- 1Faculty of Medicine, Université Libre de Bruxelles, Brussels, Belgium
- 2University Hospitals Leuven, Leuven, Belgium
- 3Joint Research Center of the European Commission, Brussels, Belgium
- 4ULB Center for Diabetes Research, Université Libre de Bruxelles, Brussels, Belgium
Rare diseases are life-threatening or chronically debilitating low-prevalent disorders caused by pathogenic mutations or particular environmental insults. Due to their high complexity and low frequency, important gaps still exist in their prevention, diagnosis, and treatment. Since new drug discovery is a very costly and time-consuming process, leading pharmaceutical companies show relatively low interest in orphan drug research and development due to the high cost of investments compared to the low market return of the product. Drug repurposing–based approaches appear then as cost- and time-saving strategies for the development of therapeutic opportunities for rare diseases. In this article, we discuss the scientific, regulatory, and economic aspects of the development of repurposed drugs for the treatment of rare neurodegenerative disorders with a particular focus on Huntington’s disease, Friedreich’s ataxia, Wolfram syndrome, and amyotrophic lateral sclerosis. The role of academia, pharmaceutical companies, patient associations, and foundations in the identification of candidate compounds and their preclinical and clinical evaluation will also be discussed.
Introduction
Rare Neurodegenerative Diseases
Rare neurodegenerative diseases are low-prevalent, life-threatening, or chronically debilitating disorders, caused by pathogenic mutations in a single gene or by particular environmental insults (e.g., pesticides, metals, air pollution, endotoxins, and prions, among others), triggering progressive neuronal dysfunction and loss of specific groups of neurons (Matilla-Duenas et al., 2017). Depending on the disease etiology, distinct parts of the central nervous system may be affected, resulting in impaired motor and cognitive function with a significant impact on the quality of life of the affected individuals (Matilla-Duenas et al., 2017). The prevalence threshold defining a disease as rare largely varies between countries, but disorders with a prevalence of five cases or less per 10,000 individuals according to the European Union (EU) are designated as such (Nguengang Wakap et al., 2020). In the last few years, the advances in next-generation sequencing (NGS) have importantly accelerated the identification of disease-causing genes. Indeed, the Online Mendelian Inheritance in Man database (https://omim.org/statistics/geneMap) of July 2021 reports 4473 genes with phenotype-causing mutations resulting in neurological and non-neurological disorders. Despite the advances in next generation sequencing (NGS), data analysis, and other technologies that importantly contribute to identify the mutated genes and understand the biology of rare diseases and their underlying pathogenic mechanisms, the diagnosis, treatment, and availability of therapeutics for these pathologies are still very limited (Fernandez-Marmiesse et al., 2018; Pogue et al., 2018). Due to their high complexity and low frequency, important gaps still exist in their prevention, diagnosis, and treatment. Most of the patients with rare diseases receive treatments intended to alleviate the disease-derived complications and improve their quality of life, but without tackling the underlying disease cause. Indeed, for most rare pathologies, including the neurodegenerative ones, there is no treatment to prevent, delay, or cure the disease (Kaufmann et al., 2018), in particular in children (Raïs Ali and Tubeuf, 2019). There is then an urgent necessity to find therapeutic opportunities to fulfill these unmet needs. Recently, gene therapies were authorized for the treatment of neurodegenerative disorders. This is the case of onasemnogene abeparvovec (Zolgensma®), which, if administered early in life seems to cure spinal muscular atrophy (Hoy, 2019). However, these technologies, even if very promising, might be very costly and time-consuming in some cases. Because of that, leading pharmaceutical companies show relatively low interest in orphan drug research and development due to the high investment compared to the low market return that may get from the developed product (Pogue et al., 2018). According to the EU, 10% of orphan drug designations have a neurological indication (Morel et al., 2016). Drug repurposing–based approaches appear then as cost- and time-saving strategies for the development of therapeutic opportunities for rare diseases (Pushpakom et al., 2019). In this article, we discuss the scientific, regulatory, and economic aspects of the repurposed drugs proposed for the treatment of rare neurodegenerative disorders using as example the approaches taken for the treatment of Huntington’s disease, Friedreich’s ataxia, Wolfram syndrome, and amyotrophic lateral sclerosis, four rare neurodegenerative disorders (Matilla-Duenas et al., 2017). The role of academia, pharmaceutical companies, patient associations, and foundations in the identification of candidate compounds and their preclinical and clinical evaluation is also discussed.
Drug Repurposing
Drug repurposing or repositioning implies the use of approved drugs or previously evaluated but unapproved active pharmaceutical compounds for the treatment of diseases or conditions different from their original medical indication (Pushpakom et al., 2019; Fetro and Scherman, 2020). In the past, this approach was only based on serendipitous findings in which a drug was found to have an additional on-target or off-target effect that could be eventually exploited for the treatment of other diseases (Ashburn and Thor, 2004). In recent years, the identification of repurposed drug candidates is based on systematic, computational, and/or experimental (patient-centered or drug-centered) approaches based on understanding the underlying pathophysiological disease mechanisms or having a better knowledge on the mechanism of the action of a given drug (Park, 2019; Pushpakom et al., 2019). The different strategies currently used for the identification of new therapeutic indications for existing drugs have been extensively reviewed by Pushpakom et al., 2019.
Compared to “de novo” drug discovery, drug repurposing constitutes a very attractive option to save time and money and reduce the risk of failure. Indeed, while some estimates find that the development of new drugs might take around 13–15 years with an overall investment of around US$ 1.3–3 billion to bring one drug to the market, it has been proposed that drug repurposing might allow to save 5–7 years, reduce the cost to around US$ 300 million per molecule, and the risk of failure from more than 95% for newly-designed drugs to around 45% for a repurposed one (Ashburn and Thor, 2004; Nosengo, 2016; Cha et al., 2018; Wouters et al., 2020). This is because the repurposed drugs have already passed preclinical testing, safety, and pharmacokinetic profiles from early-stage clinical trials, and often their formulation has already been developed (Ashburn and Thor, 2004; Nosengo, 2016; Cha et al., 2018). However, if the route of administration is changed, early phase I clinical trials need to be performed. The regulatory and phase III costs, however, are about the same as for newly developed drugs (Pushpakom et al., 2019). Even if drug repurposing offers very good advantages for the treatment of rare diseases, this approach does not always succeed. Indeed, the risk of late-stage failure is analogous to the one-off newly developed drugs, and sometimes the need for drug reformulations may be as costly as that for de novo drug development (Pushpakom et al., 2019; Fetro and Scherman, 2020).
In many European countries, the “off-label” use of repurposed drugs for rare diseases is relatively common, mostly in pediatric patients. This practice has some negative points; one is related with the liability with respect to the administration of medicinal products, since the marketing authorization holder is responsible for the adverse effects arising from the approved indication but not in the case of off-label use. In addition, the off-label administration of repurposed drugs prevents a proper documentation of their effects and safety. Therefore, it is much more advantageous and safer if the “off-label use” occurs within a clinical protocol as part of the repurposing process that will allow safe patient monitoring and a systematic data collection and analysis. For a good overview about the benefits and risks of the off-label use of repurposed medicinal products along with potential solutions to tackle the last issue, we suggest reading the study by Verbaanderd et al. (2019). According to the EU, the repurposing approval is frequently provided through a much simpler process than an initial marketing authorization. Indeed, a marketing authorization holder can apply for a type II variation of its authorized product for the addition of a new therapeutic indication under the same marketing approval (Verbaanderd et al., 2019). However, this fast procedure is only possible if no changes to the pharmaceutical form, strength, or route of the administration of the medicinal product were made. If alterations in one or more of the latter points are introduced, the marketing authorization of the repurposed drug will be considered an extension of the initial marketing authorization (Verbaanderd et al., 2019). In this case, the approval procedure will be the same as the one for newly designed drugs, and the market access for the new indication will be granted only if regulatory evidence of quality, efficacy, and safety is proven, and if the national criteria for coverage/reimbursement and pricing are satisfied (Fetro and Scherman, 2020). Regulatory approval is normally a requirement for inclusion in clinical guidelines and for reimbursement.
Additionally, even if one may think that repurposed drugs are cheaper (Chong and Sullivan, 2007), several repurposed medicinal products authorized by the European Medicines Agency (EMA) as orphan drugs are, for one reason or another, much more expensive than the original product for which the market price covered the cost for research and development. One example is mexiletine. Its price skyrocketed after receiving the European marketing authorization for the treatment of myotonia in patients with non-dystrophic myotonic disorders by the EMA. Naturally, this is only one example, and it does not imply that all drugs tend to follow the same price evolution. In fact, as described in more detail in the section “Incentives for R&D and new drug development for rare diseases”, there are several examples of drugs with a much cheaper price.
Collaborative Models: The Role of Academia, Industry, and Patient Organizations in Drug Repurposing for Rare Diseases
Academic research importantly contributes to the first steps of the discovery of “de novo” or repurposed drugs by elucidating underlying disease mechanisms and identifying therapeutic targets (Stevens et al., 2011). Indeed, a report aiming to evaluate the role of academia on the identification and development of “de novo” or repurposed transformative drugs with groundbreaking effects on patient care pointed to a key role of academic medical centers, often funded by the government and/or by patient associations or foundations, in conceptualizing therapeutic approaches based on preclinical research disease mechanisms, and providing the proof of concept for the utilization of a given molecule for a particular disease (Kesselheim et al., 2015). This study also highlighted the importance of collaborations between academia and pharmaceutical industries to perform the follow-up steps of drug development to ensure further clinical testing and formulation of the newly discovered or repurposed drug (Kesselheim et al., 2015). Besides the individual efforts taken by academic institutions or pharmaceutical companies, public–private collaborative initiatives also exist to promote the discovery of new indications for existing drugs, for example, the Medical Research Council (MRC)–AstraZeneca compound collaboration in United Kingdom (https://mrc.ukri.org/funding/browse/mrc-az-cld/mrc-astrazeneca-centre-for-lead-discovery/mrc-az-centre-for-lead-discovery-cld-faqs/), and “Discovering New Therapeutic Uses for Existing Molecules (New therapeutic uses)” program from the National Center for Advancing Translational Sciences (NCATS) of the National Institutes of Health (NIH) in the United States (https://ncats.nih.gov/ntu/about). In both models, the MRC or the NIH provide funds to academic scientists to perform research in different disease areas, including rare disorders, and pharmaceutical companies such as AstraZeneca and NIH-industry partners grant access to their compound library, and their state-of-the-art high-throughput screening facilities (Rees et al., 2016). These public–private partnerships combining biological knowledge, financial support, and screening expertise contribute to accelerate the discovery of novel targets in a collaborative setting (Simpson and Reichman, 2014; Reichman and Simpson, 2016).
The Critical Path Institute (C-Path) is a non-profit, public-private partnership organization which has been working closely with experts from the pharmaceutical industry, academia, and the FDA in the context of collaborative approaches, where both sharing of data and expertise take place. Various programs are being conducted under the C-Path which include but are not limited to Critical Path to Therapeutics for Ataxia (CPTA), Huntington’s Disease Regulatory Science Consortium (HD-RSC), Critical Path for Alzheimer’s disease (CPAD), Critical Path for Parkinson’s (CPP), and Friedreich’s Ataxia-Integrated Clinical database (FA-ICD). In 2020, a public–private partnership dedicated to advance drug repurposing – CURE Drug Repurposing Collaboratory (CDRC, https://c-path.org/programs/cdrc) has been initiated by the C-Path and the FDA in collaboration with the NCATS. The goal of this collaborative initiative is to generate a platform where all the real-world clinical outcome data are open-sourced at one place and from which knowledge can be gained to enhance drug repurposing through the identification of lead candidates. Also, the platform will provide information about unmet medical needs for diseases, assistance in regulatory roadmaps, and during clinical trials to identify safe and effective drugs for new indications.
The importance of patient associations and advocacy groups in the development of therapeutic approaches has been recognized in the last years in all disease areas, including rare diseases. These organizations are now considered an integral part in the research process, since they foster collaborations between academia, pharmaceutical companies, and clinicians and act as a link between the patients and the researchers providing useful information about patient’s expectations and needs. They are also actively involved in shaping Consortia’s research agendas and help ensure the feasibility and success of research protocols by assisting with study design and patient recruitment. In addition, besides organizing educational programs, facilitating networking amongst patient groups, and providing patient services, they also raise funds to finance academic research preclinical projects and clinical trials (Merkel et al., 2016). One example is the International Rare Diseases Research Consortium (IRDiRC) which ‘unites national and international governmental and non-profit funding bodies, companies (including pharmaceutical and biotech enterprises), umbrella patient advocacy organizations, and scientific researchers to promote international collaboration and advance rare diseases research worldwide’ (https://irdirc.org/about-us/).
Most individual patient associations or foundations are, in general, part of bigger non-profit patient organizations such as the National Organization for Rare Disorders (Nord) (https://rarediseases.org/), EURORDIS (European Organization for Rare diseases, https://www.eurordis.org) or Findacure https://www.findacure.org.uk/. The Orphanet database (https://www.orpha.net/consor/cgi-bin/index.php) provides compiled information about rare diseases and patients’ organizations registered in Europe.
Concerning rare neurodegenerative diseases, the European Reference Network for Rare Neurological Disorders (ERN-RND) http://www.ern-rnd.eu/, established by the EU supports patients and families affected by rare neurological diseases and facilitates the participation of patients in clinical trials with repurposed medicinal products. The neurodegenerative rare diseases covered by this network include several ataxias and Huntington’s disease.
Cures Within Reach (https://www.cureswithinreach.org) is another philanthropic organization dedicated to fund research projects related with drug, device, and nutraceutical repurposing to provide fast and safe treatments for unmet clinical needs in different common and rare diseases. Healx, the only commercial company with this type of model (https://healx.io/), combines artificial intelligence and collaborates with academic institutions, biotech, pharma, and patient groups to identify and progress novel therapies. Currently, Healx has 18 therapies listed in the pipeline.
Incentives for R&D and New Drug Development for Rare Diseases
Understanding the drivers of pharmaceutical research and development (R&D) is important to foster innovation in the pharmaceutical market. The pharmaceutical industry is responsive to the potential market size: when it increases, the entry of new non-generic drugs and new molecular entities (i.e., those more profitable) also increases (Acemoglu and Linn, 2004). This constitutes an issue in the case of rare diseases. In fact, the interest of pharmaceutical companies in orphan drug development is traditionally low due to the relatively high cost of investment compared to the low market return of the product, precisely because of the small market size (https://www.eurordis.org/IMG/pdf/princeps document-EN.pdf). Given the lack of competition, therapy products for rare diseases have a commercial potential, namely, when their market price is extremely high. For instance, gene therapy onasemnogene abeparvovec (Zolgensma®) has a market price of more than €2m. The justification for setting a high market price is the high costs of R&D, manufacturing and distribution, and the small market size. But by setting such a high price, potentially lifesaving therapies are prohibitive for most patients and, in practice, patients cannot have access to these medicinal products (Fischer et al., 2019).
Nevertheless, the price of drugs to treat rare diseases varies considerably. If we consider the case of repurposed drugs for Huntington’s disease currently available in Belgium, the average price is €1,280, with the cheapest drug listing a public price of €11,09 and the most expensive one listing a price equal to €4,982.
Verifying the veracity of high development costs is generally difficult. As a matter of fact, it is challenging to estimate the costs of drug development, namely, because pharmaceutical companies do not generally make the costs of drug development publicly available. Nevertheless, Dimasi et al., 2016 found that it can be more than $2b, while Wouters et al., (2020) estimated a mean of approximately $1.3b, with the latter including a large sample of orphan drugs. Jayasundara et al., (2019) found a higher clinical cost of drug development for non-orphan drugs with respect to orphan drugs. Despite the evidence of lower costs for developing orphan drugs, there is a lack of drugs to treat rare diseases.
Additionally, pharmaceutical companies might take into consideration potential competition from drugs previously adopted in the market with the same active ingredient but approved for other authorized indication or patient groups. In this situation, the drug might have a lower price or can even face competition from a generic drug. Therefore, companies could be discouraged from trying to repurpose the drug as they would pay for the research and development, while the company which has the older product in the market would take the profits. In order to quantify the extent to which this situation impacts the decision of pharmaceutical companies to try to repurpose drugs, one would need to know how many of the repurposed drugs actually have a generic competitor, for how long, and how many patients would be treated by the older drug in order to estimate potential foregone profits. In the examples considered in this article, this is almost never the case. Due to the recognition of a need to foster the development of medicinal products to treat rare diseases, different economic and regulatory incentives have been provided to the sponsors of orphan products worldwide. The first specific regulation, the Orphan Drug Act (ODA), was approved by the US in 1983, and its incentives include 1) 7 years of market exclusivity to sponsors of approved orphan products; 2) tax credits; and 3) research grants. In Europe, the regulation on orphan medicinal products (Regulation (EC) No 141/2000) provides different incentives to sponsors, such as a) scientific advice on study protocols provided by the EMA (protocol assistance); b) 10 years of market exclusivity, which can be extended by 2 years for pediatric investigation; and c) reduced fees for regulatory activities. These incentives have attracted small and medium enterprises (SMEs), academia, pharmaceutical companies, public–private partnerships, and patient advocacy groups to work in rare diseases.
In spite of these incentives, according to the EU in 2020, there were over 2,380 medicines with orphan designation but, as of August 2021, only a few more than 200 drugs had marketing authorization (https://www.ema.europa.eu/en/documents/report/annual-report-use-special-contribution-orphan-medicinal-products-2020_en.pdf). Therefore, rare diseases are still underserved in terms of drug development in comparison with non-rare diseases. Considering the need of addressing unmet needs of rare diseases’ patients, the European Commission launched an initiative which aims at revising the legislation to incentivize the development of medicines for rare diseases and children (Commission, 2021). The objective is to provide solutions to possible shortcomings of the current legislation and take into account the exclusive role of member states in crucial areas such as pricing and reimbursement of medicines. This will also be addressed within the Pharmaceutical Strategy for Europe, which has a broader set of objectives, including fostering healthcare and pharmaceutical innovation (Commission, 2020). The low number of orphan drugs authorized with respect to the large number of orphan designations is explained by the high investment needed for new drug development, and the long duration of the process that is accompanied by the regulatory hurdles and organizational issues faced while performing clinical trials in rare diseases (Ashburn and Thor, 2004; Wastfelt et al., 2006). Indeed, despite the availability of original safety data on the previous approved indication, to receive marketing authorization, the repurposed drugs need to be tested for their efficacy, safety, and tolerability in clinical trials. These clinical studies conducted to provide the benefit/risk data are expensive and complex due to the frequent complexity of the diseases, the low number of patients affected, and their wide geographical distribution (Ashburn and Thor, 2004; Wastfelt et al., 2006). Additionally, within rare diseases, pharmaceutical companies tend to target more profitable areas. In fact, R&D is higher for diseases with an older average age at death (i.e., in adulthood instead of infancy or childhood), which provides additional evidence that R&D is concentrated in more profitable areas; and in rare diseases in high-prevalence categories, which corroborates evidence that market share is a driver of R&D (Raïs Ali and Tubeuf, 2019).
Business Models for Drug Repurposing
Traditionally, the business model of leading pharmaceutical companies consisted in in-house drug development, from R&D until commercialization, and a general focus on blockbuster drugs. However, this is changing for several reasons, such as rising development costs, competition from generics, and end of patents of some blockbuster drugs (Phillips, 2013). The industry is facing an increasing productivity gap because the cost per new drug is growing while the number of new drug introductions is not accompanying this increase (Grabowski, 2004).
Drug repurposing, namely, for rare diseases can be an interesting business for pharmaceutical companies and has been considered a possible response to the productivity gap (Boguski et al., 2009). In the last 20–25 years, a number of companies and non-profit organizations devoted to drug repurposing have emerged, with a reduced number of failures (Naylor et al., 2015). Meanwhile, some of these companies were acquired by larger ones, and some leading pharmaceutical companies have created departments devoted to repurposing (Sleigh S. H. and Barton C. L., 2010). More recently, several drugs repositioned for COVID-19 are being considered, which has increased the interest in drug repurposing.
As mentioned before, from a business perspective, drug repurposing in rare diseases can be attractive, namely, because of its reduced costs compared to de novo drug development, potentially diminished risk of failure, reduced time required for approval, and higher pricing. However, a significant proportion of rare diseases affect children, which represents a challenge since in most cases the clinical trials conducted for the drugs to be repurposed only included adults. Despite drug repurposing being a viable strategy to find treatments for some rare diseases, new business models are needed to foster this approach. This includes collaborative strategies combining the strength of different agents, such as pharmaceutical and biotechnology companies, venture capitalists, and academia (Pushpakom et al., 2019). Cha et al. (2018) identified three key players in the market, each one with a different business model: academia/research institutes, repurposing technology companies, and pharmaceutical companies. The former, that usually sponsors a significant proportion of phase I and phase II trials for repurposed drugs, faces lower economic or commercial constraints while being more dependent on public funding. The repurposing technology companies are bound by their business model, which includes consulting services, offering drugs databases, and drug pipelines (Sleigh SH. and Barton CL., 2010; Sleigh S. H. and Barton C. L., 2010; Naylor et al., 2015). Some of these companies collaborate with leading pharmaceutical companies. Pharmaceutical companies have a more prominent role in drug discovery and development and tend to be profit seekers. A few might have some ethical concerns or embrace, for example, corporate social responsibility. Overall, there is no unique or widely adopted business model used by pharmaceutical companies for drug repurposing, but three business models frequently used have been identified (Naylor et al., 2015): the in-house model, in which the pharmaceutical companies have their own department or resources devoted to drug repurposing (this model has already been abandoned by some companies); the out-licensing model, in which pharmaceutical companies provide access to their compounds on an out-licensing basis (this limits exposure to risk and additional costs for the corresponding compound); and the extended profiling model, in which a drug candidate starts being evaluated for new indications immediately after a successful first-in-human study.
Drug Repurposing for Rare Neurodegenerative Diseases
Huntington’s Disease
Clinical Features and Genetic Cause
Huntington’s disease (HD) is the most common rare neurodegenerative disorder with an estimated prevalence of 1/20,000 to 1/10,000 in the Caucasian population. It was discovered by George Huntington in 1872. The disease is characterized by a triad of motor, cognitive, and psychiatric manifestations (Ghosh and Tabrizi, 2018). Motor features include involuntary choreatic movements, dystonia, and rigidity, while the behavioral and psychiatric disorders include depression, anxiety, apathy, irritability aggression, and dementia among others. The clinical manifestations usually appear during the third decade of life and become fatal after 15–20 years due to progressive neuronal dysfunction and ultimate neuronal death (Ghosh and Tabrizi, 2018). The diagnosis of this disease is usually performed by molecular genetic testing followed by computerized tomography scanning, magnetic resonance imaging (MRI), and electroencephalography. HD is an autosomal dominant disorder caused by an unstable 36–70 CAG trinucleotide expansion in exon 1 on the Huntington gene (HTT) (Snell et al., 1993) (Figure 1). The onset and the severity of the disease is dependent on the length of the CAG repeat, with longer repeats being associated with more severe phenotypes (Nance, 2017; Caron et al., 2018).
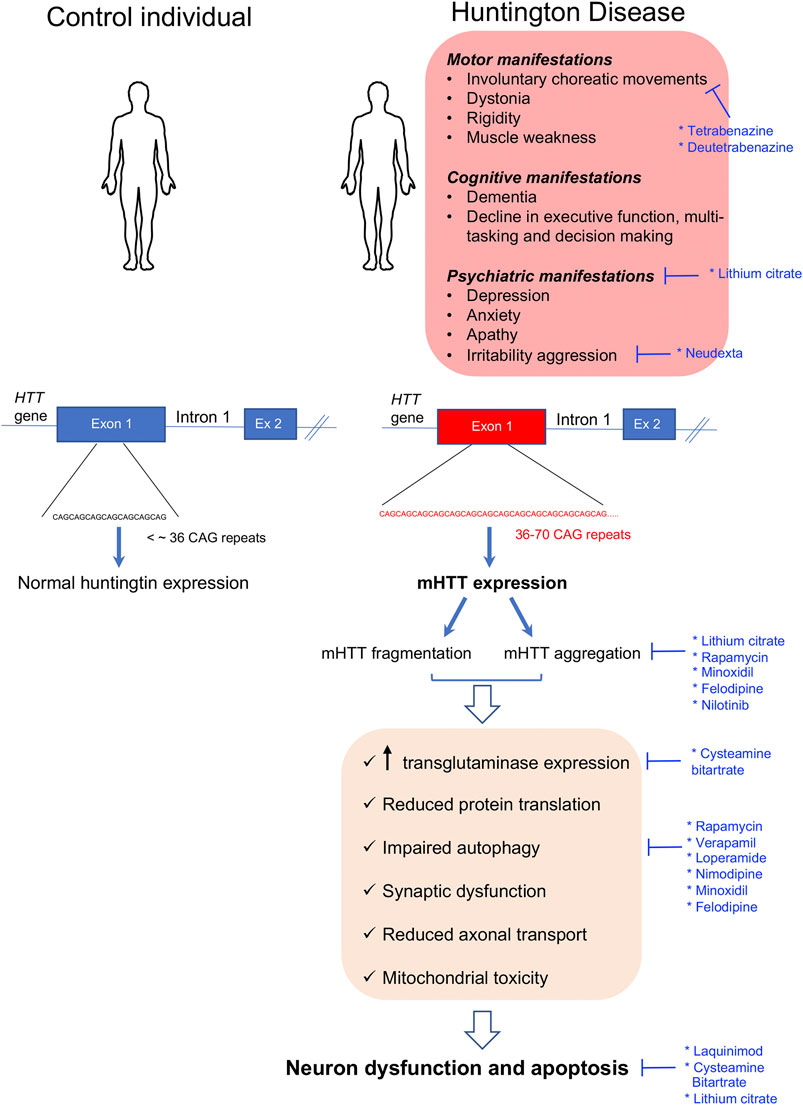
FIGURE 1. Clinical manifestations and molecular disease mechanisms in Huntington’s disease. This pathology is caused by CAG repeat expansions in the first exon of the HTT gene which result in the accumulation of mutant Huntington protein (mHTT) within the cells which causes a wide variety of cellular alterations leading to neuronal dysfunction and death. Repurposed drugs with and without orphan designation for Huntington’s disease are highlighted in blue along with the cellular dysfunctions or clinical manifestations that they tackle. Ex: exon.
