- 1Department of Biotechnology and Biomedicine, Technical University of Denmark, Kongens Lyngby, Denmark
- 2Sophion Bioscience, Ballerup, Denmark
- 3Institute for Molecular Bioscience, University of Queensland, St Lucia, QLD, Australia
- 4Australian Research Council Centre of Excellence for Innovations in Protein and Peptide Science, University of Queensland, St Lucia, QLD, Australia
Ion channels play a crucial role in diverse physiological processes, including neurotransmission and muscle contraction. Venomous creatures exploit the vital function of ion channels by producing toxins in their venoms that specifically target these ion channels to facilitate prey capture upon a bite or a sting. Envenoming can therefore lead to ion channel dysregulation, which for humans can result in severe medical complications that often necessitate interventions such as antivenom administration. Conversely, the discovery of highly potent and selective venom toxins with the capability of distinguishing between different isoforms and subtypes of ion channels has led to the development of beneficial therapeutics that are now in the clinic. This review encompasses the historical evolution of electrophysiology methodologies, highlighting their contributions to venom and antivenom research, including venom-based drug discovery and evaluation of antivenom efficacy. By discussing the applications and advancements in patch-clamp techniques, this review underscores the profound impact of electrophysiology in unravelling the intricate interplay between ion channels and venom toxins, ultimately leading to the development of drugs for envenoming and ion channel-related pathologies.
1 Introduction
Ion channels are specialized proteins that span the membrane, forming a pore or channel that allows charged ions to move across the membrane. They play a critical role in a wide range of physiological processes, including, but not limited to, neurotransmission and muscle contraction (Subramanyam and Colecraft, 2015). Activation of ion channels occurs through different mechanisms, such as in response to changes in membrane potential (voltage-gated channels) or due to binding of a ligand (ligand-gated channels). Voltage-gated ion channels come in various types, such as voltage-gated sodium (NaV) and voltage-gated potassium (KV) channels, and they are responsible for multiple physiological functions. For instance, in excitable cells, NaV channels play a crucial role in the initial depolarization of membrane and the generation of action potential, while KV channels contribute to membrane repolarization (Grider et al., 2023). Ligand-gated ion channels, on the other hand, are activated by specific ligands such as neurotransmitters. Acetylcholine, for instance, is an excitatory neurotransmitter found throughout the nervous system, which upon binding to nicotinic acetylcholine receptors (nAChRs) causes sodium and potassium ions to flow across the membrane, resulting in membrane depolarization and triggering of an action potential (Zoli et al., 2018).
Due to the multiple vital functions of most ion channels, molecules targeting these channels can interfere with and impair fundamental physiological mechanisms. This is something that venomous creatures take advantage of by producing toxins that have evolved specifically to target ion channels, aiding in prey capture and protection against predators (Osipov and Utkin, 2017). A vast array of venom toxins has been identified to interact with both voltage-gated and ligand-gated ion channels. Many of the toxins that target voltage-gated ion channels have been extracted from arthropod venoms, such as those found in scorpions and spiders (Gilchrist et al., 2014; Diochot, 2021). In terms of venom toxins affecting ligand-gated ion channels (Nasiripourdori et al., 2011), the most extensively studied toxins are those derived from cone snail venoms (Arias and Blanton, 2000) and snake venoms (Barber et al., 2013). For example, a prominent category of ion channel-targeting toxins in snake venoms are α-neurotoxins (αNTxs) that target nAChRs in the nervous system, hindering neurotransmission and muscle contraction. Thereby, snake αNTxs may cause a wide range of pathophysiological effects in humans, from itching and sweating to paralysis and respiratory arrest (Nirthanan et al., 2016).
Given the significant medical complications caused by many ion channel-targeting toxins, as well as their potential for use as human therapeutics (King, 2011), understanding their mechanism of action is critical. However, the complex structure and function of both (neuro)toxins and ion channels necessitates complex experimental setups. In this regard, a powerful tool to study toxin-ion channel interactions in real-time is electrophysiology, particularly patch-clamp methodologies. Patch-clamp electrophysiology enables direct measurement of changes in ion channel activity upon toxin exposure, and it has played a fundamental role in unraveling the mode of action of ion channel-modulating toxins, as well as in determination of their potency and selectivity. Moreover, it has recently been reported that patch-clamp electrophysiology can be used to evaluate the neutralization capacity of antibodies against scorpion (Restano-Cassulini et al., 2017) and snake venoms (Lynagh et al., 2020; Ledsgaard et al., 2022; 2023; Miersch et al., 2022), facilitating the development of efficient therapies against envenomings caused by these animals.
Although patch-clamp electrophysiology is widely recognized and utilized in the field of toxinology and venom research, there appears to be a lack of comprehensive reviews focusing on its application in antivenom research. This gap may be attributed to the relatively recent integration of patch-clamp techniques in antivenom studies, resulting in limited literature on the subject. In this review, we first present the history of electrophysiology and patch-clamp methodologies in ion channel research. We then describe the fundamental role these techniques have played in venom and antivenom research, including their role in venom-based drug discovery pipelines and assessment of the neutralizing effect of antivenoms. Finally, we discuss how patch-clamp electrophysiology can be employed in the development of new types of therapies against snakebite envenoming.
2 History of patch-clamp and ion channel discovery
In 1945, Hodgkin and Huxley were the first to demonstrate the existence of voltage-gated ion channels in cell membranes and to discover the principles of ion channel function (Hodgkin and Huxley, 1945; 1952). They used the giant axon of the squid Loligo forbesii as a model system to study the electrical activity of nerve cells. They found that nerve impulses are initiated by the movement of ions across the cell membrane, mediated by conducting structures that we now know as ion channels. Hodgkin and Huxley introduced mathematical equations that are still used for electrical membrane modelling and showed that the gating (opening and closing) of ion channels is controlled by changes in membrane potential, and that the resulting ion fluxes generate the electrical signals that underlie nerve impulses. For this discovery, they were awarded the Nobel Prize in Physiology or Medicine in 1963. Furthermore, together with Cole (Cole and Curtis, 1939), Hodgkin and Huxley developed the voltage-clamp technique, which was pivotal for the development of patch-clamp.
In the 1970s, Neher and Sakmann built on the work of Hodgkin and Huxley to introduce the patch-clamp technique (Neher et al., 1978). Using a glass pipette with an opening of a few micrometers, they were able to obtain direct measurements of ion channel currents across the cell membrane (Erwin Neher and Sakmann, 1976). By gently poking a micropipette into a cell membrane and applying a low, negative pipette pressure, they could create a high electrical resistance seal, named a giga seal as the resistance is measured in giga-ohms. Based on this cell-attached conformation (Figure 1A), further manipulation of the membrane give rise to whole-cell (Figure 1B), inside-out (Figure 1C), and outside-out (Figure 1D) configurations. In a whole-cell configuration, Neher and Sakmann applied a rapid, high-pressure suction to the pipette to rupture the membrane and create electrical contact with the intracellular environment. This allowed them to measure the electrical activity of the cell with great accuracy, manipulate the electrical activity of the cell, and control the voltage (Neher and Sakmann, 1976). The patch-clamp technique revolutionized the field of electrophysiology and won Neher and Sakmann the Nobel Prize in Physiology or Medicine in 1991.
