- 1College of Pharmacy, Southwest Minzu University, Chengdu, China
- 2School of pharmacy, Queen’s University Belfast, Belfast, United Kingdom
- 3The First Affiliated Hospital, Guizhou University of Traditional Chinese Medicine, Guiyang, China
Cancer vaccines represent among the most promising strategies in the battle against cancers. However, the clinical efficacy of current cancer vaccines is largely limited by the lack of optimized delivery systems to generate strong and persistent antitumor immune responses. Moreover, most cancer vaccines require multiple injections to boost the immune responses, leading to poor patient compliance. Controlled-release drug delivery systems are able to address these issues by presenting drugs in a controlled spatiotemporal manner, which allows co-delivery of multiple drugs, reduction of dosing frequency and avoidance of significant systemic toxicities. In this review, we outline the recent progress in cancer vaccines including subunit vaccines, genetic vaccines, dendritic cell-based vaccines, tumor cell-based vaccines and in situ vaccines. Furthermore, we highlight the efforts and challenges of controlled or sustained release drug delivery systems (e.g., microparticles, scaffolds, injectable gels, and microneedles) in ameliorating the safety, effectiveness and operability of cancer vaccines. Finally, we briefly discuss the correlations of vaccine release kinetics and the immune responses to enlighten the rational design of the next-generation platforms for cancer therapy.
Introduction
Immunotherapy was first introduced by Dr. William Coley to treat malignant tumors using intratumoral injections of live bacteria and bacterial toxins in the 1890s (Coley, 1991). Nowadays, immunotherapy has been fully embraced by the oncologists for the treatment of various tumors. Chimeric antigen receptor (CAR) T cell-based therapies as well as immune checkpoint inhibitors (ICIs), including antibodies targeting programmed cell death protein-1 (PD-1), programmed death-ligand 1 (PD-L1) and cytotoxic T-lymphocyte-associated antigen-4 (CTLA-4), have revolutionized the cancer treatments and have been approved by the U.S. Food and Drug Administration (FDA) for tackling many tumors (Allison, 2015; Sharma and Allison, 2015; Labanieh et al., 2018). However, the response rate to ICIs varies dramatically among cancers. Growing evidence supports the idea that patients lacking pre-existing tumor-infiltrating CD8+ T cells have a low response rate to ICIs, suggesting the hypothesis that ICIs need to be combined with other therapies that can stimulate potent tumor-specific T cell responses to improve the clinic outcomes (Hu et al., 2018; van der Burg, 2018; Shae et al., 2019).
One attractive strategy to mount effective antitumor responses is vaccination. Cancer vaccines can provoke antigen-specific immunity or reactivate pre-existing but quiescent tumor-reactive T cells by delivering the antigens and immunoadjuvants with the aim to prevent cancers or fight against established tumor burdens (Lybaert et al., 2018). Two prophylactic vaccines, namely the human papilloma virus (HPV) vaccine and the hepatitis B virus (HBV) vaccine, have been successfully approved for preventing cervical cancer and liver cancer, respectively (Stanley, 2017). Whereas, only one antigen-loaded therapeutic cancer vaccine, termed Sipuleucel-T (Provenge), has received FDA approval for the treatment of advanced prostate cancer so far (Shae et al., 2019). Although cancer vaccines have generated acceptable therapeutic effects in some clinic trials, their overall clinical efficacy is not encouraging especially in solid tumors (Jacobs et al., 2014; Bouzid et al., 2020). The main reasons for these disappointing outcomes include: 1) the use of suboptimal vaccine delivery systems which compromise the vaccine immunogenicity and efficacy; 2) the rapid clearance of antigens at the injection site and the inadequate delivery to lymph nodes; 3) the choice of weakly immunogenic antigens that lack variety and specificity (e.g., overexpressed self-antigens); 4) the inefficient controlling of the immunosuppressive tumor microenvironment (van der Burg, 2018; Shae et al., 2019; Bouzid et al., 2020). On the other hand, cancer vaccines usually require multiple injections to elicit effective immune responses, resulting in poor patient compliance. To surmount these issues, numerous controlled drug release technologies, which are effective in a single shot, have been developed to improve the delivery efficiency and potency of cancer vaccines. Controlled-release platforms can prolong the spatiotemporal presentation of antigens and immunomodulators to immune cells or mimic the prime-boost effect of the traditional multi-bolus vaccination schedules, thereby stimulating stronger antitumor immune responses (Ali et al., 2009b; Korupalli et al., 2019; Irvine et al., 2020). Moreover, the controlled-release systems can also improve patient compliance by eliminating the booster shots, minimize the toxic side effects by reducing drug dose and avoiding rapid drug clearance, as well as improve outcomes by integrating the synergistic effect of multiple therapeutics within one platform (Huang et al., 2019; Riley et al., 2019). Especially for in situ cancer vaccines that require intratumoral injections, this single-dose vaccine technology can improve the antitumor immunity and increase the operational feasibility by a local and sustained release of immunomodulators in vivo.
Below, we will present recent advances in the engineering of controlled-release delivery platforms for improving the safety and efficacy of cancer vaccines. Firstly, we introduce the advantages and challenges of various types of cancer vaccines. Secondly, an overview of the controlled-release drug delivery systems is provided and the limitations that should be addressed to improve the safety, effectiveness and operability of cancer vaccines are enlightened. Finally, we briefly discuss the possible relevancy between the release kinetics of controlled-release vaccines and the type and magnitude of the immune responses.
Conventional Cancer Vaccines
Conventional vaccines are composed of antigens and adjuvants. The selection of an immunogenic antigen plays a critical role in implementing the vaccine effectiveness and specificity. Tumor antigens can be broadly divided into two categories: tumor-associated antigens (TAAs) and neoantigens. TAAs are overexpressed self-antigens that are shared among many tumors and can also be found in some normal cells. TAAs have been widely used in cancer vaccines for decades but they are not tumor-specific antigens and can only trigger weak immune responses owing to thymic tolerance (Hu et al., 2018). In contrast, neoantigens are highly tumor-specific immunogens derived from somatic mutations and they are more immunogenic due to the lack of central tolerance (Chu et al., 2018). With the rapid development of next-generation sequencing and peptide immunogenicity prediction technologies, neoantigens represent the most promising candidates for the preparation of personalized cancer vaccines and the diagnosis of ICI therapy response (Desrichard et al., 2016). Tumor antigens can be presented in various forms ranging from defined proteins, peptides, protein-encoding DNA or RNA, recombinant viral or bacterial vectors, tumor cell-based preparations, to antigen-pulsed dendritic cells (DCs).
The other crucial component of a vaccine is the adjuvant that can strengthen the magnitude of the immune responses or skew the immune responses toward Th1 or Th2 immunity. There are several types of vaccine adjuvants, including toll-like receptor (TLR) agonists, e.g. unmethylated cytosine-phosphate-guanine (CpG), imiquimod and polyinosinic:polycytidylic acid (poly(I:C)); cytokines, e.g. interferon (IFN)-α and interleukin (IL)-12; bacterial derivatives, e.g. monophosphoryl lipid A (MPLA) and Bacille Calmette-Guerin (BCG); depot-like adjuvants, e.g. aluminum salts, incomplete Freund’s adjuvant (IFA) and Montanide; particle adjuvants, e.g., liposomes, virus-like particles and polymeric particles (Sivakumar et al., 2011; Pilla et al., 2018). However, only aluminum salts, three emulsions (MF59, AS03, AF03), virosomes and MPLA are approved for human vaccines (Lybaert et al., 2018). Therefore, exploring potent and safe adjuvants and identifying the optimal adjuvants for vaccines are also appealing areas of research.
Subunit Vaccines
Subunit vaccines are defined by the use of purified proteins, peptides or polysaccharides as the antigens to stimulate the immune responses. Subunit vaccines usually contain one or several well-structured antigenic components, making them easier to manufacture and much safer than live pathogen vaccines, as well as avoiding the side effects from antigen-induced unrelated immune responses (Table 1) (Riley et al., 2019). However, these vaccines can hardly conquer the tumor heterogeneity between and within tumors as a consequence of lacking antigen variety. One strategy is mixing multiple antigens in one formulation to benefit the antitumor immune responses (Fennemann et al., 2019; Noguchi et al., 2020). In comparison of whole protein-based vaccines, peptide vaccines only contain one or several epitopes, which can be easily processed by DCs (Rosalia et al., 2013). Peptide vaccines are composed of TAA peptides or neoantigen peptides which are presented in the form of short peptides (<15 amino acids) or long peptides (15–40 amino acids), and the difference between the short peptides and the long peptides has been elucidated in detail elsewhere (Lybaert et al., 2018; Bouzid et al., 2020).
Despite these excellent features, the major challenge that hinders the broad application of subunit vaccines is the low immune efficacy, which is partially due to the poor uptake of antigens and adjuvants in lymph nodes (Pilla et al., 2018; Riley et al., 2019). To overcome this issue, vaccines need to be exquisitely engineered using the following strategies. 1) Increasing the accumulation of antigens and adjuvants in lymph nodes. This can be achieved by a direct intra-lymph node injection (Ribas et al., 2011), especially when immunogenic cargos are loaded in sustained-release platforms that allow extended retention in lymph nodes (Shae et al., 2019). Another approach is the use of albumin-hitchhiking vaccines which can be constructed through conjugating an antigen (or an adjuvant) to a lipophilic albumin-binding tail (Liu et al., 2014) or to a derivative of Evans Blue (Zhu et al., 2017). Besides, nanoparticles with optimized size, surface charge and composition are more likely to be drained into lymph nodes (Jiang et al., 2017). 2) Promoting antigen uptake by DCs, such as using anchoring endocytosis molecules (e.g., mannose, fucose and N-acteylglucosamine)-decorated nanoparticles (Koerner et al., 2019) or antibody-antigen conjugates (Keler et al., 2007). 3) Improving the adjuvant effect of vaccines. This can be implemented by adding more powerful adjuvant reagents or using delivery vehicles with adjuvant activity, such as liposomes or nanoparticles (Vartak and Sucheck, 2016). 4) Promoting cross-presentation of the antigens to potentiate the Th1 immune responses. For example, pH-responsive cationic polymers can facilitate endosomal antigen escape by the “proton sponge” effect (Shae et al., 2019).
Genetic Vaccines
Genetic vaccines have emerged as promising alternatives to subunit vaccines by exploiting tumor antigen-encoding DNA or RNA sequences that require intracellular delivery into DCs to express targeted antigens (Pardi et al., 2018). Genetic vaccines have many merits (Table 1), such as cost-effectiveness, easy for mass production, allowing the delivery of multiple antigens in one platform, and avoiding human leukocyte antigen (HLA) restriction (Lybaert et al., 2018). In spite of these promising features, the clinical results of plasmid DNA vaccines for treating solid tumors have been disappointing mainly due to the barriers for nuclear delivery, low immunogenicity and the immunosuppressive factors within the tumor (Lopes et al., 2019; Riley et al., 2019). Alternatively, mRNA-based vaccines only require to cross the cell membranes to be translated in the cytoplasm, which avoids the risk of integration into the host genome (McNamara et al., 2015). Moreover, the in vivo half-life and immunogenicity of mRNA can be regulated by modification and delivery strategies (Pardi et al., 2018). However, the rapid degradation of mRNA by nucleases and the limited translocation into the cytoplasm still challenge the development of RNA-based vaccines (Lybaert et al., 2018; Lin et al., 2020).
Based on the characteristics of genetic vaccines, delivery systems with the ability to increase the drug delivery into lymph nodes, promote intracellular uptake of nucleic acids, facilitate protein translation and reduce nucleic acid degradation will greatly prompt the clinical application of genetic vaccines. These vaccines can be delivered in diverse ways, for instance, by gene gun, electroporation, ultrasound, laser, viral or bacterial vectors, liposomes, nanoparticles, autologous DCs or other carrier modalities (Guo et al., 2013). The safety and efficacy of various viral vectors, such as pox and adenovirus, have been tested in clinical trials, but the high immunogenicity of viral vectors can, on the contrary, lead to the secretion of neutralizing antibodies (Pilla et al., 2018). A heterologous prime-boost regimen can handle this dilemma. For example, PROSTVAC was a promising viral cancer vaccine, composed of a vaccinia priming vector and a fowlpox boosting vector carrying transgenes for human prostate-specific antigen (PSA) along with three costimulatory molecules (CD80, CD58, and CD54), but it failed to benefit the patients in a phase III study (Gulley et al., 2019; Zhao et al., 2019). Cationic materials are commonly used to condense nucleic acids. For example, a lipid nanoparticle with ionizable lipids was allowed to form complexation with negatively charged mRNA encoding two TAAs (gp100 and TRP2) at low pH, and this formulation could induce a strong CD8+ T cell response and remarkable tumor shrinkage (Oberli et al., 2017). Moreover, a local gene depot made of mRNA polyplex-loaded implantable porous scaffolds showed superior sustained delivery and higher local transgene expression than a bolus injection (Chen et al., 2018). This indicates that sustained delivery of mRNA may potentiate the antitumor immunity. Furthermore, the combination of immunoadjuvants or other cancer therapies may further boost the therapeutic effects of genetic vaccines.
Tumor Cell-Based Vaccines
In addition to neoantigens, another class of patient-individualized antigens is whole tumor cell derivatives including the live attenuated tumor cells, killed tumor cells, tumor lysates, tumor-derived exosomes, tumor-derived whole RNAs, tumor cell membrane-based particles, and fusions of tumor cells and DCs (Browning, 2013; Fang et al., 2014; Chiang et al., 2015). Autologous tumor cell vaccines based on patient individual-derived cancer tissues, contain the complete antigen repertoire of the tumor, thereby avoiding the costly and complex identification procedure needed for neoantigens (Table 1). Even more importantly, they are not restricted to HLA type. However, the preparation of whole tumor cell vaccines requires multiple steps: 1) obtaining the patient’s tumor cells by surgery or biopsy, 2) processing them in vitro to acquire tumor cell derivatives, 3) then loading the tumor antigens into delivery systems. These complex procedures may hinder the clinical practicality of tumor cell-based vaccines. Moreover, this autologous vaccine technology is only feasible for the patients with selected tumor types and stages that are capable of tumor biopsies or operation (Schlom et al., 2014). Alternatively, allogeneic tumor cell vaccines can benefit more patients with homologous tumors because they are prepared from two or three specific tumor cell lines which have been established and characterized (Schlom et al., 2014). In one example of this, Canvaxin containing three irradiated melanoma cell lines showed excellent therapeutic results in phase II clinical trials, but it did not meet the expectation in phase III trials (Ozao-Choy et al., 2014). This probably resulted from the use of suboptimal dosage, schedule and adjuvant. Tumor stroma-associated antigens are another kind of attractive targets for cancer vaccines as they are genetically more stable and less subjected to tumor immune evasion mechanisms in contrast to tumor cells (Chiang et al., 2015).
Although tumor cell-based vaccines have attracted enormous attention in recent years, two of the most important challenges for whole tumor cell vaccines remain the low accumulation in lymph nodes and low immunogenicity. Numerous strategies have been developed to handle these issues, such as cloaking nanoparticles with tumor cell membranes or fused cytomembranes derived from tumor cells and DCs (Liu et al., 2019b; Gan et al., 2020), or loading the immunogenically dying tumor cells with adjuvants (e.g., BCG) or adjuvant-loaded nanoparticles (Fan et al., 2017). For example, the sustained release of adjuvants and tumor cell membrane-coated nanoparticles from thermosensitive hydrogels could recruit DCs and induce a strong CD8+ T cell response, and the combination with antibodies targeting PD-1 could further prolong the survival time of tumor-bearing mice (Ye et al., 2019). It is worth noting that the immune properties of tumor-derived exosomes can be immune-activating or immunosuppressive depending on the physiological state of donor cells and exogenous factors (Théry et al., 2009; Greening et al., 2015). Fortunately, the immunosuppressive role of tumor-derived exosomes can be switched to promote the antitumor immune responses by using heat treatment, adding strong adjuvant components or using transgenic tumor cells expressing IL-2 or IL-18 (Cho et al., 2009; Théry et al., 2009). Besides, the whole tumor cell-based vaccines also contain abundant normal self-proteins which may induce autoimmunity as well as diminish the vaccine effectiveness by the dilution of the most immunogenic tumor antigens (Obeid et al., 2015; Hu et al., 2018). Using autologous induced pluripotent stem cells as the antigen resource may provide new insights to tackle these obstacles (Ouyang et al., 2019).
