- 1Department of Endocrinology, Guang’anmen Hospital, China Academy of Chinese Medical Sciences, Beijing, China
- 2Graduate School of Beijing University of Chinese Medicine, Beijing, China
Chronic low-grade inflammation affects health and is associated with aging and age-related diseases. Dysregulation of the gut flora is an important trigger for chronic low-grade inflammation. Changes in the composition of the gut flora and exposure to related metabolites have an effect on the inflammatory system of the host. This results in the development of crosstalk between the gut barrier and immune system, contributing to chronic low-grade inflammation and impairment of health. Probiotics can increase the diversity of gut microbiota, protect the gut barrier, and regulate gut immunity, thereby reducing inflammation. Therefore, the use of probiotics is a promising strategy for the beneficial immunomodulation and protection of the gut barrier through gut microbiota. These processes might positively influence inflammatory diseases, which are common in the elderly.
1 Introduction
According to the United Nations, the global population aged ≥60 years is estimated to double to nearly 2.1 billion by 2050. Hence, population aging has become a global public health concern with important socio-economic issues (Jayanama and Theou, 2020). Senescence is characterized by increased concentrations of many pro-inflammatory factors in the circulation. In addition, chronic low-grade inflammation has been identified as a key process involved in aging (López-Otín et al., 2013). Inflammation is a normal part of the immune response that defends against harmful bacteria and pathogens and plays an important role in the maintenance of tissues (van de Vyver, 2023). Chronic low-grade inflammation is influenced by changes in different tissues (muscle, adipose tissue), organs (brain, liver), systems (immune system), and ecosystems (gut flora) (Calder et al., 2017). It can indirectly trigger diseases in other organs (e.g., metabolic diseases, neuroinflammatory diseases, cardiovascular diseases, etc.) (Hutchinson et al., 2020). Chronic low-grade inflammation is one of the main contributing factors to various age-related diseases in the elderly (Warman et al., 2022).
Furthermore, it is closely related to the dysregulation of gut flora (Calder et al., 2017). Many immune cells and microbiota in the digestive tract interact with each other to maintain immune homeostasis. Intestinal microbiota plays a role in maintaining healthy levels of inflammation by integrating gastrointestinal, immune, and neurological information (Belkaid and Hand, 2014; Estrada and Contreras, 2019; Hutchinson et al., 2020). Data obtained from animal models have demonstrated that age-related microbial ecological disorders can lead to intestinal permeability, systemic inflammation, and premature death (DeJong et al., 2020). Altering the gut microbiota of older adults with wholesome bacteria exerts positive effects on the maintenance of optimal immune responses, which decline with age. Such effects include delaying the aging of T lymphocytes and increasing the number of immune cells that respond to acute antigen exposure (Moro-García et al., 2013; Hutchinson et al., 2020). This article summarizes the current knowledge on gut flora and chronic low-grade inflammation, describes the effect and possible mechanism of probiotics on chronic low-grade inflammation in old age, and focuses on their potential use to improve the health of the elderly with systemic inflammation.
2 Dysregulation of gut flora and chronic low-grade inflammation
As an important part of the human microbiome, gut flora performs necessary biological functions, such as stabilizing the immune system, regulating host metabolism, preventing pathogen invasion, and improving epithelial barrier function (Malard et al., 2021; Rastogi and Singh, 2022). Studies have found that dysregulation of gut flora plays an important role in numerous immune-mediated chronic low-grade inflammatory diseases, such as old age weakness (Xu et al., 2021), type 2 diabetes mellitus (T2DM) (Gurung et al., 2020), obesity (Gomes et al., 2018), allergy (Cukrowska et al., 2020), cognitive dysfunction (Bairamian et al., 2022), non-alcoholic fatty liver (Safari and Gérard, 2019), ulcerative colitis (Shen et al., 2018), Crohn’s disease (Agus et al., 2018), irritable bowel syndrome (Ford et al., 2020), colorectal cancer (Wong and Yu, 2019), and cardiovascular disease (Witkowski et al., 2020). The ecological dysregulation of gut flora stems from the changes in the composition of gut microorganisms and gut barrier, leading to chronic low-grade inflammation.
2.1 Changes in the composition of gut flora
The composition of gut microbiota is considered a key factor for healthy gut physiology (Warman et al., 2022). The intestinal cavity contains billions of bacteria. Phyla Firmicutes, Bacteroidetes, Actinobacteria, and Proteobacteria account for 99% of the intestinal flora, while the remaining 1% consists of flora with other functions and benefits (Tagliabue and Elli, 2013). Some of these gut bacteria possess anti- or pro-inflammatory properties. Microbial strains with anti-inflammatory properties increase the production of short-chain fatty acids (SCFAs). SCFAs (butyrate, acetate, propionate, etc.) regulate energy metabolism and act as immunomodulators to maintain the anti-inflammatory/pro-inflammatory balance (Salazar et al., 2020). Microbial flora associated with increased SCFA production includes Clostridium, Akkermansia, Lactobacillus, Lachnospira, Faecalibacterium, Bifidobacterium, Roseburia, Ruminococcus, and Dorea (Myhrstad et al., 2020; Malesza et al., 2021). Among them, Akkermansia muciniphila, which belongs to the warty microbial phyla, is a mucin degradation strain that exists in the intestinal mucus layer and improves intestinal barrier integrity by enhancing mucin production and complex interactions with other bacteria (Malesza et al., 2021). Moreover, it supports gut colonization by beneficial bacteria producing SCFAs (Bodogai et al., 2018). Bifidobacterium spp. promotes the production of anti-inflammatory cytokines, such as tumor necrosis factor (TNF) and interleukin-1 beta (IL-1β), induces the maturation of immune cells, promotes immunoglobulin A (IgA) secretion, and possesses anti-oxidant properties (Toward et al., 2012; Malesza et al., 2021). Microbial strains with pro-inflammatory properties, such as Escherichia coli, Eggerthella lenta, Streptococcus gallolyticus, and Enterococcus spp., can produce more endotoxins (Warman et al., 2022).
2.1.1 Inappropriate use of early antibiotics
Early life (including the embryonal period and infancy) is a critical time for the colonization and formation of gut flora. Disturbance of the gut flora during this period can have long-term health effects and may increase the risk of metabolic diseases such as obesity and T2DM. Intestinal flora colonization occurs mainly at birth and several days after birth as the fetus is relatively aseptic in the mother’s uterus. During delivery and breastfeeding, intestinal colonization occurs, which gradually establishes the infant’s intestinal flora (Cox and Blaser, 2015). In the first few weeks after birth, the microbiota in the body is similar to the mother’s vagina and skin flora, including Enterococcus, Streptococcus, Lactobacillaceae, Clostridae, and Bifidobacteriaceae. In addition, the flora in breast milk is also a source of intestinal flora in infants. Various dietary components, such as polysaccharides that cannot be digested by enzymes, can increase Enterococcus, Clostridium, and Ruminococcus and decrease Bifidobacterium and Enterococcus concentrations in the intestinal flora. As a result of the decrease in Bifidobacteria, the pH in the intestine increases, and the concentration of Bacillus, Clostridium, Lactobacillus, Streptococcus, and fungi increases. At the age of 1 year, the intestinal flora begins to resemble that of adults, and at the age of 3 years, it is basically similar to that of adults. Colonization of the intestinal flora resists the colonization and reproduction of pathogenic microorganisms and is essential for energy metabolism, growth and development, and maturation of the immune system in infants. The main factors affecting the establishment of gut flora early in life include intrauterine microbial exposure, delivery pattern, feeding pattern, diet, geographic environment, behavioral habits of infants and young children, and antibiotic use (Cox and Blaser, 2015). Among them, the effect of antibiotics in the resistance to pathogen invasion has been extensively examined. Additionally, antibiotic-induced microbiota interferes with host metabolism. Long-term high use of antibiotics can lead to changes in the structure of the gut symbiotic flora, leading to the growth of potentially pathogenic microorganisms, causing intestinal flora disorders and infection, thus, affecting host metabolism, resulting in the occurrence of metabolic diseases in adults (Cox and Blaser, 2015). Studies have shown that early intestinal microbial deviation precedes the development of obesity and overweight in childhood and adulthood, suggesting that changes in the composition of intestinal microbes that affect energy metabolism might begin in early life (Kalliomäki et al., 2008; Scheepers et al., 2015; Wilkins and Reimer, 2021). Studies have found that a reduction in the intestinal Bifidobacteria at the age of 3 months is associated with being overweight at the age of 10 years (Luoto et al., 2011). Aversa Z et al. have found that early antibiotic exposure was associated with an increased risk of childhood-onset asthma, allergic rhinitis, atopic dermatitis, celiac disease, overweight, obesity, and attention deficit hyperactivity disorder (Aversa et al., 2021).
Antibiotics are important for treating infectious diseases, but their long-term high use can lead to changes in the structure of the intestinal symbiotic flora, causing the growth of potentially pathogenic microbes and, thus, intestinal flora disorders and the emergence of drug-resistant strains (Wilkins and Reimer, 2021). The effect of antibiotics on the microbiome depends on the duration, dose, and frequency of treatment and age of the person (Vandenplas et al., 2020; Wilkins and Reimer, 2021). The greatest disruption to microbiota development occurs mainly in early life (McDonnell et al., 2021). According to statistics, 78% of mothers in Denmark received antibiotic treatment before, during, and 4 years after pregnancy (Stokholm et al., 2014). The use of antenatal antibiotics has been shown not only to disrupt the spread of microbiota from mother to child but also to have an impact on the birth weight of newborns associated with an increased risk of future obesity and related metabolic sequelae (Vidal et al., 2013). Antibiotics are widely used in infancy and childhood in the United States (Hicks et al., 2013). On average, American children receive nearly 3 antibiotics at age 2 years (McCaig et al., 2002). During the first 2 years of life in children exposed to antibiotics, the composition of the intestinal flora significantly changes, and the risk of obesity increases (Bailey et al., 2014; Wilkins and Reimer, 2021). In addition, because antibiotics are widely used to promote livestock growth, American infants might potentially be exposed to antibacterial agents from other sources, such as food supply chains or drinking water (Andersson and Hughes, 2014; Cox and Blaser, 2015). The extent to which these non-medical exposures affect the development of the early microbiome, as well as their impact on the health of the elderly, is an important topic for future research.
The development of microbiota in early life influences long-term metabolic function. Interference of antibiotics with the normal development of the gut microbiota can alter intestinal flora, usually only temporarily, but the metabolic consequences might persist over the long term or affect the health of the elderly, especially when animals experience obesity-causing diets, such as a diet high in fat or sucrose (Wilkins and Reimer, 2021). The use of broad-spectrum antibiotics significantly alters the composition of the gut microbiota, reducing diversity by more than 25%. However, once treatment is complete, changes in the microbiota will resume (Panda et al., 2014; Wilkins and Reimer, 2021). A worrying long-term effect of antibiotic use is their potential to produce antibiotic-resistant genes in microorganisms (Jernberg et al., 2010). Antibiotic resistance genes can alter insulin sensitivity, increase inflammatory cytokines levels, and alter SCFA metabolism and bile acid production, all of which represent potential mechanisms for microbiome-induced metabolic diseases (Montassier et al., 2021). Early use of antibiotics reduces the abundance of Bifidobacteria, the primary intestinal microorganism of newborns, and increases the ratio of Firmicutes/Bacteroidetes (Wilkins and Reimer, 2021). Increased prenatal exposure to antibiotics in infants, 3 and 12 months postpartum, is associated with childhood obesity (Tun et al., 2018). By reusing antibiotics in infants, feces collection for 24 months has found a decrease in the microbial co-abundance group (CAG) represented by Fusarium oxysporum, which is negatively associated with childhood obesity (Chen et al., 2020). A lifelong survey of antibiotic use in Finnish children has found that macrolide antibiotics were associated with changes in the composition of the microbiota, a decrease in actinomycetes, and an increase in agrobacteria and amoeba and had a positive correlation with body mass index (BMI) (Korpela et al., 2016). The gate level recovers after a year of withdrawal, but microbial richness decreases over time. In addition, the researchers have found that increased BMI was most correlated with antibiotic use when participants were exposed to prenatal antibiotics for ≥3 treatments (Zhang et al., 2019). Exposure to antibiotics early in life might lead to persistent and significant changes in the gut microbiota and alterations in metabolic function. It can be hypothesized that inappropriate use of early antibiotics leads to persistent and significant changes in the gut flora or might increase the risk of metabolic disease in adults or older adults.
2.1.2 Intestinal flora associated with aging
As aging occurs, an increased abundance of pro-inflammatory flora can inhibit the growth of beneficial flora, leading to chronic low-grade inflammation and increasing the risk of various aging-related diseases. Many studies in animals and humans have shown that the composition of the gut microbiota varies with host age, with certain microorganisms having detrimental effects on health (Table 1). Compared with young individuals, the gut microbiota of the elderly is less diverse (Mangiola et al., 2018; Xu et al., 2021). Studies have shown that the abundance of Bifidobacteria and some members of Firmicutes with anti-inflammatory properties, including Clostridium cluster IV (Ruminococcus obeum et rel., Roseburia intestinalis et rel., Eubacterium ventriosumet rel., Eubacterium rectale et rel., and Eubacterium hallii et rel.), and Clostridium cluster XIVa (Papillibacter cinnamovorans et rel. and Faecalibacterium prausnitzii et rel.), was decreased in the elderly and centenarians (Biagi et al., 2010). In Italian centenarians, the abundance of beneficial bacteria (Ruminococcaceae, Lachnospiraceae, and Bacteridaceae families) found in the gut flora was decreased with aging (Biagi et al., 2016). In addition, studies have reported that the gut microbiomes of healthy elderly and healthy young individuals from the same population are similar in terms of composition (Bian et al., 2017). Yuping Yang et al. have found that Rhodococcus spp. was significantly abundant during the middle-old age stage (Yang et al., 2021). This genus contributed greatly to L-tryptophan, catechol, and inositol degradation pathways, as well as ectoine and L-arginine biosynthesis pathways. Gut bacteria-encoded functions, such as amino acid metabolism, B vitamin metabolism, aromatic compound metabolism, and energy metabolism, varied in an age-dependent manner, and Rhodococcus spp. was the most associated functional bacteria in middle-old-aged rats (Yang et al., 2021). As shown in Table 1, health-related genera, such as Ackermanella, Bifidobacterium, and Christensonaceae, and microbial diversity are enriched in long-living populations (Biagi et al., 2016; Kong et al., 2016). Despite changes in microbes in long-lived populations, their intestinal microbial diversity and beneficial microbes are preserved to support healthy aging. Overall, the composition of gut microbes changes while microbial biodiversity decreases as potential pro-inflammatory microbes accumulate, and beneficial microbes decrease with age. Therefore, maintaining the dominant position of beneficial bacteria in the gut might prevent chronic low-grade inflammation and promote healthy aging.
