- 1Institute for Nutrition Research, School of Medical and Health Sciences, Edith Cowan University, Perth, WA, Australia
- 2Medical School, Royal Perth Hospital Research Foundation, The University of Western Australia, Perth, WA, Australia
- 3IMPACT—The Institute for Mental and Physical Health and Clinical Translation, School of Medicine, Barwon Health, Deakin University, Geelong, VIC, Australia
- 4Quadram Institute Bioscience, Norwich, United Kingdom
- 5Department of Anatomy and Physiology, Centre for Muscle Research, School of Biomedical Sciences, The University of Melbourne, Melbourne, VIC, Australia
- 6Centre for Kidney Research, Children’s Hospital at Westmead, School of Public Health, Sydney Medical School, The University of Sydney, Sydney, NSW, Australia
An increasing body of evidence highlights the strong potential for a diet rich in fruit and vegetables to delay, and often prevent, the onset of chronic diseases, including cardiometabolic, neurological, and musculoskeletal conditions, and certain cancers. A possible protective component, glucosinolates, which are phytochemicals found almost exclusively in cruciferous vegetables, have been identified from preclinical and clinical studies. Current research suggests that glucosinolates (and isothiocyanates) act via several mechanisms, ultimately exhibiting anti-inflammatory, antioxidant, and chemo-protective effects. This review summarizes the current knowledge surrounding cruciferous vegetables and their glucosinolates in relation to the specified health conditions. Although there is evidence that consumption of a high glucosinolate diet is linked with reduced incidence of chronic diseases, future large-scale placebo-controlled human trials including standardized glucosinolate supplements are needed.
Introduction
A poor diet with low amounts of fruits, vegetables, whole grains, seeds, and nuts, and excessive consumption of foods such as ultra-processed grains and sugar-sweetened beverages is the leading contributor to chronic disease risk (Afshin et al., 2019). There is increasing evidence that higher consumption of fruit and vegetables plays a central role in the prevention of non-communicable diseases (Liu, 2013). Vegetables contain a variety of different compounds that have beneficial health effects, including fiber, vitamins, minerals, and phytochemicals.
Research has shown that some types of vegetables may have greater health benefits than others for certain health conditions, such as diabetes, cardiovascular disease, and cancer (Cooper et al., 2012; Aune et al., 2017). This may be due to the presence of unique bioactive phytochemicals with potent health-related effects. Therefore, targeted recommendations surrounding the intake of specific types of vegetables with protective health benefits could assist in the reduction of non-communicable diseases.
Cruciferous vegetables include arugula (rocket), bok choy, broccoli, Brussels sprouts, cabbage, cauliflower, collard greens, daikon, horseradish, kale, kohlrabi, radish, turnips, wasabi, and watercress and are commonly consumed globally (International Agency for Research on Cancer, 2004). A number of epidemiological studies have investigated the health impact of cruciferous vegetables in humans and indicated that higher intakes of these vegetables are associated with a reduced risk of cardiometabolic diseases, musculoskeletal conditions, and cancer (Zhang et al., 2011; Liu et al., 2012; Wang et al., 2016; Aune et al., 2017; Blekkenhorst et al., 2017a; Sim et al., 2018). Although these vegetables contain a range of nutrients known to have beneficial health properties, studies have focused on the health effects of glucosinolates that are found almost exclusively in cruciferous vegetables.
Glucosinolates are phytochemicals that are proposed to be an important contributor to the health benefits of these vegetables (Manchali et al., 2012; Miękus et al., 2020). Glucosinolates (and their isothiocyanates) found in commonly consumed cruciferous vegetables include glucoraphanin (sulforaphane), sinigrin (allyl isothiocyanate), glucobrassicin, glucoraphasatin, and glucoiberin (Favela-González et al., 2020). Historically, a major research focus has been the anticancer effect of glucosinolates. However, there has been increasing evidence in recent years for the impact of cruciferous vegetables in cardiometabolic, neurological, and musculoskeletal conditions (Figure 1). Animal studies are important in identifying possible mechanisms responsible for health outcomes and provide the groundwork for future human trials. There is currently a lack of randomized controlled trials in this area, highlighting the importance of using animal data to inform trials investigating similar outcomes in humans. Therefore, the aim of this review was to provide an update of the current body of knowledge concerning the health effects of cruciferous vegetables and their glucosinolates, with a focus on cardiometabolic, neurological, and musculoskeletal conditions, and certain cancers. Electronic databases (PubMed, MEDLINE, Embase, and Google Scholar) were searched for peer-reviewed articles concerning glucosinolates and the specified health outcomes. The search was inclusive of all study designs given that this is an emerging area of research. Search terms included glucosinolates, isothiocyanates, cruciferous vegetables, cardiometabolic, hypertension, hyperglycemia, diabetes, dyslipidemia, neurological, psychiatric, musculoskeletal, muscle, bone, and cancer. Each subsection provides an overview of the epidemiological, preclinical, and clinical evidence.
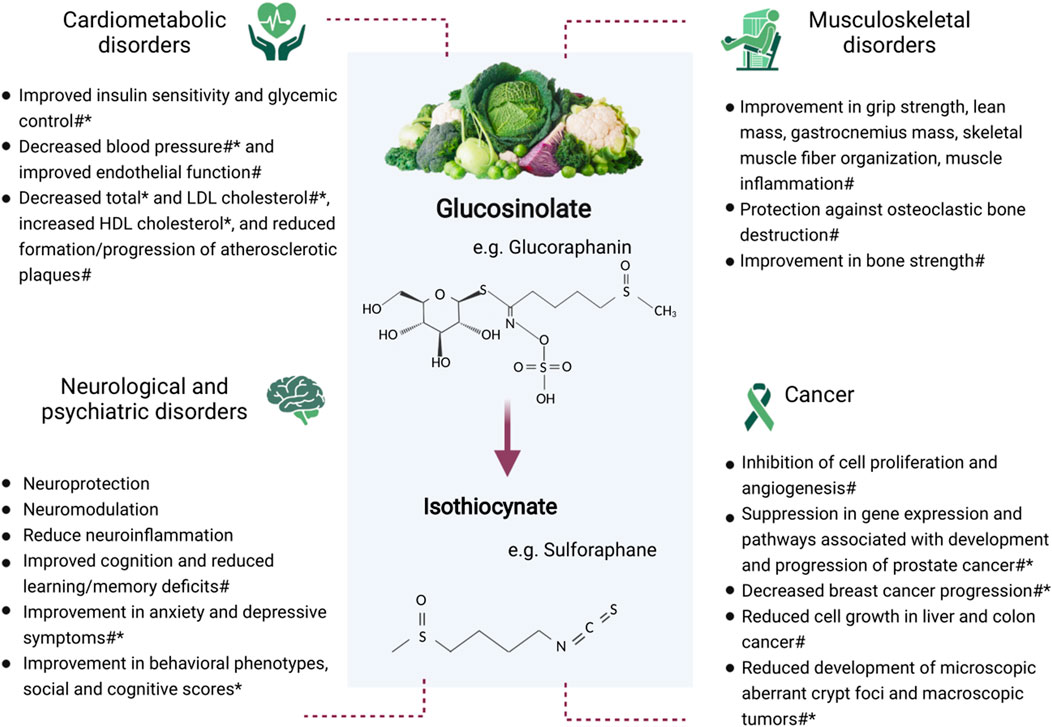
FIGURE 1. Potential benefits of glucosinolate consumption on cardiometabolic, neurological and psychiatric, and musculoskeletal conditions, and cancer. *Human study #Animal study.
Glucosinolates in Cruciferous Vegetables
Cruciferous vegetables belong to the family Brassicaceae (also known as Cruciferae) within the order Brassicales. Within this order, almost all plants contain secondary plant metabolites known as glucosinolates, with commonly consumed plants belonging to the Brassicaceae, Capparaceae, and Caricaceae families (Shakour et al., 2021). Glucosinolates are responsible for the bitter taste and pungent odor found in these vegetables (Barba et al., 2016).
The type of glucosinolate determines the compounds formed; different sidechains result in the production of different end products (Barba et al., 2016). More than 130 glucosinolates have been identified (Blažević et al., 2020), although not all are found in plants commonly consumed by humans. Glucosinolates have been commonly classified under three categories: Aliphatic, indole, and aromatic glucosinolates. However, this classification has also been disputed in a recent review where the type of degradation end product was proposed to be a more useful classification method (Blažević et al., 2020).
Upon damage to plant tissue (i.e., by chewing, cutting, or mixing), the hydrolysis of glucosinolates via enzymatic activity of myrosinases occurs due to cellular breakdown. This results in the formation of products, including isothiocyanates, nitriles, and thiocyanates (Kissen et al., 2009). Metabolism of glucosinolates can also occur by gut microbiota (Luang-In et al., 2014). If myrosinase is denatured, when ingested, glucosinolates could be partially absorbed in the stomach and the remaining intact glucosinolates transit to the small intestine and colon where they may be hydrolyzed by intestinal microbiota and absorbed as isothiocyanates (Barba et al., 2016).
The glucosinolate profile of these vegetables is an important determinant of the ultimate biological action when consumed by humans, with the beneficial health properties of these compounds largely linked to the actions of isothiocyanates (Maina et al., 2020; Shakour et al., 2021). A number of factors influence the type and concentration of glucosinolates found in these vegetables, such as genotype, cultivar, cultivation site, growth conditions (e.g., temperature, nutrient availability, water content), plant stage, plant tissue analyzed, storage conditions, and preparation and cooking methods (Maina et al., 2020).
The plant genotype determines the specific glucosinolates that are present in a particular type of cruciferous vegetable. However, the interaction between these plant genetic factors and the environment can affect the concentration of glucosinolates found within a specific sample (Björkman et al., 2011; Raiola et al., 2017). Environmental factors include sulfate and nitrogen content of the soil, water availability or drought, and seasonality (Björkman et al., 2011). Furthermore, the developmental stage and specific plant tissue also influences the concentration of glucosinolates. For example, it has been found that 3-day old broccoli and cauliflower sprouts contain 10–100 times higher glucoraphanin levels per gram compared to their mature plant forms (Fahey et al., 1997).
Seasonal variation in glucosinolate content has been recorded, with the majority of studies indicating that plants with the highest glucosinolate concentration are typically grown in spring in intermediate temperatures with high light intensity, longer days, and dry conditions (Björkman et al., 2011). However, there is variation among plants and exceptions to this. The diurnal cycle may also impact the glucosinolate profile of cruciferous vegetables. For example, Casajús and coworkers found that glucosinolate levels were optimized during postharvest storage if broccoli were harvested close to noon (Casajús et al., 2020).