DC Vaccines
DCs, as the most potent professional antigen-presenting cells (APCs), play a pivotal role in initiating and bridging the innate and adaptive immune responses. The ultimate goal of many delivery strategies of cancer vaccines is to target DCs and fully activate them. One approach for this is exogenous DC vaccines which usually rely on the isolation and differentiation of peripheral blood autologous DC precursor cells into immature DCs, followed by loading these immature DCs with a proper form of antigens (such as peptides, proteins, nucleic acids or tumor cell derivatives), then maturating these DCs with different stimuli, and finally reinfusing the manipulated DCs to the patient to implement the cancer immunotherapy (Bol et al., 2016; Sabado et al., 2017). Sipuleucel-T, prepared from autologous DCs loaded with a fusion protein (PA2024) of prostatic acid phosphatase (PAP) and granulocyte-macrophage colony-stimulating factor (GM-CSF), was approved in 2010 to treat metastatic prostate cancer on the basis of a survival benefit (Gardner et al., 2012). Although substantial clinical studies have demonstrated the safety and immunogenicity of exogenous DC vaccines, the clinical outcomes are not encouraging (Perez and De Palma, 2019). Many factors affect the efficacy of exogenous DC vaccines, including a suboptimal choice of DC subsets, limited migration to the lymph nodes and negative immune regulation within tumors. Hence, DC vaccines can be further improved by optimizing, for instance, the choice of DC type and antigen type, activation method, the design of drug delivery system, the number of DCs for injection, the vaccination schedule and the administration route (Sabado et al., 2017). Another approach to exploit natural DCs in cancer vaccines is targeting and modulating the endogenous DC subpopulations in vivo. This targeting goal can be implemented by various strategies involving GM-CSF-secreting irradiated tumor cells, conjugation of antigens or antigen-loaded nanoparticles to ligands directing against DC surface receptors (e.g., CD40, DEC205, Langerin or Clec9A), or using injectable controlled-release platforms that are engineered to release chemokines, tumor antigens and adjuvants (Sabado et al., 2017; Calmeiro et al., 2019). These in vivo targeting technologies allow to program a large scale of DC subsets without the need for a costly and labor-intensive extracorporeal training of exogenous DCs (Table 1; Sabado et al., 2017). However, the endogenous DC-targeting vaccines cannot control the extent of antigen loading and DC activation as the exogenous DC vaccines do, and DCs that are not fully activated may induce immune tolerance (Bouzid et al., 2020). Recently, artificial DCs, such as nanoparticles coated with tumor lysate-primed DC membranes or scaffolds carrying T cell activation cues and peptide antigens, can directly stimulate the activation and expansion of antigen-specific T cells (Cheung et al., 2018; Cheng et al., 2020). These artificial DCs can circumvent the short shelf-life concerns of exogenous DC vaccines and they are not vulnerable to the tumor immunosuppressive conditions (Cheng et al., 2020). Furthermore, DC vaccine-based combination therapies may provide better control over cancers (Bol et al., 2016; Tanyi et al., 2018), thus many clinical trials are exploring the synergistic effect of DC vaccines and ICI therapies (Sprooten et al., 2019).
In Situ Cancer Vaccines
Despite the fact that the substantial improvement achieved by conventional cancer vaccines, the risk of undetected contaminations during the elaborate preparation process and the high costs associated with preparation and storage remain bottlenecks limiting their broad clinical implementation (Duong et al., 2020a). In situ vaccination (ISV), without the need for previous identification and isolation of tumor antigens, is raising great attention in cancer immunotherapy. ISV is a simple, personalized and off-the-shelf cancer vaccine by in vivo transforming tumors into “antigen factories” (Table 1). As depicted in Figure 1, ISV can activate systemic antitumor immune responses by simply delivering immunomodulators (Hammerich et al., 2015; Sheen and Fiering, 2019). Taking advantage of the complete antigenic repertoire of a tumor, including all mutated antigens and unmutated antigens, ISV potentiates the immune system to recognize the evolving tumor antigen arrays, thereby overcoming the weak immunogenicity of single-antigen vaccines and minimizing immune escape.
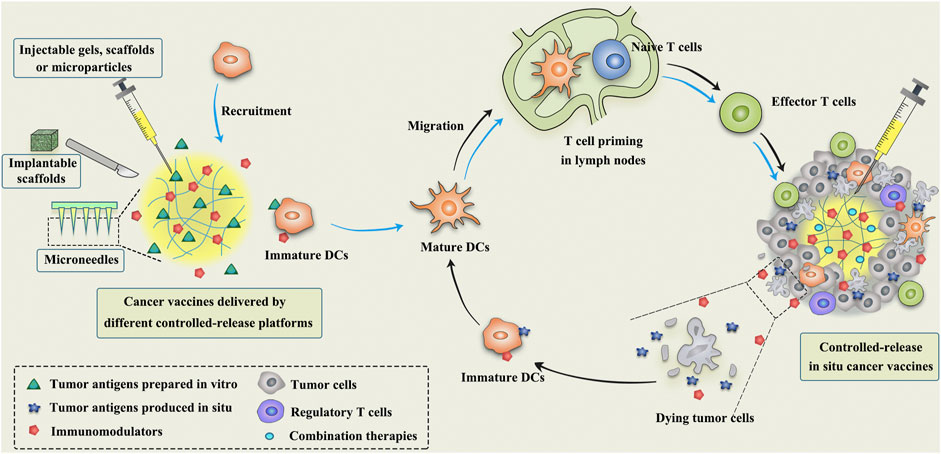
FIGURE 1. Schematic illustration of the mechanism of controlled-release cancer vaccines for cancer therapy. The blue arrows indicate the antitumor process of conventional cancer vaccines, while the black arrows indicate the mechanism of in situ cancer vaccines.
Broadly speaking, any approach that employs TAAs available at the tumor site to elicit antitumor immune responses can be termed as ISV (Hammerich et al., 2015). ISV usually refers to the approaches using intratumoral administration of immunomodulators to activate immune cells or reverse the immunosuppressive microenvironment within the tumor. The most extensively studied immunomodulators include bacteria and its derivatives (Janku et al., 2021), oncolytic viruses (Oh et al., 2017; Russell and Barber, 2018), TLR agonists (Wei et al., 2019), monoclonal antibodies (e.g., anti-PD-1, anti-CTLA-4 and anti-CD40) (Rahimian et al., 2015; Wang et al., 2016; Knorr et al., 2018), immunomodulatory cytokines (e.g., IL-2 and IL-12) (Jackaman and Nelson, 2012; Hwang et al., 2020), and immune cells (e.g., DCs) (Subbiah et al., 2018). In an example of successful ISVs, Talimogene laherparepvec (T-VEC), a genetically modified herpes simplex virus that can replicate selectively within tumors and express GM-CSF, is the first intratumoral oncolytic viral therapy approved by the FDA in 2015 for local treatment of unresectable advanced melanoma (Rehman et al., 2016).
However, a single regimen of immunomodulators is generally difficult to surmount the complexity and compensatory evolution of tumors, especially since many types of tumor cell death are nonimmunogenic, thus leading to suboptimal clinic outcomes (Sheen and Fiering, 2019). Local treatment with radiotherapy, phototherapy, oncolytic viruses, cryoablation, sonodynamic therapy, or some chemotherapeutic drugs (e.g., anthracyclines, taxane, cyclophosphamide, gemcitabine and oxaliplatin) can induce significant immunogenic cell death (ICD) of tumor cells when administrated at appropriate doses and schemes (Pol et al., 2015; Galluzzi et al., 2017; Min et al., 2017; Twumasi-Boateng et al., 2018; Yakkala et al., 2019; Zhao et al., 2020; Zhu et al., 2020). ICD can expose calreticulin on the surface of dying tumor cells to provide an “eat-me” signal for APCs, and release a large number of damage-associated molecular patterns (DAMPs) to provide adjuvant stimuli to activate APCs (Galluzzi et al., 2017). Therefore, the combination of ICD-inducing therapies with immunomodulators, such as chemoimmunotherapy, radioimmunotherapy and photoimmunotherapy, can not only directly kill tumor cells, but also turn ‘cold’ tumors into ‘hot’ tumors to elicit potent immune responses against a broad spectrum of cancers. In an elegant example of this, Patel et al. combined bacterial membrane-coated nanoparticles (BNP) with radiotherapy to treat syngeneic melanoma or neuroblastoma in mice (Patel et al., 2019). This multifunctional BNP consisted of an immunostimulatory PC7A/CpG polyplex nanocore coated with bacterial membrane and imide groups. After radiotherapy, BNP could capture TAAs released by ICD, followed by promoting the antigen uptake and cross presentation. Subsequently, a strong antitumor T-cell response was induced, leading to remarkable tumor regression alongside immunological memory.
Although ISV and its combinatorial therapies are gaining rising attention among researchers, the magnitude and durability of antitumor immune responses induced by ISV could be unfortunately impeded by the immunosuppressive tumor microenvironment (van der Burg et al., 2016). One appealing strategy is to introduce CAR-T, ICIs or other immunosuppressive signal inhibitors in the combinatorial therapies to improve the outcomes (Wang et al., 2018; Chen et al., 2019a; Song et al., 2019; Chen et al., 2020a). Moreover, approaches that loosen the desmoplastic stroma or target the tumor vasculature would help to augment the antitumor immunity of ISV by facilitating immune infiltration (Galluzzi et al., 2018). Furthermore, identifying the optimal immunoadjuvants and applying the most suitable delivery system may maximize the safety and effectiveness of ISV. Although intratumoral injection technology is widely used in accessible superficial tumors, such as skin, head and neck, and breast tumors, it remains a challenge for those deep tumors, such as brain, liver and pancreas tumors (Sheen and Fiering, 2019). Fortunately, with the help of modern imaging technologies such as ultrasound, computed tomography guidance and laparoscopy, safe and accurate injections can be performed for tumors in various locations (Sheen and Fiering, 2019). Moreover, one should take full account of the effects of injection pressure, volume, viscosity, frequency, etc. on tumor bulk, therapeutic effect and patient tolerance. Collectively, combinatorial ISV therapies have great potential to provide a comprehensive approach to treat heterogeneous cancers.
Controlled-Release Drug Delivery Systems
Early depot-like adjuvants, such as aluminum salts and IFA, are effective in promoting protective humoral immunity against pathogens, but they are not excellent candidates for cancer vaccines that rely on cellular immunity (Brito and O'Hagan, 2014; Shae et al., 2019). Therefore, the next generation of controlled-release cancer vaccines should be able to elicit efficient and durable antitumor cellular immune responses. To date, numerous controlled-release delivery platforms, such as polymeric microspheres, scaffolds, hydrogels and microneedles (MNs) have been designed for local and controlled release of multiple immunotherapeutic agents to improve antitumor efficacy and reduce off-target toxicities (Figure 1; Table 2).
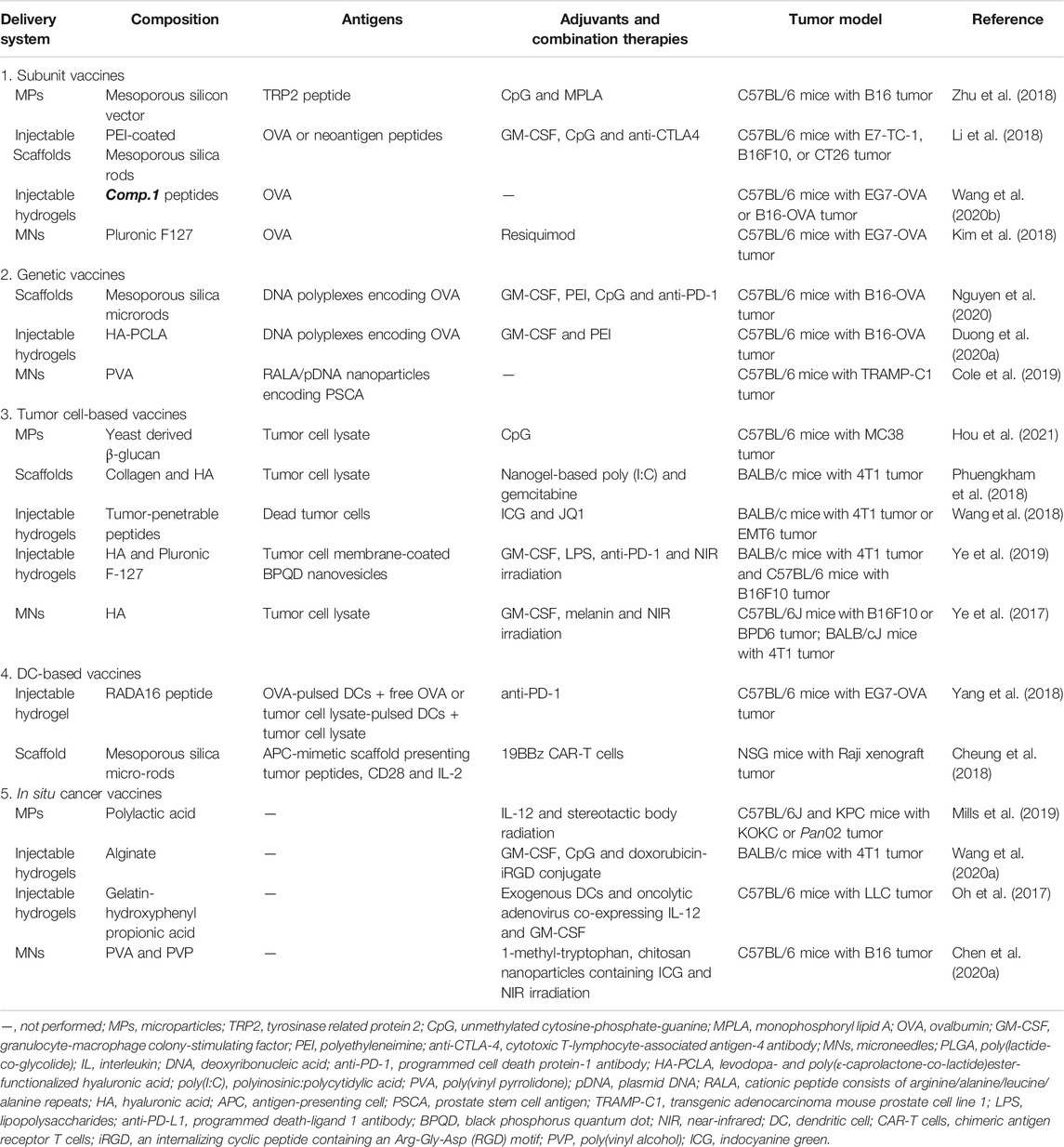
TABLE 2. Representative examples of cancer vaccines delivered by controlled-release platforms (from 2017 to 2021).
Microparticles
Peris and Langer proposed in 1979 for the first time that the release of antigens could be controlled using polymeric materials to stimulate immune responses (Peris and Langer, 1979). Numerous natural and synthetic biodegradable polymeric materials including chitosan, alginate, gelatin, poly(lactic-co-glycolic acid) (PLGA), Poly(lactic acid) (PLA), poly(ε-caprolactone) (PCL), poly(β-amino esters) (PBAE) and poly(methyl methacrylate) (PMMA), etc., as well as some inorganic materials (such as silica) have been widely used in the field of controlled release of antigens and immunomodulatory agents (Lin et al., 2015; Zhu et al., 2018; Huang et al., 2019; Koerner et al., 2019; Hou et al., 2021). Polymeric microparticles (MPs) or microspheres can induce potent antigen-specific immunity by controlling the release of antigens or mimicking the size of pathogens, but they are much safer than live pathogens (Huang et al., 2019). Different forms of antigens as mentioned above (e.g., purified proteins, peptides, nucleic acids, and cell lysates) have been successfully formulated in MPs (Joshi et al., 2014; Pradhan et al., 2014; Guarecuco et al., 2018; Zhu et al., 2018) (Table 2). As compared to bolus injections, MPs can protect the antigens and adjuvants from degradation with slow release at the injection site to prolong antigen presentation and allow simultaneous delivery of antigens and adjuvants to the same APC (Lin et al., 2015; Huang et al., 2019) (Figure 2). By modulating the polymer molecular weight, composition, preparation method, particle size and additives, etc., MPs can provide sustained or pulsatile release of entrapped antigens over periods lasting weeks to months (Sivakumar et al., 2011). Furthermore, the modification of surface physicochemical properties or decoration with ligands or antibodies can lead to different functionalized MPs (Figure 2), such as APCs-targeting MPs, immune cell-engaging particles (artificial DCs) or MPs for intranasal vaccination (Mata et al., 2011; Li et al., 2016b; Jung et al., 2019; Koerner et al., 2019; Huang et al., 2020).
