expression of TGF-β is positively affected by Dickkopf-1 (DKK1) (Fezza et al., 2019). Hence, inhibiting DKK1/TGF-β axis can lead to preventing inflammation-mediated cancer growth. ILs with anti-inflammatory roles down-regulate expression of TGF-β in suppressing inflammation. IL-22 is able to inhibit TGF-β signaling via Notch1 inhibition, leading to a decrease in inflammation and renal fibrosis (Tang et al., 2020). Although these studies demonstrate pro-inflammatory role of TGF-β, it appears that TGF-β also possesses anti-inflammatory roles. Theacrine, as an anti-inflammatory compound, prevents synovial hyperplasia and inflammatory cell infiltration in joint tissues. This anti-inflammatory effect is mediated via TGF-β induction and subsequent upregulation of Smad expression (Gao et al., 2020b). In the next sections, we investigate relationship between curcumin and TGF-β in different diseases.
Curcumin and Transforming Growth Factor-Beta in Different Diseases
Liver Diseases
The incidence of liver diseases has undergone an increase due to lifestyle. They are commonly occur in patients who suffer from obesity and alcohol abuse (Buonomo et al., 2019). The liver fibrosis is a chronic liver disease in which extracellular proteins such as collagen accumulate. In diseased liver cells, a number of cells including hepatic stellate cells, portal fibroblasts and myofibroblasts produce collagen. It is said that TGF-β1 is involved in stimulation of aforementioned cells (Komolkriengkrai et al., 2019). In rats exposed to carbon tetrachloride (CCl4), the chronic liver fibrosis occurs due to enhanced expression of TGF-β1. TGF-β is one of the signaling pathways that is down-regulated by curcumin in alleviation of liver fibrosis (Abo-Zaid et al., 2020). Liver dysfunction is a common phenomenon during DM. It is worth mentioning that TGF-β/Smad signaling pathway can lead to liver injury and inflammation in DM, and its inhibition attenuates liver damage (Zhang et al., 2019a). Incorporation of curcumin into polymeric nanoparticles significantly enhances its bioavailability and therapeutic impacts, leading to amelioration of DM-mediated liver injury by inhibition of TGF-β1 (El-Naggar et al., 2019). The paraquat is a common herbicide commonly applied in different countries. The reports display that 5–15 ml of 20% concentration of paraquat can result in moderate or severe poisoning (Wesseling et al., 2001; Baltazar et al., 2013). Several studies have shown that exposing to paraquat can induce liver injury by decreasing antioxidant capacity and stimulation of inflammation (Gao et al., 2020a; Liu et al., 2020c). Although studies have put much emphasis on the involvement of oxidative stress and inflammation in paraquat-mediated toxicity, the role of TGF-β signaling pathway is uncertain in liver toxicity. A recently published article has shown that in stimulation of liver toxicity, paraquat enhances expression of TGF-β1. The curcumin supplementation attenuates paraquat-mediated liver injury via down-regulation of TGF-β1 signaling pathway (Chen and Fu, 2018).
Cancer
It has been reported that in addition to epithelial cells, tumor microenvironment (TME) and tumor cell interaction plays a pivotal role in cancer progression (Jung and Le, 2018). The cancer associated fibroblasts (CAFs) are able to induce chemoresistance and ensure cancer progression (Houthuijzen and Jonkers, 2018; Liao et al., 2018). The different factors such as TGF-β, matrix metalloproteinases (MMPs) and so on contribute to cancer initiation via activation of CAFs (Shiga et al., 2015). The in vitro and in vivo experiments exhibit that curcumin administration is associated with inhibition of CAF-mediated cancer progression via TGF-β1 down-regulation (Jamalzaei et al., 2020). Cancer cells have higher proliferation and migration compared to normal cells. One of the factors involved in motility and metastasis of cancer cells is EMT. A number of structural and molecular alterations occurs during EMT to produce mesenchymal cells from polarized endothelial cells. In contrast to polarized and static epithelial cells, mesenchymal cells have spindle shape and are not polarized, leading to their migration capability (Lu et al., 2020; Xu et al., 2020). The TGF-β is able to enhance invasion and migration of cancer cells via stimulation of EMT (Li et al., 2019). Exposing lung cancer cells into paraquat (PQ) significantly increases their migration and invasion through TGF-β-induced EMT. The administration of curcumin inhibits TGF-β signaling to suppress EMT, leading to preserving E-cadherin and reducing cancer malignancy (Tyagi et al., 2019). Oxaliplatin (OX) is a chemotherapeutic agent for eliminating cancer cells and enhancing overall survival of patients with cancer (Li et al., 2020a). However, resistance of cancer cells has limited its efficacy. EMT is a potential factor in stimulation of chemoresistance via elevating proliferation and invasion of cancer cells (Cao et al., 2020). The TGF-β can induce EMT and its inhibition by miR-145 suppresses malignant behavior of cancer cells (Chen et al., 2020a). Besides, silencing Linc00511 inhibits EMT and metastasis of cancer cells via TGF-β signaling down-regulation (Zhong et al., 2020). The studies demonstrate that EMT regulation by TGF-β can participate in chemoresistance. Curcumin is able to inhibit nuclear translocation of Smad2/3 via suppressing TGF-β signaling pathway. This leads to a decrease in migration and proliferation of cancer cells and sensitizes them into OX chemotherapy (Yin et al., 2019). It is worth mentioning that curcumin affects TGF-β signaling pathway in cancer therapy via different pathways. In order to suppress invasion and proliferation of cervical, breast and pancreatic cancer cells, curcumin suppresses TGF-β signaling pathway through interfering with Wnt/β-catenin signaling pathway (Nna et al., 2013; Thacker and Karunagaran, 2015; Wang and Yan, 2016). However, there are controversial data showing that curcumin may stimulate TGF-β signaling pathway in inhibition of colon cancer progression (Ramamoorthi and Sivalingam, 2014). A recently published article has revealed a novel pathway of anti-tumor activity of curcumin. It is said that curcumin exerts anti-metastatic activity in pancreatic cancer cells by inhibiting canonical pathway of TGF-β signaling through androgen-dependent and independent manners (Katta et al., 2019). So, in suppressing malignant behavior of cancer cells, curcumin affects TGF-β signaling pathway via targeting another molecular signaling such as Wnt/β-catenin.
The effect of curcumin on TGF-β1 in cancer therapy is dose- and time-dependent manner (Celik et al., 2018). In suppressing malignant behavior of breast cancer cells, curcumin down-regulates expression of TGF-β1 to inactivate Smad2 and MMP-9 (Mo et al., 2012). Exposing breast cancer cells into TGF-β induces secretion of bone-resorptive peptide parathyroid hormone-related protein (PTHrP). This ensures proliferation and invasion of cancer cells. Administration of curcuminoids (25 and 50 mg/kg) suppresses breast cancer malignancy via inhibiting phosphorylation of Smad2 and Smad3 (Wright et al., 2013). It is worth mentioning that in cancer therapy, curcumin can affect upstream mediators of TGF-β1 signaling pathway. As a negative modulator of TGF-β1 signaling pathway, bone morphogenic protein -7 (BMP-7) undergoes upregulation by curcumin to inhibit TGF-β, leading to anti-metastatic activity of curcumin (Dorai et al., 2014). Curcumin can also suppress metastasis of cancer cells via inhibition of TGF-β1-mediated EMT (Xu et al., 2015). In inhibition of TGF-β1-mediated EMT, curcumin inhibits phosphorylation of Smad2 and Smad3 (Zhang et al., 2016). Another pathway in inhibition of TGF-β-mediated EMT by curcumin is that this plant derived-natural compound suppresses Smad2 phosphorylation, its nuclear translocation and interaction with promoter of Snail (Cao et al., 2017). In addition to TGF-β signaling pathway, curcumin is able to suppress receptors in this pathway. Curcumin and its derivatives inhibit ALK5 to down-regulate migration and invasion of cancer cells (Kandagalla et al., 2017).
Fibrosis
The endothelial-to-mesenchymal transition (EndMT) is a process in which endothelial cells lose their adhesion and polarity, and obtain mesenchymal phenotype, leading to enhanced cell migration and collagen secretion (Maddaluno et al., 2013). Increasing evidence demonstrates that EndMT is vital for production of myofibroblasts in fibrotic tissues or organs (Zeisberg et al., 2007; Potenta et al., 2008; Zeisberg et al., 2008; Li et al., 2009). It has been reported that TGF-β can act as an upstream modulator in stimulation of EndMT (Hou et al., 2019; Yang et al., 2019b). The enhanced expression of TGF-β1 induces an increase in inflammatory factors and asymmetric dimethylarginine (ADMA), whereas a decrease occurs in Nrf2, dimethyl arginine dimethylaminohydrolase-1 (DDAH1), VE-cadherin, secretion of nitric oxide (NO) and activity of nitric oxide synthase (NOS). These factors provide conditions for endothelial cell fibrosis via EndMT induction. It is said that curcumin supplementation down-regulates expression of TGF-β1 to enhance VE-cadherin, DDAH1 and Nrf2 levels, and diminish MMP-9 and ERK1/2 levels. Consequently, TGF-b-mediated EndMT is inhibited to suppress endothelial cell fibrosis (Chen et al., 2020b). Although chemotherapy is a common way in cancer therapy, studies have demonstrated the high adverse effects of chemotherapeutic agents. Cisplatin (CP) is a potential chemotherapeutic agent with excellent anti-tumor activity. However, accumulating data has shown that CP negatively affects kidney by stimulation of inflammation and oxidative stress (Mahran, 2020; Wang et al., 2020c). It is worth mentioning that TGF-β1 mediates nephrotoxicity of CP (Salem et al., 2018). A newly published article has examined the potential of curcumin in improving CP-mediated nephrotoxicity. It seems that a combination of curcumin and arsenic trioxide (ATO) diminishes side effects of CP on kidney and emergence of fibrosis via down-regulation of TGF-β1 (Maghmomeh et al., 2020). The peritoneal dialysis (PD) is a potential strategy of renal replacement therapy for patients who suffer from end-stage renal disease (ESRD). However, PD fluid is not completely biocompatible and has a variety of issues such as low pH, high glucose and lactate, and can lead to abnormalities of peritoneum (Cho et al., 2014). An increase in glucose levels is associated with inflammation and PD. This is mediated via TGF-β1 upregulation and results in peritoneal EMT (Yang et al., 2017). So, targeting TGF-β1 can ameliorate PD-mediated fibrosis. The administration of curcumin remarkably reduces the expression of TGF-β1 in PD animal models to improve ultrafiltration volume, diminish mass transfer of glucose and fibroproliferative response (Zhao et al., 2019a). It seems that during PD, TGF-β signaling pathway enhances migration and motility of cells via stimulation of EMT. Curcumin supplementation is associated with a decrease in migratory ability of these cells via down-regulation of TGF-β and subsequent inhibition of EMT (Zhao et al., 2019b). As a common phenomenon after joint surgery or longtime immobilization, joint contracture has significant pathological alterations including myofibroblast proliferation and enhanced deposition of ECM (Abdel et al., 2012). The prostaglandin E2 (PGE2) is formed by cyclooxygenase metabolism of arachidonic acid and inhibits the migration and proliferation of myofibroblasts, and ECM accumulation (Elias et al., 1985; Bitterman et al., 1986; Fine et al., 1989; Kolodsick et al., 2003; White et al., 2005; Huang et al., 2009). The hyaluronic acid-curcumin conjugate is beneficial in treatment of joint contracture-mediated fibrosis. It is said that hyaluronic acid-curcumin conjugate induces demethylation of prostaglandin E receptor 2 (PTGER2) to enhance its expression. Consequently, activated PTGER2 inhibits TGF-β signaling pathway to negatively affect migration and proliferation of myofibroblasts, resulting in a diminution in fibrosis (Zhang et al., 2019b).
The idiopathic pulmonary fibrosis (IPF) is a multifactorial disorder with involvement of cigarette smoking, air pollution, genetic predisposition, aging and viral infections (Gao et al., 2011; Mora et al., 2017). This chronic and progressive disorder is a form of idiopathic interstitial pneumonia (Gross and Hunninghake, 2001). The IPF interferes with pulmonary function via stimulation of inflammation (Spagnolo et al., 2015). Agents with inhibitory impact on inflammatory cytokines can attenuate IPF. It is suggested that curcumin administration can down-regulate TGF-β1 expression to partially alleviate IPF (Hu et al., 2018). We mentioned earlier that TGF-β involves in fibrosis via stimulation of EMT mechanism. In fact, TGF-β-mediated EMT occurs in a Smad-dependent manner. It is worth mentioning that TGF-β can activate EMT via Smad-independent manner. The TGF-β is able to induce EMT by stimulation of Akt/mTOR signaling pathway (Lu et al., 2019). It has been demonstrated that agents with inhibitory impact on EMT mechanism such as Dendrobium officinale can inhibit TGF-β/Akt/mTOR pathway (Xing et al., 2018; Luo et al., 2019). This shows a novel signaling pathway of TGF-β in EMT induction. The administration of curcumin ameliorates kidney fibrosis by inhibition of EMT via suppressing TGF-β/Akt/mTOR signaling pathway (Zhu et al., 2016). These studies demonstrate that in inhibition of EMT, curcumin affects various molecular pathways (Zhou et al., 2017b). The increasing evidence exhibits that TGF-β signaling pathway can contribute to pulmonary fibrosis by inducing proliferation of lung fibroblasts and their differentiation into myofibroblasts (Massagué, 1998; Hinz et al., 2007; Horbelt et al., 2012). In treatment of pulmonary fibrosis, curcumin inhibits TGF-β1 signaling pathway to suppress proliferation and differentiation of fibroblasts, leading to amelioration of pulmonary fibrosis (Saidi et al., 2019). The proliferative vitreoretinopathy (PVR) is a wound healing response that may be completed by formation of fibrotic tissues. The migration and differentiation of retinal pigment epithelial (RPE) cells play a significant role in formation of fibrotic tissues during PVR (Dartt et al., 2011; Sadaka and Giuliari, 2012). The in vitro and in vivo experiments have revealed that TGF-β-mediated EMT contributes to trans-differentiation of PRE cells into fibroblasts (Lee et al., 2007; Ali, 2011). A combination of curcumin and epigallocatechin gallate (EGCG) suppresses TGF-β1/Smad3 to inhibit EMT, leading to alleviation of PVR (Shanmuganathan et al., 2017). A same story occurs in CCl4 toxicity. The CCl4 is able to induce liver fibrosis via inflammation, oxidative stress and stimulates apoptosis (Liu et al., 2020a; Munakarmi et al., 2020; Zhang et al., 2020b). In inhibition of CCl4-mediated liver injury, curcumin down-regulates TGF-β1/Smad3 signaling pathway (Peng et al., 2018). These studies highlight the fact that hepatotoxic agents mainly exert their adverse effects via stimulation of TGF-β signaling pathway, and drugs such as curcumin that have modulatory impact on this pathway, are of importance. The important point is that in enhancing the anti-fibrotic activity of curcumin, nanoparticles are of interest, since they can remarkably enhance the bioavailability and therapeutic effect of curcumin (Charoensuk et al., 2016).
Neurological Disorders
The multiple sclerosis (MS) is an inflammatory neurological disorder negatively affecting central nervous system (CNS) (Bonetti and Raine, 1997). Due to immune attack, some degrees of axon and myelin degeneration occur in MS patients (Goldenberg, 2012). Inflammatory factors play a significant role in MS progression via stimulation of axon degeneration and neuronal dysfunction (Wujek et al., 2002). Enhancing expression of anti-inflammatory factors such as IL-4, IL-5 and TGF-β is a promising strategy in MS therapy (Soleimani et al., 2014). The administration of curcumin remarkably enhances TGF-β expression to suppress inflammation and progression in experimental encephalomyelitis (EAE) model of MS (Esmaeilzadeh et al., 2019). The spinal cord injury (SCI) is a common phenomenon that can occur after accident. It seems that during SCI, TGF-β-SOX9 signaling pathway activates inflammation factor NF-κB to induce glial scar formation. In respect to anti-inflammatory activity of curcumin, it is able to suppress glial scar formation and attenuate SCI via down-regulation of TGF-β-SOX9 axis and subsequent inhibition of NF-κB (Yuan et al., 2019). Lumbar intervertebral disc degeneration (LIDD) is a chronic and progressive disorder characterized by low back pain (Vieira et al., 2014). In respect to its high incidence rate, finding treatments for LIDD is of importance. Increasing evidence demonstrates that TGF-β has dual role in different disorders, so that it may reduce the number of cells undergoing apoptosis in a certain circumstance, while it may enhance apoptotic cell death (Hu et al., 2017). Curcumin administration alleviates LIDD by inhibition of TGF-β1 and TGF-β2 signaling pathways. In fact, studies are in agreement with neuroprotective impact of curcumin mediated by TGF-β down-regulation. It has been reported that in improving neural functionality, curcumin reduces expression of TGF-β1 and TGF-β2 (Yuan et al., 2015).
