- 1School of Basic Medicine, Health Science Center, Yangtze University, Jingzhou, China
- 2Department of Pharmacy, Faculty of Science, National University of Singapore, Singapore, Singapore
- 3Hangzhou Cancer Institute, Key Laboratory of Clinical Cancer Pharmacology and Toxicology Research of Zhejiang Province, Hangzhou, China
- 4Affiliated Hangzhou Cancer Hospital, Zhejiang University School of Medicine, Hangzhou, China
- 5Department of Pharmacology, Yong Loo Lin School of Medicine, National University of Singapore, Singapore, Singapore
- 6Cancer Science Institute of Singapore, National University of Singapore, Singapore, Singapore
- 7Department of Haematology–Oncology, National University Cancer Institute, Singapore, Singapore
Cancer has become a global health problem, accounting for one out of six deaths. Despite the recent advances in cancer therapy, there is still an ever-growing need for readily accessible new therapies. The process of drug discovery and development is arduous and takes many years, and while it is ongoing, the time for the current lead compounds to reach clinical trial phase is very long. Drug repurposing has recently gained significant attention as it expedites the process of discovering new entities for anticancer therapy. One such potential candidate is the antimalarial drug, artemisinin that has shown anticancer activities in vitro and in vivo. In this review, major molecular and cellular mechanisms underlying the anticancer effect of artemisinin and its derivatives are summarised. Furthermore, major mechanisms of action and some key signaling pathways of this group of compounds have been reviewed to explore potential targets that contribute to the proliferation and metastasis of tumor cells. Despite its established profile in malaria treatment, pharmacokinetic properties, anticancer potency, and current formulations that hinder the clinical translation of artemisinin as an anticancer agent, have been discussed. Finally, potential solutions or new strategies are identified to overcome the bottlenecks in repurposing artemisinin-type compounds as anticancer drugs.
Introduction
Cancer has been a growing challenge in the healthcare system and is one of the largest global health problems. It is the second leading cause of death worldwide following ischemic heart disease. In 2018, the disease led to approximately 9.6 million deaths (Organisation 2018). An increase in cancer cases associated with aging population can increase the strain on the healthcare system and is certainly a cause for concern (Board, 2015).
Despite significant breakthrough in cancer therapy in the past decade, chemotherapy is still the mainstay of treatment (National Cancer Institute, 2015). Novel therapies such as targeted therapy and immunotherapy are not readily accessible owing to their high cost. In addition, targeted therapy often shows efficacy in specific cancers exhibiting selected biomarkers in a small group of patients and the majority of cancer patients do not respond to immunotherapy (Ventola, 2017). Therefore, there is still an unmet demand to develop more effective and cheaper anticancer drugs and identify the lead compounds for the development of those drugs.
The cost to develop a novel cancer drug is extremely high and the process from target identification to phase III clinical trials is time-consuming. Therefore, drug repurposing is becoming an increasingly explored alternative approach to the traditional drug discovery and development pipeline (Lim et al., 2021; Ren et al., 2021). Since data on existing drugs are largely available, additional studies on its pharmacology, pharmacokinetics and safety are not required (Sleire et al., 2017). Thus, drug repurposing can greatly reduce the duration of the drug development process and time to reach the market as an oncology therapeutic (Parvathaneni et al., 2019), greatly reducing the cost and increasing the patients’ access to the treatment (Parvathaneni et al., 2019).
One group of compounds that is currently being explored for drug repurposing is artemisinin (ARS) and its derivatives (henceforth referred to as artemisinins). Artemisinins are sesquiterpene trioxanes (Figure 1A) that have been clinically used to treat malaria (Augustin et al., 2020; Wang et al., 2020; Wang et al., 2021). The maximum recommended dose is 200 mg daily for 3 days for oral therapy of uncomplicated malaria (Organization, 2015). This dosing regimen has been shown to be safe and effective for the treatment of malaria. However, cancer is a chronic condition that may require long-term treatment with artemisinins in contrast to an acute infection like malaria. In addition, cancer treatment may require a higher dose of the drug-to be effective, leading to higher levels of toxicity than that observed in malaria treatment. For the treatment of severe malaria 2.4 mg/kg IV artesunate (ART) administered at 0, 12, and 24 h for up to 7 days is recommended, which is a considerably higher than that required to treat uncomplicated malaria, and adverse reactions of delayed hemolysis at this dose have been reported (Prevention, 2020, May 28). It is unclear whether such side effects will be more prominent at doses used for cancer treatment because no dosing regimen has yet been established for cancer treatment. Therefore, the safety of artemisinins in long-term cancer therapy requires further investigation.
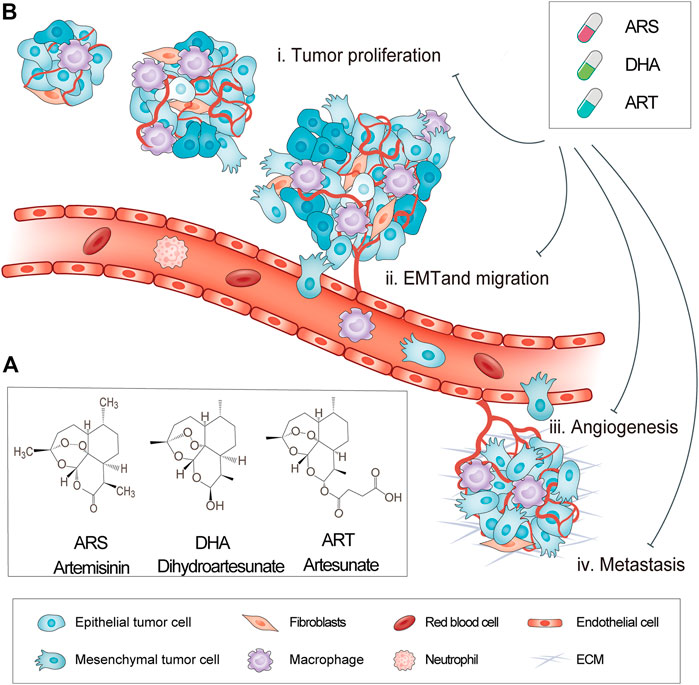
FIGURE 1. Chemical structure and anticancer activity of artemisinins. (A) Chemical structure of artemisinins (B) Multifunctional anticancer activity of artemisinins.
Artemisinins have shown potent anticancer activity in multiple cancers (Wong et al., 2017) (Figure 1B). Artemisinins, ART, and dihydroartemisinin (DHA) exhibited therapeutic effects against multiple tumor types such as breast cancer (Zhang et al., 2015; Yao Y. et al., 2018; Wen et al., 2018), prostate cancer (Xu et al., 2016; Zhou et al., 2017), ovarian cancer (Wu et al., 2012; Zhou et al., 2020), pancreatic cancer (Zhou et al., 2013), and lung cancer (Zhou et al., 2012; Zuo et al., 2014). Artemisinins acts against cancer cells via various pathways such as inducing apoptosis (Zhu et al., 2014; Zuo et al., 2014) and ferroptosis via the generation of reactive oxygen species (ROS) (Zhu et al., 2021) and causing cell cycle arrest (Willoughby Sr et al., 2009; Tin et al., 2012). Therefore, artemisinins can work on multiple targets and affect multiple signaling pathways (Wong et al., 2017). Moreover, ARS has been known to be well tolerated and safe at low doses, lowering the risk of intolerable toxicity (Efferth, 2017). Thus, artemisinins show great potential of repurposing as anticancer drugs.
While most studies showed in vitro and in vivo anticancer efficacy of artemisinins, limited clinical trials in human subjects have been conducted to date. Therefore, the practicality of clinical translation of artemisinins as anticancer agents is uncertain. This review outlines the potential anticancer activity of artemisinins. Additionally, the pharmacokinetic properties of artemisinins, one of the most important aspects in anticancer drug development are discussed in details. This review article will improve our understanding of the limitations in the development of artemisinins as anticancer drugs in human subjects and suggest potential solutions and new strategies to overcome those challenges.
Search Strategy
We performed a literature search on PubMed, Scopus, and embase. The first search aimed to identify studies on anticancer effect of artemisinins; thus the search terms (“artemisinins” [Mesh] AND “Neoplasms” [Mesh]) OR ((artemisinin [Title/Abstract]) AND (cancer [Title/Abstract]) were used. The search strategy is illustrated in Figure 2.
Another search was performed to understand the pharmacokinetic properties of artemisinins and the following search terms were used ((“artemisinins” [Mesh]) OR ((artemisinin [Title/Abstract]) AND ((“Pharmacokinetics” [Mesh]) OR (pharmacokinetic [Title/Abstract])). Duplicates were removed using Endnote and titles and abstracts were screened according to the exclusion criteria as illustrated in Figure 2.
Pharmacokinetics of Artemisinins
It is important to understand a drug’s pharmacokinetic properties to determine its potential for clinical use. Many studies have been conducted to determine the pharmacokinetic parameters of artemisinins. The main pharmacokinetic characteristics of artemisinins namely absorption, distribution, metabolism, and excretion are elaborated in Absorption of Artemisinins–Elimination of Artemisinins.
Absorption of Artemisinins
An AUC0-∞ value (area under the curve from time 0 extrapolated to infinite time) of 657 μg h L−1 was observed in a study on healthy volunteers administered orally 4 mg/kg of ART (Na-Bangchang et al., 2004). To calculate absolute bioavailability, this value was compared to that of another study on healthy volunteers administered 4 mg/kg IV dose of ART (AUC0-∞ value of 3,038 μg h L−1) (Li et al., 2009). Therefore, absolute bioavailability was estimated to be 21.6%. In contrast, the AUC0-∞ value of a group of patients with uncomplicated malaria who received 200 mg oral ART was considerably high (4,868 μg h L−1), indicating that disease condition may affect absorption (Newton et al., 2002) because patients with malaria experience greater exposure than that of healthy volunteers, as indicated by the AUC0-∞ values.
To better understand the translational potential of artemisinins as anticancer agents, maximun concentration (Cmax) values also evaluated. Cmax values of DHA ranged between 0.558–1.270 μM in healthy volunteers (Teja-isavadharm et al., 2001; Na-Bangchang et al., 2004). In healthy volunteers who received oral ART, Cmax values ranged between 0.174–1.830 μM (Teja-isavadharm et al., 2001; Batty et al., 2002; Na-Bangchang et al., 2004; Diem Thuy et al., 2008; Li et al., 2009). Moreover, Cmax values were compared with IC50 values of promising cancer cell lines obtained in vitro to understand the limitaions in clinical translation. Compared to healthy volunteers, patients with uncomplicated malaria showed high Cmax values of 3.9–4.6 μM for the use of ART (Binh et al., 2001; Newton et al., 2002) and 3.7–4.03 μM for DHA (Binh et al., 2001; Newton et al., 2002). Thus, the disease state affects the absorption of artemisinins, and further studies are required to better understand the pharmacokinetics of artemisinins in cancer patients.
Distribution of Artemisinins
Artesunate has been reported to have small volume of distribution (Vd/F) of 0.0106–0.0920 L/kg because ART has good solubility and is not lipophilic [28]. Therefore, ART would not distribute well to the tissues and might be more effective in treating cancers such as leukemia, hepatocellular carcinoma (HCC), or renal cell carcinoma because the liver and kidney are highly perfused organs. Artesunate might also be useful for the treatment of metastatic cancers. A low Vd/F also implies a short elimination half-life (t1/2). In contrast, ARS was recorded to have a much higher Vd/F ranging from 33.7 ± 16.1 to 38.4 ± 18.9 L/kg (Ashton et al., 1998) because ARS is more lipophilic and less water soluble than ART. However, ARS is converted to the active metabolite DHA in the body, which has good solubility with Vd/F of 1.46 L/kg reported in metastatic breast cancer patients (Ericsson et al., 2014).
