- 1Institute of Digestive Diseases, Longhua Hospital, Shanghai University of Traditional Chinese Medicine, Shanghai, China
- 2Institute of Interdisciplinary Integrative Biomedical Research, Shanghai University of Traditional Chinese Medicine, Shanghai, China
- 3Department of Hepatology, Longhua Hospital, Shanghai University of Traditional Chinese Medicine, Shanghai, China
Nonalcoholic steatohepatitis (NASH) is a clinical syndrome with pathological changes that are similar to those of alcoholic hepatitis without a history of excessive alcohol consumption. It is a specific form of nonalcoholic fatty liver disease (NAFLD) that is characterized by hepatocyte inflammation based on hepatocellular steatosis. Further exacerbation of NASH can lead to cirrhosis, which may then progress to hepatocellular carcinoma (HCC). There is a lack of specific and effective treatments for NASH and NASH-driven HCC, and the mechanisms of the progression of NASH to HCC are unclear. Therefore, there is a need to understand the pathogenesis and progression of these diseases to identify new therapeutic approaches. Currently, an increasing number of studies are focusing on the utility of natural products in NASH, which is likely to be a promising prospect for NASH. This paper reviews the possible mechanisms of the pathogenesis and progression of NASH and NASH-derived HCC, as well as the potential therapeutic role of natural products in NASH and NASH-derived HCC.
Introduction
Nonalcoholic fatty liver disease (NAFLD) is a chronic liver disease. It progresses from fatty infiltration of the liver, known as nonalcoholic fatty liver (NAFL), to a stage of nonalcoholic steatohepatitis (NASH) with inflammatory cell infiltration and varying degrees of fibrosis (Chalasani et al., 2018). NAFLD may eventually progress to hepatocellular carcinoma (HCC) (Vilar-Gomez et al., 2018; Younes and Bugianesi, 2018). In addition to NAFLD, a number of risk factors have been associated with the development of liver cancer, such as, hepatitis B virus (HBV) and hepatitis C virus (HCV) infection, alcohol abuse, obesity, aflatoxin B1, iron accumulation and diabetes (Chang et al., 2013). The metabolism of acetaldehyde by alcohol leads to genetic mutations that regulate the antioxidant pathway leading to the formation of reactive oxygen species and subsequent DNA damage leading to the development of hepatocellular carcinoma. HBV DNA is integrated into host DNA and the HBx viral protein leads to genetic instability and epigenetic modifications leading to genetic mutations. Replication, through various structural and non-structural proteins, HCV infection occurs through the induction of inflammation, reactive oxygen species, angiogenesis and blockage of apoptotic pathways, culminating in the development of hepatocellular carcinoma (Rawat et al., 2018).
In particular, liver-related mortality (e.g., cirrhosis and HCC) is significantly increased in the NASH population (Musso et al., 2011). Furthermore, the incidence of NASH-related hepatocellular carcinoma (NASH-HCC) or NAFLD-related hepatocellular carcinoma (NAFLD-HCC) has increased significantly worldwide and is becoming the most common cause of liver cancer (Degasperi and Colombo, 2016). NASH-HCC has a worse prognosis than other causes of liver cancer (Argyrou et al., 2017). Despite being an important cause of liver cancer, the exact mechanism by which NASH progresses to HCC remains unknown. Currently, there is a lack of effective therapeutic agents for NASH, and there is a lack of tools and indicators to prevent and monitor the progression of NASH to HCC. Therefore, it is of great practical importance to strengthen the research on the pathogenesis of NASH-HCC and find effective drugs to prevent and treat NASH-HCC.
Natural products, especially monomeric compounds isolated from herbal medicines, are active substances with well-defined molecular formulae and spatial structures. A variety of herbal monomers, such as resveratrol (Hosseini et al., 2020), berberine (Zhang et al., 2019) and curcumin (Lee et al., 2019), have been shown to have hepatoprotective, anti-inflammatory and antioxidant effects that can effectively improve NAFLD. Some drugs have been found to be associated with the inhibition of NASH-HCC progression, and thus, these drugs may be effective in treating NASH-driven HCC. This review focuses on the causal factors associated with the development of NASH and the progression of NASH to hepatocellular carcinoma, particularly those related to metabolism, and the mechanisms of action that are involved. We also highlight natural small molecule compounds that have been shown to play a role in NASH-driven HCC and some natural products that may have therapeutic promise for NASH-HCC.
Pathogenesis of NASH-Driven HCC
Insulin Resistance
Steatosis is the accumulation of fat in hepatocytes and occurs when fat input or synthesis exceeds fat output or degradation (Machado and Cortez-Pinto, 2014). The first step in the development of NAFLD/NASH is the accumulation of fat in the liver. This condition that is often associated with features of metabolic syndrome (MetS), such as obesity, type 2 diabetes, dyslipidemia and hypertension (Younossi et al., 2016). There are currently three main mechanisms that are thought to be the sources of excessive lipid accumulation in the liver. These mechanisms are an increase in visceral adipose tissue (AT) lipolysis, the activation of hepatic de novo lipogenesis (DNL) and the consumption of a diet with a high calorie and/or fat content (Donnelly et al., 2005). Triglyceride (TG) is the most obvious type of fat in adiposity (Puri et al., 2007). Donnelly et al. demonstrated that approximately 60% of the triglyceride content of the liver comes from free fatty acid (FFA) influx from AT. Additionally, 26% of the triglyceride content of the liver comes from DNL, and 15% comes from the diet (Donnelly et al., 2005).
Insulin resistance (IR) is the key pathogenic event associated with the development of hepatic steatosis. A state of IR makes AT resistant to the antilipolytic effects of insulin. This leads to the breakdown of TG and the eventual formation of FFAs and glycerol (Schweiger et al., 2017). Thus, the lack of AT lipolytic inhibition is associated with a massive release of FFAs. Then, FFAs can be taken up by the liver and accumulate as TG (Samuel and Shulman, 2012). More importantly, increased adipose tissue FFA efflux is a major mechanism of hepatic steatosis (Donnelly et al., 2005). In the presence of IR, higher insulin levels also regulate hepatic lipid metabolism by increasing TG synthesis (Vatner et al., 2015). In the liver, DNL is a key pathway that promotes lipid storage. The enzyme responsible for DNL is upregulated by insulin and glucose through the action of two transcription factors, sterol regulatory element binding protein 1 (SREBP1) and carbohydrate response element binding protein (ChREBP) (Postic and Girard, 2008). Among the insulin receptors, insulin receptor substrate 2 (IRS-2) can act as a regulator of SREBP-1c when activated, affecting DNL (Schreuder et al., 2008). In a state of IR, IRS-2 is downregulated. Therefore, SREBP-1c is overexpressed, and DNL is upregulated (Stefan et al., 2008). Unusually, activation of ChREBP occurs following an increase in glucose concentration, thereby enhancing hepatic glycolysis. Thus, intermediate and final products of glycolysis are able to activate ChREBP, which regulates the expression enzymes involved in lipogenesis, such as acetyl coenzyme A carboxylases (ACCs) and fatty acid (FA) synthases (Beaven et al., 2013). In addition, β-oxidation of FFAs is inhibited in an insulin-resistant state, which further promotes hepatic lipid accumulation (Postic and Girard, 2008).
In addition to increased efflux of FFAs from AT to the liver and increased hepatic adipogenesis in the liver, exogenous lipids are another important source of hepatic triglycerides. Thus, dietary factors are clearly critical to the development of NAFLD. High fat intake (typical of the so-called Western diet) is associated with IR, dyslipidemia and metabolic/cardiovascular diseases (Fan and Cao, 2013). Studies have found that human subjects with diets rich in saturated fat exhibit increased AT triglyceride stores and increased intrahepatic TG levels (Luukkonen et al., 2018). In addition, chronic consumption of diets containing 45%–68% energy from fat has been reported to increase intrahepatic TG in rodents (Wang et al., 2006; Koppe et al., 2009). In addition, increased intake of sugary foods and beverages has been associated with the development of NAFLD. The levels of TGs derived from adipogenesis have been reported to be elevated in subjects consuming a high-carbohydrate diet (Faeh et al., 2005; Luukkonen et al., 2018). Unlike glucose, fructose can regulate hepatic lipid metabolism by directly activating SREBP-1c and ChREBP and by decreasing mitochondrial β-oxidation. Thus, it ultimately promotes the development of steatosis, as demonstrated in dietary studies conducted in rodents fed a high-fructose diet (Kennedy et al., 2007; Caligiuri et al., 2016). By observing the key role of the Western diet in the development of NAFLD, it was shown that lifestyle changes can greatly improve metabolic abnormalities, hepatic steatosis and inflammation (Wong et al., 2013; Rodríguez-Piñeiro and Johansson, 2015; Romero-Gómez et al., 2017).
IR is also associated with the development of HCC, which has been demonstrated in mouse model of choline-deficient l-amino-acid-defined diet (CDAA)-induced NASH (De Minicis et al., 2014). To overcome IR and maintain normal metabolic functions, insulin secretion increases, resulting in compensatory hyperinsulinemia (Bugianesi et al., 2005). Previous studies have described insulin and insulin-like growth factor (IGF)-1 as growth factors leading to the inhibition of cell proliferation and apoptosis (Prisco et al., 1999). It has been elucidated that hyperinsulinemia can promote the synthesis and biological activity of IGF-1 (Le Roith, 1997). The liver is the source of over 80% of circulating IGF-1, and the primary stimulus for IGF-1 synthesis in the liver is provided by growth hormone (GH) signaling. Insulin upregulates human hepatic GH receptors (Leung et al., 2000). As a result, hyperinsulinemia produces and releases large amounts of IGF-1 from the liver and exerts growth factor-like activity on hepatocytes. This stimulates cell proliferation and inhibits apoptosis, which increases the risk of hepatocellular carcinogenesis. Moreover, insulin receptor substrate (IRS) binds to insulin or IGF to activate phosphatidylinositol 3-kinase (P13K)/protein kinase B (Akt) and mitogen-activated protein kinase (MAPK) to promote HCC occurrence (Weng et al., 2010). Not only does high glucose provide a substrate for energy metabolism in tumor cells, but sustained hyperglycemia leads to a glycosylation reaction that generates advanced glycosylation end products (AGEs). AGEs bind to the receptor for advanced glycosylation end products (RAGE), activating nuclear factor kappa-B (NF-κB) and inflammatory signaling cascades and generating ROS to induce HCC development (Jabir et al., 2018). IR may also directly accelerate hepatocarcinogenesis by stimulating hepatic neovascularization (Kaji et al., 2008).
Lipotoxicity
Lipotoxicity is defined as the dysregulation of the lipid environment and/or intracellular lipid components resulting in the accumulation of harmful lipids, which are associated with organelle dysfunction, cellular damage and death. Although triglycerides are a major component of hepatic lipids in NASH and simple steatosis, they are a safe storage lipid with little lipotoxicity (Yamaguchi et al., 2007; Wouters et al., 2010). Potential lipotoxic molecules include cholesterol (Musso et al., 2013; Hendrikx et al., 2014; Arguello et al., 2015), FFAs and their derivatives (Neuschwander-Tetri, 2010), diacylglycerols (Kumashiro et al., 2011) and ceramides (Chaurasia and Summers, 2018). There are three general mechanisms by which lipotoxicity causes cellular damage. (1) Harmful lipids alter the biology and function of intracellular organelles, such as the endoplasmic reticulum (ER) and mitochondria. (2) Direct modification of intracellular signaling pathways, such as ceramide enrichment, may regulate metabolic and inflammatory pathways (Perry et al., 2014). (3) Interactions between lipids located on the cell surface or in the cytoplasm and cellular kinases indirectly modify signaling leading to inflammation and other biological effects. For example, palmitate (PA) can activate Toll-like receptor 4 (TLR4) and thus promote inflammation (Lee et al., 2001).
Hepatocyte death caused by lipotoxicity correlates with the severity of NAFLD, and lipotoxicity plays a role in the activation of hepatic macrophages by damaged and dead hepatocytes (Malhi et al., 2006; Cazanave and Gores, 2010). Enhanced lipid peroxidation can activate macrophages by producing ligands for scavenger receptors (e.g., ox-LDL) (Terpstra et al., 2000). Stimulation of TLR4 by saturated fatty acids is another mechanism of macrophage activation that may contribute to the hepatic inflammatory response (Shi et al., 2006). Importantly, macrophage-mediated stimulation of surviving hepatocytes via NF-kB and other cell proliferation pathways is a major component of hepatocarcinogenesis (Maeda et al., 2005; Sun and Karin, 2012).
ER Stress
In eukaryotes, the ER is an intracellular organelle that supports many important cellular processes, including the folding of membranes and secreted proteins, the synthesis of lipids and sterols, and the storage of free calcium (Gething and Sambrook, 1992; Schröder and Kaufman, 2005). Disturbance of any of these processes exerts stress on the ER and interrupts the protein folding process. Normally, unfolded or misfolded proteins remain in the endoplasmic reticulum and are eventually folded or degraded. However, when the ER is stressed, unfolded or misfolded proteins can accumulate in the lumen of the ER, a condition known as ‘endoplasmic reticulum stress'. To restore homeostasis in vivo, the ER triggers a complex adaptive response collectively known as the unfolded protein response (UPR) (Ron and Walter, 2007; Hetz, 2012). The UPR is the first process used to re-establish ER homeostasis to recover from or adapt to ER stress. However, when the persistence of ER stress cannot be blocked or restored, prolonged activation of the UPR triggers the apoptotic pathway. This pathway leads to cell death to eliminate the stressed cells (Zhang and Kaufman, 2008b). The UPR is characterized by the activation of three distinct signaling pathways that are mediated by three transmembrane sensors: protein kinase RNA activation (PKR)-like ER kinase (PERK), inositol requiring enzyme (IRE)1 and activating transcription factor (ATF) 6 (Ron and Walter, 2007; Malhi and Kaufman, 2011). In the absence of ER stress, the three transmembrane UPR sensors remain inactive by binding to ER chaperone binding immunoglobulin protein (BiP) (Hotamisligil, 2010). However, under stressful conditions caused by the accumulation of misfolded or unfolded proteins, depletion of ER calcium levels or increased free cholesterol in the ER lumen, BiP segregates to activate the UPR. This leads to the activation of IRE1-, PERK- or ATF6-mediated signaling pathways (Szegezdi et al., 2006).
All three ER stress-sensing pathways (ATF6α, PERK/eukaryotic translation initiation factor 2α (eIF2α), and IRE1α/X box binding protein 1 (XBP1)) can regulate the development of steatosis in the liver (Rutkowski et al., 2008). Notably, both XBP1 and eIF2α have been shown to be involved in basal and/or diet-induced regulation of lipid metabolism (Lee et al., 2008; Oyadomari et al., 2008). Xiao et al. (Xiao et al., 2013) demonstrated that mice lacking activating transcription factor 4 (ATF4), a major ER stress mediator, were protected from high-fructose diet-induced hepatic steatosis. A study in zebrafish showed that ATF6 deficiency prevented steatosis during chronic ER stress but exacerbated steatosis during acute ER stress. These findings suggest a protective and pathological role for ATF6 in fatty liver disease (Cinaroglu et al., 2011). Another mechanism leading to hepatic steatosis is the reduction of VLDL synthesis and secretion. Packaging of TG into VLDL is a complex process that occurs in the ER lumen and requires the synthesis of apolipoproteins such as apoB100. Studies have shown that ER stress reduces apoB100 levels through the degradation of the PERK-ATF4 branch of the UPR and attenuates apoB100 translation (Qiu et al., 2009). Thus, ER stress can exacerbate hepatic steatosis. In addition, ER stress can also induce hepatic steatosis by increasing the expression of VLDL receptors, and mice lacking VLDL receptors have reduced hepatic steatosis when fed a high-fat diet (Jo et al., 2013). Recently, it has been proposed that ER stress in adipose tissue enhances lipolysis. Hepatic ER stress appears to play an important role in regulating the composition and size of lipid droplets and lipid synthesis, including cholesterol metabolism, through sterol regulatory element binding protein (SREBP) (Hotamisligil, 2010; Zámbó et al., 2013; Nakagawa et al., 2014; Zhang et al., 2014). ER stress in ob/ob mice has been shown to promote SREBP-1c activation and therefore contribute to hepatic adipogenesis. However, the overexpression of the ER chaperone BiP inhibits SREBP-1c activation and SREBP-1c target gene expression (Kammoun et al., 2009), thereby reducing hepatic steatosis.
ER stress induces apoptosis in hepatocytes through three pathways: activation of C/EBP homologous protein (CHOP), C-Jun N-terminal kinase (JNK) signaling and altered calcium homeostasis. Both the PERK and ATF6 pathways induce the activation of CHOP, which is a transcription factor with proapoptotic functions. Additionally, CHOP activation promotes cell death and induces tissue damage (Ron and Walter, 2007; Tabas and Ron, 2011). In vitro and animal studies have shown that CHOP deficiency is protective against a variety of pharmacological and physiological injuries (Tabas and Ron, 2011). Other branches of the UPR are also closely associated with cell death. Upon phosphorylation, IRE1 binds the bridging protein TNFα receptor-associated factor 2 (TRAF2) and promotes apoptosis through JNK (Urano et al., 2000) or direct activation of pro-apoptotic molecules, such as Bcl-2-like protein 4 and Bcl-2 homologous antagonists/killers (Hetz et al., 2006). Finally, alterations in calcium homeostasis in the lumen of the ER have been shown to drive ER stress and apoptosis (Zhang et al., 2014).
Finally, ER stress is inextricably linked to the development and progression of liver inflammation. Because it is a key mediator of liver inflammation, the UPR promotes the inflammatory response associated with NASH through the activation of two major inflammatory pathways (JNK)/activator protein 1 (AP1) and IkB kinase (IKK)-NF-κB (Hotamisligil, 2006; Zhang et al., 2006; Zhang and Kaufman, 2008a; Hotamisligil, 2010; Malhi and Kaufman, 2011; Morgan and Liu, 2011; Lee et al., 2012; Lee and Ozcan, 2014; Zhang et al., 2014). NF-kB is a transcription factor that is a major regulator of inflammatory activation, and its IKK2 subunit is a major component required for its activation during the acute inflammatory response (Wullaert et al., 2007). Sustained activation of the NF-κB pathway has been shown in animal models of NAFLD (Cai et al., 2005) as well as in NASH patients (Ribeiro et al., 2004). IKK2 overexpression and sustained activation of NF-kB in hepatocytes leads to a chronic inflammatory state and insulin resistance (Cai et al., 2005). The IRE1 pathway can activate JNK and IKK through their kinase structural domains and interaction with TRAF2, leading to the increased expression of proinflammatory mediators, such as TNFα, IL-1β and IL-6 and NF-κB (Urano et al., 2000; Hu et al., 2006; Zhang and Kaufman, 2008a). In turn, TNFα binding to its receptor can lead to IKK and JNK activation (Aguirre et al., 2000; Wullaert et al., 2006; Meshkani and Adeli, 2009). In addition, IL-1β, IL-6 and TNFα induce the activating element of cyclic AMP response element-binding protein H (CREBH). CREBH is a liver-specific transcription factor that is associated with ER stress. Although ER stress-induced CREBH cleavage does not lead to increased transcription of UPR genes, mature CREBH interacts with ATF-6 to synergistically induce the transcription of many acute phase response genes in the liver, such as C-reactive proteins and serum amyloid P components. Thus, it promotes and worsens the NASH-related systemic inflammatory response (Zhang et al., 2006; Zhang and Kaufman, 2008a; Zhang et al., 2014). ER stress also promotes inflammation through oxidative stress, and the mechanisms of this process are discussed later.
