- 1Graduate School, Tianjin University of Traditional Chinese Medicine, Tianjin, China
- 2Institute of TCM Ulcers, Tianjin University of Traditional Chinese Medicine, Tianjin, China
- 3Surgical Department of Traditional Chinese Medicine, Second Affiliated Hospital of Tianjin University of Traditional Chinese Medicine, Tianjin, China
Atherosclerosis (AS) is a chronic inflammatory reaction that primarily affects large and medium-sized arteries. It is a major cause of cardiovascular disease and peripheral arterial occlusive disease. The pathogenesis of AS involves specific structural and functional alterations in various populations of vascular cells at different stages of the disease. The immune response is involved throughout the entire developmental stage of AS, and targeting immune cells presents a promising avenue for its treatment. Over the past 2 decades, studies have shown that gut microbiota (GM) and its metabolites, such as trimethylamine-N-oxide, have a significant impact on the progression of AS. Interestingly, it has also been reported that there are complex mechanisms of action between GM and their metabolites, immune responses, and natural products that can have an impact on AS. GM and its metabolites regulate the functional expression of immune cells and have potential impacts on AS. Natural products have a wide range of health properties, and researchers are increasingly focusing on their role in AS. Now, there is compelling evidence that natural products provide an alternative approach to improving immune function in the AS microenvironment by modulating the GM. Natural product metabolites such as resveratrol, berberine, curcumin, and quercetin may improve the intestinal microenvironment by modulating the relative abundance of GM, which in turn influences the accumulation of GM metabolites. Natural products can delay the progression of AS by regulating the metabolism of GM, inhibiting the migration of monocytes and macrophages, promoting the polarization of the M2 phenotype of macrophages, down-regulating the level of inflammatory factors, regulating the balance of Treg/Th17, and inhibiting the formation of foam cells. Based on the above, we describe recent advances in the use of natural products that target GM and immune cells crosstalk to treat AS, which may bring some insights to guide the treatment of AS.
1 Introduction
Atherosclerosis (AS) is a chronic inflammatory disease of arterial wall thickening or functional degeneration associated with disorders of lipid metabolism and immune inflammation and is the major pathological basis of cardiovascular disease, stroke, and peripheral arterial occlusive disease (Libby, 2021). Currently, cardiovascular disease is the leading cause of death and disability for patients worldwide (Roth et al., 2020) and is a worldwide problem shared by modern society (Libby et al., 2019). According to statistics, the number of people with cardiovascular disease worldwide alone has almost doubled from 1990 to 2019, from 271 million to 523 million cases (Roth et al., 2020). Age is the largest risk factor for AS-related diseases, for example, the incidence of peripheral arterial occlusive disease is only 1% in people aged 40–49 years, whereas it is AS high as 15% in people aged 70 years and older (Aday and Matsushita, 2021). Gender is also an influencing factor in AS-related diseases. The incidence of coronary artery disease in premenopausal women is only 10%–30% of that in men, and the risk in postmenopausal women is comparable to that in men (Huang et al., 2020). By age 70, the incidence of myocardial infarction in women catches up with that in men (Man et al., 2020). With the development of the social economy and the change in dietary structure, the incidence of AS continues to rise, which further raises people’s concerns about AS. The first-line drugs for the treatment of AS, such as statins and antiplatelet drugs, have shown good efficacy, but there are some side effects (Satny et al., 2021). In addition, many patients suffer from recurrent clinical events despite achieving target therapeutic levels of low-density lipoprotein (LDL) (Doran, 2022). Therefore, the active search for new ways to treat AS is a race faced by all clinicians.
Gut microbiota (GM), known as the “second genome” of the human body, has evolved with humans for millions of years and plays an important role in regulating the development of human diseases (Chen et al., 2021). GM is interdependent and inter-constrained with the environment and host, participating in various physiological processes such as absorption, digestion, metabolism, and immunity to dynamically maintain the micro-ecological balance of the body (Adak and Khan, 2019). Everyone has a unique GM. The composition and proportion of GM are closely related to the occurrence of AS (Liu et al., 2019). Meanwhile, intermediates of GM metabolism, such as trimethylamine oxides (TMAO) and short-chain fatty acids (SCFAs), can act on many cells and molecules in the development of AS and play a disease-modifying role (Cao et al., 2023). When the quantity or quality of GM changes, the microecological balance of the body is disrupted, and the GM is disordered or dysregulated, which can lead to the occurrence and development of a variety of diseases. There is growing evidence for potential mechanisms of GM and immune response in AS-related diseases, which may provide new targets for the treatment of AS. In pathological states, GM (especially conditionally pathogenic bacteria) and their metabolites stimulate mucosal immune cell activation, hyperactivate the mucosal immune response, and mediate the production of multiple inflammation-associated cytokines (Neurath, 2019; Graham and Xavier, 2020). This link between the GM and the immune system will help to better understand the pathogenesis of AS and facilitate the development of novel therapeutic agents.
Due to their wide range of targets and low toxic effects, natural products are currently an important route for new drug discovery. Accumulating research evidence suggests that natural products can inhibit the progression of AS (Duan et al., 2021; Zhang et al., 2021). In recent years, there have been many studies on the role of GM and immune crosstalk in AS. So, could the GM play a role as a “bridge” between natural products and the immune system to inhibit the development of AS? The answer is yes. At present, some studies have provided support. Therefore, natural products with anti-AS properties may improve AS by modulating GM and immune system crosstalk, which opens up new avenues for the research and treatment of AS. We searched PubMed and Google Scholar using the keywords such as “gut microbiota”, “natural products”, “herbal medicine”, “monocytes”, “macrophages”, “dendritic cells”, “T cells”, “B cells”, and “mast cells”, and the search time was set to nearly 10 years. This article reviews the influence and mechanisms of GM and immunity in AS and summarizes the natural products that have been used as targets for treatment, to provide new insights into the prevention and treatment of AS.
2 Role of immune cells in AS
The innate immune response mainly plays a role in removing dead cells, presenting antigens, and protecting the host from external microorganisms (Zarif et al., 2016). Monocytes and macrophages are key effectors and regulators in the innate immune as well as inflammatory response (Moriya, 2019). As a bridge between innate and adaptive immunity, dendritic cells (DCs) initiates and regulate highly pathogen-specific adaptive immune responses and are central to the development of immune memory and tolerance (Liu et al., 2021). T and B lymphocytes recognize the presented antigens and dominate the development of adaptive immune responses.
The current study found that the immune and inflammatory responses have a significant impact on all stages of AS development (Zhu et al., 2018). In the early stages of AS, LDL accumulated in the intima is modified by oxidases, lipolytic enzymes, protein hydrolases, and reactive oxygen species (ROS) to form various damage-associated molecular patterns that acquire immunogenicity and the ability to elicit an immune response (Hansson and Hermansson, 2011). The entry of immunogenic oxidized low-density lipoprotein (ox-LDL) into the intima activates vascular endothelial cells, causing an inflammatory response that recruits chemotactic immune cells into the AS lesion area, such as monocytes and T cells (Abdolmaleki et al., 2019). In the pathological process of AS, the damage of vascular endothelial cells is the beginning of innate immunity, which subsequently activates the mononuclear phagocyte system, leading to the release of large amounts of inflammatory factors (van Dijk et al., 2016). Antigen-presenting cells (APCs), represented by DCs, present a series of processed antigens to T and (or) B lymphocytes to activate adaptive immunity (Roy et al., 2022). As shown in Figure 1.
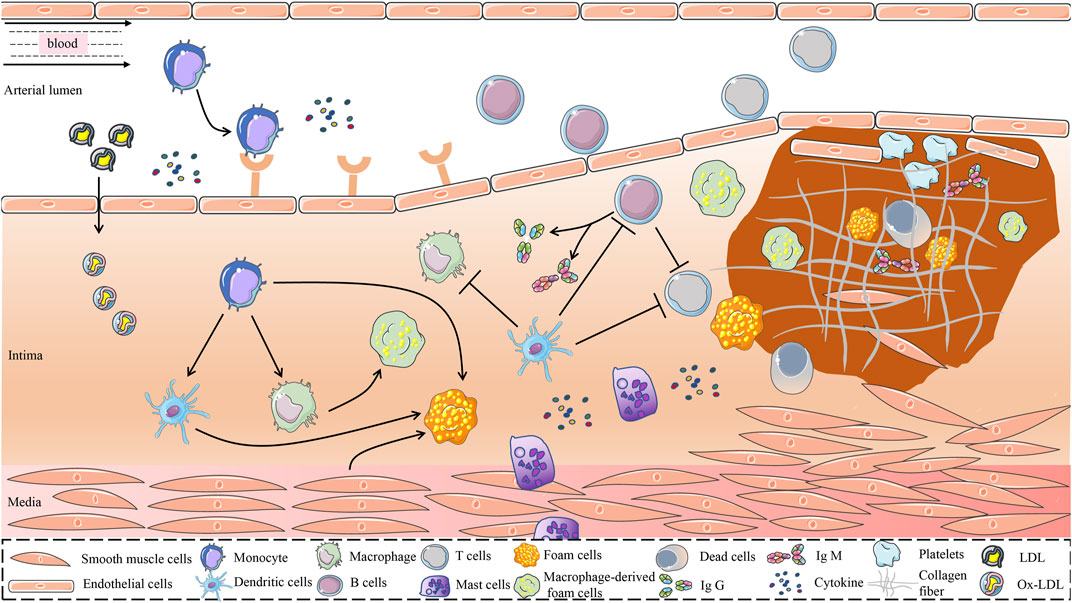
FIGURE 1. Possible mechanisms for the role of immune cells in the development of AS. The immune system is one of the major regulatory systems in the development and progression of AS. In the early stage of AS, LDL is retained in the intima of the blood vessel and is modified to form a variety of danger-associated molecular patterns mediated by a variety of risk factors, to obtain immunogenicity. Immunogenic LDL activates vascular endothelial cells and attracts immune cells such as monocytes into the vessel wall. Monocytes differentiate dendritic cells and macrophages in response to the stimulation of inflammatory mediators and cytokines. Dendritic cells recognize ox-LDL and present it to immune cells such as macrophages and T cells. Phagocytosis of lipoproteins by monocyte-derived macrophages is an early pathological event in nascent plaque formation. T cells play an important regulatory role in the development of AS. T cells recognize antigens presented by MHC class II molecules on antigen-presenting cells and MHC class I molecules on nuclear cells and activate B cells. In addition, proinflammatory cytokines and anti-inflammatory molecules secreted by T cells play an important role in the development of AS. B cells exert both proatherogenic mechanisms and antiatherogenic effects by secreting IgM and IgG, which are driven by antibodies against specific epitopes of LDL oxidation. Mast cells accelerate LDL penetration and amplify the inflammatory response by releasing histamine, an active mediator, and pro-inflammatory cytokines. Atherosclerotic plaques are formed under the combined action of multiple immune cells, vascular smooth muscle cells, and endothelial cells. AS, atherosclerosis; MHC, Major Histocompatibility Complex; LDL, Low-Density Lipoprotein; ox-LDL, Oxidized Low-Density Lipoprotein.
2.1 Monocyte
Endothelial dysfunction is the starting point for the development of AS. Endothelial injury releases adhesion factors and chemokines, such as CC chemokine ligand (CCL) 5, CXC chemokine ligand (CXCL) 1, and P-selectin, which capture circulating monocytes and cause adhesion and aggregation of monocytes (Tacke et al., 2007; Kang et al., 2021). Continuous recruitment of monocytes is necessary for the maintenance and progression of AS plaques (Potteaux et al., 2011).
The study found that monocytes are divided into three main types: classical (CD14+CD16− in humans and Ly6Chi in mice), intermediate (CD14+CD16+ in humans and Ly6C+Treml4+ in mice), and nonclassical (CD14−CD16+ in humans and Ly6Clo in mice) (Kim et al., 2020). Classic monocytes are the largest population of monocyte subsets and highly express CXC-chemokine receptor 1, CXC-chemokine receptor 2, and C-C chemokine receptor 2 (Guo et al., 2020). In response to lipopolysaccharide (LPS) stimulation, classic monocytes secrete large amounts of granulocyte colony-stimulating factor and interleukin (IL) 6 (Wong et al., 2011). In addition, classic monocytes also express phagocytosis-related proteins such as CD14, CD64, and CD36, indicating that they have a strong phagocytic capacity (Cros et al., 2010; Zawada et al., 2011). Monocytes that migrate into the plaque differentiate into monocyte-derived macrophages and monocyte-derived DCs. Monocyte-derived macrophages are the main complementary source of inflammatory macrophages in AS plaques. DCs can initiate adaptive immunity by presenting antigens to T cells (Jakubzick et al., 2017). Most non-classical monocytes mainly play a “patrolling” role and do not migrate to target vessels, maintaining the homeostasis of the vascular endothelial environment (Marcovecchio et al., 2017). If the lining of the vessel is damaged or inflamed, the “patrolling” non-classical monocytes can rapidly migrate to the appropriate site. In addition, during the progression of AS, the protective effect of non-classical monocytes is also manifested in removing damaged cells and debris and repairing blood vessels (Thomas et al., 2015). Intermediate monocytes are the transitional cells between mature monocytes and macrophages. Intermediate monocytes have greater transendothelial migration, phagocytosis, and ROS production than classic monocytes (Zawada et al., 2011). Intermediate monocytes can be rapidly recruited to sites of inflammation and secrete large amounts of C-X3-C chemokine receptor 1 and C-C chemokine receptors 2 (Guo et al., 2020), which participate in the inflammatory response.
2.2 DCs
As the most powerful APC, DCs play an important role in initiating and amplifying the immune response. Currently, the presence of classical DC (cDC) and plasmacytoid DC (pDC) has been identified in AS lesioned areas in human and AS model mice (Zernecke, 2015). Both cDC and pDC have been detected in both mouse and human AS lesions. Several cDC isoforms have been detected in arterial tissue, including CD103+ DC, CD11b+ DC, and CCL17+ DC (Gardner et al., 2020).
DCs act as central stimulators of the immune response, exerting a bidirectional pro- and anti-AS effect. Activated DCs secrete cytokines and chemokines that exert long-range effects. Such as DCs can recruit circulating leukocytes to vascular areas (Bellini et al., 2022) and regulate the differentiation of T cells into a variety of T effectors, including T helper (Th) type 1 cells, Th1, Th2, Th17, and Tregs (Markin et al., 2023). They can also activate cytotoxic T lymphocyte and B cells and polarize macrophages, leading to immune destruction of the vessel wall and induction of AS (Kloc et al., 2022). As specialized APCs, cDCs recognize antigens in the vessel wall and present them to antigen-specific naïve T cells in the lymph nodes or spleen. In addition, DCs can directly ingest lipids to form foam cells (Haka et al., 2015).
2.3 Macrophages
The origin of macrophages in AS plaques can be divided into two categories: resident macrophages derived from embryonic progenitor cells and peripheral monocyte-derived macrophages (Denardo and Ruffell, 2019; Williams et al., 2020), with monocyte-derived macrophages predominating (Shapouri-Moghaddam et al., 2018). When AS occurs, resident macrophages are replenished and self-renewed by recruiting mature peripheral monocytes into the tissue. In addition, with the development of AS, replenishment of resident macrophages within the AS plaques can also be achieved by the proliferation of macrophages in situ (Farahi et al., 2021).
Macrophages, as the main immune effector cells, are involved in all stages of AS, from the occurrence to the formation of a necrotic core (Tabas and Bornfeldt, 2016). The uptake of lipoproteins by macrophages via scavenger receptors leads to the formation of lipid-rich foam cells (Chistiakov et al., 2019). This exacerbates the development of lipid streaks. Recent studies have found that cells with foam characteristics are enriched in a large number of anti-inflammatory genes and lipid processing genes, and relatively few inflammatory genes, suggesting that the formation of foam cells may drive the regression of inflammation in the lesion area (Kim et al., 2018; Depuydt et al., 2020). The excessive accumulation of lipoproteins and aggravation of oxidative stress leads to metabolic disorders of macrophages, which trigger a series of inflammatory responses and induce the infiltration of immune cells in AS plaques (Kojima et al., 2017).
Macrophages are classically characterized by diversity and plasticity (Mantovani et al., 2013). Traditionally, macrophages are usually dichotomized as M1 and M2, but this does not encompass the multiple functions performed by macrophages (Atri et al., 2018; Moreira et al., 2020). In addition, macrophage phenotypes include Mox, HA-mac, Mhem, and M (Hb) types (Boyle et al., 2009; Marques et al., 2016; Liberale et al., 2017). Current studies mainly analyze the role of M1 and M2 macrophages in AS. Classically activated M1 macrophages are pro-inflammatory cells that are induced to polarize by granulocyte-macrophage colony-stimulating factor, LPS, tumor necrosis factor-α (TNF-α), and ox-LDL (Shapouri-Moghaddam et al., 2018; Eshghjoo et al., 2022). M1 macrophages express a series of pro-inflammatory factors, including TNF-α, IL-1β, IL-12, IL-23, chemokine ligands CXCL9, CXCL10, and CXCL11 (Yunna et al., 2020), which play an important role in the initiation of inflammation. It promotes the progression of AS and affects the stability of plaques. In contrast, alternative activated M2 macrophages are anti-inflammatory cells that can be polarized by L-4, IL-13, CCL17, IL-10, transforming growth factor-β (TGF-β), and immune complexes (Gordon and Martinez, 2010). M2 macrophages typically express high levels of IL-10 and low levels of IL-12, which effectively inhibit the inflammatory response and stabilize AS plaques (De Paoli et al., 2014). M2 macrophages are predominantly enriched in stable plaques. As the stability of plaques decreased, M1 macrophages gradually replace M2 macrophages in dominance (Stoger et al., 2012; Amengual and Barrett, 2019). The mechanism of macrophages in AS is complex. An in-depth exploration of the specific roles of macrophage subsets in AS is essential to understand their role in the progression of AS and developing novel therapeutic drugs.
2.4 Mast cells
Mast cells, as important effector cells of the innate immune response, are involved in almost all stages from the occurrence of AS to plaque rupture, and their number increases with the progress of AS (Kovanen and Bot, 2017). Histamine, the major active mediator of mast cells, can promote endothelial histamine H1 receptor expression, increase the permeability of aortic endothelium to circulating LDL, and accelerate the penetration of LDL particles into the susceptible intima (Rozenberg et al., 2010). Previous studies have shown that there is an accumulation of mast cells in human AS plaques, and the number of activated mast cells is significantly increased around the diseased vessels (Atkinson et al., 1994). Mast cells amplify the inflammatory response by releasing the pro-inflammatory cytokines IL-6 and interferon-γ (IFN-γ) (Sun et al., 2007) and increase plaque instability by releasing the chemokines CXCL1 and CXCL12 to induce neutrophil recruitment to the site of AS inflammation (Wezel et al., 2015). Generally, calcification of AS plaques is regarded as a protective factor against AS. Mast cell activation was negatively correlated with the macrocalcification of AS plaques and positively correlated with plaque fragility parameters (Skenteris et al., 2023). Stress-induced activation of mast cells has been found to cause intraplaque hemorrhage and increase plaque instability in ApoE−/− mice (Lagraauw et al., 2019). In addition, mast cells also induce apoptosis of smooth muscle cells leading to the rupture of atherosclerotic plaques (Leskinen et al., 2003). In the reprogramming of smooth muscle cells, mast cells stimulate smooth muscle cell calcification and induce their differentiation into a proinflammatory, osteochondrocyte-like phenotype (Skenteris et al., 2023). Under hyperlipidemic conditions, mast cells highly express major histocompatibility complex class II molecules, specifically activating the accumulation of CD4+ T cells within AS plaques by acting as APC (Kritikou et al., 2019).
2.5 T cells
After AS-related antigens are recognized by DCs and macrophages, MHC class molecules on the surface of APCs activate naive CD4+ and CD8+ T cells in vivo by binding to T cell surface receptors (Nilsson and Hansson, 2020). Subsequently, CD4+ and CD8+ T cells differentiate into various subsets in response to stimulation by different cytokines. For example, CD4+ T cells can differentiate into helper T cells and regulatory T cells, and CD8+ T cells can differentiate into cytotoxic T lymphocytes. T cell subsets have shown diverse roles in the complex AS microenvironment.
In different stages of AS, CD4+ T cell subsets play an immune-activating or immunosuppressive role and can assist in the activation of B cells to produce antibodies (Saigusa et al., 2020). It is generally accepted that pro-inflammatory CD4+ effector T cells are dominant during the developmental phase of AS, whereas Treg cells are dominant during the pre- or stabilization phase of AS (Buono et al., 2005; Ait-Oufella et al., 2006). T helper cell plasticity provides a challenge to our understanding of the role of Th cells in AS. Studies have found that in the late stage of AS, there are a large number of Th1 cells, which can not only enhance antigen presentation but also secrete IFN-γ, TNF, and IL to promote inflammatory response (Wolf and Ley, 2019). IFN-γ activated macrophages to pro-inflammatory polarization and promoted VSMC proliferation (Saigusa et al., 2020). In addition, IFN-γ can induce the formation of foam cells in the progressive stage of AS (Yu et al., 2015). TNF, which is expressed by a variety of effector T cells, promotes the migration of circulating leukocytes to sites of vascular endothelial injury, thereby contributing to the development of AS (Biros et al., 2022).
The role of Th2 cells in AS remains controversial, but the most convincing evidence suggests that Th2 cells primarily play an anti-AS role (Binder et al., 2004; Gronberg et al., 2017). Activated Th2 cells release IL-4, IL-5 and IL-13. IL-4 inhibits Th1 responses and is considered a protective factor against AS in ApoE−/− mice (Davenport and Tipping, 2003). However, exogenous IL-4 did not inhibit AS in ApoE−/− mice (King et al., 2007). This has led to a debate on the role of IL-4 for AS. The protective effects of IL-5 and IL-13 on AS have been relatively clear. A negative correlation between IL-5 and intima-media thickness was found in female common carotid segments, suggesting that IL-5 may be part of the protective mechanism in the early of AS (Silveira et al., 2015). Mechanistically, IL-5 stimulates B1 cells to produce IgM to prevent the uptake of ox-LDL by macrophages, thereby inhibiting the formation of necrotic cores (Munjal and Khandia, 2020). In addition, IL-5 upregulates the expression of ATP-binding cassette transporter A1 (ABCA1) by regulating the miR-211/JAK2/STAT3 signaling pathway to promote cholesterol efflux (Chen et al., 2020). In a retrospective study, lower levels of IL-13 were found to be associated with higher carotid intima-media thickness values (Boccardi et al., 2020). This suggests that IL-13 deficiency accelerates the progression of AS. IL-13 inhibits the recruitment of vascular cell adhesion molecule 1 (VCAM-1)-dependent monocytes to suppress the inflammatory response and promotes the formation of stable plaques in AS by increasing collagen content in the lesion area and modulating the macrophage phenotype (Cardilo-Reis et al., 2012).
The role of IL-17 in AS is still debated. Th17 cells secrete IL-17, IL-21, and IL-22, among which IL-17 is the signature cytokine of Th17 cells. Infiltrating Th17 cells in the arteries of patients with coronary atherosclerosis secreted large amounts of IL-17, which acted synergistically with IFN-γ to induce vascular smooth muscle cells (VSMCs) to participate in the inflammatory response (Eid et al., 2009). As a member of the IL-17 family, IL-17A promotes inflammatory responses by facilitating the recruitment of monocytes and macrophages to the AS region (Smith et al., 2010). In addition, IL-17A stimulates ox-LDL-induced foam cell formation by promoting macrophage expression of lectin-like oxidized low-density lipoprotein receptor 1 (Shiotsugu et al., 2019). The use of neutralizing antibodies against IL-17A can significantly inhibit the production of proinflammatory cytokines and chemokines (Butcher et al., 2016). However, studies in ApoE−/− IL-17A−/− mice found that IL-17A deficiency accelerated the progression of AS and promoted the formation of unstable plaques (Danzaki et al., 2012). In the hypercholesterolemic environment, promoting IL-17 expression contributes to plaque stabilization (Brauner et al., 2018). The complex target role of IL-17 provides a direction for the study of the immune mechanism of the occurrence and development of AS.