Elimination of Artemisinins
Pharmacokinetic studies showed a relatively short t1/2 of artemisinins. For ART, t1/2 was 0.41 h (Teja-isavadharm et al., 2001) after an oral dose of 100 mg in healthy volunteers. At a dose of 4 mg/kg, t1/2 of 0.74 h was reported (Na-Bangchang et al., 2004). Generally, t1/2 has been reported to be less than 1 h and dose-dependent; however, the variations in t1/2 with dose are not drastic. A low t1/2 value aligns with a low Vd/F value, which implies that a more frequent dosage regimen is required for anticancer treatment with ART because it is cleared from the body relatively quickly. The oral clearance of ART was reported to be 20.6 ± 10.6 L/h/kg (Teja-isavadharm et al., 2001) for 100 mg oral dose, which is considerably high. Because of its high solubility, ART is eliminated by the kidneys. It is important to understand the metabolism and clearance of a drug to determine the recommended dose. However, to successfully determine a dosage regimen, the desired Cmax value should be identified.
The challenges in repurposing artemisinins as anticancer drugs can be overcome by using different formulations and combination therapies based on pharmacokinetic properties of these drugs.
Mechanisms of Action Underlying Anticancer Activity of Artemisinins
Artemisinins possess anti-cancer activity, although the underlying mechanisms remain unclear. Generally, artemisinins act via similar pathways because they have a special structure called peroxide bridge, which is strongly associated with the cytotoxicity required for their antimalarial and anticancer activities (Liao et al., 2014; Tran et al., 2014; Xu et al., 2015; Tong et al., 2016). A cell death model revealed a distinguished anticancer mechanism of artemisinins through induction of ferroptotic cell death (Zhu et al., 2021). Other common mechanisms of action include induction of autophagy, cell cycle arrest, and apoptosis. Inhibition of cell proliferation and metastasis was observed in both in vitro and in vivo studies (Hou et al., 2008; Michaelis et al., 2010; Wang et al., 2012; Tran et al., 2014; Xu et al., 2015; Tong et al., 2016) (Figure 3). Hence, multiple signalling pathways are involved in anticancer activities of artemisinins in various cancer types. This section focuses on common mechanisms, which are further detailed in Table 1.
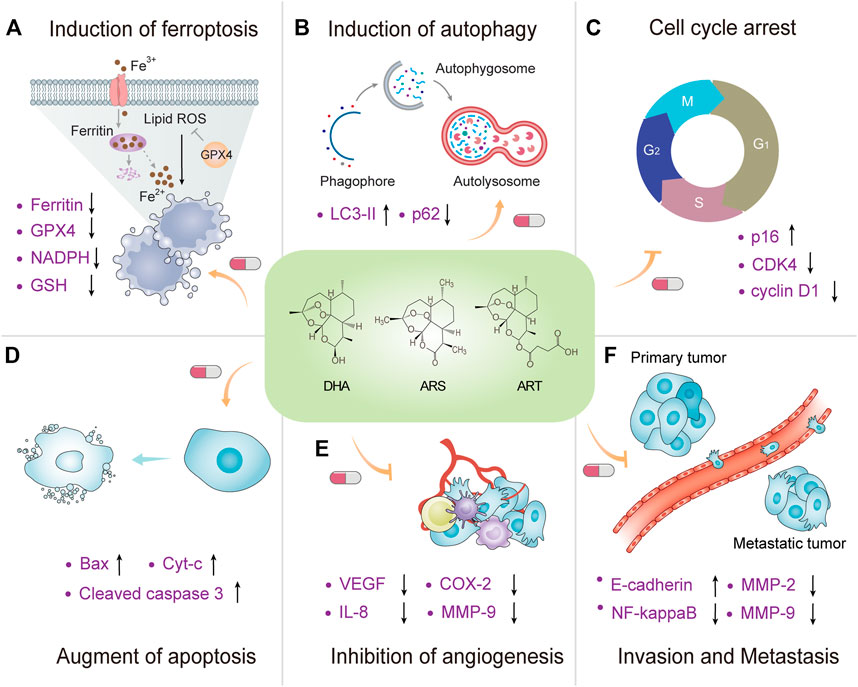
FIGURE 3. Mechanisms of action underlying anticancer activity of artemisinins. A schematic view of the molecular crosstalk pathways involved anticancer mechanisms of artemisinins, including (A) induction of ferroptosis, (B) induction of autophagy, (C) cell cycle arrest, (D) augment of apoptosis, (E) inhibition of angiogenesis, and (F) invasion and metastasis.
Induction of Ferroptosis
Ferroptosis, an oxidative, iron-dependent form of regulated cell death, is characterized by the accumulation of ROS and lipid peroxidation products to lethal levels (Stockwell et al., 2017). Emerging evidence suggests that triggering ferroptosis is a promising therapeutic strategy to kill cancer cells, particularly for eradicating aggressive malignancies that are resistant to the traditional therapies (Liang et al., 2019). Compared to normal cells, ferritin, a major iron storage protein essential for iron homeostasis, is overexpressed in many cancer cells (Buranrat and Connor, 2015). Usually, high ferritin level in blood is a poor prognostic marker in cancer patients, leading to aggressive disease. Other endogenous molecules such as glutathione, nicotinamide adenine dinucleotide phosphate, and glutathione peroxidase 4 (GPX4) have been also closely linked to the regulation of ferroptosis (Stockwell et al., 2017).
Dihydroartemisinin renders cancer cells more sensitive to ferroptosis by increasing the cellular accumulation of free ions due to its ability to induce lysosomal degradation of ferritin in an autophagy-independent manner (Chen X. et al., 2020). Dihydroartemisinin augmented GPX4 inhibition-induced ferroptosis in some cancer cells in both in vitro and in vivo models by the inducible knockout of GPX4 (Chen X. et al., 2020). Du et al. revealed that DHA, the main active metabolite of ART, could be a promising therapeutic agent to preferentially target acute myeloid leukemia cells by inducing ferroptosis (Du et al., 2019). Jiang et al. demonstrated that ART could regulate the labile iron pool (LIP) by promoting the lysosomal degradation of ferritin through lysosomal acidification, thereby inducing ROS-dependent cell death in HCC cells. The accumulation of labile iron in the endoplasmic reticulum promoted excessive ROS production and severe endoplasmic reticulum disruption, leading to cell death. These findings suggest ART is a safe anti-HCC agent that disturbs iron homeostasis (Jiang et al., 2021). Besides, artesunate greatly enhanced the anticancer effects of low dose of sorafenib against HCC by inducing oxidative stress and lysosome-mediated ferritinophagy, two essential aspects of ferroptosis (Li ZJ. et al., 2021). Furthermore, Hamacher-Brady et al. demonstrated that ART could trigger programmed cell death (PCD) in cancer cells in a manner dependent on the level of free iron and the generation of ROS (Hamacher-Brady et al., 2011). Moreover, artesunate could inhibit autophagosome turnover and cause perinuclear clustering of autophagosomes, early and late endosomes, and lysosomes. Lysosomal iron was the lethal source of ROS upstream of mitochondrial outer membrane permeabilization because lysosomal iron chelation blocked all measured parameters of ART-induced PCD, whereas lysosomal iron loading enhanced death. Two lysosomal inhibitors, chloroquine and bafilomycin A1, reduced ART-induced PCD, proving that lysosomal function is required in the process of PCD signaling (Hamacher-Brady et al., 2011). The anticancer effect of ART can be attributed, at least partially, to ferroptosis.
Induction of Autophagy
Emerging evidence suggests that autophagy induction is one of the molecular mechanisms underlying anticancer activity of artemisinins (Wang et al., 2012; Chen K. et al., 2014). Mitochondria are important molecular organelles that regulate both apoptosis and autophagy (type II PCD), and ROS generation is one of the triggering factors for mitochondrial dysfunction. DHA-induced autophagy in leukemia K562 cells, evidenced by LC3-II protein expression, was observed to be ROS-dependent (Wang et al., 2012). Inhibitory effect of DHA on the proliferation of leukemia K562 cells was also dependent upon the iron level, indicating an association between autophagy and ferroptosis (Wang et al., 2012).
In a study on breast cancer cells, ART could inhibit the proliferation of cancer cells by inducing autophagy [53]. Moreover, ART sensitized breast cancer cells to epirubicin chemotherapy. As a result, ART was regarded as a therapeutic candidate in breast cancer therapy [53]. A recent study evaluated the antineoplastic effects of ART in diffuse large B cell lymphoma cells (Chen et al., 2021). The results revealed that ART exhibited anticancer activity through multiple mechanisms of action including autophagy as evidenced by over-expression of LC3B-I/II, whereas p62 expression was downregulated in a dose dependent manner following 24 h of ART treatment. Next, Chen, et al. investigate the antitumor activity of DHA in esophagus cancer cells (Chen X. et al., 2020). The results showed that DHA could inhibit the migration capacity of Eca109 and TE-1 cells by inducing autophagy. Ma et al. also demonstrated similar results that DHA significantly reduced the viability of Eca109 cells in a dose- and time-dependent manner (Ma et al., 2020) Together, these studies indicate that autophagy is one of the key mechanisms underlying death of cancer cells treated with artemisinin and its derivatives.
Induction of Cell Cycle Arrest
Artemisinins administration resulted in cell cycle arrest in a dose-dependent manner (Willoughby Sr et al., 2009; Chen et al., 2010; Wang et al., 2011). G1-phase cell cycle arrest was observed in GBC-SD and NOZ gallbladder cancer cell lines (Jia J. et al., 2016), LNCaP, PC3, and DU145 prostate cancer cells (Steinbrück et al., 2010), A549 and H1299 lung cancer cells (Liao et al., 2014; Tong et al., 2016), BxPC-3 and AsPC-1 pancreatic cancer cells (Chen et al., 2010), human hepatoma cells (Hou et al., 2008), ovarian cancer cells (Greenshields et al., 2017) and human Ishikawa endometrial cancer cells (Tran et al., 2014).
The induction of G1-phase cell cycle arrest by artemisinins is mediated by several pathways, including downregulation of cyclin-dependent kinase 4 (CDK4) and cyclin D1 expression (Hou et al., 2008; Liao et al., 2014; Tran et al., 2014; Jia J. et al., 2016; Tong et al., 2016), both of which promote cell proliferation. Moreover, artemisinin enhanced the expression of p16 (Jia J. et al., 2016), a tumor suppressor that inhibits CDK and limits cell cycle progression. G2/M-phase cell cycle arrest was also observed in other cell lines including J-Jhan, HCT116, H69, U251 (Steinbrück et al., 2010), human osteosarcoma (Xu et al., 2011), breast cancer (Chen K. et al., 2014), and renal carcinoma (Jeong et al., 2015) cells following the administration of ART. In renal carcinoma cells and ovarian cancer cells, ART-mediated G2/M-phase cell cycle arrest was dependent on ROS generation (Jeong et al., 2015; Greenshields et al., 2017). In breast cancer cells, ART caused G2/M-phase cell cycle arrest by regulating autophagy (Chen K. et al., 2014). Cell cycle arrest is one of the key molecular mechanisms of anticancer activity of artemisinins.
Augmentation of Apoptosis
Artemisinins have been reported to induce apoptosis in J16, DU145, SK-Mel-28 (Steinbrück et al., 2010), leukaemia (Efferth et al., 2007; Zhou et al., 2007), HepG2, Hep3B hepatoma (Hou et al., 2008), ovarian cancer (Jiao et al., 2007), Kaposi’s sarcoma-IMM (Dell'Eva et al., 2004), cervical cancer (Luo et al., 2014), SKM-1 (Xu et al., 2015), glioblastoma (Berdelle et al., 2011), neuroblastoma (Michaelis et al., 2010), embryonal rhabdomyosarcoma (Beccafico et al., 2015), pancreatic cancer (Youns et al., 2009), and colorectal cancer (Li et al., 2008; Chen X. et al., 2017) cells. Similar to the cell cycle arrest, apoptosis induction was caused by a myriad of signaling pathways.