Molecular Phenotype
The HTT gene encodes for a large 350-kDa protein, also called HTT, which is ubiquitously expressed but enriched in the brain (Aronin et al., 1995; Trottier et al., 1995). The normal function of HTT is currently unknown. HTT is folded in a super helical structure containing a hydrophobic core. In the mutated HTT (mHTT), the CAG expansion results in a polyglutamine (polyQ) tract starting in residue 18 of the polypeptide. This expanded polyQ region may be proteolytically cleaved, and it has been proposed that the mHTT fragments generated may induce neurodegeneration (Halliday et al., 1998; Barbaro et al., 2015; Ghosh and Tabrizi, 2018). In addition, it was shown that the mHTT protein is highly aggregation-prone, resulting in intranuclear and intracytoplasmic inclusions throughout the brain (Difiglia et al., 1997). Many studies report that mHTT aggregates can be either toxic or protective depending on the disease stage, their subcellular localization, and their association with other partners or organelles (Saudou et al., 1998; Slow et al., 2003; Slow et al., 2005; Leitman et al., 2013). When present in the nucleus, these aggregates sequester transcription factors resulting in gene expression alterations (Labbadia and Morimoto, 2013), while when present in the cytosol, they may bind to diverse proteins resulting in an altered autophagy-lysosome pathway, reduced protein translation, synaptic dysfunction, reduced axonal transport, mitochondrial toxicity, and energy imbalance among others (Labbadia and Morimoto, 2013; McColgan and Tabrizi, 2018) (Figure 1). There is currently no treatment to prevent or delay the progression of HD; however, some pharmaceutical and non-pharmaceutical interventions (physiotherapists, psychologists, and social workers) aiming to relieve the multiple symptomatic manifestations of the disease are beneficial for some patients and contribute to improve their quality of life (Frank, 2014). Within the pharmaceutical approaches, some neuroleptics with anti-dopaminergic activity or acting as selective serotonin reuptake inhibitors (SSRIs) may be used in the management of psychosis-associated symptoms in HD-like anxiety, depression, and irritability (Mittal and Eddy, 2013; Unti et al., 2017).
Approved and Non-Approved Orphan-Designated Repurposed Drugs for Huntington’s Disease
Tetrabenazine (Xenazine®), originally indicated as an anti-psychotic drug, is a repurposed molecule approved in 2008 by the U.S. Food and Drug Administration (FDA) for the treatment of chorea in HD (Jankovic and Clarence-Smith, 2011). This authorization was based on the positive results of four controlled clinical trials performed in the United States with HD patients (Huntington Study, 2006; Frank et al., 2008; Frank, 2009). Tetrabenazine is a reversible vesicular monoamine transporter 2 (VMAT2) inhibitor which blocks the uptake of cytosolic monoamines and prevents dopamine release from synaptic vesicles (Login et al., 1982). An extensive review on the mechanism of action, pharmacodynamics, pharmacokinetics, and metabolism of this drug can be found in the study by Jankovic and Clarence-Smith, (2011). Following the positive results from a randomized double-blind, placebo-controlled trial, in April 2017, the FDA also approved AUSTEDO (deutetrabenazine), another molecule with VMAT2 inhibitor activity, to treat chorea in HD (Huntington Study et al., 2016).
Some repurposed medicinal products have received the orphan designation by the EMA for the treatment of Huntington’s disease but have not been authorized yet: for example, cysteamine bitartrate (https://www.ema.europa.eu/en/medicines/human/orphan-designations/eu3141306) and lithium citrate tetrahydrate (https://www.ema.europa.eu/en/medicines/human/orphan-designations/eu309706). In the EU, cysteamine bitartrate is used under the name of Cystagon® and Procysbi® for the treatment of nephropathic cystinosis. In HD, this drug was shown to be neuroprotective in several mouse models by improving weight loss and motor abnormalities and prolonging animal survival. It has been proposed that the drug may reduce nerve damage and improve motor function by blocking the activity of the enzyme transglutaminase, shown to be increased in HD patients and involved in nerve injury, by increasing the secretion of the brain-derived neurotrophic factor (BDNF) that improves neuron survival and function, and by other still unidentified mechanisms (Dedeoglu et al., 2002; Karpuj et al., 2002; Van Raamsdonk et al., 2005; Bailey and Johnson, 2006; Borrell-Pages et al., 2006; Arbez et al., 2019). A randomized, double-blind, placebo-controlled trial with cysteamine bitartrate showed that the drug is safe and well tolerated by HD patients, but failed to demonstrate efficacy in the full-patient cohort (Verny et al., 2017). A post hoc analysis in which patients were stratified by disease severity based on their initial motor scores suggested that the drug reduced the progression of the disease in patients with the most severe motor impairment. Further clinical studies are needed to prove the efficacy of the drug. Lithium is an inhibitor of glycogen synthase kinase-3 and inositol monophosphatase that have been used as mood stabilizers for several decades (Yatham et al., 2018). Recent studies performed in several preclinical HD models suggest that this molecule is able to increase the clearance of intracellular protein aggregates, to confer neuroprotection, and to improve motor dysfunction and coordination (Scheuing et al., 2014). Several blind and unblind clinical studies using lithium for short periods of time in HD patients showed improvement in choreatic movements, motor function, and mood stabilization in some but not all patients (Anden et al., 1973; Dalen, 1973; Mattsson, 1973; Aminoff and Marshall, 1974; Danivas et al., 2013; Raja et al., 2013). Further blinded trials with larger patient cohorts are needed to determine the effectiveness of this drug. Table 1 provides further information about the aforementioned drugs, including pharmaceutical companies or academic institutions involved in designing and running the clinical trials, whether the drugs received orphan designation from the EMA and/or the FDA, and the sponsors that made the orphan designation request.
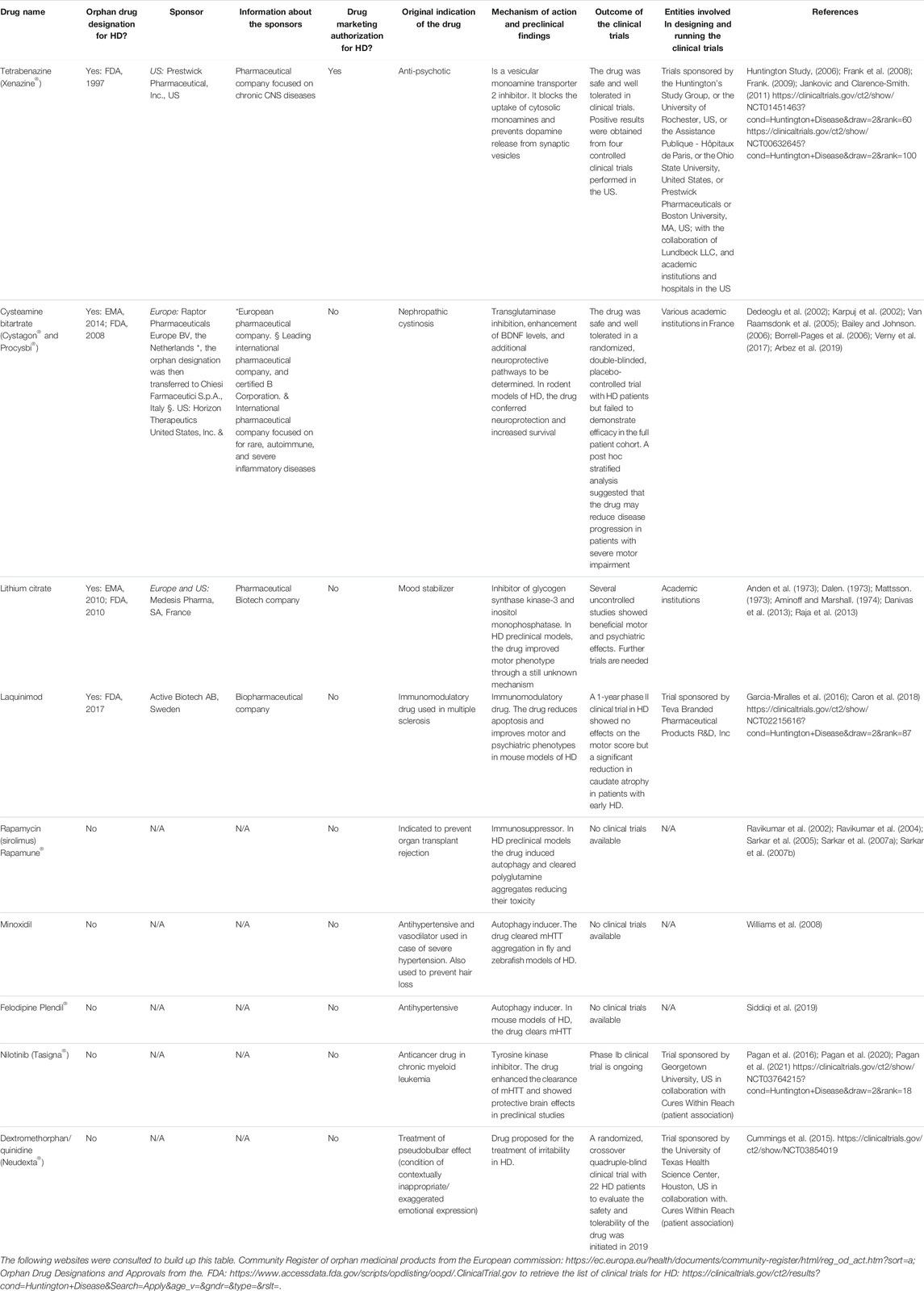
TABLE 1. List of the repurposed drugs, with and without orphan designation or drug marketing authorization for Huntington’s disease (HD) mentioned in this article. The sponsors (entities involved in making the orphan designation request to the EMA or the FDA), and the public or private organizations involved in designing and running the clinical trials are detailed. N/A = non-applicable.
Additional new and repurposed drugs that received orphan designation for HD but are not yet approved for its treatment can be found in the Orphanet portal (https://www.orpha.net/consor/cgi-bin/index.php).
Additional Drug Repurposing–Based Therapeutic Strategies Under Investigation (Non-Orphan Designated Drugs)
Without being an exhaustive list, here we will provide some examples of current drug repurposing–based approaches for HD at different stages of development (Table 1). A detailed review about all ongoing “de novo” or repurposing-based strategies for HD treatment can be found in the study by Caron et al., (2018).
As mentioned above, mHTT aggregates may compromise autophagic clearance by perturbing cargo recognition and autophagosome motility which results in cell death (Martinez-Vicente et al., 2010; Zatyka et al., 2020). In fly and mouse models of HD, rapamycin-mediated mTOR inhibition enhanced the autophagic flux and clearance of unfolded mHTT resulting in reduced toxicity (Ravikumar et al., 2002; Ravikumar et al., 2004; Sarkar et al., 2005; Sarkar et al., 2007a; Sarkar et al., 2007b). Since rapamycin has numerous side effects, Williams et al. (2008) screened a library of FDA-approved drugs looking for autophagic enhancers with mTOR-independent activity. This screening identified clonidine, verapamil, loperamide, nimodipine, and minoxidil among others as drugs with autophagic enhancing activity. In vitro assays showed that all of them were able to reduce mHTT aggregation and toxicity in neuroblastoma cells (Williams et al., 2008). The authors also showed that calpain inhibition reduced mHTT aggregation and toxicity. Similarly, the antihypertensive drug (L-type calcium channel blocker) felodipine was shown to induce autophagy and clear mHTT in a mouse model of HD (Siddiqi et al., 2019).
Abnormal immune activation and inflammatory processes resulting from the central nervous system (CNS) and peripheral immune cell dysfunction have also been highlighted as important contributors to the pathophysiology of HD (Bjorkqvist et al., 2008). Laquinimod is an immunomodulatory drug developed for the treatment of multiple sclerosis. In the context of HD, it was shown that in vitro, this molecule reduces apoptosis in primary neurons derived from YAC128 mice, a HD model (Garcia-Miralles et al., 2016). Moreover, chronic laquinimod administration in these animals improved white matter integrity, motor and psychiatric phenotypes, and reduced IL-6 serum levels (Garcia-Miralles et al., 2016). A 12-month phase II clinical trial for HD with this molecule showed no effect on the motor score but revealed a significant reduction in caudate atrophy that was more evident in patients with early HD (Caron et al., 2018), pointing to a promising role of immunomodulators for the treatment of HD. Further clinical studies are required to support the neuroprotective effect of all these drugs in HD patients.
Cures Within Reach is currently funding two clinical trials in HD with repurposed drugs. One of them, led by Dr. Anderson from the Georgetown University, will study the safety and tolerability of nilotinib, a FDA-approved cancer drug for the treatment of chronic myeloid leukemia, in 10 HD patients with early-to-moderate HD. Based on previous findings from clinical trials with nilotinib in Parkinson’s disease (Pagan et al., 2016; Pagan et al., 2020; Pagan et al., 2021) they hypothesize that this drug may contribute to reduce the accumulation of toxic mHTT and have protective brain effects in HD. The second trial is led by Dr. Furr-Stimming from the University of Texas and aims to study the safety and tolerability of Neudexta, a drug currently used for the treatment of the pseudobulbar effect. This drug was shown to importantly ameliorate agitation in patients with Alzheimer’s disease (Cummings et al., 2015). Based on that, it was hypothesized that it may be useful to treat irritability in HD.
Huntington’s Disease Patient Associations and Foundations
The International Huntington’s Disease Association http://huntington-disease.org/is a multinational federation created in 1979 that resembles 32 different Huntington’s disease societies from all over the world. The member societies promote medical professional education; provide individual and family support; and fund psychosocial, clinical, and biomedical research related with Huntington’s disease in their respective countries.
In addition, the Huntington’s disease coalition for the patient engagement (HD-COPE) is a global Huntington’s disease patient advocacy organization working in collaboration with the European Huntington’s Association (EHA), Huntington’s Disease Society of America (HDSA), and Huntington’s Society of Canada (HSC) formed in September 2017 to give patients’ voice in the clinical trials (https://hdsa.org/news/global-huntingtons-disease-patient-advocacy-organizations-unite-to-form-huntingtons-disease-coalition-for-patient-engagement-hd-cope/).
Friedreich’s Ataxia
Clinical Features and Genetic Cause
Friedreich’s ataxia (FRDA) is an autosomal recessive rare neurodegenerative disease mainly present within Caucasians. In this population, its prevalence ranges from 1/20,000 to 1/50,000, but large regional differences have been reported in Europe (Campuzano et al., 1996; Vankan, 2013). The disease is rare in sub-Saharan populations and very rare in the Far East (Vankan, 2013). The clinical manifestations include progressive limb incoordination (ataxia), gait instability, impaired vision, hearing and speech, and scoliosis and muscle weakness as a consequence of the progressive degeneration of the dorsal root ganglia neurons followed by neuronal loss in the cerebellar dentate nucleus and spinocerebellar tract degeneration (Koeppen et al., 2007; Marmolino, 2011; Koeppen et al., 2016; Selvadurai et al., 2018). In addition, as the disease progresses, non-neurological features appear, such as hypertrophic cardiomyopathy, that is the underlying cause for premature death (Harding, 1981; Pandolfo, 2009; Raman et al., 2011; Payne and Wagner, 2012; Parkinson et al., 2013), and diabetes that occurs in 30% of the patients as a result of increased pancreatic β-cell dysfunction and death in the context of insulin resistance (Finocchiaro et al., 1988; Schoenle et al., 1989; Cnop et al., 2012; Cnop et al., 2013; Igoillo-Esteve et al., 2015). In 96% of the patients, the disease is caused by homozygous trinucleotide GAA repeat expansions (from 70 to around 1700 triplets) in the first intron of the frataxin gene (FXN), while the remaining 4% have FXN point mutations (Campuzano et al., 1996; McCormack et al., 2000). The GAA repeat expansions interfere with transcription by heterochromatin silencing (Campuzano et al., 1996; Saveliev et al., 2003; Herman et al., 2006; Silva et al., 2015). The length of the GAA repeat is not identical in both alleles, and the allele with the shortest expansion size determines the residual FXN levels. Longer GAA repeats result in a more severe reduction in FXN expression (65–95% decrease with respect to healthy controls) and are associated with early disease onset and greater disease severity (Campuzano et al., 1996; Al-Mahdawi et al., 2008; Koeppen, 2011) (Figure 2).
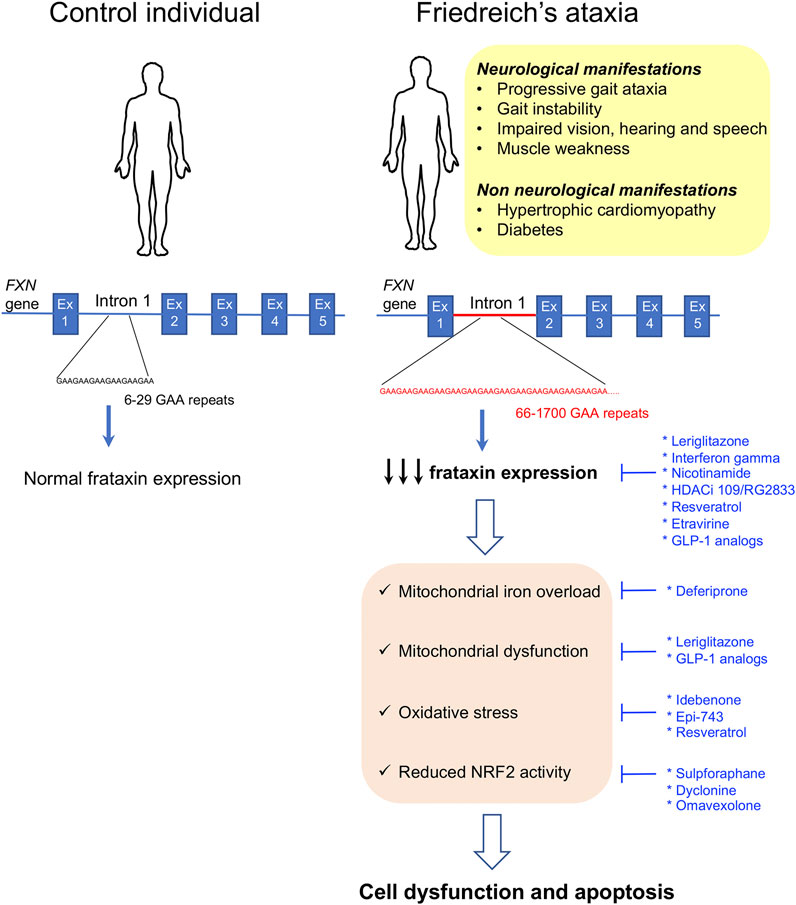
FIGURE 2. Clinical manifestations and molecular disease mechanisms in Friedreich’s ataxia. This pathology is caused by GAA repeat expansions in the first intron of the FXN gene. This results in reduced frataxin protein expression which causes a wide variety of cellular alterations leading to cell dysfunction and death. Repurposed drugs with and without orphan designation for Friedreich’s ataxia are highlighted in blue along with the cellular dysfunctions or clinical manifestations that they tackle. Ex: exon.
Molecular Phenotype
FXN encodes for a 210-amino acid mitochondrial protein called frataxin that regulates iron homeostasis by modulating iron storage, iron–sulfur cluster (Fe–S), and heme biosynthesis and iron carriage (Adamec et al., 2000; Emond et al., 2000; Schulz et al., 2000; Adinolfi et al., 2002; Becker et al., 2002; Gerber et al., 2003; Yoon and Cowan, 2003; 2004; Adinolfi et al., 2009; Paupe et al., 2009; Condo et al., 2010; Tsai and Barondeau, 2010). Accordingly, it was shown that reduced frataxin expression results in impaired function and/or expression of FeS-containing enzymes, such as catalase, and several respiratory chain proteins resulting in iron accumulation in the mitochondrial matrix, mitochondrial dysfunction, and oxidative stress (Babcock et al., 1997; Rotig et al., 1997; Puccio et al., 2001). In several FRDA models, frataxin deficiency has been associated with reduced NRF2 (nuclear factor (erythroid-derived 2) 2–like transcription factor) levels (Paupe et al., 2009; D’oria et al., 2013; Shan et al., 2013). NRF2 exhibits antioxidant properties by regulating the expression of antioxidant and cytoprotective genes (Kim et al., 2001). It also reduces inflammation, improves mitochondrial function, and maintains protein homeostasis (Dinkova-Kostova et al., 2018). Therefore, reduced NRF2 expression or activity importantly contributes to frataxin deficiency–induced cytotoxicity in FRDA (Figure 2). There is currently no approved therapy to prevent, delay, or revert the manifestations of the disease; however, the beneficial effect of many molecules targeting different aspects of the FRDA pathophysiology has been tested or is currently under study. In the beginning, the therapeutic approaches for FRDA were mostly focused on treating the downstream effects of frataxin deficiency, namely, improving iron homeostasis and mitochondrial function or reducing oxidative stress. In recent years, many of the approaches under study are centered on the upregulation of frataxin protein expression to restore frataxin levels. Indeed, increasing frataxin protein expression in FRDA patients to the levels found in carrier individuals that are asymptomatic is expected to provide a cure for the disease and stabilize disease progression. Compiling expert information on previous and current research studies for the treatment of FRDA can be found in the study by Clay et al., (2019).
Approved and Non-Approved Orphan-Designated Repurposed Drugs for Friedreich Ataxia
As mentioned above, there are currently no approved drugs for the treatment of FRDA, but several repurposed medicinal products have received orphan designation for the treatment of this disease. Deferiprone (orphan-designated by the FDA) and idebenone, omaveloxolone, alpha-tocotrienol quinone, leriglitazone, and interferon gamma (orphan-designated by both the EMA and FDA) are only some examples (https://www.orpha.net/consor/cgi-bin/index.php).
Deferiprone is a permeable iron chelator that alleviates mitochondrial iron overload and is indicated for the treatment of sickle cell disease (Pandolfo and Hausmann, 2013). Based on this feature, it was proposed that it could be beneficial for FRDA individuals. A 6-month double-blind placebo-controlled study with this molecule in pediatric and adult FRDA patients showed that deferiprone is relatively safe at the lower doses tested (20 mg/kg/day) and contributes to reduce cardiac hypertrophy. However, patients receiving higher deferiprone doses (40–60 mg/kg/day) presented worsening in their ataxic phenotype (Pandolfo et al., 2014). In this trial, the lack of deterioration in the placebo group did not allow for detection of any potential protective effect of deferiprone on the neurological manifestations of the disease. However, a post hoc subgroup analysis suggested that 20 mg/kg/day deferiprone may reduce disease progression in patients with less severe disease symptoms, pointing to the need of further clinical trials with selected patient populations to confirm or rule this beneficial effect.
The potential therapeutic benefits of idebenone, an antioxidant drug initially developed by Takeda Pharmaceutical for the treatment of Alzheimer’s disease and other cognitive defects, and currently approved to treat visual impairment in adolescents and adults with Leber’s hereditary optic neuropathy (Lyseng-Williamson, 2016), have been largely studied in preclinical models of FRDA, where it reduced apoptosis and showed cardioprotective effects (Jauslin et al., 2002; Seznec et al., 2004). Initial clinical studies suggested that this drug may have some cardioprotective effects in FRDA patients (Rustin et al., 1999; Hausse et al., 2002; Mariotti et al., 2003), but follow-up trials showed no cardioprotection (Lagedrost et al., 2011; Cook et al., 2019). In addition, these and other clinical studies (Rustin et al., 1999; Hausse et al., 2002; Buyse et al., 2003; Mariotti et al., 2003; Rustin et al., 2004; Rinaldi et al., 2009; Lynch et al., 2010) showed no neuroprotective effects. Only one open-label trial showed some neurological improvement in pediatric FRDA patients treated with this drug (Meier et al., 2012). Despite the potential cardioprotective effects of the molecule, the lack of neuroprotective properties seen in most of the studies makes this drug less attractive for FRDA treatment.