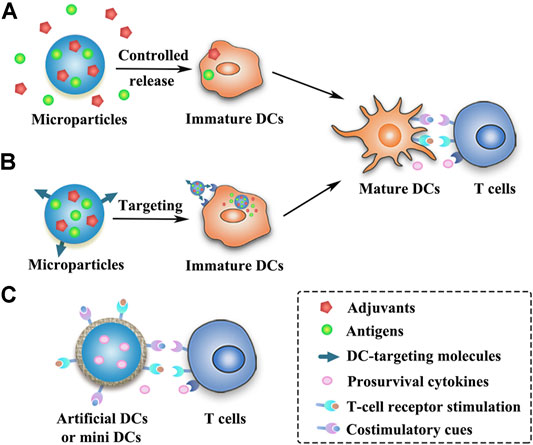
FIGURE 2. Schematic presentation of strategies of different MP-based cancer vaccines. (A) Antigens and adjuvants are released in a controlled manner from the MPs and then taken up by the immature DCs. (B) DC-targeting MPs can release antigens and adjuvants within the DCs after being taken up by the immature DCs. (C) MP-based artificial DCs or mini DCs can present T-cell activation signals to activate T cells or directly expand primary T cells.
Among the most extensively exploited synthetic polymers in many areas, PLGA has been approved by the FDA for human use in drug delivery and biomedical devices due to its biodegradability and biocompatibility (Koerner et al., 2019). PLGA micro- or nanoparticles are promising carriers with remarkable application prospects for cancer vaccines. For example, tumor lysates alongside with TLR agonists (CpG-ODN) were co-encapsulated into PLGA microspheres to induce cytotoxic T cell responses and mediate tumor shrinkage in a transgenic mouse model of prostate cancer, and these particles could be sterilized by γ-irradiation without impairing their antitumor efficacy (Mueller et al., 2012). Recently, PLGA microspheres loaded with antigens and photosensitizers facilitated an active transport of microsphere-containing APCs to draining lymph nodes after illumination, resulting in strong CD8+ T cell responses (Schineis et al., 2021). Moreover, combination therapies that attack tumors from all sides can further augment the antitumor effects of MPs-based vaccines. PLGA nanoparticles that co-deliver doxorubicin, two immune adjuvants (poly(I:C) and R848), and one chemokine (CCL20) generated superior antitumor effects than separate compounds, as demonstrated on two treatment-resistant lung and colon cancer models (Silva et al., 2019). However, the prolonged survival time requires four repeated intratumoral injections of the PLGA nanoparticles, indicating that MPs may be a preferable platform for the sustained delivery of multiple drugs.
In spite of these promising features of PLGA MPs, the encapsulation efficiency and loading efficiency of hydrophilic drugs in PLGA MPs are usually low, and antigens, especially the macromolecular proteins, have high risks of aggregation or degradation in the presence of organic solvents and high shear stresses during the preparation process, leading to impaired antigenicity and immunogenicity. Many strategies have been developed to maintain the integrity of proteins. Bailey et al. developed a “self-healing encapsulating” method to load protein antigens into pre-made porous PLGA microspheres with minimal impact on the antigens (Bailey et al., 2017). Besides, before encapsulated into the PLGA MPs, protein antigens can be previously loaded into polysaccharide (dextran) glassy particles through freezing-induced phase separation to protect antigen’s integrity (Geng et al., 2008). In another example, antigens and other immunomodulators can be efficiently and intactly loaded on the surface of PLGA MPs by conjugating short synthetic DNA scaffolds to the surface (Huang et al., 2020). More importantly, many advanced manufacturing processes, such as spray drying technology and supercritical carbon dioxide, are able to greatly improve the drug loading, protein stability, reproducibility and scaling-up production of PLGA MPs (Han et al., 2016a; Koerner et al., 2019). Another challenge limiting the broad application of PLGA MPs as vaccine carriers is high initial burst release that often consumes about 25% of the total drug in the first day (Park et al., 2019). This initial burst release, mainly resulted from the quick dissolution of adsorbed or weakly bound drugs on the surface of PLGA MPs, may lead to unintentional toxicity (Koerner et al., 2019). The release kinetics of PLGA MPs can be modulated by many approaches including multi-layered microparticles, nanoparticles-in-microparticles, hydrogel templates, coaxial electrospray and microfluidic fabrication, etc. (Han et al., 2016a) Interestingly, the initial burst can be advantageous to produce pulsatile-release MPs that mimic the prime-boost effect of conventional vaccines, showing the potential to make single-injection vaccines (Guarecuco et al., 2018). After administration, the bulk degrading PLGA MPs can generate acidic degradation products which may affect both antigen stability and release kinetics (McHugh et al., 2015). These pH issues may be alleviated by preparation of small PLGA particles which facilitate the diffusion of acidic degradation products and by addition of insoluble buffers to maintain a stable interior pH (McHugh et al., 2015).
Scaffolds
The scaffold system is commonly used in tissue engineering as the artificial extracellular matrix for the proliferation and differentiation of seeded cells or as the sustained delivery vehicles for therapeutic substances (Bessa et al., 2008; Shafiee and Atala, 2017). Nowadays, scaffolds or implants are also implemented in the field of cancer therapy (Table 2), providing new insights into the design of cancer vaccines. In order to modulate the antitumor immunity, scaffolds can be surgically implanted or low-invasively injected into the body to form localized immune niches or reservoirs for the controlled delivery of engineered immunocytes or cancer vaccines (Figures 3A–C). Various organic or inorganic materials can be used to fabricate scaffolds, such as alginate, collagen, hyaluronic acid, PLGA, and silica rods (Ali et al., 2009b; Kim et al., 2015; Stephan et al., 2015; Phuengkham et al., 2018). Early implants require surgical procedures to place the pre-formed scaffolds into the body to concentrate drug release for a long period of time at the target site. These pre-formed implantable scaffolds have a defined size and shape and can be fabricated in various sophisticated and functionalized structures to meet the needs of different medical applications. In addition, they usually exhibit an interconnected porous architecture, facilitating the diffusion of encapsulated immunomodulatory cues and providing space for the expansion and interaction of encapsulated immune cells or the incoming immune cells (Weiden et al., 2018) (Figure 3). Moreover, the release profiles of the biomolecules or immunocytes in the scaffolds can be fine-tuned to modulate the immune cell function (Ali et al., 2011b; Weiden et al., 2018). The pioneering work of scaffold vaccine was done by the Mooney group, who designed macroporous PLGA scaffolds. These scaffolds released GM-CSF in a sustained manner to recruit host DCs and subsequently presented tumor cell lysates and CpG to activate the incoming DCs (Ali et al., 2009a; Ali et al., 2009b). Implantation of this scaffold vaccine generated strong tumor-specific T cell responses, resulting in significant tumor regression and survival improvement in both melanoma and glioma mouse models (Ali et al., 2009a; Ali et al., 2009b; Ali et al., 2011a). Various chemokines, immunoadjuvants and cytokines can be also incorporated into this flexible scaffold to direct the recruitment of DC subset and augment the therapeutic activity in combination with checkpoint antibodies (Ali et al., 2013; Ali et al., 2014; Ali et al., 2016). Encouragingly, a human version of the PLGA scaffold vaccine (designated as WDVAX) is currently being evaluated in a phase I clinical trial for metastatic melanoma (US National Library of Medicine, 2021). Although the implantable scaffolds have the ability to control their structure shape and rigidity as well as prevent the diffusion of scaffold materials, the invasive surgical implantation is quite cumbersome, causing patient discomfort and increasing the infection risk (Li and Mooney, 2016; Weiden et al., 2018). These implanted scaffolds cannot be placed in areas that are surgically inaccessible or volume-sensitive due to their stiffness (Leach et al., 2019). In addition, the function of normal organs may be affected if the scaffolds are placed into the tumor resection bed or near the tumor (Leach et al., 2019).
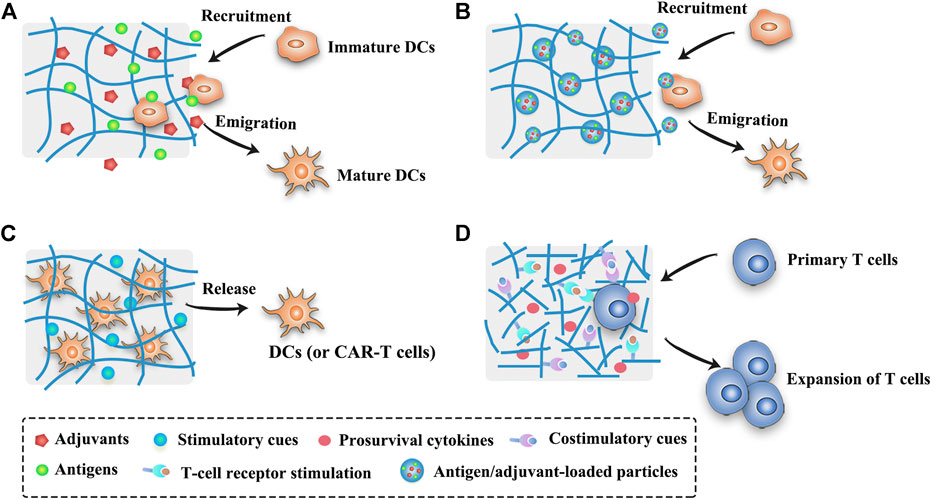
FIGURE 3. Schematic illustration of strategies of scaffold- or hydrogel-based cancer vaccines. (A) Scaffold- or hydrogel-based cancer vaccines can recruit immature DCs into the injection site and subsequently activate them into mature DCs. (B) Nanocomposite hydrogel/scaffold systems can co-deliver antigens and adjuvants into the same DCs. (C) Scaffold- or hydrogel-based adoptive cell transfer can release mature DCs or CAR-T cells in a sustained manner and maintain the viability of cells. (D) Functionalized scaffolds can mimic APCs to directly expand primary T cells.
Moving forward, the injectable scaffolds that gel or self-assemble in the body have presented highly deformable and self-organizing abilities, thus avoiding the risks associated with implant surgery. Mooney et al. designed a fascinating injectable and self-assembling scaffold vaccine which was based on mesoporous silica rods (MSRs) with high-aspect-ratio to reprogram the immune cells and enhance the vaccine efficacy (Kim et al., 2015; Li et al., 2018). Upon subcutaneous injection in mice, these MSRs spontaneously assembled themselves into three-dimensional (3D) macroporous structures for the influx and efflux of immune cells. Analogous to previous studies, this injectable inorganic scaffold extended the release of the embedded GM-CSF, CpG and model antigens to recruit and educate host immune cells, leading to significant production of systemic antibodies and cytotoxic T cells that effectively delayed the tumor growth (Kim et al., 2015). The adsorption of cationic polymer polyethyleneimine (PEI) in the previous MSR vaccine could greatly enhance the immunogenicity of neoantigens and eradicate three different established tumors (Li et al., 2018). Besides, surface modifications with poly(ethylene glycol) (PEG) or integrin-binding ligand RGD on MSR scaffolds could also regulate immune cell infiltration and activation (Li W. A. et al., 2016). Notably, these MSR scaffolds were demonstrated to be a promising platform for the delivery of DNA-based cancer vaccines (Nguyen et al., 2020). Different to those macroscopic scaffolds aiming to reprogram the incoming DCs, scaffolds that were composed of lipid bilayer-coated micro-MSRs and functionalized with T cell activation cues (anti-CD3, anti-CD28 and IL-2) could mimic APCs to directly expand primary T cells (Cheung et al., 2018) (Figure 3D). These inorganic scaffolds showed great potential in the delivery of personalized cancer vaccines, however, the biocompatibility, biodegradability and safety of injected materials need to be further evaluated before their clinical use. Other administration methods apart from surgical implantation and syringe injection are waited to be explored to broaden the application of controlled-release vaccines. In an example of this, an in situ formed fibrin gel was sprayed into the post-surgery tumor site to control the release of therapeutic antibodies (Chen et al., 2019b). In addition to these PLGA and MSR scaffolds, injectable biomaterials including hydrogels, cryogels, and other in situ forming platforms can also form the porous matrix in vivo to modulate the immune system. The opportunities and challenges of these injectable biomaterials are described below.
Injectable Gels
Injectable gels are widely applied in many areas including wound healing, bone regeneration, diabetes treatment and cosmetic surgery (Li et al., 2021). Recently, macroscopic injectable gels, especially intelligent hydrogels with the size ranging from millimetres to centimetres, have attracted enormous attention as biocompatible carriers for the spatiotemporal control over cancer vaccines involving antigens, immunomodulators and engineered immunocytes (Table 2). Injectable gels are flowable fluid before or at the time of injection. Once injected into the body, they immediately undergo “sol-to-gel” phase transition by means of chemical or physical crosslink (Li and Mooney, 2016), loss of shear force (Shear-thinning hydrogels) (Yan et al., 2010), mechanical collapse and recover (such as cryogels) (Bencherif et al., 2015), or solvent exchange (such as phospholipid-based gels) (Han et al., 2016b) to form gels. Consequently, injectable gels can be localized almost anywhere in the body by a syringe without the complicated surgical procedures of implantable scaffolds. More importantly, injectable gels have a highly deformable ability to fit the spatial structure of the injection site before they form into a persisting implant (Leach et al., 2019).
Hydrogels are crosslinked 3D polymeric networks containing a large amount of water (typically 70–99%) which enables them to mimic the extracellular matrix (Li and Mooney, 2016). Due to the excellent biocompatibility and tunable release kinetics, hydrogels are the most extensively explored gel carriers in the delivery of cancer vaccines. In addition, hydrogels have high loading capacity for hydrophilic drugs, and they are harmless to the integrity of antigens because the encapsulation process is usually carried out under mild aqueous conditions. Moreover, antigens can be protected in the hydrogels from degradation by the in vivo enzymes during the extended periods of antigen presentation. Hydrogels can be made from a variety of materials including peptides (Wang et al., 2018; Wang Z. et al., 2020), proteins (e.g., silk fibroin and gelatin) (Wu et al., 2016; Oh et al., 2017), polysaccharides (e.g., alginate, hyaluronic acid and chitosan) (Hori et al., 2009; Kordalivand et al., 2019; Ye et al., 2019), nucleic acids (e.g., DNA) (Li et al., 2020) and synthetic polymers (e.g., poloxamers and polyesters) (Cirillo et al., 2019; Leach et al., 2019). To form hydrogels, these materials can be crosslinked through different mechanisms, mainly by physical noncovalent interactions (such as hydrogen bonding, host-guest interactions, hydrophobic interactions, electrostatic interactions, etc.) and by chemical covalent bonds (such as click chemistry, Diels-Alder reactions, Michael addition, enzymatic reactions, etc.) (Yu and Ding, 2008; Li and Mooney, 2016; Lei and Tang, 2019).
Many macroscopic hydrogels seek to recruit and activate the endogenous DCs by prolonging the presentation of the antigens and immunomodulators (Figure 3A) (Bencherif et al., 2015; Ye et al., 2019; Duong et al., 2020a). In one representative study, irradiated tumor cells, GM-CSF and CpG were co-encapsulated in sponge-like macroporous cryogels which were fabricated by alginate with RGD peptide modification (Bencherif et al., 2015). After subcutaneously injected into the mice bearing melanoma, these cyrogels released the immunomodulators in a sustained and localized manner to facilitate the influx of DCs and subsequently induce robust and durable tumor-specific T cell responses. In addition, hydrogels are excellent delivery vehicles for adoptive cell therapies (such as DC vaccines and CAR-T cells, Figure 3C) due to the concentration of the engineered immunocytes at the desired site as well as the maintenance of cell viability for a long time (Hori et al., 2009; Yang et al., 2018). Furthermore, hydrogels can easily co-deliver diverse drugs using one platform for a combination of cancer treatments (Wang et al., 2018; Song et al., 2019; Wang et al., 2020b). For example, Wang et al. developed a personalized cancer vaccine (termed PVAX) by integrating JQ1 (an inhibitor of PD-L1 expression) and indocyanine green (ICG, a photosensitizer) co-loaded autologous tumor cells into a hydrogel matrix composed of tumor-penetrable peptides (Wang et al., 2018). After intratumoral administration, the PVAX was triggered by near-infrared (NIR) irradiation to release tumor antigens and JQ1, causing the full activation of the postoperative antitumor immunity that efficiently prevented tumor recurrence and metastasis.