Typically, cruciferous vegetables are not consumed immediately after harvesting. Therefore, the storage and processing of such vegetables have a significant impact on glucosinolate content and the health benefit of consumption (Björkman et al., 2011; Barba et al., 2016). This is variable depending on the cultivar, plant tissue, and growth stage of the plant (Galgano et al., 2007). Freezing has been shown to result in higher retention of glucosinolates compared to refrigeration. Storage of broccoli at 6°C for 35 days resulted in a sulforaphane loss of 29%, compared to losses of approximately 13% after freezing at −18°C for 60 days. Moreover, the reduction in glucosinolates measured after freezing was associated with steam-blanching prior to freezing (Galgano et al., 2007). Storage in darkness has been shown to decrease the content of aliphatic glucosinolates (Casajús et al., 2020).
Cooking denatures the myrosinase found in cruciferous vegetables, with high temperature (>80°C) and long cooking time increasing the intensity of denaturation (Barba et al., 2016; Raiola et al., 2017). Therefore, steaming is the preferable cooking method to maximize glucosinolate yield compared to boiling, microwaving, and pressure cooking (Shakour et al., 2021). These other methods are all associated with significant glucosinolate losses up to more than 90% (Barba et al., 2016; Wang Z. et al., 2020; Shakour et al., 2021).
Glucosinolates From Cruciferous Vegetables and Human Health
Many studies on the health effects of glucosinolates found in cruciferous vegetables have been based on laboratory or animal models. However, the number of studies involving humans is increasing. This review will focus on cardiometabolic, neurological, and musculoskeletal disorders in addition to providing a summary of the most recent literature on the effect of glucosinolates in certain cancers. Figure 1 provides an overview of the potential impact of glucosinolates on these conditions.
Cardiometabolic Disorders
Cardiometabolic disorders are a collection of interrelated conditions, mainly dyslipidemia, insulin resistance, impaired glucose tolerance, hypertension, and central adiposity (Kirk and Klein, 2009). To date, investigations into the effects of cruciferous vegetables and their glucosinolates have largely focused on hyperglycemia and diabetes, hypertension, and dyslipidemia. Epidemiological studies have indicated a potential beneficial association between cruciferous vegetables and such conditions (Zhang et al., 2011; Blekkenhorst et al., 2017a; Chen et al., 2018; Lapuente et al., 2019; Zurbau et al., 2020). The antioxidant and anti-inflammatory properties of glucosinolates have been proposed to account for some of the observed health benefits associated with cruciferous vegetable intake and cardiometabolic disorders (Esteve, 2020). The anti-inflammatory effects of sulforaphane and other isothiocyanates for these conditions may involve increased Nrf2 activity and inhibition of NF-κB (Esteve, 2020).
Hyperglycemia and Diabetes
There is increasing interest in the impact of glucosinolates on glycemic control. Isothiocyanates, such as raphasatin and sulforaphane, may prevent or reduce glycemic-related complications in animal and human studies (Maina et al., 2020). Sulforaphane was found to prevent diabetes-induced hypertension and cardiac dysfunction in a study including hyperglycemic mice treated with or without sulforaphane (0.5 mg/kg daily for 5 days/week) for 3 months and with 3 months of further observation (Bai et al., 2013).
A number of observational studies have investigated associations between cruciferous vegetable intake and glucose metabolism and risk of type 2 diabetes. A meta-analysis including 11 prospective studies (754,729 participants, 58,297 incident type 2 diabetes cases) found a 13% lower risk of type 2 diabetes with high cruciferous vegetable intake (Chen et al., 2018). Unlike other studies, in a large prospective cohort study including 200,907 adults from the United States, self-reported total cruciferous vegetable consumption was significantly associated with the development of type 2 diabetes (HR: 1.16; 95% CI: 1.07, 1.25; Ptrend: < 0.001) (Ma et al., 2018). Participants in the highest quintile of glucosinolate intake had a 19% higher risk of type 2 diabetes than those in the lowest intake quintile (Ma et al., 2018). This differing outcome may be a result of other differences in diet, as the meta-analysis included European and Asian populations (Chen et al., 2018) in addition to studies from the United States (Ma et al., 2018). Differences in other foods or beverages consumed with cruciferous vegetables as part of the diet may differ greatly between these populations. However, potential faults in the measurement of glucosinolates in the study by Ma and coworkers have been noted (Oliviero et al., 2018), which may have also affected the results (e.g., the effect of vegetable processing and preparation was not considered in the food frequency questionnaire and use of total glucosinolates instead of the bioactive isothiocyanates).
Several human intervention trials have also been performed. In a 4-week parallel, randomized, double-blind placebo-controlled study including 81 human participants with type 2 diabetes, 10 g/day broccoli sprout powder (225 μmol sulforaphane daily) decreased fasting serum insulin and insulin resistance by 18.2 and 14.2%, respectively (Bahadoran et al., 2012). Positive results were also seen in a randomized double-blind placebo-controlled study including 97 Scandinavian patients with type 2 diabetes. In this study, patients consumed broccoli sprout extract (150 μmol sulforaphane/day) or a placebo over a 12-week period. The glucoraphanin-rich broccoli sprout extract improved both fasting glucose and HbA1c (7.38–7.04%) in obese patients with dysregulated diabetes (BMI >30 kg/m2; HbA1c > 50 mmol/mol) (Axelsson et al., 2017). The authors noted that this reduction in HbA1c was likely to reflect a clinically meaningful effect, as an HbA1c of 7% is the treatment goal of the American Diabetes Association (Axelsson et al., 2017). In a recent 12-week randomized controlled parallel intervention trial, 92 patients with type 2 diabetes were randomized to one of three different diets: 500 g/day bitter and strong-tasting root vegetables and cabbages, 500 g/day mild and sweet-tasting root vegetables and cabbages, or 120 g/day mild and sweet-tasting root vegetables and cabbages (normal diet) (Thorup et al., 2021). Improvements in glycemic control were noted in both groups with increased vegetable intake; however, consumption of bitter and strong-tasting vegetables (including kale and cabbage) had the greatest improvements to fasting glucose (4-fold), total cholesterol (2-fold), and body fat mass (2-fold) (Thorup et al., 2021).
Hypertension
Positive effects of glucosinolates in relation to blood pressure have also been found in animal models (Wu et al., 2004). Dried broccoli sprouts (200 mg/day; ∼12 μmol glucoraphanin/g dry weight) administered daily to spontaneously hypertensive stroke-prone (SHRSP) rats resulted in significantly decreased measures of oxidative stress [increased glutathione (GSH) and decreased oxidized glutathione (GSSG); GSH/GSSG ratio 10.6–14.0 compared with 4.0–7.2 with SHRSP rats on control diet], which was correlated with significantly lower blood pressure (20 mmHg lower compared to control) and improved endothelial function (Wu et al., 2004). Sulforaphane, the metabolite of glucoraphanin, was considered responsible for these changes. 10 μmol/kg body weight sulforaphane administered to SHRSP rats over a 4-month period resulted in reduced blood pressure and prevented hypertension-associated vascular remodeling (Senanayake et al., 2012). It has also been hypothesized that erucin, an analog of sulforaphane, may play a role in the antihypertensive effect seen with arugula (Eruca sativa) (Salma et al., 2018). A significant reduction in mean arterial pressure was reported in salt-induced hypertensive rats administered 10 and 30 mg/kg E. sativa extract (40.33 ± 1.15 and 59.43 ± 0.77% mmHg, respectively) (Salma et al., 2018).
Eighty-six adults with type 2 diabetes and a positive H. pylori stool antigen test were included in a 4-week study and randomized to one of three groups: standard triple therapy (twice daily 20 mg omeprazole, 500 mg clarithromycin, 1,000 mg amoxicillin for 14 days), broccoli sprout powder (6 g/day), or a combination of broccoli sprout powder and standard triple therapy. Whilst there was a reduction in systolic and diastolic blood pressure in all groups, this was only significant from baseline in the group who received the standard triple therapy and broccoli sprout powder combination (14 and 9.4 mmHg reduction in systolic and diastolic blood pressure, respectively) (Mirmiran et al., 2014). In another 4-week study including 40 individuals with hypertension found that daily ingestion of 10 g dried broccoli sprouts did not improve endothelial function (Christiansen et al., 2010). Conversely, although statistically non-significant (p = 0.05), a trend towards a ∼10% decrease in diastolic blood pressure was reported in pregnant women with preeclampsia during a recent dose escalation study of activated broccoli extract (equivalent to 32 or 64 mg sulforaphane), regardless of sulforaphane dose (Langston-Cox et al., 2021). A current randomized controlled crossover trial is investigating whether regular consumption of cruciferous vegetables results in short-term improvement in cardiometabolic measures, such as ambulatory blood pressure and glycemic control (Connolly et al., 2020).
Dyslipidemia
Most of the research investigating the effects of glucosinolate consumption on lipids has been undertaken in animal models. The sprout extract of Tuscan black cabbage, a type of kale, was found to be protective against a high-fat diet in rats, restoring antioxidant and phase II enzyme levels and lowering serum lipids (total cholesterol, triacylglycerides, non-esterified fatty acids) (Melega et al., 2013). Similarly, glucosinolates may contribute to the anti-inflammatory and cholesterol-lowering effects of red cabbage. Red cabbage microgreens (equivalent to 200 g vegetables/day/person) lowered circulating LDL cholesterol, liver cholesterol, and inflammatory cytokines in a rodent model of a high fat diet (Huang et al., 2016). A systematic review and meta-analysis of rodent models found consistent significant decreases in total serum cholesterol with sulforaphane doses of more than 0.5 mg/kg/day, and a reduction in LDL cholesterol has also been identified (Du et al., 2021). Glucosinolates have also been demonstrated to reduce the formation and progression of atherosclerotic lesions in rabbit models of atherosclerosis. Rabbits fed a high cholesterol diet and 0.25 mg/kg/day sulforaphane over 4-week had improved measures of endothelial function and resistance to the development of atherosclerosis, compared to rabbits fed only a high cholesterol diet (Shehatou and Suddek, 2016). Additionally, phenethyl isothiocyanate, the product of the glucosinolate gluconasturtiin, was shown to lower atherosclerotic plaque formation and hepatic lipid accumulation in C57BL/6 mice fed a high fat/cholesterol diet with 30 or 75 mg/kg/day phenethyl isothiocyanate (Gwon et al., 2020).