As mentioned above, frataxin depletion may cause NRF2 inactivation contributing to cell death. Enhancement of NRF2 expression or activity is considered a potential therapeutic approach to prevent neurodegeneration in FRDA (Petrillo et al., 2017). In addition, it was recently found that the FXN gene contains three antioxidant-responsive element (ARE) sites in its promoter region (Sahdeo et al., 2014), suggesting that NRF2 may modulate frataxin expression by binding to these ARE elements. In line with that, several compounds with NRF2-inducing activity, such as sulforaphane, the anti-epileptic drug dyclonine, DMF, N-acetyl cysteine, and omaveloxolone among others, showed beneficial effects and frataxin-inducing activity in different models of FRDA (Petrillo et al., 2017; Clay et al., 2019; Petrillo et al., 2019). In addition, omaveloxolone was tested in a phase II clinical trial with 69 FRDA patients. In this study, the drug was overall well tolerated, but it did not change the primary outcome of the trial that is, peak workload in maximal exercise. However, it improved the modified Friedreich’s Ataxia Rating Scale (mFARS) that involves the examination of the neurological signs of the disease (Lynch et al., 2019a). In a follow-up double-blind, randomized, placebo-controlled, parallel-group, phase II trial performed with 103 FRDA patients from 11 institutions of the United States, Europe, and Australia (Trial number: NCT02255435, https://clinicaltrials.gov/ct2/show/NCT02255435), omaveloxolone significantly improved the neurological function of the FRDA patients compared to the placebo and was safe and well tolerated (Lynch et al., 2021), pointing to this drug as a potential therapeutic agent for FRDA.
Alpha-tocotrienol quinone (also known as Epi-743 or vatiquinone) is a molecule that blocks the activity of 15-lipoxygenase, an important oxidoreductase that regulates oxidative stress and neuroinflammation. Epi-743 received orphan drug designation for the treatment of mitochondrial epilepsy and other mitochondrial genetic diseases such as Leigh disease and Rett syndrome (Enns et al., 2012; Kahn-Kirby et al., 2019). Its safety and efficacy in improving visual and neurological functions in FRDA patients were also evaluated in a 6-month, double-blind, placebo-controlled, phase II clinical trial followed by 18 extra months of the open-label phase (Zesiewicz et al., 2018). In this study, the drug was shown to be safe and well tolerated by FRDA patients, but failed to improve key end points during the placebo phase. However, at the end of the 24-month intervention, EPI-743 significantly improved the neurological function and disease progression of the patients (Zesiewicz et al., 2018). In November 2020, PTC therapeutics launched MOVE-FA (Trial number: NCT04577352, https://clinicaltrials.gov/ct2/show/NCT04577352) a phase II/III double-blind, placebo-controlled trial with a follow-up open-label phase in children and adult FRDA patients from the United States, Canada, Europe, Australia, and Latin America to assess the efficacy and safety of vatiquinone. The study is currently ongoing. A total of 126 patients will be recruited.
Leriglitazone is the hydrochloride salt of the active metabolite M4 of pioglitazone. It is indicated to control glycemia in patients with type 2 diabetes. Leriglitazone, a PPAR-γ agonist with good brain penetration, is found to rescue neurodegeneration due to frataxin deficiency in the dorsal root ganglion neurons through restoration of mitochondrial membrane potential and improved mitochondrial function and calcium homeostasis (Rodriguez-Pascau et al., 2021). The drug also improved motor function in a mouse model of FRDA (Rodriguez-Pascau et al., 2021). In March 2019, leriglitazone received orphan designation from the EMA and the FDA for FRDA treatment. A phase II, randomized, double-blind, placebo-controlled clinical study called FRAMES (Trial number: NCT03917225, https://clinicaltrials.gov/ct2/show/NCT03917225?term=MIN102) has been launched to assess the efficacy and safety of leriglitazone in 36 FRDA patients. The trial is currently ongoing.
Interferon gamma is a drug currently approved in the United States for the treatment of chronic granulomatous disease and malignant osteoporosis (Britti et al., 2018). In the context of FRDA, this molecule increased frataxin expression in in vitro and in vivo models of the disease and enhanced motor function in mice (Wells et al., 2015). An initial pilot clinical trial with a small number of FRDA patients failed to detect an increase in frataxin expression but reported an improvement in the neurological outcome of the patients (Seyer et al., 2015). Nevertheless, a follow-up, double-blind, placebo-controlled study of interferon gamma performed in the US failed to replicate these findings since no difference in mFARS and frataxin levels was detected between the interferon gamma and the placebo groups (Lynch et al., 2019b).
Histone deacetylase inhibitors (HDACi) are anticancer agents that play important roles in epigenetic and non-epigenetic gene regulation. In the context of FRDA, preclinical in vitro and in vivo experiments provided proof of concept that these molecules can induce frataxin expression in different models of FRDA (Herman et al., 2006; Chan et al., 2013; Soragni et al., 2014). An initial exploratory, open-label, dose-escalation study with nicotinamide, a class III HDACi in FRDA patients, showed that the drug was safe and well tolerated. The study also showed a dose-dependent increase in frataxin mRNA expression in peripheral blood cells, together with a decrease in heterochromatin formation in the frataxin locus during the 8 weeks of daily nicotinamide dosing (Libri et al., 2014). In addition, a phase Ib clinical trial was performed with the HDACi 109/RG2833. In this study, the drug was safe and relatively well tolerated and induced a moderate increase in frataxin mRNA expression in peripheral blood cells (Soragni et al., 2014; Soragni and Gottesfeld, 2016). However, this HDAC inhibitor has poor brain penetration, and the active molecule is converted into inactive and potentially toxic metabolic products, pointing to the need of pharmacologic optimization to improve efficacy and reduce toxicity before embarking in prolonged clinical trials (Soragni and Gottesfeld, 2016).
The screening of a chemical library of 2000 FDA-approved compounds identified resveratrol, a naturally occurring antioxidant (Li et al., 2013), as a frataxin-inducing molecule with therapeutic potential for FRDA. In this study, HeLa cells expressing FXN-EGFP were used to identify molecules that are able to induce frataxin expression (Li et al., 2013). Among the 18 compounds identified as positive hits, resveratrol was the one with the best frataxin-inducing capacity in fibroblasts and lymphoblasts from FRDA patients, and the one having lesser toxic cell effects (Li et al., 2013). An open-label, non-randomized, phase I clinical trial with resveratrol failed to increase frataxin levels in FRDA patients; however, high doses of resveratrol were associated with positive clinical outcomes (Yiu et al., 2015). In May 2019, a double-blind, placebo-controlled, 2-period crossover phase 2 trial with micronized resveratrol (Trial number: NCT03933163, https://clinicaltrials.gov/ct2/show/NCT03933163) was launched to measure the neurological impact of this drug. The trial is still ongoing.
Table 2 provides additional information about the aforementioned drugs, including pharmaceutical companies or academic institutions involved in designing and running the clinical trials, and whether the drugs received orphan designation from the EMA and/or the FDA, and the sponsors that made the orphan designation request.
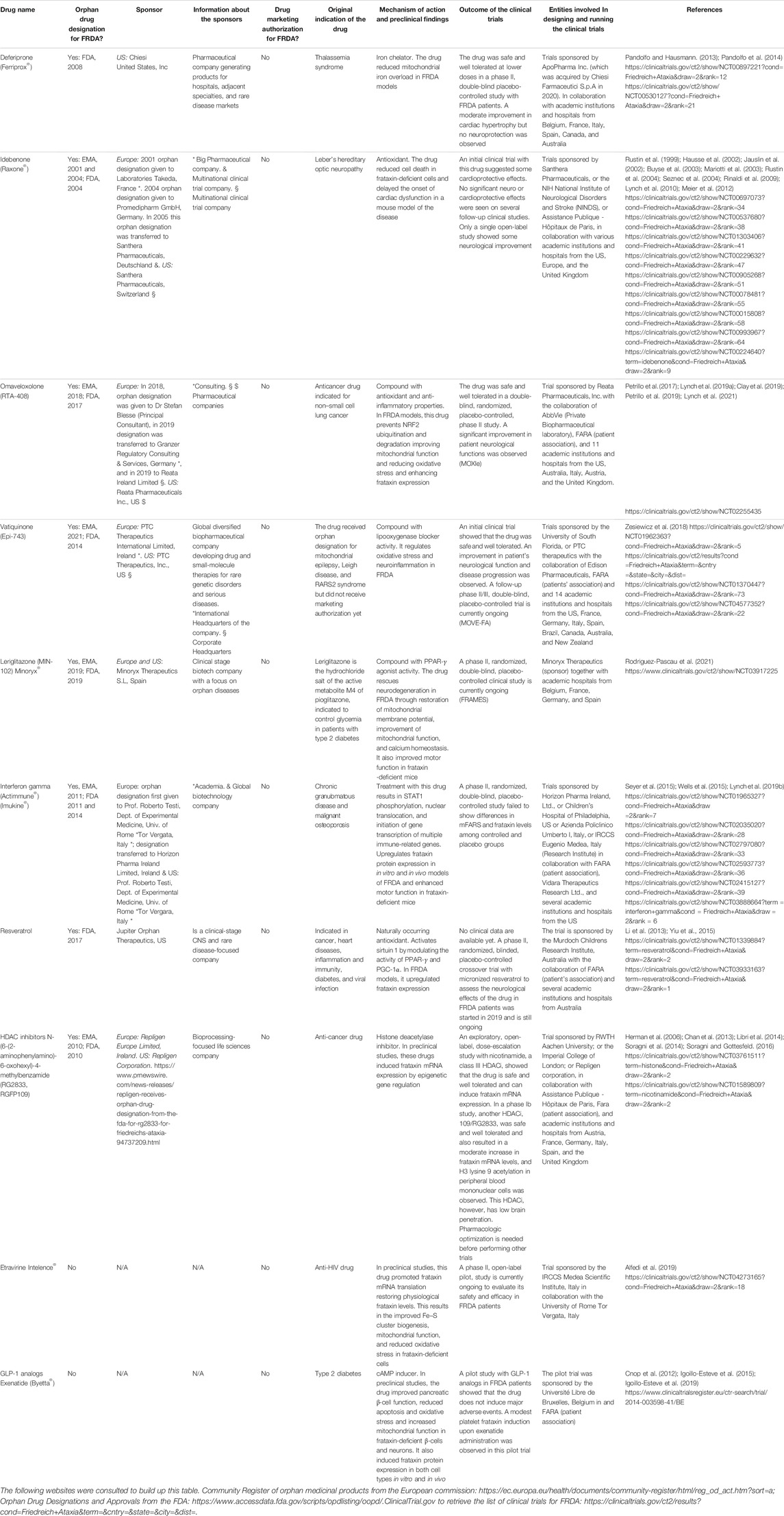
TABLE 2. List of the repurposed drugs, with and without orphan designation or drug marketing authorization for Friedreich’s ataxia (FRDA) mentioned in this article. The sponsors (entities involved in making the orphan designation request to EMA or the FDA), and the public or private organizations involved in designing and running the clinical trials are detailed. N/A = non-applicable.
Additional Drug Repurposing–Based Therapeutic Strategies Under Investigation
Etravirine, an antiviral drug currently in use as an anti-human immunodeficiency virus therapy, was identified as a potential frataxin-inducing molecule during the screening of a library of 853 US FDA–approved compounds using a high-throughput cell-based reporter assay to monitor variations in frataxin levels (Alfedi et al., 2019). Of the 853 compounds examined, 19 were able to promote at least 2-fold increase in frataxin levels. From those, etravirine was the most potent frataxin inducer in cells derived from FRDA patients (Alfedi et al., 2019). Indeed, this molecule was able to importantly induce frataxin precursor levels by selectively enhancing the translation efficiency of frataxin transcripts by promoting a shift of frataxin mRNA from silent isolated ribosomes toward translationally active polysomal subsets. This resulted in an increase in the frataxin levels to the ones present in unaffected carriers and restoration of aconitase activity (Alfedi et al., 2019). Based on these promising results, in September 2020, a phase II clinical study had been launched (Trial Number: NCT04273165, https://clinicaltrials.gov/ct2/show/NCT04273165) to evaluate the safety and efficacy of etravirine in FRDA patients.
Glucagon-like peptide 1 (GLP-1) analogs are drugs currently used for the treatment of type 2 diabetes (Vilsboll and Knop, 2008). They stimulate cAMP formation by binding to G protein-coupled receptors resulting in the activation of intracellular signaling pathways. In pancreatic β-cells, these drugs improve insulin synthesis and secretion and prevent apoptosis (Drucker et al., 1987; Yusta et al., 2006; Cunha et al., 2009). Besides being present in β-cells, GLP-1 receptors are also expressed in the heart and brain (Campbell and Drucker, 2013), and it was shown that GLP-1 analogs have cardiovascular (Ban et al., 2008) and neuroprotective actions (McClean et al., 2011). In the context of FRDA, the cAMP inducer forskolin and the GLP-1 analog exenatide were shown to reduce apoptosis in frataxin-deficient β-cells and neurons by decreasing oxidative stress and inhibiting the mitochondrial pathway of apoptosis (Cnop et al., 2012; Igoillo-Esteve et al., 2015). Besides having this protective effect, it was recently demonstrated that GLP-1 analogs and cAMP inducers also improve the functionality of pancreatic β-cells and reduce mitochondrial dysfunction in patient-derived sensory neurons (Igoillo-Esteve et al., 2019). In addition, it was demonstrated that GLP-1 analogs and cAMP inducers enhance frataxin protein expression in in vitro and in vivo FRDA models and in a pilot study with FRDA patients (Igoillo-Esteve et al., 2019). Altogether these data provide a strong rationale for the design of a long-term clinical trial to assess the disease-modifying effect of GLP-1 analogs in FRDA patients. The characteristics of these drugs have been summarized in Table 2.
Friedreich Ataxia Patient Associations and Foundations
The Friedreich’s Ataxia Research Alliance (FARA, https://www.curefa.org) is a non-profit, voluntary organization that partners with government agencies, corporations, and advocacy groups to support scientific research focused on the development of therapeutic strategies to stop the advancement of or cure FRDA.
Wolfram Syndrome
Clinical Features and Genetic Cause
Wolfram syndrome is a rare autosomal life-threatening disease with a frequency of 1/160,000 to 1/770,000 individuals in the United States and United Kingdom, respectively (Fraser and Gunn, 1977; Barrett et al., 1995). Two types of Wolfram syndrome exist that share a large number of clinical manifestations: Wolfram syndrome 1 and Wolfram syndrome 2 (Rigoli and Di Bella, 2012). The former is the most common. The majority of Wolfram syndrome 1 patients have biallelic homozygous or compound heterozygous mutations in the WFS1 gene (Inoue et al., 1998; Hardy et al., 1999; Gomez-Zaera et al., 2001; Khanim et al., 2001; Cryns et al., 2003; Hansen et al., 2005); however, autosomal dominant inherited or de novo mutations in the same gene have also been reported in some individuals (Bespalova et al., 2001; Eiberg et al., 2006; Hogewind et al., 2010; Bonnycastle et al., 2013; De Franco et al., 2017). Wolfram syndrome 2 is caused by mutations in the CISD2 gene (Rigoli and Di Bella, 2012; Mozzillo et al., 2014). Wolfram syndrome 1, also known as DIDMOAD, is characterized by non-autoimmune juvenile diabetes mellitus, diabetes insipidus, optic nerve atrophy, hearing loss, urinary tract problems, and progressive neurodegeneration that manifests principally as cerebellar ataxia, gait abnormalities, memory loss, dysphagia, speech difficulties, anxiety, and depression (Rando et al., 1992; Barrett et al., 1995; Kinsley et al., 1995; Marshall et al., 2013). Wolfram syndrome 2 patients have similar clinical manifestations with the exception that they do not develop diabetes insipidus, and they have stomach and intestine ulcers, defective platelet aggregation, and excessive bleeding (El-Shanti et al., 2000; Rigoli and Di Bella, 2012; Mozzillo et al., 2014; Rondinelli et al., 2015). Both forms of Wolfram syndrome have poor prognosis, and the patients die prematurely at a median age of 30 years due to progressive severe neurological dysfunction and respiratory failure resulting from brain stem atrophy (Urano, 2016). Both forms of the disease are progressive and exhibit a clear chronology of clinical manifestations (Figure 3).
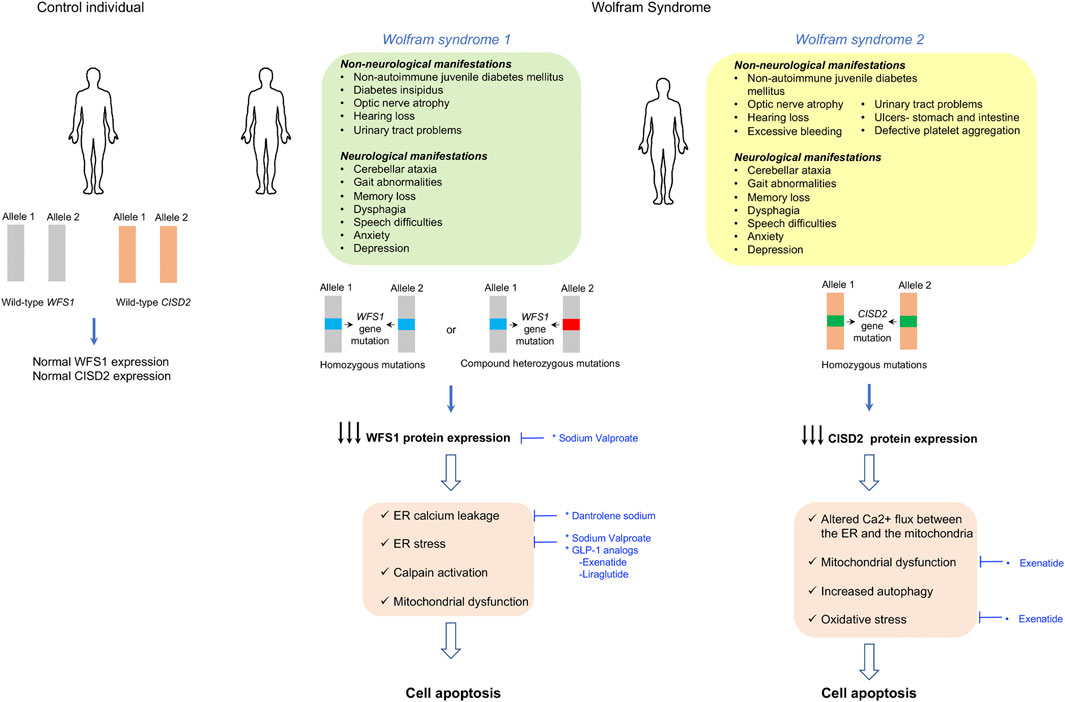
FIGURE 3. Clinical manifestations and molecular disease mechanisms in Wolfram syndrome. Wolfram syndrome 1 is caused by homozygous or heterozygous mutations in the WFS1 gene, while wolfram syndrome 2 is caused by mutations in the CISD2 gene. This results in reduced WFS1 or CISD2 protein expression, which causes several cell defects leading to cell dysfunction and apoptosis which result in the clinical manifestations of the disease. Repurposed drugs with and without orphan designation for Wolfram syndrome are highlighted in blue along with the cellular dysfunctions or clinical manifestations that they tackle. ER- endoplasmic reticulum, GLP-1- glucagon-like peptide-1.
Molecular Phenotype
The WFS1 gene encodes an endoplasmic reticulum (ER) transmembrane protein called wolframin or WFS1 that is highly expressed in the brain, pancreas, heart, lungs, liver, and kidneys (Yamada et al., 2006). The ER is an essential organelle for secretory cells, such as pancreatic β-cells and neurons, since most secretory proteins are synthesized, folded, and modified within the ER before being transported into the Golgi for secretion (Schwarz and Blower, 2016). It has been demonstrated that WFS1 deficiency causes ER stress in pancreatic β-cells, neurons, retinal ganglion cells, and oligodendrocytes, resulting in dysfunction and degeneration of the affected tissues (Ishihara et al., 2004; Fonseca et al., 2005; Riggs et al., 2005; Fonseca et al., 2010; Fonseca et al., 2011; Fischer and Ehrlich, 2020). WFS1 regulates ER calcium homeostasis and ER stress by interacting with the sarcoplasmic ER calcium (SERCA) pump and the ER stress transducer ATF6, respectively (Fonseca et al., 2005; Zatyka et al., 2008; Fonseca et al., 2009; Fonseca et al., 2010; Fonseca et al., 2011; Hatanaka et al., 2011; Lu et al., 2014; Zatyka et al., 2015). Accordingly, WFS1-deficiency results in ER calcium depletion, enhanced cytosolic Ca2+, calpain activation, and cell death (Lu et al., 2014). Moreover, it was also demonstrated that WFS1 regulates secretory granule acidification in β-cells and neurons (Gharanei et al., 2013; Sutt et al., 2015) and that the ER dysfunction caused by WFS1 deficiency is accompanied by altered mitochondrial function in β-cells and neurons contributing to diabetes and neurodegeneration (Cagalinec et al., 2016; Maxwell et al., 2020). Using a rat model of the disease, it was recently demonstrated that the lack of functional WFS1 alters calcium homeostasis in cardiac myocytes as a result of reduced expression of the plasmalemmal sodium–calcium exchanger type 1 (NCX1) (Kurekova et al., 2020).
Regarding Wolfram syndrome 2, the CISD2 gene encodes a highly conserved zinc finger Fe–S cluster containing a protein called CISD2 or Miner1. This protein is localized in the ER and in the mitochondrial-associated membranes (MAMs) (Amr et al., 2007). Its function is still unknown, but it has been proposed that it plays an important role in iron donation to the mitochondria, regulation of oxidative stress, and preservation of mitochondrial and ER Ca2+ homeostasis (Wiley et al., 2013; Wang et al., 2014). CISD2 deficiency alters the Ca2+ flux between the ER and the mitochondria resulting in mitochondrial dysfunction and reduced mitochondrial integrity, that is accompanied by an upregulation of autophagy and pro-apoptotic factors (Chen et al., 2009; Sohn et al., 2013; Danielpur et al., 2016; Holt et al., 2016). Despite the exact mechanism not being known, preclinical experiments in several Wolfram syndrome 2 cases showed that CISD2 deficiency leads to neuronal and β-cell death, a process probably mediated by calpain activation (Lu et al., 2014) (Figure 3).
There are currently no approved therapeutic options to prevent, delay, or cure Wolfram syndrome, but numerous drug repurposing–based approaches are currently under investigation to manage the clinical manifestations of the disease. Since ER stress is deleterious for pancreatic β-cells and neurons, and is a hallmark of Wolfram syndrome, it has been proposed that reducing ER stress may have beneficial outcomes in this life-threatening disease. Accordingly, different ER stress–targeting approaches are being tested, for example, ER calcium stabilizers, chemical chaperones, GLP-1 analogs, and modulators of ER stress (Urano, 2016; Abreu et al., 2021; Pallotta et al., 2019).
Approved and Non-Approved Orphan-Designated Repurposed Drugs for Wolfram Syndrome
As mentioned above, there are currently no approved drugs for the treatment of Wolfram syndrome; however, two repurposed medical compounds, namely, dantrolene sodium and sodium valproate received orphan designation for this disease (https://www.orpha.net).
Dantrolene sodium was initially approved by the FDA for malignant hyperthermia and muscle spasms derived from spinal cord injury, stroke, cerebral palsy, or multiple sclerosis. This drug acts as an ER calcium stabilizer by inhibiting ryanodine receptors on the ER (Fruen et al., 1997). In the context of Wolfram syndrome, preclinical studies using different models of the disease showed that dantrolene is able to suppress β-cell and neuronal death by preventing calcium leakage from the ER (Lu et al., 2014). This drug received orphan designation for Wolfram syndrome from EMA and the FDA. An open-label, phase Ib/IIa trial in pediatric and adult Wolfram syndrome patients with dantrolene sodium was recently performed (Abreu et al., 2021). The primary objective of the study was to assess the safety and tolerability of the molecule, and the secondary objectives were to evaluate the efficacy of the treatment in improving residual pancreatic β-cell function, visual acuity, quality of life, and measures related to vision and neurological functions. The study showed that dantrolene sodium is well tolerated by Wolfram syndrome patients, but β-cell function, visual acuity, and neurological functions were not significantly improved after 6 months of treatment (Abreu et al., 2021). However, a patient subgroup analysis revealed a significant improvement in β-cell function in subjects who possessed the greatest degree of β-cell function at the baseline. Moreover, the inflammation markers IL-1β and IL-21, that are increased in Wolfram syndrome patients as a result of ER stress (Oslowski et al., 2012), were significantly decreased in dantrolene-treated subjects (Abreu et al., 2021). These results suggest that this molecule may be beneficial in treating certain manifestations of the disease and justifies further investigation in using dantrolene sodium and other small molecules targeting the ER for the treatment of Wolfram syndrome.
