- 1Department of Pathology, Daegu Catholic University School of Medicine, Daegu, South Korea
- 2Department of Physiology, Daegu Catholic University School of Medicine, Daegu, South Korea
- 3College of Pharmacy, Chonnam National University, Gwangju, South Korea
- 4Biometrology Group, Korea Research Institute of Standards and Science, Daejeon, South Korea
- 5Department of Precision Measurement, University of Science & Technology (UST), Daejeon, South Korea
- 6Department of Biochemistry, Chungnam National University, Daejeon, South Korea
- 7Department of Biological Sciences, Chungnam National University, Daejeon, South Korea
EGFR-mediated tumors have been targeted to overcome several different malignant cancers. EGFR overexpression and mutations are directly related to the malignancy, which makes the therapy more complicated. One reason for the malignancy is the induction of AP1 followed by inflammation via IL-6 secretion. Current therapeutic strategies to overcome EGFR-mediated tumors are tyrosine kinase inhibitors (TKIs), anti-EGFR monoclonal antibodies, and the combination of these two agents with classic chemotherapy or immune checkpoint inhibitors (ICIs). Although the strategies are straightforward and have shown promising efficacy in several studies, there are still hurdles to overcoming the adverse effects and limited efficacy. This study reviews the current therapeutic strategies to target EGFR family members, how they work, and their effects and limitations. We also suggest developing novel strategies to target EGFR-mediated tumors in a novel approach. A lysosome is the main custodial staff to discard unwanted amounts of EGFR and other receptor tyrosine kinase molecules. Targeting this organelle may be a new approach to overcoming EGFR-mediated cancers.
1 Introduction
Lysosomes are acidic intracellular organelles carrying a package of hydrolytic enzymes and various membrane-associated proteins (Saftig, 2005). Lysosomes exist in all animal cells except erythrocytes, but the structure and number differ depending on the cell type and functions (Saftig, 2005; Appelqvist et al., 2013). The characteristic highly acidic pH (4.5–5.0) of lysosomes is achieved by the proton pump of endosomes, the vacuolar-type H+-ATPase (V-ATPase). The acidic environment of lysosomes enables the activity of hydrolases optimized in acidic pH (Saftig, 2005). The biogenesis of lysosomes is known to be controlled by the coordination of the regulatory gene network and the lysosomal expression, which is governed by the nuclear translocation of transcription factor EB (TFEB) (Sardiello et al., 2009; Palmieri et al., 2011; Settembre et al., 2012).
Lysosomal gene mutations can lead to reduced production or mislocalization of lysosomal enzymes and cell waste accumulation. The importance of lysosomal dysfunctions is underestimated. In cancers, nascent biomass production is required for cellular transformation, and lysosomal function has a vital role in macromolecular synthesis by precursors (Davidson and Vander Heiden, 2017). The other importance of lysosomes is the adaptation to nutrient stress, which is the further provocation by cancers, and autophagy is known to play an essential role in some tumor progressions (Levine and Kroemer, 2008; Kimmelman, 2011; White, 2015). Lysosomal enzyme activity is known to be increased in many tumors compared to neighboring normal tissue, and the lysosomal changes in cancers have been known for tens of years (Kirkegaard and Jaattela, 2009). Many cancer hallmarks lead to or are resulted from lysosomal functioning or malfunctioning (Hanahan and Weinberg, 2011). Additionally, a recent insight has suggested that the increase in lysosomal activity can lead to the downregulation of receptor tyrosine kinase activity in cancer cells (Hong et al., 2019).
Receptor tyrosine kinase (RTK) activity is well-known for the over-expression and over-activation in many cancer cells. Their implications of cancer malignancy in proliferation, metastasis, angiogenesis, and invasion have been studied for decades. RTKs are tightly related to the over-expression and exacerbated activation in cancer cells (Toulany, 2019). The epidermal growth factor receptor (EGFR) is a member of the ErbB family of RTKs and has critical roles in epithelial physiology (Schlessinger, 2014). Mutations and overexpression of EGFR in various human cancers are frequent; hence, EGFR targets several current cancer therapies (Yarden and Pines, 2012).
The EGFR pathway has been studied since the 1980s following the discovery of EGF in the 1960s. The biochemical, structural, and genetic studies of EGFR revealed the molecular mechanism of the ligand-mediated receptor transphosphorylation and serial activation of the intracellular signaling consequences. The activation of the EGFR pathway induces multiple cell proliferation pathways, survival, and differentiation from the cell surface to the nucleus through the intracellular endosomal system (Lemmon and Schlessinger, 2010; Schlessinger, 2014). The canonical EGFR pathway is activated in a ligand- and kinase-dependent manner (Lemmon and Schlessinger, 2010). Additionally, a kinase-independent pathway has been identified, which showed unexpected roles regulating autophagy and metabolism (Tan et al., 2016). The stress signals usually induce the noncanonical pathway of EGFR, and the stress signals can be easily observed in cancer cells providing survival and resistance to therapies (Jutten et al., 2013; Tan et al., 2016). These findings lead to the idea that targeting both kinase-dependent and independent pathways of EGFR can offer an additional opportunity in cancer therapies. This review aims to understand how lysosomal changes can affect EGFR-mediated cancers and suggest a new approach to developing cancer therapies targeting lysosomes and EGFR.
