- 1Graduate College of Biomedical Sciences, Western University of Health Sciences, Pomona, CA, United States
- 2Department of Psychiatry, Boonshoft School of Medicine, Dayton VA Medical Center, Wright State University, Dayton, OH, United States
- 3University of Vermont College of Medicine, Burlington, VM, United States
- 4Division of Addictive Services, Dominion Diagnostics, LLC, North Kingston, RI, United States
- 5Division of Precision Addiction Management, Geneus Health, LLC, San Antonio, TX, United States
- 6Institute of Psychology, University of Eötvös Loránd, Budapest, Hungary
- 7Department of Clinical Neurology, Path Foundation, New York, NY, United States
- 8Division of Neuroscience and Addiction Therapy, Summit Estate Recovery Center, Los Gatos, CA, United States
- 9Department of Neurogenetics Research and Addiction Therapy, The Florida House Experience, Deerfield Beach, FL, United States
- 10National Human Genome Center, Howard University, Washington, DC, United States
- 11Department of Anatomy, Howard University College of Medicine, Washington, DC, United States
- 12Behavioral Neuropharmacology and Neuroimaging Laboratory on Addictions, Clinical and Research Institute on Addictions, Department of Pharmacology and Toxicology, Jacobs School of Medicine and Biomedical Sciences, University at Buffalo, Buffalo, NY, United States
- 13Department of Psychology, University at Buffalo, Buffalo, NY, United States
- 14Department of Psychiatry, Cooper Medical School of Rowan University, Camden, NJ, United States
- 15Department of Psychiatry, Icahn School of Medicine at Mount Sinai, New York, NY, United States
Worldwide daily, millions of people are unable to combat their frustrating and even fatal romance with getting high; for some, “high” may be just experiencing feelings of well-being. The neuroscience community conducts and funds, outstanding research using sophisticated neuroimaging and molecular-genetic applied technology to improve understanding of the complex functions of brain reward circuitry that has a key role in addiction symptomatology. While it is widely accepted that dopamine is a major neurotransmitter implicated in behavioral and substance addictions, there remains controversy about how to modulate dopamine clinically to treat and prevent various types of addictive disorders. A prudent approach may be biphasic; a short-term blockade followed by long-term dopaminergic upregulation. The goal of treatment would be to enhance brain reward functional connectivity volume, and target reward deficiency and the stress-like anti reward symptomatology of addiction. Such phenotypes can be characterized using the Genetic Addiction Risk Score (GARS)®. Dopamine homeostasis may thus be achieved via “Precision Addiction Management” (PAM)®, the customization of neuronutrient supplementation based on the GARS test result, along with a behavioral intervention.
Introduction
Following almost three decades of genetic-based research related to identifying and characterizing addiction-related Reward Deficiency Syndrome (RDS), a paradigm shift is proposed in the prevention and treatment of all types of addictive behaviors. The novel adoption of “Precision Addiction Management” (PAM) based on genetic predisposition is imminent. Certainly, more research is necessary to further pinpoint the most appropriate candidate genes. However, an accelerated personalized, precision tool can be useful for the identification of highly convergent candidate gene single nucleotide polymorphisms (SNPs), associated in populations with RDS. Research directed toward improving treatment of substance use disorders in underserved populations is the basis of an NIH grant awarded to Drs. Kenneth Blum and Marjorie Gondré-Lewis. The research team is confident that eventually, the scientific community will seriously support new research directed toward the up-regulation of dopamine in mesolimbic structures with the goal of restoring homeostasis.
The interrelatedness of reward circuitry and the prefrontal cortices of the brain were not well-understood in the early sixties. The importance of the core neurotransmitters was not recognized. The functions of serotonin, GABA, dopamine, and acetylcholine were unknown, and endorphins were not even a part of our scientific acumen. The 1956 doctrine of Jellinek shocked the world when he proposed the concept of alcoholism as a disease, without much scientific evidence the idea was not generally accepted (1). However, most addiction scientists at that time agreed, in part, that deficiencies or imbalances in brain chemistry-perhaps genetic in origin –contributed to the cause of alcoholism.
Reward Deficiency Syndrome (RDS)
In the early 70s, Blum investigated the theorized neurochemical mechanisms of some psychoactive drugs (alcohol and opioids) that had been observed initially in the work of Virginia Davis, Gerald Cohen, Michael Collins and others, as being related to the interface of alcohol and opioid use disorders (2–6). Following many other foundational studies from around the world, the Royal Society of Medicine published the RDS concept in 1996 (7). To date, there have been 150 articles in PUBMED, and the SAGE Encyclopedia of Abnormal and Clinical Psychology published a definition in 2017 (8). The RDS concept arose from the findings that dysfunction in the dopaminergic system are implicated in reward mechanisms in the brain and lead to substance seeking behavior and non-substance addictive behaviors (7) like pathological gambling (9–11), Tic Disorders (12, 13), Tourette's syndrome (14), and attention deficit hyperactivity disorder (ADHD) (15–17).
Mark Gold's “Dopamine Depletion Hypothesis,” proposed an important role for dopamine in the effects of cocaine. He observed that the development of chronic cocaine use disorder (CUD) was due to the euphoric properties of cocaine and followed the acute activation of central dopamine neurons. Overstimulation of these neurons and excessive synaptic metabolism is thought to result in dopamine depletion which may underlie dysphoric aspects of cocaine abstinence and cocaine urges (18). Neurochemical disruptions caused by cocaine are consistent with the concept of “physical” rather than “psychological” addiction (19). The proposal that followed this research was to treat CUD with dopamine agonist therapy. The powerful dopamine D2 agonist bromocriptine was found to reduce cocaine craving significantly after a single dose (20). The suggestion was that bromocriptine might be an effective, non-addictive pharmacological treatment for those with CUD and open trials indicated that low-dose bromocriptine might be useful in cocaine detoxification. In 1995, Lawford et al. administered bromocriptine or placebo to subjects with alcohol use disorder (AUD), in a double-blind study, they found that the most significant improvement in craving and anxiety occurred in the bromocriptine treated subjects with the Dopamine Receptor D2 (DRD2) A1 allele and attrition was highest in the placebo-treated A1 subjects (21). Unfortunately, we now know that chronic administration of this D2 agonist induces significant down-regulation of D2 receptors, thereby making it an ineffective deterrent to relapse and preventing its clinical use (22, 23).
The Cascade of Reward Neurotransmission
The Ventral Tegmental Area (VTA)-Nucleus Accumbens (NAC) pathway is part of a series of parallel integrated circuits within the “Brain Reward Cascade,” which involves the hypothalamus, dorsal raphe and substania nigra. The net release of dopamine is due to the interrelatedness of serotonergic, endorphinergic, endocannabinoidergic, GABAergic, glutaminergic, and dopaminergic neurotransmitter signaling. The VTA is the site of dopaminergic neurons, which tell the organism whether an environmental stimulus (like natural rewards, drugs of abuse, stress) is aversive or rewarding. While a less understood brain region, the pre-frontal cortex, including the anterior cingulate cortex and orbitofrontal cortex, provides executive control of choices such drug reinstatement as being pleasurable (reward). Most recently even the dorsal raphe which contains both serotonergic and glutaminergic neurons impact the GABA input at the substania nigra (24). Therefore, for reward processing while net dopamine released at the NAC (reward site) is key, it is impacted by many neurotransmitter systems. Neurotransmitter interaction at the mesolimbic brain region induce “reward” when dopamine well-known as an anti-stress and pleasure neurotransmitter is released from the neuron and interacts with a nucleus accumbens dopamine D2 receptor. Reward produced to maintain our drives is the consequence of a cascade of neurotransmission. Initially, the release of serotonin stimulates enkephalin, then, inhibits GABA at the substantia nigra and ventral tegmental area. GABA regulates the release of DA at the nucleus accumbens. Studies indicate that balancing dopamine, possibly via dopamine D2 agonists, especially when availability of this molecule, based on genes and even epigenetics, is compromised, has important therapeutic application which has been proposed by NIDA scientists (25).
A consensus of the literature suggests dysfunction in the brain reward cascade is caused by the genetic sequence variations that cause a hypodopaminergic trait. This trait leads to multiple drug-seeking behaviors; the brain of that person requires a dopamine fix to feel good. Reduced dopamine release causes individuals to crave dopamine and have a high risk for multiple addictive, impulsive and compulsive behaviors that have been shown release dopamine (26).
Non-drug behaviors like gambling (27) and high-risk drug use are reinforced by surges of dopamine activation in the nucleus accumbens via the D1 receptors of the direct striatal pathway and inhibition of the indirect corticostriatal pathway via D2 receptors. Chronic drug administration enhances the brain's reactivity to drug cues, reduces sensitivity to non-drug rewards and causes neuroplastic changes in glutamatergic inputs to the striatum and midbrain dopamine neurons. Self-regulation is weakened, and sensitivity to stressful stimuli and dysphoria is increased. These long-lasting drug-induced impairments call for interventions designed to mitigate and if possible reverse them (28).
The Development of Precision Addiction Management
Blum et al. proposed that KB220Z; a mild neuro-nutrient formulation, can stimulate the D2 receptor (29). Blum's group advocates instigating dopamine release, to cause the induction of D2-directed mRNA to direct the proliferation of D2 receptors in the brain (30). For example, DNA-directed compensatory overexpression of the DRD2 receptors (a form of gene therapy), resulted in a significant reduction in alcohol craving behavior in alcohol-preferring rodents (31) and self-administration of cocaine (32). Thus, based on this model enhanced bioavailability of D2 receptors was shown to reduce craving.
Studies that showed rats with depleted neostriatal dopamine display increased sensitivity to dopamine agonists estimated to be 30–100 x in the 6-hydroxydopamine (6-OHDA) rotational model (33) were the basis for “denervation supersensitivity” (34). Denervation supersensitivity was identified as a putative physiological mechanism to help explain the enhanced sensitivity following intense acute dopaminergic D2 receptor activation in the face of hypodopaminergia. In contrast, promotion of long-term (chronic low vs. intense acute) dopaminergic activation by lower potency dopaminergic repletion therapy has been shown in clinical and neuro imaging studies, to be an effective modality when used to treat RDS behaviors including Substance Use Disorders (SUD), Attention Deficit Hyperactivity Disorder (ADHD), obesity and others, without side effects (35).
An unprecedented number of clinical studies validating this patented nutrigenomic technology for re-balancing brain chemistry, and optimizing dopamine sensitivity and function have been published. Here clinicians and neuroscientists are encouraged to continue to embrace the concept of “dopamine homeostasis” and search for safe, effective, validated and authentic means to achieve a lifetime of recovery, instead of reverting to anti-dopaminergic agents. Anti-dopaminergic agents are doomed to fail because chronic use continues and exacerbates hypodopaminergia while promoting powerful D2 agonists like bromocriptine and L-Dopa compromises needed balance (36). Increased resting state functional connectivity as well as an increased neuronal recruitment has been demonstrated acutely on fMRI in both animal and humans within 15 (animal) to 60 (human) minutes post administration of neuro nutrient therapy. These studies demonstrate neuronal dopamine firing in brain areas involved in reward processing and possible induced neuroplasticity and “dopamine homeostasis” (37, 38). The comprehensive role of dopamine as the mesolimbic system neurotransmitter underlying motivational function supports the low potency dopaminergic repletion therapy concept; sustainable, mild activation of D2 receptors (30).
Precision Addiction Management (PAM)
The system is a holistic therapeutic model for treating RDS that includes the Genetic Addiction Risk Score (GARS) test for genetic risk predisposition and customization of neuronutrient supplementation to target the individual genetic allele variation, based on the GARS test results, and thereby deliver (PAM)® to patients.
