- 1Department of Hematology, Affiliated Hospital of Guizhou Medical University, Guiyang, China
- 2Guizhou Province Institute of Hematology, Guizhou Province Laboratory of Hematopoietic Stem Cell Transplantation Centre, Guiyang, China
During the last decade, the underlying pathogenic mechanisms of acute myeloid leukemia (AML) have been the subject of extensive study which has considerably increased our understanding of the disease. However, both resistance to chemotherapy and disease relapse remain the principal obstacles to successful treatment. Because of acute and chronic undesirable effects frequently associated with conventional cytotoxic chemotherapy, consolidation chemotherapy is not feasible, especially for elderly patients, which has attracted a growing body of research to attempt to tackle this problem. Immunotherapies for acute myeloid leukemia, including immune checkpoint inhibitors, monoclonal antibodies, dendritic cell (DC) vaccines, together with T-cell therapy based on engineered antigen receptor have been developed recently. Our review presents the recent progress in immunotherapy for the treatment of AML and discusses effective therapies that have the most potential and major challenges.
Introduction
Acute myeloid leukemia (AML) is a form of leukemia that mostly affects the adult population with most cases occurring in this age group and is diagnosed less frequently in children. First-line chemotherapy has been the mainstay of treatment during the last four decades (Dohner et al., 2010). Despite initial response to first-line treatment and disappearance of symptoms for most patients, only a small fraction achieves prolonged survival due to chemo-resistant relapses. Superior survival has been observed in the patient group undergoing allogeneic hematopoietic stem cell transplantation (allo-HSCT), but these patients only constitute a small fraction of AML cases (Ferrara and Schiffer, 2013; Kadia et al., 2015; Coombs et al., 2016; Short et al., 2018), and the relapse does not seem to decrease with graft-versus-host disease as well as patients with active disease (Vago and Gojo, 2020). Patients who have never achieved complete remission (CR) or relapse within 6 months after achieving a CR have poorer prognosis (De Kouchkovsky et al., 2016).
Immune surveillance is pivotal in suppressing tumor growth and maturation. The immune system can identify the antigens specific to the tumor cells. Nevertheless, the tumor microenvironment fosters immunosuppressive activities and antigen loss that would eventually lead to immune evasion. On the one hand, T-cell surface checkpoint regulators such as cytotoxic T lymphocyte-associated antigen-4 (CTLA-4) and programmed cell death protein 1 (PD-1) have been demonstrated to contribute, at least in part, to these immunosuppressive activities (Gurusamy et al., 2017; Mohme et al., 2017). Enhancement of inhibitory receptors confers robust immune effector response (Deng et al., 2018). Based on this immunosuppression mechanism, CTLA-4 and PD-1 checkpoint blockade therapies have shown outstanding clinical efficacy in killing tumor cells in several solid organ malignancies (Tang et al., 2017). The continued success of immunotherapy in solid tumors has prompted its use in hematologic malignancies, and PD-1 blockade has shown promising preliminary results in classical Hodgkin lymphoma (cHL) (Hodi et al., 2010; Topalian et al., 2012; Robert et al., 2014; Younes et al., 2016). However, patients with other hematological malignancies, such as AML, have shown fewer encouraging results, underlining the need for future studies in these tumor types. On the other hand, to prevent antigen loss, a novel class of immune therapy strategies has been developed, including specific antibodies, dendritic cell (DC) vaccines, chimeric antigen receptor-engineered T cells (CAR-T), T-cell receptor-engineered T cells (TCR-T). Several antibody-based therapies have become the paradigm for treating acute lymphoblastic leukemia (ALL), and several others are being evaluated in clinical studies. Addition of Rituximab, a CD20-directed cytolytic antibody, to first line chemotherapy has demonstrated enhanced efficacy (Levato and Molica, 2018). Autologous CAR-T cell therapy targeting CD19 has shown striking responses, leading to FDA’s approval for the management of precursor B-cell ALL and diffuse large B-cell lymphoma (DLBCL) (Grupp et al., 2013).
Immune checkpoint inhibitors are capable of activating tumor-specific immune cells that are suppressed in the tumor microenvironment (TME). For hematological malignancies such as AML with a maturation and progression pattern different from solid tumors, the mechanisms underlying the activity or inactivity of the immune system may strikingly differ from previous descriptions for solid tumors. Despite the presence of mutual immune evasion pathways, hematological tumors have their unique tolerance mechanisms (Zhang et al., 2009; Zhou et al., 2009; Zhou et al., 2011; Mussai et al., 2013). Moreover, checkpoint blockade has demonstrated greater efficacy in tumors with higher mutation burdens (Ansell et al., 2015; Chalmers et al., 2017; Yarchoan et al., 2017). Characterized by a low mutation burden, AML has only 13 genetic mutations on average in newly diagnosed disease (Cancer Genome Atlas Research et al., 2013), which mean that the immune system has a smaller chance to recognize mutated coding sequences giving rise to neoantigens (Gettinger et al., 2017; Haanen, 2017; Yarchoan et al., 2017). Thus, a better insight into the mechanisms through which immune evasion occurs in AML is fundamental to help evaluate different immunologic strategies for this disease based on study data. In CAR-T therapy, genetically modified immune cells possess new tumor-targeting specificity and efficacy. While the restricted CD19 and CD20 expression profile of ALL patients facilitates successful targeting of these B cell-associated antigens, the heterogeneous tumor antigen expression in a wide array of AML makes it more difficult to select an suitable target antigen (Taussig et al., 2005; Levine et al., 2015). Leukemogenesis has been understood better in recent years (Schwartzman and Tanay, 2015; Greaves, 2016; Ferrando and Lopez-Otin, 2017), and specifically, newly discovered molecular markers have yielded new insight into the pathogenesis of AML. An increasing number of potential markers have been investigated for every immunotherapeutic strategy (Perna et al., 2017; Yang and Wang, 2018). Nevertheless, further clinical research is necessary to ascertain the clinical efficacy.
In the present review, recent advances with regard to immunotherapies for AML will be discussed, various challenges in the field will be looked at, and emerging strategies that may optimize treatment efficacy will be presented.
Checkpoint inhibitor therapy
Targeting immune checkpoints such as CTLA4, PD1 and PD-L1 has produced impressive results across a diverse variety of malignancies including AML through immunoinhibitory signal blockage and CD8+ T-cell activation to initiate a strong antitumor response (Figure 1A).
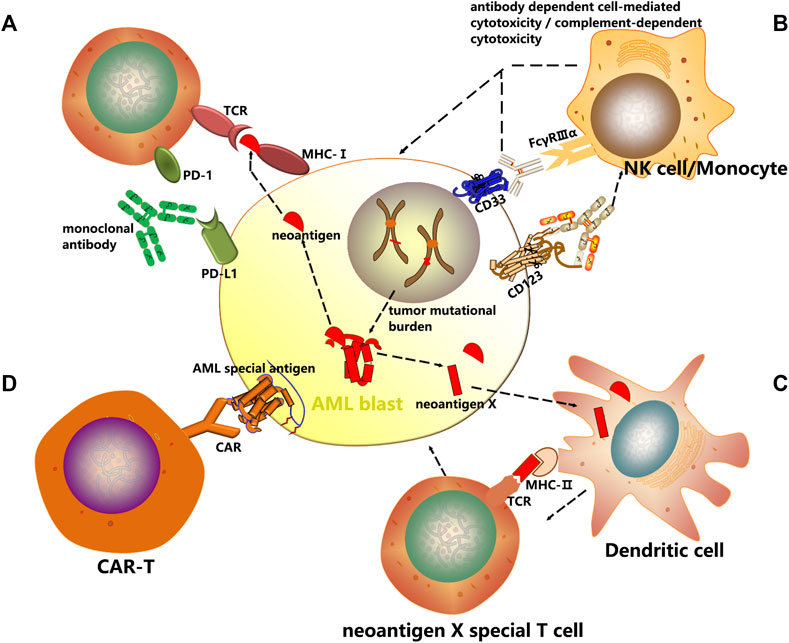
FIGURE 1. Mechanisms of cancer immunotherapy. In this review, several immunotherapeutic strategies have been discussed with an emphasis on AML treatment. (A) Checkpoint inhibitors are a new class of immunotherapy that use monoclonal antibodies to modulate inhibitory receptors on T cells to enhance T cell-mediated immune responses. (B) The antibody dependent cell mediated cytotoxicity (ADCC) and complement dependent cytotoxicity (CDC) induced by AML surface antigen-directed antibodies, as well as the effect of an internalized antibody-toxin conjugate result in AML cell death. (C) Dendritic cells are unique antigen-presenting cells (APC) and comprise the main class of APC. Autologous CD14+ monocytes derived from peripheral blood of cancer patients are differentiated with GM-CSF and IL-4/IL-13 into immature DCs in vitro. Thereafter immature DCs are supplied with tumor antigens, that is, whole cell lysates, tumor-associated antigens (TAAs) or neoantigens. These antigen-loaded DCs are further matured and then enhance vaccine-induced immune responses. (D) Chimeric antigen receptors (CARs) are genetically modified receptor proteins with both extracellular antigen binding domain and intracellular signaling domain. With their unique structure, particularly effective cytotoxic activity, as well as antigen binding in an MHC-independent fashion is possible.
CTLA-4
CTLA-4 is an immune inhibitor mainly found on the surface of T cells. Through engaging the co-stimulatory protein CD28, ligation of CTLA-4 and CD80/CD86 occurs. CTLA-4 out competes CD28 and exhibits greater affinity in binding CD80 and CD86, which results in inhibiting T cells (Friedman et al., 2016; Byun et al., 2017; Nishino et al., 2017). The higher expression of CTLA-4 on T cells was detected in peripheral blood from patients with newly diagnosed AML (Chen et al., 2020a). As the first immunosuppressive molecule identified, CTLA-4 has provided an optional treatment approach in addition to conventional chemotherapy. Ipilimumab and tremelimumab as CTLA-4 inhibitors were used to treat patients with different cancers in clinical trials and led to prolonged overall survival (Author Anonymous, 2010; Hodi et al., 2010; Kyriakidis et al., 2021), but with limited off-label use in AML. In several clinical studies, favorable anti-leukemic effect, anti-tumor reactivity for relapsed patients and durable responses were reported (Fevery et al., 2007; Davids et al., 2016). Thus, it is suggested that hematological malignancies be treated with the use of CTLA-4 inhibitor. Ipilimumab produced specific potency in treating relapsed AML patients after HSCT (NCT01822509) (Liao et al., 2019; Vago and Gojo, 2020; Penter et al., 2021) and holds promise in affecting local control for the treatment of AML-related extramedullary (Bakst et al., 2020). However, patients with AML have less response to ipilimumab. Moreover, the rational combinations has potential to improve the efficacy of drugs and ipilimumab plus nivolumab have been approved for the management of metastatic melanoma and several other malignancies (Rotte, 2019). Notwithstanding, the two drugs, when used in combination, showed no improving effect on AML (Greiner et al., 2020) and was more likely to cause infectious complications (Spallone et al., 2022). Non-etheless, clinical investigation is ongoing to determine the potent effects of CTLA-4 inhibitor for AML patients (Masarova et al., 2017).
PD-1/PD-L1
As a potent immune checkpoint protein, PD-1 receptor acts like a “brake” on the immune response (Rizvi et al., 2015; Wolchok, 2015; Im et al., 2016; Gordon et al., 2017; Kamphorst et al., 2017; Kelly, 2017; Eroglu et al., 2018). Blocking this checkpoint by PD-1 inhibitors has resulted in impressive clinical response rates in melanoma (Roboz et al., 2014) and non-small cell lung cancer (Giroux Leprieur et al., 2017), as well as in Hodgkin (Le et al., 2015; Armand et al., 2018) and non-Hodgkin lymphomas (Lesokhin et al., 2016).
AML is characterized by upregulated PD-1 levels expressed on T cells, which is closely related to the treatment and prognosis of the disease (Huang et al., 2019; Tan et al., 2020; Tang et al., 2020). Patients newly diagnosed with AML or those undergoing relapse reported elevated PD-1 levels in peripheral blood (PB) and bone marrow (BM) T cells following front-line treatment, and by contrast, patients in durable remission reported reduced expression levels (Xu et al., 2021a; Brauneck et al., 2021), and TCRβ sequencing revealed clonal expansion in PD-1 positive CD8+ T cells (Feng et al., 2020). The effector function in the BM milieu was reactivated through PD-1, CTLA-4, or TIM3 immune checkpoint pathways (Lamble et al., 2020). PD-L1/PD-L2 are found on the surface of leukemic cells, as demonstrated in several studies (Chen et al., 2008; Berthon et al., 2010). Driver mutations could regulate the expression of inhibitory proteins, for example, PD-L1/PD-L2 overexpression shown in AML cells with NRAS and ASXL1 mutations (You et al., 2022). Similarly, upregulation of PD-L1 was noted in patient cohorts with myelodysplastic syndromes (MDS) harboring TP53 mutations and secondary AML (sAML) (Sallman et al., 2020). Moreover, MDS or AML patients treated with hypomethylating agents (HMA) have also shown elevated PD-1 and PD-L1 expression in peripheral blood mononuclear cells (Yang et al., 2014). The mechanism of immune escape induced by PD-L1 may directly drive Treg cell expansion (Dong et al., 2020). PD-L1 silencing led to significant IFN-γ secretion and proliferation of MiHA-specific CD8+T cells, which was equally effective as PD-1 antibody blockade (van Ens et al., 2020).