Numerous studies are striving to develop methods for on-demand control over the release kinetics of injectable gels to improve the spatiotemporal delivery of cancer vaccines. Various intelligent hydrogels have been designed to respond to intrinsic or extrinsic stimuli such as low pH, temperature, enzymes, redox, photoirradiation, magnetic fields and ultrasound (Lu et al., 2017; Kanwar and Sinha, 2019). Duong et al. developed an injectable thermosensitive smart hydrogel by conjugating thermo-responsive poly(ε-caprolactone-co-lactide) ester and levodopa to hyaluronic acid (Duong et al., 2020a). This hydrogel exhibited sol-to-gel transition in response to the body temperature, and the levodopa moieties were introduced to strengthen the stability of the hydrogel during implantation. The sustained degradation of this smart hydrogel led to a controlled release of GM-CSF and nano-sized polyplexes expressing model protein antigen ovalbumin (OVA), and then effective inhibition of B16/OVA melanoma tumors by a single injection was observed. Furthermore, many dual-sensitive hydrogels have gained enormous attention in more precisely controlling the release profiles of drugs, such as thermo-pH dual-sensitive hydrogels (Liu et al., 2019a), thermo-reactive oxygen species (ROS) dual-sensitive hydrogels (Yu et al., 2018) and electric field-pH double-sensitive hydrogels (Qu et al., 2018). Interestingly, Brudno et al. developed a replenishable and injectable hydrogel depot system which could capture systemically administrated prodrug refills and then release them locally in a sustained manner (Brudno et al., 2018). This repeatedly refillable hydrogel can be used to prepare precisely controlled-release or pulsatile-release cancer vaccines.
The lag time during the sol to gel transformation may induce a severe initial burst release effect which is responsible for the toxic side effects of immunomodulators and even immune tolerance (Wang and Mooney, 2018; Kanwar and Sinha, 2019). Many strategies are conceived to settle this issue, for example, burst release can be alleviated by optimizing the molecular weight and the concentration of polymers, by using appropriate solvents, and by adding plasticizers or surfactants (Kanwar and Sinha, 2019). The prompt interactions of antigens (and adjuvants) with polymers can also regulate the release profiles, such as by electrostatic interaction or covalent binding (Umeki et al., 2015; Yu et al., 2018; Kordalivand et al., 2019). In one representative study, cationic OVA or cationic OVA peptide were bound to anionic CpG DNA hydrogel by electrostatic interaction, resulting in significantly slower drug release and better tumor inhibition in the group of cationic OVA hydrogel than the group of unmodified OVA hydrogel (Umeki et al., 2015). Another strategy to mitigate the burst effect is to use nanocomposite hydrogel systems (Figure 3B). For example, the incorporation of OVA and two adjuvants (MPLA and Quil A)-loaded PLGA nanoparticles into thermoresponsive pentablock copolymer (PEG-PCL-PLA-PCL-PEG) hydrogels could greatly reduce the burst release of antigens and adjuvants (Bobbala et al., 2016). In another study, OVA was encapsulated into cationic chitosan nanoparticles and then incorporated in hyaluronic acid-based hydrogels in order to achieve a prime-boost regimen (Korupalli et al., 2019). This nanocomposite hydrogel system could rapidly release the nanoparticles that had no specific interactions with the hydrogels for a priming dose, and retain the nanoparticles that were bonded to the hydrogels by covalent or electrostatic interactions for a booster dose. With the minimal burst effect, these chitosan nanoparticles could promote antigen uptake and DC activation, thus inducing higher antibody responses than soluble antigens. Moreover, an onion-structure multilayer hydrogel capsules may be another promising approach to effectively inhibit the burst release of therapeutic cargos (Zhang et al., 2019).
Despite these encouraging progresses, there still exist many hurdles lying on the road to the broad clinical application of hydrogels, including safety concerns about the elaborate materials and solvents, the difficulty in the highly precise control of the release kinetics, the inconsistent drug release resulted from the inconsistent shape of the gels formed, the challenge of in situ injectability to deep tumors, the complexity of scaling-up manufacturing, and the difficulty in storage and terminal sterilization (Kanwar and Sinha, 2019). Consequently, the influence of the polymer composition, gel stiffness, network density, porosity and drug-polymer interactions on the release kinetics, as well as the influence of combination therapies, drug doses, administration routes and times on the immune system, should be better understood and these factors should be optimized to exert the maximum efficacy of hydrogel-based cancer vaccines.
Microneedles
Most vaccines are administrated via intramuscular (IM), subcutaneous (SC) and intradermal (ID) inoculation using conventional hypodermic needles. Although the relative immunogenicity of vaccines by these three routes (IM, SC and ID) varies among individual vaccines, ID immunization usually induces more robust immune responses than IM or SC immunizations in clinical studies. This is partly because the different skin layers host a tight semi-contiguous network of immune cells that can interact with the antigens administered to direct drastically different immune responses compared to subcutaneous fat and muscle tissue (Al-Zahrani et al., 2012). The development of MNs is a great achievement to take advantage of skin immunization and to address the issues associated with vaccination by conventional needles (e.g., pain, needle-stick injuries or needle re-use) (Leone et al., 2017). MNs, arrays of sharp tiny needles at lengths varying from 100 to 2,000 μm, are designed to pierce the stratum corneum of the skin to deliver both small molecular and macromolecular drugs or even nanoparticles (NPs) to the epidermal and the dermal or even deeper layers in a safe and controlled manner (Alimardani et al., 2021). As a painless transcutaneous administration platform, MNs have been extensively explored for cancer vaccines, antiviral immunotherapies, and diabetes (Jin et al., 2018b; Chen et al., 2020b; Uppu et al., 2020). MNs can promote the deposition of the vaccines by creating micropores in the skin, facilitating vaccine transport, and hence reducing the necessary dose of antigens/vaccines for the immunological responses. A significant number of MNs mediated vaccine candidates have shown encouraging results in preclinical and clinical trials (Hossain et al., 2020).
The minimally invasive and cost-effective features of MNs have aroused their rapid development and extensive exploration in cancer vaccine delivery recently (Table 2). Various types of MNs have been investigated for cancer vaccine delivery, as depicted in Figure 4, including solid MNs (Bhowmik et al., 2011; Chablani et al., 2019), dissolving MNs (Zaric et al., 2013; Gala et al., 2018; Kim et al., 2018; Cole et al., 2019; Kim et al., 2019), coated MNs (Zeng et al., 2017; Duong et al., 2018) and hollow MNs (van der Maaden et al., 2018; Niu et al., 2019). Novel design to utilize parallel circular blades with MN on edge as electrodes was also studied for their ability to deliver siRNA targeting PD-L1 alone, or combined with anti-PD-1 antibody and immunoadjuvant CpG 2395 (Yang et al., 2021). This immunotherapy treatment has been tested in two tumor xenograft murine models and produced robust T cell immune responses and significant tumor growth inhibition as well as excellent safety profiles. Various vaccine formulations, including microparticles (Bhowmik et al., 2011), nanoparticles (Zaric et al., 2013), nanopolyplex (Duong et al., 2020b), liposomes (van der Maaden et al., 2018), and in situ generating nanomicelles (Kim et al., 2018) to ensure efficient co-encapsulation of antigens and adjuvants within the nanovaccine platforms have been delivered using MNs to improve cancer vaccine therapy. Du et al. used hollow MNs to administer a range of nanoparticle vaccine formulations loaded with OVA and poly(I:C) (Du et al., 2017). PLGA nanoparticles, liposomes, mesoporous silica nanoparticles, and gelatin nanoparticles were compared. The co-encapsulation of OVA and poly(I:C) using those formulations yielded a similar IgG1 response, but a surprisingly higher IgG2a response than OVA/poly(I:C) solution in a murine model. Particularly, PLGA nanoparticles and especially cationic liposomes, which presented sustained release profiles of both OVA and poly(I:C), elicited better immune responses (Du et al., 2017). The antigens and adjuvants were co-encapsulated in some vaccine formulations through electrostatic interaction, hydrophobic interaction as well as covalent linking interaction (Chen et al., 2021). However, when these formulations are loaded into the MNs, especially adding some excipients to form the MNs, the electrostatic interaction between the antigen and adjuvant is hampered. As a good example to address this issue, an in situ generating nanomicelle including hydrophilic tumor model antigen (OVA), hydrophobic adjuvant TLR 7/8 agonist (R848) and amphiphilic triblock copolymer has been delivered using dissolving MNs platform to form nanomicelles in situ with a size of 30–40 nm after cutaneous application (Kim et al., 2018). Antigen-specific humoral and cellular immunity protection were found to be elicited after administration, leading to a significant antitumor activity in the EG7-OVA tumor-xenograft mice. Since MNs are usually applied topically on the surface of the skin, they are very suitable to be combined with phototherapy (Ye et al., 2017; Chen et al., 2020a). One example of such combination therapies was based on the tumor lysates, melanin and GM-CSF were loaded into hyaluronic acid-based MNs that allowed sustained release of the lysates (Ye et al., 2017). After administration, the melanin converted the NIR irradiation into heat, which facilitated tumor-antigen uptake by DCs and boosted the antitumor immune responses in both B16F10 and 4T1 tumor models.
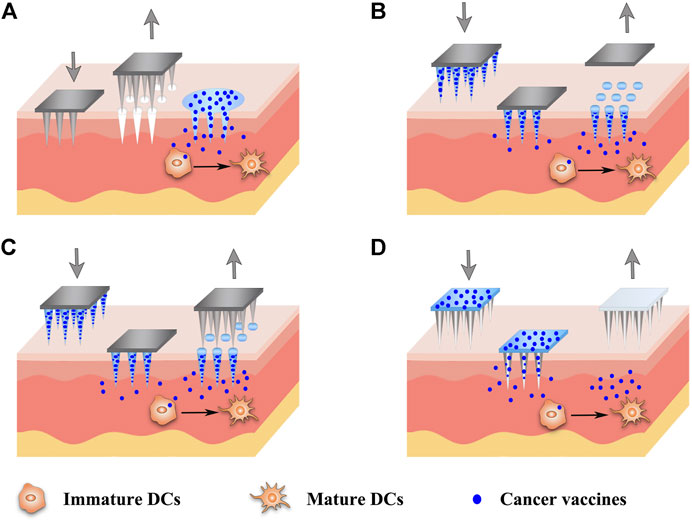
FIGURE 4. Schematic diagram of strategies of different MN-based cancer vaccines. Various types of MNs have been investigated for the delivery of cancer vaccines, including solid MNs (A), dissolving MNs (B), coated MNs (C) and hollow MNs (D).
Collectively, MNs have offered a unique set of properties for cancer vaccine delivery with improved vaccination efficacy, compliance, and coverage. The use of MNs instead of a needle injection can not only avoid pain for the patients but also provide the promise of self-vaccination as well as release the assistance from professional healthcare, which is especially suitable for mass vaccination in the case of a pandemic (Zaric et al., 2013). Although the use of MNs could be more effective than a single-needle injection as the antigens might be more evenly distributed after injection and have a more targeted delivery to APCs in the dermis and epidermis layers under the skin, MNs-based delivery may cause more frequent local reactions due to the shallow penetration (Zhang et al., 2015). MNs can be fabricated in different shapes using various materials, including stainless steel, titanium, silicon, ceramic and polymers (Alimardani et al., 2021). However, there is a possibility of needle breakage within the skin after the application of metal, silicon or glass MNs, triggering unintentional safety concerns (Jin et al., 2018a). In addition, some materials such as nickel may cause allergic reactions. Biodegradable polymeric MNs can minimize the risk of inflammation induced by undissolving materials, because they can degrade to metabolizable objects in the body (Jin et al., 2018a). On the other hand, MNs should have enough mechanical strength and sharpness to pierce the skin for transdermal applications. However, the incorporation of drugs and nanoparticles may compromise the mechanical strength of MNs (Kim et al., 2018). Therefore, the design of MNs-based cancer vaccines should be optimized with the full consideration of balancing the pain, mechanical strength, drug doses and drug release profiles for therapeutic efficacy. For a cancer vaccine, the efficacy is closely associated with the distance from the injection site to the cancer site. Most of the studies for MN-based cancer vaccines were conducted in malignant melanoma. Although they can provide invaluable information about other cancers as well, more cancer models will need testing for evaluating the systemic immune protections of MNs (Zhang et al., 2015).
Correlations of the Immune Responses and the Release Kinetics of Cancer Vaccines
It has been widely reported that controlled or sustained release of antigens and adjuvants is beneficial to generate antigen-specific antibodies, T cell responses and immunological memory responses (Kanchan et al., 2009; Ali et al., 2011b; Demento et al., 2012; Hanet al., 2016b; Irvine et al., 2020). The mechanisms underlying these benefits of controlled-release vaccines may be complex and multifactorial. Herein, we describe four major mechanisms as follows. Firstly, controlled-release platforms usually form an in situ drug depot in which antigens can maintain their intact conformation for a considerable period of time. Antigens and adjuvants can be released in a continuous or pulsatile manner to extend the antigen exposure or to mimic the traditional prime-boost regimen of vaccination (Sivakumar et al., 2011; Brito and O'Hagan, 2014). Secondly, owing to the heterogeneity and immunostimulatory activity of materials or the effect of encapsulated chemokines, most depot-like controlled-release systems can stimulate the accumulation of inflammatory cells or recruit APCs to the injection sites (Ali et al., 2009b; Brito and O'Hagan, 2014; Han et al., 2016b). Subsequently, cytokines secreted by inflammatory cells can stimulate the activation, proliferation and differentiation of immune cells. On the other hand, the antigen depot is surrounded or infiltrated by the recruited APCs, thus increasing the chance of antigen uptake. Thirdly, controlled-release systems, especially when loaded with immunoadjuvants, can further motivate the maturation of APCs and then direct the Th1 or Th2 immune responses (Li et al., 2018; Song et al., 2018). Last, but not least, some polymeric micro- or nanoparticles mimicking the size and surface characteristics of pathogens or functionalized with targeting ligands can accumulate in lymph nodes or target the specific immunocytes (Mata et al., 2011; Silva et al., 2016), thereby reducing the antigen dose and improving the magnitude of immune responses.
Although controlled-release systems have the potential to augment the vaccine efficacy with minimal toxicity, the effect of release kinetics on immune responses is complex and unclear. It is worth noting that antigen stimulation can be either immunologically activating or immunologically tolerant. For example, intravenous injection of low-dose antigens could induce immune activation, whereas intravenous injection of high-dose antigens might cause T cell exhaustion (Zinkernagel et al., 1997). Although the slow and sustained release of vaccines is demonstrated to favor the antitumor immunity, the extremely long periods of antigen presentation may, in turn, hamper the antitumor immunity by exhausting and deleting tumor-specific T cells or directing T cells to the antigen depot rather than to the target tumor (Iezzi et al., 1998; Hailemichael et al., 2013; Shae et al., 2019). On the other hand, a particularly fast release of antigens may result in rapid clearance of antigens from the lymph nodes (Shae et al., 2019; Irvine et al., 2020), necessitating repeated injections to boost the immune responses. Moreover, the release rate of adjuvants seems necessary to be coincident with that of antigens, because a fast release of adjuvants leads to early exhaustion of adjuvants, while an extremely slow release of adjuvants cannot provide sufficient activation cues to the immature DCs, thereby promoting T cell anergy and immune tolerance (Audiger et al., 2017; Wang and Mooney, 2018; Leach et al., 2019). Therefore, the release kinetics of antigens and adjuvants from the controlled-release platforms has a significant influence on the type and magnitude of immune responses. An interesting study showed that a “dose escalation” regimen with an exponentially increasing antigen dose elicited a superior CD8+ T cell response than a single bolus injection or constantly repeated dose regimen (Johansen et al., 2008). Another opinion suggested that an appropriate burst release followed by a sustained vaccine release was optimal to induce fast and powerful immune responses (Zhang et al., 2014). Consequently, a highly precisely controlled vaccine delivery system with on-demand drug release is believed to maximize the immune efficacy. Nevertheless, the optimal vaccine release kinetics for inducing the most potent and durable immunity is still not clear. It may vary among different types of controlled-release vaccines which have distinct adjuvant properties, antigen types, disease types and administration routes (Brito and O'Hagan, 2014; McHugh et al., 2015). Moreover, antigens and adjuvants can hardly share consistent release profiles due to their different physicochemical properties. Therefore, The release kinetics of antigens and adjuvants should be screened and optimized to augment the antitumor immunity. Fortunately, the release kinetics can be modulated by numerous factors, including material properties (such as chemical composition, molecular weight, hydrophobicity, crystallinity, glass transition temperature and degradation products), depot geometry (such as size, shape, porosity and network density), antigenic factors (such as antigen size, position and loading amount), and others (such as antigen-polymer interactions, additives and administration route) (McHugh et al., 2015; Li and Mooney, 2016). Furthermore, strategies that guarantee the simultaneous presentation of antigens and adjuvants to the same APCs during the long period of release may exert stronger antigen-specific CD8+ T cell responses. For example, antigens and adjuvants can be linked together by covalent or ionic bonds before loaded into controlled-release platforms, or antigens and adjuvants can be pre-encapsulated into nano- or microparticles followed by incorporating these particles into a macroscopic matrix.