See Figure 1 for an example of how simple genotyping for identified SNPs in individuals could identify targets for precision nutrigenomics treatment. Figure 1 shows the PCR amplification of four variants of dopamine receptor D4 (DRD4). Multiple repeats of DRD4 variants are associated with disorders within the RDS spectrum (39–41). In the figure, six different 48 bp repeat sequences are identified, from 2 repeats (2R) to 8R. The DRD4, DRD2, catechol-O-methyltransferase (COMT) are among genes within the mesolimbic reward pathway with SNPs that contribute to RDS, see Figure 2.
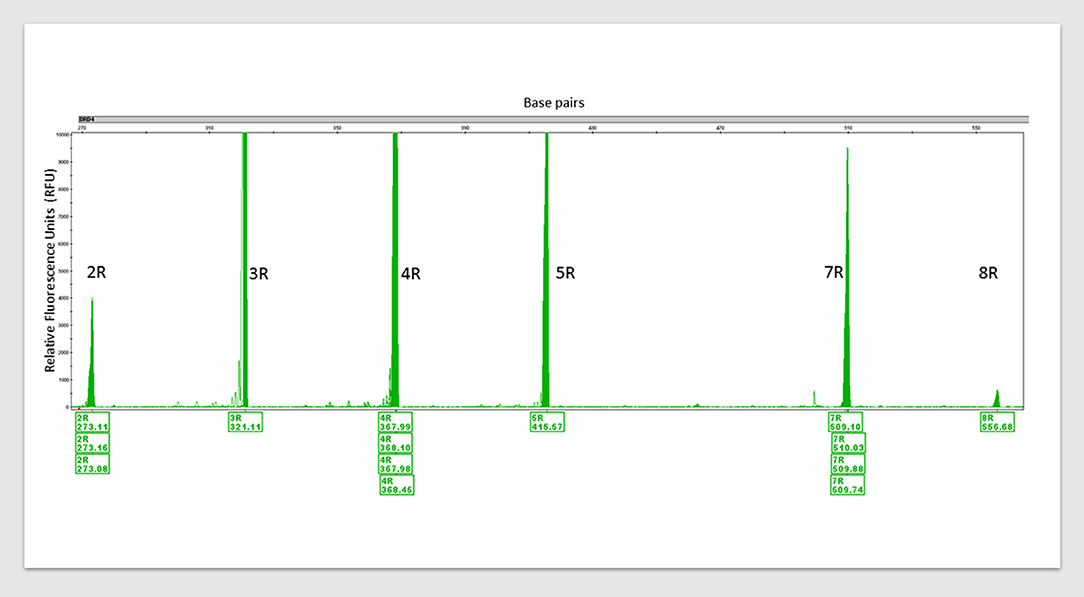
Figure 1. An example of PCR amplification of variants of dopamine receptor D4 (DRD4). DRD4 (Dopamine Receptor 4) variants detected via polymerase chain reaction (PCR) amplification with multiple control samples. 2R to 8R = six different 48 base pair (bp) repeat sequences. 2R repeats = 48 bp twice, 3R = thrice and so forth. Peak height (y-axis) indicates fluorescence signal amplitude, peak location (x-axis) indicates fragment size (bp). Fragment sizes are shown below the peaks (base pairs). Humans carry two copies of this variant and their lengths are from 2R to 11R. Carrying one or both variants at 7R+ increases the risk of developing RDS. This is one of the eleven established risk variants assessed by the GARS test.
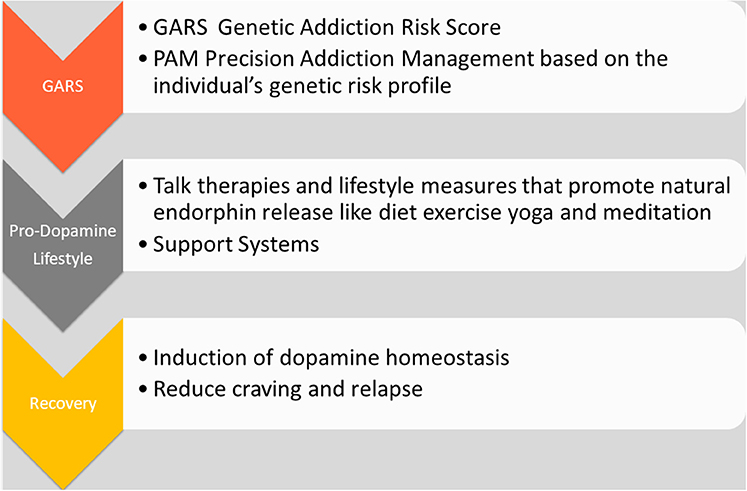
Figure 2. The Precision Addiction Management system. The schematic illustrates various elements related to “Precision Addiction Management (PAM)” and shows the interrelatedness of genetic testing, utilizing a patented Genetic Addiction Risk Score (GARS) and a customized polymorphic matched nutraceutical therapeutic adjunct KB220PAM.
The Development of the Genetic Addiction Risk Score (GARS)
There are many examples of association studies involving genes and polymorphisms especially of the ten reward genes measured in the GARS test. Alleles of genes that affect the synthesis, degradation, reception, and transport of neurotransmitters (like enkephalin, serotonin, GABA, and dopamine) and enzymes like Monoamine Oxidase (MOA) A and COMT in the reward pathway of the brain were candidates for selection for the GARS test if they contributed to hypodopaminergia. Comings and Blum proposed that functional defects in the genes for these neurotransmitters result in dopamine deficit, later identified as RDS. They suggested that individuals with hypodopaminergia are at risk for seeking reward from RDS behaviors to satisfy their lack of natural rewards (42). Some examples of functional research and studies that associated RDS behaviors with the risk alleles of the genes and second messengers that comprise the GARS test follow.
The Characterization of Phenotypes for the GARS
The Dopamine Receptor DRD1 Gene
The dopamine receptor DRD1 gene encodes the most abundant dopamine receptor in the central nervous system: the D1 subtype (43). While the D2, D3, and D4 receptor subtypes inhibit adenylyl cyclase activity, the D1 receptor stimulates a critical brain molecule adenylyl cyclase, which activates cyclic AMP (required for proper nerve brain function). The nucleotide guanidine (G) can be replaced by the adenine (A) and roughly a quarter of the human population carries one or two copies of this risk variant allele. Peer-reviewed studies involving the DRD1 gene show that carriers have an increased risk for many substance abuse and novelty seeking (NS) reward deficiency behaviors. In one of hundreds of association studies, Liu et al. looked at drug dependence and impulsive behavior and found that DRD1 gene polymorphisms are related to heroin dependence in a Chinese Han population, however, two of eight SNPs did not associate with impulsivity (44). Huang et al. found a significant association between the DRD1 and Nicotine Dependence (ND) (45). Recently they explored differential allelic expression of the DRD1 modulated by microRNA miR-504 (46). Other positive association studies on abusable drugs related to the D1 polymorphisms provide informative data indicating risk (47–50).
The Dopamine Receptor DRD2 Gene
The dopamine receptor DRD2 gene encodes the second (D2) subtype of the dopamine receptor. While the D1 receptor subtype activates, the D2 receptor inhibits adenylyl cyclase activity and reduces the intracellular concentration of cyclic AMP (51). DRD2 located near Ankyrin Repeat and Kinase Domain Containing 1 (ANKK1) at chromosome 11 q23.2, is involved in signal transduction (52). The Taq A1 genetic variant in ANKK1 equates functionally to 30–40% lower density of dopamine D2 receptors resulting in hypodopaminergic function (53). The DRD2 risk variant of interest (rs1800497) is downstream at chr11:113400106 where the nucleotide guanine (G) replaces the adenine (A). In 1986 inspired by a reported unconfirmed genetic association in depressed Amish subjects, Blum et al. discovered the first genetic association with a behavior, severe alcoholism, foundational work within the field of behavioral genetics (54). Initially, Taq 1A (rs1800497) was thought to be within the DRD2 gene, but as sequencing technology advanced and the location was corrected to exon 8 of the nearby ANKK1 gene. This risk allele is found in approximately 100 million people in the USA, with the highest frequency in Native Americans (85%) and the lowest among Western European Jews (6%).
The first of few examples of association studies is one from Singh, Ghosh, and Saraswathy. They looked at the role of dopamine receptors in susceptibility to alcohol dependence (AD) concerning three sites of the DRD2 gene (-141C Ins/Del, TaqIB, and TaqID) and TaqIA site of ANKK1 gene among Meiteis of Manipur, a Mendelian population of India, in association with AD. They found ANKK1 TaqIA polymorphism significantly associated with AD (odds ratio = 2.13, 95% confidential interval 1.04–4.39, P < 0.05), whereas a borderline significance of the −141C Del allele was observed (P = 0.059) (55).
Panduro et al. analyzed DRD2/ANKK1 genotyped a cross-section of 680 unrelated subjects including two Native Amerindians groups (87 Nahuas and 139 Huicholes), and two Mestizos groups (158 subjects from Tepic, Nayarit and 296 subjects from Guadalajara, Jalisco) by PCR-RFLP and allelic discrimination assays. Heavy drinking was considered ≥300 g alcohol/week. They concluded that the DRD2/ANKK1 A1 allele was present at a high frequency in Mexican populations and the A1/A1 genotype associated with heavy drinking in Mestizos. The highest frequencies of the A1 allele, exhibited worldwide to date were documented among the Native Amerindians (56).
Wang et al. investigated whether predisposing genetic variants and personality traits may be specific to a particular class of addiction or common to all addictions. They recruited 175 opiate-dependent patients, 102 alcohol-dependent patients, and 111 putative healthy controls diagnosed using DSM-IV criteria and assessed with Tridimensional Personality Questionnaire (TPQ). They genotyped dopamine D2 receptor (DRD2), 5-HTT-linked promoter region (5-HTTLPR), and aldehyde dehydrogenase 2 (ALDH2) genes using Polymerase Chain Reaction (PCR). They concluded that both alcohol- and opiate-dependent patients, have common genetic variants in DRD2 and 5-HTTLPR but specific for ALDH2. They found higher NS and Harm Avoidance traits in both patient groups with the interaction with DRD2, 5-HTTLPR, and ALDH2 genes (57).
Merritt and Bachtell in their non-genetic physiological study looked for the expression of the D2 dopamine receptor subtype as predictive of D2 dopamine receptor function and cocaine sensitivity that would enable cocaine abuse in rats. Quinpirole [D2 dopamine receptor agonist] was used to classify rats as high (HD2) or low responders (LD2). Results demonstrated that HD2 rats have greater cocaine conditioned place preference, enhanced sensitivity to the locomotor stimulating properties of cocaine, and self-administer more cocaine compared to LD2 animals. These findings suggested that individual differences in D2 dopamine receptor sensitivity may be predictive of cocaine sensitivity and reward (58).
Persico, Bird, Gabbay, and Uhl tested the hypothesis that a DRD2 gene variant might be more prominent in polysubstance users who preferentially use psychostimulants than in addicts who prefer opiates and those with no drug preference. Their results were consistent with the hypothesis that DRD2 gene variants marked by Taq1 A1 and B1 polymorphisms may work, probably in concert with other genetic and environmental factors, to enhance vulnerability to psychostimulant abuse (59).
Noble et al. examined the allelic prevalence of the D2 dopamine receptor (DRD2) gene in male cocaine-dependent (CD) Caucasian (non-Hispanic) subjects to determine the relationship of DRD2 alleles to family history and selected behavioral measures. The data showed a strong association of the minor alleles (A1 and B1) of DRD2 with cocaine dependence and suggested that a gene, located on the q22-q23 region of chromosome 11, confers susceptibility to this drug disorder (19).