This age-related gut microbial disorder affects host health and longevity (Du et al., 2021). In fruit flies and mice, age-related microbiota disorders could lead to gut barrier dysfunction, a pathophysiological sign of aging (Clark et al., 2015; Thevaranjan et al., 2017). In addition, the genetic composition and metabolites of microorganisms can have a positive impact on the life of the host (Langille et al., 2014; Han et al., 2017). Therefore, gut microbes might play a regulatory role in aging.
2.2 Change in intestinal barrier integrity
The intestinal barrier is one of the largest and most important internal barriers of the body. It can protect the body from harmful substances and microorganisms present in the intestinal cavity. The gut barrier consists of immune cells in the mucus layer, gut flora, intestinal epithelial cells, and intrinsic layers (Kocot et al., 2022). The mucus layer is composed of mucoglycoproteins secreted by cup cells in the intestinal epithelium to prevent contact between microorganisms in the intestinal cavity and intestinal epithelial cells (Knoop and Newberry, 2018). Intestinal epithelial cells provide a physical barrier to prevent material leakage from the intestinal cavity (Vancamelbeke and Vermeire, 2017). The paracellular gap between intestinal epithelial cells is sealed by tight junctions (TJs), adhesion junctions, and desmosomes (Vancamelbeke and Vermeire, 2017; Buckley and Turner, 2018; Kocot et al., 2022). Adhesion junctions and desmosomes adhere directly to intestinal epithelial cells, while TJs lie between the sides of intestinal epithelial cells. TJs are composed of transmembrane proteins, including claudins, occludins, and peripheral membrane proteins (zonula occludens [ZO] and regulatory protein) (Buckley and Turner, 2018; Kocot et al., 2022). Impaired inter-cell connectivity can lead to increased intestinal permeability, thereby increasing the transport of inflammatory mediators and contributing to chronic low-grade inflammation. Immune cells in the intrinsic layer can secrete immunoglobulins, such as IgA, that bind bacteria and their toxins to prevent their translocation into the body (Allaire et al., 2018). The gut microbiota protects the integrity of the gut barrier through various functions, such as combating pathogen colonization and stimulating the production of mucus, antimicrobial proteins, and regulatory T (Treg) cells (Allaire et al., 2018).
Intestinal barrier dysfunction caused by changes in the intestinal barrier integrity is the core mechanism of metabolic disease and related gastrointestinal manifestations. Man et al. (2015) have found a decrease in the transepithelial electric resistance (TEER) of the living ileal tissue in the aging population. Age-related changes in the gut flora are closely related to increased gut permeability, leading to gut barrier dysfunction. Age-related ecological disorders of the gut microbiome, thinning of the mucin layer, and increased endothelial clearance are the reasons for the increased permeability of the mucosal barrier, which allows the translocation of microbes, toxins, and antigens into the circulation (Wilson et al., 2018). Gut barrier dysfunction can lead to immune cell infiltration and chronic low-grade inflammation of the gut mucosa, activating the immune response (Singh et al., 2021; Wei et al., 2021). Some studies have shown that the gut microbiome is an important part of neuroimmune crosstalk and that some immune cells, such as muscularis macrophages (MMs), act as intermediaries between ENS and gut microbes. Hunger of microbiota, for example, in the case of reduced fiber consumption, may increase microbiota dependence on mucopolysaccharides, leading to the degradation of mucus layers and increased susceptibility to pathogens, which further results in immune activation (Desai et al., 2016). Ruminococcus gnavus, Akkermansia muciniphila, and Ruminococcus torques are mucosa-associated bacteria. Some studies have shown that their changes are related to changes in mucus and gut secretions (Paone and Cani, 2020). In addition, under susceptible conditions, the consumption of certain pathogens or certain foods can cause a tightly linked change through proteasome-mediated degradation triggered by inflammatory mediators, such as proteases, aspartons, and histamines, which can disrupt the gut barrier function (Bischoff et al., 2014). MMs are immune cells that promote bone morphogenetic protein 2 (BMP2) expression and dependence on the secretion of colony stimulant 1 receptor (CSF-1R), a cytokine receptor. BMP2 stimulates the expression of BMP receptors I and II (BMPRI and BMPRII). The development of neurons and smooth muscle cells depends on BMP receptors. Studies have shown that when mice were treated with antibiotics, the expression of CSF-1R and BMP2 and MM number decreased. Hence, the microbiome is an important part of neuroimmune crosstalk during inflammation, with immune cells acting as an important intermediary between the ENS and gut microbiome (Muller et al., 2014). Metabolic disorders are closely related to chronic low-grade inflammation. Elevated levels of pro-inflammatory cytokines (TNF- α, IL- 1β, and IL-6) have been found in the circulation of obese mice and humans, causing insulin resistance and T2DM (Olefsky and Glass, 2010). Recently, a study published in Science has found that in T2DM and obese mouse models, hyperglycemia led to intestinal barrier dysfunction through the recombination of glucose transporter 2-dependent intestinal epithelial cells, altered tight junctions, and adhesion protein integrity (Thaiss et al., 2018). As a result of the high blood sugar-mediated destruction of the epithelial barrier, systemic inflows of microbial products can enhance the distribution of gut microbiome products, leading to intestinal infections. In addition, a recent study has characterized the age-related changes that occur to septate junctions (SJ) between adjacent absorptive enterocytes (EC) in the fly intestine. The study has found that acute loss of the Drosophila tricellular junctions (TCJ) protein gliotactin (Gli) in ECs led to rapid activation of stress signaling in stem cells and an increase in intestinal stem cell (ISC) proliferation. Furthermore, a gradual disruption of the intestinal barrier was observed (Resnik-Docampo et al., 2018).
Following dysregulation of the intestinal flora, the abundance of pro-inflammatory bacteria increases, whereas that of anti-inflammatory bacteria decreases. These changes affect the integrity of the intestinal barrier and exert harmful effects on the body. Accumulating epidemiological, experimental, and clinical data indicate a strong correlation between changes in intestinal barrier integrity and chronic low-grade inflammation. A study has found that the aging-related microbiome promoted intestinal permeability and inflammation and increased the levels of pro-inflammatory cytokines, such as IL-6 and TNF (Ticinesi et al., 2019). Fransen et al. (2017) have transplanted the gut flora of elderly mice into the gut of young sterile mice. They have found that decreased levels of Akkermansia and increased levels of TM7 bacteria and amoeba phyla led to increased intestinal permeability and inflammation. In addition, some bacterial metabolites (e.g., phenylacetic acid and trimethylamine) can induce pro-inflammatory cytokines, destroy intestinal barrier integrity, and promote chronic low-grade inflammation (Tilg et al., 2020).
Pathogen-associated molecular patterns (PAMPs) are the main microbial factors contributing to chronic low-grade inflammation due to changes in intestinal barrier integrity caused by the dysregulation of gut flora. PAMPs are small molecules with conserved patterns, which are present in various microorganisms, including components that construct cell walls (bacterial peptidoglycan, lipopolysaccharide [LPS], lipoteichoic acid, and flagellate protein) and other factors common for microorganisms (viral RNA or DNA) (Dammermann et al., 2013). PAMPs are recognized by the pattern recognition receptor, which is present on the surface and in the cytosol of immune cells and intestinal epithelial cells (Schroder and Tschopp, 2010). Receptor families belonging to pattern recognition receptors include toll-like receptor (TLR), nucleotide-binding domain and leucine-rich repeat receptor (NLR), C-type lectin receptor (CLR), retinoic acid-inducing gene-I-like receptor (RLR), and absent in melanoma 2 (AIM2)-like receptor (ALR) (Potrykus et al., 2021). The binding of PAMPs to their respective specific receptors activates the innate immune system, thereby causing inflammation, enhancing damage to the intestinal barrier, and exacerbating chronic low-grade inflammation (Rajaee et al., 2018; Potrykus et al., 2021). A simplified representation of this process is shown in Figure 1.
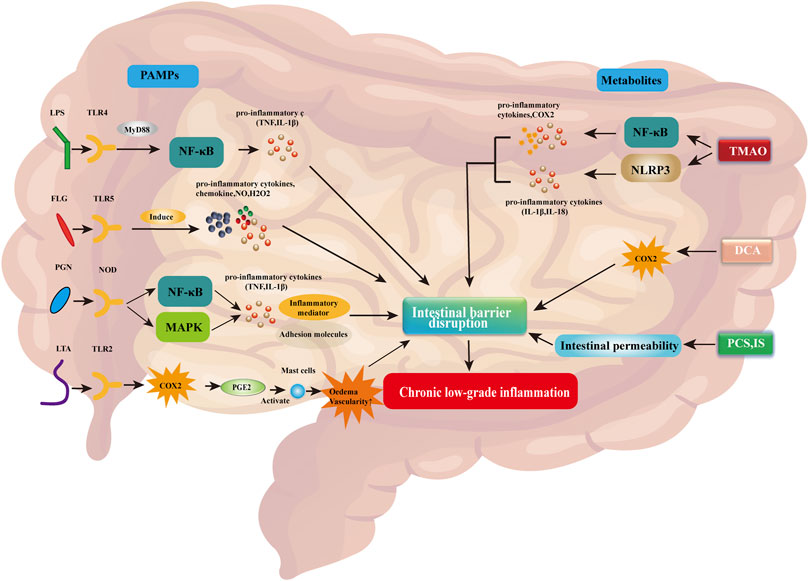
FIGURE 1. PAMPs and associated metabolites disrupt the intestinal barrier, thereby contributing to chronic low-grade inflammation. Abbreviations: COX2, cyclooxygenase 2; DCA, deoxycholic acid; FLG, flagellin; IL-1β/18, interleukin 1 beta/18; H2O2, hydrogen peroxide; IS, indoxyl sulfate; LPS, lipopolysaccharide; LTA, lipoteichoic acid; MAPK, mitogen-activated protein kinase; MyD88, myeloid differentiation factor 88; NF-κB, nuclear factor kappa B; NLRP3, NLR family pyrin domain-containing 3; NO, nitric oxide; NOD, nucleotide-binding oligomerization domain; PAMPs, pathogen-associated molecular patterns; pCS, p-cresol sulfate; PGE2, prostaglandin E2; PGN, peptidoglycan; TLR2/4/5, toll-like receptor 2/4/5; TMAO, trimethylamine N-oxide; TNF, tumor necrosis factor.
LPS is a component of the extracellular wall of Gram-negative bacteria and can be identified by TLR4. Activation of this receptor leads to the release of pro-inflammatory mediators, including myeloid differentiation factor 88 (MyD88), which stimulates the production of pro-inflammatory cytokines (e.g., TNF and IL-1β) by the nuclear factor kappa-light chain-activated B cells (NF-κB) pathway (Gnauck et al., 2016; Potrykus et al., 2021). Flagelloprotein can activate TLR5. TLR5 is mainly expressed on the basolateral side of intestinal epithelial cells and detects the translocation of bacteria through the endothelial barrier (Yang and Yan, 2017). Activation of TLR5 by flagelloprotein leads to the synthesis of chemokines, nitric oxide (NO), hydrogen peroxide (H2O2), and pro-inflammatory cytokines (Hajam et al., 2017). Excessive activation of TLR5 leads to impaired intestinal barrier integrity and causes chronic low-grade inflammation (Yang and Yan, 2017). Peptidoglycan (PGN) is an important cellular structure that protects bacteria from environmental factors. It is recognized by PGN recognition protein, which is secreted by immune and epithelial cells and can dissolve bacterial cell walls. In addition, the identification of PGNs by nucleotide-binding oligomerization domain containing 1 (NOD1) and NOD2 receptors leads to the activation of NF-κB and mitogen-activated protein kinase (MAPK) pathways (Caruso et al., 2014). Both pathways contribute to the transcription of pro-inflammatory genes, leading to the synthesis of cytokines, adhesion molecules, and inflammatory mediators. Lipoteichoic acid is a component of the cell wall of Gram-positive bacteria. It is recognized by TLR2, thereby activating the respective signaling pathway. This process induces cyclooxygenase (COX) expression, which promotes the synthesis of prostaglandin E2 (PGE2) (Tominari et al., 2021). PGE2 causes edema and vascular permeability by activating mast cells, thereby promoting inflammation (Tominari et al., 2021).
Abnormal metabolites caused by gut flora dysregulation can also contribute to inflammation, enhance damage to the gut barrier, and exacerbate chronic low-grade inflammation. Trimethylamine N-oxide is a bacterial metabolite. Elevated trimethylamine N-oxide levels can induce the activation of the NLR family bilin-containing domain 3 (NLRP3). NLRP3 belongs to the inflammasome, which is the intracellular protein complex responsible for initiating inflammatory processes. Activation of NLRP3 activates caspase 1 (CASP1) to regulate the maturation and secretion of pro-inflammatory cytokines IL1B and IL18 (Potrykus et al., 2021). In addition, trimethylamine N-oxide activates NF-κB, thereby promoting the synthesis of pro-inflammatory proteins, such as COX2, selectin E (SELE), IL6, and intracellular adhesion molecule 1 (ICAM1) (Yang et al., 2019). Intestinal microorganisms cause bile acids in the intestines to detach and form secondary bile acids, such as deoxycholic acid. Elevated deoxycholic acid levels can lead to the overproduction of COX2, which is closely related to impaired intestinal epithelial integrity and intestinal inflammation (Zhao et al., 2016). In addition, high levels of bile acids contribute to the formation of reactive oxygen species and nitrogen substances. Moreover, toxic bacterial metabolites, such as p-cresol sulfate and indoxyl sulfate, can lead to increased intestinal permeability and exacerbate chronic low-grade inflammation (Li and Tang, 2018).
2.3 Chronic low-grade inflammation in aging
Chronic low-grade inflammation can promote the onset of age-related diseases, such as T2DM, obesity, metabolic syndrome, neurodegeneration, cardiovascular disease, and decreased immunity (Figure 2) (Calder et al., 2017). There is a significant relationship between mild inflammatory status and major diseases of the elderly (e.g., cardiovascular disease and T2DM), as well as disability and mortality (Calder et al., 2017; Mengozzi et al., 2021). Age-related chronic low-level inflammation can alter the relationship between the gut-related immune system and gut microbiota, leading to changes in the latter (Mello et al., 2016). A study has investigated the relationship between the composition of the gut microbiota and the inflammatory state of centenarians. Increased levels of IL-6 and IL-8 in peripheral blood were associated with the enrichment of amoeba phyla and a decrease in some butyric acid-producing bacteria (Biagi et al., 2010). Therefore, age-related modifications in the gut microbiota might contribute to chronic low-level inflammation on one side or be affected by systemic inflammation on the other side (Mello et al., 2016).
