- 1The Key Laboratory of Biorheological Science and Technology, Ministry of Education, College of Bioengineering, Chongqing University, Chongqing, China
- 2State and Local Joint Engineering Laboratory for Vascular Implants, Chongqing, China
- 3The 111 Project Laboratory of Biomechanics and Tissue Repair, College of Bioengineering, Chongqing University, Chongqing, China
Rhodiola is an ancient wild plant that grows in rock areas in high-altitude mountains with a widespread habitat in Asia, Europe, and America. From empirical belief to research studies, Rhodiola has undergone a long history of discovery, and has been used as traditional medicine in many countries and regions for treating high-altitude sickness, anoxia, resisting stress or fatigue, and for promoting longevity. Salidroside, a phenylpropanoid glycoside, is the main active component found in all species of Rhodiola. Salidroside could enhance cell survival and angiogenesis while suppressing oxidative stress and inflammation, and thereby has been considered a potential compound for treating ischemia and ischemic injury. In this article, we highlight the recent advances in salidroside in treating ischemic diseases, such as cerebral ischemia, ischemic heart disease, liver ischemia, ischemic acute kidney injury and lower limb ischemia. Furthermore, we also discuss the pharmacological functions and underlying molecular mechanisms. To our knowledge, this review is the first one that covers the protective effects of salidroside on different ischemia-related disease.
Introduction
Rhodiola is a genus of a perennial succulent plant in the Crassulaceae family that produce flowers ranging in color from yellow to red (Galambosi et al., 2006). Having more than two hundred species in total, Rhodiola is widespread in high-altitude places, such as mountainous regions in Asian and European countries. Its habitat includes the southwestern part of China, India, Pakistan and North Korea, as well as Russia, Europe, especially in Alpen, and America (Khanum et al., 2005). Rhodiola has been used as a traditional medicine in many countries and regions for treating high-altitude sickness, anoxia, resisting stress or fatigue, and for promoting longevity (Nan et al., 2003; Chen et al., 2012; Luo et al., 2019; Zheng et al., 2019).
Previous studies have identified that Rhodiola has more than 140 components, with salidroside, tyrosol, rosavin, and triandrin as the main biologically active ones (Panossian et al., 2010). Among them, salidroside, categorized as phenylpropanoid glycoside with a molecular weight of 300.30, is the main active component found in all species of Rhodiola (Panossian et al., 2010; Qian et al., 2012; Zheng et al., 2019; Fan et al., 2020). Salidroside has been known to possess various pharmacological properties, such as resisting anoxia, anti-aging, anti-cancer, anti-inflammation, antioxidative as well as protecting the cardiovascular system (Zhang et al., 2009; Qian et al., 2012; Zheng et al., 2019; Fan et al., 2020). Accordingly, salidroside has attracted attention as a potential compound for treating ischemic diseases.
Ischemic diseases are pathological conditions caused by the obstruction of blood vessels, leading to an insufficient supply of oxygen and nutrients to organs and tissues. Ischemic diseases include ischemic stroke, myocardial infarction (MI), liver ischemia, ischemic acute kidney injury (AKI) and lower limb ischemia (Eltzschig and Eckle, 2011). Currently, standard therapeutic strategies for treating these diseases include surgery-based revascularization, organ transplantation, medication and/or application of pharmacologic agents as well as therapeutic angiogenesis (Selzner et al., 2003; Hankey, 2017). Several main components extracted from traditional herbs or plants have also been investigated for their potential for treating ischemic diseases (Han et al., 2017; Han et al., 2022). Duan et al. (2021) explored the potential of using Baicalin, the major component of the root of traditional Chinese herb Scutellaria baicalensis, for treating stroke; while Wang et al. (2021) examined the function of Danshen, the active component extracted from the root of Salvia miltiorrhiza Bunge, for treating myocardiac injury. In 2009 and 2012, Zhang et al. (2009), Chen et al. (2012) found that salidroside could ameliorate the symptoms of cardiomyopathy and hypoxia-induced neuronal damage in cardiomyocytes and mice with cortical impact injuries, respectively. Since then, more studies have revealed that salidroside possesses promising cardioprotective, anti-aging, hepatoprotective, neuroprotective and angiogenic potentials (Mao et al., 2010; Zhu et al., 2015b; Chang et al., 2016; Zhong et al., 2019). In this review, we summarize recent advances regarding the molecular mechanisms and therapeutic effects of salidroside in treating ischemic diseases, thus deepening the understanding of its therapeutic potential.
Rhodiola and its active component—Salidroside
Traditional use of Rhodiola
As a member of the Crassulaceae family, Rhodiola is an all-season succulent plant that grows flowers in various colors ranging from yellow to red. More than 200 species of Rhodiola have been found, with some of them, such as R. rosea, Rhodiola quadrifida, Rhodiola kirilowii, Rhodiola crenulate, and Rhodiola sachalinensis, have been studied (Panossian et al., 2010). Rhodiola can be found in mountainous regions with altitudes of 1,800 to 5,000 m above sea level worldwide, including the Kunlun Mountains, Himalayas, Altai Mountain and the Alps (Zhuang et al., 2019; Cunningham et al., 2020). R. rosea, also known as rose root, golden root, or arctic root, is the most extensively studied Rhodiola species, and can be found in Heilongjiang, Jilin, Tibet, Yunnan, Ningxia, Gansu, Qinghai, and Sichuan provinces in China, as well as Northern Europe, Russia, Mongolia, Korea, and Japan (Galambosi et al., 2006).
Because of its widespread habitat, Rhodiola has been used as a traditional medicine in many countries and regions (Nan et al., 2003; Chen et al., 2012; Luo et al., 2019; Zheng et al., 2019). In traditional Chinese medicine, Rhodiola has been used for increasing vital energy, invigorating blood circulation, relieving asthma, and for treating cough, diarrhea, as well as bruises (Li and Su, 2018). The Vikings used Rhodiola for strengthening the body during intense labor; while people in Ireland used it as a pain-healing and headache-curing plant (Panossian et al., 2010). In France, Rhodiola has also been used as a stimulant and astringent (Alm, 2004). Russians use R. rosea as a stimulant against fatigue and for treating nervous-mental diseases, neuroses and neurotic disorders; while in Siberia, people believed that consuming Rhodiola helped to prolong their lifespan (Panossian et al., 2010).
Use of rhodiola in the modern era
The traditional use of Rhodiola worldwide has initiated modern scientific research regarding its pharmacological activities and active components underlying its therapeutic effects (Furmanowa et al., 1995). In the 1960’s, Rhodiola was extensively studied as an adaptogen, which is defined as biochemical compounds that possess adaptive effects towards stressors, such as chemical, biological and physical factors, instead of responding to them exclusively (Lan et al., 2017), and meet the following criteria: 1) not disturbing with normal conditions, 2) non-specific actions, and 3) providing normalizing actions to recover the affected conditions (Kelly, 2001; Khanum et al., 2005). Rhodiola could function as an adaptogen that prevents high-altitude sickness by increasing adaptation potential to high-altitude places with extreme conditions, such as low oxygen content, low temperature and increased air pressure, which affect physical conditions including blood pressure, heart rate, and tissue oxygen (Basnyat and Murdoch, 2003; Nieto Estrada et al., 2017). A human study involving 15 correspondents (9 females and 6 males) revealed that Rhodiola benefited the physical conditions of ski athletes, as it increased their accuracy and balance, reduced tremors and increased heart rate (Khanum et al., 2005). A preclinical study showed that Rhodiola could also exert a stress-resistance effect by helping Cerevisiae cells undergo several adaptations against stress factors in the stationary phase and increasing cell survival at the exponential phase without activating major antioxidant enzymes (Kelly, 2001; Bayliak and Lushchak, 2011).
Early studies of the pharmaceutical use of Rhodiola in the modern era were conducted mostly using its root extract (Rhodiola root extract, RRE), which is rich in secondary metabolites. RRE is usually extracted in polar solvents, such as alcohol (Wiedenfeld et al., 2007). Since the middle of the 19th century, RRE has been studied further as an adaptogen for its antioxidant properties and effects on the central nervous system (Olsson et al., 2009; Dinel et al., 2019). Studies were then extended further for different applications, such as cardiac diseases, cancer and cerebrovascular disease (Cheng et al., 2012).
The discovery of various functions of RRE subsequently drove the exploration of its active components. Since then, several secondary metabolites from RRE have been isolated, including phenylpropanoids, phenylethanol, flavonoids, monoterpenes and phenolic acids, with salidroside, tyrosol, rosavin, and triandrin as the main active components (Ming et al., 2005). Salidroside, which could be found in all Rhodiola species with concentrations ranging from 1.3 mg/g to 11.1 mg/g, has been known to possess various pharmacological properties (Figure 1). These include resisting anoxia, anti-aging, anti-cancer, anti-inflammation, and antioxidative as well as for protecting cardiovascular system (Zhang et al., 2009; Qian et al., 2012; Zheng et al., 2019; Fan et al., 2020). Moreover, as will be discussed below, salidroside has attracted interest as a potential drug for treating ischemic disease due to its ability to reduce oxidative stress and inflammation, while promoting cell survival and improving angiogenic potentials (Figure 2) (Mao et al., 2010; Shi et al., 2012; Xu et al., 2013; Zhu et al., 2015b; Chang et al., 2016; Cai et al., 2017; Feng et al., 2017; Zhang et al., 2018; Zhong et al., 2019).
Ischemic diseases
Ischemic diseases
Normal blood perfusion provides nutrition, oxygen, and metabolic compounds systematically to the whole organs, allowing them to produce and store energy. In contrast, interruptions in the blood vessels reduce blood flow and subsequently lead to an insufficient supply of nutrition and oxygen (Eltzschig and Eckle, 2011). These conditions cause severe organ and tissue damage. Furthermore, it could be lethal for cells with a high metabolism demand, such as neuron cells, cardiac cells, liver cells and renal cells, as well as cells in the hindlimbs, the most distal organ from the heart (Eltzschig and Eckle, 2011; Tymianski, 2011).
Some ischemia-manifested diseases, including stroke, lower limb ischemia and MI, can cause cell death and even mortality. Ischemic condition typically induces cell death through apoptotic, necrotic, and autophagic pathways. The insufficiency of oxygen caused by ischemia induces oxidative stress within the cells. Continuous stress leads to more reactive oxygen species (ROS) production and assemblage in mitochondria (Fu et al., 2020). ROS then affects the mitochondrial membrane permeability transition pore, causing the secretion of cytochrome c (cyt-c) which leads to cell apoptosis (Arslan et al., 2011). Ischemic condition also induces cell necrosis, as calcium ion influx, which, together with mitochondrial membrane leakage, allow extracellular fluid to enter cells, causing cell swelling and rupture (Kristian and Siesjo, 1998; Szydlowska and Tymianski, 2010). Another cellular death pathway, autophagy, is commonly due to the massive vacuolization of cytoplasm within cells (Hotchkiss et al., 2009).