2 EGFR (ErbB) family members
EGFR family members are expressed in various cell types, such as epithelial, mesenchymal, and neuronal cells, where they show diverse roles in development, proliferation, and differentiation (Casalini et al., 2004). All four EGFR family members are RTKs. They have a highly homogeneous structure consisting of an extracellular domain for ligand binding, a hydrophobic transmembrane region, and an intracellular region for signal transduction with a conserved tyrosine kinase domain (Prenzel et al., 2001). EGFR family members bind with a ligand family of twelve polypeptides, followed by the stimulation of the homodimeric or heterodimeric interactions between family members. The interaction leads to the autophosphorylation of several intracellular tyrosine residues (Roskoski, 2004). When the tyrosine residue is phosphorylated, multiple adaptors and signaling molecules are docked to the phosphorylated site that, generate diverse responses (Hynes et al., 2001). EGFR family members are involved in several signaling pathways, such as the PI3K-Akt and RAS-ERK. EGFR (ErbB1) and ErbB4 are canonical RTK molecules interacting and signaling by specific ligands. However, ErbB2 (HER2) does not interact with any known ligand, and ErbB3 is generally accepted as missing kinase activity (Hynes et al., 2001).
Interestingly, ErbB3 has been recently known to interact with ATP inducing a low level of kinase activity (Shi et al., 2010). Because ErbB2 and ErbB3 lack the classic characteristics of EGFR family receptors, these receptors form a heterodimer with other family members. Heterodimerization of these members signals diversity and amplifies the response among EGFR family members. Among the dimers, ErbB2-ErbB3 heterodimers produce the most potent mitogenic signals (Zhang et al., 2012).
3 EGFR family overexpression and tumor inflammation
Uncontrolled activity of the EGFR family can lead to aberrant stimulation of growth and tumorigenesis in many tumor types, such as lung, brain, breast, colon, and head and neck tumors (Yarden and Pines, 2012). Abnormal EGFR and ErbB2 activation can be observed in a wide range of mechanisms like overexpression, gene mutations, and autocrinergic stimulation in cancers (Hynes and MacDonald, 2009). When EGFR and ErbB2 are mutated or overexpressed, receptors can be activated regardless of ligan-binding. In addition, these receptors show higher activity than usual with ligand binding. As stated above, ErbB3 has low kinase activity, so the oncogenic activity is observed mostly when dimerized with EGFR or ErbB2 (Hynes and MacDonald, 2009). Erb4 is a classic RTK but has multiple variants showing different activities. Some of the variants are oncogenic, while others are tumor-suppressive (Sundvall et al., 2008). However, a recent report showed that ErbB4 point mutations exist at low levels in several types of tumors, which implies that ErbB4 activation is highly related to pro-tumorigenicity (Prickett et al., 2009).
The hyperactivation of EGFR family members is not uncommon in several cancers, making EGFRs an attractive therapeutic target. Among four members of the EGFR family members, EGFR and ErbB2 are best studied and known for their roles in cancer, so most drugs developed and under clinical trials are targeting these two receptors. Most strategies targeting EGFR and ErbB2 are blocking the ligand and receptor binding or inhibiting the kinase activity of receptors. However, the combination of several antibodies or kinase inhibitors showed promising additive and synergetic therapeutic effects in studies (Tebbutt et al., 2013). In this study, we want to review and discuss new therapeutic strategies targeting EGFR families in aspects of ligand-binding, kinase, and else.
4 EGFR and inflammation
Immune cells infiltrating the tumor are not the only producers of proinflammatory cytokines but also tumor cells over-express cytokines to gather leukocytes to the tumor site. The cytokine-releasing tumor cells produce a cocktail of cytokines, such as interleukins and interferons, to maintain the tumor microenvironment to continue oncogenic signalings (Crusz and Balkwill, 2015). IL-1, IL-6, IL-11, IL-15, IL-17, IL-23, and TNF-α are representative proinflammatory cytokines promoting tumor cell differentiation, proliferation, and survival that build up the tumor microenvironment and tumor inflammation (Vendramini-Costa and Carvalho, 2012).
NF-κB activation via PI3K/Akt and MAPK pathways promotes not only the proliferation and metabolism but also inflammation signals (Figure 1). Enhanced IL-6 secretion is tightly correlated with the activation of ligand-mediated EGFR activation to promote the inflammatory tumor microenvironment of advanced-stage epithelial ovarian cancers (Alberti et al., 2012). In lung cancer, mucosa-associated lymphoid tissue 1 MALT1) cross-talks with NF-κB and STAT3 to develop an inflammatory tumor microenvironment (Pan et al., 2016). Interestingly, Erlotinib-resistant EGFR mutation in NSCLC enhanced NF-κB activity, while NF-κB inhibitors rescued the erlotinib sensitivity in resistant cells (Bivona et al., 2011). So, the inflammatory environment in tumor tissues could be mediated by EGFR via NF-κΒ and STAT3, and the blocking of EGFR signaling can suppress the promotion of tumor inflammations. This explains the importance of EGFR in tumor inflammations.