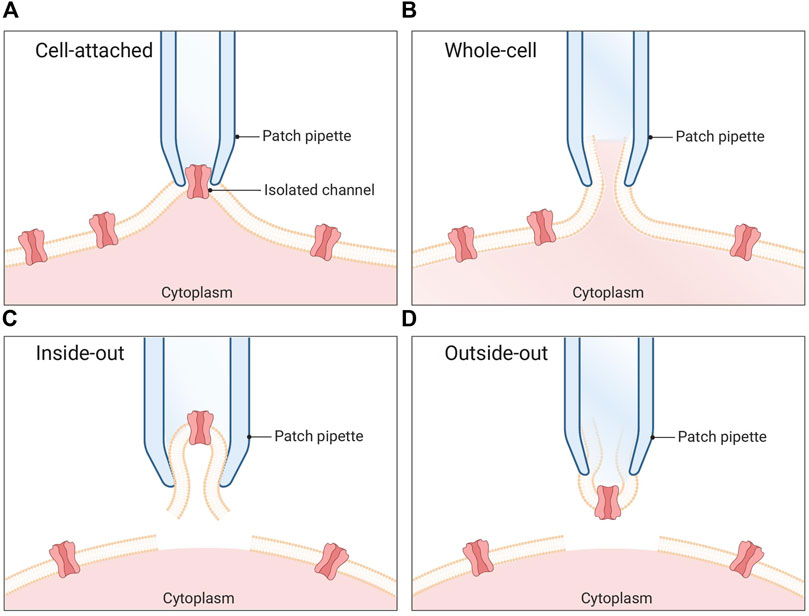
FIGURE 1. Patch clamp configurations. (A) Cell-attached mode: The pipette is positioned in proximity to the cell membrane, and gentle suction is applied to establish a tight seal between the pipette and the membrane. This configuration enables the recording of single channel currents. (B) Whole-cell mode: A pipette is utilized to apply a sufficiently strong suction force, resulting in the rupture of the cell membrane. Consequently, the pipette gains access to the cytoplasmic environment of the cell. (C) Inside-out mode: The membrane patch is carefully excised from the cell and immersed in the surrounding bath solution. In this arrangement, the seal remains intact while the rest of the cell is disrupted. (D) Outside-out mode: Initially, the whole-cell method is employed, followed by the retraction of the pipette after membrane rupture. This process causes a section of the membrane to detach from the cell and reconfigure into a small, vesicular structure, with the external side of the membrane exposed to the bath solution.
While the patch-clamp techniques were groundbreaking, they were also time-consuming and required highly skilled operators, as each cell must be individually patched with a micropipette. To increase throughput and reproducibility, automated versions of the patch-clamp technique were needed (Wang and Li, 2003). Attempts to automate the process started in the late 1990s in Denmark, where the patch clamp setup was equipped with computer-controlled patch pipettes in combination with a visual recognition system and a series of rotating patch chambers (Asmild et al., 2003; Grass et al., 2003). Pipette-based automated patch-clamp techniques continue to exist and offer the potential for streamlining the otherwise time-consuming and limited-throughput process of patch-clamp electrophysiology within tissue slices. For instance, a group at Georgia Institute of Technology has developed an automated patch clamp platform, which can patch in brain slices with intact neuronal networks (Kolb et al., 2019). Nevertheless, automated patch-clamp (APC) picked up speed in the early 2000s, with the introduction of chip-based systems, which enabled researchers to perform planar patch-clamp (Wang and Li, 2003). Here, the microchips have laser-drilled holes (1-2 µm) in a planar substrate instead of a pipette; this creates giga seals that allow recording of picoamperes of currents from a single cell (Figure 2). This technique allows to run hundreds of individual patch-clamp experiments in parallel (Brüggemann et al., 2003; Kiss et al., 2003).
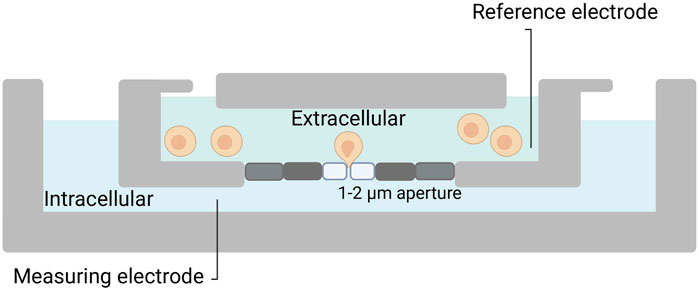
FIGURE 2. Planar patch-clamp. Silicon or plastic-based micro-fabricated substrates are utilized. Cells in suspension are added to the chip, and with a negative pressure, a cell is placed on top of the patch hole. Thereafter, the process is similar to manual patch-clamp.
The development of automated approaches has significantly increased the throughput of the patch-clamp electrophysiology, as these systems are not only automated, but can also simultaneously record measurements from multiple cells, which allows for faster and more efficient data collection. The first APC systems introduced in the late 1990s were used for drug discovery in the pharmaceutical industry. These early systems were able to measure currents from four to eight cells simultaneously, with a throughput of approximately 100–200 cells per day (Dale et al., 2007). Since then, the throughput of APC has continued to increase with advances in technology. In the early 2000s, APC systems were able to record from up to 48 cells simultaneously (Willumsen et al., 2003), and today, this number has increased to 384/768 cells (Obergrussberger et al., 2015; Chambers et al., 2016), enabling a throughput of thousands of experiments per day (Li et al., 2022). Various APC platforms are commercially available, however, a comprehensive review of such platforms falls beyond the scope of this manuscript and can be found elsewhere (Bell and Dallas, 2018).
The increased throughput of APCs has made them a valuable tool not only for drug discovery and high-throughput screening in the pharmaceutical industry, but also for basic research across several disciplines, from neurological (Rosholm et al., 2022; Ghovanloo et al., 2023) and cardiovascular sciences (Bell and Dallas, 2021) to venom (Olamendi-Portugal et al., 2008) and antivenom research (Restano-Cassulini et al., 2017; Ledsgaard et al., 2022; 2023; Miersch et al., 2022).