A previous study has reported that high levels of fibrinogen were associated with T2DM in older adults (Spazzafumo et al., 2013). A cohort study of older individuals in Italy has found that high circulating levels of inflammatory-related mediators, such as IL-6, interleukin-1 receptor antagonist (IL-1RA), and TNF receptor II (TNFR2), were associated with the occurrence of chronic diseases (i.e., hypertension, diabetes, ischemic heart disease, congestive heart failure, and cognitive impairment) (Fabbri et al., 2015). In addition, it was demonstrated that the baseline levels of IL-6 were markedly increased with aging. Furthermore, these alterations were significantly and independently correlated with a rapid increase in the incidence of multiple diseases over time (Fabbri et al., 2015). A meta-analysis of 31 cross-sectional studies has revealed significant associations of inflammatory markers, such as C-reactive protein and IL-6, elevated white blood cell counts, and fibrinogen levels with weakness as a health outcome (Soysal et al., 2017).
3 Probiotics, chronic low-grade inflammation, and aging-related diseases
The World Health Organization defines “probiotics” as living microorganisms that are beneficial to host health when present in sufficient quantities (Binda et al., 2020). Probiotic supplements directly influence the composition of gut flora through the introduction of healthy bacteria. Through the interaction with the gut microbiota, they influence the immune function of the whole body and mucosa and improve the gut barrier function (Jukic Peladic et al., 2021; Martel et al., 2022). In addition, probiotics can significantly reduce inflammatory biomarkers in middle-aged and elderly individuals (Warman et al., 2022). As mentioned above, chronic low-level inflammation is an important mechanism for age-related diseases. Studies in an elderly cohort (>65 years old) have revealed that administration of Bifidobacterium resulted in an increased abundance of this organism in stool samples, increased stool frequency, and reduced inflammatory status (Duncan and Flint, 2013). Lahtinen et al. have found that older adults who took Bifidobacterium longum, a longum fermented oat beverage for 6 months, had increased abundance of B. catenulatum, B. bifidum, and B. breve compared to the control group (Lahtinen et al., 2009). Bartosch et al.’s study has found that Bifidobacterium bifidum and B. lactis, given to the elderly population, increased Bifidobacteria abundance in the gut (Turchet et al., 2003). A recent meta-analysis has shown that probiotic interventions reduced IL-6 and C-reactive protein levels in middle-aged and older adults with chronic low inflammation (Custodero et al., 2018). Pan et al. (Pan et al., 2018) have observed that Lactobacillus murinus CR147 downregulated IL-8 production in TNF-stimulated Caco-2 cells and significantly increased the lifespan and the brood size of the C. elegans, indicating that the proliferation of Lactobacillus murinus in calorie-restricted mice causatively contributed to the attenuation of aging-associated inflammation. There is growing evidence in the literature describing the potential to regulate chronic mild inflammation through the use of probiotic supplements. Furthermore, the potential effects of these nutritional interventions on intestinal barriers and age-related diseases (e.g., metabolic diseases, cardiovascular diseases, neuroinflammation, and decreased immune function) have been emphasized.
Metabolic diseases and cardiovascular diseases frequently occur in the elderly (Khosla et al., 2020). Aging and obesity are major risk factors for chronic inflammatory diseases such as diabetes and atherosclerosis, while T2DM and its complications can seriously affect the quality of life of older persons. T2DM and cardiovascular disease are common metabolic diseases characterized by chronic low-grade inflammation. Recently, numerous studies have reported the beneficial effects of probiotics in alleviating obesity and T2DM. Probiotics can improve obesity by reducing lipid accumulation, oxidative damage, inflammation, and intestinal disorders. Lactobacillus curvatus HY7601 and Lactobacillus plantarum (L. plantarum) KY1032 have reduced overweight and fat accumulation in the high-fat diet mouse group while reducing the levels of inflammatory biomarkers (Park et al., 2013). Lactobacillus, particularly Lactobacillus casei (IMVB-7280 and IBS041), Lactobacillus paracasei (HII0 and CNCM I-4034), and Lactobacillus rhamnosus (L. rhamnosus; CGMCC1.3 and LA68), plays a positive role in reducing weight gain, cholesterol levels, obesity, and inflammation (Wiciński et al., 2020). In addition, probiotics might influence fat metabolism and the levels of alanine transaminase in obese rats (Karimi et al., 2015). Diabetes is a chronic metabolic disease characterized by a sustained increase in serum glucose. There is a significant correlation between inflammatory status and metabolic disorder in patients with diabetes (Rastogi and Singh, 2022). The consumption of probiotics has an effect on the composition of the microbiota of the intestinal tract. In turn, this reduces the intestinal epithelium and inhibits the immune response by blocking the TLR4 signaling pathway, ultimately increasing insulin sensitivity (Li et al., 2021; Rastogi and Singh, 2022). L. plantarum Y44 downregulates the expression of pro-inflammatory cytokine genes in the liver, intestine, and muscle tissue by activating the regulatory anti-inflammatory cytokine IL-10 (Liu et al., 2020). In streptozotocin-induced diabetic rats, the administration of Lactobacillus fermentum MCC 2759 was associated with decreased levels of glucose (detected through spectroscopy), pro-inflammatory cytokine IL-10, insulin sensitivity (glucagon-like peptide 1 [GLP-1], glucose transporter type 4 [GLUT-4], and adiponectin), and intestinal barrier integrity (ZO-1) and enhanced expression of TLR4 receptors (Qu et al., 2018). Although research on the role of probiotics in metabolic diseases has been mainly conducted in animal models or young people, clinical trials are still lacking, especially in older adults. However, considering obesity and T2DM as age-related diseases, probiotics should be beneficial for older patients with obesity and T2DM.
Cardiovascular disease is a major cause of death worldwide (Youssef et al., 2021). Aging is an important risk factor for cardiovascular disease. Systemic low-grade inflammation, altered gut microbiota composition, and increased gut permeability are important features of aging and are closely related to the occurrence of cardiovascular disease (Gargari et al., 2022). Probiotics enhance the basic functions of cardiovascular and metabolism-related organs by protecting the gut barrier, regulating chronic low-grade inflammation, and maintaining intestinal homeostasis. By regulating the gut microbiome in older mice (26–27 months old), consistent with that observed in younger mice (5–6 months old), arterial function was preserved, while vascular oxidative stress and inflammation weakened (Brunt et al., 2019). A study has shown that age-related ecological disorders of microorganisms contribute to vascular aging. In addition, aging is associated with a larger abundance of the genus Desulfovibrio, leading to increased plasma trimethylamine N-oxide (TMAO) levels and risk of atherosclerosis (Brunt et al., 2019). Giorgio Gargari et al. (Gargari et al., 2022) have found that polyphenols-rich diets reduce intestinal permeability, inflammation, and lipid abnormalities in older populations, thereby reducing cardiovascular risk. A study has found that Akkermansia muciniphila can effectively inhibit atherosclerotic damage in apolipoprotein E (ApoE)−/− mice by increasing the expression of TJ protein, improving restoration of the gut barrier, and reducing endotoxin-induced inflammation (Li et al., 2016). A placebo-controlled trial has shown that high doses of L. plantarum (DSM9843) effectively reduced atherosclerotic plaques by altering gut flora and increasing SCFAs (Karlsson et al., 2010). Furthermore, hypertension is an important risk factor for cardiovascular disease. Specific probiotic strains, such as Lactobacillus fermentum, Lactobacillus gasseri, and Lactobacillus coryniformis, have shown their ability to prevent hypertension and endothelial dysfunction in rat models, as well as to promote innate immunity. In addition, they can inhibit inflammation by restoring the balance between auxiliary T helper 17 (Th17) and Treg cells (Toral et al., 2018). Moreover, high-density lipoproteins reduce the risk of cardiovascular disease by limiting the accumulation of low-density lipoproteins in the blood vessel walls. L. plantarum can help reduce the levels of total cholesterol and triglycerides and improve hypercholesterolemia by increasing the concentration of high-density lipoproteins and lowering that of low-density lipoproteins (Lee et al., 2018). In general, probiotics have a significant impact on the prevention and treatment of cardiovascular diseases.
The effect of probiotics on neuroinflammation mainly depends on the exchange of nerve, hormone, and immune signals between the gastrointestinal tract and central nervous system, i.e., the “intestine-brain axis.” Among them, Alzheimer’s disease (AD) and Parkinson’s disease (PD) are the most common, as they are observed in one-tenth of people aged >65 years (Hansson, 2021). Recent advances in microbiome research help understand two-way gut-microbiome-brain communication, revealing possible links between intestinal ecological disorders and neuroinflammatory diseases (Roy Sarkar and Banerjee, 2019). There is evidence indicating that probiotics and their beneficial metabolites play a significant role in reducing neuroinflammatory and neurodegenerative diseases in experimental models or clinical settings, including multiple sclerosis, PD, and AD. AD, the most common neurodegenerative disease, is the leading cause of dementia in the elderly, accounting for about 60%–80% of total cases. The intestinal microbiota of patients with AD significantly differs from that of healthy individuals. It is characterized by a reduced abundance of Firmicutes and Bifidobacterium and an increased abundance of Bacteroidetes (Brunt et al., 2019). Similar microbiological changes have been reported in healthy older adults and might be a potential contributor to age-related cognitive impairment tendencies (Kong et al., 2019). Lactobacillus mucosae NK41, Lactobacillus reuteri NK33, Bifidobacterium longum NK46, and Bifidobacterium adolescentis NK98 can block the NF-κB pathway and reduce the levels of LPS, corticosterone, IL-6, and TNF in serum, thus, decreasing stress-induced anxiety/depression in mice (Jang et al., 2019). Additionally, Lactobacillus acidophilus, Bifidobacterium longum, and Bifidobacterium bifidum enhance spatial learning and memory in rats (Rezaei Asl et al., 2019). PD is one of the most common neuroinflammatory diseases, with aging considered the most important risk factor because the median age for PD onset is 60 years (Simon et al., 2020). Most patients with PD suffer from gastrointestinal diseases such as constipation, nausea, and vomiting, as well as increased intestinal permeability. Studies have found that giving multi-species supplements containing strains of Lactobacillus, Bifidobacterium, and Streptococcus to older adults with PD led to better bowel habits (Barichella et al., 2016) and reduced abdominal pain and bloating (Georgescu et al., 2016). Moreover, multiple sclerosis is another age-related neuroinflammatory disorder. Studies have reported that oral Lactobacillus casei, Lactobacillus acidophilus, and Lactobacillus reuteni delay the progression of multiple sclerosis by increasing the number of forkhead box P3 (Foxp3)+ and IL-10+ Treg cells and reducing pro-inflammatory Th1/Th17 polarization in the peripheral immune system and inflammatory sites (Kwon et al., 2013).
Decreased immune function is associated with chronic low-grade inflammation. This decreased immune function includes the loss of B and T lymphocytes, Th2-type cell-mediated immune response polarization, and changes in myeloid cell recruitment and phagocytosis that lead to increased susceptibility to numerous age-related diseases (Calder et al., 2017). Decreased immune function and chronic low-grade inflammation are also significant features of aging and age-related diseases, such as respiratory tract infections and cancer. Probiotics exert immunomodulatory effects, improving the decline in immune function related to those diseases. The ability of probiotics to regulate the immune system has been studied with the aim of preventing and/or limiting the effects of immune aging (Sharma et al., 2014). Several strains of Bifidobacterium and Lactobacillus probiotics have had a positive effect in reducing inflammation and the duration of winter infections in the elderly population (Ouwehand et al., 2008). Both Lactobacillus rhamnosus HN001 and Bifidobacterium lactis HN019 have increased natural killer cells and phagocytic activity in healthy elderly subjects (Mello et al., 2016). They also regulate lung immunity and promote respiratory health by regulating the intestinal-lung axis in both directions. A previous study has reported that L. rhamnosus CRL1505 increased the levels of TNF, interferon beta (IFN-β), IFN-α, and IFN-cytokine in the lungs, thereby significantly reducing respiratory inflammation (Kolling et al., 2018). In a model of allergic asthma, L. rhamnosus GR-1 has protected the gut barrier by regulating Th2-mediated immune responses and altering the composition of the gut microbiome. This probiotic passes through the gut-lung axis, thus, significantly reducing the severity of airway inflammation and hyperactivity (Spacova et al., 2020). In addition, certain strains of Lactobacillus secrete metabolites, particularly SCFAs (e.g., acetate, propionate, and butyrate), which can regulate the immune response of the host lung (Koh et al., 2016).
Cancer is the second leading cause of death worldwide after cardiovascular disease. Most patients with sporadic cancers are over 50 years old, and 75% of rectal cancer patients and 80% of colon cancer patients are over 60 years old (Haraldsdottir et al., 2014). Increased local inflammatory response, leading to the loss of intestinal barrier integrity and systemic inflammation, is one of the pathological mechanisms responsible for gastrointestinal cancer (Irrazábal et al., 2014). Intestinal microbial ecological disorders and the subsequent development of pathogenic flora may affect host metabolism or host intestinal and immune system function, leading to tumor growth (Vivarelli et al., 2019). However, some probiotic strains can directly regulate immune responses. Injection or oral administration of Bifidobacteria can effectively regulate the anti-cancer immune response in mice and inhibit cancer growth (Abdolalipour et al., 2022). Studies have shown that specific probiotic strains inhibit variants of common pathogens in the gut, such as Escherichia coli, Salmonella intestinalis, and Clostridium pneumoniae (Górska et al., 2019; Sehrawat et al., 2021). These pathogens secrete enzymes that convert pre-carcinogens into carcinogens, such as β-glucosylase, azo-reductase, and nitro-reductase (Pellegrini et al., 2020).
These studies have suggested that altering the gut microbiota of older populations by ingesting probiotics might be an effective strategy against aging and age-related diseases. At the same time, probiotics might be suitable and affordable for most older people. However, their health effects are complex, depending on the individual population and the duration of treatment. For reasons of effectiveness and safety, the development of probiotics beneficial to human health must consider possible high individual differences.
4 Mechanism of action of probiotics
Probiotics suppress chronic low-grade inflammation. The health-promoting effects of probiotics are attributed to the maintenance of a balanced abundance of Firmicutes, Bacteroidetes, Actinobacteria, and Proteobacteria, protection of the gut barrier, and achievement of an optimal immune balance (Tsai et al., 2019). Studies have shown that some probiotics can achieve effective therapeutic results by shifting the composition of microbiota to a more balanced structure (Pandey et al., 2015; Liu et al., 2018). Probiotics influence all components of the gut barrier, including the gut microbiome, mucus barrier, epithelial cells, the intrinsic layer rich in lymphocytes and plasma cells, vascular and neural components of the intrinsic layer, and mesenteric lymph nodes linked to the systemic immune system (Liu et al., 2018). Probiotics can produce key metabolites with anti-inflammatory effects, such as SCFAs, L-tryptophan (Trp) metabolites, adenosine, and histamine, that can regulate local and systemic metabolites and inhibit chronic low-grade inflammation (Figure 3).