Sodium valproate is a drug indicated to treat different neuropsychiatric disorders, such as epilepsy, bipolar disorder, and migraine. This molecule exerts its beneficial effects through multiple mechanisms of action (Johannessen and Johannessen, 2003; Rosenberg, 2007). In the context of Wolfram syndrome, sodium valproate was shown to increase WFS1 mRNA expression in neuronal cells by activating its promoter. Moreover, this drug was shown to enhance the dissociation of WFS1 from GRP94, suggesting that it may have ER stress–modulating effects (Kakiuchi et al., 2009). A recent study showed that sodium valproate also reduces ER stress and cell apoptosis in Wolfram syndrome 1 models caused by dominant WFS1 mutations (Batjargal et al., 2020). This drug received orphan designation for Wolfram syndrome from the EMA and the FDA. A phase II, placebo-controlled clinical trial with 70 pediatric and adult Wolfram syndrome patients has been launched (Trial Number: NCT03717909, https://clinicaltrials.gov/ct2/show/study/NCT03717909). The aim of this study is to assess the efficacy, safety, and tolerability of sodium valproate in the treatment of Wolfram syndrome patients. The primary outcome of this trial is visual acuity, and the secondary outcomes are safety, tolerability, and neurological outcomes.
Table 3 provides additional information about the aforementioned drugs, including pharmaceutical companies or academic institutions involved in designing and running the clinical trials, and whether the drugs received orphan designation from the EMA and/or the FDA, and the sponsors that made the orphan designation request.
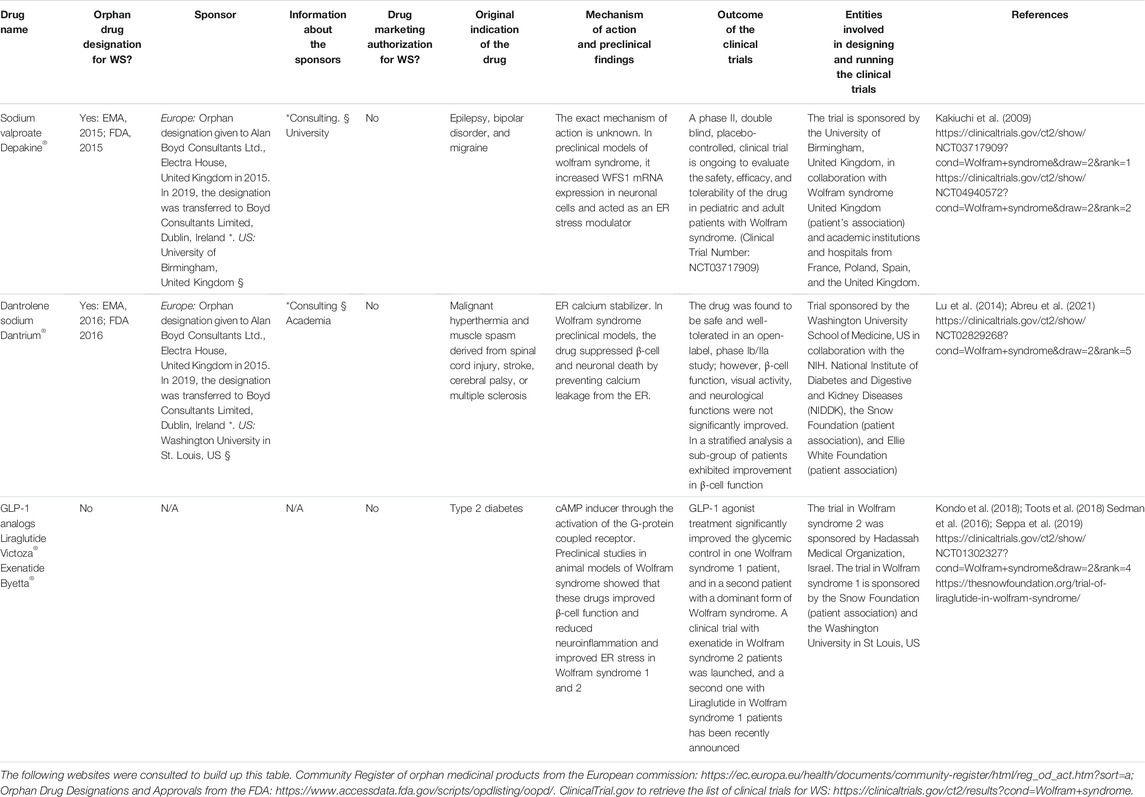
TABLE 3. List of the repurposed drugs, with and without orphan designation or drug marketing authorization for Wolfram syndrome (WS) mentioned in this article. The sponsors (entities involved in making the orphan designation request to the EMA or the FDA), and the public or private organizations involved in designing and running the clinical trials are detailed. N/A = non-applicable.
Additional Drug Repurposing–Based Therapeutic Strategies Under Investigation
GLP-1 analogs also appear as a promising therapeutic opportunity for Wolfram syndrome (Table 3). As mentioned before, these molecules are used for the treatment of type 2 diabetes and were shown to alleviate ER stress (Cunha et al., 2009; Drucker, 2018) and enhance the expression of anti-apoptotic proteins (Yusta et al., 2006; Cunha et al., 2009). Moreover, some of them cross the blood–brain barrier and confer neuroprotection (Holst et al., 2011; Hunter and Holscher, 2012; Porter et al., 2013; Zhang et al., 2018). In addition, it was shown that topical administration of GLP-1 analogs prevents retinal neurodegeneration, suggesting that these molecules may be useful to treat diabetes, neurodegeneration, and blindness in Wolfram syndrome (Hernandez et al., 2016; Hernandez et al., 2017). Accordingly, it was shown that acute exenatide injection in a mouse model of the disease enhances insulin secretion (Sedman et al., 2016) and that prolonged exenatide and liraglutide administration in WFS1-deficient mice and rats prevent glucose intolerance and improve glucose-stimulated insulin secretion by reducing cellular stress (Kondo et al., 2018; Toots et al., 2018). Additionally, a follow-up study performed with WFS1-deficient rats showed that the 6-month liraglutide treatment in these animals reduced neuroinflammation and improved ER stress in the inferior olive (Seppa et al., 2019). Moreover, this drug protected retinal ganglion cells from cell death and optic nerve axons from degeneration, suggesting that GLP-1 analogs may, indeed, be beneficial in preventing neurodegeneration and vision loss (Seppa et al., 2019). GLP-1 agonist treatment significantly improved the glycemic control in a patient with a dominant form of Wolfram syndrome, suggesting that treatment with these drugs should also be considered in patients with dominant forms of Wolfram syndrome (Scully and Wolfsdorf, 2020).
Exenatide was shown to also be beneficial in Wolfram syndrome 2. Indeed, in a β-cell model of the disease, these drugs improved glucose-stimulated insulin secretion, reduced the accumulation of labile iron in the mitochondria, and alleviated oxidative stress. Moreover, exenatide administration in one Wolfram syndrome 2 patient resulted in a 70% reduction in daily insulin requirements, improved glycemic control, and 7-fold increase in maximal insulin secretion (Danielpur et al., 2016). Altogether, these results provide evidence of the important therapeutic potential of these drugs in Wolfram syndrome. Accordingly, a clinical trial with exenatide in Wolfram syndrome 2 (Trial Number: NCT010302327) was launched, and a second one with liraglutide (Victoza®) in Wolfram syndrome 1 patients has recently been announced by Washington University with the help of the Snow Foundation (https://thesnowfoundation.org/trial-of-liraglutide-in-wolfram-syndrome/). If these drugs are shown to slowdown or revert some of the clinical manifestations of the pathology, it will constitute a great advancement in the management of this life-threatening orphan disease.
Wolfram Syndrome Patient Associations and Foundations
Many Wolfram syndrome patient associations exist that importantly contribute to fund preclinical and clinical research projects focused on the development of therapeutic opportunities for the disease. The Wolfram Syndrome Research Alliance (WSRA) (https://www.wsresearchalliance.org/foundations-supporting-ws-research.html) serves as a centralized portal to connect and coordinate the efforts of researchers, clinicians, and governmental and non-profit agencies to accelerate the development of effective treatments. This portal also provides the full list of existing Wolfram syndrome patient associations, the research groups currently working on the disease, and a pipeline of the potential therapies under study. Accordingly, different studies based on Wolfram Syndrome are funded by various patient advocacy groups. As mentioned in some examples, the clinical trial of sodium valproate (NCT03717909) initiated in October 2018, is supported by Wolfram Syndrome United Kingdom https://www.findacure.org.uk/drug-repurposing and United Kingdom Research and Innovation (UKRI) https://gtr.ukri.org/projects?ref=MR%2FP007732%2F1, the trial with dantrolene sodium, has been funded by the Snow Foundation and the Ellie White Foundation, and the Liraglutide trial (Victoza) in the United States is currently supported by the Snow Foundation https://thesnowfoundation.org/clinical-trials. The Eye Hope foundation http://www.eyehopefoundation.org/en and the Alianza de Familias Afectadas por el syndrome de wolfram https://afasw.com/have been funding research projects related with the repurposing of GLP-1 analogs for the treatment of Wolfram syndrome among others. The Association Syndrome de Wolfram (https://www.association-du-syndrome-de-wolfram.org/) organizes a family day so that families can get together and share their experiences in coping with the disease. This also provides a way for obtaining mutual support. Additionally, researchers, doctors, and psychologists are invited to provide useful information, namely, about new research and clinical trials.
Amyotrophic Lateral Sclerosis
Clinical Features and Genetic Cause
Amyotrophic Lateral Sclerosis (ALS) is a devastating, highly heterogeneous, rare motor neuron disease with an estimated prevalence in Europe of 2–3 per 100,000 individuals (Logroscino et al., 2010). The disease is characterized by progressive and selective degeneration of the upper motor neurons (projecting from the cortex to the brainstem or the spinal cord) which causes spasticity and muscle weakness and lower motor neurons (projecting from the spinal cord or the brain stem into the muscle) that causes fasciculation, cramps, and muscle wasting (Ravits et al., 2007a; Ravits et al., 2007b; Hardiman et al., 2017; Gromicho et al., 2020). Defects in neuromuscular junctions resulting in skeletal muscle denervation and progressive muscle atrophy have also been described (Cappello and Francolini, 2017). The onset of the disease usually occurs in adulthood during the sixth decade of life, and most ALS patients die from respiratory insufficiency within 2–3 years of symptom onset (Renton et al., 2014; Hardiman et al., 2017). There is currently no treatment to cure the disease. In 60% of the patients ALS has spinal onset, but in some individuals, the disease has bulbar onset that is characterized by dysarthria and dysphagia, while muscle weakness, spasticity, dysarthria, and dysphagia are the most common motor manifestations in ALS; a high proportion of the affected individuals also present cognitive and behavioral impairment (Phukan et al., 2012; Elamin et al., 2013; Al-Chalabi et al., 2017; Hardiman et al., 2017; Matilla-Duenas et al., 2017). ALS is a complex heterogeneous and multifactorial polygenic disorder with a Mendelian pattern of inheritance in 10% of the cases (familial ALS, fALS), and with no evident family history in the remaining cases (sporadic ALS, sALS) (Alsultan et al., 2016). Mostly all fALS instances are inherited in an autosomal dominant way; however, autosomal recessive and X-linked forms also exist. It has been proposed that ALS has oligogenic inheritance (meaning that a phenotypic trait is caused by mutations in more than one gene) and genetic pleiotropy (meaning that a single gene has multiple phenotypic manifestations). Indeed, mathematical models based on population-based registries suggested that ALS patients carry several risk variants that interact with environmental factors predisposing people to the disease (Hardiman et al., 2017). Accordingly, mutations in more than 50 different genes have been identified as being associated with the disease (Mejzini et al., 2019). Mutations in four of those genes, namely, C9orf72 (encoding guanine nucleotide exchange C9orf72), SOD1 (encoding superoxide dismutase 1), FUS (encoding the RNA binding protein FUS), and TARDBP (encoding TAR DNA-binding protein 43, TDP43) account for around 70% of all fALS cases (Al-Chalabi et al., 2017; Hardiman et al., 2017), and were also found to be present in 10% of the sALS cases. In the remaining cases, the actual cause of the disease remains unclear, but it has been proposed that exposure to certain environmental insults and lifestyle factors may influence the development of this disease (Bradley et al., 2013; Wang et al., 2017) (Figure 4). The full list of ALS-associated genes can be found in the study by Mejzini et al., (2019).
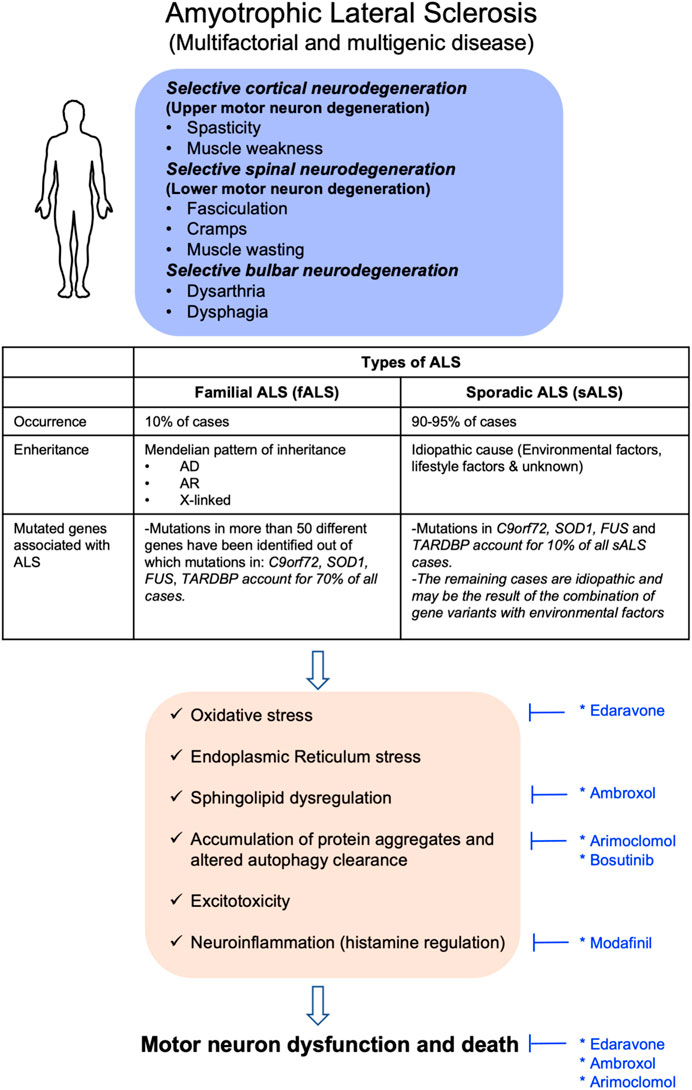
FIGURE 4. Clinical manifestations and molecular disease mechanisms in amyotrophic lateral sclerosis. This pathology may have familial origin (fALS) or sporadic origin (sALS). The first has Mendelian inheritance, while the second has mostly idiopathic causes. In both forms of ALS, diverse cellular alterations exist resulting in motor neuron dysfunction and death which cause the clinical manifestations of the disease. Repurposed drugs with and without orphan designation for ALS are highlighted in blue along with the cellular dysfunctions or clinical manifestations that they tackle. AD, autosomal dominant; AR, autosomal recessive; C9orf72, gene encoding guanine nucleotide exchange; FUS, gene encoding the RNA-binding protein FUS; SOD1, gene encoding superoxide dismutase 1; and TARDBP, gene encoding TAR DNA-binding protein 43, TDP43.
Molecular Phenotype
The pathophysiological mechanisms of the disease are not well understood; however, it is known that aggregation and accumulation of ubiquitylated protein inclusions in the cytosol of motor neurons are a neuropathological hallmark of ALS (Hardiman et al., 2017). Interestingly, in around 97% of ALS patients, these aggregates are constituted of TDP43 protein (even in the absence of TARDBP mutations). In some more rare cases, these protein aggregates are constituted of other mutated proteins, such as SOD1. Despite the protein aggregates being the hallmark of ALS, it has been proposed that the high–molecular weight protein complexes (that are present before the aggregate formation) might, in fact, be the real mediators of cell toxicity (Ross and Poirier, 2005).
Multiple factors may contribute to neuronal damage in ALS. Indeed, a large number of cellular alterations have been detected which, in most cases, are the consequence of the identified mutations. Briefly, impaired protein homeostasis and altered autophagy, aberrant RNA metabolism, increased oxidative stress, mitochondrial dysfunction, impaired DNA repair, dysregulated endosomal and vesicle transport, neuroinflammation, glial dysfunction, axonopathy, hyperexcitability, and oligodendrocyte degeneration are the main cellular disturbances detected in ALS (Rowland and Shneider, 2001; Hardiman et al., 2017; Mejzini et al., 2019) (Figure 4). For a detailed description of these mechanisms and their associated genes, refer to the articles by Hardiman et al. (2017) and Mejzini et al. (2019).
Approved and Non-Approved Orphan-Designated Repurposed Drugs for Amyotrophic Lateral Sclerosis
Currently two orphan-designated drugs received marketing authorization for the treatment of ALS. The first one, riluzole, received this authorization in the United States and Europe, while the second, edaravone, was only authorized by the FDA since the marketing authorization request to the EMA was withdrawn by the developing company in May 2019 (https://www.ema.europa.eu/en/documents/medicine-qa/questions-answers-withdrawal-marketing-authorisation-application-radicava-edaravone_fr.pdf). Riluzole is not a repurposed drug but an anti-glutamatergic compound, specifically developed for the treatment of ALS. It is shown to slow muscle strength deterioration in ALS patients and prolong their survival (Bensimon et al., 1994; Lacomblez et al., 1996a; Lacomblez et al., 1996b; Bensimon et al., 2002).
On the other hand, edaravone is a free radical scavenger that lowers neuronal damage and was initially approved in Japan for the treatment of acute cerebral infarction (Edaravone Acute Infarction Study, 2003). Administration of this molecule soon after the onset of the symptoms improved motor function and decreased SOD1 deposition in rodent ALS models (Ito et al., 2008; Aoki et al., 2011). In a phase II clinical trial with ALS patients, edaravone was relatively safe and well tolerated, and slowed the progression in motor dysfunction (Yoshino and Kimura, 2006). An initial phase III trial, however, failed to demonstrate the efficacy of edaravone to delay the disease progression (Abe et al., 2014). Nevertheless, a post hoc analysis of this trial showed that patients with early symptoms were more prone to respond to the treatment (Takei et al., 2017). Based on these results, an additional phase III clinical trial was performed in which the recruitment criteria were modified in order to work with a more clinically homogeneous patient population who were more likely to respond to the treatment (Writing and Edaravone, 2017). In this study, edaravone was shown to be effective in slowing down the decline in motor function in ALS individuals, fulfilling the criteria identified in the post hoc analysis of the previous phase III study, but not in a wider ALS population, who did not meet this criterion (Writing and Edaravone, 2017). Since all these clinical studies were performed in Japan, and the ALS genetics may vary between populations (Hardiman et al., 2017; Mejzini et al., 2019), further studies are needed to confirm the extrapolability of these findings to other ethnicities. Interestingly, a recent analysis of post-marketing clinical outcomes of edaravone in different countries showed that the drug induced a moderate reduction in disease progression in Kuwait and Korea, while no beneficial effects were reported in Italy and Israel (Ortiz et al., 2020). Further post-marketing reports on the clinical outcomes of edaravone administration are still needed to get a better view of the effectiveness of this drug. Despite riluzole and edaravone being available for the treatment of some ALS patients, there is currently no therapy that can benefit all of them. Therefore, further disease-modifying treatments are needed to handle this life-threatening disease.
Accordingly, because of preclinical and clinical research efforts, more than 30 additional repurposed and non-repurposed substances have received orphan designation for ALS without being yet authorized (https://www.orpha.net/consor/cgi-bin/index.php). Arimoclomol and ambroxol are only two examples of orphan-designated repurposed drugs.
Arimoclomol is a drug originally developed for the treatment of diabetic neuropathy and insulin resistance (Kurthy et al., 2002). This compound is a hydroxylamine derivative that works as a heat shock protein (Hsp) co-inducer in conditions of cellular stress (Hargitai et al., 2003). Treatment with this molecule results in the upregulation of several Hsps, including Hsp60, HsP70, Hsp90, and Grp94 (Vigh et al., 1997). Modulation of the Hsp response was shown to be beneficial for diseases associated with protein aggregation since it reduces the protein aggregation itself and diminishes cellular stress and apoptosis (Kalmar et al., 2005; Kalmar et al., 2014). Related with ALS, arimoclomol was shown to improve limb strength, neuron survival, and life span of SOD1G93A mice, a fALS model, while reducing the abundance of ubiquitin-positive aggregates in the motor neurons (Kieran et al., 2004; Kalmar et al., 2008). In addition, arimoclomol was shown to be safe and well tolerated in a double-blind, placebo-controlled trial performed with rapidly progressive SOD1-mutant ALS patients (Benatar et al., 2018). This initial study also suggested that the molecule could have therapeutic benefit for these patients (Benatar et al., 2018). Unfortunately, a randomized, placebo-controlled, phase III clinical trial with arimoclomol (ORARIALS-01 phase III trial, NCT03491462), that was started in 2018 and whose results were much awaited, failed to improve motor function and patient survival upon chronic treatment, as well as the ability to perform daily tasks, time to permanent assisted ventilation, and changes in lung function (defined as secondary endpoints of the study) https://alsnewstoday.com/news-posts/2021/05/11/arimoclomol-fails-phase-3-als-trial-does-not-show-efficacy-per-topline-data/).
Ambroxol, a beta-glucocerebrosidase 2 (GBA2) inhibitor originally indicated as a generic expectorant and mucolytic drug to treat respiratory tract infectious disorders (Nobata et al., 2006), has been recently found to be a potential drug candidate for ALS treatment. In a fALS transgenic mouse model, the SOD1G86R mouse, this drug delayed the disease onset, improved motor function, and rescued neuronal death by regulating the glycosphingolipid metabolism, a pathway that is importantly altered in the CNS of these mice and ALS patients (Dodge et al., 2015; Henriques et al., 2015; Henriques et al., 2017; Bouscary et al., 2019; Bouscary et al., 2020). Despite these promising results in mice, clinical trials are still needed to evaluate the safety and effectiveness of this drug in ALS patients. Table 4 provides additional information about the aforementioned drugs, including pharmaceutical companies or academic institutions involved in designing and running the clinical trials, and whether the drugs received orphan designation from the EMA and/or the FDA, and the sponsors that made the orphan designation request.
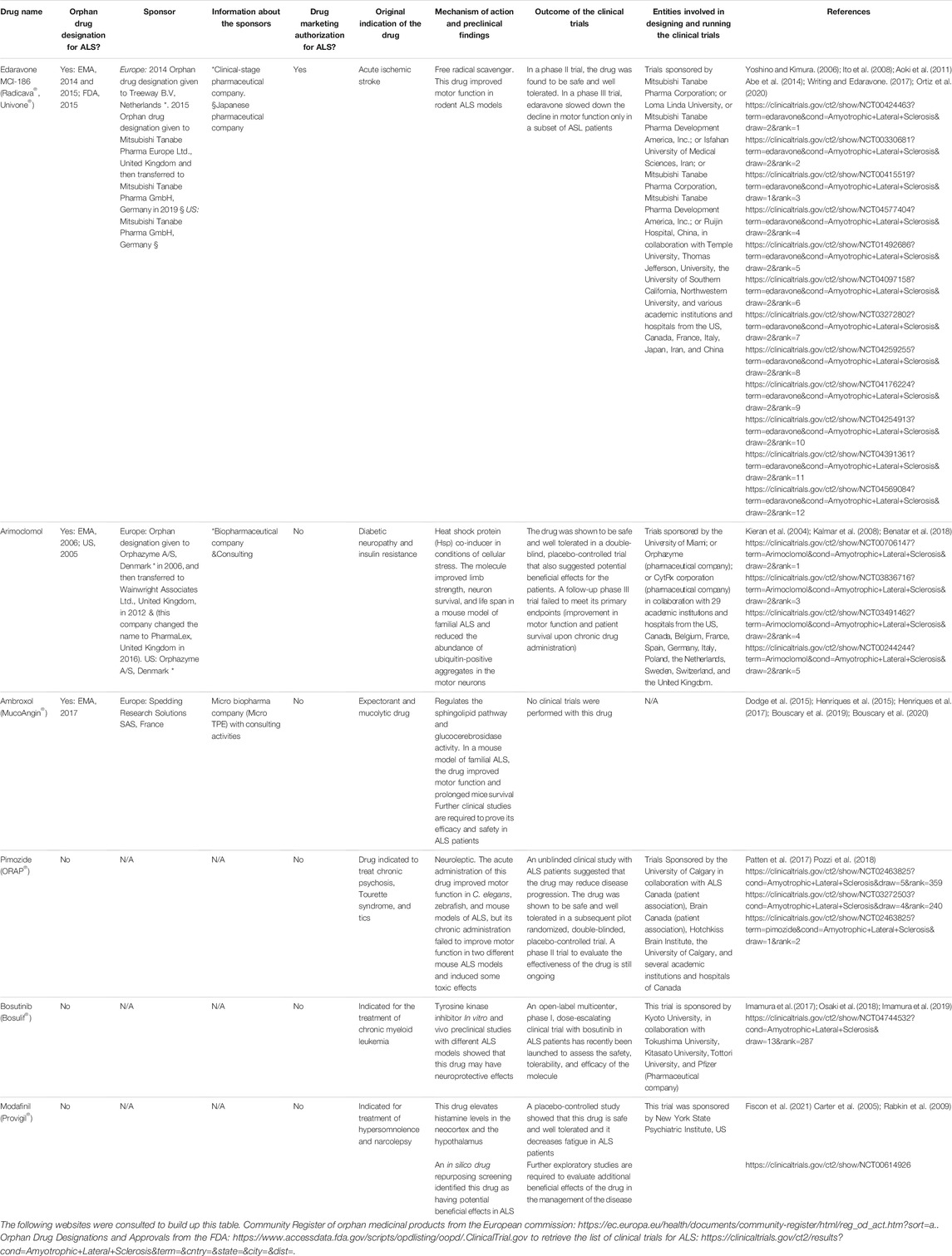
TABLE 4. List of the repurposed drugs, with and without orphan designation or drug marketing authorization for amyotrophic lateral sclerosis (ALS) mentioned in this article. The sponsors (entities involved in making the orphan designation request to the EMA or the FDA) and the public or private organizations involved in designing and running the clinical trials are detailed. N/A = non-applicable.