Few studies have been conducted in humans. In a phase I study conducted in Japan including 12 human participants (20–36 years), daily consumption of 100 g fresh broccoli sprouts was shown to improve HDL by 7.6% in female participants and reduce total cholesterol by 10% in male participants from baseline measurements (Murashima et al., 2004). Similarly, glucoraphanin-rich broccoli (400 g/week) consumed over a 12-week period was found to significantly reduce plasma LDL-C compared to consumption of standard broccoli in two randomized, double-blind parallel studies including 130 adults aged ≥50 years at risk of cardiovascular disease (Armah et al., 2015).
Neurological and Psychiatric Disorders
Preclinical evidence suggests that glucosinolates and their metabolites, particularly sulforaphane, exhibit several biological properties that may be relevant to neurological and psychiatric conditions (Wu et al., 2016; Panjwani et al., 2018). A number of potential mechanisms include the modulation of the hypothalamic-pituitary-adrenal axis, oxidative stress, and inflammatory pathways. Sulforaphane may diminish neuroinflammation (by reducing NF-κB and TNF-α, and increasing IL-10) (Folkard et al., 2014; Santín-Márquez et al., 2019), reduce beta-amyloid and tau production (Kim, 2021), increase brain derived neurotrophic factor (Kim et al., 2017), postsynaptic density protein 95, AMPA receptor 1 (GluA1), dendritic spine density (Zhang et al., 2017), and blood brain barrier integrity (Li et al., 2013). Recently, sulforaphane has revealed epigenetic properties by inhibiting DNA methyltransferases in addition to preserving proteome homeostasis, influencing increased cellular lifespan and neurodegenerative prevention (Santín-Márquez et al., 2019). Furthermore, a sulforaphane intervention study in a cohort of healthy participants augmented peripheral and brain glutathione (Sedlak et al., 2017), an antioxidant readily implicated in various neurological functions (Dwivedi et al., 2020). At present, it remains unclear whether there are numerous key mechanisms involved across various disorders or whether mechanisms are disorder specific.
Psychiatric Disorders
Due to the supportive mechanistic data, animal models and clinical trials have begun to evaluate the use of sulforaphane interventions for psychiatric outcomes, including depression, schizophrenia, and autism (Panjwani et al., 2018). Acute and chronic consumption of sulforaphane consumption ameliorated anxiety and depressive-like behaviours in ICR and C57BL/6 mouse models (assessed using the novelty suppressed feeding test, open field and tail suspension tests) (Wu et al., 2016; Yao et al., 2016). Notably, C57BL/6 male mice that received sulforaphane in conjunction with depression-inducing lipopolysaccharide, exhibited lower depressive behaviours on the tail-suspension test and forced swimming test compared to those that did not receive sulforaphane (Zhang et al., 2017).
These results are supported by a recent randomized placebo controlled trial, which demonstrated that a 6-week sulforaphane intervention (30 mg/day) safely improved depressive symptoms, as measured by the Hamilton Rating Scale for Depression, in 66 participants with a history of a cardiac procedure and presence of mild to moderate depression (Ghazizadeh-Hashemi et al., 2021). In addition, an ongoing randomized controlled trial is examining whether sulforaphane may be utilized as an adjuvant treatment in bipolar depressive disorder (Wu et al., 2020). A small (n = 10) open label study demonstrated that an 8-week sulforaphane intervention improved cognitive function in a cohort of participants with schizophrenia (Shiina et al., 2015). Conversely, a more recent 16-week randomized controlled trial (n = 58) reported that sulforaphane (approximately 45 mg/day) failed to change the severity of psychotic symptoms compared to placebo using the total Positive and Negative Syndrome Scale (Dickerson et al., 2021). A recent review identified three double blind, randomized placebo controlled trials and two open-label trials examining the effects of sulforaphane on symptoms associated with Autism spectrum disorder (McGuinness and Kim, 2020). Collectively, study results were suggestive of a significant improvement in behavioral phenotypes such as irritability and motivation, alongside social and cognitive scores during sulforaphane interventions (Singh et al., 2014; Bent et al., 2018).
Neurological Disorders
Similar to psychiatry, various animal models have suggested the efficacy of sulforaphane interventions in the treatment of neurodegenerative disease; however, clinical trials are currently lacking (Schepici et al., 2020; Kim, 2021). Various pre-clinical animal studies have demonstrated improved cognitive ability and reduced learning/memory deficits following sulforaphane interventions in Alzheimer’s disease models (Kim et al., 2013; Lee et al., 2018). Further, administration of sulforaphane in adult mice induced a variety of cognitive changes associated with memory consolidation and spatial learning (Sunkaria et al., 2018). In vitro investigations have highlighted the potential of sulforaphane to prevent the loss of oligodendrocytes and axonal damage in multiple sclerosis, by modulating neuroinflammation and oxidative stress (Lim et al., 2016). Sulforaphane administration in mice exposed to 6-hydroxydopamine has also been shown to improve motor deficits, while dietary intake of sulforaphane preserved dopaminergic neurons following induced dopaminergic neurotoxicity in mice (Morroni et al., 2013; Pu et al., 2019). The protective effects of sulforaphane have further been implicated in acute neurodegenerative conditions, such as acute ischemic brain injury and traumatic brain injury, amongst mice models (Tarozzi et al., 2013). Given the promising results in animal studies, clinical studies are required to evaluate the effect of sulforaphane on neurological outcomes in humans.
Musculoskeletal Disorders
Current epidemiological evidence suggests that cruciferous vegetables play a key role in muscle and bone health, possibly due to the presence of glucosinolates and isothiocyanates. For example, in a large cohort of older community-dwelling women (n = 1,468, ≥70 years), women with the highest cruciferous (>44 g/day) and allium (>11 g/day) vegetable intake had 28 and 34% lower relative hazards for a fracture-related hospitalization over 14.5 years, respectively (Blekkenhorst et al., 2017b). Similarly, greater cruciferous and allium vegetable intake was associated with 22 and 26% lower relative hazard ratio for an injurious fall-related hospitalization over this time, respectively (Sim et al., 2018). Although not specifically examining the role of glucosinolates, these studies demonstrate the potential importance of a diet rich in glucosinolates for musculoskeletal health.
Muscle
When considering the role of glucosinolates for musculoskeletal health, sulforaphane has been studied most extensively in animal models. Historically, the understanding of the effects of glucosinolates on skeletal muscle comes from trials of their application in animal production settings, with results indicating that although growth performance may be affected, muscle had an improved antioxidant status and fatty acid profile (Drażbo et al., 2019; Skugor et al., 2019). When sulforaphane was provided to male Wistar rats for 3 days prior to performing exhaustive exercise, sulforaphane served as an indirect antioxidant in skeletal muscle, as measured by decreased tissue total antioxidant capacity in the vastus lateralis muscle. It was concluded that sulforaphane plays a vital role in modulating the muscle redox environment; ultimately creating favorable effects on muscle (Malaguti et al., 2009). It was reported that type 2 diabetic mice receiving sulforaphane injections over 4 week had greater grip strength, lean mass, and gastrocnemius mass (Wang M. et al., 2020). These diabetic mice also presented with improved skeletal muscle fiber organization after sulforaphane treatment. It was proposed that sulforaphane may downregulate the expression of inflammatory and apoptotic associated proteins. Sulforaphane was also suggested to play a role in regulating mRNA levels of anti-inflammatory and oxidative related genes. Collectively, it was concluded that sulforaphane treatment could be protective against skeletal muscle disease in mice with type 2 diabetes.
Similar favorable results have also been reported in muscular dystrophy mice models, where sulforaphane treatment alleviated muscle inflammation in dystrophin-deficient mdx mice (Sun et al., 2015b). Three studies (from the same research group) investigated administration of sulforaphane to mdx dystrophic mice, the most widely utilised murine model of Duchenne muscular dystrophy (Swiderski and Lynch, 2021). Although there was some variation between the studies, the overall conclusion was that sulforaphane improved aspects of the dystrophic phenotype (Sun CC. et al., 2015; Sun et al., 2015c; Sun et al., 2016). Oral administration of sulforaphane (2 mg/kg/day) to 4-week-old mdx mice increased muscle mass, force production, and running distance compared to untreated mdx mice. These effects were associated with decreased expression of markers of muscle inflammation and fibrosis (Sun CC. et al., 2015; Sun et al., 2015c; Sun et al., 2016).
In the context of addressing aspects of sarcopenia or age-related impairments in skeletal muscle, 21-22-month-old mice were administered either a regular chow diet or the same diet supplemented with sulforaphane for 12 week (Bose et al., 2020). Skeletal muscle and heart function, mitochondrial function, and Nrf2 activity were assessed at the end of sulforaphane treatment. Compared to a young group of 2-month-old control mice, aged mice showed impairments in skeletal muscle and cardiac function and a decrease in Nrf2 activity. These parameters were all restored in the old mice receiving sulforaphane treatment. It was concluded that sulforaphane could be a safe and effective approach to protect against age-related impairments in skeletal muscle and the heart (Bose et al., 2020).
Bone
Sulforaphane has also been proposed to protect against osteoclastic bone destruction in vitro (Luo et al., 2021). Sulforaphane can support osteoblast differentiation (via epigenetic mechanisms) and expression of the osteoclast activator receptor activator of nuclear factor-κB ligand (RANKL) in osteocytes (Thaler et al., 2016). Here, after sulforaphane was provided over 5 week, the aforementioned effects correlated to a 20% greater bone volume in both normal and ovariectomized mouse models. Of note, no shifts in bone mineral density distribution were recorded. Ultimately, this led the authors to suggest that sulforaphane should be studied further due to its potential to counteract osteoporosis. Sulforaphane has also been studied for its potential benefits in osteoarthritis due to its reported antioxidant and anti-inflammatory properties. When a synthetic form of sulforaphane was orally supplemented to mice over 3 months, osteoarthritis-related gait asymmetry was recorded in vehicle-treated STR/Ort mice but not in sulforaphane treated mice (Javaheri et al., 2017). Sulforaphane treated mice also presented with improvements in trabecular and cortical bone, key indicators for bone strength. Noteworthy, favorable effects on bone turnover markers, such as C-terminal crosslinking telopeptide of type-I collagen (CTX-I; bone resorption marker) and total procollagen type 1 N-terminal propeptide (P1NP-bone formation marker), were recorded.
Cancer
Epidemiological studies provide evidence that a diet rich in broccoli reduces cancer risk and progression (Yagishita et al., 2019; Le et al., 2020). Much of this link has been associated with isothiocyanates, including sulforaphane (Miękus et al., 2020; Gu et al., 2021). Sulforaphane is commonly recognized for its antioxidant, anti-inflammatory, and chemo-preventive effects with numerous types of cancer, including prostate, breast, liver, and colon (Soundararajan and Kim, 2018; Livingstone et al., 2019; Mahn and Castillo, 2021).