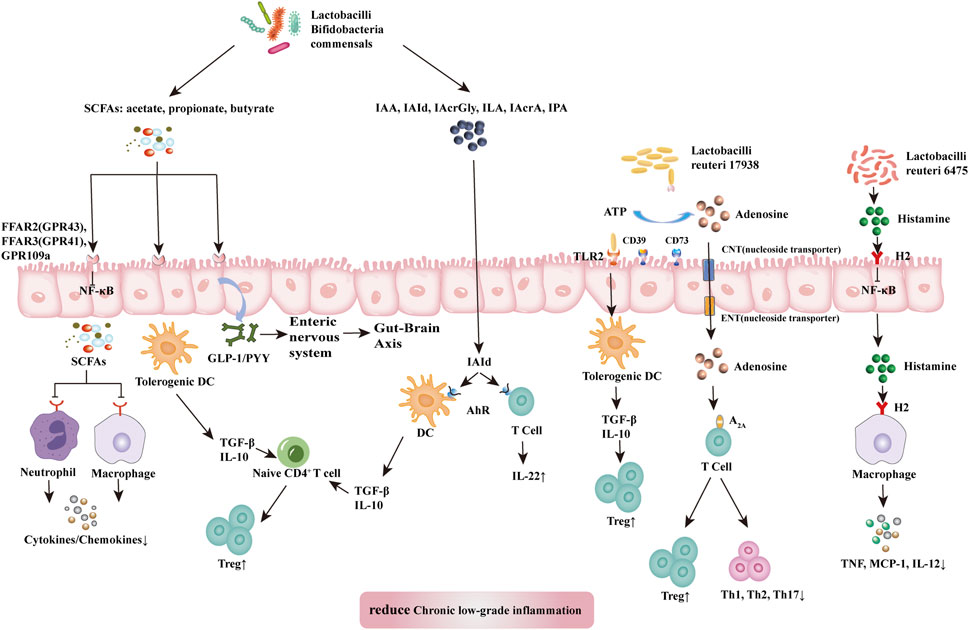
FIGURE 3. Mechanism of probiotic inhibition of chronic low-grade inflammation. SCFAs (acetate, propionate, and butyrate) produced by lactobacilli, bifidobacilli, and commensals bind and activate receptors (FFAR2, FFAR3, or GPR109a) on intestinal epithelial cells to inhibit the nuclear factor kappa-light-chain-enhancer of activated B cells (NF-κB) pathway to prevent inflammation. They also inhibit histone deacetylases from promoting the accumulation of Tregs and might release GLP1/PYY to act on the enteric and central nervous systems to influence energy homeostasis and gut motility. SCFAs also induce tolerogenic DCs, which educate naïve CD4+ T cells to differentiate into Tregs. These actions inhibit cytokine production by neutrophils and macrophages via interaction with receptors. Dietary tryptophan and probiotic-produced indole derivatives interact with AhR expressed on immune cells to produce anti-inflammatory effects. L. reuteri 17938 promotes adenosine generation, most likely by an ectonuclease present on the probiotic itself and intestinal epithelial cells. Adenosine and its derivative inosine interact with adenosine receptor-2A located on T cells to promote Treg functions and inhibit inflammatory Th1 and Th17 subsets. Histamine produced by L. reuteri 6,475 interacts with H2 presented on intestinal epithelial cells and macrophages to reduce levels of proinflammatory cytokines (TNF, MCP-1, and IL-12). In summary, the critical metabolites produced by probiotics generate anti-inflammatory effects in disease. Abbreviations: SCFAs, short-chain fatty acid; A2A, adenosine-inosine receptor 2A; AhR, aryl hydrocarbon receptor; FFARs, free fatty acid receptors; GLP-1, glucagon like protein 1; GPRs, G-binding protein receptors; H2, histamine receptor 2; IAA, indole-3-acetic acid; IAcrA, indole acrylic acid; IAcrGly, indole acryloyl glycine; IAId, indole-3-aldehyde; ILA, indole lactic acid; IL-10/12/22, interleukin 10/12/22; IPA, indolyl propionic acid; MCP-1, monocyte chemoattractant protein-1; NF-κB, nuclear factor kappa B; PYY, peptide YY; SCFAs, short-chain fatty acids; Th1/2/17, T helper 1/2/17; TNF, tumor necrosis factor.
SCFAs, specifically acetate, propionate, and butyrate, are produced by commensal bacteria (e.g., Eubacterium rectale, Facecalibacterium prausnitizii, Eubacterium hallii, and Ruminococcus bromii) and numerous probiotics (e.g., Lactobacilli and Bifidobacteria) (Liu et al., 2018). SCFAs have anti-inflammatory effects acting through a variety of mechanisms. SCFAs reduce the production of cytokines by neutrophils (Vinolo et al., 2011) while reducing macrophageal NF-κB signaling (Park et al., 2007). These effects inhibit chronic low-grade inflammation. Free fatty acid receptors (FFARs) and G protein-coupled receptors (GPRs) are SCFA receptors present in the colon. Among them, FFAR3 (GPR41) and FFAR2 (GPR43) on colon cells have been associated with movement control (Dass et al., 2007). SCFAs can bind and activate FFAR3 (GPR41) and FFAR2 (GPR43) located in the intestinal epithelium to induce the release of GLP-1 and peptide YY (PYY) into the basolateral environment (Liu et al., 2018). Released GLP-1 and PYY activate the intestinal nervous system and transmit neural information through the intestine-brain axis to the central nervous system, thereby influencing metabolic energy consumption by the host (Kuwahara, 2014). Treg cells are a subgroup of T cells with significant immunosuppressive effects that express Foxp3+, CD25+, and CD4+ as phenotypes. In addition, SCFAs (particularly butyrate) can induce the differentiation of Treg cells to inhibit chronic low-grade inflammation (Kespohl et al., 2017). Nevertheless, further study is warranted to identify the molecular mechanisms underlying these effects.
Trp metabolism plays a vital role in regulating intestinal immunity and protecting intestinal barriers (Hubbard et al., 2015). Probiotics inhibit chronic low-grade inflammation by regulating the intestinal barrier through Trp metabolites. These metabolites include bacteria-derived Trp metabolites (e.g., indole, indolic acid, skatole, and tryptamine), as well as host-derived Trp metabolites (e.g., kynurenines, serotonin, and melatonin) (Liu et al., 2018) (Gao et al., 2018). Trp metabolites can bind to aromatic hydrocarbon receptor (AhR). AhR is a cytoplasmic ligand-activated transcription factor in dendritic and T cells, which is involved in maintaining gut immune tolerance and barrier function. Host and bacterial Trp metabolites stimulate AhR and AhR-dependent gene expression, thereby producing IL-6, IL-22, vascular endothelial growth factor A (VEGFA), prostaglandin-endoperoxide synthase 2 (PTGS2), mucin 2 (MUC2), and cytochrome P450 1A1 (CYP1A1) and regulating intestinal homeostasis (Gao et al., 2018). Among them, indole acid derivatives from probiotics and symbiotics (e.g., indole-3-acetic acid, indole-3-aldehyde, indole acryloyl glycine, indole lactic acid, indole acrylic acid, and indolyl propionic acid) are considered the main metabolites involved in this process. Indole 3-propionic acid significantly enhances the production of the anti-inflammatory cytokine IL-10 after LPS stimulation and reduces TNF production (Venkatesh et al., 2014). Indole-3-aldehyde can activate group 3 innate lymphoid cells through AhR to produce IL22, thus, suppressing inflammatory responses (Zelante et al., 2013).
Probiotics inhibit the differentiation of Th1 and Th2 cells and improve chronic low-grade inflammation by altering the microbiota adenosine-inosine receptor 2A (A2A) axis (He et al., 2017a; He et al., 2017b). Aging can reduce the number of Treg cells and is closely associated with chronic low-grade inflammation. Transforming growth factor-beta (TGFβ) is a key immunomodulator in the intestinal mucosa. It can induce gene transcription in Foxp3+ thymic Treg precursor cells and convert initial T cells into induced Treg cells while protecting the latter cells from apoptosis. Lactobacillus gasseri SBT2055 activates TLR2 signaling, induces TGFB expression in dendritic cells, increases IgA production, and inhibits inflammation (Sakai et al., 2014). In addition, Lactobacillus reuteri 17938 restores the serum levels of the purine metabolite inosine and downstream products xanthine and hypoxanthine, thereby altering the metabolic spectrum that is reduced in Treg cell deficiency (He et al., 2017b). A key mechanism of Treg cells is the control of inflammatory effector memory T cells, including the Th1, Th2, and Th17 subgroups. These pro-inflammatory T cell families are controlled by the interaction of adenosine (produced by Treg cells) with receptor A2A, which is highly expressed on T cells (He et al., 2017a).
The tolerogenic effects of lactobacilli are strain- and metabolite-dependent (Liu et al., 2018). Studies have reported that L. rhamnosus strain, which secretes low levels of histamine, can suppress the immune response. Notably, Lactobacillus saerimneri strain, which secretes high levels of histamine, mediates the inflammatory response (Thomas et al., 2012; Ganesh et al., 2018). In addition, Lactobacillus reuteri 6,475 produces histamine, which acts through histidine decarboxylase, relies on histamine H-2 receptors on intestinal cells to inhibit TNF synthesis in vitro, and exert its anti-inflammatory effects (Thomas et al., 2012; Gao et al., 2017).
5 The challenges of using probiotics
Probiotics (mostly within Lactobacillus, Bifidobacterium, Lactococcus, Streptococcus, and Saccharomyces genera) are globalized, popularized, and integrated into foods, cosmetics, and supplements (Hoffmann et al., 2014). However, studies have reported highly mixed results. Research and development of probiotics inherently necessitate multiple cycles of trial and error to identify health benefits. In the absence of prior mechanistic information, the results in a plethora of literature are sometimes conflicting, thus, complicating the formulation of evidence-based clinical guidelines for the use of probiotics (Veiga et al., 2020). That merits better evidence-based proof of the impacts that probiotics have on humans and their adverse effects (Suez et al., 2019).
Probiotics are likely to be used in inflammation-related diseases as a component of various treatment regimens. Probiotic efficacy is strain- and indication-specific. Individual-specific factors can also contribute to the heterogeneity of probiotic supplementation outcomes, including diet, age, and microbiota (Veiga et al., 2020). The extent to which probiotic microorganisms can persistently or transiently colonize the gut during supplementation varies between individuals, depending (among other potential factors) on their resident microbiome (Veiga et al., 2020). Recent studies have shown that colonization-resistant microbiomes are more resilient to probiotic interventions compared to colonization-permissive individuals (Veiga et al., 2020). A precise approach to probiotics might bridge this gap by addressing heterogeneity associated with probiotic strains, individuals, and their microbiomes. If the determinants and/or mechanism of the host reaction are determined, probiotics developed through a top-down approach might eventually become precision probiotics (Veiga et al., 2020). Precision probiotics will be used as a better candidate for precision medicine and nutrition, as individuals who might respond to them will be identified based on the phenotype or target chosen for probiotics. Precision probiotics can be used to stimulate the production of beneficial microbial metabolites, inhibit the production of harmful compounds, or restore the ecological balance of metabolic networks by introducing key species damaged after intestinal inflammation or exposure to antimicrobial agents (Suez et al., 2019; Veiga et al., 2020). Furthermore, the precise method of probiotics is still subject to several challenges. First, because of the heterogeneity of probiotic strains, the ability to provide clinicians and consumers with specific guidelines for strains and/or combinations that are effective in a given medical condition is limited by a lack of research (Veiga et al., 2020). Second, individual-specific methods are used to predict efficacy, which might require extensive individualized host data (including genetics, anthropometry, and immunoassay) and microbiome data (e.g., strain level composition, transcriptomics, and metabolomics) and identify biomarkers associated with predicting colonization resistance and/or health outcomes. In addition, since fecal samples do not accurately reflect the colonization and influence of the gut microbiota along the gastrointestinal tract during probiotic replenishment, there is a strong need to design non-invasive means for identifying compatible probiotic-individual matching, such as ingestible microengineered osmotic pills. Another important factor to consider is safety, as exogenous microorganisms might have unintended effects on the microbiome and might even harm the health of vulnerable subjects and lead to bacteremia or fungemia. Therefore, understanding the mechanisms by which exogenous probiotic microorganisms (whether traditional or new) interact with the host and microbiome is important for efficacy and safety (Veiga et al., 2020).
In general, traditional, widely used probiotics, such as Bifidobacterium spp. and Lactobacillus spp., have been selected either randomly or by gathering living experiences. While most of them show biological safety and ameliorative effectiveness, the general effects and functions on the amelioration of diseases are statistically marginal (Chang et al., 2019). On the other hand, traditional probiotics are not applied to specific diseases. Therefore, the identification and characterization of novel and disease-specific next-generation probiotics (NGP) are urgently needed (Chang et al., 2019). NGPs are individual bacterial strains that scientists have screened and isolated by using rapidly evolving gene sequencing tools and bioinformatics platforms to characterize the composition and function of the gut microbiota and microbiome, as well as their relationship to the amelioration of inflammation-related diseases (Suez et al., 2020). The characteristics of NGP include a comprehensive understanding of their target disease, as well as the genetic and physiological characteristics of bacteria, including growth dynamics and antibiotic sensitivity patterns. In addition, its potential molecular improvement mechanism remains to be clarified (Chang et al., 2019). The introduction of next-generation techniques has considerably improved our ability to address the colonization question even at strain resolution to differentiate between endogenous and exogenous bacteria (Suez et al., 2020). NGP can better clarify probiotic colonization, as well as direct or microbiome-mediated effects on human hosts, and improve understanding of their mechanisms of activity, efficacy, and long-term safety.
6 Dietary modulation and gut flora
There is plenty of evidence that many foods, nutrients, and non-nutritious foods can regulate chronic low-grade inflammation by adjusting the gut flora (Minihane et al., 2015). The role of dietary patterns, specific foods, and individual nutrients and non-nutrients in influencing chronic low-grade inflammation has been extensively reviewed (Calder et al., 2011). Healthy diet patterns described by Healthy Diet Index, Alternative Healthy Diet Index, vegetarian diet, and Mediterranean diet (MD) are all associated with lower circulatory concentrations of inflammatory markers, including C-reactive protein (CRP) and several cytokines (Calder et al., 2017). Higher intake of whole grains, vegetables and fruits, nuts, and fish in healthy diets is associated with lower inflammation and overall wellbeing due to the presence of phenolic compounds and fiber (Calder et al., 2011). It is also a key feature in the prevention of non-communicable diseases (NCDs) through its impact on the microbiota. Several types of polyphenols can promote the growth of healthy gut microbial flora (e.g., Bifidobacterium, Lactobacillus, Akkermansia, Christensenellaceae, and Verrucomicrobia), and potential anti-aging effects have been reported. The intake of lemon polyphenols limits the abundance of gut flora associated with aging (Beli et al., 2018). Dietary fiber leads to the production of key metabolites, such as SCFA (good for health), with the potential to alter gut microbiota and change metabolic regulation (Henning et al., 2018). Therefore, food components and dietary habits can modulate gut microbiota composition and intestinal barrier functions (Du et al., 2021).