Now six PD-1/L1 inhibitors were approved for treatment of lung cancer, melanoma, breast cancer or lymphoma including nivolumab (Opdivo®), pembrolizumab (Keytruda®), atezolizumab (Tecentrip®), avelumab (Bavencio®), durvalumab (Imfinzi®), and cemiplimab (Libtayo®). Immune checkpoint inhibitors (ICIs) confer advantages for solid tumors that are yet to be attained for AML (Chen et al., 2020b). However, the successful therapeutic application of ICIs therapy in solid tumors has inspired its use in hematological malignancies, and the favorable clinical responses achieved in Hodgkin lymphoma are promising. Currently, PD-1 therapy is only applied for patients with relapsed/refractory AML in clinical trials. An increasing number of investigational studies are in progress to assess the efficacy of the anti-PD-1 inhibitor nivolumab. The relapsed AML patients after allo-HSCT showed good response when treated with nivolumab but paid attention to the clinical signs of graft versus-host disease (GVHD) (Albring et al., 2017; Yao et al., 2021). Another study found that HSCT with prior use of nivolumab and/or ipilimumab appears feasible in patients with AML and cyclophosphamide-based GVHD prevention is associated with improved prognosis (Oran et al., 2020).
As we mentioned earlier, combined strategies produced clinical benefits for the treatment of AML. Daver et al. reported promising preliminary results in a study combining azacitidine and nivolumab for adult cohort with relapsed and refractory AML (n = 51) (Naval Daver et al., 2016; Daver et al., 2018). Another phase II study also demonstrated the safety and efficacy of azacitidine plus nivolumab in patients with AML in first salvage status, with HMA naïvety, or with increased CD3+ BM infiltration as assessed by flow cytometry or IHC (Daver et al., 2019). The combination of the two drugs had an acceptable tolerability and produced a favorable response rate in relapsed AML patients, which is associated with the potential mechanism of adaptive T-cell plasticity and genomic alterations (Abbas et al., 2021). In addition, another clinical trial (NCT02397720) is recruiting patients to determine the therapeutic effect of azacitidine and nivolumab with ipilimumab or without ipilimumab in AML patients who were not responsive or relapsed and newly diagnosed elderly patients (>65 years). In a phase II trial (NCT02464657), the use of three drugs (nivolumab, idarubicin and cytarabine) in combination has been proved efficacious in subjects who were newly diagnosed with AML or at high risk of MDS (Ravandi et al., 2019). Moreover, several clinical studies reported the combined effect of azacitidine and other inhibitors on refractory AML, such as pembrolizumab (Zeidner et al., 2021) and avelumab (Saxena et al., 2021) with encouraging clinical activity. The therapy of pembrolizumab combined with decitabine was clinically feasible in patients who were not responding well or relapsed (NCT02996474) (Goswami et al., 2022). Given the small cohort likely to benefit from ICI therapy, further investigation is necessary to explore the mechanism of action. However, not all combinations can improve rates of durable responses for AML patients. The use of avelumab in combination with decitabine in a phase I clinical trial achieved no clinical benefit in AML patients (Zheng et al., 2021), as well as the combination of ipilimumab and nivolumab (Greiner et al., 2020). Nevertheless, several additional trials are ongoing across the globe to assess the clinical efficacy of ICIs combined with induction chemotherapy or hypomethylating agents for immunotherapy of AML (Table 1).
In addition to CTLA-4 and PD-1/PD-L1, numbers of clinical trials of TIM-3 inhibitors including sabatolimab and TQB2618 for AML are recruiting patients (NCT05426798, NCT03940352, NCT04623216, NCT05367401, NCT03066648). Similarly, TIM-3 inhibitors combined with demethylation drugs (azacitidine or decitabine) is used as the treatment strategy for AML, and one of the clinical trial adopts the combination of spartalizumab (PD-1 inhibitor) and sabatolimab (NCT03066648), which indicates that TIM3 inhibitors will occupy the market in the field of AML in the future.
Monoclonal antibodies therapy
Recent studies have identified numerous candidate AML-associated antigens that can be targeted, including CD33 and CD123. By selectively targeting AML specific antigens, monoclonal antibodies have emerged as effective therapeutic agents that can reduce morbidity and mortality. During treatment of AML, monoclonal antibodies can exert their therapeutic effects through selective drug delivery, cell-mediated and complement-mediated mechanisms, and innate immune enhancement. Besides, antibody-drug conjugation combining chemotherapeutic agents or radioactive particles with monoclonal antibodies is also being evaluated to specifically target cells expressing CD33 or CD123. Despite that the immune system is not activated, they can induce cell injuries which may initiate the innate immune system and activate immune defense (Figure 1B).
Anti-CD33
CD33 is a Siglec receptor protein primarily expressed on leukemic blasts. CD33 expression alone cannot be used as an independent prognostic marker, but low expression of CD33 is often observed in groups with complex karyotypes and translocations, and FLT3-ITD or NPM1 mutations are more prevalent in groups with higher CD33 expression level (Feldman et al., 2005; Pollard et al., 2012; Krupka et al., 2014; Khan et al., 2017). In addition, the endocytosed CD33 makes it a promising target in antibody drug conjugates (ADCs) therapy (Krupka et al., 2014; Laszlo et al., 2016).
The first approved ADC by the FDA is gemtuzumab ozogamycin (GO/Mylotarg) targeting CD33 in 2000 to treat AML (Bross et al., 2001) and was subsequently removed from market due to increased risk of hepatic veno-occlusive disease (VOD) and high mortality (Petersdorf et al., 2013). However, cytotoxic therapy utilizing CD33 has attracted enormous attention because of the remarkable responses in varying patient cohorts receiving GO. New data from a fractionated-dosing schedule for treating newly diagnosed and relapsed/refractory AML led to re-approval of GO (Jen et al., 2018). This was supported by the results that demonstrating decreased number of early deaths, bleeding, low platelet count and VOD while sustaining treatment efficacy (Baron and Wang, 2018). In the phase III ALFA-0701 trial, fractionated doses of GO in combination with daunorubicin and cytarabine (DA) chemotherapy improved event-free survival (EFS) compared to chemotherapy alone (17.3 vs 9.5 months). Moreover, a complete remission (26%) was achieved with GO in relapsed/refractory AML (Lambert et al., 2019). The use of azacitidine and GO in combination in a phase I/II trial for relapsed AML showed good tolerability and achieved a CR of 24% in 12/50 patients (Medeiros et al., 2018). In addition, the renewed knowledge and optimized management regarding VOD risk factors as well as advances in prophylaxis and treatment have promoted the application of GO (Cortes et al., 2020).
ADC linked with pyrrolobenzodiazepine (PBD) dimer is a new strategy for treatment of various cancers (Hartley, 2021). SGN-CD33A is a pyrrolobenzodiazepine (PBD) dimer-based antibody-drug conjugate targeted to CD33. Pre-clinical studies showed that SGN-CD33A was superior to GO in multi-drug resistant AML cell lines as well as in AML patients with poor risk cytogenetics (Kung Sutherland et al., 2013). In an initial phase I study (NCT01902329) designed to assess the safety of the drug, SGN-CD33A used alone resulted in a CR and CRi rate of less than 30% with minimal dose limiting toxicity (DLT) (Stein et al., 2018). SGN-CD33A in combination with a hypomethylating agent yielded a composite CR and CRi rate of 70% (Fathi et al., 2018). In addition, SGN-CD33A in combination with standard 7 + 3 chemotherapy were being assessed in a phase Ib trial (NCT02326584), achieving an encouraging composite CR and CRi rate of 78% (Erba et al., 2016). In this trial, myelosuppression-related side effects were reported in all patients. None of the patients developed VOD, and the 60-day mortality rate was less than 10%. However, due to high mortality rate in the SGN-CD33A-containing arm, the phase III CASCADE trial was discontinued (By The ASCO Post Staff, 2017).
Lintuzumab is a humanized anti-CD33 antibody with a modest single-agent activity against AML. To improve the efficacy, lintuzumab was conjugated to radioactive elements such actinium-225 and bismuth-213. The radio immune conjugates are undergoing clinical investigation in older patients newly diagnosed with AML for whom standard induction treatment is deemed unfit. Actinium-225-lintuzumab was feasible with myelosuppression and no cases of VOD (Finn LE et al., 2017; Rosenblat et al., 2022). Sequential cytarabine and bismuth-213-lintuzumab produced remissions in patients with AML (Rosenblat et al., 2010). Therefore, the conjugate of lintuzumab and actinium-225 or bismuth-213 represents a reasonable treatment option for patients ineligible for intensive chemotherapy.
Anti-CD123
CD123 is a cytokine receptor widely overexpressed in multiple hematologic cancer cells, especially in leukemic stem cells (LSCs). AML cells with FLT3-ITD or NPM1 mutations are correlated with significantly increased levels of CD123 as compared to AML cells with wild-type FLT3 or NPM1 (Ehninger et al., 2014; Cortes et al., 2018; Cruz et al., 2018). Overexpression of CD123 is associated with high-risk disease characteristics in adult and pediatric AML. CD123 as an important biomarker can be potentially targeted in relapsed or refractory AML (Lamble et al., 2022).
CSL360 is a chimeric monoclonal antibody directed against CD123 and is believed to inhibit proliferation of AML cells (Roberts et al., 2008). However, CSL360 was far less efficacious in patients with relapsed/refractory or high risk AML (He et al., 2015). CSL362 (talacotuzumab), another monoclonal antibody directed against CD123, demonstrated promising activity in AML patients (Xie et al., 2017). However, talacotuzumab alone or in combination with other drugs showed limited efficacy but considerable toxicity in AML patients in recent clinical study (Kubasch et al., 2020; Montesinos et al., 2021). Many preclinical trials have studied various CD123 antibodies, such as CD123 antagonistic peptide (Xu et al., 2021b; Xu et al., 2022) and protein-CD123 fusion antibodies (Tahk et al., 2021), which significantly reduced BM infiltration or enhanced the elimination of AML-initiating cells. SL-401 is a cytotoxin that fuses the diphtheria toxin (DT) to the IL3 ligand. It was the first treatment approved by the FDA for the management of blastic plasmacytoid dendritic cell neoplasms (BPDCN) in adult and pediatric patients 2 years and older (Jen et al., 2020), with good tolerability and a predictable and favorable safety profile (Pemmaraju et al., 2022). Clinical trials have shown that treatment with SL-401 was associated with reduced drug resistance and better prognosis in AML patients at high risk for relapse. It is worth noting that a serious adverse reaction induced by SL-401 had caused capillary leak syndrome leading to one death, which was reported among the cohort in remission with MRD (American Society of Hematology, 2016; Lane et al., 2016).
Anti-CD3/CD33 or CD3/CD123 bispecific antibody
Bispecific T-cell-engaging (BiTE) antibodies are composed of dual variable regions that simultaneously bind to CD3 on cytotoxic T lymphocytes and tumor cell antigens on malignant cells, activating the effector function, accompanied by release of cytokines and destruction of tumor cells. By means of this strategy, virtually all T lymphocytes can be recruited and directed against leukemic blasts irrespective of its specificity. The preclinical trial proved that CD3/CD33-directed antibody constructs might be utilized as an alternative treatment in AML (Reusch et al., 2016). AMG 330 has been shown to exhibit strong anti-tumor responses both in vitro and in mouse models (Laszlo et al., 2015; Krupka et al., 2016; Jitschin et al., 2018). A clinical trial (NCT02520427) designed to investigate the safety and tolerability of AMG 330 in patients with relapsed/refractory AML is in progress. CD3−CD123-BiTE antibody is another promising therapy for patients with relapsed/refractory AML (Bonnevaux et al., 2021). Additional early clinical studies are ongoing to investigate CD3−CD123-BiTE antibodies, such as XmAb14045 (NCT02730312), and flotetuzumab (MGD006; NCT02152956), a dual-affinity retargeting (DART) molecule.
Dendritic cell vaccines therapy
Cancer vaccines represent a groundbreaking therapeutic approach that employs immune effector cells to eradicate malignant cells. Upon activation of immune signaling, presentation of tumor associated antigens is increased, breaking the tolerance to tumor, and at the same time, immune regulation against autoimmunity is maintained. Dendritic cell (DC)-based vaccines offer the potential of a non-toxic and effective immunotherapeutic strategy. The four most common tumor types can be targeted include melanoma, prostate cancer, glioblastoma and renal cell carcinoma (Germeau et al., 2005; Melero et al., 2014; Anguille et al., 2015; Polyzoidis et al., 2015; Schreibelt et al., 2016). The primary types of cancer vaccines undergoing clinical trials for AML patients are DC-based vaccines (Figure 1C).