At present, it is generally believed that Treg cells play a protective role in AS (He et al., 2020). Treg cells secrete immunosuppressive cytokines such as IL-10 and TGF-β to inhibit the excessive inflammatory response, and enhance immune tolerance and immune balance through cell contact-dependent mechanism and tissue repair (Spitz et al., 2016; Sakaguchi et al., 2020). Adoptive transfer of Treg cells into the aorta of ApoE−/− mice significantly reduced macrophage infiltration and reduced AS plaque area in the aorta (Gao et al., 2020). Depletion of Treg cells in AS model mice using a CD25 monoclonal antibody affected the alternative activation of macrophages, efferocytosis, upregulation of pro-lipid regression mediators, inhibition of Th1 responses, and blocked the remodeling and contraction of AS plaques (Sharma et al., 2020). The above suggests that Treg cells play an important protective role in AS. However, the mechanisms by which Treg cells interact with various cells associated with AS are still not fully understood, and this gap needs to be filled.
Several studies (Cochain et al., 2018; Zernecke et al., 2020) have confirmed that CD8+ T cells are enriched and infiltrated in AS plaques in humans and mice. CD8+ T cells in plaques are highly activated compared to T cells in circulation (Grivel et al., 2011). CD8+ T cells in lesioned areas of AS in Ldlr−/− mice fed with a high-fat diet highly expressed IFN-γ, TNF-α, and IL-12, and antibody-mediated depletion of CD8+ T cells inhibited the development of AS (Cochain et al., 2015). However, in ApoE−/− mice lacking antigen processing-associated transporter protein-1, a significant reduction in CD8+ T cell levels did not affect the progression of AS (Kolbus et al., 2012). Notably, the increased infiltration of CD4+ T cells in AS lesions compensated for the absence of CD8+ T cells, which may mask the impact of CD8+ T cell deficiency on AS progression. In addition, CD8+ T cells can differentiate into subpopulations with specific cytokine profiles. CD8+ Tc17 T cells do not affect the development of early AS although they accumulate in the lesioned areas of mice with AS (van Duijn et al., 2021). In contrast, CD8+ IFN-γ+ T cells mediate the upregulation of immune responses and secretion of pro-inflammatory cytokines during the progressive phase of AS, exacerbating AS load and promoting the inflammatory response (Li et al., 2023). On the one hand, inflammatory cytokines produced by CD8+ T cells exacerbate the inflammatory response and drive the progression and instability of atherosclerotic lesions. On the other hand, cytotoxic activity against APCs and the presence of regulatory CD8+ T cell subsets suppress immunity and can limit AS (van Duijn et al., 2018).
2.6 B Cells
Mouse B cells can be broadly divided into B1 and B2 cells. Due to the differences in the surface markers and immune cell responses of human B cells and mice, the B cell subtypes and functions of human B cells are different from those of mice. However, the function of human B-cell subsets can be inferred from the function of mouse B-cell subsets in AS. Unlike T cells, only a small number of B cells can be found in localized lesions of AS (Poznyak et al., 2021).
At present, there is a large amount of evidence that B cell subsets play a crucial role in AS (Sage et al., 2019). B1 cells differentiated into B1a and B1b cells, and B2 cells differentiated into follicular B (FO B) cells and marginal zone B (MZ B) cells. In addition, the B cell subpopulation includes innate response activator B (IRA B) cells and Bregs. Among them, B1 cells and MZ B cells exhibit a protective role in AS (Tsiantoulas et al., 2015). In contrast to IRA B and FO B cells, which play a role in promoting AS formation (Tsiantoulas et al., 2015). Studies have found that the deficiency of B1 cells aggravates AS, while the adoptive transfer of B1 cells has an inhibitory effect on AS (Kyaw et al., 2011). The mechanism is related to the secretion and the accumulation of IgM, which inactivates oxidation-specific epitopes of LDL (Kyaw et al., 2011). In contrast, B2 cells secrete IgG and IgE that promote AS (Moriya, 2019), but this remains controversial. B2 cells differentiate into memory B cells and plasma cells, and the increase of plasma cells is associated with AS (Nutt et al., 2015). Studies have found that MZ B cells delay the progression of AS by secreting IgM and inhibiting T follicular helper cells (Nus et al., 2017). The decrease of FO B cells and the increase of Berg cells significantly attenuated AS (Douna et al., 2020). The T cell-mediated differentiation of FO B cells into plasma cells and secretion of pathogenic IgG is an important mechanism for the pro-AS effect of FO B cells (Tay et al., 2018). In addition, the pro-AS effect of FO B cells is highly dependent on their expression of MHC II, CD40, and Blimp-1 (Tay et al., 2018). Granulocyte-macrophage colony-stimulating factor-producing IRA B cells alter adaptive immune processes and shift the leukocyte response toward a Th1-associated milieu that aggravates AS (Hilgendorf et al., 2014). Breg cells have not been studied in depth yet. Breg cells can inhibit AS by secreting IL-10 (Ponnuswamy et al., 2017). Interestingly, in Ldlr−/− mice with B-cell-specific deficiency in IL-10, B-cell-derived IL-10 does not alter AS in mice (Sage et al., 2015). The research and exploration of the specific role of B cells in AS is still in its infancy, and it is necessary to continue to study the complex regulatory role of B cells and their cytokines in the environment of AS.
3 Overview of the GM
GM is mainly colonized in the gastrointestinal tract and includes bacteria such as probiotics, neutrophils, and pathogens, as well as microorganisms such as viruses that coexist with the host, 99% of which are bacteria (Neish, 2009; Jandhyala et al., 2015). The adult intestine is inhabited by at least 1,000 microorganisms, with the number increasing from the esophagus, stomach, duodenum, and small intestine to the colon (Clemente et al., 2012). The number of GM in the human body far exceeds the number of cells in the human body (Van Treuren and Dodd, 2020). The GM genome contains at least more than 9 million genes and is known as the second genome in the body (Li et al., 2014). With the development of high-throughput sequencing technologies such as macroeconomics, 16SRNA, and metabolomics, we have a more precise understanding of the classification and function of GM. The mystery of GM has been gradually unveiled. There is a delicate balance between tens of thousands of GM and their host.
The GM is inter-constrained and interdependent to maintain the balance of the intestinal environment. The balance of the microbiota is an important guarantee for the normal physiological function of the organism. The homeostasis of the bacterial microbiota is dominated by a dynamic equilibrium with interactions among its stabilizing factors, including the immune barrier, host intestinal mucosa, intestinal microecology, and metabolites (Zhou et al., 2021; Shen et al., 2022). When the balance of microbiota in the body is imbalanced, it can lead to several systemic diseases. GM is now recognized as an important factor in keeping the body healthy and influencing the development of disease (Perler et al., 2023). Therefore, effective maintenance of the dynamic balance of the GM is the key to ensuring the health of the human body and the healing of diseases.
4 GM, their metabolites, and AS
Studies have shown that GM and its metabolites may prevent or promote the progression of AS by modulating host metabolism and inflammatory responses (Brandsma et al., 2019). AS plaque itself is a microbial environment, which contains Streptococcus, Pseudomonas, Klebsiella, and Chlamydia pneumoniae (Koren et al., 2011; Lanter et al., 2014). A systematic review and Meta-analysis showed that the abundance of GM was reduced in patients with coronary artery disease and that alterations in GM composition were associated with qualitative and quantitative changes in bacterial metabolites (Choroszy et al., 2022). Compared to healthy controls, the relative abundance of GM was more significantly altered in patients with symptomatic AS, such as the higher abundance of Collinsella genus, Enterobacteriaceae, Streptococcaceae, and Klebsiella spp., and lower abundance of SCFAs-producing Eubacterium, Roseobacter, and Rumenococcus spp (Jie et al., 2017; Liu et al., 2019; Zhao et al., 2020). Fusobacterium and Proteus in the GM were found to be associated with carotid plaque, while beneficial butyrate producer Odoribacter was found to be negatively associated with plaque in people at high risk of HIV infection (Wang et al., 2022). However, another study found no significant differences in bacterial DNA amounts or microbial composition between plaques in patients with symptomatic and asymptomatic AS, or between different plaque regions (Lindskog et al., 2017). This suggests that there are other more critical factors in determining plaque susceptibility, which need further study. Pathogenic bacteria in the GM can promote the formation of plaques on the vascular wall by directly infecting the vascular wall or by causing autoimmune inflammatory responses through molecular mimicry (Epstein et al., 2000).
However, interventions with antibiotics applied to eliminate microbiota in plaques were found not to reduce the incidence of cardiovascular events (Song et al., 2008). The reasons for this result cannot be excluded to be related to the fact that the duration of the antibiotic intervention was too short and that the antibiotics applied did not target all microbiota (Jonsson and Backhed, 2017).
A synthesis of the current reports reveals that studies mainly focus on the effect of GM metabolites on AS. Such as SCFAs, secondary bile acids, and TMAO are an important part of the development of AS (Brown and Hazen, 2015; Jonsson and Backhed, 2017). The role of GM metabolites in AS has received widespread attention (Brown and Hazen, 2018), which provides a new perspective on the treatment of AS from the perspective of GM. The effect of different metabolites of the GM on AS varies. Taking TMAO and SCFAs as examples, it was reported that elevated plasma TMAO levels promote the development of AS and are associated with the risk of cardiovascular disease (Qi et al., 2018; El et al., 2023). However, SCFAs exhibit anti-AS properties (Bultman, 2018). Therefore, the effect of GM on AS needs to be discussed separately depending on the metabolite (as shown in Figure 2). This article focuses on a review of the mechanisms by which metabolites of the GM (SCFAs, secondary bile acids, and TMAO) regulate immune cells to influence the progression of AS.
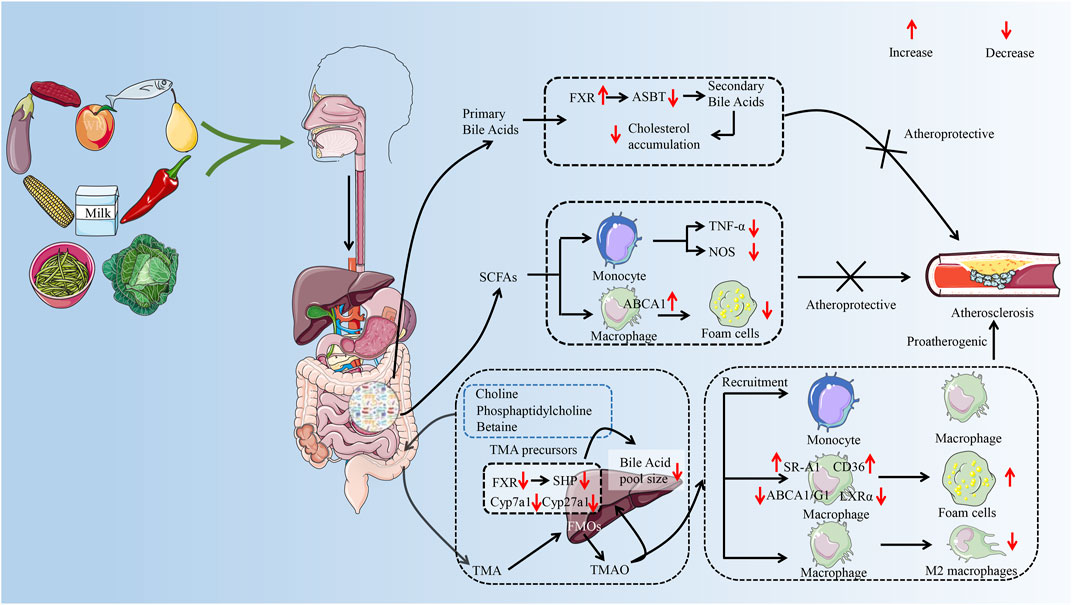
FIGURE 2. The possible mechanism of GM metabolites (TMAO, SFCAs, secondary bile acids) to exert pro-AS or anti-AS activities by regulating immune cells. The GM helps the body digest food, breaking down nondigestible cellulose and other complex carbohydrates. After entering the gut, food is metabolized by GM to primary bile acids, trimethylamine, and SCFAs. Trimethylamine is oxidized to trimethylamine oxide in the liver by FMO 3. In the progression of AS, TMAO can promote the recruitment of monocytes and macrophages and accelerate the inflammatory response. TMAO disrupted cholesterol homeostasis in macrophages by affecting the expression of ABCA 1, ABCG 1, and LXRα. It promotes the formation of macrophage-derived foam cells and accelerates the formation of atherosclerotic plaques. In addition, TMAO also prolonged the inflammatory response phase by inhibiting the polarization of the M2 phenotype of macrophages. SCFAs are considered to be protective factors for delaying the progression of AS. SCFAs can reduce foam cell formation not only by inhibiting monocyte-induced inflammatory response but also by promoting ABCA 1-mediated cholesterol efflux from macrophages. Secondary bile acids play a role in inhibiting AS by inhibiting cholesterol accumulation. GM, gut microbiota; AS, atherosclerosis; TMA, trimethylamine; SCFAs, short-chain fatty acids; FMO 3, flavin-containing monooxygenase 3; ABCA 1, ATP-binding cassette proteins A 1; ABCG 1, ATP-binding cassette proteins G 1; LXRα, liver X receptor α; FXR, farnesoid X receptor; ASBT, Apical Sodium-dependent Bile Acid Transporter; TNF-α, tumor necrosis factor-α; NOS, nitric oxide synthase; SHP, small heterodimer partner. (Some material taken from Servier Medical Art and freepik.)
5 Crosstalk between GM, its metabolites, and immune inflammatory response in AS
5.1 Bacteria and bacterial components
Under normal circumstances, beneficial and pathogenic bacteria maintain a homeostatic balance in the gut ecology and compete for nutrients to act as antagonists against foreign pathogens and prevent pathogenic bacteria from colonizing (Coyne and Comstock, 2019). Changes in the composition of GM directly affect the changes in microbiota-associated products and influence the intestinal barrier structure through various pathways. For example, when the abundance of Gram-negative bacilli increases, the LPS, the main virulence factor on the surface of the bacterial membrane, increases accordingly. Disruption of the intestinal barrier and increased permeability lead to the entry of bacteria and bacterial membrane components represented by LPS into the circulating blood (Gorabi et al., 2022). After entering the body, LPS is recognized by Toll-like receptors (TLR) and activates NF-κB to cause an immune response, leading to the aggregation of monocytes and macrophages, increased release of coagulation factors, inflammatory factors, and oxygen free radicals, and further worsening the situation of increased intestinal permeability, eventually leading to more LPS invasion of the body, forming a vicious cycle (Abdollahi et al., 2022). In AS plaques, macrophages activated by LPS acquire hyperinflammatory tissue destruction features and amplify Th1 and Th17 cell-mediated responses (Kuznetsova et al., 2020). In addition, LPS promotes the lesion of AS by enhancing the inflammatory properties of senescence and senescence-associated secretory phenotype in specific vascular cells (Suzuki et al., 2022).
In contrast, probiotics such as Bifidobacterium break down indigestible dietary fiber and resistant starch into SCFAs, and enhance the intestinal barrier through adenosine monophosphate-activated protein kinase (AMPK) (Peng et al., 2009; Parada et al., 2019). Bacteroides vulgatus and Bacteroides dorei significantly improve endotoxemia and suppress pro-inflammatory immune responses by reducing LPS production, thereby delaying the formation of AS (Yoshida et al., 2018). Recent studies have found that Lactobacillus strains enhance mucosal immunity by increasing secretory IgA in mice (Katiraei et al., 2020). In addition, Bifidobacterium reduces choline-induced plasma TMAO production by modulating the GM in mice, thereby inhibiting the progression of AS (Qiu et al., 2017; Wang et al., 2022).
In AS, the changes in GM in each phylum were similar to a large extent. At the phylum level, GM in AS patients is mainly characterized by an increased relative abundance of Firmicutes, decreased relative abundance of Bacteroidetes, and increased Firmicutes/Bacteroidetes ratio (Verhaar et al., 2020). At the genus level, it was manifested as a decrease in the relative abundance of probiotics such as Bifidobacterium and an increase in the relative abundance of pathogenic bacteria such as Klebsiella and Escherichia. Specifically, the relative abundance of Ruminococcus, Lactobacillaceae, and Streptococcus in Firmicutes increased, and the relative abundance of Aerococcaceae, Eubacterium, and Faecalibacterium decreased (van den Munckhof et al., 2018; Hashizume-Takizawa et al., 2019). The relative abundance of Bacteroides, Prevotella, Bacteroides, and Prevotella decreased in Bacteroidetes (van den Munckhof et al., 2018). In addition, the relative abundance of Collinsella and Eggerthella in Actinobacteria increased, and the levels of Bifidobacterium and Bifidobacterium genera decreased (Chen et al., 2016; Zhao et al., 2020). The relative abundance of Enterobacter aerogenes, Klebsiella, and Escherichia in Proteobacteria decreased (Lanter et al., 2014; Qiu et al., 2017). Studies have shown that Firmicutes and Proteobacteria are the majority of bacterial DNA detected in atherosclerotic plaques, which are associated with plaque formation and instability (Calandrini et al., 2014; Chen et al., 2016). It should be pointed out that the relationship between GM and AS is still under continuous research, and further in-depth scientific exploration is needed to clarify the mechanism and effect of GM regulation of AS and to clarify the close relationship between GM and AS.
5.2 Metabolites of GM
5.2.1 TMAO
Trimethylamine (TMA) is a low-boiling, nitrogen-containing small-molecule compound (Goel et al., 2016). Dietary precursors such as choline, phosphatidylcholine, and L-carnitine, which are rich in meat, dairy products, eggs, and fish, are produced TMA by TMA lyase of GM (Simo and Garcia-Canas, 2020; Luo et al., 2022). After absorption from the intestine, TMA enters the liver via the portal vein and is oxidized to TMAO by the flavin⁃containing monooxygenase (FMO) family, especially FMO3 (Gatarek and Kaluzna-Czaplinska, 2021). Nature reported in 2011 that TMAO independently predicted the risk of peripheral artery disease, coronary artery disease, and myocardial infarction (Wang et al., 2011). Since then, the relationship between TMAO and AS has attracted the attention of researchers (Ascher and Reinhardt, 2018).
A prospective nested case-control study found that long-term increases in TMAO were associated with a high risk of coronary heart disease and that long-term repeat assessment of TMAO improved the identification of people at high risk of coronary heart disease (Heianza et al., 2020). In addition, TMAO can also be used as a prognostic indicator of cardiovascular events in patients with acute coronary syndromes and stroke (Li et al., 2017; Tan et al., 2019). Plasma TMAO levels were higher in patients with healed plaques than in patients with ST-segment elevation myocardial infarction with unhealed plaques (Li et al., 2021). TMAO has been considered a risk marker for cardiovascular disease (Jaworska et al., 2019). However, a study with people aged 35–55 years showed no significant association between TMAO and the development of AS (Meyer et al., 2016). It is speculated that the reason for this result may be related to the population involved in this study, which was selected from a relatively young population, and that most of the young patients were in the early stages of AS progression, so the effect of TMAO on AS has not yet appeared. In addition, young people have better kidney function and TMAO can be excreted from the body in time. It is worth mentioning that another study found that TMAO exacerbated AS in a low-fat diet but not in a high-fat diet state (Yu et al., 2020). In summary, some progress has been made on the mechanism of TMAO in promoting AS. TMAO was found to induce the development and progression of AS in various ways, including by promoting inflammatory responses and affecting cholesterol metabolism (Zheng and He, 2022).
5.2.1.1 Promotes the recruitment of monocytes and macrophages
AS is commonly defined as a chronic inflammatory disease caused by inflammatory damage to the vascular endothelium, and damage to the vascular endothelium is the induced event of AS (Hooglugt et al., 2022). TAMO accelerates the progression of AS by damaging the vascular endothelium to recruit monocytes and macrophages to promote an inflammatory response (Seldin et al., 2016). It was found that TMAO activates the NF-κB signaling pathway by activating protein kinase C, promoting the expression of cell adhesion factor-1 (such as VCAM-1), and increasing the adhesion of monocytes, thereby accelerating endothelial dysfunction (Ma et al., 2017). In addition, TMAO can also promote inflammatory responses by enhancing MAPK/ERK activity to activate the NF-κB signaling pathway (Yang et al., 2019a).
In a study involving 5 HIV-infected and 520 HIV-uninfected subjects (217 incident plaque cases), plasma TMAO was found to be positively correlated with serum sCD14 and sCD163 levels (Shan et al., 2018). sCD14 and sCD163 are biomarkers of monocyte activation and macrophage inflammation, suggesting that TMAO is closely associated with monocyte- and macrophage-mediated inflammatory responses. In the aorta of choline-fed mice, the expression of monocyte chemotactic protein 1, macrophage inflammatory protein 2, TNF-α, ICAM-1, E-selectin, and VCAM-1 was significantly enhanced, and the macrophage marker CD68 was also highly expressed, which enhanced the recruitment of leukocytes such as monocytes and macrophages to endothelial cells (Seldin et al., 2016). The adhesion of monocytes to endothelial cells may be related to the activation of NLRP3 inflammasome by TMAO through the SIRT3-SOD3-mtROS signaling pathway (Chen et al., 2017). Previous studies have confirmed that Proline/serine-rich coiled-coil protein 1 (PSRC1) has important effects on the immune system (Tonjes et al., 2018). PSRC1 deficiency leads to a significant increase in TMAO levels, which in turn promotes infiltration of F4/80+ macrophages in AS plaques (Luo et al., 2022). At the same time, this process was accompanied by an upregulation of mRNA levels of pro-inflammatory cytokines (including IL-6, IL-10, TNF-α, and IL-17A), in contrast to a significant decrease in the expression levels of anti-inflammatory cytokines (including IL-10, IL-4, IL-13, Ym-1, and Ym-2) (Luo et al., 2022). In vitro, TMAO significantly increased ox-LDL-induced migration of macrophages, further confirming that TMAO enhanced macrophage recruitment.
5.2.1.2 Disturbance of macrophage cholesterol homeostasis and promotion of macrophage-derived foam cell formation
Cholesteryl ester-rich foam cells are an important component of AS plaques. Foam cells in AS plaques are mainly derived from macrophages and smooth muscle cells (Wang et al., 2022). Choline promotes cholesterol accumulation in the macrophages of ApoE−/− mice, which in turn accelerates the formation of macrophage-derived foam cells and ultimately exacerbates the development of AS (Wang et al., 2015). High-density lipoprotein promotes cholesterol efflux through ATP-binding cassette proteins A 1 (ABCA1), ATP-binding cassette proteins G 1, and scavenger receptor class B type I (SR-B1) protein transporters to promote cholesterol efflux and thus prevent cholesterol deposition, which is the first step of reverse cholesterol transport (RCT) (Ouimet et al., 2019). RCT transfers excess cholesterol from cells to the liver, where it is excreted from the body through transfer to bile as free cholesterol or through feces after conversion to bile acids, a process that is thought to inhibit AS (Getz and Reardon, 2018). RCT was significantly inhibited in macrophages of mice fed TMAO (Koeth et al., 2014). RCT disorders lead to the subcutaneous deposition of LDL in the arteries, which are oxidized to ox-LDL by ROS. Ox-LDL stimulates endothelial cells to secrete large amounts of inflammatory mediators, which drives inflammatory cells to infiltrate into the subendothelium leading to chronic inflammation and ultimately causing AS (Lu et al., 2022).