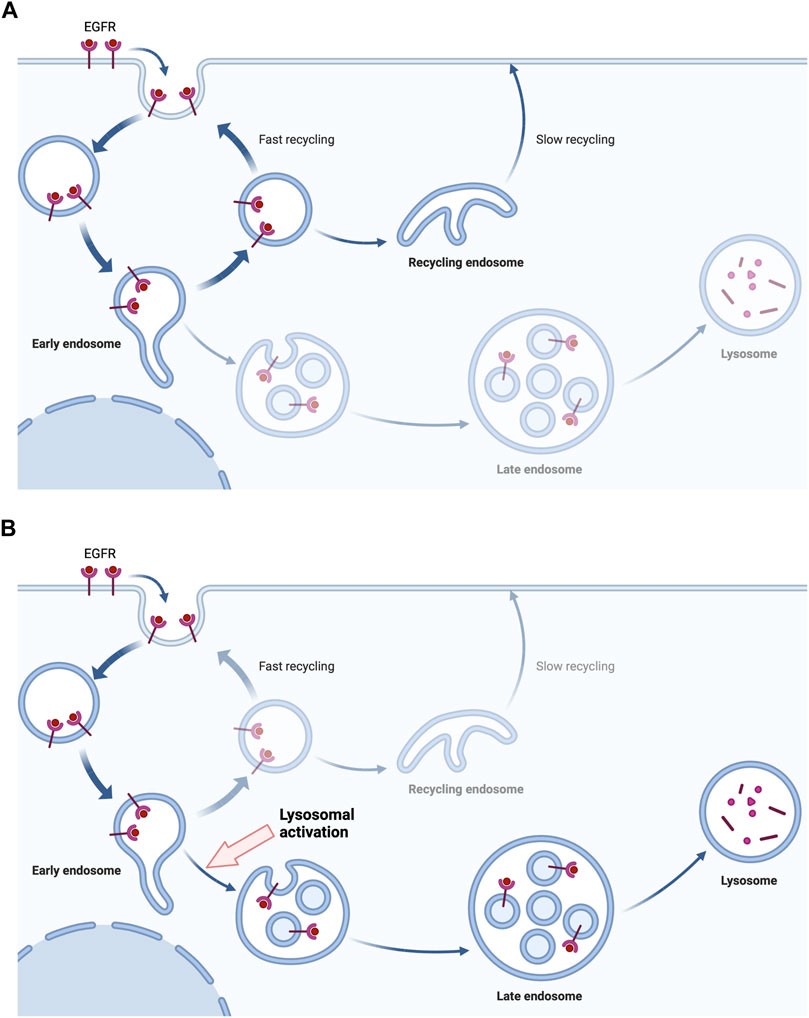
FIGURE 1. The lysosome activation drives the degradation of EGFR. The binding of EGF initiates the endocytosis of EGFR, inducing downstream signals. The endocytosed EGFR in endosomes is being recycled in tumor cells with EGFR mutation or overexpression (A). However, the activation of lysosomes gives the drive force to the lysosomal degradation of endocytosed EGFR rather than the recycling (B). Created with Biorender.com.
5 Therapies targeting EGFR and ErbB2
A number of tyrosine kinase inhibitors (TKIs) have been developed over the past 40 years, as well as still in preclinical and clinical studies (Yarden and Pines, 2012). These small molecules are considered the first choice in the treatment of solid tumors (Albanell and Gascon, 2005; Mendelsohn and Baselga, 2006). Currently, three generations of TKIs are being applied to target EGFR in practice and trials. Erlotinib and gefitinib are involved in the first-generation TKIs that reversibly competes with endogenous ATP to inhibit binding to the kinase domain (Yarden and Pines, 2012). The inhibition of ATP binding to the kinase domain leads to the suppression of tyrosine-phosphorylation, followed by the inhibition of downstream signals. These drugs are considered as the representative targeted therapy against solid tumors such as non-small-cell lung cancer (NSCLC) with kinase domain mutations, which show great response to EGFR TKIs. However, this is an exceptional example because it is well-known that a single TKI therapy usually shows poor therapeutic responses (Wheeler et al., 2010; Bria et al., 2011). The second-generation EGFR TKI includes afatinib, which irreversibly inhibits the kinase domain of EGFR and ErbB2 by binding to the free cysteine. Afatinib exhibits advantages over several platinum-based agents for the treatment of patients with EGFR mutations. However, most patients show inevitable development of acquired resistance after a median period of 10–14 months through several mechanisms (Sequist et al., 2011; Yang et al., 2012; Yu et al., 2013). Osimertinib is the third-generation TKI that targets mutations from first- and second-generation TKIs and shows high efficacy in first-line and second-line. However, resistance inevitably developed after the administration of osimertinib (Wu et al., 2020).
Antibody therapies targeting EGFR are another strategy to inactivate RTK signals. Cetuximab, panitumumab, nimotuzumab, and necitumumab prevent ligand binding and have been approved in several countries for colon cancer and head and neck cancer treatments (Mendelsohn and Baselga, 2006). In order that antibodies bind the receptors extracellularly, unlike the direct binding and inhibition of kinase, the antibodies prevent the dimerization of receptors in some cases (Mendelsohn and Baselga, 2006). The therapeutic effects of EGFR-targeting antibodies are relatively low compared to TKIs, showing only several months of survival in limited subtypes of patients (Siena et al., 2009). For example, colon cancer patients with wild-type KRAS in their tumors show good responses to EGFR-targeting antibodies (Siena et al., 2009). Recently, mixtures of new EGFR targeting antibodies like Sym004 (futuximab and modotuximab), a monoclonal antibody targeting multiple regions of EGFR extracellular domain like MM-151 and duligotuzumab as well as new monoclonal antibodies such as AMG595, anitumumab, depatuxizumab, GC118, humMR1, imagatuzumab, Mab A13, mafodotin, matuzumab, necitumumab, nimotuzumab, tomozotuximab, and zalutumumab are currently being studied (Cai et al., 2020).
6 Combination strategies targeting EGFR and ErbB2
In order to overcome the limitations of EGFR-TKIs and anti-EGFR antibodies, several combinations have been applied and are still under trial of TKIs and/or monoclonal antibodies with traditional chemotherapy, other inhibitors, and anti-angiogenic agents. The efficacy of the combination of EGFR-TKIs or monoclonal antibodies with chemotherapies shows remarkable outcomes, including in patients with head and neck tumors (Cai et al., 2020; Wu et al., 2020). However, the combination therapies also have limitations for tumors with EGFR-TKI-resistant patients in clinical trials in those with previous experience with TKIs (Laurila and Koivunen, 2015; Soria et al., 2015; Mok et al., 2017). Several combinations with platinum-based chemotherapies are under trial, but they still have a high possibility of showing resistance or other limitations (Asahina et al., 2021).
