- 1The Centre for Translational Pharmacology, Institute of Molecular, Cell and Systems Biology, College of Medical, Veterinary and Life Sciences, University of Glasgow, Glasgow, United Kingdom
- 2Sosei Heptares, Cambridge, United Kingdom
The type 5 metabotropic glutamate receptor, mGlu5, has been proposed as a potential therapeutic target for the treatment of several neurodegenerative diseases. In preclinical neurodegenerative disease models, novel allosteric modulators have been shown to improve cognitive performance and reduce disease-related pathology. A common pathological hallmark of neurodegenerative diseases is a chronic neuroinflammatory response, involving glial cells such as astrocytes and microglia. Since mGlu5 is expressed in astrocytes, targeting this receptor could provide a potential mechanism by which neuroinflammatory processes in neurodegenerative disease may be modulated. This review will discuss current evidence that highlights the potential of mGlu5 allosteric modulators to treat neurodegenerative diseases, including Alzheimer’s disease, Huntington’s disease, Parkinson’s disease, and amyotrophic lateral sclerosis. Furthermore, this review will explore the role of mGlu5 in neuroinflammatory responses, and the potential for this G protein-coupled receptor to modulate neuroinflammation.
Introduction
Neurodegenerative diseases are characterized by the progressive degeneration of neurons in the central nervous system (CNS). Common neurodegenerative diseases include Alzheimer’s disease, Huntington’s disease, amyotrophic lateral sclerosis, and Parkinson’s disease. These diseases give rise to behavioral changes and cognitive decline (Karantzoulis and Galvin, 2011). In 2019, there were over 55 million people living with dementia worldwide and it is predicted that this will increase to 83 million by 2030, and to 153 million by 2050 (Dementia Forecasting Collaborators, 2022). Most current treatments are symptomatic rather than disease-modifying in nature, and the large, predicted increase in individuals living with dementia globally means that there is an urgent need for novel therapeutic interventions.
The G protein-coupled receptor (GPCR) superfamily represents the largest group of transmembrane receptors in the human genome and are the most common target for clinically approved drugs (Santos et al., 2016; Sriram and Insel, 2018). Metabotropic glutamate receptors (mGlu), which mediate slow, long-lasting responses to the endogenous ligand, glutamate, (Conn and Pin, 1997), are members of this superfamily and can be divided into eight subtypes split further into three groups based on factors such as their sequence homology and pharmacological profile. The type 5 metabotropic glutamate receptor (mGlu5) belongs to group 1 mGlu receptors, along with the mGlu1 receptor. Although both mGlu1 and mGlu5 have been implicated in neurodegenerative processes, this review focusses on mGlu5 as a potential target for therapeutic intervention in neurodegenerative disorders.
The Type 5 Metabotropic Glutamate Receptor
Distribution
Within the brain, mGlu5 is expressed in the cerebral cortex, olfactory blub, hippocampus, striatum, and basal ganglia with highest expression rates in the hippocampus and basal ganglia where they modulate reward processing and movement (Shigemoto et al., 1993; Wong et al., 2013). It is also expressed in the spinal cord (Valerio et al., 1997). It is predominately localised on the postsynaptic membrane of glutamatergic neurons, although it can also be found on the presynaptic membrane (Luján et al., 1996) where they are suggested to play a role in autoregulation of glutamatergic exocytosis modulating the extracellular level of glutamate available to bind to postsynaptic glutamatergic receptors (Pittaluga, 2016). Postsynaptically, around 50%–80% of mGlu5 is expressed on intracellular membranes such as the nuclear membranes and endoplasmic reticulum (Hubert, Paquet and Smith, 2001; Kumar, Jong and O’Malley, 2008), where its activation results in a sustained response from extracellular signal-regulated protein kinase (ERK1/2) stimulated phosphorylation of the transcription factor Elk-1 (Jong, Kumar and O’Malley, 2009) which mediates long-term depression (LTD) (Purgert et al., 2014). Activation of mGlu5 on the plasma membrane results in a rapid calcium response (Jong, Kumar and O’Malley, 2009) which leads to both LTD and long-term potentiation (LTP) (Purgert et al., 2014). This differential expression impacts upon pharmacology, with ligands needing to either diffuse or be transported across membranes in order to reach intracellular receptors (Jong et al., 2005).
In addition to expression in neurons, mGlu5 is expressed in glial cells, including astrocytes and microglia, where its activation is important for glial cell function and their interaction with neurons (Loane et al., 2012). Astrocytes, star-like cells with processes that extend from their cell body, are the most prevalent glial cell type in the brain, accounting for between 20% and 50% of total CNS number (Hasel and Liddelow, 2021). They play many roles in the brain, including providing trophic support to neurons, mediating synapse formation, and regulating neurotransmitter uptake (Hasel and Liddelow, 2021). In astrocytes isolated from rodent tissue, mGlu5 is found in cells isolated from the hippocampus, cortex, thalamus, tegmentum and striatum, but not in the cerebellum or spinal cord (Biber et al., 1999; Silva et al., 1999). In addition, the expression of mGlu5 in astrocytes is highest during neurodevelopment in both cells isolated from rodent tissue (postnatal day 7) and in rodent brain slices (postnatal days 1–10) and then decreases with age (Cai et al., 2000), which suggests that its role may change as the brain develops. Preclinical models have identified an upregulation of astrocytic mGlu5 in several diseases including Alzheimer’s disease (Lim et al., 2013; Shrivastava et al., 2013), amyotrophic lateral sclerosis (Anneser et al., 2004; Vermeiren et al., 2006), and multiple sclerosis (Fulmer et al., 2014). Analysis of post-mortem tissue from patients have corroborated these findings, showing upregulation of astrocytic mGlu5 in and around lesions (Aronica et al., 2001; Anneser et al., 2004; Newcombe et al., 2008; Casley et al., 2009; Lim et al., 2013).
Microglia, which at rest have many ramified processes extending from their small cell body, are mediators of the brain’s innate immune response. They play a key role in maintaining homeostasis in the brain by responding to extracellular signals in their microenvironment and clearing away any toxic substances of cellular debris (Hanisch and Kettenmann, 2007). Similar to astrocytic cultures, mGlu5 is expressed in microglia isolated from rodent tissue, but at lower levels than in astrocytes (Byrnes et al., 2009). This mGlu5 expression is up-regulated in the activated microglia that surround lesions following spinal cord or brain injury in rodents (Byrnes et al., 2009; Drouin-Ouellet et al., 2011).
Pharmacological Modulation
All GPCRs have a similar core structure composed of an extracellular N-terminal tail, seven transmembrane α-helices connected by alternating intracellular and extracellular loops (7 transmembrane domain; 7TM), and an intracellular C-terminal tail (Rosenbaum, Rasmussen and Kobilka, 2009). Like all mGlu receptors, mGlu5 is organized as a dimer and has a large extracellular N-terminus (Mølck et al., 2014). This N-terminal tail is arranged as a Venus Flytrap Domain (VFTD) which forms the orthosteric binding site, where the endogenous ligand, glutamate, binds. The VFTD is connected to the 7TM by a cysteine-rich domain (Nasrallah et al., 2021) (Figure 1). In addition to binding to the orthosteric site, ligands can bind to sites that are topographically distinct from the orthosteric site, known as the allosteric site. Both group 1 mGlu receptors differ from other mGlu receptors in that they have three hydrophilic residues at the top of their allosteric binding site, which could form a bond with ligands that are group 1 specific. In mGlu5 specifically, there is a deep and narrow sub-pocket that is thought to be a key binding site for achieving mGlu5 selectivity (Harpsøe et al., 2015). Computational modelling has shown that 6 non-conserved and 4 conserved residues in the binding pocket of group 1 mGlu receptors are essential for subtype selectivity, with the non-conserved residues playing a role in ligand binding through spatial conformation (Fu et al., 2020). Using computational techniques to provide three-dimensional protein structures has paved the way for structure-based drug discovery (SBDD), a powerful method for identifying drug candidates. A highly selective ligand for mGlu5, HTL14242, was discovered using SBDD techniques (Christopher et al., 2015). For a detailed review on mGlu5 structure and its importance for the development of allosteric modulators, see Bennett et al. (2020).
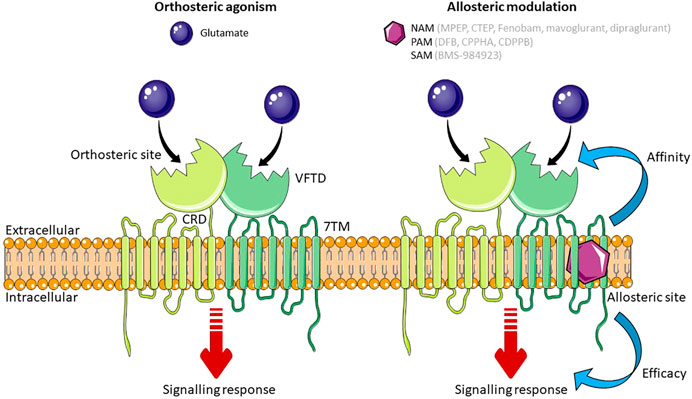
FIGURE 1. Schematic of mGlu5 activation. Glutamate, the endogenous ligand for mGlu5, binds at the orthosteric site in the VFTD. Allosteric modulators (NAMs, PAMs and SAMs) bind to a topographically distinct site in the 7TM domain. Simultaneous binding of an orthosteric agonist and an allosteric modulator effects the affinity or efficacy of the orthosteric agonist. Some allosteric modulators are able to exert their effect independent to the orthosteric agonist. Abbreviations: NAM, Negative Allosteric Modulator; PAM, Positive Allosteric Modulator; SAM, Silent Allosteric Modulator; VFTD, Venus Flytrap Domain; 7TM, 7 transmembrane domain; CRD, Cysteine Rich Domain. Figure created using Reactome Icon Library, licensed under CC BY 4.0 (Sidiropoulos et al., 2017).
Following binding of an agonist, mGlu5 receptors preferentially couple to the heterometric G protein Gαq/11 resulting in the release of Ca2+ from intracellular stores via downstream second messengers. Gαq/11 stimulates phospholipase C which cleaves phosphatidylinositol 4,5, biophosphate into diacylglycerol (DAG) and inositol 1,4,5 triphosphate (IP3). IP3 triggers the release of Ca2+ from intracellular stores by opening ligand-gated inositol phosphate receptors on the endoplasmic reticulum. Together with DAG, Ca2+ contributes to the activation of protein kinase C (PKC) (Dhami and Ferguson, 2006). As well as coupling to G proteins, GPCRs can signal via β-arrestins such as β-arrestin2 (Stoppel et al., 2017). Although there is no evidence for direct coupling of mGlu5 receptors to β-arrestins, it has been demonstrated that mGlu5 and β-arrestin co-immunoprecipitate (Eng et al., 2016) and that β-arrestin is necessary for some of the downstream signalling from mGlu5 (Stoppel et al., 2017). Stimulation of group 1 mGlu receptors can result in the activation of other downstream effector enzymes, such as extracellular regulated kinase (ERK), protein kinase B (Akt), and mammalian target of rapamycin (mTOR) (Ribeiro FM. et al., 2014).
The activity of mGlu5 can be pharmacologically manipulated by ligands that bind to either the orthosteric site or allosteric binding sites. The former either mimic the actions of glutamate by activating (agonists) or blocking receptor activity (antagonists). However, the orthosteric binding site is highly conserved between mGlu receptor subtypes (Wellendorph and Bräuner-Osborne, 2009), and so targeting an allosteric site within the 7TM domain may be more therapeutically beneficial in disease (Harpsøe et al., 2015) in part due to increased subtype-specific selectivity (May and Christopoulos, 2003). Moreover, allosteric modulators are able to induce diverse changes in GPCR signaling and can be designed to produce a biased signaling response, effecting therapeutically beneficial signaling pathways and not pathways that may cause adverse effects (Trinh et al., 2018). There are three categories of allosteric modulator: those that affect the binding affinity of the orthosteric ligand; those that affect the efficacy of the orthosteric ligand; and those that exert their effect independent of the orthosteric ligand (Langmead and Christopoulos, 2006). The affinity or efficacy of the orthosteric ligand can be altered in positive, negative, or neutral direction. Positive allosteric modulators (PAMs) increase agonist affinity and/or efficacy, negative allosteric modulators (NAMs) decrease agonist affinity and/or efficacy, and silent allosteric modulators (SAMs) have no effect on the affinity and/or efficacy of the orthosteric ligand, but act as a competitive agonist at allosteric sites, thus blocking PAM or NAM activity. Modulating the effect of the endogenous ligand at the orthosteric site is an advantage of allosteric ligands as exerting an effect only when and where glutamate is present means that signaling is altered in proportion to the physiological response (Trinh et al., 2018). In addition, glutamate shows neutral affinity cooperativity with ligands that bind to the allosteric site of mGlu5, meaning that high concentrations of glutamate do not affect the action of mGlu5 allosteric modulators, providing them with an advantage over orthosteric ligands which compete with glutamate (Bennett et al., 2020).
Allosteric Modulator Drug Discovery
Several ligands which modulate the activity of mGlu5 receptors have been developed and progressed into clinical trials for neurodegenerative disorders and in other disorders characterised by loss of brain immune function, such as mood disorders, or disorders where addiction has formed aberrant and strong cue-based associations linked to increased craving and drug use (Table 1). These disorders are not the focus of this review, however these data are relevant to mGlu5 as a target to treat neurodegenerative diseases as these neuropsychiatric symptoms can overlap with neurodegenerative disorders (Husain, 2017).
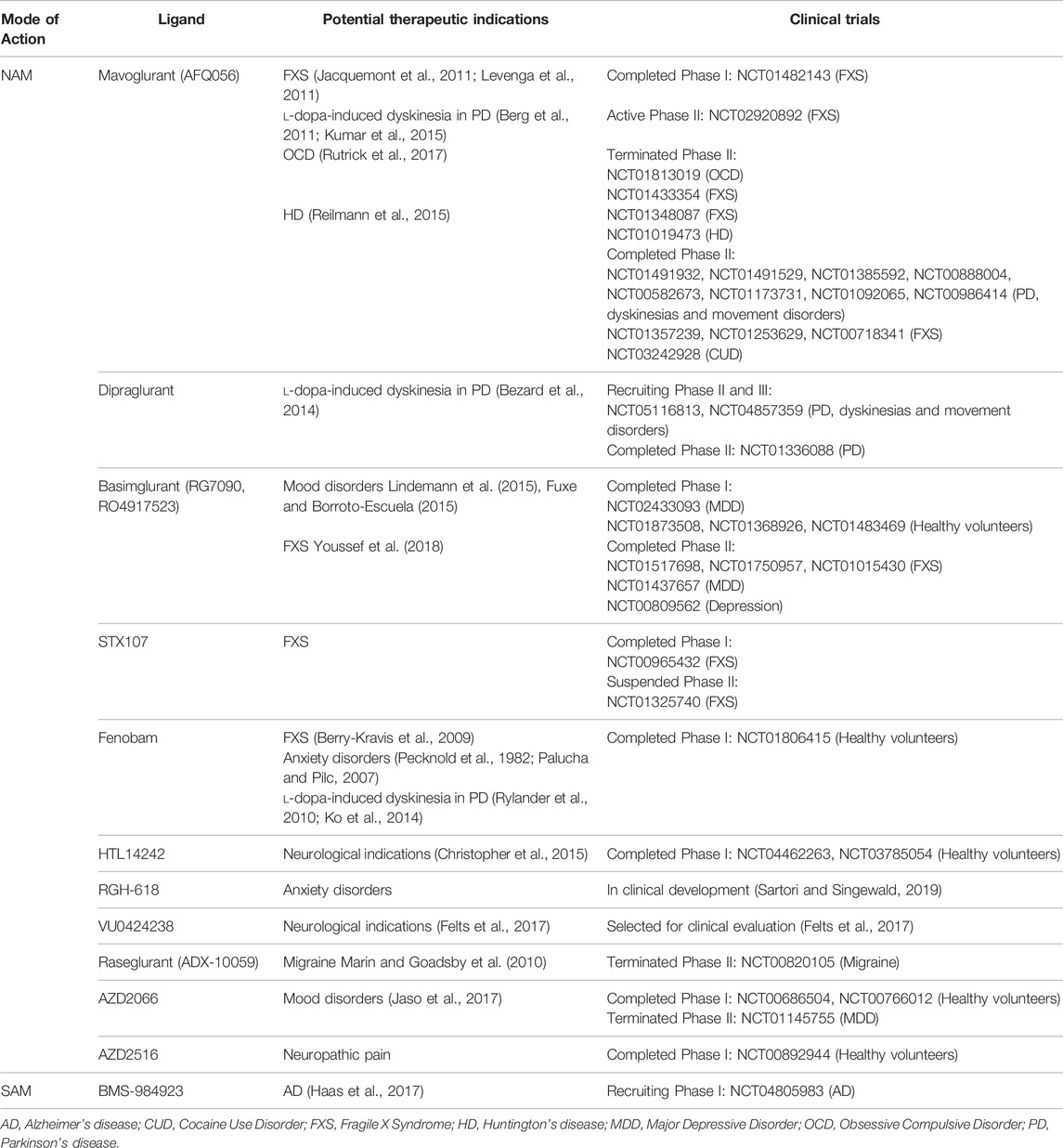
TABLE 1. Allosteric modulators of mGlu5 that have entered clinical trials for neurodegenerative and neurological disorders. All clinical trials found at www.clinicaltrials.gov. For detailed in vitro pharmacological characterization of clinically tested mGlu5 NAMs see Arsova et al. (2020).