Depiction of the drug release profiles is the inevitable step to study the controlled-release vaccines. However, most studies contain only the in vitro release data, which may not be coincident with the in vivo release due to the different release environment inside and outside the body. Unfortunately, the accurate quantitative data of in vivo release kinetics are difficult to acquire owing to the low antigen loading amount and long duration of release (McHugh et al., 2015). In vivo imaging is now commonly used to semi-quantitate the in vivo vaccine kinetics by measuring the remaining fluorescence intensity of fluorescently labeled antigens in the vaccine depot over time. However, it is unable to determine the actual amount and stability of the antigens because fluorescence quenches easily. Therefore, both the exploration of more accurate in vivo measuring technologies and the in-depth understanding of in vitro-in vivo correlations are needed to spur the development of controlled-release vaccines. By far, many controlled-release vaccine delivery systems have been using model antigens (such as OVA) and model tumors (such as immortalized tumor cell lines) to study the release kinetics and antitumor immune responses (Kim et al., 2015; Umeki et al., 2015; Han et al., 2016b; Niu et al., 2019). However, these model antigens and the model tumors cannot recapitulate the complex challenges facing the real therapeutic antigens and patient individualized tumors (Day et al., 2015; McHugh et al., 2015). For example, the model antigen ovalbumin for mouse studies is exceptionally immunogenic and may lead to overestimating vaccine efficacy. Moreover, subcutaneous injection of B16F10 cell lines into the mice is widely used to establish melanoma, but these in vitro cultured murine cancer cells cannot simulate the cancer heterogeneity and are not physiologically relevant to human cancers. Moreover, the immune system of a healthy mouse differs significantly from that of a human in both innate and adaptive immunity (Mestas and Hughes, 2004). Therefore, more data on the immune efficacy of controlled-release vaccines that are tested using clinically relevant antigens and using suitable tumor and animal models should be assessed in the future.
Conclusions and Outlook
Cancer vaccines represent the most promising and cost-effective antitumor strategy which engages the immunity of patients to target malignancies. Depending on the form and source of antigens, cancer vaccines can be briefly categorized into five types, including subunit vaccines, genetic vaccines, DC-based vaccines, tumor cell-based vaccines and in situ cancer vaccines. The pros and cons of these vaccines have been outlined in this review. Local and sustained delivery of vaccines at lower doses shows higher effectiveness and lower toxicity than bolus injections. Recently, the controlled-release drug delivery systems, such as polymeric MPs, scaffolds, hydrogels and MNs, have attracted enormous attention in the spatiotemporal delivery of cancer vaccines. However, there are still many obstacles limiting the broad application of controlled-release cancer vaccines, including the instability of antigens and adjuvants during the preparation and release period, the challenge of precisely controlling over release kinetics, safety concerns, and the difficulties in scaling-up manufacturing, storage and terminal sterilization. The mechanisms and patterns of the action of controlled-release vaccines need to be studied in-depth to develop potent, persistent, non-toxic and easily available single-shot cancer vaccines.
Despite the fact that immunotherapy have been widely studied and applied on hematological tumors and melanoma, the effect of current immunotherapies on solid tumors remains faint because of the immunosuppressive tumor microenvironments, low tumor immunogenicity and low infiltration of immune cells into tumors (Kuol et al., 2017; Galluzzi et al., 2018). Based on the unique advantages of these controlled-release systems as mentioned above, the combination of multiple cancer therapies with controlled-release cancer vaccines may be the most promising approach to treat solid tumors as well as to prevent tumor metastasis and recurrence. For example, many clinical available strategies, such as chemotherapy, radiotherapy, phototherapy, oncolytic viruses and immune checkpoint antibodies, show great therapeutic potential in combination with cancer vaccines. Moreover, targeting the microbiome in the intestine or the tumor may have a synergistic antitumor effect with cancer vaccines (Matson et al., 2018; Nejman et al., 2020). More work should be done to explore the synergistic therapeutic effects of controlled-release vaccines and other therapies. In conclusion, the next generation of cancer vaccines should focus on the following aspects: 1) the choice of antigens with high immunogenicity; 2) co-delivering antigens and appropriate adjuvants to the same APCs; 3) modulating the optimal release kinetics of the antigens and adjuvants from delivery systems to augment subsequent immune responses; 4) reshaping the immunosuppressive environment to maximize the specific cytotoxic function of T cells; 5) adopting optimal combinatorial therapeutic regimens to improve the outcomes and avoid tumor evade.
Author Contributions
All authors listed have made a substantial, direct, and intellectual contribution to the work and approved it for publication.
Funding
This work was supported by the National Natural Science Foundation of China (no. 82003683), the Science and Technology Plan of Guizhou province ((2018)2821) and the Fundamental Research Funds for the Central Universities, Southwest Minzu University (2019NQN57).
Conflict of Interest
The authors declare that the research was conducted in the absence of any commercial or financial relationships that could be construed as a potential conflict of interest.
References
Al-Zahrani, S., Zaric, M., McCrudden, C., Scott, C., Kissenpfennig, A., and Donnelly, R. F. (2012). Microneedle-mediated Vaccine Delivery: Harnessing Cutaneous Immunobiology to Improve Efficacy. Expert Opin. Drug Deliv. 9 (5), 541–550. doi:10.1517/17425247.2012.676038
Ali, O. A., Doherty, E., Bell, W. J., Fradet, T., Hudak, J., Laliberte, M.-T., et al. (2011a). The Efficacy of Intracranial PLG-Based Vaccines Is Dependent on Direct Implantation into Brain Tissue. J. Controlled Release 154 (3), 249–257. doi:10.1016/j.jconrel.2011.06.021
Ali, O. A., Doherty, E., Mooney, D. J., and Emerich, D. (2011b). Relationship of Vaccine Efficacy to the Kinetics of DC and T-Cell Responses Induced by PLG-Based Cancer Vaccines. Biomatter 1 (1), 66–75. doi:10.4161/biom.1.1.16277
Ali, O. A., Emerich, D., Dranoff, G., and Mooney, D. J. (2009a). In situ regulation of DC Subsets and T Cells Mediates Tumor Regression in Mice. Sci. Translational Med. 1 (8), 8ra19. doi:10.1126/scitranslmed.3000359
Ali, O. A., Huebsch, N., Cao, L., Dranoff, G., and Mooney, D. J. (2009b). Infection-mimicking Materials to Program Dendritic Cells In Situ. Nat. Mater 8 (2), 151–158. doi:10.1038/nmat2357
Ali, O. A., Lewin, S. A., Dranoff, G., and Mooney, D. J. (2016). Vaccines Combined with Immune Checkpoint Antibodies Promote Cytotoxic T-Cell Activity and Tumor Eradication. Cancer Immunol. Res. 4 (2), 95–100. doi:10.1158/2326-6066.Cir-14-0126
Ali, O. A., Tayalia, P., Shvartsman, D., Lewin, S., and Mooney, D. J. (2013). Inflammatory Cytokines Presented from Polymer Matrices Differentially Generate and Activate DCs In Situ. Adv. Funct. Mater. 23 (36), 4621–4628. doi:10.1002/adfm.201203859
Ali, O. A., Verbeke, C., Johnson, C., Sands, R. W., Lewin, S. A., White, D., et al. (2014). Identification of Immune Factors Regulating Antitumor Immunity Using Polymeric Vaccines with Multiple Adjuvants. Cancer Res. 74 (6), 1670–1681. doi:10.1158/0008-5472.CAN-13-0777
Alimardani, V., Abolmaali, S. S., Tamaddon, A. M., and Ashfaq, M. (2021). Recent Advances on Microneedle Arrays-Mediated Technology in Cancer Diagnosis and Therapy. Drug Deliv. Transl. Res. 11, 788–816. doi:10.1007/s13346-020-00819-z
Allison, J. P. (2015). Immune Checkpoint Blockade in Cancer Therapy. JAMA 314 (11), 1113–1114. doi:10.1001/jama.2015.11929
Audiger, C., Rahman, M. J., Yun, T. J., Tarbell, K. V., and Lesage, S. (2017). The Importance of Dendritic Cells in Maintaining Immune Tolerance. J.I. 198 (6), 2223–2231. doi:10.4049/jimmunol.1601629
Bailey, B. A., Ochyl, L. J., Schwendeman, S. P., and Moon, J. J. (2017). Toward a Single‐Dose Vaccination Strategy with Self‐Encapsulating PLGA Microspheres. Adv. Healthc. Mater. 6 (12), 1601418. doi:10.1002/adhm.201601418
Bencherif, S. A., Warren Sands, R., Ali, O. A., Li, W. A., Lewin, S. A., Braschler, T. M., et al. (2015). Injectable Cryogel-Based Whole-Cell Cancer Vaccines. Nat. Commun. 6, 7556. doi:10.1038/ncomms8556
Bessa, P. C., Casal, M., and Reis, R. L. (2008). Bone Morphogenetic Proteins in Tissue Engineering: the Road from Laboratory to Clinic, Part II (BMP Delivery). J. Tissue Eng. Regen. Med. 2 (2-3), 81–96. doi:10.1002/term.74
Bhowmik, T., D’Souza, B., Shashidharamurthy, R., Oettinger, C., Selvaraj, P., and D’Souza, M. J. (2011). A Novel Microparticulate Vaccine for Melanoma Cancer Using Transdermal Delivery. J. Microencapsulation 28 (4), 294–300. doi:10.3109/02652048.2011.559287
Bobbala, S., Tamboli, V., McDowell, A., Mitra, A. K., and Hook, S. (2016). Novel Injectable Pentablock Copolymer Based Thermoresponsive Hydrogels for Sustained Release Vaccines. AAPS J. 18 (1), 261–269. doi:10.1208/s12248-015-9843-4
Bol, K. F., Schreibelt, G., Gerritsen, W. R., de Vries, I. J. M., and Figdor, C. G. (2016). Dendritic Cell-Based Immunotherapy: State of the Art and beyond. Clin. Cancer Res. 22 (8), 1897–1906. doi:10.1158/1078-0432.CCR-15-1399
Bouzid, R., Peppelenbosch, M., and Buschow, S. I. (2020). Opportunities for Conventional and In Situ Cancer Vaccine Strategies and Combination with Immunotherapy for Gastrointestinal Cancers, a Review. Cancers 12 (5), 1121. doi:10.3390/cancers12051121
Brito, L. A., and O'Hagan, D. T. (2014). Designing and Building the Next Generation of Improved Vaccine Adjuvants. J. Controlled Release 190, 563–579. doi:10.1016/j.jconrel.2014.06.027
Browning, M. J. (2013). Antigen Presenting Cell/Tumor Cell Fusion Vaccines for Cancer Immunotherapy. Hum. Vaccin. Immunother. 9 (7), 1545–1548. doi:10.4161/hv.24235
Brudno, Y., Pezone, M. J., Snyder, T. K., Uzun, O., Moody, C. T., Aizenberg, M., et al. (2018). Replenishable Drug Depot to Combat Post-resection Cancer Recurrence. Biomaterials 178, 373–382. doi:10.1016/j.biomaterials.2018.05.005
Calmeiro, J., Carrascal, M., Gomes, C., Falcão, A., Cruz, M. T., and Neves, B. M. (2019). Biomaterial-based Platforms for In Situ Dendritic Cell Programming and Their Use in Antitumor Immunotherapy. J. Immunotherapy Cancer 7 (1), 238. doi:10.1186/s40425-019-0716-8
Chablani, L., Tawde, S. A., Akalkotkar, A., and D’Souza, M. J. (2019). Evaluation of a Particulate Breast Cancer Vaccine Delivered via Skin. AAPS J. 21 (2), 12. doi:10.1208/s12248-018-0285-7
Chen, F., Wang, Y., Gao, J., Saeed, M., Li, T., Wang, W., et al. (2021). Nanobiomaterial-based Vaccination Immunotherapy of Cancer. Biomaterials 270, 120709. doi:10.1016/j.biomaterials.2021.120709
Chen, M., Quan, G., Wen, T., Yang, P., Qin, W., Mai, H., et al. (2020a). Cold to Hot: Binary Cooperative Microneedle Array-Amplified Photoimmunotherapy for Eliciting Antitumor Immunity and the Abscopal Effect. ACS Appl. Mater. Inter. 12 (29), 32259–32269. doi:10.1021/acsami.0c05090
Chen, Q., Chen, J., Yang, Z., Xu, J., Xu, L., Liang, C., et al. (2019a). Nanoparticle-enhanced Radiotherapy to Trigger Robust Cancer Immunotherapy. Adv. Mater. 31 (10), e1802228. doi:10.1002/adma.201802228
Chen, Q., Wang, C., Zhang, X., Chen, G., Hu, Q., Li, H., et al. (2019b). In situ sprayed Bioresponsive Immunotherapeutic Gel for Post-surgical Cancer Treatment. Nat. Nanotech 14 (1), 89–97. doi:10.1038/s41565-018-0319-4
Chen, R., Zhang, H., Yan, J., and Bryers, J. D. (2018). Scaffold-mediated Delivery for Non-viral mRNA Vaccines. Gene Ther. 25 (8), 556–567. doi:10.1038/s41434-018-0040-9
Chen, S.-X., Ma, M., Xue, F., Shen, S., Chen, Q., Kuang, Y., et al. (2020b). Construction of Microneedle-Assisted Co-delivery Platform and its Combining Photodynamic/immunotherapy. J. Controlled Release 324, 218–227. doi:10.1016/j.jconrel.2020.05.006
Cheng, S., Xu, C., Jin, Y., Li, Y., Zhong, C., Ma, J., et al. (2020). Artificial Mini Dendritic Cells Boost T Cell-Based Immunotherapy for Ovarian Cancer. Adv. Sci. 7 (7), 1903301. doi:10.1002/advs.201903301
Cheung, A. S., Zhang, D. K. Y., Koshy, S. T., and Mooney, D. J. (2018). Scaffolds that Mimic Antigen-Presenting Cells Enable Ex Vivo Expansion of Primary T Cells. Nat. Biotechnol. 36 (2), 160–169. doi:10.1038/nbt.4047