3 Electrophysiology in venom research
Electrophysiology techniques have played a significant role in advancing venom research, especially for elucidating the mechanisms by which venom toxins interact with and modulate ion channels and for the identification of toxins that can be used as scaffolds for drug development. The first, and perhaps the most important, of these techniques is the two-electrode voltage clamp (TEVC) electrophysiology method, used by Hodgkin and Huxley in their study of the squid giant axon. In this technique, two intracellular electrodes (one voltage sensor and one current injector) are used to set the cell membrane potential to a desired value and to record the current across the membrane. By applying this method to oocytes from the African clawed frog, Xenopus laevis, various toxins that act on a wide range of ion channels, from the NaV and KV channels to ligand-gated nicotinic acetylcholine (nACh) and gamma aminobutyric acid (GABA) receptors (Collins et al., 2009; Kvist et al., 2011; Bertaud et al., 2022), have been studied. The results of these studies provided valuable insights into how some toxins affect ion channel conductance, activation/inactivation dynamics, and opening/closing thresholds. Additionally, because of the low abundance of endogenous ion channels within their cell membrane, X. laevis oocytes serve as a versatile model for the expression and functional characterization of exogenous ion channels (Lin-Moshier and Marchant, 2013). Beyond expression of wild type ion channels, the oocytes can be used for expression of ion channels with modified subunit compositions or mutated variants, thereby serving as a powerful tool to investigate the interaction between venom toxins and ion channels. For instance, Ellinor et al. used this system to describe the structural determinants of the blockade of N-type voltage-gated calcium (CaV) channels (i.e., CaV2.2) by ω-conotoxin-GVIA, originally described in the venom of Conus geographus (Olivera et al., 1984). Here, the researchers created chimeric channels, containing individual motifs from both a CaV2.2 channel and a ω-conotoxin-GVIA insensitive channel, and expressed these in Xenopus oocytes, which allowed them to identify the binding sites for the toxin on the ion channel (Ellinor et al., 1994). Information from such studies is important, as the identification of ion channel binding sites for a toxin can help provide an explanation for the remarkable capability of many toxins to distinguish between different isoforms and subtypes of ion channels (Chassagnon et al., 2017). In turn, discovery of toxins with high ion channel specificity can serve as a starting point for drug development efforts, possibly by using the toxin as scaffold or benchmark molecule. This approach has led to the discovery of ziconotide (Prialt®), a drug chemically identical to the ω-conotoxin-MVIIA peptide from Conus magus (Miljanich, 2004; Safavi-Hemami et al., 2019). By applying whole cell patch-clamp electrophysiology, it was demonstrated that ω-conotoxin-MVIIA, in contrast to ω-conotoxin-GVIA, can distinguish between CaV2.1 and CaV2.2 subtypes of N-type Ca2+ channels (Safavi-Hemami et al., 2019). This specificity is important since, in mammals, CaV2.1 is expressed at the neuromuscular junction, whereas CaV2.2 is exclusively expressed at the dorsal horn of the spinal cord. In this context, CaV2.2 plays an essential role in the release of neurotransmitters at the presynaptic termini of pain fibers that connect with spinal cord neurons. Consequently, when the ω-conotoxin-MVIIA peptide binds to CaV2.2, it effectively prevents the transmission of signals from the pain fibers to the central nervous system, while exerting minimal or no effect on neuromuscular junctions (Safavi-Hemami et al., 2019). Ziconotide has received approval for the treatment of chronic and cancer-related pain, as an alternative to, or in combination with, morphine (Staats et al., 2004; McGivern, 2007; Williams et al., 2008), showcasing the practical application of toxin-ion channel knowledge for therapeutic advancements.
Even though TEVC electrophysiology has played a major role in advancing our understanding of toxin-ion channel interactions, it is both time-consuming, technically challenging, and requires highly specialized operators. Therefore, automated versions of this technique have been developed that facilitate high-throughput studies of toxin-ion channel interactions. As an example from 2022, Nys et al. used automated electrophysiology, in combination with crystallography and ion channel mutagenesis, to determine the precise location of the interactions between a consensus elapid short-chain α-neurotoxin (ScNtx) and both the muscle subtype and the α7 neuronal subtype of nAChR (Nys et al., 2022). While the ScNtx showed a similar inhibitory effect on both nAChR subtypes, the recovery process after inhibition was significantly different for the two receptor subtypes. The muscle subtype exhibited a slow and partial recovery, whereas the α7 neuronal subtype displayed an immediate and complete recovery, suggesting that the ScNtx has a higher binding affinity to the muscle subtype of nAChRs compared to the α7 neuronal subtype. In addition, the combination of mutagenesis data and electrophysiology experiments yielded compelling evidence showing that point mutations at the toxin-ion channel interface had a more pronounced effect on the α7 subtype than the muscle subtype (Nys et al., 2022). Such detailed knowledge on the mechanisms of action for different toxins is central for the development of effective antivenoms, as it aids the rational assessment of whether the function of a toxin can be blocked by different types of inhibitors.
Despite its wide utility, the use of TEVC electrophysiology is limited to the measurement of macroscopic currents across the membrane of large cells, which can pose limitations to recording fast-kinetics currents, such as sodium currents (Ivorra et al., 2022). In the 1970s, patch-clamp methodologies were developed, in which the substitution of the impaling electrodes of the two-electrode voltage clamp technique with a low electrical noise borosilicate glass electrode (patch pipette) in tight contact with the cell membrane allows for the measurement of signals in the pico ampere range and makes it possible to detect currents across the membrane of small cells or even single ion channels (Petkov, 2009). Among the multiple patch-clamp configurations, including cell-attached, whole-cell, inside-out, and outside-out modes, the whole-cell voltage clamp technique is the most widely used in venom research (Figure 1).
Whole-cell voltage clamp enables the analysis of microscopic currents through ion channels in their native environment in a variety of cell types. Moreover, transfection of mammalian cell lines, such as HEK or CHO cells (Cardoso et al., 2015), with ion channel encoding mRNA or DNA enables the expression of exogenous ion channels at significantly higher levels compared to native cells (Gamper et al., 2005; Ponce et al., 2018). For instance, Cardoso et al. performed an automated whole cell patch-clamp electrophysiology experiment, using a CHO cell line expressing the NaV1.7 isoform of the human voltage-gated sodium channel. This channel isoform plays a crucial role in pain signaling in humans and is considered a prominent target for therapeutic interventions in pain management. In their experiment, Cardoso et al. used high-throughput screening to identify potential NaV1.7-specific inhibitors present in the venoms from 40 species of tarantula spiders (Theraphosidae family), which resulted in the discovery of a 33-residue peptide from the venom of Thrixopelma pruriens, named µ-TRTX-Tp1a (ProTx-III). The results obtained from this whole-cell patch-clamp experiment indicated that this peptide preferentially inhibits the NaV1.7 without causing significant alterations in the voltage dependence of activation or steady-state inactivation. Thereby, this toxin distinguishes itself from the majority of spider toxins that typically modulate NaV channels by prominently affecting the voltage dependence of activation or inactivation (Cardoso et al., 2015). The discovery of subtype-selective peptides like µ-TRTX-Tp1a is central for the identification of scaffolds that may be used as leads in drug development programs, as they are less likely to modulate other ion channel subtypes and thereby cause off-target effects.
The use of medium-throughput and high-throughput patch-clamp methods has been essential for the characterization of venom-derived drug candidates for treatment of diseases, such as epilepsy (Cardoso, 2020), irritable bowel syndrome (Cardoso et al., 2021), and endometriosis (Castro et al., 2023). Similar to the application of NaV channel-expressing cell lines for the identification of molecules that could be used for pain management, other cell lines, such as the rhabdomyosarcoma cell line, which stably expresses the muscle subtype of nAChR, have successfully been employed for patch-clamp electrophysiology in antivenom research.
4 Electrophysiology in antivenom research
Although electrophysiology techniques were swiftly integrated into venom research following their invention, it took considerably longer before they were applied in the field of antivenom research. In fact, the application of electrophysiology techniques in the antivenom field did not start until 1994, when Harvey et al. suggested that a chick biventer cervicis nerve–muscle preparation could be used for evaluating the ability of antivenoms to neutralize the neurotoxic and direct myotoxic effects of venoms (Harvey et al., 1994). In the same year, Barfaraz and Harvey successfully utilized the chick biventer cervicis nerve–muscle preparation to compare the relative potencies of six different antivenoms for neutralization of neurotoxic and myotoxic proteins present in six distinct snake venoms (Barfaraz and Harvey, 1994). The chick biventer cervicis nerve–muscle preparations, along with mouse phrenic nerve-diaphragm preparations, have since then been employed to evaluate the efficacy of antivenoms in neutralizing the neuromuscular blocking effect induced by various venoms (Fry et al., 2001; Camargo et al., 2011; Kornhauser et al., 2013; Herrera et al., 2016; Silva et al., 2017; Madhushani et al., 2020; Zukifli et al., 2022).