The first NAMs reported to be selective for mGlu5 were SIB-1757 and SIB-1893 (Varney et al., 1998). Structural rearrangement of these compounds led to the discovery of 2-methyl-6-(phenylethynyl) pyridine (MPEP) (Gasparini et al., 1999). Although MPEP is highly selective for mGlu5 as compared to other mGlu receptor subtypes, it also acts as a weak NMDA receptor antagonist (O’leary et al., 2000) and mGlu4 PAM (Mathiesen et al., 2003). NAMs for mGlu5 have been developed to show both increased selectivity and potency, including 3-[(2-methyl-1,3-thiazol-4-yl)ethynyl] pyridine (MTEP) (Cosford et al., 2003), and 2-chloro-4-((dimethyl-1-(4-(trifluoromethoxy)phenyl)-1H-imidazol-4yl)ethynyl)pyridine (CTEP), the latter of which has the highest potency and selectivity (Lindemann et al., 2011). Another potent, selective NAM for mGlu5 is fenobam, which was developed in the 1970’s but only found to target mGlu5 in 2005 (Porter et al., 2005). As well as acting as an antagonist, fenobam has inverse agonist activity, meaning it not only antagonises agonist activity, but also exerts the opposite effect by inhibiting constitutive receptor activity. Fenobam acts via the same allosteric binding site as MPEP and MTEP within in the 7TM between TM 3, 6 and 7 (Gregory et al., 2011).
NAMs of mGlu5 have shown promising results in preclinical models but many have progressed to phase II clinical trials for neurodegenerative diseases where they have failed to show efficacy in, including mavoglurant, basimglurant, STX107, fenobam, raseglurant, and AZD 2066 (Table 1). Mavoglurant met its primary endpoint in a phase II trial for cocaine use disorder and is moving into phase III (clinicaltrials.gov: NCT03242928). It was shown to reduce neuropsychiatric symptoms of addiction, with a significant reduction in the cocaine use in the group that received mavoglurant compared to patients receiving the placebo. One mGlu5 NAM, dipraglurant, has shown efficacy in phase II trials in neurodegenerative disease, specifically for levodopa-induce dyskinesia in patients with Parkinson’s disease (clinicaltrials.gov: NCT01336088). It has recently progressed to phase III (clinicaltrials.gov: NCT04857359).
The first mGlu5 PAM to be identified was 3,3′-difluorobenzaldazine (DFB) (O’Brien et al., 2003). DFB had low potency, and a year later N-[5-chloro-2-[(-1,3-dioxoisoindolin-2-yl)methyl]phenyl]-2-hydroxybenzamide (CPPHA), which exhibited greater potency, was identified (O’Brien et al., 2004). The first PAM developed with enough solubility to allow for in vivo studies was 3-cyano-N-(1,3-diphenyl-1H-pyrazol-5-yl)benzamide (CDPPB) (O’Brien et al., 2003, 2004; Lindsley et al., 2004). DFB and CDPPB both bind to the MPEP binding site in the seven transmembrane domain, whereas CPPHA binds to a distinct allosteric site.
The mGlu5 SAM BMS-984923 was identified in an attempt to identify mGlu5 PAMs for schizophrenia (Huang et al., 2016). BMS-984923 showed a therapeutic benefit in a preclinical mouse model of Alzheimer’s disease and is currently in a phase I clinical trial to determine its safety and tolerability in human Alzheimer’s disease patients (clinicaltrials.gov: NCT04805983).
Therapeutic Potential in Neurodegenerative Diseases
This review will discuss current evidence that highlights the potential of mGlu5 allosteric modulators to treat neurodegenerative diseases, including Alzheimer’s disease, Huntington’s disease, amyotrophic lateral sclerosis, and Parkinson’s disease. Additionally, this review will explore the role of mGlu5 receptors in neuroinflammatory responses, and the potential for this GPCR to modulate neuroinflammation. Neurodegenerative diseases share a number of common pathologies, including neuroinflammation, and so a ligand which targets mGlu5 on inflammatory cells, such as astrocytes and microglia, could have a therapeutic benefit across neurodegenerative diseases. However, as discussed below in this review, this is complicated by uncertainty surrounding the relationship between inflammatory cells types, their relative contribution to neurodegeneration, and whether they should be up or downregulated in order to be therapeutically beneficial.
Alzheimer’s Disease
Alzheimer’s disease (AD) is a progressive neurodegenerative disease, characterised by decline in cognitive function with attention, episodic memory, and executive function domains most affected (Giorgio et al., 2020). The disease threatens society with a substantial health and economic burden due to its increasing prevalence in line with an ageing population, and the lack of preventative treatments (Qiu et al., 2009); most current treatments for AD are symptomatic rather than disease-modifying in nature (Yiannopoulou & Papageorgiou, 2013).
The neuropathology of AD is characterized by the presence of extracellular amyloid plaques and intracellular neurofibrillary tangles (Deture and Dickson, 2019). Early AD research focused on plaques, composed primarily of β-amyloid (Aβ), as the neurotoxic species. Aβ also exists in the brain in the form of soluble Aβ oligomers, and it is thought that these oligomers are the predominant source of neurotoxicity in the Alzheimer’s brain, rather than the plaques themselves (Kayed and Lasagna-Reeves, 2013). Aβ oligomers have been shown to bind with high affinity to cellular prion protein (Laurén et al., 2009), a complex requiring mGlu5 as a co-receptor and resulting in the potentially neurotoxic release of intracellular Ca2+ (Um et al., 2013). This implicates mGlu5 as playing a role in Aβ oligomer-related pathology. For a detailed review on aberrant mGlu5 signaling following binding of Aβ oligomers see (Abd-Elrahman et al., 2022).
The genetic deletion or pharmacological blockade of mGlu5 have been shown to be neuroprotective in rodent models of AD. Genetic deletion of mGlu5 in the APPswe/PS1∆9 (APPswe) mouse model of AD was shown to reverse memory deficits in mice at both 9 and 12 months of age using a Morris Water Maze paradigm (Hamilton et al., 2014). Similarly, blockade of mGlu5 with either MTEP or CTEP improved learning and memory deficits in both APPswe and 3xTg mice mouse models (Um et al., 2013; Hamilton et al., 2016). In addition to improvements in spatial memory deficits, the chronic administration of CTEP was observed to correct deficits in episodic and recognition memory, both deficits that appear early in the disease progression of APPswe mice, similar to the early clinical presentation of AD (Hamilton et al., 2016). Strikingly, these studies found that both genetic deletion and pharmacological blockade of mGlu5 reduced the presence of AD pathology, including Aβ oligomers and plaques, (Hamilton et al., 2014; Hamilton et al., 2016), and decreased synaptic loss and increased synaptic density (Um et al., 2013). These findings are of particular interest as they indicate that mGlu5 signalling contributes directly to the establishment of AD-like pathology in APPswe and 3xTg AD mice.
Aberrant mGlu5 signaling in AD mice has been observed to inhibit autophagy, the process by which cellular organelles and protein aggregates are cleared (Nixon, 2013), via ubiquitination and proteasomal degradation of the autophagy related 14 (ATG14) protein (Zhang et al., 2015; Abd-Elrahman et al., 2017). The reduction in AD pathology and reversal of memory deficits observed in AD mice following genetic deletion or pharmacological blockade of mGlu5 is paralleled by an increase in autophagy via alterations in Zinc finger and BTB domain-containing protein 16 (ZBTB16)- and Unc-51-like kinase 1 (ULK1)-dependent pathways (Abd-Elrahman et al., 2018). Optineurin, a cytosolic protein, is essential for this regulation of mGlu5 -dependent autophagic signalling (Ibrahim et al., 2021). This has also been observed in preclinical Huntington’s Disease mouse models, indicating that mGlu5 contributes to neurodegeneration via conserved mechanisms (Abd-Elrahman et al., 2017), and suggests that antagonism of mGlu5 represents an effective approach to reverse progression of neurodegeneration by activating autophagy.
Another therapeutic target for the treatment of AD is the M1 muscarinic acetylcholine receptor (mAChR) and M1 PAMs and a partial agonist have emerged as promising strategy for improving cognitive function in AD (Scarpa, Hesse and Bradley, 2020; Abd-Elrahman and Ferguson, 2022; Nathan et al., 2022). The addition of Aβ to rodent brain slices impairs the function of M1, which is reversed by the addition of MPEP or another mGlu5 NAM, LSN (Yi et al., 2020). The authors suggest that Aβ drives M1 dysfunction via aberrantly activating mGlu5. This provides another mechanism by which mGlu5 antagonism may be beneficial in AD: the restoration of M1 mAChR function.
Whilst the use of mGlu5 NAMs to modify disease progression in AD has been promising, disrupting glutamate signaling via the antagonism or genetic deletion of mGlu5 has deleterious effects on learning and memory independent of AD (Simonyi, Schachtman and Christoffersen, 2005). A recent study suggested that the genetic deletion of mGlu5 accelerated neurodegeneration, with mGlu5 knockout mice having increased neuronal loss, astrogliosis, and microglial activation as compared to wild-type mice (Carvalho et al., 2019). Therefore, the use of mGlu5 NAMs to treat neurodegeneration could have a negative impact on brain regions that do not yet show AD pathology. However, it may also be that the use of mGlu5 NAMs to treat neurodegeneration is dependent on the presence of Aβ and tau.
Moreover, mGlu5 NAMs have been found to have psychomimetic effects in humans. For example, although fenobam has shown anxiolytic-like effects in human patients, it also induced psychomimetic effects including hallucinations and insomnia (Friedmann et al., 1980; Porter et al., 2005; Abou Farha et al., 2014). This may be due to modulation of the N-methyl-D-aspartate receptors (NMDARs) which are coupled to mGlu5 both structurally and functionally. Inhibition of NMDARs induces psychomimetic effects in both rodents and humans, which may be enhanced by inhibition of mGlu5 (Marino and Conn, 2002). Additionally, some mGlu5 NAMs have inverse agonist activity, which may also contribute to psychotic side effects in human patients (Porter et al., 2005; Keck et al., 2012). These side effects may limit the therapeutic window of mGlu5 NAMs, although no psychotomimetic effects were seen at the efficacious dose of mavoglurant in patients with cocaine use disorders in safety and tolerability studies (Ufer et al., 2016).
It may be important to elucidate mechanisms of blocking Aβ-mediated mGlu5 signaling, without blocking glutamate signalling. Unlike NAMs, mGlu5 SAMs do not alter levels of glutamate-mediated Ca2+ signaling (Haas et al., 2017). Silent allosteric modulation of mGlu5 using the mGlu5 SAM BMS-984923 has been shown to reduce the interaction between Aβ oligomers and cellular prion protein, resulting in an improvement in cognitive deficits and synaptic loss in APPswe mice without an alteration in physiological glutamate signaling (Haas et al., 2017). No improvements in Aβ plaque load were observed, suggesting that BMS-984923 acted to block processes downstream of mGlu5, rather than altering Aβ accumulation. The treatment of these mice began after Aβ plaques, synapse loss, and memory impairment has developed, suggesting that the use of SAMs to treat AD could slow or stop disease progression in advanced stages.
Perhaps surprisingly, considering the potential for mGlu5 NAMs in the treatment of AD, there is evidence to suggest that the agonism of mGlu5 may also be neuroprotective in AD. In both sexes of T41 mice, which overexpress mutant human amyloid precursor protein, and in wild-type mice injected with Aβ aggregates, neuronal loss was prevented by treatment with the mGlu5 PAM CDPBB. Moreover, elevated levels of microglial and astrocytic markers observed in the T41 mice were partially reversed in the CA1 hippocampal region. However, despite the reversal in Aβ-mediated neurotoxicity, CDPBB was not able to promote cognitive improvement in the T41 mice. The authors suggest that this could be due to the age at which the mice were treated; at 14 months the animals already show severe AD pathology and cognitive decline (Bellozi et al., 2019). Interestingly, the APPswe and 3xTg mice used in the previously discussed mGlu5 NAM experiments were treated with MTEP or CTEP months after the development of Aβ pathology and memory deficits, yet an improvement in cognition was still observed (Um et al., 2013; Hamilton et al., 2014; Hamilton et al., 2016; Abd-Elrahman et al., 2018).
The binding of Aβ oligomers to mGlu5 in AD not only activates mGlu5, but it has also been shown to induce the clustering of mGlu5 at the surface of synapses (Renner et al., 2010). Increased cell surface expression of mGlu5 has been observed in a number of mouse AD models (Um et al., 2013; Hamilton et al., 2014; Abd-Elrahman et al., 2018). Hamilton et al. (2014), for example, observed a 4.4 fold increase in mGlu5 cell surface expression in APPswe mice as compared to control mice, without a change in total cellular mGlu5 level. However, it should be noted that a recent study using highly sensitive, ultrastructural techniques such as SDS-FRL in the same mouse model at the same time point as Hamilton and colleagues demonstrated that, although the total level of mGlu5 was unchanged in APPswe mice as compared to control mice, the localisation of mGlu5 was significantly reduced along the cell surface of certain hippocampal cells, including pyramidal cells in the CA1 region and granule cells in the dentate gyrus (Martín-Belmonte et al., 2021). In addition, PET imaging in 5xFAD mice found that mGlu5 was reduced in the hippocampus and striatum of diseased animals as compared to controls (Lee et al., 2018). These findings were corroborated by a human PET study showing a 43% reduction of mGlu5 expression in the hippocampus of patients with mild AD (Mecca et al., 2020).
In models where mGlu5 is recruited to the cell surface, it is thought that Aβ oligomers act as an extracellular scaffold thus reducing the lateral diffusion of mGlu5 receptors (Renner et al., 2010). This means that the receptor is more readily accessible for activation by both Aβ oligomers and glutamate, thereby increasing Ca2+ levels (Renner et al., 2010) and potentially excitotoxicity. Therefore, pharmacologically activating mGlu5 to treat AD could be neurotoxic in the long term. A mGlu5 PAM 5PAM523 given orally to wild-type rats resulted in indications of neurotoxicity, including convulsions and excitability, which occurred in an mGlu5-dependent manner (Parmentier-Batteur et al., 2014). Similar to mGlu5 NAMs, it may be that this mGlu5 PAM-induced toxicity is via modulation of NMDAR currents (Conde-Ceide et al., 2015). Of note, Abd-Elrahman et al. (2018) found that the elevation in cell surface expression observed in both APPswe and 3xTg mice as compared to wild-type mice was reversed in animals treated with CTEP. Chronic administration of an mGlu5 NAM may slow the progression of AD pathology by disrupting the Aβ oligomer-mediated increase in mGlu5 cell surface expression.
In humans, PET imaging has been used to investigate changes in mGlu5 expression in healthy patients as they age. A recent study found no significant change in mGlu5 expression across brain regions when their analysis was corrected to include brain atrophy with age (Mecca et al., 2021). In AD patients, PET imaging studies have shown a significant decrease in mGlu5 expression in the hippocampus and amygdala, which remained after correcting to brain atrophy (Mecca et al., 2020; Treyer et al., 2020). Conversely, a post-mortem study of mGlu5 binding saw increases in mGlu5 expression in the frontal cortex and hippocampus of severe AD patients as compared to controls (5.2-fold and 2.5-fold respectively) (Müller Herde et al., 2019). This study, however, was underpowered with only 2 AD patients and 4 controls assessed and no supporting demographic data to assess the degree to which patient and control samples were matched giving a high probability of a type 1 error. Notwithstanding, it cannot be ruled out that there may be variation in mGlu5 up-regulation at different disease-stages, with mGlu5 up-regulation potentially only occurring in later-stage disease. Further longitudinal research is required to fully understand changes in mGlu5 expression in human AD.
Further thought and investigation are needed to reconcile the findings that both mGlu5 NAMs and PAMs can be neuroprotective in rodent models of AD, but it may be that antagonism and agonism of mGlu5 are neuroprotective at different stages of disease progression. Recently, it has been suggested that the contribution of mGlu5 to AD neuropathology is disease-stage dependent (Abd-Elrahman KS. et al., 2020). APPswe mice were given CTEP from the age of 6 months for either 24 or 36 weeks. When administered for 24 weeks, CTEP reversed memory deficits, increased autophagy and reduced AD pathology, including Aβ plagues and neuroinflammation. However, when treatment was extended to 36 weeks, CTEP was found to be ineffective at reversing memory deficits or AD pathology. The authors suggest that this may be due to reduced contribution of mGlu5 to AD-related pathology at advanced disease-stages. Going forward, it will be important to study the long-term efficacy of potential therapeutic targets related to disease trajectory and known risk factors, such as APOE status (Evans et al., 2019). Taken together, these data support a role for targeting mGlu5 in early stages of AD, perhaps in combination with the current standard of care (i.e., acetylcholinesterase inhibitors) to slow disease progression and/or improve the treatment response to the standard of care.
Most research into the effect of mGlu5 antagonism on AD mouse models has used male mice. However, recent investigations into whether the observed changes are conserved in female AD mice have suggested that mGlu5 may not make a significant contribution to AD pathology in female AD mice (Abd-Elrahman KS. et al., 2020). As discussed, Aβ oligomers bind to mGlu5 in a complex with cellular prion protein. Using radioligand binding assays, it was demonstrated that this complex formed only in the brains of male, but not female, mice. The same finding was observed in human brain tissue. Moreover, treating primary cortical neurons with an mGlu5 agonist or with Aβ oligomers only activated neuroprotective autophagy pathways in neurons derived from male, but not female, mouse embryos. Taken together, these data indicate that there are sex-specific differences in mGlu5 receptor signaling. In addition, it was found that the cell surface expression of mGlu5 differed between male and female mice. As previously mentioned, Aβ oligomers promote the clustering of mGlu5 receptors at the cell surface, however Abd-Elrahman and colleagues found that this increase in cell surface expression occurred only in male APPswe mice. Conversely, there was little mGlu5 cell surface expression in the cortex and hippocampus of female APPswe mice. Both sexes of APPswe mice have comparable levels of Aβ oligomers, neuroinflammatory markers, and cognitive decline, however chronic treatment of these mice with CTEP was found to improve cognition and reduce AD pathology in male mice only. These findings provide evidence that the contribution of mGlu5 receptors to AD-related neuropathology in APPswe mice is sex-dependent. This must be taken into consideration when investigating the potential of mGlu5 as a drug target for AD, and perhaps other diseases, too.
Huntington’s Disease
Huntington’s disease (HD) is an autosomal dominant neurodegenerative disease characterized by motor deficits including chorea and loss of coordination, cognitive decline, and psychiatric changes that ultimately results in death (McColgan and Tabrizi, 2018). Like Alzheimer’s disease, there are currently no disease-modifying treatments (McColgan and Tabrizi, 2018).