Chiang, C., Coukos, G., and Kandalaft, L. (2015). Whole Tumor Antigen Vaccines: where Are We?. Vaccines 3 (2), 344–372. doi:10.3390/vaccines3020344
Cho, J.-a., Lee, Y.-S., Kim, S.-H., Ko, J.-K., and Kim, C.-W. (2009). MHC Independent Anti-tumor Immune Responses Induced by Hsp70-Enriched Exosomes Generate Tumor Regression in Murine Models. Cancer Lett. 275 (2), 256–265. doi:10.1016/j.canlet.2008.10.021
Chu, Y., Liu, Q., Wei, J., and Liu, B. (2018). Personalized Cancer Neoantigen Vaccines Come of Age. Theranostics 8 (15), 4238–4246. doi:10.7150/thno.24387
Cirillo, G., Curcio, U. G., Nicoletta, M., Iemma, F. P., and Iemma, F. (2019). Injectable Hydrogels for Cancer Therapy over the Last Decade. Pharmaceutics 11 (9), 486. doi:10.3390/pharmaceutics11090486
Cole, G., Ali, A. A., McErlean, E., Mulholland, E. J., Short, A., McCrudden, C. M., et al. (2019). DNA Vaccination via RALA Nanoparticles in a Microneedle Delivery System Induces a Potent Immune Response against the Endogenous Prostate Cancer Stem Cell Antigen. Acta Biomater. 96, 480–490. doi:10.1016/j.actbio.2019.07.003
Coley, W. B. (1991). The Classic. Clin. Orthopaedics Relat. Res. 262, 3–11. doi:10.1097/00003086-199101000-00002
Da Silva, C. G., Camps, M. G. M., Li, T. M. W. Y., Zerrillo, L., Löwik, C. W., Ossendorp, F., et al. (2019). Effective Chemoimmunotherapy by Co-delivery of Doxorubicin and Immune Adjuvants in Biodegradable Nanoparticles. Theranostics 9 (22), 6485–6500. doi:10.7150/thno.34429
Day, C.-P., Merlino, G., and Van Dyke, T. (2015). Preclinical Mouse Cancer Models: a Maze of Opportunities and Challenges. Cell 163 (1), 39–53. doi:10.1016/j.cell.2015.08.068
Demento, S. L., Cui, W., Criscione, J. M., Stern, E., Tulipan, J., Kaech, S. M., et al. (2012). Role of Sustained Antigen Release from Nanoparticle Vaccines in Shaping the T Cell Memory Phenotype. Biomaterials 33 (19), 4957–4964. doi:10.1016/j.biomaterials.2012.03.041
Desrichard, A., Snyder, A., and Chan, T. A. (2016). Cancer Neoantigens and Applications for Immunotherapy. Clin. Cancer Res. 22 (4), 807–812. doi:10.1158/1078-0432.CCR-14-3175
Du, G., Hathout, R. M., Nasr, M., Nejadnik, M. R., Tu, J., Koning, R. I., et al. (2017). Intradermal Vaccination with Hollow Microneedles: a Comparative Study of Various Protein Antigen and Adjuvant Encapsulated Nanoparticles. J. Controlled Release 266, 109–118. doi:10.1016/j.jconrel.2017.09.021
Duong, H. T. T., Thambi, T., Yin, Y., Kim, S. H., Nguyen, T. L., Phan, V. H. G., et al. (2020a). Degradation-regulated Architecture of Injectable Smart Hydrogels Enhances Humoral Immune Response and Potentiates Antitumor Activity in Human Lung Carcinoma. Biomaterials 230, 119599. doi:10.1016/j.biomaterials.2019.119599
Duong, H. T. T., Yin, Y., Thambi, T., Kim, B. S., Jeong, J. H., and Lee, D. S. (2020b). Highly Potent Intradermal Vaccination by an Array of Dissolving Microneedle Polypeptide Cocktails for Cancer Immunotherapy. J. Mater. Chem. B 8 (6), 1171–1181. doi:10.1039/c9tb02175b
Duong, H. T. T., Yin, Y., Thambi, T., Nguyen, T. L., Giang Phan, V. H., Lee, M. S., et al. (2018). Smart Vaccine Delivery Based on Microneedle Arrays Decorated with Ultra-pH-responsive Copolymers for Cancer Immunotherapy. Biomaterials 185, 13–24. doi:10.1016/j.biomaterials.2018.09.008
Fan, Y., Kuai, R., Xu, Y., Ochyl, L. J., Irvine, D. J., and Moon, J. J. (2017). Immunogenic Cell Death Amplified by Co-localized Adjuvant Delivery for Cancer Immunotherapy. Nano Lett. 17 (12), 7387–7393. doi:10.1021/acs.nanolett.7b03218
Fang, R. H., Hu, C.-M. J., Luk, B. T., Gao, W., Copp, J. A., Tai, Y., et al. (2014). Cancer Cell Membrane-Coated Nanoparticles for Anticancer Vaccination and Drug Delivery. Nano Lett. 14 (4), 2181–2188. doi:10.1021/nl500618u
Fennemann, F. L., de Vries, I. J. M., Figdor, C. G., and Verdoes, M. (2019). Attacking Tumors from All Sides: Personalized Multiplex Vaccines to Tackle Intratumor Heterogeneity. Front. Immunol. 10, 824–832. doi:10.3389/fimmu.2019.00824
Gala, R., Zaman, R., D’Souza, M., and Zughaier, S. (2018). Novel Whole-Cell Inactivated neisseria Gonorrhoeae Microparticles as Vaccine Formulation in Microneedle-Based Transdermal Immunization. Vaccines 6 (3), 60. doi:10.3390/vaccines6030060
Galluzzi, L., Buqué, A., Kepp, O., Zitvogel, L., and Kroemer, G. (2017). Immunogenic Cell Death in Cancer and Infectious Disease. Nat. Rev. Immunol. 17 (2), 97–111. doi:10.1038/nri.2016.107
Galluzzi, L., Chan, T. A., Kroemer, G., Wolchok, J. D., and López-Soto, A. (2018). The Hallmarks of Successful Anticancer Immunotherapy. Sci. Transl. Med. 10 (459), eaat7807. doi:10.1126/scitranslmed.aat7807
Gan, J., Du, G., He, C., Jiang, M., Mou, X., Xue, J., et al. (2020). Tumor Cell Membrane Enveloped Aluminum Phosphate Nanoparticles for Enhanced Cancer Vaccination. J. Controlled Release 326, 297–309. doi:10.1016/j.jconrel.2020.07.008
Gardner, T., Elzey, B., and Hahn, N. M. (2012). Sipuleucel-T (Provenge) Autologous Vaccine Approved for Treatment of Men with Asymptomatic or Minimally Symptomatic Castrate-Resistant Metastatic Prostate Cancer. Hum. Vaccin. Immunother. 8 (4), 534–539. doi:10.4161/hv.19795
Geng, Y., Yuan, W., Wu, F., Chen, J., He, M., and Jin, T. (2008). Formulating Erythropoietin-Loaded Sustained-Release PLGA Microspheres without Protein Aggregation. J. Controlled Release 130 (3), 259–265. doi:10.1016/j.jconrel.2008.06.011
Greening, D. W., Gopal, S. K., Xu, R., Simpson, R. J., and Chen, W. (2015). Exosomes and Their Roles in Immune Regulation and Cancer. Semin. Cel Dev. Biol. 40, 72–81. doi:10.1016/j.semcdb.2015.02.009
Guarecuco, R., Lu, J., McHugh, K. J., Norman, J. J., Thapa, L. S., Lydon, E., et al. (2018). Immunogenicity of Pulsatile-Release PLGA Microspheres for Single-Injection Vaccination. Vaccine 36 (22), 3161–3168. doi:10.1016/j.vaccine.2017.05.094
Gulley, J. L., Borre, M., Vogelzang, N. J., Ng, S., Agarwal, N., Parker, C. C., et al. (2019). Phase III Trial of PROSTVAC in Asymptomatic or Minimally Symptomatic Metastatic Castration-Resistant Prostate Cancer. Jco 37 (13), 1051–1061. doi:10.1200/jco.18.02031
Guo, C., Manjili, M. H., Subjeck, J. R., Sarkar, D., Fisher, P. B., and Wang, X.-Y. (2013). Therapeutic Cancer Vaccines. Adv. Cancer Res. 119, 421–475. doi:10.1016/B978-0-12-407190-2.00007-1
Hailemichael, Y., Dai, Z., Jaffarzad, N., Ye, Y., Medina, M. A., Huang, X.-F., et al. (2013). Persistent Antigen at Vaccination Sites Induces Tumor-specific CD8+ T Cell Sequestration, Dysfunction and Deletion. Nat. Med. 19 (4), 465–472. doi:10.1038/nm.3105
Hammerich, L., Binder, A., and Brody, J. D. (2015). In Situvaccination: Cancer Immunotherapy Both Personalizedandoff-The-Shelf. Mol. Oncol. 9 (10), 1966–1981. doi:10.1016/j.molonc.2015.10.016
Han, F. Y., Thurecht, K. J., Whittaker, A. K., and Smith, M. T. (2016a). Bioerodable PLGA-Based Microparticles for Producing Sustained-Release Drug Formulations and Strategies for Improving Drug Loading. Front. Pharmacol. 7, 185. doi:10.3389/fphar.2016.00185
Han, L., Xue, J., Wang, L., Peng, K., Zhang, Z., Gong, T., et al. (2016b). An Injectable, Low-Toxicity Phospholipid-Based Phase Separation Gel that Induces Strong and Persistent Immune Responses in Mice. Biomaterials 105, 185–194. doi:10.1016/j.biomaterials.2016.08.007
Hori, Y., Stern, P. J., Hynes, R. O., and Irvine, D. J. (2009). Engulfing Tumors with Synthetic Extracellular Matrices for Cancer Immunotherapy. Biomaterials 30 (35), 6757–6767. doi:10.1016/j.biomaterials.2009.08.037
Hossain, M. K., Ahmed, T., Bhusal, P., Subedi, R. K., Salahshoori, I., Soltani, M., et al. (2020). Microneedle Systems for Vaccine Delivery: the Story So Far. Expert Rev. Vaccin. 19 (12), 1153–1166. doi:10.1080/14760584.2020.1874928
Hou, Y., Liu, R., Hong, X., Zhang, Y., Bai, S., Luo, X., et al. (2021). Engineering a Sustained Release Vaccine with a Pathogen-Mimicking Manner for Robust and Durable Immune Responses. J. Controlled Release 333, 162–175. doi:10.1016/j.jconrel.2021.03.037
Hu, Z., Ott, P. A., and Wu, C. J. (2018). Towards Personalized, Tumour-specific, Therapeutic Vaccines for Cancer. Nat. Rev. Immunol. 18 (3), 168–182. doi:10.1038/nri.2017.131
Huang, P., Wang, X., Liang, X., Yang, J., Zhang, C., Kong, D., et al. (2019). Nano-, Micro-, and Macroscale Drug Delivery Systems for Cancer Immunotherapy. Acta Biomater. 85, 1–26. doi:10.1016/j.actbio.2018.12.028
Huang, X., Williams, J. Z., Chang, R., Li, Z., Burnett, C. E., Hernandez-Lopez, R., et al. (2020). DNA Scaffolds Enable Efficient and Tunable Functionalization of Biomaterials for Immune Cell Modulation. Nat. Nanotechnol. 16 (2), 214–223. doi:10.1038/s41565-020-00813-z
Hwang, M. P., Fecek, R. J., Qin, T., Storkus, W. J., and Wang, Y. (2020). Single Injection of IL-12 Coacervate as an Effective Therapy against B16-F10 Melanoma in Mice. J. Controlled Release 318, 270–278. doi:10.1016/j.jconrel.2019.12.035
Iezzi, G., Karjalainen, K., and Lanzavecchia, A. (1998). The Duration of Antigenic Stimulation Determines the Fate of Naive and Effector T Cells. Immunity 8 (1), 89–95. doi:10.1016/s1074-7613(00)80461-6
Irvine, D. J., Aung, A., and Silva, M. (2020). Controlling Timing and Location in Vaccines. Adv. Drug Deliv. Rev. 158, 91–115. doi:10.1016/j.addr.2020.06.019
Jackaman, C., and Nelson, D. J. (2012). Intratumoral Interleukin-2/agonist CD40 Antibody Drives CD4+-independent Resolution of Treated-Tumors and CD4+-dependent Systemic and Memory Responses. Cancer Immunol. Immunother. 61 (4), 549–560. doi:10.1007/s00262-011-1120-5
Jacobs, J. J., Snackey, C., Geldof, A. A., Characiejus, D., Van Moorselaar, R. J., and Den Otter, W. (2014). Inefficacy of Therapeutic Cancer Vaccines and Proposed Improvements. Casus of Prostate Cancer. Anticancer Res. 34 (6), 2689–2700.
Janku, F., Zhang, H. H., Pezeshki, A., Goel, S., Murthy, R., Wang-Gillam, A., et al. (2021). Intratumoral Injection of Clostridium Novyi-NT Spores in Patients with Treatment-Refractory Advanced Solid Tumors. Clin. Cancer Res. 27 (1), 96–106. doi:10.1158/1078-0432.CCR-20-2065
Jiang, H., Wang, Q., and Sun, X. (2017). Lymph Node Targeting Strategies to Improve Vaccination Efficacy. J. Controlled Release 267, 47–56. doi:10.1016/j.jconrel.2017.08.009
Jin, X., Zhu, D. D., Chen, B. Z., Ashfaq, M., and Guo, X. D. (2018a). Insulin Delivery Systems Combined with Microneedle Technology. Adv. Drug Deliv. Rev. 127, 119–137. doi:10.1016/j.addr.2018.03.011
Jin, X., Zhu, D. D., Chen, B. Z., Ashfaq, M., and Guo, X. D. (2018b). Insulin Delivery Systems Combined with Microneedle Technology. Adv. Drug Deliv. Rev. 127, 119–137. doi:10.1016/j.addr.2018.03.011
Johansen, P., Storni, T., Rettig, L., Qiu, Z., Der-Sarkissian, A., Smith, K. A., et al. (2008). Antigen Kinetics Determines Immune Reactivity. Pnas 105 (13), 5189–5194. doi:10.1073/pnas.0706296105
Joshi, V. B., Geary, S. M., Gross, B. P., Wongrakpanich, A., Norian, L. A., and Salem, A. K. (2014). Tumor Lysate-Loaded Biodegradable Microparticles as Cancer Vaccines. Expert Rev. Vaccin. 13 (1), 9–15. doi:10.1586/14760584.2014.851606
Jung, H., Jang, H.-E., Kang, Y. Y., Song, J., and Mok, H. (2019). PLGA Microspheres Coated with Cancer Cell-Derived Vesicles for Improved Internalization into Antigen-Presenting Cells and Immune Stimulation. Bioconjug. Chem. 30 (6), 1690–1701. doi:10.1021/acs.bioconjchem.9b00240
Kanchan, V., Katare, Y. K., and Panda, A. K. (2009). Memory Antibody Response from Antigen Loaded Polymer Particles and the Effect of Antigen Release Kinetics. Biomaterials 30 (27), 4763–4776. doi:10.1016/j.biomaterials.2009.05.075
Kanwar, N., and Sinha, V. R. (2019). In situ forming Depot as Sustained-Release Drug Delivery Systems. Crit. Rev. Ther. Drug Carrier Syst. 36 (2), 93–136. doi:10.1615/CritRevTherDrugCarrierSyst.2018025013
Keler, T., He, L., Ramakrishna, V., and Champion, B. (2007). Antibody-targeted Vaccines. Oncogene 26 (25), 3758–3767. doi:10.1038/sj.onc.1210375
Kim, H., Seong, K.-Y., Lee, J. H., Park, W., Yang, S. Y., and Hahn, S. K. (2019). Biodegradable Microneedle Patch Delivering Antigenic Peptide-Hyaluronate Conjugate for Cancer Immunotherapy. ACS Biomater. Sci. Eng. 5 (10), 5150–5158. doi:10.1021/acsbiomaterials.9b00961
Kim, J., Li, W. A., Choi, Y., Lewin, S. A., Verbeke, C. S., Dranoff, G., et al. (2015). Injectable, Spontaneously Assembling, Inorganic Scaffolds Modulate Immune Cells in vivo and Increase Vaccine Efficacy. Nat. Biotechnol. 33 (1), 64–72. doi:10.1038/nbt.3071
Kim, N. W., Kim, S.-Y., Lee, J. E., Yin, Y., Lee, J. H., Lim, S. Y., et al. (2018). Enhanced Cancer Vaccination by In Situ Nanomicelle-Generating Dissolving Microneedles. ACS Nano 12 (10), 9702–9713. doi:10.1021/acsnano.8b04146
Knorr, D. A., Dahan, R., and Ravetch, J. V. (2018). Toxicity of an Fc-Engineered Anti-CD40 Antibody Is Abrogated by Intratumoral Injection and Results in Durable Antitumor Immunity. Proc. Natl. Acad. Sci. USA 115 (43), 11048–11053. doi:10.1073/pnas.1810566115