ER stress was identified as a mediator of NAFLD-promoted HCC in one study (Nakagawa et al., 2014). More importantly, spontaneous typical steatohepatitis HCC was observed in these mice even in the absence of carcinogen treatment, suggesting that ER stress is sufficient to induce HCC in the NAFLD setting. This is most likely because enhanced ER stress increases TNF production by macrophages. TNF activates the tumor necrosis factor receptor 1 (TNFR1)-IKKβ-NF-kB pathway in HCC progenitor cells (HcPC) and leads to tumor formation (Nakagawa et al., 2014). The tumor-promoting effect of ER stress was also found in melatonin-accelerated DEN-induced HCC in rats (Moreira et al., 2015). These results suggest that ER stress can act as a trigger for malignant transformation during the progression of NAFLD to HCC. Obesity induces AT macrophages to polarize toward a proinflammatory phenotype (Promrat et al., 2010). These macrophages secrete pro-inflammatory cytokines that, together with excess FFAs, travel toward the liver. In the liver, proinflammatory cytokines and FFAs activate the hepatic macrophage pool. These macrophages, in turn, secrete proinflammatory signals, including the chemokine CCL2. These signals attract monocytes (MOs) from the circulation and thereby maintain liver inflammation (Chalasani et al., 2009). Tumor-associated macrophages (TAMs) contribute to tumor development and metastasis. TAMs secrete cytokines and growth factors and induce cancer stem cell-like properties (Angulo et al., 2007; Malik et al., 2009). Macrophage activation is regulated by several proteins, including IRE1α. IRE1α activation in macrophages is associated with the TLR-mediated production of proinflammatory cytokines and may therefore regulate the inflammatory milieu (Bhala et al., 2011; White et al., 2012). More importantly, IRE1α has been shown to be associated with proinflammatory macrophage polarization (Bhala et al., 2011). Thus, given the role of IRE1α in the inflammatory milieu, IRE1α may also be involved in the formation of specific TAMs in inflammation-induced cancer development.
Oxidative Stress
Oxidative stress refers to various detrimental processes that are due to an imbalance between the excessive formation of prooxidants (ROS and/or reactive nitrogen species (RNS)) and limited antioxidant defenses (Robertson et al., 2001). This imbalance is common in various pathological processes, and in these processes, oxidative damage leads to cell death and tissue damage (Matés et al., 2008). Mitochondria are not only an important source of intracellular ROS production but also a major target of ROS-induced damage. Thus, they are prone to dysfunction (Turrens, 2003). Moreover, decreased mitochondrial function may lead to enhanced ROS production, which further increases mitochondrial dysfunction (Turrens, 2003; Malhotra and Kaufman, 2007; Zhang, 2010). In NAFLD, there are at least three main mechanisms for the production of mitochondrial ROS. The first mechanism involves the reduction of mitochondrial GSH (Marí et al., 2006). The second mechanism involves the impaired mitochondrial respiratory chain (MRC), and the third involves increased mitochondrial cytochrome P450 2E1 (CYP2E1) levels/activity.
GSH depletion during the UPR drives mitochondrial production of ROS, which leads to cell death. Additionally, the accumulation of unfolded proteins in the ER leads to Ca2+ leakage into the cytoplasm, which increases the production of ROS in mitochondria (Malhotra and Kaufman, 2007). Both mechanisms ultimately lead to increased production of ROS in mitochondria. This affects the mitochondrial electron transport chain (ETC) and ultimately affects ATP production. Since protein folding is an ATP-dependent process, it leads to protein misfolding, increases ROS production, and perturbs cellular homeostasis. Therefore, there is clear crosstalk between the ER and mitochondria. CYP2E1 is capable of directly producing ROS. Although its relative contribution to oxidative stress has not been conclusively determined, increased CYP2E1 activity has been observed in NASH patients (Chalasani et al., 2003). Additionally, animal studies using a methionine choline deficient (MCD) diet to induce NASH reported increased CYP2E1 activity in a rat model (Weltman et al., 1996). A recent study using CYP2E1 knockout mice found that CYP2E1 is required for NASH development (Abdelmegeed et al., 2012). The authors noted that high-fat-fed CYP2E1 knockout and WT mice had higher levels of TNF-α in the liver compared to chow (low fat) animals; the levels were lower in CYP2E1 knockouts compared to WT mice (Abdelmegeed et al., 2012). In addition, TNF-α (Day, 2002; Haque and Sanyal, 2002) and lipid peroxidation products (Angulo and Lindor, 2001) inhibit the mitochondrial electron transport chain, damage mitochondrial DNA and deplete mitochondrial cytochrome C (Pessayre et al., 2002). Thus, mitochondrial dysfunction and ROS production are exacerbated. Mitochondrial damage leads to a secondary inhibition of lipid β-oxidation, further increasing steatosis (Day, 2002; Haque and Sanyal, 2002) and initiating a vicious cycle.
Kupffer cells and hepatic stellate cells (HSCs) are nonparenchymal cells that account for almost 40% of all hepatocytes, and they play an important role in the progression of chronic liver inflammation and fibrosis (Rolo et al., 2012). Excessive fatty acid accumulation in hepatocytes causes oxidative stress not only in mitochondria but also in peroxisomes or microsomes. These cytotoxic ROS and lipid peroxidation products can diffuse into the extracellular space, affecting Kupffer cells and HSCs. This cellular oxidative stress from hepatocytes and the direct uptake of free fatty acids or free cholesterol in Kupffer cells induce the activation of NF-κB. NF-κB in turn induces the synthesis of TNF-α and several proinflammatory cytokines, such as IL-6 or IL-8 (Hui et al., 2004). Moreover, inflammation and hepatocellular injury drive the activation of regenerative pathways and the proliferation of fibrotic cells, mainly HSCs. The ability of HSCs to remodel the extracellular matrix (ECM) places them in a strategic position for a microenvironment conducive to cell survival and proliferation. In this environment of continuous regeneration and repair, some hepatocytes may evolve adaptive mechanisms of cell survival and proliferation that promote precancerous transformation and/or tumor growth (Stickel and Hellerbrand, 2010).
Genetic and Epigenetic Factors
A range of single nucleotide polymorphisms (SNPs) are known to be associated with the presence of NAFLD and the risk of disease progression to advanced fibrosis (Anstee and Day, 2015). Of greatest interest are the genes encoding the patatin-like phospholipase domain-containing protein 3 (PNPLA3; rs738409c.444C > Gp. I148M) and transmembrane 6 superfamily member 2 (TM6SF2. rs58542926c.449C > T, p. E167K), which are strongly associated with steatosis, NASH and the severity of fibrosis or cirrhosis (Romeo et al., 2008; Valenti et al., 2010; Liu et al., 2014a; Kozlitina et al., 2014; Dongiovanni et al., 2015). The PNPLA3 gene is a known determinant of liver fat content, and the PNPLA3 rs738409 C > G SNP leads to the replacement of isoleucine next to the catalytic structural domain with methionine, resulting in the PNPLA3 I148M variant (Banini and Sanyal, 2021). Overexpression of the I148M variant in mouse liver promotes triglyceride accumulation, increases fatty acid synthesis, and impairs triglyceride hydrolysis (Smagris et al., 2015). The I148M variant has also been shown to predispose to HCC in the presence of metabolic risk factors such as T2DM and obesity (Valenti et al., 2013; Ueyama et al., 2016). Patients carrying the PNPLA3 polymorphism not only have a higher risk of steatohepatitis and fibrosis, but also have a more than threefold increased risk of HCC (Liu et al., 2014b; Singal et al., 2014).
Another SNP associated with an increased risk of HCC in NAFLD patients is the TM6SF2 gene. TM6SF2 is thought to be a lipid transporter protein that interacts with proteins involved in intestinal absorption (Mahdessian et al., 2014). Knockdown of TM6SF2 has been shown in vitro to reduce secretion of triglyceride-rich lipoproteins and Apo-B (Li BT. et al., 2020). Knockdown of TM6SF2 in vitro showed a reduction in secretion of triglyceride-rich lipoproteins and Apo-B (Li BT. et al., 2020). TM6SF2 rs58542926 C > T gene variants leading to substitution of glutamate for lysine result in increased steatosis and advanced fibrosis in NASH patients (Liu et al., 2014a; Kozlitina et al., 2014) and are associated with NASH- HCC (Chen et al., 2015), independent of diabetes, obesity or PNPLA3 genotype. Despite conflicting data on its role in HCC progression, the TM6SF2 variant is thought to be associated with liver injury in the pathogenesis of NASH-associated HCC (Chen et al., 2015). Other genetic abnormalities that may contribute to the development of HCC in NAFLD patients include the C282Y and H63D mutations in the human haemostatic iron regulator (HFE) gene (Bugianesi et al., 2002; Bugianesi et al., 2004; Nelson et al., 2007) and the membrane bound O-acyltransferase structural domain 7 (MBOAT7) gene rs641738 C > T variant (Donati et al., 2017). In particular, the H63D mutation has been identified in non-cirrhotic HCC and leads to liver inflammation, fibrosis and carcinogenesis due to increased iron loading in these patients (Ye et al., 2016). More recently, the rs641738 genotype encoding MBOAT7 has been associated with an increased risk of more severe liver injury and fibrosis in patients with NASH; however, these findings require further investigation into HCC progression (Mancina et al., 2016; Thabet et al., 2017).
Epigenetic changes leading to aberrant DNA methylation are thought to be another important mechanism in the progression of NASH (Iacobazzi et al., 2013). It occurs through the enzyme methyltransferase (DNMT) and leads to gene silencing associated with DNA damage and repair, lipid and glucose metabolism, and fibrosis progression (Tryndyak et al., 2011). In humans, DNMT levels have been found to be higher in NASH patients than in NAFL patients (Pirola et al., 2013). However, the only epigenetic change clearly associated with NASH-related HCC is the gene encoding the chromodomain helicase DNA-binding protein 1 (CHD1) (Liu et al., 2015). NAFLD-related HCC is associated with hypermethylation of the glycine N-methyltransferase (GNMT) promoter, resulting in reduced expression of this gene. GNMT inhibits HCC growth through an unknown mechanism and has been noted to be absent in fast-growing HCC and present at low levels in slow-growing HCC (Simile et al., 2018).
In addition, exome sequencing analysis of HCC revealed the highest mutation rates in oncogenes such as CTNNB1, AXIN1 (involved in the β-catenin/WNT signalling pathway), albumin (ALB), TP53 and CDKN2A (Schulze et al., 2015). In addition, a number of microRNAs (miRNAs) appear to play a role in NAFLD-associated HCC, including miR-21, miR-23, miR-29, miR-93, miR-106, miR-155, miR-221, miR-222 and miR-519 (de Conti et al., 2017). Compelling evidence suggests that miRNA expression is dysregulated in many cancers through a variety of mechanisms and that they may function as oncogenes or tumour suppressors under certain conditions (Erstad et al., 2018). Among these, liver-specific miR-122 expression is reduced in NASH patients and therefore negatively regulates hepatic adipogenesis (Cheung et al., 2008). Downregulation of miR-122 has also been demonstrated in a mouse model of NASH-HCC, suggesting a direct role for this miRNA in NASH-associated HCC (Takaki et al., 2014).
Gut Microbiota
Under physiological conditions, the gut microbiota regulates hepatic lipogenesis, bile acid metabolism, oxidation and the levels of hepatic inflammatory mediators. In turn, the liver regulates the gut microbiota through the secretion of bile (Jiang et al., 2019). The gut microbiota is thought to be a driver of hepatic steatosis and inflammation (Moschen et al., 2013). Dysbiosis of the gut flora is involved in the development of several liver diseases, including simple steatosis, NASH and NAFLD-HCC. Studies have found dysbiosis of the gut microflora in patients with NAFLD and NASH; these patients have a significantly lower abundance of Clostridium than healthy individuals (Mouzaki et al., 2013). The proportion of alcohol-producing bacteria in the gut is also increased in patients with NASH, leading to increased blood ethanol concentrations. Ethanol can induce chronic inflammatory damage to hepatocytes. Therefore, it is likely that alcohol-producing bacteria are involved in the pathogenesis of NASH by participating in oxidative stress and liver inflammation through ethanol metabolism (Zhu et al., 2013). The abundance of Bacteroides and Ruminococcaceae were increased in the gut of NASH-HCC patients, and the abundance of Bifidobacterium was reduced in these patients (Ponziani et al., 2019). Both Akkermansia and Bifidobacterium play a protective role against liver injury. Thus, their depletion may enhance inflammation in the gut and liver, thereby promoting the process of NASH-HCC (Fang et al., 2017; Wu et al., 2017).
In addition to changes in the gut microbiota itself, changes in intestinal permeability are also important factors in the development of NASH and NASH-HCC. The intestinal barrier is a multilayered defense system against external pathogens. This barrier includes the intestinal epithelium, the mucus layer, and the immune system associated with the mucosa. These structures maintain a delicate symbiotic balance between the gut-dwelling microbiota and the host—primarily by preventing them from coming into direct contact with each other (Peterson and Artis, 2014). Alterations in gut permeability help activate local immune responses that lead to tissue inflammation and excessive scarring. These alterations provide a source of oxidative stress that promotes the progression from steatosis to steatohepatitis and subsequently to cirrhosis or ultimately to HCC (Arab et al., 2018). In a previous clinical study, NASH patients had higher intestinal permeability than simple steatosis patients and healthy individuals (Luther et al., 2015). Using fecal microbiota transplantation (FMT), the study found that transplanting the microbiome of high-fat diet-fed mice into germ-free recipient mice induced gut barrier disruption, suggesting that gut barrier disruption in NASH is caused by abnormal gut microbiota (Mouries et al., 2019). Increased intestinal permeability is caused by decreased expression of zonula occludens-1 (ZO-1), a representative tight junction protein (Miele et al., 2009; Giorgio et al., 2014; Luther et al., 2015). More importantly, the expression of ZO-1 and junctional adhesion molecule A (JAM-A) were reduced in NAFLD patients (Miele et al., 2009; Rahman et al., 2016).
Studies have found that many bacteria in the gut are associated with the expression of ZO-1. For example, Lactobacillus, Bifidobacterium spp. and A. muciniphila induce ZO-1 expression to promote intestinal barrier integrity (Lam et al., 2012; Ling et al., 2016; Wu et al., 2017). However, Desulfovibrio spp. produce genotoxic hydrogen sulfide (H2S), which increases intestinal permeability (Lam et al., 2015). Following disruption of the intestinal barrier, bacteria and pathogen-associated molecular patterns (PAMPs), including lipopolysaccharides (LPS), can be transferred to the liver via the leaky gut. Then, the pathogen recognition receptors are activated, and the immune responses promote the progression of NASH and HCC (Dapito et al., 2012; Chopyk and Grakoui, 2020). LPS-induced liver inflammation occurs through activation of TLR4 in a variety of cell types, including Kupffer cells, hepatocytes, HSCs and liver sinusoidal endothelial cells (LSECs). In Kupffer cells, the activation of TLR4 signaling through myeloid differentiation primary response 88 (MyD88) induces TNF-α and ROS, further enhancing hepatic inflammation. LPS-induced TLR4 production in HSCs induces the production of various chemokines and adhesion molecules, which in turn induce Kupffer cell chemotaxis. Moreover, the activation of TLR4 on hepatocytes induces hepcidin production through the MyD88/c-JNK pathway, which is associated with hepatic lipid accumulation (Kolodziejczyk et al., 2019). In addition to LPS, other PAMPs, including peptidoglycan, flagellin, and bacterial RNA and DNA, can enter the liver due to increased intestinal permeability and trigger an inflammatory response. Activation of TLR9 by bacterial DNA further induces IL-1β production by Kupffer cells, which leads to hepatic steatosis, inflammation and fibrosis (Miura et al., 2010). In addition to TLRs, inflammasome proteins activated and assembled by the nucleotide-binding oligomerization domain (NOD)-like receptor (NLR) recognize PAMPs. This leads to IL-1 and IL-18 production and further inflammation (Szabo and Petrasek, 2015). A previous study showed that the NLR family pyrin domain containing 3 (NLRP3) inflammasome components were significantly increased in NASH patients compared to non-NASH/NAFLD patients (Wree et al., 2014). These results suggest an association between liver inflammation and NLRP3 inflammatory vesicles. PAMPs also activate the HSC senescence-associated secretory phenotype (SASP) and induce epiregulin production through TLRs, further promoting the development of fibrosis (Dapito et al., 2012; Schwabe and Greten, 2020).
Finally, in addition to the gut microbes themselves, another interesting link between the microbiome and NAFLD is microbial metabolites (Zhao M. et al., 2020). Several metabolites, including amino acids, SCFAs, and bile acids, in the gut, circulation, and liver tissues have been identified to promote NAFLD and NAFLD-HCC. In particular, bile acids are closely associated with NASH and NASH-HCC. Bile acids are another important metabolite that link the gut microbiota to liver disease. Bile acids can alter their receptors, the farnesoid X receptor (FXR), to modulate the development of NASH (Jiao et al., 2018). FXR activation is known to reduce triglyceride levels and inhibit fatty acid synthesis and uptake in the liver (Zhu et al., 2011). In addition, a role for FXR in reducing inflammation has emerged (Shaik et al., 2015). The FXR activator obeticholic acid is known to significantly improve fibrosis and disease severity in patients with NASH (Younossi et al., 2019). In general, primary bile acids secreted by the liver are not bound to intestinal microorganisms. Thus, these unbound bile acids are reabsorbed to form secondary bile acids and returned to the liver for detoxification (Jia et al., 2018). Dysregulated bile acid-microbiome crosstalk can impair this process, thereby contributing to inflammation and HCC development. Secondary bile acids have also been shown to regulate immune functions and HCC development. For example, deoxycholic acid (DCA) induces NASH-related HCC by activating mTOR (Yamada et al., 2018). Increased DCA in the liver can also trigger the SASP phenotype in HSCs. This, in turn, leads to the production of proinflammatory cytokines and protumor factors in the liver, thereby promoting HCC (Yamada et al., 2018). Antibiotic treatment reduces the production of DCA-producing bacteria, which in turn reduces the development of HCC (Schwabe and Jobin, 2013). This suggests that the DCA-SASP axis in HSCs promotes the development of obesity-associated HCC. Importantly, blocking DCA production or depleting the gut microbiota may reduce the development of HCC (Ray, 2013).
The role of natural products in NASH-driven HCC:
Curcumin
One of the bioactive molecules in turmeric is curcumin. Curcumin is a diarylheptane compound with significant anticancer effects due to its interaction with various cell signaling proteins, particularly protein kinases, cytokine signaling receptors, adhesion molecules, and transcription and growth factors (Song et al., 2013). It also exerts potent lipid-regulating, anti-inflammatory, antioxidant and anticancer properties, which are important for the prevention of NASH. The preventive effects of curcumin on NASH have been identified in previous studies. In a methionine choline-deficient (MCD)-induced NASH mouse model, curcumin treatment significantly reduced MCD-induced inflammation but did not affect steatosis or hepatic lipid peroxide levels. In addition, curcumin prevented the activation of NF-kB in these NASH mice (Leclercq et al., 2004), thereby alleviating the progression of NASH. Subsequently, a study using a rabbit NASH model induced by a HFD further confirmed the significant effect of curcumin on the treatment of NASH. It was found that curcumin significantly reduced aminotransferase levels, TNF-α protein levels and mitochondrial reactive oxygen species in rabbits with NASH. Thus, it could increase mitochondrial antioxidant levels and improve mitochondrial function. The investigators suggested that curcumin may regulate NASH through mechanisms involving the antioxidant, anti-inflammatory and mitochondrial protective effects (Ramirez-Tortosa et al., 2009).