WT1 DC vaccine
AML cells have been shown to express the Wilms’ tumor 1 (WT1) protein and upregulation of this protein is a predictor of poor prognosis in AML and MDS patients (Bergmann et al., 1997; Brayer et al., 2015). The specific antibody targeting WT1 shows a good inhibitory effect on AML, which prompted the conduct of a clinical trial for relapsed/refractory AML (NCT0458012) (Augsberger et al., 2021). In addition, TCR-T cell therapy or CRISPR-Cas9 genome editing tools has a potential strategy for WT1-exprssing AML (Tawara et al., 2017; Chapuis et al., 2019; Ruggiero et al., 2022). WT1 is one principal protein studied for vaccination potential and is currently in the clinical investigation phase. In a phase I/II trial, Tendeloo et al. assessed the DC-WT1 vaccine in 10 AML patients who achieved CR or PR following chemotherapy and were at a high risk of relapse (Van Tendeloo et al., 2010). Subsequently, the clinical results of 66 cancer patients after DC-WT1 vaccination, including thirty AML patients who were at very high risk of relapse, were reported (Table 2, NCT00965224), which proved that WT1-targeted DC-based vaccination is an effective immunotherapeutic strategy to prevent relapse in AML patients (Anguille et al., 2017). In another phase I/II trial (NCT01051063), 5 elderly AML patients observed clinical benefit after receiving WT1 recombinant protein vaccination (Kreutmair, 2022). Moreover, clinical trial found the decrease of the regulatory T cells in 8 AML patients following WT1 peptide-loaded DC vaccination (Ogasawara et al., 2022).
Human telomerase reverse transcriptase (hTERT) DC vaccine
Telomerase is a ribonucleoprotein enzyme that positively expressed in most malignant tumor cells and is critical for increasing proliferative potential of cancer cells (Counter et al., 1995; Zhang et al., 1996; Ohyashiki et al., 1997; Stewart and Weinberg, 2000). Telomerase can be used as an important predictor for AML due to its strong expression in AML patients, particularly in high-risk cytogenetic AML subtypes. A phase II clinical trial (NCT00510133) enrolled a total of 22 patients achieving early or subsequent complete remission at intermediate risk or high risk of AML (Khoury et al., 2017). The relapse free survival achieved in this trial is superior to that reported in previous trials, but further clinical validation of these results is needed. Preclinical models confirmed the improved survival outcome and showed that telomerase was upregulated in AML blasts, and reduced telomerase activity thwarts leukemic cell proliferation and disease relapse after chemotherapy (Bruedigam et al., 2014). The TERT gene variability and telomere length affect risk and overall survival in AML (Dratwa et al., 2021). It was reported that diverse short telomere-related somatic mutations promote transformation to MDS/AML (Schratz et al., 2021). If the same results can be obtained from further clinical trials, hTERT-DCs should soon become a form of safe and effective immunotherapy that can be used to extend relapse-free survival for AML patients in complete remission. The approach would provide promising treatment options that bring new hope to AML patients.
DC-AML fusion vaccine
An individualized cancer vaccine strategy was developed by Jacalyn Rosenblatt et al. in which fusion hybrids of autologous dendritic cells and patient-derived AML cells are used to generate antileukemic responses (Rosenblatt et al., 2016). In this phase I/II clinical trial (NCT01096602), a cohort of 17 subjects were responding well to front-line treatment and subsequently received vaccination to target MRD and prevent relapse. The vaccine regimens induced strong T-cell expansion in both PB and BM. Meanwhile, 12 subjects in the cohort undergoing vaccination achieved prolonged survival when followed up to 5 years. These results show that individualized vaccine regimens for AML patients in remission can enhance leukemia-specific T-cell responses and prevent disease relapse.
Cancer vaccines are used to confer protective immunity and enhance tumor antigen-specific T-cell and antibodies. However, clinical outcomes have shown little promise to date. This is largely due to immune suppression in the process of tumor growth, which affects the vaccines’ capability to induce strong and systemic immune responses and functionality of immune responses at the tumor site. Therefore, new approaches have been developed, supported by findings demonstrating that human dendritic cells (DCs) can be cultured in vitro (Steinman and Banchereau, 2007). The success of DC vaccines generated enormous enthusiasm in DC vaccines employing the latest technology. Major efforts have often directed to the origin of DCs. For example, specific subsets of naturally occurring DCs are superior to monocyte-derived DCs in terms of potency and cost-effectiveness. Results from preliminary trials demonstrated safety and feasibility of next-generation DC vaccines employing naturally occurring DC subsets; furthermore, this approach has resulted in superior efficacy. A phase I trial showed that 12 elderly patients with late-stage AML receiving a DC vaccine generate both cellular and humoral immune response (van de Loosdrecht et al., 2018). A phase I/II trial (NCT01146262) showed DC-vaccines with leukemic apoptotic bodies produced a safety and efficacy for the AML patients (Chevallier et al., 2021). On the other hand, a major impediment with regard to DC vaccination is to pinpoint the right antigen to be vaccinated for maximal T-cell effector function, stimulation of memory T-cells, and reducing the expansion of inhibitory subsets. Normal cancer antigens such as WT1 and hTERT loading to AML patients’ DCs are currently under clinical investigation. Another method to improve the immunogenicity of DC is to combine with hypomethylating agents (Nahas et al., 2019) or immune checkpoint inhibitors (Stroopinsky et al., 2021). Future development of cancer vaccines would rely more on multiple antigens or individualized neoantigens to maximize vaccine-based immune responses.
CAR-T cell therapy
CARs, unlike TCRs which interact with HLA molecules, do not need antigen processing or presentation by the HLA peptidome (June et al., 2018), and therefore broadly recognize target antigens independent of HLA background (Figure 1D).
CD19-specific CAR-T cells represent a well-established CAR-T cell therapy with restrictive expression pattern and favorable safety outcome. The promise of anti-CD19 CAR-T cells was immense in ALL, achieving CR in over 90% of B-ALL patients (Davila et al., 2014; Maude et al., 2014). Considering the intense prior treatment: a median of 3 prior intensive chemo regimens with over 1/3 relapsed following allogeneic HSCT, these clinical results are impressive. Anti-CD19 CD28-based CAR-T therapy induced a CR rate of 57% in seven patients with DLBCL with no response to three prior lines of therapy (Locke et al., 2017). The modified CD19 CAR-T cells using the FasT system displayed favorable responses for B-cell ALL in both preclinical study and a phase I trial (NCT03825718) (Yang et al., 2022). CAR-T cell therapy targeting CD19 induces high response rates in B-ALL with central nervous system leukemia (NCT02782351) (Qi et al., 2022). In the context of AML, however, the putative antigens targeted by CAR-T cells must have a restrictive expression pattern to avert destruction of normal myeloid cells. Some targets have been identified in preclinical trials, including natural killer group 2 member D (NKG2D), CD123, CD33, Lewis Y (LeY), CLL-1, etc.
In a phase I study, four patients infused with anti-LeY CAR-T cells were examined to determine the safety and clinical efficacy of adoptive transfer of T cells. The CAR T cells sustained efficacy for up to 10 months post infusion, confirming the feasibility and safety of anti-LeY CAR-T cells in patients with high-risk AML, and demonstrating long-term efficacy (Ritchie et al., 2013). CLL-1 is strongly expressed on AML cells and not detectable on normal HSCs, making it an ideal target in immunotherapy for AML. In a phase I trial that included 10 adult participants with relapsed or refractory AML, chemotherapy coupled with CLL-1 CAR-T cell therapy achieved a CR rate of 70% when followed up to a median period of 173 days, proving that CLL-1 CAR-T cell therapy can mitigate the risk of relapse and improve patient survival (Jin et al., 2022). CLL-1 CAR-T cells with PD-1 knockdown show a successful therapy in patients and represent a feasible immunotherapeutic alternative for treating relapsed or refractory AML (Lin et al., 2021; Ma et al., 2022). Recently, CAR-T cell treatments targeting new potential protein markers (e.g., Siglec-6 and CD70) have been reported. Siglec-6 and CD70 are found on the surface of most AML cells, but are rare or absent in normal BM. Experiments on Siglec-6 and CD70 CAR-T cells in both human and animal models show anti-AML activity and ability to expand and persist (Jetani et al., 2021; Sauer et al., 2021). Based on the CD70 target immunotherapy, the modified CD70-targeted CAR by CD8 non-cleavable hinge method enhance binding capability and expansion, resulting in higher antitumor potency (Leick et al., 2022). Despite the growing number of early-phase studies showing potent antitumor responses of multiple CAR-T cells in recent years, translation into clinical practice and benefits has been slow and challenging. In several early phase clinical trials (Table 3), some adults have received AML CAR-T cell immunotherapy. All NKG2D, CD123, and CD33 have been studied extensively, which are described below.
NKG2D CAR-T
NKG2D ligands are selectively expressed on monoblastic cells in AML, but absent or weakly expressed on myeloblastic cells and chemotherapy-resistant leukemic stem cells in AML, which avoid NK-mediated killing and cause immune evasion (Diermayr et al., 2008; Paczulla et al., 2019). Therefore, inhibitor-mediated activation of surface NKG2D ligands is a useful approach to immunotherapy of AML. Based on this, bispecific FLT3 antibody system (scFv)/NKG2D-CAR T cells have been designed, effectively eradicating AML cells in preclinical study (Li et al., 2022). NKG2D CAR and IL-15 constitutively expressed enhance the efficacy and significantly prolong the mouse survival in the KG-1 AML model.
The safety and lymphodepleting conditioning chemotherapy in participants with AML/MDS or relapsed/refractory multiple myeloma were assessed in a phase I trial (NCT02203825) with dose escalation design (Baumeister et al., 2019). A NKG2D CAR was constructed by human NKG2D linked to CD3ζ signaling domain. Following the treatment, no dose-limiting toxicities, cytokine release syndrome, or neurological toxicities associated with CAR T cells were reported. No autoimmune disorders or serious adverse reactions were found to be associated with NKG2D-based CAR-T cells. NKG2D CAR-T cells were not expanded or persisted in the majority of patients, which is in agreement with murine models in which repeat infusions are needed to eliminate the tumor completely (Barber et al., 2011). As a highly conserved receptor, NKG2D is less likely to elicit immune responses. Additional CAR trials (NCT03018405) assessing multiple infusions and higher doses are underway to further establish the safety profile of NKG2D CAR therapy (Sallman et al., 2018).
CD123 CAR-T
CD123 is a poor prognostic antigen marker found on AML cells and chemo-resistant leukemic stem cells. Anti-CD123 CAR-T therapy was able to eradicate AML blasts in experiments (Arcangeli et al., 2017). Gene-edited CAR-T cells targeting CD123 are primarily directed against AML cells, with tolerable toxicity to normal cells (Sugita et al., 2022).
An early phase I pilot study sponsored by the University of Pennsylvania (NCT02623582) sought to evaluate autologous T lymphocytes containing anti-CD123 linked to TCR/4-1BB domains in AML patients (Tasian, 2018). Five adult subjects with relapsed/refractory AML were given lymphodepleting chemotherapy prior to the CD123 CAR-T cells infusion. However, this trial was eventually terminated because the regimen had induced little anti-leukemic efficacy and on target/off tumor toxicologic effects had been reported. Another phase I trial also reported preliminary results using lentiviral infected T cells to express a CAR targeting CD123 (NCT02159495). CD123-based CAR-T cell treatment induced complete remission in some participants, who became eligible for a second HSCT. Moreover, other drugs such as demethylating agents can augment immune responses and facilitate clearance of tumor cells by CD123-targeting CAR-T cells (El Khawanky et al., 2021). New CD123-directed CAR-T cells were engineered by the rapidly switchable universal CAR-T platform and retain complete anti-AML potency as well as ensuring improved safety of CD123-based immunotherapy in different applications. Currently, a dose-escalating clinical trial is ongoing to assess the therapeutic benefit of this new study drug (NCT04230265) (Loff et al., 2020). Other clinical trials for CD123 CAR-T cells are ongoing including NCT03766126, NCT03114670, NCT03190278, and NCT03631576.
CD33 CAR-T
The CD33 antigen exhibits high expression levels in AML blasts; however, normal myeloid progenitors also express CD33 antigen, limiting its potential as an immunotherapeutic target for AML (Ehninger et al., 2014). An in vivo NSG mice model experimenting with AML xenotransplantation has reported significant reduction of leukemic burden and prolonged survival (O'Hear et al., 2015).
Worldwide, several other trials of anti-CD33 CAR T cells for the treatment of AML are underway. A safety test of varying doses of CD33-CAR-T cells in patients with CD33+ AML was conducted by the MD Anderson Cancer Center (MDACC) (NCT03126864). The results found that CAR-T cell production was only possible in cohorts with increased lymphocyte levels and decreased blood cells, but along with systemic inflammatory syndrome and neurotoxic effects (Tambaro et al., 2021). Chinese PLA General Hospital is conducting an early phase I trial (NCT01864902) and will assess the safety and feasibility of CD33-engineered lymphocyte therapy in patients with AML that is relapsed or not responding to chemotherapy. After initial treatment, one participant with relapsed AML who had previously received an infusion of CD33 CAR T cells had a noticeable decrease in blast count in BM; however these AML blasts subsequently recovered, and relapse occurred 2 months post infusion (Wang et al., 2015). A preclinical experiment evaluated six CD33 CAR constructs using scFv containing CD3ζ domain and showed antileukemia activity, which results in a clinical trial for relapsed/refractory childhood AML (NCT03971799) (Qin et al., 2021). Additional clinical trials with CD33-CAR-T cell strategies are underway (NCT03927261).