On the other hand, ischemia and reperfusion also contribute to vascular dysfunction. The most common cause is through increasing vascular cells permeability and endothelial inflammation. The hypoxic state caused by less oxygen availability stimulates reduced intracellular activities of adenylate cyclase activity and the level of AMP, interrupting vascular balance permeability and leading to vascular leakage (Perrot et al., 2018). Meanwhile, dead cells that have undergone apoptosis or necrosis release ATP, which can trigger endothelial inflammation by direct binding to the NOD-like receptor family pyrin domain containing 3 (NLRP3) inflammasome and/or to endothelial inflammation receptors such as pyrimidinergic receptors (Figure 3) (Riegel et al., 2011).
Current therapeutic strategies for ischemic diseases
Current therapeutic strategies for ischemic diseases include therapeutic angiogenesis, surgery-based revascularization, organ transplantation, medication and/or application of pharmacologic agents (Selzner et al., 2003; Hankey, 2017; Deng et al., 2020; Han et al., 2022; Tarantul and Gavrilenko, 2022). Revascularization surgery usually builds blood vessel bypasses to promote blood flow, or applies special balloons and bionic stents to expand blocked blood vessels. Another surgery-based approach is organ transplantation to replace damaged organs (Thorgersen et al., 2019). For medication treatment, drugs such as rivaroxaban and statins have been recently shown as potential drugs for treating ischemic diseases owing to their function as anti-platelet aggregation and anti-platelet thrombosis as well as for relaxing peripheral blood vessels and vasodilator (Investigators et al., 2010; Oesterle et al., 2017). Therapeutic angiogenesis, which aims to induce intrinsic angiogenic potential, promote the sprouting of new vasculature, and subsequently recover blood perfusion in the ischemic site, has emerged as one of the most promising strategies for treating ischemic diseases in the past two decades (Annex, 2013; Ko and Bandyk, 2014).
Pharmacological studies of salidroside in ischemic diseases
Cerebral ischemia
Cerebral ischemia is a condition where blood flow to the brain is insufficient to meet the metabolic demand, thus causing energy metabolism disorder in brain tissues. Furthermore, it might also cause oxidative stress due to excessive inflammatory cytokines production (Siesjo, 1988; Liu et al., 2011). The most well-known manifestation of cerebral ischemia is ischemic stroke, which could be caused by hypertension, atherosclerosis, smoking and complication of diabetes (Szeto et al., 2018; Ajoolabady et al., 2021; Paul and Candelario-Jalil, 2021). Ischemic stroke could lead to harmful outcomes, such as death, dementia, and disability (Ghosh et al., 2019). Decreased oxygen and glucose supply, in turn, causes insufficient metabolism and ATP production, which subsequently triggers cell death in the area of extreme ischemia. In areas with less acute ischemia, the secretion of toxic substances, such as extracellular glutamate and other excitatory neurotransmitters, leads to excitotoxicity (Iadecola and Anrather, 2011). This then drives the activation of the inflammatory response, mitochondrial dysfunction, and oxidative stress-activated programmed cell death. Therefore, therapeutic strategies for cerebral ischemia mainly aim to protect neuron functions by suppressing excitotoxicity, inflammation, apoptosis, and oxidative stress (Iadecola and Anrather, 2011).
Zhang et al. (2018) revealed that salidroside could upregulate the expressions of brain-derived neurotrophic factor (BDNF) and tyrosine kinase receptor B (TrκB), whose binding activates phosphoinositide 3-kinase (PI3K), which in turn phosphorylates phosphatidylinositol-4,5-bisphosphate (PIP2) to phosphatidylinositol-4,5,-triphosphate (PIP3). Then, PIP3 activates the mammalian target of rapamycin complex 2 (mTORC2), which, in turn, phosphorylates and activates protein kinase B (Akt) protein (Sarbassov et al., 2005). Activation of the BDNF/PI3K/Akt pathway is crucial to inhibit excitotoxicity (Dar et al., 2018). Excitotoxicity inhibition then triggers the activation of the mammalian target of rapamycin complex 1 (mTORC1), which promotes cell survival and proliferation by stimulating lipid and protein synthesis (Valvezan et al., 2017). Furthermore, animal studies with the middle cerebral artery occlusion (MCAO) mice model showed that salidroside treatment lowered ischemia/reperfusion (I/R) injury-induced neuron cell apoptosis by promoting PI3K and Akt expression levels, resulting in the recovery of mice brain post-stroke injury (Chen et al., 2012; Zhang et al., 2018; Yin et al., 2021). Moreover, salidroside could also suppress ischemia-induced apoptosis by inhibiting apoptotic proteins, such as B-cell lymphoma 2 (Bcl-2) and Bcl-2-associated protein (Bax) (Shi et al., 2012).
Microglia are the major macrophages that reside in the brain to react during brain damage (Figure 3). There are two phenotypes of microglia, M1 and M2. M1 microglia are responsible for secreting inflammatory cytokines, such as tumor necrosis factor α (TNF-α), interleukin-6 (IL-6) and interleukin-1β (IL-1β), while M2 microglia release anti-inflammatory cytokines, such as interleukin-10 (IL-10) and transforming growth factor-β (TGF-β) (Tan et al., 2021). Upon exposure to ischemic condition, these two microglia regulate the inflammatory response in opposite ways: M1 microglia induce inflammation and impair neurogenesis, while M2 microglia protect the brain damage by restoring the neurogenesis (Tan et al., 2021). Salidroside was found to stimulate the polarization of M1 and M2 microglia cells in the MCAO mouse model, resulting in decreased pro-inflammatory cytokines secretion from M1 cells and increased phagocytosis function of M1 cells. Moreover, salidroside also indulged in the phenotypic change of M1 cells to M2 cells, eventually increasing the number of M2 cells in the ischemic area of the brain (Liu et al., 2018). Salidroside has also been proven to reduce lipopolysaccharide (LPS)-induced microglia apoptosis by suppressing the nuclear factor kappa-light-chain-enhancer of activated B (NF-κB) and mitogen-activated protein kinase (MAPK) pathways (Hu et al., 2014).
Recruitment of T-cells and B-cells as immunosuppressor is another mechanism involved in inflammatory response triggered by cerebral ischemia. Salidroside could restore the balance of Th17/Treg cells, which is disrupted upon exposure to ischemia, thereby maintaining the peripheral neurons stability after ischemia (Yin et al., 2021).
Another mechanism of neuroprotection by salidroside is by maintaining monoamine neurotransmitters in the dopaminergic system. Monoamine oxidase (MAO) is an enzyme rich in the striatum and hypothalamus that could catalyze the oxidation of monoamines, such as dopamine, serotonin, adrenaline, non-adrenaline and histamine (Youdim et al., 2006). Dopamine plays a crucial role in preventing the delayed calcium deregulation caused by excitotoxicity (Vaarmann et al., 2013; Dar et al., 2018). Zhong et al. reported that salidroside administered intraperitoneally could increase the levels of MAO, dopamine, 3,4-dihydroxyphenylacetic acid, and homovanillic acid in the striatum of a focal brain ischemic injury rat model. Salidroside could also induce the expression of tyrosine hydroxylase (TH), an important enzyme in dopamine synthesis (Zhong et al., 2019).
Increased oxidative stress is a crucial factor that induces ischemic cell death. Salidroside could also ameliorate post-ischemic neuronal injury by promoting the expression of nuclear erythroid 2-related factor 2 (Nrf2), a transcription factor that binds to the antioxidant response element (ARE) and induces the expression of several antioxidant enzymes involved in ROS scavenging, such as catalase (CAT), superoxide dismutase (SOD), glutathione (GSH), and glutathione peroxidase (GSH-px). Salidroside elevated the expression of Nrf2 and protein deglycase DJ-1 (Li et al., 2019). From these downstream and upstream mechanisms, ischemic injury is ameliorated through ROS scavenging (Han et al., 2015).
Ischemic heart disease
Blood flow in the human body is rhythmical blood distribution within the capillary beds throughout each tissue (Xu, 2020). In addition to being responsible for pumping blood, the heart itself could also suffer severe damage when the blood flow rhythm is disturbed. Ischemic heart disease (IHD) is a type of cardiovascular disease characterized by stenosis or arterial obstruction, which leads to insufficiency of blood flowing through the heart (Wong, 2014). The acute or chronic manifestations of this condition are MI, heart failure and cardiac death (Guo et al., 2018).
Salidroside could prevent the progress of myocardial ischemia from the early stage, as it could reduce exacerbation of atherosclerosis in blood vessels by inhibiting plaque formation and reducing the expression of vascular cell adhesion molecule (VCAM), intercellular adhesion molecule (ICAM), and monocyte chemoattractant protein-1 (MCP-1), which are inflammatory mediators involved in inflammatory cascade and in the pathogenesis of atherosclerosis and plaque destabilization (Figure 3) (Zhang et al., 2012).
Ischemia can lead to cardiomyocyte cell death. Two types of apoptosis are involved in post-ischemic heart failure progression: intrinsic apoptosis, which occurs in the infarct zone within 24 h of ischemia; and extrinsic apoptosis, which occurs in the myocardium under persistent ischemic conditions (Olivetti et al., 1997; Crow et al., 2004; Ben-Shahar et al., 2021). The intrinsic apoptotic pathway, also known as the mitochondrial pathway, is also engaged in myocardium apoptosis around the infarction area. This type of apoptosis involved both pro- and anti-apoptotic proteins of the Bcl-2 family, for example, Bax and Bcl-2, respectively. Upon the occurrence of MI, Bax is highly accumulated around the infarction area, while Bcl-2 is absent in this area. In contrast, an increase in Bcl-2 could be detected in non-infarcted cardiomyocytes surrounding the infarcted cells (Misao et al., 1996; Krijnen et al., 2002). Meanwhile, in the persistent-ischemic myocardium, the death receptor, which induces an extrinsic apoptotic signaling cascade, is activated (Fan et al., 2013). For example, the death receptor Fas can bind to Fas-associated protein with the death domain (FADD), which in turn directly binds to caspase 8, one of the initial caspases, and activates it by triggering its cleavage. Activated caspase 8 then cleaves and activates caspase 3, an executor caspase, which subsequently stimulates apoptosis (Figure 4) (Hotchkiss et al., 2009). Lai et al. (2014), Li et al. (2016). reported that salidroside demonstrated a cardioprotective effect by preventing cardiomyocyte apoptosis in cardiac disease mouse and rat models, respectively. Orally-administered salidroside significantly affected the expression levels of both extrinsic and intrinsic apoptotic-related factors, as it suppressed pro-apoptotic factors FasL, Fas, FADD, and Bax, respectively, while increasing the level of anti-apoptotic factors, such as Bcl-2 and Bcl-xl (Lai et al., 2014; Li et al., 2016). Furthermore, intragastric salidroside treatment in rats with I/R injury suppressed myocardial apoptosis after 30 min of left anterior descending occlusion due to an increased Bcl-2/Bax ratio and decreased caspase 3 and caspase 9 (Zhu et al., 2015b).