More importantly, because of the anticancer effects of curcumin, studies have begun to examine its potential therapeutic role in NASH-HCC. A NASH-HCC model was successfully simulated using streptozotocin (STZ) injections and HFD-fed mice. This model showed 100% reproducibility for hepatocellular carcinoma, and this model demonstrated the progression from fatty liver disease to NASH, liver fibrosis and hepatocellular carcinoma in a diabetic background (Fujii et al., 2013). Interestingly, this study found that the protein expression levels of TLR4 and cytoplasmic translocation of HMGB1 were significantly reduced in the livers of mice in the curcumin-treated NASH group compared to the NASH-only group (Afrin et al., 2017). High-mobility group box 1 (HMGB1) is a DNA-binding nuclear protein, a proinflammatory cytokine without precursors, and an important member of the inflammatory cascade response (Sims et al., 2010). Extracellular HMGB1 can bind to different cell surface receptors, including TLR2, TLR4 and the receptor for advanced glycosylation end products (RAGE), to act on target cells and promote inflammation (Karuppagounder et al., 2015). Activation of these receptors leads to the activation of the NF-κB and extracellular signal-regulated kinase (ERK) signaling pathways, which trigger a variety of inflammatory and pro-inflammatory cytokines. These proinflammatory factors further promote the development and progression of HCC, and curcumin reverses the release of these proinflammatory factors. Moreover, the protein expression levels of SREBP-1c and ADRP, which are associated with DNL, were significantly lower in the NASH-curcumin group. However, Nrf2 expression was significantly higher in the liver, suggesting that curcumin may also enhance the antioxidant capacity of the body (Afrin et al., 2017). However, little research has been done on curcumin, especially in the progression of NASH to HCC, so the specific anticancer mechanism it exerts in the liver in the transition from inflammation to cancer is not well understood. Thus, this will be a focus of subsequent studies.
Silibinin
Silibinin is derived from milk thistle seeds and is involved in targeting proinflammatory markers as well as reducing lipogenesis (Deep and Agarwal, 2010; Lin et al., 2012; Ghasemi et al., 2013). It has a wide range of pharmacological activities, such as hepatoprotective, antioxidant, and antitumour activities. It maintains hepatocyte membrane stability and is reported to be the most potent flavonoid against liver disease (Muthumani and Prabu, 2012; Bosch-Barrera and Menendez, 2015). Silibinin has been reported to improve dyslipidemia and insulin resistance in HFD-fed Sprague–Dawley (SD) rats by modulating the IRS-1/PI3K/Akt pathway (Zhang et al., 2013). Additionally, silymarin-phospholipid complexes prevented mitochondrial dysfunction in MCD diet-fed Wistar rats (Serviddio et al., 2010), and a mixture of silymarin, phosphatidylcholine and vitamin E has been used clinically to treat NASH patients (Loguercio et al., 2012). Caspase 8 and Fas-related proteins with death domain-like apoptosis regulator (CFLAR) is known to reverse the course of NASH (Wang et al., 2017). Recent studies have shown that CFLAR inhibits the phosphorylation of JNK, thereby ameliorating the pathological features of NASH, such as disorders of glucolipid metabolism, oxidative stress, inflammation and liver fibrosis (Wang et al., 2017). Importantly, silymarin activates CFLAR and inhibits the downstream pJNK/JNK expression in vivo and in vitro. Furthermore, silymarin has an antagonistic effect on the inhibition of the expression of key genes involved in lipid acid β-oxidation, such as Ppar α, Fabp5, Cpt1α and Acox, that is induced by feeding the MCD diet. These findings suggest that silymarin may promote hepatic fatty acid β-oxidation. Finally, silymarin may also improve NASH-related oxidative stress damage by increasing hepatic antioxidant enzyme activity and inhibiting free radical-generating enzyme activity (Liu Y. et al., 2019). The above findings, especially the modulation of CFLAR by silymarin, provide us with further insight into its potential for the treatment of NASH.
Silymarin has been shown to have anti-inflammatory and antitumour properties. While there are no studies that clearly show that silymarin can halt this progression in NASH-driven liver cancer, similar studies may serve as supporting evidence for this. Previous reports have shown that obesity promotes fatty acid accumulation and inflammation in the liver, leading to liver injury (Marengo et al., 2016). Obesity is associated with an increased risk of liver cancer development and progression (Federico et al., 2015). Serum levels of pro-tumor cytokines and adipokines (e.g., leptin, IL-6, VEGF, IL-8, resistin and endolipin) are higher in obese males than in overweight (OW) and normal weight (NW) males (Steppan et al., 2001; Ramirez et al., 2012). The administration of silymarin significantly inhibited the obesity-induced secretion of MMP-9, a marker of an aggressive phenotype. MMP-9 and obesity further promote the invasive ability of hepatocellular carcinoma cells. The administration of silymarin also significantly reduced ROS production in the OB group and restored these levels to levels close to those of the NW group. Silymarin inhibited obesity-induced ROS production and prevented DNA damage (Miethe et al., 2017). Previous studies have shown that obesity induces adipogenesis, which can lead to steatosis and the progression to hepatocellular carcinoma (Donohoe et al., 2017). Similarly, this study found a significant increase in DNL in OB sera compared to OW and NW sera in hepatocellular carcinoma cells. In addition, the addition of silymarin to OB serum had a significant protective effect on DNL. These data suggest that silymarin may prevent the accumulation of fatty acids in the liver, thereby preventing local inflammation and tumor growth (Miethe et al., 2017). While we cannot be sure whether obesity-induced liver cancer is NASH-driven liver cancer, there are many similarities. Therefore, further validation in animal models is needed.
Berberine
Berberine is an isoquinoline quaternary alkaloid that is derived from Coptis chinensis and has been reported to be an effective drug with anti-inflammatory and antitumour pharmacological effects (Sun et al., 2009). In previous studies, berberine was shown to ameliorate high-fat, high-cholesterol (HFHC) diet-induced NASH in APOE−/− mice (Yang et al., 2017). The latest study found that berberine also had a therapeutic effect in NASH-HCC mice. This study used STZ injections in combination with a HFHC diet to establish a mouse model of NASH-HCC (Fujii et al., 2013). The model was able to reflect the pathological progression from fatty liver, steatohepatitis and fibrosis to HCC. Phellodendron was found to significantly reduce TC, LDL and HDL levels in NASH-HCC mice, suggesting that berberine could lower cholesterol and lipid levels. Moreover, increased expression of adipogenic genes, such as FASN, SCD1 and SREBP-1c, in STZ-HFHC mice was significantly inhibited by berberine, suggesting that berberine may attenuate lipid accumulation (Luo Y. et al., 2019). Previous studies have shown that berberine can inhibit the overexpression of fibrotic genes, such as α-SMA, fibronectin, and collagen I and III (Chitra et al., 2015). In addition, berberine has been shown to improve liver fibrosis by inhibiting hepatic oxidative stress and the fibrotic potential (Domitrović et al., 2013). Berberine was also found to inhibit the activation of blast cells in the liver of NASH-HCC mice, thereby exerting an antitumour effect. Increased expression of COX2 in NASH-HCC mice was inhibited by berberine, possibly because berberine inhibits inflammation and angiogenesis via the p38MAPK/ERK-COX2 pathway (Luo Y. et al., 2019). It is known that abnormally elevated COX2 levels are associated with inflammation, angiogenesis and the promotion of tumor formation (Möbius et al., 2005; Harris, 2007). In another model of NASH-HCC induced by deoxycholic acid, COX2 overexpression was detected and exerted a protumor effect by suppressing antitumor immunity (Loo et al., 2017). However, given the clinical application of berberine, the elevated COX2 levels in NASH-HCC mice may be due to changes in the intestinal flora that are induced by the HFHC diet. Previous studies have mentioned the effect of berberine on NASH via the gut-liver axis (Cao et al., 2016). Berberine may affect the intestinal microbiota and thus have multiple effects in NASH-HCC. Therefore, further research on this topic is needed.
Green Tea Catechin
The results from a population-based case–control study suggest that long-term green tea consumption is associated with a lower risk of HCC (Li et al., 2011). These benefits may be attributed to epigallocatechin gallate (EGCG), the major catechin in green tea. EGCG selectively induces apoptosis and cell cycle arrest in HCC cell lines but not in noncancerous hepatocytes (Zhang et al., 2015). In addition, catechin-rich green tea extract (GTE) has been shown to protect obese mice from NASH by inhibiting liver inflammation. This finding is consistent with a mechanism involving the gut-liver axis that limits metabolic endotoxemia and hepatic TLR4/NFκB activation (Li et al., 2018). Many in vitro studies have demonstrated the chemopreventive and antitumour properties of green tea in hepatocellular carcinoma (Darvesh and Bishayee, 2013). EGCG inhibits the growth and proliferation of hepatocellular carcinoma cells by inducing apoptosis (Kuo and Lin, 2003). EGCG also inhibited the growth of the human hepatoma cell line HepG2 by inhibiting the phosphorylation of insulin-like growth factor-1 receptor (IGF-1R) and reducing the activation of its signaling molecules, such as ERK, STAT-3, Akt and GSK-3β (Shimizu et al., 2008). However, it is not clear whether its reported anti-inflammatory activity also prevents NASH-induced HCC progression. A recent study found that in a NASH-HCC model (Wang et al., 2009) established by the administration of DEN to mice fed a HFD, GTE inhibited the signal transducer (gp130, tumor suppressor M) that promotes STAT3 activation (Stephens and Elks, 2017). It also downregulated STAT3-dependent genes (c-Fos, c-Myc, survivin) that promote tumorigenesis (Dey et al., 2019). DEN effectively induces fibrosis and hepatocyte proliferation in a STAT3-dependent manner (Ding et al., 2017). Therefore, we have reason to believe that GTE prevents NASH-related HCC in a STAT3-dependent manner, but this requires further study.
The role of potential natural products in NASH-driven HCC:
Regulating Lipid Metabolism
Changes in lipid metabolism affect the progression of many tumor cells by affecting the processes of cell growth, proliferation, differentiation and migration (Santos and Schulze, 2012; Luo X. et al., 2019). Many natural compounds have been reported to regulate lipid metabolism, particularly through the expression of adipogenesis-related genes. Luteolin (1), tomatidine (2), oxymatrine (3) and oleanolic acid (4) have been reported to regulate glucose homeostasis and lipid synthesis by reducing SREBP-1c, ACC and FAS expression (Liu et al., 2011; Gamede et al., 2019; Kusu et al., 2019; Zhang et al., 2020). Betaine (5) significantly improved hepatic steatosis in C57BL/6J mice by activating AMPK and downregulating SREBP-1c (Xu et al., 2018). It also attenuated hepatic triglyceride accumulation by reducing methylation of the PPARα promoter and upregulating PPARα expression (Wang et al., 2013). Nuciferine (6) treatment essentially reversed the HFD-induced increase in hepatic protein levels of SREBP-1c and fatty acid synthase (FASN), suggesting that nuciferine could attenuate the development of HFD-induced hepatic steatosis (Guo et al., 2013). In addition, baicalein (7) partially reduced methionine and choline-deficient diet-induced hepatic lipid accumulation in mice by modulating the expression of SREBP-1 c, FAS, PPARα and CPT1a (Zhang et al., 2018). Puerarin (8) inhibits FAS and activates AMPK expression, thereby preventing SREBP-1c translocation to the nucleus. Thus, it ultimately reduces lipid synthesis and increases fatty acid oxidation (Kang et al., 2015). Emodin (9) can improve hepatic steatosis in rats with liquid fructose-induced NAFLD by inhibiting the adipogenic transcription factor SREBP-1c and its downstream proteins FAS and stearoyl coenzyme A desaturase 1 (SCD1) (Li et al., 2016). In addition to affecting DNL, some natural products are thought to enhance fatty acid oxidation and thus reduce NASH. For example, nordihydroguaiaretic acid (10) or schizandrin A (11) supplements may reduce obesity, in part by increasing fatty acid oxidation (Li et al., 2016; Jeong et al., 2019).
Regulating Inflammation
Inflammation is a very important manifestation of NASH and is thought to be an important factor in the progression of NASH to HCC. The discovery of the anti-inflammatory effects of natural products is therefore necessary and is likely to be an important tool in the treatment of NASH and NASH-HCC. Resveratrol (12) can reduce the secretion and expression of proinflammatory cytokines (Cheng et al., 2019). Further studies have shown that resveratrol improves hepatic steatosis by activating the AMPKα-SIRT1 pathway to enhance AMPKα phosphorylation and inhibit the NF-κB inflammatory pathway (Tian et al., 2016). Celastrol (13) significantly reduced TG accumulation in steatotic HepG2 cells and downregulated the expression levels of TLR4, MyD88, phosphorylated-NF-κBp65, TNF-α and IL-1β. In addition, the combination of celastrol and TLR4 siRNA further attenuated the expression of inflammatory mediators. This finding suggests that celastrol may exert a protective effect by inhibiting TLR4-mediated inflammatory responses (Han et al., 2018). Nuciferine (6) ameliorates NAFLD by reducing necroinflammation (IL-1β, IL-6 and TNF-α) and reversing serum markers of MS (TC, TG and LDL-c) (Cui et al., 2020). Emodin (9) downregulates serum IL-1 and TNF-α secretion and exerts anti-NAFLD effects by inhibiting the hepatic TLR4 signaling pathway (Tao et al., 2017). Emodin also inhibits proinflammatory cytokine expression in LPS-induced macrophages by suppressing ERK1/2 and p38 signaling. Thus it reduces inflammatory cell infiltration in the liver and impairs liver function (Jia et al., 2014).
Isoorientin (14) (a flavonoid isolated from several edible plants) significantly improved inflammation by enhancing antioxidant enzyme activity and inhibiting the secretion of inflammatory cytokines (TNF-α, IL-1, IL-6) in high fructose-induced obesity in mice. Thus, it suppressed hyperlipidemia and liver injury (Yuan et al., 2016). Geraniol (15), a monoterpene alcohol isolated from rose and lemon essential oils, prevented increases in serum IL-1β and TNF-α levels and hepatic nitric oxide levels and modulated fructose-induced inflammation and oxidative stress (Ibrahim et al., 2015). Astaxanthin (16), a lutein-like carotenoid isolated from Haemococcus pluvialis, has been reported to inhibit the phosphorylation of JNK1 and IκB-β, ROS production and the nuclear translocation of NF-κB p65 in the liver. Thus, it can reduce inflammation in mice fed a high-fructose diet (Bhuvaneswari et al., 2014). In the NASH mouse model, treatment with schisandrin B (17) or kukoamine B (18) downregulated TNF-α, IL-1β and IL-6 levels (Leong et al., 2016; Zhao Q. et al., 2020). Geraniol (15) or betaine (5) supplements have shown anti-inflammatory properties by improving mitochondrial dysfunction in rats and mice fed an MCD diet (Chen et al., 2016). Genistein (19) is a phytoestrogen that is mainly found in soy and has antioxidant properties (Chen et al., 2016). Genistein (19) treatment reduced liver inflammation and fibrosis through a decrease in TNF-α and IL-1β levels in mice with MCD diet-induced NASH (Yoo et al., 2015). Similarly, naringenin (20) and scopolamine (21) were found to significantly inhibit liver inflammation in MCD-fed mice (Liu et al., 2020; Wang et al., 2020).
Regulating Fibrosis
Liver fibrosis is a major determinant of clinical disease progression and outcome in patients with NASH (Angulo et al., 2015). When excessive fibrosis occurs, the liver structure is inevitably damaged, and fibrosis usually indicates a higher likelihood of progression to cirrhosis and other end-stage liver diseases in NASH (Angulo et al., 2015). The fibrotic process in the liver is thought to be regulated primarily by HSCs, a type of hepatic progenitor cell that is in a quiescent state under physiological conditions (Tsuchida and Friedman, 2017). If liver injury is not resolved, the continued supply of inflammatory cytokines and apoptotic cell bodies will perpetuate the fibrotic effects of HSC and promote further tissue remodelling (Bachem et al., 1992). The disease has formally progressed from NASH to cirrhosis when collagen deposits are present in the majority of liver tissue (Kleiner et al., 2005). Furthermore, results from longitudinal studies have shown that patients with NAFLD/NASH who have severe liver fibrosis are at increased risk of HCC and mortality compared to patients with mild liver fibrosis (Ekstedt et al., 2015; Alexander et al., 2019). However, a significant number of patients with NAFLD-associated HCC (more than one third) are reported to have no extensive fibrosis at the time of presentation (Baffy et al., 2012). An interesting conceptual possibility is the malignant transformation of hepatocellular adenomas (HCA) in non-cirrhotic NAFLD patients. A correlation between malignant transformation of HCA and the metabolic syndrome has been described in a series of US patients (Farges et al., 2011). These data suggest that NAFLD, a hepatic manifestation of the metabolic syndrome, may lead to the development of HCC in the absence of cirrhotic HCA. In addition, inflammatory HCA, a subtype associated with an increased risk of malignant transformation, is associated with obesity, further supporting this association (Chang et al., 2013). However additional epidemiological and pathophysiological data are needed to demonstrate this correlation.
As described earlier, although liver fibrosis is not necessarily a necessary process for the progression of NASH to HCC, once excessive fibrosis has occurred, the liver structure will inevitably be damaged. Therefore, it is particularly important to prevent and control the development of liver fibrosis. Isorhamnetin (22) treatment showed a protective effect against fibrosis by reducing the expression of fibrosis markers in mice with steatosis induced by a combination of a HFD and CCl4 (Ganbold et al., 2019). Astragaloside (23) is the main active component of Radix Astragali that significantly ameliorated liver fibrosis by inhibiting the TGFβ1/Smad pathway in a CCl4-induced mouse model of fibrosis (Yuan et al., 2018). Astragaloside has been shown to have a variety of pharmacological activities, including antioxidative stress, anti-inflammatory, anticancer and anti-fibrotic activities (Rajput et al., 2011; Gui et al., 2013). Salvianolic acid B (SalB) is a polyphenolic antioxidant component extracted from Salvia miltiorrhiza (Zhai et al., 2019). SalB (24) can inhibit NAFLD/NASH by regulating cellular oxidative stress and lipid metabolism and exerting anti-inflammatory and anti-fibrosis effects to improve liver fibrosis (Hong et al., 2017). Pycnogenol (PYC) is a group of flavonoids isolated from French maritime pine bark (Pinus pinaster Aiton, synonym Pinus maritima Mill.) and is a mixture of proanthocyanidins with potent antioxidant and ROS scavenging properties. PYC (25) improved fibrosis and cirrhosis in NASH rats, and these results suggest that PYC delayed the progression of fatty liver to fibrosis (Mei et al., 2012). Calycosin (26) significantly reduced liver fibrosis in NASH mice by activating FXR and inhibiting HSC activation (Duan et al., 2017). In MCD diet-fed mice, glycyrrhetinic acid (27) exerted several hepatoprotective effects, including improving inflammation and regulating lipid metabolism, in addition to limiting pericellular fibrosis and collagen deposition (Wang et al., 2016). Xanthohumol (28) is a promising compound for the treatment of fibrosis in NASH. It induces the apoptosis of activated HSCs in vitro in a dose-dependent manner without impairing the viability of human primary hepatocytes at high doses (Dorn et al., 2010). Thymoquinone (29) has the potential to ameliorate hepatic steatosis, inflammation, apoptotic state and fibrosis in rats fed a HFHC diet (Awad et al., 2016)[136]. Furthermore, in a mouse model induced by an MCD diet, isochlorogenic acid B (30) exhibited a comprehensive antifibrotic effect by downregulating genes involved in fibrosis and inhibiting several profibrotic factors (Liu X. et al., 2019).