To achieve optimal therapeutic effect, the preferred marker in CAR-T treatment should be present in the majority of cancer cells including the subpopulation of cancer stem cells in a substantial percentage of patients. Moreover, to avoid undesired toxicity, the candidate target should not be present on normal tissues or on CAR T cells to avert unwanted self-elimination of CAR T cells. Without a favorable target such as CD19, it is of critical importance to develop a generalizable and combinatorial targeting approach that allows identification of several promising target pairings in AML CAR-T therapy. It was found that AML cells were associated with overexpression of CD33/TIM3 and CLL1/TIM3 as compared to normal tissues when analyzing the density of CD33, CD123, TIM3, among other antigens, on AML bulk and LSCs at initial diagnosis and relapse (Haubner et al., 2019). Like bispecific antibody, CD33/TIM3 or CLL1/TIM3 may serve as viable targets to improve treatment efficiency while limiting toxicity during CAR-T therapy in AML patients. Meanwhile, CD33/CLL-1 is a preferential generic combinatorial immunotarget in pediatric AML (Willier et al., 2021). Moreover, the combination of CAR-T cells and other drugs such as targeted/chemotherapy agents has synergistic effect on AML (El Khawanky et al., 2021; Li et al., 2022). Also, the feasibility of constructing an antigen specific to AML can be considered by removing CD33 from normal cells to overcome resistance to CD33-directed therapy and realize on-target effects with CAR-T cells (Kim et al., 2018).
TCR-T cell therapy
Similar to CAR-T cells, TCR-T cells is belonged to adoptive cell therapy with antigen-specific T cells, and was successfully adopted and transferred in mice in 1986. TCR-T cell therapy targeting New York esophageal squamous cell carcinoma (NY-ESO)-1 has achieved remarkable clinical benefits in the treatment of solid tumors (D'Angelo et al., 2018; Robbins et al., 2011). TCR-T cell specific for MAGE-A4, HA-1H, WT1 are potential strategies for hematological malignancies (Kageyama et al., 2015; Tawara et al., 2017; van Balen et al., 2020; Vasileiou et al., 2021).
In several clinical trials, WT1-specific TCR-T cells has shown strong clinical responses with tolerated safety for AML patients (Tawara, I. et al. S, 2017). Moreover, numerous clinical trials are underway to evaluate other antigen-specific TCR-T cells in AML. Recently, Kang et al. reviewed the update and challenges of TCR-T for AML. TCR-T exhibits a promising tool for AML patients according to the benefit efficacy of clinical trials. However, the toxicity produced in the treatment process and the persistence of in vivo TCR-T cells should be the urgent problems of TCR-T immunotherapy.
Conclusion
In this review, we summarized the recent advances for AML immunotherapies and noted the representative emerging strategies. The immunotherapy as a treatment option for AML patients with relapse has shown great promise. However, several limitations to the AML immunotherapy are limited its application in AML. Immune evasion remains a clinical challenge in effectively implementing immunotherapeutic strategies. AML is a complex disorder associated with distinctive genetic features and accumulation of genetic aberrations. The lower level of mutation load in AML would lead to higher chance of immune escape of the immune system and less neoantigen epitopes when compared to melanoma and other types of solid cancers such as lung cancer. Considering reduced mutation load, a core issue around AML requiring further exploration is the extent of immunogenicity. Therefore, clinical success of immune therapy in AML will depend on at least the following efforts: exploiting immune escape mechanism and identifying specific targets.
AML has a distinct pattern of development and progression, and there is a paucity of data on the mechanisms of immune activation or tolerance. The mechanisms underlying antitumor immune responses are well understood and the strategies of immune evasion employed by solid tumors have been studied extensively. Accumulating evidence suggests that certain immune evasion mechanisms are exclusive to AML despite the fact that PD1/L1, among other immune escape pathways, are shared with solid malignancies. Although currently encountering various obstacles, the progress in exploring the mechanisms by which immune surveillance and evasion take place in AML can be attained via genetically modified AML models reconstructing the disease. Moreover, integrated data on antigens coupled with analytical platforms to screen out truly AML-specific antigen markers are required. Up till now, transcriptome analyses are mainly used for screening candidate AML targets assuming a direct link between mRNA abundance and protein expression. This complex correlation cannot represent the complete set of proteins expressed by the cell, due to numerous factors such as variations in drug metabolism and mechanisms of post-transcriptional regulation. Therefore, integrative analysis of RNA transcripts and proteins should be performed to complement mRNA or protein expression analysis. In addition, coupling advanced proteomics analytical approaches with plasma membrane proteome enrichment will facilitate direct assessment of surface proteins. In summary, because of the heterogeneous nature of AML, each patient may present distinct cytogenetic aberrations and chromosomal rearrangements that require biomarker-specific individualized treatment. Therefore, tailored treatments combining different forms of immunotherapy with chemotherapy and autologous or allogeneic SCT hold immense promise.
Author contributions
YC wrote the manuscript. JW designed the structure of the paper. FZ drawed the figure and tables. PL proofread the paper. All authors read and approved the final manuscript.
Funding
This work was supported by the National Natural Science Foundation of China (No. 81960032 and 82170168). Science and Technology Program of Guizhou Province Health Committee (No. gzwkj 2019-2-11 and gzwkj 2021-158). Translational Research Grant of NCRCH (2021WWB01).
Conflict of interest
The authors declare that the research was conducted in the absence of any commercial or financial relationships that could be construed as a potential conflict of interest.
Publisher’s note
All claims expressed in this article are solely those of the authors and do not necessarily represent those of their affiliated organizations, or those of the publisher, the editors and the reviewers. Any product that may be evaluated in this article, or claim that may be made by its manufacturer, is not guaranteed or endorsed by the publisher.
References
Abbas, H. A., Hao, D., Tomczak, K., Barrodia, P., Im, J. S., Reville, P. K., et al. (2021). Single cell T cell landscape and T cell receptor repertoire profiling of AML in context of PD-1 blockade therapy. Nat. Commun. 12, 6071. doi:10.1038/s41467-021-26282-z
Albring, J. C., Inselmann, S., Sauer, T., Schliemann, C., Altvater, B., Kailayangiri, S., et al. (2017). PD-1 checkpoint blockade in patients with relapsed AML after allogeneic stem cell transplantation. Bone Marrow Transplant. 52, 317–320. doi:10.1038/bmt.2016.274
American Society of Hematology (2016). Third patient death reported in SL-401 drug trial for patients with BPDCN and AML. San Diego: ASH Clinical News.
Anguille, S., Smits, E. L., Bryant, C., Van Acker, H. H., Goossens, H., Lion, E., et al. (2015). Dendritic cells as pharmacological tools for cancer immunotherapy. Pharmacol. Rev. 67, 731–753. doi:10.1124/pr.114.009456
Anguille, S., Van de Velde, A. L., Smits, E. L., Van Tendeloo, V. F., Juliusson, G., Cools, N., et al. (2017). Dendritic cell vaccination as postremission treatment to prevent or delay relapse in acute myeloid leukemia. Blood 130, 1713–1721. doi:10.1182/blood-2017-04-780155
Ansell, S. M., Lesokhin, A. M., Borrello, I., Halwani, A., Scott, E. C., Gutierrez, M., et al. (2015). PD-1 blockade with nivolumab in relapsed or refractory Hodgkin's lymphoma. N. Engl. J. Med. 372, 311–319. doi:10.1056/nejmoa1411087
Arcangeli, S., Rotiroti, M. C., Bardelli, M., Simonelli, L., Magnani, C. F., Biondi, A., et al. (2017). Balance of anti-cd123 chimeric antigen receptor binding affinity and density for the targeting of acute myeloid leukemia. Mol. Ther. 25, 1933–1945. doi:10.1016/j.ymthe.2017.04.017
Armand, P., Engert, A., Younes, A., Fanale, M., Santoro, A., Zinzani, P. L., et al. (2018). Nivolumab for relapsed/refractory classic Hodgkin lymphoma after failure of autologous hematopoietic cell transplantation: Extended follow-up of the multicohort single-arm phase II CheckMate 205 trial. J. Clin. Oncol. 36, 1428–1439. doi:10.1200/JCO.2017.76.0793
Augsberger, C., Hänel, G., Xu, W., Pulko, V., Hanisch, L. J., Augustin, A., et al. (2021). Targeting intracellular WT1 in AML with a novel RMF-peptide-MHC-specific T-cell bispecific antibody. Blood 138, 2655–2669. doi:10.1182/blood.2020010477
Bakst, R., Powers, A., and Yahalom, J. (2020). Diagnostic and therapeutic considerations for extramedullary leukemia. Curr. Oncol. Rep. 22, 75. doi:10.1007/s11912-020-00919-6
Barber, A., Meehan, K. R., and Sentman, C. L. (2011). Treatment of multiple myeloma with adoptively transferred chimeric NKG2D receptor-expressing T cells. Gene Ther. 18, 509–516. doi:10.1038/gt.2010.174
Baron, J., and Wang, E. S. (2018). Gemtuzumab ozogamicin for the treatment of acute myeloid leukemia. Expert Rev. Clin. Pharmacol. 11, 549–559. doi:10.1080/17512433.2018.1478725
Baumeister, S. H., Murad, J., Werner, L., Daley, H., Trebeden-Negre, H., Gicobi, J. K., et al. (2019). Phase I trial of autologous CAR T cells targeting NKG2D ligands in patients with AML/MDS and multiple myeloma. Cancer Immunol. Res. 7, 100–112. doi:10.1158/2326-6066.cir-18-0307
Bergmann, L., Miething, C., Maurer, U., Brieger, J., Karakas, T., Weidmann, E., et al. (1997). High levels of Wilms' tumor gene (wt1) mRNA in acute myeloid leukemias are associated with a worse long-term outcome. Blood 90, 1217–1225. doi:10.1182/blood.v90.3.1217.1217_1217_1225
Berthon, C., Driss, V., Liu, J., Kuranda, K., Leleu, X., Jouy, N., et al. (2010). In acute myeloid leukemia, B7-H1 (PD-L1) protection of blasts from cytotoxic T cells is induced by TLR ligands and interferon-gamma and can be reversed using MEK inhibitors. Cancer Immunol. Immunother. 59, 1839–1849. doi:10.1007/s00262-010-0909-y
Bonnevaux, H., Guerif, S., Albrecht, J., Jouannot, E., De Gallier, T., Beil, C., et al. (2021). Pre-clinical development of a novel CD3-CD123 bispecific T-cell engager using cross-over dual-variable domain (CODV) format for acute myeloid leukemia (AML) treatment. Oncoimmunology 10, 1945803. doi:10.1080/2162402X.2021.1945803
Brauneck, F., Haag, F., Woost, R., Wildner, N., Tolosa, E., Rissiek, A., et al. (2021). Increased frequency of TIGIT(+)CD73-CD8(+) T cells with a TOX(+) TCF-1low profile in patients with newly diagnosed and relapsed AML. Oncoimmunology 10, 1930391. doi:10.1080/2162402X.2021.1930391
Brayer, J., Lancet, J. E., Powers, J., List, A., Balducci, L., Komrokji, R., et al. (2015). WT1 vaccination in AML and MDS: A pilot trial with synthetic analog peptides. Am. J. Hematol. 90, 602–607. doi:10.1002/ajh.24014
Bross, P. F., Beitz, J., Chen, G., Chen, X. H., Duffy, E., Kieffer, L., et al. (2001). Approval summary: Gemtuzumab ozogamicin in relapsed acute myeloid leukemia. Clin. Cancer Res. 7, 1490–1496.
Bruedigam, C., Bagger, F. O., Heidel, F. H., Paine Kuhn, C., Guignes, S., Song, A., et al. (2014). Telomerase inhibition effectively targets mouse and human AML stem cells and delays relapse following chemotherapy. Cell stem Cell 15, 775–790. doi:10.1016/j.stem.2014.11.010
By The ASCO Post Staff (2017). Phase III CASCADE trial of vadastuximab talirine in front-line AML discontinued. Chicago: ASCO Post.