One common pathway by which artemisinins induced apoptosis is the generation of ROS which in turn damages organelles, DNA, and proteins, eventually leading to the death of cancer cells (Efferth et al., 2007; Beccafico et al., 2015; Pang et al., 2016; Chen X. et al., 2017). ROS-dependent apoptosis caused by Bax-mediated intrinsic pathway has been observed in Huh-7 and Hep3B cells following treatment with ART (Pang et al., 2016), in which caused mitochondrial activation, and release of cytochrome c and subsequent activation of caspase-9. leading to activation of caspase-3, an executioner caspase that destroys cellular structures such as poly (ADP-ribose) polymerase, an enzyme involved in DNA repair, causing cell death (Hou et al., 2008; Jia J. et al., 2016; Chen X. et al., 2017). In another study, exposure to artemisinins led to a dose dependent increase in caspase-3 cleavage in HepG2 cells (Hou et al., 2008). This process was also evident in K562 leukemia (Zhou et al., 2007) and pancreatic cancer cells (Youns et al., 2009). However, activation of caspase-3 is not always ROS-dependent. Both in vitro and in vivo studies have also shown that ART could induce ROS-independent apoptosis in HepG2 cells (Pang et al., 2016).
Inhibition of Angiogenesis
Angiogenesis is a key factor in tumor growth, invasion and metastasis. It is partly mediated by the transcription factor NF-κB and pro-angiogenic factors (including VEGF, IL-8, COX-2 and MMP-9) (Ferrara and Kerbel, 2005; Liu et al., 2021). Dihydroartemisinin showed anti-angiogenic effect in both in vitro angiogenesis models and in vivo pancreatic cancer-derived tumor models (Wang et al., 2011). These effects were likely to be mediated by inhibiting the NF-κB pathway and its downstream pro-angiogenic growth factors. In this study, the results showed that treatment of human umbilical vein endothelial cells with DHA resulted in a dose-dependent inhibition of cell proliferation and capillary tube formation.
The pleiotropic transcription factor NF-κB regulates the expression of multiple genes, including VEGF and IL-8 (Huang et al., 2000). The constitutive NF-κB activity drives the constitutive overexpression of VEGF and IL-8, which contributes to the angiogenic phenotype of human pancreatic cancer. After DHA treatment, decreased expression of VEGF and IL-8 in vitro and in vivo is associated with decreased proliferation and neovascularization.
Artesunate can inhibit the expression of VEGF, which is closely related to the level of VEGF secreted in the conditioned medium. Artesunate has potential anti-leukemia effects for the treatment for cronic myeloid leukemia or as an adjunct to standard chemotherapy regimens (Zhou et al., 2007). Using KS-IMM cells derived from Kaposi’s sarcoma lesions of kidney transplant patients, Dell E’va et al. proved that ART could inhibit the growth of cancer cells and normal human umbilical cord endothelial cells (Dell'Eva et al., 2004) ART also reduces angiogenesis in vivo in terms of vascularization of Matrigel plugs injected subcutaneously into syngeneic mice. In summary, ART is a promising low-cost drug candidate for the treatment of hyper vascularized Kaposi’s sarcoma. and for preventing tumor angiogenesis.
Inhibition of the Key Signaling Pathways
NF-κB is a transcription factor that regulates apoptosis, and promotes tumorigenesis, cell proliferation, metastasis, and angiogenesis upon activation (Chen H. et al., 2009). Hence, inhibition of the NF-κB pathway may block these processes and result in cell apoptosis. In BxPC-3 and PANC-1 pancreatic cancer cells, DHA inhibited NF-κB and decreased the production of vascular endothelial growth factor (VEGF), IL-8, COX-2, and MMP-9 (Wang et al., 2011), promoting angiogenesis. NF-κB activates cyclin D1 and Bcl-2 transcription. DHA inhibited both Bcl-2 and cyclin D1 (Chen H. et al., 2009), which are the downstream gene products of NF-κB. The disruption of the NF- B pathway at different points was also observed in HCT116 (Chen X. et al., 2017) and lung cancer cells (Rasheed et al., 2010), after ART administration, HT-1080 cells (Hwang et al., 2010) after DHA administration, and human Ishikawa endometrial cancer cells (Tran et al., 2014) after ARS administration.
Tong et al. demonstrated that ARS, DHA and ART induced cell cycle arrest in the G1 phase, thereby inhibiting the proliferation of A549 and H1299 cells. Moreover, artemisinins inhibited other malignant tumor markers by migration, invasion, cancer stem cells and epthelial-mesenchymal transition (EMT) and decreased tumor growth in xenograft mouse model. Using IWP-2, Wnt/β-catenin pathway inhibitor and Wnt5a siRNA, Tong et al. showed that anticancer effect of artemisinins partly depends on the inactivation of the Wnt/β-catenin signaling. Artemisinin significantly reduced the protein levels of Wnt5-a/b, and increased the levels of NKD2 and Axin2, and ultimately inhibited the Wnt/β-catenin pathway (Tong et al., 2016). Xu et al. demonstrated that ART induced SKM-1 cell apoptosis in a dose- and time-dependent manner by inhibiting the hyperactive β-catenin signaling pathway (Xu et al., 2015).
Artemisinins inhibit cell proliferation and metastasis (Hou et al., 2008; Xu et al., 2015; Tong et al., 2016). Inhibition of the Wnt/β-catenin pathway in lung cancer by DHA and SKM-1 cells by ART led to increased E-cadherin expression (Xu et al., 2015; Tong et al., 2016), which mediates cell-cell adhesion. The increased cell-cell adhesion suppressed tumor metastasis (Xu et al., 2015). In a human fibrosarcoma HT-1080 cell model, anti-invasive effect of DHA was caused by inhibiting the phosphorylation of PKCalpha/Raf/ERK and JNK and reducing the activation of NF-κB and AP-1, thereby leading to the down-regulation of MMP-9 expression. Therefore, DHA is an effective anti-metastatic agent that works by down-regulating MMP-9 expression (Hwang et al., 2010). In another study on HepG2 cells, ARS activated Cdc42, promoting E-cadherin action which is necessary for cell adhesion (Weifeng et al., 2011). Additionally, artemisinins administration downregulated proliferating cell nuclear antigen gene expression, MMP2, p-p38, p-ERK1/2, CSC markers, and EMT-related proteins, which promote tumor growth, proliferation, and metastasis in lung cancer and HCC cells and their downregulation would inhibit tumor growth (Rasheed et al., 2010; Weifeng et al., 2011; Liao et al., 2014; Tong et al., 2016). Artemisinins inhibited proliferation in prostate cancer, human osteosarcoma, HepG2, and pancreatic cancer cells (Willoughby Sr et al., 2009; Youns et al., 2009; Xu et al., 2011; Zeng and Zhang, 2011).
Overall, artemisinins act via multipe pathways by regulating the key targets of suppression of cell cycle, induction of apoptosis, inhibition of NF-κB signalling pathway, and suppression of mitogen-activated protein kinase (MAPK) signaling.
Anticancer Efficacy of Artemisinins in Vitro and in Vivo Models
Artemisinins have been recognized as antimalarials, but they have demonstrated great anticancer potential in in vitro and in vivo studies (Table 2).
In vitro Anticancer Efficacy
Several studies have been conducted to assess the effect-of artemisinins against different types of cancer. For DHA, IC50 values ranged between 1.20–15.2 μM (Jiao et al., 2007; Hou et al., 2008; Chen T. et al., 2009; Michaelis et al., 2010; Wang et al., 2012), with the exception of BxPC-3 pancreatic cancer cells (Chen et al., 2010; Wang et al., 2011), TE671 rhabdomyosarcoma cells (Beccafico et al., 2015) and Fadu, Hep-2, and Cal-27 head and neck squamous cancer cells (Jia L. et al., 2016) which were highly resistant. This IC50 range was considerably higher than that of Cmax in healthy volunteers (0.558–1.27 μM). Only HCT116, HT29, SW480, and LOVO colon cancer cell lines showed IC50 values within the Cmax range (Yao Z. et al., 2018; Chen GQ. et al., 2020). For ART, IC50 values range bewteen 2.0–39.4 μM (Efferth et al., 2007; Hou et al., 2008; Li et al., 2008; Youns et al., 2009; Du et al., 2010; Michaelis et al., 2010; Rasheed et al., 2010; Steinbrück et al., 2010; Zeng and Zhang, 2011; Luo et al., 2014; Beccafico et al., 2015; Jeong et al., 2015; Xu et al., 2015; Pang et al., 2016; Greenshields et al., 2017; Chen GQ. et al., 2020). Inconsistent with the range of Cmax values (0.174–1.83 μM) except in CEM, J-Jhan and Molt-4 leukemia cells (Efferth et al., 2007; Steinbrück et al., 2010), and TOV-112D ovarian cancer cells (Greenshields et al., 2017) which are within range. High IC50 value is a significant barrier in the clinical application of use of artemisinins in humans because high doses in vivo may lead to toxicity problems. Combination therapy can also be considered as a therapeutic option because artemisinins can synergize with other drugs to increase efficacy.
In Vivo Anticancer Efficacy
Several studies demonstrated the efficacy of artemisinins in tumor-bearing animal models. The cancer types identified in vitro have been effectively treated by artemisinins in vivo. The in vivo studies used more aggressive dosage regimens of artemisinins with effective doses ranging from 50 to 100 mg/kg/dand showed little toxicity in animals (Hou et al., 2008; Willoughby Sr et al., 2009; Du et al., 2010; Wang et al., 2011; Weifeng et al., 2011; Xu et al., 2011; Jeong et al., 2015; Jia L. et al., 2016; Jia et al., 2016b; Tong et al., 2016). In HepG2 HCC xenografts, tumor inhibition rates of up to 79.6% was observed after administration of 100 mg/kg/d of ARS (Weifeng et al., 2011). Another study repoted 60.6% inhibition of tumor growth after administration of 100 mg/kg/d of DHA (Hou et al., 2008). Since HCC cell lines were not highlighted in previous in vitro studies, the underlying mechanism of the efficacy of DHA observed in HCC xenografts in vivo should be further explored.
At this dosage range, artemisinins showed a significant and conclusive effect on the inhibition of tumor growth. However, 100 mg/kg/d dose would translate to 3 g/d for a 60 kg adult, which is significantly greater than the safe and effective dose established for the treatment of malaria (200 mg/d) (Organization, 2015). Another promising result was observed in LOVO colorectal cancer xenografts where the tumor growth inhibition rate was 52.2% (Li et al., 2008) at a dose of 300 mg/kg twice a week. This discrepancy in dosage regimens between malaria cases and in vivo studies in xenograft mouse models can make clinical translation challenging.
Notably, among many derivatives of artemisinin, ART has the most extensive data, thus, it has the greatest potential to be developed for future use in cancer treatment in humans.
Clinical Application of Artemisinins in Cancer Therapy
A few clinical trials conducted were using ART to understand the efficacy of artemisinins in breast cancer, colorectal cancer, and other solid tumors (Table 3) (Krishna et al., 2015; von Hagens et al., 2017; Deeken et al., 2018). The effective dose of ART ranged up to 200 mg/d, which was safe and well tolerated (von Hagens et al., 2017; von Hagens et al., 2019).
A clinical trial conductedin patients with solid tumors revealed the maximum tolerated dose of IV ART as 18 mg/kg in a Day 1/Day 8 regimen with a 3-week administration cycle with dose-limiting toxicities such as myelosuppression, liver dysfunction, and uncontrolled nausea and vomiting (Deeken et al., 2018). Other side effects included anemia, fatigue, dizziness, and anorexia (Deeken et al., 2018) at a much lower dose than the effective dose used in in vivo studies. This result indicates that in vivo studies do not accurately represent toxicity data in humans. While effective therapeutic range in vivo can be as high as 200 mg/kg/d, the same dose cannot be used in humans. Caution should be exercised in proceeding with higher doses of ART that are likely to be more efficacious but less safe.