Wound Healing
During wound healing, a variety of cells such as inflammatory cells, fibroblasts, keratinocytes, endothelial cells, and growth factors as well as enzymes are involved (Velnar et al., 2009; Abdel-Ghani et al., 2019; Makvandi et al., 2019). The presence of other diseases such as DM impairs wound healing, demanding novel intervention to improve wound process by enhancing growth factor production, induction of angiogenesis, elevating collagen accumulation and macrophage function (Falanga, 2005; Campos et al., 2008). Multiple studies have evaluated the role of TGF-β during wound healing. It seems that upregulation of TGF-β induces angiogenesis to improve wound healing (Zong et al., 2020). Impairment of TGF-β signaling pathway inhibits adaptive response for tissue repair (Jiang et al., 2020). So, restoring expression of TGF-β is a promising strategy in wound healing. Loading a combination of curcumin and lithospermi vadix extract on nanofibrous scaffolds improve wound healing in DM rats partially by stimulation of TGF-β signaling pathway (Yang et al., 2019a). Increasing evidence demonstrates that expression of TGF-β3 undergoes upregulation in scar-less wound healing (Chen et al., 2005). It is held that TGF-β3 induces Smad2/3 phosphorylation at epidermal cells compared to dermal cells (Bandyopadhyay et al., 2006). The TβRII (involved in canonical pathway of TGF-β signaling) demonstrates differential expression during wound healing. The Smad anchor for receptor activation (SARA) attaches into MAD homolog 2 (MH2) of Smad2/3 to regulate nuclear translocation of Smad in TGF-β signaling pathway (Tsukazaki et al., 1998). In amelioration of acute burn injury and accelerating wound healing, Zno-curcumin nanocomposite loaded in hybrid collagen scaffolds stimulates TGF-β3 signaling pathway by upregulation of TβRII and SARA (Kalirajan and Palanisamy, 2019). Tissue engineering has helped us in accelerating wound healing. Using chitosan- and collagen-scaffold is considered as a promising strategy in wound healing (Ramasamy et al., 2014). It seems that chitosan can facilitate wound healing through ameliorating functions of fibroblasts, macrophages and inflammatory cells (Dai et al., 2009). In order to promote functionality of scaffold, collagen can be synergistically used with chitosan, and then, other agents with capability of improving wound healing can be loaded on this scaffold (Dai et al., 2004; Gopinath et al., 2004; Sionkowska et al., 2004). It was shown that curcumin-nanoparticles (CNs) incorporated in collagen-chitosan scaffold are able to remarkably improve wound healing via inhibition of TGF-β1/Smad7 axis to reduce inflammation and pave the road for wound healing (Rezaii et al., 2019). These examples show the significance of curcumin for soft tissue regeneration.
Asthma
Asthma is a multifactorial disorder with involvement of inflammation, pulmonary edema, airflow obstruction and environmental factors (Jolliffe et al., 2020). This disorder affects a high number of people worldwide (Engelkes et al., 2020; Shinan-Altman and Katzav, 2020), resulting in much attraction into identification its cause and finding novel treatments. It has been demonstrated that pro-inflammatory and pro-fibrotic factors such as TGF-β and TNF-α play a considerable role in asthma pathogenesis (Janulaityte et al., 2020; Liu and Shang, 2020). Agents with inhibitory impact on the expression and level of TGF-β are of considerable importance in asthma therapy. In respect to excellent anti-inflammatory activity of curcumin, it diminishes expression of TGF-β as a pro-fibrotic cytokine to abate airway inflammation and pulmonary edema (Shahid et al., 2019).
Arthritis
The rheumatoid arthritis (RA) is a joint swelling abnormality that is characterized with synovial inflammation (Mao et al., 2020). Due to the involvement of inflammatory cytokines in RA, anti-inflammatory agents have been of interest in treatment of this disorder. For instance, Brb is able to inhibit RA development via inhibition of IL-21-mediated proliferation of fibroblast like synoviocytes (Dinesh and Rasool, 2019). A newly published article also demonstrates that curcumin can ameliorate RA by targeting inflammation. As a pro-inflammatory cytokine, the expression of TGF-b undergoes down-regulation in rat exposed to curcumin (200 mg/kg), leading to amelioration of inflammation (Wang et al., 2019a).
Diabetes
The diabetic cardiomyopathy (DCM) is a major complication of both DM type I (DMI) and DM type II (DMII). It seems that DCM affects 12% of patients with DM and can lead to death (Bugger and Abel, 2014; Lorenzo-Almoros et al., 2017). Interestingly, Janus kinase/signal transducer and activator of transcription (JAK/STAT) is involved in intracellular signaling pathways and mechanisms such as proliferation, differentiation and so on by translocation at the route of cytoplasm to nucleus and affecting down-stream targets (Losuwannarak et al., 2019; Wang et al., 2020b). The JAK/STAT signaling pathway can participate in inflammation via stimulation of TGF-β1 (Boengler et al., 2008). In enhancing the ameliorative impact of metformin in DCM, curcumin down-regulates the expression of TGF-β1 via inhibition of JAK/STAT signaling pathway, leading to reducing inflammation and improving DCM (Abdelsamia et al., 2019). Cardiac fibrosis is a common phenomenon during DCM. It has been demonstrated that enhanced accumulation of ECM commonly occurs in cardiac fibrosis. The collagen type I and III are main elements of ECM (Abdel et al., 2012; Russo and Frangogiannis, 2016). So, reducing the level of these components can pave the road into cardiac fibrosis treatment during ECM. The in vivo experiment on animal model of DM (rat) demonstrates that curcumin administration (300 mg/kg) for 16 weeks improves cardiac fibrosis via decreasing accumulation of collagen type I and II in ECM. The investigation of molecular pathways reveals that in attenuation of cardiac fibrosis, curcumin down-regulates TGF-β1, TβRII and Smad2/3, while it induces Smad7 expression (Guo et al., 2018).
Infection
The candida albicans is a commensal yeast of genital and intestinal tracts. The increasing evidence has shown that candida albicans is a pathogenic yeast in women and can induce vulvovaginal candidiasis (VVC) in the presence of other diseases such as DM and immune disorders (Deorukhkar and Saini, 2013). In respect to immunomodulatory impact of curcumin, its administration can be beneficial in VVC treatment. By reducing the level of IL-1β (pro-inflammatory factor) compared to TGF-β (anti-inflammatory factor), an amelioration occurs in VVC and paves the road for efficient treatment of this infection (Rodero et al., 2018).
Clinical Studies
Nowadays, we are witnessing that a high number of studies evaluate the efficiency of drugs in both in vitro and in vivo experiments. However, clinical translation of these studies is of importance in directing into commercial application. Notably, the effect of curcumin on TGF-β level has been evaluated in clinical trials (Panahi et al., 2016). In this study, 117 patients were enrolled and they were randomly divided into two groups including placebo (n = 58) and treatment (n = 59). The treatment group received curcumin daily at the dose of 1 g for 8 weeks. The results of this study revealed that curcumin is advantageous in treatment of metabolic syndrome. This plant derived-natural compound is able to diminish serum levels of pro-inflammatory cytokines, and among them, TGF-β level demonstrates a remarkable decrease after curcumin supplementation, showing the potential of curcumin in treatment of metabolic syndrome. The oral squamous cell carcinoma (OSCC) is one of the malignancies affecting high number of people worldwide (Saranath et al., 1999). A variety of factors contribute to OSCC development, and among them, oral submucous fibrosis (OSMF) is of importance (Lippman and Hong, 2001; Reibel, 2003). The stimulation of inflammation by myofibroblasts enhances levels of TGF-β that subsequently, promotes deposition and generation of ECM (Khan et al., 2012). A pilot study has been performed on 28 patients (23 males and five females) to evaluate the efficiency of curcumin in decreasing TGF-β levels. The treatment group received a mixture of curcumin and piperine (300 mg) twice daily in a period of 9 months. The findings revealed that curcumin is able to considerably diminish TGF-β expression by 32.1% (Gupta et al., 2017), showing that curcumin can be applied as a potent chemopreventive agent.
We previously discussed curcumin and its effect on TGF-β in MS treatment. Regulatory T cells (Treg cells) are key players in MS. Normally, Treg cells contribute to self-tolerance preservation and regulation of immune responses against infections and cancer cells (Fujio et al., 2010). Treg cells are capable of secretion of TGF-β. Noteworthy, TGF-β is vital for differentiation of Treg cells (Sakaguchi and Sakaguchi, 2005). In preventing inflammation and immune responses, Treg cells secrete TGF-β (Wan and Flavell, 2008). So, there is a dual relationship between Treg cells and TGF-β, so that Treg cells exert their anti-inflammatory action through TGF-β secrion, and also, TGF-β is necessary for Treg cell differentiation. Any impairment in this interaction can predispose to development of inflammatory diseases such as MS. Recently, nanocurcumin has been developed for treatment of MS patients. As curcumin suffers from poor bioavailability, loading it on nanoparticles promotes its therapeutic effects. Nanocurcumin administration significantly enhances TGF-β expression and also, its secretion levels. Based on interaction between TGF-β and Treg cells, enhanced expression and secretion of TGF-β by nanocurcumin result in an improvement in function of Treg cells, and alleviation of MS (Dolati et al., 2019). Table 1 summarizes the therapeutic effects of curcumin mediated by its effect of TGF-β. Figures 3 and 4 summarize the therapeutic impacts of curcumin mediated by its effect on TGF-β signaling pathway.
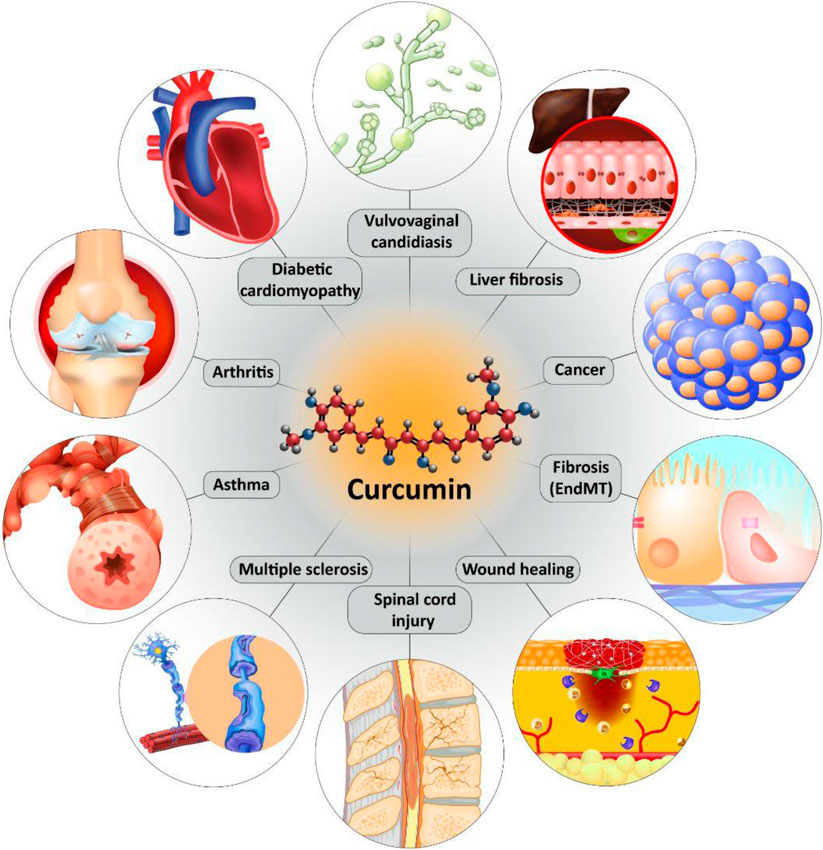
FIGURE 4. The protective effects of curcumin in different diseases mediated by its effect on TGF-β signaling pathway.
Conclusion and Remarks
Curcumin is a naturally occurring nutraceutical compound with excellent therapeutic and biological activities. A look at PubMed demonstrates that annually, a high number of studies investigate the protective effects of curcumin against various diseases with a focus on underlying molecular pathways. In the present review, we comprehensively discussed the role of TGF-β in protective effects of curcumin. Noteworthy, curcumin both upregulates/down-regulates TGF-β signaling pathway in diseases therapy. The most studied therapeutic effect of curcumin mediated by TGF-β regulation is anti-fibrotic. Different studies have shown that curcumin inhibits migration and proliferation of fibroblasts and their differentiation by down-regulation of TGF-β. Curcumin inhibits EMT by suppressing TGF-β to ameliorate collagen synthesis and cell migration during fibrosis. It is worth mentioning that curcumin inhibits chemotherapy-mediated fibrosis via down-regulation of TGF-β. In alleviation of fibrosis, curcumin reduces ECM deposition and accumulation by targeting TGF-β. The interesting point is that curcumin inhibits EMT-mediated fibrosis. In this way, curcumin is able to target down-stream mediators of TGF-β such as PI3K/Akt/mTOR pathway. Another potential therapeutic effect of curcumin mediated by its effect of TGF-β is anti-tumor activity. In spite of great advances in medicine, cancer is still a big challenge for scientists. Curcumin exerts anti-metastatic activity via inhibition of TGF-β-mediated EMT. It also inhibits progression of cancer cells by suppressing CAFs via TGF-β down-regulation. By inhibition of TGF-β, curcumin protects liver cells against toxic agents. In neurological disorders and arthritis as well as asthma, curcumin exerts anti-inflammatory activity via targeting TGF-β signaling pathway. In accelerating wound healing, curcumin inhibits TGF-β1/Smad7 axis, while it induces TGF-β3. It is held that using nanoparticles enhances bioavailability and capability of curcumin in affecting TGF-β signaling pathway. In diabetes, fibrosis is a common phenomenon due to increased accumulation of collagen type I and III that is inhibited by curcumin via down-regulation of TGF-β. The important point is that clinical trials have shown that efficacy of curcumin in regulation of TGF-β in treatment of metabolic syndrome. All of the studies are in line with modulatory impact of curcumin on TGF-β in different diseases. However, more studies are required to clarify mentioned discussions.
Author Contributions
HM and MN contributed in conception, design, statistical analysis and drafting of the manuscript. MA, AZ, KH, FH, VZ, ERM, SS, and PM contributed in data collection and manuscript drafting. All authors approved the final version for submission.
Conflict of Interest
The authors declare that the research was conducted in the absence of any commercial or financial relationships that could be construed as a potential conflict of interest.
Glossary
TGF-β, transforming growth factor-β;
DMC, demethoxycurcumin;
BDMC, bis-demethoxycurcumin;
Nrf2, nuclear factor erythroid 2-related factor 2;
HO-1, heme oxygenase-1;
SOD, superoxide dismutase;
NQO1, NADPH quinone reductase 1;
DM, diabetes mellitus;
CAVD, calcified aortic valve disease;
NF-κB, nuclear factor-kappaB;
ER, endoplasmic reticulum;
GRP78, glucose-regulated protein 78;
CHOP, CCAAT-enhancer-binding protein homologous protein;
ATF4, activating transcription factor 4;
UPR, unfolded protein response;
BPA, bisphenol A;
LAP, latency-associated peptide;
TβRII, TGF-β type II;
ALK5, TGF-β type I;
TGIF, tumor growth-interacting factor;
I-Smads, inhibitor Smads;
MAPK, mitogen-activated protein kinase;
PI3K, phosphatidylinositol 3-kinase;
FAPs, fibroadipogenic progenitors;
TRIM33, tripartite motif-containing 33;
ECM, extracellular matrix;
miR, microRNA;
EMT, epithelial-to-mesenchymal transition;
Brb, berberine; Res, resveratrol;
CCl4, carbon tetrachloride;
TIME, carbon tetrachloride;
TME, tumor microenvironment;
CAFs, cancer-associated fibroblasts;
MMPs, matrix metalloproteinases;
PQ, paraquat;
OX, oxaliplatin;
PTHrP, peptide parathyroid hormone-related protein;
BMP-7, bone morphogenic protein-7;
EndMT, endothelial-to-mesenchymal transition;
ADMA, asymmetric dimethylarginine;
DDAH1, dimethylaminohydrolase-1;
NO, nitric oxide;
NOS, nitric oxide synthase;
CP, cisplatin;
ATO, arsenic trioxide;
PD, peritoneal dialysis;
ESRD, end-stage renal disease;
PGE2, prostaglandin E2;
PTGER2, prostaglandin E receptor 2;
IPF, idiopathic pulmonary fibrosis;
PVR, proliferative vitroretinopathy;
RPE, retinal pigment epithelial;
EGCG, epigallocatechin gallate;
MS, multiple sclerosis;
CNS, central nervous system;
SCI, spinal cord injury;
LIDD, lumbar intervertebral disc degeneration;
MH2, MAD homolog 2;
SARA, Smad anchor for receptor activation,
CNs, curcumin-nanoparticles;
RA, rheumatoid arthritis;
DCM, diabetic cardiomyopathy;
DMI, DM type I,
DMII, DM type II;
JAK/STAT, Janus kinase/signal transducer and activator of transcription;
VVC, vulvovaginal candidiasis;
OSCC, oral squamous cell carcinoma;
OSMF, oral submucous fibrosis.