While assays involving nerve-muscle preparations have proven to be highly valuable and effective, their implementation and integration into routine quality control analysis of antivenoms is hindered by their time-consuming and labor intensive nature (Gutiérrez et al., 2021). Therefore, other electrophysiological approaches, such as different patch-clamp methodologies, may be more attractive for researchers, as they offer more adaptability and versatility for evaluating the efficacy of antivenoms. As an example, in 2017, Restano-Cassulini et al. demonstrated that whole cell patch-clamp using cell lines with heterologous expression of human NaV and nAchR could be used for validation of the neutralizing efficacy of scorpion antivenoms (Restano-Cassulini et al., 2017). Approaches like this hold the potential to be employed for the quality control of scorpion antivenoms and might provide an ethically preferable alternative over animal experiments, as they conform with the 3Rs principle of replacement, reduction, and refinement of animal experiments, encouraged by the World Health Organization (Gutiérrez et al., 2021). Another advantage of patch-clamp approaches is that cell lines that express human ion channels, either endogenously or via heterologous expression, can be used. Thus, data obtained from these approaches may prove to be clinically even more relevant than animal models, since there can be slight, yet potentially significant, differences between human ion channels and those found in animal cells. While it is necessary to further substantiate the results from the in vitro patch-clamp protocol suggested by Restano-Cassulini et al. and benchmark these quantitatively with the existing in vivo models, the in vitro patch-clamp technique could potentially be implemented as a high-throughput, standard approach for quality control of scorpion antivenoms and may find utility for the evaluation of other types of antivenoms as well.
Patch-clamp techniques not only facilitate the evaluation of the neutralization capacity of existing antivenoms, but can also aid in the development of new and improved types of antivenoms by enabling the functional characterization of recombinant and/or consensus toxins. One example of such an application is related to one of the most toxic super-families of snake venom toxins for mammals, i.e., the three finger-toxin family. A subgroup of 3FTxs is the α-neurotoxins, which bind to nAChRs. Thereby, these toxins cause post-synaptic neurotoxicity through non-depolarizing blockade of the nAChRs and impair neuromuscular transmission. Due to their small size (6–8 kDa), low immunogenicity (Laustsen et al., 2017), and relatively low abundance in most venoms in which they occur, α-neurotoxins are often unable to provoke a strong immune response. As a result, they may not effectively be neutralized by current snake antivenoms, as these antivenoms are produced through hyper-immunization of animals (Laustsen et al., 2017). One approach for improving antivenoms against α-neurotoxins is thus by using designed consensus toxins with selected amino acid substitutions to enhance immunogenicity, which elicit an antibody response with broader cross-reactivity against multiple (related) toxins. As an example of this approach, de la Rosa et al. designed, expressed, and purified a consensus toxin, referred to as ScNtx, based on sequences from twelve short-chain α-neurotoxins found in the venoms of medically important snake species (de la Rosa et al., 2018). The pharmacological properties of ScNtx were thereafter assessed by voltage clamping, using X. laevis oocytes, which demonstrated the ability of ScNtx to antagonize the nAChR receptor. ScNTx was then used as an immunogen for the immunization of rabbits, whereupon their sera could recognize native short-chain α-neurotoxins present in various elapid venoms in an in vitro binding assay. This example demonstrates how patch-clamp can be used to establish the functionality of a recombinant (consensus) toxin and further facilitate the development of efficacious antivenoms derived through animal immunization.
Another approach that is being pursued to improve therapy against α-neurotoxins is the development of recombinant antivenoms (Kini et al., 2018). Here, monoclonal antibodies or nanobodies are typically discovered via in vitro display technologies using purified toxins (Ledsgaard et al., 2022; 2023; Miersch et al., 2022). However, the purification of low-abundance snake toxins, such as α-neurotoxins, from crude venoms is a challenging process involving intensive manual labor. Fortunately, it has recently been shown that this toxin purification process can be circumvented by using recombinant DNA technology. In 2023, Rimbault et al. cloned, expressed, and purified recombinant α-cobratoxin, a potent long-chain α-neurotoxins from Naja kaouthia venom (Rimbault et al., 2023), and demonstrated its ability to inhibit the ion flux through the muscular nAChR using a high-throughput automated electrophysiology platform, thereby confirming its correct folding. In brief, the human-derived rhabdomyosarcoma RD cell line with endogenous expression of αβγδ nAChR was used, and the current mediated by nAChR was elicited by the addition of acetylcholine. Afterwards, both recombinant and native α-cobratoxin were applied to the system, and it was shown that both could inhibit the acetylcholine-induced current in the cells. After confirming the functionality of the recombinant α-cobratoxin, the researchers used it as antigen in a phage display-based antibody discovery campaign and discovered three antibody fragments capable of blocking the ability of the native toxin to bind to the nAChR.
Although the use of recombinant toxins offers several benefits, the technical challenges associated with their expression and purification (Rivera-de-Torre et al., 2022) hinder their widespread adoption. Therefore, crude venoms remain the primary sources of toxins used in antivenom research. Nevertheless, patch-clamp techniques still play an important role, particularly in the analysis of the neutralizing capacities of recombinant antibodies and other inhibitory agents discovered against ion channel-targeting toxins. For example, whole-cell patch-clamp techniques have been used to evaluate the functional inhibitory effect of synthetic antibodies (Miersch et al., 2022), chain-shuffled antibodies with native domains (Ledsgaard et al., 2022; 2023), and peptides (Lynagh et al., 2020) selected against native α-cobratoxin using phage display technology. In all these studies, the same principle was used for assessing the neutralizing potency, i.e., the cell membrane current in a nAChR-expressing cell line was measured in three different scenarios: first, in the presence of acetylcholine alone (Figure 3A); second, after the addition of acetylcholine and toxin (Figure 3B); and finally, after the addition of toxin pre-incubated with the selected antibodies or peptides (Figure 3C).
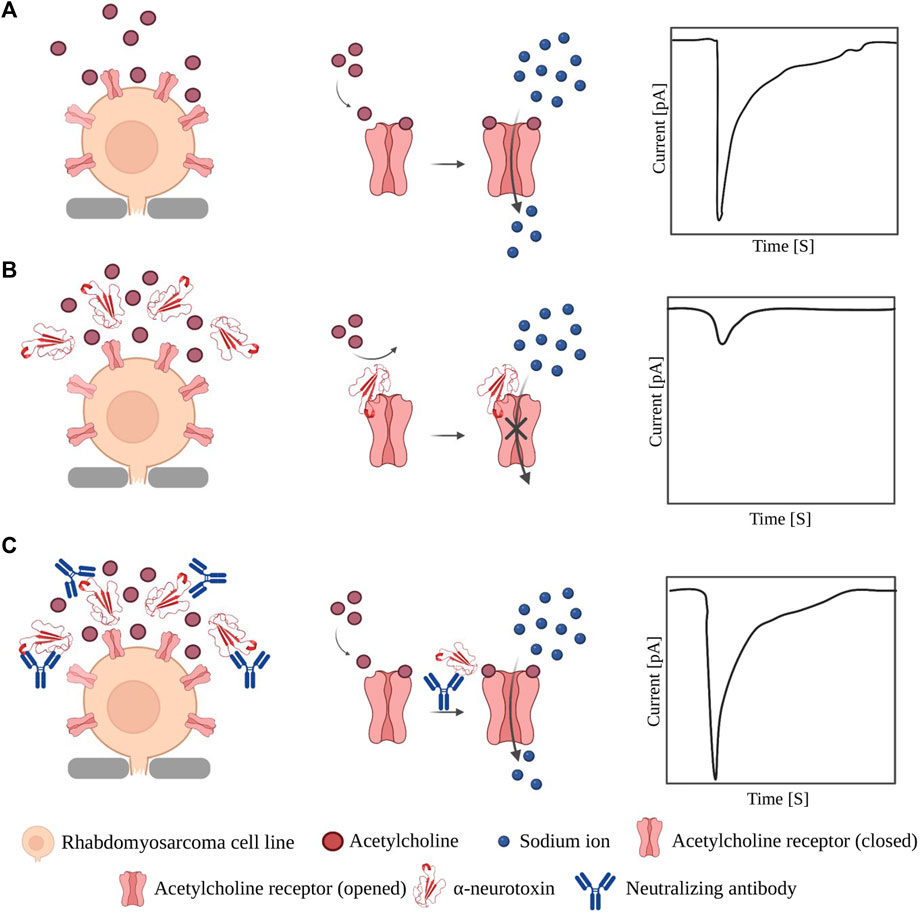
FIGURE 3. Application of patch-clamp in evaluating neutralization effect of binders against α-NTx. (A) Opening of nAChR upon binding of acetylcholine allows influx of sodium ions which can be measured as a current by patch-clamp instruments. (B) When α-NTxs are added to the cells, the pore and the influx of sodium ions are blocked, which results in a small current difference between the inside and outside of the cell. (C) When a mixture of pre-incubated α-NTx and a neutralizing molecule is added, α-NTxs cannot bind to the receptor, and the influx of sodium ions is not affected. Note: the relative sizes of the components in the figure are not accurately depicted.