Regulating the Gut Microbiota
The key role of the gut microbiota in NASH and NASH-HCC has been discussed previously. Although the exact mechanisms by which the gut microbiota plays a role in both conditions are currently unknown, studies have identified natural products that may inhibit the development of NASH by modulating gut microbiota. Thus, they have the potential to hinder the progression of NASH to HCC. Salidroside (31) reduced hepatic lipid accumulation, the inflammatory response and insulin resistance in NAFLD rats (Li et al., 2017). In an HFD-induced NASH model in mice, salidroside (31) treatment significantly improved HFD-induced intestinal bacterial and bile acid disturbances, thus demonstrating the potential role of salidroside (31) in NASH treatment via the intestinal microbial-bile acid-FXR axis (Li H. et al., 2020). In addition, resveratrol (12) has been reported to maintain intestinal barrier integrity and limit intestinal inflammation. Treatment with resveratrol (12) resulted in reduced metabolic endotoxemia and altered the intestinal microbial distribution in SD rats fed a HFD (Chen et al., 2020). In a mouse model of HFD-induced NAFLD, quercetin (32) was shown to reverse intestinal flora imbalance and inhibit endotoxemia-mediated TLR4 signaling. Thus, it was able to suppress the inflammatory response and activate reticulo-stress pathways, which ultimately prevented the dysregulation of lipid metabolism-related gene expression (Porras et al., 2017). A subsequent study reaffirmed that the regulation of the gut microbiota and transplantation of gut microbiota by quercetin (32) can influence the development of NAFLD (Porras et al., 2019). This suggests that the prebiotic capacity of quercetin and the gut microbiota transplantation could be used as a protective strategy against obesity-associated NAFLD.
Conclusion and Perspectives
Based on our account above, it is clear that the current research is primarily focused on NASH. However, there is little research on the progression of NASH to HCC, in terms of both the specific mechanisms involved in the transformation process and its prevention or treatment. The development of NAFLD is clearly associated with metabolic dysregulation as a result of excess carbohydrates and fat leading to insulin resistance, obesity and fatty acid accumulation. These processes upregulate various pathways, including lipotoxicity, oxidative stress, ER stress and innate immune responses, among others. More importantly, a growing number of studies have found that changes in the gut microbiota also play a large role in the development and progression of NASH. The chronic inflammation, regeneration and fibrotic remodeling that follows this predisposes hepatocytes to cellular transformation and chromosomal aberrations, leading to hepatocarcinogenesis (Figure 1). We focused on the process of NASH-driven HCC because the transition from inflammation to cancer is common and can be actively intervened upon to delay or even hinder the development of cancer. Gastritis and colorectal inflammation, if left unchecked, can progress to gastric and colorectal cancer, as it does in the liver. The importance of liver inflammation in the development and progression of cancer has been well documented.
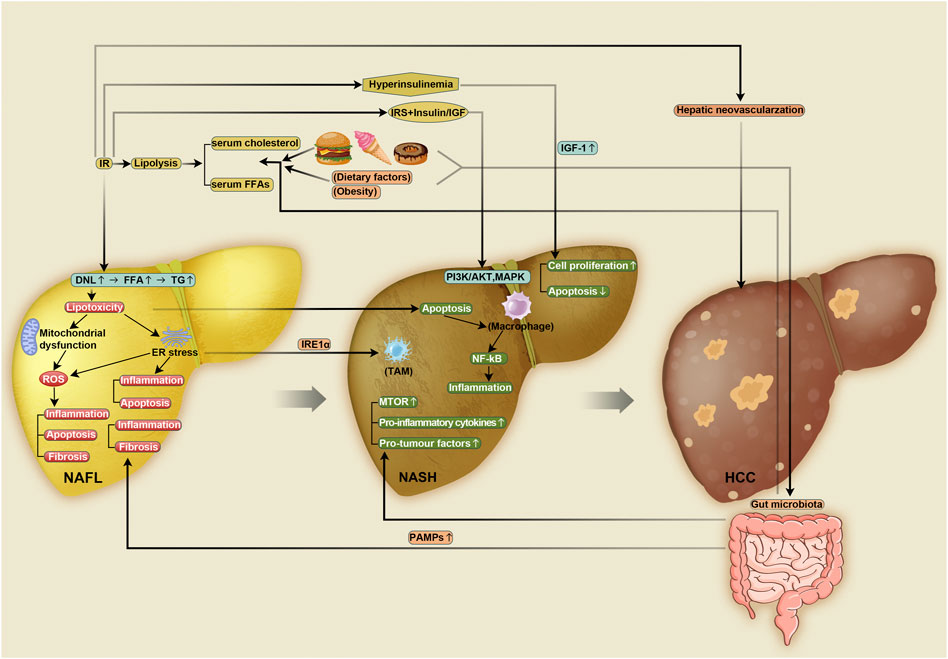
FIGURE 1. Proposed mechanism of NASH and NASH-driven HCC. Insulin resistance causes a compensatory increase in insulin in the body, leading to hyperinsulinemia and a marked increase in IGF-1 levels. These conditions can promote hepatocyte proliferation and inhibit apoptosis. Therefore, they can exacerbate the development of HCC. Insulin resistance also directly triggers HCC by promoting hepatic neovascularization. When insulin resistance occurs, IRS binds to insulin or IGF and induces HCC through the PI3K/Akt and MAPK pathways. Insulin resistance promotes lipolysis in adipose tissue, which leads to increased serum cholesterol and FFA levels. High levels of FFA in the serum travel to the liver, causing a significant increase in FFA in the liver. Insulin resistance can also directly promote DNL, which leads to an increase in hepatic FFA levels. Increased FFA levels in the liver promotes the synthesis of TG and triggers lipotoxicity. Lipotoxicity induces apoptosis, which activates liver macrophages and triggers inflammation through the NF-kB pathway, further inducing the development of HCC. Lipotoxicity also leads to mitochondrial dysfunction and ER stress, both of which further induce ROS production. This further promotes inflammation, apoptosis and fibrosis, thereby contributing to the development of NASH and NASH-HCC. ER stress can also directly promote inflammation and apoptosis. Dietary factors, obesity and the gut microbiota also contribute to elevated serum cholesterol and FFA levels, and diet and obesity alter the gut microbiota. The gut microbiota promotes inflammation and fibrosis through PAMPs, which further induce NASH and NASH-HCC. Changes in the gut microbiota can also directly trigger HCC through elevated levels of MTOR, proinflammatory factors and protumor factors. IGF: insulin-like growth factor, HCC: hepatocellular carcinoma, PI3K: phosphatidylinositol 3-kinase, Akt: protein kinase B, MAPK: mitogen-activated protein kinase, FFA: free fatty acid, DNL: de novo lipogenesis, TG: triglyceride, NF-kB: nuclear factor-jB kinase-b, ER: endoplasmic reticulum, ROS: reactive oxygen species, NASH: nonalcoholic steatohepatitis, PAMPs: pathogen-associated molecular patterns, MTOR: mammalian target of rapamycin.
This review highlights several natural products that have been shown to inhibit the conversion of NASH to HCC, as well as a wide range of natural compounds with great potential to play a role in this process (Table 1). Natural products are derived from components or metabolites in plants and animals and play an important role in the exploration of new structural compounds and drug discovery. The use of plant products for the treatment of cancer has a long history; approximately 60% of the anticancer agents currently in use are of natural origin. Herbs and their derived plant compounds are useful in cancer treatment. Due to their low toxicity, easy availability and low cost, natural compounds are a possible therapeutic agent for the field of anticancer research. An increasing number of natural products have been found to act on NASH. More importantly, research is beginning to focus on the role of natural products in inhibiting the conversion of NASH to HCC. Silymarin, berberine, curcumin and green tea catechin are four natural small molecule compounds that have been shown to impede the conversion of NASH to HCC (Figure 2), although the exact mechanisms of their roles in this process requires further investigation. An increasing number of studies have focused on the effects of natural products on the development of NASH. These effects may act mainly in the following ways: modulating lipid metabolism, anti-inflammatory activities, anti-fibrotic activities, and modulating the gut microbiota. These processes can combat NASH and impede its subsequent progression. Disturbances in lipid metabolism, inflammation, fibrosis and changes in the gut microbiota are all potential factors contributing to the progression of NASH to HCC. It is therefore reasonable to assume that these natural small molecule compounds are likely to be effective drugs for the treatment of NASH-HCC in the future. However, the hepatotoxicity of Chinese herbal medicines has always been a concern. Therefore, while studying the inhibition of HCC by natural products derived from Chinese herbal medicines, we also need to pay attention to the toxicity of HCC. In addition to obtaining evidence of efficacy, comprehensive studies on the genotoxicity, carcinogenicity, and systemic and reproductive toxicity of candidate anti-HCC drugs are urgently needed.
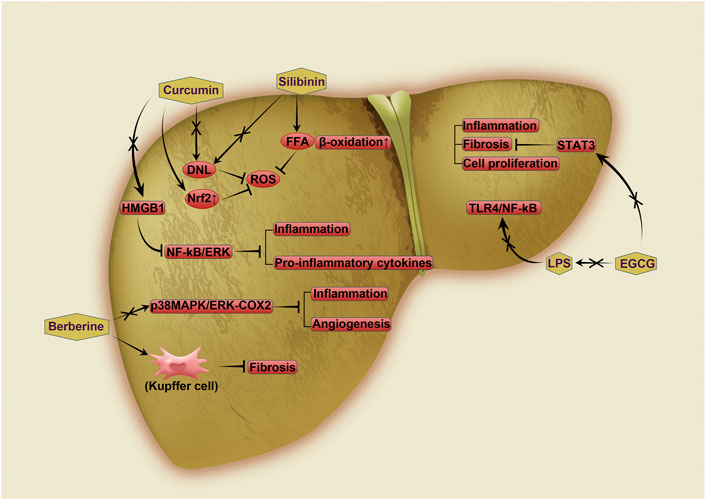
FIGURE 2. Mechanisms of inhibition of NASH-driven HCC by four natural products. Curcumin can reduce the expression of HMGB1. This can in turn reduce inflammation and the production of proinflammatory factors by inhibiting both the NF-kB and ERK pathways, thereby inhibiting the development of HCC. Curcumin also inhibits NASH and NASH-HCC by downregulating DNL and upregulating Nrf2 expression, respectively, thereby suppressing ROS production. Silibinin also inhibits DNL and, importantly, enhances β-oxidation of FFAs, thereby reducing ROS production. Berberine directly attenuates ROS production and hinders the development of HCC by upregulating p38MAPK/ERK-COX2. Thus, it reduces inflammation and inhibits hepatocyte apoptosis. EGCG regulates the development of NASH-HCC in two ways. First, it inhibits the progression of NASH to HCC by reducing the level of LPS in the blood, thereby inhibiting the TLR4/NF-kB pathway. Additionally, it inhibits HCC development by reducing STAT3 expression and suppressing inflammation, fibrosis and cell proliferation. HMGB1: high-mobility group box 1, Nf-kB: nuclear factor-jB kinase-b, ERK: extracellular signal-regulated kinase, HCC: hepatocellular carcinoma, NASH: nonalcoholic steatohepatitis, DNL: de novo lipogenesis, Nrf2: nuclear factor erythroid 2-related 2, ROS: reactive oxygen species, MAPK: mitogen-activated protein kinase, COX2: cyclooxygenase 2, EGCG: epigallocatechin gallate, TLR4: toll-like receptor 4, STAT3: signal transducer and activator of transcription 3.
Author Contributions
HX and GJ proposed the topic and made the frame. GS, YL and LL contributed to original draft preparation. GZ, LW and WZ participated in part of text arrangement and literature collection. LL and GS participated in the conception and drawing of the image. HX, TW and GJ revised the manuscript.
Funding
This work was supported by National Nature Science Foundation of China, No. 81874206, 82104466; Shanghai Frontiers Science Center of Disease and Syndrome Biology of Inflammatory Cancer Transformation (2021KJ03-12); Shanghai Rising-Star Program, No. 20QA1409300; and the Program for Young Eastern Scholar at Shanghai Institutions of Higher Learning, No. QD2019034; Young Elite Scientists Sponsorship Program by CACM.
Conflict of Interest
The authors declare that the research was conducted in the absence of any commercial or financial relationships that could be construed as a potential conflict of interest.
Publisher’s Note
All claims expressed in this article are solely those of the authors and do not necessarily represent those of their affiliated organizations, or those of the publisher, the editors and the reviewers. Any product that may be evaluated in this article, or claim that may be made by its manufacturer, is not guaranteed or endorsed by the publisher.
References
Abdelmegeed, M. A., Banerjee, A., Yoo, S. H., Jang, S., Gonzalez, F. J., and Song, B. J. (2012). Critical Role of Cytochrome P450 2E1 (CYP2E1) in the Development of High Fat-Induced Non-alcoholic Steatohepatitis. J. Hepatol. 57 (4), 860–866. doi:10.1016/j.jhep.2012.05.019
Afrin, R., Arumugam, S., Rahman, A., Wahed, M. I., Karuppagounder, V., Harima, M., et al. (2017). Curcumin Ameliorates Liver Damage and Progression of NASH in NASH-HCC Mouse Model Possibly by Modulating HMGB1-NF-Κb Translocation. Int. Immunopharmacol. 44, 174–182. doi:10.1016/j.intimp.2017.01.016
Aguirre, V., Uchida, T., Yenush, L., Davis, R., and White, M. F. (2000). The C-Jun NH(2)-terminal Kinase Promotes Insulin Resistance during Association with Insulin Receptor Substrate-1 and Phosphorylation of Ser(307). J. Biol. Chem. 275 (12), 9047–9054. doi:10.1074/jbc.275.12.9047
Alexander, M., Loomis, A. K., van der Lei, J., Duarte-Salles, T., Prieto-Alhambra, D., Ansell, D., et al. (2019). Risks and Clinical Predictors of Cirrhosis and Hepatocellular Carcinoma Diagnoses in Adults with Diagnosed NAFLD: Real-World Study of 18 Million Patients in Four European Cohorts. BMC Med. 17 (1), 95. doi:10.1186/s12916-019-1321-x
Angulo, P., Hui, J. M., Marchesini, G., Bugianesi, E., George, J., Farrell, G. C., et al. (2007). The NAFLD Fibrosis Score: a Noninvasive System that Identifies Liver Fibrosis in Patients with NAFLD. Hepatology 45 (4), 846–854. doi:10.1002/hep.21496
Angulo, P., Kleiner, D. E., Dam-Larsen, S., Adams, L. A., Bjornsson, E. S., Charatcharoenwitthaya, P., et al. (2015). Liver Fibrosis, but No Other Histologic Features, Is Associated with Long-Term Outcomes of Patients with Nonalcoholic Fatty Liver Disease. Gastroenterology 149 (2), 389–e10. doi:10.1053/j.gastro.2015.04.043
Angulo, P., and Lindor, K. D. (2001). Insulin Resistance and Mitochondrial Abnormalities in NASH: a Cool Look into a Burning Issue. Gastroenterology 120 (5), 1281–1285. doi:10.1053/gast.2001.23591
Anstee, Q. M., and Day, C. P. (2015). The Genetics of Nonalcoholic Fatty Liver Disease: Spotlight on PNPLA3 and TM6SF2. Semin. Liver Dis. 35 (3), 270–290. doi:10.1055/s-0035-1562947
Arab, J. P., Martin-Mateos, R. M., and Shah, V. H. (2018). Gut-liver axis, Cirrhosis and Portal Hypertension: the Chicken and the Egg. Hepatol. Int. 12 (Suppl. 1), 24–33. doi:10.1007/s12072-017-9798-x
Arguello, G., Balboa, E., Arrese, M., and Zanlungo, S. (2015). Recent Insights on the Role of Cholesterol in Non-alcoholic Fatty Liver Disease. Biochim. Biophys. Acta 1852 (9), 1765–1778. doi:10.1016/j.bbadis.2015.05.015
Argyrou, C., Moris, D., and Vernadakis, S. (2017). Hepatocellular Carcinoma Development in Non-alcoholic Fatty Liver Disease and Non-alcoholic Steatohepatitis. Is it Going to Be the "Plague" of the 21st Century? A Literature Review Focusing on Pathogenesis, Prevention and Treatment. J. BUON 22 (1), 6–20.
Awad, A. S., Abd Al Haleem, E. N., El-Bakly, W. M., and Sherief, M. A. (2016). Thymoquinone Alleviates Nonalcoholic Fatty Liver Disease in Rats via Suppression of Oxidative Stress, Inflammation, Apoptosis. Naunyn Schmiedeb. Arch. Pharmacol. 389 (4), 381–391. doi:10.1007/s00210-015-1207-1
Bachem, M. G., Meyer, D., Melchior, R., Sell, K. M., and Gressner, A. M. (1992). Activation of rat liver perisinusoidal lipocytes by transforming growth factors derived from myofibroblastlike cells. A potential mechanism of self perpetuation in liver fibrogenesis. J. Clin. Invest 89 (1), 19–27. doi:10.1172/JCI115561
Baffy, G., Brunt, E. M., and Caldwell, S. H. (2012). Hepatocellular carcinoma in non-alcoholic fatty liver disease: an emerging menace. J. Hepatol. 56 (6), 1384–1391. doi:10.1016/j.jhep.2011.10.027
Banini, B. A., and Sanyal, A. J. (2021). NAFLD-related HCC. Adv. Cancer Res. 149, 143–169. doi:10.1016/bs.acr.2020.11.001
Beaven, S. W., Matveyenko, A., Wroblewski, K., Chao, L., Wilpitz, D., Hsu, T. W., et al. (2013). Reciprocal regulation of hepatic and adipose lipogenesis by liver X receptors in obesity and insulin resistance. Cell Metab. 18 (1), 106–117. doi:10.1016/j.cmet.2013.04.021
Bhala, N., Angulo, P., van der Poorten, D., Lee, E., Hui, J. M., Saracco, G., et al. (2011). The natural history of nonalcoholic fatty liver disease with advanced fibrosis or cirrhosis: an international collaborative study. Hepatology 54 (4), 1208–1216. doi:10.1002/hep.24491
Bhuvaneswari, S., Yogalakshmi, B., Sreeja, S., and Anuradha, C. V. (2014). Astaxanthin reduces hepatic endoplasmic reticulum stress and nuclear factor-κB-mediated inflammation in high fructose and high fat diet-fed mice. Cell Stress Chaperones 19 (2), 183–191. doi:10.1007/s12192-013-0443-x
Bosch-Barrera, J., and Menendez, J. A. (2015). Silibinin and STAT3: A natural way of targeting transcription factors for cancer therapy. Cancer Treat. Rev. 41 (6), 540–546. doi:10.1016/j.ctrv.2015.04.008
Bugianesi, E., Leone, N., Vanni, E., Marchesini, G., Brunello, F., Carucci, P., et al. (2002). Expanding the natural history of nonalcoholic steatohepatitis: from cryptogenic cirrhosis to hepatocellular carcinoma. Gastroenterology 123 (1), 134–140. doi:10.1053/gast.2002.34168
Bugianesi, E., Manzini, P., D'Antico, S., Vanni, E., Longo, F., Leone, N., et al. (2004). Relative contribution of iron burden, HFE mutations, and insulin resistance to fibrosis in nonalcoholic fatty liver. Hepatology 39 (1), 179–187. doi:10.1002/hep.20023
Bugianesi, E., McCullough, A. J., and Marchesini, G. (2005). Insulin resistance: a metabolic pathway to chronic liver disease. Hepatology 42 (5), 987–1000. doi:10.1002/hep.20920
Cai, D., Yuan, M., Frantz, D. F., Melendez, P. A., Hansen, L., Lee, J., et al. (2005). Local and systemic insulin resistance resulting from hepatic activation of IKK-beta and NF-kappaB. Nat. Med. 11 (2), 183–190. doi:10.1038/nm1166
Caligiuri, A., Gentilini, A., and Marra, F. (2016). Molecular Pathogenesis of NASH. Int. J. Mol. Sci. 17 (9). doi:10.3390/ijms17091575
Cao, Y., Pan, Q., Cai, W., Shen, F., Chen, G. Y., Xu, L. M., et al. (2016). Modulation of Gut Microbiota by Berberine Improves Steatohepatitis in High-Fat Diet-Fed BALB/C Mice. Arch. Iran. Med. 19 (3), 197–203.
