- 1Department of Pharmacy, Baotou Medical College, Baotou, China
- 2Xilinguole Meng Mongolian General Hospital, Xilinhaote, China
- 3Pharmaceutical Laboratory, Inner Mongolia Institute of Traditional Chinese Medicine, Hohhot, China
- 4Inner Mongolia Key Laboratory of Characteristic Geoherbs Resources and Utilization, Baotou Medical College, Baotou, China
- 5Office of Academic Research, Qiqihar Medical University, Qiqihar, China
Cardiovascular disease is the main cause of death worldwide, and traditional cardiovascular risk factors cannot fully explain the occurrence of the disease. In recent years, the relationship between gut microbiota and its metabolites and cardiovascular disease has been a hot study topic. The changes in gut microbiota and its metabolites are related to the occurrence and development of atherosclerosis, myocardial infarction, heart failure, and hypertension. The mechanisms by which gut microbiota and its metabolites influence cardiovascular disease have been reported, although not comprehensively. Additionally, following ingestion, flavonoids are decomposed into phenolic acids that are more easily absorbed by the body after being processed by enzymes produced by intestinal microorganisms, which increases flavonoid bioavailability and activity, consequently affecting the onset of cardiovascular disease. However, flavonoids can also inhibit the growth of harmful microorganisms, promote the proliferation of beneficial microorganisms, and maintain the balance of gut microbiota. Hence, it is important to study the relationship between gut microbiota and flavonoids to elucidate the protective effects of flavonoids in cardiovascular diseases. This article will review the role and mechanism of gut microbiota and its metabolites in the occurrence and development of atherosclerosis, myocardial infarction, heart failure, and hypertension. It also discusses the potential value of flavonoids in the prevention and treatment of cardiovascular disease following their transformation through gut microbiota metabolism.
Introduction
Cardiovascular disease (CVD) is the largest contributor to mortality and morbidity worldwide, accounting for 31% of all global deaths (Who, 2017). CVD is a manifestation of both a systemic circulatory disease and a problem localized to the heart. There are four main causes: 1) vascular factors, such as atherosclerosis (Stefanadis, 2013), hypertensive arteriosclerosis, and arteritis; 2) hemodynamic factors, such as hypertension (Sanchez-Rodriguez et al., 2020); 3) abnormal hemorheology, such as hyperlipidemia (Ma et al., 2019) and diabetes; and 4) blood composition factors, such as leukemia, anemia, and thrombocytosis. Presently, statins play a very important role in controlling CVD development by effectively controlling the blood lipid level, thus, alleviating the rapid progression of CVD. However, in some patients this effective control could not be observed even after the use of high-intensity statins. Additionally, statins have certain side effects, most commonly gastrointestinal reactions and possibly, individual allergic reactions to the different drugs that constitute statins. In serious cases, liver damage may occur. Hence, there is an urgent need to identify new effective targets for CVD and to investigate new therapeutic approaches.
Gut microbiota (GM) is a complex ecosystem of trillions of microorganisms (Qin et al., 2010; Man et al., 2020). Upon being affected by external environmental changes, the balance between the host and the flora can be broken, resulting in an imbalance in the intestinal micro-ecosystem, thus leading to impaired body functions and disease (Lau et al., 2017). With increasing developments in scientific research, the important role of GM in CVD development has been discovered. GM can improve CVD prognosis by reducing several risk factors, and its regulation is expected to become a new therapeutic target for CVDs. Close information exchange between GM and the host plays a vital role in digestion, immune defense, and nervous system regulation, especially cardiovascular protection, maintaining a delicate balance between itself and the human host (Guo and Wu, 2017; Jia et al., 2019b). Furthermore, it is important to study the relationship between GM and flavonoids for the protection of flavonoids to CVDs.
Flavonoids are the most common group of dietary polyphenolic compounds that are present in their glycosidic forms and found in a wide variety of foodstuffs of plant origin (Manach et al., 2004; Lu et al., 2017; Bi, 2018). They have a variety of biological activities (in vitro and in vivo) (Gabriele, 2015), including antibacterial, anti-inflammatory (Gabriele, 2015), antitumor, and antioxidant activities (Gabriele, 2015), as well as significant therapeutic effects on CVD (Manson, 2002; Bazzano et al., 2003; Gong et al., 2019). Flavonoids are recognized as xenobiotics and most glycosides are not easily transported to the target site because of their low lipophilicity, resulting in poor absorption and relatively low bioavailability (Karlsson et al., 2012; Kumar and Pandey, 2013; Man et al., 2020). Therefore, it is important to note that during GM growth and metabolism, GM can produce an array of metabolic enzymes, such as α-rhamnosidase, β-glucuronidase, β-glucosidase, β-galactosidase, nitroreductase, azoreductase, 7-α hydrolase, protease, and various carbohydrate metabolism-related enzymes (Gong et al., 2020). Flavonoids are metabolized by these GM enzymes and can be converted into their corresponding phenolic acids, thus affecting the bioavailability of flavonoids in the human body (Pan et al., 2013) and increasing the bioactivity of some flavonoid metabolites. On the other hand, the composition of GM can also be modulated by flavonoids toward homeostasis recovery (Akyildiz et al., 2010). Hence, it is important to study the relationship between GM and flavonoids to elucidate the protective effects of flavonoids in CVDs.
In this review, we comprehensively evaluate recently published scientific literature on the interaction between GM and flavonoids, with particular emphasis on the metabolized and transformed pathways involved. Further, we explain how flavonoids prevent CVDs through GM and discuss the roles of the GM in CVDs, including atherosclerosis, hypertension, myocardial ischemia (MI), and heart failure (HF), as well as the molecular mechanisms underlying its effects.
Interaction Between Flavonoids and Gut Microbiota
Flavonoid metabolism by human GM and the effect of flavonoids on the growth of GM are mutually causal. On the one hand, when flavonoids enter the intestinal tract, they inevitably become metabolized and transformed through the action of various drug-metabolizing enzymes produced by the GM, leading to the formation of various bioactive metabolites, which have a positive effect in improving CVDs. On the other hand, flavonoids affect GM balance (Gil-Cardoso et al., 2016), which is closely related to human health. Thus, the interaction between flavonoids and GM is of great significance for intestinal health.
Role of Gut Microbiota in Flavonoid Metabolism
In vivo, flavonoid metabolism is closely associated with GM. Microorganisms interact and cooperate with each other for the completion of complex metabolic functions. Under the action of GM, flavonoid glycosides are first hydrolyzed to aglycones in the intestinal tract, then reduced, with the hydrogenation of the C-ring, after which O–C2 bonds in the C-ring are cleaved to form phenolic ketones and phenolic acids (Figure 1). Therefore, hydrolysis and reduction are the main reactions of flavonoids in intestinal metabolism. In addition, GM can perform degradation of glycoside flavonoid macromolecules O-glycoside and C-glycoside, ester hydrolysis, hydrolysis (Jin et al., 2007), and deglucuronylation; partial dehydrogenation (Hor-Gil and Fatemeh, 2000), dimethoxylation (Shi et al., 2014), and demethylation of aglycones; and hydrogenation, α-oxidation, β-oxidation, and cracking of aromatic rings of substituted aliphatic groups. After the aromatic ring of the flavonoid aglycone is cracked, the hydroxylated forms of phloroglucinol and phenylacetate or phenylpropionate are produced.
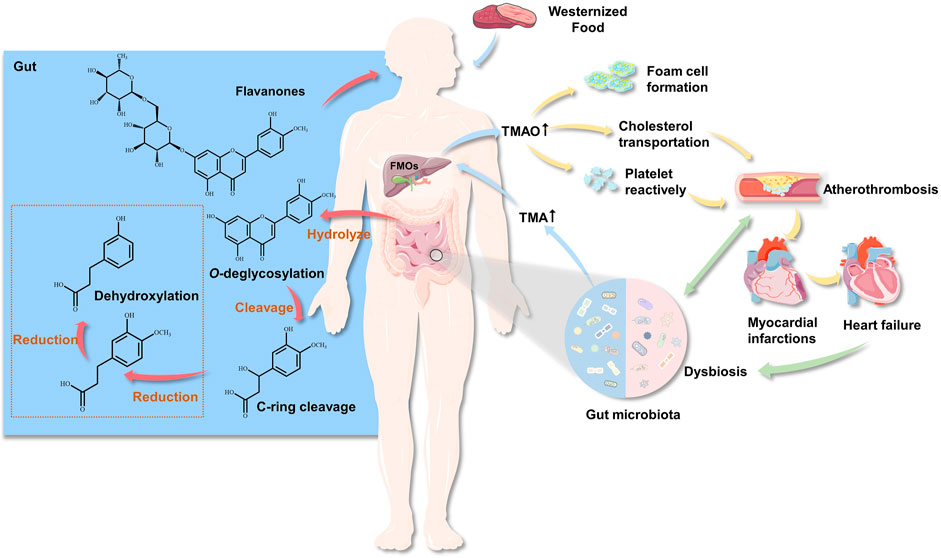
FIGURE 1. The role of gut microbiota in cardiovascular disease and flavonoid metabolism. The GM produces TMA by ingesting choline (such as choline, phosphatidylcholine and l–carnitine) from food. TMA enters the liver through the hepatointestinal circulation, and forms TMAO through the oxidation of flavin monooxygenase, then enters the systemic circulation. Circulating TMAO levels increase, causing foam cells and platelets to accumulate, while affecting cholesterol transport, thereby promoting atheromatous plaque formation.