The characteristics of the MD include (a) high consumption of vegetables, fruits, cereals (mainly whole grains), nuts, and legumes; (b) low consumption of saturated fats, sweets, and meat; (c) high intake of unsaturated fats (especially olive oil); (d) medium-high fish consumption; (e) drinking wine in moderation; (f) medium-low intake of dairy products (mainly yogurt and cheese) (Klement and Pazienza, 2019). A study has found higher levels of SCFA in subjects who adhered to MD patterns better (Garcia-Mantrana et al., 2018). In addition, the abundance of lactobacillus in MD-fed monkeys has increased tenfold compared to Western diet-fed monkeys, which was accompanied by an increase in bile acid metabolites and a decrease in reactive oxygen metabolites (Shively et al., 2018). Thus, MD patterns can regulate chronic low-grade inflammation by regulating local microbiota.
A low-carbohydrate diet is an additional dietary approach associated with weight loss and improved health markers. In overweight individuals, a low-carbohydrate, high-protein weight loss diet has had no effect on the proportion of different bacterial phyla but triggered significant decreases in Collinsella aerofaciens and E. rectale relatives (Klement and Pazienza, 2019). A diet rich in complex carbohydrates increases levels of beneficial Bifidobacteria, such as subspecies Bifidobacterium aureus, Bifidobacterium short, and Bifidobacteria polyformis; on the other hand, it reduces levels of opportunistic pathogens, such as Mycobacterium avium subspecies paratuberculosis and Enterobacteriaceae (Walker et al., 2011). Low-carbohydrate diet facilitates the growth of anti-inflammatory microorganisms, such as Lachnospiraceae, while reducing pro-inflammatory microbes, such as Bacteroides acidifaciens, Escherichia coli, Ruminococcus gnavus, and Clostridium cocleatum. Hence, the low-carbohydrate diet has a positive effect on the gut microbial community, modulates chronic low-grade inflammation, and improves age-related diseases.
Ketogenic diets (KDs) are a special type of low-carbohydrate diet that reduce carbohydrate content to such an extent (usually <50 g/day) that the corresponding low insulin levels and mildly elevated cortisol levels induce the production of ketone bodies in the liver (Klement and Pazienza, 2019). In the mouse model of autism spectrum disorder, KDs increased the level of bacterium A. muciniphila and significantly increased the Firmicutes/Bacteroidetes ratio (Newell et al., 2016). In infants with refractory epilepsy, KD significantly alters the composition of gut microbes, bringing them close to healthy controls: Bacteroides and Prevotella increased, while Cronobacter levels decreased by approximately 50% (Xie et al., 2017).
Evolutional medicine argues that inadequate adaptation to modern lifestyles can lead to non-communicable diseases, and the paleolithic diet concept has been then introduced (Klement and Pazienza, 2019). The paleolithic diet refers to the modern diet that mimics the diet of our ancestors during the Paleolithic, which spans the majority of human existence in chronological order (Klement and Pazienza, 2019). Paleolithic diet typically consists of the following modes: (a) high consumption of fruits, herbs, spices, and vegetables; (b) moderate-to-high consumption of lean meat, organs, fish, and eggs; (c) appropriate consumption of nuts and seeds; (d) exclusion of all processed foods, legumes, cereals, dairy products, and vegetable oils (except for olive and coconut oils) (Klement and Pazienza, 2019). Healthy Italians, after more than a year of the modern paleolithic diet, have had much higher microbiome diversity than Italians who adhered to MD (Barone et al., 2019). The association with high microbiome diversity and its presumed anti-inflammatory properties will make the paleolithic diet an important complementary treatment in future clinical studies.
7 Conclusion
Chronic low-grade inflammation is thought to be responsible for many declining functions associated with aging and age-related diseases. Therefore, preventing, mitigating, or reversing inflammatory processes is highly correlated with healthy aging and improved wellbeing. It is important to understand the triggers of chronic low-grade inflammation and identify strategies to prevent, slow, or even reverse its development. Dysregulation of the gut flora is an important trigger of chronic low-grade inflammation. Changes in the composition of the gut flora and exposure to related metabolites directly interact with the inflammatory system of the host and develop crosstalk between the gut barrier and the systemic immune system. These processes contribute to chronic low-grade inflammation, resulting in poor health and impaired wellbeing associated with aging. Probiotics can effectively assist in maintaining the balance in the composition of gut microbial flora, thereby protecting the gut barrier and regulating gut immunity. Increasing scientific evidence has shown that probiotics exert a positive effect on chronic low-grade inflammation and play a key role in healthy aging and improving age-related diseases. Probiotics might be an important therapeutic strategy for the prevention, delay, or even reversal of low-grade inflammation in old age. However, robust controlled clinical trials are warranted to further validate this hypothesis.
Author contributions
QW and JW designed the review, HL, GZ, and BP searched literatures, JR collected literature data, wrote the manuscript, drew the figure; contributed to scientific writing of the manuscript; JR, QW, and JW revised the manuscript. All authors contributed to the article and approved the submitted version.
Funding
This study was supported by the Scientific and Technological Innovation Project of China Academy of Chinese Medical Sciences (Grant Nos. CI 2021A01617 and CI 2021A01612), Chinese Medicine Prevention and Treatment of Type 2 Diabetes Evidence-based Capacity-building Projects (Grant No. 60104), Young Elite Scientists Sponsorship Program by CAST (Grant No. 2019QNRC001), and the National Natural Science Foundation of China (grant No. 82074412), but this study did not receive any public funding from commercial.
Conflict of interest
The authors declare that the research was conducted in the absence of any commercial or financial relationships that could be construed as a potential conflict of interest.
Publisher’s note
All claims expressed in this article are solely those of the authors and do not necessarily represent those of their affiliated organizations, or those of the publisher, the editors and the reviewers. Any product that may be evaluated in this article, or claim that may be made by its manufacturer, is not guaranteed or endorsed by the publisher.
References
Abdolalipour, E., Mahooti, M., Gorji, A., and Ghaemi, A. (2022). Synergistic therapeutic effects of probiotic lactobacillus casei TD-2 consumption on GM-CSF-induced immune responses in a murine model of cervical cancer. Nutr. Cancer 74 (1), 372–382. doi:10.1080/01635581.2020.1865419
Agus, A., Planchais, J., and Sokol, H. (2018). Gut microbiota regulation of tryptophan metabolism in health and disease. Cell Host Microbe 23 (6), 716–724. doi:10.1016/j.chom.2018.05.003
Allaire, J. M., Crowley, S. M., Law, H. T., Chang, S.-Y., Ko, H.-J., and Vallance, B. A. (2018). The intestinal epithelium: Central coordinator of mucosal immunity. Trends Immunol. 39 (9), 677–696. doi:10.1016/j.it.2018.04.002
Andersson, D. I., and Hughes, D. (2014). Microbiological effects of sublethal levels of antibiotics. Nat. Rev. Microbiol. 12 (7), 465–478. doi:10.1038/nrmicro3270
Aversa, Z., Atkinson, E. J., Schafer, M. J., Theiler, R. N., Rocca, W. A., Blaser, M. J., et al. (2021). Association of infant antibiotic exposure with childhood health outcomes. Mayo Clin. Proc. 96 (1), 66–77. doi:10.1016/j.mayocp.2020.07.019
Bailey, L. C., Forrest, C. B., Zhang, P., Richards, T. M., Livshits, A., and DeRusso, P. A. (2014). Association of antibiotics in infancy with early childhood obesity. JAMA Pediatr. 168 (11), 1063–1069. doi:10.1001/jamapediatrics.2014.1539
Bairamian, D., Sha, S., Rolhion, N., Sokol, H., Dorothée, G., Lemere, C. A., et al. (2022). Microbiota in neuroinflammation and synaptic dysfunction: A focus on Alzheimer's disease. Mol. Neurodegener. 17 (1), 19. doi:10.1186/s13024-022-00522-2
Barichella, M., Pacchetti, C., Bolliri, C., Cassani, E., Iorio, L., Pusani, C., et al. (2016). Probiotics and prebiotic fiber for constipation associated with Parkinson disease: An RCT. Neurology 87 (12), 1274–1280. doi:10.1212/WNL.0000000000003127
Barone, M., Turroni, S., Rampelli, S., Soverini, M., D'Amico, F., Biagi, E., et al. (2019). Gut microbiome response to a modern Paleolithic diet in a Western lifestyle context. PloS One 14 (8), 0220619. doi:10.1371/journal.pone.0220619
Beli, E., Yan, Y., Moldovan, L., Vieira, C. P., Gao, R., Duan, Y., et al. (2018). Restructuring of the gut microbiome by intermittent fasting prevents retinopathy and prolongs survival in db/db mice. Diabetes 67 (9), 1867–1879. doi:10.2337/db18-0158
Belkaid, Y., and Hand, T. W. (2014). Role of the microbiota in immunity and inflammation. Cell 157 (1), 121–141. doi:10.1016/j.cell.2014.03.011
Biagi, E., Franceschi, C., Rampelli, S., Severgnini, M., Ostan, R., Turroni, S., et al. (2016). Gut microbiota and extreme longevity. Curr. Biol. CB 26 (11), 1480–1485. doi:10.1016/j.cub.2016.04.016
Biagi, E., Nylund, L., Candela, M., Ostan, R., Bucci, L., Pini, E., et al. (2010). Through ageing, and beyond: Gut microbiota and inflammatory status in seniors and centenarians. PloS One 5 (5), 10667. doi:10.1371/journal.pone.0010667
Bian, G., Gloor, G. B., Gong, A., Jia, C., Zhang, W., Hu, J., et al. (2017). The gut microbiota of healthy aged Chinese is similar to that of the healthy young. MSphere 2 (5). doi:10.1128/mSphere.00327-17
Binda, S., Hill, C., Johansen, E., Obis, D., Pot, B., Sanders, M. E., et al. (2020). Criteria to qualify microorganisms as "probiotic" in foods and dietary supplements. Front. Microbiol. 11, 1662. doi:10.3389/fmicb.2020.01662
Bischoff, S. C., Barbara, G., Buurman, W., Ockhuizen, T., Schulzke, J.-D., Serino, M., et al. (2014). Intestinal permeability-a new target for disease prevention and therapy. BMC Gastroenterol. 14, 189. doi:10.1186/s12876-014-0189-7
Bodogai, M., O'Connell, J., Kim, K., Kim, Y., Moritoh, K., Chen, C., et al. (2018). Commensal bacteria contribute to insulin resistance in aging by activating innate B1a cells. Sci. Transl. Med. 10 (467), 4271. doi:10.1126/scitranslmed.aat4271
Brunt, V. E., Gioscia-Ryan, R. A., Richey, J. J., Zigler, M. C., Cuevas, L. M., Gonzalez, A., et al. (2019). Suppression of the gut microbiome ameliorates age-related arterial dysfunction and oxidative stress in mice. J. Physiology 597 (9), 2361–2378. doi:10.1113/JP277336
Buckley, A., and Turner, J. R. (2018). Cell Biology of tight junction barrier regulation and mucosal disease. Cold Spring Harb. Perspect. Biol. 10 (1), 029314. doi:10.1101/cshperspect.a029314
Calder, P. C., Ahluwalia, N., Brouns, F., Buetler, T., Clement, K., Cunningham, K., et al. (2011). Dietary factors and low-grade inflammation in relation to overweight and obesity. Br. J. Nutr. 106 (3), S5–S78. doi:10.1017/S0007114511005460
Calder, P. C., Bosco, N., Bourdet-Sicard, R., Capuron, L., Delzenne, N., Doré, J., et al. (2017). Health relevance of the modification of low grade inflammation in ageing (inflammageing) and the role of nutrition. Ageing Res. Rev. 40, 95–119. doi:10.1016/j.arr.2017.09.001
Caruso, R., Warner, N., Inohara, N., and Núñez, G. (2014). NOD1 and NOD2: Signaling, host defense, and inflammatory disease. Immunity 41 (6), 898–908. doi:10.1016/j.immuni.2014.12.010
Chang, C.-J., Lin, T.-L., Tsai, Y.-L., Wu, T.-R., Lai, W.-F., Lu, C.-C., et al. (2019). Next generation probiotics in disease amelioration. J. Food Drug Analysis 27 (3), 615–622. doi:10.1016/j.jfda.2018.12.011
Chen, L.-W., Xu, J., Soh, S. E., Aris, I. M., Tint, M.-T., Gluckman, P. D., et al. (2020). Implication of gut microbiota in the association between infant antibiotic exposure and childhood obesity and adiposity accumulation. Int. J. Obes. (2005) 44 (7), 1508–1520. doi:10.1038/s41366-020-0572-0
Claesson, M. J., Cusack, S., O'Sullivan, O., Greene-Diniz, R., de Weerd, H., Flannery, E., et al. (2011). Composition, variability, and temporal stability of the intestinal microbiota of the elderly. Proc. Natl. Acad. Sci. U. S. A. 108 (1), 4586–4591. doi:10.1073/pnas.1000097107
Clark, R. I., Salazar, A., Yamada, R., Fitz-Gibbon, S., Morselli, M., Alcaraz, J., et al. (2015). Distinct shifts in microbiota composition during Drosophila aging impair intestinal function and drive mortality. Cell Rep. 12 (10), 1656–1667. doi:10.1016/j.celrep.2015.08.004