Another study showed anticancer activity of ART in colorectal cancer patients, which is consistent with the previous in vitro and in vivo studies (Li et al., 2008; Chen GQ. et al., 2020). Treatment with 200 mg oral ART increased recurrence-free survival rate compared to placebo after 3 years (Krishna et al., 2015), but two patients at the lower weight limit developed leukopenia.
The ARCTIC M33/2 study conducted in patients with metastatic breast cancer used ART as an adjuvant to the patients’ guideline-based cancer therapy for 4 weeks; 10 out of 23 patients had stable disease, whereas five patients experienced disease progression (von Hagens et al., 2017). Therefore, while 200 mg oral ART has been established as a relatively safe dose, efficacy at this dose remains inconclusive. The ARCTIC M33/2 study was extended for long-term compassionate use in 13 patients who did not experience any clinically relevant adverse events in the original phase I study. Results from the follow-up study suggested the dose dependent effects of ART; a greater number of patients adminitered lower dose (100 mg/kg/d) experienced disease progression than patients administered higher doses (von Hagens et al., 2019). In some patients, up to 37 months of use of ART has been reported, demonstrating the safety of the long-term use of oral ART at this dosage range.
Few clinical trials that have been conducted to date are limited to phase I trials which involved relatively small study populations. Hence, phase II trials are required to investigate the effect of artemisinins on a larger number of patientsand gain better insight into the safety and efficacy of the use of artemisinins, in particular ART, as potential anticancer agents in large populations.
Future Perspectives
Artemisinins, in particular ART, have been proven to promising drugs to repurpose for cancer treatment. Additional phase II and III trials should be conducted in future to gain a better understanding of the long-term safety and efficacy profile of artemisinins in large populations. Further strategies should be explored to expedite the development of artemisinins as anticancer agents.
Combination Therapy
Combination therapy makes use of multiple agents to treat a single condition, a strategy that is commonly employed in cancer treatment. The use of combination therapy has advantages of synergistic and additive effects because different drugs can work on different molecular pathways to exert a greater anticancer effect, thereby leading to greater efficacy. Since IC50 values of artemisinins cancer treatment are relatively high, combination therapy can be used to take advantage of the synergistic effect and lower IC50, and minimise any dose-related toxicities because combination therapy allows the use of lower doses of multiple agents.
Several drugs have demonstrated synergistic effects in vitro when administered in combination with either DHA or ART or both (Table 4). Many studies also reported that the use of artemisinins sensitized cancer cells to conventional chemotherapy and exerted a synergistic effect on apoptosis, inhibition of cell growth, and a reduction of cell viability, leading to a lower IC50 value (Chen T. et al., 2009; Zhang YJ. et al., 2013; Liu and Cui, 2013; Shen et al., 2016; Tai et al., 2016; Chen H. et al., 2017; Wang et al., 2017; Yang et al., 2019a; Yang et al., 2019b; Hu et al., 2019). Combination index, which measures the degree of drug interactions (Zhang JL. et al., 2013) was used to understand the potential of combination therapy. The combination of DHA with cisplatin (Zhang YJ. et al., 2013), DHA with onconase (Shen et al., 2016), DHA with gemcitabine (Yang et al., 2019a), DHA with Apo2L/TRAIL (Kong et al., 2012), and ART with sorafenib (Yao et al., 2020), which were used to treat lung, lung, ovarian, pancreatic, and liver cancer, had combination index values < 1, which indicates synergism.
Animal xenograft models showed that the combination of artemisinins with onconase (Shen et al., 2016), gemcitabine (Yang et al., 2019a), carboplatin (Chen T. et al., 2009), cisplatin (Zhang YJ. et al., 2013; Li W. et al., 2021), doxorubicin (Tai et al., 2016), Apo2L/TRAIL (Kong et al., 2012), allicin (Jiang et al., 2013), cytarabine (Drenberg et al., 2016), sorafenib (Jeong et al., 2015; Jing et al., 2019), triptolide [17], and temozolomide (Berte et al., 2016) can exert a synergistic effect on leukemia (Drenberg et al., 2016), renal cell carcinoma (Jeong et al., 2015), glioblastoma (Berte et al., 2016), lung (Zhang YJ. et al., 2013; Shen et al., 2016; Li W. et al., 2021), ovarian (Chen T. et al., 2009; Yang et al., 2019a), cervical (Tai et al., 2016), pancreatic (Kong et al., 2012; Liu and Cui, 2013), and liver (Jing et al., 2019) cancer. Many studies reported the synergistic effect of ART with conventional chemotherapy on the inhibition of tumor growth without a significant decrease in body weight (Kong et al., 2012; Liu and Cui, 2013; Shen et al., 2016; Tai et al., 2016; Yang et al., 2019a; Li W. et al., 2021), suggesting improved efficacy without an overt increase in toxicity. The complete elimination of an ovarian cancer tumor was observed in a study that used DHA and gemcitabine combination therapy.
In summary, combination therapy is a promising strategy to advance the repurposing of artemisinins as anticancer therapeutics. Since more combination therapy studies have been conducted for DHA than for ART, the use of DHA in human clinical trials should also be explored in future research. Clinical trials exploring ART or DHA as an adjuvant to the conventional chemotherapy should also be conducted.
Nanoformulation
To overcome the limitations that result from poor pharmacokinetic properties of artemisinins, novel delivery methods that could improve the absorption and elimination profile of artemisinins should be explored. Several in vitro and in vivo studies have been conducted to investigate the use of nanoparticles, nanocarriers, and liposomes as carriers for ARS, ART, and DHA to improve their delivery to the cancer cells. These new formulations improved solubility, exposure, and stability, increased cellular uptake, and enhanced permeability and retention in breast, colorectal, liver, lung, and cervical cancer cells (Chen J. et al., 2014; Chen et al., 2015; Tran et al., 2015; Leto et al., 2016; Liu et al., 2016; Tran et al., 2016; Tran et al., 2017; Wang et al., 2018; Wang et al., 2019; Phung et al., 2020). Both in vitro and in vivo studies revealed promising results with low IC50 values (Zhang et al., 2015; Leto et al., 2016) and high rates of tumor inhibition (Jin et al., 2013; Chen et al., 2015; Wang et al., 2016b; Liu et al., 2016; Dong et al., 2019; Wang et al., 2019; Li et al., 2020).
In a study conducted on BT474 (HER2+) breast tumor cells made using liposomal nanoparticles for drug delivery, IC50 values ranged between 0.07–0.39 µM (Zhang YJ. et al., 2013), indicating high potency. In another study, IC50 values decreased from 127 ± 8.5 µM when free ARS was administered to 69 ± 23 µM when liposomes were administered (Leto et al., 2016), demonstrating the ability of liposomes to increase the efficacy of ARS. Many formulations used pH-dependent drug release in the slightly acidic environment of tumor cells (Wang et al., 2016a; Wang et al., 2016b; Dong et al., 2019; Wan et al., 2019; Wang et al., 2019) for targeted drug delivery and increased accumulation of the drug in the tumor cells while simultaneously reducing unintended off-target interactions. This might have contributed to the greater cytotoxicity observed with the use of novel nanoformulations than with the use of free drug (Chen J. et al., 2014; Chen et al., 2015; Tran et al., 2015; Wang et al., 2016b; Tran et al., 2017; Dong et al., 2019).
After nanoformulation administration, the same efficacy was demonstrated in in vivo studies, whereas an increase in antitumor effect was observed in tumor-bearing mice models (Jin et al., 2013; Chen et al., 2015; Zhang et al., 2015; Wang et al., 2016a; Wang et al., 2016b; Liu et al., 2016; Wang et al., 2018; Dong et al., 2019; Wang et al., 2019; Li et al., 2020; Phung et al., 2020). Antitumor effect was measured by using the tumor volume and tumor growth inhibition rate. In a study that used nanoconjugates, breast tumor volume was 989 ± 164 mm3 after treatment with nanoconjugate formulation compared to 1,417 ± 148 mm3 after treatment with the free drug (Li et al., 2020). Another study conducted on Lewis lung carcinoma tumor bearing mice model reported a tumor growth inhibition rate of 84.6% after treatment with polyethylene DHA nanoparticles compared to 29.9% after treatment with free DHA. Survival rate was also markedly higher (83.3%) than that of free DHA (16.7%) (Liu et al., 2016).
In the future research, combination therapy and nanotechnology should be further explored. The combinations of DHA with oxaliplatin (Duan et al., 2019), DHA with sorafenib (Wang et al., 2019), DHA with docetaxel (Li et al., 2020), and DHA with paclitaxel (Phung et al., 2020) along with the use of nanoparticles have been studied, and in vitro and in vivo data are promising, implying their viability for human trials.
Concluding Remarks
Despite challenges, repurposing artemisinins for cancer treatmentis possible. Artemisinin and its derivatives have anticancer effects against multiple cancer types. because they act through various pathways, although their potency varies across cancer types. Their efficacy has also been demonstrated in in vivo studies with evidence of inhibition of tumor growth in tumor bearing mice models. A few human trials have also shown promising results that artemisinins, in particular ART, are safe for use, although their efficacy is still relatively limited. The limitations due to their pharmacokinetic properties such as low tissue distribution, short half-life, and unpredictable toxicity at high doses hinder their clinical translation. However, there are viable options such as the use of combination therapy and nanoformulations that can overcome the pharmacokinetic barriers of artemisinins. At high doses of artemisinins are used in cancer treatment, toxicity prediction models should be used to ensure that severe toxicity is controlled (Li S. et al., 2021). Although artemisinins have great potential as anticancer agents, additional extensive human trials are required before the drug can be established as an anticancer agent.
Data Availability Statement
The raw data supporting the conclusions of this article will be made available by the authors, without undue reservation.
Author Contributions
ZM and CW equally contributed to drafting the article; C-GL, J-TC, MY, GS, and AW contributed to acquisition of data, figure preparation, analysis and interpretation of data, and manuscript revision. PH, DZ, PO, LW, B-CG contributed to the structure design and the conception and design of the study.
Funding
This work was supported by the following research grants from The National Medical Research Council, Singapore (NMRC/CSASI/0006/2016 (B-CG) and NMRC/CG/M005/2017_NCIS (LW)). Joint NCIS and NUS Cancer Program Seed Funding Grants (NUHSRO/2020/122/MSC/07/Cancer) (B-CG and LW).
Conflict of Interest
The authors declare that the research was conducted in the absence of any commercial or financial relationships that could be construed as a potential conflict of interest.
Publisher’s Note
All claims expressed in this article are solely those of the authors and do not necessarily represent those of their affiliated organizations, or those of the publisher, the editors and the reviewers. Any product that may be evaluated in this article, or claim that may be made by its manufacturer, is not guaranteed or endorsed by the publisher.