Knockout of FMO3 in mice stimulates liver X receptor α to induce macrophage-mediated RCT, which in turn inhibits intestinal cholesterol absorption and improves cholesterol homeostasis (Warrier et al., 2015). TMAO elevates serum cholesterol levels by decreasing the expression of SR-B1, Abcg5/g8, and Cyp7a1, which in turn promotes the development of AS (Zhu et al., 2016; Canyelles et al., 2018). The scavenger receptor A 1 (SR-A1) and CD36 are not subject to negative feedback regulation from intracellular cholesterol and can sustain cholesterol uptake to induce macrophage transformation into foam cells (Okazaki et al., 2015; Xia et al., 2020). TMAO mediates the phagocytosis of large amounts of cholesterol by macrophages by up-regulating the expression of CD36 and SR-A1 (Wang et al., 2011), and significantly enhances the intracellular load of cholesterol in macrophages. TMAO inhibits cholesterol transport by downregulating the expression of ABCA1 in macrophages to promote lipid accumulation (Mohammadi et al., 2016). In addition, the CD36/MAPK/JNK pathway may play an important role in TMAO-induced lipid accumulation as well as the formation of foam cells (Geng et al., 2018; Shi et al., 2021).
The human body converts about 0.5 g of cholesterol into bile acids every day, which is almost half of the cholesterol consumed by the body every day (Li and Dawson, 2019). The synthesis of bile acids is the main pathway of cholesterol catabolism (Hu et al., 2022). Bile acid metabolism has an important role in cholesterol transport, and the upregulation of bile acid synthase expression helps to enlarge the bile acid pool to increase cholesterol transport. The downregulation of bile acid synthase (Cyp7a1 and Cyp27a1) and bile acid transport protein expression (Oatp1, Oatp4, Mrp2, and Ntcp) inhibits the transportation of cholesterol, resulting in intracellular accumulation of cholesterol and the formation of foam cells, which leads to the development of AS (Koeth et al., 2013; Xu et al., 2021). The decrease in bile acid synthesis leads to the accumulation of large amounts of cholesterol in the body (Bove et al., 2004). TMAO further inhibits bile acid synthesis by activating the nuclear receptor farnesoid X receptor (FXR) and small heterodimer partner (SHP) and inhibiting the expression of Cyp7a1 (Ding et al., 2018), thereby accelerating the formation of aortic lesions in mice. In conclusion, the above studies suggest that TMAO affects cholesterol metabolism through multiple pathways, promoting lipid accumulation and the formation of macrophage-derived foam cells.
5.2.1.3 Other
Macrophage polarization is an activity in which macrophages change phenotype and show differential functions under the influence of complex plaque microenvironments to adapt to the adjustment of the surrounding environment. In the plaque microenvironment, macrophages are stimulated by multiple signals simultaneously and polarized into different subtypes. M1 macrophages are mainly pro-inflammatory and accelerate the development of AS (Stoger et al., 2012). In contrast, M2 macrophages are mainly anti-inflammatory and promote tissue repair, which can inhibit the development of lesions to some extent (Shapouri-Moghaddam et al., 2018). The imbalance between the proportion of M1 and M2 macrophages affects the development of AS. A study conducted in a C57/BL6 mouse model of unstable carotid plaques found that TMAO inhibited the cytostatic effect of macrophages and polarization to the M2 phenotype, which reduced the stability of AS plaques (Shi et al., 2021). However, it was found that the downregulation of TMAO levels had no significant effect on the polarization of M1 macrophages (Shi et al., 2021). In addition, TMAO induces endoplasmic reticulum stress involved in foam cell formation and abnormal macrophage activation by promoting the expression of heat shock proteins and glucose-regulated proteins, such as HSP70, HSP60, GRP94, and GRP78 (Yang et al., 2019b).
Oxidative stress refers to the imbalance between the production of intracellular oxides and the antioxidant effect when the body is stimulated by various risk factors, which ultimately damage the biological system of the organism (Koutsaliaris et al., 2022). Elevated levels of TMAO inhibit the expression of Sirtuin 1 and thus promote oxidative stress (Ke et al., 2018). TMAO activates oxidative stress and increases the levels of interleukin-1β and interleukin-18 by inhibiting the activation of endothelial NOS and the expression of nitric oxide (Sun et al., 2016). NLRP3 inflammatory vesicle activation and oxidative stress were significantly attenuated in carotid ligation mice fed TMAO inhibitors, which inhibited vascular remodeling (Chen et al., 2023).
5.2.2 SCFAs
SCFAs are mainly produced by the fermentation of undigested dietary fiber in the cecal and large intestinal by anaerobic microbiota and include acetate, butyrate, propionate, and so on (Macfarlane and Macfarlane, 2003). SCFAs are key regulators of lipid metabolism and inhibition of inflammatory responses (Brown and Hazen, 2018). SCFAs are produced by the metabolism of different GM. For example, Bacteroides produce acetate and propionate, while Firmicutes produce butyrate (Jia et al., 2023).
Current studies suggest that SCFAs have a potential modulatory effect on AS and are considered to be protective factors for AS. SCFAs are strongly associated with inhibition of intestinal inflammation, protection of intestinal barrier function, and improvement of lipid metabolism. Supplementation with a diet containing 1% butyrate reduced the size of aortic lesions by 50% in ApoE−/− mice (Aguilar et al., 2014). SCFAs play a beneficial role in preventing vascular inflammation not only by activating G-protein coupled receptor 41/43 and inhibiting histone deacetylases to alleviate the endothelial inflammatory response (Li et al., 2018) but also by blocking the NLRP3/Caspase-1 pathway to inhibit the activation of macrophage (Yi et al., 2022). Propionate and butyrate alleviate the inflammatory response and exert immunomodulatory effects by inhibiting LPS-induced expression of TNF-α and nitric oxide synthase (NOS) by monocytes (Vinolo et al., 2011). Propionate also inhibits LPS or TNF-α-induced secretion of IL-6 and IL-8 from endothelial cells and reduces the expression of VCAM-1 (Li et al., 2018). In addition, in ApoE−/− mice fed a high-fat diet (HFD), propionate suppressed the expression of Niemann-Pick C1-like 1 by increasing the number of regulatory T cells and IL-10 levels in the intestinal microenvironment and reduced intestinal cholesterol absorption, which in turn inhibited AS progression (Haghikia et al., 2022). A high-fiber diet alters GM by increasing the abundance of acetate-producing bacteria, which in turn protects against the development of cardiovascular disease (Marques et al., 2017). Transcriptome analysis showed that the protective effects of high fiber and acetate were associated with the downregulation of Egr1, a master regulator of cardiovascular inflammation (Marques et al., 2017). In mouse models of hypertensive cardiac injury and AS, propionate significantly reduced the lesion size in AS and attenuated systemic inflammation, as evidenced by reductions in splenic effector memory T-cell frequency and splenic T-helper 17 cells, as well as reductions in localized cardiac immune cell infiltration in mice in both models (Bartolomaeus et al., 2019). Moreover, due to their antioxidative properties, SCFAs can attenuate the oxidative and pro-inflammatory characteristics of AS. Butyrate attenuated endothelial dysfunction at AS lesion sites by inhibiting CCL-2 and superoxide ion productions and reducing the NADPH oxidase subunit p22phox (Aguilar et al., 2016). Co-culture with macrophages significantly reduced NOS, NO, and iNOS production, which effectively inhibited macrophage migration and activation (Aguilar et al., 2016). Butyrate also prevented endothelial dysfunction in AS by reducing endothelial NOX2 expression and ROS production via the PPARδ/miR-181b pathway and rescuing impaired endothelium-dependent relaxations in IL-1β-treated thoracic aorta ring (Tian et al., 2021). The above indicates that inhibition of oxidative stress may be one of the mechanisms of SCFAs-mediated anti-AS.
Additionally, modulation of lipid metabolism is another pathway of SCFAs against AS. Hypercholesterolemia is one of the risk factors for AS, and ABCA1 mediates the binding of cholesterol to apolipoprotein A-I (ApoA-I) to produce HDL (Li et al., 2020). This process is the main function of ABCA1 and a key step in HDL synthesis in the blood. Butyrate significantly inhibits HFD-induced AS and hepatic steatosis by a mechanism related to the promotion of ABCA1-mediated cholesterol efflux from macrophages (Du Y et al., 2020). SCFAs with two to four carbons lower cholesterol levels by up-regulating hepatic gene expression of sterol-regulatory-element-binding protein 2, low-density-lipoprotein receptor, and cholesterol 7α-hydroxylase to promote hepatic uptake of cholesterol from the blood and accelerate the excretion of bile acids in the feces (Zhao et al., 2017). The concentration of ApoA-I is reduced by inflammation, which may lead to HDL dysfunction and induce cardiovascular disease. SCFAs inhibit NF-κB activation through the peroxisome proliferator-activated receptor-alpha (PPARα) pathway, thereby rescuing the transcriptional function of ApoA-I under inflammatory conditions (Tayyeb et al., 2020). SCFAs have evolved as beneficial microbial products for the prevention or treatment of AS. The risk of AS can be reduced by increasing dietary fiber or direct SCFA supplementation, or the GM microenvironment can be improved by supplementing or transplanting SCFA-producing GM with increased SCFA-producing capacity.
5.3 Secondary bile acids
Bile acids are receiving increasing attention and focus from the medical community as important signaling factors produced by GM. Primary bile acids that escape the enterohepatic circulation are metabolized by GM into secondary bile acids (Sun et al., 2019). Secondary bile acids mainly include deoxycholic acid, litho biliary acid, and ursodeoxycholic acid. In mice, secondary bile acids have been shown to regulate lipid metabolism by modulating specific genes in the liver or small intestine (Mills et al., 2019). A study have found that under the same dietary conditions, the bile acid excretion ability of patients with a high-cholesterol diet is significantly weaker than that of healthy controls, the content of total bile acids in feces is significantly less than that of controls, and DCA and LCA are less (Charach et al., 2018), which suggest that the inability to excrete bile acids effectively accelerates the development of AS.
Secondary bile acids act as important signaling factors that regulate lipid, glucose, and energy metabolism, inflammatory responses, and immune responses mainly through the regulation of FXR and G-protein-coupled bile acid receptor 5 (TGR5) (Kawamata et al., 2003; Chavez-Talavera et al., 2017). Activation of FXR promotes RCT, which has been shown to increase fecal cholesterol excretion and downregulate proinflammatory cytokine levels (Hageman et al., 2010). After feeding on a high-fat diet, the area of AS lesions in mice deficient in both FXR and ApoE-deficient mice was approximately twice as large as in ApoE-deficient mice only (Hanniman et al., 2005). This suggests that the expression of FXR has a protective role in AS. In addition, FXR also inhibits the activation of NF-κB and the expression of inducible NOS, thereby inhibiting vascular smooth muscle migration, preventing vascular remodeling, and enhancing the stability of AS plaques (Li et al., 2007). However, another study found that FXR deficiency reduced the area of AS lesions in male Ldlr−/− mice and hypothesized that the mechanism was related to the inhibition of CD36-dependent lipid uptake in macrophages (Zhang et al., 2006). Notably, the expression of FOM3 is regulated by FXR, indicating that the metabolic pathways of TMAO and bile acids may interact (Bennett et al., 2013). TGR5 is an important receptor for bile acid metabolism (Chiang and Ferrell, 2019). Bile acids reduce the transcriptional activity of NF-κB by activating the cell surface membrane receptor TGR5 to downregulate the levels of pro-inflammatory cytokines, which in turn inhibits inflammation in AS plaques of Ldlr−/− mice and reduces the content of macrophages and lipid load in the plaques (Pols et al., 2011). In addition, TGR5 agonism induces NO production via Akt activation and intracellular Ca2+ increase, and this function inhibits the adhesion of monocytes to endothelial cells in response to inflammatory stimuli (Kida et al., 2013).
6 Natural products modulate the crosstalk between GM and immune response in AS
Natural products have been the subject of many studies and they are increasingly being used as a complementary therapy in clinical practice. It is well known that most natural products are chemically diverse and bioavailable. To maximize the therapeutic potential of natural products, a large number of trials are still in progress or preparation. Natural products have been shown to have multi-pathway and multi-target therapeutic effects in the treatment of AS (Liu et al., 2022). In recent years, some scholars have turned their attention to the study of natural products and GM and tried to acknowledge the pharmacological activities of natural products from the perspective of GM. The role of GM in AS has been gradually unveiled (Verhaar et al., 2020). So, is the specific mechanism of natural products in the treatment of AS by targeting GM and immune response crosstalk? Recent studies have provided an answer. As shown in Table 1.
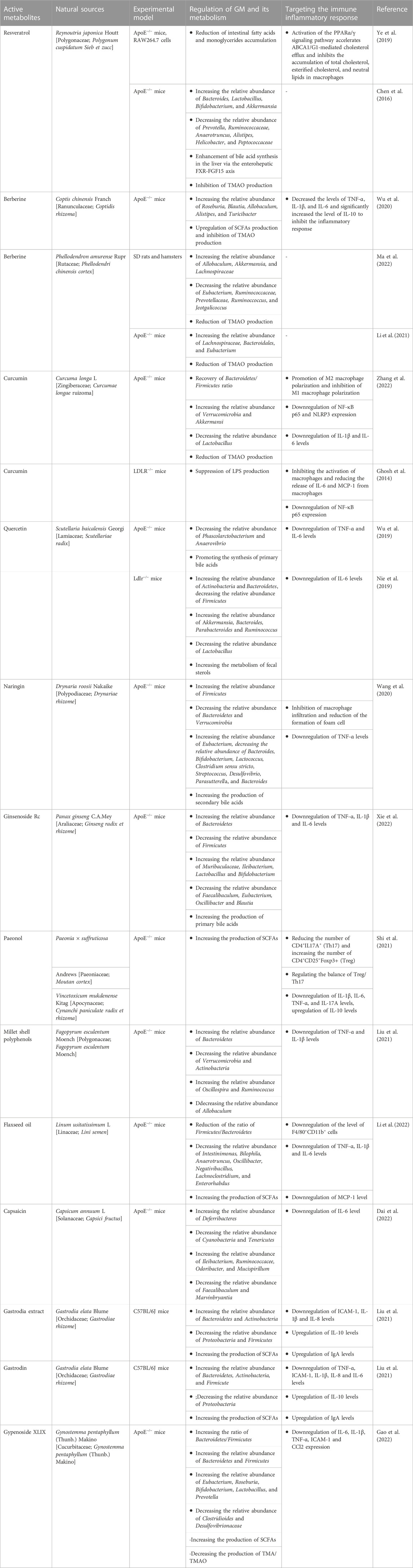
TABLE 1. Mechanism of natural products targeting the GM-immune response axis in the treatment of AS.
6.1 Resveratrol
Resveratrol (RSV) is an antitoxin produced by plants in nature when they are stimulated. It belongs to a polyphenolic organic metabolite and is widely found in plants such as Polygonum cuspidatum (Meng et al., 2023). Resveratrol has various pharmacological effects such as anti-inflammatory, antibacterial, antioxidant, and tumor-inhibiting activities (Koushki et al., 2018). The protective effect of resveratrol in AS-related diseases has been demonstrated (Koushki et al., 2018). RSV increases the levels of Lactobacillus and Bifidobacterium in the intestine of C57BL/6J and ApoE−/− mice, thereby enhancing the activity of bile saline lyase and promoting the unbinding of bile acids and fecal excretion (Chen et al., 2016). In addition, RSV reduces the GM metabolism of TMAO and enhances bile acid synthesis in the liver via the enterohepatic FXR-FGF15 axis (Chen et al., 2016). The intestinal carbohydrate and amino acid metabolism were effectively improved and the accumulation of fatty acids and monoglycerides was significantly reduced in AS mice successfully modeled by a high-fat diet and fed RSV (Ye et al., 2019). Peroxisome proliferator-activated receptors (PPARs) play a key role in cholesterol homeostasis and are an important way to treat dyslipidemia in AS (Hu et al., 2022). RSV promotes ABCA1/G1-mediated cholesterol efflux through activation of the PPARα/γ signaling pathway and inhibits the oleic acid-induced accumulation of total cholesterol, esterified cholesterol, and neutral lipids in macrophages (Ye et al., 2019). These results suggest that RSV interacts with GM and thus plays a regulatory role in AS.
6.2 Berberine
Berberine (BBR) is a traditional Chinese medicinal preparation containing isoquinoline alkaloids extracted from plants, which has a variety of pharmacological properties (Wang et al., 2019). In China, it has been used to treat diabetes, obesity, AS, and metabolic diseases (Zhang et al., 2020). BBR was found to inhibit Streptococcus, Staphylococcus epidermidis, and Shigella dysenteriae (Wang et al., 2009; Yan et al., 2014), and increase the abundance of Lactobacillus and mucinophilic Ackermannia in the intestine of mice (Zhang et al., 2019). Therefore, BBR is considered to have antibiotic-like effects and a broad antibacterial spectrum.
The metabolite of BBR, dihydro flavopiridol, inhibits TMAO production by blocking the Choline-TMA-TMAO production pathway through vitamin-like effects, leading to a decrease in serum TMAO levels and contributing to the inhibition of plaque formation in blood vessels (Ma et al., 2022). Clinical trials have confirmed that both TMA and TMAO in the feces of patients were significantly reduced after 4 months of oral BBR, and plaque scores were significantly decreased (Ma et al., 2022). The mechanism is related to the reduction of cutC and cntA, key functional genes of the TMA synthesis pathway by BBR, as a way to reshape the GM and thus inhibit the development of AS (Li et al., 2021). BBR showed a dose-dependent approach in reducing plaque area and improving the inflammatory response (Wang et al., 2009). Compared to low-dose (50 mg/kg) BBR, high-dose (100 mg/kg) BBR significantly reduced plaque size, significantly reduced the levels of TNF-α, IL-1β, and IL-6, and significantly increased the levels of IL-10. However, low-dose BBR had no significant effect on IL-6 and IL-10 levels. In addition, alterations in the composition and function of the GM showed different sensitivities to BBR doses. For example, Alistipes and Roseburia were significantly enriched in the high-dose BBR group, while Blautia and Allobaculum were more enriched in the low-dose group.
6.3 Curcumin
Curcumin has multiple pharmacological mechanisms such as anti-inflammatory, antioxidant, and anti-free radical (Peng et al., 2021). Numerous studies have shown that curcumin can play a positive role in the prevention and treatment of many metabolic diseases by interacting with GM to achieve beneficial effects on the health of the host organism (Pluta et al., 2020). The study showed found that after 15 days of continuous gavage of C57BL/6 mice with curcumin (100 mg/kg), the composition of the GM of mice was significantly altered, with a significant decrease in the abundance of pathogenic bacteria such as Prevoteltellaceae, Bacteroidaceae and Rikenellaceae (Shen et al., 2017). An 8-week randomized, double-blind, and placebo-controlled trial (Peterson et al., 2018) showed an overall decrease of 15% in bacterial species in the placebo group after treatment, compared to an increase of 7% and 69% in the turmeric and curcumin-treated groups, respectively. The above suggests that curcumin has a significant modulating effect on the composition of GM.
Curcumin has been confirmed to directly affect GM and restore cadmium-induced metabolic disorders of GM, such as restoring the Bacteroidetes-to-Firmicutes ratio, reducing the abundance of Lactobacillus and increasing the abundance of Verrucomicrobia (Zhang et al., 2022). Curcumin restored the polarization of macrophages by remodeling the GM, that is, promoting M2-type polarization and inhibiting M1-type polarization (Zhang et al., 2022). In addition, in the AS region of the aorta, curcumin downregulated the expression of the inflammatory proteins NF-κB p65 and NLRP3 and decreased the levels of the inflammatory factors IL-1β and IL-6 (Zhang et al., 2022). The pathological increase of LPS can promote the hyperactivation of macrophages and activate the expression of NF-κB, upregulate the levels of chemokines and proinflammatory cytokines, which in turn lead to inflammation-associated diseases (Sieve et al., 2018). The disruption of the intestinal barrier loses the ability to limit the release of LPS from bacteria into circulation (Wang et al., 2017). Curcumin inhibited LPS-induced translocation of [3H]-mannitol and FITC dextran by increasing the activity of intestinal mucosal defense factor intestinal alkaline phosphatase (IAP), which in turn improved intestinal barrier function (Ghosh et al., 2014). The mechanism by which curcumin inhibits AS may be through reducing the release of LPS and inhibiting the release of proinflammatory factors such as IL-6 and MCP-1 after excessive activation of macrophages, and reducing the expression of pro-inflammatory transcription factor NF-κB.
Curcumin not only achieves its broad biological functions by regulating the level of structural abundance of the host GM but also is metabolized and catabolized by the microbiota in the host gut to produce a variety of active metabolites that participate in the regulation of various physiological processes in the host (Tsuda, 2018). The human GM catabolizes curcumin mainly through several pathways such as reduction, demethylation, acetylation, and hydroxylation, producing a mixture of demethoxycurcumin and bisdemethoxycurcumin (Lou et al., 2015). Among them, bisdemethoxycurcumin can significantly increase the relative abundance of E. faecalis and decrease the relative abundance of Bacteroides and Subdoligranulum in the broiler intestine, as well as significantly improve the morphology of the intestinal mucosa, maintain the integrity of the intestinal barrier, inhibit the secretion of pro-inflammatory factors, and promote the recovery of GM dysbiosis, thereby alleviating LPS-induced intestinal inflammatory damage in broilers (Zhang et al., 2021). This suggests that the interaction between curcumin and GM is an important link in regulating the physiological functions of the organism.
6.4 Quercetin
Numerous in vivo and in vitro studies have shown that quercetin exhibits good protective effects on several key aspects of AS development, such as reducing oxidative stress levels, antagonizing inflammation, and restoring vascular endothelial function (Xiao et al., 2017; Patel et al., 2018). Pharmacokinetic studies show that the bioavailability of oral quercetin is only 5.3%, of which about 93.3% is metabolized in the gut (Chen et al., 2005).
Quercetin can selectively promote the proliferation of beneficial intestinal bacteria and inhibit the colonization of harmful bacteria in the gut, thereby optimizing the intestinal microecological environment to maintain the health of the gut and the body. Quercetin significantly increased the alpha diversity of GM. At the phylum level, quercetin increased the relative abundance of Actinobacteria, Cyanobacteria, and Firmicutes and decreased the relative abundance of Verrocomicrobia (Nie et al., 2019; Wu et al., 2019). At the genus level, the abundance of Ackermannia, Bacillus, Paramecium, and Ruminalis was significantly increased by quercetin treatment, while the abundance of Lactobacillus was significantly decreased (Nie et al., 2019).
Quercetin not only regulates the abundance of GM but also influences the production of metabolites of GM. Quercetin promotes the synthesis of primary bile acids, which in turn consumes more cholesterol and reduces the clinical risk of HFD-induced AS (Wu et al., 2019). Furthermore, reducing the abundance of Actinobacteria and increasing the abundance of Firmicutes increased the level of IL-6 and inhibited the expression of malondialdehyde (MDA), which in turn had a positive regulatory effect on oxidative stress, immunity, and inflammatory response (Nie et al., 2019). The above studies suggest that quercetin plays a beneficial role in GM and immune response crosstalk, but the specific mechanisms still need to be further studied.