Prostate Cancer
Sulforaphane has been indicated to have multiple roles in a variety of key metabolic pathways involved in prostate cancer development including inhibition of angiogenesis and cell proliferation and initiating apoptosis (Traka et al., 2014; Soundararajan and Kim, 2018; Livingstone et al., 2019). Treatment with sulforaphane significantly reduced expression of key glycolytic genes, including hexokinase II and pyruvate kinase M2, in prostate cancer cell lines, LNCaP, 22RV1, and PC-3, and in transgenic mouse models, TRAMP and Hi-Myc (Singh et al., 2019). Sulforaphane also induced S-phase and G2/M-phase cell cycle arrest, enhanced histone acetylation, and up-regulated cell cycle proteins in the prostate cancer cell lines, DU145 and PC-3 (Rutz et al., 2020), suggesting that sulforaphane treatment leads to reduced cell proliferation activity.
There have been numerous human studies investigating the influence of sulforaphane on prostate cancer. Traka and coworkers evaluated the effect of a once-a-week consumption of broccoli soups with increasing concentration of glucoraphanin in a prostate cancer cohort on active surveillance. They indicated a dose-dependent suppression in gene expression and pathways associated with the development and progression of prostate cancer (Traka et al., 2019). Consistent with this study, Zhang and coworkers assessed the influence of daily consumption of either 200 µmol broccoli sprout extract or placebo in men scheduled for prostate biopsy and demonstrated changes in gene expression with the downregulation of genes associated with prostate cancer progression (AMACR and ARLNC1) (Zhang et al., 2020).
Breast Cancer
Previous cell-based and human studies have indicated that sulforaphane exposure is associated with decreased breast cancer progression, including initiating cell cycle arrest and apoptosis (Kuran et al., 2020). Sulforaphane treatment led to the inhibition of cell proliferation of breast cancer cells lines, MCF-7 and MDA-MB-231 and provoked cytotoxic activity by changing cysteine residues of the promyelocytic leukemia protein, known to promote proliferation of the MCF-7 breast cancer cell line (Alhazmi et al., 2020). Sulforaphane has also been shown to modify cell migration and expression of epithelial mesenchymal transition markers that play a large role in breast cancer metastasis (Bagheri et al., 2020).
There are limited human studies that have evaluated the role of sulforaphane on breast cancer. Atwell and coworkers evaluated the effect of either a supplement containing standardized concentration of glucoraphanin with myrosinase enzyme (BroccoMax) or placebo for a minimum of 2-week in women with abnormal mammograms and booked for breast biopsies. They indicated that BroccoMax supplementation significantly reduced the tissue biomarkers, Ki-67 and HDAC3, in benign tissue but not in ductal carcinoma in situ (DCIS) or invasive ductal carcinoma breast tissues (Atwell et al., 2015). Zhang and coworkers evaluated the interaction between cruciferous vegetable intake and biomarkers in women scheduled for breast biopsies and found a negative association with total cruciferous vegetable intake and cell proliferation in DCIS breast tissue (Zhang et al., 2016), suggesting a role of sulforaphane in chemoprevention against breast cancer.
Liver Cancer
Treatment with sulforaphane significantly reduced migration and adhesion and inhibited expression of key molecules in angiogenesis, VEGF, STAT3, and HIF-1α, in liver cancer cell line, HepG2 (Liu et al., 2017). Sulforaphane exposure led to reduction in cell growth, by significantly downregulating key cell cycle-related genes in HepG2 and Huh7 cell lines, and also within a xenograft mouse model (Sato et al., 2018). Sulforaphane decreased secretion of the pro-inflammatory cytokine, interleukin-6, and was not linked with cellular toxicity in HepG2 cells (Al-Bakheit and Abu-Qatouseh, 2020), suggesting that sulforaphane could have anti-inflammatory properties and may modulate liver carcinogenesis.
Whilst there is much cell-based evidence for the role of sulforaphane on liver cancer, there are limited animal and no human studies. Chen and coworkers assessed the effect on mice given diethylnitrosamine before being placed on a Western diet or Western diet supplemented with 10% w/w freeze-dried broccoli powder. The broccoli diet decreased liver damage and fatty liver progression but did not reduce liver cancer development (Chen et al., 2016a). However, in another study, mice were given a control or Western diet with or without the addition of 10% w/w freeze-dried broccoli before being treated with diethylnitrosamine. The initiation and progression of liver cancer was reduced in mice receiving the freeze-dried broccoli (Chen et al., 2016b). Thus, further animal and human studies are required to delineate the effect of sulforaphane on liver cancer.
Colon Cancer
Colon cancer incidence is heavily associated with dietary habits; diets rich in red meat are associated with an increased risk, whilst those high in fruit and vegetables are associated with a reduced risk (Veettil et al., 2021). In human colon cancer cell lines, HCT116 and SW480, sulforaphane diminished cell growth in a dose-dependent way, and increased apoptosis induced by Lactobacillus through the TNFα pathway (Yasuda et al., 2019). Sulforaphane treatment also led to suppression of key colorectal cancer stem cell markers, such as CD44 and CD133 in HCT116 and SW480 spheroids, facilitated by the TAp63α/Lgr5/β-catenin pathway (Chen et al., 2020), suggesting that exposure to sulforaphane could lead to lowered cell proliferation activity. On the contrary, sulforaphane treatment (<10 µM) led to increased cell proliferation and also lowered expression of key apoptotic proteins Bcl-2 and Bax, but concentrations of greater than 10 µM caused cell death in p53-wild-type HCT116 cells (Wang et al., 2021). There are few animal or human studies that have assessed the role of sulforaphane on colon cancer. However, Suzuki and coworkers found that sulforaphane intake led to reduced development of microscopic aberrant crypt foci and macroscopic tumors in mice or patients with colon cancer (Suzuki et al., 2019), suggesting that consumption of broccoli could prevent colon cancer progression.
Conclusion and Future Directions
The evidence presented in this review suggests that glucosinolates and their isothiocyanate metabolites found in cruciferous vegetables are important components in the prevention and treatment of multiple chronic diseases. Studies indicate that cruciferous vegetables and their glucosinolates may have an impact on a number of cardiometabolic disorders. Of note, improvements in glycemic control, blood pressure, and lipid profile have been identified, which may lead to a reduction or delay in disease progression. Likewise, glucosinolate metabolites, particularly sulforaphane, may exert a beneficial effect on neurological and psychiatric conditions, such as depression, schizophrenia, autism, Alzheimer’s disease, and multiple sclerosis. Sulforaphane has also been indicated as an important dietary component for musculoskeletal disorders, with studies reporting improvements in measures for both muscle and bone. To date, however, most of the aforementioned results have come from animal models, with limited human randomized controlled trials. Although there are limitations in the extrapolation of animal data directly to humans, animal studies provide an important insight into the mechanisms by which glucosinolates may also exert outcomes in humans.
Moreover, cruciferous vegetables also contain a variety of other nutrients known to have important health effects (e.g., vitamin C, vitamin K, carotenoids, and flavonoids) (Manchali et al., 2012). As such, it is difficult to separate the action of glucosinolates from these other compounds when determining the results of food-based cruciferous vegetable interventions. Further, when these vegetables are consumed as part of a particular dietary pattern, there may be other factors influencing the effect of glucosinolates consumed (e.g., influences on metabolism and gut microbiota). This idea is also relevant to observational studies, where other diet components could influence the observed associations between glucosinolate-rich vegetables and particular health outcomes. Therefore, future large-scale human trials utilizing standardized, reproducible protocols, and appropriate glucosinolate doses that reflect realistic habitual intake are needed to further elucidate the benefits of cruciferous vegetables and related compounds.
Whilst there is also evidence that sulforaphane reduces cancer development, future research on the underlying molecular mechanisms is also warranted to further understand the role of sulforaphane in the metabolic rewiring of cancer progression. Interestingly, recent studies have indicated that sulforaphane treatment in conjunction with anti-cancer treatments such as chemotherapy, increases cancer cell sensitivity (Jabbarzadeh Kaboli et al., 2020), lessens their toxic side effects (Calcabrini et al., 2020), and inhibits key survival pathways in cancer progression (Mokhtari et al., 2021). This suggests that sulforaphane could not only be a potential drug candidate but also be used in combination with current anti-cancer treatments.
A number of different factors can influence the glucosinolate content of vegetables (e.g., cultivar, growth conditions, storage, and cooking method), and this variation in glucosinolate content may be partially responsible for differing results from studies utilizing the same vegetables (e.g., broccoli) across different locations and with varying preparation and cooking methods. Further studies are also needed to determine and utilize the optimal dose and delivery of sulforaphane for treating different aspects of the dystrophic pathology and for its potential future application as a nutraceutical for treating neuromuscular disorders. Additionally, future studies should examine whether sulforaphane could address different aspects of age-related impairments in skeletal muscle function, including injury susceptibility, fatigue, and muscle regenerative capacity.
Given the positive actions of glucosinolates are largely related to anti-inflammatory and antioxidant mechanisms, the consumption of cruciferous vegetables and their glucosinolates may also have beneficial outcomes on other health conditions. Although there are few studies investigating the impact of certain cruciferous vegetables on other chronic conditions, such as obesity, future studies are needed to further investigate the role of glucosinolates.
Author Contributions
LB conceptualized the manuscript. EC wrote the first draft of the manuscript and the cardiometabolic disorders section. NT and WM wrote the neurological and psychiatric disorders section, MS wrote the musculoskeletal disorders section with input from GL and GB wrote the cancer section. GB, CB, and EC constructed the figure. All authors critically revised and approved the final version of the manuscript.
Funding
The salary of LB is supported by a National Health and Medical Research Council of Australia Emerging Leadership Investigator Grant (ID: 1172987) and a National Heart Foundation of Australia Post-Doctoral Research Fellowship (ID: 102498). EC is supported by an Australian Government Research Training Program Scholarship at Edith Cowan University. WM is currently funded by an Alfred Deakin Postdoctoral Research Fellowship and a Multiple Sclerosis Research Australia early-career fellowship. The salary of MS is supported by a Royal Perth Hospital Research Foundation Career Advancement Fellowship (ID: 00/2020). GB is supported by the Big C Scientific/Clinical Research Grant 11-13R. The salary of JL is supported by a National Heart Foundation of Australia Future Leader Fellowship (ID: 102817). The salary of JH is supported by a National Health and Medical Research Council of Australia Senior Research Fellowship (Grant number 1116973).