HD is caused by an inherited CAG trinucleotide repeat expansion in the gene that codes for the huntingtin protein (Htt), resulting in a mutant version of the protein that has an abnormally long polyglutamine repeat (mHtt) (MacDonald et al., 1993). This elongated protein can be cleaved into fragments which then aggregate to form neuronal intranuclear inclusions (NII), the presence of which correlates with disease progression (Miller et al., 2010).
The most striking pathological feature of Huntington’s disease is the loss of striatal neurons, although degeneration is not confined to these cells (Masnata & Cicchetti, 2017). There is high expression of mGlu5 in the striatum (Shigemoto et al., 1993) where it is enriched in medium-sized striatal cells (Testa et al., 1995). It has been shown that mGlu5 interacts directly with both Htt and mHtt, and that this interaction uncouples mGlu5 receptor signaling (Anborgh et al., 2005) and leads to increased intracellular Ca2+ levels, which is associated with excitotoxic cell death (Tang et al., 2003). This dysregulation of Ca2+ signalling is a feature of HD mouse models (Ribeiro et al., 2010). In addition, mGlu5 is thought to play a role in motor control as mGlu5 knockout mice have increased locomotor activity (Ribeiro F. M. et al., 2014). Taken together, these observations suggest that mGlu5 is a potential target in the treatment of Huntington’s disease.
Longitudinal PET imaging studies carried out in the zQ175 HD model showed a significant reduction in mGlu5 binding in the striatum and cortex as compared to wild-type mice over time (Bertoglio et al., 2018). There was no significant decrease in neuronal density in these mice, suggesting that the reduction in mGlu5 was not due to neuronal loss. Examination of post-mortem human HD tissue showed a decrease in mGlu5 expression in the caudate and putamen. However, as neuronal cell density was also significantly reduced, it may be that the reductions observed were due to neuronal loss (Gulyás et al., 2015). To date, there is no in vivo mGlu5 PET imaging data available in human HD patients to assess these changes. Notwithstanding, there are in vivo indications of altered cholinergic function in HD which may be related to altered mGlu5 function as previously discussed (D’Souza and Waldvogel et al., 2016). This is an important area of research to understand novel treatment strategies for this disease.
It has been shown that the blockade of mGlu5 is neuroprotective in mouse models of HD. Treatment with the mGlu5 NAM MPEP was shown to slightly increase survival and reverse the loss of motor coordination in R6/2 HD mice. However, this was not paralleled by a reduction in NII formation. Interestingly, mice treated with MPEP were observed to have larger NIIs in their cortical neurons (Schiefer et al., 2004). Other studies have found the neuroprotective effects of mGlu5 blockade to be paralleled by a reduction in NII formation. The genetic deletion of mGlu5 in HdhQ111/Q111 HD mice improved motor coordination and reduced the formation of NIIs (Ribeiro FM. et al., 2014). Likewise, the chronic administration of CTEP to 12-month-old zQ175 huntingtin knockin (zQ175) mice improved both motor and cognitive deficits, and significantly reduced mHtt aggregates and neuronal cell death (Abd-Elrahman et al., 2017). Interestingly, this study found that acute blockade of mGlu5 (1 week) was effective at ameliorating motor and cognitive deficits in heterozygous, but not homozygous, zQ175 mice.
A key regulator of HTT-mediated gene expression is the repressor element 1-silencing transcription factor/neuron restrictive silencer factor (REST/NRSF) (Rigamonti et al., 2009). In HD, REST/NRSF leads to the decreased transcription of several neuronal genes (such as brain-derived neurotrophic factor, BDNF) in the nucleus of diseased cells. Using primary neuronal cultures, mGlu5 was shown to regulate REST/NRSF expression via modulating N-cadherin/β-catenin interactions (De Souza et al., 2020); the activation of mGlu5 with 3,5-dihydroxyphenylglycine (DHPG) resulted in increased REST/NRSF expression, whilst the inhibition of mGlu5 with CTEP resulted in decreased REST/NRSF expression. This was paralleled by changes in SNAP-25 expression. These findings were also observed in vitro using zQ175 mice chronically treated with CTEP, and in BACHD mGlu5 KO mice, confirming that mGlu5 modulates REST/NSRF signaling in HD. The authors suggest that the improvements in motor function and reduction in disease pathology after the pharmacological and genetic blockade of mGlu5 in HD mouse models may be due to a reduction in aberrant mGlu5 -regulated REST/NRSF signaling.
Similar to AD, it has been suggested that mGlu5 antagonism may improve cognitive function in HD mice by promoting the increased removal of aggregated mHtt via autophagy. The presence of mHtt aggregates correlates highly with HD progression, and the number and size of these aggregates is reduced via mGlu5 deletion or antagonism in HD mice models (Ribeiro F. M. et al., 2014; Abd-Elrahman et al., 2017). This observation is linked to increased autophagy via the same ZBTB16- and ULK-1-dependent mechanisms observed to be altered in AD (Abd-Elrahman et al., 2017, 2018). The chronic antagonism of mGlu5 promotes a reduction in ZBTB16 expression, which, in turn, leads to the rescue of ATG14, a key autophagy adapter. In addition, mGlu5 inhibition reduces the inhibitory phosphorylation of ULK1, resulting in its activation which is essential for the phosphorylation of ATG13 (Abd-Elrahman et al., 2017). This ULK-1 activation is triggered by the normalization of aberrant mammalian target of rapamycin (mTOR) activation (Abd-Elrahman and Ferguson, 2019). ATG13 is required for the formation of the autophagosome, which is a critical autophagy process (Abd-Elrahman and Ferguson, 2019). Therefore, targeting mGlu5 with NAMs represents an effective approach to slow the progression of HD by promoting autophagy to reduce the aggregation of mHtt aggregates.
Apoptosis is another process that is altered by mHtt (Steffan et al., 2000). Chronic mGlu5 antagonism in partially reduced neuronal apoptosis, and increased cell survival in zQ175 mice (Abd-Elrahman et al., 2017). Further examination of these mice revealed that chronic CTEP administration enhanced CREB-dependent BDNF transcription, which reduces apoptosis and promotes neuronal survival (Abd-Elrahman et al., 2019). It is likely that the promotion of autophagy to clear mHtt facilitates this increase in CREB expression and BNDF production; this can be confirmed by blocking autophagy in measuring BNDF synthesis in a HD mouse model. Taken together, these findings suggest that targeting mGlu5 with a NAM may be beneficial in treating neurodegeneration by promoting autophagy and reducing apoptotic neuronal cell loss.
As in AD, the effects of mGlu5 antagonism on HD pathology have been studied predominantly in male HD mice. Recent work compared the response of male and female zQ175 mice to chronic treatment with CTEP (Li et al., 2022). This study showed that improvements in motor and cognitive skills differed between the sexes. Chronic mGlu5 antagonism improved the motor performance of male HD mice in grip strength and rotarod tests after both 4 and 12 weeks of CTEP administration. In comparison, female mice only saw an improvement in rotarod performance after 12 weeks of treatment. In regards to cognition, only male HD mice regained their cognitive ability in a novel object recognition test. Despite differences in motor and cognitive improvements, both sexes of HD mice had an attenuation of HD pathology, including reduced mHtt aggregates and neuronal cell death. These findings suggest that antagonism of mGlu5 could be neuroprotective in both male and female rodents, but further work is required to understand any differences in the efficacy of mGlu5 NAMs between the sexes.
The agonism of mGlu5 using PAMs may also be neuroprotective in the treatment of HD. DFB, CDPPB, and VU1545 were shown to be neuroprotective in primary striatal culture, all preventing excitotoxic cell death caused by elevated glutamate or NMDA concentrations (Doria et al., 2013). The chronic administration of CDPBB has been shown to reverse motor and cognitive deficits in the BACHD mouse model of HD (Doria et al., 2013, 2015). This cognitive improvement is paralleled by the increased activation of neuroprotective pathways, including Akt, ERK1/2, and BDNF expression, and a reduction in the formation of mHtt aggregates (Doria et al., 2015). Sub-chronic administration (8 days) of another mGlu5 PAM, VU0409551, was shown to ameliorate memory impairments in the same HD mouse model (Doria et al., 2018). These mice had a significant increase in mGlu5 cell surface expression, especially in the hippocampus and striatum, and showed an increase in the regulation of several genes that are known to play a role in synaptic plasticity, such as BDNF, c-Fos and PSD95. BACHD mice exhibit motor and cognitive impairments from 6 months of age, yet mHtt aggregates and neuronal cell death are only apparent at 12 months of age. In the studies mentioned here, mice were treated after the appearance of behavioral deficits, but before the appearance of HD neuropathology. Thus, the authors suggest that CDPPB and VU0409551 may enhance the memory of BACHD mice independent of a neuroprotective effect, by activating synaptic plasticity pathways (Doria et al., 2018).
The signalling of mGlu5 is altered in neuronal cultures and brain slices from pre-symptomatic HdhQ111/Q111 HD mice, with mGlu5 agonism leading to a reduction in mGlu5 -mediated IP3 formation due to an increase in PKC-mediated mGlu5 receptor desensitisation (Ribeiro et al., 2010). This desensitisation may be neuroprotective, resulting in a reduction in toxic Ca2+ signaling. Moreover, mGlu5 activation in these cultures resulted in an increase in Akt and ERK activation, both of which can be neuroprotective. These observations were present only in pre-symptomatic HdhQ111/Q111 HD mice, and it may be that the neuroprotection provided by mGlu5 agonism may be lost at later disease stages. Moreover, despite a reduction in IP3 formation after mGlu5 agonism in the diseased neuronal cultures, the authors saw an increase in Ca2+ release, which suggests that the neuroprotective benefit of mGlu5 agonism in these cells may not be sufficient to reduce excitotoxic levels of Ca2+ signaling.
Amyotrophic Lateral Sclerosis
Amyotrophic Lateral Sclerosis (ALS) is a fatal neurodegenerative disease characterized by the progressive loss of motor neurons in the motor cortex, brainstem, and spinal cord which results in progressive weakness and muscle atrophy (Mejzini et al., 2019). Most cases of ALS are sporadic, but around 10% of affected individuals have familial ALS which is inherited in an autosomal dominant manner (Kirby et al., 2016). The most widely used treatment for ALS, Riluzole, is symptomatic in nature, rather than working to halt disease progression (Mejzini et al., 2019). Riluzole modulates the glutamatergic system, but its effects are modest and wear off over time (Cetin et al., 2015), and thus targeting mGlu5 may be a more effective strategy.
Altered excitatory neurotransmission plays a key role in the progression of ALS. SOD1G93A mice and human ALS patients have increased concentrations of extracellular glutamate in their spinal cord plasms and cerebrospinal fluid (Shaw et al., 1995; Alexander et al., 2000; Wuolikainen et al., 2011). Different mechanisms by which glutamate levels are sustained have been proposed, such as an increased glutamate release into, and insufficient clearance of glutamate from, the synaptic cleft (King et al., 2016). In SOD1G93A mice, mGlu5 activation results in the abnormal release of glutamate which occurs in both pre-symptomatic and late-stage disease (Giribaldi et al., 2013; Bonifacino et al., 2019). Moreover, mGlu5 expression is increased in the brain of SOD1G93A mice as compared to wild-type mice. This upregulation is observed in the hippocampus, striatum, cortex, and spinal cord and is found to increase in line with disease progression in the hippocampus, spinal cord and cortex (Giribaldi et al., 2013; Brownell et al., 2015; Bonifacino et al., 2019). Autoradiography studies in human post-mortem ALS brain tissue showed that mGlu5 was upregulated in diseased brains as compared to controls, particularly in the motor, frontal and temporal cortices and basal ganglia (Müller Herde et al., 2019).
Reducing mGlu5 expression in ALS SOD1G93A mice by crossing the animals with mice heterozygous for the mGlu5 knockout mutation (Grm5+/−) has been shown to delay disease onset and prolong survival. This was paralleled by a reduction in a number of histological characteristics; motor neurons were preserved and astrogliosis and microgliosis were reduced (Bonifacino et al., 2017). Complete knockout of mGlu5 in SOD1G93A mice (SOD1G93A/Grm5−/−) resulted in an enhanced improvement in delayed disease onset and survival (Bonifacino et al., 2019). In SOD1G93A/Grm5+/− mice, with reduced mGlu5 expression, motor improvements were observed solely in male mice. When mGlu5 was completely ablated, no difference was seen between male and female mice; both sexes showed significant improvements in motor coordination and muscle strength, although the improvements seen in the male mice were slightly more pronounced. Perhaps confusingly, the same group treated SOD1G93A mice with the mGlu5 NAM CTEP and found that low doses improved motor skills and prolonged survival in female mice only. With an increased CTEP dose, improvements were seen in both sexes, but with improvements in the female mice being more pronounced (Milanese et al., 2021). Why genetic blockade of mGlu5 and blockade with a NAM showed differences in sex-specific responses requires further thought. Also of note, similar to findings in AD models (Abd-Elrahman K. S. et al., 2020), the authors found that CTEP had a disease-stage dependent effect, with improvements in specific motor skills (muscle force) being seen during early disease stages only. Despite complexity surrounding sex- and disease-stage specific effects of mGlu5 antagonism on ALS, mGlu5 NAMs represent a potentially promising avenue for ALS future research.
Parkinson’s Disease
Parkinson’s disease (PD) is a progressive neurodegenerative disease characterized by the death of dopaminergic neurons in the substantia nigra and the abnormal accumulation of the intracellular protein α-synuclein. Clinically, patients display motor symptoms such as bradykinesia (slow movements), shaking, and rigidity, and non-motor symptoms such as difficulty sleeping, mood disorders, and dementia (Poewe et al., 2017). There is currently no cure, but the most effective drug for treating PD is dopamine-replacement therapy using L-3,4-dihydroxyphenylalanine (L-DOPA). However, the long-term use of L-DOPA is associated with L-DOPA-induced dyskinesia (LID) which involves movement disorders such as chorea and dystonia (Thanvi, Lo and Robinson, 2007).
It is well documented that increased glutamate signaling, particularly in the basal ganglia where mGlu5 is highly expressed, is associated with the neuropathology of PD and LID (DeLong and Wichmann, 2015). It has been suggested that the mGlu5 NAMs might be an effective mechanism by which excessive glutamate transmission in PD could be decreased. As previously discussed, cellular prion protein has been shown to act as a co-receptor for mGlu5 and Aβ oligomers (Laurén et al., 2009). Similarly, α-synuclein oligomers have been observed to interact physically with cellular prion protein via mGlu5. This interaction phosphorylates Fyn kinase and results in the subsequent release of intracellular calcium (Ferreira et al., 2017), thus implicating mGlu5 as playing a role in α-synuclein oligomer-related pathology.
The two most widely used PD animal models are the 6-hydroxydopamine (6-OHDA)-lesioned model, which involves destroying nigrostriatal dopaminergic neurons by unilaterally injecting 6-OHDA into the forebrain (Simola, Morelli and Carta, 2007), and the 1-methyl-4-phenyl-1,2,3,6-tetrahydropyridine (MPTP)-lesioned model, which involves the administration of MPTP, a neurotoxin that causes an acute loss of striatal dopamine neurons (Meredith and Rademacher, 2011; Porras et al., 2012). A large number of studies have demonstrated that mGlu5 antagonists are able to ameliorate motor symptoms in these animal models of PD.
Early studies showed that the antagonism of mGlu5 was able to reduce motor deficits in PD animal models, with the acute administration of MPEP resulting in an attenuation in unilateral rotating behavior in the rat 6-OHDA-lesioned model (Spooren et al., 2000). The effect seen was small, and the authors suggested that mGlu5 antagonists may not be sufficiently effective to use alone in the treatment of PD. v (Breysse et al., 2002).
The administration of MPEP, and the genetic deletion of mGlu5, have both been shown to reduce nigrostriatal damage and increase survival in MPTP-lesioned rats (Battaglia et al., 2004). Furthermore, the chronic administration of MPEP improves non-motor deficits, including working and recognition memory in preclinical rodent models (Hsieh et al., 2012). This improvement in cognition was paralleled by a reduction in lesion-induced dopaminergic degeneration. Similar results have been observed in MPTP-lesioned monkeys after L-DOPA and acute or chronic MPEP treatment (Morin et al., 2010; Morin et al., 2013a). Strikingly, MPTP-lesioned monkeys treated with both MPEP and L-DOPA developed 72% less dyskinesia compared to those treated with L-DOPA alone (Morin et al., 2013b).
Similarly, acute administration of MTEP has been observed to maintain anti-parkinsonian effects in L-DOPA-treated MPTP-lesioned monkeys and reduced peak dose LID by 96% (Johnston et al., 2010). Moreover, monkeys treated with both MPTP and MTEP for 18–21 weeks did not develop parkinsonian symptoms compared to monkeys treated with MPTP alone (Masilamoni et al., 2011). Furthermore, acute MTEP administration reduced catalepsy and muscle rigidity in a rat model of parkinsonism (Ossowska et al., 2005).
The 6-ODHA model of PD is thought to be more representative of the degeneration that occurs in human PD patients, as the loss of dopaminergic neurons occurs more slowly than in MPTP models (Schober, 2004). Knockout mGlu5 mice showed a reduction in 6-ODHA-induced neuronal loss, similar to findings in MPTP-lesioned rats (Black et al., 2010; Battaglia et al., 2004). Moreover, 6-ODHA-lesion mGlu5 knockout mice performed better than wild-type mice at certain motor tests, such as the pole and motor asymmetry tests. The inhibition of mGlu5 using CTEP in this model showed a duration-dependent improvement in motor deficits, with a partial reversal in deficits seen after 1 week of administration, and a complete attenuation of certain deficits, such as coordination, after 12 weeks of treatment (Farmer et al., 2020). These improvements were paralleled by the activation of the mTOR pathway, an increase in striatal BDNF levels, and the re-innervation of dopaminergic terminals in the striatum. The administration of other mGlu5 NAMs, including MPEP, fenobam, mavoglurant and dipraglurant, have been shown to reduce LID severity and enhance the anti-parkinsonian effects of L-DOPA treatment in 6-OHDA-lesioned rats (Rylander et al., 2010; Huang et al., 2018).
