- 1Department of Pharmaceutics, College of Pharmacy, King Saud University, Riyadh, Saudi Arabia
- 2Nanobiotechnology Unit, College of Pharmacy, King Saud University, Riyadh, Saudi Arabia
- 3Department of Pharmacology and Toxicology, College of Pharmacy, King Saud University, Riyadh, Saudi Arabia
- 4Department of Clinical Pharmacy, College of Pharmacy, King Saud University, Riyadh, Saudi Arabia
- 5Department of Pharmaceutics and Pharmaceutical Technology, College of Pharmacy, Taif University, Taif, Saudi Arabia
Pancreatic cancer (PC) remains one of the most lethal and incurable forms of cancer and has a poor prognosis. One of the significant therapeutic challenges in PC is multidrug resistance (MDR), a phenomenon in which cancer cells develop resistance toward administered therapy. Development of novel therapeutic platforms that could overcome MDR in PC is crucial for improving therapeutic outcomes. Nanotechnology is emerging as a promising tool to enhance drug efficacy and minimize off-target responses via passive and/or active targeting mechanisms. Over the past decade, tremendous efforts have been made to utilize nanocarriers capable of targeting PC cells while minimizing off-target effects. In this review article, we first give an overview of PC and the major molecular mechanisms of MDR, and then we discuss recent advancements in the development of nanocarriers used to overcome PC drug resistance. In doing so, we explore the developmental stages of this research in both pre-clinical and clinical settings. Lastly, we discuss current challenges and gaps in the literature as well as potential future directions in the field.
Introduction
Pancreatic cancer (PC) accounts for 2.5% of all cancer cases worldwide, making it the fourth leading cause of cancer mortality (Siegel et al., 2019). An estimated 495,773 patients were diagnosed with PC in 2021 (Cancer.Net, 2022), with approximately 50% of the cases were diagnosed with metastatic disease (Bray et al., 2018). The location of the tumor within the pancreas can influence the symptoms and clinical presentation (Ryan et al., 2014; Ducreux et al., 2015; Tempero et al., 2019). Almost 65% of PC tumors develop in the head and the neck of the pancreas, and patients commonly present with jaundice and abdominal pain due to bile obstruction (Ducreux et al., 2015; Tempero et al., 2019). In some cases, PC can either be located in the pancreas body (15%), and the tail (10%), or can be a multifocal tumor (2%). Furthermore, patients can present with other non-specific symptoms, including late-onset type II diabetes, abdominal pain, weight loss, and steatorrhea (Gheorghe et al., 2020).
Pathologically, PC originates from two types of cells: exocrine or endocrine cells (Bardeesy and DePinho, 2002; Ghaneh et al., 2007; Corbo et al., 2012; Aguirre and Collisson, 2017). Over 95% of all PC types are of exocrine origin, where the majority of these cases are pancreatic adenocarcinomas (PDAC) (Cowgill and Muscarella, 2003; Li and Jiao, 2003). Less common cells are those of acinar and cystadenocarcinoma origin (Table 1). The endocrine tumors include neuroendocrine tumors (PNET), which account for 5% of all PC cases (Hackeng et al., 2016). PNETs grow slowly and are less aggressive than the PDAC (Bardeesy and DePinho, 2002; Ghaneh et al., 2007). PDAC is a complex disease with poorly differentiated histological features. The high intertumoral heterogeneity, genomic instability, and stromal desmoplasia formation cause significant challenges in the early diagnosis and treatment of PDAC (Ghaneh et al., 2007; Corbo et al., 2012; Aguirre and Collisson, 2017). Pancreatic intraepithelial neoplasia (PanIN) is the most common early non-invasive precursor lesion of PDAC, in addition to mucinous cystic neoplasm and intraductal papillary mucinous neoplasm. The precursor’s progression from low-grade to high-grade is due to genetic and epigenetic alterations that lead to the formation of invasive PDAC. When PDAC lesions form, the genetic mutations will continue to progress beyond primary mutations (Ghaneh et al., 2007; Rishi et al., 2015; Hackeng et al., 2016; Dreyer et al., 2017).

TABLE 1. Pancreatic cancer pathological types. Pancreatic cancer pathologically originates from exocrine or endocrine cells. The prevalence and common mutations are different depending on the tumor type and cell origin. Invasive ductal adenocarcinoma is a common type of PC in cells with an exocrine origin.
Drug resistance, both intrinsic (innate) and acquired (in response to drug therapy), is a key contributing factor to the poor prognosis of PC (Binenbaum et al., 2015). The survival rate of PC has remained almost unchanged for several decades, and it is considered among the lowest, with a 5-year survival rate for a maximum of 9% of cases for all stages combined (Rawla et al., 2019; Siegel et al., 2019). Such a low rate of survival is attributed to two major factors: 1) late diagnosis of typically advanced/metastasized and unresectable PC due to a lack of early diagnostic biomarkers; and 2) lack of effective therapeutic intervention (Siegel et al., 2019). There are fundamental reasons why pancreatic tumors are difficult to treat in PC. Firstly, pancreatic tumors, such as PDAC, are solid tumors comprised of a dense stromal environment of cancerous cells, non-cancerous cells such as fibroblasts, and a dense extracellular matrix. The density of these arrangements impedes drug permeation. Additionally, if a drug can permeate the cancerous stroma, the drug molecules are typically unable to differentiate between cancerous and non-cancerous cells due to the dense tumor stroma (Heidemann and Kirschner, 1978; Sofuni et al., 2005). An important determinant of peritoneal metastasis is the anatomical position of the primary tumor (Baretti et al., 2019). In some cases, cells directly attach to and invade organs and tissues in the peritoneal cavity (Avula et al., 2020) or result in intraperitoneal metastases via blood vessels or lymphatic absorption through the hematogenous route (Ge et al., 2017). In most cases of PC, metastasis has already occurred by the time of diagnosis. Furthermore, even when surgical intervention is applicable, following chemoradiotherapy, rapid relapse is often seen due to the presence of pancreatic satellite cells that promote carcinogenesis (Hosoki, 1983; Sofuni et al., 2005; Zhi et al., 2014; Binenbaum et al., 2015).
The stromal desmoplastic reactions induced by the pancreatic stellate cells (PSC), when activated by growth factors, lead to the secretion of collagen, hyaluronic acid, and other components of the extracellular matrix. Thus, PSCs induce stromal fibrosis, reduce cellular vascularity, and induce hypoxia. The stromal barrier created by PSC elevates the interstitial fluid pressure and compresses the blood vessels, preventing passive transportation of chemotherapeutic agents and eventually leading to treatment failure (Erkan et al., 2012; Hackeng et al., 2016). Efficacy of Trans-arterial chemoembolization (TACE) with TACE + radiofrequency ablation (RFA) and/or 125I radioactive seed implantation for unresectable pancreatic cancer are other approaches that have been studied retrospectively and TACE combined with either 125I seed implantation and/or RFA, was shown to have improved treatment response and overall survival rate compared with TACE alone (Das et al., 2019). However, in the same study, the overall survival rates were better in the case of RFA. To summarize, based on these previous data, the sequence of use and the best combination therapy in the case of PC remains to be investigated.
While there are numerous drugs available for the treatment of PC, two nanoparticle formulations, Abraxane® and Onivyde® (irinotecan liposome injection) have been approved as of July 2022 by the US FDA for the treatment of metastasized cancers, in combination with gemcitabine. Abraxane® is a Paclitaxel Albumin-stabilized Nanoparticle Formulation, approved for the treatment of metastasized PC in combination with gemcitabine hydrochloride as a first-line treatment. More recently, the use of nanotechnology for delivering drugs to targeted sites in the body is an inexpensive and effective system for treating diseases and other conditions. Indeed, the use of nanotechnology can revolutionize the treatment of cancer, by enabling diagnostic tools for early detection of the disease (Hu et al., 2021) as well as improving drug delivery. In PC, the delivery and distribution of drugs to the tumor are compromised due to intrinsic physical and biochemical barriers, which result in increased interstitial fluid pressure, vascular compression, and hypoxia. Moreover, therapies based on targeting immune responses including therapeutic vaccines, immune checkpoint inhibition, and CAR-T cell therapy often do not show expected responses due to a highly immunosuppressive tumor microenvironment. These two factors present as a major challenge for developing effective therapies against PC. Nanoparticles have been extensively studied as delivery platforms and adjuvants for cancer and other disease therapies. Knowledge gained through using nanocarrier-based systems in other cancer types, combined with the ability to modulate nanocarriers toward targeting multiple MDR mechanisms simultaneously provides an opportunity to enable improvement in drug delivery and enhancing therapeutic outcomes for PC.
Mechanisms of resistance in pancreatic cancer
Numerous alterations at the genetic, epigenetic, and protein levels are implicated in PC drug resistance (Binenbaum et al., 2015). Although different therapy regimens exist, the current standard of care regimen therapy for PC remains largely dependent on gemcitabine (GEM), which is considered a gold standard in chemotherapy. GEM is a nucleoside analog, which provides only a modest clinical benefit (Burris et al., 1997). Multiple combination regimens of chemotherapeutics such as GEM with 5-fluorouracil (5-FU), cisplatin or paclitaxel (PTX), or FOLFIRINOX (i.e., fluorouracil, leucovorin, irinotecan, and oxaliplatin alone or alongside targeted therapies [e.g., cetuximab and bevacizumab]) have failed to demonstrate significant clinical benefits (Berlin et al., 2002; Cascinu et al., 2006; Heinemann et al., 2006). Despite the initial response to chemotherapy in the different forms of PC, the rapid development of drug resistance remains a major challenge in the treatment of PC (Binenbaum et al., 2015).
It is now well established that a variety of cancers mediate their aggressiveness and resistance to chemoradiotherapy via modulating key cellular regulatory pathways that control cell proliferation and differentiation, inflammation, and programmed cell death pathways, including apoptosis and autophagy (Xia et al., 2014). PC shows a significant up-regulation of ATP binding cassette (ABC) transporters ABCB4, ABCB11, ABCC1, ABCC3, ABCC5, ABCC10, and ABCG2 at the RNA level in tumors relative to the normal pancreas (Mohelnikova-Duchonova et al., 2013). Additionally, drug efflux pump MDR1/P-gp is highly expressed in PC cells (O'Driscoll et al., 2007), which may play a critical role in the development of resistance to chemotherapeutic agents. Therefore, it is equally important to understand the underlying molecular mechanisms of PC drug resistance for unraveling novel therapeutic interventions with improved efficacy. In addition, somatic mutations in key genes such as many proto-oncogenes (e.g., Ras, Myc, Cdk4) are critical in the initiation and progression of malignant tumors. However, cancer treatment is even more challenging because tumor exposure to therapy, including chemoradiotherapy and targeted therapy, is often associated with further mutations and the development of compensatory mechanisms that render cancer refractoriness to therapy and increased aggressiveness and metastasis (Binenbaum et al., 2015). Therefore, understanding the molecular mechanisms of drug resistance is crucial in order to intervene and eventually win the battle against cancer.