6.5 Naringin
Naringin is a natural dihydro flavonoid mainly derived from the peel and pulp of Rutaceae. Naringin has been reported to inhibit AS by inhibiting the adhesion of monocytes and endothelial cells (Hsueh et al., 2016), inhibiting macrophage infiltration, and regulating cholesterol metabolism in macrophages (Xu et al., 2019). Naringin has low bioavailability and is difficult to digest and absorb in the small intestine, thus reaching the colon where the GM colonizes (Mulvihill et al., 2016). Therefore, oral administration of naringin may affect the composition and metabolism of GM. Naringin significantly reduced the serum levels of total cholesterol, low-density lipoprotein cholesterol, very-low-density lipoprotein cholesterol, and free cholesterol in AS model mice and reduced the area of AS plaques (Wang et al., 2020). Meanwhile, naringin also promoted the diversity and stability of GM, reduced the number of harmful microbiota, and increased the number of beneficial microbiota. For example, increasing the abundance of Firmicutes and reducing the abundance of Bacteroidetes and Verrucomirobia, thus promoting the synthesis of secondary bile acids. In terms of lipid metabolism, naringin regulated cholesterol metabolism by inhibiting the liver X receptor and the steroid transporter protein ABCG5/G8. In addition, naringin inhibited macrophage infiltration in AS plaques, which in turn alleviated the inflammatory response.
6.6 Ginsenoside Rc
Ginsenoside Rc is one of the bioactive components in ginseng with a wide range of physiological activities, such as inhibition of oxidative stress, anti-inflammation, and regulation of glucose metabolism (Lee et al., 2010; Kim et al., 2014; Yu et al., 2017). Ginsenoside Rc also showed anti-atherosclerosis activity. Ginsenoside Rc significantly influenced the composition of GM and fecal metabolites in mice with high-fat diet-induced AS, thereby improving the condition of AS. Specifically, ginsenoside Rc increased the number of beneficial bacteria such as Lactobacillus and Bifidobacterium, while decreasing the number of harmful microbiota such as Faecalibaculum (Xie et al., 2022). In addition, ginsenoside Rc increases the metabolism of primary bile acids by remodeling the GM, which in turn inhibits the expression of the pro-inflammatory cytokines TNF-α, IL-6, and IL-1β to alleviate inflammatory response (Xie et al., 2022).
6.7 Paeonol
Paeonol is a bioactive substance extracted from the root bark of a Paeonia ×suffruticosa Andrews in Ranunculaceae or the dried root of Cynanchum paniculatum in Metaplexy. It has analgesic, anti-inflammatory, and anti-tumor pharmacological effects (Zhang et al., 2019). Current studies found that Paeonol inhibits the progression of AS by downregulating the inflammatory response of vascular endothelial cells (Yu et al., 2020), inhibiting excessive proliferation of VSMCs (Zhang et al., 2018), and regulating macrophage function (Xu et al., 2018). Paeonol can alleviate AS in a GM-dependent manner. Paeonol inhibits plaque formation and vascular fibrosis in a high-fat diet-induced AS mouse model (Shi et al., 2021). Paeonol selectively upregulates the proportion of Treg cells and reduces the number of Th17 by remodeling the GM, such as increasing the metabolism of SCFAs and reducing the accumulation of LPS (Shi et al., 2021), and inhibits the abnormal proliferation of VSMCs caused by the high expression of osteopontin in monocytes (Shi et al., 2022). In addition, Paeonol significantly inhibited the expression of inflammatory cytokines IL-1β, IL-6, TNF-α, and IL-17A and increased the level of anti-inflammatory cytokine IL10 in the aorta of AS mice (Shi et al., 2022).
6.8 Millet shell polyphenols
As an important component of cereals, the active polyphenols in millet shells have hypolipidemic and antioxidant effects as well as inhibiting inflammatory responses (Khare et al., 2020). These functions are closely related to their anti-AS effects. Millet shell polyphenols inhibited aortic lesions in ApoE−/− mice (Liu et al., 2021). Its mechanism is to regulate the relative abundance of GM, such as reducing the abundance of Verrurella and Actinomyces and increasing the abundance of Bacteroidetes, which in turn exerts a barrier-protective effect and inhibits the expression of LPS and the pro-inflammatory cytokines TNF-α and IL-1β (Liu et al., 2021). In addition, Millet shell polyphenols block the expression of STAT1 and NF-κB in macrophages and inhibit the formation of macrophage-derived foam cells (Liu et al., 2023).
6.9 Flaxseed oil
Flaxseed oil, one of the major edible oils worldwide, is the main component extracted from Linum usitatissimum L and an important source of α-linolenic acid (Xu et al., 2012). As an important nutrient for humans, flaxseed oil is closely related to vision, brain development, and behavioral development. In addition, flaxseed oil plays an active role in lowering blood lipids and blood glucose, antioxidant, antibacterial and anti-inflammatory, anti-cancer, and anti-AS (Dupasquier et al., 2007; Zhu et al., 2020; Bao et al., 2022). Flaxseed oil reduced the relative abundance of Enterobacteriaceae, Biliophilus, Anaerobacter, Oscillibacter, and Negatibacillus in the GM of AS model mice and significantly reduced the proportion of the Firmicutes/Bacteroidetes ratio (Li et al., 2022). The production of SCFAs, a metabolite of GM in mice, was also promoted by flaxseed oil (Li et al., 2022).
By modulating the GM of AS model mice, flaxseed oil downregulated the levels of F4/80+CD11b+ cells in the aortic region and suppressed the inflammatory response (such as TNF-α and IL-1β), thus exerting an anti-AS effect (Li et al., 2022).
6.10 Capsaicin
Capsaicin is a vanilla amide-containing alkaloid and the natural capsaicin consists of a series of similar substances such as dihydrocapsaicin, hypo-dihydrocapsaicin, and hypercapsaicin (Wang et al., 2022). Among them, capsaicin which can be absorbed and metabolized by the gastrointestinal tract accounts for 80%–90%. In recent years, studies have shown that capsaicin has a wide range of pharmacological effects, including analgesia, regulation of blood glucose metabolism, and antioxidant and anti-AS effects (Munjuluri et al., 2021; Wang et al., 2022). It was found that capsaicin exerts anti-AS effects by modulating the GM of ApoE−/− mice as well as metabolites such as increasing the abundance of Turicibacter, Odoribacter, and Ileibacterium in the feces and reducing the metabolism of deoxycholic acid, bile acids, hypoxanthine, and fecal bile in the cecum, thereby inhibiting the inflammatory response (Dai et al., 2022).
6.11 Gastrodia extract and gastrodin
Gastrodia elata is a traditional Chinese medicine with a long history of application in China. Studies have shown that Gastrodia elata has good efficacy in the treatment of cardiovascular diseases (Sun et al., 2023). Gastrodin is the most important active component of Gastrodia elata. Gastrodin and gastrodin extracts reduce lipid deposition in early AS mice in a dose-dependent manner and slow down the progression of AS (Liu et al., 2021b; Liu et al., 2021). The mechanism is to increase the diversity of GM, regulate the relative abundance of GM (such as increasing the relative abundance of Bacteroidetes and reducing the relative abundance of Proteobacteria), and increase the production of SCFAs, thereby inhibiting the secretion of the pro-inflammatory cytokines IL-1β and IL-6 and promoting the expression of the anti-inflammatory cytokine IL-10, and ultimately alleviating the inflammatory response in AS mice (Liu et al., 2021b; Liu et al., 2021). Asparagine and asparagine extracts also increase sIgA levels and downregulate D-LA expression, which in turn promote the repair of GM and reduce permeability (Liu et al., 2021). This also helps to delay the progression of AS. In addition, gastrodin reduces the formation of foam cells and improves inflammation by inhibiting the NF-κB signaling pathway to anti-AS (Xue et al., 2023).
6.12 Gypenoside XLIX
Gynostemma pentaphyllum (Thunb.) Makino is a perennial prostrate plant in the Cucurbitaceae family, which is mainly distributed in China, Korea, and Japan. Gynostemma pentaphyllum (Thunb.) Makino has been used as a Chinese herbal medicine in ancient times, which has the functions of clearing heat and detoxifying, invigorating the spleen and calming the mind, invigorating qi, and promoting body fluid (Nguyen et al., 2021). With the discovery of modern research on herbal medicine, Gynostemma pentaphyllum (Thunb.) Makino leaves have been widely used as vegetables, dietary supplements, and herbal teas for their potential in reducing hyperlipidemia, malignancy, diabetes, chronic inflammation, and anti-aging (Liao et al., 2021; Weng et al., 2021). As the main chemical component of Gynostemma pentaphyllum (Thunb.) Makino, Gypenoside XLIX inhibits the inflammatory response in AS mice by modulating the GM (such as increasing the relative abundance of Firmicutes, Bacteroidetes, Bifidobacteria, and decreasing the relative abundance of Clostridioides and Desulfovibrionaceae), inhibiting the production of TMAO and promoting the synthesis of SCFAs (Gao et al., 2022).
7 Oral probiotics modulate the crosstalk between GM and immune responses in AS
Probiotics colonized in the gut, such as Lactic Acid Bacteria and Bifidobacterium, can positively affect human health by improving GM balance, enhancing immune function, and regulating metabolism (Hill et al., 2014). GM imbalance can lead to intestinal environment disorder, resulting in the reduction of the total amount of probiotics and causing a series of diseases. Current studies have shown that probiotic supplementation can effectively improve the risk factors of AS, such as lipid metabolism disorders, hypertension, and persistent inflammation. The intervention of probiotics provides a new direction and idea for the regulation and remodeling of intestinal microorganisms. Oral probiotics have opened new avenues for the treatment of AS.
The interactions between probiotics and the host immune system are highly complex and are still being explored. Treatment of ApoE−/− mice with the probiotic lactic acid bacterium Pediococcus acidilactici R037 found that the number of CD4+T cells producing interferon-γ in the spleen and the production of proinflammatory cytokines by splenic lymphocytes were significantly reduced (Mizoguchi et al., 2017). In vitro, experiments further confirmed that R037 alleviated AS by inducing tolerogenic DCs, thereby inhibiting Th1-driven inflammation and the proliferation of CD4+T cells (Mizoguchi et al., 2017). Lab4 is a probiotic consortium composed of Lactobacillus acidophilus CUL21 (NCIMB 30156) and CUL60 (NCIMB 30157), Bifidobacterium bifidum CUL20 (NCIMB 30153) and Bifidobacterium animalis subsp. lactis CUL34 (NCIMB 30172). The combination of Lab4 with L. plantarum CUL66 (called Lab4P) significantly reduced LDL and very low-density lipoprotein levels in HFD-fed LDL receptor-deficient mice. It alleviates inflammation by inhibiting monocyte migration, the proliferation of monocytes and macrophages, and reduces the formation of foam cells (O'Morain et al., 2021). In addition, Lab4P can increase the number of VSMCs, which is a marker of plaque stability (O'Morain et al., 2021). VSL#3 is a well-studied probiotic mixture comprised of 8 strains, showing similar efficacy to telmisartan in inhibiting vascular inflammation and reducing markers of AS development, and increasing the diversity of GM (Chan et al., 2016). A recent clinical study showed that supplementation with Lactobacillus paracasei TISTR 2593 significantly reduced MDA and TNF-α levels in AS patients, thereby inhibiting oxidative stress and inflammation (Khongrum et al., 2023). It also significantly increased serum ApoE and adiponectin levels (Khongrum et al., 2023). It should be noted that not all probiotics have anti-AS effects, such AS Lactobacillus reuteri, which does no effect on AS in ApoE−/− mice (Fak and Backhed, 2012).
In addition, probiotics have been shown to exert positive effects by influencing the production of gut microbiota metabolites. Bifidobacterium animalis subsp. lactis F1-three to two, Bifidobacterium animalis subsp. lactis F1-7, Lactobacillus plantarum F3-2 reduce the content of TMA in the intestine and downregulate the levels of TMA and TMAO in serum, and the mechanism is not related to the regulation of FMO3 function (Liang et al., 2020). The mechanism of action of this probiotic may be directly degrading TMA or changing the structure of GM. Similarly, Bifidobacterium breve Bb4 and Bifidobacterium longum BL1 and BL7 also reduced plasma TMAO and plasma and cecal TMA concentrations. However, it did not affect the protein expression of FMO, FMO3, and FXR and the excretion of TMAO (Wang et al., 2022). A Pilot Study found that short-term supplementation with probiotics Streptococcus thermophilus (KB19), Lactobacillus acidophilus (KB27), and Bifidobacteria longum (KB31) did not affect plasma TMAO levels in hemodialysis patients (Borges et al., 2019). Food-derived probiotics can increase the number of SCFAs-producing bacteria and promote the synthesis of SCFAs, which can delay the progression of AS (Derrien and van Hylckama, 2015). Lactobacillus fermentum ZJUIDS06 supplementation upregulated colon SCFA levels and increased the relative abundance of Paracbacteroides in the cecum of hyperlipidemic hamsters. This is a biomarker of colonic SCFA production and improved serum cholesterol levels (Yang et al., 2021). Lactobacillus reuteri CCFM8631 increased the contents of acetate, propionate, and butyrate and decreased the level of TMAO in the feces of AS model mice. At the same time, it improved the GM microenvironment (an increase in the relative abundance of faecal Deferribacteres, Lachnospiraceae NK4A136 group, Lactobacillus, and Dubosiella; a decrease in the relative abundance of Erysipelatoclostridium and Romboutsia) (Wang et al., 2022). The application of probiotics to improve health or treat diseases by affecting the physiological functions of the host has become a popular research area in medical disciplines. The current research has made preliminary progress, but limited by the current technology and the lack of prior knowledge, scientists have not yet fully revealed the interaction mechanism between each strain and between the microbiota and the host, which is the focus of GM research.
8 Dietary fiber in the prevention of AS
Dietary fiber is a non-digestible and non-absorbable carbohydrate polymer that can regulate the physiological health of the host by regulating the GM, promoting the dynamic balance of the intestinal microbiota, and then influencing the production of intestinal microbial metabolites. Consuming an abundance of fibrous foods, such as vegetables, fruits, grains, and legumes, is beneficial to gut health and overall health, and is thought to prevent cardiovascular disease. A cross-sectional study in Korea showed that total fiber and fruit fiber intake in men were inversely associated with metabolic syndrome, and increased total fruit fiber intake in women contributed to lower rates of obesity and abdominal obesity (Song and Song, 2021). Clinical studies have found that dietary fiber consumption can help regulate lipid metabolism and slow the progression of AS (Kameyama et al., 2020; Munoz-Cabrejas et al., 2021).
The fermentation of dietary fiber by GM produces large amounts of SCFAs, and the amount and type of SCFAs produced may vary due to different fermentation characteristics. For example, pea fiber significantly increases butyrate levels in humans (Guo et al., 2021). Whole wheat diets, on the other hand, have been associated with an increase in propionate (Vetrani et al., 2016). Dietary fiber may exert beneficial effects against AS by increasing the production of SCFAs. Soluble dietary fiber was found to remodel the microenvironment of GM by increasing beneficial intestinal bacteria and inhibiting TMAO production by activating AMPK (Li et al., 2017). In addition, the intake of dietary fiber helps to expand the size of the bile acid pool. Cereal fiber intake significantly increased serum bile acid concentrations in obese but not overweight individuals, which may be related to a compensatory increase in the bile acid pool (Weickert et al., 2018). Arabinoxylan and β-glucan significantly increased the number of bacilli in the intestinal tract of mice and significantly upregulated various genes involved in cholesterol and bile acid metabolism in the liver, which effectively promoted bile acid excretion and attenuated hepatic steatosis (Raza et al., 2019). Numerous current studies have confirmed the need for appropriate dietary modifications. Different dietary fibers have different regulatory effects on GM and their metabolites, requiring individualized programs for different populations and different diets.
9 Discussion
At present, although the mechanisms underlying the development of AS have not been elucidated, there has been some progress in related studies. The important role of immune response in AS has been widely recognized (Wolf and Ley, 2019). The immune response has a very complex biological characteristic, involving a variety of cell types, multiple pathway regulation, and diverse response types. The occurrence of immune responses depends on the recognition and activation of immune cells and the immune response. During the development and progression of AS, a complex series of changes in the local microenvironment occurs with the infiltration of multiple immune cells (Herrero-Fernandez et al., 2019). So, who can play a role in activating the immune response in AS? Numerous studies have found that GM and its metabolites are closely associated with AS (El et al., 2023). The GM constitutes a complex and vast microenvironment in the human body, and the composition and function of the microbiota affect the balance of health and disease.
Current research has shown that intervention with GM is an effective strategy for the prevention and treatment of AS. Firstly, targeting the GM itself, various compounds including natural product supplements have the potential to cause GM remodeling due to the sensitivity of the microbiota to changes in the chemical environment in the gut. Therefore, targeting GM remodeling is a way to explore the therapeutic potential of GM. As mentioned above, probiotics may have therapeutic potential by inhibiting inflammatory responses, maintaining the gut barrier, and modulating lipid metabolism. The use of probiotics to maintain as well as reshape the balance of GM is an important development in the treatment of cardiovascular diseases such as AS. However, it should be noted that studies on probiotics for the treatment of AS are still relatively limited and the results are inconsistent. Due to species diversity and individual differences, as well as the survival and stability of probiotics, further breakthroughs are needed. In addition, dietary fiber intake and application of natural products are also potential options for modulating the GM to intervene in AS. Natural products, usually of plant and animal origin, have a wide range of diverse biological activities and pharmacological effects and have a wide range of applications and research values in the medical field. In the treatment of AS, natural products have shown great potential (Cheng et al., 2022). The search for effective new drugs from natural products and extracts for the treatment of AS has become a hot research topic in recent years. Natural products, probiotic interventions, fecal microbial transplants, and other therapeutic modalities based on the regulation of intestinal microecology have been proposed and some have been successfully applied in the treatment of AS (Ji et al., 2020; Kim et al., 2022). The advantages of natural products, such as wide sources, low toxicity, and diverse immunomodulatory activities, suggest that natural products have a promising future in the treatment of AS. Through in-depth research on natural products, we can search and discover new compounds with biological activities, which can not only expand the application fields of natural products but also meet human needs for treating diseases and improving the quality of life. Although natural products have shown convincing results in several studies as AS therapies. However, there is still a lack of research on the effects of natural products on the immune system through GM and their metabolites. As mentioned above, in terms of modulating the immune response, current studies have focused on how GM and its metabolites promote or inhibit the development of AS by regulating macrophage infiltration, polarization, cholesterol metabolism, and the associated inflammatory response. There are few studies on how GM affects other immune cells (such as T cells, B cells, mast cells, etc.). In addition, the mechanism of production of metabolites associated with GM is unclear, such as how bacteria use dietary fiber to produce SCFAs. This is a gap that needs to be filled in future research. Further research is needed to improve the use of natural products in the treatment of AS.
In addition, there are some limitations in the current studies on the relationship between GM and the immune system, which need further research and exploration. Although many studies have shown that there is an interaction between GM and the immune system, the correlation and causality have not been clearly explained. Most of the current studies are still observational, and it is difficult to determine causality. The interaction between GM and the immune system involves complex biological mechanisms, such as signal transduction, the influence of metabolites, and immune cell interactions. Our current understanding of these mechanisms is relatively limited, and further studies are needed to reveal the details. In addition, there is a lack of consistency in the results obtained from different studies, which may be due to reasons such as study design, sample size, and population differences. There is also the inevitable problem that there are significant differences in GM composition and immune systems between individuals, making the generalization of the findings somewhat challenging.
Future studies should explore the association between different microbiota composition, function, and metabolites and the immune system, as well as their impact on health and disease states, by analyzing large-scale gut microbiota data. In addition, it focuses on the deeper interaction mechanism between gut microbiota and the immune system, including immune regulation, signal transduction, immune cell development, and function. This helps to develop precise regulatory strategies to adjust the structure and function of gut microbiota, thereby affecting the state of the immune system. The differences in gut microbiota and immune system among different individuals cannot be ignored, and their relationship with individual health, susceptibility, and response to treatment can be explored. Considering the great interindividual differences in people’s microbiota composition and immune response, this research can help to achieve personalized medicine, customized nutrition, and treatment programs. In terms of drug development, focusing on how the active components of natural products are metabolized by GM, and whether these metabolites have synergistic or antagonistic effects on atherosclerotic diseases, and then finding new favorable metabolites of GM will provide a new direction for the clinical drug treatment of AS.
In summary, the emerging role of natural products in the treatment of AS still has greater potential and deserves attention. Natural products improve the immune response in AS by modulating the GM and its metabolites, which in turn inhibit the progression of AS. The role of natural products in targeting gut microbiota and immune response provides unique insights into the gut arterial axis and broadens our understanding of natural products.
Author contributions
JJ: Conceptualization, Investigation, Writing—original draft. JG: Writing—review and editing. RD: Investigation. CZ Supervision, Conceptualization, Writing—review and editing. ZZ: Supervision, Editing the final manuscript, Participating in discussion. All authors contributed to the article and approved the submitted version.
Funding
This work was supported by the Science and Technology Project of Tianjin Municipal Education Commission (No. 2021ZD020) and the Tianjin Famous Chinese Medicine Inheritance Studio Construction Project (Jin Wei Zhong (2022) No. 303).
Conflict of interest
The authors declare that the research was conducted in the absence of any commercial or financial relationships that could be construed as a potential conflict of interest.
Publisher’s note
All claims expressed in this article are solely those of the authors and do not necessarily represent those of their affiliated organizations, or those of the publisher, the editors and the reviewers. Any product that may be evaluated in this article, or claim that may be made by its manufacturer, is not guaranteed or endorsed by the publisher.