Byun, D. J., Wolchok, J. D., Rosenberg, L. M., and Girotra, M. (2017). Cancer immunotherapy - immune checkpoint blockade and associated endocrinopathies. Nat. Rev. Endocrinol. 13, 195–207. doi:10.1038/nrendo.2016.205
Cancer Genome Atlas Research, N., Ley, T. J., Miller, C., Ding, L., Raphael, B. J., Mungall, A. J., et al. (2013). Genomic and epigenomic landscapes of adult de novo acute myeloid leukemia. N. Engl. J. Med. 368, 2059–2074. doi:10.1056/NEJMoa1301689
Chalmers, Z. R., Connelly, C. F., Fabrizio, D., Gay, L., Ali, S. M., Ennis, R., et al. (2017). Analysis of 100,000 human cancer genomes reveals the landscape of tumor mutational burden. Genome Med. 9, 34. doi:10.1186/s13073-017-0424-2
Chapuis, A. G., Egan, D. N., Bar, M., Schmitt, T. M., McAfee, M. S., Paulson, K. G., et al. (2019). T cell receptor gene therapy targeting WT1 prevents acute myeloid leukemia relapse post-transplant. Nat. Med. 25, 1064–1072. doi:10.1038/s41591-019-0472-9
Chen, C., Liang, C., Wang, S., Chio, C. L., Zhang, Y., Zeng, C., et al. (2020). Expression patterns of immune checkpoints in acute myeloid leukemia. J. Hematol. Oncol. 13, 28. doi:10.1186/s13045-020-00853-x
Chen, X., Liu, S., Wang, L., Zhang, W., Ji, Y., and Ma, X. (2008). Clinical significance of B7-H1 (PD-L1) expression in human acute leukemia. Cancer Biol. Ther. 7, 622–627. doi:10.4161/cbt.7.5.5689
Chen, Y., Tan, J., Huang, S., Huang, X., Huang, J., Chen, J., et al. (2020). Higher frequency of the CTLA-4(+) LAG-3(+) T-cell subset in patients with newly diagnosed acute myeloid leukemia. Asia-Pacific J. Clin. Oncol. 16, e12–e18. doi:10.1111/ajco.13236
Chevallier, P., Saiagh, S., Dehame, V., Guillaume, T., Peterlin, P., Bercegeay, S., et al. (2021). A phase I/II feasibility vaccine study by autologous leukemic apoptotic corpse-pulsed dendritic cells for elderly AML patients. Hum. Vaccines Immunother. 17, 3511–3514. doi:10.1080/21645515.2021.1943991
Coombs, C. C., Tallman, M. S., and Levine, R. L. (2016). Molecular therapy for acute myeloid leukaemia. Nat. Rev. Clin. Oncol. 13, 305–318. doi:10.1038/nrclinonc.2015.210
Cortes, J. E., Tallman, M. S., Schiller, G. J., Trone, D., Gammon, G., Goldberg, S. L., et al. (2018). Phase 2b study of 2 dosing regimens of quizartinib monotherapy in FLT3-ITD-mutated, relapsed or refractory AML. Blood 132, 598–607. doi:10.1182/blood-2018-01-821629
Cortes, J. E., de Lima, M., Dombret, H., Estey, E. H., Giralt, S. A., Montesinos, P., et al. (2020). Prevention, recognition, and management of adverse events associated with gemtuzumab ozogamicin use in acute myeloid leukemia. J. Hematol. Oncol. 13, 137. doi:10.1186/s13045-020-00975-2
Counter, C. M., Gupta, J., Harley, C. B., Leber, B., and Bacchetti, S. (1995). Telomerase activity in normal leukocytes and in hematologic malignancies. Blood 85, 2315–2320. doi:10.1182/blood.v85.9.2315.bloodjournal8592315
Cruz, N. M., Sugita, M., Ewing-Crystal, N., Lam, L., Galetto, R., Gouble, A., et al. (2018). Selection and characterization of antibody clones are critical for accurate flow cytometry-based monitoring of CD123 in acute myeloid leukemia. Leukemia lymphoma 59, 978–982. doi:10.1080/10428194.2017.1361023
D'Angelo, S. P., Melchiori, L., Merchant, M. S., Bernstein, D., Glod, J., Kaplan, R., et al. (2018). Antitumor activity associated with prolonged persistence of adoptively transferred NY-ESO-1 (c259)T cells in synovial sarcoma. Cancer Discov. 8, 944–957. doi:10.1158/2159-8290.CD-17-1417
Daver, N., Garcia-Manero, G., Basu, S., Boddu, P. C., Alfayez, M., Cortes, J. E., et al. (2018). Efficacy, safety, and biomarkers of response to azacitidine and nivolumab in relapsed/refractory acute myeloid leukemia: A nonrandomized, open-label, phase II study. Cancer Discov. 9, 370–383. doi:10.1158/2159-8290.CD-18-0774
Daver, N., Garcia-Manero, G., Basu, S., Boddu, P. C., Alfayez, M., Cortes, J. E., et al. (2019). Efficacy, safety, and biomarkers of response to azacitidine and nivolumab in relapsed/refractory acute myeloid leukemia: A nonrandomized, open-label, phase II study. Cancer Discov. 9, 370–383. doi:10.1158/2159-8290.CD-18-0774
Davids, M. S., Kim, H. T., Bachireddy, P., Costello, C., Liguori, R., Savell, A., et al. (2016). Ipilimumab for patients with relapse after allogeneic transplantation. N. Engl. J. Med. 375, 143–153. doi:10.1056/NEJMoa1601202
Davila, M. L., Riviere, I., Wang, X., Bartido, S., Park, J., Curran, K., et al. (2014). Efficacy and toxicity management of 19-28z CAR T cell therapy in B cell acute lymphoblastic leukemia. Sci. Transl. Med. 6, 224ra225. doi:10.1126/scitranslmed.3008226
De Kouchkovsky, I., and Abdul-Hay, M.' (2016). Acute myeloid leukemia: A comprehensive review and 2016 update. Blood cancer J. 6, e441. doi:10.1038/bcj.2016.50
Deng, M., Gui, X., Kim, J., Xie, L., Chen, W., Li, Z., et al. (2018). LILRB4 signalling in leukaemia cells mediates T cell suppression and tumour infiltration. Nature 562, 605–609. doi:10.1038/s41586-018-0615-z
Diermayr, S., Himmelreich, H., Durovic, B., Mathys-Schneeberger, A., Siegler, U., Langenkamp, U., et al. (2008). NKG2D ligand expression in AML increases in response to HDAC inhibitor valproic acid and contributes to allorecognition by NK-cell lines with single KIR-HLA class I specificities. Blood 111, 1428–1436. doi:10.1182/blood-2007-07-101311
Dohner, H., Estey, E. H., Amadori, S., Appelbaum, F. R., Büchner, T., Burnett, A. K., et al. (2010). Diagnosis and management of acute myeloid leukemia in adults: Recommendations from an international expert panel, on behalf of the European LeukemiaNet. Blood 115, 453–474. doi:10.1182/blood-2009-07-235358
Dong, Y., Han, Y., Huang, Y., Jiang, S., Huang, Z., Chen, R., et al. (2020). PD-L1 is expressed and promotes the expansion of regulatory T cells in acute myeloid leukemia. Front. Immunol. 11, 1710. doi:10.3389/fimmu.2020.01710
Dratwa, M., Wysoczańska, B., Butrym, A., Łacina, P., Mazur, G., and Bogunia-Kubik, K. (2021). TERT genetic variability and telomere length as factors affecting survival and risk in acute myeloid leukaemia. Sci. Rep. 11, 23301. doi:10.1038/s41598-021-02767-1
Ehninger, A., Kramer, M., Röllig, C., Thiede, C., Bornhäuser, M., von Bonin, M., et al. (2014). Distribution and levels of cell surface expression of CD33 and CD123 in acute myeloid leukemia. Blood Cancer J. 4, e218. doi:10.1038/bcj.2014.39
El Khawanky, N., Hughes, A., Yu, W., Myburgh, R., Matschulla, T., Taromi, S., et al. (2021). Demethylating therapy increases anti-CD123 CAR T cell cytotoxicity against acute myeloid leukemia. Nat. Commun. 12, 6436. doi:10.1038/s41467-021-26683-0
Erba, H. P., Levy, M. Y., Vasu, S., Stein, A. S., Fathi, A. T., Maris, M. B., et al. (2016). A phase 1b study of vadastuximab talirine in combination with 7+3 induction therapy for patients with newly diagnosed acute myeloid leukemia (AML). Blood 128 (22), 211. doi:10.1182/blood.v128.22.211.211
Eroglu, Z., Zaretsky, J. M., Hu-Lieskovan, S., Kim, D. W., Algazi, A., Johnson, D. B., et al. (2018). High response rate to PD-1 blockade in desmoplastic melanomas. Nature 553, 347–350. doi:10.1038/nature25187
Fathi, A. T., Erba, H. P., Lancet, J. E., Stein, E. M., Ravandi, F., Faderl, S., et al. (2018). A phase 1 trial of vadastuximab talirine combined with hypomethylating agents in patients with CD33-positive AML. Blood 132, 1125–1133. doi:10.1182/blood-2018-03-841171
Feldman, E. J., Brandwein, J., Stone, R., Kalaycio, M., Moore, J., O'Connor, J., et al. (2005). Phase III randomized multicenter study of a humanized anti-CD33 monoclonal antibody, lintuzumab, in combination with chemotherapy, versus chemotherapy alone in patients with refractory or first-relapsed acute myeloid leukemia. J. Clin. Oncol. 23, 4110–4116. doi:10.1200/jco.2005.09.133
Feng, Z., Fang, Q., Kuang, X., Liu, X., Chen, Y., Ma, D., et al. (2020). Clonal expansion of bone marrow CD8(+) T cells in acute myeloid leukemia patients at new diagnosis and after chemotherapy. Am. J. Cancer Res. 10, 3973–3989.
Ferrando, A. A., and Lopez-Otin, C. (2017). Clonal evolution in leukemia. Nat. Med. 23, 1135–1145. doi:10.1038/nm.4410
Ferrara, F., and Schiffer, C. A. (2013). Acute myeloid leukaemia in adults. Lancet 381, 484–495. doi:10.1016/s0140-6736(12)61727-9
Fevery, S., Billiau, A. D., Sprangers, B., Rutgeerts, O., Lenaerts, C., Goebels, J., et al. (2007). CTLA-4 blockade in murine bone marrow chimeras induces a host-derived antileukemic effect without graft-versus-host disease. Leukemia 21, 1451–1459. doi:10.1038/sj.leu.2404720
Finn Le, L. M., Orozco, J. J., Park, J. H., Atallah, E., and Craig, M. (2017). A phase 2 study of actinium-225-lintuzumab in older patients with previously untreated acute myeloid leukemia (AML) unfit for intensive chemotherapy. Blood 130 (1), 2638.
Friedman, C. F., Proverbs-Singh, T. A., and Postow, M. A. (2016). Treatment of the immune-related adverse effects of immune checkpoint inhibitors: A review. JAMA Oncol. 2, 1346–1353. doi:10.1001/jamaoncol.2016.1051
Germeau, C., Ma, W., Schiavetti, F., Lurquin, C., Henry, E., Vigneron, N., et al. (2005). High frequency of antitumor T cells in the blood of melanoma patients before and after vaccination with tumor antigens. J. Exp. Med. 201, 241–248. doi:10.1084/jem.20041379
Gettinger, S., Choi, J., Hastings, K., Truini, A., Datar, I., Sowell, R., et al. (2017). Impaired HLA class I antigen processing and presentation as a mechanism of acquired resistance to immune checkpoint inhibitors in lung cancer. Cancer Discov. 7, 1420–1435. doi:10.1158/2159-8290.CD-17-0593
Giroux Leprieur, E., Dumenil, C., Julie, C., Giraud, V., Dumoulin, J., Labrune, S., et al. (2017). Immunotherapy revolutionises non-small-cell lung cancer therapy: Results, perspectives and new challenges. Eur. J. Cancer 78, 16–23. doi:10.1016/j.ejca.2016.12.041
Gordon, S. R., Maute, R. L., Dulken, B. W., Hutter, G., George, B. M., McCracken, M. N., et al. (2017). PD-1 expression by tumour-associated macrophages inhibits phagocytosis and tumour immunity. Nature 545, 495–499. doi:10.1038/nature22396
Goswami, M., Gui, G., Dillon, L. W., Lindblad, K. E., Thompson, J., Valdez, J., et al. (2022). Pembrolizumab and decitabine for refractory or relapsed acute myeloid leukemia. J. Immunother. Cancer 10, e003392. doi:10.1136/jitc-2021-003392
Greaves, M. (2016). Leukaemia 'firsts' in cancer research and treatment. Nat. Rev. Cancer 16, 163–172. doi:10.1038/nrc.2016.3
Greiner, J., Götz, M., Hofmann, S., Schrezenmeier, H., Wiesneth, M., Bullinger, L., et al. (2020). Specific T-cell immune responses against colony-forming cells including leukemic progenitor cells of AML patients were increased by immune checkpoint inhibition. Cancer Immunol. Immunother. 69, 629–640. doi:10.1007/s00262-020-02490-2
Grupp, S. A., Kalos, M., Barrett, D., Aplenc, R., Porter, D. L., Rheingold, S. R., et al. (2013). Chimeric antigen receptor-modified T cells for acute lymphoid leukemia. N. Engl. J. Med. 368, 1509–1518. doi:10.1056/NEJMoa1215134