The main mutations of PDAC include KRAS, CDKN2A, TP53, and SMAD4 (Hackeng et al., 2016). Over 90% of PC is associated with KRAS mutations, most commonly KRASG12D, during both the initial stage (i.e., precursor lesions that develop into invasive pancreatic ductal adenocarcinoma, also known as pancreatic intraepithelial neoplasia) and progression stage (di Magliano and Logsdon, 2013). There have been efforts to discover new PDAC targeting agents. A phase 1 clinical trial (NCT04117087) used a long peptide vaccine combined with Nivolumab and Ipilimumab for resected MMR-p colorectal and pancreatic cancer patients. Interestingly, Govindan et al. discovered that AMG 510 is a novel small molecule that can bind specifically and irreversibly in KRASG12C (Govindan et al., 2019; Alzhrani et al., 2021). KRASG12C is a mutation that is predominantly found in non-small lung cancer. However, the use of AMG 510 is limited in PDAC because KRASG12C mutation only accounts for 2%. These findings will motivate the scientific community to develop new drugs that can target inactive KRASG12D and KRASG12V, which account for 80% of PDAC (Govindan et al., 2019; Alzhrani et al., 2021).
It is worth noting that tremendous efforts over the past years have revealed numerous molecular components and intricate signaling networks that are deregulated in PC, contributing to chemoresistance. These include, but are not limited, drug transporters (e.g., hENT and hCNT), intracellular enzymes (e.g., deoxycytidine kinase), dCK, which is critical for GEM bioactivation, DNA repair mechanisms (e.g., excision repair cross-complementation 1), ERCC1, antioxidant response (e.g., Nrf and HSPs), signaling pathways that regulate cell-cycle and programmed cell death (e.g., Nuclear Factor κB [NFκB], MAPK, PI3K/Akt and p53), epigenetic components (e.g., histone deacetylase), HDAC and more recently, noncoding RNAs (ncRNAs) [e.g., microRNAs (miRNAs), long noncoding RNAs (lncRNAs) and circular RNAs (circRNAs)] (Binenbaum et al., 2015; Xie et al., 2020a; Lin et al., 2020; Pandya et al., 2020). More recently, differential upregulation and functional role of the transmembrane mucin MUC4 in PC is an attractive target for immunotherapy and MUC4β encapsulation in polyanhydride nanoparticles has been shown to provide long-term protection against rapid phagocytic and proteolytic clearance in circulation. Stable MUC4β release from these nanoparticles and its immunogenic capacity has recently been demonstrated by Liu and colleagues in mice models (Liu et al., 2021). Discussing the specific molecular mechanism is beyond the scope of this review and is discussed in detail elsewhere, but here we give an overall overview and describe three key themes involved in PC drug resistance. These are epithelial-mesenchymal transition (EMT), expansion of pancreatic cancer stem cells (PCSCs), and dynamic state of the tumor microenvironment (TME), as illustrated in (Figure 1) (Binenbaum et al., 2015; Zeng et al., 2019).
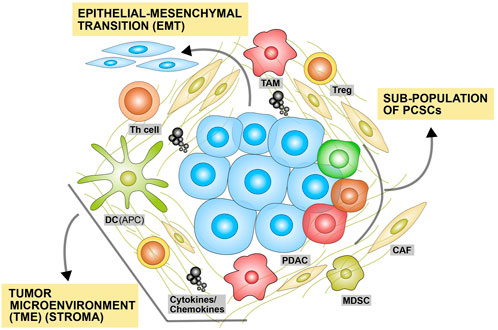
FIGURE 1. Major molecular contexts underlying drug resistance in pancreatic cancer. This figure illustrates the three major molecular contexts underlying drug resistance in pancreatic cancer. These include: 1) the process of epithelial-mesenchymal transition (EMT) in which cancer cells lose their epithelial phenotype (such as cell-to-cell contact) to gain more aggressive and metastatic mesenchymal phenotypes; 2) expansion of subpopulation of pancreatic cancer stem cells (PCSCs); and 3) dynamic tumor microenvironment (TME). The tumor stroma surrounding cancer cells and PCSCs consist non-cellular components including extracellular matrix (ECM) and multiple cell types including CAF, MDSC, Th-cells, Treg, TAM and DC. Cytokines released from the different cell types help maintaining the TME in dynamic state that supports tumor growth and metastasis. Th-cells exist in TME but are suppressed. DCs, which are key in the processing and presentation of cancer neoantigens, exist in TME but they are suppressed as well. EMT: epithelial-mesenchymal transition; PCSC: pancreatic cancer stem cell; TME: tumor microenvironment; CAF: Cancer-associated fibroblast; MDSC: myeloid-derived suppressor cell; Th-cells: Helper T cells; Treg: Regulatory T-cell; TAM: tumor-associated macrophage; DC: Dendritic cell.
Epithelial-mesenchymal transition (EMT) is a dynamic process that involves the transitioning of differentiated epithelial cells into the mesenchymal cell phenotype. During this transformation, cells lose key features of epithelial cells such as intercellular junctions while simultaneously undergoing cytoskeletal rearrangement of the mesenchymal cell phenotype (Nieto et al., 2016). EMT is particularly critical during cancer progression and the generation of stem-like cells with a high capacity for metastasis (Nieto et al., 2016). Notably, previous literature has demonstrated the key role of EMT in conferring drug resistance against chemoradiotherapy and targeted therapy in a variety of solid tumors, including PC (Shah et al., 2007; Wang et al., 2009; Singh and Settleman, 2010; Nieto et al., 2016). For instance, chemoresistance to GEM in PC cells has been associated with increased expression of mesenchymal markers such as vimentin and ZEB1, in contrast to GEM-sensitive cells with a high E-cadherin expression: a common epithelial phenotype maker (Li et al., 2009). Similar findings have been found with other chemotherapeutics, such as 5-FU and cisplatin (Arumugam et al., 2009). Although the underlying molecular mechanisms of EMT-induced chemoresistance are not fully understood, accumulating evidence suggests the involvement of multiple signaling pathways, including NFκB, TGFβ, and Notch pathways (Ellenrieder et al., 2001; Min et al., 2008; Wang et al., 2009). Another key molecular regulator of EMT in PC cells is miRNAs: small non-coding RNAs that regulate gene expression (Yonemori et al., 2017). A multitude of miRNAs has been shown to be implicated in regulating major cellular signaling pathways involved in the development of PC (Yonemori et al., 2017). For instance, a low expression of miR-200 was found in the GEM-resistant but not in the GEM-sensitive PC cell lines (Li et al., 2009). Together, these findings indicate the critical role of EMT in the development of PC drug resistance in transformed cells.
Previous evidence has demonstrated that cancers are heterogenous in nature, with subpopulations of cells of varying phenotypes. Cancer stem cells (CSCs) represent a small subpopulation of tumor cells with a high capacity for self-renewing, differentiation, and tumor progression (Yu et al., 2012). Indeed, CSCs display a high capacity for tumorigenesis when transplanted into a host compared to other tumor subpopulations (Yu et al., 2012). Several cellular surface markers have been identified to be expressed by CSCs in various cancers, including CD44, CD24, CD133, aldehyde dehydrogenase1 (ALDH1), and epithelial-specific antigen (ESA) (Yu et al., 2012). In PC, previous work has demonstrated that CD44, CD24, and ESA-expressing PCSCs, represented only by a small percentage of the cancer cell population (∼1%), were associated with high tumorigenic potential, and it is likely that PCSCs at least partially mediate chemoradiotherapy-induced drug resistance, as evident in PCSC enrichment in response to chemoradiotherapy treatment (Du et al., 2011). Similar findings were also found in another report, demonstrating that within a pancreatic tumor, a subset of undifferentiated, stem-like cells expressing the surface marker CD133, was associated with high tumorigenic potential and chemoresistance (Hermann et al., 2007). Although there is an overlap between the two subpopulations of PCSCs in the two reports (CD44/CD24/ESA-vs. CD133-expressing PCSCs), they were not identical (Hermann et al., 2007). Additionally, another subset of cells with exclusive migratory properties expressing CD133 and CXCR4 were identified at the invasive front of pancreatic tumors. It was found that without these CD133+/CXCR4+ cells, metastasis was almost completely abrogated (Hermann et al., 2007). Together, these findings indicate the existence of different subsets of PCSCs within pancreatic tumors with a high capacity for tumorigenesis and drug resistance. Such findings demonstrate the heterogenic nature of cancers which has been long disregarded in cancer research. It is worth noting that EMT transformed cells can share cellular and molecular features of CSCs, which could explain an increased tumorigenic profile of EMT transformed cells as well as drug resistance. Nonetheless, these cells, unlike PCSCs, were sensitive to conventional chemotherapy. Furthermore, it is now widely accepted that subpopulations of CSCs exist in a dynamic state (quiescent vs. slow-vs. rapid-cycling CSCs), which is constantly influenced by signals and cues from their surroundings (the tumor microenvironment (TME). With their large plasticity and ability to switch to a quiescent state, CSCs can resist chemoradiotherapy (Batlle and Clevers, 2017).
The tumor microenvironment (TME) consists of cellular and non-cellular components (extracellular matrix (ECM)) and plays a crucial role in drug resistance. Indeed, one major obstacle in the treatment of PC is the dense fibrotic stroma, also known as desmoplastic stroma, surrounding tumor cells that act as a physical barrier. As such, they prevent drugs from infiltrating tumor core cells (Dauer et al., 2017). Thus, the dense fibrotic stroma is considered a histopathological hallmark of PC. It is worth noting that TME is composed of several types of cells, including fibroblasts, pancreatic stellate cells (myofibroblast-like cells), and a variety of immune cells and despite the considerable efforts made to target a multitude of cellular and noncellular components within TME, yet it was associated with limited clinical success (Ho et al., 2020). For instance, within the stromal cells, cancer-associated fibroblasts (CAFs), which are the major source of ECM, are associated with immunosuppressive properties (Kraman et al., 2010). Depletion of CAFs was associated with enhanced efficacy of immune checkpoint inhibitors (ICIs) (Feig et al., 2013). In addition to CAFs, other cell types within the TME including regulatory T-cells (Treg), myeloid-derived suppressor cell (MDSC), and tumor-associated macrophage (TAM) also possess immunosuppressive properties (Ho et al., 2020). Cancer immunotherapy has revolutionized the field over the past couple of decades. Notable success has been made against multiple solid cancers, particularly those with high tumor mutational burden (TMB) within the tumor genome, such as melanoma and lung cancer (Goodman et al., 2017; Yousefi et al., 2017). This success has not been evident in PC (Schizas et al., 2020), which has largely been attributed to the non-immunogenic nature of most types of PC. In other words, most PC types are not readily recognized by the immune cells due to low TMB and thus a low number of neoantigens, a tumor-associated antigen, and subsequent presences of TILs (Bailey et al., 2016; Danilova et al., 2019). Additionally, as mentioned earlier, PC is typically contained in a highly dense fibrotic stroma consisting of multiple cell types with immunosuppressive properties (Ho et al., 2020; Schizas et al., 2020). Unsurprisingly, together, these two factors make cancer immunotherapy less effective in PC. Utilizing the properties of TME for controlling local therapeutic delivery is an area of active research (Alshememry et al., 2017).