References
Abdollahi, E., Keyhanfar, F., Delbandi, A. A., Falak, R., Hajimiresmaiel, S. J., and Shafiei, M. (2022). Dapagliflozin exerts anti-inflammatory effects via inhibition of LPS-induced TLR-4 overexpression and NF-κB activation in human endothelial cells and differentiated macrophages. Eur. J. Pharmacol. 918, 174715. doi:10.1016/j.ejphar.2021.174715
Abdolmaleki, F., Gheibi, H. S., Bianconi, V., Johnston, T. P., and Sahebkar, A. (2019). Atherosclerosis and immunity: a perspective. Trends. cardiovasc. Med. 29 (6), 363–371. doi:10.1016/j.tcm.2018.09.017
Adak, A., and Khan, M. R. (2019). An insight into gut microbiota and its functionalities. Cell. Mol. Life Sci. 76 (3), 473–493. doi:10.1007/s00018-018-2943-4
Aday, A. W., and Matsushita, K. (2021). Epidemiology of peripheral artery disease and polyvascular disease. Circ. Res. 128 (12), 1818–1832. doi:10.1161/CIRCRESAHA.121.318535
Aguilar, E. C., Leonel, A. J., Teixeira, L. G., Silva, A. R., Silva, J. F., Pelaez, J. M., et al. (2014). Butyrate impairs atherogenesis by reducing plaque inflammation and vulnerability and decreasing NFκB activation. Nutr. Metab. Cardiovas.c Dis. 24 (6), 606–613. doi:10.1016/j.numecd.2014.01.002
Aguilar, E. C., Santos, L. C., Leonel, A. J., de Oliveira, J. S., Santos, E. A., Navia-Pelaez, J. M., et al. (2016). Oral butyrate reduces oxidative stress in atherosclerotic lesion sites by a mechanism involving NADPH oxidase down-regulation in endothelial cells. J. Nutr. Biochem. 34, 99–105. doi:10.1016/j.jnutbio.2016.05.002
Ait-Oufella, H., Salomon, B. L., Potteaux, S., Robertson, A. K., Gourdy, P., Zoll, J., et al. (2006). Natural regulatory T cells control the development of atherosclerosis in mice. Nat. Med. 12 (2), 178–180. doi:10.1038/nm1343
Amengual, J., and Barrett, T. J. (2019). Monocytes and macrophages in atherogenesis. Curr. Opin. Lipidol. 30 (5), 401–408. doi:10.1097/MOL.0000000000000634
Ascher, S., and Reinhardt, C. (2018). The gut microbiota: an emerging risk factor for cardiovascular and cerebrovascular disease. Eur. J. Immunol. 48 (4), 564–575. doi:10.1002/eji.201646879
Atkinson, J. B., Harlan, C. W., Harlan, G. C., and Virmani, R. (1994). The association of mast cells and atherosclerosis: a morphologic study of early atherosclerotic lesions in young people. Hum. Pathol. 25 (2), 154–159. doi:10.1016/0046-8177(94)90271-2
Atri, C., Guerfali, F. Z., and Laouini, D. (2018). Role of human macrophage polarization in inflammation during infectious diseases. Int. J. Mol. Sci. 19 (6), 1801. doi:10.3390/ijms19061801
Bao, X., Yuan, X., Li, X., and Liu, X. (2022). Flaxseed-derived peptide, IPPF, inhibits intestinal cholesterol absorption in Caco-2 cells and hepatic cholesterol synthesis in HepG2 cells. J. Food Biochem. 46 (1), e14031. doi:10.1111/jfbc.14031
Bartolomaeus, H., Balogh, A., Yakoub, M., Homann, S., Marko, L., Hoges, S., et al. (2019). Short-chain fatty acid propionate protects from hypertensive cardiovascular damage. Circulation 139 (11), 1407–1421. doi:10.1161/CIRCULATIONAHA.118.036652
Bellini, R., Bonacina, F., and Norata, G. D. (2022). Crosstalk between dendritic cells and T lymphocytes during atherogenesis: focus on antigen presentation and break of tolerance. Front. Cardiovasc. Med. 9, 934314. doi:10.3389/fcvm.2022.934314
Bennett, B. J., de Aguiar, V. T., Wang, Z., Shih, D. M., Meng, Y., Gregory, J., et al. (2013). Trimethylamine-N-oxide, a metabolite associated with atherosclerosis, exhibits complex genetic and dietary regulation. Cell. Metab. 17 (1), 49–60. doi:10.1016/j.cmet.2012.12.011
Binder, C. J., Hartvigsen, K., Chang, M. K., Miller, M., Broide, D., Palinski, W., et al. (2004). IL-5 links adaptive and natural immunity specific for epitopes of oxidized LDL and protects from atherosclerosis. J. Clin. Investig. 114 (3), 427–437. doi:10.1172/JCI20479
Biros, E., Reznik, J. E., and Moran, C. S. (2022). Role of inflammatory cytokines in genesis and treatment of atherosclerosis. Trends. cardiovasc. Med. 32 (3), 138–142. doi:10.1016/j.tcm.2021.02.001
Boccardi, V., Paolacci, L., Croce, M. F., Baroni, M., Ercolani, S., Cecchetti, R., et al. (2020). Lower serum levels of IL-13 is associated with increased carotid intima-media thickness in old age subjects. Aging Clin. Exp. Res. 32 (7), 1289–1294. doi:10.1007/s40520-019-01313-4
Borges, N. A., Stenvinkel, P., Bergman, P., Qureshi, A. R., Lindholm, B., Moraes, C., et al. (2019). Effects of probiotic supplementation on trimethylamine-N-oxide plasma levels in hemodialysis patients: a Pilot study. Probiotics Antimicrob. Proteins 11 (2), 648–654. doi:10.1007/s12602-018-9411-1
Bove, K. E., Heubi, J. E., Balistreri, W. F., and Setchell, K. D. (2004). Bile acid synthetic defects and liver disease: a comprehensive review. Pediatr. Dev. Pathol. 7 (4), 315–334. doi:10.1007/s10024-002-1201-8
Boyle, J. J., Harrington, H. A., Piper, E., Elderfield, K., Stark, J., Landis, R. C., et al. (2009). Coronary intraplaque hemorrhage evokes a novel atheroprotective macrophage phenotype. Am. J. Pathol. 174 (3), 1097–1108. doi:10.2353/ajpath.2009.080431
Brandsma, E., Kloosterhuis, N. J., Koster, M., Dekker, D. C., Gijbels, M., van der Velden, S., et al. (2019). A proinflammatory gut microbiota increases systemic inflammation and accelerates atherosclerosis. Circ. Res. 124 (1), 94–100. doi:10.1161/CIRCRESAHA.118.313234
Brauner, S., Jiang, X., Thorlacius, G. E., Lundberg, A. M., Ostberg, T., Yan, Z. Q., et al. (2018). Augmented Th17 differentiation in Trim21 deficiency promotes a stable phenotype of atherosclerotic plaques with high collagen content. Cardiovasc. Res. 114 (1), 158–167. doi:10.1093/cvr/cvx181
Brown, J. M., and Hazen, S. L. (2018). Microbial modulation of cardiovascular disease. Nat. Rev. Microbiol. 16 (3), 171–181. doi:10.1038/nrmicro.2017.149
Brown, J. M., and Hazen, S. L. (2015). The gut microbial endocrine organ: bacterially derived signals driving cardiometabolic diseases. Annu. Rev. Med. 66, 343–359. doi:10.1146/annurev-med-060513-093205
Bultman, S. J. (2018). Bacterial butyrate prevents atherosclerosis. Nat. Microbiol. 3 (12), 1332–1333. doi:10.1038/s41564-018-0299-z
Buono, C., Binder, C. J., Stavrakis, G., Witztum, J. L., Glimcher, L. H., and Lichtman, A. H. (2005). T-bet deficiency reduces atherosclerosis and alters plaque antigen-specific immune responses. Proc. Natl. Acad. Sci. U. S. A. 102 (5), 1596–1601. doi:10.1073/pnas.0409015102
Butcher, M. J., Wu, C. I., Waseem, T., and Galkina, E. V. (2016). CXCR6 regulates the recruitment of pro-inflammatory IL-17A-producing T cells into atherosclerotic aortas. Int. Immunol. 28 (5), 255–261. doi:10.1093/intimm/dxv068
Calandrini, C. A., Ribeiro, A. C., Gonnelli, A. C., Ota-Tsuzuki, C., Rangel, L. P., Saba-Chujfi, E., et al. (2014). Microbial composition of atherosclerotic plaques. Oral Dis. 20 (3), e128–e134. doi:10.1111/odi.12205
Canyelles, M., Tondo, M., Cedo, L., Farras, M., Escola-Gil, J. C., and Blanco-Vaca, F. (2018). Trimethylamine N-oxide: a link among diet, gut microbiota, gene regulation of liver and intestine cholesterol homeostasis and HDL function. Int. J. Mol. Sci. 19 (10), 3228. doi:10.3390/ijms19103228
Cao, H., Zhu, Y., Hu, G., Zhang, Q., and Zheng, L. (2023). Gut microbiome and metabolites, the future direction of diagnosis and treatment of atherosclerosis?. Pharmacol. Res. 187, 106586. doi:10.1016/j.phrs.2022.106586
Cardilo-Reis, L., Gruber, S., Schreier, S. M., Drechsler, M., Papac-Milicevic, N., Weber, C., et al. (2012). Interleukin-13 protects from atherosclerosis and modulates plaque composition by skewing the macrophage phenotype. EMBO Mol. Med. 4 (10), 1072–1086. doi:10.1002/emmm.201201374
Chan, Y. K., El-Nezami, H., Chen, Y., Kinnunen, K., and Kirjavainen, P. V. (2016). Probiotic mixture VSL#3 reduce high fat diet induced vascular inflammation and atherosclerosis in ApoE(-/-) mice. Amb. Express 6 (1), 61. doi:10.1186/s13568-016-0229-5
Charach, G., Argov, O., Geiger, K., Charach, L., Rogowski, O., and Grosskopf, I. (2018). Diminished bile acids excretion is a risk factor for coronary artery disease: 20-year follow up and long-term outcome. Ther. Adv. Gastroenterol. 11, 1756283X17743420. doi:10.1177/1756283X17743420
Chavez-Talavera, O., Tailleux, A., Lefebvre, P., and Staels, B. (2017). Bile acid control of metabolism and inflammation in obesity, type 2 diabetes, dyslipidemia, and nonalcoholic fatty liver disease. Gastroenterology 152 (7), 1679–1694. doi:10.1053/j.gastro.2017.01.055
Chen, C. Y., Leu, H. B., Wang, S. C., Tsai, S. H., Chou, R. H., Lu, Y. W., et al. (2023). Inhibition of trimethylamine N-oxide attenuates neointimal formation through reduction of inflammasome and oxidative stress in a mouse model of carotid artery ligation. Antioxid. Redox Signal. 38 (1-3), 215–233. doi:10.1089/ars.2021.0115
Chen, C., Zhou, F., Hu, Z., Zeng, C., Hu, Z., et al. (2016). Zonulin regulates intestinal permeability and facilitates enteric bacteria permeation in coronary artery disease. Sci. Rep. 6, 29142. doi:10.1038/srep29142
Chen, K., Zhao, Z., Wang, G., Zou, J., Yu, X., Zhang, D., et al. (2020). Interleukin-5 promotes ATP-binding cassette transporter A1 expression through miR-211/JAK2/STAT3 pathways in THP-1-dervied macrophages. Acta. Biochim. Biophys. Sin. (Shanghai). 52 (8), 832–841. doi:10.1093/abbs/gmaa071
Chen, M. L., Yi, L., Zhang, Y., Zhou, X., Ran, L., Yang, J., et al. (2016). Resveratrol attenuates trimethylamine-N-oxide (TMAO)-Induced atherosclerosis by regulating TMAO synthesis and bile acid metabolism via remodeling of the gut microbiota. mBio 7 (2), e02210–e02215. doi:10.1128/mBio.02210-15
Chen, M. L., Zhu, X. H., Ran, L., Lang, H. D., Yi, L., and Mi, M. T. (2017). Trimethylamine-N-Oxide induces vascular inflammation by activating the NLRP3 inflammasome through the SIRT3-SOD2-mtROS signaling pathway. J. Am. Heart Assoc. 6 (9), e006347. doi:10.1161/JAHA.117.006347
Chen, X., Yin, O. Q., Zuo, Z., and Chow, M. S. (2005). Pharmacokinetics and modeling of quercetin and metabolites. Pharm. Res. 22 (6), 892–901. doi:10.1007/s11095-005-4584-1
Chen, Y., Zhou, J., and Wang, L. (2021). Role and mechanism of gut microbiota in human disease. Front. Cell. Infect. Microbiol. 11, 625913. doi:10.3389/fcimb.2021.625913
Cheng, X., Zhao, C., Jin, Z., Hu, J., Zhang, Z., and Zhang, C. (2022). Natural products: potential therapeutic agents for atherosclerosis. Chin. J. Nat. Med. 20 (11), 830–845. doi:10.1016/S1875-5364(22)60219-X
Chiang, J., and Ferrell, J. M. (2019). Bile acids as metabolic regulators and nutrient sensors. Annu. Rev. Nutr. 39, 175–200. doi:10.1146/annurev-nutr-082018-124344
Chistiakov, D. A., Kashirskikh, D. A., Khotina, V. A., Grechko, A. V., and Orekhov, A. N. (2019). Immune-inflammatory responses in atherosclerosis: the role of myeloid cells. J. Clin. Med. 8 (11), 1798. doi:10.3390/jcm8111798
Choroszy, M., Litwinowicz, K., Bednarz, R., Roleder, T., Lerman, A., Toya, T., et al. (2022). Human gut microbiota in coronary artery disease: A systematic review and meta-analysis. Metabolites 12 (12), 1165. doi:10.3390/metabo12121165
Clemente, J. C., Ursell, L. K., Parfrey, L. W., and Knight, R. (2012). The impact of the gut microbiota on human health: an integrative view. Cell. 148 (6), 1258–1270. doi:10.1016/j.cell.2012.01.035
Cochain, C., Koch, M., Chaudhari, S. M., Busch, M., Pelisek, J., Boon, L., et al. (2015). CD8+ T cells regulate monopoiesis and circulating Ly6C-high monocyte levels in atherosclerosis in mice. Circ. Res. 117 (3), 244–253. doi:10.1161/CIRCRESAHA.117.304611
Cochain, C., Vafadarnejad, E., Arampatzi, P., Pelisek, J., Winkels, H., Ley, K., et al. (2018). Single-cell RNA-seq reveals the transcriptional landscape and heterogeneity of aortic macrophages in murine atherosclerosis. Circ. Res. 122 (12), 1661–1674. doi:10.1161/CIRCRESAHA.117.312509
Coyne, M. J., and Comstock, L. E. (2019). Type VI secretion systems and the gut microbiota. Microbiol. Spectr. 7 (2), 7. doi:10.1128/microbiolspec.PSIB-0009-2018
Cros, J., Cagnard, N., Woollard, K., Patey, N., Zhang, S. Y., Senechal, B., et al. (2010). Human CD14dim monocytes patrol and sense nucleic acids and viruses via TLR7 and TLR8 receptors. Immunity 33 (3), 375–386. doi:10.1016/j.immuni.2010.08.012
Dai, Z., Li, S., Meng, Y., Zhao, Q., Zhang, Y., Suonan, Z., et al. (2022). Capsaicin ameliorates high-fat diet-induced atherosclerosis in ApoE(-/-) mice via remodeling gut microbiota. Nutrients 14 (20), 4334. doi:10.3390/nu14204334
Danzaki, K., Matsui, Y., Ikesue, M., Ohta, D., Ito, K., Kanayama, M., et al. (2012). Interleukin-17A deficiency accelerates unstable atherosclerotic plaque formation in apolipoprotein E-deficient mice. Arterioscler. Thromb. Vasc. Biol. 32 (2), 273–280. doi:10.1161/ATVBAHA.111.229997
Davenport, P., and Tipping, P. G. (2003). The role of interleukin-4 and interleukin-12 in the progression of atherosclerosis in apolipoprotein E-deficient mice. Am. J. Pathol. 163 (3), 1117–1125. doi:10.1016/S0002-9440(10)63471-2
De Paoli, F., Staels, B., and Chinetti-Gbaguidi, G. (2014). Macrophage phenotypes and their modulation in atherosclerosis. Circ. J. 78 (8), 1775–1781. doi:10.1253/circj.cj-14-0621
Denardo, D. G., and Ruffell, B. (2019). Macrophages as regulators of tumour immunity and immunotherapy. Nat. Rev. Immunol. 19 (6), 369–382. doi:10.1038/s41577-019-0127-6
Depuydt, M., Prange, K., Slenders, L., Ord, T., Elbersen, D., Boltjes, A., et al. (2020). Microanatomy of the human atherosclerotic plaque by single-cell transcriptomics. Circ. Res. 127 (11), 1437–1455. doi:10.1161/CIRCRESAHA.120.316770
Derrien, M., and van Hylckama, V. J. (2015). Fate, activity, and impact of ingested bacteria within the human gut microbiota. Trends Microbiol. 23 (6), 354–366. doi:10.1016/j.tim.2015.03.002
Ding, L., Chang, M., Guo, Y., Zhang, L., Xue, C., Yanagita, T., et al. (2018). Trimethylamine-N-oxide (TMAO)-induced atherosclerosis is associated with bile acid metabolism. Lipids Health Dis. 17 (1), 286. doi:10.1186/s12944-018-0939-6
Doran, A. C. (2022). Inflammation resolution: implications for atherosclerosis. Circ. Res. 130 (1), 130–148. doi:10.1161/CIRCRESAHA.121.319822
Douna, H., Amersfoort, J., Schaftenaar, F. H., Kroner, M. J., Kiss, M. G., Slutter, B., et al. (2020). B- and T-lymphocyte attenuator stimulation protects against atherosclerosis by regulating follicular B cells. Cardiovasc. Res. 116 (2), 295–305. doi:10.1093/cvr/cvz129
Du, Y., Li, X., Su, C., Xi, M., Zhang, X., Jiang, Z., et al. (2020). Butyrate protects against high-fat diet-induced atherosclerosis via up-regulating ABCA1 expression in apolipoprotein E-deficiency mice. Br. J. Pharmacol. 177 (8), 1754–1772. doi:10.1111/bph.14933
Duan, H., Zhang, Q., Liu, J., Li, R., Wang, D., Peng, W., et al. (2021). Suppression of apoptosis in vascular endothelial cell, the promising way for natural medicines to treat atherosclerosis. Pharmacol. Res. 168, 105599. doi:10.1016/j.phrs.2021.105599
Dupasquier, C. M., Dibrov, E., Kneesh, A. L., Cheung, P. K., Lee, K. G., Alexander, H. K., et al. (2007). Dietary flaxseed inhibits atherosclerosis in the LDL receptor-deficient mouse in part through antiproliferative and anti-inflammatory actions. Am. J. Physiol. Heart Circ. Physiol. 293 (4), H2394–H2402. doi:10.1152/ajpheart.01104.2006
Eid, R. E., Rao, D. A., Zhou, J., Lo, S. F., Ranjbaran, H., Gallo, A., et al. (2009). Interleukin-17 and interferon-gamma are produced concomitantly by human coronary artery-infiltrating T cells and act synergistically on vascular smooth muscle cells. Circulation 119 (10), 1424–1432. doi:10.1161/CIRCULATIONAHA.108.827618
El, H. R., Al-Arawe, N., and Hinterseher, I. (2023). The role of the gut microbiome and trimethylamine oxide in atherosclerosis and age-related disease. Int. J. Mol. Sci. 24 (3), 2399. doi:10.3390/ijms24032399
Epstein, S. E., Zhu, J., Burnett, M. S., Zhou, Y. F., Vercellotti, G., and Hajjar, D. (2000). Infection and atherosclerosis: potential roles of pathogen burden and molecular mimicry. Arterioscler. Thromb. Vasc. Biol. 20 (6), 1417–1420. doi:10.1161/01.atv.20.6.1417
Eshghjoo, S., Kim, D. M., Jayaraman, A., Sun, Y., and Alaniz, R. C. (2022). Macrophage polarization in atherosclerosis. Genes. (Basel) 13 (5), 756. doi:10.3390/genes13050756
Fak, F., and Backhed, F. (2012). Lactobacillus reuteri prevents diet-induced obesity, but not atherosclerosis, in a strain dependent fashion in Apoe-/- mice. PLoS One 7 (10), e46837. doi:10.1371/journal.pone.0046837
Farahi, L., Sinha, S. K., and Lusis, A. J. (2021). Roles of macrophages in atherogenesis. Front. Pharmacol. 12, 785220. doi:10.3389/fphar.2021.785220
Gao, M., Heng, X., Jin, J., and Chu, W. (2022). Gypenoside XLIX ameliorate high-fat diet-induced atherosclerosis via regulating intestinal microbiota, alleviating inflammatory response and restraining oxidative stress in ApoE(-/-) mice. Pharm. (Basel) 15 (9), 1056. doi:10.3390/ph15091056
Gao, X., Lin, J., Zheng, Y., Liu, S., Liu, C., Liu, T., et al. (2020). Type 2 innate lymphoid cells regulation by regulatory T cells attenuates atherosclerosis. J. Mol. Cell. Cardiol. 145, 99–111. doi:10.1016/j.yjmcc.2020.05.017
Gardner, A., de Mingo, P. A., and Ruffell, B. (2020). Dendritic cells and their role in immunotherapy. Front. Immunol. 11, 924. doi:10.3389/fimmu.2020.00924
Gatarek, P., and Kaluzna-Czaplinska, J. (2021). Trimethylamine N-oxide (TMAO) in human health. EXCLI J. 20, 301–319. doi:10.17179/excli2020-3239
Geng, J., Yang, C., Wang, B., Zhang, X., Hu, T., Gu, Y., et al. (2018). Trimethylamine N-oxide promotes atherosclerosis via CD36-dependent MAPK/JNK pathway. Biomed. Pharmacother. 97, 941–947. doi:10.1016/j.biopha.2017.11.016
Getz, G. S., and Reardon, C. A. (2018). Apoprotein E and reverse cholesterol transport. Int. J. Mol. Sci. 19 (11), 3479. doi:10.3390/ijms19113479
Ghosh, S. S., Bie, J., Wang, J., and Ghosh, S. (2014). Oral supplementation with non-absorbable antibiotics or curcumin attenuates western diet-induced atherosclerosis and glucose intolerance in LDLR-/- mice--role of intestinal permeability and macrophage activation. PLoS One 9 (9), e108577. doi:10.1371/journal.pone.0108577
Goel, J. C., Gaur, A., Singhal, V., Parakh, N., Bhargava, B., and Sharma, A. (2016). The complex metabolism of trimethylamine in humans: endogenous and exogenous sources-CORRIGENDUM. Expert Rev. Mol. Med. 18, e19. doi:10.1017/erm.2016.19
Gorabi, A. M., Kiaie, N., Khosrojerdi, A., Jamialahmadi, T., Al-Rasadi, K., Johnston, T. P., et al. (2022). Implications for the role of lipopolysaccharide in the development of atherosclerosis. Trends cardiovasc. Med. 32 (8), 525–533. doi:10.1016/j.tcm.2021.08.015
Gordon, S., and Martinez, F. O. (2010). Alternative activation of macrophages: mechanism and functions. Immunity 32 (5), 593–604. doi:10.1016/j.immuni.2010.05.007
Graham, D. B., and Xavier, R. J. (2020). Pathway paradigms revealed from the genetics of inflammatory bowel disease. Nature 578 (7796), 527–539. doi:10.1038/s41586-020-2025-2
Grivel, J. C., Ivanova, O., Pinegina, N., Blank, P. S., Shpektor, A., Margolis, L. B., et al. (2011). Activation of T lymphocytes in atherosclerotic plaques. Arterioscler. Thromb. Vas.c Biol. 31 (12), 2929–2937. doi:10.1161/ATVBAHA.111.237081
Gronberg, C., Nilsson, J., and Wigren, M. (2017). Recent advances on CD4(+) T cells in atherosclerosis and its implications for therapy. Eur. J. Pharmacol. 816, 58–66. doi:10.1016/j.ejphar.2017.04.029
Guo, N., Chen, Y., Su, B., Yang, X., Zhang, Q., Song, T., et al. (2020). Alterations of CCR2 and CX3CR1 on three monocyte subsets during HIV-1/Treponema pallidum coinfection. Front. Med. (Lausanne). 7, 272. doi:10.3389/fmed.2020.00272