For example, the main components of Scutellaria baicalensis Georgi include baicalin, wogonoside, and xyloside. These glycosides are converted by GM into the corresponding aglycones, such as baicalein, wogonin, and xyloside A, respectively, with a higher anti-complement activity than that of the prototypes. Antibacterial activity evaluation showed that the activities of all aglycones were stronger than those of the corresponding glycosides (Xing et al., 2014). With respect to anti-platelet aggregation, baicalein activity was significantly stronger than that of baicalin, which has a positive effect on improving atherosclerosis. It has been found that baicalin is first transformed into its aglycone baicalein in the intestinal tract of rats, absorbed, then converted into baicalin in the small intestine and liver (Akao et al., 2000). These findings suggest that the insoluble glycosides may be hydrolyzed into easily absorbed aglycones to make them better absorbed by the small intestine, and then converting them back to glycosides using host enzymes, thereby promoting glycoside absorption. Hesperidin, a flavanone glycoside, is a natural phenolic compound with a wide range of biological effects (Hajialyani et al., 2019). To clarify hesperidin metabolism by GM, hesperidin was incubated with human and rat fecal suspensions. By comparison, when hesperidin was orally administered to rats, hesperetin was detected in the urine, but hesperidin was not, indicating that hesperidin was metabolized to hesperetin, which was the main metabolite during incubation (Lee et al., 2004). Hesperidin can dilate blood vessels, and reduce blood lipid levels, capillary permeability, and vascular wall fragility. Protocatechuic acid is the main metabolite of anthocyanin fermented by human GM. It exhibits obvious antioxidant activity, scavenging of free radicals, inhibition of platelet aggregation, and anti-hypoxia activity, which are significant for improving CVDs. The appearance of low molecular weight metabolites indicates that anthocyanin has been transformed by GM (He et al., 2005). Kaempferitrin is a flavonoid glycoside isolated from Siraitia grosvenorii (Swingle) C. Jeffrey ex Lu and Zhang, which is incubated and transformed by the bacteria in human intestines to produce afzelin, kaempferol, p-hydroxybenzoic acid, and kaempferol-7-O-α-L-rhamnoside (Yang et al., 2005). It has various pharmacological activities, such as lowering of blood pressure, reduction of blood lipid levels, and inhibition of platelet aggregation.
Effect of Flavonoids on Gut Microbiota
Flavonoids can promote the growth of beneficial intestinal microorganisms. Flavonoids, such as hesperidin and quercetin have been reported to modulate the composition of GM by promoting the growth of probiotic bacteria and effecting a reduction in the growth of pathogens. A study conducted by Simmering (Rainer et al., 2002) examined the influence of flavonoids on the population of Eubacterium ramulus in the human intestinal tract. A controlled study was performed with human subjects who received a flavonoid-free diet and a single high dose of quercetin, rutin, or buckwheat. Results showed that oral quercetin and rutin intake significantly increased the bacterial load of E. ramulus in humans. Metabolomic analyses have also shown that grape seed extract, rich in flavanols, had a strong value-added effect on the proliferation of Lactobacillus plantarum, Lactobacillus casei, and Lactobacillus bulgaricus (Tabasco et al., 2011).
Moreover, flavonoids have anti-microbial activity, and a large number of studies have shown that they can inhibit the proliferation of symbiotic bacteria and pathogenic microorganisms, and thus have a certain impact on CVD. The quantity of Lactobacillus and Escherichia coli per gram of fecal contents in rats fed soybean isoflavones was calculated using the bacterial culture method. The results showed that the erobic E. coli numbers in the intestinal tract of rats fed soybean isoflavone decreased significantly, while the count of the facultative anaerobic Lactobacillus spp. increased significantly (Zhu et al., 2013). This is similar to the results of a study by Duda-Chodak (Duda-Chodak, 2012) in which the liquid medium was supplemented with various polyphenol monomers, whose effects on GM were evaluated by measuring the turbidity of the medium after 24 h. It was found that quercetin significantly inhibited the growth of Bacteroides galactosus and Lactobacillus. Furthermore, Gwiazdowska (Daniela et al., 2015) studied the effects of different polyphenol monomers (2, 20, and 100 μg/ml) on the growth of Bifidobacterium Bifidum using the 96-well microtitration plate method. The results showed that rutin and quercetin promoted the growth of B. Bifidum, but high-dose quercetin inhibited the growth of B. Bifidum. These observations indicate that the effect of flavonoids on GM depends on the dosage of flavonoids, and different concentrations of flavonoids have different inhibitory effects on different bacterial strains. Currently, flavonoids, as antibacterial drugs, have been widely used in the medical and food preservative industries.
Flavonoids Prevent Cardiovascular Disease Through Gut Microbiota
Flavonoid intake is closely associated with CVD. The interaction between flavonoid glycosides and GM can produce a new type of microbial flavonoid transformation with significantly increased biological activity and bioavailability, thus promoting the full use of flavonoids in cardiovascular protection (Table 1).
On the one hand, because of their low lipophilicity, most flavonoid glycosides are difficult to transport to the target site, resulting in poor absorption of flavonoid glycosides in the digestive tract. However, after biotransformation of some flavonoids by GM, the flavonoid metabolites produced show a greater biological activity than the parent flavonoid. For example, a large number of epidemiological studies have shown that soybean isoflavones have many physiological functions, such as anti-cancer, anti-oxidation, and anti-inflammatory properties, cardiovascular and cerebrovascular protection, and osteoporosis prevention. Soybean isoflavones ingested by humans and other mammals can be degraded into dihydrodaidzein, dihydrogenistein, O-desmethylangolensin, estriol, and other metabolites by GM. In vivo and in vitro, the results showed that soybean isoflavone metabolites had higher and wider biological activity than soybean isoflavone. Besides, estriol has a higher affinity for thromboxane receptors in human platelets than soybean isoflavones; therefore, it can be used clinically to regulate platelet function and prevent thrombus. Compared with isoflavones, the conformation of estriol is more flexible, allowing its easier penetration into the cell membrane. Its antioxidant activity plays an important role in preventing lipid peroxidation and has positive effects on the treatment and prevention of chronic diseases, preventing atherosclerosis, and reducing the risk of CVD.
On the other hand, there are substantial differences in the efficacies of some flavonoids in vitro and in vivo. One of the important reasons is the low solubility or poor absorption in vivo, which leads to poor bioavailability. The metabolism of flavonoids by GM can improve their water solubility and bioavailability. For example, rutin is a flavonoid obtained from a wide range of sources. It can prevent and treat hemorrhage caused by capillary embrittlement. It is used clinically in the auxiliary treatment of hypertension and it performs anti-capillary fragility activity. However, rutin is poorly soluble in water and fat, with low bioavailability after oral administration, which limits its clinical application. Rutin can be hydrolyzed to quercetin by GM and finally converted to 3,4-dihydroxyphenylacetic acid, the antiplatelet activity of which is greater than that of rutin and quercetin, and also it can be absorbed into the blood circulation system. Consequently, in the preparation of rutin as the main effective ingredient, in addition to the dosage form, we can also consider increasing the residence time of rutin in the intestinal tract, in order to enhance its metabolism by GM and its cardiovascular protection efficacy.
Mechanisms Underlying the Improvement of Cardiovascular Diseases by Gut Microbiota
Trimethylamine N-oxide (TMAO) is formed from carnitine-, choline-, and betaine-rich foods, such as egg yolk, red meat, and some fish, through the action of trimethylamine (TMA) lyase in intestinal microorganisms, followed by oxidation by liver flavin monooxygenases (FMOs; mainly FMO3). Table 2 lists the microorganisms involved in metabolizing carnitine, choline, and betaine (Aura, 2008). Additionally, short-chain fatty acids (SCFAs) are organic acids with aliphatic terminal carboxylic acids, which are mainly produced by anaerobic bacteria in the cecum and proximal colon through the fermentation of dietary fiber, with a small portion produced by the decomposition of proteins and peptides (Pingitore et al., 2017). SCFAs play a regulatory role in energy homeostasis, insulin sensitivity, and glucose and lipid metabolism. It is generally accepted that these regulatory roles of SCFAs can be used to reduce the risk of cardiovascular and metabolic diseases (Donohoe et al., 2018). Biologically active GM-derived metabolites, such as TMAO and SCFAs, are bioactive molecules and a promoter of chronic CVDs (Romano et al., 2015; Roberts et al., 2018). Moreover, alterations in the human gut-associated microbiome have been implicated in the pathogenesis of several chronic conditions ranging from atherosclerosis and thrombosis to hypertension and HF (Tang and Hazen, 2017) (Figure 1). The following sections will elaborate on the effect of GM and its metabolites on atherosclerosis, MI, HF, hypertension, and related mechanisms (Figure 2).