Nanotechnology for the treatment of pancreatic cancer
Nanomaterials and nanoparticles (NPs) are extremely small (1–100 nm) in size and hence can directly interact with biological molecules (Hosein et al., 2013). Engineered NPs are an excellent tool for drug delivery due to their unique structural properties which include a large surface-to-mass ratio, capacity to be modulated to bind different cellular targets, and ability to carry different cargo including proteins, nucleotides, and drugs. Advances in the field of nanotechnology have created tremendous prospects for improving therapeutic drug delivery (Schroeder et al., 2011; Melancon et al., 2012; Prabhu and Patravale, 2012). Currently, the use of nanotechnology in drug delivery typically involves a combination of nanomaterials and a drug of interest, and a significant number of nanoplatforms are being employed and are under testing in the different phases of clinical trials (Ogawa and Miura, 2014; Rebelo et al., 2017). These combinations utilize different types of nanomaterials such as polymeric NPs, liposomes, amphiphilic polymer NPs, small interfering RNA (siRNA), graft polymers, dendrimers, thermo-responsive polymers, mixed micelles, ultrasound-responsive nano-emulsions, carbon nanotubes, quantum dots and inorganic NPs (magnetic-hybrid NPs, and gold NPs) (Manzur et al., 2017; Rebelo et al., 2017; Sielaff and Mousa, 2018).
Nanotechnology has been largely utilized in cancer research to improve the delivery of drugs to the tumor site exploiting the leaky vasculature of the tumor via passive or enhanced permeability and retention (EPR) effect. However, a major challenge in PC is its hypovascularization, and hence, other strategies must be utilized, such as using targeted (active) delivery (Xie et al., 2020b). As a result of this insufficient vascularization, ineffective distribution of drugs may account for much of the chemotherapy resistance seen in PC treatment. The use of nanotherapy may bypass the inefficient vascularization issues by delivering chemotherapeutic drugs directly to the pancreatic tissue, for example, by targeting stromal hedgehog receptors rather than relying on blood flow, a methodology that provides a tunable distribution of chemotherapeutic agents throughout pancreatic tumor tissue, thereby increasing drug solubility, half-life, and stability (Jiang et al., 2020). Moreover, NPs delivering chemotherapeutic drugs can bypass multidrug-resistant (MDR) efflux pumps present on the surface of most tumor cells (Lobato-Mendizabal and Ruiz-Arguelles, 1990; Blanco et al., 2011; McCarroll et al., 2014). This, among other reasons that will be discussed in later sections, supports the use of nanotechnology in PC treatment.
Nano-based intervention to overcome MDR-PC in pre-clinical and clinical settings: Key examples
The use of NPs has several advantages, including minimizing MDR and drug-related toxicities. Nanoparticles can target a wide range of physiological and metabolic characteristics of the targeted tissues, thereby increasing biodistribution and bioavailability of drugs and enhancing their plasma half-life and EPR (Parhi et al., 2012; Poon et al., 2015; Gad et al., 2016; Liu et al., 2022). Recently, the potency of the chemotherapeutic agents for the treatment of PC has been improved through the use of RNA interference (RNAi) technologies, including miRNA and siRNA, which selectively suppress the expression of target genes leading to increased drug efficacy and enhancing anti-cancer activity (Tang et al., 2021). Integrating RNAi with NPs can therefore be extremely effective at treating PC (Hiss et al., 2007; Gurunathan et al., 2018). Studies have recently shown that nano-sized exosomes are an efficient RNAi carrier, making them an attractive delivery cargo to cancerous cells (Farran and Nagaraju, 2020) Exosomes have intrinsic advantages over liposomes, as they are less toxic and can be dosed at higher concentrations in the blood to work as molecular cargos which could be used to inhibit oncogenes, activate tumor suppressor genes and modulate immune responses to control tumor cell growth (Oliveira et al., 2021) (Zhao et al., 2021b). As researchers continue to uncover the cellular and molecular basis of PC drug resistance, nanotechnology used for the delivery of drugs can be further engineered to provide effective solutions for mitigating MDR in the treatment of PC (Zhang et al., 2017). Table 2 summarizes key nano-based systems used for the delivery of antitumor drugs to mitigate MDR.
Albumin-based nanoparticles in PC
Albumin-based nanoparticles can be utilized as theranostics (i.e., to deliver therapeutic agents and simultaneously used for diagnosis). Albumin is the most abundant plasma protein and known ligand to be associated with a caveolae-mediated endocytosis mechanism. Albumin-based nanoparticles when internalized by the cell via caveolae-mediated endocytosis, can overcome the issue of MDR by bypassing and evading ATP-binding cassette (ABC) transporters, which are responsible for the efflux of anticancer drugs and subsequent MDR once released into the cytoplasm (Yuan et al., 2016). Nanoparticle albumin (Nab) is made by mixing human albumin in an aqueous medium under high pressure to form 100–200 nm albumin NPs. These NPs are mixed with chemotherapeutic alkaloids such as PTX (Macarulla et al., 2019). As shown in Table 3, Nab-based delivery systems are the most extensively studied nanocarrier system in the treatment of PC in human clinical trials (Von Hoff et al., 2011; Hosein et al., 2013; Von Hoff et al., 2013; Goldstein et al., 2015; Vogel et al., 2016; Macarulla et al., 2019). A common example of albumin-based nanoparticles is Nab-PTX, an albumin-binding PTX and a microtubule-stabilizing agent known to enhance microtubule polymerization during mitosis leading to cell cycle arrest in the G2/M phases. Based on these properties, Nab-PTX can stop rapid and uncontrollable cell division and help overcome MDR receptor-mediated endocytosis (Demidenko et al., 2008; Guo et al., 2018). Importantly, Nab-PTX has been shown to mitigate MDR in PC (Guo et al., 2018). In 2018, Guo et al. created GEM-resistant pancreatic cells by inducing lower rates of hENT1 expression. These cells were then exposed to free GEM or GEM delivered using human-serum albumin nanoparticles (HSA-NPs). Their results showed that GEM-HSA-NPs was more effective at slowing pancreatic cell proliferation and triggering apoptosis in comparison to free GEM alone, without any increase in toxicity, as shown in vivo studies. In phase III clinical trial (MPACT) on previously untreated patients with metastatic PC, a combination of Nab-PTX and GEM increased the median OS of patients receiving nab-PTX and gemcitabine to 8.7 vs. 6.6 months in patients treated with gemcitabine alone (p < 0.0001) (van Horssen et al., 2006; Libutti et al., 2010). Furthermore, the combination showed an increase in the cumulative delivery of gemcitabine by 2.8-fold compared to gemcitabine alone (Von Hoff et al., 2011; Von Hoff et al., 2013; Goldstein et al., 2015). Based on the findings of the trial, GEM plus nab-PTX became the first therapy recommended by the National Institute for Health and Care Excellence in the United Kingdom for the management of previously untreated metastatic PC (Von Hoff et al., 2011).
Metal-based nanoparticles in PC
Metal-based NPs such as gold, silver, iron, platinum, and titanium are commonly used for imaging, as drug delivery carriers, and as radiosensitizers in radiation, proton, or photodynamic therapy (Klebowski et al., 2018). Due to their inherent physicochemical properties, metal NPs could overcome MDR via different mechanisms (Sharma et al., 2018), as shown in Figure 2. The endosomal-based cellular uptake mechanism can be considered one of the main advantages of metal-based NPs in overcoming MDR. This is achieved by selectively releasing intracellular drugs after evading the membrane-embedded multidrug efflux pumps (Ayers and Nasti, 2012). Khan et al. developed a nanoformulation of superparamagnetic iron oxide nanoparticles (SPION) loaded with curcumin (SP-CUR), an anti-inflammatory and anti-tumorigenic compound. They tested the activity of this nanoformulation in combination with GEM on GEM-resistant pancreatic cells. Efficient delivery of curcumin was achieved using their formulation, and they found that SP-CUR increased the effectiveness of GEM therapy through suppressing two signal transduction pathways that are implemented in MDR: 1) sonic hedgehog (SHH) and 2) oncogenic CXCR4/CXCL12. Following their endosomal escape, SPION particles showed increased cellular internalization where they were observed to be more closely associated with cytosol/mitochondria to prevent lysosomal degradation (Khan et al., 2019). In 2010, Libutti et al. used a novel drug delivery system, CYT-6091, surface-modified colloidal gold nanoparticles, to increase tumor levels of rhTNF and reduce its systemic metabolism and toxicity (Libutti et al., 2010). CYT-6091 is constructed by combining rhTNF and thiolyated glycol to the surface of 27 nm gold colloidal particles. CYT9061 was studied at doses between 50 mg/m2 to 600 mg/m2 in a phase I clinical trial conducted with three patients with pancreatic adenocarcinoma. Using the gold colloidal nanoparticles, researchers were able to administer extremely high concentrations of rhTNF compared to when given in isolation: the highest isolated tolerated concentration of pure rhTNF is 1 mg per cycle. After treatment, examination by electron microscope of normal and tumor tissues was carried out and found gold particles isolated within tumor tissues or at anticipated clearance sites (Ladd et al., 2017). These findings suggest that gold-based, colloidal nano-delivery systems can be used to deliver chemotherapy drugs to target tissues. However, subsequent clinical trials remain to be conducted to prove their efficacy and safety.