Myocardial ischemia could also trigger inflammation, as energy crisis and oxidative stress due to persistent I/R injury elevate the expression levels of pro-inflammatory cytokines and inflammatory transcription factors, such as NF-ĸB, IL-6, IL-1β, and TNF-α (Xie et al., 2011; Ma et al., 2015; Subramani et al., 2021). Zhu et al. found that subcutaneously injected salidroside could prevent autophagy in an isoproterenol (IOS)-induced myocardial injury rat model by suppressing the levels of NF-ĸB, IL-6, IL-1β, and TNF-α proteins (Zhu et al., 2015a). Moreover, activator protein 1 (AP1), a transcription factor that regulates inflammation similar to NF-ĸB, was also reduced in the salidroside-treated ISO-induced myocardial injury rat model. Meanwhile, Chen et al. (2017) demonstrated that oral administration of salidroside could also attenuate myocardial inflammation and promote angiogenesis in LPS-induced myocardial ischemia rat model.
The antioxidant potential of salidroside also plays a critical role in its cardioprotective effect. Besides suppressing ROS levels, intragastrically or subcutaneously administered salidroside could suppress the expression of non-mitochondrial ROS sources, such as NADPH oxidase 2 and NADPH oxidase 4, which are members of the NADPH oxidases (Noxs) protein family, while increasing the levels of antioxidant enzymes that could act as free radicals scavenger, such as CAT, SOD, GSH, and GSH-px in LPS-induced myocardial ischemic rat models and ISO-induced myocardial injury rat model (Zhu et al., 2015a; Chen et al., 2017).
Endoplasmic reticulum (ER) stress is also one of the complications of stress and extreme factors, such as ischemia. Unfolded protein response mediates ER stress and serves a role in the activation of three primary signaling pathways, including protein kinase RNA-like ER kinase (PERK), inositol-requiring enzyme-1α (IRE1α) and activating transcription factor 6. Salidroside mitigated hypoxia/reoxygenation injury by alleviating ER stress-induced apoptosis through declining the phosphorylation of PERK and IRE1α pathways in H9c2 cardiomyocytes (Sun M. Y. et al., 2018). Furthermore, Tian et al. (2022) found that salidroside protected against myocardial I/R by inhibiting ER stress in myocardial I/R rat model.
Not only protecting cardiomyocytes but salidroside could also benefit myocardial treatment by promoting angiogenesis via PI3K/Akt/mTOR pathway. Intragastrical, oral, or intracoronary administration of salidroside restored the expression of PI3K, Akt, and mTOR in myocardial ischemia in both rat and rabbit models (Xu et al., 2013; Chen et al., 2017; Chen et al., 2019). It could also promote angiogenesis by increasing HIF-1α expression and vascular endothelial growth factor (VEGF) secretion in H9c2 cells (Zhang et al., 2009), as VEGF is a growth factor transcriptionally regulated by HIF-1α and could promote the formation of the tube-like structure by endothelial cells. Furthermore, it could stimulate pericytes to express matrix metalloproteinases, leading to vasodilatation and increased permeability of the wall as well as macrophage and neutrophil chemotaxis, thereby restoring the blood vessel function and integrity (Ferrara, 2004). Oral salidroside treatment improved the hypoperfusion state in the early stages of myocardial ischemia, controlled the decline in myocardial hypertrophy and contractile force, and effectively prevented heart failure by elevating VEGF and CD34 expression levels in myocardial tissues (Chen et al., 2019).
Liver ischemia
Unlike other organs, liver ischemia is mostly affected by external causes during surgical procedures, such as liver resection, liver transplantation and trauma (Peralta et al., 2013). However, liver ischemia can lead to fatal outcomes, such as liver dysfunction or even mortality in patients. After the injury, Kupffer cells or resident macrophages in the liver are recruited to activate the inflammatory response. These cells also release ROS and induce oxidative stress at the injured site. Furthermore, I/R injury in the liver triggers both apoptosis and necrosis in hepatocytes (Gujral et al., 2001; Guicciardi et al., 2013). Hence, targeting these types of cellular damage or death is a promising approach for treating liver ischemia injury (Konishi and Lentsch, 2017).
Several factors, such as oxidative stress, heat shock, mitogens, osmotic stress and pro-inflammatory cytokines, are activated in liver ischemia. Feng et al. (2017) found that salidroside treatment prior to liver ischemia in a rat model could attenuate liver damage. Expression levels of apoptotic factor Bax and pro-inflammatory cytokines, such as TNF-α and IL-6, were also downregulated in salidroside-pretreated rats, while that of Bcl2 was elevated. Another study conducted by the same group using concanavalin A-induced acute liver injury mice showed that intraperitoneal injection of salidroside activated PI3K/Akt signaling pathway, thus suppressing the level of pro-inflammatory cytokines as well as apoptosis- and autophagy-associated marker proteins in serum and liver tissues (Feng et al., 2018).
Non-alcoholic fatty liver disease (NAFLD) could induce liver steatosis, a major risk factor for liver ischemia. NAFLD usually implies fatty acid infiltration, inflammation, cell death and collagen deposition in liver tissues (Cotter and Rinella, 2020). Zheng et al. (2018) found that oral administration of salidroside reduced oxidative stress and alleviated NAFLD in the livers of mice with NAFLD. In addition, salidroside also downregulated the inflammatory pathway by decreasing the expression of Toll-like receptor 4 (TLR4) and NLRP3 as well as pro-inflammatory cytokines.
Ischemic acute kidney injury
Renal I/R injury causes structural and functional damage to the renal tubules by directly inducing tubular cell death. These dying cells, in turn, trigger renal mucosal injury, tubulointerstitial nephritis and cortical fibrosis (Li et al., 2017). Thus, renal I/R injury is a major cause of AKI, which often arises from hypovolemic conditions, septic shock, surgery and transplantation. AKI can lead to chronic kidney disease and, subsequently, end-stage renal disease (Wang et al., 2014). Insufficient oxygen and nutrition supply might induce ATP depletion in kidney epithelial and endothelial cells, resulting in their cytoskeletal changes and eventually leading to their apoptosis or even necrosis (Sharfuddin and Molitoris, 2011). Furthermore, damage to endothelial cells could also worsen ischemic injury, as it contributes to hypoperfusion and eventually results in more cell death (Sharfuddin and Molitoris, 2011). In addition to cell death, similar to ischemic injury in other organs, oxidative stress and inflammation are also critical factors that could induce AKI (Ghasemzadeh et al., 2016; Nazir et al., 2017).
Sun et al. (2018b) discovered that salidroside decreased ROS levels and promoted SOD activity in human renal tubular epithelial cells (HK-2) under I/R conditions, thus promoting their viability. Salidroside also inhibited inflammation by reducing the levels of TNF-α, IL-1β and IL-6. It could also inhibit apoptosis in HK-2 cells by increasing Bcl-2 expression while decreasing Bax expression. Fan et al. (2022) established AKI septic rat models and found that salidroside injected via the tail vein of rats significantly reduced the plasma TNF-α, IL-1β, and IL-17A levels. Furthermore, salidroside reduced the mRNA level of Bax while increasing that of Bcl-2.
I/R injury leads to hypoxic conditions in the kidneys. The HIF protein family is a transcription factor regulated according to oxygen concentration (Semenza, 2001; Pugh and Ratcliffe, 2003). In normoxic conditions, HIF-1α protein is hydroxylated and degraded (Maxwell and Eckardt, 2016; Voit and Sankaran, 2020); however, under hypoxia, HIF-1α is stabilized and penetrates to the nucleus, where it binds to the promoters of hypoxia-activated genes, such as VEGF-A, platelet-derived growth factor B (PDGF-B), stromal cell-derived factor-1 (SDF-1), endothelial nitric oxide synthase (eNOS), erythropoietin (EPO), and heme oxygenase-1 (HO-1), and activates their transcription (Cao et al., 2003; Rajagopalan et al., 2007; Semenza, 2009; Zheng et al., 2012; Jain et al., 2018; Kuan et al., 2021; Nakashima et al., 2021). Zheng et al. (2012) found that salidroside treatment stimulated the accumulation of hypoxia-inducible factor-1α (HIF-1 α) protein by reducing HIF-1α protein degradation, thus promoting EPO expression in human embryonic kidney fibroblast (HEK293T).
Nephropathy is a microvascular disease in the kidney caused by endothelial cell injury and dysfunction, and is one of the major complications of diabetes (Tervaert et al., 2010; Navarro-Gonzalez et al., 2011; Flyvbjerg, 2017). Pathological conditions in diabetes contribute to ischemia, and inflammation induced by ischemia worsens diabetic nephropathy. Xie et al. (2019) showed that salidroside alleviated glomerular endothelial cell injury in diabetic nephropathy by upregulating the expression level of HIF protein. Guo et al. (2018a) found that salidroside administered via gavage inhibited proximal renal tubule cell apoptosis by suppressing Bax expression in diabetic rats undergoing uninephrectomy.
Lower limb ischemia
Peripheral artery disease (PAD) is a pathological condition that affects a wide range of the world’s population (Fowkes et al., 2013). PAD is caused by an obstruction in blood flow, mainly by the formation of plague and/or damage to blood vessels (Creager et al., 2008). Lower limb ischemia, which is caused mainly by damage, stenosis, or blockage of the lower extremity blood vessels, is a common form of PAD. This results in insufficient blood supply to the lower extremities, which are the most distanced tissues from the heart, leaving the lower extremity in an environment of ischemia, hypoxia and nutritional deficiency (Norgren et al., 2007a; Farber and Eberhardt, 2016; Fowkes et al., 2017). Critical limb ischemia (CLI) is the most severe clinical manifestation of PAD (Criqui et al., 2021). Percutaneous revascularization or vascular surgery is the standard of immediate aid for PAD (Weinberg et al., 2011). However, revascularization approach is largely inappropriate for patients with CLI, the most severe clinical manifestation of PAD, due to severe damages in their vessels (Norgren et al., 2007b, Gerhard-Herman et al., 2017). Therapeutic angiogenesis, which aims to induce the formation of new functional blood vessels, is one of the most studied and potential therapeutic strategies to treat lower limb ischemia, including CLI patients, who are inappropriate for surgical-based therapies and are recognized as “no-option” patients (Annex, 2013).
Neoangiogenesis requires a myriad of angiogenic factors and involves different types of cells and cellular mechanisms to stimulate and promote neovascularization. PI3K can phosphorylate phosphatidylinositol (PI) to activate Akt, which in turn activates mTORC1. Activation of mTORC1 induces HIF-1α expression, which subsequently promotes angiogenesis by inducing the expression and secretion of various angiogenic factors, including VEGF and PDGF-BB (Karar and Maity, 2011).