Cazanave, S. C., and Gores, G. J. (2010). Mechanisms and clinical implications of hepatocyte lipoapoptosis. Clin. Lipidol. 5 (1), 71–85. doi:10.2217/clp.09.85
Chalasani, N., Gorski, J. C., Asghar, M. S., Asghar, A., Foresman, B., Hall, S. D., et al. (2003). Hepatic cytochrome P450 2E1 activity in nondiabetic patients with nonalcoholic steatohepatitis. Hepatology 37 (3), 544–550. doi:10.1053/jhep.2003.50095
Chalasani, N., Younossi, Z., Lavine, J. E., Charlton, M., Cusi, K., Rinella, M., et al. (2018). The diagnosis and management of nonalcoholic fatty liver disease: Practice guidance from the American Association for the Study of Liver Diseases. Hepatology 67 (1), 328–357. doi:10.1002/hep.29367
Chalasani, N. P., Sanyal, A. J., Kowdley, K. V., Robuck, P. R., Hoofnagle, J., Kleiner, D. E., et al. (2009). Pioglitazone versus vitamin E versus placebo for the treatment of non-diabetic patients with non-alcoholic steatohepatitis: PIVENS trial design. Contemp. Clin. Trials 30 (1), 88–96. doi:10.1016/j.cct.2008.09.003
Chan, J. K. W., Bittner, S., Bittner, A., Atwal, S., Shen, W. J., Inayathullah, M., et al. (2018). Nordihydroguaiaretic Acid, a Lignan from Larrea tridentata (Creosote Bush), Protects Against American Lifestyle-Induced Obesity Syndrome Diet-Induced Metabolic Dysfunction in Mice. J. Pharmacol. Exp. Ther. 365 (2), 281–290. doi:10.1124/jpet.117.243733
Chang, C. Y., Hernandez-Prera, J. C., Roayaie, S., Schwartz, M., and Thung, S. N. (2013). Changing epidemiology of hepatocellular adenoma in the United States: review of the literature. Int. J. Hepatol. 2013, 604860. doi:10.1155/2013/604860
Chaurasia, B., and Summers, S. A. (20182015). Ceramides - Lipotoxic Inducers of Metabolic Disorders: (Trends in Endocrinology and Metabolism 26, 538-550; 2015). Trends Endocrinol. Metab. 29 (1), 53866–55067. doi:10.1016/j.tem.2017.09.005
Chen, J., Fan, X., Zhou, L., and Gao, X. (2016). Treatment with geraniol ameliorates methionine-choline-deficient diet-induced non-alcoholic steatohepatitis in rats. J. Gastroenterol. Hepatol. 31 (7), 1357–1365. doi:10.1111/jgh.13272
Chen, L. Z., Xia, H. H., Xin, Y. N., Lin, Z. H., and Xuan, S. Y. (2015). TM6SF2 E167K Variant, a Novel Genetic Susceptibility Variant, Contributing to Nonalcoholic Fatty Liver Disease. J. Clin. Transl. Hepatol. 3 (4), 265–270. doi:10.14218/JCTH.2015.00023
Chen, M., Hou, P., Zhou, M., Ren, Q., Wang, X., Huang, L., et al. (2020). Resveratrol attenuates high-fat diet-induced non-alcoholic steatohepatitis by maintaining gut barrier integrity and inhibiting gut inflammation through regulation of the endocannabinoid system. Clin. Nutr. 39 (4), 1264–1275. doi:10.1016/j.clnu.2019.05.020
Cheng, K., Song, Z., Zhang, H., Li, S., Wang, C., Zhang, L., et al. (2019). The therapeutic effects of resveratrol on hepatic steatosis in high-fat diet-induced obese mice by improving oxidative stress, inflammation and lipid-related gene transcriptional expression. Med. Mol. Morphol. 52 (4), 187–197. doi:10.1007/s00795-019-00216-7
Cheung, O., Puri, P., Eicken, C., Contos, M. J., Mirshahi, F., Maher, J. W., et al. (2008). Nonalcoholic steatohepatitis is associated with altered hepatic MicroRNA expression. Hepatology 48 (6), 1810–1820. doi:10.1002/hep.22569
Chitra, P., Saiprasad, G., Manikandan, R., and Sudhandiran, G. (2015). Berberine inhibits Smad and non-Smad signaling cascades and enhances autophagy against pulmonary fibrosis. J. Mol. Med. Berl. 93 (9), 1015–1031. doi:10.1007/s00109-015-1283-1
Chopyk, D. M., and Grakoui, A. (2020). Contribution of the Intestinal Microbiome and Gut Barrier to Hepatic Disorders. Gastroenterology 159 (3), 849–863. doi:10.1053/j.gastro.2020.04.077
Cinaroglu, A., Gao, C., Imrie, D., and Sadler, K. C. (2011). Activating transcription factor 6 plays protective and pathological roles in steatosis due to endoplasmic reticulum stress in zebrafish. Hepatology 54 (2), 495–508. doi:10.1002/hep.24396
Cui, H., Li, Y., Cao, M., Liao, J., Liu, X., Miao, J., et al. (2020). Untargeted Metabolomic Analysis of the Effects and Mechanism of Nuciferine Treatment on Rats With Nonalcoholic Fatty Liver Disease. Front. Pharmacol. 11, 858. doi:10.3389/fphar.2020.00858
Dapito, D. H., Mencin, A., Gwak, G. Y., Pradere, J. P., Jang, M. K., Mederacke, I., et al. (2012). Promotion of hepatocellular carcinoma by the intestinal microbiota and TLR4. Cancer Cell 21 (4), 504–516. doi:10.1016/j.ccr.2012.02.007
Darvesh, A. S., and Bishayee, A. (2013). Chemopreventive and therapeutic potential of tea polyphenols in hepatocellular cancer. Nutr. Cancer 65 (3), 329–344. doi:10.1080/01635581.2013.767367
Day, C. P. (2002). Pathogenesis of steatohepatitis. Best. Pract. Res. Clin. Gastroenterol. 16 (5), 663–678. doi:10.1053/bega.2002.0333
de Conti, A., Ortega, J. F., Tryndyak, V., Dreval, K., Moreno, F. S., Rusyn, I., et al. (2017). MicroRNA deregulation in nonalcoholic steatohepatitis-associated liver carcinogenesis. Oncotarget 8 (51), 88517–88528. doi:10.18632/oncotarget.19774
De Minicis, S., Agostinelli, L., Rychlicki, C., Sorice, G. P., Saccomanno, S., Candelaresi, C., et al. (2014). HCC development is associated to peripheral insulin resistance in a mouse model of NASH. PloS One 9 (5), e97136. doi:10.1371/journal.pone.0097136
Deep, G., and Agarwal, R. (2010). Antimetastatic efficacy of silibinin: molecular mechanisms and therapeutic potential against cancer. Cancer Metastasis Rev. 29 (3), 447–463. doi:10.1007/s10555-010-9237-0
Degasperi, E., and Colombo, M. (2016). Distinctive features of hepatocellular carcinoma in non-alcoholic fatty liver disease. Lancet Gastroenterol. Hepatol. 1 (2), 156–164. doi:10.1016/S2468-1253(16)30018-8
Dey, P., Kim, J. B., Chitchumroonchokchai, C., Li, J., Sasaki, G. Y., Olmstead, B. D., et al. (2019). Green tea extract inhibits early oncogenic responses in mice with nonalcoholic steatohepatitis. Food Funct. 10 (10), 6351–6361. doi:10.1039/c9fo01199d
Ding, Y., Liu, P., Chen, Z. L., Zhang, S. J., Wang, Y. Q., Cai, X., et al. (2018). Emodin Attenuates Lipopolysaccharide-Induced Acute Liver Injury via Inhibiting the TLR4 Signaling Pathway In Vitro and In Vivo. Front. Pharmacol. 9, 962. doi:10.3389/fphar.2018.00962
Ding, Y. F., Wu, Z. H., Wei, Y. J., Shu, L., and Peng, Y. R. (2017). Hepatic inflammation-fibrosis-cancer axis in the rat hepatocellular carcinoma induced by diethylnitrosamine. J. Cancer Res. Clin. Oncol. 143 (5), 821–834. doi:10.1007/s00432-017-2364-z
Domitrović, R., Jakovac, H., Marchesi, V. V., and Blažeković, B. (2013). Resolution of liver fibrosis by isoquinoline alkaloid berberine in CCl₄-intoxicated mice is mediated by suppression of oxidative stress and upregulation of MMP-2 expression. J. Med. Food 16 (6), 518–528. doi:10.1089/jmf.2012.0175
Donati, B., Dongiovanni, P., Romeo, S., Meroni, M., McCain, M., Miele, L., et al. (2017). MBOAT7 rs641738 variant and hepatocellular carcinoma in non-cirrhotic individuals. Sci. Rep. 7 (1), 4492. doi:10.1038/s41598-017-04991-0
Dongiovanni, P., Petta, S., Maglio, C., Fracanzani, A. L., Pipitone, R., Mozzi, E., et al. (2015). Transmembrane 6 superfamily member 2 gene variant disentangles nonalcoholic steatohepatitis from cardiovascular disease. Hepatology 61 (2), 506–514. doi:10.1002/hep.27490
Donnelly, K. L., Smith, C. I., Schwarzenberg, S. J., Jessurun, J., Boldt, M. D., and Parks, E. J. (2005). Sources of fatty acids stored in liver and secreted via lipoproteins in patients with nonalcoholic fatty liver disease. J. Clin. Invest 115 (5), 1343–1351. doi:10.1172/JCI23621
Donohoe, C. L., Lysaght, J., O'Sullivan, J., and Reynolds, J. V. (2017). Emerging Concepts Linking Obesity with the Hallmarks of Cancer. Trends Endocrinol. Metab. 28 (1), 46–62. doi:10.1016/j.tem.2016.08.004
Dorn, C., Kraus, B., Motyl, M., Weiss, T. S., Gehrig, M., Schölmerich, J., et al. (2010). Xanthohumol, a chalcon derived from hops, inhibits hepatic inflammation and fibrosis. Mol. Nutr. Food Res. 54 (Suppl. 2), S205–S213. doi:10.1002/mnfr.200900314
Duan, X., Meng, Q., Wang, C., Liu, Z., Liu, Q., Sun, H., et al. (2017). Calycosin attenuates triglyceride accumulation and hepatic fibrosis in murine model of non-alcoholic steatohepatitis via activating farnesoid X receptor. Phytomedicine 25, 83–92. doi:10.1016/j.phymed.2016.12.006
Ekstedt, M., Hagström, H., Nasr, P., Fredrikson, M., Stål, P., Kechagias, S., et al. (2015). Fibrosis stage is the strongest predictor for disease-specific mortality in NAFLD after up to 33 years of follow-up. Hepatology 61 (5), 1547–1554. doi:10.1002/hep.27368
Erstad, D. J., Fuchs, B. C., and Tanabe, K. K. (2018). Molecular signatures in hepatocellular carcinoma: A step toward rationally designed cancer therapy. Cancer 124 (15), 3084–3104. doi:10.1002/cncr.31257
Faeh, D., Minehira, K., Schwarz, J. M., Periasamy, R., Periasami, R., Park, S., et al. (2005). Effect of fructose overfeeding and fish oil administration on hepatic De Novo lipogenesis and insulin sensitivity in healthy men. Diabetes 54 (7), 1907–1913. doi:10.2337/diabetes.54.7.1907
Fan, J. G., and Cao, H. X. (2013). Role of diet and nutritional management in non-alcoholic fatty liver disease. J. Gastroenterol. Hepatol. 28 Suppl 4 (Suppl. 4), 81–87. doi:10.1111/jgh.12244
Fang, D., Shi, D., Lv, L., Gu, S., Wu, W., Chen, Y., et al. (2017). Bifidobacterium pseudocatenulatum LI09 and Bifidobacterium catenulatum LI10 attenuate D-galactosamine-induced liver injury by modifying the gut microbiota. Sci. Rep. 7 (1), 8770. doi:10.1038/s41598-017-09395-8
Farges, O., Ferreira, N., Dokmak, S., Belghiti, J., Bedossa, P., and Paradis, V. (2011). Changing trends in malignant transformation of hepatocellular adenoma. Gut 60 (1), 85–89. doi:10.1136/gut.2010.222109
Federico, A., Dallio, M., Di Fabio, G., Zarrelli, A., Zappavigna, S., Stiuso, P., et al. (2015). Silybin-Phosphatidylcholine Complex Protects Human Gastric and Liver Cells from Oxidative Stress. Vivo 29 (5), 569–575.
Fujii, M., Shibazaki, Y., Wakamatsu, K., Honda, Y., Kawauchi, Y., Suzuki, K., et al. (2013). A murine model for non-alcoholic steatohepatitis showing evidence of association between diabetes and hepatocellular carcinoma. Med. Mol. Morphol. 46 (3), 141–152. doi:10.1007/s00795-013-0016-1
Gamede, M., Mabuza, L., Ngubane, P., and Khathi, A. (2019). Plant-derived oleanolic acid ameliorates markers associated with non-alcoholic fatty liver disease in a diet-induced pre-diabetes rat model. Diabetes Metab. Syndr. Obes. 12, 1953–1962. doi:10.2147/DMSO.S218626
Ganbold, M., Owada, Y., Ozawa, Y., Shimamoto, Y., Ferdousi, F., Tominaga, K., et al. (2019). Isorhamnetin Alleviates Steatosis and Fibrosis in Mice with Nonalcoholic Steatohepatitis. Sci. Rep. 9 (1), 16210. doi:10.1038/s41598-019-52736-y
Gething, M. J., and Sambrook, J. (1992). Protein folding in the cell. Nature 355 (6355), 33–45. doi:10.1038/355033a0
Ghasemi, R., Ghaffari, S. H., Momeny, M., Pirouzpanah, S., Yousefi, M., Malehmir, M., et al. (2013). Multitargeting and antimetastatic potentials of silibinin in human HepG-2 and PLC/PRF/5 hepatoma cells. Nutr. Cancer 65 (4), 590–599. doi:10.1080/01635581.2013.770043
Giorgio, V., Miele, L., Principessa, L., Ferretti, F., Villa, M. P., Negro, V., et al. (2014). Intestinal permeability is increased in children with non-alcoholic fatty liver disease, and correlates with liver disease severity. Dig. Liver Dis. 46 (6), 556–560. doi:10.1016/j.dld.2014.02.010
Gui, D., Huang, J., Guo, Y., Chen, J., Chen, Y., Xiao, W., et al. (2013). Astragaloside IV ameliorates renal injury in streptozotocin-induced diabetic rats through inhibiting NF-κB-mediated inflammatory genes expression. Cytokine 61 (3), 970–977. doi:10.1016/j.cyto.2013.01.008
Guo, F., Yang, X., Li, X., Feng, R., Guan, C., Wang, Y., et al. (2013). Nuciferine prevents hepatic steatosis and injury induced by a high-fat diet in hamsters. PloS One 8 (5), e63770. doi:10.1371/journal.pone.0063770
Han, L. P., Sun, B., Li, C. J., Xie, Y., and Chen, L. M. (2018). Effect of celastrol on toll-like receptor 4-mediated inflammatory response in free fatty acid-induced HepG2 cells. Int. J. Mol. Med. 42 (4), 2053–2061. doi:10.3892/ijmm.2018.3775
Haque, M., and Sanyal, A. J. (2002). The metabolic abnormalities associated with non-alcoholic fatty liver disease. Best. Pract. Res. Clin. Gastroenterol. 16 (5), 709–731. doi:10.1053/bega.2002.0325
Harris, R. E. (2007). Cyclooxygenase-2 (cox-2) and the inflammogenesis of cancer. Subcell. Biochem. 42, 93–126. doi:10.1007/1-4020-5688-5_4
Hendrikx, T., Walenbergh, S. M., Hofker, M. H., and Shiri-Sverdlov, R. (2014). Lysosomal cholesterol accumulation: driver on the road to inflammation during atherosclerosis and non-alcoholic steatohepatitis. Obes. Rev. 15 (5), 424–433. doi:10.1111/obr.12159
Hetz, C., Bernasconi, P., Fisher, J., Lee, A. H., Bassik, M. C., Antonsson, B., et al. (2006). Proapoptotic BAX and BAK modulate the unfolded protein response by a direct interaction with IRE1alpha. Science 312 (5773), 572–576. doi:10.1126/science.1123480
Hetz, C. (2012). The unfolded protein response: controlling cell fate decisions under ER stress and beyond. Nat. Rev. Mol. Cell Biol. 13 (2), 89–102. doi:10.1038/nrm3270
Hong, M., Li, S., Wang, N., Tan, H. Y., Cheung, F., and Feng, Y. (2017). A Biomedical Investigation of the Hepatoprotective Effect of Radix salviae miltiorrhizae and Network Pharmacology-Based Prediction of the Active Compounds and Molecular Targets. Int. J. Mol. Sci. 18 (3). doi:10.3390/ijms18030620
Hosseini, H., Teimouri, M., Shabani, M., Koushki, M., Babaei Khorzoughi, R., Namvarjah, F., et al. (2020). Resveratrol alleviates non-alcoholic fatty liver disease through epigenetic modification of the Nrf2 signaling pathway. Int. J. Biochem. Cell Biol. 119, 105667. doi:10.1016/j.biocel.2019.105667
Hotamisligil, G. S. (2010). Endoplasmic reticulum stress and the inflammatory basis of metabolic disease. Cell 140 (6), 900–917. doi:10.1016/j.cell.2010.02.034
Hotamisligil, G. S. (2006). Inflammation and metabolic disorders. Nature 444 (7121), 860–867. doi:10.1038/nature05485
Hu, P., Han, Z., Couvillon, A. D., Kaufman, R. J., and Exton, J. H. (2006). Autocrine tumor necrosis factor alpha links endoplasmic reticulum stress to the membrane death receptor pathway through IRE1alpha-mediated NF-kappaB activation and down-regulation of TRAF2 expression. Mol. Cell Biol. 26 (8), 3071–3084. doi:10.1128/MCB.26.8.3071-3084.2006
Hui, J. M., Hodge, A., Farrell, G. C., Kench, J. G., Kriketos, A., and George, J. (2004). Beyond insulin resistance in NASH: TNF-alpha or adiponectin? Hepatology 40 (1), 46–54. doi:10.1002/hep.20280
Iacobazzi, V., Castegna, A., Infantino, V., and Andria, G. (2013). Mitochondrial DNA methylation as a next-generation biomarker and diagnostic tool. Mol. Genet. Metab. 110 (1-2), 25–34. doi:10.1016/j.ymgme.2013.07.012
Ibrahim, S. M., El-Denshary, E. S., and Abdallah, D. M. (2015). Geraniol, alone and in combination with pioglitazone, ameliorates fructose-induced metabolic syndrome in rats via the modulation of Both inflammatory and oxidative stress status. PloS One 10 (2), e0117516. doi:10.1371/journal.pone.0117516
Jabir, N. R., Ahmad, S., and Tabrez, S. (2018). An insight on the association of glycation with hepatocellular carcinoma. Semin. Cancer Biol. 49, 56–63. doi:10.1016/j.semcancer.2017.06.005
Jeong, M. J., Kim, S. R., and Jung, U. J. (2019). Schizandrin A supplementation improves nonalcoholic fatty liver disease in mice fed a high-fat and high-cholesterol diet. Nutr. Res. 64, 64–71. doi:10.1016/j.nutres.2019.01.001
Jia, W., Xie, G., and Jia, W. (2018). Bile acid-microbiota crosstalk in gastrointestinal inflammation and carcinogenesis. Nat. Rev. Gastroenterol. Hepatol. 15 (2), 111–128. doi:10.1038/nrgastro.2017.119
Jia, X., Iwanowycz, S., Wang, J., Saaoud, F., Yu, F., Wang, Y., et al. (2014). Emodin attenuates systemic and liver inflammation in hyperlipidemic mice administrated with lipopolysaccharides. Exp. Biol. Med. (Maywood) 239 (8), 1025–1035. doi:10.1177/1535370214530247
Jiang, J. W., Chen, X. H., Ren, Z., and Zheng, S. S. (2019). Gut microbial dysbiosis associates hepatocellular carcinoma via the gut-liver axis. Hepatobiliary Pancreat. Dis. Int. 18 (1), 19–27. doi:10.1016/j.hbpd.2018.11.002
Jiao, N., Baker, S. S., Chapa-Rodriguez, A., Liu, W., Nugent, C. A., Tsompana, M., et al. (2018). Suppressed hepatic bile acid signalling despite elevated production of primary and secondary bile acids in NAFLD. Gut 67 (10), 1881–1891. doi:10.1136/gutjnl-2017-314307
Jo, H., Choe, S. S., Shin, K. C., Jang, H., Lee, J. H., Seong, J. K., et al. (2013). Endoplasmic reticulum stress induces hepatic steatosis via increased expression of the hepatic very low-density lipoprotein receptor. Hepatology 57 (4), 1366–1377. doi:10.1002/hep.26126
Kaji, K., Yoshiji, H., Kitade, M., Ikenaka, Y., Noguchi, R., Yoshii, J., et al. (2008). Impact of insulin resistance on the progression of chronic liver diseases. Int. J. Mol. Med. 22 (6), 801–808.