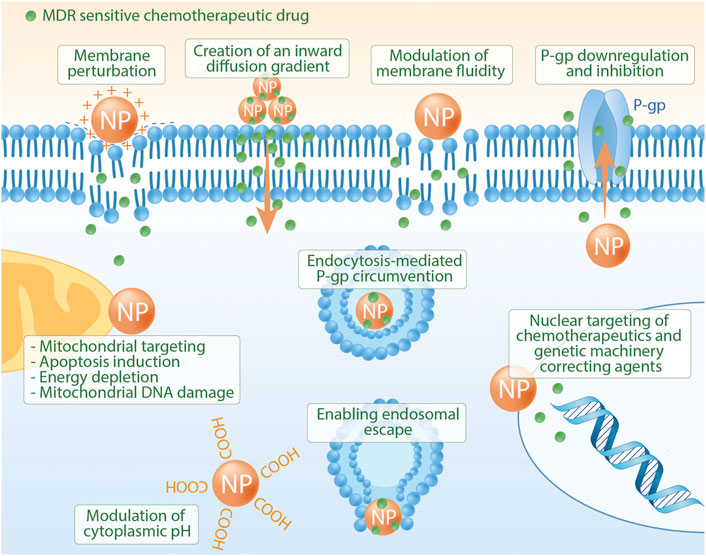
FIGURE 2. Common nanomedicine strategies to overcome multidrug-resistant tumors. Reprinted from Pharmacological Research, 126, Manu S. Singh, Salma N. Tammam, Maryam A. Shetab Boushehri, Alf Lamprecht, MDR in cancer: Addressing the underlying cellular alterations with the use of nanocarriers, 2-30, Copyright (2017), with permission from Elsevier.
Polymeric micelles in PC
Polymeric micelles, including both hydrophobic and hydrophilic moieties (Manzur et al., 2017), are the largest class of nanomaterials being investigated for PC treatment in pre-clinical settings. These nanoparticles contain linear or highly branched and symmetrical polymers, with hydrophilic dendritic extensions and a hydrophobic core. The terminal groups at the dendritic extensions can be adjusted to allow for better solubility depending upon the carrier medium and allow for superior anchorage and permeability into target tissues (Chiba and Twyman, 2017; Ladd et al., 2017). Polymeric micelles are NPs formed by the self-assembly of amphiphilic block copolymers when present in certain solvents with a surfactant concentration above a critical micelle concentration (c.m.c). They are efficient drug delivery systems for cancer treatment with the ability to inhibit P-gp action, alter drug internalization, enable selective drug targeting, and enable subcellular localization. Polymeric micelles can circumvent MDR through a combination of mechanisms, including the EPR effect, endosomal-triggered active internalization, and drug escape (Kapse-Mistry et al., 2014). In our literature review, we found at least seven published studies using polymeric micelles for the delivery of anti-tumor drugs in clinical trials, including Genexol®-PM, NK105, NC-4016, NK012, NC-6004, NK911, and SP1049C (Gong et al., 2012). NK105 is a nanoparticle formulation that incorporates PTX into a “core-shell-type” polymeric micelle. In a phase I trial conducted by Hamaguchi et al. (2007), the pharmacokinetics of NK105, a micelle carrier for PTX, was studied. The study included 19 patients with advanced PC who were given an initial dose of 10 mg/m2 of NK105; this dose increased successively. The size of metastatic liver tumors was reduced by 90% in patients given a 150 mg/m2 dose of the drug (Hamaguchi et al., 2007).
Lipid-based nanoformulations in PC
Lipid-based nanoformulations have been extensively studied for the delivery of antitumor agents, including natural products (Kashyap et al., 2019). Liposomes are lipid bilayer systems that can cross the lipid bilayer to selectively target cancer cells based on biomarkers, overly expressed on PC cells (Urey et al., 2017). There are several strategies whereby liposomes can enhance drug bioavailability and efficacy in drug-resistant cancer. These include 1) liposomes modified for controlled and on-demand release; and 2) ligand-targeted liposomes such as immunoliposomes, which facilitate intracellular drug delivery into tumor cells. Liposomes can also directly inhibit P-gp through endocytosis and consequently enhance intracellular drug accumulation (Kapse-Mistry et al., 2014). In 2012, the use of liposomes in PC treatment was explored by Yoshida et al. They targeted fucosylated antigens that are highly expressed on the surface of PC cells. They engineered l-fucose-bound liposomes that encapsulated Cy5.5 or cisplatin. In vitro studies on CA19-9 expressing PC cells showed that l-fucose-bound liposomes encapsulating either Cy5.5 or cisplatin were effectively delivered and in mouse xenograft models, cisplatin-loaded liposomes were successfully delivered to PC cells and inhibited tumor growth (Yoshida et al., 2012). Furthermore, in the second-line setting of metastatic PDAC following administration of GEM-based regimens, nanoliposomes irinotecan (nal-IRI) was approved by the FDA in combination with 5-FU and leucovorin (5-FU/LV) in 2015. In phase III, the NAPOLI-1 trial, the median progression-free survival (PFS) was 3.1 and 1.5 months (p < 0.001) in patients who received nal-IRI + 5-FU/LV and patient’s 5-FU/LV alone, respectively (Wang-Gillam et al., 2016). In the final OS analysis of the NAPOLI-1 trial, the median OS was increased by 2 months (p = 0.042) in the nal-IRI + 5-FU/LV group (Wang-Gillam et al., 2016).
Nanogels in PC
Nanogels can utilize the unique characteristics of tumor microenvironments such as pH and temperature, to release drugs within the cell, resulting in efficient drug delivery (Damaghi et al., 2013). In a study by Damaghi et al., a nanogel-based platform for PC therapy was reported (Soni et al., 2019). They developed a cisplatin-loaded, mAb-coated nanogel for targeted delivery and used it in combination with GEM. In vitro results revealed an increase in drug efficacy. Additionally, enhanced drug delivery and synergistic cytotoxic effect were observed after sequential exposure of PC cells to GEM. Together, these studies have all demonstrated the advantage and improved therapeutic outcomes with the use of nanomaterials and nano-drug platforms, particularly and most importantly against MDR in experimental models and clinical trials (Kesharwani et al., 2015; Borsoi et al., 2017; Guo et al., 2018; Kong et al., 2019). Such advantage to the use of nanomaterials is mediated through a wide range of mechanisms, including enhanced cellular uptake, evading endosomal-lysosomal drug breakdown, inhibition of drug efflux, and increasing plasma half-life.
Key limitations on the clinical translation of nanomedicine in PC
There has been a tremendous effort to understand the structural and functional properties of nanoparticles directed against cancer but their translation to clinical practice has been largely limited. This can primarily be attributed to a poor understanding of the biological barriers and nanomaterial behavior inside the body and cells, as well as the overemphasis and relying on animal models during pre-clinical evaluation, which does not necessarily represent the same disease phenotype in humans (Gonzalez-Valdivieso et al., 2021).
There are several major challenges in the treatment of PC, which need to be overcome to make the use of nanotherapies a success against PCs. These include off-target toxicity, low bioavailability of chemotherapeutic drugs, and undesirable pharmacokinetics. One way to address these obstacles is through the use of nanotechnology as an effective vehicle for chemotherapeutic drugs. Currently, only 16 nano-based cancer drugs are approved by FDA and around 75 nanoformulations are being investigated in clinical trials (He et al., 2019). It is extremely important to narrow the gap between preclinical toxicity studies and toxicity studies in patients, as nanomedicines have been shown to exert additional unintended and often toxic effects on normal cellular function. Moreover, there has been a lack of convincing data on the process of excretion of nanomedicines from the human system, as most data is available from animal disease models. Nanomedicines can pose safety issues at different levels (apart from the intrinsic toxicity of the API itself). Furthermore, the biodistribution of nanoparticles changes unpredictably resulting in uptake and accumulation in certain organs, which may result in target off-target effects and local overexposure. Indeed, some nanoparticles have a known tendency to accumulate in lymphoid organs and kidneys (for some polymer-bound drugs) (Metselaar and Lammers, 2020).
Furthermore, there remains a general lack of understanding on the cost-effectiveness, manufacturing, and scaling up, as well as regulation with regard to using nanomedicines for cancer (van der Meel et al., 2017). As the science behind the structural-functional relationship provides clarity on the interaction of nanomedicines in vivo, the regulatory challenges must be addressed simultaneously to bring these potentially game-changing therapeutics to the frontline against fighting pancreatic cancer.
Conclusion and future directions
Numerous noncarriers have been developed and investigated for the treatment of PC to overcome the problem of MDR seen with chemotherapy and other therapeutic options, however, with limited success Even though nano-based carriers show great promise in treating various cancers, they have several limitations, including potential toxicity, difficult scalability, and low loading efficiency that could be responsible for their low success rate reaching clinical settings. Notably, the albumin-based nanocarrier was the most successful in clinical studies for PC. This is mainly because albumin nanoparticles were successful in encapsulating widely used chemotherapeutics that are less soluble/insoluble in water. Additionally, albumin is highly biocompatibility and biodegradability, making it an attractive material for drug delivery applications. The development of future therapies for cancer and nano-based therapeutics should not be limited by designing nanocarriers only for passive targeting of cancerous cells. Internalization of chemotherapeutic agents into tumor cells can be further improved via the utilization of an active targeting approach to enhance drug delivery efficacy. Additionally, research into novel biomarkers to enable active targeting will empower delivery strategies of nanocarriers to combat cancer resistance.
While we have certainly made huge progress in understanding the drug resistance mechanisms in PC and the signaling pathways responsible for PC cell metastases, investigations on the use of nanomedicine in this field lag behind. Currently, the majority of work in the field of nanomedicine is largely focused on increasing drug stability, accumulation, and targeting, which is yet of critical importance, particularly in nucleoside transporter (e.g., ENT1 and CNT1)-mediated drug resistance against GEM (Hung et al., 2015; Poon et al., 2015). Future studies utilizing nanotechnology against MDR pancreatic cancers should integrate multiple modalities and exploit the rapidly accumulating mechanistic knowledge in this cancer model (e.g., targeting PCSCs, dual delivery of potential drug modalities, etc.). In addition, utilizing the endogenous properties of the TME to trigger the release of cancer therapeutics from nanocarriers should be considered during the delivery system design. Such a design will add another dimension of controlled release that can impact clinical efficacy, where adverse effects can be minimized while retaining therapeutic benefits.
The main goal of PC treatment is to enhance the efficiency of drug delivery and minimize drug resistance. Despite the tremendous effort in making novel nanocarriers in pre-clinical settings, the development of clinical translation to the bedside remains laborious. Extrapolation of scientific findings from animals to humans is extremely challenging, mainly due to differences in physiology and anatomy between species, making direct extrapolation unreliable. Furthermore, unlike the experimental settings in clinical studies, animals are designed with syngeneic backgrounds, and disease models are designed to produce as homogenous a population as possible. On the other hand, heterogeneity is the basis of ineffectiveness in clinical trials. Moreover, individual variability in lifestyle and disease progression plays key roles in the overall efficacy, unlike the well-controlled animal experiments.
The promise of nanomedicine will be realized by moving away from designing a targeting strategy against a single target to including targeting approaches that address multiple signaling mechanisms and molecular targets, considering the complexity of both the human physiology and the tumor microenvironment, including the development of MDR mechanisms.
Author contributions
Conception of the work, draft, and revision of the work: AKA, NBA, NA, RA, and AA.