As mentioned previously, vascular dysfunction is an outcome of an ischemic condition. However, the human body also has a homeostatic system that counteracts persistent ischemic conditions. Under ischemia, the enzymatic activity of prolyl hydroxylase domain (PHD) family to hydroxylate prolines in HIF-1α is suppressed due to the lack of oxygen as the substrate of this enzymatic reaction, thus promoting HIF-1α accumulation and allowing the body to adapt to hypoxia (Eltzschig and Carmeliet, 2011). Salidroside could be applied in therapeutic angiogenesis strategies, as Zhang et al. (2017) and Ariyanti et al., 2017 found that salidroside could act as a PHD3-specific inhibitor, thus stabilizes HIF-1α and promotes the secretory functions of skeletal muscle cells (Figure 4). This in turn elevates neovascularization through cell-cell communications between skeletal muscle cells and endothelial and/or smooth muscle cells, which are mediated by muscle-secreted multiple angiogenic factors. The skeletal muscle cell-mediated therapeutic angiogenic effect of salidroside was further confirmed in hindlimb ischemia (HLI) model mice, as intramuscular injection of salidroside enhanced neoangiogenesis and recovered blood perfusion in the HLI mice model by inhibiting PHD3, thus stabilizing HIF-1α and promoting the expression of various angiogenic factors (Ariyanti et al., 2017).
Diabetes mellitus is one of the main causes and risk factors of lower extremity ischemic disease (Cryer, 2009). Diabetes-induced systemic damage leads to lesions of blood vessels, which in turn induces the development of diabetic complications, such as diabetic lower limb ischemia and diabetic foot ulcers (Frier, 2014). Meanwhile, accumulated advanced glycation end-products (AGEs) and excessive ROS levels caused by the pathological environment of diabetes could lead to defective angiogenic potential, activation of pro-inflammatory, apoptotic and/or autophagic pathways, thus further worsening the condition of diabetic lower limb ischemia patient (Bentzon et al., 2014; Steven et al., 2017; Allahverdian et al., 2018; Grootaert et al., 2018). AGE is a covalent compound that binds to its receptor, RAGE, and is highly provoked in diabetes. Their interplays can stimulate oxidative stress by increasing NOS, causing endothelial inflammation and cell death (Figure 4). Hu et al. (2020) showed that salidroside could protect endothelial cells by suppressing the level of NF-κB, thus preventing the AGE/RAGE-stimulated NF-ĸB pathway. Furthermore, salidroside also exerts its endothelial cell protective effect by suppressing the level of NLRP3, leading to the reduction of the levels of inflammatory factors released from NLRP3 inflammasome, such as IL-6, IL-1β and TNF-β. Upregulation of AMP-activated protein kinase (AMPK) upon AGE induction is another mechanism by which salidroside protects endothelial cells (Hu et al., 2020). AMPK is crucial for endothelial cell survival, as it could exert anti-inflammatory and anti-oxidant properties by enhancing eNOS expression level through activating PI3K/Akt pathway (Zheng et al., 2019). Furthermore, it could suppress the activation of the NLRP3 inflammasome by blocking the thioredoxin-interacting protein (TXNIP).
Salidroside not only plays a direct role against ischemia but also provides synergistic effects along with stem cell treatment. Mesenchymal stem cells (MSCs) are widely used in regenerative medicine, as they are relatively easy to isolate and ideal for allogeneic transplantation without the need for immunosuppression (Hedhli et al., 2017). Moreover, MSCs can also be genetically modified to deliver specific genes required for neovascularization (Luo et al., 2019). MSCs have been used to treat ischemic diseases, such as MI and CLI, due to their wound healing and anti-inflammation properties (Gupta et al., 2017). However, poor MSCs survival in transplanted cells due to the lack of proper stem cell niches has become a hurdle for the clinical application of this strategy (Huang et al., 2019; Mohamed et al., 2020). Therefore, improving the post-transplantation survival rate is the key to MSCs’ clinical application. HO-1 is an antioxidant and a cell survival factor whose expression could be induced by several factors, including inflammatory cytokines and oxidative stress. HO-1 expression is downregulated under ischemic conditions, leading to a decrease in cell survival (Di Filippo et al., 2005). Ariyanti et al. (2019) demonstrated that salidroside pre-treatment could enhance MSC wound healing potential in diabetic mice by elevating the expression of crucial wound healing factors, such as HO-1, fibroblast growth factor-2 (FGF2), and hepatocyte growth factor (HGF). Although further investigation is needed to confirm the therapeutic effect of this combinatorial strategy in therapeutic angiogenesis, this finding indicates the potential of combining salidroside with stem cell therapy for therapeutic angiogenesis in both lower limb ischemia and CLI.
Discussion
The utilization of Rhodiola has emerged from its part or whole plants in the ancient era to RRE and, further, to the use of its active component, salidroside, in the modern era (Kelly, 2001; Ming et al., 2005; Panossian et al., 2010; Bayliak and Lushchak, 2011; Wang et al., 2013; Chen et al., 2017; Zheng et al., 2019; Zhuang et al., 2019). Pharmacological research has led to the discovery of new functions of salidroside, as well as the molecular mechanisms underlying these functions. In recent years, studies have revealed the anti-aging, anti-oxidative, anti-stress, and anoxia-resisting properties of salidroside, indicating that it has a wide-range potential use both as a supplement and as a drug (Nan et al., 2003; Chen et al., 2012; Luo et al., 2019; Zheng et al., 2019). Owing to its anti-oxidative, anti-apoptotic, anti-inflammatory, angiogenic, and cell-protective properties, salidroside has been identified as a potential compound for ischemic diseases (Table 1).
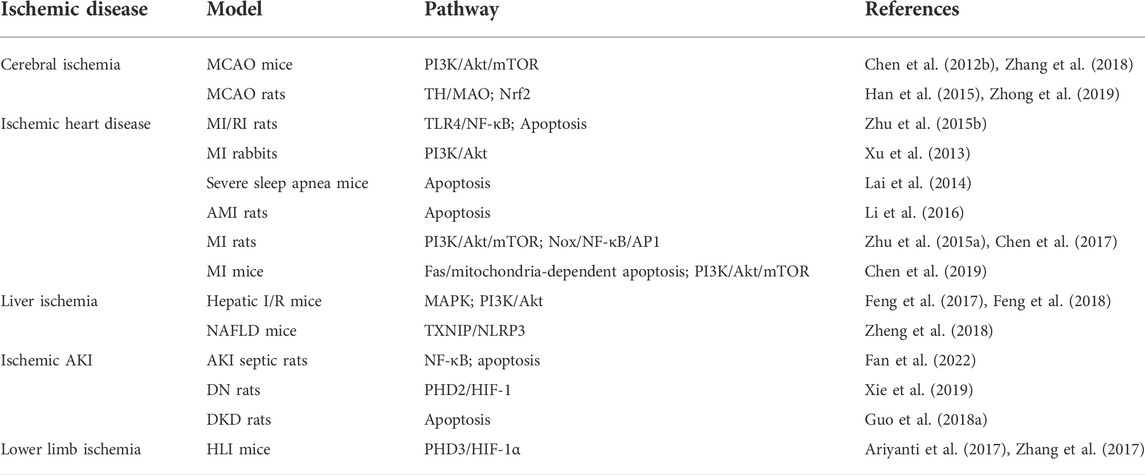
TABLE 1. Therapeutic potential and molecular mechanisms of salidroside in animal models with ischemic diseases.
However, studies regarding the use of salidroside for treating PAD were still limited at the cellular and animal models at current, and have not progressed into clinical trials yet. One of the most important obstacles might be the concentration of salidroside needed for effective treatment of ischemic disease was high, most plausibly due to the active hydrogen atoms in its phenolic hydroxyl group. Furthermore, a high dosage of salidroside increases the risk of side effects, thus impeding its clinical application. To optimize its drug-likeness, further intensive studies regarding the structure of salidroside, as well as its possible direct target in cells, which has not yet been identified, are necessary. Recently, Liu et al. (2022) synthesized more than 30 salidroside analogues and performed a structure-activity relationship study. The most optimized compound in this study could induce better neovascularization and blood perfusion recovery than salidroside in both non-diabetic and diabetic HLI mice at a significantly lower dose, suggesting the potential of applying structure-modified analogues of salidroside for clinical applications.
Several concerns need to be overcome before applying salidroside and/or its analogues for clinical use. For example, the optimal administration method, dosage, and form of the drugs might be different between patients with different types of PAD, and between patients with or without comorbid. Furthermore, preclinical and clinical systematic examinations of their side-effects as well as efficacies involving larger sample sizes are absolutely needed. Moreover, besides structural optimization as described above, combining salidroside with nanomaterials or controlled-release drug administration system could also be considered for improving its stability and efficacy.
Taken together, while further structural optimization, preclinical, and clinical studies are necessary, salidroside might become a potential drug for treating ischemic diseases.
Author contributions
VK and SW contributed to all the conceptions. JH, LL, and YW did the systematic literature search. JH, LL, SW, and VK wrote the manuscript. LL contributed to creating visual aids. VK and SW supervised the article writing and editing. All authors critically reviewed and revised the manuscript. All authors have approved the final version of the manuscript.
Funding
This work was supported by grants from the National Natural Science Foundation of China (31871367 and 81872273).
Acknowledgments
Our intention is to summarize the state of art. However, due to space limitations, we would like to apologize to authors whose works are not cited here. Their contributions should not be considered less important than those that are cited.
Conflict of interest
The authors declare that the research was conducted in the absence of any commercial or financial relationships that could be construed as a potential conflict of interest.
Publisher’s note
All claims expressed in this article are solely those of the authors and do not necessarily represent those of their affiliated organizations, or those of the publisher, the editors and the reviewers. Any product that may be evaluated in this article, or claim that may be made by its manufacturer, is not guaranteed or endorsed by the publisher.
References
Ajoolabady, A., Wang, S., Kroemer, G., Penninger, J. M., Uversky, V. N., Pratico, D., et al. (2021). Targeting autophagy in ischemic stroke: From molecular mechanisms to clinical therapeutics. Pharmacol. Ther. 225, 107848. doi:10.1016/j.pharmthera.2021.107848
Allahverdian, S., Chaabane, C., Boukais, K., Francis, G. A., and Bochaton-Piallat, M. L. (2018). Smooth muscle cell fate and plasticity in atherosclerosis. Cardiovasc Res. 114, 540–550. doi:10.1093/cvr/cvy022
Alm, T. (2004). Ethnobotany of Rhodiola rosea (Crassulaceae) in Norway. SIDA, Contributions Bot. 21, 321–344.