PET imaging in human PD patients has shown that mGlu5 binding is slightly increased in the brains of PD patients, specifically in the putamen, hippocampus, and amygdala (Kang et al., 2019). The administration of L-DOPA has been shown to enhance mGlu5 expression in the striatum, and co-administration with MPEP in 6-OHDA-lesioned rats is able to reduce this striatal overexpression (Huang et al., 2018; Zhang et al., 2021). Similarly, the L-DOPA treatment of MPTP monkeys has been observed to increase mGlu5 receptor-specific binding in the basal ganglia as compared to control animals, but not in animals who had been co-administered L-DOPA and MPEP (Morin et al., 2013a). This suggests that L-DOPA-induced motor complications are associated with enhanced mGlu5 expression, which can be reduced using an mGlu5 antagonist.
The accumulation of misfolded proteins in the endoplasmic reticulum (ER) leads to ER stress in a pathway known as the unfolded protein response (UPR) which has been suggested to play a role in the pathogenesis of PD (Mercado et al., 2016). The upregulation of mGlu5 has been observed to induce ER stress (Gu et al., 2021). Overexpression or activation of mGlu5 with an agonist increased ER stress and DNA damage in primary neurons by activating ERK and JNK signaling pathways. This resulted in neuronal damage which was attenuated by pre-treatment with MPEP. These findings link the upregulation of mGlu5 to neurotoxicity and provide a mechanism by which mGlu5 antagonism may be neuroprotective in PD.
Another key process in the pathogenesis of PD is axonal degeneration (Salvadores et al., 2017). Dopaminergic neurons in the putamen are almost completely lost from 4 years after a PD diagnosis (Kordower et al., 2013). These dopaminergic neurons play as essential role in motor control and their degeneration leads to the profound motor impairments seen in PD patients (Howe and Dombeck, 2016). The downregulation of mGlu5 expression in 6-OHDA-lesioned primary neurons and rats treated with MPEP resulted in a reduction in axonal degeneration and attenuated 6-ODHA-activated Ca2+ increases (Zhang et al., 2021). In addition, this study demonstrated ERK phosphorylation and the activation of calpain play a key role in the axonal degeneration that 6-ODHA induces, both of which are also inhibited by mGlu5 antagonism.
Due to the therapeutic potential of mGlu5 antagonism in animal models of PD, several clinical trials have been carried out to investigate the efficacy of mGlu5 NAMs in LID PD patients. Both mavoglurant (Berg et al., 2011; clinicaltrials.gov: NCT00582673, NCT00888004; Stocchi et al., 2013; clinicaltrials.gov: NCT00986414) and dipraglurant (Tison et al., 2016; clinicaltrials.gov: NCT01336088) have been shown to have anti-dyskinetic activity in patients, without any worsening of motor symptoms. However, a meta-analysis comparing mavoglurant to placebo across randomized controlled trials found mavoglurant to be inconsistent in reducing off time in LID in PD patients, but treatment was associated with lower abnormal involuntary movements (Negida et al., 2021). To date, the only approved treatment for PD-LID is amantadine, a weak NMDA antagonist that gives increases in glutamate suggesting that in more severe PD patients that develop LID this is a more effective treatment strategy. Whether chronic mGlu5 NAM treatment is neuroprotective and could delay the onset LID still needs to be assessed empirically in PD.
Neuroinflammation
A chronic neuroinflammatory response is a common pathological hallmark found across neurodegenerative diseases, including those discussed in this review. This inflammatory response is often characterized by the upregulation of neuroinflammatory cell markers, such as ionized calcium-binding adapter molecule 1 (Iba-1) and glial fibrillary acidic protein (GFAP). In addition, glial cells undergo changes in morphology and gene expression after injury or during disease (Hasel and Liddelow, 2021). These responses to pathological insult are rapid and profound, and are diverse in regards to space, time, sex and cell-subtype (Liddelow and Barres, 2017). Until recently, it was thought that this neuroinflammatory response was secondary to neuronal loss. However, there is now accumulating evidence to suggest that neurodegeneration can occur, at least in part, due to neuroinflammation (Ransohoff, 2016).
As previously mentioned, mGlu5 is expressed on both astrocytes and microglia. Astrocytic mGlu5 plays a key role in regulating excitatory transmission. Upon activation of mGlu5 on astrocytes, calcium oscillations are induced (Bradley et al., 2011) and glutamate is released, which subsequently induces a slow, intracellular current in neurons (Loane et al., 2012). Additionally, activation of astrocytic mGlu5 results in increased astrocyte proliferation (Kanumilli and Roberts, 2006), the release neurotrophic factors such as BNDF (Jean et al., 2008), and increases the activity of the glutamate transporter GLT1 leading to rapid glutamate uptake in astrocytes (Vermeiren et al., 2005). The physiological role of mGlu5 in microglia is less clear. This review focusses specifically on therapeutic potential of targeting of astrocytic and microglial mGlu5 in neurodegenerative disease (Figure 2).
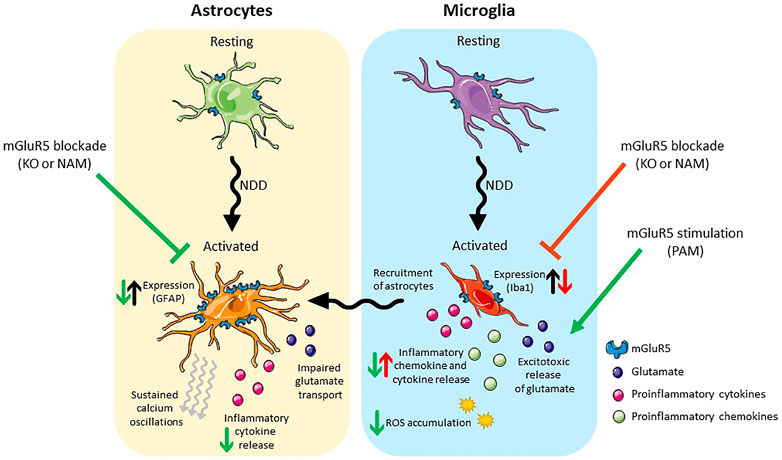
FIGURE 2. Schematic presentation of the effects of mGlu5 allosteric modulation on astrocytes (left) and microglia (right). Under pathological conditions both cell types take on an activated phenotype. Activated astrocytes have an increase in mGlu5 expression, sustained calcium oscillations, impaired glutamate transport and release pro-inflammatory cytokines. Blockade of mGlu5, either genetically or pharmacologically, is neuroprotective, reducing GFAP expression and the release of inflammatory cytokines. Activated microglia release inflammatory cytokines/chemokines and excitotoxic levels of glutamate and result in the ROS accumulation. In addition, they recruit activated astrocytes. Blockade of mGlu5, either genetically or pharmacologically reduces the expression of Iba-1 but is neurotoxic in that it results in an increase in inflammatory chemokine/cytokine release. Stimulation of mGlu5 with a PAM, on the other hand, is neuroprotective and reduces the release of inflammatory cytokines/chemokines and the accumulation of ROS. Abbreviations: NDD, neurodegenerative disease; KO, knockout; NAM, negative allosteric modulator; PAM, positive allosteric modulator; GFAP, Glial fibrillary acidic protein; Iba-1, Ionised calcium binding adaptor molecule 1; ROS, reactive oxygen species. Figure created using Reactome Icon Library, licensed under CC BY 4.0 (Sidiropoulos et al., 2017).
Astrocytes
In neurodegenerative diseases (as well as after infection or injury), astrocytes undergo a transition from a resting state to a reactive state (for an in-depth review see Escartin et al., 2021). The up-regulation of GFAP is a key feature of the majority of reactive astrocytes, but other markers are also upregulated such as nestin (Moreels et al., 2008), vimentin (Yamada et al., 1992), and aldolase-C (Halford et al., 2017). In addition, reactive astrocytes take on an altered morphology, with their star-like processes becoming elongated and stretching towards injury sites (Schiweck et al.,2018). Reactive astrocytes are diverse in their response to insult and take on specific states in different models of disease. It had been proposed that astrocytes took on one of two phenotypes, labelled “A1” and “A2” (Zamanian et al., 2012; Liddelow and Barres, 2017), with the former being found in chronic neuroinflammatory conditions, and the latter being found after ischemic stroke. However, the field is now moving away from these terms as it is evident that there is a large amount of heterogeneity in the responses of astrocytes to pathological conditions (Hasel and Liddelow, 2021).
Under pathophysiological conditions, mGlu5 is overexpressed in reactive astrocytes in AD (Shrivastava et al., 2013), ALS (Aronica et al., 2001), and epilepsy (Aronica et al., 2001). Similarly, in vitro, mGlu5 has been observed to be upregulated in cultured astrocytes after exposure to Aβ oligomers (Casley, et al., 2009), and in diseased astrocytes isolated from SODG93A rodents as compared to controls (Vermeiren et al., 2006). Upon activation, astrocytic mGlu5 initiates Ca2+ oscillations (Bradley et al., 2011). Aβ oligomers in AD and α-synuclein in PD are able to bind to and activate mGlu5, which could initiate sustained Ca2+ oscillations and lead to excitotoxicity (Price et al., 2010; Spampinato et al., 2018). Furthermore, in activated astrocytes prepared from SOD1G93A mice, the overexpression of mGlu5 significantly impaired glutamate transport and resulted in glutamate-induced excitotoxicity (Vermeiren et al., 2006). In a similar ALS primary astrocyte culture, the typical mGlu5-induced Ca2+ oscillations showed a shift to a sustained plateau at the peak of the first oscillation (Vergouts et al., 2018). This was shown to be regulated by protein kinase C epsilon (PKCε) as increasing PKCε expression restored the mGlu5-induced Ca2+ oscillations. Moreover, astrocytes derived from ALS mice are highly vulnerable to glutamate as compared to wild-type astrocytes. This increased vulnerability is mediated via the activation of mGlu5 and results in astrocyte degeneration which is reversed by the blockade of mGlu5 signaling in vivo using MPEP (Rossi et al., 2008). Calcium signaling and glutamate transport play key roles in astrocyte-neuron communication, and their disruption may play a key role in the pathology of neurodegenerative diseases (Thibault et al., 2007; Pekny and Pekna, 2014).
The elevated expression of astrocytic markers seen in AD, HD and ALS mice is reduced with the genetic and pharmacological blockade of mGlu5 (Hamilton et al., 2016; Abd-Elrahman et al., 2017; Bonifacino et al., 2017; Abd-Elrahman KS. et al., 2020). In addition, pharmacological inhibition of mGlu5 prevents the secretion of inflammatory cytokines from astrocytes (Shah et al., 2012). Taken together, these findings suggest that the inhibition of astrocytic mGlu5 may lead to a reduction in the neurotoxic inflammatory state seen in neurodegenerative disease, thereby contributing to the disease-modifying effects seen in rodent models after mGlu5 NAM treatment.
Microglia
Like astrocytes, microglia undergo a transformation to a reactive phenotype after pathological insult. Reactive microglia have retracted processes and an enlarged nucleus. Traditionally, reactive microglia were also characterized into two groups: “M1” and “M2”. M1 was considered a pro-inflammatory state, whereas M2 microglia were thought to be anti-inflammatory. Microglia activation is now known to be broader and more diverse (Bachiller et al., 2018). For example, a unique subtype of reactive microglia, diseased-associated microglia (DAM), has been observed in AD and ALS models, and is protective in nature (Keren-Shaul et al., 2017).
As with astrogliosis, the genetic or pharmacological blockade of mGlu5 in AD and ALS rodent models led to a reduction in microgliosis (Bonifacino et al., 2017; Abd-Elrahman K. S. et al., 2020; Milanese et al., 2021). As reactive microglia have a neurotoxic effect mediated by the secretion of proinflammatory cytokines and chemokines and the release of excitotoxic levels of glutamate (Barger and Basile, 2001; Hemonnot et al., 2019), it could be assumed that reducing their levels would be neuroprotective. To the contrary, the inhibition of mGlu5 in microglia drives them towards a pro-inflammatory state (Chantong et al., 2014). In cultured microglia activated by the overexpression of α-synuclein, the addition of an mGlu5 NAM enhanced inflammation in these cells by exacerbating inflammatory signaling pathways (Zhang et al., 2021).
There is mounting evidence to suggest that activation of microglial mGlu5 could be important for counteracting the neurotoxic effect of microglia in neurodegenerative disease. Activation of mGlu5 with an agonist or PAM has been shown to consistently reduce microglial activation and the associated inflammation in primary microglial cultures and cells lines challenged with pro-inflammatory molecules, including Aβ and α-synuclein (Byrnes et al., 2009; Farso, O’Shea and Beart, 2009; Loane et al., 2009, 2013, 2014; Piers, Heales and Pocock, 2011). This effect was not observed in primary cultures from mGlu5 knockout mice nor in the presence of an mGlu5 antagonist. Moreover, activating microglial mGlu5 reduces apoptosis, reduced reactive oxygen species accumulation, and increases the production of brain-derived neurotrophic factor (Ye et al., 2017). In vivo, mGlu5 PAM administration reduces neuronal loss in a mouse model of traumatic brain injury by reducing the inflammation induced by microglia (Loane et al., 2014). Whilst it is clear that mGlu5 signaling in astrocytes can contribute to neurotoxicity, these data suggest that mGlu5 signaling in microglia may be neuroprotective.
It is important to consider the differing effects of mGlu5 agonists, antagonists, NAMs and PAMs on glial cells when developing ligands for the treatment of neurodegeneration. In addition, the activation of microglia in disease results in the recruitment of astrocytes and induces their conversion into a reactive phenotype (Liddelow and Barres, 2017). Thus, the benefit of activating microglia in disease with an mGlu5 PAM may be limited by the subsequent neurotoxic response from astrocytes. Similarly, a NAM that is neuroprotective in pre-clinical and clinical models may have a detrimental effect by driving a proinflammatory microglial response, even though it may reduce astrocyte-induced excitotoxicity. As targeting neuroinflammatory processes may be of benefit across multiple neurodegenerative diseases and targeting mGlu5 modulates inflammation, it will be important to better understand the relative contribution of each cell type to pathology in order to select the most effective ligands to treat disease. It is not yet clear whether mGlu5 will need to be activated or inhibited in order to most effectively modulate disease pathology and progression via its effect on neuroinflammation.
Conclusion
Much of the research into the role of mGlu5 receptors in neurodegenerative diseases has focused on the use of specific allosteric modulators, both positive and negative. The neuroprotective effects of both the up and downregulation of mGlu5 receptor signaling emphasise the role mGlu5 plays in the pathology of these diseases and highlights its potential as a therapeutic target. In Alzheimer’s disease, the genetic deletion or pharmacological blockade of mGlu5 improved cognition and reduced disease-related pathology in rodent models of disease. These benefits were paralleled by an increase in autophagy and a reduction in neuroinflammation. However, the genetic deletion of mGlu5 in healthy mice worsens learning and memory processes and accelerates neurodegeneration. Enhancing mGlu5 signaling with mGlu5 PAMs has also been beneficial in rodent models of AD, preventing neuronal loss but not improving cognition. Similarly, both NAMs and PAMs have been neuroprotective in rodent models of Huntington’s disease. However, PAMs may have a potential excitotoxic effect. In ALS and PD, it is mGlu5 NAMs that have been associated with improved disease pathology and cognition. However, no drug has yet received regulatory approval for these indications.
It is evident that glial mGlu5, specifically mGlu5 receptors expressed on astrocytes and microglia, plays a role in neurodegeneration. Astrocytes are upregulated in the brains of patients and rodent models and mGlu5 activation contributes to the neurotoxic effect they exert. Microglial activation, on the other hand, is neuroprotective against inflammation. Fundamental questions remain surrounding the opposing role of mGlu5 in astrocytes and microglia and the precise contribution of mGlu5 signaling in these cells to inflammation.
In conclusion, the neuroprotective effect of modulating mGlu5 signaling highlights this receptor as a promising therapeutic target for the treatment of neurodegenerative diseases. Furthermore, its expression on glial cells and ability to modulate inflammation suggests that it play a neuroprotective role by resolving common pathologies across diseases. Ongoing clinical trials using mGlu5 modulators in neurodegenerative diseases, including AD and PD, as well as other neurological conditions, such as FXS, will help elucidate the role of the mGlu5 in different disease mechanisms and its therapeutic potential in neurodegenerative disorders. The outcomes of these studies will have a major impact on completing the picture of the role of mGlu5 in neurodegeneration and its potential as a drug target for the treatment of neurodegenerative diseases.
Author Contributions
RB, GB, ES, KB, and SB contributed to writing this review.
Funding
This research was funded by Medical Research Scotland.
Conflict of Interest
GB, ES, KB, and SB are all employees of Sosei Heptares.
The remaining author declares that the research was conducted in the absence of any commercial or financial relationships that could be construed as a potential conflict of interest.
Publisher’s Note
All claims expressed in this article are solely those of the authors and do not necessarily represent those of their affiliated organizations, or those of the publisher, the editors and the reviewers. Any product that may be evaluated in this article, or claim that may be made by its manufacturer, is not guaranteed or endorsed by the publisher.