Acknowledgments
The authors are thankful to the Research Center, College of Pharmacy, and the Deanship of Scientific Research, King Saud University for their support to conduct this work.
Conflict of interest
The authors declare that the research was conducted in the absence of any commercial or financial relationships that could be construed as a potential conflict of interest.
Publisher’s note
All claims expressed in this article are solely those of the authors and do not necessarily represent those of their affiliated organizations, or those of the publisher, the editors, and the reviewers. Any product that may be evaluated in this article, or claim that may be made by its manufacturer, is not guaranteed or endorsed by the publisher.
References
Aguirre, A. J., and Collisson, E. A. (2017). Advances in the genetics and biology of pancreatic cancer. Cancer J. 23 (6), 315–320. doi:10.1097/PPO.0000000000000286
Alshememry, A. K., El-Tokhy, S. S., and Unsworth, L. D. (2017). Using properties of tumor microenvironments for controlling local, on-demand delivery from biopolymer-based nanocarriers. Curr. Pharm. Des. 23 (35), 5358–5391. doi:10.2174/1381612823666170522100545
Alzhrani, R., Alsaab, H. O., Vanamala, K., Bhise, K., Tatiparti, K., Barari, A., et al. (2021). Overcoming the tumor microenvironmental barriers of pancreatic ductal adenocarcinomas for achieving better treatment outcomes. Adv. Ther. 4 (6), 2000262. doi:10.1002/adtp.202000262
Arumugam, T., Ramachandran, V., Fournier, K. F., Wang, H., Marquis, L., Abbruzzese, J. L., et al. (2009). Epithelial to mesenchymal transition contributes to drug resistance in pancreatic cancer. Cancer Res. 69 (14), 5820–5828. doi:10.1158/0008-5472.CAN-08-2819
Avula, L. R., Hagerty, B., and Alewine, C. (2020). Molecular mediators of peritoneal metastasis in pancreatic cancer. Cancer Metastasis Rev. 39 (4), 1223–1243. doi:10.1007/s10555-020-09924-4
Ayers, D., and Nasti, A. (2012). Utilisation of nanoparticle technology in cancer chemoresistance. J. Drug Deliv. 2012, 265691. doi:10.1155/2012/265691
Bailey, P., Chang, D. K., Nones, K., Johns, A. L., Patch, A. M., Gingras, M. C., et al. (2016). Genomic analyses identify molecular subtypes of pancreatic cancer. Nature 531 (7592), 47–52. doi:10.1038/nature16965
Bardeesy, N., and DePinho, R. A. (2002). Pancreatic cancer biology and genetics. Nat. Rev. Cancer 2 (12), 897–909. doi:10.1038/nrc949
Baretti, M., Pulluri, B., Tsai, H. L., Blackford, A. L., Wolfgang, C. L., Laheru, D., et al. (2019). The significance of ascites in patients with pancreatic ductal adenocarcinoma: A case-control study. Pancreas 48 (4), 585–589. doi:10.1097/mpa.0000000000001262
Batlle, E., and Clevers, H. (2017). Cancer stem cells revisited. Nat. Med. 23 (10), 1124–1134. doi:10.1038/nm.4409
Berlin, J. D., Catalano, P., Thomas, J. P., Kugler, J. W., Haller, D. G., and Benson, A. B. (2002). Phase III study of gemcitabine in combination with fluorouracil versus gemcitabine alone in patients with advanced pancreatic carcinoma: Eastern Cooperative Oncology Group Trial E2297. J. Clin. Oncol. 20 (15), 3270–3275. doi:10.1200/JCO.2002.11.149
Binenbaum, Y., Na'ara, S., and Gil, Z. (2015). Gemcitabine resistance in pancreatic ductal adenocarcinoma. Drug resist. updat. 23, 55–68. doi:10.1016/j.drup.2015.10.002
Blanco, E., Hsiao, A., Mann, A. P., Landry, M. G., Meric-Bernstam, F., and Ferrari, M. (2011). Nanomedicine in cancer therapy: Innovative trends and prospects. Cancer Sci. 102 (7), 1247–1252. doi:10.1111/j.1349-7006.2011.01941.x
Borsoi, C., Leonard, F., Lee, Y., Zaid, M., Elganainy, D., Alexander, J. F., et al. (2017). Gemcitabine enhances the transport of nanovector-albumin-bound paclitaxel in gemcitabine-resistant pancreatic ductal adenocarcinoma. Cancer Lett. 403, 296–304. doi:10.1016/j.canlet.2017.06.026
Bray, F., Ferlay, J., Soerjomataram, I., Siegel, R. L., Torre, L. A., and Jemal, A. (2018). Global cancer statistics 2018: GLOBOCAN estimates of incidence and mortality worldwide for 36 cancers in 185 countries. Ca. Cancer J. Clin. 68 (6), 394–424. doi:10.3322/caac.21492
Burris, H. A., Moore, M. J., Andersen, J., Green, M. R., Rothenberg, M. L., Modiano, M. R., et al. (1997). Improvements in survival and clinical benefit with gemcitabine as first-line therapy for patients with advanced pancreas cancer: A randomized trial. J. Clin. Oncol. 15 (6), 2403–2413. doi:10.1200/JCO.1997.15.6.2403
Cancer.Net (2022). Pancreatic Cancer: Statistics [Online]. Available: https://www.cancer.net/cancer-types/pancreatic-cancer/statistics (Accessed July 15, 2022).
Cascinu, S., Verdecchia, L., Valeri, N., Berardi, R., and Scartozzi, M. (2006). New target therapies in advanced pancreatic cancer. Ann. Oncol. 17 (5), v148–152. doi:10.1093/annonc/mdj971
Chen, H., Guo, Q., Chu, Y., Li, C., Zhang, Y., Liu, P., et al. (2022). Smart hypoxia-responsive transformable and charge-reversible nanoparticles for the deep penetration and tumor microenvironment modulation of pancreatic cancer. Biomaterials 287, 121599. doi:10.1016/j.biomaterials.2022.121599
Chiba, F., and Twyman, L. J. (2017). Effect of terminal-group functionality on the ability of dendrimers to bind proteins. Bioconjug. Chem. 28 (8), 2046–2050. doi:10.1021/acs.bioconjchem.7b00350
Corbo, V., Tortora, G., and Scarpa, A. (2012). Molecular pathology of pancreatic cancer: From bench-to-bedside translation. Curr. Drug Targets 13 (6), 744–752. doi:10.2174/138945012800564103
Cowgill, S. M., and Muscarella, P. (2003). The genetics of pancreatic cancer. Am. J. Surg. 186 (3), 279–286. doi:10.1016/s0002-9610(03)00226-5
Damaghi, M., Wojtkowiak, J. W., and Gillies, R. J. (2013). pH sensing and regulation in cancer. Front. Physiol. 4, 370. doi:10.3389/fphys.2013.00370
Danilova, L., Ho, W. J., Zhu, Q., Vithayathil, T., De Jesus-Acosta, A., Azad, N. S., et al. (2019). Programmed cell death ligand-1 (PD-L1) and CD8 expression profiling identify an immunologic subtype of pancreatic ductal adenocarcinomas with favorable survival. Cancer Immunol. Res. 7 (6), 886–895. doi:10.1158/2326-6066.CIR-18-0822
Das, S. K., Wang, J. L., Li, B., Zhang, C., and Yang, H. F. (2019). Clinical effectiveness of combined interventional therapy as a salvage modality for unresectable pancreatic carcinoma. Oncol. Lett. 18 (1), 375–385. doi:10.3892/ol.2019.10323
Dauer, P., Nomura, A., Saluja, A., and Banerjee, S. (2017). Microenvironment in determining chemo-resistance in pancreatic cancer: Neighborhood matters. Pancreatology 17 (1), 7–12. doi:10.1016/j.pan.2016.12.010
Demidenko, Z. N., Kalurupalle, S., Hanko, C., Lim, C. U., Broude, E., and Blagosklonny, M. V. (2008). Mechanism of G1-like arrest by low concentrations of paclitaxel: Next cell cycle p53-dependent arrest with sub G1 DNA content mediated by prolonged mitosis. Oncogene 27 (32), 4402–4410. doi:10.1038/onc.2008.82
di Magliano, M. P., and Logsdon, C. D. (2013). Roles for KRAS in pancreatic tumor development and progression. Gastroenterology 144 (6), 1220–1229. doi:10.1053/j.gastro.2013.01.071
Dreyer, S. B., Chang, D. K., Bailey, P., and Biankin, A. V. (2017). Pancreatic cancer genomes: Implications for clinical management and therapeutic development. Clin. Cancer Res. 23 (7), 1638–1646. doi:10.1158/1078-0432.CCR-16-2411
Du, Z., Qin, R., Wei, C., Wang, M., Shi, C., Tian, R., et al. (2011). Pancreatic cancer cells resistant to chemoradiotherapy rich in "stem-cell-like" tumor cells. Dig. Dis. Sci. 56 (3), 741–750. doi:10.1007/s10620-010-1340-0
Ducreux, M., Cuhna, A. S., Caramella, C., Hollebecque, A., Burtin, P., Goere, D., et al. (2015). Cancer of the pancreas: ESMO clinical practice guidelines for diagnosis, treatment and follow-up. Ann. Oncol. 26 (5), v56–68. doi:10.1093/annonc/mdv295
Elgogary, A., Xu, Q., Poore, B., Alt, J., Zimmermann, S. C., Zhao, L., et al. (2016). Combination therapy with BPTES nanoparticles and metformin targets the metabolic heterogeneity of pancreatic cancer. Proc. Natl. Acad. Sci. U. S. A. 113 (36), E5328–E5336. doi:10.1073/pnas.1611406113
Ellenrieder, V., Hendler, S. F., Boeck, W., Seufferlein, T., Menke, A., Ruhland, C., et al. (2001). Transforming growth factor beta1 treatment leads to an epithelial-mesenchymal transdifferentiation of pancreatic cancer cells requiring extracellular signal-regulated kinase 2 activation. Cancer Res. 61 (10), 4222–4228.