Wang et al. used the MassARRAY system to identify markers that contribute to the genetic susceptibility to heroin addiction. They compared 334 patients with heroin dependence, and 299 healthy controls who participated in the research. Their findings indicated a role for DRD2 polymorphism in heroin dependence in the Chinese Han population and may be helpful for future genetic or neurobiological studies on heroin dependence (60). Most recently, Zhang et al. (61) found a 35.8 kilobases haplotype spanning ANKK1 and DRD2 is associated with heroin dependence in Han Chinese males (61).
The Dopamine Receptor DRD3 Gene
The dopamine receptor DRD3 gene encodes the D3 subtype of the five (D1–D5) dopamine receptors. As with D2 receptors, the activated D3 receptor inhibits adenylyl cyclase DRD3. Having a cytosine (C) instead of thymine (T) intensifies the effect of dopamine, magnifying the “high” observed with alcohol and cocaine to dangerous levels (62). The location of this receptor is within the older, more emotionally bent, limbic areas of the brain, including the pituitary gland, the olfactory bulb (smell) and the nucleus accumbens (cravings, aversions, reward). This gene demonstrates increased risks for alcohol, cocaine, and heroin dependence as well as RDS behaviors including ADHD, OCD, and even pathological aggression.
Thome et al. investigated the distribution of a dopamine D3 receptor gene polymorphism (Ball) in patients suffering from AD and compared with non-dependent controls. The allele A1 occurred significantly more frequently among patients compared to controls. Patients with the genotype A1/A2 showed significantly higher defined NS scores in the tridimensional personality questionnaire (TPQ) than patients with the genotype A1/A1. While this seems counter intuitive it can be explained by heterosis a well-known genetic phenomenon extensively discussed in the literature. There were significantly more individuals with higher NS scores and fewer individuals with lower NS scores than expected. The results of this study support the hypothesis of a genetically determined involvement of the dopaminergic system in AD (63).
Another study by Huang et al. investigated 13 single nucleotide polymorphisms (SNPs) spanning a region of the dopamine D(3) receptor gene (DRD3) to determine whether DRD3 is associated with ND. They studied a set of 2,037 subjects in 602 nuclear families representing two distinct American populations using three ND measures, namely, smoking quantity (SQ), the Heaviness of Smoking Index (HSI), and the Fagerström Test for ND (FTND). The results indicate that DRD3 associated significantly with ND in the European American cohort, and that rs6280, a functional polymorphism causing an amino acid change of serine to glycine (Ser9Gly) in the N-terminal extracellular domain of the D(3) receptor, likely is causative of the association between DRD3 and ND (64). Other positive association studies involving avoidant and obsessive personality traits and disorders, violent behavior and response to morphine reveal the importance of DRD3 polymorphisms in RDS (65–67).
The Dopamine Receptor DRD4 Gene
The dopamine receptor DRD4 gene that encodes for the receptor (subtype) D4. Dopamine receptors are responsible for neuronal signaling in the old reptilian mesolimbic system of the brain, which is an area that regulates emotions as well as RDS addictive behaviors. The DRD4 located on chromosome 11 at 11p15.5., is a G-protein receptor which is activated by the chemical messenger dopamine. Two different variants are measured in the GARS test. The first is a SNP located at rs1800955, −521 C>T and the risk allele is C. The second, is a Variable Number of Tandem Repeats (VNTR) located in intron 3, 48. The base–pair repeat; <7 is short, while if there are >7R, the risk allele is long VNTR equal or >7–11 repeats (68, 69). There are hundreds of studies of the DRD4 gene, and many of these studies have linked these risk variants to neurological and psychiatric conditions including schizophrenia, bipolar disorder, anhedonia, ADHD, Addictive behaviors, Parkinson's disease, eating disorders, and even anorexia nervosa (a non-eating repetitive RDS behavior). The underlying brain mechanism is D2-like in which the activated receptor inhibits the enzyme adenylate cyclase, thereby reducing the intracellular concentration of the second messenger cyclic AMP (required for cell function). The 7R allele appears to have been selected, for providing a survival advantage, about 40,000 years ago. It has been shown that compared to sedentary populations, the frequency of the 7R variant of DRD4, is much higher in nomadic populations suggesting its association with modern day “NS.” One important feature is that good parenting (epigenetic) was associated with appropriate decision making even at the age of four. Thus, early knowledge of this 7R or greater risk variant could be very helpful to prevent risk for substance use: opiate, alcohol, cannabis, glucose, and nicotine as well non-substance RDS behaviors: ADHD, Novelty seeking, Conduct Disorder (CD), hypersexuality, pathological aggression, and others.
Multiple repeats of DRD4 variants are associated with disorders within the RDS spectrum. Gervasini et al. conducted research to determine the effect of functional polymorphisms and haplotypes of the DRD4 gene on general psychopathological symptoms of 273 eating disorder (ED) patients [199 with Anorexia Nervosa (AN) and 74 with Bulimia Nervosa (BN)] who completed the SCL-90R symptom inventory. They found that certain combinations of DRD4 variants haplotype *2 (non7R-TR long-C-C) associated with higher scores in the three global SCL-90R indices the Global Severity Index (GSI), the Positive Symptom Distress Index, (PSDI), and the Positive Symptom Total (PST) after Bonferroni correction (p ≤ 0.01 in all instances). They also found that these polymorphisms may contribute to psychopathological features like Somatization, Obsessive-Compulsive, Anxiety, Phobic anxiety, Paranoid ideation, in BN patients (41).
In another example Dragan and Oniszczenko analyzed the association between the variable number tandem repeat (VNTR) DRD4 exon III polymorphism and intensity of PTSD symptoms in 107 (57 women and 50 men) survivors of a flood aged 14–62. PTSD symptoms were more intense for participants with at least one copy of the long (seven or eight repetitions) DRD4 allele than participants who did not have these alleles (40). Huang et al. used a transmission disequilibrium test of DRD4 exon III 48 bp variant-number-tandem-repeat polymorphism and tic disorder. Their results revealed an association between the longer alleles of DRD4 exon III 48 bp VNTR polymorphism and tic disorder accompanied with ADHD, thus suggesting a possible genetic risk factor of tic disorder with ADHD in Chinese (39). One interesting recent study by Ji et al. (70) involving epigenetics revealed that DNA methylation of DRD4 may be responsible for the pathophysiology of drug addiction (70).
The Dopamine Transporter Gene
The dopamine transporter gene (DAT1/SLC6A3) gives instructions for the production of a membrane-spanning protein that controls the reuptake (recycling) of dopamine from the synapse. The dopamine transporter helps regulate the level of neurotransmitter present in the synapse and controls how long a signal resulting from neurotransmitter release lasts (71).
The function of DAT1 is to clear excess dopamine released from the pre-neuron into the synapse and prevent uptake into the receptors on the next neuron. Much research, both biochemical and structural, has been performed to obtain clues about the mechanism of reuptake.The activity of clearing dopamine from the synapse is dependent on the variant form of this gene. So under normal conditions, the dopamine active transporter protein pumps the chemical messenger dopamine out of the synaptic cleft back into the cytosol of the pre-neuron cell. The DAT1 gene is located on chromosome 5 at p15. The gene has a variable number tandem repeats (VNTR) at the 3′end of the gene and another in the intron 8 region (72). The importance here is that differences in the VNTR, for example, 10R vs. 9R have been shown to effect the basal level of expression (activity) of the transporter. Indeed it has been demonstrated that the 9R is a risk form because it has a much higher ability to clear DA from the synaptic cleft compared to the 10R allele (73). Therefore, carriers of the 9R are more prone to both substance and non-substance RDS addictive behaviors due to hypodopaminergia (low dopamine function). The regional brain distribution of the DAT includes high dopamine-containing neurons in the old reptilian limbic system similar to the DRD2 receptor distribution (74). The maximum expression of the DAT1 gene is found in a parts of the brain called the substantia nigra and ventral tegmentum area (75) [brain regions containing large amounts of the inhibitory chemical messenger GABA that fine-tunes dopamine release at the reward site]. It is also interesting that DAT is co-localized with the D2 receptors (76).
There exists over 2,700 studies (PUBMED 7-14-18) concerning the role of the DAT1 gene and predisposition for the use of substances: particularly, heroin, alcohol, cocaine, and nicotine dependence; and non-substance RDS behaviors: ADHD, depression (Anhedonia), and PTSD are included.
Sullivan et al. focus on the role of aberrant dopaminergic signaling, interaction with dopamine transporter DAT, a cocaine target, and the dopamine D2 receptor in subjects and controls from the Miami Dade County Brain Bank splicing polymorphism rs2283265 of DRD2, encoding D2 receptors, was shown to confer risk (odds ratio ~3) of cocaine overdose/death. This risk was attributable to the minor allele of rs2283265 enhanced significantly in homozygous carriers of the main 6-repeat allele of DAT rs3836790to OR = 7.5 (P = 0.0008). In contrast, no significant risk to carriers of the minor 5-repeat DAT allele was demonstrated. The results demonstrated gene-gene-drug interaction affecting the risk of fatal cocaine intoxication (77).
Cinque et al. used transporter (DAT) knockout (KO) and heterozygous (HET) mice to investigate diseases with altered dopamine transmission such as attention-deficit/hyperactivity disorder (ADHD) and obsessive-compulsive disorder (OCD). These diseases characterized by poor decision making and executive function have been studied using many animal models. DAT KO rats appeared less sensitive to rewarding stimuli than wild-type (WT) and HET rats: they also showed a prominent hyperactive behavior with a rigid choice pattern and a wide number of compulsive stereotypies. Moreover, when the effects of amphetamine (AMPH) and RO-5203648 were tested, the AMPH accentuated impulsive behaviors in WT and HET rats, it did not affect in DAT KO rats (78).
Monoamine Oxidase A Gene
Monoamine oxidase A, (MAO-A), an enzyme, is encoded by the MAOA gene in humans. Two neighboring gene family members MAOA and MAOB encode mitochondrial enzymes which catalyze the oxidative deamination (breaks down the molecules) of catecholamines, like dopamine, norepinephrine, and indoleamines like serotonin. This gene is associated with a variety of psychiatric disorders, including antisocial behavior. The MAOA gene located on only the X chromosome at Xp11.3, the short (p) arm of the X chromosome, at position 11.3. a crucial enzyme for healthy brain function degrades chemical messengers like dopamine and serotonin (79–82).
The risk variant is 4R found at the 3′ 30 base-pair Repeat (R) on X chromosome only. The 3.5R and 4R variants have been found to be more highly active than 3R or 5R. Carriers of the 3.5 and 4R may display hypodopaminergia (low dopamine function) whereby too much of dopamine is broken down in the presynaptic neuron which may result is less dopamine to be released into the synaptic cleft. The 4R has been associated with Alzheimer's disease, aggression, panic disorder, bipolar affective disorder, major depressive disorder, and ADHD. There are over 900 studies on the MAO-A gene showing risk for substance use disorder: alcohol, opioid, and nicotine dependence, as well as obesity, and RDS behaviors: harm avoidance, NS, and ADHD.
Regarding catabolism the studies reveal risk for MAOA, Wang et al. conducted a family-based association analysis of AD in the COGA sample in the Australian twin-family study and found support for an association of MAOA gene (P = 4.14 × 10(−4) for rs979606) and AD (83).
Ducci et al. investigated the interaction between a functional monoamine oxidase A (MAOA) locus and childhood sexual abuse (CSA). They tested whether MAOA-LPR influences the impact of CSA on alcoholism and antisocial personality disorder (ASPD) in a sample of 291 women, 50% of whom had experienced CSA. They also tested whether haplotypes covering the location of both MAOA and MAOB) genes predict risk for alcoholism and ASPD better than the MAOA-LPR locus alone. Three haplotypes showed the MAOA-LPR low activity allele. The most abundant was among alcoholics (P = 0.008) and antisocial alcoholics (P = 0.001). Independently from ASPD the MAOB haplotype associated significantly with alcoholism (P = 0.006), and antisocial alcoholism (P = 0.03) (84).