Cox, L. M., and Blaser, M. J. (2015). Antibiotics in early life and obesity. Nat. Rev. Endocrinol. 11 (3), 182–190. doi:10.1038/nrendo.2014.210
Cukrowska, B., Bierła, J. B., Zakrzewska, M., Klukowski, M., and Maciorkowska, E. (2020). The relationship between the infant gut microbiota and allergy. The role of Bifidobacterium breve and prebiotic oligosaccharides in the activation of anti-allergic mechanisms in early life. Nutrients 12 (4), 946. doi:10.3390/nu12040946
Custodero, C., Mankowski, R. T., Lee, S. A., Chen, Z., Wu, S., Manini, T. M., et al. (2018). Evidence-based nutritional and pharmacological interventions targeting chronic low-grade inflammation in middle-age and older adults: A systematic review and meta-analysis. Ageing Res. Rev. 46, 42–59. doi:10.1016/j.arr.2018.05.004
Dammermann, W., Wollenberg, L., Bentzien, F., Lohse, A., and Lüth, S. (2013). Toll like receptor 2 agonists lipoteichoic acid and peptidoglycan are able to enhance antigen specific IFNγ release in whole blood during recall antigen responses. J. Immunol. Methods 396 (1-2), 107–115. doi:10.1016/j.jim.2013.08.004
Dass, N. B., John, A. K., Bassil, A. K., Crumbley, C. W., Shehee, W. R., Maurio, F. P., et al. (2007). The relationship between the effects of short-chain fatty acids on intestinal motility in vitro and GPR43 receptor activation. Neurogastroenterol. Motil. Official J. Eur. Gastrointest. Motil. Soc. 19 (1), 66–74. doi:10.1111/j.1365-2982.2006.00853.x
DeJong, E. N., Surette, M. G., and Bowdish, D. M. E. (2020). The gut microbiota and unhealthy aging: Disentangling cause from consequence. Cell Host Microbe 28 (2), 180–189. doi:10.1016/j.chom.2020.07.013
Desai, M. S., Seekatz, A. M., Koropatkin, N. M., Kamada, N., Hickey, C. A., Wolter, M., et al. (2016). A dietary fiber-deprived gut microbiota degrades the colonic mucus barrier and enhances pathogen susceptibility. Cell 167 (5), 1339–1353. doi:10.1016/j.cell.2016.10.043
Du, Y., Gao, Y., Zeng, B., Fan, X., Yang, D., and Yang, M. (2021). Effects of anti-aging interventions on intestinal microbiota. Gut Microbes 13 (1), 1994835. doi:10.1080/19490976.2021.1994835
Duncan, S. H., and Flint, H. J. (2013). Probiotics and prebiotics and health in ageing populations. Maturitas 75 (1), 44–50. doi:10.1016/j.maturitas.2013.02.004
Estrada, J. A., and Contreras, I. (2019). Nutritional modulation of immune and central nervous system homeostasis: The role of diet in development of neuroinflammation and neurological disease. Nutrients 11 (5), 1076. doi:10.3390/nu11051076
Fabbri, E., An, Y., Zoli, M., Simonsick, E. M., Guralnik, J. M., Bandinelli, S., et al. (2015). Aging and the burden of multimorbidity: Associations with inflammatory and anabolic hormonal biomarkers. Journals Gerontology. Ser. A, Biol. Sci. Med. Sci. 70 (1), 63–70. doi:10.1093/gerona/glu127
Flemer, B., Gaci, N., Borrel, G., Sanderson, I. R., Chaudhary, P. P., Tottey, W., et al. (2017). Fecal microbiota variation across the lifespan of the healthy laboratory rat. Gut Microbes 8 (5), 428–439. doi:10.1080/19490976.2017.1334033
Ford, A. C., Sperber, A. D., Corsetti, M., and Camilleri, M. (2020). Irritable bowel syndrome. Lancet (London, Engl. 396 (10263), 1675–1688. doi:10.1016/S0140-6736(20)31548-8
Ganesh, B. P., Hall, A., Ayyaswamy, S., Nelson, J. W., Fultz, R., Major, A., et al. (2018). Diacylglycerol kinase synthesized by commensal Lactobacillus reuteri diminishes protein kinase C phosphorylation and histamine-mediated signaling in the mammalian intestinal epithelium. Mucosal Immunol. 11 (2), 380–393. doi:10.1038/mi.2017.58
Gao, C., Ganesh, B. P., Shi, Z., Shah, R. R., Fultz, R., Major, A., et al. (2017). Gut microbe-mediated suppression of inflammation-associated colon carcinogenesis by luminal histamine production. Am. J. Pathology 187 (10), 2323–2336. doi:10.1016/j.ajpath.2017.06.011
Gao, J., Xu, K., Liu, H., Liu, G., Bai, M., Peng, C., et al. (2018). Impact of the gut microbiota on intestinal immunity mediated by tryptophan metabolism. Front. Cell. Infect. Microbiol. 8, 13. doi:10.3389/fcimb.2018.00013
Garcia-Mantrana, I., Selma-Royo, M., Alcantara, C., and Collado, M. C. (2018). Shifts on gut microbiota associated to mediterranean diet adherence and specific dietary intakes on general adult population. Front. Microbiol. 9, 890. doi:10.3389/fmicb.2018.00890
Gargari, G., Taverniti, V., Del Bo, C., Bernardi, S., Hidalgo-Liberona, N., Meroño, T., et al. (2022). Higher bacterial DNAemia can affect the impact of a polyphenol-rich dietary pattern on biomarkers of intestinal permeability and cardiovascular risk in older subjects. Eur. J. Nutr. 61 (3), 1209–1220. doi:10.1007/s00394-021-02680-3
Georgescu, D., Ancusa, O. E., Georgescu, L. A., Ionita, I., and Reisz, D. (2016). Nonmotor gastrointestinal disorders in older patients with Parkinson's disease: Is there hope? Clin. Interventions Aging 11, 1601–1608. doi:10.2147/CIA.S106284
Gnauck, A., Lentle, R. G., and Kruger, M. C. (2016). The characteristics and function of bacterial lipopolysaccharides and their endotoxic potential in humans. Int. Rev. Immunol. 35 (3), 189–218. doi:10.3109/08830185.2015.1087518
Gomes, A. C., Hoffmann, C., and Mota, J. F. (2018). The human gut microbiota: Metabolism and perspective in obesity. Gut Microbes 9 (4), 308–325. doi:10.1080/19490976.2018.1465157
Górska, A., Przystupski, D., Niemczura, M. J., and Kulbacka, J. (2019). Probiotic bacteria: A promising tool in cancer prevention and therapy. Curr. Microbiol. 76 (8), 939–949. doi:10.1007/s00284-019-01679-8
Gurung, M., Li, Z., You, H., Rodrigues, R., Jump, D. B., Morgun, A., et al. (2020). Role of gut microbiota in type 2 diabetes pathophysiology. EBioMedicine 51, 102590. doi:10.1016/j.ebiom.2019.11.051
Hajam, I. A., Dar, P. A., Shahnawaz, I., Jaume, J. C., and Lee, J. H. (2017). Bacterial flagellin-a potent immunomodulatory agent. Exp. Mol. Med. 49 (9), e373. doi:10.1038/emm.2017.172
Han, B., Sivaramakrishnan, P., Lin, C.-C. J., Neve, I. A. A., He, J., Tay, L. W. R., et al. (2017). Microbial genetic composition tunes host longevity. Cell 169 (7), 1249–1262. doi:10.1016/j.cell.2017.05.036
Hansson, O. (2021). Biomarkers for neurodegenerative diseases. Nat. Med. 27 (6), 954–963. doi:10.1038/s41591-021-01382-x
Haraldsdottir, S., Einarsdottir, H. M., Smaradottir, A., Gunnlaugsson, A., and Halfdanarson, T. R. (2014). Colorectal cancer - review. Laeknabladid 100 (2), 75–82. doi:10.17992/lbl.2014.02.531
He, B., Hoang, T. K., Tran, D. Q., Rhoads, J. M., and Liu, Y. (2017a). Adenosine A2A receptor deletion blocks the beneficial effects of lactobacillus reuteri in regulatory T-deficient scurfy mice. Front. Immunol. 8, 1680. doi:10.3389/fimmu.2017.01680
He, B., Hoang, T. K., Wang, T., Ferris, M., Taylor, C. M., Tian, X., et al. (2017b). Resetting microbiota by Lactobacillus reuteri inhibits T reg deficiency-induced autoimmunity via adenosine A2A receptors. J. Exp. Med. 214 (1), 107–123. doi:10.1084/jem.20160961
Henning, S. M., Yang, J., Hsu, M., Lee, R.-P., Grojean, E. M., Ly, A., et al. (2018). Decaffeinated green and black tea polyphenols decrease weight gain and alter microbiome populations and function in diet-induced obese mice. Eur. J. Nutr. 57 (8), 2759–2769. doi:10.1007/s00394-017-1542-8
Hicks, L. A., Taylor, T. H., and Hunkler, R. J. (2013). U.S. outpatient antibiotic prescribing, 2010. N. Engl. J. Med. 368 (15), 1461–1462. doi:10.1056/NEJMc1212055
Hoffmann, D. E., Fraser, C. M., Palumbo, F., Ravel, J., Rowthorn, V., and Schwartz, J. (2014). Probiotics: Achieving a better regulatory fit. Food Drug Law J. 69 (2), 237–272.
Hubbard, T. D., Murray, I. A., Bisson, W. H., Lahoti, T. S., Gowda, K., Amin, S. G., et al. (2015). Adaptation of the human aryl hydrocarbon receptor to sense microbiota-derived indoles. Sci. Rep. 5, 12689. doi:10.1038/srep12689
Hutchinson, A. N., Tingö, L., and Brummer, R. J. (2020). The potential effects of probiotics and ω-3 fatty acids on chronic low-grade inflammation. Nutrients 12 (8), 2402. doi:10.3390/nu12082402
Irrazábal, T., Belcheva, A., Girardin, S. E., Martin, A., and Philpott, D. J. (2014). The multifaceted role of the intestinal microbiota in colon cancer. Mol. Cell 54 (2), 309–320. doi:10.1016/j.molcel.2014.03.039
Jang, H.-M., Lee, K.-E., and Kim, D.-H. (2019). The preventive and curative effects of lactobacillus reuteri NK33 and Bifidobacterium adolescentis NK98 on immobilization stress-induced anxiety/depression and colitis in mice. Nutrients 11 (4), 819. doi:10.3390/nu11040819
Jayanama, K., and Theou, O. (2020). Effects of probiotics and prebiotics on frailty and ageing: A narrative review. Curr. Clin. Pharmacol. 15 (3), 183–192. doi:10.2174/1574884714666191120124548
Jernberg, C., Löfmark, S., Edlund, C., and Jansson, J. K. (2010). Long-term impacts of antibiotic exposure on the human intestinal microbiota. Microbiol. Read. Engl. 156 (11), 3216–3223. doi:10.1099/mic.0.040618-0
Jukic Peladic, N., Dell'Aquila, G., Carrieri, B., Maggio, M., Cherubini, A., and Orlandoni, P. (2021). Potential role of probiotics for inflammaging: A narrative review. Nutrients 13 (9), 2919. doi:10.3390/nu13092919
Kalliomäki, M., Collado, M. C., Salminen, S., and Isolauri, E. (2008). Early differences in fecal microbiota composition in children may predict overweight. Am. J. Clin. Nutr. 87 (3), 534–538. doi:10.1093/ajcn/87.3.534
Karimi, G., Sabran, M. R., Jamaluddin, R., Parvaneh, K., Mohtarrudin, N., Ahmad, Z., et al. (2015). The anti-obesity effects of Lactobacillus casei strain Shirota versus Orlistat on high fat diet-induced obese rats. Food & Nutr. Res. 59, 29273. doi:10.3402/fnr.v59.29273
Karlsson, C., Ahrné, S., Molin, G., Berggren, A., Palmquist, I., Fredrikson, G. N., et al. (2010). Probiotic therapy to men with incipient arteriosclerosis initiates increased bacterial diversity in colon: A randomized controlled trial. Atherosclerosis 208 (1), 228–233. doi:10.1016/j.atherosclerosis.2009.06.019
Kespohl, M., Vachharajani, N., Luu, M., Harb, H., Pautz, S., Wolff, S., et al. (2017). The microbial metabolite butyrate induces expression of Th1-associated factors in CD4+ T cells. Front. Immunol. 8, 1036. doi:10.3389/fimmu.2017.01036
Khosla, S., Farr, J. N., Tchkonia, T., and Kirkland, J. L. (2020). The role of cellular senescence in ageing and endocrine disease. Nat. Rev. Endocrinol. 16 (5), 263–275. doi:10.1038/s41574-020-0335-y
Kim, K.-A., Jeong, J.-J., Yoo, S.-Y., and Kim, D.-H. (2016). Gut microbiota lipopolysaccharide accelerates inflamm-aging in mice. BMC Microbiol. 16, 9. doi:10.1186/s12866-016-0625-7
Klement, R. J., and Pazienza, V. (2019). Impact of different types of diet on gut microbiota profiles and cancer prevention and treatment. Med. Kaunas. Lith. 55 (4), 84. doi:10.3390/medicina55040084
Knoop, K. A., and Newberry, R. D. (2018). Goblet cells: Multifaceted players in immunity at mucosal surfaces. Mucosal Immunol. 11 (6), 1551–1557. doi:10.1038/s41385-018-0039-y
Kocot, A. M., Jarocka-Cyrta, E., and Drabińska, N. (2022). Overview of the importance of biotics in gut barrier integrity. Int. J. Mol. Sci. 23 (5), 2896. doi:10.3390/ijms23052896
Koh, A., De Vadder, F., Kovatcheva-Datchary, P., and Bäckhed, F. (2016). From dietary fiber to host physiology: Short-chain fatty acids as key bacterial metabolites. Cell 165 (6), 1332–1345. doi:10.1016/j.cell.2016.05.041
Kolling, Y., Salva, S., Villena, J., and Alvarez, S. (2018). Are the immunomodulatory properties of Lactobacillus rhamnosus CRL1505 peptidoglycan common for all Lactobacilli during respiratory infection in malnourished mice? PloS One 13 (3), 0194034. doi:10.1371/journal.pone.0194034
Kong, F., Deng, F., Li, Y., and Zhao, J. (2019). Identification of gut microbiome signatures associated with longevity provides a promising modulation target for healthy aging. Gut Microbes 10 (2), 210–215. doi:10.1080/19490976.2018.1494102
Kong, F., Hua, Y., Zeng, B., Ning, R., Li, Y., and Zhao, J. (2016). Gut microbiota signatures of longevity. Curr. Biol. CB 26 (18), R832–R833. doi:10.1016/j.cub.2016.08.015
Korpela, K., Salonen, A., Virta, L. J., Kekkonen, R. A., Forslund, K., Bork, P., et al. (2016). Intestinal microbiome is related to lifetime antibiotic use in Finnish pre-school children. Nat. Commun. 7, 10410. doi:10.1038/ncomms10410
Kuwahara, A. (2014). Contributions of colonic short-chain Fatty Acid receptors in energy homeostasis. Front. Endocrinol. 5, 144. doi:10.3389/fendo.2014.00144
Kwon, H.-K., Kim, G.-C., Kim, Y., Hwang, W., Jash, A., Sahoo, A., et al. (2013). Amelioration of experimental autoimmune encephalomyelitis by probiotic mixture is mediated by a shift in T helper cell immune response. Clin. Immunol. Orl. Fla 146 (3), 217–227. doi:10.1016/j.clim.2013.01.001