In summary, these studies demonstrate that patch-clamp techniques not only facilitate the evaluation of the neutralization capacity of existing antivenoms, but also enable functional characterization of the native and recombinant toxins used for antivenom development, as well as high-throughput functional screening of monoclonal antibodies and other toxin-neutralizing agents.
5 Discussion
The application of electrophysiology, particularly APCs, for the study of venom toxins has significantly contributed to our understanding of ion channel pharmacology and function. Additionally, the utilization of diverse electrophysiology techniques to study toxin-ion channel interactions and screen venoms for specific ion channel-targeting toxins has played a crucial role in the discovery of venom-based therapeutic lead molecules for ion channel-related diseases, such as chronic pain, epilepsy, and irritable bowel syndrome. Moreover, within the field of envenoming therapy, electrophysiology techniques, such as patch-clamp, are now playing a pivotal role in advancing the development of novel types of antivenoms that can neutralize ion channel-targeting toxins.
It is, however, important to highlight that although APC systems have significantly advanced venom and antivenom research, they have not entirely replaced the need for manual patch clamp techniques. Rather, these two methods should be considered complementary. Manual patch clamp remains essential for successfully patching cells in some cell cultures and tissue preparations, such as organoids and brain slides with intact neural networks. On the other hand, planar automated patch clamp is well suited for cells in suspension, where the cell selection is unbiased, and operators cannot visually examine the cells as in manual patch clamp. Additionally, substantial cell line and assay optimization is often required before conducting high-throughput screens on APCs. In cases where the project’s throughput requirements do not justify the initial investment for an APC system, manual patch clamp offers a more accessible starting point (Dunlop et al., 2008; Bell and Dallas, 2018). In the field of venom research, a common limitation of employing manual patch clamp techniques arises from the scarcity and costliness of toxins. Meeting the substantial quantities required for perfusion systems used in manual patch clamp setups can be problematic. Conversely, numerous APC systems utilize microfluidics, necessitating a smaller volume and thereby low amount of materials per experiment. However, this reduction in liquid volume leads to a significant increase in the liquid-to-surface ratio, potentially giving rise to a challenge through nonspecific polyreactivity, “stickiness,” of some toxins (Bridgland-Taylor et al., 2006; Gillie et al., 2013; Yu et al., 2016).
In general, a limitation of electrophysiology techniques arises from their prerequisite for an intact cell membrane. This poses challenges to their application in venom and antivenom research, especially when studying animal venoms that contain membrane-disrupting components, such as cytotoxins and phospholipases. Consequently, certain patch-clamp configurations may not be immediately suitable for studying complex whole venoms, such as those found in most snakes, but require that laborious toxin expression and/or purification protocols are used to produce or isolate the ion channel-targeting components from the whole venom. In contrast, venoms with no or a low abundance of membrane-disrupting toxins, like scorpion venoms that mainly contain ion channel-targeting toxins, are more amenable to patch-clamp. This makes patch-clamp a valuable tool for evaluating the efficacy of scorpion antivenoms and may position patch-clamp as a potential alternative to animal experiments for quality control of antivenoms. The substitution of animal models with in vitro methodologies remains a topic of debate. It is challenging to fully assess how results obtained from in vitro patch-clamp experiments will translate to the clinical setting in terms of treatment outcomes. Therefore, it is crucial to comprehend the advantages and limitations of electrophysiological techniques in the context of envenoming treatment and ascertain the extent to which their results can be extrapolated. Despite the inherent challenges, electrophysiology in general, and patch-clamp methods in particular, remain powerful assets in venom and antivenom research, where we predict that they will continue to provide valuable molecular insights that can be exploited in the development of effective treatments for ion channel-related pathologies.
Author contributions
SA, MB-V, KB, FC, GK, AHL, and AL wrote the manuscript. All authors contributed to the article and approved the submitted version.
Funding
AHL is supported by a grant from the European Research Council (ERC) under the European Union’s Horizon 2020 research and innovation program [850974], by a grant from the Villum Foundation [00025302], and a grant from the Wellcome Trust [221702/Z/20/Z]. GK is supported by a Principal Research Fellowship [APP1136889] from the Australian National Health and Medical Research Council and a Centre of Excellence Grant [CE200100012] from the Australian Research Council. FC is supported by a CDMRP from the U.S.A. Department of Defense [HT9425-23-1-0146] and an Ideas Grant from the Australian National Health and Medical Research Council [APP1188959]. MB-V is supported by a Eurotech postdoctoral fellow from the European Union’s Horizon 2020 research and innovation programme under the Marie Skłodowska-Curie grant agreement [899987].
Conflict of interest
KB is employed by Sophion Bioscience.
The remaining authors declare that the research was conducted in the absence of any commercial or financial relationships that could be construed as a potential conflict of interest.
Publisher’s note
All claims expressed in this article are solely those of the authors and do not necessarily represent those of their affiliated organizations, or those of the publisher, the editors and the reviewers. Any product that may be evaluated in this article, or claim that may be made by its manufacturer, is not guaranteed or endorsed by the publisher.