Guo, Y., Yu, Y., Li, H., Ding, X., Li, X., Jing, X., et al. (2021). Inulin supplementation ameliorates hyperuricemia and modulates gut microbiota in Uox-knockout mice. Eur. J. Nutr. 60 (4), 2217–2230. doi:10.1007/s00394-020-02414-x
Hageman, J., Herrema, H., Groen, A. K., and Kuipers, F. (2010). A role of the bile salt receptor FXR in atherosclerosis. Arterioscler. Thromb. Vasc. Biol. 30 (8), 1519–1528. doi:10.1161/ATVBAHA.109.197897:
Haghikia, A., Zimmermann, F., Schumann, P., Jasina, A., Roessler, J., Schmidt, D., et al. (2022). Propionate attenuates atherosclerosis by immune-dependent regulation of intestinal cholesterol metabolism. Eur. Heart J. 43 (6), 518–533. doi:10.1093/eurheartj/ehab644
Haka, A. S., Singh, R. K., Grosheva, I., Hoffner, H., Capetillo-Zarate, E., Chin, H. F., et al. (2015). Monocyte-derived dendritic cells upregulate extracellular catabolism of aggregated low-density lipoprotein on maturation, leading to foam cell formation. Arterioscler. Thromb. Vasc. Biol. 35 (10), 2092–2103. doi:10.1161/ATVBAHA.115.305843
Hanniman, E. A., Lambert, G., Mccarthy, T. C., and Sinal, C. J. (2005). Loss of functional farnesoid X receptor increases atherosclerotic lesions in apolipoprotein E-deficient mice. J. Lipid Res. 46 (12), 2595–2604. doi:10.1194/jlr.M500390-JLR200
Hansson, G. K., and Hermansson, A. (2011). The immune system in atherosclerosis. Nat. Immunol. 12 (3), 204–212. doi:10.1038/ni.2001
Hashizume-Takizawa, T., Yamaguchi, Y., Kobayashi, R., Shinozaki-Kuwahara, N., Saito, M., and Kurita-Ochiai, T. (2019). Oral challenge with Streptococcus sanguinis induces aortic inflammation and accelerates atherosclerosis in spontaneously hyperlipidemic mice. Biochem. Biophys. Res. Commun. 520 (3), 507–513. doi:10.1016/j.bbrc.2019.10.057
He, X., Liang, B., and Gu, N. (2020). Th17/Treg imbalance and atherosclerosis. Dis. Markers 2020, 8821029. doi:10.1155/2020/8821029
Heianza, Y., Ma, W., Didonato, J. A., Sun, Q., Rimm, E. B., Hu, F. B., et al. (2020). Long-term changes in gut microbial metabolite trimethylamine N-oxide and coronary heart disease risk. J. Am. Coll. Cardiol. 75 (7), 763–772. doi:10.1016/j.jacc.2019.11.060
Herrero-Fernandez, B., Gomez-Bris, R., Somovilla-Crespo, B., and Gonzalez-Granado, J. M. (2019). Immunobiology of atherosclerosis: a complex net of interactions. Int. J. Mol. Sci. 20 (21), 5293. doi:10.3390/ijms20215293
Hilgendorf, I., Theurl, I., Gerhardt, L. M., Robbins, C. S., Weber, G. F., Gonen, A., et al. (2014). Innate response activator B cells aggravate atherosclerosis by stimulating T helper-1 adaptive immunity. Circulation 129 (16), 1677–1687. doi:10.1161/CIRCULATIONAHA.113.006381
Hill, C., Guarner, F., Reid, G., Gibson, G. R., Merenstein, D. J., Pot, B., et al. (2014). Expert consensus document. The International Scientific Association for Probiotics and Prebiotics consensus statement on the scope and appropriate use of the term probiotic. Nat. Rev. Gastroenterol. Hepatol. 11 (8), 506–514. doi:10.1038/nrgastro.2014.66
Hooglugt, A., Klatt, O., and Huveneers, S. (2022). Vascular stiffening and endothelial dysfunction in atherosclerosis. Curr. Opin. Lipidol. 33 (6), 353–363. doi:10.1097/MOL.0000000000000852
Hsueh, T. P., Sheen, J. M., Pang, J. H., Bi, K. W., Huang, C. C., Wu, H. T., et al. (2016). The anti-atherosclerotic effect of naringin is associated with reduced expressions of cell adhesion molecules and chemokines through NF-κB pathway. Molecules 21 (2), 195. doi:10.3390/molecules21020195
Hu, H. J., Wang, X. H., Zhang, T. Q., Liu, Y., Chen, Z. R., Zhang, Z. Z., et al. (2022). PLK1 promotes cholesterol efflux and alleviates atherosclerosis by up-regulating ABCA1 and ABCG1 expression via the AMPK/PPARγ/LXRα pathway. Biochim. Biophys. Acta. Mol. Cell. Biol. Lipids 1867 (12), 159221. doi:10.1016/j.bbalip.2022.159221
Hu, H., Shao, W., Liu, Q., Liu, N., Wang, Q., Xu, J., et al. (2022). Gut microbiota promotes cholesterol gallstone formation by modulating bile acid composition and biliary cholesterol secretion. Nat. Commun. 13 (1), 252. doi:10.1038/s41467-021-27758-8
Huang, D., Jia, Z., Chang, L., Zhou, H., Wu, X., and Wei, C. (2020). The role of collateral disease theory in the prevention and treatment of atherosclerosis in post-menopausal women: a narrative review. Ann. Palliat. Med. 9 (4), 2314–2322. doi:10.21037/apm-20-1257
Jakubzick, C. V., Randolph, G. J., and Henson, P. M. (2017). Monocyte differentiation and antigen-presenting functions. Nat. Rev. Immunol. 17 (6), 349–362. doi:10.1038/nri.2017.28
Jandhyala, S. M., Talukdar, R., Subramanyam, C., Vuyyuru, H., Sasikala, M., and Nageshwar, R. D. (2015). Role of the normal gut microbiota. World J. Gastroenterol. 21 (29), 8787–8803. doi:10.3748/wjg.v21.i29.8787
Jaworska, K., Hering, D., Mosieniak, G., Bielak-Zmijewska, A., Pilz, M., Konwerski, M., et al. (2019). TMA, A forgotten uremic toxin, but not TMAO, is involved in cardiovascular pathology. Toxins (Basel) 11 (9), 490. doi:10.3390/toxins11090490
Ji, W., Jiang, T., Sun, Z., Teng, F., Ma, C., Huang, S., et al. (2020). The enhanced pharmacological effects of modified traditional Chinese medicine in attenuation of atherosclerosis is driven by modulation of gut microbiota. Front. Pharmacol. 11, 546589. doi:10.3389/fphar.2020.546589
Jia, B., Zou, Y., Han, X., Bae, J. W., and Jeon, C. O. (2023). Gut microbiome-mediated mechanisms for reducing cholesterol levels: implications for ameliorating cardiovascular disease. Trends Microbiol. 31 (1), 76–91. doi:10.1016/j.tim.2022.08.003
Jie, Z., Xia, H., Zhong, S. L., Feng, Q., Li, S., Liang, S., et al. (2017). The gut microbiome in atherosclerotic cardiovascular disease. Nat. Commun. 8 (1), 845. doi:10.1038/s41467-017-00900-1
Jonsson, A. L., and Backhed, F. (2017). Role of gut microbiota in atherosclerosis. Nat. Rev. Cardiol. 14 (2), 79–87. doi:10.1038/nrcardio.2016.183
Kameyama, N., Maruyama, C., Shijo, Y., Umezawa, A., Sato, A., Ayaori, M., et al. (2020). Comparison of food and nutrient intakes between Japanese dyslipidemic patients with and without low-density lipoprotein cholesterol lowering drug therapy: a cross-sectional study. J. Atheroscler. Thromb. 27 (7), 683–694. doi:10.5551/jat.52316
Kang, H., Li, X., Xiong, K., Song, Z., Tian, J., Wen, Y., et al. (2021). The entry and egress of monocytes in atherosclerosis: a biochemical and biomechanical driven process. Cardiovasc. Ther. 2021, 6642927. doi:10.1155/2021/6642927
Katiraei, S., de Vries, M. R., Costain, A. H., Thiem, K., Hoving, L. R., van Diepen, J. A., et al. (2020). Akkermansia muciniphila exerts lipid-lowering and immunomodulatory effects without affecting neointima formation in hyperlipidemic APOE*3-Leiden.CETP mice. Mol. Nutr. Food Res. 64 (15), e1900732. doi:10.1002/mnfr.201900732
Kawamata, Y., Fujii, R., Hosoya, M., Harada, M., Yoshida, H., Miwa, M., et al. (2003). A G protein-coupled receptor responsive to bile acids. J. Biol. Chem. 278 (11), 9435–9440. doi:10.1074/jbc.M209706200
Ke, Y., Li, D., Zhao, M., Liu, C., Liu, J., Zeng, A., et al. (2018). Gut flora-dependent metabolite Trimethylamine-N-oxide accelerates endothelial cell senescence and vascular aging through oxidative stress. Free Radic. Biol. Med. 116, 88–100. doi:10.1016/j.freeradbiomed.2018.01.007
Khare, P., Maurya, R., Bhatia, R., Mangal, P., Singh, J., Podili, K., et al. (2020). Polyphenol rich extracts of finger millet and kodo millet ameliorate high fat diet-induced metabolic alterations. Food Funct. 11 (11), 9833–9847. doi:10.1039/d0fo01643h
Khongrum, J., Yingthongchai, P., Boonyapranai, K., Wongtanasarasin, W., Aobchecy, P., Tateing, S., et al. (2023). Safety and effects of Lactobacillus paracasei TISTR 2593 supplementation on improving cholesterol metabolism and atherosclerosis-related parameters in subjects with hypercholesterolemia: a randomized, double-blind, placebo-controlled clinical trial. Nutrients 15 (3), 661. doi:10.3390/nu15030661
Kida, T., Tsubosaka, Y., Hori, M., Ozaki, H., and Murata, T. (2013). Bile acid receptor TGR5 agonism induces NO production and reduces monocyte adhesion in vascular endothelial cells. Arterioscler. Thromb. Vasc. Biol. 33 (7), 1663–1669. doi:10.1161/ATVBAHA.113.301565
Kim, D. H., Park, C. H., Park, D., Choi, Y. J., Park, M. H., Chung, K. W., et al. (2014). Ginsenoside Rc modulates Akt/FoxO1 pathways and suppresses oxidative stress. Arch. Pharm. Res. 37 (6), 813–820. doi:10.1007/s12272-013-0223-2
Kim, E. S., Yoon, B. H., Lee, S. M., Choi, M., Kim, E. H., Lee, B. W., et al. (2022). Fecal microbiota transplantation ameliorates atherosclerosis in mice with C1q/TNF-related protein 9 genetic deficiency. Exp. Mol. Med. 54 (2), 103–114. doi:10.1038/s12276-022-00728-w
Kim, K., Shim, D., Lee, J. S., Zaitsev, K., Williams, J. W., Kim, K. W., et al. (2018). Transcriptome analysis reveals nonfoamy rather than foamy plaque macrophages are proinflammatory in atherosclerotic murine models. Circ. Res. 123 (10), 1127–1142. doi:10.1161/CIRCRESAHA.118.312804
Kim, K. W., Ivanov, S., and Williams, J. W. (2020). Monocyte recruitment, specification, and function in atherosclerosis. Cells 10 (1), 15. doi:10.3390/cells10010015
King, V. L., Cassis, L. A., and Daugherty, A. (2007). Interleukin-4 does not influence development of hypercholesterolemia or angiotensin II-induced atherosclerotic lesions in mice. Am. J. Pathol. 171 (6), 2040–2047. doi:10.2353/ajpath.2007.060857
Kloc, M., Kubiak, J. Z., and Ghobrial, R. M. (2022). Macrophage-dendritic-smooth muscle-endothelium-and stem cells-derived foam cells in atherosclerosis. Int. J. Mol. Sci. 23 (22), 14154. doi:10.3390/ijms232214154
Koeth, R. A., Levison, B. S., Culley, M. K., Buffa, J. A., Wang, Z., Gregory, J. C., et al. (2014). γ-Butyrobetaine is a proatherogenic intermediate in gut microbial metabolism of L-carnitine to TMAO. Cell. Metab. 20 (5), 799–812. doi:10.1016/j.cmet.2014.10.006
Koeth, R. A., Wang, Z., Levison, B. S., Buffa, J. A., Org, E., Sheehy, B. T., et al. (2013). Intestinal microbiota metabolism of L-carnitine, a nutrient in red meat, promotes atherosclerosis. Nat. Med. 19 (5), 576–585. doi:10.1038/nm.3145
Kojima, Y., Weissman, I. L., and Leeper, N. J. (2017). The role of efferocytosis in atherosclerosis. Circulation 135 (5), 476–489. doi:10.1161/CIRCULATIONAHA.116.025684
Kolbus, D., Ljungcrantz, I., Soderberg, I., Alm, R., Bjorkbacka, H., Nilsson, J., et al. (2012). TAP1-deficiency does not alter atherosclerosis development in Apoe-/- mice. PLoS One 7 (3), e33932. doi:10.1371/journal.pone.0033932
Koren, O., Spor, A., Felin, J., Fak, F., Stombaugh, J., Tremaroli, V., et al. (2011). Human oral, gut, and plaque microbiota in patients with atherosclerosis. Proc. Natl. Acad. Sci. U. S. A. 108(1), 4592–4598. doi:10.1073/pnas.1011383107
Koushki, M., Amiri-Dashatan, N., Ahmadi, N., Abbaszadeh, H. A., and Rezaei-Tavirani, M. (2018). Resveratrol: a miraculous natural compound for diseases treatment. Food Sci. Nutr. 6 (8), 2473–2490. doi:10.1002/fsn3.855
Koutsaliaris, I. K., Moschonas, I. C., Pechlivani, L. M., Tsouka, A. N., and Tselepis, A. D. (2022). Inflammation, oxidative stress, vascular aging and atherosclerotic ischemic stroke. Curr. Med. Chem. 29 (34), 5496–5509. doi:10.2174/0929867328666210921161711
Kovanen, P. T., and Bot, I. (2017). Mast cells in atherosclerotic cardiovascular disease - activators and actions. Eur. J. Pharmacol. 816, 37–46. doi:10.1016/j.ejphar.2017.10.013
Kritikou, E., van der Heijden, T., Swart, M., van Duijn, J., Slutter, B., Wezel, A., et al. (2019). Hypercholesterolemia induces a mast cell-CD4(+) T cell interaction in atherosclerosis. J. Immunol. 202 (5), 1531–1539. doi:10.4049/jimmunol.1800648
Kuznetsova, T., Prange, K., Glass, C. K., and de Winther, M. (2020). Transcriptional and epigenetic regulation of macrophages in atherosclerosis. Nat. Rev. Cardiol. 17 (4), 216–228. doi:10.1038/s41569-019-0265-3
Kyaw, T., Tay, C., Krishnamurthi, S., Kanellakis, P., Agrotis, A., Tipping, P., et al. (2011). B1a B lymphocytes are atheroprotective by secreting natural IgM that increases IgM deposits and reduces necrotic cores in atherosclerotic lesions. Circ. Res. 109 (8), 830–840. doi:10.1161/CIRCRESAHA.111.248542
Lagraauw, H. M., Wezel, A., van der Velden, D., Kuiper, J., and Bot, I. (2019). Stress-induced mast cell activation contributes to atherosclerotic plaque destabilization. Sci. Rep. 9 (1), 2134. doi:10.1038/s41598-019-38679-4
Lanter, B. B., Sauer, K., and Davies, D. G. (2014). Bacteria present in carotid arterial plaques are found as biofilm deposits which may contribute to enhanced risk of plaque rupture. mBio 5 (3), e01206–e01214. doi:10.1128/mBio.01206-14
Lee, M. S., Hwang, J. T., Kim, S. H., Yoon, S., Kim, M. S., Yang, H. J., et al. (2010). Ginsenoside Rc, an active component of Panax ginseng, stimulates glucose uptake in C2C12 myotubes through an AMPK-dependent mechanism. J. Ethnopharmacol. 127 (3), 771–776. doi:10.1016/j.jep.2009.11.022
Leskinen, M. J., Kovanen, P. T., and Lindstedt, K. A. (2003). Regulation of smooth muscle cell growth, function and death in vitro by activated mast cells--a potential mechanism for the weakening and rupture of atherosclerotic plaques. Biochem. Pharmacol. 66 (8), 1493–1498. doi:10.1016/s0006-2952(03)00503-3
Li, J., and Dawson, P. A. (2019). Animal models to study bile acid metabolism. Biochim. Biophys. Acta Mol. Basis Dis. 1865 (5), 895–911. doi:10.1016/j.bbadis.2018.05.011
Li, J., Jia, H., Cai, X., Zhong, H., Feng, Q., Sunagawa, S., et al. (2014). An integrated catalog of reference genes in the human gut microbiome. Nat. Biotechnol. 32 (8), 834–841. doi:10.1038/nbt.2942
Li, J., Sheng, Z., Tan, Y., Zhou, P., Liu, C., Zhao, H., et al. (2021). Association of plasma trimethylamine N-Oxide level with healed culprit plaques examined by optical coherence tomography in patients with ST-Segment elevation myocardial infarction. Nutr. Metab. Cardiovasc. Dis. 31 (1), 145–152. doi:10.1016/j.numecd.2020.06.016
Li, L., Li, R., Zacharek, A., Wang, F., Landschoot-Ward, J., Chopp, M., et al. (2020). ABCA1/ApoE/HDL signaling pathway facilitates myelination and oligodendrogenesis after stroke. Int. J. Mol. Sci. 21 (12), 4369. doi:10.3390/ijms21124369
Li, M., van Esch, B., Henricks, P., Folkerts, G., and Garssen, J. (2018). The anti-inflammatory effects of short chain fatty acids on lipopolysaccharide- or tumor necrosis factor alpha-stimulated endothelial cells via activation of GPR41/43 and inhibition of HDACs. Front. Pharmacol. 9, 533. doi:10.3389/fphar.2018.00533
Li, M., van Esch, B., Henricks, P., Garssen, J., and Folkerts, G. (2018). Time and concentration dependent effects of short chain fatty acids on lipopolysaccharide- or tumor necrosis factor alpha-induced endothelial activation. Front. Pharmacol. 9, 233. doi:10.3389/fphar.2018.00233
Li, Q., Wei, S., Li, Y., Wu, F., Qin, X., Li, Z., et al. (2023). Blocking of programmed cell death-ligand 1 (PD-L1) expressed on endothelial cells promoted the recruitment of CD8+IFN-γ+ T cells in atherosclerosis. Inflamm. Res. 72 (4), 783–796. doi:10.1007/s00011-023-01703-5
Li, Q., Wu, T., Liu, R., Zhang, M., and Wang, R. (2017). Soluble dietary fiber reduces trimethylamine metabolism via gut microbiota and Co-regulates host AMPK pathways. Mol. Nutr. Food Res. 61 (12), 1700473. doi:10.1002/mnfr.201700473
Li, X. S., Obeid, S., Klingenberg, R., Gencer, B., Mach, F., Raber, L., et al. (2017). Gut microbiota-dependent trimethylamine N-oxide in acute coronary syndromes: a prognostic marker for incident cardiovascular events beyond traditional risk factors. Eur. Heart J. 38 (11), 814–824. doi:10.1093/eurheartj/ehw582
Li, X., Su, C., Jiang, Z., Yang, Y., Zhang, Y., Yang, M., et al. (2021). Berberine attenuates choline-induced atherosclerosis by inhibiting trimethylamine and trimethylamine-N-oxide production via manipulating the gut microbiome. NPJ Biofilms Microbiomes 7 (1), 36. doi:10.1038/s41522-021-00205-8
Li, Y. T., Swales, K. E., Thomas, G. J., Warner, T. D., and Bishop-Bailey, D. (2007). Farnesoid x receptor ligands inhibit vascular smooth muscle cell inflammation and migration. Arterioscler. Thromb. Vasc. Biol. 27 (12), 2606–2611. doi:10.1161/ATVBAHA.107.152694
Li, Y., Yu, Z., Liu, Y., Wang, T., Liu, Y., Bai, Z., et al. (2022). Dietary α-linolenic acid-rich flaxseed oil ameliorates high-fat diet-induced atherosclerosis via gut microbiota-inflammation-artery Axis in ApoE-/- mice. Front. Cardiovasc. Med. 9, 830781. doi:10.3389/fcvm.2022.830781
Liang, X., Zhang, Z., Lv, Y., Tong, L., Liu, T., Yi, H., et al. (2020). Reduction of intestinal trimethylamine by probiotics ameliorated lipid metabolic disorders associated with atherosclerosis. Nutrition 79-80, 110941. doi:10.1016/j.nut.2020.110941
Liao, W., Khan, I., Huang, G., Chen, S., Liu, L., Leong, W. K., et al. (2021). Bifidobacterium animalis: the missing link for the cancer-preventive effect of Gynostemma pentaphyllum. Gut Microbes 13 (1)–14. doi:10.1080/19490976.2020.1847629
Libby, P., Buring, J. E., Badimon, L., Hansson, G. K., Deanfield, J., Bittencourt, M. S., et al. (2019). Atheroscler. Nat. Rev. Dis. Prim. 5 (1), 56. doi:10.1038/s41572-019-0106-z
Libby, P. (2021). The changing landscape of atherosclerosis. Nature 592 (7855), 524–533. doi:10.1038/s41586-021-03392-8
Liberale, L., Dallegri, F., Montecucco, F., and Carbone, F. (2017). Pathophysiological relevance of macrophage subsets in atherogenesis. Thromb. Haemost. 117 (1), 7–18. doi:10.1160/TH16-08-0593
Lindskog, J. A., Hallenius, F. F., Akrami, R., Johansson, E., Wester, P., Arnerlov, C., et al. (2017). Bacterial profile in human atherosclerotic plaques. Atherosclerosis 263, 177–183. doi:10.1016/j.atherosclerosis.2017.06.016
Liu, F., Shan, S., Li, H., Shi, J., Hao, R., Yang, R., et al. (2021). Millet shell polyphenols prevent atherosclerosis by protecting the gut barrier and remodeling the gut microbiota in ApoE(-/-) mice. Food Funct. 12 (16), 7298–7309. doi:10.1039/d1fo00991e
Liu, F., Shan, S., Li, H., Shi, J., Yang, R., and Li, Z. (2023). Millet shell polyphenols ameliorate atherosclerosis development by suppressing foam cell formation. J. Nutr. Biochem. 115, 109271. doi:10.1016/j.jnutbio.2023.109271
Liu, F. Y., Wen, J., Hou, J., Zhang, S. Q., Sun, C. B., Zhou, L. C., et al. (2021). Gastrodia remodels intestinal microflora to suppress inflammation in mice with early atherosclerosis. Int. Immunopharmacol. 96, 107758. doi:10.1016/j.intimp.2021.107758
Liu, H., Chen, X., Hu, X., Niu, H., Tian, R., Wang, H., et al. (2019). Alterations in the gut microbiome and metabolism with coronary artery disease severity. Microbiome 7 (1), 68. doi:10.1186/s40168-019-0683-9
Liu, H., Zhu, L., Chen, L., and Li, L. (2022). Therapeutic potential of traditional Chinese medicine in atherosclerosis: a review. Phytother. Res. 36 (11), 4080–4100. doi:10.1002/ptr.7590
Liu, J., Zhang, X., Cheng, Y., and Cao, X. (2021). Dendritic cell migration in inflammation and immunity. Cell. Mol. Immunol. 18 (11), 2461–2471. doi:10.1038/s41423-021-00726-4
Lou, Y., Zheng, J., Hu, H., Lee, J., and Zeng, S. (2015). Application of ultra-performance liquid chromatography coupled with quadrupole time-of-flight mass spectrometry to identify curcumin metabolites produced by human intestinal bacteria. J. Chromatogr. B. Anal. Technol. Biomed. Life Sci. 985, 38–47. doi:10.1016/j.jchromb.2015.01.014
Lu, Y., Cui, X., Zhang, L., Wang, X., Xu, Y., Qin, Z., et al. (2022). The functional role of lipoproteins in atherosclerosis: novel directions for diagnosis and targeting therapy. Aging Dis. 13 (2), 491–520. doi:10.14336/AD.2021.0929