Erkan, M., Hausmann, S., Michalski, C. W., Fingerle, A. A., Dobritz, M., Kleeff, J., et al. (2012). The role of stroma in pancreatic cancer: Diagnostic and therapeutic implications. Nat. Rev. Gastroenterol. Hepatol. 9 (8), 454–467. doi:10.1038/nrgastro.2012.115
Farran, B., and Nagaraju, G. P. (2020). Exosomes as therapeutic solutions for pancreatic cancer. Drug Discov. Today 25 (12), 2245–2256. doi:10.1016/j.drudis.2020.09.037
Feig, C., Jones, J. O., Kraman, M., Wells, R. J., Deonarine, A., Chan, D. S., et al. (2013). Targeting CXCL12 from FAP-expressing carcinoma-associated fibroblasts synergizes with anti-PD-L1 immunotherapy in pancreatic cancer. Proc. Natl. Acad. Sci. U. S. A. 110 (50), 20212–20217. doi:10.1073/pnas.1320318110
Gad, A., Kydd, J., Piel, B., and Rai, P. (2016). Targeting cancer using polymeric nanoparticle mediated combination chemotherapy. Int. J. Nanomed. Nanosurg. 2 (3), 10.16966/2470-3206.116. doi:10.16966/2470-3206.116
Ge, W., Chen, G., and Fan, X. S. (2017). Pathway of peritoneal carcinomatosis maybe hematogenous metastasis rather than peritoneal seeding. Oncotarget 8 (25), 41549–41554. doi:10.18632/oncotarget.14607
Ghaneh, P., Costello, E., and Neoptolemos, J. P. (2007). Biology and management of pancreatic cancer. Postgrad. Med. J. 56 (8), 478–497. doi:10.1136/gut.2006.103333
Gheorghe, G., Bungau, S., Ilie, M., Behl, T., Vesa, C. M., Brisc, C., et al. (2020). Early diagnosis of pancreatic cancer: The key for survival. Diagn. (Basel) 10 (11), E869. doi:10.3390/diagnostics10110869
Goldstein, D., El-Maraghi, R. H., Hammel, P., Heinemann, V., Kunzmann, V., Sastre, J., et al. (2015). nab-Paclitaxel plus gemcitabine for metastatic pancreatic cancer: long-term survival from a phase III trial. J. Natl. Cancer Inst. 107 (2), dju413. doi:10.1093/jnci/dju413
Gong, J., Chen, M., Zheng, Y., Wang, S., and Wang, Y. (2012). Polymeric micelles drug delivery system in oncology. J. Control. Release 159 (3), 312–323. doi:10.1016/j.jconrel.2011.12.012
Gonzalez-Valdivieso, J., Girotti, A., Schneider, J., and Arias, F. J. (2021). Advanced nanomedicine and cancer: Challenges and opportunities in clinical translation. Int. J. Pharm. 599, 120438. doi:10.1016/j.ijpharm.2021.120438
Goodman, A. M., Kato, S., Bazhenova, L., Patel, S. P., Frampton, G. M., Miller, V., et al. (2017). Tumor mutational burden as an independent predictor of response to immunotherapy in diverse cancers. Mol. Cancer Ther. 16 (11), 2598–2608. doi:10.1158/1535-7163.MCT-17-0386
Govindan, R., Fakih, M., Price, T., Falchook, G., Desai, J., Kuo, J., et al. (2019). OA02. 02 Phase 1 study of safety, tolerability, PK and efficacy of AMG 510, a novel KRASG12C inhibitor, evaluated in NSCLC. J. Thorac. Oncol. 14 (10), S208. doi:10.1016/j.jtho.2019.08.412
Guo, Z., Wang, F., Di, Y., Yao, L., Yu, X., Fu, D., et al. (2018). Antitumor effect of gemcitabine-loaded albumin nanoparticle on gemcitabine-resistant pancreatic cancer induced by low hENT1 expression. Int. J. Nanomedicine 13, 4869–4880. doi:10.2147/IJN.S166769
Gurunathan, S., Kang, M. H., Qasim, M., and Kim, J. H. (2018). Nanoparticle-mediated combination therapy: Two-in-One approach for cancer. Int. J. Mol. Sci. 19 (10), E3264. doi:10.3390/ijms19103264
Hackeng, W. M., Hruban, R. H., Offerhaus, G. J., and Brosens, L. A. (2016). Surgical and molecular pathology of pancreatic neoplasms. Diagn. Pathol. 11 (1), 47. doi:10.1186/s13000-016-0497-z
Hamaguchi, T., Kato, K., Yasui, H., Morizane, C., Ikeda, M., Ueno, H., et al. (2007). A phase I and pharmacokinetic study of NK105, a paclitaxel-incorporating micellar nanoparticle formulation. Br. J. Cancer 97 (2), 170–176. doi:10.1038/sj.bjc.6603855
Han, H., Wang, J., Chen, T., Yin, L., Jin, Q., and Ji, J. (2017). Enzyme-sensitive gemcitabine conjugated albumin nanoparticles as a versatile theranostic nanoplatform for pancreatic cancer treatment. J. Colloid Interface Sci. 507, 217–224. doi:10.1016/j.jcis.2017.07.047
He, H., Liu, L., Morin, E. E., Liu, M., and Schwendeman, A. (2019). Survey of clinical translation of cancer nanomedicines-lessons learned from successes and failures. Acc. Chem. Res. 52 (9), 2445–2461. doi:10.1021/acs.accounts.9b00228
He, X., Chen, X., Liu, L., Zhang, Y., Lu, Y., Zhang, Y., et al. (2018). Sequentially triggered nanoparticles with tumor penetration and intelligent drug release for pancreatic cancer therapy. Adv. Sci. 5 (5), 1701070. doi:10.1002/advs.201701070
Heidemann, S. R., and Kirschner, M. W. (1978). Induced formation of asters and cleavage furrows in oocytes of Xenopus laevis during in vitro maturation. J. Exp. Zool. 204 (3), 431–444. doi:10.1002/jez.1402040314
Heinemann, V., Quietzsch, D., Gieseler, F., Gonnermann, M., Schonekas, H., Rost, A., et al. (2006). Randomized phase III trial of gemcitabine plus cisplatin compared with gemcitabine alone in advanced pancreatic cancer. J. Clin. Oncol. 24 (24), 3946–3952. doi:10.1200/JCO.2005.05.1490
Hermann, P. C., Huber, S. L., Herrler, T., Aicher, A., Ellwart, J. W., Guba, M., et al. (2007). Distinct populations of cancer stem cells determine tumor growth and metastatic activity in human pancreatic cancer. Cell Stem Cell 1 (3), 313–323. doi:10.1016/j.stem.2007.06.002
Hiss, D. C., Gabriels, G. A., and Folb, P. I. (2007). Combination of tunicamycin with anticancer drugs synergistically enhances their toxicity in multidrug-resistant human ovarian cystadenocarcinoma cells. Cancer Cell Int. 7, 5. doi:10.1186/1475-2867-7-5
Ho, W. J., Jaffee, E. M., and Zheng, L. (2020). The tumour microenvironment in pancreatic cancer - clinical challenges and opportunities. Nat. Rev. Clin. Oncol. 17 (9), 527–540. doi:10.1038/s41571-020-0363-5
Hosein, P. J., de Lima Lopes, G., Pastorini, V. H., Gomez, C., Macintyre, J., Zayas, G., et al. (2013). A phase II trial of nab-Paclitaxel as second-line therapy in patients with advanced pancreatic cancer. Am. J. Clin. Oncol. 36 (2), 151–156. doi:10.1097/COC.0b013e3182436e8c
Hosoki, T. (1983). Dynamic CT of pancreatic tumors. AJR. Am. J. Roentgenol. 140 (5), 959–965. doi:10.2214/ajr.140.5.959
Hu, X., Xia, F., Lee, J., Li, F., Lu, X., Zhuo, X., et al. (2021). Tailor-made nanomaterials for diagnosis and therapy of pancreatic ductal adenocarcinoma. Adv. Sci. 8 (7), 2002545. doi:10.1002/advs.202002545
Hung, S. W., Marrache, S., Cummins, S., Bhutia, Y. D., Mody, H., Hooks, S. B., et al. (2015). Defective hCNT1 transport contributes to gemcitabine chemoresistance in ovarian cancer subtypes: Overcoming transport defects using a nanoparticle approach. Cancer Lett. 359 (2), 233–240. doi:10.1016/j.canlet.2015.01.017
Jiang, B., Zhou, L., Lu, J., Wang, Y., Liu, C., You, L., et al. (2020). Stroma-targeting therapy in pancreatic cancer: One coin with two sides? Front. Oncol. 10, 576399. doi:10.3389/fonc.2020.576399
Kapse-Mistry, S., Govender, T., Srivastava, R., and Yergeri, M. (2014). Nanodrug delivery in reversing multidrug resistance in cancer cells. Front. Pharmacol. 5, 159. doi:10.3389/fphar.2014.00159
Kashyap, D., Tuli, H. S., Yerer, M. B., Sharma, A., Sak, K., Srivastava, S., et al. (2019). Natural product-based nanoformulations for cancer therapy: Opportunities and challenges. Semin. Cancer Biol. 69, 5–23. doi:10.1016/j.semcancer.2019.08.014
Kesharwani, P., Banerjee, S., Padhye, S., Sarkar, F. H., and Iyer, A. K. (2015). Hyaluronic acid engineered nanomicelles loaded with 3, 4-difluorobenzylidene curcumin for targeted killing of CD44+ stem-like pancreatic cancer cells. Biomacromolecules 16 (9), 3042–3053. doi:10.1021/acs.biomac.5b00941
Khan, S., Chauhan, N., Yallapu, M. M., Ebeling, M. C., Balakrishna, S., Ellis, R. T., et al. (2015). Nanoparticle formulation of ormeloxifene for pancreatic cancer. Biomaterials 53, 731–743. doi:10.1016/j.biomaterials.2015.02.082
Khan, S., Setua, S., Kumari, S., Dan, N., Massey, A., Hafeez, B. B., et al. (2019). Superparamagnetic iron oxide nanoparticles of curcumin enhance gemcitabine therapeutic response in pancreatic cancer. Biomaterials 208, 83–97. doi:10.1016/j.biomaterials.2019.04.005
Klebowski, B., Depciuch, J., Parlinska-Wojtan, M., and Baran, J. (2018). Applications of noble metal-based nanoparticles in medicine. Int. J. Mol. Sci. 19 (12), E4031. doi:10.3390/ijms19124031
Kong, C., Li, Y., Liu, Z., Ye, J., Wang, Z., Zhang, L., et al. (2019). Targeting the oncogene KRAS mutant pancreatic cancer by synergistic blocking of lysosomal acidification and rapid drug release. ACS Nano 13 (4), 4049–4063. doi:10.1021/acsnano.8b08246
Kraman, M., Bambrough, P. J., Arnold, J. N., Roberts, E. W., Magiera, L., Jones, J. O., et al. (2010). Suppression of antitumor immunity by stromal cells expressing fibroblast activation protein-alpha. Science 330 (6005), 827–830. doi:10.1126/science.1195300
Ladd, E., Sheikhi, A., Li, N., van de Ven, T. G. M., and Kakkar, A. (2017). Design and synthesis of dendrimers with facile surface group functionalization, and an evaluation of their bactericidal efficacy. Molecules 22 (6), E868. doi:10.3390/molecules22060868
Li, D., and Jiao, L. (2003). Molecular epidemiology of pancreatic cancer. Int. J. Gastrointest. Cancer 33 (1), 3–14. doi:10.1385/IJGC:33:1:3
Li, Y., VandenBoom, T. G., Kong, D., Wang, Z., Ali, S., Philip, P. A., et al. (2009). Up-regulation of miR-200 and let-7 by natural agents leads to the reversal of epithelial-to-mesenchymal transition in gemcitabine-resistant pancreatic cancer cells. Cancer Res. 69 (16), 6704–6712. doi:10.1158/0008-5472.CAN-09-1298
Libutti, S. K., Paciotti, G. F., Byrnes, A. A., Alexander, H. R., Gannon, W. E., Walker, M., et al. (2010). Phase I and pharmacokinetic studies of CYT-6091, a novel PEGylated colloidal gold-rhTNF nanomedicine. Clin. Cancer Res. 16 (24), 6139–6149. doi:10.1158/1078-0432.CCR-10-0978
Libutti, S. K., Tamarkin, L., and Nilubol, N. (2018). Targeting the invincible barrier for drug delivery in solid cancers: Interstitial fluid pressure. Oncotarget 9 (87), 35723–35725. doi:10.18632/oncotarget.26267
Lin, Z., Lu, S., Xie, X., Yi, X., and Huang, H. (2020). Noncoding RNAs in drug-resistant pancreatic cancer: A review. Biomed. Pharmacother. 131, 110768. doi:10.1016/j.biopha.2020.110768
Liu, L., Kshirsagar, P., Christiansen, J., Gautam, S. K., Aithal, A., Gulati, M., et al. (2021). Polyanhydride nanoparticles stabilize pancreatic cancer antigen MUC4β. J. Biomed. Mat. Res. A 109 (6), 893–902. doi:10.1002/jbm.a.37080
Liu, L., Kshirsagar, P. G., Gautam, S. K., Gulati, M., Wafa, E. I., Christiansen, J. C., et al. (2022). Nanocarriers for pancreatic cancer imaging, treatments, and immunotherapies. Theranostics 12 (3), 1030–1060. doi:10.7150/thno.64805
Lobato-Mendizabal, E., and Ruiz-Arguelles, G. J. (1990). Leukemia and malnutrition. II. The magnitude of maintenance chemotherapy as a prognostic factor in the survival of patients with standard-risk acute lymphoblastic leukemia. Rev. Invest. Clin. 42 (2), 81–87.