References
Abarikwu, S. O., Akiri, O. F., Durojaiye, M. A., and Alabi, A. F. (2014). Combined administration of curcumin and gallic acid inhibits gallic acid-induced suppression of steroidogenesis, sperm output, antioxidant defenses and inflammatory responsive genes. J. Steroid Biochem. Mol. Biol. 143, 49–60. doi:10.1016/j.jsbmb.2014.02.008
Abdel, M. P., Morrey, M. E., Barlow, J. D., Kreofsky, C. R., An, K.-N., Steinmann, S. P., et al. (2012). Myofibroblast cells are preferentially expressed early in a rabbit model of joint contracture. J. Orthop. Res. 30, 713–719. doi:10.1002/jor.21588
Abdel-Ghani, L. M., Rahmy, T. R., Tawfik, M. M., Kaziri, I., Al-Obaidi, A., Rowan, E. G., et al. (2019). Cytotoxicity of Nubein6.8 peptide isolated from the snake venom of Naja nubiae on melanoma and ovarian carcinoma cell lines. Toxicon 168, 22–31. doi:10.1016/j.toxicon.2019.06.220
Abdelsamia, E. M., Khaleel, S. A., Balah, A., and Abdel Baky, N. A. (2019). Curcumin augments the cardioprotective effect of metformin in an experimental model of type I diabetes mellitus; Impact of Nrf2/HO-1 and JAK/STAT pathways. Biomed. Pharmacother. 109, 2136–2144. doi:10.1016/j.biopha.2018.11.064
Abo-Zaid, M. A., Shaheen, E. S., and Ismail, A. H. (2020). Immunomodulatory effect of curcumin on hepatic cirrhosis in experimental rats. J. Food Biochem. 44 (6). doi:10.1111/jfbc.13219
Abouzied, M. M. M., Eltahir, H. M., Abdel Aziz, M. A., Ahmed, N. S., Abd El-Ghany, A. A., Abd El-Aziz, E. A., et al. (2015). Curcumin ameliorate DENA-induced HCC via modulating TGF-β, AKT, and caspase-3 expression in experimental rat model. Tumor Biol. 36, 1763–1771. doi:10.1007/s13277-014-2778-z
Aggarwal, B. B., Sundaram, C., Malani, N., and Ichikawa, H. (2007). Curcumin: the Indian solid gold. The molecular targets and therapeutic uses of curcumin in health and disease. Adv. Exp. Med. Biol. 595, 1–75. doi:10.1007/978-0-387-46401-5_1
Aggarwal, B. B., and Harikumar, K. B. (2009). Potential therapeutic effects of curcumin, the anti-inflammatory agent, against neurodegenerative, cardiovascular, pulmonary, metabolic, autoimmune and neoplastic diseases. Int. J. Biochem. Cell Biol. 41, 40–59. doi:10.1016/j.biocel.2008.06.010
Aggarwal, B. B., Ichikawa, H., Garodia, P., Weerasinghe, P., Sethi, G., Bhatt, I. D., et al. (2006). From traditional Ayurvedic medicine to modern medicine: identification of therapeutic targets for suppression of inflammation and cancer. Expert Opin. Ther. Targets 10, 87–118. doi:10.1517/14728222.10.1.87
Ahmadi, A., Najafi, M., Farhood, B., and Mortezaee, K. (2019). Transforming growth factor‐β signaling: tumorigenesis and targeting for cancer therapy. J. Cell. Physiol. 234, 12173–12187. doi:10.1002/jcp.27955
Ahmed, S., Khan, H., and Mirzaei, H. (2019). Mechanics insights of curcumin in myocardial ischemia: where are we standing?. Eur. J. Med. Chem. 183, 111658. doi:10.1016/j.ejmech.2019.111658
Ak, T., and Gülçin, İ. (2008). Antioxidant and radical scavenging properties of curcumin. Chem. Biol. Interact. 174, 27–37. doi:10.1016/j.cbi.2008.05.003
Al-Saud, N. B. S. (2020). Impact of curcumin treatment on diabetic albino rats. Saudi J. Biol. Sci. 27, 689–694. doi:10.1016/j.sjbs.2019.11.037
Ali, I. (2011). Editorial [hot topic: nano drugs: novel agents for cancer chemo-therapy (guest editor: imran Ali)]. Ccdt 11, 130. doi:10.2174/156800911794328466
Amalraj, A., Pius, A., Gopi, S., and Gopi, S. (2017). Biological activities of curcuminoids, other biomolecules from turmeric and their derivatives-a review. J. Tradit. Complement. Med. 7, 205–233. doi:10.1016/j.jtcme.2016.05.005
Bagherian, A., Mardani, R., Roudi, B., Taghizadeh, M., Banfshe, H. R., Ghaderi, A., et al. 2020. Combination therapy with nanomicellar-curcumin and temozolomide for in vitro therapy of glioblastoma multiforme via Wnt signaling pathways. J. Mol. Neurosci. 70, 1471–1483. doi:10.1007/s12031-020-01639-z
Bahrami, A., Majeed, M., and Sahebkar, A. (2019). Curcumin: a potent agent to reverse epithelial-to-mesenchymal transition. Cell. Oncol. 42, 405–421. doi:10.1007/s13402-019-00442-2
Bai, M., Pan, C. L., Jiang, G. X., and Zhang, Y. M. (2020). CircRNA 010567 improves myocardial infarction rats through inhibiting TGF-β1. Eur. Rev. Med. Pharmacol. Sci. 24, 369–375. doi:10.26355/eurrev_202001_19935
Bai, X., Yi, M., Jiao, Y., Chu, Q., and Wu, K. (2019). Blocking TGF-β signaling to enhance the efficacy of immune checkpoint inhibitor. OncoTargets Ther. 12, 9527. doi:10.2147/ott.s224013
Baldi, A., De Luca, A., Maiorano, P., D’Angelo, C., and Giordano, A. (2020). Curcumin as an anticancer agent in malignant mesothelioma: a review. Int. J. Mol. Sci. 21 (5), 1839. doi:10.3390/ijms21051839
Baltazar, T., Dinis-Oliveira, R. J., Duarte, J. A., de Lourdes Bastos, M., and Carvalho, F. (2013). Paraquat research: do recent advances in limiting its toxicity make its use safer?. Br. J. Pharmacol. 168 (1), 44–45. doi:10.1111/j.1476-5381.2012.02017.x
Bandyopadhyay, B., Fan, J., Guan, S., Li, Y., Chen, M., Woodley, D. T., et al. (2006). A "traffic control" role for TGFbeta3: orchestrating dermal and epidermal cell motility during wound healing. J. Cell Biol. 172 (7), 1093–1105. doi:10.1083/jcb.200507111
Bian, Y., and Guo, D. (2020). Targeted therapy for hepatocellular carcinoma: Co-delivery of sorafenib and curcumin using lactosylated pH-responsive nanoparticles. Drug Des. Dev. Ther. 14, 647–659. doi:10.2147/dddt.s238955
Bitterman, P., Wewers, M. D., Rennard, S. I., Adelberg, S., and Crystal, R. G. (1986). Modulation of alveolar macrophage-driven fibroblast proliferation by alternative macrophage mediators. J. Clin. Invest. 77 (3), 700–708. doi:10.1172/jci112364
Boengler, K., Hilfikerkleiner, D., Drexler, H., Heusch, G., and Schulz, R. (2008). The myocardial JAK/STAT pathway: from protection to failure. Pharmacol. Ther. 120 (2), 172–185. doi:10.1016/j.pharmthera.2008.08.002
Boguslawska, J., Kryst, P., Poletajew, S., and Piekielko-Witkowska, A. (2019). TGF-β and microRNA interplay in genitourinary cancers. Cells 8 (12), 1619. doi:10.3390/cells8121619
Bonetti, B., and Raine, C. S. (1997). Multiple sclerosis: oligodendrocytes display cell death–related molecules in situ but do not undergo apoptosis. Ann. Neurol. 42, 74–84. doi:10.1002/ana.410420113
Boutanquoi, P. M., Burgy, O., Beltramo, G., Bellaye, P.-S., Dondaine, L., Marcion, G., et al. (2020). TRIM33 prevents pulmonary fibrosis by impairing TGF-beta1 signaling. Eur. Respir. J. 55 (6), 1901346. doi:10.1183/13993003.01346-2019
Bugger, H., and Abel, E. D. (2014). Molecular mechanisms of diabetic cardiomyopathy. Diabetologia 57, 660–671. doi:10.1007/s00125-014-3171-6
Buhrmann, C., Kraehe, P., Lueders, C., Shayan, P., Goel, A., and Shakibaei, M. (2014). Curcumin suppresses crosstalk between colon cancer stem cells and stromal fibroblasts in the tumor microenvironment: potential role of EMT. PLoS One 9 (9), e107514. doi:10.1371/journal.pone.0107514
Buonomo, A. R., Scotto, R., Nappa, S., Arcopinto, M., Salzano, A., Maria Marra, A., et al. (2019). The role of curcumin in liver diseases. Arch. Med. Sci.: AMS 15 (6), 1608. doi:10.5114/aoms.2018.73596
Campos, A. C., Groth, A. K., and Branco, A. B. (2008). Assessment and nutritional aspects of wound healing. Curr. Opin. Clin. Nutr. Metab. Care 11 (3), 281–288. doi:10.1097/mco.0b013e3282fbd35a
Cao, C., Lin, S., Zhi, W., Lazare, C., Meng, Y., Wu, P., et al. (2020). LOXL2 expression status is correlated with molecular characterizations of cervical carcinoma and associated with poor cancer survival via epithelial-mesenchymal transition (EMT) phenotype. Front. Oncol. 10, 284. doi:10.3389/fonc.2020.00284
Cao, M. T., Liu, H. F., Liu, Z. G., Xiao, P., Chen, J. J., Tan, Y., et al. (2017). Curcumin downregulates the expression of Snail via suppressing Smad2 pathway to inhibit TGF-beta1-induced epithelial-mesenchymal transitions in hepatoma cells. Oncotarget 8 (65), 108498–108508. doi:10.18632/oncotarget.22590
Celik, H., Aydin, T., Solak, K., Khalid, S., and Farooqi, A. A. (2018). Curcumin on the “flying carpets” to modulate different signal transduction cascades in cancers: next‐generation approach to bridge translational gaps. J. Cell. Biochem. 119 (6), 4293–4303. doi:10.1002/jcb.26749
Chainoglou, E., and Hadjipavlou-Litina, D. (2020). Curcumin in health and diseases: alzheimer’s disease and curcumin analogues, derivatives, and hybrids. Int. J. Mol. Sci. 21, 1975. doi:10.3390/ijms21061975
Charoensuk, L., Pinlaor, P., Wanichwecharungruang, S., Intuyod, K., Vaeteewoottacharn, K., Chaidee, A., et al. (2016). Nanoencapsulated curcumin and praziquantel treatment reduces periductal fibrosis and attenuates bile canalicular abnormalities in Opisthorchis viverrini-infected hamsters. Nanomed. Nanotechnol. Biol. Med. 12 (1), 21–32. doi:10.1016/j.nano.2015.10.005
Chen, H., Yang, R., Tang, Y., Xu, J., Feng, Y., Liu, S., et al. (2017). Effects of curcumin on pulmonary fibrosis and functions of paraquat-challenged rats. Zhonghua Wei Zhong Bing Ji Jiu Yi Xue 29, 973–976. doi:10.3760/cma.j.issn.2095-4352.2017.11.003
Chen, H., and Fu, X. (2018). Dynamics study on the role of curcumin on TGF-β1 expression and pathological changes in acute paraquat poisoned rats. Exp. Ther. Med. 16, 3841–3846. doi:10.3892/etm.2018.6667
Chen, J. T., Wang, C. Y., and Chen, M. H. (2018). Curcumin inhibits TGF-beta1-induced connective tissue growth factor expression through the interruption of Smad2 signaling in human gingival fibroblasts. J. Formos. Med. Assoc. 117 (12), 1115–1123. doi:10.1016/j.jfma.2017.12.014
Chen, S., Xu, J., Su, Y., Hua, L., Feng, C., Lin, Z., et al. (2020a). MicroRNA-145 suppresses epithelial to mesenchymal transition in pancreatic cancer cells by inhibiting TGF-beta signaling pathway. J. Canc. 11 (9), 2716–2723. doi:10.7150/jca.34902
Chen, W.-C., Lai, Y.-A., Lin, Y.-C., Ma, J.-W., Huang, L.-F., Yang, N.-S., et al. (2013). Curcumin suppresses doxorubicin-induced epithelial–mesenchymal transition via the inhibition of TGF-β and PI3K/AKT signaling pathways in triple-negative breast cancer cells. J. Agric. Food Chem. 61 (48), 11817–11824. doi:10.1021/jf404092f
Chen, W., Fu, X., Ge, S., Sun, T., Zhou, G., Jiang, D., et al. (2005). Ontogeny of expression of transforming growth factor-β and its receptors and their possible relationship with scarless healing in human fetal skin. Wound Repair Regen. 13 (1), 68–75. doi:10.1111/j.1067-1927.2005.130109.x
Chen, X., Chen, X., Shi, X., Gao, Z., and Guo, Z. (2020b). Curcumin attenuates endothelial cell fibrosis through inhibiting endothelial‐interstitial transformation. Clin. Exp. Pharmacol. Physiol. 47 (7), 1182–1192. doi:10.1111/1440-1681.13271
Chen, X., Feng, L., Li, S., Long, D., Shan, J., and Li, Y. (2020c). TGF-β1 maintains Foxp3 expression and inhibits glycolysis in natural regulatory T cells via PP2A-mediated suppression of mTOR signaling. Immunol. Lett. 226, 31–37. doi:10.1016/j.imlet.2020.06.016
Cho, Y., Johnson, D. W., Craig, J. C., Strippoli, G. F. M., Badve, S. V., and Wiggins, K. J. (2014). Biocompatible dialysis fluids for peritoneal dialysis. Cochrane Database Syst. Rev. 10 (10), CD007554. doi:10.1002/14651858.cd007554.pub2
Chung, C.-C., Kao, Y.-H., Liou, J.-P., and Chen, Y.-J. (2014). Curcumin suppress cardiac fibroblasts activities by regulating proliferation, migration, and the extracellular matrix. Acta Cardiol. Sin. 30 (5), 474–482.
Dai, M., Zheng, X., Xu, X., Zheng, X., Li, X., Guo, G., et al. (2009). Chitosan-alginate sponge: preparation and application in curcumin delivery for dermal wound healing in rat. BioMed Res. Int. 2009, 1–8. doi:10.1155/2009/595126
Dai, N.-T., Williamson, M. R., Khammo, N., Adams, E. F., and Coombes, A. G. A. (2004). Composite cell support membranes based on collagen and polycaprolactone for tissue engineering of skin. Biomaterials 25, 4263–4271.doi:10.1016/j.biomaterials.2003.11.022
Dartt, D. A., Dana, R., Amore, P., and Niederkorn, J. (2011). Immunology, inflammation and diseases of the eye. Cambridge, MA: Academic Press.