References
An, F. F., Liu, Y. C., Zhang, W. W., and Liang, L. (2013). Dihydroartemisinine Enhances Dictamnine-Induced Apoptosis via a Caspase Dependent Pathway in Human Lung Adenocarcinoma A549 Cells. Asian Pac. J. Cancer Prev. 14 (10), 5895–5900. doi:10.7314/APJCP.2013.14.10.5895
Ashton, M., Gordi, T., Trinh, N. H., Nguyen, V. H., Nguyen, D. S., Nguyen, T. N., et al. (1998). Artemisinin Pharmacokinetics in Healthy Adults after 250, 500 and 1000 Mg Single Oral Doses. Biopharm. Drug Dispos 19 (4), 245–250. doi:10.1002/(sici)1099-081x(199805)19:4<245:aid-bdd99>3.0.co;2-z
Augustin, Y., Staines, H. M., and Krishna, S. (2020). Artemisinins as a Novel Anti-cancer Therapy: Targeting a Global Cancer Pandemic through Drug Repurposing. Pharmacol. Ther. 216, 107706. doi:10.1016/j.pharmthera.2020.107706
Batty, K. T., Iletr, K. E., Powell, S. M., Martin, J., and Davis, T. M. (2002). Relative Bioavailability of Artesunate and Dihydroartemisinin: Investigations in the Isolated Perfused Rat Liver and in Healthy Caucasian Volunteers. Am. J. Trop. Med. Hyg. 66 (2), 130–136. doi:10.4269/ajtmh.2002.66.130
Beccafico, S., Morozzi, G., Marchetti, M. C., Riccardi, C., Sidoni, A., Donato, R., et al. (2015). Artesunate Induces ROS- and P38 MAPK-Mediated Apoptosis and Counteracts Tumor Growth In Vivo in Embryonal Rhabdomyosarcoma Cells. Carcinogenesis 36 (9), 1071–1083. doi:10.1093/carcin/bgv098
Berdelle, N., Nikolova, T., Quiros, S., Efferth, T., and Kaina, B. (2011). Artesunate Induces Oxidative DNA Damage, Sustained DNA Double-Strand Breaks, and the ATM/ATR Damage Response in Cancer Cells. Mol. Cancer Ther. 10 (12), 2224–2233. doi:10.1158/1535-7163.MCT-11-0534
Berger, T. G., Dieckmann, D., Efferth, T., Schultz, E. S., Funk, J. O., Baur, A., et al. (2005). Artesunate in the Treatment of Metastatic Uveal Melanoma-Ffirst Experiences. Oncol. Rep. 14 (6), 1599–1603. doi:10.3892/or.14.6.1599
Berte, N., Lokan, S., Eich, M., Kim, E., and Kaina, B. (2016). Artesunate Enhances the Therapeutic Response of Glioma Cells to Temozolomide by Inhibition of Homologous Recombination and Senescence. Oncotarget 7 (41), 67235–67250. doi:10.18632/oncotarget.11972
Binh, T. Q., Ilett, K. F., Batty, K. T., Davis, T. M., Hung, N. C., Powell, S. M., et al. (2001). Oral Bioavailability of Dihydroartemisinin in Vietnamese Volunteers and in Patients with Falciparum Malaria. Br. J. Clin. Pharmacol. 51 (6), 541–546. doi:10.1046/j.1365-2125.2001.01395.x
Buranrat, B., and Connor, J. R. (2015). Cytoprotective Effects of Ferritin on Doxorubicin-Induced Breast Cancer Cell Death. Oncol. Rep. 34 (5), 2790–2796. doi:10.3892/or.2015.4250
Chen, G. Q., Benthani, F. A., Wu, J., Liang, D., Bian, Z. X., and Jiang, X. (2020a). Artemisinin Compounds Sensitize Cancer Cells to Ferroptosis by Regulating Iron Homeostasis. Cell Death Differ 27 (1), 242–254. doi:10.1038/s41418-019-0352-3
Chen, H., Gu, S., Dai, H., Li, X., and Zhang, Z. (2017a). Dihydroartemisinin Sensitizes Human Lung Adenocarcinoma A549 Cells to Arsenic Trioxide via Apoptosis. Biol. Trace Elem. Res. 179 (2), 203–212. doi:10.1007/s12011-017-0975-5
Chen, H., Sun, B., Wang, S., Pan, S., Gao, Y., Bai, X., et al. (2010). Growth Inhibitory Effects of Dihydroartemisinin on Pancreatic Cancer Cells: Involvement of Cell Cycle Arrest and Inactivation of Nuclear Factor-kappaB. J. Cancer Res. Clin. Oncol. 136 (6), 897–903. doi:10.1007/s00432-009-0731-0
Chen, H., Sun, B., Wang, S., Pan, S., Gao, Y., Bai, X., et al. (2009a). Growth Inhibitory Effects of Dihydroartemisinin on Pancreatic Cancer Cells: Involvement of Cell Cycle Arrest and Inactivation of Nuclear Factor-Κb. J. Cancer Res. Clin. Oncol. 136, 897–903. doi:10.1007/s00432-009-0731-0
Chen, J., Guo, Z., Wang, H. B., Zhou, J. J., Zhang, W. J., and Chen, Q. W. (2014a). Multifunctional Mesoporous Nanoparticles as pH-Responsive Fe(2+) Reservoirs and Artemisinin Vehicles for Synergistic Inhibition of Tumor Growth. Biomaterials 35 (24), 6498–6507. doi:10.1016/j.biomaterials.2014.04.028
Chen, J., Zhang, W., Zhang, M., Guo, Z., Wang, H., He, M., et al. (2015). Mn(II) Mediated Degradation of Artemisinin Based on Fe3O4@MnSiO3-FA Nanospheres for Cancer Therapy In Vivo. Nanoscale 7 (29), 12542–12551. doi:10.1039/c5nr02402a
Chen, K., Shou, L. M., Lin, F., Duan, W. M., Wu, M. Y., Xie, X., et al. (2014b). Artesunate Induces G2/M Cell Cycle Arrest through Autophagy Induction in Breast Cancer Cells. Anticancer Drugs 25 (6), 652–662. doi:10.1097/CAD.0000000000000089
Chen, T., Li, M., Zhang, R., and Wang, H. (2009b). Dihydroartemisinin Induces Apoptosis and Sensitizes Human Ovarian Cancer Cells to Carboplatin Therapy. J. Cel Mol Med 13 (7), 1358–1370. doi:10.1111/j.1582-4934.2008.00360.x
Chen, X., He, L. Y., Lai, S., and He, Y. (2020b). Dihydroartemisinin Inhibits the Migration of Esophageal Cancer Cells by Inducing Autophagy. Oncol. Lett. 20 (4), 94. doi:10.3892/ol.2020.11955
Chen, X., Wong, Y. K., Lim, T. K., Lim, W. H., Lin, Q., Wang, J., et al. (2017b). Artesunate Activates the Intrinsic Apoptosis of HCT116 Cells through the Suppression of Fatty Acid Synthesis and the NF-Κb Pathway. Molecules 22 (8). doi:10.3390/molecules22081272
Chen, Y., Wang, F., Wu, P., Gong, S., Gao, J., Tao, H., et al. (2021). Artesunate Induces Apoptosis, Autophagy and Ferroptosis in Diffuse Large B Cell Lymphoma Cells by Impairing STAT3 Signaling. Cell Signal 88, 110167. doi:10.1016/j.cellsig.2021.110167
Deeken, J. F., Wang, H., Hartley, M., Cheema, A. K., Smaglo, B., Hwang, J. J., et al. (2018). A Phase I Study of Intravenous Artesunate in Patients with Advanced Solid Tumor Malignancies. Cancer Chemother. Pharmacol. 81 (3), 587–596. doi:10.1007/s00280-018-3533-8
Dell'Eva, R., Pfeffer, U., Vené, R., Anfosso, L., Forlani, A., Albini, A., et al. (2004). Inhibition of Angiogenesis In Vivo and Growth of Kaposi's Sarcoma Xenograft Tumors by the Anti-malarial Artesunate. Biochem. Pharmacol. 68 (12), 2359–2366. doi:10.1016/j.bcp.2004.08.021
Diem Thuy, L. T., Ngoc Hung, L., Danh, P. T., and Na-Bangchang, K. (2008). Absence of Time-dependent Artesunate Pharmacokinetics in Healthy Subjects during 5-day Oral Administration. Eur. J. Clin. Pharmacol. 64 (10), 993–998. doi:10.1007/s00228-008-0506-6
Dong, L., Wang, C., Zhen, W., Jia, X., An, S., Xu, Z., et al. (2019). Biodegradable Iron-Coordinated Hollow Polydopamine Nanospheres for Dihydroartemisinin Delivery and Selectively Enhanced Therapy in Tumor Cells. J. Mater. Chem. B 7 (40), 6172–6180. doi:10.1039/c9tb01397k
Drenberg, C. D., Buaboonnam, J., Orwick, S. J., Hu, S., Li, L., Fan, Y., et al. (2016). Evaluation of Artemisinins for the Treatment of Acute Myeloid Leukemia. Cancer Chemother. Pharmacol. 77 (6), 1231–1243. doi:10.1007/s00280-016-3038-2
Du, J., Wang, T., Li, Y., Zhou, Y., Wang, X., Yu, X., et al. (2019). DHA Inhibits Proliferation and Induces Ferroptosis of Leukemia Cells through Autophagy Dependent Degradation of Ferritin. Free Radic. Biol. Med. 131, 356–369. doi:10.1016/j.freeradbiomed.2018.12.011
Du, J. H., Zhang, H. D., Ma, Z. J., and Ji, K. M. (2010). Artesunate Induces Oncosis-like Cell Death In Vitro and Has Antitumor Activity against Pancreatic Cancer Xenografts In Vivo. Cancer Chemother. Pharmacol. 65 (5), 895–902. doi:10.1007/s00280-009-1095-5
Duan, X., Chan, C., Han, W., Guo, N., Weichselbaum, R. R., and Lin, W. (2019). Immunostimulatory Nanomedicines Synergize with Checkpoint Blockade Immunotherapy to Eradicate Colorectal Tumors. Nat. Commun. 10 (1), 1899. doi:10.1038/s41467-019-09221-x
Efferth, T. (2017). From Ancient Herb to Modern Drug: Artemisia Annua and Artemisinin for Cancer Therapy. Semin. Cancer Biol. 46, 65–83. doi:10.1016/j.semcancer.2017.02.009
Efferth, T., Giaisi, M., Merling, A., Krammer, P. H., and Li-Weber, M. (2007). Artesunate Induces ROS-Mediated Apoptosis in Doxorubicin-Resistant T Leukemia Cells. PLoS ONE 2 (8), e693. doi:10.1371/journal.pone.0000693
Ericsson, T., Blank, A., von Hagens, C., Ashton, M., and Äbelö, A. (2014). Population Pharmacokinetics of Artesunate and Dihydroartemisinin during Long-Term Oral Administration of Artesunate to Patients with Metastatic Breast Cancer. Eur. J. Clin. Pharmacol. 70 (12), 1453–1463. doi:10.1007/s00228-014-1754-2
Ferrara, N., and Kerbel, R. S. (2005). Angiogenesis as a Therapeutic Target. Nature 438 (7070), 967–974. doi:10.1038/nature04483
Greenshields, A. L., Shepherd, T. G., and Hoskin, D. W. (2017). Contribution of Reactive Oxygen Species to Ovarian Cancer Cell Growth Arrest and Killing by the Anti-malarial Drug Artesunate. Mol. Carcinog 56 (1), 75–93. doi:10.1002/mc.22474
Hamacher-Brady, A., Stein, H. A., Turschner, S., Toegel, I., Mora, R., Jennewein, N., et al. (2011). Artesunate Activates Mitochondrial Apoptosis in Breast Cancer Cells via Iron-Catalyzed Lysosomal Reactive Oxygen Species Production. J. Biol. Chem. 286 (8), 6587–6601. doi:10.1074/jbc.M110.210047
Hou, J., Wang, D., Zhang, R., and Wang, H. (2008). Experimental Therapy of Hepatoma with Artemisinin and its Derivatives: In Vitro and In Vivo Activity, Chemosensitization, and Mechanisms of Action. Clin. Cancer Res. 14 (17), 5519–5530. doi:10.1158/1078-0432.CCR-08-0197
Hu, L. J., Jiang, T., Wang, F. J., Huang, S. H., Cheng, X. M., and Jia, Y. Q. (2019). Effects of Artesunate Combined with Bortezomib on Apoptosis and Autophagy of Acute Myeloid Leukemia Cells In Vitro and its Mechanism. Zhonghua Xue Ye Xue Za Zhi 40 (3), 204–208. doi:10.3760/cma.j.issn.0253-2727.2019.03.008
Huang, S., Robinson, J. B., Deguzman, A., Bucana, C. D., and Fidler, I. J. (2000). Blockade of Nuclear Factor-kappaB Signaling Inhibits Angiogenesis and Tumorigenicity of Human Ovarian Cancer Cells by Suppressing Expression of Vascular Endothelial Growth Factor and Interleukin 8. Cancer Res. 60 (19), 5334–5339.