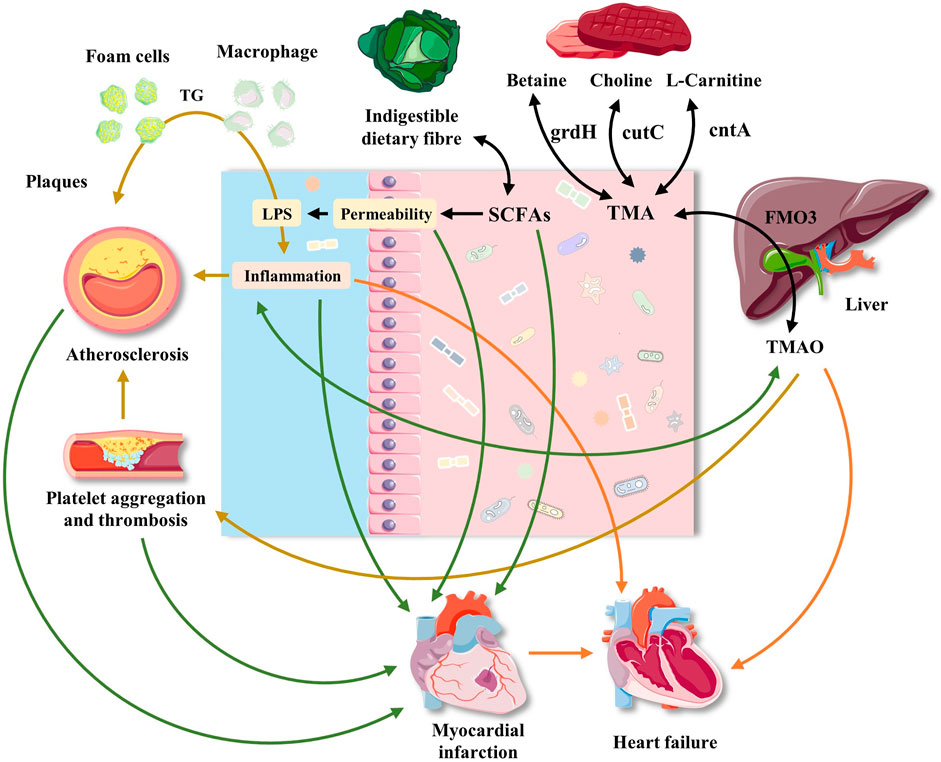
FIGURE 2. Effects of gut microbiota and its metabolites on atherosclerosis, myocardial infarction, heart failure and hypertension. The interaction between diet and gut microbiota may promote the development of cardiovascular disease through common and different mechanisms. Western food rich in red meat promotes the production of TMA by bacteria, which is oxidized to TMAO in liver. TMAO may participate in atherosclerosis by interfering with cholesterol transport, foam cell formation and platelet aggregation. The effect of TMAO on blood pressure was mainly manifested in its enhancement of pressure-raizing effect of Ang II. Platelet aggregation also plays a role in atherosclerosis. The decrease of dietary fiber is related to the decrease of bacterial production of SCFAs, which play an immunomodulatory role in intestinal mucosa. The decrease of SCFAs level can promote local inflammation, aggravate intestinal ecological imbalance, and lead to the damage of intestinal barrier function. The damage of intestinal barrier function leads to the leakage of bacterial toxins, which further aggravates local and systemic inflammation. In addition, dysbiosis destroys the intestinal mucosal barrier, leading to gut microbiota entering the systemic circulation, which increases the incidence of adverse cardiovascular events after MI and aggravates the progress of HF. The roles of gut microbiota and its metabolites in hypertension.
Hypertension is one of the most common CVDs and its incidence rate increases with age. Its pathogenesis is complex and has many influencing factors (Li et al., 2017). More recently, a limited number of studies have indicated a direct association between GM and blood pressure control in animal models (Yang et al., 2015). GM produces unique metabolites that are potentially important for hypertension control. It was found that the diversity, abundance, and uniformity of GM decreased in the animal model of spontaneous hypertension, while the ratio of Firmicutes to Bacteroidetes increased (Yang et al., 2015; Karbach et al., 2016). In one study, the GM of 41 healthy volunteers, 56 patients with prehypertension, and 99 patients with essential hypertension were analyzed. Additionally, the researchers transplanted the fecal microbiota from the patient into the intestines of sterile mice and observed a significant increase in mice blood pressure after transplantation (Li et al., 2017).
In recent years, SCFAs have been shown to act on G-protein-coupled receptors (GPCRs), including free fatty acid receptor 3 (Gpr41) and olfactory receptor 78 (Olfr78), to regulate blood pressure. Short-chain fatty acids (SCFA) activate three independent GPCRs, namely GPR41, free fatty acid receptor 2 (GPR43), and Olfr78. SCFA can activate Olfr78, which makes renin released from renal arterioles increase hypertension, (Pluznick, 2017), while GPR41 shows an antagonistic effect (Natarajan et al., 2016). In contrast, SCFA activates GPR43 to induce vasodilatation to counteract increased blood pressure induced by Olfr78-mediated renin release (Chiou and Burotto, 2015). However, it has also been suggested that GPR41 and vascular Olfr78 have opposite effects on blood pressure regulation after propionate reaction (Pluznick et al., 2013; Jia et al., 2019a). In addition, compared with the traditional culture mice, the administration of angiotensin II in aseptic mice can reduce the formation of vasoactive oxygen species, decrease the levels of monocyte chemoattractant protein, and reduce the adhesion of vascular leukocytes, neutrophils, and monocytes to the vascular wall, thereby reducing vascular endothelial dysfunction and increasing blood pressure.
Further, the 3-years incidence of cardiovascular events is significantly higher in patients with high plasma TMAO concentration (Tang et al., 2013), which might be related to the increased degree of atherosclerosis associated with TMAO. Animal experiments identified elevated TMAO levels in mice accompanied by significantly increased areas of plaque and a higher number of foam cells (Wang et al., 2011; Koeth et al., 2015). However, the effect of TMAO on blood pressure mainly manifests as an enhanced pressor effect of angiotensin II (Ang II). Although simple infusion of Ang II only significantly increases the systolic and diastolic blood pressure of rats, the pressor effect can last until the end of the experiment when TMAO is injected simultaneously. Similarly, TMAO alone had no effect on the blood pressure of rats, whereas its combination with low-dose Ang II prolonged the Ang II-mediated pressor effect (Ufnal et al., 2014). A possible mechanism might involve TMAO participation in the protein processing of some Ang II receptors or Ang II-like peptide hormones, which improves the efficiency of protein production and thereby prolongs the Ang II-mediated pressor effect (Wu et al., 2016b).
The Roles of Gut Microbiota and its Metabolites in Atherosclerosis
Atherosclerosis, one of the main diseases that harm human cardiovascular and cerebrovascular health is mainly caused by lipid metabolism disorder and vascular endothelial inflammation (Chistiakov et al., 2015). It is a chronic inflammatory reaction of the arterial wall (Wu M. Y. et al., 2017), mainly manifested by the formation of atherosclerotic plaques on the arterial wall, resulting in loss of arterial elasticity and eventually leading to adverse cardiovascular events (Greaves and Gordon, 2013; Mailer et al., 2017; Peter, 2017). Recent studies show that the changes in GM composition and diversity are closely related to the occurrence of chronic low-grade inflammation and atherosclerosis (Jones et al., 2013; Witjes et al., 2015); it was observed that the Yersinia and Rhodobacter population decreased, while that of Collinsella erofaciens and monocytes increased (Svetel et al., 2013). This was illustrated in a study of the feces of 12 patients with obvious symptoms of carotid atherosclerotic plaques and 12 healthy individuals matched according to gender and age (Karlsson et al., 2012). The data showed that the number of coliforms in the intestinal tract of the patients increased, while the number of Rhodobacter and Eubacterium increased in the healthy controls. Further, in a metagenomic association study on feces from 218 individuals with atherosclerotic CVD and 187 healthy controls (Jie et al., 2017), significant differences in GM were found between individuals with atherosclerotic CVD and the control group, including a relative reduction in Bacteroides and Prevotella populations, and the enrichment of Streptococcus and E. coli.
GM can affect the development of atherosclerosis through a variety of ways. It can not only promote an inflammatory reaction, aggravate the development of atherosclerotic plaque, or lead to plaque rupture but also affect the occurrence and development of atherosclerotic plaque by regulating host cholesterol and lipid metabolism. It is generally believed that GM is an activator of inflammation, especially that related to lipopolysaccharide (LPS) synthesized by Gram-negative bacteria and a strong inflammatory trigger (Du et al., 2017). LPS produced by GM can promote the progression of chronic low-grade inflammation, which is recognized as a risk factor for atherosclerosis. GM dysbiosis is accompanied by increased intestinal permeability, which leads to increase in endotoxin levels in the blood. Additionally, high-dose LPS can significantly increase the expression of nuclear factor kappaB (NF-κB) and other inflammatory factors, whereas low-dose LPS can induce macrophage differentiation into a mild proinflammatory state through Toll-like receptor 4, interleukin-1 receptor-related kinase-1, and Toll-interacting protein on the cell surface and promote the occurrence of atherosclerosis (Maitra et al., 2012). Moreover, GM inhibits the expression of fasting-induced adipocyte factor, increases triglycerides in blood circulation, and promotes deposition of adipocytes, resulting in increased lipid levels and leading to dyslipidemia and induction of atherosclerosis (Backhed et al., 2004). Furthermore, the signaling molecules produced by GM significantly influence cholesterol reverse transport. For example, low-dose endotoxin LPS can significantly reduce the expression of ATP-binding cassette (ABC) subfamily A member 1 and ABC subfamily G member 1 by cholesterol-reversion factor and inhibit the reverse process of cholesterol accumulation in macrophages, which promotes foam-cell accumulation in the vascular endothelium to form plaques and the occurrence of arteriosclerosis (Maitra and Li, 2013).