Unlike the combination of TKIs with classic chemotherapies, immune checkpoint inhibitors (ICIs) have shown promising therapeutic options in the second and third lines. EGFR mutations and EGFR TKI treatment had relevance with the increased PD-L1 levels, which suggests the combination of anti-PD-1/PD-K1 with TKIs may be synergistically effective to NSCLC (D’Incecco et al., 2015). However, the TATTON study revealed that Osimertinib treatment with durvalumab in patients with EGFR mutations shows higher risk of adverse effects (Oxnard et al., 2020). The CAUREL phase III clinical trial showed the effects of Osimertinib with or without nivolumab administration in T790M point mutation-bearing NSCLC patients who had previous TKI treatment. This study found patients with the combination of nivolumab and TKI showed markedly higher onset of interstitial pneumonitis than TKI single agent-treated patients (Oshima et al., 2018). Furthermore, other combination strategies of ICIs and TKIs also showed increased onset of adverse effects without significant improvement of efficacy (Oshima et al., 2018; Yang et al., 2018; Latif and Liu, 2019). Additionally, the combination of erlotinib with nivolumab showed tolerance and durable responses in EGFR mutation from clinical trials on TKI-treated NSCLC patients (Rizvi et al., 2016; Gettinger et al., 2018). In a nutshell, the combination therapy of TKIs and ICIs is in too early a stage, and further studies and assessments are required to treat cancer patients with EGFR overexpression or mutations. So, clinicians have to consider weighing the advantages and disadvantages, such as adverse reactions and therapeutic efficacy, when they make decisions to apply the agents. And therefore, more mechanistic studies of EGFR-mediated tumors are still going on to target the receptors and their roles in cancer.
7 Lysosomes
The lysosome is an acidic intracellular organelle containing hydrolases active in an acidic environment and specific membrane proteins. The lysosome lacks the mannose-6-phosphate receptor (M6PR), which is distinct from late-stage endosomes. These characteristics are shared by cell type-specific lysosomes such as melanosomes in melanocytes, delta granules in platelets, lamellar bodies in lung endothelial cells, lytic granules in lymphocytes, and other lysosomes in different cell types (Huizing et al., 2008).
Lysosomes exist in all animal cells except erythrocytes. Under the microscope, lysosomes are dense bodies in cytosol residing mostly in the perinuclear region. The shape of lysosomes ranges from spherical to tubular, and the size is different depending on the cell type from ∼ 1 μm through several μm. The shape, size, and number of lysosomes can be changed remarkably depending on the accumulation of internal undigested materials (Dingle and Shaw, 1979).
A phospholipid-bilayer surrounds lysosomes with 7–10 nm (Saftig et al., 2010). The difference between lysosomal membranes from other membranes is the high carbohydrate contents because 25 lysosomal membrane proteins are highly glycosylated (Lubke et al., 2009). The most profound membrane proteins are lysosome-associated membrane protein (LAMP)-1 and -2, lysosomal integral membrane protein (LIMP)-2, and CD63 (LAMP-3) (Eskelinen et al., 2003). The lysosomal structure is protected by glycocalyx formed by glycosylations at luminal domains of lysosomes from lysosomal hydrolases (Granger et al., 1990). In addition to the lysosomal membrane, lysosomes have intralysosomal membranes representing the primary membrane degradation site within lysosomes (Schulze et al., 2009). The intralysosomal membranes have abundant phospholipid bis (monoacylglycerol-phosphate (BMP), also known as lyso-bis-phosphatidic acid (LBPA). BMP is an exclusive marker of lysosomes and late-stage endosomes (Kobayashi et al., 1998).
Lysosomes enclose up to 600 μM of calcium content, a concentration similar to the classic calcium storage organelle, the endoplasmic reticulum (Bygrave and Benedetti, 1996; Christensen et al., 2002; Lloyd-Evans et al., 2008). Endosomal calcium has important roles in maintaining normal trafficking, recycling, and vesicular fusion (Lloyd-Evans and Platt, 2011). Lysosomal calcium release is obtained by nicotinic acid adenine dinucleotide phosphate (NAADP), a strong intracellular calcium-releasing secondary messenger, through its interaction with the two-pore channel (TPC) family (Churchill et al., 2002).
The finding of the coordinated lysosomal expression and regulation (CLEAR), a specific gene network of lysosomes, revealed the lysosomal biogenesis and regulation machinery. Many lysosomal genes interact with a CLEAR sequence (GTCACGTGAC) near the transcription initiation site (Sardiello et al., 2009). TFEB enters the nucleus, binds to the CLEAR site, and then induces lysosomal gene transcription. Nearly 500 direct TFEB target genes are known, including the lysosomal biogenesis and autophagy-related genes (Palmieri et al., 2011; Settembre et al., 2011). TFEB is the primary regulator of lysosomal function and orchestrates the function of the lysosomal gene network fulfilling the cellular needs of lysosomal degradations.
The lysosome is the major place of EGFR proteolysis, as well as other RTK molecules (Wiley and Burke, 2001). When EGF is bound to EGFR, the receptor is rapidly internalized by the kinase activity of the receptor and the specific motifs of the C-terminus. After the internalization of the receptor, EGFR is sorted into early endosomes and followed by late endosomes and lysosomes (Kil et al., 1999). RTKs share the common sorting and degradation paths, while the degree of regulation is different depending on the receptor. In the case of platelet-derived growth factor (PDGF) targets lysosome even without the presence of the specific ligand (Sorkin et al., 1991). This degradation process of RTKs, including EGFR can be a new therapeutic target against EGFR-mediated tumor cells. Since the regulation of lysosomal activity is distinct from the inhibition of the ligand-binding or kinase inhibition, lysosomal activation can be the new therapeutic approach as a combination therapy with EGFR antibodies or TKIs. There are additional roles of endosomes and lysosomes in immune cells. Lysosomes process antigens in antigen-presenting cells (APCs) for host-defense mechanisms. Granulocytes and monocytic cells degrade endocytosed or phagocytosed pathogens or tumor antigens. They also present processed antigens on MHCs for further process by T cells (Watts, 2022).