References
Abd-Elrahman, K. S., Albaker, A., de Souza, J. M., Ribeiro, F. M., Schlossmacher, M. G., Tiberi, M., et al. (2020a). Aβ Oligomers Induce Pathophysiological mGluR5 Signaling in Alzheimer's Disease Model Mice in a Sex-Selective Manner. Sci. Signal 13 (662). doi:10.1126/scisignal.abd2494
Abd-Elrahman, K. S., and Ferguson, S. S. G. (2019). Modulation of mTOR and CREB Pathways Following mGluR5 Blockade Contribute to Improved Huntington's Pathology in zQ175 Mice. Mol. Brain 12 (1), 35–39. doi:10.1186/s13041-019-0456-1
Abd-Elrahman, K. S., Hamilton, A., Hutchinson, S. R., Liu, F., Russell, R. C., and Ferguson, S. S. G. (2017). mGluR5 Antagonism Increases Autophagy and Prevents Disease Progression in the zQ175 Mouse Model of Huntington's Disease. Sci. Signal 10 (510), 1–12. doi:10.1126/scisignal.aan6387
Abd-Elrahman, K. S., Hamilton, A., Vasefi, M., and Ferguson, S. S. G. (2018). Autophagy Is Increased Following Either Pharmacological or Genetic Silencing of mGluR5 Signaling in Alzheimer's Disease Mouse Models. Mol. Brain 11 (1), 19–11. doi:10.1186/s13041-018-0364-9
Abd-Elrahman, K. S., Sarasija, S., Colson, T. L., and Ferguson, S. S. G. (2022). A Positive Allosteric Modulator for the Muscarinic Receptor (M1 mAChR) Improves Pathology and Cognitive Deficits in Female APPswe/PSEN1ΔE9 Mice. Br. J. Pharmacol. 179 (8), 1769–1783. doi:10.1111/BPH.15750
Abd-Elrahman, K. S., Albaker, A., de Souza, J. M., Ribeiro, F. M., Schlossmacher, M. G., Tiberi, M., et al. (2019). Aβ Oligomers Induce Sex-Selective Differences in mGluR5 Pharmacology and Pathophysiological Signaling in Alzheimer Mice. bioRxiv. doi:10.1101/803262
Abd-Elrahman, K. S., and Ferguson, S. S. G. (2022). Noncanonical Metabotropic Glutamate Receptor 5 Signaling in Alzheimer's Disease. Annu. Rev. Pharmacol. Toxicol. 62, 235–254. doi:10.1146/ANNUREV-PHARMTOX-021821-091747
Abd-Elrahman, K. S., Hamilton, A., Albaker, A., and Ferguson, S. S. G. (2020b). mGluR5 Contribution to Neuropathology in Alzheimer Mice Is Disease Stage-dependent. ACS Pharmacol. Transl. Sci. 3, 334–344. doi:10.1021/acsptsci.0c00013
Abou Farha, K., Bruggeman, R., and Baljé-Volkers, C. (2014). Metabotropic Glutamate Receptor 5 Negative Modulation in Phase I Clinical Trial: Potential Impact of Circadian Rhythm on the Neuropsychiatric Adverse Reactions-Do Hallucinations Matter? ISRN Psychiatry 2014, 652750–652758. doi:10.1155/2014/652750
Alexander, G. M., Deitch, J. S., Seeburger, J. L., Del Valle, L., and Heiman-Patterson, T. D. (2000). Elevated Cortical Extracellular Fluid Glutamate in Transgenic Mice Expressing Human Mutant (G93A) Cu/Zn Superoxide Dismutase. J. Neurochem. 74 (4), 1666–1673. doi:10.1046/j.1471-4159.2000.0741666.x
Anborgh, P. H., Godin, C., Pampillo, M., Dhami, G. K., Dale, L. B., Cregan, S. P., et al. (2005). Inhibition of Metabotropic Glutamate Receptor Signaling by the Huntingtin-Binding Protein Optineurin. J. Biol. Chem. 280 (41), 34840–34848. doi:10.1074/jbc.M504508200
Anneser, J. M., Ince, P. G., Shaw, P. J., and Borasio, G. D. (2004). Differential Expression of mGluR5 in Human Lumbosacral Motoneurons. Neuroreport 15 (2), 271–273. doi:10.1097/00001756-200402090-00012
Aronica, E., Catania, M. V., Geurts, J., Yankaya, B., and Troost, D. (2001). Immunohistochemical Localization of Group I and II Metabotropic Glutamate Receptors in Control and Amyotrophic Lateral Sclerosis Human Spinal Cord: Upregulation in Reactive Astrocytes. Neuroscience 105 (2), 509–520. doi:10.1016/S0306-4522(01)00181-6
Arsova, A., Møller, T. C., Vedel, L., Hansen, J. L., Foster, S. R., Gregory, K. J., et al. (2020). Detailed In Vitro Pharmacological Characterization of Clinically Tested Negative Allosteric Modulators of the Metabotropic Glutamate Receptor 5. Mol. Pharmacol. 98 (1), 49–60. doi:10.1124/MOL.119.119032/-/DC1
Bachiller, S., Jiménez-Ferrer, I., Paulus, A., Yang, Y., Swanberg, M., Deierborg, T., et al. (2018). Microglia in Neurological Diseases: A Road Map to Brain-Disease Dependent-Inflammatory Response. Front. Cell Neurosci. 12, 488. doi:10.3389/FNCEL.2018.00488/BIBTEX
Barger, S. W., and Basile, A. S. (2001). Activation of Microglia by Secreted Amyloid Precursor Protein Evokes Release of Glutamate by Cystine Exchange and Attenuates Synaptic Function. J. Neurochem. 76 (3), 846–854. doi:10.1046/J.1471-4159.2001.00075.X
Battaglia, G., Busceti, C. L., Molinaro, G., Biagioni, F., Storto, M., Fornai, F., et al. (2004). Endogenous Activation of mGlu5 Metabotropic Glutamate Receptors Contributes to the Development of Nigro-Striatal Damage Induced by 1-Methyl-4-Phenyl-1,2,3,6-Tetrahydropyridine in Mice. J. Neurosci. 24 (4), 828–835. doi:10.1523/JNEUROSCI.3831-03.2004
Bellozi, P. M. Q., Gomes, G. F., da Silva, M. C. M., Lima, I. V. A., Batista, C. R. Á., Carneiro Junior, W. O., et al. (2019). A Positive Allosteric Modulator of mGluR5 Promotes Neuroprotective Effects in Mouse Models of Alzheimer's Disease. Neuropharmacology 160 (September), 107785. doi:10.1016/j.neuropharm.2019.107785
Bennett, K. A., Christopher, J. A., and Tehan, B. G. (2020). Structure-based Discovery and Development of Metabotropic Glutamate Receptor 5 Negative Allosteric Modulators. Adv. Pharmacol. 88, 35–58. doi:10.1016/BS.APHA.2020.03.001
Berg, D., Godau, J., Trenkwalder, C., Eggert, K., Csoti, I., Storch, A., et al. (2011). AFQ056 Treatment of Levodopa-Induced Dyskinesias: Results of 2 Randomized Controlled Trials. Mov. Disord. 26 (7), 1243–1250. doi:10.1002/MDS.23616
Berry-Kravis, E., Hessl, D., Coffey, S., Hervey, C., Schneider, A., Yuhas, J., et al. (2009). A Pilot Open Label, Single Dose Trial of Fenobam in Adults with Fragile X Syndrome. J. Med. Genet. 46 (4), 266–271. doi:10.1136/JMG.2008.063701
Bertoglio, D., Kosten, L., Verhaeghe, J., Thomae, D., Wyffels, L., Stroobants, S., et al. (2018). Longitudinal Characterization of mGluR5 Using 11C-Abp688 PET Imaging in the Q175 Mouse Model of Huntington Disease. J. Nucl. Med. 59 (11), 1722–1727. doi:10.2967/JNUMED.118.210658
Bezard, E., Pioli, E. Y., Li, Q., Girard, F., Mutel, V., Keywood, C., et al. (2014). The mGluR5 Negative Allosteric Modulator Dipraglurant Reduces Dyskinesia in the MPTP Macaque Model. Mov. Disord. 29 (8), 1074–1079. doi:10.1002/MDS.25920
Biber, K., Laurie, D. J., Berthele, A., Sommer, B., Tölle, T. R., Gebicke-Härter, P.-J., et al. (1999). Expression and Signaling of Group I Metabotropic Glutamate Receptors in Astrocytes and Microglia. undefined 72 (4), 1671–1680. doi:10.1046/J.1471-4159.1999.721671.X
Black, Y. D., Xiao, D., Pellegrino, D., Kachroo, A., Brownell, A. L., and Schwarzschild, M. A. (2010). Protective Effect of Metabotropic Glutamate mGluR5 Receptor Elimination in a 6-hydroxydopamine Model of Parkinson's Disease. Neurosci. Lett. 486 (3), 161–165. doi:10.1016/J.NEULET.2010.09.043
Bonifacino, T., Cattaneo, L., Gallia, E., Puliti, A., Melone, M., Provenzano, F., et al. (2017). In-vivo Effects of Knocking-Down Metabotropic Glutamate Receptor 5 in the SOD1G93A Mouse Model of Amyotrophic Lateral Sclerosis. Neuropharmacology 123, 433–445. doi:10.1016/J.NEUROPHARM.2017.06.020
Bonifacino, T., Provenzano, F., Gallia, E., Ravera, S., Torazza, C., Bossi, S., et al. (2019). In-vivo Genetic Ablation of Metabotropic Glutamate Receptor Type 5 Slows Down Disease Progression in the SOD1G93A Mouse Model of Amyotrophic Lateral Sclerosis. Neurobiol. Dis. 129 (January), 79–92. doi:10.1016/j.nbd.2019.05.007
Bradley, S. J., Langmead, C. J., Watson, J. M., and Challiss, R. A. (2011). Quantitative Analysis Reveals Multiple Mechanisms of Allosteric Modulation of the mGlu5 Receptor in Rat Astroglia. Mol. Pharmacol. 79 (5), 874–885. doi:10.1124/MOL.110.068882
Breysse, N., Baunez, C., Spooren, W., Gasparini, F., and Amalric, M. (2002). Chronic but Not Acute Treatment with a Metabotropic Glutamate 5 Receptor Antagonist Reverses the Akinetic Deficits in a Rat Model of Parkinsonism. J. Neurosci. 22 (13), 5669–5678. doi:10.1523/jneurosci.22-13-05669.200220026513
Brownell, A. L., Kuruppu, D., Kil, K. E., Jokivarsi, K., Poutiainen, P., Zhu, A., et al. (2015). PET Imaging Studies Show Enhanced Expression of mGluR5 and Inflammatory Response during Progressive Degeneration in ALS Mouse Model Expressing SOD1-G93a Gene. J. Neuroinflammation 12 (1), 217. doi:10.1186/s12974-015-0439-9
Byrnes, K. R., Stoica, B., Loane, D. J., Riccio, A., Davis, M. I., and Faden, A. I. (2009). Metabotropic Glutamate Receptor 5 Activation Inhibits Microglial Associated Inflammation and Neurotoxicity. Glia 57 (5), 550–560. doi:10.1002/GLIA.20783
Cai, Z., Schools, G. P., and Kimelberg, H. K. (2000). Metabotropic Glutamate Receptors in Acutely Isolated Hippocampal Astrocytes: Developmental Changes of mGluR5 mRNA and Functional Expression. Glia 29 (1), 70–80. doi:10.1002/(sici)1098-1136(20000101)29:1<70::aid-glia7>3.0.co;2-v
Carvalho, T. G., Alves-Silva, J., de Souza, J. M., Real, A. L. C. V., Doria, J. G., Vieira, E. L. M., et al. (2019). Metabotropic Glutamate Receptor 5 Ablation Accelerates Age-Related Neurodegeneration and Neuroinflammation. Neurochem. Int. 126, 218–228. doi:10.1016/J.NEUINT.2019.03.020
Casley, C. S., Lakics, V., Lee, H. G., Broad, L. M., Day, T. A., Cluett, T., et al. (2009). Up-regulation of Astrocyte Metabotropic Glutamate Receptor 5 by Amyloid-β Peptide. Brain Res. 1260, 65–75. doi:10.1016/J.BRAINRES.2008.12.082
Cetin, H., Rath, J., Füzi, J., Reichardt, B., Fülöp, G., Koppi, S., et al. (2015). Epidemiology of Amyotrophic Lateral Sclerosis and Effect of Riluzole on Disease Course. Neuroepidemiology 44 (1), 6–15. doi:10.1159/000369813
Chantong, B., Kratschmar, D. V., Lister, A., and Odermatt, A. (2014). Inhibition of Metabotropic Glutamate Receptor 5 Induces Cellular Stress through Pertussis Toxin-Sensitive Gi-Proteins in Murine BV-2 Microglia Cells. J. Neuroinflammation 11 (190), 1–16. doi:10.1186/S12974-014-0190-7/FIGURES/8
Christopher, J. A., Aves, S. J., Bennett, K. A., Doré, A. S., Errey, J. C., Jazayeri, A., et al. (2015). Fragment and Structure-Based Drug Discovery for a Class C GPCR: Discovery of the mGlu5 Negative Allosteric Modulator HTL14242 (3-Chloro-5-[6-(5-Fluoropyridin-2-Yl)pyrimidin-4-Yl]benzonitrile). J. Med. Chem. 58 (16), 6653–6664. doi:10.1021/ACS.JMEDCHEM.5B00892
Conde-Ceide, S., Martínez-Viturro, C. M., Alcázar, J., Garcia-Barrantes, P. M., Lavreysen, H., Mackie, C., et al. (2015). Discovery of VU0409551/JNJ-46778212: An mGlu5 Positive Allosteric Modulator Clinical Candidate Targeting Schizophrenia. ACS Med. Chem. Lett. 6 (6), 716–720. doi:10.1021/ACSMEDCHEMLETT.5B00181
Conn, P. J., and Pin, J. P. (1997). Pharmacology and Functions of Metabotropic Glutamate Receptors. Annu. Rev. Pharmacol. Toxicol. 37, 205–237. doi:10.1146/annurev.pharmtox.37.1.205
Cosford, N. D., Roppe, J., Tehrani, L., Schweiger, E. J., Seiders, T. J., Chaudary, A., et al. (2003). [3H]-methoxymethyl-MTEP and [3H]-Methoxy-PEPy: Potent and Selective Radioligands for the Metabotropic Glutamate Subtype 5 (mGlu5) Receptor. Bioorg Med. Chem. Lett. 13 (3), 351–354. doi:10.1016/S0960-894X(02)00997-6
De Souza, J. M., Abd-Elrahman, K. S., Ribeiro, F. M., and Ferguson, S. S. G. (2020). mGluR5 Regulates REST/NRSF Signaling through N-Cadherin/β-Catenin Complex in Huntington's Disease. Mol. Brain 13 (1), 118. doi:10.1186/S13041-020-00657-7
DeLong, M. R., and Wichmann, T. (2015). Basal Ganglia Circuits as Targets for Neuromodulation in Parkinson Disease. JAMA Neurol. 72, 1354–1360. doi:10.1001/jamaneurol.2015.2397
Dementia Forecasting Collaborators (2022). Estimation of the Global Prevalence of Dementia in 2019 and Forecasted Prevalence in 2050: an Analysis for the Global Burden of Disease Study 2019. Lancet Public Health 7 (0), e105–e125. doi:10.1016/S2468-2667(21)00249-8
Deture, M. A., and Dickson, D. W. (2019). The Neuropathological Diagnosis of Alzheimer's Disease. Mol. Neurodegener. 14, 1–18. doi:10.1186/s13024-019-0333-5
Dhami, G. K., and Ferguson, S. S. G. (2006). Regulation of Metabotropic Glutamate Receptor Signaling, Desensitization and Endocytosis. Pharmacol. Ther. 111, 260–271. doi:10.1016/j.pharmthera.2005.01.008
Doria, J. G., de Souza, J. M., Andrade, J. N., Rodrigues, H. A., Guimaraes, I. M., Carvalho, T. G., et al. (2015). The mGluR5 Positive Allosteric Modulator, CDPPB, Ameliorates Pathology and Phenotypic Signs of a Mouse Model of Huntington's Disease. Neurobiol. Dis. 73, 163–173. doi:10.1016/j.nbd.2014.08.021
Doria, J. G., de Souza, J. M., Silva, F. R., Olmo, I. G., Carvalho, T. G., Alves-Silva, J., et al. (2018). The mGluR5 Positive Allosteric Modulator VU0409551 Improves Synaptic Plasticity and Memory of a Mouse Model of Huntington's Disease. J. Neurochem. 147 (2), 222–239. doi:10.1111/jnc.14555
Doria, J. G., Silva, F. R., de Souza, J. M., Vieira, L. B., Carvalho, T. G., Reis, H. J., et al. (2013). Metabotropic Glutamate Receptor 5 Positive Allosteric Modulators Are Neuroprotective in a Mouse Model of Huntington's Disease. Br. J. Pharmacol. 169 (4), 909–921. doi:10.1111/bph.12164
Drouin-Ouellet, J., Brownell, A. L., Saint-Pierre, M., Fasano, C., Emond, V., Trudeau, L. E., et al. (2011). Neuroinflammation Is Associated with Changes in Glial mGluR5 Expression and the Development of Neonatal Excitotoxic Lesions. Glia 59 (2), 188–199. doi:10.1002/GLIA.21086
D’Souza, G. X., and Waldvogel, H. J. (2016). Targeting the Cholinergic System to Develop a Novel Therapy for Huntington's Disease. Jhd 5 (4), 333–342. doi:10.3233/JHD-160200
Eng, A. G., Kelver, D. A., Hedrick, T. P., and Swanson, G. T. (2016). Transduction of Group I mGluR-Mediated Synaptic Plasticity by β-arrestin2 Signalling. Nat. Commun. 7, 13571. doi:10.1038/ncomms13571
Escartin, C., Galea, E., Lakatos, A., O'Callaghan, J. P., Petzold, G. C., Serrano-Pozo, A., et al. (2021). Reactive Astrocyte Nomenclature, Definitions, and Future Directions. Nat. Neurosci. 24 (3), 312–325. doi:10.1038/S41593-020-00783-4
Evans, S., McRae‐McKee, K., Hadjichrysanthou, C., Wong, M. M., Ames, D., Lopez, O., et al. (2019). Alzheimer's Disease Progression and Risk Factors: A Standardized Comparison between Six Large Data Sets. Alzheimer’s dementia (New York, N. Y.) 5, 515–523. doi:10.1016/J.TRCI.2019.04.005
Farmer, K., Abd-Elrahman, K. S., Derksen, A., Rowe, E. M., Thompson, A. M., Rudyk, C. A., et al. (2020). mGluR5 Allosteric Modulation Promotes Neurorecovery in a 6-OHDA-Toxicant Model of Parkinson's Disease. Mol. Neurobiol. 57 (3), 1418–1431. doi:10.1007/S12035-019-01818-Z/FIGURES/8
Farso, M. C., O'Shea, R. D., and Beart, P. M. (2009). Evidence Group I mGluR Drugs Modulate the Activation Profile of Lipopolysaccharide-Exposed Microglia in Culture. Neurochem. Res. 34 (10), 1721–1728. doi:10.1007/S11064-009-9999-3/FIGURES/2
Felts, A. S., Rodriguez, A. L., Blobaum, A. L., Morrison, R. D., Bates, B. S., Thompson Gray, A., et al. (2017). Discovery of N-(5-Fluoropyridin-2-yl)-6-methyl-4-(pyrimidin-5-yloxy)picolinamide (VU0424238): A Novel Negative Allosteric Modulator of Metabotropic Glutamate Receptor Subtype 5 Selected for Clinical Evaluation. J. Med. Chem. 60 (12), 5072–5085. doi:10.1021/ACS.JMEDCHEM.7B00410
Ferreira, D. G., Temido-Ferreira, M., Vicente Miranda, H., Batalha, V. L., Coelho, J. E., Szegö, É. M., et al. (2017). α-Synuclein Interacts with PrPC to Induce Cognitive Impairment through mGluR5 and NMDAR2B. Nat. Neurosci. 20 (11), 1569–1579. doi:10.1038/nn.4648
Friedmann, C. T. H., Davis, L. J., Ciccone, P. E., Rubin, R. T., et al. (1980). Phase II Double-Blind Controlled Study of a New Anxiolytic, Fenobam (McN-3377) vs Placebo. Curr. Ther. Res. - Clin. Exp. 27 (2), 144–151.