Lahtinen, S. J., Tammela, L., Korpela, J., Parhiala, R., Ahokoski, H., Mykkänen, H., et al. (2009). Probiotics modulate the Bifidobacterium microbiota of elderly nursing home residents. Age Dordr. Neth. 31 (1), 59–66. doi:10.1007/s11357-008-9081-0
Langille, M. G., Meehan, C. J., Koenig, J. E., Dhanani, A. S., Rose, R. A., Howlett, S. E., et al. (2014). Microbial shifts in the aging mouse gut. Microbiome 2 (1), 50. doi:10.1186/s40168-014-0050-9
Lee, E., Jung, S.-R., Lee, S.-Y., Lee, N.-K., Paik, H.-D., and Lim, S.-I. (2018). Lactobacillus plantarum strain Ln4 attenuates diet-induced obesity, insulin resistance, and changes in hepatic mRNA levels associated with glucose and lipid metabolism. Nutrients 10 (5), 643. doi:10.3390/nu10050643
Li, D. Y., and Tang, W. H. W. (2018). Contributory role of gut microbiota and their metabolites toward cardiovascular complications in chronic kidney disease. Seminars Nephrol. 38 (2), 193–205. doi:10.1016/j.semnephrol.2018.01.008
Li, H.-Y., Zhou, D.-D., Gan, R.-Y., Huang, S.-Y., Zhao, C.-N., Shang, A., et al. (2021). Effects and mechanisms of probiotics, prebiotics, synbiotics, and postbiotics on metabolic diseases targeting gut microbiota: A narrative review. Nutrients 13 (9), 3211. doi:10.3390/nu13093211
Li, J., Lin, S., Vanhoutte, P. M., Woo, C. W., and Xu, A. (2016). Akkermansia muciniphila protects against atherosclerosis by preventing metabolic endotoxemia-induced inflammation in apoe-/- mice. Circulation 133 (24), 2434–2446. doi:10.1161/CIRCULATIONAHA.115.019645
Liu, Y., Alookaran, J. J., and Rhoads, J. M. (2018). Probiotics in autoimmune and inflammatory disorders. Nutrients 10 (10), 1537. doi:10.3390/nu10101537
Liu, Y., Gao, Y., Ma, F., Sun, M., Mu, G., and Tuo, Y. (2020). The ameliorative effect of Lactobacillus plantarum Y44 oral administration on inflammation and lipid metabolism in obese mice fed with a high fat diet. Food & Funct. 11 (6), 5024–5039. doi:10.1039/d0fo00439a
López-Otín, C., Blasco, M. A., Partridge, L., Serrano, M., and Kroemer, G. (2013). The hallmarks of aging. Cell 153 (6), 1194–1217. doi:10.1016/j.cell.2013.05.039
Luo, D., Chen, K., Li, J., Fang, Z., Pang, H., Yin, Y., et al. (2020). Gut microbiota combined with metabolomics reveals the metabolic profile of the normal aging process and the anti-aging effect of FuFang Zhenshu TiaoZhi(FTZ) in mice. Biomed. Pharmacother. = Biomedecine Pharmacother. 121, 109550. doi:10.1016/j.biopha.2019.109550
Luoto, R., Kalliomäki, M., Laitinen, K., Delzenne, N. M., Cani, P. D., Salminen, S., et al. (2011). Initial dietary and microbiological environments deviate in normal-weight compared to overweight children at 10 years of age. J. Pediatr. Gastroenterology Nutr. 52 (1), 90–95. doi:10.1097/MPG.0b013e3181f3457f
Malard, F., Dore, J., Gaugler, B., and Mohty, M. (2021). Introduction to host microbiome symbiosis in health and disease. Mucosal Immunol. 14 (3), 547–554. doi:10.1038/s41385-020-00365-4
Malesza, I. J., Malesza, M., Walkowiak, J., Mussin, N., Walkowiak, D., Aringazina, R., et al. (2021). High-fat, western-style diet, systemic inflammation, and gut microbiota: A narrative review. Cells 10 (11), 3164. doi:10.3390/cells10113164
Man, A. L., Bertelli, E., Rentini, S., Regoli, M., Briars, G., Marini, M., et al. (2015). Age-associated modifications of intestinal permeability and innate immunity in human small intestine. Clin. Sci. Lond. Engl. 1979) 129 (7), 515–527. doi:10.1042/CS20150046
Mangiola, F., Nicoletti, A., Gasbarrini, A., and Ponziani, F. R. (2018). Gut microbiota and aging. Eur. Rev. For Med. Pharmacol. Sci. 22 (21), 7404–7413. doi:10.26355/eurrev_201811_16280
Martel, J., Chang, S.-H., Ko, Y.-F., Hwang, T.-L., Young, J. D., and Ojcius, D. M. (2022). Gut barrier disruption and chronic disease. Trends Endocrinol. Metabolism TEM 33 (4), 247–265. doi:10.1016/j.tem.2022.01.002
McCaig, L. F., Besser, R. E., and Hughes, J. M. (2002). Trends in antimicrobial prescribing rates for children and adolescents. JAMA 287 (23), 3096–3102. doi:10.1001/jama.287.23.3096
McDonnell, L., Gilkes, A., Ashworth, M., Rowland, V., Harries, T. H., Armstrong, D., et al. (2021). Association between antibiotics and gut microbiome dysbiosis in children: Systematic review and meta-analysis. Gut Microbes 13 (1), 1–18. doi:10.1080/19490976.2020.1870402
Mello, A. M., Paroni, G., Daragjati, J., and Pilotto, A. (2016). Gastrointestinal microbiota and their contribution to healthy aging. Dig. Dis. (Basel, Switz. 34 (3), 194–201. doi:10.1159/000443350
Mengozzi, A., Pugliese, N. R., Chiriacò, M., Masi, S., Virdis, A., and Taddei, S. (2021). Microvascular ageing links metabolic disease to age-related disorders: The role of oxidative stress and inflammation in promoting microvascular dysfunction. J. Cardiovasc. Pharmacol. 78 (6), S78–S87. doi:10.1097/FJC.0000000000001109
Minihane, A. M., Vinoy, S., Russell, W. R., Baka, A., Roche, H. M., Tuohy, K. M., et al. (2015). Low-grade inflammation, diet composition and health: Current research evidence and its translation. Br. J. Nutr. 114 (7), 999–1012. doi:10.1017/S0007114515002093
Montassier, E., Valdés-Mas, R., Batard, E., Zmora, N., Dori-Bachash, M., Suez, J., et al. (2021). Probiotics impact the antibiotic resistance gene reservoir along the human GI tract in a person-specific and antibiotic-dependent manner. Nat. Microbiol. 6 (8), 1043–1054. doi:10.1038/s41564-021-00920-0
Moro-García, M. A., Alonso-Arias, R., Baltadjieva, M., Fernández Benítez, C., Fernández Barrial, M. A., Díaz Ruisánchez, E., et al. (2013). Oral supplementation with Lactobacillus delbrueckii subsp. bulgaricus 8481 enhances systemic immunity in elderly subjects. Age Dordr. Neth. 35 (4), 1311–1326. doi:10.1007/s11357-012-9434-6
Muller, P. A., Koscsó, B., Rajani, G. M., Stevanovic, K., Berres, M.-L., Hashimoto, D., et al. (2014). Crosstalk between muscularis macrophages and enteric neurons regulates gastrointestinal motility. Cell 158 (2), 300–313. doi:10.1016/j.cell.2014.04.050
Myhrstad, M. C. W., Tunsjø, H., Charnock, C., and Telle-Hansen, V. H. (2020). Dietary fiber, gut microbiota, and metabolic regulation-current status in human randomized trials. Nutrients 12 (3), 859. doi:10.3390/nu12030859
Newell, C., Bomhof, M. R., Reimer, R. A., Hittel, D. S., Rho, J. M., and Shearer, J. (2016). Ketogenic diet modifies the gut microbiota in a murine model of autism spectrum disorder. Mol. Autism 7 (1), 37. doi:10.1186/s13229-016-0099-3
Odamaki, T., Kato, K., Sugahara, H., Hashikura, N., Takahashi, S., Xiao, J.-Z., et al. (2016). Age-related changes in gut microbiota composition from newborn to centenarian: A cross-sectional study. BMC Microbiol. 16, 90. doi:10.1186/s12866-016-0708-5
Olefsky, J. M., and Glass, C. K. (2010). Macrophages, inflammation, and insulin resistance. Annu. Rev. Physiology 72, 219–246. doi:10.1146/annurev-physiol-021909-135846
Ouwehand, A. C., Bergsma, N., Parhiala, R., Lahtinen, S., Gueimonde, M., Finne-Soveri, H., et al. (2008). Bifidobacterium microbiota and parameters of immune function in elderly subjects. FEMS Immunol. Med. Microbiol. 53 (1), 18–25. doi:10.1111/j.1574-695X.2008.00392.x
Pan, F., Zhang, L., Li, M., Hu, Y., Zeng, B., Yuan, H., et al. (2018). Predominant gut Lactobacillus murinus strain mediates anti-inflammaging effects in calorie-restricted mice. Microbiome 6 (1), 54. doi:10.1186/s40168-018-0440-5
Panda, S., El khader, I., Casellas, F., López Vivancos, J., García Cors, M., Santiago, A., et al. (2014). Short-term effect of antibiotics on human gut microbiota. PloS One 9 (4), 95476. doi:10.1371/journal.pone.0095476
Pandey, K. R., Naik, S. R., and Vakil, B. V. (2015). Probiotics, prebiotics and synbiotics-a review. J. Food Sci. Technol. 52 (12), 7577–7587. doi:10.1007/s13197-015-1921-1
Paone, P., and Cani, P. D. (2020). Mucus barrier, mucins and gut microbiota: The expected slimy partners? Gut 69 (12), 2232–2243. doi:10.1136/gutjnl-2020-322260
Park, D.-Y., Ahn, Y.-T., Park, S.-H., Huh, C.-S., Yoo, S.-R., Yu, R., et al. (2013). Supplementation of Lactobacillus curvatus HY7601 and Lactobacillus plantarum KY1032 in diet-induced obese mice is associated with gut microbial changes and reduction in obesity. PloS One 8 (3), 59470. doi:10.1371/journal.pone.0059470
Park, J.-S., Lee, E.-J., Lee, J.-C., Kim, W.-K., and Kim, H.-S. (2007). Anti-inflammatory effects of short chain fatty acids in IFN-gamma-stimulated RAW 264.7 murine macrophage cells: Involvement of NF-kappaB and ERK signaling pathways. Int. Immunopharmacol. 7 (1), 70–77. doi:10.1016/j.intimp.2006.08.015
Pellegrini, M., Ippolito, M., Monge, T., Violi, R., Cappello, P., Ferrocino, I., et al. (2020). Gut microbiota composition after diet and probiotics in overweight breast cancer survivors: A randomized open-label pilot intervention trial. Nutr. (Burbank, Los Angel. Cty. Calif.) 74, 110749. doi:10.1016/j.nut.2020.110749
Potrykus, M., Czaja-Stolc, S., Stankiewicz, M., Kaska, Ł., and Małgorzewicz, S. (2021). Intestinal microbiota as a contributor to chronic inflammation and its potential modifications. Nutrients 13 (11), 3839. doi:10.3390/nu13113839
Qu, L., Ren, J., Huang, L., Pang, B., Liu, X., Liu, X., et al. (2018). Antidiabetic effects of lactobacillus casei fermented yogurt through reshaping gut microbiota structure in type 2 diabetic rats. J. Agric. Food Chem. 66 (48), 12696–12705. doi:10.1021/acs.jafc.8b04874
Rajaee, A., Barnett, R., and Cheadle, W. G. (2018). Pathogen- and danger-associated molecular patterns and the cytokine response in sepsis. Surg. Infect. 19 (2), 107–116. doi:10.1089/sur.2017.264
Rastogi, S., and Singh, A. (2022). Gut microbiome and human health: Exploring how the probiotic genus Lactobacillus modulate immune responses. Front. Pharmacol. 13, 1042189. doi:10.3389/fphar.2022.1042189
Resnik-Docampo, M., Sauer, V., Schinaman, J. M., Clark, R. I., Walker, D. W., and Jones, D. L. (2018). Keeping it tight: The relationship between bacterial dysbiosis, septate junctions, and the intestinal barrier in Drosophila. Fly 12 (1), 34–40. doi:10.1080/19336934.2018.1441651
Rezaei Asl, Z., Sepehri, G., and Salami, M. (2019). Probiotic treatment improves the impaired spatial cognitive performance and restores synaptic plasticity in an animal model of Alzheimer's disease. Behav. Brain Res. 376, 112183. doi:10.1016/j.bbr.2019.112183
Rondanelli, M., Giacosa, A., Faliva, M. A., Perna, S., Allieri, F., and Castellazzi, A. M. (2015). Review on microbiota and effectiveness of probiotics use in older. World J. Clin. Cases 3 (2), 156–162. doi:10.12998/wjcc.v3.i2.156
Roy Sarkar, S., and Banerjee, S. (2019). Gut microbiota in neurodegenerative disorders. J. Neuroimmunol. 328, 98–104. doi:10.1016/j.jneuroim.2019.01.004
Safari, Z., and Gérard, P. (2019). The links between the gut microbiome and non-alcoholic fatty liver disease (NAFLD). Cell. Mol. Life Sci. CMLS 76 (8), 1541–1558. doi:10.1007/s00018-019-03011-w
Sakai, F., Hosoya, T., Ono-Ohmachi, A., Ukibe, K., Ogawa, A., Moriya, T., et al. (2014). Lactobacillus gasseri SBT2055 induces TGF-β expression in dendritic cells and activates TLR2 signal to produce IgA in the small intestine. PloS One 9 (8), e105370. doi:10.1371/journal.pone.0105370
Salazar, J., Angarita, L., Morillo, V., Navarro, C., Martínez, M. S., Chacín, M., et al. (2020). Microbiota and diabetes mellitus: Role of lipid mediators. Nutrients 12 (10), 3039. doi:10.3390/nu12103039
Scheepers, L. E. J. M., Penders, J., Mbakwa, C. A., Thijs, C., Mommers, M., and Arts, I. C. W. (2015). The intestinal microbiota composition and weight development in children: The KOALA birth cohort study. Int. J. Obes. (2005) 39(1), 16–25. doi:10.1038/ijo.2014.178
Schroder, K., and Tschopp, J. (2010). The inflammasomes. Cell 140 (6), 821–832. doi:10.1016/j.cell.2010.01.040
Sehrawat, N., Yadav, M., Singh, M., Kumar, V., Sharma, V. R., and Sharma, A. K. (2021). Probiotics in microbiome ecological balance providing a therapeutic window against cancer. Seminars Cancer Biol. 70, 24–36. doi:10.1016/j.semcancer.2020.06.009
Sharma, R., Kapila, R., Kapasiya, M., Saliganti, V., Dass, G., and Kapila, S. (2014). Dietary supplementation of milk fermented with probiotic Lactobacillus fermentum enhances systemic immune response and antioxidant capacity in aging mice. Nutr. Res. (New York, N.Y.) 34 (11), 968–981. doi:10.1016/j.nutres.2014.09.006
Shen, Z.-H., Zhu, C.-X., Quan, Y.-S., Yang, Z.-Y., Wu, S., Luo, W.-W., et al. (2018). Relationship between intestinal microbiota and ulcerative colitis: Mechanisms and clinical application of probiotics and fecal microbiota transplantation. World J. Gastroenterology 24 (1), 5–14. doi:10.3748/wjg.v24.i1.5