8 Conclusion and perspectives
The combination of anti-cancer agents is inevitable as the continuous mutations and overexpression is making the tumors more resistant to single-agent therapies. The limitations and adverse effects are current obstacles in EGFR targeting therapies. However, this will be a powerful strategy to overcome a huge portion of current cancers. In a recent study, lysosome activation leads to the vigorous degradation of RTKs, including EGFR (Hong et al., 2019). As RTKs are given with the driving force to be degraded rather than recycled, the downstream signal of EGFR will inevitably be decreased. This may be one of the new therapeutic strategies to target EGFR family molecules in combination with TKIs or anti-EGFR antibodies (Figure 2). As lysosomal degradation is the further later step in the pathway of EGFR signals, targeting lysosomes may show a good synergistic effect with TKIs and anti-EGFR antibodies. Experimentally, the synergistic effect of lysosomal activation and an anti-EGFR monoclonal antibody has been observed (Hong et al., 2019), so further study to discover a specific lysosome-activating agent or gene-therapy targeting tumor cells may be the next field to overcome EGFR-mediated tumors. Currently, lysosome-activating agents are not available for both therapeutic and experimental usages and we believe the development of specific agents to target lysosomes will be much helpful for future cancer treatments.
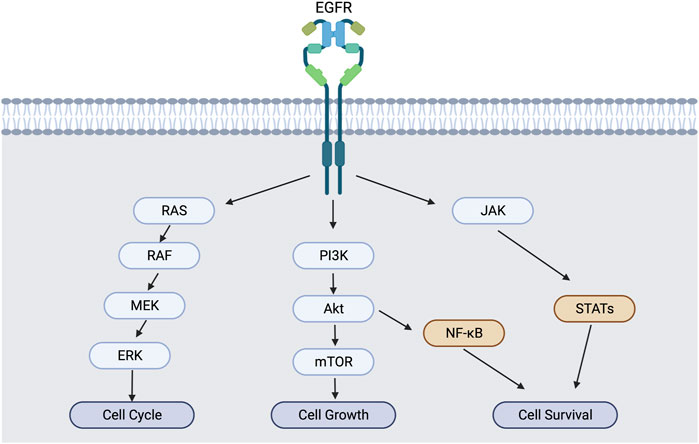
FIGURE 2. EGFR activation and downstream signaling cascade. EGFR activation induces not only MAPK/PI3K pathways, but also NF-κB and STAT pathways for cell survival and inflammation.
Author contributions
WJS, DK and AZ wrote the original draft and equally contributed to this work. NC, HMY, JHN, KMK and H-SL collected and curated the data. JH supervised and finished the manuscript. All authors contributed to the article and approved the submitted version.
Funding
This research was supported by Basic Science Research Program through the National Research Foundation of Korea (NRF), funded by the Ministry of Education (No. 2021R1I1A3043909).
Conflict of interest
The authors declare that the research was conducted in the absence of any commercial or financial relationships that could be construed as a potential conflict of interest.
Publisher’s note
All claims expressed in this article are solely those of the authors and do not necessarily represent those of their affiliated organizations, or those of the publisher, the editors and the reviewers. Any product that may be evaluated in this article, or claim that may be made by its manufacturer, is not guaranteed or endorsed by the publisher.
References
Albanell, J., and Gascon, P. (2005). Small molecules with EGFR-TK inhibitor activity. Curr. Drug Targets 6, 259–274. doi:10.2174/1389450053765888
Alberti, C., Pinciroli, P., Valeri, B., Ferri, R., Ditto, A., Umezawa, K., et al. (2012). Ligand-dependent EGFR activation induces the co-expression of IL-6 and PAI-1 via the NFkB pathway in advanced-stage epithelial ovarian cancer. Oncogene 31, 4139–4149. doi:10.1038/onc.2011.572
Appelqvist, H., Waster, P., Kagedal, K., and Ollinger, K. (2013). The lysosome: From waste bag to potential therapeutic target. J. Mol. Cell. Biol. 5, 214–226. doi:10.1093/jmcb/mjt022
Asahina, H., Tanaka, K., Morita, S., Maemondo, M., Seike, M., Okamoto, I., et al. (2021). A phase II study of osimertinib combined with platinum plus pemetrexed in patients with EGFR-mutated advanced non-small-cell lung cancer: The OPAL study (NEJ032C/LOGIK1801). Clin. Lung Cancer 22, 147–151. doi:10.1016/j.cllc.2020.09.023
Bivona, T. G., Hieronymus, H., Parker, J., Chang, K., Taron, M., Rosell, R., et al. (2011). FAS and NF-κB signalling modulate dependence of lung cancers on mutant EGFR. Nature 471, 523–526. doi:10.1038/nature09870
Bria, E., Milella, M., Cuppone, F., Novello, S., Ceribelli, A., Vaccaro, V., et al. (2011). Outcome of advanced NSCLC patients harboring sensitizing EGFR mutations randomized to EGFR tyrosine kinase inhibitors or chemotherapy as first-line treatment: A meta-analysis. Ann. Oncol. 22, 2277–2285. doi:10.1093/annonc/mdq742
Bygrave, F. L., and Benedetti, A. (1996). What is the concentration of calcium ions in the endoplasmic reticulum? Cell. Calcium 19, 547–551. doi:10.1016/s0143-4160(96)90064-0
Cai, W. Q., Zeng, L. S., Wang, L. F., Wang, Y. Y., Cheng, J. T., Zhang, Y., et al. (2020). The latest battles between EGFR monoclonal antibodies and resistant tumor cells. Front. Oncol. 10, 1249. doi:10.3389/fonc.2020.01249
Casalini, P., Iorio, M. V., Galmozzi, E., and Menard, S. (2004). Role of HER receptors family in development and differentiation. J. Cell. Physiol. 200, 343–350. doi:10.1002/jcp.20007
Christensen, K. A., Myers, J. T., and Swanson, J. A. (2002). pH-dependent regulation of lysosomal calcium in macrophages. J. Cell. Sci. 115, 599–607. doi:10.1242/jcs.115.3.599
Churchill, G. C., Okada, Y., Thomas, J. M., Genazzani, A. A., Patel, S., and Galione, A. (2002). NAADP mobilizes Ca(2+) from reserve granules, lysosome-related organelles, in sea urchin eggs. Cell. 111, 703–708. doi:10.1016/s0092-8674(02)01082-6
Crusz, S. M., and Balkwill, F. R. (2015). Inflammation and cancer: Advances and new agents. Nat. Rev. Clin. Oncol. 12, 584–596. doi:10.1038/nrclinonc.2015.105
D'Incecco, A., Andreozzi, M., Ludovini, V., Rossi, E., Capodanno, A., Landi, L., et al. (2015). PD-1 and PD-L1 expression in molecularly selected non-small-cell lung cancer patients. Br. J. Cancer 112, 95–102. doi:10.1038/bjc.2014.555
Davidson, S. M., and Vander Heiden, M. G. (2017). Critical functions of the lysosome in cancer biology. Annu. Rev. Pharmacol. Toxicol. 57, 481–507. doi:10.1146/annurev-pharmtox-010715-103101
Dingle, J. T. J., and Shaw, I. H. (1979). Lysosomes in applied biology and therapeutics, lysosomes in biology and patholigy. North-HollandAmsterdam: Wiley, 719.