As a potential promoter of atherosclerosis and cardiac metabolic diseases, TMAO significantly affects the development of atherosclerotic lesions, resulting in increased attention being given to its molecular mechanisms in the human body (Tang et al., 2013). TMAO can not only significantly accelerate the development of atherosclerosis in apolipoprotein E-deficient mice but also activate the mitogen-activated protein kinase and NF-κB signaling pathways in endothelial cells and smooth muscle cells, thereby triggering inflammatory reactions in vessels and causing increased accumulation of foam cells in the vascular wall and atherosclerosis (Zeisel and Warrier, 2017). These findings suggest that reducing in vivo TMAO levels by inhibiting the proliferation of TMA-producing bacteria might improve the symptoms of atherosclerosis. One study reported that TMAO can inhibit the reverse transport of cholesterol and regulate the activity of cholesterol transporters in macrophages, thus leading to atherosclerosis (Koeth et al., 2015). Conversely, another study aimed at the key TMA enzyme (cutC/D) produced by bacteria and developed two strong inhibitors that can permanently inactivate cutC/D without affecting the survival ability of the symbiotic bacteria. After oral administration, the selective accumulation of the inhibitor in the intestinal symbiotic bacteria can almost completely inhibit the production of TMA and TMAO caused by hypercholinergic food, reduce platelet aggregation and thrombosis, and thus reduce the risk of atherosclerosis. Simultaneously, it reverses the changes in the intestinal bacterial composition induced by high choline foods, and increases the levels of Akkermansia muciniphila (Roberts et al., 2018). The TMA/TMAO pathway likely represents only one of many microbe-dependent pathways that will ultimately be linked to CVD pathogenesis. Early intervention of GM and its metabolites can help to better control the occurrence and progression of atherosclerosis, stabilize atherosclerotic plaques, and reduce the risk of MI.
The Roles of Gut Microbiota and its Metabolites in Myocardial Ischemia
MI, caused by the necrosis of myocardium due to prolonged ischemia, was one of the most critical stress to the patients (Wu Z. X. et al., 2017). Nevertheless, different from other chronic CVDs, MI is a kind of severe stress injury. Under this kind of trauma, the structure of GM and its metabolites undergo stress changes. Patients with MI will have a typical GM dysbiosis, with a significant reduction of probiotics, a large number of pathogens, impaired intestinal barrier function, increased intestinal permeability and a series of changes. Based on the analysis of the blood microflora of 49 healthy controls, 50 patients with coronary heart disease, and 100 patients with MI, it was found that the diversity and richness of the blood microflora in patients with MI increased, and more than 12% of the bacteria in the blood was derived from GM (Zhou et al., 2018). Studies based on MI rat models have shown that the GM abundance was significantly higher in rats with MI than in the sham operation group, upon comparing the intestinal barrier injury of both rat groups (Wu Z. X. et al., 2017). Further, MI led to a decrease in Lactobacillus, and SCFAs were found to alleviate MI, indicating that GM and its metabolites have a significant repair effect on the cardiac tissue after MI. Similar studies have confirmed that the dysbacteriosis destroys the intestinal mucosal barrier, causing GM to enter systemic circulation, thus increasing the incidence of adverse cardiovascular events after MI. The incidence of adverse cardiovascular events increased significantly in patients with MI who had significantly increased GM (Lactobacillus, Bacteroides, and Streptococcus) populations (Org et al., 2015). Another study also found that the use of antibiotics to reduce the bacterial translocation in mice after MI can reduce the systemic inflammatory response and myocardial damage (Zhou et al., 2018).
Additionally, GM and its metabolites can, not only decrease the risk of MI by affecting intestinal barrier function, but also influence MI occurrence and development in other ways. Studies have shown that bacterial DNA found in arterial plaques is similar to that found in GM, and these bacteria may be related to plaque stability, implying that unstable atherosclerotic plaques increase the risk of MI (Koren et al., 2011). Elevated TMAO levels can affect the repair of mitochondria and myocardial metabolism and increase the risk and severity of MI (Makrecka-Kuka et al., 2017). TMAO can also promote the release of calcium ions into the cells through adenosine diphosphate, thrombin, and collagen, improve the sensitivity of platelets, and then promote thrombosis, which may directly stimulate the occurrence of MI (Zhu et al., 2016). Further experiments showed that antibiotics can reduce the MI area of acute myocardial infarction in mice, with the mechanism possibly related to the regulation of intestinal microorganisms (Lam et al., 2016). Furthermore, a recent study found that before myocardial infarction, probiotics supplementation in mice treated with antibiotics was beneficial at restoring cardiac function and prolonging survival time. That study found that GM-derived SCFAs played an important role in maintaining host immune components and repair ability after myocardial infarction, suggesting that regulation of intestinal flora might provide opportunities for rehabilitation after myocardial infarction (Tang et al., 2019); however, their ability to prevent coronary atherosclerosis and reduce the incidence of acute MI remains unclear. Thus, the mechanism by which GM and its metabolites influence the occurrence and development of MI is extremely complex, and needs to be studied further.
The Roles of Gut Microbiota and Its Metabolites in Heart Failure
HF is the ultimate stage after MI. The structure and function of the heart are seriously damaged. Currently, surgery and drug treatment can delay the progress of HF to some extent, but the mortality rate is still very high. In recent years, great advances have taken place in understanding the pathophysiological mechanism of HF, from hemodynamics to neurohumoral immune regulation. The role of GM in HF has attracted widespread attention. In patients with HF, due to volume overload, intestinal congestion and ischemia could occur, leading to the dysbiosis of GM and an increase in intestinal permeability (Pasini et al., 2015). This will allow too many toxins to enter into blood circulation, activate cytokines, generate a systemic inflammatory response, and aggravate the progress of HF (Krack et al., 2004; Cannon and Mcmurray, 2014; Cui et al., 2018). Similar results were observed in a more recent study of patients with HF who were more likely to be infected with pathogenic bacteria than those in the control group (Pasini et al., 2015). Candida, Campylobacter, and Shigella were positively correlated with disease severity. Compared with the control group, 78.3% of the patients with HF had increased intestinal permeability, which was higher in patients with moderate and severe HF than in patients with mild HF. These results show that HF destroys GM balance, and the destruction of the intestinal mucosal barrier leads to bacterial translocation, an increase in systemic endotoxins, potential inflammation, and aggravation of HF.
Additionally, GM metabolites are closely related to HF. Recent studies have shown that TMAO is directly involved in the development of HF. TMAO levels were significantly higher in the blood of patients with HF than in that of healthy controls, and the prognosis of patients with high TMAO levels was poor (Tang et al., 2014). For instance, results from a study of a large number of chronic HF patients undergoing treatment show that increased TMAO content is positively correlated with the probability of death, worsening of patients’ condition, and probability of relapse in recovered patients within a short period of time (Chun and Rui, 2017). Further, Organ et al. (Organ et al., 2016) established a mouse HF model by surgery and found that mice fed TMAO showed more severe symptoms and signs of HF than mice fed a normal diet. Because 3,3-dimethyl-1-butanol can reduce the level of plasma TMAO, another animal study found that feeding 3,3-dimethyl-1-butanol to mice with HF induced by aortic coarctation significantly improved cardiac structural remodeling and electrical remodeling, suggesting a relationship between low levels of plasma TMAO and these improvements (Wang et al., 2020). Moreover, TMAO directly leads to progressive tubulointerstitial fibrosis and dysfunction, which might represent a potential mechanism associated with HF progression (Tang et al., 2015). Although there are several studies on the mechanisms underlying the effects of metabolites on HF, the GM composition and the specific mechanism by which the metabolites affect HF still need to be further studied.
Conclusion and Future Prospects
In recent years, interest in studying the interaction between flavonoids and GM has increased. When flavonoids enter the intestinal tract, they inevitably become metabolized and transformed under the action of various drug-metabolizing enzymes produced by the GM, and produce bioactive metabolites, which can promote cardiovascular health by exerting systemic and local effects. A large number of microorganisms in the intestine can metabolize flavonoids that cannot be metabolized by the gastrointestinal tract. The metabolic products are absorbed by the body or help in resisting CVDs and exhibit certain biological activities. Meanwhile, flavonoids from different sources are beneficial for GM and also affect GM balance. They interact with GM, potentially inhibiting the growth of harmful microorganisms, promoting the proliferation of beneficial microorganisms, and maintaining GM balance. Therefore, GM, as an integral part of the human body, actively participates in the biotransformation process of flavonoids, thereby influencing the cardiovascular system. Further, GM metabolites also affect CVD.
Although the research on the relationship between GM and its metabolites and atherosclerosis, MI, HF and hypertension is growing rapidly. Further researches, however, are encouraged to focus on the host-microbiota interactions and related effects during the treatment of flavonoids on CVD. Potential challenges and problems in the future can be summarized as follows: 1) Limited development and application of flavonoids due to the lack of specificity and selectivity for CVDs because of their complex structure and multiple targets. 2) The difficulty in excluding individual GM differences, such as age, environment, diet, and other influencing factors. 3) Most of the data were obtained from rodents. Due to the differences in intestinal and body functions between rodents and humans, many research results cannot be directly applied to humans. 4) The effects of bacterial imbalance, intestinal barrier damage, and chronic inflammation associated with GM translocation, TMAO, SCFAs, etc., on CVD have been confirmed, but the mechanisms of action remain to be further studied.