Conflict of Interest
WM has previously received funding from the NHMRC, Clifford Craig Foundation, Cancer Council Queensland and university grants/fellowships from La Trobe University, Deakin University, University of Queensland, and Bond University, received industry funding and has attended events funded by Cobram Estate Pty. Ltd., received travel funding from Nutrition Society of Australia, received consultancy funding from Nutrition Research Australia, and has received speakers honoraria from The Cancer Council Queensland and the Princess Alexandra Research Foundation. NT has received funding from Deakin University for an Executive Dean’s Post-Doctoral Fellowship.
The remaining authors declare that the research was conducted in the absence of any commercial or financial relationships that could be construed as a potential conflict of interest.
Publisher’s Note
All claims expressed in this article are solely those of the authors and do not necessarily represent those of their affiliated organizations, or those of the publisher, the editors and the reviewers. Any product that may be evaluated in this article, or claim that may be made by its manufacturer, is not guaranteed or endorsed by the publisher.
References
Afshin, A., Sur, P. J., Fay, K. A., Cornaby, L., Ferrara, G., Salama, J. S., et al. (2019). Health Effects of Dietary Risks in 195 Countries, 1990-2017: a Systematic Analysis for the Global Burden of Disease Study 2017. Lancet 393 (10184), 1958–1972. doi:10.1016/S0140-6736(19)30041-8
Al-Bakheit, A. A., and Abu-Qatouseh, L. (2020). Sulforaphane from Broccoli Attenuates Inflammatory Hepcidin by Reducing IL-6 Secretion in Human HepG2 Cells. J. Funct. Foods 75, 104210. doi:10.1016/j.jff.2020.104210
Alhazmi, N., Pai, C. P., Albaqami, A., Wang, H., Zhao, X., Chen, M., et al. (2020). The Promyelocytic Leukemia Protein Isoform PML1 Is an Oncoprotein and a Direct Target of the Antioxidant Sulforaphane (SFN). Biochim. Biophys. Acta Mol. Cel Res 1867 (8), 118707. doi:10.1016/j.bbamcr.2020.118707
Armah, C. N., Derdemezis, C., Traka, M. H., Dainty, J. R., Doleman, J. F., Saha, S., et al. (2015). Diet Rich in High Glucoraphanin Broccoli Reduces Plasma LDL Cholesterol: Evidence from Randomised Controlled Trials. Mol. Nutr. Food Res. 59 (5), 918–926. doi:10.1002/mnfr.201400863
Atwell, L. L., Zhang, Z., Mori, M., Farris, P., Vetto, J. T., Naik, A. M., et al. (2015). Sulforaphane Bioavailability and Chemopreventive Activity in Women Scheduled for Breast Biopsy. Cancer Prev. Res. (Phila) 8 (12), 1184–1191. doi:10.1158/1940-6207.Capr-15-0119
Aune, D., Giovannucci, E., Boffetta, P., Fadnes, L. T., Keum, N., Norat, T., et al. (2017). Fruit and Vegetable Intake and the Risk of Cardiovascular Disease, Total Cancer and All-Cause Mortality-A Systematic Review and Dose-Response Meta-Analysis of Prospective Studies. Int. J. Epidemiol. 46 (3), 1029–1056. doi:10.1093/ije/dyw319
Axelsson, A. S., Tubbs, E., Mecham, B., Chacko, S., Nenonen, H. A., Tang, Y., et al. (2017). Sulforaphane Reduces Hepatic Glucose Production and Improves Glucose Control in Patients with Type 2 Diabetes. Sci. Transl Med. 9 (394), 4477. doi:10.1126/scitranslmed.aah4477
Bagheri, M., Fazli, M., Saeednia, S., Gholami Kharanagh, M., and Ahmadiankia, N. (2020). Sulforaphane Modulates Cell Migration and Expression of β-Catenin and Epithelial Mesenchymal Transition Markers in Breast Cancer Cells. Iran J. Public Health 49 (1), 77–85. doi:10.18502/ijph.v49i1.3054
Bahadoran, Z., Tohidi, M., Nazeri, P., Mehran, M., Azizi, F., and Mirmiran, P. (2012). Effect of Broccoli Sprouts on Insulin Resistance in Type 2 Diabetic Patients: a Randomized Double-Blind Clinical Trial. Int. J. Food Sci. Nutr. 63 (7), 767–771. doi:10.3109/09637486.2012.665043
Bai, Y., Cui, W., Xin, Y., Miao, X., Barati, M. T., Zhang, C., et al. (2013). Prevention by Sulforaphane of Diabetic Cardiomyopathy Is Associated with Up-Regulation of Nrf2 Expression and Transcription Activation. J. Mol. Cel Cardiol 57, 82–95. doi:10.1016/j.yjmcc.2013.01.008
Barba, F. J., Nikmaram, N., Roohinejad, S., Khelfa, A., Zhu, Z., and Koubaa, M. (2016). Bioavailability of Glucosinolates and Their Breakdown Products: Impact of Processing. Front. Nutr. 3, 24. doi:10.3389/fnut.2016.00024
Bent, S., Lawton, B., Warren, T., Widjaja, F., Dang, K., Fahey, J. W., et al. (2018). Identification of Urinary Metabolites that Correlate with Clinical Improvements in Children with Autism Treated with Sulforaphane from Broccoli. Mol. Autism 9 (1), 35–12. doi:10.1186/s13229-018-0218-4
Björkman, M., Klingen, I., Birch, A. N., Bones, A. M., Bruce, T. J., Johansen, T. J., et al. (2011). Phytochemicals of Brassicaceae in Plant protection and Human Health-Iinfluences of Climate, Environment and Agronomic Practice. Phytochemistry 72 (7), 538–556. doi:10.1016/j.phytochem.2011.01.014
Blažević, I., Montaut, S., Burčul, F., Olsen, C. E., Burow, M., Rollin, P., et al. (2020). Glucosinolate Structural Diversity, Identification, Chemical Synthesis and Metabolism in Plants. Phytochemistry 169, 112100. doi:10.1016/j.phytochem.2019.112100
Blekkenhorst, L. C., Bondonno, C. P., Lewis, J. R., Devine, A., Zhu, K., Lim, W. H., et al. (2017a). Cruciferous and Allium Vegetable Intakes Are Inversely Associated with 15-Year Atherosclerotic Vascular Disease Deaths in Older Adult Women. J. Am. Heart Assoc. 6 (10), e006558. doi:10.1161/jaha.117.006558
Blekkenhorst, L. C., Hodgson, J. M., Lewis, J. R., Devine, A., Woodman, R. J., Lim, W. H., et al. (2017b). Vegetable and Fruit Intake and Fracture-Related Hospitalisations: A Prospective Study of Older Women. Nutrients 9 (5), 511. doi:10.3390/nu9050511
Bose, C., Alves, I., Singh, P., Palade, P. T., Carvalho, E., Børsheim, E., et al. (2020). Sulforaphane Prevents Age-Associated Cardiac and Muscular Dysfunction through Nrf2 Signaling. Aging Cell 19 (11), e13261. doi:10.1111/acel.13261
Calcabrini, C., Maffei, F., Turrini, E., and Fimognari, C. (2020). Sulforaphane Potentiates Anticancer Effects of Doxorubicin and Cisplatin and Mitigates Their Toxic Effects. Front. Pharmacol. 11, 567. doi:10.3389/fphar.2020.00567
Casajús, V., Demkura, P., Civello, P., Gómez Lobato, M., and Martínez, G. (2020). Harvesting at Different Time-Points of Day Affects Glucosinolate Metabolism during Postharvest Storage of Broccoli. Food Res. Int. 136, 109529. doi:10.1016/j.foodres.2020.109529
Chen, G. C., Koh, W. P., Yuan, J. M., Qin, L. Q., and van Dam, R. M. (2018). Green Leafy and Cruciferous Vegetable Consumption and Risk of Type 2 Diabetes: Results from the Singapore Chinese Health Study and Meta-Analysis. Br. J. Nutr. 119 (9), 1057–1067. doi:10.1017/s0007114518000119
Chen, Y., Wang, M. H., Zhu, J. Y., Xie, C. F., Li, X. T., Wu, J. S., et al. (2020). TAp63α Targeting of Lgr5 Mediates Colorectal Cancer Stem Cell Properties and Sulforaphane Inhibition. Oncogenesis 9 (10), 89. doi:10.1038/s41389-020-00273-z
Chen, Y. J., Myracle, A. D., Wallig, M. A., and Jeffery, E. H. (2016a). Dietary Broccoli Protects against Fatty Liver Development but Not against Progression of Liver Cancer in Mice Pretreated with Diethylnitrosamine. J. Funct. Foods 24, 57–62. doi:10.1016/j.jff.2016.03.028
Chen, Y. J., Wallig, M. A., and Jeffery, E. H. (2016b). Dietary Broccoli Lessens Development of Fatty Liver and Liver Cancer in Mice Given Diethylnitrosamine and Fed a Western or Control Diet. J. Nutr. 146 (3), 542–550. doi:10.3945/jn.115.228148
Christiansen, B., Bellostas Muguerza, N., Petersen, A. M., Kveiborg, B., Madsen, C. R., Thomas, H., et al. (2010). Ingestion of Broccoli Sprouts Does Not Improve Endothelial Function in Humans with Hypertension. PLoS One 5 (8), e12461. doi:10.1371/journal.pone.0012461
Connolly, E. L., Bondonno, C. P., Sim, M., Radavelli-Bagatini, S., Croft, K. D., Boyce, M. C., et al. (2020). A Randomised Controlled Crossover Trial Investigating the Short-Term Effects of Different Types of Vegetables on Vascular and Metabolic Function in Middle-Aged and Older Adults with Mildly Elevated Blood Pressure: the VEgetableS for vaScular hEaLth (VESSEL) Study Protocol. Nutr. J. 19 (1), 41. doi:10.1186/s12937-020-00559-3
Cooper, A. J., Forouhi, N. G., Ye, Z., Buijsse, B., Arriola, L., Balkau, B., et al. (2012). Fruit and Vegetable Intake and Type 2 Diabetes: EPIC-InterAct Prospective Study and Meta-Analysis. Eur. J. Clin. Nutr. 66 (10), 1082–1092. doi:10.1038/ejcn.2012.85