Shenghua, P., Ziqin, Z., Shuyu, T., Huixia, Z., Xianglu, R., and Jiao, G. (2020). An integrated fecal microbiome and metabolome in the aged mice reveal anti-aging effects from the intestines and biochemical mechanism of FuFang zhenshu TiaoZhi(FTZ). Biomed. Pharmacother. = Biomedecine Pharmacother. 121, 109421. doi:10.1016/j.biopha.2019.109421
Shively, C. A., Register, T. C., Appt, S. E., Clarkson, T. B., Uberseder, B., Clear, K. Y. J., et al. (2018). Consumption of mediterranean versus western diet leads to distinct mammary gland microbiome populations. Cell Rep. 25 (1), 47–56. doi:10.1016/j.celrep.2018.08.078
Simon, D. K., Tanner, C. M., and Brundin, P. (2020). Parkinson disease epidemiology, pathology, genetics, and pathophysiology. Clin. Geriatric Med. 36 (1), 1–12. doi:10.1016/j.cger.2019.08.002
Singh, R., Zogg, H., Wei, L., Bartlett, A., Ghoshal, U. C., Rajender, S., et al. (2021). Gut microbial dysbiosis in the pathogenesis of gastrointestinal dysmotility and metabolic disorders. J. Neurogastroenterol. Motil. 27 (1), 19–34. doi:10.5056/jnm20149
Smith, P., Willemsen, D., Popkes, M., Metge, F., Gandiwa, E., Reichard, M., et al. (2017). Regulation of life span by the gut microbiota in the short-lived African turquoise killifish. ELife 6, 27014. doi:10.7554/eLife.27014
Soysal, P., Stubbs, B., Lucato, P., Luchini, C., Solmi, M., Peluso, R., et al. (2017). Corrigendum to "inflammation and frailty in the elderly: A systematic review and meta-analysis" [ageing res rev. 31 (2016) 1-8] Ageing Res. Rev. 35, 364–365. doi:10.1016/j.arr.2016.12.007
Spacova, I., Van Beeck, W., Seys, S., Devos, F., Vanoirbeek, J., Vanderleyden, J., et al. (2020). Lactobacillus rhamnosus probiotic prevents airway function deterioration and promotes gut microbiome resilience in a murine asthma model. Gut Microbes 11 (6), 1729–1744. doi:10.1080/19490976.2020.1766345
Spazzafumo, L., Olivieri, F., Abbatecola, A. M., Castellani, G., Monti, D., Lisa, R., et al. (2013). Remodelling of biological parameters during human ageing: Evidence for complex regulation in longevity and in type 2 diabetes. Age Dordr. Neth. 35 (2), 419–429. doi:10.1007/s11357-011-9348-8
Stokholm, J., Sevelsted, A., Bønnelykke, K., and Bisgaard, H. (2014). Maternal propensity for infections and risk of childhood asthma: A registry-based cohort study. Lancet. Respir. Med. 2 (8), 631–637. doi:10.1016/S2213-2600(14)70152-3
Suez, J., Zmora, N., and Elinav, E. (2020). Probiotics in the next-generation sequencing era. Gut Microbes 11 (1), 77–93. doi:10.1080/19490976.2019.1586039
Suez, J., Zmora, N., Segal, E., and Elinav, E. (2019). The pros, cons, and many unknowns of probiotics. Nat. Med. 25 (5), 716–729. doi:10.1038/s41591-019-0439-x
Tagliabue, A., and Elli, M. (2013). The role of gut microbiota in human obesity: Recent findings and future perspectives. Nutr. Metabolism, Cardiovasc. Dis. NMCD 23 (3), 160–168. doi:10.1016/j.numecd.2012.09.002
Thaiss, C. A., Levy, M., Grosheva, I., Zheng, D., Soffer, E., Blacher, E., et al. (2018). Hyperglycemia drives intestinal barrier dysfunction and risk for enteric infection. Sci. (New York, N.Y.) 359 (6382), 1376–1383. doi:10.1126/science.aar3318
Thevaranjan, N., Puchta, A., Schulz, C., Naidoo, A., Szamosi, J. C., Verschoor, C. P., et al. (2017). Age-associated microbial dysbiosis promotes intestinal permeability, systemic inflammation, and macrophage dysfunction. Cell Host Microbe 21 (4), 455–466. doi:10.1016/j.chom.2017.03.002
Thomas, C. M., Hong, T., van Pijkeren, J. P., Hemarajata, P., Trinh, D. V., Hu, W., et al. (2012). Histamine derived from probiotic Lactobacillus reuteri suppresses TNF via modulation of PKA and ERK signaling. PloS One 7 (2), 31951. doi:10.1371/journal.pone.0031951
Ticinesi, A., Nouvenne, A., Cerundolo, N., Catania, P., Prati, B., Tana, C., et al. (2019). Gut microbiota, muscle mass and function in aging: A focus on physical frailty and sarcopenia. Nutrients 11 (7), 1633. doi:10.3390/nu11071633
Tilg, H., Zmora, N., Adolph, T. E., and Elinav, E. (2020). The intestinal microbiota fuelling metabolic inflammation. Nat. Rev. Immunol. 20 (1), 40–54. doi:10.1038/s41577-019-0198-4
Tominari, T., Sanada, A., Ichimaru, R., Matsumoto, C., Hirata, M., Itoh, Y., et al. (2021). Gram-positive bacteria cell wall-derived lipoteichoic acid induces inflammatory alveolar bone loss through prostaglandin E production in osteoblasts. Sci. Rep. 11 (1), 13353. doi:10.1038/s41598-021-92744-5
Toral, M., Romero, M., Rodríguez-Nogales, A., Jiménez, R., Robles-Vera, I., Algieri, F., et al. (2018). Lactobacillus fermentum improves tacrolimus-induced hypertension by restoring vascular redox state and improving eNOS coupling. Mol. Nutr. Food Res. 62 (14), 1800033. doi:10.1002/mnfr.201800033
Toward, R., Montandon, S., Walton, G., and Gibson, G. R. (2012). Effect of prebiotics on the human gut microbiota of elderly persons. Gut Microbes 3 (1), 57–60. doi:10.4161/gmic.19411
Tsai, Y.-L., Lin, T.-L., Chang, C.-J., Wu, T.-R., Lai, W.-F., Lu, C.-C., et al. (2019). Probiotics, prebiotics and amelioration of diseases. J. Biomed. Sci. 26 (1), 3. doi:10.1186/s12929-018-0493-6
Tun, M. H., Tun, H. M., Mahoney, J. J., Konya, T. B., Guttman, D. S., Becker, A. B., et al. (2018). Postnatal exposure to household disinfectants, infant gut microbiota and subsequent risk of overweight in children. CMAJ Can. Med. Assoc. J. = J. de L'Association Medicale Can. 190 (37), 1097–1107. doi:10.1503/cmaj.170809
Turchet, P., Laurenzano, M., Auboiron, S., and Antoine, J. M. (2003). Effect of fermented milk containing the probiotic lactobacillus casei DN-114001 on winter infections in free-living elderly subjects: A randomised, controlled pilot study. J. Nutr. Health & Aging 7 (2), 75–77.
van de Vyver, M. (2023). Immunology of chronic low-grade inflammation: Relationship with metabolic function. J. Endocrinol. 257, 220271. doi:10.1530/JOE-22-0271
Vancamelbeke, M., and Vermeire, S. (2017). The intestinal barrier: A fundamental role in health and disease. Expert Rev. Gastroenterology Hepatology 11 (9), 821–834. doi:10.1080/17474124.2017.1343143
Vandenplas, Y., Carnielli, V. P., Ksiazyk, J., Luna, M. S., Migacheva, N., Mosselmans, J. M., et al. (2020). Factors affecting early-life intestinal microbiota development. Nutr. (Burbank, Los Angel. Cty. Calif.) 78, 110812. doi:10.1016/j.nut.2020.110812
Veiga, P., Suez, J., Derrien, M., and Elinav, E. (2020). Moving from probiotics to precision probiotics. Nat. Microbiol. 5 (7), 878–880. doi:10.1038/s41564-020-0721-1
Vemuri, R., Shinde, T., Gundamaraju, R., Gondalia, S. V., Karpe, A. V., Beale, D. J., et al. (2018). Lactobacillus acidophilus DDS-1 modulates the gut microbiota and improves metabolic profiles in aging mice. Nutrients 10 (9), 1255. doi:10.3390/nu10091255
Venkatesh, M., Mukherjee, S., Wang, H., Li, H., Sun, K., Benechet, A. P., et al. (2014). Symbiotic bacterial metabolites regulate gastrointestinal barrier function via the xenobiotic sensor PXR and Toll-like receptor 4. Immunity 41 (2), 296–310. doi:10.1016/j.immuni.2014.06.014
Vidal, A. C., Murphy, S. K., Murtha, A. P., Schildkraut, J. M., Soubry, A., Huang, Z., et al. (2013). Associations between antibiotic exposure during pregnancy, birth weight and aberrant methylation at imprinted genes among offspring. Int. J. Obes. (2005) 37 (7), 907–913. doi:10.1038/ijo.2013.47
Vinolo, M. A. R., Rodrigues, H. G., Hatanaka, E., Sato, F. T., Sampaio, S. C., and Curi, R. (2011). Suppressive effect of short-chain fatty acids on production of proinflammatory mediators by neutrophils. J. Nutr. Biochem. 22 (9), 849–855. doi:10.1016/j.jnutbio.2010.07.009
Vivarelli, S., Salemi, R., Candido, S., Falzone, L., Santagati, M., Stefani, S., et al. (2019). Gut microbiota and cancer: From pathogenesis to therapy. Cancers 11 (1), 38. doi:10.3390/cancers11010038
Walker, A. W., Ince, J., Duncan, S. H., Webster, L. M., Holtrop, G., Ze, X., et al. (2011). Dominant and diet-responsive groups of bacteria within the human colonic microbiota. ISME J. 5 (2), 220–230. doi:10.1038/ismej.2010.118
Warman, D. J., Jia, H., and Kato, H. (2022). The potential roles of probiotics, resistant starch, and resistant proteins in ameliorating inflammation during aging (inflammaging). Nutrients 14 (4), 747. doi:10.3390/nu14040747
Wei, L., Singh, R., Ro, S., and Ghoshal, U. C. (2021). Gut microbiota dysbiosis in functional gastrointestinal disorders: Underpinning the symptoms and pathophysiology. JGH Open Open Access J. Gastroenterology Hepatology 5 (9), 976–987. doi:10.1002/jgh3.12528
Wiciński, M., Gębalski, J., Gołębiewski, J., and Malinowski, B. (2020). Probiotics for the treatment of overweight and obesity in humans-A review of clinical trials. Microorganisms 8 (8), 1148. doi:10.3390/microorganisms8081148
Wilkins, A. T., and Reimer, R. A. (2021). Obesity, early life gut microbiota, and antibiotics. Microorganisms 9 (2), 413. doi:10.3390/microorganisms9020413
Wilson, Q. N., Wells, M., Davis, A. T., Sherrill, C., Tsilimigras, M. C. B., Jones, R. B., et al. (2018). Greater microbial translocation and vulnerability to metabolic disease in healthy aged female monkeys. Sci. Rep. 8 (1), 11373. doi:10.1038/s41598-018-29473-9
Witkowski, M., Weeks, T. L., and Hazen, S. L. (2020). Gut microbiota and cardiovascular disease. Circulation Res. 127 (4), 553–570. doi:10.1161/CIRCRESAHA.120.316242
Wong, S. H., and Yu, J. (2019). Gut microbiota in colorectal cancer: Mechanisms of action and clinical applications. Nat. Rev. Gastroenterology Hepatology 16 (11), 690–704. doi:10.1038/s41575-019-0209-8
Xie, G., Zhou, Q., Qiu, C.-Z., Dai, W.-K., Wang, H.-P., Li, Y.-H., et al. (2017). Ketogenic diet poses a significant effect on imbalanced gut microbiota in infants with refractory epilepsy. World J. Gastroenterology 23 (33), 6164–6171. doi:10.3748/wjg.v23.i33.6164
Xu, Y., Liu, X., Liu, X., Chen, D., Wang, M., Jiang, X., et al. (2021). The roles of the gut microbiota and chronic low-grade inflammation in older adults with frailty. Front. Cell. Infect. Microbiol. 11, 675414. doi:10.3389/fcimb.2021.675414
Yang, J., and Yan, H. (2017). TLR5: Beyond the recognition of flagellin. Cell. Mol. Immunol. 14 (12), 1017–1019. doi:10.1038/cmi.2017.122
Yang, S., Li, X., Yang, F., Zhao, R., Pan, X., Liang, J., et al. (2019). Gut microbiota-dependent marker TMAO in promoting cardiovascular disease: Inflammation mechanism, clinical prognostic, and potential as a therapeutic target. Front. Pharmacol. 10, 1360. doi:10.3389/fphar.2019.01360
Yang, Y., Chen, T., Zhang, X., and Wang, X. (2021). Age-related functional changes of intestinal flora in rats. FEMS Microbiol. Lett. 368 (10), 051. doi:10.1093/femsle/fnab051
Youssef, M., Ahmed, H. Y., Zongo, A., Korin, A., Zhan, F., Hady, E., et al. (2021). Probiotic supplements: Their strategies in the therapeutic and prophylactic of human life-threatening diseases. Int. J. Mol. Sci. 22 (20), 11290. doi:10.3390/ijms222011290
Yu, H. J., Jing, C., Xiao, N., Zang, X. M., Zhang, C. Y., Zhang, X., et al. (2020). Structural difference analysis of adult's intestinal flora basing on the 16S rDNA gene sequencing technology. Eur. Rev. For Med. Pharmacol. Sci. 24 (24), 12983–12992. doi:10.26355/eurrev_202012_24203
Zelante, T., Iannitti, R. G., Cunha, C., De Luca, A., Giovannini, G., Pieraccini, G., et al. (2013). Tryptophan catabolites from microbiota engage aryl hydrocarbon receptor and balance mucosal reactivity via interleukin-22. Immunity 39 (2), 372–385. doi:10.1016/j.immuni.2013.08.003
Zhang, M., Differding, M. K., Benjamin-Neelon, S. E., Østbye, T., Hoyo, C., and Mueller, N. T. (2019). Association of prenatal antibiotics with measures of infant adiposity and the gut microbiome. Ann. Clin. Microbiol. Antimicrob. 18 (1), 18. doi:10.1186/s12941-019-0318-9
Keywords: probiotics, inflammation, gut microbiota, intestinal barrier, dysbiosis, elderly
Citation: Ren J, Li H, Zeng G, Pang B, Wang Q and Wei J (2023) Gut microbiome-mediated mechanisms in aging-related diseases: are probiotics ready for prime time?. Front. Pharmacol. 14:1178596. doi: 10.3389/fphar.2023.1178596
Received: 03 March 2023; Accepted: 24 May 2023;
Published: 01 June 2023.
Edited by:
Siomar De Castro Soares, Universidade Federal do Triângulo Mineiro, BrazilReviewed by:
Vanessa D’Antongiovanni, University of Pisa, ItalyRajan Singh, University of Nevada, United States
Copyright © 2023 Ren, Li, Zeng, Pang, Wang and Wei. This is an open-access article distributed under the terms of the Creative Commons Attribution License (CC BY). The use, distribution or reproduction in other forums is permitted, provided the original author(s) and the copyright owner(s) are credited and that the original publication in this journal is cited, in accordance with accepted academic practice. No use, distribution or reproduction is permitted which does not comply with these terms.
*Correspondence: Qiuhong Wang, qiuhongfortune@126.com; Junping Wei, weijunping@126.com