In summary, this review shows that the intake of flavonoids could increase the proliferation of certain gut bacteria that attenuate CVDs, and the relationships among flavonoids, GM, and CVD could offer a new perspective to understanding the mechanisms underlying the effects of flavonoids on CVD. It should be noted that research on the absorption, distribution, and metabolism of flavonoids in the intestinal tract is still at the initial stage globally. After the metabolic transformation of flavonoids in GM, their effects on CVDs are unstable and the mechanism of action is unclear, which is one of the difficulties in its development and utilization. Therefore, it is necessary to further enrich and strengthen research on the in vivo analysis of the action mechanism of flavonoids, which is of great significance for guiding rational diets and improving the use of functional foods.
Author Contributions
Conceptualization, QL and RZ; methodology, QL, QH and BG; writing—original draft preparation, QL and XM; writing—review and editing, QL and NhZ; visualization, QL; supervision, NaZ and ML; project administration, BS, NaZ and ML; funding acquisition, BS, NaZ and ML. All authors have read and agreed to the published version of the manuscript.
Funding
This work was supported by the National Natural Science Foundation of China (No. 81903925) and Science and Technology Program in Inner Mongolia (No. 201802097).
Conflict of Interest
The authors declare that the research was conducted in the absence of any commercial or financial relationships that could be construed as a potential conflict of interest.
Acknowledgments
Figures 1, 2 were modified from Servier Medical Art (http://smart.servier.com/), licensed under a Creative Common Attribution 3.0 Generic License. (https://creativecommons.org/licenses/by/3.0/).
Reference
Akao, T., Kawabata, K., Yanagisawa, E., Ishihara, K., Mizuhara, Y., Wakui, Y., et al. (2010). Balicalin, the Predominant Flavone Glucuronide of Scutellariae Radix, is Absorbed from the Rat Gastrointestinal Tract as the Aglycone and Restored to its Original Form. Journal of Pharmacy & Pharmacology 52, 1563–1568. doi:10.1211/0022357001777621
Akyildiz, B. N., Kurtoğlu, S., Kondolot, M., and Tunç, A. (2010). Cyanide poisoning caused by ingestion of apricot seeds. Annals of Tropical Paediatrics 30, 39–43. doi:10.1179/146532810X12637745451951
Assini, J. M., Mulvihill, E. E., and Huff, M. W. (2013). Citrus flavonoids and lipid metabolism. Curr. Opin. Lipidol. 24, 34–40. doi:10.1097/MOL.0b013e32835c07fd
Aura, A.-M. (2008). Microbial Metabolism of Dietary Phenolic Compounds in the Colon. Phytochem. Rev. 7, 407–429. doi:10.1007/s11101-008-9095-3
Backhed, F., Ding, H., Wang, T., Hooper, L. V., Koh, G. Y., Nagy, A., et al. (2004). The gut microbiota as an environmental factor that regulates fat storage. Proceedings of the National Academy of Sciences 101 (44), 15718–15723. doi:10.1073/pnas.0407076101
Bazzano, L. A., He, J., Ogden, L. G., Loria, C. M., and Whelton, P. K. (2003). Dietary Fiber Intake and Reduced Risk of Coronary Heart Disease in US Men and Women. Arch Intern Med 163, 1897. doi:10.1001/archinte.163.16.1897
Bi, K.S (2018). Bioactive flavonoids in medicinal plants:Structure, activity and biological fate. Asian Journal of Pharmaceutical Sciences 13, 14–25. doi:10.1016/j.ajps.2017.08.004
Bowey, E., Adlercreutz, H., and Rowland, I. (2003). Metabolism of Isoflavones and Lignans by the Gut Microflora: A Study in Germ-Free and Human Flora Associated Rats. Food Chem. Toxicol. 41, 631–636. doi:10.1016/S0278-6915(02)00324-1
Braune, A., and Blaut, M. (2011). Deglycosylation of Puerarin and Other Aromatic C-Glucosides by a Newly Isolated Human Intestinal Bacterium. Environ. Microbiol. 13, 482–494. doi:10.1111/j.1462-2920.2010.02352.x
Cannon, J. A., and Mcmurray, J. J. V. (2014). Gut Feelings About Heart Failure∗. Journal of the american college of cardiology 64, 1915–1916. doi:10.1016/j.jacc.2014.04.088
Cassidy, A., Hanley, B., and Lamuelaraventos, R. (2010). Isoflavones, Lignans and Stilbenes - Origins, Metabolism and Potential Importance to Human Health. J. Ence Food Agri. 80, 1044–1062. doi:10.1002/(SICI)1097-0010(20000515)80:7<1044::AID-JSFA586>3.0.CO;2
Chang, P., Xia, W., Zhang, Y., Zhang, Y., and Zhao, X. (2008). Effect of Gut Microbiota on Apigenin Metabolism in Rats. Chin. Pub. Health 24, 382–383.
Chen, Y., Patel, N. A., Crombie, A., Scrivens, J. H., and Murrell, J. C. (2011). Bacterial flavin-containing monooxygenase is trimethylamine monooxygenase. Proc. Natl. Acad. Sci. U. A. S. 108, 17791–17796. doi:10.1073/pnas.1112928108
Chiou, V. L., and Burotto, M. (2015). Pseudoprogression and Immune-Related Response in Solid Tumors. Jco 33, 3541–3543. doi:10.1200/jco.2015.61.6870
Chistiakov, D. A., Bobryshev, Y. V., Kozarov, E., Sobenin, I. A., and Orekhov, A. N. (2015). Role of gut microbiota in the modulation of atherosclerosis-associated immune response. Front. Microbiol. 6, 671. doi:10.3389/fmicb.2015.00671
Chun, H.Y., and Rui, T.X (2017). Association of gut microbiota with cardiovascular diseases:Present and future. World Chinese Journal of Digestology 025, 31–42. doi:10.11569/wcjd.v25.i1.31
Zhu, C. M., Chen, Z. L., Pan, K. C., and Shen, Y. B. (2013). Preliminary study for the effect of soybean isoflavones on the flora of intestinal contents of rats. Heilongjiang Anim. Sci. and Vet. Med. 2013.
Craciun, S., and Balskus, E. P. (2012). Microbial Conversion of Choline to Trimethylamine Requires a Glycyl Radical Enzyme. Proc. Natl. Acad. Sci. U. S. A. 109, 21307–21312. doi:10.1073/pnas.1215689109
Cruden, D. L., and Galask, R. P. (1988). Reduction of Trimethylamine Oxide to Trimethylamine by Mobiluncus Strains Isolated from Patients with Bacterial Vaginosis. Microb. Ecol. Health Dis. 1, 95–100. doi:10.3402/mehd.v1i2.7400
Cui, X., Ye, L., Li, J., Jin, L., Wang, W., Li, S., et al. (2018). Metagenomic and metabolomic analyses unveil dysbiosis of gut microbiota in chronic heart failure patients. Sci Rep 8, 635. doi:10.1038/s41598-017-18756-2
Daniela, G., Krzysztof, J., Joanna, J.M.E., and Katarzyna, K (2015). The impact of polyphenols on Bifidobacterium growth. Acta Biochimica Polonica 62, 895–901. doi:10.18388/abp.2015_1154
Denby, K. J., Rolfe, M. D., Crick, E., Sanguinetti, G., Poole, R. K., and Green, J. (2015). Adaptation of Anaerobic Cultures of Escherichia coli K-12 in Response to Environmental Trimethylamine-N-Oxide. Environ. Microbiol. 17, 2477–2491. doi:10.1111/1462-2920.12726
Donohoe, DR, Garge, N, Zhang, X, Sun, W, O'Connell, TM, Bunger, MK, et al. (2018). The Microbiome and butyrate regulate energy metabolism and autophagy in the mammalian colon. Cell Metab 13 (5), 517–26. doi:10.1016/j.cmet.2011.02.018
Du, M., Yuan, L., Tan, X., Huang, D., Wang, X., Zheng, Z., et al. (2017). The LPS-inducible lncRNA Mirt2 is a negative regulator of inflammation. Nat Commun 8 (1), 2049. doi:10.1038/s41467-017-02229-1
Duda-Chodak, A (2012). The inhibitory effect of polyphenols on human gut microbiota. J Physiol Pharmacol 63, 497–503. doi:10.1002/cphy.c110065
Fennema, D., Phillips, I. R., and Shephard, E. A. (2016). Trimethylamine and Trimethylamine N-Oxide, a Flavin-Containing Monooxygenase 3 (FMO3)-Mediated Host-Microbiome Metabolic Axis Implicated in Health and Disease. Drug Metab. Dispos. 44 (11), 1839–1850. doi:10.1124/dmd.116.070615
Gabriele, D.A (2015). Quercetin: A flavonol with multifaceted therapeutic applications?. Fitoterapia 106, 256–271. doi:10.1016/j.fitote.2015.09.018
Gil-Cardoso, K., Ginés, I., Pinent, M., Ardévol, A., Blay, M., and Terra, X. (2016). Effects of flavonoids on intestinal inflammation, barrier integrity and changes in gut microbiota during diet-induced obesity. Nutr. Res. Rev. 29, 234–248. doi:10.1017/S0954422416000159
Gong, X., Ji, M. Y., Xu, J. P., Zhang, C. H., and Li, M. H. (2019). Hypoglycemic effects of bioactive ingredients from medicine food homology and medicinal health food species used in China. Critical Reviews in Food Science and Nutrition 60, 2303–2326. doi:10.1080/10408398.2019.1634517
Gong, X., Li, X., Bo, A., Shi, R.-Y., Li, Q.-Y., Lei, L.-J., et al. (2020). The interactions between gut microbiota and bioactive ingredients of traditional Chinese medicines: A review. Pharmacological Research 157, 104824. doi:10.1016/j.phrs.2020.104824
Greaves, D.R., and Gordon, S (2013). Immunity, atherosclerosis and cardiovascular disease. rends in Immunology 11, 117. doi:10.1186/1741-7015-11-117
Guo, P., and Wu, C. M. (2017). Gut Microbiota Brings a Novel Way to Illuminate In Vivo Mechanisms of Natural Products. Chin. Herb. Med. 9, 301–306. doi:10.1016/S1674-6384(17)60109-6
Guo, Q., Wang, W., and Guo, W. (2014). Advances in Pharmacological Effects of Gentiopicrin. Guangdong Chem. Ind. 41, 121.