Additional Drug Repurposing–Based Therapeutic Strategies Under Investigation
Besides the molecules described above, a large number of additional repurposed drugs are currently being tested as potential therapeutic agents for ALS. Between them, neuroleptics have been identified as lead compounds for the management of the disease. Recently Patten et al (2017) performed a phenotypic drug screening of an approved drug compound library containing 3,850 molecules on C. elegans transgenics-expressing mutant TDP-43 (a protein mutated in ALS patients). Since the worms expressing the mutated protein exhibit paralysis phenotypes, this model allows the identification of compounds with beneficial effects in motor function. From the 24 compounds identified as potentially beneficial, 13 were neuroleptics. The positive hits were retested in worms and in zebrafish, expressing mutated TDP-43, FUS, and SOD1. These studies identified pimozide, an FDA-approved neuroleptic used to treat chronic psychosis, Tourette syndrome, and tics (Shapiro et al., 1987), as the drug with the strongest beneficial effect in motor function in these two models and a rodent model of fALS—the SOD1G37R mouse (Patten et al., 2017). Despite these promising results, a recent study with two other ALS mouse models showed that the chronic administration of pimozide does not attenuate motor and pathological deficits and, in some cases, had deleterious effects (Pozzi et al., 2018). Regarding the effect of the drug in humans, an initial clinical study analyzing the effect of pimozide in ALS patients compared to other potentially neuroprotective compounds suggested that this drug may reduce the patient’s disease progression (Szczudlik et al., 1998). Since this trial was unblinded, a factor that may importantly influence the results, two additional trials were performed: a pilot randomized, double-blinded, placebo-controlled trial with ALS patients to assess the safety and tolerability of pimozide, and phase II randomized, placebo-controlled, double-blinded, multi-centre clinical trial of pimozide in 100 ALS patients to evaluate the effectiveness of the drug in slowing ALS progression. The former showed that the drug is safe and well tolerated in doses up to 4 mg/day (Patten et al., 2017), while the latter, initiated in October 2017, has not been finished yet.
An inducible drug repurposing screening using pluripotent stem cell (iPSC)–derived motor neurons from an ALS patient with an SOD1 mutation identified bosutinib, a tyrosine kinase inhibitor indicated for the treatment of chronic myeloid leukemia, as a drug with potential therapeutic benefits for ALS (Imamura et al., 2017). This molecule was not only beneficial for the cells mentioned before but also increased the survival of iPSC-derived motor neurons from patients with sALS or other forms of fALS (Imamura et al., 2017), and the contractability of iPSC-derived skeletal muscle cells from ALS patients (Osaki et al., 2018). In addition, bosutinib modestly extended the survival of an ALS mouse model with SOD1 mutation (Imamura et al., 2017). Based on these promising findings, an open-label, multicenter, phase I, dose-escalating clinical trial with bosutinib in ALS patients (iDReAM study) has recently been designed to assess the safety, tolerability, and efficacy of the molecule (Imamura et al., 2019). No results are yet available for this trial.
Besides these efforts, in silico approaches are currently being actively used for the identification of repurposed drug candidates with potential beneficial effects in ALS. These strategies that exploit current knowledge on disease-associated genes and disease mechanisms, protein–protein interactions, signaling networks, and drug-target interactions allow to save time and resources on the identification of candidate compounds. Accordingly, Fiscon et al. (2021) exploited SAveRUNNER, a recently developed network-based algorithm for drug repurposing, which quantifies the proximity of disease-associated genes to drug targets to identify drug candidates for ALS. This approach allowed to identify 403 repurposable drugs that were strongly associated with the disease. Most of these compounds belonged to drug families already identified as having disease-modifying potential, but some were non-customary ALS drugs (Fiscon et al., 2021). Among the latter, modafinil, a compound that elevates histamine levels in the neocortex and the hypothalamus and which is currently indicated for the treatment of hypersomnolence and narcolepsy (Ishizuka et al., 2010), was identified as the drug with the highest predictive score for ALS alone or combined with other drugs that are currently being tested in clinical trials (Fiscon et al., 2021). In the past, modafinil was proposed as a drug used to treat fatigue in ALS patients. An open-label, control study and a placebo-controlled trial with modafinil showed that this drug is safe and well tolerated and may, indeed, decrease fatigue in ALS patients (Carter et al., 2005; Rabkin et al., 2009). These results and the ones from the in silico prediction study (Fiscon et al., 2021) point to modafinil as a promising drug for ALS treatment whose beneficial effect needs to be proved in further clinical trials.
The main characteristics of the drugs mentioned above are summarized in Table 4.
Amyotrophic Lateral Sclerosis Patient Associations and Foundations
There are a large number of ALS patient associations and foundations that are compiled in the North database (https://rarediseases.org/rare-diseases/amyotrophic-lateral-sclerosis/). The ALS association (https://www.als.org/), ALS Canada (https://www.als.ca/), the International Alliance of ALS/MND associations (https://www.als-mnd.org/), and ALS Liga België Vzw–Ligue SLA Belgique Asbl (https://als.be/fr/qui-sommes-nous) are just a few examples of non-profit organizations that provide assistance to people with ALS, coordinate multidisciplinary care, and fund research programs all over the word to discover treatments and a cure for ALS.
Conclusion
The four rare neurodegenerative diseases taken as example in this study clearly highlight the limited availability of approved therapies for the management of rare pathologies, harnessing the importance of drug repurposing–based approaches to fill these gaps. Indeed, drug repurposing appears to be a cost- and time saving procedure in the research and development of orphan drugs. The identification of repurposed drugs with potential therapeutic benefit involves experimental or in silico approaches that depend on a good understanding of the molecular disease mechanisms, highlighting the key role of fundamental research in the process. During the last years, the utilization of patient stem cell–based high-throughput screening approaches and in silico prediction tools such as network mapping, genome-wide association studies, (GWAS) and rare variant association studies (RVAS) significantly accelerated the identification of candidate drugs for disease treatment. However, despite these very good advances that helped identify promising drugs for the treatments of different rare diseases, including HD, FRDA, Wolfram syndrome, and ALS, there is still an important translational gap between the volume of preclinical and clinical research conducted and the number of repurposed approved drugs. This is the consequence of several factors, for example, the complexity of organizing clinical trials in rare diseases due to reduced patient number, their wide geographical distribution, their short life span, and the severity of the diseases that complicate the study design, the determination of the relevant clinical endpoints, and the ethical concerns in introducing a placebo group in the trial which, if present, may discourage the patients to participate in the study, and if absent will reduce the validity of the trial. Moreover, the important rate of late-stage failure of clinical studies, as observed for the rare neurodegenerative diseases described in this article is another important factor contributing to the reduced number of repurposed approved drugs. Indeed, HD, Wolfram syndrome, FRDA, and ALS are largely heterogenous diseases with varying severity. Clinical trials performed in large heterogenous patient cohorts often failed to demonstrate the benefits of the drugs under investigation despite very promising preclinical and pilot clinical data. This points to the need for a better clinical trial design to evaluate drug effects in specific patient cohorts as highlighted by several post hoc data analyses.
Taking all this into consideration, further collaborative initiatives between pharmaceutical companies, small- and medium-sized enterprises, academic researchers, patient associations, and regulatory authorities are needed, which not only accelerate the identification and validation of repurposed compounds but also improve the design and organization of clinical trials. Indeed, a more systematic interaction between the clinical trial organizers and the regulatory authorities in terms of scientific advice or protocol assistance appears as the key to ameliorate the trial design by defining valid endpoints, the minimum number of patients to be enrolled, and the study format (e.g., placebo-controlled trial or N-of-1 trials). All this is expected to improve the rate of the approval of new candidate compounds and accelerate their access to the affected patients.
In conclusion, drug repurposing appears as an excellent platform to accelerate drug discovery and availability of therapeutics not only for rare neurodegenerative diseases but also for other rare pathologies. This approach can be fostered by the implementation of better economic incentives by the governments in collaboration with public–private partnerships.
Author Contributions
SS, SA-G, MD, and MI-E performed the bibliographic research. SS, SA-G, and MI-E wrote the article. All the authors revised the manuscript and approved the final version. SA-G, MD, and MI-E are guarantors of this study.
Funding
This study was supported by the Brussels Region Innoviris (Bridge) project DiaType, the Eye Hope Foundation, and the Alianza de familias afectadas por el syndrome de Wolfram (AFASW).
Conflict of Interest
The authors declare that the research was conducted in the absence of any commercial or financial relationships that could be construed as a potential conflict of interest.
Publisher’s Note
All claims expressed in this article are solely those of the authors and do not necessarily represent those of their affiliated organizations, or those of the publisher, the editors, and the reviewers. Any product that may be evaluated in this article, or claim that may be made by its manufacturer, is not guaranteed or endorsed by the publisher.
References
Abe, K., Itoyama, Y., Sobue, G., Tsuji, S., Aoki, M., Doyu, M., et al. (2014). Confirmatory Double-Blind, Parallel-Group, Placebo-Controlled Study of Efficacy and Safety of Edaravone (MCI-186) in Amyotrophic Lateral Sclerosis Patients. Amyotroph. Lateral Scler. Frontotemporal Degener 15, 610–617. doi:10.3109/21678421.2014.959024
Abreu, D., Stone, S. I., Pearson, T. S., Bucelli, R. C., Simpson, A. N., Hurst, S., et al. (2021). A Phase 1b/2a Clinical Trial of Dantrolene Sodium in Patients with Wolfram Syndrome. JCI Insight. doi:10.1172/jci.insight.145188
Acemoglu, D., and Linn, J. (2004). Market Size in Innovation: Theory and Evidence from the Pharmaceutical Industry. Q. J. Econ. 119, 1049–1090. doi:10.1162/0033553041502144
Adamec, J., Rusnak, F., Owen, W. G., Naylor, S., Benson, L. M., Gacy, A. M., et al. (2000). Iron-dependent Self-Assembly of Recombinant Yeast Frataxin: Implications for Friedreich Ataxia. Am. J. Hum. Genet. 67, 549–562. doi:10.1086/303056
Adinolfi, S., Iannuzzi, C., Prischi, F., Pastore, C., Iametti, S., Martin, S. R., et al. (2009). Bacterial Frataxin CyaY Is the Gatekeeper of Iron-Sulfur Cluster Formation Catalyzed by IscS. Nat. Struct. Mol. Biol. 16, 390–396. doi:10.1038/nsmb.1579
Adinolfi, S., Trifuoggi, M., Politou, A. S., Martin, S., and Pastore, A. (2002). A Structural Approach to Understanding the Iron-Binding Properties of Phylogenetically Different Frataxins. Hum. Mol. Genet. 11, 1865–1877. doi:10.1093/hmg/11.16.1865
Al-Chalabi, A., Van Den Berg, L. H., and Veldink, J. (2017). Gene Discovery in Amyotrophic Lateral Sclerosis: Implications for Clinical Management. Nat. Rev. Neurol. 13, 96–104. doi:10.1038/nrneurol.2016.182
Al-Mahdawi, S., Pinto, R. M., Ismail, O., Varshney, D., Lymperi, S., Sandi, C., et al. (2008). The Friedreich Ataxia GAA Repeat Expansion Mutation Induces Comparable Epigenetic Changes in Human and Transgenic Mouse Brain and Heart Tissues. Hum. Mol. Genet. 17, 735–746. doi:10.1093/hmg/ddm346
Alfedi, G., Luffarelli, R., Condò, I., Pedini, G., Mannucci, L., Massaro, D. S., et al. (2019). Drug Repositioning Screening Identifies Etravirine as a Potential Therapeutic for Friedreich's Ataxia. Mov Disord. 34, 323–334. doi:10.1002/mds.27604
Alsultan, A. A., Waller, R., Heath, P. R., and Kirby, J. (2016). The Genetics of Amyotrophic Lateral Sclerosis: Current Insights. Degener Neurol. Neuromuscul. Dis. 6, 49–64. doi:10.2147/DNND.S84956
Aminoff, M. J., and Marshall, J. (1974). Treatment of Huntington's Chorea with Lithium Carbonate. A Double-Blind Trial. Lancet 1, 107–109. doi:10.1016/s0140-6736(74)92339-3
Amr, S., Heisey, C., Zhang, M., Xia, X. J., Shows, K. H., Ajlouni, K., et al. (2007). A Homozygous Mutation in a Novel Zinc-finger Protein, ERIS, Is Responsible for Wolfram Syndrome 2. Am. J. Hum. Genet. 81, 673–683. doi:10.1086/520961
Andén, N. E., Dalén, P., and Johansson, B. (1973). Baclofen and Lithium in Huntington's Chorea. Lancet 2, 93. doi:10.1016/s0140-6736(73)93285-6
Aoki, M., Warita, H., Mizuno, H., Suzuki, N., Yuki, S., and Itoyama, Y. (2011). Feasibility Study for Functional Test Battery of SOD Transgenic Rat (H46R) and Evaluation of Edaravone, a Free Radical Scavenger. Brain Res. 1382, 321–325. doi:10.1016/j.brainres.2011.01.058
Arbez, N., Roby, E., Akimov, S., Eddings, C., Ren, M., Wang, X., et al. (2019). Cysteamine Protects Neurons from Mutant Huntingtin Toxicity. J. Huntingtons Dis. 8, 129–143. doi:10.3233/JHD-180312
Aronin, N., Chase, K., Young, C., Sapp, E., Schwarz, C., Matta, N., et al. (1995). CAG Expansion Affects the Expression of Mutant Huntingtin in the Huntington's Disease Brain. Neuron 15, 1193–1201. doi:10.1016/0896-6273(95)90106-x
Ashburn, T. T., and Thor, K. B. (2004). Drug Repositioning: Identifying and Developing New Uses for Existing Drugs. Nat. Rev. Drug Discov. 3, 673–683. doi:10.1038/nrd1468
Babcock, M., De Silva, D., Oaks, R., Davis-Kaplan, S., Jiralerspong, S., Montermini, L., et al. (1997). Regulation of Mitochondrial Iron Accumulation by Yfh1p, a Putative Homolog of Frataxin. Science 276, 1709–1712. doi:10.1126/science.276.5319.1709
Bailey, C. D., and Johnson, G. V. (2006). The Protective Effects of Cystamine in the R6/2 Huntington's Disease Mouse Involve Mechanisms Other Than the Inhibition of Tissue Transglutaminase. Neurobiol. Aging 27, 871–879. doi:10.1016/j.neurobiolaging.2005.04.001
Ban, K., Noyan-Ashraf, M. H., Hoefer, J., Bolz, S. S., Drucker, D. J., and Husain, M. (2008). Cardioprotective and Vasodilatory Actions of Glucagon-like Peptide 1 Receptor Are Mediated through Both Glucagon-like Peptide 1 Receptor-dependent and -independent Pathways. Circulation 117, 2340–2350. doi:10.1161/CIRCULATIONAHA.107.739938
Barbaro, B. A., Lukacsovich, T., Agrawal, N., Burke, J., Bornemann, D. J., Purcell, J. M., et al. (2015). Comparative Study of Naturally Occurring Huntingtin Fragments in Drosophila Points to Exon 1 as the Most Pathogenic Species in Huntington's Disease. Hum. Mol. Genet. 24, 913–925. doi:10.1093/hmg/ddu504
Barrett, T. G., Bundey, S. E., and Macleod, A. F. (1995). Neurodegeneration and Diabetes: UK Nationwide Study of Wolfram (DIDMOAD) Syndrome. Lancet 346, 1458–1463. doi:10.1016/s0140-6736(95)92473-6
Batjargal, K., Tajima, T., Jimbo, E. F., and Yamagata, T. (2020). Effect of 4-phenylbutyrate and Valproate on Dominant Mutations of WFS1 Gene in Wolfram Syndrome. J. Endocrinol. Invest. 43, 1317–1325. doi:10.1007/s40618-020-01228-2
Becker, E. M., Greer, J. M., Ponka, P., and Richardson, D. R. (2002). Erythroid Differentiation and Protoporphyrin IX Down-Regulate Frataxin Expression in Friend Cells: Characterization of Frataxin Expression Compared to Molecules Involved in Iron Metabolism and Hemoglobinization. Blood 99, 3813–3822. doi:10.1182/blood.v99.10.3813
Benatar, M., Wuu, J., Andersen, P. M., Atassi, N., David, W., Cudkowicz, M., et al. (2018). Randomized, Double-Blind, Placebo-Controlled Trial of Arimoclomol in Rapidly Progressive SOD1 ALS. Neurology 90, e565–e574. doi:10.1212/WNL.0000000000004960
Bensimon, G., Lacomblez, L., Delumeau, J. C., Bejuit, R., Truffinet, P., Meininger, V., et al. (2002). A Study of Riluzole in the Treatment of Advanced Stage or Elderly Patients with Amyotrophic Lateral Sclerosis. J. Neurol. 249, 609–615. doi:10.1007/s004150200071
Bensimon, G., Lacomblez, L., and Meininger, V. (1994). A Controlled Trial of Riluzole in Amyotrophic Lateral Sclerosis. ALS/Riluzole Study Group. N. Engl. J. Med. 330, 585–591. doi:10.1056/NEJM199403033300901
Bespalova, I. N., Van Camp, G., Bom, S. J., Brown, D. J., Cryns, K., Dewan, A. T., et al. (2001). Mutations in the Wolfram Syndrome 1 Gene (WFS1) Are a Common Cause of Low Frequency Sensorineural Hearing Loss. Hum. Mol. Genet. 10, 2501–2508. doi:10.1093/hmg/10.22.2501
Björkqvist, M., Wild, E. J., Thiele, J., Silvestroni, A., Andre, R., Lahiri, N., et al. (2008). A Novel Pathogenic Pathway of Immune Activation Detectable before Clinical Onset in Huntington's Disease. J. Exp. Med. 205, 1869–1877. doi:10.1084/jem.20080178
Boguski, M. S., Mandl, K. D., and Sukhatme, V. P. (2009). Drug Discovery. Repurposing with a Difference. Science 324, 1394–1395. doi:10.1126/science10.1126/science.1169920
Bonnycastle, L. L., Chines, P. S., Hara, T., Huyghe, J. R., Swift, A. J., Heikinheimo, P., et al. (2013). Autosomal Dominant Diabetes Arising from a Wolfram Syndrome 1 Mutation. Diabetes 62, 3943–3950. doi:10.2337/db13-0571
Borrell-Pagès, M., Canals, J. M., Cordelières, F. P., Parker, J. A., Pineda, J. R., Grange, G., et al. (2006). Cystamine and Cysteamine Increase Brain Levels of BDNF in Huntington Disease via HSJ1b and Transglutaminase. J. Clin. Invest. 116, 1410–1424. doi:10.1172/JCI27607
Bouscary, A., Quessada, C., Mosbach, A., Callizot, N., Spedding, M., Loeffler, J. P., et al. (2019). Ambroxol Hydrochloride Improves Motor Functions and Extends Survival in a Mouse Model of Familial Amyotrophic Lateral Sclerosis. Front. Pharmacol. 10, 883. doi:10.3389/fphar.2019.00883
Bouscary, A., Quessada, C., René, F., Spedding, M., Henriques, A., Ngo, S., et al. (2020). Drug Repositioning in Neurodegeneration: An Overview of the Use of Ambroxol in Neurodegenerative Diseases. Eur. J. Pharmacol. 884, 173446. doi:10.1016/j.ejphar.2020.173446
Bradley, W. G., Borenstein, A. R., Nelson, L. M., Codd, G. A., Rosen, B. H., Stommel, E. W., et al. (2013). Is Exposure to Cyanobacteria an Environmental Risk Factor for Amyotrophic Lateral Sclerosis and Other Neurodegenerative Diseases? Amyotroph. Lateral Scler. Frontotemporal Degener 14, 325–333. doi:10.3109/21678421.2012.750364
Britti, E., Delaspre, F., Feldman, A., Osborne, M., Greif, H., Tamarit, J., et al. (2018). Frataxin-deficient Neurons and Mice Models of Friedreich Ataxia Are Improved by TAT-MTScs-FXN Treatment. J. Cel Mol Med 22, 834–848. doi:10.1111/jcmm.13365
Buyse, G., Mertens, L., Di Salvo, G., Matthijs, I., Weidemann, F., Eyskens, B., et al. (2003). Idebenone Treatment in Friedreich's Ataxia: Neurological, Cardiac, and Biochemical Monitoring. Neurology 60, 1679–1681. doi:10.1212/01.wnl.0000068549.52812.0f
Cagalinec, M., Liiv, M., Hodurova, Z., Hickey, M. A., Vaarmann, A., Mandel, M., et al. (2016). Role of Mitochondrial Dynamics in Neuronal Development: Mechanism for Wolfram Syndrome. Plos Biol. 14, e1002511. doi:10.1371/journal.pbio.1002511
Campbell, J. E., and Drucker, D. J. (2013). Pharmacology, Physiology, and Mechanisms of Incretin Hormone Action. Cell Metab 17, 819–837. doi:10.1016/j.cmet.2013.04.008
Campuzano, V., Montermini, L., Moltò, M. D., Pianese, L., Cossée, M., Cavalcanti, F., et al. (1996). Friedreich's Ataxia: Autosomal Recessive Disease Caused by an Intronic GAA Triplet Repeat Expansion. Science 271, 1423–1427. doi:10.1126/science.271.5254.1423
Cappello, V., and Francolini, M. (2017). Neuromuscular Junction Dismantling in Amyotrophic Lateral Sclerosis. Int. J. Mol. Sci. 18. doi:10.3390/ijms18102092
Caron, N. S., Dorsey, E. R., and Hayden, M. R. (2018). Therapeutic Approaches to Huntington Disease: from the Bench to the Clinic. Nat. Rev. Drug Discov. 17, 729–750. doi:10.1038/nrd.2018.133
Carter, G. T., Weiss, M. D., Lou, J. S., Jensen, M. P., Abresch, R. T., Martin, T. K., et al. (2005). Modafinil to Treat Fatigue in Amyotrophic Lateral Sclerosis: an Open Label Pilot Study. Am. J. Hosp. Palliat. Care 22, 55–59. doi:10.1177/104990910502200112
Cha, Y., Erez, T., Reynolds, I. J., Kumar, D., Ross, J., Koytiger, G., et al. (2018). Drug Repurposing from the Perspective of Pharmaceutical Companies. Br. J. Pharmacol. 175, 168–180. doi:10.1111/bph.13798
Chan, P. K., Torres, R., Yandim, C., Law, P. P., Khadayate, S., Mauri, M., et al. (2013). Heterochromatinization Induced by GAA-Repeat Hyperexpansion in Friedreich's Ataxia Can Be Reduced upon HDAC Inhibition by Vitamin B3. Hum. Mol. Genet. 22, 2662–2675. doi:10.1093/hmg/ddt115
Chen, Y. F., Kao, C. H., Kirby, R., and Tsai, T. F. (2009). Cisd2 Mediates Mitochondrial Integrity and Life Span in Mammals. Autophagy 5, 1043–1045. doi:10.4161/auto.5.7.9351
Chong, C. R., and Sullivan, D. J. (2007). New Uses for Old Drugs. Nature 448, 645–646. doi:10.1038/448645a
Clay, A., Hearle, P., Schadt, K., and Lynch, D. R. (2019). New Developments in Pharmacotherapy for Friedreich Ataxia. Expert Opin. Pharmacother. 20, 1855–1867. doi:10.1080/14656566.2019.1639671
Cnop, M., Igoillo-Esteve, M., Rai, M., Begu, A., Serroukh, Y., Depondt, C., et al. (2012). Central Role and Mechanisms of β-cell Dysfunction and Death in Friedreich Ataxia-Associated Diabetes. Ann. Neurol. 72, 971–982. doi:10.1002/ana.23698
Cnop, M., Mulder, H., and Igoillo-Esteve, M. (2013). Diabetes in Friedreich Ataxia. J. Neurochem. 126 Suppl 1 (Suppl. 1), 94–102. doi:10.1111/jnc.12216
Commission, E. (2021). Health and Food Safety Directorate-General, STAMP Unmet Medical Need - Revision of the Orphan and Paediatric Medicines Legislation. Brussels: European Commission.