Tikkanen et al. found that the PCL-R symptom inventory total score predicts impulsive reconvictions among offenders with high-activity MAOA (6.8% risk increase for every one-point increase in PCL-R total score, P = 0.015) but not among offenders with low-activity MAOA. Antisocial behavior and attitudes were found to predict reconvictions in both high and low activity MAOA genotypes a17% risk increase and a 12.8% increase, respectively. In a meta-analysis, Yang et al. found an association between MAO gene polymorphisms and smoking behavior. The meta-analysis showed the T allele in MAO-A C1460T reduced the risk of heavy smoking (OR = 0.66, 95% CI: 0.52–0.84; I(2) = 0.0%), especially in Caucasians. However, the active group in MAO-A VNTR increased the likelihood of smoking cessation failure in males (OR = 1.49, 95% CI: 1.01–2.22; I(2) = 0.0%) and the A allele in MAO-B G644A reduced the risk of heavy smoking in males (OR = 0.20, 95% CI: 0.04–0.98) (85).
Gorodetsky et al. investigated the interactive effect of MAOA-LPR genotype and a history of childhood trauma in predicting aggressive behaviors in a population of 692 male prisoners. Within the group not exposed to physical neglect (PN), carriers of the MAOA-LPR high-activity variant were more aggressive: (tR = 2.47, P < 0.014). They observed a crossover effect in that the increase in aggression scores with PN was greater in low-activity individuals (tR = 5.55, P < 0.0001) than in high-activity individuals (tR = 4.18, P < 0.0001). These findings suggested that childhood trauma and the functional MAOA-LPR polymorphism may interact to specifically increase the risk for over-aggressive behavior but not impulsivity or hostility. The MAOA-LPR low-activity variant may be protective against the development of aggressive behavior under low-stress conditions (86).
Vanyukov et al. looked for evidence of an association of a dinucleotide repeat polymorphism at the MAOA gene with early onset alcoholism/substance abuse. They found a correlation between the presence/absence of the disorder and the length of the MAOCA-1 repeat was significant in males only, with both increased risk for the disorder and lower age of onset of substance abuse associated with “long” alleles (repeat length above 115 bp) (87).
Tikkanen et al. looked at the relationship between MAOA the effects of heavy drinking and childhood physical abuse (CPA) on risk for severe impulsive acts of violence among violent alcoholic offenders. The sample population of 174 male impulsive, alcoholic, Finnish, violent offenders assessed after 8 years of non-incarcerated follow-up mostly exhibited antisocial (ASPD) or borderline personality disorder (BPD) or both. Logistic regression analyses demonstrated that both CPA and heavy drinking were significant independent predictors of recidivism in violent behavior (OR 5.2, p = 0.004 and OR 5.3, p = 0.003) among offenders having the high MAOA activity genotype (MAOA-H), but these predictors showed no effect among offenders carrying the low MAOA activity genotype (MAOA-L) (88). The work of Huang et al. (89) suggested the interaction of DRD2 rs1079597 and MAOA rs309850 3-repeat affects smoking intensity in young Taiwanese men (89).
The Catechol-O-methyltransferase Gene
The COMT gene (location 22q11.2) provides instructions for making an enzyme called COMT. Enzymes facilitate chemical reactions without the enzyme being changed (90). The gene makes two versions of COMT enzyme. The longer form, called membrane-bound catechol-O-methyltransferase (MB-COMT), is chiefly produced by nerve cells in the brain. The COMT enzyme destroys the dopamine molecule in the synapse and helps maintain appropriate levels of neurotransmitters (dopamine and norepinephrine) in the brain. In some people, there is a variation of a single protein building block (amino acid) in the enzyme (91). The amino acid valine (Val) replaces the amino acid methionine (Met), expressed as Val108/158Met. Carriers of two copies of MET have very heightened dopamine function because COMT activity is low and less dopamine is broken down, while two copies of Val, destroy more dopamine, and lower dopamine function (hypodopaminergia) is the result. The double copy (homozygote) of Val variant metabolizes dopamine at up to four times the rate of its Met counterpart. There have been over 2,400 studies involving the COMT gene. Val carriers are at increased risk for substance-related reward deficiency behaviors: including dependence on alcohol, cannabis, glucose; opiates/opioids, stimulants, and nicotine; or non-substance-related: ADHD, Oppositional Defiant Disorder, pathological aggression, panic disorder, anxiety, Obsessive-Compulsive Disorder (OCD) (92).
Hill et al. investigated caudate volume and working memory with brain scans and fMRI in offspring with childhood disorders followed until adolescence. They were tested for genotypic variation in the COMT and DRD2 genes. Caudate volume and working memory were reduced in association with externalizing disorders of childhood-adolescence and associated with variation in COMT and DRD2 genes (93).
Sery et al. performed a restriction analysis for the detection of the Val158Met polymorphism to look at the association between high-activity COMT allele and alcoholism in DNA samples from 799 subjects in total (279 male alcoholics and 120 female alcoholics, 151 male controls and 249 female controls). They found a significant difference between male alcoholics and male controls in allele and genotype frequencies (p < 0.007; and p < 0.04, respectively) (94).
Guillot et al. examined associations of COMT rs4680 with dimensionally and categorically measured gambling and drinking problems in a non-clinical sample (139 Caucasian adults). They found that COMT rs4680 was related to both dimensionally and categorically measured gambling and drinking problems and may be a genetic risk factor that contributes to the development of both problems 36 (95).
Enoch et al. looked for sex differences in the influence of COMT Val158Met on alcoholism and smoking in plains American Indians. They found that both male and female alcoholics were more likely to have at least 1 Val158 allele compared with non-alcoholics (0.95 vs. 0.88, p < 0.05). Approximately 30% of all participants were long-term, non-addicted light, social smokers (3.6±1.7 cigarettes/d); they had the same Val158Met frequencies as non-smokers (96).
The Opioid Receptor Genes
Three opioid receptors, mu, delta and kappa exert their pharmacological actions through the Oprm1, Oprd1, and Oprk1 genes, respectively, and these genes have been cloned. A family of natural opiate-like endogenous peptides, (enkephalins, dynorphins, and endorphin), which are released by neurons activate opioid receptors in the brain. The mu (μ) opioid receptors (MOR), located on chromosome 6 at q24-q25, have a high affinity for the enkephalins and beta-endorphin, found in the brain. The prototypical μ-opioid receptor activator is the mu agonist morphine, the primary psychoactive alkaloid in opium. The primary purpose of the mu opiate receptor is the control of pain; it is also very involved in the regulation of dopamine release in the reward site (nucleus accumbens) of the brain. One function of MOR, when activated by either endogenous natural opioids like enkephalins or opioid compounds like Fentanyl or Oxycontin, is to suppress the inhibitory chemical messenger GABA allowing for dopamine release at the reward site. These potent activators of MOR can cause overdose and death by blocking breathing. The risk variant of the MOR is the G allele 118A>G (p.Asn40Asp); SNP rs1799971). The definition of opioid is any synthetic narcotic that has opiate-like activities not derived from opium. The over-prescription of opioids (297 million in 2016) for pain relief, has been linked to the unwanted deaths (one person every 17 min dying from an overdose in America). Studies on the MOR variants are primarily related to substance use disorder: alcohol, food, opiate/opioid, and nicotine dependence; and RDS behaviors: overeating, inability to cope with stress, and PTSD. Carriers of the G allele have a reduced response to opioids (97) and exhibit hypodopaminergia.
Published studies reveal that polymorphisms in the Mu opioid receptor associate with drug dependence. A meta-analysis from Haerian and Haerian is a good example. They sort to resolve the question of whether the OPRM1 rs1799971 polymorphism associated with opioid dependence by evaluating evidence from 13 studies (n = 9,385), comprising 4,601 opioid dependents and 4,784 controls. These studies evaluated the association of the OPRM1 rs1799971 polymorphism with susceptibility to opioids. The analysis showed a significant association between this polymorphism and susceptibility to opioid dependence in overall studies under a codominant model, as well as susceptibility to opioid dependence or heroin dependence in Asians under an autosomal dominant model. They concluded OPRM1 rs1799971 might be a risk factor for addiction to opioids or heroin in an Asian population (98).
In another study Marini et al. examined the involvement of the mu-opioid receptor gene polymorphism A118G in the efficacy of detoxification of alcohol-dependent patients. They found that alcohol-dependent patients with the A/A genotype could have a faster restoration of their liver function than those with the A/G genotype: it might be possible that the presence of G allele confers on these patients a reduced ability in abstaining from drinking alcohol (99).
Wang et al. looked at genetic polymorphisms in the OPRM1 gene to determine if in methadone maintenance they are associated with changes in libido and insomnia in patients. The results obtained using dominant model analysis indicate that the OPRM1 SNPs rs1074287, rs6912029, rs12209447, rs510769, rs3798676, rs7748401, rs495491, rs10457090, rs589046, rs3778152, rs563649, and rs2075572 are significantly associated with change-in-libido side effects (adjusted p < 0.042). A recessive model analysis, of these SNPs, were found to be significantly associated with insomnia side effects in this cohort (p < 0.009). A systematic review and meta-analysis of six previous studies from Chamorro, et al. that analyzed the role of A118G polymorphism in response to naltrexone for AD. After meta-analysis, they found lower relapse rates in patients treated with naltrexone who carried the G allele, than patients who were homozygous for the A allele (OR: 2.02, 95% CI 1.26–3.22; P = 0.003) (100).
Bond et al. examined alterations in beta-endorphin binding and activity and the possible implications for opiate addiction. Their results show that SNPs in the mu opioid receptor gene can alter binding and signal transduction in the resulting receptor. This finding may have implications for normal physiology, therapeutics, and vulnerability to develop or be protected from diverse diseases including the addictive diseases. The object of this review is the mu opioid receptor gene, Oprm1 that generates 3 sets of proteins, each containing many variants. The review suggests these variants might be targeted to generate safer, effective analgesic drugs lacking respiratory depression, physical dependence, and reward behavior (101).
The Serotonin Transporter Gene
The serotonin transporter gene encoded by (SLC6A4) located with the 5-HTTLPR polymorphism on chromosome 17, occurs in the promoter region of the gene. Researchers report two variations in humans; a short (s) and a long (l). The 5-HTTLPR (serotonin-transporter-linked polymorphic region) is a degenerate repeat polymorphic region. There have been thousands of reports many related to behavioral, pharmacogenetic and RDS behaviors since the identification of the polymorphism in the 1990's. The risk variant involves a 43 base –pair 5″ insertion/deletion, S' at SNP rs25531. Researchers found that long allele results in higher serotonin transporter mRNA transcription in human cell lines. Thus, the serotonin is swiftly released into the synaptic cleft; eliminated from the synapse into the pre-nerve cell resulting in low serotonin content in the synapse leading to reduced function. Sometimes the long A allele of SNP rs25531 is written LA. Some studies revealed that the risk form signifies a predisposition to affective disorders, depressive responses to life stress, hyperactivity, and slowing of the electroactivity (speed) of the brain. Carriers of the S' or LA variant are at risk for substance-related: alcohol, opiate/ opioid, nicotine, cocaine, cannabis, and glucose dependence; RDS addictive behaviors: ADHD, PTSD, and pathological gambling and even pain response (102–105).