Gurusamy, D., Clever, D., Eil, R., and Restifo, N. P. (2017). Novel "elements" of immune suppression within the tumor microenvironment. Cancer Immunol. Res. 5, 426–433. doi:10.1158/2326-6066.CIR-17-0117
Haanen, J. (2017). Converting cold into hot tumors by combining immunotherapies. Cell 170, 1055–1056. doi:10.1016/j.cell.2017.08.031
Hartley, J. A. (2021). Antibody-drug conjugates (ADCs) delivering pyrrolobenzodiazepine (PBD) dimers for cancer therapy. Expert Opin. Biol. Ther. 21, 931–943. doi:10.1080/14712598.2020.1776255
Haubner, S., Perna, F., Köhnke, T., Schmidt, C., Berman, S., Augsberger, C., et al. (2019). Coexpression profile of leukemic stem cell markers for combinatorial targeted therapy in AML. Leukemia 33, 64–74. doi:10.1038/s41375-018-0180-3
He, S. Z., Busfield, S., Ritchie, D. S., Hertzberg, M. S., Durrant, S., Lewis, I. D., et al. (2015). A Phase 1 study of the safety, pharmacokinetics and anti-leukemic activity of the anti-CD123 monoclonal antibody CSL360 in relapsed, refractory or high-risk acute myeloid leukemia. Leukemia lymphoma 56, 1406–1415. doi:10.3109/10428194.2014.956316
Hodi, F. S., O'Day, S. J., McDermott, D. F., Weber, R. W., Sosman, J. A., Haanen, J. B., et al. (2010). Improved survival with ipilimumab in patients with metastatic melanoma. N. Engl. J. Med. 363, 711–723. doi:10.1056/nejmoa1003466
Huang, J., Tan, J., Chen, Y., Huang, S., Xu, L., Zhang, Y., et al. (2019). A skewed distribution and increased PD-1+Vβ+CD4+/CD8+ T cells in patients with acute myeloid leukemia. J. Leukoc. Biol. 106, 725–732. doi:10.1002/jlb.ma0119-021r
Im, S. J., Hashimoto, M., Gerner, M. Y., Lee, J., Kissick, H. T., Burger, M. C., et al. (2016). Defining CD8+ T cells that provide the proliferative burst after PD-1 therapy. Nature 537, 417–421. doi:10.1038/nature19330
Jen, E. Y., Gao, X., Li, L., Zhuang, L., Simpson, N. E., Aryal, B., et al. (2020). FDA approval summary: Tagraxofusp-erzs for treatment of blastic plasmacytoid dendritic cell neoplasm. Clin. Cancer Res. 26, 532–536. doi:10.1158/1078-0432.CCR-19-2329
Jen, E. Y., Ko, C. W., Lee, J. E., Del Valle, P. L., Aydanian, A., Jewell, C., et al. (2018). FDA approval: Gemtuzumab ozogamicin for the treatment of adults with newly diagnosed CD33-positive acute myeloid leukemia. Clin. Cancer Res. 24, 3242–3246. doi:10.1158/1078-0432.CCR-17-3179
Jetani, H., Navarro-Bailón, A., Maucher, M., Frenz, S., Verbruggen, C., Yeguas, A., et al. (2021). Siglec-6 is a novel target for CAR T-cell therapy in acute myeloid leukemia. Blood 138, 1830–1842. doi:10.1182/blood.2020009192
Jin, X., Zhang, M., Sun, R., Lyu, H., Xiao, X., Zhang, X., et al. (2022). First-in-human phase I study of CLL-1 CAR-T cells in adults with relapsed/refractory acute myeloid leukemia. J. Hematol. Oncol. 15, 88. doi:10.1186/s13045-022-01308-1
Jitschin, R., Saul, D., Braun, M., Tohumeken, S., Völkl, S., Kischel, R., et al. (2018). CD33/CD3-bispecific T-cell engaging (BiTE®) antibody construct targets monocytic AML myeloid-derived suppressor cells. J. Immunother. Cancer 6, 116. doi:10.1186/s40425-018-0432-9
June, C. H., O'Connor, R. S., Kawalekar, O. U., Ghassemi, S., and Milone, M. C. (2018). CAR T cell immunotherapy for human cancer. Science 359, 1361–1365. doi:10.1126/science.aar6711
Kadia, T. M., Ravandi, F., Cortes, J., and Kantarjian, H. (2015). Toward individualized therapy in acute myeloid leukemia: A contemporary review. JAMA Oncol. 1, 820–828. doi:10.1001/jamaoncol.2015.0617
Kageyama, S., Ikeda, H., Miyahara, Y., Imai, N., Ishihara, M., Saito, K., et al. (2015). Adoptive transfer of MAGE-A4 T-cell receptor gene-transduced lymphocytes in patients with recurrent esophageal cancer. Clin. Cancer Res. 21, 2268–2277. doi:10.1158/1078-0432.CCR-14-1559
Kamphorst, A. O., Wieland, A., Nasti, T., Yang, S., Zhang, R., Barber, D. L., et al. (2017). Rescue of exhausted CD8 T cells by PD-1-targeted therapies is CD28-dependent. Science 355, 1423–1427. doi:10.1126/science.aaf0683
Kelly, P. N. (2017). CD28 is a critical target for PD-1 blockade. Science 355, 1386. doi:10.1126/science.355.6332.1386-b
Khan, N., Hills, R. K., Virgo, P., Couzens, S., Clark, N., Gilkes, A., et al. (2017). Expression of CD33 is a predictive factor for effect of gemtuzumab ozogamicin at different doses in adult acute myeloid leukaemia. Leukemia 31, 1059–1068. doi:10.1038/leu.2016.309
Khoury, H. J., Collins, R. H., Blum, W., Stiff, P. S., Elias, L., Lebkowski, J. S., et al. (2017). Immune responses and long-term disease recurrence status after telomerase-based dendritic cell immunotherapy in patients with acute myeloid leukemia. Cancer 123, 3061–3072. doi:10.1002/cncr.30696
Kim, M. Y., Yu, K. R., Kenderian, S. S., Ruella, M., Chen, S., Shin, T. H., et al. (2018). Genetic inactivation of CD33 in hematopoietic stem cells to enable CAR T cell immunotherapy for acute myeloid leukemia. Cell 173, 1439–1453. doi:10.1016/j.cell.2018.05.013
Kreutmair, S. (2022). First-in-human study of WT1 recombinant protein vaccination in elderly patients with AML in remission: A single-center experience. Cancer Immunol. Immunother. 71 (12), 2913–2928. doi:10.1007/s00262-022-03202-8
Krupka, C., Kufer, P., Kischel, R., Zugmaier, G., Bögeholz, J., Köhnke, T., et al. (2014). CD33 target validation and sustained depletion of AML blasts in long-term cultures by the bispecific T-cell-engaging antibody AMG 330. Blood 123, 356–365. doi:10.1182/blood-2013-08-523548
Krupka, C., Kufer, P., Kischel, R., Zugmaier, G., Lichtenegger, F. S., Köhnke, T., et al. (2016). Blockade of the PD-1/PD-L1 axis augments lysis of AML cells by the CD33/CD3 BiTE antibody construct AMG 330: Reversing a T-cell-induced immune escape mechanism. Leukemia 30, 484–491. doi:10.1038/leu.2015.214
Kubasch, A. S., Schulze, F., Giagounidis, A., Götze, K. S., Krönke, J., Sockel, K., et al. (2020). Single agent talacotuzumab demonstrates limited efficacy but considerable toxicity in elderly high-risk MDS or AML patients failing hypomethylating agents. Leukemia 34, 1182–1186. doi:10.1038/s41375-019-0645-z
Kung Sutherland, M. S., Walter, R. B., Jeffrey, S. C., Burke, P. J., Yu, C., Kostner, H., et al. (2013). SGN-CD33A: A novel CD33-targeting antibody-drug conjugate using a pyrrolobenzodiazepine dimer is active in models of drug-resistant AML. Blood 122, 1455–1463. doi:10.1182/blood-2013-03-491506
Kyriakidis, I., Vasileiou, E., Rossig, C., Roilides, E., Groll, A. H., and Tragiannidis, A. (2021). Invasive fungal diseases in children with hematological malignancies treated with therapies that target cell surface antigens: Monoclonal antibodies, immune checkpoint inhibitors and CAR T-cell therapies. J. Fungi (Basel) 7, 186. doi:10.3390/jof7030186
Lambert, J., Pautas, C., Terré, C., Raffoux, E., Turlure, P., Caillot, D., et al. (2019). Gemtuzumab ozogamicin for de novo acute myeloid leukemia: Final efficacy and safety updates from the open-label, phase III ALFA-0701 trial. Haematologica 104, 113–119. doi:10.3324/haematol.2018.188888
Lamble, A. J., Eidenschink Brodersen, L., Alonzo, T. A., Wang, J., Pardo, L., Sung, L., et al. (2022). CD123 expression is associated with high-risk disease characteristics in childhood acute myeloid leukemia: A report from the children's oncology group. J. Clin. Oncol. 40, 252–261. doi:10.1200/JCO.21.01595
Lamble, A. J., Kosaka, Y., Laderas, T., Maffit, A., Kaempf, A., Brady, L. K., et al. (2020). Reversible suppression of T cell function in the bone marrow microenvironment of acute myeloid leukemia. Proc. Natl. Acad. Sci. U. S. A. 117, 14331–14341. doi:10.1073/pnas.1916206117
Lane, A. A., Sweet, K. L., Wang, E. S., Donnellan, W. B., Walter, R. B., Stein, A. S., et al. (2016). Results from ongoing phase 2 trial of SL-401 as consolidation therapy in patients with acute myeloid leukemia (AML) in remission with high relapse risk including minimal residual disease (MRD). Blood 128 (22), 215. doi:10.1182/blood.v128.22.215.215
Laszlo, G. S., Gudgeon, C. J., Harrington, K. H., and Walter, R. B. (2015). T-cell ligands modulate the cytolytic activity of the CD33/CD3 BiTE antibody construct, AMG 330. Blood Cancer J. 5, e340. doi:10.1038/bcj.2015.68
Laszlo, G. S., Harrington, K. H., Gudgeon, C. J., Beddoe, M. E., Fitzgibbon, M. P., Ries, R. E., et al. (2016). Expression and functional characterization of CD33 transcript variants in human acute myeloid leukemia. Oncotarget 7, 43281–43294. doi:10.18632/oncotarget.9674
Le, D. T., Uram, J. N., Wang, H., Bartlett, B., Kemberling, H., Eyring, A., et al. (2015). PD-1 blockade in tumors with mismatch-repair deficiency. N. Engl. J. Med. 372, LBA100–2520. doi:10.1200/jco.2015.33.18_suppl.lba100
Leick, M. B., Silva, H., Scarfò, I., Larson, R., Choi, B. D., Bouffard, A. A., et al. (2022). Non-cleavable hinge enhances avidity and expansion of CAR-T cells for acute myeloid leukemia. Cancer Cell 40, 494–508.e5. doi:10.1016/j.ccell.2022.04.001
Lesokhin, A. M., Ansell, S. M., Armand, P., Scott, E. C., Halwani, A., Gutierrez, M., et al. (2016). Nivolumab in patients with relapsed or refractory hematologic malignancy: Preliminary results of a phase Ib study. J. Clin. Oncol. 34, 2698–2704. doi:10.1200/jco.2015.65.9789
Levato, L., and Molica, S. (2018). Rituximab in the management of acute lymphoblastic leukemia. Expert Opin. Biol. Ther. 18, 221–226. doi:10.1080/14712598.2018.1425389
Levine, J. H., Simonds, E. F., Bendall, S. C., Davis, K. L., Amir, E. a. D., Tadmor, M. D., et al. (2015). Data-driven phenotypic dissection of AML reveals progenitor-like cells that correlate with prognosis. Cell 162, 184–197. doi:10.1016/j.cell.2015.05.047
Li, K. X., Wu, H. Y., Pan, W. Y., Guo, M. Q., Qiu, D. Z., He, Y. J., et al. (2022). A novel approach for relapsed/refractory FLT3(mut+) acute myeloid leukaemia: Synergistic effect of the combination of bispecific FLT3scFv/nkg2d-CAR T cells and gilteritinib. Mol. Cancer 21, 66. doi:10.1186/s12943-022-01541-9
Liao, D., Wang, M., Liao, Y., Li, J., and Niu, T. (2019). A review of efficacy and safety of checkpoint inhibitor for the treatment of acute myeloid leukemia. Front. Pharmacol. 10, 609. doi:10.3389/fphar.2019.00609
Lin, G., Zhang, Y., Yu, L., and Wu, D. (2021). Cytotoxic effect of CLL-1 CAR-T cell immunotherapy with PD-1 silencing on relapsed/refractory acute myeloid leukemia. Mol. Med. Rep. 23, 208. doi:10.3892/mmr.2021.11847
Locke, F. L., Neelapu, S. S., Bartlett, N. L., Siddiqi, T., Chavez, J. C., Hosing, C. M., et al. (2017). Phase 1 results of ZUMA-1: A multicenter study of kte-C19 anti-CD19 CAR T cell therapy in refractory aggressive lymphoma. Mol. Ther. 25, 285–295. doi:10.1016/j.ymthe.2016.10.020
Loff, S., Dietrich, J., Meyer, J. E., Riewaldt, J., Spehr, J., von Bonin, M., et al. (2020). Rapidly switchable universal CAR-T cells for treatment of cd123-positive leukemia. Mol. Ther. Oncolytics 17, 408–420. doi:10.1016/j.omto.2020.04.009
Ma, Y. J., Dai, H. P., Cui, Q. Y., Cui, W., Zhu, W. J., Qu, C. J., et al. (2022). Successful application of PD-1 knockdown CLL-1 CAR-T therapy in two AML patients with post-transplant relapse and failure of anti-CD38 CAR-T cell treatment. Am. J. Cancer Res. 12, 615–621.