Fu, T. T., Tu, G., Ping, M., Zheng, G. X., Yang, F. Y., Yang, J. Y., et al. (20202020). Subtype-selective Mechanisms of Negative Allosteric Modulators Binding to Group I Metabotropic Glutamate Receptors. Acta Pharmacol. Sin. 42 (8428), 1354–1367. doi:10.1038/S41401-020-00541-z
Fulmer, C. G., VonDran, M. W., Stillman, A. A., Huang, Y., Hempstead, B. L., and Dreyfus, C. F. (2014). Astrocyte-Derived BDNF Supports Myelin Protein Synthesis after Cuprizone-Induced Demyelination. J. Neurosci. 34 (24), 8186–8196. doi:10.1523/JNEUROSCI.4267-13.2014
Fuxe, K., and Borroto-Escuela, D. O. (2015). Basimglurant for Treatment of Major Depressive Disorder: a Novel Negative Allosteric Modulator of Metabotropic Glutamate Receptor 5. Expert Opin. Investig. Drugs 24 (9), 1247–1260. doi:10.1517/13543784.2015.1074175
Gasparini, F., Lingenhöhl, K., Stoehr, N., Flor, P. J., Heinrich, M., Vranesic, I., et al. (1999). 2-Methyl-6-(phenylethynyl)-pyridine (MPEP), a Potent, Selective and Systemically Active mGlu5 Receptor Antagonist. Neuropharmacology 38 (10), 1493–1503. doi:10.1016/S0028-3908(99)00082-9
Giorgio, J., Landau, S. M., Jagust, W. J., Tino, P., and Kourtzi, Z. (2020). Modelling Prognostic Trajectories of Cognitive Decline Due to Alzheimer's Disease. Neuroimage Clin. 26, 102199. doi:10.1016/J.NICL.2020.102199
Giribaldi, F., Milanese, M., Bonifacino, T., Anna Rossi, P. I., Di Prisco, S., Pittaluga, A., et al. (2013). Group I Metabotropic Glutamate Autoreceptors Induce Abnormal Glutamate Exocytosis in a Mouse Model of Amyotrophic Lateral Sclerosis. Neuropharmacology 66, 253–263. doi:10.1016/J.NEUROPHARM.2012.05.018
Gregory, K. J., Dong, E. N., Meiler, J., and Conn, P. J. (2011). Allosteric Modulation of Metabotropic Glutamate Receptors: Structural Insights and Therapeutic Potential. Neuropharmacology 60 (1), 66–81. doi:10.1016/J.NEUROPHARM.2010.07.007
Gu, L., Luo, W.-Y., Xia, N., Zhang, J.-N., Fan, J.-K., Yang, H.-M., et al. (2021). Upregulated mGluR5 Induces ER Stress and DNA Damage by Regulating the NMDA Receptor Subunit NR2B. J. Biochem. 171, 349–359. [Preprint]. doi:10.1093/JB/MVAB140
Gulyás, B., Sovago, J., Gomez-Mancilla, B., Jia, Z., Szigeti, C., Gulya, K., et al. (2015). Decrease of mGluR5 Receptor Density Goes Parallel with Changes in Enkephalin and Substance P Immunoreactivity in Huntington’s Disease: a Preliminary Investigation in the Postmortem Human Brain. Brain Struct. Funct. 220 (5), 3043–3051. doi:10.1007/S00429-014-0812-Y/FIGURES/4
Haas, L. T., Salazar, S. V., Smith, L. M., Zhao, H. R., Cox, T. O., Herber, C. S., et al. (2017). Silent Allosteric Modulation of mGluR5 Maintains Glutamate Signaling while Rescuing Alzheimer's Mouse Phenotypes. Cell Rep. 20 (1), 76–88. doi:10.1016/j.celrep.2017.06.023.Silent
Halford, J., Shen, S., Itamura, K., Levine, J., Chong, A. C., Czerwieniec, G., et al. (2017). New Astroglial Injury-Defined Biomarkers for Neurotrauma Assessment. J. Cereb. Blood Flow. Metab. 37 (10), 3278–3299. doi:10.1177/0271678X17724681
Hamilton, A., Esseltine, J. L., DeVries, R. A., Cregan, S. P., and Ferguson, S. S. (2014). Metabotropic Glutamate Receptor 5 Knockout Reduces Cognitive Impairment and Pathogenesis in a Mouse Model of Alzheimer's Disease. Mol. Brain 7 (1), 40–12. doi:10.1186/1756-6606-7-40
Hamilton, A., Vasefi, M., Vander Tuin, C., McQuaid, R. J., Anisman, H., and Ferguson, S. S. (2016). Chronic Pharmacological mGluR5 Inhibition Prevents Cognitive Impairment and Reduces Pathogenesis in an Alzheimer Disease Mouse Model. Cell Rep. 15 (9), 1859–1865. doi:10.1016/j.celrep.2016.04.077
Hanisch, U. K., and Kettenmann, H. (2007). Microglia: Active Sensor and Versatile Effector Cells in the Normal and Pathologic Brain. Nat. Neurosci. 10 (11), 1387–1394. doi:10.1038/NN1997
Harpsøe, K., Isberg, V., Tehan, B. G., Weiss, D., Arsova, A., Marshall, F. H., et al. (2015). Selective Negative Allosteric Modulation of Metabotropic Glutamate Receptors - A Structural Perspective of Ligands and Mutants. Sci. Rep. 5. doi:10.1038/SREP13869
Hasel, P., and Liddelow, S. A. (2021). Astrocytes. Curr. Biol. 31 (7), R326–R327. doi:10.1016/J.CUB.2021.01.056
Hemonnot, A. L., Hua, J., Ulmann, L., and Hirbec, H. (2019). Microglia in Alzheimer Disease: Well-Known Targets and New Opportunities. Front. Aging Neurosci. 11 (JUL), 233. doi:10.3389/FNAGI.2019.00233/BIBTEX
Howe, M. W., and Dombeck, D. A. (20162016). Rapid Signalling in Distinct Dopaminergic Axons during Locomotion and Reward. Nature 535, 505–510. doi:10.1038/nature18942
Hsieh, M. H., Ho, S. C., Yeh, K. Y., Pawlak, C. R., Chang, H. M., Ho, Y. J., et al. (2012). Blockade of Metabotropic Glutamate Receptors Inhibits Cognition and Neurodegeneration in an MPTP-Induced Parkinson's Disease Rat Model. Pharmacol. Biochem. Behav. 102 (1), 64–71. doi:10.1016/j.pbb.2012.03.022
Huang, H., Degnan, A. P., Balakrishnan, A., Easton, A., Gulianello, M., Huang, Y., et al. (2016). Oxazolidinone-based Allosteric Modulators of mGluR5: Defining Molecular Switches to Create a Pharmacological Tool Box. Bioorg Med. Chem. Lett. 26 (17), 4165–4169. doi:10.1016/J.BMCL.2016.07.065
Huang, Y., Shu, H., Li, L., Zhen, T., Zhao, J., Zhou, X., et al. (2018). L-DOPA-Induced Motor Impairment and Overexpression of Corticostriatal Synaptic Components Are Improved by the mGluR5 Antagonist MPEP in 6-OHDA-Lesioned Rats. ASN Neuro 10, 1759091418811021. doi:10.1177/1759091418811021
Hubert, G. W., Paquet, M., and Smith, Y. (2001). Differential Subcellular Localization of mGluR1a and mGluR5 in the Rat and Monkey Substantia Nigra. J. Neurosci. 21 (6), 1838–1847. doi:10.1523/JNEUROSCI.21-06-01838.2001
Husain, M. (2017). Transdiagnostic Neurology: Neuropsychiatric Symptoms in Neurodegenerative Diseases. Brain 140 (6), 1535–1536. doi:10.1093/BRAIN/AWX115
Ibrahim, K. S., McLaren, C. J., Abd-Elrahman, K. S., and Ferguson, S. S. G. (2021). Optineurin Deletion Disrupts Metabotropic Glutamate Receptor 5-mediated Regulation of ERK1/2, GSK3β/ZBTB16, mTOR/ULK1 Signaling in Autophagy. Biochem. Pharmacol. 185, 114427. doi:10.1016/J.BCP.2021.114427
Jacquemont, S., Curie, A., des Portes, V., Torrioli, M. G., Berry-Kravis, E., Hagerman, R. J., et al. (2011). Epigenetic Modification of the FMR1 Gene in Fragile X Syndrome Is Associated with Differential Response to the mGluR5 Antagonist AFQ056. Sci. Transl. Med. 3 (64), 64ra1. doi:10.1126/SCITRANSLMED.3001708
Jaso, B. A., Niciu, M. J., Iadarola, N. D., Lally, N., Richards, E. M., Park, M., et al. (2017). Therapeutic Modulation of Glutamate Receptors in Major Depressive Disorder. Curr. Neuropharmacol. 15 (1), 57–70. doi:10.2174/1570159X14666160321123221
Jean, Y. Y., Lercher, L. D., and Dreyfus, C. F. (2008). Glutamate Elicits Release of BDNF from Basal Forebrain Astrocytes in a Process Dependent on Metabotropic Receptors and the PLC Pathway. Neuron Glia Biol. 4 (1), 35–42. doi:10.1017/S1740925X09000052
Johnston, T. H., Fox, S. H., McIldowie, M. J., Piggott, M. J., and Brotchie, J. M. (2010). Reduction of L-DOPA-Induced Dyskinesia by the Selective Metabotropic Glutamate Receptor 5 Antagonist 3-[(2-Methyl-1,3-Thiazol-4-Yl)ethynyl]pyridine in the 1-Methyl-4-Phenyl-1,2,3,6-Tetrahydropyridine-Lesioned Macaque Model of Parkinson's Disease. J. Pharmacol. Exp. Ther. 333 (3), 865–873. doi:10.1124/jpet.110.166629
Jong, Y. J., Kumar, V., Kingston, A. E., Romano, C., and O'Malley, K. L. (2005). Functional Metabotropic Glutamate Receptors on Nuclei from Brain and Primary Cultured Striatal Neurons. Role of Transporters in Delivering Ligand. J. Biol. Chem. 280 (34), 30469–30480. doi:10.1074/JBC.M501775200
Jong, Y. J., Kumar, V., and O'Malley, K. L. (2009). Intracellular Metabotropic Glutamate Receptor 5 (mGluR5) Activates Signaling Cascades Distinct from Cell Surface Counterparts. J. Biol. Chem. 284 (51), 35827–35838. doi:10.1074/JBC.M109.046276
Kang, Y., Henchcliffe, C., Verma, A., Vallabhajosula, S., He, B., Kothari, P. J., et al. (2019). 18F-FPEB PET/CT Shows mGluR5 Upregulation in Parkinson's Disease. J. Neuroimaging 29 (1), 97–103. doi:10.1111/JON.12563
Kanumilli, S., and Roberts, P. J. (2006). Mechanisms of Glutamate Receptor Induced Proliferation of Astrocytes. NeuroReport 17 (18), 1877–1881. doi:10.1097/WNR.0B013E3280102EE6
Karantzoulis, S., and Galvin, J. E. (2011). Distinguishing Alzheimer's Disease from Other Major Forms of Dementia. Expert Rev. Neurother. 11 (11), 1579–1591. doi:10.1586/ERN.11.155
Kayed, R., and Lasagna-Reeves, C. A. (2012). Molecular Mechanisms of Amyloid Oligomers Toxicity. Jad 33, S67–S78. doi:10.3233/JAD-2012-129001
Keck, T. M., Zou, M. F., Zhang, P., Rutledge, R. P., and Newman, A. H. (2012). Metabotropic Glutamate Receptor 5 Negative Allosteric Modulators as Novel Tools for In Vivo Investigation. ACS Med. Chem. Lett. 3 (7), 544–549. doi:10.1021/ML3000726
Keren-Shaul, H., Spinrad, A., Weiner, A., Matcovitch-Natan, O., Dvir-Szternfeld, R., Ulland, T. K., et al. (2017). A Unique Microglia Type Associated with Restricting Development of Alzheimer's Disease. Cell 169 (7), 1276–e17. e17. doi:10.1016/J.CELL.2017.05.018
King, A. E., Woodhouse, A., Kirkcaldie, M. T. K., and Vickers, J. C. (2016). Excitotoxicity in ALS: Overstimulation, or Overreaction? Exp. Neurol. 275, 162–171. doi:10.1016/j.expneurol.2015.09.019
Kirby, J., Al Sultan, A., Waller, R., and Heath, P. (2016). The Genetics of Amyotrophic Lateral Sclerosis: Current Insights. Dnnd 6, 49. doi:10.2147/dnnd.s84956
Ko, W. K., Pioli, E., Li, Q., McGuire, S., Dufour, A., Sherer, T. B., et al. (2014). Combined Fenobam and Amantadine Treatment Promotes Robust Antidyskinetic Effects in the 1-Methyl-4-Phenyl-1,2,3,6-Tetrahydropyridine (MPTP)-Lesioned Primate Model of Parkinson's Disease. Mov. Disord. 29 (6), 772–779. doi:10.1002/MDS.25859
Kordower, J. H., Olanow, C. W., Dodiya, H. B., Chu, Y., Beach, T. G., Adler, C. H., et al. (2013). Disease Duration and the Integrity of the Nigrostriatal System in Parkinson's Disease. Brain 136 (8), 2419–2431. doi:10.1093/BRAIN/AWT192
Kumar, V., Jong, Y. J., and O'Malley, K. L. (2008). Activated Nuclear Metabotropic Glutamate Receptor mGlu5 Couples to Nuclear Gq/11 Proteins to Generate Inositol 1,4,5-Trisphosphate-Mediated Nuclear Ca2+ Release. J. Biol. Chem. 283 (20), 14072–14083. doi:10.1074/JBC.M708551200
Kumar, R., Hauser, R. A., Mostillo, J., Dronamraju, N., Graf, A., Merschhemke, M., et al. (2015). Mavoglurant (AFQ056) in Combination with Increased Levodopa Dosages in Parkinson’s Disease Patients. Int. J. Neurosci. 126 (1), 20–24. doi:10.3109/00207454.2013.841685
Langmead, C. J., and Christopoulos, A. (2006). Allosteric Agonists of 7TM Receptors: Expanding the Pharmacological Toolbox. Trends Pharmacol. Sci. 27, 475–481. doi:10.1016/j.tips.2006.07.009
Laurén, J., Gimbel, D. A., Nygaard, H. B., Gilbert, J. W., and Strittmatter, S. M. (2009). Cellular Prion Protein Mediates Impairment of Synaptic Plasticity by Amyloid-β Oligomers. Nature 457 (7233), 1128–1132. doi:10.1038/nature07761
Lee, M., Lee, H. J., Park, I. S., Park, J. A., Kwon, Y. J., Ryu, Y. H., et al. (2018). Aβ Pathology Downregulates Brain mGluR5 Density in a Mouse Model of Alzheimer. Neuropharmacology 133, 512–517. doi:10.1016/J.NEUROPHARM.2018.02.003
Levenga, J., Hayashi, S., de Vrij, F. M., Koekkoek, S. K., van der Linde, H. C., Nieuwenhuizen, I., et al. (2011). AFQ056, a New mGluR5 Antagonist for Treatment of Fragile X Syndrome. Neurobiol. Dis. 42 (3), 311–317. doi:10.1016/J.NBD.2011.01.022
Li, S. H., Colson, T.-L. L., Abd-Elrahman, K. S., and Ferguson, S. S. G. (2022). Metabotropic Glutamate Receptor 5 Antagonism Reduces Pathology and Differentially Improves Symptoms in Male and Female Heterozygous zQ175 Huntington's Mice. Front. Mol. Neurosci. 15, 6. doi:10.3389/FNMOL.2022.801757
Liddelow, S. A., and Barres, B. A. (2017). Reactive Astrocytes: Production, Function, and Therapeutic Potential. Immunity 46 (6), 957–967. doi:10.1016/J.IMMUNI.2017.06.006
Lim, D., Iyer, A., Ronco, V., Grolla, A. A., Canonico, P. L., Aronica, E., et al. (2013). Amyloid Beta Deregulates Astroglial mGluR5-Mediated Calcium Signaling via Calcineurin and Nf-kB. Glia 61 (7), 1134–1145. doi:10.1002/GLIA.22502
Lindemann, L., Jaeschke, G., Michalon, A., Vieira, E., Honer, M., Spooren, W., et al. (2011). CTEP: A Novel, Potent, Long-Acting, and Orally Bioavailable Metabotropic Glutamate Receptor 5 Inhibitor. J. Pharmacol. Exp. Ther. 339 (2), 474–486. doi:10.1124/jpet.111.185660
Lindemann, L., Porter, R. H., Scharf, S. H., Kuennecke, B., Bruns, A., von Kienlin, M., et al. (2015). Pharmacology of Basimglurant (RO4917523, RG7090), a Unique Metabotropic Glutamate Receptor 5 Negative Allosteric Modulator in Clinical Development for Depression. J. Pharmacol. Exp. Ther. 353 (1), 213–233. doi:10.1124/JPET.114.222463
Lindsley, C. W., Wisnoski, D. D., Leister, W. H., O'brien, J. A., Lemaire, W., Williams, D. L., et al. (2004). Discovery of Positive Allosteric Modulators for the Metabotropic Glutamate Receptor Subtype 5 from a Series of N-(1,3-diphenyl-1H- Pyrazol-5-Yl)benzamides that Potentiate Receptor Function In Vivo. J. Med. Chem. 47 (24), 5825–5828. doi:10.1021/jm049400d
Loane, D. J., Stoica, B. A., Byrnes, K. R., Jeong, W., and Faden, A. I. (2013). Activation of mGluR5 and Inhibition of NADPH Oxidase Improves Functional Recovery after Traumatic Brain Injury. J. Neurotrauma 30 (5), 403–412. doi:10.1089/NEU.2012.2589
Loane, D. J., Stoica, B. A., and Faden, A. I. (2012). Metabotropic Glutamate Receptor-Mediated Signaling in Neuroglia. Wiley Interdiscip. Rev. Membr. Transp. Signal 1 (2), 136–150. doi:10.1002/wmts.30