The established role of the serotonin transporter and associated polymorphisms across the Brain Reward Cascade and addiction liability is exemplified by this study from Herman et al. They examined the association between a measure of sociopathy and 5-HTTLPR genotype in a sample of individuals from a multi-center alcohol treatment trial [Project MATCH]. Regression analysis revealed that males with the L'L' genotype (i.e., those homozygous for the L(A) allele) had lower socialization scores (i.e., greater sociopathy) than males who were carriers of the S' allele (P = 0.03). In contrast, women with the S'S' genotype had lower socialization scores than women with two L' alleles (P = 0.002) and tended to have lower Socialization Index of the California Psychological Inventory scores than women with one copy of the L' allele (P = 0.07). Among individuals with AUDs, the tri-allelic 5-HTTLPR polymorphism had opposite effects on socialization scores in men than women. The basis for this finding is unknown, but it may have implications for sub-typing alcoholics (106). Herman and Balogh explored polymorphisms of the serotonin transporter and receptor genes and susceptibility to substance abuse. They suggested that Genetic variations in the 5-HT system, such as SLC6A4, HTR1B, HTR2A, HTR2C, HTR3 (HTR3A, HTR3B, HTR3C, HTR3D, and HTR3E), likely play a role contributing to SUD patient heterogeneity (107).
Polsinelli, Levitan, and De Luca used a multiple-model meta-analysis to clarify the association between BN and 5-HTTLPR using statistical models not used by previous meta-analyses and extend upon previous meta-analyses by including new samples. Data were pooled using dominant and additive models. Both models showed an association between the 5-HTTLPR polymorphism and BN that was non-significant (108).
Harkness et al. examined the moderating role of childhood emotional, physical and sexual maltreatment and the serotonin-transporter-linked promoter region (5-HTTLPR) polymorphism to stress generation in a cross-sectional community sample of 297 adolescents and young adults. Individuals with the risk s-allele of the serotonin transporter gene and a history of maternal emotional maltreatment or sexual maltreatment reported higher rates of dependent and dependent-interpersonal life events than those homozygous for the l-allele (109).
Liu et al. investigated the relationship between the serotonin transporter gene (SLC6A4) 5-HTTLPR genotypes, cocaine-dependence, and impulsivity in 98 healthy control and 243 treatment-seeking, cocaine-dependent subjects. They found that the impulsivity BIS-11 total score was associated positively with years of cocaine use for S'-allele carriers (r = 0.26, P = 0.0006, Pearson's correlation analysis), but not for L'L' genotype subjects (r = 0.02, P = 0.87) (110). It is noteworthy that Garbarino et al. (111), suggested that extreme bidirectional perturbations of serotonin signaling during development of one's DNA likely compound or synergize to facilitate enduring neurochemical changes resulting in insufficient or excessive 5-HT signaling, that could underlie the persistent behavioral characteristics of autism spectrum disorder (111).
The Gamma-aminobutyric Acid Receptor Subunits
GABA a neurotransmitter mediates neuronal inhibition by binding to the GABA/benzodiazepine receptor and opening an integral chloride channel. Gamma-aminobutyric acid receptor subunit alpha-3 (GABRA3) is a human protein that is encoded by the GABRA3 gene. GABA is the major inhibitory neurotransmitter that acts at GABAA receptors to fine-tune dopamine release in the reward site of the brain. Of the 16 identified and distinct subunits of GABA-A receptors, the GABRA3 gene located on Xq28, and the risk variant is CA-Repeat (171-201) whereby allele 181 results in higher activity. This risk allele if overexpressed will cause low dopamine function (hypodopaminergia) leading to SUD: including AD, and other RDS non-substance addictive behaviors and PTSD (112–117).
There are also association studies involving various polymorphisms of GABA receptors and RDS behaviors including alcoholism. An example is an extensive investigation, by Enoch et al. research to identify the functional locus of GABRA2 genotyped 24 SNPs across GABRG1 (gamma subunit) and GABRA2 in 547 Finnish Caucasian men (266 alcoholics), and 311 community-derived Plains Indian men and women (181 alcoholics). GABRG1 haplotypes and SNPs associated significantly with AUD whereas there was no association between AUD and GABRA2 haplotypes. Although of several common (>or = 0.05) haplotypes that spanned GABRG1 and GABRA2 (341 kb) emerged, three of which were present in both populations. One associated with AUD, the other two were more common in non-alcoholics determined by GABRG1. Three less-common extended haplotypes (<0.05) in the Finns, associated with AUD that was determined by GABRA2. These results suggest that there are likely to be independent, complex contributions from both GABRG1 and GABRA2 to alcoholism vulnerability (118).
Terranova et al. analyze the connection between AD and criminal behavior and GABA receptors by an integrated genetic-environmental approach that examined 186 alcohol-dependent males; group 1 (N = 47 convicted subjects) compared with group 2 (N = 139 no previous criminal records). Genetic results highlighted group 1 differences in genotype distribution (p = 0.0067) for SNP rs3780428, found on the intronic region of subunit 2 of the GABA B receptor gene (GABBR2) (119).
Massat et al. (120) found that the GABRA3 polymorphism may confer susceptibility to or may be in linkage disequilibrium with another gene involved in the genetic etiology of bi-polar depression (120). Cui et al. looked at the genetics of GABAergic signaling in nicotine and AD. Human genetic studies support the involvement of genes and variants in the GABAergic signaling system in the etiology of nicotine dependence and alcoholism based on linkage, association, and gene-by-gene interaction studies (121).
Finally, there are multitudes of genetic studies that associate specific behaviors with identified reward gene alleles (specific SNPs) within the mesolimbic pathway. These descriptions of the contribution made by polymorphisms (sequence variations) that effect the healthy function of reward genes, and this small sampling of association studies illustrate why the presence of these alleles in a gene panel indicate genetic risk for associated behaviors.
Promoting a Pro-dopamine Lifestyle
A comprehensive treatment program that teaches a pro-dopamine lifestyle and uses urine drug screens like the Comprehensive Analysis of Reported Drugs (CARD) to monitor outcomes, and as a basis for therapeutic interactions, is suggested. Can a pro-dopamine lifestyle with gentle prolonged D2 agonist therapy overcome DNA polymorphisms by promoting positive epigenetic effects which can be transferred from generation to generation (32, 122, 123)? Holistic modalities like exercise (124), low glycemic index diet (125), mindfulness training, neurofeedback, yoga, and meditation are known to support reward neurotramsmission and naturally release dopamine the product of reward neurotransmission (126, 127). These holistic pro dopamine modalities supported by the 12 step fellowship, might induce feelings of well-being and thereby reduce craving and relapse. With this in mind, we wonder if we have been “licking our pups” enough? Could substance and non-substance seeking- behaviors be attenuated through nurturing (128) as suggested by David E. Smith in the late 60's “love needs care” (129)?
Summary
These basic concepts underpin translational addiction-related research that can help the multitude of victims of genetically induced RDS become the recipients of better therapeutic relapse-preventive tactics.
Finally, as neuroscientists and psychiatrists, working in the “addiction space” we encourage the global scientific community to take heed and reconsider the current utilization of dopaminergic blockade and instead adopt the goal of regaining dopamine homeostasis. Optimistically, early predisposition diagnosis through genetic testing; including pharmacogenetic and pharmacogenomic monitoring, with appropriate urine drug screening, and treatment with pro-dopamine regulators could conceivably reduce stress, craving, and relapse and enhance well-being in the recovery community. Following required basic and clinically directed research, the notion of genetically guided therapy may become a front-line technology with the potential to overcome, in part, the current heightened rates of substance abuse.
Author Contributions
The original concept was developed by KB, DB, JN, PT, and RDB. The original draft was provided by KB and MG-L. The entire paper was carefully vetted by RDB, IE, MG-L, KB, EB, PT, DB, JN, and approved.
Funding
KB and EB the recipient of a grant awarded to PATH Foundation NY from the Life Extension Foundation, Ft Lauderdale, Florida. The work of RDB was partially supported by the National Institutes of Health grants 1R01NS073884 and 1R21MH073624. MG-L is the recipient of R01 AA021262/AA/NIAAA NIH HHS/United States. KB and MG-L are the recipients of R41 MD012318/NIMHD NIH HHS/United States. PT is the recipient of R01HD70888-01A1. The work of IE is supported by the Veterans Health Administration; Merit Review number is 1 I01 CX001118–01A2. The work PT is funded by the NY Research Foundation (RIAQ0940) and the NIH (DA035923 and DA035949).
Conflict of Interest Statement
KB owns stock in some companies holding patents on genetic testing and KB220PAM and is Chairman of the Genus Health LLC scientific advisory board. MG-L, DB, PT, IE, and RDB are members of the Genus Health LLC scientific advisory board.
The remaining authors declare that the research was conducted in the absence of any commercial or financial relationships that could be construed as a potential conflict of interest.
Acknowledgments
The authors appreciate the edits by Margaret A. Madigan and the support of Mary Hauser and the staff of Dominion Diagnostics, LLC and Geneus Health LLC, especially Justin Jones, Erin Gallager, David Swicki, Lisa Lott and Jessica Ponce-Rodriguez.
References
1. Jellinek E. The Disease Concept of Alcoholism. New Heaven, CT: College and University Press (1960).
2. Cohen G, Collins M. Alkaloids from catecholamines in adrenal tissue: possible role in alcoholism. Science (1970) 167:1749–51. doi: 10.1126/science.167.3926.1749
4. Blum K, Hamilton MG, Hirst M, Wallace JE. Putative role of isoquinoline alkaloids in alcoholism: a link to opiates. Alcohol Clin Exp Res. (1978) 2:113–20. doi: 10.1111/j.1530-0277.1978.tb04710.x
5. Hamilton MG, Blum K, Hirst M. Identification of an isoquinoline alkaloid after chronic exposure to ethanol. Alcohol Clin Exp Res. (1978) 2:133–7. doi: 10.1111/j.1530-0277.1978.tb04713.x
6. Collins MA, Kahn AJ. Attraction to ethanol solutions in mice: induction by a tetrahydroisoquinoline derivative of L-DOPA. Subst Alcohol Actions Misuse (1982) 3:299–302.
7. Blum K, Sheridan PJ, Wood RC, Braverman ER, Chen TJ, Cull JG, et al. The D2 dopamine receptor gene as a determinant of reward deficiency syndrome. J R Soc Med. (1996) 89:396–400. doi: 10.1177/014107689608900711
8. Blum K. Reward deficiency syndrome. In: Wenzel A, editor. The Sage Encyclopedia of Abnormal Clinical Psychology. Sage Publications (2017).