Condò, I., Malisan, F., Guccini, I., Serio, D., Rufini, A., and Testi, R. (2010). Molecular Control of the Cytosolic aconitase/IRP1 Switch by Extramitochondrial Frataxin. Hum. Mol. Genet. 19, 1221–1229. doi:10.1093/hmg/ddp592
Cook, A., Boesch, S., Heck, S., Brunt, E., Klockgether, T., Schöls, L., et al. (2019). Patient-reported Outcomes in Friedreich's Ataxia after Withdrawal from Idebenone. Acta Neurol. Scand. 139, 533–539. doi:10.1111/ane.13088
Cryns, K., Sivakumaran, T. A., Van Den Ouweland, J. M., Pennings, R. J., Cremers, C. W., Flothmann, K., et al. (2003). Mutational Spectrum of the WFS1 Gene in Wolfram Syndrome, Nonsyndromic Hearing Impairment, Diabetes Mellitus, and Psychiatric Disease. Hum. Mutat. 22, 275–287. doi:10.1002/humu.10258
Cummings, J. L., Lyketsos, C. G., Peskind, E. R., Porsteinsson, A. P., Mintzer, J. E., Scharre, D. W., et al. (2015). Effect of Dextromethorphan-Quinidine on Agitation in Patients with Alzheimer Disease Dementia: A Randomized Clinical Trial. JAMA 314, 1242–1254. doi:10.1001/jama.2015.10214
Cunha, D. A., Ladrière, L., Ortis, F., Igoillo-Esteve, M., Gurzov, E. N., Lupi, R., et al. (2009). Glucagon-like Peptide-1 Agonists Protect Pancreatic Beta-Cells from Lipotoxic Endoplasmic Reticulum Stress through Upregulation of BiP and JunB. Diabetes 58, 2851–2862. doi:10.2337/db09-0685
D'oria, V., Petrini, S., Travaglini, L., Priori, C., Piermarini, E., Petrillo, S., et al. (2013). Frataxin Deficiency Leads to Reduced Expression and Impaired Translocation of NF-E2-Related Factor (Nrf2) in Cultured Motor Neurons. Int. J. Mol. Sci. 14, 7853–7865. doi:10.3390/ijms14047853
Dalén, P. (1973). Lithium Therapy in Huntington's Chorea and Tardive Dyskinesia. Lancet 1, 107–108. doi:10.1016/s0140-6736(73)90510-2
Danielpur, L., Sohn, Y. S., Karmi, O., Fogel, C., Zinger, A., Abu-Libdeh, A., et al. (2016). GLP-1-RA Corrects Mitochondrial Labile Iron Accumulation and Improves β-Cell Function in Type 2 Wolfram Syndrome. J. Clin. Endocrinol. Metab. 101, 3592–3599. doi:10.1210/jc.2016-2240
Danivas, V., Moily, N. S., Thimmaiah, R., Muralidharan, K., Purushotham, M., Muthane, U., et al. (2013). Off Label Use of Lithium in the Treatment of Huntington's Disease: A Case Series. Indian J. Psychiatry 55, 81–83. doi:10.4103/0019-5545.105522
De Franco, E., Flanagan, S. E., Yagi, T., Abreu, D., Mahadevan, J., Johnson, M. B., et al. (2017). Dominant ER Stress-Inducing WFS1 Mutations Underlie a Genetic Syndrome of Neonatal/Infancy-Onset Diabetes, Congenital Sensorineural Deafness, and Congenital Cataracts. Diabetes 66, 2044–2053. doi:10.2337/db16-1296
Dedeoglu, A., Kubilus, J. K., Jeitner, T. M., Matson, S. A., Bogdanov, M., Kowall, N. W., et al. (2002). Therapeutic Effects of Cystamine in a Murine Model of Huntington's Disease. J. Neurosci. 22, 8942–8950. doi:10.1523/jneurosci.22-20-08942.2002
Difiglia, M., Sapp, E., Chase, K. O., Davies, S. W., Bates, G. P., Vonsattel, J. P., et al. (1997). Aggregation of Huntingtin in Neuronal Intranuclear Inclusions and Dystrophic Neurites in Brain. Science 277, 1990–1993. doi:10.1126/science.277.5334.1990
Dimasi, J. A., Grabowski, H. G., and Hansen, R. W. (2016). Innovation in the Pharmaceutical Industry: New Estimates of R&D Costs. J. Health Econ. 47, 20–33. doi:10.1016/j.jhealeco.2016.01.012
Dinkova-Kostova, A. T., Kostov, R. V., and Kazantsev, A. G. (2018). The Role of Nrf2 Signaling in Counteracting Neurodegenerative Diseases. FEBS J. 285, 3576–3590. doi:10.1111/febs.14379
Dodge, J. C., Treleaven, C. M., Pacheco, J., Cooper, S., Bao, C., Abraham, M., et al. (2015). Glycosphingolipids Are Modulators of Disease Pathogenesis in Amyotrophic Lateral Sclerosis. Proc. Natl. Acad. Sci. U S A. 112, 8100–8105. doi:10.1073/pnas.1508767112
Drucker, D. J. (2018). Mechanisms of Action and Therapeutic Application of Glucagon-like Peptide-1. Cel Metab 27, 740–756. doi:10.1016/j.cmet.2018.03.001
Drucker, D. J., Philippe, J., Mojsov, S., Chick, W. L., and Habener, J. F. (1987). Glucagon-like Peptide I Stimulates Insulin Gene Expression and Increases Cyclic AMP Levels in a Rat Islet Cell Line. Proc. Natl. Acad. Sci. U S A. 84, 3434–3438. doi:10.1073/pnas.84.10.3434
Edaravone Acute Infarction Study, G. (2003). Effect of a Novel Free Radical Scavenger, Edaravone (MCI-186), on Acute Brain Infarction. Randomized, Placebo-Controlled, Double-Blind Study at Multicenters. Cerebrovasc. Dis. 15, 222–229. doi:10.1159/000069318
Eiberg, H., Hansen, L., Kjer, B., Hansen, T., Pedersen, O., Bille, M., et al. (2006). Autosomal Dominant Optic Atrophy Associated with Hearing Impairment and Impaired Glucose Regulation Caused by a Missense Mutation in the WFS1 Gene. J. Med. Genet. 43, 435–440. doi:10.1136/jmg.2005.034892
El-Shanti, H., Lidral, A. C., Jarrah, N., Druhan, L., and Ajlouni, K. (2000). Homozygosity Mapping Identifies an Additional Locus for Wolfram Syndrome on Chromosome 4q. Am. J. Hum. Genet. 66, 1229–1236. doi:10.1086/302858
Elamin, M., Bede, P., Byrne, S., Jordan, N., Gallagher, L., Wynne, B., et al. (2013). Cognitive Changes Predict Functional Decline in ALS: a Population-Based Longitudinal Study. Neurology 80, 1590–1597. doi:10.1212/WNL.0b013e31828f18ac
Emond, M., Lepage, G., Vanasse, M., and Pandolfo, M. (2000). Increased Levels of Plasma Malondialdehyde in Friedreich Ataxia. Neurology 55, 1752–1753. doi:10.1212/wnl.55.11.1752
Enns, G. M., Kinsman, S. L., Perlman, S. L., Spicer, K. M., Abdenur, J. E., Cohen, B. H., et al. (2012). Initial Experience in the Treatment of Inherited Mitochondrial Disease with EPI-743. Mol. Genet. Metab. 105, 91–102. doi:10.1016/j.ymgme.2011.10.009
Fernandez-Marmiesse, A., Gouveia, S., and Couce, M. L. (2018). NGS Technologies as a Turning Point in Rare Disease Research , Diagnosis and Treatment. Curr. Med. Chem. 25, 404–432. doi:10.2174/0929867324666170718101946
Fetro, C., and Scherman, D. (2020). Drug Repurposing in Rare Diseases: Myths and Reality. Therapie 75, 157–160. doi:10.1016/j.therap.2020.02.006
Finocchiaro, G., Baio, G., Micossi, P., Pozza, G., and Di Donato, S. (1988). Glucose Metabolism Alterations in Friedreich's Ataxia. Neurology 38, 1292–1296. doi:10.1212/wnl.38.8.1292
Fischer, A., Dewatripont, M., and Goldman, M. (2019). Benefit Corporation: a Path to Affordable Gene Therapies? Nat. Med. 25, 1813–1814. doi:10.1038/s41591-019-0676-z
Fischer, T. T., and Ehrlich, B. E. (2020). Wolfram Syndrome: a Monogenic Model to Study Diabetes Mellitus and Neurodegeneration. Curr. Opin. Physiol. 17, 115–123. doi:10.1016/j.cophys.2020.07.009
Fiscon, G., Conte, F., Amadio, S., Volonté, C., and Paci, P. (2021). Drug Repurposing: A Network-Based Approach to Amyotrophic Lateral Sclerosis. Neurotherapeutics. doi:10.1007/s13311-021-01064-z
Fonseca, S. G., Burcin, M., Gromada, J., and Urano, F. (2009). Endoplasmic Reticulum Stress in Beta-Cells and Development of Diabetes. Curr. Opin. Pharmacol. 9, 763–770. doi:10.1016/j.coph.2009.07.003
Fonseca, S. G., Fukuma, M., Lipson, K. L., Nguyen, L. X., Allen, J. R., Oka, Y., et al. (2005). WFS1 Is a Novel Component of the Unfolded Protein Response and Maintains Homeostasis of the Endoplasmic Reticulum in Pancreatic Beta-Cells. J. Biol. Chem. 280, 39609–39615. doi:10.1074/jbc.M507426200
Fonseca, S. G., Gromada, J., and Urano, F. (2011). Endoplasmic Reticulum Stress and Pancreatic β-cell Death. Trends Endocrinol. Metab. 22, 266–274. doi:10.1016/j.tem.2011.02.008
Fonseca, S. G., Ishigaki, S., Oslowski, C. M., Lu, S., Lipson, K. L., Ghosh, R., et al. (2010). Wolfram Syndrome 1 Gene Negatively Regulates ER Stress Signaling in Rodent and Human Cells. J. Clin. Invest. 120, 744–755. doi:10.1172/JCI39678
Frank, S., Ondo, W., Fahn, S., Hunter, C., Oakes, D., Plumb, S., et al. (2008). A Study of Chorea after Tetrabenazine Withdrawal in Patients with Huntington Disease. Clin. Neuropharmacol 31, 127–133. doi:10.1097/WNF.0b013e3180ca77ea
Frank, S. (2009). Tetrabenazine as Anti-chorea Therapy in Huntington Disease: an Open-Label Continuation Study. Huntington Study Group/TETRA-HD Investigators. BMC Neurol. 9, 62. doi:10.1186/1471-2377-9-62
Frank, S. (2014). Treatment of Huntington's Disease. Neurotherapeutics 11, 153–160. doi:10.1007/s13311-013-0244-z
Fraser, F. C., and Gunn, T. (1977). Diabetes Mellitus, Diabetes Insipidus, and Optic Atrophy. An Autosomal Recessive Syndrome? J. Med. Genet. 14, 190–193. doi:10.1136/jmg.14.3.190
Fruen, B. R., Mickelson, J. R., and Louis, C. F. (1997). Dantrolene Inhibition of Sarcoplasmic Reticulum Ca2+ Release by Direct and Specific Action at Skeletal Muscle Ryanodine Receptors. J. Biol. Chem. 272, 26965–26971. doi:10.1074/jbc.272.43.26965
Garcia-Miralles, M., Hong, X., Tan, L. J., Caron, N. S., Huang, Y., To, X. V., et al. (2016). Laquinimod Rescues Striatal, Cortical and white Matter Pathology and Results in Modest Behavioural Improvements in the YAC128 Model of Huntington Disease. Sci. Rep. 6, 31652. doi:10.1038/srep31652
Gerber, J., Mühlenhoff, U., and Lill, R. (2003). An Interaction between Frataxin and Isu1/Nfs1 that Is Crucial for Fe/S Cluster Synthesis on Isu1. EMBO Rep. 4, 906–911. doi:10.1038/sj.embor.embor918
Gharanei, S., Zatyka, M., Astuti, D., Fenton, J., Sik, A., Nagy, Z., et al. (2013). Vacuolar-type H+-ATPase V1A Subunit Is a Molecular Partner of Wolfram Syndrome 1 (WFS1) Protein, Which Regulates its Expression and Stability. Hum. Mol. Genet. 22, 203–217. doi:10.1093/hmg/dds400
Ghosh, R., and Tabrizi, S. J. (2018). Clinical Features of Huntington's Disease. Adv. Exp. Med. Biol. 1049, 1–28. doi:10.1007/978-3-319-71779-1_1
Gómez-Zaera, M., Strom, T. M., Rodríguez, B., Estivill, X., Meitinger, T., and Nunes, V. (2001). Presence of a Major WFS1 Mutation in Spanish Wolfram Syndrome Pedigrees. Mol. Genet. Metab. 72, 72–81. doi:10.1006/mgme.2000.3107
Grabowski, H. (2004). Are the Economics of Pharmaceutical Research and Development Changing? Productivity, Patents and Political Pressures. Pharmacoeconomics 22, 15–24. doi:10.2165/00019053-200422002-00003
Gromicho, M., Figueiral, M., Uysal, H., Grosskreutz, J., Kuzma-Kozakiewicz, M., Pinto, S., et al. (2020). Spreading in ALS: The Relative Impact of Upper and Lower Motor Neuron Involvement. Ann. Clin. Transl Neurol. 7, 1181–1192. doi:10.1002/acn3.51098
Halliday, G. M., Mcritchie, D. A., Macdonald, V., Double, K. L., Trent, R. J., and Mccusker, E. (1998). Regional Specificity of Brain Atrophy in Huntington's Disease. Exp. Neurol. 154, 663–672. doi:10.1006/exnr.1998.6919
Hansen, L., Eiberg, H., Barrett, T., Bek, T., Kjaersgaard, P., Tranebjaerg, L., et al. (2005). Mutation Analysis of the WFS1 Gene in Seven Danish Wolfram Syndrome Families; Four New Mutations Identified. Eur. J. Hum. Genet. 13, 1275–1284. doi:10.1038/sj.ejhg.5201491
Hardiman, O., Al-Chalabi, A., Chio, A., Corr, E. M., Logroscino, G., Robberecht, W., et al. (2017). Amyotrophic Lateral Sclerosis. Nat. Rev. Dis. Primers 3, 17071. doi:10.1038/nrdp.2017.71
Harding, A. E. (1981). Friedreich's Ataxia: a Clinical and Genetic Study of 90 Families with an Analysis of Early Diagnostic Criteria and Intrafamilial Clustering of Clinical Features. Brain 104, 589–620. doi:10.1093/brain/104.3.589
Hardy, C., Khanim, F., Torres, R., Scott-Brown, M., Seller, A., Poulton, J., et al. (1999). Clinical and Molecular Genetic Analysis of 19 Wolfram Syndrome Kindreds Demonstrating a Wide Spectrum of Mutations in WFS1. Am. J. Hum. Genet. 65, 1279–1290. doi:10.1086/302609
Hargitai, J., Lewis, H., Boros, I., Rácz, T., Fiser, A., Kurucz, I., et al. (2003). Bimoclomol, a Heat Shock Protein Co-inducer, Acts by the Prolonged Activation of Heat Shock Factor-1. Biochem. Biophys. Res. Commun. 307, 689–695. doi:10.1016/s0006-291x(03)01254-3
Hatanaka, M., Tanabe, K., Yanai, A., Ohta, Y., Kondo, M., Akiyama, M., et al. (2011). Wolfram Syndrome 1 Gene (WFS1) Product Localizes to Secretory Granules and Determines Granule Acidification in Pancreatic Beta-Cells. Hum. Mol. Genet. 20, 1274–1284. doi:10.1093/hmg/ddq568
Hausse, A. O., Aggoun, Y., Bonnet, D., Sidi, D., Munnich, A., Rötig, A., et al. (2002). Idebenone and Reduced Cardiac Hypertrophy in Friedreich's Ataxia. Heart 87, 346–349. doi:10.1136/heart.87.4.346
Henriques, A., Croixmarie, V., Bouscary, A., Mosbach, A., Keime, C., Boursier-Neyret, C., et al. (2017). Sphingolipid Metabolism Is Dysregulated at Transcriptomic and Metabolic Levels in the Spinal Cord of an Animal Model of Amyotrophic Lateral Sclerosis. Front. Mol. Neurosci. 10, 433. doi:10.3389/fnmol.2017.00433
Henriques, A., Croixmarie, V., Priestman, D. A., Rosenbohm, A., Dirrig-Grosch, S., D'ambra, E., et al. (2015). Amyotrophic Lateral Sclerosis and Denervation Alter Sphingolipids and Up-Regulate Glucosylceramide Synthase. Hum. Mol. Genet. 24, 7390–7405. doi:10.1093/hmg/ddv439
Herman, D., Jenssen, K., Burnett, R., Soragni, E., Perlman, S. L., and Gottesfeld, J. M. (2006). Histone Deacetylase Inhibitors Reverse Gene Silencing in Friedreich's Ataxia. Nat. Chem. Biol. 2, 551–558. doi:10.1038/nchembio815
Hernández, C., Bogdanov, P., Corraliza, L., García-Ramírez, M., Solà-Adell, C., Arranz, J. A., et al. (2016). Topical Administration of GLP-1 Receptor Agonists Prevents Retinal Neurodegeneration in Experimental Diabetes. Diabetes 65, 172–187. doi:10.2337/db15-0443
Hernández, C., Bogdanov, P., Solà-Adell, C., Sampedro, J., Valeri, M., Genís, X., et al. (2017). Topical Administration of DPP-IV Inhibitors Prevents Retinal Neurodegeneration in Experimental Diabetes. Diabetologia 60, 2285–2298. doi:10.1007/s00125-017-4388-y
Hogewind, B. F., Pennings, R. J., Hol, F. A., Kunst, H. P., Hoefsloot, E. H., Cruysberg, J. R., et al. (2010). Autosomal Dominant Optic Neuropathy and Sensorineual Hearing Loss Associated with a Novel Mutation of WFS1. Mol. Vis. 16, 26–35.