Hwang, Y. P., Yun, H. J., Kim, H. G., Han, E. H., Lee, G. W., and Jeong, H. G. (2010). Suppression of PMA-Induced Tumor Cell Invasion by Dihydroartemisinin via Inhibition of PKCalpha/Raf/MAPKs and NF-kappaB/AP-1-dependent Mechanisms. Biochem. Pharmacol. 79 (12), 1714–1726. doi:10.1016/j.bcp.2010.02.003
Jeong, D. E., Song, H. J., Lim, S., Lee, S. J., Lim, J. E., Nam, D. H., et al. (2015). Repurposing the Anti-malarial Drug Artesunate as a Novel Therapeutic Agent for Metastatic Renal Cell Carcinoma Due to its Attenuation of Tumor Growth, Metastasis, and Angiogenesis. Oncotarget 6 (32), 33046–33064. doi:10.18632/oncotarget.5422
Jia, J., Qin, Y., Zhang, L., Guo, C., Wang, Y., Yue, X., et al. (2016a). Artemisinin Inhibits Gallbladder Cancer Cell Lines through Triggering Cell Cycle Arrest and Apoptosis. Mol. Med. Rep. 13 (5), 4461–4468. doi:10.3892/mmr.2016.5073
Jia, L., Song, Q., Zhou, C., Li, X., Pi, L., Ma, X., et al. (2016b). Dihydroartemisinin as a Putative STAT3 Inhibitor, Suppresses the Growth of Head and Neck Squamous Cell Carcinoma by Targeting Jak2/STAT3 Signaling. PLoS ONE 11 (1), e0147157. doi:10.1371/journal.pone.0147157
Jiang, W., Huang, Y., Wang, J. P., Yu, X. Y., and Zhang, L. Y. (2013). The Synergistic Anticancer Effect of Artesunate Combined with Allicin in Osteosarcoma Cell Line In Vitro and In Vivo. Asian Pac. J. Cancer Prev. 14 (8), 4615–4619. doi:10.7314/APJCP.2013.14.8.4615
Jiang, Z., Wang, Z., Chen, L., Zhang, C., Liao, F., Wang, Y., et al. (2021). Artesunate Induces ER-Derived-ROS-Mediated Cell Death by Disrupting Labile Iron Pool and Iron Redistribution in Hepatocellular Carcinoma Cells. Am. J. Cancer Res. 11 (3), 691–711.
Jiao, Y., Ge, C. M., Meng, Q. H., Cao, J. P., Tong, J., and Fan, S. J. (2007). Dihydroartemisinin Is an Inhibitor of Ovarian Cancer Cell Growth. Acta Pharmacol. Sin 28 (7), 1045–1056. doi:10.1111/j.1745-7254.2007.00612.x
Jin, H., Jiang, A. Y., Wang, H., Cao, Y., Wu, Y., and Jiang, X. F. (2017). Dihydroartemisinin and Gefitinib Synergistically Inhibit NSCLC Cell Growth and Promote Apoptosis via the Akt/mTOR/STAT3 Pathway. Mol. Med. Rep. 16 (3), 3475–3481. doi:10.3892/mmr.2017.6989
Jin, M., Shen, X., Zhao, C., Qin, X., Liu, H., Huang, L., et al. (2013). In Vivo study of Effects of Artesunate Nanoliposomes on Human Hepatocellular Carcinoma Xenografts in Nude Mice. Drug Deliv. 20 (3-4), 127–133. doi:10.3109/10717544.2013.801047
Jing, W., Shuo, L., Yingru, X., Min, M., Runpeng, Z., Jun, X., et al. (2019). Artesunate Promotes Sensitivity to Sorafenib in Hepatocellular Carcinoma. Biochem. Biophys. Res. Commun. 519 (1), 41–45. doi:10.1016/j.bbrc.2019.08.115
Kim, C., Lee, J. H., Kim, S. H., Sethi, G., and Ahn, K. S. (2015). Artesunate Suppresses Tumor Growth and Induces Apoptosis through the Modulation of Multiple Oncogenic Cascades in a Chronic Myeloid Leukemia Xenograft Mouse Model. Oncotarget 6 (6), 4020–4035. doi:10.18632/oncotarget.3004
Kong, R., Jia, G., Cheng, Z. X., Wang, Y. W., Mu, M., Wang, S. J., et al. (2012). Dihydroartemisinin Enhances Apo2l/TRAIL-Mediated Apoptosis in Pancreatic Cancer Cells via ROS-Mediated Up-Regulation of Death Receptor 5. PLoS ONE 7 (5), e37222. doi:10.1371/journal.pone.0037222
Krishna, S., Ganapathi, S., Ster, I. C., Saeed, M. E., Cowan, M., Finlayson, C., et al. (2015). A Randomised, Double Blind, Placebo-Controlled Pilot Study of Oral Artesunate Therapy for Colorectal Cancer. EBioMedicine 2 (1), 82–90. doi:10.1016/j.ebiom.2014.11.010
Leto, I., Coronnello, M., Righeschi, C., Bergonzi, M. C., Mini, E., and Bilia, A. R. (2016). Enhanced Efficacy of Artemisinin Loaded in Transferrin-Conjugated Liposomes versus Stealth Liposomes against HCT-8 Colon Cancer Cells. ChemMedChem 11, 1745–1751. doi:10.1002/cmdc.201500586
Li, L. N., Zhang, H. D., Yuan, S. J., Yang, D. X., Wang, L., and Sun, Z. X. (2008). Differential Sensitivity of Colorectal Cancer Cell Lines to Artesunate Is Associated with Expression of Beta-Catenin and E-Cadherin. Eur. J. Pharmacol. 588 (1), 1–8. doi:10.1016/j.ejphar.2008.03.041
Li, N., Guo, W., Li, Y., Zuo, H., Zhang, H., Wang, Z., et al. (2020). Construction and Anti-tumor Activities of Disulfide-Linked Docetaxel-Dihydroartemisinin Nanoconjugates. Colloids Surf. B Biointerfaces 191, 111018. doi:10.1016/j.colsurfb.2020.111018
Li, Q., Cantilena, L. R., Leary, K. J., Saviolakis, G. A., Miller, R. S., Melendez, V., et al. (2009). Pharmacokinetic Profiles of Artesunate after Single Intravenous Doses at 0.5, 1, 2, 4, and 8 Mg/kg in Healthy Volunteers: a Phase I Study. Am. J. Trop. Med. Hyg. 81 (4), 615–621. doi:10.4269/ajtmh.2009.09-0150
Li, S., Yu, Y., Bian, X., Yao, L., Li, M., Lou, Y. R., et al. (2021a). Prediction of Oral Hepatotoxic Dose of Natural Products Derived from Traditional Chinese Medicines Based on SVM Classifier and PBPK Modeling. Arch. Toxicol. 95 (5), 1683–1701. doi:10.1007/s00204-021-03023-1
Li, W., Ma, G., Deng, Y., Wu, Q., Wang, Z., and Zhou, Q. (2021b). Artesunate Exhibits Synergistic Anti-cancer Effects with Cisplatin on Lung Cancer A549 Cells by Inhibiting MAPK Pathway. Gene 766, 145134. doi:10.1016/j.gene.2020.145134
Li, Z. J., Dai, H. Q., Huang, X. W., Feng, J., Deng, J. H., Wang, Z. X., et al. (2021c). Artesunate Synergizes with Sorafenib to Induce Ferroptosis in Hepatocellular Carcinoma. Acta Pharmacol. Sin 42 (2), 301–310. doi:10.1038/s41401-020-0478-3
Liang, C., Zhang, X., Yang, M., and Dong, X. (2019). Recent Progress in Ferroptosis Inducers for Cancer Therapy. Adv. Mater. 31 (51), e1904197. doi:10.1002/adma.201904197
Liao, K., Li, J., and Wang, Z. (2014). Dihydroartemisinin Inhibits Cell Proliferation via AKT/GSK3β/cyclinD1 Pathway and Induces Apoptosis in A549 Lung Cancer Cells. Int. J. Clin. Exp. Pathol. 7 (12), 8684–8691.
Lim, H. Y., Ong, P. S., Wang, L., Goel, A., Ding, L., Li-Ann Wong, A., et al. (2021). Celastrol in Cancer Therapy: Recent Developments, Challenges and Prospects. Cancer Lett. 521, 252–267. doi:10.1016/j.canlet.2021.08.030
Liu, C. G., Li, J., Xu, Y., Li, W., Fang, S. X., Zhang, Q., et al. (2021). Long Non-coding RNAs and Circular RNAs in Tumor Angiogenesis: From Mechanisms to Clinical Significance. Mol. Ther. Oncolytics 22, 336–354. doi:10.1016/j.omto.2021.07.001
Liu, K., Dai, L., Li, C., Liu, J., Wang, L., and Lei, J. (2016). Self-assembled Targeted Nanoparticles Based on Transferrin-Modified Eight-Arm-Polyethylene Glycol-Dihydroartemisinin Conjugate. Sci. Rep. 6, 29461. doi:10.1038/srep29461
Liu, W. M., Gravett, A. M., and Dalgleish, A. G. (2011). The Antimalarial Agent Artesunate Possesses Anticancer Properties that Can Be Enhanced by Combination Strategies. Int. J. Cancer 128 (6), 1471–1480. doi:10.1002/ijc.25707
Liu, Y., and Cui, Y. F. (2013). Synergism of Cytotoxicity Effects of Triptolide and Artesunate Combination Treatment in Pancreatic Cancer Cell Lines. Asian Pac. J. Cancer Prev. 14 (9), 5243–5248. doi:10.7314/APJCP.2013.14.9.5243
Luo, J., Zhu, W., Tang, Y., Cao, H., Zhou, Y., Ji, R., et al. (2014). Artemisinin Derivative Artesunate Induces Radiosensitivity in Cervical Cancer Cells In Vitro and In Vivo. Radiat. Oncol. 9 (1), 84. doi:10.1186/1748-717X-9-84
Ma, Q., Liao, H., Xu, L., Li, Q., Zou, J., Sun, R., et al. (2020). Autophagy-dependent Cell Cycle Arrest in Esophageal Cancer Cells Exposed to Dihydroartemisinin. Chin. Med. 15, 37. doi:10.1186/s13020-020-00318-w
Michaelis, M., Kleinschmidt, M. C., Barth, S., Rothweiler, F., Geiler, J., Breitling, R., et al. (2010). Anti-cancer Effects of Artesunate in a Panel of Chemoresistant Neuroblastoma Cell Lines. Biochem. Pharmacol. 79 (2), 130–136. doi:10.1016/j.bcp.2009.08.013
Na-Bangchang, K., Krudsood, S., Silachamroon, U., Molunto, P., Tasanor, O., Chalermrut, K., et al. (2004). The Pharmacokinetics of Oral Dihydroartemisinin and Artesunate in Healthy Thai Volunteers. Southeast. Asian J. Trop. Med. Public Health 35 (3), 575–582.
National Cancer Institute. (2015). Chemotherapy to Treat Cancer. Available: https://www.cancer.gov/about-cancer/treatment/types/chemotherapy.
Newton, P. N., van Vugt, M., Teja-Isavadharm, P., Siriyanonda, D., Rasameesoroj, M., Teerapong, P., et al. (2002). Comparison of Oral Artesunate and Dihydroartemisinin Antimalarial Bioavailabilities in Acute Falciparum Malaria. Antimicrob. Agents Chemother. 46 (4), 1125–1127. doi:10.1128/aac.46.4.1125-1127.2002
Organisation, W. H. (2018). Cancer. Available: https://www.who.int/news-room/fact-sheets/detail/cancer.