9. Comings DE, Rosenthal RJ, Lesieur HR, Rugle LJ, Muhleman D, Chiu C, et al. A study of the dopamine D2 receptor gene in pathological gambling. Pharmacogenetics (1996) 6:223–34. doi: 10.1097/00008571-199606000-00004
10. Comings DE, Gonzalez N, Wu S, Gade R, Muhleman D, Saucier G, et al. (1999). Studies of the 48 bp repeat polymorphism of the DRD4 gene in impulsive, compulsive, addictive behaviors: tourette syndrome, ADHD, pathological gambling, and substance abuse. Am J Med Genet. 88, 358–368. doi: 10.1002/(SICI)1096-8628(19990820)88:4<358::AID-AJMG13>3.0.CO;2-G
11. Joutsa J, Hirvonen MM, Arponen E, Hietala J, Kaasinen V. DRD2-related TaqIA genotype is associated with dopamine release during a gambling task. J Addict Med. (2014) 8:294–5. doi: 10.1097/ADM.0000000000000037
12. Comings DE. Clinical and molecular genetics of ADHD and Tourette syndrome. Two related polygenic disorders. Ann N Y Acad Sci. (2001) 931:50–83. doi: 10.1111/j.1749-6632.2001.tb05773.x
13. Nespoli E, Rizzo F, Boeckers T, Schulze U, Hengerer B. Altered dopaminergic regulation of the dorsal striatum is able to induce tic-like movements in juvenile rats. PLoS ONE (2018) 13:e0196515. doi: 10.1371/journal.pone.0196515
14. Yuan A, Su L, Yu S, Li C, Yu T, Sun J. Association between DRD2/ANKK1 TaqIA polymorphism and susceptibility with tourette syndrome: a meta-analysis. PLoS ONE (2015) 10:e0131060. doi: 10.1371/journal.pone.0131060
15. Spencer TJ, Biederman J, Madras BK, Dougherty DD, Bonab AA, Livni E, et al. Further evidence of dopamine transporter dysregulation in ADHD: a controlled PET imaging study using altropane. Biol Psychiatry (2007) 62:1059–61. doi: 10.1016/j.biopsych.2006.12.008
16. Ghosh P, Sarkar K, Bhaduri N, Ray A, Sarkar K, Sinha S, et al. Catecholaminergic gene variants: contribution in ADHD and associated comorbid attributes in the eastern Indian probands. Biomed Res Int. (2013) 2013:918410. doi: 10.1155/2013/918410
17. Spencer TJ, Biederman J, Faraone SV, Madras BK, Bonab AA, Dougherty DD, et al. Functional genomics of attention-deficit/hyperactivity disorder (ADHD) risk alleles on dopamine transporter binding in ADHD and healthy control subjects. Biol Psychiatry (2013) 74:84–9. doi: 10.1016/j.biopsych.2012.11.010
18. Dackis CA, Gold MS. New concepts in cocaine addiction: the dopamine depletion hypothesis. Neurosci Biobehav Rev. (1985) 9:469–77. doi: 10.1016/0149-7634(85)90022-3
19. Noble EP, Blum K, Khalsa ME, Ritchie T, Montgomery A, Wood RC, et al. Allelic association of the D2 dopamine receptor gene with cocaine dependence. Drug Alcohol Depend (1993) 33:271–85. doi: 10.1016/0376-8716(93)90113-5
20. Dackis CA, Gold MS, Sweeney DR, Byron JPJr, Climko R. Single-dose bromocriptine reverses cocaine craving. Psychiatry Res. (1987) 20:261–4. doi: 10.1016/0165-1781(87)90086-2
21. Lawford BR, Young RM, Rowell JA, Qualichefski J, Fletcher BH, Syndulko K, et al. Bromocriptine in the treatment of alcoholics with the D2 dopamine receptor A1 allele. Nat Med. (1995) 1:337–41. doi: 10.1038/nm0495-337
22. Rouillard C, Bedard PJ, Falardeau P, Dipaolo T. Behavioral and biochemical evidence for a different effect of repeated administration of L-dopa and bromocriptine on denervated versus non-denervated striatal dopamine receptors. Neuropharmacology (1987) 26:1601–6. doi: 10.1016/0028-3908(87)90008-6
23. Bogomolova EV, Rauschenbach IY, Adonyeva NV, Alekseev AA, Faddeeva NV, Gruntenko NE. Dopamine down-regulates activity of alkaline phosphatase in Drosophila: the role of D2-like receptors. J Insect Physiol. (2010) 56:1155–9. doi: 10.1016/j.jinsphys.2010.03.014
24. Morales M, Root DH. Glutamate neurons within the midbrain dopamine regions. Neuroscience (2014) 282c:60–8. doi: 10.1016/j.neuroscience.2014.05.032
25. Rothman RB, Blough BE, Baumann MH. Dual dopamine/serotonin releasers: potential treatment agents for stimulant addiction. Exp Clin Psychopharmacol. (2008) 16:458–74. doi: 10.1037/a0014103
26. Blum K, Braverman ER, Holder JM, Lubar JF, Monastra VJ, Miller D, et al. Reward deficiency syndrome: a biogenetic model for the diagnosis and treatment of impulsive, addictive, and compulsive behaviors. J Psychoactive Drugs (2000) 32(Suppl. i-iv):1–112. doi: 10.1080/02791072.2000.10736099
27. Heiden P, Heinz A, Romanczuk-Seiferth N. Pathological gambling in Parkinson's disease: what are the risk factors and what is the role of impulsivity? Eur J Neurosci. (2017) 45:67–72. doi: 10.1111/ejn.13396
28. Volkow ND, Morales M. The brain on drugs: from reward to addiction. Cell (2015) 162:712–25. doi: 10.1016/j.cell.2015.07.046
29. Blum K, Chen AL, Chen TJ, Braverman ER, Reinking J, Blum SH, et al. Activation instead of blocking mesolimbic dopaminergic reward circuitry is a preferred modality in the long term treatment of reward deficiency syndrome (RDS): a commentary. Theor Biol Med Model. (2008) 5:24. doi: 10.1186/1742-4682-5-24
30. Blum K, Oscar-Berman M, Stuller E, Miller D, Giordano J, Morse S, et al. Neurogenetics and nutrigenomics of neuro-nutrient therapy for reward deficiency syndrome (RDS): clinical ramifications as a function of molecular neurobiological mechanisms. J Addict Res Ther. (2012) 3:139. doi: 10.4172/2155-6105.1000139
31. Thanos PK, Rivera SN, Weaver K, Grandy DK, Rubinstein M, Umegaki H, et al. Dopamine D2R DNA transfer in dopamine D2 receptor-deficient mice: effects on ethanol drinking. Life Sci. (2005) 77:130–9. doi: 10.1016/j.lfs.2004.10.061
32. Thanos PK, Michaelides M, Umegaki H, Volkow ND. D2R DNA transfer into the nucleus accumbens attenuates cocaine self-administration in rats. Synapse (2008) 62:481–6. doi: 10.1002/syn.20523
33. Mandel RJ, Hartgraves SL, Severson JA, Woodward JJ, Wilcox RE, Randall PK. A quantitative estimate of the role of striatal D-2 receptor proliferation in dopaminergic behavioral supersensitivity: the contribution of mesolimbic dopamine to the magnitude of 6-OHDA lesion-induced agonist sensitivity in the rat. Behav Brain Res. (1993) 59:53–64. doi: 10.1016/0166-4328(93)90151-F
34. Blum K, Chen TJ, Downs BW, Bowirrat A, Waite RL, Braverman ER, et al. Neurogenetics of dopaminergic receptor supersensitivity in activation of brain reward circuitry and relapse: proposing “deprivation-amplification relapse therapy” (DART). Postgrad Med. (2009) 121:176–96. doi: 10.3810/pgm.2009.11.2087
35. Blum K, Febo M, Badgaiyan RD. Fifty Years in the development of a glutaminergic-dopaminergic optimization complex (KB220) to balance brain reward circuitry in reward deficiency syndrome: a pictorial. Austin Addict Sci (2016) 1:1006.
36. Blum K, Downs, B.w, Baron D, Steinberg B, Siwicki D, Giordano J, et al. “Dopamine homeostasis” requires balanced polypharmacy: Issue with destructive, powerful dopamine agents to combat America's drug epidemic. J Syst Integr Neurosci. (2017) 3:6. doi: 10.15761/JSIN.1000183
37. Blum K, Liu Y, Wang W, Wang Y, Zhang Y, Oscar-Berman M, et al. rsfMRI effects of KB220Z on neural pathways in reward circuitry of abstinent genotyped heroin addicts. Postgrad Med. (2015) 127:232–41. doi: 10.1080/00325481.2015.994879
38. Febo M, Blum K, Badgaiyan RD, Baron D, Thanos PK, Colon-Perez LM, et al. Dopamine homeostasis: brain functional connectivity in reward deficiency syndrome. Front Biosci. (Landmark Ed.) (2017) 22:669–91. doi: 10.2741/4509
39. Huang Y, Liu X, Li T, Guo L, Ma X, Yuan G, et al. Transmission disequilibrium test of DRD4 exon III 48bp variant number tandem repeat polymorphism and tic disorder. Zhonghua Yi Xue Yi Chuan Xue Za Zhi (2002) 19:100–3.
40. Dragan WL, Oniszczenko W. The association between dopamine D4 receptor exon III polymorphism and intensity of PTSD symptoms among flood survivors. Anxiety Stress Coping (2009) 22:483–95. doi: 10.1080/10615800802419407
41. Gervasini G, Gonzalez LM, Gamero-Villarroel C, Mota-Zamorano S, Carrillo JA, Flores I, et al. Effect of dopamine receptor D4 (DRD4) haplotypes on general psychopathology in patients with eating disorders. Gene (2018). doi: 10.1016/j.gene.2018.02.035
42. Comings DE, Blum K. Reward deficiency syndrome: genetic aspects of behavioral disorders. Prog Brain Res. (2000) 126:325–41. doi: 10.1016/S0079-6123(00)26022-6
43. Ren K, Guo B, Dai C, Yao H, Sun T, Liu X, et al. Striatal distribution and cytoarchitecture of dopamine receptor subtype 1 and 2: evidence from double-labeling transgenic mice. Front Neural Circuits (2017) 11:57. doi: 10.3389/fncir.2017.00057
44. Liu JH, Zhong HJ, Dang J, Peng L, Zhu YS. Single-nucleotide polymorphisms in dopamine receptor D1 are associated with heroin dependence but not impulsive behavior. Genet Mol Res. (2015a) 14:4041–50. doi: 10.4238/2015
45. Huang W, Ma JZ, Payne TJ, Beuten J, Dupont RT, Li MD. Significant association of DRD1 with nicotine dependence. Hum Genet. (2008a) 123:133–40. doi: 10.1007/s00439-007-0453-9
46. Huang W, Li MD. Differential allelic expression of dopamine D1 receptor gene (DRD1) is modulated by microRNA miR-504. Biol Psychiatry (2009) 65:702–5. doi: 10.1016/j.biopsych.2008.11.024
47. Kim DJ, Park BL, Yoon S, Lee HK, Joe KH, Cheon YH, et al. 5' UTR polymorphism of dopamine receptor D1 (DRD1) associated with severity and temperament of alcoholism. Biochem Biophys Res Commun. (2007) 357:1135–41. doi: 10.1016/j.bbrc.2007.04.074
48. Batel P, Houchi H, Daoust M, Ramoz N, Naassila M, Gorwood P. A haplotype of the DRD1 gene is associated with alcohol dependence. Alcohol Clin Exp Res. (2008) 32:567–72. doi: 10.1111/j.1530-0277.2008.00618.x
49. Jacobs MM, Okvist A, Horvath M, Keller E, Bannon MJ, Morgello S, et al. Dopamine receptor D1 and postsynaptic density gene variants associate with opiate abuse and striatal expression levels. Mol Psychiatry (2013) 18:1205–10. doi: 10.1038/mp.2012.140
50. Peng S, Du J, Jiang H, Fu Y, Chen H, Sun H, et al. The dopamine receptor D1 gene is associated with the length of interval between first heroin use and onset of dependence in Chinese Han heroin addicts. J Neural Transm (Vienna) (2013) 120:1591–8. doi: 10.1007/s00702-013-1029-6
51. Grandy DK, Litt M, Allen L, Bunzow JR, Marchionni M, Makam H, et al. The human dopamine D2 receptor gene is located on chromosome 11 at q22-q23 and identifies a TaqI RFLP. Am J Hum Genet. (1989) 45:778–85.