Dees, C., Pötter, S., Zhang, Y., Bergmann, C., Zhou, X., Luber, M., et al. (2020). TGF-β-induced epigenetic deregulation of SOCS3 facilitates STAT3 signaling to promote fibrosis. J. Clin. Invest. 130 (5), 2347–2363. doi:10.1172/JCI122462
Deljoo, S., Rabiee, N., and Rabiee, M. (2019). Curcumin-hybrid nanoparticles in drug delivery system. Asian J. Nanosci. Mater. 2 (1), 66–91. doi:10.26655/AJNANOMAT.2019.1.5
Deorukhkar, S., and Saini, S. (2013). Vulvovaginal candidiasis due to non albicans Candida: its species distribution and antifungal susceptibility profile. Int. J. Curr. Microbiol. App. Sci. 2 (3), 323–328. doi:10.5455/ijmsph.2013.080320131
Derynck, R., and Budi, E. H. (2019). Specificity, versatility, and control of TGF-β family signaling. Sci. Signal. 12 (570), eaav5183. doi:10.1126/scisignal.aav5183
Derynck, R., and Zhang, Y. E. (2003). Smad-dependent and Smad-independent pathways in TGF-β family signalling. Nature 425 (6958), 577–584. doi:10.1038/nature02006
Di Conza, G., and Ho, P. C. (2020). ER stress responses: an emerging modulator for innate immunity. Cells 9 (3), 695. doi:10.3390/cells9030695
Dinesh, P., and Rasool, M. (2019). Berberine mitigates IL-21/IL-21R mediated autophagic influx in fibroblast-like synoviocytes and regulates Th17/Treg imbalance in rheumatoid arthritis. Apoptosis 24 (7-8), 644–661. doi:10.1007/s10495-019-01548-6
Dogaru, G., Elena Bulboaca, A., Gheban, D., Mihai Boarescu, P., Rus, V., Festila, D., et al. (2020). Effect of liposomal curcumin on acetaminophen hepatotoxicity by down-regulation of oxidative stress and matrix metalloproteinases. Vivo 34 (2), 569–582. doi:10.21873/invivo.11809
Dolati, S., Babaloo, Z., Ayromlou, H., Ahmadi, M., Rikhtegar, R., Rostamzadeh, D., et al. (2019). Nanocurcumin improves regulatory T-cell frequency and function in patients with multiple sclerosis. J. Neuroimmunol. 327, 15–21. doi:10.1016/j.jneuroim.2019.01.007
Dorai, T., Diouri, J., O’Shea, O., and Doty, S. B. (2014). Curcumin inhibits prostate cancer bone metastasis by up-regulating bone morphogenic protein-7 in vivo. J. Canc. Ther. 05 (04), 369. doi:10.4236/jct.2014.54044
Eatemadi, A., Daraee, H., Karimkhanloo, H., Kouhi, M., Zarghami, N., Akbarzadeh, A., et al. (2014). Carbon nanotubes: properties, synthesis, purification, and medical applications. Nanoscale Res. Lett. 9, 393. doi:10.1186/1556-276x-9-393
El-Naggar, M. E., Al-Joufi, F., Anwar, M., Attia, M. F., and El-Bana, M. A. (2019). Curcumin-loaded PLA-PEG copolymer nanoparticles for treatment of liver inflammation in streptozotocin-induced diabetic rats. Colloids Surf. B Biointerfaces 177, 389–398. doi:10.1016/j.colsurfb.2019.02.024
Elias, J. A., Rossman, M. D., Zurier, R. B., and Daniele, R. P. (1985). Human alveolar macrophage inhibition of lung fibroblast growth: a prostaglandin-dependent process. Am. Rev. Respir. Dis. 131 (1), 94–99. doi:10.1164/arrd.1985.131.1.94
Engelkes, M., de Ridder, M. A. J., Svensson, E., Berencsi, K., Prieto-Alhambra, D., Lapi, F., et al. (2020). Multinational cohort study of mortality in patients with asthma and severe asthma. Respir. Med. 165, 105919. doi:10.1016/j.rmed.2020.105919
Eshaghian, A., Al-Joufi, F., Anwar, M., Attia, M. F., and El-Bana, M. A. (2018). Curcumin attenuates hepatic fibrosis and insulin resistance induced by bile duct ligation in rats. Br. J. Nutr. 120, 393–403. doi:10.1017/s0007114518001095
Esmaeilzadeh, E., Soleimani, M., Zare‐Abdollahi, D., Jameie, B., and Khorram Kohrshid, H. R. (2019). Curcumin ameliorates experimental autoimmune encephalomyelitis in a C57BL/6 mouse model. Drug Dev. Res. 80, 629–636. doi:10.1002/ddr.21540
Esposito, T., Lucariello, A., Hay, E., Contieri, M., Tammaro, P., Varriale, B., et al. (2019). Effects of curcumin and its adjuvant on TPC1 thyroid cell line. Chem. Biol. Interact. 305, 112–118. doi:10.1016/j.cbi.2019.03.031
Falanga, V. (2005). Wound healing and its impairment in the diabetic foot. Lancet 366 (9498), 1736–1743. doi:10.1016/s0140-6736(05)67700-8
Fang, L., Li, Y., Wang, S., Li, Y., Chang, H.-M., Yi, Y., et al. (2020). TGF-beta1 induces VEGF expression in human granulosa-lutein cells: a potential mechanism for the pathogenesis of ovarian hyperstimulation syndrome. Exp. Mol. Med. 52 (3), 450–460. doi:10.1038/s12276-020-0396-y
Farhood, B., Mortezaee, K., Goradel, N. H., Khanlarkhani, N., Salehi, E., Nashtaei, M. S., et al. (2019b). Curcumin as an anti‐inflammatory agent: implications to radiotherapy and chemotherapy. J. Cell. Physiol. 234 (5), 5728–5740. doi:10.1002/jcp.27442
Farhood, B., Goradel, N. H., Mortezaee, K., Khanlarkhani, N., Najafi, M., and Sahebkar, A. (2019a). Melatonin and cancer: from the promotion of genomic stability to use in cancer treatment. J. Cell. Physiol. 234 (5), 5613–5627. doi:10.1002/jcp.27391
Farhood, B., Khodamoradi, E., Hoseini-Ghahfarokhi, M., Motevaseli, E., Mirtavoos-Mahyari, H., Musa, A. E., et al. (2020). TGF-β in radiotherapy: mechanisms of tumor resistance and normal tissues injury. Pharmacol. Res. 155, 104745. doi:10.1016/j.phrs.2020.104745
Farooqi, A. A., Fuentes-Mattei, E., Fayyaz, S., Raj, P., Goblirsch, M., Poltronieri, P., et al. (2019b). Interplay between epigenetic abnormalities and deregulated expression of microRNAs in cancer. Semin. Cancer Biol. 58, 47–55. doi:10.1016/j.semcancer.2019.02.003
Farooqi, A. A., de la Roche, M., Djamgoz, M. B. A., and Siddik, Z. H. (2019a). Overview of the oncogenic signaling pathways in colorectal cancer: mechanistic insights. Semin. Cancer Biol. 58, 65–79. doi:10.1016/j.semcancer.2019.01.001
Farooqi, A. A., Adylova, A., Sabitaliyevich, U. Y., Attar, R., Sohail, M. I., and Yilmaz, S. (2020). Recent updates on true potential of an anesthetic Agent as a regulator of cell signaling pathways and non-coding RNAs in different cancers: focusing on the brighter side of propofol. Gene 737, 144452. doi:10.1016/j.gene.2020.144452
Fayyaz, S., Javed, Z., Attar, R., Farooqi, A. A., Yaylim, I., and Ahmad, A. (2019). MicroRNA regulation of TRAIL mediated signaling in different cancers: control of micro steering wheels during the journey from bench-top to the bedside. Semin. Cancer Biol. 58, 56–64. doi:10.1016/j.semcancer.2019.01.007
Fezza, M., Moussa, M., Aoun, R., Haber, R., and Hilal, G. (2019). DKK1 promotes hepatocellular carcinoma inflammation, migration and invasion: implication of TGF-β1. PLoS One 14 (9), e0223252. doi:10.1371/journal.pone.0223252
Fine, A., Poliks, C. F., Donahue, L. P., Smith, B. D., and Goldstein, R. H. (1989). The differential effect of prostaglandin E2 on transforming growth factor-beta and insulin-induced collagen formation in lung fibroblasts. J. Biol. Chem. 264 (29), 16988–16991
Finnson, K., Parker, W. L., Chi, Y., Hoemann, C. D., Goldring, M. B., Antoniou, J., et al. (2010). Endoglin differentially regulates TGF-β-induced Smad2/3 and Smad1/5 signalling and its expression correlates with extracellular matrix production and cellular differentiation state in human chondrocytes. Osteoarthr. Cartil. 18 (11), 1518–1527. doi:10.1016/j.joca.2010.09.002
Finnson, K. W., Parker, W. L., Ten Dijke, P., Thorikay, M., and Philip, A. (2008). ALK1 opposes ALK5/Smad3 signaling and expression of extracellular matrix components in human chondrocytes. J. Bone Miner. Res. 23 (6), 896–906. doi:10.1359/jbmr.080209
Finnson, K. W., Almadani, Y., and Philip, A. (2019). Non-canonical (non-SMAD2/3) TGF-β signaling in fibrosis: mechanisms and targets. Semin. Cell Dev. Biol. 101, 115–122. doi:10.1016/j.semcdb.2019.11.013
Fionda, C., Stabile, H., Cerboni, C., Soriani, A., Gismondi, A., Cippitelli, M., et al. (2020). Hitting more birds with a stone: impact of TGF-beta on ILC activity in cancer. J. Clin. Med. 9 (1), 143. doi:10.3390/jcm9010143
Fu, X.-y., Zhang, D.-w., Li, Y.-d., Zhao, P.-w., Tang, Y.-q., Niu, J.-z., et al. (2015). Curcumin treatment suppresses CCR7 expression and the differentiation and migration of human circulating fibrocytes. Cell. Physiol. Biochem. 35 (2), 489–498. doi:10.1159/000369714
Fujio, K., Okamura, T., and Yamamoto, K. (2010). “Chapter 4 - the family of IL-10-secreting CD4+ T cells,” in Advances in immunology. Editor F. W. Alt (Cambridge, MA: Academic Press), Vol. 105, 99–130. doi:10.1016/S0065-2776(10)05004-2
Funamoto, M., Shimizu, K., Sunagawa, Y., Katanasaka, Y., Miyazaki, Y., Kakeya, H., et al. (2019). Effects of highly absorbable curcumin in patients with impaired glucose tolerance and non-insulin-dependent diabetes mellitus. J Diabetes Res. 2019, 1–7. doi:10.1155/2019/8208237
Gao, J., Huang, Y., Li, P., Xu, D., Li, J., Liu, Y., et al. (2011). Antifibrosis effects of total glucosides of Danggui–Buxue–Tang in a rat model of bleomycin-induced pulmonary fibrosis. J. Ethnopharmacol. 136 (1), 21–26. doi:10.1016/j.jep.2011.03.013
Gao, L., Yuan, H., Xu, E., and Liu, J. (2020a). Toxicology of paraquat and pharmacology of the protective effect of 5-hydroxy-1-methylhydantoin on lung injury caused by paraquat based on metabolomics. Sci. Rep. 10 (1), 1790. doi:10.1038/s41598-020-58599-y
Gao, M., Zheng, J., Zheng, C., Huang, Z., and Huang, Q. (2020b). Theacrine alleviates chronic inflammation by enhancing TGF-β-mediated shifts via TGF-β/SMAD pathway in Freund's incomplete adjuvant-induced rats. Biochem. Biophys. Res. Commun. 522 (3), 743–748. doi:10.1016/j.bbrc.2019.11.126
Ge, S., Zhang, H., Deng, T., Sun, W., Ning, T., Fan, Q., et al. (2019). MiR-181a, a new regulator of TGF-β signaling, can promote cell migration and proliferation in gastric cancer. Invest. N. Drugs 37 (5), 923–934. doi:10.1007/s10637-018-0695-5
Ghasemi, F., Shafiee, M., Banikazemi, Z., Pourhanifeh, M. H., Khanbabaei, H., Shamshirian, A., et al. (2019). Curcumin inhibits NF-kB and Wnt/β-catenin pathways in cervical cancer cells. Pathol. Res. Pract. 215 (10), 152556. doi:10.1016/j.prp.2019.152556
Godwin, M. A., Mahithashri, K., Jeba Shiney, O., Bhagat, M., and Praseetha, P. K. (2019). Metal incorporated g-C3N4 nanosheets as potential cytotoxic agents for promoting free radical scavenging in cancer cell lines. J. Nanosci. Nanotechnol. 19 (9), 5448–5455. doi:10.1166/jnn.2019.16572
Gopinath, D., Rafiuddin Ahmed, M., Gomathi, K., Chitra, K., Sehgal, P. K., and Jayakumar, R. (2004). Dermal wound healing processes with curcumin incorporated collagen films. Biomaterials 25 (10), 1911–1917. doi:10.1016/s0142-9612(03)00625-2
Gross, T. J., and Hunninghake, G. W. (2001). Idiopathic pulmonary fibrosis. N. Engl. J. Med. 345, 517–525. doi:10.1056/nejmra003200
Guan, S., Yu, P., Cao, J., Xi, X., Zhang, Q., Zhu, C., et al. (2020). Ginsenoside Rg1 protects against cigarette smoke-induced airway remodeling by suppressing the TGF-beta1/Smad3 signaling pathway. Am. J. Transl. Res. 12 (2), 493–506.