Dickerson, F., Origoni, A., Katsafanas, E., Squire, A., Newman, T., Fahey, J., et al. (2021). Randomized Controlled Trial of an Adjunctive Sulforaphane Nutraceutical in Schizophrenia. Schizophr Res. 231, 142–144. doi:10.1016/j.schres.2021.03.018
Drażbo, A., Kozłowski, K., Ognik, K., Zaworska, A., and Jankowski, J. (2019). The Effect of Raw and Fermented Rapeseed Cake on Growth Performance, Carcass Traits, and Breast Meat Quality in turkey. Poult. Sci. 98 (11), 6161–6169. doi:10.3382/ps/pez322
Du, K., Fan, Y., and Li, D. (2021). Sulforaphane Ameliorates Lipid Profile in Rodents: an Updated Systematic Review and Meta-Analysis. Sci. Rep. 11 (1), 7804. doi:10.1038/s41598-021-87367-9
Dwivedi, D., Megha, K., Mishra, R., and Mandal, P. K. (2020). Glutathione in Brain: Overview of its Conformations, Functions, Biochemical Characteristics, Quantitation and Potential Therapeutic Role in Brain Disorders. Neurochem. Res. 45 (7), 1461–1480. doi:10.1007/s11064-020-03030-1
Esteve, M. (2020). Mechanisms Underlying Biological Effects of Cruciferous Glucosinolate-Derived Isothiocyanates/Indoles: A Focus on Metabolic Syndrome. Front. Nutr. 7, 111. doi:10.3389/fnut.2020.00111
Fahey, J. W., Zhang, Y., and Talalay, P. (1997). Broccoli Sprouts: an Exceptionally Rich Source of Inducers of Enzymes that Protect against Chemical Carcinogens. Proc. Natl. Acad. Sci. U S A. 94 (19), 10367–10372. doi:10.1073/pnas.94.19.10367
Favela-González, K. M., Hernández-Almanza, A. Y., and De la Fuente-Salcido, N. M. (2020). The Value of Bioactive Compounds of Cruciferous Vegetables (Brassica) as Antimicrobials and Antioxidants: A Review. J. Food Biochem. 44 (10), e13414. doi:10.1111/jfbc.13414
Folkard, D. L., Melchini, A., Traka, M. H., Al-Bakheit, A., Saha, S., Mulholland, F., et al. (2014). Suppression of LPS-Induced Transcription and Cytokine Secretion by the Dietary Isothiocyanate Sulforaphane. Mol. Nutr. Food Res. 58 (12), 2286–2296. doi:10.1002/mnfr.201400550
Galgano, F., Favati, F., Caruso, M., Pietrafesa, A., and Natella, S. (2007). The Influence of Processing and Preservation on the Retention of Health-Promoting Compounds in Broccoli. J. Food Sci. 72 (2), S130–S135. doi:10.1111/j.1750-3841.2006.00258.x
Ghazizadeh-Hashemi, F., Bagheri, S., Ashraf-Ganjouei, A., Moradi, K., Shahmansouri, N., Mehrpooya, M., et al. (2021). Efficacy and Safety of Sulforaphane for Treatment of Mild to Moderate Depression in Patients with History of Cardiac Interventions: A Randomized, Double-Blind, Placebo-Controlled Clinical Trial. Psychiatry Clin. Neurosciences 75 (8), 250–255. doi:10.1111/pcn.13276
Gu, H. F., Mao, X. Y., and Du, M. (2021). Metabolism, Absorption, and Anti-cancer Effects of Sulforaphane: an Update. Crit. Rev. Food Sci. Nutr., 1–17. doi:10.1080/10408398.2020.1865871
Gwon, M. H., Im, Y. S., Seo, A. R., Kim, K. Y., Moon, H. R., and Yun, J. M. (2020). Phenethyl Isothiocyanate Protects against High Fat/Cholesterol Diet-Induced Obesity and Atherosclerosis in C57BL/6 Mice. Nutrients 12 (12), 3657. doi:10.3390/nu12123657
Huang, H., Jiang, X., Xiao, Z., Yu, L., Pham, Q., Sun, J., et al. (2016). Red Cabbage Microgreens Lower Circulating Low-Density Lipoprotein (LDL), Liver Cholesterol, and Inflammatory Cytokines in Mice Fed a High-Fat Diet. J. Agric. Food Chem. 64 (48), 9161–9171. doi:10.1021/acs.jafc.6b03805
International Agency for Research on Cancer (2004). "Cruciferous Vegetables," in IARC Handbooks Of Cancer Prevention: Cruciferous Vegetables, Isothiocyanates And Indoles. Lyon, France: International Agency for Research on Cancer.
Jabbarzadeh Kaboli, P., Afzalipour Khoshkbejari, M., Mohammadi, M., Abiri, A., Mokhtarian, R., Vazifemand, R., et al. (2020). Targets and Mechanisms of Sulforaphane Derivatives Obtained from Cruciferous Plants with Special Focus on Breast Cancer - Contradictory Effects and Future Perspectives. Biomed. Pharmacother. 121, 109635. doi:10.1016/j.biopha.2019.109635
Javaheri, B., Poulet, B., Aljazzar, A., de Souza, R., Piles, M., Hopkinson, M., et al. (2017). Stable Sulforaphane Protects against Gait Anomalies and Modifies Bone Microarchitecture in the Spontaneous STR/Ort Model of Osteoarthritis. Bone 103, 308–317. doi:10.1016/j.bone.2017.07.028
Kim, H. V., Kim, H. Y., Ehrlich, H. Y., Choi, S. Y., Kim, D. J., and Kim, Y. (2013). Amelioration of Alzheimer's Disease by Neuroprotective Effect of Sulforaphane in Animal Model. Amyloid 20 (1), 7–12. doi:10.3109/13506129.2012.751367
Kim, J., Lee, S., Choi, B. R., Yang, H., Hwang, Y., Park, J. H., et al. (2017). Sulforaphane Epigenetically Enhances Neuronal BDNF Expression and TrkB Signaling Pathways. Mol. Nutr. Food Res. 61 (2), 1600194. doi:10.1002/mnfr.201600194
Kim, J. (2021). Pre-Clinical Neuroprotective Evidences and Plausible Mechanisms of Sulforaphane in Alzheimer's Disease. Int. J. Mol. Sci. 22 (6), 2929. doi:10.3390/ijms22062929
Kirk, E. P., and Klein, S. (2009). Pathogenesis and Pathophysiology of the Cardiometabolic Syndrome. J. Clin. Hypertens. (Greenwich) 11 (12), 761–765. doi:10.1111/j.1559-4572.2009.00054.x
Kissen, R., Rossiter, J. T., and Bones, A. M. (2009). The 'mustard Oil Bomb': Not So Easy to Assemble? Localization, Expression and Distribution of the Components of the Myrosinase Enzyme System. Phytochem. Rev. 8 (1), 69–86. doi:10.1007/s11101-008-9109-1
Kuran, D., Pogorzelska, A., and Wiktorska, K. (2020). Breast Cancer Prevention-Is There a Future for Sulforaphane and its Analogs? Nutrients 12 (6), 1559. doi:10.3390/nu12061559
Langston-Cox, A. G., Anderson, D., Creek, D. J., Palmer, K. R., Marshall, S. A., and Wallace, E. M. (2021). Sulforaphane Bioavailability and Effects on Blood Pressure in Women with Pregnancy Hypertension. Reprod. Sci. 28 (5), 1489–1497. doi:10.1007/s43032-020-00439-5
Lapuente, M., Estruch, R., Shahbaz, M., and Casas, R. (2019). Relation of Fruits and Vegetables with Major Cardiometabolic Risk Factors, Markers of Oxidation, and Inflammation. Nutrients 11 (10), 2381. doi:10.3390/nu11102381
Le, T. N., Chiu, C. H., and Hsieh, P. C. (2020). Bioactive Compounds and Bioactivities of Brassica oleracea L. Var. Italica Sprouts and Microgreens: An Updated Overview from a Nutraceutical Perspective. Plants (Basel)9 (8), 946. doi:10.3390/plants9080946
Lee, S., Choi, B. R., Kim, J., LaFerla, F. M., Park, J. H. Y., Han, J. S., et al. (2018). Sulforaphane Upregulates the Heat Shock Protein Co-chaperone CHIP and Clears Amyloid-β and Tau in a Mouse Model of Alzheimer's Disease. Mol. Nutr. Food Res. 62 (12), e1800240. doi:10.1002/mnfr.201800240
Li, B., Cui, W., Liu, J., Li, R., Liu, Q., Xie, X. H., et al. (2013). Sulforaphane Ameliorates the Development of Experimental Autoimmune Encephalomyelitis by Antagonizing Oxidative Stress and Th17-Related Inflammation in Mice. Exp. Neurol. 250, 239–249. doi:10.1016/j.expneurol.2013.10.002
Lim, J. L., van der Pol, S. M., Baron, W., McCord, J. M., de Vries, H. E., van Horssen, J., et al. (2016). Protandim Protects Oligodendrocytes against an Oxidative Insult. Antioxidants (Basel) 5 (3), 30. doi:10.3390/antiox5030030
Liu, B., Mao, Q., Cao, M., and Xie, L. (2012). Cruciferous Vegetables Intake and Risk of Prostate Cancer: A Meta-Analysis. Int. J. Urol. 19 (2), 134–141. doi:10.1111/j.1442-2042.2011.02906.x
Liu, P., Atkinson, S. J., Akbareian, S. E., Zhou, Z., Munsterberg, A., Robinson, S. D., et al. (2017). Sulforaphane Exerts Anti-angiogenesis Effects against Hepatocellular Carcinoma through Inhibition of STAT3/HIF-1α/VEGF Signalling. Sci. Rep. 7 (1), 12651. doi:10.1038/s41598-017-12855-w
Liu, R. H. (2013). Health-promoting Components of Fruits and Vegetables in the Diet. Adv. Nutr. 4 (3), 384S–92Ss 392s. doi:10.3945/an.112.003517
Livingstone, T. L., Beasy, G., Mills, R. D., Plumb, J., Needs, P. W., Mithen, R., et al. (2019). Plant Bioactives and the Prevention of Prostate Cancer: Evidence from Human Studies. Nutrients 11 (9), 2245. doi:10.3390/nu11092245
Luang-In, V., Narbad, A., Nueno-Palop, C., Mithen, R., Bennett, M., and Rossiter, J. T. (2014). The Metabolism of Methylsulfinylalkyl- and Methylthioalkyl-Glucosinolates by a Selection of Human Gut Bacteria. Mol. Nutr. Food Res. 58 (4), 875–883. doi:10.1002/mnfr.201300377