Loane, D. J., Stoica, B. A., Pajoohesh-Ganji, A., Byrnes, K. R., and Faden, A. I. (2009). Activation of Metabotropic Glutamate Receptor 5 Modulates Microglial Reactivity and Neurotoxicity by Inhibiting NADPH Oxidase. J. Biol. Chem. 284 (23), 15629–15639. doi:10.1074/JBC.M806139200
Loane, D. J., Stoica, B. A., Tchantchou, F., Kumar, A., Barrett, J. P., Akintola, T., et al. (2014). Novel mGluR5 Positive Allosteric Modulator Improves Functional Recovery, Attenuates Neurodegeneration, and Alters Microglial Polarization after Experimental Traumatic Brain Injury. Neurotherapeutics 11 (4), 857–869. doi:10.1007/s13311-014-0298-6
Luján, R., Nusser, Z., Roberts, J. D. B., Shigemoto, R., and Somogyi, P. (1996). Perisynaptic Location of Metabotropic Glutamate Receptors mGluR1 and mGluR5 on Dendrites and Dendritic Spines in the Rat Hippocampus. Eur. J. Neurosci. 8 (7), 1488–1500. doi:10.1111/j.1460-9568.1996.tb01611.x
MacDonald, M. E., Ambrose, C. M., Duyao, M. P., Myers, R. H., Lin, C., Srinidhi, L., et al. (1993). A Novel Gene Containing a Trinucleotide Repeat that Is Expanded and Unstable on Huntington's Disease Chromosomes. The Huntington's Disease Collaborative Research Group. Cell 72 (6), 971–983. doi:10.1016/0092-8674(93)90585-E
Marin, J. C., and Goadsby, P. J. (2010). Glutamatergic Fine Tuning with ADX-10059: a Novel Therapeutic Approach for Migraine? Expert Opin. Investig. Drugs 19 (4), 555–561. doi:10.1517/13543781003691832
Marino, M. J., and Conn, P. J. (2002). Direct and Indirect Modulation of the N-Methyl D-Aspartate Receptor. Curr. Drug Targets CNS Neurol. Disord. 1 (1), 1–16. doi:10.2174/1568007023339544
Martín-Belmonte, A., Aguado, C., Alfaro-Ruiz, R., Albasanz, J. L., Martín, M., Moreno-Martínez, A. E., et al. (2021). The Density of Group I Mglu5 Receptors Is Reduced along the Neuronal Surface of Hippocampal Cells in a Mouse Model of Alzheimer’s Disease. Int. J. Mol. Sci. 22 (11). doi:10.3390/IJMS22115867/S1
Masilamoni, G. J., Bogenpohl, J. W., Alagille, D., Delevich, K., Tamagnan, G., Votaw, J. R., et al. (2011). Metabotropic Glutamate Receptor 5 Antagonist Protects Dopaminergic and Noradrenergic Neurons from Degeneration in MPTP-Treated Monkeys. Brain 134, 2057–2073. [Preprint]. doi:10.1093/brain/awr137
Masnata, M., and Cicchetti, F. (2017). The Evidence for the Spread and Seeding Capacities of the Mutant Huntingtin Protein in In Vitro Systems and Their Therapeutic Implications. Front. Neurosci. 11 (NOV), 647. doi:10.3389/FNINS.2017.00647
Mathiesen, J. M., Svendsen, N., Bräuner-Osborne, H., Thomsen, C., and Ramirez, M. T. (2003). Positive Allosteric Modulation of the Human Metabotropic Glutamate Receptor 4 (hmGluR4) by SIB-1893 and MPEP. Br. J. Pharmacol. 138 (6), 1026–1030. doi:10.1038/sj.bjp.0705159
May, L., and Christopoulos, A. (2003). Allosteric Modulators of G-Protein-Coupled Receptors. Curr. Opin. Pharmacol. 3, 551–556. doi:10.1016/S1471-4892(03)00107-3
McColgan, P., and Tabrizi, S. J. (2018). Huntington's Disease: a Clinical Review. Eur. J. Neurol. 25 (1), 24–34. doi:10.1111/ene.13413
Mecca, A. P., McDonald, J. W., Michalak, H. R., Godek, T. A., Harris, J. E., Pugh, E. A., et al. (2020). PET Imaging of mGluR5 in Alzheimer's Disease. Alzheimers Res. Ther. 12 (1), 15. doi:10.1186/S13195-020-0582-0
Mecca, A. P., Rogers, K., Jacobs, Z., McDonald, J. W., Michalak, H. R., DellaGioia, N., et al. (2021). Effect of Age on Brain Metabotropic Glutamate Receptor Subtype 5 Measured with [18F]FPEB PET. Neuroimage 238, 118217. doi:10.1016/J.NEUROIMAGE.2021.118217
Mejzini, R., Flynn, L. L., Pitout, I. L., Fletcher, S., Wilton, S. D., and Akkari, P. A. (2019). ALS Genetics, Mechanisms, and Therapeutics: Where Are We Now? Front. Neurosci. 13, 1310. doi:10.3389/fnins.2019.01310
Mercado, G., Castillo, V., Soto, P., and Sidhu, A. (2016). ER Stress and Parkinson's Disease: Pathological Inputs that Converge into the Secretory Pathway. Brain Res. 1648 (Pt B), 626–632. doi:10.1016/J.BRAINRES.2016.04.042
Meredith, G. E., and Rademacher, D. J. (2011). MPTP Mouse Models of Parkinson's Disease: an Update. J. Park. Dis. 1, 19–33. doi:10.3233/JPD-2011-11023
Milanese, M., Bonifacino, T., Torazza, C., Provenzano, F., Kumar, M., Ravera, S., et al. (2021). Blocking Glutamate mGlu5 Receptors with the Negative Allosteric Modulator CTEP Improves Disease Course in SOD1G93A Mouse Model of Amyotrophic Lateral Sclerosis. Br. J. Pharmacol. 178 (18), 3747–3764. doi:10.1111/BPH.15515
Miller, J., Arrasate, M., Shaby, B. A., Mitra, S., Masliah, E., and Finkbeiner, S. (2010). Quantitative Relationships between Huntingtin Levels, Polyglutamine Length, Inclusion Body Formation, and Neuronal Death Provide Novel Insight into Huntington's Disease Molecular Pathogenesis. J. Neurosci. 30 (31), 10541–10550. doi:10.1523/JNEUROSCI.0146-10.2010
Mølck, C., Harpsøe, K., Gloriam, D. E., Mathiesen, J. M., Nielsen, S. M., and Bräuner-Osborne, H. (2014). mGluR5: Exploration of Orthosteric and Allosteric Ligand Binding Pockets and Their Applications to Drug Discovery. Neurochem. Res. 39 (10), 1862–1875. doi:10.1007/s11064-014-1248-8
Moreels, M., Vandenabeele, F., Dumont, D., Robben, J., and Lambrichts, I. (2008). Alpha-smooth Muscle Actin (Alpha-SMA) and Nestin Expression in Reactive Astrocytes in Multiple Sclerosis Lesions: Potential Regulatory Role of Transforming Growth Factor-Beta 1 (TGF-Beta1). Neuropathol. Appl. Neurobiol. 34 (5), 532–546. doi:10.1111/J.1365-2990.2007.00910.X
Morin, N., Grégoire, L., Gomez-Mancilla, B., Gasparini, F., and Di Paolo, T. (2010). Effect of the Metabotropic Glutamate Receptor Type 5 Antagonists MPEP and MTEP in Parkinsonian Monkeys. Neuropharmacology 58 (7), 981–986. doi:10.1016/j.neuropharm.2009.12.024
Morin, N., Grégoire, L., Morissette, M., Desrayaud, S., Gomez-Mancilla, B., Gasparini, F., et al. (2013b). MPEP, an mGlu5 Receptor Antagonist, Reduces the Development of L-DOPA-Induced Motor Complications in De Novo Parkinsonian Monkeys: Biochemical Correlates. Neuropharmacology 66, 355–364. doi:10.1016/j.neuropharm.2012.07.036
Morin, N., Morissette, M., Grégoire, L., Gomez-Mancilla, B., Gasparini, F., and Di Paolo, T. (2013a). Chronic Treatment with MPEP, an mGlu5 Receptor Antagonist, Normalizes Basal Ganglia Glutamate Neurotransmission in L-DOPA-Treated Parkinsonian Monkeys. Neuropharmacology 73, 216–231. doi:10.1016/j.neuropharm.2013.05.028
Müller Herde, A., Schibli, R., Weber, M., and Ametamey, S. M. (2019). Metabotropic Glutamate Receptor Subtype 5 Is Altered in LPS-Induced Murine Neuroinflammation Model and in the Brains of AD and ALS Patients. Eur. J. Nucl. Med. Mol. Imaging 46 (2), 407–420. doi:10.1007/S00259-018-4179-9/FIGURES/6
Nasrallah, C., Cannone, G., Briot, J., Rottier, K., Berizzi, A. E., Huang, C. Y., et al. (2021). Agonists and Allosteric Modulators Promote Signaling from Different Metabotropic Glutamate Receptor 5 Conformations. Cell Rep. 36 (9), 109648. doi:10.1016/J.CELREP.2021.109648
Nathan, P. J., Millais, S. B., Godwood, A., Dewit, O., Cross, D. M., Liptrot, J., et al. (2022). A Phase 1b/2a Multicentre Study of the Safety and Preliminary Pharmacodynamic Effects of the Selective Muscarinic M1 Receptor Agonist HTL0018318 in Patients with Mild-To-Moderate Alzheimer’s Disease. Alzheimer’s Dementia 8, e12273. doi:10.1002/trc2.12273
Negida, A., Ghaith, H. S., Fala, S. Y., Ahmed, H., Bahbah, E. I., Ebada, M. A., et al. (2021). Mavoglurant (AFQ056) for the Treatment of Levodopa-Induced Dyskinesia in Patients with Parkinson's Disease: a Meta-Analysis. Neurol. Sci. 42 (8), 3135–3143. doi:10.1007/S10072-021-05319-7
Newcombe, J., Uddin, A., Dove, R., Patel, B., Turski, L., Nishizawa, Y., et al. (2008). Glutamate Receptor Expression in Multiple Sclerosis Lesions. Brain Pathol. 18 (1), 52–61. doi:10.1111/J.1750-3639.2007.00101.X
Nixon, R. A. (2013). The Role of Autophagy in Neurodegenerative Disease. Nat. Med. 19, 983–997. doi:10.1038/nm.3232
O'Brien, J. A., Lemaire, W., Chen, T.-B., Chang, R. S. L., Jacobson, M. A., Ha, S. N., et al. (2003). A Family of Highly Selective Allosteric Modulators of the Metabotropic Glutamate Receptor Subtype 5. Mol. Pharmacol. 64 (3), 731–740. doi:10.1124/mol.64.3.731
O'Brien, J. A., Lemaire, W., Wittmann, M., Jacobson, M. A., Ha, S. N., Wisnoski, D. D., et al. (2004). A Novel Selective Allosteric Modulator Potentiates the Activity of Native Metabotropic Glutamate Receptor Subtype 5 in Rat Forebrain. J. Pharmacol. Exp. Ther. 309 (2), 568–577. doi:10.1124/jpet.103.061747
O'Leary, D. M., Movsesyan, V., Vicini, S., and Faden, A. I. (2000). Selective mGluR5 Antagonists MPEP and SIB-1893 Decrease NMDA or Glutamate-Mediated Neuronal Toxicity through Actions that Reflect NMDA Receptor Antagonism. Br. J. Pharmacol. 131 (7), 1429–1437. doi:10.1038/sj.bjp.0703715
Ossowska, K., Konieczny, J., Wolfarth, S., and Pilc, A. (2005). MTEP, a New Selective Antagonist of the Metabotropic Glutamate Receptor Subtype 5 (mGluR5), Produces Antiparkinsonian-like Effects in Rats. Neuropharmacology 49 (4), 447–455. doi:10.1016/j.neuropharm.2005.04.002
Palucha, A., and Pilc, A. (2007). Metabotropic Glutamate Receptor Ligands as Possible Anxiolytic and Antidepressant Drugs. Pharmacol. Ther. 115 (1), 116–147. doi:10.1016/J.PHARMTHERA.2007.04.007
Parmentier-Batteur, S., Hutson, P. H., Menzel, K., Uslaner, J. M., Mattson, B. A., O'Brien, J. A., et al. (2014). Mechanism Based Neurotoxicity of mGlu5 Positive Allosteric Modulators-Ddevelopment Challenges for a Promising Novel Antipsychotic Target. Neuropharmacology 82, 161–173. doi:10.1016/J.NEUROPHARM.2012.12.003
Pecknold, J. C., McClure, D. J., Appeltauer, L., Wrzesinski, L., and Allan, T. (1982). Treatment of Anxiety Using Fenobam (A Nonbenzodiazepine) in a Double-Blind Standard (Diazepam) Placebo-Controlled Study. J. Clin. Psychopharmacol. 2 (2), 129–133. doi:10.1097/00004714-198204000-00010
Pekny, M., and Pekna, M. (2014). Astrocyte Reactivity and Reactive Astrogliosis: Costs and Benefits. Physiol. Rev. 94 (4), 1077–1098. doi:10.1152/PHYSREV.00041.2013
Piers, T. M., Heales, S. J., and Pocock, J. M. (2011). Positive Allosteric Modulation of Metabotropic Glutamate Receptor 5 Down-Regulates Fibrinogen-Activated Microglia Providing Neuronal Protection. Neurosci. Lett. 505 (2), 140–145. doi:10.1016/J.NEULET.2011.10.007
Pittaluga, A. (2016). Presynaptic Release-Regulating mGlu1 Receptors in Central Nervous System. Front. Pharmacol. 7, 295. doi:10.3389/fphar.2016.00295
Poewe, W., Seppi, K., Tanner, C. M., Halliday, G. M., Brundin, P., Volkmann, J., et al. (2017). Parkinson Disease. Nat. Rev. Dis. Prim. 3 (1), 1–21. doi:10.1038/nrdp.2017.13
Porras, G., Li, Q., and Bezard, E. (2012). Modeling Parkinson's Disease in Primates: The MPTP Model. Cold Spring Harb. Perspect. Med. 2 (3), a009308. doi:10.1101/cshperspect.a009308
Porter, R. H., Jaeschke, G., Spooren, W., Ballard, T. M., Büttelmann, B., Kolczewski, S., et al. (2005). Fenobam: A Clinically Validated Nonbenzodiazepine Anxiolytic Is a Potent, Selective, and Noncompetitive mGlu5 Receptor Antagonist with Inverse Agonist Activity. J. Pharmacol. Exp. Ther. 315 (2), 711–721. doi:10.1124/jpet.105.089839
Price, D. L., Rockenstein, E., Ubhi, K., Phung, V., MacLean-Lewis, N., Askay, D., et al. (2010). Alterations in mGluR5 Expression and Signaling in Lewy Body Disease and in Transgenic Models of Alpha-Synucleinopathy-Iimplications for Excitotoxicity. PloS one 5 (11), e14020. doi:10.1371/JOURNAL.PONE.0014020
Purgert, C. A., Izumi, Y., Jong, Y. J., Kumar, V., Zorumski, C. F., and O'Malley, K. L. (2014). Intracellular mGluR5 Can Mediate Synaptic Plasticity in the hippocampus. J. Neurosci. 34 (13), 4589–4598. doi:10.1523/JNEUROSCI.3451-13.2014
Qiu, C., Kivipelto, M., and von Strauss, E. (2009). Epidemiology of Alzheimer's Disease: Occurrence, Determinants, and Strategies toward Intervention. Dialogues Clin. Neurosci. 11 (2), 111–128. doi:10.31887/DCNS.2009.11.2/CQIU
Ransohoff, R. M. (2016). How Neuroinflammation Contributes to Neurodegeneration. Science 353 (6301), 777–783. doi:10.1126/SCIENCE.AAG2590
Reilmann, R., Rouzade-Dominguez, M. L., Saft, C., Süssmuth, S. D., Priller, J., Rosser, A., et al. (2015). A Randomized, Placebo-Controlled Trial of AFQ056 for the Treatment of Chorea in Huntington's Disease. Mov. Disord. 30 (3), 427–431. doi:10.1002/MDS.26174
Renner, M., Lacor, P. N., Velasco, P. T., Xu, J., Contractor, A., Klein, W. L., et al. (2010). Deleterious Effects of Amyloid Beta Oligomers Acting as an Extracellular Scaffold for mGluR5. Neuron 66 (5), 739–754. doi:10.1016/j.neuron.2010.04.029
Ribeiro, F. M., Hamilton, A., Doria, J. G., Guimaraes, I. M., Cregan, S. P., and Ferguson, S. S. (2014a). Metabotropic Glutamate Receptor 5 as a Potential Therapeutic Target in Huntington's Disease. Expert Opin. Ther. Targets 18 (11), 1293–1304. doi:10.1517/14728222.2014.948419
Ribeiro, F. M., Paquet, M., Ferreira, L. T., Cregan, T., Swan, P., Cregan, S. P., et al. (2010). Metabotropic Glutamate Receptor-Mediated Cell Signaling Pathways Are Altered in a Mouse Model of Huntington's Disease. J. Neurosci. 30 (1), 316–324. doi:10.1523/JNEUROSCI.4974-09.2010
Ribeiro, F. M., DeVries, R. A., Hamilton, A., Guimaraes, I. M., Cregan, S. P., Pires, R. G. W., et al. (2014b). Metabotropic Glutamate Receptor 5 Knockout Promotes Motor and Biochemical Alterations in a Mouse Model of Huntington's Disease. Hum. Mol. Genet. 23, 2030–2042. doi:10.1093/hmg/ddt598
Rigamonti, D., Mutti, C., Zuccato, C., Cattaneo, E., and Contini, A. (2009). Turning REST/NRSF Dysfunction in Huntington's Disease into a Pharmaceutical Target. Curr. Pharm. Des. 15 (34), 3958–3967. doi:10.2174/138161209789649303