Gui, Y., Sun, J., You, W., Wei, Y., Tian, H., and Jiang, S. (2020). Glycyrrhizin suppresses epithelial-mesenchymal transition by inhibiting high-mobility group box1 via the TGF-beta1/Smad2/3 pathway in lung epithelial cells. PeerJ 8, e8514. doi:10.7717/peerj.8514
Guimaraes-Stabili, M. R., de Aquino, S. G., de Almeida Curylofo, F., Olga Tasso, C., Godoy Rocha, F. R., de Medeiros, M. C., et al. (2019). Systemic administration of curcumin or piperine enhances the periodontal repair: a preliminary study in rats. Clin. Oral Invest. 23 (8), 3297–3306. doi:10.1007/s00784-018-2755-9
Guo, J.-m., Makvandi, P., Wei, C.-c., Chen, J.-h., Xu, H.-k., Breschi, L., et al. (2019). Polymer conjugation optimizes EDTA as a calcium-chelating agent that exclusively removes extrafibrillar minerals from mineralized collagen. Acta Biomater. 90, 424–440. doi:10.1016/j.actbio.2019.04.011
Guo, S., Meng, X.-w., Yang, X.-s., Liu, X.-f., Ou-Yang, C.-h., and Liu, C. (2018). Curcumin administration suppresses collagen synthesis in the hearts of rats with experimental diabetes. Acta Pharmacol. Sin. 39 (2), 195–204. doi:10.1038/aps.2017.92
Gupta, S. C., Patchva, S., Koh, W., and Aggarwal, B. B. (2012). Discovery of curcumin, a component of golden spice, and its miraculous biological activities. Clin. Exp. Pharmacol. Physiol. 39, 283–299. doi:10.1111/j.1440-1681.2011.05648.x
Gupta, S., Ghosh, S., Gupta, S., and Sakhuja, P. (2017). Effect of curcumin on the expression of p53, transforming growth factor‐β, and inducible nitric oxide synthase in oral submucous fibrosis: a pilot study. J Investig. Clin. Dent. 8, e12252. doi:10.1111/jicd.12252
Hadi, A., Pourmasoumi, M., Ghaedi, E., and Sahebkar, A. (2019). The effect of curcumin/turmeric on blood pressure modulation: a systematic review and meta-analysis. Pharmacol. Res. 150, 104505. doi:10.1016/j.phrs.2019.104505
Hallajzadeh, J., Milajerdi, A., Kolahdooz, F., Amirani, E., Mirzaei, H., and Asemi, Z. (2019). The effects of curcumin supplementation on endothelial function: a systematic review and meta‐analysis of randomized controlled trials. Phytother Res. 33, 2989–2995. doi:10.1002/ptr.6477
Hata, A., Lagna, G., Massague, J., and Hemmati-Brivanlou, A. (1998). Smad6 inhibits BMP/Smad1 signaling by specifically competing with the Smad4 tumor suppressor. Genes Dev. 12, 186–197. doi:10.1101/gad.12.2.186
Hatcher, H., Planalp, R., Cho, J., Torti, F. M., and Torti, S. V. (2008). Curcumin: from ancient medicine to current clinical trials. Cell. Mol. Life Sci. 65, 1631–1652. doi:10.1007/s00018-008-7452-4
Hay, E., Lucariello, A., Contieri, M., Esposito, T., De Luca, A., Guerra, G., et al. (2019). Therapeutic effects of turmeric in several diseases: an overview. Chem. Biol. Interact. 310, 108729. doi:10.1016/j.cbi.2019.108729
Heldin, C.-H., and Moustakas, A. (2016). Signaling receptors for TGF-β family members. Cold Spring Harb. Perspect. Biol. 8, a005900. doi:10.1101/cshperspect.a005900
Hesari, A., Azizian, M., Sheikhi, A., Nesaei, A., Sanaei, S., Mahinparvar, N., et al. (2019). Chemopreventive and therapeutic potential of curcumin in esophageal cancer: current and future status, Int. J. Cancer 144, 1215–1226. doi:10.1002/ijc.31947
Hill, C. S. (2009). Nucleocytoplasmic shuttling of Smad proteins. Cell Res. 19, 36–46. doi:10.1038/cr.2008.325
Hinz, B., Phan, S. H., Thannickal, V. J., Galli, A., Bochaton-Piallat, M.-L., and Gabbiani, G. (2007). The myofibroblast. Am. J. Pathol. 170, 1807–1816. doi:10.2353/ajpath.2007.070112
Ho, C., Hsu, Y.-C., Lei, C.-C., Mau, S.-C., Shih, Y.-H., and Lin, C.-L. (2016). Curcumin rescues diabetic renal fibrosis by targeting superoxide-mediated Wnt signaling pathways. Am. J. Med. Sci. 351, 286–295. doi:10.1016/j.amjms.2015.12.017
Horbelt, D., Denkis, A., and Knaus, P. (2012). A portrait of Transforming Growth Factor β superfamily signalling: background matters. Int. J. Biochem. Cell Biol. 44, 469–474. doi:10.1016/j.biocel.2011.12.013
Horn, L. A., Riskin, J., Hempel, H. A., Fousek, K., Lind, H., Hamilton, D. H., et al. (2020). Simultaneous inhibition of CXCR1/2, TGF-beta, and PD-L1 remodels the tumor and its microenvironment to drive antitumor immunity. J. Immunother. Cancer 8 (1). doi:10.1136/jitc-2019-000326
Hou, Z., Yan, W., Li, T., Wu, W., Cui, Y., Zhang, X., et al. (2019). Lactic acid-mediated endothelial to mesenchymal transition through TGF-beta1 contributes to in-stent stenosis in poly-L-lactic acid stent. Int. J. Biol. Macromol. 155, 1589–1598. doi:10.1016/j.ijbiomac.2019.11.136
Houthuijzen, J. M., and Jonkers, J. (2018). Cancer-associated fibroblasts as key regulators of the breast cancer tumor microenvironment. Canc. Metastasis Rev. 37, 577–597. doi:10.1007/s10555-018-9768-3
Hu, Y., He, Y., Ji, J., Zheng, S., and Cheng, Y. (2020). Tumor targeted curcumin delivery by folate-modified MPEG-PCL self-assembly micelles for colorectal cancer therapy. Ijn 15, 1239–1252. doi:10.2147/ijn.s232777
Hu, Y., Li, M., Zhang, M., and Jin, Y. (2018). Inhalation treatment of idiopathic pulmonary fibrosis with curcumin large porous microparticles. Int. J. Pharm. 551, 212–222. doi:10.1016/j.ijpharm.2018.09.031
Hu, Y., Tang, J.-S., Hou, S.-X., Shi, X.-X., Qin, J., Zhang, T.-S., et al. (2017). Neuroprotective effects of curcumin alleviate lumbar intervertebral disc degeneration through regulating the expression of iNOS, COX-2, TGF-β1/2, MMP-9 and BDNF in a rat model. Mol. Med. Rep. 16, 6864–6869. doi:10.3892/mmr.2017.7464
Huang, S. K., White, E. S., Wettlaufer, S. H., Grifka, H., Hogaboam, C. M., Thannickal, V. J., et al. (2009). Prostaglandin E 2 induces fibroblast apoptosis by modulating multiple survival pathways. FASEB J. 23, 4317–4326. doi:10.1096/fj.08-128801
Jamalzaei, P., Valojerdi, M. R., Montazeri, L., and Baharvand, H. (2020). Effects of alginate concentration and ovarian cells on in vitro development of mouse preantral follicles: a factorial study. Int. J. Fertil. Steril. 13 (4), 330–338. doi:10.22074/ijfs.2020.5746
Janulaityte, I., Januskevicius, A., Kalinauskaite-Zukauske, V., Bajoriuniene, I., and Malakauskas, K. (2020). In Vivo allergen-activated eosinophils promote collagen I and fibronectin gene expression in airway smooth muscle cells via TGF-beta1 signaling pathway in asthma. Int. J. Mol. Sci. 21 (5). doi:10.3390/ijms21051837
Jiang, D., Singh, K., Muschhammer, J., Schatz, S., Sindrilaru, A., Makrantonaki, E., et al. (2020). MSCs rescue impaired wound healing in a murine LAD1 model by adaptive responses to low TGF-β1 levels. EMBO Rep., 21 (4), e49115. doi:10.15252/embr.201949115
Jin, S., Gao, J., Qi, Y., Hao, Y., Li, X., Liu, Q., et al. (2020). TGF-β1 fucosylation enhances the autophagy and mitophagy via PI3K/Akt and Ras-Raf-MEK-ERK in ovarian carcinoma. Biochem. Biophys. Res. Commun. 524, 970–976. doi:10.1016/j.bbrc.2020.02.028
Jolliffe, D. A., Stefanidis, C., Wang, Z., Kermani, N. Z., Dimitrov, V., White, J. H., et al. (2020). Vitamin D metabolism is dysregulated in asthma and chronic obstructive pulmonary disease. Am. J. Respir. Crit. Care Med. 202 (3), 371–382. doi:10.1164/rccm.201909-1867OC
Jung, J. G., and Le, A. (2018). Targeting metabolic cross talk between cancer cells and cancer-associated fibroblasts. Adv. Exp. Med. Biol. 1063, 167–178. doi:10.1007/978-3-319-77736-8_12
Kalirajan, C., and Palanisamy, T. (2019). A ZnO-curcumin nanocomposite embedded hybrid collagen scaffold for effective scarless skin regeneration in acute burn injury. J. Mater. Chem. B 7, 5873–5886. doi:10.1039/c9tb01097a
Kandagalla, S., Sharath, B. S., Bharath, B. R., Hani, U., and Manjunatha, H. (2017). Molecular docking analysis of curcumin analogues against kinase domain of ALK5. Silico Pharmacol. 5, 15. doi:10.1007/s40203-017-0034-0
Kant, V., Gopal, A., Kumar, D., Pathak, N. N., Ram, M., Jangir, B. L., et al. (2015). Curcumin-induced angiogenesis hastens wound healing in diabetic rats. J. Surg. Res. 193, 978–988. doi:10.1016/j.jss.2014.10.019
Katta, S., Srivastava, A., Thangapazham, R. L., Rosner, I. L., Cullen, J., Li, H., et al. (2019). Curcumin-gene expression response in hormone dependent and independent metastatic prostate cancer cells. Int. J. Mol. Sci. 20 (19), 4891. doi:10.3390/ijms20194891
Keshk, W. A., Elseady, W. S., Sarhan, N. I., and Zineldeen, D. H. (2020). Curcumin attenuates cytoplasmic/endoplasmic reticulum stress, apoptosis and cholinergic dysfunction in diabetic rat hippocampus. Metab. Brain Dis. 35 (4), 637–647. doi:10.1007/s11011-020-00551-0
Khan, I., Kumar, N., Pant, I., Narra, S., and Kondaiah, P. (2012). Activation of TGF-β pathway by areca nut constituents: a possible cause of oral submucous fibrosis. PLoS One 7 (12), e51806. doi:10.1371/journal.pone.0051806
Kharat, M., Skrzynski, M., Decker, E. A., and McClements, D. J. (2020). Enhancement of chemical stability of curcumin-enriched oil-in-water emulsions: impact of antioxidant type and concentration. Food Chem. 320, 126653. doi:10.1016/j.foodchem.2020.126653
Kim, S. J., Park, J.-H., Lee, S.-A., Lee, J.-G., Shin, J.-M., and Lee, H.-M. (2020). All-trans retinoic acid regulates TGF-beta1-induced extracellular matrix production via p38, JNK, and NF-kappaB-signaling pathways in nasal polyp-derived fibroblasts. Int. Forum Allergy Rhinol. 10 (5), 636–645. doi:10.1002/alr.22525
Kim, S. K., Seok, H., Park, H. J., Jeon, H. S., Kang, S. W., Lee, B.-C., et al. (2015). Inhibitory effect of curcumin on testosterone induced benign prostatic hyperplasia rat model. BMC Complementary and Alternative Medicine 15 (1), 380. doi:10.1186/s12906-015-0825-y
Kirchhof, P., Benussi, S., Kotecha, D., Ahlsson, A., Atar, D., Casadei, B., et al. (2016). 2016 ESC Guidelines for the management of atrial fibrillation developed in collaboration with EACTS. Eur. J. Cardio. Thorac. Surg. 50, e1–e88. doi:10.1093/ejcts/ezw313
Kocaadam, B., and Şanlier, N. (2017). Curcumin, an active component of turmeric (Curcuma longa), and its effects on health. Crit. Rev. Food Sci. Nutr. 57, 2889–2895. doi:10.1080/10408398.2015.1077195
Kolodsick, J. E., Peters-Golden, M., Larios, J., Toews, G. B., Thannickal, V. J., and Moore, B. B. (2003). Prostaglandin E2Inhibits fibroblast to myofibroblast transition via E. prostanoid receptor 2 signaling and cyclic adenosine monophosphate elevation. Am. J. Respir. Cell Mol. Biol. 29, 537–544. doi:10.1165/rcmb.2002-0243oc
Komolkriengkrai, M., Nopparat, J., Vongvatcharanon, U., Anupunpisit, V., and Khimmaktong, W. (2019). Effect of glabridin on collagen deposition in liver and amelioration of hepatocyte destruction in diabetes rats. Exp. Ther. Med. 18, 1164–1174. doi:10.3892/etm.2019.7664
Kong, D., Zhang, F., Shao, J., Wu, L., Zhang, X., Chen, L., et al. (2015). Curcumin inhibits cobalt chloride-induced epithelial-to-mesenchymal transition associated with interference with TGF-β/Smad signaling in hepatocytes. Lab. Invest. 95, 1234–1245. doi:10.1038/labinvest.2015.107
Kumari, A., Dash, D., and Singh, R. (2017). Curcumin inhibits lipopolysaccharide (LPS)-induced endotoxemia and airway inflammation through modulation of sequential release of inflammatory mediators (TNF-α and TGF-β1) in murine model. Inflammopharmacol. 25, 329–341. doi:10.1007/s10787-017-0334-3
Kumari, A., Singh, D. K., Dash, D., and Singh, R. (2019). Intranasal curcumin protects against LPS-induced airway remodeling by modulating toll-like receptor-4 (TLR-4) and matrixmetalloproteinase-9 (MMP-9) expression via affecting MAP kinases in mouse model. Inflammopharmacol. 27, 731–748. doi:10.1007/s10787-018-0544-3
Kunnumakkara, A. B., Bordoloi, D., Padmavathi, G., Monisha, J., Roy, N. K., Prasad, S., et al. (2017). Curcumin, the golden nutraceutical: multitargeting for multiple chronic diseases. Br. J. Pharmacol. 174, 1325–1348. doi:10.1111/bph.13621
Kuszewski, J. C., Wong, R. H. X., Wood, L. G., and Howe, P. R. C. (2019). Effects of fish oil and curcumin supplementation on cerebrovascular function in older adults: a randomized controlled trial. Nutr. Metabol. Cardiovasc. Dis. 30 (4), 625–633. doi:10.1016/j.numecd.2019.12.010
Lai, X.-N., Li, J., Tang, L.-B., Chen, W.-T., Zhang, L., and Xiong, L.-X. (2020). MiRNAs and LncRNAs: dual roles in TGF-β signaling-regulated metastasis in lung cancer. Ijms 21, 1193. doi:10.3390/ijms21041193
Lampe, V., and Milobedzka, J. (1913). Studien über Curcumin. Ber. Dtsch. Chem. Ges. 46, 2235–2240. doi:10.1002/cber.191304602149
Lee, H., O'Meara, S. J., O'Brien, C., and Kane, R. (2007). The role of gremlin, a BMP antagonist, and epithelial-to-mesenchymal transition in proliferative vitreoretinopathy. Invest. Ophthalmol. Vis. Sci. 48, 4291–4299. doi:10.1167/iovs.07-0086
Lee, J.-E., Yoon, S. S., Lee, J.-W., and Moon, E.-Y. (2020a). Curcumin-induced cell death depends on the level of autophagic flux in A172 and U87MG human glioblastoma cells. Chin. J. Nat. Med. 18, 114–122. doi:10.1016/s1875-5364(20)30012-1
Lee, J. H., Mohan, C. D., Deivasigamani, A., Jung, Y. Y., Rangappa, S., Basappa, S., et al. (2020d). Brusatol suppresses STAT3-driven metastasis by downregulating epithelial-mesenchymal transition in hepatocellular carcinoma. J. Adv. Res. 26, 83–94. doi:10.1016/j.jare.2020.07.004