Pang, Y., Qin, G., Wu, L., Wang, X., and Chen, T. (2016). Artesunate Induces ROS-dependent Apoptosis via a Bax-Mediated Intrinsic Pathway in Huh-7 and Hep3B Cells. Exp. Cel Res 347 (2), 251–260. doi:10.1016/j.yexcr.2016.06.012
Parvathaneni, V., Kulkarni, N. S., Muth, A., and Gupta, V. (2019). Drug Repurposing: a Promising Tool to Accelerate the Drug Discovery Process. Drug Discov. Today 24 (10), 2076–2085. doi:10.1016/j.drudis.2019.06.014
Phung, C. D., Le, T. G., Nguyen, V. H., Vu, T. T., Nguyen, H. Q., Kim, J. O., et al. (2020). PEGylated-Paclitaxel and Dihydroartemisinin Nanoparticles for Simultaneously Delivering Paclitaxel and Dihydroartemisinin to Colorectal Cancer. Pharm. Res. 37 (7), 129. doi:10.1007/s11095-020-02819-7
Prevention, C. F. D. C. A. (2020). FDA Approval of Artesunate for Injection for Treatment of Severe Malaria. Available: https://www.cdc.gov/malaria/new_info/2020/artesunate_approval.html (Accessed May 28, 2020).
Rasheed, S. A., Efferth, T., Asangani, I. A., and Allgayer, H. (2010). First Evidence that the Antimalarial Drug Artesunate Inhibits Invasion and In Vivo Metastasis in Lung Cancer by Targeting Essential Extracellular Proteases. Int. J. Cancer 127 (6), 1475–1485. doi:10.1002/ijc.25315
Ren, B., Kwah, M. X., Liu, C., Ma, Z., Shanmugam, M. K., Ding, L., et al. (2021). Resveratrol for Cancer Therapy: Challenges and Future Perspectives. Cancer Lett. 515, 63–72. doi:10.1016/j.canlet.2021.05.001
Roh, J. L., Kim, E. H., Jang, H., and Shin, D. (2017). Nrf2 Inhibition Reverses the Resistance of Cisplatin-Resistant Head and Neck Cancer Cells to Artesunate-Induced Ferroptosis. Redox Biol. 11, 254–262. doi:10.1016/j.redox.2016.12.010
Shen, R., Li, J., Ye, D., Wang, Q., and Fei, J. (2016). Combination of Onconase and Dihydroartemisinin Synergistically Suppresses Growth and Angiogenesis of Non-small-cell Lung Carcinoma and Malignant Mesothelioma. Acta Biochim. Biophys. Sin (Shanghai) 48 (10), 894–901. doi:10.1093/abbs/gmw082
Sieber, S., Gdynia, G., Roth, W., Bonavida, B., and Efferth, T. (2009). Combination Treatment of Malignant B Cells Using the Anti-CD20 Antibody Rituximab and the Anti-malarial Artesunate. Int. J. Oncol. 35 (1), 149–158. doi:10.3892/ijo_00000323
Sleire, L., Førde, H. E., Netland, I. A., Leiss, L., Skeie, B. S., and Enger, P. Ø. (2017). Drug Repurposing in Cancer. Pharmacol. Res. 124, 74–91. doi:10.1016/j.phrs.2017.07.013
Steinbrück, L., Pereira, G., and Efferth, T. (2010). Effects of Artesunate on Cytokinesis and G₂/M Cell Cycle Progression of Tumour Cells and Budding Yeast. Cancer Genomics Proteomics 7 (6), 337–346.
Stockwell, B. R., Friedmann Angeli, J. P., Bayir, H., Bush, A. I., Conrad, M., Dixon, S. J., et al. (2017). Ferroptosis: A Regulated Cell Death Nexus Linking Metabolism, Redox Biology, and Disease. Cell 171 (2), 273–285. doi:10.1016/j.cell.2017.09.021
Tai, X., Cai, X. B., Zhang, Z., and Wei, R. (2016). In Vitro and In Vivo Inhibition of Tumor Cell Viability by Combined Dihydroartemisinin and Doxorubicin Treatment, and the Underlying Mechanism. Oncol. Lett. 12 (5), 3701–3706. doi:10.3892/ol.2016.5187
Teja-isavadharm, P., Watt, G., Eamsila, C., Jongsakul, K., Li, Q., Keeratithakul, G., et al. (2001). Comparative Pharmacokinetics and Effect Kinetics of Orally Administered Artesunate in Healthy Volunteers and Patients with Uncomplicated Falciparum Malaria. Am. J. Trop. Med. Hyg. 65 (6), 717–721. doi:10.4269/ajtmh.2001.65.717
Tin, A. S., Sundar, S. N., Tran, K. Q., Park, A. H., Poindexter, K. M., and Firestone, G. L. (2012). Antiproliferative Effects of Artemisinin on Human Breast Cancer Cells Requires the Downregulated Expression of the E2F1 Transcription Factor and Loss of E2F1-Target Cell Cycle Genes. Anticancer Drugs 23 (4), 370–379. doi:10.1097/CAD.0b013e32834f6ea8
Tong, Y., Liu, Y., Zheng, H., Zheng, L., Liu, W., Wu, J., et al. (2016). Artemisinin and its Derivatives Can Significantly Inhibit Lung Tumorigenesis and Tumor Metastasis through Wnt/β-Catenin Signaling. Oncotarget 7 (21), 31413–31428. doi:10.18632/oncotarget.8920
Tran, B. N., Nguyen, H. T., Kim, J. O., Yong, C. S., and Nguyen, C. N. (2017). Developing Combination of Artesunate with Paclitaxel Loaded into Poly-D,l-Lactic-Co-Glycolic Acid Nanoparticle for Systemic Delivery to Exhibit Synergic Chemotherapeutic Response. Drug Dev. Ind. Pharm. 43 (12), 1952–1962. doi:10.1080/03639045.2017.1357729
Tran, K. Q., Tin, A. S., and Firestone, G. L. (2014). Artemisinin Triggers a G1 Cell Cycle Arrest of Human Ishikawa Endometrial Cancer Cells and Inhibits Cyclin-dependent Kinase-4 Promoter Activity and Expression by Disrupting Nuclear Factor-Κb Transcriptional Signaling. Anticancer Drugs 25 (3), 270–281. doi:10.1097/CAD.0000000000000054
Tran, T. H., Nguyen, A. N., Kim, J. O., Yong, C. S., and Nguyen, C. N. (2016). Enhancing Activity of Artesunate against Breast Cancer Cells via Induced-Apoptosis Pathway by Loading into Lipid Carriers. Artif. Cell Nanomed Biotechnol 44 (8), 1979–1987. doi:10.3109/21691401.2015.1129616
Tran, T. H., Nguyen, T. D., Poudel, B. K., Nguyen, H. T., Kim, J. O., Yong, C. S., et al. (2015). Development and Evaluation of Artesunate-Loaded Chitosan-Coated Lipid Nanocapsule as a Potential Drug Delivery System against Breast Cancer. AAPS PharmSciTech 16 (6), 1307–1316. doi:10.1208/s12249-015-0311-3
Trimble, C. L., Levinson, K., Maldonado, L., Donovan, M. J., Clark, K. T., Fu, J., et al. (2020). A First-In-Human Proof-Of-Concept Trial of Intravaginal Artesunate to Treat Cervical Intraepithelial Neoplasia 2/3 (CIN2/3). Gynecol. Oncol. 157 (1), 188–194. doi:10.1016/j.ygyno.2019.12.035
Ventola, C. L. (2017). Cancer Immunotherapy, Part 3: Challenges and Future Trends. P t 42 (8), 514–521.
von Hagens, C., Walter-Sack, I., Goeckenjan, M., Osburg, J., Storch-Hagenlocher, B., Sertel, S., et al. (2017). Prospective Open Uncontrolled Phase I Study to Define a Well-Tolerated Dose of Oral Artesunate as Add-On Therapy in Patients with Metastatic Breast Cancer (ARTIC M33/2). Breast Cancer Res. Treat. 164 (2), 359–369. doi:10.1007/s10549-017-4261-1
von Hagens, C., Walter-Sack, I., Goeckenjan, M., Storch-Hagenlocher, B., Sertel, S., Elsässer, M., et al. (2019). Long-term Add-On Therapy (Compassionate Use) with Oral Artesunate in Patients with Metastatic Breast Cancer after Participating in a Phase I Study (ARTIC M33/2). Phytomedicine 54, 140–148. doi:10.1016/j.phymed.2018.09.178
Wan, X., Zhong, H., Pan, W., Li, Y., Chen, Y., Li, N., et al. (2019). Programmed Release of Dihydroartemisinin for Synergistic Cancer Therapy Using a CaCO3 Mineralized Metal-Organic Framework. Angew. Chem. Int. Ed. Engl. 58 (40), 14134–14139. doi:10.1002/anie.201907388
Wang, B., Hou, D., Liu, Q., Wu, T., Guo, H., Zhang, X., et al. (2015). Artesunate Sensitizes Ovarian Cancer Cells to Cisplatin by Downregulating RAD51. Cancer Biol. Ther. 16 (10), 1548–1556. doi:10.1080/15384047.2015.1071738
Wang, D., Zhou, J., Chen, R., Shi, R., Xia, G., Zhou, S., et al. (2016a). Magnetically Guided Delivery of DHA and Fe Ions for Enhanced Cancer Therapy Based on pH-Responsive Degradation of DHA-Loaded Fe3O4@C@MIL-100(Fe) Nanoparticles. Biomaterials 107, 88–101. doi:10.1016/j.biomaterials.2016.08.039
Wang, D., Zhou, J., Chen, R., Shi, R., Zhao, G., Xia, G., et al. (2016b). Controllable Synthesis of Dual-MOFs Nanostructures for pH-Responsive Artemisinin Delivery, Magnetic Resonance and Optical Dual-Model Imaging-Guided Chemo/photothermal Combinational Cancer Therapy. Biomaterials 100, 27–40. doi:10.1016/j.biomaterials.2016.05.027
Wang, J., Xu, C., Wong, Y. K., Liao, F. L., Jiang, T., and Tu, Y. (2020). Malaria Eradication. Lancet 395 (10233), e69. doi:10.1016/S0140-6736(20)30223-3
Wang, J., Xu, C., Wong, Y. K., Ma, N., Liao, F. L., Jiang, T., et al. (2021). Triple Artemisinin-Based Combination Therapies for Malaria: Proceed with Caution. Lancet 396 (10267), 1976. doi:10.1016/S0140-6736(20)32400-4
Wang, L., Hu, Y., Hao, Y., Li, L., Zheng, C., Zhao, H., et al. (2018). Tumor-targeting Core-Shell Structured Nanoparticles for Drug Procedural Controlled Release and Cancer Sonodynamic Combined Therapy. J. Control. Release 286, 74–84. doi:10.1016/j.jconrel.2018.07.028
Wang, S. J., Gao, Y., Chen, H., Kong, R., Jiang, H. C., Pan, S. H., et al. (2010a). Dihydroartemisinin Inactivates NF-kappaB and Potentiates the Anti-tumor Effect of Gemcitabine on Pancreatic Cancer Both In Vitro and In Vivo. Cancer Lett. 293 (1), 99–108. doi:10.1016/j.canlet.2010.01.001
Wang, S. J., Sun, B., Cheng, Z. X., Zhou, H. X., Gao, Y., Kong, R., et al. (2011). Dihydroartemisinin Inhibits Angiogenesis in Pancreatic Cancer by Targeting the NF-Κb Pathway. Cancer Chemother. Pharmacol. 68 (6), 1421–1430. doi:10.1007/s00280-011-1643-7
Wang, S. J., Sun, B., Pan, S. H., Chen, H., Kong, R., Li, J., et al. (2010b). Experimental Study of the Function and Mechanism Combining Dihydroartemisinin and Gemcitabine in Treating Pancreatic Cancer. Zhonghua Wai Ke Za Zhi 48 (7), 530–534.