References
Arias, H. R., and Blanton, M. P. (2000). Alpha-conotoxins. Int. J. Biochem. Cell. Biol. 32 (10), 1017–1028. doi:10.1016/S1357-2725(00)00051-0
Asmild, M., Oswald, N., Krzywkowski, K. M., Friis, S., Jacobsen, R. B., Reuter, D., et al. (2003). Upscaling and automation of electrophysiology: toward high throughput screening in ion channel drug discovery. Recept. Channels 9 (1), 49–58. doi:10.3109/10606820308258
Barber, C. M., Isbister, G. K., and Hodgson, W. C. (2013). Alpha neurotoxins. Toxicon 66, 47–58. doi:10.1016/j.toxicon.2013.01.019
Barfaraz, A., and Harvey, A. L. (1994). The use of the chick biventer cervicis preparation to assess the protective activity of six international reference antivenoms on the neuromuscular effects of snake venoms in vitro. Toxicon 32 (3), 267–272. doi:10.1016/0041-0101(94)90079-5
Bell, D. C., and Dallas, M. L. (2021). “Advancing ion channel research with automated patch clamp (APC) electrophysiology platforms,” in Ion channels in biophysics and Physiology. Editor L. Zhou (Singapore: Springer Nature Advances in Experimental Medicine and Biology), 21–32. doi:10.1007/978-981-16-4254-8_2
Bell, D. C., and Dallas, M. L. (2018). Using automated patch clamp electrophysiology platforms in pain-related ion channel research: insights from industry and academia. Br. J. Pharmacol. 175 (12), 2312–2321. doi:10.1111/bph.13916
Bertaud, A., Cens, T., Mary, R., Rousset, M., Arel, E., Thibaud, J. B., et al. (2022). Xenopus oocytes: a tool to decipher molecular specificity of insecticides towards mammalian and insect GABA— receptors. Membranes 12 (5), 440. doi:10.3390/membranes12050440
Bridgland-Taylor, M. H., Hargreaves, A. C., Easter, A., Orme, A., Henthorn, D. C., Ding, M., et al. (2006). Optimisation and validation of a medium-throughput electrophysiology-based hERG assay using IonWorksTM HT. J. Pharmacol. Toxicol. Methods 54 (2), 189–199. doi:10.1016/j.vascn.2006.02.003
Brüggemann, A., George, M., Klau, M., Beckler, M., Steindl, J., Behrends, J. C., et al. (2003). High quality ion channel analysis on a chip with the NPC© technology. ASSAY Drug Dev. Technol. 1 (5), 665–673. doi:10.1089/154065803770381020
Camargo, T. M., de Roodt, A. R., da Cruz-Höfling, M. A., and Rodrigues-Simioni, L. (2011). The neuromuscular activity of Micrurus pyrrhocryptus venom and its neutralization by commercial and specific coral snake antivenoms. J. Venom Res. 2, 24–31.
Cardoso, F. C., Castro, J., Grundy, L., Schober, G., Garcia-Caraballo, S., Zhao, T., et al. (2021). A spider-venom peptide with multitarget activity on sodium and calcium channels alleviates chronic visceral pain in a model of irritable bowel syndrome. PAIN 162 (2), 569–581. doi:10.1097/j.pain.0000000000002041
Cardoso, F. C., Dekan, Z., Rosengren, K. J., Erickson, A., Vetter, I., Deuis, J. R., et al. (2015). Identification and characterization of Protx-III [μ-Trtx-Tp1a], a new voltage-gated sodium channel inhibitor from venom of the Tarantula thrixopelma pruriens. Mol. Pharmacol. 88 (2), 291–303. doi:10.1124/mol.115.098178
Cardoso, F. C. (2020). Multi-targeting sodium and calcium channels using venom peptides for the treatment of complex ion channels-related diseases. Biochem. Pharmacol. 181, 114107. doi:10.1016/j.bcp.2020.114107
Castro, J., Maddern, J., Chow, C. Y., Tran, P., Vetter, I., King, G. F., et al. (2023). The voltage-gated sodium channel NaV1.7 underlies endometriosis-associated chronic pelvic pain. J. Neurochem. doi:10.1111/jnc.15795
Chambers, C., Witton, I., Adams, C., Marrington, L., and Kammonen, J. (2016). High-throughput screening of NaV1.7 modulators using a giga-seal automated patch clamp instrument. ASSAY Drug Dev. Technol. 14 (2), 93–108. doi:10.1089/adt.2016.700
Chassagnon, I. R., McCarthy, C. A., Chin, Y. K. Y., Pineda, S. S., Keramidas, A., Mobli, M., et al. (2017). Potent neuroprotection after stroke afforded by a double-knot spider-venom peptide that inhibits acid-sensing ion channel 1a. Proc. Natl. Acad. Sci. U. S. A. 114 (14), 3750–3755. doi:10.1073/pnas.1614728114
Cole, K. S., and Curtis, H. J. (1939). Electric impedance of the squid giant axon during activity. J. General Physiology 22 (5), 649–670. doi:10.1085/jgp.22.5.649
Collins, A. C., Salminen, O., Marks, M. J., Whiteaker, P., and Grady, S. R. (2009). The road to discovery of neuronal nicotinic cholinergic receptor subtypes. Handb. Exp. Pharmacol. 192, 85–112. doi:10.1007/978-3-540-69248-5_4
Dale, T. J., Townsend, C., Hollands, E. C., and Trezise, D. J. (2007). Population patch clamp electrophysiology: a breakthrough technology for ion channel screening. Mol. Biosyst. 3 (10), 714–722. doi:10.1039/B706152H
de la Rosa, G., Corrales-García, L. L., Rodriguez-Ruiz, X., López-Vera, E., and Corzo, G. (2018). Short-chain consensus alpha-neurotoxin: a synthetic 60-mer peptide with generic traits and enhanced immunogenic properties. Amino Acids 50 (7), 885–895. doi:10.1007/s00726-018-2556-0
Diochot, S. (2021). Pain-related toxins in scorpion and spider venoms: a face to face with ion channels. J. Venom. Animals Toxins Incl. Trop. Dis. 27, e20210026. doi:10.1590/1678-9199-JVATITD-2021-0026
Dunlop, J., Bowlby, M., Peri, R., Vasilyev, D., and Arias, R. (2008). High-throughput electrophysiology: an emerging paradigm for ion-channel screening and physiology. Nat. Rev. Drug Discov. 7 (4), 358–368. doi:10.1038/nrd2552
Ellinor, P. T., Zhang, J. F., Horne, W. A., and Tsien, R. W. (1994). Structural determinants of the blockade of N-type calcium channels by a peptide neurotoxin. Nature 372 (6503), 272–275. doi:10.1038/372272a0
Fry, B. G., Wickramaratna, J. C., Jones, A., Alewood, P. F., and Hodgson, W. C. (2001). Species and regional variations in the effectiveness of antivenom against the in vitro neurotoxicity of death adder (Acanthophis) venoms. Toxicol. Appl. Pharmacol. 175 (2), 140–148. doi:10.1006/taap.2001.9233
Gamper, N., Stockand, J. D., and Shapiro, M. S. (2005). The use of Chinese hamster ovary (CHO) cells in the study of ion channels. J. Pharmacol. Toxicol. Methods 51 (3), 177–185. doi:10.1016/j.vascn.2004.08.008
Ghovanloo, M.-R., Tyagi, S., Zhao, P., Kiziltug, E., Estacion, M., Dib-Hajj, S. D., et al. (2023). High-throughput combined voltage-clamp/current-clamp analysis of freshly isolated neurons. Cell. Rep. Methods 3 (1), 100385. doi:10.1016/j.crmeth.2022.100385
Gilchrist, J., Olivera, B. M., and Bosmans, F. (2014). Animal toxins influence voltage-gated sodium channel function. Handb. Exp. Pharmacol. 221, 203–229. doi:10.1007/978-3-642-41588-3_10
Gillie, D. J., Novick, S. J., Donovan, B. T., Payne, L. A., and Townsend, C. (2013). Development of a high-throughput electrophysiological assay for the human ether-à-go-go related potassium channel hERG. J. Pharmacol. Toxicol. Methods 67 (1), 33–44. doi:10.1016/j.vascn.2012.10.002
Grass, G. M. (2003). Pharmacokinetic-based drug design tool and method. Available at: https://www.freepatentsonline.com/6647358.html.
Grider, M. H., Jessu, R., and Kabir, R. (2023). Physiology, action potential. Treasure Island (FL): StatPearls Publishing.