Holst, J. J., Burcelin, R., and Nathanson, E. (2011). Neuroprotective Properties of GLP-1: Theoretical and Practical Applications. Curr. Med. Res. Opin. 27, 547–558. doi:10.1185/03007995.2010.549466
Holt, S. H., Darash-Yahana, M., Sohn, Y. S., Song, L., Karmi, O., Tamir, S., et al. (2016). Activation of Apoptosis in NAF-1-Deficient Human Epithelial Breast Cancer Cells. J. Cel Sci 129, 155–165. doi:10.1242/jcs.178293
Hoy, S. M. (2019). Onasemnogene Abeparvovec: First Global Approval. Drugs 79, 1255–1262. doi:10.1007/s40265-019-01162-5
Hunter, K., and Hölscher, C. (2012). Drugs Developed to Treat Diabetes, Liraglutide and Lixisenatide, Cross the Blood Brain Barrier and Enhance Neurogenesis. BMC Neurosci. 13, 33. doi:10.1186/1471-2202-13-33
Huntington Study, G., Frank, S., Testa, C. M., Stamler, D., Kayson, E., Davis, C., et al. (2016). Effect of Deutetrabenazine on Chorea Among Patients with Huntington Disease: A Randomized Clinical Trial. JAMA 316, 40–50. doi:10.1001/jama.2016.8655
Huntington Study, G. (2006). Tetrabenazine as Antichorea Therapy in Huntington Disease: a Randomized Controlled Trial. Neurology 66, 366–372. doi:10.1212/01.wnl.0000198586.85250.13
Igoillo-Esteve, M., Gurgul-Convey, E., Hu, A., Romagueira Bichara Dos Santos, L., Abdulkarim, B., Chintawar, S., et al. (2015). Unveiling a Common Mechanism of Apoptosis in β-cells and Neurons in Friedreich's Ataxia. Hum. Mol. Genet. 24, 2274–2286. doi:10.1093/hmg/ddu745
Igoillo-Esteve, M., Oliveira, A. F., Cosentino, C., Fantuzzi, F., Demarez, C., Toivonen, S., et al. (2020). Exenatide Induces Frataxin Expression and Improves Mitochondrial Function in Friedreich Ataxia. JCI Insight 5. doi:10.1172/jci.insight.134221
Imamura, K., Izumi, Y., Banno, H., Uozumi, R., Morita, S., Egawa, N., et al. (2019). Induced Pluripotent Stem Cell-Based Drug Repurposing for Amyotrophic Lateral Sclerosis Medicine (iDReAM) Study: Protocol for a Phase I Dose Escalation Study of Bosutinib for Amyotrophic Lateral Sclerosis Patients. BMJ Open 9, e033131. doi:10.1136/bmjopen-2019-033131
Imamura, K., Izumi, Y., Watanabe, A., Tsukita, K., Woltjen, K., Yamamoto, T., et al. (2017). The Src/c-Abl Pathway Is a Potential Therapeutic Target in Amyotrophic Lateral Sclerosis. Sci. Transl Med. 9. doi:10.1126/scitranslmed.aaf3962
Inoue, H., Tanizawa, Y., Wasson, J., Behn, P., Kalidas, K., Bernal-Mizrachi, E., et al. (1998). A Gene Encoding a Transmembrane Protein Is Mutated in Patients with Diabetes Mellitus and Optic Atrophy (Wolfram Syndrome). Nat. Genet. 20, 143–148. doi:10.1038/2441
Ishihara, H., Takeda, S., Tamura, A., Takahashi, R., Yamaguchi, S., Takei, D., et al. (2004). Disruption of the WFS1 Gene in Mice Causes Progressive Beta-Cell Loss and Impaired Stimulus-Secretion Coupling in Insulin Secretion. Hum. Mol. Genet. 13, 1159–1170. doi:10.1093/hmg/ddh125
Ishizuka, T., Murotani, T., and Yamatodani, A. (2010). Modanifil Activates the Histaminergic System through the Orexinergic Neurons. Neurosci. Lett. 483, 193–196. doi:10.1016/j.neulet.2010.08.005
Ito, H., Wate, R., Zhang, J., Ohnishi, S., Kaneko, S., Ito, H., et al. (2008). Treatment with Edaravone, Initiated at Symptom Onset, Slows Motor Decline and Decreases SOD1 Deposition in ALS Mice. Exp. Neurol. 213, 448–455. doi:10.1016/j.expneurol.2008.07.017
Jankovic, J., and Clarence-Smith, K. (2011). Tetrabenazine for the Treatment of Chorea and Other Hyperkinetic Movement Disorders. Expert Rev. Neurother 11, 1509–1523. doi:10.1586/ern.11.149
Jauslin, M. L., Wirth, T., Meier, T., and Schoumacher, F. (2002). A Cellular Model for Friedreich Ataxia Reveals Small-Molecule Glutathione Peroxidase Mimetics as Novel Treatment Strategy. Hum. Mol. Genet. 11, 3055–3063. doi:10.1093/hmg/11.24.3055
Jayasundara, K., Hollis, A., Krahn, M., Mamdani, M., Hoch, J. S., and Grootendorst, P. (2019). Estimating the Clinical Cost of Drug Development for Orphan versus Non-orphan Drugs. Orphanet J. Rare Dis. 14, 12. doi:10.1186/s13023-018-0990-4
Johannessen, C. U., and Johannessen, S. I. (2003). Valproate: Past, Present, and Future. CNS Drug Rev. 9, 199–216. doi:10.1111/j.1527-3458.2003.tb00249.x
Kahn-Kirby, A. H., Amagata, A., Maeder, C. I., Mei, J. J., Sideris, S., Kosaka, Y., et al. (2019). Targeting Ferroptosis: A Novel Therapeutic Strategy for the Treatment of Mitochondrial Disease-Related Epilepsy. PLoS One 14, e0214250. doi:10.1371/journal.pone.0214250
Kakiuchi, C., Ishigaki, S., Oslowski, C. M., Fonseca, S. G., Kato, T., and Urano, F. (2009). Valproate, a Mood Stabilizer, Induces WFS1 Expression and Modulates its Interaction with ER Stress Protein GRP94. PLoS One 4, e4134. doi:10.1371/journal.pone.0004134
Kalmar, B., Kieran, D., and Greensmith, L. (2005). Molecular Chaperones as Therapeutic Targets in Amyotrophic Lateral Sclerosis. Biochem. Soc. Trans. 33, 551–552. doi:10.1042/BST0330551
Kalmar, B., Lu, C. H., and Greensmith, L. (2014). The Role of Heat Shock Proteins in Amyotrophic Lateral Sclerosis: The Therapeutic Potential of Arimoclomol. Pharmacol. Ther. 141, 40–54. doi:10.1016/j.pharmthera.2013.08.003
Kalmar, B., Novoselov, S., Gray, A., Cheetham, M. E., Margulis, B., and Greensmith, L. (2008). Late Stage Treatment with Arimoclomol Delays Disease Progression and Prevents Protein Aggregation in the SOD1 Mouse Model of ALS. J. Neurochem. 107, 339–350. doi:10.1111/j.1471-4159.2008.05595.x
Karpuj, M. V., Becher, M. W., and Steinman, L. (2002). Evidence for a Role for Transglutaminase in Huntington's Disease and the Potential Therapeutic Implications. Neurochem. Int. 40, 31–36. doi:10.1016/s0197-0186(01)00060-2
Kaufmann, P., Pariser, A. R., and Austin, C. (2018). From Scientific Discovery to Treatments for Rare Diseases - the View from the National Center for Advancing Translational Sciences - Office of Rare Diseases Research. Orphanet J. Rare Dis. 13, 196. doi:10.1186/s13023-018-0936-x
Kesselheim, A. S., Tan, Y. T., and Avorn, J. (2015). The Roles of Academia, Rare Diseases, and Repurposing in the Development of the Most Transformative Drugs. Health Aff. (Millwood) 34, 286–293. doi:10.1377/hlthaff.2014.1038
Khanim, F., Kirk, J., Latif, F., and Barrett, T. G. (2001). WFS1/wolframin Mutations, Wolfram Syndrome, and Associated Diseases. Hum. Mutat. 17, 357–367. doi:10.1002/humu.1110
Kieran, D., Kalmar, B., Dick, J. R., Riddoch-Contreras, J., Burnstock, G., and Greensmith, L. (2004). Treatment with Arimoclomol, a Coinducer of Heat Shock Proteins, Delays Disease Progression in ALS Mice. Nat. Med. 10, 402–405. doi:10.1038/nm1021
Kim, Y. C., Masutani, H., Yamaguchi, Y., Itoh, K., Yamamoto, M., and Yodoi, J. (2001). Hemin-induced Activation of the Thioredoxin Gene by Nrf2. A Differential Regulation of the Antioxidant Responsive Element by a Switch of its Binding Factors. J. Biol. Chem. 276, 18399–18406. doi:10.1074/jbc.M100103200
Kinsley, B. T., Swift, M., Dumont, R. H., and Swift, R. G. (1995). Morbidity and Mortality in the Wolfram Syndrome. Diabetes Care 18, 1566–1570. doi:10.2337/diacare.18.12.1566
Koeppen, A. H. (2011). Friedreich's Ataxia: Pathology, Pathogenesis, and Molecular Genetics. J. Neurol. Sci. 303, 1–12. doi:10.1016/j.jns.2011.01.010
Koeppen, A. H., Michael, S. C., Knutson, M. D., Haile, D. J., Qian, J., Levi, S., et al. (2007). The Dentate Nucleus in Friedreich's Ataxia: the Role of Iron-Responsive Proteins. Acta Neuropathol. 114, 163–173. doi:10.1007/s00401-007-0220-y
Koeppen, A. H., Ramirez, R. L., Becker, A. B., and Mazurkiewicz, J. E. (2016). Dorsal Root Ganglia in Friedreich Ataxia: Satellite Cell Proliferation and Inflammation. Acta Neuropathol. Commun. 4, 46. doi:10.1186/s40478-016-0288-5
Kondo, M., Tanabe, K., Amo-Shiinoki, K., Hatanaka, M., Morii, T., Takahashi, H., et al. (2018). Activation of GLP-1 Receptor Signalling Alleviates Cellular Stresses and Improves Beta Cell Function in a Mouse Model of Wolfram Syndrome. Diabetologia 61, 2189–2201. doi:10.1007/s00125-018-4679-y
Kureková, S., Plaas, M., and Cagalinec, M. (2020). Lack of Functional Wolframin Causes Drop in Plasmalemmal Sodium-Calcium Exchanger Type 1 Expression at Early Stage in Rat Model of Wolfram Syndrome. Gen. Physiol. Biophys. 39, 499–503. doi:10.4149/gpb_2020017
Kürthy, M., Mogyorósi, T., Nagy, K., Kukorelli, T., Jednákovits, A., Tálosi, L., et al. (2002). Effect of BRX-220 against Peripheral Neuropathy and Insulin Resistance in Diabetic Rat Models. Ann. N. Y Acad. Sci. 967, 482–489. doi:10.1111/j.1749-6632.2002.tb04306.x
Labbadia, J., and Morimoto, R. I. (2013). Huntington's Disease: Underlying Molecular Mechanisms and Emerging Concepts. Trends Biochem. Sci. 38, 378–385. doi:10.1016/j.tibs.2013.05.003
Lacomblez, L., Bensimon, G., Leigh, P. N., Guillet, P., and Meininger, V. (1996a). Dose-ranging Study of Riluzole in Amyotrophic Lateral Sclerosis. Amyotrophic Lateral Sclerosis/Riluzole Study Group II. Lancet 347, 1425–1431. doi:10.1016/s0140-6736(96)91680-3
Lacomblez, L., Bensimon, G., Leigh, P. N., Guillet, P., Powe, L., Durrleman, S., et al. (1996b). A Confirmatory Dose-Ranging Study of Riluzole in ALS. ALS/Riluzole Study Group-II. Neurology 47, S242–S250. doi:10.1212/wnl.47.6_suppl_4.242s
Lagedrost, S. J., Sutton, M. S., Cohen, M. S., Satou, G. M., Kaufman, B. D., Perlman, S. L., et al. (2011). Idebenone in Friedreich Ataxia Cardiomyopathy-Results from a 6-month Phase III Study (IONIA). Am. Heart J. 161, 639–e1. doi:10.1016/j.ahj.2010.10.038
Leitman, J., Ulrich Hartl, F., and Lederkremer, G. Z. (2013). Soluble Forms of polyQ-Expanded Huntingtin rather Than Large Aggregates Cause Endoplasmic Reticulum Stress. Nat. Commun. 4, 2753. doi:10.1038/ncomms3753
Li, L., Voullaire, L., Sandi, C., Pook, M. A., Ioannou, P. A., Delatycki, M. B., et al. (2013). Pharmacological Screening Using an FXN-EGFP Cellular Genomic Reporter Assay for the Therapy of Friedreich Ataxia. PLoS One 8, e55940. doi:10.1371/journal.pone.0055940
Libri, V., Yandim, C., Athanasopoulos, S., Loyse, N., Natisvili, T., Law, P. P., et al. (2014). Epigenetic and Neurological Effects and Safety of High-Dose Nicotinamide in Patients with Friedreich's Ataxia: an Exploratory, Open-Label, Dose-Escalation Study. Lancet 384, 504–513. doi:10.1016/S0140-6736(14)60382-2
Login, I. S., Cronin, M. J., and Macleod, R. M. (1982). Tetrabenazine Has Properties of a Dopamine Receptor Antagonist. Ann. Neurol. 12, 257–262. doi:10.1002/ana.410120308
Logroscino, G., Traynor, B. J., Hardiman, O., Chiò, A., Mitchell, D., Swingler, R. J., et al. (2010). Incidence of Amyotrophic Lateral Sclerosis in Europe. J. Neurol. Neurosurg. Psychiatry 81, 385–390. doi:10.1136/jnnp.2009.183525
Lu, S., Kanekura, K., Hara, T., Mahadevan, J., Spears, L. D., Oslowski, C. M., et al. (2014). A Calcium-dependent Protease as a Potential Therapeutic Target for Wolfram Syndrome. Proc. Natl. Acad. Sci. U S A. 111, E5292–E5301. doi:10.1073/pnas.1421055111
Lynch, D. R., Chin, M. P., Delatycki, M. B., Subramony, S. H., Corti, M., Hoyle, J. C., et al. (2021). Safety and Efficacy of Omaveloxolone in Friedreich Ataxia (MOXIe Study). Ann. Neurol. 89, 212–225. doi:10.1002/ana.25934
Lynch, D. R., Farmer, J., Hauser, L., Blair, I. A., Wang, Q. Q., Mesaros, C., et al. (2019a). Safety, Pharmacodynamics, and Potential Benefit of Omaveloxolone in Friedreich Ataxia. Ann. Clin. Transl Neurol. 6, 15–26. doi:10.1002/acn3.660
Lynch, D. R., Hauser, L., Mccormick, A., Wells, M., Dong, Y. N., Mccormack, S., et al. (2019b). Randomized, Double-Blind, Placebo-Controlled Study of Interferon-γ 1b in Friedreich Ataxia. Ann. Clin. Transl Neurol. 6, 546–553. doi:10.1002/acn3.731
Lynch, D. R., Perlman, S. L., and Meier, T. (2010). A Phase 3, Double-Blind, Placebo-Controlled Trial of Idebenone in Friedreich Ataxia. Arch. Neurol. 67, 941–947. doi:10.1001/archneurol.2010.168
Lyseng-Williamson, K. A. (2016). Idebenone: A Review in Leber's Hereditary Optic Neuropathy. Drugs 76, 805–813. doi:10.1007/s40265-016-0574-3
Mariotti, C., Solari, A., Torta, D., Marano, L., Fiorentini, C., and Di Donato, S. (2003). Idebenone Treatment in Friedreich Patients: One-Year-Long Randomized Placebo-Controlled Trial. Neurology 60, 1676–1679. doi:10.1212/01.wnl.0000055872.50364.fc
Marmolino, D. (2011). Friedreich's Ataxia: Past, Present and Future. Brain Res. Rev. 67, 311–330. doi:10.1016/j.brainresrev.2011.04.001
Marshall, B. A., Permutt, M. A., Paciorkowski, A. R., Hoekel, J., Karzon, R., Wasson, J., et al. (2013). Phenotypic Characteristics of Early Wolfram Syndrome. Orphanet J. Rare Dis. 8, 64. doi:10.1186/1750-1172-8-64
Martinez-Vicente, M., Talloczy, Z., Wong, E., Tang, G., Koga, H., Kaushik, S., et al. (2010). Cargo Recognition Failure Is Responsible for Inefficient Autophagy in Huntington's Disease. Nat. Neurosci. 13, 567–576. doi:10.1038/nn.2528
Matilla-Dueñas, A., Corral-Juan, M., Rodríguez-Palmero Seuma, A., Vilas, D., Ispierto, L., Morais, S., et al. (2017). Rare Neurodegenerative Diseases: Clinical and Genetic Update. Adv. Exp. Med. Biol. 1031, 443–496. doi:10.1007/978-3-319-67144-4_25
Mattsson, B., and Persson, S. A. (1973). Huntington's Chorea, Lithium, and G.A.B.A. Lancet 2, 684–719. doi:10.1016/s0140-6736(73)91500-610.1016/s0140-6736(73)92529-4
Maxwell, K. G., Augsornworawat, P., Velazco-Cruz, L., Kim, M. H., Asada, R., Hogrebe, N. J., et al. (2020). Gene-edited Human Stem Cell-Derived β Cells from a Patient with Monogenic Diabetes Reverse Preexisting Diabetes in Mice. Sci. Transl Med. 12. doi:10.1126/scitranslmed.aax9106
Mcclean, P. L., Parthsarathy, V., Faivre, E., and Hölscher, C. (2011). The Diabetes Drug Liraglutide Prevents Degenerative Processes in a Mouse Model of Alzheimer's Disease. J. Neurosci. 31, 6587–6594. doi:10.1523/JNEUROSCI.0529-11.2011
Mccolgan, P., and Tabrizi, S. J. (2018). Huntington's Disease: a Clinical Review. Eur. J. Neurol. 25, 24–34. doi:10.1111/ene.13413
Mccormack, M. L., Guttmann, R. P., Schumann, M., Farmer, J. M., Stolle, C. A., Campuzano, V., et al. (2000). Frataxin point Mutations in Two Patients with Friedreich's Ataxia and Unusual Clinical Features. J. Neurol. Neurosurg. Psychiatry 68, 661–664. doi:10.1136/jnnp.68.5.661
Meier, T., Perlman, S. L., Rummey, C., Coppard, N. J., and Lynch, D. R. (2012). Assessment of Neurological Efficacy of Idebenone in Pediatric Patients with Friedreich's Ataxia: Data from a 6-month Controlled Study Followed by a 12-month Open-Label Extension Study. J. Neurol. 259, 284–291. doi:10.1007/s00415-011-6174-y
Mejzini, R., Flynn, L. L., Pitout, I. L., Fletcher, S., Wilton, S. D., and Akkari, P. A. (2019). ALS Genetics, Mechanisms, and Therapeutics: Where Are We Now? Front. Neurosci. 13, 1310. doi:10.3389/fnins.2019.01310
Merkel, P. A., Manion, M., Gopal-Srivastava, R., Groft, S., Jinnah, H. A., Robertson, D., et al. (2016). The Partnership of Patient Advocacy Groups and Clinical Investigators in the Rare Diseases Clinical Research Network. Orphanet J. Rare Dis. 11, 66. doi:10.1186/s13023-016-0445-8
Mittal, S. K., and Eddy, C. (2013). The Role of Dopamine and Glutamate Modulation in Huntington Disease. Behav. Neurol. 26, 255–263. doi:10.3233/BEN-2012-120268
Morel, T., Lhoir, A., Picavet, E., Mariz, S., Sepodes, B., Llinares, J., et al. (2016). Regulatory Watch: The Orphan Drug Pipeline in Europe. Nat. Rev. Drug Discov. 15, 376. doi:10.1038/nrd.2016.96
Mozzillo, E., Delvecchio, M., Carella, M., Grandone, E., Palumbo, P., Salina, A., et al. (2014). A Novel CISD2 Intragenic Deletion, Optic Neuropathy and Platelet Aggregation Defect in Wolfram Syndrome Type 2. BMC Med. Genet. 15, 88. doi:10.1186/1471-2350-15-88
Nance, M. A. (2017). Genetics of Huntington Disease. Handb Clin. Neurol. 144, 3–14. doi:10.1016/B978-0-12-801893-4.00001-8
Naylor, S., Kauppi, D. M., and Schonfeld, J. M. (2015). Therapeutic Drug Repurposing, Repositioning and Rescue, Part II: Business Review. Drug Discov. World 16, 57–72.
Nguengang Wakap, S., Lambert, D. M., Olry, A., Rodwell, C., Gueydan, C., Lanneau, V., et al. (2020). Estimating Cumulative point Prevalence of Rare Diseases: Analysis of the Orphanet Database. Eur. J. Hum. Genet. 28, 165–173. doi:10.1038/s41431-019-0508-0
Nobata, K., Fujimura, M., Ishiura, Y., Myou, S., and Nakao, S. (2006). Ambroxol for the Prevention of Acute Upper Respiratory Disease. Clin. Exp. Med. 6, 79–83. doi:10.1007/s10238-006-0099-2
Ortiz, J. F., Khan, S. A., Salem, A., Lin, Z., Iqbal, Z., and Jahan, N. (2020). Post-marketing Experience of Edaravone in Amyotrophic Lateral Sclerosis: A Clinical Perspective and Comparison with the Clinical Trials of the Drug. Cureus 12, e10818. doi:10.7759/cureus.10818
Osaki, T., Uzel, S. G. M., and Kamm, R. D. (2018). Microphysiological 3D Model of Amyotrophic Lateral Sclerosis (ALS) from Human iPS-Derived Muscle Cells and Optogenetic Motor Neurons. Sci. Adv. 4, eaat5847. doi:10.1126/sciadv.aat5847
Oslowski, C. M., Hara, T., O'sullivan-Murphy, B., Kanekura, K., Lu, S., Hara, M., et al. (2012). Thioredoxin-interacting Protein Mediates ER Stress-Induced β Cell Death through Initiation of the Inflammasome. Cel Metab 16, 265–273. doi:10.1016/j.cmet.2012.07.005
Pagan, F., Hebron, M., Valadez, E. H., Torres-Yaghi, Y., Huang, X., Mills, R. R., et al. (2016). Nilotinib Effects in Parkinson's Disease and Dementia with Lewy Bodies. J. Parkinsons Dis. 6, 503–517. doi:10.3233/JPD-160867
Pagan, F. L., Hebron, M. L., Wilmarth, B., Torres-Yaghi, Y., Lawler, A., Mundel, E. E., et al. (2020). Nilotinib Effects on Safety, Tolerability, and Potential Biomarkers in Parkinson Disease: A Phase 2 Randomized Clinical Trial. JAMA Neurol. 77, 309–317. doi:10.1001/jamaneurol.2019.4200
Pagan, F. L., Wilmarth, B., Torres-Yaghi, Y., Hebron, M. L., Mulki, S., Ferrante, D., et al. (2021). Long-Term Safety and Clinical Effects of Nilotinib in Parkinson's Disease. Mov Disord. 36, 740–749. doi:10.1002/mds.28389
Pandolfo, M., Arpa, J., Delatycki, M. B., Le Quan Sang, K. H., Mariotti, C., Munnich, A., et al. (2014). Deferiprone in Friedreich Ataxia: a 6-month Randomized Controlled Trial. Ann. Neurol. 76, 509–521. doi:10.1002/ana.24248
Pandolfo, M. (2009). Friedreich Ataxia: the Clinical Picture. J. Neurol. 256 Suppl 1 (Suppl. 1), 3–8. doi:10.1007/s00415-009-1002-3
Pandolfo, M., and Hausmann, L. (2013). Deferiprone for the Treatment of Friedreich's Ataxia. J. Neurochem. 126 Suppl 1 (Suppl. 1), 142–146. doi:10.1111/jnc.12300
Park, K. (2019). A Review of Computational Drug Repurposing. Transl Clin. Pharmacol. 27, 59–63. doi:10.12793/tcp.2019.27.2.59
Parkinson, M. H., Boesch, S., Nachbauer, W., Mariotti, C., and Giunti, P. (2013). Clinical Features of Friedreich's Ataxia: Classical and Atypical Phenotypes. J. Neurochem. 126 Suppl 1 (Suppl. 1), 103–117. doi:10.1111/jnc.12317
Patten, S. A., Aggad, D., Martinez, J., Tremblay, E., Petrillo, J., Armstrong, G. A., et al. (2017). Neuroleptics as Therapeutic Compounds Stabilizing Neuromuscular Transmission in Amyotrophic Lateral Sclerosis. JCI Insight 2. doi:10.1172/jci.insight.97152
Paupe, V., Dassa, E. P., Goncalves, S., Auchère, F., Lönn, M., Holmgren, A., et al. (2009). Impaired Nuclear Nrf2 Translocation Undermines the Oxidative Stress Response in Friedreich Ataxia. PLoS One 4, e4253. doi:10.1371/journal.pone.0004253
Payne, R. M., and Wagner, G. R. (2012). Cardiomyopathy in Friedreich Ataxia: Clinical Findings and Research. J. Child. Neurol. 27, 1179–1186. doi:10.1177/0883073812448535
Petrillo, S., D'amico, J., La Rosa, P., Bertini, E. S., and Piemonte, F. (2019). Targeting NRF2 for the Treatment of Friedreich's Ataxia: A Comparison Among Drugs. Int. J. Mol. Sci. 20. doi:10.3390/ijms20205211
Petrillo, S., Piermarini, E., Pastore, A., Vasco, G., Schirinzi, T., Carrozzo, R., et al. (2017). Nrf2-Inducers Counteract Neurodegeneration in Frataxin-Silenced Motor Neurons: Disclosing New Therapeutic Targets for Friedreich's Ataxia. Int. J. Mol. Sci. 18. doi:10.3390/ijms18102173
Phukan, J., Elamin, M., Bede, P., Jordan, N., Gallagher, L., Byrne, S., et al. (2012). The Syndrome of Cognitive Impairment in Amyotrophic Lateral Sclerosis: a Population-Based Study. J. Neurol. Neurosurg. Psychiatry 83, 102–108. doi:10.1136/jnnp-2011-300188
Pogue, R. E., Cavalcanti, D. P., Shanker, S., Andrade, R. V., Aguiar, L. R., De Carvalho, J. L., et al. (2018). Rare Genetic Diseases: Update on Diagnosis, Treatment and Online Resources. Drug Discov. Today 23, 187–195. doi:10.1016/j.drudis.2017.11.002
Porter, W. D., Flatt, P. R., Hölscher, C., and Gault, V. A. (2013). Liraglutide Improves Hippocampal Synaptic Plasticity Associated with Increased Expression of Mash1 in Ob/ob Mice. Int. J. Obes. (Lond) 37, 678–684. doi:10.1038/ijo.2012.91
Pozzi, S., Thammisetty, S. S., and Julien, J. P. (2018). Chronic Administration of Pimozide Fails to Attenuate Motor and Pathological Deficits in Two Mouse Models of Amyotrophic Lateral Sclerosis. Neurotherapeutics 15, 715–727. doi:10.1007/s13311-018-0634-3
Puccio, H., Simon, D., Cossée, M., Criqui-Filipe, P., Tiziano, F., Melki, J., et al. (2001). Mouse Models for Friedreich Ataxia Exhibit Cardiomyopathy, Sensory Nerve Defect and Fe-S Enzyme Deficiency Followed by Intramitochondrial Iron Deposits. Nat. Genet. 27, 181–186. doi:10.1038/84818
Pushpakom, S., Iorio, F., Eyers, P. A., Escott, K. J., Hopper, S., Wells, A., et al. (2019). Drug Repurposing: Progress, Challenges and Recommendations. Nat. Rev. Drug Discov. 18, 41–58. doi:10.1038/nrd.2018.168
Rabkin, J. G., Gordon, P. H., Mcelhiney, M., Rabkin, R., Chew, S., and Mitsumoto, H. (2009). Modafinil Treatment of Fatigue in Patients with ALS: a Placebo-Controlled Study. Muscle Nerve 39, 297–303. doi:10.1002/mus.21245
Raïs Ali, S., and Tubeuf, S. (2019). (In)-Equality in the Allocation of R&D Resources for Rare Diseases. Soc. Just Res. 32, 277–317. doi:10.1007/s11211-019-00332-w
Raja, M., Soleti, F., and Bentivoglio, A. R. (2013). Lithium Treatment in Patients with Huntington Disease and Suicidal Behavior. J. Clin. Psychopharmacol. 33, 819–821. doi:10.1097/JCP.0b013e31829c9748