Masarova, L., Kantarjian, H., Garcia-Mannero, G., Ravandi, F., Sharma, P., and Daver, N. (2017). Harnessing the immune system against leukemia: Monoclonal antibodies and checkpoint strategies for AML. Adv. Exp. Med. Biol. 995, 73–95. doi:10.1007/978-3-319-53156-4_4
Maude, S. L., Frey, N., Shaw, P. A., Aplenc, R., Barrett, D. M., Bunin, N. J., et al. (2014). Chimeric antigen receptor T cells for sustained remissions in leukemia. N. Engl. J. Med. 371, 1507–1517. doi:10.1056/NEJMoa1407222
Medeiros, B. C., Tanaka, T. N., Balaian, L., Bashey, A., Guzdar, A., Li, H., et al. (2018). A phase I/II trial of the combination of azacitidine and gemtuzumab ozogamicin for treatment of relapsed acute myeloid leukemia. Clin. Lymphoma Myeloma Leuk 18, 346–352.e5. doi:10.1016/j.clml.2018.02.017
Melero, I., Gaudernack, G., Gerritsen, W., Huber, C., Parmiani, G., Scholl, S., et al. (2014). Therapeutic vaccines for cancer: An overview of clinical trials. Nat. Rev. Clin. Oncol. 11, 509–524. doi:10.1038/nrclinonc.2014.111
Mohme, M., Riethdorf, S., and Pantel, K. (2017). Circulating and disseminated tumour cells - mechanisms of immune surveillance and escape. Nat. Rev. Clin. Oncol. 14, 155–167. doi:10.1038/nrclinonc.2016.144
Montesinos, P., Roboz, G. J., Bulabois, C. E., Subklewe, M., Platzbecker, U., Ofran, Y., et al. (2021). Safety and efficacy of talacotuzumab plus decitabine or decitabine alone in patients with acute myeloid leukemia not eligible for chemotherapy: Results from a multicenter, randomized, phase 2/3 study. Leukemia 35, 62–74. doi:10.1038/s41375-020-0773-5
Mussai, F., De Santo, C., Abu-Dayyeh, I., Booth, S., Quek, L., McEwen-Smith, R. M., et al. (2013). Acute myeloid leukemia creates an arginase-dependent immunosuppressive microenvironment. Blood 122, 749–758. doi:10.1182/blood-2013-01-480129
Nahas, M. R., Stroopinsky, D., Rosenblatt, J., Cole, L., Pyzer, A. R., Anastasiadou, E., et al. (2019). Hypomethylating agent alters the immune microenvironment in acute myeloid leukaemia (AML) and enhances the immunogenicity of a dendritic cell/AML vaccine. Br. J. Haematol. 185, 679–690. doi:10.1111/bjh.15818
Naval Daver, S. B., Garcia-Manero, G., Cortes, J. E., Ravandi, F., Jabbour, E. J., Hendrickson, S., et al. (2016). Phase IB/II study of nivolumab in combination with azacytidine (AZA) in patients (pts) with relapsed acute myeloid leukemia (AML). Blood 128, 763. doi:10.1182/blood.v128.22.763.763
Nishino, M., Ramaiya, N. H., Hatabu, H., and Hodi, F. S. (2017). Monitoring immune-checkpoint blockade: Response evaluation and biomarker development. Nat. Rev. Clin. Oncol. 14, 655–668. doi:10.1038/nrclinonc.2017.88
O'Hear, C., Heiber, J. F., Schubert, I., Fey, G., and Geiger, T. L. (2015). Anti-CD33 chimeric antigen receptor targeting of acute myeloid leukemia. Haematologica 100, 336–344. doi:10.3324/haematol.2014.112748
Ogasawara, M., Miyashita, M., Yamagishi, Y., and Ota, S. (2022). Wilms' tumor 1 peptide-loaded dendritic cell vaccination in patients with relapsed or refractory acute leukemia. Ther. Apher. Dial. 26, 537–547. doi:10.1111/1744-9987.13828
Ohyashiki, J. H., Ohyashiki, K., Iwama, H., Hayashi, S., Toyama, K., and Shay, J. W. (1997). Clinical implications of telomerase activity levels in acute leukemia. Clin. Cancer Res. 3, 619–625.
Oran, B., Garcia-Manero, G., Saliba, R. M., Alfayez, M., Al-Atrash, G., Ciurea, S. O., et al. (2020). Posttransplantation cyclophosphamide improves transplantation outcomes in patients with AML/MDS who are treated with checkpoint inhibitors. Cancer 126, 2193–2205. doi:10.1002/cncr.32796
Paczulla, A. M., Rothfelder, K., Raffel, S., Konantz, M., Steinbacher, J., Wang, H., et al. (2019). Absence of NKG2D ligands defines leukaemia stem cells and mediates their immune evasion. Nature 572, 254–259. doi:10.1038/s41586-019-1410-1
Pemmaraju, N., Sweet, K. L., Stein, A. S., Wang, E. S., Rizzieri, D. A., Vasu, S., et al. (2022). Long-term benefits of tagraxofusp for patients with blastic plasmacytoid dendritic cell neoplasm. J. Clin. Oncol. 40, 3032–3036. doi:10.1200/JCO.22.00034
Penter, L., Zhang, Y., Savell, A., Huang, T., Cieri, N., Thrash, E. M., et al. (2021). Molecular and cellular features of CTLA-4 blockade for relapsed myeloid malignancies after transplantation. Blood 137, 3212–3217. doi:10.1182/blood.2021010867
Perna, F., Berman, S. H., Soni, R. K., Mansilla-Soto, J., Eyquem, J., Hamieh, M., et al. (2017). Integrating proteomics and transcriptomics for systematic combinatorial chimeric antigen receptor therapy of AML. Cancer Cell 32, 506–519.e5. doi:10.1016/j.ccell.2017.09.004
Petersdorf, S. H., Kopecky, K. J., Slovak, M., Willman, C., Nevill, T., Brandwein, J., et al. (2013). A phase 3 study of gemtuzumab ozogamicin during induction and postconsolidation therapy in younger patients with acute myeloid leukemia. Blood 121, 4854–4860. doi:10.1182/blood-2013-01-466706
Pollard, J. A., Alonzo, T. A., Loken, M., Gerbing, R. B., Ho, P. A., Bernstein, I. D., et al. (2012). Correlation of CD33 expression level with disease characteristics and response to gemtuzumab ozogamicin containing chemotherapy in childhood AML. Blood 119, 3705–3711. doi:10.1182/blood-2011-12-398370
Polyzoidis, S., Tuazon, J., Brazil, L., Beaney, R., Al-Sarraj, S. T., Doey, L., et al. (2015). Active dendritic cell immunotherapy for glioblastoma: Current status and challenges. Br. J. Neurosurg. 29, 197–205. doi:10.3109/02688697.2014.994473
Qi, Y., Zhao, M., Hu, Y., Wang, Y., Li, P., Cao, J., et al. (2022). Efficacy and safety of CD19-specific CAR T cell-based therapy in B-cell acute lymphoblastic leukemia patients with CNSL. Blood 139, 3376–3386. doi:10.1182/blood.2021013733
Qin, H., Yang, L., Chukinas, J. A., Shah, N., Tarun, S., Pouzolles, M., et al. (2021). Systematic preclinical evaluation of CD33-directed chimeric antigen receptor T cell immunotherapy for acute myeloid leukemia defines optimized construct design. J. Immunother. Cancer 9, e003149. doi:10.1136/jitc-2021-003149
Ravandi, F., Assi, R., Daver, N., Benton, C. B., Kadia, T., Thompson, P. A., et al. (2019). Idarubicin, cytarabine, and nivolumab in patients with newly diagnosed acute myeloid leukaemia or high-risk myelodysplastic syndrome: A single-arm, phase 2 study. Lancet. Haematol. 6, e480–e488. doi:10.1016/S2352-3026(19)30114-0
Reusch, U., Harrington, K. H., Gudgeon, C. J., Fucek, I., Ellwanger, K., Weichel, M., et al. (2016). Characterization of CD33/CD3 tetravalent bispecific tandem diabodies (TandAbs) for the treatment of acute myeloid leukemia. Clin. Cancer Res. 22, 5829–5838. doi:10.1158/1078-0432.CCR-16-0350
Ritchie, D. S., Neeson, P. J., Khot, A., Peinert, S., Tai, T., Tainton, K., et al. (2013). Persistence and efficacy of second generation CAR T cell against the LeY antigen in acute myeloid leukemia. Mol. Ther. J. Am. Soc. Gene Ther. 21, 2122–2129. doi:10.1038/mt.2013.154
Rizvi, N. A., Hellmann, M. D., Snyder, A., Kvistborg, P., Makarov, V., Havel, J. J., et al. (2015). Mutational landscape determines sensitivity to PD-1 blockade in non–small cell lung cancer. Science 348, 124–128. doi:10.1126/science.aaa1348
Robbins, P. F., Morgan, R. A., Feldman, S. A., Yang, J. C., Sherry, R. M., Dudley, M. E., et al. (2011). Tumor regression in patients with metastatic synovial cell sarcoma and melanoma using genetically engineered lymphocytes reactive with NY-ESO-1. J. Clin. Oncol. 29, 917–924. doi:10.1200/JCO.2010.32.2537
Robert, C., Ribas, A., Wolchok, J. D., Hodi, F. S., Hamid, O., Kefford, R., et al. (2014). Anti-programmed-death-receptor-1 treatment with pembrolizumab in ipilimumab-refractory advanced melanoma: A randomised dose-comparison cohort of a phase 1 trial. Lancet 384, 1109–1117. doi:10.1016/S0140-6736(14)60958-2
Roberts, A. W., He, S., Bradstock, K. F., Hertzberg, M. S., Durrant, S. T. S., Ritchie, D., et al. (2008). A phase 1 and correlative biological study of CSL360 (anti-CD123 mAb) in AML. Blood 112 (11), 2956. doi:10.1182/blood.v112.11.2956.2956
Roboz, G. J., Rosenblat, T., Arellano, M., Gobbi, M., Altman, J. K., Montesinos, P., et al. (2014). International randomized phase III study of elacytarabine versus investigator choice in patients with relapsed/refractory acute myeloid leukemia. J. Clin. Oncol. 32, 1919–1926. doi:10.1200/JCO.2013.52.8562
Rosenblat, T. L., McDevitt, M. R., Carrasquillo, J. A., Pandit-Taskar, N., Frattini, M. G., Maslak, P. G., et al. (2022). Treatment of patients with acute myeloid leukemia with the targeted alpha-particle nanogenerator actinium-225-lintuzumab. Clin. Cancer Res. 28, 2030–2037. doi:10.1158/1078-0432.CCR-21-3712
Rosenblat, T. L., McDevitt, M. R., Mulford, D. A., Pandit-Taskar, N., Divgi, C. R., Panageas, K. S., et al. (2010). Sequential cytarabine and alpha-particle immunotherapy with bismuth-213-lintuzumab (HuM195) for acute myeloid leukemia. Clin. Cancer Res. 16, 5303–5311. doi:10.1158/1078-0432.CCR-10-0382
Rosenblatt, J., Stone, R. M., Uhl, L., Neuberg, D., Joyce, R., Levine, J. D., et al. (2016). Individualized vaccination of AML patients in remission is associated with induction of antileukemia immunity and prolonged remissions. Sci. Transl. Med. 8, 368ra171. doi:10.1126/scitranslmed.aag1298
Rotte, A. (2019). Combination of CTLA-4 and PD-1 blockers for treatment of cancer. J. Exp. Clin. Cancer Res. 38, 255. doi:10.1186/s13046-019-1259-z
Ruggiero, E., Carnevale, E., Prodeus, A., Magnani, Z. I., Camisa, B., Merelli, I., et al. (2022). CRISPR-based gene disruption and integration of high-avidity, WT1-specific T cell receptors improve antitumor T cell function. Sci. Transl. Med. 14, eabg8027. doi:10.1126/scitranslmed.abg8027
Sallman, D. A., Brayer, J., Sagatys, E. M., Lonez, C., Breman, E., Agaugué, S., et al. (2018). NKG2D-based chimeric antigen receptor therapy induced remission in a relapsed/refractory acute myeloid leukemia patient. Haematologica 103, e424–e426. doi:10.3324/haematol.2017.186742
Sallman, D. A., McLemore, A. F., Aldrich, A. L., Komrokji, R. S., McGraw, K. L., Dhawan, A., et al. (2020). TP53 mutations in myelodysplastic syndromes and secondary AML confer an immunosuppressive phenotype. Blood 136, 2812–2823. doi:10.1182/blood.2020006158
Sauer, T., Parikh, K., Sharma, S., Omer, B., Sedloev, D., Chen, Q., et al. (2021). CD70-specific CAR T cells have potent activity against acute myeloid leukemia without HSC toxicity. Blood 138, 318–330. doi:10.1182/blood.2020008221
Saxena, K., Herbrich, S. M., Pemmaraju, N., Kadia, T. M., DiNardo, C. D., Borthakur, G., et al. (2021). A phase 1b/2 study of azacitidine with PD-L1 antibody avelumab in relapsed/refractory acute myeloid leukemia. Cancer 127, 3761–3771. doi:10.1002/cncr.33690
Schratz, K. E., Gaysinskaya, V., Cosner, Z. L., DeBoy, E. A., Xiang, Z., Kasch-Semenza, L., et al. (2021). Somatic reversion impacts myelodysplastic syndromes and acute myeloid leukemia evolution in the short telomere disorders. J. Clin. Investigation 131, e147598. doi:10.1172/JCI147598
Schreibelt, G., Bol, K. F., Westdorp, H., Wimmers, F., Aarntzen, E. H. J. G., Duiveman-de Boer, T., et al. (2016). Effective clinical responses in metastatic melanoma patients after vaccination with primary myeloid dendritic cells. Clin. Cancer Res. 22, 2155–2166. doi:10.1158/1078-0432.CCR-15-2205