Rosenbaum, D. M., Rasmussen, S. G., and Kobilka, B. K. (2009). The Structure and Function of G-Protein-Coupled Receptors. Nature 459, 356–363. doi:10.1038/nature08144
Rossi, D., Brambilla, L., Valori, C. F., Roncoroni, C., Crugnola, A., Yokota, T., et al. (20082008). Focal Degeneration of Astrocytes in Amyotrophic Lateral Sclerosis. Cell Death Differ. 15 (11), 111691–111700. doi:10.1038/cdd.2008.99
Rutrick, D., Stein, D. J., Subramanian, G., Smith, B., Fava, M., Hasler, G., et al. (2017). Mavoglurant Augmentation in OCD Patients Resistant to Selective Serotonin Reuptake Inhibitors: A Proof-Of-Concept, Randomized, Placebo-Controlled, Phase 2 Study. Adv. Ther. 34 (2), 524–541. doi:10.1007/S12325-016-0468-5
Rylander, D., Iderberg, H., Li, Q., Dekundy, A., Zhang, J., Li, H., et al. (2010). A mGluR5 Antagonist under Clinical Development Improves L-DOPA-Induced Dyskinesia in Parkinsonian Rats and Monkeys. Neurobiol. Dis. 39 (3), 352–361. doi:10.1016/j.nbd.2010.05.001
Salvadores, N., Sanhueza, M., Manque, P., and Court, F. A. (2017). Axonal Degeneration during Aging and its Functional Role in Neurodegenerative Disorders. Front. Neurosci. 11 (SEP), 451. doi:10.3389/FNINS.2017.00451/BIBTEX
Santos, R., Ursu, O., Gaulton, A., Bento, A. P., Donadi, R. S., Bologa, C. G., et al. (2016). A Comprehensive Map of Molecular Drug Targets. Nat. Rev. Drug Discov. 16 (1), 19–34. doi:10.1038/nrd.2016.230
Sartori, S. B., and Singewald, N. (2019). Novel Pharmacological Targets in Drug Development for the Treatment of Anxiety and Anxiety-Related Disorders. Pharmacol. Ther. 204, 107402. doi:10.1016/J.PHARMTHERA.2019.107402
Scarpa, M., Hesse, S., and Bradley, S. J. (2020). M1 Muscarinic Acetylcholine Receptors: A Therapeutic Strategy for Symptomatic and Disease-Modifying Effects in Alzheimer's Disease? Adv. Pharmacol. 88, 277–310. doi:10.1016/BS.APHA.2019.12.003
Schiefer, J., Sprünken, A., Puls, C., Lüesse, H. G., Milkereit, A., Milkereit, E., et al. (2004). The Metabotropic Glutamate Receptor 5 Antagonist MPEP and the mGluR2 Agonist LY379268 Modify Disease Progression in a Transgenic Mouse Model of Huntington's Disease. Brain Res. 1019 (1–2), 246–254. doi:10.1016/j.brainres.2004.06.005
Schiweck, J., Eickholt, B. J., and Murk, K. (2018). Important Shapeshifter: Mechanisms Allowing Astrocytes to Respond to the Changing Nervous System during Development, Injury and Disease. Front. Cell Neurosci. 12, 261. doi:10.3389/FNCEL.2018.00261
Schober, A. (2004). Classic Toxin-Induced Animal Models of Parkinson's Disease: 6-OHDA and MPTP. Cell Tissue Res. 318, 215–224. doi:10.1007/s00441-004-0938-y
Shah, A., Silverstein, P. S., Singh, D. P., and Kumar, A. (2012). Involvement of Metabotropic Glutamate Receptor 5, AKT/PI3K Signaling and NF-κB Pathway in Methamphetamine-Mediated Increase in IL-6 and IL-8 Expression in Astrocytes. J. Neuroinflammation 9 (1), 52–10. doi:10.1186/1742-2094-9-52/FIGURES/5_547
Shaw, P. J., Forrest, V., Ince, P. G., Richardson, J. P., and Wastell, H. J. (1995). CSF and Plasma Amino Acid Levels in Motor Neuron Disease: Elevation of CSF Glutamate in a Subset of Patients. Neurodegeneration 4 (2), 209–216. doi:10.1006/neur.1995.0026
Shigemoto, R., Nomura, S., Ohishi, H., Sugihara, H., Nakanishi, S., and Mizuno, N. (1993). Immunohistochemical Localization of a Metabotropic Glutamate Receptor, mGluR5, in the Rat Brain. Neurosci. Lett. 163 (1), 53–57. doi:10.1016/0304-3940(93)90227-C
Shrivastava, A. N., Kowalewski, J. M., Renner, M., Bousset, L., Koulakoff, A., Melki, R., et al. (2013). β-Amyloid and ATP-Induced Diffusional Trapping of Astrocyte and Neuronal Metabotropic Glutamate Type-5 Receptors. Glia 61 (10), 1673–1686. doi:10.1002/GLIA.22548
Sidiropoulos, K., Viteri, G., Sevilla, C., Jupe, S., Webber, M., Orlic-Milacic, M., et al. (2017). Reactome enhanced pathway visualization. Bioinform. 33 (21), 3461–3467. doi:10.1093/bioinformatics/btx441
Silva, G. A., Theriault, E., Mills, L. R., Pennefather, P. S., and Feeney, C. J. (1999). Group I and II Metabotropic Glutamate Receptor Expression in Cultured Rat Spinal Cord Astrocytes. Neurosci. Lett. 263 (2–3), 117–120. doi:10.1016/S0304-3940(99)00145-7
Simola, N., Morelli, M., and Carta, A. R. (2007). The 6-Hydroxydopamine Model of Parkinson's Disease. Neurotox. Res. 11, 151–167. doi:10.1007/BF03033565
Simonyi, A., Schachtman, T. R., and Christoffersen, G. R. J. (2005). The Role of Metabotropic Glutamate Receptor 5 in Learning and Memory Processes. Drug News Perspect. 18, 353–361. doi:10.1358/dnp.2005.18.6.927927
Spampinato, S. F., Copani, A., Nicoletti, F., Sortino, M. A., and Caraci, F. (2018). Metabotropic Glutamate Receptors in Glial Cells: A New Potential Target for Neuroprotection? Front. Mol. Neurosci. 11, 414. doi:10.3389/FNMOL.2018.00414/BIBTEX
Spooren, W. P., Gasparini, F., Bergmann, R., and Kuhn, R. (2000). Effects of the Prototypical mGlu(5) Receptor Antagonist 2-Methyl-6-(phenylethynyl)-Pyridine on Rotarod, Locomotor Activity and Rotational Responses in Unilateral 6-OHDA-Lesioned Rats. Eur. J. Pharmacol. 406 (3), 403–410. doi:10.1016/S0014-2999(00)00697-X
Sriram, K., and Insel, P. A. (2018). G Protein-Coupled Receptors as Targets for Approved Drugs: How Many Targets and How Many Drugs? Mol. Pharmacol. 93, 251–258. doi:10.1124/mol.117.111062
Steffan, J. S., Kazantsev, A., Spasic-Boskovic, O., Greenwald, M., Zhu, Y. Z., Gohler, H., et al. (2000). The Huntington's Disease Protein Interacts with P53 and CREB-Binding Protein and Represses Transcription. Proc. Natl. Acad. Sci. U. S. A. 97 (12), 6763–6768. doi:10.1073/PNAS.100110097
Stocchi, F., Rascol, O., Destee, A., Hattori, N., Hauser, R. A., Lang, A. E., et al. (2013). AFQ056 in Parkinson Patients with Levodopa-Induced Dyskinesia: 13-week, Randomized, Dose-Finding Study. Mov. Disord. 28 (13), 1838–1846. doi:10.1002/mds.25561
Stoppel, L. J., Auerbach, B. D., Senter, R. K., Preza, A. R., Lefkowitz, R. J., and Bear, M. F. (2017). β-Arrestin2 Couples Metabotropic Glutamate Receptor 5 to Neuronal Protein Synthesis and Is a Potential Target to Treat Fragile X. Cell Rep. 18 (12), 2807–2814. doi:10.1016/j.celrep.2017.02.075
Tang, T. S., Tu, H., Chan, E. Y., Maximov, A., Wang, Z., Wellington, C. L., et al. (2003). Huntingtin and Huntingtin-Associated Protein 1 Influence Neuronal Calcium Signaling Mediated by Inositol-(1,4,5) Triphosphate Receptor Type 1. Neuron 39 (2), 227–239. doi:10.1016/S0896-6273(03)00366-0
Testa, C. M., Standaert, D. G., Landwehrmeyer, G. B., Penney, J. B., and Young, A. B. (1995). Differential Expression of mGluR5 Metabotropic Glutamate Receptor mRNA by Rat Striatal Neurons. J. Comp. Neurol. 354 (2), 241–252. doi:10.1002/CNE.903540207
Thanvi, B., Lo, N., and Robinson, T. (2007). Levodopa-induced Dyskinesia in Parkinson's Disease: Clinical Features, Pathogenesis, Prevention and Treatment. Postgrad. Med. J. 83, 384–388. doi:10.1136/pgmj.2006.054759
Thibault, O., Gant, J. C., and Landfield, P. W. (2007). Expansion of the Calcium Hypothesis of Brain Aging and Alzheimer's Disease: Minding the Store. Aging cell 6 (3), 307–317. doi:10.1111/J.1474-9726.2007.00295.X
Tison, F., Keywood, C., Wakefield, M., Durif, F., Corvol, J. C., Eggert, K., et al. (2016). A Phase 2A Trial of the Novel mGluR5-Negative Allosteric Modulator Dipraglurant for Levodopa-Induced Dyskinesia in Parkinson's Disease. Mov. Disord. 31 (9), 1373–1380. doi:10.1002/mds.26659
Treyer, V., Gietl, A. F., Suliman, H., Gruber, E., Meyer, R., Buchmann, A., et al. (2020). Reduced Uptake of [11C]-Abp688, a PET Tracer for Metabolic Glutamate Receptor 5 in hippocampus and Amygdala in Alzheimer's Dementia. Brain Behav. 10 (6), e01632. doi:10.1002/BRB3.1632
Trinh, P. N. H., May, L. T., Leach, K., and Gregory, K. J. (2018). Biased Agonism and Allosteric Modulation of Metabotropic Glutamate Receptor 5. Clin. Sci. (Lond) 132 (21), 2323–2338. doi:10.1042/CS20180374
Ufer, M., Sagkriotis, A., Salunke, A., Ganesan, S., Tisserant, A., Dodman, A., et al. (2016). Intravenous Dosing as an Alternate Approach to Safely Achieve Supratherapeutic Exposure for Assessments of Cardiac Repolarization: A Randomized Clinical Trial with Mavoglurant (AFQ056). Clin. Ther. 38, 2589–2597. doi:10.1016/j.clinthera.2016.10.007
Um, J. W., Kaufman, A. C., Kostylev, M., Heiss, J. K., Stagi, M., Takahashi, H., et al. (2013). Metabotropic Glutamate Receptor 5 Is a Coreceptor for Alzheimer Aβ Oligomer Bound to Cellular Prion Protein. Neuron 79 (5), 887–902. doi:10.1016/j.neuron.2013.06.036
Valerio, A., Rizzonelli, P., Paterlini, M., Moretto, G., Knöpfel, T., Kuhn, R., et al. (1997). mGluR5 Metabotropic Glutamate Receptor Distribution in Rat and Human Spinal Cord: a Developmental Study. Neurosci. Res. 28 (1), 49–57. doi:10.1016/S0168-0102(97)01175-9
Varney, M. A., Rao, S. P., Jachec, C., Deal, C., Hess, S. D., Daggett, L. P., et al. (1998). Pharmacological Characterization of the Human Ionotropic Glutamate Receptor Subtype GluR3 Stably Expressed in Mammalian Cells. J. Pharmacol. Exp. Ther. 285 (1), 358–370.
Vergouts, M., Doyen, P. J., Peeters, M., Opsomer, R., and Hermans, E. (2018). Constitutive Downregulation Protein Kinase C Epsilon in hSOD1G93A Astrocytes Influences mGluR5 Signaling and the Regulation of Glutamate Uptake. Glia 66 (4), 749–761. doi:10.1002/GLIA.23279
Vermeiren, C., Hemptinne, I., Vanhoutte, N., Tilleux, S., Maloteaux, J. M., and Hermans, E. (2006). Loss of Metabotropic Glutamate Receptor-Mediated Regulation of Glutamate Transport in Chemically Activated Astrocytes in a Rat Model of Amyotrophic Lateral Sclerosis. J. Neurochem. 96 (3), 719–731. doi:10.1111/J.1471-4159.2005.03577.X
Vermeiren, C., Najimi, M., Vanhoutte, N., Tilleux, S., de Hemptinne, I., Maloteaux, J. M., et al. (2005). Acute Up-Regulation of Glutamate Uptake Mediated by mGluR5a in Reactive Astrocytes. J. Neurochem. 94 (2), 405–416. doi:10.1111/J.1471-4159.2005.03216.X
Wellendorph, P., and Bräuner-Osborne, H. (2009). Molecular Basis for Amino Acid Sensing by Family C G-Protein-Coupled Receptors. Br. J. Pharmacol. 156 (6), 869–884. doi:10.1111/J.1476-5381.2008.00078.X
Wong, D. F., Waterhouse, R., Kuwabara, H., Kim, J., Brašić, J. R., Chamroonrat, W., et al. (2013). 18F-FPEB, a PET Radiopharmaceutical for Quantifying Metabotropic Glutamate 5 Receptors: A First-In-Human Study of Radiochemical Safety, Biokinetics, and Radiation Dosimetry. J. Nucl. Med. 54 (3), 388–396. doi:10.2967/JNUMED.112.107995
Wuolikainen, A., Moritz, T., Marklund, S. L., Antti, H., and Andersen, P. M. (2011). Disease-related Changes in the Cerebrospinal Fluid Metabolome in Amyotrophic Lateral Sclerosis Detected by GC/TOFMS. PLoS ONE 6 (4), e17947. doi:10.1371/journal.pone.0017947
Yamada, T., Kawamata, T., Walker, D. G., and McGeer, P. L. (1992). Vimentin Immunoreactivity in Normal and Pathological Human Brain Tissue. Acta Neuropathol. 84 (2), 157–162. doi:10.1007/BF00311389
Ye, X., Yu, L., Zuo, D., Zhang, L., Zu, J., Hu, J., et al. (2017). Activated mGluR5 Protects BV2 Cells against OGD/R Induced Cytotoxicity by Modulating BDNF-TrkB Pathway. Neurosci. Lett. 654, 70–79. doi:10.1016/J.NEULET.2017.06.029
Yi, J. H., Whitcomb, D. J., Park, S. J., Martinez-Perez, C., Barbati, S. A., Mitchell, S. J., et al. (2020). M1 Muscarinic Acetylcholine Receptor Dysfunction in Moderate Alzheimer's Disease Pathology. Brain Commun. 2 (2), fcaa058. doi:10.1093/BRAINCOMMS/FCAA058
Yiannopoulou, K. G., and Papageorgiou, S. G. (2013). Current and Future Treatments for Alzheimer's Disease. Ther. Adv. Neurol. Disord. 6 (1), 19–33. doi:10.1177/1756285612461679
Youssef, E. A., Berry-Kravis, E., Czech, C., Hagerman, R. J., Hessl, D., Wong, C. Y., et al. (2018). Effect of the mGluR5-NAM Basimglurant on Behavior in Adolescents and Adults with Fragile X Syndrome in a Randomized, Double-Blind, Placebo-Controlled Trial: FragXis Phase 2 Results. Neuropsychopharmacology 43 (3), 503–512. doi:10.1038/NPP.2017.177
Zamanian, J. L., Xu, L., Foo, L. C., Nouri, N., Zhou, L., Giffard, R. G., et al. (2012). Genomic Analysis of Reactive Astrogliosis. J. Neurosci. 32 (18), 6391–6410. doi:10.1523/JNEUROSCI.6221-11.2012
Zhang, T., Dong, K., Liang, W., Xu, D., Xia, H., Geng, J., et al. (2015). G-protein-coupled Receptors Regulate Autophagy by ZBTB16-Mediated Ubiquitination and Proteasomal Degradation of Atg14L. Elife 4, e06734. doi:10.7554/eLife.06734.001
Keywords: GPCR, Alzheimer’s disease, neurodegenerative disease, neuroinflammation, G protein coupled receptors, drug discovery
Citation: Budgett RF, Bakker G, Sergeev E, Bennett KA and Bradley SJ (2022) Targeting the Type 5 Metabotropic Glutamate Receptor: A Potential Therapeutic Strategy for Neurodegenerative Diseases?. Front. Pharmacol. 13:893422. doi: 10.3389/fphar.2022.893422
Received: 10 March 2022; Accepted: 18 April 2022;
Published: 11 May 2022.
Edited by:
Nina Vardjans, University of Ljubljana, SloveniaReviewed by:
Stephen Ferguson, University of Ottawa, CanadaTiziana Bonifacino, University of Genoa, Italy
Copyright © 2022 Budgett, Bakker, Sergeev, Bennett and Bradley. This is an open-access article distributed under the terms of the Creative Commons Attribution License (CC BY). The use, distribution or reproduction in other forums is permitted, provided the original author(s) and the copyright owner(s) are credited and that the original publication in this journal is cited, in accordance with accepted academic practice. No use, distribution or reproduction is permitted which does not comply with these terms.
*Correspondence: Sophie J. Bradley, sophie.bradley@soseiheptares.com