Lee, J. H., Mellado-Gil, J. M., Bahn, Y. J., Pathy, S. M., Zhang, Y. E., and Rane, S. G. (2020b). Protection from beta-cell apoptosis by inhibition of TGF-beta/Smad3 signaling. Cell Death Dis. 11 (3), 184. doi:10.1038/s41419-020-2365-8
Lee, J. H., Mellado-Gil, J. M., Bahn, Y. J., Pathy, S. M., Zhang, Y. E., and Rane, S. G. (2020c). Protection from β-cell apoptosis by inhibition of TGF-β/Smad3 signaling. Cell Death Dis. 11 (3), 184. doi:10.1038/s41419-020-2365-8
Lee, S. H., and Choi, J.-H. (2018). Involvement of inflammatory responses in the early development of calcific aortic valve disease: lessons from statin therapy. Anim. Cell Syst. 22, 390–399. doi:10.1080/19768354.2018.1528175
Li, C., Ao, H., Chen, G., Wang, F., and Li, F. (2019). The interaction of CDH20 with β-catenin inhibits cervical cancer cell migration and invasion via TGF-β/smad/SNAIL mediated EMT. Front. Oncol. 9, 1481. doi:10.3389/fonc.2019.01481
Li, J., Qin, X., Wu, R., Wan, L., Zhang, L., and Liu, R. (2020a). Circular RNA circFBXO11 modulates hepatocellular carcinoma progress and oxaliplatin resistance through miR-605/FOXO3/ABCB1 axis. J. Cell Mol. Med. 24 (9), 5152–5161. doi:10.1111/jcmm.15162
Li, J., Qu, X., and Bertram, J. F. (2009). Endothelial-myofibroblast transition contributes to the early development of diabetic renal interstitial fibrosis in streptozotocin-induced diabetic mice. Am. J. Pathol. 175, 1380–1388. doi:10.2353/ajpath.2009.090096
Li, R., Lin, Z., Zhang, Q., Zhang, Y., Liu, Y., Lyu, Y., et al. (2020b). Injectable and in-situ formable thiolated chitosan coated liposomal hydrogels as curcumin carriers for prevention of in vivo breast cancer recurrence. ACS Appl. Mater. Interfaces 12 (15), 17936–17948. doi:10.1021/acsami.9b21528
Li, S.-N., and Wu, J.-F. (2020). TGF-β/SMAD signaling regulation of mesenchymal stem cells in adipocyte commitment. Stem Cell Res. Ther. 11, 41. doi:10.1186/s13287-020-1552-y
Liao, J.-k., Zhou, B., Zhuang, X.-m., Zhuang, P.-l., Zhang, D.-m., and Chen, W.-l. (2018). Cancer-associated fibroblasts confer cisplatin resistance of tongue cancer via autophagy activation. Biomed. Pharmacother. 97, 1341–1348. doi:10.1016/j.biopha.2017.11.024
Liczbiński, P., Michałowicz, J., and Bukowska, B. (2020). Molecular mechanism of curcumin action in signaling pathways: review of the latest research. Phytother Res. 34 (8), 1992–2005. doi:10.1002/ptr.6663
Lin, Y.-T., and Wu, K.-J. (2020). Epigenetic regulation of epithelial-mesenchymal transition: focusing on hypoxia and TGF-β signaling. J. Biomed. Sci. 27, 1–10. doi:10.1186/s12929-020-00632-3
Lippman, S. M., and Hong, W. K. (2001). Molecular markers of the risk of oral cancer. N. Engl. J. Med. 344 (17), 1323–1326. doi:10.1056/nejm200104263441710
Liu, D., Gong, L., Zhu, H., Pu, S., Wu, Y., Zhang, W., et al. (2016a). Curcumin inhibits transforming growth factor beta induced differentiation of mouse lung fibroblasts to myofibroblasts. Front. Pharmacol. 7, 419. doi:10.3389/fphar.2016.00419
Liu, D., Qin, H., Yang, B., Du, B., and Yun, X. (2020a). Oridonin ameliorates carbon tetrachloride-induced liver fibrosis in mice through inhibition of the NLRP3 inflammasome. Drug Dev. Res. 81 (4), 526–533. doi:10.1002/ddr.21649
Liu, F., and Shang, Y.-X. (2020). Sirtuin 6 attenuates epithelial-mesenchymal transition by suppressing the TGF-β1/Smad3 pathway and c-Jun in asthma models. Int. Immunopharm. 82, 106333. doi:10.1016/j.intimp.2020.106333
Liu, H., Liu, A., Shi, C., and Li, B. (2016b). Curcumin suppresses transforming growth factor-β1-induced cardiac fibroblast differentiation via inhibition of Smad-2 and p38 MAPK signaling pathways. Exp. Ther. Med. 11, 998–1004. doi:10.3892/etm.2016.2969
Liu, Q., Zhu, L.-J., Waaga-Gasser, A. M., Ding, Y., Cao, M., Jadhav, S. J., et al. (2019). The axis of local cardiac endogenous Klotho-TGF-β1-Wnt signaling mediates cardiac fibrosis in human. J. Mol. Cell. Cardiol. 136, 113–124. doi:10.1016/j.yjmcc.2019.09.004
Liu, R., Wang, Q., Ding, Z., Zhang, X., Li, Y., Zang, Y., et al. (2020b). Silibinin augments the antifibrotic effect of valsartan through inactivation of TGF-beta1 signaling in kidney. Drug Des. Dev. Ther. 14, 603–611. doi:10.2147/dddt.s224308
Liu, T., and Feng, X.-H. (2010). Regulation of TGF-β signalling by protein phosphatases. Biochem. J. 430, 191–198. doi:10.1042/bj20100427
Liu, X., Ma, J., Dong, X.-D., Xiang, L.-T., Gu, Q.-R., Du, Z.-Y., et al. (2017). Protective effects of curcumin analogue L6H4 on kidney from type 2 diabetic rats. Zhongguo Ying Yong Sheng Li Xue Za Zhi 33, 11–15. doi:10.12047/j.cjap.5448.2017.003
Liu, Z., Wang, X., Li, L., Wei, G., and Zhao, M. (2020c). Hydrogen sulfide protects against paraquat-induced acute liver injury in rats by regulating oxidative stress, mitochondrial function, and inflammation. Oxid. Med. Cell Longev. 2020, 1–16. doi:10.1155/2020/6325378
Lorenzo-Almoros, A., Tuñón, J., Orejas, M., Cortés, M., Egido, J., and Lorenzo, Ó. (2017). Diagnostic approaches for diabetic cardiomyopathy. Cardiovasc. Diabetol. 16, 28. doi:10.1186/s12933-017-0506-x
Losuwannarak, N., Maiuthed, A., Kitkumthorn, N., Leelahavanichkul, A., Roytrakul, S., and Chanvorachote, P. (2019). Gigantol targets cancer stem cells and destabilizes tumors via the suppression of the PI3K/AKT and JAK/STAT pathways in ectopic lung cancer xenografts. Cancers 11 (12), 2032. doi:10.3390/cancers11122032
Lu, A., Wang, W., Wang-Renault, S.-F., Ring, B. Z., Tanaka, Y., Weng, J., et al. (2020). 5-Aza-2'-deoxycytidine advances EMT of breast cancer cells by demethylating Sipa1 promoter-proximal elements. J. Cell Sci. 133 (9), jcs236125. doi:10.1242/jcs.236125
Lu, Q., Wang, W.-W., Zhang, M.-Z., Ma, Z.-X., Qiu, X.-R., Shen, M., et al. (2019). ROS induces epithelial-mesenchymal transition via the TGF-beta1/PI3K/Akt/mTOR pathway in diabetic nephropathy. Exp. Ther. Med. 17, 835–846. doi:10.3892/etm.2018.7014
Lu, W., Jiang, J.-P., Hu, J., Wang, J., and Zheng, M.-Z. (2015). Curcumin protects against lipopolysaccharide-induced vasoconstriction dysfunction via inhibition of thrombospondin-1 and transforming growth factor-β1. Exp. Ther. Med. 9 (2), 377–383. doi:10.3892/etm.2014.2105
Lucariello, A., Trabucco, E., Boccia, O., and Perna, A. (2015). Small leucine rich proteoglycans are differently distributed in normal and pathological endometrium. Vivo 29 (2), 217–222
Luo, Y., Ren, Z., Du, B., Xing, S., Huang, S., Li, Y., et al. (2019). Structure identification of ViceninII extracted from Dendrobium officinale and the reversal of TGF-beta1-induced Epithelial(-)Mesenchymal transition in lung adenocarcinoma cells through TGF-beta/smad and PI3K/Akt/mTOR signaling pathways. Molecules 24 (1), 144. doi:10.3390/molecules24010144
Ma, J., Ma, S.-y., and Ding, C.-h. (2017). Curcumin reduces cardiac fibrosis by inhibiting myofibroblast differentiation and decreasing transforming growth factor β1 and matrix metalloproteinase 9/tissue inhibitor of metalloproteinase 1. Chin. J. Integr. Med. 23 (5), 362–369. doi:10.1007/s11655-015-2159-5
Maddaluno, L., Rudini, N., Cuttano, R., Bravi, L., Giampietro, C., Corada, M., et al. (2013). EndMT contributes to the onset and progression of cerebral cavernous malformations. Nature 498 (7455), 492–496. doi:10.1038/nature12207
Maghmomeh, A. O., El-Gayar, A. M., El-Karef, A., and Abdel-Rahman, N. (2020). Arsenic trioxide and curcumin attenuate cisplatin-induced renal fibrosis in rats through targeting hedgehog signaling. N. Schmied. Arch. Pharmacol. 393 (3), 303–313. doi:10.1007/s00210-019-01734-y
Mahran, Y. F. (2020). New insights into the protection of growth hormone in cisplatin-induced nephrotoxicity: the impact of IGF-1 on the Keap1-Nrf2/HO-1 signaling. Life Sci. 253, 117581. doi:10.1016/j.lfs.2020.117581
Makvandi, P., Ali, G. W., Sala, F. D., Abdel-Fattah, W. I., and Borzacchiello, A. (2019). Biosynthesis and characterization of antibacterial thermosensitive hydrogels based on corn silk extract, hyaluronic acid and nanosilver for potential wound healing. Carbohydr. Polym. 223, 115023–115034. doi:10.1016/j.carbpol.2019.115023
Manning, G. (2002). The protein kinase complement of the human genome. Science 298 (5600), 1912–1934. doi:10.1126/science.1075762
Mao, X., Li, W., Chen, W., Li, Y., Wang, Q., Wang, X., et al. (2020). Exploring and characterizing a novel combination of paeoniflorin and talatizidine for the treatment of rheumatoid arthritis. Pharmacol. Res. 153, 104658. doi:10.1016/j.phrs.2020.104658
Mao, X., Xu, Z., Xu, X., Zeng, M., Zhao, Z., Zhang, Z., et al. (2019). TGF-β1 inhibits the autophagy of podocytes by activating mTORC1 in IgA nephropathy. Exp. Cell Res. 385 (1), 111670. doi:10.1016/j.yexcr.2019.111670
Mardani, R., Hamblin, M. R., Taghizadeh, M., Banafshe, H. R., Nejati, M., Mokhtari, M., et al. (2020). Nanomicellar-curcumin exerts its therapeutic effects via affecting angiogenesis, apoptosis, and T cells in a mouse model of melanoma lung metastasis. Pathol. Res. Pract. 216 (9), 153082. doi:10.1016/j.prp.2020.153082
Massagué, J. (1998). “TGF-β signal transduction.” in Annual reviews 4139 el camino way. Palo Alto, CA.
Mazala, D. A., Novak, J. S., Hogarth, M. W., Nearing, M., Adusumalli, P., Tully, C. B., et al. (2020). TGF-beta-driven muscle degeneration and failed regeneration underlie disease onset in a DMD mouse model. JCI Insight 5 (6). doi:10.1172/jci.insight.135703
Mehanny, M., Hathout, R. M., Geneidi, A. S., and Mansour, S. (2016). Exploring the use of nanocarrier systems to deliver the magical molecule; curcumin and its derivatives. J. Contr. Release 225, 1–30. doi:10.1016/j.jconrel.2016.01.018
Ming, J., Ye, J., Zhang, Y., Xu, Q., Yang, X., Shao, X., et al. (2020). Optimal dietary curcumin improved growth performance, and modulated innate immunity, antioxidant capacity and related genes expression of NF-κB and Nrf2 signaling pathways in grass carp (Ctenopharyngodon idella) after infection with Aeromonas hydrophila. Fish Shellfish Immunol. 97, 540–553. doi:10.1016/j.fsi.2019.12.074
Miyazono, K., and Heldin, C.-H. (1989). Role for carbohydrate structures inTGF-β1 latency. Nature 338, 158–160. doi:10.1038/338158a0
Mo, N., Li, Z.-Q., Li, J., and Cao, Y.-D. (2012). Curcumin inhibits TGF-β1-induced MMP-9 and invasion through ERK and Smad signaling in breast cancer MDA-MB-231 cells. Asian Pac. J. Cancer Prev. APJCP 13 (11), 5709–5714. doi:10.7314/apjcp.2012.13.11.5709
Moeini, A., Pedram, P., Makvandi, P., Malinconico, M., and d'Ayala, G. G. (2020). Wound healing and antimicrobial effect of active secondary metabolites in chitosan-based wound dressings: a review. Carbohydr. Polym. 233, 115839. doi:10.1016/j.carbpol.2020.115839
Mohajeri, M., Bianconi, V., Ávila-Rodriguez, M. F., Barreto, G. E., Jamialahmadi, T., Pirro, M., et al. (2020). Curcumin: a phytochemical modulator of estrogens and androgens in tumors of the reproductive system. Pharmacol. Res. 156, 104765. doi:10.1016/j.phrs.2020.104765
Mohankumar, K., Sridharan, S., Pajaniradje, S., Singh, V. K., Ronsard, L., Banerjea, A. C., et al. (2015). BDMC-A, an analog of curcumin, inhibits markers of invasion, angiogenesis, and metastasis in breast cancer cells via NF-κB pathway—a comparative study with curcumin. Biomed. Pharmacother. 74, 178–186. doi:10.1016/j.biopha.2015.07.024
Mora, A. L., Rojas, M., Pardo, A., and Selman, M. (2017). Emerging therapies for idiopathic pulmonary fibrosis, a progressive age-related disease. Nat. Rev. Drug Discov. 16 (11), 755. doi:10.1038/nrd.2017.170
Mortezaee, K., Potes, Y., Mirtavoos-Mahyari, H., Motevaseli, E., Shabeeb, D., Musa, A. E., et al. (2019b). Boosting immune system against cancer by melatonin: a mechanistic viewpoint. Life Sci. 238, 116960. doi:10.1016/j.lfs.2019.116960
Mortezaee, K., Salehi, E., Mirtavoos‐mahyari, H., Motevaseli, E., Najafi, M., Farhood, B., et al. (2019c). Mechanisms of apoptosis modulation by curcumin: implications for cancer therapy. J. Cell. Physiol. 234, 12537–12550. doi:10.1002/jcp.28122
Mortezaee, K., Najafi, M., Farhood, B., Ahmadi, A., Potes, Y., Shabeeb, D., et al. (2019a). Modulation of apoptosis by melatonin for improving cancer treatment efficiency: an updated review. Life Sci. 228, 228–241. doi:10.1016/j.lfs.2019.05.009
Mortezaee, K., Najafi, M., Farhood, B., Ahmadi, A., Shabeeb, D., and Musa, A. E. (2020). Resveratrol as an adjuvant for normal tissues protection and tumor sensitization. Curr. Cancer Drug Targets 20, 130–145. doi:10.2174/1568009619666191019143539
Moustakas, A., and Heldin, C.-H. (2005). Non-Smad TGF-β signals. J. Cell Sci. 118, 3573–3584. doi:10.1242/jcs.02554
Munakarmi, S., Chand, L., Shin, H. B., Jang, K. Y., and Jeong, Y. J. (2020). Indole-3-Carbinol derivative DIM mitigates carbon tetrachloride-induced acute liver injury in mice by inhibiting inflammatory response, apoptosis and regulating oxidative stress. Int. J. Mol. Sci. 21 (6), 2048. doi:10.3390/ijms21062048
Munoz, M. D., de la Fuente, N., and Sánchez-Capelo, A. (2020). TGF-beta/Smad3 signalling modulates GABA neurotransmission: implications in Parkinson's disease. Int. J. Mol. Sci. 21 (2), 590. doi:10.3390/ijms21020590