Kammoun, H. L., Chabanon, H., Hainault, I., Luquet, S., Magnan, C., Koike, T., et al. (2009). GRP78 expression inhibits insulin and ER stress-induced SREBP-1c activation and reduces hepatic steatosis in mice. J. Clin. Invest 119 (5), 1201–1215. doi:10.1172/JCI37007
Kang, O. H., Kim, S. B., Mun, S. H., Seo, Y. S., Hwang, H. C., Lee, Y. M., et al. (2015). Puerarin ameliorates hepatic steatosis by activating the PPARα and AMPK signaling pathways in hepatocytes. Int. J. Mol. Med. 35 (3), 803–809. doi:10.3892/ijmm.2015.2074
Karuppagounder, V., Arumugam, S., Thandavarayan, R. A., Pitchaimani, V., Sreedhar, R., Afrin, R., et al. (2015). Modulation of HMGB1 translocation and RAGE/NFκB cascade by quercetin treatment mitigates atopic dermatitis in NC/Nga transgenic mice. Exp. Dermatol 24 (6), 418–423. doi:10.1111/exd.12685
Kennedy, A. R., Pissios, P., Otu, H., Roberson, R., Xue, B., Asakura, K., et al. (2007). A high-fat, ketogenic diet induces a unique metabolic state in mice. Am. J. Physiol. Endocrinol. Metab. 292 (6), E1724–E1739. doi:10.1152/ajpendo.00717.2006
Kleiner, D. E., Brunt, E. M., Van Natta, M., Behling, C., Contos, M. J., Cummings, O. W., et al. (2005). Design and validation of a histological scoring system for nonalcoholic fatty liver disease. Hepatology 41 (6), 1313–1321. doi:10.1002/hep.20701
Kolodziejczyk, A. A., Zheng, D., Shibolet, O., and Elinav, E. (2019). The role of the microbiome in NAFLD and NASH. EMBO Mol. Med. 11 (2). doi:10.15252/emmm.201809302
Koppe, S. W., Elias, M., Moseley, R. H., and Green, R. M. (2009). Trans fat feeding results in higher serum alanine aminotransferase and increased insulin resistance compared with a standard murine high-fat diet. Am. J. Physiol. Gastrointest. Liver Physiol. 297 (2), G378–G384. doi:10.1152/ajpgi.90543.2008
Kozlitina, J., Smagris, E., Stender, S., Nordestgaard, B. G., Zhou, H. H., Tybjærg-Hansen, A., et al. (2014). Exome-wide association study identifies a TM6SF2 variant that confers susceptibility to nonalcoholic fatty liver disease. Nat. Genet. 46 (4), 352–356. doi:10.1038/ng.2901
Kumashiro, N., Erion, D. M., Zhang, D., Kahn, M., Beddow, S. A., Chu, X., et al. (2011). Cellular mechanism of insulin resistance in nonalcoholic fatty liver disease. Proc. Natl. Acad. Sci. U. S. A. 108 (39), 16381–16385. doi:10.1073/pnas.1113359108
Kuo, P. L., and Lin, C. C. (2003). Green tea constituent (-)-epigallocatechin-3-gallate inhibits Hep G2 cell proliferation and induces apoptosis through p53-dependent and Fas-mediated pathways. J. Biomed. Sci. 10 (2), 219–227. doi:10.1007/BF02256057
Kusu, H., Yoshida, H., Kudo, M., Okuyama, M., Harada, N., Tsuji-Naito, K., et al. (2019). Tomatidine Reduces Palmitate-Induced Lipid Accumulation by Activating AMPK via Vitamin D Receptor-Mediated Signaling in Human HepG2 Hepatocytes. Mol. Nutr. Food Res. 63 (22), e1801377. doi:10.1002/mnfr.201801377
Lam, Y. Y., Ha, C. W., Campbell, C. R., Mitchell, A. J., Dinudom, A., Oscarsson, J., et al. (2012). Increased gut permeability and microbiota change associate with mesenteric fat inflammation and metabolic dysfunction in diet-induced obese mice. PloS One 7 (3), e34233. doi:10.1371/journal.pone.0034233
Lam, Y. Y., Ha, C. W., Hoffmann, J. M., Oscarsson, J., Dinudom, A., Mather, T. J., et al. (2015). Effects of dietary fat profile on gut permeability and microbiota and their relationships with metabolic changes in mice. Obes. (Silver Spring) 23 (7), 1429–1439. doi:10.1002/oby.21122
Le Roith, D. (1997). Seminars in medicine of the Beth Israel Deaconess Medical Center. Insulin-like growth factors. N. Engl. J. Med. 336 (9), 633–640. doi:10.1056/NEJM199702273360907
Leclercq, I. A., Farrell, G. C., Sempoux, C., dela Peña, A., and Horsmans, Y. (2004). Curcumin inhibits NF-kappaB activation and reduces the severity of experimental steatohepatitis in mice. J. Hepatol. 41 (6), 926–934. doi:10.1016/j.jhep.2004.08.010
Lee, A. H., Scapa, E. F., Cohen, D. E., and Glimcher, L. H. (2008). Regulation of hepatic lipogenesis by the transcription factor XBP1. Science 320 (5882), 1492–1496. doi:10.1126/science.1158042
Lee, D. E., Lee, S. J., Kim, S. J., Lee, H. S., and Kwon, O. S. (2019). Curcumin Ameliorates Nonalcoholic Fatty Liver Disease through Inhibition of O-GlcNAcylation. Nutrients 11 (11). doi:10.3390/nu11112702
Lee, J., and Ozcan, U. (2014). Unfolded protein response signaling and metabolic diseases. J. Biol. Chem. 289 (3), 1203–1211. doi:10.1074/jbc.R113.534743
Lee, J. S., Zheng, Z., Mendez, R., Ha, S. W., Xie, Y., and Zhang, K. (2012). Pharmacologic ER stress induces non-alcoholic steatohepatitis in an animal model. Toxicol. Lett. 211 (1), 29–38. doi:10.1016/j.toxlet.2012.02.017
Lee, J. Y., Sohn, K. H., Rhee, S. H., and Hwang, D. (2001). Saturated fatty acids, but Not unsaturated fatty acids, induce the expression of cyclooxygenase-2 mediated through Toll-like receptor 4. J. Biol. Chem. 276 (20), 16683–16689. doi:10.1074/jbc.M011695200
Leong, P. K., Wong, H. S., Chen, J., Chan, W. M., Leung, H. Y., and Ko, K. M. (2016). Differential Action between Schisandrin A and Schisandrin B in Eliciting an Anti-Inflammatory Action: The Depletion of Reduced Glutathione and the Induction of an Antioxidant Response. PloS One 11 (5), e0155879. doi:10.1371/journal.pone.0155879
Leung, K. C., Doyle, N., Ballesteros, M., Waters, M. J., and Ho, K. K. (2000). Insulin regulation of human hepatic growth hormone receptors: divergent effects on biosynthesis and surface translocation. J. Clin. Endocrinol. Metab. 85 (12), 4712–4720. doi:10.1210/jcem.85.12.7017
Li, B. T., Sun, M., Li, Y. F., Wang, J. Q., Zhou, Z. M., Song, B. L., et al. (2020a). Disruption of the ERLIN-TM6SF2-APOB complex destabilizes APOB and contributes to non-alcoholic fatty liver disease. PLoS Genet. 16 (8), e1008955. doi:10.1371/journal.pgen.1008955
Li, H., Xi, Y., Xin, X., Tian, H., and Hu, Y. (2020b). Salidroside improves high-fat diet-induced non-alcoholic steatohepatitis by regulating the gut microbiota-bile acid-farnesoid X receptor axis. Biomed. Pharmacother. 124, 109915. doi:10.1016/j.biopha.2020.109915
Li, H., Ying, H., Hu, A., Li, D., and Hu, Y. (2017). Salidroside Modulates Insulin Signaling in a Rat Model of Nonalcoholic Steatohepatitis. Evid. Based Complement. Altern. MedECAM 2017, 9651371. doi:10.1155/2017/9651371
Li, J., Sasaki, G. Y., Dey, P., Chitchumroonchokchai, C., Labyk, A. N., McDonald, J. D., et al. (2018). Green tea extract protects against hepatic NFκB activation along the gut-liver axis in diet-induced obese mice with nonalcoholic steatohepatitis by reducing endotoxin and TLR4/MyD88 signaling. J. Nutr. Biochem. 53, 58–65. doi:10.1016/j.jnutbio.2017.10.016
Li, X., Xu, Z., Wang, S., Guo, H., Dong, S., Wang, T., et al. (2016). Emodin ameliorates hepatic steatosis through endoplasmic reticulum-stress sterol regulatory element-binding protein 1c pathway in liquid fructose-feeding rats. Hepatol. Res. 46 (3), E105–E117. doi:10.1111/hepr.12538
Li, Y., Chang, S. C., Goldstein, B. Y., Scheider, W. L., Cai, L., You, N. C., et al. (2011). Green tea consumption, inflammation and the risk of primary hepatocellular carcinoma in a Chinese population. Cancer Epidemiol. 35 (4), 362–368. doi:10.1016/j.canep.2011.01.005
Lin, C. M., Chen, Y. H., Ma, H. P., Wang, B. W., Chiu, J. H., Chua, S. K., et al. (2012). Silibinin inhibits the invasion of IL-6-stimulated colon cancer cells via selective JNK/AP-1/MMP-2 modulation In Vitro. J. Agric. Food Chem. 60 (51), 12451–12457. doi:10.1021/jf300964f
Ling, X., Linglong, P., Weixia, D., and Hong, W. (2016). Protective Effects of Bifidobacterium on Intestinal Barrier Function in LPS-Induced Enterocyte Barrier Injury of Caco-2 Monolayers and in a Rat NEC Model. PloS One 11 (8), e0161635. doi:10.1371/journal.pone.0161635
Liu, B., Deng, X., Jiang, Q., Li, G., Zhang, J., Zhang, N., et al. (2020). Scoparone improves hepatic inflammation and autophagy in mice with nonalcoholic steatohepatitis by regulating the ROS/P38/Nrf2 axis and PI3K/AKT/mTOR pathway in macrophages. Biomed. Pharmacother. 125, 109895. doi:10.1016/j.biopha.2020.109895
Liu, F., Li, H., Chang, H., Wang, J., and Lu, J. (2015). Identification of hepatocellular carcinoma-associated hub genes and pathways by integrated microarray analysis. Tumori 101 (2), 206–214. doi:10.5301/tj.5000241
Liu, J. F., Ma, Y., Wang, Y., Du, Z. Y., Shen, J. K., and Peng, H. L. (2011). Reduction of lipid accumulation in HepG2 cells by luteolin is associated with activation of AMPK and mitigation of oxidative stress. Phytother. Res. 25 (4), 588–596. doi:10.1002/ptr.3305
Liu, X., Huang, K., Niu, Z., Mei, D., and Zhang, B. (2019a). Protective effect of isochlorogenic acid B on liver fibrosis in non-alcoholic steatohepatitis of mice. Basic Clin. Pharmacol. Toxicol. 124 (2), 144–153. doi:10.1111/bcpt.13122
Liu, Y., Xu, W., Zhai, T., You, J., and Chen, Y. (2019b). Silibinin ameliorates hepatic lipid accumulation and oxidative stress in mice with non-alcoholic steatohepatitis by regulating CFLAR-JNK pathway. Acta Pharm. Sin. B 9 (4), 745–757. doi:10.1016/j.apsb.2019.02.006
Liu, Y. L., Patman, G. L., Leathart, J. B., Piguet, A. C., Burt, A. D., Dufour, J. F., et al. (2014b). Carriage of the PNPLA3 rs738409 C >G polymorphism confers an increased risk of non-alcoholic fatty liver disease associated hepatocellular carcinoma. J. Hepatol. 61 (1), 75–81. doi:10.1016/j.jhep.2014.02.030
Liu, Y. L., Reeves, H. L., Burt, A. D., Tiniakos, D., McPherson, S., Leathart, J. B., et al. (2014a). TM6SF2 rs58542926 influences hepatic fibrosis progression in patients with non-alcoholic fatty liver disease. Nat. Commun. 5, 4309. doi:10.1038/ncomms5309
Loguercio, C., Andreone, P., Brisc, C., Brisc, M. C., Bugianesi, E., Chiaramonte, M., et al. (2012). Silybin combined with phosphatidylcholine and vitamin E in patients with nonalcoholic fatty liver disease: a randomized controlled trial. Free Radic. Biol. Med. 52 (9), 1658–1665. doi:10.1016/j.freeradbiomed.2012.02.008
Loo, T. M., Kamachi, F., Watanabe, Y., Yoshimoto, S., Kanda, H., Arai, Y., et al. (2017). Gut Microbiota Promotes Obesity-Associated Liver Cancer through PGE2-Mediated Suppression of Antitumor Immunity. Cancer Discov. 7 (5), 522–538. doi:10.1158/2159-8290.CD-16-0932
Luo, X., Li, N., Zhao, X., Liao, C., Ye, R., Cheng, C., et al. (2019a). DHRS2 mediates cell growth inhibition induced by Trichothecin in nasopharyngeal carcinoma. J. Exp. Clin. Cancer Res. 38 (1), 300. doi:10.1186/s13046-019-1301-1
Luo, Y., Tian, G., Zhuang, Z., Chen, J., You, N., Zhuo, L., et al. (2019b). Berberine prevents non-alcoholic steatohepatitis-derived hepatocellular carcinoma by inhibiting inflammation and angiogenesis in mice. Am. J. Transl. Res. 11 (5), 2668–2682.