Annex, B. H. (2013). Therapeutic angiogenesis for critical limb ischaemia. Nat. Rev. Cardiol. 10, 387–396. doi:10.1038/nrcardio.2013.70
Ariyanti, A. D., Sisjayawan, J., Zhang, J., Zhang, J. Q., Wang, G. X., Miyagishi, M., et al. (2017). Elevating vegf-a and pdgf-bb secretion by salidroside enhances neoangiogenesis in diabetic hind-limb ischemia. Oncotarget 8, 97187–97205. doi:10.18632/oncotarget.21907
Ariyanti, A. D., Zhang, J., Marcelina, O., Nugrahaningrum, D. A., Wang, G., Kasim, V., et al. (2019). Salidroside-pretreated mesenchymal stem cells enhance diabetic wound healing by promoting paracrine function and survival of mesenchymal stem cells under hyperglycemia. Stem Cells Transl. Med. 8, 404–414. doi:10.1002/sctm.18-0143
Arslan, F., De Kleijn, D. P., and Pasterkamp, G. (2011). Innate immune signaling in cardiac ischemia. Nat. Rev. Cardiol. 8, 292–300. doi:10.1038/nrcardio.2011.38
Basnyat, B., and Murdoch, D. R. (2003). High-altitude illness. Lancet 361, 1967–1974. doi:10.1016/S0140-6736(03)13591-X
Bayliak, M. M., and Lushchak, V. I. (2011). The golden root, Rhodiola rosea, prolongs lifespan but decreases oxidative stress resistance in yeast Saccharomyces Cerevisiae. Phytomedicine 18, 1262–1268. doi:10.1016/j.phymed.2011.06.010
Ben-Shahar, Y., Abassi, Z., Pollak, Y., Koppelmann, T., Gorelik, G., and Sukhotnik, I. (2021). Cell death induction (extrinsic versus intrinsic apoptotic pathway) by intestinal ischemia-reperfusion injury in rats is time-depended. Pediatr. Surg. Int. 37, 369–376. doi:10.1007/s00383-020-04817-7
Bentzon, J. F., Otsuka, F., Virmani, R., and Falk, E. (2014). Mechanisms of plaque formation and rupture. Circ. Res. 114, 1852–1866. doi:10.1161/CIRCRESAHA.114.302721
Cai, L., Li, Y., Zhang, Q., Sun, H., Yan, X., Hua, T., et al. (2017). Salidroside protects rat liver against ischemia/reperfusion injury by regulating the gsk-3beta/nrf2-dependent antioxidant response and mitochondrial permeability transition. Eur. J. Pharmacol. 806, 32–42. doi:10.1016/j.ejphar.2017.04.011
Cao, R., Brakenhielm, E., Pawliuk, R., Wariaro, D., Post, M. J., Wahlberg, E., et al. (2003). Angiogenic synergism, vascular stability and improvement of hind-limb ischemia by a combination of pdgf-bb and fgf-2. Nat. Med. 9, 604–613. doi:10.1038/nm848
Chang, X., Zhang, K., Zhou, R., Luo, F., Zhu, L., Gao, J., et al. (2016). Cardioprotective effects of salidroside on myocardial ischemia-reperfusion injury in coronary artery occlusion-induced rats and langendorff-perfused rat hearts. Int. J. Cardiol. 215, 532–544. doi:10.1016/j.ijcard.2016.04.108
Chen, D., Fan, J., Wang, P., Zhu, L., Jin, Y., Peng, Y., et al. (2012a). Isolation, identification and antioxidative capacity of water-soluble phenylpropanoid compounds from Rhodiola crenulata. Food Chem. 134, 2126–2133. doi:10.1016/j.foodchem.2012.04.011
Chen, L., Liu, P., Feng, X., and Ma, C. (2017). Salidroside suppressing lps-induced myocardial injury by inhibiting ros-mediated pi3k/akt/mtor pathway in vitro and in vivo. J. Cell Mol. Med. 21, 3178–3189. doi:10.1111/jcmm.12871
Chen, P., Liu, J., Ruan, H., Zhang, M., Wu, P., Yimei, D., et al. (2019). Protective effects of salidroside on cardiac function in mice with myocardial infarction. Sci. Rep. 9, 18127. doi:10.1038/s41598-019-54713-x
Chen, S. F., Tsai, H. J., Hung, T. H., Chen, C. C., Lee, C. Y., Wu, C. H., et al. (2012b). Salidroside improves behavioral and histological outcomes and reduces apoptosis via pi3k/akt signaling after experimental traumatic brain injury. PLoS One 7, e45763. doi:10.1371/journal.pone.0045763
Cheng, Y. Z., Chen, L. J., Lee, W. J., Chen, M. F., Jung Lin, H., and Cheng, J. T. (2012). Increase of myocardial performance by rhodiola-ethanol extract in diabetic rats. J. Ethnopharmacol. 144, 234–239. doi:10.1016/j.jep.2012.08.029
Cotter, T. G., and Rinella, M. (2020). Nonalcoholic fatty liver disease 2020: The state of the disease. Gastroenterology 158, 1851–1864. doi:10.1053/j.gastro.2020.01.052
Creager, M. A., White, C. J., Hiatt, W. R., Criqui, M. H., Josephs, S. C., Alberts, M. J., et al. (2008). Atherosclerotic peripheral vascular disease symposium ii: Executive summary. Circulation 118, 2811–2825. doi:10.1161/CIRCULATIONAHA.108.191170
Criqui, M. H., Matsushita, K., Aboyans, V., Hess, C. N., Hicks, C. W., Kwan, T. W., et al. (2021). Lower extremity peripheral artery disease: Contemporary epidemiology, management gaps, and future directions: A scientific statement from the American heart association. Circulation 144, e171–e191. doi:10.1161/CIR.0000000000001005
Crow, M. T., Mani, K., Nam, Y. J., and Kitsis, R. N. (2004). The mitochondrial death pathway and cardiac myocyte apoptosis. Circ. Res. 95, 957–970. doi:10.1161/01.RES.0000148632.35500.d9
Cryer, P. E. (2009). Preventing hypoglycaemia: What is the appropriate glucose alert value? Diabetologia 52, 35–37. doi:10.1007/s00125-008-1205-7
Cunningham, A. B., Li, H. L., Luo, P., Zhao, W. J., Long, X. C., and Brinckmann, J. A. (2020). There "ain't No mountain high enough"?: The drivers, diversity and sustainability of China's Rhodiola trade. J. Ethnopharmacol. 252, 112379. doi:10.1016/j.jep.2019.112379
Dar, N. J., Satti, N. K., Dutt, P., Hamid, A., and Ahmad, M. (2018). Attenuation of glutamate-induced excitotoxicity by withanolide-a in neuron-like cells: Role for pi3k/akt/mapk signaling pathway. Mol. Neurobiol. 55, 2725–2739. doi:10.1007/s12035-017-0515-5
Deng, J., Guo, M., Li, G., and Xiao, J. (2020). Gene therapy for cardiovascular diseases in China: Basic research. Gene Ther. 27, 360–369. doi:10.1038/s41434-020-0148-6
Di Filippo, C., Marfella, R., Cuzzocrea, S., Piegari, E., Petronella, P., Giugliano, D., et al. (2005). Hyperglycemia in streptozotocin-induced diabetic rat increases infarct size associated with low levels of myocardial Ho-1 during ischemia/reperfusion. Diabetes 54, 803–810. doi:10.2337/diabetes.54.3.803
Dinel, A. L., Guinobert, I., Lucas, C., Blondeau, C., Bardot, V., Ripoche, I., et al. (2019). Reduction of acute mild stress corticosterone response and changes in stress-responsive gene expression in male balb/C mice after repeated administration of a Rhodiola rosea L. Root extract. Food Sci. Nutr. 7, 3827–3841. doi:10.1002/fsn3.1249
Duan, L., Zhang, Y., Yang, Y., Su, S., Zhou, L., Lo, P. C., et al. (2021). Baicalin inhibits ferroptosis in intracerebral hemorrhage. Front. Pharmacol. 12, 629379. doi:10.3389/fphar.2021.629379
Eltzschig, H. K., and Carmeliet, P. (2011). Hypoxia and inflammation. N. Engl. J. Med. 364, 656–665. doi:10.1056/NEJMra0910283
Eltzschig, H. K., and Eckle, T. (2011). Ischemia and reperfusion--from mechanism to translation. Nat. Med. 17, 1391–1401. doi:10.1038/nm.2507
Fan, F., Yang, L., Li, R., Zou, X., Li, N., Meng, X., et al. (2020). Salidroside as a potential neuroprotective agent for ischemic stroke: A review of sources, pharmacokinetics, mechanism and safety. Biomed. Pharmacother. 129, 110458. doi:10.1016/j.biopha.2020.110458
Fan, H., Su, B. J., Le, J. W., and Zhu, J. H. (2022). Salidroside protects acute kidney injury in septic rats by inhibiting inflammation and apoptosis. Drug Des. Devel Ther. 16, 899–907. doi:10.2147/DDDT.S361972
Fan, Q., Huang, Z. M., Boucher, M., Shang, X., Zuo, L., Brinks, H., et al. (2013). Inhibition of fas-associated death domain-containing protein (fadd) protects against myocardial ischemia/reperfusion injury in a heart failure mouse model. PLoS One 8, e73537. doi:10.1371/journal.pone.0073537
Farber, A., and Eberhardt, R. T. (2016). The current state of critical limb ischemia: A systematic review. JAMA Surg. 151, 1070–1077. doi:10.1001/jamasurg.2016.2018
Feng, J., Niu, P., Chen, K., Wu, L., Liu, T., Xu, S., et al. (2018). Salidroside mediates apoptosis and autophagy inhibition in concanavalin a-induced liver injury. Exp. Ther. Med. 15, 4599–4614. doi:10.3892/etm.2018.6053
Feng, J., Zhang, Q., Mo, W., Wu, L., Li, S., Li, J., et al. (2017). Salidroside pretreatment attenuates apoptosis and autophagy during hepatic ischemia-reperfusion injury by inhibiting the mitogen-activated protein kinase pathway in mice. Drug Des. Devel Ther. 11, 1989–2006. doi:10.2147/DDDT.S136792
Ferrara, N. (2004). Vascular endothelial growth factor: Basic science and clinical progress. Endocr. Rev. 25, 581–611. doi:10.1210/er.2003-0027
Flyvbjerg, A. (2017). The role of the complement system in diabetic nephropathy. Nat. Rev. Nephrol. 13, 311–318. doi:10.1038/nrneph.2017.31
Fowkes, F. G., Aboyans, V., Fowkes, F. J., Mcdermott, M. M., Sampson, U. K., and Criqui, M. H. (2017). Peripheral artery disease: Epidemiology and global perspectives. Nat. Rev. Cardiol. 14, 156–170. doi:10.1038/nrcardio.2016.179
Fowkes, F. G., Rudan, D., Rudan, I., Aboyans, V., Denenberg, J. O., Mcdermott, M. M., et al. (2013). Comparison of global estimates of prevalence and risk factors for peripheral artery disease in 2000 and 2010: A systematic review and analysis. Lancet 382, 1329–1340. doi:10.1016/S0140-6736(13)61249-0
Frier, B. M. (2014). Hypoglycaemia in diabetes mellitus: Epidemiology and clinical implications. Nat. Rev. Endocrinol. 10, 711–722. doi:10.1038/nrendo.2014.170
Fu, Z. J., Wang, Z. Y., Xu, L., Chen, X. H., Li, X. X., Liao, W. T., et al. (2020). Hif-1alpha-Bnip3-Mediated mitophagy in tubular cells protects against renal ischemia/reperfusion injury. Redox Biol. 36, 101671. doi:10.1016/j.redox.2020.101671
Furmanowa, M., Oledzka, H., Michalska, M., Sokolnicka, I., and Radomska, D. (1995). “Rhodiola rosea L. (Roseroot),” in Vitroregeneration and the biological activity of roots (Berlin, Heidelberg: Springer). doi:10.1007/978-3-662-08612-4_23
Galambosi, B., Gerhard-Herman, M. D., Gornik, H. L., Barrett, C., Barshes, N. R., Corriere, M. A., et al. (2017). 2016 aha/acc guideline on the management of patients with lower extremity peripheral artery disease: Executive summary: A report of the American College of cardiology/American heart association task force on clinical practice guidelines. Circulation 135, e686–e725. doi:10.1161/CIR.0000000000000470
Ghasemzadeh, P., Rezayat, S. M., Adeli, S., and Rahbar-Roshandel, N. (2016). Protective effect of 25mg-porphyrin-fullerene nanoparticles on oxygen-glucose deprivation/reperfusion injury in Pc12 cells. Acta Med. Iran. 54, 478–484.