Raman, R., Vaitheeswaran, K., Vinita, K., and Sharma, T. (2011). Is Prevalence of Retinopathy Related to the Age of Onset of Diabetes? Sankara Nethralaya Diabetic Retinopathy Epidemiology and Molecular Genetic Report No. 5. Ophthalmic Res. 45, 36–41. doi:10.1159/000314720
Rando, T. A., Horton, J. C., and Layzer, R. B. (1992). Wolfram Syndrome: Evidence of a Diffuse Neurodegenerative Disease by Magnetic Resonance Imaging. Neurology 42, 1220–1224. doi:10.1212/wnl.42.6.1220
Ravikumar, B., Duden, R., and Rubinsztein, D. C. (2002). Aggregate-prone Proteins with Polyglutamine and Polyalanine Expansions Are Degraded by Autophagy. Hum. Mol. Genet. 11, 1107–1117. doi:10.1093/hmg/11.9.1107
Ravikumar, B., Vacher, C., Berger, Z., Davies, J. E., Luo, S., Oroz, L. G., et al. (2004). Inhibition of mTOR Induces Autophagy and Reduces Toxicity of Polyglutamine Expansions in Fly and Mouse Models of Huntington Disease. Nat. Genet. 36, 585–595. doi:10.1038/ng1362
Ravits, J., Laurie, P., Fan, Y., and Moore, D. H. (2007a). Implications of ALS Focality: Rostral-Caudal Distribution of Lower Motor Neuron Loss Postmortem. Neurology 68, 1576–1582. doi:10.1212/01.wnl.0000261045.57095.56
Ravits, J., Paul, P., and Jorg, C. (2007b). Focality of Upper and Lower Motor Neuron Degeneration at the Clinical Onset of ALS. Neurology 68, 1571–1575. doi:10.1212/01.wnl.0000260965.20021.47
Rees, S., Gribbon, P., Birmingham, K., Janzen, W. P., and Pairaudeau, G. (2016). Towards a Hit for Every Target. Nat. Rev. Drug Discov. 15, 1–2. doi:10.1038/nrd.2015.19
Reichman, M., and Simpson, P. B. (2016). Open Innovation in Early Drug Discovery: Roadmaps and Roadblocks. Drug Discov. Today 21, 779–788. doi:10.1016/j.drudis.2015.12.008
Renton, A. E., Chiò, A., and Traynor, B. J. (2014). State of Play in Amyotrophic Lateral Sclerosis Genetics. Nat. Neurosci. 17, 17–23. doi:10.1038/nn.3584
Riggs, A. C., Bernal-Mizrachi, E., Ohsugi, M., Wasson, J., Fatrai, S., Welling, C., et al. (2005). Mice Conditionally Lacking the Wolfram Gene in Pancreatic Islet Beta Cells Exhibit Diabetes as a Result of Enhanced Endoplasmic Reticulum Stress and Apoptosis. Diabetologia 48, 2313–2321. doi:10.1007/s00125-005-1947-4
Rigoli, L., and Di Bella, C. (2012). Wolfram Syndrome 1 and Wolfram Syndrome 2. Curr. Opin. Pediatr. 24, 512–517. doi:10.1097/MOP.0b013e328354ccdf
Rinaldi, C., Tucci, T., Maione, S., Giunta, A., De Michele, G., and Filla, A. (2009). Low-dose Idebenone Treatment in Friedreich's Ataxia with and without Cardiac Hypertrophy. J. Neurol. 256, 1434–1437. doi:10.1007/s00415-009-5130-6
Rodríguez-Pascau, L., Britti, E., Calap-Quintana, P., Dong, Y. N., Vergara, C., Delaspre, F., et al. (2021). PPAR Gamma Agonist Leriglitazone Improves Frataxin-Loss Impairments in Cellular and Animal Models of Friedreich Ataxia. Neurobiol. Dis. 148, 105162. doi:10.1016/j.nbd.2020.105162
Rondinelli, M., Novara, F., Calcaterra, V., Zuffardi, O., and Genovese, S. (2015). Wolfram Syndrome 2: a Novel CISD2 Mutation Identified in Italian Siblings. Acta Diabetol. 52, 175–178. doi:10.1007/s00592-014-0648-1
Rosenberg, G. (2007). The Mechanisms of Action of Valproate in Neuropsychiatric Disorders: Can We See the forest for the Trees? Cell Mol Life Sci 64, 2090–2103. doi:10.1007/s00018-007-7079-x
Ross, C. A., and Poirier, M. A. (2005). Opinion: What Is the Role of Protein Aggregation in Neurodegeneration? Nat. Rev. Mol. Cel Biol 6, 891–898. doi:10.1038/nrm1742
Rötig, A., De Lonlay, P., Chretien, D., Foury, F., Koenig, M., Sidi, D., et al. (1997). Aconitase and Mitochondrial Iron-sulphur Protein Deficiency in Friedreich Ataxia. Nat. Genet. 17, 215–217. doi:10.1038/ng1097-215
Rowland, L. P., and Shneider, N. A. (2001). Amyotrophic Lateral Sclerosis. N. Engl. J. Med. 344, 1688–1700. doi:10.1056/NEJM200105313442207
Rustin, P., Bonnet, D., Rötig, A., Munnich, A., and Sidi, D. (2004). Idebenone Treatment in Friedreich Patients: One-Year-Long Randomized Placebo-Controlled Trial. Neurology 62, 524–525. doi:10.1212/wnl.62.3.524
Rustin, P., Von Kleist-Retzow, J. C., Chantrel-Groussard, K., Sidi, D., Munnich, A., and Rötig, A. (1999). Effect of Idebenone on Cardiomyopathy in Friedreich's Ataxia: a Preliminary Study. Lancet 354, 477–479. doi:10.1016/S0140-6736(99)01341-0
Sahdeo, S., Scott, B. D., Mcmackin, M. Z., Jasoliya, M., Brown, B., Wulff, H., et al. (2014). Dyclonine Rescues Frataxin Deficiency in Animal Models and Buccal Cells of Patients with Friedreich's Ataxia. Hum. Mol. Genet. 23, 6848–6862. doi:10.1093/hmg/ddu408
Sarkar, S., Davies, J. E., Huang, Z., Tunnacliffe, A., and Rubinsztein, D. C. (2007a). Trehalose, a Novel mTOR-independent Autophagy Enhancer, Accelerates the Clearance of Mutant Huntingtin and Alpha-Synuclein. J. Biol. Chem. 282, 5641–5652. doi:10.1074/jbc.M609532200
Sarkar, S., Floto, R. A., Berger, Z., Imarisio, S., Cordenier, A., Pasco, M., et al. (2005). Lithium Induces Autophagy by Inhibiting Inositol Monophosphatase. J. Cel Biol 170, 1101–1111. doi:10.1083/jcb.200504035
Sarkar, S., Perlstein, E. O., Imarisio, S., Pineau, S., Cordenier, A., Maglathlin, R. L., et al. (2007b). Small Molecules Enhance Autophagy and Reduce Toxicity in Huntington's Disease Models. Nat. Chem. Biol. 3, 331–338. doi:10.1038/nchembio883
Saudou, F., Finkbeiner, S., Devys, D., and Greenberg, M. E. (1998). Huntingtin Acts in the Nucleus to Induce Apoptosis but Death Does Not Correlate with the Formation of Intranuclear Inclusions. Cell 95, 55–66. doi:10.1016/s0092-8674(00)81782-1
Saveliev, A., Everett, C., Sharpe, T., Webster, Z., and Festenstein, R. (2003). DNA Triplet Repeats Mediate Heterochromatin-Protein-1-Sensitive Variegated Gene Silencing. Nature 422, 909–913. doi:10.1038/nature01596
Scheuing, L., Chiu, C. T., Liao, H. M., Linares, G. R., and Chuang, D. M. (2014). Preclinical and Clinical Investigations of Mood Stabilizers for Huntington's Disease: what Have We Learned? Int. J. Biol. Sci. 10, 1024–1038. doi:10.7150/ijbs.9898
Schoenle, E. J., Boltshauser, E. J., Baekkeskov, S., Landin Olsson, M., Torresani, T., and Von Felten, A. (1989). Preclinical and Manifest Diabetes Mellitus in Young Patients with Friedreich's Ataxia: No Evidence of Immune Process behind the Islet Cell Destruction. Diabetologia 32, 378–381. doi:10.1007/BF00277262
Schulz, J. B., Dehmer, T., Schöls, L., Mende, H., Hardt, C., Vorgerd, M., et al. (2000). Oxidative Stress in Patients with Friedreich Ataxia. Neurology 55, 1719–1721. doi:10.1212/wnl.55.11.1719
Schwarz, D. S., and Blower, M. D. (2016). The Endoplasmic Reticulum: Structure, Function and Response to Cellular Signaling. Cel Mol Life Sci 73, 79–94. doi:10.1007/s00018-015-2052-6
Scully, K. J., and Wolfsdorf, J. I. (2020). Efficacy of GLP-1 Agonist Therapy in Autosomal Dominant WFS1-Related Disorder: A Case Report. Horm. Res. Paediatr. 93, 1–6. doi:10.1159/000510852
Sedman, T., Rünkorg, K., Krass, M., Luuk, H., Plaas, M., Vasar, E., et al. (2016). Exenatide Is an Effective Antihyperglycaemic Agent in a Mouse Model of Wolfram Syndrome 1. J. Diabetes Res. 2016, 9239530. doi:10.1155/2016/9239530
Selvadurai, L. P., Harding, I. H., Corben, L. A., and Georgiou-Karistianis, N. (2018). Cerebral Abnormalities in Friedreich Ataxia: A Review. Neurosci. Biobehav Rev. 84, 394–406. doi:10.1016/j.neubiorev.2017.08.006
Seppa, K., Toots, M., Reimets, R., Jagomäe, T., Koppel, T., Pallase, M., et al. (2019). GLP-1 Receptor Agonist Liraglutide Has a Neuroprotective Effect on an Aged Rat Model of Wolfram Syndrome. Sci. Rep. 9, 15742. doi:10.1038/s41598-019-52295-2
Seyer, L., Greeley, N., Foerster, D., Strawser, C., Gelbard, S., Dong, Y., et al. (2015). Open-label Pilot Study of Interferon Gamma-1b in Friedreich Ataxia. Acta Neurol. Scand. 132, 7–15. doi:10.1111/ane.12337
Seznec, H., Simon, D., Monassier, L., Criqui-Filipe, P., Gansmuller, A., Rustin, P., et al. (2004). Idebenone Delays the Onset of Cardiac Functional Alteration without Correction of Fe-S Enzymes Deficit in a Mouse Model for Friedreich Ataxia. Hum. Mol. Genet. 13, 1017–1024. doi:10.1093/hmg/ddh114
Shan, Y., Schoenfeld, R. A., Hayashi, G., Napoli, E., Akiyama, T., Iodi Carstens, M., et al. (2013). Frataxin Deficiency Leads to Defects in Expression of Antioxidants and Nrf2 Expression in Dorsal Root Ganglia of the Friedreich's Ataxia YG8R Mouse Model. Antioxid. Redox Signal. 19, 1481–1493. doi:10.1089/ars.2012.4537
Shapiro, A. K., Shapiro, E., and Fulop, G. (1987). Pimozide Treatment of Tic and Tourette Disorders. Pediatrics 79, 1032–1039.
Siddiqi, F. H., Menzies, F. M., Lopez, A., Stamatakou, E., Karabiyik, C., Ureshino, R., et al. (2019). Felodipine Induces Autophagy in Mouse Brains with Pharmacokinetics Amenable to Repurposing. Nat. Commun. 10, 1817. doi:10.1038/s41467-019-09494-2
Silva, A. M., Brown, J. M., Buckle, V. J., Wade-Martins, R., and Lufino, M. M. (2015). Expanded GAA Repeats Impair FXN Gene Expression and Reposition the FXN Locus to the Nuclear Lamina in Single Cells. Hum. Mol. Genet. 24, 3457–3471. doi:10.1093/hmg/ddv096
Simpson, P. B., and Reichman, M. (2014). Opening the lead Generation Toolbox. Nat. Rev. Drug Discov. 13, 3–4. doi:10.1038/nrd4202
Sleigh, S. H., and Barton, C. L. (2010a). Realizing the Potential of Therapeutic Stem Cells with Effective Delivery. Ther. Deliv. 1, 11–15. doi:10.4155/tde.10.12
Sleigh, S. H., and Barton, C. L. (2010b). Repurposing Strategies for Therapeutics. Pharm. Med. 24, 151–159. doi:10.1007/BF03256811
Slow, E. J., Graham, R. K., Osmand, A. P., Devon, R. S., Lu, G., Deng, Y., et al. (2005). Absence of Behavioral Abnormalities and Neurodegeneration In Vivo Despite Widespread Neuronal Huntingtin Inclusions. Proc. Natl. Acad. Sci. U S A. 102, 11402–11407. doi:10.1073/pnas.0503634102
Slow, E. J., Van Raamsdonk, J., Rogers, D., Coleman, S. H., Graham, R. K., Deng, Y., et al. (2003). Selective Striatal Neuronal Loss in a YAC128 Mouse Model of Huntington Disease. Hum. Mol. Genet. 12, 1555–1567. doi:10.1093/hmg/ddg169
Snell, R. G., Macmillan, J. C., Cheadle, J. P., Fenton, I., Lazarou, L. P., Davies, P., et al. (1993). Relationship between Trinucleotide Repeat Expansion and Phenotypic Variation in Huntington's Disease. Nat. Genet. 4, 393–397. doi:10.1038/ng0893-393
Sohn, Y. S., Tamir, S., Song, L., Michaeli, D., Matouk, I., Conlan, A. R., et al. (2013). NAF-1 and mitoNEET Are central to Human Breast Cancer Proliferation by Maintaining Mitochondrial Homeostasis and Promoting Tumor Growth. Proc. Natl. Acad. Sci. U S A. 110, 14676–14681. doi:10.1073/pnas.1313198110
Soragni, E., and Gottesfeld, J. M. (2016). Translating HDAC Inhibitors in Friedreich's Ataxia. Expert Opin. Orphan Drugs 4, 961–970. doi:10.1080/21678707.2016.1215910
Soragni, E., Miao, W., Iudicello, M., Jacoby, D., De Mercanti, S., Clerico, M., et al. (2014). Epigenetic Therapy for Friedreich Ataxia. Ann. Neurol. 76, 489–508. doi:10.1002/ana.24260
Stevens, A. J., Jensen, J. J., Wyller, K., Kilgore, P. C., Chatterjee, S., and Rohrbaugh, M. L. (2011). The Role of Public-Sector Research in the Discovery of Drugs and Vaccines. N. Engl. J. Med. 364, 535–541. doi:10.1056/NEJMsa1008268
Sütt, S., Altpere, A., Reimets, R., Visnapuu, T., Loomets, M., Raud, S., et al. (2015). Wfs1-deficient Animals Have Brain-region-specific Changes of Na+, K+-ATPase Activity and mRNA Expression of α1 and β1 Subunits. J. Neurosci. Res. 93, 530–537. doi:10.1002/jnr.23508
Szczudlik, A., Tomik, B., Słowik, A., and Kasprzyk, K. (1998). Assessment of the Efficacy of Treatment with Pimozide in Patients with Amyotrophic Lateral Sclerosis. Introductory Notes. Neurol. Neurochir Pol. 32, 821–829.
Takei, K., Takahashi, F., Liu, S., Tsuda, K., and Palumbo, J. (2017). Post-hoc Analysis of Randomised, Placebo-Controlled, Double-Blind Study (MCI186-19) of Edaravone (MCI-186) in Amyotrophic Lateral Sclerosis. Amyotroph. Lateral Scler. Frontotemporal Degener 18, 49–54. doi:10.1080/21678421.2017.1361443
Toots, M., Seppa, K., Jagomäe, T., Koppel, T., Pallase, M., Heinla, I., et al. (2018). Preventive Treatment with Liraglutide Protects against Development of Glucose Intolerance in a Rat Model of Wolfram Syndrome. Sci. Rep. 8, 10183. doi:10.1038/s41598-018-28314-z
Trottier, Y., Devys, D., Imbert, G., Saudou, F., An, I., Lutz, Y., et al. (1995). Cellular Localization of the Huntington's Disease Protein and Discrimination of the normal and Mutated Form. Nat. Genet. 10, 104–110. doi:10.1038/ng0595-104
Tsai, C. L., and Barondeau, D. P. (2010). Human Frataxin Is an Allosteric Switch that Activates the Fe-S Cluster Biosynthetic Complex. Biochemistry 49, 9132–9139. doi:10.1021/bi1013062
Unti, E., Mazzucchi, S., Palermo, G., Bonuccelli, U., and Ceravolo, R. (2017). Antipsychotic Drugs in Huntington's Disease. Expert Rev. Neurother 17, 227–237. doi:10.1080/14737175.2016.1226134
Urano, F. (2016). Wolfram Syndrome: Diagnosis, Management, and Treatment. Curr. Diab Rep. 16, 6. doi:10.1007/s11892-015-0702-6
Van Raamsdonk, J. M., Pearson, J., Bailey, C. D., Rogers, D. A., Johnson, G. V., Hayden, M. R., et al. (2005). Cystamine Treatment Is Neuroprotective in the YAC128 Mouse Model of Huntington Disease. J. Neurochem. 95, 210–220. doi:10.1111/j.1471-4159.2005.03357.x
Vankan, P. (2013). Prevalence Gradients of Friedreich's Ataxia and R1b Haplotype in Europe Co-localize, Suggesting a Common Palaeolithic Origin in the Franco-Cantabrian Ice Age Refuge. J. Neurochem. 126 Suppl 1 (Suppl. 1), 11–20. doi:10.1111/jnc.12215
Verbaanderd, C., Rooman, I., Meheus, L., and Huys, I. (2019). On-Label or Off-Label? Overcoming Regulatory and Financial Barriers to Bring Repurposed Medicines to Cancer Patients. Front. Pharmacol. 10, 1664. doi:10.3389/fphar.2019.01664
Verny, C., Bachoud-Lévi, A. C., Durr, A., Goizet, C., Azulay, J. P., Simonin, C., et al. (2017). A Randomized, Double-Blind, Placebo-Controlled Trial Evaluating Cysteamine in Huntington's Disease. Mov Disord. 32, 932–936. doi:10.1002/mds.27010
Vígh, L., Literáti, P. N., Horváth, I., Török, Z., Balogh, G., Glatz, A., et al. (1997). Bimoclomol: a Nontoxic, Hydroxylamine Derivative with Stress Protein-Inducing Activity and Cytoprotective Effects. Nat. Med. 3, 1150–1154. doi:10.1038/nm1097-1150
Vilsbøll, T., and Knop, F. K. (2008). Long-acting GLP-1 Analogs for the Treatment of Type 2 Diabetes Mellitus. BioDrugs 22, 251–257. doi:10.2165/00063030-200822040-00004
Wang, C. H., Kao, C. H., Chen, Y. F., Wei, Y. H., and Tsai, T. F. (2014). Cisd2 Mediates Lifespan: Is There an Interconnection Among Ca²⁺ Homeostasis, Autophagy, and Lifespan? Free Radic. Res. 48, 1109–1114. doi:10.3109/10715762.2014.936431
Wang, M. D., Little, J., Gomes, J., Cashman, N. R., and Krewski, D. (2017). Identification of Risk Factors Associated with Onset and Progression of Amyotrophic Lateral Sclerosis Using Systematic Review and Meta-Analysis. Neurotoxicology 61, 101–130. doi:10.1016/j.neuro.2016.06.015
Wästfelt, M., Fadeel, B., and Henter, J. I. (2006). A Journey of hope: Lessons Learned from Studies on Rare Diseases and Orphan Drugs. J. Intern. Med. 260, 1–10. doi:10.1111/j.1365-2796.2006.01666.x
Wells, M., Seyer, L., Schadt, K., and Lynch, D. R. (2015). IFN-γ for Friedreich Ataxia: Present Evidence. Neurodegener Dis. Manag. 5, 497–504. doi:10.2217/nmt.15.52
Wiley, S. E., Andreyev, A. Y., Divakaruni, A. S., Karisch, R., Perkins, G., Wall, E. A., et al. (2013). Wolfram Syndrome Protein, Miner1, Regulates Sulphydryl Redox Status, the Unfolded Protein Response, and Ca2+ Homeostasis. EMBO Mol. Med. 5, 904–918. doi:10.1002/emmm.201201429
Williams, A., Sarkar, S., Cuddon, P., Ttofi, E. K., Saiki, S., Siddiqi, F. H., et al. (2008). Novel Targets for Huntington's Disease in an mTOR-independent Autophagy Pathway. Nat. Chem. Biol. 4, 295–305. doi:10.1038/nchembio.79
Wouters, O. J., Mckee, M., and Luyten, J. (2020). Estimated Research and Development Investment Needed to Bring a New Medicine to Market, 2009-2018. JAMA 323, 844–853. doi:10.1001/jama.2020.1166
Writing, G., and Edaravone, A. L. S. S. G. (2017). Safety and Efficacy of Edaravone in Well Defined Patients with Amyotrophic Lateral Sclerosis: a Randomised, Double-Blind, Placebo-Controlled Trial. Lancet Neurol. 16, 505–512. doi:10.1016/S1474-4422(17)30115-1
Yamada, T., Ishihara, H., Tamura, A., Takahashi, R., Yamaguchi, S., Takei, D., et al. (2006). WFS1-deficiency Increases Endoplasmic Reticulum Stress, Impairs Cell Cycle Progression and Triggers the Apoptotic Pathway Specifically in Pancreatic Beta-Cells. Hum. Mol. Genet. 15, 1600–1609. doi:10.1093/hmg/ddl081
Yatham, L. N., Kennedy, S. H., Parikh, S. V., Schaffer, A., Bond, D. J., Frey, B. N., et al. (2018). Canadian Network for Mood and Anxiety Treatments (CANMAT) and International Society for Bipolar Disorders (ISBD) 2018 Guidelines for the Management of Patients with Bipolar Disorder. Bipolar Disord. 20, 97–170. doi:10.1111/bdi.12609
Yoon, T., and Cowan, J. A. (2004). Frataxin-mediated Iron Delivery to Ferrochelatase in the Final Step of Heme Biosynthesis. J. Biol. Chem. 279, 25943–25946. doi:10.1074/jbc.C400107200
Yoon, T., and Cowan, J. A. (2003). Iron-sulfur Cluster Biosynthesis. Characterization of Frataxin as an Iron Donor for Assembly of [2Fe-2S] Clusters in ISU-type Proteins. J. Am. Chem. Soc. 125, 6078–6084. doi:10.1021/ja027967i
Yoshino, H., and Kimura, A. (2006). Investigation of the Therapeutic Effects of Edaravone, a Free Radical Scavenger, on Amyotrophic Lateral Sclerosis (Phase II Study). Amyotroph. Lateral Scler. 7, 241–245. doi:10.1080/17482960600881870
Yusta, B., Baggio, L. L., Estall, J. L., Koehler, J. A., Holland, D. P., Li, H., et al. (2006). GLP-1 Receptor Activation Improves Beta Cell Function and Survival Following Induction of Endoplasmic Reticulum Stress. Cel Metab 4, 391–406. doi:10.1016/j.cmet.2006.10.001
Zatyka, M., Da Silva Xavier, G., Bellomo, E. A., Leadbeater, W., Astuti, D., Smith, J., et al. (2015). Sarco(endo)plasmic Reticulum ATPase Is a Molecular Partner of Wolfram Syndrome 1 Protein, Which Negatively Regulates its Expression. Hum. Mol. Genet. 24, 814–827. doi:10.1093/hmg/ddu499
Zatyka, M., Ricketts, C., Da Silva Xavier, G., Minton, J., Fenton, S., Hofmann-Thiel, S., et al. (2008). Sodium-potassium ATPase 1 Subunit Is a Molecular Partner of Wolframin, an Endoplasmic Reticulum Protein Involved in ER Stress. Hum. Mol. Genet. 17, 190–200. doi:10.1093/hmg/ddm296
Zatyka, M., Sarkar, S., and Barrett, T. (2020). Autophagy in Rare (NonLysosomal) Neurodegenerative Diseases. J. Mol. Biol. 432, 2735–2753. doi:10.1016/j.jmb.2020.02.012
Zesiewicz, T., Salemi, J. L., Perlman, S., Sullivan, K. L., Shaw, J. D., Huang, Y., et al. (2018). Double-blind, Randomized and Controlled Trial of EPI-743 in Friedreich's Ataxia. Neurodegener Dis. Manag. 8, 233–242. doi:10.2217/nmt-2018-0013
Zhang, L., Zhang, L., Hölscher, C., and Holscher, C. (2018). Neuroprotective Effects of the Novel GLP-1 Long Acting Analogue Semaglutide in the MPTP Parkinson's Disease Mouse Model. Neuropeptides 71, 70–80. doi:10.1016/j.npep.2018.07.003
Glossary
ALS Amyotrophic lateral sclerosis
BDNF Brain-derived neurotrophic factor
CDRC CURE Drug Repurposing Collaboratory
CNS Central nervous system
CPAD Critical path for Alzheimer’s disease
CPP Critical Path for Parkinson’s
CPTA Critical Path to Therapeutics for Ataxia
EMA European Medicines Agency
ER Endoplasmic reticulum
ERN-RND European Reference Network for Rare Neurological Disorders
EU European Union
EHA European Huntington’s Association
EURORDIS European Organization for Rare Diseases
FA-ICD Friedreich’s Ataxia-Integrated Clinical Database
fALS familial ALS
FDA Food and Drug Administration
FXN Frataxin
FRDA Friedreich’s ataxia
GBA2 Beta-glucocerebrosidase 2
GLP-1 Glucagon-like peptide-1
HD Huntington’s disease
HDAC Histone deacetylase
HD-RSC Huntington’s Disease Regulatory Science Consortium
HDSA Huntington’s Disease Society of America
Hsp Heat shock protein
HSC Huntington’s Society of Canada
HTT Huntington
IRDiRC International Rare Diseases Research Consortium
iPSC Inducible pluripotent stem cell
mHTT mutated Huntington
MRC Medical Research Council
MRI Magnetic resonance imaging
mTOR Mammalian target of rapamycin
NCATS National Center for Advancing Translational Sciences
NGS Next-generation sequencing
NIH National Institutes of Health
NORD National Organization of Rare Disorders
ODA Orphan Drug Act
R&D Research and Development
sALS Sporadic ALS
SME Small- and medium-sized enterprise
VMAT Vesicular monoamine transporter
UK United Kingdom
US United States
Keywords: drug repurposing, wolfram syndrome, amyotrophic lateral sclerosis, Huntington’s disease, Friedreich’s ataxia, European Medicines Agency (EMA), Food and Drug Administration (FDA, US), orphanet
Citation: Shah S, Dooms MM, Amaral-Garcia S and Igoillo-Esteve M (2021) Current Drug Repurposing Strategies for Rare Neurodegenerative Disorders. Front. Pharmacol. 12:768023. doi: 10.3389/fphar.2021.768023
Received: 31 August 2021; Accepted: 10 November 2021;
Published: 21 December 2021.
Edited by:
Maria Dimitrova, Medical University Sofia, BulgariaReviewed by:
François Houÿez, European Organisation for Rare Diseases (EURORDIS), FranceDaniel O’'Connor, Medicines and Healthcare products Regulatory Agency, United Kingdom
Copyright © 2021 Shah, Dooms, Amaral-Garcia and Igoillo-Esteve. This is an open-access article distributed under the terms of the Creative Commons Attribution License (CC BY). The use, distribution or reproduction in other forums is permitted, provided the original author(s) and the copyright owner(s) are credited and that the original publication in this journal is cited, in accordance with accepted academic practice. No use, distribution or reproduction is permitted which does not comply with these terms.
*Correspondence: Marc Marie Dooms, bWFyYy5kb29tc0B1emxldXZlbi5iZQ==; Mariana Igoillo-Esteve, bWFyaWFuYS5pZ29pbGxvLmVzdGV2ZUB1bGIuYmU=