Eskelinen, E. L., Tanaka, Y., and Saftig, P. (2003). At the acidic edge: Emerging functions for lysosomal membrane proteins. Trends Cell. Biol. 13, 137–145. doi:10.1016/s0962-8924(03)00005-9
Gettinger, S., Hellmann, M. D., Chow, L. Q. M., Borghaei, H., Antonia, S., Brahmer, J. R., et al. (2018). Nivolumab plus erlotinib in patients with EGFR-mutant advanced NSCLC. J. Thorac. Oncol. 13, 1363–1372. doi:10.1016/j.jtho.2018.05.015
Granger, B. L., Green, S. A., Gabel, C. A., Howe, C. L., Mellman, I., and Helenius, A. (1990). Characterization and cloning of lgp110, a lysosomal membrane glycoprotein from mouse and rat cells. J. Biol. Chem. 265, 12036–12043. doi:10.1016/s0021-9258(19)38504-7
Hanahan, D., and Weinberg, R. A. (2011). Hallmarks of cancer: The next generation. Cell. 144, 646–674. doi:10.1016/j.cell.2011.02.013
Hong, J., Wuest, T. R., Min, Y., and Lin, P. C. (2019). Oxygen tension regulates lysosomal activation and receptor tyrosine kinase degradation. Cancers (Basel) 11, E1653. doi:10.3390/cancers11111653
Huizing, M., Helip-Wooley, A., Westbroek, W., Gunay-Aygun, M., and Gahl, W. A. (2008). Disorders of lysosome-related organelle biogenesis: Clinical and molecular genetics. Annu. Rev. Genomics Hum. Genet. 9, 359–386. doi:10.1146/annurev.genom.9.081307.164303
Hynes, N. E., Horsch, K., Olayioye, M. A., and Badache, A. (2001). The ErbB receptor tyrosine family as signal integrators. Endocr. Relat. Cancer 8, 151–159. doi:10.1677/erc.0.0080151
Hynes, N. E., and MacDonald, G. (2009). ErbB receptors and signaling pathways in cancer. Curr. Opin. Cell. Biol. 21, 177–184. doi:10.1016/j.ceb.2008.12.010
Jutten, B., Keulers, T. G., Schaaf, M. B., Savelkouls, K., Theys, J., Span, P. N., et al. (2013). EGFR overexpressing cells and tumors are dependent on autophagy for growth and survival. Radiother. Oncol. 108, 479–483. doi:10.1016/j.radonc.2013.06.033
Kil, S. J., Hobert, M., and Carlin, C. (1999). A leucine-based determinant in the epidermal growth factor receptor juxtamembrane domain is required for the efficient transport of ligand-receptor complexes to lysosomes. J. Biol. Chem. 274, 3141–3150. doi:10.1074/jbc.274.5.3141
Kimmelman, A. C. (2011). The dynamic nature of autophagy in cancer. Genes. Dev. 25, 1999–2010. doi:10.1101/gad.17558811
Kirkegaard, T., and Jaattela, M. (2009). Lysosomal involvement in cell death and cancer. Biochim. Biophys. Acta 1793, 746–754. doi:10.1016/j.bbamcr.2008.09.008
Kobayashi, T., Stang, E., Fang, K. S., de Moerloose, P., Parton, R. G., and Gruenberg, J. (1998). A lipid associated with the antiphospholipid syndrome regulates endosome structure and function. Nature 392, 193–197. doi:10.1038/32440
Latif, H., and Liu, S. V. (2019). Combining immunotherapy and epidermal growth factor receptor kinase inhibitors: Worth the risk? Ann. Transl. Med. 7, S76. doi:10.21037/atm.2019.03.6
Laurila, N., and Koivunen, J. P. (2015). EGFR inhibitor and chemotherapy combinations for acquired TKI resistance in EGFR-mutant NSCLC models. Med. Oncol. 32, 205. doi:10.1007/s12032-015-0627-6
Lemmon, M. A., and Schlessinger, J. (2010). Cell signaling by receptor tyrosine kinases. Cell. 141, 1117–1134. doi:10.1016/j.cell.2010.06.011
Levine, B., and Kroemer, G. (2008). Autophagy in the pathogenesis of disease. Cell. 132, 27–42. doi:10.1016/j.cell.2007.12.018
Lloyd-Evans, E., Morgan, A. J., He, X., Smith, D. A., Elliot-Smith, E., Sillence, D. J., et al. (2008). Niemann-Pick disease type C1 is a sphingosine storage disease that causes deregulation of lysosomal calcium. Nat. Med. 14, 1247–1255. doi:10.1038/nm.1876
Lloyd-Evans, E., and Platt, F. M. (2011). Lysosomal Ca(2+) homeostasis: Role in pathogenesis of lysosomal storage diseases. Cell. Calcium 50, 200–205. doi:10.1016/j.ceca.2011.03.010
Lubke, T., Lobel, P., and Sleat, D. E. (2009). Proteomics of the lysosome. Biochim. Biophys. Acta 1793, 625–635. doi:10.1016/j.bbamcr.2008.09.018
Mendelsohn, J., and Baselga, J. (2006). Epidermal growth factor receptor targeting in cancer. Semin. Oncol. 33, 369–385. doi:10.1053/j.seminoncol.2006.04.003
Mok, T. S. K., Kim, S. W., Wu, Y. L., Nakagawa, K., Yang, J. J., Ahn, M. J., et al. (2017). Gefitinib plus chemotherapy versus chemotherapy in epidermal growth factor receptor mutation-positive non-small-cell lung cancer resistant to first-line gefitinib (IMPRESS): Overall survival and biomarker analyses. J. Clin. Oncol. 35, 4027–4034. doi:10.1200/JCO.2017.73.9250