Ghosh, M. K., Chakraborty, D., Sarkar, S., Bhowmik, A., and Basu, M. (2019). The interrelationship between cerebral ischemic stroke and glioma: A comprehensive study of recent reports. Signal Transduct. Target Ther. 4, 42. doi:10.1038/s41392-019-0075-4
Grootaert, M. O. J., Moulis, M., Roth, L., Martinet, W., Vindis, C., Bennett, M. R., et al. (2018). Vascular smooth muscle cell death, autophagy and senescence in atherosclerosis. Cardiovasc Res. 114, 622–634. doi:10.1093/cvr/cvy007
Guicciardi, M. E., Malhi, H., Mott, J. L., and Gores, G. J. (2013). Apoptosis and necrosis in the liver. Compr. Physiol. 3, 977–1010. doi:10.1002/cphy.c120020
Gujral, J. S., Bucci, T. J., Farhood, A., and Jaeschke, H. (2001). Mechanism of cell death during warm hepatic ischemia-reperfusion in rats: Apoptosis or necrosis? Hepatology 33, 397–405. doi:10.1053/jhep.2001.22002
Guo, C., Li, Y., Zhang, R., Zhang, Y., Zhao, J., Yao, J., et al. (2018a). Protective effect of salidroside against diabetic kidney disease through inhibiting bim-mediated apoptosis of proximal renal tubular cells in rats. Front. Pharmacol. 9, 1433. doi:10.3389/fphar.2018.01433
Guo, D., Murdoch, C. E., Liu, T., Qu, J., Jiao, S., Wang, Y., et al. (2018b). Therapeutic angiogenesis of Chinese herbal medicines in ischemic heart disease: A review. Front. Pharmacol. 9, 428. doi:10.3389/fphar.2018.00428
Gupta, P. K., Krishna, M., Chullikana, A., Desai, S., Murugesan, R., Dutta, S., et al. (2017). Administration of adult human bone marrow-derived, cultured, pooled, allogeneic mesenchymal stromal cells in critical limb ischemia due to buerger's disease: Phase ii study report suggests clinical efficacy. Stem Cells Transl. Med. 6, 689–699. doi:10.5966/sctm.2016-0237
Han, J., Luo, L., Marcelina, O., Kasim, V., and Wu, S. (2022). Therapeutic angiogenesis-based strategy for peripheral artery disease. Theranostics 12, 5015–5033. doi:10.7150/thno.74785
Han, J., Xiao, Q., Lin, Y. H., Zheng, Z. Z., He, Z. D., Hu, J., et al. (2015). Neuroprotective effects of salidroside on focal cerebral ischemia/reperfusion injury involve the nuclear erythroid 2-related factor 2 pathway. Neural Regen. Res. 10, 1989–1996. doi:10.4103/1673-5374.172317
Han, J. Y., Li, Q., Ma, Z. Z., and Fan, J. Y. (2017). Effects and mechanisms of compound Chinese medicine and major ingredients on microcirculatory dysfunction and organ injury induced by ischemia/reperfusion. Pharmacol. Ther. 177, 146–173. doi:10.1016/j.pharmthera.2017.03.005
Hedhli, J., Konopka, C. J., Schuh, S., Bouvin, H., Cole, J. A., Huntsman, H. D., et al. (2017). Multimodal assessment of mesenchymal stem cell therapy for diabetic vascular complications. Theranostics 7, 3876–3888. doi:10.7150/thno.19547
Hotchkiss, R. S., Strasser, A., Mcdunn, J. E., and Swanson, P. E. (2009). Cell death. N. Engl. J. Med. 361, 1570–1583. doi:10.1056/NEJMra0901217
Hu, H., Li, Z., Zhu, X., Lin, R., and Chen, L. (2014). Salidroside reduces cell mobility via nf- kappa B and mapk signaling in lps-induced Bv2 microglial cells. Evid. Based Complement. Altern. Med. 2014, 383821. doi:10.1155/2014/383821
Hu, R., Wang, M. Q., Ni, S. H., Wang, M., Liu, L. Y., You, H. Y., et al. (2020). Salidroside ameliorates endothelial inflammation and oxidative stress by regulating the ampk/nf-kappab/nlrp3 signaling pathway in ages-induced huvecs. Eur. J. Pharmacol. 867, 172797. doi:10.1016/j.ejphar.2019.172797
Huang, A., Liu, D., Qi, X., Yue, Z., Cao, H., Zhang, K., et al. (2019). Self-assembled gffyk peptide hydrogel enhances the therapeutic efficacy of mesenchymal stem cells in a mouse hindlimb ischemia model. Acta Biomater. 85, 94–105. doi:10.1016/j.actbio.2018.12.015
Iadecola, C., and Anrather, J. (2011). Stroke research at a crossroad: Asking the brain for directions. Nat. Neurosci. 14, 1363–1368. doi:10.1038/nn.2953
Investigators, E., Bauersachs, R., Berkowitz, S. D., Brenner, B., Buller, H. R., Decousus, H., et al. (2010). Oral rivaroxaban for symptomatic venous thromboembolism. N. Engl. J. Med. 363, 2499–2510. doi:10.1056/NEJMoa1007903
Jain, T., Nikolopoulou, E. A., Xu, Q., and Qu, A. (2018). Hypoxia inducible factor as a therapeutic target for atherosclerosis. Pharmacol. Ther. 183, 22–33. doi:10.1016/j.pharmthera.2017.09.003
Karar, J., and Maity, A. (2011). Pi3k/Akt/Mtor pathway in angiogenesis. Front. Mol. Neurosci. 4, 51. doi:10.3389/fnmol.2011.00051
Khanum, F., Bawa, A. S., and Singh, B. (2005). Rhodiola rosea: A versatile adaptogen. Compr. Rev. Food Sci. Food Saf. 4, 55–62. doi:10.1111/j.1541-4337.2005.tb00073.x
Ko, S. H., and Bandyk, D. F. (2014). Therapeutic angiogenesis for critical limb ischemia. Semin. Vasc. Surg. 27, 23–31. doi:10.1053/j.semvascsurg.2014.10.001
Konishi, T., and Lentsch, A. B. (2017). Hepatic ischemia/reperfusion: Mechanisms of tissue injury, repair, and regeneration. Gene Expr. 17, 277–287. doi:10.3727/105221617X15042750874156
Krijnen, P. A., Nijmeijer, R., Meijer, C. J., Visser, C. A., Hack, C. E., and Niessen, H. W. (2002). Apoptosis in myocardial ischaemia and infarction. J. Clin. Pathol. 55, 801–811. doi:10.1136/jcp.55.11.801
Kristian, T., and Siesjo, B. K. (1998). Calcium in ischemic cell death. Stroke 29, 705–718. doi:10.1161/01.str.29.3.705
Kuan, C. Y., Chen, H. R., Gao, N., Kuo, Y. M., Chen, C. W., Yang, D., et al. (2021). Brain-targeted hypoxia-inducible factor stabilization reduces neonatal hypoxic-ischemic brain injury. Neurobiol. Dis. 148, 105200. doi:10.1016/j.nbd.2020.105200
Lai, M. C., Lin, J. G., Pai, P. Y., Lai, M. H., Lin, Y. M., Yeh, Y. L., et al. (2014). Protective effect of salidroside on cardiac apoptosis in mice with chronic intermittent hypoxia. Int. J. Cardiol. 174, 565–573. doi:10.1016/j.ijcard.2014.04.132
Lan, K. C., Chao, S. C., Wu, H. Y., Chiang, C. L., Wang, C. C., Liu, S. H., et al. (2017). Salidroside ameliorates sepsis-induced acute lung injury and mortality via downregulating nf-kappab and Hmgb1 pathways through the upregulation of Sirt1. Sci. Rep. 7, 12026. doi:10.1038/s41598-017-12285-8
Li, B., Haridas, B., Jackson, A. R., Cortado, H., Mayne, N., Kohnken, R., et al. (2017). Inflammation drives renal scarring in experimental pyelonephritis. Am. J. Physiol. Ren. Physiol. 312, F43–F53. doi:10.1152/ajprenal.00471.2016
Li, J., Li, J.-F., Wei, T.-T., and Li, J.-H. (2016). Effects of salidroside on myocardial cell apoptosis in acute myocardial ischemia rats and its mechanism. J. Xiangya Med. 1, 1.