Najafi, M., Salehi, E., Farhood, B., Nashtaei, M. S., Goradel, N. H., Khanlarkhani, N., et al. (2019a). Adjuvant chemotherapy with melatonin for targeting human cancers: a review. J. Cell. Physiol. 234 (3), 2356–2372. doi:10.1002/jcp.27259
Najafi, M., Hooshangi Shayesteh, M. R., Mortezaee, K., Farhood, B., and Haghi-Aminjan, H. (2019b). The role of melatonin on doxorubicin-induced cardiotoxicity: a systematic review. Life Sci. 241, 117173. doi:10.1016/j.lfs.2019.117173
Nna, E., Madukwe, J., Egbujo, E., Obiorah, C., Okolie, C., Echejoh, G., et al. (2013). Gene expression of aurora kinases in prostate cancer and nodular hyperplasia tissues. Med. Princ. Pract. 22 (2), 138–143. doi:10.1159/000342679
Panahi, Y., Hosseini, M. S., Khalili, N., Naimi, E., Simental-Mendía, L. E., Majeed, M., et al. (2016). Effects of curcumin on serum cytokine concentrations in subjects with metabolic syndrome: a post-hoc analysis of a randomized controlled trial. Biomed. Pharmacother. 82, 578–582. doi:10.1016/j.biopha.2016.05.037
Pardali, E., Goumans, M.-J., and ten Dijke, P. (2010). Signaling by members of the TGF-β family in vascular morphogenesis and disease. Trends Cell Biol. 20 (9), 556–567. doi:10.1016/j.tcb.2010.06.006
Peng, X., Dai, C., Liu, Q., Li, J., and Qiu, J. (2018). Curcumin attenuates on carbon tetrachloride-induced acute liver injury in mice via modulation of the Nrf2/HO-1 and TGF-beta1/smad3 pathway. Molecules 23 (1), 215. doi:10.3390/molecules23010215
Perna, A., Luca, A. D., Adelfi, L., Pasquale, T., Varriale, B., and Esposito, T. (2018). Effects of different extracts of curcumin on TPC1 papillary thyroid cancer cell line. BMC Complement Altern. Med. 18 (1), 63. doi:10.1186/s12906-018-2125-9
Potenta, S., Zeisberg, E., and Kalluri, R. (2008). The role of endothelial-to-mesenchymal transition in cancer progression. Br. J. Canc. 99 (9), 1375–1379. doi:10.1038/sj.bjc.6604662
Prasad, S., Tyagi, A. K., and Aggarwal, B. B. (2014). Recent developments in delivery, bioavailability, absorption and metabolism of curcumin: the golden pigment from golden spice. Cancer Res. Treat. 46 (1), 2–18. doi:10.4143/crt.2014.46.1.2
Qin, F., Liu, X., Chen, J., Huang, S., Wei, W., Zou, Y., et al. (2020). Anti-TGF-beta attenuates tumor growth via polarization of tumor associated neutrophils towards an anti-tumor phenotype in colorectal cancer. J. Canc. 11, 2580–2592. doi:10.7150/jca.38179
Qin, F., Huang, X., Zhang, H.-M., and Ren, P. (2009). Pharmacokinetic comparison of puerarin after oral administration of Jiawei‐Xiaoyao‐San to healthy volunteers and patients with functional dyspepsia: influence of disease state. J. Pharm. Pharmacol. 61 (1), 125–129. doi:10.1211/jpp.61.01.0018
Rahmani, A. H., Alsahli, M. A., Aly, S. M., Khan, M. A., and Aldebasi, Y. H. (2018). Role of curcumin in disease prevention and treatment. Adv. Biomed. Res. 7 (1), 38. doi:10.4103/abr.abr_147_16
Rai, M., Ingle, A. P., Pandit, R., Paralikar, P., Anasane, N., and Dos Santos, C. A. (2020). Curcumin and curcumin-loaded nanoparticles: antipathogenic and antiparasitic activities. Expert Rev. Anti Infect. Ther. 18 (4), 367–379. doi:10.1080/14787210.2020.1730815
Ramamoorthi, G., and Sivalingam, N. (2014). Molecular mechanism of TGF-beta signaling pathway in colon carcinogenesis and status of curcumin as chemopreventive strategy. Tumour Biol. 35, 7295–7305. doi:10.1007/s13277-014-1840-1
Ramasamy, P., Subhapradha, N., Shanmugam, V., and Shanmugam, A. (2014). Protective effect of chitosan from Sepia kobiensis (Hoyle 1885) cuttlebone against CCl4 induced hepatic injury. Int. J. Biol. Macromol. 65, 559–563. doi:10.1016/j.ijbiomac.2014.02.009
Regis, S., Dondero, A., Caliendo, F., Bottino, C., and Castriconi, R. (2020). NK cell function regulation by TGF-beta-induced epigenetic mechanisms. Front. Immunol. 11, 311. doi:10.3389/fimmu.2020.00311
Reibel, J. (2003). Prognosis of oral pre-malignant lesions: significance of clinical, histopathological, and molecular biological characteristics. Crit. Rev. Oral Biol. Med. 14, 47–62. doi:10.1177/154411130301400105
Rezaii, M., Oryan, S., and Javeri, A. (2019). Curcumin nanoparticles incorporated collagen-chitosan scaffold promotes cutaneous wound healing through regulation of TGF-β1/Smad7 gene expression. Mater. Sci. Eng. C 98, 347–357. doi:10.1016/j.msec.2018.12.143
Rodero, C. F., Fioramonti Calixto, G. M., dos Santos, K. C., Sato, M. R., dos Santos Ramos, M. A., Miró, M. S., et al. (2018). Curcumin-loaded liquid crystalline systems for controlled drug release and improved treatment of vulvovaginal candidiasis. Mol. Pharm. 15 (10), 4491–4504. doi:10.1021/acs.molpharmaceut.8b00507
Roxo, D. F., Arcaro, C. A., Gutierres, V. O., Costa, M. C., Oriel Oliveira, J., Oliveira Lima, T. F., et al. (2019). Curcumin combined with metformin decreases glycemia and dyslipidemia, and increases paraoxonase activity in diabetic rats. Diabetol. Metab. Syndrome 11, 33. doi:10.1186/s13098-019-0431-0
Russo, I., and Frangogiannis, N. G. (2016). Diabetes-associated cardiac fibrosis: cellular effectors, molecular mechanisms and therapeutic opportunities. J. Mol. Cell. Cardiol. 90, 84–93. doi:10.1016/j.yjmcc.2015.12.011
Sadaka, A., and Giuliari, G. P. (2012). Proliferative vitreoretinopathy: current and emerging treatments. Clin. Ophthalmol. 6, 1325. doi:10.2147/opth.s27896
Saidi, A., Kasabova, M., Vanderlynden, L., Wartenberg, M., Kara-Ali, G. H., Marc, D., et al. (2019). Curcumin inhibits the TGF-β1-dependent differentiation of lung fibroblasts via PPARγ-driven upregulation of cathepsins B and L. Sci. Rep. 9, 1–15. doi:10.1038/s41598-018-36858-3
Sakaguchi, S., and Sakaguchi, N. (2005). Regulatory T cells in immunologic self-tolerance and autoimmune disease. Int. Rev. Immunol. 24, 211–226. doi:10.1080/08830180590934976
Salehi, B., Prado-Audelo, M. L. D., Cortés, H., Leyva-Gómez, G., Stojanović-Radić, Z., Singh, Y. D., et al. (2020a). Therapeutic applications of curcumin nanomedicine formulations in cardiovascular diseases. J. Clin. Med. 9 (3), 746. doi:10.3390/jcm9030746
Salehi, M., Movahedpour, A., Tayarani, A., Shabaninejad, Z., Pourhanifeh, M. H., Mortezapour, E., et al. , 2020b. Therapeutic potentials of curcumin in the treatment of non-small-cell lung carcinoma. Phytother. Res. 34 (10), 2557–2576. doi:10.1002/ptr.6704
Salem, N., Helmi, N., and Assaf, N. (2018). Renoprotective effect of platelet-rich plasma on cisplatin-induced nephrotoxicity in rats. Oxid. Med. Cell Longev. 2018, 1–10. doi:10.1155/2018/9658230
Saranath, D., Tandle, A. T., Teni, T. R., Dedhia, P. M., Borges, A. M., Parikh, D., et al. (1999). p53 inactivation in chewing tobacco-induced oral cancers and leukoplakias from India. Oral Oncol. 35 (3), 242–250. doi:10.1016/s1368-8375(98)00110-9
Schmierer, B., and Hill, C. S. (2007). TGFβ–SMAD signal transduction: molecular specificity and functional flexibility. Nat. Rev. Mol. Cell Biol. 8, 970–982. doi:10.1038/nrm2297
Shabaninejad, Z., Pourhanifeh, M. H., Movahedpour, A., Mottaghi, R., Nickdasti, A., Mortezapour, E., et al. (2020). Therapeutic potentials of curcumin in the treatment of glioblstoma. Eur. J. Med. Chem. 188, 112040. doi:10.1016/j.ejmech.2020.112040
Shahid, H., Shahzad, M., Shabbir, A., and Saghir, G. (2019). Immunomodulatory and anti-inflammatory potential of curcumin for the treatment of allergic asthma: effects on expression levels of pro-inflammatory cytokines and aquaporins. Inflammation 42 (6), 2037–2047. doi:10.1007/s10753-019-01066-2
Shanmuganathan, S., Shanmuganathan, S., and Sumantran, V. (2017). Epigallocatechin gallate & curcumin prevent transforming growth factor beta 1-induced epithelial to mesenchymal transition in ARPE-19 cells. Indian J. Med. Res. 146, S85. doi:10.4103/ijmr.ijmr_1583_15
Sharifi, S., Fathi, N., Memar, M. Y., Khatibi, S. M. H., Khalilov, R., Negahdari, R., et al. (2020). Anti‐microbial activity of curcumin nanoformulations: new trends and future perspectives. Phytother Res. 34 (8), 1926–1946. doi:10.1002/ptr.6658
Sharma, R., Gescher, A. J., and Steward, W. P. (2005). Curcumin: the story so far. Eur. J. Canc. 41 (13), 1955–1968. doi:10.1016/j.ejca.2005.05.009
Shiga, K., Hara, M., Nagasaki, T., Sato, T., Takahashi, H., and Takeyama, H. (2015). Cancer-associated fibroblasts: their characteristics and their roles in tumor growth. Cancers 7 (4), 2443–2458. doi:10.3390/cancers7040902
Shinan-Altman, S., and Katzav, K. O. (2020). The relationship between illness representations, alexithymia, coping strategies and subjective well-being among persons with asthma. J. Asthma, 1–7. doi:10.1080/02770903.2020.1741610
Singh, S. (2007). From exotic spice to modern drug?. Cell 130, 765–768. doi:10.1016/j.cell.2007.08.024
Sinjari, B., Pizzicannella, J., D’Aurora, M., Zappacosta, R., Gatta, V., Fontana, A., et al. (2019). Curcumin/liposome nanotechnology as delivery platform for anti-inflammatory activities via NFkB/ERK/pERK pathway in human dental pulp treated with 2-HydroxyEthyl MethAcrylate (HEMA). Front. Physiol. 10, 633. doi:10.3389/fphys.2019.00633
Sionkowska, A. (2004). Molecular interactions in collagen and chitosan blends. Biomaterials 25 (5), 795–801. doi:10.1016/s0142-9612(03)00595-7
Sneharani, A. H. (2019). Curcumin-sunflower protein nanoparticles-a potential antiinflammatory agent. J. Food Biochem. 43, e12909. doi:10.1111/jfbc.12909
Soleimani, A., Khazaei, M., Ferns, G. A., Ryzhikov, M., Avan, A., and Hassanian, S. M. (2019). Role of TGF-β signaling regulatory microRNAs in the pathogenesis of colorectal cancer. J. Cell. Physiol. 234, 14574–14580. doi:10.1002/jcp.28169
Soleimani, M., Jameie, S. B., Barati, M., Mehdizadeh, M., and Kerdari, M. (2014). Effects of coenzyme Q10 on the ratio of TH1/TH2 in experimental autoimmune encephalomyelitis model of multiple sclerosis in C57bl/6. Iran. Biomed. J. 18 (4), 203–211. doi:10.6091/ibj.13362.2014
Soliman, M. M., Baiomy, A. A., and Yassin, M. H., (2015). Molecular and histopathological study on the ameliorative effects of curcumin against lead acetate-induced hepatotoxicity and nephrototoxicity in Wistar rats. Biol. Trace Elem. Res. 167 (1), 91–102. doi:10.1007/s12011-015-0280-0
Song, Y., Wu, W., Sheng, L., Jiang, B., Li, X., and Cai, K. (2020). Chrysin ameliorates hepatic steatosis induced by a diet deficient in methionine and choline by inducing the secretion of hepatocyte nuclear factor 4α-dependent very low-density lipoprotein. J. Biochem. Mol. Toxicol. 34 (7), e22497. doi:10.1002/jbt.22497
Spagnolo, P., Sverzellati, N., Rossi, G., Cavazza, A., Tzouvelekis, A., Crestani, B., et al. (2015). Idiopathic pulmonary fibrosis: an update. Ann. Med. 47 (1), 15–27. doi:10.3109/07853890.2014.982165
Stohs, S. J., Chen, O., Ray, S. D., Ji, J., Bucci, L. R., and Preuss, H. G. (2020). Highly bioavailable forms of curcumin and promising avenues for curcumin-based research and application: a review. Molecules 25 (6), 1397. doi:10.3390/molecules25061397
Sturzu, A., Sheikh, S., Kalbacher, H., Nägele, T., Weidenmaier, C., Wegenast-Braun, B. M., et al. (2019). Synthesis of a novel curcumin derivative as a potential imaging probe in alzheimer's disease imaging. Curr. Alzheimer Res. 16 (8), 723–731. doi:10.2174/1567205016666190816130516
Tandon, A., Singh, S. J., Gupta, M., Singh, N., Shankar, J., Arjaria, N., et al. (2020). Notch pathway up-regulation via curcumin mitigates bisphenol-A (BPA) induced alterations in hippocampal oligodendrogenesis. J. Hazard Mater. 392, 122052. doi:10.1016/j.jhazmat.2020.122052
Tang, F., Wang, H., Chen, E., Bian, E., Xu, Y., Ji, X., et al. (2019). LncRNA-ATB promotes TGF-β-induced glioma cells invasion through NF-κB and P38/MAPK pathway. J. Cell. Physiol. 234 (12), 23302–23314. doi:10.1002/jcp.28898
Tang, R., Xiao, X., Lu, Y., Li, H., Zhou, Q., Nuro-Gyina, P. K., et al. (2020). Interleukin-22 attenuates renal tubular cells inflammation and fibrosis induced by TGF-β1 through Notch1 signaling pathway. Ren. Fail. 42 (1), 381–390. doi:10.1080/0886022X.2020.1753538
Ten Dijke, P., and Arthur, H. M. (2007). Extracellular control of TGFβ signalling in vascular development and disease. Nat. Rev. Mol. Cell Biol. 8, 857–869. doi:10.1038/nrm2262
Thacker, P. C., and Karunagaran, D. (2015). Curcumin and emodin down-regulate TGF-beta signaling pathway in human cervical cancer cells. PLoS One 10, e0120045. doi:10.1371/journal.pone.0120045
Tsukazaki, T., Chiang, T. A., Davison, A. F., Attisano, L., and Wrana, J. L. (1998). SARA, a FYVE domain protein that recruits Smad2 to the TGFβ receptor. Cell 95 (6), 779–791. doi:10.1016/s0092-8674(00)81701-8
Tyagi, N., Singh, D. K., Dash, D., and Singh, R. (2019). Curcumin modulates paraquat-induced epithelial to mesenchymal transition by regulating transforming growth factor-beta (TGF-beta) in A549 cells. Inflammation 42, 1441–1455. doi:10.1007/s10753-019-01006-0
Tzavlaki, K., and Moustakas, A. (2020). TGF-β signaling. Biomolecules 10, 487. doi:10.3390/biom10030487
Unlu, A., Nayir, E., Kalenderoglu, M. D., Kirca, O., and Ozdogan, M. (2016). Curcumin (turmeric) and cancer. J. Buon. 21 (5), 1050–1060.