Lucero-Acuna, A., Jeffery, J. J., Abril, E. R., Nagle, R. B., Guzman, R., Pagel, M. D., et al. (2014). Nanoparticle delivery of an AKT/PDK1 inhibitor improves the therapeutic effect in pancreatic cancer. Int. J. Nanomedicine 9, 5653–5665. doi:10.2147/IJN.S68511
Macarulla, T., Pazo-Cid, R., Guillen-Ponce, C., Lopez, R., Vera, R., Reboredo, M., et al. (2019). Phase I/II trial to evaluate the efficacy and safety of nanoparticle albumin-bound paclitaxel in combination with gemcitabine in patients with pancreatic cancer and an ECOG performance status of 2. J. Clin. Oncol. 37 (3), 230–238. doi:10.1200/JCO.18.00089
Manzur, A., Oluwasanmi, A., Moss, D., Curtis, A., and Hoskins, C. (2017). Nanotechnologies in pancreatic cancer therapy. Pharmaceutics 9 (4), E39. doi:10.3390/pharmaceutics9040039
McCarroll, J., Teo, J., Boyer, C., Goldstein, D., Kavallaris, M., and Phillips, P. A. (2014). Potential applications of nanotechnology for the diagnosis and treatment of pancreatic cancer. Front. Physiol. 5, 2. doi:10.3389/fphys.2014.00002
Melancon, M. P., Stafford, R. J., and Li, C. (2012). Challenges to effective cancer nanotheranostics. J. Control. Release 164 (2), 177–182. doi:10.1016/j.jconrel.2012.07.045
Metselaar, J. M., and Lammers, T. (2020). Challenges in nanomedicine clinical translation. Drug Deliv. Transl. Res. 10 (3), 721–725. doi:10.1007/s13346-020-00740-5
Min, C., Eddy, S. F., Sherr, D. H., and Sonenshein, G. E. (2008). NF-kappaB and epithelial to mesenchymal transition of cancer. J. Cell. Biochem. 104 (3), 733–744. doi:10.1002/jcb.21695
Mohelnikova-Duchonova, B., Brynychova, V., Oliverius, M., Honsova, E., Kala, Z., Muckova, K., et al. (2013). Differences in transcript levels of ABC transporters between pancreatic adenocarcinoma and nonneoplastic tissues. Pancreas 42 (4), 707–716. doi:10.1097/MPA.0b013e318279b861
Nieto, M. A., Huang, R. Y., Jackson, R. A., and Thiery, J. P. (2016). Emt: 2016. Cell 166 (1), 21–45. doi:10.1016/j.cell.2016.06.028
O'Driscoll, L., Walsh, N., Larkin, A., Ballot, J., Ooi, W. S., Gullo, G., et al. (2007). MDR1/P-glycoprotein and MRP-1 drug efflux pumps in pancreatic carcinoma. Anticancer Res. 27 (4B), 2115–2120.
Obaid, G., Bano, S., Thomsen, H., Callaghan, S., Shah, N., Swain, J. W. R., et al. (2022). Remediating desmoplasia with EGFR-targeted photoactivable multi-inhibitor liposomes doubles overall survival in pancreatic cancer. Adv. Sci. (Weinh)., 24, e2104594. doi:10.1002/advs.202104594
Ogawa, K., and Miura, T. (2014). Aphid polyphenisms: trans-generational developmental regulation through viviparity. Front. Physiol. 5, 1. doi:10.3389/fphys.2014.00001
Oliveira, C., Calmeiro, J., Carrascal, M. A., Falcão, A., Gomes, C., Miguel Neves, B., et al. (2021). Exosomes as new therapeutic vectors for pancreatic cancer treatment. Eur. J. Pharm. Biopharm. 161, 4–14. doi:10.1016/j.ejpb.2021.02.002
Pandya, G., Kirtonia, A., Sethi, G., Pandey, A. K., and Garg, M. (2020). The implication of long non-coding RNAs in the diagnosis, pathogenesis and drug resistance of pancreatic ductal adenocarcinoma and their possible therapeutic potential. Biochim. Biophys. Acta. Rev. Cancer 1874 (2), 188423. doi:10.1016/j.bbcan.2020.188423
Parhi, P., Mohanty, C., and Sahoo, S. K. (2012). Nanotechnology-based combinational drug delivery: An emerging approach for cancer therapy. Drug Discov. Today 17 (17-18), 1044–1052. doi:10.1016/j.drudis.2012.05.010
Poon, C., He, C., Liu, D., Lu, K., and Lin, W. (2015). Self-assembled nanoscale coordination polymers carrying oxaliplatin and gemcitabine for synergistic combination therapy of pancreatic cancer. J. Control. Release 201, 90–99. doi:10.1016/j.jconrel.2015.01.026
Prabhu, P., and Patravale, V. (2012). The upcoming field of theranostic nanomedicine: An overview. J. Biomed. Nanotechnol. 8 (6), 859–882. doi:10.1166/jbn.2012.1459
Rawla, P., Sunkara, T., and Gaduputi, V. (2019). Epidemiology of pancreatic cancer: Global trends, etiology and risk factors. World J. Oncol. 10 (1), 10–27. doi:10.14740/wjon1166
Rebelo, A., Molpeceres, J., Rijo, P., and Reis, C. P. (2017). Pancreatic cancer therapy review: From classic therapeutic agents to modern nanotechnologies. Curr. Drug Metab. 18 (4), 346–359. doi:10.2174/1389200218666170201151135
Rishi, A., Goggins, M., Wood, L. D., and Hruban, R. H. (2015). Pathological and molecular evaluation of pancreatic neoplasms. Semin. Oncol. 42 (1), 28–39. doi:10.1053/j.seminoncol.2014.12.004
Ryan, D. P., Hong, T. S., and Bardeesy, N. (2014). Pancreatic adenocarcinoma. N. Engl. J. Med. 371 (11), 1039–1049. doi:10.1056/NEJMra1404198
Schizas, D., Charalampakis, N., Kole, C., Economopoulou, P., Koustas, E., Gkotsis, E., et al. (2020). Immunotherapy for pancreatic cancer: A 2020 update. Cancer Treat. Rev. 86, 102016. doi:10.1016/j.ctrv.2020.102016
Schroeder, A., Heller, D. A., Winslow, M. M., Dahlman, J. E., Pratt, G. W., Langer, R., et al. (2011). Treating metastatic cancer with nanotechnology. Nat. Rev. Cancer 12 (1), 39–50. doi:10.1038/nrc3180
Shah, A. N., Summy, J. M., Zhang, J., Park, S. I., Parikh, N. U., and Gallick, G. E. (2007). Development and characterization of gemcitabine-resistant pancreatic tumor cells. Ann. Surg. Oncol. 14 (12), 3629–3637. doi:10.1245/s10434-007-9583-5
Sharma, A., Goyal, A. K., and Rath, G. (2018). Recent advances in metal nanoparticles in cancer therapy. J. Drug Target. 26 (8), 617–632. doi:10.1080/1061186X.2017.1400553
Siegel, R. L., Miller, K. D., and Jemal, A. (2019). Cancer statistics, 2019. Ca. Cancer J. Clin. 69 (1), 7–34. doi:10.3322/caac.21551
Sielaff, C. M., and Mousa, S. A. (2018). Status and future directions in the management of pancreatic cancer: Potential impact of nanotechnology. J. Cancer Res. Clin. Oncol. 144 (7), 1205–1217. doi:10.1007/s00432-018-2651-3
Singh, A., and Settleman, J. (2010). EMT, cancer stem cells and drug resistance: An emerging axis of evil in the war on cancer. Oncogene 29 (34), 4741–4751. doi:10.1038/onc.2010.215
Sofuni, A., Iijima, H., Moriyasu, F., Nakayama, D., Shimizu, M., Nakamura, K., et al. (2005). Differential diagnosis of pancreatic tumors using ultrasound contrast imaging. J. Gastroenterol. 40 (5), 518–525. doi:10.1007/s00535-005-1578-z
Soni, K. S., Thomas, D., Caffrey, T., Mehla, K., Lei, F., O'Connell, K. A., et al. (2019). A polymeric nanogel-based treatment regimen for enhanced efficacy and sequential administration of synergistic drug combination in pancreatic cancer. J. Pharmacol. Exp. Ther. 370 (3), 894–901. doi:10.1124/jpet.118.255372
Stathopoulos, G. P., Boulikas, T., Vougiouka, M., Deliconstantinos, G., Rigatos, S., Darli, E., et al. (2005). Pharmacokinetics and adverse reactions of a new liposomal cisplatin (Lipoplatin): phase I study. Oncol. Rep. 13 (4), 589–595. doi:10.3892/or.13.4.589
Syrigos, K. N., Michalaki, B., Alevyzaki, F., Machairas, A., Mandrekas, D., Kindilidis, K., et al. (2002). A phase-II study of liposomal doxorubicin and docetaxel in patients with advanced pancreatic cancer. Anticancer Res. 22 (6B), 3583–3588.