Li, R., Wang, S., Li, T., Wu, L., Fang, Y., Feng, Y., et al. (2019). Salidroside protects dopaminergic neurons by preserving complex I activity via dj-1/nrf2-mediated antioxidant pathway. Park. Dis. 2019, 6073496. doi:10.1155/2019/6073496
Li, T., and Su, C. (2018). Authenticity identification and classification of Rhodiola species in traditional Tibetan medicine based on fourier transform near-infrared spectroscopy and chemometrics analysis. Spectrochim. Acta A Mol. Biomol. Spectrosc. 204, 131–140. doi:10.1016/j.saa.2018.06.004
Liu, C., Han, J., Marcelina, O., Nugrahaningrum, D. A., Huang, S., Zou, M., et al. (2022). Discovery of salidroside-derivated glycoside analogues as novel angiogenesis agents to treat diabetic hind limb ischemia. J. Med. Chem. 65, 135–162. doi:10.1021/acs.jmedchem.1c00947
Liu, X., Wen, S., Yan, F., Liu, K., Liu, L., Wang, L., et al. (2018). Salidroside provides neuroprotection by modulating microglial polarization after cerebral ischemia. J. Neuroinflammation 15, 39. doi:10.1186/s12974-018-1081-0
Liu, Z., Li, P., Zhao, D., Tang, H., and Guo, J. (2011). Anti-inflammation effects of cordyceps sinensis mycelium in focal cerebral ischemic injury rats. Inflammation 34, 639–644. doi:10.1007/s10753-010-9273-5
Luo, C., Xu, X., Wei, X., Feng, W., Huang, H., Liu, H., et al. (2019a). Natural medicines for the treatment of fatigue: Bioactive components, Pharmacology, and mechanisms. Pharmacol. Res. 148, 104409. doi:10.1016/j.phrs.2019.104409
Luo, R., Lu, Y., Liu, J., Cheng, J., and Chen, Y. (2019b). Enhancement of the efficacy of mesenchymal stem cells in the treatment of ischemic diseases. Biomed. Pharmacother. 109, 2022–2034. doi:10.1016/j.biopha.2018.11.068
Ma, S., Wang, Y., Chen, Y., and Cao, F. (2015). The role of the autophagy in myocardial ischemia/reperfusion injury. Biochim. Biophys. Acta 1852, 271–276. doi:10.1016/j.bbadis.2014.05.010
Mao, G. X., Deng, H. B., Yuan, L. G., Li, D. D., Li, Y. Y., and Wang, Z. (2010). Protective role of salidroside against aging in a mouse model induced by D-galactose. Biomed. Environ. Sci. 23, 161–166. doi:10.1016/s0895-3988(10)60047-5
Maxwell, P. H., and Eckardt, K. U. (2016). Hif prolyl hydroxylase inhibitors for the treatment of renal anaemia and beyond. Nat. Rev. Nephrol. 12, 157–168. doi:10.1038/nrneph.2015.193
Ming, D. S., Hillhouse, B. J., Guns, E. S., Eberding, A., Xie, S., Vimalanathan, S., et al. (2005). Bioactive compounds from Rhodiola rosea (Crassulaceae). Phytother. Res. 19, 740–743. doi:10.1002/ptr.1597
Misao, J., Hayakawa, Y., Ohno, M., Kato, S., Fujiwara, T., and Fujiwara, H. (1996). Expression of bcl-2 protein, an inhibitor of apoptosis, and Bax, an accelerator of apoptosis, in ventricular myocytes of human hearts with myocardial infarction. Circulation 94, 1506–1512. doi:10.1161/01.cir.94.7.1506
Mohamed, S. A., Howard, L., Mcinerney, V., Hayat, A., Krawczyk, J., Naughton, S., et al. (2020). Autologous bone marrow mesenchymal stromal cell therapy for "No-option" critical limb ischemia is limited by karyotype Abnormalities. Cytotherapy 22, 313–321. doi:10.1016/j.jcyt.2020.02.007
Nakashima, M., Watanabe, M., Nakano, K., Uchimaru, K., and Horie, R. (2021). Differentiation of hodgkin lymphoma cells by reactive oxygen species and regulation by heme oxygenase-1 through hif-1alpha. Cancer Sci. 112, 2542–2555. doi:10.1111/cas.14890
Nan, J. X., Jiang, Y. Z., Park, E. J., Ko, G., Kim, Y. C., and Sohn, D. H. (2003). Protective effect of Rhodiola sachalinensis extract on carbon tetrachloride-induced liver injury in rats. J. Ethnopharmacol. 84, 143–148. doi:10.1016/s0378-8741(02)00293-3
Navarro-Gonzalez, J. F., Mora-Fernandez, C., Muros De Fuentes, M., and Garcia-Perez, J. (2011). Inflammatory molecules and pathways in the pathogenesis of diabetic nephropathy. Nat. Rev. Nephrol. 7, 327–340. doi:10.1038/nrneph.2011.51
Nazir, S., Gadi, I., Al-Dabet, M. M., Elwakiel, A., Kohli, S., Ghosh, S., et al. (2017). Cytoprotective activated protein C averts Nlrp3 inflammasome-induced ischemia-reperfusion injury via Mtorc1 inhibition. Blood 130, 2664–2677. doi:10.1182/blood-2017-05-782102
Nieto Estrada, V. H., Molano Franco, D., Medina, R. D., Gonzalez Garay, A. G., Marti-Carvajal, A. J., and Arevalo-Rodriguez, I. (2017). Interventions for preventing high altitude illness: Part 1. Commonly-used classes of drugs. Cochrane Database Syst. Rev. 6, CD009761. doi:10.1002/14651858.CD009761.pub2
Norgren, L., Hiatt, W. R., Dormandy, J. A., Nehler, M. R., Harris, K. A., Fowkes, F. G., et al. (2007a). Inter-society consensus for the management of peripheral arterial disease (tasc ii). J. Vasc. Surg. 45 (1), S5–S67. doi:10.1016/j.jvs.2006.12.037
Norgren, L., Hiatt, W. R., Dormandy, J. A., Nehler, M. R., Harris, K. A., Fowkes, F. G., et al. (2007b). Inter-society consensus for the management of peripheral arterial disease (tasc ii). Eur. J. Vasc. Endovasc. Surg. 33 (1), S1–S75. doi:10.1016/j.ejvs.2006.09.024
Oesterle, A., Laufs, U., and Liao, J. K. (2017). Pleiotropic effects of statins on the cardiovascular system. Circ. Res. 120, 229–243. doi:10.1161/CIRCRESAHA.116.308537
Olivetti, G., Abbi, R., Quaini, F., Kajstura, J., Cheng, W., Nitahara, J. A., et al. (1997). Apoptosis in the failing human heart. N. Engl. J. Med. 336, 1131–1141. doi:10.1056/NEJM199704173361603
Olsson, E. M., Von Scheele, B., and Panossian, A. G. (2009). A randomised, double-blind, placebo-controlled, parallel-group study of the standardised extract shr-5 of the roots of Rhodiola rosea in the treatment of subjects with stress-related fatigue. Planta Med. 75, 105–112. doi:10.1055/s-0028-1088346
Panossian, A., Wikman, G., and Sarris, J. (2010). Rosenroot (Rhodiola rosea): Traditional use, chemical composition, Pharmacology and clinical efficacy. Phytomedicine 17, 481–493. doi:10.1016/j.phymed.2010.02.002
Paul, S., and Candelario-Jalil, E. (2021). Emerging neuroprotective strategies for the treatment of ischemic stroke: An overview of clinical and preclinical studies. Exp. Neurol. 335, 113518. doi:10.1016/j.expneurol.2020.113518
Peralta, C., Jimenez-Castro, M. B., and Gracia-Sancho, J. (2013). Hepatic ischemia and reperfusion injury: Effects on the liver sinusoidal milieu. J. Hepatol. 59, 1094–1106. doi:10.1016/j.jhep.2013.06.017
Perrot, C. Y., Sawada, J., and Komatsu, M. (2018). Prolonged activation of camp signaling leads to endothelial barrier disruption via transcriptional repression of rras. FASEB J. 32, fj201700818RRR. doi:10.1096/fj.201700818RRR
Pugh, C. W., and Ratcliffe, P. J. (2003). Regulation of angiogenesis by hypoxia: Role of the hif system. Nat. Med. 9, 677–684. doi:10.1038/nm0603-677
Qian, E. W., Ge, D. T., and Kong, S. K. (2012). Salidroside protects human erythrocytes against hydrogen peroxide-induced apoptosis. J. Nat. Prod. 75, 531–537. doi:10.1021/np200555s
Rajagopalan, S., Olin, J., Deitcher, S., Pieczek, A., Laird, J., Grossman, P. M., et al. (2007). Use of a constitutively active hypoxia-inducible factor-1alpha transgene as a therapeutic strategy in No-option critical limb ischemia patients: Phase I dose-escalation experience. Circulation 115, 1234–1243. doi:10.1161/CIRCULATIONAHA.106.607994
Riegel, A. K., Faigle, M., Zug, S., Rosenberger, P., Robaye, B., Boeynaems, J. M., et al. (2011). Selective induction of endothelial P2y6 nucleotide receptor promotes vascular inflammation. Blood 117, 2548–2555. doi:10.1182/blood-2010-10-313957
Sarbassov, D. D., Guertin, D. A., Ali, S. M., and Sabatini, D. M. (2005). Phosphorylation and regulation of akt/pkb by the rictor-mtor complex. Science 307, 1098–1101. doi:10.1126/science.1106148
Selzner, N., Rudiger, H., Graf, R., and Clavien, P. A. (2003). Protective strategies against ischemic injury of the liver. Gastroenterology 125, 917–936. doi:10.1016/s0016-5085(03)01048-5
Semenza, G. L. (2001). Hif-1 and mechanisms of hypoxia sensing. Curr. Opin. Cell Biol. 13, 167–171. doi:10.1016/s0955-0674(00)00194-0
Semenza, G. L. (2009). Regulation of vascularization by hypoxia-inducible factor 1. Ann. N. Y. Acad. Sci. 1177, 2–8. doi:10.1111/j.1749-6632.2009.05032.x
Sharfuddin, A. A., and Molitoris, B. A. (2011). Pathophysiology of ischemic acute kidney injury. Nat. Rev. Nephrol. 7, 189–200. doi:10.1038/nrneph.2011.16
Shi, T. Y., Feng, S. F., Xing, J. H., Wu, Y. M., Li, X. Q., Zhang, N., et al. (2012). Neuroprotective effects of salidroside and its analogue tyrosol galactoside against focal cerebral ischemia in vivo and H2o2-induced neurotoxicity in vitro. Neurotox. Res. 21, 358–367. doi:10.1007/s12640-011-9290-7
Siesjo, B. K. (1988). Historical overview. Calcium, ischemia, and death of brain cells. Ann. N. Y. Acad. Sci. 522, 638–661. doi:10.1111/j.1749-6632.1988.tb33410.x
Steven, S., Daiber, A., Dopheide, J. F., Munzel, T., and Espinola-Klein, C. (2017). Peripheral artery disease, redox signaling, oxidative stress - basic and clinical aspects. Redox Biol. 12, 787–797. doi:10.1016/j.redox.2017.04.017
Subramani, J., Kundumani-Sridharan, V., and Das, K. C. (2021). Chaperone-mediated autophagy of enos in myocardial ischemia-reperfusion injury. Circ. Res. 129, 930–945. doi:10.1161/CIRCRESAHA.120.317921