Luo, T., Fu, X., Liu, Y., Ji, Y., and Shang, Z. (2021). Sulforaphane Inhibits Osteoclastogenesis via Suppression of the Autophagic Pathway. Molecules 26 (2), 347. doi:10.3390/molecules26020347
Ma, L., Liu, G., Sampson, L., Willett, W. C., Hu, F. B., and Sun, Q. (2018). Dietary Glucosinolates and Risk of Type 2 Diabetes in 3 Prospective Cohort Studies. Am. J. Clin. Nutr. 107 (4), 617–625. doi:10.1093/ajcn/nqy003
Mahn, A., and Castillo, A. (2021). Potential of Sulforaphane as a Natural Immune System Enhancer: A Review. Molecules 26 (3), 752. doi:10.3390/molecules26030752
Maina, S., Misinzo, G., Bakari, G., and Kim, H. Y. (2020). Human, Animal and Plant Health Benefits of Glucosinolates and Strategies for Enhanced Bioactivity: A Systematic Review. Molecules 25 (16), 3682. doi:10.3390/molecules25163682
Malaguti, M., Angeloni, C., Garatachea, N., Baldini, M., Leoncini, E., Collado, P. S., et al. (2009). Sulforaphane Treatment Protects Skeletal Muscle against Damage Induced by Exhaustive Exercise in Rats. J. Appl. Physiol. (1985) 107 (4), 1028–1036. doi:10.1152/japplphysiol.00293.2009
Manchali, S., Chidambara Murthy, K. N., and Patil, B. S. (2012). Crucial Facts about Health Benefits of Popular Cruciferous Vegetables. J. Funct. Foods 4 (1), 94–106. doi:10.1016/j.jff.2011.08.004
McGuinness, G., and Kim, Y. (2020). Sulforaphane Treatment for Autism Spectrum Disorder: A Systematic Review. EXCLI J. 19, 892–903. doi:10.17179/excli2020-2487
Melega, S., Canistro, D., De Nicola, G. R., Lazzeri, L., Sapone, A., and Paolini, M. (2013). Protective Effect of Tuscan Black Cabbage Sprout Extract against Serum Lipid Increase and Perturbations of Liver Antioxidant and Detoxifying Enzymes in Rats Fed a High-Fat Diet. Br. J. Nutr. 110 (6), 988–997. doi:10.1017/S0007114513000068
Miękus, N., Marszałek, K., Podlacha, M., Iqbal, A., Puchalski, C., and Świergiel, A. H. (2020). Health Benefits of Plant-Derived Sulfur Compounds, Glucosinolates, and Organosulfur Compounds. Molecules (Basel, Switzerland) 25 (17), 3804. doi:10.3390/molecules25173804
Mirmiran, P., Bahadoran, Z., Golzarand, M., Zojaji, H., and Azizi, F. (2014). A Comparative Study of Broccoli Sprouts Powder and Standard Triple Therapy on Cardiovascular Risk Factors Following H.Pylori Eradication: a Randomized Clinical Trial in Patients with Type 2 Diabetes. J. Diabetes Metab. Disord. 13, 64. doi:10.1186/2251-6581-13-64
Mokhtari, R. B., Qorri, B., Baluch, N., Sparaneo, A., Fabrizio, F. P., Muscarella, L. A., et al. (2021). Next-generation Multimodality of Nutrigenomic Cancer Therapy: Sulforaphane in Combination with Acetazolamide Actively Target Bronchial Carcinoid Cancer in Disabling the PI3K/Akt/mTOR Survival Pathway and Inducing Apoptosis. Oncotarget 12 (15), 1470–1489. doi:10.18632/oncotarget.28011
Morroni, F., Tarozzi, A., Sita, G., Bolondi, C., Zolezzi Moraga, J. M., Cantelli-Forti, G., et al. (2013). Neuroprotective Effect of Sulforaphane in 6-Hydroxydopamine-Lesioned Mouse Model of Parkinson's Disease. Neurotoxicology 36, 63–71. doi:10.1016/j.neuro.2013.03.004
Murashima, M., Watanabe, S., Zhuo, X. G., Uehara, M., and Kurashige, A. (2004). Phase 1 Study of Multiple Biomarkers for Metabolism and Oxidative Stress after One-Week Intake of Broccoli Sprouts. Biofactors 22 (1-4), 271–275. doi:10.1002/biof.5520220154
Oliviero, T., Verkerk, R., and Dekker, M. (2018). Reply to “Dietary Glucosinolates and Risk of Type 2 Diabetes in 3 Prospective Cohort Studies”. Am. J. Clin. Nutr. 108 (2), 425. doi:10.1093/ajcn/nqy126
Panjwani, A. A., Liu, H., and Fahey, J. W. (2018). Crucifers and Related Vegetables and Supplements for Neurologic Disorders: what Is the Evidence? Curr. Opin. Clin. Nutr. Metab. Care 21 (6), 451–457. doi:10.1097/MCO.0000000000000511
Pu, Y., Qu, Y., Chang, L., Wang, S. M., Zhang, K., Ushida, Y., et al. (2019). Dietary Intake of Glucoraphanin Prevents the Reduction of Dopamine Transporter in the Mouse Striatum after Repeated Administration of MPTP. Neuropsychopharmacol. Rep. 39 (3), 247–251. doi:10.1002/npr2.12060
Raiola, A., Errico, A., Petruk, G., Monti, D. M., Barone, A., and Rigano, M. M. (2017). Bioactive Compounds in Brassicaceae Vegetables with a Role in the Prevention of Chronic Diseases. Molecules 23 (1), 15. doi:10.3390/molecules23010015
Rutz, J., Thaler, S., Maxeiner, S., Chun, F. K., and Blaheta, R. A. (2020). Sulforaphane Reduces Prostate Cancer Cell Growth and Proliferation In Vitro by Modulating the Cdk-Cyclin Axis and Expression of the CD44 Variants 4, 5, and 7. Int. J. Mol. Sci. 21 (22), 8724. doi:10.3390/ijms21228724
Salma, U., Khan, T., and Shah, A. J. (2018). Antihypertensive Effect of the Methanolic Extract from Eruca Sativa Mill., (Brassicaceae) in Rats: Muscarinic Receptor-Linked Vasorelaxant and Cardiotonic Effects. J. Ethnopharmacol 224, 409–420. doi:10.1016/j.jep.2018.06.013
Santín-Márquez, R., Alarcón-Aguilar, A., López-Diazguerrero, N. E., Chondrogianni, N., and Königsberg, M. (2019). Sulforaphane - Role in Aging and Neurodegeneration. GeroScience 41 (5), 655–670. doi:10.1007/s11357-019-00061-7
Sato, S., Moriya, K., Furukawa, M., Saikawa, S., Namisaki, T., Kitade, M., et al. (2018). Sulforaphane Inhibits Liver Cancer Cell Growth and Angiogenesis. Arch. Cancer Res. 6 (4), 23.
Schepici, G., Bramanti, P., and Mazzon, E. (2020). Efficacy of Sulforaphane in Neurodegenerative Diseases. Int. J. Mol. Sci. 21 (22), 8637. doi:10.3390/ijms21228637
Sedlak, T. W., Nucifora, L. G., Koga, M., Shaffer, L. S., Higgs, C., Tanaka, T., et al. (2017). Sulforaphane Augments Glutathione and Influences Brain Metabolites in Human Subjects: a Clinical Pilot Study. Mol. Neuropsychiatry 3 (4), 214–222. doi:10.1159/000487639
Senanayake, G. V., Banigesh, A., Wu, L., Lee, P., and Juurlink, B. H. (2012). The Dietary Phase 2 Protein Inducer Sulforaphane Can Normalize the Kidney Epigenome and Improve Blood Pressure in Hypertensive Rats. Am. J. Hypertens. 25 (2), 229–235. doi:10.1038/ajh.2011.200
Shakour, Z. T., Shehab, N. G., Gomaa, A. S., Wessjohann, L. A., and Farag, M. A. (2021). Metabolic and Biotransformation Effects on Dietary Glucosinolates, Their Bioavailability, Catabolism and Biological Effects in Different Organisms. Biotechnol. Adv. 6, 107784. doi:10.1016/j.biotechadv.2021.107784
Shehatou, G. S., and Suddek, G. M. (2016). Sulforaphane Attenuates the Development of Atherosclerosis and Improves Endothelial Dysfunction in Hypercholesterolemic Rabbits. Exp. Biol. Med. (Maywood) 241 (4), 426–436. doi:10.1177/1535370215609695
Shiina, A., Kanahara, N., Sasaki, T., Oda, Y., Hashimoto, T., Hasegawa, T., et al. (2015). An Open Study of Sulforaphane-Rich Broccoli Sprout Extract in Patients with Schizophrenia. Clin. Psychopharmacol. Neurosci. 13 (1), 62–67. doi:10.9758/cpn.2015.13.1.62
Sim, M., Blekkenhorst, L. C., Lewis, J. R., Bondonno, C. P., Devine, A., Zhu, K., et al. (2018). Vegetable and Fruit Intake and Injurious Falls Risk in Older Women: a Prospective Cohort Study. Br. J. Nutr. 120 (8), 925–934. doi:10.1017/s0007114518002155
Singh, K., Connors, S. L., Macklin, E. A., Smith, K. D., Fahey, J. W., Talalay, P., et al. (2014). Sulforaphane Treatment of Autism Spectrum Disorder (ASD). Proc. Natl. Acad. Sci. U S A. 111 (43), 15550–15555. doi:10.1073/pnas.1416940111
Singh, K. B., Hahm, E. R., Alumkal, J. J., Foley, L. M., Hitchens, T. K., Shiva, S. S., et al. (2019). Reversal of the Warburg Phenomenon in Chemoprevention of Prostate Cancer by Sulforaphane. Carcinogenesis 40 (12), 1545–1556. doi:10.1093/carcin/bgz155
Skugor, A., Kjos, N. P., Sundaram, A. Y. M., Mydland, L. T., Ånestad, R., Tauson, A.-H., et al. (2019). Effects of Long-Term Feeding of Rapeseed Meal on Skeletal Muscle Transcriptome, Production Efficiency and Meat Quality Traits in Norwegian Landrace Growing-Finishing Pigs. Plos One 14 (8), e0220441. doi:10.1371/journal.pone.0220441
Soundararajan, P., and Kim, J. S. (2018). Anti-Carcinogenic Glucosinolates in Cruciferous Vegetables and Their Antagonistic Effects on Prevention of Cancers. Molecules 23 (11), 2983. doi:10.3390/molecules23112983
Sun, C., Li, S., and Li, D. (2016). Sulforaphane Mitigates Muscle Fibrosis in Mdx Mice via Nrf2-Mediated Inhibition of TGF-β/Smad Signaling. J. Appl. Physiol. (1985) 120 (4), 377–390. doi:10.1152/japplphysiol.00721.2015