Schwartzman, O., and Tanay, A. (2015). Single-cell epigenomics: Techniques and emerging applications. Nat. Rev. Genet. 16, 716–726. doi:10.1038/nrg3980
Short, N. J., Rytting, M. E., and Cortes, J. E. (2018). Acute myeloid leukaemia. Lancet 392, 593–606. doi:10.1016/s0140-6736(18)31041-9
Spallone, A., Alotaibi, A. S., Jiang, Y., Daver, N., and Kontoyiannis, D. P. (2022). Infectious complications among patients with AML treated with immune checkpoint inhibitors. Clin. lymphoma, myeloma and leukemia 22, 305–310. doi:10.1016/j.clml.2021.10.012
Stein, E. M., Walter, R. B., Erba, H. P., Fathi, A. T., Advani, A. S., Lancet, J. E., et al. (2018). A phase 1 trial of vadastuximab talirine as monotherapy in patients with CD33-positive acute myeloid leukemia. Blood 131, 387–396. doi:10.1182/blood-2017-06-789800
Steinman, R. M., and Banchereau, J. (2007). Taking dendritic cells into medicine. Nature 449, 419–426. doi:10.1038/nature06175
Stewart, S. A., and Weinberg, R. A. (2000). Telomerase and human tumorigenesis. Seminars Cancer Biol. 10, 399–406. doi:10.1006/scbi.2000.0339
Stroopinsky, D., Liegel, J., Bhasin, M., Cheloni, G., Thomas, B., Bhasin, S., et al. (2021). Leukemia vaccine overcomes limitations of checkpoint blockade by evoking clonal T cell responses in a murine acute myeloid leukemia model. Haematologica 106, 1330–1342. doi:10.3324/haematol.2020.259457
Sugita, M., Galetto, R., Zong, H., Ewing-Crystal, N., Trujillo-Alonso, V., Mencia-Trinchant, N., et al. (2022). Allogeneic TCRαβ deficient CAR T-cells targeting CD123 in acute myeloid leukemia. Nat. Commun. 13, 2227. doi:10.1038/s41467-022-29668-9
Tahk, S., Vick, B., Hiller, B., Schmitt, S., Marcinek, A., Perini, E. D., et al. (2021). SIRPα-αCD123 fusion antibodies targeting CD123 in conjunction with CD47 blockade enhance the clearance of AML-initiating cells. J. Hematol. Oncol. 14, 155. doi:10.1186/s13045-021-01163-6
Tambaro, F. P., Singh, H., Jones, E., Rytting, M., Mahadeo, K. M., Thompson, P., et al. (2021). Autologous CD33-CAR-T cells for treatment of relapsed/refractory acute myelogenous leukemia. Leukemia 35, 3282–3286. doi:10.1038/s41375-021-01232-2
Tan, J., Yu, Z., Huang, J., Chen, Y., Huang, S., Yao, D., et al. (2020). Increased PD-1+Tim-3+ exhausted T cells in bone marrow may influence the clinical outcome of patients with AML. Biomark. Res. 8, 6. doi:10.1186/s40364-020-0185-8
Tang, J., Shalabi, A., and Hubbard-Lucey, V. M. (2017). Comprehensive analysis of the clinical immuno-oncology landscape. Ann. Oncol. 29, 84–91. doi:10.1093/annonc/mdx755
Tang, L., Wu, J., Li, C. G., Jiang, H. W., Xu, M., Du, M., et al. (2020). Characterization of immune dysfunction and identification of prognostic immune-related risk factors in acute myeloid leukemia. Clin. Cancer Res. 26, 1763–1772. doi:10.1158/1078-0432.CCR-19-3003
Tasian, S. K. (2018). Acute myeloid leukemia chimeric antigen receptor T-cell immunotherapy: How far up the road have we traveled? Ther. Adv. Hematol. 9 (6), 135–148. doi:10.1177/2040620718774268
Taussig, D. C., Pearce, D. J., Simpson, C., Rohatiner, A. Z., Lister, T. A., Kelly, G., et al. (2005). Hematopoietic stem cells express multiple myeloid markers: Implications for the origin and targeted therapy of acute myeloid leukemia. Blood 106, 4086–4092. doi:10.1182/blood-2005-03-1072
Tawara, I., Kageyama, S., Miyahara, Y., Fujiwara, H., Nishida, T., Akatsuka, Y., et al. (2017). Safety and persistence of WT1-specific T-cell receptor gene-transduced lymphocytes in patients with AML and MDS. Blood 130, 1985–1994. doi:10.1182/blood-2017-06-791202
Topalian, S. L., Hodi, F. S., Brahmer, J. R., Gettinger, S. N., Smith, D. C., McDermott, D. F., et al. (2012). Safety, activity, and immune correlates of anti-PD-1 antibody in cancer. N. Engl. J. Med. 366, 2443–2454. doi:10.1056/NEJMoa1200690
Vago, L., and Gojo, I. (2020). Immune escape and immunotherapy of acute myeloid leukemia. J. Clin. Investigation 130, 1552–1564. doi:10.1172/jci129204
van Balen, P., Jedema, I., van Loenen, M. M., de Boer, R., van Egmond, H. M., Hagedoorn, R. S., et al. (2020). HA-1H T-cell receptor gene transfer to redirect virus-specific T cells for treatment of hematological malignancies after allogeneic stem cell transplantation: A phase 1 clinical study. Front. Immunol. 11, 1804. doi:10.3389/fimmu.2020.01804
van de Loosdrecht, A. A., van Wetering, S., Santegoets, S. J. A. M., Singh, S. K., Eeltink, C. M., den Hartog, Y., et al. (2018). A novel allogeneic off-the-shelf dendritic cell vaccine for post-remission treatment of elderly patients with acute myeloid leukemia. Cancer Immunol. Immunother. 67, 1505–1518. doi:10.1007/s00262-018-2198-9
van Ens, D., Mousset, C. M., Hutten, T. J. A., van der Waart, A. B., Campillo-Davo, D., van der Heijden, S., et al. (2020). PD-L1 siRNA-mediated silencing in acute myeloid leukemia enhances anti-leukemic T cell reactivity. Bone Marrow Transplant. 55, 2308–2318. doi:10.1038/s41409-020-0966-6
Van Tendeloo, V. F., Van de Velde, A., Van Driessche, A., Cools, N., Anguille, S., Ladell, K., et al. (2010). Induction of complete and molecular remissions in acute myeloid leukemia by Wilms' tumor 1 antigen-targeted dendritic cell vaccination. Proc. Natl. Acad. Sci. U. S. A. 107, 13824–13829. doi:10.1073/pnas.1008051107
Vasileiou, S., Lulla, P. D., Tzannou, I., Watanabe, A., Kuvalekar, M., Callejas, W. L., et al. (2021). T-cell therapy for lymphoma using nonengineered multiantigen-targeted T cells is safe and produces durable clinical effects. J. Clin. Oncol. 39, 1415–1425. doi:10.1200/JCO.20.02224
Wang, Q. S., Wang, Y., Lv, H. y., Han, Q. w., Fan, H., Guo, B., et al. (2015). Treatment of CD33-directed chimeric antigen receptor-modified T cells in one patient with relapsed and refractory acute myeloid leukemia. Mol. Ther. 23, 184–191. doi:10.1038/mt.2014.164
Willier, S., Rothämel, P., Hastreiter, M., Wilhelm, J., Stenger, D., Blaeschke, F., et al. (2021). CLEC12A and CD33 coexpression as a preferential target for pediatric AML combinatorial immunotherapy. Blood 137, 1037–1049. doi:10.1182/blood.2020006921
Xie, L. H., Biondo, M., Busfield, S. J., Arruda, A., Yang, X., Vairo, G., et al. (2017). CD123 target validation and preclinical evaluation of ADCC activity of anti-CD123 antibody CSL362 in combination with NKs from AML patients in remission. Blood Cancer J. 7, e567. doi:10.1038/bcj.2017.52
Xu, L., Liu, L., Yao, D., Zeng, X., Zhang, Y., Lai, J., et al. (2021). PD-1 and TIGIT are highly Co-expressed on CD8(+) T cells in AML patient bone marrow. Front. Oncol. 11, 686156. doi:10.3389/fonc.2021.686156
Xu, S., Zhang, M., Fang, X., Hu, X., Xing, H., Yang, Y., et al. (2022). CD123 antagonistic peptides assembled with nanomicelles act as monotherapeutics to combat refractory acute myeloid leukemia. ACS Appl. Mater. interfaces 14, 38584–38593. doi:10.1021/acsami.2c11538
Xu, S., Zhang, M., Fang, X., Meng, J., Xing, H., Yan, D., et al. (2021). A novel CD123-targeted therapeutic peptide loaded by micellar delivery system combats refractory acute myeloid leukemia. J. Hematol. Oncol. 14, 193. doi:10.1186/s13045-021-01206-y
Yang, H., Bueso-Ramos, C., DiNardo, C., Estecio, M. R., Davanlou, M., Geng, Q. R., et al. (2014). Expression of PD-L1, PD-L2, PD-1 and CTLA4 in myelodysplastic syndromes is enhanced by treatment with hypomethylating agents. Leukemia 28, 1280–1288. doi:10.1038/leu.2013.355
Yang, J., He, J., Zhang, X., Li, J., Wang, Z., Zhang, Y., et al. (2022). Next-day manufacture of a novel anti-CD19 CAR-T therapy for B-cell acute lymphoblastic leukemia: First-in-human clinical study. Blood cancer J. 12, 104. doi:10.1038/s41408-022-00694-6
Yang, X., and Wang, J. (2018). Precision therapy for acute myeloid leukemia. J. Hematol. Oncol. 11, 3. doi:10.1186/s13045-017-0543-7
Yao, S., Jianlin, C., Zhuoqing, Q., Yuhang, L., Jiangwei, H., Guoliang, H., et al. (2021). Case report: Combination therapy with PD-1 blockade for acute myeloid leukemia after allogeneic hematopoietic stem cell transplantation resulted in fatal GVHD. Front. Immunol. 12, 639217. doi:10.3389/fimmu.2021.639217
Yarchoan, M., Hopkins, A., and Jaffee, E. M. (2017). Tumor mutational burden and response rate to PD-1 inhibition. N. Engl. J. Med. 377, 2500–2501. doi:10.1056/NEJMc1713444
You, X., Liu, F., Binder, M., Vedder, A., Lasho, T., Wen, Z., et al. (2022). Asxl1 loss cooperates with oncogenic Nras in mice to reprogram the immune microenvironment and drive leukemic transformation. Blood 139, 1066–1079. doi:10.1182/blood.2021012519
Younes, A., Santoro, A., Shipp, M., Zinzani, P. L., Timmerman, J. M., Ansell, S., et al. (2016). Nivolumab for classical hodgkin's lymphoma after failure of both autologous stem-cell transplantation and brentuximab vedotin: A multicentre, multicohort, single-arm phase 2 trial. Lancet. Oncol. 17, 1283–1294. doi:10.1016/S1470-2045(16)30167-X
Zeidner, J. F., Vincent, B. G., Ivanova, A., Moore, D., McKinnon, K. P., Wilkinson, A. D., et al. (2021). Phase II trial of pembrolizumab after high-dose cytarabine in relapsed/refractory acute myeloid leukemia. Blood Cancer Discov. 2, 616–629. doi:10.1158/2643-3230.BCD-21-0070
Zhang, L., Gajewski, T. F., and Kline, J. (2009). PD-1/PD-L1 interactions inhibit antitumor immune responses in a murine acute myeloid leukemia model. Blood 114, 1545–1552. doi:10.1182/blood-2009-03-206672
Zhang, W., Piatyszek, M. A., Kobayashi, T., Estey, E., Andreeff, M., Deisseroth, A. B., et al. (1996). Telomerase activity in human acute myelogenous leukemia: Inhibition of telomerase activity by differentiation-inducing agents. Clin. Cancer Res. 2, 799–803.
Zheng, H., Mineishi, S., Claxton, D., Zhu, J., Zhao, C., Jia, B., et al. (2021). A phase I clinical trial of avelumab in combination with decitabine as first line treatment of unfit patients with acute myeloid leukemia. Am. J. Hematol. 96, E46–e50. doi:10.1002/ajh.26043
Zhou, Q., Bucher, C., Munger, M. E., Highfill, S. L., Tolar, J., Munn, D. H., et al. (2009). Depletion of endogenous tumor-associated regulatory T cells improves the efficacy of adoptive cytotoxic T-cell immunotherapy in murine acute myeloid leukemia. Blood 114, 3793–3802. doi:10.1182/blood-2009-03-208181
Keywords: acute myeloid leukemia, immunotherapy, checkpoint inhibitor, car-t, DC vaccines
Citation: Chen Y, Wang J, Zhang F and Liu P (2023) A perspective of immunotherapy for acute myeloid leukemia: Current advances and challenges. Front. Pharmacol. 14:1151032. doi: 10.3389/fphar.2023.1151032
Received: 25 January 2023; Accepted: 24 March 2023;
Published: 19 April 2023.
Edited by:
Ouyang Chen, Duke University, United StatesCopyright © 2023 Chen, Wang, Zhang and Liu. This is an open-access article distributed under the terms of the Creative Commons Attribution License (CC BY). The use, distribution or reproduction in other forums is permitted, provided the original author(s) and the copyright owner(s) are credited and that the original publication in this journal is cited, in accordance with accepted academic practice. No use, distribution or reproduction is permitted which does not comply with these terms.
*Correspondence: Jishi Wang, d2FuZ2ppc2hpOTY0NkAxNjMuY29t