Luo, T., Guo, Z., Liu, D., Guo, Z., Wu, Q., Li, Q., et al. (2022). Deficiency of PSRC1 accelerates atherosclerosis by increasing TMAO production via manipulating gut microbiota and flavin monooxygenase 3. Gut Microbes 14 (1), 2077602. doi:10.1080/19490976.2022.2077602
Ma, G., Pan, B., Chen, Y., Guo, C., Zhao, M., Zheng, L., et al. (2017). Trimethylamine N-oxide in atherogenesis: impairing endothelial self-repair capacity and enhancing monocyte adhesion. Biosci. Rep. 37 (2). doi:10.1042/BSR20160244
Ma, S. R., Tong, Q., Lin, Y., Pan, L. B., Fu, J., Peng, R., et al. (2022). Berberine treats atherosclerosis via a vitamine-like effect down-regulating Choline-TMA-TMAO production pathway in gut microbiota. Signal Transduct. Target. Ther. 7 (1), 207. doi:10.1038/s41392-022-01027-6
Macfarlane, S., and Macfarlane, G. T. (2003). Regulation of short-chain fatty acid production. Proc. Nutr. Soc. 62 (1), 67–72. doi:10.1079/PNS2002207
Man, J. J., Beckman, J. A., and Jaffe, I. Z. (2020). Sex as a biological variable in atherosclerosis. Circ. Res. 126 (9), 1297–1319. doi:10.1161/CIRCRESAHA.120.315930
Mantovani, A., Biswas, S. K., Galdiero, M. R., Sica, A., and Locati, M. (2013). Macrophage plasticity and polarization in tissue repair and remodelling. J. Pathol. 229 (2), 176–185. doi:10.1002/path.4133
Marcovecchio, P. M., Thomas, G. D., Mikulski, Z., Ehinger, E., Mueller, K., Blatchley, A., et al. (2017). Scavenger receptor CD36 directs nonclassical monocyte patrolling along the endothelium during early atherogenesis. Arterioscler. Thromb. Vasc. Biol. 37 (11), 2043–2052. doi:10.1161/ATVBAHA.117.309123
Markin, A. M., Markina, Y. V., Bogatyreva, A. I., Tolstik, T. V., Chakal, D. A., Breshenkov, D. G., et al. (2023). The role of cytokines in cholesterol accumulation in cells and atherosclerosis progression. Int. J. Mol. Sci. 24 (7), 6426. doi:10.3390/ijms24076426
Marques, F. Z., Nelson, E., Chu, P. Y., Horlock, D., Fiedler, A., Ziemann, M., et al. (2017). High-fiber diet and acetate supplementation change the gut microbiota and prevent the development of hypertension and heart failure in hypertensive mice. Circulation 135 (10), 964–977. doi:10.1161/CIRCULATIONAHA.116.024545
Marques, L., Negre-Salvayre, A., Costa, L., and Canonne-Hergaux, F. (2016). Iron gene expression profile in atherogenic Mox macrophages. Biochim. Biophys. Acta. 1862 (6), 1137–1146. doi:10.1016/j.bbadis.2016.03.004
Meng, Q., Li, J., Wang, C., and Shan, A. (2023). Biological function of resveratrol and its application in animal production: a review. J. Anim. Sci. Biotechnol. 14 (1), 25. doi:10.1186/s40104-022-00822-z
Meyer, K. A., Benton, T. Z., Bennett, B. J., Jacobs, D. J., Lloyd-Jones, D. M., Gross, M. D., et al. (2016). Microbiota-dependent metabolite trimethylamine N-oxide and coronary artery calcium in the coronary artery risk development in young adults study (CARDIA). J. Am. Heart Assoc. 5 (10), e003970. doi:10.1161/JAHA.116.003970
Mills, S., Stanton, C., Lane, J. A., Smith, G. J., and Ross, R. P. (2019). Precision nutrition and the microbiome, Part I: current state of the science. Nutrients 11 (4), 923. doi:10.3390/nu11040923
Mizoguchi, T., Kasahara, K., Yamashita, T., Sasaki, N., Yodoi, K., Matsumoto, T., et al. (2017). Oral administration of the lactic acid bacterium Pediococcus acidilactici attenuates atherosclerosis in mice by inducing tolerogenic dendritic cells. Heart Vessels 32 (6), 768–776. doi:10.1007/s00380-017-0949-8
Mohammadi, A., Najar, A. G., Yaghoobi, M. M., Jahani, Y., and Vahabzadeh, Z. (2016). Trimethylamine-N-Oxide treatment induces changes in the ATP-binding cassette transporter A1 and scavenger receptor A1 in murine macrophage J774A.1 cells. Inflammation 39 (1), 393–404. doi:10.1007/s10753-015-0261-7
Moreira, L. T., Mosser, D. M., and Goncalves, R. (2020). Macrophage polarization in intestinal inflammation and gut homeostasis. Inflamm. Res. 69 (12), 1163–1172. doi:10.1007/s00011-020-01398-y
Moriya, J. (2019). Critical roles of inflammation in atherosclerosis. Cardiol. 73 (1), 22–27. doi:10.1016/j.jjcc.2018.05.010
Mulvihill, E. E., Burke, A. C., and Huff, M. W. (2016). Citrus flavonoids as regulators of lipoprotein metabolism and atherosclerosis. Annu. Rev. Nutr. 36, 275–299. doi:10.1146/annurev-nutr-071715-050718
Munjal, A., and Khandia, R. (2020). Atherosclerosis: orchestrating cells and biomolecules involved in its activation and inhibition. Adv. Protein Chem. Struct. Biol. 120, 85–122. doi:10.1016/bs.apcsb.2019.11.002
Munjuluri, S., Wilkerson, D. A., Sooch, G., Chen, X., White, F. A., and Obukhov, A. G. (2021). Capsaicin and TRPV1 channels in the cardiovascular system: the role of inflammation. Cells 11 (1), 18. doi:10.3390/cells11010018
Munoz-Cabrejas, A., Laclaustra, M., Guallar-Castillon, P., Casasnovas, J. A., Jarauta, E., Sandoval-Insausti, H., et al. (2021). High-quality intake of carbohydrates is associated with lower prevalence of subclinical atherosclerosis in femoral arteries: the AWHS study. Clin. Nutr. 40 (6), 3883–3889. doi:10.1016/j.clnu.2021.04.049
Neish, A. S. (2009). Microbes in gastrointestinal health and disease. Gastroenterology 136 (1), 65–80. doi:10.1053/j.gastro.2008.10.080
Neurath, M. F. (2019). Targeting immune cell circuits and trafficking in inflammatory bowel disease. Nat. Immunol. 20 (8), 970–979. doi:10.1038/s41590-019-0415-0
Nguyen, N. H., Ha, T., Yang, J. L., Pham, H., and Oh, W. K. (2021). Triterpenoids from the genus Gynostemma: chemistry and pharmacological activities. J. Ethnopharmacol. 268, 113574. doi:10.1016/j.jep.2020.113574
Nie, J., Zhang, L., Zhao, G., and Du, X. (2019). Quercetin reduces atherosclerotic lesions by altering the gut microbiota and reducing atherogenic lipid metabolites. J. Appl. Microbiol. 127 (6), 1824–1834. doi:10.1111/jam.14441
Nilsson, J., and Hansson, G. K. (2020). Vaccination strategies and immune modulation of atherosclerosis. Circ. Res. 126 (9), 1281–1296. doi:10.1161/CIRCRESAHA.120.315942
Nus, M., Sage, A. P., Lu, Y., Masters, L., Lam, B., Newland, S., et al. (2017). Marginal zone B cells control the response of follicular helper T cells to a high-cholesterol diet. Nat. Med. 23 (5), 601–610. doi:10.1038/nm.4315
Nutt, S. L., Hodgkin, P. D., Tarlinton, D. M., and Corcoran, L. M. (2015). The generation of antibody-secreting plasma cells. Nat. Rev. Immunol. 15 (3), 160–171. doi:10.1038/nri3795
O'Morain, V. L., Chan, Y. H., Williams, J. O., Alotibi, R., Alahmadi, A., Rodrigues, N. P., et al. (2021). The Lab4P consortium of probiotics attenuates atherosclerosis in LDL receptor deficient mice fed a high fat diet and causes plaque stabilization by inhibiting inflammation and several pro-atherogenic processes. Mol. Nutr. Food Res. 65 (17), e2100214. doi:10.1002/mnfr.202100214
Okazaki, R., Takahashi, T., Ueno, K., Takahashi, K., Ishitobi, M., Kikuchi, M., et al. (2015). Changes in EEG complexity with electroconvulsive therapy in a patient with autism spectrum disorders: a multiscale entropy approach. Front. Hum. Neurosci. 9, 106. doi:10.3389/fnhum.2015.00106
Ouimet, M., Barrett, T. J., and Fisher, E. A. (2019). HDL and reverse cholesterol transport. Circ. Res. 124 (10), 1505–1518. doi:10.1161/CIRCRESAHA.119.312617
Parada, V. D., De la Fuente, M. K., Landskron, G., Gonzalez, M. J., Quera, R., Dijkstra, G., et al. (2019). Corrigendum: short chain fatty acids (SCFAs)-Mediated gut epithelial and immune regulation and its relevance for inflammatory bowel diseases. Front. Immunol. 10, 1486. doi:10.3389/fimmu.2019.01486
Patel, R. V., Mistry, B. M., Shinde, S. K., Syed, R., Singh, V., and Shin, H. S. (2018). Therapeutic potential of quercetin as a cardiovascular agent. Eur. J. Med. Chem. 155, 889–904. doi:10.1016/j.ejmech.2018.06.053
Peng, L., Li, Z. R., Green, R. S., Holzman, I. R., and Lin, J. (2009). Butyrate enhances the intestinal barrier by facilitating tight junction assembly via activation of AMP-activated protein kinase in Caco-2 cell monolayers. J. Nutr. 139 (9), 1619–1625. doi:10.3945/jn.109.104638
Peng, Y., Ao, M., Dong, B., Jiang, Y., Yu, L., Chen, Z., et al. (2021). Anti-inflammatory effects of curcumin in the inflammatory diseases: status, limitations and countermeasures. Drug Des. devel. Ther. 15, 4503–4525. doi:10.2147/DDDT.S327378
Perler, B. K., Friedman, E. S., and Wu, G. D. (2023). The role of the gut microbiota in the relationship between diet and human health. Annu. Rev. Physiol. 85, 449–468. doi:10.1146/annurev-physiol-031522-092054
Peterson, C. T., Vaughn, A. R., Sharma, V., Chopra, D., Mills, P. J., Peterson, S. N., et al. (2018). Effects of turmeric and curcumin dietary supplementation on human gut microbiota: a double-blind, randomized, placebo-controlled Pilot study. J. Evid. Based. Integr. Med. 23, 2515690X18790725. doi:10.1177/2515690X18790725
Pluta, R., Januszewski, S., and Ulamek-Koziol, M. (2020). Mutual two-way interactions of curcumin and gut microbiota. Int. J. Mol. Sci. 21 (3), 1055. doi:10.3390/ijms21031055
Pols, T. W., Nomura, M., Harach, T., Lo, S. G., Oosterveer, M. H., Thomas, C., et al. (2011). TGR5 activation inhibits atherosclerosis by reducing macrophage inflammation and lipid loading. Cell. Metab. 14 (6), 747–757. doi:10.1016/j.cmet.2011.11.006
Ponnuswamy, P., Joffre, J., Herbin, O., Esposito, B., Laurans, L., Binder, C. J., et al. (2017). Angiotensin II synergizes with BAFF to promote atheroprotective regulatory B cells. Sci. Rep. 7 (1), 4111. doi:10.1038/s41598-017-04438-6
Potteaux, S., Gautier, E. L., Hutchison, S. B., van Rooijen, N., Rader, D. J., Thomas, M. J., et al. (2011). Suppressed monocyte recruitment drives macrophage removal from atherosclerotic plaques of Apoe-/- mice during disease regression. J. Clin. Investig. 121 (5), 2025–2036. doi:10.1172/JCI43802
Poznyak, A. V., Bezsonov, E. E., Popkova, T. V., Starodubova, A. V., and Orekhov, A. N. (2021). Immunity in atherosclerosis: focusing on T and B cells. Int. J. Mol. Sci. 22 (16), 8379. doi:10.3390/ijms22168379
Qi, J., You, T., Li, J., Pan, T., Xiang, L., Han, Y., et al. (2018). Circulating trimethylamine N-oxide and the risk of cardiovascular diseases: a systematic review and meta-analysis of 11 prospective cohort studies. J. Cell. Mol. Med. 22 (1), 185–194. doi:10.1111/jcmm.13307
Qiu, L., Yang, D., Tao, X., Yu, J., Xiong, H., and Wei, H. (2017). Enterobacter aerogenes ZDY01 attenuates choline-induced trimethylamine N-oxide levels by remodeling gut microbiota in mice. J. Microbiol. Biotechnol. 27 (8), 1491–1499. doi:10.4014/jmb.1703.03039
Raza, G. S., Maukonen, J., Makinen, M., Niemi, P., Niiranen, L., Hibberd, A. A., et al. (2019). Hypocholesterolemic effect of the lignin-rich insoluble residue of brewer's spent grain in mice fed a high-fat diet. J. Agric. Food Chem. 67 (4), 1104–1114. doi:10.1021/acs.jafc.8b05770
Roth, G. A., Mensah, G. A., and Fuster, V. (2020). The global burden of cardiovascular diseases and risks: a compass for global action. J. Am. Coll. Cardiol. 76 (25), 2980–2981. doi:10.1016/j.jacc.2020.11.021
Roth, G. A., Mensah, G. A., Johnson, C. O., Addolorato, G., Ammirati, E., Baddour, L. M., et al. (2020). Global burden of cardiovascular diseases and risk factors, 1990-2019: update from the GBD 2019 study. J. Am. Coll. Cardiol. 76 (25), 2982–3021. doi:10.1016/j.jacc.2020.11.010
Roy, P., Orecchioni, M., and Ley, K. (2022). How the immune system shapes atherosclerosis: roles of innate and adaptive immunity. Nat. Rev. Immunol. 22 (4), 251–265. doi:10.1038/s41577-021-00584-1
Rozenberg, I., Sluka, S. H., Rohrer, L., Hofmann, J., Becher, B., Akhmedov, A., et al. (2010). Histamine H1 receptor promotes atherosclerotic lesion formation by increasing vascular permeability for low-density lipoproteins. Arterioscler. Thromb. Vasc. Biol. 30 (5), 923–930. doi:10.1161/ATVBAHA.109.201079
Sage, A. P., Nus, M., Baker, L. L., Finigan, A. J., Masters, L. M., and Mallat, Z. (2015). Regulatory B cell-specific interleukin-10 is dispensable for atherosclerosis development in mice. Arterioscler. Thromb. Vasc. Biol. 35 (8), 1770–1773. doi:10.1161/ATVBAHA.115.305568
Sage, A. P., Tsiantoulas, D., Binder, C. J., and Mallat, Z. (2019). The role of B cells in atherosclerosis. Nat. Rev. Cardiol. 16 (3), 180–196. doi:10.1038/s41569-018-0106-9
Saigusa, R., Winkels, H., and Ley, K. (2020). T cell subsets and functions in atherosclerosis. Nat. Rev. Cardiol. 17 (7), 387–401. doi:10.1038/s41569-020-0352-5
Sakaguchi, S., Mikami, N., Wing, J. B., Tanaka, A., Ichiyama, K., and Ohkura, N. (2020). Regulatory T cells and human disease. Annu. Rev. Immunol. 38, 541–566. doi:10.1146/annurev-immunol-042718-041717
Satny, M., Hubacek, J. A., and Vrablik, M. (2021). Statins and inflammation. Curr. Atheroscler. Rep. 23 (12), 80. doi:10.1007/s11883-021-00977-6
Seldin, M. M., Meng, Y., Qi, H., Zhu, W., Wang, Z., Hazen, S. L., et al. (2016). Trimethylamine N-oxide promotes vascular inflammation through signaling of mitogen-activated protein kinase and nuclear factor-κb. J. Am. Heart Assoc. 5 (2), e002767. doi:10.1161/JAHA.115.002767
Shan, Z., Clish, C. B., Hua, S., Scott, J. M., Hanna, D. B., Burk, R. D., et al. (2018). Gut microbial-related choline metabolite trimethylamine-N-oxide is associated with progression of carotid artery atherosclerosis in HIV infection. J. Infect. Dis. 218 (9), 1474–1479. doi:10.1093/infdis/jiy356
Shapouri-Moghaddam, A., Mohammadian, S., Vazini, H., Taghadosi, M., Esmaeili, S. A., Mardani, F., et al. (2018). Macrophage plasticity, polarization, and function in health and disease. J. Cell. Physiol. 233 (9), 6425–6440. doi:10.1002/jcp.26429
Sharma, M., Schlegel, M. P., Afonso, M. S., Brown, E. J., Rahman, K., Weinstock, A., et al. (2020). Regulatory T cells license macrophage pro-resolving functions during atherosclerosis regression. Circ. Res. 127 (3), 335–353. doi:10.1161/CIRCRESAHA.119.316461
Shen, H., Zhao, Z., Zhao, Z., Chen, Y., and Zhang, L. (2022). Native and engineered probiotics: promising agents against related systemic and intestinal diseases. Int. J. Mol. Sci. 23 (2), 594. doi:10.3390/ijms23020594
Shen, L., Liu, L., and Ji, H. F. (2017). Regulative effects of curcumin spice administration on gut microbiota and its pharmacological implications. Food Nutr. Res. 61 (1), 1361780. doi:10.1080/16546628.2017.1361780
Shi, W., Huang, Y., Yang, Z., Zhu, L., and Yu, B. (2021). Reduction of TMAO level enhances the stability of carotid atherosclerotic plaque through promoting macrophage M2 polarization and efferocytosis. Biosci. Rep. 41 (6), BSR20204250. doi:10.1042/BSR20204250
Shi, X., Huang, H., Zhou, M., Liu, Y., Wu, H., and Dai, M. (2021). Paeonol attenuated vascular fibrosis through regulating Treg/Th17 balance in a gut microbiota-dependent manner. Front. Pharmacol. 12, 765482. doi:10.3389/fphar.2021.765482
Shi, X., Wu, H., Liu, Y., Huang, H., Liu, L., Yang, Y., et al. (2022). Inhibiting vascular smooth muscle cell proliferation mediated by osteopontin via regulating gut microbial lipopolysaccharide: a novel mechanism for paeonol in atherosclerosis treatment. Front. Pharmacol. 13, 936677. doi:10.3389/fphar.2022.936677
Shiotsugu, S., Okinaga, T., Habu, M., Yoshiga, D., Yoshioka, I., Nishihara, T., et al. (2019). The biological effects of interleukin-17a on adhesion molecules expression and foam cell formation in atherosclerotic lesions. J. Interferon Cytokine Res. 39 (11), 694–702. doi:10.1089/jir.2019.0034
Sieve, I., Ricke-Hoch, M., Kasten, M., Battmer, K., Stapel, B., Falk, C. S., et al. (2018). A positive feedback loop between IL-1β, LPS and NEU1 may promote atherosclerosis by enhancing a pro-inflammatory state in monocytes and macrophages. Vasc. Pharmacol. 103-105, 16–28. doi:10.1016/j.vph.2018.01.005
Silveira, A., Mcleod, O., Strawbridge, R. J., Gertow, K., Sennblad, B., Baldassarre, D., et al. (2015). Plasma IL-5 concentration and subclinical carotid atherosclerosis. Atherosclerosis 239 (1), 125–130. doi:10.1016/j.atherosclerosis.2014.12.046
Simo, C., and Garcia-Canas, V. (2020). Dietary bioactive ingredients to modulate the gut microbiota-derived metabolite TMAO. New opportunities for functional food development. Food Funct. 11 (8), 6745–6776. doi:10.1039/d0fo01237h
Skenteris, N. T., Hemme, E., Delfos, L., Karadimou, G., Karlof, E., Lengquist, M., et al. (2023). Mast cells participate in smooth muscle cell reprogramming and atherosclerotic plaque calcification. Vasc. Pharmacol. 150, 107167. doi:10.1016/j.vph.2023.107167
Smith, E., Prasad, K. M., Butcher, M., Dobrian, A., Kolls, J. K., Ley, K., et al. (2010). Blockade of interleukin-17A results in reduced atherosclerosis in apolipoprotein E-deficient mice. Circulation 121 (15), 1746–1755. doi:10.1161/CIRCULATIONAHA.109.924886
Song, S., and Song, Y. (2021). Dietary fiber and its source are associated with cardiovascular risk factors in Korean adults. Nutrients 13 (1), 160. doi:10.3390/nu13010160
Song, Z., Brassard, P., and Brophy, J. M. (2008). A meta-analysis of antibiotic use for the secondary prevention of cardiovascular diseases. J. Cardiol. 24 (5), 391–395. doi:10.1016/s0828-282x(08)70603-2
Spitz, C., Winkels, H., Burger, C., Weber, C., Lutgens, E., Hansson, G. K., et al. (2016). Regulatory T cells in atherosclerosis: critical immune regulatory function and therapeutic potential. Cell. Mol. Life Sci. 73 (5), 901–922. doi:10.1007/s00018-015-2080-2
Stoger, J. L., Gijbels, M. J., van der Velden, S., Manca, M., van der Loos, C. M., Biessen, E. A., et al. (2012). Distribution of macrophage polarization markers in human atherosclerosis. Atherosclerosis 225 (2), 461–468. doi:10.1016/j.atherosclerosis.2012.09.013
Sun, J., Sukhova, G. K., Wolters, P. J., Yang, M., Kitamoto, S., Libby, P., et al. (2007). Mast cells promote atherosclerosis by releasing proinflammatory cytokines. Nat. Med. 13 (6), 719–724. doi:10.1038/nm1601
Sun, L., Pang, Y., Wang, X., Wu, Q., Liu, H., Liu, B., et al. (2019). Ablation of gut microbiota alleviates obesity-induced hepatic steatosis and glucose intolerance by modulating bile acid metabolism in hamsters. Acta. Pharm. Sin. B 9 (4), 702–710. doi:10.1016/j.apsb.2019.02.004
Sun, X., Jia, B., Sun, J., Lin, J., Lu, B., Duan, J., et al. (2023). Gastrodia elata blume: a review of its mechanisms and functions on cardiovascular systems. Fitoterapia 167, 105511. doi:10.1016/j.fitote.2023.105511
Sun, X., Jiao, X., Ma, Y., Liu, Y., Zhang, L., He, Y., et al. (2016). Trimethylamine N-oxide induces inflammation and endothelial dysfunction in human umbilical vein endothelial cells via activating ROS-TXNIP-NLRP3 inflammasome. Biochem. Biophys. Res. Commun. 481 (1-2), 63–70. doi:10.1016/j.bbrc.2016.11.017
Suzuki, K., Susaki, E. A., and Nagaoka, I. (2022). Lipopolysaccharides and cellular senescence: involvement in atherosclerosis. Int. J. Mol. Sci. 23 (19), 11148. doi:10.3390/ijms231911148
Tabas, I., and Bornfeldt, K. E. (2016). Macrophage phenotype and function in different stages of atherosclerosis. Circ. Res. 118 (4), 653–667. doi:10.1161/CIRCRESAHA.115.306256
Tacke, F., Alvarez, D., Kaplan, T. J., Jakubzick, C., Spanbroek, R., Llodra, J., et al. (2007). Monocyte subsets differentially employ CCR2, CCR5, and CX3CR1 to accumulate within atherosclerotic plaques. J. Clin. Investig. 117 (1), 185–194. doi:10.1172/JCI28549
Tan, Y., Sheng, Z., Zhou, P., Liu, C., Zhao, H., Song, L., et al. (2019). Plasma trimethylamine N-oxide as a novel biomarker for plaque rupture in patients with ST-segment-elevation myocardial infarction. Circ. Cardiovasc. Interv. 12 (1), e007281. doi:10.1161/CIRCINTERVENTIONS.118.007281