Hajialyani, M., Hosein Farzaei, M., Echeverría, J., Nabavi, S., Uriarte, E., and Sobarzo-Sánchez, E. (2019). Hesperidin as a Neuroprotective Agent: A Review of Animal and Clinical Evidence. Molecules 24, 648. doi:10.3390/molecules24030648
Hanske, L., Loh, G., Sczesny, S., Blaut, M., and Braune, A. (2009). The Bioavailability of Apigenin-7-Glucoside Is Influenced by Human Intestinal Microbiota in Rats. J. Nutr. 139, 1095–1102. doi:10.3945/jn.108.102814
He, C., Wang, Z., and Shi, J. (2020). Pharmacological effects of icariin. Advances in pharmacology 87, 179–203. doi:10.1016/bs.apha.2019.10.004
He, J., Magnuson, B. A., and Giusti, M. M. (2005). Analysis of Anthocyanins in Rat Intestinal ContentsImpact of Anthocyanin Chemical Structure on Fecal Excretion. J. Agric. Food Chem. 53, 2859–2866. doi:10.1021/jf0479923
Hollman, P. C. H., and Arts, I. C. W. (2000). Flavonols, flavones and flavanols - nature, occurrence and dietary burden. J. Sci. Food Agric. 80, 1081–1093. doi:10.1002/(sici)1097-0010(20000515)80:7<1081::aid-jsfa566>3.0.co;2-g
Hor-Gil, H., and Fatemeh, R (2000). Biotransformation of the isoflavonoids biochanin A, formononetin, and glycitein by Eubacterium limosum. Fems Microbiology Letters 192, 21–25. doi:10.1016/S0378-1097(00)00404-3
Hormann, K., and Andreesen, J. R. (1989). Reductive cleavage of sarcosine and betaine by Eubacterium acidaminophilum via enzyme systems different from glycine reductase. Arch. Microbiol. 153, 50–59. doi:10.1007/BF00277541
Hur, H.-G., Beger, R., Heinze, T., Lay, J., Freeman, J., Dore, J., et al. (2002). Isolation of an Anaerobic Intestinal Bacterium Capable of Cleaving the C-Ring of the Isoflavonoid Daidzein. Arch. Microbiol. 178, 75. doi:10.1007/s00203-002-0437-z
Jia, Q., Xie, Y., Lu, C., Zhang, A., Lu, Y., Lv, S., et al. (2019b). Endocrine organs of cardiovascular diseases: Gut microbiota. J Cell Mol Med 23, 2314–2323. doi:10.1111/jcmm.14164
Jia, Q., Li, H., Zhou, H., Zhang, X., Zhang, A., Xie, Y., et al. (2019a). Role and Effective Therapeutic Target of Gut Microbiota in Heart Failure. Cardiovascular Therapeutics 2019, 1–10. doi:10.1155/2019/5164298
Jie, Z. Y., Xia, H. H., Zhong, S. L., Feng, Q., Li, S. H., Liang, S. S., et al. (2017). The gut microbiome in atherosclerotic cardiovascular disease. Nat Commun 8. doi:10.1038/s41467-017-00900-1
Jin, J. S., Kakiuchi, N., and Hattori, M. (2007). Enantioselective oxidation of enterodiol to enterolactone by human intestinal bacteria. Biol. Pharm. Bull. 30 (11), 2204. doi:10.1248/bpb.30.2204
Jones, M. L., Tomaro-Duchesneau, C., Martoni, C. J., and Prakash, S. (2013). Cholesterol lowering with bile salt hydrolase-active probiotic bacteria, mechanism of action, clinical evidence, and future direction for heart health applications. Expert Opinion on Biological Therapy 13, 631–642. doi:10.1517/14712598.2013.758706
Karbach, S. H., Schönfelder, T., Brandão, I., Wilms, E., Hörmann, N., Jäckel, S., et al. (2016). Gut Microbiota Promote Angiotensin II-Induced Arterial Hypertension and Vascular Dysfunction. Jaha 5, e003698. doi:10.1161/JAHA.116.003698
Karlsson, F. H., Fåk, F., Nookaew, I., Tremaroli, V., Fagerberg, B., Petranovic, D., et al. (2012). Symptomatic atherosclerosis is associated with an altered gut metagenome. Nat Commun 3, 1245. doi:10.1038/ncomms2266
Kim, D.-H., Yu, K.-U., Bae, E.-A., and Han, M. J. (1998). Metabolism of Puerarin and Daidzin by Human Intestinal Bacteria and Their Relation to In Vitro Cytotoxicity. Biol. Pharm. Bull. 21, 628–630. doi:10.2165/00063030-199809060-00006
Koeth, R. A., Wang, Z., Levison, B. S., Buffa, J. A., Org, E., Sheehy, B. T., et al. (2013). Intestinal microbiota metabolism of L-carnitine, a nutrient in red meat, promotes atherosclerosis. Nat Med 19, 576–585. doi:10.1038/nm.3145
Koren, O, Spor, A, Felin, J, Fåk, F, Stombaugh, J, Tremaroli, V, et al. (2011). Human oral, gut, and plaque microbiota in patients with atherosclerosis. Proc Natl Acad Sci U S A 108, 4592–8. doi:10.1002/cphy.c110065
Krack, A., Richartz, B. M., Gastmann, A., Greim, K., Lotze, U., Anker, S. D., et al. (2004). Studies on intragastric PCO2at rest and during exercise as a marker of intestinal perfusion in patients with chronic heart failure. European Journal of Heart Failure 6, 403–407. doi:10.1016/j.ejheart.2004.03.002
Kumar, S., and Pandey, A. K. (2013). Chemistry and biological activities of flavonoids: An overview. The Scientific World Journal 2013, 1–16. doi:10.1155/2013/162750
Lam, V, Su, J, Hsu, A, Gross, G.J., and Baker, J.E (2016). Intestinal Microbial Metabolites Are Linked to Severity of Myocardial Infarction in Rats. PLoS One 11 (8), e0160840. doi:10.1371/journal.pone.0160840
Lau, K., Srivatsav, V., Rizwan, A., Nashed, A., Liu, R., Shen, R., et al. (2017). Bridging the Gap between Gut Microbial Dysbiosis and Cardiovascular Diseases. Nutrients 9, 859. doi:10.1186/s40168-016-0222-x10.3390/nu9080859
Lee, N. K., Choi, S. H., Park, S. H., Park, E. K., and Kim, D. H. (2004). Antiallergic Activity of Hesperidin Is Activated by Intestinal Microflora. Pharmacology 71, 174–180. doi:10.1159/000078083
Li, J., Zhao, F., Wang, Y., Chen, J., Tao, J., Tian, G., et al. (2017). Gut microbiota dysbiosis contributes to the development of hypertension. Microbiome 5, 14. doi:10.1186/s40168-016-0222-x
Lilian, S., Ruchika, M., Annett, B., Marc, B., and Michael, B. (2002). Anaerobic C-ring Cleavage of Genistein and Daidzein by Eubacterium Ramulus. Fems Microbiol. Lett. 208, 197–202. doi:10.1016/S0378-1097(02)00458-5
Liu, X., and Zhao, Y. (2004). Antidepressant Effects of Apigenin and 2,4,5-trimethoxycinnamic Acid in Perilla frutescens. Trad. Chin. Med. 26, 303–304.