Sun, M. Y., Ma, D. S., Zhao, S., Wang, L., Ma, C. Y., and Bai, Y. (2018a). Salidroside mitigates hypoxia/reoxygenation injury by alleviating endoplasmic reticulum stressinduced apoptosis in H9c2 cardiomyocytes. Mol. Med. Rep. 18, 3760–3768. doi:10.3892/mmr.2018.9403
Sun, Y., Xun, L., Jin, G., and Shi, L. (2018b). Salidroside protects renal tubular epithelial cells from hypoxia/reoxygenation injury in vitro. J. Pharmacol. Sci. 137, 170–176. doi:10.1016/j.jphs.2018.05.011
Szeto, V., Chen, N. H., Sun, H. S., and Feng, Z. P. (2018). The role of katp channels in cerebral ischemic stroke and diabetes. Acta Pharmacol. Sin. 39, 683–694. doi:10.1038/aps.2018.10
Szydlowska, K., and Tymianski, M. (2010). Calcium, ischemia and excitotoxicity. Cell Calcium 47, 122–129. doi:10.1016/j.ceca.2010.01.003
Tan, R. P., Ryder, I., Yang, N., Lam, Y. T., Santos, M., Michael, P. L., et al. (2021). Macrophage polarization as a novel therapeutic target for endovascular intervention in peripheral artery disease. JACC Basic Transl. Sci. 6, 693–704. doi:10.1016/j.jacbts.2021.04.008
Tarantul, V. Z., and Gavrilenko, A. V. (2022). Gene therapy for critical limb ischemia: Per aspera ad astra. Curr. Gene Ther. 22, 214–227. doi:10.2174/1566523221666210712185742
Tervaert, T. W., Mooyaart, A. L., Amann, K., Cohen, A. H., Cook, H. T., Drachenberg, C. B., et al. (2010). Pathologic classification of diabetic nephropathy. J. Am. Soc. Nephrol. 21, 556–563. doi:10.1681/ASN.2010010010
Thorgersen, E. B., Barratt-Due, A., Haugaa, H., Harboe, M., Pischke, S. E., Nilsson, P. H., et al. (2019). The role of complement in liver injury, regeneration, and transplantation. Hepatology 70, 725–736. doi:10.1002/hep.30508
Tian, X., Huang, Y., Zhang, X., Fang, R., Feng, Y., Zhang, W., et al. (2022). Salidroside attenuates myocardial ischemia/reperfusion injury via ampk-induced suppression of endoplasmic reticulum stress and mitochondrial fission. Toxicol. Appl. Pharmacol. 448, 116093. doi:10.1016/j.taap.2022.116093
Tymianski, M. (2011). Emerging mechanisms of disrupted cellular signaling in brain ischemia. Nat. Neurosci. 14, 1369–1373. doi:10.1038/nn.2951
Vaarmann, A., Kovac, S., Holmstrom, K. M., Gandhi, S., and Abramov, A. Y. (2013). Dopamine protects neurons against glutamate-induced excitotoxicity. Cell Death Dis. 4, e455. doi:10.1038/cddis.2012.194
Valvezan, A. J., Turner, M., Belaid, A., Lam, H. C., Miller, S. K., Mcnamara, M. C., et al. (2017). Mtorc1 couples nucleotide synthesis to nucleotide demand resulting in a targetable metabolic vulnerability. Cancer Cell 32, 624–638. e625. doi:10.1016/j.ccell.2017.09.013
Voit, R. A., and Sankaran, V. G. (2020). Stabilizing hif to ameliorate anemia. Cell 180, 6. doi:10.1016/j.cell.2019.12.010
Wang, H., Pang, W., Xu, X., You, B., Zhang, C., and Li, D. (2021). Cryptotanshinone attenuates ischemia/reperfusion-induced apoptosis in myocardium by upregulating Mapk3. J. Cardiovasc Pharmacol. 77, 370–377. doi:10.1097/FJC.0000000000000971
Wang, L., Chen, H., Liu, X. H., Chen, Z. Y., Weng, X. D., Qiu, T., et al. (2014). Ozone oxidative preconditioning inhibits renal fibrosis induced by ischemia and reperfusion injury in rats. Exp. Ther. Med. 8, 1764–1768. doi:10.3892/etm.2014.2004
Wang, Y., Xu, P., Wang, Y., Liu, H., Zhou, Y., and Cao, X. (2013). The protection of salidroside of the heart against acute exhaustive injury and molecular mechanism in rat. Oxid. Med. Cell Longev. 2013, 507832. doi:10.1155/2013/507832
Weinberg, M. D., Lau, J. F., Rosenfield, K., and Olin, J. W. (2011). Peripheral artery disease. Part 2: Medical and endovascular treatment. Nat. Rev. Cardiol. 8, 429–441. doi:10.1038/nrcardio.2011.81
Wiedenfeld, H., Dumaa, M., Malinowski, M., Furmanowa, M., and Narantuya, S. (2007). Phytochemical and analytical studies of extracts from Rhodiola rosea and Rhodiola quadrifida. Pharmazie 62, 308–311.
Wong, N. D. (2014). Epidemiological studies of chd and the evolution of preventive cardiology. Nat. Rev. Cardiol. 11, 276–289. doi:10.1038/nrcardio.2014.26
Xie, M., Morales, C. R., Lavandero, S., and Hill, J. A. (2011). Tuning flux: Autophagy as a target of heart disease therapy. Curr. Opin. Cardiol. 26, 216–222. doi:10.1097/HCO.0b013e328345980a
Xie, R. Y., Fang, X. L., Zheng, X. B., Lv, W. Z., Li, Y. J., Ibrahim Rage, H., et al. (2019). Salidroside and fg-4592 ameliorate high glucose-induced glomerular endothelial cells injury via hif upregulation. Biomed. Pharmacother. 118, 109175. doi:10.1016/j.biopha.2019.109175
Xu, M. C., Shi, H. M., Gao, X. F., and Wang, H. (2013). Salidroside attenuates myocardial ischemia-reperfusion injury via pi3k/akt signaling pathway. J. Asian Nat. Prod. Res. 15, 244–252. doi:10.1080/10286020.2012.762358
Xu, S. (2020). Therapeutic potential of blood flow mimetic compounds in preventing endothelial dysfunction and atherosclerosis. Pharmacol. Res. 155, 104737. doi:10.1016/j.phrs.2020.104737
Yin, L., Ouyang, D., Lin, L., Xin, X., and Ji, Y. (2021). Salidroside regulates imbalance of Th17/treg and promotes ischemic tolerance by targeting stat-3 in cerebral ischemia-reperfusion injury. Arch. Med. Sci. 17, 523–534. doi:10.5114/aoms.2019.85349
Youdim, M. B., Edmondson, D., and Tipton, K. F. (2006). The therapeutic potential of monoamine oxidase inhibitors. Nat. Rev. Neurosci. 7, 295–309. doi:10.1038/nrn1883
Zhang, B. C., Li, W. M., Guo, R., and Xu, Y. W. (2012). Salidroside decreases atherosclerotic plaque formation in low-density lipoprotein receptor-deficient mice. Evid. Based Complement. Altern. Med. 2012, 607508. doi:10.1155/2012/607508
Zhang, J., Kasim, V., Xie, Y. D., Huang, C., Sisjayawan, J., Dwi Ariyanti, A., et al. (2017). Inhibition of phd3 by salidroside promotes neovascularization through cell-cell communications mediated by muscle-secreted angiogenic factors. Sci. Rep. 7, 43935. doi:10.1038/srep43935
Zhang, J., Liu, A., Hou, R., Zhang, J., Jia, X., Jiang, W., et al. (2009). Salidroside protects cardiomyocyte against hypoxia-induced death: A hif-1alpha-activated and vegf-mediated pathway. Eur. J. Pharmacol. 607, 6–14. doi:10.1016/j.ejphar.2009.01.046
Zhang, X., Du, Q., Yang, Y., Wang, J., Liu, Y., Zhao, Z., et al. (2018). Salidroside alleviates ischemic brain injury in mice with ischemic stroke through regulating bdnk mediated pi3k/akt pathway. Biochem. Pharmacol. 156, 99–108. doi:10.1016/j.bcp.2018.08.015
Zheng, K. Y., Zhang, Z. X., Guo, A. J., Bi, C. W., Zhu, K. Y., Xu, S. L., et al. (2012). Salidroside stimulates the accumulation of hif-1alpha protein resulted in the induction of epo expression: A signaling via blocking the degradation pathway in kidney and liver cells. Eur. J. Pharmacol. 679, 34–39. doi:10.1016/j.ejphar.2012.01.027
Zheng, T., Bian, F., Chen, L., Wang, Q., and Jin, S. (2019). Beneficial effects of Rhodiola and salidroside in diabetes: Potential role of amp-activated protein kinase. Mol. Diagn Ther. 23, 489–498. doi:10.1007/s40291-019-00402-4
Zheng, T., Yang, X., Li, W., Wang, Q., Chen, L., Wu, D., et al. (2018). Salidroside attenuates high-fat diet-induced nonalcoholic fatty liver disease via ampk-dependent txnip/nlrp3 pathway. Oxid. Med. Cell Longev. 2018, 8597897. doi:10.1155/2018/8597897
Zhong, Z. F., Han, J., Zhang, J. Z., Xiao, Q., Chen, J. Y., Zhang, K., et al. (2019). Neuroprotective effects of salidroside on cerebral ischemia/reperfusion-induced behavioral impairment involves the dopaminergic system. Front. Pharmacol. 10, 1433. doi:10.3389/fphar.2019.01433
Zhu, L., Wei, T., Chang, X., He, H., Gao, J., Wen, Z., et al. (2015a). Effects of salidroside on myocardial injury in vivo in vitro via regulation of nox/nf-kappab/ap1 pathway. Inflammation 38, 1589–1598. doi:10.1007/s10753-015-0134-0
Zhu, L., Wei, T., Gao, J., Chang, X., He, H., Luo, F., et al. (2015b). The cardioprotective effect of salidroside against myocardial ischemia reperfusion injury in rats by inhibiting apoptosis and inflammation. Apoptosis 20, 1433–1443. doi:10.1007/s10495-015-1174-5
Keywords: Rhodiola, salidroside, ischemic diseases, hypoxia, angiogenesis
Citation: Han J, Luo L, Wang Y, Wu S and Kasim V (2022) Therapeutic potential and molecular mechanisms of salidroside in ischemic diseases. Front. Pharmacol. 13:974775. doi: 10.3389/fphar.2022.974775
Received: 21 June 2022; Accepted: 29 July 2022;
Published: 19 August 2022.
Edited by:
Xuelin Zhou, Capital Medical University, ChinaReviewed by:
Haibo Yang, Harvard Medical School, United StatesYijun Shen, Fudan University, China
Marta Grech-Baran, Institute of Biochemistry and Biophysics (PAN), Poland
Zhou Xunian, MD Anderson Cancer Center, United States
Copyright © 2022 Han, Luo, Wang, Wu and Kasim. This is an open-access article distributed under the terms of the Creative Commons Attribution License (CC BY). The use, distribution or reproduction in other forums is permitted, provided the original author(s) and the copyright owner(s) are credited and that the original publication in this journal is cited, in accordance with accepted academic practice. No use, distribution or reproduction is permitted which does not comply with these terms.
*Correspondence: Shourong Wu, c2hvdXJvbmd3dUBjcXUuZWR1LmNu; Vivi Kasim, dml2aWthc2ltQGNxdS5lZHUuY24=
†These authors have contributed equally to this work