Valencia, G. A., Zare, E. N., Makvandi, P., and Gutiérrez, T. J. (2019). Self‐assembled carbohydrate polymers for food applications: a review. Compr. Rev. Food Sci. Food Saf. 18 (6), 2009–2024. doi:10.1111/1541-4337.12499
van der Kraan, P. M., Blaney Davidson, E. N., and van den Berg, W. B. (2010). A role for age-related changes in TGFβ signaling in aberrant chondrocyte differentiation and osteoarthritis. Arthritis Research & Therapy 12 (1), 201. doi:10.1186/ar2896
Varshosaz, J., Jandaghian, S., Mirian, M., and Sajjadi, S. E. (2020). Co-delivery of rituximab targeted curcumin and imatinib nanostructured lipid carriers in non-Hodgkin lymphoma cells. J. Liposome Res., 1–15. doi:10.1080/08982104.2020.1720718
Vasanthkumar, T., Hanumanthappa, M., and Lakshminarayana, R. (2019). Curcumin and capsaicin modulates LPS induced expression of COX-2, IL-6 and TGF-β in human peripheral blood mononuclear cells. Cytotechnology 71, 963–976. doi:10.1007/s10616-019-00338-x
Velnar, T., Bailey, T., and Smrkolj, V. (2009). The wound healing process: an overview of the cellular and molecular mechanisms. J. Int. Med. Res. 37, 1528–1542. doi:10.1177/147323000903700531
Vieira, L. A., De Marchi, P. L., dos Santos, A. A., Christofolini, D. M., Barbosa, C. P., Fonseca, F. L. A., et al. (2014). Analysis of FokI polymorphism of vitamin D receptor gene in intervertebral disc degeneration. Genet. Test. Mol. Biomarkers 18, 625–629. doi:10.1089/gtmb.2014.0030
Wan, R., Xu, X., Ma, L., Chen, Y., Tang, L., and Feng, J. (2020). Novel alternatively spliced variants of Smad4 expressed in TGF-beta-induced EMT regulating proliferation and migration of A549 cells. OncoTargets Ther. 13, 2203–2213. doi:10.2147/ott.s247015
Wan, Y. Y., and Flavell, R. A. (2008). TGF-β and regulatory T cell in immunity and autoimmunity. J. Clin. Immunol. 28, 647–659. doi:10.1007/s10875-008-9251-y
Wang, B., Cao, C., Liu, X., He, X., Zhuang, H., Wang, D., et al. (2020a). BRCA1-associated protein inhibits glioma cell proliferation and migration and glioma stem cell self-renewal via the TGF-β/PI3K/AKT/mTOR signalling pathway. Cell. Oncol. 43 (2), 223–235. doi:10.1007/s13402-019-00482-8
Wang, H., and Li, G. (2020b). LncRNA-UCA1 inhibits the astrocyte activation in the temporal lobe epilepsy via regulating the JAK/STAT signaling pathway. J. Cell. Biochem. doi:10.1007/s00210-020-01808-2
Wang, Q., Ye, C., Sun, S., Li, R., Shi, X., Wang, S., et al. (2019a). Curcumin attenuates collagen-induced rat arthritis via anti-inflammatory and apoptotic effects. Int. Immunopharm. 72, 292–300. doi:10.1016/j.intimp.2019.04.027
Wang, Y., Chen, L., Wang, K., Da, Y., Zhou, M., Yan, H., et al. (2019b). Suppression of TRPM2 reduces renal fibrosis and inflammation through blocking TGF-β1-regulated JNK activation. Biomed. Pharmacother. 120, 109556. doi:10.1016/j.biopha.2019.109556
Wang, Z., Sun, W., Sun, X., Wang, Y., and Zhou, M. (2020c). Kaempferol ameliorates Cisplatin induced nephrotoxicity by modulating oxidative stress, inflammation and apoptosis via ERK and NF-kappaB pathways. Amb. Express 10, 58. doi:10.1186/s13568-020-00993-w
Wang, Z., and Yan, C. (2016). Emerging roles of ATF3 in the suppression of prostate cancer. Mol. Cell Oncol. 3, e1010948. doi:10.1080/23723556.2015.1010948
Wardhani, B. W., Puteri, M. U., Watanabe, Y., Louisa, M., Setiabudy, R., and Kato, M. (2020). TGF-beta-Induced TMEPAI attenuates the response of triple-negative breast cancer cells to doxorubicin and paclitaxel. J. Exp. Pharmacol. 12, 17–26. doi:10.2147/jep.s235233
Weng, W., and Goel, A. (2020). Curcumin and colorectal cancer: an update and current perspective on this natural medicine. Semin Cancer Biol. doi:10.1016/j.semcancer.2020.02.011
Wesseling, C., van Wendel de Joode, B., Ruepert, C., León, C., Monge, P., Hermosillo, H., et al. (2001). Paraquat in developing countries. Int. J. Occup. Environ. Health 7, 275–286. doi:10.1179/oeh.2001.7.4.275
White, E. S., Atrasz, R. G., Dickie, E. G., Aronoff, D. M., Stambolic, V., Mak, T. W., et al. (2005). Prostaglandin E2 inhibits fibroblast migration by E-prostanoid 2 receptor–mediated increase in PTEN activity. Am. J. Respir. Cell Mol. Biol. 32, 135–141. doi:10.1165/rcmb.2004-0126oc
Wright, L. E., Frye, J. B., Lukefahr, A. L., Timmermann, B. N., Mohammad, K. S., Guise, T. A., et al. (2013). Curcuminoids block TGF-β signaling in human breast cancer cells and limit osteolysis in a murine model of breast cancer bone metastasis. J. Nat. Prod. 76 (3), 316–321. doi:10.1021/np300663v
Wrighton, K. H., Lin, X., and Feng, X.-H. (2009). Phospho-control of TGF-β superfamily signaling. Cell Res. 19 (1), 8–20. doi:10.1038/cr.2008.327
Wujek, J. R., Bjartmar, C., Richer, E., Ransohoff, R. M., Yu, M., Tuohy, V. K., et al. (2002). Axon loss in the spinal cord determines permanent neurological disability in an animal model of multiple sclerosis. J. Neuropathol. Exp. Neurol. 61 (1), 23–32. doi:10.1093/jnen/61.1.23
Xia, Z. H., Jiang, X., Li, K., Li, L.-x., Chen, W.-b., Wang, Y.-x., et al. (2020). Curcumin inhibits alloxan-induced pancreatic islet cell damage via antioxidation and antiapoptosis. J. Biochem. Mol. Toxicol. 34 (7), e22499. doi:10.1002/jbt.22499
Xiao, Y., Zhou, L., Zhang, T., Qin, C., Wei, P., Luo, L., et al. (2020). Anti-fibrosis activity of quercetin attenuates rabbit tracheal stenosis via the TGF-beta/AKT/mTOR signaling pathway. Life Sci. 250, 117552. doi:10.1016/j.lfs.2020.117552
Xing, S., Yu, W., Zhang, X., Luo, Y., Lei, Z., Huang, D., et al. (2018). Isoviolanthin extracted from Dendrobium officinale reverses TGF-beta1-mediated Epithelial(-)Mesenchymal transition in hepatocellular carcinoma cells via deactivating the TGF-beta/smad and PI3K/Akt/mTOR signaling pathways. Int. J. Mol. Sci. 19 (6), 1556. doi:10.3390/ijms19061556
Xiong, R., Yin, T., Gao, J.-l., and Yuan, Y.-f. (2020). HOXD9 activates the TGF-beta/smad signaling pathway to promote gastric cancer. OncoTargets Ther. 13, 2163–2172. doi:10.2147/ott.s234829
Xu, F., Xu, Y., Xiong, J.-H., Zhang, J.-H., Wu, J., Luo, J., et al. (2020). AOC1 contributes to tumor progression by promoting the AKT and EMT pathways in gastric cancer. Canc. Manag. Res. 12, 1789–1798. doi:10.2147/cmar.s225229
Xu, J.-H., Yang, H.-P., Zhou, X.-D., Wang, H.-J., Gong, L., and Tang, C.-L. (2015). Role of Wnt inhibitory factor-1 in inhibition of bisdemethoxycurcumin mediated epithelial-to-mesenchymal transition in highly metastatic lung cancer 95D cells. Chin. Med. J. 128 (10), 1376. doi:10.4103/0366-6999.156795
Xu, S., Jiang, B., Wang, H., Shen, C., Chen, H., and Zeng, L. (2017). Curcumin suppresses intestinal fibrosis by inhibition of PPARgamma-mediated epithelial-mesenchymal transition. Evid. Based Complement. Alternat. Med. 2017, 1–12. doi:10.1155/2017/7876064
Yan, X., Liu, Z., and Chen, Y. (2009). Regulation of TGF-β signaling by Smad7. Acta Biochim. Biophys. Sin. 41 (4), 263–272. doi:10.1093/abbs/gmp018
Yang, B.-Y., Hu, C.-H., Huang, W.-C., Ho, C.-Y., Yao, C.-H., and Huang, C.-H. (2019a). Effects of bilayer nanofibrous scaffolds containing curcumin/lithospermi radix extract on wound healing in streptozotocin-induced diabetic rats. Polymers 11 (11), 1745. doi:10.3390/polym11111745
Yang, L., Wu, L., Zhang, X., Hu, Y., Fan, Y., and Ma, J. (2017). 1, 25 (OH) 2D3/VDR attenuates high glucose-induced epithelial-mesenchymal transition in human peritoneal mesothelial cells via the TGFβ/Smad3 pathway. Mol. Med. Rep. 15 (4), 2273–2279. doi:10.3892/mmr.2017.6276
Yang, P., Dong, X., and Zhang, Y. (2020). MicroRNA profiles in plasma samples from young metabolically healthy obese patients and miRNA-21 are associated with diastolic dysfunction via TGF-beta1/Smad pathway. J. Clin. Lab. Anal. 34 (6) e23246. doi:10.1002/jcla.23246
Yang, R., Yang, F., Hu, Y., Chen, M., Liu, Y., Li, J., et al. (2019b). Hepatocyte growth factor attenuates the development of TGF-beta1-induced EndMT through down-regulating the Notch signaling. Endocr. Metab. Immune Disord. Drug Targets 20 (5), 781–787. doi:10.2174/1871530319666191023141638
Yeung, A. W. K., Horbańczuk, M., Tzvetkov, N. T., Mocan, A., Carradori, S., Maggi, F., et al. (2019). Curcumin: total-scale analysis of the scientific literature. Molecules 24 (7), 1393. doi:10.3390/molecules24071393
Yin, J., Wang, L., Wang, Y., Shen, H., Wang, X., and Wu, L. (2019). Curcumin reverses oxaliplatin resistance in human colorectal cancer via regulation of TGF-β/Smad2/3 signaling pathway. OncoTargets Ther. 12, 3893. doi:10.2147/ott.s199601
Yuan, J., Botchway, B. O. A., Zhang, Y., Tan, X., Wang, X., and Liu, X., (2019). Curcumin can improve spinal cord injury by inhibiting TGF-β-SOX9 signaling pathway. Cell. Mol. Neurobiol. 39 (5), 569–575. doi:10.1007/s10571-019-00671-x
Yuan, J., Zou, M., Xiang, X., Zhu, H., Chu, W., Liu, W., et al. (2015). Curcumin improves neural function after spinal cord injury by the joint inhibition of the intracellular and extracellular components of glial scar. J. Surg. Res. 195 (1), 235–245. doi:10.1016/j.jss.2014.12.055
Zare, E. N., Makvandi, P., Borzacchiello, A., Tay, F. R., Ashtari, B., and Padil, V. V. T. (2019a). Antimicrobial gum bio-based nanocomposites and their industrial and biomedical applications. Chem. Commun. 55 (99), 14871–14885. doi:10.1039/c9cc08207g
Zare, E. N., Makvandi, P., and Tay, F. R. (2019b). Recent progress in the industrial and biomedical applications of tragacanth gum: a review. Carbohydr. Polym. 212, 450–467. doi:10.1016/j.carbpol.2019.02.076
Zeisberg, E. M., Tarnavski, O., Zeisberg, M., Dorfman, A. L., McMullen, J. R., Gustafsson, E., et al. (2007). Endothelial-to-mesenchymal transition contributes to cardiac fibrosis. Nat. Med. 13, 952–961. doi:10.1038/nm1613
Zeisberg, E. M., Potenta, S. E., Sugimoto, H., Zeisberg, M., and Kalluri, R. (2008). Fibroblasts in kidney fibrosis emerge via endothelial-to-mesenchymal transition. J. Am. Soc. Nephrol. 19 (12), 2282–2287. doi:10.1681/asn.2008050513
Zhang, L., Zhang, J., Yan, E., He, J., Zhong, X., Zhang, L., et al. (2020a). Dietary supplemented curcumin improves meat quality and antioxidant status of intrauterine growth retardation growing pigs via Nrf2 signal pathway. Animals 10 (3), 539. doi:10.3390/ani10030539
Zhang, L., Cheng, X., Gao, Y., Zhang, C., Bao, J., Guan, H., et al. (2016). Curcumin inhibits metastasis in human papillary thyroid carcinoma BCPAP cells via down-regulation of the TGF-β/Smad2/3 signaling pathway. Exp. Cell Res. 341 (2), 157–165. doi:10.1016/j.yexcr.2016.01.006
Zhang, L., Su, S., Zhu, Y., Guo, J., Guo, S., Qian, D., et al. (2019a). Mulberry leaf active components alleviate type 2 diabetes and its liver and kidney injury in db/db mice through insulin receptor and TGF-beta/Smads signaling pathway. Biomed. Pharmacother. 112, 108675. doi:10.1016/j.biopha.2019.108675
Zhang, M. Q., Ren, X., Zhao, Q., Yue, S.-J., Fu, X.-M., Li, X., et al. (2020b). Hepatoprotective effects of total phenylethanoid glycosides from Acanthus ilicifolius L. against carbon tetrachloride-induced hepatotoxicity. J. Ethnopharmacol. 256, 112795. doi:10.1016/j.jep.2020.112795
Zhang, P., Fang, J., Zhang, J., Ding, S., and Gan, D. (2020c). Curcumin inhibited podocyte cell apoptosis and accelerated cell autophagy in diabetic nephropathy via regulating Beclin1/UVRAG/Bcl2. Diabetes Metab. Syndr. Obes. 13, 641–652. doi:10.2147/dmso.s237451
Zhang, Y. E. (2009). Non-Smad pathways in TGF-β signaling. Cell Res. 19, 128–139. doi:10.1038/cr.2008.328
Zhang, Y., Chen, F., Xiao, X., Pan, W., Yuan, Q., and Cao, J. (2019b). Chrysin inhibits sphere formation in SMMC-7721 cells via modulation of SHP-1/STAT3 signaling pathway. Canc. Manag. Res. 11, 2977–2985. doi:10.2147/cmar.s193647
Zhao, H.-M., Xu, R., Huang, X.-Y., Cheng, S.-M., Huang, M.-F., Yue, H.-Y., et al. (2016). Curcumin suppressed activation of dendritic cells via JAK/STAT/SOCS signal in mice with experimental colitis. Front. Pharmacol. 7, 455. doi:10.3389/fphar.2016.00455
Zhao, H. M., Han, F., Xu, R., Huang, X.-Y., Cheng, S.-M., Huang, M.-F., et al. (2017). Therapeutic effect of curcumin on experimental colitis mediated by inhibiting CD8(+)CD11c(+) cells. World J. Gastroenterol. 23 (10), 1804–1815. doi:10.3748/wjg.v23.i10.1804
Zhao, J.-L., Zhang, T., Shao, X., Zhu, J.-J., and Guo, M.-Z. (2019a). Curcumin ameliorates peritoneal fibrosis via inhibition of transforming growth factor-activated kinase 1 (TAK1) pathway in a rat model of peritoneal dialysis. BMC Complement. Altern. Med. 19, 1–11. doi:10.1186/s12906-019-2702-6
Zhao, J. L., Guo, M.-Z., Zhu, J.-J., Zhang, T., and Min, D.-Y. (2019b). Curcumin suppresses epithelial-to-mesenchymal transition of peritoneal mesothelial cells (HMrSV5) through regulation of transforming growth factor-activated kinase 1 (TAK1). Cell. Mol. Biol. Lett. 24 (1), 32. doi:10.1186/s11658-019-0157-x
Zhao, R., Du, S., Liu, Y., Lv, C., Song, Y., Chen, X., et al. (2020). Mucoadhesive-to-penetrating controllable peptosomes-in-microspheres co-loaded with anti-miR-31 oligonucleotide and curcumin for targeted colorectal cancer therapy. Theranostics 10, 3594–3611. doi:10.7150/thno.40318
Zheng, J., Cheng, J., Zheng, S., Feng, Q., and Xiao, X. (2018). Curcumin, a polyphenolic curcuminoid with its protective effects and molecular mechanisms in diabetes and diabetic cardiomyopathy. Front. Pharmacol. 9, 472. doi:10.3389/fphar.2018.00472
Zhong, X., Liu, D., Jiang, Z., Li, C., Chen, L., Xia, Y., et al. (2020). Chrysin induced cell apoptosis and inhibited invasion through regulation of TET1 expression in gastric cancer cells. OncoTargets Ther. 13, 3277–3287. doi:10.2147/ott.s246031
Zhou, H., Beevers, C. S., and Huang, S. (2011). The targets of curcumin. Curr. Drug Targets 12 (3), 332–347. doi:10.2174/138945011794815356
Zhou, J., Miao, H., Li, X., Hu, Y., Sun, H., and Hou, Y. (2017a). Curcumin inhibits placental inflammation to ameliorate LPS-induced adverse pregnancy outcomes in mice via upregulation of phosphorylated Akt. Inflamm. Res. 66 (2), 177–185. doi:10.1007/s00011-016-1004-4
Zhou, T., Wang, Y., Liu, M., Huang, Y., Shi, J., Dong, N., et al. (2020). Curcumin inhibits calcification of human aortic valve interstitial cells by interfering NF-kappaB, AKT, and ERK pathways. Phytother Res. 34 (8), 2074–2081. doi:10.1002/ptr.6674
Zhou, X., Zhang, J., Xu, C., and Wang, W. (2014). Curcumin ameliorates renal fibrosis by inhibiting local fibroblast proliferation and extracellular matrix deposition. J. Pharmacol. Sci. 126 (4), 344–350. doi:10.1254/jphs.14173fp
Zhou, X., Kuang, X., Long, C., Liu, W., Tang, Y., Liu, L., et al. (2017b). Curcumin inhibits proliferation and epithelial-mesenchymal transition of retinal pigment epithelial cells via multiple pathways. Curr. Mol. Med. 17 (4), 312–319. doi:10.2174/1566524017666171106115655
Zhu, F.-q., Chen, M.-j., Zhu, M., Zhao, R.-s., Qiu, W., Xu, X., et al. (2016). Curcumin suppresses epithelial-mesenchymal transition of renal tubular epithelial cells through the inhibition of Akt/mTOR pathway. Biol. Pharm. Bull. 40 (1), 17–24. doi:10.1248/bpb.b16-00364
Keywords: curcumin, transforming growth factor-β, diseases, antioxiants, natural compound
Citation: Ashrafizadeh M, Zarrabi A, Hushmandi K, Zarrin V, Moghadam ER, Hashemi F, Makvandi P, Samarghandian S, Khan H, Hashemi F, Najafi M and Mirzaei H (2020) Toward Regulatory Effects of Curcumin on Transforming Growth Factor-Beta Across Different Diseases: A Review. Front. Pharmacol. 11:585413. doi: 10.3389/fphar.2020.585413
Received: 20 July 2020; Accepted: 12 October 2020;
Published: 14 December 2020.
Edited by:
Stefania Tacconelli, University of Studies G. d’Annunzio Chieti and Pescara, ItalyReviewed by:
Marina Korotkova, Karolinska Institutet (KI), SwedenWeicang Wang, University of California, Davis, United States
Copyright © 2020 Ashrafizadeh, Zarrabi, Hushmandi, Zarrin, Moghadam, Hashemi, Makvandi, Samarghandian, Khan, Najafi and Mirzaei. This is an open-access article distributed under the terms of the Creative Commons Attribution License (CC BY). The use, distribution or reproduction in other forums is permitted, provided the original author(s) and the copyright owner(s) are credited and that the original publication in this journal is cited, in accordance with accepted academic practice. No use, distribution or reproduction is permitted which does not comply with these terms.
*Correspondence: Masoud Najafi, TmFqYWZpX21hQHlhaG9vLmNvbQ==; Hamed Mirzaei, aC5taXJ6YWVpMjAwMkBnbWFpbC5jb20=