Lu, N., Han, J., Ren, B., Li, D., and Zheng, Q (2017). Antioxidation effect of dihydroquercetin pretreatment in isolated rat hearts during myocardial ischemia reperfusion injury. Chinese Pharmacological Bulletin 33, 487–492. doi:10.3969/j.issn.1001-1978.2017.04.009
Luo, M. X., Luo, D., and Zhao, W. H. (2014). Research Progress on Pharmacological Action of Quercetin. Chinese J. Ethnomedicine Ethnopharmacy. doi:10.1109/ccdc.2014.6852143
Ma, H., Zhang, B., Hu, Y., Wang, J., Liu, J., Qin, R., et al. (2019). Correlation analysis of intestinal redox state with the gut microbiota reveals the positive intervention of tea polyphenols on hyperlipidemia in high fat diet fed mice. J. Agric. Food Chem. 67, 7325–7335. doi:10.1021/acs.jafc.9b02211
Mailer, R. K. W., Gisterå, A., Polyzos, K. A., Ketelhuth, D. F. J., and Hansson, G. K. (2017). Hypercholesterolemia Induces Differentiation of Regulatory T Cells in the Liver. Circ Res 120, 1740–1753. doi:10.1161/CIRCRESAHA.116.310054
Maitra, U., and Li, L. (2013). Molecular Mechanisms Responsible for the Reduced Expression of Cholesterol Transporters From Macrophages by Low-Dose Endotoxin. Arterioscler Thromb Vasc Biol. 33 (1), 24–33. doi:10.1161/ATVBAHA.112.300049
Maitra, U., Deng, H., Glaros, T., Baker, B., Capelluto, D. G. S., Li, Z., et al. (2012). Molecular mechanisms responsible for the selective and low-grade induction of proinflammatory mediators in murine macrophages by lipopolysaccharide. J.I. 189, 1014–1023. doi:10.4049/jimmunol.1200857
Makrecka-Kuka, M., Volska, K., Antone, U., Vilskersts, R., Grinberga, S., Bandere, D., et al. (2017). Trimethylamine N-oxide impairs pyruvate and fatty acid oxidation in cardiac mitochondria. Toxicology Letters 267, 32–38. doi:10.1016/j.toxlet.2016.12.017
Man, A. W. C., Xia, N., Daiber, A., and Li, H. (2020). The roles of gut microbiota and circadian rhythm in the cardiovascular protective effects of polyphenols. Br J Pharmacol 177, 1278–1293. doi:10.1111/bph.14850
Manach, C., Scalbert, A., Morand, C., Rémésy, C., and Jiménez, L. (2004). Polyphenols: food sources and bioavailability. American Journal of Clinical Nutrition 79, 727–747. doi:10.1079/PHN200355510.1093/ajcn/79.5.727
Manson, J.a.E. (2002). A prospective study of dietary fiber intake and risk of cardiovascular disease among women. Journal of the American College of Cardiology 39, 49–56. doi:10.1016/S0735-1097(01)01695-3
Martínez-del Campo, A., Campo, S., Hamer, H. A., Marks, J. A., Haiser, H. J., Turnbaugh, P. J., et al. (2015). Characterization and Detection of a Widely Distributed Gene Cluster That Predicts Anaerobic Choline Utilization by Human Gut Bacteria. MBio 6, e00042–15. doi:10.1128/mBio.00042-15
Meadows, J. A., and Wargo, M. J. (2015). Carnitine in Bacterial Physiology and Metabolism. J. Microbiol. 161, 1161–1174. doi:10.1099/mic.0.000080
Mengyu, W., Chiajung, L., Mingfeng, H., and Peiyi, C (2017). New insights into the role of inflammation in the pathogenesis of atherosclerosis. International Journal of Molecular Sciences 18, 2034.
Natarajan, N., Hori, D., Flavahan, S., Steppan, J., Flavahan, N. A., Berkowitz, D. E., et al. (2016). Microbial short chain fatty acid metabolites lower blood pressure via endothelial G protein-coupled receptor 41. Physiological Genomics 48, 826–834. doi:10.1152/physiolgenomics.00089.2016
Org, E., Mehrabian, M., and Lusis, A. J. (2015). Unraveling the environmental and genetic interactions in atherosclerosis: Central role of the gut microbiota. Atherosclerosis 241, 387–399. doi:10.1016/j.atherosclerosis.2015.05.035
Organ, C. L., Otsuka, H., Bhushan, S., Wang, Z., Bradley, J., Trivedi, R., et al. (2016). Choline Diet and Its Gut Microbe-Derived Metabolite, Trimethylamine N-Oxide, Exacerbate Pressure Overload-Induced Heart Failure. Circ Heart Fail 9. doi:10.1161/CIRCHEARTFAILURE.115.002314
Pasini, E., Aquilani, R., Testa, C., Baiardi, P., Angioletti, S., Boschi, F., et al. (2016). Pathogenic Gut Flora in Patients With Chronic Heart Failure. JACC: Heart Failure 4, 220–227. doi:10.1016/j.jchf.2015.10.009
Peter, L (2017). Superficial erosion and the precision management of acute coronary syndromes: not one-size-fits-all. European Heart Journal 38, 801–803. doi:10.1093/eurheartj/ehw599
Pingitore, A., Chambers, E. S., Hill, T., Maldonado, I. R., Liu, B., Morrison, D. J., et al. (2017). The diet-derived short chain fatty acid propionate improves beta-cell function in humans and stimulates insulin secretion from human islets in vitro. Diabetes Obes Metab 19 (2), 257–265. doi:10.1111/dom.12811
Pluznick, J. L. (2017). Microbial short-chain fatty acids and blood pressure regulation. Curr Hypertens Rep 19, 25. doi:10.1007/s11906-017-0722-5
Pluznick, J. L., Protzko, R. J., Gevorgyan, H., Peterlin, Z., Sipos, A., Han, J., et al. (2013). Olfactory receptor responding to gut microbiota-derived signals plays a role in renin secretion and blood pressure regulation. Proc Natl Acad Sci USA 110, 4410–4415. doi:10.1073/pnas.1215927110
Qin, J., Li, R., Li, R., Raes, J., Arumugam, M., Burgdorf, K. S., et al. (2010). A human gut microbial gene catalogue established by metagenomic sequencing. Nature 464, 59–65. doi:10.1038/nature08821
Rainer, S, Pforte, H, Jacobasch, G, and Blaut, M (2002). The growth of the flavonoid-degrading intestinal bacterium, Eubacterium ramulus, is stimulated by dietary flavonoids in vivo. FEMS Microbiol Ecol 40, 243–8. doi:10.1016/S0168-6496(02)00238-6
Rath, S., Heidrich, B., Pieper, D. H., and Vital, M. (2017). Uncovering the Trimethylamine-Producing Bacteria of the Human Gut Microbiota. Microbiome 5 (1), 54. doi:10.1186/s40168-017-0271-9
Roberts, A. B., Gu, X., Buffa, J. A., Hurd, A. G., Wang, Z., Zhu, W., et al. (2018). Development of a gut microbe-targeted nonlethal therapeutic to inhibit thrombosis potential. Nat Med 24, 1407–1417. doi:10.1038/s41591-018-0128-1
Robinson, J., Gibbons, N. E., and Thatcher, F. S. (1952). A Mechanism of Halophilism in Micrococcus Halodenitrificans1. J. Bacteriol. 64, 69–77. doi:10.1128/JB.64.1.69-77.1952
Romano, K. A., Vivas, E. I., Amador-Noguez, D., and Rey, F. E. (2015). Intestinal Microbiota Composition Modulates Choline Bioavailability from Diet and Accumulation of the Proatherogenic Metabolite Trimethylamine-N-Oxide. mBio 6. doi:10.1128/mBio.02481-14
Sanchez-Rodriguez, E., Egea-Zorrilla, A., Plaza-Díaz, J., Aragón-Vela, J., Muñoz-Quezada, S., Tercedor-Sánchez, L., et al. (2020). The gut microbiota and its implication in the development of atherosclerosis and related cardiovascular diseases. Nutrients 12, 605. doi:10.3390/nu12030605
Sellars, M. J., Hall, S. J., and Kelly, D. J. (2002). Growth of Campylobacter Jejuni Supported by Respiration of Fumarate, Nitrate, Nitrite, Trimethylamine-N-Oxide, or Dimethyl Sulfoxide Requires Oxygen. J. Bacteriol. 184, 4187–4196. doi:10.1128/JB.184.15.4187-4196.2002
Serra, A., Macià, A., Romero, M.-P., Reguant, J., Ortega, N., and Motilva, M.-J. (2012). Metabolic Pathways of the Colonic Metabolism of Flavonoids (Flavonols, Flavones and Flavanones) and Phenolic Acids. Food Chem. 130, 383–393. doi:10.1016/j.foodchem.2011.07.055
Simpson, F. J., Talbot, G., and Westlake, D. W. S. (1960). Production of Carbon Monoxide in the Enzymatic Degradation of Rutin. Biochem. Biophys. Res. Commun. 2, 15–18. doi:10.1016/0006-291X(60)90255-2
Stefanadis, C (2013). Flavonoids in Atherosclerosis: An Overview of Their Mechanisms of Action. Current Medicinal Chemistry 20, 2641–2660. doi:10.2174/0929867311320210003
Svetel, M., Pekmezovic, T., Markovic, V., Novaković, I., Dobričić, V., Djuric, G., et al. (2013). No Association between Brain-Derived Neurotrophic Factor G196A Polymorphism and Clinical Features of Parkinson's Disease. Eur Neurol 70, 257–262. doi:10.1159/000352033