Luther, J., Garber, J. J., Khalili, H., Dave, M., Bale, S. S., Jindal, R., et al. (2015). Hepatic Injury in Nonalcoholic Steatohepatitis Contributes to Altered Intestinal Permeability. Cell Mol. Gastroenterol. Hepatol. 1 (2), 222–232. doi:10.1016/j.jcmgh.2015.01.001
Luukkonen, P. K., Sädevirta, S., Zhou, Y., Kayser, B., Ali, A., Ahonen, L., et al. (2018). Saturated Fat Is More Metabolically Harmful for the Human Liver Than Unsaturated Fat or Simple Sugars. Diabetes Care 41 (8), 1732–1739. doi:10.2337/dc18-0071
Machado, M. V., and Cortez-Pinto, H. (2014). Non-alcoholic fatty liver disease: what the clinician needs to know. World J. Gastroenterol. 20 (36), 12956–12980. doi:10.3748/wjg.v20.i36.12956
Maeda, S., Kamata, H., Luo, J. L., Leffert, H., and Karin, M. (2005). IKKbeta couples hepatocyte death to cytokine-driven compensatory proliferation that promotes chemical hepatocarcinogenesis. Cell 121 (7), 977–990. doi:10.1016/j.cell.2005.04.014
Mahdessian, H., Taxiarchis, A., Popov, S., Silveira, A., Franco-Cereceda, A., Hamsten, A., et al. (2014). TM6SF2 is a regulator of liver fat metabolism influencing triglyceride secretion and hepatic lipid droplet content. Proc. Natl. Acad. Sci. U. S. A. 111 (24), 8913–8918. doi:10.1073/pnas.1323785111
Malhi, H., Bronk, S. F., Werneburg, N. W., and Gores, G. J. (2006). Free fatty acids induce JNK-dependent hepatocyte lipoapoptosis. J. Biol. Chem. 281 (17), 12093–12101. doi:10.1074/jbc.M510660200
Malhi, H., and Kaufman, R. J. (2011). Endoplasmic reticulum stress in liver disease. J. Hepatol. 54 (4), 795–809. doi:10.1016/j.jhep.2010.11.005
Malhotra, J. D., and Kaufman, R. J. (2007). Endoplasmic reticulum stress and oxidative stress: a vicious cycle or a double-edged sword? Antioxid. Redox Signal 9 (12), 2277–2293. doi:10.1089/ars.2007.1782
Malik, R., Chang, M., Bhaskar, K., Nasser, I., Curry, M., Schuppan, D., et al. (2009). The clinical utility of biomarkers and the nonalcoholic steatohepatitis CRN liver biopsy scoring system in patients with nonalcoholic fatty liver disease. J. Gastroenterol. Hepatol. 24 (4), 564–568. doi:10.1111/j.1440-1746.2008.05731.x
Mancina, R. M., Dongiovanni, P., Petta, S., Pingitore, P., Meroni, M., Rametta, R., et al. (2016). The MBOAT7-TMC4 Variant rs641738 Increases Risk of Nonalcoholic Fatty Liver Disease in Individuals of European Descent. Gastroenterology 150 (5), 1219–e6. doi:10.1053/j.gastro.2016.01.032
Marengo, A., Rosso, C., and Bugianesi, E. (2016). Liver Cancer: Connections with Obesity, Fatty Liver, and Cirrhosis. Annu. Rev. Med. 67, 103–117. doi:10.1146/annurev-med-090514-013832
Marí, M., Caballero, F., Colell, A., Morales, A., Caballeria, J., Fernandez, A., et al. (2006). Mitochondrial free cholesterol loading sensitizes to TNF- and Fas-mediated steatohepatitis. Cell Metab. 4 (3), 185–198. doi:10.1016/j.cmet.2006.07.006
Matés, J. M., Segura, J. A., Alonso, F. J., and Márquez, J. (2008). Intracellular redox status and oxidative stress: implications for cell proliferation, apoptosis, and carcinogenesis. Arch. Toxicol. 82 (5), 273–299. doi:10.1007/s00204-008-0304-z
Mei, L., Mochizuki, M., and Hasegawa, N. (2012). Hepatoprotective effects of pycnogenol in a rat model of non-alcoholic steatohepatitis. Phytother. Res. 26 (10), 1572–1574. doi:10.1002/ptr.4602
Meshkani, R., and Adeli, K. (2009). Hepatic insulin resistance, metabolic syndrome and cardiovascular disease. Clin. Biochem. 42 (13-14), 1331–1346. doi:10.1016/j.clinbiochem.2009.05.018
Miele, L., Valenza, V., La Torre, G., Montalto, M., Cammarota, G., Ricci, R., et al. (2009). Increased intestinal permeability and tight junction alterations in nonalcoholic fatty liver disease. Hepatology 49 (6), 1877–1887. doi:10.1002/hep.22848
Miethe, C., Nix, H., Martin, R., Hernandez, A. R., and Price, R. S. (2017). Silibinin Reduces the Impact of Obesity on Invasive Liver Cancer. Nutr. Cancer 69 (8), 1272–1280. doi:10.1080/01635581.2017.1367935
Miura, K., Kodama, Y., Inokuchi, S., Schnabl, B., Aoyama, T., Ohnishi, H., et al. (2010). Toll-like receptor 9 promotes steatohepatitis by induction of interleukin-1beta in mice. Gastroenterology 139 (1), 323–e7. doi:10.1053/j.gastro.2010.03.052
Möbius, C., Stein, H. J., Spiess, C., Becker, I., Feith, M., Theisen, J., et al. (2005). COX2 expression, angiogenesis, proliferation and survival in Barrett's cancer. Eur. J. Surg. Oncol. 31 (7), 755–759. doi:10.1016/j.ejso.2005.01.006
Moreira, A. J., Ordoñez, R., Cerski, C. T., Picada, J. N., García-Palomo, A., Marroni, N. P., et al. (2015). Melatonin Activates Endoplasmic Reticulum Stress and Apoptosis in Rats with Diethylnitrosamine-Induced Hepatocarcinogenesis. PloS One 10 (12), e0144517. doi:10.1371/journal.pone.0144517
Morgan, M. J., and Liu, Z. G. (2011). Crosstalk of reactive oxygen species and NF-κB signaling. Cell Res. 21 (1), 103–115. doi:10.1038/cr.2010.178
Moschen, A. R., Kaser, S., and Tilg, H. (2013). Non-alcoholic steatohepatitis: a microbiota-driven disease. Trends Endocrinol. Metab. 24 (11), 537–545. doi:10.1016/j.tem.2013.05.009
Mouries, J., Brescia, P., Silvestri, A., Spadoni, I., Sorribas, M., Wiest, R., et al. (2019). Microbiota-driven gut vascular barrier disruption is a prerequisite for non-alcoholic steatohepatitis development. J. Hepatol. 71 (6), 1216–1228. doi:10.1016/j.jhep.2019.08.005
Mouzaki, M., Comelli, E. M., Arendt, B. M., Bonengel, J., Fung, S. K., Fischer, S. E., et al. (2013). Intestinal microbiota in patients with nonalcoholic fatty liver disease. Hepatology 58 (1), 120–127. doi:10.1002/hep.26319
Musso, G., Gambino, R., and Cassader, M. (2013). Cholesterol metabolism and the pathogenesis of non-alcoholic steatohepatitis. Prog. Lipid Res. 52 (1), 175–191. doi:10.1016/j.plipres.2012.11.002
Musso, G., Gambino, R., Cassader, M., and Pagano, G. (2011). Meta-analysis: natural history of non-alcoholic fatty liver disease (NAFLD) and diagnostic accuracy of non-invasive tests for liver disease severity. Ann. Med. 43 (8), 617–649. doi:10.3109/07853890.2010.518623
Muthumani, M., and Prabu, S. M. (2012). Silibinin potentially protects arsenic-induced oxidative hepatic dysfunction in rats. Toxicol. Mech. Methods 22 (4), 277–288. doi:10.3109/15376516.2011.647113
Nakagawa, H., Umemura, A., Taniguchi, K., Font-Burgada, J., Dhar, D., Ogata, H., et al. (2014). ER stress cooperates with hypernutrition to trigger TNF-dependent spontaneous HCC development. Cancer Cell 26 (3), 331–343. doi:10.1016/j.ccr.2014.07.001
Nelson, J. E., Bhattacharya, R., Lindor, K. D., Chalasani, N., Raaka, S., Heathcote, E. J., et al. (2007). HFE C282Y mutations are associated with advanced hepatic fibrosis in Caucasians with nonalcoholic steatohepatitis. Hepatology 46 (3), 723–729. doi:10.1002/hep.21742
Neuschwander-Tetri, B. A. (2010). Hepatic lipotoxicity and the pathogenesis of nonalcoholic steatohepatitis: the central role of nontriglyceride fatty acid metabolites. Hepatology 52 (2), 774–788. doi:10.1002/hep.23719
Oyadomari, S., Harding, H. P., Zhang, Y., Oyadomari, M., and Ron, D. (2008). Dephosphorylation of translation initiation factor 2alpha enhances glucose tolerance and attenuates hepatosteatosis in mice. Cell Metab. 7 (6), 520–532. doi:10.1016/j.cmet.2008.04.011
Perry, R. J., Samuel, V. T., Petersen, K. F., and Shulman, G. I. (2014). The role of hepatic lipids in hepatic insulin resistance and type 2 diabetes. Nature 510 (7503), 84–91. doi:10.1038/nature13478
Pessayre, D., Mansouri, A., and Fromenty, B. (2002). Nonalcoholic steatosis and steatohepatitis. V. Mitochondrial dysfunction in steatohepatitis. Am. J. Physiol. Gastrointest. Liver Physiol. 282 (2), G193–G199. doi:10.1152/ajpgi.00426.2001
Peterson, L. W., and Artis, D. (2014). Intestinal epithelial cells: regulators of barrier function and immune homeostasis. Nat. Rev. Immunol. 14 (3), 141–153. doi:10.1038/nri3608
Pirola, C. J., Gianotti, T. F., Burgueño, A. L., Rey-Funes, M., Loidl, C. F., Mallardi, P., et al. (2013). Epigenetic modification of liver mitochondrial DNA is associated with histological severity of nonalcoholic fatty liver disease. Gut 62 (9), 1356–1363. doi:10.1136/gutjnl-2012-302962
Ponziani, F. R., Bhoori, S., Castelli, C., Putignani, L., Rivoltini, L., Del Chierico, F., et al. (2019). Hepatocellular Carcinoma Is Associated With Gut Microbiota Profile and Inflammation in Nonalcoholic Fatty Liver Disease. Hepatology 69 (1), 107–120. doi:10.1002/hep.30036
Porras, D., Nistal, E., Martínez-Flórez, S., Olcoz, J. L., Jover, R., Jorquera, F., et al. (2019). Functional Interactions between Gut Microbiota Transplantation, Quercetin, and High-Fat Diet Determine Non-Alcoholic Fatty Liver Disease Development in Germ-Free Mice. Mol. Nutr. Food Res. 63 (8), e1800930. doi:10.1002/mnfr.201800930
Porras, D., Nistal, E., Martínez-Flórez, S., Pisonero-Vaquero, S., Olcoz, J. L., Jover, R., et al. (2017). Protective effect of quercetin on high-fat diet-induced non-alcoholic fatty liver disease in mice is mediated by modulating intestinal microbiota imbalance and related gut-liver axis activation. Free Radic. Biol. Med. 102, 188–202. doi:10.1016/j.freeradbiomed.2016.11.037
Postic, C., and Girard, J. (2008). Contribution of De Novo fatty acid synthesis to hepatic steatosis and insulin resistance: lessons from genetically engineered mice. J. Clin. Invest 118 (3), 829–838. doi:10.1172/JCI34275
Prisco, M., Romano, G., Peruzzi, F., Valentinis, B., and Baserga, R. (1999). Insulin and IGF-I receptors signaling in protection from apoptosis. Horm. Metab. Res. 31 (2-3), 80–89. doi:10.1055/s-2007-978703
Promrat, K., Kleiner, D. E., Niemeier, H. M., Jackvony, E., Kearns, M., Wands, J. R., et al. (2010). Randomized controlled trial testing the effects of weight loss on nonalcoholic steatohepatitis. Hepatology 51 (1), 121–129. doi:10.1002/hep.23276
Puri, P., Baillie, R. A., Wiest, M. M., Mirshahi, F., Choudhury, J., Cheung, O., et al. (2007). A lipidomic analysis of nonalcoholic fatty liver disease. Hepatology 46 (4), 1081–1090. doi:10.1002/hep.21763
Qiu, W., Su, Q., Rutledge, A. C., Zhang, J., and Adeli, K. (2009). Glucosamine-induced endoplasmic reticulum stress attenuates apolipoprotein B100 synthesis via PERK signaling. J. Lipid Res. 50 (9), 1814–1823. doi:10.1194/jlr.M800343-JLR200
Rahman, K., Desai, C., Iyer, S. S., Thorn, N. E., Kumar, P., Liu, Y., et al. (2016). Loss of Junctional Adhesion Molecule A Promotes Severe Steatohepatitis in Mice on a Diet High in Saturated Fat, Fructose, and Cholesterol. Gastroenterology 151 (4), 733–e12. doi:10.1053/j.gastro.2016.06.022
Rajput, S. K., Siddiqui, M. A., Kumar, V., Meena, C. L., Pant, A. B., Jain, R., et al. (2011). Protective effects of L-pGlu-(2-propyl)-L-His-L-ProNH2, a newer thyrotropin releasing hormone analog in In Vitro and In Vivo models of cerebral ischemia. Peptides 32 (6), 1225–1231. doi:10.1016/j.peptides.2011.04.006
Ramirez, A. G., Weiss, N. S., Holden, A. E., Suarez, L., Cooper, S. P., Munoz, E., et al. (2012). Incidence and risk factors for hepatocellular carcinoma in Texas Latinos: implications for prevention research. PloS One 7 (4), e35573. doi:10.1371/journal.pone.0035573
Ramirez-Tortosa, M. C., Ramirez-Tortosa, C. L., Mesa, M. D., Granados, S., Gil, A., and Quiles, J. L. (2009). Curcumin ameliorates rabbits's steatohepatitis via respiratory chain, oxidative stress, and TNF-alpha. Free Radic. Biol. Med. 47 (7), 924–931. doi:10.1016/j.freeradbiomed.2009.06.015
Rawat, D., Shrivastava, S., Naik, R. A., Chhonker, S. K., Mehrotra, A., and Koiri, R. K. (2018). An Overview of Natural Plant Products in the Treatment of Hepatocellular Carcinoma. Anti-cancer Agents Med. Chem. 18, 1838–1859.
Ray, K. (2013). Gut microbiota: Obesity-induced microbial metabolite promotes HCC. Nat. Rev. Gastroenterol. Hepatol. 10 (8), 442. doi:10.1038/nrgastro.2013.121
Ribeiro, P. S., Cortez-Pinto, H., Solá, S., Castro, R. E., Ramalho, R. M., Baptista, A., et al. (2004). Hepatocyte apoptosis, expression of death receptors, and activation of NF-kappaB in the liver of nonalcoholic and alcoholic steatohepatitis patients. Am. J. Gastroenterol. 99 (9), 1708–1717. doi:10.1111/j.1572-0241.2004.40009.x
Robertson, G., Leclercq, I., and Farrell, G. C. (2001). Nonalcoholic steatosis and steatohepatitis. II. Cytochrome P-450 enzymes and oxidative stress. Am. J. Physiol. Gastrointest. Liver Physiol. 281 (5), G1135–G1139. doi:10.1152/ajpgi.2001.281.5.G1135
Rodríguez-Piñeiro, A. M., and Johansson, M. E. V. (2015). The colonic mucus protection depends on the microbiota. Gut Microbes 6 (5), 326–330. doi:10.1080/19490976.2015.1086057
Rolo, A. P., Teodoro, J. S., and Palmeira, C. M. (2012). Role of oxidative stress in the pathogenesis of nonalcoholic steatohepatitis. Free Radic. Biol. Med. 52 (1), 59–69. doi:10.1016/j.freeradbiomed.2011.10.003
Romeo, S., Kozlitina, J., Xing, C., Pertsemlidis, A., Cox, D., Pennacchio, L. A., et al. (2008). Genetic variation in PNPLA3 confers susceptibility to nonalcoholic fatty liver disease. Nat. Genet. 40 (12), 1461–1465. doi:10.1038/ng.257
Romero-Gómez, M., Zelber-Sagi, S., and Trenell, M. (2017). Treatment of NAFLD with diet, physical activity and exercise. J. Hepatology 67 (4), 829–846. doi:10.1016/j.jhep.2017.05.016
Ron, D., and Walter, P. (2007). Signal integration in the endoplasmic reticulum unfolded protein response. Nat. Rev. Mol. Cell Biol. 8 (7), 519–529. doi:10.1038/nrm2199
Rutkowski, D. T., Wu, J., Back, S. H., Callaghan, M. U., Ferris, S. P., Iqbal, J., et al. (2008). UPR pathways combine to prevent hepatic steatosis caused by ER stress-mediated suppression of transcriptional master regulators. Dev. Cell 15 (6), 829–840. doi:10.1016/j.devcel.2008.10.015
Samuel, V. T., and Shulman, G. I. (2012). Mechanisms for insulin resistance: common threads and missing links. Cell 148 (5), 852–871. doi:10.1016/j.cell.2012.02.017
Santos, C. R., and Schulze, A. (2012). Lipid metabolism in cancer. FEBS J. 279 (15), 2610–2623. doi:10.1111/j.1742-4658.2012.08644.x
Schreuder, T. C., Verwer, B. J., van Nieuwkerk, C. M., and Mulder, C. J. (2008). Nonalcoholic fatty liver disease: an overview of current insights in pathogenesis, diagnosis and treatment. World J. Gastroenterol. 14 (16), 2474–2486. doi:10.3748/wjg.14.2474
Schröder, M., and Kaufman, R. J. (2005). The mammalian unfolded protein response. Annu. Rev. Biochem. 74, 739–789.
Schulze, K., Imbeaud, S., Letouzé, E., Alexandrov, L. B., Calderaro, J., Rebouissou, S., et al. (2015). Exome sequencing of hepatocellular carcinomas identifies new mutational signatures and potential therapeutic targets. Nat. Genet. 47 (5), 505–511. doi:10.1038/ng.3252
Schwabe, R. F., and Greten, T. F. (2020). Gut microbiome in HCC - Mechanisms, diagnosis and therapy. J. Hepatol. 72 (2), 230–238. doi:10.1016/j.jhep.2019.08.016
Schwabe, R. F., and Jobin, C. (2013). The microbiome and cancer. Nat. Rev. Cancer 13 (11), 800–812. doi:10.1038/nrc3610
Schweiger, M., Romauch, M., Schreiber, R., Grabner, G. F., Hütter, S., Kotzbeck, P., et al. (2017). Pharmacological inhibition of adipose triglyceride lipase corrects high-fat diet-induced insulin resistance and hepatosteatosis in mice. Nat. Commun. 8, 14859. doi:10.1038/ncomms14859
Serviddio, G., Bellanti, F., Giudetti, A. M., Gnoni, G. V., Petrella, A., Tamborra, R., et al. (2010). A silybin-phospholipid complex prevents mitochondrial dysfunction in a rodent model of nonalcoholic steatohepatitis. J. Pharmacol. Exp. Ther. 332 (3), 922–932. doi:10.1124/jpet.109.161612
Shaik, F. B., Prasad, D. V., and Narala, V. R. (2015). Role of farnesoid X receptor in inflammation and resolution. Inflamm. Res. 64 (1), 9–20. doi:10.1007/s00011-014-0780-y
Shi, H., Kokoeva, M. V., Inouye, K., Tzameli, I., Yin, H., and Flier, J. S. (2006). TLR4 links innate immunity and fatty acid-induced insulin resistance. J. Clin. Invest 116 (11), 3015–3025. doi:10.1172/JCI28898
Shimizu, M., Shirakami, Y., Sakai, H., Tatebe, H., Nakagawa, T., Hara, Y., et al. (2008). EGCG inhibits activation of the insulin-like growth factor (IGF)/IGF-1 receptor axis in human hepatocellular carcinoma cells. Cancer Lett. 262 (1), 10–18. doi:10.1016/j.canlet.2007.11.026
Simile, M. M., Latte, G., Feo, C. F., Feo, F., Calvisi, D. F., and Pascale, R. M. (2018). Alterations of methionine metabolism in hepatocarcinogenesis: the emergent role of glycine N-methyltransferase in liver injury. Ann. Gastroenterol. 31 (5), 552–560. doi:10.20524/aog.2018.0288
Sims, G. P., Rowe, D. C., Rietdijk, S. T., Herbst, R., and Coyle, A. J. (2010). HMGB1 and RAGE in inflammation and cancer. Annu. Rev. Immunol. 28, 367–388. doi:10.1146/annurev.immunol.021908.132603
Singal, A. G., Manjunath, H., Yopp, A. C., Beg, M. S., Marrero, J. A., Gopal, P., et al. (2014). The effect of PNPLA3 on fibrosis progression and development of hepatocellular carcinoma: a meta-analysis. Am. J. Gastroenterol. 109 (3), 325–334. doi:10.1038/ajg.2013.476
Smagris, E., BasuRay, S., Li, J., Huang, Y., Lai, K. M., Gromada, J., et al. (2015). Pnpla3I148M knockin mice accumulate PNPLA3 on lipid droplets and develop hepatic steatosis. Hepatology 61 (1), 108–118. doi:10.1002/hep.27242
Song, Y. N., Sun, J. J., Lu, Y. Y., Xu, L. M., Gao, Y. Q., Zhang, W., et al. (2013). Therapeutic efficacy of fuzheng-huayu tablet based traditional chinese medicine syndrome differentiation on hepatitis-B-caused cirrhosis: a multicenter double-blind randomized controlled trail. Evid. Based Complement. Altern. Med. 2013, 709305. doi:10.1155/2013/709305