Oshima, Y., Tanimoto, T., Yuji, K., and Tojo, A. (2018). EGFR-TKI-Associated interstitial pneumonitis in nivolumab-treated patients with non-small cell lung cancer. JAMA Oncol. 4, 1112–1115. doi:10.1001/jamaoncol.2017.4526
Oxnard, G. R., Yang, J. C., Yu, H., Kim, S. W., Saka, H., Horn, L., et al. (2020). Tatton: A multi-arm, phase ib trial of osimertinib combined with selumetinib, savolitinib, or durvalumab in EGFR-mutant lung cancer. Ann. Oncol. 31, 507–516. doi:10.1016/j.annonc.2020.01.013
Palmieri, M., Impey, S., Kang, H., di Ronza, A., Pelz, C., Sardiello, M., et al. (2011). Characterization of the CLEAR network reveals an integrated control of cellular clearance pathways. Hum. Mol. Genet. 20, 3852–3866. doi:10.1093/hmg/ddr306
Pan, D., Jiang, C., Ma, Z., Blonska, M., You, M. J., and Lin, X. (2016). MALT1 is required for EGFR-induced NF-κB activation and contributes to EGFR-driven lung cancer progression. Oncogene 35, 919–928. doi:10.1038/onc.2015.146
Prenzel, N., Fischer, O. M., Streit, S., Hart, S., and Ullrich, A. (2001). The epidermal growth factor receptor family as a central element for cellular signal transduction and diversification. Endocr. Relat. Cancer 8, 11–31. doi:10.1677/erc.0.0080011
Prickett, T. D., Agrawal, N. S., Wei, X., Yates, K. E., Lin, J. C., Wunderlich, J. R., et al. (2009). Analysis of the tyrosine kinome in melanoma reveals recurrent mutations in ERBB4. Nat. Genet. 41, 1127–1132. doi:10.1038/ng.438
Rizvi, N. A., Hellmann, M. D., Brahmer, J. R., Juergens, R. A., Borghaei, H., Gettinger, S., et al. (2016). Nivolumab in combination with platinum-based doublet chemotherapy for first-line treatment of advanced non-small-cell lung cancer. J. Clin. Oncol. 34, 2969–2979. doi:10.1200/JCO.2016.66.9861
Roskoski, R. (2004). The ErbB/HER receptor protein-tyrosine kinases and cancer. Biochem. Biophys. Res. Commun. 319, 1–11. doi:10.1016/j.bbrc.2004.04.150
Saftig, P., Schroder, B., and Blanz, J. (2010). Lysosomal membrane proteins: Life between acid and neutral conditions. Biochem. Soc. Trans. 38, 1420–1423. doi:10.1042/BST0381420
Sardiello, M., Palmieri, M., di Ronza, A., Medina, D. L., Valenza, M., Gennarino, V. A., et al. (2009). A gene network regulating lysosomal biogenesis and function. Science 325, 473–477. doi:10.1126/science.1174447
Schlessinger, J. (2014). Receptor tyrosine kinases: Legacy of the first two decades. Cold Spring Harb. Perspect. Biol. 6, a008912. doi:10.1101/cshperspect.a008912
Schulze, H., Kolter, T., and Sandhoff, K. (2009). Principles of lysosomal membrane degradation: Cellular topology and biochemistry of lysosomal lipid degradation. Biochim. Biophys. Acta 1793, 674–683. doi:10.1016/j.bbamcr.2008.09.020
Sequist, L. V., Waltman, B. A., Dias-Santagata, D., Digumarthy, S., Turke, A. B., Fidias, P., et al. (2011). Genotypic and histological evolution of lung cancers acquiring resistance to EGFR inhibitors. Sci. Transl. Med. 3, 75ra26. 75ra26. doi:10.1126/scitranslmed.3002003
Settembre, C., Di Malta, C., Polito, V. A., Garcia Arencibia, M., Vetrini, F., Erdin, S., et al. (2011). TFEB links autophagy to lysosomal biogenesis. Science 332, 1429–1433. doi:10.1126/science.1204592
Settembre, C., Zoncu, R., Medina, D. L., Vetrini, F., Erdin, S., Erdin, S., et al. (2012). A lysosome-to-nucleus signalling mechanism senses and regulates the lysosome via mTOR and TFEB. EMBO J. 31, 1095–1108. doi:10.1038/emboj.2012.32
Shi, F., Telesco, S. E., Liu, Y., Radhakrishnan, R., and Lemmon, M. A. (2010). ErbB3/HER3 intracellular domain is competent to bind ATP and catalyze autophosphorylation. Proc. Natl. Acad. Sci. U. S. A. 107, 7692–7697. doi:10.1073/pnas.1002753107
Siena, S., Sartore-Bianchi, A., Di Nicolantonio, F., Balfour, J., and Bardelli, A. (2009). Biomarkers predicting clinical outcome of epidermal growth factor receptor-targeted therapy in metastatic colorectal cancer. J. Natl. Cancer Inst. 101, 1308–1324. doi:10.1093/jnci/djp280