52. Neville MJ, Johnstone EC, Walton RT. Identification and characterization of ANKK1: a novel kinase gene closely linked to DRD2 on chromosome band 11q23.1. Hum Mutat (2004) 23:540–5. doi: 10.1002/humu.20039
53. Noble EP, Blum K, Ritchie T, Montgomery A, Sheridan PJ. Allelic association of the D2 dopamine receptor gene with receptor-binding characteristics in alcoholism. Arch Gen Psychiatry (1991) 48:648–54. doi: 10.1001/archpsyc.1991.01810310066012
54. Blum K, Noble EP, Sheridan PJ, Montgomery A, Ritchie T, Jagadeeswaran P, et al. Allelic association of human dopamine D2 receptor gene in alcoholism. JAMA (1990) 263:2055–60. doi: 10.1001/jama.1990.03440150063027
55. Suraj Singh H, Ghosh PK, Saraswathy KN. DRD2 and ANKK1 gene polymorphisms and alcohol dependence: a case-control study among a Mendelian population of East Asian ancestry. Alcohol Alcohol (2013) 48:409–14. doi: 10.1093/alcalc/agt014
56. Panduro A, Ramos-Lopez O, Campollo O, Zepeda-Carrillo EA, Gonzalez-Aldaco K, Torres-Valadez R, et al. High frequency of the DRD2/ANKK1 A1 allele in Mexican Native Amerindians and Mestizos and its association with alcohol consumption. Drug Alcohol Depend (2017) 172:66–72. doi: 10.1016/j.drugalcdep.2016.12.006
57. Wang TY, Lee SY, Chen SL, Huang SY, Chang YH, Tzeng NS, et al. Association between DRD2, 5-HTTLPR, and ALDH2 genes and specific personality traits in alcohol- and opiate-dependent patients. Behav Brain Res. (2013) 250:285–92. doi: 10.1016/j.bbr.2013.05.015
58. Merritt KE, Bachtell RK. Initial d2 dopamine receptor sensitivity predicts cocaine sensitivity and reward in rats. PLoS ONE (2013) 8:e78258. doi: 10.1371/journal.pone.0078258
59. Persico AM, Bird G, Gabbay FH, Uhl GR. D2 dopamine receptor gene TaqI A1 and B1 restriction fragment length polymorphisms: enhanced frequencies in psychostimulant-preferring polysubstance abusers. Biol Psychiatry (1996) 40:776–84. doi: 10.1016/0006-3223(95)00483-1
60. Wang N, Zhang JB, Zhao J, Cai XT, Zhu YS, Li SB. Association between dopamine D2 receptor gene polymorphisms and the risk of heroin dependence. Genet Mol Res. (2016) 15:4. doi: 10.4238/gmr15048772
61. Zhang J, Yan P, Li Y, Cai X, Yang Z, Miao X, et al. A 35.8 kilobases haplotype spanning ANKK1 and DRD2 is associated with heroin dependence in Han Chinese males. Brain Res (2018) 1688:54–64. doi: 10.1016/j.brainres.2018.03.017
62. Vengeliene V, Leonardi-Essmann F, Perreau-Lenz S, Gebicke-Haerter P, Drescher K, Gross G, et al. The dopamine D3 receptor plays an essential role in alcohol-seeking and relapse. Faseb J. (2006) 20:2223–33. doi: 10.1096/fj.06-6110com
63. Thome J, Weijers HG, Wiesbeck GA, Sian J, Nara K, Boning J, et al. Dopamine D3 receptor gene polymorphism and alcohol dependence: relation to personality rating. Psychiatr Genet. (1999) 9:17–21. doi: 10.1097/00041444-199903000-00004
64. Huang W, Payne TJ, Ma JZ, Li MD. A functional polymorphism, rs6280, in DRD3 is significantly associated with nicotine dependence in European-American smokers. Am J Med Genet B Neuropsychiatr Genet (2008b) 147b:1109–15. doi: 10.1002/ajmg.b.30731
65. Joyce PR, Rogers GR, Miller AL, Mulder RT, Luty SE, Kennedy MA. Polymorphisms of DRD4 and DRD3 and risk of avoidant and obsessive personality traits and disorders. Psychiatry Res. (2003) 119:1–10. doi: 10.1016/S0165-1781(03)00124-0
66. Retz W, Rosler M, Supprian T, Retz-Junginger P, Thome J. Dopamine D3 receptor gene polymorphism and violent behavior: relation to impulsiveness and ADHD-related psychopathology. J Neural Transm (Vienna) (2003) 110:561–72. doi: 10.1007/s00702-002-0805-5
67. Spangler R, Goddard NL, Avena NM, Hoebel BG, Leibowitz SF. Elevated D3 dopamine receptor mRNA in dopaminergic and dopaminoceptive regions of the rat brain in response to morphine. Brain Res Mol Brain Res. (2003) 111:74–83. doi: 10.1016/S0169-328X(02)00671-X
68. Chen C, Burton M, Greenberger E, Dmitrieva J. Population migration and the variation of dopamine D4 receptor (DRD4) allele frequencies around the globe. Evol Hum Behav. (1999) 20:309–24. doi: 10.1016/S1090-5138(99)00015-X
69. Wang E, Ding YC, Flodman P, Kidd JR, Kidd KK, Grady DL, et al. The genetic architecture of selection at the human dopamine receptor D4 (DRD4) gene locus. Am J Hum Genet. (2004) 74:931–44. doi: 10.1086/420854
70. Ji H, Xu X, Liu G, Liu H, Wang Q, Shen W, et al. Dopamine receptor D4 promoter hypermethylation increases the risk of drug addiction. Exp Ther Med. (2018) 15:2128–33. doi: 10.3892/etm.2017.5615
71. Kawarai T, Kawakami H, Yamamura Y, Nakamura S. Structure and organization of the gene encoding human dopamine transporter. Gene (1997) 195:11–8. doi: 10.1016/S0378-1119(97)00131-5
72. Moron JA, Brockington A, Wise RA, Rocha BA, Hope BT. Dopamine uptake through the norepinephrine transporter in brain regions with low levels of the dopamine transporter: evidence from knock-out mouse lines. J Neurosci. (2002) 22:389–95. doi: 10.1523/JNEUROSCI.22-02-00389.2002
73. Faraone SV, Spencer TJ, Madras BK, Zhang-James Y, Biederman J. Functional effects of dopamine transporter gene genotypes on in vivo dopamine transporter functioning: a meta-analysis. Mol Psychiatry (2014) 19:880–9. doi: 10.1038/mp.2013.126
74. Wheeler DD, Edwards AM, Chapman BM, Ondo JG. Effects of cocaine on sodium dependent dopamine uptake in rat striatal synaptosomes. Neurochem Res. (1994) 19:49–56. doi: 10.1007/BF00966728
75. Ciliax BJ, Drash GW, Staley JK, Haber S, Mobley CJ, Miller GW, et al. Immunocytochemical localization of the dopamine transporter in human brain. J Comp Neurol. (1999) 409:38–56. doi: 10.1002/(SICI)1096-9861(19990621)409:1<38::AID-CNE4>3.0.CO;2-1
76. Liu Z, Yan SF, Walker JR, Zwingman TA, Jiang T, Li J, et al. Study of gene function based on spatial co-expression in a high-resolution mouse brain atlas. BMC Syst Biol. (2007) 1:19. doi: 10.1186/1752-0509-1-19
77. Sullivan D, Pinsonneault JK, Papp AC, Zhu H, Lemeshow S, Mash DC, et al. Dopamine transporter DAT and receptor DRD2 variants affect risk of lethal cocaine abuse: a gene-gene-environment interaction. Transl Psychiatry (2013) 3:e222. doi: 10.1038/tp.2012.146
78. Cinque S, Zoratto F, Poleggi A, Leo D, Cerniglia L, Cimino S, et al. Behavioral phenotyping of dopamine transporter knockout rats: compulsive traits, motor stereotypies, and anhedonia. Front Psychiatry (2018) 9:43. doi: 10.3389/fpsyt.2018.00043
80. Zhu Q, Shih JC. An extensive repeat structure down-regulates human monoamine oxidase A promoter activity independent of an initiator-like sequence. J Neurochem. (1997) 69:1368–73. doi: 10.1046/j.1471-4159.1997.69041368.x
81. Shih JC, Chen K, Ridd MJ. Role of MAO A and B in neurotransmitter metabolism and behavior. Pol J Pharmacol. (1999) 51:25–9.
82. Brummett BH, Krystal AD, Siegler IC, Kuhn C, Surwit RS, Zuchner S, et al. Associations of a regulatory polymorphism of monoamine oxidase-A gene promoter (MAOA-uVNTR) with symptoms of depression and sleep quality. Psychosom Med. (2007) 69:396–401. doi: 10.1097/PSY.0b013e31806d040b
83. Wang KS, Liu X, Aragam N, Jian X, Mullersman JE, Liu Y, et al. Family-based association analysis of alcohol dependence in the COGA sample and replication in the Australian twin-family study. J Neural Transm (Vienna) (2011) 118:1293–9. doi: 10.1007/s00702-011-0628-3
84. Ducci F, Enoch MA, Hodgkinson C, Xu K, Catena M, Robin RW, et al. Interaction between a functional MAOA locus and childhood sexual abuse predicts alcoholism and antisocial personality disorder in adult women. Mol Psychiatry (2008) 13:334–47. doi: 10.1038/sj.mp.4002034
85. Tikkanen R, Auvinen-Lintunen L, Ducci F, Sjoberg RL, Goldman D, Tiihonen J, et al. Psychopathy, PCL-R, and MAOA genotype as predictors of violent reconvictions. Psychiatry Res. (2011) 185:382–6. doi: 10.1016/j.psychres.2010.08.026
86. Gorodetsky E, Bevilacqua L, Carli V, Sarchiapone M, Roy A, Goldman D, et al. The interactive effect of MAOA-LPR genotype and childhood physical neglect on aggressive behaviors in Italian male prisoners. Genes Brain Behav. (2014) 13:543–9. doi: 10.1111/gbb.12140
87. Vanyukov MM, Moss HB, Yu LM, Tarter RE, Deka R. Preliminary evidence for an association of a dinucleotide repeat polymorphism at the MAOA gene with early onset alcoholism/substance abuse. Am J Med Genet. (1995) 60:122–6. doi: 10.1002/ajmg.1320600207
88. Tikkanen R, Ducci F, Goldman D, Holi M, Lindberg N, Tiihonen J, et al. MAOA alters the effects of heavy drinking and childhood physical abuse on risk for severe impulsive acts of violence among alcoholic violent offenders. Alcohol Clin Exp Res. (2010) 34:853–60. doi: 10.1111/j.1530-0277.2010.01157.x
89. Huang CL, Ou WC, Chen PL, Liu CN, Chen MC, Lu CC, et al. Effects of interaction between dopamine D2 receptor and monoamine oxidase a genes on smoking status in young men. Biol Res Nurs. (2015) 17:422–8. doi: 10.1177/1099800415589366
90. Labrosse EH, Axelrod J, Kety SS. O-Methylation, the principal route of metabolism of epinephrine in man. Science (1958) 128:593–4. doi: 10.1126/science.128.3324.593
91. Wilson AF, Elston RC, Siervogel RM, Weinshilboum R, Ward LJ. Linkage relationships between a major gene for catechol-o-methyltransferase activity and 25 polymorphic marker systems. Am J Med Genet. (1984) 19:525–32. doi: 10.1002/ajmg.1320190314
92. Vandenbergh DJ, Rodriguez LA, Hivert E, Schiller JH, Villareal G, Pugh EW, et al. Long forms of the dopamine receptor (DRD4) gene VNTR are more prevalent in substance abusers: no interaction with functional alleles of the catechol-o-methyltransferase (COMT) gene. Am J Med Genet. (2000) 96:678–83. doi: 10.1002/1096-8628(20001009)96:5<678::AID-AJMG15>3.0.CO;2-8
93. Hill SY, Lichenstein S, Wang S, Carter H, Mcdermott M. Caudate volume in offspring at ultra high risk for alcohol dependence: COMT Val158Met, DRD2, externalizing disorders, and working memory. Adv J Mol Imaging (2013) 3:43–54. doi: 10.4236/ami.2013.34007
94. Sery O, Didden W, Mikes V, Pitelova R, Znojil V, Zvolsky P. The association between high-activity COMT allele and alcoholism. Neuro Endocrinol Lett. (2006) 27:231–5.