Sun, C., Yang, C., Xue, R., Li, S., Zhang, T., Pan, L., et al. (2015c). Sulforaphane Alleviates Muscular Dystrophy in Mdx Mice by Activation of Nrf2. J. Appl. Physiol. (1985) 118 (2), 224–237. doi:10.1152/japplphysiol.00744.2014
Sun, C. C., Li, S. J., Yang, C. L., Xue, R. L., Xi, Y. Y., Wang, L., et al. (2015a). Sulforaphane Attenuates Muscle Inflammation in Dystrophin-Deficient Mdx Mice via NF-E2-Related Factor 2 (Nrf2)-Mediated Inhibition of NF-Κb Signaling Pathway. J. Biol. Chem. 290, 17784–17795. doi:10.1074/jbc.M115.655019
Sun, C., Pan, J.-Y., Li, S.-J., and Li, D.-J. (2015b). The Role of Sulforaphane on Duchenne Muscular Dystrophy by Activation of Nrf2. Integr. Mol. Med. 2, 359–361. doi:10.15761/IMM.1000167
Sunkaria, A., Bhardwaj, S., Yadav, A., Halder, A., and Sandhir, R. (2018). Sulforaphane Attenuates Postnatal Proteasome Inhibition and Improves Spatial Learning in Adult Mice. J. Nutr. Biochem. 51, 69–79. doi:10.1016/j.jnutbio.2017.09.016
Suzuki, H., Mutoh, M., Kamoshida, T., Kakinoki, N., Yoshida, S., Ebihara, T., et al. (2019). Chemoprevention against colon Cancer by Dietary Intake of Sulforaphane. Funct. Foods Health Dis. 9 (6), 392–411. doi:10.1080/10715760600722643
Swiderski, K., and Lynch, G. S. (2021). Murine Models of Duchenne Muscular Dystrophy: Is There a Best Model? Am. J. Physiol. Cel Physiol 321 (2), C409–C412. doi:10.1152/ajpcell.00212.2021
Tarozzi, A., Angeloni, C., Malaguti, M., Morroni, F., Hrelia, S., and Hrelia, P. (2013). Sulforaphane as a Potential Protective Phytochemical against Neurodegenerative Diseases. Oxidative Med. Cell. longevity 2013, 415078. doi:10.1155/2013/415078
Thaler, R., Maurizi, A., Roschger, P., Sturmlechner, I., Khani, F., Spitzer, S., et al. (2016). Anabolic and Antiresorptive Modulation of Bone Homeostasis by the Epigenetic Modulator Sulforaphane, a Naturally Occurring Isothiocyanate. J. Biol. Chem. 291 (13), 6754–6771. doi:10.1074/jbc.M115.678235
Thorup, A. C., Kristensen, H. L., Kidmose, U., Lambert, M. N. T., Christensen, L. P., Fretté, X., et al. (2021). Strong and Bitter Vegetables from Traditional Cultivars and Cropping Methods Improve the Health Status of Type 2 Diabetics: A Randomized Control Trial. Nutrients 13 (6), 1813. doi:10.3390/nu13061813
Traka, M. H., Melchini, A., Coode-Bate, J., Al Kadhi, O., Saha, S., Defernez, M., et al. (2019). Transcriptional Changes in Prostate of Men on Active Surveillance after a 12-mo Glucoraphanin-Rich Broccoli Intervention-Results from the Effect of Sulforaphane on Prostate CAncer PrEvention (ESCAPE) Randomized Controlled Trial. Am. J. Clin. Nutr. 109 (4), 1133–1144. doi:10.1093/ajcn/nqz012
Traka, M. H., Melchini, A., and Mithen, R. F. (2014). Sulforaphane and Prostate Cancer Interception. Drug Discov. Today 19 (9), 1488–1492. doi:10.1016/j.drudis.2014.07.007
Veettil, S. K., Wong, T. Y., Loo, Y. S., Playdon, M. C., Lai, N. M., Giovannucci, E. L., et al. (2021). Role of Diet in Colorectal Cancer Incidence: Umbrella Review of Meta-Analyses of Prospective Observational Studies. JAMA Netw. Open 4 (2), e2037341. doi:10.1001/jamanetworkopen.2020.37341
Wang, M., Pu, D., Zhao, Y., Chen, J., Zhu, S., Lu, A., et al. (2020a). Sulforaphane Protects against Skeletal Muscle Dysfunction in Spontaneous Type 2 Diabetic Db/db Mice. Life Sci. 255, 117823. doi:10.1016/j.lfs.2020.117823
Wang, P. Y., Fang, J. C., Gao, Z. H., Zhang, C., and Xie, S. Y. (2016). Higher Intake of Fruits, Vegetables or Their Fiber Reduces the Risk of Type 2 Diabetes: A Meta-Analysis. J. Diabetes Investig. 7 (1), 56–69. doi:10.1111/jdi.12376
Wang, Y., Wu, H., Dong, N., Su, X., Duan, M., Wei, Y., et al. (2021). Sulforaphane Induces S-phase Arrest and Apoptosis via P53-dependent Manner in Gastric Cancer Cells. Sci. Rep. 11 (1), 2504. doi:10.1038/s41598-021-81815-2
Wang, Z., Kwan, M. L., Pratt, R., Roh, J. M., Kushi, L. H., Danforth, K. N., et al. (2020b). Effects of Cooking Methods on Total Isothiocyanate Yield from Cruciferous Vegetables. Food Sci. Nutr. 8 (10), 5673–5682. doi:10.1002/fsn3.1836
Wu, C., Chen, X., Lai, J., Xu, Y., and Hu, S. (2020). The Efficacy and Safety of Sulforaphane as an Adjuvant in the Treatment of Bipolar Depressive Disorder: Study Protocol for a Randomized, Double-Blinded, Placebo-Controlled, Parallel-Group Clinical Trial. Medicine 99 (26), e20981. doi:10.1097/MD.0000000000020981
Wu, L., Noyan Ashraf, M. H., Facci, M., Wang, R., Paterson, P. G., Ferrie, A., et al. (2004). Dietary Approach to Attenuate Oxidative Stress, Hypertension, and Inflammation in the Cardiovascular System. Proc. Natl. Acad. Sci. U S A. 101 (18), 7094–7099. doi:10.1073/pnas.0402004101
Wu, S., Gao, Q., Zhao, P., Gao, Y., Xi, Y., Wang, X., et al. (2016). Sulforaphane Produces Antidepressant- and Anxiolytic-like Effects in Adult Mice. Behav. Brain Res. 301, 55–62. doi:10.1016/j.bbr.2015.12.030
Yagishita, Y., Fahey, J. W., Dinkova-Kostova, A. T., and Kensler, T. W. (2019). Broccoli or Sulforaphane: Is it the Source or Dose that Matters? Molecules 24 (19), 3593. doi:10.3390/molecules24193593
Yao, W., Zhang, J. C., Ishima, T., Dong, C., Yang, C., Ren, Q., et al. (2016). Role of Keap1-Nrf2 Signaling in Depression and Dietary Intake of Glucoraphanin Confers Stress Resilience in Mice. Sci. Rep. 6 (1), 30659. doi:10.1038/srep30659
Yasuda, S., Horinaka, M., and Sakai, T. (2019). Sulforaphane Enhances Apoptosis Induced by Lactobacillus Pentosus Strain S-PT84 via the TNFα Pathway in Human colon Cancer Cells. Oncol. Lett. 18 (4), 4253–4261. doi:10.3892/ol.2019.10739
Zhang, J. C., Yao, W., Dong, C., Yang, C., Ren, Q., Ma, M., et al. (2017). Prophylactic Effects of Sulforaphane on Depression-like Behavior and Dendritic Changes in Mice after Inflammation. J. Nutr. Biochem. 39, 134–144. doi:10.1016/j.jnutbio.2016.10.004
Zhang, X., Shu, X. O., Xiang, Y. B., Yang, G., Li, H., Gao, J., et al. (2011). Cruciferous Vegetable Consumption Is Associated with a Reduced Risk of Total and Cardiovascular Disease Mortality. Am. J. Clin. Nutr. 94 (1), 240–246. doi:10.3945/ajcn.110.009340
Zhang, Z., Atwell, L. L., Farris, P. E., Ho, E., and Shannon, J. (2016). Associations between Cruciferous Vegetable Intake and Selected Biomarkers Among Women Scheduled for Breast Biopsies. Public Health Nutr. 19 (7), 1288–1295. doi:10.1017/s136898001500244x
Zhang, Z., Garzotto, M., Davis, E. W., Mori, M., Stoller, W. A., Farris, P. E., et al. (2020). Sulforaphane Bioavailability and Chemopreventive Activity in Men Presenting for Biopsy of the Prostate Gland: A Randomized Controlled Trial. Nutr. Cancer 72 (1), 74–87. doi:10.1080/01635581.2019.1619783
Zurbau, A., Au-Yeung, F., Mejia, S. B., Khan, T. A., Vuksan, V., Jovanovski, E., et al. (2020). Relation of Different Fruit and Vegetable Sources with Incident Cardiovascular Outcomes: A Systematic Review and Meta‐Analysis of Prospective Cohort Studies. J. Am. Heart Assoc. 9 (19), e017728. doi:10.1161/jaha.120.017728
Keywords: glucosinolates, isothiocyanates, cardiometabolic disorders, neurological disorders, musculoskeletal health, cancer, cruciferous vegetables
Citation: Connolly EL, Sim M, Travica N, Marx W, Beasy G, Lynch GS, Bondonno CP, Lewis JR, Hodgson JM and Blekkenhorst LC (2021) Glucosinolates From Cruciferous Vegetables and Their Potential Role in Chronic Disease: Investigating the Preclinical and Clinical Evidence. Front. Pharmacol. 12:767975. doi: 10.3389/fphar.2021.767975
Received: 02 September 2021; Accepted: 11 October 2021;
Published: 26 October 2021.
Edited by:
Diego A. Moreno, Food Science and Technology Department (CSIC), SpainReviewed by:
Antonio José Ruiz-Alcaraz, University of Murcia, SpainElizabeth H. Jeffery, University of Illinois at Urbana-Champaign, United States
Debora Esposito, North Carolina State University, United States
Copyright © 2021 Connolly, Sim, Travica, Marx, Beasy, Lynch, Bondonno, Lewis, Hodgson and Blekkenhorst. This is an open-access article distributed under the terms of the Creative Commons Attribution License (CC BY). The use, distribution or reproduction in other forums is permitted, provided the original author(s) and the copyright owner(s) are credited and that the original publication in this journal is cited, in accordance with accepted academic practice. No use, distribution or reproduction is permitted which does not comply with these terms.
*Correspondence: Lauren C. Blekkenhorst, bC5ibGVra2VuaG9yc3RAZWN1LmVkdS5hdQ==