Gutiérrez, J. M., Vargas, M., Segura, Á., Herrera, M., Villalta, M., Solano, G., et al. (2021). In vitro tests for assessing the neutralizing ability of snake antivenoms: toward the 3Rs principles. Front. Immunol. 11, 617429. doi:10.3389/fimmu.2020.617429
Harvey, A. L., Barfaraz, A., Thomson, E., Faiz, A., Preston, S., and Harris, J. B. (1994). Screening of snake venoms for neurotoxic and myotoxic effects using simple in vitro preparations from rodents and chicks. Toxicon 32 (3), 257–265. doi:10.1016/0041-0101(94)90078-7
Herrera, M., de Cássia de O Collaço, R., Villalta, M., Segura, Á., Vargas, M., Wright, C. E., et al. (2016). Neutralization of the neuromuscular inhibition of venom and taipoxin from the taipan (Oxyuranus scutellatus) by F(ab′)2 and whole IgG antivenoms. Toxicol. Lett. 241, 175–183. doi:10.1016/j.toxlet.2015.11.020
Hodgkin, A. L., and Huxley, A. F. (1952). A quantitative description of membrane current and its application to conduction and excitation in nerve. J. Physiology 117 (4), 500–544. doi:10.1113/jphysiol.1952.sp004764
Hodgkin, A. L., and Huxley, A. F. (1945). Resting and action potentials in single nerve fibres. J. Physiology 104 (2), 176–195. doi:10.1113/jphysiol.1945.sp004114
Ivorra, I., Alberola-Die, A., Cobo, R., González-Ros, J. M., and Morales, A. (2022). Xenopus oocytes as a powerful cellular model to study foreign fully-processed membrane proteins. Membranes 12 (10), 986. doi:10.3390/membranes12100986
King, G. F. (2011). Venoms as a platform for human drugs: translating toxins into therapeutics. Expert Opin. Biol. Ther. 11 (11), 1469–1484. doi:10.1517/14712598.2011.621940
Kini, R. M., Sidhu, S. S., and Laustsen, A. H. (2018). Biosynthetic oligoclonal antivenom (BOA) for snakebite and next-generation treatments for snakebite victims. Toxins 10 (12), 534. doi:10.3390/toxins10120534
Kiss, L., Bennett, P. B., Uebele, V. N., Koblan, K. S., Kane, S. A., Neagle, B., et al. (2003). High throughput ion-channel pharmacology: planar-array-based voltage clamp. ASSAY Drug Dev. Technol. 1 (Suppl. 2), 127–135. doi:10.1089/154065803321537845
Kolb, I., Landry, C. R., Yip, M. C., Lewallen, C. F., Stoy, W. A., Lee, J., et al. (2019). PatcherBot: a single-cell electrophysiology robot for adherent cells and brain slices. J. Neural Eng. 16 (4), 046003. doi:10.1088/1741-2552/ab1834
Kornhauser, R., Isbister, G. K., O'Leary, M. A., Mirtschin, P., Dunstan, N., and Hodgson, W. C. (2013). Cross-neutralisation of the neurotoxic effects of Egyptian cobra venom with commercial tiger snake antivenom. Basic and Clin. Pharmacol. Toxicol. 112 (2), 138–143. doi:10.1111/j.1742-7843.2012.00925.x
Kvist, T., Hansen, K. B., and Bräuner-Osborne, H. (2011). The use of Xenopus oocytes in drug screening. Expert Opin. Drug Discov. 6 (2), 141–153. doi:10.1517/17460441.2011.546396
Laustsen, A. H., Engmark, M., Clouser, C., Timberlake, S., Vigneault, F., Gutiérrez, J. M., et al. (2017). Exploration of immunoglobulin transcriptomes from mice immunized with three-finger toxins and phospholipases A2 from the Central American coral snake. Micrurus nigrocinctus 5, e2924. doi:10.7717/peerj.2924
Ledsgaard, L., Laustsen, A. H., Pus, U., Wade, J., Villar, P., Boddum, K., et al. (2022). In vitro discovery of a human monoclonal antibody that neutralizes lethality of cobra snake venom. mAbs 14 (1), 2085536. doi:10.1080/19420862.2022.2085536
Ledsgaard, L., Wade, J., Jenkins, T. P., Boddum, K., Oganesyan, I., Harrison, J. A., et al. (2023). Discovery and optimization of a broadly-neutralizing human monoclonal antibody against long-chain α-neurotoxins from snakes. Nat. Commun. 14 (1), 682. doi:10.1038/s41467-023-36393-4
Li, T., Ginkel, M., Yee, A. X., Foster, L., Chen, J., Heyse, S., et al. (2022). An efficient and scalable data analysis solution for automated electrophysiology platforms. SLAS Discov. 27 (4), 278–285. doi:10.1016/j.slasd.2021.11.001
Lin-Moshier, Y., and Marchant, J. S. (2013). The Xenopus oocyte: a single-cell model for studying Ca2+ signaling. Cold Spring Harb. Protoc. 2013 (3), top066308. doi:10.1101/pdb.top066308
Lynagh, T., Kiontke, S., Meyhoff-Madsen, M., Gless, B. H., Johannesen, J., Kattelmann, S., et al. (2020). Peptide inhibitors of the α-cobratoxin-nicotinic acetylcholine receptor interaction. J. Med. Chem. 63 (22), 13709–13718. doi:10.1021/acs.jmedchem.0c01202
Madhushani, U., Isbister, G. K., Tasoulis, T., Hodgson, W. C., and Silva, A. (2020). In vitro neutralization of the neurotoxicity of coastal taipan venom by Australian polyvalent antivenom: the window of opportunity. Toxins 12 (11), 690. doi:10.3390/toxins12110690
McGivern, J. G. (2007). Ziconotide: a review of its pharmacology and use in the treatment of pain. Neuropsychiatric Dis. Treat. 3 (1), 69–85. doi:10.2147/nedt.2007.3.1.69
Miersch, S., de la Rosa, G., Friis, R., Ledsgaard, L., Boddum, K., Laustsen, A. H., et al. (2022). Synthetic antibodies block receptor binding and current-inhibiting effects of α-cobratoxin from Naja kaouthia. Protein Sci. 31 (5), e4296. doi:10.1002/pro.4296
Miljanich, G. P. (2004). Ziconotide: neuronal calcium channel blocker for treating severe chronic pain. Curr. Med. Chem. 11 (23), 3029–3040. doi:10.2174/0929867043363884
Nasiripourdori, A., Taly, V., Grutter, T., and Taly, A. (2011). From toxins targeting ligand gated ion channels to therapeutic molecules. Toxins 3 (3), 260–293. doi:10.3390/toxins3030260
Neher, E., and Sakmann, B. (1976). Single-channel currents recorded from membrane of denervated frog muscle fibres. Nature 260 (5554), 799–802. doi:10.1038/260799a0
Neher, E., Sakmann, B., and Steinbach, J. H. (1978). The extracellular patch clamp: a method for resolving currents through individual open channels in biological membranes. Pflugers Archiv Eur. J. Physiology 375 (2), 219–228. doi:10.1007/BF00584247
Nirthanan, S., Awal, W., and Niranjan, N. R. (2016). “Snake α-neurotoxins and the nicotinic acetylcholine receptor,” in Snake venoms. Editors Gopalakrishnakone, P., Inagaki, H., Mukherjee, A., Rahmy, T., and Vogel, C. W. (Dordrecht: Springer Netherlands, Toxinology). doi:10.1007/978-94-007-6648-8_29-1