Stefan, N., Kantartzis, K., and Häring, H. U. (2008). Causes and metabolic consequences of Fatty liver. Endocr. Rev. 29 (7), 939–960. doi:10.1210/er.2008-0009
Stephens, J. M., and Elks, C. M. (2017). Oncostatin M: Potential Implications for Malignancy and Metabolism. Curr. Pharm. Des. 23 (25), 3645–3657. doi:10.2174/1381612823666170704122559
Steppan, C. M., Bailey, S. T., Bhat, S., Brown, E. J., Banerjee, R. R., Wright, C. M., et al. (2001). The hormone resistin links obesity to diabetes. Nature 409 (6818), 307–312. doi:10.1038/35053000
Stickel, F., and Hellerbrand, C. (2010). Non-alcoholic fatty liver disease as a risk factor for hepatocellular carcinoma: mechanisms and implications. Gut 59 (10), 1303–1307. doi:10.1136/gut.2009.199661
Sun, B., and Karin, M. (2012). Obesity, inflammation, and liver cancer. J. Hepatol. 56 (3), 704–713. doi:10.1016/j.jhep.2011.09.020
Sun, Y., Xun, K., Wang, Y., and Chen, X. (2009). A systematic review of the anticancer properties of berberine, a natural product from Chinese herbs. Anticancer Drugs 20 (9), 757–769. doi:10.1097/CAD.0b013e328330d95b
Szabo, G., and Petrasek, J. (2015). Inflammasome activation and function in liver disease. Nat. Rev. Gastroenterol. Hepatol. 12 (7), 387–400. doi:10.1038/nrgastro.2015.94
Szegezdi, E., Logue, S. E., Gorman, A. M., and Samali, A. (2006). Mediators of endoplasmic reticulum stress-induced apoptosis. EMBO Rep. 7 (9), 880–885. doi:10.1038/sj.embor.7400779
Tabas, I., and Ron, D. (2011). Integrating the mechanisms of apoptosis induced by endoplasmic reticulum stress. Nat. Cell Biol. 13 (3), 184–190. doi:10.1038/ncb0311-184
Takaki, Y., Saito, Y., Takasugi, A., Toshimitsu, K., Yamada, S., Muramatsu, T., et al. (2014). Silencing of microRNA-122 is an early event during hepatocarcinogenesis from non-alcoholic steatohepatitis. Cancer Sci. 105 (10), 1254–1260. doi:10.1111/cas.12498
Tao, L., Cao, F., Xu, G., Xie, H., Zhang, M., and Zhang, C. (2017). Mogroside IIIE Attenuates LPS-Induced Acute Lung Injury in Mice Partly Through Regulation of the TLR4/MAPK/NF-κB Axis via AMPK Activation. Phytother. Res. 31 (7), 1097–1106. doi:10.1002/ptr.5833
Terpstra, V., van Amersfoort, E. S., van Velzen, A. G., Kuiper, J., and van Berkel, T. J. (2000). Hepatic and extrahepatic scavenger receptors: function in relation to disease. Arterioscler. Thromb. Vasc. Biol. 20 (8), 1860–1872. doi:10.1161/01.atv.20.8.1860
Thabet, K., Chan, H. L. Y., Petta, S., Mangia, A., Berg, T., Boonstra, A., et al. (2017). The membrane-bound O-acyltransferase domain-containing 7 variant rs641738 increases inflammation and fibrosis in chronic hepatitis B. Hepatology 65 (6), 1840–1850. doi:10.1002/hep.29064
Tian, Y., Ma, J., Wang, W., Zhang, L., Xu, J., Wang, K., et al. (2016). Resveratrol supplement inhibited the NF-κB inflammation pathway through activating AMPKα-SIRT1 pathway in mice with fatty liver. Mol. Cell Biochem. 422 (1-2), 75–84. doi:10.1007/s11010-016-2807-x
Tryndyak, V. P., Han, T., Muskhelishvili, L., Fuscoe, J. C., Ross, S. A., Beland, F. A., et al. (2011). Coupling global methylation and gene expression profiles reveal key pathophysiological events in liver injury induced by a methyl-deficient diet. Mol. Nutr. Food Res. 55 (3), 411–418. doi:10.1002/mnfr.201000300
Tsuchida, T., and Friedman, S. L. (2017). Mechanisms of hepatic stellate cell activation. Nat. Rev. Gastroenterol. Hepatol. 14 (7), 397–411. doi:10.1038/nrgastro.2017.38
Turrens, J. F. (2003). Mitochondrial formation of reactive oxygen species. J. Physiol. 552 (Pt 2), 335–344. doi:10.1113/jphysiol.2003.049478
Ueyama, M., Nishida, N., Korenaga, M., Korenaga, K., Kumagai, E., Yanai, H., et al. (2016). The impact of PNPLA3 and JAZF1 on hepatocellular carcinoma in non-viral hepatitis patients with type 2 diabetes mellitus. J. Gastroenterol. 51 (4), 370–379. doi:10.1007/s00535-015-1116-6
Urano, F., Wang, X., Bertolotti, A., Zhang, Y., Chung, P., Harding, H. P., et al. (2000). Coupling of stress in the ER to activation of JNK protein kinases by transmembrane protein kinase IRE1. Science 287 (5453), 664–666. doi:10.1126/science.287.5453.664
Valenti, L., Al-Serri, A., Daly, A. K., Galmozzi, E., Rametta, R., Dongiovanni, P., et al. (2010). Homozygosity for the patatin-like phospholipase-3/adiponutrin I148M polymorphism influences liver fibrosis in patients with nonalcoholic fatty liver disease. Hepatology 51 (4), 1209–1217. doi:10.1002/hep.23622
Valenti, L., Dongiovanni, P., Ginanni Corradini, S., Burza, M. A., and Romeo, S. (2013). PNPLA3 I148M variant and hepatocellular carcinoma: a common genetic variant for a rare disease. Dig. Liver Dis. 45 (8), 619–624. doi:10.1016/j.dld.2012.12.006
Vatner, D. F., Majumdar, S. K., Kumashiro, N., Petersen, M. C., Rahimi, Y., Gattu, A. K., et al. (2015). Insulin-independent regulation of hepatic triglyceride synthesis by fatty acids. Proc. Natl. Acad. Sci. U. S. A. 112 (4), 1143–1148. doi:10.1073/pnas.1423952112
Vilar-Gomez, E., Calzadilla-Bertot, L., Wai-Sun Wong, V., Castellanos, M., Aller-de la Fuente, R., Metwally, M., et al. (2018). Fibrosis Severity as a Determinant of Cause-Specific Mortality in Patients With Advanced Nonalcoholic Fatty Liver Disease: A Multi-National Cohort Study. Gastroenterology 155 (2), 443–e17. doi:10.1053/j.gastro.2018.04.034
Wang, C., Duan, X., Sun, X., Liu, Z., Sun, P., Yang, X., et al. (2016). Protective effects of glycyrrhizic acid from edible botanical glycyrrhiza glabra against non-alcoholic steatohepatitis in mice. Food Funct. 7 (9), 3716–3723. doi:10.1039/c6fo00773b
Wang, D., Wei, Y., and Pagliassotti, M. J. (2006). Saturated fatty acids promote endoplasmic reticulum stress and liver injury in rats with hepatic steatosis. Endocrinology 147 (2), 943–951. doi:10.1210/en.2005-0570
Wang, L., Chen, L., Tan, Y., Wei, J., Chang, Y., Jin, T., et al. (2013). Betaine supplement alleviates hepatic triglyceride accumulation of apolipoprotein E deficient mice via reducing methylation of peroxisomal proliferator-activated receptor alpha promoter. Lipids Health Dis. 12, 34. doi:10.1186/1476-511X-12-34
Wang, P. X., Ji, Y. X., Zhang, X. J., Zhao, L. P., Yan, Z. Z., Zhang, P., et al. (2017). Targeting CASP8 and FADD-like apoptosis regulator ameliorates nonalcoholic steatohepatitis in mice and nonhuman primates. Nat. Med. 23 (4), 439–449. doi:10.1038/nm.4290
Wang, Q., Ou, Y., Hu, G., Wen, C., Yue, S., Chen, C., et al. (2020). Naringenin attenuates non-alcoholic fatty liver disease by down-regulating the NLRP3/NF-κB pathway in mice. Br. J. Pharmacol. 177 (8), 1806–1821. doi:10.1111/bph.14938
Wang, Y., Ausman, L. M., Greenberg, A. S., Russell, R. M., and Wang, X. D. (2009). Nonalcoholic steatohepatitis induced by a high-fat diet promotes diethylnitrosamine-initiated early hepatocarcinogenesis in rats. Int. J. Cancer 124 (3), 540–546. doi:10.1002/ijc.23995
Weltman, M. D., Farrell, G. C., and Liddle, C. (1996). Increased hepatocyte CYP2E1 expression in a rat nutritional model of hepatic steatosis with inflammation. Gastroenterology 111 (6), 1645–1653. doi:10.1016/s0016-5085(96)70028-8
Weng, C. J., Hsieh, Y. H., Tsai, C. M., Chu, Y. H., Ueng, K. C., Liu, Y. F., et al. (2010). Relationship of insulin-like growth factors system gene polymorphisms with the susceptibility and pathological development of hepatocellular carcinoma. Ann. Surg. Oncol. 17 (7), 1808–1815. doi:10.1245/s10434-009-0904-8
White, D. L., Kanwal, F., and El-Serag, H. B. (2012). Association between nonalcoholic fatty liver disease and risk for hepatocellular cancer, based on systematic review. Clin. Gastroenterol. Hepatol. 10 (12), 1342–e2. doi:10.1016/j.cgh.2012.10.001
Wong, V. W., Chan, R. S., Wong, G. L., Cheung, B. H., Chu, W. C., Yeung, D. K., et al. (2013). Community-based lifestyle modification programme for non-alcoholic fatty liver disease: a randomized controlled trial. J. Hepatol. 59 (3), 536–542. doi:10.1016/j.jhep.2013.04.013
Wouters, K., van Bilsen, M., van Gorp, P. J., Bieghs, V., Lütjohann, D., Kerksiek, A., et al. (2010). Intrahepatic cholesterol influences progression, inhibition and reversal of non-alcoholic steatohepatitis in hyperlipidemic mice. FEBS Lett. 584 (5), 1001–1005. doi:10.1016/j.febslet.2010.01.046
Wree, A., McGeough, M. D., Peña, C. A., Schlattjan, M., Li, H., Inzaugarat, M. E., et al. (2014). NLRP3 inflammasome activation is required for fibrosis development in NAFLD. J. Mol. Med. Berl. 92 (10), 1069–1082. doi:10.1007/s00109-014-1170-1
Wu, W., Lv, L., Shi, D., Ye, J., Fang, D., Guo, F., et al. (2017). Protective Effect of Akkermansia muciniphila against Immune-Mediated Liver Injury in a Mouse Model. Front. Microbiol. 8, 1804. doi:10.3389/fmicb.2017.01804
Wullaert, A., Heyninck, K., and Beyaert, R. (2006). Mechanisms of crosstalk between TNF-induced NF-kappaB and JNK activation in hepatocytes. Biochem. Pharmacol. 72 (9), 1090–1101. doi:10.1016/j.bcp.2006.07.003
Wullaert, A., van Loo, G., Heyninck, K., and Beyaert, R. (2007). Hepatic tumor necrosis factor signaling and nuclear factor-kappaB: effects on liver homeostasis and beyond. Endocr. Rev. 28 (4), 365–386. doi:10.1210/er.2006-0031
Xiao, G., Zhang, T., Yu, S., Lee, S., Calabuig-Navarro, V., Yamauchi, J., et al. (2013). ATF4 protein deficiency protects against high fructose-induced hypertriglyceridemia in mice. J. Biol. Chem. 288 (35), 25350–25361. doi:10.1074/jbc.M113.470526
Xu, G., Huang, K., and Zhou, J. (2018). Hepatic AMP Kinase as a Potential Target for Treating Nonalcoholic Fatty Liver Disease: Evidence from Studies of Natural Products. Curr. Med. Chem. 25 (8), 889–907. doi:10.2174/0929867324666170404142450
Yamada, S., Takashina, Y., Watanabe, M., Nagamine, R., Saito, Y., Kamada, N., et al. (2018). Bile acid metabolism regulated by the gut microbiota promotes non-alcoholic steatohepatitis-associated hepatocellular carcinoma in mice. Oncotarget 9 (11), 9925–9939. doi:10.18632/oncotarget.24066
Yamaguchi, K., Yang, L., McCall, S., Huang, J., Yu, X. X., Pandey, S. K., et al. (2007). Inhibiting triglyceride synthesis improves hepatic steatosis but exacerbates liver damage and fibrosis in obese mice with nonalcoholic steatohepatitis. Hepatology 45 (6), 1366–1374. doi:10.1002/hep.21655
Yang, J., Ma, X. J., Li, L., Wang, L., Chen, Y. G., Liu, J., et al. (2017). Berberine ameliorates non-alcoholic steatohepatitis in ApoE-/- mice. Exp. Ther. Med. 14 (5), 4134–4140. doi:10.3892/etm.2017.5051
Ye, Q., Qian, B. X., Yin, W. L., Wang, F. M., and Han, T. (2016). Association between the HFE C282Y, H63D Polymorphisms and the Risks of Non-Alcoholic Fatty Liver Disease, Liver Cirrhosis and Hepatocellular Carcinoma: An Updated Systematic Review and Meta-Analysis of 5,758 Cases and 14,741 Controls. PloS One 11 (9), e0163423. doi:10.1371/journal.pone.0163423
Yoo, N. Y., Jeon, S., Nam, Y., Park, Y. J., Won, S. B., and Kwon, Y. H. (2015). Dietary Supplementation of Genistein Alleviates Liver Inflammation and Fibrosis Mediated by a Methionine-Choline-Deficient Diet in db/db Mice. J. Agric. Food Chem. 63 (17), 4305–4311. doi:10.1021/acs.jafc.5b00398
Younes, R., and Bugianesi, E. (2018). Should we undertake surveillance for HCC in patients with NAFLD? J. Hepatol. 68 (2), 326–334. doi:10.1016/j.jhep.2017.10.006
Younossi, Z. M., Koenig, A. B., Abdelatif, D., Fazel, Y., Henry, L., and Wymer, M. (2016). Global epidemiology of nonalcoholic fatty liver disease-Meta-analytic assessment of prevalence, incidence, and outcomes. Hepatology 64 (1), 73–84. doi:10.1002/hep.28431
Younossi, Z. M., Ratziu, V., Loomba, R., Rinella, M., Anstee, Q. M., Goodman, Z., et al. (2019). Obeticholic acid for the treatment of non-alcoholic steatohepatitis: interim analysis from a multicentre, randomised, placebo-controlled phase 3 trial. Lancet 394 (10215), 2184–2196. doi:10.1016/S0140-6736(19)33041-7
Yuan, L., Han, X., Li, W., Ren, D., and Yang, X. (2016). Isoorientin Prevents Hyperlipidemia and Liver Injury by Regulating Lipid Metabolism, Antioxidant Capability, and Inflammatory Cytokine Release in High-Fructose-Fed Mice. J. Agric. Food Chem. 64 (13), 2682–2689. doi:10.1021/acs.jafc.6b00290
Yuan, X., Gong, Z., Wang, B., Guo, X., Yang, L., Li, D., et al. (2018). Astragaloside Inhibits Hepatic Fibrosis by Modulation of TGF-β1/Smad Signaling Pathway. Evid. Based Complement. Altern. Med. 2018, 3231647. doi:10.1155/2018/3231647
Zámbó, V., Simon-Szabó, L., Szelényi, P., Kereszturi, É., Bánhegyi, G., and Csala, M. (2013). Lipotoxicity in the liver. Wjh 5 (10), 550–557. doi:10.4254/wjh.v5.i10.550
Zhai, J., Tao, L., Zhang, Y., Gao, H., Qu, X., Song, Y., et al. (2019). Salvianolic Acid B Attenuates Apoptosis of HUVEC Cells Treated with High Glucose or High Fat via Sirt1 Activation. Evid. Based Complement. Altern. MedECAM 2019, 9846325. doi:10.1155/2019/9846325
Zhang, H., Yang, L., Wang, Y., Huang, W., Li, Y., Chen, S., et al. (2020). Oxymatrine alleviated hepatic lipid metabolism via regulating miR-182 in non-alcoholic fatty liver disease. Life Sci. 257, 118090. doi:10.1016/j.lfs.2020.118090
Zhang, J., Zhang, H., Deng, X., Zhang, N., Liu, B., Xin, S., et al. (2018). Baicalin attenuates non-alcoholic steatohepatitis by suppressing key regulators of lipid metabolism, inflammation and fibrosis in mice. Life Sci. 192, 46–54. doi:10.1016/j.lfs.2017.11.027
Zhang, K. (2010). Integration of ER stress, oxidative stress and the inflammatory response in health and disease. Int. J. Clin. Exp. Med. 3 (1), 33–40.
Zhang, K., and Kaufman, R. J. (2008a). From endoplasmic-reticulum stress to the inflammatory response. Nature 454 (7203), 455–462. doi:10.1038/nature07203
Zhang, K., and Kaufman, R. J. (2008b). Identification and characterization of endoplasmic reticulum stress-induced apoptosis In Vivo. Methods Enzymol. 442, 395–419. doi:10.1016/S0076-6879(08)01420-1
Zhang, K., Shen, X., Wu, J., Sakaki, K., Saunders, T., Rutkowski, D. T., et al. (2006). Endoplasmic reticulum stress activates cleavage of CREBH to induce a systemic inflammatory response. Cell 124 (3), 587–599. doi:10.1016/j.cell.2005.11.040
Zhang, X. Q., Xu, C. F., Yu, C. H., Chen, W. X., and Li, Y. M. (2014). Role of endoplasmic reticulum stress in the pathogenesis of nonalcoholic fatty liver disease. World J. Gastroenterol. 20 (7), 1768–1776. doi:10.3748/wjg.v20.i7.1768
Zhang, Y., Duan, W., Owusu, L., Wu, D., and Xin, Y. (2015). Epigallocatechin-3-gallate induces the apoptosis of hepatocellular carcinoma LM6 cells but Not non-cancerous liver cells. Int. J. Mol. Med. 35 (1), 117–124. doi:10.3892/ijmm.2014.1988
Zhang, Y., Hai, J., Cao, M., Zhang, Y., Pei, S., Wang, J., et al. (2013). Silibinin ameliorates steatosis and insulin resistance during non-alcoholic fatty liver disease development partly through targeting IRS-1/PI3K/Akt pathway. Int. Immunopharmacol. 17 (3), 714–720. doi:10.1016/j.intimp.2013.08.019
Zhang, Y. P., Deng, Y. J., Tang, K. R., Chen, R. S., Liang, S., Liang, Y. J., et al. (2019). Berberine Ameliorates High-Fat Diet-Induced Non-Alcoholic Fatty Liver Disease in Rats via Activation of SIRT3/AMPK/ACC Pathway. Curr. Med. Sci. 39 (1), 37–43. doi:10.1007/s11596-019-1997-3
Zhao, M., Zhao, L., Xiong, X., He, Y., Huang, W., Liu, Z., et al. (2020a). TMAVA, a Metabolite of Intestinal Microbes, Is Increased in Plasma From Patients With Liver Steatosis, Inhibits γ-Butyrobetaine Hydroxylase, and Exacerbates Fatty Liver in Mice. Gastroenterology 158 (8), 2266–e27. doi:10.1053/j.gastro.2020.02.033
Zhao, Q., Li, L., Zhu, Y., Hou, D., Li, Y., Guo, X., et al. (2020b). Kukoamine B Ameliorate Insulin Resistance, Oxidative Stress, Inflammation and Other Metabolic Abnormalities in High-Fat/High-Fructose-Fed Rats. Diabetes Metab. Syndr. Obes. 13, 1843–1853. doi:10.2147/DMSO.S247844
Zhu, L., Baker, S. S., Gill, C., Liu, W., Alkhouri, R., Baker, R. D., et al. (2013). Characterization of gut microbiomes in nonalcoholic steatohepatitis (NASH) patients: a connection between endogenous alcohol and NASH. Hepatology 57 (2), 601–609. doi:10.1002/hep.26093
Keywords: nonalcoholic steatohepatitis, hepatocellular carcinoma, natural products, pathogenesis, therapeutic strategies
Citation: Shao G, Liu Y, Lu L, Zhang G, Zhou W, Wu T, Wang L, Xu H and Ji G (2022) The Pathogenesis of HCC Driven by NASH and the Preventive and Therapeutic Effects of Natural Products. Front. Pharmacol. 13:944088. doi: 10.3389/fphar.2022.944088
Received: 14 May 2022; Accepted: 20 June 2022;
Published: 07 July 2022.
Edited by:
Wei Zhao, City University of Hong Kong, Hong Kong SAR, ChinaReviewed by:
Jian-gao Fan, Shanghai Jiao Tong University, ChinaDewei Ye, Guangdong Pharmaceutical University, China
Copyright © 2022 Shao, Liu, Lu, Zhang, Zhou, Wu, Wang, Xu and Ji. This is an open-access article distributed under the terms of the Creative Commons Attribution License (CC BY). The use, distribution or reproduction in other forums is permitted, provided the original author(s) and the copyright owner(s) are credited and that the original publication in this journal is cited, in accordance with accepted academic practice. No use, distribution or reproduction is permitted which does not comply with these terms.
*Correspondence: Hanchen Xu, aGFuc29uMDcwMkAxMjYuY29t, aGN4dUBzaHV0Y20uZWR1LmNu; Guang Ji, amlsaXZlckB2aXAuc2luYS5jb20=, amdAc2h1dGNtLmVkdS5jbg==