Tay, C., Liu, Y. H., Kanellakis, P., Kallies, A., Li, Y., Cao, A., et al. (2018). Follicular B cells promote atherosclerosis via T cell-mediated differentiation into plasma cells and secreting pathogenic immunoglobulin G. Arterioscler. Thromb. Vasc. Biol. 38 (5), e71–e84. doi:10.1161/ATVBAHA.117.310678
Tayyeb, J. Z., Popeijus, H. E., Mensink, R. P., Konings, M., Mokhtar, F., and Plat, J. (2020). Short-chain fatty acids (except hexanoic acid) lower NF-kB transactivation, which rescues inflammation-induced decreased apolipoprotein A-I transcription in HepG2 cells. Int. J. Mol. Sci. 21 (14), 5088. doi:10.3390/ijms21145088
Thomas, G., Tacke, R., Hedrick, C. C., and Hanna, R. N. (2015). Nonclassical patrolling monocyte function in the vasculature. Arterioscler. Thromb. Vasc. Biol. 35 (6), 1306–1316. doi:10.1161/ATVBAHA.114.304650
Tian, Q., Leung, F. P., Chen, F. M., Tian, X. Y., Chen, Z., Tse, G., et al. (2021). Butyrate protects endothelial function through PPARδ/miR-181b signaling. Pharmacol. Res. 169, 105681. doi:10.1016/j.phrs.2021.105681
Tonjes, A., Scholz, M., Kruger, J., Krause, K., Schleinitz, D., Kirsten, H., et al. (2018). Genome-wide meta-analysis identifies novel determinants of circulating serum progranulin. Hum. Mol. Genet. 27 (3), 546–558. doi:10.1093/hmg/ddx413
Tsiantoulas, D., Sage, A. P., Mallat, Z., and Binder, C. J. (2015). Targeting B cells in atherosclerosis: closing the gap from bench to bedside. Arterioscler. Thromb. Vasc. Biol. 35 (2), 296–302. doi:10.1161/ATVBAHA.114.303569
Tsuda, T. (2018). Curcumin as a functional food-derived factor: degradation products, metabolites, bioactivity, and future perspectives. Food Funct. 9 (2), 705–714. doi:10.1039/c7fo01242j
van den Munckhof, I., Kurilshikov, A., Ter Horst, R., Riksen, N. P., Joosten, L., Zhernakova, A., et al. (2018). Role of gut microbiota in chronic low-grade inflammation as potential driver for atherosclerotic cardiovascular disease: A systematic review of human studies. Obes. Rev. 19 (12), 1719–1734. doi:10.1111/obr.12750
van Dijk, R. A., Rijs, K., Wezel, A., Hamming, J. F., Kolodgie, F. D., Virmani, R., et al. (2016). Systematic evaluation of the cellular innate immune response during the process of human atherosclerosis. J. Am. Heart Assoc. 5 (6), e002860. doi:10.1161/JAHA.115.002860
van Duijn, J., de Jong, M., Benne, N., Leboux, R., van Ooijen, M. E., Kruit, N., et al. (2021). Tc17 CD8+ T cells accumulate in murine atherosclerotic lesions, but do not contribute to early atherosclerosis development. Cardiovasc. Res. 117 (14), 2755–2766. doi:10.1093/cvr/cvaa286
van Duijn, J., Kuiper, J., and Slutter, B. (2018). The many faces of CD8+ T cells in atherosclerosis. Opin. Lipidol. 29 (5), 411–416. doi:10.1097/MOL.0000000000000541
Van Treuren, W., and Dodd, D. (2020). Microbial contribution to the human metabolome: implications for health and disease. Annu. Rev. Pathol. 15, 345–369. doi:10.1146/annurev-pathol-020117-043559
Verhaar, B., Prodan, A., Nieuwdorp, M., and Muller, M. (2020). Gut microbiota in hypertension and atherosclerosis: a review. Nutrients 12 (10), 2982. doi:10.3390/nu12102982
Vetrani, C., Costabile, G., Luongo, D., Naviglio, D., Rivellese, A. A., Riccardi, G., et al. (2016). Effects of whole-grain cereal foods on plasma short chain fatty acid concentrations in individuals with the metabolic syndrome. Nutrition 32 (2), 217–221. doi:10.1016/j.nut.2015.08.006
Vinolo, M. A., Rodrigues, H. G., Hatanaka, E., Sato, F. T., Sampaio, S. C., and Curi, R. (2011). Suppressive effect of short-chain fatty acids on production of proinflammatory mediators by neutrophils. Nutr. Biochem. 22 (9), 849–855. doi:10.1016/j.jnutbio.2010.07.009
Wang, B., Tang, X., Yao, L., Wang, Y., Chen, Z., Li, M., et al. (2022). Disruption of USP9X in macrophages promotes foam cell formation and atherosclerosis. J. Clin. Investig. 132 (10), e154217. doi:10.1172/JCI154217
Wang, F., Xue, Y., Fu, L., Wang, Y., He, M., Zhao, L., et al. (2022). Extraction, purification, bioactivity and pharmacological effects of capsaicin: a review. Crit. Rev. Food Sci. Nutr. 62 (19), 5322–5348. doi:10.1080/10408398.2021.1884840
Wang, F., Zhao, C., Tian, G., Wei, X., Ma, Z., Cui, J., et al. (2020). Naringin alleviates atherosclerosis in ApoE(-/-) mice by regulating cholesterol metabolism involved in gut microbiota remodeling. J. Agric. Food Chem. 68 (45), 12651–12660. doi:10.1021/acs.jafc.0c05800
Wang, J., Ghosh, S. S., and Ghosh, S. (2017). Curcumin improves intestinal barrier function: modulation of intracellular signaling, and organization of tight junctions. Am. J. Physiol. Cell. Physiol. 312 (4), C438-C445–C445. doi:10.1152/ajpcell.00235.2016
Wang, J., Wang, L., Lou, G. H., Zeng, H. R., Hu, J., Huang, Q. W., et al. (2019). Coptidis rhizoma: a comprehensive review of its traditional uses, botany, phytochemistry, pharmacology and toxicology. Pharm. Biol. 57 (1), 193–225. doi:10.1080/13880209.2019.1577466
Wang, Q., Guo, M., Liu, Y., Xu, M., Shi, L., Li, X., et al. (2022). Bifidobacterium breve and Bifidobacterium longum attenuate choline-induced plasma trimethylamine N-oxide production by modulating gut microbiota in mice. Nutrients 14 (6), 1222. doi:10.3390/nu14061222
Wang, Q., He, Y., Li, X., Zhang, T., Liang, M., Wang, G., et al. (2022). Lactobacillus reuteri CCFM8631 alleviates hypercholesterolaemia caused by the paigen atherogenic diet by regulating the gut microbiota. Nutrients 14 (6), 1272. doi:10.3390/nu14061272
Wang, Q., Zhang, C., Yang, C., Sun, Y., Chen, K., and Lu, Y. (2022). Capsaicin alleviates vascular endothelial dysfunction and cardiomyopathy via TRPV1/eNOS pathway in diabetic rats. Oxid. Med. Cell. Longev. 2022, 6482363. doi:10.1155/2022/6482363
Wang, X., Yao, X., Zhu, Z., Tang, T., Dai, K., Sadovskaya, I., et al. (2009). Effect of berberine on Staphylococcus epidermidis biofilm formation. Int. J. Antimicrob. Agents. 34 (1), 60–66. doi:10.1016/j.ijantimicag.2008.10.033
Wang, Z., Klipfell, E., Bennett, B. J., Koeth, R., Levison, B. S., Dugar, B., et al. (2011). Gut flora metabolism of phosphatidylcholine promotes cardiovascular disease. Nature 472 (7341), 57–63. doi:10.1038/nature09922
Wang, Z., Peters, B. A., Usyk, M., Xing, J., Hanna, D. B., Wang, T., et al. (2022). Gut microbiota, plasma metabolomic profiles, and carotid artery atherosclerosis in HIV infection. Arterioscler. Thromb. Vasc. Biol. 42 (8), 1081–1093. doi:10.1161/ATVBAHA.121.317276
Wang, Z., Roberts, A. B., Buffa, J. A., Levison, B. S., Zhu, W., Org, E., et al. (2015). Non-lethal inhibition of gut microbial trimethylamine production for the treatment of atherosclerosis. Cell. 163 (7), 1585–1595. doi:10.1016/j.cell.2015.11.055
Warrier, M., Shih, D. M., Burrows, A. C., Ferguson, D., Gromovsky, A. D., Brown, A. L., et al. (2015). The TMAO-generating enzyme flavin monooxygenase 3 is a central regulator of cholesterol balance. Cell. Rep. 10 (3), 326–338. doi:10.1016/j.celrep.2014.12.036
Weickert, M. O., Hattersley, J. G., Kyrou, I., Arafat, A. M., Rudovich, N., Roden, M., et al. (2018). Effects of supplemented isoenergetic diets varying in cereal fiber and protein content on the bile acid metabolic signature and relation to insulin resistance. Nutr. Diabetes. 8 (1), 11. doi:10.1038/s41387-018-0020-6
Weng, X., Lou, Y. Y., Wang, Y. S., Huang, Y. P., Zhang, J., Yin, Z. Q., et al. (2021). New dammarane-type glycosides from Gynostemma pentaphyllum and their lipid-lowering activity. Bioorg. Chem. 111, 104843. doi:10.1016/j.bioorg.2021.104843
Wezel, A., Lagraauw, H. M., van der Velden, D., de Jager, S. C., Quax, P. H., Kuiper, J., et al. (2015). Mast cells mediate neutrophil recruitment during atherosclerotic plaque progression. Atherosclerosis 241 (2), 289–296. doi:10.1016/j.atherosclerosis.2015.05.028
Williams, J. W., Zaitsev, K., Kim, K. W., Ivanov, S., Saunders, B. T., Schrank, P. R., et al. (2020). Limited proliferation capacity of aortic intima resident macrophages requires monocyte recruitment for atherosclerotic plaque progression. Nat. Immunol. 21 (10), 1194–1204. doi:10.1038/s41590-020-0768-4
Wolf, D., and Ley, K. (2019). Immunity and inflammation in atherosclerosis. Circ. Res. 124 (2), 315–327. doi:10.1161/CIRCRESAHA.118.313591
Wong, K. L., Tai, J. J., Wong, W. C., Han, H., Sem, X., Yeap, W. H., et al. (2011). Gene expression profiling reveals the defining features of the classical, intermediate, and nonclassical human monocyte subsets. Blood 118 (5), e16–e31. doi:10.1182/blood-2010-12-326355
Wu, D. N., Guan, L., Jiang, Y. X., Ma, S. H., Sun, Y. N., Lei, H. T., et al. (2019). Microbiome and metabonomics study of quercetin for the treatment of atherosclerosis. Cardiovasc. Diagn. Ther. 9 (6), 545–560. doi:10.21037/cdt.2019.12.04
Wu, M., Yang, S., Wang, S., Cao, Y., Zhao, R., Li, X., et al. (2020). Effect of berberine on atherosclerosis and gut microbiota modulation and their correlation in high-fat diet-fed ApoE-/- mice. Front. Pharmacol. 11, 223. doi:10.3389/fphar.2020.00223
Xia, X., Hu, T., He, J., Xu, Q., Yu, C., Liu, X., et al. (2020). USP10 deletion inhibits macrophage-derived foam cell formation and cellular-oxidized low density lipoprotein uptake by promoting the degradation of CD36. Aging (Albany NY) 12 (22), 22892–22905. doi:10.18632/aging.104003
Xiao, L., Liu, L., Guo, X., Zhang, S., Wang, J., Zhou, F., et al. (2017). Quercetin attenuates high fat diet-induced atherosclerosis in apolipoprotein E knockout mice: a critical role of NADPH oxidase. Food Chem. Toxicol. 105, 22–33. doi:10.1016/j.fct.2017.03.048
Xie, B., Zu, X., Wang, Z., Xu, X., Liu, G., and Liu, R. (2022). Ginsenoside Rc ameliorated atherosclerosis via regulating gut microbiota and fecal metabolites. Front. Pharmacol. 13, 990476. doi:10.3389/fphar.2022.990476
Xu, J., Yang, W., Deng, Q., Huang, Q., Yang, J., and Huang, F. (2012). Flaxseed oil and alpha-lipoic acid combination reduces atherosclerosis risk factors in rats fed a high-fat diet. Lipids Health Dis. 11, 148. doi:10.1186/1476-511X-11-148
Xu, Q., Liu, X., Mei, L., Wen, Q., Chen, J., Miao, J., et al. (2018). Paeonol reduces the nucleocytoplasmic transportation of HMGB1 by upregulating HDAC3 in LPS-induced RAW264.7 cells. Inflammation 41 (4), 1536–1545. doi:10.1007/s10753-018-0800-0
Xu, X., Lei, T., Li, W., and Ou, H. (2019). Enhanced cellular cholesterol efflux by naringenin is mediated through inhibiting endoplasmic reticulum stress - ATF6 activity in macrophages. Biochim. Biophys. Acta. Mol. Cell. Biol. Lipids 1864 (10), 1472–1482. doi:10.1016/j.bbalip.2019.06.005
Xu, Y., Li, Y., Jadhav, K., Pan, X., Zhu, Y., Hu, S., et al. (2021). Hepatocyte ATF3 protects against atherosclerosis by regulating HDL and bile acid metabolism. Nat. Metab. 3 (1), 59–74. doi:10.1038/s42255-020-00331-1
Xue, X., Li, F., Xu, M., Chen, B., Zhao, Y., Wang, M., et al. (2023). Gastrodin ameliorates atherosclerosis by inhibiting foam cells formation and inflammation through down-regulating NF-κB pathway. Nutr. Metab. (Lond). 20 (1), 9. doi:10.1186/s12986-022-00722-z
Yan, D., Li, J., Xiong, Y., Zhang, C., Luo, J., Han, Y., et al. (2014). Promotion of quality standard of herbal medicine by constituent removing and adding. Sci. Rep. 4, 3668. doi:10.1038/srep03668
Yang, D., Lyu, W., Hu, Z., Gao, J., Zheng, Z., Wang, W., et al. (2021). Probiotic effects of Lactobacillus fermentum ZJUIDS06 and Lactobacillus plantarum ZY08 on hypercholesteremic golden hamsters. Front. Nutr. 8, 705763. doi:10.3389/fnut.2021.705763
Yang, G., Lin, C. C., Yang, Y., Yuan, L., Wang, P., Wen, X., et al. (2019a). Nobiletin prevents trimethylamine oxide-induced vascular inflammation via inhibition of the NF-κB/MAPK pathways. J. Agric. Food Chem. 67 (22), 6169–6176. doi:10.1021/acs.jafc.9b01270
Yang, S., Li, X., Yang, F., Zhao, R., Pan, X., Liang, J., et al. (2019b). Gut microbiota dependent marker TMAO in promoting cardiovascular disease: inflammation mechanism, clinical prognostic, and potential as a therapeutic target. Front. Pharmacol. 10, 1360. doi:10.3389/fphar.2019.01360
Ye, G., Chen, G., Gao, H., Lin, Y., Liao, X., Zhang, H., et al. (2019). Resveratrol inhibits lipid accumulation in the intestine of atherosclerotic mice and macrophages. J. Cell. Mol. Med. 23 (6), 4313–4325. doi:10.1111/jcmm.14323
Yi, C., Sun, W., Ding, L., Yan, M., Sun, C., Qiu, C., et al. (2022). Short-chain fatty acids weaken ox-LDL-induced cell inflammatory injury by inhibiting the NLRP3/caspase-1 pathway and affecting cellular metabolism in THP-1 cells. Molecules 27 (24), 8801. doi:10.3390/molecules27248801
Yoshida, N., Emoto, T., Yamashita, T., Watanabe, H., Hayashi, T., Tabata, T., et al. (2018). Bacteroides vulgatus and Bacteroides dorei reduce gut microbial lipopolysaccharide production and inhibit atherosclerosis. Circulation 138 (22), 2486–2498. doi:10.1161/CIRCULATIONAHA.118.033714
Yu, T., Yang, Y., Kwak, Y. S., Song, G. G., Kim, M. Y., Rhee, M. H., et al. (2017). Ginsenoside Rc from Panax ginseng exerts anti-inflammatory activity by targeting TANK-binding kinase 1/interferon regulatory factor-3 and p38/ATF-2. J. Ginseng Res. 41 (2), 127–133. doi:10.1016/j.jgr.2016.02.001
Yu, X. H., Zhang, J., Zheng, X. L., Yang, Y. H., and Tang, C. K. (2015). Interferon-gamma in foam cell formation and progression of atherosclerosis. Clin. Chim. Acta. 441, 33–43. doi:10.1016/j.cca.2014.12.007
Yu, Y., Yan, R., Chen, X., Sun, T., and Yan, J. (2020). Paeonol suppresses the effect of ox-LDL on mice vascular endothelial cells by regulating miR-338-3p/TET2 axis in atherosclerosis. Mol. Cell. Biochem. 475 (1-2), 127–135. doi:10.1007/s11010-020-03865-w
Yu, Z. L., Ding, L., Xue, C. H., Zhang, T. T., and Wang, Y. M. (2020). Dietary trimethylamine N-oxide exacerbated atherosclerosis under a low-fat rather than high-fat diet. J. Agric. Food Chem. 68 (25), 6789–6791. doi:10.1021/acs.jafc.0c03190
Yunna, C., Mengru, H., Lei, W., and Weidong, C. (2020). Macrophage M1/M2 polarization. Eur. J. Pharmacol. 877, 173090. doi:10.1016/j.ejphar.2020.173090
Zarif, J. C., Hernandez, J. R., Verdone, J. E., Campbell, S. P., Drake, C. G., and Pienta, K. J. (2016). A phased strategy to differentiate human CD14+monocytes into classically and alternatively activated macrophages and dendritic cells. Biotechniques 61 (1), 33–41. doi:10.2144/000114435
Zawada, A. M., Rogacev, K. S., Rotter, B., Winter, P., Marell, R. R., Fliser, D., et al. (2011). SuperSAGE evidence for CD14++CD16+ monocytes as a third monocyte subset. Blood 118 (12), e50–e61. doi:10.1182/blood-2011-01-326827
Zernecke, A. (2015). Dendritic cells in atherosclerosis: evidence in mice and humans. Arterioscler. Thromb. Vasc. Biol. 35 (4), 763–770. doi:10.1161/ATVBAHA.114.303566
Zernecke, A., Winkels, H., Cochain, C., Williams, J. W., Wolf, D., Soehnlein, O., et al. (2020). Meta-analysis of leukocyte diversity in atherosclerotic mouse aortas. Circ. Res. 127 (3), 402–426. doi:10.1161/CIRCRESAHA.120.316903
Zhang, J., Ou, C., and Chen, M. (2022). Curcumin attenuates cadmium-induced atherosclerosis by regulating trimethylamine-N-oxide synthesis and macrophage polarization through remodeling the gut microbiota. Ecotoxicol. Environ. Saf. 244, 114057. doi:10.1016/j.ecoenv.2022.114057
Zhang, J., Yang, Y., Han, H., Zhang, L., and Wang, T. (2021). Bisdemethoxycurcumin attenuates lipopolysaccharide-induced intestinal damage through improving barrier integrity, suppressing inflammation, and modulating gut microbiota in broilers. J. Anim. Sci. 99 (11), skab296. doi:10.1093/jas/skab296
Zhang, L., Li, D. C., and Liu, L. F. (2019). Paeonol: pharmacological effects and mechanisms of action. Int. Immunopharmacol. 72, 413–421. doi:10.1016/j.intimp.2019.04.033
Zhang, L., Ma, C., Gu, R., Zhang, M., Wang, X., Yang, L., et al. (2018). Paeonol regulates hypoxia-induced proliferation of pulmonary artery smooth muscle cells via EKR 1/2 signalling. Eur. J. Pharmacol. 834, 257–265. doi:10.1016/j.ejphar.2018.07.017
Zhang, L., Wu, X., Yang, R., Chen, F., Liao, Y., Zhu, Z., et al. (2020). Effects of berberine on the gastrointestinal microbiota. Front. Cell. Infect. Microbiol. 10, 588517. doi:10.3389/fcimb.2020.588517
Zhang, S., Li, L., Chen, W., Xu, S., Feng, X., and Zhang, L. (2021). Natural products: the role and mechanism in low-density lipoprotein oxidation and atherosclerosis. Phytother. Res. 35 (6), 2945–2967. doi:10.1002/ptr.7002
Zhang, W., Xu, J. H., Yu, T., and Chen, Q. K. (2019). Effects of berberine and metformin on intestinal inflammation and gut microbiome composition in db/db mice. Biomed. Pharmacother. 118, 109131. doi:10.1016/j.biopha.2019.109131
Zhang, Y., Wang, X., Vales, C., Lee, F. Y., Lee, H., Lusis, A. J., et al. (2006). FXR deficiency causes reduced atherosclerosis in Ldlr-/- mice. Arterioscler. Thromb. Vasc. Biol. 26 (10), 2316–2321. doi:10.1161/01.ATV.0000235697.35431.05
Zhao, H., Chen, L., He, C., Li, S., Yang, H., Xu, X., et al. (2020). Chronic Staphylococcus aureus superantigen toxic shock syndrome toxin-1 exposure accelerates the progression of atherosclerosis in rabbits. Acta Cardiol. Sin. 36 (1), 24–32. doi:10.6515/ACS.202001_36(1).20190611B
Zhao, Y., Liu, J., Hao, W., Zhu, H., Liang, N., He, Z., et al. (2017). Structure-specific effects of short-chain fatty acids on plasma cholesterol concentration in male Syrian hamsters. J. Agric. Food Chem. 65 (50), 10984–10992. doi:10.1021/acs.jafc.7b04666
Zheng, Y., and He, J. Q. (2022). Pathogenic mechanisms of trimethylamine N-Oxide-induced atherosclerosis and cardiomyopathy. Curr. Vasc. Pharmacol. 20 (1), 29–36. doi:10.2174/1570161119666210812152802
Zhou, C. B., Zhou, Y. L., and Fang, J. Y. (2021). Gut microbiota in cancer immune response and immunotherapy. Trends Cancer 7 (7), 647–660. doi:10.1016/j.trecan.2021.01.010
Zhu, L., Sha, L., Li, K., Wang, Z., Wang, T., Li, Y., et al. (2020). Dietary flaxseed oil rich in omega-3 suppresses severity of type 2 diabetes mellitus via anti-inflammation and modulating gut microbiota in rats. Lipids Health Dis. 19 (1), 20. doi:10.1186/s12944-019-1167-4
Zhu, W., Gregory, J. C., Org, E., Buffa, J. A., Gupta, N., Wang, Z., et al. (2016). Gut microbial metabolite TMAO enhances platelet hyperreactivity and thrombosis risk. Cell. 165 (1), 111–124. doi:10.1016/j.cell.2016.02.011
Zhu, Y., Xian, X., Wang, Z., Bi, Y., Chen, Q., Han, X., et al. (2018). Research progress on the relationship between atherosclerosis and inflammation. Biomolecules 8 (3), 80. doi:10.3390/biom8030080
Glossary
Keywords: gut microbiota, immune cells, natural products, atherosclerosis, probiotics
Citation: Jing J, Guo J, Dai R, Zhu C and Zhang Z (2023) Targeting gut microbiota and immune crosstalk: potential mechanisms of natural products in the treatment of atherosclerosis. Front. Pharmacol. 14:1252907. doi: 10.3389/fphar.2023.1252907
Received: 04 July 2023; Accepted: 21 August 2023;
Published: 01 September 2023.
Edited by:
Hammad Ullah, University of Naples Federico II, ItalyReviewed by:
Stanislav Kotlyarov, Ryazan State Medical University named after academician I.P. Pavlov, RussiaMuhammad Usman, COMSATS University Islamabad, Pakistan
Copyright © 2023 Jing, Guo, Dai, Zhu and Zhang. This is an open-access article distributed under the terms of the Creative Commons Attribution License (CC BY). The use, distribution or reproduction in other forums is permitted, provided the original author(s) and the copyright owner(s) are credited and that the original publication in this journal is cited, in accordance with accepted academic practice. No use, distribution or reproduction is permitted which does not comply with these terms.
*Correspondence: Chaojun Zhu, emNqMDcwNkAxMjYuY29t; Zhaohui Zhang, emh6aDA0MDVAMTYzLmNvbQ==
†These authors have contributed equally to this work