Nys, M., Zarkadas, E., Brams, M., Mehregan, A., Kambara, K., Kool, J., et al. (2022). The molecular mechanism of snake short-chain α-neurotoxin binding to muscle-type nicotinic acetylcholine receptors. Nat. Commun. 13 (1), 4543. doi:10.1038/s41467-022-32174-7
Obergrussberger, A., Stölzle-Feix, S., Becker, N., Brüggemann, A., Fertig, N., and Möller, C. (2015). Novel screening techniques for ion channel targeting drugs. Channels 9 (6), 367–375. doi:10.1080/19336950.2015.1079675
Olamendi-Portugal, T., Batista, C. V. F., Restano-Cassulini, R., Pando, V., Villa-Hernandez, O., Zavaleta-Martínez-Vargas, A., et al. (2008). Proteomic analysis of the venom from the fish eating coral snake Micrurus surinamensis: novel toxins, their function and phylogeny. Proteomics 8 (9), 1919–1932. doi:10.1002/pmic.200700668
Olivera, B. M., McIntosh, J. M., Cruz, L. J., Luque, F. A., and Gray, W. R. (1984). Purification and sequence of a presynaptic peptide toxin from Conus geographus venom. Biochemistry 23 (22), 5087–5090. doi:10.1021/bi00317a001
Osipov, A. V., and Utkin, Y. N. (2017). “Snake venom toxins targeted at the nervous system,” in Snake venoms. Editor H. Inagaki (Dordrecht: Springer Netherlands Toxinology), 189–214. doi:10.1007/978-94-007-6410-1_23
Petkov, G. V. (2009). in Chapter 16 - ion channels. Pharmacology. Editors M. Hacker, W. Messer, and K. Bachmann (San Diego: Academic Press), 387–427. doi:10.1016/B978-0-12-369521-5.00016-6
Ponce, A., Castillo, A., Hinojosa, L., Martinez-Rendon, J., and Cereijido, M. (2018). The expression of endogenous voltage-gated potassium channels in HEK293 cells is affected by culture conditions. Physiol. Rep. 6 (8), e13663. doi:10.14814/phy2.13663
Restano-Cassulini, R., Garcia, W., Paniagua-Solís, J. F., and Possani, L. D. (2017). Antivenom evaluation by electrophysiological analysis. Toxins 9 (3), 74. doi:10.3390/toxins9030074
Rimbault, C., Knudsen, P. D., Damsbo, A., Boddum, K., Ali, H., Hackney, C. M., et al. (2023). A single-chain variable fragment selected against a conformational epitope of a recombinantly produced snake toxin using phage display. New Biotechnol. 76 (25), 23–32. doi:10.1016/j.nbt.2023.04.002
Rivera-de-Torre, E., Rimbault, C., Jenkins, T. P., Sørensen, C. V., Damsbo, A., Saez, N. J., et al. (2022). Strategies for heterologous expression, synthesis, and purification of animal venom toxins. Front. Bioeng. Biotechnol. 9, 811905. doi:10.3389/fbioe.2021.811905
Rosholm, K. R., Badone, B., Karatsiompani, S., Nagy, D., Seibertz, F., Voigt, N., et al. (2022). Adventures and advances in time travel with induced pluripotent stem cells and automated patch clamp. Front. Mol. Neurosci. 15, 898717. doi:10.3389/fnmol.2022.898717
Safavi-Hemami, H., Brogan, S. E., and Olivera, B. M. (2019). Pain therapeutics from cone snail venoms: from ziconotide to novel non-opioid pathways. J. Proteomics 190, 12–20. doi:10.1016/j.jprot.2018.05.009
Silva, A., Kuruppu, S., Othman, I., Goode, R. J. A., Hodgson, W. C., and Isbister, G. K. (2017). Neurotoxicity in Sri Lankan Russell’s viper (Daboia russelii) envenoming is primarily due to U1-viperitoxin-Dr1a, a pre-synaptic neurotoxin. Neurotox. Res. 31 (1), 11–19. doi:10.1007/s12640-016-9650-4
Staats, P. S., Yearwood, T., Charapata, S. G., Presley, R. W., Wallace, M. S., Byas-Smith, M., et al. (2004). Intrathecal Ziconotide in the treatment of refractory pain in patients with cancer or AIDSA randomized controlled trial. JAMA 291 (1), 63–70. doi:10.1001/jama.291.1.63
Subramanyam, P., and Colecraft, H. M. (2015). Ion channel engineering: perspectives and strategies. J. Mol. Biol. 427 (1), 190–204. doi:10.1016/j.jmb.2014.09.001
Wang, X., and Li, M. (2003). Automated electrophysiology: high throughput of art. ASSAY Drug Dev. Technol. 1 (5), 695–708. doi:10.1089/154065803770381057
Williams, J. A., Day, M., and Heavner, J. E. (2008). Ziconotide: an update and review. Expert Opin. Pharmacother. 9 (9), 1575–1583. doi:10.1517/14656566.9.9.1575
Willumsen, N. J., Bech, M., Olesen, S. P., Jensen, B. S., Korsgaard, M. P. G., and Christophersen, P. (2003). High throughput electrophysiology: new perspectives for ion channel drug discovery. Recept. Channels 9 (1), 3–12. doi:10.3109/10606820308259
Yu, H., Li, M., Wang, W. p., and Wang, X. l. (2016). High throughput screening technologies for ion channels. Acta Pharmacol. Sin. 37 (1), 34–43. doi:10.1038/aps.2015.108
Zoli, M., Pucci, S., Vilella, A., and Gotti, C. (2018). Neuronal and extraneuronal nicotinic acetylcholine receptors. Curr. Neuropharmacol. 16 (4), 338–349. doi:10.2174/1570159X15666170912110450
Zukifli, N. A., Ibrahim, Z., Othman, I., Ismail, A. K., Chaisakul, J., Hodgson, W. C., et al. (2022). In vitro neurotoxicity and myotoxicity of Malaysian Naja sumatrana and Naja kaouthia venoms: neutralization by monovalent and neuro polyvalent antivenoms from Thailand. PLOS ONE 17 (9), e0274488. doi:10.1371/journal.pone.0274488
Keywords: electrophysiology, patch-clamp, ion channel, venom, antivenom, drug discovery, neurotoxin
Citation: Ahmadi S, Benard-Valle M, Boddum K, Cardoso FC, King GF, Laustsen AH and Ljungars A (2023) From squid giant axon to automated patch-clamp: electrophysiology in venom and antivenom research. Front. Pharmacol. 14:1249336. doi: 10.3389/fphar.2023.1249336
Received: 28 June 2023; Accepted: 11 August 2023;
Published: 24 August 2023.
Edited by:
Conor McClenaghan, The State University of New Jersey, United StatesReviewed by:
Christian Legros, Université d'Angers, FranceHai Minh Nguyen, University of California, Davis, United States
Copyright © 2023 Ahmadi, Benard-Valle, Boddum, Cardoso, King, Laustsen and Ljungars. This is an open-access article distributed under the terms of the Creative Commons Attribution License (CC BY). The use, distribution or reproduction in other forums is permitted, provided the original author(s) and the copyright owner(s) are credited and that the original publication in this journal is cited, in accordance with accepted academic practice. No use, distribution or reproduction is permitted which does not comply with these terms.
*Correspondence: Andreas Hougaard Laustsen, ahola@bio.dtu.dk; Anne Ljungars, aellj@dtu.dk
†These authors share last authorship