Tang, S., Hang, Y., Ding, L., Tang, W., Yu, A., Zhang, C., et al. (2021). Intraperitoneal siRNA nanoparticles for augmentation of gemcitabine efficacy in the treatment of pancreatic cancer. Mol. Pharm. 18 (12), 4448–4458. doi:10.1021/acs.molpharmaceut.1c00653
Tarannum, M., Holtzman, K., Dreau, D., Mukherjee, P., and Vivero-Escoto, J. L. (2022). Nanoparticle combination for precise stroma modulation and improved delivery for pancreatic cancer. J. Control. Release 347, 425–434. doi:10.1016/j.jconrel.2022.05.019
Tempero, M. A., Malafa, M. P., Chiorean, E. G., Czito, B., Scaife, C., Narang, A. K., et al. (2019). Pancreatic adenocarcinoma, version 1.2019. J. Natl. Compr. Canc. Netw. 17 (3), 202–210. doi:10.6004/jnccn.2019.0014
Thakkar, A., Desai, P., Chenreddy, S., Modi, J., Thio, A., Khamas, W., et al. (2018). Novel nano-drug combination therapeutic regimen demonstrates significant efficacy in the transgenic mouse model of pancreatic ductal adenocarcinoma. Am. J. Cancer Res. 8 (10), 2005–2019.
Thapa, R. K., Ku, S. K., Choi, H. G., Yong, C. S., Byeon, J. H., and Kim, J. O. (2018). Vibrating droplet generation to assemble zwitterion-coated gold-graphene oxide stealth nanovesicles for effective pancreatic cancer chemo-phototherapy. Nanoscale 10 (4), 1742–1749. doi:10.1039/c7nr07603g
Urey, C., Hilmersson, K. S., Andersson, B., Ansari, D., and Andersson, R. (2017). Development and in vitro characterization of a gemcitabine-loaded MUC4-targeted immunoliposome against pancreatic ductal adenocarcinoma. Anticancer Res. 37 (11), 6031–6039. doi:10.21873/anticanres.12050
van der Meel, R., Lammers, T., and Hennink, W. E. (2017). Cancer nanomedicines: Oversold or underappreciated? Expert Opin. Drug Deliv. 14 (1), 1–5. doi:10.1080/17425247.2017.1262346
van Horssen, R., Ten Hagen, T. L., and Eggermont, A. M. (2006). TNF-Alpha in cancer treatment: Molecular insights, antitumor effects, and clinical utility. Oncologist 11 (4), 397–408. doi:10.1634/theoncologist.11-4-397
Vogel, A., Rommler-Zehrer, J., Li, J. S., McGovern, D., Romano, A., and Stahl, M. (2016). Efficacy and safety profile of nab-paclitaxel plus gemcitabine in patients with metastatic pancreatic cancer treated to disease progression: A subanalysis from a phase 3 trial (MPACT). BMC Cancer 16 (1), 817. doi:10.1186/s12885-016-2798-8
Von Hoff, D. D., Ervin, T., Arena, F. P., Chiorean, E. G., Infante, J., Moore, M., et al. (2013). Increased survival in pancreatic cancer with nab-paclitaxel plus gemcitabine. N. Engl. J. Med. 369 (18), 1691–1703. doi:10.1056/NEJMoa1304369
Von Hoff, D. D., Ramanathan, R. K., Borad, M. J., Laheru, D. A., Smith, L. S., Wood, T. E., et al. (2011). Gemcitabine plus nab-paclitaxel is an active regimen in patients with advanced pancreatic cancer: A phase I/II trial. J. Clin. Oncol. 29 (34), 4548–4554. doi:10.1200/JCO.2011.36.5742
Wang, L., Liu, X., Zhou, Q., Sui, M., Lu, Z., Zhou, Z., et al. (2017). Terminating the criminal collaboration in pancreatic cancer: Nanoparticle-based synergistic therapy for overcoming fibroblast-induced drug resistance. Biomaterials 144, 105–118. doi:10.1016/j.biomaterials.2017.08.002
Wang, Z., Li, Y., Kong, D., Banerjee, S., Ahmad, A., Azmi, A. S., et al. (2009). Acquisition of epithelial-mesenchymal transition phenotype of gemcitabine-resistant pancreatic cancer cells is linked with activation of the notch signaling pathway. Cancer Res. 69 (6), 2400–2407. doi:10.1158/0008-5472.CAN-08-4312
Wang-Gillam, A., Li, C. P., Bodoky, G., Dean, A., Shan, Y. S., Jameson, G., et al. (2016). Nanoliposomal irinotecan with fluorouracil and folinic acid in metastatic pancreatic cancer after previous gemcitabine-based therapy (NAPOLI-1): A global, randomised, open-label, phase 3 trial. Lancet 387 (10018), 545–557. doi:10.1016/S0140-6736(15)00986-1
Xia, Y., Shen, S., and Verma, I. M. (2014). NF-κB, an active player in human cancers. Cancer Immunol. Res. 2 (9), 823–830. doi:10.1158/2326-6066.CIR-14-0112
Xie, V. K., He, J., and Xie, K. (2020a). Protein arginine methylation promotes therapeutic resistance in human pancreatic cancer. Cytokine Growth Factor Rev. 55, 58–69. doi:10.1016/j.cytogfr.2020.07.011
Xie, Y., Hang, Y., Wang, Y., Sleightholm, R., Prajapati, D. R., Bader, J., et al. (2020b). Stromal modulation and treatment of metastatic pancreatic cancer with local intraperitoneal triple miRNA/siRNA nanotherapy. ACS Nano 14 (1), 255–271. doi:10.1021/acsnano.9b03978
Xu, Y., Meng, H., Du, F., Lu, W., Liu, S., Huang, J., et al. (2015). Preparation of intravenous injection nanoformulation of VESylated gemcitabine by co-assembly with TPGS and its anti-tumor activity in pancreatic tumor-bearing mice. Int. J. Pharm. 495 (2), 792–797. doi:10.1016/j.ijpharm.2015.09.030
Yonemori, K., Kurahara, H., Maemura, K., and Natsugoe, S. (2017). MicroRNA in pancreatic cancer. J. Hum. Genet. 62 (1), 33–40. doi:10.1038/jhg.2016.59
Yoshida, M., Takimoto, R., Murase, K., Sato, Y., Hirakawa, M., Tamura, F., et al. (2012). Targeting anticancer drug delivery to pancreatic cancer cells using a fucose-bound nanoparticle approach. PLoS One 7 (7), e39545. doi:10.1371/journal.pone.0039545
Yousefi, H., Yuan, J., Keshavarz-Fathi, M., Murphy, J. F., and Rezaei, N. (2017). Immunotherapy of cancers comes of age. Expert Rev. Clin. Immunol. 13 (10), 1001–1015. doi:10.1080/1744666X.2017.1366315
Yu, X., Zhu, W., Di, Y., Gu, J., Guo, Z., Li, H., et al. (2017). Triple-functional albumin-based nanoparticles for combined chemotherapy and photodynamic therapy of pancreatic cancer with lymphatic metastases. Int. J. Nanomedicine 12, 6771–6785. doi:10.2147/IJN.S131295
Yu, Z., Pestell, T. G., Lisanti, M. P., and Pestell, R. G. (2012). Cancer stem cells. Int. J. Biochem. Cell Biol. 44 (12), 2144–2151. doi:10.1016/j.biocel.2012.08.022
Yuan, Y., Cai, T., Xia, X., Zhang, R., Chiba, P., and Cai, Y. (2016). Nanoparticle delivery of anticancer drugs overcomes multidrug resistance in breast cancer. Drug Deliv. 23 (9), 3350–3357. doi:10.1080/10717544.2016.1178825
Zeng, S., Pottler, M., Lan, B., Grutzmann, R., Pilarsky, C., and Yang, H. (2019). Chemoresistance in pancreatic cancer. Int. J. Mol. Sci. 20 (18), E4504. doi:10.3390/ijms20184504
Zhang, M., Liu, E., Cui, Y., and Huang, Y. (2017). Nanotechnology-based combination therapy for overcoming multidrug-resistant cancer. Cancer Biol. Med. 14 (3), 212–227. doi:10.20892/j.issn.2095-3941.2017.0054
Zhao, Y., Zheng, Y., Zhu, Y., Zhang, Y., Zhu, H., and Liu, T. (2021a). M1 macrophage-derived exosomes loaded with Gemcitabine and deferasirox against chemoresistant pancreatic cancer. Pharmaceutics 13 (9), 1493. doi:10.3390/pharmaceutics13091493
Zhao, Y., Zheng, Y., Zhu, Y., Zhang, Y., Zhu, H., and Liu, T. (2021b). M1 macrophage-derived exosomes loaded with gemcitabine and deferasirox against chemoresistant pancreatic cancer. Pharmaceutics 13 (9), 1493. doi:10.3390/pharmaceutics13091493
Keywords: multidrug resistance, drug delivery, paclitaxel, tumor microenvironment, pancreatic cancer, non-clinical studies, clinical studies
Citation: Alshememry AK, Alsaleh NB, Alkhudair N, Alzhrani R and Alshamsan A (2022) Recent nanotechnology advancements to treat multidrug-resistance pancreatic cancer: Pre-clinical and clinical overview. Front. Pharmacol. 13:933457. doi: 10.3389/fphar.2022.933457
Received: 30 April 2022; Accepted: 29 July 2022;
Published: 24 August 2022.
Edited by:
Saeed Ahmad Khan, Kohat University of Science and Technology, PakistanReviewed by:
Riya Biswas, Auckland University of Technology, New ZealandAbimanyu Sugumaran, SRM Institute of Science and Technology, India
Shibing Wang, Zhejiang Provincial People’s Hospital, China
Copyright © 2022 Alshememry, Alsaleh, Alkhudair, Alzhrani and Alshamsan. This is an open-access article distributed under the terms of the Creative Commons Attribution License (CC BY). The use, distribution or reproduction in other forums is permitted, provided the original author(s) and the copyright owner(s) are credited and that the original publication in this journal is cited, in accordance with accepted academic practice. No use, distribution or reproduction is permitted which does not comply with these terms.
*Correspondence: Aws Alshamsan, YWFsc2hhbXNhbkBrc3UuZWR1LnNh,
†These authors have contributed equally to this work