95. Guillot CR, Fanning JR, Liang T, Berman ME. COMT Associations with disordered gambling and drinking measures. J Gambl Stud. (2015) 31:513–24. doi: 10.1007/s10899-013-9434-1
96. Enoch MA, Waheed JF, Harris CR, Albaugh B, Goldman D. Sex differences in the influence of COMT Val158Met on alcoholism and smoking in plains American Indians. Alcohol Clin Exp Res. (2006) 30:399–406. doi: 10.1111/j.1530-0277.2006.00045.x
97. Blum K, Chen ALC, Thanos PK, Febo M, Demetrovics Z, Dushaj K, et al. Genetic addiction risk score (GARS), a predictor of vulnerability to opioid dependence. Front Biosci. (Elite Ed.) (2018) 10:175–96. doi: 10.2741/e816
98. Haerian BS, Haerian MS. OPRM1 rs1799971 polymorphism and opioid dependence: evidence from a meta-analysis. Pharmacogenomics (2013) 14:813–24. doi: 10.2217/pgs.13.57
99. Marini V, Fucile C, Zuccoli ML, Testino G, Sumberaz A, Robbiano L, et al. Involvement of the mu-opioid receptor gene polymorphism A118G in the efficacy of detoxification of alcohol dependent patients. Addict Behav. (2013) 38:1669–71. doi: 10.1016/j.addbeh.2012.09.015
100. Wang SC, Tsou HH, Chen CH, Chen YT, Ho IK, Hsiao CF, et al. Genetic polymorphisms in the opioid receptor mu1 gene are associated with changes in libido and insomnia in methadone maintenance patients. Eur Neuropsychopharmacol. (2012) 22:695–703. doi: 10.1016/j.euroneuro.2012.02.002
101. Bond C, Laforge KS, Tian M, Melia D, Zhang S, Borg L, et al. Single-nucleotide polymorphism in the human mu opioid receptor gene alters beta-endorphin binding and activity: possible implications for opiate addiction. Proc Natl Acad Sci USA. (1998) 95:9608–13. doi: 10.1073/pnas.95.16.9608
102. Pinto E, Reggers J, Gorwood P, Boni C, Scantamburlo G, Pitchot W, et al. The short allele of the serotonin transporter promoter polymorphism influences relapse in alcohol dependence. Alcohol Alcohol (2008) 43:398–400. doi: 10.1093/alcalc/agn015
103. Kosek E, Jensen KB, Lonsdorf TB, Schalling M, Ingvar M. Genetic variation in the serotonin transporter gene (5-HTTLPR, rs25531) influences the analgesic response to the short acting opioid Remifentanil in humans. Mol Pain (2009) 5:37. doi: 10.1186/1744-8069-5-37
104. Treister R, Pud D, Ebstein RP, Laiba E, Raz Y, Gershon E, et al. Association between polymorphisms in serotonin and dopamine-related genes and endogenous pain modulation. J Pain (2011) 12:875–83. doi: 10.1016/j.jpain.2011.02.348
105. Otten R, Engels RC. Testing bidirectional effects between cannabis use and depressive symptoms: moderation by the serotonin transporter gene. Addict Biol. (2013) 18:826–35. doi: 10.1111/j.1369-1600.2011.00380.x
106. Herman AI, Conner TS, Anton RF, Gelernter J, Kranzler HR, Covault J. Variation in the gene encoding the serotonin transporter is associated with a measure of sociopathy in alcoholics. Addict Biol. (2011) 16:124–32. doi: 10.1111/j.1369-1600.2009.00197.x
107. Herman AI, Balogh KN. Polymorphisms of the serotonin transporter and receptor genes: susceptibility to substance abuse. Subst Abuse Rehabil. (2012) 3:49–57. doi: 10.2147/SAR.S25864
108. Polsinelli GN, Levitan RN, De Luca V. 5-HTTLPR polymorphism in bulimia nervosa: a multiple-model meta-analysis. Psychiatr Genet. (2012) 22:219–25. doi: 10.1097/YPG.0b013e32835669b3
109. Harkness KL, Bagby RM, Stewart JG, Larocque CL, Mazurka R, Strauss JS, et al. Childhood emotional and sexual maltreatment moderate the relation of the serotonin transporter gene to stress generation. J Abnorm Psychol. (2015) 124:275–87. doi: 10.1037/abn0000034
110. Liu S, Maili L, Lane SD, Schmitz JM, Spellicy CJ, Cunningham KA, et al. Serotonin transporter gene promoter polymorphism predicts relationship between years of cocaine use and impulsivity. Psychiatr Genet. (2015b) 25:213–4. doi: 10.1097/YPG.0000000000000094
111. Garbarino VR, Gilman TL, Daws LC, Gould GG. Extreme enhancement or depletion of serotonin transporter function and serotonin availability in autism spectrum disorder. Pharmacol Res. (2018). doi: 10.1016/j.phrs.2018.07.010
112. Noble EP, Zhang X, Ritchie T, Lawford BR, Grosser SC, Young RM, et al. D2 dopamine receptor and GABA(A) receptor beta3 subunit genes and alcoholism. Psychiatry Res. (1998) 81:133–47. doi: 10.1016/S0165-1781(98)00084-5
113. Edenberg HJ, Dick DM, Xuei X, Tian H, Almasy L, Bauer LO, et al. Variations in GABRA2, encoding the alpha 2 subunit of the GABA(A) receptor, are associated with alcohol dependence and with brain oscillations. Am J Hum Genet. (2004) 74:705–14. doi: 10.1086/383283
114. Konishi T, Calvillo M, Leng AS, Lin KM, Wan YJ. Polymorphisms of the dopamine D2 receptor, serotonin transporter, and GABA(A) receptor beta(3) subunit genes and alcoholism in Mexican-Americans. Alcohol (2004) 32:45–52. doi: 10.1016/j.alcohol.2003.11.002
115. Han DH, Bolo N, Daniels MA, Lyoo IK, Min KJ, Kim CH, et al. Craving for alcohol and food during treatment for alcohol dependence: modulation by T allele of 1519T>C GABAAalpha6. Alcohol Clin Exp Res. (2008) 32:1593–9. doi: 10.1111/j.1530-0277.2008.00734.x
116. Namkoong K, Cheon KA, Kim JW, Jun JY, Lee JY. Association study of dopamine D2, D4 receptor gene, GABAA receptor beta subunit gene, serotonin transporter gene polymorphism with children of alcoholics in Korea: a preliminary study. Alcohol (2008) 42:77–81. doi: 10.1016/j.alcohol.2008.01.004
117. Enoch MA, Baghal B, Yuan Q, Goldman D. A factor analysis of global GABAergic gene expression in human brain identifies specificity in response to chronic alcohol and cocaine exposure. PLoS ONE (2013) 8:e64014. doi: 10.1371/journal.pone.0064014
118. Enoch MA, Hodgkinson CA, Yuan Q, Albaugh B, Virkkunen M, Goldman D. GABRG1 and GABRA2 as independent predictors for alcoholism in two populations. Neuropsychopharmacology (2009) 34:1245–54. doi: 10.1038/npp.2008.171
119. Terranova C, Tucci M, Sartore D, Cavarzeran F, Di Pietra L, Barzon L, et al. GABA receptors, alcohol dependence and criminal behavior. J Forensic Sci. (2013) 58:1227–32. doi: 10.1111/1556-4029.12201
120. Massat I, Souery D, Del-Favero J, Oruc L, Noethen MM, Blackwood D, et al. Excess of allele1 for alpha3 subunit GABA receptor gene (GABRA3) in bipolar patients: a multicentric association study. Mol Psychiatry (2002) 7:201–7. doi: 10.1038/sj.mp.4000953
121. Cui WY, Seneviratne C, Gu J, Li MD. Genetics of GABAergic signaling in nicotine and alcohol dependence. Hum Genet. (2012) 131:843–55. doi: 10.1007/s00439-011-1108-4
122. Szutorisz H, Dinieri JA, Sweet E, Egervari G, Michaelides M, Carter JM, et al. Parental THC exposure leads to compulsive heroin-seeking and altered striatal synaptic plasticity in the subsequent generation. Neuropsychopharmacology (2014) 39:1315–23. doi: 10.1038/npp.2013.352
123. Mclaughlin T, Blum K, Oscar-Berman M, Febo M, Agan G, Fratantonio JL, et al. Putative dopamine agonist (KB220Z) attenuates lucid nightmares in PTSD patients: role of enhanced brain reward functional connectivity and homeostasis redeeming joy. J Behav Addict. (2015) 4:106–15. doi: 10.1556/2006.4.2015.008
124. Arida RM, Gomes Da Silva S, De Almeida AA, Cavalheiro EA, Zavala-Tecuapetla C, Brand S, et al. Differential effects of exercise on brain opioid receptor binding and activation in rats. J Neurochem. (2015) 132:206–17. doi: 10.1111/jnc.12976
125. Newquist G, Gardner RA. Reconsidering food reward, brain stimulation, and dopamine: incentives act forward. Am J Psychol. (2015) 128:431–44. doi: 10.5406/amerjpsyc.128.4.0431
126. Kjaer TW, Bertelsen C, Piccini P, Brooks D, Alving J, Lou HC. Increased dopamine tone during meditation-induced change of consciousness. Brain Res Cogn Brain Res. (2002) 13:255–9. doi: 10.1016/S0926-6410(01)00106-9
127. Blum K, Chen TJ, Morse S, Giordano J, Chen AL, Thompson J, et al. Overcoming qEEG abnormalities and reward gene deficits during protracted abstinence in male psychostimulant and polydrug abusers utilizing putative dopamine D(2) agonist therapy: part 2. Postgrad Med. (2010) 122:214–26. doi: 10.3810/pgm.2010.11.2237
128. Starkman BG, Sakharkar AJ, Pandey SC. Epigenetics—beyond the genome in alcoholism. Alcohol Res. (2012) 34:293–305.
Keywords: reward deficiency syndrome (RDS), dopamine homeostasis, genetic addiction risk score (GARS), comprehensive analysis of reported drugs (CARD), precision addiction management (PAM)
Citation: Blum K, Gondré-Lewis MC, Baron D, Thanos PK, Braverman ER, Neary J, Elman I and Badgaiyan RD (2018) Introducing Precision Addiction Management of Reward Deficiency Syndrome, the Construct That Underpins All Addictive Behaviors. Front. Psychiatry 9:548. doi: 10.3389/fpsyt.2018.00548
Received: 18 March 2018; Accepted: 12 October 2018;
Published: 27 November 2018.
Edited by:
Jung-Seok Choi, SMG-SNU Boramae Medical Center, South KoreaReviewed by:
Yeun-Jun Chung, Catholic University of Korea, South KoreaMartin Zack, Centre for Addiction and Mental Health (CAMH), Canada
Copyright © 2018 Blum, Gondré-Lewis, Baron, Thanos, Braverman, Neary, Elman and Badgaiyan. This is an open-access article distributed under the terms of the Creative Commons Attribution License (CC BY). The use, distribution or reproduction in other forums is permitted, provided the original author(s) and the copyright owner(s) are credited and that the original publication in this journal is cited, in accordance with accepted academic practice. No use, distribution or reproduction is permitted which does not comply with these terms.
*Correspondence: Kenneth Blum, ZHJkMmdlbmVAZ21haWwuY29t