Koerner, J., Horvath, D., and Groettrup, M. (2019). Harnessing Dendritic Cells for Poly (D,L-lactide-co-glycolide) Microspheres (PLGA MS)-mediated Anti-tumor Therapy. Front. Immunol. 10, 707. doi:10.3389/fimmu.2019.00707
Kordalivand, N., Tondini, E., Lau, C. Y. J., Vermonden, T., Mastrobattista, E., Hennink, W. E., et al. (2019). Cationic Synthetic Long Peptides-Loaded Nanogels: an Efficient Therapeutic Vaccine Formulation for Induction of T-Cell Responses. J. Controlled Release 315, 114–125. doi:10.1016/j.jconrel.2019.10.048
Korupalli, C., Pan, W.-Y., Yeh, C.-Y., Chen, P.-M., Mi, F.-L., Tsai, H.-W., et al. (2019). Single-injecting, Bioinspired Nanocomposite Hydrogel that Can Recruit Host Immune Cells In Situ to Elicit Potent and Long-Lasting Humoral Immune Responses. Biomaterials 216, 119268. doi:10.1016/j.biomaterials.2019.119268
Kuol, N., Stojanovska, L., Nurgali, K., and Apostolopoulos, V. (2017). The Mechanisms Tumor Cells Utilize to Evade the Host's Immune System. Maturitas 105, 8–15. doi:10.1016/j.maturitas.2017.04.014
Labanieh, L., Majzner, R. G., and Mackall, C. L. (2018). Programming CAR-T Cells to Kill Cancer. Nat. Biomed. Eng. 2 (6), 377–391. doi:10.1038/s41551-018-0235-9
Leach, D. G., Young, S., and Hartgerink, J. D. (2019). Advances in Immunotherapy Delivery from Implantable and Injectable Biomaterials. Acta Biomater. 88, 15–31. doi:10.1016/j.actbio.2019.02.016
Lei, K., and Tang, L. (2019). Surgery-free Injectable Macroscale Biomaterials for Local Cancer Immunotherapy. Biomater. Sci. 7 (3), 733–749. doi:10.1039/c8bm01470a
Leone, M., Mönkäre, J., Bouwstra, J. A., and Kersten, G. (2017). Dissolving Microneedle Patches for Dermal Vaccination. Pharm. Res. 34 (11), 2223–2240. doi:10.1007/s11095-017-2223-2
Li, A. W., Sobral, M. C., Badrinath, S., Choi, Y., Graveline, A., Stafford, A. G., et al. (2018). A Facile Approach to Enhance Antigen Response for Personalized Cancer Vaccination. Nat. Mater 17 (6), 528–534. doi:10.1038/s41563-018-0028-2
Li, F., Lyu, D., Liu, S., and Guo, W. (2020). DNA Hydrogels and Microgels for Biosensing and Biomedical Applications. Adv. Mater. 32 (3), e1806538. doi:10.1002/adma.201806538
Li, J., and Mooney, D. J. (2016). Designing Hydrogels for Controlled Drug Delivery. Nat. Rev. Mater. 1 (12), 16071. doi:10.1038/natrevmats.2016.71
Li, W. A., Lu, B. Y., Gu, L., Choi, Y., Kim, J., and Mooney, D. J. (2016a). The Effect of Surface Modification of Mesoporous Silica Micro-rod Scaffold on Immune Cell Activation and Infiltration. Biomaterials 83, 249–256. doi:10.1016/j.biomaterials.2016.01.026
Li, Y., Yang, H. Y., and Lee, D. S. (2021). Advances in Biodegradable and Injectable Hydrogels for Biomedical Applications. J. Controlled Release 330, 151–160. doi:10.1016/j.jconrel.2020.12.008
Li, Z., Xiong, F., He, J., Dai, X., and Wang, G. (2016b). Surface-functionalized, pH-Responsive Poly(lactic-Co-Glycolic Acid)-Based Microparticles for Intranasal Vaccine Delivery: Effect of Surface Modification with Chitosan and Mannan. Eur. J. Pharmaceutics Biopharmaceutics 109, 24–34. doi:10.1016/j.ejpb.2016.08.012
Lin, C.-Y., Lin, S.-J., Yang, Y.-C., Wang, D.-Y., Cheng, H.-F., and Yeh, M.-K. (2015). Biodegradable Polymeric Microsphere-Based Vaccines and Their Applications in Infectious Diseases. Hum. Vaccin. Immunother. 11 (3), 650–656. doi:10.1080/21645515.2015.1009345
Lin, Y.-X., Wang, Y., Blake, S., Yu, M., Mei, L., Wang, H., et al. (2020). RNA Nanotechnology-Mediated Cancer Immunotherapy. Theranostics 10 (1), 281–299. doi:10.7150/thno.35568
Liu, H., Moynihan, K. D., Zheng, Y., Szeto, G. L., Li, A. V., Huang, B., et al. (2014). Structure-based Programming of Lymph-Node Targeting in Molecular Vaccines. Nature 507 (7493), 519–522. doi:10.1038/nature12978
Liu, H., Shi, X., Wu, D., Kahsay Khshen, F., Deng, L., Dong, A., et al. (2019a). Injectable, Biodegradable, Thermosensitive Nanoparticles-Aggregated Hydrogel with Tumor-specific Targeting, Penetration, and Release for Efficient Postsurgical Prevention of Tumor Recurrence. ACS Appl. Mater. Inter. 11 (22), 19700–19711. doi:10.1021/acsami.9b01987
Liu, W.-L., Zou, M.-Z., Liu, T., Zeng, J.-Y., Li, X., Yu, W.-Y., et al. (2019b). Cytomembrane Nanovaccines Show Therapeutic Effects by Mimicking Tumor Cells and Antigen Presenting Cells. Nat. Commun. 10 (1), 3199. doi:10.1038/s41467-019-11157-1
Lopes, A., Vandermeulen, G., and Préat, V. (2019). Cancer DNA Vaccines: Current Preclinical and Clinical Developments and Future Perspectives. J. Exp. Clin. Cancer Res. 38 (1), 146. doi:10.1186/s13046-019-1154-7
Lu, Y., Aimetti, A. A., Langer, R., and Gu, Z. (2017). Bioresponsive Materials. Nat. Rev. Mater. 2, 16075. doi:10.1038/natrevmats.2016.75
Lybaert, L., Vermaelen, K., De Geest, B. G., and Nuhn, L. (2018). Immunoengineering through Cancer Vaccines - A Personalized and Multi-step Vaccine Approach towards Precise Cancer Immunity. J. Controlled Release 289, 125–145. doi:10.1016/j.jconrel.2018.09.009
Mata, E., Igartua, M., Patarroyo, M. E., Pedraz, J. L., and Hernández, R. M. (2011). Enhancing Immunogenicity to PLGA Microparticulate Systems by Incorporation of Alginate and RGD-Modified Alginate. Eur. J. Pharm. Sci. 44 (1-2), 32–40. doi:10.1016/j.ejps.2011.05.015
Matson, V., Fessler, J., Bao, R., Chongsuwat, T., Zha, Y., Alegre, M.-L., et al. (2018). The Commensal Microbiome Is Associated with Anti-PD-1 Efficacy in Metastatic Melanoma Patients. Science 359 (6371), 104–108. doi:10.1126/science.aao3290
McHugh, K. J., Guarecuco, R., Langer, R., and Jaklenec, A. (2015). Single-injection Vaccines: Progress, Challenges, and Opportunities. J. Controlled Release 219, 596–609. doi:10.1016/j.jconrel.2015.07.029
McNamara, M. A., Nair, S. K., and Holl, E. K. (2015). RNA-based Vaccines in Cancer Immunotherapy. J. Immunol. Res. 2015, 794528. doi:10.1155/2015/794528
Mestas, J., and Hughes, C. C. W. (2004). Of Mice and Not Men: Differences between Mouse and Human Immunology. J. Immunol. 172 (5), 2731–2738. doi:10.4049/jimmunol.172.5.2731
Mills, B. N., Connolly, K. A., Ye, J., Murphy, J. D., Uccello, T. P., Han, B. J., et al. (2019). Stereotactic Body Radiation and Interleukin-12 Combination Therapy Eradicates Pancreatic Tumors by Repolarizing the Immune Microenvironment. Cel Rep. 29 (2), 406–421. e405. doi:10.1016/j.celrep.2019.08.095
Min, Y., Roche, K. C., Tian, S., Eblan, M. J., McKinnon, K. P., Caster, J. M., et al. (2017). Antigen-capturing Nanoparticles Improve the Abscopal Effect and Cancer Immunotherapy. Nat. Nanotech 12 (9), 877–882. doi:10.1038/nnano.2017.113
Mueller, M., Reichardt, W., Koerner, J., and Groettrup, M. (2012). Coencapsulation of Tumor Lysate and CpG-ODN in PLGA-Microspheres Enables Successful Immunotherapy of Prostate Carcinoma in TRAMP Mice. J. Controlled Release 162 (1), 159–166. doi:10.1016/j.jconrel.2012.06.015
Nejman, D., Livyatan, I., Fuks, G., Gavert, N., Zwang, Y., Geller, L. T., et al. (2020). The Human Tumor Microbiome Is Composed of Tumor Type-specific Intracellular Bacteria. Science 368 (6494), 973–980. doi:10.1126/science.aay9189
Nguyen, T. L., Yin, Y., Choi, Y., Jeong, J. H., and Kim, J. (2020). Enhanced Cancer DNA Vaccine via Direct Transfection to Host Dendritic Cells Recruited in Injectable Scaffolds. ACS Nano 14 (9), 11623–11636. doi:10.1021/acsnano.0c04188
Niu, L., Chu, L. Y., Burton, S. A., Hansen, K. J., and Panyam, J. (2019). Intradermal Delivery of Vaccine Nanoparticles Using Hollow Microneedle Array Generates Enhanced and Balanced Immune Response. J. Controlled Release 294, 268–278. doi:10.1016/j.jconrel.2018.12.026
Noguchi, M., Arai, G., Egawa, S., Ohyama, C., Naito, S., Matsumoto, K., et al. (2020). Mixed 20-peptide Cancer Vaccine in Combination with Docetaxel and Dexamethasone for Castration-Resistant Prostate Cancer: a Randomized Phase II Trial. Cancer Immunol. Immunother. 69 (5), 847–857. doi:10.1007/s00262-020-02498-8
Obeid, J., Hu, Y., and Slingluff, C. L. (2015). Vaccines, Adjuvants, and Dendritic Cell Activators-Current Status and Future Challenges. Semin. Oncol. 42 (4), 549–561. doi:10.1053/j.seminoncol.2015.05.006
Oberli, M. A., Reichmuth, A. M., Dorkin, J. R., Mitchell, M. J., Fenton, O. S., Jaklenec, A., et al. (2017). Lipid Nanoparticle Assisted mRNA Delivery for Potent Cancer Immunotherapy. Nano Lett. 17 (3), 1326–1335. doi:10.1021/acs.nanolett.6b03329
Oh, E., Oh, J.-E., Hong, J., Chung, Y., Lee, Y., Park, K. D., et al. (2017). Optimized Biodegradable Polymeric Reservoir-Mediated Local and Sustained Co-delivery of Dendritic Cells and Oncolytic Adenovirus Co-expressing IL-12 and GM-CSF for Cancer Immunotherapy. J. Controlled Release 259, 115–127. doi:10.1016/j.jconrel.2017.03.028
Ouyang, X., Telli, M. L., and Wu, J. C. (2019). Induced Pluripotent Stem Cell-Based Cancer Vaccines. Front. Immunol. 10, 1510. doi:10.3389/fimmu.2019.01510
Ozao-Choy, J., Lee, D. J., and Faries, M. B. (2014). Melanoma Vaccines. Surg. Clin. North America 94 (5), 1017–1030. doi:10.1016/j.suc.2014.07.005
Pardi, N., Hogan, M. J., Porter, F. W., and Weissman, D. (2018). mRNA Vaccines - a New Era in Vaccinology. Nat. Rev. Drug Discov. 17 (4), 261–279. doi:10.1038/nrd.2017.243
Park, K., Skidmore, S., Hadar, J., Garner, J., Park, H., Otte, A., et al. (2019). Injectable, Long-Acting PLGA Formulations: Analyzing PLGA and Understanding Microparticle Formation. J. Controlled Release 304, 125–134. doi:10.1016/j.jconrel.2019.05.003
Patel, R. B., Ye, M., Carlson, P. M., Jaquish, A., Zangl, L., Ma, B., et al. (2019). Development of an In Situ Cancer Vaccine via Combinational Radiation and Bacterial‐Membrane‐Coated Nanoparticles. Adv. Mater. 31 (43), e1902626. doi:10.1002/adma.201902626
Perez, C. R., and De Palma, M. (2019). Engineering Dendritic Cell Vaccines to Improve Cancer Immunotherapy. Nat. Commun. 10 (1), 5408. doi:10.1038/s41467-019-13368-y
Peris, I., and Langer, R. S. (1979). A Single-step Immunization by Sustained Antigen Release. J. Immunol. Methods 28 (1), 193–197. doi:10.1016/0022-1759(79)90341-7
Phuengkham, H., Song, C., Um, S. H., and Lim, Y. T. (2018). Implantable Synthetic Immune Niche for Spatiotemporal Modulation of Tumor-Derived Immunosuppression and Systemic Antitumor Immunity: Postoperative Immunotherapy. Adv. Mater. 30 (18), e1706719. doi:10.1002/adma.201706719
Pilla, L., Ferrone, S., and Maccalli, C. (2018). Methods for Improving the Immunogenicity and Efficacy of Cancer Vaccines. Expert Opin. Biol. Ther. 18 (7), 765–784. doi:10.1080/14712598.2018.1485649
Pol, J., Vacchelli, E., Aranda, F., Castoldi, F., Eggermont, A., Cremer, I., et al. (2015). Trial Watch: Immunogenic Cell Death Inducers for Anticancer Chemotherapy. Oncoimmunol. 4 (4), e1008866. doi:10.1080/2162402X.2015.1008866
Pradhan, P., Qin, H., Leleux, J. A., Gwak, D., Sakamaki, I., Kwak, L. W., et al. (2014). The Effect of Combined IL10 siRNA and CpG ODN as Pathogen-Mimicking Microparticles on Th1/Th2 Cytokine Balance in Dendritic Cells and Protective Immunity against B Cell Lymphoma. Biomaterials 35 (21), 5491–5504. doi:10.1016/j.biomaterials.2014.03.039
Qu, J., Zhao, X., Ma, P. X., and Guo, B. (2018). Injectable Antibacterial Conductive Hydrogels with Dual Response to an Electric Field and pH for Localized "smart" Drug Release. Acta Biomater. 72, 55–69. doi:10.1016/j.actbio.2018.03.018
Rahimian, S., Fransen, M. F., Kleinovink, J. W., Amidi, M., Ossendorp, F., and Hennink, W. E. (2015). Polymeric Microparticles for Sustained and Local Delivery of antiCD40 and antiCTLA-4 in Immunotherapy of Cancer. Biomaterials 61, 33–40. doi:10.1016/j.biomaterials.2015.04.043
Rehman, H., Silk, A. W., Kane, M. P., and Kaufman, H. L. (2016). Into the Clinic: Talimogene Laherparepvec (T-VEC), a First-In-Class Intratumoral Oncolytic Viral Therapy. J. Immunotherapy Cancer 4, 53. doi:10.1186/s40425-016-0158-5
Ribas, A., Weber, J. S., Chmielowski, B., Comin-Anduix, B., Lu, D., Douek, M., et al. (2011). Intra-lymph Node Prime-Boost Vaccination against Melan A and Tyrosinase for the Treatment of Metastatic Melanoma: Results of a Phase 1 Clinical Trial. Clin. Cancer Res. 17 (9), 2987–2996. doi:10.1158/1078-0432.Ccr-10-3272
Riley, R. S., June, C. H., Langer, R., and Mitchell, M. J. (2019). Delivery Technologies for Cancer Immunotherapy. Nat. Rev. Drug Discov. 18 (3), 175–196. doi:10.1038/s41573-018-0006-z
Rosalia, R. A., Quakkelaar, E. D., Redeker, A., Khan, S., Camps, M., Drijfhout, J. W., et al. (2013). Dendritic Cells Process Synthetic Long Peptides Better Than Whole Protein, Improving Antigen Presentation and T-Cell Activation. Eur. J. Immunol. 43 (10), 2554–2565. doi:10.1002/eji.201343324
Russell, S. J., and Barber, G. N. (2018). Oncolytic Viruses as Antigen-Agnostic Cancer Vaccines. Cancer Cell 33 (4), 599–605. doi:10.1016/j.ccell.2018.03.011
Sabado, R. L., Balan, S., and Bhardwaj, N. (2017). Dendritic Cell-Based Immunotherapy. Cell Res 27 (1), 74–95. doi:10.1038/cr.2016.157