Shi, T.R., Liu, Y., Wang, S., Wang, Y., Li, D., and Zhu, D.D (2014). Intestinal flora and metabolism of effective components of Chinese herbal medicine. Chin. J. Microecol 26, 479–482. doi:10.13381/j.cnki.cjm.201404030
Tabasco, R., Sánchez-Patán, F., Monagas, M., Bartolomé, B., Victoria Moreno-Arribas, M., Peláez, C., et al. (2011). Effect of grape polyphenols on lactic acid bacteria and bifidobacteria growth: Resistance and metabolism. Food Microbiology 28, 1345–1352. doi:10.1016/j.fm.2011.06.005
Tang, T. W. H., Chen, H.-C., Chen, C.-Y., Yen, C. Y. T., Lin, C.-J., Prajnamitra, R. P., et al. (2019). Loss of Gut Microbiota Alters Immune System Composition and Cripples Postinfarction Cardiac Repair. Circulation 139 (5), 647–659. doi:10.1161/circulationaha.118.035235
Tang, W. H. W., Wang, Z., Kennedy, D. J., Wu, Y., Buffa, J. A., Agatisa-Boyle, B., et al. (2015). Gut Microbiota-Dependent TrimethylamineN-Oxide (TMAO) Pathway Contributes to Both Development of Renal Insufficiency and Mortality Risk in Chronic Kidney Disease. Circ Res 116 (3), 448–455. doi:10.1161/CIRCRESAHA.116.305360
Tang, W. H. W., Wang, Z., Levison, B. S., Koeth, R. A., Britt, E. B., Fu, X., et al. (2013). Intestinal microbial metabolism of phosphatidylcholine and cardiovascular risk. N Engl J Med 368 (17), 1575–1584. doi:10.1056/nejmoa1109400
Tang, W. H. W., and Hazen, S. L. (2017). The Gut Microbiome and Its Role in Cardiovascular Diseases. Circulation 135, 1008–1010. doi:10.1161/CIRCULATIONAHA.116.024251
Tang, W. H. W., Wang, Z., Fan, Y., Levison, B., Hazen, J. E., Donahue, L. M., et al. (2014). Prognostic Value of Elevated Levels of Intestinal Microbe-Generated Metabolite Trimethylamine-N-Oxide in Patients With Heart Failure. Journal of the American College of Cardiology 64, 1908–1914. doi:10.1016/j.jacc.2014.02.617
Tomás-Barberán, F. A., and Clifford, M. N. (2000). Flavanones, Chalcones and Dihydrochalcones—Nature, Occurrence and Dietary Burden. J. Sci. Food Agri. 80, 1073–1080. doi:10.1002/(sici)1097-0010(20000515)80:7<1073::aid-jsfa568>3.0.co;2
Tzounis, X., Vulevic, J., Kuhnle, G. G. C., George, T., Leonczak, J., Gibson, G. R., et al. (2008). Flavanol Monomer-Induced Changes to the Human Faecal Microflora. Br. J. Nutr. 99, 782–792. doi:10.1017/S0007114507853384
Ufnal, M., Jazwiec, R., Dadlez, M., Drapala, A., Sikora, M., and Skrzypecki, J. (2014). Trimethylamine-N-oxide: a carnitine-derived metabolite that prolongs the hypertensive effect of angiotensin II in rats. Canadian Journal of Cardiology 30, 1700–1705. doi:10.1016/j.cjca.2014.09.010
Villarreal, D. T., Morales, A. C., García, H. C., López, R. O., and Garza, A. L. D. L (2019). Anti-Obesity Effects of Kaempferol by Inhibiting Adipogenesis and Increasing Lipolysis in 3T3-L1 Cells. J. Physiol. Biochem. 75, 83–88. doi:10.1007/s13105-018-0659-4
Wang, G., Kong, B., Shuai, W., Fu, H., Jiang, X., and Huang, H. (2020). 3,3-Dimethyl-1-butanol attenuates cardiac remodeling in pressure-overload-induced heart failure mice. The Journal of Nutritional Biochemistry 78, 108341. doi:10.1016/j.jnutbio.2020.108341
Wang, Z., Klipfell, E., Bennett, B. J., Koeth, R., Levison, B. S., Dugar, B., et al. (2011). Gut flora metabolism of phosphatidylcholine promotes cardiovascular disease. Nature 472 (7341), 57–63. doi:10.1038/nature09922
Wang, J., He, Y., and Zhang, W. (2013). Advances in Pharmacological Effects of Luteolin. Life Sci. 6, 148–150.
Who (2017). Cardiovascular diseases (CVDs): fact sheets. Available at: https://www.who.int/en/news-room/fact-sheets/detail/cardiovascular-diseases-(cvds).
Winter, J., Moore, L. H., Dowell, V. R., and Bokkenheuser, V. D. (1989). C-Ring Cleavage of Flavonoids by Human Intestinal Bacteria. Appl. Environ. Microbiol. 55, 1203–1208. doi:10.1016/0167-7799(89)90027-910.1128/aem.55.5.1203-1208.1989
Witjes, J. J., van Raalte, D. H., and Nieuwdorp, M. (2015). About the gut microbiome as a pharmacological target in atherosclerosis. European Journal of Pharmacology 763 (Pt A), 75–78. doi:10.1016/j.ejphar.2015.06.023
Wu, H., Xie, Y. Q., and Zhang, Y. C. (2016a). Research Progress of Intestinal Microbial Metabolite TMAO and Cardiovascular Diseases. J. Clin. Cardiol. 32 (1), 86–90.
Wu, H., Kim, M., and Han, J. (2016b). Icariin Metabolism by Human Intestinal Microflora. Molecules 21, 1158. doi:10.3390/molecules21091158
Wu, Z. X., Li, S. F., Chen, H., Song, J. X., and Cao, C. F. (2017). The changes of gut microbiota after acute myocardial infarction in rats. PLoS ONE 12, e0180717. doi:10.1371/journal.pone.0180717
Xing, S., Wang, M., Peng, Y., Chen, D., and Li, X. (2014). Simulated gastrointestinal tract metabolism and pharmacological activities of water extract of Scutellaria baicalensis roots. Journal of Ethnopharmacology 152, 183–189. doi:10.1016/j.jep.2013.12.056
Yang, X. W., Zhang, J. Y., Xu, W., and Zhang, W. Q. (2005). The biotransformation of kaempferitrin by human intestinal flora. Acta Pharmaceutica Sinica 40 (8): 717–721. doi:10.3321/j.issn:0513-4870.2005.08.009
Yang, T., Santisteban, M. M., Rodriguez, V., Li, E., Ahmari, N., Carvajal, J. M., et al. (2015). Gut dysbiosis is linked to hypertension. Hypertension 65, 1331–1340. doi:10.1161/HYPERTENSIONAHA.115.05315
Pan, Y. P., Zhang, Z. H., Ding, D. M., and Jia, X. B. (2013). Advance in studies on biotransformation of flavonoids by intestinal bacteria. China J. Chin. Materia Med. 038, 3239–3245. doi:10.4268/cjcmm20131904
Yin, L. B., Xia, Q. L., Zhao, L. Z., and Zhang, C. F. (2016). Research Progress of Pharmacological Effects of Puerarin. Modern Agri. Ence Technol.
Zeisel, S. H., and Warrier, M. (2017). TrimethylamineN-Oxide, the Microbiome, and Heart and Kidney Disease. Annu. Rev. Nutr. 37 (1), 157–181. doi:10.1146/annurev-nutr-071816-064732
Zhou, X., Li, J., Guo, J., Geng, B., Ji, W., Zhao, Q., et al. (2018). Gut-dependent microbial translocation induces inflammation and cardiovascular events after ST-elevation myocardial infarction. Microbiome 6, 66. doi:10.1186/s40168-018-0441-4
Zhu, W., Gregory, J. C., Org, E., Buffa, J. A., Gupta, N., Wang, Z., et al. (2016). Gut Microbial Metabolite TMAO Enhances Platelet Hyperreactivity and Thrombosis Risk. J. Cell 165, 111–124. doi:10.1016/j.cell.2016.02.011
Keywords: gut microbiota, cardiovascular disease, interactions, metabolite, flavonoid
Citation: Li Q, Gao B, Siqin B, He Q, Zhang R, Meng X, Zhang N, Zhang N and Li M (2021) Gut Microbiota: A Novel Regulator of Cardiovascular Disease and Key Factor in the Therapeutic Effects of Flavonoids. Front. Pharmacol. 12:651926. doi: 10.3389/fphar.2021.651926
Received: 11 January 2021; Accepted: 23 April 2021;
Published: 16 June 2021.
Edited by:
Augusto Montezano, University of Glasgow, United KingdomReviewed by:
Debabrata Chanda, Council of Scientific and Industrial Research (CSIR), IndiaInKyeom Kim, Kyungpook National University, South Korea
Copyright © 2021 Li, Gao, Siqin, He, Zhang, Meng, Zhang, Zhang and Li. This is an open-access article distributed under the terms of the Creative Commons Attribution License (CC BY). The use, distribution or reproduction in other forums is permitted, provided the original author(s) and the copyright owner(s) are credited and that the original publication in this journal is cited, in accordance with accepted academic practice. No use, distribution or reproduction is permitted which does not comply with these terms.
*Correspondence: Na Zhang, emhhbmduYTU2NzdAMTYzLmNvbQ==; Minhui Li, cHJvZl9saW1pbmh1aUB5ZWFoLm5ldA==
†These authors have contributed equally to this work