Soria, J. C., Wu, Y. L., Nakagawa, K., Kim, S. W., Yang, J. J., Ahn, M. J., et al. (2015). Gefitinib plus chemotherapy versus placebo plus chemotherapy in EGFR-mutation-positive non-small-cell lung cancer after progression on first-line gefitinib (IMPRESS): A phase 3 randomised trial. Lancet. Oncol. 16, 990–998. doi:10.1016/S1470-2045(15)00121-7
Sorkin, A., Westermark, B., Heldin, C. H., and Claesson-Welsh, L. (1991). Effect of receptor kinase inactivation on the rate of internalization and degradation of PDGF and the PDGF beta-receptor. J. Cell. Biol. 112, 469–478. doi:10.1083/jcb.112.3.469
Sundvall, M., Iljin, K., Kilpinen, S., Sara, H., Kallioniemi, O. P., and Elenius, K. (2008). Role of ErbB4 in breast cancer. J. Mammary Gland. Biol. Neoplasia 13, 259–268. doi:10.1007/s10911-008-9079-3
Tan, X., Lambert, P. F., Rapraeger, A. C., and Anderson, R. A. (2016). Stress-Induced EGFR trafficking: Mechanisms, functions, and therapeutic implications. Trends Cell. Biol. 26, 352–366. doi:10.1016/j.tcb.2015.12.006
Tebbutt, N., Pedersen, M. W., and Johns, T. G. (2013). Targeting the ERBB family in cancer: Couples therapy. Nat. Rev. Cancer 13, 663–673. doi:10.1038/nrc3559
Toulany, M. (2019). Targeting DNA double-strand break repair pathways to improve radiotherapy response. Genes. (Basel) 10, E25. doi:10.3390/genes10010025
Vendramini-Costa, D. B., and Carvalho, J. E. (2012). Molecular link mechanisms between inflammation and cancer. Curr. Pharm. Des. 18, 3831–3852. doi:10.2174/138161212802083707
Watts, C. (2022). Lysosomes and lysosome-related organelles in immune responses. FEBS Open Bio 12, 678–693. doi:10.1002/2211-5463.13388
Wheeler, D. L., Dunn, E. F., and Harari, P. M. (2010). Understanding resistance to EGFR inhibitors-impact on future treatment strategies. Nat. Rev. Clin. Oncol. 7, 493–507. doi:10.1038/nrclinonc.2010.97
White, E. (2015). The role for autophagy in cancer. J. Clin. Investig. 125, 42–46. doi:10.1172/jci73941
Wiley, H. S., and Burke, P. M. (2001). Regulation of receptor tyrosine kinase signaling by endocytic trafficking. Traffic 2, 12–18. doi:10.1034/j.1600-0854.2001.020103.x
Wu, L., Ke, L., Zhang, Z., Yu, J., and Meng, X. (2020). Development of EGFR TKIs and options to manage resistance of third-generation EGFR TKI osimertinib: Conventional ways and immune checkpoint inhibitors. Front. Oncol. 10, 602762. doi:10.3389/fonc.2020.602762
Yang, H., Shen, K., Zhu, C., Li, Q., Zhao, Y., and Ma, X. (2018). Safety and efficacy of durvalumab (MEDI4736) in various solid tumors. Drug Des. devel. Ther. 12, 2085–2096. doi:10.2147/DDDT.S162214
Yang, J. C., Shih, J. Y., Su, W. C., Hsia, T. C., Tsai, C. M., Ou, S. H., et al. (2012). Afatinib for patients with lung adenocarcinoma and epidermal growth factor receptor mutations (LUX-Lung 2): A phase 2 trial. Lancet. Oncol. 13, 539–548. doi:10.1016/S1470-2045(12)70086-4
Yarden, Y., and Pines, G. (2012). The ERBB network: At last, cancer therapy meets systems biology. Nat. Rev. Cancer 12, 553–563. doi:10.1038/nrc3309
Yu, H. A., Arcila, M. E., Rekhtman, N., Sima, C. S., Zakowski, M. F., Pao, W., et al. (2013). Analysis of tumor specimens at the time of acquired resistance to EGFR-TKI therapy in 155 patients with EGFR-mutant lung cancers. Clin. Cancer Res. 19, 2240–2247. doi:10.1158/1078-0432.CCR-12-2246
Keywords: ErbB, EGFR, TKI, lysosome, combination therapy
Citation: Sung WJ, Kim D, Zhu A, Cho N, Yoo HM, Noh JH, Kim KM, Lee H-S and Hong J (2022) The lysosome as a novel therapeutic target of EGFR-mediated tumor inflammation. Front. Pharmacol. 13:1050758. doi: 10.3389/fphar.2022.1050758
Received: 22 September 2022; Accepted: 01 November 2022;
Published: 11 November 2022.
Edited by:
Ning Ji, China Academy of Chinese Medical Sciences, ChinaReviewed by:
Shaoxun Wang, Yale University, United StatesCopyright © 2022 Sung, Kim, Zhu, Cho, Yoo, Noh, Kim, Lee and Hong. This is an open-access article distributed under the terms of the Creative Commons Attribution License (CC BY). The use, distribution or reproduction in other forums is permitted, provided the original author(s) and the copyright owner(s) are credited and that the original publication in this journal is cited, in accordance with accepted academic practice. No use, distribution or reproduction is permitted which does not comply with these terms.
*Correspondence: Jaewoo Hong, jhong@cu.ac.kr
†These authors have contributed equally to this work