Wang, X., Du, Q., Mao, Z., Fan, X., Hu, B., Wang, Z., et al. (2017). Combined Treatment with Artesunate and Bromocriptine Has Synergistic Anticancer Effects in Pituitary Adenoma Cell Lines. Oncotarget 8 (28), 45874–45887. doi:10.18632/oncotarget.17437
Wang, Z., Duan, X., Lv, Y., and Zhao, Y. (2019). Low Density Lipoprotein Receptor (LDLR)-targeted Lipid Nanoparticles for the Delivery of Sorafenib and Dihydroartemisinin in Liver Cancers. Life Sci. 239, 117013. doi:10.1016/j.lfs.2019.117013
Wang, Z., Hu, W., Zhang, J. L., Wu, X. H., and Zhou, H. J. (2012). Dihydroartemisinin Induces Autophagy and Inhibits the Growth of Iron-Loaded Human Myeloid Leukemia K562 Cells via ROS Toxicity. FEBS Open Bio 2, 103–112. doi:10.1016/j.fob.2012.05.002
Weifeng, T., Feng, S., Xiangji, L., Changqing, S., Zhiquan, Q., Huazhong, Z., et al. (2011). Artemisinin Inhibits In Vitro and In Vivo Invasion and Metastasis of Human Hepatocellular Carcinoma Cells. Phytomedicine 18 (2-3), 158–162. doi:10.1016/j.phymed.2010.07.003
Wen, L., Liu, L., Wen, L., Yu, T., and Wei, F. (2018). Artesunate Promotes G2/M Cell Cycle Arrest in MCF7 Breast Cancer Cells through ATM Activation. Breast Cancer 25 (6), 681–686. doi:10.1007/s12282-018-0873-5
Willoughby, J. A., Sundar, S. N., Cheung, M., Tin, A. S., Modiano, J., and Firestone, G. L. (2009). Artemisinin Blocks Prostate Cancer Growth and Cell Cycle Progression by Disrupting Sp1 Interactions with the Cyclin-dependent Kinase-4 (CDK4) Promoter and Inhibiting CDK4 Gene Expression. J. Biol. Chem. 284 (4), 2203–2213. doi:10.1074/jbc.M804491200
Wong, Y. K., Xu, C., Kalesh, K. A., He, Y., Lin, Q., Wong, W. S. F., et al. (2017). Artemisinin as an Anticancer Drug: Recent Advances in Target Profiling and Mechanisms of Action. Med. Res. Rev. 37 (6), 1492–1517. doi:10.1002/med.21446
Wu, B., Hu, K., Li, S., Zhu, J., Gu, L., Shen, H., et al. (2012). Dihydroartiminisin Inhibits the Growth and Metastasis of Epithelial Ovarian Cancer. Oncol. Rep. 27 (1), 101–108. doi:10.3892/or.2011.1505
Wu, G. S., Lu, J. J., Guo, J. J., Huang, M. Q., Gan, L., Chen, X. P., et al. (2013). Synergistic Anti-cancer Activity of the Combination of Dihydroartemisinin and Doxorubicin in Breast Cancer Cells. Pharmacol. Rep. 65 (2), 453–459. doi:10.1016/s1734-1140(13)71021-1
Xu, G., Zou, W. Q., Du, S. J., Wu, M. J., Xiang, T. X., and Luo, Z. G. (2016). Mechanism of Dihydroartemisinin-Induced Apoptosis in Prostate Cancer PC3 Cells: An iTRAQ-Based Proteomic Analysis. Life Sci. 157, 1–11. doi:10.1016/j.lfs.2016.05.033
Xu, N., Zhou, X., Wang, S., Xu, L. L., Zhou, H. S., and Liu, X. L. (2015). Artesunate Induces SKM-1 Cells Apoptosis by Inhibiting Hyperactive β-catenin Signaling Pathway. Int. J. Med. Sci. 12 (6), 524–529. doi:10.7150/ijms.11352
Xu, Q., Li, Z. X., Peng, H. Q., Sun, Z. W., Cheng, R. L., Ye, Z. M., et al. (2011). Artesunate Inhibits Growth and Induces Apoptosis in Human Osteosarcoma HOS Cell Line In Vitro and In Vivo. J. Zhejiang Univ. Sci. B 12 (4), 247–255. doi:10.1631/jzus.B1000373
Yang, S., Zhang, D., Shen, N., Wang, G., Tang, Z., and Chen, X. (2019a). Dihydroartemisinin Increases Gemcitabine Therapeutic Efficacy in Ovarian Cancer by Inducing Reactive Oxygen Species. J. Cel Biochem 120 (1), 634–644. doi:10.1002/jcb.27421
Yang, Y., He, J., Chen, J., Lin, L., Liu, Y., Zhou, C., et al. (2019b2019). Dihydroartemisinin Sensitizes Mutant P53 (R248Q)-Expressing Hepatocellular Carcinoma Cells to Doxorubicin by Inhibiting P-Gp Expression. Biomed. Res. Int. 2019, 1–10. doi:10.1155/2019/8207056
Yao, X., Zhao, C. R., Yin, H., Wang, K., and Gao, J. J. (2020). Synergistic Antitumor Activity of Sorafenib and Artesunate in Hepatocellular Carcinoma Cells. Acta Pharmacol. Sin 41 (12), 1609–1620. doi:10.1038/s41401-020-0395-5
Yao, Y., Guo, Q., Cao, Y., Qiu, Y., Tan, R., Yu, Z., et al. (2018a). Artemisinin Derivatives Inactivate Cancer-Associated Fibroblasts through Suppressing TGF-β Signaling in Breast Cancer. J. Exp. Clin. Cancer Res. 37 (1), 282–314. doi:10.1186/s13046-018-0960-7
Yao, Z., Bhandari, A., Wang, Y., Pan, Y., Yang, F., Chen, R., et al. (2018b). Dihydroartemisinin Potentiates Antitumor Activity of 5-fluorouracil against a Resistant Colorectal Cancer Cell Line. Biochem. Biophys. Res. Commun. 501 (3), 636–642. doi:10.1016/j.bbrc.2018.05.026
Youns, M., Efferth, T., Reichling, J., Fellenberg, K., Bauer, A., and Hoheisel, J. D. (2009). Gene Expression Profiling Identifies Novel Key Players Involved in the Cytotoxic Effect of Artesunate on Pancreatic Cancer Cells. Biochem. Pharmacol. 78 (3), 273–283. doi:10.1016/j.bcp.2009.04.014
Zeng, Q. P., and Zhang, P. Z. (2011). Artesunate Mitigates Proliferation of Tumor Cells by Alkylating Heme-Harboring Nitric Oxide Synthase. Nitric Oxide 24 (2), 110–112. doi:10.1016/j.niox.2010.12.005
Zhang, J. L., Wang, Z., Hu, W., Chen, S. S., Lou, X. E., and Zhou, H. J. (2013a). DHA Regulates Angiogenesis and Improves the Efficiency of CDDP for the Treatment of Lung Carcinoma. Microvasc. Res. 87, 14–24. doi:10.1016/j.mvr.2013.02.006
Zhang, Y. J., Gallis, B., Taya, M., Wang, S., Ho, R. J., and Sasaki, T. (2013b). pH-Responsive Artemisinin Derivatives and Lipid Nanoparticle Formulations Inhibit Growth of Breast Cancer Cells In Vitro and Induce Down-Regulation of HER Family Members. PLoS ONE 8 (3), e59086. doi:10.1371/journal.pone.0059086
Zhang, Y. J., Zhan, X., Wang, L., Ho, R. J., and Sasaki, T. (2015). pH-responsive Artemisinin Dimer in Lipid Nanoparticles Are Effective against Human Breast Cancer in a Xenograft Model. J. Pharm. Sci. 104 (5), 1815–1824. doi:10.1002/jps.24407
Zhang, Z. Y., Yu, S. Q., Miao, L. Y., Huang, X. Y., Zhang, X. P., Zhu, Y. P., et al. (2008). Artesunate Combined with Vinorelbine Plus Cisplatin in Treatment of Advanced Non-small Cell Lung Cancer: a Randomized Controlled Trial. Zhong Xi Yi Jie He Xue Bao 6 (2), 134–138. doi:10.3736/jcim20080206
Zhou, C., Pan, W., Wang, X. P., and Chen, T. S. (2012). Artesunate Induces Apoptosis via a Bak-Mediated Caspase-independent Intrinsic Pathway in Human Lung Adenocarcinoma Cells. J. Cel Physiol 227 (12), 3778–3786. doi:10.1002/jcp.24086
Zhou, H. J., Wang, W. Q., Wu, G. D., Lee, J., and Li, A. (2007). Artesunate Inhibits Angiogenesis and Downregulates Vascular Endothelial Growth Factor Expression in Chronic Myeloid Leukemia K562 Cells. Vascul Pharmacol. 47 (2-3), 131–138. doi:10.1016/j.vph.2007.05.002
Zhou, Y., Wang, X., Zhang, J., He, A., Wang, Y. L., Han, K., et al. (2017). Artesunate Suppresses the Viability and Mobility of Prostate Cancer Cells through UCA1, the Sponge of miR-184. Oncotarget 8 (11), 18260–18270. doi:10.18632/oncotarget.15353
Zhou, Y., Li, X., Chen, K., Ba, Q., Zhang, X., Li, J., et al. (2021). Structural Optimization and Biological Evaluation for Novel Artemisinin Derivatives against Liver and Ovarian Cancers. Eur. J. Med. Chem. 211, 113000. doi:10.1016/j.ejmech.2020.113000
Zhou, Z. H., Chen, F. X., Xu, W. R., Qian, H., Sun, L. Q., Lü, X. T., et al. (2013). Enhancement Effect of Dihydroartemisinin on Human γδ T Cell Proliferation and Killing Pancreatic Cancer Cells. Int. Immunopharmacol 17 (3), 850–857. doi:10.1016/j.intimp.2013.09.015
Zhu, S., Liu, W., Ke, X., Li, J., Hu, R., Cui, H., et al. (2014). Artemisinin Reduces Cell Proliferation and Induces Apoptosis in Neuroblastoma. Oncol. Rep. 32 (3), 1094–1100. doi:10.3892/or.2014.3323
Zhu, S., Yu, Q., Huo, C., Li, Y., He, L., Ran, B., et al. (2021). Ferroptosis: A Novel Mechanism of Artemisinin and its Derivatives in Cancer Therapy. Curr. Med. Chem. 28 (2), 329–345. doi:10.2174/0929867327666200121124404
Keywords: artemisinin, artemisinin derivatives, drug repurposing, anticancer therapy, pharmacokinetics, signalling pathways
Citation: Ma Z, Woon CY-N, Liu C-G, Cheng J-T, You M, Sethi G, Wong AL-A, Ho PC-L, Zhang D, Ong P, Wang L and Goh B-C (2021) Repurposing Artemisinin and its Derivatives as Anticancer Drugs: A Chance or Challenge?. Front. Pharmacol. 12:828856. doi: 10.3389/fphar.2021.828856
Received: 04 December 2021; Accepted: 13 December 2021;
Published: 31 December 2021.
Edited by:
Haishu Lin, Shenzhen Technology University, ChinaReviewed by:
Qingyu Zhou, University of South Florida, United StatesLiming Wang, Hunan University, China
Copyright © 2021 Ma, Woon, Liu, Cheng, You, Sethi, Wong, Ho, Zhang, Ong, Wang and Goh. This is an open-access article distributed under the terms of the Creative Commons Attribution License (CC BY). The use, distribution or reproduction in other forums is permitted, provided the original author(s) and the copyright owner(s) are credited and that the original publication in this journal is cited, in accordance with accepted academic practice. No use, distribution or reproduction is permitted which does not comply with these terms.
*Correspondence: Daping Zhang, RGFwaW5nWmhAMTYzLmNvbQ==; Peishi Ong, cGhhb3BzQG51cy5lZHUuc2c=; Lingzhi Wang, Y3Npd2xAbnVzLmVkdS5zZw==
†These authors have contributed equally to this work