Schineis, P., Kotkowska, Z. K., Vogel-Kindgen, S., Friess, M. C., Theisen, M., Schwyter, D., et al. (2021). Photochemical Internalization (PCI)-mediated Activation of CD8 T Cells Involves Antigen Uptake and CCR7-Mediated Transport by Migratory Dendritic Cells to Draining Lymph Nodes. J. Controlled Release 332, 96–108. doi:10.1016/j.jconrel.2021.02.014
Schlom, J., Hodge, J. W., Palena, C., Tsang, K.-Y., Jochems, C., Greiner, J. W., et al. (2014). Therapeutic Cancer Vaccines. Adv. Cancer Res. 121, 67–124. doi:10.1016/B978-0-12-800249-0.00002-0
Shae, D., Baljon, J. J., Wehbe, M., Becker, K. W., Sheehy, T. L., and Wilson, J. T. (2019). At the Bench: Engineering the Next Generation of Cancer Vaccines. J. Leukoc. Biol. 108 (4), 1435–1453. doi:10.1002/jlb.5bt0119-016r
Shafiee, A., and Atala, A. (2017). Tissue Engineering: toward a New Era of Medicine. Annu. Rev. Med. 68, 29–40. doi:10.1146/annurev-med-102715-092331
Sharma, P., and Allison, J. P. (2015). Immune Checkpoint Targeting in Cancer Therapy: toward Combination Strategies with Curative Potential. Cell 161 (2), 205–214. doi:10.1016/j.cell.2015.03.030
Sheen, M. R., and Fiering, S. (2019). In situ vaccination: Harvesting Low Hanging Fruit on the Cancer Immunotherapy Tree. WIREs Nanomed Nanobiotechnol 11 (1), e1524. doi:10.1002/wnan.1524
Silva, A. L., Soema, P. C., Slütter, B., Ossendorp, F., and Jiskoot, W. (2016). PLGA Particulate Delivery Systems for Subunit Vaccines: Linking Particle Properties to Immunogenicity. Hum. Vaccin. Immunother. 12 (4), 1056–1069. doi:10.1080/21645515.2015.1117714
Sivakumar, S. M., Safhi, M. M., Kannadasan, M., and Sukumaran, N. (2011). Vaccine Adjuvants - Current Status and Prospects on Controlled Release Adjuvancity. Saudi Pharm. J. 19 (4), 197–206. doi:10.1016/j.jsps.2011.06.003
Song, C., Phuengkham, H., Kim, Y. S., Dinh, V. V., Lee, I., Shin, I. W., et al. (2019). Syringeable Immunotherapeutic Nanogel Reshapes Tumor Microenvironment and Prevents Tumor Metastasis and Recurrence. Nat. Commun. 10 (1), 3745. doi:10.1038/s41467-019-11730-8
Song, H., Huang, P., Niu, J., Shi, G., Zhang, C., Kong, D., et al. (2018). Injectable Polypeptide Hydrogel for Dual-Delivery of Antigen and TLR3 Agonist to Modulate Dendritic Cells in vivo and Enhance Potent Cytotoxic T-Lymphocyte Response against Melanoma. Biomaterials 159, 119–129. doi:10.1016/j.biomaterials.2018.01.004
Sprooten, J., Ceusters, J., Coosemans, A., Agostinis, P., De Vleeschouwer, S., Zitvogel, L., et al. (2019). Trial Watch: Dendritic Cell Vaccination for Cancer Immunotherapy. Oncoimmunol. 8 (11), e1638212. doi:10.1080/2162402x.2019.1638212
Stanley, M. (2017). Tumour Virus Vaccines: Hepatitis B Virus and Human Papillomavirus. Phil. Trans. R. Soc. B 372 (1732), 20160268. doi:10.1098/rstb.2016.0268
Stephan, S. B., Taber, A. M., Jileaeva, I., Pegues, E. P., Sentman, C. L., and Stephan, M. T. (2015). Biopolymer Implants Enhance the Efficacy of Adoptive T-Cell Therapy. Nat. Biotechnol. 33 (1), 97–101. doi:10.1038/nbt.3104
Subbiah, V., Murthy, R., Hong, D. S., Prins, R. M., Hosing, C., Hendricks, K., et al. (2018). Cytokines Produced by Dendritic Cells Administered Intratumorally Correlate with Clinical Outcome in Patients with Diverse Cancers. Clin. Cancer Res. 24 (16), 3845–3856. doi:10.1158/1078-0432.CCR-17-2707
Tanyi, J. L., Bobisse, S., Ophir, E., Tuyaerts, S., Roberti, A., Genolet, R., et al. (2018). Personalized Cancer Vaccine Effectively Mobilizes Antitumor T Cell Immunity in Ovarian Cancer. Sci. Transl. Med. 10 (436), eaao5931. doi:10.1126/scitranslmed.aao5931
Théry, C., Ostrowski, M., and Segura, E. (2009). Membrane Vesicles as Conveyors of Immune Responses. Nat. Rev. Immunol. 9 (8), 581–593. doi:10.1038/nri2567
Twumasi-Boateng, K., Pettigrew, J. L., Kwok, Y. Y. E., Bell, J. C., and Nelson, B. H. (2018). Oncolytic Viruses as Engineering Platforms for Combination Immunotherapy. Nat. Rev. Cancer 18 (7), 419–432. doi:10.1038/s41568-018-0009-4
Umeki, Y., Mohri, K., Kawasaki, Y., Watanabe, H., Takahashi, R., Takahashi, Y., et al. (2015). Induction of Potent Antitumor Immunity by Sustained Release of Cationic Antigen from a DNA-Based Hydrogel with Adjuvant Activity. Adv. Funct. Mater. 25 (36), 5758–5767. doi:10.1002/adfm.201502139
Uppu, D. S. S. M., Turvey, M. E., Sharif, A. R. M., Bidet, K., He, Y., Ho, V., et al. (2020). Temporal Release of a Three-Component Protein Subunit Vaccine from Polymer Multilayers. J. Controlled Release 317, 130–141. doi:10.1016/j.jconrel.2019.11.022
US National Library of Medicine (2021). Dendritic Cell Activating Scaffold in Melanoma. [Online]. Available: https://clinicaltrials.gov/ct2/show/NCT01753089 (Accessed March 10, 2021).
van der Burg, S. H., Arens, R., Ossendorp, F., van Hall, T., and Melief, C. J. M. (2016). Vaccines for Established Cancer: Overcoming the Challenges Posed by Immune Evasion. Nat. Rev. Cancer 16 (4), 219–233. doi:10.1038/nrc.2016.16
van der Burg, S. H. (2018). Correlates of Immune and Clinical Activity of Novel Cancer Vaccines. Semin. Immunol. 39, 119–136. doi:10.1016/j.smim.2018.04.001
van der Maaden, K., Heuts, J., Camps, M., Pontier, M., Terwisscha van Scheltinga, A., Jiskoot, W., et al. (2018). Hollow Microneedle-Mediated Micro-injections of a Liposomal HPV E743-63 Synthetic Long Peptide Vaccine for Efficient Induction of Cytotoxic and T-Helper Responses. J. Controlled Release 269, 347–354. doi:10.1016/j.jconrel.2017.11.035
Vartak, A., and Sucheck, S. (2016). Recent Advances in Subunit Vaccine Carriers. Vaccines 4 (2), 12. doi:10.3390/vaccines4020012
Wang, C., Ye, Y., Hochu, G. M., Sadeghifar, H., and Gu, Z. (2016). Enhanced Cancer Immunotherapy by Microneedle Patch-Assisted Delivery of Anti-PD1 Antibody. Nano Lett. 16 (4), 2334–2340. doi:10.1021/acs.nanolett.5b05030
Wang, H., and Mooney, D. J. (2018). Biomaterial-assisted Targeted Modulation of Immune Cells in Cancer Treatment. Nat. Mater 17 (9), 761–772. doi:10.1038/s41563-018-0147-9
Wang, H., Najibi, A. J., Sobral, M. C., Seo, B. R., Lee, J. Y., Wu, D., et al. (2020a). Biomaterial-based Scaffold for In Situ Chemo-Immunotherapy to Treat Poorly Immunogenic Tumors. Nat. Commun. 11 (1), 5696. doi:10.1038/s41467-020-19540-z
Wang, T., Wang, D., Yu, H., Feng, B., Zhou, F., Zhang, H., et al. (2018). A Cancer Vaccine-Mediated Postoperative Immunotherapy for Recurrent and Metastatic Tumors. Nat. Commun. 9 (1), 1532. doi:10.1038/s41467-018-03915-4
Wang, Z., Shang, Y., Tan, Z., Li, X., Li, G., Ren, C., et al. (2020b). A Supramolecular Protein Chaperone for Vaccine Delivery. Theranostics 10 (2), 657–670. doi:10.7150/thno.39132
Wei, X., Liu, L., Li, X., Wang, Y., Guo, X., Zhao, J., et al. (2019). Selectively Targeting Tumor-Associated Macrophages and Tumor Cells with Polymeric Micelles for Enhanced Cancer Chemo-Immunotherapy. J. Controlled Release 313, 42–53. doi:10.1016/j.jconrel.2019.09.021
Weiden, J., Tel, J., and Figdor, C. G. (2018). Synthetic Immune Niches for Cancer Immunotherapy. Nat. Rev. Immunol. 18 (3), 212–219. doi:10.1038/nri.2017.89
Wu, H., Liu, S., Xiao, L., Dong, X., Lu, Q., and Kaplan, D. L. (2016). Injectable and pH-Responsive Silk Nanofiber Hydrogels for Sustained Anticancer Drug Delivery. ACS Appl. Mater. Inter. 8 (27), 17118–17126. doi:10.1021/acsami.6b04424
Yakkala, C., Chiang, C. L.-L., Kandalaft, L., Denys, A., and Duran, R. (2019). Cryoablation and Immunotherapy: an Enthralling Synergy to Confront the Tumors. Front. Immunol. 10, 2283. doi:10.3389/fimmu.2019.02283
Yan, C., Altunbas, A., Yucel, T., Nagarkar, R. P., Schneider, J. P., and Pochan, D. J. (2010). Injectable Solid Hydrogel: Mechanism of Shear-Thinning and Immediate Recovery of Injectable β-hairpin Peptide Hydrogels. Soft Matter 6 (20), 5143–5156. doi:10.1039/c0sm00642d
Yang, P., Song, H., Qin, Y., Huang, P., Zhang, C., Kong, D., et al. (2018). Engineering Dendritic-Cell-Based Vaccines and PD-1 Blockade in Self-Assembled Peptide Nanofibrous Hydrogel to Amplify Antitumor T-Cell Immunity. Nano Lett. 18 (7), 4377–4385. doi:10.1021/acs.nanolett.8b01406
Yang, T., Huang, D., Li, C., Zhao, D., Li, J., Zhang, M., et al. (2021). Rolling Microneedle Electrode Array (RoMEA) Empowered Nucleic Acid Delivery and Cancer Immunotherapy. Nano Today 36, 101017. doi:10.1016/j.nantod.2020.101017
Ye, X., Liang, X., Chen, Q., Miao, Q., Chen, X., Zhang, X., et al. (2019). Surgical Tumor-Derived Personalized Photothermal Vaccine Formulation for Cancer Immunotherapy. ACS Nano 13 (3), 2956–2968. doi:10.1021/acsnano.8b07371
Ye, Y., Wang, C., Zhang, X., Hu, Q., Zhang, Y., Liu, Q., et al. (2017). A Melanin-Mediated Cancer Immunotherapy Patch. Sci. Immunol. 2 (17), eaan5692. doi:10.1126/sciimmunol.aan5692
Yu, L., and Ding, J. (2008). Injectable Hydrogels as Unique Biomedical Materials. Chem. Soc. Rev. 37 (8), 1473–1481. doi:10.1039/b713009k
Yu, S., Wang, C., Yu, J., Wang, J., Lu, Y., Zhang, Y., et al. (2018). Injectable Bioresponsive Gel Depot for Enhanced Immune Checkpoint Blockade. Adv. Mater. 30 (28), e1801527. doi:10.1002/adma.201801527
Zaric, M., Lyubomska, O., Touzelet, O., Poux, C., Al-Zahrani, S., Fay, F., et al. (2013). Skin Dendritic Cell Targeting via Microneedle Arrays Laden with Antigen-Encapsulated Poly-D,l-Lactide-Co-Glycolide Nanoparticles Induces Efficient Antitumor and Antiviral Immune Responses. ACS Nano 7 (3), 2042–2055. doi:10.1021/nn304235j
Zeng, Q., Gammon, J. M., Tostanoski, L. H., Chiu, Y.-C., and Jewell, C. M. (2017). In vivo expansion of Melanoma-specific T Cells Using Microneedle Arrays Coated with Immune-Polyelectrolyte Multilayers. ACS Biomater. Sci. Eng. 3 (2), 195–205. doi:10.1021/acsbiomaterials.6b00414
Zhang, L., Wang, W., and Wang, S. (2015). Effect of Vaccine Administration Modality on Immunogenicity and Efficacy. Expert Rev. Vaccin. 14 (11), 1509–1523. doi:10.1586/14760584.2015.1081067
Zhang, W., Jin, X., Li, H., Wei, C.-x., and Wu, C.-w. (2019). Onion-structure Bionic Hydrogel Capsules Based on Chitosan for Regulating Doxorubicin Release. Carbohydr. Polym. 209, 152–160. doi:10.1016/j.carbpol.2019.01.028
Zhang, W., Wang, L., Liu, Y., Chen, X., Liu, Q., Jia, J., et al. (2014). Immune Responses to Vaccines Involving a Combined Antigen-Nanoparticle Mixture and Nanoparticle-Encapsulated Antigen Formulation. Biomaterials 35 (23), 6086–6097. doi:10.1016/j.biomaterials.2014.04.022
Zhao, H., Zhao, B., Li, L., Ding, K., Xiao, H., Zheng, C., et al. (2020). Biomimetic Decoy Inhibits Tumor Growth and Lung Metastasis by Reversing the Drawbacks of Sonodynamic Therapy. Adv. Healthc. Mater. 9 (1), e1901335. doi:10.1002/adhm.201901335
Zhao, J., Chen, Y., Ding, Z.-Y., and Liu, J.-Y. (2019). Safety and Efficacy of Therapeutic Cancer Vaccines Alone or in Combination with Immune Checkpoint Inhibitors in Cancer Treatment. Front. Pharmacol. 10, 1184. doi:10.3389/fphar.2019.01184
Zhu, G., Lynn, G. M., Jacobson, O., Chen, K., Liu, Y., Zhang, H., et al. (2017). Albumin/vaccine Nanocomplexes that Assemble in vivo for Combination Cancer Immunotherapy. Nat. Commun. 8 (1), 1954. doi:10.1038/s41467-017-02191-y
Zhu, M., Ding, X., Zhao, R., Liu, X., Shen, H., Cai, C., et al. (2018). Co-delivery of Tumor Antigen and Dual Toll-like Receptor Ligands into Dendritic Cell by Silicon Microparticle Enables Efficient Immunotherapy against Melanoma. J. Controlled Release 272, 72–82. doi:10.1016/j.jconrel.2018.01.004
Zhu, Y., Xue, J., Chen, W., Bai, S., Zheng, T., He, C., et al. (2020). Albumin-biomineralized Nanoparticles to Synergize Phototherapy and Immunotherapy against Melanoma. J. Controlled Release 322, 300–311. doi:10.1016/j.jconrel.2020.03.045
Keywords: cancer vaccine, in situ vaccination, drug delivery system, controlled release, sustained release, hydrogel, microneedle
Citation: Han L, Peng K, Qiu L-Y, Li M, Ruan J-H, He L-L and Yuan Z-X (2021) Hitchhiking on Controlled-Release Drug Delivery Systems: Opportunities and Challenges for Cancer Vaccines. Front. Pharmacol. 12:679602. doi: 10.3389/fphar.2021.679602
Received: 12 March 2021; Accepted: 28 April 2021;
Published: 10 May 2021.
Edited by:
Peixin Dong, Hokkaido University, JapanReviewed by:
Guangbao Yang, Soochow University, ChinaThomas Kieber-Emmons, University of Arkansas for Medical Sciences, United States
Jian You, Zhejiang University, China
Copyright © 2021 Han, Peng, Qiu, Li, Ruan, He and Yuan. This is an open-access article distributed under the terms of the Creative Commons Attribution License (CC BY). The use, distribution or reproduction in other forums is permitted, provided the original author(s) and the copyright owner(s) are credited and that the original publication in this journal is cited, in accordance with accepted academic practice. No use, distribution or reproduction is permitted which does not comply with these terms.
*Correspondence: Jing-Hua Ruan, 1438274952@qq.com; Li-Li He, lilihes@163.comlilihes@163.com; Zhi-Xiang Yuan, Zhixiang-yuan@hotmail.com